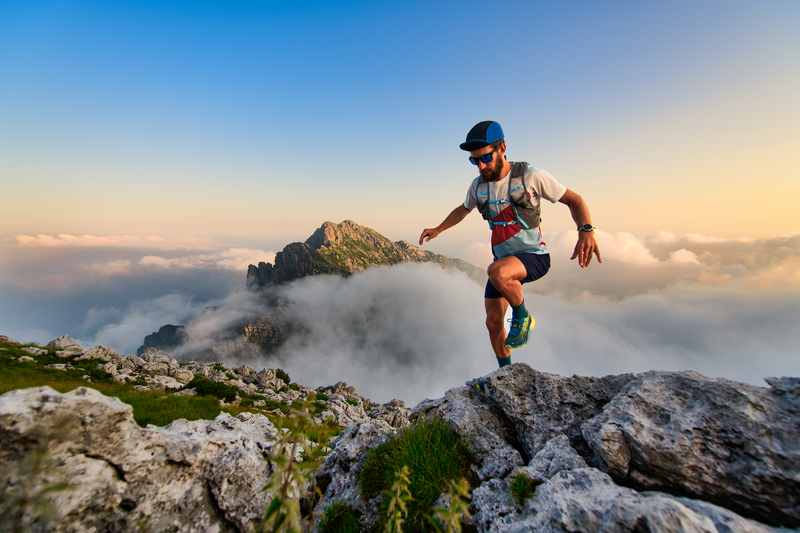
95% of researchers rate our articles as excellent or good
Learn more about the work of our research integrity team to safeguard the quality of each article we publish.
Find out more
REVIEW article
Front. Cell Dev. Biol. , 13 January 2022
Sec. Stem Cell Research
Volume 9 - 2021 | https://doi.org/10.3389/fcell.2021.793363
Intervertebral disc degeneration (IVDD) is the leading cause of low back pain related to degradation of cartilaginous tissues, mainly resulting from oxidative stress, cell apoptosis, and extracellular matrix degradation. Extracellular vesicles (EVs) exist in all bodily fluids and can be produced by all types of cells. Stem cell-derived EVs (SC-EVs), which are the main paracrine components of stem cells, have gained significant attention in the field of regenerative medicine. Over the past years, accumulating evidence indicates the therapeutic and diagnostic potentials of EVs in IVDD. The main mechanisms involve the induction of regenerative phenotypes, apoptosis alleviation, and immune modulation. In addition, the efficiency of SC-EVs can be enhanced by choosing appropriate donor cells and cell phenotypes, optimizing cell culture conditions, or engineering EVs to deliver drugs and targeting molecules. Given the importance and novelty of SC-EVs, we give an overview of SC-EVs and discuss the roles of SC-EVs in IVDD.
Intervertebral disc degeneration (IVDD) is an age-related disease associated with various factors. The pathology involves the decrease of nucleus pulposus cells (NPCs) and extracellular matrix (ECM), aging of the annulus fibrosus (AF), and calcification of cartilage endplates (CEPs) (Feng et al., 2016; Vo et al., 2016). The NPCs maintain homeostasis by producing components of ECM, such as proteoglycan and type II collagen (Zhang et al., 2021a). The highly specialized ECM is essential for stabilizing the biomechanical equilibrium and structure of IVD. Furthermore, mechanical damage and inflammation are involved in IVDD, disturbing ECM metabolism (Ruiz-Fernández et al., 2019; Zhang et al., 2021b). Hence, the dysfunction or loss of such cells could disrupt the ECM formation, which is significantly related to the degradation of cartilaginous tissues.
IVDD is a leading chronic joint disease among the elderly population, seriously affecting the life quality of people (Goh et al., 2019; Tryfonidou et al., 2020). Despite augmented studies focused on investigating the pathogenesis of the disease, many of the underlying mechanisms involved in IVDD remain largely unknown. Compared to terminally differentiated cells, stem cells (SCs) exhibit beneficial characteristics including the capability of self-renewal and the potential to differentiate towards multilineage cells. However, despite the promises and benefits, there are still many challenges facing SC applications, such as their variability, scalability, and delivery, as well as ethical and safety issues (Sakai and Andersson, 2015; Sakai and Schol, 2017; Clouet et al., 2019). Extracellular vesicles (EVs) are particles released from cells that are delimited by a lipid bilayer and cannot replicate (Théry et al., 2018). Recently, SC-derived EVs (SC-EVs), preventing safety concerns associated with cell therapy, have emerged as novel drug and gene delivery tools. SC-EVs have been implicated in good therapeutic effects against several types of musculoskeletal disorders including osteoarthritis, congenital myopathies, IVDD, and so on (Bari et al., 2018; Bier et al., 2018; Murphy et al., 2018; Zhang et al., 2020a). In the present review, we provide an overview of EVs and summarize emerging literature to discuss the roles of SC-EVs in IVDD.
EVs are an umbrella definition of lipid bilayer-delimited particles that are naturally released or shed from almost all organisms and cell types studied. Also, EVs are not cells. Therefore, they cannot replicate because they are not living. Since EV release was first observed in sheep reticulocytes in 1983, a growing number of studies have shown EVs as a pivotal mechanism of the intercellular communication network (Pan and Johnstone, 1983).
The exact classification of EVs is still evolving. In studies on degenerative joint diseases, the term exosome is commonly used. Exosomes were deemed to have an endocytic origin and their diameters between 30 and 150 nm (Hessvik and Llorente, 2018). However, some vesicles with diameters larger than 150 nm can also be generated by the endosomal pathway (Ronquist and Brody, 1985), and some with diameters smaller than 150 nm can bleb directly from the plasma membrane (Nabhan et al., 2012). Therefore, the classification of EVs based on size must be considered carefully. Due to significant size overlap, similarities in composition, and lack of specific markers, terms for EV subtypes are recommended to refer to physical traits such as size [for instance, respectively, <100 or <200 nm (small EVs), or >200 nm (large and/or medium EVs)], density (low, middle, high), biochemical composition, or descriptions of conditions or cell of origin according to minimal information for studies of extracellular vesicles (MISEV) 2018 (Théry et al., 2018).
Isolation of EVs from specific tissues, biological fluids, or cells is the first step carried out in all studies on EVs. However, it is not realistic to achieve absolute purification or complete isolation of EVs from other entities. Therefore, it is recommended to use the terms “separation” and “enrichment”.
Hitherto, there is no consensus on a “gold standard” method for EV separation (Gardiner et al., 2016). The optimal method of separation should be chosen based on the downstream applications and scientific issues. According to a worldwide survey published in 2016, the most common downstream applications were in vitro functional analyses, transcriptome analysis, proteomic analysis, in vivo functional analyses, and lipidomic analysis (Gardiner et al., 2016). In the survey, researchers coping with complex biological fluids and/or performing proteomic analysis usually tend to utilize more elaborate strategies than those who separate EVs from culture media and/or use flow cytometry for EVs analysis.
Ultracentrifugation (UC) remains the most utilized primary separation technique for EVs, regardless of the starting material used (Gardiner et al., 2016). However, there still exist some problems and drawbacks such as lengthy timescales, the requirement of a large number of cells or biological fluids, and non-vesicular macromolecule contamination (Webber and Clayton, 2013; Gupta et al., 2018). Also, UC can induce aggregation of EVs, which may lead to erroneous interpretation during analysis (Linares et al., 2015). EVs are considered a drug delivery tool and gain considerable interest. However, the efficacy of EVs depends greatly on biodistribution and clearance. Interestingly, different separation methods may result in different results. For instance, some studies revealed that UC-separated EVs have a higher rate of accumulation, affecting the biodistribution and delivery efficiency (Nordin et al., 2015; Smyth et al., 2015).
To overcome the limitations of conventional techniques, recently emerging EV separation techniques have been developed including size-, charge-, and affinity-based techniques. For instance, size-exclusion chromatography (SEC) applications have become more prominent since 2010 (Liangsupree et al., 2021). SEC has been utilized for the separation of EVs from a large variety of origins from both prokaryotes and eukaryotes, involving cells, plasma and serum, urine, synovial fluid, cerebrospinal fluids, and others (Foers et al., 2018; Guan et al., 2020; Povero et al., 2020). Several studies have also shown the superiority of SEC, allowing purer EV preparations with easy implementation (Allelein et al., 2021; Tsai et al., 2021). For instance, when EVs were separated from plasma via the UC technique, high-density lipoproteins (HDLs) were co-separated, because HDLs share a similar density range with EVs. With the SEC technique, this issue could be addressed due to HDLs being smaller than EVs. However, this method is time-consuming and not suitable for large sample processing.
Other methods include ultrafiltration, flow field-flow fractionation, anion-exchange chromatography, electrophoresis, dielectrophoresis, affinity chromatography, immunocapture, and so on (Heath et al., 2018; Stranska et al., 2018; Lewis et al., 2019; Logozzi et al., 2020; Wu et al., 2020; Zhang et al., 2021c). In the included studies, the most commonly used method is ultracentrifugation. Among the included studies, one study reported a pilot production process for a freeze-dried, “ready-off-the-shelf” and free soluble powder containing EVs and proteins via ultrafiltration (Bari et al., 2018). This may contribute to transforming MSC-secretome into a pharmaceutical product for its large-scale production. Of note, it is still recommended to use combinations of methods, which may outperform single-method approaches.
After separation, it is extremely important to assess EV characterization via multiple, complementary approaches, confirming that biomarkers or functions are related to EVs rather than other co-separated materials. So far, there is no single perfect quantification approach for EVs. The most frequently used are total particle number and total protein amount. Alternatively, quantification of total lipid can also be considered. Nanoparticle tracking analysis (NTA) is developed to assess particle concentration and size distribution. Unlike measuring bulk scattered light from EVs via dynamic light scattering (DLS), NTA measures individual particle scattering (Shao et al., 2018). For accurate quantification, however, it requires accurate optimization of camera and analysis settings. Of note, large EVs (>400 nm) and very small EVs (<50 nm) are not well quantified by all NTA. Tunable resistive pulse sensing can be a substitute to NTA for a wide range of sizes, depending on pore diameter (Vogel et al., 2016; Maas et al., 2017). According to the size of EVs, standard or high-resolution flow cytometry can also be applied (Nolan and Duggan, 2018; Tertel et al., 2020).
MISEV 2018 highlights three categories of markers (transmembrane or GPI-anchored proteins associated with the plasma membrane and/or endosomes; cytosolic proteins recovered in EVs; major components of non-EV co-isolated structures), which investigated necessarily in all bulk EV preparations to show the presence of EVs and evaluate their purity from common contaminants (Théry et al., 2018) (Figure 1). However, there are no suggestions for universal “negative controls” related to a particular subtype of EVs. Western blotting is the most common method to evaluate the presence of proteins in EV preparations. However, it is easier applied for cells than biofluids. Alternatively, flow cytometry of EVs decorating beads of bulk EV populations can be utilized, but with appropriate negative controls including antibodies alone or isotype controls used with caution (Pospichalova et al., 2015). In addition, mass spectrometry is also an economical and accessible approach, enabling the assessment of many proteins at once (Bandu et al., 2019).
FIGURE 1. Schematic diagram of components and markers of extracellular vesicles. EVs are particles naturally released from the cell that are delimited by a lipid bilayer and cannot replicate. The components of EVs contain DNAs, RNAs, proteins, amino acids, metabolites, and so on. Regarding protein markers of EVs, at least one protein of transmembrane or GPI-anchored proteins associated to plasma membrane and/or endosomes, cytosolic proteins recovered in EVs, and major components of non-EV co-isolated structures must be analyzed to demonstrate the EV nature and the degree of purity of EV preparation. Note: EVs, extracellular vesicles; GPI, glycosylphosphatidylinositol.
Techniques allowing visualization of single vesicles at high resolution are recommended to verify the characterization of EVs. Transmission electron microscopy (TEM) is a popular technique for characterizing EVs. It could provide superior resolution, with capabilities to image less than 1 nm objects (Mohammadian et al., 2020). Moreover, contrasting and embedding in heavy metal stains such as osmium tetroxide and uranyl acetate can be used to maintain the morphology of the lipid membrane. Also, TEM can be combined with immunogold labeling (immuno-EM) to visualize molecular characterization (Street et al., 2012). Other similar methods include scanning electron microscopy, cryo-electron microscopy, and atomic force microscopy (Shao et al., 2018).
Importantly, an increasing number of studies found that the topology of EV components can fundamentally influence the biological functions of EVs (Cvjetkovic et al., 2016; De la Torre-Escudero et al., 2019). Some active components of EVs are localized on the EV surface and sensitive to digestion. Hence, it is recommended to perform mild digestions, permeabilizations, or antibody studies to determine the actual topology of active components. Alternatively, flow cytometry and fluorescence microscopy with antibodies targeting EV membranal or cytoplasmic epitopes. In addition, as mentioned above, TEM with immuno-EM can also be applied to aid in determining differences in topology among different EVs. In the included studies, the most commonly used methods are TEM and NTA combined with western blotting.
Depending on the cell of origin, EVs can contain various components of cells, including DNA, RNA, lipids, metabolites, and proteins (Kalluri and LeBleu, 2020). The cargo carried by EVs may mirror the parental cell physiological or pathological conditions, involving different diseases as well as stages of a disease. Such intrinsic properties of EVs have facilitated their potential utility in therapy and diagnosis for many diseases, including degenerative joint diseases. Also, EVs can be engineered to deliver many effective constituents to target cells, including short interfering RNAs, antisense oligonucleotides, chemotherapeutic agents, and immune modulators. (Figure 1).
Since the first public online database of EVs, Exocarta (http://www.exocarta.org), is accessible, a growing number of databases focused on EVs have been established (Keerthikumar et al., 2016). For instance, ESBL (https://hpcwebapps.cit.nih.gov/ESBL/Database/Exosome) is a database of urinary EVs proteins based on published protein mass spectrometry data (Pisitkun et al., 2004). Vesiclepedia (http://www.microvesicles.org) is another database of RNA, proteins, lipids, and metabolites identified in EVs from both published and unpublished studies (Pathan et al., 2019). Now, Vesiclepedia holds data obtained from 1254 EV studies, 38,146 RNA entries, 349,988 protein entries, and 639 lipid/metabolite entries. Recently, ISEV established an updatable tool, the EV-TRACK knowledgebase, to encourage researchers to report details of EVs studies, ensuring the transparency and reproducibility of procedures (EV-TRACK ConsortiumVan Deun et al., 2017). In this way, it may promote researchers to put MISEV 2018 guidelines into practice.
With characteristics of self-renewal and differentiation potentials, SCs have been applied successfully for treating a wide range of musculoskeletal disorders in preclinical and clinical studies (Alcaraz et al., 2019). However, some issues for direct SC applications still exist. For instance, cell survival is unpredictable after cell injection, which is of importance to sustain the effects due to intercellular interactions. It has been reported that mesenchymal SCs (MSCs) inoculated into the damage site demonstrated low survival incidence and loss of function after only 1 week (Rani et al., 2015). Also, the longevity issue of SCs may be related to the status of donors and the microenvironment for SC survival, resulting in unstable effects in treatment. Other possible encountered issues include storage, immunoreaction, occlusion, gene mutation, and tumorigenesis or tumor promotion (Kim et al., 2021).
SC-EVs, as the main paracrine mediators, overcome most limitations of SCs. SC-EVs, an approach of cell-free therapy, different from therapy based on whole cells, are much easier to manage and safer because of lower quantities of membrane-bound proteins such as MHC molecules and their inability of tumorigenesis directly (Keshtkar et al., 2018). Previous studies have shown SC-EVs to traffic SC associated transcription factors including Nanog, Oct-4, HoxB4, and Rex-1 operating at the level of pluripotent SCs (Ratajczak et al., 2006). Furthermore, direct effectors of SC phenotype such as Wnt, ß-catenin, and Hedgehog have also been identified on SC-EVs besides several other components (Nawaz et al., 2016). These are considered to be potential factors in SCs biology. As a result, SC-EVs have been used for the treatment of various diseases in preclinical and clinical studies via induction of regenerative phenotypes, apoptosis alleviation, and immune modulation (Yin et al., 2020). In addition, the efficiency of SC-EVs can be enhanced by choosing appropriate donor cells and cell phenotypes, optimizing cell culture conditions, or engineering EVs to deliver drugs and targeting molecules.
MscMSC is one of the main cell sources of tissue regeneration because of its potential for multidirectional differentiation and self-renewal. MSCs can produce various kinds of EVs, potentially restoring an extensive range of damaged or diseased tissues and organs, such as lungs, blood vessels, esophagus bowel, as well as bone reconstruction after injury (Tsiapalis and O'Driscoll, 2020). For instance, it was reported that miR-155–5 p in MSC-EVs could enhance proliferation and migration, attenuate apoptosis, and modulate ECM secretion in chondrocytes (Wang et al., 2021). In vivo, MSC-EVs can effectively attenuate osteoarthritis progression and protect cartilage from degeneration (Woo et al., 2020). Herein, the most investigated type of MSCs to separate EVs to treat IVDD is bone marrow-derived MSCs (BM-MSCs) due to their easy accessibility. Other sources include the umbilical cord, adipose tissue, placenta, urine, cartilage endplate, and nucleus pulposus (Figure 2).
FIGURE 2. Schematic diagram of stem cell-derived extracellular vesicle separation and delivery. Stem cells are isolated and cultured from different origins. Optionally, stem cells are preconditioned or modified, especially genetically engineered. Then, EVs are separated and enriched, and delivered to the IVD for treatment. Note: SCs, stem cells; EVs, extracellular vesicles; IVD, intervertebral disc.
Excessive apoptosis and senescence of NPCs have been proven to be involved in the process of IVDD, considered as the potential therapeutic target (Chen et al., 2016). Recently, SC-EVs have been applied to deliver EV cargos to suppress apoptosis and senescence as well as promote proliferation both in vitro and in vivo (Lu et al., 2017; Cheng et al., 2018). It was reported that the beneficial effects of SC-EVs were mainly mediated by phosphoinositide 3-kinase (PI3K)-protein kinase B (Akt) pathway, mitogen-activated protein kinase (MAPK)-extracellular signal-regulated kinase (ERK) pathway, Wnt pathway, and transforming growth factor-beta (TGF-β) pathway (Cheng et al., 2018; Liao et al., 2019; Qi et al., 2019; Li et al., 2020a; Zhu et al., 2020a; Xiang et al., 2020; Luo et al., 2021a; Guo et al., 2021). For instance, SC-EVs resisted senescence and promoted NPC proliferation by delivery of matrilin-3 (MATN3) activating TGF-β (Guo et al., 2021). After intradiscal injection of SC-EVs in an animal IVDD model, NPCs apoptosis, senescence, and IVDD were significantly alleviated (Cheng et al., 2018; Sun et al., 2021). In addition, using a 3D in vitro model, Hingert et al. explored the effects of SC-EVs on degenerated disc cells (DCs), and the results demonstrated an over 50% increase in cell proliferation and decrease in cellular apoptosis (Hingert et al., 2020).
Previous studies have shown the important role of lactic acid and abnormal PH levels in IVD, leading to decreased permeability of CEPs (Malandrino et al., 2014; Xiao et al., 2020). Compared with NPCs cultured at pH 7.1–7.3, proliferation activity of counterparts cultured at pH 5.9–6.7 decreased significantly (Li et al., 2020b). However, SC-EVs reversed the adverse effects of acidic PH on proliferation and apoptosis (Li et al., 2020b). Until now, it is not clear whether the acidic microenvironment is a result or a cause of the degeneration. Hence, further studies need to investigate the relationship between PH and IVDD as well as the mechanism of beneficial effects of SC-EVs.
Interestingly, as the source of EVs, cartilage endplate-derived SCs (CESCs) could not only inhibit apoptosis via activation of the PI3K/AKT/autophagy signaling pathway but also promote the invasion, migration, and differentiation of themselves via the hypoxia-inducible factor 1-alpha (HIF-1α)/Wnt pathway, increasing expression of GATA binding protein 4 (GATA4) and TGF-β (Luo et al., 2021a; Luo et al., 2021b). In addition, the apoptosis of NPCs and IVDD was more attenuated when stimulated by normal CESC-derived EVs than degenerated ones in vitro and in vivo (Luo et al., 2021a). Of note, these studies did not conduct any sorting analysis to remove CESCs from general CEs. Additionally, IVD stem and progenitor cells are still debated (Wang et al., 2015). The evidence underlines the critical role of the state of cells (Table 1).
ECM provides mechanical and chemical support to cells, contributing to the structural framework and homeostasis of connective tissues. The current evidence has indicated that intercellular interactions could be disrupted physically and functionally via EV-mediated proteolytic activities. EVs have been reported to contain matrix-remodeling enzymes including matrix metalloproteinases (MMPs), heparanases, hyaluronidases, and extracellular matrix metalloproteinase inducer, as well as aggrecanases such as adamalysin metalloproteinases having disintegrin and thrombospondin domains and tissue inhibitors of metalloproteinases (TIMPs), among others (Nawaz et al., 2018). It has been found that after simulation of SC-EVs, extracellular gene expression in degenerate NPCs significantly increases, such as Aggrecan, Collagen I or II, and SRY-Box transcription factor 9 (SOX9) (Lu et al., 2017; Zhu et al., 2020b). Also, SC-EVs downregulate the expression of MMPs, whereas upregulating TIMP-1, suggesting a more balanced anabolism/catabolism. In addition to regulating the proliferation activity of NPCs negatively, acidic pH disturbs the metabolic balance of ECM (Ohshima and Urban, 1992). In the pathological acid environment, SC-EVs promoted ECM synthesis and reduced ECM degradation by downregulating related enzymes (Li et al., 2020b). For instance, EVs derived from BM-MSCs or umbilical cord MSCs or were found to be able to protect NPCs or nucleus pulposus-derived MSCs (NP-MSCs) from high glucose-induced ECM degradation via the p38 MAPK pathway (Qi et al., 2019; Cui and Zhang, 2021).
EV therapy is a promising therapeutic approach for IVDD. However, the rapid clearance and disruption of EVs are the two primary challenges for the application of EV therapy in IVDD. Recently, a thermosensitive acellular ECM hydrogel coupled with adipose-derived MSCs (ASC) EVs were found to be able to regulate matrix synthesis and degradation, mitigating the inflammatory response, and ameliorating IVDD both in vitro and in vivo (Xing et al., 2021). Moreover, in a 3D pellet culture of DCs, after stimulation of SC-EVs, the researchers observed ECM production as early as day 7 and more than three times higher than the control group (Hingert et al., 2020). In addition, SC-EVs inhibited the secretion of MMP-1 in DCs. Interestingly, EVs derived from NPCs could recruit BM-MSCs and induce them to differentiate toward NP-like cells (Lu et al., 2017). In addition, there may be other interactions between NPCs and other cells inside the IVD such as AF or CEPs. These findings give an insight into new tissue regeneration treatment strategies to be developed for IVDD. Further studies should be focused on uncovering the role of EVs in matrix synthesis and degradation.
Previous studies have shown that oxidative stress (OS) and inflammation are extensively presented in IVDD (Feng et al., 20172017; Chao-Yang et al., 2021). For instance, inflammation was shown to increase in a rat IVDD model, which could be mitigated by SC-EVs via maintaining microenvironment hemostasis (Xing et al., 2021). Advanced glycation end products (AGEs) usually accumulate in aging and degenerative tissues, especially in collagen-rich ones, and are closely associated with endoplasmic reticulum (ER) stress and apoptosis (Liao et al., 2019). It was found that SC-EVs could reduce NPC AGE-induced ER stress and inhibit excessive activation of the unfolded protein response (UPR), subsequently alleviating apoptosis (Liao et al., 2019). The main pathways involved are reported to be AKT and ERK pathways. In another study, a pneumatic pressure model was constructed to mimic pressure-associated IVD tissue damage (Xiang et al., 2020). In this study, urine-derived SCs were utilized to separate EVs, inhibiting NPC ER stress-induced cell apoptosis in a dose-dependent manner. Of note, the study did not conduct any sorting analysis to remove urine-derived SCs from general urine-derived cells, which may affect the results (Ji et al., 20172017). Similarly, a study on lyophilized MSC-EVs showed that the EVs could counteract the OS damage induced by H2O2 on NPCs at concentrations between 5 and 50 mg/ml but become cytotoxic to NPCs at a concentration of over 50 mg/ml (Bari et al., 2018).
Mitochondria is an important organelle for cellular homeostasis, playing a crucial role in aging and disease development (Kang et al., 2020). Also, it is the predominant place to generate reactive oxygen species (ROS), which are also a source of organelle injury that can bring about cell damage as a secondary consequence of OS. It was reported that SC-EVs could not only suppress ROS production and NLRP3 inflammasome activation but also replenish mitochondrial-related proteins and attenuate mitochondrial dysfunction in NPCs (Xia et al., 2019; Zhang et al., 2020b).
Autophagy is the main intracellular degradation system that transports cellular components into autophagosomes for degradation, recycling, and reuse to keep renovation and homeostasis (Levine and Kroemer, 2019). Under physiological conditions, autophagy is found to be at a low level in NPCs and AF cells which are not degenerative. This suggests that autophagy is involved in the progress of IVDD. When IL-1β was applied to induce inflammation and apoptosis of AF cells, SC-EVs significantly inhibited the expression of autophagy-related proteins (Beclin-1 and LC3-II/I) by activating PI3K/AKT/mTOR signaling pathway (Li et al., 2020a). The beneficial effects could be reversed by rapamycin, an activator of autophagy. The results confirm OS and inflammation as potential targets, and SC-EVs could be a promising therapeutic strategy.
As the crucial mediators for the benefits of SC-EVs, miRNAs are known to impact the expression of 30% of protein-coding genes mainly at the post-transcriptional level and numerous intracellular processes (Cazzanelli and Wuertz-Kozak, 2020). miRNAs can produce a sustained therapeutic effect and fundamental changes of the local microenvironment. In a study investigating EVs derived from umbilical cord MSCs, at least 100 kinds of miRNAs were found in EVs, among which the top 10 miRNAs with the highest abundance were miR-221–3p/222–3p, miR-574–3p, let-7a-5p, miR-23a-3p, miR-146a-5p, miR-320a, miR-193b-3p, miR-125a-5p, and miR-21–5p (Qi et al., 2019). However, the authors did not explore the functions of certain miRNAs further. Recently, many studies have investigated the role of miRNAs in SC-EVs for IVDD (Cheng et al., 2018; Zhu et al., 2020a; Zhu et al., 2020b; Xie et al., 2020; Yuan et al., 2020; Cui and Zhang, 2021; Sun et al., 2021; Wen et al., 2021). Anti-apoptotic miRNAs in SC-EVs include miR-105–5p, miR-129–5p, miR-199a, miR-21, miR-31–5p, miR-4450 inhibitor, miR-532–5p, and miR-142–3p for IVDD.
As mentioned above, some pathways mediate the beneficial effects of SC-EVs, while miRNAs regulate the expression of target genes in these pathways. For instance, miR-21 in SC-EVs suppressed NPC apoptosis by targeting phosphatase and tensin homolog (PTEN) through the PI3K-AKT pathway (Cheng et al., 2018). SC-EVs miR-142–3p alleviated NPCs injury by suppressing MAPK signaling by targeting MLK3 (Zhu et al., 2020a). Induced pluripotent stem cell-derived EVs exerted anti-aging effects by delivering miR-105–5p to senescent NPCs and activating the Sirt6 pathway (Sun et al., 2021). BM-MSC-EVs promoted proliferation and inhibited apoptosis and senescence via miR-199a targeting Gremlin 1 (GREM1) to downregulate the TGF-βpathway (Wen et al., 2021). Of note, through autocrine EVs, TGF-β was upregulated in CESCs, indicating different roles of TGF-β in different cells.
The inflammatory factors, such as tumor necrosis factor-alpha (TNF-α), played an important role in the pathological processes of IVDD. It has been reported that miR-532–5p is significantly downregulated in IVDD patients (Zhu et al., 2020b). BM-MSC-derived EVs transfer miR-532–5p to NPCs, suppressing apoptosis, ECM degradation, and fibrosis deposition via targeting RASSF5 (Zhu et al., 2020b). Macrophages play crucial roles in physiological conditions and inflammatory responses (Cunha et al., 2018). Polarization of M1 macrophages can precipitate local inflammation and structural change in IVDD. Interestingly, delivery of miR-129–5p to NPCs by BM-MSC-derived EVs led to a decline in apoptosis, ECM degradation, and M1 polarization of macrophages via targeting LRG1 and suppressing the p38 MAPK signaling pathway (Cui and Zhang, 2021).
Compared to the BM-MSCs, placenta-derived MSCs (PLMSCs) were shown to be more unlimited differentiation potential, therapeutic effects, and lower immunogenicity (Ni et al., 2014; Yuan et al., 2020). Therefore, PLMSC-derived EVs were used as nanocarriers to deliver miR-4450 inhibitors to increase NPC proliferation and migration while decreasing apoptosis by upregulating zinc finger protein 121 (ZNF121) (Yuan et al., 2020). Consistent with in vitro studies, in vivo experiments displayed the inhibitory effect of miR-4450 inhibitor in PLMSC-derived EVs, retarding IVDD damage.
As the nutrition channel, CEPs play a significant role as a barrier to nutrient transport in the endplate. In IVDD, CEPs degeneration results in malnutrition, leading to a degeneration of the NPCs and failed endogenous repair subsequently. To explore potential therapeutic methods, miR-31–5p in SC-EVs was found to be able to inhibit apoptosis and calcification under OS by targeting ATF6 in endplate chondrocytes (Xie et al., 2020). Similarly, EVs secreted by NPCs could promote NP-MSCs chondrogenic differentiation by delivery of miR-15a, downregulating MMP-3 through PI3K/AKT, and Wnt3a/β-catenin axis (Zhang et al., 2021d).
As a form of programmed cell death, pyroptosis, triggered by various inflammasomes such as NLRP3 or NLRP1, is also found in IVDD (Zhao et al., 2021). Recently, miR-410 in SC-EVs was proven to be a regulator of NLRP3. After delivery of miR-410 in SC-EVs, pyroptosis was significantly inhibited in IVDD (Zhang et al., 2020b). Similarly, miR-26a-5p in SC-EVs was reported to be another regulator of NLRP3, which targets METTL3, a methyltransferase mediating N6-methyladenosine (m6A), the most common endogenous RNA modification in mammalian cells (Yuan et al., 2021).
Recipient cells accept exogenous EVs usually via an endocytosis route (Mulcahy et al., 2014). However, the mechanism for EVs engulfed by NPCs is unknown. Recently, a study found that the EV uptake in NPCs is mediated by caveolae-dependent endocytosis, and caveolae-associated protein 2 (Cavin-2) plays a critical role in the process (Liao et al., 2021a). Also, Cavin-2-engineered EVs could not only protect against TNF-α-induced NPCs pyroptosis in vitro but also retard the progression of IVDD in the ex vivo disc culture model.
Metformin is the most commonly prescribed drug for type 2 diabetes (Flory and Lipska, 2019). Besides, it is an AMPK activator and is closed to aging diseases and autophagy (Wang et al., 2018). Recently, a study found that metformin could not only promote MSC-derived EVs secretion but also yield better quality (Liao et al., 2021b). The positive was found to be associated with the autophagy-related pathway. Furthermore, metformin induced the phosphorylation of synaptosome-associated protein 29 (SNAP29), a critical soluble N-ethylmaleimide sensitive fusion factor attachment protein receptor (SNARE) protein that mediates the fusion of multivesicular bodies with the plasma membrane, facilitating the release of EVs. Through functional analysis, EVs derived from metformin-treated BM-MSCs significantly ameliorated NPCs senescence (Figure 3).
FIGURE 3. Roles of stem cell-derived extracellular vesicles in intervertebral disc degeneration. SC-EVs ameliorate IVDD via suppressing apoptosis and senescence as well as promoting proliferation. They also promote ECM synthesis and inhibit degradation. In addition, oxidative stress and inflammation are reduced after SC-EVs delivered. Note: red arrow, upregulated; green arrow, downregulated; SC-EVs, stem cell-derived extracellular vesicles; IVDD, intervertebral disc degeneration; ECM, extracellular matrix.
The present review reveals overall positive findings and may result in an increasing interest in the current emerging field. Although SC-EVs represent a more accessible and cell-free therapy, some challenges should be taken into consideration before translation to clinical applications such as production, safety, long-term durability, and regulation (Bari et al., 2018; Xing et al., 2021). First of all, many different procedures to separate EVs have been described in the literature. Unfortunately, a standardized separation process is still lacking, leading to uncertainty about EVs biological effects and safety, which is strongly influenced by the preparation methods (Théry et al., 2018). Future studies should focus on improving separation and enrichment techniques, formulating standardized procedures for the large-scale production of SC-EVs, ready-to-use products, suitable to replace SCs in regenerative nanomedicine. Also, it is of importance to differentiate the different subtypes of EVs in heterogeneous samples qualitatively and quantitatively such as chromatography techniques, tangential flow, and microfluidics. A series of procedures and regulations need to be formulated and carried out based on MISEV 2018.
Secondly, the rapid clearance and disruption of EVs are two major challenges when applying SC-EVs in IVDD. Also, the mode of administration is also of importance for the application of SC-EVs including systematic or local injections. One-shot may not ensure the alleviation of the degeneration process for a long time, whereas repeated injections may destroy the structure of the intervertebral disc. Recently, a thermosensitive acellular ECM hydrogel was reported to serve as a powerful platform for IVDD drug or EV delivery (Xing et al., 2021). Future studies could focus on different materials combined with EVs to be a biotherapy and an alternative therapy for IVDD. Preconditions and modified EVs, especially genetically engineered EVs, could be another method to target specific genes to treat IVDD safely and efficiently.
Thirdly, although EVs have been administered to humans already in the early 2000s to treat cancer patients, there are still no standard techniques established for the clinical-grade production and quality control of EV-based therapeutics so far. Hence, another critical need will be to develop assays that predict the therapeutic potency of SC-EVs in quantifiable, robust, and reproducible parameters for clinical applications. Previous studies have emphasized quantifiable assays to define the identity of SC-EVs and requirements for prospective potency assays to predict the effectiveness and safety (Lener et al., 2015; Witwer et al., 2019; Gimona et al., 2021). Although all the included studies rest on the preclinical stage, current evidence for cell-free therapy reveals the feasibility of revolutionizing traditional therapeutic alternatives for IVDD in the future.
WS supervised the study and critically reviewed the paper; XW directed the project and wrote the paper.
This study was funded by the National Natural Science Foundation of China (Grant Nos. 82072524), the Natural Science Foundation of Beijing Municipality (Grant No. 7182146), and Innovation Fund for Outstanding Doctoral Candidates of Peking University Health Science Center.
The authors declare that the research was conducted in the absence of any commercial or financial relationships that could be construed as a potential conflict of interest.
All claims expressed in this article are solely those of the authors and do not necessarily represent those of their affiliated organizations, or those of the publisher, the editors and the reviewers. Any product that may be evaluated in this article, or claim that may be made by its manufacturer, is not guaranteed or endorsed by the publisher.
Alcaraz, M. J., Compañ, A., and Guillén, M. I. (2019). Extracellular Vesicles from Mesenchymal Stem Cells as Novel Treatments for Musculoskeletal Diseases. Cells 9 (1). doi:10.3390/cells9010098
Allelein, S., Medina-Perez, P., Lopes, A. L. H., Rau, S., Hause, G., Kölsch, A., et al. (2021). Potential and Challenges of Specifically Isolating Extracellular Vesicles from Heterogeneous Populations. Sci. Rep. 11 (1), 11585. doi:10.1038/s41598-021-91129-y
Bandu, R., Oh, J. W., and Kim, K. P. (2019). Mass Spectrometry-Based Proteome Profiling of Extracellular Vesicles and Their Roles in Cancer Biology. Exp. Mol. Med. 51 (3), 1–10. doi:10.1038/s12276-019-0218-2
Bari, E., Perteghella, S., Di Silvestre, D., Sorlini, M., Catenacci, L., Sorrenti, M., et al. (2018). Pilot Production of Mesenchymal Stem/Stromal Freeze-Dried Secretome for Cell-free Regenerative Nanomedicine: A Validated GMP-Compliant Process. Cells 7 (11), E190. doi:10.3390/cells7110190
Bier, A., Berenstein, P., Kronfeld, N., Morgoulis, D., Ziv-Av, A., Goldstein, H., et al. (2018). Placenta-derived Mesenchymal Stromal Cells and Their Exosomes Exert Therapeutic Effects in Duchenne Muscular Dystrophy. Biomaterials 174, 67–78. doi:10.1016/j.biomaterials.2018.04.055
Cazzanelli, P., and Wuertz-Kozak, K. (2020). MicroRNAs in Intervertebral Disc Degeneration, Apoptosis, Inflammation, and Mechanobiology. Int. J. Mol. Sci. 21 (10), E3601. doi:10.3390/ijms21103601
Chao-Yang, G., Peng, C., and Hai-Hong, Z. (2021). Roles of NLRP3 Inflammasome in Intervertebral Disc Degeneration. Osteoarthritis and Cartilage 29 (6), 793–801. doi:10.1016/j.joca.2021.02.204
Chen, D., Xia, D., Pan, Z., Xu, D., Zhou, Y., Wu, Y., et al. (2016). Metformin Protects against Apoptosis and Senescence in Nucleus Pulposus Cells and Ameliorates Disc Degeneration In Vivo. Cell Death Dis. 7 (10), e2441. doi:10.1038/cddis.2016.334
Cheng, X., Zhang, G., Zhang, L., Hu, Y., Zhang, K., Sun, X., et al. (2018). Mesenchymal Stem Cells Deliver Exogenous miR-21viaexosomes to Inhibit Nucleus Pulposus Cell Apoptosis and Reduce Intervertebral Disc Degeneration. J. Cel. Mol. Med. 22 (1), 261–276. doi:10.1111/jcmm.13316
Clouet, J., Fusellier, M., Camus, A., Le Visage, C., and Guicheux, J. (2019). Intervertebral Disc Regeneration: From Cell Therapy to the Development of Novel Bioinspired Endogenous Repair Strategies. Adv. Drug Deliv. Rev. 146, 306–324. doi:10.1016/j.addr.2018.04.017
Cui, S., and Zhang, L. (2021). microRNA-129-5p Shuttled by Mesenchymal Stem Cell-Derived Extracellular Vesicles Alleviates Intervertebral Disc Degeneration via Blockade of LRG1-Mediated P38 MAPK Activation. J. Tissue Eng. 12, 20417314211021680. doi:10.1177/20417314211021679
Cunha, C., Silva, A. J., Pereira, P., Vaz, R., Gonçalves, R. M., and Barbosa, M. A. (2018). The Inflammatory Response in the Regression of Lumbar Disc Herniation. Arthritis Res. Ther. 20 (1), 251. doi:10.1186/s13075-018-1743-4
Cvjetkovic, A., Jang, S. C., Konečná, B., Höög, J. L., Sihlbom, C., Lässer, C., et al. (2016). Detailed Analysis of Protein Topology of Extracellular Vesicles-Evidence of Unconventional Membrane Protein Orientation. Sci. Rep. 6, 36338. doi:10.1038/srep36338
De la Torre-Escudero, E., Gerlach, J. Q., Bennett, A. P. S., Cwiklinski, K., Jewhurst, H. L., Huson, K. M., et al. (2019). Surface Molecules of Extracellular Vesicles Secreted by the Helminth Pathogen Fasciola Hepatica Direct Their Internalisation by Host Cells. Plos Negl. Trop. Dis. 13 (1), e0007087. doi:10.1371/journal.pntd.0007087
Ev-Track Consortium, , Van Deun, J., Mestdagh, P., Agostinis, P., Akay, Ö., Anand, S., et al. (2017). EV-TRACK: Transparent Reporting and Centralizing Knowledge in Extracellular Vesicle Research. Nat. Methods 14 (3), 228–232. doi:10.1038/nmeth.4185
Feng, C., Yang, M., Lan, M., Liu, C., Zhang, Y., Huang, B., et al. (2017). ROS: Crucial Intermediators in the Pathogenesis of Intervertebral Disc Degeneration. Oxid Med. Cel Longev 2017, 5601593. doi:10.1155/2017/5601593
Feng, C., Liu, H., Yang, M., Zhang, Y., Huang, B., and Zhou, Y. (2016). Disc Cell Senescence in Intervertebral Disc Degeneration: Causes and Molecular Pathways. Cell Cycle 15 (13), 1674–1684. doi:10.1080/15384101.2016.1152433
Flory, J., and Lipska, K. (2019). Metformin in 2019. JAMA 321 (19), 1926–1927. doi:10.1001/jama.2019.3805
Foers, A. D., Chatfield, S., Dagley, L. F., Scicluna, B. J., Webb, A. I., Cheng, L., et al. (2018). Enrichment of Extracellular Vesicles from Human Synovial Fluid Using Size Exclusion Chromatography. J. Extracellular Vesicles 7 (1), 1490145. doi:10.1080/20013078.2018.1490145
Gardiner, C., Vizio, D. D., Sahoo, S., Théry, C., Witwer, K. W., Wauben, M., et al. (2016). Techniques Used for the Isolation and Characterization of Extracellular Vesicles: Results of a Worldwide Survey. J. Extracellular Vesicles 5, 32945. doi:10.3402/jev.v5.32945
Gimona, M., Brizzi, M. F., Choo, A. B. H., Dominici, M., Davidson, S. M., Grillari, J., et al. (2021). Critical Considerations for the Development of Potency Tests for Therapeutic Applications of Mesenchymal Stromal Cell-Derived Small Extracellular Vesicles. Cytotherapy. 23 (5), 373–380. doi:10.1016/j.jcyt.2021.01.001
Goh, S.-L., Persson, M. S. M., Stocks, J., Hou, Y., Welton, N. J., Lin, J., et al. (2019). Relative Efficacy of Different Exercises for Pain, Function, Performance and Quality of Life in Knee and Hip Osteoarthritis: Systematic Review and Network Meta-Analysis. Sports Med. 49 (5), 743–761. doi:10.1007/s40279-019-01082-0
Guan, S., Yu, H., Yan, G., Gao, M., Sun, W., and Zhang, X. (2020). Characterization of Urinary Exosomes Purified with Size Exclusion Chromatography and Ultracentrifugation. J. Proteome Res. 19 (6), 2217–2225. doi:10.1021/acs.jproteome.9b00693
Guo, Z., Su, W., Zhou, R., Zhang, G., Yang, S., Wu, X., et al. (2021). Exosomal MATN3 of Urine-Derived Stem Cells Ameliorates Intervertebral Disc Degeneration by Antisenescence Effects and Promotes NPC Proliferation and ECM Synthesis by Activating TGF-β. Oxid Med. Cel Longev 2021, 5542241. doi:10.1155/2021/5542241
Gupta, S., Rawat, S., Arora, V., Kottarath, S. K., Dinda, A. K., Vaishnav, P. K., et al. (2018). An Improvised One-step Sucrose Cushion Ultracentrifugation Method for Exosome Isolation from Culture Supernatants of Mesenchymal Stem Cells. Stem Cel Res Ther. 9 (1), 180. doi:10.1186/s13287-018-0923-0
Heath, N., Grant, L., De Oliveira, T. M., Rowlinson, R., Osteikoetxea, X., Dekker, N., et al. (2018). Rapid Isolation and Enrichment of Extracellular Vesicle Preparations Using Anion Exchange Chromatography. Sci. Rep. 8 (1), 5730. doi:10.1038/s41598-018-24163-y
Hessvik, N. P., and Llorente, A. (2018). Current Knowledge on Exosome Biogenesis and Release. Cell. Mol. Life Sci. 75 (2), 193–208. doi:10.1007/s00018-017-2595-9
Hingert, D., Ekström, K., Aldridge, J., Crescitelli, R., and Brisby, H. (2020). Extracellular Vesicles from Human Mesenchymal Stem Cells Expedite Chondrogenesis in 3D Human Degenerative Disc Cell Cultures. Stem Cel Res Ther. 11 (1), 323. doi:10.1186/s13287-020-01832-2
Ji, X., Wang, M., Chen, F., and Zhou, J. (2017). Urine-Derived Stem Cells: The Present and the Future. Stem Cell Int 2017, 4378947. doi:10.1155/2017/4378947
Kalluri, R., and LeBleu, V. S. (2020). The Biology, Function, and Biomedical Applications of Exosomes. Science 367 (6478), eaau6977. doi:10.1126/science.aau6977
Kang, L., Liu, S., Li, J., Tian, Y., Xue, Y., and Liu, X. (2020). The Mitochondria-Targeted Anti-oxidant MitoQ Protects against Intervertebral Disc Degeneration by Ameliorating Mitochondrial Dysfunction and Redox Imbalance. Cell Prolif. 53 (3), e12779. doi:10.1111/cpr.12779
Keerthikumar, S., Chisanga, D., Ariyaratne, D., Al Saffar, H., Anand, S., Zhao, K., et al. (2016). ExoCarta: A Web-Based Compendium of Exosomal Cargo. J. Mol. Biol. 428 (4), 688–692. doi:10.1016/j.jmb.2015.09.019
Keshtkar, S., Azarpira, N., and Ghahremani, M. H. (2018). Mesenchymal Stem Cell-Derived Extracellular Vesicles: Novel Frontiers in Regenerative Medicine. Stem Cel Res Ther. 9 (1), 63. doi:10.1186/s13287-018-0791-7
Kim, G. B., Shon, O.-J., Seo, M.-S., Choi, Y., Park, W. T., and Lee, G. W. (2021). Mesenchymal Stem Cell-Derived Exosomes and Their Therapeutic Potential for Osteoarthritis. Biology (Basel) 10 (4). doi:10.3390/biology10040285
Lener, T., Gimona, M., Aigner, L., Börger, V., Buzas, E., Camussi, G., et al. (2015). Applying Extracellular Vesicles Based Therapeutics in Clinical Trials - an ISEV Position Paper. J. Extracellular Vesicles 4, 30087. doi:10.3402/jev.v4.30087
Levine, B., and Kroemer, G. (2019). Biological Functions of Autophagy Genes: A Disease Perspective. Cell. 176 (1–2), 11–42. doi:10.1016/j.cell.2018.09.048
Lewis, J., Alattar, A. A., Akers, J., Carter, B. S., Heller, M., and Chen, C. C. (2019). A Pilot Proof-Of-Principle Analysis Demonstrating Dielectrophoresis (DEP) as a Glioblastoma Biomarker Platform. Sci. Rep. 9 (1), 10279. doi:10.1038/s41598-019-46311-8
Li, M., Li, R., Yang, S., Yang, D., Gao, X., Sun, J., et al. (2020). Exosomes Derived from Bone Marrow Mesenchymal Stem Cells Prevent Acidic pH-Induced Damage in Human Nucleus Pulposus Cells. Med. Sci. Monit. 26, e922928. doi:10.12659/MSM.922928
Li, Z.-Q., Kong, L., Liu, C., and Xu, H.-G. (2020). Human Bone Marrow Mesenchymal Stem Cell-Derived Exosomes Attenuate IL-1β-induced Annulus Fibrosus Cell Damage. Am. J. Med. Sci. 360 (6), 693–700. doi:10.1016/j.amjms.2020.07.025
Liangsupree, T., Multia, E., and Riekkola, M.-L. (2021). Modern Isolation and Separation Techniques for Extracellular Vesicles. J. Chromatogr. A 1636, 461773. doi:10.1016/j.chroma.2020.461773
Liao, Z., Li, S., Lu, S., Liu, H., Li, G., Ma, L., et al. (2021). Metformin Facilitates Mesenchymal Stem Cell-Derived Extracellular Nanovesicles Release and Optimizes Therapeutic Efficacy in Intervertebral Disc Degeneration. Biomaterials 274, 120850. doi:10.1016/j.biomaterials.2021.120850
Liao, Z., Liu, H., Ma, L., Lei, J., Tong, B., Li, G., et al. (2021). Engineering Extracellular Vesicles Restore the Impaired Cellular Uptake and Attenuate Intervertebral Disc Degeneration. ACS Nano. doi:10.1021/acsnano.1c04514
Liao, Z., Luo, R., Li, G., Song, Y., Zhan, S., Zhao, K., et al. (2019). Exosomes from Mesenchymal Stem Cells Modulate Endoplasmic Reticulum Stress to Protect against Nucleus Pulposus Cell Death and Ameliorate Intervertebral Disc Degeneration In Vivo. Theranostics. 9 (14), 4084–4100. doi:10.7150/thno.33638
Linares, R., Tan, S., Gounou, C., Arraud, N., and Brisson, A. R. (2015). High-speed Centrifugation Induces Aggregation of Extracellular Vesicles. J. Extracellular Vesicles 4, 29509. doi:10.3402/jev.v4.29509
Logozzi, M., Di Raimo, R., Mizzoni, D., and Fais, S. (2020). Immunocapture-based ELISA to Characterize and Quantify Exosomes in Both Cell Culture Supernatants and Body Fluids. Methods Enzymol. 645, 155–180. doi:10.1016/bs.mie.2020.06.011
Lu, K., Li, H.-Y., Yang, K., Wu, J.-L., Cai, X.-W., Zhou, Y., et al. (2017). Exosomes as Potential Alternatives to Stem Cell Therapy for Intervertebral Disc Degeneration: In-Vitro Study on Exosomes in Interaction of Nucleus Pulposus Cells and Bone Marrow Mesenchymal Stem Cells. Stem Cel Res Ther. 8 (1), 108. doi:10.1186/s13287-017-0563-9
Luo, L., Gong, J., Zhang, H., Qin, J., Li, C., Zhang, J., et al. (2021). Cartilage Endplate Stem Cells Transdifferentiate into Nucleus Pulposus Cells via Autocrine Exosomes. Front. Cel Dev. Biol. 9, 648201. doi:10.3389/fcell.2021.648201
Luo, L., Jian, X., Sun, H., Qin, J., Wang, Y., Zhang, J., et al. (2021). Cartilage Endplate Stem Cells Inhibit Intervertebral Disc Degeneration by Releasing Exosomes to Nucleus Pulposus Cells to Activate Akt/autophagy. Stem Cells 39 (4), 467–481. doi:10.1002/stem.3322
Maas, S. L. N., Broekman, M. L. D., and de Vrij, J. (2017). Tunable Resistive Pulse Sensing for the Characterization of Extracellular Vesicles. Methods Mol. Biol. 1545, 21–33. doi:10.1007/978-1-4939-6728-5_2
Malandrino, A., Lacroix, D., Hellmich, C., Ito, K., Ferguson, S. J., and Noailly, J. (2014). The Role of Endplate Poromechanical Properties on the Nutrient Availability in the Intervertebral Disc. Osteoarthritis and Cartilage 22 (7), 1053–1060. doi:10.1016/j.joca.2014.05.005
Mohammadian, S., Agronskaia, A. V., Blab, G. A., van Donselaar, E. G., de Heus, C., Liv, N., et al. (2020). Integrated Super Resolution Fluorescence Microscopy and Transmission Electron Microscopy. Ultramicroscopy 215, 113007. doi:10.1016/j.ultramic.2020.113007
Mulcahy, L. A., Pink, R. C., and Carter, D. R. (2014). Routes and Mechanisms of Extracellular Vesicle Uptake. J. Extracell Vesicles 3. doi:10.3402/jev.v3.24641
Murphy, C., Withrow, J., Hunter, M., Liu, Y., Tang, Y. L., Fulzele, S., et al. (2018). Emerging Role of Extracellular Vesicles in Musculoskeletal Diseases. Mol. Aspects Med. 60, 123–128. doi:10.1016/j.mam.2017.09.006
Nabhan, J. F., Hu, R., Oh, R. S., Cohen, S. N., and Lu, Q. (2012). Formation and Release of Arrestin Domain-Containing Protein 1-mediated Microvesicles (ARMMs) at Plasma Membrane by Recruitment of TSG101 Protein. Proc. Natl. Acad. Sci. 109 (11), 4146–4151. doi:10.1073/pnas.1200448109
Nawaz, M., Fatima, F., Vallabhaneni, K. C., Penfornis, P., Valadi, H., Ekström, K., et al. (2016). Extracellular Vesicles: Evolving Factors in Stem Cell Biology. Stem Cell Int. 2016, 1073140. doi:10.1155/2016/1073140
Nawaz, M., Shah, N., Zanetti, B. R., Maugeri, M., Silvestre, R. N., Fatima, F., et al. (2018). Extracellular Vesicles and Matrix Remodeling Enzymes: The Emerging Roles in Extracellular Matrix Remodeling, Progression of Diseases and Tissue Repair. Cells 7 (10), E167. doi:10.3390/cells7100167
Ni, L., Liu, X., Sochacki, K. R., Ebraheim, M., Fahrenkopf, M., Shi, Q., et al. (2014). Effects of Hypoxia on Differentiation from Human Placenta-Derived Mesenchymal Stem Cells to Nucleus Pulposus-like Cells. Spine J. 14 (10), 2451–2458. doi:10.1016/j.spinee.2014.03.028
Nolan, J. P., and Duggan, E. (2018). Analysis of Individual Extracellular Vesicles by Flow Cytometry. Methods Mol. Biol. 1678, 79–92. doi:10.1007/978-1-4939-7346-0_5
Nordin, J. Z., Lee, Y., Vader, P., Mäger, I., Johansson, H. J., Heusermann, W., et al. (2015). Ultrafiltration with Size-Exclusion Liquid Chromatography for High Yield Isolation of Extracellular Vesicles Preserving Intact Biophysical and Functional Properties. Nanomedicine: Nanotechnology, Biol. Med. 11 (4), 879–883. doi:10.1016/j.nano.2015.01.003
Ohshima, H., and Urban, J. P. (1992). The Effect of Lactate and pH on Proteoglycan and Protein Synthesis Rates in the Intervertebral Disc. Spine (Phila Pa 1976) 17 (9), 1079–1082. doi:10.1097/00007632-199209000-00012
Pan, B.-T., and Johnstone, R. M. (1983). Fate of the Transferrin Receptor during Maturation of Sheep Reticulocytes In Vitro: Selective Externalization of the Receptor. Cell. 33 (3), 967–978. doi:10.1016/0092-8674(83)90040-5
Pathan, M., Fonseka, P., Chitti, S. V., Kang, T., Sanwlani, R., Van Deun, J., et al. (2019). Vesiclepedia 2019: a Compendium of RNA, Proteins, Lipids and Metabolites in Extracellular Vesicles. Nucleic Acids Res. 47 (D1), D516–D519. doi:10.1093/nar/gky1029
Pisitkun, T., Shen, R.-F., and Knepper, M. A. (2004). Identification and Proteomic Profiling of Exosomes in Human Urine. Proc. Natl. Acad. Sci. 101 (36), 13368–13373. doi:10.1073/pnas.0403453101
Pospichalova, V., Svoboda, J., Dave, Z., Kotrbova, A., Kaiser, K., Klemova, D., et al. (2015). Simplified Protocol for Flow Cytometry Analysis of Fluorescently Labeled Exosomes and Microvesicles Using Dedicated Flow Cytometer. J. Extracellular Vesicles 4, 25530. doi:10.3402/jev.v4.25530
Povero, D., Yamashita, H., Ren, W., Subramanian, M. G., Myers, R. P., Eguchi, A., et al. (2020). Characterization and Proteome of Circulating Extracellular Vesicles as Potential Biomarkers for NASH. Hepatol. Commun. 4 (9), 1263–1278. doi:10.1002/hep4.1556
Qi, L., Wang, R., Shi, Q., Yuan, M., Jin, M., and Li, D. (2019). Umbilical Cord Mesenchymal Stem Cell Conditioned Medium Restored the Expression of Collagen II and Aggrecan in Nucleus Pulposus Mesenchymal Stem Cells Exposed to High Glucose. J. Bone Miner Metab. 37 (3), 455–466. doi:10.1007/s00774-018-0953-9
Rani, S., Ryan, A. E., Griffin, M. D., and Ritter, T. (2015). Mesenchymal Stem Cell-Derived Extracellular Vesicles: Toward Cell-free Therapeutic Applications. Mol. Ther. 23 (5), 812–823. doi:10.1038/mt.2015.44
Ratajczak, J., Miekus, K., Kucia, M., Zhang, J., Reca, R., Dvorak, P., et al. (2006). Embryonic Stem Cell-Derived Microvesicles Reprogram Hematopoietic Progenitors: Evidence for Horizontal Transfer of mRNA and Protein Delivery. Leukemia 20 (5), 847–856. doi:10.1038/sj.leu.2404132
Ronquist, G., and Brody, I. (1985). The Prostasome: its Secretion and Function in Man. Biochim. Biophys. Acta (Bba) - Rev. Biomembranes 822 (2), 203–218. doi:10.1016/0304-4157(85)90008-5
Ruiz-Fernández, C., Francisco, V., Pino, J., Mera, A., González-Gay, M. A., Gómez, R., et al. (2019). Molecular Relationships Among Obesity, Inflammation and Intervertebral Disc Degeneration: Are Adipokines the Common Link? Int. J. Mol. Sci. 20 (8), E2030.
Sakai, D., and Andersson, G. B. J. (2015). Stem Cell Therapy for Intervertebral Disc Regeneration: Obstacles and Solutions. Nat. Rev. Rheumatol. 11 (4), 243–256. doi:10.1038/nrrheum.2015.13
Sakai, D., and Schol, J. (2017). Cell Therapy for Intervertebral Disc Repair: Clinical Perspective. J. Orthopaedic Translation 9, 8–18. doi:10.1016/j.jot.2017.02.002
Shao, H., Im, H., Castro, C. M., Breakefield, X., Weissleder, R., and Lee, H. (2018). New Technologies for Analysis of Extracellular Vesicles. Chem. Rev. 118 (4), 1917–1950. doi:10.1021/acs.chemrev.7b00534
Smyth, T., Kullberg, M., Malik, N., Smith-Jones, P., Graner, M. W., and Anchordoquy, T. J. (2015). Biodistribution and Delivery Efficiency of Unmodified Tumor-Derived Exosomes. J. Controlled Release 199, 145–155. doi:10.1016/j.jconrel.2014.12.013
Stranska, R., Gysbrechts, L., Wouters, J., Vermeersch, P., Bloch, K., Dierickx, D., et al. (2018). Comparison of Membrane Affinity-Based Method with Size-Exclusion Chromatography for Isolation of Exosome-like Vesicles from Human Plasma. J. Transl Med. 16 (1), 1. doi:10.1186/s12967-017-1374-6
Street, J. M., Barran, P. E., Mackay, C. L., Weidt, S., Balmforth, C., Walsh, T. S., et al. (2012). Identification and Proteomic Profiling of Exosomes in Human Cerebrospinal Fluid. J. Transl Med. 10 (5), 5. doi:10.1186/1479-5876-10-5
Sun, Y., Zhang, W., and Li, X. (2021). Induced Pluripotent Stem Cell-Derived Mesenchymal Stem Cells Deliver Exogenous miR-105-5p via Small Extracellular Vesicles to Rejuvenate Senescent Nucleus Pulposus Cells and Attenuate Intervertebral Disc Degeneration. Stem Cel Res Ther. 12 (1), 286. doi:10.1186/s13287-021-02362-1
Tertel, T., Bremer, M., Maire, C., Lamszus, K., Peine, S., Jawad, R., et al. (2020). High‐Resolution Imaging Flow Cytometry Reveals Impact of Incubation Temperature on Labeling of Extracellular Vesicles with Antibodies. Cytometry 97 (6), 602–609. doi:10.1002/cyto.a.24034
Théry, C., Witwer, K. W., Aikawa, E., Alcaraz, M. J., Anderson, J. D., Andriantsitohaina, R., et al. (2018). Minimal Information for Studies of Extracellular Vesicles 2018 (MISEV2018): a Position Statement of the International Society for Extracellular Vesicles and Update of the MISEV2014 Guidelines. J. Extracell Vesicles 7 (1), 1535750.
Tryfonidou, M. A., de Vries, G., Hennink, W. E., and Creemers, L. B. (2020). "Old Drugs, New Tricks" - Local Controlled Drug Release Systems for Treatment of Degenerative Joint Disease. Adv. Drug Deliv. Rev. 160, 170–185. doi:10.1016/j.addr.2020.10.012
Tsai, B. W., Lau, S., Chen, Q., and Chamley, L. W. (2021). Comparison of Methods for Separating Fluorescently Labelled Placental Extracellular Vesicles from Free Stain. Placenta. 109, 1–3. doi:10.1016/j.placenta.2021.04.001
Tsiapalis, D., and O'Driscoll, L. (2020). Mesenchymal Stem Cell Derived Extracellular Vesicles for Tissue Engineering and Regenerative Medicine Applications. Cells 9 (4). doi:10.3390/cells9040991
Vo, N. V., Hartman, R. A., Patil, P. R., Risbud, M. V., Kletsas, D., Iatridis, J. C., et al. (2016). Molecular Mechanisms of Biological Aging in Intervertebral Discs. J. Orthop. Res. 34 (8), 1289–1306. doi:10.1002/jor.23195
Vogel, R., Coumans, F. A. W., Maltesen, R. G., Böing, A. N., Bonnington, K. E., Broekman, M. L., et al. (2016). A Standardized Method to Determine the Concentration of Extracellular Vesicles Using Tunable Resistive Pulse Sensing. J. Extracellular Vesicles 5, 31242. doi:10.3402/jev.v5.31242
Wang, F., Shi, R., Cai, F., Wang, Y.-T., and Wu, X.-T. (2015). Stem Cell Approaches to Intervertebral Disc Regeneration: Obstacles from the Disc Microenvironment. Stem Cell Development 24 (21), 2479–2495. doi:10.1089/scd.2015.0158
Wang, Y., Xu, W., Yan, Z., Zhao, W., Mi, J., Li, J., et al. (2018). Metformin Induces Autophagy and G0/G1 Phase Cell Cycle Arrest in Myeloma by Targeting the AMPK/mTORC1 and mTORC2 Pathways. J. Exp. Clin. Cancer Res. 37 (1), 63. doi:10.1186/s13046-018-0731-5
Wang, Z., Yan, K., Ge, G., Zhang, D., Bai, J., Guo, X., et al. (2021). Exosomes Derived from miR-155-5p-Overexpressing Synovial Mesenchymal Stem Cells Prevent Osteoarthritis via Enhancing Proliferation and Migration, Attenuating Apoptosis, and Modulating Extracellular Matrix Secretion in Chondrocytes. Cell Biol Toxicol. 37 (1), 85–96. doi:10.1007/s10565-020-09559-9
Webber, J., and Clayton, A. (2013). How Pure Are Your Vesicles? J. Extracell Vesicles 2. doi:10.3402/jev.v2i0.19861
Wen, T., Wang, H., Li, Y., Lin, Y., Zhao, S., Liu, J., et al. (2021). Bone Mesenchymal Stem Cell-Derived Extracellular Vesicles Promote the Repair of Intervertebral Disc Degeneration by Transferring microRNA-199a. Cell Cycle 20 (3), 256–270. doi:10.1080/15384101.2020.1863682
Witwer, K. W., Van Balkom, B. W. M., Bruno, S., Choo, A., Dominici, M., Gimona, M., et al. (2019). Defining Mesenchymal Stromal Cell (MSC)-derived Small Extracellular Vesicles for Therapeutic Applications. J. Extracellular Vesicles 8 (1), 1609206. doi:10.1080/20013078.2019.1609206
Woo, C. H., Kim, H. K., Jung, G. Y., Jung, Y. J., Lee, K. S., Yun, Y. E., et al. (2020). Small Extracellular Vesicles from Human Adipose‐derived Stem Cells Attenuate Cartilage Degeneration. J. Extracellular Vesicles 9 (1), 1735249. doi:10.1080/20013078.2020.1735249
Wu, B., Chen, X., Wang, J., Qing, X., Wang, Z., Ding, X., et al. (2020). Separation and Characterization of Extracellular Vesicles from Human Plasma by Asymmetrical Flow Field-Flow Fractionation. Analytica Chim. Acta 1127, 234–245. doi:10.1016/j.aca.2020.06.071
Xia, C., Zeng, Z., Fang, B., Tao, M., Gu, C., Zheng, L., et al. (2019). Mesenchymal Stem Cell-Derived Exosomes Ameliorate Intervertebral Disc Degeneration via Anti-oxidant and Anti-inflammatory Effects. Free Radic. Biol. Med. 143, 1–15. doi:10.1016/j.freeradbiomed.2019.07.026
Xiang, H., Su, W., Wu, X., Chen, W., Cong, W., Yang, S., et al. (2020). Exosomes Derived from Human Urine-Derived Stem Cells Inhibit Intervertebral Disc Degeneration by Ameliorating Endoplasmic Reticulum Stress. Oxid Med. Cel Longev 2020, 6697577. doi:10.1155/2020/6697577
Xiao, L., Zhao, Q., Hu, B., Wang, J., Liu, C., and Xu, H. (2020). METTL3 Promotes IL‐1β-induced Degeneration of Endplate Chondrocytes by Driving m6A‐dependent Maturation of miR‐126‐5p. J. Cel. Mol. Med. 24 (23), 14013–14025. doi:10.1111/jcmm.16012
Xie, L., Chen, Z., Liu, M., Huang, W., Zou, F., Ma, X., et al. (2020). MSC-derived Exosomes Protect Vertebral Endplate Chondrocytes against Apoptosis and Calcification via the miR-31-5p/ATF6 Axis. Mol. Ther. - Nucleic Acids 22, 601–614. doi:10.1016/j.omtn.2020.09.026
Xing, H., Zhang, Z., Mao, Q., Wang, C., Zhou, Y., Zhou, X., et al. (2021). Injectable Exosome-Functionalized Extracellular Matrix Hydrogel for Metabolism Balance and Pyroptosis Regulation in Intervertebral Disc Degeneration. J. Nanobiotechnol 19 (1), 264. doi:10.1186/s12951-021-00991-5
Yin, L., Liu, X., Shi, Y., Ocansey, D. K. W., Hu, Y., Li, X., et al. (2020). Therapeutic Advances of Stem Cell-Derived Extracellular Vesicles in Regenerative Medicine. Cells 9 (3). doi:10.3390/cells9030707
Yuan, Q., Wang, X., Liu, L., Cai, Y., Zhao, X., Ma, H., et al. (2020). Exosomes Derived from Human Placental Mesenchymal Stromal Cells Carrying AntagomiR-4450 Alleviate Intervertebral Disc Degeneration through Upregulation of ZNF121. Stem Cell Development 29 (16), 1038–1058. doi:10.1089/scd.2020.0083
Yuan, X., Li, T., Shi, L., Miao, J., Guo, Y., and Chen, Y. (2021). Human Umbilical Cord Mesenchymal Stem Cells Deliver Exogenous miR-26a-5p via Exosomes to Inhibit Nucleus Pulposus Cell Pyroptosis through METTL14/NLRP3. Mol. Med. 27 (1), 91. doi:10.1186/s10020-021-00355-7
Zhang, F., Lin, F., Xu, Z., and Huang, Z. (2021). Circular RNA ITCH Promotes Extracellular Matrix Degradation via Activating Wnt/β-Catenin Signaling in Intervertebral Disc Degeneration. Aging (Albany NY) 13. doi:10.18632/aging.203036
Zhang, J., Nguyen, L. T. H., Hickey, R., Walters, N., Wang, X., Kwak, K. J., et al. (2021). Immunomagnetic Sequential Ultrafiltration (iSUF) Platform for Enrichment and Purification of Extracellular Vesicles from Biofluids. Sci. Rep. 11 (1), 8034. doi:10.1038/s41598-021-86910-y
Zhang, J., Rong, Y., Luo, C., and Cui, W. (2020). Bone Marrow Mesenchymal Stem Cell-Derived Exosomes Prevent Osteoarthritis by Regulating Synovial Macrophage Polarization. Aging 12 (24), 25138–25152. doi:10.18632/aging.104110
Zhang, J., Zhang, J., Zhang, Y., Liu, W., Ni, W., Huang, X., et al. (2020). Mesenchymal Stem Cells‐derived Exosomes Ameliorate Intervertebral Disc Degeneration through Inhibiting Pyroptosis. J. Cel. Mol. Med. 24 (20), 11742–11754. doi:10.1111/jcmm.15784
Zhang, Q., Shen, Y., Zhao, S., Jiang, Y., Zhou, D., and Zhang, Y. (2021). Exosomes miR-15a Promotes Nucleus Pulposus-Mesenchymal Stem Cells Chondrogenic Differentiation by Targeting MMP-3. Cell Signal. 86, 110083. doi:10.1016/j.cellsig.2021.110083
Zhang, S., Hu, B., Liu, W., Wang, P., Lv, X., Chen, S., et al. (2021). The Role of Structure and Function Changes of Sensory Nervous System in Intervertebral Disc-Related Low Back Pain. Osteoarthritis and Cartilage 29 (1), 17–27. doi:10.1016/j.joca.2020.09.002
Zhao, K., An, R., Xiang, Q., Li, G., Wang, K., Song, Y., et al. (2021). Acid-sensing Ion Channels Regulate Nucleus Pulposus Cell Inflammation and Pyroptosis via the NLRP3 Inflammasome in Intervertebral Disc Degeneration. Cel Prolif. 54 (1), e12941. doi:10.1111/cpr.12941
Zhu, G., Yang, X., Peng, C., Yu, L., and Hao, Y. (2020). Exosomal miR-532-5p from Bone Marrow Mesenchymal Stem Cells Reduce Intervertebral Disc Degeneration by Targeting RASSF5. Exp. Cel Res. 393 (2), 112109. doi:10.1016/j.yexcr.2020.112109
Zhu, L., Shi, Y., Liu, L., Wang, H., Shen, P., and Yang, H. (2020). Mesenchymal Stem Cells-Derived Exosomes Ameliorate Nucleus Pulposus Cells Apoptosis via Delivering miR-142-3p: Therapeutic Potential for Intervertebral Disc Degenerative Diseases. Cell Cycle 19 (14), 1727–1739. doi:10.1080/15384101.2020.1769301
Keywords: nucleus pulposus cells, annulus fibrosus, cartilage endplate, intervertebral disc degeneration, extracellular vesicles
Citation: Wu X and Sun W (2022) Extracellular Vesicles Derived From Stem Cells in Intervertebral Disc Degeneration. Front. Cell Dev. Biol. 9:793363. doi: 10.3389/fcell.2021.793363
Received: 12 October 2021; Accepted: 06 December 2021;
Published: 13 January 2022.
Edited by:
Selim Kuci, University Hospital Frankfurt, GermanyReviewed by:
Muhammad Nawaz, University of Gothenburg, SwedenCopyright © 2022 Wu and Sun. This is an open-access article distributed under the terms of the Creative Commons Attribution License (CC BY). The use, distribution or reproduction in other forums is permitted, provided the original author(s) and the copyright owner(s) are credited and that the original publication in this journal is cited, in accordance with accepted academic practice. No use, distribution or reproduction is permitted which does not comply with these terms.
*Correspondence: Wei Sun, ZHJzdW53ZWlAMTI2LmNvbQ==
Disclaimer: All claims expressed in this article are solely those of the authors and do not necessarily represent those of their affiliated organizations, or those of the publisher, the editors and the reviewers. Any product that may be evaluated in this article or claim that may be made by its manufacturer is not guaranteed or endorsed by the publisher.
Research integrity at Frontiers
Learn more about the work of our research integrity team to safeguard the quality of each article we publish.