- 1State Key Laboratory of Ecological Pest Control for Fujian and Taiwan Crops, Fujian Agriculture and Forestry University, Fuzhou, China
- 2Institute of Plant Virology, Fujian Agriculture and Forestry University, Fuzhou, China
Programmed cell death (PCD) in animals mainly refers to lytic and non-lytic forms. Disruption and integrity of the plasma membrane are considered as hallmarks of lytic and apoptotic cell death, respectively. These lytic cell death programs can prevent the hosts from microbial pathogens. The key to our understanding of these cases is pattern recognition receptors, such as TLRs in animals and LRR-RLKs in plants, and nod-like receptors (NLRs). Herein, we emphatically discuss the biochemical and structural studies that have clarified the anti-apoptotic and pro-apoptotic functions of Bcl-2 family proteins during intrinsic apoptosis and how caspase-8 among apoptosis, necroptosis, and pyroptosis sets the switchable threshold and integrates innate immune signaling, and that have compared the similarity and distinctness of the apoptosome, necroptosome, and inflammasome. We recapitulate that the necroptotic MLKL pore, pyroptotic gasdermin pore, HR-inducing resistosome, and mitochondrial Bcl-2 family all can form ion channels, which all directly boost membrane disruption. Comparing the conservation and unique aspects of PCD including ferrroptosis among bacteria, animals, and plants, the commonly shared immune domains including TIR-like, gasdermin-like, caspase-like, and MLKL/CC-like domains act as arsenal modules to restructure the diverse architecture to commit PCD suicide upon stresses/stimuli for host community.
Introduction
Programmed cell death (PCD) is clearly characterized in animals and contains several types. Of PCDs, apoptosis is required for tissue development, maintenance of the homeostasis of proliferating cells, and multicellular morphogenesis in plants and animals. The conceptual proposal of apoptosis started from 1965 (Kerr, 1965). Australian scientists discovered that some scattered dead cells from the liver parenchyma were present when observed under an electron microscope after ligation of the rat portal vein. The lysosomes from these cells seemed not to be damaged and were kept in an intact situation. These cells, featured by morphological shrinkage and chromatin aggregations, fall off from their surrounding tissues and were ultimately engulfed. Kerr and other three scientists formally put forward the concept of apoptosis in 1972 (Kerr et al., 1972). The molecular progresses on apoptosis per se began with a good model organism Caenorhabditis elegans. Sydney Brenner first determined the C. elegans cell development lineage (Brenner, 1973). John Sulston discovered the specific cell division and differentiation during the nematode developmental process and identified that nematode apoptosis is dictated by alternate gene expressions. Robert Horvitz found more than 20 genes regulating apoptosis (Lettre and Hengartner, 2006; Ellis and Horvitz, 1986). These come in (at least) two distinct flavors, containing either ones responsible for initiating or executing cell death or others involved in inhibition of cell death. The four genes that regulate all somatic cell deaths in C. elegans are CED-3, CED-4, CED-9, and EGL-1 genes. CED-9 is an anti-apoptotic Bcl-2 homolog with four Bcl-2 homology (BH) domains, whereas EGL-1 acts as a pro-apoptotic BH3-only domain protein (Hengartner et al., 1992) (Figure 1A); CED-3 and CED-4 are pro-apoptotic (Lettre and Hengartner, 2006). CED-3 is homologous to mammalian caspases (cysteinyl aspartic acid–specific proteases) formerly known as interleukin-1B–converting enzyme in animals (Yuan et al., 1993), and CED-4 is an adaptor protein that is orthologous to mammalian apoptotic protease–activating factor-1 (Apaf-1) being the main scaffold protein of apoptosome for caspase-9 activation in the intrinsic pathway (Lettre and Hengartner, 2006) (Figure 1A). Although the three conserved gene-encoding proteins regulate apoptosis in animals, no corresponding orthologous proteins have been found in plants.
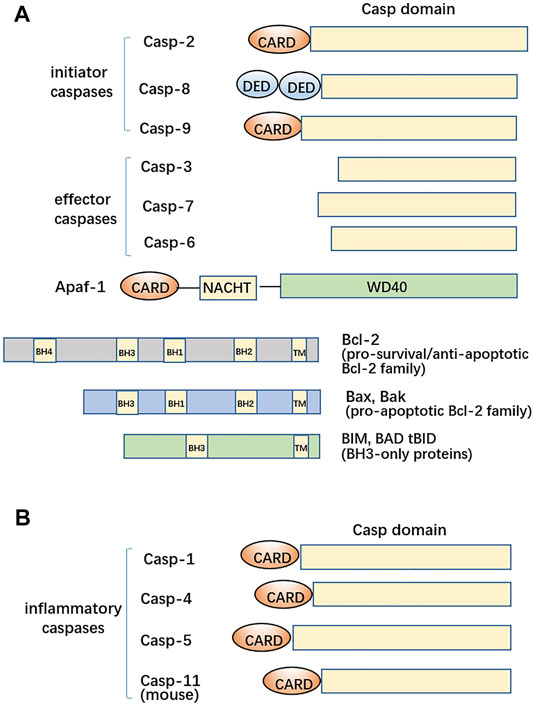
FIGURE 1. Structural features of caspases, Apaf-1, and the Bcl-2 family. (A) Master initiator caspases, caspase-2, caspase-8, and caspase-9, are characterized by N-terminal domain(s) including DED or CARD. The effector caspases have caspase-3, caspase-6, and caspase-7 for apoptosis. Apaf-1 is a main scaffold protein for recruitment of Cyt c and caspase-9. Bcl-2 itself plays anti-apoptotic roles whereas the Bax/Bak members of the Bcl-2 family are pro-apoptotic. BH3-only protein is an intrinsic apoptosis initiator. (B) Another clade contains inflammatory caspases, including human caspase-1, -4, and -5 and mouse caspase-11, have the N-terminal CARD domains.
Mammalian Apoptosis Mediated by Caspases
During the process of apoptosis, the central hub is the activation of caspases. Human caspases have 11 members and are categorized into 3 subclasses (Figures 1A,B). Different clades largely correspond to distinct physiological functions. Not all caspases are involved in apoptotic regulation, albeit caspases dictate the destiny of apoptotic cells. Caspase-2, -8, -9, and -10 are involved in the initiation of apoptosis (as initiators). Upon dimerization, procaspase-2 becomes active and can process cytosolic Bid to trigger the release of Cyt c (Baliga et al., 2004; Guo et al., 2002); caspase-8 and caspase-9 individually initiate the extrinsic and intrinsic mammalian apoptosis (Chai and Shi, 2014), or caspase-8 has an N-terminal tandem death effector domain (DED) and is coordinated with the death receptor TNFR for perception of extracellular death signals. Hence, recruitment of caspase-8 forms a death-inducing signaling complex (DISC) and then activates caspase-8 (Schleich et al., 2013). The Apaf-1 apoptosome assembles into a heptameric wheel-like complex with cytochrome c (Cyt c) and caspase-9 having the N-terminal caspase recruitment domain (CARD) (Li et al., 1998). The dome of apoptosome was identified to be the oligomer of CARDs from Apaf-1 and caspase-9 (7:3-4 stoichiometry); then, procaspase-9 undergoes conversion into caspase-9 (Figure 2A). However, the DARK-DRONC apoptosome (8:8 stoichiometry) complex found in flies and the C. elegans octameric CED-4 apoptosome interacting with CED-3 (8:2 stoichiometry) does not require cytochrome c to assemble, as it does in humans. This activating ligand Cyt c for Apaf-1 can be released from the perforated mitochondria by pro-death Bad/Bax, which is being initiated via activation of Bid by caspase-8 (Li et al., 1998; Antignani and Youle, 2006). The plasma membrane of apoptotic cells keeps basically intact; however, the perforated mitochondrial outer membrane by Bax/Bak alternates the mitochondrial outer membrane permeabilization (MOMP) during intrinsic apoptosis. Then, endonuclease G (Endo G) is released from the disrupted mitochondria and enters the nucleus, resulting in DNA cleavage to form DNA ladders, which can also be contributed by DNase γ (Li et al., 2001; Shiokawa et al., 2002). Additionally, the active caspase-8 sequentially activates effector caspase(s) (Stennicke et al., 1998). The activated caspase-3 and -7 have similar cleavage profiles of substrates. The degradations of poly (ADP-ribose) polymerase (PARP) and DNA fragmentation factor-45 (DFF-45) by caspase-3 and caspase-7 give rise to failures in DNA repair and initiation of DNA degradation (Jänicke et al., 1998; Tang and Kidd, 1998; Wride et al., 1999). The lamin A critical for nuclear architecture acts as the substrate of activated caspase-6 (Ruchaud et al., 2002). Degraded lamin A and other cellular skeletal proteins lead to cellular shrinkages and chromatin condensations. However, the cytokines cannot leak out from apoptotic cells to the bystander cells due to the integrity of the plasma membrane during apoptosis genesis. Therefore, apoptosis without the release of cytokines cannot sequentially induce inflammation.
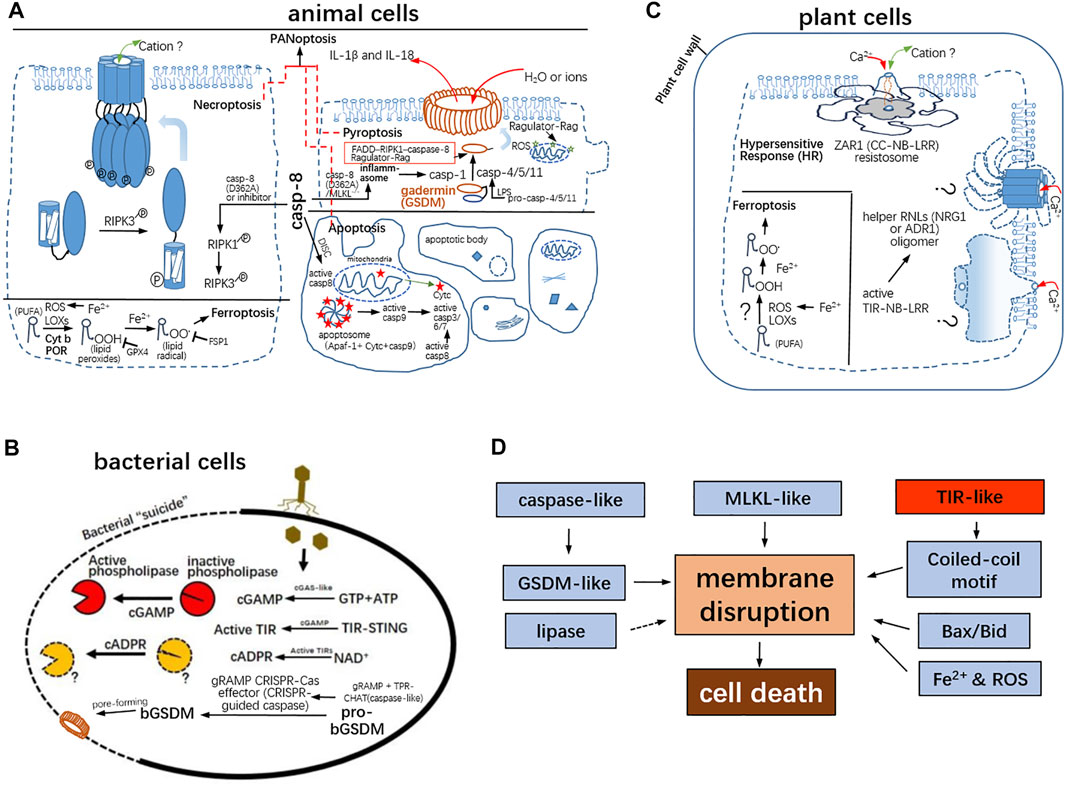
FIGURE 2. Commonality of various PCDs among bacteria, animals, and plants. (A) Necroptosis is mediated by RIPK3-phosphorylated MLKL. The oligomerization of the 4-helice bundle in the N-terminal region of MLKL should form a cation channel in the plasma membrane and lead to disruption of membrane integrity. Inflammatory caspases were activated by canonical and/or non-canonical inflammosomes. The active inflammatory caspases can cleave gasdermins. The resulting N-gasdermins insert the plasma membrane and form a membrane pore with 18–21 nm in inner diameter. The gasdermin pore prefers the release of IL-1β and IL-18. Apoptosis is a non-lytic cell death. In the instinct pathway, the initiator caspase-9 is activated by the apoptosome consisting of apaf-1, Cyt c, and caspase-9; caspase-8 is activated by the death-inducing signaling complex (DISC) for extrinsic apoptosis. Caspase-8 is the molecular switch for apoptosis, for necroptosis, and pyroptosis. Ferroptosis is required for iron and is mediated by LOXs and ROS and POR and Cyt b. The lipid peroxides and lipid radicals are capable of being sequestered by GPX4 and FSP1, respectively. Upon infection, PANoptosis co-featured by pyroptosis, apoptosis, and necroptosis is present as well. (B) The bacterial gasdermins are conserved and commit cell death via pore-forming as well. cGAMP as the elicitor activates the phospholipase, which perturb membrane integrity and result in cell death. gRAMP, the giant repeat–associated mysterious protein from CRISPR-Cas type III effectors; TPR-CHAT, caspase HetF associated with the tetratricopeptide repeat. proteases (C) Resistance (R) protein-mediated HR and ferroptosis-like in the plant cell. The R protein ZAR1 (CC-NBS-LRR) with RKS1 and PBL2 UMP ligands form the pentameric complex. The funnel-like channel (∼0.5 nm in narrowest diameter) is a non-selective, Ca2+ influx, cation channel. Likewise, helper NLRs required for TLR (TIR-NB-LRR)-mediated HR constitute a Ca2+ influx, cation channel. But the definitive 3D models of helper NLRs remain elusive; other CNL resistosomes may be cation channels, but their 3D structures are not be determined. And, ferroptosis-like cell death was also observed in plant cells. (D) In total, GSDM and MLKL domains directly target the plasma membrane; the Bcl-2 family is responsible for mitochondrial membrane damages; and TIR-like sense and monitor energy deficit to produce a second messenger for downstream signaling.
The Emerging Roles of Mammalian Bcl-2 Family Proteins in Apoptosis
The protein members of the B-cell lymphoma 2 (Bcl-2) family residing at the outer mitochondrial membrane may be classified into three functionally and structurally distinct subgroups, such as BH3 (the Bcl-2 homology 3)-only proteins (which communicate signals to initiate intrinsic apoptosis), the Bcl-2 itself as the pro-survival cell guardian, and the pro-apoptotic effector proteins BAX (Bcl-2–associated X protein) and BAK (Bcl-2 antagonist/killer) (Lindsten et al., 2000; Czabotar et al., 2014; Ke et al., 2012; Naim and Kaufmann, 2020) (Figure 1A). The anti- and pro-apoptotic ones both balance the mitochondrial membrane potential, and their interplay sets the apoptotic threshold in the mitochondrial outer membrane. With respect to the BH3-only protein, there are activator BH3-only proteins including BIM and the truncated form of BID (tBID) that can directly bind and activate BAX or BAK and sensitizer BH3-only proteins, such as BAD, which indirectly activate BAX or BAK by neutralizing pro-survival Bcl-2 family members (Czabotar et al., 2014). Overexpressed BH3-only proteins, particularly those (BIM, tBID, and PUMA) that target all pro-survival Bcl-2 family members, can trigger apoptosis. Diverse cell types from Bax−/−Bak−/− mice are completely resistant to multiple apoptotic stimuli, including the enforced expression of BH3-only proteins (Wei et al., 2001; Cheng et al., 2001; Zong et al., 2001) and are required for normal tissue development (Rathmell et al., 2002; Mason et al., 2013). Thus, the BH3-only proteins function upstream of BAX and BAK (Czabotar et al., 2014) and cannot cause cell death unless BAX or BAK is present. In transgenic mice, all of the pro-survival Bcl-2 family members endow much cell type resistance against diverse apoptotic stimuli. The apoptosis of autoreactive BIM −/− B cells and T cells negatively regulated by Bcl-2 act as one important checkpoint for preventing autoimmune kidney disease that resembles human systemic lupus erythematosus. BH3-only proteins or BH3-mimicking small molecules (BH3 mimetics) might promote apoptosis and improve the cancer therapy effect. Accordingly, the Bcl-2 overexpression in B cells of mice, loss of BIM, or loss of both BAX and BAK can provoke a fatal autoimmune kidney disease and might improve the treatment of diverse types of cancer (Adams and Cory, 2018).
MLKL-Mediated Necroptosis in Mammalian Cells
As known, non-lytic apoptosis is a non-inflammatory form of cell death. On the contrary, necroptosis and pyroptosis belong to lytic PCDs along with inflammatory exudates but differ for distinguishably lytic phenotypes. The necroptotic feature corresponds to cellular explosion, and pyroptosis is characterized by osmotic swelling/balloon-like protrusions. Necroptotic stimuli (including Z-DNA, Z-DNA binding protein 1 (ZBP1), and TNF-α) initiate a supramolecular organizing center (SMOC), called the necrosome RIPK1-RIPK3 core, as a hetero-amyloid signaling complex to perform autophosphorylations (Zhang, et al., 2009; Sun et al., 2012; Sun and Wang, 2014) (Figure 2A). The activated RIPK3 phosphorylates the necroptosis effector, as a pseudokinase—mixed lineage kinase domain-like (MLKL). And, the phosphorylated MLKLs undergo conformational alternation and initiatively bind with IP6 and recognize the anionic phospholipids (such as phosphatidylinositol-4-phosphate (PtIns4P)) of the inner leaflet of the plasma membrane, finally oligomerize and perforate the plasma membrane to form a non-selective cation channel (Huang et al., 2017; Su et al., 2014; Wang et al., 2014) (Figure 2A). Necroptosis would occur upon death of stimuli when caspase-8 is inactive after genetic depletion or chemical inhibition by the Z-VAD-FMK inhibitor (He, et al., 2009) (Figure 2A). In the more complex necroptotic pathway, tumor necrosis factor receptor 1 (TNFR1), toll-like receptor 3 (TLR3)–TRIF, and TLR4–TRIF signal via RIPK1 to activate NF-κB, but RIPK1 is not required for the TRIF-type I IFN response (Fitzgerald and Kagan, 2020).
Gasdermin-Mediated Pyroptosis in Animals and Bacterial Cells
Like apoptosomes and necroptosomes, pyroptotic inflammasomes are responsible for the activation of inflammatory caspase-1 (Ding and Shao, 2017). How are the other inflammatory caspase-4/5/11 activated upon bacterial infections? Shao Lab identified that caspase-4/5/11 can directly recognize the cytosolic lipopolysaccharides (LPS) to aggregate into non-canonical inflammasomes and commit self-cleavage to form active caspases (Shi et al., 2014). Once activated, caspase-1, 4/5/11 are capable of cleaving gasdermin D (GSDMD), being a bipartite protein whose amino-terminal and carboxy-terminal domains are connected by a linker and the free N-terminal fragment of GSDMD to induce 31∼34-fold symmetry gasdermin pore forming and pyroptosis (Shi et al., 2015; Ding et al., 2016; Ding and Shao, 2017; Ruan et al., 2018; Xia et al., 2021) (Figure 2A). The mature forms of pro–interleukin-1β (IL-1β) and pro–IL-18 processed by caspase-1 are feasible to outflow through the GSDMD pore (∼21 nm in internal diameter), while large amounts of sodium and water enter, and increasing expansion of pores finally lead to osmotic swelling and cell death (Xia et al., 2021) (Figure 2A).
The gasdermin subfamily has six members GSDMA-GASDME and GSDMF (DFNB59, also called PJVK) in humans. GSDMF is highly similar to GSDME, and its mutation is associated with autosomal recessive deafness (Broz et al., 2020). Different gasdermin members are activated by different protease(s) including caspase(s) or other proteases. The activation of GSDME was licensed to caspase-3, and activated gasdermin E assemblies (pore rings) have 26∼28-fold symmetry and may drive chemotherapy-induced pyroptosis (Wang et al., 2017; Broz et al., 2020; Zhang et al., 2020; Xia et al., 2021). And, gasdermin B can also be functionally cleaved by granzyme(s) from lymphocytes and NK cells for activation (Zhou et al., 2020). Apart from targeting the plasma membrane, active gasdermin B prefers to bind bacterial phospholipids and has strong bactericidal activities rather than cellular toxicity for NK cells (Hansen et al., 2021).
Importantly, oligomerization of the released N-lobe of gasdermins is a requisite for pyroptotic cell death. Pyroptosis is defined as gasdermin-mediated programmed necrotic cell death (Broz et al., 2020). As known, pyroptosis accompanies mitochondrial damages. And, the mitochondrial tricarboxylic acid (TCA) cycle metabolites (namely, fumarate and its derivatives) can modify a reactive cysteine of GSDMD by succination, which results in significantly decreased cleavage and pore formation (Humphries et al., 2020). Nevertheless, GSDMD cleavage by proteases appears to be not equivalent to pore formation. Recent studies identified that the lysosome-locating Ragulator-Rag complex involved in the mTORC1 pathway maintaining metabolic homeostasis may control the pore formation of gasdermins in the plasma membrane and/or serve as a scaffold for the activation of the FADD–RIPK1–caspase-8 complex to induce pyroptosis (Evavold et al., 2021; Zheng et al., 2021) (Figure 2A). The mTORC1 sensitize and control mitochondrial dysfunction and promote mitochondrial ROS generation, which boost gasdermin oligomerization in vivo (Evavold et al., 2021). The Rag-Ragulator complex surveils both metabolism and infection to act as a molecular hub dictating the living or death fate of infected cells (Zheng et al., 2021).
During the last stage of pyroptotic cell death, the plasma membrane rupture (PMR) usually occurs in dying cells. PMR previously is considered as a passive process following pore formation. But Kayagaki et al. (2021) recently identified that PMR may be a positive event mediated by the protein NINJ1, which is a 16-kDa protein with two transmembrane motifs and juxtaposed to the plasma membrane with both termini outside the cytoplasm. In addition to pyroptosis, other programmed lytic cell deaths all undergo PMR as well. Therefore, NINJ1 may act downstream of the formations of the GSDMD pore or MLML channel to elicit PMR.
Apart from mammalian gasdermins, the only CsGSDME from aquatic teleost Cynoglossus semilaevis can be cleaved by Cscaspase-1, -3, and -7 to elicit pyroptosis (Jiang et al., 2019). Additionally, the GSDME homolog also exists in the marine invertebrate coral Orbicella faveolata, and Ofcaspase-3 is capable of cleaving OfGSDME to induce pyroptosis upon the infection by the bacterial pathogen Vibrio coralliilyticus (Jiang S et al., 2020). These important findings of GSDME-mediated pyroptosis in aquatic animals shed light on the activation mode of gasdermin during pyroptosis and broaden the evolutionary insights into pyroptosis-related immunological stresses upon bacterial invasions. In contrast to animals’ gasdermins, the uncharacterized proteins with predicted homology to gasdermin domains were identified after bioinformatical analyses of bacterial anti-phage defense islands. The majority are encoded adjacent to one or more genes with a predicted protease domain through examining the genomic neighborhood of bacterial gasdermin-likes (Johnson et al., 2022). Some of the GSDM-associated proteases are fused to repeat domains including leucine-rich repeats, tetratricopeptide repeats, WD40 repeats, or NACHT domains frequently involved in prokaryotic samples. In addition, the gRAMP CRISPR-Cas effector is an RNA endonuclease complex with a caspase-like peptidase (van Beljouw et al., 2021), regardless of the structure and substrate specificity of bacterial caspase-like proteases temporarily named ‘‘orthocaspases’’ (Minina et al., 2020). While breaking of viral RNAs is inadequate to escape infections, bacteria would switch on suicide as a consequence of activation of caspase-likes by sensing viral RNAs. Another recent report claimed that the activated Runella gasdermin-likes after removal of the short C-terminal region (about 20 AAs) by the associated caspase-like protease (or a certain orthocaspase) has the capability to form mesh-like membrane pores (average 28 nm in inner diameter) and displays bactericidal activity via non-selective leakage (Johnson et al., 2022) (Figure 2B), although how caspase-like protease becomes active was not documented. Collectively, it uncovered that the conserved gasdermin-like pore is an ancient conduit for the cellular content efflux in prokaryotes and eukaryotes.
The Switch of Apoptosis, Necroptosis, and Pyroptosis by Caspase-8
In response to influenza A virus (IAV) infection, the induced pro-death complex encompasses a plethora of proteins: RIPK1, apoptosis-associated speck-like protein containing a caspase recruitment domain (ASC), nucleotide-binding oligomerization domain NOD-like receptor pyrin domain-containing 3 (NLRP3), and caspase-8, RIPK3, ZBP1, and caspase-1 (Samir et al., 2020). In addition, the AIM2 sensitizing dsDNA sense double-stranded DNA (dsDNA) forms the inflammasome being an important sentinel of the innate immune defense and has essential roles in development of infectious diseases. However, AIM2 beyond its canonical role in inflammasome formation and observed pyroptosis cannot explain the outcome resulted from the AIM2 inflammasome. During infections by dsDNA herpes simplex virus 1 (HSV1) and the Gram-negative bacterium Francisella novicida, AIM2, pyrin, and ZBP1 were constituents of a large multiplex complex concomitant with ASC,caspase-1, caspase-8, RIPK3, RIPK1, and FADD, that led to PANoptosis, an inflammatory cell death pluralized by apoptosis, pyroptosis, and necroptosis (Lee et al., 2021) (Figure 2A). The confluence of critical molecules for apoptosis, pyroptosis, and necroptosis could explain why the different types of cell death can exchange under certain conditions (Schwarzer et al., 2020). Caspase-8 is the initiator caspase of extrinsic apoptosis and cleaves RIPK1/3 to restrict necroptosis (Frank and Vince, 2019). Therefore, caspase-8 deficiency in mice causes embryonic lethality which can be rescued by deletion of either RIPK3 or MLKL (Fritsch et al., 2019; Newton et al., 2019). MLKL deficiency rescues the cardiovascular defect phenotype but unexpectedly causes necroptosis-independent death. When necroptosis is blocked, the expression of non-catalytic caspase-8 triggered the formation of ASC-associated inflammasomes and resulted in pyroptosis in mice. Genetic analyses confirmed that caspase-8 serves as the molecular switch for hierarchical activation of apoptotic, necroptotic, and pyroptotic signaling pathways (Orning et al., 2018; Fritsch et al., 2019; Newton et al., 2019) (Figure 2A).
Animal and Plant Bcl-2–Associated Athanogene Proteins in Cell Death Regulation and Stress Responses
The extrinsic apoptosis pathway and other types of PCD are orchestrated by caspase-8, whereas MOMP during intrinsic apoptosis is positively and negatively regulated by Bcl-2 family proteins. To identify Bcl-2 partner(s), the Bcl-2–associated athanogene (BAG) family genes were initially found via a yeast two-hybrid screening (Kabbage and Dickman, 2008). The BAG1 gene was shown to enhance the anti-apoptotic activity of Bcl-2, which seemed to be indicative of its involvement in the apoptotic pathway(s) (Takayama et al., 1995; Brive et al., 2001; Takayama and Reed, 2001). The BAG family is a phylogenetically conserved group of proteins with orthologues widely across organisms from plants to metazoans including humans. The C-terminal BAG domain (BD) from all BAG proteins directly interact with the heat shock protein 70 (HSP70) chaperone (Takayama and Reed, 2001). The BAG proteins serve as co-chaperones that function as molecular switches associating with HSP70 and other substrates and maintain protein homeostasis and modulate cell death. In fact, BAG1 itself functions as a substrate of E3 ligase (i.e., the C terminus of HSC70-interacting protein (CHIP)), and the formation of the BAG1-CHIP ternary complex targets proteins for degradation (Kabbage et al., 2017). Reversely, BAG2 associating with CHIP inhibits the E3 ligase activity. BAG3 has roles in protein quality control to sustain cell survival and was indicative of the antagonistic effect against chemotherapy (Behl 2016). BAG4 has been considered to act as a negative regulator of the TNF superfamily. BAG5 has been relevant to neurodegeneration (such as Parkinson’s disease) and was discovered to suppress both parkin E3 ligase and HSP70 chaperone activities (Kalia et al., 2004). BAG6 ablation might contribute to increased lethality and severe developmental abnormality in various organs (Kabbage et al., 2017).
Arabidopsis BAG proteins may be categorized into two sub-groups according to their featured domain: AtBAG1–4 having a UBL motif similar to human BAG1 besides the BD, and AtBAG5–7 containing a calmodulin (CaM)–binding motif nearby the BD. The AtBAG1–3 keeps functionally unknown. BAG4 binds to HSP70 chaperones and is related to cell death inhibition upon abiotic stress. AtBAG5 constitutes a complex with CaM/HSC70 and is involved in plant senescence (Kabbage et al., 2017). AtBAG6 is functionally activated through aspartyl protease processing and coordinates with chitin perception to inducible autophagy (Kang et al., 2006). The ER-locating AtBAG7, as an essential component of the unfolded protein response, recognizes the molecular chaperone BIP2. Upon ER stresses, AtBAG7 can translocate to the nucleus, where it interplays with the transcription factor WRKY29 related to stress response and/or immunity (Li et al., 2017). Due to BAGs’ association with HSP70 partially and their multiplex targets, the conservation of BAG molecular regulations and contributive properties in immunity-associated cell death was discovered in plants and animals.
Ferroptosis in Animal and Plant Cells
Along with the discovery of apoptosis, necroptosis, pyroptosis, and immune cell death, ferroptosis dependent of iron was proposed in 2012 (Dixon et al., 2012). Ferroptosis is characterized by the peroxidation of polyunsaturated fatty acids (PUFAs) from membrane lipids by lipoxygenases (LOXs) being non-heme iron oxidases and reactive oxygen species (ROS) from the Fe2+-directed Fenton reaction (Yang et al., 2016). Recently, lipid peroxidation during ferroptosis may be mainly catalyzed by oxidoreductases POR and cytochrome b5 reductase 1 (CYB5R1) other than LOXs (Yan et al., 2021). Importantly, ferroptosis can be hindered by glutathione peroxidase GPX4 for depletion of lipid peroxide and coenzyme Q oxidoreductase FSP1 and mitochondrial dihydrooratic acid dehydrogenase (DHODH) for neutralization of the lipid peroxide free radical (Bersuker et al., 2019; Mao et al., 2021) (Figure 2A). Hence, lipoperoxides cannot be excessively aggregated to disrupt the integrity of the plasma membrane. Due to the prevalence of the conserved cytochromes, LOXs, and other popular oxidoreductases and dehydrogenases in plants, the induced ferroptosis-like cell death may contribute to immune responses in plants upon biotic stresses (Figure 2C) (Distéfano et al., 2017; Dangol et al., 2019; Distéfano et al., 2021). Also, it has been reported that iron-dependent death regulates conidiospore development of pathogen fungi ——Magnaporthe grisea (Shen et al., 2020). The mechanistic insights into inhibition of ferroptosis in plants remain to be further elucidated by biochemical and genetic analyses.
CNL Resistosome and Helper Nod-Like Receptors Mediate Ca2+ Influx Required for Programmed Cell Death in Plant Cells
The LRR-NBS domains from R proteins are largely similar to the LRR-NACHT domain in the inflammatory NOD-like receptor protein 3 (NLRP3) for caspase-1 activation. The N-terminal domains of R proteins can be divided into three categories: TIR-NBS-LRR (TNLs), CC-NBS-LRR (CNLs), and CCR-NB-LRR (RNLs). The three subfamilies are collectively referred to as Nod-like receptors (NLRs). NLRs evolved from a common primordial prokaryotic adenosine triphosphatase (ATPase), which is classified into two distinct derivatives: NACHT and NB-ARC type NBDs. The NB-ARC type is found in plant NLRs and NACHT in animal NLRs (Jones et al., 2016). Animal NLRs with cognate ligands can oligomerize into wheel-like complexes as inflammasomes upon stimuli (Hu et al., 2015). Similarly, the Arabidopsis ZAR1 protein (a CNL) initially confers resistance to P. syringae carrying the effector protein HopZ1a and sensitizes the alteration of the host sensory protein PBS1-LIKE 2 (PBL2) upon Xanthomonas campestris pv. campestris (Xcc) effector AvrAC (Bi and Zhou, 2021). The cryo-EM structures of the ZAR1 resistosome in resting and activated states were reported. ZAR1 interacts with the plant protein pseudokinase RKS1 (a receptor-like cytoplasmic kinase (RLCK), belonging to the RLCK-XII subfamily) and remained at the resting state. Upon infection, AvrAC uridylates PBS1-like protein 2 (a member of RLCK-VII subfamily) to generate PBL2UMP, which is recruited to the ZAR1-RKS1 complex to form the ZA1-RKS1- PBL2UMP complex in a primed state lacking ATP or dATP (Wang et al., 2019a; Wang et al., 2019b). PBL2UMP binding activates the nucleotide exchange factor activity of RKS1. Once activated, RKS1 facilitates ADP release from ZAR1 by inducing conformation changes in the NBD domain of ZAR, which enables ZAR1 to go through the fold switch of its CC domain and leads to the formation of a pentameric ZA1-RKS1- PBL2UMP structure (Wang et al., 2019a) (Figure 2C). The funnel-shaped architecture constituted by the N-terminal alpha-helices of ZAR1 in the resistosome promotes ZAR1 integration into the plasma membrane. It may perturb the membrane integrity or ionic homeostasis. Subsequent studies showed that this funnel-like structure of helices in the N-terminal region is featured by a Ca2+ channel, being responsible for the Ca2+ influx, which is required for resistance (Bi et al., 2021). It is reminiscent that the activation of the inflammasome NLRP3 requires the serine/threonine kinase NEK7, and NLRP3-NEK7 modules constitute a disk-like structure (He et al., 2016; Sharif et al., 2019). This indicated the convergence on activation strategies of diverse varieties of NLRs. NLRs from animals and plants are all capable of being activated by kinase(s), which may possess nucleotide exchange activities and elicit the allosteric effect of NLRs to oligomerize into active resistosomes (Wang et al., 2019a).
TNLs RPP1 and roq1 recognize the bacterial effector ATR1 and Xanthomonas effector RPP1 of oomycetes, respectively. The direct binding of ATR1/RPP1 to a C-terminal jelly roll/Ig-like domain and LRR domain leads to induce the tetrameric assembly. The two catalytic centers of NADase are composed of asymmetric homodimers in tetrameric TIR domains, which define the formation of active holoenzyme (Martin et al., 2020; Ma et al., 2020). TNLs and CNLs directly or indirectly sensitize pathogen effectors. But RNLs are not conferred to perceive microbial avirulent factors but acts downstream of TNL-mediated signaling. Therefore, it is called helper NLR, which contains two types: NRG1 and ADR1. EDS1 and PAD4 are plant effector proteins with lipase-like domains, which aggregate to promote cell death. Additionally, NRG1 involves the downstream cascade of TNL-mediated cell death. Structural data and electrophysiological experiments also corroborated that the self-activating mutant of NRG1 is a calcium-permeable but non-selective cation channel. Arabidopsis NRG1 CCR was structurally similar to pseudokinase MLKL as a cationic channel causing cell necrosis in animals (Jacob et al., 2021) (Figure 2C). Both self-activating mutants expressing NRG1 and ADR1 in tobacco and human cancer cells can give rise to cell death. And, the death phenotype depends on plasma membrane localization of helper NLRs and the Ca2+influx (Jacob et al., 2021). Collectively, the activation of NLR in CNL and TNL resistosomes both converge on the Ca2+ influx–mediated cation channels formed by NLRs. We have not yet understood how to trigger plant cell death after inducing the Ca2+ influx. According to previous reports, the appearance of HR required the plasma membrane fusion with a plant vacuole, in which cysteine proteases (including vacuolar processing enzymes (VPEs), and/or RD19) are required for cytolysis (Hatsugai et al., 2004; Bernoux et al., 2008).
TIR as NADase for Producing Cyclic ADPR Essential for TNL-Mediated Death Signal Cascading and Activated Myd88-5 Inducing Neuron Death
The plant TNLs RPP1 and ROQ1 recognize their respective cognate effector ATR1. The complexes both individually oligomerize into tetramers, and the tetrameric wheel-like resistosomes have NADase holoenzyme activity through adjacent TIR–TIR close contact. The oligomerization of the TIR-domain is essential for its NAD+-catalyzing activity for variant cADPR (v-cADPR) production (Wan et al., 2019). TIR domains unlike other effector domains (such as Bcl-2, MLKL, and gasdermin) directly target the plasma membrane and perceive and/or amplify signals to trigger downstream immune responses (Figure 2D). TIR domains from plant TNLs have NADase activities versus TLR-containing TIR domains devoid of catalytic activities and are paralogous to the TIR domain of SARM1 protein, namely MyD88-5 (Figures 3A,B). MyD88 is an adaptor molecule involved in the signaling through the IL-1R and TLR families and is essential for the response to IL-1, IL-18, LPS, and many other bacterial cell-wall components. Otherwise, SARM1/MyD88-5 is the critical mediator of axon degeneration upon energy deficit (Jiang S et al., 2020).
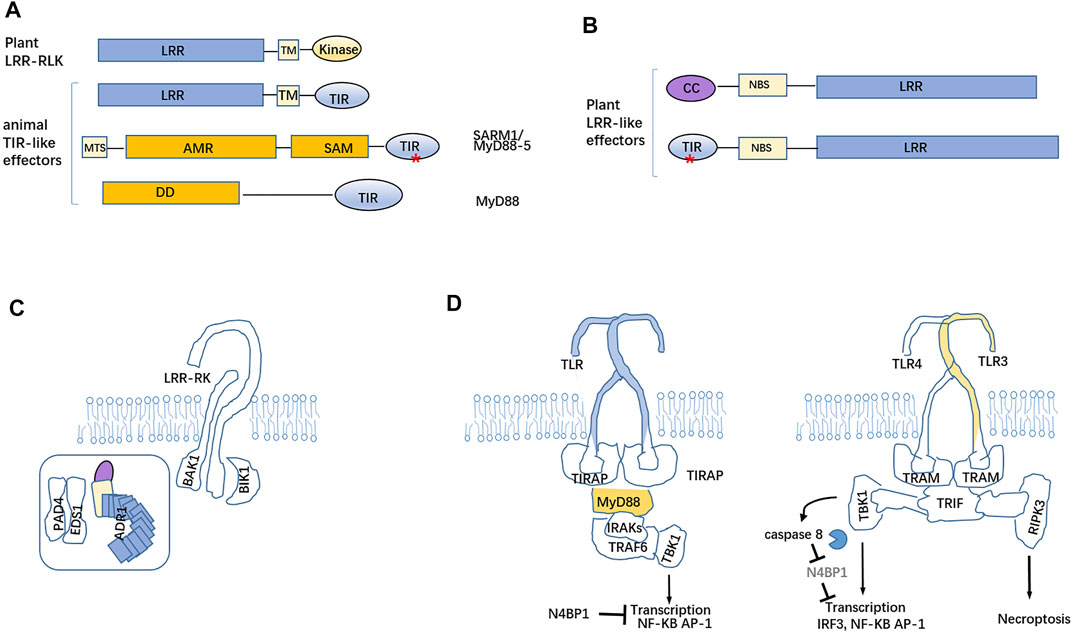
FIGURE 3. Mutual potentiation and regulation of TLR-mediated immunity and NLR-mediated immunity in plants and animals. (A) LRR-RLK (receptor-like kinase) confer to pattern-triggered immunity, functionally similar to animal Toll-like on the cell surface. SARM1 has a mitochondria-targeting signal (MTS), AMR, SAM, and C-terminal TIR domain, where there being conserved Asp/Glu residues, which endow the NADase activity. (B) Cytosolic CNL and TNL are composed of NBS and LRR domains with a distinct N-terminal region (coiled coil or TIR domain). (C) The PAD4-EDS1 complex and ADR1 together locate the inner leaflet of the plasma membrane and enhance PRR (LRR-RK)- and RLCK (BIK1)-dependent PTI. (D) Cytosolic toll and interleukin 1 receptor (TIR) of TLR4 can interplay with the TIR domain(s) of four adaptor molecules MyD88, TIRAP, TRIF, and TRAM to transmit the cascade reaction and promote the secretion of inflammatory factors and interferons. The capase-8 substrate, N4BP, act as a suppressor of cytokine responses. Asterisk (*) represents the catalytic activities of TIR domains.
The cryo-EM structure of full-length human SARM1 revealed that it bound NAD+ constitutes, an octamer in its inactive state, which inhibit its TIR NADase activity under high NAD+ levels (Bratkowski et al., 2020; Jiang Y et al., 2020; Sporny et al., 2020). The human SARM1 with a nicotinamide mononucleotide (NMN) octamer undergoes a conformational change disrupting NAD+binding sites of the ARM domains to enable TIR–TIR dimerization. NAD+deficit upon severe damages of the mitochondria from axons may result in SARM1 TIR-TIR associations and then produce cADPR to trigger axon death (Jiang Y et al., 2020). The ectopic expression of the TIR domain of human SARM1 in tobacco for cADPR generation may trigger cell death independent of EDS1 (Horsefield et al., 2019). The amount of cADPR dramatically increases upon accumulation of the senescence-related phytohormone ABA in plants and ultimately elicits cell death (Wu et al., 1997). It should be presumed that cADPR as a candidate common second messenger conveys death signal to the cascading pathways in plants and animals. In bacteria, cyclic oligonucleotide sensor(s) conjugated with effectors (such as lipase or transmembrane protein or other effectors) triggers enough bacterial cell disruption in response to phage invasion and results in the abortive infection (Severin et al., 2018; Morehouse et al., 2020). The infected bacterial cells commit suicide prior to the performance of the phage replication cycle. This strategy eliminates infected cells from the bacterial community and protects the bacterial population from a phagic epidemic (Hampton et al., 2020). Some bacteria exploit TIR-STING fusion protein to inhibit phage infections (Cohen et al., 2019; Eaglesham et al., 2019). Bacterial STING coupling cyclic dinucleotide recognition forms filaments to drive TIR oligomerization for cADPR production (Morehouse et al., 2020). This strategy is used to remove infected cells from the bacterial community and protect the population from a phage epidemic.
Plant Immune Response Coordinated by PTI and ETI Versus TLR- and Nod-Like Receptor-Mediated Immune Responses in Animals
Upon pathogen infection, plants utilize cell-surface pattern-recognition receptors (PRRs) to rapidly recognize pathogen/damage-associated molecular patterns (PAMPs/DAMPs) and then bind and phosphorylate co-receptors—receptor-like cytoplasmic kinases (RLCKs) and phosphorylated RLCKs sequentially activate MAPK cascade signaling, Ca2+-dependent protein kinases (CDPKs), and reactive oxygen species (ROS) burst (Tang et al., 2017; Liang and Zhou, 2018). For examples, the NO burst was induced in Arabidopsis suspension cells in response to bacterial LPS. LPS treatment not only induces the expression of Arabidopsis NO synthase (AtNOS1) but also activates the defense genes (Zeidler et al., 2004). Sequentially, the Arabidopsis LPS receptor was identified to be lectin S-domain-1 receptor–like kinase LORE (Ranf et al., 2015). AtNOS1-deficient and LPS-insensitive LORE mutants are hypersusceptible to the pathogen Pseudomonas syringae (Zeidler et al., 2004; Ranf et al., 2015). Plant LysM domain proteins have been widely implicated in the recognition of GlcNAc-containing glycans. CERK1, a lysin-motif (LysM) receptor kinase (LYK) can recognize fungal MAMP chitin (Miya et al., 2007). LYK5 and LYK4 are also identified to be components of a tripartite chitin receptor complex (Cao et al., 2014; Xue et al., 2019). The glycosylphosphatidylinositol-anchored LysM proteins (LYM1 and LYM3) sense PGNs (Willmann et al., 2011). LRR-RK MIK2 recognizes multiple plant endogenous peptides of SCOOP family members, leading to a series of PTI responses, including the cytosolic Ca2+ influx, ROS burst, MAPK activation, ethylene production, and defense-related gene expression (Hou et al., 2021; Rhodes et al., 2021). The LRR receptor kinase HPCA1(other name CARD1) as the receptor(s) of DAMPs hydrogen peroxide and quinone perceives H2O2 and host-derived quinone DMBQ to activate the Ca2+ influx and MAPK pathway (Laohavisit et al., 2020; Wu et al., 2020).
These responses are collectively called pattern-triggered immunity (PTI), which can impede further invasion at the pre-infection phase. But, successful pathogens exploit secreted effectors dedicates to pathogen virulence to intervene with PTI; this leads to effector-triggered susceptibility (ETS); then, the host continuously evolves the novel NB-LRR protein(s) to specifically recognize/sequester pathogen effector(s), inducing effector-triggered immunity (ETI) (Jones and Dangl, 2006). R protein-mediated ETI accelerates and amplifies immune responses, leading to resistance against diseases, usually, a hypersensitive cell death response (HR) at infection sites (Jones and Dangl, 2006). Certainly, ETI has two-branched responses: promotion of the resistance-related gene expression and HR. Recent advances confirmed that ETI also robustly boosted the expression of PTI-involved genes; meanwhile, the sole activation of NLR-mediated resistance in absence of PTI is insufficient to safeguard the host against bacterial infections (Yuan et al., 2021). PTI can enhance resistance from PTI. The PTI and ETI comprising the two-tiered plant immune system that monitors threats from pathogens are not separable and collaboratively contribute to the reciprocal enhancement of plant immunity (Ngou et al., 2021; Pruitt et al., 2021; Yuan et al., 2021; Tian et al., 2021). HR as one canonical type of PCD in plants is beneficial to the host likely by eliminating the intracellular niche for proliferation of certain pathogens. Furthermore, the resulting cellular debris coordinates a systemic immune response to promote the resolution of infection. As known, HR cannot represent the whole resistance in plants. Reprogramming of immune genes enforces resistance. The ADR1-EDS1-PAD4 module can be polymerized with the complex formed by LRR-RP-SOBIR1 and PBL31 of the RLCK family to form a supramolecular complex, which not only binds the inner leaflet of the plasma membrane to mediate the response of PTI but ADR1 is contributive to TNL-directed resistance signaling involved in reprogrammed transcription of pathogenesis-responsive genes (Pruitt et al., 2021) (Figure 3C).
Likewise, the Toll-like receptor (TLR) families in animals are phylogenetically conserved mediators of innate immunity and are responsible for microbial recognition on cellular surfaces. TLRs consist of a large family with extracellular/ectodomain leucine-rich repeats (LRRs) and a cytoplasmic Toll/interleukin (IL)-1 receptor (TIR) homology domain (Fitzgerald and Kagan, 2020). TLRs occupy the plasma membranes and detect the microbial conserved components present on the host cell surface. TLRs sensitize peptidoglycan (TLR2), dsRNA (TLR3), lipopolysaccharide (LPS) (TLR4), flagellin (TLR5), unmethylated CpG DNAs (TLR9), and other PAMPs. In virtue of the unraveled extracellular receptors for molecular patterns in plants, more diverse motifs present in the extracellular space are used for sensing various molecular patterns including MAMPs and/or other stimuli. Moreover, the commonalities between receptors in plants and animals are single-pass transmembrane proteins which recruit cytosolic kinase(s) to activate phosphorylation-cascading pathway(s) and induce the expression of stress-related genes. In virtue of alien dsRNA or ssDNA being conserved signatures, plant hosts should license PRRs to perceive the immune signal, although the potent extracellular dsDNA/RNA receptors located at the plant cell surface are yet to be validated.
Upon recognition of PAMPs, the cytoplasmic TIR domains of dimerized TLRs located at the plasma membrane recruit TIR-containing TIRAP and Myd88 to assembly Myddosome containing TRAF6, which functions to stimulate TBK1 to drive IKK- and MAPK-dependent transcription and cytokine releases (Fitzgerald and Kagan, 2020). On endosomes, TLR4 and TLR3 are capable of engaging a SMOC called the triffosome. TRIF is present in this complex. TRIF encompasses a pLxIS motif that increases the TBK1-regulated gene expression and an RHIM domain to promote RIPK3-mediated necroptosis (He et al., 2011) (Figure 3D). Under certain conditions, the TLR3 and TLR4 pair activates caspase-8 through the adaptor TRIF, but generally TLR3/4 signaling does elicit apoptosis. Caspase-8 cleaves N4BP1, which inhibits cytokine responses and suppression of the LPS-stimulated gene expression (Gitlin et al., 2020) (Figure 3D). Functionally, TLRs from animals may be regarded as the counterpart of LRR-RLKs involved in PTI in plants. The cytoplasmic NOD-like receptors (NLRs) monitored the intracellular environment for an alternative sign upon pathogen infection and then fulfill the assembly of inflammasomes. In parallel, plants have evolved intracellular R proteins to intercept the activities of effector proteins that are delivered inside the host cell and activate defenses, complementing the ETI, which resembles inflammasome-mediated innate immunity in animals. Despite many breakthroughs on the understandings of PTI and ETI from plants and the proposed conceptual resistosome in parallel with the inflammasome, the direct executioners of HR in plants need to be further investigated and explored by the state-of-the-art and maneuverable technology.
Outlook
Mammalian intrinsic apoptosis is marked by the mitochondrial membrane rupture, which resulted from Bax/Bak oligomerization in mitochondrial membranes. The mitochondria branches within alphaproteobacteria. And, the pore-forming domains of bacterial toxins such as colicins A1 and E1 and diphtheria toxin structurally are similar to mitochondrial Bcl-2 (Kelekar and Thompson, 1998). Like bacterial toxins, Bcl-2, Bcl-xL, and Bax can insert into synthetic lipid vesicles and planar lipid bilayers and form ion-conducting channels (Schendel et al., 1997). This suggested that the Bcl-2 family responsible for mitochondrial “suicide” might have ancestral origins from bacteria. Why PCD in host cells requires that semi-parasitic mitochondria commit self-death in advance? Apoptosomes sense oxidation–reduction potential to activate major initiator caspase-9 for intrinsic apoptosis. Indeed, pyroptosis is also concomitant with damaged mitochondria. The elevated mitochondrial ROS promote gasdermin pore formation for pyroptosis.
Similarly, the plant NLRs activate downstream immune responses, which escalate the expression level of crucial immune proteins, such as BIK1 and NADPH oxidase RBOHD. A plethora of heme-containing RBOHD located in the plasma membrane boost ROS burst (Yuan et al., 2021). R protein-mediated HR coincides with the coexistence of Fe2+ and ROS, which both being requisite for ferroptosis. As aforementioned, NLRs (CC- and CCR-types) in plants possess similar structural features of inflammatory NLRs and necroptotic MLKL in animals. These appeared to be indicative of HR in plants as PCD was featured by the chimeric/promiscuous combination of pyroptotic, necroptotic, and ferroptotic forms. According to the above descriptions and comparisons, the PRRs (such as LRR-RK) and R proteins (TNLs and CNLs with LRR motifs) from plants structurally and evolutionarily correspond to TLRs and NLRs (both comprising LRR motifs) in animals. Evidently, the common superhelical conformation of distinct LRR motifs is selected to recognize the diverse molecular patterns derived from pathogens or hosts in plants and animals. Second, ROS act as common inducers for PCD in plants and animals. Third, the serious damage/disruption of biomembranes (the plasma membrane and/or mitochondria/plastid membrane) is essential for PCD in plants and animals. Considering the extreme diversity of bacteriophage species and their tremendous amounts, bacteria hosts exert utmost efforts on the development of immune arsenal including caspase-like,TIR-like, cGAS-like, STING-like, and lipase-like domains shared by prokaryotes and eukaryotes (Figure 2B). Based on the subsequent discoveries of the primordial signature of bacterial immune proteins orthologous to counterparts in animals, there are grounds to believe that plants have uncharacterized immune effectors which might contribute to conserved PCDs and which might be evolved from the common ancestors/progenitors shared by bacteria and animals.
Relative to the clear mechanistic insights into animal PCDs, in plant PCDs there are many important unsettled problems: 1) the final executioner of plant HR death upon infections; 2) the exact substrates of cysteine proteases or other proteases involved in PCD; 3) the role of the Ca2+ influx. 4) the definite signalings conjugated with the second messengers; 5) the linkage of various types of plant PCDs upon infections; 6) the necessary roles of lipase-like activities in plant HR. Additionally, plant cells have rigid cell walls surrounding the plasma membrane. The question as to whether or not the cell death of plant cells required the degradation of cell wall components such as cellulose, hemicellulose, and pectin remains unknown. We should recapitulate the cellular states including transcriptional and translational profiles through single-cell multi-omic analyses. Collectively, these embodies that the uniform but diversity in all organism PCDs involved in immunity.
Author Contributions
JZ wrote this manuscript. LX and LZ collected references specifically related with the Bcl-2 family and discussed the whole manuscript.
Funding
This work was supported by the National Natural Science Foundation of China (No. 31301641 to JZ) and the FAFU Science developmental funding (KFA20015A to JZ and CXZX2018094 to LZ).
Conflict of Interest
The authors declare that the research was conducted in the absence of any commercial or financial relationships that could be construed as a potential conflict of interest.
Publisher’s Note
All claims expressed in this article are solely those of the authors and do not necessarily represent those of their affiliated organizations, or those of the publisher, the editors, and the reviewers. Any product that may be evaluated in this article, or claim that may be made by its manufacturer, is not guaranteed or endorsed by the publisher.
References
Adams, J. M., and Cory, S. (2018). The BCL-2 Arbiters of Apoptosis and Their Growing Role as Cancer Targets. Cell Death Differ 25, 27–36. doi:10.1038/cdd.2017.161
Antignani, A., and Youle, R. J. (2006). How Do Bax and Bak lead to Permeabilization of the Outer Mitochondrial Membrane? Curr. Opin. Cell Biol. 18, 685–689. doi:10.1016/j.ceb.2006.10.004
Baliga, B. C., Read, S. H., and Kumar, S. (2004). The Biochemical Mechanism of Caspase-2 Activation. Cell Death Differ 11, 1234–1241. doi:10.1038/sj.cdd.4401492
Behl, C. (2016). Breaking BAG: the Co-chaperone BAG3 in Health and Disease. Trends Pharmacol. Sci. 37, 672–688. doi:10.1016/j.tips.2016.04.007
Bernoux, M., Timmers, T., Jauneau, A., Brière, C., de Wit, P. J. G. M., Marco, Y., et al. (2008). RD19, an Arabidopsis Cysteine Protease Required for RRS1-R-Mediated Resistance, Is Relocalized to the Nucleus by the Ralstonia Solanacearum PopP2 Effector. Plant Cell 20, 2252–2264. doi:10.1105/tpc.108.058685
Bersuker, K., Hendricks, J. M., Li, Z., Magtanong, L., Ford, B., Tang, P. H., et al. (2019). The CoQ Oxidoreductase FSP1 Acts Parallel to GPX4 to Inhibit Ferroptosis. Nature 575, 688–692. doi:10.1038/s41586-019-1705-2
Bi, G., Su, M., Li, N., Liang, Y., Dang, S., Xu, J., et al. (2021). The ZAR1 Resistosome Is a Calcium-Permeable Channel Triggering Plant Immune Signaling. Cell 184 (13), 3528–3541. doi:10.1016/j.cell.2021.05.003
Bi, G., and Zhou, J.-M. (2021). Regulation of Cell Death and Signaling by Pore-Forming Resistosomes. Annu. Rev. Phytopathol. 59, 239–263. doi:10.1146/annurev-phyto-020620-095952
Bratkowski, M., Xie, T., Thayer, D. A., Lad, S., Mathur, P., Yang, Y.-S., et al. (2020). Structural and Mechanistic Regulation of the Pro-degenerative NAD Hydrolase SARM1. Cell Rep. 32, 107999. doi:10.1016/j.celrep.2020.107999
Brenner, S. (1973). The Genetics of Caenorhabditis elegans. Genetics 77, 71–94. doi:10.1093/genetics/77.1.71
Brive, L., Takayama, S., Briknarová, K., Homma, S., Ishida, S. K., Reed, J. C., et al. (2001). The Carboxyl-Terminal Lobe of Hsc70 ATPase Domain Is Sufficient for Binding to BAG1. Biochem. Biophysical Res. Commun. 289, 1099–1105. doi:10.1006/bbrc.2001.6087
Broz, P., Pelegrín, P., and Shao, F. (2020). The Gasdermins, a Protein Family Executing Cell Death and Inflammation. Nat. Rev. Immunol. 20, 143–157. doi:10.1038/s41577-019-0228-2
Cao, Y., Liang, Y., Tanaka, K., Nguyen, C. T., Jedrzejczak, R. P., Joachimiak, A., et al. (2014). The Kinase LYK5 Is a Major Chitin Receptor in Arabidopsis and Forms a Chitin-Induced Complex with Related Kinase CERK1. elife 3, e03766. doi:10.7554/eLife.03766
Chai, J., and Shi, Y. (2014). Apoptosome and Inflammasome: Conserved Machineries for Caspase Activation. Nat. Sci. Rev. 1, 101–118. doi:10.1093/nsr/nwt025
Cheng, E. H., Wei, M. C., Weiler, S., Flavell, R. A., Mak, T. W., Lindsten, T., et al. (2001). BCL-2, BCL-X(L) Sequester BH3 Domain-Only Molecules Preventing BAX- and BAK-Mediated Mitochondrial Apoptosis. Mol. Cell 8, 705–711. doi:10.1016/s1097-2765(01)00320-3
Cohen, D., Melamed, S., Millman, A., Shulman, G., Oppenheimer-Shaanan, Y., Kacen, A., et al. (2019). Cyclic GMP-AMP Signalling Protects Bacteria against Viral Infection. Nature 574, 691–695. doi:10.1038/s41586-019-1605-5
Czabotar, P. E., Lessene, G., Strasser, A., and Adams, J. M. (2014). Control of Apoptosis by the BCL-2 Protein Family: Implications for Physiology and Therapy. Nat. Rev. Mol. Cell Biol. 15, 49–63. doi:10.1038/nrm3722
Dangol, S., Chen, Y., Hwang, B. K., and Jwa, N.-S. (2019). Iron- and Reactive Oxygen Species-dependent Ferroptotic Cell Death in Rice-Magnaporthe Oryzae Interactions. Plant Cell 31, 189–209. doi:10.1105/tpc.18.00535
Ding, J., and Shao, F. (2017). SnapShot: The Noncanonical Inflammasome. Cell 168, 544. doi:10.1016/j.cell.2017.01.008
Ding, J., Wang, K., Liu, W., She, Y., Sun, Q., Shi, J., et al. (2016). Pore-forming Activity and Structural Autoinhibition of the Gasdermin Family. Nature 535, 111–116. doi:10.1038/nature18590
Distéfano, A. M., Martin, M. V., Córdoba, J. P., Bellido, A. M., D'Ippólito, S., Colman, S. L., et al. (2017). Heat Stress Induces Ferroptosis-like Cell Death in Plants. J. Cell Biol 216, 463–476. doi:10.1083/jcb.201605110
Distéfano, A. M., López, G. A., Setzes, N., Marchetti, F., Cainzos, M., Cascallares, M., et al. (2021). Ferroptosis in Plants: Triggers, Proposed Mechanisms, and the Role of Iron in Modulating Cell Death. J. Exp. Bot. 72, 2125–2135. doi:10.1093/jxb/eraa425
Dixon, S. J., Lemberg, K. M., Lamprecht, M. R., Skouta, R., Zaitsev, E. M., Gleason, C. E., et al. (2012). Ferroptosis: an Iron-dependent Form of Nonapoptotic Cell Death. Cell 149, 1060–1072. doi:10.1016/j.cell.2012.03.042
Eaglesham, J. B., Pan, Y., Kupper, T. S., and Kranzusch, P. J. (2019). Viral and Metazoan Poxins Are cGAMP-specific Nucleases that Restrict cGAS-STING Signalling. Nature 566, 259–263. doi:10.1038/s41586-019-0928-6
Ellis, H., and Horvitz, H. R. (1986). Genetic Control of Programmed Cell Death in the Nematode C. elegans. Cell 44, 817–829. doi:10.1016/0092-8674(86)90004-8
Evavold, C. L., Hafner-Bratkovič, I., Devant, P., D’Andrea, J. M., Ngwa, E. M., Boršić, E., et al. (2021). Control of Gasdermin D Oligomerization and Pyroptosis by the Ragulator-Rag-mTORC1 Pathway. Cell 184, 4495–4511. doi:10.1016/j.cell.2021.06.028
Fitzgerald, K. A., and Kagan, J. C. (2020). Toll-like Receptors and the Control of Immunity. Cell 180, 1044–1066. doi:10.1016/j.cell.2020.02.041
Frank, D., and Vince, J. E. (2019). Pyroptosis versus Necroptosis: Similarities, Differences, and Crosstalk. Cell Death Differ 26, 99–114. doi:10.1038/s41418-018-0212-6
Fritsch, M., Günther, S. D., Schwarzer, R., Albert, M.-C., Schorn, F., Werthenbach, J. P., et al. (2019). Caspase-8 Is the Molecular Switch for Apoptosis, Necroptosis and Pyroptosis. Nature 575, 683–687. doi:10.1038/s41586-019-1770-6
Gitlin, A. D., Heger, K., Schubert, A. F., Reja, R., Yan, D., Pham, V. C., et al. (2020). Integration of Innate Immune Signalling by Caspase-8 Cleavage of N4BP1. Nature 587, 275–280. doi:10.1038/s41586-020-2796-5
Gu, L., Surolia, R., Larson-Casey, J. L., He, C., Davis, D., Kang, J., et al. (2021). Targeting Cpt1a-Bcl-2 Interaction Modulates Apoptosis Resistance and Fibrotic Remodeling. Cell Death Differ 1-15. doi:10.1038/s41418-021-00840-w
Guo, Y., Srinivasula, S. M., Druilhe, A., Fernandes-Alnemri, T., and Alnemri, E. S. (2002). Caspase-2 Induces Apoptosis by Releasing Proapoptotic Proteins from Mitochondria. J. Biol. Chem. 277 (16), 13430–13437. doi:10.1074/jbc.m108029200
Hampton, H. G., Watson, B. N. J., and Fineran, P. C. (2020). The Arms Race between Bacteria and Their Phage Foes. Nature 577, 327–336. doi:10.1038/s41586-019-1894-8
Hansen, J. M., de Jong, M. F., Wu, Q., Zhang, L.-S., Heisler, D. B., Alto, L. T., et al. (2021). Pathogenic Ubiquitination of GSDMB Inhibits NK Cell Bactericidal Functions. Cell 184, 3178–3191. doi:10.1016/j.cell.2021.04.036
Hatsugai, N., Kuroyanagi, M., Yamada, K., Meshi, T., Tsuda, S., Kondo, M., et al. (2004). A Plant Vacuolar Protease, VPE, Mediates Virus-Induced Hypersensitive Cell Death. Science 305, 855–858. doi:10.1126/science.1099859
He, S., Liang, Y., Shao, F., and Wang, X. (2011). Toll-like Receptors Activate Programmed Necrosis in Macrophages through a Receptor-Interacting Kinase-3-Mediated Pathway. Proc. Natl. Acad. Sci. 108, 20054–20059. doi:10.1073/pnas.1116302108
He, S., Wang, L., Miao, L., Wang, T., Du, F., Zhao, L., et al. (2009). Receptor Interacting Protein Kinase-3 Determines Cellular Necrotic Response to TNF-α. Cell 137, 1100–1111. doi:10.1016/j.cell.2009.05.021
He, Y., Zeng, M. Y., Yang, D., Motro, B., and Núñez, G. (2016). NEK7 Is an Essential Mediator of NLRP3 Activation Downstream of Potassium Efflux. Nature 530, 354–357. doi:10.1038/nature16959
Hengartner, M. O., Ellis, R., and Horvitz, R. (1992). Caenorhabditis elegans Gene Ced-9 Protects Cells from Programmed Cell Death. Nature 356, 494–499. doi:10.1038/356494a0
Horsefield, S., Burdett, H., Zhang, X., Manik, M. K., Shi, Y., Chen, J., et al. (2019). NAD + Cleavage Activity by Animal and Plant TIR Domains in Cell Death Pathways. Science 365, 793–799. doi:10.1126/science.aax1911
Hou, S., Liu, D., Huang, S., Luo, D., Liu, Z., Xiang, Q., et al. (2021). The Arabidopsis MIK2 Receptor Elicits Immunity by Sensing a Conserved Signature from Phytocytokines and Microbes. Nat. comm 12, 1–15. doi:10.1038/s41467-021-25580-w
Hu, Z., Zhou, Q., Zhang, C., Fan, S., Cheng, W., Zhao, Y., et al. (2015). Structural and Biochemical Basis for Induced Self-Propagation of NLRC4. Science 350, 399–404. doi:10.1126/science.aac5489
Huang, D., Zheng, X., Wang, Z. A., Chen, X., He, W. T., Zhang, Y., et al. (2017). The MLKL Channel in Necroptosis Is an Octamer Formed by Tetramers in a Dyadic Process. Mol. Cell Biol. 37, e00497–16. doi:10.1128/MCB.00497-16
Humphries, F., Shmuel-Galia, L., Ketelut-Carneiro, N., Li, S., Wang, B., Nemmara, V. V., et al. (2020). Succination Inactivates Gasdermin D and Blocks Pyroptosis. Science 369, 1633–1637. doi:10.1126/science.abb9818
Jacob, P., Kim, N. H., Wu, F., El-Kasmi, F., Chi, Y., Walton, W. G., et al. (2021). Plant "helper" Immune Receptors Are Ca 2+ -permeable Nonselective Cation Channels. Science 373, 420–425. doi:10.1126/science.abg7917
Jänicke, R. U., Ng, P., Sprengart, M. L., and Porter, A. G. (1998). Caspase-3 Is Required for Alpha-Fodrin Cleavage but Dispensable for Cleavage of Other Death Substrates in Apoptosis. J. Biol. Chem. 273, 15540–15545. doi:10.1074/jbc.273.25.15540
Jiang S, S., Zhou, Z., Sun, Y., Zhang, T., and Sun, L. (2020). Coral Gasdermin Triggers Pyroptosis. Sci. Immunol. 5, eabd2591. doi:10.1126/sciimmunol.abd2591
Jiang, S., Gu, H., Zhao, Y., and Sun, L. (2019). Teleost Gasdermin E Is Cleaved by Caspase 1, 3, and 7 and Induces Pyroptosis. J.I. 203, 1369–1382. doi:10.4049/jimmunol.1900383
Jiang Y, Y., Liu, T., Lee, C.-H., Chang, Q., Yang, J., and Zhang, Z. (2020). The NAD+-mediated Self-Inhibition Mechanism of Pro-neurodegenerative SARM1. Nature 588, 658–663. doi:10.1038/s41586-020-2862-z
Johnson, A. G., Wein, T., Mayer, M. L., Duncan-Lowey, B., Yirmiya, E., Oppenheimer-Shaanan, Y., et al. (2022). Bacterial Gasdermins Reveal an Ancient Mechanism of Cell Death. Science 375, 221–225. doi:10.1126/science.abj8432
Jones, J. D., Vance, R. E., and Dangl, J. L. (2016). Intracellular Innate Immune Surveillance Devices in Plants and Animals. Science 354, aaf6395. doi:10.1126/science.aaf6395
Jones, J. D. G., and Dangl, J. L. (2006). The Plant Immune System. Nature 444, 323–329. doi:10.1038/nature05286
Kabbage, M., Kessens, R., Bartholomay, L. C., and Williams, B. (2017). The Life and Death of a Plant Cell. Annu. Rev. Plant Biol. 68, 375–404. doi:10.1146/annurev-arplant-043015-111655
Kabbage, M., and Dickman, M. B. (2008). The BAG Proteins: a Ubiquitous Family of Chaperone Regulators. Cell. Mol. Life Sci. 65, 1390–1402. doi:10.1007/s00018-008-7535-2
Kalia, S. K., Lee, S., Smith, P. D., Liu, L., Crocker, S. J., Thorarinsdottir, T. E., et al. (2004). BAG5 Inhibits Parkin and Enhances Dopaminergic Neuron Degeneration. Neuron 44, 931–945. doi:10.1016/j.neuron.2004.11.026
Kang, C. H., Jung, W. Y., Kang, Y. H., Kim, J. Y., Kim, D. G., Jeong, J. C., et al. (2006). AtBAG6, a Novel Calmodulin-Binding Protein, Induces Programmed Cell Death in Yeast and Plants. Cell Death Differ 13, 84–95. doi:10.1038/sj.cdd.4401712
Kayagaki, N., Kornfeld, O. S., Lee, B. L., Stowe, I. B., O’Rourke, K., Li, Q., et al. (2021). NINJ1 Mediates Plasma Membrane Rupture during Lytic Cell Death. Nature 591, 131–136. doi:10.1038/s41586-021-03218-7
Ke, F., Voss, A., Kerr, J. B., O'Reilly, L. A., Tai, L., Echeverry, N., et al. (2012). BCL-2 Family Member BOK Is Widely Expressed but its Loss Has Only Minimal Impact in Mice. Cell Death Differ 19, 915–925. doi:10.1038/cdd.2011.210
Kelekar, A., and Thompson, C. B. (1998). Bcl-2-family Proteins: the Role of the BH3 Domain in Apoptosis. Trends Cell Biol. 8, 324–330. doi:10.1016/s0962-8924(98)01321-x
Kerr, J. F. R. (1965). A Histochemical Study of Hypertrophy and Ischaemic Injury of Rat Liver with Special Reference to Changes in Lysosomes. J. Pathol. 90, 419–435. doi:10.1002/path.1700900210
Kerr, J. F. R., Wyllie, A. H., and Currie, A. R. (1972). Apoptosis: A Basic Biological Phenomenon with Wideranging Implications in Tissue Kinetics. Br. J. Cancer 26, 239–257. doi:10.1038/bjc.1972.33
Laohavisit, A., Wakatake, T., Ishihama, N., Mulvey, H., Takizawa, K., Suzuki, T., et al. (2020). Quinone Perception in Plants via Leucine-Rich-Repeat Receptor-like Kinases. Nature 587, 92–97. doi:10.1038/s41586-020-2655-4
Lee, S., Karki, R., Wang, Y., Nguyen, L. N., Kalathur, R. C., and Kanneganti, T.-D. (2021). AIM2 Forms a Complex with Pyrin and ZBP1 to Drive PANoptosis and Host Defence. Nature 597, 415–419. doi:10.1038/s41586-021-03875-8
Lettre, G., and Hengartner, M. O. (2006). Developmental Apoptosis in C. elegans: a Complex CEDnario. Nat. Rev. Mol. Cell Biol. 7, 97–108. doi:10.1038/nrm1836
Li, H., Zhu, H., Xu, C.-j., and Yuan, J. (1998). Cleavage of BID by Caspase 8 Mediates the Mitochondrial Damage in the Fas Pathway of Apoptosis. Cell 94, 491–501. doi:10.1016/s0092-8674(00)81590-1
Li, L. Y., Luo, X., and Wang, X. (2001). Endonuclease G Is an Apoptotic DNase when Released from Mitochondria. Nature 412, 95–99. doi:10.1038/35083620
Li, P., Nijhawan, D., Budihardjo, I., Srinivasula, S. M., Ahmad, M., Alnemri, E. S., et al. (1997). Cytochrome C and dATP-dependent Formation of Apaf-1/caspase-9 Complex Initiates an Apoptotic Protease cascade. Cell 91 (4), 479–489. doi:10.1016/s0092-8674(00)80434-1
Li, Y., Williams, B., and Dickman, M. (2017). Arabidopsis B‐cell Lymphoma2 (Bcl‐2)‐associated Athanogene 7 ( BAG 7)‐mediated Heat Tolerance Requires Translocation, Sumoylation and Binding to WRKY 29. New Phytol. 2142, 695–705. doi:10.1111/nph.14388
Liang, X., and Zhou, J.-M. (2018). Receptor-Like Cytoplasmic Kinases: Central Players in Plant Receptor Kinase-Mediated Signaling. Annu. Rev. Plant Biol. 69, 267–299. doi:10.1146/annurev-arplant-042817-040540
Lindsten, T., Ross, A. J., King, A., Zong, W.-X., Rathmell, J. C., Shiels, H. A., et al. (2000). The Combined Functions of Proapoptotic Bcl-2 Family Members Bak and Bax Are Essential for normal Development of Multiple Tissues. Mol. Cell 6, 1389–1399. doi:10.1016/s1097-2765(00)00136-2
Ma, S., Lapin, D., Liu, L., Sun, Y., Song, W., Zhang, X., et al. (2020). Direct Pathogen-Induced Assembly of an NLR Immune Receptor Complex to Form a Holoenzyme. Science 370, eabe3069. doi:10.1126/science.abe3069
Mao, C., Liu, X., Zhang, Y., Lei, G., Yan, Y., Lee, H., et al. (2021). DHODH-mediated Ferroptosis Defence Is a Targetable Vulnerability in Cancer. Nature 593, 586–590. doi:10.1038/s41586-021-03539-7
Martin, R., Qi, T., Zhang, H., Liu, F., King, M., Toth, C., et al. (2020). Structure of the Activated ROQ1 Resistosome Directly Recognizing the Pathogen Effector XopQ. Science 370, eabd9993. doi:10.1126/science.abd9993
Mason, K. D., Lin, A., Robb, L., Josefsson, E. C., Henley, K. J., Gray, D. H. D., et al. (2013). Proapoptotic Bak and Bax Guard against Fatal Systemic and Organ-specific Autoimmune Disease. Proc. Natl. Acad. Sci. 110, 2599–2604. doi:10.1073/pnas.1215097110
Minina, E. A., Staal, J., Alvarez, V. E., Berges, J. A., Berman-Frank, I., Beyaert, R., et al. (2020). Classification and Nomenclature of Metacaspases and Paracaspases: No More Confusion with Caspases. Mol. Cell 77 (5), 927–929. doi:10.1016/j.molcel.2019.12.020
Miya, A., Albert, P., Shinya, T., Desaki, Y., Ichimura, K., Shirasu, K., et al. (2007). CERK1, a LysM Receptor Kinase, Is Essential for Chitin Elicitor Signaling in Arabidopsis. Proc. Natl. Acad. Sci. 104, 19613–19618. doi:10.1073/pnas.0705147104
Morehouse, B. R., Govande, A. A., Millman, A., Keszei, A. F. A., Lowey, B., Ofir, G., et al. (2020). STING Cyclic Dinucleotide Sensing Originated in Bacteria. Nature 586, 429–433. doi:10.1038/s41586-020-2719-5
Naim, S., and Kaufmann, T. (2020). The Multifaceted Roles of the Bcl-2 Family Member Bok. Front. Cell Dev. Biol. 8, 574338. doi:10.3389/fcell.2020.574338
Newton, K., Wickliffe, K. E., Maltzman, A., Dugger, D. L., Reja, R., Zhang, Y., et al. (2019). Activity of Caspase-8 Determines Plasticity between Cell Death Pathways. Nature 575, 679–682. doi:10.1038/s41586-019-1752-8
Ngou, B. P. M., Ahn, H. K., Ding, P., and Jones, J. D. G. (2021). Mutual Potentiation of Plant Immunity by Cell-Surface and Intracellular Receptors. Nature 592, 110–115. doi:10.1038/s41586-021-03315-7
Orning, P., Weng, D., Starheim, K., Ratner, D., Best, Z., Lee, B., et al. (2018). Pathogen Blockade of TAK1 Triggers Caspase-8-dependent Cleavage of Gasdermin D and Cell Death. Science 362, 1064–1069. doi:10.1126/science.aau2818
Pruitt, R. N., Locci, F., Wanke, F., Zhang, L., Saile, S. C., Joe, A., et al. (2021). The EDS1–PAD4–ADR1 Node Mediates Arabidopsis Pattern-Triggered Immunity. Nature 598, 495–499. doi:10.1038/s41586-021-03829-0
Ranf, S., Gisch, N., Schäffer, M., Illig, T., Westphal, L., Knirel, Y. A., et al. (2015). A Lectin S-Domain Receptor Kinase Mediates Lipopolysaccharide Sensing in Arabidopsis thaliana. Nat. Immunol. 16, 426–433. doi:10.1038/ni.3124
Rathmell, J. C., Lindsten, T., Zong, W.-X., Cinalli, R. M., and Thompson, C. B. (2002). Deficiency in Bak and Bax Perturbs Thymic Selection and Lymphoid Homeostasis. Nat. Immunol. 3, 932–939. doi:10.1038/ni834
Rhodes, J., Yang, H., Moussu, S., Boutrot, F., Santiago, J., and Zipfel, C. (2021). Perception of a Divergent Family of Phytocytokines by the Arabidopsis Receptor Kinase MIK2. Nat. comm 12, 1–10. doi:10.1038/s41467-021-20932-y
Ruan, J., Xia, S., Liu, X., Lieberman, J., and Wu, H. (2018). Cryo-EM Structure of the Gasdermin A3 Membrane Pore. Nature 557, 62–67. doi:10.1038/s41586-018-0058-6
Ruchaud, S., Korfali, N., Villa, P., Kottke, T. J., Dingwall, C., Kaufmann, S. H., et al. (2002). Caspase-6 Gene Disruption Reveals a Requirement for Lamin A Cleavage in Apoptotic Chromatin Condensation. EMBO J. 21 (8), 1967–1977. doi:10.1093/emboj/21.8.1967
Samir, P., Malireddi, R. K. S., and Kanneganti, T.-D. (2020). The PANoptosome: a Deadly Protein Complex Driving Pyroptosis, Apoptosis, and Necroptosis (PANoptosis). Front. Cell. Infect. Microbiol. 10, 238. doi:10.3389/fcimb.2020.00238
Schendel, S. L., Xie, Z., Montal, M. O., Matsuyama, S., Montal, M., and Reed, J. C. (1997). Channel Formation by Antiapoptotic Protein Bcl-2. Proc. Natl. Acad. Sci. 94, 5113–5118. doi:10.1073/pnas.94.10.5113
Schleich, K., Krammer, P. H., and Lavrik, I. N. (2013). The Chains of Death. Cell Cycle 12, 193–194. doi:10.4161/cc.23464
Schwarzer, R., Laurien, L., and Pasparakis, M. (2020). New Insights into the Regulation of Apoptosis, Necroptosis, and Pyroptosis by Receptor Interacting Protein Kinase 1 and Caspase-8. Curr. Opin. Cell Biol. 63, 186–193. doi:10.1016/j.ceb.2020.02.004
Severin, G. B., Ramliden, M. S., Hawver, L. A., Wang, K., Pell, M. E., Kieninger, A.-K., et al. (2018). Direct Activation of a Phospholipase by Cyclic GMP-AMP in El TorVibrio Cholerae. Proc. Natl. Acad. Sci. USA 115, E6048–E6055. doi:10.1073/pnas.1801233115
Sharif, H., Wang, L., Wang, W. L., Magupalli, V. G., Andreeva, L., Qiao, Q., et al. (2019). Structural Mechanism for NEK7-Licensed Activation of NLRP3 Inflammasome. Nature 570, 338–343. doi:10.1038/s41586-019-1295-z
Shen, Q., Liang, M., Yang, F., Deng, Y. Z., and Naqvi, N. I. (2020). Ferroptosis Contributes to Developmental Cell Death in rice Blast. New Phytol. 227 (6), 1831–1846. doi:10.1111/nph.16636
Shi, J., Zhao, Y., Wang, K., Shi, X., Wang, Y., Huang, H., et al. (2015). Cleavage of GSDMD by Inflammatory Caspases Determines Pyroptotic Cell Death. Nature 526, 660–665. doi:10.1038/nature15514
Shi, J., Zhao, Y., Wang, Y., Gao, W., Ding, J., Li, P., et al. (2014). Inflammatory Caspases Are Innate Immune Receptors for Intracellular LPS. Nature 514 (7521), 187–192. doi:10.1038/nature13683
Sporny, M., Guez-Haddad, J., Khazma, T., Yaron, A., Dessau, M., Shkolnisky, Y., et al. (2020). Structural Basis for SARM1 Inhibition and Activation under Energetic Stress. Elife 9, e62021. doi:10.7554/eLife.62021
Stennicke, H. R., Jürgensmeier, J. M., Shin, H., Deveraux, Q., Wolf, B. B., Yang, X., et al. (1998). Pro-caspase-3 Is a Major Physiologic Target of Caspase-8. J. Biol. Chem. 273, 27084–27090. doi:10.1074/jbc.273.42.27084
Su, L., Quade, B., Wang, H., Sun, L., Wang, X., and Rizo, J. (2014). A Plug Release Mechanism for Membrane Permeation by MLKL. Structure 22, 1489–1500. doi:10.1016/j.str.2014.07.014
Sun, L., Wang, H., Wang, Z., He, S., Chen, S., Liao, D., et al. (2012). Mixed Lineage Kinase Domain-like Protein Mediates Necrosis Signaling Downstream of RIP3 Kinase. Cell 148, 213–227. doi:10.1016/j.cell.2011.11.031
Sun, L., and Wang, X. (2014). A New Kind of Cell Suicide: Mechanisms and Functions of Programmed Necrosis. Trends Biochem. Sci. 39, 587–593. doi:10.1016/j.tibs.2014.10.003
Takayama, S., and Reed, J. C. (2001). Molecular Chaperone Targeting and Regulation by BAG Family Proteins. Nat. Cell Biol. 3, E237–E241. doi:10.1038/ncb1001-e237
Takayama, S., Sato, T., Krajewski, S., Kochel, K., Irie, S., Milian, J. A., et al. (1995). Cloning and Functional Analysis of BAG-1: A Novel Bcl-2-Binding Protein with Anti-cell Death Activity. Cell 80, 279–284. doi:10.1016/0092-8674(95)90410-7
Tang, D., and Kidd, V. J. (1998). Cleavage of DFF-45/ICAD by Multiple Caspases Is Essential for its Function during Apoptosis. J. Biol. Chem. 273, 28549–28552. doi:10.1074/jbc.273.44.28549
Tang, D., Wang, G., and Zhou, J.-M. (2017). Receptor Kinases in Plant-Pathogen Interactions: More Than Pattern Recognition. Plant Cell 29, 618–637. doi:10.1105/tpc.16.00891
Tian, H., Wu, Z., Chen, S., Ao, K., Huang, W., Yaghmaiean, H., et al. (2021). Activation of TIR Signalling Boosts Pattern-Triggered Immunity. Nature 1-7. doi:10.1038/s41586-021-03987-1
van Beljouw, S. P., Haagsma, A. C., Rodríguez-Molina, A., van den Berg, D. F., Vink, J. N., and Brouns, S. J. (2021). The gRAMP CRISPR-Cas Effector Is an RNA Endonuclease Complexed with a Caspase-like Peptidase. Science 373, eabk2718. doi:10.1126/science.abk2718
Wan, L., Essuman, K., Anderson, R. G., Sasaki, Y., Monteiro, F., Chung, E.-H., et al. (2019). TIR Domains of Plant Immune Receptors Are NAD + -cleaving Enzymes that Promote Cell Death. Science 365, 799–803. doi:10.1126/science.aax1771
Wang, H., Sun, L., Su, L., Rizo, J., Liu, L., Wang, L.-F., et al. (2014). Mixed Lineage Kinase Domain-like Protein MLKL Causes Necrotic Membrane Disruption upon Phosphorylation by RIP3. Mol. Cell 54, 133–146. doi:10.1016/j.molcel.2014.03.003
Wang, J., Hu, M., Wang, J., Qi, J., Han, Z., Wang, G., et al. (2019a). Reconstitution and Structure of a Plant NLR Resistosome Conferring Immunity. Science 364, eaav5870. doi:10.1126/science.aav5870
Wang, J., Wang, J., Hu, M., Wu, S., Qi, J., Wang, G., et al. (2019b). Ligand-triggered Allosteric ADP Release Primes a Plant NLR Complex. Science 364, eaav5868. doi:10.1126/science.aav5868
Wang, Y., Gao, W., Shi, X., Ding, J., Liu, W., He, H., et al. (2017). Chemotherapy Drugs Induce Pyroptosis through Caspase-3 Cleavage of a Gasdermin. Nature 547, 99–103. doi:10.1038/nature22393
Wei, M. C., Zong, W.-X., Cheng, E. H.-Y., Lindsten, T., Panoutsakopoulou, V., Ross, A. J., et al. (2001). Proapoptotic BAX and BAK: a Requisite Gateway to Mitochondrial Dysfunction and Death. Science 292, 727–730. doi:10.1126/science.1059108
Willmann, R., Lajunen, H. M., Erbs, G., Newman, M. A., Kolb, D., Tsuda, K., et al. (2011). Arabidopsis Lysin-Motif Proteins LYM1 LYM3 CERK1 Mediate Bacterial Peptidoglycan Sensing and Immunity to Bacterial Infection. Proc. Natl. Acad. Sci. U S A. 108, 19824–19829. doi:10.1073/pnas.1112862108
Wride, M. A., Parker, E., and Sanders, E. J. (1999). Members of the Bcl-2 and Caspase Families Regulate Nuclear Degeneration during Chick Lens Fibre Differentiation. Developmental Biol. 213, 142–156. doi:10.1006/dbio.1999.9375
Wu, F., Chi, Y., Jiang, Z., Xu, Y., Xie, L., Huang, F., et al. (2020). Hydrogen Peroxide Sensor HPCA1 Is an LRR Receptor Kinase in Arabidopsis. Nature 578, 577–581. doi:10.1038/s41586-020-2032-3
Wu, Y., Kuzma, J., Maréchal, E., Graeff, R., Lee, H. C., Foster, R., et al. (1997). Abscisic Acid Signaling through Cyclic ADP-Ribose in Plants. Science 278 (5346), 2126–2130. doi:10.1126/science.278.5346.2126
Xia, S., Zhang, Z., Magupalli, V. G., Pablo, J. L., Dong, Y., Vora, S. M., et al. (2021). Gasdermin D Pore Structure Reveals Preferential Release of Mature Interleukin-1. Nature 593, 607–611. doi:10.1038/s41586-021-03478-3
Xue, D.-X., Li, C.-L., Xie, Z.-P., and Staehelin, C. (2019). LYK4 Is a Component of a Tripartite Chitin Receptor Complex in Arabidopsis thaliana. J. Exp. Bot. 70, 5507–5516. doi:10.1093/jxb/erz313
Yan, B., Ai, Y., Sun, Q., Ma, Y., Cao, Y., Wang, J., et al. (2021). Membrane Damage during Ferroptosis Is Caused by Oxidation of Phospholipids Catalyzed by the Oxidoreductases POR and CYB5R1. Mol. Cell 81, 355–369. doi:10.1016/j.molcel.2020.11.024
Yang, W. S., Kim, K. J., Gaschler, M. M., Patel, M., Shchepinov, M. S., and Stockwell, B. R. (2016). Peroxidation of Polyunsaturated Fatty Acids by Lipoxygenases Drives Ferroptosis. Proc. Natl. Acad. Sci. USA 113, E4966–E4975. doi:10.1073/pnas.1603244113
Yuan, J., Shaham, S., Ledoux, S., Ellis, H. M., and Horvitz, H. R. (1993). The C. elegans Cell Death Gene Ced-3 Encodes a Protein Similar to Mammalian Interleukin-1β-Converting Enzyme. Cell 75, 641–652. doi:10.1016/0092-8674(93)90485-9
Yuan, M., Jiang, Z., Bi, G., Nomura, K., Liu, M., Wang, Y., et al. (2021). Pattern-recognition Receptors Are Required for NLR-Mediated Plant Immunity. Nature 592, 105–109. doi:10.1038/s41586-021-03316-6
Zeidler, D., Zahringer, U., Gerber, I., Dubery, I., Hartung, T., Bors, W., et al. (2004). From the Cover: Innate Immunity in Arabidopsis thaliana: Lipopolysaccharides Activate Nitric Oxide Synthase (NOS) and Induce Defense Genes. Proc. Natl. Acad. Sci. 101, 15811–15816. doi:10.1073/pnas.0404536101
Zhang, D.-W., Shao, J., Lin, J., Zhang, N., Lu, B.-J., Lin, S.-C., et al. (2009). RIP3, an Energy Metabolism Regulator that Switches TNF-Induced Cell Death from Apoptosis to Necrosis. Science 325, 332–336. doi:10.1126/science.1172308
Zhang, Z., Zhang, Y., Xia, S., Kong, Q., Li, S., Liu, X., et al. (2020). Gasdermin E Suppresses Tumour Growth by Activating Anti-tumour Immunity. Nature 579, 415–420. doi:10.1038/s41586-020-2071-9
Zheng, Z., Deng, W., Bai, Y., Miao, R., Mei, S., Zhang, Z., et al. (2021). The Lysosomal Rag-Ragulator Complex Licenses RIPK1–And Caspase-8–Mediated Pyroptosis by Yersinia. Science 372 (6549), eabg0269. doi:10.1126/science.abg0269
Zhou, Z., He, H., Wang, K., Shi, X., Wang, Y., Su, Y., et al. (2020). Granzyme A from Cytotoxic Lymphocytes Cleaves GSDMB to Trigger Pyroptosis in Target Cells. Science 368, eaaz7548. doi:10.1126/science.aaz7548
Keywords: pyroptosis, necroptosis, apoptosis, hypersensitive cell death response, ferroptosis, bacterial PCD
Citation: Zhuang J, Xie L and Zheng L (2022) A Glimpse of Programmed Cell Death Among Bacteria, Animals, and Plants. Front. Cell Dev. Biol. 9:790117. doi: 10.3389/fcell.2021.790117
Received: 06 October 2021; Accepted: 24 November 2021;
Published: 10 February 2022.
Edited by:
Shuai Jiang, Institute of Oceanology, Chinese Academy of Sciences (CAS), ChinaReviewed by:
Jens Staal, Ghent University, BelgiumFaheem Ahmed Khan, Huazhong Agricultural University, China
Copyright © 2022 Zhuang, Xie and Zheng. This is an open-access article distributed under the terms of the Creative Commons Attribution License (CC BY). The use, distribution or reproduction in other forums is permitted, provided the original author(s) and the copyright owner(s) are credited and that the original publication in this journal is cited, in accordance with accepted academic practice. No use, distribution or reproduction is permitted which does not comply with these terms.
*Correspondence: Jun Zhuang, ZmFmdXpqQDEyNi5jb20=