- 1Department of Rehabilitation, Tongji Hospital, Tongji Medical College, Huazhong University of Science and Technology, Wuhan, China
- 2Stem Cell Research Center, Tongji Hospital, Tongji Medical College, Huazhong University of Science and Technology, Wuhan, China
Neurodegenerative diseases (NDDs) are disorders in which neurons are lost owing to various factors, resulting in a series of dysfunctions. Their rising prevalence and irreversibility have brought physical pain to patients and economic pressure to both individuals and society. However, the pathogenesis of NDDs has not yet been fully elucidated, hampering the use of precise medication. Induced pluripotent stem cell (IPSC) modeling provides a new method for drug discovery, and exploring the early pathological mechanisms including mitochondrial dysfunction, which is not only an early but a prominent pathological feature of NDDs. In this review, we summarize the iPSC modeling approach of Alzheimer’s disease, Parkinson’s disease, and Amyotrophic lateral sclerosis, as well as outline typical mitochondrial dysfunction and recapitulate corresponding therapeutic strategies.
1 Introduction
With the aging of the population, neurodegenerative diseases (NDDs) are affecting an increasing number of people. By 2030, seventy-eight million individuals would have been affected by dementia (predominantly Alzheimer’s disease), with up to 55 million cases by 2021 (WHO, 2021). NDDs are characterized by the progressive loss of motor, sensory, and/or cognitive functions as a result of neuronal cell death (Pasteuning-Vuhman et al., 2021). Several common NDDs, including Alzheimer’s disease (AD), Parkinson’s disease (PD), and amyotrophic lateral sclerosis (ALS), have a remarkably complex pathogenesis. Due to its high etiologic heterogeneity, interplayed with diverse genetic backgrounds, and environmental factors, there are few target strategies despite decades of research (Giacomelli et al., 2022). Diving into the pathogenesis of NDDs remains a prioritized step toward their understanding and treatment.
Although several models have been developed to aid mechanistic research, much remains elusive (Ke et al., 2021; Okano and Morimoto, 2022). The autopsy sample serves as an ideal model for investigation; however, it is featured as the late stage of NDDs, which is unfit for early pathophysiological studies. Animal models have been utilized in numerous studies, but the translational clinic applications remain a long way off (Okano and Morimoto, 2022). With such a predicament, it is essential to develop accurate models that reflect the early stages of the pathological processes in NDDs (Israel and Goldstein, 2011; Grekhnev et al., 2022).
Induced pluripotent stem cells (iPSCs) are pluripotent, which indicates their capacity to differentiate into almost all human cell types with identical genetic background of the donor patient (Li and Fraenkel, 2021). This process provides developmental cues to areas of interest. Moreover, patient-specific iPSCs combined with gene editing technology can also be used for the development of therapeutic targets and clinical drug screening (Okano and Yamanaka, 2014; Park et al., 2014). NDDs affect specific types of neurons; therefore, the in vitro modeling of patient-specific iPSCs to thoroughly understand the pathological processes of NDDs has significantly furthered the exploration of their mechanism.
Mitochondria are organelles that continuously undergo fusion and fission in eukaryotic cells and play a crucial role in bioenergetic and biosynthetic pathways, calcium homeostasis management, and the regulation of programmed cell death (Friedman and Nunnari, 2014). Mitochondrial dysfunction has emerged as one of the major participants in NDDs (Magrané et al., 2012; Shaltouki et al., 2015; Fang et al., 2019). For instance, mitochondrial transport disturbances were found in ALS SOD1G93AMN (Magrané et al., 2012). Fang and his team observed the accumulation of damaged mitochondria in the hippocampus of AD patients due to defective mitophagy (Fang et al., 2019). This evidence indicates that different types of mitochondrial dysfunction occur in NDDs. Although it is still unknown whether mitochondrial dysfunction is the cause or functional outcome of NDDs, reversing mitochondrial dysfunction can be an effective means of treating the disease (Burtscher et al., 2022). IPSC modeling shows great potential in figuring out the occurrence of mitochondrial dysfunction in NDDs. In this review, we summarize several differentiation methods that induce iPSCs into characteristic neurons for modeling NDDs such as AD, PD, and ALS. We also recapitulated the mitochondrial abnormalities in differentiated neurons including mitochondrial dynamics and transport, mitochondrial reactive oxygen species (ROS) and mitophagy, mitochondrial energy metabolism dysfunction, and treatment approaches targeting mitochondrial abnormalities.
2 IPSC differentiation protocols in neurodegenerative diseases
AD, a common neurodegenerative disease, is the major cause of dementia (Ayodele et al., 2021). The neuropathology of AD is characterized by the progressive accumulation of parenchymal amyloid-β (Aβ) and by the hyperphosphorylation and aggregation of tau protein into neurofibrillary tangles (Venkatramani and Panda, 2019; Pardo-Moreno et al., 2022). Thus, researchers have differentiated hiPSCs into specific neurons, including neural stem cells, cortical neurons, and astrocytes, to explore the pathological changes in AD (Emdad et al., 2011; Krencik and Zhang, 2011; Roybon et al., 2013; Tcw et al., 2017). Dual SMAD signaling was inhibited by SB431542 (SB, an inhibitor of activin-nodal signaling) and LDN193189 (LDN, an inhibitor of BMP signaling) at the first neural induction step (Chambers et al., 2009), following which several small-molecule compounds were used to obtain cortical tissue. For instance, Neurobasal and B27 supplements replaced NPC culture medium at day 10 and were supplemented with 20 ng/ml brain-derived neurotrophic factor (BDNF) to induce final neural maturation at day 30 (Martín-Maestro et al., 2017). For astrocyte differentiation, after the NPCs were obtained, the cells were cultured in suspension with an astrocyte differentiation medium supplemented with basic fibroblast growth factor (bFGF or FGF2) and epidermal growth factor (EGF). The spheres were maintained in suspension for 5–7 months, after which they were associated and plated on Matrigel-coated dishes using ciliary neurotrophic factor (CNTF) and bone morphogenetic protein 4 (BMP4) for maturation (Oksanen et al., 2017). Because the differentiation process of astrocytes can take months, some innovative techniques have been created. Li et al. found that CRISPR‒Cas9-mediated expression of the transcription factors nuclear factor I A (NFIA) or NFIA plus SRY-box transcription factor 9 (SOX9) enables the rapid acquisition of astrocytes from hPSCs within 4–7 weeks, which undoubtedly speeds up the iPSC-derived astrocyte modeling of diseases (Li X. et al., 2018).
PD is the second most common neurodegenerative disease. It is predicted that by 2040, there will be 14.2 million persons with PD, up from 6.9 million in 2015 (Dorsey and Bloem, 2018). Patients diagnosed with PD always have movement and physical problems, such as tremors, stiffness, slowness, and imbalance (Armstrong and Okun, 2020). The pathological hallmark of PD is the Lewy body consisting largely of α-synuclein protein aggregations and loss of dopaminergic neurons in the substantia nigra (Armstrong and Okun, 2020). The floor plate (FP) cells are located at the ventral midline of the neural tube and are the source of midbrain dopamine neurons (DA neurons) (Placzek and Briscoe, 2005; Ono et al., 2007). The protocols to obtain DA neurons are also being modified (Cooper et al., 2010; Kriks et al., 2011; Xi et al., 2012; Wang et al., 2015; Kim et al., 2021). Christopher A. Fasano induced hiPSC to FP cells using noggin with SB431542 to inhibit dual SMAD signaling and Sonic C25II to activate sonic hedgehog (SHH) signaling; treatment with FGF8 or Wnt1 led to a conversion of FP identity into a caudal, midbrain-like identity (Fasano et al., 2010). Subsequently, Kriks et al. demonstrated that the simultaneous use of LSB (LSN + SB)/FGF8/CHIR (GSK3 inhibitor) increases the yield of FOXA2+/TH+ expressing DA neurons from FP cells, and the DA neurons generated with their protocol possess the essential physiological traits of mature substantia nigra pars compacta DA neurons and the viability of transplantation to the animal models (Kriks et al., 2011). Modification of CHIR concentration to a narrow range was reported to play an important role in inducing DA neurons from iPSC (Xi et al., 2012). They used 0.4 uM CHIR and 500 ng/ml SHH to treat iPSC for 12 days, and then added 20 ng/ml SHH with 100 ng/ml FGF8 at day 13–28, resulting in TH+/En1+ co-expressing DA neurons.
ALS is a fatal neurodegenerative disease that causes selective degeneration of motor neurons (MNs) in the motor cortex, brainstem, and spinal cord (Vandoorne et al., 2019). ALS patients always suffer from progressive muscle weakness and respiratory failure and ultimately die within 3–5 years (Brown and Al-Chalabi, 2017). Since MNs were used to study the pathology of ALS in recent years, the differentiation protocol of IPSCs to MNs is constantly being refined (Hu and Zhang, 2009; Egawa et al., 2012; Qu et al., 2014; Du et al., 2015; Dafinca et al., 2020). In 2006, Hu and Zhang published a protocol that can generate more than 50% of HB9-expressing motor neurons within 5 weeks. They induced iPSCs into neuroepithelial (NE) with a neural differentiation medium including instantly added cAMP, ascorbic acid, BDNF, glial-derived neurotrophic factor (GDNF), and insulin-like growth factor-1 (IGF1). The authors used 100 nM retinoic acid (RA) on day 10 and 1 µM purmorphamine (targeting smoothened of the SHH signaling pathway, pur) at day 15 to pattern NE cells to ventral spinal progenitor fate, which expresses Olig2. After plating spheres on substrates, several neurotrophic factors and cAMP are added to support the survival and process outgrowth of motor neurons expressing HB9 (Hu and Zhang, 2009). Although later reports added dual inhibition of SMAD signaling and smoothened agonist (SAG) or compound C (AMPK inhibitor) on the basis of a previous protocol (Amoroso et al., 2013; Qu et al., 2014), significantly shortening the differentiation time, the purity of motor neurons still requires improvement. Surprisingly, Du and his colleagues (Du et al., 2015) used a small-molecule cocktail including SB, DMH1 (SMAD inhibitor), CHIR, RA, and pur to obtain a near-pure population of OLIG2+ MNPs in 12 days. Then, they added compound E (a NOTCH inhibitor) during MN maturation to improve the purity of motor neurons, resulting in the generation of highly pure (>90%) motor neurons expressing CHAT in 16 days. Because of the shorter time for differentiation into highly pure motor neurons, the protocol has been widely applied in the disease modeling research (Ding et al., 2022; Liu et al., 2022).
AD, PD, and ALS involve corresponding neurons, thereby accurate differentiation of iPSC is significant for research. For instance, mature dopamine neuron subtypes co-expressing TH, NR4A2, and FOXA2 have different gene expression profiles and have differential responses to oxidative stress (Fernandes et al., 2020), emphasizing that there remains a need for referring to the protocol established by other laboratories using small chemical molecules to generate more mature and specific neurons. Table 1 summarizes the mainstream protocols of astrocytes, DA neurons, and MNs differentiation. The most widely applied protocols were featured as less time-consuming and with high purity. The cost of small molecule compounds applied in the protocol also needs to be considered.
3 IPSC modeling in neurodegenerative diseases
AD is divided into familial AD (FAD) and sporadic AD (sAD), and FAD constitutes less than 3% of all AD subtypes. The APP gene and the proteolytic enzymes that produce peptide A, presenilin 1 and 2, are the two primary genes that are mutated in FAD (García-Morales et al., 2021). IPSC modeling demonstrates that there are some common pathological features in FAD and sAD (Bélanger et al., 2011; Piers et al., 2020). Jones et al. induced astrocytes derived from AD iPSC have been reported to display cellular atrophy and to have aberrant expression and localization of S100B, EAAT1 and GS, which are cell-autonomous instead of compromised neuronal intermediates, supporting the conjecture that astrocyte dysfunction is an early hallmark of AD (Jones et al., 2017). Moreover, iPSC modeling provides a comprehensive phenotype study of AD. PSEN1 iPSC-derived astrocytes displayed robust accumulation of full-length presenilin-1, increased secretion and compromised uptake of Aβ1–42, disturbed Ca2+ signaling, and altered metabolism (Oksanen et al., 2017). In addition, astrocytes derived from AD iPSC co-cultured with healthy astrocytes provide an opportunity to observe the pathological microenvironment, including the effects of AD astrocytes and autonomic response of healthy astrocytes.
Likewise, SNCA (α-synuclein), PARK2 (parkin), PARK7 (DJ-1), PINK1 and LRRK2 are the mutated genes of parkinsonism, among which mutations in both PARK2 (parkin) and LRRK2 are the most common genetic causes (Healy et al., 2008). However, the cause of PD remains unclear at the molecular level. IPSC modeling of mutant genes, including PARK2 and LRRK2, helps to investigate PD occurrence and development, and can target a pathway to delay disease progression (Cooper et al., 2012; Wasner et al., 2022). In PD iPSC-derived DA neurons, Jian Feng found higher protein level of tyrosine hydroxylase (TH) and high ROS levels. Moreover, there is no significant alterations in DA levels, which might be due to homeostatic regulation (Ren et al., 2022). However, decreased TH mRNA level and DA release were reported in postmortem brain tissues and cerebrospinal fluid of PD patients respectively (Goldstein et al., 2012; Zhang et al., 2022). The inconsistent phenotype between iPSC modeling and postmortem tissues may precisely account for the transition from early to late stage of diseases. Young-onset PD iPSC-derived DA neurons displayed increased accumulation of soluble α-synuclein protein and phosphorylated protein kinase Cα, as well as dysregulated of lysosomal biogenesis and function, establishing a highly predictive phenotype of young-onset PD (Laperle et al., 2020).
ALS familial ALS (fALS) and sporadic ALS (sALS). In fALS, mutations in the “superoxide dismutase 1” (SOD1) gene are the most popular genetic factor, and the remaining common mutation genes are “fused in sarcoma” (FUS), “TAR DNA binding protein” (TARDBP), or a hexanucleotide repeat expansion in the “chromosome nine open reading frame 72” (C9ORF72) (Taylor et al., 2016). ALS-related iPSC modeling is able to reveal the sequence of its occurrence and development and provide a platform to explore the molecular mechanism of its pathology (Chen et al., 2014; Kiskinis et al., 2014; Lopez-Gonzalez et al., 2016; Dafinca et al., 2020; Altman et al., 2021). Chen et al. generated MNs and non-MNs derived from ALS SOD1 iPSC to reveal that neurofilament aggregation is an early event in ALS pathogenesis, resulting from the dysregulation of NF subunit proportion caused by SOD1 mutant binding NF-L mRNA in MNs (Chen et al., 2014). Likewise, iPSC modeling undoubtedly provides a good research tool for studying of sporadic ALS (Burkhardt et al., 2013; Fujimori et al., 2018a; Fujimori et al., 2018b; Coyne et al., 2021). Moreover, it has been found that intranuclear TDP-43 aggregation in iPSC-derived MNs reprogrammed from three sALS patients and firstly validated it in postmortem tissue from one of the previous sALS patients, which highlights the importance of iPSC modeling in sALS (Burkhardt et al., 2013).
4 Advantages and disadvantages of iPSC modeling
IPSC modeling of different phenotypes using mature protocols showcased stability and scalability, which together with its high yield facilitates high-throughput screening of clinical drugs (Pasteuning-Vuhman et al., 2021). In addition, iPSC modeling overcame the problem of clinical drug screening caused by heterogeneity of NDDs patients. Okano et al. used two types of MNs carrying FUS and TDP43 to screen 1,232 drugs. They ultimately selected ropinirole as the candidate, which is ineffective on the phenotypes of SOD1-mutant iPSC-derived MNs (Fujimori et al., 2018a). Thus, IPSC-derived neurons carrying patients’ specific genetic background help to identify drug responders, contributing to patient stratification in clinical studies (Haston and Finkbeiner, 2016).
In addition, iPSC-based disease modeling in vitro is different from animal models, including the neuronal physiological morphology and function and the molecular characteristics of pathological states. In AD, human protoplasmic astrocytes are bigger and more complex than their rodent counterparts, and the calcium wave in mouse astrocytes transmitted much more slowly than in human astrocytes (Oberheim et al., 2009). Chen et al. reported that there was no SOD1 aggregation or mitochondrial swelling in human ALS MNs but these appeared in SOD1 transgenic mice (Chen et al., 2014). Most importantly, mouse models fail to mimic sporadic neurodegenerative diseases, which account for most NDDs (Hawrot et al., 2020). However, mouse models are advantageous because they exhibit complex interactions between cells and the microenvironment, as well as provide behavioral assessment and pharmacokinetics features (Ke et al., 2021). Therefore, a promising way with the combination of advantages in both iPSC modeling and mouse models would contribute to a complementary and comprehensive view of NDD researches.
There are still some limitations for iPSC remodeling NDDs, such as the lack of aging-signature. Lorenz Studer and his lab overexpressed progeria with the treatment of modified-RNAs in PD iPSC-derived DA neurons, and found a significant reduction in dendrite length and downregulation of AKT signaling (Miller et al., 2013). Moreover, chemical pretreatment also accelerates the maturation of neurons (Fujimori et al., 2018a). Thus, progerin-induction or chemical pretreatment combing with iPSC-modeling overcomes the problem of iPSCs taking a long time to naturally develop into the degenerative disease stage, which mainly includes the expression of aging signatures. Another modeling method is direct neuronal conversion to induced neurons (iNs), which maintains the aging signature and epigenetic status of neurons (Vierbuchen et al., 2010; Mertens et al., 2015). However, the high cost and low cell output limit its application; future improvements should address these issues.
5 Normal mitochondrial function
IPSC modeling has undoubtedly greatly contributed to the knowledge of degenerative diseases, including mitochondrial dysfunction. Healthy mitochondria can maintain their own homeostasis through mitochondrial dynamics and autophagy even in the face of external stimuli, thereby exerting stable functions (Figure 1).
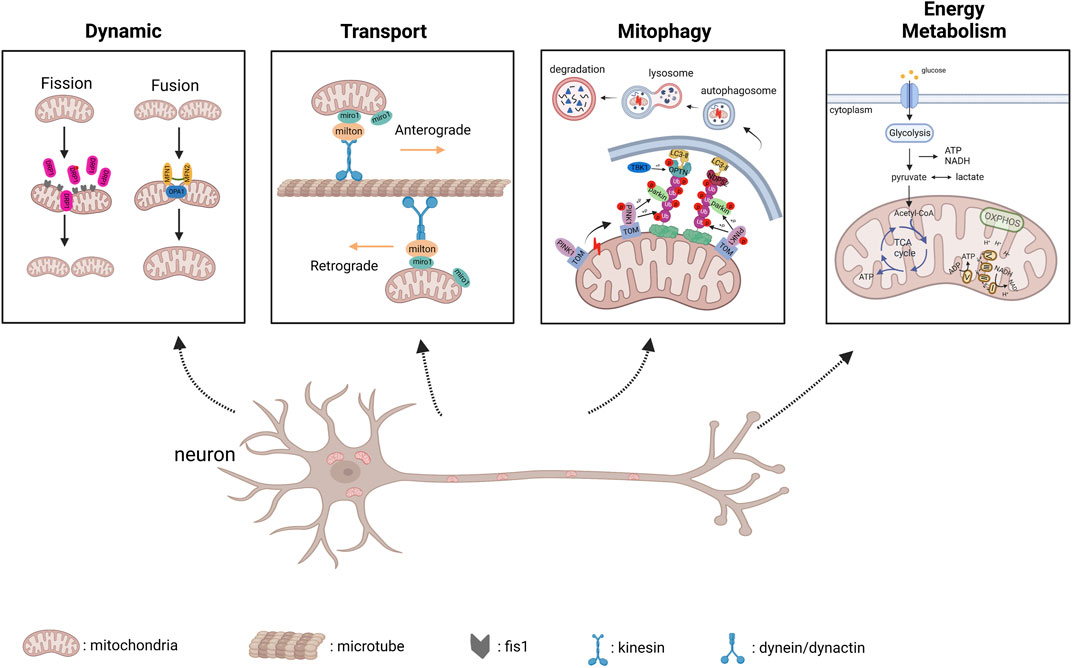
FIGURE 1. Physiological progress of mitochondrial dynamics, transport, PINK1/parkin-mediated mitophagy and energy metabolism (created with BioRender.com). 1) Dynamic: DRP1 is recruited to mitochondrial membrane along with Fis1 to regulate mitochondrial fission; MFN1/2 mediates fusion of mitochondrial outer membrane and OPA1 mediates fusion of inter membrane. 2) Transport: Anterograde transport is mediated by kinesin motor and Miro/Milton adaptors, and retrograde transport is mediated by dynein/dynactin and Miro/Milton adaptors. 3) PINK1/parkin-mediated Mitophagy: Once mitophagy starts, PINK1 accumulates in mitochondria, and in turn results in PINK1 autophosphorylation, which subsequently triggers ubiquitin phosphorylation, and recruits parkin to damaged mitochondria; Parkin ubiquitinates mitochondrial outer membrane proteins, and Optineurin interacts with these proteins tagged by ubiquitin and targets the isolation membrane to damaged mitochondria, which are eventually sent to lysosomes for degradation. 4) Energy Metabolism: Glycolysis occurs in cytoplasm, and produce ATP, NADH as by-product; The pyruvate molecules derived from glycolysis are imported to mitochondria and generate acetyl-CoA molecules, which will enter the tricarboxylic acid (TCA) cycle. Finally, OXPHOS complexes located in mitochondrial inter membrane uses NADH and FADH2 to generate ATP.
5.1 Mitochondrial dynamics and transport
Mitochondria continuously undergo fusion and fission, which are known as mitochondrial dynamics, to exchange metabolites (Schrepfer and Scorrano, 2016). MFN1 (membrane protein mitofusin 1) and MFN2 (membrane protein mitofusin 2) are fusion-related proteins, while DRP1 (dynamin-related protein 1) and Fis1 (mitochondrial fission one protein) are responsible for mitochondrial fission. Mitochondrial transport between Soma and distal axonal was mediated by motor proteins kinesin and dynein, which correspond to anterograde and retrograde transport respectively (Lin and Sheng, 2015). Moreover, miro and Milton serve as motor adaptors involved in mitochondrial transport (Lin and Sheng, 2015). It is reported that defective mitochondrial transport has been associated with NDDs (Cozzolino et al., 2013; Cai and Tammineni, 2017; Prots et al., 2018).
5.2 Mitochondrial ROS and mitophagy
Reactive oxygen species (ROS) include free radicals such as superoxide anion (O2−), hydroxyl radical (HO−), and non-radical hydrogen peroxide (H2O2), which are the endogenous sources of a mitochondrial respiratory chain under the high demand of neurons for oxygen and ATP (Chen et al., 2012). There is a hypothesis that mutant SOD1 has the ability to generate HO− from H2O2, thus creating an oxidative environment and toxic aggregates in MNs (Julien, 2001). As for PINK1/parkin-mediated mitophagy, When the mitochondrial membrane potential decreases, PINK1 accumulates in mitochondria and gets autophosphorylation, which subsequently triggers ubiquitin phosphorylation, and recruits parkin to damaged mitochondria. Subsequently, Parkin ubiquitinates mitochondrial outer membrane proteins and Optineurin interacts with these proteins tagged by ubiquitin and targets the isolation membrane to damaged mitochondria, which are eventually sent to lysosomes for degradation (Wei et al., 2015; Rasool et al., 2018; Goiran et al., 2022; Pradeepkiran et al., 2022).
5.3 Mitochondrial energy metabolism
Neurons in the brain need energy expenditure to maintain various functional activities, including synaptic transmission processes (Faria-Pereira and Morais, 2022). The major metabolic precursors for biosynthesis and energy generation come from the TCA cycle and glycolysis (Zheng et al., 2016). The pyruvate molecules produced in cytosolic glycolysis can be imported into the mitochondria and generate acetyl-CoA molecules, which will enter the tricarboxylic acid (TCA) cycle. Finally, OXPHOS complexes use NADH and FADH2 to generate ATP from this cycle (Faria-Pereira and Morais, 2022). Neurons tend to rely on mitochondrial OXPHOS to meet their energy demands, thereby dysfunction of this process would cause NDDs (Breuer et al., 2013; Koopman et al., 2013).
6 Mitochondrial dysfunction and therapeutic strategies in iPSC modeling
It is widely recognized that neurodegenerative diseases are closely related to mitochondrial dysfunction (Wang et al., 2009; Queliconi et al., 2021; Mehta et al., 2022). Just as the same disease may manifest in different ways, so the same disease modeling will also have different representations of mitochondrial dysfunction. Tables 2–4 summarizes the iPSC-based modeling of AD, PD, ALS, and the associated characterization of mitochondrial dysfunction. As for neurons derived from AD or PD iPSC, they mostly exhibit impaired mitophagy and dysregulated mitochondrial dynamics including increased mitochondrial fragments, while aberrant mitochondrial transport and reduced MMP are more common in ALS iPSC derived MNs (Dafinca et al., 2016; Zanon et al., 2017; Naumann et al., 2018; Martín-Maestro et al., 2019; Li et al., 2020). Also, PD iPSC modeling has shown abnormal mitochondrial morphology and increased ROS level (Imaizumi et al., 2012; Shaltouki et al., 2015; Chung et al., 2016). Furthermore, neurons from iPSC of NDDs manifest abnormal energy metabolism, showing decreased basal glycolysis and ATP level in AD iPSC modeling (Bélanger et al., 2011; Konttinen et al., 2019), reduced of complex I activity and decreased basal respiration in PD iPSC modeling (Zanon et al., 2017; Zambon et al., 2019), as well as impaired basal respiration and increased glycolysis in ALS iPSC modeling (Hor et al., 2021; Mehta et al., 2021). Sometimes neurons carrying the same mutant gene displayed different even contradictory energy metabolism, which may be attributed to the culture state and sensible to testing environment. Moreover, these pathological mechanisms are interconnected. Many studies have confirmed that the mitochondrial fission and fusion machinery is coupled with mitochondrial transport and mitophagy (Misko et al., 2010; Wu et al., 2016; Gao et al., 2017). Mitophagy regulates the mitochondrial energetic status in neurons (Han et al., 2021), thus damage to any link may lead to the occurrence and development of NDDs. Next, we describe mitochondrial dysfunctions of mitochondrial dynamic, transport, mitophagy, and energy metabolism abnormalities in AD, PD, ALS, and their corresponding treatment strategies.
6.1 Alzheimer’s disease
In AD, a study reported that the balance of mitochondrial fusion and fission was disrupted in PSEN1-E120K iPSC-derived cortical neurons, with an increase in DRP1 and a decrease in MFN1 (Li L. et al., 2018). After 2 years, this team found the same phenomenon in PSEN1-S170F iPSC-derived cortical neurons (Li et al., 2020). Aβ and phosphorylated tau interact with DRP1, which activates DRP1 by different pathways, causing impairments in mitochondrial dynamics (Manczak et al., 2011; Kandimalla et al., 2016). Since Aβ is produced by the sequential cleavage of APP via ß-site APP cleaving enzyme 1 (BACE1), so γ-secretase, an inhibitor of BACE1 (BSI and 5 μmol/L LY2884721), significantly reduced the levels of Aβ and p-Tau (Li et al., 2020). However, Fang explained that defective mitophagy induces AMPK activation (p-AMPK), which leads to excessive mitochondrial fragmentation. They used two mitophagy detection nematode lines to screen the enhancer for mitophagy, which ameliorated neuronal pathology and mitochondrial fragments in AD iPSC-derived cortical neurons (Fang et al., 2019).
Mitophagy plays an important role in maintaining cell homeostasis, including the removal of damaged mitochondria and resistance to oxidative stress (Scheibye-Knudsen et al., 2015). Martín-Maestro et al. demonstrated dysregulation of mitophagy because of deficient lysosomal function in iPSC-derived cortical neurons from FAD1 patients harboring PSEN1 A246E mutation (Martín-Maestro et al., 2017). After 2 years, they found the same problem in PSEN1 M146L iPSC-derived NSCs, along with the downregulation of oxidative phosphorylation (OXPHOS)-related proteins, suggesting an impaired mitochondrial respiratory chain (Martín-Maestro et al., 2019). Deficiency in autophagy induction and lysosomal acidification was confirmed as the main reason for mitophagy failure, and that was corrected by using bexarotene, an FDA-approved retinoid X receptor (RXR) agonist, whose therapeutic efficacy stems from increased mitophagy flux (Martín-Maestro et al., 2019). Another study reported that the abnormal accumulation of Aβ and p-Tau decreases the levels of activated mitophagy proteins in AD iPSC-derived cortical neurons, leading to the accumulation of damaged mitochondria and the elevation of ROS levels (Fang et al., 2019). The authors screened two mitophagy inducers (urolithin A and actinonin) to restore mitophagy, further improving mitochondrial function by reducing mitochondrial ROS and ameliorating AD’s pathology. As mentioned above, treatments targeting impaired mitophagy display a significant role in rescuing neuronal apoptosis (Shaltouki et al., 2018; Fang et al., 2019). In addition, Kshirsagar et al. found that several mitophagy enhancers, especially UA were able to rescue mitochondrial dysfunction and increase cell survival in AD HT22 cell models, indicating that mitophagy enhancers could developed as drugs to delay neurodegenerative pathology in clinical patients (Kshirsagar et al., 2021, 2022).
With regard to energy metabolism, Minna Oksanen et al. reported increased basal respiration and decreased basal glycolysis in PSEN1 iPSC-derived astrocytes, as typical astrocytes rely more on glycolysis (Bélanger et al., 2011). Additionally, the authors detected more cellular ROS and less lactate secretion in these astrocytes, suggesting that the energy metabolism activity of the PSEN1 mutation made a switch from glycolysis to OXPHOS (Oksanen et al., 2017). Given that neurons may sustain themselves with lactate generated by glycolysis, this alteration may not be advantageous to astrocytes (Figley, 2011). Similarly, another study conducted by the same laboratory reported impaired fatty acid oxidation (FAO) except for the same changes in basal respiration and basal glycolysis in PSEN1 iPSC-derived astrocytes, and using a PPARβ/δ-agonist is beneficial for impaired FAO and memory deficits in APP/PSEN1 mice (Konttinen et al., 2019). Ryu et al. published a more specific metabolism study of AD astrocytes and NPC. Their results showed increased mitochondrial respiration, energy (ATP) output, and elevated glycolytic activity, which are partly different from the results of previous studies. Considering the reduced ability to absorb glucose, as well as the deficiencies in the generation and transfer of reducing agents throughout the glycolytic process and the mitochondrial respiratory chain, upregulating OXPHOS and glycolysis is the way for AD astrocytes and NPCs overcompensate. Moreover, the levels of NAD+ and NADH are significantly decreased in AD astrocytes in NPC, despite the general biochemical reducing power in LOAD not being compromised, which reflects aberrant energy metabolic activity in AD astrocytes and NPC(Ryu et al., 2021).
6.2 Parkinson’s disease
Regarding PD, its main mutated genes, PARK2 and PINK1, are involved in mitochondrial functions, and there is no doubt that mitochondrial dysfunction is one of the pathological features of Zanon reported that DA neurons derived from the PARK2 mutation carrier iPS-B125 showed fragmented mitochondria, which was compensated by SLP-2 overexpression (Zanon et al., 2017). Parkin and SLP-2 are able to interact functionally to maintain mitochondrial function (Zanon et al., 2017). Ke et al. detected upregulation of the fission proteins DRP1 and Fis1 and downregulation of the fusion protein MFN1 in PLA2G6 mutant DA neurons (Ke et al., 2020). The PLA2G6 mutant induced ER stress, which facilitated the unfolded protein response (UPR), resulting in decreased signaling of CREB and final mitochondrial fragments. Thus, they used azoramide (a small-molecule modulator) to enhance the CERB signaling, and rescue mitochondrial function, thereby preventing the apoptosis of DA neurons (Ke et al., 2020). Given that the PD-associated LRRK2 mutant interacts with DRP1 to recruit and phosphorylate mitochondrial DRP1 (Wang et al., 2012), it is not surprising that Su and Qi found increased mitochondrial fragmentation in LRRK2 G2019S-derived DA neurons, and used P110 to inhibit the hyperactivation of DRP1 to reduce mitochondrial fragments (Su and Qi, 2013).
Mitophagy is closely related to the pathological mechanism of PD. Aberrant elimination of mitochondria treated with carbonyl cyanide m-chlorophenyl hydrazine (CCCP) was reported in PARK2 iPSC-derived neurons (Imaizumi et al., 2012). Mitophagy plays an important role in maintaining cell homeostasis, including the removal of damaged mitochondria and resistance to oxidative stress (Scheibye-Knudsen et al., 2015). Yu-Chin Su and colleagues detected increased mitochondrial fragmentation, which resulted in a defective ETC complex and an elevated level of mitochondrial ROS by DRP1 hyperactivation in LRRK2 G2019S DA neurons (Su and Qi, 2013). Subsequently, increased mitochondrial fragmentation simulates excessive mitophagy, which causes a loss of mitochondrial mass. This mitochondrial dysfunction was solved by P110 treatment which inhibits DRP1 hyperactivation (Su and Qi, 2013). Some studies focusing on PARK2 and PINK1 iPSC-derived DA neurons respectively reported higher mitochondrial ROS levels and defective mitophagy (Chung et al., 2016; Oh et al., 2017), among which the author found the PINK1(C568A) mutant could be able to mimic PINK1 S-nitrosylated caused by endogenous NOS, and this PINK1(C568A) mutant prevented parkin from translocating to mitochondrial membrane, thus resulting in impaired mitophagy and neuronal death (Oh et al., 2017). Treatment of these cells with l-NAME could be able to ameliorate damaged mitophagy and neuronal death by inhibiting the production of NOS(Oh et al., 2017). Miro, a protein that anchors mitochondria to microtubule motors, should be removed first, then mitochondria stop trafficking and mitophagy starts (Glater et al., 2006; Wang et al., 2011). Shaltouki and Hsieh found that accumulation of miro1 delayed the initiation of mitophagy in both LRRK2 G2019S and SCNA A53T iPSC-derived DA neurons, and decreased the level of miro1 protein by RNAi promoted mitophagy, and further rescued neurodegeneration (Hsieh et al., 2016; Shaltouki et al., 2018).
In PD SNCA iPSC-derived DA neurons, Zambon et al. found a decrease in basal respiration, spare capacity, ATP and lactate production, and no significant differences in glycolytic activity, suggesting deficits in mitochondrial energy metabolism (Zambon et al., 2019). This suggests that the association between α Syn and TOM20 leads to mitochondrial metabolic dysfunction (Di Maio et al., 2016; Zambon et al., 2019). PINK1 mutant iPSC-derived DA neurons were found to have reduced levels of ATP production, which was improved by treatment with cerulenin to rescue PINK1 deficiency (Vos et al., 2017). Fernandes5 reported downregulation of OXPHOS-related gene expression and upregulation of glycolysis-related gene expression under oxidative stress conditions in one type of SNCA DA neurons (Fernandes et al., 2020). PARK2 knockout DA neurons were reported to have reduced basal respiration and ATP production under only lactate respiration, along with decreased glycolysis and accumulation of lactate, which was confirmed by proteomic and metabolomic analysis of dysregulated key factors and enzymes in mitochondrial energy metabolism (Bogetofte et al., 2019; Okarmus et al., 2021).
6.3 Amyotrophic lateral sclerosis
Impaired mitochondrial dynamics can also be observed in ALS-related MN. It was reported that activation of PP1 dephosphorylates DRP1 S616, resulting in increased mitochondrial fission in TDP43-derived MNs. PP1 suppression by treatment with I-2 or okadaic acid (OA) significantly inhibited excessive mitochondrial fission (Choi et al., 2020). Interestingly, although there are numerous reports of impaired mitochondrial dynamics in ALS patients and animals, this has been less reported in ALS-iPSC modeling (Wang et al., 2013; Méndez-López et al., 2021). Deficits in mitochondrial transport along the axon are more common in ALS iPSC-derived MNs, and mitochondrial transport plays an important role in maintaining normal functioning of MNs. Whether it is mitochondrial dynamics or axonal transport, its damage has an important impact on neuron survival and disease progression. Thus, therapeutic strategies targeting both can effectively alleviate disease progression. A study by Guo and colleagues reported that there were fewer moving mitochondria than stationary mitochondria, while the total number of mitochondria remained unaltered in mutant FUS MNs (Guo et al., 2017). The same phenomenon was found in mutant TDP43 MNs (Fazal et al., 2021). However, it remains unclear why mitochondrial transport is impaired in mutated gene-derived MNs. One inference is that cytosolic aggregation or aberrant TDP-43 causes a reduction in mitochondria-associated ER membranes (MAM), and the mitochondrial movement-related protein miro1 preferentially localizes in MAM sites; thus, mitochondrial transport will decrease (Macaskill et al., 2009; Stoica et al., 2014). Interestingly, both different MNs’ mitochondrial transport defects can be solved by HDAC inhibitors, possibly because a rise in the acetylation of α-tubulin increases the number of motor proteins binding to the microtubules, which leads to an increase in mitochondrial transport (Guo et al., 2017).
Surprisingly, mutant forms of proteins (such as optineurin OPTN and TBK1) involved in mediating mitophagy are associated with ALS (Wong and Holzbaur, 2014; Moore and Holzbaur, 2016; Harding et al., 2021); however, there are few articles related to iPSC-based ALS modeling that characterized by defects in mitophagy. Moreover, ALS animals and other cells which transfected the mutated gene of ALS were used to report mitophagy defects (Palomo et al., 2018; Foster et al., 2020). For instance, the mitochondria in N2A cells transfected with SOD1 displayed low levels of mitophagy and high levels of ROS. This can be explained by the fact that mutated SOD1 binds to OPTN and sequesters it in N2A cells, thereby disrupting mitophagy, which in turn leads to increased ROS release (Tak et al., 2020). Overexpression of OPTN reduces the cytotoxicity of mutant SOD1, thus improving mitophagy and decreasing ROS levels (Tak et al., 2020). In addition, there are paths to control mitochondrial quality except for mitophagy (Lin et al., 2017; Gautam et al., 2019). Mukesh Gautam et al. found a unique self-destructive path for mitochondria, and the morphology of defective mitochondria changed including being elongated and curling themselves, then beginning to disintegrate from the inner membrane and the cristae, and finally being eliminated (Gautam et al., 2019). Therefore, whether mitophagy disorder occurs in iPSC-derived MNs carrying mutated ALS-related genes warrants further investigation.
Mitochondrial energy metabolic dysfunction is also a pathologic characteristic of ALS. There are diverse, specialized cell populations in the mammalian brain, and related studies have also suggested that bioenergetic genes and metabolic patterns in different cell types are differentially altered in developing cells or diseases, such as astrocytes and MNs (Qi et al., 2019). Vandoorne et al. reported an interesting finding that when iPSC is differentiated into MNs, its metabolic pattern shifts from glycolytic to oxidative metabolism, along with reduced glycolytic flux and elevated TCA cycle activity (Vandoorne et al., 2019). In addition, the ALS-related FUS mutant does not change the energy metabolism of MNs. Another study focusing on MNs carrying the C9orf72 mutant reported impaired basal and maximal mitochondrial respiration; however, glycolytic function was not affected (Mehta et al., 2021). Moreover, the transcriptomic analysis showed decreased gene expression of the mitochondrial ETC, which was restored by overexpression of PGC1α to stimulate mitochondrial biogenesis and improve mitochondrial function (Mehta et al., 2021). Günther et al. reported that SOD1 mutant iPSC-derived MNs displayed low levels of ATP without other mitochondrial metabolic changes (Günther et al., 2022). Hor and colleagues used three sALS iPSC line-derived MNs and three fALS iPSC line-derived MNs carrying SOD1, TDP43, and C9ORF72 mutants to find reductions in basal respiration, ATP production, and spare respiratory capacity, causing increased glycolysis and lactate. These abnormal changes illustrate impaired mitochondrial respiration and a compensatory response by ALS MNs (Hor et al., 2021). Notably, treatment with C12 ameliorated the mitochondrial energy metabolism via SIRT3 activation and mitochondrial deacetylation. The improvement of neuronal mitochondrial energetics through the restoration of OXPHOS and glycolysis as well as the correction of mitochondrial dysfunctions have become increasingly important therapeutic targets for delaying the progression of NDDs (Cunnane et al., 2020).
7 Discussion
NDDs are one of the most widespread diseases with a low cure rate, bringing enormous physical pain and economic pressure to patients and a heavy burden to society. At present, our exploration of the NDD is not thorough yet. IPSC modeling provides a panoramic view of the pathophysiology of NDDs. Unlike other models, this type of modeling enables the early-phase mechanism to be uncovered. Moreover, in the era of precise medication, it is a promising method for the personalized identification of unique molecular features in NDDs of either the sporadic or the familiar type.
This review showed different mitochondrial dysfunction in AD, PD, and ALS, along with feasible treatments ameliorating these mitochondrial defects or even inhibiting disease progress (Figures 2–4). Mitochondrial dysfunction is one of the early pathological features of NDDs (Lin and Beal, 2006). Moreover, the impairment of mitochondria can directly threaten neural survival and trigger neurodegeneration, and the deteriorating microenvironment of neurodegeneration in turn exacerbates mitochondrial dysfunction, leading to the continued progression of NDDs (Onishi et al., 2021; Jetto et al., 2022). Therapeutic measures are divided into two types those that target pathways and those that target sources—for example, targeting a molecule in the disease signaling pathway or targeting compensation for damaged mitochondria to correct mitochondrial dysfunction. However, extensive animal experiments and preclinical trials are required to confirm the safety and efficacy of drugs before these therapeutic strategies can be translated into clinical drugs.
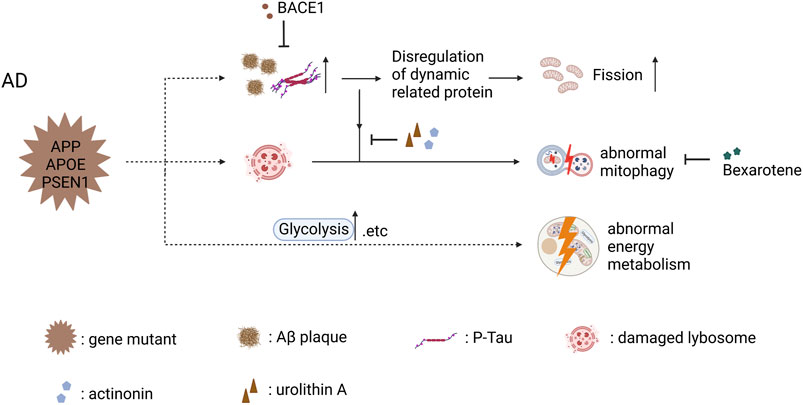
FIGURE 2. Mitochondrial dysfunction in AD iPSC-derived neurons (carrying APP, APOE, and PSEN1 mutant) and therapeutic strategies (created with BioRender.com). Abnormal accumulation of Aβ and p-Tau disrupted the balance of mitochondrial fusion and fission and increased mitochondrial fission in cortical neuron carrying PSEN1 mutant, and BACE1 inhibited the abnormal accumulation of Aβ and p-Tau to ameliorate mitochondrial fission. Abnormal accumulation of Aβ and p-Tau decreased the levels of activated mitophagy proteins, leading to the abnormal mitophagy in AD iPSC-derived cortical neurons; actinonin, urolithin A and bexarotene are able to restore abnormal mitophagy through their own mechanism. AD iPSC-derived astrocytes showed abnormal energy metabolism including increased mitochondrial respiration and elevated glycolytic activity.
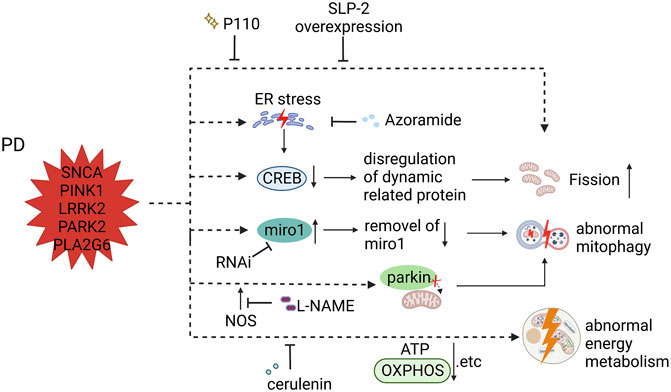
FIGURE 3. Mitochondrial dysfunction in PD iPSC-derived DA neurons (carrying SNCA, PINK1, LRRK2, PARK2, and PLA2G6 mutant) and therapeutic strategies (created with BioRender.com). DA neurons carrying PARK2 and LRRK2 mutants showed fragmented mitochondria, which was compensated by SLP-2 overexpression and P110. DA neurons with PLA2G6 mutation induced ER stress that facilitated the unfolded protein response (UPR), resulting in decreased signaling of CREB and final mitochondrial fragments; treatment with azoramide alleviated mitochondrial fission by enhancing the CERB signaling. PINK1 mutant iPSC-derived DA neurons prevented parkin from translocating to mitochondrial membrane, resulting in impaired mitophagy; L-NAME ameliorated damaged mitophagy by inhibiting the production of endogenous NOS. Accumulation of miro1 delayed the initiation of mitophagy in both LRRK2 and SCNA iPSC-derived DA neurons, and decreasing the level of miro1 protein by RNAi promotes mitophagy. DA neurons carrying PINK1 mutant displayed reduced levels of ATP production, which was improved by cerulenin through rescuing PINK1 deficiency.
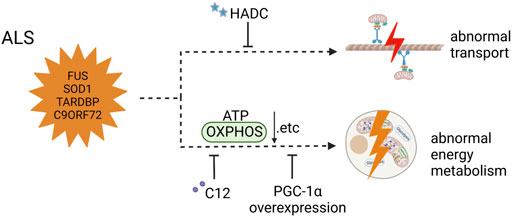
FIGURE 4. Mitochondrial dysfunction in ALS iPSC-derived neurons (carrying FUS, SOD1, and TARDBP mutant and C90ORF72 expanded GGGGCC repeats) and therapeutic strategies (created with BioRender.com). Mitochondrial transport defects were solved by HDAC inhibitors in MNs carrying FUS mutant. MNs with C9orf72 mutation showed impaired basal and maximal mitochondrial respiration, which was restored by overexpression of PGC1α. MNs carrying SOD1, TDP43, and C9ORF72 mutants and 3sALS mutants had reductions in basal respiration, ATP production, and spare respiratory, and treatment with C12 ameliorated the abnormal energy metabolism.
In conclusion, the use of iPSC-based modeling to characterize mitochondrial dysfunction in NDDs reviewed in this paper can provide a reference for other research studies, and these modeling methods provide an effective platform for mechanistic research and drug screening in NDDs. In the future, developing more effective and affordable treatment strategies may improve the quality of life for the majority of NDD patients.
Author contributions
H-ML designed and wrote the manuscript. D-XH, Y-QC, Y-ZL, Y-JL searched and interpret the relevant literature. JX, HC supervised and revised the paper. All authors have read and agreed to the published version of the manuscript.
Funding
This work was supported by the National Natural Science Foundation of China (82171422); Key Research and Development Project of Hubei Province of China (2022BCA028).
Conflict of interest
The authors declare that the research was conducted in the absence of any commercial or financial relationships that could be construed as a potential conflict of interest.
Publisher’s note
All claims expressed in this article are solely those of the authors and do not necessarily represent those of their affiliated organizations, or those of the publisher, the editors and the reviewers. Any product that may be evaluated in this article, or claim that may be made by its manufacturer, is not guaranteed or endorsed by the publisher.
References
Altman, T., Ionescu, A., Ibraheem, A., Priesmann, D., Gradus-Pery, T., Farberov, L., et al. (2021). Axonal TDP-43 condensates drive neuromuscular junction disruption through inhibition of local synthesis of nuclear encoded mitochondrial proteins. Nat. Commun. 12 (1), 6914. doi:10.1038/s41467-021-27221-8
Amoroso, M. W., Croft, G. F., Williams, D. J., O'Keeffe, S., Carrasco, M. A., Davis, A. R., et al. (2013). Accelerated high-yield generation of limb-innervating motor neurons from human stem cells. J. Neurosci. 33 (2), 574–586. doi:10.1523/jneurosci.0906-12.2013
Armstrong, M. J., and Okun, M. S. (2020). Diagnosis and treatment of Parkinson disease: A review. Jama 323 (6), 548–560. doi:10.1001/jama.2019.22360
Ayodele, T., Rogaeva, E., Kurup, J. T., Beecham, G., and Reitz, C. (2021). Early-onset Alzheimer's disease: What is missing in research? Curr. Neurol. Neurosci. Rep. 21 (2), 4. doi:10.1007/s11910-020-01090-y
Bélanger, M., Allaman, I., and Magistretti, P. J. (2011). Brain energy metabolism: Focus on astrocyte-neuron metabolic cooperation. Cell Metab. 14 (6), 724–738. doi:10.1016/j.cmet.2011.08.016
Bogetofte, H., Jensen, P., Ryding, M., Schmidt, S. I., Okarmus, J., Ritter, L., et al. (2019). PARK2 mutation causes metabolic disturbances and impaired survival of human iPSC-derived neurons. Front. Cell. Neurosci. 13, 297. doi:10.3389/fncel.2019.00297
Breuer, M. E., Koopman, W. J., Koene, S., Nooteboom, M., Rodenburg, R. J., Willems, P. H., et al. (2013). The role of mitochondrial OXPHOS dysfunction in the development of neurologic diseases. Neurobiol. Dis. 51, 27–34. doi:10.1016/j.nbd.2012.03.007
Brown, R. H., and Al-Chalabi, A. (2017). Amyotrophic lateral sclerosis. N. Engl. J. Med. 377 (2), 162–172. doi:10.1056/NEJMra1603471
Burkhardt, M. F., Martinez, F. J., Wright, S., Ramos, C., Volfson, D., Mason, M., et al. (2013). A cellular model for sporadic ALS using patient-derived induced pluripotent stem cells. Mol. Cell. Neurosci. 56, 355–364. doi:10.1016/j.mcn.2013.07.007
Burtscher, J., Romani, M., Bernardo, G., Popa, T., Ziviani, E., Hummel, F. C., et al. (2022). Boosting mitochondrial health to counteract neurodegeneration. Prog. Neurobiol. 215, 102289. doi:10.1016/j.pneurobio.2022.102289
Cai, Q., and Tammineni, P. (2017). Mitochondrial aspects of synaptic dysfunction in Alzheimer's disease. J. Alzheimers Dis. 57 (4), 1087–1103. doi:10.3233/jad-160726
Chambers, S. M., Fasano, C. A., Papapetrou, E. P., Tomishima, M., Sadelain, M., and Studer, L. (2009). Highly efficient neural conversion of human ES and iPS cells by dual inhibition of SMAD signaling. Nat. Biotechnol. 27 (3), 275–280. doi:10.1038/nbt.1529
Chen, H., Qian, K., Du, Z., Cao, J., Petersen, A., Liu, H., et al. (2014). Modeling ALS with iPSCs reveals that mutant SOD1 misregulates neurofilament balance in motor neurons. Cell Stem Cell 14 (6), 796–809. doi:10.1016/j.stem.2014.02.004
Chen, X., Guo, C., and Kong, J. (2012). Oxidative stress in neurodegenerative diseases. Neural Regen. Res. 7 (5), 376–385. doi:10.3969/j.issn.1673-5374.2012.05.009
Choi, S. Y., Lee, J. H., Chung, A. Y., Jo, Y., Shin, J. H., Park, H. C., et al. (2020). Prevention of mitochondrial impairment by inhibition of protein phosphatase 1 activity in amyotrophic lateral sclerosis. Cell Death Dis. 11 (10), 888. doi:10.1038/s41419-020-03102-8
Chung, S. Y., Kishinevsky, S., Mazzulli, J. R., Graziotto, J., Mrejeru, A., Mosharov, E. V., et al. (2016). Parkin and PINK1 patient iPSC-derived midbrain dopamine neurons exhibit mitochondrial dysfunction and α-synuclein accumulation. Stem Cell Rep. 7 (4), 664–677. doi:10.1016/j.stemcr.2016.08.012
Cooper, O., Hargus, G., Deleidi, M., Blak, A., Osborn, T., Marlow, E., et al. (2010). Differentiation of human ES and Parkinson's disease iPS cells into ventral midbrain dopaminergic neurons requires a high activity form of SHH, FGF8a and specific regionalization by retinoic acid. Mol. Cell. Neurosci. 45 (3), 258–266. doi:10.1016/j.mcn.2010.06.017
Cooper, O., Seo, H., Andrabi, S., Guardia-Laguarta, C., Graziotto, J., Sundberg, M., et al. (2012). Pharmacological rescue of mitochondrial deficits in iPSC-derived neural cells from patients with familial Parkinson's disease. Sci. Transl. Med. 4 (141), 141ra90. doi:10.1126/scitranslmed.3003985
Coyne, A. N., Baskerville, V., Zaepfel, B. L., Dickson, D. W., Rigo, F., Bennett, F., et al. (2021). Nuclear accumulation of CHMP7 initiates nuclear pore complex injury and subsequent TDP-43 dysfunction in sporadic and familial ALS. Sci. Transl. Med. 13 (604), eabe1923. doi:10.1126/scitranslmed.abe1923
Cozzolino, M., Ferri, A., Valle, C., and Carrì, M. T. (2013). Mitochondria and ALS: Implications from novel genes and pathways. Mol. Cell. Neurosci. 55, 44–49. doi:10.1016/j.mcn.2012.06.001
Cunnane, S. C., Trushina, E., Morland, C., Prigione, A., Casadesus, G., Andrews, Z. B., et al. (2020). Brain energy rescue: An emerging therapeutic concept for neurodegenerative disorders of ageing. Nat. Rev. Drug Discov. 19 (9), 609–633. doi:10.1038/s41573-020-0072-x
Dafinca, R., Barbagallo, P., Farrimond, L., Candalija, A., Scaber, J., Ababneh, N. A., et al. (2020). Impairment of mitochondrial calcium buffering links mutations in C9ORF72 and TARDBP in iPS-derived motor neurons from patients with ALS/FTD. Stem Cell Rep. 14 (5), 892–908. doi:10.1016/j.stemcr.2020.03.023
Dafinca, R., Scaber, J., Ababneh, N., Lalic, T., Weir, G., Christian, H., et al. (2016). C9orf72 hexanucleotide expansions are associated with altered endoplasmic reticulum calcium homeostasis and stress granule formation in induced pluripotent stem cell-derived neurons from patients with amyotrophic lateral sclerosis and frontotemporal dementia. Stem Cells 34 (8), 2063–2078. doi:10.1002/stem.2388
Di Maio, R., Barrett, P. J., Hoffman, E. K., Barrett, C. W., Zharikov, A., Borah, A., et al. (2016). α-Synuclein binds to TOM20 and inhibits mitochondrial protein import in Parkinson's disease. Sci. Transl. Med. 8 (342), 342ra78. doi:10.1126/scitranslmed.aaf3634
Ding, Q., Kesavan, K., Lee, K. M., Wimberger, E., Robertson, T., Gill, M., et al. (2022). Impaired signaling for neuromuscular synaptic maintenance is a feature of Motor Neuron Disease. Acta Neuropathol. Commun. 10 (1), 61. doi:10.1186/s40478-022-01360-5
Dorsey, E. R., and Bloem, B. R. (2018). The Parkinson pandemic-A call to action. JAMA Neurol. 75 (1), 9–10. doi:10.1001/jamaneurol.2017.3299
Du, Z. W., Chen, H., Liu, H., Lu, J., Qian, K., Huang, C. L., et al. (2015). Generation and expansion of highly pure motor neuron progenitors from human pluripotent stem cells. Nat. Commun. 6, 6626. doi:10.1038/ncomms7626
Egawa, N., Kitaoka, S., Tsukita, K., Naitoh, M., Takahashi, K., Yamamoto, T., et al. (2012). Drug screening for ALS using patient-specific induced pluripotent stem cells. Sci. Transl. Med. 4 (145), 145ra104. doi:10.1126/scitranslmed.3004052
Emdad, L., D'Souza, S. L., Kothari, H. P., Qadeer, Z. A., and Germano, I. M. (2011). Efficient differentiation of human embryonic and induced pluripotent stem cells into functional astrocytes. Stem Cells Dev. 21 (3), 404–410. doi:10.1089/scd.2010.0560
Fang, E. F., Hou, Y., Palikaras, K., Adriaanse, B. A., Kerr, J. S., Yang, B., et al. (2019). Mitophagy inhibits amyloid-β and tau pathology and reverses cognitive deficits in models of Alzheimer’s disease. Nat. Neurosci. 22 (3), 401–412. doi:10.1038/s41593-018-0332-9
Faria-Pereira, A., and Morais, V. A. (2022). Synapses: The brain's energy-demanding sites. Int. J. Mol. Sci. 23 (7), 3627. doi:10.3390/ijms23073627
Fasano, C. A., Chambers, S. M., Lee, G., Tomishima, M. J., and Studer, L. (2010). Efficient derivation of functional floor plate tissue from human embryonic stem cells. Cell Stem Cell 6 (4), 336–347. doi:10.1016/j.stem.2010.03.001
Fazal, R., Boeynaems, S., Swijsen, A., De Decker, M., Fumagalli, L., Moisse, M., et al. (2021). HDAC6 inhibition restores TDP-43 pathology and axonal transport defects in human motor neurons with TARDBP mutations. Embo J. 40 (7), e106177. doi:10.15252/embj.2020106177
Fernandes, H. J. R., Patikas, N., Foskolou, S., Field, S. F., Park, J. E., Byrne, M. L., et al. (2020). Single-cell transcriptomics of Parkinson's disease human in vitro models reveals dopamine neuron-specific stress responses. Cell Rep. 33 (2), 108263. doi:10.1016/j.celrep.2020.108263
Figley, C. R. (2011). Lactate transport and metabolism in the human brain: Implications for the astrocyte-neuron lactate shuttle hypothesis. J. Neurosci. 31 (13), 4768–4770. doi:10.1523/jneurosci.6612-10.2011
Foster, A. D., Downing, P., Figredo, E., Polain, N., Stott, A., Layfield, R., et al. (2020). ALS-associated TBK1 variant p.G175S is defective in phosphorylation of p62 and impacts TBK1-mediated signalling and TDP-43 autophagic degradation. Mol. Cell. Neurosci. 108, 103539. doi:10.1016/j.mcn.2020.103539
Friedman, J. R., and Nunnari, J. (2014). Mitochondrial form and function. Nature 505 (7483), 335–343. doi:10.1038/nature12985
Fujimori, K., Ishikawa, M., Otomo, A., Atsuta, N., Nakamura, R., Akiyama, T., et al. (2018a). Modeling sporadic ALS in iPSC-derived motor neurons identifies a potential therapeutic agent. Nat. Med. 24 (10), 1579–1589. doi:10.1038/s41591-018-0140-5
Fujimori, K., Ishikawa, M., Otomo, A., Atsuta, N., Nakamura, R., Akiyama, T., et al. (2018b). Modeling sporadic ALS in iPSC-derived motor neurons identifies a potential therapeutic agent. Nat. Med. 24 (10), 1579–1589. doi:10.1038/s41591-018-0140-5
Gao, J., Wang, L., Liu, J., Xie, F., Su, B., and Wang, X. (2017). Abnormalities of mitochondrial dynamics in neurodegenerative diseases. Antioxidants (Basel) 6 (2), E25. doi:10.3390/antiox6020025
García-Morales, V., González-Acedo, A., Melguizo-Rodríguez, L., Pardo-Moreno, T., Costela-Ruiz, V. J., Montiel-Troya, M., et al. (2021). Current understanding of the physiopathology, diagnosis and therapeutic approach to Alzheimer's disease. Biomedicines 9 (12), 1910. doi:10.3390/biomedicines9121910
Gautam, M., Xie, E. F., Kocak, N., and Ozdinler, P. H. (2019). Mitoautophagy: A unique self-destructive path mitochondria of upper motor neurons with TDP-43 pathology take, very early in ALS. Front. Cell. Neurosci. 13, 489. doi:10.3389/fncel.2019.00489
Giacomelli, E., Vahsen, B. F., Calder, E. L., Xu, Y., Scaber, J., Gray, E., et al. (2022). Human stem cell models of neurodegeneration: From basic science of amyotrophic lateral sclerosis to clinical translation. Cell Stem Cell 29 (1), 11–35. doi:10.1016/j.stem.2021.12.008
Glater, E. E., Megeath, L. J., Stowers, R. S., and Schwarz, T. L. (2006). Axonal transport of mitochondria requires milton to recruit kinesin heavy chain and is light chain independent. J. Cell Biol. 173 (4), 545–557. doi:10.1083/jcb.200601067
Goiran, T., Eldeeb, M. A., Zorca, C. E., and Fon, E. A. (2022). Hallmarks and molecular tools for the study of mitophagy in Parkinson's disease. Cells 11 (13), 2097. doi:10.3390/cells11132097
Goldstein, D. S., Holmes, C., and Sharabi, Y. (2012). Cerebrospinal fluid biomarkers of central catecholamine deficiency in Parkinson's disease and other synucleinopathies. Brain 135 (6), 1900–1913. doi:10.1093/brain/aws055
Grekhnev, D. A., Kaznacheyeva, E. V., and Vigont, V. A. (2022). Patient-specific iPSCs-based models of neurodegenerative diseases: Focus on aberrant calcium signaling. Int. J. Mol. Sci. 23 (2), 624. doi:10.3390/ijms23020624
Günther, R., Pal, A., Williams, C., Zimyanin, V. L., Liehr, M., von Neubeck, C., et al. (2022). Alteration of mitochondrial integrity as upstream event in the pathophysiology of SOD1-ALS. Cells 11 (7), 1246. doi:10.3390/cells11071246
Guo, W., Naujock, M., Fumagalli, L., Vandoorne, T., Baatsen, P., Boon, R., et al. (2017). HDAC6 inhibition reverses axonal transport defects in motor neurons derived from FUS-ALS patients. Nat. Commun. 8 (1), 861. doi:10.1038/s41467-017-00911-y
Han, S., Zhang, M., Jeong, Y. Y., Margolis, D. J., and Cai, Q. (2021). The role of mitophagy in the regulation of mitochondrial energetic status in neurons. Autophagy 17 (12), 4182–4201. doi:10.1080/15548627.2021.1907167
Harding, O., Evans, C. S., Ye, J., Cheung, J., Maniatis, T., and Holzbaur, E. L. F. (2021). ALS- and FTD-associated missense mutations in TBK1 differentially disrupt mitophagy. Proc. Natl. Acad. Sci. U. S. A. 118 (24), e2025053118. doi:10.1073/pnas.2025053118
Haston, K. M., and Finkbeiner, S. (2016). Clinical trials in a dish: The potential of pluripotent stem cells to develop therapies for neurodegenerative diseases. Annu. Rev. Pharmacol. Toxicol. 56, 489–510. doi:10.1146/annurev-pharmtox-010715-103548
Hawrot, J., Imhof, S., and Wainger, B. J. (2020). Modeling cell-autonomous motor neuron phenotypes in ALS using iPSCs. Neurobiol. Dis. 134, 104680. doi:10.1016/j.nbd.2019.104680
Healy, D. G., Falchi, M., O'Sullivan, S. S., Bonifati, V., Durr, A., Bressman, S., et al. (2008). Phenotype, genotype, and worldwide genetic penetrance of LRRK2-associated Parkinson's disease: A case-control study. Lancet. Neurol. 7 (7), 583–590. doi:10.1016/s1474-4422(08)70117-0
Hor, J. H., Santosa, M. M., Lim, V. J. W., Ho, B. X., Taylor, A., Khong, Z. J., et al. (2021). ALS motor neurons exhibit hallmark metabolic defects that are rescued by SIRT3 activation. Cell Death Differ. 28 (4), 1379–1397. doi:10.1038/s41418-020-00664-0
Hsieh, C. H., Shaltouki, A., Gonzalez, A. E., Bettencourt da Cruz, A., Burbulla, L. F., St Lawrence, E., et al. (2016). Functional impairment in miro degradation and mitophagy is a shared feature in familial and sporadic Parkinson's disease. Cell Stem Cell 19 (6), 709–724. doi:10.1016/j.stem.2016.08.002
Hu, B. Y., and Zhang, S. C. (2009). Differentiation of spinal motor neurons from pluripotent human stem cells. Nat. Protoc. 4 (9), 1295–1304. doi:10.1038/nprot.2009.127
Imaizumi, Y., Okada, Y., Akamatsu, W., Koike, M., Kuzumaki, N., Hayakawa, H., et al. (2012). Mitochondrial dysfunction associated with increased oxidative stress and α-synuclein accumulation in PARK2 iPSC-derived neurons and postmortem brain tissue. Mol. Brain 5, 35. doi:10.1186/1756-6606-5-35
Israel, M. A., and Goldstein, L. S. (2011). Capturing Alzheimer's disease genomes with induced pluripotent stem cells: Prospects and challenges. Genome Med. 3 (7), 49. doi:10.1186/gm265
Jetto, C. T., Nambiar, A., and Manjithaya, R. (2022). Mitophagy and neurodegeneration: Between the knowns and the unknowns. Front. Cell Dev. Biol. 10, 837337. doi:10.3389/fcell.2022.837337
Jones, V. C., Atkinson-Dell, R., Verkhratsky, A., and Mohamet, L. (2017). Aberrant iPSC-derived human astrocytes in Alzheimer's disease. Cell Death Dis. 8 (3), e2696. doi:10.1038/cddis.2017.89
Julien, J. P. (2001). Amyotrophic lateral sclerosis. unfolding the toxicity of the misfolded. Cell 104 (4), 581–591. doi:10.1016/s0092-8674(01)00244-6
Kandimalla, R., Manczak, M., Fry, D., Suneetha, Y., Sesaki, H., and Reddy, P. H. (2016). Reduced dynamin-related protein 1 protects against phosphorylated Tau-induced mitochondrial dysfunction and synaptic damage in Alzheimer's disease. Hum. Mol. Genet. 25 (22), 4881–4897. doi:10.1093/hmg/ddw312
Ke, M., Chong, C. M., Zeng, H., Huang, M., Huang, Z., Zhang, K., et al. (2020). Azoramide protects iPSC-derived dopaminergic neurons with PLA2G6 D331Y mutation through restoring ER function and CREB signaling. Cell Death Dis. 11 (2), 130. doi:10.1038/s41419-020-2312-8
Ke, M., Chong, C. M., Zhu, Q., Zhang, K., Cai, C. Z., Lu, J. H., et al. (2021). Comprehensive perspectives on experimental models for Parkinson's disease. Aging Dis. 12 (1), 223–246. doi:10.14336/ad.2020.0331
Kim, T. W., Piao, J., Koo, S. Y., Kriks, S., Chung, S. Y., Betel, D., et al. (2021). Biphasic activation of WNT signaling facilitates the derivation of midbrain dopamine neurons from hESCs for translational use. Cell Stem Cell 28 (2), 343–355.e345. doi:10.1016/j.stem.2021.01.005
Kiskinis, E., Sandoe, J., Williams, L. A., Boulting, G. L., Moccia, R., Wainger, B. J., et al. (2014). Pathways disrupted in human ALS motor neurons identified through genetic correction of mutant SOD1. Cell Stem Cell 14 (6), 781–795. doi:10.1016/j.stem.2014.03.004
Konttinen, H., Gureviciene, I., Oksanen, M., Grubman, A., Loppi, S., Huuskonen, M. T., et al. (2019). PPARβ/δ-agonist GW0742 ameliorates dysfunction in fatty acid oxidation in PSEN1ΔE9 astrocytes. Glia 67 (1), 146–159. doi:10.1002/glia.23534
Koopman, W. J., Distelmaier, F., Smeitink, J. A., and Willems, P. H. (2013). OXPHOS mutations and neurodegeneration. Embo J. 32 (1), 9–29. doi:10.1038/emboj.2012.300
Krencik, R., and Zhang, S. C. (2011). Directed differentiation of functional astroglial subtypes from human pluripotent stem cells. Nat. Protoc. 6 (11), 1710–1717. doi:10.1038/nprot.2011.405
Kriks, S., Shim, J. W., Piao, J., Ganat, Y. M., Wakeman, D. R., Xie, Z., et al. (2011). Dopamine neurons derived from human ES cells efficiently engraft in animal models of Parkinson's disease. Nature 480 (7378), 547–551. doi:10.1038/nature10648
Kshirsagar, S., Sawant, N., Morton, H., Reddy, A. P., and Reddy, P. H. (2021). Mitophagy enhancers against phosphorylated Tau-induced mitochondrial and synaptic toxicities in Alzheimer disease. Pharmacol. Res. 174, 105973. doi:10.1016/j.phrs.2021.105973
Kshirsagar, S., Sawant, N., Morton, H., Reddy, A. P., and Reddy, P. H. (2022). Protective effects of mitophagy enhancers against amyloid beta-induced mitochondrial and synaptic toxicities in Alzheimer disease. Hum. Mol. Genet. 31 (3), 423–439. doi:10.1093/hmg/ddab262
Laperle, A. H., Sances, S., Yucer, N., Dardov, V. J., Garcia, V. J., Ho, R., et al. (2020). iPSC modeling of young-onset Parkinson’s disease reveals a molecular signature of disease and novel therapeutic candidates. Nat. Med. 26 (2), 289–299. doi:10.1038/s41591-019-0739-1
Li, J., and Fraenkel, E. (2021). Phenotyping neurodegeneration in human iPSCs. Annu. Rev. Biomed. Data Sci. 4, 83–100. doi:10.1146/annurev-biodatasci-092820-025214
Li, L., Kim, H. J., Roh, J. H., Kim, M., Koh, W., Kim, Y., et al. (2020). Pathological manifestation of the induced pluripotent stem cell-derived cortical neurons from an early-onset Alzheimer's disease patient carrying a presenilin-1 mutation (S170F). Cell Prolif. 53 (4), e12798. doi:10.1111/cpr.12798
Li, L., Roh, J. H., Chang, E. H., Lee, Y., Lee, S., Kim, M., et al. (2018a). iPSC modeling of Presenilin1 mutation in Alzheimer's disease with cerebellar ataxia. Exp. Neurobiol. 27 (5), 350–364. doi:10.5607/en.2018.27.5.350
Li, X., Tao, Y., Bradley, R., Du, Z., Tao, Y., Kong, L., et al. (2018b). Fast generation of functional subtype Astrocytes from human pluripotent stem cells. Stem Cell Rep. 11 (4), 998–1008. doi:10.1016/j.stemcr.2018.08.019
Lin, M. T., and Beal, M. F. (2006). Mitochondrial dysfunction and oxidative stress in neurodegenerative diseases. Nature 443 (7113), 787–795. doi:10.1038/nature05292
Lin, M. Y., Cheng, X. T., Xie, Y., Cai, Q., and Sheng, Z. H. (2017). Removing dysfunctional mitochondria from axons independent of mitophagy under pathophysiological conditions. Autophagy 13 (10), 1792–1794. doi:10.1080/15548627.2017.1356552
Lin, M. Y., and Sheng, Z. H. (2015). Regulation of mitochondrial transport in neurons. Exp. Cell Res. 334 (1), 35–44. doi:10.1016/j.yexcr.2015.01.004
Liu, Y., Andreucci, A., Iwamoto, N., Yin, Y., Yang, H., Liu, F., et al. (2022). Preclinical evaluation of WVE-004, aninvestigational stereopure oligonucleotide forthe treatment of C9orf72-associated ALS or FTD. Mol. Ther. Nucleic Acids 28, 558–570. doi:10.1016/j.omtn.2022.04.007
Macaskill, A. F., Rinholm, J. E., Twelvetrees, A. E., Arancibia-Carcamo, I. L., Muir, J., Fransson, A., et al. (2009). Miro1 is a calcium sensor for glutamate receptor-dependent localization of mitochondria at synapses. Neuron 61 (4), 541–555. doi:10.1016/j.neuron.2009.01.030
Lopez-Gonzalez, R., Lu, Y., Gendron, T. F., Karydas, A., Tran, H., Yang, D., et al. (2016). Poly(GR) in C9ORF72-related ALS/FTD compromises mitochondrial function and increases oxidative stress and DNA damage in iPSC-derived motor neurons. Neuron 92 (2), 383–391. doi:10.1016/j.neuron.2016.09.015
Magrané, J., Sahawneh, M. A., Przedborski, S., Estévez, Á. G., and Manfredi, G. (2012). Mitochondrial dynamics and bioenergetic dysfunction is associated with synaptic alterations in mutant SOD1 motor neurons. J. Neurosci. 32 (1), 229–242. doi:10.1523/JNEUROSCI.1233-11.2012
Manczak, M., Calkins, M. J., and Reddy, P. H. (2011). Impaired mitochondrial dynamics and abnormal interaction of amyloid beta with mitochondrial protein Drp1 in neurons from patients with Alzheimer's disease: Implications for neuronal damage. Hum. Mol. Genet. 20 (13), 2495–2509. doi:10.1093/hmg/ddr139
Martín-Maestro, P., Gargini, R., A Sproul, A., García, E., Antón, L. C., Noggle, S., et al. (2017). Mitophagy failure in fibroblasts and iPSC-derived neurons of Alzheimer's disease-associated presenilin 1 mutation. Front. Mol. Neurosci. 10, 291. doi:10.3389/fnmol.2017.00291
Martín-Maestro, P., Sproul, A., Martinez, H., Paquet, D., Gerges, M., Noggle, S., et al. (2019). Autophagy induction by bexarotene promotes mitophagy in presenilin 1 familial Alzheimer's disease iPSC-derived neural stem cells. Mol. Neurobiol. 56 (12), 8220–8236. doi:10.1007/s12035-019-01665-y
Mehta, A. R., Chandran, S., and Selvaraj, B. T. (2022). Assessment of mitochondrial trafficking as a surrogate for fast axonal transport in human induced pluripotent stem cell-derived spinal motor neurons. Methods Mol. Biol. 2431, 311–322. doi:10.1007/978-1-0716-1990-2_16
Mehta, A. R., Gregory, J. M., Dando, O., Carter, R. N., Burr, K., Nanda, J., et al. (2021). Mitochondrial bioenergetic deficits in C9orf72 amyotrophic lateral sclerosis motor neurons cause dysfunctional axonal homeostasis. Acta Neuropathol. 141 (2), 257–279. doi:10.1007/s00401-020-02252-5
Méndez-López, I., Sancho-Bielsa, F. J., Engel, T., García, A. G., and Padín, J. F. (2021). Progressive mitochondrial SOD1(g93a) accumulation causes severe structural, metabolic and functional aberrations through OPA1 down-regulation in a mouse model of amyotrophic lateral sclerosis. Int. J. Mol. Sci. 22 (15), 8194. doi:10.3390/ijms22158194
Mertens, J., Paquola, A. C. M., Ku, M., Hatch, E., Böhnke, L., Ladjevardi, S., et al. (2015). Directly reprogrammed human neurons retain aging-associated transcriptomic signatures and reveal age-related nucleocytoplasmic defects. Cell Stem Cell 17 (6), 705–718. doi:10.1016/j.stem.2015.09.001
Miller, J. D., Ganat, Y. M., Kishinevsky, S., Bowman, R. L., Liu, B., Tu, E. Y., et al. (2013). Human iPSC-based modeling of late-onset disease via progerin-induced aging. Cell Stem Cell 13 (6), 691–705. doi:10.1016/j.stem.2013.11.006
Misko, A., Jiang, S., Wegorzewska, I., Milbrandt, J., and Baloh, R. H. (2010). Mitofusin 2 is necessary for transport of axonal mitochondria and interacts with the Miro/Milton complex. J. Neurosci. 30 (12), 4232–4240. doi:10.1523/jneurosci.6248-09.2010
Moore, A. S., and Holzbaur, E. L. (2016). Dynamic recruitment and activation of ALS-associated TBK1 with its target optineurin are required for efficient mitophagy. Proc. Natl. Acad. Sci. U. S. A. 113 (24), E3349–E3358. doi:10.1073/pnas.1523810113
Naumann, M., Pal, A., Goswami, A., Lojewski, X., Japtok, J., Vehlow, A., et al. (2018). Impaired DNA damage response signaling by FUS-NLS mutations leads to neurodegeneration and FUS aggregate formation. Nat. Commun. 9 (1), 335. doi:10.1038/s41467-017-02299-1
Oberheim, N. A., Takano, T., Han, X., He, W., Lin, J. H., Wang, F., et al. (2009). Uniquely hominid features of adult human astrocytes. J. Neurosci. 29 (10), 3276–3287. doi:10.1523/jneurosci.4707-08.2009
Oh, C. K., Sultan, A., Platzer, J., Dolatabadi, N., Soldner, F., McClatchy, D. B., et al. (2017). S-nitrosylation of PINK1 attenuates PINK1/parkin-dependent mitophagy in hiPSC-based Parkinson's disease models. Cell Rep. 21 (8), 2171–2182. doi:10.1016/j.celrep.2017.10.068
Okano, H., and Morimoto, S. (2022). iPSC-based disease modeling and drug discovery in cardinal neurodegenerative disorders. Cell Stem Cell 29 (2), 189–208. doi:10.1016/j.stem.2022.01.007
Okano, H., and Yamanaka, S. (2014). IPS cell technologies: Significance and applications to CNS regeneration and disease. Mol. Brain 7 (1), 22. doi:10.1186/1756-6606-7-22
Okarmus, J., Havelund, J. F., Ryding, M., Schmidt, S. I., Bogetofte, H., Heon-Roberts, R., et al. (2021). Identification of bioactive metabolites in human iPSC-derived dopaminergic neurons with PARK2 mutation: Altered mitochondrial and energy metabolism. Stem Cell Rep. 16 (6), 1510–1526. doi:10.1016/j.stemcr.2021.04.022
Oksanen, M., Petersen, A. J., Naumenko, N., Puttonen, K., Lehtonen, Š., Gubert Olivé, M., et al. (2017). PSEN1 mutant iPSC-derived model reveals severe astrocyte pathology in Alzheimer's disease. Stem Cell Rep. 9 (6), 1885–1897. doi:10.1016/j.stemcr.2017.10.016
Onishi, M., Yamano, K., Sato, M., Matsuda, N., and Okamoto, K. (2021). Molecular mechanisms and physiological functions of mitophagy. Embo J. 40 (3), e104705. doi:10.15252/embj.2020104705
Ono, Y., Nakatani, T., Sakamoto, Y., Mizuhara, E., Minaki, Y., Kumai, M., et al. (2007). Differences in neurogenic potential in floor plate cells along an anteroposterior location: Midbrain dopaminergic neurons originate from mesencephalic floor plate cells. Development 134 (17), 3213–3225. doi:10.1242/dev.02879
Palomo, G. M., Granatiero, V., Kawamata, H., Konrad, C., Kim, M., Arreguin, A. J., et al. (2018). Parkin is a disease modifier in the mutant SOD1 mouse model of ALS. EMBO Mol. Med. 10 (10), e8888. doi:10.15252/emmm.201808888
Pardo-Moreno, T., González-Acedo, A., Rivas-Domínguez, A., García-Morales, V., García-Cozar, F. J., et al. (2022). Therapeutic approach to alzheimer’s disease: Current treatments and new perspectives. Pharmaceutics 14 (6). doi:10.3390/pharmaceutics14061117
Park, C.-Y., Kim, J., Kweon, J., Son Jeong, S., Lee Jae, S., Yoo, J.-E., et al. (2014). Targeted inversion and reversion of the blood coagulation factor 8 gene in human iPS cells using TALENs. Proc. Natl. Acad. Sci. U. S. A. 111 (25), 9253–9258. doi:10.1073/pnas.1323941111
Pasteuning-Vuhman, S., de Jongh, R., Timmers, A., and Pasterkamp, R. J. (2021). Towards advanced iPSC-based drug development for neurodegenerative disease. Trends Mol. Med. 27 (3), 263–279. doi:10.1016/j.molmed.2020.09.013
Piers, T. M., Cosker, K., Mallach, A., Johnson, G. T., Guerreiro, R., Hardy, J., et al. (2020). A locked immunometabolic switch underlies TREM2 R47H loss of function in human iPSC-derived microglia. Faseb J. 34 (2), 2436–2450. doi:10.1096/fj.201902447R
Placzek, M., and Briscoe, J. (2005). The floor plate: Multiple cells, multiple signals. Nat. Rev. Neurosci. 6 (3), 230–240. doi:10.1038/nrn1628
Pradeepkiran, J. A., Hindle, A., Kshirsagar, S., and Reddy, P. H. (2022). Are mitophagy enhancers therapeutic targets for Alzheimer's disease? Biomed. Pharmacother. 149, 112918. doi:10.1016/j.biopha.2022.112918
Prots, I., Grosch, J., Brazdis, R. M., Simmnacher, K., Veber, V., Havlicek, S., et al. (2018). α-Synuclein oligomers induce early axonal dysfunction in human iPSC-based models of synucleinopathies. Proc. Natl. Acad. Sci. U. S. A. 115 (30), 7813–7818. doi:10.1073/pnas.1713129115
Qi, G., Mi, Y., and Yin, F. (2019). Cellular specificity and inter-cellular coordination in the brain bioenergetic system: Implications for aging and neurodegeneration. Front. Physiol. 10, 1531. doi:10.3389/fphys.2019.01531
Qu, Q., Li, D., Louis, K. R., Li, X., Yang, H., Sun, Q., et al. (2014). High-efficiency motor neuron differentiation from human pluripotent stem cells and the function of Islet-1. Nat. Commun. 5 (1), 3449. doi:10.1038/ncomms4449
Queliconi, B. B., Kojima, W., Kimura, M., Imai, K., Udagawa, C., Motono, C., et al. (2021). Unfolding is the driving force for mitochondrial import and degradation of the Parkinson's disease-related protein DJ-1. J. Cell Sci. 134 (22), jcs258653. doi:10.1242/jcs.258653
Rasool, S., Soya, N., Truong, L., Croteau, N., Lukacs, G. L., and Trempe, J. F. (2018). PINK1 autophosphorylation is required for ubiquitin recognition. EMBO Rep. 19 (4), e44981. doi:10.15252/embr.201744981
Ren, Y., Jiang, H., Pu, J., Li, L., Wu, J., Yan, Y., et al. (2022). Molecular features of Parkinson's disease in patient-derived midbrain dopaminergic neurons. Mov. Disord. 37 (1), 70–79. doi:10.1002/mds.28786
Roybon, L., Lamas, N. J., Garcia-Diaz, A., Yang, E. J., Sattler, R., Jackson-Lewis, V., et al. (2013). Human stem cell-derived spinal cord astrocytes with defined mature or reactive phenotypes. Cell Rep. 4 (5), 1035–1048. doi:10.1016/j.celrep.2013.06.021
Ryu, W. I., Bormann, M. K., Shen, M., Kim, D., Forester, B., Park, Y., et al. (2021). Brain cells derived from Alzheimer's disease patients have multiple specific innate abnormalities in energy metabolism. Mol. Psychiatry 26 (10), 5702–5714. doi:10.1038/s41380-021-01068-3
Scheibye-Knudsen, M., Fang, E. F., Croteau, D. L., Wilson, D. M., and Bohr, V. A. (2015). Protecting the mitochondrial powerhouse. Trends Cell Biol. 25 (3), 158–170. doi:10.1016/j.tcb.2014.11.002
Schrepfer, E., and Scorrano, L. (2016). Mitofusins, from mitochondria to metabolism. Mol. Cell 61 (5), 683–694. doi:10.1016/j.molcel.2016.02.022
Shaltouki, A., Hsieh, C. H., Kim, M. J., and Wang, X. (2018). Alpha-synuclein delays mitophagy and targeting Miro rescues neuron loss in Parkinson's models. Acta Neuropathol. 136 (4), 607–620. doi:10.1007/s00401-018-1873-4
Shaltouki, A., Sivapatham, R., Pei, Y., Gerencser, A. A., Momčilović, O., Rao, M. S., et al. (2015). Mitochondrial alterations by PARKIN in dopaminergic neurons using PARK2 patient-specific and PARK2 knockout isogenic iPSC lines. Stem Cell Rep. 4 (5), 847–859. doi:10.1016/j.stemcr.2015.02.019
Stoica, R., De Vos, K. J., Paillusson, S., Mueller, S., Sancho, R. M., Lau, K. F., et al. (2014). ER-mitochondria associations are regulated by the VAPB-PTPIP51 interaction and are disrupted by ALS/FTD-associated TDP-43. Nat. Commun. 5, 3996. doi:10.1038/ncomms4996
Su, Y.-C., and Qi, X. (2013). Inhibition of excessive mitochondrial fission reduced aberrant autophagy and neuronal damage caused by LRRK2 G2019S mutation. Hum. Mol. Genet. 22 (22), 4545–4561. doi:10.1093/hmg/ddt301
Tak, Y. J., Park, J. H., Rhim, H., and Kang, S. (2020). ALS-related mutant SOD1 aggregates interfere with mitophagy by sequestering the autophagy receptor optineurin. Int. J. Mol. Sci. 21 (20), E7525. doi:10.3390/ijms21207525
Taylor, J. P., Brown, R. H., and Cleveland, D. W. (2016). Decoding ALS: From genes to mechanism. Nature 539 (7628), 197–206. doi:10.1038/nature20413
Tcw, J., Wang, M., Pimenova, A. A., Bowles, K. R., Hartley, B. J., Lacin, E., et al. (2017). An efficient platform for astrocyte differentiation from human induced pluripotent stem cells. Stem Cell Rep. 9 (2), 600–614. doi:10.1016/j.stemcr.2017.06.018
Vandoorne, T., Veys, K., Guo, W., Sicart, A., Vints, K., Swijsen, A., et al. (2019). Differentiation but not ALS mutations in FUS rewires motor neuron metabolism. Nat. Commun. 10 (1), 4147. doi:10.1038/s41467-019-12099-4
Venkatramani, A., and Panda, D. (2019). Regulation of neuronal microtubule dynamics by tau: Implications for tauopathies. Int. J. Biol. Macromol. 133, 473–483. doi:10.1016/j.ijbiomac.2019.04.120
Vierbuchen, T., Ostermeier, A., Pang, Z. P., Kokubu, Y., Südhof, T. C., and Wernig, M. (2010). Direct conversion of fibroblasts to functional neurons by defined factors. Nature 463 (7284), 1035–1041. doi:10.1038/nature08797
Vos, M., Geens, A., Böhm, C., Deaulmerie, L., Swerts, J., Rossi, M., et al. (2017). Cardiolipin promotes electron transport between ubiquinone and complex I to rescue PINK1 deficiency. J. Cell Biol. 216 (3), 695–708. doi:10.1083/jcb.201511044
Wang, S., Zou, C., Fu, L., Wang, B., An, J., Song, G., et al. (2015). Autologous iPSC-derived dopamine neuron transplantation in a nonhuman primate Parkinson's disease model. Cell Discov. 1, 15012. doi:10.1038/celldisc.2015.12
Wang, W., Li, L., Lin, W. L., Dickson, D. W., Petrucelli, L., Zhang, T., et al. (2013). The ALS disease-associated mutant TDP-43 impairs mitochondrial dynamics and function in motor neurons. Hum. Mol. Genet. 22 (23), 4706–4719. doi:10.1093/hmg/ddt319
Wang, X., Su, B., Zheng, L., Perry, G., Smith, M. A., and Zhu, X. (2009). The role of abnormal mitochondrial dynamics in the pathogenesis of Alzheimer's disease. J. Neurochem. 109 (1), 153–159. doi:10.1111/j.1471-4159.2009.05867.x
Wang, X., Winter, D., Ashrafi, G., Schlehe, J., Wong, Y. L., Selkoe, D., et al. (2011). PINK1 and Parkin target Miro for phosphorylation and degradation to arrest mitochondrial motility. Cell 147 (4), 893–906. doi:10.1016/j.cell.2011.10.018
Wang, X., Yan, M. H., Fujioka, H., Liu, J., Wilson-Delfosse, A., Chen, S. G., et al. (2012). LRRK2 regulates mitochondrial dynamics and function through direct interaction with DLP1. Hum. Mol. Genet. 21 (9), 1931–1944. doi:10.1093/hmg/dds003
Wasner, K., Smajic, S., Ghelfi, J., Delcambre, S., Prada-Medina, C. A., Knappe, E., et al. (2022). Parkin deficiency impairs mitochondrial DNA dynamics and propagates inflammation. Mov. Disord. 37 (7), 1405–1415. doi:10.1002/mds.29025
Wei, H., Liu, L., and Chen, Q. (2015). Selective removal of mitochondria via mitophagy: Distinct pathways for different mitochondrial stresses. Biochim. Biophys. Acta 1853 (10), 2784–2790. doi:10.1016/j.bbamcr.2015.03.013
WHO (2021). World Health organization dementia factsheet [online]. Available at: https://www.who.int/en/news-room/fact-sheets/detail/dementia (Accessed 8 28, 2022).
Wong, Y. C., and Holzbaur, E. L. (2014). Optineurin is an autophagy receptor for damaged mitochondria in parkin-mediated mitophagy that is disrupted by an ALS-linked mutation. Proc. Natl. Acad. Sci. U. S. A. 111 (42), E4439–E4448. doi:10.1073/pnas.1405752111
Wu, H., Wei, H., Sehgal, S. A., Liu, L., and Chen, Q. (2016). Mitophagy receptors sense stress signals and couple mitochondrial dynamic machinery for mitochondrial quality control. Free Radic. Biol. Med. 100, 199–209. doi:10.1016/j.freeradbiomed.2016.03.030
Xi, J., Liu, Y., Liu, H., Chen, H., Emborg, M. E., and Zhang, S. C. (2012). Specification of midbrain dopamine neurons from primate pluripotent stem cells. Stem Cells 30 (8), 1655–1663. doi:10.1002/stem.1152
Zambon, F., Cherubini, M., Fernandes, H. J. R., Lang, C., Ryan, B. J., Volpato, V., et al. (2019). Cellular α-synuclein pathology is associated with bioenergetic dysfunction in Parkinson's iPSC-derived dopamine neurons. Hum. Mol. Genet. 28 (12), 2001–2013. doi:10.1093/hmg/ddz038
Zanon, A., Kalvakuri, S., Rakovic, A., Foco, L., Guida, M., Schwienbacher, C., et al. (2017). SLP-2 interacts with Parkin in mitochondria and prevents mitochondrial dysfunction in Parkin-deficient human iPSC-derived neurons and Drosophila. Hum. Mol. Genet. 26 (13), 2412–2425. doi:10.1093/hmg/ddx132
Zhang, Z. W., Tu, H., Jiang, M., Vanan, S., Chia, S. Y., Jang, S. E., et al. (2022). The APP intracellular domain promotes LRRK2 expression to enable feed-forward neurodegenerative mechanisms in Parkinson's disease. Sci. Signal. 15 (748), eabk3411. doi:10.1126/scisignal.abk3411
Keywords: neurodegenerative diseases, mitochondrial dysfunction, therapeutic strategy, IPSCs (induced pluripotent stem cells), mitochondrial dynamic, mitochondrial transport, mitochondrial energy metabolism
Citation: Luo H-M, Xu J, Huang D-X, Chen Y-Q, Liu Y-Z, Li Y-J and Chen H (2022) Mitochondrial dysfunction of induced pluripotent stem cells-based neurodegenerative disease modeling and therapeutic strategy. Front. Cell Dev. Biol. 10:1030390. doi: 10.3389/fcell.2022.1030390
Received: 28 August 2022; Accepted: 31 October 2022;
Published: 21 November 2022.
Edited by:
Prasad Tammineni, University of Hyderabad, IndiaReviewed by:
Rajesh Angireddy, Children’s Hospital of Philadelphia, United StatesP. Hemachandra Reddy, Texas Tech University Health Sciences Center, United States
Daria Diodato, Bambino Gesù Children’s Hospital (IRCCS), Italy
Copyright © 2022 Luo, Xu, Huang, Chen, Liu, Li and Chen. This is an open-access article distributed under the terms of the Creative Commons Attribution License (CC BY). The use, distribution or reproduction in other forums is permitted, provided the original author(s) and the copyright owner(s) are credited and that the original publication in this journal is cited, in accordance with accepted academic practice. No use, distribution or reproduction is permitted which does not comply with these terms.
*Correspondence: Hong Chen, Y2hlbmhvbmcxMTI5QGhvdG1haWwuY29t