- 1Clinical Medical College, Yangzhou University, Yangzhou, China
- 2Department of General Surgery, Northern Jiangsu People’s Hospital, Clinical Medical College, Institute of General Surgery, Yangzhou University, Yangzhou, China
Pancreatic cancer is associated with highly malignant tumors and poor prognosis due to strong therapeutic resistance. Accumulating evidence shows that activated pancreatic stellate cells (PSC) play an important role in the malignant progression of pancreatic cancer. In recent years, the rapid development of single-cell sequencing technology has facilitated the analysis of PSC population heterogeneity, allowing for the elucidation of the relationship between different subsets of cells with tumor development and therapeutic resistance. Researchers have identified two spatially separated, functionally complementary, and reversible subtypes, namely myofibroblastic and inflammatory PSC. Myofibroblastic PSC produce large amounts of pro-fibroproliferative collagen fibers, whereas inflammatory PSC express large amounts of inflammatory cytokines. These distinct cell subtypes cooperate to create a microenvironment suitable for cancer cell survival. Therefore, further understanding of the differentiation of PSC and their distinct functions will provide insight into more effective treatment options for pancreatic cancer patients.
1 Introduction
Pancreatic cancer is among the most deadly forms of cancer, with a mortality-to-incidence ratio of 0.82 in 2020 and a 5 year survival rate of about 9% (Mizrahi et al., 2020). Associated symptoms typically go undetected until the advanced stages of the disease, resulting in great difficulty in early diagnosis (Kamisawa et al., 2016). Although surgical resection remains the preferred treatment option for pancreatic cancer patients, it is not effective in cases involving distant metastases. Interstitial cells, especially pancreatic stellate cells (PSC), play an important role in the malignant progression and treatment resistance observed in pancreatic cancer patients. PSC promote the fibrosis and inflammatory response of the tumor microenvironment by producing a large number of collagen fibers, exosomes, and soluble factors, which provide the basis for the proliferation, migration, and immune escape of pancreatic cancer cells (Wang et al., 2020), (Wang et al., 2017). More importantly, PSC play an extremely critical role in promoting the epithelial-mesenchymal transition (EMT) of cancer cells and enhancing cancer cell stemness. PSC promote EMT in pancreatic cancer cells by secreting exosomal microRNA-21 (Ma et al., 2020). It was shown that PSC also induce cancer stem cell-like properties in cancer cells through the secretion of osteopontin (Cao et al., 2019). Moreover, PSC also promote EMT in cancer cells by secreting hepatocyte growth factor (Xu et al., 2020), and the hepatocyte growth factor also promotes the expression of cancer stem cell pluripotency markers in cancer cells (Yan et al., 2018).
With the rapid development of single-cell sequencing technology, the heterogeneity of PSC populations has been recently investigated and attracted the attention of researchers, as this may provide a potential therapeutic route to targeting the tumor microenvironment (Pothula et al., 2020). Recently, researchers have identified two spatially separated, functionally complementary, and reversible subtypes of PSC in pancreatic cancer tissues, namely myofibroblastic and inflammatory PSC. Myofibroblastic PSC, characterized by elevated a-smooth muscle actin (α-SMA) expression, produce large amounts of pro-fibroproliferative collagen fibers, whereas inflammatory PSC express large amounts of inflammatory cytokines. Importantly, these two subtypes of PSC have been shown to operate synergistically to promote the progression of pancreatic cancer (Öhlund et al., 2017). Other PSC subtypes have also been identified. Cluster of differentiation (CD) 10-positive PSC assists in cancer cell invasion (Ikenaga et al., 2010). Bcl2‐associated athanogene- or fibroblast activation protein (FAP)-positive PSC not only enhance cancer cell migration but also promote fibrosis (Yuan et al., 2019), (Feig et al., 2013). There are even examples of differentiation among tumor suppressor subtypes, such as subsets expressing CD271 or Meflin (Nielsen et al., 2018), (Mizutani et al., 2019). However, improving patient survival requires a more detailed understanding of the mechanisms underlying PSC differentiation and their role in tumor malignancy. In the present review, we discuss the differentiation mechanism and cancer-promoting functions of myofibroblastic and inflammatory PSC. Moreover, we propose a more effective approach to manage treatment resistance in pancreatic cancer, at the level of PSC heterogeneity.
Article Category: Review
Differentiation Mechanism of Myofibroblastic and Inflammatory PSC
Cancer-associated fibroblasts (CAF) that are induced by cancer cells are mesenchymal-derived cells and play an active role in promoting pancreatic cancer progression. CAF in the pancreas are highly heterogeneous, and researchers have recently identified cancer-promoting inflammatory CAF and myofibroblastic CAF as well as cancer-suppressing CAF that exert antigen-presenting ability (Hosein et al., 2020). Different groups of CAF have very different functions, for example, inflammatory CAF mainly lead to the inflammatory response of cancer stroma, while myofibroblastic CAF mainly lead to stroma fibrosis. apCAF, which play an antigen-presenting role, can inhibit tumorigenesis (Sahai et al., 2020). In addition, the sources of CAF are again extensive. Currently, a prevailing view is that the main source of CAF is the quiescent PSC in the pancreas. However, it has also been shown that adipose-derived mesenchymal stem cells can differentiate into CAF in vitro and in vivo (Miyazaki et al., 2020), (Miyazaki et al., 2021). Moreover, bone marrow-derived macrophages also can differentiate into CAF (Iwamoto et al., 2021). Whereas the diverse source of CAF may lead to functional differences, it is necessary to study PSC as a well-defined concept.
How Do Cancer Cells Activate PSC?
PSC are stellate stromal cells unique to the pancreas and are usually located in a quiescent state on the outer side of the acinus (Pothula et al., 2016). In addition to storing vitamin A lipid droplets, quiescent PSC also express several protein markers such as synemin and desmin (Roife et al., 2020). Accumulating evidence shows that pancreatic cancer cells can recruit and activate PSC. The activity of PSC isolated from pancreatic cancer tissue is much higher than those associated with chronic pancreatitis (Lenggenhager et al., 2019). Pancreatic cancer cells secrete a large number of paracrine cytokines, as summarized in Table 1, such as transforming growth factor-beta (TGF-β), Sonic hedgehog (Shh), and Interleukin-1 (IL-1) alpha to activate PSC (Sherman, 2018), while direct contact between pancreatic cancer cells and PSC, stimulating the Notch signaling pathway in PSC, leads to the activation of PSC (Fujita et al., 2009). The Notch receptors Notch1 and Notch3 are known to be highly expressed in the pancreatic cancer stroma and are accompanied by a rise in delta-like ligands Dll1, Dll3, and Dll4. Binding of the receptor to the ligand leads to the entry of the intracellular portion of the receptor into the nucleus, which activates the CSL/RBPJ protein, to promote the transcription of collagen fibers (Song and Zhang, 2018), (Procopio et al., 2015). Studies suggest that Notch3 is involved in the activation of PSC (Procopio et al., 2015). Activated PSC proliferate and secrete a large number of growth factors, inflammatory mediators, and collagen fibers that reshape the tumor microenvironment.
Additionally, the rapid proliferation of cancer cells imposes significant pressure on the surroundings, including mechanical pressure which promotes the activation of PSC through a positive feedback loop (Sahai et al., 2020). G protein-coupled estrogen receptors, located within PSC membranes, sense interstitial mechanical signals and activate Ras homolog family member A (RhoA) (Cortes et al., 2019a). Both cell contraction and mechanosensation are dependent on RhoA activity, which regulates cell contractility and maintains the activated phenotype of PSC by regulating actomyosin (Cortes et al., 2019b), (Rodriguez-Hernandez et al., 2016). G protein-coupled estrogen receptor expression in the mesenchyme promotes stiffening and remodeling of the extracellular matrix (ECM), which also enhances the transmission of mechanical signals (Cortes et al., 2019c). PSC can maintain their activation state by autocrine TGF-β and other cytokines (Apte and Wilson, 2012). These cytokines effectively promote collagen synthesis and proliferation of PSC (Wu et al., 2020). Furthermore, pressure may be involved in the maintenance and enhancement of the activated state of PSC. Under conditions of high mechanical pressure, PSC will activate the injury-related stress response and synthesize a large number of reactive oxygen species (ROS) (Asaumi et al., 2007), which have been associated with the production of various cytokines and growth factors that facilitate the continuous activation of PSC (Richter et al., 2015). Importantly, PSC can activate the ROS system under a diverse array of conditions, including inflammation, hypoxia, and a high glucose environment, which seem to be key mechanisms for the maintenance of PSC activity (Hu et al., 2007; Lei et al., 2014; Kim et al., 2016). Furthermore, the researchers identified a key role for the Keap1-Nrf2 signalling axis in influencing the functional status of PSC in pancreatic cancer through the regulation of ROS production (Shao et al., 2019).
The Driving Mechanism of PSC Differentiation Into Myofibroblastic and Inflammatory Subtypes
In mice and human pancreatic cancer tissues, researchers have identified two distinct subgroups of PSC. The first, myofibroblastic PSC, are associated with elevated a-SMA expression in immediately surrounding of cancer cells, and produce large amounts of pro-fibroproliferative collagen fibers, while the second type, inflammatory PSC, are not associated with elevated a-SMA expression, but are known to secrete large amounts of inflammatory cytokines further away from the cancer cells (Öhlund et al., 2017). Studies have shown that soluble cytokines secreted by cancer cells dominate this process and the differentiation of PSC into these two subtypes involves an antagonistic mechanism (Figure 1 and Figure 2). Specifically, TGF-β activates Smads signaling to drive the expression of downstream target genes, including a-SMA and type 1 collagen (Col1), promoting the differentiation of myofibroblastic PSC. In contrast, IL-1α activates JAK-STAT signaling to mediate mass production of Interleukin-6 (IL-6) and leukemia inhibitory factor (LIF), promoting the differentiation of inflammatory PSC (Biffi et al., 2019). IL-1α-activated PSC can assist cancer cell migration, which is known to be inhibited by TGF-β via blocking IL-1α-mediated secretion of hepatocyte growth factor and reducing IL-1 receptor expression (Tjomsland et al., 2016a). These factors also act antagonistically in regulating the matrix metalloproteinase profile of PSC (Tjomsland et al., 2016b). Additionally, Shh protein shows a similar regulatory effect, as it promotes the differentiation of myofibroblastic PSC and inhibits the differentiation of inflammatory PSC in the tumor microenvironment, (Steele et al., 2021). Furthermore, Shh activates Hedgehog signaling, in a dose-dependent manner, to enhance the proliferation of PSC and induce the expression of a-SMA (Bailey et al., 2008). Moreover, the differentiation of PSC is also regulated by mechanical signal transduction, and the mechanical pressure caused by glandular dilatation is known to induce the expression of a-SMA in surrounding stromal cells (Miyai et al., 2020).
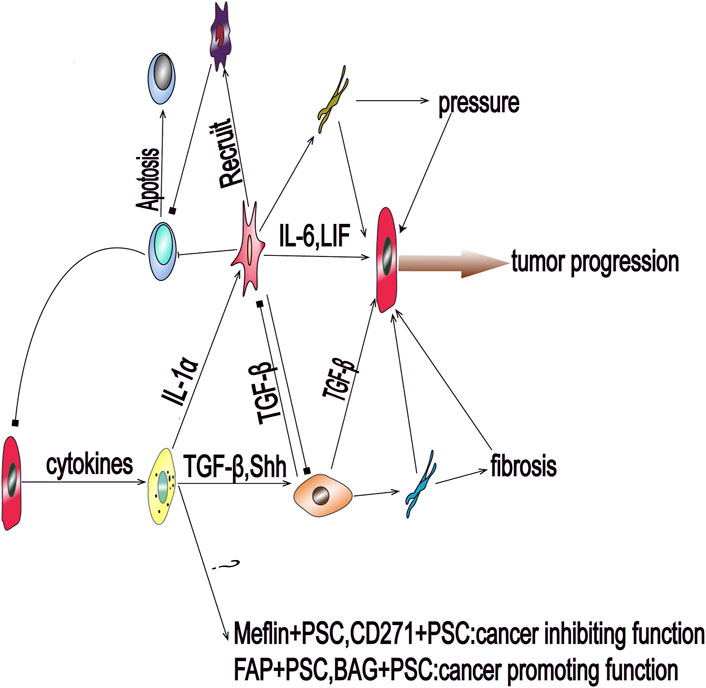
FIGURE 1. Pancreatic cancer cells can send activation signals to quiescent pancreatic stellate cells (PSCs) via paracrine factors, resulting in the activation of PSCs and differentiation into multiple subsets that participate in the progression of pancreatic cancer. Transforming growth factor beta and Sonic hedgehog can promote the activation of PSCs to myofibroblastic PSCs. The cells of this subgroup mainly secrete collagen fibers and mediate environmental fibrosis and hypoxia. Interleukin-1 alpha can promote the differentiation of PSCs into inflammatory PSCs to induce the inflammatory response and interstitial hypertension. Inflammatory PSCs also induce immunosuppression by recruiting immunosuppressive cells; BAG: Bcl2‐associated athanogene; FAP: fibroblast activation protein; IL-6: Interleukin-6; LIF: leukemia inhibitory factor; PSC: pancreatic stellate cell; Shh: Sonic hedgehog; TGF-β: transforming growth factor beta.
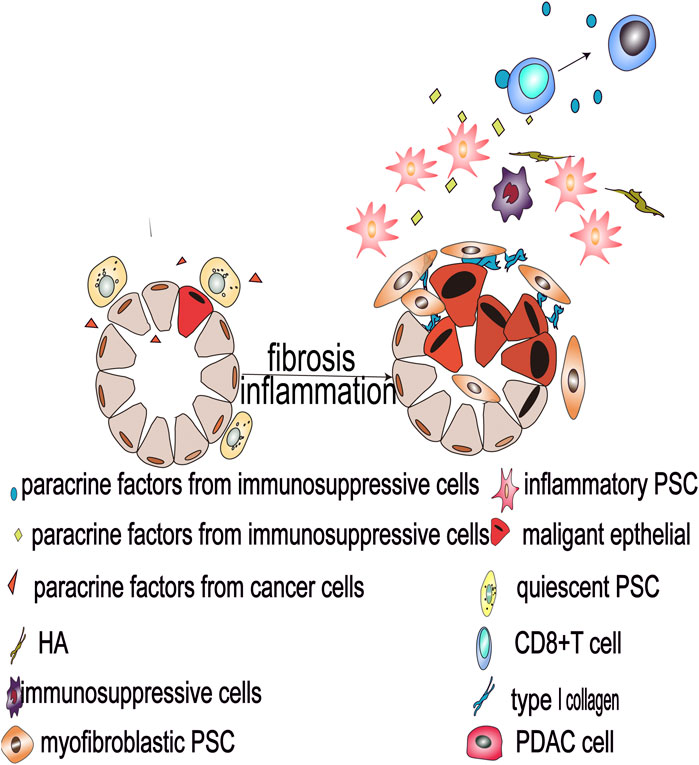
FIGURE 2. Malignant pancreatic epithelial cells activate PSCs and secrete oncogenic factors that drive PSC differentiation into myofibroblastic and inflammatory PSC subtypes, and such heterogeneous PSCs reshape the tumor microenvironment and promote the development of pancreatic cancer.
Indeed, whereas Öhlund identified two typical PSC subgroups, myofibroblastic PSC and inflammatory PSC, other researchers revealed the mechanism of differentiation of the two subgroups (Pothula et al., 2020), (Biffi et al., 2019). But subgroups are not stable and can re-differentiate into other types under certain conditions, including the rapid re-differentiation of inflammatory PSC into myofibroblastic PSC, in the case of monolayer distribution (Öhlund et al., 2017). This work also implies that the differentiation of PSC is dominated by cancer cells and PSC present different phenotypes according to the spatial and biochemical ecological niches in the PDA microenvironment. For myofibroblastic PSC and inflammatory PSC, the essential difference is whether a-SMA is expressed at high levels or not. Whether this marking method is reasonable or not needs to be further examined. Additional markers are available to differentiate diverse subgroups of PSC, such as FAP, Platelet-derived growth factor receptors, and Vimentin (Nurmik et al., 2020). It was shown that the FAP-positive PSC may produce both ECM and inflammatory cytokines (Wen et al., 2019), and the ecological niche of FAP-positive PSC in the PDA microenvironment is located between myofibroblastic PSC and inflammatory PSC (Feig et al., 2013). Thus, FAP-positive PSC may be an intermediate state between the differentiation of myofibroblastic PSC and inflammatory PSC. We need more authoritative classification criteria for the study of subgroups of PSC, which is crucial for us to further investigate the functions of PSC. Different activators, markers, and ecological niche characteristics are key factors for further identification of PSC subgroups in the future. Based on existing studies, the following section will focus on the known features and functions of myofibroblastic PSC and inflammatory PSC to further explore the functions of PSC in pancreatic cancer.
The Function of Myofibroblastic and Inflammatory PSC
Myofibroblastic PSC Mediate Characteristic Desmoplasia in Pancreatic Cancer
Pancreatic cancer has a high degree of desmoplasia, which is characterized by the differentiation of PSC into myofibroblastic PSC and overexpression of ECM proteins (Incio et al., 2016). Myofibroblastic PSC dominate the desmoplasia and form a physically protective barrier outside pancreatic cancer cells, protecting them from drug intervention and immune recognition, representing a significant impediment to the treatment of pancreatic cancer, (Schnittert et al., 2019). This process is regulated by many myofibroblastic PSC differentiation drivers, such as TGF- ß and Shh. Targeting TGF-β can improve patient prognosis, whereas targeting Shh has no observable effect (Melisi et al., 2019), (Kim et al., 2014). Alternatively, the depletion of the ECM, to dissolve the fibrous barrier, was conjectured, but its application to the treatment in pancreatic cancer has not been successful. Knockout a-SMA transgenic mice successfully deplete a-SMA positive PSC in the stroma, which was associated with earlier metastasis, a high inflammatory response, and immunosuppression of pancreatic cancer (Özdemir et al., 2014), while silencing Shh in mice leads to depletion of stromal a-SMA+ cells, revealing a similar course of malignant progression (Rhim et al., 2014). Similarly, the depletion of Col1, which is the main component of the ECM, leads to immunosuppression and premature death in mice (Chen et al., 2021). Whether it is depletion of myofibroblast PSC or COL1, these are some drastic approaches. This does not directly confirm that they have a cancer-suppressive function. However, there are several possible reasons for immunosuppression and malignant progression: (Mizrahi et al., 2020): the self-protection mechanisms in pancreatic cancer cells were activated. (Kamisawa et al., 2016). the conditions created by inducing cell death in the cancer microenvironment may have primary responsibility for the adverse effects. This inspires us that targeting the mesenchymal component of pancreatic cancer requires more caution.
A novel treatment approach involves inducing PSC quiescence, which can not only effectively prevent the proliferation of connective tissue in pancreatic cancer, but also prevent the side effects caused by the depletion of the ECM. Calcipotriol, a ligand of the vitamin D receptor, is used to induce PSC quiescence and effectively reduce the degree of inflammation and fibrosis associated with pancreatic cancer in mice. The survival time of mice treated with calcipotriol chemotherapy increased by 57% (Sherman et al., 2014). All-trans retinoic acid (ATRA), an active metabolite of vitamin A, restores mechanical quiescence of PSC through a mechanism dependent on the contractile downregulation of actomyosin (Chronopoulos et al., 2016). ATRA also inhibits the ability of PSC to mechanically release active TGF-β, blocking the capacity of TGF-β to maintain the activity of PSC in an autocrine manner (Sarper et al., 2016). Recent studies have shown that ATRA-targeted stroma reduces pancreatic cancer aggressiveness (Froeling et al., 2011). A phase I clinical trial of ATRA-induced PSC quiescence demonstrates that patients tolerate ATRA well (Kocher et al., 2020); however, further clinical trials are required to verify the effectiveness of ATRA.
As the depletion of a-SMA positive stromal cells leads to the malignant progression of pancreatic cancer, an important question arises as to whether myofibroblastic PSC can inhibit tumor progression. This is likely not the case, and the expression of a-SMA in pancreatic cancer tissues is unstable and provides no predictive value in the prognosis of patients (Erkan et al., 2008; Moffitt et al., 2015; Haeberle et al., 2018). Studies have shown that the depletion of myofibroblastic PSC will lead to a substantial expansion of the number of inflammatory PSC, which then become the dominant subgroup in the tumor microenvironment, leading to the malignant progression of pancreatic cancer. This implies that an increase in the number of inflammatory PSC may be the main reason for the deterioration of a-SMA- mice (Steele et al., 2021). In addition, Col1 plays an important role in sending malignant signals to cancer cells (Grzesiak et al., 2007). Studies show that patients with low Col1 have a median survival time of 14.6 months, in comparison to 6.4 months for patients with high levels (Whatcott et al., 2015). α1β2 integrin is an α/β heterodimer membrane protein and the main receptor mediating the adhesion of cancer cells to the surrounding Col1 (Naci et al., 2015). Once these two elements combine to form adhesion plaques on the cell surface, they provide an important medium for the ECM to transmit signals into the cell, activating downstream Src family kinases, focal adhesion kinase, and extracellular regulated kinase signaling pathways (Ivaska and Heino, 2011), (Cooper and Giancotti, 2019). Discoidin domain receptor 1 (DDR1) is a member of the receptor tyrosine kinase family and acts as an important receptor for Col1 (Baltes et al., 2020). DDR1 is widely expressed in epithelial cells, involved in the regulation of multiple signaling pathways, and is known to have a strong association with cancer progression (Henriet et al., 2018). Col1 can transmit a variety of carcinogenic signals to cells, through the two pathways described above, to promote the migration, proliferation, and drug resistance of pancreatic cancer.
Col1 makes an outstanding contribution in promoting the malignant procession of cancer (Figure 3). Col1 signals the p130Crk-associated substrate and activates the JNK signaling pathway to increase N-cadherin expression (Huang et al., 2016), (Shintani et al., 2006) DDR1 also activates downstream Src to decrease the expression of E-cadherin (Sun et al., 2008), (Chen et al., 2016). These factors reduce the adhesion of cancer cells to the adjacent extracellular matrix and help them to complete their EMT, greatly enhancing their ability to migrate. In addition, Col1 can engage in crosstalk with transforming growth factor-beta (TGF-β) signaling to promote EMT in cancer cells. Studies have shown that Col1 increases the expression of transcription factor Snail by interacting with TGF-β-Smads. Moreover, the up-regulation of Snail can not only promote the occurrence of EMT but also increase the expression of membrane-type matrix metalloproteinase-1, which further helps pancreatic cancer cells to dissolve collagen fibers and achieve metastasis (Shields et al., 2011). Furthermore, Col1 positively regulates the transcription of Snail via the Smad interacting protein 1, a positive regulator of Smads signal (Imamichi et al., 2007). Col1 also induces the proliferation of pancreatic cancer cells by disrupting the E-cadherin-Col1 complex, leading to the accumulation of ß-catenin in the nucleus and activating the transcription of the oncogene c-Myc (Koenig et al., 2006; Katoh, 2018). Overexpression of c-Myc promotes the immortalization of cancer cells (Dang, 2012) and alters the original signal transduction mode of cells, resulting in malignant cell proliferation, anti-apoptosis, and chromosome instability (Kolenda et al., 2020). Moreover, the α1β2 integrin pathway can also assist cells to acquire a cancer stem cell phenotype (Begum et al., 2017). Finally, Col1 mediates drug resistance by activating MT1-MMP to increase the expression of high mobility group A2, a non-histone DNA-binding nuclear protein involved in chromatin remodeling and gene transcription (Dangi-Garimella et al., 2011).
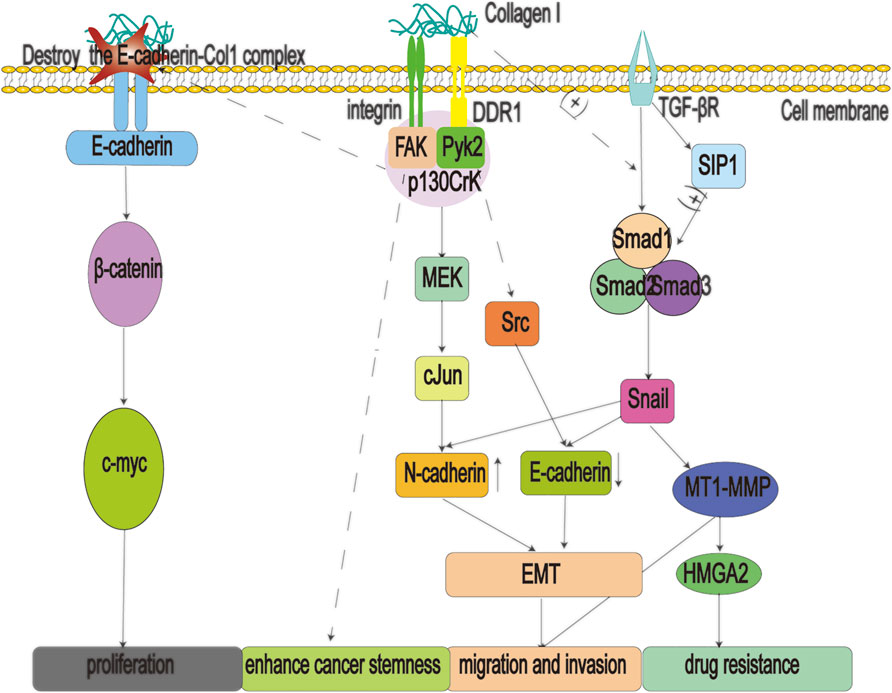
FIGURE 3. Type 1 collagen (Col1) fibers are secreted by myofibroblastic pancreatic stellate cells (PSCs), which can promote multiple processes such as migration, proliferation, drug resistance, and stemness enhancement of pancreatic cancer cells, by binding to α1β2 integrins and discoidin domain receptors 1 (DDR1). In addition, when the E-cadherin-Col1 complex is destroyed, it will lead to the accumulation of ß-catenin in the nucleus, activate the oncogene c-myc, and eventually lead to the proliferation of pancreatic cancer cells; Col1: type 1 collagen; DDR1: Discoidin domain receptors one; EMT: epithelial mesenchymal transition; FAK: focal adhesion kinase; MET: metformin; HMGA2: high mobility group A2; MMPs: matrix metalloproteinases; MT1-MMP: membrane-type matrix metalloproteinase-1; SIP1: smad interacting protein one; TGF-βR: transforming growth factor beta receptor.
Interstitial Hypertension Dominated by Inflammatory PSC Is a Natural Protective Barrier for Pancreatic Cancer
Hyaluronic acid (HA) is a linear glycosaminoglycan macromolecule composed of repeating units that are synthesized by hyaluronan synthase enzymes HAS1, HAS2, and HAS3, and is the main source of external pressure in the cancer stroma. HA can also specifically bind to the CD44 protein to affect the physiological activity of cancer cells (Sato et al., 2016; Caon et al., 2020). Studies have shown that the content of HA in pancreatic cancer is higher than in other cancer tissues, which leads to the characteristic interstitial hypertension observed in pancreatic cancer (Jacobetz et al., 2013). The content of HA in pancreatic cancer is 12 times higher than that in a healthy pancreas (Theocharis et al., 2000). Studies show that activated PSCs are the main source of HA (Junliang et al., 2019). Surprisingly, HA is not synthesized by myofibroblastic PSC, and their depletion does not affect the expression of HA in pancreatic cancer stroma (Özdemir et al., 2014). In contrast, inflammatory PSC express HAS1 and HAS2, suggesting that these cells represent the main source of HA (Elyada et al., 2019). Pancreatic cancer has extremely high external pressure in the interstitium, which contributes to difficulties associated with treatment (DuFort et al., 2016). HA-induced external pressure forms a physical barrier to help pancreatic cancer cells resist the effects of therapeutic intervention (Jacobetz et al., 2013). Studies have shown that low molecular weight HA enhances the migration of cancer cells (Junliang et al., 2019), whereas high molecular weight HA is the main cause of external pressure, leading to interstitial pressures of up to 100 mmHg, resulting in vascular collapse, impeding the delivery of nutrients, oxygen, and drugs, and reducing the infiltration of immune cells (Chauhan et al., 2014). A recent study shows a median survival time of 24.3 months for patients with low levels of HA, in comparison to 9.3 months for patients with high levels (Whatcott et al., 2015). The degradation of HA in pancreatic cancer tissue by administering halofuginone can significantly weaken the effect of the physical barrier, resulting in prolonged survival time in mice (Elahi-Gedwillo et al., 2019).
Dissolving HA with drugs represents a viable treatment option for pancreatic cancer patients (Sato et al., 2016). Compounds capable of dissolving HA in pancreatic tumor-bearing mice include 4-methylumbelliferone (Nagy et al., 2015), Minnelide (Banerjee et al., 2016), and PEGylated human recombinant hyaluronidase (PEGPH20) (Hingorani et al., 2016). PEGPH20 has been shown to significantly prolong disease-free survival of pancreatic cancer patients, especially in patients with high HA expression. Maximum survival was observed upon treatment with a combination therapy regimen including PEGPH20, albumin-bound paclitaxel, and gemcitabine (Hingorani et al., 2018; Ramanathan et al., 2019). Unfortunately, clinical trials of PEGPH20 were eventually discontinued due to pharmacological toxicity.
Several pancreatic cancer cell lines with HA receptor expression exhibit potential for hypo-differentiation and high migration (Abetamann et al., 1996; Sugahara et al., 2008). Research has shown that high levels of HA can lead to the malignant progression of pancreatic cancer. HA binding to the CD44 receptor mediates cancer cell EMT, drug resistance, and proliferation through both RAS/ERK and PI3K/AKT signaling pathway activation (Sato et al., 2016). Recently, researchers have developed a therapeutic approach involving a combination of HA and CD44 targeting. Specifically, the drug is modified by HA and targeted to CD44-positive tumor cells to improve the efficiency of drug utilization (Mattheolabakis et al., 2015). Many preclinical trials have revealed positive results using HA-modified drugs, as shown in Table 2.
Inflammatory PSC Lead to Malignant Inflammation of Pancreatic Cancer
Inflammation in the tumor microenvironment assists in the process of drug resistance, proliferation, metastasis, and immunosuppression in pancreatic cancer (Jarrin Jara et al., 2020). However, IL-6, secreted by inflammatory PSC, plays a significant role in mediating inflammation-related malignant progression of pancreatic cancer (Figure 4). Research has shown that IL-6 expression levels are significantly higher in patients with systemic metastases, and high levels of IL-6 reliably predict poor prognosis in patients treated with surgery (Palmquist et al., 2020). Additionally, high levels of IL-6 are often accompanied by large tumor size and distant metastases (Miura et al., 2015). IL-6 activates the JAK-STAT3 signaling pathway in cancer cells to exert its carcinogenic effect (Nagathihalli et al., 2016) and is regulated by both classical and trans-signaling pathways. In classical signaling, IL-6 can bind to the IL-6 receptor and induce a conformational change that triggers glycoprotein (gp) 130 dimerization. Subsequently, two IL-6-IL-R molecules bind to a gp130 dimer forming a 6-membered complex resulting in the activation of downstream JAK (Yu et al., 2014). In trans-signaling, the cleavage of IL-6R by specific enzymes, or alternative splicing of IL6R mRNA, produces soluble IL-6R (sIL-6R) (Johnson et al., 2018). IL-6R binds sIL-6R and forms an L-6-sIL-6R complex with the ability to induce gp130 dimerization to activate downstream JAK (104). Both types of regulation result in JAK activation and phosphorylation of the transcription factor STAT3, which regulates gene expression in cancer cells (van Duijneveldt et al., 19792020). Studies have shown that activating the JAK-STAT3 pathway enhances migration (Okitsu et al., 2010), drug resistance, proliferation (Zhang et al., 2017), and stemness (Alcalá et al., 2019) of pancreatic cancer cells. Inhibition of STAT3 signaling leads to apoptosis of pancreatic cancer cells (Nagathihalli et al., 2015). IL-6-receptor blockers enhance chemotherapeutic efficacy in KPC (LSL-Kras G12D/+; LSL-Trp53 R172H/+; Pdx-1Cre) mice (Long et al., 2017).
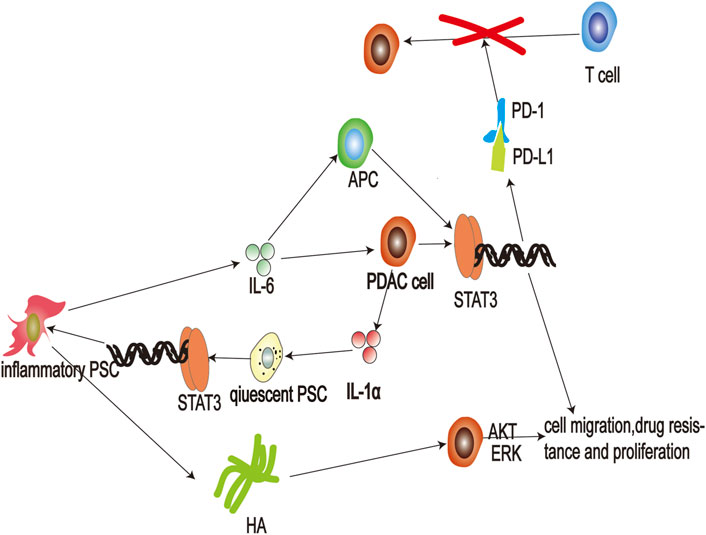
FIGURE 4. Schematic representation of the interaction between pancreatic cancer cells and inflammatory pancreatic stellate cells (PSCs). Secretion of interleukin (IL)-1α by pancreatic cancer cells stimulates activation of JAK-STAT3 signaling in quiescent PSCs, and leads to differentiation of inflammatory PSCs. Thereafter, inflammatory PSCs produce hyaluronic acid that then binds to cluster of differentiation (CD) 44 to activate AKT and ERK signaling pathways and promote the malignant process of pancreatic cancer. Additionally, inflammatory PSCs can also secrete large amounts of the cytokine IL-6, which activates JAK-STAT3 signaling pathways in cancer cells, promoting their proliferation and invasion. In addition, IL-6 also promotes the transcription of PD-L1 in antigen-presenting cells and cancer cells, leading to suppression of T-cell immunity; APC: antigen-presenting cell; ERK: extracellular regulated kinase; HA: hyaluronic acid; HAS: hyaluronan synthase; IL-1: Interleukin-1 IL-6: Interleukin-6; PD-1: programmed cell death one; PD-L1: protein programmed cell death one ligand one; PSC: pancreatic stellate cell.
Furthermore, inflammatory PSC also secrete IL-6 resulting in immunosuppression. PD-1 is one of the co-suppressor receptors of activated immune cells, and PD-L1 is mainly expressed in tumor cells and antigen-presenting cells (Ai et al., 2020). When PD-L1 binds to PD-1, this leads to suppression of activated immune cells and assists in the immune escape of tumor cells (Patel and Kurzrock, 2015). Anti-PD-1 targeted therapy has been successfully used in many cancers, but not in pancreatic cancer (Tsoukalas et al., 2019). This may be due to the persistent inflammatory response in pancreatic cancer (Antonangeli et al., 2020). Research has shown that transcription of PD-L1 is regulated by IL-6 in pancreatic cancer (Tsukamoto et al., 2018). IL-6 accelerates bone marrow-derived myeloid-derived suppressor cell differentiation and leads to increased levels of PD-L1 expression on myeloid-derived suppressor cells (Marigo et al., 2010; Weber et al., 2021). IL-6 also induces apoptosis of conventional type 1 dendritic cells to prevent antigen presentation (Lin et al., 2020). Moreover, antibody blockade of IL-6 reduces the expression of PD-L1 in dendritic cells (Eriksson et al., 2019). JAK-STAT3 activation in pancreatic tumor-bearing mice inhibits the effect of anti-PD-1 treatment (Lu et al., 2017). Therefore, blocking IL-6 may represent an effective adjuvant method for anti-PD-1 therapy. The combination of anti-IL-6 and anti-PD-L1 therapy effectively increases the survival rates of pancreatic cancer mice (Mace et al., 2018).
Studies focused on blocking IL-6-mediated JAK-STAT3 signaling activation have been conducted in the following categories ((Kaur et al., 2020): (Mizrahi et al., 2020) IL-6 receptor antagonists: tocilizumab, sarilumab; (Kamisawa et al., 2016); IL-6 production inhibitors: olokizumab, sirukumab, siltuximab, clazakizumab, PF-423691; and (Wang et al., 2020) JAK1/2 and STAT3 inhibitors: ruxolitinib, momelotinib. Despite the success of blocking IL-6 in pancreatic cancer animal models, this approach was virtually ineffective in a clinical setting (Ng et al., 2019). Anti-IL-6 therapy as an adjuvant in combination with other targeted therapies may represent a novel area worthy of exploration in the treatment of pancreatic cancer.
Outlook: The diversity of PSC subsets represents an important factor in the therapeutic intervention of pancreatic cancer.
We have shown that different groups of PSC play different roles in the progression of pancreatic cancer. The discovery of PSC heterogeneity will provide the basis for the development of novel approaches for the treatment of pancreatic cancer. Finding therapeutic targets for the treatment of pancreatic cancer at the level of PSC heterogeneity represents an unexplored potential therapeutic strategy. Although most of our regimens targeting myofibroblastic PSC for pancreatic cancer (including depletion of a-SMA + PSC and blockade of Shh) have failed, we have still achieved some successes such as blocking TGF-β or inducing PSC quiescence. Simultaneous inhibition of inflammatory responses and fibroplasia caused by different PSC subgroups significantly prevented pancreatic cancer progression in mice with no side effects (Khan et al., 2020). These reveal that targeting PSC for pancreatic cancer is an extremely valuable idea, but it should be done rationally. Future research should focus on the following aspects: 1) Induction of PSC quiescence to effectively block the progression of pancreatic cancer, 2) Evaluation of how pancreatic cancer cells produce and regulate the production of PSC subsets, and how PSC tumor suppressor subsets, specifically CD271 + PSC (Nielsen et al., 2020) and Meflin + PSC (Mizutani et al., 2019), are formed, and 3) Simultaneous blockade of the oncogenic effects of different PSC subgroups to treat pancreatic cancer more effectively.
Author Contributions
ZZ drafted the manuscript. HZ researched the literature and drafted figures. TL and TC counted and plotted the tables. DT and DW critically revised the article for important intellectual content. All authors read and approved the final manuscript.
Funding
This work was supported by grants from the Training Project of Key Talents of Youth Medicine in Jiangsu province, China (No. QNRC2016330), the Social Development-Health Care Project of Yangzhou, Jiangsu Province (No. YZ2018087), the Social Development-Health Care Project of Yangzhou, Jiangsu Province (No. YZ2021075), and High-level talent “six one projects” top talent scientific research project of Jiangsu Province (No. LGY2019034) the Graduate Research-Innovation Project in Jiangsu province (No. SJCX21_1644). The funding bodies had no role in the design of the study; in the collection, analysis, and interpretation of the data; and in writing the manuscript.
Conflict of Interest
The authors declare that the research was conducted in the absence of any commercial or financial relationships that could be construed as a potential conflict of interest.
Publisher’s Note
All claims expressed in this article are solely those of the authors and do not necessarily represent those of their affiliated organizations, or those of the publisher, the editors and the reviewers. Any product that may be evaluated in this article, or claim that may be made by its manufacturer, is not guaranteed or endorsed by the publisher.
References
Abetamann, V., Kern, H. F., and Elsässer, H. P. (1996). Differential Expression of the Hyaluronan Receptors CD44 and RHAMM in Human Pancreatic Cancer Cells. Clin. Cancer Res. 2 (9), 1607–1618. Epub 1996/09/01. PubMed PMID: 9816340.
Ai, L., Xu, A., and Xu, J. (2020). Roles of PD-1/pd-L1 Pathway: Signaling, Cancer, and beyond. Adv. Exp. Med. Biol. 1248, 33–59. Epub 2020/03/19PubMed PMID: 32185706. doi:10.1007/978-981-15-3266-5_3
Alcalá, S., Martinelli, P., Hermann, P. C., Heeschen, C., and Sainz, B. (2019). The Anthrax Toxin Receptor 1 (ANTXR1) Is Enriched in Pancreatic Cancer Stem Cells Derived from Primary Tumor Cultures. Stem Cell Int. 2019, 1–13. Epub 2019/06/14PubMed PMID: 31191663; PubMed Central PMCID: PMCPMC6525821. doi:10.1155/2019/1378639
Antonangeli, F., Natalini, A., Garassino, M. C., Sica, A., Santoni, A., and Di Rosa, F. (2020). Regulation of PD-L1 Expression by NF-Κb in Cancer. Front. Immunol. 11, 584626. Epub 2020/12/17PubMed PMID: 33324403; PubMed Central PMCID: PMCPMC7724774. doi:10.3389/fimmu.2020.584626
Apte, M. V., Haber, P. S., Darby, S. J., Rodgers, S. C., McCaughan, G. W., Korsten, M. A., et al. (1999). Pancreatic Stellate Cells Are Activated by Proinflammatory Cytokines: Implications for Pancreatic Fibrogenesis. Gut 44 (4), 534–541. Epub 1999/03/17PubMed PMID: 10075961; PubMed Central PMCID: PMCPMC1727467. doi:10.1136/gut.44.4.534
Apte, M. V., and Wilson, J. S. (2012). Dangerous Liaisons: Pancreatic Stellate Cells and Pancreatic Cancer Cells. J. Gastroenterol. Hepatol. 27 (Suppl. 2), 69–74. Epub 2012/02/15PubMed PMID: 22320920. doi:10.1111/j.1440-1746.2011.07000.x
Asaumi, H., Watanabe, S., Taguchi, M., Tashiro, M., and Otsuki, M. (2007). Externally Applied Pressure Activates Pancreatic Stellate Cells through the Generation of Intracellular Reactive Oxygen Species. Am. J. Physiology-Gastrointestinal Liver Physiol. 293 (5), G972–G978. Epub 2007/09/01PubMed PMID: 17761838. doi:10.1152/ajpgi.00018.2007
Bailey, J. M., Swanson, B. J., Hamada, T., Eggers, J. P., Singh, P. K., Caffery, T., et al. (2008). Sonic Hedgehog Promotes Desmoplasia in Pancreatic Cancer. Clin. Cancer Res. 14 (19), 5995–6004. Epub 2008/10/03PubMed PMID: 18829478; PubMed Central PMCID: PMCPMC2782957. doi:10.1158/1078-0432.ccr-08-0291
Baltes, F., Caspers, J., Henze, S., Schlesinger, M., and Bendas, G. (2020). Targeting Discoidin Domain Receptor 1 (DDR1) Signaling and its Crosstalk with β1-Integrin Emerges as a Key Factor for Breast Cancer Chemosensitization upon Collagen Type 1 Binding. Ijms 21 (14), 4956. Epub 2020/07/17PubMed PMID: 32668815; PubMed Central PMCID: PMCPMC7404217. doi:10.3390/ijms21144956
Banerjee, S., Modi, S., McGinn, O., Zhao, X., Dudeja, V., Ramakrishnan, S., et al. (2016). Impaired Synthesis of Stromal Components in Response to Minnelide Improves Vascular Function, Drug Delivery, and Survival in Pancreatic Cancer. Clin. Cancer Res. 22 (2), 415–425. Epub 2015/09/26PubMed PMID: 26405195; PubMed Central PMCID: PMCPMC4716007. doi:10.1158/1078-0432.ccr-15-1155
Begum, A., Ewachiw, T., Jung, C., Huang, A., Norberg, K. J., Marchionni, L., et al. (2017). The Extracellular Matrix and Focal Adhesion Kinase Signaling Regulate Cancer Stem Cell Function in Pancreatic Ductal Adenocarcinoma. PloS one 12 (7), e0180181. Epub 2017/07/12PubMed PMID: 28692661. doi:10.1371/journal.pone.0180181
Biffi, G., Oni, T. E., Spielman, B., Hao, Y., Elyada, E., Park, Y., et al. (2019). IL1-Induced JAK/STAT Signaling Is Antagonized by TGFβ to Shape CAF Heterogeneity in Pancreatic Ductal Adenocarcinoma. Cancer Discov. 9 (2), 282–301. Epub 2018/10/28PubMed PMID: 30366930; PubMed Central PMCID: PMCPMC6368881. doi:10.1158/2159-8290.cd-18-0710
Cao, J., Li, J., Sun, L., Qin, T., Xiao, Y., Chen, K., et al. (2019). Hypoxia‐driven Paracrine Osteopontin/integrin αvβ3 Signaling Promotes Pancreatic Cancer Cell Epithelial-Mesenchymal Transition and Cancer Stem Cell‐like Properties by Modulating Forkhead Box Protein M1. Mol. Oncol. 13 (2), 228–245. Epub 2018/10/28PubMed PMID: 30367545; PubMed Central PMCID: PMCPMC6360359. doi:10.1002/1878-0261.12399
Caon, I., Bartolini, B., Parnigoni, A., Caravà, E., Moretto, P., Viola, M., et al. (2020). Revisiting the Hallmarks of Cancer: The Role of Hyaluronan. Semin. Cancer Biol. 62, 9–19. Epub 2019/07/19PubMed PMID: 31319162. doi:10.1016/j.semcancer.2019.07.007
Chauhan, V. P., Boucher, Y., Ferrone, C. R., Roberge, S., Martin, J. D., Stylianopoulos, T., et al. (2014). Compression of Pancreatic Tumor Blood Vessels by Hyaluronan Is Caused by Solid Stress and Not Interstitial Fluid Pressure. Cancer cell 26 (1), 14–15. Epub 2014/07/16PubMed PMID: 25026209; PubMed Central PMCID: PMCPMC4381566. doi:10.1016/j.ccr.2014.06.003
Chen, H.-R., Yeh, Y.-C., Liu, C.-Y., Wu, Y.-T., Lo, F.-Y., Tang, M.-J., et al. (2016). DDR1 Promotes E-Cadherin Stability via Inhibition of Integrin-Β1-Src Activation-Mediated E-Cadherin Endocytosis. Sci. Rep. 6, 36336. Epub 2016/11/09PubMed PMID: 27824116; PubMed Central PMCID: PMCPMC5099905. doi:10.1038/srep36336
Chen, Y.-T., Chen, F.-W., Chang, T.-H., Wang, T.-W., Hsu, T.-P., Chi, J.-Y., et al. (2019). Hepatoma-derived Growth Factor Supports the Antiapoptosis and Profibrosis of Pancreatic Stellate Cells. Cancer Lett. 457, 180–190. Epub 2019/05/13PubMed PMID: 31078734. doi:10.1016/j.canlet.2019.05.001
Chen, Y., Kim, J., Yang, S., Wang, H., Wu, C.-J., Sugimoto, H., et al. (2021). Type I Collagen Deletion in αSMA+ Myofibroblasts Augments Immune Suppression and Accelerates Progression of Pancreatic Cancer. Cancer cell 39 (4), 548–565. e6. Epub 2021/03/06PubMed PMID: 33667385; PubMed Central PMCID: PMCPMC8423173. doi:10.1016/j.ccell.2021.02.007
Chronopoulos, A., Robinson, B., Sarper, M., Cortes, E., Auernheimer, V., Lachowski, D., et al. (2016). ATRA Mechanically Reprograms Pancreatic Stellate Cells to Suppress Matrix Remodelling and Inhibit Cancer Cell Invasion. Nat. Commun. 7, 12630. Epub 2016/09/08PubMed PMID: 27600527; PubMed Central PMCID: PMCPMC5023948. doi:10.1038/ncomms12630
Cooper, J., and Giancotti, F. G. (2019). Integrin Signaling in Cancer: Mechanotransduction, Stemness, Epithelial Plasticity, and Therapeutic Resistance. Cancer cell 35 (3), 347–367. Epub 2019/03/20PubMed PMID: 30889378; PubMed Central PMCID: PMCPMC6684107. doi:10.1016/j.ccell.2019.01.007
Cortes, E., Lachowski, D., Rice, A., Thorpe, S. D., Robinson, B., Yeldag, G., et al. (2019). Tamoxifen Mechanically Deactivates Hepatic Stellate Cells via the G Protein-Coupled Estrogen Receptor. Oncogene 38 (16), 2910–2922. Epub 2018/12/24PubMed PMID: 30575816; PubMed Central PMCID: PMCPMC6755965. doi:10.1038/s41388-018-0631-3
Cortes, E., Lachowski, D., Robinson, B., Sarper, M., Teppo, J. S., Thorpe, S. D., et al. (2019). Tamoxifen Mechanically Reprograms the Tumor Microenvironment via HIF ‐1A and Reduces Cancer Cell Survival. EMBO Rep. 20 (1), e46557. Epub 2018/12/13PubMed PMID: 30538116; PubMed Central PMCID: PMCPMC6322388. doi:10.15252/embr.201846557
Cortes, E., Sarper, M., Robinson, B., Lachowski, D., Chronopoulos, A., Thorpe, S. D., et al. (2019). GPER Is a Mechanoregulator of Pancreatic Stellate Cells and the Tumor Microenvironment. EMBO Rep. 20 (1), e46556. Epub 2018/12/13PubMed PMID: 30538117; PubMed Central PMCID: PMCPMC6322386. doi:10.15252/embr.201846556
Dalla Pozza, E., Lerda, C., Costanzo, C., Donadelli, M., Dando, I., Zoratti, E., et al. (2013). Targeting Gemcitabine Containing Liposomes to CD44 Expressing Pancreatic Adenocarcinoma Cells Causes an Increase in the Antitumoral Activity. Biochim. Biophys. Acta (Bba) - Biomembranes 1828 (5), 1396–1404. Epub 2013/02/07PubMed PMID: 23384419. doi:10.1016/j.bbamem.2013.01.020
Dang, C. V. (2012). MYC on the Path to Cancer. Cell 149 (1), 22–35. Epub 2012/04/03PubMed PMID: 22464321; PubMed Central PMCID: PMCPMC3345192. doi:10.1016/j.cell.2012.03.003
Dangi-Garimella, S., Krantz, S. B., Barron, M. R., Shields, M. A., Heiferman, M. J., Grippo, P. J., et al. (2011). Three-dimensional Collagen I Promotes Gemcitabine Resistance in Pancreatic Cancer through MT1-MMP-mediated Expression of HMGA2. Cancer Res. 71 (3), 1019–1028. Epub 2010/12/15PubMed PMID: 21148071; PubMed Central PMCID: PMCPMC3076124. doi:10.1158/0008-5472.can-10-1855
Das, S., Shapiro, B., Vucic, E. A., Vogt, S., and Bar-Sagi, D. (2020). Tumor Cell-Derived IL1β Promotes Desmoplasia and Immune Suppression in Pancreatic Cancer. Cancer Res. 80 (5), 1088–1101. Epub 2020/01/10PubMed PMID: 31915130; PubMed Central PMCID: PMCPMC7302116. doi:10.1158/0008-5472.can-19-2080
DuFort, C. C., DelGiorno, K. E., Carlson, M. A., Osgood, R. J., Zhao, C., Huang, Z., et al. (2016). Interstitial Pressure in Pancreatic Ductal Adenocarcinoma Is Dominated by a Gel-Fluid Phase. Biophysical J. 110 (9), 2106–2119. Epub 2016/05/12PubMed PMID: 27166818; PubMed Central PMCID: PMCPMC4939548. doi:10.1016/j.bpj.2016.03.040
Elahi-Gedwillo, K. Y., Carlson, M., Zettervall, J., and Provenzano, P. P. (2019). Antifibrotic Therapy Disrupts Stromal Barriers and Modulates the Immune Landscape in Pancreatic Ductal Adenocarcinoma. Cancer Res. 79 (2), 372–386. Epub 2018/11/08PubMed PMID: 30401713; PubMed Central PMCID: PMCPMC6335156. doi:10.1158/0008-5472.can-18-1334
Elyada, E., Bolisetty, M., Laise, P., Flynn, W. F., Courtois, E. T., Burkhart, R. A., et al. (2019). Cross-Species Single-Cell Analysis of Pancreatic Ductal Adenocarcinoma Reveals Antigen-Presenting Cancer-Associated Fibroblasts. Cancer Discov. 9 (8), 1102–1123. Epub 2019/06/15PubMed PMID: 31197017; PubMed Central PMCID: PMCPMC6727976. doi:10.1158/2159-8290.cd-19-0094
Eriksson, E., Milenova, I., Wenthe, J., Moreno, R., Alemany, R., and Loskog, A. (2019). IL-6 Signaling Blockade during CD40-Mediated Immune Activation Favors Antitumor Factors by Reducing TGF-β, Collagen Type I, and PD-L1/pd-1. J.I. Baltimore, Md, 202(3), 787–798. Epub 2019/01/09PubMed PMID: 30617223. doi:10.4049/jimmunol.1800717
Erkan, M., Michalski, C. W., Rieder, S., Reiser–Erkan, C., Abiatari, I., Kolb, A., et al. (2008). The Activated Stroma index Is a Novel and Independent Prognostic Marker in Pancreatic Ductal Adenocarcinoma. Clin. Gastroenterol. Hepatol. 6 (10), 1155–1161. Epub 2008/07/22PubMed PMID: 18639493. doi:10.1016/j.cgh.2008.05.006
Farag, M. M., Abd El Malak, N. S., Yehia, S. A., and Ahmed, M. A. (2021). Hyaluronic Acid Conjugated Metformin-Phospholipid Sonocomplex: A Biphasic Complexation Approach to Correct Hypoxic Tumour Microenvironment. Ijn 16, 1005–1019. Epub 2021/02/20PubMed PMID: 33603365; PubMed Central PMCID: PMCPMC7885809. doi:10.2147/ijn.s297634
Feig, C., Jones, J. O., Kraman, M., Wells, R. J. B., Deonarine, A., Chan, D. S., et al. (2013). Targeting CXCL12 from FAP-Expressing Carcinoma-Associated Fibroblasts Synergizes with Anti-PD-L1 Immunotherapy in Pancreatic Cancer. Proc. Natl. Acad. Sci. 110 (50), 20212–20217. Epub 2013/11/28PubMed PMID: 24277834; PubMed Central PMCID: PMCPMC3864274. doi:10.1073/pnas.1320318110
Froeling, F. E. M., Feig, C., Chelala, C., Dobson, R., Mein, C. E., Tuveson, D. A., et al. (2011). Retinoic Acid-Induced Pancreatic Stellate Cell Quiescence Reduces Paracrine Wnt-β-Catenin Signaling to Slow Tumor Progression. Gastroenterology 141 (4), 1486–1497. Epub 2011/06/28PubMed PMID: 21704588. doi:10.1053/j.gastro.2011.06.047
Fujita, H., Ohuchida, K., Mizumoto, K., Egami, T., Miyoshi, K., Moriyama, T., et al. (2009). Tumor-stromal Interactions with Direct Cell Contacts Enhance Proliferation of Human Pancreatic Carcinoma Cells. Cancer Sci. 100 (12), 2309–2317. Epub 2009/09/09PubMed PMID: 19735487. doi:10.1111/j.1349-7006.2009.01317.x
Grzesiak, J. J., Ho, J. C., Moossa, A. R., and Bouvet, M. (2007). The Integrin-Extracellular Matrix axis in Pancreatic Cancer. Pancreas 35 (4), 293–301. Epub 2007/12/20PubMed PMID: 18090233. doi:10.1097/mpa.0b013e31811f4526
Haeberle, L., Steiger, K., Schlitter, A. M., Safi, S. A., Knoefel, W. T., Erkan, M., et al. (2018). Stromal Heterogeneity in Pancreatic Cancer and Chronic Pancreatitis. Pancreatology 18 (5), 536–549. Epub 2018/05/21PubMed PMID: 29778400. doi:10.1016/j.pan.2018.05.004
Hama, K., Ohnishi, H., Aoki, H., Kita, H., Yamamoto, H., Osawa, H., et al. (2006). Angiotensin II Promotes the Proliferation of Activated Pancreatic Stellate Cells by Smad7 Induction through a Protein Kinase C Pathway. Biochem. biophysical Res. Commun. 340 (3), 742–750. Epub 2005/12/29PubMed PMID: 16380081. doi:10.1016/j.bbrc.2005.12.069
Henriet, E., Sala, M., Abou Hammoud, A., Tuariihionoa, A., Di Martino, J., Ros, M., et al. (2018). Multitasking Discoidin Domain Receptors Are Involved in Several and Specific Hallmarks of Cancer. Cell Adhes. Migration 12 (4), 1–15. Epub 2018/04/28PubMed PMID: 29701112; PubMed Central PMCID: PMCPMC6411096. doi:10.1080/19336918.2018.1465156
Hingorani, S. R., Harris, W. P., Beck, J. T., Berdov, B. A., Wagner, S. A., Pshevlotsky, E. M., et al. (2016). Phase Ib Study of PEGylated Recombinant Human Hyaluronidase and Gemcitabine in Patients with Advanced Pancreatic Cancer. Clin. Cancer Res. 22 (12), 2848–2854. Epub 2016/01/28PubMed PMID: 26813359; PubMed Central PMCID: PMCPMC7787348. doi:10.1158/1078-0432.ccr-15-2010
Hingorani, S. R., Zheng, L., Bullock, A. J., Seery, T. E., Harris, W. P., Sigal, D. S., et al. (2018). HALO 202: Randomized Phase II Study of PEGPH20 Plus Nab-Paclitaxel/Gemcitabine versus Nab-Paclitaxel/Gemcitabine in Patients with Untreated, Metastatic Pancreatic Ductal Adenocarcinoma. Jco 36 (4), 359–366. Epub 2017/12/13PubMed PMID: 29232172. doi:10.1200/jco.2017.74.9564
Hosein, A. N., Brekken, R. A., and Maitra, A. (2020). Pancreatic Cancer Stroma: an Update on Therapeutic Targeting Strategies. Nat. Rev. Gastroenterol. Hepatol. 17 (8), 487–505. Epub 2020/05/13PubMed PMID: 32393771; PubMed Central PMCID: PMCPMC8284850. doi:10.1038/s41575-020-0300-1
Hu, R., Wang, Y.-L., Edderkaoui, M., Lugea, A., Apte, M. V., and Pandol, S. J. (2007). Ethanol Augments PDGF-Induced NADPH Oxidase Activity and Proliferation in Rat Pancreatic Stellate Cells. Pancreatology 7 (4), 332–340. Epub 2007/07/14PubMed PMID: 17627098; PubMed Central PMCID: PMCPMC2826430. doi:10.1159/000105499
Huang, H., Svoboda, R. A., Lazenby, A. J., Saowapa, J., Chaika, N., Ding, K., et al. (2016). Up-regulation of N-Cadherin by Collagen I-Activated Discoidin Domain Receptor 1 in Pancreatic Cancer Requires the Adaptor Molecule Shc1. J. Biol. Chem. 291 (44), 23208–23223. Epub 2016/10/30PubMed PMID: 27605668; PubMed Central PMCID: PMCPMC5087738. doi:10.1074/jbc.M116.740605
Ikenaga, N., Ohuchida, K., Mizumoto, K., Cui, L., Kayashima, T., Morimatsu, K., et al. (2010). CD10+ Pancreatic Stellate Cells Enhance the Progression of Pancreatic Cancer. Gastroenterology 139 (3), 1041–1051. PubMed PMID: 20685603. doi:10.1053/j.gastro.2010.05.084
Imamichi, Y., König, A., Gress, T., and Menke, A. (2007). Collagen Type I-Induced Smad-Interacting Protein 1 Expression Downregulates E-Cadherin in Pancreatic Cancer. Oncogene 26 (16), 2381–2385. Epub 2006/10/18PubMed PMID: 17043655. doi:10.1038/sj.onc.1210012
Incio, J., Liu, H., Suboj, P., Chin, S. M., Chen, I. X., Pinter, M., et al. (2016). Obesity-Induced Inflammation and Desmoplasia Promote Pancreatic Cancer Progression and Resistance to Chemotherapy. Cancer Discov. 6 (8), 852–869. Epub 2016/06/02PubMed PMID: 27246539; PubMed Central PMCID: PMCPMC4972679. doi:10.1158/2159-8290.cd-15-1177
Ivaska, J., and Heino, J. (2011). Cooperation between Integrins and Growth Factor Receptors in Signaling and Endocytosis. Annu. Rev. Cel Dev. Biol. 27, 291–320. Epub 2011/06/15PubMed PMID: 21663443. doi:10.1146/annurev-cellbio-092910-154017
Iwamoto, C., Ohuchida, K., Shinkawa, T., Okuda, S., Otsubo, Y., Okumura, T., et al. (2021). Bone Marrow-Derived Macrophages Converted into Cancer-Associated Fibroblast-like Cells Promote Pancreatic Cancer Progression. Cancer Lett. 512, 15–27. Epub 2021/05/08PubMed PMID: 33961925. doi:10.1016/j.canlet.2021.04.013
Jacobetz, M. A., Chan, D. S., Neesse, A., Bapiro, T. E., Cook, N., Frese, K. K., et al. (2013). Hyaluronan Impairs Vascular Function and Drug Delivery in a Mouse Model of Pancreatic Cancer. Gut 62 (1), 112–120. Epub 2012/04/03PubMed PMID: 22466618; PubMed Central PMCID: PMCPMC3551211. doi:10.1136/gutjnl-2012-302529
Jarrin Jara, M. D., Gautam, A. S., Peesapati, V. S. R., Sadik, M., and Khan, S. (2020). The Role of Interleukin-6 and Inflammatory Cytokines in Pancreatic Cancer-Associated Depression. Cureus 12 (8), e9969. Epub 2020/08/28PubMed PMID: 32850269; PubMed Central PMCID: PMCPMC7444958. doi:10.7759/cureus.9969
Johnson, D. E., O'Keefe, R. A., and Grandis, J. R. (2018). Targeting the IL-6/JAK/STAT3 Signalling axis in Cancer. Nat. Rev. Clin. Oncol. 15 (4), 234–248. Epub 2018/02/07PubMed PMID: 29405201; PubMed Central PMCID: PMCPMC5858971. doi:10.1038/nrclinonc.2018.8
Junliang, L., Lili, W., Xiaolong, L., Xuguang, L., Huanwen, W., and Zhiyong, L. (2019). High-molecular-weight Hyaluronan Produced by Activated Pancreatic Stellate Cells Promotes Pancreatic Cancer Cell Migration via Paracrine Signaling. Biochem. biophysical Res. Commun. 515 (3), 493–498. Epub 2019/06/07PubMed PMID: 31171359. doi:10.1016/j.bbrc.2019.05.167
Kamisawa, T., Wood, L. D., Itoi, T., and Takaori, K. (2016). Pancreatic Cancer. The Lancet 388 (10039), 73–85. Epub 2016/02/03PubMed PMID: 26830752. doi:10.1016/s0140-6736(16)00141-0
Katoh, M. (2018). Multi-layered P-revention and T-reatment of C-hronic I-nflammation, O-rgan F-ibrosis and C-ancer A-ssociated with C-anonical WNT/β-catenin S-ignaling A-ctivation (Review). Int. J. Mol. Med. 42 (2), 713–725. Epub 2018/05/23PubMed PMID: 29786110; PubMed Central PMCID: PMCPMC6034925. doi:10.3892/ijmm.2018.3689
Kaur, S., Bansal, Y., Kumar, R., and Bansal, G. (2020). A Panoramic Review of IL-6: Structure, Pathophysiological Roles and Inhibitors. Bioorg. Med. Chem. 28 (5), 115327. Epub 2020/01/30PubMed PMID: 31992476. doi:10.1016/j.bmc.2020.115327
Kesharwani, P., Banerjee, S., Padhye, S., Sarkar, F. H., and Iyer, A. K. (2015). Hyaluronic Acid Engineered Nanomicelles Loaded with 3,4-Difluorobenzylidene Curcumin for Targeted Killing of CD44+ Stem-like Pancreatic Cancer Cells. Biomacromolecules 16 (9), 3042–3053. Epub 2015/08/25PubMed PMID: 26302089. doi:10.1021/acs.biomac.5b00941
Kesharwani, P., Xie, L., Banerjee, S., Mao, G., Padhye, S., Sarkar, F. H., et al. (2015). Hyaluronic Acid-Conjugated Polyamidoamine Dendrimers for Targeted Delivery of 3,4-difluorobenzylidene Curcumin to CD44 Overexpressing Pancreatic Cancer Cells. Colloids Surf. B: BiointerfacesBiointerfaces 136, 413–423. Epub 2015/10/07PubMed PMID: 26440757. doi:10.1016/j.colsurfb.2015.09.043
Khan, M. A., Srivastava, S. K., Zubair, H., Patel, G. K., Arora, S., Khushman, M. d., et al. (2020). Co-targeting of CXCR4 and Hedgehog Pathways Disrupts Tumor-Stromal Crosstalk and Improves Chemotherapeutic Efficacy in Pancreatic Cancer. J. Biol. Chem. 295 (25), 8413–8424. Epub 2020/05/03PubMed PMID: 32358063; PubMed Central PMCID: PMCPMC7307206. doi:10.1074/jbc.RA119.011748
Kim, E. J., Sahai, V., Abel, E. V., Griffith, K. A., Greenson, J. K., Takebe, N., et al. (2014). Pilot Clinical Trial of Hedgehog Pathway Inhibitor GDC-0449 (Vismodegib) in Combination with Gemcitabine in Patients with Metastatic Pancreatic Adenocarcinoma. Clin. Cancer Res. 20 (23), 5937–5945. Epub 2014/10/04PubMed PMID: 25278454; PubMed Central PMCID: PMCPMC4254161. doi:10.1158/1078-0432.ccr-14-1269
Kim, J.-W., Park, S.-Y., You, Y.-H., Ham, D.-S., Lee, S.-H., Yang, H. K., et al. (2016). Suppression of ROS Production by Exendin-4 in PSC Attenuates the High Glucose-Induced Islet Fibrosis. PloS one 11 (12), e0163187. Epub 2016/12/16PubMed PMID: 27977690; PubMed Central PMCID: PMCPMC5157943. doi:10.1371/journal.pone.0163187
Kocher, H. M., Basu, B., Froeling, F. E. M., Sarker, D., Slater, S., Carlin, D., et al. (2020). Phase I Clinical Trial Repurposing All-Trans Retinoic Acid as a Stromal Targeting Agent for Pancreatic Cancer. Nat. Commun. 11 (1), 4841. Epub 2020/09/26PubMed PMID: 32973176. doi:10.1038/s41467-020-18636-w
Koenig, A., Mueller, C., Hasel, C., Adler, G., and Menke, A. (2006). Collagen Type I Induces Disruption of E-Cadherin-Mediated Cell-Cell Contacts and Promotes Proliferation of Pancreatic Carcinoma Cells. Cancer Res. 66 (9), 4662–4671. Epub 2006/05/03PubMed PMID: 16651417. doi:10.1158/0008-5472.can-05-2804
Kolenda, T., Guglas, K., Kopczyńska, M., Sobocińska, J., Teresiak, A., Bliźniak, R., et al. (2020). Good or Not Good: Role of miR-18a in Cancer Biology. Rep. Pract. Oncol. Radiother. 25 (5), 808–819. Epub 2020/09/05PubMed PMID: 32884453; PubMed Central PMCID: PMCPMC7451592. doi:10.1016/j.rpor.2020.07.006
Lei, J., Huo, X., Duan, W., Xu, Q., Li, R., Ma, J., et al. (2014). α-Mangostin Inhibits Hypoxia-Driven ROS-Induced PSC Activation and Pancreatic Cancer Cell Invasion. Cancer Lett. 347 (1), 129–138. Epub 2014/02/12PubMed PMID: 24513179; PubMed Central PMCID: PMCPMC4005872. doi:10.1016/j.canlet.2014.02.003
Lenggenhager, D., Amrutkar, M., Sántha, P., Aasrum, M., Löhr, J.-M., Gladhaug, I. P., et al. (2019). Commonly Used Pancreatic Stellate Cell Cultures Differ Phenotypically and in Their Interactions with Pancreatic Cancer Cells. Cells 8 (1), 23. Epub 2019/01/10PubMed PMID: 30621293; PubMed Central PMCID: PMCPMC6356867. doi:10.3390/cells8010023
Lin, J. H., Huffman, A. P., Wattenberg, M. M., Walter, D. M., Carpenter, E. L., Feldser, D. M., et al. (2020). Type 1 Conventional Dendritic Cells Are Systemically Dysregulated Early in Pancreatic Carcinogenesis. J. Exp. Med. 217 (8). Epub 2020/05/27PubMed PMID: 32453421. doi:10.1084/jem.20190673
Long, K. B., Tooker, G., Tooker, E., Luque, S. L., Lee, J. W., Pan, X., et al. (2017). IL6 Receptor Blockade Enhances Chemotherapy Efficacy in Pancreatic Ductal Adenocarcinoma. Mol. Cancer Ther. 16 (9), 1898–1908. Epub 2017/06/15PubMed PMID: 28611107; PubMed Central PMCID: PMCPMC5587413. doi:10.1158/1535-7163.mct-16-0899
Lu, C., Talukder, A., Savage, N. M., Singh, N., and Liu, K. (2017). JAK-STAT-mediated Chronic Inflammation Impairs Cytotoxic T Lymphocyte Activation to Decrease Anti-PD-1 Immunotherapy Efficacy in Pancreatic Cancer. Oncoimmunology 6 (3), e1291106. Epub 2017/04/14PubMed PMID: 28405527; PubMed Central PMCID: PMCPMC5384417. doi:10.1080/2162402x.2017.1291106
Ma, Q., Wu, H., Xiao, Y., Liang, Z., and Liu, T. (2020). Upregulation of Exosomal microRNA-21 in P-ancreatic S-tellate C-ells P-romotes P-ancreatic C-ancer C-ell M-igration and E-nhances Ras/ERK P-athway A-ctivity. Int. J. Oncol. 56 (4), 1025–1033. Epub 2020/04/23PubMed PMID: 32319558. doi:10.3892/ijo.2020.4986
Mace, T. A., Shakya, R., Pitarresi, J. R., Swanson, B., McQuinn, C. W., Loftus, S., et al. (2018). IL-6 and PD-L1 Antibody Blockade Combination Therapy Reduces Tumour Progression in Murine Models of Pancreatic Cancer. Gut 67 (2), 320–332. Epub 2016/11/01PubMed PMID: 27797936; PubMed Central PMCID: PMCPMC5406266. doi:10.1136/gutjnl-2016-311585
Marengo, A., Forciniti, S., Dando, I., Dalla Pozza, E., Stella, B., Tsapis, N., et al. (2019). Pancreatic Cancer Stem Cell Proliferation Is Strongly Inhibited by Diethyldithiocarbamate-Copper Complex Loaded into Hyaluronic Acid Decorated Liposomes. Biochim. Biophys. Acta (Bba) - Gen. Subjects 1863 (1), 61–72. Epub 2018/09/30PubMed PMID: 30267751. doi:10.1016/j.bbagen.2018.09.018
Marigo, I., Bosio, E., Solito, S., Mesa, C., Fernandez, A., Dolcetti, L., et al. (2010). Tumor-Induced Tolerance and Immune Suppression Depend on the C/EBPβ Transcription Factor. Immunity 32 (6), 790–802. Epub 2010/07/08PubMed PMID: 20605485. doi:10.1016/j.immuni.2010.05.010
Masamune, A., Kikuta, K., Watanabe, T., Satoh, K., Hirota, M., Hamada, S., et al. (2009). Fibrinogen Induces Cytokine and Collagen Production in Pancreatic Stellate Cells. Gut 58 (4), 550–559. Epub 2008/12/05PubMed PMID: 19052021. doi:10.1136/gut.2008.154401
Masamune, A., Satoh, M., Hirabayashi, J., Kasai, K., Satoh, K., and Shimosegawa, T. (2006). Galectin-1 Induces Chemokine Production and Proliferation in Pancreatic Stellate Cells. Am. J. Physiology-Gastrointestinal Liver Physiol. 290 (4), G729–G736. Epub 2005/12/24PubMed PMID: 16373424. doi:10.1152/ajpgi.00511.2005
Mattheolabakis, G., Milane, L., Singh, A., and Amiji, M. M. (2015). Hyaluronic Acid Targeting of CD44 for Cancer Therapy: from Receptor Biology to Nanomedicine. J. Drug Target. 23 (7-8), 605–618. Epub 2015/10/11PubMed PMID: 26453158. doi:10.3109/1061186x.2015.1052072
Melisi, D., Garcia-Carbonero, R., Macarulla, T., Pezet, D., Deplanque, G., Fuchs, M., et al. (2019). TGFβ Receptor Inhibitor Galunisertib Is Linked to Inflammation- and Remodeling-Related Proteins in Patients with Pancreatic Cancer. Cancer Chemother. Pharmacol. 83 (5), 975–991. Epub 2019/03/20PubMed PMID: 30887178. doi:10.1007/s00280-019-03807-4
Mews, P., Phillips, P., Fahmy, R., Korsten, M., Pirola, R., Wilson, J., et al. (2002). Pancreatic Stellate Cells Respond to Inflammatory Cytokines: Potential Role in Chronic Pancreatitis. Gut 50 (4), 535–541. Epub 2002/03/13PubMed PMID: 11889076; PubMed Central PMCID: PMCPMC1773172. doi:10.1136/gut.50.4.535
Miura, T., Mitsunaga, S., Ikeda, M., Shimizu, S., Ohno, I., Takahashi, H., et al. (2015). Characterization of Patients with Advanced Pancreatic Cancer and High Serum Interleukin-6 Levels. Pancreas 44 (5), 756–763. Epub 2015/05/02PubMed PMID: 25931255. doi:10.1097/mpa.0000000000000335
Miyai, Y., Esaki, N., Takahashi, M., and Enomoto, A. (2020). Cancer‐associated Fibroblasts that Restrain Cancer Progression: Hypotheses and Perspectives. Cancer Sci. 111 (4), 1047–1057. Epub 2020/02/16PubMed PMID: 32060987; PubMed Central PMCID: PMCPMC7156845. doi:10.1111/cas.14346
Miyazaki, Y., Oda, T., Inagaki, Y., Kushige, H., Saito, Y., Mori, N., et al. (2021). Adipose-derived Mesenchymal Stem Cells Differentiate into Heterogeneous Cancer-Associated Fibroblasts in a Stroma-Rich Xenograft Model. Sci. Rep. 11 (1), 4690. Epub 2021/02/27PubMed PMID: 33633222; PubMed Central PMCID: PMCPMC7907195. doi:10.1038/s41598-021-84058-3
Miyazaki, Y., Oda, T., Mori, N., and Kida, Y. S. (2020). Adipose‐derived Mesenchymal Stem Cells Differentiate into Pancreatic Cancer‐associated Fibroblasts In Vitro. FEBS open bio 10 (11), 2268–2281. Epub 2020/09/16PubMed PMID: 32931156; PubMed Central PMCID: PMCPMC7609785. doi:10.1002/2211-5463.12976
Mizrahi, J. D., Surana, R., Valle, J. W., and Shroff, R. T. (2020). Pancreatic Cancer. Lancet Lond. Engl. 395 (10242), 2008–2020. Epub 2020/07/01PubMed PMID: 32593337. doi:10.1016/s0140-6736(20)30974-0
Mizutani, Y., Kobayashi, H., Iida, T., Asai, N., Masamune, A., Hara, A., et al. (2019). Meflin-Positive Cancer-Associated Fibroblasts Inhibit Pancreatic Carcinogenesis. Cancer Res. 79 (20), 5367–5381. Epub 2019/08/24PubMed PMID: 31439548. doi:10.1158/0008-5472.can-19-0454
Moffitt, R. A., Marayati, R., Flate, E. L., Volmar, K. E., Loeza, S. G. H., Hoadley, K. A., et al. (2015). Virtual Microdissection Identifies Distinct Tumor- and Stroma-specific Subtypes of Pancreatic Ductal Adenocarcinoma. Nat. Genet. 47 (10), 1168–1178. Epub 2015/09/08PubMed PMID: 26343385; PubMed Central PMCID: PMCPMC4912058. doi:10.1038/ng.3398
Naci, D., Vuori, K., and Aoudjit, F. (2015). Alpha2beta1 Integrin in Cancer Development and Chemoresistance. Semin. Cancer Biol. 35, 145–153. Epub 2015/08/25PubMed PMID: 26297892. doi:10.1016/j.semcancer.2015.08.004
Nagathihalli, N. S., Castellanos, J. A., Shi, C., Beesetty, Y., Reyzer, M. L., Caprioli, R., et al. (2015). Signal Transducer and Activator of Transcription 3, Mediated Remodeling of the Tumor Microenvironment Results in Enhanced Tumor Drug Delivery in a Mouse Model of Pancreatic Cancer. Gastroenterology 149 (7), 1932–1943. e9. Epub 2015/08/11PubMed PMID: 26255562; PubMed Central PMCID: PMCPMC4863449. doi:10.1053/j.gastro.2015.07.058
Nagathihalli, N. S., Castellanos, J. A., VanSaun, M. N., Dai, X., Ambrose, M., Guo, Q., et al. (2016). Pancreatic Stellate Cell Secreted IL-6 Stimulates STAT3 Dependent Invasiveness of Pancreatic Intraepithelial Neoplasia and Cancer Cells. Oncotarget 7 (40), 65982–65992. Epub 2016/09/08PubMed PMID: 27602757; PubMed Central PMCID: PMCPMC5323208. doi:10.18632/oncotarget.11786
Nagy, N., Kuipers, H. F., Frymoyer, A. R., Ishak, H. D., Bollyky, J. B., Wight, T. N., et al. (2015). 4-methylumbelliferone Treatment and Hyaluronan Inhibition as a Therapeutic Strategy in Inflammation, Autoimmunity, and Cancer. Front. Immunol. 6, 123. Epub 2015/04/09PubMed PMID: 25852691; PubMed Central PMCID: PMCPMC4369655. doi:10.3389/fimmu.2015.00123
Ng, K., Hendifar, A., Starodub, A., Chaves, J., Yang, Y., Koh, B., et al. (2019). Phase 1 Dose-Escalation Study of Momelotinib, a Janus Kinase 1/2 Inhibitor, Combined with Gemcitabine and Nab-Paclitaxel in Patients with Previously Untreated Metastatic Pancreatic Ductal Adenocarcinoma. Invest. New Drugs 37 (1), 159–165. Epub 2018/08/15PubMed PMID: 30105668; PubMed Central PMCID: PMCPMC6510909. doi:10.1007/s10637-018-0650-5
Nielsen, M. F. B., Mortensen, M. B., Sørensen, M. D., Wirenfeldt, M., Kristensen, B. W., Schrøder, H. D., et al. (2020). Spatial and Phenotypic Characterization of Pancreatic Cancer-Associated Fibroblasts after Neoadjuvant Treatment. Histol. Histopathol 35 (8), 811–825. Epub 2020/01/22PubMed PMID: 31960942. doi:10.14670/hh-18-201
Nielsen, M. F. B., Mortensen, M. B., and Detlefsen, S. (2018). Typing of Pancreatic Cancer-Associated Fibroblasts Identifies Different Subpopulations. Wjg 24 (41), 4663–4678. Epub 2018/11/13PubMed PMID: 30416314; PubMed Central PMCID: PMCPMC6224473. doi:10.3748/wjg.v24.i41.4663
Nigam Joshi, P., Agawane, S., Athalye, M. C., Jadhav, V., Sarkar, D., and Prakash, R. (2017). Multifunctional Inulin Tethered Silver-Graphene Quantum Dots Nanotheranostic Module for Pancreatic Cancer Therapy. Mater. Sci. Eng. C 78, 1203–1211. Epub 2017/06/04PubMed PMID: 28575959. doi:10.1016/j.msec.2017.03.176
Nurmik, M., Ullmann, P., Rodriguez, F., Haan, S., and Letellier, E. (2020). In Search of Definitions: Cancer‐associated Fibroblasts and Their Markers. Int. J. Cancer 146 (4), 895–905. Epub 2019/02/09PubMed PMID: 30734283; PubMed Central PMCID: PMCPMC6972582. doi:10.1002/ijc.32193
Öhlund, D., Handly-Santana, A., Biffi, G., Elyada, E., Almeida, A. S., Ponz-Sarvise, M., et al. (2017). Distinct Populations of Inflammatory Fibroblasts and Myofibroblasts in Pancreatic Cancer. J. Exp. Med. 214 (3), 579–596. Epub 2017/02/25PubMed PMID: 28232471; PubMed Central PMCID: PMCPMC5339682. doi:10.1084/jem.20162024
Okitsu, K., Kanda, T., Imazeki, F., Yonemitsu, Y., Ray, R. B., Chang, C., et al. (2010). Involvement of Interleukin-6 and Androgen Receptor Signaling in Pancreatic Cancer. Genes & Cancer 1 (8), 859–867. Epub 2011/07/23PubMed PMID: 21779469; PubMed Central PMCID: PMCPMC3092246. doi:10.1177/1947601910383417
Özdemir, B. C., Pentcheva-Hoang, T., Carstens, J. L., Zheng, X., Wu, C.-C., Simpson, T. R., et al. (2014). Depletion of Carcinoma-Associated Fibroblasts and Fibrosis Induces Immunosuppression and Accelerates Pancreas Cancer with Reduced Survival. Cancer cell 25 (6), 719–734. Epub 2014/05/27PubMed PMID: 24856586; PubMed Central PMCID: PMCPMC4180632. doi:10.1016/j.ccr.2014.04.005
Palmquist, C., Dehlendorff, C., Calatayud, D., Hansen, C. P., Hasselby, J. P., and Johansen, J. S. (2020). Prediction of Unresectability and Prognosis in Patients Undergoing Surgery on Suspicion of Pancreatic Cancer Using Carbohydrate Antigen 19-9, Interleukin 6, and YKL-40. Pancreas 49 (1), 53–61. Epub 2019/12/20PubMed PMID: 31856080. doi:10.1097/mpa.0000000000001466
Patel, S. P., and Kurzrock, R. (2015). PD-L1 Expression as a Predictive Biomarker in Cancer Immunotherapy. Mol. Cancer Ther. 14 (4), 847–856. Epub 2015/02/20PubMed PMID: 25695955. doi:10.1158/1535-7163.mct-14-0983
Pothula, S. P., Pirola, R. C., Wilson, J. S., and Apte, M. V. (2020). Pancreatic Stellate Cells: Aiding and Abetting Pancreatic Cancer Progression. Pancreatology 20 (3), 409–418. Epub 2020/01/14PubMed PMID: 31928917. doi:10.1016/j.pan.2020.01.003
Pothula, S. P., Xu, Z., Goldstein, D., Pirola, R. C., Wilson, J. S., and Apte, M. V. (2016). Key Role of Pancreatic Stellate Cells in Pancreatic Cancer. Cancer Lett. 381 (1), 194–200. Epub 2015/11/17PubMed PMID: 26571462. doi:10.1016/j.canlet.2015.10.035
Procopio, M.-G., Laszlo, C., Al Labban, D., Kim, D. E., Bordignon, P., Jo, S.-H., et al. (2015). Combined CSL and P53 Downregulation Promotes Cancer-Associated Fibroblast Activation. Nat. Cel Biol 17 (9), 1193–1204. Epub 2015/08/25PubMed PMID: 26302407; PubMed Central PMCID: PMCPMC4699446. doi:10.1038/ncb3228
Qian, Z. Y., Peng, Q., Zhang, Z. W., Zhou, L. A., and Miao, Y. (2010). Roles of Smad3 and Smad7 in Rat Pancreatic Stellate Cells Activated by Transforming Growth Factor-Beta 1. Hepatobiliary Pancreat. Dis. Int. 9 (5), 531–536. Epub 2010/10/15. PubMed PMID: 20943464.
Ramanathan, R. K., McDonough, S. L., Philip, P. A., Hingorani, S. R., Lacy, J., Kortmansky, J. S., et al. (2019). Phase IB/II Randomized Study of FOLFIRINOX Plus Pegylated Recombinant Human Hyaluronidase versus FOLFIRINOX Alone in Patients with Metastatic Pancreatic Adenocarcinoma: SWOG S1313. Jco 37 (13), 1062–1069. Epub 2019/03/01PubMed PMID: 30817250; PubMed Central PMCID: PMCPMC6494359. doi:10.1200/jco.18.01295
Rhim, A. D., Oberstein, P. E., Thomas, D. H., Mirek, E. T., Palermo, C. F., Sastra, S. A., et al. (2014). Stromal Elements Act to Restrain, rather Than Support, Pancreatic Ductal Adenocarcinoma. Cancer cell 25 (6), 735–747. Epub 2014/05/27PubMed PMID: 24856585; PubMed Central PMCID: PMCPMC4096698. doi:10.1016/j.ccr.2014.04.021
Richter, K., Konzack, A., Pihlajaniemi, T., Heljasvaara, R., and Kietzmann, T. (2015). Redox-fibrosis: Impact of TGFβ1 on ROS Generators, Mediators and Functional Consequences. Redox Biol. 6, 344–352. Epub 2015/09/04PubMed PMID: 26335400; PubMed Central PMCID: PMCPMC4565043. doi:10.1016/j.redox.2015.08.015
Rodriguez-Hernandez, I., Cantelli, G., Bruce, F., and Sanz-Moreno, V. (2016). Rho, ROCK and Actomyosin Contractility in Metastasis as Drug Targets. F1000Res 5, 783. Epub 2016/05/10PubMed PMID: 27158478; PubMed Central PMCID: PMCPMC4856114. doi:10.12688/f1000research.7909.1
Roife, D., Sarcar, B., and Fleming, J. B. (2020). Stellate Cells in the Tumor Microenvironment. Adv. Exp. Med. Biol. 1263, 67–84. Epub 2020/06/27PubMed PMID: 32588324. doi:10.1007/978-3-030-44518-8_6
Roy, I., Boyle, K. A., Vonderhaar, E. P., Zimmerman, N. P., Gorse, E., Mackinnon, A. C., et al. (2017). Cancer Cell Chemokines Direct Chemotaxis of Activated Stellate Cells in Pancreatic Ductal Adenocarcinoma. Lab. Invest. 97 (3), 302–317. Epub 2017/01/17PubMed PMID: 28092365; PubMed Central PMCID: PMCPMC5334280. doi:10.1038/labinvest.2016.146
Sahai, E., Astsaturov, I., Cukierman, E., DeNardo, D. G., Egeblad, M., Evans, R. M., et al. (2020). A Framework for Advancing Our Understanding of Cancer-Associated Fibroblasts. Nat. Rev. Cancer 20 (3), 174–186. Epub 2020/01/26PubMed PMID: 31980749. doi:10.1038/s41568-019-0238-1
Sarper, M., Cortes, E., Lieberthal, T. J., and del Río Hernández, A. (2016). ATRA Modulates Mechanical Activation of TGF-β by Pancreatic Stellate Cells. Sci. Rep. 6, 27639. Epub 2016/07/05PubMed PMID: 27375161; PubMed Central PMCID: PMCPMC4931506. doi:10.1038/srep27639
Sato, N., Kohi, S., Hirata, K., and Goggins, M. (2016). Role of Hyaluronan in Pancreatic Cancer Biology and Therapy: Once Again in the Spotlight. Cancer Sci. 107 (5), 569–575. Epub 2016/02/27PubMed PMID: 26918382; PubMed Central PMCID: PMCPMC4970823. doi:10.1111/cas.12913
Schnittert, J., Bansal, R., and Prakash, J. (2019). Targeting Pancreatic Stellate Cells in Cancer. Trends Cancer 5 (2), 128–142. Epub 2019/02/14PubMed PMID: 30755305. doi:10.1016/j.trecan.2019.01.001
Serri, C., Quagliariello, V., Iaffaioli, R. V., Fusco, S., Botti, G., Mayol, L., et al. (2019). Combination Therapy for the Treatment of Pancreatic Cancer through Hyaluronic Acid‐decorated Nanoparticles Loaded with Quercetin and Gemcitabine: A Preliminary In Vitro Study. J. Cel Physiol 234 (4), 4959–4969. Epub 2018/10/20PubMed PMID: 30334571. doi:10.1002/jcp.27297
Shao, C., Tu, C., Cheng, X., Xu, Z., Wang, X., Shen, J., et al. (2019). Inflammatory and Senescent Phenotype of Pancreatic Stellate Cells Induced by Sqstm1 Downregulation Facilitates Pancreatic Cancer Progression. Int. J. Biol. Sci. 15 (5), 1020–1029. Epub 2019/06/12PubMed PMID: 31182922; PubMed Central PMCID: PMCPMC6535784. doi:10.7150/ijbs.27825
Sherman, M. H. (2018). Stellate Cells in Tissue Repair, Inflammation, and Cancer. Annu. Rev. Cel Dev. Biol. 34, 333–355. Epub 2018/07/22PubMed PMID: 30028641. doi:10.1146/annurev-cellbio-100617-062855
Sherman, M. H., Yu, R. T., Engle, D. D., Ding, N., Atkins, A. R., Tiriac, H., et al. (2014). Vitamin D Receptor-Mediated Stromal Reprogramming Suppresses Pancreatitis and Enhances Pancreatic Cancer Therapy. Cell 159 (1), 80–93. Epub 2014/09/27PubMed PMID: 25259922; PubMed Central PMCID: PMCPMC4177038. doi:10.1016/j.cell.2014.08.007
Shields, M. A., Dangi-Garimella, S., Krantz, S. B., Bentrem, D. J., and Munshi, H. G. (2011). Pancreatic Cancer Cells Respond to Type I Collagen by Inducing Snail Expression to Promote Membrane Type 1 Matrix Metalloproteinase-dependent Collagen Invasion. J. Biol. Chem. 286 (12), 10495–10504. Epub 2011/02/04PubMed PMID: 21288898; PubMed Central PMCID: PMCPMC3060503. doi:10.1074/jbc.M110.195628
Shintani, Y., Hollingsworth, M. A., Wheelock, M. J., and Johnson, K. R. (2006). Collagen I Promotes Metastasis in Pancreatic Cancer by Activating C-Jun NH2-Terminal Kinase 1 and Up-Regulating N-Cadherin Expression. Cancer Res. 66 (24), 11745–11753. Epub 2006/12/21PubMed PMID: 17178870. doi:10.1158/0008-5472.can-06-2322
Song, H., and Zhang, Y. (2018). Regulation of Pancreatic Stellate Cell Activation by Notch3. BMC cancer 18 (1), 36. Epub 2018/01/07PubMed PMID: 29304760; PubMed Central PMCID: PMCPMC5756326. doi:10.1186/s12885-017-3957-2
Steele, N. G., Biffi, G., Kemp, S. B., Zhang, Y., Drouillard, D., Syu, L., et al. (2021). Inhibition of Hedgehog Signaling Alters Fibroblast Composition in Pancreatic Cancer. Clin. Cancer Res. 27 (7), 2023–2037. Epub 2021/01/27PubMed PMID: 33495315; PubMed Central PMCID: PMCPMC8026631. doi:10.1158/1078-0432.ccr-20-3715
Sugahara, K. N., Hirata, T., Tanaka, T., Ogino, S., Takeda, M., Terasawa, H., et al. (2008). Chondroitin Sulfate E Fragments Enhance CD44 Cleavage and CD44-dependent Motility in Tumor Cells. Cancer Res. 68 (17), 7191–7199. Epub 2008/09/02PubMed PMID: 18757435. doi:10.1158/0008-5472.can-07-6198
Sun, C. K., Man, K., Ng, K. T., Ho, J. W., Lim, Z. X., Cheng, Q., et al. (2008). Proline-rich Tyrosine Kinase 2 (Pyk2) Promotes Proliferation and Invasiveness of Hepatocellular Carcinoma Cells through C-Src/ERK Activation. Carcinogenesis 29 (11), 2096–2105. Epub 2008/09/04PubMed PMID: 18765415. doi:10.1093/carcin/bgn203
Tahara, H., Sato, K., Yamazaki, Y., Ohyama, T., Horiguchi, N., Hashizume, H., et al. (2013). Transforming Growth Factor-α Activates Pancreatic Stellate Cells and May Be Involved in Matrix Metalloproteinase-1 Upregulation. Lab. Invest. 93 (6), 720–732. Epub 2013/04/24PubMed PMID: 23608755. doi:10.1038/labinvest.2013.59
Theocharis, A. D., Tsara, M. E., Papageorgacopoulou, N., Karavias, D. D., and Theocharis, D. A. (2000). Pancreatic Carcinoma Is Characterized by Elevated Content of Hyaluronan and Chondroitin Sulfate with Altered Disaccharide Composition. Biochim. Biophys. Acta (Bba) - Mol. Basis Dis. 1502 (2), 201–206. Epub 2000/10/21PubMed PMID: 11040445. doi:10.1016/s0925-4439(00)00051-x
Tjomsland, V., Pomianowska, E., Aasrum, M., Sandnes, D., Verbeke, C. S., and Gladhaug, I. P. (2016). Profile of MMP and TIMP Expression in Human Pancreatic Stellate Cells: Regulation by IL-1α and TGFβ and Implications for Migration of Pancreatic Cancer Cells. Neoplasia New York, NY 18 (7), 447–456. Epub 2016/07/21PubMed PMID: 27435927; PubMed Central PMCID: PMCPMC4954934. doi:10.1016/j.neo.2016.06.003
Tjomsland, V., Sandnes, D., Pomianowska, E., Cizmovic, S. T., Aasrum, M., Brusevold, I. J., et al. (2016). The TGFβ-SMAD3 Pathway Inhibits IL-1α Induced Interactions between Human Pancreatic Stellate Cells and Pancreatic Carcinoma Cells and Restricts Cancer Cell Migration. J. Exp. Clin. Cancer Res. 35 (1), 122. Epub 2016/07/31PubMed PMID: 27473228; PubMed Central PMCID: PMCPMC4966589. doi:10.1186/s13046-016-0400-5
Tjomsland, V., Spångeus, A., Välilä, J., Sandström, P., Borch, K., Druid, H., et al. (2011). Interleukin 1α Sustains the Expression of Inflammatory Factors in Human Pancreatic Cancer Microenvironment by Targeting Cancer-Associated Fibroblasts. Neoplasia New York, NY 13 (8), 664–IN3. Epub 2011/08/19PubMed PMID: 21847358; PubMed Central PMCID: PMCPMC3156657. doi:10.1593/neo.11332
Tsoukalas, N., Kiakou, M., Tsapakidis, K., Tolia, M., Aravantinou-Fatorou, E., Baxevanos, P., et al. (2019). PD-1 and PD-L1 as Immunotherapy Targets and Biomarkers in Non-small Cell Lung Cancer. J. BUON 24 (3), 883–888. Epub 2019/08/20. PubMed PMID: 31424637.
Tsukamoto, H., Fujieda, K., Miyashita, A., Fukushima, S., Ikeda, T., Kubo, Y., et al. (2018). Combined Blockade of IL6 and PD-1/pd-L1 Signaling Abrogates Mutual Regulation of Their Immunosuppressive Effects in the Tumor Microenvironment. Cancer Res. 78 (17), 5011–5022. Epub 2018/07/04PubMed PMID: 29967259. doi:10.1158/0008-5472.can-18-0118
van Duijneveldt, G., Griffin, M. D. W., and Putoczki, T. L. (19792020). Emerging Roles for the IL-6 Family of Cytokines in Pancreatic Cancer. Clin. Sci. Lond. Engl. 134 (16), 2091–2115. Epub 2020/08/19PubMed PMID: 32808663; PubMed Central PMCID: PMCPMC7434989. doi:10.1042/cs20191211
Wang, H.-C., Lin, Y.-L., Hsu, C.-C., Chao, Y.-J., Hou, Y.-C., Chiu, T.-J., et al. (2019). Pancreatic Stellate Cells Activated by Mutant KRAS-Mediated PAI-1 Upregulation foster Pancreatic Cancer Progression via IL-8. Theranostics 9 (24), 7168–7183. Epub 2019/11/07PubMed PMID: 31695760; PubMed Central PMCID: PMCPMC6831292. doi:10.7150/thno.36830
Wang, L., Wu, H., Wang, L., Zhang, H., Lu, J., Liang, Z., et al. (2017). Asporin Promotes Pancreatic Cancer Cell Invasion and Migration by Regulating the Epithelial-To-Mesenchymal Transition (EMT) through Both Autocrine and Paracrine Mechanisms. Cancer Lett. 398, 24–36. Epub 2017/04/13PubMed PMID: 28400334. doi:10.1016/j.canlet.2017.04.001
Wang, S., Li, Y., Xing, C., Ding, C., Zhang, H., Chen, L., et al. (2020). Tumor Microenvironment in Chemoresistance, Metastasis and Immunotherapy of Pancreatic Cancer. Am. J. Cancer Res. 10 (7), 1937–1953. Epub 2020/08/11. PubMed PMID: 32774994; PubMed Central PMCID: PMCPMC7407356.
Weber, R., Groth, C., Lasser, S., Arkhypov, I., Petrova, V., Altevogt, P., et al. (2021). IL-6 as a Major Regulator of MDSC Activity and Possible Target for Cancer Immunotherapy. Cell Immunol. 359, 104254. Epub 2020/12/10PubMed PMID: 33296753. doi:10.1016/j.cellimm.2020.104254
Wei, X., Senanayake, T. H., Warren, G., and Vinogradov, S. V. (2013). Hyaluronic Acid-Based Nanogel-Drug Conjugates with Enhanced Anticancer Activity Designed for the Targeting of CD44-Positive and Drug-Resistant Tumors. Bioconjug. Chem. 24 (4), 658–668. Epub 2013/04/04PubMed PMID: 23547842; PubMed Central PMCID: PMCPMC3677708. doi:10.1021/bc300632w
Wen, Z., Liu, Q., Wu, J., Xu, B., Wang, J., Liang, L., et al. (2019). Fibroblast Activation Protein α-positive Pancreatic Stellate Cells Promote the Migration and Invasion of Pancreatic Cancer by CXCL1-Mediated Akt Phosphorylation. Ann. Transl. Med. 7 (20), 532. Epub 2019/12/07PubMed PMID: 31807514; PubMed Central PMCID: PMCPMC6861799. doi:10.21037/atm.2019.09.164
Whatcott, C. J., Diep, C. H., Jiang, P., Watanabe, A., LoBello, J., Sima, C., et al. (2015). Desmoplasia in Primary Tumors and Metastatic Lesions of Pancreatic Cancer. Clin. Cancer Res. 21 (15), 3561–3568. Epub 2015/02/20PubMed PMID: 25695692; PubMed Central PMCID: PMCPMC4526394. doi:10.1158/1078-0432.ccr-14-1051
Wu, Y., Zhang, C., Jiang, K., Werner, J., Bazhin, A. V., and D’Haese, J. G. (2020). The Role of Stellate Cells in Pancreatic Ductal Adenocarcinoma: Targeting Perspectives. Front. Oncol. 10, 621937. Epub 2021/02/02PubMed PMID: 33520728; PubMed Central PMCID: PMCPMC7841014. doi:10.3389/fonc.2020.621937
Xu, J., Liu, S., Yang, X., Cao, S., and Zhou, Y. (2020). Paracrine HGF Promotes EMT and Mediates the Effects of PSC on Chemoresistance by Activating C-Met/PI3K/Akt Signaling in Pancreatic Cancer In Vitro. Life Sci. 263, 118523. Epub 2020/10/12PubMed PMID: 33039386. doi:10.1016/j.lfs.2020.118523
Yan, B., Jiang, Z., Cheng, L., Chen, K., Zhou, C., Sun, L., et al. (2018). Paracrine HGF/c-MET Enhances the Stem Cell-like Potential and Glycolysis of Pancreatic Cancer Cells via Activation of YAP/HIF-1α. Exp. Cel. Res. 371 (1), 63–71. Epub 2018/07/30PubMed PMID: 30056064. doi:10.1016/j.yexcr.2018.07.041
Yu, H., Lee, H., Herrmann, A., Buettner, R., and Jove, R. (2014). Revisiting STAT3 Signalling in Cancer: New and Unexpected Biological Functions. Nat. Rev. Cancer 14 (11), 736–746. Epub 2014/10/25PubMed PMID: 25342631. doi:10.1038/nrc3818
Yuan, Y., Jiang, J. Y., Wang, J. M., Sun, J., Li, C., Liu, B. Q., et al. (2019). BAG3‐positive Pancreatic Stellate Cells Promote Migration and Invasion of Pancreatic Ductal Adenocarcinoma. J. Cel Mol Med 23 (8), 5006–5016. Epub 2019/05/24PubMed PMID: 31119886; PubMed Central PMCID: PMCPMC6653255. doi:10.1111/jcmm.14352
Zhang, Z., Wang, F., Du, C., Guo, H., Ma, L., Liu, X., et al. (2017). BRM/SMARCA2 Promotes the Proliferation and Chemoresistance of Pancreatic Cancer Cells by Targeting JAK2/STAT3 Signaling. Cancer Lett. 402, 213–224. Epub 2017/06/13PubMed PMID: 28602977. doi:10.1016/j.canlet.2017.05.006
Zhao, W., Ajani, J. A., Sushovan, G., Ochi, N., Hwang, R., Hafley, M., et al. (2018). Galectin-3 Mediates Tumor Cell-Stroma Interactions by Activating Pancreatic Stellate Cells to Produce Cytokines via Integrin Signaling. Gastroenterology 154 (5), 1524–1537. e6. Epub 2017/12/25PubMed PMID: 29274868. doi:10.1053/j.gastro.2017.12.014
Glossary
ATRA: All-trans retinoic acid
CAF: Cancer-associated fibroblasts
Coll: type 1 collagen.
DDR1: Discoidin domain receptors one
ECM: extracellular matrix.
FAP: fibroblast activation protein
HA: hyaluronic acid.
HAS: hyaluronan synthase.
IL-1: Interleukin-1.
IL-6: Interleukin-6.
IL-6R: Interleukin-6 receptor.
LIF: leukemia inhibitory factor.
PD-1: programmed death-ligand 1.
PD-L1: protein programmed cell death one ligand 1.
PEGPH20: PEGylated human recombinant hyaluronidase.
PDGF: platelet derived growth factor.
PSC: pancreatic stellate cell.
a-SMA: a-smooth muscle actin.
RhoA: Ras homolog family member A
ROS: reactive oxygen species
Shh: Sonic hedgehog.
TGF-β: transforming growth factor beta
Keywords: inflammation, fibrosis, pancreatic stellate cells, pancreatic neoplasms, antineoplastic protocols
Citation: Zhang Z, Zhang H, Liu T, Chen T, Wang D and Tang D (2021) Heterogeneous Pancreatic Stellate Cells Are Powerful Contributors to the Malignant Progression of Pancreatic Cancer. Front. Cell Dev. Biol. 9:783617. doi: 10.3389/fcell.2021.783617
Received: 26 September 2021; Accepted: 24 November 2021;
Published: 20 December 2021.
Edited by:
Francesca Pirini, Istituto Scientifico Romagnolo per lo Studio e la Cura dei Tumori (IRCCS), ItalyReviewed by:
Yuan Seng Wu, Sunway University, MalaysiaShin Hamada, Tohoku University, Japan
Richard T. Waldron, Cedars Sinai Medical Center, United States
Copyright © 2021 Zhang, Zhang, Liu, Chen, Wang and Tang. This is an open-access article distributed under the terms of the Creative Commons Attribution License (CC BY). The use, distribution or reproduction in other forums is permitted, provided the original author(s) and the copyright owner(s) are credited and that the original publication in this journal is cited, in accordance with accepted academic practice. No use, distribution or reproduction is permitted which does not comply with these terms.
*Correspondence: Dong Tang, 83392785@qq.com
†Present address:Dong Tang, Department of General Surgery, Northern Jiangsu People's Hospital, Clinical Medical College, Institute of General Surgery, Yangzhou University, Yangzhou, China
‡These authors have contributed equally to this work