- Department of Biology, University of Puerto Rico, Río Piedras Campus, San Juan, Puerto Rico
The microbiota, the set of microorganisms associated with a particular environment or host, has acquired a prominent role in the study of many physiological and developmental processes. Among these, is the relationship between the microbiota and regenerative processes in various organisms. Here we introduce the concept of the microbiota and its involvement in regeneration-related cellular events. We then review the role of the microbiota in regenerative models that extend from the repair of tissue layers to the regeneration of complete organs or animals. We highlight the role of the microbiota in the digestive tract, since it accounts for a significant percentage of an animal microbiota, and at the same time provides an outstanding system to study microbiota effects on regeneration. Lastly, while this review serves to highlight echinoderms, primarily holothuroids, as models for regeneration studies, it also provides multiple examples of microbiota-related interactions in other processes in different organisms.
Introduction
Microorganisms evolved billions of years before animals (reviewed in Knoll, 2003). It is now widely accepted that these microorganisms shaped the environment in which animals evolved (Szathmáry and Smith, 1995; Narbonne, 2005; Knoll, 2011). As a result, animals have conserved close associations with microorganisms, making the microbes an integral part of the animal’s environment. In recent years our understanding of the relationship between animals and microorganisms has advanced greatly, thanks in part to new technologies, such as sequencing technologies and mass spectrometers. These advances have brought with them new or redefined terms to describe the participants and/or relationships (Turnbaugh et al., 2007). Terms such as “microbiota” to describe the microbial taxa composition that are found within a certain environment, and “microbiome” to describe the collective genome of such symbionts (Turnbaugh et al., 2007) are now commonly used, and will be part of the terminology used in this review. Naturally, the impacts of the microorganisms have been, for many centuries, associated with disease. However, during the last decades, many studies have shown hitherto unrecognized roles, such as, protecting against pathogens (Iacob et al., 2019), modulating host metabolism, digestion, and nutrition (Kellow et al., 2013; Vijay-Kumar et al., 2010; Turnbaugh et al., 2006; O’Hara and Shanahan, 2006; Turnbaugh et al., 2009; Long et al., 2017), and immune system response (Neish, 2009; Round and Mazmanian, 2009; Bäckhed and Crawford, 2010; Fraune and Bosch, 2010). For example, it is now well established that an altered gut microbial ecosystem impairs gut homeostasis and health. Accordingly, an imbalance (dysbiosis) in the gut microbial community has been associated to diseases such as obesity (Ley et al., 2006; Turnbaugh et al., 2008), malnutrition (Kau et al., 2011), atherosclerosis (Karlsson et al., 2012), and diabetes type 2 (Qin et al., 2012) demonstrating the importance of the gut microbiota composition.
Genomic and molecular approaches, and the characterization of the microbiota role have allowed for new discoveries that extend beyond host health/disease issues (Weinstock, 2012). Recently, the microbiota has been associated with host development, including processes that were thought to be dependent on the host’s genetic program, such as morphogenesis and organ development (Sommer and Bäckhed, 2013). Moreover, it has been proposed that the microbiota might play roles in behavior, reproduction, and even in degenerative diseases, among others (Wang et al., 2017).
The present review focuses on the relationship between the microbiota and the process of regeneration. This is a relatively new area of research that explores how the associated microbial taxa within a particular host might modulate the regeneration of a particular tissue, organ or even the whole-body of the host species. We include a summary of models that have been used to study the role of the gut microbiota during intestinal regeneration and associated processes (Table 1). Therefore, for the writing of this review, we screened for articles relevant to our topics in the search engine PubMed (pubmed.ncbi.nlm.nih.gov) using keywords such as “microbiota”, “microbiome” and “regeneration”, among others, and included information considered pertinent. Specifically, we have highlighted research done in animals that belong to the phylum Echinodermata, a phylum known for extraordinary regeneration abilities such as partial or total re-growth of different appendages or internal organs (García-Arrarás and Dolmatov, 2010). In particular, many of them are able to regenerate their digestive tract, thus providing the venue to study the effect of the microbiota in one of the organs best known for microbiota-host associations. This review serves to present a group of echinoderms, the holothurians or sea cucumbers, as excellent models to study microbiome-host associations and their impact on regenerative processes.
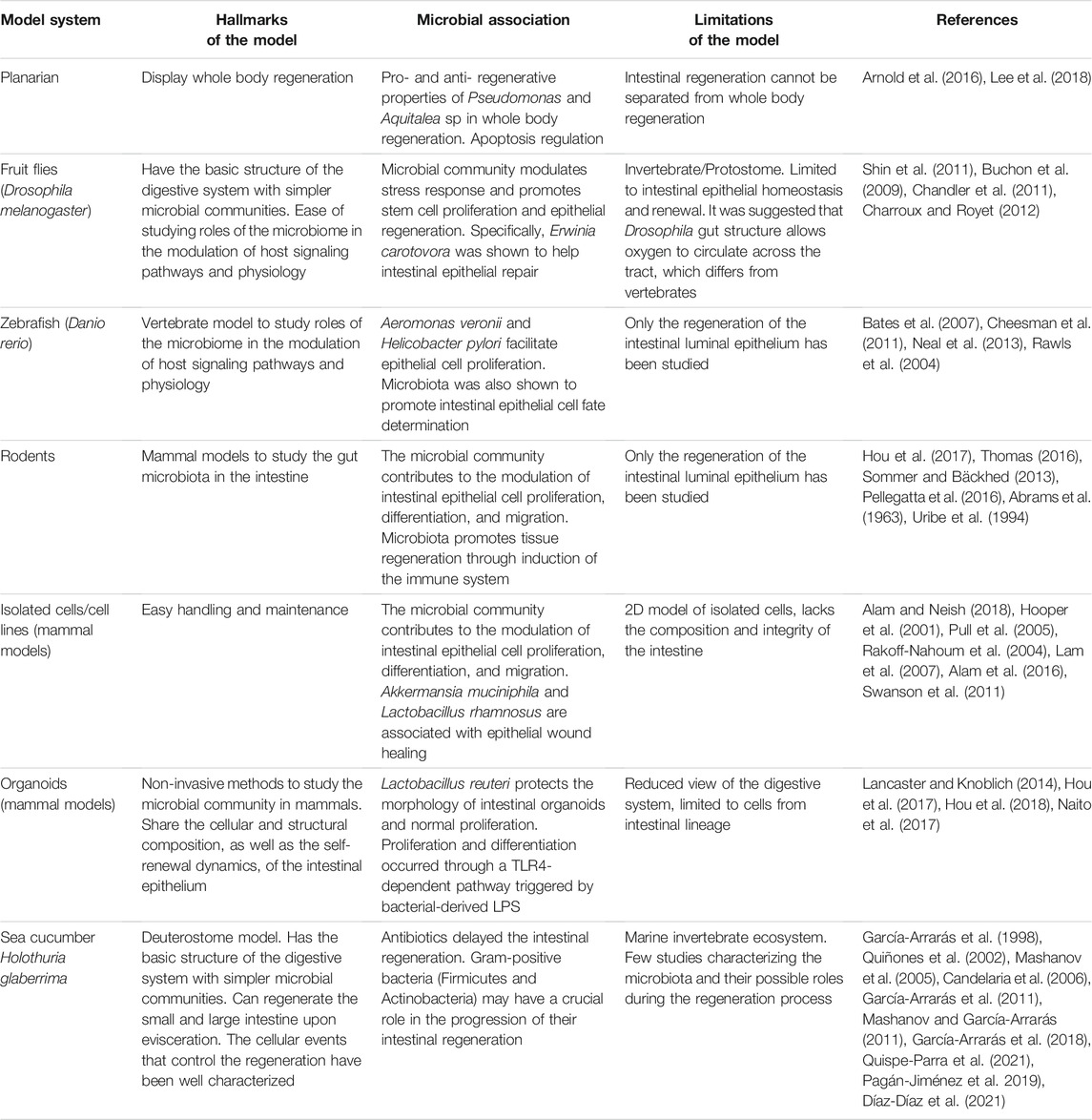
TABLE 1. Model systems used to decipher the associations between the microbiota and the intestinal regeneration in biomedical research.
Prior to delving into microbiome-regeneration studies, we begin by reviewing some findings from three regeneration-related fields where microbiome associations are important to the host. These are the association of the microbiota with: 1) the host metabolic/digestive processes, 2) embryonic developmental processes, and 3) wound healing (Figure 1). These three processes play important roles in regeneration and two of them (wound healing and embryonic development), share key mechanisms with regeneration, thus, the particular interest in singling them out.
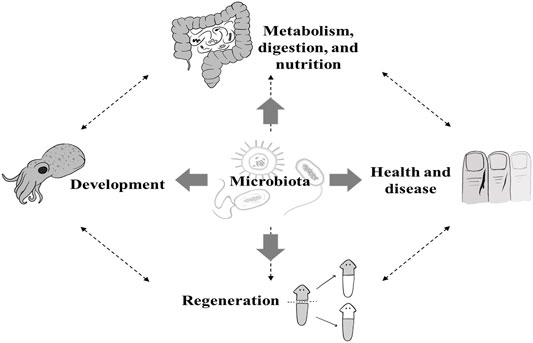
FIGURE 1. The influence of microbiota on host physiology. This figure outlines the aim of this review where we describe the role of the microbial composition associated with an animal host. In this review we focus on the regeneration process. However, we incorporated studies that link the microbiota to the metabolism, digestion and nutrition, health, and development of animal hosts to point out the interconnection between all these processes (dashed arrows).
Microbiota is Essential for Host Metabolism, Digestion, and Nutrition
From the roles ascribed to the microbiota, probably the best understood is their importance on host metabolism, which impacts their digestion and nutrition, by the assimilation of the digested food for the host physiological process. Multiple studies have shown the involvement of the mammalian gut microbiota in metabolic processes and energy homeostasis of host animals (O’Hara and Shanahan, 2006; Turnbaugh et al., 2006; Turnbaugh et al., 2009; Vijay-Kumar et al., 2010; Long et al., 2017). The gut microbiome was found to be crucial in processing non-digestible substrates that are necessary for host health maintenance and thus physiology (Gill et al., 2006). For example, the fermentation of dietary fibers and endogenous intestinal mucus, ensured by the intestinal microbiota, allows the growth of microorganisms that produce short-chain fatty acids (SCFAs) and gases (Wong et al., 2006). Acetate, the most abundant SCFA, is used in cholesterol metabolism and lipogenesis in the peripheral tissues (Frost et al., 2014). Butyrate, another major SCFA, is the main energy source for human luminal cells in the colon (De Vadder et al., 2014), and is key for generating a hypoxia state in epithelial cells, oxygen balance, and prevention of gut microbiota dysbiosis (Byndloss et al., 2017). The butyrate producer Faecalibacterium prausnitzii, one of the most represented bacteria in the intestine of healthy human adults, has exhibited anti-inflammatory effects in a colitis-mouse model (Miquel et al., 2013). Propionate, another dominant SCFA, regulates gluconeogenesis and satiety signaling through interaction with the gut fatty acid receptors in the liver (De Vadder et al., 2014). Another example of bacteria metabolites that can alter a host’s physiology is polyhydroxybutyrate (PHB), which is a polyhydroxylkanoate that comprises the primary product of carbon assimilation from glucose and starch. Microorganisms retain PHB and metabolize it when other common energy sources are not available, principally when carbon concentration is higher than nitrogen’s (Madison and Huisman, 1999; Jendrossek and Pfeiffer, 2014). Moreover, PHBs are used for host development both in fish and crustacean aquaculture (De Schryver et al., 2010; Nhan et al., 2010; Najdegerami et al., 2012).
The data shown above, focusing on a minor subset of gut bacterial products, clearly present the interdependence of the microbiota with its host highlighting how bacterial metabolites are not only essential for the host physiological processes but are also needed for the growth of other bacteria.
Microbiota Role in Development: Focus on Immune System and Organ Formation
Immune system development and activation- The actions of some symbionts go well beyond localized functions and are crucial for the overall development of the host. This provides a useful background for our discussion of microbiota effects on regeneration, specifically because of the links between embryological development and regeneration. Multiple studies from different organisms have demonstrated that the cellular and molecular mechanisms used in regenerative processes are similar, and in many cases identical, to those that take place during development (Arvizu et al., 2006; Yokoyama et al., 2008; Petersen and Reddien, 2009; Tu and Johnson, 2011; Tischer et al., 2013; Bryant et al., 2017; Reddy et al., 2019).
Therefore, the role of the microbiota during an organism’s developmental history can lead to important insights on a possible role during regeneration processes in the same or closely related organisms. A classic example of the effect of microbiota during embryonic or postnatal development is the development of the immune system in vertebrates (Round and Mazmanian, 2009; Bäckhed and Crawford, 2010; Fraune and Bosch, 2010). Studies have revealed that mutualistic or commensal microbe colonization are pivotal for the development, maturation, and activation of the immune system. Developmental effects of the microbiota in vertebrate species have usually been studied using germ-free models. In some of the key studies, germ-free reared animals presented deficient development of the immune system, including underdeveloped lymphatic organs (Falk et al., 1998; Macpherson and Harris, 2004; Bouskra et al., 2008), and defects in T cell regulation and B cells antibody production (Round and Mazmanian, 2009). In addition to the direct effects of these symbionts through the production of antimicrobial substances, immune response in germ-free animals lacked a priori instruction, induced by commensals (Hansen et al., 2014). This was confirmed with the propensity to infections when microbes were reintroduced to germ-free animals.
The study of Toll-like receptor (TLR) signaling in host-microbiome models has shown the mechanism by which the microbiota interacts with immune system activation and maturation (Akira and Takeda, 2004). This pathway is highly conserved in metazoans (Khalturin et al., 2004; Iwanaga and Lee, 2005; Roach et al., 2005; Satake and Sekiguchi, 2012; Nie et al., 2018), increasing the number of possible models in which to examine the relationship between microbiota and host immunity. The Toll pathway is activated by the binding of various microbe-associated molecular patterns (MAMPs) to the Toll-like receptors (TLRs) (Janssens and Beyaert, 2003; Kawai and Akira, 2010; Narayanan and Park, 2015; Wang et al., 2020). In some invertebrates, the pathway is activated indirectly, when the cytokine-like endogenous molecule Spätzle detects the microorganisms and activates the Toll receptors (Kawai and Akira, 2010). The activation of Toll pathway provokes the secretion of toxic molecules, such as antimicrobial peptides and reactive oxygen species (ROS) (Tzou et al., 2000; Ha, EM, et al., 2005).
Studies in mice have identified possible mediators of the microbiota-host immune response. These studies revealed that mice harbor specific Firmicutes, Candidatus arthromitus (Snel et al., 1995), that influence the innate immune system maturation (Suzuki et al., 2004; Gaboriau-Routhiau et al., 2009; Ivanov, II, et al., 2009). This suggests that Fusobacteria and Firmicutes may be important in the regulation of immune system development, immune-inflammatory response, and gut homeostasis. However, these filamentous bacteria have only been found in some infants younger than 3 years old (Yin et al., 2013), and a similar role in immune maturation in humans remains to be discovered. Moreover, recent studies have evidenced that metabolite generation, including SCFAs and adenosine triphosphate, influences the host’s immunity (Atarashi et al., 2008; Furusawa et al., 2013).
Organ morphogenesis- That the microbiota is involved in the process of immune system development and maturation might be expected, since after all, one of the system’s main functions involves the direct interaction of immune cells with the environmental bacteria. Other findings that associate the microbiota with an organism’s development are somewhat more surprising. One such study is the symbiotic association between the marine bobtail squid Euprymna scolopes and bioluminescent Vibrio fischeri. This model has arguably played a pivotal role in advancing the field of host-microbe associations involved in developmental processes (Nyholm and McFall-Ngai, 2004). This model provides an interesting phenomenon where the host-microbiome interaction is crucial to the formation of an anatomical complex structure and at the same time is not associated with health/disease issues, as are most other cases involving the microbiota. In this system, during development, the squid forms a structure named “the light organ” which helps in the protection of the host from predators (Boettcher and Ruby, 1990; McFall-Ngai and Ruby, 1998; Jones and Nishiguchi, 2004). This organ is colonized by bacteria during the day, the photosynthetic bacterium camouflages the squid from predators at night, and then at dawn, the squid ejects the light organ bacteria into the ocean, a cycle that is repeated daily.
Researchers have described in detail the process of host colonization and bacterial interactions (Nyholm and McFall-Ngai, 2004). Newly hatched juveniles are born with fields of ciliated epithelia on the nascent squid rudimentary light organ (McFall-Ngai and Ruby EG, 1991). They acquire the bacteria from the ocean environment (Ruby and Lee, 1998). When the host is exposed to bacterial peptidoglycan, the epithelial cells produce mucus that promotes the aggregation of bacteria (Nyholm et al., 2000; Nyholm et al., 2002). The symbiont then moves in the mucus to the crypt spaces of the light organ and colonizes it. As a result, it triggers developmental changes of the squid light organ (Doino and McFall-Ngai, 1995). Some of these adaptations include constriction of the ducts that lead to the crypt space delimitation, suspension of mucus secretion, and a regression of the ciliated epithelium, which might prevent further colonization of environmental symbionts. Other changes include trafficking of hemocytes into the blood of the ciliated epithelium (Nyholm and McFall-Ngai, 2004) facilitating the retrogression of the ciliated epithelium (Koropatnick et al., 2007), and increasing the density of microvilli in crypt cells (Nyholm and McFall-Ngai, 2004) which increases the surface area of interaction between the bacteria and the crypt cells (Lamarcq and McFall-Ngai, 1998). In addition to morphological and mechanical adaptations, chemical changes also take place. For example, following colonization by V. fischeri during crypt metamorphosis, a decrease in nitric oxide (NO) production is observed (Davidson et al., 2004). All these events favor the V. fischeri selection and proliferation to ensure mature organ light formation and bioluminescence (Nyholm and McFall-Ngai, 2004).
Antibiotic induced V. fischeri clearance from the crypts produces some irreversible developmental changes, such as the permanent loss of the surface ciliated epithelium and the attenuation of NO in the ducts (Nyholm and McFall-Ngai, 2004). Mutant V. fischeri that are defective in producing luminescence because of a mutation in the luxA gene (Visick et al., 2000) or deletion of lux operon do not persist in the crypts (Bose et al., 2008). Apart from not producing the required luminescence, these mutants cause developmental effects on the host, which fail to appropriately induce swelling of the crypt epithelial cells, hemocyte trafficking, and apoptosis of cells of the epithelial fields (McFall-Ngai et al., 2012). The mutant bacteria also have an altered expression of lipopolysaccharide (LPS) lipid A and peptidoglycan (PGN) tracheal cytotoxin (TCT) monomer. This correlates with observed changes in squids exposed to mutant bacteria that have a different expression of their LPS-binding proteins and peptidoglycan-recognition proteins. Thus, V. fischeri’s luminescence is somehow dependent on the expression of MAMPs and host pattern-recognition receptors to induce the immune system to cause the developmental changes in E. scolopes (McFall-Ngai et al., 2012).
Another interesting aspect of this symbiosis is the fact that V. fischeri that colonize the light organ are not eliminated by the immune system of E. scolopes (McFall-Ngai et al., 2012). It is thought that the recognition of V. fischeri molecules play a pivotal role in the selection of bacterial species by the immune system and therefore, the morphogenesis of the light organ of the host (Koropatnick et al., 2004; Troll et al., 2010).
Other developmental effects- Microbiota effects on embryonic development have been studied in other invertebrates (these are usually chosen because they generally have simpler microbial communities). The Drosophila-Acetobacter system has been a convenient model for understanding the genetic and functional roles of the microbiome in the modulation of host signaling pathways and physiology. Extensive studies in Drosophila and its symbiont Acetobacter pomorum showed that this gut bacteria impacts not only the metabolism of its hosts, but the growth, body size gain, and stem cellular activity (Shin et al., 2011). An A. pomorum mutant library has been used to decipher their beneficial role on host’s developmental homeostasis. This has led to the finding that the periplasmic pyrroloquinoline quinone–dependent alcohol dehydrogenase (PQQ-ADH)–dependent oxidative respiratory chain of the A. pomorum interaction with the insulin/insulin-like growth factor (IGF)-1 signaling (IIS) of the host is necessary for the maintenance of the gut mutualism. However, the sole bacterial PQQ-ADH is insufficient to promote the A. pomorum–mediated effects on host physiology, suggesting that the host genetic program and gut bacteria regulate each other. Additional studies using multiple insect models have confirmed the role of the hindgut bacteria in various aspects of digestion and host development. These cases include digestive efficiency of soluble plant polysaccharides and growth rate in crickets (Kaufman and Klug, 1991), insect generation time, adult body weight gain, and methane production in cockroaches (Gijzen and Barugahare, 1992), cellulose breakdown and nitrogen fixation in beetles (Morales-Jiménez et al., 2009), and potential proteolytic activity in aphids (Wang and Zhang, 2015).
Many studies performed in germ-free mammals have shown that the intestinal microbiota influences the postnatal development of the gastrointestinal tract in these organisms. For example, in mice, successions in the microbiota composition during development were shown to lead to gastrointestinal maturation (Wagner et al., 2008; Reinhardt et al., 2009). The intestine of an adult mouse accommodates a sophisticated vascular network that originates from a system of vessels that form postnatally in small intestinal villi. The formation of this network occurs concurrent with the assembly of the microbiota. Comparative studies of the capillary networks of germ-free mice versus animals colonized (ex-germ-free) during or after gut development demonstrated abnormalities in the capillary network of adult germ-free mice (Stappenbeck et al., 2002). However, colonization either with conventionalized mice microbiota or with Bacteroides thetaiotaomicron restarted and completed the developmental program. Other studies, using germ-free transgenic mice lacking Paneth cells (which secrete antibacterial peptides that affect luminal microbial ecology) in the intestinal epithelium, showed that this angiogenesis was regulated by B. thetaiotaomicron colonization of the mucosal surface (Stappenbeck et al., 2002). In addition, the associated microbial community contributes to the modulation of intestinal epithelial cell proliferation, as evidenced by the scarcity of proliferating cells in the intestines of germ-free rodents (Abrams et al., 1963; Uribe et al., 1994) and zebrafish (Rawls et al., 2004).
Further information on the role of the microbiota on vertebrate developmental processes has been obtained using germ-free and gnotobiotic zebrafish (Milligan-Myhre et al., 2011). Both zebrafish and murine germ-free models presented significant differences in the intestinal morphology in comparison with conventional controls, including reduced cell division, decreased number of goblet cells and intestinal associated immune cells, and perturbed expression of genes involved in metabolism and innate immunity (Savage et al., 1981; Kandori et al., 1996; Cebra et al., 1998; Hooper et al., 2001; Rawls et al., 2004; Bates et al., 2007; Bouskra et al., 2008; Cheesman et al., 2011; Kanther et al., 2011).
A New Role for Microbiota as Regulator of Regenerative Processes
The role of the microbiota has been studied, quite extensively, in processes associated with wound healing. These processes are usually the initial steps in more complex regenerative events, and will be briefly reviewed here, prior to discussing the role of the microbiota in overall regeneration of tissues and organs.
Wound healing following injury - The first response after a trauma or injury to an organism is the wound healing cascade which ensures the repair of the wound and avoids the colonization or translocation of pathogens. This takes place prior to the reorganization of the injured tissue (Guo and Dipietro, 2010) and might involve the microbiota (Thomas, 2016; Maheswary et al., 2021) as shown by studies of human skin microbiota during wound healing processes. Many of the findings on the role of microbiota in wound healing were facilitated by studies of chronic wounds, such as diabetic foot ulcers and non-healing surgical wounds, which represent major healthcare problems. These studies provided valuable data on how the microbiota can shape the process of wound healing and perhaps other processes related to regeneration.
Chronic wounds are caused by a disruption of the cutaneous wound healing process, preventing the restoration of the skin barrier. The main bacterial phyla identified in acute and chronic wounds are also found in healthy skin, however wounds are characterized by skin dysbiosis where their relative abundance differs significantly by wound type (Ammons et al., 2015; Loesche et al., 2017). Pseudomonas and Staphylococcus dominate in all types of chronic wounds (Dowd et al., 2008; James et al., 2008; Gardner et al., 2013; Wolcott et al., 2016; Gardiner et al., 2017), and usually are present in acute wounds created by blunt or penetrating trauma (Hannigan et al., 2014; Bartow-McKenney et al., 2018), burns (Hannigan et al., 2014; Liu et al., 2018), or atopic dermatitis (Seite et al., 2014). However, higher levels of anaerobic bacteria are present in chronic wounds and are commonly associated with worse prognosis (Loesche et al., 2017).
Moreover, pathogenic microorganisms are suspected of playing a substantial role in delayed wound healing. Hence, perturbations of microbial communities that are not promoting cutaneous wound healing may be beneficial. As shown by Loesche et al. the use of antibiotics to destabilize pathogenic wound microbiomes, resulted in faster wound healing (Loesche et al., 2017). In other studies, when probiotic bacteria were applied to a rodent wound, the bacterial load was decreased and tissue repair was promoted (Rodrigues et al., 2005; Valdez et al., 2005; Huseini et al., 2012). Similarly, wounded dermal tissues of mice showed improved proliferation of epidermal cells, vascularization, and re-epithelialization after inoculation with Pseudomonas aeruginosa strain PAO1 (Kanno et al., 2011). Also, in humans, topical application of probiotics exerted positive wound healing properties for chronic venous ulcers infected with Staphylococcus aureus and Pseudomonas aeruginosa (Peral et al., 2009). Consequently, microbial communities may be useful for the diagnosis of wound healing progresses (by predicting those wounds that will experience infectious complications). Hence, studies in skin microbiota provide an example of interactions between host and microbiomes with biomedical relevance to health issues.
In addition, the gut microbiota has been implicated in intestinal epithelial repair. This is highlighted by recent studies on intestinal wounds where gut microbiota enhanced epithelial wound repair (Alam and Neish, 2018). Specifically, intestinal commensal bacteria have been found to regulate the proliferation, migration, and survival of host epithelial cells, as well as promote barrier function and resolution of epithelial wounds (Hooper et al., 2001; Rakoff-Nahoum et al., 2004; Pull et al., 2005; Lam et al., 2007). One of these commensals is Akkermansia muciniphila, which is enriched in healing mucosal wounds and dominates the wound-mucosa-associated microbiota (Alam et al., 2016). When mice are treated with exogenous A. muciniphila to treat colonic mucosal wounds enhanced mucosal closure occurs. The bacterial treatment stimulates the mice intestinal cellular proliferation and enterocyte migration from the crypt apparently through the generation of ROS when the bacteria colonize the wounded area. The possibility that ROS might be the mediator in this phenomenon is strengthened by experiments with another gut commensal, Lactobacillus rhamnosus. This bacterium has also been associated with intestinal epithelium repair by experiments showing that the sole contact of intestinal epithelial cells (IECs) with L. rhamnosus strain GG (LGG) induces ROS accumulation, consequently stimulating cellular proliferation and migration (Swanson et al., 2011).
Metazoans have different regeneration capabilities. Since mammals are not well know for their regenerative potential, the roles of microbiota in the regeneration of tissues or organs have been focused on particular model organisms. Various species, well known for their regenerative responses, such as planarians, salamanders, and zebrafish have been used to study whether the microbiome can regulate the regeneration potential of their hosts or are directly involved in the regeneration process (Figure 2). Some of these roles will be discussed below.
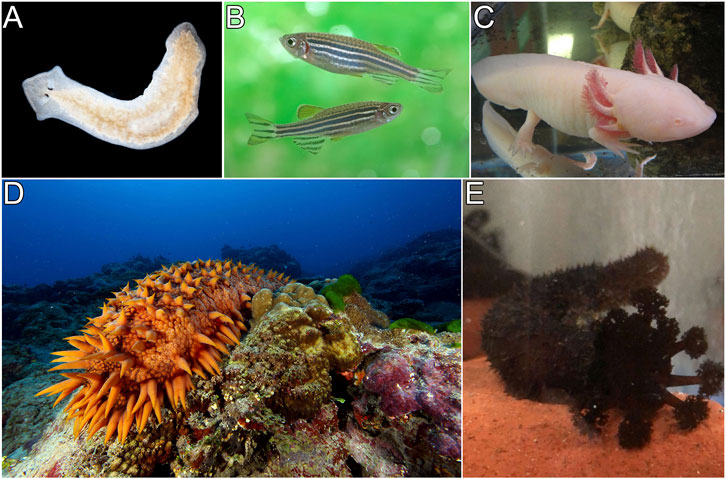
FIGURE 2. Models of regeneration. This figure portrays organisms that are used as regeneration models: planaria (A), zebrafish (B), axolotl (C), and two holothurian species, Apostichopus japonicus (D) and Holothuria glaberrima (E).
Whole body regeneration in planarians- Two studies in the planaria Schmidtea mediterranea have shown that bacteria can influence whole body regeneration. In the first study, the microbiome of healthy planarians was characterized, revealing a high Bacteroidetes to Proteobacteria ratio (Arnold et al., 2016). Animal manipulations such as tissue amputations and changes in culturing conditions (which elicits a relative increase of Proteobacteria) and cultures with a strain of Pseudomonas, produced ectopic lesions and progressive tissue degeneration. Furthermore, infection with the Pseudomonas strain enhanced apoptosis, in contrast to what occurs in the absence of infection where regeneration represses apoptosis. To explain this phenomenon, Arnold et al. suggested that activation of an innate immunity signaling (TAK1/MKK/p38) pathway had an opposite role in host immunity versus normal regeneration. In a second study, a different group studied the impact of bacterial metabolites on the regeneration of planarians (Lee et al., 2018). They described the microbial community of Dugesia japonica, a close relative to S. mediterranea, and inoculated tail and head-amputated antibiotic-treated organisms with representative bacteria species. Lee and colleagues found that regeneration was compromised in animals inoculated with an indole producing bacteria, Aquitalea sp., and tail and head formation was delayed. To test whether the production of indole (which is formed from tryptophan by bacterial enzymatic action) was the causative agent, amputated trunks were incubated with Aquitalea sp. in tryptophan supplemented media. Animals exposed to both tryptophan and indole producing bacteria presented a delayed regeneration in comparison to controls. These experiments demonstrated a direct effect of an indole-producing bacteria on the regenerative properties of planarians.
Limb regeneration in salamanders- A possible association between bacteria and regeneration has also been observed in one of the best studied vertebrate regeneration models, the Mexican axolotl Ambystoma mexicanum (Demircan et al., 2019). This amphibian is capable of regenerating internal organs such as heart, brain, and lungs and external organs such as limbs, gills, and tail (Vieira et al., 2020). In Demircan and colleagues work, a 16S rRNA amplicon dataset was obtained from limbs at different days post amputation (dpa) and correlated with axolotl limb regeneration stages; the stages (0-, 1-, 4-, 7-, 30-, and 60- dpa) (Demircan et al., 2019). Although the study was purely correlative, it showed changes in the microbiota during regeneration, suggesting that certain bacterial groups might be associated with the regenerating tissues. At the phylum level, the bacterial communities in normal animals were dominated by Bacteroidetes, Firmicutes, Proteobacteria, Actinobacteria, and Verrucomicrobia. In regenerating limbs, a temporal shift in bacterial composition was observed, which included differential phylum abundances at certain limb regeneration stages. Post-amputated groups had different microbial communities compared to aquarium control groups, since there was a shift from Firmicutes-enriched (controls) to Proteobacteria-enriched (regenerating) relative abundance. The significant differences observed between the water and the regenerating limb microbiotas suggested selective colonization of axolotl limb tissues and that substantial restructuring of bacterial communities occur in regenerating tissues. Moreover, a comparison of the microbial community demonstrated less variation in the relative abundance of bacterial communities between samples at the same stage of regeneration, and higher variation between groups at different stages. Also, they found differences between limb microbial communities among the regeneration phases: the 0- and 1- dpa samples, 4- and 7- dpa samples, and 30- and 60- dpa samples all differed between them in the measures of beta-diversity. That different bacterial communities were found at specific limb regeneration stages, such as wound healing, dedifferentiation, and re-development, could indicate that specific bacterial groups have specific roles in these processes.
Tissue layer (luminal epithelium regeneration) in vertebrates- Many investigators have studied the regeneration of the luminal epithelial layer (Figure 3A) of the vertebrate digestive tract (see Santos et al., 2018 for review). This tissue layer is continuously being formed as the cells undergo damage by the exposure to the digestive lumen content and the digestive process itself (Barker et al., 2007; Sailaja et al., 2016; Santos et al., 2018). In addition to the ongoing epithelial turnover to achieve gut homeostasis, this tissue can undergo regeneration if injured by exposure to factors such as toxins, radiation or others (Metcalfe et al., 2014; Beumer and Clevers, 2016). Homeostatic maintenance of the luminal epithelium is well understood and has been well described particularly in the mammalian intestine (Barker et al., 2007). The renovation of the layer is dependent on the intestinal stem cells (ISC) and their associated environment (ISC niche). These cells are found within the luminal crypts and give rise to the different cell types in the epithelium. The stem cells divide within the crypts and their progeny continue this division as they transit to the intestinal villi where they differentiate into the intestinal luminal epithelial phenotypes. As cells reach the tip of the intestinal villi, they are shed into the lumen, maintaining a continuous migration of cells from the crypts to the villi.
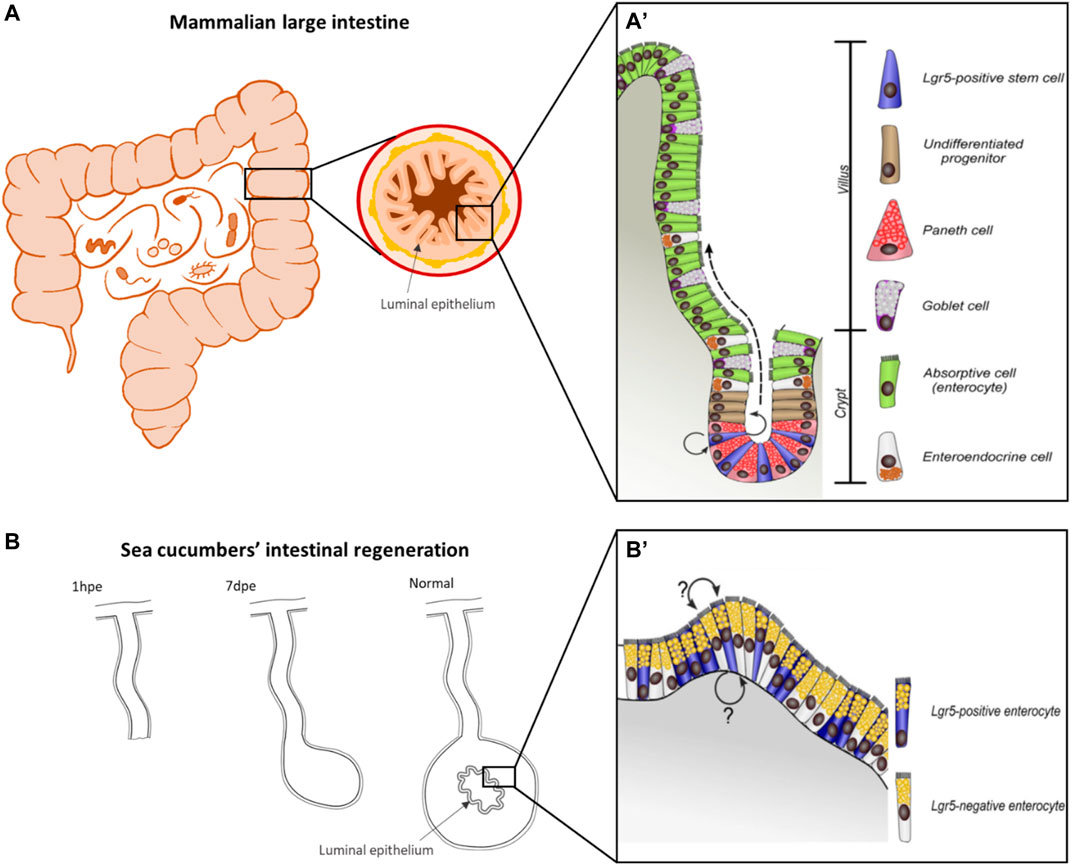
FIGURE 3. Comparison of mammalian and holothurian intestinal epithelium anatomy and renewal. Representative organization of the luminal epithelium of mammal intestine (A) and the mucosal epithelium in the digestive tube of sea cucumbers (B), highlighting the difference in cell renewal mechanisms. (A) In mammals, Lgr5-positive intestinal stem cells are localized at the bottom of the crypt, which self-renew and produce dividing transit-amplifying progenitors (arrows), which gradually migrate apically and form the villus (dashed arrow), where are localized the specialized cells. Paneth cells (which appear to be unique in mammals) are the only differentiated cell type that remains in the stem cell niche. (B) In the digestive epithelium of echinoderms, the spatial organization of mammals is not present, instead Lgr5-positive cells are interspersed among Lgr5-negative or differentiated cells, but the lineage of these cells is not well understood. (A′,B′) were retrieved from Mashanov et al., 2014 and modified by LD-D, (A,B) are drawings by the authors of this article (AR-V and LD-D, respectively) for the purposes of this comparison.
As response to injury, the ISC niche adapts to ensure epithelial regeneration beyond the homeostatic state (Beumer and Clevers, 2016). The epithelial restitution is achieved either by proliferation of active ISCs (Lgr5+ ISCs) or by mature cells dedifferentiated to ISC. This regeneration of mucosal epithelia has been found to be modulated by the microbiota (Thomas, 2016; Hou et al., 2017). Also, the microbiota has been suggested to promote gut healing regeneration through induction of immune responses (Sommer and Bäckhed, 2013; Pellegatta et al., 2016; Thomas, 2016; Hou et al., 2017).
Various experiments demonstrate a similar role of microbiota on luminal epithelium regeneration in zebrafish. For one, in the developing zebrafish intestine, epithelial cell proliferation was shown to be facilitated by their symbiont bacteria, Aeromonas veronii (Cheesman et al., 2011). In other studies, the virulence factor CagA from Helicobacter pylori also promoted intestinal cell proliferation through Wnt pathway signaling (Neal et al., 2013). Lastly, microbiota was also shown to promote intestinal epithelial cell fate determination via the Notch-MyD88 signaling (Bates et al., 2007; Cheesman et al., 2011).
Additional model systems, mainly in vitro models comprising cell cultures, tissue explants, and organoids, have been developed to decipher the microbiota’s influence on the homeostasis and regeneration of mammalian intestines. Among these, organoids have been used to understand the effects that the commensal microbiota, or a particular microorganism, might have on intestinal epithelium homeostasis (Peck et al., 2017; Blutt et al., 2019). Organoids are three-dimensional tissue structures obtained from stem cells in culture, that are differentiated into multiple organ-specific cell types. Thus, cells in these structures acquire some of the organ or tissue organization and functions (Lancaster and Knoblich, 2014). Small intestinal organoids share the cell and structural composition of the small intestinal epithelium, as well as the self-renewal dynamics. (Sato et al., 2009; Sato et al., 2011). Using organoids, it was shown that live Lactobacillus reuteri protected the morphology of intestinal organoids and normal proliferation (Hou et al., 2017; Hou et al., 2018). The protection of the intestinal barrier and activation of intestinal epithelial proliferation seemed to control intestinal inflammation.
A possible mechanism for the bacterial effect was described in a recent work showing that the ISC expresses nucleotide binding oligomerization domain-containing protein 2 (NOD2). This protein interacts with a peptidoglycan motif expressed on most bacterial organisms, suggesting a putative pathway for communication between the microbiome and the ISC niche (Nigro et al., 2014). Treatment of organoids with ligands for NOD2 resulted in an increase in their number and size, indicating that these ligands induce epithelial proliferation. Additional support that bacterial species have a role in the ISC niche comes from studies in mice, where a crypt-specific microbiome has been associated with homeostatic proliferation. This finding led to organoid studies showing that modulation of the colonic epithelial balance between proliferation and differentiation occurred through a TLR4-dependent pathway triggered by bacterial-derived LPS (Naito et al., 2017). Other work showed that colonic crypts from mice devoid of microbiota lose their regenerative capacity, as assessed by the ability to form organoids (Zaborin et al., 2017). There, the regenerative capacity was recovered by fecal microbiota transplantation that restored the crypt microbial communities. Furthermore, in recent studies, lactate derived from bacteria was shown to mediate small intestinal epithelial proliferation through stimulation of the stem cells in murine organoid cultures (Lee et al., 2018), suggesting there may be specific bacteria-derived factors that interact with the host cells to modulate the ISC response. These findings provide strong evidence for a microbiome role in homeostasis of the ISC niche.
Although the day-to-day regeneration of the luminal epithelium has been well studied and has provided important information, as described above, there is a “catch” to these studies that must be addressed. This regeneration is considered to be homeostatic, meaning that it is an ongoing replacement of the lost cells and whose mechanism is deeply embedded within the physiology of the organ in order to maintain its function. Many researchers differentiate this type of regeneration from the one that takes place following injury to the organ or tissue. Available data support the notion that the mechanisms by which homeostatic regeneration takes place differ from the regeneration that follows injury (Beumer and Clevers, 2016). In this respect, the data shown above relates to the microbiota role in homeostatic regeneration and might not apply to the regeneration of the luminal epithelium under injury or to massive loss due to other manipulations.
In an attempt to understand the ongoing interactions within the digestive tract, invertebrates have been used as simplified organisms. The understanding of the impact of gut microbiota on host physiology has been limited, due to restricted in-depth integrated genetic analysis of both the microbes and the host. In this respect, the study of insect non-binary, yet simpler bacterial communities than mammals, is noteworthy. Intestinal bacterial communities of insects have been widely studied, and the amenability of Drosophila melanogaster, allowed its implementation to study animal symbioses. Numerous studies have shown that Drosophila’s bacterial communities are simpler than mammals; hundreds of species are present in humans (Qin, et al., 2010), while the adult Drosophila midgut symbiotic commensal community is composed of 5–20 different microbial species (Corby-Harris et al., 2007; Cox and Gilmore, 2007; Ren et al., 2007; Ryu et al., 2008; Chandler et al., 2011; Wong et al., 2011). Among them, the families of Acetobacteraceae, Lactobacillales, and Enterobacteriaceae are the most prevalent microbes identified in the Drosophila gut microbiota (Ryu et al., 2008; Roh et al., 2008; Chandler, et al., 2011). The simplicity of their microbial communities have made them attractive models for host-microbe studies. Thus, the microbiota effect on intestinal epithelial renewal was studied in Drosophila. A crosstalk between the gut and its microbial community was demonstrated to modulate stress response and promote stem cell proliferation and epithelial regeneration (Buchon et al., 2009). Specifically, the pathogenic bacterium Erwinia carotovora was shown to be important to undergo intestinal epithelial repairs. This result supports the influence of gut microbiota in epithelial healing, as seen in mammal models. However, unlike vertebrate gut, in Drosophila, the intestinal microbiota is composed of either aerotolerant or obligate aerobes, suggesting that oxygen is able to circulate across the Drosophila gut (Chandler et al., 2011; Charroux and Royet, 2012). This provides a limitation when comparing the essential compartmentalization that drives the complex ecosystem in humans and non-human vertebrate bodies.
Leading Studies in Echinoderm Microbial Community
Microbiota has also been associated in echinoderms with other processes important for regeneration such as metabolism and growth. In an early study focused on another echinoderm group, brittle stars, it was suggested that subcuticular and intestinal bacteria could metabolize dissolved organic matter and use it as a significant carbon source (Fielman et al., 1991; Hoskins et al., 2003). These products from microorganisms such as Pseudoalteromonas atlantica are proposed to be important for echinoderm physiology, including regeneration processes. The link between microbiota and nutrient availability has also been studied in sea stars, where the need for symbionts’ assistance to ingest structurally complex polysaccharides or require detoxification of dietary products has been suggested (Douglas, 2009). These organic compounds produced by symbionts are potentially used as energy to promote growth and regeneration (Kelly et al., 1995). In another echinoderm species, the purple sea urchin Strongylocentrotus purpuratus, studies have also suggested that fasting reduces bacteremia in the coelomic fluid and increases spine regeneration (Scholnick and Winslow, 2020).
Organ regeneration in echinoderms- While regeneration of the digestive tract luminal layer has been studied in several model systems, regeneration of the complete intestinal organ has been the focus of work in an understudied group of animals: the Holothuroidea (Echinodermata) (Figure 3B). Several factors make holothurians or sea cucumbers the ideal model system to study the role of the microbiota on regenerative processes. The main one is their ability to eject their digestive tract in a process named evisceration, and to regenerate the entire organ in a period of about a month (Hyman, 1955; Byrne, 2001; Wilkie, 2001; Carnevali, 2006; García-Arrarás et al., 2018). This autotomy, with the subsequent regeneration, provides a unique “natural” model system where the process is part of the animal biology. Moreover, the cellular events that take place during the regeneration of the intestine in these animals have been well studied (García-Arrarás et al., 1998; Quiñones et al., 2002; Mashanov et al., 2005; Candelaria et al., 2006; García-Arrarás et a., 2011; Mashanov and García-Arrarás, 2011; García-Arrarás et al., 2018) and the molecular basis for the regeneration is being actively investigated (Mashanov and García-Arrarás, 2011; García-Arrarás et al., 2018; Quispe-Parra et al., 2021). Echinoderms, being basal deuterostomes, occupy a key branch together with the chordate evolutionary tree, while at the same time are close to most other invertebrates. Moreover, the digestive tube is one of the best conserved organs, common to most metazoans. Thus, these animals can provide useful evolutionary insights into microbiome-host associations. Probably unknown to many, sea cucumbers also have a huge economic value, as part of an aquaculture industry centered in Asia. Thus, the microbiota-host relationships of these animals extend beyond the regenerative process and are studied in terms of health, growth, and other issues related to their nutritional value.
Comparison of Microbiota Structure Among Sea Cucumber Species
To study the role of the microbiota in intestinal regeneration, we need to first determine the components of the microbiota of our model organisms. Holothurians, as documented in all echinoderms studied to date, have a microbial diversity that is both relatively low and dominated by Proteobacteria. This has been shown in the sub-cuticle of the brittle stars Ophiactis balli and Amphipholis squamate (Burnett and McKenzie, 1997; Morrow et al., 2018), in the body wall, gonads, pyloric caeca, and coelomic fluid of multiple sea star species (Jackson et al., 2018), in the coelomic fluid, intestines, pharynx, and gut digesta of the sea urchin Lytechinus variegatus (Hakim et al., 2015; Hakim et al., 2016; Brothers et al., 2018) and in the intestine of the sea cucumbers Apostichopus japonicus and Holothuria glaberrima (Gao et al., 2014a; Gao et al., 2014b; Pagán-Jiménez et al., 2019).
The gut commensal microbes of sea cucumbers have been a focus of study during the last decade. The intestinal microbiota of three sea cucumber species: A. japonicus, H. glaberrima, and Sclerodactyla briareus have been described using 16S rRNA gene amplicon sequencing (Gao et al., 2014a; Gao et al., 2014b; Wang et al., 2018; Pagán-Jiménez et al., 2019; Weigel, 2020). Though the Proteobacteria and Bacteroidetes are among the most abundant phyla in all sea cucumber species (Figure 4), a difference in relative representation is seen among different species. Proteobacteria was the predominant phylum within the gut of the holothurian A. japonicus, while Gammaproteobacteria was the predominant bacterial class (Gao et al., 2014a; Gao et al., 2014b). A recent study in S. briareus supported these findings (Weigel, 2020). In the latter work, the taxonomic representation in the stomach and intestine from animals that were collected from different ponds or aquaria were evaluated and found that the mature intestine microbiota was composed primarily of Proteobacteria. In contrast, our group found that in the intestine of H. glaberrima, Firmicutes was the dominant phylum followed by Bacteroidetes, and then Proteobacteria (Pagán-Jiménez et al., 2019). The higher abundance of Firmicutes in H. glaberrima may be a key difference with other holothurians, however microbiota differences among holothurians are probably determined by the differences in habitat and/or feeding behaviors (Table 2).
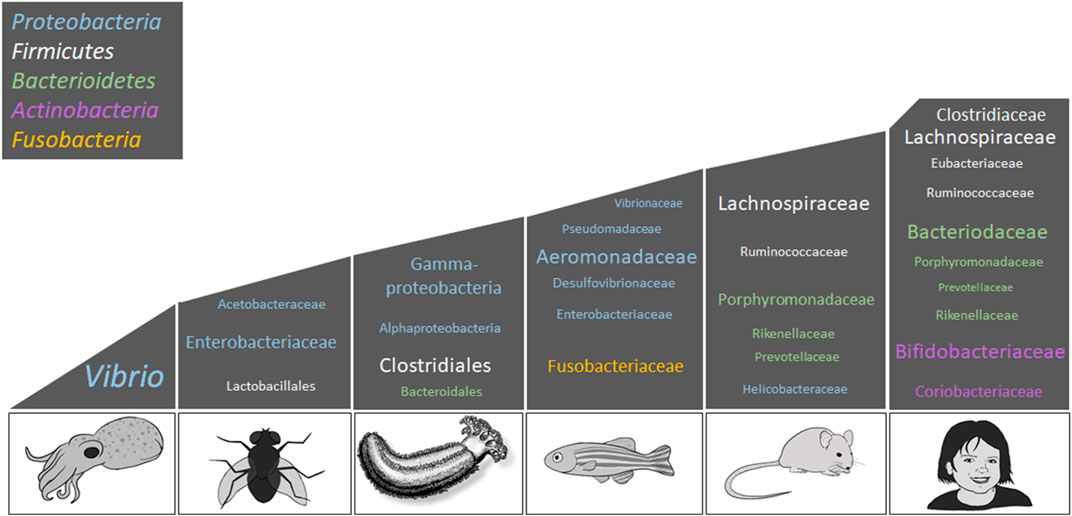
FIGURE 4. Bacterial composition associated with animal hosts. This scheme presents the most representative taxa among the microbiota of E. scolopes, D. melanogaster, H. glaberrima, D. rerio, M. musculus, and H. sapiens; however, relative representation of these taxa may vary per individual. Top phyla among the animal kingdom includes Proteobacteria (blue), Firmicutes (white), Bacteroidetes (green), Actinobacteria (lilac), and Fusobacteria (yellow). The font size represents the relative abundance of the lower taxonomic levels. This figure is an adaptation of Kostic et al. (2013), and contains information from the following studies: Arumugam et al. (2011), Brinkman et al. (2011), Chandler et al. (2011), Roeselers et al. (2011), and Pagán-Jiménez et al. (2019). Images are original drawings by LD-D and AR-V.
Apart from differences among the gut microbiota of various species, discrepancies in the microbiota between areas of the gut have been observed. Some of these differences are seen in the relative abundances of the microbial community in various segments of the digestive tract. In H. glaberrima dissected in situ (as soon as collected from the intertidal space), both areas of the small intestine (comprised of anterior and the medial) showed a similar microbiota, composed mostly by members of the phylum Proteobacteria (Pagán-Jiménez et al., 2019). However, the large (posterior) intestine contained mostly Firmicutes. Beta analysis supported these results, revealing that the anterior, medial, and posterior intestine samples had significantly different microbial communities. In addition, differences between environmental microbiota and gut microbiota were documented. Both seawater microbial communities (collected in situ and the aquarium water) were more similar to the communities of the anterior and medial intestine, than that of the posterior intestine. These data suggested a distinctive microbiota in the large intestine. In species where the digestive tract includes a stomach, different bacterial communities were found between the stomach and the intestine (Weigel, 2020). Similarly, different microbiotas were found between the water and the internal organs. Thus, all published studies of sea cucumbers microbiota show significant differences between the seawater and the intestinal communities, and differences among the digestive tract structures themselves (Gao et al., 2014a; Gao et al., 2014b; León-Palmero et al., 2018; Pagan-Jimenez et al., 2019; Weigel, 2020). This contrast between marine animal organs and seawater microbial communities was found in other organisms including corals, sea urchins, sea stars and sea anemones (Ainsworth et al., 2015; Brothers et al., 2018; Jackson et al., 2018; León-Palmero et al., 2018).
It is imperative to mention that the experimental design for studies of the microbial communities in holothurians, including the dissections and tissue collections varied among the different studies. In some, the viscera were processed individually, while in others the intestine was not separated from the cloaca. Thus, in Table 2 we summarized the similarities and contrast of holothurian microbiota studies.
Examining the Microbe-Echinoderm Associations
In A. japonicus, the link between microbial diversity and animal growth has been examined (Yamazaki et al., 2016). The metagenomes of feces of large and small sea cucumbers were sequenced, showing that larger and smaller animals had different microbiota, and that while the alpha diversity was similar, the relative abundance differed. The orders Rhodobacterales, Oceanospirillales, and Desulfobacterales were more abundant in larger animals. The long-term effects, in terms of growth and disease resistance, of disrupting the bacterial community of A. japonicus sea cucumbers by using antibiotics was also explored (Zhao et al., 2019). Interestingly, after administering different antibiotics (tetracycline, erythromycin, or norfloxacin), it was observed that some antibiotics increased the growth of the animals yet weakened their immunological system. In a different study, Yamazaki and colleagues found that Rhodobacterales are the third most abundant order in the fecal microbiota of A. japonicus, and the relative abundances were significantly higher in larger animals than in smaller individuals (Yamazaki, et al., 2016). However, the subsequent article by Zhao and colleagues reported a decreased relative abundance of Rhodobacteraceae in A. japonicus juveniles when treated with either tetracycline or erythromycin, but an increase in sea cucumber survival and body weight (Zhao et al., 2019). The above summarized studies demonstrate how bacteria metabolic activity might play a key role in providing the energy source to hosts to facilitate or activate their cellular and molecular process.
Is the Echinoderm’s Regenerative Capacity Influenced by the Microbiota?
Two types of studies explore if the microbiome influences the intestinal regeneration of holothurians. The first group of studies focuses on correlating changes in the microbiome with different stages of intestinal regeneration. The regenerating gut microbiome of A. japonicus was characterized, showing that Proteobacteria (Wang et al., 2018) or Actinobacteria (Zhang et al., 2020) were the dominant phyla from wound healing stage to lumen formation (early in their regeneration process), and at later stages of regeneration, Proteobacteria and Bacteroidetes became the dominant phyla (Wang et al., 2018; Zhang et al., 2020). This change suggested that during early stages of the regeneration process, the gut was populated mostly by the bacteria from the sediments and water, and then was gradually replaced by digestive-associated microbiota.
An expanded analysis of regenerating intestine microbiota in a different holothurian species, S. briareus, documented higher richness (on day 20 after evisceration) and evenness (on day 13–20 after evisceration), when compared to mature intestines (Weigel 2020). Moreover, an Alphaproteobacteria species abundant in mature intestine samples was not found in regenerating intestines. Regenerating stomachs were found to be more diverse in comparison to mature ones. Interestingly, beta-analysis plots showed that regenerating stomach and regenerating intestine were similar. Taxonomic representation and alpha diversity analysis revealed that the regeneration process was associated with a change in microbial community that recovered at the end of the regeneration process. In addition, tank residence, but not collection site, were suggested to affect gut microbial community, however changes in the regenerating microbes were not simply due to tank effects.
It is important to highlight these studies because they propose a correlation between the gut microbiota and the regeneration process. However, as mentioned before, these findings were shown mainly by using functional inferences from genomic data which do not strongly establish that the microbial community causes a particular effect ruling the intestinal regeneration associated events. For example, genomic data cannot distinguish if the organisms found in this community were even alive or if they were transient (ingested debris or indiscriminate colonization). Thus, the future of this field is beyond correlative analysis, and it requires experimentation that delves directly into the microbial community influence and if its modulation alters the effects on host’s regeneration.
The second type of study, which precisely examined the role of the microbiome in holothurian gut regeneration, was recently published by our group (Díaz-Díaz, et al., 2021). Here, different antibiotic cocktails were used to cause dysbiosis and study the influence of the commensal community in the intestinal regeneration process. We observed that antibiotic treatments altered cellular processes associated with regeneration such as cellular dedifferentiation, extracellular matrix remodeling, and cell proliferation. To rule out that the antibiotics were exerting a direct effect on the holothurian tissues, we performed MTT assays on dissociated cells and explant cultures. Ex vivo experiments suggested that the antibiotics used did not directly alter the holothurian tissue metabolic activity, while being capable of inhibiting gut bacterial populations in vitro. Therefore, we proposed that the antibiotics are influencing H. glaberrima regeneration via the dysbiosis of the gut microbiota. Moreover, because H. glaberrima microbiota is mainly composed of Firmicutes (mostly Gram-positive bacteria) and Proteobacteria (mostly Gram-negative bacteria) (Pagán-Jiménez et al., 2019), and the cocktails targeting mostly the Gram-positive bacteria had the most detrimental effects over the intestinal regeneration, we suggest that Firmicutes may have a crucial role in the progression of the intestinal regeneration. Antibiotics have also been shown to have long-term effects on holothurian growth and disease resistance. In an experiment where antibiotics (tetracycline, erythromycin, or norfloxacin) were administered to disrupt the bacterial community, some antibiotics increased the growth of sea cucumbers, yet appear to inhibit the animal immune system (Zhao et al., 2019).
Furthermore, the role of the microbiota during regeneration could be addressed using other echinoderms. The crinoids, which are well known for their potential to regenerate their arms, can also lose and renew their entire digestive system (Dendy, 1886; Meyer, 1985; Meyer, 1988; Mozzi et al., 2006; Bobrovskaya and Dolmatov, 2014; Kalacheva et al., 2017). These studies describe the fast visceral regeneration potential in crinoids, such as Antedon mediterranea, Antedon rosaceus, Himerometra robustipinna and Lamprometra palmata, through histological and cytological analysis but were neglected for many years. We propose that echinoderms are promising models to elucidate if, and how, the regeneration events in the digestive system are influenced by the gut microbiota. Moreover, these organisms provide models whose findings on the whole organ regrowth are not limited solely to the study of the repair of the luminal epithelium layer of the intestine.
Nevertheless, we acknowledge that these models have some disadvantages. First, one deficiency for many of the echinoderms members is the lack of genomic and metagenomic data available. Second, as they are marine invertebrates, the structure and function of their microbiota might be very distinctive, in comparison to humans; colonized by species that are not observed in terrestrial vertebrates.
Conclusion
Microbiota effects on regenerating tissues are just beginning to be investigated. The initial findings strongly suggest that, indeed, bacterial species composition is an important factor in the timing and effectiveness of the regenerative process. However, most of the available data is correlative and needs to be backed by functional studies. These correlative studies on microbial successions and the regeneration process do not demonstrate a causal effect on the intestinal regeneration exerted by the gut microbiota. Nonetheless they do provide some evidence that supports the hypothesis that the microbiota may be influencing regenerative events.
The challenge for future investigations is to identify the specific roles of the microbiota and the signaling pathways or physiological processes by which they might modulate regeneration. Central to this issue is the use of appropriate model systems in which to decipher the specifics of the microbe associations. We consider that in vivo examinations where the use of agents that modulate the microbiota, such as prebiotic, probiotic or antibiotics, will be crucial to understand the role of these microorganisms during gut repair mechanisms. Here, we have described various promising echinoderm models to decipher the role of the microbiota during intestinal regeneration, that encompasses the whole organ formation beyond the luminal epithelium repair and homeostasis. We propose that this need may be fulfilled in part by the sea cucumber intestinal regeneration model. The fact that the regenerating organ is a structure present in most metazoans and is the one organ where most microbiome studies have been made, makes this model particularly attractive to study host-microbiome interactions. Thus, we expect that studies with holothurians will provide groundbreaking knowledge on the field of microbiome-host associations and their impact on regenerative processes. However, this model also has some limitations. Among them, the need to improve the molecular tools available to study the specific functions of certain genes as well as the present limitations on identifying and characterizing many bacteria (and other components of the microbiota) that are difficult or impossible to grow in the laboratory. Nonetheless, we believe that comparative studies using the sea cucumber, as well as other models, will be transformative in defining the interactions of host-microbiome in regenerative processes.
Author Contributions
LD-D and AR-V acquisition, analysis or interpretation of data for the work; LD-D and AR-V drafting the work, LD-D, AR-V, and JG-A revision and edition.
Funding
This research was funded by the National Science Foundation grant (NSFIOS-2100494) and the Puerto Rico Science, Technology and Research Trust (PRSTRT) grant 20270.001.000.XXXX.220.206350070017.00. LD-D and AR-V were funded by Research Training Initiative for Student Enhancement (RISE) program grant 5R25GM061151.
Conflict of Interest
The authors declare that the research was conducted in the absence of any commercial or financial relationships that could be construed as a potential conflict of interest.
Publisher’s Note
All claims expressed in this article are solely those of the authors and do not necessarily represent those of their affiliated organizations, or those of the publisher, the editors and the reviewers. Any product that may be evaluated in this article, or claim that may be made by its manufacturer, is not guaranteed or endorsed by the publisher.
Acknowledgments
We would like to acknowledge the people who kindly allow us to use their pictures in this review: Figure 2A: planaria’s picture by Sr. Waldo Nell (retrieved form: https://www.flickr.com/photos/pwnell/33110930070/in/photolist-SrUaWE-7Tb3yC-dYwSwt-7e1Z8C-pydNx-DXtNWx-eR3dS4-2faoBGD-TS8NDw-ar6gCS-98Gf7j-2iUc5S-RBf6ic-2kErabH-cCvnr1-2ivr1Lb-2kErG19-eF21pi-2iPML2-2iUcio-T6b7c9-2kFfwPJ-2gieQcL-q4sX2j-rTbgJN-oY7DDk-LkhrbL-cwHeq9-7R8JV5-9MuHFM-9MuFUB-9Mxqe7-QYfTcJ-8QVidv-7R5sQV-q7qYC4-ppgA8R-qkXUUU-2kFbUJR-pp341C-bUQucV-uAw1fQ-rms2vX-5heWp1-nmZhDm-2f52EPc-5tH2Yj-azz8F1-eN3vNh-eiA7TD), Figure 2B: Adult zebrafish (Danio rerio) of AB strain. Top: female, bottom: male. Photography and postwork by Tohru Murakami, Gunma University Graduate School of Medicine, Japan (retrieved from: https://www.flickr.com/photos/8659392@N07/13896905021/in/dateposted-public/), Figure 2C: Axolotl photographed by David Shane, professor of Physics, Lansing Community College (retrieved from: https://www.flickr.com/photos/david_shane/6151631980/in/photolist-eSE6YY-2RxHYB-anAHSm-6owNsm-6owN3Q-5aUjGL-DUgzM-5xwbz9-RR7cMG-6k8wXU-cjsomA-8XHiw2-JSVwLA-b1cPhH-acM7Qx-22gq9BQ-jKo6Ag-5ejWZG-7W814u-b5BZ6a-Aw3CAA-dbFVVr-ga3Q52-PbKTj-o8fRou-9Gxggp-PbKSN-6L58D3-6qUJW3-9pG7Ro-8rLdzs-Przrow-NAGBT-JXMBsF-9g9tAJ-Ktf9Y7-4w5Kxe-4tdERU-521zsc-4eXZ48-4UNP9a-bfuKNB-5RY3qa-PbKT9-PbKSQ-aq317b-9KW5UH-23WC4PS-7dtcVs-7zCNbM). Figure 2D was assessed from NOAA Office of National Marine Sanctuaries (Public Domain, retrieved from https://www.flickr.com/photos/onms/27984972905/in/photolist-2jngRaw-a8nsFL-a8nsBd-JCWisR-2hJt2YU-fZaNi-at6gX5-GniC3z-2hjNEaC-29Py1gc). Figure 2E was taken by one of the authors of this review (LD-D).
References
Abrams, G. D., Bauer, H., and Sprinz, H. (1963). Influence of the normal flora on Mucosal Morphology and Cellular Renewal in the Ileum. A Comparison of Germ-free and Conventional Mice. Lab. Invest. 12, 355–364.
Ainsworth, T. D., Krause, L., Bridge, T., Torda, G., Raina, J.-B., Zakrzewski, M., et al. (2015). The Coral Core Microbiome Identifies Rare Bacterial Taxa as Ubiquitous Endosymbionts. Isme J. 9, 2261–2274. doi:10.1038/ismej.2015.39
Akira, S., and Takeda, K. (2004). Toll-like Receptor Signalling. Nat. Rev. Immunol. 4, 499–511. doi:10.1038/nri1391
Alam, A., Leoni, G., Quiros, M., Wu, H., Desai, C., Nishio, H., et al. (2016). The Microenvironment of Injured Murine Gut Elicits a Local Pro-restitutive Microbiota. Nat. Microbiol. 1, 15021. doi:10.1038/nmicrobiol.2015.21
Alam, A., and Neish, A. (2018). Role of Gut Microbiota in Intestinal Wound Healing and Barrier Function. Tissue Barriers 6, e1539595. doi:10.1080/21688370.2018.1539595
Ammons, M. C. B., Morrissey, K., Tripet, B. P., Van Leuven, J. T., Han, A., Lazarus, G. S., et al. (2015). Biochemical Association of Metabolic Profile and Microbiome in Chronic Pressure Ulcer Wounds. PLoS ONE 10, e0126735. doi:10.1371/journal.pone.0126735
Arnold, C. P., Merryman, M. S., Harris-Arnold, A., McKinney, S. A., Seidel, C. W., Loethen, S., et al. (2016). Pathogenic Shifts in Endogenous Microbiota Impede Tissue Regeneration via Distinct Activation of TAK1/MKK/P38. eLife 5, e16793. doi:10.7554/eLife.16793
Arumugam, M., Raes, J., Raes, J., Pelletier, E., Le Paslier, D., Yamada, T., et al. MetaHIT Consortium (2011). Enterotypes of the Human Gut Microbiome. Nature 473, 174–180. doi:10.1038/nature09944
Arvizu, F., Aguilera, A., and Salgado, L. M. (2006). Activities of the Protein Kinases STK, PI3K, MEK, and ERK Are Required for the Development of the Head Organizer in Hydra Magnipapillata. Differentiation 74 (6), 305–312. doi:10.1111/j.1432-0436.2006.00078.x
Atarashi, K., Nishimura, J., Shima, T., Umesaki, Y., Yamamoto, M., Onoue, M., et al. (2008). ATP Drives Lamina Propria TH17 Cell Differentiation. Nature 455, 808–812. doi:10.1038/nature07240
Bäckhed, F., and Crawford, P. A. (2010). Coordinated Regulation of the Metabolome and Lipidome at the Host-Microbial Interface. Biochim. Biophys. Acta (Bba) - Mol. Cel Biol. Lipids 1801 (3), 240–245. doi:10.1016/j.bbalip.2009.09.009
Barker, N., van Es, J. H., Kuipers, J., Kujala, P., van den Born, M., Cozijnsen, M., et al. (2007). Identification of Stem Cells in Small Intestine and colon by Marker Gene Lgr5. Nature 449 (7165), 1003–1007. doi:10.1038/nature06196
Bartow-McKenney, C., Hannigan, G. D., Horwinski, J., Hesketh, P., Horan, A. D., Mehta, S., et al. (2018). The Microbiota of Traumatic, Open Fracture Wounds Is Associated with Mechanism of Injury. Wound Rep. Reg. 26 (2), 127–135. doi:10.1111/wrr.12642
Bates, J. M., Akerlund, J., Mittge, E., and Guillemin, K. (2007). Intestinal Alkaline Phosphatase Detoxifies Lipopolysaccharide and Prevents Inflammation in Zebrafish in Response to the Gut Microbiota. Cell Host & Microbe 2 (6), 371–382. doi:10.1016/j.chom.2007.10.010
Beumer, J., and Clevers, H. (2016). Regulation and Plasticity of Intestinal Stem Cells during Homeostasis and Regeneration. Development 143 (20), 3639–3649. doi:10.1242/dev.133132
Blutt, S. E., Klein, O. D., Donowitz, M., Shroyer, N., Guha, C., and Estes, M. K. (2019). Use of Organoids to Study Regenerative Responses to Intestinal Damage. Am. J. Physiology-Gastrointestinal Liver Physiol. 317 (6), G845–G852. doi:10.1152/ajpgi.00346.2018
Bobrovskaya, N. V., and Dolmatov, I. Y. (2014). Autotomy of the Visceral Mass in the Feather star Himerometra Robustipinna (Crinoidea, Comatulida). Biol. Bull. 226, 81–91. doi:10.1086/BBLv226n2p81
Boettcher, K. J., and Ruby, E. G. (1990). Depressed Light Emission by Symbiotic Vibrio Fischeri of the Sepiolid Squid Euprymna scolopes. J. Bacteriol. 172, 3701–3706. Accessed January 19, 2013, Available at: http://www.ncbi.nlm.nih.gov/pmc/articles/PMC213346/pdf/jbacter00121-0177.pdf. doi:10.1128/jb.172.7.3701-3706.1990
Bose, J. L., Rosenberg, C. S., and Stabb, E. V. (2008). Effects of luxCDABEG Induction in Vibrio Fischeri: Enhancement of Symbiotic Colonization and Conditional Attenuation of Growth in Culture. Arch. Microbiol. 190, 169–183. doi:10.1007/s00203-008-0387-1
Bouskra, D., Brézillon, C., Bérard, M., Werts, C., Varona, R., Boneca, I. G., et al. (2008). Lymphoid Tissue Genesis Induced by Commensals through NOD1 Regulates Intestinal Homeostasis. Nature 456 (7221), 507–510. doi:10.1038/nature07450
Brinkman, B. M., Hildebrand, F., Kubica, M., Goosens, D., Del Favero, J., Declercq, W., et al. (2011). Caspase Deficiency Alters the Murine Gut Microbiome. Cell Death Dis 2 (10), e220. doi:10.1038/cddis.2011.101
Brothers, C. J., van der Pol, W. J., Morrow, C. D., Hakim, J. A., Koo, H., and McClintock, J. B. (2018). Ocean Warming Alters Predicted Microbiome Functionality in a Common Sea Urchin. Proc. R. Soc. B. 285, 20180340. doi:10.1098/rspb.2018.0340
Bryant, D. M., Sousounis, K., Payzin-Dogru, D., Bryant, S., Sandoval, A. G. W., Martinez Fernandez, J., et al. (2017). Identification of Regenerative Roadblocks via Repeat Deployment of Limb Regeneration in Axolotls. Npj Regen. Med. 2 (1), 30. doi:10.1038/s41536-017-0034-z
Buchon, N., Broderick, N. A., Poidevin, M., Pradervand, S., and Lemaitre, B. (2009). Drosophila Intestinal Response to Bacterial Infection: Activation of Host Defense and Stem Cell Proliferation. Cell Host & Microbe 5, 200–211. doi:10.1016/j.chom.2009.01.003
Burnett, W. J., and McKenzie, J. D. (1997). Subcuticular Bacteria from the Brittle star Ophiactis Balli (Echinodermata: Ophiuroidea) Represent a New Lineage of Extracellular marine Symbionts in the Alpha Subdivision of the Class Proteobacteria. Appl. Environ. Microbiol. 63 (5), 1721–1724. doi:10.1128/aem.63.5.1721-1724.1997
Byndloss, M. X., Olsan, E. E., Rivera-Chávez, F., Tiffany, C. R., Cevallos, S. A., Lokken, K. L., et al. (2017). Microbiota-activated PPAR-γ Signaling Inhibits Dysbiotic Enterobacteriaceae Expansion. Science 357 (6351), 570–575. doi:10.1126/science.aam10.1126/science.aam9949
Byrne, M. (2001). The Morphology of Autotomy Structures in the Sea Cucumber Eupentacta Quinquesemita before and during Evisceration. J. Exp. Biol. 204, 849–863. doi:10.1242/jeb.204.5.849
Candelaria, A. G., Murray, G., File, S. K., and García-Arrarás, J. E. (2006). Contribution of Mesenterial Muscle Dedifferentiation to Intestine Regeneration in the Sea Cucumber Holothuria Glaberrima. Cell Tissue Res 325 (1), 55–65. doi:10.1007/s00441-006-0170-z
Carnevali, M. D. C. (2006). Regeneration in Echinoderms: Repair, Regrowth, Cloning. ISJ 3, 64–76. https://www.isj.unimore.it/index.php/ISJ/article/view/124.
Cebra, J. J., Periwal, S. B., Lee, G., Lee, F., and Shroff, K. E. (1998). Development and Maintenance of the Gut-Associated Lymphoid Tissue (GALT): the Roles of Enteric Bacteria and Viruses. Develop. Immunol. 6, 13–18. doi:10.1155/1998/68382
Chandler, J. A., Morgan Lang, J., Bhatnagar, S., Eisen, J. A., and Kopp, A. (2011). Bacterial Communities of Diverse Drosophila Species: Ecological Context of a Host-Microbe Model System. Plos Genet. 7, e1002272. doi:10.1371/journal.pgen.1002272
Charroux, B., and Royet, J. (2012). Gut-microbiota Interactions in Non-mammals: what Can We Learn from Drosophila? Semin. Immunol. 24 (1), 17–24. doi:10.1016/j.smim.2011.11.003
Cheesman, S. E., Neal, J. T., Mittge, E., Seredick, B. M., and Guillemin, K. (2011). Epithelial Cell Proliferation in the Developing Zebrafish Intestine Is Regulated by the Wnt Pathway and Microbial Signaling via Myd88. Proc. Natl. Acad. Sci. 108, 4570–4577. doi:10.1073/pnas.1000072107
Corby-Harris, V., Pontaroli, A. C., Shimkets, L. J., Bennetzen, J. L., Habel, K. E., and Promislow, D. E. L. (2007). Geographical Distribution and Diversity of Bacteria Associated with Natural Populations of Drosophila melanogaster. Appl. Environ. Microbiol. 73, 3470–3479. doi:10.1128/aem.02120-06
Cox, C. R., and Gilmore, M. S. (2007). Native Microbial Colonization of Drosophila melanogaster and its Use as a Model of Enterococcus faecalis Pathogenesis. Infect. Immun. 75, 1565–1576. doi:10.1128/iai.01496-06
Davidson, S. K., Koropatnick, T. A., Kossmehl, R., Sycuro, L., and McFall-Ngai, M. J. (2004). NO Means 'yes' in the Squid-vibrio Symbiosis: Nitric Oxide (NO) during the Initial Stages of a Beneficial Association. Cell Microbiol 6 (12), 1139–1151. doi:10.1111/j.1462-5822.2004.00429.x
De Schryver, P., Sinha, A. K., Kunwar, P. S., Baruah, K., Verstraete, W., Boon, N., et al. (2010). Poly-β-hydroxybutyrate (PHB) Increases Growth Performance and Intestinal Bacterial Range-Weighted Richness in Juvenile European Sea Bass, Dicentrarchus labrax. Appl. Microbiol. Biotechnol. 86, 1535–1541. doi:10.1007/s00253-009-2414-9
De Vadder, F., Kovatcheva-Datchary, P., Goncalves, D., Vinera, J., Zitoun, C., Duchampt, A., et al. (2014). Microbiota-generated Metabolites Promote Metabolic Benefits via Gut-Brain Neural Circuits. Cell 156 (1-2), 84–96. doi:10.1016/j.cell.2013.12.016
Demircan, T., İlhan, A. E., Ovezmyradov, G., Öztürk, G., and Yıldırım, S. (2019). Longitudinal 16S rRNA Data Derived from Limb Regenerative Tissue Samples of Axolotl Ambystoma mexicanum. Sci. Data 6 (1), 70. doi:10.1038/s41597-019-0077-7
Dendy, A. (1886). On the Regeneration of the Visceral Mass in Antedon Rosaceus. Stud. Biol. laboratories Owens Coll. 1, 299–312.
Díaz-Díaz, L. M., Rosario-Meléndez, N., Rodríguez-Villafañe, A., Figueroa-Vega, Y. Y., Pérez-Villafañe, O. A., Colón-Cruz, A. M., et al. (2021). Antibiotics Modulate Intestinal Regeneration. Biology 10 (3), 236. doi:10.3390/biology10030236
Doino, J. A., and McFall-Ngai, M. J. (1995). A Transient Exposure to Symbiosis-Competent Bacteria Induces Light Organ Morphogenesis in the Host Squid. Biol. Bull. 189, 347–355. doi:10.2307/1542152
Douglas, A. E. (2009). The Microbial Dimension in Insect Nutritional Ecology. Funct. Ecol. 23, 38–47. doi:10.1111/j.1365-2435.2008.01442.x
Dowd, S. E., Sun, Y., Secor, P. R., Rhoads, D. D., Wolcott, B. M., James, G. A., et al. (2008). Survey of Bacterial Diversity in Chronic Wounds Using Pyrosequencing, DGGE, and Full Ribosome Shotgun Sequencing. BMC Microbiol. 8, 43. doi:10.1186/1471-2180-8-43
Falk, P. G., Hooper, L. V., Midtvedt, T., and Gordon, J. I. (1998). Creating and Maintaining the Gastrointestinal Ecosystem: what We Know and Need to Know from Gnotobiology. Microbiol. Mol. Biol. Rev. 62, 1157–1170. doi:10.1128/MMBR.62.4.1157-1170.1998
Fielman, K. T., Stancyk, S. E., Dobson, W. E., and Jerome Clements, L. A. (1991). Effects of Disc and Arm Loss on Regeneration byMicrophiopholis Gracillima (Echinodermata: Ophiuroidea) in Nutrient-free Seawater. Mar. Biol. 111, 121–127. doi:10.1007/bf01986353
Fraune, S., and Bosch, T. C. G. (2010). Why Bacteria Matter in Animal Development and Evolution. Bioessays 32 (7), 571–580. doi:10.1002/bies.200900192
Frost, G., Sleeth, M. L., Sahuri-Arisoylu, M., Lizarbe, B., Cerdan, S., Brody, L., et al. (2014). The Short-Chain Fatty Acid Acetate Reduces Appetite via a central Homeostatic Mechanism. Nat. Commun. 5, 3611. doi:10.1038/ncomms4611
Furusawa, Y., Obata, Y., Fukuda, S., Endo, T. A., Nakato, G., Takahashi, D., et al. (2013). Commensal Microbe-Derived Butyrate Induces the Differentiation of Colonic Regulatory T Cells. Nature 504, 446–450. doi:10.1038/nature12721
Gaboriau-Routhiau, V., Rakotobe, S., Lécuyer, E., Mulder, I., Lan, A., Bridonneau, C., et al. (2009). The Key Role of Segmented Filamentous Bacteria in the Coordinated Maturation of Gut Helper T Cell Responses. Immunity 31 (4), 677–689. doi:10.1016/j.immuni.2009.08.020
Gao, F., Li, F., Tan, J., Yan, J., and Sun, H. (2014a). Bacterial Community Composition in the Gut Content and Ambient Sediment of Sea Cucumber Apostichopus Japonicus Revealed by 16S rRNA Gene Pyrosequencing. PLoS ONE 9, e100092. doi:10.1371/journal.pone.0100092
Gao, F., Tan, J., Sun, H., and Yan, J. (2014b). Bacterial Diversity of Gut Content in Sea Cucumber (Apostichopus Japonicus) and its Habitat Surface Sediment. J. Ocean Univ. China 13, 303–310. doi:10.1007/s11802-014-2078-7
Garcia-Arraras, J., and Dolmatov, I. (2010). Echinoderms: Potential Model Systems for Studies on Muscle Regeneration. Cpd 16 (8), 942–955. doi:10.2174/138161210790883426
García-Arrarás, J. E., Estrada-Rodgers, L., Santiago, R., Torres, I. I., Díaz-Miranda, L., and Torres-Avillán, I. (1998). Cellular Mechanisms of Intestine Regeneration in the Sea Cucumber, Holothuria Glaberrima Selenka (Holothuroidea:Echinodermata). J. Exp. Zool 281, 288–304. doi:10.1002/(sici)1097-010x(19980701)281:4<288::aid-jez5>3.0.co;2-k
García-Arrarás, J. E., Lázaro-Peña, M. I., and Díaz-Balzac, C. A. (2018). Holothurians as a Model System to Study Regeneration. Results Probl. Cel Differ 65, 255–283. doi:10.1007/978-3-319-92486-1_13
García-Arrarás, J. E., Valentín-Tirado, G., Flores, J. E., Rosa, R. J., Rivera-Cruz, A., San Miguel-Ruiz, J. E., et al. (2011). Cell Dedifferentiation and Epithelial to Mesenchymal Transitions during Intestinal Regeneration in H. Glaberrima. BMC Develop. Biol. 11, 61. doi:10.1186/1471-213X-11-61
Gardiner, M., Vicaretti, M., Sparks, J., Bansal, S., Bush, S., Liu, M., et al. (2017). A Longitudinal Study of the Diabetic Skin and Wound Microbiome. PeerJ 5, e3543. doi:10.7717/peerj.3543
Gardner, S. E., Hillis, S. L., Heilmann, K., Segre, J. A., and Grice, E. A. (2013). The Neuropathic Diabetic Foot Ulcer Microbiome Is Associated with Clinical Factors. Diabetes 62 (3), 923–930. doi:10.2337/db12-0771
Gijzen, H. J., and Barugahare, M. (1992). Contribution of Anaerobic Protozoa and Methanogens to Hindgut Metabolic Activities of the American Cockroach, Periplaneta americana. Appl. Environ. Microbiol. 58 (8), 2565–2570. doi:10.1128/aem.58.8.2565-2570.1992
Gill, S. R., Pop, M., Deboy, R. T., Eckburg, P. B., Turnbaugh, P. J., Samuel, B. S., et al. (2006). Metagenomic Analysis of the Human Distal Gut Microbiome. Science 312 (5778), 1355–1359. doi:10.1126/science.1124234
Guo, S., and Dipietro, L. A. (2010). Factors Affecting Wound Healing. J. Dent Res. 89 (3), 219–229. doi:10.1177/0022034509359125
Ha, E.-M., Oh, C.-T., Ryu, J.-H., Bae, Y.-S., Kang, S.-W., Jang, I.-h., et al. (2005). An Antioxidant System Required for Host protection against Gut Infection in Drosophila. Develop. Cel 8 (1), 125–132. doi:10.1016/j.devcel.2004.11.007
Hakim, J. A., Koo, H., Dennis, L. N., Kumar, R., Ptacek, T., Morrow, C. D., et al. (2015). An Abundance of Epsilonproteobacteria Revealed in the Gut Microbiome of the Laboratory Cultured Sea Urchin, Lytechinus variegatus. Front. Microbiol. 6, 343. doi:10.3389/fmicb.2015.01047
Hakim, J. A., Koo, H., Kumar, R., Lefkowitz, E. J., Morrow, C. D., Powell, M. L., et al. (2016). The Gut Microbiome of the Sea urchin,Lytechinus variegatus, from its Natural Habitat Demonstrates Selective Attributes of Microbial Taxa and Predictive Metabolic Profiles. FEMS Microbiol. Ecol. 92, fiw146. doi:10.1093/femsec/fiw146
Hannigan, G. D., Hodkinson, B. P., McGinnis, K., Tyldsley, A. S., Anari, J. B., Horan, A. D., et al. (2014). Culture-independent Pilot Study of Microbiota Colonizing Open Fractures and Association with Severity, Mechanism, Location, and Complication from Presentation to Early Outpatient Follow-Up. J. Orthop. Res. 32 (4), 597–605. doi:10.1002/jor.22578
Hansen, A. K., Hansen, C. H. F., Krych, L., and Nielsen, D. S. (2014). Impact of the Gut Microbiota on Rodent Models of Human Disease. Wjg 20 (47), 17727–17736. doi:10.3748/wjg.v20.i47.17727
Hooper, L. V., Wong, M. H., Thelin, A., Hansson, L., Falk, P. G., and Gordon, J. I. (2001). Molecular Analysis of Commensal Host-Microbial Relationships in the Intestine. Science 291, 881–884. doi:10.1126/science.291.5505.881
Hoskins, D., Stancyk, S., and Decho, A. (2003). Utilization of Algal and Bacterial Extracellular Polymeric Secretions (EPS) by the deposit-feeding Brittlestar Amphipholis Gracillima (Echinodermata). Mar. Ecol. Prog. Ser. 247, 93–101. doi:10.3354/meps247093
Hou, Q., Ye, L., Huang, L., and Yu, Q. (2017). The Research Progress on Intestinal Stem Cells and its Relationship with Intestinal Microbiota. Front. Immunol. 8, 599. doi:10.3389/fimmu.2017.00599
Hou, Q., Ye, L., Liu, H., Huang, L., Yang, Q., Turner, J., et al. (2018). Lactobacillus Accelerates ISCs Regeneration to Protect the Integrity of Intestinal Mucosa through Activation of STAT3 Signaling Pathway Induced by LPLs Secretion of IL-22. Cell Death Differ 25 (9), 1657–1670. doi:10.1038/s41418-018-0070-2
Huseini, H. F., Rahimzadeh, G., Fazeli, M. R., Mehrazma, M., and Salehi, M. (2012). Evaluation of Wound Healing Activities of Kefir Products. Burns 38 (5), 719–723. doi:10.1016/j.burns.2011.12.005
Hyman, L. (1955). The Invertebrates. IV. Echinodermata. New York: The Celomate Bilateria McGraw-Hill Book Co. Inc.
Iacob, S., Iacob, D. G., and Luminos, L. M. (2019). Intestinal Microbiota as a Host Defense Mechanism to Infectious Threats. Front. Microbiol. 9, 3328. doi:10.3389/fmicb.2018.03328
Ivanov, I. I., Atarashi, K., Manel, N., Brodie, E. L., Shima, T., Karaoz, U., et al. (2009). Induction of Intestinal Th17 Cells by Segmented Filamentous Bacteria. Cell 139 (3), 485–498. doi:10.1016/j.cell.2009.09.033
Iwanaga, S., and Lee, B.-L. (2005). Recent Advances in the Innate Immunity of Invertebrate Animals. BMB Rep. 38, 128–150. doi:10.5483/bmbrep.2005.38.2.128
Jackson, E. W., Pepe-Ranney, C., Debenport, S. J., Buckley, D. H., and Hewson, I. (2018). The Microbial Landscape of Sea Stars and the Anatomical and Interspecies Variability of Their Microbiome. Front. Microbiol. 9, 1829. doi:10.3389/fmicb.2018.01829
James, G. A., Swogger, E., Wolcott, R., Pulcini, E. d., Secor, P., Sestrich, J., et al. (2008). Biofilms in Chronic Wounds. Wound Repair Regen. 16, 37–44. doi:10.1111/j.1524-475X.2007.00321.x
Janssens, S., and Beyaert, R. (2003). Role of Toll-like Receptors in Pathogen Recognition. Clin. Microbiol. Rev. 16 (4), 637–646. doi:10.1128/CMR.16.4.637-646.2003
Jendrossek, D., and Pfeiffer, D. (2014). New Insights in the Formation of Polyhydroxyalkanoate Granules (Carbonosomes) and Novel Functions of Poly(3-Hydroxybutyrate). Environ. Microbiol. 16, 2357–2373. doi:10.1111/1462-2920.12356
Jones, B. W., and Nishiguchi, M. K. (2004). Counterillumination in the Hawaiian Bobtail Squid, Euprymna scolopes Berry (Mollusca: Cephalopoda). Mar. Biol. 144, 1151–1155. Accessed January 19, 2013. Available at: http://www.medmicro.wisc.edu/labs/mcfall_ruby_papers/pdf/2004/Jones_Nishiguchi_2004_Biol.pdf. doi:10.1007/s00227-003-1285-3
Kalacheva, N. V., Eliseikina, M. G., Frolova, L. T., and Dolmatov, I. Y. (2017). Regeneration of the Digestive System in the Crinoid Himerometra Robustipinna Occurs by Transdifferentiation of Neurosecretory-like Cells. PLOS ONE 12 (7), e0182001. doi:10.1371/journal.pone.01820010.1371/journal.pone.0182001
Kandori, H., Hirayama, K., Takeda, M., and Doi, K. (1996). Histochemical, Lectin-Histochemical and Morphometrical Characteristics of Intestinal Goblet Cells of Germfree and Conventional Mice. Exp. Anim. 45, 155–160. doi:10.1538/expanim.45.155
Kanno, A., Ozawa, T., and Umezawa, Y. (2011). Detection of Protein-Protein Interactions in Bacteria by GFP-Fragment Reconstitution. Methods Mol. Biol. 705, 251–258. doi:10.1007/978-1-61737-967-3_15
Kanther, M., Sun, X., Mühlbauer, M., Mackey, L. C., Flynn, E. J., Bagnat, M., et al. (2011). Microbial Colonization Induces Dynamic Temporal and Spatial Patterns of NF-Κb Activation in the Zebrafish Digestive Tract. Gastroenterology 141, 197–207. doi:10.1053/j.gastro.2011.03.042
Karlsson, F. H., Fåk, F., Nookaew, I., Tremaroli, V., Fagerberg, B., Petranovic, D., et al. (2012). Symptomatic Atherosclerosis Is Associated with an Altered Gut Metagenome. Nat. Commun. 3, 1245. doi:10.1038/ncomms2266
Kau, A. L., Ahern, P. P., Griffin, N. W., Goodman, A. L., and Gordon, J. I. (2011). Human Nutrition, the Gut Microbiome and the Immune System. Nature 474, 327–336. doi:10.1038/nature10213
Kaufman, M. G., and Klug, M. J. (1991). The Contribution of Hindgut Bacteria to Dietary Carbohydrate Utilization by Crickets (Orthoptera: Gryllidae). Comp. Biochem. Physiol. A: Physiol. 98 (1), 117–123. doi:10.1016/0300-9629(91)90588-4
Kawai, T., and Akira, S. (2010). The Role of Pattern-Recognition Receptors in Innate Immunity: Update on Toll-like Receptors. Nat. Immunol. 11, 373–384. doi:10.1038/ni.1863
Kellow, N. J., Coughlan, M. T., and Reid, C. M. (2013). Metabolic Benefits of Dietary Prebiotics in Human Subjects: A Systematic Review of Randomised Controlled Trials. Br. J. Nutr. 111, 1147–1161. doi:10.1017/S0007114513003607
Kelly, M. S., Barker, M. F., McKenzie, J. D., and Powell, J. (1995). The Incidence and Morphology of Subcuticular Bacteria in the Echinoderm Fauna of New Zealand. Biol. Bull. 189 (2), 91–105. doi:10.2307/1542459
Khalturin, K., Panzer, Z., Cooper, M., and Bosch, T. (2004). Recognition Strategies in the Innate Immune System of Ancestral Chordates. Mol. Immunol. 41, 1077–1087. doi:10.1016/j.molimm.2004.06.010
Knoll, A. H. (2003). The First Three Billion Years of Evolution on Earth. Princeton/Oxford: Princeton Univ. Press, 277.Life on a Young Planet
Knoll, A. H. (2011). The Multiple Origins of Complex Multicellularity. Annu. Rev. Earth Planet. Sci. 39, 217–239. doi:10.1146/annurev.earth.031208.100209
Koropatnick, T. A., Engle, J. T., Apicella, M. A., Stabb, E. V., Goldman, W. E., and McFall-Ngai, M. J. (2004). Microbial Factor-Mediated Development in a Host-Bacterial Mutualism. Science 306, 1186–1188. doi:10.1126/science.1102218
Koropatnick, T. A., Kimbell, J. R., and McFall-Ngai, M. J. (2007). Responses of Host Hemocytes during the Initiation of the Squid-Vibrio Symbiosis. Biol. Bull. 212 (1), 29–39. doi:10.2307/25066578
Kostic, A. D., Howitt, M. R., and Garrett, W. S. (2013). Exploring Host-Microbiota Interactions in Animal Models and Humans. Genes Dev. 27 (7), 701–718. doi:10.1101/gad.212522.112
Lam, E. K. Y., Yu, L., Wong, H. P. S., Wu, W. K. K., Shin, V. Y., Tai, E. K. K., et al. (2007). Probiotic Lactobacillus Rhamnosus GG Enhances Gastric Ulcer Healing in Rats. Eur. J. Pharmacol. 565, 171–179. doi:10.1016/j.ejphar.2007.02.050
Lamarcq, L. H., and McFall-Ngai, M. J. (1998). Induction of a Gradual, Reversible Morphogenesis of its Host's Epithelial brush Border by Vibrio Fischeri. Infect. Immun. 66 (2), 777–785. doi:10.1128/IAI.66.2.777-785.1998
Lancaster, M. A., and Knoblich, J. A. (2014). Organogenesis in a Dish: Modeling Development and Disease Using Organoid Technologies. Science 345 (6194), 1247125. doi:10.1126/science.1247125
Lee, Y.-S., Kim, T.-Y., Kim, Y., Lee, S.-H., Kim, S., Kang, S. W., et al. (2018). Microbiota-derived Lactate Accelerates Intestinal Stem-Cell-Mediated Epithelial Development. Cell Host & Microbe 24, 833–846. e6. doi:10.1016/j.chom.2018.11.002
León-Palmero, E., Joglar, V., Álvarez, P. A., Martín-Platero, A., Llamas, I., and Reche, I. (2018). Diversity and Antimicrobial Potential in Sea Anemone and Holothurian Microbiomes. PLoS One 13, e0196178. doi:10.1371/journal.pone.0196178
Ley, R. E., Turnbaugh, P. J., Klein, S., and Gordon, J. I. (2006). Human Gut Microbes Associated with Obesity. Nature 444 (7122), 1022–1023. doi:10.1038/4441022a
Liu, S.-H., Huang, Y.-C., Chen, L. Y., Yu, S.-C., Yu, H.-Y., and Chuang, S.-S. (2018). The Skin Microbiome of Wound Scars and Unaffected Skin in Patients with Moderate to Severe burns in the Subacute Phase. Wound Rep. Reg. 26 (2), 182–191. doi:10.1111/wrr.12632
Loesche, M., Gardner, S. E., Kalan, L., Horwinski, J., Zheng, Q., Hodkinson, B. P., et al. (2017). Temporal Stability in Chronic Wound Microbiota Is Associated with Poor Healing. J. Invest. Dermatol. 137, 237–244. doi:10.1016/j.jid.2016.08.009
Long, S. L., Gahan, C. G. M., and Joyce, S. A. (2017). Interactions between Gut Bacteria and Bile in Health and Disease. Mol. Aspects Med. 56, 54–65. doi:10.1016/j.mam.2017.06.002
Macpherson, A. J., and Harris, N. L. (2004). Interactions between Commensal Intestinal Bacteria and the Immune System. Nat. Rev. Immunol. 4, 478–485. doi:10.1038/nri1373
Madison, L. L., and Huisman, G. W. (1999). Metabolic Engineering of Poly(3-Hydroxyalkanoates): From DNA to Plastic. Microbiol. Mol. Biol. Rev. 63, 21–53. doi:10.1128/MMBR.63.1.21-53.1999
Maheswary, T., Nurul, A. A., and Fauzi, M. B. (2021). The Insights of Microbes' Roles in Wound Healing: A Comprehensive Review. Pharmaceutics 13 (7), 981. doi:10.3390/pharmaceutics13070981
Mashanov, V. S., Dolmatov, I. Y., and Heinzeller, T. (2005). Transdifferentiation in Holothurian Gut Regeneration. Biol. Bull. 209 (3), 184–193. doi:10.2307/3593108
Mashanov, V. S., and García-Arrarás, J. E. (2011). Gut Regeneration in Holothurians: a Snapshot of Recent Developments. Biol. Bull. 221 (1), 93–109. doi:10.1086/BBLv221n1p93
Mashanov, V. S., Zueva, O., and García-Arrarás, J. E. (2014). Postembryonic Organogenesis of the Digestive Tube: Why Does It Occur in Worms and Sea Cucumbers but Fail in Humans?. Develop. Biol. 108, 185–216. doi:10.1016/B978-0-12-391498-9.00006-1
McFall-Ngai, M., Heath-Heckman, E. A. C., Gillette, A. A., Peyer, S. M., and Harvie, E. A. (2012). The Secret Languages of Coevolved Symbioses: Insights from the Euprymna Scolopes-Vibrio Fischeri Symbiosis. Semin. Immunol. 24, 3–8. doi:10.1016/j.smim.2011.11.006
McFall-Ngai, M. J., and Ruby, E. G. (1998). Sepiolids and Vibrios: When First They Meet. BioScience 48, 257–265. doi:10.2307/1313352
McFall-Ngai, M. J., and Ruby, E. G. (1991). Symbiont Recognition and Subsequent Morphogenesis as Early Events in an Animal-Bacterial Mutualism. Science 254 (5037), 1491–1494. doi:10.1126/science.1962208
Metcalfe, C., Kljavin, N. M., Ybarra, R., and de Sauvage, F. J. (2014). Lgr5+ Stem Cells Are Indispensable for Radiation-Induced Intestinal Regeneration. Cell Stem Cell 14 (2), 149–159. doi:10.1016/j.stem.2013.11.008
Meyer, D. L. (1988). “Crinoids as Renewable Resource: Rapid Regeneration of the Visceral Mass in a Tropical Reef-Dwelling Crinoid from Australia,” in Echinoderm Biology. Editors RD Burke, PV Mladenov, P Lambert, and RL Parsley (Rotterdam: Balkema), 519–522.
Meyer, D. L. (1985). Evolutionary Implications of Predation on Recent Comatulid Crinoids from the Great Barrier Reef. Paleobiology 11, 154–164. doi:10.1017/s0094837300011477
Milligan-Myhre, K., Charette, J. R., Phennicie, R. T., Stephens, W. Z., Rawls, J. F., Guillemin, K., et al. (2011). Study of Host-Microbe Interactions in Zebrafish. Methods Cel Biol 105, 87–116. doi:10.1016/B978-0-12-381320-6.00004-7
Miquel, S., Martín, R., Rossi, O., Bermúdez-Humarán, L., Chatel, J., Sokol, H., et al. (2013). Faecalibacterium Prausnitzii and Human Intestinal Health. Curr. Opin. Microbiol. 16 (3), 255–261. PMID 23831042. doi:10.1016/j.mib.2013.06.003
Morales-Jiménez, J., Zúñiga, G., Villa-Tanaca, L., and Hernández-Rodríguez, C. (2009). Bacterial Community and Nitrogen Fixation in the Red Turpentine Beetle, Dendroctonus Valens LeConte (Coleoptera: Curculionidae: Scolytinae). Microb. Ecol. 58, 879–891. doi:10.1007/s00248-009-9548-2
Morrow, K. M., Tedford, A. R., Pankey, M. S., and Lesser, M. P. (2018). A Member of the Roseobacter Clade, Octadecabacter sp., Is the Dominant Symbiont in the Brittle star Amphipholis Squamata. FEMS Microbiol. Ecol. 94, 1967. doi:10.1093/femsec/fiy030
Mozzi, D., Dolmatov, I., Bonasoro, F., and Carnevali, M. (2006). Visceral Regeneration in the Crinoid Antedon Mediterranea: Basic Mechanisms, Tissues and Cells Involved in Gut Regrowth. Cent. Eur. J. Biol. 1, 609–635. doi:10.2478/s11535-006-0042-2
Naito, T., Mulet, C., De Castro, C., Molinaro, A., Saffarian, A., Nigro, G., et al. (2017). Lipopolysaccharide from Crypt-specific Core Microbiota Modulates the Colonic Epithelial Proliferation-To-Differentiation Balance. MBio 8, e01680–17. doi:10.1128/mBio.01680-17
Najdegerami, E. H., Tran, T. N., Defoirdt, T., Marzorati, M., Sorgeloos, P., Boon, N., et al. (2012). Effects of Poly-β-Hydroxybutyrate (PHB) on Siberian sturgeon (Acipenser baerii) Fingerlings Performance and its Gastrointestinal Tract Microbial Community. FEMS Microbiol. Ecol. 79, 25–33. doi:10.1111/j.1574-6941.2011.01194.x
Narayanan, K. B., and Park, H. H. (2015). Toll/interleukin-1 Receptor (TIR) Domain-Mediated Cellular Signaling Pathways. Apoptosis 20, 196–209. PMID: 25563856. doi:10.1007/s10495-014-1073-1
Narbonne, G. M. (2005). The Ediacara Biota: Neoproterozoic Origin of Animals and Their Ecosystems. Annu. Rev. Earth Planet. Sci. 33, 421–442. doi:10.1146/annurev.earth.33.092203.122519
Neal, J. T., Peterson, T. S., Kent, M. L., and Guillemin, K. (2013). H. pylori Virulence Factor CagA Increases Intestinal Cell Proliferation by Wnt Pathway Activation in a Transgenic Zebrafish Model. Dis. Model. Mech. 6 (3), 802–810. doi:10.1242/dmm.011163
Neish, A. S. (2009). Microbes in Gastrointestinal Health and Disease. Gastroenterology 136 (1), 65–80. doi:10.1053/j.gastro.2008.10.080
Nhan, D. T., Wille, M., De Schryver, P., Defoirdt, T., Bossier, P., and Sorgeloos, P. (2010). The Effect of Poly β-hydroxybutyrate on Larviculture of the Giant Freshwater Prawn Macrobrachium Rosenbergii. Aquaculture 302, 76–81. doi:10.1016/j.aquaculture.2010.02.011
Nie, L., Cai, S.-Y., Shao, J.-Z., and Chen, J. (2018). Toll-Like Receptors, Associated Biological Roles, and Signaling Networks in Non-mammals. Front. Immunol. 9, 1523. doi:10.3389/fimmu.2018.01523
Nigro, G., Rossi, R., Commere, P.-H., Jay, P., and Sansonetti, P. J. (2014). The Cytosolic Bacterial Peptidoglycan Sensor Nod2 Affords Stem Cell protection and Links Microbes to Gut Epithelial Regeneration. Cell Host & Microbe 15, 792–798. doi:10.1016/j.chom.2014.05.003
Nyholm, S. V., Deplancke, B., Gaskins, H. R., Apicella, M. A., and McFall-Ngai, M. J. (2002). Roles of Vibrio Fischeri and Nonsymbiotic Bacteria in the Dynamics of Mucus Secretion during Symbiont Colonization of the Euprymna scolopes Light Organ. Appl. Environ. Microbiol. 68, 5113–5122. doi:10.1128/aem.68.10.5113-5122.2002
Nyholm, S. V., and McFall-Ngai, M. (2004). The Winnowing: Establishing the Squid-Vibrio Symbiosis. Nat. Rev. Microbiol. 2, 632–642. doi:10.1038/nrmicro957
Nyholm, S. V., Stabb, E. V., Ruby, E. G., and McFall-Ngai, M. J. (2000). Establishment of an Animal-Bacterial Association: Recruiting Symbiotic Vibrios from the Environment. Proc. Natl. Acad. Sci. 97, 10231–10235. doi:10.1073/pnas.97.18.10231
O'Hara, A. M., and Shanahan, F. (2006). The Gut flora as a Forgotten Organ. EMBO Rep. 7 (7), 688–693. doi:10.1038/sj.embor.7400731
Pagán-Jiménez, M., Ruiz-Calderón, J. F., Domínguez-Bello, M. G., and García-Arrarás, J. E. (2019). Characterization of the Intestinal Microbiota of the Sea Cucumber Holothuria Glaberrima. PLoS ONE 14 (1), e0208011. doi:10.1371/journal.pone.0208011
Peck, B. C. E., Shanahan, M. T., Singh, A. P., and Sethupathy, P. (20172017). Gut Microbial Influences on the Mammalian Intestinal Stem Cell Niche. Stem Cell Int. 2017, 1–17. doi:10.1155/2017/5604727
Pellegatta, T., Saler, M., Bonfanti, V., Nicoletti, G., and Faga, A. (2016). Novel Perspectives on the Role of the Human Microbiota in Regenerative Medicine and Surgery. Biomed. Rep. 5, 519–524. doi:10.3892/br.2016.778
Peral, M. C., Martinez, M. A. H., and Valdez, J. C. (2009). Bacteriotherapy withLactobacillus Plantarumin burns. Int. Wound J. 6 (1), 73–81. doi:10.1111/j.1742-481X.2008.00577.x
Petersen, C. P., and Reddien, P. W. (2009). A Wound-Induced Wnt Expression Program Controls Planarian Regeneration Polarity. Proc. Natl. Acad. Sci. 106 (40), 17061–17066. doi:10.1073/pnas.0906823106
Pull, S. L., Doherty, J. M., Mills, J. C., Gordon, J. I., and Stappenbeck, T. S. (2005). Activated Macrophages Are an Adaptive Element of the Colonic Epithelial Progenitor Niche Necessary for Regenerative Responses to Injury. Proc. Natl. Acad. Sci. 102, 99–104. doi:10.1073/pnas.0405979102
Qin, J., Li, R., Li, R., Raes, J., Arumugam, M., Burgdorf, K. S., et al. MetaHIT Consortium (2010). A Human Gut Microbial Gene Catalogue Established by Metagenomic Sequencing. Nature 464, 59–65. doi:10.1038/nature08821
Qin, J., Li, Y., Cai, Z., Li, S., Zhu, J., Zhang, F., et al. (2012). A Metagenome-wide Association Study of Gut Microbiota in Type 2 Diabetes. Nature 490, 55–60. doi:10.1038/nature11450
Quiñones, J. L., Rosa, R., Ruiz, D. L., and García-Arrarás, J. E. (2002). Extracellular Matrix Remodeling and Metalloproteinase Involvement During Intestine Regeneration in the Sea Cucumber Holothuria glaberrima. Develop. Biol. 250 (1), 181–197. doi:10.1006/dbio.2002.0778
Quispe-Parra, D. J., Medina-Feliciano, J. G., Cruz-González, S., Ortiz-Zuazaga, H., and García-Arrarás, J. E. (2021). Transcriptomic Analysis of Early Stages of Intestinal Regeneration in Holothuria Glaberrima. Sci. Rep. 11 (1), 346. doi:10.1038/s41598-020-79436-2
Rakoff-Nahoum, S., Paglino, J., Eslami-Varzaneh, F., Edberg, S., and Medzhitov, R. (2004). Recognition of Commensal Microflora by Toll-like Receptors Is Required for Intestinal Homeostasis. Cell 118, 229–241. doi:10.1016/j.cell.2004.07.002
Rawls, J. F., Samuel, B. S., and Gordon, J. I. (2004). From the Cover: Gnotobiotic Zebrafish Reveal Evolutionarily Conserved Responses to the Gut Microbiota. Proc. Natl. Acad. Sci. 101, 4596–4601. doi:10.1073/pnas.0400706101
Reddy, P. C., Gungi, A., and Unni, M. (2019). Cellular and Molecular Mechanisms of Hydra Regeneration. Results Probl. Cel. Differ. 68, 259–290. doi:10.1007/978-3-030-23459-1_12
Reinhardt, C., Reigstad, C. S., and Bäckhed, F. (2009). Intestinal Microbiota during Infancy and its Implications for Obesity. J. Pediatr. Gastroenterol. Nutr. 48 (3), 249–256. doi:10.1097/mpg.0b013e318183187c
Ren, C., Webster, P., Finkel, S. E., and Tower, J. (2007). Increased Internal and External Bacterial Load during Drosophila Aging without Life-Span Trade-Off. Cel Metab. 6, 144–152. doi:10.1016/j.cmet.2007.06.006
Roach, J. C., Glusman, G., Rowen, L., Kaur, A., Purcell, M. K., Smith, K. D., et al. (2005). The Evolution of Vertebrate Toll-like Receptors. Proc. Natl. Acad. Sci. 102, 9577. doi:10.1073/pnas.0502272102
Rodrigues, J. C. V., Locali, E. C., Freitas-Astua, J., and Kitajima, E. W. (2005). Transmissibility of Citrus Leprosis Virus by Brevipalpus Phoenicis to Solanum Violaefolium. Plant Dis. 89 (8), 911. doi:10.1094/PD-89-0911B
Roeselers, G., Mittge, E. K., Stephens, W. Z., Parichy, D. M., Cavanaugh, C. M., Guillemin, K., et al. (2011). Evidence for a Core Gut Microbiota in the Zebrafish. ISME J. 5, 1595–1608. doi:10.1038/ismej.2011.38
Roh, S. W., Sung, Y., Nam, Y.-D., Chang, H.-W., Kim, K.-H., Yoon, J.-H., et al. (2008). Arthrobacter Soli Sp. nov., a Novel Bacterium Isolated from Wastewater Reservoir Sediment. J. Microbiol. 46, 40–44. doi:10.1007/s12275-007-0239-8
Round, J. L., and Mazmanian, S. K. (2009). The Gut Microbiota Shapes Intestinal Immune Responses during Health and Disease. Nat. Rev. Immunol. 9 (5), 313–323. doi:10.1038/nri2515
Ruby, E. G., and Lee, K.-H. (1998). The Vibrio Fischeri-Euprymna scolopes Light Organ Association: Current Ecological Paradigms. Appl. Environ. Microbiol. 64 (3), 805–812. doi:10.1128/AEM.64.3.805-812.1998
Ryu, J.-H., Kim, S.-H., Lee, H.-Y., Bai, J. Y., Nam, Y.-D., Bae, J.-W., et al. (2008). Innate Immune Homeostasis by the Homeobox Gene Caudal and Commensal-Gut Mutualism in Drosophila. Science 319 (5864), 777–782. doi:10.1126/science.1149357
Sailaja, B. S., He, X. C., and Li, L. (2016). The Regulatory Niche of Intestinal Stem Cells. J. Physiol. 594 (17), 4827–4836. doi:10.1113/jp271931
Santos, A. J. M., Lo, Y.-H., Mah, A. T., and Kuo, C. J. (2018). The Intestinal Stem Cell Niche: Homeostasis and Adaptations. Trends Cell Biology 28 (12), 1062–1078. doi:10.1016/j.tcb.2018.08.001
Satake, H., and Sekiguchi, T. (2012). Toll-like Receptors of Deuterostome Invertebrates. Front. Immun. 3, 34. doi:10.3389/fimmu.2012.00034
Sato, T., Stange, D. E., Ferrante, M., Vries, R. G. J., Van Es, J. H., Van den Brink, S., et al. (2011). Long-term Expansion of Epithelial Organoids from Human Colon, Adenoma, Adenocarcinoma, and Barrett's Epithelium. Gastroenterology 141, 1762–1772. doi:10.1053/j.gastro.2011.07.050
Sato, T., Vries, R. G., Snippert, H. J., van de Wetering, M., Barker, N., Stange, D. E., et al. (2009). Single Lgr5 Stem Cells Build Crypt-Villus Structures In Vitro without a Mesenchymal Niche. Nature 459, 262–265. doi:10.1038/nature07935
Savage, D. C., Siegel, J. E., Snellen, J. E., and Whitt, D. D. (1981). Transit Time of Epithelial Cells in the Small Intestines of Germfree Mice and Ex-Germfree Mice Associated with Indigenous Microorganisms. Appl. Environ. Microbiol. 42, 996–1001. doi:10.1128/aem.42.6.996-1001.1981
Scholnick, D. A., and Winslow, A. E. (2020). The Role of Fasting on Spine Regeneration and Bacteremia in the Purple Sea Urchin Strongylocentrotus purpuratus. PLOS ONE 15 (2), e0228711. doi:10.1371/journal.pone.0228711
Seite, S., Flores, G. E., Henley, J. B., Martin, R., Zelenkova, H., Aguilar, L., et al. (2014). Microbiome of Affected and Unaffected Skin of Patients with Atopic Dermatitis before and after Emollient Treatment. J. Drugs Dermatol. 13, 1365–1372.
Shin, S. C., Kim, S.-H., You, H., Kim, B., Kim, A. C., Lee, K.-A., et al. (2011). Drosophila Microbiome Modulates Host Developmental and Metabolic Homeostasis via Insulin Signaling. Science 334 (6056), 670–674. doi:10.1126/science.1212782
Snel, J., Heinen, P. P., Blok, H. J., Carman, R. J., Duncan, A. J., Allen, P. C., et al. (1995). Comparison of 16S rRNA Sequences of Segmented Filamentous Bacteria Isolated from Mice, Rats, and Chickens and Proposal of "Candidatus Arthromitus". Int. J. Syst. Bacteriol. 45 (4), 780–782. doi:10.1099/00207713-45-4-780
Sommer, F., and Bäckhed, F. (2013). The Gut Microbiota - Masters of Host Development and Physiology. Nat. Rev. Microbiol. 11, 227–238. doi:10.1038/nrmicro2974
Stappenbeck, T. S., Hooper, L. V., and Gordon, J. I. (2002). Nonlinear Partial Differential Equations and Applications: Developmental Regulation of Intestinal Angiogenesis by Indigenous Microbes via Paneth Cells. Proc. Natl. Acad. Sci. 99 (24), 15451–15455. doi:10.1073/pnas.202604299
Suzuki, K., Meek, B., Doi, Y., Muramatsu, M., Chiba, T., Honjo, T., et al. (2004). Aberrant Expansion of Segmented Filamentous Bacteria in IgA-Deficient Gut. Pnas 101 (7), 1981–1986. doi:10.1073/pnas.0307317101
Swanson, P. A., Kumar, A., Samarin, S., Vijay-Kumar, M., Kundu, K., Murthy, N., et al. (2011). Enteric Commensal Bacteria Potentiate Epithelial Restitution via Reactive Oxygen Species-Mediated Inactivation of Focal Adhesion Kinase Phosphatases. Proc. Natl. Acad. Sci. 108 (21), 8803–8808. doi:10.1073/pnas.1010042108
Thomas, H. (2016). Microbiota Promote Gut Healing. Nat. Rev. Gastroenterol. Hepatol. 13, 189. doi:10.1038/nrgastro.2016.31
Tischer, S., Reineck, M., Söding, J., Münder, S., and Böttger, A. (2013). Eph Receptors and Ephrin Class B Ligands Are Expressed at Tissue Boundaries in Hydra vulgaris. Int. J. Dev. Biol. 57 (9-10), 759–765. doi:10.1387/ijdb.130158ab
Troll, J. V., Bent, E. H., Pacquette, N., Wier, A. M., Goldman, W. E., Silverman, N., et al. (2010). Taming the Symbiont for Coexistence: A Host PGRP Neutralizes a Bacterial Symbiont Toxin. Environ. Microbiol. 12 (8), 2190–2203. doi:10.1111/j.1462-2920.2009.02121.x
Tu, S., and Johnson, S. L. (2011). Fate Restriction in the Growing and Regenerating Zebrafish Fin. Develop. Cel. 20 (5), 725–732. doi:10.1016/j.devcel.2011.04.013
Turnbaugh, P. J., Ley, R. E., Hamady, M., Fraser-Liggett, C. M., Knight, R., Gordon, J. I., et al. (2007). The Human Microbiome Project. Nature 449 (7164), 804–810. doi:10.1038/nature06244
Turnbaugh, P. J., Bäckhed, F., Fulton, L., and Gordon, J. I. (2008). Diet-induced Obesity Is Linked to Marked but Reversible Alterations in the Mouse Distal Gut Microbiome. Cell Host & Microbe 3, 213–223. doi:10.1016/j.chom.2008.02.015
Turnbaugh, P. J., Hamady, M., Yatsunenko, T., Cantarel, B. L., Duncan, A., Ley, R. E., et al. (2009). A Core Gut Microbiome in Obese and Lean Twins. Nature 457 (7228), 480–484. doi:10.1038/nature07540
Turnbaugh, P. J., Ley, R. E., Mahowald, M. A., Magrini, V., Mardis, E. R., and Gordon, J. I. (2006). An Obesity-Associated Gut Microbiome with Increased Capacity for Energy Harvest. Nature 444 (7122), 1027–1031. doi:10.1155/2015/80624810.1038/nature05414
Tzou, P., Ohresser, S., Ferrandon, D., Capovilla, M., Reichhart, J.-M., Lemaitre, B., et al. (2000). Tissue-specific Inducible Expression of Antimicrobial Peptide Genes in Drosophila Surface Epithelia. Immunity 13 (5), 737–748. doi:10.1016/s1074-7613(00)00072-8
Uribe, A., Alam, M., Johansson, O., Midtvedt, T., and Theodorsson, E. (1994). Microflora Modulates Endocrine Cells in the Gastrointestinal Mucosa of the Rat. Gastroenterology 107, 1259–1269. doi:10.1016/0016-5085(94)90526-6
Valdéz, J. C., Peral, M. C., Rachid, M., Santana, M., and Perdigón, G. (2005). Interference of Lactobacillus Plantarum with Pseudomonas aeruginosa In Vitro and in Infected burns: the Potential Use of Probiotics in Wound Treatment. Clin. Microbiol. Infect. 11 (6), 472–479. doi:10.1111/j.1469-0691.2005.01142.x
Vieira, W. A., Wells, K. M., and McCusker, C. D. (2020). Advancements to the Axolotl Model for Regeneration and Aging. Gerontology 66, 212–222. doi:10.1159/000504294
Vijay-Kumar, M., Aitken, J. D., Carvalho, F. A., Cullender, T. C., Mwangi, S., Srinivasan, S., et al. (2010). Metabolic Syndrome and Altered Gut Microbiota in Mice Lacking Toll-like Receptor 5. Science 328 (5975), 228–231. doi:10.1126/science.1179721
Visick, K. L., Foster, J., Doino, J., McFall-Ngai, M., and Ruby, E. G. (2000). Vibrio Fischeri Lux Genes Play an Important Role in Colonization and Development of the Host Light Organ. J. Bacteriol. 182, 4578–4586. doi:10.1128/JB.182.16.4578-4586.2000
Wagner, C. L., Taylor, S. N., and Johnson, D. (2008). Host Factors in Amniotic Fluid and Breast Milk that Contribute to Gut Maturation. Clinic Rev. Allerg Immunol. 34, 191–204. doi:10.1007/s12016-007-8032-3
Wang, B., Yao, M., Lv, L., Ling, Z., and Li, L. (2017). The Human Microbiota in Health and Disease. Engineering 3 (1), 71–82. doi:10.1016/J.ENG.2017.01.008
Wang, L., Zhao, X., Xu, H., Bao, X., Liu, X., Chang, Y., et al. (2018). Characterization of the Bacterial Community in Different Parts of the Gut of Sea Cucumber (Apostichopus Japonicus ) and its Variation during Gut Regeneration. Aquac. Res. 49, 1987–1996. doi:10.1111/are.13654
Wang, Y., Zhang, S., Li, H., Wang, H., Zhang, T., Hutchinson, M. R., et al. (2020). Small-Molecule Modulators of Toll-like Receptors. Acc. Chem. Res. 20 (5), 1046–1055. doi:10.1021/acs.accounts.9b00631
Wang, Y., and Zhang, Y. (2015). Investigation of Gut-Associated Bacteria inTenebrio molitor(Coleoptera: Tenebrionidae) Larvae Using Culture-dependent and DGGE Methods. Ann. Entomol. Soc. Am. 108 (5), 941–949. doi:10.1093/aesa/sav079
Weigel, B. L. (2020). Sea Cucumber Intestinal Regeneration Reveals Deterministic Assembly of the Gut Microbiome. Appl. Environ. Microbiol. 86, e00489–20. doi:10.1128/AEM.00489-20
Weinstock, G. M. (2012). Genomic Approaches to Studying the Human Microbiota. Nature 489 (7415), 250–256. doi:10.1038/nature11553
Wilkie, I. C. (2001). Autotomy as a Prelude to Regeneration in Echinoderms. Microsc. Res. Tech. 55, 369–396. doi:10.1002/jemt.1185
Wolcott, R. D., Hanson, J. D., Rees, E. J., Koenig, L. D., Phillips, C. D., Wolcott, R. A., et al. (2016). Analysis of the Chronic Wound Microbiota of 2,963 Patients by 16S rDNA Pyrosequencing. Wound Rep. Reg. 24, 163–174. doi:10.1111/wrr.12370
Wong, C. N. A., Ng, P., and Douglas, A. E. (2011). Low-diversity Bacterial Community in the Gut of the Fruitfly Drosophila melanogaster. Environ. Microbiol. 13 (7), 1889–1900. doi:10.1111/j.1462-2920.2011.02511.x
Wong, J. M. W., de Souza, R., Kendall, C. W. C., Emam, A., and Jenkins, D. J. A. (2006). Colonic Health: Fermentation and Short Chain Fatty Acids. J. Clin. Gastroenterol. 40, 235–243. doi:10.1097/00004836-200603000-00015
Yamazaki, Y., Meirelles, P. M., Mino, S., Suda, W., Oshima, K., Hattori, M., et al. (2016). Individual Apostichopus Japonicus Fecal Microbiome Reveals a Link with Polyhydroxybutyrate Producers in Host Growth Gaps. Sci. Rep. 6, 21631. doi:10.1038/srep21631
Yin, Y., Wang, Y., Zhu, L., Liu, W., Liao, N., Jiang, M., et al. (2013). Comparative Analysis of the Distribution of Segmented Filamentous Bacteria in Humans, Mice and Chickens. ISME J. 7 (3), 615–621. doi:10.1038/ismej.2012.128
Yokoyama, H. (2008). Initiation of Limb Regeneration: the Critical Steps for Regenerative Capacity. Dev. Growth Differ. 50 (1), 13–22. doi:10.1111/j.1440-169X.2007.00973.x
Zaborin, A., Krezalek, M., Hyoju, S., Defazio, J. R., Setia, N., Belogortseva, N., et al. (2017). Critical Role of Microbiota within Cecal Crypts on the Regenerative Capacity of the Intestinal Epithelium Following Surgical Stress. Am. J. Physiol. Gastrointest. Liver Physiol. 312, G112–G122. doi:10.1152/ajpgi.00294.2016
Zhang, H., Wang, Q., Zhao, J., Liu, S., Zhang, L., Zhao, Y., et al. (2020). Quantitative Microbiome Profiling Links Microbial Community Variation to the Intestine Regeneration Rate of the Sea Cucumber Apostichopus Japonicus. Genomics 112, 5012–5020. doi:10.1016/j.ygeno.2020.09.017
Keywords: regeneration, echinoderm, development, symbiosis, microbiota, microbiome, sea cucumber
Citation: Díaz-Díaz LM, Rodríguez-Villafañe A and García-Arrarás JE (2022) The Role of the Microbiota in Regeneration-Associated Processes. Front. Cell Dev. Biol. 9:768783. doi: 10.3389/fcell.2021.768783
Received: 01 September 2021; Accepted: 03 December 2021;
Published: 26 January 2022.
Edited by:
Igor Schneider, Federal University of Pará, BrazilReviewed by:
Julia Ganz, Michigan State University, United StatesSuleyman Yildirim, Istanbul Medipol University, Turkey
Brooke Weigel, University of Washington, United States
Copyright © 2022 Díaz-Díaz, Rodríguez-Villafañe and García-Arrarás. This is an open-access article distributed under the terms of the Creative Commons Attribution License (CC BY). The use, distribution or reproduction in other forums is permitted, provided the original author(s) and the copyright owner(s) are credited and that the original publication in this journal is cited, in accordance with accepted academic practice. No use, distribution or reproduction is permitted which does not comply with these terms.
*Correspondence: José E. García-Arrarás, amVnYXJjaWFAaHBjZi51cHIuZWR1