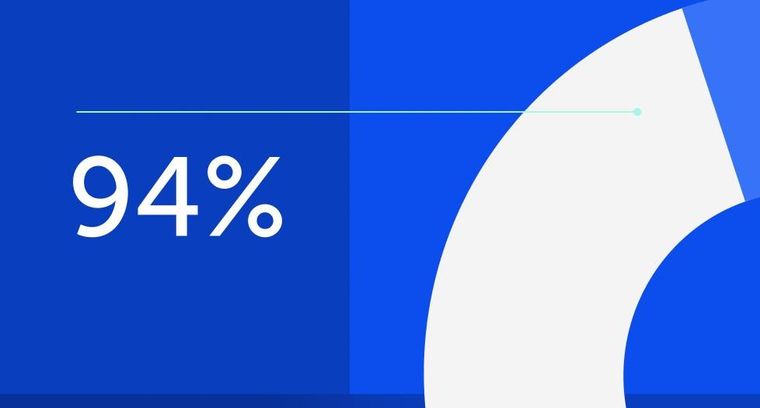
94% of researchers rate our articles as excellent or good
Learn more about the work of our research integrity team to safeguard the quality of each article we publish.
Find out more
REVIEW article
Front. Cell Dev. Biol., 21 December 2021
Sec. Signaling
Volume 9 - 2021 | https://doi.org/10.3389/fcell.2021.786129
This article is part of the Research Topic17th Edition of the Spanish Society for Developmental Biology Meeting: New Trends in Developmental BiologyView all 15 articles
Adipose tissue is a dynamic organ, well known for its function in energy storage and mobilization according to nutrient availability and body needs, in charge of keeping the energetic balance of the organism. During the last decades, adipose tissue has emerged as the largest endocrine organ in the human body, being able to secrete hormones as well as inflammatory molecules and having an important impact in multiple processes such as adipogenesis, metabolism and chronic inflammation. However, the cellular progenitors, development, homeostasis and metabolism of the different types of adipose tissue are not fully known. During the last decade, Drosophila melanogaster has demonstrated to be an excellent model to tackle some of the open questions in the field of metabolism and development of endocrine/metabolic organs. Discoveries ranged from new hormones regulating obesity to subcellular mechanisms that regulate lipogenesis and lipolysis. Here, we review the available evidences on the development, types and functions of adipose tissue in Drosophila and identify some gaps for future research. This may help to understand the cellular and molecular mechanism underlying the pathophysiology of this fascinating key tissue, contributing to establish this organ as a therapeutic target.
In this manuscript we review the past and recent literature on the origin, development, types and function of mammalian adipose tissue and put it in relation to physiological and disease conditions such as obesity, diabetes, lipodystrophies or cancer-associated cachexia. We identify the gaps that need to be addressed regarding the origin and development of this tissue and propose Drosophila as a suitable model organism to explore those open questions. We review the existing evidences on the origin, development and function of the adipose tissue (AT) of this organism, making a clear distinction between the embryonic and larval stages and the adulthood, when the developmental programmes are finished. As we considered there are two different scenarios where play different actors and also where same actors could play different roles. We also review the available studies in Drosophila on the above-mentioned metabolic diseases.
The lifestyle of developed countries, where population have an easy access to high caloric food and decreased physical exercise, has played important roles into the rise of obesity and Type 2 Diabetes Mellitus (T2DM) to the category of pandemics (Doria et al., 2008; Guilherme et al., 2008; Saltiel, 2012). Global prevalence of overweight and obesity combined has risen by 27.5% for adults and 47.1% for children between 1980 and 2013 (Ng et al., 2014). Furthermore, excess body weight is one of the major risk factors contributing to the global incidence of disease worldwide. According to the International Diabetes Federation (IDF), more than 371 million people across the globe have diabetes and this number is predicted to rise to over 550 million by 2030. Adipose tissue (AT) plays also important roles in other diseases. For instance, lipid storages at AT are susceptible to be wasted by tumour-secreted molecules, a fact known as cancer-associated cachexia (Rydén and Arner, 2007; Tsoli et al., 2016). This process is featured by increased systemic inflammation, general metabolic dysfunction, and elevated resting energy expenditure (Fearon et al., 2011). Cachexia affects 50–80% of cancer patients and accounts for up to 20% of cancer deaths. It is estimated that death normally ensues when weight loss exceeds 30–40% (Arthur et al., 2014). In this scenario, the understanding of adipocytes’ development will improve our knowledge on metabolic diseases such as obesity and T2DM, as well as on anomalous metabolic states that lead to chronic inflammation. (Stephens, 2012; Wu et al., 2013; Hotamisligil, 2017). Understanding in deep the biology of the AT will allow the development of potential therapies targeting thermogenesis as a means of increasing energy expenditure.
Energy is fundamental for life. Therefore, storage and homeostasis of energy are key processes for any organism. In the mammalian body, including that of humans, the energy that is neither consumed nor converted into glycogen is stored in form of neutral lipids in the adipocytes of the AT, more specifically in the lipid droplets (LDs). The LDs are key organelles controlling fat storage and mobilization (Olofsson et al., 2008; Beller et al., 2010). They consist of a core of neutral lipids (triglyceride and cholesterol esters) surrounded by a monolayer of phospholipid and cholesterol in which several proteins are embedded (Thiam and Beller, 2017).
Importantly, the AT is a plastic organ able to adapt to different physiological circumstances to ensure energy distribution among the different needs: metabolism, thermogenesis and lactation (Cinti, 2018). AT can grow by either increasing the number of adipocytes, which depends on adipocyte stem cells (ASC) or increasing the LDs size. In fat oxidizing tissues, LDs expansion is supported by specific mitochondria, known as peridroplet mitochondria. Those remain bound to the LD even after the homogenization the tissue and show specific features such as enhanced bioenergetic capacity, reduced β-oxidation capacity, supported LD expansion by providing ATP for triacylglycerides (TAG) synthesis, and maintenance of a distinct protein composition due to low fusion-fission dynamics (Benador et al., 2018).
In mammals there are different types of ATs: white AT (WAT), designed for energy storage, and brown AT (BAT), intended to dissipate energy and generate heat (Berry et al., 2013; Gupta, 2014). WAT is classified in subcutaneous or visceral WAT, depending on its anatomical location (Cleal et al., 2017). The two types of WATs have distinct developmental timing, microscopic appearance, molecular signature and certainly biological function. Subcutaneous WAT may protect against certain aspects of metabolic dysfunction (Snijder et al., 2003a; Snijder et al., 2003b). Visceral WAT is associated with metabolic complications and appears to increase the risk of T2DM, hyperlipidemia and cardiovascular disease (Grauer et al., 1984). Increasing number of evidences converge on to the idea that within mammalian bodies there are different AT depots, which present a vast heterogeneity among them, and contain the ASC that support their homeostasis (Cleal et al., 2017).
Until recently, the functions of the BAT were associated to the neonatal period (Novak et al., 1971) and the scientific community thought that this type of fat was not present during the adulthood. A bit more a decade ago, BAT was identified in adult humans and it was found to be reduced, on mass and activity, in obese and diabetic patients (Cypess et al., 2009; Cypess et al., 2013; Lidell et al., 2013; Alcalá et al., 2019). This finding pointed out to the idea of enhancement of BAT preadipocyte differentiation and proliferation as a therapeutic strategy to fight obesity (Alcalá et al., 2019). Later evidences converged on to the idea that adult human BAT shares molecular characteristics with murine “beige” cells rather than classical brown cells (Wu et al., 2012; Cannon et al., 2020).
“Beige” or “brite” adipocytes, also known as Beige Adipose Tissue (BeAT), are energy-burning adipocytes, with “brown-like’ features, such as increased mitochondrial content, multilocular storing of LDs, and the ability to burn off lipids as heat (Ikeda et al., 2018). BeAT is found in different spots of the adult body within the WAT (Cypess et al., 2009; Cypess et al., 2013; Lidell et al., 2013; Wu et al., 2012; Whittle et al., 2011). Although beige adipocytes share some markers with brown adipocytes, they also show specific markers different from those of both brown and white adipocytes (Table 1) (Sharp et al., 2012; Waldén et al., 2012; Ussar et al., 2014).
In spite of their distinct functions, WAT and “Beige AT” (BeAT) share the ability for reciprocal, reversible transdifferentiation to tackle special physiologic needs. Thus, chronic need for thermogenesis induces browning and chronic positive energy balance induces whitening (Cohen and Spiegelman, 2016; Cinti, 2018). Different signals, after birth and adult state, will determine BeAT differentiation. While Platelet activating factor and Interleukin 6 (IL-6) are determining factors in BeAT development after birth, β-adrenergic stimulation and IL-4 are active during adulthood (Chung et al., 2017; Finlin et al., 2017; Babaei et al., 2018; Yu et al., 2019; Hoang et al., 2021). Interestingly, a switch from BeAT to WAT underlies cancer-associated cachexia, triggered by the parathyroid-hormone-related protein (Kir et al., 2014; Petruzzelli and Wagner, 2016).
AT is a large endocrine organ, insulin sensitive, that secretes around 600 different adipokines, the hormones that act on distant organs (Table 2) (Lehr et al., 2012a; Cinti, 2018) as well as a vast diversity of other signalling molecules, such as metabolites, lipids, non-coding RNAs or extracellular vesicles (Funcke and Scherer, 2019). Leptin and adiponectin are well known hormones secreted by adipocytes (Cinti, 2018). Leptin inhibits appetite, stimulate thermogenesis, enhance fatty acid oxidation, decrease glucose, and reduce body weight and fat. Adiponectin mediates the insulin-sensitizing effect (Yadav et al., 2013). Omentin, secreted by non-adipocyte cells in the AT, acts as insulin-sensitizing factor and it has been reported to have anti-inflammatory, anti-atherogenic and anti-cardiovascular disease properties (Tan et al., 2010). Dipeptidyl peptidase IV, secreted by visceral white adipocytes from obese individuals, seems to be associated to insulin-resistance development (Lamers et al., 2011; Lehr et al., 2012b).
AT is not only composed by adipocytes but also comprises as well other cell types such as preadipocytes, fibroblasts, stromal cells, T-cells, granulocytes, macrophages and monocytes (Hotamisligil, 2017). During the last years, several adipokines and cytokines secreted by AT and also other molecules of signalling pathways linking AT metabolism and immune system, have been identified. For example resistin, a hormone secreted by macrophages M1, pro-inflammatory phenotype, that infiltrate obese adipoctyes, produces a notable effect on systemic metabolism by acting as a crosstalk between obesity-inflammation and metabolic diseases (Schwartz and Lazar, 2011). Likewise, the dysfunction of AT is associated with the secretion of multiple molecules that mediate the inflammatory response. In fact, stressed adipocytes from obese ATs activate the inflammasome system, which could induce a chronic low-grade inflammation. Inflammation appears in other tissues besides AT, including brain, liver, airways and pancreatic islets. This inflammatory state is known as “metaflammation” because it contributes to several immunometabolic diseases, including T2DM, cardiovascular disease, asthma, neurodegenerative disease, cancer and lipodystrophies (Hotamisligil, 2017; Cinti, 2018).
A great number of studies, most of them carried out in vitro, shed light on the factors involved in differentiation of white, brown and beige adipocytes, such as peroxisome proliferator-activated receptor gamma (PPARγ), CCAAT/enhancer binding proteins (C/EPB), krupper-like factors (KLFs), peroxisome proliferator-activated receptor gamma coactivator-1 alpha (PGC1a) or nuclear factor I A (NFIA) (reviewed at (Hiraike et al., 2017; Cinti, 2018; Hiraike et al., 2020). However, the initial commitment of mesenchymal progenitors to the adipocyte lineage remains less explored (Billon et al., 2007).
A comprehensive understanding of the origin of white/brown/beige adipocytes differentiation is of upmost interest given the potential to induce browning AT in obese patients (Cypess and Kahn, 2010). It is accepted that animals with more BAT are more resistant not only to obesity but also to T2DM (Kopecký et al., 1996; Collins et al., 1997; Guerra et al., 1998; Almind et al., 2007). Conversely, animals without functional BAT are prone to obesity and T2DM (Lowell et al., 1993; Bachman et al., 2002; Feldmann et al., 2009). Attempts to block lipid storage or inhibiting WAT development failed, as several studies have shown that this strategy drives fat accumulation in organs not specialized for fat storage. This condition is known as “ectopic lipid” and has been associated with insulin resistance and the development of T2DM (Gastaldelli, 2011).
BAT, WAT and BeAT can develop from both neural crest and mesoderm, specifically BAT develops from paraxial mesoderm and WAT and Beige AT does from lateral plate mesoderm (Billon et al., 2007). Periaortic arch adipose tissue (PAAT), which is composed of both BAT and WAT, is derived from multiple cell lines, being neural crest cells the main contributors (Fu et al., 2019). Furthermore, brown adipocytes could arise from Myogenic Factor 5 (MYF5)-expressing myogenic precursor cells by the action of PRDM16 (PRD1-BF1-RIZ1 homologous domain containing 16). PRDM16 controls a bidirectional cell fate switch between skeletal myoblasts and BeAT cells and it is also responsible for beige cells found within WAT depots (Seale et al., 2008; Enerbäck, 2009; Lshibashi and Seale, 2010; Petrovic et al., 2010; Seale et al., 2011). PRDM16 gene expression is downregulated by miR-149-3p during fasting conditions allowing the switch from subcutaneous to visceral AT (Ding et al., 2016).
The specific origin of the beige adipocytes remains to be clarified. Some evidences support the idea that they arise from unique precursor cells (Wu et al., 2012), but others suggest that they may arise from white adipocytes in a process referred to as transdifferentiation (Cinti, 2012; Cinti, 2018).
Moreover, developed spots of both BAT and WAT present a remarkable adipocytes’ heterogeneity. Low- and high-thermogenic brown adipocytes with distinct features and functions coexist in BAT. Low-thermogenic brown adipocytes, unlike the high-thermogenic ones, show low Ucp1 and Adipoq expression, larger lipid droplets, lower mitochondrial content and are functionally specialized in fatty acid uptake (Song et al., 2020). Similarly, functional heterogeneity is found when comparing subcutaneous and omental preadipocytes, which show distinct capacities for replication, adipogenesis and apoptosis (Tchkonia et al., 2005).
To study in deep all aspects of adipocyte biology is required to know the molecular properties of adipose precursor cells and the ontogeny of fat cells in vivo (Gupta, 2014). Understanding the origin and differentiation of the different types of adipocytes might pave the way for future therapies for obesity, T2DM, cancer-associate cachexia and immunometabolic diseases (Stephens, 2012; Jung et al., 2019).
One of the most important open questions is the origin of the AT: which factor(s) are required for the specification of the AT primordium, how proliferation of ASC is regulated and how ASC differentiate into adipocytes. The identification and characterization of the ASC population is fundamental to understand AT development, formation and maintenance.
Equally important is to study how the ASC contribute to the homeostasis and maintenance of the AT under both normal energy intake and excess nutrient load. In the same way, it would be critical to know the growth factors and developmental signalling pathways altering ASC behaviour and adipocyte formation. Furthermore, although some factors determining fat cells fate has been described, most of these studies have been carried out in vitro and their role in vivo has not been explored (Berry et al., 2013; Gupta, 2014; Jung et al., 2019).
Last but not least, the growing number of functions in which the mammalian AT is involved require further studies, being the use of model systems an important tool to be exploited (Hotamisligil, 2017).
The ability to store nutrients, mainly in form of TAG, is conserved from yeast to human (Kadereit et al., 2008) (Figure 1). Even though a better knowledge on AT from an evolutionary perspective could improve our understanding on the development, function and dysfunction of this organ, this theme remain as a neglected enigma (Ottaviani et al., 2011). TAG synthesis and lipolysis related genes are already present in unicellular organisms such as Saccharomyces cerevisiae or Candida parapsilosis (Zweytick et al., 2000; Neugnot et al., 2002; Daum et al., 2007). Caenorhabiditis elegans also stores TAG in form of LDs in the intestinal epithelial cells (Watts, 2009).
FIGURE 1. Evolution of the adipose tissue (AT). Presence of fat cells, fat organs and proper AT is indicated in different phyla and species throughout evolution.
The ability to, not only store fat, but also to secrete endocrine factors is already present in molluscs. Haliotis fulgens or Helix aspersa store TAG in the midintestinal gland known as hepatopancreas. This also secretes a glucose lowering level hormone, Phe-Met-Arg-Phe-amide (FMRFa), belonging to the evolutionarily conserved RF-amide neuropeptide family (Rőszer and Kiss-Tóth, 2014). Neuropeptide FF (NPFF) an anorexigenic peptide, also member of this family, is critical to keep a basal NPY gene expression at arcuate nucleus and promote diet-induced thermogenesis, coupling energy homeostasis with energy partitioning to AT and bone tissue (Zhang et al., 2018). Moreover, NPFF is able to promote macrophage M2, anti-inflammatory phenotype, activation and increase the proliferation of murine and human adipose tissue macrophages (Waqas et al., 2017). Latter in evolution, the AT, in addition to serve as a fat storage and endocrine tissue, is also involved in immunity, as is the case of insects. The lipid storage tissue in insects is known as fat body (FB), which is an endocrine-secreting organ involved in nutrient sensing, development, metabolism, immunity and reproduction (Doane, 1960; Dean et al., 1985; Charroux and Royet, 2010).
WAT is present in most of vertebrate taxa, including fish, amphibian, reptiles and mammals (Gesta et al., 2007; Todorčević et al., 2009; Imrie and Sadler, 2010). Lampreys, at the base of vertebrates’ evolution, present fat cells with similar morphological characteristic to white and brown adipocytes (Müller, 1968), which is in conflict with the generally accepted idea about BAT is not present in cold-blooded vertebrates.
BAT is larger in small mammals, such as mouse, than in human. A recent debate points out to mice BAT, rather than the human adult BAT, as a classic defined BAT and, therefore, considers mouse the best model to study the development of this tissue (Cannon et al., 2020).
However, considering the complexity of the AT in mammals, a simpler model than mouse is required to study the development and determination of this tissue. A model organism that allows performing genetics analysis in vivo would be suitable to address those open questions. In this regard, Drosophila represents a good model for the study of AT based on its genetics accessibility, the lower complexity of the AT and the functional conservation of this tissue along evolution.
Drosophila melanogaster has been used for more than 100 years to study conserved biological processes and decipher the molecular and genetic basis of multicellular organisms, as well as a vast number of human diseases (Yamaguchi and Yoshida, 2018). Several studies have established Drosophila as a model to study obesity and metabolic diseases [reviewed at (Musselman and Kühnlein, 2018)]. Importantly, molecules and signalling pathways involved in the regulation of metabolism and physiology of the AT in mammals are conserved in Drosophila. For instance, Drosophila insulin/insulin like growth factor signalling (IIS) acts as a conserved satiety pathway promoting glucose uptake by peripheral tissues (Saltiel and Kahn, 2001) and sustaining sugar and lipid anabolic processes (Kim and Rulifson, 2004; Buch et al., 2008). Glucagon-like peptide adipokinetic hormone (Akh) signalling, conversely, is activated in response to reduced nutrient availability and promotes mobilization of energy reserves (Kim and Rulifson, 2004; Lee and Park, 2004; Bharucha et al., 2008). Short neuropeptide F (sNPF) is a functional homolog of mammalian orexigenic Neuropeptide Y (Nässel and Wegener, 2011), and its overexpression in sNPF-producing neurons causes hyperphagia and body fat accumulation in flies (Baumbach et al., 2014). Conversely, downregulation of this gene in sNPF-positive neurons reduces food intake (Lee et al., 2004). More recently, Drosophila has been demonstrated to be a good model to study T2DM. Flies fed on high sugar diet (HSD) develop diabetes showing increased levels of glucose in their hemolymph (blood-like system), insulin resistance and heart dysfunction (Palanker Musselman et al., 2011; Pasco and Léopold, 2012; Na et al., 2013). Drosophila has also been used as a model to identify new regulators of mammalian glucose metabolism (Ugrankar et al., 2015) and has made important contributions to understand the main components of signalling pathways involved in tumour development, including the cancer associated cachexia (Figueroa-Clarevega and Bilder, 2015; Enomoto et al., 2018; Saavedra and Perrimon, 2019).
Studies to fully understand the regulation of lipid metabolism in Drosophila are ongoing. It is well known that lipids are taken by adipocytes from the hemolymph, and are esterified and stored as TAGs and cholesterol esters. Moreover, the Drosophila FB, functionally equivalent to mammalian AT and liver, carries out glycolysis and lipogenesis using carbohydrates (Figure 2) [reviewed at (Arrese and Soulages, 2010)]. Also, cellular lipid uptake as well as lipid transport and lipoprotein metabolism has been well studied in Drosophila (Parra-Peralbo and Culi, 2011; Palm et al., 2012; Rodríguez-Vázquez et al., 2015; Yin et al., 2021). Furthermore, fly mutants in Lipin, a phosphatidate phosphatase required for normal insulin pathway signalling that plays a central role in FB function and energy metabolism, Seipin, a transmembrane protein with roles in ER calcium homeostasis and lipid storage, or Sik3 (Salt-inducible kinase 3), a kinase involved in lipid catabolism by regulating bmm gene expression show reduced lipid content and lipodystrophy (Li et al., 2019). All together make this model organism suitable to study different types of lipodystrophies.
FIGURE 2. Drosophila melanogaster fat body and mammalian functional orthologous organs. Left, schematic representation of Drosophila melanogaster adult animal. Fat body is in orange, heat in red and digestive system in green. *Adult Drosophila FB is represented here using a composition made by repeating a confocal image of a single panicle of one the dorsal abdominal segments from an adult female fly. Confocal image shows adipocytes in yellow (Nile Red staining) and oenocyte nuclei in blue (DAPI staining). Right, illustrations show mammalian liver and adipose tissue (cells in orange) infiltrated with immune cells (blue and purple cells).
As Edward O. Wilson said in “Letters to a young Scientist” for, each biological question there is a suitable system for discovering the answer (Wilson, 2013). In that case, we, as Azeez and collaborators, think that Drosophila melanogaster is a suitable system to identify the primordium of AT and the population of ASCs in adults, as well as to characterize the adult AT in order to understand the adipocyte biology (Azeez et al., 2014). As a proof of principle, Pospisilik et al (Pospisilik et al., 2010) found Hedgehog as a determinant of Brown versus White adipose cell fate, using Drosophila as a model system. In addition, a well-established cell linage tracing system, G-TRACE, has been a key tool for exploring origin, development and differentiation of tissues in Drosophila (Evans et al., 2009), only very recently available in mammalian model systems (Berry et al., 2013; Jung et al., 2019).
The Drosophila FB arises from the embryonic mesoderm (Hartenstein and Jan 1992). At stage 11, the progenitor fat cells arise from nine bilateral clusters of cells in the inner mesodermal layer that span the parasegments 4 through 12 and the mesoderm separates in the splanchnopleure and somatopleure. The somatopleure will give rise to the FB, somatic musculature and other cell types (Campos-Ortega and Hartenstein, 1985). FB cells’ lineage can be traced by analyzing the expression patterns of the genes Alcohol dehydrogenase (Adh), Collagen type IV alpha 1 (Col4a1), the steroid hormone receptor seven up (svp) and serpent (srp), as well as the enhancer-trap line 29D that exhibits an expression pattern restricted to developing embryonic fat cells (Hoshizaki et al., 1994) (Tables 3, 4). This enhancer-trap line allowed tracing the fat-cell lineage to nine bilateral cluster of cells within the emerging mesoderm, representing the progenitor fat cells. The svp-positive cells at stage 12 identified early precursor fat cells, and the expression of Adh and Col4a1 was used to identify the terminal fat cell differentiation at stage 15. By late stage 15/16 embryo, mature fat cells coalesce into a single cell thick FB layer throughout the abdomen and form three domains: the lateral FB, the dorsal fat cell projection, and the ventral collar (Miller et al., 2002). Finally, the expression of the GATA-like transcription factor Srp is a marker for the early stages of fat cell development (Sam et al., 1996). Other enhancer traps-lines that drive expression in fat cells at larval and adult stages are 3-76a, X8-157a, and l(3)2E2. Interestingly, l(3)2E2 regulates svp gene expression (Hoshizaki et al., 1995). Therefore, svp and the gene(s) near to the enhancer trap 29D were suggested to be key factors for determination and differentiation of embryonic FB (Tables 3, 4) (Hoshizaki et al., 1994).
The development of the FB requires the GATA-like transcription factor Srp, necessary and sufficient for the progression through the early stages and development of fat cells (Saltiel and Kahn, 2001; Buch et al., 2008; Musselman and Kühnlein, 2018). In fact, FB and gonads derive from mesoderm and abdA allows gonadal mesoderm to develop by repressing Srp function in this region (Moore et al., 1998).
The larval FB is a single cell layer that spreads along the larval body cavity, surrounding the gut and reproductive organs and being exposed to the hemolymph (TM. (1978); Dean et al., 1985). Larval FB contains 2200 cells, a number that remains constant throughout FB development. At larval stages, the FB growth is achieved by increasing cell size through endoreplication cycles, with successive rounds of DNA synthesis without mitosis (Butterworth et al., 1988; Britton and Edgar, 1998). Cell size changes are associated with the accumulation of LDs, glycogen deposits and protein granules. The endocycling progression in the FB cells requires the heteromeric transcription factor complex E2f1/E2f2/DP to repress telomere fusion (tefu) and suppress DNA damage responses (Guarner et al., 2017). In addition, endoreplication in the FB cells is tightly regulated in response to nutrition and depends on IIS (Britton et al., 2002).
Evidences suggest that the development of larval FB might require the expression of various unidentified genes, revealed by the expression of a number of enhancer traps (Table 4) including 3-76a, X8-157a, l(3)2E2. Specifically, the last one regulates the gene expression of svp, suggesting that Svp activity might be involved in that process. kruppel (kr) expression is not detected in fat cells during embryogenesis, nor during the first- and second-instar stages. However, Kr is expressed in fat cells at the stage previous to metamorphosis and in adults (Table 4). It is possible that Kr serves as a transcriptional regulator in the FB in this last larval instar (Table 3) (Hoshizaki, 1994; Hoshizaki et al., 1994). According to that, it has been found that Kruppel-like factor 11 (KLF11) is a novel browning transcription factor in human adipocytes (Loft et al., 2015).
At the end of the larval development, the FB undergoes a remodelling process with massive autophagy that initiates the pupal transition. The larval FB decreases gradually throughout metamorphosis, and during the first 3 days of adulthood, until no more cells can be observed.
The Drosophila larval FB is involved in multiple functions that allow the coordination of the metabolic homeostasis. Larval FB extends as a longitudinal fat sheet at each larval body side. Salivary glands present also an associated-FB whose function is unknown. The most important functions of this tissue include the storage and release of energy, the nutrient sensing function, and the role in the systemic immunity (Figure 3). These functions are regulated by hormones and require the crosstalk of the FB with other tissues. The pathways that adjust the growth rate to the nutritional conditions are the IIS and the target of rapamycin (TOR) pathways, and those involved in the systemic immunity are the Toll and Immune deficiency (Imd) pathways. In the next sections, we review the current knowledge about the role of these signalling pathways and the main factors involved in the different functions of the larval FB and in its communication with other tissues.
FIGURE 3. Schematic representation of the diverse functions of Drosophila fat body in larvae. Top, larvae brain represented in soft purple. Abbreviations: Akh Adipokinetic hormone; AkhR, Akh receptor; AMPK, AMP-activated protein kinase; CC, corpora cardiaca; CCHa2, CCHamide-2; Daw, Dawdle; Ec, ecdysone; EcR, ecdysone receptor; Egr, Eiger; GBP1/2, Growth blocking peptide 1/2; ImpL2, Imaginal morphogenesis protein-late 2; Imd, Immune deficiency; Ilps, insulin-like peptides; Ilp6, Insulin-like peptide 6; IPC, insulin producing cells; IR, insulin receptor; miR-8, mir-8 stem loop; PG, prothoracic gland; Sun, Stunted; Svp, Seven up; TOR, target of rapamycin; Upd2, Unpaired 2.
Similar to the mammalian WAT, the Drosophila larval FB stores and releases energy in response to the organism energetic demands. The energy is stored mainly in the form of glycogen and of TAGs, the lipolysis products of those being transported to other tissues to support growth and survival.
In Drosophila, glycogen is the main storage form of carbohydrates and is found in the body wall muscles and in the FB in late larval stages (Baker and Thummel, 2007; Garrido et al., 2015). In addition to glycogen, trehalose is synthesized in the FB and released into the hemolymph. Upon starvation, glycogen is mobilized to maintain the circulating sugar levels (Mattila et al., 2015; Yamada et al., 2018). In mammals, the sensing of sugar at intracellular levels is mediated by the heterodimer formed by the conserved bHLH-Zip transcription factors ChREBP (Carbohydrate Response Element Binding Protein) and MondoA, together with their common partner Mlx (Max-like protein X), which are activated by sugars and promote the conversion of sugars to lipids. They control most of the sugar-responsive genes as well as carbohydrate, amino acid and lipid metabolism (Havula and Hietakangas, 2012; Mattila et al., 2015). In Drosophila, the single orthologs of ChREBP/Mondo and Mlx are Mondo and Bigmax, respectively, and this transcriptional network is essential for sugar tolerance also in this organism. Accordingly, the Mondo-Bigmax deficient Drosophila larvae presents lethality on any diet containing high levels of sucrose, glucose or fructose (Havula et al., 2013). In addition to the regulation of metabolic genes, Mondo-Bigmax regulate the expression of the TGFβ/Activin ligand Dawdle (Daw), the Gli-similar transcription factor Sugarbabe and the orthologue of mammalian Kruppel-like factors 10 and 11, Cabut (Bartok et al., 2015; Mattila et al., 2015). As detailed in next sections, the intracellular glucose sensing by Mondo-Bigmax is coupled to systemic growth through Daw. Other nutrient sensors involved in sugar tolerance are the nuclear receptor Hnf4 (Hepatocyte nuclear factor 4) and the NAD+-dependent deacetylase Sirtuin 1 and 2 (Sirt1, Sirt2). Hnf4 plays a critical role in carbohydrate metabolism as shown by the Hnf4 mutant larvae, which display highly elevated circulating glucose and trehalose levels and defects in lipid homeostasis (Palanker et al., 2009; Palu and Thummel, 2016). Sirt2, is required in the FB to maintain glucose homeostasis and peripheral insulin sensitivity by deacetylating and stabilizing Hnf4 through protein interactions (Palu and Thummel, 2016). Moreover, Sirt1 negatively regulates TAG accumulation in the larval FB (Reis et al., 2010).
TAG is the main lipid form in the FB, which is synthesized from dietary carbohydrates, fatty acids or proteins and is stored in intracellular LDs. Similarly to mammals, LDs of different sizes belong to distinct functional classes, which differ in their properties owing to differential association with particular sets of LD-associated proteins (Wilfling et al., 2013). Characterization of the LD proteome at different stages uncovered that LD-associated proteins are different according to the functional complexity among LDs (Cermelli et al., 2006; Beller et al., 2010; Walther and Farese, 2012; Krahmer et al., 2013). The best characterized LD proteins in the FB during the larval life are Lsd-1 and Lsd-2 (Lipid storage droplet-1 and -2), homologous to the mammalian PAT domain protein family (Perilipin, ADRP, and TIP47) (Grönke et al., 2003; Teixeira et al., 2003). Lsd-2 is required for storage of TAG, whereas Lsd-1 stimulates TAG hydrolysis (Bi et al., 2012). The subproteome analysis of LDs of Drosophila FB identified 248 proteins (Beller et al., 2006). Most of them were involved in cellular metabolism but proteins have been identified with diverse biological functions, including intracellular transport, cell organization and cell biogenesis. For instance, the droplet-associated protein Sturkopf has a role in endocrine physiology regulation (Werthebach et al., 2019). The sturkopf mutant adults show a mild decrease in TAG storage levels. However, they fail to adjust their developmental rate to dietary yeast-to-sugar ratio changes, suggesting a function in insulin and juvenile hormone signalling activities. Moreover, distinct spatially LD populations have been described in Drosophila FB: the peripheral LDs, in contact with the plasma membrane, and the larger cytoplasmic medial LDs. The peripheral LD homeostasis is regulated by Snazarus (Snz), which binds to LDs and promotes TAG storage (Ugrankar et al., 2019).
Interestingly, the regulation of lipid homeostasis is coupled to FB development and growth. For example, Lipin, which converts phosphatidate to diacylglycerol, is required for normal FB development and TAG storage (Ugrankar et al., 2011). Loss of Lipin in Drosophila leads to severe defects in the development of the FB with changes in cell nucleus, mitochondria, autophagosome formation and size of LDs. Similarly, the Drosophila BMP-5,7 orthologue, glass bottom boat (gbb), is also required for the development of the larval FB and for maintaining proper metabolism. gbb mutants exhibit developmental delay and altered FB morphology with reduced total lipid, glucose and trehalose levels (Ballard et al., 2010). A recent study shows that the FB expression of the atypical MAP kinase, Erk7 (Extracellularly regulated kinase 7), inhibits cell autonomous and systemic growth and lipid storage. Erk7 expression is upregulated by fasting and, therefore, contributes to the adaptation to nutrient shortage. Erk7 regulates the subcellular localization of the chromatin binding protein No child left behind (Nclb), a growth-promoting downstream effector of mTOR, and inhibits the expression of the lipogenic transcription factor gene sugarbabe (Hasygar et al., 2021).
The energy storage in the FB during the larval development is required during low nutrient conditions and for the survival during the non-feeding periods, such as before and during metamorphosis and during the early stages of adulthood. The maintenance of the metabolic homeostasis requires the communication between the nutrient-storing FB and the consuming tissues.
In mammals, the main hormones that regulate the mobilization of fat and glucose are insulin and glucagon (Freychet P. 1990). Insulin is secreted by pancreatic β cells in response to high blood sugar levels, which triggers glycogen synthesis. Under low sugar levels pancreatic α cells release glucagon and triggers the breakdown of glycogen. Glucagon is also a lipolytic hormone that regulates fatty acids, ketone bodies and TAG.
In Drosophila, the insulin/glucagon axis is well conserved and involves the insulin-like peptides (Ilps) and the glucagon-like peptide Akh (Schaffer et al., 1990; Semaniuk et al., 2021). The mobilization of carbohydrate and lipid energy reserves from the FB in response to starvation is regulated by Akh/AkhR, which is produced by the neurosecretory cells of the corpora cardiaca (Kim and Rulifson, 2004; Lee and Park, 2004; Isabel et al., 2005). For carbohydrate mobilization, Akh/AkhR stimulates, through glycogen phosphorylase, the conversion of stored glycogen to hemolymph trehalose, which is important during the nonfeeding periods and during adult flight. The lipid mobilization through the action of Akh/AkhR, led to the phosphorylation of Lsd-1, which activates the Triglyceride Lipase (TGL) (Arrese et al., 2006; Arrese and Soulages, 2010). However, the role of Akh/AkhR is not completely elucidated as some reports suggest that Akh/AkhR is dispensable for lipid homeostasis in third instar larvae (Lee and Park, 2004; Gáliková et al., 2015). A recent report shows that, although in nutrient abundant conditions Akh/AkhR is dispensable during larval development, in low nutrient stress conditions Akh/AkhR signalling alters larval development and the adult metabolism and behaviour (Hughson et al., 2021).
In mammals, the mobilization of fatty acids from TAG storage is coordinated by the hormone-sensitive lipase (HSL) and the Patatin Like phospholipase Domain Containing 2 (PNPLA2, also known as ATGL) (Zimmermann et al., 2004; Young and Zechner, 2013). Interestingly, ATGL-dependent lipolysis of WAT triggers a systemic insulin release, which is essential for the replenishment of BAT energy storage in mice (Heine et al., 2018). In Drosophila, independently of Akh/AkhR signalling, the Brummer (Bmm) lipase, homolog of mammalian ATGL, converts the accumulated TAG to fatty acids (Grönke et al., 2005).
The FB acts as a sensing organ that coordinates the metabolic and physiological responses to the nutrient status of the organism. The FB relays the nutrient information through the secretion of humoral factors to the insulin-producing cells (IPCs), which secrete Ilps to control the systemic ISS.
In Drosophila FB, the IIS and the TOR pathways regulate nutrient uptake, storage and metabolism. In addition, there is a crosstalk between the steroid hormone 20-hydroxyecdysone (ecdysone) and those pathways. Furthermore, the FB is the main sensor of internal oxygen levels that control organismal growth.
The Drosophila genome encodes eight Ilps (Grönke et al., 2010): Ilp, 2, 3 and 5 are produced by IPCs in the brain and are functionally comparable to insulin; Ilp6, produced by the FB, is related to mammalian Insulin Growth Factors, IGFs (Okamoto et al., 2009; Slaidina et al., 2009); Ilp7 and Ilp8 are relaxin-like peptides (Grönke et al., 2010). Similar to mammalian insulin, Ilps are able to regulate circulating levels of carbohydrates in the hemolymph. Insulin is a positive regulator of fat cell mass, acting through changes in both cell number and lipid storage (DiAngelo and Birnbaum, 2009). Ilp2 and Ilp5 regulate glycogen deposition, Ilp3 is responsible for the synthesis and release of trehalose into hemolymph and Ilp5 and Ilp7 regulate the synthesis of TAG (Kim and Rulifson, 2004; Post et al., 2018; Semaniuk et al., 2021). In addition, IIS/PI3K (Phosphatidylinositol 3-kinase) signalling coordinates nutritional status with endoreplicating tissues metabolism and growth (Britton et al., 2002). Thus, insulin regulates the critical weight, a checkpoint that occurs early in third instar larvae that determines the final body size (Mirth and Riddiford, 2007).
Mammals and Drosophila use the TOR pathway for cellular nutrient sensing, playing an important role in the balance of energy storage. The TOR kinase activity depends on amino acid availability and mediates protein synthesis, amino acid import, ribosome biogenesis and autophagy (Saxton and Sabatini, 2017). Consequently, Tor mutant larvae show reduced size and glucose and lipid storage levels, larvae showing a transparent phenotype (Colombani et al., 2003; Luong et al., 2006).
In addition, there is crosstalk between IIS and ecdysone. Ecdysone signalling in the FB antagonizes IIS and promotes autophagy (Rusten et al., 2004; Colombani et al., 2005). Furthermore, ecdysone modulates organismal growth through a FB relay that attenuates systemic insulin signalling (Colombani et al., 2005; Arquier et al., 2008; Honegger et al., 2008; Jin et al., 2012; Lee et al., 2018).
In Drosophila and other animals, the organisms require sensing the levels of oxygen to adapt their systemic growth to the environmental conditions. A central regulator for the maintenance of oxygen homeostasis is the hypoxia-inducible factor 1 (HIF-1), a heterodimeric transcription factor composed of the oxygen regulated HIF-1α and the constitutively expressed HIF-1β. In presence of oxygen, HIF-1α is hydroxylated by HIF prolyl hydroxylase (Hph), targeting it for ubiquitin-dependent proteasomal degradation. In hypoxia, HIF-1α is stabilized and induces the expression of target genes that regulate growth and metabolism (Semenza, 2014).
To link the organismal growth to the nutrient availability, the FB produces signalling molecules that promote or inhibit the insulin secretion from IPCs (Figure 3 and Table 3). Some of these factors and neuropeptides are secreted in response to dietary fats and/or sugars such as Unpaired 2 (Upd2), Daw and CCHamide-2 (CCHa2).
Upd2, a JAK/STAT cytokine (Rajan and Perrimon, 2012), binds to its receptor Dome (Domeless) on GABAergic neurons, releases the inhibition of IPCs and promotes Ilp secretion. Recently, an essential role for adipose p53 in sensing nutrient stress and maintaining metabolic homeostasis has been reported (Ingaramo et al., 2020). Under nutrient deprivation and high-sugar diet, p53 is activated in the FB and represses the expression of Upd2. This AMP-activated protein kinase (AMPK)-dependent p53 activation leads to modulation of Ilp2 levels, systemic insulin/TOR signalling and autophagy induction (Ingaramo et al., 2020). Another response to the consumption of sugar is the release by the FB of the activin-like factor Daw, which promotes the secretion of Ilps through the TGF-β/activin receptor Baboon (Babo) (Ghosh and O’Connor, 2014). In addition, Daw released from the FB signals to the intestine where inhibits the expression of carbohydrases and lipases by enhancing Smad on X (Smox) levels (Chng et al., 2014). The sugar induced gene expression of Daw is mediated by Mondo-Bigmax, whereas Foxo (forkhead box, sub-group O) negatively regulates its expression (Bai et al., 2013; Mattila et al., 2015). A third mechanism by which carbohydrates promote Ilp expression and secretion is through CCHa2, a neuropeptide induced in the FB by proteins and sugars. When released, the CCHa2 peptide promotes the secretion of Ilp2 and Ilp5 via its receptor, CCHa2R, expressed in the IPCs (Ren et al., 2015; Sano et al., 2015).
TOR-dependent FB humoral signals couple Ilp2 and Ilp5 secretion from the IPCs with amino acid intake and some humoral factors are secreted in response to dietary amino acids such as Stunted (Sun), Eiger (Egr) and the Growth blocking peptides GBP1 and GBP2 (Colombani et al., 2003; Honegger et al., 2008; Géminard et al., 2009; Rajan and Perrimon, 2012; Ghosh and O’Connor, 2014; Sano et al., 2015; Agrawal et al., 2016; Delanoue et al., 2016; Koyama and Mirth, 2016). Interestingly, amino acid-dependent TOR signalling derived from the FB controls neural stem cell proliferation independent from IPCs-derived Ilps. In the developing central nervous system, embryonic and larval neuroblasts undergo proliferative phases, intercalated with periods of a quiescent state, that is reversible by dietary amino acids (Britton and Edgar, 1998). The TOR-mediated amino acid sensing induces a secreted FB signal that activates the expression of Ilps in glial cells. The local glial Ilps signal on adjacent neuroblasts via the IIS/PI3K/TOR pathway and control their reactivation (Chell and Brand, 2010; Sousa-Nunes et al., 2011).
Furthermore, ecdysone signalling in the FB modulates insulin dependent systemic growth through the regulation of Myc, microRNA miR-8 and ImpL2 (Ecdysone-inducible gene L2), a member of the immunoglobulin superfamily homolog to the Insulin-Like Growth Factor Binding Protein 7, IGFBP7 (Arquier et al., 2008; Honegger et al., 2008; Hyun et al., 2009; Delanoue et al., 2010; Jin et al., 2012; Lee et al., 2018).
The FB is a sensor tissue for amino acid levels and coordinates growth of peripheral tissues through a humoral mechanism (Colombani et al., 2003). Hence, the downregulation of the Slimfast (Slif) amino acid transporter within the FB is sufficient to induce a general reduction in the rate of larval growth (Colombani et al., 2003). In response to dietary amino acids, the peptide Sun is released from the FB (Delanoue et al., 2016). Sun binds to Methuselah (Mth), a secretin-incretin receptor on IPCs, and stimulates the secretion of Ilps. On the other hand, under conditions of low amino-acid concentrations, Egr, a Drosophila tumor necrosis factor alpha (TNF-alpha) orthologue is released from the larval FB (Agrawal et al., 2016). This cytokine signals through its receptor Grindelwald (Grnd) on the larval IPCs to activate the JNK-dependent inhibition of Ilps production. The expression of the endoplasmic reticulum (ER) degradation enhancing α-mannosidase-like protein 1 (Edem1) in the FB is also crucial for maintaining systemic insulin signalling, since its down-regulation results in the accumulation of Ilp2 in the IPCs and reduced systemic insulin signalling. The reduction in Edem1 levels is crucial for survival during starvation as lowering edem1 expression levels facilitates the activation Eiger on IPCs and the reduction in ISS. In addition, Edem1 regulates Upd2 to manage the metabolic status (Pathak and Varghese, 2021). Moreover, Growth-blocking peptides 1 and 2 (GBP1 and GBP2) are epidermal growth factors-like cytokines secreted by the FB upon availability of dietary amino acids (Koyama and Mirth, 2016). Recently, it was shown that these adipose tissue factors regulate Ilps secretion by silencing a pair of inhibitory neurons that synapse with IPCs (Meschi et al., 2019).
During late larval life, increased levels of ecdysone affect also systemic growth. Myc expression in the Drosophila FB triggers a cell autonomous mechanism that controls glucose and lipid metabolism to favour the storage of nutrients (Parisi et al., 2013). During the late third instar, ecdysone signalling represses Myc function inhibiting systemic growth. This suggests a humoral factor released downstream of Myc that relays information to control IIS (Delanoue et al., 2010). The ability of FB Myc activity to affect IPC Ilp2 secretion depends on stearoyl-CoA desaturase (Desat1) activity, an enzyme necessary for production of fatty acids and lipid biosynthesis (Parisi et al., 2013). The increased levels of ecdysone suppress the body growth also through the regulation of FB microRNA miR-8 (Hyun et al., 2009; Jin et al., 2012). Multiple peptide hormones regulated by miR-8 may contribute to Drosophila growth (Lee et al., 2015). Among them, the IGF-like factor Ilp6 and the Imaginal morphogenesis protein Late 2 (ImpL2) are upregulated in the FB of miR-8 null mutant larvae. Ilp6 expression from larval FB represses secretion of Ilp2 from IPCs and extends lifespan (Bai et al., 2012). Before and during pupariation or in response to starvation, Ilp6 communicates the FB with other organs. For example, it promotes the growth of imaginal discs, which gives rise to adult organs, and the lipid uptake in oenocytes, cell clusters of ectodermal origin that regulate lipid metabolism (Chatterjee et al., 2014). Nutritional restriction also increases the levels of ecdysone, which triggers the production of ImpL2 in the FB (Lee et al., 2018). In response to nutrient limitation, the FB nutrient sensor function, which restricts the growth of peripheral tissues, is complemented by the release of nutrients through autophagic degradation of the FB cytoplasm. This provides other tissues with a source of nutrients necessary for survival. Thus, under conditions of low TOR signalling, autophagy promotes normal cell function and survival (Scott et al., 2004).
To adapt the systemic growth to the environmental conditions, the FB integrates the oxygen and amino acids levels through the Hph/HIF-1α and Hph/TOR pathways. In hypoxia, the FB release HIF-1α-dependent humoral factors that inhibit Ilps expression and secretion from the IPCs, thereby restricting the systemic growth. Moreover, independently of HIF-1α, Hph is required for nutrient-dependent TOR activation (Texada et al., 2019). To allow adults viability in hypoxia, the larval FB inhibits TORC1 signalling and reorganizes the lipid storage (Lee et al., 2019). A recent study showed that FOXO is a hypoxia inducible factor that mediates tolerance to low oxygen by inducing immune-like responses in the FB (Barretto et al., 2020).
The Drosophila FB coordinates not only the nutrient storage and the animal growth but also the humoral immune response. In Drosophila, the infection by microbes induces the secretion of antimicrobial peptides (AMP) by the FB, which are controlled by the Toll and Imd pathways (De Gregorio et al., 2002). The Toll-NF-kB signalling, which triggers the nuclear translocation of Dif (Dorsal-related immunity factor) and Dorsal, is induced by fungi and Gram-positive bacteria, whereas infection by Gram-negative bacteria leads to the processing and transport or Relish via the Imd pathway. To support the immune activation, the FB increases its volume, expands the ER and alters its metabolism, shifting from lipid metabolism to membrane phospholipid synthesis (Martínez et al., 2020). These changes, induced by Toll signalling to sustain AMP synthesis and secretion, may become detrimental if maintained over long periods due to insufficient nutrient storage. Thus, the expression of a constitutively active Toll receptor in the larval FB inhibits the whole organismal growth, disrupts the insulin signalling in the FB and reduces the TAG storage (DiAngelo and Birnbaum, 2009; Roth et al., 2018; Suzawa et al., 2019). Similarly, persistent activation of the Imd pathway in the larval FB diminished IIS/TOR activity, which resulted in decreased TAG levels and impaired whole animal growth (Davoodi et al., 2019). Moreover, increasing insulin signalling in the FB leads to decreased immune gene expression and, vice versa, decreasing insulin signalling leads to increased immune gene expression and increased resistance to infection (Musselman and Kühnlein, 2018). This supports a model in which insulin signalling and the immune response negatively regulate each other to maintain the energy balance.
The origin of the adult FB, in invertebrate, as in mammals, remains elusive and unexplored due to the difficulty in its manipulation (The mesoderm and its derivatives and BM, 1993; Hoshizaki et al., 1995; Berry et al., 2013). Although both larval and adult FBs play a role as energy storage organs and nutrient availability sensing, they show different features. For example, contrary to the larval FB, adult FB is able to expand by increasing the number of adipocytes. Moreover, they might not share a common origin: while larval FB derives from the nine embryonic bilateral primordia, the origin of the adult FB has not been identified (Hoshizaki et al., 1994; Hoshizaki et al., 1995; Aguila et al., 2007).
During metamorphosis, unlike most larval tissues that undergo histolysis, some of the larval FB cells persist and are found in the newly eclosed adult, free floating as single cells or small clusters. These larval fat cells are refractive to the autophagic cell death that removes most of the larval cells during metamorphosis. It has been shown that these larval adipocytes, now dissociated, are a source of nutrients during the non-feeding stage of adulthood, approximately the first 3 days after eclosion (Aguila et al., 2007). Three to five days after eclosion these cells are replaced by the adult fat adipocytes (Johnson and Butterworth, 1985), which accumulate lipid reserves through feeding and de novo lipid synthesis during those days. The myokine Pvf1 (PDGF- and VEGF-related factor 1) represses lipid synthesis at the end of the adult FB lipid build-up phase by activating TOR pathway specifically in the oenocytes (Ghosh et al., 2020). Adult adipocytes must develop from some pupal progenitors, the specific cells that give rise to the adult fat cells have not been identified (The mesoderm and its derivatives and BM, 1993).
The development of adult FB might require the expression of genes driven by a number of enhancers that are identified through enhancer traps (Table 4). Most of them, except for 29D and RD61, drive the expression in larval FB as well as in adult one 3-76a, X8-157a and l(3)2E2 driving the expression in fat cells of all stages. As l(3)2E2 is an enhancer of the srp gene, the activity of Srp might be also involved in fat cell decision or/and differentiation programmes at adult stage (Tables 3, 4) (Hoshizaki et al., 1995).
Although the cells that give rise to the adult fat cells have not been identified, two fundamentally different mechanisms have been suggested to explain how the adult FB arises: 1) cell remodelling, a process in which larval FB tissue is dissociated into isolated cells that later associate to form the adult FB (Larsen, 1976), or 2) the complete destruction of larval FB and simultaneous synthesis of adult FB from undifferentiated ASC (Haunerland and Shirk, 1995).
It has been shown that adult FB derives from the mesoderm (Lawrence and Johnston, 1986). However, the ASC population that maintain the adult FB has not been identified. Hoshizaki et al. suggested that a subset of adepithelial cells, precursors of adult thoracic muscles, might be as well the precursors of adult adipocytes (Hoshizaki et al., 1995). Adepithelial cells are in fact a plausible source of ASCs, since two of the fat cells-specific enhancer traps mentioned above, 3-76a and X8-157a, are also active in the adepithelial cells. This suggests a possible lineage connexion for fat cells from embryo to adult, including the adepithelial cells during larval stage. Furthermore, adepithelial cells are the precursors of adult muscles (Holz et al., 1997) and a subset of these cells expressing Breathless are the precursors of the adult tracheal air sacs (Sato and Kornberg, 2002). This might suggest that adepithelial cells could potentially be the pluripotent stem cell population in the adult stage.
In spite of the fact that most of the functional studies in Drosophila are conducted at larval stages, there are enough evidences to ensure that the adult FB carries out liver, adipose, and immune functions (Hotamisligil, 2017; Arrese and Soulages, 2010). Oenocytes, specialized hepatocyte-like cells, are closely associated to adipocytes, specifically at the subcuticular FB (Figure 2) (Gutierrez et al., 2007). In fact, very recently, the role of oenocytes regulating lipid synthesis and content in the adipose tissue has been described, a role-played by hepatocytes in mammals. Furthermore, loss of function of TOR pathway in adult oenocytes leads to obesity (Ghosh et al., 2020).
Although further studies would be necessary to prove the equivalence of these organs, there is a subcuticular FB that is extended through the whole Drosophila adult body (Figure 2), and also a FB wrapping some organs such as the heart, intestine, spermatheca and brain, and these could be the equivalent to mammalian subcutaneous and visceral WAT, respectively.
There are not many evidences indicating the existence of a BAT or beige adipocytes tissue in Drosophila. However, a set of genes coding for Uncouple proteins, including UCP1 that is a marker for BAT in mammalian systems, are conserved in Drosophila (Harms and Seale, 2013). Similarly to UCP1, Drosophila Ucp4C has been involved into the dissipation of energy in the mitochondria (Cannon and Nedergaard, 2004; Da-Ré et al., 2014).
Adult FB has an important role in physiology, longevity as well as disease, e.g., cancer (Figure 4).
FIGURE 4. Schematic representation of the diverse functions of Drosophila fat body in adult fly. Left, adult digestive system represented in green; top, adult brain represented in soft purpure. Abbreviations: Akh Adipokinetic hormone; AkhR, Akh receptor; bmm, brummer lipase gene; Burs, Bursicon receptor; CC, corpora cardiaca; Dlgr2, leucine rich repeat containing G protein-coupled receptor 2; HDAC4, Histone desacetylase 4; ImpL2, Imaginal morphogenesis protein-late 2; Ilps, insulin-like peptides; IPC, insulin producing cells; IR, insulin receptor; Lkb1, Liver kinase B1; oe, oenocytes; Pvf1, PDGF- and VEGF-related factor 1; PvR, Pvf1 receptor; Sik3, Salt-inducible kinase 3; Svp, Seven up; TOR, target of rapamycin.
Similarly to the larval one, the adult FB is the central metabolic organ involved in the accumulation of fat and glycogen from caloric overload and in the mobilization of the stored fat during starvation or egg production (Lee and Park, 2004; Parra-Peralbo and Culi, 2011; Musselman et al., 2013; Mattila and Hietakangas, 2017; Zhao and Karpac, 2017; Weaver and Drummond-Barbosa, 2019). Not surprisingly, in females the FB has higher proportion of lipids than that in males (Johnson and Butterworth, 1985). In contrast to larval FB there are evidences suggesting the ability of adult FB to grow in order to accumulate lipids, in obese flies (DiAngelo and Birnbaum, 2009).
In the adult FB, Akh/AkhR signalling activates cAMP-responsive element binding (CREB) transcription factor (Song et al., 2017). CREB downregulation was shown to promote overeating and obesity in adult flies (Iijima et al., 2009). In addition, Akh/AkhR signalling modulates TAG content in adult FB through the store-operated calcium entry (SOCE) (Baumbach et al., 2014). Under short-term fasting conditions, Akh/AkhR signalling promotes lipase bmm gene expression by reducing Lkb1-Sik3 (Salt-inducible kinase 3)-HDAC4 (Histone deacetylase 4) signalling axis, probably through Foxo (Wang et al., 2011; Choi et al., 2015). Under long-term fasting conditions, however, the reduction of the Lkb1-Sik3 pathway to induce the lipolytic response is independent of Akh/AkhR. Conversely, insulin pathway induces Sik3 activity under feeding conditions, independently of Lkb1 (Choi et al., 2015) (Figure 4).
Recently, Relish known to be part of Imd pathway, as mentioned above, has been identified as a repressor of bmm gene expression through FOXO, by fasting-dependent histone deacetylation, during metabolic adaptation to fasting (Molaei et al., 2019).
Recently, an intestinal/neuronal/FB inter-organ communication has been described in adults to preserve energy homeostasis. In response to nutrients, enteroendocrine cells secrete systemically the hormone Bursicon α (Bursα), which binds to its neural receptor DLgr2. Bursα/DLgr2 signalling regulates energy metabolism through a neuronal relay that repress AKH production and, therefore, the subsequent modulation of AKHR signalling within the FB. The reduction of systemic Bursα/DLgr2 signalling leads to exacerbated glucose oxidation, strong lipodystrophy and depletion of energy stores with the consequent reduced organismal resistance to nutrient deprivation conditions (Scopelliti et al., 2019). Therefore, Bursicon inhibits the mobilization of glycogen storage under nutrient availability (Figure 4).
The overexpression in the adult FB of the gene foxo, encoding for the key target of the IIS pathway, leads to increased life span (Giannakou et al., 2004). Similarly, overexpression of the gene Sirt2 in adult FB increases longevity in both sexes. It also modulates the composition of the LD proteome, a plausible mechanism underlying extended longevity by Sirt2, as LDs regulate aging processes (Goldberg et al., 2009; Hoffmann et al., 2013). All these evidences point to a role of the adult AT in controlling longevity.
Tumors and their microenvironment can produce different circulating factors that cause cachexia, the wasting syndrome observed in advance cancer patients which is characterized by a general metabolic dysfunction that includes systemic inflammation, increased catabolism and lipolysis or proteolysis in muscles and AT (Ebadi and Mazurak, 2014). In Drosophila, two main models of cachexia have been described which show similarities with human patients (Figueroa-Clarevega and Bilder, 2015; Kwon et al., 2015; Song et al., 2019). One of the models is induced by activation of Yorkie (Yki), the Yap1 oncogen ortholog, in intestine stem cells that secrete a PDGF-and VEGF-related factor 1 (PvF1) ligand. Pvf1 leads to the pathological activation of ERK/MAPK signalling in peripheral tissues and induce wasting of muscles and AT (Song et al., 2019). The other model consists of the transplantation, in adult flies, of clones of eye disc cells mutant for the polarity gene scribble and ectopically expressing an activated form of Ras (V12) (RasV12, scrib−/−). Interestingly, both tumor models secrete high levels of ImpL2 (Figueroa-Clarevega and Bilder, 2015; Kwon et al., 2015). Increased levels of circulating ImpL2 reduce systemic insulin signalling, which leads to reduction of nutrients uptake by muscle and adipose tissue, driving organ wasting. The RasV12, scrib−/− tumors also induce a systemic autophagy stress response in muscles and AT that mediates organ wasting (Katheder et al., 2017; Khezri et al., 20215). Recently, another wasting model in Drosophila, relates the FB remodelling and muscle detachment to the tumor-secreted matrix metalloproteinase 1 (Mmp1). Mmp1 can modulate TGFβ signalling in the FB and disrupts the basement membrane/extracellular matrix in FB and muscle (Lodge et al., 2021). All theses studies show that the conservation of the signalling pathways and the existing genetic tools, make of Drosophila an important model to study the process of organ wasting and to identify new molecular mechanisms involved in this process.
The FB acts as a detoxifying tissue based on the expression of members of the Cytochrome P450 (Cyp450) superfamily of monooxygenases. These are enzymes involved in metabolizing foreign substances and drugs implicated in resistance to insecticides (Feyereisen, 1999; Chung et al., 20097; Terhzaz et al., 2015).
Recently, Weaver and Drummond-Barbosa showed that the nuclear receptor Svp regulates a number of factors involved in immunity and xenobiotic detoxification responses in adult female FB (Weaver and Drummond-Barbosa, 2020). Specifically, Svp would acts as the first line of defence against infections, regulating genes involved in the capture and elimination of foreign pathogens. Svp also regulates the expression of genes encoding members of the CYP450 family involved in the initiation of phase I of the xenobiotic detoxification response. Reduction of svp expression results in the upregulation of genes encoding Metallothionein A and B (MtnA and MtnB) (Weaver and Drummond-Barbosa, 2020). MtnA and B are enzymes involved in heavy metal detoxification and protection against free radicals and have been involved in the response to xenobiotic and immune stress (Bonneton et al., 1996). It has been suggested that a reduced activity of Svp could lead to a toxic scenario, which would need MtnA and B activity to eliminate this toxicity (Weaver and Drummond-Barbosa, 2020) (Figure 4).
The AT is a central organ, which regulates metabolism and immune responses, as inflammation, so that it has a major impact on human physiology. AT dysfunction associates to metabolic diseases such as: obesity, diabetes, lipodystrophies and cancer-associated cachexia.
Despite of the advance in the knowledge in the last years, still there are many open questions that need to address about the functions and development of AT.
However, the knowledge at this moment can only be obtained through the studies of animal models. Drosophila can be a good model for the study of AT based on the possibility of the genetics analysis that can be performed in vivo, the lower complexity of the tissue and the functional conservation of this tissue along the evolution.
Further studies focused on tracing the cell lineages expressing the transcription factors Svp, Srp and Kr, involved in the determination and differentiation and maintenance of fat cells during embryonic and larval stages, would shed light on how those processes develop and what are the actors involved. Similarly, it would be very interesting to trace the cells showing expression driven by the enhancer traps l(3)2E2, 3-76a and X8-157, which show expression in embryonic, larval and adult fat cells.
Adepithelial cells could potentially represent the ASC population of adult FB in Drosophila. Future characterization of the gene expression profile of this population will help to understand the origin and cellular differentiation of adult adipocytes. Also, it will reveal the mechanisms leading to the different adipocyte fates as well as putative fate-switching factors.
Most of the studies that shed light on the functions carried out by FB were conducted at larval stages. Therefore, further studies are needed to characterize and identify potentially new functions of the adult AT related to regulation of energy homeostasis and immunity that may be conserved in mammals. The identification of adult FB-secreted derived signals would drive to a comprehensive understanding of the roles that this organ is playing in inter-organ communication and in AT wasting, which would help to understand human cancer-associated cachexia and other diseases like obesity and DMT2.
EP-P conceived the idea, wrote the manuscript and elaborated the figures and tables. AT wrote the manuscript and reviewed the figures and tables. RB wrote/reviewed the manuscript, reviewed the figure and reviewed/elaborated the tables.
EP-P acknowledges the funding from European University to support this work. RB acknowledges funding by grants BFU 2017-84653-P and PID 2020-114178GB-I00 (MINECO/MICINN/FEDER, EU), SEV-2016-0644 (Severo Ochoa Excellence Program), SAF 2017-90900-REDT (UBIRed Program), 765445-EU (UbiCODE Program) and IT1165-19 (Basque Country Government). Additional support was provided by the Department of Industry, Tourism, and Trade of the Basque Country Government (Elkartek Research Programs) and by the Innovation Technology Department of the Bizkaia County.
The authors declare that the research was conducted in the absence of any commercial or financial relationships that could be construed as a potential conflict of interest.
All claims expressed in this article are solely those of the authors and do not necessarily represent those of their affiliated organizations, or those of the publisher, the editors, and the reviewers. Any product that may be evaluated in this article, or claim that may be made by its manufacturer, is not guaranteed or endorsed by the publisher.
Authors would like to thank the reviewers for their thoughtful comments and efforts towards improving our manuscript.
Agrawal, N., Delanoue, R., Mauri, A., Basco, D., Pasco, M., Thorens, B., et al. (2016). The Drosophila TNF Eiger Is an Adipokine that Acts on Insulin-Producing Cells to Mediate Nutrient Response. Cell Metab 23 (4), 675–684. doi:10.1016/j.cmet.2016.03.003
Aguila, J. R., Suszko, J., Gibbs, A. G., and Hoshizaki, D. K. (2007). The Role of Larval Fat Cells in Adult Drosophila melanogaster. J. Exp. Biol. 210 (6), 956–963. doi:10.1242/jeb.001586
Alcalá, M., Calderon-Dominguez, M., Serra, D., Herrero, L., and Viana, M. (2019). Mechanisms of Impaired Brown Adipose Tissue Recruitment in Obesity. Front. Physiol. Vol. 10.
Almind, K., Manieri, M., Sivitz, W. I., Cinti, S., and Kahn, C. R. (2007). Ectopic Brown Adipose Tissue in Muscle Provides a Mechanism for Differences in Risk of Metabolic Syndrome in Mice. Proc. Natl. Acad. Sci. 104 (7), 2366–2371. doi:10.1073/pnas.0610416104
Arquier, N., Géminard, C., Bourouis, M., Jarretou, G., Honegger, B., Paix, A., et al. (2008). Drosophila ALS Regulates Growth and Metabolism through Functional Interaction with Insulin-like Peptides. Cel Metab 7 (4), 333–338. doi:10.1016/j.cmet.2008.02.003
Arrese, E. L., Patel, R. T., and Soulages, J. L. (2006). The Main Triglyceride-Lipase from the Insect Fat Body Is an Active Phospholipase A1: Identification and Characterization. J. Lipid Res. 47 (12), 2656–2667. doi:10.1194/jlr.m600161-jlr200
Arrese, E. L., and Soulages, J. L. (2010). Insect Fat Body: Energy, Metabolism, and Regulation. Annu. Rev. Entomol. 55, 207–225. doi:10.1146/annurev-ento-112408-085356
Arthur, S. T., Noone, J. M., Van Doren, B. A., Roy, D., and Blanchette, C. M. (2014). One-year Prevalence, Comorbidities and Cost of Cachexia-Related Inpatient Admissions in the USA. Drugs Context 3, 212265. doi:10.7573/dic.212265
Azeez, O. I., Meintjes, R., and Chamunorwa, J. P. (2014). Fat Body, Fat Pad and Adipose Tissues in Invertebrates and Vertebrates: The Nexus. Lipids Health Dis. Vol. 13. doi:10.1186/1476-511x-13-71
Babaei, R., Schuster, M., Meln, I., Lerch, S., Ghandour, R. A., Pisani, D. F., et al. (2018). Jak-Tgfβ Cross-Talk Links Transient Adipose Tissue Inflammation to Beige Adipogenesis. Sci. Signal. 11 (527), 11. doi:10.1126/scisignal.aai7838
Bachman, E. S., Dhillon, H., Zhang, C. Y., Cinti, S., Bianco, A. C., Kobilka, B. K., et al. (2002). betaAR Signaling Required for Diet-Induced Thermogenesis and Obesity Resistance. Science 297 (5582), 843–845. doi:10.1126/science.1073160
Bai, H., Kang, P., Hernandez, A. M., and Tatar, M. (2013). Activin Signaling Targeted by Insulin/dFOXO Regulates Aging and Muscle Proteostasis in Drosophila. Plos Genet. 9 (11), e1003941. doi:10.1371/journal.pgen.1003941
Bai, H., Kang, P., and Tatar, M. (2012). Drosophila Insulin-like Peptide-6 (Dilp6) Expression from Fat Body Extends Lifespan and Represses Secretion of Drosophila Insulin-like Peptide-2 from the Brain. Aging Cell 11 (6), 978–985. doi:10.1111/acel.12000
Baker, K. D., and Thummel, C. S. (2007). Diabetic Larvae and Obese Flies-Emerging Studies of Metabolism in Drosophila. Cel Metab. Vol. 6, 257–266. doi:10.1016/j.cmet.2007.09.002
Ballard, S. L., Jarolimova, J., and Wharton, K. A. (2010). Gbb/BMP Signaling Is Required to Maintain Energy Homeostasis in Drosophila. Dev. Biol. 337 (2), 375–385. doi:10.1016/j.ydbio.2009.11.011
Barretto, E. C., Polan, D. M., Beevor-Potts, A. N., Lee, B., and Grewal, S. S. (2020). Tolerance to Hypoxia Is Promoted by FOXO Regulation of the Innate Immunity Transcription Factor NF-kB/relish in Drosophila. Genetics 215 (4), 1013–1025. doi:10.1534/genetics.120.303219
Bartok, O., Teesalu, M., Ashwall‐Fluss, R., Pandey, V., Hanan, M., Rovenko, B. M., et al. (2015). The Transcription Factor Cabut Coordinates Energy Metabolism and the Circadian Clock in Response to Sugar Sensing. EMBO J. 34 (11), 1538–1553. doi:10.15252/embj.201591385
Baumbach, J., Hummel, P., Bickmeyer, I., Kowalczyk, K. M., Frank, M., Knorr, K., et al. (2014). A drosophila In Vivo Screen Identifies Store-Operated Calcium Entry as a Key Regulator of Adiposity. Cel Metab. 19 (2), 331–343. doi:10.1016/j.cmet.2013.12.004
Beller, M., Riedel, D., Jänsch, L., Dieterich, G., Wehland, J., Jäckle, H., et al. (2006). Characterization of the Drosophila Lipid Droplet Subproteome. Mol. Cel Proteomics 5 (6), 1082–1094. doi:10.1074/mcp.m600011-mcp200
Beller, M., Bulankina, A. V., Hsiao, H.-H., Urlaub, H., Jäckle, H., and Kühnlein, R. P. (2010). PERILIPIN-dependent Control of Lipid Droplet Structure and Fat Storage in Drosophila. Cel Metab. 12 (5), 521–532. doi:10.1016/j.cmet.2010.10.001
Benador, I. Y., Veliova, M., Mahdaviani, K., Petcherski, A., Wikstrom, J. D., Assali, E. A., et al. (2018). Mitochondria Bound to Lipid Droplets Have Unique Bioenergetics, Composition, and Dynamics that Support Lipid Droplet Expansion. Cel Metab. 27 (4), 869–885. e6. doi:10.1016/j.cmet.2018.03.003
Berry, D. C., Stenesen, D., Zeve, D., and Graff, J. M. (2013). The Developmental Origins of Adipose Tissue, 140. Cambridge): DevelopmentDevelopment, 3939–3949. doi:10.1242/dev.080549
Bharucha, K. N., Tarr, P., and Zipursky, S. L. (2008). A Glucagon-like Endocrine Pathway inDrosophilamodulates Both Lipid and Carbohydrate Homeostasis. J. Exp. Biol. 211 (19), 3103–3110. doi:10.1242/jeb.016451
Bi, J., Xiang, Y., Chen, H., Liu, Z., Grönke, S., Kühnlein, R. P., et al. (2012). Opposite and Redundant Roles of the Two Drosophila: Perilipins in Lipid Mobilization. J. Cel Sci 125 (15), 3568–3577. doi:10.1242/jcs.101329
Billon, N., Iannarelli, P., Monteiro, M. C., Glavieux-Pardanaud, C., Richardson, W. D., Kessaris, N., et al. (2007). The Generation of Adipocytes by the Neural Crest. Development 134 (12), 2283–2292. doi:10.1242/dev.002642
Bonneton, F., Théodore, L., Silar, P., Maroni, G., and Wegnez, M. (1996). Response of Drosophila Metallothionein Promoters to Metallic, Heat Shock and Oxidative Stresses. FEBS Lett. 380 (1–2), 33–38. doi:10.1016/0014-5793(95)01544-2
Britton, J. S., and Edgar, B. A. (1998). Environmental Control of the Cell Cycle in Drosophila: Nutrition Activates Mitotic and Endoreplicative Cells by Distinct Mechanisms. Development 125 (11), 2149–2158. doi:10.1242/dev.125.11.2149
Britton, J. S., Lockwood, W. K., Li, L., Cohen, S. M., and Edgar, B. A. (2002). Drosophila’s insulin/PI3-Kinase Pathway Coordinates Cellular Metabolism with Nutritional Conditions. Dev. Cel 2 (2), 239–249. doi:10.1016/s1534-5807(02)00117-x
Buch, S., Melcher, C., Bauer, M., Katzenberger, J., and Pankratz, M. J. (2008). Opposing Effects of Dietary Protein and Sugar Regulate a Transcriptional Target of Drosophila Insulin-like Peptide Signaling. Cel Metab. 7 (4), 321–332. doi:10.1016/j.cmet.2008.02.012
Butterworth, F. M., Emerson, L., and Rasch, E. M. (1988). Maturation and Degeneration of the Fat Body in the Drosophila Larva and Pupa as Revealed by Morphometric Analysis. Tissue Cell 20 (2), 255–268. doi:10.1016/0040-8166(88)90047-x
Campos-Ortega, J. A., and Hartenstein, V. (1985). The Embryonic Development of Drosophila melanogaster. Springer Berlin Heidelberg.
Cannon, B., and Nedergaard, J. (2004). Brown Adipose Tissue: Function and Physiological Significance. Physiol. Rev. Vol. 84, 277–359. doi:10.1152/physrev.00015.2003
Cannon, B., Jong, J. M. A., Fischer, A. W., Nedergaard, J., and Petrovic, N. (2020). Human Brown Adipose Tissue: Classical Brown rather Than Brite/beige? Exp. Physiol. 105 (8), 1191–1200. doi:10.1113/ep087875
Cermelli, S., Guo, Y., Gross, S. P., and Welte, M. A. (2006). The Lipid-Droplet Proteome Reveals that Droplets Are a Protein-Storage Depot. Curr. Biol. 16 (18), 1783–1795. doi:10.1016/j.cub.2006.07.062
Charroux, B., and Royet, J. (2010). Drosophila Immune Response: From Systemic Antimicrobial Peptide Production in Fat Body Cells to Local Defense in the Intestinal Tract. Fly 4 (1), 40–47. doi:10.4161/fly.4.1.10810
Chatterjee, D., Katewa, S. D., Qi, Y., Jackson, S. A., Kapahi, P., and Jasper, H. (2014). Control of Metabolic Adaptation to Fasting by dILP6-Induced Insulin Signaling in Drosophila Oenocytes. Proc. Natl. Acad. Sci. U S A. 111 (50), 17959–17964. doi:10.1073/pnas.1409241111
Chell, J. M., and Brand, A. H. (2010). Nutrition-responsive Glia Control Exit of Neural Stem Cells from Quiescence. Cell 143 (7), 1161–1173. doi:10.1016/j.cell.2010.12.007
Chng, W. bin. A., Sleiman, M. S. B., Schüpfer, F., and Lemaitre, B. (2014). Transforming Growth Factor β/activin Signaling Functions as a Sugar-Sensing Feedback Loop to Regulate Digestive Enzyme Expression. Cell Rep 9 (1), 336–348. doi:10.1016/j.celrep.2014.08.064
Choi, S., Lim, D. S., and Chung, J. (2015). Feeding and Fasting Signals Converge on the LKB1-SIK3 Pathway to Regulate Lipid Metabolism in Drosophila. Plos Genet. 11 (5). doi:10.1371/journal.pgen.1005263
Chung, H., Sztal, T., Pasricha, S., Sridhar, M., Batterham, P., and Daborn, P. J. (2009). Characterization of Drosophila melanogaster Cytochrome P450 Genes. Proc. Natl. Acad. Sci. U S A. 106 (14), 5731–5736. doi:10.1073/pnas.0812141106
Chung, K.-J., Chatzigeorgiou, A., Economopoulou, M., Garcia-Martin, R., Alexaki, V. I., Mitroulis, I., et al. (2017). A Self-Sustained Loop of Inflammation-Driven Inhibition of Beige Adipogenesis in Obesity. Nat. Immunol. 18 (6), 654–664. doi:10.1038/ni.3728
Cinti, S. (2018). Adipose Organ Development and Remodeling. Compr. Physiol. Vol. 8, 1357–1431. doi:10.1002/cphy.c170042
Cinti, S. (2012). The Adipose Organ at a Glance. Dis. Model. Mech. 5, 588–594. doi:10.1242/dmm.009662
Cleal, L., Aldea, T., and Chau, Y.-Y. (2017). Fifty Shades of white: Understanding Heterogeneity in white Adipose Stem Cells. Adipocyte 6, 205–216. doi:10.1080/21623945.2017.1372871
Cohen, P., and Spiegelman, B. M. (2016). Cell Biology of Fat Storage. MBoC 27, 2523–2527. doi:10.1091/mbc.e15-10-0749
Collins, S., Daniel, K. W., Petro, A. E., and Surwit, R. S. (1997). Strain-Specific Response Toβ3-Adrenergic Receptor Agonist Treatment of Diet-Induced Obesity in Mice1. Endocrinology 138 (1), 405–413. doi:10.1210/endo.138.1.4829
Colombani, J., Bianchini, L., Layalle, S., Pondeville, E., Dauphin-Villemant, C., Antoniewski, C., et al. (2005). Antagonistic Actions of Ecdysone and Insulins Determine Final Size in Drosophila. Science 310 (5748), 667–670. doi:10.1126/science.1119432
Colombani, J., Raisin, S., Pantalacci, S., Radimerski, T., Montagne, J., and Léopold, P. (2003). A Nutrient Sensor Mechanism Controls Drosophila Growth. Cell 114 (6), 739–749. doi:10.1016/s0092-8674(03)00713-x
Cypess, A. M., and Kahn, C. R. (2010). Brown Fat as a Therapy for Obesity and Diabetes. Curr. Opin. Endocrinol. Diabetes Obes. 17, 143–149. doi:10.1097/med.0b013e328337a81f
Cypess, A. M., Lehman, S., Williams, G., Tal, I., Rodman, D., Goldfine, A. B., et al. (2009). Identification and Importance of Brown Adipose Tissue in Adult Humans. N. Engl. J. Med. 360 (15), 1509–1517. doi:10.1056/nejmoa0810780
Cypess, A. M., White, A. P., Vernochet, C., Schulz, T. J., Xue, R., Sass, C. A., et al. (2013). Anatomical Localization, Gene Expression Profiling and Functional Characterization of Adult Human Neck Brown Fat. Nat. Med. 19 (5), 635–639. doi:10.1038/nm.3112
Da-Ré, C., De Pittà, C., Zordan, M. A., Teza, G., Nestola, F., Zeviani, M., et al. (2014). UCP4C Mediates Uncoupled Respiration in Larvae of Drosophila melanogaster. EMBO Rep. 15 (5), 586–591.
Daum, G., Wagner, A., Czabany, T., and Athenstaedt, K. (2007). Dynamics of Neutral Lipid Storage and Mobilization in Yeast. Biochimie 89, 243–248. doi:10.1016/j.biochi.2006.06.018
Davoodi, S., Galenza, A., Panteluk, A., Deshpande, R., Ferguson, M., Grewal, S., et al. (2019). The Immune Deficiency Pathway Regulates Metabolic Homeostasis in Drosophila. J. Immunol. 202 (9), 2747–2759. doi:10.4049/jimmunol.1801632
De Gregorio, E., Spellman, P. T., Tzou, P., Rubin, G. M., and Lemaitre, B. (2002). The Toll and Imd Pathways Are the Major Regulators of the Immune Response in Drosophila. EMBO J. 21 (11), 2568–2579. doi:10.1093/emboj/21.11.2568
Dean, R. L., Locke, M., and Collins, J. V. (1985). “Structure of the Fat Body,” in Comprehensive Insect Physiology, Biochemistry, and Pharmacology. Editors G. A. Kerkut, and L. I. Gilbert (Oxford: Pergamon Press), Vol. 3, 155–210. doi:10.1016/b978-0-08-030804-3.50011-x
Delanoue, R., Meschi, E., Agrawal, N., Mauri, A., Tsatskis, Y., McNeill, H., et al. (2016). Drosophila Insulin Release Is Triggered by Adipose Stunted Ligand to Brain Methuselah Receptor. Science 353 (6307), 1553–1556. doi:10.1126/science.aaf8430
Delanoue, R., Slaidina, M., and Léopold, P. (2010). The Steroid Hormone Ecdysone Controls Systemic Growth by Repressing dMyc Function in drosophila Fat Cells. Dev. Cel 18 (6), 1012–1021. doi:10.1016/j.devcel.2010.05.007
DiAngelo, J. R., and Birnbaum, M. J. (2009). Regulation of Fat Cell Mass by Insulin in Drosophila melanogaster. Mol. Cel Biol 29 (24), 6341–6352. doi:10.1128/mcb.00675-09
Ding, H., Zheng, S., Garcia-Ruiz, D., Hou, D., Wei, Z., Liao, Z., et al. (2016). Fasting Induces a Subcutaneous-To-Visceral Fat Switch Mediated by microRNA-149-3p and Suppression of PRDM16. Nat. Commun. 7, 11533. doi:10.1038/ncomms11533
Doane, W. W. (1960). Developmental Physiology of the Mutantfemale Sterile(2)adipose ofDrosophila Melanogaster. I. Adult Morphology, Longevity, Egg Production, and Egg Lethality. J. Exp. Zool. 145 (1), 1–21. doi:10.1002/jez.1401450102
Doria, A., Patti, M.-E., and Kahn, C. R. (2008). The Emerging Genetic Architecture of Type 2 Diabetes. Cel Metab. 8, 186–200. doi:10.1016/j.cmet.2008.08.006
Ebadi, M., and Mazurak, V. C. (2014). Evidence and Mechanisms of Fat Depletion in Cancer. Nutrients Vol. 6, 5280–5297. doi:10.3390/nu6115280
Enomoto, M., Siow, C., and Igaki, T. (2018). Drosophila as a Cancer Model. Adv. Exp. Med. Biol., 173–194. doi:10.1007/978-981-13-0529-0_10
Evans, C. J., Olson, J. M., Ngo, K. T., Kim, E., Lee, N. E., Kuoy, E., et al. (2009). G-TRACE: Rapid Gal4-Based Cell Lineage Analysis in Drosophila. Nat. Methods 6 (8), 603–605. doi:10.1038/nmeth.1356
Fearon, K., Strasser, F., Anker, S. D., Bosaeus, I., Bruera, E., Fainsinger, R. L., et al. (2011). Definition and Classification of Cancer Cachexia: An International Consensus. Lancet Oncol. 12, 489–495. doi:10.1016/s1470-2045(10)70218-7
Feldmann, H. M., Golozoubova, V., Cannon, B., and Nedergaard, J. (2009). UCP1 Ablation Induces Obesity and Abolishes Diet-Induced Thermogenesis in Mice Exempt from Thermal Stress by Living at Thermoneutrality. Cel Metab. 9 (2), 203–209. doi:10.1016/j.cmet.2008.12.014
Feyereisen, R. (1999). Insect P450 Enzymes. Annu. Rev. Entomol. Vol. 44, 507–533. doi:10.1146/annurev.ento.44.1.507
Figueroa-Clarevega, A., and Bilder, D. (2015). Malignant drosophila Tumors Interrupt Insulin Signaling to Induce Cachexia-like Wasting. Dev. Cel 33 (1), 47–55. doi:10.1016/j.devcel.2015.03.001
Finlin, B. S., Zhu, B., Confides, A. L., Westgate, P. M., Harfmann, B. D., Dupont-Versteegden, E. E., et al. (2017). Mast Cells Promote Seasonal white Adipose Beiging in Humans. Diabetes 66 (5), 1237–1246. doi:10.2337/db16-1057
Freychet, P. (1990). Pancreatic hormones. Hormones: from molecules to disease. Editors E.-E. Baulieu, and P. A. Kelly (Chapman and Hall: New York.
Fu, M., Xu, L., Chen, X., Han, W., Ruan, C., Li, J., et al. (2019). Neural Crest Cells Differentiate into Brown Adipocytes and Contribute to Periaortic Arch Adipose Tissue Formation. Atvb 39 (8), 1629–1644. doi:10.1161/atvbaha.119.312838
Funcke, J.-B., and Scherer, P. E. (2019). Beyond Adiponectin and Leptin: Adipose Tissue-Derived Mediators of Inter-organ Communication. J. Lipid Res. 60, 1648–1697. doi:10.1194/jlr.r094060
Gáliková, M., Diesner, M., Klepsatel, P., Hehlert, P., Xu, Y., Bickmeyer, I., et al. (2015). Energy Homeostasis Control in drosophila Adipokinetic Hormone Mutants. Genetics 201 (2), 665–683. doi:10.1534/genetics.115.178897
Garrido, D., Rubin, T., Poidevin, M., Maroni, B., Le Rouzic, A., Parvy, J. P., et al. (2015). Fatty Acid Synthase Cooperates with Glyoxalase 1 to Protect against Sugar Toxicity. Plos Genet. 11 (2), 1–26. doi:10.1371/journal.pgen.1004995
Gastaldelli, A. (2011). Role of Beta-Cell Dysfunction, Ectopic Fat Accumulation and Insulin Resistance in the Pathogenesis of Type 2 Diabetes Mellitus. Diabetes Res. Clin. Pract. 93 Suppl 1 (Suppl. 1), S60–S65. doi:10.1016/S0168-8227(11)70015-8
Géminard, C., Rulifson, E. J., and Léopold, P. (2009). Remote Control of Insulin Secretion by Fat Cells in Drosophila. Cel Metab 10 (3), 199–207.
Gesta, S., Tseng, Y.-H., and Kahn, C. R. (2007). Developmental Origin of Fat: Tracking Obesity to its Source. Cell 131, 242–256. doi:10.1016/j.cell.2007.10.004
Ghosh, A. C., and O’Connor, M. B. (2014). Systemic Activin Signaling Independently Regulates Sugar Homeostasis, Cellular Metabolism, and pH Balance in Drosophila melanogaster. Proc. Natl. Acad. Sci. U S A. 111 (15), 5729–5734. doi:10.1073/pnas.1319116111
Ghosh, A. C., Tattikota, S. G., Liu, Y., Comjean, A., Hu, Y., Barrera, V., et al. (2020). Drosophila Pdgf/vegf Signaling from Muscles to Hepatocyte-like Cells Protects against Obesity. Elife 9, 1–61. doi:10.7554/eLife.56969
Giannakou, M. E., Goss, M., Jünger, M. A., Hafen, E., Leevers, S. J., and Partridge, L. (2004). Long-lived Drosophila with Over-expressed dFOXO in Adult Fat Body. Science 305 (5682), 361. doi:10.1126/science.1098219
Goldberg, A. A., Bourque, S. D., Kyryakov, P., Boukh-Viner, T., Gregg, C., Beach, A., et al. (2009). A Novel Function of Lipid Droplets in Regulating Longevity. Biochem. Soc. Trans., 1050. doi:10.1042/bst0371050
Grauer, W. O., Moss, A. A., Cann, C. E., and Goldberg, H. I. (1984). Quantification of Body Fat Distribution in the Abdomen Using Computed Tomography. Am. J. Clin. Nutr. 39 (4), 631–637. doi:10.1093/ajcn/39.4.631
Grönke, S., Beller, M., Fellert, S., Ramakrishnan, H., Jäckle, H., and Kühnlein, R. P. (2003). Control of Fat Storage by a Drosophila PAT Domain Protein. Curr. Biol. 13 (7), 603–606. doi:10.1016/s0960-9822(03)00175-1
Grönke, S., Clarke, D. F., Broughton, S., Andrews, T. D., and Partridge, L. (2010). Molecular Evolution and Functional Characterization of Drosophila Insulin-like Peptides. Plos Genet. 6 (2). doi:10.1371/journal.pgen.1000857
Grönke, S., Mildner, A., Fellert, S., Tennagels, N., Petry, S., Müller, G., et al. (2005). Brummer Lipase Is an Evolutionary Conserved Fat Storage Regulator in Drosophila. Cel Metab 1 (5), 323–330. doi:10.1016/j.cmet.2005.04.003
Guarner, A., Morris, R., Korenjak, M., Boukhali, M., Zappia, M. P., Van Rechem, C., et al. (2017). E2F/DP Prevents Cell-Cycle Progression in Endocycling Fat Body Cells by Suppressing dATM Expression. Dev. Cel 43 (6), 689–703. doi:10.1016/j.devcel.2017.11.008
Guerra, C., Koza, R. A., Yamashita, H., Walsh, K., and Kozak, L. P. (1998). Emergence of Brown Adipocytes in white Fat in Mice Is under Genetic Control. Effects on Body Weight and Adiposity. J. Clin. Invest. 102 (2), 412–420. doi:10.1172/jci3155
Guilherme, A., Virbasius, J. V., Puri, V., and Czech, M. P. (2008). Adipocyte Dysfunctions Linking Obesity to Insulin Resistance and Type 2 Diabetes. Nat. Rev. Mol. Cel Biol 9, 367–377. doi:10.1038/nrm2391
Gupta, R. K. (2014). AdipocytesCurrent Biology. Curr. Biol. Vol. 24, R988. doi:10.1016/j.cub.2014.09.003
Gutierrez, E., Wiggins, D., Fielding, B., and Gould, A. P. (2007). Specialized Hepatocyte-like Cells Regulate Drosophila Lipid Metabolism. Nature 445 (7125), 275–280. doi:10.1038/nature05382
Harms, M., and Seale, P. (2013). Brown and Beige Fat: Development, Function and Therapeutic Potential. Nat. Med., Vol. 19, 1252–1263. doi:10.1038/nm.3361
Hartenstein, V., and Jan, Y. N. (1992). Studying Drosophila Embryogenesis with P-lacZ Enhancer Trap Lines. Roux's Arch. Dev. Biol. 201 (4), 194–220. doi:10.1007/bf00188752
Hasygar, K., Deniz, O., Liu, Y., Gullmets, J., Hynynen, R., Ruhanen, H., et al. (2021). Coordinated Control of Adiposity and Growth by Anti‐anabolic Kinase ERK7. EMBO Rep. 22 (2), 1–16. doi:10.15252/embr.201949602
Haunerland, N. H., and Shirk, P. D. (1995). Regional and Functional Differentiation in the Insect Fat Body. Annu. Rev. Entomol. 40 (1), 121–145. doi:10.1146/annurev.en.40.010195.001005
Havula, E., and Hietakangas, V. (2012). Glucose Sensing by ChREBP/MondoA-Mlx Transcription Factors. Vol. 23, Seminars in Cell and Developmental Biology. Semin. Cel Dev Biol, 640–647. doi:10.1016/j.semcdb.2012.02.007
Havula, E., Teesalu, M., Hyötyläinen, T., Seppälä, H., Hasygar, K., Auvinen, P., et al. (2013). Mondo/ChREBP-Mlx-Regulated Transcriptional Network Is Essential for Dietary Sugar Tolerance in Drosophila. Plos Genet. 9 (4). doi:10.1371/journal.pgen.1003438
Heine, M., Fischer, A. W., Schlein, C., Jung, C., Straub, L. G., Gottschling, K., et al. (2018). Lipolysis Triggers a Systemic Insulin Response Essential for Efficient Energy Replenishment of Activated Brown Adipose Tissue in Mice. Cel Metab 28 (4), 644–655. doi:10.1016/j.cmet.2018.06.020
Hiraike, Y., Waki, H., Miyake, K., Wada, T., Oguchi, M., Saito, K., et al. (2020). NFIA Differentially Controls Adipogenic and Myogenic Gene Program through Distinct Pathways to Ensure Brown and Beige Adipocyte Differentiation. Plos Genet. 16 (9), e1009044. doi:10.1371/journal.pgen.1009044
Hiraike, Y., Waki, H., Yu, J., Nakamura, M., Miyake, K., Nagano, G., et al. (2017). NFIA Co-localizes with PPARγ and Transcriptionally Controls the Brown Fat Gene Program. Nat. Cel Biol 19 (9), 1081–1092. doi:10.1038/ncb3590
Hoang, A. C., Yu, H., and Röszer, T. (2021). Transcriptional Landscaping Identifies a Beige Adipocyte Depot in the Newborn Mouse. Cells 10 (9). doi:10.3390/cells10092368
Hoffmann, J., Romey, R., Fink, C., Yong, L., and Roeder, T. (2013). Overexpression of Sir2 in the Adult Fat Body Is Sufficient to Extend Lifespan of Male and Female Drosophila. Aging (Albany NY) 5 (4), 315–327. doi:10.18632/aging.100553
Holz, A., Meise, M., and Janning, W. (1997). Adepithelial Cells in Drosophila melanogaster: Origin and Cell Lineage. Mech. Dev. 62 (1), 93–101. doi:10.1016/s0925-4773(97)00654-0
Honegger, B., Galic, M., Köhler, K., Wittwer, F., Brogiolo, W., Hafen, E., et al. (2008). Imp-L2, a Putative Homolog of Vertebrate IGF-Binding Protein 7, Counteracts Insulin Signaling in Drosophila and Is Essential for Starvation Resistance. J. Biol. 7 (3), 172. doi:10.1186/jbiol72
Hoshizaki, D. K. (1994). Krüppel Expression during Postembryonic Development of Drosophila. Dev. Biol. 163 (1), 133–140. doi:10.1006/dbio.1994.1129
Hoshizaki, D. K., Blackburn, T., Price, C., Ghosh, M., Miles, K., Ragucci, M., et al. (1994). Embryonic Fat-Cell Lineage in Drosophila melanogaster. Development 120 (9), 2489–2499. doi:10.1242/dev.120.9.2489
Hoshizaki, D. K., Lunz, R., Johnson, W., and Ghosh, M. (1995). Identification of Fat-Cell Enhancer Activity inDrosophila Melanogasterusing P-Element Enhancer Traps. Genome 38 (3), 497–506. doi:10.1139/g95-065
Hotamisligil, G. S. (2017). Inflammation, Metaflammation and Immunometabolic Disorders. Nature 542, 177–185. doi:10.1038/nature21363
Hughson, B. N., Shimell, M. J., and O’Connor, M. B. (2021). AKH Signaling in D. melanogaster Alters Larval Development in a Nutrient-dependent Manner that Influences Adult Metabolism. Front. Physiol., 12. doi:10.3389/fphys.2021.619219
Hyun, S., Lee, J. H., Jin, H., Nam, J. W., Namkoong, B., Lee, G., et al. (2009). Conserved MicroRNA miR-8/miR-200 and its Target USH/FOG2 Control Growth by Regulating PI3K. Cell 139 (6), 1096–1108. doi:10.1016/j.cell.2009.11.020
Iijima, K., Zhao, L., Shenton, C., and Iijima-Ando, K. (2009). Regulation of Energy Stores and Feeding by Neuronal and Peripheral CREB Activity in Drosophila. PLoS One 4 (12). doi:10.1371/journal.pone.0008498
Ikeda, K., Maretich, P., and Kajimura, S. (2018). The Common and Distinct Features of Brown and Beige Adipocytes. Trends Endocrinol Metab. 29 (3), 191–200.
Imrie, D., and Sadler, K. C. (2010). White Adipose Tissue Development in Zebrafish Is Regulated by Both Developmental Time and Fish Size. Dev. Dyn. 239 (11), 3013–3023. doi:10.1002/dvdy.22443
Ingaramo, M. C., Sánchez, J. A., Perrimon, N., and Dekanty, A. (2020). Fat Body P53 Regulates Systemic Insulin Signaling and Autophagy under Nutrient Stress via Drosophila Upd2 Repression. Cel Rep 33 (4), 321. doi:10.1016/j.celrep.2020.108321
Isabel, G., Martin, J. R., Chidami, S., Veenstra, J. A., and Rosay, P. (2005). AKH-producing Neuroendocrine Cell Ablation Decreases Trehalose and Induces Behavioral Changes in Drosophila. Am. J. Physiol. - Regul. Integr. Comp. Physiol. 288 (2 57-2). 2004. doi:10.1152/ajpregu.00158.2004
Jin, H., Narry Kim, V., and Hyun, S. (2012). Conserved microRNA miR-8 Controls Body Size in Response to Steroid Signaling in Drosophila. Genes Dev. 26 (13), 1427–1432. doi:10.1101/gad.192872.112
Johnson, M. B., and Butterworth, F. M. (1985). Maturation and Aging of Adult Fat Body and Oenocytes in Drosophila as Revealed by Light Microscopic Morphometry. J. Morphol. 184 (1), 51–59. doi:10.1002/jmor.1051840106
Jung, S. M., Sanchez-Gurmaches, J., and Guertin, D. A. (2019). Brown Adipose Tissue Development and Metabolism. Handb Exp. Pharmacol. 251, 3–36. doi:10.1007/164_2018_168
Kadereit, B., Kumar, P., Wang, W.-J., Miranda, D., Snapp, E. L., Severina, N., et al. (2008). Evolutionarily Conserved Gene Family Important for Fat Storage. Proc. Natl. Acad. Sci. 105 (1), 94–99. doi:10.1073/pnas.0708579105
Katheder, N. S., Khezri, R., O’Farrell, F., Schultz, S. W., Jain, A., Schink, M. K. O., et al. (2017). Microenvironmental Autophagy Promotes Tumour Growth. Nature 541 (7637), 417–420. doi:10.1038/nature20815
Khezri, R., Holland, P., Schoborg, T. A., Abramovich, I., Takáts, S., Dillard, C., et al. (2021). Host Autophagy Mediates Organ Wasting and Nutrient Mobilization for Tumor Growth. EMBO J. 40 (18), e107336. doi:10.15252/embj.2020107336
Kim, S. K., and Rulifson, E. J. (20041670). Conserved Mechanisms of Glucose Sensing and Regulation by Drosophila Corpora Cardiaca Cells. Nature 431, 431316–431320. doi:10.1038/nature02897
Kir, S., White, J. P., Kleiner, S., Kazak, L., Cohen, P., Baracos, V. E., et al. (2014). Tumour-derived PTH-Related Protein Triggers Adipose Tissue browning and Cancer Cachexia. Nature 513 (7516), 100–104. doi:10.1038/nature13528
Kopecký, J., Hodný, Z., Rossmeisl, M., Syrový, I., and Kozak, L. P. (1996). Reduction of Dietary Obesity in aP2-Ucp Transgenic Mice: Physiology and Adipose Tissue Distribution. Am. J. Physiol. - Endocrinol. Metab. 270 (5 33-5).
Koyama, T., and Mirth, C. K. (2016). Growth-Blocking Peptides as Nutrition-Sensitive Signals for Insulin Secretion and Body Size Regulation. Plos Biol. 14 (2). doi:10.1371/journal.pbio.1002392
Krahmer, N., Hilger, M., Kory, N., Wilfling, F., Stoehr, G., Mann, M., et al. (2013). Protein Correlation Profiles Identify Lipid Droplet Proteins with High Confidence. Mol. Cel Proteomics 12 (5), 1115–1126. doi:10.1074/mcp.m112.020230
Kwon, H. J., Waghmare, I., Verghese, S., Singh, A., Singh, A., and Kango-Singh, M. (2015). Drosophila C-Terminal Src Kinase Regulates Growth via the Hippo Signaling Pathway. Dev. Biol. 397 (1), 67–76. doi:10.1016/j.ydbio.2014.10.010
Lamers, D., Famulla, S., Wronkowitz, N., Hartwig, S., Lehr, S., Ouwens, D. M., et al. (2011). Dipeptidyl Peptidase 4 Is a Novel Adipokine Potentially Linking Obesity to the Metabolic Syndrome. Diabetes 60 (7), 1917–1925. doi:10.2337/db10-1707
Larsen, W. J. (1976). Cell Remodeling in the Fat Body of an Insect. Tissue Cell 8 (1), 73–92. doi:10.1016/0040-8166(76)90021-5
Lawrence, P. A., and Johnston, P. (1986). Observations on Cell Lineage of Internal Organs of Drosophila. J. Embryol. Exp. Morphol. 91, 251–266. doi:10.1242/dev.91.1.251
Lee, B., Barretto, E. C., and Grewal, S. S. (2019). TORC1 Modulation in Adipose Tissue Is Required for Organismal Adaptation to Hypoxia in Drosophila. Nat. Commun. 10 (1). doi:10.1038/s41467-019-09643-7
Lee, G. J., Han, G., Yun, H. M., Lim, J. J., Noh, S., Lee, J., et al. (2018). Steroid Signaling Mediates Nutritional Regulation of Juvenile Body Growth via IGF-Binding Protein in Drosophila. Proc. Natl. Acad. Sci. U S A. 115 (23), 5992–5997. doi:10.1073/pnas.1718834115
Lee, G. J., Jun, J. W., and Hyun, S. (2015). MicroRNA miR-8 Regulates Multiple Growth Factor Hormones Produced from Drosophila Fat Cells. Insect Mol. Biol. 24 (3), 311–318. doi:10.1111/imb.12156
Lee, G., and Park, J. H. (2004). Hemolymph Sugar Homeostasis and Starvation-Induced Hyperactivity Affected by Genetic Manipulations of the Adipokinetic Hormone-Encoding Gene in Drosophila melanogaster. Genetics 167 (1), 311–323. doi:10.1534/genetics.167.1.311
Lee, K.-S., You, K.-H., Choo, J.-K., Han, Y.-M., and Yu, K. (2004). Drosophila Short Neuropeptide F Regulates Food Intake and Body Size. J. Biol. Chem. 279 (49), 50781–50789. doi:10.1074/jbc.m407842200
Lehr, S., Hartwig, S., Lamers, D., Famulla, S., Müller, S., Hanisch, F. G., et al. (2012). Identification and Validation of Novel Adipokines Released from Primary Human Adipocytes. Mol. Cel Proteomics 11 (1), M111–M010504. doi:10.1074/mcp.M111.010504
Lehr, S., Hartwig, S., and Sell, H. (2012). Adipokines: A Treasure Trove for the Discovery of Biomarkers for Metabolic Disorders. Prot. Clin. Appl. 6, 91–101. doi:10.1002/prca.201100052
Li, S., Yu, X., and Feng, Q. (2019). Fat Body Biology in the Last Decade. Annu. Rev. Entomol. 64, 315–333. doi:10.1146/annurev-ento-011118-112007
Lidell, M. E., Betz, M. J., Leinhard, O. D., Heglind, M., Elander, L., Slawik, M., et al. (2013). Evidence for Two Types of Brown Adipose Tissue in Humans. Nat. Med. 19 (5), 631–634. doi:10.1038/nm.3017
Lodge, W., Zavortink, M., Golenkina, S., Froldi, F., Dark, C., Cheung, S., et al. (2021). Tumor-derived MMPs Regulate Cachexia in a Drosophila Cancer Model. Dev. Cel 56 (18), 2664–2680. doi:10.1016/j.devcel.2021.08.008
Loft, A., Forss, I., Siersbæk, M. S., Schmidt, S. F., Larsen, A. S. B., Madsen, J. G. S., et al. (2015). Browning of Human Adipocytes Requires KLF11 and Reprogramming of PPARγ Superenhancers. Genes Dev. 29 (1), 7–22. doi:10.1101/gad.250829.114
Lowell, B. B., S-Susulic, V., Hamann, A., Lawitts, J. A., Himms-Hagen, J., Boyer, B. B., et al. (1993). Development of Obesity in Transgenic Mice after Genetic Ablation of Brown Adipose Tissue. Nature 366 (6457), 740–742. doi:10.1038/366740a0
Lshibashi, J., and Seale, P. (2010). Beige Can Be Slimming. Sci. Sci. Vol. 328, 1113–1114. doi:10.1126/science.1190816
Luong, N., Davies, C. R., Wessells, R. J., Graham, S. M., King, M. T., Veech, R., et al. (2006). Activated FOXO-Mediated Insulin Resistance Is Blocked by Reduction of TOR Activity. Cel Metab 4 (2), 133–142. doi:10.1016/j.cmet.2006.05.013
Martínez, B. A., Hoyle, R. G., Yeudall, S., Granade, M. E., Harris, T. E., David Castle, J., et al. (2020). Innate Immune Signaling in Drosophila Shifts Anabolic Lipid Metabolism from Triglyceride Storage to Phospholipid Synthesis to Support Immune Function. Plos Genet. (11), 16. doi:10.1371/journal.pgen.1009192
Mattila, J., Havula, E., Suominen, E., Teesalu, M., Surakka, I., Hynynen, R., et al. (2015). Mondo-Mlx Mediates Organismal Sugar Sensing through the Gli-Similar Transcription Factor Sugarbabe. Cel Rep 13 (2), 350–364. doi:10.1016/j.celrep.2015.08.081
Mattila, J., and Hietakangas, V. (2017). Regulation of Carbohydrate Energy Metabolism in Drosophila melanogaster. Genetics 207 (4), 1231–1253. doi:10.1534/genetics.117.199885
Meschi, E., Léopold, P., and Delanoue, R. (2019). An EGF-Responsive Neural Circuit Couples Insulin Secretion with Nutrition in Drosophila. Dev. Cel 48 (1), 76–86. doi:10.1016/j.devcel.2018.11.029
Miller, J. M., Oligino, T., Pazdera, M., López, A. J., and Hoshizaki, D. K. (2002). Identification of Fat-Cell Enhancer Regions inDrosophila Melanogaster. Insect Mol. Biol. 11 (1), 67–77. doi:10.1046/j.0962-1075.2001.00310.x
Mirth, C. K., and Riddiford, L. M. (2007). Size Assessment and Growth Control: How Adult Size Is Determined in Insects. BioEssays Vol. 29, 344–355. doi:10.1002/bies.20552
Molaei, M., Vandehoef, C., and Karpac, J. (2019). NF-κB Shapes Metabolic Adaptation by Attenuating Foxo-Mediated Lipolysis in Drosophila. Dev. Cel 49 (5), 802–810.e6. doi:10.1016/j.devcel.2019.04.009
Moore, L. A., Broihier, H. T., Van Doren, M., and Lehmann, R. (1998). Gonadal Mesoderm and Fat Body Initially Follow a Common Developmental Path in Drosophila. Development 125 (5), 837–844. doi:10.1242/dev.125.5.837
Müller, H. (1968). Fine Structure and Lipid Formation in Fat Cells of the Perimeningeal Tissue of Lampreys under normal and Experimental Conditions. Z. Zellforsch Mikrosk Anat. 84 (4), 585–608.
Musselman, L. P., Fink, J. L., Ramachandran, P. V., Patterson, B. W., Okunade, A. L., Maier, E., et al. (2013). Role of Fat Body Lipogenesis in protection against the Effects of Caloric Overload in drosophila. J. Biol. Chem. 288 (12), 8028–8042. doi:10.1074/jbc.m112.371047
Musselman, L. P., and Kühnlein, R. P. (2018). Drosophila as a Model to Study Obesity and Metabolic Disease. Vol. 121. J. Exp. BiologyJ Exp Biol.
Na, J., Musselman, L. P., Pendse, J., Baranski, T. J., Bodmer, R., Ocorr, K., et al. (2013). A Drosophila Model of High Sugar Diet-Induced Cardiomyopathy. Plos Genet. 9 (1), e1003175. doi:10.1371/journal.pgen.1003175
Nässel, D. R., and Wegener, C. (2011). A Comparative Review of Short and Long Neuropeptide F Signaling in Invertebrates: Any Similarities to Vertebrate Neuropeptide Y Signaling. Peptides 32, 1335–1355. doi:10.1016/j.peptides.2011.03.013
Neugnot, V., Moulin, G., Dubreucq, E., and Bigey, F. (2002). The Lipase/acyltransferase fromCandida Parapsilosis. Eur. J. Biochem. 269 (6), 1734–1745. doi:10.1046/j.1432-1327.2002.02828.x
Ng, M., Fleming, T., Robinson, M., Thomson, B., Graetz, N., Margono, C., et al. (2014). Global, Regional, and National Prevalence of Overweight and Obesity in Children and Adults during 1980-2013: A Systematic Analysis for the Global Burden of Disease Study 2013. Lancet 384 (9945), 766–781. doi:10.1016/S0140-6736(14)60460-8
Novak, M., Monkus, E., and Pardo, V. (1971). Human Neonatal Subcutaneous Adipose Tissue. Function and Ultrastructure. Biol. Neonate 19 (4–6), 306–321. doi:10.1159/000240425
Okamoto, N., Yamanaka, N., Yagi, Y., Nishida, Y., Kataoka, H., O’Connor, M. B., et al. (2009). A Fat Body-Derived IGF-like Peptide Regulates Postfeeding Growth in Drosophila. Dev. Cel 17 (6), 885–891. doi:10.1016/j.devcel.2009.10.008
Olofsson, S.-O., Boström, P., Andersson, L., Rutberg, M., Levin, M., Perman, J., et al. (2008). Triglyceride Containing Lipid Droplets and Lipid Droplet-Associated Proteins. Curr. Opin. Lipidol. 19, 441–447. doi:10.1097/mol.0b013e32830dd09b
Ottaviani, E., Malagoli, D., and Franceschi, C. (2011). The Evolution of the Adipose Tissue: A Neglected enigma. Gen. Comp. Endocrinol. 174, 1–4. doi:10.1016/j.ygcen.2011.06.018
Palanker, L., Tennessen, J. M., Lam, G., and Thummel, C. S. (2009). Drosophila HNF4 Regulates Lipid Mobilization and β-Oxidation. Cel Metab 9 (3), 228–239. doi:10.1016/j.cmet.2009.01.009
Palanker Musselman, L., Fink, J. L., Narzinski, K., Ramachandran, P. V., Sukumar Hathiramani, S., Cagan, R. L., et al. (2011). A High-Sugar Diet Produces Obesity and Insulin Resistance in Wild-type Drosophila. DMM Dis. Model. Mech. 4 (6), 842–849. doi:10.1242/dmm.007948
Palm, W., Sampaio, J. L., Brankatschk, M., Carvalho, M., Mahmoud, A., Shevchenko, A., et al. (2012). Lipoproteins in Drosophila Melanogaster-Aassembly, Function, and Influence on Tissue Lipid Composition. Plos Genet. 8 (7), e1002828. doi:10.1371/journal.pgen.1002828
Palu, R. A. S., and Thummel, C. S. (2016). Sir2 Acts through Hepatocyte Nuclear Factor 4 to Maintain Insulin Signaling and Metabolic Homeostasis in Drosophila. Plos Genet. 12 (4). doi:10.1371/journal.pgen.1005978
Parisi, F., Riccardo, S., Zola, S., Lora, C., Grifoni, D., Brown, L. M., et al. (2013). DMyc Expression in the Fat Body Affects DILP2 Release and Increases the Expression of the Fat Desaturase Desat1 Resulting in Organismal Growth. Dev. Biol. 379 (1), 64–75. doi:10.1016/j.ydbio.2013.04.008
Parra-Peralbo, E., and Culi, J. (2011). Drosophila Lipophorin Receptors Mediate the Uptake of Neutral Lipids in Oocytes and Imaginal Disc Cells by an Endocytosis-independent Mechanism. Plos Genet. 7 (2), e1001297. doi:10.1371/journal.pgen.1001297
Pasco, M. Y., and Léopold, P. (2012). High Sugar-Induced Insulin Resistance in Drosophila Relies on the Lipocalin Neural Lazarillo. PLoS One 7 (5), e36583. doi:10.1371/journal.pone.0036583
Pathak, H., and Varghese, J. (2021). Edem1 Activity in the Fat Body Regulates Insulin Signalling and Metabolic Homeostasis in Drosophila. Life Sci. Alliance 4 (8), 1079. doi:10.26508/lsa.202101079
Petrovic, N., Walden, T. B., Shabalina, I. G., Timmons, J. A., Cannon, B., and Nedergaard, J. (2010). Chronic Peroxisome Proliferator-Activated Receptor γ (PPARγ) Activation of Epididymally Derived White Adipocyte Cultures Reveals a Population of Thermogenically Competent, UCP1-Containing Adipocytes Molecularly Distinct from Classic Brown Adipocytes. J. Biol. Chem. 285 (10), 7153–7164. doi:10.1074/jbc.m109.053942
Petruzzelli, M., and Wagner, E. F. (2016). Mechanisms of Metabolic Dysfunction in Cancer-Associated Cachexia. Genes Dev. 30, 489–501. doi:10.1101/gad.276733.115
Pospisilik, J. A., Schramek, D., Schnidar, H., Cronin, S. J. F., Nehme, N. T., Zhang, X., et al. (2010). Drosophila Genome-wide Obesity Screen Reveals Hedgehog as a Determinant of Brown versus White Adipose Cell Fate. Cell 140 (1), 148–160. doi:10.1016/j.cell.2009.12.027
Post, S., Karashchuk, G., Wade, J. D., Sajid, W., De Meyts, P., and Tatar, M. (2018). Drosophila Insulin-like Peptides DILP2 and DILP5 Differentially Stimulate Cell Signaling and Glycogen Phosphorylase to Regulate Longevity. Front. Endocrinol. (Lausanne) 9 (MAY). 245. doi:10.3389/fendo.2018.00245
Rajan, A., and Perrimon, N. (2012). Drosophila Cytokine Unpaired 2 Regulates Physiological Homeostasis by Remotely Controlling Insulin Secretion. Cell 151 (1), 123–137. doi:10.1016/j.cell.2012.08.019
Reis, T., van Gilst, M. R., and Hariharan, I. K. (2010). A Buoyancy-Based Screen of drosophila Larvae for Fat- Storage Mutants Reveals a Role for Sir2 in Coupling Fat Storage to Nutrient Availability. Plos Genet. 6 (11), 206. doi:10.1371/journal.pgen.1001206
Ren, G. R., Hauser, F., Rewitz, K. F., Kondo, S., Engelbrecht, A. F., Didriksen, A. K., et al. (2015). CCHamide-2 Is an Orexigenic Brain-Gut Peptide in Drosophila. PLoS One 10 (7). doi:10.1371/journal.pone.0133017
Riechmann, V., and Rehorn, K. P. (1998). The Genetic Control of the Distinction between Fat Body and Gonadal Mesoderm in Drosophila. Development 125(4):713–723.
Rodríguez-Vázquez, M., Vaquero, D., Parra-Peralbo, E., Mejía-Morales, J. E., and Culi, J. (2015). Drosophila Lipophorin Receptors Recruit the Lipoprotein LTP to the Plasma Membrane to Mediate Lipid Uptake. Plos Genet. 11 (6). doi:10.1371/journal.pgen.1005356
Rőszer, T., and Kiss-Tóth, É. D. (2014). FMRF-amide Is a Glucose-Lowering Hormone in the Snail Helix Aspersa. Cell Tissue Res 358 (2), 371–383. doi:10.1007/s00441-014-1966-x
Roth, S. W., Bitterman, M. D., Birnbaum, M. J., and Bland, M. L. (2018). Innate Immune Signaling in Drosophila Blocks Insulin Signaling by Uncoupling PI(3,4,5)P 3 Production and Akt Activation. Cel Rep 22 (10), 2550–2556. doi:10.1016/j.celrep.2018.02.033
Rusten, T. E., Lindmo, K., Juhász, G., Sass, M., Seglen, P. O., Brech, A., et al. (2004). Programmed Autophagy in the Drosophila Fat Body Is Induced by Ecdysone through Regulation of the PI3K Pathway. Dev. Cel 7 (2), 179–192. doi:10.1016/j.devcel.2004.07.005
Rydén, M., and Arner, P. (2007). Fat Loss in Cachexia-Is There a Role for Adipocyte Lipolysis? Clin. Nutr. Vol. 26, 1–6.
Saavedra, P., and Perrimon, N. (2019). Drosophila as a Model for Tumor-Induced Organ Wasting. Adv. Exp. Med. Biol., 191–205. doi:10.1007/978-3-030-23629-8_11
Saltiel, A. R. (2012). Insulin Resistance in the Defense against Obesity. Cel Metab. 15, 798–804. doi:10.1016/j.cmet.2012.03.001
Saltiel, A. R., and Kahn, C. R. (2001). Insulin Signalling and the Regulation of Glucose and Lipid Metabolism. Nature 414, 799–806. doi:10.1038/414799a
Sam, S., Leise, W., and Keiko Hoshizaki, D. (1996). The Serpent Gene Is Necessary for Progression through the Early Stages of Fat-Body Development. Mech. Dev. 60 (2), 197–205. doi:10.1016/s0925-4773(96)00615-6
Sano, H., Nakamura, A., Texada, M. J., Truman, J. W., Ishimoto, H., Kamikouchi, A., et al. (2015). The Nutrient-Responsive Hormone CCHamide-2 Controls Growth by Regulating Insulin-like Peptides in the Brain of Drosophila melanogaster. Plos Genet. 11 (5). doi:10.1371/journal.pgen.1005209
Sato, M., and Kornberg, T. B. (2002). FGF Is an Essential Mitogen and Chemoattractant for the Air Sacs of the Drosophila Tracheal System. Dev. Cel 3 (2), 195–207. doi:10.1016/s1534-5807(02)00202-2
Saxton, R. A., and Sabatini, D. M. (2017). mTOR Signaling in Growth, Metabolism, and Disease. Cell Vol. 168, 960–976. doi:10.1016/j.cell.2017.02.004
Schaffer, M. H., Noyes, B. E., Slaughter, C. A., Thorne, G. C., and Gaskell, S. J. (1990). The Fruitfly Drosophila melanogaster Contains a Novel Charged Adipokinetic-Hormone-Family Peptide. Biochem. J. 269 (2), 315–320. doi:10.1042/bj2690315
Schwartz, D. R., and Lazar, M. A. (2011). Human Resistin: Found in Translation from Mouse to Man. Trends Endocrinol. Metab. 22, 259–265. doi:10.1016/j.tem.2011.03.005
Scopelliti, A., Bauer, C., Yu, Y., Zhang, T., Kruspig, B., Murphy, D. J., et al. (2019). A Neuronal Relay Mediates a Nutrient Responsive Gut/Fat Body Axis Regulating Energy Homeostasis in Adult Drosophila. Cel Metab 29 (2), 269–284. doi:10.1016/j.cmet.2018.09.021
Scott, R. C., Schuldiner, O., and Neufeld, T. P. (2004). Role and Regulation of Starvation-Induced Autophagy in the Drosophila Fat Body. Dev. Cel 7 (2), 167–178. doi:10.1016/j.devcel.2004.07.009
Seale, P., Bjork, B., Yang, W., Kajimura, S., Chin, S., Kuang, S., et al. (2008). PRDM16 Controls a Brown Fat/skeletal Muscle Switch. Nature 454 (7207), 961–967. doi:10.1038/nature07182
Seale, P., Conroe, H. M., Estall, J., Kajimura, S., Frontini, A., Ishibashi, J., et al. (2011). Prdm16 Determines the Thermogenic Program of Subcutaneous white Adipose Tissue in Mice. J. Clin. Invest. 121 (1), 96–105. doi:10.1172/jci44271
Semaniuk, U., Piskovatska, V., Strilbytska, O., Strutynska, T., Burdyliuk, N., Vaiserman, A., et al. (2021). Drosophila Insulin-like Peptides: From Expression to Functions – a Review Entomologia Experimentalis et Applicata (John Wiley & Sons), Vol. 169, 195–208. doi:10.1111/eea.12981
Semenza, G. L. (2014). Oxygen Sensing, Hypoxia-Inducible Factors, and Disease Pathophysiology. Annu. Rev. Pathol. Mech. Dis. 9, 47–71. doi:10.1146/annurev-pathol-012513-104720
Sharp, L. Z., Shinoda, K., Ohno, H., Scheel, D. W., Tomoda, E., Ruiz, L., et al. (2012). Human BAT Possesses Molecular Signatures that Resemble Beige/Brite Cells. PLoS One 7 (11), e49452. doi:10.1371/journal.pone.0049452
Slaidina, M., Delanoue, R., Gronke, S., Partridge, L., and Léopold, P. (2009). A Drosophila Insulin-like Peptide Promotes Growth during Nonfeeding States. Dev. Cel 17 (6), 874–884. doi:10.1016/j.devcel.2009.10.009
Snijder, M. B., Dekker, J. M., Visser, M., Bouter, L. M., Stehouwer, C. D., Kostense, P. J., et al. (2003). Associations of Hip and Thigh Circumferences Independent of Waist Circumference with the Incidence of Type 2 Diabetes: The Hoorn Study. Am. J. Clin. Nutr. 77 (5), 1192–1197. doi:10.1093/ajcn/77.5.1192
Snijder, M. B., Dekker, J. M., Visser, M., Yudkin, J. S., Stehouwer, C. D. A., Bouter, L. M., et al. (2003). Larger Thigh and Hip Circumferences Are Associated with Better Glucose Tolerance: The Hoorn Study. Obes. Res. 11 (1), 104–111. doi:10.1038/oby.2003.18
Song, A., Dai, W., Jang, M. J., Medrano, L., Li, Z., Zhao, H., et al. (2020). Low- and High-Thermogenic Brown Adipocyte Subpopulations Coexist in Murine Adipose Tissue. J. Clin. Invest. 130 (1), 247–257. doi:10.1172/JCI129167
Song, W., Cheng, D., Hong, S., Sappe, B., Hu, Y., Wei, N., et al. (2017). Midgut-Derived Activin Regulates Glucagon-like Action in the Fat Body and Glycemic Control. Cel Metab 25 (2), 386–399. doi:10.1016/j.cmet.2017.01.002
Song, W., Kir, S., Hong, S., Hu, Y., Wang, X., Binari, R., et al. (2019). Tumor-Derived Ligands Trigger Tumor Growth and Host Wasting via Differential MEK Activation. Dev. Cel 48 (2), 277–286. doi:10.1016/j.devcel.2018.12.003
Sousa-Nunes, R., Yee, L. L., and Gould, A. P. (2011). Fat Cells Reactivate Quiescent Neuroblasts via TOR and Glial Insulin Relays in Drosophila. Nature 471 (7339), 508–513. doi:10.1038/nature09867
Stephens, J. M. (2012). The Fat Controller: Adipocyte Development. Plos Biol. 10 (11), e1001436. doi:10.1371/journal.pbio.1001436
Suzawa, M., Muhammad, N. M., Joseph, B. S., and Bland, M. L. (2019). The Toll Signaling Pathway Targets the Insulin-like Peptide Dilp6 to Inhibit Growth in Drosophila. Cel Rep 28 (6), 1439–1446. doi:10.1016/j.celrep.2019.07.015
Tan, B. K., Adya, R., and Randeva, H. S. (2010). Omentin: A Novel Link between Inflammation, Diabesity, and Cardiovascular Disease. Trends Cardiovasc. Med. 20, 143–148. doi:10.1016/j.tcm.2010.12.002
Tchkonia, T., Tchoukalova, Y. D., Giorgadze, N., Pirtskhalava, T., Karagiannides, I., Forse, R. A., et al. (2005). Abundance of Two Human Preadipocyte Subtypes with Distinct Capacities for Replication, Adipogenesis, and Apoptosis Varies Among Fat Depots. Am. J. Physiol. Endocrinol. Metab. 288 (1 51-1), E267–E277. doi:10.1152/ajpendo.00265.2004
Teixeira, L., Rabouille, C., Rørth, P., Ephrussi, A., and Vanzo, N. F. (2003). Drosophila Perilipin/ADRP Homologue Lsd2 Regulates Lipid Metabolism. Mech. Dev. 120 (9), 1071–1081. doi:10.1016/s0925-4773(03)00158-8
Terhzaz, S., Cabrero, P., Brinzer, R. A., Halberg, K. A., Dow, J. A. T., and Davies, S. A. (2015). A novel role of Drosophila cytochrome P450-4e3 in permethrin insecticide tolerance. Insect Biochem. Mol. Biol. 67, 38–46. doi:10.1016/j.ibmb.2015.06.002
Texada, M. J., Jørgensen, A. F., Christensen, C. F., Koyama, T., Malita, A., Smith, D. K., et al. (2019). A Fat-Tissue Sensor Couples Growth to Oxygen Availability by Remotely Controlling Insulin Secretion. Nat. Commun. 10 (1), 1–16. doi:10.1038/s41467-019-09943-y
The mesoderm and its derivatives, (1993). in The Development of Drosophila melanogasterCold Spring Harbor. Editors A. BM (New York: Cold Spring Harbor Laboratory Press).
Thiam, A. R., and Beller, M. (2017). The Why, when and How of Lipid Droplet Diversity, J. Cel Sci, 130. 315–324. doi:10.1242/jcs.192021
TM, R. (1978). “Fat Body,” in The Genetics and Biology of Drosophila. Editors M. Ashburner, and T. R. F. Wright (London: Academic Press), 561–601.
Todorčević, M., Kjær, M. A., Djaković, N., Vegusdal, A., Torstensen, B. E., and Ruyter, B. (2009). N-3 HUFAs Affect Fat Deposition, Susceptibility to Oxidative Stress, and Apoptosis in Atlantic salmon Visceral Adipose Tissue. Comp. Biochem. Physiol. - B Biochem. Mol. Biol. 152 (2), 135–143.
Tsoli, M., Swarbrick, M. M., and Robertson, G. R. (2016). Lipolytic and Thermogenic Depletion of Adipose Tissue in Cancer Cachexia. Semin. Cel Dev. Biol. 54, 68–81. doi:10.1016/j.semcdb.2015.10.039
Ugrankar, R., Berglund, E., Akdemir, F., Tran, C., Kim, M. S., Noh, J., et al. (2015). Drosophila Glucome Screening Identifies Ck1alpha as a Regulator of Mammalian Glucose Metabolism. Nat. Commun. 6, 7102. doi:10.1038/ncomms8102
Ugrankar, R., Bowerman, J., Hariri, H., Chandra, M., Chen, K., Bossanyi, M. F., et al. (2019). Drosophila Snazarus Regulates a Lipid Droplet Population at Plasma Membrane-Droplet Contacts in Adipocytes. Dev. Cel 50 (5), 557–572. doi:10.1016/j.devcel.2019.07.021
Ugrankar, R., Liu, Y., Provaznik, J., Schmitt, S., and Lehmann, M. (2011). Lipin Is a Central Regulator of Adipose Tissue Development and Function in Drosophila melanogaster. Mol. Cel Biol 31 (8), 1646–1656. doi:10.1128/mcb.01335-10
Ussar, S., Lee, K. Y., Dankel, S. N., Boucher, J., Haering, M. F., Kleinridders, A., et al. (2014). ASC-1, PAT2, and P2RX5 Are Cell Surface Markers for white, Beige, and Brown Adipocytes. Sci. Transl Med. 6 (247), 247ra103. doi:10.1126/scitranslmed.3008490
Waldén, T. B., Hansen, I. R., Timmons, J. A., Cannon, B., and Nedergaard, J. (2012). Recruited vs. Nonrecruited Molecular Signatures of Brown, "brite," and white Adipose Tissues. Am. J. Physiol. Endocrinol. Metab. 302 (1), E19–E31. doi:10.1152/ajpendo.00249.2011
Walther, T. C., and Farese, R. V. (2012). Lipid Droplets and Cellular Lipid Metabolism. Annu. Rev. Biochem. 81, 687–714. doi:10.1146/annurev-biochem-061009-102430
Wang, B., Moya, N., Niessen, S., Hoover, H., Mihaylova, M. M., Shaw, R. J., et al. (2011). A Hormone-dependent Module Regulating Energy Balance. Cell 145 (4), 596–606. doi:10.1016/j.cell.2011.04.013
Waqas, S. F. H., Hoang, A. C., Lin, Y.-T., Ampem, G., Azegrouz, H., Balogh, L., et al. (2017). Neuropeptide FF Increases M2 Activation and Self-Renewal of Adipose Tissue Macrophages. J. Clin. Invest. 127 (7), 2842–2854. doi:10.1172/jci90152
Watts, J. L. (2009). Fat Synthesis and Adiposity Regulation in Caenorhabditis elegans. Trends Endocrinol. Metab. 20, 58–65. doi:10.1016/j.tem.2008.11.002
Weaver, L. N., and Drummond-Barbosa, D. (2019). The Nuclear Receptor Seven up Functions in Adipocytes and Oenocytes to Control Distinct Steps of Drosophila Oogenesis. Dev. Biol. 456 (2), 179–189. doi:10.1016/j.ydbio.2019.08.015
Weaver, L. N., and Drummond-Barbosa, D. (2020). The Nuclear Receptor Seven up Regulates Genes Involved in Immunity and Xenobiotic Response in the Adult drosophila Female Fat Body. G3 Genes, Genomes, Genet. 10 (12), 4625–4635. doi:10.1534/g3.120.401745
Werthebach, M., Stewart, F. A., Gahlen, A., Mettler-Altmann, T., Akhtar, I., Maas-Enriquez, K., et al. (2019). Control of Drosophila Growth and Survival by the Lipid Droplet-Associated Protein CG9186/Sturkopf. Cel Rep 26 (13), 3726–3740.doi:10.1016/j.celrep.2019.02.110
Whittle, A. J., López, M., and Vidal-Puig, A. (2011). Using Brown Adipose Tissue to Treat Obesity - the central Issue. Trends Mol. Med. 17, 405–411. doi:10.1016/j.molmed.2011.04.001
Wilfling, F., Wang, H., Haas, J. T., Krahmer, N., Gould, T. J., Uchida, A., et al. (2013). Triacylglycerol Synthesis Enzymes Mediate Lipid Droplet Growth by Relocalizing from the ER to Lipid Droplets. Dev. Cel 24 (4), 384–399. doi:10.1016/j.devcel.2013.01.013
Wu, J., Boström, P., Sparks, L. M., Ye, L., Choi, J. H., Giang, A.-H., et al. (2012). Beige Adipocytes Are a Distinct Type of Thermogenic Fat Cell in Mouse and Human. Cell 150 (2), 366–376. doi:10.1016/j.cell.2012.05.016
Wu, J., Cohen, P., and Spiegelman, B. M. (2013). Adaptive Thermogenesis in Adipocytes: Is Beige the New Brown? Genes Dev. 27, 234–250. doi:10.1101/gad.211649.112
Yadav, A., Kataria, M. A., Saini, V., and Yadav, A. (2013). Role of Leptin and Adiponectin in Insulin Resistance. Clinica Chim. Acta 417, 80–84. doi:10.1016/j.cca.2012.12.007
Yamada, T., Habara, O., Kubo, H., and Nishimura, T. (2018). Fat Body Glycogen Serves as a Metabolic Safeguard for the Maintenance of Sugar Levels in drosophila. Dev 145 (6), 65. doi:10.1242/dev.158865
Yamaguchi, M., and Yoshida, H. (2018). Drosophila as a Model Organism. Adv. Exp. Med. Biol.. 1076, 1–10. doi:10.1007/978-981-13-0529-0_1
Yin, J., Spillman, E., Cheng, E. S., Short, J., Chen, Y., Lei, J., et al. (2021). Brain-specific Lipoprotein Receptors Interact with Astrocyte Derived Apolipoprotein and Mediate Neuron-Glia Lipid Shuttling. Nat. Commun. 12 (1), 7. doi:10.1038/s41467-021-22751-7
Young, S. G., and Zechner, R. (2013). Biochemistry and Pathophysiology of Intravascular and Intracellular Lipolysis. Genes Develop. Genes Dev Vol. 27, 459–484. doi:10.1101/gad.209296.112
Yu, H., Dilbaz, S., Coßmann, J., Hoang, A. C., Diedrich, V., Herwig, A., et al. (2019). Breast Milk Alkylglycerols Sustain Beige Adipocytes through Adipose Tissue Macrophages. J. Clin. Invest. 129 (6), 2485–2499. doi:10.1172/jci125646
Zhang, L., Ip, C. K., Lee, I. J., Qi, Y., Reed, F., Karl, T., et al. (2018). Diet-induced Adaptive Thermogenesis Requires Neuropeptide FF Receptor-2 Signalling. Nat. Commun. 9 (1), 4722. doi:10.1038/s41467-018-06462-0
Zhao, X., and Karpac, J. (2017). Muscle Directs Diurnal Energy Homeostasis through a Myokine-dependent Hormone Module in Drosophila. Curr. Biol. 27 (13), 1941–1955.e6. doi:10.1016/j.cub.2017.06.004
Zimmermann, R., Strauss, J. G., Haemmerle, G., Schoiswohl, G., Birner-Gruenberger, R., Riederer, M., et al. (2004). Fat Mobilization in Adipose Tissue Is Promoted by Adipose Triglyceride Lipase. Science 306 (5700), 1383–1386. doi:10.1126/science.1100747
Keywords: WAT, BAT, beige adipocytes, adipose tissue, adipose stem cells, drosophila, fat body development, adepithelial cells
Citation: Parra-Peralbo E, Talamillo A and Barrio R (2021) Origin and Development of the Adipose Tissue, a Key Organ in Physiology and Disease. Front. Cell Dev. Biol. 9:786129. doi: 10.3389/fcell.2021.786129
Received: 29 September 2021; Accepted: 01 December 2021;
Published: 21 December 2021.
Edited by:
Juan Jose Sanz-Ezquerro, National Center for Biotechnology (CSIC), SpainReviewed by:
Tamás Röszer, University of Ulm, GermanyCopyright © 2021 Parra-Peralbo, Talamillo and Barrio. This is an open-access article distributed under the terms of the Creative Commons Attribution License (CC BY). The use, distribution or reproduction in other forums is permitted, provided the original author(s) and the copyright owner(s) are credited and that the original publication in this journal is cited, in accordance with accepted academic practice. No use, distribution or reproduction is permitted which does not comply with these terms.
*Correspondence: Esmeralda Parra-Peralbo, ZXNtZXJhbGRhLnBhcnJhQHVuaXZlcnNpZGFkZXVyb3BlYS5lcw==; Ana Talamillo, YXRhbGFtaWxsb0BjaWNiaW9ndW5lLmVz
Disclaimer: All claims expressed in this article are solely those of the authors and do not necessarily represent those of their affiliated organizations, or those of the publisher, the editors and the reviewers. Any product that may be evaluated in this article or claim that may be made by its manufacturer is not guaranteed or endorsed by the publisher.
Research integrity at Frontiers
Learn more about the work of our research integrity team to safeguard the quality of each article we publish.