- 1Department of Biology, McGill University, Montreal, QC, Canada
- 2Department of Biology, Concordia University, Montreal, QC, Canada
Cytokinesis is required to physically cleave a cell into two daughters at the end of mitosis. Decades of research have led to a comprehensive understanding of the core cytokinesis machinery and how it is regulated in animal cells, however this knowledge was generated using single cells cultured in vitro, or in early embryos before tissues develop. This raises the question of how cytokinesis is regulated in diverse animal cell types and developmental contexts. Recent studies of distinct cell types in the same organism or in similar cell types from different organisms have revealed striking differences in how cytokinesis is regulated, which includes different threshold requirements for the structural components and the mechanisms that regulate them. In this review, we highlight these differences with an emphasis on pathways that are independent of the mitotic spindle, and operate through signals associated with the cortex, kinetochores, or chromatin.
Introduction
Overview of cytokinesis in animal cells
Cytokinesis must occur with high fidelity to prevent pathologies, and multiple pathways create a robust system to accommodate perturbations. While the relative role of these pathways likely varies with cell fate, ploidy and size, we lack knowledge of how they function in most cell types and tissues. Since several reviews describe the core cytokinesis machinery in depth, we will emphasize differences in cytokinesis among animal cell types (e.g., Green et al., 2012; Basant and Glotzer, 2018; Leite et al., 2019; Pintard and Bowerman, 2019; Pollard and O'Shaughnessy, 2019; Nguyen and Robinson, 2020; Sugioka, 2022).
Cytokinesis occurs by the ingression of an actomyosin ring that constricts to pinch in the membrane (Figure 1A). The anaphase spindle provides cues for RhoA-dependent ring assembly in the equatorial plane (Figure 1B and Figure 2A; Rappaport, 1986; Bement et al., 2005). RhoA-GDP is inactive, while RhoA-GTP binds to effectors including formins and Rho-kinase (ROCK) to generate linear actomyosin filaments (Figure 1B; Piekny et al., 2005; Green et al., 2012). The GTPase activating protein (GAP) MP-GAP (CeRGA-3/4) globally inactivates RhoA by stimulating GTP hydrolysis, while the guanine nucleotide exchange factor (GEF) Ect2 (CeECT-2, DmPbl) activates RhoA by exchanging GDP for GTP (Figure 1B; Tatsumoto et al., 1999; Yuce et al., 2005; Zanin et al., 2013). Ect2 activity is spatiotemporally controlled by centralspindlin (Cyk4/MgcRacGAP, CeCYK-4, DmRacGAP50C and MKLP1/KIF23, CeZEN-4, DmPav), which bundles microtubules to form the central spindle during anaphase (Mishima et al., 2002; Yuce et al., 2005; Hara et al., 2006; Niiya et al., 2006). Cyk4-binding recruits Ect2 to the central spindle (Figure 1B; Yuce et al., 2005; Petronczki et al., 2007; Wolfe et al., 2009). Cyk4 also requires Plk1 phosphorylation for Ect2-binding, and the loss or inhibition of Plk1 or Cyk4, and/or blocking Cyk4 phosphorylation prevents ring assembly and phenocopies Ect2 depletion (Somers and Saint, 2003; Zhao and Fang, 2005; Burkard et al., 2007; Miller and Bement, 2009; Wolfe et al., 2009; Gomez-Cavazos et al., 2020). Plk1-phosphorylation could reduce the affinity of centralspindlin for microtubules, causing its release to the overlying membrane where it activates Ect2 and is regulated by Aurora B kinase (Petronczki et al., 2007; Wolfe et al., 2009; Frenette et al., 2012; Lekomtsev et al., 2012; Adriaans et al., 2019). RhoA-GTP also recruits anillin (CeANI-1), which crosslinks F-actin and myosin with phospholipids for ring positioning, and forms complexes with septins to facilitate ingression (Figure 1B; Piekny and Maddox, 2010; Carim et al., 2020). Anillin also feeds back to facilitate RhoA-GTP effector binding (Budnar et al., 2019). As linear filaments are generated in the equatorial plane, their alignment is facilitated by cortical flow and/or crosslinkers in the C. elegans zygote (Reymann et al., 2016; Khaliullin et al., 2018; Leite et al., 2020). Constriction then occurs by the myosin-dependent binding and/or sliding of actin filaments (e.g., Ma et al., 2012; Osorio et al., 2019). In addition, a hypothesis paper proposed that anillin-septin membrane microdomains are shed from the ring to relieve tension and mediate ring closure (Carim et al., 2020).
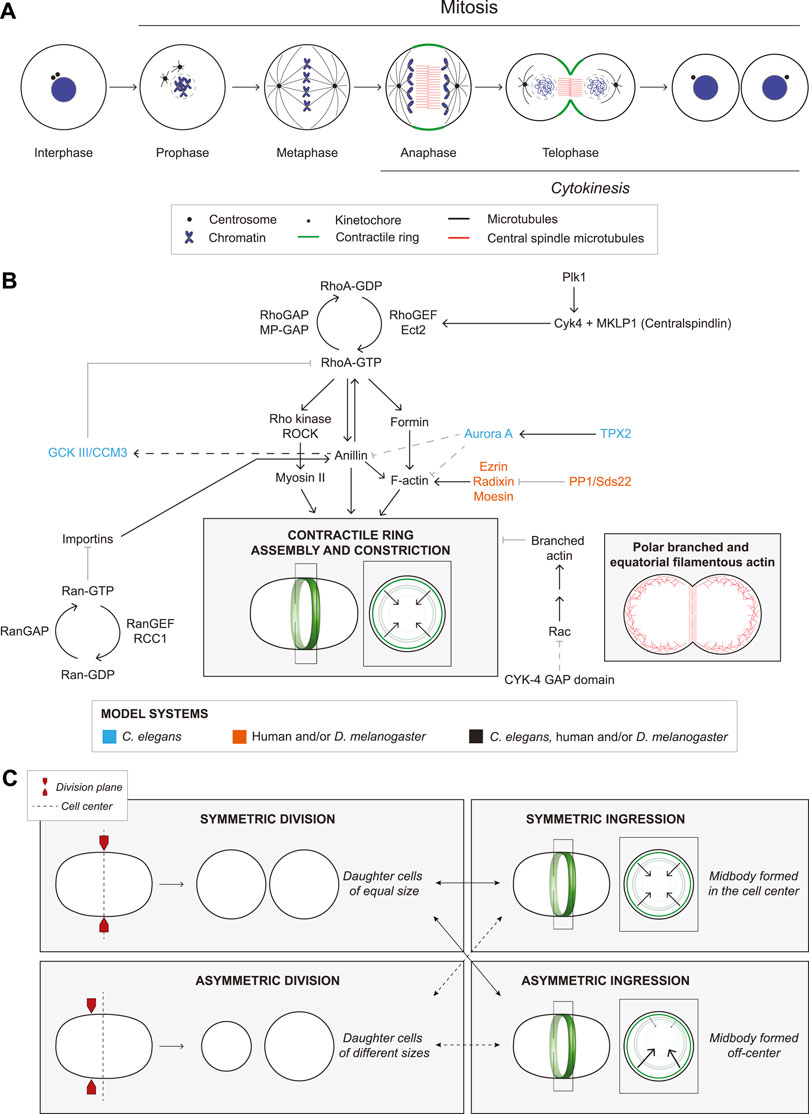
FIGURE 1. The molecular regulation of ring assembly and constriction. (A) The cartoon schematic shows a cell undergoing mitosis. Chromosome condensation and nuclear envelope breakdown occur during prophase. In metaphase, centrosomes (black circles) form a bipolar spindle (black) that aligns the sister chromatids (blue). During anaphase, the central spindle forms, consisting of anti-parallel bundled microtubules (red). A contractile ring (green) assembles between the segregating chromosomes and in a plane that bisects the central spindle. During telophase, the ring constricts to divide the cytosol, and the nuclear membrane reassembles. After the ring ingresses, a midbody forms that controls abscission to separate the two daughter cells. (B) Multiple proteins regulate cytokinesis as indicated by the arrows (solid lines are established interactions, while dashed lines are hypothetical). These pathways culminate in the assembly and constriction of an actomyosin ring (cartoon cell, ring in green). To the right, another cell shows the location of polar, branched F-actin (red branches) and linear F-actin (red lines) during cytokinesis. Font colors indicate whether studies were performed in C. elegans (blue), human cells and/or D. melanogaster (orange), or all three (black). (C) Cartoon schematics show cells undergoing symmetric division (top left), where two daughter cells of equal sizes are generated, and an asymmetric division (bottom left) forming daughter cells of different sizes. In either type of division, the ring can ingress symmetrically (top right) or asymmetrically (bottom right) where there is more ‘pull’, from one side of the ring.
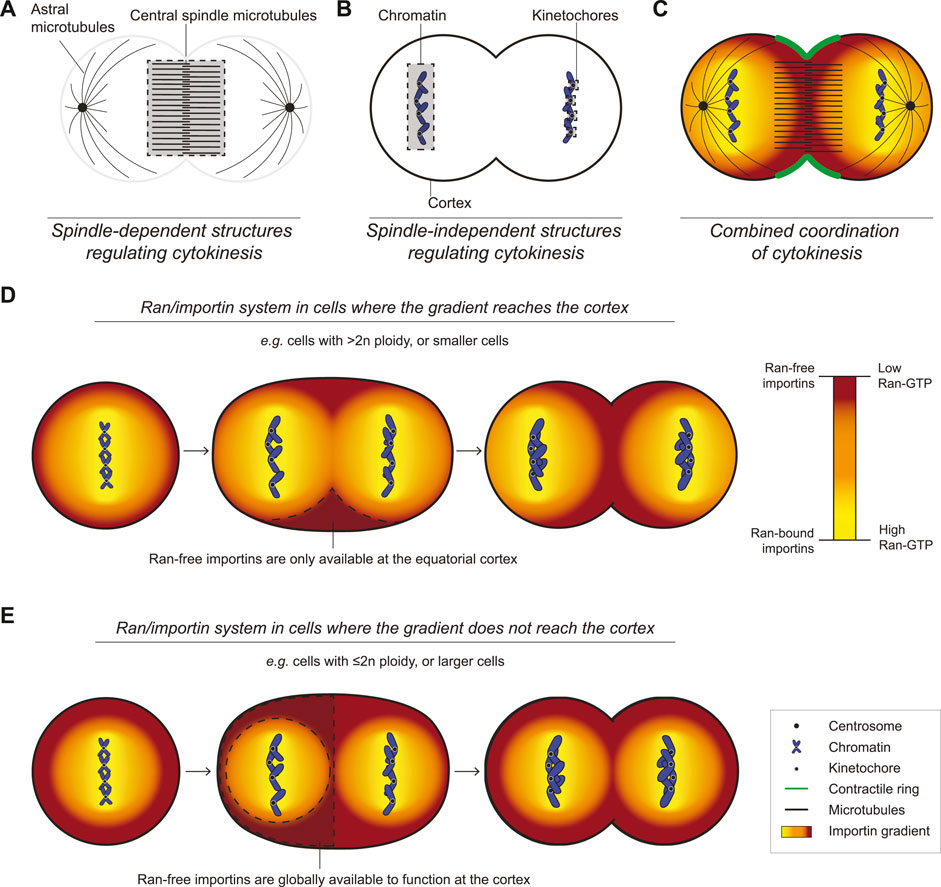
FIGURE 2. An overview of the mechanisms regulating cytokinesis. (A) A cartoon cell shows the spindle components relative to the overlying cortex in late anaphase/early telophase. (B) A similarly staged cell shows the relative locations of the chromatin, kinetochores, and cortex. (C) All of the key components from (A) and (B) are shown together in a one cell, which work together to ensure successful cytokinesis (Ran-free importins in dark orange, Ran-GTP in yellow, scale below). (D) Cartoons show how in small cells or in cells with high ploidy, Ran-GTP could restrict cortical importins, which only reach sufficient levels to recruit anillin to the equatorial cortex during anaphase as a dominant mechanism to control ring positioning. (E) Cartoons show how in large cells or in cells with low ploidy, Ran-GTP may not reach the cortex and importins would be able to recruit anillin uniformly to the cortex in metaphase and anaphase. These cells would require other mechanisms to control ring positioning. The legend indicates the relevant components for all cells (A–E).
Despite our extensive knowledge of cytokinesis, studies suggest that the core structural components and their regulators do not play the same role in all cells. For example, differences in the organization, levels and threshold requirements of F-actin (e.g., Davies et al., 2018), myosin (e.g., Ozugergin et al., 2022), and formin (e.g., Davies et al., 2018; Higashi et al., 2019) would cause different cortical properties that affect ring closure kinetics (Leite et al., 2019).
Differences in cytokinesis among animal cell types
Cytokinesis is influenced by intrinsic and extrinsic factors that affect filament alignment for constriction and include polarity, cell–substrate adhesion and adherens junctions (Higashi et al., 2016; Pinheiro et al., 2017; Dix et al., 2018; Chaigne et al., 2021; Gupta et al., 2021; Ozugergin et al., 2022; Paim and FitzHarris, 2022). Along with causing different rates of ingression, these factors can also cause ingression to be more asymmetric (Figure 1C). Here, we will describe differences in the core structural components and upstream regulators of the ring.
Differences in structural ring components
Differences in the ring components can affect ring kinetics. Distinct actin and myosin isoforms can have different biochemical properties, while actin can form branched or unbranched filaments with different rates of assembly or disassembly. For example, distinct actin and myosin isoforms are differentially enriched in the equatorial plane compared to the polar cortex (Maupin et al., 1994; Dugina et al., 2009; Po’uha and Kavallaris, 2015; Chen et al., 2017; Yamamoto et al., 2019; Shagieva et al., 2020; Taneja et al., 2020; Chen et al., 2021). Different actins assemble into distinct linear or branched filaments via different formins or Arp2/3 (Figure 1B), while myosin isoforms have different crosslinking or motor activities (Bao et al., 2005; Chen et al., 2017; Taneja et al., 2020; Wang et al., 2020; Chen et al., 2021). In C. elegans, aligned actin filaments in the equatorial plane facilitate the assembly of new filaments (Li and Munro, 2021). The requirement for myosin’s function as a motor or crosslinker also differs between cell types in mice and C. elegans (Ma et al., 2012; Osorio et al., 2019). As mentioned earlier, levels could also affect ring kinetics. Partial depletion of ARX-2 (CeArp2) or CYK-1 (Ceformin) can alter ring dynamics by changing the levels of equatorial F-actin (Chan et al., 2019). Germline-fated cells in C. elegans embryos have less linear F-actin and myosin and slower ring assembly compared to somatic cells, and they operate closer to threshold requirements (Davies et al., 2018; Ozugergin et al., 2022). A prior study proposed that larger cells have more contractile units in the ring than smaller cells to coordinate ingression (Carvalho et al., 2009). However, ring closure has distinct phases that may or may not correlate with size (Davies et al., 2018; Ozugergin et al., 2022). The amount of actomyosin could cause different tension or flow rates that influence ring closure, which could be crucial during development. In C. elegans, signalling between P2 and EMS cells regulates their fate, and their relative positions are controlled by coordinating division at the two-cell stage (Rose and Gonczy, 2014; Davies et al., 2018).
Differences in ring closure symmetry
Asymmetric ring ingression is more extreme in cells with apicobasal polarity or that contact other cells (Figure 1C). Symmetry breaking is modeled to occur through the positive feedback of membrane curvature-dependent filament alignment (Dorn et al., 2016). The mechanisms that control filament alignment could be influenced intrinsically or extrinsically as described earlier (Maddox et al., 2007; Singh and Pohl, 2014; Reymann et al., 2016; Spira et al., 2017; Khaliullin et al., 2018). Asymmetric alignment could cause higher contractility and/or different tension in part of the ring. However, the molecular regulation of asymmetric closure is not clear. CYK-1, ANI-1 and septins control asymmetric ingression in the C. elegans zygote (Maddox et al., 2007; Chan et al., 2019). However, in the vulval precursor cells, tissue geometry and adhesion play a stronger role (Maddox et al., 2007; Bourdages et al., 2014). PARD6B is required for apicobasal polarity and asymmetric ingression in the early mouse embryo, and the localization of anillin and myosin is mutually exclusive with apically-enriched PARD6B (Paim and FitzHarris, 2022). This mechanism differs from Drosophila epithelial cells where ingression is influenced by extrinsic forces transmitted through adhesion junctions (Herszterg et al., 2014; Osswald and Morais-de-Sa, 2019; Buckley and St Johnston, 2022).
Differences in ring regulators
Differences in the upstream regulators can also affect ring kinetics. Ect2 and Pbl localize to microtubules and the equatorial cortex in HeLa cells, Drosophila embryos and S2 cells (Prokopenko et al., 1999; Yuce et al., 2005; Verma and Maresca, 2019), but ECT-2 is cortical in the C. elegans zygote (Gomez-Cavazos et al., 2020). Both Cyk4 and Ect2 require membrane localization to generate active RhoA for cytokinesis (Su et al., 2011; Frenette et al., 2012; Lekomtsev et al., 2012; Basant et al., 2015). Thus, the requirement for cortical centralspindlin and/or Ect2 could be higher in cells where the central spindle is far from the cortex. There is also a debate (Basant and Glotzer, 2017; Zhuravlev et al., 2017) about whether Cyk4 activates RhoA, or functions as a GAP for Rac. Point mutations that disrupt GAP activity cause cytokinesis phenotypes, and Rac depletion suppresses phenotypes caused by the loss of CYK-4 or ECT-2 in C. elegans embryos (Canman et al., 2008; Zhuravlev et al., 2017). CYK-4 was proposed to downregulate Arp2/3-mediated branched F-actin and decrease cortical stiffness in the equatorial plane (Figure 1B; Canman et al., 2008; Bastos et al., 2012; Zhuravlev et al., 2017). However, an alternative interpretation is that Rac globally regulates cortical stiffness and its depletion makes it easier for weakly formed rings to ingress (Loria et al., 2012; Basant and Glotzer, 2017). In HeLa cells, Cyk4 regulates RhoA, but it could also regulate Rac1 to control effectors for adhesion (Yuce et al., 2005; Bastos et al., 2012). Further research is needed to clarify the role of Cyk4 in cytokinesis in additional cell types.
Anillin also varies between cells. Anillin is cytosolic in interphase C. elegans and Drosophila embryonic cells, but is nuclear in cultured Drosophila and human cells (Piekny and Maddox, 2010). Anillin depletion causes cytokinesis failure in C. elegans neuroblasts, Xenopus embryos, Drosophila S2 and HeLa cells, but not in the C. elegans zygote, despite a ∼97% reduction in anillin levels (Maddox et al., 2005; Straight et al., 2005; Hickson and O'Farrell, 2008; Piekny and Glotzer, 2008; Piekny and Maddox, 2010; Fotopoulos et al., 2013; Reyes et al., 2014). Dalmatians with an early nonsense mutation in anillin were born, albeit with developmental defects, suggesting that anillin is not required for cytokinesis in most cells (Holopainen et al., 2017). However, alternative splicing, initiation codons or translation could still produce functional protein depending on the cell type. Anillin also plays multiple roles in cytokinesis, including ring positioning, ingression and midbody formation, which could require different threshold levels (Hickson and O'Farrell, 2008; Piekny and Glotzer, 2008). In the C. elegans zygote, ANI-1 controls ingression through negative feedback by recruiting GCK-1 and its cofactor CCM-3 to inactivate RhoA through RGA-3/4 for RhoA inactivation (Figure 1B; Rehain-Bell et al., 2017; Bell et al., 2020), while anillin controls RhoA-GTP signaling by facilitating its interaction with effectors in mammalian cells (Budnar et al., 2019). Anillin’s crosslinking function can also slide actin filaments and generate force in vitro without myosin (Kucera et al., 2021). The variable threshold requirements for anillin could reflect its different interactions and functions.
Spindle-independent regulation of cytokinesis in animal cells
Spindle-independent pathways also regulate cytokinesis, and their requirement likely varies with cell fate, ploidy or size (Figure 2B). These pathways would contribute to the cytokinetic diversity of cells with different developmental paths, providing a robust system that precludes cytokinesis failure (Figure 2C).
Cortical mechanisms
Aligned actomyosin filaments generate force for ring constriction. The ring forms within a continuous, cortical network that spans the cell, and actin-binding proteins that control cortical connectivity such as plastin and spectrin can influence this meshwork and stabilize the ring (Turlier et al., 2014; Ding et al., 2017; Leite et al., 2019; Sobral et al., 2021). Excess cytoplasmic pressure may arise in the polar cortex as the ring constricts, which is released by blebs that form from localized changes in the cortex (Sedzinski et al., 2011). For example, RhoA is typically inactive at the polar cortex, and blebs occur more frequently after MP-GAP depletion (Sedzinski et al., 2011; Zanin et al., 2013). Blebbing can vary among cell types, reflecting differences in their cortical properties; e.g., HeLa cells display more prominent blebbing than C. elegans embryos (Zanin et al., 2013).
Cortical pathways facilitate ring positioning in asymmetrically dividing cells (Figure 1C). Drosophila neuroblasts have apicobasal polarity and divide asymmetrically to produce daughter cells with different sizes and fates. The ring assembles closer to the basal pole where myosin enrichment is controlled by Pins and Dlg (Cabernard et al., 2010; Connell et al., 2011). In the C. elegans zygote, actomyosin contractility is enriched at the anterior cortex via feedback mechanisms that establish anterior-posterior polarity through the localization of distinct PAR (partitioning defective) complexes (Lang and Munro, 2017; Delattre and Goehring, 2021). The contractile ring aligns with the anterior-posterior boundary, but it is unclear how PAR proteins control ring position (Schenk et al., 2010; Pittman and Skop, 2012). One model is that anterior actomyosin competes for ANI-1, restricting its levels in the ring (Jordan et al., 2016).
Chromatin sensing via kinetochores
Kinetochores regulate cytokinesis by promoting the removal of F-actin from the polar cortex (Figure 2B). Kinetochores are crucial for chromosome segregation by stably attaching chromosomes to the mitotic spindle (Musacchio and Desai, 2017; Lara-Gonzalez et al., 2021; Navarro and Cheeseman, 2021). Ezrin-Radixin-Moesin (ERM) proteins crosslink F-actin to the membrane to regulate cortical properties (Carreno et al., 2008; Kunda et al., 2008). As chromosomes segregate, kinetochore-associated PP1 phosphatase and Sds22 inactivate moesin, causing a decrease in polar F-actin in Drosophila S2 and HeLa cells (Figure 1B; Roubinet et al., 2011; Kunda et al., 2012; Rodrigues et al., 2015). While PP1/Sds22 and moesin are not required for cytokinesis, their depletion causes cell shape changes and membrane protrusions, respectively (Carreno et al., 2008; Rodrigues et al., 2015). The chloride channel CLIC4 also controls polar cortical stability through ezrin-binding, but it is not clear if CLIC4 is part of the kinetochore pathway (Peterman et al., 2020; Uretmen Kagiali et al., 2020).
Polar relaxation occurs through other mechanisms when kinetochores are far from the cortex. In C. elegans zygotes, astral microtubules regulate the polar cortex through AIR-1 (Aurora A kinase) and TPXL-1 (HsTPX2), which inhibits the polar accumulation of ANI-1 and F-actin (Figure 1B; Mangal et al., 2018). More recent work in C. elegans revealed that astral microtubules control the dynein-dependent removal of myosin from the polar cortex (Chapa et al., 2020). Other studies showed that in C. elegans and cultured human cells, ANI-1/anillin binds to astral microtubules in cortical regions where RhoA-GTP is low, and astral microtubules cause a decrease in formin activity and γ-actin at the polar cortex (Tse et al., 2011; van Oostende Triplet et al., 2014; Chen et al., 2021). It is not clear if these mechanisms are related, and studies are needed to reveal how their requirement varies with cell type.
Chromatin sensing via Ran signaling
Other chromatin sensing pathways regulate cytokinesis. Lagging chromosomes delay cytokinesis, likely to prevent aneuploidy (Steigemann et al., 2009; Kotadia et al., 2012; Montembault et al., 2017). In Drosophila neuroblasts, trailing chromatids correlate with broad myosin accumulation, cell elongation and delayed completion of cytokinesis (Kotadia et al., 2012). This phenotype is associated with delayed nuclear envelope assembly, leaving Pbl at the midzone where it could cause persistent RhoA activation (Montembault et al., 2017). While the chromatin-associated signal is not known, a likely candidate is Ran GTPase.
Active Ran forms an inverse gradient with importins to control ring positioning (Figure 2C; Kiyomitsu and Cheeseman, 2013; Beaudet et al., 2017; Beaudet et al., 2020). Importin-α and -β bind to nuclear localization signals (NLSs) in proteins and Ran-GTP dissociates this complex (Xu and Massague, 2004; Lange et al., 2007; Clarke and Zhang, 2008; Ozugergin and Piekny, 2021). Ran-GTP is generated by histone-tethered RCC1 (RanGEF), while cytosolic RanGAP negatively regulates Ran, causing active Ran to be highest around chromatin and lowest near the cortex (Figure 1B; Kalab et al., 2002; Kalab et al., 2006). In anaphase, the segregating chromosomes could lead to the equatorial enrichment of importins where they control the localization and function of anillin (Hinkle et al., 2002; Kiyomitsu and Cheeseman, 2013; Beaudet et al., 2017). In meiosis, active Ran functions as a ruler to control formation of an F-actin cap for polar body extrusion in mouse oocytes (Deng et al., 2007). Although the cortical targets of Ran signaling in meiosis are not known, they regulate branched F-actin (Yi et al., 2011; Dehapiot et al., 2013; Burdyniuk et al., 2018). Importins also regulate cellularization of the syncytial Drosophila embryo, where ingressing membranes partition nuclei into individual cells (Lecuit, 2004). Silverman-Gavrila et al. (2008) showed that importin-α overexpression causes a decrease in anillin and Peanut (DmSeptin) localization and prevents cellularization, because importins compete with Peanut for anillin-binding. Importin-β overexpression also decreases anillin’s cortical localization in HeLa cells, supporting the ruler model where different levels of importins promote or inhibit function. This model is supported by the molecular regulation of anillin; the RhoA-GTP binding domain autoinhibits a neighbouring domain with overlapping NLS and phospholipid-binding sites, and RhoA-GTP relieves this autoinhibition, permitting importin-binding to stabilize anillin for recruitment to the overlying phospholipids (Beaudet et al., 2017; Beaudet et al., 2020). We propose that importins are sufficiently enriched only between the segregating chromosomes in cells where Ran-GTP reaches the cortex (e.g., higher ploidy; Figure 2D), while in cells where cortical importins are uniform, other mechanisms would control ring positioning (e.g., lower ploidy; Figure 2E).
The Ran pathway could control cortical targets other than anillin (Ozugergin et al., 2022). In C. elegans embryos, importin-β (IMB-1) facilitates the equatorial enrichment of ANI-1 in a somatic cell, while importin-α (IMA-3) and/or -β control ring assembly in a germline-fated cell through unknown targets. Also, importins could bind as homo- or heterodimers which could differently impact protein function (Ozugergin and Piekny, 2021). An exciting hypothesis is that the Ran pathway has multiple targets that respond to different importin levels to confer the cortical properties controlling cytokinesis in diverse cell types.
Discussion
After a century of research, our understanding of cytokinesis is extensive. However, there is considerable diversity in how the core machinery is expressed and regulated, and in the number of mechanisms that control cytokinesis. The differences we reviewed here are just the tip of the iceberg, reflecting the need to break away from the ‘one-size-fits-all’ approach. Novel research exploring differences among diverse cell types is crucial to reveal how cytokinesis can be ‘personalized’, and to gain an appreciation of its diversity.
Author contributions
IO drafted the manuscript and prepared the figures, AP made critical revisions of the manuscript.
Funding
This work was funded by the Natural Sciences and Engineering Research Council of Canada (NSERC) [RGPIN-04161-2017] and [CREATE-511601-2018].
Acknowledgments
We apologize to colleagues whose valuable work could not be discussed due to space limitations.
Conflict of interest
The authors declare that the research was conducted in the absence of any commercial or financial relationships that could be construed as a potential conflict of interest.
Publisher’s note
All claims expressed in this article are solely those of the authors and do not necessarily represent those of their affiliated organizations, or those of the publisher, the editors and the reviewers. Any product that may be evaluated in this article, or claim that may be made by its manufacturer, is not guaranteed or endorsed by the publisher.
References
Adriaans, I. E., Basant, A., Ponsioen, B., Glotzer, M., and Lens, S. M. A. (2019). Plk1 plays dual roles in centralspindlin regulation during cytokinesis. J. Cell Biol. 218, 1250–1264. doi:10.1083/jcb.201805036
Bao, J., Jana, S. S., and Adelstein, R. S. (2005). Vertebrate nonmuscle myosin ii isoforms rescue small interfering RNA-induced defects in COS-7 cell cytokinesis. J. Biol. Chem. 280, 19594–19599. doi:10.1074/jbc.M501573200
Basant, A., and Glotzer, M. (2017). A GAP that Divides. F1000res 6, 1788. doi:10.12688/f1000research.12064.1
Basant, A., and Glotzer, M. (2018). Spatiotemporal regulation of Rhoa during Cytokinesis. Curr. Biol. 28, R570–R580. doi:10.1016/j.cub.2018.03.045
Basant, A., Lekomtsev, S., Tse, Y. C., Zhang, D., Longhini, K. M., Petronczki, M., et al. (2015). Aurora B kinase promotes cytokinesis by inducing centralspindlin oligomers that associate with the plasma membrane. Dev. Cell 33, 204–215. doi:10.1016/j.devcel.2015.03.015
Bastos, R. N., Penate, X., Bates, M., Hammond, D., and Barr, F. A. (2012). Cyk4 inhibits Rac1-dependent PAK1 and ARHGEF7 effector pathways during cytokinesis. J. Cell Biol. 198, 865–880. doi:10.1083/jcb.201204107
Beaudet, D., Akhshi, T., Phillipp, J., Law, C., and Piekny, A. (2017). Active Ran regulates anillin function during cytokinesis. Mol. Biol. Cell 28, 3517–3531. doi:10.1091/mbc.E17-04-0253
Beaudet, D., Pham, N., Skaik, N., and Piekny, A. (2020). Importin binding mediates the intramolecular regulation of anillin during cytokinesis. Mol. Biol. Cell 31, 1124–1139. doi:10.1091/mbc.E20-01-0006
Bell, K. R., Werner, M. E., Doshi, A., Cortes, D. B., Sattler, A., Vuong-Brender, T., et al. (2020). Novel cytokinetic ring components drive negative feedback in cortical contractility. Mol. Biol. Cell 31, 1623–1636. doi:10.1091/mbc.E20-05-0304
Bement, W. M., Benink, H. A., and Von Dassow, G. (2005). A microtubule-dependent zone of active Rhoa during cleavage plane specification. J. Cell Biol. 170, 91–101. doi:10.1083/jcb.200501131
Bourdages, K. G., Lacroix, B., Dorn, J. F., Descovich, C. P., and Maddox, A. S. (2014). Quantitative analysis of cytokinesis in situ during C. Elegans postembryonic development. Plos One 9, E110689. doi:10.1371/journal.pone.0110689
Buckley, C. E., and St Johnston, D. (2022). Apical-basal polarity and the control of epithelial form and function. Nat. Rev. Mol. Cell Biol. 23, 559–577. doi:10.1038/s41580-022-00465-y
Budnar, S., Husain, K. B., Gomez, G. A., Naghibosadat, M., Varma, A., Verma, S., et al. (2019). Anillin promotes cell contractility by cyclic resetting of Rhoa residence kinetics. Dev. Cell 49, 894–906. doi:10.1016/j.devcel.2019.04.031
Burdyniuk, M., Callegari, A., Mori, M., Nedelec, F., and Lenart, P. (2018). F-actin nucleated on chromosomes coordinates their capture by microtubules in oocyte meiosis. J. Cell Biol. 217, 2661–2674. doi:10.1083/jcb.201802080
Burkard, M. E., Randall, C. L., Larochelle, S., Zhang, C., Shokat, K. M., Fisher, R. P., et al. (2007). Chemical genetics reveals the requirement for Polo-like kinase 1 activity in positioning Rhoa and triggering cytokinesis in human cells. Proc. Natl. Acad. Sci. U. S. A. 104, 4383–4388. doi:10.1073/pnas.0701140104
Cabernard, C., Prehoda, K. E., and Doe, C. Q. (2010). A spindle-independent cleavage furrow positioning pathway. Nature 467, 91–94. doi:10.1038/nature09334
Canman, J. C., Lewellyn, L., Laband, K., Smerdon, S. J., Desai, A., Bowerman, B., et al. (2008). Inhibition of Rac by the GAP activity of centralspindlin is essential for cytokinesis. Science 322, 1543–1546. doi:10.1126/science.1163086
Carim, S. C., Kechad, A., and Hickson, G. R. X. (2020). Animal Cell Cytokinesis: The Rho-dependent Actomyosin-Anilloseptin Contractile ring as A membrane microdomain gathering, compressing, and sorting machine. Front. Cell Dev. Biol. 8, 575226. doi:10.3389/fcell.2020.575226
Carreno, S., Kouranti, I., Glusman, E. S., Fuller, M. T., Echard, A., and Payre, F. (2008). Moesin and its activating kinase Slik are required for cortical stability and microtubule organization in mitotic cells. J. Cell Biol. 180, 739–746. doi:10.1083/jcb.200709161
Carvalho, A., Desai, A., and Oegema, K. (2009). Structural memory in the contractile ring makes the duration of cytokinesis independent of cell size. Cell 137, 926–937. doi:10.1016/j.cell.2009.03.021
Chaigne, A., Smith, M. B., Lopez Cavestany, R., Hannezo, E., Chalut, K. J., and Paluch, E. K. (2021). Three-dimensional geometry controls division symmetry in stem cell colonies. J. Cell Sci. 134, jcs255018. doi:10.1242/jcs.255018
Chan, F. Y., Silva, A. M., Saramago, J., Pereira-Sousa, J., Brighton, H. E., Pereira, M., et al. (2019). The ARP2/3 complex prevents excessive formin activity during cytokinesis. Mol. Biol. Cell 30, 96–107. doi:10.1091/mbc.E18-07-0471
Chapa, Y. L. B., Hamanaka, M., Wray, A., Balasubramanian, M. K., and Mishima, M. (2020). Polar relaxation by dynein-mediated removal of cortical myosin ii. J. Cell Biol. 219, e201903080. doi:10.1083/jcb.201903080
Chen, A., Arora, P. D., Mcculloch, C. A., and Wilde, A. (2017). Cytokinesis requires localized beta-actin filament production by an actin isoform specific nucleator. Nat. Commun. 8, 1530. doi:10.1038/s41467-017-01231-x
Chen, A., Ulloa Severino, L., Panagiotou, T. C., Moraes, T. F., Yuen, D. A., Lavoie, B. D., et al. (2021). Inhibition of polar actin assembly by astral microtubules is required for cytokinesis. Nat. Commun. 12, 2409. doi:10.1038/s41467-021-22677-0
Clarke, P. R., and Zhang, C. (2008). Spatial and temporal coordination of mitosis by Ran GTPase. Nat. Rev. Mol. Cell Biol. 9, 464–477. doi:10.1038/nrm2410
Connell, M., Cabernard, C., Ricketson, D., Doe, C. Q., and Prehoda, K. E. (2011). Asymmetric cortical extension shifts cleavage furrow position in Drosophila neuroblasts. Mol. Biol. Cell 22, 4220–4226. doi:10.1091/mbc.E11-02-0173
Davies, T., Kim, H. X., Romano Spica, N., Lesea-Pringle, B. J., Dumont, J., Shirasu-Hiza, M., et al. (2018). Cell-intrinsic and -extrinsic mechanisms promote cell-type-specific cytokinetic diversity. Elife 7, e36204. doi:10.7554/eLife.36204
Dehapiot, B., Carriere, V., Carroll, J., and Halet, G. (2013). Polarized Cdc42 activation promotes polar body protrusion and asymmetric division in mouse oocytes. Dev. Biol. 377, 202–212. doi:10.1016/j.ydbio.2013.01.029
Delattre, M., and Goehring, N. W. (2021). The first steps in the life of A worm: Themes and variations in asymmetric division in C. Elegans and other nematodes. Curr. Top. Dev. Biol. 144, 269–308. doi:10.1016/bs.ctdb.2020.12.006
Deng, M., Suraneni, P., Schultz, R. M., and Li, R. (2007). The Ran GTPase mediates chromatin signaling to control cortical polarity during polar body extrusion in mouse oocytes. Dev. Cell 12, 301–308. doi:10.1016/j.devcel.2006.11.008
Ding, W. Y., Ong, H. T., Hara, Y., Wongsantichon, J., Toyama, Y., Robinson, R. C., et al. (2017). Plastin increases cortical connectivity to facilitate robust polarization and timely cytokinesis. J. Cell Biol. 216, 1371–1386. doi:10.1083/jcb.201603070
Dix, C. L., Matthews, H. K., Uroz, M., Mclaren, S., Wolf, L., Heatley, N., et al. (2018). The role of Mitotic Cell-Substrate Adhesion Re-Modeling in Animal Cell Division. Dev. Cell 45, 132–145. doi:10.1016/j.devcel.2018.03.009
Dorn, J. F., Zhang, L., Phi, T. T., Lacroix, B., Maddox, P. S., Liu, J., et al. (2016). A theoretical model of cytokinesis implicates feedback between membrane curvature and cytoskeletal organization in asymmetric cytokinetic furrowing. Mol. Biol. Cell 27, 1286–1299. doi:10.1091/mbc.E15-06-0374
Dugina, V., Zwaenepoel, I., Gabbiani, G., Clement, S., and Chaponnier, C. (2009). Beta and gamma-cytoplasmic actins display distinct distribution and functional diversity. J. Cell Sci. 122, 2980–2988. doi:10.1242/jcs.041970
Fotopoulos, N., Wernike, D., Chen, Y., Makil, N., Marte, A., and Piekny, A. (2013). Caenorhabditis elegans anillin (Ani-1) regulates neuroblast cytokinesis and epidermal morphogenesis during embryonic development. Dev. Biol. 383, 61–74. doi:10.1016/j.ydbio.2013.08.024
Frenette, P., Haines, E., Loloyan, M., Kinal, M., Pakarian, P., and Piekny, A. (2012). An anillin-Ect2 complex stabilizes central spindle microtubules at the cortex during cytokinesis. Plos One 7, E34888. doi:10.1371/journal.pone.0034888
Gomez-Cavazos, J. S., Lee, K. Y., Lara-Gonzalez, P., Li, Y., Desai, A., Shiau, A. K., et al. (2020). A non-canonical BRCT-Phosphopeptide recognition mechanism underlies Rhoa Activation in Cytokinesis. Curr. Biol. 30, 3101–3115. doi:10.1016/j.cub.2020.05.090
Green, R. A., Paluch, E., and Oegema, K. (2012). Cytokinesis in animal cells. Annu. Rev. Cell Dev. Biol. 28, 29–58. doi:10.1146/annurev-cellbio-101011-155718
Gupta, V. K., Nam, S., Yim, D., Camuglia, J., Martin, J. L., Sanders, E. N., et al. (2021). The nature of cell division forces in epithelial monolayers. J. Cell Biol. 220, e202011106. doi:10.1083/jcb.202011106
Hara, T., Abe, M., Inoue, H., Yu, L. R., Veenstra, T. D., Kang, Y. H., et al. (2006). Cytokinesis regulator ECT2 changes its conformation through phosphorylation at thr-341 in G2/M phase. Oncogene 25, 566–578. doi:10.1038/sj.onc.1209078
Herszterg, S., Pinheiro, D., and Bellaiche, Y. (2014). A multicellular view of cytokinesis in epithelial tissue. Trends Cell Biol. 24, 285–293. doi:10.1016/j.tcb.2013.11.009
Hickson, G. R., and O'farrell, P. H. (2008). Rho-dependent control of anillin behavior during cytokinesis. J. Cell Biol. 180, 285–294. doi:10.1083/jcb.200709005
Higashi, T., Arnold, T. R., Stephenson, R. E., Dinshaw, K. M., and Miller, A. L. (2016). Maintenance of the epithelial barrier and remodeling of cell-cell junctions during cytokinesis. Curr. Biol. 26, 1829–1842. doi:10.1016/j.cub.2016.05.036
Higashi, T., Stephenson, R. E., and Miller, A. L. (2019). Comprehensive analysis of formin localization in Xenopus epithelial cells. Mol. Biol. Cell 30, 82–95. doi:10.1091/mbc.E18-02-0133
Hinkle, B., Slepchenko, B., Rolls, M. M., Walther, T. C., Stein, P. A., Mehlmann, L. M., et al. (2002). Chromosomal association of ran during meiotic and mitotic divisions. J. Cell Sci. 115, 4685–4693. doi:10.1242/jcs.00136
Holopainen, S., Hytonen, M. K., Syrja, P., Arumilli, M., Jarvinen, A. K., Rajamaki, M., et al. (2017). ANLN truncation causes A familial fatal acute respiratory distress syndrome in Dalmatian dogs. PLoS Genet. 13, E1006625. doi:10.1371/journal.pgen.1006625
Jordan, S. N., Davies, T., Zhuravlev, Y., Dumont, J., Shirasu-Hiza, M., Canman, J. C., et al. (2016). Cortical PAR polarity proteins promote robust cytokinesis during asymmetric cell division. J. Cell Biol. 212 (1), 39–49.
Kalab, P., Pralle, A., Isacoff, E. Y., Heald, R., and Weis, K. (2006). Analysis of A RanGTP-regulated gradient in mitotic somatic cells. Nature 440, 697–701. doi:10.1038/nature04589
Kalab, P., Weis, K., and Heald, R. (2002). Visualization of A Ran-GTP gradient in interphase And mitotic Xenopus egg extracts. Science 295, 2452–2456. doi:10.1126/science.1068798
Khaliullin, R. N., Green, R. A., Shi, L. Z., Gomez-Cavazos, J. S., Berns, M. W., Desai, A., et al. (2018). A positive-feedback-based mechanism for constriction rate acceleration during cytokinesis in Caenorhabditis elegans. Elife 7, e36073. doi:10.7554/eLife.36073
Kiyomitsu, T., and Cheeseman, I. M. (2013). Cortical dynein and asymmetric membrane elongation coordinately position the spindle in anaphase. Cell 154, 391–402. doi:10.1016/j.cell.2013.06.010
Kotadia, S., Montembault, E., Sullivan, W., and Royou, A. (2012). Cell elongation is an adaptive response for clearing long chromatid arms from the cleavage plane. J. Cell Biol. 199, 745–753. doi:10.1083/jcb.201208041
Kucera, O., Siahaan, V., Janda, D., Dijkstra, S. H., Pilatova, E., Zatecka, E., et al. (2021). Anillin propels myosin-independent constriction of actin rings. Nat. Commun. 12, 4595. doi:10.1038/s41467-021-24474-1
Kunda, P., Pelling, A. E., Liu, T., and Baum, B. (2008). Moesin controls cortical rigidity, cell rounding, and spindle morphogenesis during mitosis. Curr. Biol. 18, 91–101. doi:10.1016/j.cub.2007.12.051
Kunda, P., Rodrigues, N. T., Moeendarbary, E., Liu, T., Ivetic, A., Charras, G., et al. (2012). PP1-mediated moesin dephosphorylation couples polar relaxation to mitotic exit. Curr. Biol. 22, 231–236. doi:10.1016/j.cub.2011.12.016
Lang, C. F., and Munro, E. (2017). The PAR proteins: From molecular circuits to dynamic self-stabilizing cell polarity. Development 144, 3405–3416. doi:10.1242/dev.139063
Lange, A., Mills, R. E., Lange, C. J., Stewart, M., Devine, S. E., and Corbett, A. H. (2007). Classical nuclear localization signals: Definition, function, and interaction with importin alpha. J. Biol. Chem. 282, 5101–5105. doi:10.1074/jbc.R600026200
Lara-Gonzalez, P., Pines, J., and Desai, A. (2021). Spindle assembly checkpoint Activation and silencing at kinetochores. Semin. Cell Dev. Biol. 117, 86–98. doi:10.1016/j.semcdb.2021.06.009
Lecuit, T. (2004). Junctions and vesicular trafficking during Drosophila cellularization. J. Cell Sci. 117, 3427–3433. doi:10.1242/jcs.01312
Leite, J., Chan, F. Y., Osorio, D. S., Saramago, J., Sobral, A. F., Silva, A. M., et al. (2020). Equatorial non-muscle myosin ii and plastin cooperate to align and compact F-actin bundles in the cytokinetic ring. Front. Cell Dev. Biol. 8, 573393. doi:10.3389/fcell.2020.573393
Leite, J., Osorio, D. S., Sobral, A. F., Silva, A. M., and Carvalho, A. X. (2019). Network contractility during cytokinesis-from molecular to global views. Biomolecules 9, E194. doi:10.3390/biom9050194
Lekomtsev, S., Su, K. C., Pye, V. E., Blight, K., Sundaramoorthy, S., Takaki, T., et al. (2012). Centralspindlin links the mitotic spindle to the plasma membrane during cytokinesis. Nature 492, 276–279. doi:10.1038/nature11773
Li, Y., and Munro, E. (2021). Filament-guided filament assembly provides structural memory of filament alignment during cytokinesis. Dev. Cell 56, 2486–2500 E6. doi:10.1016/j.devcel.2021.08.009
Loria, A., Longhini, K. M., and Glotzer, M. (2012). The RhoGAP domain of CYK-4 has an essential role in Rhoa activation. Curr. Biol. 22, 213–219. doi:10.1016/j.cub.2011.12.019
Ma, X., Kovacs, M., Conti, M. A., Wang, A., Zhang, Y., Sellers, J. R., et al. (2012). Nonmuscle myosin ii exerts tension but does not translocate actin in vertebrate cytokinesis. Proc. Natl. Acad. Sci. U. S. A. 109, 4509–4514. doi:10.1073/pnas.1116268109
Maddox, A. S., Habermann, B., Desai, A., and Oegema, K. (2005). Distinct roles for two C. Elegans anillins in the gonad and early embryo. Development 132, 2837–2848. doi:10.1242/dev.01828
Maddox, A. S., Lewellyn, L., Desai, A., and Oegema, K. (2007). Anillin and the septins promote asymmetric ingression of the cytokinetic furrow. Dev. Cell 12, 827–835. doi:10.1016/j.devcel.2007.02.018
Mangal, S., Sacher, J., Kim, T., Osorio, D. S., Motegi, F., Carvalho, A. X., et al. (2018). TPXl-1 activates Aurora A to clear contractile ring components from the polar cortex during cytokinesis. J. Cell Biol. 217, 837–848. doi:10.1083/jcb.201706021
Maupin, P., Phillips, C. L., Adelstein, R. S., and Pollard, T. D. (1994). Differential localization of myosin-ii isozymes in human cultured cells and blood cells. J. Cell Sci. 107 (11), 3077–3090. doi:10.1242/jcs.107.11.3077
Miller, A. L., and Bement, W. M. (2009). Regulation of cytokinesis by Rho GTPase flux. Nat. Cell Biol. 11, 71–77. doi:10.1038/ncb1814
Mishima, M., Kaitna, S., and Glotzer, M. (2002). Central spindle assembly and cytokinesis require A kinesin-like protein/RhoGAP complex with microtubule bundling activity.
Montembault, E., Claverie, M. C., Bouit, L., Landmann, C., Jenkins, J., Tsankova, A., et al. (2017). Myosin efflux promotes cell elongation to coordinate chromosome segregation with cell cleavage. Nat. Commun. 8, 326. doi:10.1038/s41467-017-00337-6
Musacchio, A., and Desai, A. (2017). A molecular view of kinetochore assembly and function. Biology (Basel), 6.
Navarro, A. P., and Cheeseman, I. M. (2021). Kinetochore assembly throughout the cell cycle. Semin. Cell Dev. Biol. 117, 62–74. doi:10.1016/j.semcdb.2021.03.008
Nguyen, L. T. S., and Robinson, D. N. (2020). The unusual suspects in cytokinesis: Fitting the pieces together. Front. Cell Dev. Biol. 8, 441. doi:10.3389/fcell.2020.00441
Niiya, F., Tatsumoto, T., Lee, K. S., and Miki, T. (2006). Phosphorylation of the cytokinesis regulator ECT2 at G2/M phase stimulates association of the mitotic kinase PLK1 and accumulation of GTP-bound Rhoa. Oncogene 25, 827–837. doi:10.1038/sj.onc.1209124
Osorio, D. S., Chan, F. Y., Saramago, J., Leite, J., Silva, A. M., Sobral, A. F., et al. (2019). Crosslinking activity of non-muscle myosin II is not sufficient for embryonic cytokinesis. C. Elegans. Development, 146, dev179150. doi:10.1242/dev.179150
Osswald, M., and Morais-De-Sa, E. (2019). Dealing with apical-basal polarity and intercellular junctions: A multidimensional challenge for epithelial cell division. Curr. Opin. Cell Biol. 60, 75–83. doi:10.1016/j.ceb.2019.04.006
Ozugergin, I., Mastronardi, K., Law, C., and Piekny, A. (2022). Diverse mechanisms regulate contractile ring assembly for cytokinesis in the two-cell Caenorhabditis elegans embryo. J. Cell Sci. 135, jcs258921. doi:10.1242/jcs.258921
Ozugergin, I., and Piekny, A. (2021). Complementary functions for the Ran gradient during division. Small Gtpases 12, 177–187. doi:10.1080/21541248.2020.1725371
Paim, L. M. G., and Fitzharris, G. (2022). Cell size and polarization determine cytokinesis furrow ingression dynamics in mouse embryos. Proc. Natl. Acad. Sci. U. S. A. 119, E2119381119. doi:10.1073/pnas.2119381119
Peterman, E., Valius, M., and Prekeris, R. (2020). CLIC4 is A cytokinetic cleavage furrow protein that regulates cortical cytoskeleton stability during cell division. J. Cell Sci. 133, jcs241117. doi:10.1242/jcs.241117
Petronczki, M., Glotzer, M., Kraut, N., and Peters, J. M. (2007). Polo-like kinase 1 triggers the initiation of cytokinesis in human cells by promoting recruitment of the RhoGEF Ect2 to the central spindle. Dev. Cell 12, 713–725. doi:10.1016/j.devcel.2007.03.013
Piekny, A. J., and Glotzer, M. (2008). Anillin is A scaffold protein that links Rhoa, actin, and myosin during cytokinesis. Curr. Biol. 18, 30–36. doi:10.1016/j.cub.2007.11.068
Piekny, A. J., and Maddox, A. S. (2010). The myriad roles of anillin during cytokinesis. Semin. Cell Dev. Biol. 21, 881–891. doi:10.1016/j.semcdb.2010.08.002
Piekny, A., Werner, M., and Glotzer, M. (2005). Cytokinesis: Welcome to the Rho zone. Trends Cell Biol. 15, 651–658. doi:10.1016/j.tcb.2005.10.006
Pinheiro, D., Hannezo, E., Herszterg, S., Bosveld, F., Gaugue, I., Balakireva, M., et al. (2017). Transmission of cytokinesis forces via E-cadherin dilution and actomyosin flows. Nature 545, 103–107. doi:10.1038/nature22041
Pintard, L., and Bowerman, B. (2019). Mitotic cell division in Caenorhabditis elegans. Genetics 211, 35–73. doi:10.1534/genetics.118.301367
Pittman, K. J., and Skop, A. R. (2012). Anterior par proteins function during cytokinesis and maintain dYN-1 at the cleavage furrow in Caenorhabditis elegans. Cytoskelet. Hob. 69, 826–839. doi:10.1002/cm.21053
Po'uha, S. T., and Kavallaris, M. (2015). Gamma-actin is involved in regulating centrosome function and mitotic progression in cancer cells. Cell Cycle 14, 3908–3919. doi:10.1080/15384101.2015.1120920
Pollard, T. D., and O'shaughnessy, B. (2019). Molecular Mechanism of Cytokinesis. Annu. Rev. Biochem. 88, 661–689. doi:10.1146/annurev-biochem-062917-012530
Prokopenko, S. N., Brumby, A., O'keefe, L., Prior, L., He, Y., Saint, R., et al. (1999). A putative exchange factor for Rho1 GTPase is required for initiation of cytokinesis in Drosophila. Genes Dev. 13, 2301–2314. doi:10.1101/gad.13.17.2301
Rappaport, R. (1986). Establishment of the mechanism of cytokinesis in animal cells. Int. Rev. Cytol. 105, 245–281. doi:10.1016/s0074-7696(08)61065-7
Rehain-Bell, K., Love, A., Werner, M. E., Macleod, I., Yates, J. R., and Maddox, A. S. (2017). A sterile 20 family kinase and its Co-factor CCM-3 regulate contractile ring proteins on germline intercellular bridges. Curr. Biol. 27, 860–867. doi:10.1016/j.cub.2017.01.058
Reyes, C. C., Jin, M., Breznau, E. B., Espino, R., Delgado-Gonzalo, R., Goryachev, A. B., et al. (2014). Anillin regulates cell-cell junction integrity by organizing junctional accumulation of Rho-GTP and actomyosin. Curr. Biol. 24, 1263–1270. doi:10.1016/j.cub.2014.04.021
Reymann, A. C., Staniscia, F., Erzberger, A., Salbreux, G., and Grill, S. W. (2016). Cortical flow aligns actin filaments to form A furrow. Elife 5, e17807. doi:10.7554/eLife.17807
Rodrigues, N. T., Lekomtsev, S., Jananji, S., Kriston-Vizi, J., Hickson, G. R., and Baum, B. (2015). Kinetochore-localized PP1-Sds22 couples chromosome segregation to polar relaxation. Nature 524, 489–492. doi:10.1038/nature14496
Rose, L., and Gonczy, P. (2014). Polarity establishment, asymmetric division and segregation of fate determinants in early C. Elegans embryos. Pasadena, CA: Wormbook, 1–43.
Roubinet, C., Decelle, B., Chicanne, G., Dorn, J. F., Payrastre, B., Payre, F., et al. (2011). Molecular networks linked by Moesin drive remodeling of the cell cortex during mitosis. J. Cell Biol. 195, 99–112. doi:10.1083/jcb.201106048
Schenk, C., Bringmann, H., Hyman, A. A., and Cowan, C. R. (2010). Cortical domain correction repositions the polarity boundary to match the cytokinesis furrow in C. Elegans embryos. Development 137, 1743–1753. doi:10.1242/dev.040436
Sedzinski, J., Biro, M., Oswald, A., Tinevez, J. Y., Salbreux, G., and Paluch, E. (2011). Polar actomyosin contractility destabilizes the position of the cytokinetic furrow. Nature 476, 462–466. doi:10.1038/nature10286
Shagieva, G. S., Alieva, I. B., Chaponnier, C., and Dugina, V. B. (2020). Divergent impact of actin isoforms on division of epithelial cells. Biochemistry. 85, 1072–1063. doi:10.1134/S0006297920090072
Silverman-Gavrila, R. V., Hales, K. G., and Wilde, A. (2008). Anillin-mediated targeting of Peanut to pseudocleavage furrows is regulated by the gtpase ran. Mol. Biol. Cell 19, 3735–3744. doi:10.1091/mbc.e08-01-0049
Singh, D., and Pohl, C. (2014). Coupling of rotational cortical flow, asymmetric midbody positioning, and spindle rotation mediates dorsoventral Axis formation in C. Elegans. Dev. Cell 28, 253–267. doi:10.1016/j.devcel.2014.01.002
Sobral, A. F., Chan, F. Y., Norman, M. J., Osorio, D. S., Dias, A. B., Ferreira, V., et al. (2021). Plastin and spectrin cooperate to stabilize the actomyosin cortex during cytokinesis. Curr. Biol. 31, 5415–5428 E10. doi:10.1016/j.cub.2021.09.055
Somers, W. G., and Saint, R. (2003). A RhoGEF and Rho family GTPase-Activating protein complex links the contractile ring to cortical microtubules at the onset of cytokinesis. Dev. Cell 4, 29–39. doi:10.1016/s1534-5807(02)00402-1
Spira, F., Cuylen-Haering, S., Mehta, S., Samwer, M., Reversat, A., Verma, A., et al. (2017). Cytokinesis in vertebrate cells initiates by contraction of an equatorial actomyosin network composed of randomly oriented filaments. Elife 6, e30867. doi:10.7554/eLife.30867
Steigemann, P., Wurzenberger, C., Schmitz, M. H., Held, M., Guizetti, J., Maar, S., et al. (2009). Aurora B-mediated abscission checkpoint protects against tetraploidization. Cell 136, 473–484. doi:10.1016/j.cell.2008.12.020
Straight, A. F., Field, C. M., and Mitchison, T. J. (2005). Anillin binds nonmuscle myosin II and regulates the contractile ring. Mol. Biol. Cell 16, 193–201. doi:10.1091/mbc.e04-08-0758
Su, K. C., Takaki, T., and Petronczki, M. (2011). Targeting of the RhoGEF Ect2 to the equatorial membrane controls cleavage furrow formation during cytokinesis. Dev. Cell 21, 1104–1115. doi:10.1016/j.devcel.2011.11.003
Sugioka, K. (2022). Symmetry-breaking of animal cytokinesis. Semin. Cell Dev. Biol. 127, 100–109. doi:10.1016/j.semcdb.2021.12.008
Taneja, N., Bersi, M. R., Baillargeon, S. M., Fenix, A. M., Cooper, J. A., Ohi, R., et al. (2020). Precise tuning of cortical contractility regulates cell shape during cytokinesis. Cell Rep. 31, 107477. doi:10.1016/j.celrep.2020.03.041
Tatsumoto, T., Xie, X., Blumenthal, R., Okamoto, I., and Miki, T. (1999). Human ECT2 is an exchange factor for Rho GTPases, phosphorylated in G2/M phases, and involved in cytokinesis. J. Cell Biol. 147, 921–928. doi:10.1083/jcb.147.5.921
Tse, Y. C., Piekny, A., and Glotzer, M. (2011). Anillin promotes astral microtubule-directed cortical myosin polarization. Mol. Biol. Cell 22, 3165–3175. doi:10.1091/mbc.E11-05-0399
Turlier, H., Audoly, B., Prost, J., and Joanny, J. F. (2014). Furrow constriction in animal cell cytokinesis. Biophys. J. 106, 114–123. doi:10.1016/j.bpj.2013.11.014
Uretmen Kagiali, Z. C., Saner, N., Akdag, M., Sanal, E., Degirmenci, B. S., Mollaoglu, G., et al. (2020). CLIC4 and CLIC1 bridge plasma membrane and cortical actin network for A successful cytokinesis. Life Sci. Alliance 3.
Van Oostende Triplet, C., Jaramillo Garcia, M., Haji Bik, H., Beaudet, D., and Piekny, A. (2014). Anillin interacts with microtubules and is Part Of the astral pathway that defines cortical domains. J. Cell Sci. 127, 3699–3710. doi:10.1242/jcs.147504
Verma, V., and Maresca, T. J. (2019). Microtubule plus-ends act as physical signaling hubs to activate RhoA during cytokinesis. Elife 8, e38968. doi:10.7554/eLife.38968
Wang, K., Okada, H., and Bi, E. (2020). Comparative analysis of the roles of non-muscle myosin-IIs in cytokinesis in budding yeast, fission yeast, and mammalian cells. Front. Cell Dev. Biol. 8, 593400. doi:10.3389/fcell.2020.593400
Wolfe, B. A., Takaki, T., Petronczki, M., and Glotzer, M. (2009). Polo-like kinase 1 directs assembly of the HsCyk-4 RhoGAP/Ect2 RhoGEF complex to initiate cleavage furrow formation. PLoS Biol. 7, E1000110. doi:10.1371/journal.pbio.1000110
Xu, L., and Massague, J. (2004). Nucleocytoplasmic shuttling of signal transducers. Nat. Rev. Mol. Cell Biol. 5, 209–219. doi:10.1038/nrm1331
Yamamoto, K., Otomo, K., Nemoto, T., Ishihara, S., Haga, H., Nagasaki, A., et al. (2019). Differential contributions of nonmuscle myosin IIA and IIB to cytokinesis in human immortalized fibroblasts. Exp. Cell Res. 376, 67–76. doi:10.1016/j.yexcr.2019.01.020
Yi, K., Unruh, J. R., Deng, M., Slaughter, B. D., Rubinstein, B., and Li, R. (2011). Dynamic maintenance of asymmetric meiotic spindle position through Arp2/3-complex-driven cytoplasmic streaming in mouse oocytes. Nat. Cell Biol. 13, 1252–1258. doi:10.1038/ncb2320
Yuce, O., Piekny, A., and Glotzer, M. (2005). An ECT2-centralspindlin complex regulates the localization and function of RhoA. J. Cell Biol. 170, 571–582. doi:10.1083/jcb.200501097
Zanin, E., Desai, A., Poser, I., Toyoda, Y., Andree, C., Moebius, C., et al. (2013). A conserved RhoGAP limits M phase contractility and coordinates with microtubule asters to confine RhoA during cytokinesis. Dev. Cell 26, 496–510. doi:10.1016/j.devcel.2013.08.005
Zhao, W. M., and Fang, G. (2005). MgcRacGAP controls the assembly of the contractile ring and the initiation of cytokinesis. Proc. Natl. Acad. Sci. U. S. A. 102, 13158–13163. doi:10.1073/pnas.0504145102
Keywords: mitosis, cytokinesis, RhoA, actomyosin, mitotic spindle, chromatin
Citation: Ozugergin I and Piekny A (2022) Diversity is the spice of life: An overview of how cytokinesis regulation varies with cell type. Front. Cell Dev. Biol. 10:1007614. doi: 10.3389/fcell.2022.1007614
Received: 30 July 2022; Accepted: 24 October 2022;
Published: 07 November 2022.
Edited by:
Paola Vagnarelli, Brunel University London, United KingdomReviewed by:
Gang Zhang, Qingdao University, ChinaAna Xavier De Carvalho, Universidade do Porto, Portugal
Copyright © 2022 Ozugergin and Piekny. This is an open-access article distributed under the terms of the Creative Commons Attribution License (CC BY). The use, distribution or reproduction in other forums is permitted, provided the original author(s) and the copyright owner(s) are credited and that the original publication in this journal is cited, in accordance with accepted academic practice. No use, distribution or reproduction is permitted which does not comply with these terms.
*Correspondence: Alisa Piekny, YWxpc2EucGlla255QGNvbmNvcmRpYS5jYQ==