- 1Departamento de Biología Celular, Facultad de Ciencias Biológicas, Universidad de Concepción, Concepción, Chile
- 2Grupo de Procesos en Biología del Desarrollo (GDeP), Facultad de Ciencias Biológicas, Universidad de Concepción, Concepción, Chile
- 3Núcleo de Investigaciones Aplicadas en Ciencias Veterinarias y Agronómicas, Universidad de las Américas, Concepción, Chile
- 4Departamento de Bioquímica y Biología Molecular, Facultad de Ciencias Biológicas, Universidad de Concepción, Concepción, Chile
The vertebral column, or spine, provides mechanical support and determines body axis posture and motion. The most common malformation altering spine morphology and function is adolescent idiopathic scoliosis (AIS), a three-dimensional spinal deformity that affects approximately 4% of the population worldwide. Due to AIS genetic heterogenicity and the lack of suitable animal models for its study, the etiology of this condition remains unclear, thus limiting treatment options. We here review current advances in zebrafish phenogenetics concerning AIS-like models and highlight the recently discovered biological processes leading to spine malformations. First, we focus on gene functions and phenotypes controlling critical aspects of postembryonic aspects that prime in spine architecture development and straightening. Second, we summarize how primary cilia assembly and biomechanical stimulus transduction, cerebrospinal fluid components and flow driven by motile cilia have been implicated in the pathogenesis of AIS-like phenotypes. Third, we highlight the inflammatory responses associated with scoliosis. We finally discuss recent innovations and methodologies for morphometrically characterize and analyze the zebrafish spine. Ongoing phenotyping projects are expected to identify novel and unprecedented postembryonic gene functions controlling spine morphology and mutant models of AIS. Importantly, imaging and gene editing technologies are allowing deep phenotyping studies in the zebrafish, opening new experimental paradigms in the morphometric and three-dimensional assessment of spinal malformations. In the future, fully elucidating the phenogenetic underpinnings of AIS etiology in zebrafish and humans will undoubtedly lead to innovative pharmacological treatments against spinal deformities.
Introduction
The vertebral column (VC) or spine is the main feature that defines vertebrate animals. It extends from the head to the pelvis and consists in a straight segmented structure formed by a series of stacked bones or building blocks called vertebrae. These bones are separated and supported by intervertebral disks, muscles and ligaments. Morphologically, each vertebra is composed of a vertebral body or centrum, which wraps the notochord, and vertebral arches (Fleming et al., 2015). This structure provides support and flexibility along the body axis, allowing the symmetric distribution of the body weight and giving stability in both normal posture and corporal movement (Rockwell et al., 1938). Additionally, VC protects the spinal cord and vascular elements and supports the thoracic cage, providing a scaffold for lungs and heart protection (Rockwell et al., 1938).
Along the antero-posterior axis, the human spine is divided into four portions or curves: cervical, thoracic, lumbar, and sacral. The thoracic and sacral portions are formed during the early prenatal period as a continuous curve, while the cervical and lumbar curves appear in the fetal stage. Thus, completing their development after birth in response to head movements and to sitting and walking positions (Bagnall et al., 1977; Panattoni and Todros, 1988; Choufani et al., 2009). Abnormalities in the development of these curves lead to the three most common congenital VC deformities: kyphosis (excessive outward curve of the thoracic region), lordosis (abnormal lumbar curvature), and scoliosis [a three-dimensional (3D) torsion deformity of the spine and trunk] (Lonstein, 1999; Good et al., 2011).
Scoliosis is the most common deformity of the VC in humans and represents a major public health issue (Lonner et al., 2013). In human patients, this malformation is characterized by a lateral curvature greater than 10° (measured by the Cobb angle on X-ray image) and, in some cases, rotational defects (Negrini et al., 2012). This pathology develops before birth as a failure in vertebral formation or segmentation (congenital scoliosis), or later during postnatal growth (i.e., neuromuscular or idiopathic). Among the different types of scoliosis, the adolescent idiopathic scoliosis (AIS) is the most common, representing 80% of cases worldwide. This deformity arises in healthy individuals, with the absence of structural spine defects. The term “idiopathic” means that the etiology under scoliosis is unknown, and “adolescent” indicates that this condition emerges in children older than 10 years old (Hresko, 2013).
While developmental and morphological differences exist between spines from distinct vertebrate species, a common evolutionary origin has been proposed for this skeletal structure. Indeed, the straight morphology of the VC is conferred by conserved molecules and signaling pathways orchestrating the establishment and maintenance of the spine 3D axial plane (Brand-Saberi and Christ, 2000; Alejevski et al., 2021). However, our understanding of the identity and function of the molecules regulating spine morphology remains elusive because of the lack of tools to develop genetically tractable models to link genomic sequences with AIS.
In this review, we will discuss how the current emergence of zebrafish (Danio rerio) phenotyping data is providing a comprehensive collection of tools and information to understand spinal deformities in vertebrates, such as AIS. Mutant phenotypes displaying body axis curves are valuable genetic resources to identify molecular and cellular mechanisms that shape spine formation and patterning. Human genomic resources, coupled with phenogenetic information generated by gene function studies in zebrafish, will reveal novel genotype-phenotype correlations during spinal architecture morphogenesis and maintenance.
1 Human Adolescent Idiopathic Scoliosis: Genetics of a Mysterious Early and Defective Phenotype of the Spine
Scoliosis affects 0.47–5.2% of the population depending on the geographic location, ethnicity and sex, showing a marked bias to females, both in prevalence and severity. The risk indicators reveal a 2:1 prevalence ratio between girls and boys, and a 10-fold greater chance of developing curves that require surgical treatment (Karol et al., 1993; Konieczny et al., 2013). Severe spine curves in adolescence lead to health problems in adulthood, such as aesthetic issues, quality of life, disability, back pain, breathing function, among others (Negrini et al., 2006). Depending on the severity of the curve, scoliosis can be treated with observation, physical therapy, bracing, or invasive surgical corrections (Rose and Lenke, 2007). Because the underlying biological cause of AIS is poorly understood, effective treatment options are limited.
Genetic and environmental factors contribute to causing AIS. Also, the sexual dimorphism observed in this pathology suggest a model in which hereditary factors are involved. Despite nearly five decades of investigations on AIS supporting the idea that this condition has a genetic basis, surprisingly little is known about the genetic variants involved in its etiology. Thus, our ability to link genomic sequences with AIS has been hampered by the lack of tools to develop genetically tractable models. Such a resource would allow to identify scoliosis heritability; thus, contributing to the variability in limited treatment options.
In 1968, idiopathic scoliosis (IS) was described as a familial condition, because of its high incidence among relatives (Wynne-Davies, 1968). Studies accomplished on the Europeans twin population, revealed a higher concordance of AIS in monozygotes compared to dizygotes. This concordance for AIS in twins, strongly suggests a genetic contribution to the disease. Additionally, it has been shown that 38% of the variance in the risk to develop scoliosis is explained by additive genetic effects (Andersen et al., 2007; Grauers et al., 2012). In the recent years, several studies have focused on discovering the genetic variants associated with AIS, either by identifying mutations that segregate with the disease or polymorphic loci in affected population studies. Thus, genome-wide association studies (GWAS) and whole exome sequencing (WES) have greatly contributed to understanding the etiology of AIS by identifying single nucleotide polymorphisms (SNPs) and gene variants in several loci, respectively (Gao et al., 2007; Takahashi et al., 2011; Kou et al., 2013; Baschal et al., 2014; Ogura et al., 2015; Patten et al., 2015; Sharma et al., 2015; Zhu et al., 2015; Zhu et al., 2017; Khanshour et al., 2018; Kou et al., 2019; Liu et al., 2019; Wang et al., 2020; Terhune et al., 2021b). These efforts have been pivotal to characterize genotype-AIS associations and expand our understanding of the genetic regulation behind scoliosis in humans. However, these strategies are imprecise to identify the phenotypic consequences of genetic variants (Chanock et al., 2007; Linghu and DeLisi, 2010). Therefore, and considering that humans are unsuitable for robust functional interrogation of the largely unknown functions of AIS-associated genes at large scale, there is a need for model systems that enable the generation of mutants to study genotype-phenotype interactions. Indeed, the function of AIS risk genes identified by GWAS and WES studies, including LBX1, DNAAF1, ZMYND10, MAPK7, KIF6, and PAX1, has successfully been studied in a vertebrate mutant system (Figure 1A) (Adham et al., 2005; Guo et al., 2016; Gao et al., 2017; Konjikusic et al., 2018; Wang et al., 2020).
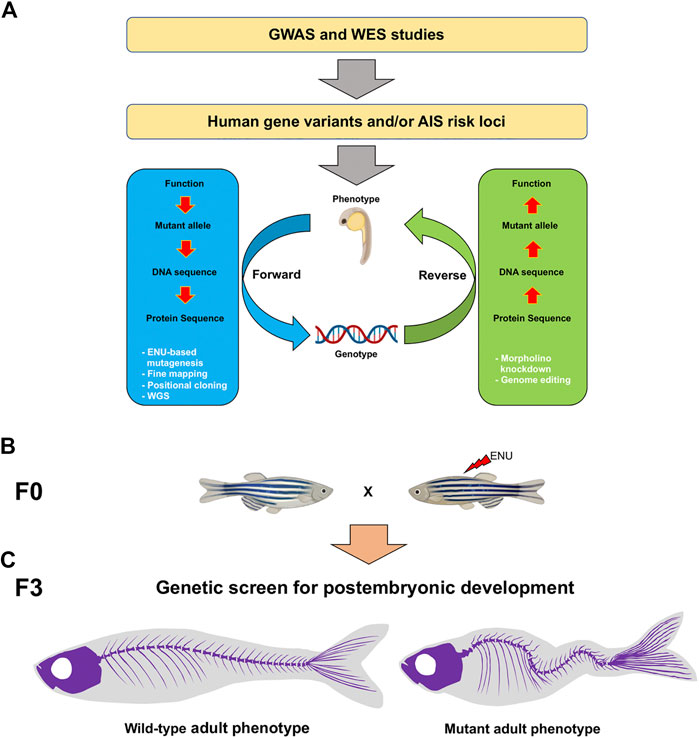
FIGURE 1. Schematic of the genotype-phenotype association and genetic approaches to study AIS candidate genes in the zebrafish. (A) To identify AIS risk loci, GWAS and WES strategies can be performed. Forward (blue) and reverse (green) genetic strategies (white) are powerful tools to study mutant genotypes and phenotypes associated with AIS. (B) In a forward genetic screen, male founders (F0) are mutagenized with ENU and then outcrossed to generate F2 families. To screen for postembryonic gene functions in late larval to adult stages after 5 dpf, F3 families are generated either through incrossing F2 heterozygous fish or through in vitro fertilization (IVF) using cryopreserved sperm and wild-type females, and raised to adulthood. (C) By using a combination of morphological, tomographic, molecular and cellular approaches, F3 individuals are screened for adult spine alterations. AIS, adolescent idiopathic scoliosis; GWAS, genome-wide association studies; WES, whole exome sequencing; ENU, N-ethyl-N-nitrosourea; WGS, whole genome sequencing.
Although the idea that genetic factors contribute to scoliosis has strong supporting evidence, AIS inheritance does not behave as a simple Mendelian mechanism. It is thought that AIS is a polygenic condition with genetic heterogenicity, variable patterns of inheritance, and a mode of genetic transmission that still remains to be identified (Cheng et al., 2015). Even considering the last advances in genomic technologies applied to discover human AIS-related genes, these findings represent statistical association rather than causality. Currently, the available genetic tests are inaccurate enough to predict the prognosis of AIS; therefore, further investigation is needed to develop genetic tools for early detection, which would allow physicians to design better management plans for this condition (Zamborsky et al., 2019).
2 Zebrafish as a Model System to Understand Body Axis Linearity Maintenance and Human Adolescent Idiopathic Scoliosis
To study the causes of AIS in humans, diverse animal model systems have been used to date. Due to the low natural incidence of scoliosis in quadrupedal organisms, it has been proposed that upright position is critical in the development of spinal malformation (Machida et al., 1999; Castelein et al., 2005). Also, the mechanical load distribution along the VC differs between bipedal and quadrupedal animals, which impacts spine anatomy. However, how bipedal behaviors and adaptations are linked to scoliosis pathogenesis remains mysterious.
Because of the lack of non-human bipedal mammals and the low rate of spinal deformities in quadrupedal vertebrates, researchers have developed several procedures to induce bipedalism and scoliosis-like phenotypes in experimental animals. These include amputation of forelimbs and tail, or pinealectomy (reviewed in Ouellet and Odent, 2013). These approaches have also been applied to primates without any success (Robin and Stein, 1975). In our knowledge, many of these procedures aim to reproduce the curvatures of human scoliosis rather than its pathogenesis, thus contributing to testing corrective surgeries or mechanical treatments, instead of finding genetic causes underlying AIS in humans (Bobyn et al., 2015). As none of these experimental animals can recapitulate all the features of the pathogenic phenotype, it is paramount the emergence of additional model systems to model genotype-phenotype relationships across AIS development.
Recently, the molecular mechanisms underlying AIS have been better understood with the emergence of zebrafish as a suitable model for recapitulating human diseases. Using zebrafish and other teleost fish such as medaka and guppy, several research groups have achieved important advances in our understanding of AIS phenogenetics (Gorman et al., 2007; Hayes et al., 2014; Grimes et al., 2016; Kobayashi et al., 2017; Cantaut-Belarif et al., 2018; Van Gennip et al., 2018; Zhang et al., 2018; Rose et al., 2020). Unlike classical quadruped animal model systems; whose spinal loading and center of mass are different compared to humans (Gorman and Breden, 2009), zebrafish generates craniocaudal forces because of swimming, similar to those exerted by humans standing upright. These physicomechanical features, in addition to emerging genetic and phenotypic evidence from zebrafish, pose this animal model as suitable to study the role of molecular, cellular and physiological processes implicated in the etiopathogenesis of AIS (Buchan et al., 2014; Grimes et al., 2016; Van Gennip et al., 2018; Zhang et al., 2018; Rose et al., 2020). All these aspects will be discussed in the next sections.
3 Cellular Identity and Mechanisms of the Spine Axis Forming Activity in Zebrafish
In teleostean fishes, the building blocks of the spine arise from two embryonic structures: the notochord and somites. During early embryogenesis, somites are formed sequentially along the anterior-posterior (AP) axis from the paraxial mesoderm, which undergoes mesenchymal-to-epithelial transition, and is patterned by maternally defined signaling morphogen gradients such as Nodal, Wnt, and FGF (Dequeant and Pourquie, 2008; Tuazon and Mullins, 2015; Fuentes et al., 2020). Somites are sphere-like structures formed by epithelial cells, which localize on both sides of the neural tube in the developing embryo. Then, each somite subdivides into dermomyotome (precursors of the dermis and skeletal muscle) and the sclerotome (precursor of most ligaments, bones and cartilages of the spine) (Fleming et al., 2015). Alternatively, the notochord is also formed by AP patterning cues during early development, supporting the body axis and providing flexibility during locomotion until the spine is structured (Stemple, 2005). The notochord is formed by inner vacuolated cells called chondrocytes, and by an external layer of chondroblasts that secretes the notochordal sheath (a thin membrane with high elastin content enveloping a collagenous layer). This extracellular matrix is essential for the hydrostatic properties of the notochord, and forms a basal lamina on which chondroblasts rest (Grotmol et al., 2005).
The first step during zebrafish spine development is the formation of mineralized rings (called chordacentra) that surround the notochord sheath (Arratia et al., 2001; Fleming et al., 2015). The chordacentra mineralizes in the absence of osteoblasts, and serves as a scaffold for the developing autocentrum, a layer of perinotochordal bone formed at the periphery of the notochord by direct ossification (Arratia et al., 2001; Fleming et al., 2004; Peskin et al., 2020). During this process, the notochordal sheath is segmented, from a cartilage-like structure to alternated mineralized domains (Forero et al., 2018; Wopat et al., 2018). This transition occurs through the spatially restricted expression of ectonucleoside triphosphate diphosphohydrolase 5 (entpd5) gene in the notochord sheath cells, and relies on the Notch pathway. Entpd5 functions to recruit osteoblasts to the mineralized zone and ultimately form the centra (Forero et al., 2018; Wopat et al., 2018). Depending on the position along the spine, the ventral and neural arches can develop from either cartilage or membrane bone. The most anterior (Weberian apparatus) and posterior (caudal fin) arches have a cartilaginous precursor, which is formed by metametric aggregations of sclerotomal mesenchyme (sclerotome). Central arches are formed by membranous ossification or by a combination of both (Bird and Mabee, 2003; Grotmol et al., 2003).
Although the spine is formed in all vertebrates from the sclerotome and notochord, there are important differences concerning the contribution of each embryonic tissue and the concomitantly spine formation between species. Some of these include the proportion of the somites, the segmentation pattern of the spine that relies on the segmentation clock patterning, and the segmental periodicity of vertebrae that is determined by the sclerotome instead of the notochord in some species. However, in teleost fish, the segmentation of the spine relies on the notochord, with contribution from the paraxial mesoderm (Morin-Kensicki and Eisen, 1997). Despite these differences, the general architecture of the spine is evolutionarily conserved among vertebrate groups and suggests the existence of multiscale and spatially defined signatures of its morphogenesis at the phenogenetic level (Boswell and Ciruna, 2017). Therefore, this evolutionary conservation opens the possibility to establish new model systems for investigating genotype-phenotype correlations at large-scale during spine formation. In this sense, the zebrafish emerges as an attractive model system for studying scoliosis and interrogating specific gene functions underpinning spine axis morphogenesis (Figure 1). Discovering novel genes involved in biological processes associated with the generation of scoliosis will also allow us not only to study this pathology in real-time, also to develop efficient genetic-based diagnostic or prognostic tools.
4 Emerging Roles of Genetic Factors Related to Scoliosis Development in Zebrafish
Phenotyping efforts in zebrafish based on forward and reverse genetic approaches (Figure 1A), along with recent advances in sequencing and genome-editing technologies, represent promising strategies to reveal the underlying causes of AIS in humans (Buchan et al., 2014; Grimes et al., 2016; Zhang et al., 2018; Rose et al., 2020). Thus, zebrafish mutants have revealed several genes related to scoliosis, most of these regulating three main and apparently linked biological processes: skeleton development, ciliogenesis [that impacts on cerebrospinal fluid (CSF) dynamics], and immune response.
4.1 Molecular Factors Involved in Spine Patterning and Maintenance
In the zebrafish early embryo, notochord formation is critical for the AP extension of the body axis, and ultimately, spine biogenesis. Signaling pathways, such as Notch, regulate notochord-lineage cell fate by determining if these differentiate into chondrocytes or chondroblasts (Yamamoto et al., 2010). As aforementioned, Notch signaling activation induces the transition from cartilage-like to mineralized domains by inducing the expression of Entpd5 as an essential factor for the notochord ossification (Huitema et al., 2012; Wopat et al., 2018). While the role of the Notch signaling in determining the onset of spine formation has been established, much less is known about the genetic program controlling how the spine develops from embryo to juvenile stages, and maintains its morphology and linearity in the adult individual.
The study of a collection of zebrafish mutants displaying scoliosis-like phenotypes has significantly increased our knowledge regarding the genetic regulation of spine development (Figures 1B,C). A forward genetic approach led to the identification of dozens of genes involved in axial skeleton morphogenesis during early embryogenesis (Brand et al., 1996; Sun et al., 2004; Henke et al., 2017; Gray et al., 2020). Regardless of the great contribution in the understanding of spine formation in the early embryo, postembryonic genetic analysis of this structure has received much less attention; thus constituting a gap in our knowledge of human pathologies, including AIS.
Pioneering large-scale screens in zebrafish have identified several adult mutant phenotypes (Driever et al., 1996; Haffter et al., 1996). Since then, phenotyping approaches have allowed the identification and characterization of a large number of genes functioning in axial skeleton development (Haffter et al., 1996). For instance, Stemple and colleagues reported the mutant known as leviathan, which shows a dorsoventral and lateral folded notochord (Stemple et al., 1996). Almost two decades later, three different recessive mutations in the col8a1a gene were associated with the leviathan genetic lesion (Gray et al., 2014). These mutant alleles also display vertebral malformations in the adult stage, becoming a suitable model for congenital scoliosis.
Unlike leviathan mutant, skolios, another zebrafish mutant with skeletal abnormalities, shares several features with human IS as it develops a curved body axis without vertebral deformations and bone homeostasis alterations. A nonsense mutation in the kinesin family member 6 (kif6) gene, which functions in the cilia assembly, was identified in skolios mutants (Buchan et al., 2014). However, no typical ciliary dysfunctions such as hydrocephalus, kidney cyst or fluid flow at the central canal were observed in kif6 mutants (Buchan et al., 2014), perhaps reflecting a highly tissue-specific function. Additionally, the molecular characterization of the zebrafish protein tyrosine kinase 7 (ptk7) gene indicates a role of the Wnt/β-catenin and planar cell polarity signaling in patterning, establishing and maintaining axial coordinates during the juvenile-to-adult transition (Hayes et al., 2013; Grimes et al., 2016). skolios/kif6 and ptk7 were the first identified mutant genes to disrupt spine linearity; thus being pioneers in recapitulating AIS in zebrafish (Buchan et al., 2014; Grimes et al., 2016). Recently, it has been reported that a deficiency of somite shorten (smt) gene leads to failure in notochord function, which generates a scoliosis-like phenotype (Sun et al., 2020). The molecular identification of the zebrafish smt gene as dual serine/threonine and tyrosine protein kinase (dstyk), indicates a role of Smt/Dstyk in establishing spine axial coordinates through a lysosome-to-nucleus signaling mechanism (Sun et al., 2020). Altogether, these findings highlight the potential of zebrafish as a model system for understanding the onset of scoliosis, but there is much yet to be discovered at the genetic and molecular level.
4.2 Cilia Function and Spinal Curvature in Zebrafish
Cilia are evolutionarily conserved hair-like organelles, protruding from the cell membrane to the extracellular space. These cellular structures are present in unicellular and multicellular organisms, and can be found in many vertebrate cell types (Satir et al., 2008; Ishikawa and Marshall, 2011; Malicki and Johnson, 2017). At the structural level, cilia consist of a microtubule (MT) skeleton called axoneme that is surrounded by and docks to the plasma membrane through the basal body (Ishikawa and Marshall, 2011; Malicki and Johnson, 2017). Based on MT organization, cilia are classified either as motile or non-motile. The axoneme of motile cilia contains nine doublets surrounding a central pair of singlet MTs (9 + 2), and axonemal dynein arms that allow cilia movements catalyzed by ATP hydrolysis. Non-motile or primary cilia lack the central pair of singlet MTs (9 + 0) and do not contain dynein arms (Wang and Dynlacht, 2018). From a functional perspective, motile cilia generate forces for cell motility and can rhythmically beat to drive fluids to flow over epithelial cells, and/or generate signaling gradients. Primary, or non-motile, cilia act as sensors transducing chemical or physical signals from the pericellular environment into the cell (Wang and Dynlacht, 2018). Interestingly, cilia biology is critical for spine maintenance and impaired function is associated with scoliosis (reviewed in Boswell and Ciruna, 2017; Grimes, 2019; Bearce and Grimes, 2021).
The relevance of cilia in the maintenance of body axis straightness was initially described in the 1990s in large-scale N-ethyl-Nitrosourea (ENU)-based mutagenesis screens and more recently, by specifically mutating cilia-related genes (Driever et al., 1996; Haffter et al., 1996; Grimes, 2019). Thus, most of the curly tail down phenotypes have alterations in their cilia. Also, motile cilia have been described as critical for normal CSF flow (Kramer-Zucker et al., 2005; Grimes et al., 2016; Thouvenin et al., 2020), which is an important corporal fluid for vertebrate development (Lun et al., 2015), and required for early body axis straightening and its maintenance during zebrafish development (see Section 5.3) (Grimes et al., 2016; Cantaut-Belarif et al., 2018; Zhang et al., 2018; Troutwine et al., 2020).
One of the most remarkable associations between motile ciliopathy and scoliosis was conducted by Grimes et al. (2016). These authors analyzed the body curvature phenotype in five different cilia-related zebrafish mutants. They also generated two complementary strategies to avoid the early lethality caused by aberrant cilia motility in other mutants during the first weeks of development (Grimes et al., 2016). First, they used a temperature-sensitive mutation (kurly/cfap298 mutant), allowing the embryo to develop normally when the temperature is shifted from a warmer to cooler conditions. These animals appear wild-type through the first development stages but develop spinal curves during late larval stages. The second strategy consisted of injecting wild-type RNA at 1-cell stage in animals from three different mutant backgrounds showing defective cilia motility. This rescue approach allowed early development to proceed normally, and revealed that older embryos developed curved spines (Grimes et al., 2016). One year later, Oliazadeh et al. (2017) studied the organization and morphology of primary cilia in bone cells from patients with AIS, describing an increase in the length with cellular consequences related to mechanotransduction, that in turn affected bone formation and development (Nguyen and Jacobs, 2013; Oliazadeh et al., 2017).
In addition to ptk7 (see Section 4.1), several motile ciliary zebrafish genes loss-of-function resemble human ciliopathy phenotypes, including curved body axis (reviewed in Song et al., 2016). Alternatively, it is also well known that the sensory primary cilia function determines the coordinated activation of signaling pathways such as Hedgehog, WNT, TGF-β/BMP, among others (Anvarian et al., 2019). However, how mutations in cilia structure protein-coding genes may generate alterations in spine biology remains an unsolved problem. In addition, while these results put in evidence the direct relationship between cilia function perturbance and scoliosis, the involved cilia (primary cilia, motile cilia or both) remains controversial.
For example, Cep290 (centrosomal protein 290) promotes primary cilia assembly and localizes at centrioles and basal bodies. Mutations in this gene have been implicated in different human ciliopathies, including Joubert syndrome, which is characterized by hindbrain malformation, and motor and cognitive impairments (Saraiva and Baraitser, 1992). Zebrafish cep290 morphants resemble human ciliopathies such as body axis curvature, defects in cerebellar development, cystic kidney and reduced vision (Sayer et al., 2006; Baye et al., 2011). Additionally, adult cep290 mutants exhibit a severe spine malformation and are unable to breed (Lessieur et al., 2019), thus suggesting an important role of primary cilia in zebrafish reproduction.
Additionally, the human Armc9 (armadillo repeat containing 9) protein has recently been associated with primary cilia function and ciliopathies (Breslow et al., 2018). Armc9 localizes at the proximal region of cilia and regulates their length in mammalian cells. On the other hand, Van De Weghe et al. (2017) found that this protein is also mutated in individuals with Joubert syndrome. Remarkably, zebrafish armc9 crispant shows a decreased number of cilia in brain ventricles, and typical ciliopathy phenotypes, including scoliosis-like spinal curvature (Van De Weghe et al., 2017).
The role of cilia in spine coordinate establishment and morphology is increasing the spectrum of genotype-phenotype relationships in vertebrate post-embryonic development so far. The use of zebrafish seems advantageous in the study of primary and motile cilia structure and how their functions contribute to body axis development and the maintenance of its straightening. Ongoing phenotyping projects in zebrafish are expected to provide unprecedented genetic entry points to cilia biology and spine morphogenesis.
4.3 Mechanism of Cerebrospinal Fluid Flow Establishment: A Hydrodynamic Model Predicting the Spine Axial Coordinates and Curvature
CSF is a clear liquid filling the cerebral ventricles and central canal of the spinal cord. This fluid also surrounds the central nervous system (CNS) in the subarachnoid space (Brinker et al., 2014). CSF functions: a) providing buoyancy and protection to the brain and spinal cord, and b) transporting several signals along with the central nervous system (Brinker et al., 2014; Fame and Lehtinen, 2020). The CSF and its correct circulation are also crucial for normal CNS development, providing growth factors and signaling molecules that impact neuroepithelium proliferation and differentiation.
The “classic CSF circulation theory” presumes a constant downward flow from the lateral ventricles toward the subarachnoid space. This theory describes CSF flow as unidirectional, circulating from the lateral to the third ventricle via the foramen of Monro, and then through the Sylvian aqueduct to the fourth ventricle. Subsequently, the CSF flows caudally along the central canal of the spinal cord or circulates through the foramens of Luschka and Magendie into the subarachnoid space of the brain and spinal cord, where a portion of CSF drains to the blood via arachnoid granulation, via spinal nerve roots and the remainder via the olfactory tracts (Brodbelt and Stoodley, 2007).
Recently, the use of molecular, cell biology, and neuroimaging techniques has revealed that CSF flow is not as simple as previously thought. This flow depends on multiple factors including cilia motility, hearth beat, and pulsatile and local exchange between interstitial fluid, blood, and CSF (Brinker et al., 2014; Matsumae et al., 2019; Fame and Lehtinen, 2020). Although most of the current knowledge about CSF flow corresponds to its dynamics in the brain, much less is known about its behavior within the central canal of the spinal cord.
In vertebrates, the central canal of the spinal cord is a narrow cylindrical cavity surrounded by ependymal cells. It extends caudally from the floor of the fourth ventricle to the terminal ventricle or ampulla caudalis, at the conus medularis (Storer et al., 1998). The central canal is limited by ependyma ciliated cells whose asymmetric distribution generates a recently described bidirectional fluid flow (Thouvenin et al., 2020). At 30 h post-fertilization, zebrafish embryos display a density of ventral motile cilia, which is four times higher than the dorsal ones and beat with higher frequency (Troutwine et al., 2020). This asymmetry generates a dorsocaudal CSF flow at the ventral region of the central canal and the opposite direction at the dorsal region. In the absence of cilia motility, the central canal collapses, indicating that the ciliary activity is necessary to maintain its morphology and the transport of molecules between the brain and spinal cord (Troutwine et al., 2020).
In addition to the CSF, the central canal contains a threadlike structure, called Reissner fiber (RF) (Figure 2A). This fiber is formed from the early stages of development in most vertebrates, although its presence in man and bats is still controversial (Rodriguez et al., 1992). Over the last years, and using the zebrafish as an animal model system, several studies have identified genetic factors associated with body axis patterning and defective functions of the CSF flow, motile cilia or RF formation. These findings are starting to fill the gap in our knowledge about the link between CNS functionality and scoliosis progression, as reviewed below.
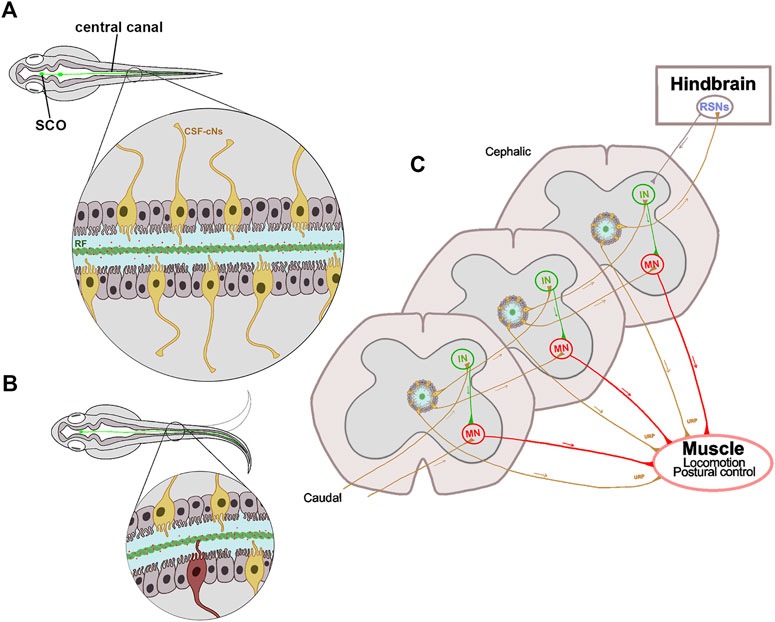
FIGURE 2. Schematic representation of the proprioceptive organ conformed by RF and CSF-cNs. (A) The central canal in a zebrafish embryo is surrounded by ependymal ciliated cells (gray) necessary for the CSF flow, and by sensory CSF-cNs (yellow). The RF (green cord) runs from the SCO to the entire spinal cord inside the central canal. The RF binds and transports epinephrine and norepinephrine molecules (red dots) that trigger Urp1 and Urp2 expression in mechanosensory CSF-cNs. (B) The curvature of the spinal cord during locomotion, allows the interaction between RF and CSF-cNs (highlighted in red); thus, transferring information through a spinal circuit and shaping the spinal curvature. (C) CSF-cNs project an ipsilateral ascending axon that synapses onto premotor INs, onto primary MNs or directly onto muscle that expresses the Urp receptor. Additionally, the most cephalic CSF-cNs send their axons to RSNs in the hindbrain, that in turn send descending motor commands to the spinal cord. All these projections participate in the correct muscle contraction and postural control. SCO, subcommissural organ; CSF, cerebrospinal fluid; CSF-cNs, CSF contacting neurons; RF, Reissner fiber; Urp, urotensin neuropeptides; IN, interneuron; MN, motor neuron; RSN, reticulospinal neuron.
4.3.1 Relevance of Cerebrospinal Fluid Flow, Reissner Fiber Biogenesis and Spine Morphogenesis in Zebrafish
In zebrafish, CSF circulation in the spinal cord is critical for spine morphogenesis, and disturbances in its dynamics have been associated with scoliosis (Grimes et al., 2016; Boswell and Ciruna, 2017; Zhang et al., 2018; Grimes, 2019; Thouvenin et al., 2020). The relevance of a normal CSF flow inside the central canal to keep a straight body axis is emerging from the zebrafish model, but how do CSF flow perturbances generate scoliosis? To address this question, there are at least two non-exclusive hypotheses that will be discussed here.
Firstly, the CSF provides signaling resources necessary for correct body axis maintenance. CSF flow is driven by motile cilia on ependymal cells of brain ventricles and central canal. As discussed in Section 4.2, these MT-based structures emerge from the apical cell surface and, by a synchronized beat, generate a wave-like movement of the extracellular fluid (Figure 2). In this way, the injection of fluorescent dyes in the brain ventricles of zebrafish embryos led to a rapidly dispersion of fluorescence along the spinal canal. However, dye transport is impaired in ciliary mutants, and correlates with the severity of body curvature (Zhang et al., 2018). The ptk7 mutant shows abnormal cilia structure and organization, as well as CSF flow defects, hydrocephalus and scoliosis (Grimes et al., 2016). These defective phenotypes can be rescued when expressing the Ptk7 wild-type version in motile ciliated cell lineages. Thus, these findings indicate that motile cilia function is necessary to maintain a correct CSF flow and right body axis (Grimes et al., 2016).
To characterize the molecular pathways affected by the CSF flow perturbance, Zhang and colleagues elegantly compared gene-expression patterns between control and zmynd10 mutant embryos (Zhang et al., 2018). The Zmynd10 protein functions assembling the arms of axonemal dynein and mutations on its coding gene cause ciliopathy primary ciliary dyskinesia (PCD) in humans (Zariwala et al., 2013). The results showed that two genes, urotensin-related peptide 1 and 2 (Urp1 and Urp2) were downregulated in the zmynd10 mutant, as well as in other cilia mutants displaying a body curvature phenotype (Zhang et al., 2018). The relevance of these peptides was then analyzed by gain- and loss-of-function assays. In gain-of-function assays, these researchers achieved to rescue body axis curvature in different cilia mutants by overexpressing Urp1 (or Urp2). In loss-of-function assays, the generation of urp1 morphant embryos develop a body-curvature defect, and the phenotype was more pronounced when urp2 morpholine was co-injected. These results show that body curvature alteration found in motile cilia mutants is caused by downregulation of Urps. These peptides are secreted by CSF-contacting neurons (CSF-cNs) (Figure 2C), and activate the Uts2ra receptor in somitic muscle cells; thus, regulating body axis extension. In this way, norepinephrine can restore the expression of Urp1 and right body axis in motile cilia mutants but not in urp1 or utsra receptor morphants (Zhang et al., 2018). These findings indicate that the transport of molecules by the CSF is relevant to maintain the straight body axis (Figures 2A,B).
Secondly, CSF flow is necessary for the correct aggregation of RF components. RF is an elastic extracellular structure suspended in the CSF and extended from the diencephalon to the entire length of the central canal of the spinal cord (Figure 2A) (Rodriguez et al., 1998; Troutwine et al., 2020). RF is evolutionarily conserved, and its main component is a protein secreted to the CSF from early stages of development by the subcommissural organ (SCO), a brain gland located at the diencephalic-mesencephalic boundary in all vertebrates (Rodriguez et al., 1992) (Figure 2A). This protein is known as the SCO-spondin, a glycoprotein with a high molecular mass (540.67 KDa), and broadly conserved across vertebrates (Meiniel and Meiniel, 2007; Munoz et al., 2019). Once released into the CSF, SCO-spondin undergoes a progressive process of aggregation. Primarily, as pre-RF by forming a mesh of fibrils on the entire surface of the SCO and then into the RF (Sterba et al., 1967; Rodriguez et al., 1992). Then, RF continuously grows by the addition of new SCO-spondin molecules at the distal end, which disassembles at the caudal region of the spinal cord where it forms a flocculent material that enters into the local blood vessels (Rodriguez et al., 1992; Molina et al., 2001; Troutwine et al., 2020).
The role of RF in body axis architecture determination was first proposed more than a century ago by Nicholls (1917) after the observation that RF incision in fish generates its retraction and abnormal posture (Nicholls, 1917). This relationship was also observed in lordotic fish that present anomalies in the central canal, RF, and SCO secretory activity (Andrades et al., 1994). The use of zebrafish mutants has confirmed the crucial role of RF in body axis maintenance. By using CRISPR/Cas9-mediated genome editing, Cantaut-Belarif et al. (2018) generated a scospondin (sspo) mutant that cannot assemble the RF. Such a mutant embryo develops a curly tail down phenotype, which gradually worsens until death occurs 10 days after fertilization (Cantaut-Belarif et al., 2018). However, removing the chorion of the sspo null mutant early embryos allows the survival of 30% of them, which mature into adult fish with a severe curved body axis (Lu et al., 2020).
Although the sspo mutants resemble the curly tail down phenotype seen in cilia motility mutants, the cilia activity and CSF flow are normal in sspo mutants (Cantaut-Belarif et al., 2018). By contrast, the secretion of SCO-spondin is apparently normal in mutants with defective cilia, but the RF fails to develop (Troutwine et al., 2020). Altogether, this data suggests a crucial role of motile cilia in SCO-spondin aggregation and RF formation.
Since the sspo null mutation generates a fully penetrant curvy body axis phenotype, complementary studies have focused on the use of mutants showing incomplete penetrance (Table 1). In a zebrafish forward genetic screen for adult viable scoliosis mutants, Troutwine et al. (2020) studied two recessive hypomorphic alleles harboring missense mutations in sspo. In contrast to null sspo alleles, the RF of hypomorphic mutants appeared devoid of defects during the initial assembly phase, but it progressively became abnormal until its disappearance at 10 days post-fertilization (dpf), at the same time that appears the curved axis phenotype (Troutwine et al., 2020). In addition, the generation of a SCO-spondin-GFP fusion transgenic line has facilitated genetic and phenotyping efforts, allowing to monitor in vivo the dynamic properties of RF formation in developing zebrafish embryos (Troutwine et al., 2020). This transgenic line has revealed unprecedented features of the initial SCO-spondin secretion and RF formation at the rostral portions of the spinal canal. Taken together, the analysis of SCO-spondin zebrafish mutants, displaying curvy tail down phenotypes, shows that the RF disassembly coincides with the development of an axial curvature of the spine (Cantaut-Belarif et al., 2018; Troutwine et al., 2020). The relevance of RF in the adult has also been reported in sspo null mutants that manage to survive after dechorionization, and exhibited a strong curvature in the spine (Lu et al., 2020; Rose et al., 2020). Also, in heterozygous sspodmh4/+ mutants that, as well as AIS patients, develop the spinal curvature during juvenile stages (Rose et al., 2020). These findings support the relevance of RF to maintain right body axis, and open the question about the function exerted by this fiber in the central canal of the spinal cord.
4.3.2 The Link Between Reissner Fiber and Cerebrospinal Fluid Contacting Neurons to Conform a Proprioceptive Organ in the Zebrafish Spinal Cord
As stated before, the neuropeptides Urp1 and Urp2 are expressed in CSF-cNs, which are localized in the zebrafish spinal cord (Zhang et al., 2018) (Figure 2A). Interestingly, these molecules are down-regulated in the zmynd10 mutant and other cilia zebrafish mutants (Zhang et al., 2018) and their overexpression can rescue body axis curvature in different cilia mutants. These findings indicate that the cilia-driven CSF flow would be necessary to trigger Urp signaling in CSF-cNs and these molecules then induce body straightening in zebrafish embryos. Additionally, a transcriptomic analysis of sspo mutants revealed a high down-regulation of Urp2, and in a lesser extent of Urp1(Cantaut-Belarif et al., 2020; Rose et al., 2020); and the restoration of Urp expression in CSF-cNs of sspo mutants showed a significant rescue of embryonic body curvature (Lu et al., 2020). These antecedents show that the expression of Urps in CSF-cNs is sufficient to restore axial deformities in sspo mutant and in motile cilia mutants (Zhang et al., 2018; Lu et al., 2020).
CSF-cNs are sensitive ciliated cells surrounding the central canal of the vertebrate ventral spinal cord (Jalalvand et al., 2014; Djenoune et al., 2017). These GABAergic neurons detect local spinal bending and relay this mechanosensory feedback information to motor circuits involved in locomotion and postural control (Bohm et al., 2016). In this way, the genetic blockage of neurotransmission in CSF-cNs by expression of botulinum toxin light chain under the specific promoter of the Pkd2l1 channel, reduces locomotor frequency and hampers postural control (Hubbard et al., 2016). In zebrafish larvae, CSF-cNs send an ipsilateral ascending axon from two to six segments that synapse onto premotor interneurons involved in slow locomotion, onto primary motor neurons that innervate the entire ventral musculature, and interneurons involved in sensory-motor gating (Figure 2C). Moreover, the rostral most CSF-cNs contact large reticulospinal neurons of the hindbrain, that in turn send descending motor commands to the spinal cord (Figure 2C) (Wu et al., 2021), suggesting that the contractile activity of these structures promotes proper axial morphogenesis (Zhang et al., 2018). Additionally, adult pkd2l1 zebrafish mutant develop a moderate spine curvature but did not resemble the motile cilia mutant phenotype. The opening of Pkd2l1 channel is regulated by mechanical pressure applied against the membranes of CSF-cNs (Cantaut-Belarif et al., 2018), that may reflect the CSF flow or even the RF contact. Changes in CSF pH and osmolarity can also affect the functionality of these neurons, impacting on the posture through spinal curvatures due to inadequate muscle contraction (Orts-Del’Immagine et al., 2014; Bohm et al., 2016; Jalalvand et al., 2016a; Jalalvand et al., 2016b). These antecedents reveal that these CSF-cNs contribute to the maintenance of natural curvature of the spine (Sternberg et al., 2018).
Morphologically, CSF-cNs project a ciliated apical extension in the central canal that may contact the RF and detect spinal curvature in a directionally motion-directed manner (Orts-Del’Immagine et al., 2020). Using a hypomorphic mutant of the sspo gene, Orts-Del’Immagine et al. (2020) revealed that in the absence of RF, CSF-cNs reduce their ability to allow calcium into these cells. In addition to mechanical stimuli, this neural population is also chemosensitive since it responds indirectly to CSF norepinephrine (Orts-Del’Immagine et al., 2020). Interestingly, it has been reported that RF binds and transports norepinephrine (Figure 2A) (Caprile et al., 2003), likely linking the mechanosensitive and chemosensitive responses of CSF-cNs. However, the role of Urp2 expression in these neurons is unclear. Rose et al. (2020) emphasized that this peptide is down-regulated in sspo mutants, but that its expression levels were not back to normal by inhibiting the pro-inflammatory factor COX-2 that rescues the mutant axial development (Rose et al., 2020). In addition, overexpression of Urp2 in CSF-cNs of sspo mutants rescued curvature phenotypes during the larval-to-adult transition, thus concluding that its expression is sufficient to restore body axis straightening of zebrafish individuals showing both ciliopathy and RF abnormalities (Lu et al., 2020). Additionally, the expression of Urp2 in sspo mutants can be restored by the exposition to adrenaline and noradrenaline (Cantaut-Belarif et al., 2020; Lu et al., 2020), neurotransmitters that are normally attached to the RF (Caprile et al., 2003).
Altogether, these findings indicate that, at least in zebrafish, the maintenance of the spinal right axis requires the cilia motility to promote a correct CSF flow, which in turn is necessary for the RF assembly to transport different signaling molecules (i.e., norepinephrine) along the entire nervous system (Figure 2A). Depending on the spinal position, RF would contact the cilia of the CSF-cNs (Figure 2B), which finally synapse onto motor neurons, premotor excitatory or reticulospinal neurons; thus, modulating the excitability of spinal circuits underlying locomotion and posture (Figure 2C). Ultimately, mutations of any gen that perturbs this cascade of events will lead to spinal curvature. Having this information, the question that arises is whether these phenomena also occur in humans, and their possible connection with AIS.
4.3.3 Human Idiopathic Scoliosis and Its Link With Cerebrospinal Fluid Flow, Ciliopathies, and an Altered Proprioception
The transparency of the zebrafish larvae facilitates the visualization of CSF flow, RF dynamics and CSF-cNs connectivity, elements that conform a proprioceptive organ involved in locomotion and postural control and is also required for the maintenance of natural curvature of the spine (Figure 2). The presence of this spinal proprioceptive organ in other vertebrates has not yet been proved. However, the literature suggests that, except for humans, similar processes may be occurring in vertebrates, as they have the same anatomical elements. Thus, it has been described in most vertebrates the presence of a central canal in the spinal cord, surrounded by ciliary ependyma cells and CSF-cNs (Djenoune and Wyart, 2017), and containing the RF inside (Rodriguez et al., 1984).
On the other hand, there are arguments suggesting that in humans this proprioceptive organ would not be present, or it will have some differences. Firstly, the central canal of human spinal cord, in embryos and children, is similar to the one described in other vertebrates (Sakakibara et al., 2007). After this period, it becomes discontinuous and from the second decade of life it becomes obliterated and contains a morphologically heterogeneous accumulation of cells (Garcia-Ovejero et al., 2015). In this way, it is important to highlight that the biological material under study is obtained from autopsies, in which the spinal cord can only be dissected several hours or days after death. For this reason, the occlusion of the canal central has been largely interpreted as postmortem artifact. However, magnetic resonance imaging (MRI) studies of more than hundred individuals confirms that the central canal is absent from the vast majority of individuals beyond 18 years old, gender-independently and throughout the entire length of the spinal cord (Garcia-Ovejero et al., 2015). Secondly, although the principal component of the RF, SCO-spondin, has been described solubilized in human fetal CSF (Ortega et al., 2016), there are not reports showing the RF in the human spinal cord. In fact, the gland that secretes this protein, the SCO, suffers a progressive atrophy after childhood and is only vestigial in adult individuals (Rodriguez et al., 2001). Finally, the presence of CSF-cNs has been described in more than 200 vertebrates (reviewed in Djenoune and Wyart, 2017), but to our knowledge there are no reports showing these cells in the human spinal cord.
In spite of these differences between humans and other vertebrate organisms, there are also antecedents suggesting that cilia and spinal CSF flow are important to maintain the spinal curvature and that disorders in the proprioception are a cause of AIS. A recent study using a noninvasive and radiation-free MRI revealed disturbances in the CSF flow into the spinal cord in scoliotic individuals, especially at the thoracic level (Algin et al., 2021). This finding is promissory, although these authors analyzed the velocity of the subarachnoid CSF. Additionally, the authors mention that these alterations in the CSF flow in patients with scoliosis may be related to changes in the thoracic cavity or respiratory disturbances. It will be interesting to increase the number of individuals analyzed to know if subarachnoid CSF disturbances described into the spinal cord is the cause or a secondary effect of scoliosis.
A compromised CSF flow in human scoliosis has also been suggested in individuals with Chiari malformation type I (CM-I) with or without syringomyelia (Pinna et al., 2000; Panigrahi et al., 2004). Indeed, scoliosis is the most common deformity found in these patients, with an incidence of up to 20% (Colombo and Motta, 2011; Kelly et al., 2015). CM-I is characterized by descent protrusion of the cerebellar tonsils into the cervical spinal canal, generating a partial obliteration and impeding the free CSF movement at the cranio-cervical region (Buell et al., 2015). The prevalence of scoliosis in these patients depends on the degree of CSF flow obstruction, independent of the size of the cerebellar tonsil herniation (Shaffer et al., 2014). The current treatment proposed for CM-I patients consists in correcting the CSF flow by decompression of the cranio-cervical region, leading to the reestablishment of the normal body axis in young patients (Kelly et al., 2015). It is important to highlight that the obstruction of the CSF flow in CM-I individuals has also been studied at the subarachnoid level instead of at the central canal as performed in zebrafish studies.
In relation with the link between cilia and scoliosis in humans, is important to separate between motile cilia and primary cilia disturbances. In this way, PCD is characterized by an alteration in motile cilia, leading to chronic respiratory tract infections, abnormally positioned internal organs, and infertility (reviewed in Mirra et al., 2017). However, this pathology is not clearly associated with body axis maintenance. WES studies from two women of consanguineous families with PCD revealed that the mutation of the dynein axonemal assembly factor 2 (DNAAF2) gene may also be associated with spine malformation (Lu et al., 2021). Both patients exhibited bronchiectasis, sinusitis and infertility, classical disorders in PCD, and one of them also presented situs inversus and scoliosis. However, the spinal alteration found in this patient seems to be a consequence of the situs inversus (Schlosser et al., 2017), and not a direct consequence of the cilia motility or alterations in CSF flow.
Regarding to primary cilia function, the first WES analysis performed in diverse families affected with IS identified three different variants of the POC5 gene, which co-segregated with the disease (Patten et al., 2015). To analyze the function of this gene, a mutant version of the poc5 transcript was injected into zebrafish one- or two-cell stage, generating an overt axial phenotype after 72 hpf, ranging from mild to severe curvature of the body axis in approximately 50% of the injected embryos (Patten et al., 2015). In situ hybridization analysis performed in these embryos revealed that at 72 hpf stage, when the curved phenotype appears, this gene is expressed mainly in the brain but not in the spinal cord. The authors suggested that this mutation may be impairing the function of primary cilia, and it was confirmed in a posterior analysis in which cells that express the mutated poc5 gene have shorter cilia (Hassan et al., 2019).
In a similar report, WES analysis from patients with IS with a familiar history revealed a heterozygous variant within the ciliary kinesin family member 7 (KIF7) gene (Terhune et al., 2021a). This gene was studied in control and other IS patients, showing a different mutation in affected individuals with IS only. This finding leaded to the generation of a kif7 zebrafish crispant, which displayed severe scoliosis, present in juvenile individuals and progressing through adulthood. It is important to mention that these animals had an intact CSF flow and RF structure (Terhune et al., 2021a). By contrast, the expression of some genes related to the hedgehog (Hh) signaling is altered in kif7 mutants, suggesting a connection between the kif7 gene function and Hh pathway. Another gene, also linked to AIS susceptibility and primary cilia function, is the tubulin tyrosine ligase like gene member 11 (TTLL11) (Mathieu et al., 2021). This protein induces tubulin glutamylation, required for cilia elongation. The function of this gene was studied in a zebrafish model, and the resulting phenotype showed spinal deformity at larvae and adult stages (Mathieu et al., 2021).
In summary, sequence alterations of these three genes (POC5, KIF7 and TTLL11) found by WES analysis from scoliotic individuals suggest disturbances of primary cilia function. The posterior generation of zebrafish mutants confirmed the relevance of these genes in the maintenance of a straight body axis. In this way, the massification of WES analysis and the ease performing of CRISPR/Cas9-mediated targeted mutagenesis in zebrafish, are providing us with a powerful tool to understand the pathophysiology of human diseases, particularly scoliosis.
Other mutations affecting primary cilia function (revised in Oliazadeh et al., 2017) have been described testing the association between rare variants and scoliotic phenotypes in GWAS studies. As mentioned before, the primary cilia is present in many cell types, sensing their environment, and capable of responding to fluid flow dynamics. For example, primary cilia participate in mechanotransduction and are a crucial part of the vestibular region in the inner ear (Whitfield, 2020), a proprioceptive organ capable of detecting gravity, acceleration, and orientation of the body (similar to the proprioceptive organ formed by CSF-cNs and RF in zebrafish). This detection requires the tethering of calcified masses to the cilia of mechanosensory hair cells. The mammalian ear contains thousands of biomineralized particles called otoconias, whereas the inner ear of zebrafish contains three large ear stones called otoliths that execute a similar function (Petko et al., 2008). A defect in the otolith formation is characteristic in most of the zebrafish ciliary mutants and morphants (Whitfield, 2020).
The link between AIS and vestibular disturbances has been well documented (Wiener-Vacher and Mazda, 1998; Pollak et al., 2013; Hitier et al., 2015; Antoniadou et al., 2018; Hatzilazaridis et al., 2019), suggesting that in response to the asymmetry between the left and right vestibular organs, the individuals adopt an asymmetric posture and activation of the axial muscles that in turn generates a spine deformity. This bilateral organ is highly conserved in vertebrates and projects to various spinal cord level, forming the lateral and medial vestibule-spinal tracts, the tangential vestibular nucleus and vestibule-reticule-spinal projections, controlling motor targets at different spinal levels (reviewed in Deliagina et al., 2014; Gordy and Straka, 2021). The relevance of this proprioceptive organ in the maintenance of the body axis has been demonstrated in different vertebrates, such as Xenopus, in which the unilateral removal of this organ generates a severe curvature in the spinal cord (Lambert et al., 2009). In this way, the zebrafish has been proposed as a suitable model to study the vestibular function (Bagnall and Schoppik, 2018). Finally, it is interesting to note that the expression of Otoc1, a protein required for the correct otolith formation, coincides almost exactly with the expression pattern of SCO-spondin (Petko et al., 2008). Also, Otogelin, a protein required for otoliths tethering to the hair cells has identical SCO-spondin’s functional domains, as revealed by comparing their amino acid sequences (VS and TC, unpublished).
5 Mechanisms of Inflammation and Scoliosis: Emerging Models of an Immune Response-Mediated Spine Curvature Regulation
Inflammation is a physiological process responsible for restoring structural and functional homeostasis in tissues altered by different harmful stimuli, including infectious, mechanical, chemical, or physical factors (Serhan et al., 2007; Takeuchi and Akira, 2010; Klein-Wieringa et al., 2016). Also, inflammation is characterized by classic symptoms such as heat, pain, swelling and redness, among others (Klein-Wieringa et al., 2016). Although AIS causes remain controversial, studies into the molecular mechanisms of spine formation have revealed an active involvement of the inflammatory response in the development of this highly prevalent structural disorder (Table 2). However, while the influence of inflammation as a trigger of AIS development remains unclear, some studies indicate that infection and altered immune mechanisms might be correlated with this pathology (Papanastasiou et al., 2010; Sanders et al., 2012; Van Gennip et al., 2018), as well as the appearance of scoliotic phenotypes (Klein-Wieringa et al., 2016). In the next section, we will discuss how infectious agents, external stressors, and inflammatory responses promote scoliosis and spine malformations in zebrafish.
5.1 Infectious Agents and Spine Malformations
It has been shown that infections by pathogens, such as the fungus Pseudoloma neurophilia, affect the nervous and muscular system, generating scoliosis in zebrafish (Sanders et al., 2012). The infection produced by P. neurophilia is chronic and difficult to control. Indeed, when the inflammatory process is perpetuated, the action of immune cells to eliminate this fungus through phagocytosis releases oxidative substances and hydrolytic enzymes, which can harm the zebrafish body (Nathan, 2002; Camicia and de Larranaga, 2013; Serhan, 2014). Ultimately, generates an abnormal phenotype consisting of evident signs of spinal deformities and slimming (Matthews et al., 2001).
On the other hand, the Hyper-Immunoglobulin E immune disease, which is characterized by excessive production of E antibodies and recurrent infections by the Staphylococcus aureus, has non-immune symptoms associated with the development of scoliosis (Grimbacher et al., 1999). However, recent pathophysiological studies of this disease have shown an alteration in the binding capacity of the signal transducer and activator of transcription 3 (STAT3) to target DNA sequences, thus limiting the response to the stimulation of interleukin 6 (Papanastasiou et al., 2010). STAT3 regulates the passage of the inflammatory to an anti-inflammatory response (Levy and Lee, 2002; Hillmer et al., 2016; Sorg et al., 2020) and zebrafish mutants show a dysfunction expression of collagen genes during the pathogenesis of scoliosis (Xiong et al., 2017). This highlights the importance of STAT3 as a regulator of various responses, including inflammation, for the correct development of the vertebral axis.
5.2 Inflammation, Oxidative Stressors, and Neurodegenerative Processes Involved in Zebrafish Scoliosis
In addition to pathogens, oxidative stressors are involved in scoliosis generation. For instance, Tenor et al. (2002) showed that up-regulation of phosphodiesterase 4 (PDE4) in chondrocytes influenced by an inflammatory environment led to increased nitric oxide (NO) levels. The degradation of cartilage, and a subsequent spine malformation, was accelerated in response to interleukin-1β in vitro through known mediators, including prostaglandin E2, NO, and metalloproteinases (Tenor et al., 2002; Tenor et al., 2011). In zebrafish, recent studies have found that ptk7 mutants also exhibit up-regulation of reactive oxygen species-related genes, suggesting a link between oxidative stressors and scoliosis. Interestingly, when juvenile zebrafish ptk7 mutants were treated with the antioxidant agent N-acetylcysteine (NAC), the incidence of scoliosis and the severity of spinal curvature were considerably reduced (Van Gennip et al., 2018). This confirms the interplay between oxidative stressors and the development, as well as the severity, of spine malformations. In addition, the study of zebrafish sspo mutant larvae has provided significant progress in our understanding of the link between oxidative stressors and scoliosis. In sspo mutants, the high mortality due to the development of a curly tail down phenotype is largely rescued by NAC ethyl ester (NACET) treatment, suggesting that an oxidative stress-dependent immune response controls the degree of scoliosis in zebrafish (Rose et al., 2020). Remarkably, the use of antioxidants and anti-inflammatory agents reverse the morphological alterations of the body axis. Therefore, we foresee that future efforts will be crucial to better understand the effect of oxidizing agents in scoliosis development.
Several studies have also indicated that neurodegenerative demyelination processes occur with episodes of stress and chronic inflammation (Carter et al., 1995; Feehan and Gilroy, 2019; Desplats et al., 2020). For instance, failures in myelination increase microglial activation, inflammation, and spine malformation in vertebrates (Monk et al., 2011; Mogha et al., 2013). Low levels of Leptin, an enzyme involved in myelination, have been linked to a high risk of scoliosis in adolescents (Qiu et al., 2007; Liang et al., 2012; Liu et al., 2012). Additionally, Leptin participates in the differentiation and mineralization of the bone matrix, promoting the proliferation of osteoblasts and chondrocytes, and inhibiting osteoclastic activity (Wu et al., 2012; Man et al., 2019). Increases in osteoclast differentiation and functionality have been observed in cases of scoliosis, also associated with the incorrect activation of STAT3 (Minegishi et al., 2007).
There is a growing interest in studying the effect of oxidizing agents in scoliosis development. This, can be conducted by using genetic and pharmacological approaches. Thus, the identification of oxidative and neurodegenerative stress markers in zebrafish models of AIS emerges as a valuable tool to investigate how stress-responsive mechanisms are involved in spine morphogenesis.
5.3 Cerebrospinal Fluid and Inflammatory Response Involved in Zebrafish Scoliosis
As discussed earlier, the etiology of AIS has been associated with functional disturbances in genes related to cilia motility, CSF flow, and inflammatory response (Van Gennip et al., 2018). Over-expression of proinflammatory factors, such as tumor necrosis factor alpha (TNF-α), confirmed a neuroinflammatory response in sspo zebrafish mutants, both at embryonic (3 dpf showing a curvy tail down phenotype) and post-embryonic stages (Figure 3) (Rose et al., 2020). In vivo monitoring of immune cells showed that the accumulation of macrophages in the curved areas, specifically within the medullary zone (Figure 3). Conversely, the migration of macrophages and neutrophils to the telencephalon was visualized in the zebrafish sspo mutant larvae in association with high expression of the tnf-α gene (Rose et al., 2020). In addition, transcriptomic and differential gene expression analysis of brains isolated from 21 dpf heterozygous sspo mutants revealed that inflammation-related genes were up-regulated compared to wild-type siblings (Rose et al., 2020). Similar results were found in the ptk7 mutant (Van Gennip et al., 2018).
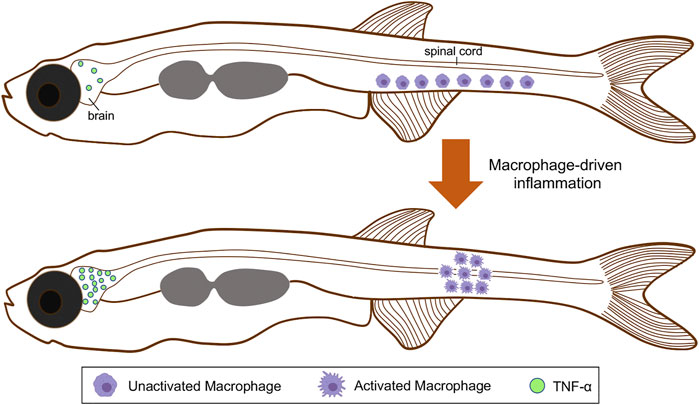
FIGURE 3. Schematic representation of macrophages activation and spatial distribution, and the regionalized inflammatory response triggered during scoliosis development. Colored macrophages (purple) are shown. At the onset of the spinal curve formation, immature macrophages are distributed along the ventral side of the tail. After their activation, these cells move and accumulate within the spinal cord. Macrophages maturation also triggers a neuroinflammatory response associated with TNF-α overexpression in the brain. TNF-α, tumor necrosis factor alpha.
Furthermore, the relevance of the inflammation response triggered by a spinal curvature was demonstrated through anti-inflammatory drug treatment, which suppressed the severity and incidence of spine defects in cilia ptk7 mutants (Van Gennip et al., 2018). In the same way, the treatment of zebrafish sspo mutant embryos with a cyclooxygenase-2 (COX-2) inhibitor can fully rescue normal axial development, even though the same animals developed scoliosis during early juvenile stages (Rose et al., 2020).
Also, the zebrafish ptk7 mutant displaying impaired CSF movement and ciliary structure also exhibits inflammatory and demyelinating events (Hayes et al., 2014). These data support the idea that an inflammatory response is activated in the development of scoliosis caused by the alteration of CSF flow (Van Gennip et al., 2018; Rose et al., 2020). On the other hand, the arrival of cellular reinforcements and other mediators during inflammation can alter the properties of the CSF. Thus, changes in the osmolarity between CSF interstitial and blood fluids occur depending on the proximity to the inflammatory focus, negatively affecting communication signals necessary for the correct posture of the vertebral axis (Klarica et al., 2013; Bohm et al., 2016).
Altogether, these studies indicate that inflammation play an important role in disturbing the homeostasis of various signaling pathways in generating spinal curvatures. Therefore, it is necessary to pursue the study of inflammation pathways during the development of scoliosis. Such an approach will undoubtedly identify novel factors controlling spinal morphology, coupled with functional monogenic interrogation in vertebrate animals (e.g., zebrafish) will provide new ways of preventing and treatment for AIS in humans.
6 Phenogenetic Data Integration and Scoliosis Modeling in the Post-Genomic Era
Currently, the availability of complete genome sequences of many model organisms is allowing us to evaluate gene function and decipher the complex crosstalk between genomics and phenomics. In this quest, deep phenotyping is a valuable approach to capture subtle and dynamic cellular and molecular variations (Bilder et al., 2009; Brown et al., 2018; Fuentes et al., 2018). This consists of a multi-scale experimental approach aiming at searching, measuring, and analyzing the biological origins of quantifiable traits; thus, unraveling genotype-phenotype associations and explaining how genomic information is expressed to determine complex morphological features. In this sense, zebrafish is amenable for gene function discovery, and efficient and rapid phenotyping; therefore, it represents an ideal multicellular model for deep phenotyping (Fuentes et al., 2018).
Traditional and automated histological techniques such as high-throughput histology and micron-scale computed tomography (microCT) have recently been used to describe mutant phenotypes, including those showing alterations in skeleton morphogenesis. Thus, whole-mount staining and high-resolution imaging of juvenile and adult animals are being exploited to generate precise phenogenetic information of organ systems and tissue morphology as well as physiological defects at cell resolution level, including the spine architecture (Sabaliauskas et al., 2006; Charles et al., 2017; Henke et al., 2017; Hur et al., 2017; Weinhardt et al., 2018; Ding et al., 2019; Kwon et al., 2019; Marie-Hardy et al., 2019). This, has been pivotal to understand gene function and AIS etiology caused by single gene lesions (Grimes et al., 2016; Gistelinck et al., 2018; Zhang et al., 2018; Gray et al., 2020; Lu et al., 2020; Rose et al., 2020; Sun et al., 2020; Troutwine et al., 2020).
Although microCT imaging has allowed quantitative morphometric analysis of the spine defects in zebrafish AIS-like models, it has also revealed subtle phenotypic deviations that would not be observable by the naked eye. The study of post-embryonic features by using deep skeletal phenotyping identified spatial bone mineralization patterns within the axial skeleton (Hur et al., 2017). For instance, computational-based morphological and densitometric analysis of mutant phenotypes demonstrated the value of linking interrogation of genes requirements and phenomic profiling (Hur et al., 2017).
In addition to mutagenesis approaches, complementary high-resolution and -quality 3D axial skeleton reconstructions will help determine the morphological effects of manifested mutations generating spine malformations (Hur et al., 2017; Weinhardt et al., 2018; Ding et al., 2019; Kwon et al., 2019; Marie-Hardy et al., 2019). Importantly, sequencing technologies will reveal a list of zebrafish genes and genetic pathways for phenomic analysis to systematically describe and define their function. In this context, the zebrafish model is advantageous because: i) it is genetically tractable and there is a broad range of manipulation methods, most of them relatively easy to perform, and ii) it is possible to conduct molecular manipulations that can be followed over time through geometric morphometrics approaches. Thus, patterns of deformations can be detected and deliver data to model spine biomechanical performance in the context of a living vertebrate organism for being extrapolated to human AIS studies.
7 Discussion
Scoliosis is the most common deformity of the spine in humans. Among the different types of scoliosis, AIS represents a predominant spine deformity worldwide. Since the first report of AIS in humans (Wynne-Davies, 1968), our knowledge of such spine malformation has increased considerably, especially in the cellular, molecular, and genetic aspects. On the other hand, with the dawn of the genomic revolution, there has been a substantial increase in our knowledge of the molecular nature of genes and their function associated with scoliosis. Despite the use of a wide diversity of animal model systems used to study AIS, the etiology of this spine deformity is far away to be understood. This, has been partially caused by the lack of animal models that recapitulate the genotype-phenotype relationships to unravel the etiology of AIS. With the aim to identify current knowledge gaps around AIS and propose future animal model systems to investigate this condition, we here focused on what is known about the cellular, molecular, and functional processes underlying spine malformations in zebrafish. We highlighted the potential of zebrafish as a phenogenetic platform to understand the functional and molecular program underlying AIS-like phenotypes.
Several mechanisms and features of zebrafish spine deformity, such as the role of ciliogenesis, CSF flow, and inflammation, have been investigated in mutants to particular human AIS-related genes. Although the contribution of zebrafish as a genetic system has been crucial to study spine malformation, it is unclear if this animal model recapitulates the pathogenesis cause for all known human AIS risk loci. Further knowledge of the genetic factors controlling the spine formation is critical to understand the etiology of AIS. By doing so, it will reveal candidate genes associated to this malformation and allow the development of new therapeutic alternatives to improve the quality of life of humans worldwide.
The progress in the study and definition of genetic risk factors for human scoliosis appears to be limited in the ability to link specific genomic sequences with the disease, which could increase clinical biases and imprecise genotype-phenotype associations. High throughput next-generation sequencing has unveiled the dysregulation of thousands of non-coding RNAs, including microRNAs (miRNAs) and long non-coding RNAs (lncRNAs), linked to scoliosis (Garcia-Gimenez et al., 2018; Li et al., 2020). Indeed, recent studies using blood samples of patients with AIS have detected dysregulated miRNAs levels in humans (Ogura et al., 2017; Garcia-Gimenez et al., 2018). Further analysis of their targets and activation of cellular pathways have indicated that miRNA imbalance could change the homeostasis of bone formation and destruction, and by doing so, ultimately contributing to the AIS pathology (Garcia-Gimenez et al., 2018). Another type of non-coding RNAs with a putative role in scoliosis corresponds to lncRNAs. A comprehensive screening of lncRNA and mRNAs in AIS patients showed that 139 lncRNAs were differentially expressed compared with healthy controls (Liu et al., 2015). The aforementioned link between ncRNAs and scoliosis has revealed new information from AIS patients. Thus, a great number of these dysregulated molecules could represent an additional layer of regulation and potential diagnostic biomarkers of this multifactorial pathology. However, genetically tractable models to understand molecular genetics and identify conserved ncRNAs linked to AIS in humans are needed. Therefore, future phenotyping efforts are urgently needed to explore the role of these non-coding RNAs during spine zebrafish morphogenesis, which in turn will help determine the importance of these molecules in AIS.
In addition, the integration of disciplines, such as genomics and phenomics, into the study of zebrafish spine malformations will provide a description of the molecular, morphological, physiological and behavioral underpinning scoliosis/AIS. This will allow us to construct molecular genotype-phenotype maps that would be valuable resources to investigate not only this condition, but also other human diseases. In light of this, further efforts to construct molecular genotype-phenotype maps in zebrafish will provide guiding principles into human scoliosis/AIS. Taken together, we foresee that by using zebrafish, along with phenogenetic approaches would then in turn allow to progressively conduct novel therapies to treat scoliosis in humans.
Author Contributions
CM-M, AR, VS, MV, KF, SM, FA, TC and RF contributed to conception and design of the article. CM-M, MV and RF prepared the figures. CM-M, TC and RF wrote the first draft of the manuscript. CM-M, AR, VS, KF, SM, FA, TC and RF wrote sections of the manuscript. All authors contributed to manuscript revision, read, and approved the submitted version.
Funding
This work was supported by Agencia Nacional de Investigación y Desarrollo (ANID) Beca Magister Nacional (22191268) to CM-M, ANID Proyecto PAI Inserción en la Academia (PAI79170033) and ANID Proyecto Fondecyt de Iniciación (11180084) to FA, ANID Proyecto Fondecyt Regular (1191860) to TC, and Apoyo FCBI 2019-01, Facultad de Ciencias Biológicas, Universidad de Concepción, and ANID Proyecto Fondecyt de Iniciación (11201118) to RF.
Conflict of Interest
The authors declare that the research was conducted in the absence of any commercial or financial relationships that could be construed as a potential conflict of interest.
Publisher’s Note
All claims expressed in this article are solely those of the authors and do not necessarily represent those of their affiliated organizations, or those of the publisher, the editors and the reviewers. Any product that may be evaluated in this article, or claim that may be made by its manufacturer, is not guaranteed or endorsed by the publisher.
Acknowledgments
We thank Constanza Aguirre and Claudia Montecinos for providing figures from Biorender.com and figures drawing, respectively. We apologize not to have been able to include many interesting articles. Fortunately, these are easily accessible in well-known resources.
References
Acaroglu, R. E., Akel, A., Alanay, M., Yazici, M., and Marcucio, R. (2009). Comparison of the melatonin and calmodulin in paravertebral muscle and platelets of patients with or without adolescent ıdiopathic scoliosis. Spine J. 18, E659-63.
Adham, I. M., Gille, M., Gamel, A. J., Reis, A., Dressel, R., Steding, G., et al. (2005). The Scoliosis (Sco) Mouse: a New Allele of Pax1. Cytogenet. Genome Res. 111 (1), 16–26. doi:10.1159/000085665
Alejevski, F., Leemans, M., Gaillard, A.-L., Leistenschneider, D., de Flori, C., Bougerol, M., et al. (2021). Conserved Role of the Urotensin II Receptor 4 Signalling Pathway to Control Body Straightness in a Tetrapod. Open Biol. 11 (8), 210065. doi:10.1098/rsob.210065
Algin, O., Koc, U., and Yalcin, N. (2021). Cerebrospinal Fluid Velocity Changes of Idiopathic Scoliosis: a Preliminary Study on 3-T PC-MRI and 3D-SPACE-VFAM Data. Childs Nerv Syst. doi:10.1007/s00381-021-05339-w
Andersen, M. O., Thomsen, K., and Kyvik, K. O. (20071976). Adolescent Idiopathic Scoliosis in Twins. Spine 32 (8), 927–930. doi:10.1097/01.brs.0000259865.08984.00
Andrades, J. A., Becerra, J., and Fernández-Llebrez, P. (1994). Skeletal Deformities of the Gilthead Sea Bream (Spams Aurata, L.): Study of the Subcommissural Organ (SCO) and Reissner’s Fiber (RF). Ann. Anat. - Anatomischer Anzeiger 176 (4), 381–383. doi:10.1016/s0940-9602(11)80524-8
Antoniadou, N., Hatzitaki, V., Stavridis, S. I., and Samoladas, E. (2018). Verticality Perception Reveals a Vestibular Deficit in Adolescents with Idiopathic Scoliosis. Exp. Brain Res. 236 (6), 1725–1734. doi:10.1007/s00221-018-5256-9
Anvarian, Z., Mykytyn, K., Mukhopadhyay, S., Pedersen, L. B., and Christensen, S. T. (2019). Cellular Signalling by Primary Cilia in Development, Organ Function and Disease. Nat. Rev. Nephrol. 15 (4), 199–219. doi:10.1038/s41581-019-0116-9
Arratia, G., Schultze, H.-P., and Casciotta, J. (2001). Vertebral Column and Associated Elements in Dipnoans and Comparison with Other Fishes: Development and Homology. J. Morphol. 250 (2), 101–172. doi:10.1002/jmor.1062
Bagnall, K. M., Harris, P. F., and Jones, P. R. (1977). A Radiographic Study of the Human Fetal Spine. 1. The Development of the Secondary Cervical Curvature. J. Anat. 123 (Pt 3), 777–782.
Bagnall, M. W., and Schoppik, D. (2018). Development of Vestibular Behaviors in Zebrafish. Curr. Opin. Neurobiol. 53, 83–89. doi:10.1016/j.conb.2018.06.004
Baschal, E. E., Wethey, C. I., Swindle, K., Baschal, R. M., Gowan, K., Tang, N. L., et al. (2014). Exome Sequencing Identifies a Rare HSPG2 Variant Associated with Familial Idiopathic Scoliosis. G3 (Bethesda) 5 (2), 167–174. doi:10.1534/g3.114.015669
Baye, L. M., Patrinostro, X., Swaminathan, S., Beck, J. S., Zhang, Y., Stone, E. M., et al. (2011). The N-Terminal Region of Centrosomal Protein 290 (CEP290) Restores Vision in a Zebrafish Model of Human Blindness. Hum. Mol. Genet. 20 (8), 1467–1477. doi:10.1093/hmg/ddr025
Bearce, E. A., and Grimes, D. T. (2021). On Being the Right Shape: Roles for Motile Cilia and Cerebrospinal Fluid Flow in Body and Spine Morphology. Semin. Cel Develop. Biol. 110, 104–112. doi:10.1016/j.semcdb.2020.07.005
Bilder, R. M., Sabb, F. W., Cannon, T. D., London, E. D., Jentsch, J. D., Parker, D. S., et al. (2009). Phenomics: the Systematic Study of Phenotypes on a Genome-wide Scale. Neuroscience 164 (1), 30–42. doi:10.1016/j.neuroscience.2009.01.027
Bird, N. C., and Mabee, P. M. (2003). Developmental Morphology of the Axial Skeleton of the Zebrafish, Danio rerio (Ostariophysi: Cyprinidae). Dev. Dyn. 228 (3), 337–357. doi:10.1002/dvdy.10387
Bobyn, J. D., Little, D. G., Gray, R., and Schindeler, A. (2015). Animal Models of Scoliosis. J. Orthop. Res. 33 (4), 458–467. doi:10.1002/jor.22797
Böhm, U. L., Prendergast, A., Djenoune, L., Nunes Figueiredo, S., Gomez, J., Stokes, C., et al. (2016). CSF-contacting Neurons Regulate Locomotion by Relaying Mechanical Stimuli to Spinal Circuits. Nat. Commun. 7, 10866. doi:10.1038/ncomms10866
Boswell, C. W., and Ciruna, B. (2017). Understanding Idiopathic Scoliosis: A New Zebrafish School of Thought. Trends Genet. 33 (3), 183–196. doi:10.1016/j.tig.2017.01.001
Brand, M., Heisenberg, C. P., Warga, R. M., Pelegri, F., Karlstrom, R. O., Beuchle, D., et al. (1996). Mutations Affecting Development of the Midline and General Body Shape during Zebrafish Embryogenesis. Development 123, 129–142. doi:10.1242/dev.123.1.129
Brand-Saberi, B., and Christ, B. (2000). Evolution and Development of Distinct Cell Lineages Derived from Somites. Curr. Top. Dev. Biol. 48, 1–42. doi:10.1016/s0070-2153(08)60753-x
Breslow, D. K., Hoogendoorn, S., Kopp, A. R., Morgens, D. W., Vu, B. K., Kennedy, M. C., et al. (2018). A CRISPR-Based Screen for Hedgehog Signaling Provides Insights into Ciliary Function and Ciliopathies. Nat. Genet. 50 (3), 460–471. doi:10.1038/s41588-018-0054-7
Brinker, T., Stopa, E., Morrison, J., and Klinge, P. (2014). A New Look at Cerebrospinal Fluid Circulation. Fluids Barriers CNS 11, 10. doi:10.1186/2045-8118-11-10
Brodbelt, A., and Stoodley, M. (2007). CSF Pathways: a Review. Br. J. Neurosurg. 21 (5), 510–520. doi:10.1080/02688690701447420
Brown, S. D. M., Holmes, C. C., Mallon, A.-M., Meehan, T. F., Smedley, D., and Wells, S. (2018). High-throughput Mouse Phenomics for Characterizing Mammalian Gene Function. Nat. Rev. Genet. 19 (6), 357–370. doi:10.1038/s41576-018-0005-2
Buchan, J. G., Gray, R. S., Gansner, J. M., Alvarado, D. M., Burgert, L., Gitlin, J. D., et al. (2014). Kinesin Family Member 6 (Kif6) Is Necessary for Spine Development in Zebrafish. Dev. Dyn. 243 (12), 1646–1657. doi:10.1002/dvdy.24208
Buell, T. J., Heiss, J. D., and Oldfield, E. H. (2015). Pathogenesis and Cerebrospinal Fluid Hydrodynamics of the Chiari I Malformation. Neurosurg. Clin. North America 26 (4), 495–499. doi:10.1016/j.nec.2015.06.003
Camicia, G., and de Larrañaga, G. (2013). Trampas extracelulares de neutrófilos: un mecanismo de defensa con dos caras. Medicina Clínica 140 (2), 70–75. doi:10.1016/j.medcli.2012.04.022
Cantaut-Belarif, Y., Orts Del’Immagine, A., Penru, M., Pézeron, G., Wyart, C., and Bardet, P. L. (2020). Adrenergic Activation Modulates the Signal from the Reissner Fiber to Cerebrospinal Fluid-Contacting Neurons during Development. Elife 9. doi:10.7554/eLife.59469
Cantaut-Belarif, Y., Sternberg, J. R., Thouvenin, O., Wyart, C., and Bardet, P.-L. (2018). The Reissner Fiber in the Cerebrospinal Fluid Controls Morphogenesis of the Body Axis. Curr. Biol. 28 (15), 2479–2486. e2474. doi:10.1016/j.cub.2018.05.079
Caprile, T., Hein, S., Rodríguez, S., Montecinos, H., and Rodríguez, E. (2003). Reissner Fiber Binds and Transports Away Monoamines Present in the Cerebrospinal Fluid. Mol. Brain Res. 110 (2), 177–192. doi:10.1016/s0169-328x(02)00565-x
Carter, G. T., Abresch, R. T., Fowler, W. M., Johnson, E. R., Kilmer, D. D., and McDonald, C. M. (1995). Profiles of Neuromuscular Diseases. Hereditary Motor and Sensory Neuropathy, Types I and II. Am. J. Phys. Med. Rehabil. 74 (5 Suppl. l), S140–S149. doi:10.1097/00002060-199509001-00008
Castelein, R. M., Dieën, J. H. v., and Smit, T. H. (2005). The Role of Dorsal Shear Forces in the Pathogenesis of Adolescent Idiopathic Scoliosis - A Hypothesis. Med. Hypotheses 65 (3), 501–508. doi:10.1016/j.mehy.2005.03.025
Chanock, S. J., Manolio, T., Boehnke, M., Boerwinkle, E., Hunter, D. J., Thomas, G., et al. NCI-NHGRI Working Group on Replication in Association Studies (2007). Replicating Genotype-Phenotype Associations. Nature 447 (7145), 655–660. doi:10.1038/447655a
Charles, J. F., Sury, M., Tsang, K., Urso, K., Henke, K., Huang, Y., et al. (2017). Utility of Quantitative Micro-computed Tomographic Analysis in Zebrafish to Define Gene Function during Skeletogenesis. Bone 101, 162–171. doi:10.1016/j.bone.2017.05.001
Cheng, J. C., Castelein, R. M., Chu, W. C., Danielsson, A. J., Dobbs, M. B., Grivas, T. B., et al. (2015). Adolescent Idiopathic Scoliosis. Nat. Rev. Dis. Primers 1, 15030. doi:10.1038/nrdp.2015.30
Choufani, E., Jouve, J.-L., Pomero, V., Adalian, P., Chaumoitre, K., and Panuel, M. (2009). Lumbosacral Lordosis in Fetal Spine: Genetic or Mechanic Parameter. Eur. Spine J. 18 (9), 1342–1348. doi:10.1007/s00586-009-1012-y
Colombo, L. F., and Motta, F. (2011). Consensus Conference on Chiari: a Malformation or an Anomaly? Scoliosis and Others Orthopaedic Deformities Related to Chiari 1 Malformation. Neurol. Sci. 32 (Suppl. 3), S341–S343. doi:10.1007/s10072-011-0689-y
Deliagina, T. G., Beloozerova, I. N., Orlovsky, G. N., and Zelenin, P. V. (2014). Contribution of Supraspinal Systems to Generation of Automatic Postural Responses. Front. Integr. Neurosci. 8, 76. doi:10.3389/fnint.2014.00076
Dequéant, M.-L., and Pourquié, O. (2008). Segmental Patterning of the Vertebrate Embryonic axis. Nat. Rev. Genet. 9 (5), 370–382. doi:10.1038/nrg2320
Desplats, P., Gutierrez, A. M., Antonelli, M. C., and Frasch, M. G. (2020). Microglial Memory of Early Life Stress and Inflammation: Susceptibility to Neurodegeneration in Adulthood. Neurosci. Biobehavioral Rev. 117, 232–242. doi:10.1016/j.neubiorev.2019.10.013
Ding, Y., Vanselow, D. J., Yakovlev, M. A., Katz, S. R., Lin, A. Y., Clark, D. P., et al. (2019). Computational 3D Histological Phenotyping of Whole Zebrafish by X-ray Histotomography. Elife 8, e44898. doi:10.7554/eLife.44898
Djenoune, L., Desban, L., Gomez, J., Sternberg, J. R., Prendergast, A., Langui, D., et al. (2017). The Dual Developmental Origin of Spinal Cerebrospinal Fluid-Contacting Neurons Gives Rise to Distinct Functional Subtypes. Sci. Rep. 7 (1), 719. doi:10.1038/s41598-017-00350-1
Djenoune, L., and Wyart, C. (2017). Light on a Sensory Interface Linking the Cerebrospinal Fluid to Motor Circuits in Vertebrates. J. Neurogenet. 31 (3), 113–127. doi:10.1080/01677063.2017.1359833
Driever, W., Solnica-Krezel, L., Schier, A. F., Neuhauss, S. C., Malicki, J., Stemple, D. L., et al. (1996). A Genetic Screen for Mutations Affecting Embryogenesis in Zebrafish. Development 123, 37–46. doi:10.1242/dev.123.1.37
Fame, R. M., and Lehtinen, M. K. (2020). Emergence and Developmental Roles of the Cerebrospinal Fluid System. Develop. Cel 52 (3), 261–275. doi:10.1016/j.devcel.2020.01.027
Feehan, K. T., and Gilroy, D. W. (2019). Is Resolution the End of Inflammation? Trends Mol. Med. 25 (3), 198–214. doi:10.1016/j.molmed.2019.01.006
Fleming, A., Keynes, R., and Tannahill, D. (2004). A central Role for the Notochord in Vertebral Patterning. Development 131 (4), 873–880. doi:10.1242/dev.00952
Fleming, A., Kishida, M. G., Kimmel, C. B., and Keynes, R. J. (2015). Building the Backbone: the Development and Evolution of Vertebral Patterning. Development 142 (10), 1733–1744. doi:10.1242/dev.118950
Forero, L. L., Narayanan, R., Huitema, L. F., VanBergen, M., Apschner, A., Peterson-Maduro, J., et al. (2018). Segmentation of the Zebrafish Axial Skeleton Relies on Notochord Sheath Cells and Not on the Segmentation Clock. Elife 7, e33843. doi:10.7554/eLife.33843
Fuentes, R., Letelier, J., Tajer, B., Valdivia, L. E., and Mullins, M. C. (2018). Fishing Forward and Reverse: Advances in Zebrafish Phenomics. Mech. Develop. 154, 296–308. doi:10.1016/j.mod.2018.08.007
Fuentes, R., Tajer, B., Kobayashi, M., Pelliccia, J. L., Langdon, Y., Abrams, E. W., et al. (2020). The Maternal Coordinate System: Molecular-Genetics of Embryonic axis Formation and Patterning in the Zebrafish. Curr. Top. Dev. Biol. 140, 341–389. doi:10.1016/bs.ctdb.2020.05.002
Gao, W., Chen, C., Zhou, T., Yang, S., Gao, B., Zhou, H., et al. (2017). Rare Coding Variants in MAPK7 Predispose to Adolescent Idiopathic Scoliosis. Hum. Mutat. 38 (11), 1500–1510. doi:10.1002/humu.23296
Gao, X., Gordon, D., Zhang, D., Browne, R., Helms, C., Gillum, J., et al. (2007). CHD7 Gene Polymorphisms Are Associated with Susceptibility to Idiopathic Scoliosis. Am. J. Hum. Genet. 80 (5), 957–965. doi:10.1086/513571
García-Giménez, J. L., Rubio-Belmar, P. A., Peiró-Chova, L., Hervás, D., González-Rodríguez, D., Ibañez-Cabellos, J. S., et al. (2018). Circulating miRNAs as Diagnostic Biomarkers for Adolescent Idiopathic Scoliosis. Sci. Rep. 8 (1), 2646. doi:10.1038/s41598-018-21146-x
Garcia-Ovejero, D., Arevalo-Martin, A., Paniagua-Torija, B., Florensa-Vila, J., Ferrer, I., Grassner, L., et al. (2015). The Ependymal Region of the Adult Human Spinal Cord Differs from Other Species and Shows Ependymoma-like Features. Brain 138 (Pt 6), 1583–1597. doi:10.1093/brain/awv089
Gistelinck, C., Kwon, R. Y., Malfait, F., Symoens, S., Harris, M. P., Henke, K., et al. (2018). Zebrafish Type I Collagen Mutants Faithfully Recapitulate Human Type I Collagenopathies. Proc. Natl. Acad. Sci. USA 115 (34), E8037–E8046. doi:10.1073/pnas.1722200115
Good, C. R., Auerbach, J. D., O’Leary, P. T., and Schuler, T. C. (2011). Adult Spine Deformity. Curr. Rev. Musculoskelet. Med. 4 (4), 159–167. doi:10.1007/s12178-011-9101-z
Gordy, C., and Straka, H. (2021). Vestibular Influence on Vertebrate Skeletal Symmetry and Body Shape. Front. Syst. Neurosci. 15, 753207. doi:10.3389/fnsys.2021.753207
Gorman, K. F., and Breden, F. (2009). Idiopathic-type Scoliosis Is Not Exclusive to Bipedalism. Med. Hypotheses 72 (3), 348–352. doi:10.1016/j.mehy.2008.09.052
Gorman, K. F., Tredwell, S. J., and Breden, F. (20071976). The Mutant Guppy Syndrome Curveback as a Model for Human Heritable Spinal Curvature. Spine 32 (7), 735–741. doi:10.1097/01.brs.0000259081.40354.e2
Grauers, A., Rahman, I., and Gerdhem, P. (2012). Heritability of Scoliosis. Eur. Spine J. 21 (6), 1069–1074. doi:10.1007/s00586-011-2074-1
Gray, R. S., Gonzalez, R., Ackerman, S. D., Minowa, R., Griest, J. F., Bayrak, M. N., et al. (2020). Postembryonic Screen for Mutations Affecting Spine Development in Zebrafish. Dev. Biol. 471, 18–33. doi:10.1016/j.ydbio.2020.11.009
Gray, R. S., Wilm, T. P., Smith, J., Bagnat, M., Dale, R. M., Topczewski, J., et al. (2014). Loss of Col8a1a Function during Zebrafish Embryogenesis Results in Congenital Vertebral Malformations. Develop. Biol. 386 (1), 72–85. doi:10.1016/j.ydbio.2013.11.028
Grimbacher, B., Holland, S. M., Gallin, J. I., Greenberg, F., Hill, S. C., Malech, H. L., et al. (1999). Hyper-IgE Syndrome with Recurrent Infections - an Autosomal Dominant Multisystem Disorder. N. Engl. J. Med. 340 (9), 692–702. doi:10.1056/nejm199903043400904
Grimes, D. T., Boswell, C. W., Morante, N. F. C., Henkelman, R. M., Burdine, R. D., and Ciruna, B. (2016). Zebrafish Models of Idiopathic Scoliosis Link Cerebrospinal Fluid Flow Defects to Spine Curvature. Science 352 (6291), 1341–1344. doi:10.1126/science.aaf6419
Grimes, D. T. (2019). Developmental Biology: Go with the Flow to Keep the Body Straight. Curr. Biol. 29 (3), R101–R103. doi:10.1016/j.cub.2018.12.011
Grotmol, S., Kryvi, H., Nordvik, K., and Totland, G. K. (2003). Notochord Segmentation May Lay Down the Pathway for the Development of the Vertebral Bodies in the Atlantic salmon. Anat. Embryol. (Berl) 207 (4-5), 263–272. doi:10.1007/s00429-003-0349-y
Grotmol, S., Nordvik, K., Kryvi, H., and Totland, G. K. (2005). A Segmental Pattern of Alkaline Phosphatase Activity within the Notochord Coincides with the Initial Formation of the Vertebral Bodies. J. Anat. 206 (5), 427–436. doi:10.1111/j.1469-7580.2005.00408.x
Guo, L., Yamashita, H., Kou, I., Takimoto, A., Meguro-Horike, M., Horike, S.-i., et al. (2016). Functional Investigation of a Non-coding Variant Associated with Adolescent Idiopathic Scoliosis in Zebrafish: Elevated Expression of the Ladybird Homeobox Gene Causes Body Axis Deformation. Plos Genet. 12 (1), e1005802. doi:10.1371/journal.pgen.1005802
Haffter, P., Odenthal, J. r., Mullins, M. C., Lin, S., Farrell, M. J., Vogelsang, E., et al. (1996). Mutations Affecting Pigmentation and Shape of the Adult Zebrafish. Develop. Genes Evol. 206 (4), 260–276. doi:10.1007/s004270050051
Haller, G., Alvarado, D., Mccall, K., Yang, P., Cruchaga, C., Harms, M., and Goate, A. (2016). A polygenic burden of rare variants across extracelular matrix genes among individuals with adolescent idiopathic scoliosis. Human Molecular Genetics 25, 202-209.
Hassan, A., Parent, S., Mathieu, H., Zaouter, C., Molidperee, S., Bagu, E. T., et al. (2019). Adolescent Idiopathic Scoliosis Associated POC5 Mutation Impairs Cell Cycle, Cilia Length and Centrosome Protein Interactions. PLoS One 14 (3), e0213269. doi:10.1371/journal.pone.0213269
Hatzilazaridis, I., Hatzitaki, V., Antoniadou, N., and Samoladas, E. (2019). Postural and Muscle Responses to Galvanic Vestibular Stimulation Reveal a Vestibular Deficit in Adolescents with Idiopathic Scoliosis. Eur. J. Neurosci. 50 (10), 3614–3626. doi:10.1111/ejn.14525
Hayes, M., Gao, X., Yu, L. X., Paria, N., Henkelman, R. M., Wise, C. A., et al. (2014). ptk7 Mutant Zebrafish Models of Congenital and Idiopathic Scoliosis Implicate Dysregulated Wnt Signalling in Disease. Nat. Commun. 5, 4777. doi:10.1038/ncomms5777
Hayes, M., Naito, M., Daulat, A., Angers, S., and Ciruna, B. (2013). Ptk7 Promotes Non-canonical Wnt/PCP-Mediated Morphogenesis and Inhibits Wnt/β-catenin-dependent Cell Fate Decisions during Vertebrate Development. Development 140 (8), 1807–1818. doi:10.1242/dev.090183
Henke, K., Daane, J. M., Hawkins, M. B., Dooley, C. M., Busch-Nentwich, E. M., Stemple, D. L., et al. (2017). Genetic Screen for Postembryonic Development in the Zebrafish (Danio rerio): Dominant Mutations Affecting Adult Form. Genetics 207 (2), 609–623. doi:10.1534/genetics.117.300187
Hillmer, E. J., Zhang, H., Li, H. S., and Watowich, S. S. (2016). STAT3 Signaling in Immunity. Cytokine Growth Factor. Rev. 31, 1–15. doi:10.1016/j.cytogfr.2016.05.001
Hitier, M., Hamon, M., Denise, P., Lacoudre, J., Thenint, M.-A., Mallet, J.-F., et al. (2015). Lateral Semicircular Canal Asymmetry in Idiopathic Scoliosis: An Early Link between Biomechanical, Hormonal and Neurosensory Theories? PLoS One 10 (7), e0131120. doi:10.1371/journal.pone.0131120
Hresko, M. T. (2013). Idiopathic Scoliosis in Adolescents. N. Engl. J. Med. 368 (9), 834–841. doi:10.1056/nejmcp1209063
Hubbard, J. M., Böhm, U. L., Prendergast, A., Tseng, P.-E. B., Newman, M., Stokes, C., et al. (2016). Intraspinal Sensory Neurons Provide Powerful Inhibition to Motor Circuits Ensuring Postural Control during Locomotion. Curr. Biol. 26 (21), 2841–2853. doi:10.1016/j.cub.2016.08.026
Huitema, L. F. A., Apschner, A., Logister, I., Spoorendonk, K. M., Bussmann, J., Hammond, C. L., et al. (2012). Entpd5 Is Essential for Skeletal Mineralization and Regulates Phosphate Homeostasis in Zebrafish. Proc. Natl. Acad. Sci. 109 (52), 21372–21377. doi:10.1073/pnas.1214231110
Hur, M., Gistelinck, C. A., Huber, P., Lee, J., Thompson, M. H., Monstad-Rios, A. T., et al. (2017). MicroCT-based Phenomics in the Zebrafish Skeleton Reveals Virtues of Deep Phenotyping in a Distributed Organ System. Elife 6, e26014. doi:10.7554/eLife.26014
Ishikawa, H., and Marshall, W. F. (2011). Ciliogenesis: Building the Cell’s Antenna. Nat. Rev. Mol. Cel Biol 12 (4), 222–234. doi:10.1038/nrm3085
Jalalvand, E., Robertson, B., Tostivint, H., Wallén, P., and Grillner, S. (2016a). The Spinal Cord Has an Intrinsic System for the Control of pH. Curr. Biol. 26 (10), 1346–1351. doi:10.1016/j.cub.2016.03.048
Jalalvand, E., Robertson, B., Wallén, P., and Grillner, S. (2016b). Ciliated Neurons Lining the central Canal Sense Both Fluid Movement and pH through ASIC3. Nat. Commun. 7, 10002. doi:10.1038/ncomms10002
Jalalvand, E., Robertson, B., Wallén, P., Hill, R. H., and Grillner, S. (2014). Laterally Projecting Cerebrospinal Fluid-Contacting Cells in the Lamprey Spinal Cord Are of Two Distinct Types. J. Comp. Neurol. 522 (8), Spc1. doi:10.1002/cne.23584
Karner, C. M., Long, F., Solnica-Krezel, L., Monk, K. R., and Gray, R. S. (2015). Gpr126/Adgrg6 deletion in cartilage models idiopathic scoliosis and pectus excavatum in micedoi. Human Molecular Genetics. doi:10.1093/hmg/ddv170
Karol, L. A., Johnston, C. E., Browne, R. H., and Madison, M. (1993). Progression of the Curve in Boys Who Have Idiopathic Scoliosis. J. Bone Jt. Surg. 75 (12), 1804–1810. doi:10.2106/00004623-199312000-00010
Kelly, M. P., Guillaume, T. J., and Lenke, L. G. (2015). Spinal Deformity Associated with Chiari Malformation. Neurosurg. Clin. North America 26 (4), 579–585. doi:10.1016/j.nec.2015.06.005
Khanshour, A. M., Kou, I., Fan, Y., Einarsdottir, E., Makki, N., Kidane, Y. H., et al. (2018). Genome-wide Meta-Analysis and Replication Studies in Multiple Ethnicities Identify Novel Adolescent Idiopathic Scoliosis Susceptibility Loci. Hum. Mol. Genet. 27 (22), 3986–3998. doi:10.1093/hmg/ddy306
Klarica, M., Miše, B., Vladić, A., Orešković, M., and Oreskovic, D. (2013). "Compensated Hyperosmolarity" of Cerebrospinal Fluid and the Development of Hydrocephalus. Neuroscience 248, 278–289. doi:10.1016/j.neuroscience.2013.06.022
Klein-Wieringa, I. R., de Lange-Brokaar, B. J. E., Yusuf, E., Andersen, S. N., Kwekkeboom, J. C., Kroon, H. M., et al. (2016). Inflammatory Cells in Patients with Endstage Knee Osteoarthritis: A Comparison between the Synovium and the Infrapatellar Fat Pad. J. Rheumatol. 43 (4), 771–778. doi:10.3899/jrheum.151068
Kobayashi, D., Asano-Hoshino, A., Nakakura, T., Nishimaki, T., Ansai, S., Kinoshita, M., et al. (2017). Loss of Zinc finger MYND-type Containing 10 (Zmynd10) Affects Cilia Integrity and Axonemal Localization of Dynein Arms, Resulting in Ciliary Dysmotility, Polycystic Kidney and Scoliosis in Medaka (Oryzias latipes). Develop. Biol. 430 (1), 69–79. doi:10.1016/j.ydbio.2017.08.016
Konieczny, M. R., Senyurt, H., and Krauspe, R. (2013). Epidemiology of Adolescent Idiopathic Scoliosis. J. Child. Orthop. 7 (1), 3–9. doi:10.1007/s11832-012-0457-4
Konjikusic, M. J., Yeetong, P., Boswell, C. W., Lee, C., Roberson, E. C., Ittiwut, R., et al. (2018). Mutations in Kinesin Family Member 6 Reveal Specific Role in Ependymal Cell Ciliogenesis and Human Neurological Development. Plos Genet. 14 (11), e1007817. doi:10.1371/journal.pgen.1007817
Kou, I., Otomo, N., Takeda, K., Momozawa, Y., Lu, H.-F., Kubo, M., et al. (2019). Genome-wide Association Study Identifies 14 Previously Unreported Susceptibility Loci for Adolescent Idiopathic Scoliosis in Japanese. Nat. Commun. 10 (1), 3685. doi:10.1038/s41467-019-11596-w
Kou, I., Takahashi, Y., Johnson, T. A., Takahashi, A., Guo, L., Dai, J., et al. (2013). Genetic Variants in GPR126 Are Associated with Adolescent Idiopathic Scoliosis. Nat. Genet. 45 (6), 676–679. doi:10.1038/ng.2639
Kramer-Zucker, A. G., Olale, F., Haycraft, C. J., Yoder, B. K., Schier, A. F., and Drummond, I. A. (2005). Cilia-driven Fluid Flow in the Zebrafish Pronephros, Brain and Kupffer’s Vesicle Is Required for normal Organogenesis. Development 132 (8), 1907–1921. doi:10.1242/dev.01772
Kwon, R. Y., Watson, C. J., and Karasik, D. (2019). Using Zebrafish to Study Skeletal Genomics. Bone 126, 37–50. doi:10.1016/j.bone.2019.02.009
Lambert, F. M., Malinvaud, D., Glaunes, J., Bergot, C., Straka, H., and Vidal, P.-P. (2009). Vestibular Asymmetry as the Cause of Idiopathic Scoliosis: a Possible Answer from Xenopus. J. Neurosci. 29 (40), 12477–12483. doi:10.1523/jneurosci.2583-09.2009
Lessieur, E. M., Song, P., Nivar, G. C., Piccillo, E. M., Fogerty, J., Rozic, R., et al. (2019). Ciliary Genes Arl13b, Ahi1 and Cc2d2a Differentially Modify Expression of Visual Acuity Phenotypes but Do Not Enhance Retinal Degeneration Due to Mutation of Cep290 in Zebrafish. PLoS One 14 (4), e0213960. doi:10.1371/journal.pone.0213960
Levy, D. E., and Lee, C.-k. (2002). What Does Stat3 Do? J. Clin. Invest. 109 (9), 1143–1148. doi:10.1172/jci0215650
Li, Z., Li, X., Shen, J., Zhang, L., Chan, M. T. V., and Wu, W. K. K. (2020). Emerging Roles of Non-coding RNAs in Scoliosis. Cell Prolif 53 (2), e12736. doi:10.1111/cpr.12736
Liang, G., Gao, W., Liang, A., Ye, W., Peng, Y., Zhang, L., et al. (2012). Normal Leptin Expression, Lower Adipogenic Ability, Decreased Leptin Receptor and Hyposensitivity to Leptin in Adolescent Idiopathic Scoliosis. PLoS One 7 (5), e36648. doi:10.1371/journal.pone.0036648
Linghu, B., and DeLisi, C. (2010). Phenotypic Connections in Surprising Places. Genome Biol. 11 (4), 116. doi:10.1186/gb-2010-11-4-116
Liu, X. Y., Wang, L., Yu, B., Zhuang, Q. Y., Wang, Y. P., et al. (2015). Expression Signatures of Long Noncoding RNAs in Adolescent Idiopathic Scoliosis. BioMed Research International, 2015. doi:10.1155/2015/276049
Liu, G., Wang, L., Wang, X., Yan, Z., Yang, X., Lin, M., et al. (2019). Whole-Genome Methylation Analysis of Phenotype Discordant Monozygotic Twins Reveals Novel Epigenetic Perturbation Contributing to the Pathogenesis of Adolescent Idiopathic Scoliosis. Front. Bioeng. Biotechnol. 7, 364. doi:10.3389/fbioe.2019.00364
Liu, Z., Tam, E. M. S., Sun, G.-Q., Lam, T.-P., Zhu, Z.-Z., Sun, X., et al. (20121976). Abnormal Leptin Bioavailability in Adolescent Idiopathic Scoliosis. Spine 37 (7), 599–604. doi:10.1097/brs.0b013e318227dd0c
Lonner, B., Yoo, A., Terran, J. S., Sponseller, P., Samdani, A., Betz, R., et al. (20131976). Effect of Spinal Deformity on Adolescent Quality of Life. Spine 38 (12), 1049–1055. doi:10.1097/brs.0b013e3182893c01
Lonstein, J. E. (1999)., 30. viii, 387–405. doi:10.1016/s0030-5898(05)70094-8Congenital Spine DeformitiesOrthop. Clin. North America3
Lu, C., Yang, D., Lei, C., Wang, R., Guo, T., and Luo, H. (2021). Identification of Two Novel DNAAF2 Variants in Two Consanguineous Families with Primary Ciliary Dyskinesia. Pgpm Vol. 14, 1415–1423. doi:10.2147/pgpm.s338981
Lu, H., Shagirova, A., Goggi, J. L., Yeo, H. L., and Roy, S. (2020). Reissner Fibre-Induced Urotensin Signalling from Cerebrospinal Fluid-Contacting Neurons Prevents Scoliosis of the Vertebrate Spine. Biol. Open 9 (5), 52027. doi:10.1242/bio.052027
Lun, M. P., Monuki, E. S., and Lehtinen, M. K. (2015). Development and Functions of the Choroid Plexus-Cerebrospinal Fluid System. Nat. Rev. Neurosci. 16 (8), 445–457. doi:10.1038/nrn3921
Machida, M., Murai, I., Miyashita, Y., Dubousset, J., Yamada, T., and Kimura, J. (19991976). Pathogenesis of Idiopathic Scoliosis. Spine 24 (19), 1985–1989. doi:10.1097/00007632-199910010-00004
Malicki, J. J., and Johnson, C. A. (2017). The Cilium: Cellular Antenna and Central Processing Unit. Trends Cel Biol. 27 (2), 126–140. doi:10.1016/j.tcb.2016.08.002
Man, G. C.-W., Tam, E. M.-S., Wong, Y. S., Hung, V. W.-Y., Hu, Z., Lam, T. P., et al. (2019). Abnormal Osteoblastic Response to Leptin in Patients with Adolescent Idiopathic Scoliosis. Sci. Rep. 9 (1), 17128. doi:10.1038/s41598-019-53757-3
Marie-Hardy, L., Khalifé, M., Slimani, L., and Pascal-Moussellard, H. (2019). Computed Tomography Method for Characterising the Zebrafish Spine. Orthopaedics Traumatol. Surg. Res. 105 (2), 361–367. doi:10.1016/j.otsr.2018.12.008
Mathieu, H., Patten, S. A., Aragon-Martin, J. A., Ocaka, L., Simpson, M., Child, A., et al. (2021). Genetic Variant of TTLL11 Gene and Subsequent Ciliary Defects Are Associated with Idiopathic Scoliosis in a 5-generation UK Family. Sci. Rep. 11 (1), 11026. doi:10.1038/s41598-021-90155-0
Matsumae, M., Kuroda, K., Yatsushiro, S., Hirayama, A., Hayashi, N., Takizawa, K., et al. (2019). Changing the Currently Held Concept of Cerebrospinal Fluid Dynamics Based on Shared Findings of Cerebrospinal Fluid Motion in the Cranial Cavity Using Various Types of Magnetic Resonance Imaging Techniques. Neurol. Med. Chir.(Tokyo) 59 (4), 133–146. doi:10.2176/nmc.ra.2018-0272
Matthews, J. L., Brown, A. M. V., Larison, K., Bishop-Stewart, J. K., Rogers, P., and Kent, M. L. (2001). Pseudoloma Neurophilia N. g., N. sp., a New Microsporidium from the central Nervous System of the Zebrafish (Danio rerio). J. Eukaryot. Microbiol. 48 (2), 227–233. doi:10.1111/j.1550-7408.2001.tb00307.x
Meiniel, O., and Meiniel, A. (2007). The Complex Multidomain Organization of SCO-Spondin Protein Is Highly Conserved in Mammals. Brain Res. Rev. 53 (2), 321–327. doi:10.1016/j.brainresrev.2006.09.007
Minegishi, Y., Saito, M., Tsuchiya, S., Tsuge, I., Takada, H., Hara, T., et al. (2007). Dominant-negative Mutations in the DNA-Binding Domain of STAT3 Cause Hyper-IgE Syndrome. Nature 448 (7157), 1058–1062. doi:10.1038/nature06096
Mirra, V., Werner, C., and Santamaria, F. (2017). Primary Ciliary Dyskinesia: An Update on Clinical Aspects, Genetics, Diagnosis, and Future Treatment Strategies. Front. Pediatr. 5, 135. doi:10.3389/fped.2017.00135
Mogha, A., Benesh, A. E., Patra, C., Engel, F. B., Schoneberg, T., Liebscher, I., et al. (2013). Gpr126 Functions in Schwann Cells to Control Differentiation and Myelination via G-Protein Activation. J. Neurosci. 33 (46), 17976–17985. doi:10.1523/jneurosci.1809-13.2013
Molina, B., Rodríguez, E. M., Peruzzo, B., Caprile, T., and Nualart, F. (2001). Spatial Distribution of Reissner’s Fiber Glycoproteins in the Filum Terminale of the Rat and Rabbit. Microsc. Res. Tech. 52 (5), 552–563. doi:10.1002/1097-0029(20010301)52:5<552::aid-jemt1040>3.0.co;2-h
Monk, K. R., Oshima, K., Jörs, S., Heller, S., and Talbot, W. S. (2011). Gpr126 Is Essential for Peripheral Nerve Development and Myelination in Mammals. Development 138 (13), 2673–2680. doi:10.1242/dev.062224
Morin-Kensicki, E. M., and Eisen, J. S. (1997). Sclerotome Development and Peripheral Nervous System Segmentation in Embryonic Zebrafish. Development 124 (1), 159–167. doi:10.1242/dev.124.1.159
Muñoz, R. I., Kähne, T., Herrera, H., Rodríguez, S., Guerra, M. M., Vío, K., et al. (2019). The Subcommissural Organ and the Reissner Fiber: Old Friends Revisited. Cell Tissue Res 375 (2), 507–529. doi:10.1007/s00441-018-2917-8
Nathan, C. (2002). Points of Control in Inflammation. Nature 420 (6917), 846–852. doi:10.1038/nature01320
Negrini, S., Aulisa, A. G., Aulisa, L., Circo, A. B., de Mauroy, J. C., Durmala, J., et al. (2012). 2011 SOSORT Guidelines: Orthopaedic and Rehabilitation Treatment of Idiopathic Scoliosis during Growth. Scoliosis 7 (1), 3. doi:10.1186/1748-7161-7-3
Negrini, S., Grivas, T. B., Kotwicki, T., Maruyama, T., Rigo, M., and Weiss, H. R.Members of the Scientific society On Scoliosis Orthopaedic and Rehabilitation Treatment (SOSORT) (2006). Members of the Scientific Society on Scoliosis and T. RehabilitationWhy Do We Treat Adolescent Idiopathic Scoliosis? what We Want to Obtain and to Avoid for Our Patients. SOSORT 2005 Consensus Paper. Scoliosis 1, 4. doi:10.1186/1748-7161-1-4
Nguyen, A. M., and Jacobs, C. R. (2013). Emerging Role of Primary Cilia as Mechanosensors in Osteocytes. Bone 54 (2), 196–204. doi:10.1016/j.bone.2012.11.016
Nicholls, G. E. (1917). Some Experiments on the Nature and Function of Reissner’s Fiber. J. Comp. Neurol. 27 (2), 117–199. doi:10.1002/cne.900270202
Ogura, Y., Kou, I., Miura, S., Takahashi, A., Xu, L., Takeda, K., et al. (2015). A Functional SNP in BNC2 Is Associated with Adolescent Idiopathic Scoliosis. Am. J. Hum. Genet. 97 (2), 337–342. doi:10.1016/j.ajhg.2015.06.012
Ogura, Y., Kou, I., Takahashi, Y., Takeda, K., Minami, S., Kawakami, N., et al. (2017). A Functional Variant in MIR4300HG, the Host Gene of microRNA MIR4300 Is Associated with Progression of Adolescent Idiopathic Scoliosis. Hum. Mol. Genet. 26 (20), 4086–4092. doi:10.1093/hmg/ddx291
Oliazadeh, N., Gorman, K. F., Eveleigh, R., Bourque, G., and Moreau, A. (2017). Identification of Elongated Primary Cilia with Impaired Mechanotransduction in Idiopathic Scoliosis Patients. Sci. Rep. 7, 44260. doi:10.1038/srep44260
Ortega, E., Muñoz, R. I., Luza, N., Guerra, F., Guerra, M., Vio, K., et al. (2016). The Value of Early and Comprehensive Diagnoses in a Human Fetus with Hydrocephalus and Progressive Obliteration of the Aqueduct of Sylvius: Case Report. BMC Neurol. 16, 45. doi:10.1186/s12883-016-0566-7
Orts-Del’Immagine, A., Cantaut-Belarif, Y., Thouvenin, O., Roussel, J., Baskaran, A., Langui, D., et al. (2020). Sensory Neurons Contacting the Cerebrospinal Fluid Require the Reissner Fiber to Detect Spinal Curvature In Vivo. Curr. Biol. 30 (5), 827–e4. doi:10.1016/j.cub.2019.12.071
Orts-Del’Immagine, A., Kastner, A., Tillement, V., Tardivel, C., Trouslard, J., and Wanaverbecq, N. (2014). Morphology, Distribution and Phenotype of Polycystin Kidney Disease 2-like 1-positive Cerebrospinal Fluid Contacting Neurons in the Brainstem of Adult Mice. PLoS One 9 (2), e87748. doi:10.1371/journal.pone.0087748
Ouellet, J., and Odent, T. (2013). Animal Models for Scoliosis Research: State of the Art, Current Concepts and Future Perspective Applications. Eur. Spine J. 22 (Suppl. 2), S81–S95. doi:10.1007/s00586-012-2396-7
Panattoni, G. L., and Todros, T. (1988). Postural Aspects of the Human Fetal Spine. Morphometric and Functional Study. Panminerva Med. 30 (4), 250–253.
Panigrahi, M., Reddy, B. P., Reddy, A. K., and Reddy, J. J. M. (2004). CSF Flow Study in Chiari I Malformation. Child’s Nervous Syst. 20 (5), 336–340. doi:10.1007/s00381-003-0881-3
Papanastasiou, A. D., Mantagos, S., Papanastasiou, D. A., and Zarkadis, I. K. (2010). A Novel Mutation in the Signal Transducer and Activator of Transcription 3 (STAT3) Gene, in Hyper-IgE Syndrome. Mol. Immunol. 47 (7-8), 1629–1634. doi:10.1016/j.molimm.2010.01.010
Patten, S. A., Margaritte-Jeannin, P., Bernard, J.-C., Alix, E., Labalme, A., Besson, A., et al. (2015). Functional Variants of POC5 Identified in Patients with Idiopathic Scoliosis. J. Clin. Invest. 125 (3), 1124–1128. doi:10.1172/jci77262
Peskin, B., Henke, K., Cumplido, N., Treaster, S., Harris, M. P., Bagnat, M., et al. (2020). Notochordal Signals Establish Phylogenetic Identity of the Teleost Spine. Curr. Biol. 30 (14), 2805–2814. e2803. doi:10.1016/j.cub.2020.05.037
Petko, J. A., Millimaki, B. B., Canfield, V. A., Riley, B. B., and Levenson, R. (2008). Otoc1: a Novel Otoconin-90 Ortholog Required for Otolith Mineralization in Zebrafish. Devel Neurobio 68 (2), 209–222. doi:10.1002/dneu.20587
Pinna, G., Alessandrini, F., Alfieri, A., Rossi, M., and Bricolo, A. (2000). Cerebrospinal Fluid Flow Dynamics Study in Chiari I Malformation: Implications for Syrinx Formation. Neurosurg. Focus 8 (3), E3. doi:10.3171/foc.2000.8.3.3
Pollak, L., Shlamkovic, N., Minewicz, A., and Mirovsky, Y. (2013). Otolith Dysfunction as a Possible Cause for the Development of Idiopathic Scoliosis. J. Pediatr. Orthop. 33 (3), 293–297. doi:10.1097/bpo.0b013e31827c0643
Qiu, Y., Sun, X., Qiu, X., Li, W., Zhu, Z., Zhu, F., et al. (20071976). Decreased Circulating Leptin Level and its Association with Body and Bone Mass in Girls with Adolescent Idiopathic Scoliosis. Spine 32 (24), 2703–2710. doi:10.1097/brs.0b013e31815a59e5
Robin, G. C., and Stein, H. (1975). Experimental Scoliosis in Primates. The J. Bone Jt. Surg. Br. volume 57-B (2), 142–145. doi:10.1302/0301-620x.57b2.142
Rockwell, H., Evans, F. G., and Pheasant, H. C. (1938). The Comparative Morphology of the Vertebrate Spinal Column. Its Form as Related to Function. J. Morphol. 63 (1), 87–117. doi:10.1002/jmor.1050630105
Rodríguez, E. M., Oksche, A., Hein, S., Rodríguez, S. R., and Yulis, R. (1984). Comparative Immunocytochemical Study of the Subcommissural Organ. Cel Tissue Res 237 (3), 427–441. doi:10.1007/BF00228427
Rodríguez, E. M., Oksche, A., and Montecinos, H. (2001). Human Subcommissural Organ, with Particular Emphasis on its Secretory Activity during the Fetal Life. Microsc. Res. Tech. 52 (5), 573–590. doi:10.1002/1097-0029(20010301)52:5<573::AID-JEMT1042>3.0.CO;2-6
Rodríguez, E. M., Oksche, A., Hein, S., and Yulis, C. R. (1992). Cell Biology of the Subcommissural Organ. Int. Rev. Cytol. 135, 39–121. Elsevier. doi:10.1016/s0074-7696(08)62038-0
Rodríguez, E. M., Oksche, A., Hein, S., and Yulis, C. R. (1992). Cell Biology of the Subcommissural Organ. Int. Rev. Cytol. 135, 39–121. doi:10.1016/s0074-7696(08)62038-0
Rodríguez, E. M., Rodríguez, S., and Hein, S. (1998). The Subcommissural Organ. Microsc. Res. Tech. 41 (2), 98–123. doi:10.1002/(sici)1097-0029(19980415)41:2<98::aid-jemt2>3.0.co;2-m
Rose, C. D., Pompili, D., Henke, K., Van Gennip, J. L. M., Meyer-Miner, A., Rana, R., et al. (2020). SCO-spondin Defects and Neuroinflammation Are Conserved Mechanisms Driving Spinal Deformity across Genetic Models of Idiopathic Scoliosis. Curr. Biol. 30 (12), 2363–2373. e2366. doi:10.1016/j.cub.2020.04.020
Rose, P. S., and Lenke, L. G. (2007). Classification of Operative Adolescent Idiopathic Scoliosis: Treatment Guidelines. Orthop. Clin. North America 38 (4), 521–529. vi. doi:10.1016/j.ocl.2007.06.001
Sabaliauskas, N. A., Foutz, C. A., Mest, J. R., Budgeon, L. R., Sidor, A. T., Gershenson, J. A., et al. (2006). High-throughput Zebrafish Histology. Methods 39 (3), 246–254. doi:10.1016/j.ymeth.2006.03.001
Sakakibara, A., Aoki, E., Hashizume, Y., Mori, N., and Nakayama, A. (2007). Distribution of Nestin and Other Stem Cell-Related Molecules in Developing and Diseased Human Spinal Cord. Pathol. Int. 57 (6), 358–368. doi:10.1111/j.1440-1827.2007.02108.x
Sanders, J. L., Watral, V., and Kent, M. L. (2012). Microsporidiosis in Zebrafish Research Facilities. ILAR J. 53 (2), 106–113. doi:10.1093/ilar.53.2.106
Saraiva, J. M., and Baraitser, M. (1992). Joubert Syndrome: a Review. Am. J. Med. Genet. 43 (4), 726–731. doi:10.1002/ajmg.1320430415
Satir, P., Mitchell, D. R., and Jékely, G. (2008). Chapter 3 How Did the Cilium Evolve? Curr. Top. Dev. Biol. 85, 63–82. doi:10.1016/s0070-2153(08)00803-x
Sayer, J. A., Otto, E. A., O’Toole, J. F., Nurnberg, G., Kennedy, M. A., Becker, C., et al. (2006). The Centrosomal Protein Nephrocystin-6 Is Mutated in Joubert Syndrome and Activates Transcription Factor ATF4. Nat. Genet. 38 (6), 674–681. doi:10.1038/ng1786
Schlösser, T. P. C., Semple, T., Carr, S. B., Padley, S., Loebinger, M. R., Hogg, C., et al. (2017). Scoliosis Convexity and Organ Anatomy Are Related. Eur. Spine J. 26 (6), 1595–1599. doi:10.1007/s00586-017-4970-5
Serhan, C. N., Brain, S. D., Buckley, C. D., Gilroy, D. W., Haslett, C., O’Neill, L. A. J., et al. (2007). Resolution of in Flammation: State of the Art, Definitions and Terms. FASEB j. 21 (2), 325–332. doi:10.1096/fj.06-7227rev
Serhan, C. N. (2014). Pro-resolving Lipid Mediators Are Leads for Resolution Physiology. Nature 510 (7503), 92–101. doi:10.1038/nature13479
Shaffer, N., Martin, B. A., Rocque, B., Madura, C., Wieben, O., Iskandar, B. J., et al. (2014). Cerebrospinal Fluid Flow Impedance Is Elevated in Type I Chiari Malformation. J. Biomech. Eng. 136 (2), 021012. doi:10.1115/1.4026316
Sharma, S., Londono, D., Londono, D., Eckalbar, W. L., Gao, X., Zhang, D., et al. (2015). A PAX1 Enhancer Locus Is Associated with Susceptibility to Idiopathic Scoliosis in Females. Nat. Commun. 6, 6452. doi:10.1038/ncomms7452
Song, Z., Zhang, X., Jia, S., Yelick, P. C., and Zhao, C. (2016). Zebrafish as a Model for Human Ciliopathies. J. Genet. Genomics 43 (3), 107–120. doi:10.1016/j.jgg.2016.02.001
Sorg, I., Schmutz, C., Lu, Y.-Y., Fromm, K., Siewert, L. K., Bögli, A., et al. (2020). A Bartonella Effector Acts as Signaling Hub for Intrinsic STAT3 Activation to Trigger Anti-inflammatory Responses. Cell Host & Microbe 27 (3), 476–485. doi:10.1016/j.chom.2020.01.015
Stemple, D. L., Solnica-Krezel, L., Zwartkruis, F., Neuhauss, S. C., Schier, A. F., Malicki, J., et al. (1996). Mutations Affecting Development of the Notochord in Zebrafish. Development 123, 117–128. doi:10.1242/dev.123.1.117
Stemple, D. L. (2005). Structure and Function of the Notochord: an Essential Organ for Chordate Development. Development 132 (11), 2503–2512. doi:10.1242/dev.01812
Sterba, G., Ermisch, A., Freyer, K., and Hartmann, G. (1967). Incorporation of sulphur-35 into the Subcommissural Organ and Reissner’s Fibre. Nature 216 (5114), 504. doi:10.1038/216504a0
Sternberg, J. R., Prendergast, A. E., Brosse, L., Cantaut-Belarif, Y., Thouvenin, O., Orts-Del’Immagine, A., et al. (2018). Pkd2l1 Is Required for Mechanoception in Cerebrospinal Fluid-Contacting Neurons and Maintenance of Spine Curvature. Nat. Commun. 9 (1), 3804. doi:10.1038/s41467-018-06225-x
Storer, K. P., Toh, J., Stoodley, M. A., and Jones, N. R. (1998). The central Canal of the Human Spinal Cord: a Computerised 3-D Study. J. Anat. 192 (Pt 4), 565–572. doi:10.1046/j.1469-7580.1998.19240565.x
Sun, X., Zhou, Y., Zhang, R., Wang, Z., Xu, M., Zhang, D., et al. (2020). Dstyk Mutation Leads to Congenital Scoliosis-like Vertebral Malformations in Zebrafish via Dysregulated mTORC1/TFEB Pathway. Nat. Commun. 11 (1), 479. doi:10.1038/s41467-019-14169-z
Sun, Z., Amsterdam, A., Pazour, G. J., Cole, D. G., Miller, M. S., and Hopkins, N. (2004). A Genetic Screen in Zebrafish Identifies Cilia Genes as a Principal Cause of Cystic Kidney. Development 131 (16), 4085–4093. doi:10.1242/dev.01240
Takahashi, Y., Kou, I., Takahashi, A., Johnson, T. A., Kono, K., Kawakami, N., et al. (2011). A Genome-wide Association Study Identifies Common Variants Near LBX1 Associated with Adolescent Idiopathic Scoliosis. Nat. Genet. 43 (12), 1237–1240. doi:10.1038/ng.974
Takeuchi, O., and Akira, S. (2010). Pattern Recognition Receptors and Inflammation. Cell 140 (6), 805–820. doi:10.1016/j.cell.2010.01.022
Tenor, H., Hatzelmann, A., Beume, R., Lahu, G., Zech, K., and Bethke, T. D. (2011). Pharmacology, Clinical Efficacy, and Tolerability of Phosphodiesterase-4 Inhibitors: Impact of Human Pharmacokinetics. Handb Exp. Pharmacol. 204, 85–119. doi:10.1007/978-3-642-17969-3_3
Tenor, H., Hedbom, E., Häuselmann, H.-J., Schudt, C., and Hatzelmann, A. (2002). Phosphodiesterase Isoenzyme Families in Human Osteoarthritis Chondrocytes - Functional Importance of Phosphodiesterase 4. Br. J. Pharmacol. 135 (3), 609–618. doi:10.1038/sj.bjp.0704480
Terhune, E. A., Cuevas, M. T., Monley, A. M., Wethey, C. I., Chen, X., Cattell, M. V., et al. (2021a). Mutations in KIF7 Implicated in Idiopathic Scoliosis in Humans and Axial Curvatures in Zebrafish. Hum. Mutat. 42 (4), 392–407. doi:10.1002/humu.24162
Terhune, E. A., Wethey, C. I., Cuevas, M. T., Monley, A. M., Baschal, E. E., Bland, M. R., et al. (2021b). Whole Exome Sequencing of 23 Multigeneration Idiopathic Scoliosis Families Reveals Enrichments in Cytoskeletal Variants, Suggests Highly Polygenic Disease. Genes (Basel) 12 (6), 922. doi:10.3390/genes12060922
Thouvenin, O., Keiser, L., Cantaut-Belarif, Y., Carbo-Tano, M., Verweij, F., Jurisch-Yaksi, N., et al. (2020). Origin and Role of the Cerebrospinal Fluid Bidirectional Flow in the central Canal. Elife 9, e47699. doi:10.7554/eLife.47699
Troutwine, B. R., Gontarz, P., Konjikusic, M. J., Minowa, R., Monstad-Rios, A., Sepich, D. S., et al. (2020). The Reissner Fiber Is Highly Dynamic In Vivo and Controls Morphogenesis of the Spine. Curr. Biol. 30 (12), 2353–2362. e2353. doi:10.1016/j.cub.2020.04.015
Tuazon, F. B., and Mullins, M. C. (2015). Temporally Coordinated Signals Progressively Pattern the Anteroposterior and Dorsoventral Body Axes. Semin. Cel Develop. Biol. 42, 118–133. doi:10.1016/j.semcdb.2015.06.003
Van De Weghe, J. C., Rusterholz, T. D. S., Latour, B., Grout, M. E., Aldinger, K. A., Shaheen, R., et al. (2017). Mutations in ARMC9 , Which Encodes a Basal Body Protein, Cause Joubert Syndrome in Humans and Ciliopathy Phenotypes in Zebrafish. Am. J. Hum. Genet. 101 (1), 23–36. doi:10.1016/j.ajhg.2017.05.010
Van Gennip, J. L. M., Boswell, C. W., and Ciruna, B. (2018). Neuroinflammatory Signals Drive Spinal Curve Formation in Zebrafish Models of Idiopathic Scoliosis. Sci. Adv. 4 (12), eaav1781. doi:10.1126/sciadv.aav1781
Wang, L., and Dynlacht, B. D. (2018). The Regulation of Cilium Assembly and Disassembly in Development and Disease. Development 145 (18), dev151407. doi:10.1242/dev.151407
Wang, Y., Liu, Z., Yang, G., Gao, Q., Xiao, L., Li, J., et al. (2020). Coding Variants Coupled with Rapid Modeling in Zebrafish Implicate Dynein Genes, Dnaaf1 and Zmynd10, as Adolescent Idiopathic Scoliosis Candidate Genes. Front. Cel Dev. Biol. 8, 582255. doi:10.3389/fcell.2020.582255
Weinhardt, V., Shkarin, R., Wernet, T., Wittbrodt, J., Baumbach, T., and Loosli, F. (2018). Quantitative Morphometric Analysis of Adult Teleost Fish by X-ray Computed Tomography. Sci. Rep. 8 (1), 16531. doi:10.1038/s41598-018-34848-z
Whitfield, T. T. (20201792). Cilia in the Developing Zebrafish Ear. Phil. Trans. R. Soc. B 375, 20190163. doi:10.1098/rstb.2019.0163
Wiener-Vacher, S. R., and Mazda, K. (1998). Asymmetric Otolith Vestibulo-Ocular Responses in Children with Idiopathic Scoliosis. J. Pediatr. 132 (6), 1028–1032. doi:10.1016/s0022-3476(98)70403-2
Wopat, S., Bagwell, J., Sumigray, K. D., Dickson, A. L., Huitema, L. F. A., Poss, K. D., et al. (2018). Spine Patterning Is Guided by Segmentation of the Notochord Sheath. Cel Rep. 22 (8), 2026–2038. doi:10.1016/j.celrep.2018.01.084
Wu, M.-Y., Carbo-Tano, M., Mirat, O., Lejeune, F.-X., Roussel, J., Quan, F. B., et al. (2021). Spinal Sensory Neurons Project onto the Hindbrain to Stabilize Posture and Enhance Locomotor Speed. Curr. Biol. 31 (15), 3315–3329. e3315. doi:10.1016/j.cub.2021.05.042
Wu, T., Sun, X., Zhu, Z., Zheng, X., Qian, B., Zhu, F., et al. (2012). Role of High central Leptin Activity in a Scoliosis Model Created in Bipedal Amputated Mice. Stud. Health Technol. Inform. 176, 31–35. doi:10.3233/978-1-61499-067-3-31
Wynne-Davies, R. (1968). Familial (Idiopathic) Scoliosis. J. Bone Jt. Surg. Br. volume 50-B (1), 24–30. doi:10.1302/0301-620x.50b1.24
Xiong, S., Wu, J., Jing, J., Huang, P., Li, Z., Mei, J., et al. (2017). Loss of Stat3 Function Leads to Spine Malformation and Immune Disorder in Zebrafish. Sci. Bull. 62 (3), 185–196. doi:10.1016/j.scib.2017.01.008
Yamamoto, M., Morita, R., Mizoguchi, T., Matsuo, H., Isoda, M., Ishitani, T., et al. (2010). Mib-Jag1-Notch Signalling Regulates Patterning and Structural Roles of the Notochord by Controlling Cell-Fate Decisions. Development 137 (15), 2527–2537. doi:10.1242/dev.051011
Zamborský, R., Liščák, B., Trepáč, M., Danisovič, A. Ľ., and Danisovic, L. (2019). Adolescent Idiopathic Scoliosis - Future Molecular-Based Diagnostic and Prognostic Testing. Ortop Traumatol. Rehabil. 21 (4), 253–260. doi:10.5604/01.3001.0013.5070
Zariwala, M. A., Gee, H. Y., Kurkowiak, M., Al-Mutairi, D. A., Leigh, M. W., Hurd, T. W., et al. (2013). ZMYND10 Is Mutated in Primary Ciliary Dyskinesia and Interacts with LRRC6. Am. J. Hum. Genet. 93 (2), 336–345. doi:10.1016/j.ajhg.2013.06.007
Zhang, X., Jia, S., Chen, Z., Chong, Y. L., Xie, H., Feng, D., et al. (2018). Cilia-driven Cerebrospinal Fluid Flow Directs Expression of Urotensin Neuropeptides to Straighten the Vertebrate Body axis. Nat. Genet. 50 (12), 1666–1673. doi:10.1038/s41588-018-0260-3
Zhu, Z., Tang, N. L.-S., Xu, L., Qin, X., Mao, S., Song, Y., et al. (2015). Genome-wide Association Study Identifies New Susceptibility Loci for Adolescent Idiopathic Scoliosis in Chinese Girls. Nat. Commun. 6, 8355. doi:10.1038/ncomms9355
Keywords: spine, scoliosis, zebrafish, cilia, cerebrospinal fluid, Reissner fiber, CSF-cNs, inflammation
Citation: Muñoz-Montecinos C, Romero A, Sepúlveda V, Vira MÁ, Fehrmann-Cartes K, Marcellini S, Aguilera F, Caprile T and Fuentes R (2022) Turning the Curve Into Straight: Phenogenetics of the Spine Morphology and Coordinate Maintenance in the Zebrafish. Front. Cell Dev. Biol. 9:801652. doi: 10.3389/fcell.2021.801652
Received: 25 October 2021; Accepted: 31 December 2021;
Published: 26 January 2022.
Edited by:
Con Sullivan, University of Maine at Augusta, United StatesReviewed by:
Daniel Grimes, University of Oregon, United StatesBo Gao, The University of Hong Kong, Hong Kong, SAR China
Copyright © 2022 Muñoz-Montecinos, Romero, Sepúlveda, Vira, Fehrmann-Cartes, Marcellini, Aguilera, Caprile and Fuentes. This is an open-access article distributed under the terms of the Creative Commons Attribution License (CC BY). The use, distribution or reproduction in other forums is permitted, provided the original author(s) and the copyright owner(s) are credited and that the original publication in this journal is cited, in accordance with accepted academic practice. No use, distribution or reproduction is permitted which does not comply with these terms.
*Correspondence: Teresa Caprile, dGNhcHJpbGVAdWRlYy5jbA==; Ricardo Fuentes, cmljZnVlbnRlc0B1ZGVjLmNs