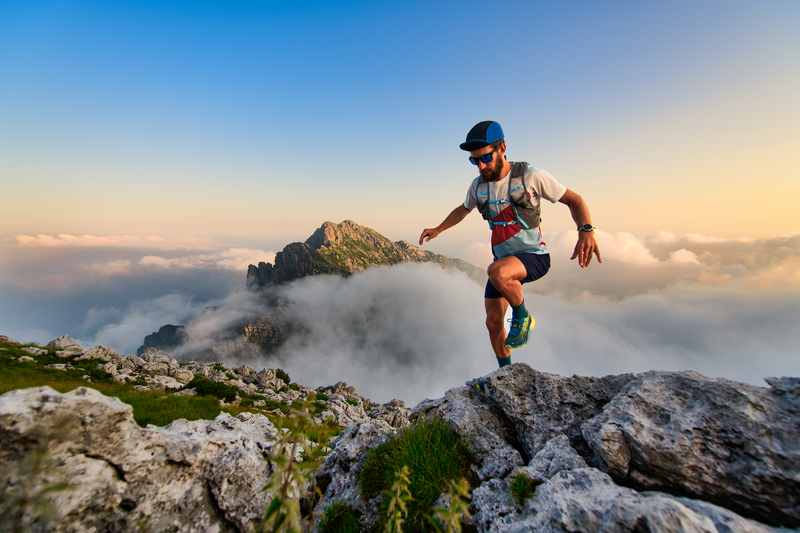
95% of researchers rate our articles as excellent or good
Learn more about the work of our research integrity team to safeguard the quality of each article we publish.
Find out more
REVIEW article
Front. Cell Dev. Biol. , 14 December 2021
Sec. Membrane Traffic and Organelle Dynamics
Volume 9 - 2021 | https://doi.org/10.3389/fcell.2021.774587
This article is part of the Research Topic Molecular Basis of Membrane Dynamics in Health and Disease View all 8 articles
Clathrin is a cytosolic protein involved in the intracellular trafficking of a wide range of cargo. It is composed of three heavy chains and three light chains that together form a triskelion, the subunit that polymerizes to form a clathrin coated vesicle. In addition to its role in membrane trafficking, clathrin is also involved in various cellular and biological processes such as chromosomal segregation during mitosis and organelle biogenesis. Although the role of the heavy chains in regulating important physiological processes has been well documented, we still lack a complete understanding of how clathrin light chains regulate membrane traffic and cell signaling. This review highlights the importance and contributions of clathrin light chains in regulating clathrin assembly, vesicle formation, endocytosis of selective receptors and physiological and developmental processes.
Endocytosis is a process carried out by eukaryotic cells to internalize extracellular molecules, plasma membrane proteins and lipids (Doherty and McMahon, 2009). While several other pathways for endocytosis such as caveolin-mediated endocytosis, phagocytosis and macropinocytosis have been described, clathrin mediated endocytosis (CME) remains the major route for internalization of many membrane lipids and proteins (Kaksonen and Roux, 2018).
CME was first observed by Roth and Porter in 1964 where they found uptake of yolk-containing bristled-coated pits in the mosquito oocyte (Roth and Porter, 1964). Later these bristled-coated structures isolated from pig brain were identified as coat proteins and named ‘Clathrin’ by Barbara Pearse (1975, 1976). Since then, this process has been extensively studied and although we have a fairly good understanding of the process itself, many unanswered questions still remain about how over 50 molecules that take part in this molecular process (Haucke and Kozlov, 2018), come together in a highly coordinated manner.
CME is characterized by the recruitment of clathrin and its associated molecules to the plasma membrane allowing the formation of clathrin-coated vesicles (CCVs). The formation of CCVs involves the polymerization of ‘clathrin triskelia’, which are the basic building blocks of the clathrin coats (Kaksonen and Roux, 2018). A triskelion is composed of three clathrin heavy chains (CHC) (∼190 kDa) each of which is associated with a smaller clathrin light chain (CLC) (∼25 kDa). While the major role of the clathrin heavy chain is in intracellular trafficking, it is also involved in several other processes including chromosomal segregation during mitosis (Royle et al., 2005), regulation of basal NF-κB activity in epithelial cells (Kim et al., 2011), control of neuropeptide degradation and secretion during neuronal development (Nahorski et al., 2018), and maintenance of mouse embryonic stem cell pluripotency (Narayana et al., 2019; Mote et al., 2020).
Variations in the clathrin heavy and light chains alter the biophysical properties of the clathrin lattice, in turn affecting trafficking of receptors and thereby several physiological functions of the cell. The heavy chain is essential for triskelion assembly and for all clathrin-dependent endocytic events, with a number of excellent reviews highlighting the function of this protein (Kirchhausen, 2000; Brodsky, 2012; Kirchhausen et al., 2014; Brodsky, 2016; Kaksonen and Roux, 2018; Briant et al., 2020). In contrast, the role of the clathrin light chains remains relatively under-explored. In this review, we look at how the clathrin light chains affect clathrin polymerization, vesicle formation, receptor trafficking and cell signaling.
In metazoans, the clathrin heavy chain protein is encoded by a single gene, Cltc. In humans, due to large-scale gene duplications during chordate evolution, there are two CHC paralogs, CHC17 (encoded by Cltc) and CHC22 (encoded by Cltcl1) based on their location on chromosome 17 and 22, respectively. Although Cltcl1 is found in several other vertebrate species, it is functional only in humans. In mice, only a pseudogene for CHC22 is present (Wakeham et al., 2005). In yeast and invertebrates such as Drosophila and Caenorhabditis elegans, the CHC protein is encoded by a single gene. Plants have two genes for the clathrin heavy chain, CHC1 and CHC2 (Baisa et al., 2013).
In invertebrates, the clathrin light chain is encoded by a single gene. However, as a result of local gene duplication, higher eukaryotes have two light chains, CLCa and CLCb encoded by the genes Clta and Cltb, respectively (Wakeham et al., 2005). They both share 60% homology in their amino acid sequence but are expressed at different levels in various vertebrate tissues (Wu et al., 2016). Despite having considerable divergence in sequence, the single light chain from yeast shares various physical properties with mammalian light chains (Silveira et al., 1990). In plants, the three clathrin light chain genes CLC1, CLC2 and CLC3 (Scheele and Holstein, 2002; Baisa et al., 2013) share at least 30% sequence homology with mammalian CLCs (Wang et al., 2013).
Vertebrate CLCs contain a consensus region of 22 amino acids shared by both CLCa and CLCb. Additionally, they also include distinct domains for binding to calcium, clathrin heavy chain, calmodulin and a neuron-specific insertion sequence (Brodsky, 2012). CLCa contains a unique Hsc70 binding region (DeLuca-Flaherty et al., 1990). However, functions have been attributed to only some of these domains. A detailed representation of CLC domain organization can be found in Figure 1.
FIGURE 1. CLC protein domains: Domain maps of the vertebrate CLCs, CLCa and CLCb. Common functional domains indicated include the consensus sequence (CON) shared by all vertebrate CLCs, the calcium-binding sequence (Ca++), the heavy chain-binding region (HC), the neuronal inserts of 18(N-18) and 12(N-12) residues, and the calmodulin-binding domain (CBD). Unique to CLCa is a region that can stimulate the uncoating ATPase, HSC70, in vitro.
In mammals, at the N-terminus, a 22 amino acid conserved sequence is shared by CLCa (residues 28–49) and CLCb (residues 20–41) with the negatively charged residues, EED responsible for CLC binding to the CHC knee (Brodsky, 2012). This conserved sequence is also the binding site for the Huntington interacting protein (HIP) family (Chen and Brodsky, 2005), and plays a role in regulating clathrin self-assembly (Legendre-Guillemin et al., 2005; Ybe et al., 2007a).
CLCa has an Hsc70 binding sequence that was shown to stimulate uncoating in vitro (DeLuca-Flaherty et al., 1990). However later studies suggested that uncoating of vesicles could also be done in the absence of CLCs in vitro (Ungewickell et al., 1995) Both the light chains also have a calcium binding region (Nathke et al., 1990) and calmodulin binding domain (Pley et al., 1995) present at the centre and C-terminal, respectively. While these domains have been found to play a role in in vitro studies, no function has been attributed to them in vivo.
In Dictyostelium, overexpression of the C-terminal fragment of CLCa in clc null cells produced dynamic punctae distribution along the plasma membrane and within the cytoplasm, similar to full-length CLCa (Wang et al., 2006), indicating that its function can be attributed almost entirely to the C-terminal domain.
In vitro assembly of the clathrin hub, and CHC trimer stability is enhanced by the C-terminal domain of the light chain (Ybe et al., 2007b). Co-expressing the trimer-defective hub heavy chain mutant C1573A, along with the light chain C-terminal domain construct could achieve approximately 67% of wild-type clathrin assembly.
CLCs undergo alternate mRNA splicing in vertebrates, giving rise to four isoforms for CLCa and two for CLCb (Blue et al., 2018a). Exons 5 and 6, encoding 18 and 12 amino acids respectively, in the Clta gene are alternatively spliced resulting in four isoforms. These are: 1) neuronal CLCa (nCLCa) containing both 18 and 12 amino acid residue insertions; 2) a splice variant containing only the 18 residue-insertion found only in brain; 3) a splice variant containing only the 12 residue-insertion found in brain, heart and skeletal muscle; and 4) a splice variant without either insertion.
In vertebrates, the two splice variants for CLCb include an isoform having an insert of 18 residues present in neurons (nCLCb) and another that lacks the insert in non-neuronal tissues (Wong et al., 1990; Blue et al., 2018a). In rats, the CLCb gene contains six exons. The isoform containing all exons is brain-specific (LCB2), while the isoform lacking exon 5 (LCB3) is present in other tissues. LCB2 is predominantly present in primary rat neuronal cultures, whereas LCB3 is present in primary rat glial cultures (Stamm et al., 1992).
Due to the insertions mentioned above, neuronal splice variants have a higher molecular mass than CLCs in other cell types. Under oxidizing conditions, CLC isoforms can form internal disulfide bonds. Both brain-specific CLCa isoforms, contain the 12 residue insert in exon 5, allowing the formation of internal disulfide bonds between two cysteine residues in vitro, while the smallest CLCa isoform only has a single cysteine residue. Both CLCb isoforms have two cysteine residues present at the C-terminal. In vitro purification of CLCs in the presence or absence of thiols or alkylating agents caused an alteration in electrophoretic mobility depending on the formation of disulfide bonds (Parham et al., 1989). The electrophoretic mobility change of CLCs was also found to be species- and tissue-specific. This could be due to the presence of different isoforms (Ungewickell, 1983), disulfide bond formation (Parham et al., 1989), or other post-translational modifications such as phosphorylation (Ferreira et al., 2012).
At all developmental stages, the Clta transcript in the mouse heart does not include exon 5. However, due to alternative splicing, Clta exon 6 is included at a low level at birth with its inclusion increasing in adulthood (Giudice et al., 2014; Blue et al., 2018b). This suggests that expression of CLC splice variants is variable and can change with the stage of development, and in a tissue-specific manner.
Both the CLCs are developmentally and tissue-specifically regulated by alternative splicing but the physiological and functional implications of different splice variants of CLCs still remain unexplored. Although we still lack complete understanding of tissue-specific expression patterns of alternatively spliced variants of CLCs, one can speculate that the presence or absence of particular exons may lead to a change in interacting partners thereby influencing function.
Despite having 60% sequence similarity, the two light chains are diverse in nature due to alternative splicing, internal disulfide bond formation and tissue specific expression patterns, allowing speculation that their ability to perform distinct physiological and functional roles could be attributed to such differences.
The phosphorylation of clathrin light chains was initially identified in vitro in coated vesicles isolated from bovine brains (Usami et al., 1985). Using rat reticulocytes, Bar-Zvi et al. (1998), then demonstrated that unassembled pools of CLCb were highly phosphorylated. Further investigations revealed that CLCb underwent phosphorylation mediated by Casein Kinase 2 at the N-terminal serine residues at positions 11 and 13. These residues are unique to CLCb and absent in CLCa (Hill et al., 1988). However both CLCa and CLCb contain the phosphorylation site for G-Protein coupled receptor kinase 2 (GRK2) at Ser204. Mutation of all phosphorylation sites in CLCb impeded internalization of purinergic GPCRs, P2Y1 and P2Y12, with phosphorylation of Ser204 being specific for P2Y12 uptake (Ferreira et al., 2012). Phosphorylation of CLCb at Ser204 was also required for lattice rearrangement and curvature generation by regulating clathrin exchange in a cargo-dependent manner (Maib et al., 2018). Together these findings indicate a role for phosphorylated forms of clathrin light chains in regulating the uptake of specific membrane-resident proteins.
Both CLCs can bind and regulate CHC17, but do not functionally interact with CHC22 (Towler et al., 2004). Previous studies have shown that CLCs bind to the proximal leg of the heavy chain via their central region (Kirchhausen et al., 1983; Brodsky et al., 1987; Jackson et al., 1987; Nathke et al., 1992; Liu et al., 1995). Using a yeast-two hybrid system it was shown that the core interaction occurs between CHC residues 1,267–1,522, and CLCb residues 90–157 (Chen et al., 2002). Mutations in the central region (residues 90–157) of CLCb disrupt the alpha-helical structure suggesting that this region is crucial for interaction with CHC. Cryo-EM based structural analysis revealed that two tryptophan residues (W105 and W127) were required for light chain binding to the heavy chain. Mutation of W105 to arginine disrupted CLC-CHC binding, but could be rescued by mutation of lysine to glutamate at residue position 1,326 of the heavy chain. Additionally, two helices present in the CLC trimerization domain (TxD) tended to form stable association with two heavy chain TxDs in trans conformation, connecting adjacent legs and forming the triskelion vertex (Morris et al., 2019).
Studies done in yeast suggest that light chains affect the trimerization and stability of the heavy chain (Silveira et al., 1990; Huang et al., 1997). The amount of heavy chain in light chain-deficient strains is reduced to 20–25% of their wild-type counterparts, most of which are not trimerized (Huang et al., 1997). CLC-deficient strains have also been known to show a slow-growth phenotype, similar to CHC deficient strains (Silveira et al., 1990), indicative of the fact that the light chain in yeast is essential for heavy chain trimerization and stability. In Dictyostelium however, the light chains do not contribute to heavy chain trimerization or stability, but affect the assembly of triskelia onto intracellular membranes (Wang et al., 2003). This indicates a species-specific role for light chains in conferring stability to triskelia. The reason behind this is not completely understood as the domain structure of CLCs across species remains conserved, despite having little similarity in amino acid sequence (Wang et al., 2003).
CLCs stabilize the triskelion via their C-terminal region which interacts with the vertex and reduces the flexibility of the legs to produce triskelia with uniform vertex geometry (Ybe et al., 2007b). In the absence of light chains, the legs can adopt various geometries due to increased flexibility at the vertex (Ybe et al., 2007b). These changes in triskelion structure can affect cage and lattice forming properties of clathrin, which is discussed in the next section.
The role of clathrin light chains in cage assembly and disassembly has been studied extensively since their identification in 1981. Early studies reported that treatment of clathrin with elastase, which selectively digests the light chains, renders the triskelion incapable of correctly assembling and forming cages (Kirchhausen and Harrison, 1981; Schmid et al., 1982). However, it was later shown that heavy chain trimers can reassemble into polygonal cages even in the absence of light chains (Winkler and Stanley, 1983). We now know that the light chains function as negative regulators of cage assembly, as shown by the following studies.
In vitro studies using recombinant hubs (trimeric clathrin heavy chain structures without the distal domain and the N-terminal region) have shown that while hubs lacking light chains can self-assemble reversibly at a physiological pH, they can self-assemble only at a pH below 6.5 in the presence of light chains (Liu et al., 1995). They also require the presence of adaptor proteins such as AP-1, AP-2 or the neuron-specific AP-180 to assemble at physiological pH (Ahle and Ungewickell, 1986; Keen, 1990; Pearse and Robinson, 1990; Lindner and Ungewickell, 1992). These reports show that light chains regulate cage assembly by preventing unnecessary polymerization of clathrin triskelia and allowing regulated assembly by adaptor molecules.
Light chains have a negatively charged EED domain which can bind to the positively charged KR loop present in the crease of the heavy chain (Wilbur et al., 2010). As mentioned earlier, this interaction influences the flexibility at the knee, which affects lattice assembly. If CLC is bound, the knee is straight and the triskelion is more rigid. Such a conformation inhibits cage assembly. The retraction of light chain produces more compact and flexible triskelia and allows the triskelia to form clathrin cages (Wilbur et al., 2010).
Adaptors can overcome the effect of light chains by introducing competing positively charged residues that can free up the heavy chain to polymerize (Greene et al., 2000). AP-2 can directly bind to the clathrin heavy chain (Owen et al., 2000). By aligning the distal regions of the heavy chain with the proximal hub segments it provides the competing residues required to reverse the effect of light chains (Greene et al., 2000). It has recently been shown that these interactions between adaptors and the clathrin coat also regulate cargo binding and coat curvature, by reconfiguring low-affinity, high-avidity interactions (Kovtun et al., 2020).
The presence of light chains also increases the stiffness of clathrin lattices which increases the ability of clathrin to deform liposomal membranes into buds (Dannhauser et al., 2015). Budding efficiency has also been shown to vary with different CLC isoforms. Lattices containing neuronal isoforms of the light chains exhibit a poorer lattice quality and a lower budding efficiency compared to lattices with non-neuronal isoforms (Redlingshöfer et al., 2020).
Hsc70, like most chaperone proteins, requires cofactors to recruit the chaperone to the target site (Böcking et al., 2011). Auxilin 1 and GAK (also known as Auxilin 2) are two cofactors of Hsc70 belonging to the DnaJ family of chaperones (Jiang et al., 1997; Umeda et al., 2000). While Auxilin 1 is expressed only in the brain (Böcking et al., 2011), GAK is expressed in several other tissues (Kanaoka et al., 1997; Kimura et al., 1997). Whether the light chains directly affect Auxilin and GAK mediated uncoating is still unclear. According to Ungewickell et al. (1995), CLCs are dispensable for Auxilin-mediated uncoating of clathrin-coated vesicles. Later studies suggest that although the light chains are not essential for uncoating, their removal significantly reduces the efficiency with which Auxilin facilitates disassembly (Young et al., 2013). A study by Ferreira et al. (2012) suggests that clathrin light chain B can modulate the interaction between auxilin and clathrin heavy chain, thereby regulating the process of vesicle uncoating.
The physiological importance of light chains has mostly been studied using knockdown or knockouts of the light chains, or through the use of mutant forms to study receptor trafficking (Huang et al., 2004; Poupon et al., 2008; Majeed et al., 2014; Wu et al., 2016; Redlingshöfer et al., 2020). Knockout of CLCa in mice hampered the internalization of Transforming growth factor β receptor2 (TGFβR2), affecting antibody isotype switching in B lymphocytes (Wu et al., 2016). Knockdown of both light chains in mammalian HeLa cells did not affect the internalization of β1 integrin but disrupted its recycling back to the plasma membrane (Majeed et al., 2014). CLC knockdown (KD) also altered the targeting of cation-independent mannose-6 phosphate receptor (CI-MPR) to the endosome, resulting in clustering of the receptor near the trans-Golgi network, leading to a delay in processing of the lysosomal hydrolase cathepsin D in HeLa and Cos7 cells (Poupon et al., 2008).
Internalization of GPCRs was also shown to be dependent on CLCb phosphorylation (Ferreira et al., 2012). Internalization of P2Y12 receptor, a member of a family of purinergic GPCRs, in 1321N1 astrocytoma cells is regulated by phosphorylation of CLCb. Trafficking of low-density lipoprotein on the other hand, is not affected by the removal of light chains (Poupon et al., 2008). Furthermore, internalization of EGFR was also not affected by siRNA-mediated knockdown of both the light chains in HeLa cells (Huang et al., 2004). However, a study using single light chain-expressing H1299 cells, a non-small cell lung cancer cell line, showed accelerated internalization of EGFR in cells that expressed only CLCb in contrast to wild type and CLCa-only expressing cells (Chen et al., 2017). Similar observations have been made with respect to internalization of transferrin receptor (Tfr) (Huang et al., 2004; Chen et al., 2017). Tfr internalization can also be dependent on the phosphorylation status of CLCb. When transferrin receptor is clustered with other cargo, its uptake can become sensitive to the status of CLCb phosphorylation. In 1321N1 human astrocytoma cells, packaging of Tfr with P2Y12 receptor resulted in delayed internalization of Tfr in presence of a phosphorylation-deficient mutant of CLCb (Maib et al., 2018), with similar results also observed in HeLa cells. Knockdown of both the light chains attenuated Tfr recycling in HeLa cells (Majeed et al., 2014), which remains unaffected in single light chain expressing H1299 cells (Chen et al., 2017).
From these studies one can infer that i) dependence of receptor trafficking on light chains is influenced by other factors such as the cell type and presence of other cargo; ii) CLCa and CLCb can differentially affect cargo uptake; and iii) phosphorylation status of CLCb can potentially be a method of regulating receptor trafficking.
Altered trafficking of receptors can compromise cell signaling, which is an important regulator of several physiological functions. The sections below discuss how light chains regulate important biological functions and pathological conditions. These are also summarized in Table 1.
Mammalian development is dependent on the presence and action of light chains, especially in the context of B-cell development (Wu et al., 2016). The lymphoid tissue shows an almost exclusive expression of CLCa. Germinal centres in CLCa knockout mice have fewer B cells, which predominantly produce IgA antibodies. This increased IgA production is attributed to enhanced signaling by the TGFβR2 receptor due to its defective endocytosis (Wu et al., 2016).
Normal eye development in Drosophila is dependent on clathrin light chains (Schreij et al., 2015). LRRK2, a high molecular weight Ras GTPase, directly binds to light chains present on the endosomes. CLC and LRRK2 interact to inhibit Rac1 activation, with disruption in this pathway resulting in altered eye development in Drosophila (Schreij et al., 2015).
Clathrin light chains also play an important role in plant development. The loss of light chains, CLC2 and CLC3 affect auxin-regulated endocytosis, resulting in multiple developmental defects in Arabidopsis thaliana (Wang et al., 2013). Additionally CLC1 mutant pollen also display reduced viability (Wang et al., 2013), suggesting that the three light chains have specific and independent roles in gamete formation and development, and that the loss of a single light chain may not be compensated for by the presence of the other two.
Dictyostelium CLC null-mutants show defects in development as demonstrated by their inability to form fruiting bodies (Wang et al., 2003). Overexpression of the C-terminal domain of CLC rescues this phenotype with robust fruiting body formation indistinguishable from wild type fruiting bodies (Wang et al., 2006). Loss of CLC results in larger vacuoles in Dictyostelium, indicative of disruption of osmoregulation (Stavrou and O’Halloran, 2006). Together, these studies from different species indicate that clathrin light chains perform distinct and diverse functions during development.
As mentioned above, depletion of both the light chains reduced the surface expression of β1 integrin due to altered recycling, which decreased cell migration in both HeLa and H1299 cells (Majeed et al., 2014). Migratory displacement of HeLa cells was reduced by 22% in contrast to H1299 cells whose displacement was reduced by 41% upon loss of light chains (Majeed et al., 2014). Non-small cell lung cancers expressed elevated levels of CLCb resulting in increased activation of Dynamin1 via a pathway involving Akt/GSK3β phosphorylation. This resulted in abnormal EGFR trafficking and signaling, leading to increased migration and metastasis (Chen et al., 2017). Another recent study showed that CLCa and not CLCb was important for focal adhesion (FA) maturation, cell spreading and migration, with CLCa targeting FAKs to nascent FAs. In the absence of CLCa these transient nascent structures were unable to mature to radially elongated FAs due to reduction in integrin-mediated activation of Src and Rac (Tsygankova and Keen, 2019).
In U373 astrocytes, overexpression of a dominant negative CLCb mutant which could bind to the heavy chain but not to Hip1/R, resulted in increased motility due to reduction in plaque formation (Saffarian et al., 2009).
Recently, it has also been shown that light chains are involved in invadopodia formation in HEK293T cells (Mukenhirn et al., 2021). Deletion of both light chains resulted in increased recycling of MMP14, a matrix metalloproteinase protein whose increased surface expression has been known to coincide with malignant cancer progression. Furthermore, loss of the light chains caused actin to polymerize and form patches on the plasma membrane. These actin structures along with MMP14 clusters on the plasma membrane formed mature invadopodia. Invadopodia are important for embryonic development, bone remodeling and cancer metastasis (Mukenhirn et al., 2021). Altered invadopodia formation could possibly affect these important physiological processes.
Together these studies demonstrate that individual clathrin light chains regulate migration and invasion differentially depending on the cell type. Additionally, these phenotypes may also be a reflection of their interaction with specific proteins that are also expressed in a cell-type dependent manner.
Besides playing an important role in cell-signaling by regulating receptor trafficking, clathrin also plays an important role in neuron-specific functions such as synaptic vesicle recycling and neurotransmitter receptor trafficking. Photo-inactivation of the clathrin light chain in Drosophila at neuromuscular junctions (NMJ) resulted in a block in synaptic vesicle re-formation. Although clathrin-independent mechanisms of membrane internalization do exist at the Drosophila NMJ, these were unable to generate fusion-competent vesicles, indicating a specificity for the light chain in this context (Heerssen et al., 2008). A similar phenotype was also seen in CLCa and CLCb knockout mice. Knockout of individual light chains in mice showed electrophysiological defects, indicative of impaired synaptic vesicle recycling (Redlingshöfer et al., 2020). Interestingly, CLCa and CLCb knockout mice exhibited different phenotypes. In the synapses of cerebellar neurons, CLCa knockout mice showed reduced number of synaptic vesicles whereas CLCb knockout mice did not show any decrease compared to wild type mice. However, in hippocampal neurons, CLCa knockout mice showed a decrease in the number of synaptic vesicles, while CLCb knockout mice showed almost twice the number of vesicles relative to wild type mice. CLCa knockout mice also showed defects in motor function (Redlingshöfer et al., 2020). This highlights the fact that CLCa and CLCb have distinct roles in synaptic vesicle recycling and also indicates that the same paralog can differentially affect function in neurons from different regions of the brain.
Altered endocytosis is also associated with several neurodegenerative disorders. Immunohistochemical analysis of the hippocampus from individuals with Alzheimer’s disease show an abnormal distribution of clathrin light chains, with a high concentration of CLCb detected in neurofibrillary tangles (Nakamura et al., 1994a). Under normal circumstances, clathrin is concentrated at the synaptic terminals. However, in patients with Alzheimer’s disease, CLCb is reduced at the synapse indicating that the normal transport of clathrin from the neuronal perikarya to the axon terminals is hampered (Nakamura et al., 1994a). The implications, if any, of this abnormal distribution of light chains are still not understood. Proteomic analysis of the hippocampus of the Alzheimer’s disease mice models showed that CLCb and Dynamin 1 were upregulated in diseased mice compared to wild type mice. Interestingly, no significant difference was observed in the expression of CLCa (Takano et al., 2013). Abnormal distribution of clathrin light chains was also observed in the brains of patients with Pick’s disease. Immunohistochemical analysis showed a high concentration of light chains in Pick’s bodies. In neurons of the dentate gyrus of Pick’s disease patients, light chains were also found in increased amounts in the neuronal perikarya compared to healthy individuals (Nakamura et al., 1994b). While the cause and implications of the increase in CLCs are still not understood, one can speculate that the altered expression and localization may contribute to the disease phenotype.
Clathrin-mediated endocytosis is a continuous event in non-dividing cells. However, in cells undergoing mitosis, endocytic events stop (Fielding et al., 2012), and clathrin accumulates at the spindle apparatus carrying out an important function, independent of trafficking (Royle, 2012). It functions by crosslinking the microtubules of the kinetochore to stabilize the mitotic spindle (Royle et al., 2005). CHC also promotes centrosome maturation by stabilizing the microtubule-binding protein ch-TOG (colonic, hepatic tumor overexpressed gene) (Foraker et al., 2012).
A study in Arabidopsis thaliana has shown that CLCs accumulate at the mitotic spindle during cell division. GFP-tagged CLC has been shown to be associated with the distal plasma membrane in expanding root hairs, and at the cell plate in dividing root cells (Konopka et al., 2008).
In Dictyostelium, CLC null mutants display a defect in cytokinesis, which can be rescued by overexpression of the C-terminal domain-containing CLC construct (residues 124–194) (Wang et al., 2006).
Another important protein involved in mitosis is the mitotic arrest deficient protein, MAD2B which binds to, and inhibits the anaphase promoting complex (APC) (Chen and Fang, 2001). Depletion of MAD2B in renal carcinoma cells caused a significant increase in the number of misaligned chromosomes. MAD2B interacts with the C-terminus of CLCa during the G2/M phase of the cell cycle, with knockdown of MAD2B resulting in redistribution of CLCa away from the mitotic spindle (Medendorp et al., 2010). The functional relevance of this interaction is as yet unexplored. It should also be noted that heavy chain distribution remained unaffected upon depletion of MAD2B (Medendorp et al., 2010).
The role of actin in endocytosis is well established. Actin is recruited to sites of endocytosis and helps the membrane to invaginate and form coated pits (Engqvist-Goldstein and Drubin, 2003; Mooren et al., 2012). Clathrin light chains can bind to Hip1/R proteins through a conserved domain present at their N-terminus, which in turn binds to actin (Chen and Brodsky, 2005). The light chains therefore act as a connecting link between the endocytic machinery and the cytoskeleton.
Hip1/R proteins can bind to actin through their THATCH domain independent of CLCs. Binding of the light chains to the coiled-coil domains of Hip1 and Hip1R reduce their actin-binding activity. This suggests that Hip proteins do not interact with actin while incorporated into the clathrin coat. Instead Hip proteins interact with actin at the neck of the budding vesicle or edge of the clathrin coat, promoting development of a budding vesicle (Wilbur et al., 2008; Boettner et al., 2011). Hip1 binding to CLC is necessary for its targeting to clathrin-coated pits (Legendre-Guillemin et al., 2005) and loss of CLCs result in mislocalization of Hip1R and overassembly of actin patches (Poupon et al., 2008), further emphasizing the point that light chains are essential for recruiting actin to sites of endocytosis by interacting with Hip1/R proteins.
In yeast, all clathrin-dependent endocytic events are actin-dependent and therefore, light chain-dependent (Chu et al., 1996). In mammalian cells however, the light chains and actin are not essential for CME to occur.
In what context is an endocytic event light chain-dependent or -independent? The factor that dictates the requirement of light chains is the amount of force that is required for the membrane to invaginate. Membrane tension opposes membrane deformation. Invagination of membranes with high tension require greater force. Boulant et al. (2011) showed that actin was recruited by the light chains to counteract membrane tension in polarized MDCK cells. It is plausible that in instances where clathrin polymerization does not produce enough energy to bend the membrane, the light chains recruit actin, which polymerizes and provides energy for membrane invagination. Membrane tension may differ between cell types. This explains why the uptake of the same receptor may be light chain dependent in one type of cell, and independent in another. Another factor that opposes membrane budding is turgor pressure. Turgor pressure of yeast is higher than that of mammalian cells (Aghamohammadzadeh and Ayscough, 2009), which may explain why all clathrin-dependent endocytic events in yeast are light chain and actin-dependent (Goode et al., 2015). It is important to note however, that in plants, which have a similarly high turgor pressure as yeast, actin is not required for endocytosis (Baisa et al., 2013), allowing speculation that other proteins may be involved in this process.
Recruiting actin to provide energy for membrane invagination is not the only way light chains help in vesicle formation. As mentioned above, light chains are also involved in lattice rearrangement which introduces membrane curvature in flat clathrin lattices as they transform into shallow pits (Maib et al., 2018). However, clathrin does not always assemble as a flat lattice first. This happens only when the constant area model of membrane invagination is followed. According to this model the clathrin coat assembles into a flat lattice of a given area. The lattice is then remodeled by inserting pentagons to introduce curvature without changing the area. Another proposed model is the constant curvature model for membrane invagination according to which clathrin assembles directly into a bud of constant curvature. As a spherical vesicle is formed from a shallow pit, the clathrin coated area increases (Figure 2). Both these models have been shown to exist in vitro (Scott et al., 2018). An increase in membrane tension also increases the number of flat clathrin lattices (Bucher et al., 2018). Based on these studies, Maib et al. (2018) hypothesized that in cases where the polymerization energy of the clathrin triskelia is not sufficient to deform the membrane directly, it will initially assemble as a flat lattice, whereas membranes that are easier to deform might follow the path of constant curvature, directly polymerizing into spherical vesicles.
FIGURE 2. Interaction of clathrin light chains with actin: When forces opposing membrane invagination (such as membrane tension and membrane rigidity) are high, clathrin first assembles as a flat lattice, and light chain-dependent rearrangement takes place to introduce curvature (constant area model). Since the polymerization energy of clathrin is insufficient to deform the membrane, the actin cytoskeleton is recruited by light chains to further counteract these opposing forces. On the other hand, when forces opposing membrane invagination are low, the polymerization energy of clathrin is sufficient to deform the membrane. Clathrin directly polymerizes onto the budding membrane (constant curvature model) and clathrin light chains and the actin cytoskeleton are not required.
To summarize, when there is low membrane tension, clathrin may directly polymerize onto the budding surface and light chains will not be required to rearrange the lattice and recruit actin. When there is high membrane tension clathrin may first assemble as a flat lattice which can then be rearranged with the help of light chains and actin to provide energy for membrane invagination.
This ability of the light chains to bind to Hip1/R protein and recruit actin is often exploited by bacteria and viruses to facilitate their entry into cells. Listeria monocytogenes for example, binds to cadherin through internalin, a protein which induces phosphorylation of the heavy chain. This phosphorylation recruits actin through the Hip1/R binding domain of the light chains to surround clathrin at the membrane and facilitate the entry of the pathogen into the cell (Bonazzi et al., 2011).
Five decades of research has provided a huge amount of insight into the complex process of CME (Kaksonen and Roux, 2018; Briant et al., 2020). While studies reveal the role of clathrin light chains in regulating clathrin assembly and several physiological processes, a number of open questions remain unanswered. For example, the specific roles of CLCa and CLCb and their splice variants are not completely understood. While recent studies have shed some light on their specific functions (Wu et al., 2016; Maib et al., 2018; Tsygankova and Keen, 2019; Redlingshöfer et al., 2020), we are only beginning to appreciate the role of each paralog. Apart from this, there is little clarity on why the requirement for light chains is different between different species and cell types. Other questions that need to be answered include the role of light chains in auxilin and GAK-mediated uncoating, mitosis, cell migration and neurodegeneration.
The molecular complexity and dynamic nature of endocytosis make it a difficult process to study. The presence of two paralogs further complicates the problem of elucidating the role of light chains. Small interfering RNA (siRNA)-mediated knockdown of CLCb is often compensated by increased expression of CLCa, whereas knockdown of CLCa is often accompanied by decrease in CHC expression (Majeed et al., 2014). This can lead to inconclusive and confounding results. Use of molecular techniques such as CRISPR-Cas9 genome editing can help overcome these problems by generating single isoform expressing cells. Spatio-temporal deletion of CLCs can further be instrumental in understanding their function in regulating physiological processes.
JD, MT, and DS wrote the first draft of the review together. They were all involved in subsequent edits and modifications to the review. All authors have read and approved the final draft.
This work was supported by funds to DS from the Department of Biotechnology (DBT) India (BT/PR25883/GET/119/105/2017), Indian Council of Medical Research (2020-3076/SCR-ADHOC-BMS) and NCCS intramural funding. JD and MT are recipients of Senior Research Fellowships from UGC, India.
The authors declare that the research was conducted in the absence of any commercial or financial relationships that could be construed as a potential conflict of interest.
All claims expressed in this article are solely those of the authors and do not necessarily represent those of their affiliated organizations, or those of the publisher, the editors and the reviewers. Any product that may be evaluated in this article, or claim that may be made by its manufacturer, is not guaranteed or endorsed by the publisher.
We thank Prof. Frances M. Brodsky for her valuable inputs and guidance in putting together this review.
Aghamohammadzadeh, S., and Ayscough, K. R. (2009). Differential Requirements for Actin during Yeast and Mammalian Endocytosis. Nat. Cell Biol 11 (8), 1039–1042. doi:10.1038/ncb1918
Ahle, S., and Ungewickell, E. (1986). Purification and Properties of a New Clathrin Assembly Protein. EMBO J. 5 (12), 3143–3149. doi:10.1002/j.1460-2075.1986.tb04621.x
Baisa, G. A., Mayers, J. R., and Bednarek, S. Y. (2013). Budding and Braking News about Clathrin-Mediated Endocytosis. Curr. Opin. Plant Biol. 16 (6), 718–725. doi:10.1016/j.pbi.2013.09.005
Bar-Zvi, D., Mosley, S. T., and Branton, D. (1988). In Vivo phosphorylation of Clathrin-Coated Vesicle Proteins from Rat Reticulocytes. J. Biol. Chem. 263 (9), 4408–4415. doi:10.1016/S0021-9258(18)68941-0
Blue, R. E., Curry, E. G., Engels, N. M., Lee, E. Y., and Giudice, J. (2018a). How Alternative Splicing Affects Membrane-Trafficking Dynamics. J. Cell. Sci. 131 (10), jcs216465. doi:10.1242/jcs.216465
Blue, R. E., Koushik, A., Engels, N. M., Wiedner, H. J., Cooper, T. A., and Giudice, J. (2018b). Modulation of Alternative Splicing of Trafficking Genes by Genome Editing Reveals Functional Consequences in Muscle Biology. Int. J. Biochem. Cell Biol. 105, 134–143. doi:10.1016/j.biocel.2018.10.004
Böcking, T., Aguet, F., Harrison, S. C., and Kirchhausen, T. (2011). Single-molecule Analysis of a Molecular Disassemblase Reveals the Mechanism of Hsc70-Driven Clathrin Uncoating. Nat. Struct. Mol. Biol. 18 (3), 295–301. doi:10.1038/nsmb.1985
Boettner, D. R., Friesen, H., Andrews, B., and Lemmon, S. K. (2011). Clathrin Light Chain Directs Endocytosis by Influencing the Binding of the Yeast Hip1R Homologue, Sla2, to F-Actin. MBoC 22 (19), 3699–3714. doi:10.1091/mbc.e11-07-0628
Bonazzi, M., Vasudevan, L., Mallet, A., Sachse, M., Sartori, A., Prevost, M.-C., et al. (2011). Clathrin Phosphorylation Is Required for Actin Recruitment at Sites of Bacterial Adhesion and Internalization. J. Cell Biol. 195 (3), 525–536. doi:10.1083/jcb.201105152
Boulant, S., Kural, C., Zeeh, J.-C., Ubelmann, F., and Kirchhausen, T. (2011). Actin Dynamics Counteract Membrane Tension during Clathrin-Mediated Endocytosis. Nat. Cell Biol 13 (9), 1124–1131. doi:10.1038/ncb2307
Briant, K., Redlingshöfer, L., and Brodsky, F. M. (2020). Clathrin's Life beyond 40: Connecting Biochemistry with Physiology and Disease. Curr. Opin. Cell Biol. 65, 141–149. doi:10.1016/j.ceb.2020.06.004
Brodsky, F. M., Galloway, C. J., Blank, G. S., Jackson, A. P., Seow, H.-F., Drickamer, K., et al. (1987). Localization of Clathrin Light-Chain Sequences Mediating Heavy-Chain Binding and Coated Vesicle Diversity. Nature 326 (6109), 203–205. doi:10.1038/326203a0
Brodsky, F. M. (2012). Diversity of Clathrin Function: New Tricks for an Old Protein. Annu. Rev. Cell Dev. Biol. 28, 309–336. doi:10.1146/annurev-cellbio-101011-155716
Brodsky, F. M. (2016). Clathrin and Clathrin-dependent Endocytosis. Encyclopedia Cell Biol. 2, 384–393. doi:10.1016/b978-0-12-394447-4.20038-2
Bucher, D., Frey, F., Sochacki, K. A., Kummer, S., Bergeest, J.-P., Godinez, W. J., et al. (2018). Clathrin-adaptor Ratio and Membrane Tension Regulate the Flat-To-Curved Transition of the Clathrin Coat during Endocytosis. Nat. Commun. 9 (1), 1–13. doi:10.1038/s41467-018-03533-0
Chen, C.-Y., and Brodsky, F. M. (2005). Huntingtin-interacting Protein 1 (Hip1) and Hip1-Related Protein (Hip1R) Bind the Conserved Sequence of Clathrin Light Chains and Thereby Influence Clathrin Assembly In Vitro and Actin Distribution In Vivo. J. Biol. Chem. 280 (7), 6109–6117. doi:10.1074/jbc.M408454200
Chen, J., and Fang, G. (2001). MAD2B Is an Inhibitor of the Anaphase-Promoting Complex. Genes Dev. 15 (14), 1765–1770. doi:10.1101/gad.898701
Chen, C.-Y., Reese, M. L., Hwang, P. K., Ota, N., Agard, D., and Brodsky, F. M. (2002). Clathrin Light and Heavy Chain Interface: α-helix Binding Superhelix Loops via Critical Tryptophans. Embo J. 21 (22), 6072–6082. doi:10.1093/emboj/cdf594
Chen, P.-H., Bendris, N., Hsiao, Y.-J., Reis, C. R., Mettlen, M., Chen, H.-Y., et al. (2017). Crosstalk between CLCb/Dyn1-Mediated Adaptive Clathrin-Mediated Endocytosis and Epidermal Growth Factor Receptor Signaling Increases Metastasis. Dev. Cell 40 (3), 278–288. doi:10.1016/j.devcel.2017.01.007
Chu, D. S., Pishvaee, B., and Payne, G. S. (1996). The Light Chain Subunit Is Required for Clathrin Function in Saccharomyces cerevisiae. J. Biol. Chem. 271 (51), 33123–33130. doi:10.1074/jbc.271.51.33123
Dannhauser, P. N., Platen, M., Böning, H., Ungewickell, H., Schaap, I. A. T., and Ungewickell, E. J. (2015). Effect of Clathrin Light Chains on the Stiffness of Clathrin Lattices and Membrane Budding. Traffic 16 (5), 519–533. doi:10.1111/tra.12263
DeLuca-Flaherty, C., McKay, D. B., Parham, P., and Hill, B. L. (1990). Uncoating Protein (Hsc70) Binds a Conformationally Labile Domain of Clathrin Light Chain LCa to Stimulate ATP Hydrolysis. Cell 62 (5), 875–887. doi:10.1016/0092-8674(90)90263-E
Doherty, G. J., and McMahon, H. T. (2009). Mechanisms of Endocytosis. Annu. Rev. Biochem. 78, 857–902. doi:10.1146/annurev.biochem.78.081307.110540
Engqvist-Goldstein, Å. E. Y., and Drubin, D. G. (2003). Actin Assembly and Endocytosis: from Yeast to Mammals. Annu. Rev. Cell Dev. Biol. 19 (1), 287–332. doi:10.1146/annurev.cellbio.19.111401.093127
Ferreira, F., Foley, M., Cooke, A., Cunningham, M., Smith, G., Woolley, R., et al. (2012). Endocytosis of G Protein-Coupled Receptors Is Regulated by Clathrin Light Chain Phosphorylation. Curr. Biol. 22 (15), 1361–1370. doi:10.1016/j.cub.2012.05.034
Fielding, A. B., Willox, A. K., Okeke, E., and Royle, S. J. (2012). Clathrin-mediated Endocytosis Is Inhibited during Mitosis. Proc. Natl. Acad. Sci. 109 (17), 6572–6577. doi:10.1073/pnas.1117401109
Foraker, A. B., Camus, S. M., Evans, T. M., Majeed, S. R., Chen, C.-Y., Taner, S. B., et al. (2012). Clathrin Promotes Centrosome Integrity in Early Mitosis through Stabilization of Centrosomal Ch-TOG. J. Cell Biol. 198 (4), 591–605. doi:10.1083/jcb.201205116
Giudice, J., Xia, Z., Wang, E. T., Scavuzzo, M. A., Ward, A. J., Kalsotra, A., et al. (2014). Alternative Splicing Regulates Vesicular Trafficking Genes in Cardiomyocytes during Postnatal Heart Development. Nat. Commun. 5 (1), 1–15. doi:10.1038/ncomms4603
Goode, B. L., Eskin, J. A., and Wendland, B. (2015). Actin and Endocytosis in Budding Yeast. Genetics 199 (2), 315–358. doi:10.1534/genetics.112.145540
Greene, B., Liu, S.-H., Wilde, A., and Brodsky, F. M. (2000). Complete Reconstitution of Clathrin Basket Formation with Recombinant Protein Fragments: Adaptor Control of Clathrin Self-Assembly. traffic 1 (1), 69–75. doi:10.1034/j.1600-0854.2000.010110.x
Haucke, V., and Kozlov, M. M. (2018). Membrane Remodeling in Clathrin-Mediated Endocytosis. J. Cell Sci 131 (17), jcs216812. doi:10.1242/jcs.216812
Heerssen, H., Fetter, R. D., and Davis, G. W. (2008). Clathrin Dependence of Synaptic-Vesicle Formation at the Drosophila Neuromuscular junction. Curr. Biol. 18 (6), 401–409. doi:10.1016/j.cub.2008.02.055
Hill, B. L., Drickamer, K., Brodsky, F. M., and Parham, P. (1988). Identification of the Phosphorylation Sites of Clathrin Light Chain LCb. J. Biol. Chem. 263 (12), 5499–5501. doi:10.1016/S0021-9258(18)60591-5
Huang, K. M., Gullberg, L., Nelson, K. K., Stefan, C. J., Blumer, K., and Lemmon, S. K. (1997). Novel Functions of Clathrin Light Chains: Clathrin Heavy Chain Trimerization Is Defective in Light Chain-Deficient Yeast. J. Cell Sci. 110 (7), 899–910. doi:10.1242/jcs.110.7.899
Huang, F., Khvorova, A., Marshall, W., and Sorkin, A. (2004). Analysis of Clathrin-Mediated Endocytosis of Epidermal Growth Factor Receptor by RNA Interference. J. Biol. Chem. 279 (16), 16657–16661. doi:10.1074/jbc.C400046200
Jackson, A. P., Seow, H. F., Holmes, N., Drickamer, K., and Parham, P. (1987). Clathrin Light Chains Contain Brain-Specific Insertion Sequences and a Region of Homology With Intermediate Filaments. Nature 326 (6109), 154–159. doi:10.1038/326154a0
Jiang, R.-F., Greener, T., Barouch, W., Greene, L., and Eisenberg, E. (1997). Interaction of Auxilin with the Molecular Chaperone, Hsc70. J. Biol. Chem. 272 (10), 6141–6145. doi:10.1074/jbc.272.10.6141
Kaksonen, M., and Roux, A. (2018). Mechanisms of Clathrin-Mediated Endocytosis. Nat. Rev. Mol. Cell Biol 19 (5), 313–326. doi:10.1038/nrm.2017.132
Kanaoka, Y., Kimura, S. H., Okazaki, I., Ikeda, M., and Nojima, H. (1997). GAK: a Cyclin G Associated Kinase Contains a Tensin/auxilin-like Domain 1. FEBS Lett. 402 (1), 73–80. doi:10.1016/S0014-5793(96)01484-6
Keen, J. H. (1990). Clathrin and Associated Assembly and Disassembly Proteins. Annu. Rev. Biochem. 59 (1), 415–438. doi:10.1146/annurev.bi.59.070190.002215
Kim, M. L., Sorg, I., and Arrieumerlou, C. (2011). Endocytosis-Independent Function of Clathrin Heavy Chain in the Control of Basal NF-Κb Activation. PLoS One 6 (2), e17158. doi:10.1371/journal.pone.0017158
Kimura, S. H., Tsuruga, H., Yabuta, N., Endo, Y., and Nojima, H. (1997). Structure, Expression, and Chromosomal Localization of Human GAK. Genomics 44 (2), 179–187. doi:10.1006/geno.1997.4873
Kirchhausen, T., and Harrison, S. C. (1981). Protein Organization in Clathrin Trimers. Cell 23 (3), 755–761. doi:10.1016/0092-8674(81)90439-6
Kirchhausen, T., Harrison, S. C., Parham, P., and Brodsky, F. M. (1983). Location and Distribution of the Light Chains in Clathrin Trimers. Proc. Natl. Acad. Sci. 80 (9), 2481–2485. doi:10.1073/pnas.80.9.2481
Kirchhausen, T., Owen, D., and Harrison, S. C. (2014). Molecular Structure, Function, and Dynamics of Clathrin-Mediated Membrane Traffic. Cold Spring Harbor Perspect. Biol. 6 (5), a016725. doi:10.1101/cshperspect.a016725
Kirchhausen, T. (2000). Clathrin. Annu. Rev. Biochem. 69 (1), 699–727. doi:10.1146/annurev.biochem.69.1.699
Konopka, C. A., Backues, S. K., and Bednarek, S. Y. (2008). Dynamics of Arabidopsis Dynamin-Related Protein 1C and a Clathrin Light Chain at the Plasma Membrane. The Plant Cell 20 (5), 1363–1380. doi:10.1105/tpc.108.059428
Kovtun, O., Dickson, V. K., Kelly, B. T., Owen, D. J., and Briggs, J. A. G. (2020). Architecture of the AP2/clathrin Coat on the Membranes of Clathrin-Coated Vesicles. Sci. Adv. 6 (30), eaba8381. doi:10.1126/sciadv.aba8381
Legendre-Guillemin, V., Metzler, M., Lemaire, J.-F., Philie, J., Gan, L., Hayden, M. R., et al. (2005). Huntingtin Interacting Protein 1 (HIP1) Regulates Clathrin Assembly through Direct Binding to the Regulatory Region of the Clathrin Light Chain. J. Biol. Chem. 280 (7), 6101–6108. doi:10.1074/jbc.M408430200
Lindner, R., and Ungewickell, E. (1992). Clathrin-associated Proteins of Bovine Brain Coated Vesicles. An Analysis of Their Number and Assembly-Promoting Activity. J. Biol. Chem. 267 (23), 16567–16573. doi:10.1016/S0021-9258(18)42040-6
Liu, S.-H., Wong, M. L., Craik, C. S., and Brodsky, F. M. (1995). Regulation of Clathrin Assembly and Trimerization Defined Using Recombinant Triskelion Hubs. Cell 83 (2), 257–267. doi:10.1016/0092-8674(95)90167-1
Maib, H., Ferreira, F., Vassilopoulos, S., and Smythe, E. (2018). Cargo Regulates Clathrin-Coated Pit Invagination via Clathrin Light Chain Phosphorylation. J. Cell Biol. 217 (12), 4253–4266. doi:10.1083/jcb.201805005
Majeed, S. R., Vasudevan, L., Chen, C.-Y., Luo, Y., Torres, J. A., Evans, T. M., et al. (2014). Clathrin Light Chains Are Required for the Gyrating-Clathrin Recycling Pathway and Thereby Promote Cell Migration. Nat. Commun. 5 (1), 1–14. doi:10.1038/ncomms4891
Medendorp, K., Vreede, L., van Groningen, J. J. M., Hetterschijt, L., Brugmans, L., Jansen, P. A. M., et al. (2010). The Mitotic Arrest Deficient Protein MAD2B Interacts with the Clathrin Light Chain A during Mitosis. PloS one 5 (11), e15128. doi:10.1371/journal.pone.0015128
Mooren, O. L., Galletta, B. J., and Cooper, J. A. (2012). Roles for Actin Assembly in Endocytosis. Annu. Rev. Biochem. 81, 661–686. doi:10.1146/annurev-biochem-060910-094416
Morris, K. L., Jones, J. R., Halebian, M., Wu, S., Baker, M., Armache, J.-P., et al. (2019). Cryo-EM of Multiple Cage Architectures Reveals a Universal Mode of Clathrin Self-Assembly. Nat. Struct. Mol. Biol. 26 (10), 890–898. doi:10.1038/s41594-019-0292-0
Mote, R. D., Yadav, J., Singh, S. B., Tiwari, M., V, S. L., Patil, S., et al. (2020). Pluripotency of Embryonic Stem Cells Lacking Clathrin-Mediated Endocytosis Cannot Be Rescued by Restoring Cellular Stiffness. J. Biol. Chem. 295 (49), 16888–16896. doi:10.1074/jbc.AC120.014343
Mukenhirn, M., Muraca, F., Bucher, D., Asberger, E., Cappio Barazzone, E., Cavalcanti-Adam, E. A., et al. (2021). Role of Clathrin Light Chains in Regulating Invadopodia Formation. Cells 10 (2), 451. doi:10.3390/cells10020451
Näthke, I., Hill, B. L., Parham, P., and Brodsky, F. M. (1990). The Calcium-Binding Site of Clathrin Light Chains. J. Biol. Chem. 265 (30), 18621–18627. doi:10.1016/S0021-9258(17)44797-1
Näthke, I. S., Heuser, J., Lupas, A., Stock, J., Turck, C. W., and Brodsky, F. M. (1992). Folding and Trimerization of Clathrin Subunits at the Triskelion Hub. Cell 68 (5), 899–910. doi:10.1016/0092-8674(92)90033-9
Nahorski, M. S., Borner, G. H. H., Shaikh, S. S., Davies, A. K., Al-Gazali, L., Antrobus, R., et al. (2018). Clathrin Heavy Chain 22 Contributes to the Control of Neuropeptide Degradation and Secretion during Neuronal Development. Sci. Rep. 8 (1), 1–11. doi:10.1038/s41598-018-19980-0
Nakamura, Y., Takeda, M., Yoshimi, K., Hattori, H., Hariguchi, S., Kitajima, S., et al. (1994a). Involvement of Clathrin Light Chains in the Pathology of Alzheimer's Disease. Acta Neuropathol. 87 (1), 23–31. doi:10.1007/BF00386251
Nakamura, Y., Takeda, M., Yoshimi, K., Hattori, H., Hariguchi, S., Hashimoto, S., et al. (1994b). Involvement of Clathrin Light Chains in the Pathology of Pick's Disease; Impilication for Impairment of Axonal Transport. Neurosci. Lett. 180 (1), 25–28. doi:10.1016/0304-3940(94)90905-9
Narayana, Y. V., Gadgil, C., Mote, R. D., Rajan, R., and Subramanyam, D. (2019). Clathrin-mediated Endocytosis Regulates a Balance between Opposing Signals to Maintain the Pluripotent State of Embryonic Stem Cells. Stem Cell Rep. 12 (1), 152–164. doi:10.1016/j.stemcr.2018.11.018
Owen, D. J., Vallis, Y., Pearse, B. M. F., McMahon, H. T., and Evans, P. R. (2000). The Structure and Function of the Beta2-Adaptin Appendage Domain. EMBO J. 19 (16), 4216–4227. doi:10.1093/emboj/19.16.4216
Parham, P., Brodsky, F. M., and Drickamer, K. (1989). The Occurrence of Disulphide Bonds in Purified Clathrin Light Chains. Biochem. J. 257 (3), 775–781. doi:10.1042/bj2570775
Pearse, B. M. F., and Robinson, M. S. (1990). Clathrin, Adaptors, and Sorting. Annu. Rev. Cell. Biol. 6 (1), 151–171. doi:10.1146/annurev.cb.06.110190.001055
Pearse, B. M. F. (1975). Coated Vesicles from Pig Brain: Purification and Biochemical Characterization. J. Mol. Biol. 97 (1), 93–98. doi:10.1016/s0022-2836(75)80024-6
Pearse, B. M. (1976). Clathrin: a Unique Protein Associated with Intracellular Transfer of Membrane by Coated Vesicles. Proc. Natl. Acad. Sci. 73 (4), 1255–1259. doi:10.1073/pnas.73.4.1255
Pley, U. M., Hill, B. L., Alibert, C., Brodsky, F. M., and Parham, P. (1995). The Interaction of Calmodulin with Clathrin-Coated Vesicles, Triskelions, and Light Chains. J. Biol. Chem. 270 (5), 2395–2402. doi:10.1074/jbc.270.5.2395
Poupon, V., Girard, M., Legendre-Guillemin, V., Thomas, S., Bourbonniere, L., Philie, J., et al. (2008). Clathrin Light Chains Function in Mannose Phosphate Receptor Trafficking via Regulation of Actin Assembly. Proc. Natl. Acad. Sci. 105 (1), 168–173. doi:10.1073/pnas.0707269105
Redlingshöfer, L., McLeod, F., Chen, Y., Camus, M. D., Burden, J. J., Palomer, E., et al. (2020). Clathrin Light Chain Diversity Regulates Membrane Deformation In Vitro and Synaptic Vesicle Formation In Vivo. Proc. Natl. Acad. Sci. USA 117 (38), 23527–23538. doi:10.1073/pnas.2003662117
Roth, T. F., and Porter, K. R. (1964). Yolk Protein Uptake in the Oocyte of the Mosquito Aedes aegypti. L. Journal of Cell Biology 20 (2), 313–332. doi:10.1083/jcb.20.2.313
Royle, S. J., Bright, N. A., and Lagnado, L. (2005). Clathrin Is Required for the Function of the Mitotic Spindle. Nature 434 (7037), 1152–1157. doi:10.1038/nature03502
Royle, S. J. (2012). The Role of Clathrin in Mitotic Spindle Organisation. J. Cell Sci. 125 (1), 19–28. doi:10.1242/jcs.094607
Saffarian, S., Cocucci, E., and Kirchhausen, T. (2009). Distinct Dynamics of Endocytic Clathrin-Coated Pits and Coated Plaques. Plos Biol. 7 (9), e1000191. doi:10.1371/journal.pbio.1000191
Scheele, U., and Holstein, S. E. (2002). Functional Evidence for the Identification of an Arabidopsis Clathrin Light Chain Polypeptide. FEBS Lett. 514 (2-3), 355–360. doi:10.1016/S0014-5793(02)02439-0
Schmid, S. L., Matsumoto, A. K., and Rothman, J. E. (1982). A Domain of Clathrin that Forms coats. Proc. Natl. Acad. Sci. 79 (1), 91–95. doi:10.1073/pnas.79.1.91
Schreij, A. M., Chaineau, M., Ruan, W., Lin, S., Barker, P. A., Fon, E. A., et al. (2015). LRRK 2 Localizes to Endosomes and Interacts with Clathrin‐light Chains to Limit Rac1 Activation. EMBO Rep. 16 (1), 79–86. doi:10.15252/embr.201438714
Scott, B. L., Sochacki, K. A., Low-Nam, S. T., Bailey, E. M., Luu, Q., Hor, A., et al. (2018). Membrane Bending Occurs at All Stages of Clathrin-Coat Assembly and Defines Endocytic Dynamics. Nat. Commun. 9 (1), 1–9. doi:10.1038/s41467-018-02818-8
Silveira, L. A., Wong, D. H., Masiarz, F. R., and Schekman, R. (1990). Yeast Clathrin Has a Distinctive Light Chain that Is Important for Cell Growth. J. Ccell Biol. 111 (4), 1437–1449. doi:10.1083/jcb.111.4.1437
Stamm, S., Casper, D., Dinsmore, J., Kaufmann, C. A., Brosius, J., and Helfman, D. M. (1992). Clathrin Light Chain B: Gene Structure and Neuron-specific Splicing. Nucl. Acids Res. 20 (19), 5097–5103. doi:10.1093/nar/20.19.5097
Stavrou, I., and O'Halloran, T. J. (2006). The Monomeric Clathrin Assembly Protein, AP180, Regulates Contractile Vacuole Size inDictyostelium Discoideum. MBoC 17 (12), 5381–5389. doi:10.1091/mbc.e06-06-0531
Takano, M., Yamashita, T., Nagano, K., Otani, M., Maekura, K., Kamada, H., et al. (2013). Proteomic Analysis of the hippocampus in Alzheimer's Disease Model Mice by Using Two-Dimensional Fluorescence Difference in Gel Electrophoresis. Neurosci. Lett. 534, 85–89. doi:10.1016/j.neulet.2012.11.010
Towler, M. C., Gleeson, P. A., Hoshino, S., Rahkila, P., Manalo, V., Ohkoshi, N., et al. (2004). Clathrin Isoform CHC22, a Component of Neuromuscular and Myotendinous Junctions, Binds Sorting Nexin 5 and Has Increased Expression during Myogenesis and Muscle Regeneration. MBoC 15 (7), 3181–3195. doi:10.1091/mbc.e04-03-0249
Tsygankova, O. M., and Keen, J. H. (2019). A Unique Role for Clathrin Light Chain A in Cell Spreading and Migration. J. cell Sci. 132 (10). doi:10.1242/jcs.224030
Umeda, A., Meyerholz, A., and Ungewickell, E. (2000). Identification of the Universal Cofactor (Auxilin 2) in Clathrin Coat Dissociation. Eur. J.Cell Biol. 79 (5), 336–342. doi:10.1078/S0171-9335(04)70037-0
Ungewickell, E., Ungewickell, H., Holstein, S. E. H., Lindner, R., Prasad, K., Barouch, W., et al. (1995). Role of Auxilin in Uncoating Clathrin-Coated Vesicles. Nature 378 (6557), 632–635. doi:10.1038/378632a0
Ungewickell, E. (1983). Biochemical and Immunological Studies on Clathrin Light Chains and Their Binding Sites on Clathrin Triskelions. EMBO J. 2 (8), 1401–1408. doi:10.1002/j.1460-2075.1983.tb01598.x
Usami, M., Takahashi, A., Kadota, T., and Katoda, K. (1985). Phosphorylation of a Clathrin light Chain of Coated Vesicles in the Presence of Histones. J. Biochem. 97 (6), 1819–1822. doi:10.1093/oxfordjournals.jbchem.a135243
Wakeham, D. E., Abi-Rached, L., Towler, M. C., Wilbur, J. D., Parham, P., and Brodsky, F. M. (2005). Clathrin Heavy and Light Chain Isoforms Originated by Independent Mechanisms of Gene Duplication during Chordate Evolution. Proc. Natl. Acad. Sci. 102 (20), 7209–7214. doi:10.1073/pnas.0502058102
Wang, J., Virta, V. C., Riddelle-Spencer, K., and O'Halloran, T. J. (2003). Compromise of Clathrin Function and Membrane Association by Clathrin Light Chain Deletion. Traffic 4 (12), 891–901. doi:10.1046/j.1600-0854.2003.00144.x
Wang, J., Wang, Y., and O'Halloran, T. J. (2006). Clathrin Light Chain: Importance of the Conserved Carboxy Terminal Domain to Function in Living Cells. Traffic 7 (7), 824–832. doi:10.1111/j.1600-0854.2006.00438.x
Wang, C., Yan, X., Chen, Q., Jiang, N., Fu, W., Ma, B., et al. (2013). Clathrin Light Chains Regulate Clathrin-Mediated Trafficking, Auxin Signaling, and Development in Arabidopsis. The Plant Cell 25 (2), 499–516. doi:10.1105/tpc.112.108373
Wilbur, J. D., Chen, C.-Y., Manalo, V., Hwang, P. K., Fletterick, R. J., and Brodsky, F. M. (2008). Actin Binding by Hip1 (Huntingtin-interacting Protein 1) and Hip1R (Hip1-Related Protein) Is Regulated by Clathrin Light Chain. J. Biol. Chem. 283 (47), 32870–32879. doi:10.1074/jbc.M802863200
Wilbur, J. D., Hwang, P. K., Ybe, J. A., Lane, M., Sellers, B. D., Jacobson, M. P., et al. (2010). Conformation Switching of Clathrin Light Chain Regulates Clathrin Lattice Assembly. Dev. Cell 18 (5), 854–861. doi:10.1016/j.devcel.2010.04.007
Winkler, F. K., and Stanley, K. K. (1983). Clathrin Heavy Chain, Light Chain Interactions. EMBO J. 2 (8), 1393–1400. doi:10.1002/j.1460-2075.1983.tb01597.x
Wong, D., Ignatius, M., Parosky, G., Parham, P., Trojanowski, J., and Brodsky, F. (1990). Neuron-specific Expression of High-Molecular-Weight Clathrin Light Chain. J. Neurosci. 10 (9), 3025–3031. doi:10.1523/JNEUROSCI.10-09-03025.1990
Wu, S., Majeed, S. R., Evans, T. M., Camus, M. D., Wong, N. M. L., Schollmeier, Y., et al. (2016). Clathrin Light Chains' Role in Selective Endocytosis Influences Antibody Isotype Switching. Proc. Natl. Acad. Sci. USA 113 (35), 9816–9821. doi:10.1073/pnas.1611189113
Ybe, J. A., Mishra, S., Helms, S., and Nix, J. (2007a). Crystal Structure at 2.8 Å of the DLLRKN-Containing Coiled-Coil Domain of Huntingtin-Interacting Protein 1 (HIP1) Reveals a Surface Suitable for Clathrin Light Chain Binding. J. Mol. Biol. 367 (1), 8–15. doi:10.1016/j.jmb.2006.12.052
Ybe, J. A., Perez-Miller, S., Niu, Q., Coates, D. A., Drazer, M. W., and Clegg, M. E. (2007b). Light Chain C-Terminal Region Reinforces the Stability of Clathrin Heavy Chain Trimers. Traffic 8 (8), 1101–1110. doi:10.1111/j.1600-0854.2007.00597.x
Keywords: clathrin, membrane trafficking, endocytosis, triskelion, physiology, actin
Citation: Das J, Tiwari M and Subramanyam D (2021) Clathrin Light Chains: Not to Be Taken so Lightly. Front. Cell Dev. Biol. 9:774587. doi: 10.3389/fcell.2021.774587
Received: 12 September 2021; Accepted: 12 November 2021;
Published: 14 December 2021.
Edited by:
Huijie Bian, Fourth Military Medical University, ChinaReviewed by:
Derek Prosser, Virginia Commonwealth University, United StatesCopyright © 2021 Das, Tiwari and Subramanyam. This is an open-access article distributed under the terms of the Creative Commons Attribution License (CC BY). The use, distribution or reproduction in other forums is permitted, provided the original author(s) and the copyright owner(s) are credited and that the original publication in this journal is cited, in accordance with accepted academic practice. No use, distribution or reproduction is permitted which does not comply with these terms.
*Correspondence: Deepa Subramanyam, ZGVlcGFAbmNjcy5yZXMuaW4=
Disclaimer: All claims expressed in this article are solely those of the authors and do not necessarily represent those of their affiliated organizations, or those of the publisher, the editors and the reviewers. Any product that may be evaluated in this article or claim that may be made by its manufacturer is not guaranteed or endorsed by the publisher.
Research integrity at Frontiers
Learn more about the work of our research integrity team to safeguard the quality of each article we publish.