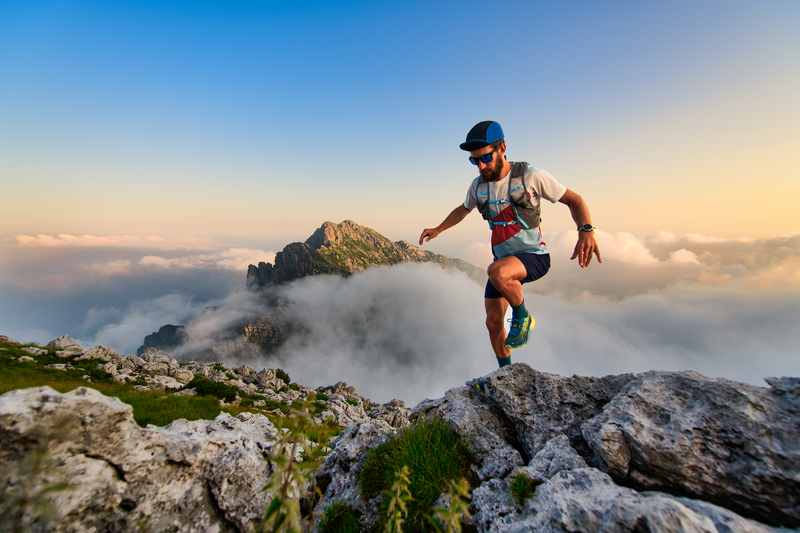
94% of researchers rate our articles as excellent or good
Learn more about the work of our research integrity team to safeguard the quality of each article we publish.
Find out more
PERSPECTIVE article
Front. Cell Dev. Biol. , 28 September 2022
Sec. Cellular Biochemistry
Volume 10 - 2022 | https://doi.org/10.3389/fcell.2022.1003030
This article is part of the Research Topic Healthy Adipose Tissue Expansion View all 7 articles
It is believed that insulin regulates metabolic functions of white adipose tissue primarily at the post-translational level via the PI3K-Akt-mediated pathway. Still, changes in transcription also play an important role in the response of white adipocytes to insulin and environmental signals. One transcription factor that is dramatically and rapidly induced in adipocytes by insulin and nutrients is called Early Growth Response 1, or Egr1. Among other functions, it directly binds to promoters of leptin and ATGL stimulating the former and inhibiting the latter. Furthermore, expression of Egr1 in adipocytes demonstrates cell autonomous circadian pattern suggesting that Egr1 not only mediates the effect of insulin and nutrients on lipolysis and leptin production but also, coordinates insulin action with endogenous circadian rhythms of adipose tissue.
White adipose tissue (WAT) plays a key role in mammalian physiology and pathophysiology (Sakers et al., 2022). There are at least three highly important metabolic functions in the body that are attributed primarily (but not exclusively) to WAT: dynamic storage of triglycerides (TG), secretion of adipokines, such as leptin, and regulated glucose uptake. Each of these functions is controlled by insulin at the level of transcription and translation as well as by post-translational mechanisms (Kandror, 2015; Grabner et al., 2021). Although glucose transporter four mediated glucose uptake is believed to be up-regulated by insulin exclusively at a post-translational level (Calejman et al., 2022), preservation of the low level of glucose uptake in basal adipocytes not treated with insulin requires continuous RNA- and protein biosynthesis de novo (Meriin et al., in press). The details of the transcriptional control of glucose homeostasis in adipocytes are not yet known; however, it has been established that effects of insulin on lipolysis and leptin expression are mediated at least in part, by Early Growth Response transcription factor, Egr1.
Egr1 (a.k.a. NGFI-A, Zif268, TIS8, and Krox24) is a zinc finger transcription factor that belongs to the family of primary response genes (Fowler et al., 2011). Like other members of this family, Egr1 participates in growth control, differentiation, and cancer progression (Thiel et al., 2010; Pagel and Deindl, 2011; Wang et al., 2021). The role of Egr1 in regulation of metabolism remains poorly explored and will be the focus of this review.
Basal adipocytes maintain low, almost undetectable levels of Egr1. Treatment of adipocytes with insulin causes a dramatic induction of both Egr1 mRNA (Alexander-Bridges et al., 1992; Sartipy and Loskutoff, 2003) and protein (Chakrabarti et al., 2013; Mohtar et al., 2019) both in vivo and in vitro. Incubation of cultured adipocytes with glucose (Supplementary Image S1) or high fat feeding of mice in vivo (Chakrabarti et al., 2013) also elevate expression of Egr1.
Similar to other primary response genes (Fowler et al., 2011), expression of Egr1 in various cell types is regulated at the level of transcription (Thiel and Cibelli, 2002; Thiel et al., 2010; Pagel and Deindl, 2011). In adipocytes, the effect of insulin on the Egr1 mRNA is mediated by Erk (Singh et al., 2015). Interestingly, this increase in Egr1 mRNA contributes relatively little to insulin-triggered up-regulation of the Egr1 protein in adipocytes. The latter takes place primarily at the level of translation via the mTORC1-4E-BP-mediated axis (Singh et al., 2015) and depends on the highly structured 5′-UTR of the Egr1 mRNA. To this end, we have deleted the 5′-UTR of the Egr1 mRNA using the CRISPR/Cas9 technique. This procedure alone brings up expression of the Egr1 protein to the maximum, so that insulin does not have any additional stimulatory effect (Mohtar et al., 2019).
Expression of the Egr1 protein reaches its maximum after 1 h of insulin stimulation and goes back to the basal level after approximately 4 h (Mohtar et al., 2019). Still, insulin does not significantly stimulate degradation of Egr1 (Supplementary Figure S2) and its rapid decline is most likely explained by inherent instability of the Egr1 mRNA (Singh et al., 2015) and protein.
In mammalian organism, most energy is stored in adipose tissue in a form of TG in distinct intracellular organelles called lipid droplets (LDs). Upon TG hydrolysis, FA are circulated in the blood to cells and tissues where they are taken up and used for energy production and synthesis of complex lipids. Despite their fundamental physiological importance, an oversupply of FA is highly detrimental as it causes abnormal lipid partitioning and lipotoxicity which in turn, impairs membrane function, induces ER stress, mitochondrial dysfunction, inflammation, cell death, insulin resistance, and other metabolic disease (Unger et al., 2010). The fine balance between healthy and unhealthy levels of circulating FA is maintained via a tight control of lipolysis coordinated with food intake. Thus, an increase in circulating levels of FA after food intake is normally compensated by insulin-mediated suppression of lipolysis in adipose tissue. This may be crucial for at least two reasons. First, dietary FAs in combination with those FAs produced endogenously by lipolysis may overcome all existing defense mechanisms of the body and impose a significant nutritional stress on cells and tissues leading to lipotoxicity and metabolic disease. Second, arrest of lipolysis when nutrients are abundant protects valuable fat reserves from unnecessary depletion. Failure of insulin to restrain lipolysis is a serious metabolic defect that leads to T2D and other health problems (McGarry, 1992; McGarry, 2002).
Complete lipolysis, i.e., hydrolysis of TG to glycerol and FA, is performed jointly by tri-, di-, and monoacylglyceride lipases (Grabner et al., 2021). The rate-limiting lipolytic enzyme, ATGL, is responsible for the bulk of triacylglycerol hydrolase activity in various cells. In other words, in every experimental model tested thus far, elevated ATGL expression increases, while attenuated ATGL expression decreases, both basal and cAMP-stimulated lipolysis (Jenkins et al., 2004; Villena et al., 2004; Zimmermann et al., 2004; Gronke et al., 2005; Haemmerle et al., 2006; Kershaw et al., 2006; Kurat et al., 2006; Smirnova et al., 2006; Miyoshi et al., 2008; Bezaire et al., 2009; Chakrabarti et al., 2010). ATGL has low affinity for di- and monoacylglycerides (Grabner et al., 2021). The major diacylglyceride lipase in adipocytes is hormone-sensitive lipase, or HSL and monoacylglyceride products of HSL are hydrolyzed by monoacylglyceride lipase (Grabner et al., 2021).
According to current views, lipolysis is regulated by catecholamines primarily at the post-translational level with the cAMP/cGMP-mediated signaling pathways playing the key role in this process. Briefly, phosphorylation of the lipid droplet protein perilipin and HSL by PKA and/or PKG leads to the recruitment of HSL to lipid droplets and activation of the enzyme. At the same time, a protein co-factor of ATGL, Abhd5 (a.k.a. CGI-58) dissociates from phosphorylated perilipin and activates ATGL (Lafontan and Langin, 2009; Grabner et al., 2021). Jointly, both processes rapidly and significantly stimulate lipolysis. On the contrary, insulin inhibits lipolysis and promotes accumulation of TG. Within this model, the effect of insulin is attributed primarily to the inhibition of cAMP-mediated signaling via Akt-dependent (Kitamura et al., 1999; Duncan et al., 2007) and independent (Choi et al., 2010) mechanisms.
In addition, regulation of lipolysis in vivo by such physiological stimuli as feeding, fasting, hypoxia, and physical exercise is accompanied and likely mediated by changes in the ATGL expression (Fortier et al., 2004; Villena et al., 2004; Lake et al., 2005; Kershaw et al., 2006; Kim et al., 2006; Alsted et al., 2009; Nielsen et al., 2011; Han et al., 2019). In particular, Supplementary Figure S3 shows that insulin rapidly and completely shuts ATGL transcription in cultured adipocytes. Expression of the ATGL protein follows the levels of cognate mRNA suggesting that expression of ATGL is controlled primarily at the level of transcription.
Thus, not only post-translational regulation of the enzymatic activity but also, precise control of the ATGL transcription defines the rates of lipolysis and FA homeostasis. However, unlike post-translational regulation that has been studied in much detail, little has been known about regulation of ATGL expression.
To this end, we have initiated a search for the pathways that regulate transcription of ATGL by nutrients and insulin. We have found two pathways: the Egr1-mediated pathway that inhibits lipolysis by decreasing transcription of ATGL (Chakrabarti et al., 2010; Chakrabarti et al., 2013; Singh et al., 2014) and the Sirt1/FoxO1-mediated pathway that activates lipolysis by increasing transcription of ATGL (Chakrabarti and Kandror, 2009; Chakrabarti et al., 2011; Jung et al., 2019). Both Egr1 (but not its close relative, Egr2) and FoxO1 directly bind to the ATGL promoter with different outcomes: Egr1 inhibits while FoxO1 stimulates its activity (Chakrabarti and Kandror, 2009; Chakrabarti et al., 2013; Singh et al., 2015) leading to corresponding changes in the ATGL expression and lipolysis. Importantly, regulation of ATGL expression by Egr1 is conserved in evolution from yeast to mammals and thus should be essential for metabolic control (Chakrabarti et al., 2013).
Expression of ATGL can be regulated by other transcription factors as well. Thus, early experiments have demonstrated that expression of ATGL is stimulated by PPARγ (Kim et al., 2006; Kershaw et al., 2007). Furthermore, interferon regulatory factor 4 induced in adipocytes by starvation via FoxO1 up-regulates transcription of ATGL (Eguchi et al., 2011), while insulin-induced transcription factor Snail1 suppresses its transcription (Sun et al., 2016).
Apparently, transcriptional control of lipolysis works on a different time scale, than the previously established mechanism of the short-term insulin action by inhibition of cAMP-mediated signaling to HSL and perilipin. The first one takes 4–6 h while the latter occurs within minutes. Both types of regulation seem essential for the physiological control of circulating FA.
Leptin, a 16 kDa product of the ob gene (Zhang et al., 1994), is synthesized predominantly in adipocytes and targets the central nervous system. It has been established as a major metabolic regulator that controls food intake, energy expenditure, neuroendocrine functions, carbohydrate and lipid metabolism, and several other important physiological functions of the mammalian organism (Ahima and Flier, 2000; Friedman, 2009; Dalamaga et al., 2013; Zeng et al., 2015). The discovery of leptin over two decades ago has completely changed the landscape of metabolic research and opened a new era in obesity studies.
Regardless of how leptin exerts its biological activity, it is essential that leptin production in adipocytes is coupled to nutrient uptake and energy status of the body. As circulating leptin and insulin levels increase after feeding and decrease after food deprivation (Frederich et al., 1995; Levy et al., 1997; Ahima and Flier, 2000), the predominant hypothesis in the field has been that leptin expression is controlled by insulin. Indeed, multiple studies have shown that insulin increases leptin production by adipose cells both in vivo and in vitro (Ahima and Flier, 2000). Although this regulatory connection is central to all proposed mechanisms of leptin action, its mechanism has remained unknown. Recently, we have found that insulin and nutrients activate leptin transcription in adipocytes via the same mTORC1-Egr1 axis that plays the central role in downregulation of ATGL (Mohtar et al., 2019). Very briefly, Egr1 directly interacts not only with the ATGL promoter (see above) but also, with the leptin promoter, suppressing the former and activating the latter. This mechanism may explain the long-known connection between food intake and circulating leptin (Frederich et al., 1995; Levy et al., 1997; Ahima and Flier, 2000).
Multiple lines of evidence demonstrate that insulin and nutrients control expression of leptin not exclusively at the level of transcription but also, at the level of translation, secretion, and even degradation (Lee and Fried, 2006; Lee and Fried, 2009; Kandror, 2015). It has been shown that mTORC1 plays a major role in the translation control of leptin (Roh et al., 2003; Lee and Fried, 2006), but its input into leptin secretion and degradation has not yet been studied.
In any case, a reverse regulation of ATGL and leptin by the same mTORC1-Egr1 axis may help to coordinate and even to synchronize changes in lipolysis (via ATGL) with food intake and energy expenditure (via leptin). Counter-regulation of leptin production and lipolysis may be maintained by other mechanisms as well. Thus, it has been demonstrated that hypoleptinemia may activate hypothalamic-pituitary-adrenal axis to promote lipolysis in fat (Perry et al., 2018).
Two polymorphisms in Egr1 have been associated with impaired lipid metabolism in humans (Brand et al., 2000), and several recent reports have confirmed that Egr1 is intimately involved in the regulation of lipid metabolism. Thus, in addition to the regulation of ATGL and leptin (Chakrabarti et al., 2013; Mohtar et al., 2019), Egr1 has been implicated in adipogenesis (Boyle et al., 2009) and browning of white adipocytes (Milet et al., 2017). Interestingly, Egr1 has a negative effect on adipose differentiation, while Egr2 is pro-adipogenic (Boyle et al., 2009). It has also been reported that Egr1 regulates insulin biosynthesis (Muller et al., 2012) and resistance (Shen et al., 2011), and cholesterol biosynthesis (Gokey et al., 2011). A comprehensive and balanced picture of all metabolic effects of Egr1 and Egr2 has yet to be established.
Circadian patterns of circulating free fatty acids in humans have been known for a long time (Schlierf and Raetzer, 1972); more recently, they have been attributed to oscillations of ATGL- and less so, HSL-mediated lipolysis in white adipose tissue (Shostak et al., 2013b). It has been also shown that in both diurnal (humans, monkeys) and nocturnal (rats, mice) animals food intake is regulated by circadian changes in plasma leptin levels (reviewed in (Froy and Garaulet, 2018). In line with these experiments, we have found that expression of ATGL and leptin oscillates in synchronized adipocytes cultured in serum-free media in the absence of any putative light or food entrainable oscillator (Figures 1A,B).
FIGURE 1. Cell autonomous oscillations in synchronized 3T3-L1 adipocytes. Cells were serum starved for 2 h, treated with 50% horse serum for 2 h, washed, and monitored for the next 48 h under normal culturing conditions without serum. Panels (A–D): levels of various mRNAs were determined by qPCR data in three independent experiments; mean values ± SE are shown. Panel (E): lysates of two biological replicates were analyzed by Western blotting; both are shown. The following antibodies from Cell Signaling Technology have been used: rabbit monoclonal antibody against Egr1 (Catalog #4153), mouse monoclonal antibody against β-Actin (Catalog #3700), rabbit monoclonal antibody against phospho-MEK1/2 (Catalog #9154), rabbit monoclonal antibody against MEK1/2 (Catalog #8727), rabbit monoclonal antibody against phospho-Erk1/2 (Catalog #4370), rabbit monoclonal antibody against Erk1/2 (Catalog # 4695), rabbit monoclonal antibody against phospho-S6 Kinase (Catalog # 9208), rabbit monoclonal antibody against S6 Kinase (Catalog # 2708).
In diverse organisms, the circadian clock coordinates metabolism with day/night cycles. The core mechanism of the mammalian clock consists of heteromeric transcription complex BMAL1:CLOCK that transcribes cryptochrome (Cry1 & Cry2) and period (Per1, Per2, and Per3) genes. CRYs and PERs heterodimerize, translocate to the nucleus, repress BMAL1:CLOCK transcriptional activity and undergo proteasomal degradation. This transcription-translation feedback loop takes about 24 h. In addition, BMAL1:CLOCK drive expression of REV-ERBα/β that inhibit transcription of Bmal1.
As is seen in Figure 1C, expression of Bmal1 and Per1 oscillates in synchronized cultured adipocytes in a cell autonomous fashion, and their phases are, as expected, reverse. Expression of Egr1 also demonstrates self-sustained circadian pattern in synchronized cultured 3T3-L1 adipocytes (Figure 1D). Noteworthy, circadian phases of Bmal1 and Per1, are different from that of Egr1. Clearly, there should be other factors that are responsible for the circadian rhythm of the Egr1 expression. To this end, various actinomycin D- and α-Amanitin-resistant (i.e., non-transcriptional) circadian clocks have been described by many research groups in the course of the years [reviewed in (Reddy and Rey, 2014)].
We have found that oscillations in the Egr1 mRNA and protein in synchronized adipocytes correlate with rhythmic changes in the activity of the MEK/Erk and mTORC1 pathways (Figure 1E). Indeed, there are distinct peaks in phosphorylation of MEK, Erk, and S6K1 at 6, 18, and 36–42 h that overlap with or slightly precede peaks of the Egr1 mRNA and protein. Since both MEK/Erk & mTORC1 pathways directly control expression of Egr1, a close correlation between these events may prove to have a causative connection. Furthermore, oscillations of the MEK/Erk and/or mTORC1 pathways may represent a totally novel type of an endogenous circadian regulator or may be linked to the autonomous cycling of the known “core” clock genes via an as yet unknown mechanism. In any case, understanding their molecular nature seems warranted.
Strong evidence supports the idea that BMAL1 contributes to the circadian pattern of the ATGL (Shostak et al., 2013b) and leptin (Paschos et al., 2012) expression in adipocytes. An interesting question is whether and to what extent Egr1 can also regulate insulin-independent circadian expression of its direct transcriptional targets, ATGL and leptin. Oscillation patterns of either leptin or ATGL mRNA do not apparently overlap with cycling of Egr1 or Bmal1. This is to be expected as both leptin and ATGL promoters are regulated by various transcription factors with their own cycling patterns, so the resulting picture may be complex. Most genes in various tissues demonstrate the same phenomenon (Fang et al., 2014; Shostak and Brunner, 2019), and its biological sense is not completely understood.
Importantly, both FA and leptin represent signaling molecules that work on hypothalamic neurons to regulate physiological rhythms of the whole organism (Ahima and Flier, 2000; Lam et al., 2005). Therefore, further studies of the cell autonomous biological clock in adipocytes that regulate expression of FA and leptin should have a global physiological significance.
As is pointed out in the previous section, both lipolysis and food intake are regulated by nutrients/insulin as well as by endogenous circadian rhythms. It is essential to inhibit lipolysis at the time of food abundance and to activate lipolysis upon fasting and to coordinate these responses with endogenous circadian rhythm that adapts the organism to cyclic changes of the environment.
Disruption of insulin (McGarry, 2002) and circadian (Shostak et al., 2013a; Froy and Garaulet, 2018; Kolbe et al., 2018; Lemmer and Oster, 2018; Pilorz et al., 2018) regulation of lipolysis and food intake is associated with obesity, insulin resistance, and metabolic diseases. Although a temporal misalignment of feeding time and circadian rhythms may be metabolically acceptable, there is no question that systemic ignoring and abuse of circadian rhythms disrupts metabolic homeostasis (Cederroth et al., 2019). At present, there is little understanding of the interplay between circadian rhythms and metabolic regulation. This question is directly related to human health and thus represents a high priority direction of research (Eckel-Mahan et al., 2013; Asher and Sassone-Corsi, 2015; Cederroth et al., 2019; Duglan and Lamia, 2019).
Since Egr1 responds not only to metabolic signals (i.e., nutrients and insulin) but also, to an endogenous circadian pacemaker in adipocytes, it is well suited to coordinate metabolic and circadian regulation of lipolysis, food intake, and energy expenditure (Figure 2). This robust system may have evolved to provide metabolic stability to the organism under unpredictable life conditions. For example, in the past, animals used to live in the same time zone, but food was scarce and its availability was random. Therefore, strong circadian regulation of Egr1 in both nocturnal and diurnal animals could play the primary role in the adjustment of their metabolism to dark/light cycles regardless of food supply.
FIGURE 2. In fat tissue, Egr1 receives regulatory inputs from nutrients/insulin and circadian regulator and maintains metabolic health and longevity by suppressing ATGL and activating leptin expression.
In modern humans the situation is reversed. Counter to our diurnal nature, we often work night shifts or travel through multiple time zones. At the same time, food has become more available, and we can take advantage of time-restricted feeding to correct negative metabolic consequences of disordered molecular clock (Vollmers et al., 2009; Hatori et al., 2012; Sherman et al., 2012; Chaix et al., 2014). Thus, dysregulation of the circadian pattern of Egr1 expression may be compensated by strengthening the nutrient-based regulatory axis (in particular, by time-restricted feeding) and vice versa.
The original contributions presented in the study are included in the article/Supplementary Materials, further inquiries can be directed to the corresponding author.
AM, NZ, and DR: Methodology, investigation, and editing. KK: Conceptualization, methodology, writing—reviewing, and editing.
This work was supported by research grant RO1DK52057 from the NIH to KK.
The authors declare that the research was conducted in the absence of any commercial or financial relationships that could be construed as a potential conflict of interest.
All claims expressed in this article are solely those of the authors and do not necessarily represent those of their affiliated organizations, or those of the publisher, the editors and the reviewers. Any product that may be evaluated in this article, or claim that may be made by its manufacturer, is not guaranteed or endorsed by the publisher.
The Supplementary Material for this article can be found online at: https://www.frontiersin.org/articles/10.3389/fcell.2022.1003030/full#supplementary-material
SUPPLEMENTARY FIGURE S1 | Incubation of cultured 3T3-L1 adipocytes with glucose increases expression of Egr1. Panel (A): Cells were incubated in the glucose-free medium for 2.5 h, then glucose (20 mM) or insulin (100 nM) were added for 1.5 h and the Egr1 mRNA was measured by RT-qPCR. Panel (B): Cells were incubated in the glucose-free medium for 6 h, then glucose (Glu, 20 mM) or insulin (Ins, 100 nM) were added for the indicated time, and total cell extracts were analyzed by Western blotting. Dotted lines indicate that irrelevant bands have been spliced out. The experiment was repeated twice. The following antibodies from Cell Signaling Technology have been used: rabbit monoclonal antibody against Egr1 (Catalog #4153), mouse monoclonal antibody against β-Actin (Catalog #3700).
SUPPLEMENTARY FIGURE S2 | Insulin does not stimulate degradation of Egr1. 3T3-L1 adipocytes were labeled with a mixture of [35S]-labeled cysteine and methionine for 1 h, after which the cells were harvested or chased with 2 mM of unlabeled amino acids in the absence and in the presence of 100 nM insulin for the indicated amounts of time. Panel (A): Egr1 was immunoprecipitated from cell lysates using rabbit monoclonal antibody against Egr1 (Cell Signaling Technology, Catalog #4153) or normal rabbit IgG (Cell Signaling Technology, Catalog #2729) (CON) and analyzed by SDS electrophoresis and autoradiography. Panel (B): The membrane shown in Panel (A) was further blotted with HRP-linked secondary anti-rabbit IgG (Cell Signaling Technology, Catalog #7074) for the IgG Heavy Chain to confirm that equal amounts of the anti-Egr1 antibodies were used in the experiment. Panel (C): Prior to immunoprecipitation, total cell extracts (TE) were analyzed by SDS electrophoresis and autoradiography.
SUPPLEMENTARY FIGURE S3 | The inhibitory effects of insulin and Actinomycin D on ATGL transcription in cultured 3T3-L1 adipocytes. Differentiated 3T3-L1 adipocytes were incubated with or without insulin (100 nM) and Actinomycin D (5 μM) for the indicated amounts of time. Panel (A): Atgl mRNA was measured by RT-qPCR in three independent experiments; mean values +/− SE are shown. Panel (B): Total cell extracts were analyzed by Western blotting with rabbit polyclonal antibody against ATGL (Cell Signaling Technology, Catalog #2138) and mouse monoclonal antibody against β-Actin (Cell Signaling Technology, Catalog #3700).
Ahima, R. S., and Flier, J. S. (2000). Leptin. Annu. Rev. Physiol. 62, 413–437. doi:10.1146/annurev.physiol.62.1.413
Alexander-Bridges, M., Buggs, C., Giere, L., Denaro, M., Kahn, B., White, M., et al. (1992). Models of insulin action on metabolic and growth response genes. Mol. Cell. Biochem. 109, 99–105. doi:10.1007/BF00229762
Alsted, T. J., Nybo, L., Schweiger, M., Fledelius, C., Jacobsen, P., Zimmermann, R., et al. (2009). Adipose triglyceride lipase in human skeletal muscle is upregulated by exercise training. Am. J. Physiol. Endocrinol. Metab. 296, E445–E453. doi:10.1152/ajpendo.90912.2008
Asher, G., and Sassone-Corsi, P. (2015). Time for food: The intimate interplay between nutrition, metabolism, and the circadian clock. Cell 161, 84–92. doi:10.1016/j.cell.2015.03.015
Bezaire, V., Mairal, A., Ribet, C., Lefort, C., Girousse, A., Jocken, J., et al. (2009). Contribution of adipose triglyceride lipase and hormone-sensitive lipase to lipolysis in hMADS adipocytes. J. Biol. Chem. 284, 18282–18291. doi:10.1074/jbc.M109.008631
Boyle, K. B., Hadaschik, D., Virtue, S., Cawthorn, W. P., Ridley, S. H., O'rahilly, S., et al. (2009). The transcription factors Egr1 and Egr2 have opposing influences on adipocyte differentiation. Cell Death Differ. 16, 782–789. doi:10.1038/cdd.2009.11
Brand, E., Herrmann, S. M., Nicaud, V., Evans, A., Ruidavets, J. B., Arveiler, D., et al. (2000). Identification of two polymorphisms in the early growth response protein-1 gene: Possible association with lipid variables. J. Mol. Med. 78, 81–86. doi:10.1007/s001090000074
Calejman, C. M., Doxsey, W. G., Fazakerley, D. J., and Guertin, D. A. (2022). Integrating adipocyte insulin signaling and metabolism in the multi-omics era. Trends biochem. Sci. 47, 531–546. doi:10.1016/j.tibs.2022.02.009
Cederroth, C. R., Albrecht, U., Bass, J., Brown, S. A., Dyhrfjeld-Johnsen, J., Gachon, F., et al. (2019). Medicine in the fourth dimension. Cell Metab. 30, 238–250. doi:10.1016/j.cmet.2019.06.019
Chaix, A., Zarrinpar, A., Miu, P., and Panda, S. (2014). Time-restricted feeding is a preventative and therapeutic intervention against diverse nutritional challenges. Cell Metab. 20, 991–1005. doi:10.1016/j.cmet.2014.11.001
Chakrabarti, P., English, T., Karki, S., Qiang, L., Tao, R., Kim, J., et al. (2011). SIRT1 controls lipolysis in adipocytes via FOXO1-mediated expression of ATGL. J. Lipid Res. 52, 1693–1701. doi:10.1194/jlr.M014647
Chakrabarti, P., English, T., Shi, J., Smas, C. M., and Kandror, K. V. (2010). Mammalian target of rapamycin complex 1 suppresses lipolysis, stimulates lipogenesis, and promotes fat storage. Diabetes 59, 775–781. doi:10.2337/db09-1602
Chakrabarti, P., and Kandror, K. V. (2009). FoxO1 controls insulin-dependent adipose triglyceride lipase (ATGL) expression and lipolysis in adipocytes. J. Biol. Chem. 284, 13296–13300. doi:10.1074/jbc.C800241200
Chakrabarti, P., Kim, J. Y., Singh, M., Shin, Y. K., Kim, J., Kumbrink, J., et al. (2013). Insulin inhibits lipolysis in adipocytes via the evolutionarily conserved mTORC1-Egr1-ATGL-mediated pathway. Mol. Cell. Biol. 33, 3659–3666. doi:10.1128/MCB.01584-12
Choi, S. M., Tucker, D. F., Gross, D. N., Easton, R. M., Dipilato, L. M., Dean, A. S., et al. (2010). Insulin regulates adipocyte lipolysis via an Akt-independent signaling pathway. Mol. Cell. Biol. 30, 5009–5020. doi:10.1128/MCB.00797-10
Dalamaga, M., Chou, S. H., Shields, K., Papageorgiou, P., Polyzos, S. A., and Mantzoros, C. S. (2013). Leptin at the intersection of neuroendocrinology and metabolism: Current evidence and therapeutic perspectives. Cell Metab. 18, 29–42. doi:10.1016/j.cmet.2013.05.010
Duglan, D., and Lamia, K. A. (2019). Clocking in, working out: Circadian regulation of exercise physiology. Trends Endocrinol. Metab. 30, 347–356. doi:10.1016/j.tem.2019.04.003
Duncan, R. E., Ahmadian, M., Jaworski, K., Sarkadi-Nagy, E., and Sul, H. S. (2007). Regulation of lipolysis in adipocytes. Annu. Rev. Nutr. 27, 79–101. doi:10.1146/annurev.nutr.27.061406.093734
Eckel-Mahan, K. L., Patel, V. R., De Mateo, S., Orozco-Solis, R., Ceglia, N. J., Sahar, S., et al. (2013). Reprogramming of the circadian clock by nutritional challenge. Cell 155, 1464–1478. doi:10.1016/j.cell.2013.11.034
Eguchi, J., Wang, X., Yu, S., Kershaw, E. E., Chiu, P. C., Dushay, J., et al. (2011). Transcriptional control of adipose lipid handling by IRF4. Cell Metab. 13, 249–259. doi:10.1016/j.cmet.2011.02.005
Fang, B., Everett, L. J., Jager, J., Briggs, E., Armour, S. M., Feng, D., et al. (2014). Circadian enhancers coordinate multiple phases of rhythmic gene transcription in vivo. Cell 159, 1140–1152. doi:10.1016/j.cell.2014.10.022
Fortier, M., Wang, S. P., Mauriege, P., Semache, M., Mfuma, L., Li, H., et al. (2004). Hormone-sensitive lipase-independent adipocyte lipolysis during beta-adrenergic stimulation, fasting, and dietary fat loading. Am. J. Physiol. Endocrinol. Metab. 287, E282–E288. doi:10.1152/ajpendo.00203.2003
Fowler, T., Sen, R., and Roy, A. L. (2011). Regulation of primary response genes. Mol. Cell 44, 348–360. doi:10.1016/j.molcel.2011.09.014
Frederich, R. C., Lollmann, B., Hamann, A., Napolitano-Rosen, A., Kahn, B. B., Lowell, B. B., et al. (1995). Expression of ob mRNA and its encoded protein in rodents. Impact of nutrition and obesity. J. Clin. Invest. 96, 1658–1663. doi:10.1172/JCI118206
Friedman, J. M. (2009). Leptin at 14 y of age: An ongoing story. Am. J. Clin. Nutr. 89, 973S–979S. doi:10.3945/ajcn.2008.26788B
Froy, O., and Garaulet, M. (2018). The circadian clock in white and Brown adipose tissue: Mechanistic, endocrine, and clinical aspects. Endocr. Rev. 39, 261–273. doi:10.1210/er.2017-00193
Gokey, N. G., Lopez-Anido, C., Gillian-Daniel, A. L., and Svaren, J. (2011). Early growth response 1 (egr1) regulates cholesterol biosynthetic gene expression. J. Biol. Chem. 286, 29501–29510. doi:10.1074/jbc.M111.263509
Grabner, G. F., Xie, H., Schweiger, M., and Zechner, R. (2021). Lipolysis: Cellular mechanisms for lipid mobilization from fat stores. Nat. Metab. 3, 1445–1465. doi:10.1038/s42255-021-00493-6
Gronke, S., Mildner, A., Fellert, S., Tennagels, N., Petry, S., Muller, G., et al. (2005). Brummer lipase is an evolutionary conserved fat storage regulator in Drosophila. Cell Metab. 1, 323–330. doi:10.1016/j.cmet.2005.04.003
Haemmerle, G., Lass, A., Zimmermann, R., Gorkiewicz, G., Meyer, C., Rozman, J., et al. (2006). Defective lipolysis and altered energy metabolism in mice lacking adipose triglyceride lipase. Science 312, 734–737. doi:10.1126/science.1123965
Han, J. S., Lee, J. H., Kong, J., Ji, Y., Kim, J., Choe, S. S., et al. (2019). Hypoxia restrains lipid utilization via protein kinase A and adipose triglyceride lipase downregulation through hypoxia-inducible factor. Mol. Cell. Biol. 39 (2), e00390–18. doi:10.1128/MCB.00390-18
Hatori, M., Vollmers, C., Zarrinpar, A., Ditacchio, L., Bushong, E. A., Gill, S., et al. (2012). Time-restricted feeding without reducing caloric intake prevents metabolic diseases in mice fed a high-fat diet. Cell Metab. 15, 848–860. doi:10.1016/j.cmet.2012.04.019
Jenkins, C. M., Mancuso, D. J., Yan, W., Sims, H. F., Gibson, B., and Gross, R. W. (2004). Identification, cloning, expression, and purification of three novel human calcium-independent phospholipase A2 family members possessing triacylglycerol lipase and acylglycerol transacylase activities. J. Biol. Chem. 279, 48968–48975. doi:10.1074/jbc.M407841200
Jung, S. M., Hung, C. M., Hildebrand, S. R., Sanchez-Gurmaches, J., Martinez-Pastor, B., Gengatharan, J. M., et al. (2019). Non-canonical mTORC2 signaling regulates Brown adipocyte lipid catabolism through SIRT6-FoxO1. Mol. Cell 75, 807–822. doi:10.1016/j.molcel.2019.07.023
Kandror, K. V. (2015). “Translational and post-translational control of leptin production by fat cells,” in Post-transcriptional mechanisms in endocrine regulation. Editors K. M. J. Menon, and Aaron C. Goldstrohm (Springer), 221–233.
Kershaw, E. E., Hamm, J. K., Verhagen, L. A., Peroni, O., Katic, M., and Flier, J. S. (2006). Adipose triglyceride lipase: Function, regulation by insulin, and comparison with adiponutrin. Diabetes 55, 148–157. doi:10.2337/diabetes.55.01.06.db05-0982
Kershaw, E. E., Schupp, M., Guan, H. P., Gardner, N. P., Lazar, M. A., and Flier, J. S. (2007). PPARgamma regulates adipose triglyceride lipase in adipocytes in vitro and in vivo. Am. J. Physiol. Endocrinol. Metab. 293, E1736–E1745. doi:10.1152/ajpendo.00122.2007
Kim, J. Y., Tillison, K., Lee, J. H., Rearick, D. A., and Smas, C. M. (2006). The adipose tissue triglyceride lipase ATGL/PNPLA2 is downregulated by insulin and TNF-alpha in 3T3-L1 adipocytes and is a target for transactivation by PPARgamma. Am. J. Physiol. Endocrinol. Metab. 291, E115–E127. doi:10.1152/ajpendo.00317.2005
Kitamura, T., Kitamura, Y., Kuroda, S., Hino, Y., Ando, M., Kotani, K., et al. (1999). Insulin-induced phosphorylation and activation of cyclic nucleotide phosphodiesterase 3B by the serine-threonine kinase Akt. Mol. Cell. Biol. 19, 6286–6296. doi:10.1128/mcb.19.9.6286
Kolbe, I., Brehm, N., and Oster, H. (2018). Interplay of central and peripheral circadian clocks in energy metabolism regulation. J. Neuroendocrinol. 31, e12659. doi:10.1111/jne.12659
Kurat, C. F., Natter, K., Petschnigg, J., Wolinski, H., Scheuringer, K., Scholz, H., et al. (2006). Obese yeast: Triglyceride lipolysis is functionally conserved from mammals to yeast. J. Biol. Chem. 281, 491–500. doi:10.1074/jbc.M508414200
Lafontan, M., and Langin, D. (2009). Lipolysis and lipid mobilization in human adipose tissue. Prog. Lipid Res. 48, 275–297. doi:10.1016/j.plipres.2009.05.001
Lake, A. C., Sun, Y., Li, J. L., Kim, J. E., Johnson, J. W., Li, D., et al. (2005). Expression, regulation, and triglyceride hydrolase activity of Adiponutrin family members. J. Lipid Res. 46, 2477–2487. doi:10.1194/jlr.M500290-JLR200
Lam, T. K., Pocai, A., Gutierrez-Juarez, R., Obici, S., Bryan, J., Aguilar-Bryan, L., et al. (2005). Hypothalamic sensing of circulating fatty acids is required for glucose homeostasis. Nat. Med. 11, 320–327. doi:10.1038/nm1201
Lee, M. J., and Fried, S. K. (2009). Integration of hormonal and nutrient signals that regulate leptin synthesis and secretion. Am. J. Physiol. Endocrinol. Metab. 296, E1230–E1238. doi:10.1152/ajpendo.90927.2008
Lee, M. J., and Fried, S. K. (2006). Multilevel regulation of leptin storage, turnover, and secretion by feeding and insulin in rat adipose tissue. J. Lipid Res. 47, 1984–1993. doi:10.1194/jlr.M600065-JLR200
Lemmer, B., and Oster, H. (2018). The role of circadian rhythms in the hypertension of diabetes mellitus and the metabolic syndrome. Curr. Hypertens. Rep. 20, 43. doi:10.1007/s11906-018-0843-5
Levy, J. R., Legall-Salmon, E., Santos, M., Pandak, W. M., and Stevens, W. (1997). Effect of enteral versus parenteral nutrition on leptin gene expression and release into the circulation. Biochem. Biophys. Res. Commun. 237, 98–102. doi:10.1006/bbrc.1997.7086
Mcgarry, J. D. (2002). Banting lecture 2001: Dysregulation of fatty acid metabolism in the etiology of type 2 diabetes. Diabetes 51, 7–18. doi:10.2337/diabetes.51.1.7
Mcgarry, J. D. (1992). What if minkowski had been ageusic? An alternative angle on diabetes. Science 258, 766–770. doi:10.1126/science.1439783
Milet, C., Bleher, M., Allbright, K., Orgeur, M., Coulpier, F., Duprez, D., et al. (2017). Egr1 deficiency induces browning of inguinal subcutaneous white adipose tissue in mice. Sci. Rep. 7, 16153. doi:10.1038/s41598-017-16543-7
Miyoshi, H., Perfield, J. W., Obin, M. S., and Greenberg, A. S. (2008). Adipose triglyceride lipase regulates basal lipolysis and lipid droplet size in adipocytes. J. Cell. Biochem. 105, 1430–1436. doi:10.1002/jcb.21964
Mohtar, O., Ozdemir, C., Roy, D., Shantaram, D., Emili, A., and Kandror, K. V. (2019). Egr1 mediates the effect of insulin on leptin transcription in adipocytes. J. Biol. Chem. 294, 5784–5789. doi:10.1074/jbc.AC119.007855
Muller, I., Rossler, O. G., Wittig, C., Menger, M. D., and Thiel, G. (2012). Critical role of Egr transcription factors in regulating insulin biosynthesis, blood glucose homeostasis, and islet size. Endocrinology 153, 3040–3053. doi:10.1210/en.2012-1064
Nielsen, T. S., Vendelbo, M. H., Jessen, N., Pedersen, S. B., Jorgensen, J. O., Lund, S., et al. (2011). Fasting, but not exercise, increases adipose triglyceride lipase (ATGL) protein and reduces G(0)/G(1) switch gene 2 (G0S2) protein and mRNA content in human adipose tissue. J. Clin. Endocrinol. Metab. 96, E1293–E1297. doi:10.1210/jc.2011-0149
Pagel, J. I., and Deindl, E. (2011). Early growth response 1--a transcription factor in the crossfire of signal transduction cascades. Indian J. biochem. Biophys. 48, 226–235.
Paschos, G. K., Ibrahim, S., Song, W. L., Kunieda, T., Grant, G., Reyes, T. M., et al. (2012). Obesity in mice with adipocyte-specific deletion of clock component Arntl. Nat. Med. 18, 1768–1777. doi:10.1038/nm.2979
Perry, R. J., Wang, Y., Cline, G. W., Rabin-Court, A., Song, J. D., Dufour, S., et al. (2018). Leptin mediates a glucose-fatty acid cycle to maintain glucose homeostasis in starvation. Cell 172, 234–248. doi:10.1016/j.cell.2017.12.001
Pilorz, V., Helfrich-Forster, C., and Oster, H. (2018). The role of the circadian clock system in physiology. Pflugers Arch. 470, 227–239. doi:10.1007/s00424-017-2103-y
Reddy, A. B., and Rey, G. (2014). Metabolic and nontranscriptional circadian clocks: Eukaryotes. Annu. Rev. Biochem. 83, 165–189. doi:10.1146/annurev-biochem-060713-035623
Roh, C., Han, J., Tzatsos, A., and Kandror, K. V. (2003). Nutrient-sensing mTOR-mediated pathway regulates leptin production in isolated rat adipocytes. Am. J. Physiol. Endocrinol. Metab. 284, E322–E330. doi:10.1152/ajpendo.00230.2002
Sakers, A., De Siqueira, M. K., Seale, P., and Villanueva, C. J. (2022). Adipose-tissue plasticity in health and disease. Cell 185, 419–446. doi:10.1016/j.cell.2021.12.016
Sartipy, P., and Loskutoff, D. J. (2003). Expression profiling identifies genes that continue to respond to insulin in adipocytes made insulin-resistant by treatment with tumor necrosis factor-alpha. J. Biol. Chem. 278, 52298–52306. doi:10.1074/jbc.M306922200
Schlierf, G., and Raetzer, H. (1972). Diurnal patterns of blood sugar, plasma insulin, free fatty acid and triglyceride levels in normal subjects and in patients with type IV hyperlipoproteinemia and the effect of meal frequency. Nutr. Metab. 14, 113–126. doi:10.1159/000175372
Shen, N., Yu, X., Pan, F. Y., Gao, X., Xue, B., and Li, C. J. (2011). An early response transcription factor, egr-1, enhances insulin resistance in type 2 diabetes with chronic hyperinsulinism. J. Biol. Chem. 286, 14508–14515. doi:10.1074/jbc.M110.190165
Sherman, H., Genzer, Y., Cohen, R., Chapnik, N., Madar, Z., and Froy, O. (2012). Timed high-fat diet resets circadian metabolism and prevents obesity. FASEB J. 26, 3493–3502. doi:10.1096/fj.12-208868
Shostak, A., and Brunner, M. (2019). Help from my friends-cooperation of BMAL1 with noncircadian transcription factors. Genes Dev. 33, 255–257. doi:10.1101/gad.324046.119
Shostak, A., Husse, J., and Oster, H. (2013a). Circadian regulation of adipose function. Adipocyte 2, 201–206. doi:10.4161/adip.26007
Shostak, A., Meyer-Kovac, J., and Oster, H. (2013b). Circadian regulation of lipid mobilization in white adipose tissues. Diabetes 62, 2195–2203. doi:10.2337/db12-1449
Singh, M., Kaur, R., Lee, M. J., Pickering, R. T., Sharma, V. M., Puri, V., et al. (2014). Fat-specific protein 27 inhibits lipolysis by facilitating the inhibitory effect of transcription factor Egr1 on transcription of adipose triglyceride lipase. J. Biol. Chem. 289, 14481–14487. doi:10.1074/jbc.C114.563080
Singh, M., Shin, Y. K., Yang, X., Zehr, B., Chakrabarti, P., and Kandror, K. V. (2015). 4E-BPs control fat storage by regulating the expression of Egr1 and ATGL. J. Biol. Chem. 290, 17331–17338. doi:10.1074/jbc.M114.631895
Smirnova, E., Goldberg, E. B., Makarova, K. S., Lin, L., Brown, W. J., and Jackson, C. L. (2006). ATGL has a key role in lipid droplet/adiposome degradation in mammalian cells. EMBO Rep. 7, 106–113. doi:10.1038/sj.embor.7400559
Sun, C., Jiang, L., Liu, Y., Shen, H., Weiss, S. J., Zhou, Y., et al. (2016). Adipose Snail1 regulates lipolysis and lipid partitioning by suppressing adipose triacylglycerol lipase expression. Cell Rep. 17, 2015–2027. doi:10.1016/j.celrep.2016.10.070
Thiel, G., and Cibelli, G. (2002). Regulation of life and death by the zinc finger transcription factor Egr-1. J. Cell. Physiol. 193, 287–292. doi:10.1002/jcp.10178
Thiel, G., Mayer, S. I., Muller, I., Stefano, L., and Rossler, O. G. (2010). Egr-1-A Ca(2+)-regulated transcription factor. Cell Calcium 47, 397–403. doi:10.1016/j.ceca.2010.02.005
Unger, R. H., Clark, G. O., Scherer, P. E., and Orci, L. (2010). Lipid homeostasis, lipotoxicity and the metabolic syndrome. Biochim. Biophys. Acta 1801, 209–214. doi:10.1016/j.bbalip.2009.10.006
Villena, J. A., Roy, S., Sarkadi-Nagy, E., Kim, K. H., and Sul, H. S. (2004). Desnutrin, an adipocyte gene encoding a novel patatin domain-containing protein, is induced by fasting and glucocorticoids: Ectopic expression of desnutrin increases triglyceride hydrolysis. J. Biol. Chem. 279, 47066–47075. doi:10.1074/jbc.M403855200
Vollmers, C., Gill, S., Ditacchio, L., Pulivarthy, S. R., Le, H. D., and Panda, S. (2009). Time of feeding and the intrinsic circadian clock drive rhythms in hepatic gene expression. Proc. Natl. Acad. Sci. U. S. A. 106, 21453–21458. doi:10.1073/pnas.0909591106
Wang, B., Guo, H., Yu, H., Chen, Y., Xu, H., and Zhao, G. (2021). The role of the transcription factor EGR1 in cancer. Front. Oncol. 11, 642547. doi:10.3389/fonc.2021.642547
Zeng, W., Pirzgalska, R. M., Pereira, M. M., Kubasova, N., Barateiro, A., Seixas, E., et al. (2015). Sympathetic neuro-adipose connections mediate leptin-driven lipolysis. Cell 163, 84–94. doi:10.1016/j.cell.2015.08.055
Zhang, Y., Proenca, R., Maffei, M., Barone, M., Leopold, L., and Friedman, J. M. (1994). Positional cloning of the mouse obese gene and its human homologue. Nature 372, 425–432. doi:10.1038/372425a0
Keywords: Egr1, insulin, adipocytes, ATGL, leptin, circadian rhythms
Citation: Meriin AB, Zaarur N, Roy D and Kandror KV (2022) Egr1 plays a major role in the transcriptional response of white adipocytes to insulin and environmental cues. Front. Cell Dev. Biol. 10:1003030. doi: 10.3389/fcell.2022.1003030
Received: 25 July 2022; Accepted: 08 September 2022;
Published: 28 September 2022.
Edited by:
Kevin Lee, Ohio University, United StatesReviewed by:
Aijun Qiao, University of Alabama at Birmingham, United StatesCopyright © 2022 Meriin, Zaarur, Roy and Kandror. This is an open-access article distributed under the terms of the Creative Commons Attribution License (CC BY). The use, distribution or reproduction in other forums is permitted, provided the original author(s) and the copyright owner(s) are credited and that the original publication in this journal is cited, in accordance with accepted academic practice. No use, distribution or reproduction is permitted which does not comply with these terms.
*Correspondence: K. V. Kandror, a2thbmRyb3JAYnUuZWR1
†These authors have contributed equally to this work and share first authorship
Disclaimer: All claims expressed in this article are solely those of the authors and do not necessarily represent those of their affiliated organizations, or those of the publisher, the editors and the reviewers. Any product that may be evaluated in this article or claim that may be made by its manufacturer is not guaranteed or endorsed by the publisher.
Research integrity at Frontiers
Learn more about the work of our research integrity team to safeguard the quality of each article we publish.