- Department of Cardiology, The First Affiliated Hospital of Nanjing Medical University, Nanjing, China
Abnormalities in programmed cell death (PCD) signaling cascades can be observed in the development and progression of various cardiovascular diseases, such as apoptosis, necrosis, pyroptosis, ferroptosis, and cell death associated with autophagy. Aberrant activation of PCD pathways is a common feature leading to excessive cardiac remodeling and heart failure, involved in the pathogenesis of various cardiovascular diseases. Conversely, timely activation of PCD remodels cardiac structure and function after injury in a spatially or temporally restricted manner and corrects cardiac development similarly. As many cardiovascular diseases exhibit abnormalities in PCD pathways, drugs that can inhibit or modulate PCD may be critical in future therapeutic strategies. In this review, we briefly describe the process of various types of PCD and their roles in the occurrence and development of cardiovascular diseases. We also discuss the interplay between different cell death signaling cascades and summarize pharmaceutical agents targeting key players in cell death signaling pathways that have progressed to clinical trials. Ultimately a better understanding of PCD involved in cardiovascular diseases may lead to new avenues for therapy.
Background
The development and homeostasis of multicellular organisms depend not only on the regulation of cell survival and renewal, but also on the processing of those cells that are no longer needed or pose a potential danger to the organism. This includes the removal of cells at risk of neoplastic transformation or those infected by pathogens (Kramer et al., 2012; Saunders et al., 2015; Zhu and Sun, 2018). Programmed cell death (PCD) is the primary approach organisms adapt to eliminate these abnormal cells, which stimulates membrane-bound and cytoplasmic proteins by developmental programs and stress-induced signaling, triggering cell death through complex cascade transcriptional and post-translational protein modifications (Shi et al., 2017). Several types of PCD have been discovered in the past 3 decades: autophagy, necrosis, ferroptosis, apoptosis, and pyroptosis (Bai et al., 2017). Each type of PCD has its unique characteristics. Apoptosis represents the coordinated disassembly of dead cells and typical “immune silencing” clearance, while pyroptosis and necrosis refer to the relatively “violent” type of cell death, characterized by the rupture of dead cells, from which effective inflammatory inducers are released (Kung et al., 2011). Autophagy is an evolutionarily conserved catabolic process that begins with forming autophagosomes, a double-membrane-bound structure surrounding cytoplasmic macromolecules and organelles which are ultimately recycled (Nishida and Otsu, 2008; Oka et al., 2012). In addition, another novel iron-dependent PCD mode, ferroptosis, is mainly caused by the peroxidation of unsaturated fatty acids highly expressed on the cell membrane under the action of divalent iron or ester oxygenase inducing cell death (Dixon et al., 2012; Ma et al., 2017). At present, research on PCD is generally limited to a single type, and reports on synergistic or antagonistic effects between different types of PCD are rare.
At present, increasing studies suggest that PCD is a significant cause of cardiovascular diseases such as ischemia/reperfusion (I/R) injury, myocardial infarction (MI) and cardiomyopathy (Tower, 2015; Yang et al., 2016). Each PCD plays a vital role in maintaining cellular homeostasis. Apoptosis ensures the normal development and tissue homeostasis of mature organisms, as well as pyroptosis, ferroptosis, and necrosis protect the host from pathogens and other external threats (Kung et al., 2011; Dixon et al., 2012; Corbalan et al., 2016; Vande Walle and Lamkanfi, 2016). Unlike apoptosis and other PCD, autophagy may commit suicide by undergoing cell death and responding to excessive stress. This view is supported by the analysis of different PCD pathways (Sciarretta et al., 2012). Increasing evidence indicates that different PCD pathways in cardiovascular diseases are associated and interplayed at multiple levels. Still, no article had made a systematic study and report on this issue.
Moreover, many clinical trials have found that the application of appropriate drugs could target cell death signaling pathways in cardiovascular diseases. A clinical phase 2 trial of simvastatin found that targeting inhibition of apoptosis through miR-15a-5p could protect the myocardium in non-coronary surgery, and another trial of berberine found that it could regulate myocardial autophagy through AMPK/mTOR pathway to promote myocardial protection in postoperative patients (Qing et al., 2018; Zhou et al., 2018). However, all the existing studies focus on the targeted therapy through a single type of PCD signaling pathway in cardiovascular diseases. Hitherto, there are no relevant reviews systematically analyzing and collating the interplay of different drugs in cardiovascular diseases through crosstalk between different types of PCD signaling pathways.
Here, we discuss the role of PCD in cardiovascular disease, the interactions between different PCD signaling cascades, and describe the application of drugs targeting cell death signaling pathways in cardiovascular pathology. Moreover, we summarize the latest progress on the relevant studies that have progressed to clinical trials and put forward a novel understanding for the better transformation of PCD pathways in cardiovascular medicine.
Different Types of PCD and Molecular Mechanisms in Cardiovascular Diseases
Apoptosis Pathways in Cardiovascular Diseases
Apoptosis is usually induced by intrinsic and extrinsic (also known as death receptor-mediated) triggers. The intrinsic pathway, also called the mitochondrial or Bcl-2-mediated pathway, is activated by Bh3 proteins (Bim, Puma, Bid, Bmf, Bad, Hrk, Bik, Noxa) to initiate apoptosis when facing intracellular stress caused by various physicochemical factors (Dong et al., 2019). Activated Bax and Bak then form oligomers that promote the activation of the caspase cascade by releasing downstream apoptotic factors such as cytochrome c and Smac/DIABLO from mitochondria, resulting in ultimate protein cleavage and cell death. Extrinsic pathway, also known as death receptor pathway, is the binding and activation of death receptors such as tumor necrosis factor receptor (TNFR) superfamily members, Fas/Fas ligand (FasL) system members and death receptor family members (DR3, DR4 and DR5) with corresponding ligands to form intracellular death-inducing signaling complexes, which activate caspase-8 and its effector (Wang et al., 2021a). However, in normal cells, the Bcl-2 protein family (Bcl-2, Bcl-xl, Mcl-1, Bcl-W, and A1/BFL1) protects cell survival by inhibiting Bax and Bak (Figure 1).
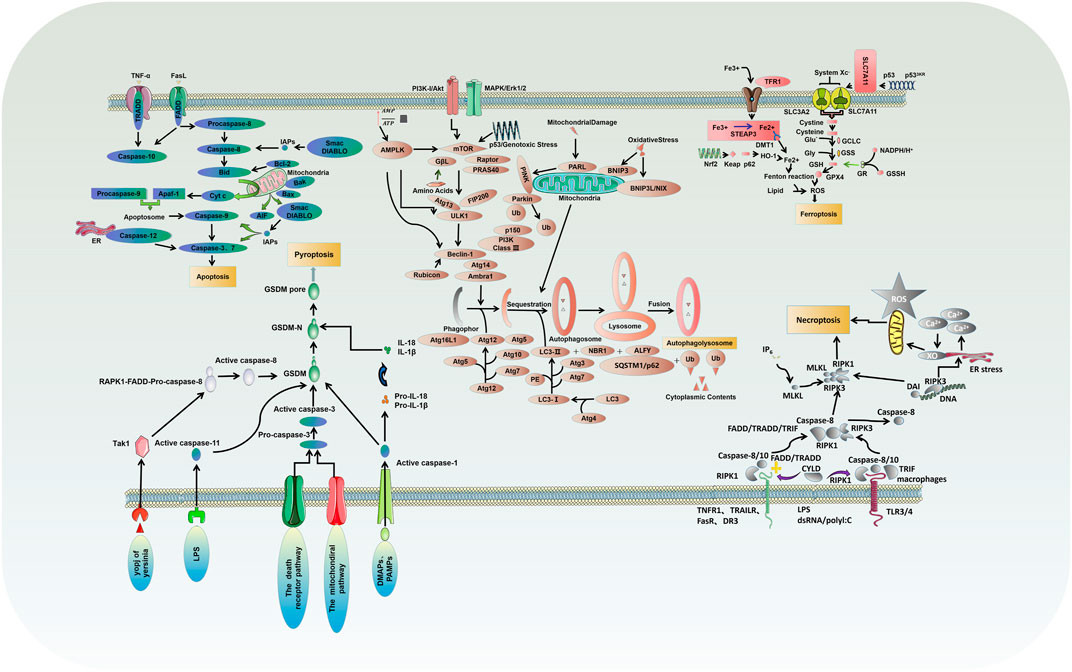
FIGURE 1. Molecular mechanisms of different types of PCD: critical molecular pathways of autophagy, apoptosis, necrosis, pyroptosis and ferroptosis.
It has been found that myocardial apoptosis can simultaneously exert detrimental effects on cardiac function. For instance, Wu et al. found that bradykinin (BK) could reduce myocardial apoptosis by reducing caspase-3 expression in a human cardiac c-Kit + progenitor cell (hCPCs) myocardial infarction model, thereby improving ischemic heart disease (Wu et al., 2021a), Uberti F et al. showed that levosimendan could interfere with mitochondrial function by regulating mitoK (ATP) channels and NO, and could control the interaction between autophagy and apoptosis to protect H9c2 cells against oxidative damage (Uberti et al., 2011), Nie et al. reported that lncRNA AK006774 could reduce I/R-induced infarct size and myocardial apoptosis by regulating Bcl-2 expression through miR-448 in a mouse I/R model (Nie et al., 2021), Xu et al. found that GSK-3β upregulated CD47 expression in cardiac tissues after MI by activating NF-κb, and then regulated apoptosis leading to myocardial injury (Xu et al., 2021). Besides, AKT1/GSK3β, NF-κb, and other related signaling molecules can also regulate apoptosis in cardiovascular diseases (Supplementary Table S1) (Shen et al., 2020; Jiang et al., 2021a; Wang et al., 2021a; Jiang et al., 2021b; He et al., 2021; Lu et al., 2021; Sun et al., 2021; Xiong et al., 2021; Xu et al., 2021).
Necrosis Pathways in Cardiovascular Diseases
Necrosis is a type of PCD characterized by lytic necrosis and could drive inflammation, in which the RIPK1/RIPK3/MLKL axis may play an essential role (Chen et al., 2020; She et al., 2019). Receptor-interacting protein kinase1 (RIPK1) autophosphorylation is activated by RIPK3/MLKL sequence, induces membrane permeability and cell destruction to release cell damage-associated molecular pattern (cDAMP), and finally leads to the occurrence of cell necrosis (Zhu and Sun, 2018). Caspase-8 activation, on the other hand, could inhibit cell necrosis by cleaving RIPK1 and RIPK3 (Figure 1). In their study, M.I. Oerlemans et al. demonstrated that RIPK1, RIPK3, and mixed lineage kinase domain-like (MLKL) expression was up-regulated in the myocardium after reperfusion, and the necrosis inhibitor Necrostatin-1 could reduce the phosphorylation levels of RIPK1 and RIPK3 and the recruitment of MLKL, thus inhibiting cell necrosis and reducing myocardial infarct size (Oerlemans et al., 2012). Currently, I/R models are preferred to elucidate the effect of different factors on cardiomyocyte necrosis through various pathways such as mechanistic target of rapamycin (mTOR), rate-oxygenation (ROX) and MLKL (Supplementary Table S2) (Yang et al., 2018a; Zhu et al., 2018; She et al., 2019; Chen et al., 2020; Jiang et al., 2021c; Han et al., 2021; Liu et al., 2021; Pullaiah et al., 2021; Zhang et al., 2021).
Ferroptosis Pathways in Cardiovascular Diseases
Ferroptosis, characterized by mitochondrial atrophy, increased membrane density, and reduced mitochondrial cristae, is a new form of PCD discovered recently, which proceeds with cell morphology and function different from the various modes of death described above (Dixon et al., 2012). Ferroptosis is usually executed by compressive lipid peroxidation and inhibited by iron chelators. In recent years, research has gradually unveiled its mystery, and many characteristic molecules are identified: acyl-CoA synthetase long-chain family 4 and lysophosphatidylcholine acyltransferase 3 (ACSL4 and LPCAT3) of membrane lipids susceptible to oxidation, xCT of the glutamate-cystine reverse transport system, glutathione peroxidase 4 (GPX4), and ferroptosis inhibitor protein 1, etc. (Figure 1) (Chen et al., 2019; Li et al., 2019).
Current studies have found that cardiovascular diseases caused by high iron levels are associated with ferroptosis (Wu et al., 2021b). Baba et al. have demonstrated that rapamycin could protect cardiomyocytes from excess iron-induced ferroptosis by reacting with mechanistic targets (Baba et al., 2018). Ferrostatin-1 (Fer-1) is an inhibitor of ferroptosis and can mediate the death of cardiomyocytes and neutrophil recruitment after heart transplantation through the toll-like receptor 4/interferon-β (TLR4/TRIF) signaling pathway (Li et al., 2019). Studies also indicate that other signaling molecules such as NADPH oxidase 4 (NOX4) and GPX4 regulate ferroptosis and thus affect heart disease (Supplementary Table S3). Ferroptosis is increasingly considered a potential cause of cardiovascular morbidity, and inhibition of ferroptosis represents a new strategy for these patients (Liu et al., 2018a; Bai et al., 2018; Chen et al., 2019; Fang et al., 2019; Feng et al., 2019; Fan et al., 2021).
Autophagy Pathways in Cardiovascular Diseases
Autophagy is a highly conserved catalytic process that leads to the autolysosomal degradation of primary cytoplasmic contents, removing abnormally aggregated proteins and excess organelles. Generally, the activation of autophagy is triggered by undernutrition and oxidative stress (Dong et al., 2019). It is also associated with many other physiological and pathological processes, such as development, differentiation, neurodegenerative diseases, stress, infection, and cancer. Autophagy can be classified into three types: macroautophagy, microautophagy, and partner-mediated autophagy (Moujalled et al., 2021). mTOR is an important regulatory molecule that induces autophagy, and activating mTOR by phosphatidylinositol 3-kinase (PI3K) and mitogen-activated protein kinase (MARK) transduction can inhibit autophagy. In contrast, the negative regulation of mTOR by adenosine monophosphate-activated protein kinase (AMPK) and p53 signal transduction promotes autophagy (Dong et al., 2019; Shi et al., 2020). The formed mTOR complex is extensively linked to the downstream autophagy-related gene (ATG) family to construct the autophagosomes and ultimately complete the process of ubiquitin-like reactions. There is an extensive interaction between autophagy and apoptosis, which can be positively or negatively connected. Some studies have suggested the apoptosis-protective factor Bcl-2 could inhibit Beclin-1 dependent autophagy and function as its anti-autophagy regulatory molecule (Figure 1) (Oka et al., 2012; Grootaert et al., 2015; Dong et al., 2019).
In the normal physiological state of the heart, cardiomyocytes, vascular epithelial cells, and smooth muscle cells, autophagy plays an essential role in organelle function and cell renewal. Autophagy is also activated in response to cardiovascular stress, including I/R and heart failure. Sciarretta S et al. found that in ischemic stress, Rheb, as a GTP-binding protein, could inhibit autophagy by activating mTORC1, thereby aggravating post-ischemic infarct size (Sciarretta et al., 2012). During heart failure and aortic atherosclerosis, miR-212/132, laminar flow and Danqi pills regulated autophagy by modulating autophagy-related pathways and molecules, affecting cardiac function (Supplementary Table S4) (Oka et al., 2012; Shi et al., 2020; Grootaert et al., 2015; Ucar et al., 2012; Yuan et al., 2020; Wang et al., 2021b). As mentioned before, autophagy restricted to an appropriate extent protects against ischemic injury via maintaining cardiomyocyte homeostasis, degrading organelles, or misfolding proteins that produce adenosine triphosphate (ATP). However, it is also reported that overwhelming cardiac autophagy induction may also promote cell death and worsen cardiac function in the setting of severe ischemia. Some studies have found that knockdown of TLR4 or NOX4 in rats with heart failure significantly inhibited autophagy activation, thereby improving cardiac function. In addition, the PI3K/AKT/mTOR pathway is indicated to be involved in regulating the excessive autophagy process to protect cardiomyocytes (Supplementary Table S5) (Xuan and Jian, 2016; Xuan et al., 2017; Liu et al., 2018b; Chen et al., 2019; Zhang et al., 2020).
Pyroptosis Pathways in Cardiovascular Diseases
Pyroptosis is an inflammatory PCD process that rapidly initiates innate immune responses through pattern recognition receptors (PRRs), and different inflammatory bodies can sense different pathogen-associated molecular patterns and damage-associated molecular patterns (PAMPs and DAMPs) that induce pyroptosis (Zhu and Sun, 2018). Classical pyroptosis induction requires activating caspase-1, which cleaves and activates inflammatory cytokines (IL-1b, IL-18), caspase-1 cleaves and activates Gasdermin D (GSDMD), which is also key to the occurrence of pyroptosis (Figure 1) (Zhang et al., 2018a; Yue et al., 2019).
An increasing number of studies have shown that cardiovascular risk factors can activate nod-like receptor protein 3 (NLRP3) inflammasomes in cells. In addition, NLRP3 inflammasomes mediated pyroptosis was observed in various cardiovascular diseases. Studies suggested that NLRP3 inflammasomes and related pyrogenic signaling molecules played an essential role in the progression of cardiovascular diseases (Supplementary Table S6) (Liu et al., 2013; Jeyabal et al., 2016; Zhou et al., 2016; Huang et al., 2017; Nazir et al., 2017; Zhang et al., 2018a; Yang et al., 2018b; Li et al., 2018; Yue et al., 2019; Ding et al., 2020; Li et al., 2020). For example: in myocardial I/R model mice, Ding et al. found that Mi-29a expression was up-regulated and Sirtuin-1 (SIRT1) expression was down-regulated, which in turn regulated NLRP3 to attenuate myocardial I/R injury and enhanced cardiomyocytes survival (Ding et al., 2020).
Interconnections Between Different PCD Pathways
There is now increasing evidence that different PCD pathways in cardiovascular diseases are interconnected and affect each other on multiple levels. Studies have found that the PI3K/AKT pathway can regulate autophagy by targeting mTOR, and can also regulate apoptosis through the Bad pathway (Liu et al., 2018b; Wu et al., 2021a). mTOR kinase is an important regulatory molecule that induces PCD. Studies have found that the AMPK/mTOR pathway is not only involved in necrosis and pyroptosis, but can also regulate autophagy through Beclin-1 (Alers et al., 2012; Nazir et al., 2017; Han et al., 2021). Caspase-3 and caspase-7 act as executors and directly degrade structural and functional proteins, whereas caspase-8 and caspase-10 act as initiators and cause the caspase cascade after being activated by signaling stimuli (Kurokawa and Kornbluth, 2009). The caspase family is involved in the regulation of apoptosis and mediates necrosis and pyroptosis (Martin and Henry, 2013; Galluzzi et al., 2017; Yuan et al., 2018). Wu C et al. found that BK effectively improves cardiac function by reducing cardiomyocyte apoptosis, inflammatory infiltration, and myocardial fibrosis in the infarcted heart by reducing cleaved caspase-3 expression (Wu et al., 2021a). The study carried by Wang K et al. showed that miR-874 could regulate cardiomyocytes necrosis by affecting caspase-8 activity (Wang et al., 2013). Zheng X et al. found that BNIP3 mediated Doxorubicin-induced cardiomyocyte pyroptosis through activation of caspase-3 and cleavage of gasdermin D (GSDMD) (Zheng et al., 2020). In addition, it has been found that there is an interaction between TNF-α/TNFαR, PAMP/TLR, and DAMP/TLR that can activate NF-κB through the IKK pathway. Activation of NF-κb induces the expression of pro-survival genes, including RIPK1 and Pro-IL-1β (Wajant and Scheurich, 2011; Vande Walle and Lamkanfi, 2016). RIPK1 has been implicated in the regulation of necroptosis, whereas Pro-IL-1β mediated pyroptosis (Van Herreweghe et al., 2010; Yuan et al., 2018). An interaction between ferroptosis and apoptosis in cardiovascular diseases was also found in the present study (Figure 2).
Different Types of PCD and Clinical Drug Therapy
Targeting Apoptosis in Clinical Practice
Currently, interventions targeting apoptosis may have a significant impact on the treatment of cardiovascular diseases. Bcl-2 family, p53 gene, IEG family, ICE gene family, and Fasl-Fas system play essential roles in initiating and developing apoptosis (Dispersyn and Borgers, 2001). Accumulating evidence from clinical trials suggested that modulation of the Bcl-2 family and the Fasl-Fas system as a novel intervention could improve myocardial function (Corbalan et al., 2016). Zhou et al. and Li et al. reported that statins could regulate the cardiomyocytes apoptosis in patients by regulating the expression of Bcl-2 and Bax, thereby protecting the cardiac function (Zhou et al., 2018; Li et al., 2016); Adamopoulos S et al. and Skudicky D et al. demonstrated that growth hormone (GH) and pentoxifylline could alleviate cardiac insults by modulating the apoptosis of cardiomyocytes and the level of soluble Fasl/Fas system (Adamopoulos et al., 2002; Skudicky et al., 2000). Other agents were also found to achieve the efficacy of myocardial protection in apoptosis-targeted ways (Supplementary Table S7) (Rössig et al., 2001; Zhang et al., 2005; Zhang et al., 2018b; Qing et al., 2018). Considering that apoptosis plays a vital role in cardiovascular pathologies, elucidating the underlying therapeutic mechanisms of these drugs in clinical trials provides us with a brand-new concept and idea for the occurrence and development of cardiovascular diseases.
Targeting Necrosis in Clinical Practice
Necrosis is a class of cell death initiated by death receptor ligands, mediated through death receptors, occurs in the inhibition of apoptotic pathways. The death receptor-receptor interaction protein-mitochondria-oxygen free radical pathway is proved to be an essential regulator of cardiac necrosis, and current clinical trials have found that drugs targeting this pathway can reduce cellular necrosis and improve cardiac function (Kim et al., 2011; Vandenabeele et al., 2010; Thévenod and Lee, 2013). Verma S et al. verified the effect of PZ-128 in a phase 2 clinical trial. The results indicated that it could reduce cyclic myocardial necrosis by targeting protease-activated receptor-1 (PAR1) on vascular cells on the inner surface of recipient cells, thereby further improving the outcome of patients with myocardial infarction (Wang, 2018); Silvain J et al. validated the combined efficacy of ticagrelor and clopidogrel on patients undergoing percutaneous coronary intervention (PCI) in a phase 3b clinical trial and found that apart from their anti-platelet aggregation and effect, it could also reduce perioperative myocardial necrosis by inhibiting NF-κb pathway and further improve the postoperative recovery of patients with myocardial infarction (Silvain et al., 2020). Other studies found that some drugs could regulate cell necrosis by anti-aggregation, effect or reducing cell calcium overload, thereby improving myocardial necrosis in patients before and after surgery (Supplementary Table S8) (Alexander et al., 2008; Talarico et al., 2009; Lader et al., 2016; Nauck et al., 2018; Liu et al., 2019; Mohammad et al., 2020; Tcheng et al., 2020). At present, more and more attention has been focused on the clinical trials evaluating anti-necrosis therapies in cardiovascular diseases. The deepening of its targeted treatment in cardiovascular diseases will undoubtedly profoundly impact the clinical treatment of cardiovascular diseases.
Targeting Ferroptosis in Clinical Practice
Unlike classical apoptosis, there is no hallmark of apoptosis such as chromatin condensation during ferroptosis, but it is accompanied by mitochondrial shrinkage and accumulation of lipid oxides. Studies have revealed that overloaded iron would cause irreparable damage to multiple organs. The use of inhibitors of apoptosis in clinical trials does not also inhibit ferroptosis, while the use of iron chelators can inhibit the ferroptosis process of cells. Wongjaikam S et al. demonstrated that a combination of lamotrigine and desferrioxamine as chelation therapy could reduce the level of myocardial iron by restoring sarco/endoplasmic reticulum calcium ATPase (SERCA) levels (Wongjaikam et al., 2017). Farmaki K et al. also found that the combined chelation therapy of desferrioxamine and desferrioxone can prevent and reverse the cardiac complications caused by excessive iron in blood transfusion (Farmaki et al., 2010). Other clinical trials concerning iron chelators in cardiovascular diseases are shown in Supplementary Table S9 (Penckofer and Schwertz, 2000; Al-Rousan et al., 2009; Farmaki et al., 2010; Lal et al., 2013; Jansová et al., 2014; Shakoor et al., 2014; Pennell et al., 2015; Ho et al., 2017; Eghbali et al., 2019). Although iron ion chelators are manifested as a promising drug target in clinical trials, their potential molecular signaling pathways and networks remain to be explored. Besides, the metabolism of iron at the systemic level is another challenge. Iron metabolism forms homeostasis between the body and target organs to regulate iron death in the clinical treatment of cardiovascular diseases.
Targeting Autophagy in Clinical Practice
Autophagy plays a broad role in developing cardiovascular pathology and can act as both a suppressor and a promoting factor of cardiovascular disease. Although some drugs and regimens can modulate autophagy, few clinical trials have assessed their role in the cardiovascular system. Among them, Qing et al. conducted a phase 2 clinical trial of berberine and found that it could reduce myocardial injury by regulating the AMPK/mTOR pathway; Hua et al. elucidated that simvastatin could reduce myocardial injury by regulating the expression of LC3-II/LC3-I, Beclin- 1, and AMPK phosphorylation, which in turn improved cardiac function (Supplementary Table S10) (Hua et al., 2017; Qing et al., 2018). Another significant limitation in this field is the lack of spatially specific delivery of exogenous autophagy modulators for cardiac or vascular repair, so there is still a long way to go for effective autophagy therapy. However, the insights gained from these works will inspire future clinical trials assessing autophagic responses to achieve therapeutic efficacies.
Targeting Pyroptosis in Clinical Practice
Pyroptosis is a new programmed inflammatory death mainly regulated by classical and non-classical pathways, the classical pathway of which is mediated by caspase-1 dependence, and the non-classical inflammasome pathway is mediated by caspase-4/5/11 (Li et al., 2020). Numerous studies have shown that pyroptosis is generally involved in the development and outcome of various cardiovascular diseases such as myocardial infarction, heart failure, and myocardial I/R (Zhu and Sun, 2018; Wang and Kanneganti, 2021). To date, few clinical trials have assessed its role in cardiovascular disease, mainly due to the lack of cell/organ-targeted delivery of exogenous pyroptosis modulators for cardiac or vascular repair. However, since pyroptosis is involved in the development of a variety of cardiovascular diseases, the subsequent in-depth study of cell/organ targeted delivery of apoptosis modulators will provide a new direction and effective target for the therapeutic research of related diseases.
Summary and Discussion
While research into cardiovascular disease has focused on the molecular mechanisms of individual PCD types, it is now clear that different PCD pathways do not operate in isolation. Indeed, a more likely explanation basing on current knowledge about the various possibilities of triggering and reconnecting PCD signaling cascades suggests that autophagy, apoptosis, ferroptosis, pyroptosis, and necrosis constitute a pluralistic, coordinated cell death system in which one pathway can flexibly compensate for the other (Konstantinidis et al., 2012; Tower, 2015; Wang and Kanneganti, 2021). For example, it is demonstrated that in I/R injury models, both pyroptosis and necrosis could affect cardiovascular disease through AMPK/mTOR and other pathways (Oerlemans et al., 2013; Nazir et al., 2017). In this review, we describe the characteristics and molecular mechanisms of individual PCD types and discuss the interactions between different cell death signaling cascades to provide a better understanding of the relationship between PCD and cardiovascular diseases.
Up to now, while the increasingly important role of different PCD is unveiled, few clinical trial studies are conducted to treat cardiovascular diseases by pharmacological intervention to PCD (Mani, 2008). Therefore, we describe the critical role of drugs targeting cell death signaling pathways and their efficacy in various cardiovascular diseases by collecting currently available drugs studied in clinical trials. Multiple medications are targeted in cardiovascular disease by modulating different types of PCD in many clinical trials. For instance, statins can regulate myocardial apoptosis in patients by regulating the expression of Bcl-2 and Bax (Zhou et al., 2018). Simvastatin can regulate the autophagy of cardiomyocytes by regulating the expression of LC3-I/II, Beclin-1, and other factors (Hua et al., 2017). Drugs such as liraglutide and pyridoxal 5′-phosphate (MC-1) can modulate the necrosis of cardiomyocytes by intervening calcium ions (Nauck et al., 2018; Alexander et al., 2008). Amlodipine and pantoprazole regulate ferroptosis in cardiomyocytes by affecting iron absorption (Shakoor et al., 2014; Eghbali et al., 2019) (Figure 3). Therefore, more work is needed to explore whether drugs can target therapy in various cardiac cells by handling multiple types of PCD. Most previous drug studies reported only a single PCD type, while recent studies found that drugs could act simultaneously by regulating multiple different types of PCD. For example, Qing et al., in phase 2 of clinical trials, found that berberine could reduce cardiomyocyte autophagy and apoptosis by regulating the AMPK/mTOR pathway, thereby reducing myocardial injury in PCI patients (Qing et al., 2018). In general, treatment for any type of PCD can reverse further damage to the heart to some extent (Moujalled et al., 2021). More research is needed to clarify whether PCD acts as an initiator or a critical node in the injury path when studying drugs acting through the PCD pathway.
Apart from cardiovascular disorders, PCD is also involved in other diseases, including respiratory and digestive tumors (Venderova and Park, 2012; Tai et al., 2018). However, current studies reported only PCD on a single type of cell, and the effects and differences between different types of cells are poorly informed. Therefore, in future work, we can start from various cell types and study the impact of drugs on cardiovascular diseases by intervening in various types of PCD in different cell types, which can not only reduce the adverse events of PCD-targeted therapy but also more accurately treat various types of cardiovascular diseases.
While the underlying biological mechanisms regarding the PCD remain uncovered, therapeutic manipulation of cell death pathways holds great promise. At present, among various types of diseases, the most studied is the intervention of drugs in various tumors: recent clinical trials reported that the combination of deferoxamine (DFO) and deferiprone (DFP) effectively improves hematologic malignancies (Wongjaikam et al., 2017). The combined therapy of drugs regulates ferroptosis in the clinical treatment of various diseases by affecting iron metabolism at the systemic level and allowing iron metabolism to form homeostasis between the body and target organs. Based on this, recently, Farmaki K et al. found that combination therapy with desferrioxamine drugs also improved prognosis in cardiovascular disease and has been initiated in clinical trials (Farmaki et al., 2010). Moreover, combination therapy with DFO and DFP can improve cardiac function by inhibiting ferroptosis by reducing iron levels in the heart (Wongjaikam et al., 2017). Studies in future work can be carried out in different types of cardiovascular diseases based on drug-mediated signaling pathways.
Therefore, our review may pave the way for further novel research in the future. On the one hand, we hope to uncover complex signaling pathways between different PCD and clarify their functions in cardiovascular pathologies. On the other hand, acceleration in clinical transformation is warranted to enable PCD-targeted agents to be applied to detect, prevent, and treat cardiovascular diseases.
Author Contributions
LW and JS designed the review; LZ and JS wrote the first draft of the manuscript; LZ and JS prepared the figures; All authors contributed to the editing of the review and approved the final version of the manuscript for submission.
Funding
This work was supported by grants from the National Natural Science Foundation of China (No. 81770361 and 82070367).
Conflict of Interest
The authors declare that the research was conducted in the absence of any commercial or financial relationships that could be construed as a potential conflict of interest.
Publisher’s Note
All claims expressed in this article are solely those of the authors and do not necessarily represent those of their affiliated organizations, or those of the publisher, the editors and the reviewers. Any product that may be evaluated in this article, or claim that may be made by its manufacturer, is not guaranteed or endorsed by the publisher.
Acknowledgments
We thank for the technical assistance support from Jiangsu Province Collaborative Innovation Center for Cardiovascular Disease Translational Medicine. Figures was modified from Servier Medical Art (http://smart.servier.com/), licensed under a Creative Common Attribution 3.0 Generic License (https://creativecommons.org/licenses/by/3.0/).
Supplementary Material
The Supplementary Material for this article can be found online at: https://www.frontiersin.org/articles/10.3389/fcell.2021.794879/full#supplementary-material
References
Adamopoulos, S., Parissis, J. T., Georgiadis, M., Karatzas, D., Paraskevaidis, J., Kroupis, C., et al. (2002). Growth Hormone Administration Reduces Circulating Proinflammatory Cytokines and Soluble Fas/soluble Fas Ligand System in Patients with Chronic Heart Failure Secondary to Idiopathic Dilated Cardiomyopathy. Am. Heart J. 144 (2), 359–364. doi:10.1067/mhj.2002.124052
Al-Rousan, R. M., Paturi, S., Laurino, J. P., Kakarla, S. K., Gutta, A. K., Walker, E. M., et al. (2009). Deferasirox Removes Cardiac Iron and Attenuates Oxidative Stress in the Iron-Overloaded Gerbil. Am. J. Hematol. 84 (9), 565–570. doi:10.1002/ajh.21487
Alers, S., Löffler, A. S., Wesselborg, S., and Stork, B. (2012). Role of AMPK-mTOR-Ulk1/2 in the Regulation of Autophagy: Cross Talk, Shortcuts, and Feedbacks. Mol. Cel Biol 32 (1), 2–11. doi:10.1128/mcb.06159-11
Alexander, J. H., Alexander, J. H., Emery, R. W., Carrier, M., Ellis, S. J., Mehta, R. H., et al. (2008). Efficacy and Safety of Pyridoxal 5'-phosphate (MC-1) in High-Risk Patients Undergoing Coronary Artery Bypass Graft Surgery: the MEND-CABG II Randomized Clinical Trial. Jama 299 (15), 1777–1787. doi:10.1001/jama.299.15.joc80027
Baba, Y., Higa, J. K., Shimada, B. K., Horiuchi, K. M., Suhara, T., Kobayashi, M., et al. (2018). Protective Effects of the Mechanistic Target of Rapamycin against Excess Iron and Ferroptosis in Cardiomyocytes. Am. J. Physiology-Heart Circulatory Physiol. 314 (3), H659–h668. doi:10.1152/ajpheart.00452.2017
Bai, L., Mei, X., Wang, Y., Yuan, Y., Bi, Y., Li, G., et al. (2017). The Role of Netrin-1 in Improving Functional Recovery through Autophagy Stimulation Following Spinal Cord Injury in Rats. Front. Cel. Neurosci. 11, 350. doi:10.3389/fncel.2017.00350
Bai, Y.-T., Chang, R., Wang, H., Xiao, F.-J., Ge, R.-L., and Wang, L.-S. (2018). ENPP2 Protects Cardiomyocytes from Erastin-Induced Ferroptosis. Biochem. Biophysical Res. Commun. 499 (1), 44–51. doi:10.1016/j.bbrc.2018.03.113
Chen, H., Tang, L.-J., Tu, H., Zhou, Y.-J., Li, N.-S., Luo, X.-J., et al. (2020). Arctiin Protects Rat Heart against Ischemia/reperfusion Injury via a Mechanism Involving Reduction of Necroptosis. Eur. J. Pharmacol. 875, 173053. doi:10.1016/j.ejphar.2020.173053
Chen, X., Xu, S., Zhao, C., and Liu, B. (2019). Role of TLR4/NADPH Oxidase 4 Pathway in Promoting Cell Death through Autophagy and Ferroptosis during Heart Failure. Biochem. Biophysical Res. Commun. 516 (1), 37–43. doi:10.1016/j.bbrc.2019.06.015
Corbalan, J. J., Vatner, D. E., and Vatner, S. F. (2016). Myocardial Apoptosis in Heart Disease: Does the Emperor Have Clothes? Basic Res. Cardiol. 111 (3), 31. doi:10.1007/s00395-016-0549-2
Ding, S., Liu, D., Wang, L., Wang, G., and Zhu, Y. (2020). Inhibiting MicroRNA-29a Protects Myocardial Ischemia-Reperfusion Injury by Targeting SIRT1 and Suppressing Oxidative Stress and NLRP3-Mediated Pyroptosis Pathway. J. Pharmacol. Exp. Ther. 372 (1), 128–135. doi:10.1124/jpet.119.256982
Dispersyn, G. D., and Borgers, M. (2001). Apoptosis in the Heart: about Programmed Cell Death and Survival. Physiology 16, 41–47. doi:10.1152/physiologyonline.2001.16.1.41
Dixon, S. J., Lemberg, K. M., Lamprecht, M. R., Skouta, R., Zaitsev, E. M., Gleason, C. E., et al. (2012). Ferroptosis: an Iron-dependent Form of Nonapoptotic Cell Death. Cell 149 (5), 1060–1072. doi:10.1016/j.cell.2012.03.042
Dong, Y., Chen, H., Gao, J., Liu, Y., Li, J., and Wang, J. (2019). Molecular Machinery and Interplay of Apoptosis and Autophagy in Coronary Heart Disease. J. Mol. Cell Cardiol. 136, 27–41. doi:10.1016/j.yjmcc.2019.09.001
Eghbali, A., Khalilpour, A., Taherahmadi, H., and Bagheri, B. (2019). Pantoprazole Reduces Serum Ferritin in Patients with Thalassemia Major and Intermedia: A Randomized, Controlled Study. Therapies 74 (5), 507–512. doi:10.1016/j.therap.2018.11.013
Fan, K., Huang, W., Qi, H., Song, C., He, C., Liu, Y., et al. (2021). The Egr-1/miR-15a-5p/GPX4 axis Regulates Ferroptosis in Acute Myocardial Infarction. Eur. J. Pharmacol. 909, 174403. doi:10.1016/j.ejphar.2021.174403
Fang, X., Wang, H., Han, D., Xie, E., Yang, X., Wei, J., et al. (2019). Ferroptosis as a Target for protection against Cardiomyopathy. Proc. Natl. Acad. Sci. USA 116 (7), 2672–2680. doi:10.1073/pnas.1821022116
Farmaki, K., Tzoumari, I., Pappa, C., Chouliaras, G., and Berdoukas, V. (2010). Normalisation of Total Body Iron Load with Very Intensive Combined Chelation Reverses Cardiac and Endocrine Complications of Thalassaemia Major. Br. J. Haematol. 148 (3), 466–475. doi:10.1111/j.1365-2141.2009.07970.x
Feng, Y., Madungwe, N. B., Imam Aliagan, A. D., Tombo, N., and Bopassa, J. C. (2019). Liproxstatin-1 Protects the Mouse Myocardium against Ischemia/reperfusion Injury by Decreasing VDAC1 Levels and Restoring GPX4 Levels. Biochem. Biophysical Res. Commun. 520 (3), 606–611. doi:10.1016/j.bbrc.2019.10.006
Galluzzi, L., Kepp, O., Chan, F. K.-M., and Kroemer, G. (2017). Necroptosis: Mechanisms and Relevance to Disease. Annu. Rev. Pathol. Mech. Dis. 12, 103–130. doi:10.1146/annurev-pathol-052016-100247
Grootaert, M. O., da Costa Martins, P. A., Bitsch, N., Pintelon, I., De Meyer, G. R., Martinet, W., et al. (2015). Defective Autophagy in Vascular Smooth Muscle Cells Accelerates Senescence and Promotes Neointima Formation and Atherogenesis. Autophagy 11 (11), 2014–2032. doi:10.1080/15548627.2015.1096485
Han, Y. L., Chen, S., and Peng, X. (2021). Electroacupuncture Pretreatment at Neiguan (PC6) Attenuates Autophagy in Rats with Myocardial Ischemia Reperfusion through the Phosphatidylinositol 3-Kinase-Akt-Mammalian Target of Rapamycin Pathway. J. Tradit Chin. Med. 41 (3), 455–462. doi:10.19852/j.cnki.jtcm.2021.03.014
He, Z., Zeng, X., Zhou, D., Liu, P., Han, D., Xu, L., et al. (2021). LncRNA Chaer Prevents Cardiomyocyte Apoptosis from Acute Myocardial Infarction through AMPK Activation. Front. Pharmacol. 12, 649398. doi:10.3389/fphar.2021.649398
Ho, P. J., Tay, L., Teo, J., Marlton, P., Grigg, A., St Pierre, T., et al. (2017). Cardiac Iron Load and Function in Transfused Patients Treated with Deferasirox (The MILE Study). Eur. J. Haematol. 98 (2), 97–105. doi:10.1111/ejh.12793
Hua, P., Liu, J., Tao, J., Zou, R., Lin, X., Zhang, D., et al. (2017). Efficacy and Mechanism of Preoperative Simvastatin Therapy on Myocardial Protection after Extracorporeal Circulation. Biomed. Res. Int. 2017, 6082430. doi:10.1155/2017/6082430
Huang, W.-Q., Wei, P., Lin, R.-Q., and Huang, F. (2017). Protective Effects of Microrna-22 against Endothelial Cell Injury by Targeting NLRP3 through Suppression of the Inflammasome Signaling Pathway in a Rat Model of Coronary Heart Disease. Cell Physiol Biochem 43 (4), 1346–1358. doi:10.1159/000481846
Jansová, H., Macháček, M., Wang, Q., Hašková, P., Jirkovská, A., Potůčková, E., et al. (2014). Comparison of Various Iron Chelators and Prochelators as Protective Agents against Cardiomyocyte Oxidative Injury. Free Radic. Biol. Med. 74, 210–221. doi:10.1016/j.freeradbiomed.2014.06.019
Jeyabal, P., Thandavarayan, R. A., Joladarashi, D., Suresh Babu, S., Krishnamurthy, S., Bhimaraj, A., et al. (2016). MicroRNA-9 Inhibits Hyperglycemia-Induced Pyroptosis in Human Ventricular Cardiomyocytes by Targeting ELAVL1. Biochem. Biophysical Res. Commun. 471 (4), 423–429. doi:10.1016/j.bbrc.2016.02.065
Jiang, C., Zhao, Q., Wang, C., Peng, M., Hao, G., Liu, Z., et al. (2021). Downregulation of Long Noncoding RNA LINC00261 Attenuates Myocardial Infarction through the miR-522-3p/Trinucleotide Repeat-Containing Gene 6a (TNRC6A) Axis. Cardiovasc. Ther. 2021, 6628194. doi:10.1155/2021/6628194
Jiang, W., Song, J., Zhang, S., Ye, Y., Wang, J., and Zhang, Y. (2021). CTRP13 Protects H9c2 Cells against Hypoxia/Reoxygenation (H/R)-Induced Injury via Regulating the AMPK/Nrf2/ARE Signaling Pathway. Cel Transpl. 30, 9636897211033275. doi:10.1177/09636897211033275
Jiang, X., Wu, D., Jiang, Z., Ling, W., and Qian, G. (2021). Protective Effect of Nicorandil on Cardiac Microvascular Injury: Role of Mitochondrial Integrity. Oxid Med. Cel Longev 2021, 4665632. doi:10.1155/2021/4665632
Kim, J.-Y., Kim, Y.-J., Lee, S., and Park, J.-H. (2011). BNip3 Is a Mediator of TNF-Induced Necrotic Cell Death. Apoptosis 16 (2), 114–126. doi:10.1007/s10495-010-0550-4
Konstantinidis, K., Whelan, R. S., and Kitsis, R. N. (2012). Mechanisms of Cell Death in Heart Disease. Atvb 32 (7), 1552–1562. doi:10.1161/atvbaha.111.224915
Kramer, J. L. K., Lammertse, D. P., Schubert, M., Curt, A., and Steeves, J. D. (2012). Relationship between Motor Recovery and independence after Sensorimotor-Complete Cervical Spinal Cord Injury. Neurorehabil. Neural Repair 26 (9), 1064–1071. doi:10.1177/1545968312447306
Kung, G., Konstantinidis, K., and Kitsis, R. N. (2011). Programmed Necrosis, Not Apoptosis, in the Heart. Circ. Res. 108 (8), 1017–1036. doi:10.1161/circresaha.110.225730
Kurokawa, M., and Kornbluth, S. (2009). Caspases and Kinases in a Death Grip. Cell 138 (5), 838–854. doi:10.1016/j.cell.2009.08.021
Lader, A. S., Baguisi, A., Casale, R., Kates, S. A., and Beeuwkes, R. (2016). CMX-2043 Mechanisms of Action In Vitro. J. Cardiovasc. Pharmacol. 68 (3), 241–247. doi:10.1097/fjc.0000000000000408
Lal, A., Porter, J., Sweeters, N., Ng, V., Evans, P., Neumayr, L., et al. (2013). Combined Chelation Therapy with Deferasirox and Deferoxamine in Thalassemia. Blood Cell Mol. Dis. 50 (2), 99–104. doi:10.1016/j.bcmd.2012.10.006
Li, M., Xue, L., Sun, H., and Xu, S. (2016). Myocardial Protective Effects of L-Carnitine on Ischemia-Reperfusion Injury in Patients with Rheumatic Valvular Heart Disease Undergoing Cardiac Surgery. J. Cardiothorac. Vasc. Anesth. 30 (6), 1485–1493. doi:10.1053/j.jvca.2016.06.006
Li, P., Zhong, X., Li, J., Liu, H., Ma, X., He, R., et al. (2018). MicroRNA-30c-5p Inhibits NLRP3 Inflammasome-Mediated Endothelial Cell Pyroptosis through FOXO3 Down-Regulation in Atherosclerosis. Biochem. Biophysical Res. Commun. 503 (4), 2833–2840. doi:10.1016/j.bbrc.2018.08.049
Li, W., Feng, G., Gauthier, J. M., Lokshina, I., Higashikubo, R., Evans, S., et al. (2019). Ferroptotic Cell Death and TLR4/Trif Signaling Initiate Neutrophil Recruitment after Heart Transplantation. J. Clin. Invest. 129 (6), 2293–2304. doi:10.1172/jci126428
Li, Y., Niu, X., Xu, H., Li, Q., Meng, L., He, M., et al. (2020). VX-765 Attenuates Atherosclerosis in ApoE Deficient Mice by Modulating VSMCs Pyroptosis. Exp. Cel Res. 389 (1), 111847. doi:10.1016/j.yexcr.2020.111847
Liu, A., Gao, X., Zhang, Q., and Cui, L. (2013). Cathepsin B Inhibition Attenuates Cardiac Dysfunction and Remodeling Following Myocardial Infarction by Inhibiting the NLRP3 Pathway. Mol. Med. Rep. 8 (2), 361–366. doi:10.3892/mmr.2013.1507
Liu, B., Zhao, C., Li, H., Chen, X., Ding, Y., and Xu, S. (2018). Puerarin Protects against Heart Failure Induced by Pressure Overload through Mitigation of Ferroptosis. Biochem. Biophysical Res. Commun. 497 (1), 233–240. doi:10.1016/j.bbrc.2018.02.061
Liu, X., Deng, Y., Xu, Y., Jin, W., and Li, H. (2018). MicroRNA-223 Protects Neonatal Rat Cardiomyocytes and H9c2 Cells from Hypoxia-Induced Apoptosis and Excessive Autophagy via the Akt/mTOR Pathway by Targeting PARP-1. J. Mol. Cell Cardiol. 118, 133–146. doi:10.1016/j.yjmcc.2018.03.018
Liu, X., Wang, Y., Zhang, M., Liu, Y., Hu, L., and Gu, Y. (2019). Ticagrelor Reduces Ischemia-Reperfusion Injury through the NF-κb-dependent Pathway in Rats. J. Cardiovasc. Pharmacol. 74 (1), 13–19. doi:10.1097/fjc.0000000000000675
Liu, Y., Chen, J., Xia, P., Stratakis, C. A., and Cheng, Z. (2021). Loss of PKA Regulatory Subunit 1α Aggravates Cardiomyocyte Necrosis and Myocardial Ischemia/reperfusion Injury. J. Biol. Chem. 297 (1), 100850. doi:10.1016/j.jbc.2021.100850
Lu, C., Liu, L., Chen, S., Niu, J., Li, S., Xie, W., et al. (2021). Azathioprine Pretreatment Ameliorates Myocardial Ischaemia Reperfusion Injury in Diabetic Rats by Reducing Oxidative Stress, Apoptosis, and Inflammation. Clin. Exp. Pharmacol. Physiol. 48, 1621–1632. doi:10.1111/1440-1681.13569
Ma, S., Dielschneider, R. F., Henson, E. S., Xiao, W., Choquette, T. R., Blankstein, A. R., et al. (2017). Ferroptosis and Autophagy Induced Cell Death Occur Independently after Siramesine and Lapatinib Treatment in Breast Cancer Cells. PLoS One 12 (8), e0182921. doi:10.1371/journal.pone.0182921
Mani, K. (2008). Programmed Cell Death in Cardiac Myocytes: Strategies to Maximize post-ischemic Salvage. Heart Fail. Rev. 13 (2), 193–209. doi:10.1007/s10741-007-9073-7
Martin, S. J., and Henry, C. M. (2013). Distinguishing between Apoptosis, Necrosis, Necroptosis and Other Cell Death Modalities. Methods 61 (2), 87–89. doi:10.1016/j.ymeth.2013.06.001
Mohammad, H. M. F., Makary, S., Atef, H., El-Sherbiny, M., Atteia, H. H., Ibrahim, G. A., et al. (2020). Clopidogrel or Prasugrel Reduces Mortality and Lessens Cardiovascular Damage from Acute Myocardial Infarction in Hypercholesterolemic Male Rats. Life Sci. 247, 117429. doi:10.1016/j.lfs.2020.117429
Moujalled, D., Strasser, A., and Liddell, J. R. (2021). Molecular Mechanisms of Cell Death in Neurological Diseases. Cell Death Differ 28 (7), 2029–2044. doi:10.1038/s41418-021-00814-y
Nauck, M. A., Tornøe, K., Rasmussen, S., Treppendahl, M. B., and Marso, S. P. (2018). Cardiovascular Outcomes in Patients Who Experienced a Myocardial Infarction while Treated with Liraglutide versus Placebo in the LEADER Trial. Diabetes Vasc. Dis. Res. 15 (5), 465–468. doi:10.1177/1479164118783935
Nazir, S., Gadi, I., Al-Dabet, M. d. M., Elwakiel, A., Kohli, S., Ghosh, S., et al. (2017). Cytoprotective Activated Protein C Averts Nlrp3 Inflammasome-Induced Ischemia-Reperfusion Injury via mTORC1 Inhibition. Blood 130 (24), 2664–2677. doi:10.1182/blood-2017-05-782102
Nie, S., Cui, X., Guo, J., Ma, X., Zhi, H., Li, S., et al. (2021). Long Non-coding RNA AK006774 Inhibits Cardiac Ischemia-Reperfusion Injury via Sponging miR-448. Bioengineered 12 (1), 4972–4982. doi:10.1080/21655979.2021.1954135
Nishida, K., and Otsu, K. (2008). Cell Death in Heart Failure. Circ. J. 72 Suppl A (Suppl. A), A17–A21. doi:10.1253/circj.cj-08-0669
Oerlemans, M. I. F. J., Koudstaal, S., Chamuleau, S. A., de Kleijn, D. P., Doevendans, P. A., and Sluijter, J. P. G. (2013). Targeting Cell Death in the Reperfused Heart: Pharmacological Approaches for Cardioprotection. Int. J. Cardiol. 165 (3), 410–422. doi:10.1016/j.ijcard.2012.03.055
Oerlemans, M. I. F. J., Liu, J., Arslan, F., Ouden, K., Middelaar, B. J., Doevendans, P. A., et al. (2012). Inhibition of RIP1-dependent Necrosis Prevents Adverse Cardiac Remodeling after Myocardial Ischemia-Reperfusion In Vivo. Basic Res. Cardiol. 107 (4), 270. doi:10.1007/s00395-012-0270-8
Oka, T., Hikoso, S., Yamaguchi, O., Taneike, M., Takeda, T., Tamai, T., et al. (2012). Mitochondrial DNA that Escapes from Autophagy Causes Inflammation and Heart Failure. Nature 485 (7397), 251–255. doi:10.1038/nature10992
Penckofer, S., and Schwertz, D. (2000). Improved Iron Status Parameters May Be a Benefit of Hormone Replacement Therapy. J. Women's Health Gender-Based Med. 9 (2), 141–151. doi:10.1089/152460900318632
Pennell, D. J., Porter, J. B., Piga, A., Lai, Y. R., El‐Beshlawy, A., Elalfy, M., et al. (2015). Sustained Improvements in Myocardial T2* over 2 Years in Severely Iron‐overloaded Patients with Beta Thalassemia Major Treated with Deferasirox or Deferoxamine. Am. J. Hematol. 90 (2), 91–96. doi:10.1002/ajh.23876
Pullaiah, C. P., Nelson, V. K., Rayapu, S., G v, N. K., and Kedam, T. (2021). Exploring Cardioprotective Potential of Esculetin against Isoproterenol Induced Myocardial Toxicity in Rats: In Vivo and In Vitro Evidence. BMC Pharmacol. Toxicol. 22 (1), 43. doi:10.1186/s40360-021-00510-0
Qing, Y., Dong, X., Hongli, L., and Yanhui, L. (2018). Berberine Promoted Myocardial protection of Postoperative Patients through Regulating Myocardial Autophagy. Biomed. Pharmacother. 105, 1050–1053. doi:10.1016/j.biopha.2018.06.088
Rössig, L., Hoffmann, J., Hugel, B., Mallat, Z., Haase, A., Freyssinet, J. M., et al. (2001). Vitamin C Inhibits Endothelial Cell Apoptosis in Congestive Heart Failure. Circulation 104 (18), 2182–2187. doi:10.1161/hc4301.098284
Saunders, L. L., Clarke, A., Tate, D. G., Forchheimer, M., and Krause, J. S. (2015). Lifetime Prevalence of Chronic Health Conditions Among Persons with Spinal Cord Injury. Arch. Phys. Med. Rehabil. 96 (4), 673–679. doi:10.1016/j.apmr.2014.11.019
Sciarretta, S., Zhai, P., Shao, D., Maejima, Y., Robbins, J., Volpe, M., et al. (2012). Rheb Is a Critical Regulator of Autophagy during Myocardial Ischemia. Circulation 125 (9), 1134–1146. doi:10.1161/circulationaha.111.078212
Shakoor, A., Zahoor, M., Sadaf, A., Alvi, N., Fadoo, Z., Rizvi, A., et al. (2014). Effect of L-type Calcium Channel Blocker (Amlodipine) on Myocardial Iron Deposition in Patients with Thalassaemia with Moderate-To-Severe Myocardial Iron Deposition: Protocol for a Randomised, Controlled Trial. BMJ Open 4 (12), e005360. doi:10.1136/bmjopen-2014-005360
She, L., Tu, H., Zhang, Y.-Z., Tang, L.-J., Li, N.-S., Ma, Q.-L., et al. (2019). Inhibition of Phosphoglycerate Mutase 5 Reduces Necroptosis in Rat Hearts Following Ischemia/Reperfusion through Suppression of Dynamin-Related Protein 1. Cardiovasc. Drugs Ther. 33 (1), 13–23. doi:10.1007/s10557-018-06848-8
Shen, Z., Shen, A., Chen, X., Wu, X., Chu, J., Cheng, Y., et al. (2020). Huoxin Pill Attenuates Myocardial Infarction-Induced Apoptosis and Fibrosis via Suppression of P53 and TGF-β1/Smad2/3 Pathways. Biomed. Pharmacother. 130, 110618. doi:10.1016/j.biopha.2020.110618
Shi, B., Huang, Y., Ni, J., Chen, J., Wei, J., Gao, H., et al. (2020). Qi Dan Li Xin Pill Improves Chronic Heart Failure by Regulating mTOR/p70S6k-Mediated Autophagy and Inhibiting Apoptosis. Sci. Rep. 10 (1), 6105. doi:10.1038/s41598-020-63090-9
Shi, Z., Zhou, H., Lu, L., Li, X., Fu, Z., Liu, J., et al. (2017). The Roles of microRNAs in Spinal Cord Injury. Int. J. Neurosci. 127 (12), 1104–1115. doi:10.1080/00207454.2017.1323208
Silvain, J., Lattuca, B., Beygui, F., Rangé, G., Motovska, Z., Dillinger, J. G., et al. (2020). Ticagrelor versus Clopidogrel in Elective Percutaneous Coronary Intervention (ALPHEUS): a Randomised, Open-Label, Phase 3b Trial. Lancet 396 (10264), 1737–1744. doi:10.1016/S0140-6736(20)32236-4
Skudicky, D., Sliwa, K., Bergemann, A., Candy, G., and Sareli, P. (2000). Reduction in Fas/APO-1 Plasma Concentrations Correlates With Improvement in Left Ventricular Function in Patients With Idiopathic Dilated Cardiomyopathy Treated with Pentoxifylline. Heart 84 (4), 438–441. doi:10.1136/heart.84.4.438
Sun, M., Zhang, W., Bi, Y., Xu, H., Abudureyimu, M., Peng, H., et al. (2021). NDP52 Protects against Myocardial Infarction-Provoked Cardiac Anomalies through Promoting Autophagosome-Lysosome Fusion via Recruiting TBK1 and RAB7. Antioxid. Redox Signal. doi:10.1089/ars.2020.8253
Tai, Y.-T., Cho, K. C., and Anderson, K. C. (2018). Osteoclast Immunosuppressive Effects in Multiple Myeloma: Role of Programmed Cell Death Ligand 1. Front. Immunol. 9, 1822. doi:10.3389/fimmu.2018.01822
Talarico, G. P., Brancati, M., Burzotta, F., Porto, I., Trani, C., De Vita, M., et al. (2009). Glycoprotein IIB/IIIA Inhibitor to Reduce Postpercutaneous Coronary Intervention Myonecrosis and Improve Coronary Flow in Diabetics: the ‘OPTIMIZE-IT’ Pilot Randomized Study. J. Cardiovasc. Med. (Hagerstown) 10 (3), 245–251. doi:10.2459/jcm.0b013e32832180d9
Tcheng, J. E., Gibson, M., Krucoff, M. W., Patel, M. R., Ajit, M., Hiremath, J., et al. (2020). SUPPORT-1 (Subjects Undergoing PCI and Perioperative Reperfusion Treatment): A Prospective, Randomized Trial of CMX-2043 in Patients Undergoing Elective Percutaneous Coronary Intervention. J. Cardiovasc. Pharmacol. 76 (2), 189–196. doi:10.1097/fjc.0000000000000830
Thévenod, F., and Lee, W.-K. (2013). Cadmium and Cellular Signaling Cascades: Interactions between Cell Death and Survival Pathways. Arch. Toxicol. 87 (10), 1743–1786. doi:10.1007/s00204-013-1110-9
Tower, J. (2015). Programmed Cell Death in Aging. Ageing Res. Rev. 23 (Pt), 90–100. doi:10.1016/j.arr.2015.04.002
Uberti, F., Caimmi, P. P., Molinari, C., Mary, D., Vacca, G., and Grossini, E. (2011). Levosimendan Modulates Programmed Forms of Cell Death through KATP Channels and Nitric Oxide. J. Cardiovasc. Pharmacol. 57 (2), 246–258. doi:10.1097/fjc.0b013e318204bb55
Ucar, A., Gupta, S. K., Fiedler, J., Erikci, E., Kardasinski, M., Batkai, S., et al. (2012). The miRNA-212/132 Family Regulates Both Cardiac Hypertrophy and Cardiomyocyte Autophagy. Nat. Commun. 3, 1078. doi:10.1038/ncomms2090
Van Herreweghe, F., Festjens, N., Declercq, W., and Vandenabeele, P. (2010). Tumor Necrosis Factor-Mediated Cell Death: to Break or to Burst, That's the Question. Cell. Mol. Life Sci. 67 (10), 1567–1579. doi:10.1007/s00018-010-0283-0
Vande Walle, L., and Lamkanfi, M. (2016). Pyroptosis. Curr. Biol. 26 (13), R568–r572. doi:10.1016/j.cub.2016.02.019
Vandenabeele, P., Declercq, W., Van Herreweghe, F., and Vanden Berghe, T. (2010). The Role of the Kinases RIP1 and RIP3 in TNF-Induced Necrosis. Sci. Signal. 3 (115), re4. doi:10.1126/scisignal.3115re4
Venderova, K., and Park, D. S. (2012). Programmed Cell Death in Parkinson's Disease. Cold Spring Harb Perspect. Med. 2 (8). doi:10.1101/cshperspect.a009365
Wajant, H., and Scheurich, P. (2011). TNFR1-induced Activation of the Classical NF-Κb Pathway. Febs j 278 (6), 862–876. doi:10.1111/j.1742-4658.2011.08015.x
Wang, B., Xu, H., Kong, J., Liu, D., Qin, W. D., Bai, W., et al. (2021). Krüppel-like Factor 15 Reduces Ischemia-Induced Apoptosis Involving Regulation of P38/MAPK Signaling. Hum. Gene Ther. doi:10.1089/hum.2021.075
Wang, K., Liu, F., Zhou, L.-Y., Ding, S.-L., Long, B., Liu, C.-Y., et al. (2013). miR-874 Regulates Myocardial Necrosis by Targeting Caspase-8. Cell Death Dis 4, e709. doi:10.1038/cddis.2013.233
Wang, X., Jiang, Y., Zhang, Q., Tian, X., Sun, Q., Guo, D., et al. (2021). Autophagy as a Novel Insight into Mechanism of Danqi Pill against post-acute Myocardial Infarction Heart Failure. J. Ethnopharmacology 266, 113404. doi:10.1016/j.jep.2020.113404
Wang, Y., and Kanneganti, T.-D. (2021). From Pyroptosis, Apoptosis and Necroptosis to PANoptosis: A Mechanistic Compendium of Programmed Cell Death Pathways. Comput. Struct. Biotechnol. J. 19, 4641–4657. doi:10.1016/j.csbj.2021.07.038
Wang, Z., Wang, M., Hu, X., Li, Y., Ma, D., Li, S., et al. (2019). Liraglutide, a Glucagon-like Peptide-1 Receptor Agonist, Attenuates Development of Cardiac Allograft Vasculopathy in a Murine Heart Transplant Model. Transplantation 103 (3), 502–511. doi:10.1097/TP.0000000000002448
Wongjaikam, S., Kumfu, S., Khamseekaew, J., Chattipakorn, S. C., and Chattipakorn, N. (2017). Restoring the Impaired Cardiac Calcium Homeostasis and Cardiac Function in Iron Overload Rats by the Combined Deferiprone and N-Acetyl Cysteine. Sci. Rep. 7, 44460. doi:10.1038/srep44460
Wu, C., Zhou, X.-X., Li, J.-Z., Qiang, H.-F., Wang, Y., and Li, G. (2021). Pretreatment of Cardiac Progenitor Cells with Bradykinin Attenuates H2O2-Induced Cell Apoptosis and Improves Cardiac Function in Rats by Regulating Autophagy. Stem Cel Res Ther 12 (1), 437. doi:10.1186/s13287-021-02503-6
Wu, X., Li, Y., Zhang, S., and Zhou, X. (2021). Ferroptosis as a Novel Therapeutic Target for Cardiovascular Disease. Theranostics 11 (7), 3052–3059. doi:10.7150/thno.54113
Xiong, X., Liu, J., He, Q., Dai, R., Zhang, H., Cao, Z., et al. (2021). Long Non‐coding RNA NORAD Aggravates Acute Myocardial Infarction by Promoting Fibrosis and Apoptosis via miR ‐577/ COBLL1 axis. Environ. Toxicol. 36 (11), 2256–2265. doi:10.1002/tox.23339
Xu, L.-N., Wang, S.-H., Su, X.-L., Komal, S., Fan, H.-K., Xia, L., et al. (2021). Targeting Glycogen Synthase Kinase 3 Beta Regulates CD47 Expression after Myocardial Infarction in Rats via the NF-Κb Signaling Pathway. Front. Pharmacol. 12, 662726. doi:10.3389/fphar.2021.662726
Xuan, F., and Jian, J. (2016). Epigallocatechin Gallate Exerts Protective Effects against Myocardial Ischemia/reperfusion Injury through the PI3K/Akt Pathway-Mediated Inhibition of Apoptosis and the Restoration of the Autophagic Flux. Int. J. Mol. Med. 38 (1), 328–336. doi:10.3892/ijmm.2016.2615
Xuan, F., Jian, J., Lin, X., Huang, J., Jiao, Y., Huang, W., et al. (2017). 17-Methoxyl-7-Hydroxy-Benzene-Furanchalcone Ameliorates Myocardial Ischemia/Reperfusion Injury in Rat by Inhibiting Apoptosis and Autophagy via the PI3K-Akt Signal Pathway. Cardiovasc. Toxicol. 17 (1), 79–87. doi:10.1007/s12012-016-9358-y
Yang, F., Qin, Y., Lv, J., Wang, Y., Che, H., Chen, X., et al. (2018). Silencing Long Non-coding RNA Kcnq1ot1 Alleviates Pyroptosis and Fibrosis in Diabetic Cardiomyopathy. Cel Death Dis 9 (10), 1000. doi:10.1038/s41419-018-1029-4
Yang, L., Zhang, Y., Zhu, M., Zhang, Q., Wang, X., Wang, Y., et al. (2016). Resveratrol Attenuates Myocardial Ischemia/reperfusion Injury through Up-Regulation of Vascular Endothelial Growth Factor B. Free Radic. Biol. Med. 101, 1–9. doi:10.1016/j.freeradbiomed.2016.09.016
Yang, Z., Li, C., Wang, Y., Yang, J., Yin, Y., Liu, M., et al. (2018). Melatonin Attenuates Chronic Pain Related Myocardial Ischemic Susceptibility through Inhibiting RIP3-MLKL/CaMKII Dependent Necroptosis. J. Mol. Cell Cardiol. 125, 185–194. doi:10.1016/j.yjmcc.2018.10.018
Yuan, P., Hu, Q., He, X., Long, Y., Song, X., Wu, F., et al. (2020). Laminar Flow Inhibits the Hippo/YAP Pathway via Autophagy and SIRT1-Mediated Deacetylation against Atherosclerosis. Cel Death Dis 11 (2), 141. doi:10.1038/s41419-020-2343-1
Yuan, Y.-Y., Xie, K.-X., Wang, S.-L., and Yuan, L.-W. (2018). Inflammatory Caspase-Related Pyroptosis: Mechanism, Regulation and Therapeutic Potential for Inflammatory Bowel Disease. Gastroenterol. Rep. (Oxf) 6 (3), 167–176. doi:10.1093/gastro/goy011
Yue, R.-C., Lu, S.-Z., Luo, Y., Wang, T., Liang, H., Zeng, J., et al. (2019). Calpain Silencing Alleviates Myocardial Ischemia-Reperfusion Injury through the NLRP3/ASC/Caspase-1 axis in Mice. Life Sci. 233, 116631. doi:10.1016/j.lfs.2019.116631
Zhang, B., Zhang, Y., Liu, B., Fang, L., Li, Y., Meng, S., et al. (2018). So-Osmolar Iodixanol Induces Less Increase in Circulating Endothelial Microparticles In Vivo and Less Endothelial Apoptosis In Vitro Compared with Low-Osmolar Iohexo. Contrast Media Mol. Imaging. l, 2018, 8303609. doi:10.1155/2018/8303609I
Zhang, H., Zhang, R.-H., Liao, X.-M., Yang, D., Wang, Y.-C., Zhao, Y.-L., et al. (2021). Discovery of β-Carboline Derivatives as a Highly Potent Cardioprotectant against Myocardial Ischemia-Reperfusion Injury. J. Med. Chem. 64 (13), 9166–9181. doi:10.1021/acs.jmedchem.1c00384
Zhang, J. G., Yang, N., He, H., Wei, G. H., Gao, D. S., Wang, X. L., et al. (2005). Effect of Astragalus Injection on Plasma Levels of Apoptosis-Related Factors in Aged Patients with Chronic Heart Failure. Chin. J. Integr. Med. 11 (3), 187–190. doi:10.1007/BF02836502
Zhang, X., Li, Y., Wang, Y., Zhuang, Y., Ren, X., Yang, K., et al. (2020). Dexmedetomidine Postconditioning Suppresses Myocardial Ischemia/reperfusion Injury by Activating the SIRT1/mTOR axis. Biosci. Rep. 40 (5). doi:10.1042/BSR20194030
Zhang, Y., Liu, X., Bai, X., Lin, Y., Li, Z., Fu, J., et al. (2018). Melatonin Prevents Endothelial Cell Pyroptosis via Regulation of Long Noncoding RNA MEG3/miR-223/NLRP3 axis. J. Pineal Res. 64 (2). doi:10.1111/jpi.12449
Zheng, X., Zhong, T., Ma, Y., Wan, X., Qin, A., Yao, B., et al. (2020). Bnip3 Mediates Doxorubicin-Induced Cardiomyocyte Pyroptosis via Caspase-3/GSDME. Life Sci. 242, 117186. doi:10.1016/j.lfs.2019.117186
Zhou, L., Liu, X., Wang, Z.-Q., Li, Y., Shi, M.-M., Xu, Z., et al. (2018). Simvastatin Treatment Protects Myocardium in Noncoronary Artery Cardiac Surgery by Inhibiting Apoptosis through miR-15a-5p Targeting. J. Cardiovasc. Pharmacol. 72 (4), 176–185. doi:10.1097/fjc.0000000000000611
Zhou, Z., Wang, Z., Guan, Q., Qiu, F., Li, Y., Liu, Z., et al. (2016). PEDF Inhibits the Activation of NLRP3 Inflammasome in Hypoxia Cardiomyocytes through PEDF Receptor/Phospholipase A2. Int. J. Mol. Sci. 17 (12). doi:10.3390/ijms17122064
Zhu, H., and Sun, A. (2018). Programmed Necrosis in Heart Disease: Molecular Mechanisms and Clinical Implications. J. Mol. Cell Cardiol. 116, 125–134. doi:10.1016/j.yjmcc.2018.01.018
Keywords: cardiovascular diseases, programmed cell death, apoptosis, necrosis, autophagy, pyroptosis, ferroptosis, drug therapy
Citation: Zhou L, Sun J, Gu L, Wang S, Yang T, Wei T, Shan T, Wang H and Wang L (2021) Programmed Cell Death: Complex Regulatory Networks in Cardiovascular Disease. Front. Cell Dev. Biol. 9:794879. doi: 10.3389/fcell.2021.794879
Received: 14 October 2021; Accepted: 15 November 2021;
Published: 26 November 2021.
Edited by:
Zhi Qi, Nankai University, ChinaReviewed by:
Yaozu Xiang, Tongji University, ChinaElena Grossini, University of Eastern Piedmont, Italy
Copyright © 2021 Zhou, Sun, Gu, Wang, Yang, Wei, Shan, Wang and Wang. This is an open-access article distributed under the terms of the Creative Commons Attribution License (CC BY). The use, distribution or reproduction in other forums is permitted, provided the original author(s) and the copyright owner(s) are credited and that the original publication in this journal is cited, in accordance with accepted academic practice. No use, distribution or reproduction is permitted which does not comply with these terms.
*Correspondence: Liansheng Wang, ZHJsc3dhbmdAbmptdS5lZHUuY24=
†These authors have contributed equally to this work and share the first authorship