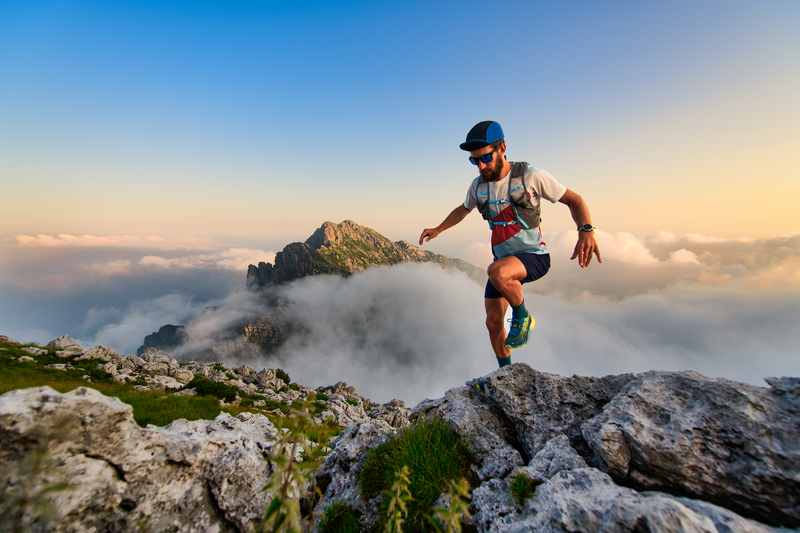
94% of researchers rate our articles as excellent or good
Learn more about the work of our research integrity team to safeguard the quality of each article we publish.
Find out more
REVIEW article
Front. Cell Dev. Biol. , 14 December 2022
Sec. Signaling
Volume 10 - 2022 | https://doi.org/10.3389/fcell.2022.1068213
This article is part of the Research Topic Novel Strategies to Target Cell Death Signaling in Cancer and Neurodegenerative Diseases: New Findings and Mechanistic Studies View all 7 articles
Glaucoma is a common blinding eye disease characterized by progressive loss of retinal ganglion cells (RGCs) and their axons, progressive loss of visual field, and optic nerve atrophy. Autophagy plays a pivotal role in the pathophysiology of glaucoma and is closely related to its pathogenesis. Targeting autophagy and blocking the apoptosis of RGCs provides emerging guidance for the treatment of glaucoma. Here, we provide a systematic review of the mechanisms and targets of interventions related to autophagy in glaucoma and discuss the outlook of emerging ideas, techniques, and multidisciplinary combinations to provide a new basis for further research and the prevention of glaucomatous visual impairment.
Glaucoma is the most common irreversible eye disease, the second leading cause of blindness, and one of the four leading causes of moderate and severe vision impairment (MSVI) GBD 2019 Blindness and Vision Impairment Collaborators;Vision Loss Expert Group of the Global Burden of Disease Study, 2021. The number of people (aged 40–80 years) with glaucoma worldwide is expected to reach 111.8 million by 2040 (Tham et al., 2014). Recently, attention has been paid to the pathogenesis of glaucoma, providing novel insight into therapeutic strategies. Glaucoma mainly affects the retina and optic nerve. The optic nerve originates from the diencephalon and is part of the central nervous system (CNS), combined with the pathological characteristics of glaucoma, which is classified as a neurodegenerative disease. Current glaucoma treatments are based on lowering intraocular pressure (IOP) through pharmacological and surgical control. These treatments can prevent the loss of retinal ganglion cells (RGCs) and visual impairment in the early stages of the disease to some extent. Unfortunately, as visual impairment is a late symptom of glaucomatous optic neuropathy (2021), most patients with visual impairment have experienced significant RGC loss by the time of diagnosis, rendering conventional treatments futile at later stages of the disease.
Autophagy is a highly conserved catabolic pathway in organisms that promotes the degradation and recycling of cellular components and protects damaged cells by removing excess/impaired organelles and metabolites from the cytoplasm, regulating the constant renewal of peroxisomes, mitochondria, and endoplasmic reticulum, and performing subcellular level remodeling (He et al., 2019). Autophagy has recently attracted considerable attention as a vital mechanism for maintaining neuronal homeostasis, and defects in autophagy have been implicated in various neurodegenerative disorders (Frake et al., 2015; Menzies et al., 2017). Autophagy dysfunction has been implicated in chronic neurodegenerative diseases such as Alzheimer’s disease (Nixon et al., 2005), Parkinson’s disease (PD) (Pan et al., 2008), and Huntington’s disease (Ravikumar et al., 2004) and in acute diseases such as cerebral hypoxia/ischemia and trauma (Wong and Cuervo, 2010). Autophagy plays a key regulatory role in the development and progression of glaucoma and is a potential new therapeutic target. In recent years, numerous studies have demonstrated that autophagy plays an important role in the development of glaucoma and that dysfunctional autophagy is closely associated with this disease (Villarejo-Zori et al., 2021). In addition, glaucoma is thought to be associated with mutations in autophagy-related genes (Mizushima and Levine, 2020). Autophagy is a particularly crucial 'clean-up station' in neurons efficiently removes misfolded proteins and damaged or aged organelles to safeguard cellular homeostasis (Chen et al., 2019); if not removed effectively, these waste products can accumulate and lead to neuronal degeneration and death (Chen et al., 2019), causing permanent damage to the retina. Research on autophagy in glaucomatous optic nerve injury mainly focuses on organismal stress in response to high IOP (HIOP), optic nerve protection, immune regulation, trabecular meshwork (TM) dysfunction, and scar modulation (Ishikawa et al., 2021). These studies suggest that autophagy is dysfunctional in glaucoma, i.e., impaired autophagy is part of the pathogenesis.
Herein, this review focuses on the role of autophagy in the pathogenesis of glaucoma and the therapeutic potential of targeting the autophagy pathway. The normal function of autophagy is first described first, followed by a discussion of the major roles of autophagy in some of the pathogenic mechanisms of glaucoma. Finally, the current and promising approaches to modulating autophagy for glaucoma treatment are highlighted, to provide a new basis for further investigation and the prevention of glaucomatous visual impairment.
The word “autophagy” is derived from Greek, meaning “self-eating” and refers to the metabolic process of cellular degradation and the recycling of cellular components within lysosomes (Galluzzi et al., 2017). There are three subtypes of autophagy, macroautophagy, chaperone-mediated autophagy, and microautophagy, which are divided depending on the mechanism by which the material destined for degradation enters the lysosome. Macroautophagy is accompanied by the emergence of a phagophore (a small bilayer of membranes) in the cytoplasm. Proteins and organelles are then encapsulated in a bilayer membrane structure that closes to form an organelle referred to as the autophagosome, which later fuses with the lysosome to enable the degradation of the enclosed material by lysosomal hydrolases (Figure 1). In chaperone-mediated autophagy, heat shock cognate 70 and several accessory chaperone proteins specifically recognize the KFERQ-like region and are transported to the lysosome for degradation (Kaushik and Cuervo, 2018). In microautophagy, the least characterized form of autophagy, the cytoplasmic cargo is destined for degradation through lysosomal or late endosomal membranes that directly engulfs cytoplasmic cargo (Schuck, 2020). Macroautophagy (hereafter referred to as autophagy) is the most widely studied of the three forms.
FIGURE 1. Molecular mechanisms during macroautophagy processes. Autophagy is a multistep process that includes induction, initiation, elongation, maturation, fusion with lysosomes, and degradation. The autophagy process is regulated by the mTOR and AMPK signaling pathways, which are responsible for monitoring the nutritional status of the cell. AMPK is an activator and mTOR acts as an inhibitor through the phosphorylation of ULK1 at distinct residues. The ULK1-containing initiation complex triggers phagophore formation by phosphorylating components of the class III Ptdlns3K nucleation complex. During autophagosome biogenesis, after ATG5 binds ATG12, the ATG5-ATG12 conjugate binds to ATG16L1 to generate the autophagy elongation complex (ATG5-12/16L1), which regulates lipidation of LC3. LC3, a ubiquitin-like modifier, is cleaved by the protease ATG4 to form LC3-I. LC3-I conjugates with PE to form LC3-II, which incorporates into autophagosomal membranes and is critical for the formation of autophagosomes. Autophagy receptor (e.g., P62, OPTN) serves as a link between LC3 and ubiquitinated proteins or organelles targeted for degradation. Autophagosomes are eventually fused with lysosomes to form autolysosomes. The cargos in autolysosomes are degraded by lysosomal enzymes, and nutrients and metabolites are recycled. Metformin induces autophagy by targeting AMPK. MTOR signals can be inhibited directly by rapamycin. PI3K and AKT are elevated by acteoside. 3-Methyladenine(3-MA) can specifically block autophagosome formation. BAFA1 inhibits the acidification of the autolysosome by blocking the V-ATPase while chloroquine (CQ) and 3-hydroxychloroquine (HCQ) impair the maturation of autolysosomes. Drugs are depicted in red font.
Although autophagy was originally thought to be a nonselective process, recent evidence suggests that it is highly selective (Villarejo-Zori et al., 2021). This selectivity means that specific types of cytoplasmic cargos have been identified (Johansen and Lamark, 2020) that are transported to the autophagosome for degradation, where they bind LC3 (light chain 3 protein) via LC3-interacting regions. Depending on the nature of the specific cargo, selective autophagy can be classified as heterogeneous phagocytosis (pathogen degradation), lipophagy (lipid droplet degradation), or aggregative phagocytosis (protein aggregate degradation) (Johansen and Lamark, 2020). Similarly, organelle-specific autophagy can be divided into endoplasmic reticulum, peroxisomal, mitochondrial and lysosomal autophagy (Johansen and Lamark, 2020). Recent studies have shown that the Golgi apparatus may degrade proteins by retrograde trafficking to the ER, suggesting Golgi might be a target for selective autophagy degradation (Benyair et al., 2022) (Nthiga et al., 2021).
Autophagy is primarily a cellular stress response and plays an important role in biological cells. Depending on the autophagy state, theprocess can be divided into several sequential stages: induction, initiation/vesicle nucleation, phagophore formation, elongation, autophagosome formation, maturation/fusion, and degradation (Kiriyama and Nochi, 2015).
Autophagy regulation is dependent on all phases. Autophagy occurs at a low basal level under physiological conditions, but it can be rapidly induced in certain states of cellular stress, such as hypoxia, glucose deficiency (Kim et al., 2011), starvation (Kuma et al., 2004) protein aggregates (Kraft et al., 2010), damaged organelles (e.g. mitochondria, endoplasmic reticulum, and peroxisomes) and intracellular pathogens infections (Glick et al., 2010). Autophagy is induced by these stresses and is regulated extensively by posttranslational mechanisms. Among all stresses, a lack of nutrients (especially amino acids) is the most important factor in the induction of autophagy. This process is mediated by upstream regulators of autophagy including mTOR and AMPK, which are responsible for monitoring nutritional status (Wong et al., 2013; Parzych and Klionsky, 2014). Phagophore formation is a key step that follows autophagy induction and is important for all phases. Autophagy initiation is controlled in part by the ULK complex (autophagy related gene (ATG)13, ULK1, FIP200), which is regulated by cellular energy sensors (AMPK and mTOR) (Bressan and Saghatelyan, 2020). After the autophagy-initiating kinase ULK1 is phosphorylated, a phagophore forms via phosphatidylinositol 3-phosphate (PI3P) generation (Metaxakis et al., 2018). Elongation is a crucial step in the complete autophagosomes (Ebrahimi-Fakhari et al., 2012). Tow ATG 7-catalyzed ubiquitin-like reactions control the elongation, one is to form autophagy elongation complex (ATG5-12/16L1), and the other is phosphatidylethanolamine (PE) -LC3I conjugated to form LC3-II, (Ebrahimi-Fakhari et al., 2012). Membrane elongation closure to maturation, which fuse with lysosomes to form autolysosomes and degrade proteins and organelles (Yim and Mizushima, 2020). Molecular mechanisms during macroautophagic processes are shown in Figure 1.
Autophagy is primarily regulated at the posttranslational level; various stressors can induce autophagy, and stress can also stimulate the upregulation of autophagy genes (Feng et al., 2015). The transcription factor EB (TFEB) is the main regulator of autophagy genes (Puertollano et al., 2018). Phosphorylation retains TFEB in the cytoplasm, but when dephosphorylated by stress, TFEB translocates to the nucleus where it promotes the transcription of its target genes (Puertollano et al., 2018). Optineurin (OPTN) is a gene linked to glaucoma (Shen et al., 2011), and its encoded protein is an autophagy receptor, which targets specific cargo for degradation and is itself degraded by autophagy (Mijaljica et al., 2012; Klionsky et al., 2021). Recently, at least 42 genes associated with the autophagy pathway (Mizushima, 2020), and more genes will be identified as research progresses.
Autophagy levels decline during normal human aging in all tissues examined to date, including the brain and retina, which contributes to the pathogenesis of several neurodegenerative diseases, such as glaucoma (Boya, 2017; Wong et al., 2020). Altered selective autophagy also has important pathological implications in the pathogenesis of neurodegenerative diseases. For example, inadequate removal of pathogenic protein aggregates is associated with neurodegenerative diseases (Conway et al., 2020), and dysregulation of mitochondrial autophagy, i.e., the selective removal of unnecessary or damaged mitochondria is impaired, constitutes a severe redox imbalance that is detrimental to neurons (Teodorof-Diedrich and Spector, 2018) and is common in neurodegenerative diseases.
Glaucoma is a multifactorial progressive optic neuropathy characterized by axonal degeneration and loss of RGCs, leading to severe and irreversible blindness (Gupta and Yücel, 2007; Tham et al., 2014). Studies have shown that the underlying mechanisms of glaucomatous neurodegeneration are not the result of a single factor, but rather a causal and interactive effect of various factors, including HIOP (mean IOP increases with age) (Bonomi et al., 1998), oxidative stress (Kimura et al., 2017), neurotrophic factor deprivation (Harvey et al., 2012), mitochondrial dysfunction (Ito and Di Polo, 2017), autoimmune dysregulation (Ito and Di Polo, 2017), age (Ko et al., 2016), and gene mutation. HIOP and aging are considered the main risk factors for glaucoma (Chang and Goldberg, 2012) (Weinreb and Khaw, 2004). Previous studies have suggested that autophagy is involved in the pathophysiology of glaucoma along with these key pathogenic triggers and is closely related to glaucoma pathogenesis. We next elaborate on the pathological mechanisms of autophagy and HIOP, mitochondrial dysfunction, oxidative stress, aging, and neuroinflammation in glaucoma (key molecules are depicted in Table 1 and Figure 2).
FIGURE 2. Timeline of autophagy regulation in glaucoma pathogenesis and treatment. The underlying mechanisms of glaucomatous neurodegeneration involve multiple factors, including HIOP, mitochondrial dysfunction, oxidative stress, immune-mediated neuroinflammatory responses, aging, and gene mutation. Strategies targeting the known mechanisms of autophagy might be neuroprotective and, thus, work for treating glaucoma. Abbreviations: HIOP, high intraocular pressure; TNF-α, tumor necrosis factor-alpha; IL-1β, interleukin-1 beta; IL-6, interleukin-6; TGF-β, transforming growth factor-β; MMP, matrix metalloproteinase.
Autophagy activation in HIOP (acute or chronic) has been observed in different experimental models of glaucoma (Piras et al., 2011; Hirt et al., 2018). IOP is exerted by the aqueous humor (AH) on the inner surface of the anterior segment of the eye; normal is approximately 16 mmHg (Carreon et al., 2017). AH is a clear fluid produced by filtering blood through the ciliary body, which enters the anterior chamber of the eye at a relatively constant rate. AH nourishes the cornea, lens, and TM in the anterior chamber and exits mainly through the TM/Schlemm’s canal (SC). Elevated IOP is caused by the failure of the TM/SC, a conventional outflow tract, leading to an inability to maintain an adequate level of AH outflow resistance (i.e., impaired outflow) (Stamer and Acott, 2012). Thus, the TM is therefore particularly important for maintaining normal IOP as it is the main outflow pathway for AH. Dysregulation of autophagy in the retina and TM has been demonstrated in the HIOP model of glaucoma (Nettesheim et al., 2020).
Numerous studies have demonstrated that cells in the outflow pathway are subjected and responsive to fluctuating IOP and mechanical loads (Hirt and Liton, 2017). Eleven known mechanotransduction channel proteins were detected in TM tissue and isolated TM cell cultures (Tran et al., 2014). Experiments conducted in in vitro perfusion eyes have demonstrated the presence of a mechanism regulating IOP homeostasis in TM/SC outflow pathway tissues (Acott et al., 2014). After the perfusion rate increased, resulting in a short IOP increase, even if the inflow was maintained at high pressure, the IOP gradually returned to the baseline level in a few days (Bradley et al., 2001). This observation has been confirmed by various researchers, suggesting that a compensatory mechanism helps the maintenance of IOP homeostasis (Borrás, 2003; Baetz et al., 2009; Porter et al., 2014). Cells in the outflow channel sense pressure imbalance in the form of mechanical stretching, which is thought to trigger a response (Hirt and Liton, 2017). TM cells are prone to stretch injury after long-term exposure to tension; thus, they must be guided to adapt to this mechanical force and repair the damage caused by potential stretching. One study suggested that autophagy may be a possible cellular adaptation mechanism in response to this stretch (Shim et al., 2020). A recent study has shown that primary cilia (PC) are mechanosensory for stretch-induced autophagy in TM cells, and PC-mediated autophagy is critical for IOP homeostasis (Shim et al., 2021). Stretch-induced autophagy under normal physiological forces is helpful to eliminate damaged components and accelerate the renewal of proteins and organelles, resulting in increased cytoskeletal and cortical stiffness in TM cells (Liton, 2016), maintaining cellular homeostasis, and inhibiting cell death (Porter et al., 2014). Conversely, autophagy dysregulation may trigger cell death and lead to disease if the mechanical forces exceed the physiological limits or if the conditions are pathological (Hirt and Liton, 2017). Overactivation of autophagy is a potential cellular mechanism leading to optic nerve degeneration in DBA/2J mice (Hirt et al., 2018).
Mitochondria are exquisitely dynamic organelles that move along axons and dendrites in neurons and constantly respond to endogenous and exogenous stimuli, to satisfy the energy requirements of neuronal networks and to maintain neuronal Ca2+ homeostasis and synaptic plasticity (Mattson et al., 2008). The highly coordinated regulation of mitochondria is essential for maintaining the energy in RGC dendrites and axon terminals for optimal neurotransmission (Ito and Di Polo, 2017). To date, several studies have shown that oxidative stress damage and mitochondrial dysfunction are involved in the pathogenesis of glaucoma and are tightly linked to autophagy pathways (Chang et al., 2022).
Studies have shown that overexpression of oxidative stress markers including lipid peroxidation, and oxidative DNA damage is present in various ocular tissues in glaucoma patients (Fernández-Durango et al., 2008). Oxidative stress results from the overproduction of reactive oxygen species (ROS) (⋅O2−, ⋅OH, and H2O2), which are byproducts of cellular respiration, particularly during mitochondrial oxidative phosphorylation, and are produced by the electron transport chain in the inner mitochondrial membrane (Shadel and Horvath, 2015). Although low ROS at physiological functions in redox signaling, excessive ROS produced by mitochondrial malfunction can lead to the oxidative damage of cellular components, comprising lipids, proteins, and DNA (Shim and Liton, 2022). One is in the mitochondria ROS in the form of superoxide and H2O2 mediates the sustained activation of nuclear factor kappa-B (NF-κB) and the upregulation of inflammatory markers like interleukin-1α (IL-1α) and endothelial leukocyte adhesion molecule-1 (ELAM-1) (Li et al., 2007). Another crucial source is the production of ROS through the iron-catalyzed Fenton/Haber-Weiss reaction (Kruszewski, 2003; Yauger et al., 2019). The Fenton/Haber-Weiss reaction results in the production of highly oxidized hydroxyl radicals, which induce lipid and protein peroxidation and cause oxidative damage to DNA (Kruszewski, 2003). Among other things, the increase in lipid peroxidation may be attributed to a deficiency in the antioxidant system (Fernández-Durango et al., 2008). ROS exert two regulatory effects on autophagy, inducing autophagy through the activation of transcription factors (HIF-1α, NRF2, P53, and FOXO3) and by driving the expression of genes required for autophagy induction (including BECN1, LC3, SQSTM1) via posttranslational regulation (Chang et al., 2022). Autophagy can also be inhibited by the oxidation of ATG proteins (ATG7 and ATG10) or by the inactivation of autophagy regulators (TFEB and PTEN) (Su et al., 2018; Chang et al., 2022). In turn, autophagy can reduce ROS levels and alleviate oxidative damage by eliminating damaged molecules (e.g., protein aggregates) and damaged organelles (e.g., mitochondrial cartilage), thus maintaining proper redox signaling and DNA damage repair systems (Li et al., 2015). These findings suggests that mitochondrial autophagy induction may improve the pathogenesis of glaucoma.
The main neuroglia present in the retina are microglia, astrocytes, and Müller cells (Vecino et al., 2016). Of these, microglia play a crucial role in neuroinflammation (Zhang W. et al., 2021), which is a critical process in glaucoma (Guzman-Martinez et al., 2019). Microglia in the retina are resident innate immune cells in neural tissue that perform as neuropathological receptors and are also the primary immune defense against retinal damage (Chen et al., 2022). Under physiological conditions, glial cells secrete neurotrophic factors to promote nerve cell survival and tissue regeneration and perform phagocytosis to eliminate foreign components (Campagno et al., 2021). Microglia activated by stimulation [such as HIOP (Campagno et al., 2021)] secrete inflammatory cytokines to induce neuronal apoptosis, which further contributes to neuroinflammation. Activated microglia can be polarized into either an M1 proinflammatory phenotype or an M2 anti-inflammatory phenotype, and the two can switch between each other in response to different microenvironmental disorders, known as classical activation and alternative activation respectively (Lan et al., 2017). M1 polarized microglia activate the neuroinflammatory response and exacerbate neuronal damage via the synthesis and release of injury-mediating cytokines (TNFα, IL-1β, IL-6, IL-12), protein hydrolases (MMPs), and the expression of MHC-II (Ramirez et al., 2017). M2 polarized microglia, secrete anti-inflammatory cytokines and growth factors and play the opposite role of M1 microglia (Lan et al., 2017), promoting phagocytosis and waste removal, neurogenesis, axonal remodeling, or myelin regeneration after injury (Liu et al., 2018). Activated neuroinflammatory pathways may initially restore homeostasis between RGCs and the extracellular environment, with protective effects. However, the overproduction of chemokines, and cytokines and the excessive activation of retina-resident microglia and other innate immune cells, may prompt RGC degeneration (Quaranta et al., 2021). Neuroinflammation may be an important cause of RGC degeneration and targeting M2 microglial polarization could be a potential therapeutic option to treat glaucoma (Cherry et al., 2014; Reboussin et al., 2022).
The proinflammatory cytokine TNF-α produced by M1 microglia was demonstrated to inhibit autophagic flux in neurons and microglia, which further drives M1 polarization in microglia via activation of the Akt/mTOR pathway (Jin et al., 2018). Conversely, IL-4, which promotes microglial polarization to the M2 subtype, activates autophagic flux and exerts a protective effect (Tang et al., 2019). Autophagy has been reported to direct microglial activation to the M2 subtype, and consistently, autophagy inhibition promotes polarization toward the proinflammatory M1 subtype (Jin et al., 2018). The M2 subtype is maintained by high autophagic flux, and disruption of autophagic flux results in a shift in the M1-related subtype (Zubova et al., 2022). In conclusion, autophagy degrades proinflammatory proteins, limits the inflammatory process, and exerts a protective effect.
In most tissues, autophagy declines with age, which can lead to the accumulation of certain types of cellular components at potentially toxic levels that can be harmful to cells (Hansen et al., 2018). Dysregulation of autophagy, a cellular catabolic mechanism that removes misfolded proteins, is responsible for a range of neurodegenerative diseases (Lipinski et al., 2010). Autophagy activity is impaired as the brain ages in different species (Hansen et al., 2018). For example, autophagy is reduced in mouse hypothalamic neurons (Kaushik et al., 2012) and the mRNA levels of many ATG are reduced in the aging human brain (Lipinski et al., 2010). Studies on the retina have determined that glaucoma is also a neurodegenerative disease, and the mRNA expression of autophagy regulators (e.g., ATG and Beclin) is reduced (Rodríguez-Muela et al., 2013). In an optic nerve crush model, autophagy-deficiency in aged mice (Ambra1+/gt) increases axonal damage susceptibility (Bell et al., 2020). Autophagy in the retina decreases with age and activation of autophagy shows neuroprotective effects in vivo experimental of glaucoma (Bell et al., 2020). Dysregulation of TM autophagy associated with elevated IOP also occurs during aging (Hirt et al., 2018). Overall, there is growing evidence to support the beneficial role of autophagy in the fight against aging and age-related diseases.
OPTN, TANK binding kinase 1 (TBK1), and Myocilin (MYOC) are genes linked to glaucoma (Sears et al., 2019). Mutation in each of these genes may cause this major blinding disease (Sears et al., 2019). E50K and M98K mutants induced excess cell death than wild-type OPTN (Sirohi et al., 2013; Sirohi and Swarup, 2016). E50K mutation causes retinal cell death in the cell culture model and transgenic mouse model by inhibition of autophagy (Chalasani et al., 2014; Zhang S. et al., 2021). M98K is a gain-of-function mutation, overexpression of M98K shows enhanced autophagic signaling leading to endocytic recycling of transferrin receptor (TFRC) degradation and causing cell death (Sirohi et al., 2013). Uncontrolled activation of TBK1 by the mutation that A 2-bp insertion in OPTN leads to impaired autophagy that results in the accumulation of LC3-positive aggregates, which induces ER stress and a retinal cell line, 661 W, cell death (Medchalmi et al., 2021). In addition, duplication of TBK1 can cause glaucoma, for the reason that increased transcription of TBK1 encodes a kinase that phosphorylates OPTN, which recruits LC3B and initiates autophagy (Tucker et al., 2014). The mutant MYOC-induced impaired autophagy leads to TM cell death and HIOP, which is associated with the induction of transcription factor, CCAAT/enhancer-binding protein homologous protein (Stothert et al., 2016; Kasetti et al., 2021; Yan et al., 2022). These evidences indicated that some glaucoma caused by gene mutation is related to impaired autophagy.
Glaucoma is a complex disease with no effective cure. Glaucoma is usually treated with prescription eye drops that function via different molecular mechanisms, including prostaglandin analogs (latanoprost, travoprost, bimatoprost, and tafluprost) (Aihara, 2021), β-blockers (Skov et al., 2021), α-adrenergic agonists (Nocentini and Supuran, 2019), cholinergic inhibitors, carbonic anhydrase inhibitors (Jansook et al., 2021) and cholinergic drugs (Faiq et al., 2019). These eye drops reduce IOP by improving the outflow of AH through the trabecular drainage system or by reducing the volume of AH produced by the eye. However, the absorption of eye drop molecules into the bloodstream can result in side effects (Xu et al., 2017). By way of explanation, beta-blockers are connected with cardiac arrhythmia, congestive heart failure, and airway obstruction (Argulian et al., 2019), and carbonic anhydrase inhibitors may provoke thrombocytopenia (Xu et al., 2017). In some patients with advanced glaucoma, eye drops cannot lower the IOP to the required level and surgery or alternative treatment is needed. Surgical treatment to increase AH flow is currently the main method used to reduce IOP. The first laser treatment to enhance the outflow system was performed approximately 50 years ago (Wise and Witter, 1979). At present, the main types of surgery are selective laser trabeculoplasty (Töteberg-Harms and Meier-Gibbons, 2021), glaucoma filtration surgery(Wolters et al., 2021), Ahmed glaucoma valve implantation(a popular glaucoma drainage implant) (Riva et al., 2017), or minimally invasive glaucoma surgery (Kan et al., 2021).
Therapy to lower IOP has proven successful in preserving vision in some glaucoma patients (Heijl et al., 2002; Lichter, 2002), but not all. There is growing evidence that regulation of autophagy to degrade misfolded proteins and remove damaged organelles may be an ideal option for glaucoma treatment (Tsai et al., 2022). However, autophagy can be a "double-edged sword" in various animal models and experimental approaches and requires to be precisely regulated; thus, it is particularly crucial to better perceive the molecular pathways and targeted regulation of autophagy (Park et al., 2018; Ishikawa et al., 2021). Autophagy is a highly conservative, highly dynamic, and complex regulatory process, which can theoretically be stimulated at multiple levels, providing a variety of pharmacological targets for the development of corresponding agonists or antagonists (Zhang Z. et al., 2021). Below, we will discuss the potential mechanisms of various Western and Chinese therapies, emerging biological agents, and stem cell interventions for autophagy in glaucoma treatment as well as their effectiveness in animal models and clinical trials.
In recent years, Chinese medicine has been effective for treating glaucoma in combination with Western medicine or surgery (Wen-bo et al., 2020; Xirui et al., 2021). In Chinese medicine, it is considered that the pathogenesis of glaucoma is based on “stasis” and “deficiency”, and treatment should be based on activating blood circulation, removing blood stasis, moving qi, and opening depression, which corresponds to the “clearing” effect of autophagy. Autophagy, a current research hotspot, is closely related to glaucomatous optic neuropathy in many ways and has led to initial research progress in using Chinese medicine therapies to regulate autophagy to protect the optic nerve.
In research on Chinese medicine/Chinese herbal extracts, in a rat model of chronic hypertensive glaucoma established by the extrascleral vein cautery (EVC) method, autophagy was significantly activated in retinal cells and the number of autophagic vesicles increased, while chuanxiongzin completely reversed this change in rats by activating the PI3K-Akt/mTOR pathway to inhibit autophagy, acting as a protective agent (Du et al., 2021). The possible signaling pathways through which each herbal medicine/Chinese medicine extract exerts its protective effect by regulating autophagy are shown in Table 2.
Clinical data show that acupuncture combined with conventional Western medicine can effectively reduce intraocular pressure and significantly improve the degree of optic nerve atrophy in patients with glaucoma (Wang, 2017). Acupuncture also reduces visual field defects and improves ocular artery hemodynamics and visual acuity (Leszczynska et al., 2018; Lan and Xiaojie, 2020). At present, studies involving the optic neuroprotective effects of acupuncture therapy have been limited to clinical data analysis, but in the neuroprotective effect of cranial nerves, acupuncture can play a protective role by inhibiting autophagy through the mTORC1–ULK complex–Beclin1pathway (Liu et al., 2016).
Enhanced autophagy efficacy may reduce the number of toxic protein aggregates, provide an effective stress response, and prevent or reduce cell death by degrading inessential components for energy and supporting adaptive protein synthesis. Allogeneic progesterone (Allop) increases autophagy vesicles, activates autophagy, and protects RGCs via GABR/GABAA receptors (Ishikawa et al., 2021). Rapamycin inhibits mTOR and therefore induces autophagy in peripheral organs and the CNS. Using an optic nerve transection model, rapamycin-treated mice showed a 40% improvement in RGC survival 10 days after axotomy, exerting a beneficial effect (Rodríguez-Muela et al., 2012). Rapamycin can also increase the RGC number and visual function, and reduce apoptosis of E50K-OPTN mice (Shen et al., 2011; Zhang S. et al., 2021). Stimulation of autophagy via tat-beclin 1 peptide or Torin 2 rescued MYOC-associated transgenic mice model (Kasetti et al., 2021). In various in vivo experimental models of glaucoma, the induction of autophagy by pharmacological or genetic manipulation can improve RGC survival (Table 2).
Evidence suggests that autophagy induction has a neuroprotective effect on glaucoma-damaged RGCs; however, autophagy induction cannot be directly said to be a panacea for neurodegenerative disease, and the induction of autophagosome biogenesis may be harmful if autophagosome accumulation is not effectively cleared. It has been shown that autophagy inhibition also has neuroprotective effect on glaucoma-damaged RGCs. In a rat model of chronic HIOP glaucoma, autophagy was significantly activated in RGCs (Deng et al., 2013). The number of TUNEL-positive cells was reduced in the GCL since autophagy inhibition by the autophagy inhibitor 3-Methyladenine (3-MA), indicating that apoptosis occurred in RGCs after autophagy-induced chronic IOP elevation (Park et al., 2012). M98K-OPTN potentiates the degradation of TFRC by autophagy and contributes to the death of RGCs; chloroquine (CQ) or the knockdown of Atg5 by shRNA significantly reduced cell death mediated by M98K (Sirohi et al., 2013; Swarup and Sayyad, 2018). The possible signaling pathways through which each Western drug exerts its protective effects by modulating autophagy are shown in Table 2.
Currently the role of autophagy in glaucoma is still controversial; it relies on the type of glaucoma model, the target of pharmacological treatment, and the period of drug administration (Wang et al., 2015; Russo et al., 2018). Studies have shown that is chemic/reperfusion can trigger autophagy in the first few hours; although RGC loss at this time, autophagy as the mechanism of self-adaptation under stress conditions might maintain cellular homeostasis and reduce the harm (Russo et al., 2018). The subsequent protective effect of rapamycin also confirmed this point (Russo et al., 2018). However, it is demonstrated that rapamycin aggravated RGC loss and axon demyelination at day 14 of reperfusion, as mTOR activity plays a crucial role in determining neuronal survival (Chen et al., 2016). The role of autophagy in glaucoma is multifunctional and dynamic. Consequently, a better comprehension of the underlying mechanisms of autophagy dysfunction in glaucoma is essential for developing therapeutic approaches.
RGCs degenerate in glaucoma, resulting in permanent vision loss. Cell replacement strategies are considered potential therapies for RGC loss. Researchers have recently explored a potential replacement therapy based on human stem cells that can differentiate into RGC cells (Nuzzi and Tridico, 2017; Chang et al., 2019). However, scaling up donor cells, advancing cell differentiation, and promoting cell durability remain challenges in replacement therapies.
Elaborate mechanisms render mesenchymal stem cells (MSCs) neuroprotective, including modulating neuroinflammation, enhancing cell survival, increasing neurogenesis, and regulating ubiquitinated proteins (Shin et al., 2014). It has been documented that hUC-MSCs have a protective effect on retinal neurons (Wang et al., 2021), and MSC-EVs also exert a neuroprotective effect on the retina by reducing neuroinflammation and neuronal apoptosis (Mathew et al., 2019). At present, studies involving the optic neuroprotective effects of MSCs and MSC-EVs are more limited to phenotypic analysis, and in the brain, MSCs can improve neuronal survival by activating autophagy (Shin et al., 2014; Ceccariglia et al., 2020).
In summary, autophagy is an important metabolic process that maintains cellular homeostasis and autophagy dysfunction influences in the pathogenesis of glaucoma. Although the relationship between autophagy and glaucoma remains controversial and it is uncertain whether autophagy dysfunction plays a determinant role in disease progression, increasing evidence suggests that autophagy induction has a neuroprotective effect in glaucoma (Russo et al., 2018). Overactivated autophagy may also cause the development of autophagic cell death (Shi et al., 2012). In any case, since autophagy has a waste-disposal function, its activation and inhibition may be a novel therapeutic strategy in the context of glaucoma.
It has been shown that autophagy activity may not be simply positive or negative for cell survival, but that its effects depend on the timing, amplitude and target of autophagy activation (Ferrucci et al., 2018). Thus, more detailed studies are required to determine the timing and dosage of autophagy modulators that moderately activate autophagy and maintain it at relatively normal metabolic levels. It has been found that the induction of acute autophagy in a retinal ischemia-reperfusion model continues for the first few hours and then declines (Russo et al., 2018; Tang et al., 2021). While the activation of autophagy has been mentioned as having a protective effect, inhibition of autophagy has also exhibited a protective effect. The initial increase in autophagy may be compensatory effect, while activating autophagy with drugs can only have protective effect after autophagy subsequently decreases. Thus, it is necessary to perform a precise assessment of autophagy at various time points in the disease model and make minor adjustments to drug concentrations to characterize the potential time-dependent changes in autophagy and the optimal concentration of drugs for obtaining accurate data on the protective effects of modulating autophagy.
There have been many studies on the role of autophagy in the pathogenesis and treatment of glaucoma in the experiment. HIOP, oxidative stress, neuroinflammation, aging and gene mutation may all lead to the dysregulation of autophagy, and regulating autophagy is also a target for the treatment of glaucoma. However, progress in evaluating the characteristics of autophagy in the pathogenesis of human glaucoma and therapeutic options depend heavily on the advancement of methods to monitor autophagy activity in humans. For retina, it is not as easy to obtain human fresh biopsies as blood, muscle, and adipose. Autopsy materials are heterogeneity and can only be measured statically (Klionsky et al., 2021). Monitoring autophagy dynamic activity in glaucoma and truly autophagy fluxes of some human tissues remain relatively challenging. For example, it is not rigorous to simply assay levels of endogenous LC3-II in retinal tissue samples and use this as a proxy for autophagy flux. Because there is no real effective degradation of LC3II, if there is an impaired fusion of autophagosomes with lysosomes or impaired lysosomal activity late in the autophagy pathway, an increase in LC3II does not represent increased autophagy activity (Mizushima et al., 2010). There are studies suggest that the total cellular expression level of p62 is generally considered inversely proportional to autophagy activity (Klionsky et al., 2021), but it is unclear whether p62 is degraded only or partially by the ubiquitin-proteasome pathway of autophagy (Mizushima et al., 2010). This also means that most experts in the field do not consider some of the methods used in the literature to be appropriate measures of autophagic flux (or autophagic activity). It is essential to develop real-time monitoring methods for autophagy and measurement of biomarkers that can be applied in clinical practice.
Autophagy-oriented strategies may offer a new and promising alternative for the development of drugs for glaucoma. In addition, it would be interesting to investigate emerging targeted delivery molecules to modulate autophagy and reduce side effects, and to investigate the use of biological agents such as stem cell extracellular vesicles or engineered exosomes to modulate autophagy and achieve a protective effect consistent with that of stem cells.
ML and Z-LG were the major contributors to reviewing the literature, writing the manuscript, and creating descriptive figures. Q-PZ provided supervision. A-XL and W-YX were major contributors to editing the tables and figures. T-QD and X-PW assisted in the literature review. J-FH was a major contributor to editing the manuscript. All authors read and approved the final manuscript.
This research was supported by the Fundamental Research FUNDS FOR THE Central Universities of Central South University (2021XQLH083, 2021XQLH091, 2021XQLH092, 2021XQLH093).
We would like to thank the English language editing service of AJE.
The authors declare that the research was conducted in the absence of any commercial or financial relationships that could be construed as a potential conflict of interest.
All claims expressed in this article are solely those of the authors and do not necessarily represent those of their affiliated organizations, or those of the publisher, the editors and the reviewers. Any product that may be evaluated in this article, or claim that may be made by its manufacturer, is not guaranteed or endorsed by the publisher.
RGCs, retinal ganglion cells; MSVI, moderate and severe vision impairment; CNS, central nervous system; IOP, intraocular pressure; PD, Parkinson’s disease; HIOP, high IOP; TM, trabecular meshwork; LC3, light chain 3 protein; ATG, autophagy related genes; PI3K, phosphatidylinositol 3-kinase; PI3P, phosphatidylinositol 3-phosphate; ATG5-12/16L1, ATG5-ATG12 conjugate binds to ATG16L1 to generate the autophagy elongation complex; PE, phosphatidylethanolamine; TFEB, transcription factor EB; OPTN, optineurin; AH, aqueous humor; SC, Schlemm’s canal; PC, primary cilia; ROS, reactive oxygen species; NF-κB, nuclear factor kappa-B; IL-1α, interleukin-1α; ELAM-1, endothelial leukocyte adhesion molecule-1; TBK1, TANK binding kinase 1, MYOC, Myocilin; TFRC, transferrin receptor; EVC, extra-scleral vein cautery; Allop, Allogeneic progesterone; 3-MA, 3-Methyladenine; CQ, chloroquine; MSCs, Mesenchymal stem cells.
Acott, T. S., Kelley, M. J., Keller, K. E., Vranka, J. A., Abu-Hassan, D. W., Li, X., et al. (2014). Intraocular pressure homeostasis: Maintaining balance in a high-pressure environment. J. Ocul. Pharmacol. Ther. 30 (2-3), 94–101. doi:10.1089/jop.2013.0185
Aihara, M. (2021). Prostanoid receptor agonists for glaucoma treatment. Jpn. J. Ophthalmol. 65 (5), 581–590. doi:10.1007/s10384-021-00844-6
Argulian, E., Bangalore, S., and Messerli, F. H. (2019). Misconceptions and facts about beta-blockers. Am. J. Med. 132 (7), 816–819. doi:10.1016/j.amjmed.2019.01.039
Baetz, N. W., Hoffman, E. A., Yool, A. J., and Stamer, W. D. (2009). Role of aquaporin-1 in trabecular meshwork cell homeostasis during mechanical strain. Exp. Eye Res. 89 (1), 95–100. doi:10.1016/j.exer.2009.02.018
Bell, K., Rosignol, I., Sierra-Filardi, E., Rodriguez-Muela, N., Schmelter, C., Cecconi, F., et al. (2020). Age related retinal Ganglion cell susceptibility in context of autophagy deficiency. Cell Death Discov. 6, 21. doi:10.1038/s41420-020-0257-4
Benyair, R., Eisenberg-Lerner, A., and Merbl, Y. (2022). Maintaining Golgi homeostasis: A balancing act of two proteolytic pathways. Cells 11 (5), 780. doi:10.3390/cells11050780
Bonomi, L., Marchini, G., Marraffa, M., Bernardi, P., De Franco, I., Perfetti, S., et al. (1998). Prevalence of glaucoma and intraocular pressure distribution in a defined population. The Egna-Neumarkt Study. Ophthalmology 105 (2), 209–215. doi:10.1016/s0161-6420(98)92665-3
Borrás, T. (2003). Gene expression in the trabecular meshwork and the influence of intraocular pressure. Prog. Retin. Eye Res. 22 (4), 435–463. doi:10.1016/s1350-9462(03)00018-1
Boya, P. (2017). Why autophagy is good for retinal ganglion cells? Eye (Lond) 31 (2), 185–190. doi:10.1038/eye.2016.278
Bradley, J. M., Kelley, M. J., Zhu, X., Anderssohn, A. M., Alexander, J. P., and Acott, T. S. (2001). Effects of mechanical stretching on trabecular matrix metalloproteinases. Invest. Ophthalmol. Vis. Sci. 42 (7), 1505–1513.
Bressan, C., Saghatelyan, A., Czyzewska, M. M., Brodzki, M., and Mozrzymas, J. W. (2020). GABAA receptor β2E155 residue located at the agonist-binding site is involved in the receptor gating. Front. Cell. Neurosci. 14, 2. doi:10.3389/fncel.2020.00002
Campagno, K. E., Lu, W., Jassim, A. H., Albalawi, F., Cenaj, A., Tso, H. Y., et al. (2021). Rapid morphologic changes to microglial cells and upregulation of mixed microglial activation state markers induced by P2X7 receptor stimulation and increased intraocular pressure. J. Neuroinflammation 18 (1), 217. doi:10.1186/s12974-021-02251-7
Carreon, T., van der Merwe, E., Fellman, R. L., Johnstone, M., and Bhattacharya, S. K. (2017). Aqueous outflow - a continuum from trabecular meshwork to episcleral veins. Prog. Retin. Eye Res. 57, 108–133. doi:10.1016/j.preteyeres.2016.12.004
Ceccariglia, S., Cargnoni, A., Silini, A. R., and Parolini, O. (2020). Autophagy: A potential key contributor to the therapeutic action of mesenchymal stem cells. Autophagy 16 (1), 28–37. doi:10.1080/15548627.2019.1630223
Chalasani, M. L., Kumari, A., Radha, V., and Swarup, G. (2014). E50K-OPTN-induced retinal cell death involves the Rab GTPase-activating protein, TBC1D17 mediated block in autophagy. PLoS One 9 (4), e95758. doi:10.1371/journal.pone.0095758
Chang, E. E., and Goldberg, J. L. (2012). Glaucoma 2.0: Neuroprotection, neuroregeneration, neuroenhancement. Ophthalmology 119 (5), 979–986. doi:10.1016/j.ophtha.2011.11.003
Chang, K. C., Liu, P. F., Chang, C. H., Lin, Y. C., Chen, Y. J., and Shu, C. W. (2022). The interplay of autophagy and oxidative stress in the pathogenesis and therapy of retinal degenerative diseases. Cell Biosci. 12 (1), 1. doi:10.1186/s13578-021-00736-9
Chang, K. C., Sun, C., Cameron, E. G., Madaan, A., Wu, S., Xia, X., et al. (2019). Opposing effects of growth and differentiation factors in cell-fate specification. Curr. Biol. 29 (12), 1963–1975. doi:10.1016/j.cub.2019.05.011
Chen, D., Peng, C., Ding, X. M., Wu, Y., Zeng, C. J., Xu, L., et al. (2022). Interleukin-4 promotes microglial polarization toward a neuroprotective phenotype after retinal ischemia/reperfusion injury. Neural Regen. Res. 17 (12), 2755–2760. doi:10.4103/1673-5374.339500
Chen, G., Tang, L., Wei, W., Li, Z., Li, Y., Duan, X., et al. (2016). mTOR regulates neuroprotective effect of immunized CD4+Foxp3+ T cells in optic nerve ischemia. Sci. Rep. 6, 37805. doi:10.1038/srep37805
Chen, Q., Xi, X., Zeng, Y., He, Z., Zhao, J., and Li, Y. (2019). Acteoside inhibits autophagic apoptosis of retinal ganglion cells to rescue glaucoma-induced optic atrophy. J. Cell. Biochem. 120 (8), 13133–13140. doi:10.1002/jcb.28586
Cherry, J. D., Olschowka, J. A., and O'Banion, M. K. (2014). Neuroinflammation and M2 microglia: The good, the bad, and the inflamed. J. Neuroinflammation 11, 98. doi:10.1186/1742-2094-11-98
Conway, O., Akpinar, H. A., Rogov, V. V., and Kirkin, V. (2020). Selective autophagy receptors in neuronal Health and disease. J. Mol. Biol. 432 (8), 2483–2509. doi:10.1016/j.jmb.2019.10.013
Deng, S., Wang, M., Yan, Z., Tian, Z., Chen, H., Yang, X., et al. (2013). Autophagy in retinal ganglion cells in a rhesus monkey chronic hypertensive glaucoma model. PLoS One 8 (10), e77100. doi:10.1371/journal.pone.0077100
Du, H. Y., Wang, R., Li, J. L., Luo, H., Xie, X. Y., Yan, R., et al. (2021). Ligustrazine protects against chronic hypertensive glaucoma in rats by inhibiting autophagy via the PI3K-Akt/mTOR pathway. Mol. Vis. 27, 725–733.
Ebrahimi-Fakhari, D., Wahlster, L., and McLean, P. J. (2012). Protein degradation pathways in Parkinson's disease: Curse or blessing. Acta Neuropathol. 124 (2), 153–172. doi:10.1007/s00401-012-1004-6
Faiq, M. A., Wollstein, G., Schuman, J. S., and Chan, K. C. (2019). Cholinergic nervous system and glaucoma: From basic science to clinical applications. Prog. Retin. Eye Res. 72, 100767. doi:10.1016/j.preteyeres.2019.06.003
Feng, Y., Yao, Z., and Klionsky, D. J. (2015). How to control self-digestion: Transcriptional, post-transcriptional, and post-translational regulation of autophagy. Trends Cell Biol. 25 (6), 354–363. doi:10.1016/j.tcb.2015.02.002
Fernández-Durango, R., Fernández-Martínez, A., García-Feijoo, J., Castillo, A., de la Casa, J. M., García-Bueno, B., et al. (2008). Expression of nitrotyrosine and oxidative consequences in the trabecular meshwork of patients with primary open-angle glaucoma. Invest. Ophthalmol. Vis. Sci. 49 (6), 2506–2511. doi:10.1167/iovs.07-1363
Ferrucci, M., Biagioni, F., Ryskalin, L., Limanaqi, F., Gambardella, S., Frati, A., et al. (2018). Ambiguous effects of autophagy activation following hypoperfusion/ischemia. Int. J. Mol. Sci. 19 (9), 2756. doi:10.3390/ijms19092756
Frake, R. A., Ricketts, T., Menzies, F. M., and Rubinsztein, D. C. (2015). Autophagy and neurodegeneration. J. Clin. Invest. 125 (1), 65–74. doi:10.1172/jci73944
Galluzzi, L., Baehrecke, E. H., Ballabio, A., Boya, P., Bravo-San Pedro, J. M., Cecconi, F., et al. (2017). Molecular definitions of autophagy and related processes. Embo J. 36 (13), 1811–1836. doi:10.15252/embj.201796697
GBD 2019 Blindness and Vision Impairment Collaborators; Vision Loss Expert Group of the Global Burden of Disease Study (2021). Causes of blindness and vision impairment in 2020 and trends over 30 years, and prevalence of avoidable blindness in relation to VISION 2020: The right to sight: An analysis for the global burden of disease study. Lancet. Glob. Health 9 (2), e144–e160. doi:10.1016/s2214-109x(20)30489-7
Glick, D., Barth, S., and Macleod, K. F. (2010). Autophagy: Cellular and molecular mechanisms. J. Pathol. 221 (1), 3–12. doi:10.1002/path.2697
Gupta, N., and Yücel, Y. H. (2007). Glaucoma as a neurodegenerative disease. Curr. Opin. Ophthalmol. 18 (2), 110–114. doi:10.1097/ICU.0b013e3280895aea
Guzman-Martinez, L., Maccioni, R. B., Andrade, V., Navarrete, L. P., Pastor, M. G., and Ramos-Escobar, N. (2019). Neuroinflammation as a common feature of neurodegenerative disorders. Front. Pharmacol. 10, 1008. doi:10.3389/fphar.2019.01008
Hansen, M., Rubinsztein, D. C., and Walker, D. W. (2018). Autophagy as a promoter of longevity: Insights from model organisms. Nat. Rev. Mol. Cell Biol. 19 (9), 579–593. doi:10.1038/s41580-018-0033-y
Harvey, A. R., Ooi, J. W., and Rodger, J. (2012). Neurotrophic factors and the regeneration of adult retinal ganglion cell axons. Int. Rev. Neurobiol. 106, 1–33. doi:10.1016/b978-0-12-407178-0.00002-8
He, J., Deng, L., Liu, H., Chen, T., Chen, S., Xia, S., et al. (2019). BCL2L10/BECN1 modulates hepatoma cells autophagy by regulating PI3K/AKT signaling pathway. Aging (Albany NY) 11 (2), 350–370. doi:10.18632/aging.101737
Heijl, A., Leske, M. C., Bengtsson, B., Hyman, L., Bengtsson, B., Hussein, M., et al. (2002). Reduction of intraocular pressure and glaucoma progression: Results from the early manifest glaucoma trial. Arch. Ophthalmol. 120 (10), 1268–1279. doi:10.1001/archopht.120.10.1268
Hirt, J., and Liton, P. B. (2017). Autophagy and mechanotransduction in outflow pathway cells. Exp. Eye Res. 158, 146–153. doi:10.1016/j.exer.2016.06.021
Hirt, J., Porter, K., Dixon, A., McKinnon, S., and Liton, P. B. (2018). Contribution of autophagy to ocular hypertension and neurodegeneration in the DBA/2J spontaneous glaucoma mouse model. Cell Death Discov. 4, 14. doi:10.1038/s41420-018-0077-y
Ishikawa, M., Takaseki, S., Yoshitomi, T., Covey, D. F., Zorumski, C. F., and Izumi, Y. (2021). The neurosteroid allopregnanolone protects retinal neurons by effects on autophagy and GABRs/GABA(A) receptors in rat glaucoma models. Autophagy 17 (3), 743–760. doi:10.1080/15548627.2020.1731270
Ito, Y. A., and Di Polo, A. (2017). Mitochondrial dynamics, transport, and quality control: A bottleneck for retinal ganglion cell viability in optic neuropathies. Mitochondrion 36, 186–192. doi:10.1016/j.mito.2017.08.014
Jansook, P., Hnin, H. M., Loftsson, T., and Stefánsson, E. (2021). Cyclodextrin-based formulation of carbonic anhydrase inhibitors for ocular delivery - a review. Int. J. Pharm. 606, 120955. doi:10.1016/j.ijpharm.2021.120955
Jin, M. M., Wang, F., Qi, D., Liu, W. W., Gu, C., Mao, C. J., et al. (2018). A critical role of autophagy in regulating microglia polarization in neurodegeneration. Front. Aging Neurosci. 10, 378. doi:10.3389/fnagi.2018.00378
Johansen, T., and Lamark, T. (2020). Selective autophagy: ATG8 family proteins, LIR motifs and cargo receptors. J. Mol. Biol. 432 (1), 80–103. doi:10.1016/j.jmb.2019.07.016
Kan, J. T., Betzler, B. K., Lim, S. Y., and Ang, B. C. H. (2021). Anterior segment imaging in minimally invasive glaucoma surgery - a systematic review. Acta Ophthalmol. 100, e617–e634. doi:10.1111/aos.14962
Kasetti, R. B., Maddineni, P., Kiehlbauch, C., Patil, S., Searby, C. C., Levine, B., et al. (2021). Autophagy stimulation reduces ocular hypertension in a murine glaucoma model via autophagic degradation of mutant myocilin. JCI Insight 6 (5), e143359. doi:10.1172/jci.insight.143359
Kaushik, S., Arias, E., Kwon, H., Lopez, N. M., Athonvarangkul, D., Sahu, S., et al. (2012). Loss of autophagy in hypothalamic POMC neurons impairs lipolysis. EMBO Rep. 13 (3), 258–265. doi:10.1038/embor.2011.260
Kaushik, S., and Cuervo, A. M. (2018). The coming of age of chaperone-mediated autophagy. Nat. Rev. Mol. Cell Biol. 19 (6), 365–381. doi:10.1038/s41580-018-0001-6
Kim, J., Kundu, M., Viollet, B., and Guan, K. L. (2011). AMPK and mTOR regulate autophagy through direct phosphorylation of Ulk1. Nat. Cell Biol. 13 (2), 132–141. doi:10.1038/ncb2152
Kimura, A., Namekata, K., Guo, X., Noro, T., Harada, C., and Harada, T. (2017). Targeting oxidative stress for treatment of glaucoma and optic neuritis. Oxid. Med. Cell. Longev. 2017, 2817252. doi:10.1155/2017/2817252
Kiriyama, Y., and Nochi, H. (2015). The function of autophagy in neurodegenerative diseases. Int. J. Mol. Sci. 16 (11), 26797–26812. doi:10.3390/ijms161125990
Klionsky, D. J., Abdel-Aziz, A. K., Abdelfatah, S., Abdellatif, M., Abdoli, A., Abel, S., et al. (2021). Guidelines for the use and interpretation of assays for monitoring autophagy (4th edition)1. Autophagy 17 (1), 1–382. doi:10.1080/15548627.2020.1797280
Ko, F., Boland, M. V., Gupta, P., Gadkaree, S. K., Vitale, S., Guallar, E., et al. (2016). Diabetes, triglyceride levels, and other risk factors for glaucoma in the national Health and nutrition examination survey 2005-2008. Invest. Ophthalmol. Vis. Sci. 57 (4), 2152–2157. doi:10.1167/iovs.15-18373
Kraft, C., Peter, M., and Hofmann, K. (2010). Selective autophagy: Ubiquitin-mediated recognition and beyond. Nat. Cell Biol. 12 (9), 836–841. doi:10.1038/ncb0910-836
Kruszewski, M. (2003). Labile iron pool: The main determinant of cellular response to oxidative stress. Mutat. Res. 531 (1-2), 81–92. doi:10.1016/j.mrfmmm.2003.08.004
Kuma, A., Hatano, M., Matsui, M., Yamamoto, A., Nakaya, H., Yoshimori, T., et al. (2004). The role of autophagy during the early neonatal starvation period. Nature 432 (7020), 1032–1036. doi:10.1038/nature03029
Lan, J., and Xiaojie, M. (2020). Effect of acupuncture on vision recovery and ocular hemodynamics in patients with glaucomatous optic atrophy. J Mod. J. Integr. Traditional Chin. West. Med. 29 (27), 3003–3007.
Lan, X., Han, X., Li, Q., Li, Q., Gao, Y., Cheng, T., et al. (2017). Pinocembrin protects hemorrhagic brain primarily by inhibiting toll-like receptor 4 and reducing M1 phenotype microglia. Brain Behav. Immun. 61, 326–339. doi:10.1016/j.bbi.2016.12.012
Leszczynska, A., Ramm, L., Spoerl, E., Pillunat, L. E., and Terai, N. (2018). The short-term effect of acupuncture on different ocular blood flow parameters in patients with primary open-angle glaucoma: A randomized, clinical study. Clin. Ophthalmol. 12, 1285–1291. doi:10.2147/opth.S170396
Li, G., Luna, C., Liton, P. B., Navarro, I., Epstein, D. L., and Gonzalez, P. (2007). Sustained stress response after oxidative stress in trabecular meshwork cells. Mol. Vis. 13, 2282–2288.
Li, L., Tan, J., Miao, Y., Lei, P., and Zhang, Q. (2015). ROS and autophagy: Interactions and molecular regulatory mechanisms. Cell. Mol. Neurobiol. 35 (5), 615–621. doi:10.1007/s10571-015-0166-x
Li, X., Leng, Y., Jiang, Q., Wang, Z., Luo, P., Zhang, C., et al. (2020). Eye drops of metformin prevents fibrosis after glaucoma filtration surgery in rats via activating AMPK/Nrf2 signaling pathway. Front. Pharmacol. 11, 1038. doi:10.3389/fphar.2020.01038
Lichter, P. R. (2002). Impact of intraocular pressure reduction on glaucoma progression. Jama 288 (20), 2607–2608. doi:10.1001/jama.288.20.2607
Lipinski, M. M., Zheng, B., Lu, T., Yan, Z., Py, B. F., Ng, A., et al. (2010). Genome-wide analysis reveals mechanisms modulating autophagy in normal brain aging and in Alzheimer's disease. Proc. Natl. Acad. Sci. U. S. A. 107 (32), 14164–14169. doi:10.1073/pnas.1009485107
Liton, P. B. (2016). The autophagic lysosomal system in outflow pathway physiology and pathophysiology. Exp. Eye Res. 144, 29–37. doi:10.1016/j.exer.2015.07.013
Liu, W., Shang, G., Yang, S., Huang, J., Xue, X., Lin, Y., et al. (2016). Electroacupuncture protects against ischemic stroke by reducing autophagosome formation and inhibiting autophagy through the mTORC1-ULK1 complex-Beclin1 pathway. Int. J. Mol. Med. 37 (2), 309–318. doi:10.3892/ijmm.2015.2425
Liu, X., Wen, S., Yan, F., Liu, K., Liu, L., Wang, L., et al. (2018). Salidroside provides neuroprotection by modulating microglial polarization after cerebral ischemia. J. Neuroinflammation 15 (1), 39. doi:10.1186/s12974-018-1081-0
Madhu, L. N., Kodali, M., and Shetty, A. K. (2022). Promise of metformin for preventing age-related cognitive dysfunction. Neural Regen. Res. 17 (3), 503–507. doi:10.4103/1673-5374.320971
Mathew, B., Ravindran, S., Liu, X., Torres, L., Chennakesavalu, M., Huang, C. C., et al. (2019). Mesenchymal stem cell-derived extracellular vesicles and retinal ischemia-reperfusion. Biomaterials 197, 146–160. doi:10.1016/j.biomaterials.2019.01.016
Mattson, M. P., Gleichmann, M., and Cheng, A. (2008). Mitochondria in neuroplasticity and neurological disorders. Neuron 60 (5), 748–766. doi:10.1016/j.neuron.2008.10.010
Medchalmi, S., Tare, P., Sayyad, Z., and Swarup, G. (2021). A glaucoma- and ALS-associated mutant of OPTN induces neuronal cell death dependent on Tbk1 activity, autophagy and ER stress. Febs J. 288 (15), 4576–4595. doi:10.1111/febs.15752
Menzies, F. M., Fleming, A., Caricasole, A., Bento, C. F., Andrews, S. P., Ashkenazi, A., et al. (2017). Autophagy and neurodegeneration: Pathogenic mechanisms and therapeutic opportunities. Neuron 93 (5), 1015–1034. doi:10.1016/j.neuron.2017.01.022
Metaxakis, A., Ploumi, C., and Tavernarakis, N. (2018). Autophagy in age-associated neurodegeneration. Cells 7 (5), 37. doi:10.3390/cells7050037
Mijaljica, D., Nazarko, T. Y., Brumell, J. H., Huang, W. P., Komatsu, M., Prescott, M., et al. (2012). Receptor protein complexes are in control of autophagy. Autophagy 8 (11), 1701–1705. doi:10.4161/auto.21332
Mizushima, N., and Levine, B. (2020). Autophagy in human diseases. N. Engl. J. Med. 383 (16), 1564–1576. doi:10.1056/NEJMra2022774
Mizushima, N. (2020). The ATG conjugation systems in autophagy. Curr. Opin. Cell Biol. 63, 1–10. doi:10.1016/j.ceb.2019.12.001
Mizushima, N., Yoshimori, T., and Levine, B. (2010). Methods in mammalian autophagy research. Cell 140 (3), 313–326. doi:10.1016/j.cell.2010.01.028
Nettesheim, A., Shim, M. S., Dixon, A., Raychaudhuri, U., Gong, H., and Liton, P. B. (2020). Cathepsin B localizes in the caveolae and participates in the proteolytic cascade in trabecular meshwork cells. Potential new drug target for the treatment of glaucoma. J. Clin. Med. 10 (1), 78. doi:10.3390/jcm10010078
Nixon, R. A., Wegiel, J., Kumar, A., Yu, W. H., Peterhoff, C., Cataldo, A., et al. (2005). Extensive involvement of autophagy in alzheimer disease: An immuno-electron microscopy study. J. Neuropathol. Exp. Neurol. 64 (2), 113–122. doi:10.1093/jnen/64.2.113
Nocentini, A., and Supuran, C. T. (2019). Adrenergic agonists and antagonists as antiglaucoma agents: A literature and patent review (2013-2019). Expert Opin. Ther. Pat. 29 (10), 805–815. doi:10.1080/13543776.2019.1665023
Nthiga, T. M., Shrestha, B. K., Bruun, J. A., Larsen, K. B., Lamark, T., and Johansen, T. (2021). Regulation of Golgi turnover by CALCOCO1-mediated selective autophagy. J. Cell Biol. 220 (6), e202006128. doi:10.1083/jcb.202006128
Nuzzi, R., and Tridico, F. (2017). Glaucoma: Biological trabecular and neuroretinal pathology with perspectives of therapy innovation and preventive diagnosis. Front. Neurosci. 11, 494. doi:10.3389/fnins.2017.00494
Pan, T., Kondo, S., Le, W., and Jankovic, J. (2008). The role of autophagy-lysosome pathway in neurodegeneration associated with Parkinson's disease. Brain 131 (8), 1969–1978. doi:10.1093/brain/awm318
Park, H. L., Kim, J. H., and Park, C. K. (2018). Different contributions of autophagy to retinal ganglion cell death in the diabetic and glaucomatous retinas. Sci. Rep. 8 (1), 13321. doi:10.1038/s41598-018-30165-7
Park, H. Y., Kim, J. H., and Park, C. K. (2012). Activation of autophagy induces retinal ganglion cell death in a chronic hypertensive glaucoma model. Cell Death Dis. 3 (4), e290. doi:10.1038/cddis.2012.26
Parzych, K. R., and Klionsky, D. J. (2014). An overview of autophagy: Morphology, mechanism, and regulation. Antioxid. Redox Signal. 20 (3), 460–473. doi:10.1089/ars.2013.5371
Piras, A., Gianetto, D., Conte, D., Bosone, A., and Vercelli, A. (2011). Activation of autophagy in a rat model of retinal ischemia following high intraocular pressure. PLoS One 6 (7), e22514. doi:10.1371/journal.pone.0022514
Porter, K. M., Jeyabalan, N., and Liton, P. B. (2014). MTOR-independent induction of autophagy in trabecular meshwork cells subjected to biaxial stretch. Biochim. Biophys. Acta 1843 (6), 1054–1062. doi:10.1016/j.bbamcr.2014.02.010
Puertollano, R., Ferguson, S. M., Brugarolas, J., and Ballabio, A. (2018). The complex relationship between TFEB transcription factor phosphorylation and subcellular localization. Embo J. 37 (11), e98804. doi:10.15252/embj.201798804
Quaranta, L., Bruttini, C., Micheletti, E., Konstas, A. G. P., Michelessi, M., Oddone, F., et al. (2021). Glaucoma and neuroinflammation: An overview. Surv. Ophthalmol. 66 (5), 693–713. doi:10.1016/j.survophthal.2021.02.003
Ramirez, A. I., de Hoz, R., Salobrar-Garcia, E., Salazar, J. J., Rojas, B., Ajoy, D., et al. (2017). The role of microglia in retinal neurodegeneration: Alzheimer's disease, Parkinson, and glaucoma. Front. Aging Neurosci. 9, 214. doi:10.3389/fnagi.2017.00214
Ravikumar, B., Vacher, C., Berger, Z., Davies, J. E., Luo, S., Oroz, L. G., et al. (2004). Inhibition of mTOR induces autophagy and reduces toxicity of polyglutamine expansions in fly and mouse models of Huntington disease. Nat. Genet. 36 (6), 585–595. doi:10.1038/ng1362
Reboussin, É., Buffault, J., Brignole-Baudouin, F., Réaux-Le Goazigo, A., Riancho, L., Olmiere, C., et al. (2022). Evaluation of neuroprotective and immunomodulatory properties of mesenchymal stem cells in an ex vivo retinal explant model. J. Neuroinflammation 19 (1), 63. doi:10.1186/s12974-022-02418-w
Riva, I., Roberti, G., Katsanos, A., Oddone, F., and Quaranta, L. (2017). A review of the ahmed glaucoma valve implant and comparison with other surgical operations. Adv. Ther. 34 (4), 834–847. doi:10.1007/s12325-017-0503-1
Rodríguez-Muela, N., Germain, F., Mariño, G., Fitze, P. S., and Boya, P. (2012). Autophagy promotes survival of retinal ganglion cells after optic nerve axotomy in mice. Cell Death Differ. 19 (1), 162–169. doi:10.1038/cdd.2011.88
Rodríguez-Muela, N., Koga, H., García-Ledo, L., de la Villa, P., de la Rosa, E. J., Cuervo, A. M., et al. (2013). Balance between autophagic pathways preserves retinal homeostasis. Aging Cell 12 (3), 478–488. doi:10.1111/acel.12072
Russo, R., Varano, G. P., Adornetto, A., Nazio, F., Tettamanti, G., Girardello, R., et al. (2018). Rapamycin and fasting sustain autophagy response activated by ischemia/reperfusion injury and promote retinal ganglion cell survival. Cell Death Dis. 9 (10), 981. doi:10.1038/s41419-018-1044-5
Schuck, S. (2020). Microautophagy - distinct molecular mechanisms handle cargoes of many sizes. J. Cell Sci. 133 (17), jcs246322. doi:10.1242/jcs.246322
Sears, N. C., Boese, E. A., Miller, M. A., and Fingert, J. H. (2019). Mendelian genes in primary open angle glaucoma. Exp. Eye Res. 186, 107702. doi:10.1016/j.exer.2019.107702
Shadel, G. S., and Horvath, T. L. (2015). Mitochondrial ROS signaling in organismal homeostasis. Cell 163 (3), 560–569. doi:10.1016/j.cell.2015.10.001
Shen, X., Ying, H., Qiu, Y., Park, J. S., Shyam, R., Chi, Z. L., et al. (2011). Processing of optineurin in neuronal cells. J. Biol. Chem. 286 (5), 3618–3629. doi:10.1074/jbc.M110.175810
Shi, R., Weng, J., Zhao, L., Li, X. M., Gao, T. M., and Kong, J. (2012). Excessive autophagy contributes to neuron death in cerebral ischemia. CNS Neurosci. Ther. 18 (3), 250–260. doi:10.1111/j.1755-5949.2012.00295.x
Shim, M. S., and Liton, P. B. (2022). The physiological and pathophysiological roles of the autophagy lysosomal system in the conventional aqueous humor outflow pathway: More than cellular clean up. Prog. Retin. Eye Res. 90, 101064. doi:10.1016/j.preteyeres.2022.101064
Shim, M. S., Nettesheim, A., Dixon, A., and Liton, P. B. (2021). Primary cilia and the reciprocal activation of AKT and SMAD2/3 regulate stretch-induced autophagy in trabecular meshwork cells. Proc. Natl. Acad. Sci. U. S. A. 118 (13), e2021942118. doi:10.1073/pnas.2021942118
Shim, M. S., Nettesheim, A., Hirt, J., and Liton, P. B. (2020). The autophagic protein LC3 translocates to the nucleus and localizes in the nucleolus associated to NUFIP1 in response to cyclic mechanical stress. Autophagy 16 (7), 1248–1261. doi:10.1080/15548627.2019.1662584
Shin, J. Y., Park, H. J., Kim, H. N., Oh, S. H., Bae, J. S., Ha, H. J., et al. (2014). Mesenchymal stem cells enhance autophagy and increase β-amyloid clearance in Alzheimer disease models. Autophagy 10 (1), 32–44. doi:10.4161/auto.26508
Sirohi, K., Chalasani, M. L., Sudhakar, C., Kumari, A., Radha, V., and Swarup, G. (2013). M98K-OPTN induces transferrin receptor degradation and RAB12-mediated autophagic death in retinal ganglion cells. Autophagy 9 (4), 510–527. doi:10.4161/auto.23458
Sirohi, K., and Swarup, G. (2016). Defects in autophagy caused by glaucoma-associated mutations in optineurin. Exp. Eye Res. 144, 54–63. doi:10.1016/j.exer.2015.08.020
Skov, A. G., Rives, A. S., Freiberg, J., Virgili, G., Azuara-Blanco, A., and Kolko, M. (2021). Comparative efficacy and safety of preserved versus preservative-free beta-blockers in patients with glaucoma or ocular hypertension: A systematic review. Acta Ophthalmol. 100, 253–261. doi:10.1111/aos.14926
Stamer, W. D., and Acott, T. S. (2012). Current understanding of conventional outflow dysfunction in glaucoma. Curr. Opin. Ophthalmol. 23 (2), 135–143. doi:10.1097/ICU.0b013e32834ff23e
Stothert, A. R., Fontaine, S. N., Sabbagh, J. J., and Dickey, C. A. (2016). Targeting the ER-autophagy system in the trabecular meshwork to treat glaucoma. Exp. Eye Res. 144, 38–45. doi:10.1016/j.exer.2015.08.017
Su, Q., Zheng, B., Wang, C. Y., Yang, Y. Z., Luo, W. W., Ma, S. M., et al. (2018). Oxidative stress induces neuronal apoptosis through suppressing transcription factor EB phosphorylation at Ser467. Cell. Physiol. biochem. 46 (4), 1536–1554. doi:10.1159/000489198
Swarup, G., and Sayyad, Z. (2018). Altered functions and interactions of glaucoma-associated mutants of optineurin. Front. Immunol. 9, 1287. doi:10.3389/fimmu.2018.01287
Tang, L. H. C., Fung, F. K. C., Lai, A. K. W., Wong, I. Y. H., Shih, K. C., and Lo, A. C. Y. (2021). Autophagic upregulation is cytoprotective in ischemia/reperfusion-injured retina and retinal progenitor cells. Int. J. Mol. Sci. 22 (16), 8446. doi:10.3390/ijms22168446
Tang, R. H., Qi, R. Q., and Liu, H. Y. (2019). Interleukin-4 affects microglial autophagic flux. Neural Regen. Res. 14 (9), 1594–1602. doi:10.4103/1673-5374.255975
Teodorof-Diedrich, C., and Spector, S. A. (2018). Human immunodeficiency virus type 1 gp120 and tat induce mitochondrial fragmentation and incomplete mitophagy in human neurons. J. Virol. 92 (22), 1–16. doi:10.1128/jvi.00993-18
Tham, Y. C., Li, X., Wong, T. Y., Quigley, H. A., Aung, T., and Cheng, C. Y. (2014). Global prevalence of glaucoma and projections of glaucoma burden through 2040: A systematic review and meta-analysis. Ophthalmology 121 (11), 2081–2090. doi:10.1016/j.ophtha.2014.05.013
Töteberg-Harms, M., and Meier-Gibbons, F. (2021). Is laser trabeculoplasty the new star in glaucoma treatment? Curr. Opin. Ophthalmol. 32 (2), 141–147. doi:10.1097/icu.0000000000000732
Tran, V. T., Ho, P. T., Cabrera, L., Torres, J. E., and Bhattacharya, S. K. (2014). Mechanotransduction channels of the trabecular meshwork. Curr. Eye Res. 39 (3), 291–303. doi:10.3109/02713683.2013.842593
Tsai, H. Y., Lai, H., Chen, Z. Y., Lin, T. C., Khor, W., Kuo, W. C., et al. (2022). Inhibition of DUSP6 activates autophagy and rescues the retinal pigment epithelium in sodium iodate-induced retinal degeneration models in vivo and in vitro. Biomedicines 10 (1), 159. doi:10.3390/biomedicines10010159
Tucker, B. A., Solivan-Timpe, F., Roos, B. R., Anfinson, K. R., Robin, A. L., Wiley, L. A., et al. (2014). Duplication of TBK1 stimulates autophagy in iPSC-derived retinal cells from a patient with normal tension glaucoma. J. Stem Cell Res. Ther. 3 (5), 161. doi:10.4172/2157-7633.1000161
Vecino, E., Rodriguez, F. D., Ruzafa, N., Pereiro, X., and Sharma, S. C. (2016). Glia-neuron interactions in the mammalian retina. Prog. Retin. Eye Res. 51, 1–40. doi:10.1016/j.preteyeres.2015.06.003
Villarejo-Zori, B., Jiménez-Loygorri, J. I., Zapata-Muñoz, J., Bell, K., and Boya, P. (2021). New insights into the role of autophagy in retinal and eye diseases. Mol. Asp. Med. 82, 101038. doi:10.1016/j.mam.2021.101038
Wang, R. (2017). Effect of acupuncture for intraocular pressure control and eye function in patients w ith glaucoma. J Int. Eye Sci. 17 (05), 958–960.
Wang, Y., Huang, C., Zhang, H., and Wu, R. (2015). Autophagy in glaucoma: Crosstalk with apoptosis and its implications. Brain Res. Bull. 117, 1–9. doi:10.1016/j.brainresbull.2015.06.001
Wang, Y., Lv, J., Huang, C., Li, X., Chen, Y., Wu, W., et al. (2021). Human umbilical cord-mesenchymal stem cells survive and migrate within the vitreous cavity and ameliorate retinal damage in a novel rat model of chronic glaucoma. Stem Cells Int. 2021, 8852517. doi:10.1155/2021/8852517
Weinreb, R. N., and Khaw, P. T. (2004). Primary open-angle glaucoma. Lancet 363 (9422), 1711–1720. doi:10.1016/s0140-6736(04)16257-0
Wen-bo, H., Jun, F., Jie, C., and Wei, W. (2020). Effect of tiluo yangxue mingmu prescription on visual function, vessel density and thickness of retinal nerve fiber layer of optic nerve in glaucoma patients. J Chin. J. Exp. Traditional Med. Formulae 26 (24), 108–115. doi:10.13422/j.cnki.syfjx.20202213
Wise, J. B., and Witter, S. L. (1979). Argon laser therapy for open-angle glaucoma: A pilot study. Arch. Ophthalmol. 97 (2), 319–322. doi:10.1001/archopht.1979.01020010165017
Wolters, J. E. J., van Mechelen, R. J. S., Al Majidi, R., Pinchuk, L., Webers, C. A. B., Beckers, H. J. M., et al. (2021). History, presence, and future of mitomycin C in glaucoma filtration surgery. Curr. Opin. Ophthalmol. 32 (2), 148–159. doi:10.1097/icu.0000000000000729
Wong, E., and Cuervo, A. M. (2010). Autophagy gone awry in neurodegenerative diseases. Nat. Neurosci. 13 (7), 805–811. doi:10.1038/nn.2575
Wong, P. M., Puente, C., Ganley, I. G., and Jiang, X. (2013). The ULK1 complex: Sensing nutrient signals for autophagy activation. Autophagy 9 (2), 124–137. doi:10.4161/auto.23323
Wong, S. Q., Kumar, A. V., Mills, J., and Lapierre, L. R. (2020). Autophagy in aging and longevity. Hum. Genet. 139 (3), 277–290. doi:10.1007/s00439-019-02031-7
Xirui, Y., Jixue, W., Feixue, D., and He, S. (2021). The treatment of glaucoma with liver depression A systematic review and meta-analysis of randomized controlled trials. J J. Traditional Chin. Ophthalmol. 31 (03), 218–223. doi:10.13444/j.cnki.zgzyykzz.2021.03.016
Xu, L., Wang, X., and Wu, M. (2017). Topical medication instillation techniques for glaucoma. Cochrane Database Syst. Rev. 2 (2), Cd010520. doi:10.1002/14651858.CD010520.pub2
Yan, X., Wu, S., Liu, Q., Cheng, Y., Zhang, J., and Wang, N. (2022). Myocilin gene mutation induced autophagy activation causes dysfunction of trabecular meshwork cells. Front. Cell Dev. Biol. 10, 900777. doi:10.3389/fcell.2022.900777
Yauger, Y. J., Bermudez, S., Moritz, K. E., Glaser, E., Stoica, B., and Byrnes, K. R. (2019). Iron accentuated reactive oxygen species release by NADPH oxidase in activated microglia contributes to oxidative stress in vitro. J. Neuroinflammation 16 (1), 41. doi:10.1186/s12974-019-1430-7
Yim, W. W., and Mizushima, N. (2020). Lysosome biology in autophagy. Cell Discov. 6, 6. doi:10.1038/s41421-020-0141-7
Zhang, M. L., Zhao, G. L., Hou, Y., Zhong, S. M., Xu, L. J., Li, F., et al. (2020). Rac1 conditional deletion attenuates retinal ganglion cell apoptosis by accelerating autophagic flux in a mouse model of chronic ocular hypertension. Cell Death Dis. 11 (9), 734. doi:10.1038/s41419-020-02951-7
Zhang, Q., He, C., Li, R., Ke, Y., Sun, K., and Wang, J. (2021a). miR-708 and miR-335-3p inhibit the apoptosis of retinal ganglion cells through suppressing autophagy. J. Mol. Neurosci. 71 (2), 284–292. doi:10.1007/s12031-020-01648-y
Zhang, S., Shao, Z., Liu, X., Hou, M., Cheng, F., Lei, D., et al. (2021b). The E50K optineurin mutation impacts autophagy-mediated degradation of TDP-43 and leads to RGC apoptosis in vivo and in vitro. Cell Death Discov. 7 (1), 49. doi:10.1038/s41420-021-00432-0
Zhang, W., Tian, T., Gong, S. X., Huang, W. Q., Zhou, Q. Y., Wang, A. P., et al. (2021c). Microglia-associated neuroinflammation is a potential therapeutic target for ischemic stroke. Neural Regen. Res. 16 (1), 6–11. doi:10.4103/1673-5374.286954
Zhang, Z., Yang, X., Song, Y. Q., and Tu, J. (2021d). Autophagy in Alzheimer's disease pathogenesis: Therapeutic potential and future perspectives. Ageing Res. Rev. 72, 101464. doi:10.1016/j.arr.2021.101464
Keywords: autophagy, glaucoma, therapeutic strategies, retinal ganglion cells, neurodegenerative
Citation: Li M, Gao Z-L, Zhang Q-P, Luo A-X, Xu W-Y, Duan T-Q, Wen X-P, Zhang R-Q, Zeng R and Huang J-F (2022) Autophagy in glaucoma pathogenesis: Therapeutic potential and future perspectives. Front. Cell Dev. Biol. 10:1068213. doi: 10.3389/fcell.2022.1068213
Received: 12 October 2022; Accepted: 02 December 2022;
Published: 14 December 2022.
Edited by:
Degui Wang, Lanzhou University, ChinaReviewed by:
Ghanshyam Swarup, Centre for Cellular & Molecular Biology (CCMB), IndiaCopyright © 2022 Li, Gao, Zhang, Luo, Xu, Duan, Wen, Zhang, Zeng and Huang. This is an open-access article distributed under the terms of the Creative Commons Attribution License (CC BY). The use, distribution or reproduction in other forums is permitted, provided the original author(s) and the copyright owner(s) are credited and that the original publication in this journal is cited, in accordance with accepted academic practice. No use, distribution or reproduction is permitted which does not comply with these terms.
*Correspondence: Ju-Fang Huang, aHVhbmdqdWZhbmdAY3N1LmVkdS5jbg==
†These authors have contributed equally to this work and share first authorship
Disclaimer: All claims expressed in this article are solely those of the authors and do not necessarily represent those of their affiliated organizations, or those of the publisher, the editors and the reviewers. Any product that may be evaluated in this article or claim that may be made by its manufacturer is not guaranteed or endorsed by the publisher.
Research integrity at Frontiers
Learn more about the work of our research integrity team to safeguard the quality of each article we publish.