- Department of Biology, College of Science and Mathematics, University of Massachusetts Boston, Boston, MA, United States
The size and shape of the tetrapod limb play central roles in their functionality and the overall physiology of the organism. In this minireview we will discuss observations on mutant animal models and humans, which show that the growth and final size of the limb is most impacted by factors that regulate either limb bud patterning or the elongation of the long bones. We will also apply the lessons that have been learned from embryos to how growth could be regulated in regenerating limb structures and outline the challenges that are unique to regenerating animals.
Introduction
Although the underlying anatomy is shared, the scale and shape of limbs vary greatly among tetrapod species. The batwing is optimized for flying, horse legs are optimized for running, and snake legs have all but disappeared to allow for the serpentine movements of the body. Beyond the various impacts on locomotive abilities, limb sizing also plays key roles in activities such as eating, mating, and communication. Thus, the development of limbs that are the proportionally appropriate size for each species is essential for the functionality of these structures and the overall physiology of these animals. This review will focus on the molecular mechanisms that regulate limb growth, which will ultimately impact the overall size and functionality of the limb structures that form.
Limb formation in all tetrapod species begins with the development of a structure known as the limb bud. The limb bud is composed of an ectodermal signaling center that covers a cluster of mesodermal cells which will proliferate, pattern, and differentiate into the tissues that compose the basic blueprint of the tetrapod limb. Therefore, alterations that impact limb development, such as those involved in pattern formation and physiology in the limb bud cells, will greatly impact subsequent steps that also influence limb length. As the limb tissues continue to mature, the limb elongates through the growth of the long bones to the length that is uniquely appropriate to the body size in each species. The process by which the limb grows in relation to the rest of the organism’s body is called ontogenetic allometric growth, and alterations to this growth can greatly impact the size and functionality of the limbs.
Although the mechanisms regulating limb growth are not fully elucidated, studies on developing embryonic limbs in model organisms as well as genetic characterization of humans with limb length pathologies, indicate that factors that regulate limb bud development, cell and tissue physiology, and the activity of the growth plates in the limb long bones all play important roles (Figure 1). The impact that the alteration of these different factors can have on limb size varies depending on the stage of development and whether the animal is a determinant or indeterminately growing species (Figure 1). Determinant species cease growing once they reach adulthood, whereases indeterminant species continue to grow throughout their lifecycle. Some indeterminant tetrapods, such as Urodele amphibians, retain the ability to regenerate complete limbs through adulthood, and thus require specialized regulation of the regenerating structure. In this review we will discuss the various molecular factors that contribute to limb growth (Table 1). Because most of the studies that have identified these factors were performed in mammals and birds, the focus will be on determinant species. We will then draw parallels with what is known about the mechanisms that regulate sizing during limb regeneration in Urodeles.
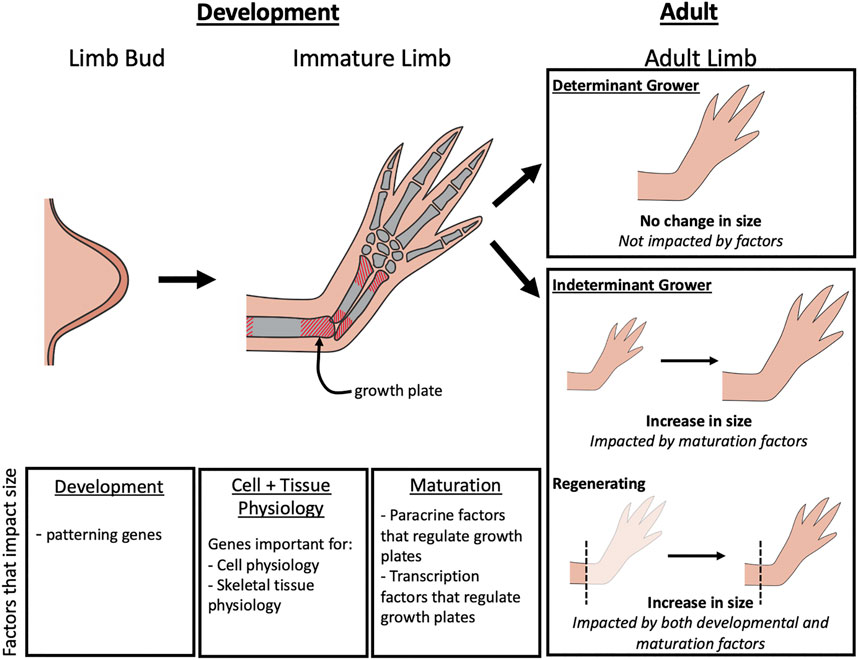
FIGURE 1. Factors that impact limb growth. During limb bud development, changes in limb patterning genes can lead to differences in the overall size of the adult limb. As the immature limb elongates, size is controlled by paracrine factors and transcription factors that regulate growth of the growth plates. In adult limbs, the factors that influence size is dependent on the type of organismal growth type (determinant or indeterminant) and whether regeneration is occurring. Limb size on determinant growers will not be impacted by regulation during adulthood, but in indeterminant growers, limb size can be impacted by maturation factors that alter growth plate activity. During regeneration, both development and maturation factors can influence limb size.
Appendage Size Regulation During Limb Bud Development
Transcription Factors
The alteration of a number of transcription factors have been found to impact limb length in mammals through their roles in patterning and differentiation of the limb bud. For example, Paired Related Homeobox 1 (Prx1 or Prrx1) is a homeobox transcription factor known for its role in mesodermal cell proliferation and fate in the developing limb. In an elegant experiment, the limb specific transcriptional enhancer of mouse Prx1 was replaced by the orthologous enhancer from bat, Carollia perspicillata (Cretekos et al., 2008). This manipulation resulted in increased expression and an expansion of the expression domains of mouse Prx1, and an increase in the overall length of the mutant mouse limbs (Cretekos et al., 2008).
HOX genes, a group of highly conserved transcription factors that are essential for limb patterning also impact the length of the limb structures (Zakany and Duboule, 2007). Mouse knockouts of HoxD13, HoxA13, or HoxD12 result in both the truncation of the limb pattern and reduction of the overall limb size (Fromental-Ramain et al., 1996; Hérault et al., 1996; Cho et al., 2008). Increased and sustained expression of the HoxD locus occurs in the developing forelimb buds in bats. While these differences in expression do not result in noticeable differences in the growth and size of the fore and hind limb buds at the early stages, once differentiated, the skeletal elements in the autopod segment of bat forelimbs undergo a dramatic elongation, resulting in their proportionally larger size. Thus, loss of limb specific Hox genes appear to result in shortened limbs by negatively impacting pattern formation, while increased Hox expression positively correlates with limb size by increasing growth during the elongation stage of limb development.
Sox9 and paralogs Sox5 and Sox6 are members of the SRY-related HMG-box family of transcription factors and effect limb size through their regulation on chondrogenesis (Liu and Lefebvre, 2015). During embryonic limb development, Sox9 is considered the master chondrogenic factor, required for differentiation of mesenchymal precursor cells into chondrocytes (Lefebvre et al., 2001; Liu and Lefebvre, 2015). Sox9 then works in concert with Sox5 and Sox6 to drive differentiation and proliferation of chondrocytes (Lefebvre et al., 2001; Liu and Lefebvre, 2015). Activating mutations in Sox9 in mice results in a long limb phenotype (Long et al., 2020), while inhibiting mutations in the same gene results in short limb phenotypes (Akiyama et al., 2002, 2007). Furthermore, mouse knockouts of Sox5 and Sox6 in the limb bud mesenchyme results in chondrodysplasia with shortened limbs (Smits et al., 2001; Dy et al., 2008). These observations highlight the importance of this family of transcription factors on the regulation of growth during limb development.
Genes Involved With Limb Skeletal Maturation
Once the limb bud is patterned and the skeletal tissues have differentiated, the regulation of the long bone growth plates greatly contributes to the overall size of the adult limb. The genes that regulate limb growth at this stage are involved with paracrine factor signaling. One example of this is Indian hedgehog (Ihh) signaling, which positively regulates cell proliferation within the growth plates of the long bones. When Ihh signaling is inactivated, through null Ihh or mutations in Ihh transducers or effectors, the resulting mammalian limbs are severely shortened (Mo et al., 1997; St-Jacques et al., 1999; Long et al., 2001; Razzaque et al., 2005; Ruiz-Perez et al., 2007; Sohaskey et al., 2008; Joeng and Long, 2009; Caparrós-Martín et al., 2013; Yoshida et al., 2015; Zhang et al., 2015; Kim et al., 2018). In contrast, overexpressing Ihh in the developing chick limb through viral transfection resulted in increased limb length (Bren-Mattison et al., 2011). These effects on limb size are generally tied to altered Ihh signaling during the processes of chondrocyte proliferation and differentiation and osteoblast differentiation in the growth plates in the long bones (Minina et al., 2002).
Interestingly, FGF activity has a differential impact on cell division depending on the stage of limb development. Studies in mammals and amphibians have shown that FGF signaling is essential for proliferation in the limb bud mesenchyme, while during post-embryonic limb maturation, FGFs participate in a negative feedback loop with Ihh in the growth plates (Coffin et al., 1995; Mancilla et al., 1998; Minina et al., 2002; Purushothaman et al., 2019). Gain-of-function mutations in both human and mice FGFR3 result in achondroplasia characterized by a short limb phenotype (Iwata et al., 2000, 2001; Lee et al., 2017; Segev et al., 2000), while knockout of FGFR3 in mice produces a long limb phenotype (Eswarakumar and Schlessinger, 2007; Toydemir et al., 2006; Tseng et al., 2010; Wen et al., 2016) (Table 1). Furthermore, knockout of FGFR3 has been directly tied to increased Ihh and BMP signaling within the elongating skeletal tissue in mice (Wen et al., 2016).
BMPs also participate in a negative feedback loop with FGFs in the developing limb, and the inhibition of FGFs by BMPs is particularly important for the activation of Sox9 expression, which is essential for chondrogenesis of the developing skeletal tissue in avian and mammalian limb buds (Chimal-Monroy et al., 2003; Yoon et al., 2006; Norrie et al., 2014). The negative feedback between BMP and FGF signaling is also present in the growth pates of mammalian long bones (Olsen et al., 2000; Chen et al., 2012; Studer et al., 2012; Wei et al., 2016). Overexpression of BMP2 and BMP4 ligands increases skeletal element size during chick limb development (Duprez et al., 1996). Moreover, inhibiting BMP signaling, via mutations in the receptors or downstream genes, leads to a shortened limb phenotype in mouse models (Evers et al., 1996; Barna et al., 2000; Settle et al., 2003; Klüppel et al., 2005; Lallemand et al., 2005; Aizawa et al., 2012; Bhattacharyya et al., 2015; Zhang et al., 2020). BMP signaling is essential for chondrocyte proliferation and differentiation in mouse growth plates (Yoon et al., 2006). Additionally, when BMP signaling is not present, FGFR1 expression is elevated, which further represses the elongation of the long bones in both chicken and mouse models (Chimal-Monroy et al., 2003; Yoon et al., 2006; Norrie et al., 2014).
Both long and short limb phenotypes are additionally observed in mutations that affect TGFβ signaling. TGFβ’s regulate the construction and destruction of skeletal tissue by modulating the activity of osteoblasts and osteoclasts, respectively (Tang et al., 2009). Mutations in the human TGFβ1 gene causes Camurati-Engelmann disease, one characteristic of which is elongated limbs (Kinoshita et al., 2000; Janssens et al., 2003). Additionally, mutations in Fibrillin1 (FBN1), a TGFβ-binding partner, can lead to congenital syndromes (Marfan syndrome and Weill-Marchesani) in humans that result in either elongated or shortened limbs (Goff et al., 2011; Quarto et al., 2012). Fibrillin1 is an extracellular matrix glycoprotein necessary for microfibril associated signal transduction (Goff et al., 2011; Quarto et al., 2012). The human mutations largely reside in the TGFβ-binding domain, decreasing FBN1’s ability to sequester TGFβ ligands in the extracellular matrix, and increasing the bioavailability of TGFβ ligands (Goff et al., 2011; Quarto et al., 2012). It is unknown how the increased TGFβ activity observed in both Marfan syndrome and Weill-Marchesani syndromes lead to long and short limbs respectively, but the key difference might rely on the cell types that TGFβ signaling is hyperactivated in.
C-type natriuretic peptides, mostly known for their role in kidney function, also play a crucial role in limb sizing through chondrocyte regulation (Potter et al., 2009). Natriuretic peptide type C (NPC) activates the receptor (NPR-B or NPR2) to drive the synthesis of the second messenger, cGMP (Potter et al., 2009). In human patients, loss-of-function mutations in NPR2 result in shortened limbs, while gain-of-function mutations cause Acromesomelic Dysplasia, Maroteaux Type, characterized by elongated limbs (Bartels et al., 2004; Faivre et al., 2000; Hannema et al., 2013; Ianakiev et al., 2000; Jiao et al., 2007; Kant et al., 1998; Lane and Dickie, 1968; Miura et al., 2012, 2014). The limb length phenotypes due to these mutations appear to be caused by effects on chondrocyte proliferation and differentiation, supporting the hypothesis that the regulation of the long bone growth pates is critical in determining overall scaling of the limb (Lane and Dickie, 1968; Kant et al., 1998; Faivre et al., 2000; Ianakiev et al., 2000; Bartels et al., 2004; Jiao et al., 2007; Potter et al., 2009; Miura et al., 2012, 2014; Hannema et al., 2013).
Cell and Tissue Physiology Genes
Limb development and elongation requires that the cells are healthy enough to respond to the factors that regulate allometric growth. Thus, it is not surprising that gene mutations that negatively impact various aspects of cell physiology in the limb bud and immature limb will ultimately impact limb size. All the genes that fall under this category, including those that regulate lipid biosynthesis (Wanders et al., 1992; Clayton et al., 1994; Ofman et al., 1998; Thai et al., 2001; Rodemer et al., 2003; Nimmo et al., 2010; Itzkovitz et al., 2012), ion transport (Li et al., 1999; Kornak et al., 2001; Neutzsky-Wulff et al., 2008; Camacho et al., 2010; Weinstein et al., 2014), cell proliferation, and DNA damage repair (Vernersson Lindahl et al., 2013) have only been found to negatively impact limb size in mice and humans, suggesting that these factors may play permissive rather than instructive roles.
Post-Embryonic Size Regulation
Homeostasis
The maintenance of the appropriate limb size during tissue homeostasis depends on both the developmental stage of the animal, and whether it is a determinant or indeterminately growing species (Figure 1). In animals that have determinant growth, the lenth of limbs can be impacted up until the initiation of adulthood. In humans, limb elongation ends in late puberty, when the growth plates fuse and are no longer susceptible to the signals that promote their growth (reviewed in Shim, 2015). For example, altered nerve signaling in the limbs of pre-adult humans can result in a phenomenon known as macrodactyly, where one or more digits grows disproportionally larger than the other (Tsuge and Ikuta, 1973; Frykman and Wood, 1978; Razzaghi and Anastakis, 2005). In contrast, indeterminately growing species grow throughout their entire lives, and thus maintain active growth plates as adults (Riquelme-Guzmán et al., 2021). This indicates that growth plate activity must be continuously regulated in these limbs to maintain a size that is proportionally appropriate.
Regeneration
Regeneration of adult limbs presents additional challenges that are nonexistent during embryonic/larval development. The injured limb is much larger than it was during embryonic development, and this larger size must be re-established to regain full function. While humans cannot regenerate their limbs, researchers are actively working to understand the mechanisms by which other species, such as the mouse and the Mexican axolotl (Ambystoma mexicanum), are capable of regenerating with the hopes that the knowledge is transferable to humans. While mice regenerate digit tips, the axolotl are able to regenerate complete limb structures (McCusker et al., 2015; Dolan et al., 2018). Thus, the factors that regulate the growth of the regenerate can have a large impact on the overall size of the limb in the axolotl model. Axolotl are also an indeterminately growing species. This creates an interesting paradigm since the regenerating limb must grow to a size larger than it was at the time of amputation to accommodate the animal’s growing body length. How this growth is regulated is unknown, and studies on this aspect of regeneration in the axolotl are challenging because of the extended period it takes for a regenerated limb to reach its “completed” size.
Blastema Development
Limb regeneration begins with the formation of a transient organ known as the limb blastema, which shares many molecular and functional similarities with the embryonic limb bud. Thus, it is reasonable to postulate that the modulation of factors that influence growth at this early stage in limb regeneration are conserved between limb development and regeneration. Because of the ease of loss of function approaches in the regenerating system, most of the manipulations that have led to sizing defects are a result of inhibition of signaling pathways that are essential during the early steps of blastema development. For example, pharmaceutical inhibition of FGF, BMP, or TGFβ signaling in the blastema all result in smaller limbs by impacting patterning, tissue differentiation, or the overall physiology in the blastema (Lévesque et al., 2007; Purushothaman et al., 2019; Vincent et al., 2020). Recently, it was observed that the repeated removal of the axolotl limb bud resulted in the formation of permanently miniaturized limbs (Bryant et al., 2017). Interestingly, these miniaturized limbs have a decreased abundance of limb nerves, which play a central role in the activation of key paracrine signals, such as FGFS and BMPs, during blastemal development (Makanae et al., 2014; Satoh et al., 2016; Bryant et al., 2017). Thus, the formation of the miniaturized size following limb amputation is likely related, in part, to diminished activation of these essential pathways.
To date, the only known signal that has been shown to positively influence the length of the regenerating limb is Retinoic Acid (RA). RA signaling is essential for pattern formation in both the embryonic and regenerating limb. Treatment of the regenerating limb with exogenous RA results in the elongation of the skeletal elements, and at high levels, causes the duplication of proximal/distal limb elements (Maden, 1983; Niazi et al., 1985). These phenotypes could be linked to the effect of RA on multiple transcription factors including HOXs that are essential in limb pattern formation (Gardiner and Bryant, 1996).
We have recently focused on the regulation of sizing of the axolotl limb regenerate during the maturation stages (Wells et al., 2021). Following the blastema stage of development, the regenerated limb is patterned and differentiated, yet is proportionally small. The regenerating limb then undergoes a phase of rapid growth until it reaches the size that is proportionally appropriate to the body size and is indistinguishable in length to the unamputated limb. Once the appropriate size is reached, the regenerated limb slows its rate of growth to match that of the rest of the animal (Wells et al., 2021). How the growth of the regenerating limb is regulated is only beginning to be elucidated, and our lab has recently discovered that signaling from the limb nerves play a key role in this process (Wells et al., 2021). Although the molecular mechanisms by which nerves control growth in the regenerate remain unknown, we speculate based on the above-described observations from developing limbs that they may impact the activity of the long bone growth plates. Additionally, one fascinating outstanding question is how the growth of the limb regenerate slows once the proportionally appropriate size has been reached.
Summary
Tetrapods exhibit beautiful diversity in the proportionality, shape, and functionality of their limbs. Despite this, the underlying mechanisms that regulate limb growth, whether it is occurring in developing or regenerating limbs, appears to be well conserved. Mutant analyses indicate that factors that impact either limb bud patterning or the elongation of the long bones play the most important roles in limb size within an individual tetrapod species. So far, the limited data in regenerating limbs appears to follow the same rules, and thus studies in developing limbs can provide clues to better understanding post-embryonic limb growth. However, multiple aspects of regenerating limbs, such as how growth can be differentially regulated in a regenerating and non-injured limb on the same animal, and what the role of the nerves is in this regulation will likely only be resolved in regenerating species.
Author Contributions
KW contributed to the conception, research of the primary literature, and writing of the article. MB contributed to the conception and research of the primary literature for the article. CM contributed to the conception, research of the primary literature, and writing of the article.
Funding
The McCusker lab is supported by the Eunice Kennedy Shriver National Institute Of Child Health and Human Development of the National Institutes of Health under Award Number R15HD092180.
Conflict of Interest
The authors declare that the research was conducted in the absence of any commercial or financial relationships that could be construed as a potential conflict of interest.
Publisher’s Note
All claims expressed in this article are solely those of the authors and do not necessarily represent those of their affiliated organizations, or those of the publisher, the editors and the reviewers. Any product that may be evaluated in this article, or claim that may be made by its manufacturer, is not guaranteed or endorsed by the publisher.
Supplementary Material
The Supplementary Material for this article can be found online at: https://www.frontiersin.org/articles/10.3389/fcell.2021.768505/full#supplementary-material
References
Aizawa, R., Yamada, A., Suzuki, D., Iimura, T., Kassai, H., Harada, T., et al. (2012). Cdc42 Is Required for Chondrogenesis and Interdigital Programmed Cell Death during Limb Development. Mech. Dev. 129, 38–50. doi:10.1016/j.mod.2012.02.002
Akiyama, H., Chaboissier, M. C., Martin, J. F., Schedl, A., and De Crombrugghe, B. (2002). The Transcription Factor Sox9 Has Essential Roles in Successive Steps of the Chondrocyte Differentiation Pathway and Is Required for Expression of Sox5 and Sox6. Genes Dev. 16, 2813–2828. doi:10.1101/gad.1017802
Akiyama, H., Stadler, H. S., Martin, J. F., Ishii, T. M., Beachy, P. A., Nakamura, T., et al. (2007). Misexpression of Sox9 in Mouse Limb Bud Mesenchyme Induces Polydactyly and Rescues Hypodactyly Mice. Matrix Biol. 26, 224–233. doi:10.1016/j.matbio.2006.12.002
Barna, M., Hawe, N., Niswander, L., and Pandolfi, P. P. (2000). Plzf Regulates Limb and Axial Skeletal Patterning. Nat. Genet. 25, 166–172. doi:10.1038/76014
Bartels, C. F., Bükülmez, H., Padayatti, P., Rhee, D. K., Van Ravenswaaij-Arts, C., Pauli, R. M., et al. (2004). Mutations in the Transmembrane Natriuretic Peptide Receptor NPR-B Impair Skeletal Growth and Cause Acromesomelic Dysplasia, Type Maroteaux. Am. J. Hum. Genet. 75, 27–34. doi:10.1086/422013
Bhattacharyya, S., Feferman, L., and Tobacman, J. K. (2015). Regulation of Chondroitin-4-Sulfotransferase (CHST11) Expression by Opposing Effects of Arylsulfatase B on BMP4 and Wnt9A. Biochim. Biophys. Acta - Gene Regul. Mech. 1849, 342–352. doi:10.1016/j.bbagrm.2014.12.009
Bren-Mattison, Y., Hausburg, M., and Olwin, B. B. (2011). Growth of Limb Muscle Is Dependent on Skeletal-Derived Indian Hedgehog. Dev. Biol. 356, 486–495. doi:10.1016/j.ydbio.2011.06.002
Bryant, D. M., Sousounis, K., Farkas, J. E., Bryant, S., Thao, N., Guzikowski, A. R., et al. (2017). Repeated Removal of Developing Limb Buds Permanently Reduces Appendage Size in the Highly-Regenerative Axolotl. Dev. Biol. 424, 1–9. doi:10.1016/j.ydbio.2017.02.013
Camacho, N., Krakow, D., Johnykutty, S., Katzman, P. J., Pepkowitz, S., Vriens, J., et al. (2010). Dominant TRPV4 Mutations in Nonlethal and Lethal Metatropic Dysplasia. Am. J. Med. Genet. A. 152A, 1169–1177. doi:10.1002/ajmg.a.33392
Caparrós-Martín, J. A., Valencia, M., Reytor, E., Pacheco, M., Fernandez, M., Perez-Aytes, A., et al. (2013). The Ciliary EVC/EVC2 Complex Interacts with Smo and Controls Hedgehog Pathway Activity in Chondrocytes by Regulating Sufu/Gli3 Dissociation and Gli3 Trafficking in Primary Cilia. Hum. Mol. Genet. 22, 124–139. doi:10.1093/hmg/dds409
Chen, G., Deng, C., and Li, Y. P. (2012). TGF-β and BMP Signaling in Osteoblast Differentiation and Bone Formation. Int. J. Biol. Sci. 8, 272–288. doi:10.7150/ijbs.2929
Chimal-Monroy, J., Rodriguez-Leon, J., Montero, J. A., Gañan, Y., Macias, D., Merino, R., et al. (2003). Analysis of the Molecular cascade Responsible for Mesodermal Limb Chondrogenesis: Sox Genes and BMP Signaling. Dev. Biol. 257, 292–301. doi:10.1016/s0012-1606(03)00066-6
Cho, K. W., Kim, J. Y., Cho, J. W., Cho, K. H., Song, C. W., and Jung, H. S. (2008). Point Mutation of Hoxd12 in Mice. Yonsei Med. J. 49, 965–972. doi:10.3349/ymj.2008.49.6.965
Clayton, P. T., Eckhardt, S., Wilson, J., Hall, C. M., Yousuf, Y., Wanders, R. J., et al. (1994). Isolated Dihydroxyacetonephosphate Acyltransferase Deficiency Presenting with Developmental Delay. J. Inherit. Metab. Dis. 17, 533–540. doi:10.1007/BF00711587
Coffin, J. D., Florkiewicz, R. Z., Neumann, J., Mort-Hopkins, T., Dorn, G. W., Lightfoot, P., et al. (1995). Abnormal Bone Growth and Selective Translational Regulation in Basic Fibroblast Growth Factor (FGF-2) Transgenic Mice. Mol. Biol. Cel 6, 1861–1873. doi:10.1091/mbc.6.12.1861
Cretekos, C. J., Wang, Y., Green, E. D., Martin, J. F., Rasweiler, J. J., and Behringer, R. R. (2008). Regulatory Divergence Modifies Limb Length between Mammals. Genes Dev. 22, 141–151. doi:10.1101/gad.1620408
Dolan, C. P., Dawson, L. A., and Muneoka, K. (2018). Digit Tip Regeneration: Merging Regeneration Biology with Regenerative Medicine. Stem Cell Transl Med 7, 262–270. doi:10.1002/sctm.17-0236
Duprez, D., Bell, E. J., Richardson, M. K., Archer, C. W., Wolpert, L., Brickell, P. M., et al. (1996). Overexpression of BMP-2 and BMP-4 Alters the Size and Shape of Developing Skeletal Elements in the Chick Limb. Mech. Dev. 57, 145–157. doi:10.1016/0925-4773(96)00540-0
Dy, P., Han, Y., and Lefebvre, V. (2008). Generation of Mice Harboring a Sox5 Conditional Null Allele. Genesis 46, 294–299. doi:10.1002/dvg.20392
Eswarakumar, V. P., and Schlessinger, J. (2007). Skeletal Overgrowth Is Mediated by Deficiency in a Specific Isoform of Fibroblast Growth Factor Receptor 3. Proc. Natl. Acad. Sci. U. S. A. 104, 3937–3942. doi:10.1073/pnas.0700012104
Evers, M., Saftig, P., Schmidt, P., Hafner, A., McLoghlin, D. B., Schmahl, W., et al. (1996). Targeted Disruption of the Arylsulfatase B Gene Results in Mice Resembling the Phenotype of Mucopolysaccharidosis VI. Proc. Natl. Acad. Sci. U. S. A. 93, 8214–8219. doi:10.1073/pnas.93.16.8214
Faivre, L., Le Merrer, M., Megarbane, A., Gilbert, B., Mortier, G., Cusin, V., et al. (2000). Exclusion of Chromosome 9 Helps to Identify Mild Variants of Acromesomelic Dysplasia Maroteaux Type. J. Med. Genet. 37, 52–54. doi:10.1136/jmg.37.1.52
Fromental-Ramain, C., Warot, X., Messadecq, N., LeMeur, M., Dollé, P., and Chambon, P. (1996). Hoxa-13 and Hoxd-13 Play a Crucial Role in the Patterning of the Limb Autopod. Development 122, 2997–3011. doi:10.1242/dev.122.10.2997
Frykman, G. K., and Wood, V. E. (1978). Peripheral Nerve Hamartoma with Macrodactyly in the Hand: Report of Three Cases and Review of the Literature. J. Hand Surg. 3, 307–312. doi:10.1016/s0363-5023(78)80029-x
Gardiner, D. M., and Bryant, S. V. (1996). Molecular Mechanisms in the Control of Limb Regeneration: The Role of Homeobox Genes. Int. J. Dev. Biol. 40, 797–805.
Hannema, S. E., Van Duyvenvoorde, H. A., Premsler, T., Yang, R. B., Mueller, T. D., Gassner, B., et al. (2013). An Activating Mutation in the Kinase Homology Domain of the Natriuretic Peptide Receptor-2 Causes Extremely Tall Stature without Skeletal Deformities. J. Clin. Endocrinol. Metab. 98, E1988–E1998. doi:10.1210/jc.2013-2358
Hérault, Y., Hraba-Renevey, S., Van Der Hoeven, F., and Duboule, D. (1996). Function of the Evx-2 Gene in the Morphogenesis of Vertebrate Limbs. EMBO J. 15, 6727–6738. doi:10.1002/j.1460-2075.1996.tb01062.x
Ianakiev, P., Kilpatrick, M. W., Daly, M. J., Zolindaki, A., Bagley, D., Beighton, G., et al. (2000). Localization of an Acromesomelic Dysplasia on Chromosome 9 by Homozygosity Mapping. Clin. Genet. 57, 278–283. doi:10.1034/j.1399-0004.2000.570406.x
Itzkovitz, B., Jiralerspong, S., Nimmo, G., Loscalzo, M., Horovitz, D. D., Snowden, A., et al. (2012). Functional Characterization of Novel Mutations in GNPAT and AGPS, Causing Rhizomelic Chondrodysplasia Punctata (RCDP) Types 2 and 3. Hum. Mutat. 33, 189–197. doi:10.1002/humu.21623
Iwata, T., Chen, L., Li, C., Ovchinnikov, D. A., Behringer, R. R., Francomano, C. A., et al. (2000). A Neonatal Lethal Mutation in FGFR3 Uncouples Proliferation and Differentiation of Growth Plate Chondrocytes in Embryos. Hum. Mol. Genet. 9, 1603–1613. doi:10.1093/hmg/9.11.1603
Iwata, T., Li, C. L., Deng, C. X., and Francomano, C. A. (2001). Highly Activated Fgfr3 with the K644M Mutation Causes Prolonged Survival in Severe dwarf Mice. Hum. Mol. Genet. 10, 1255–1264. doi:10.1093/hmg/10.12.1255
Janssens, K., Ten Dijke, P., Ralston, S. H., Bergmann, C., and Van Hul, W. (2003). Transforming Growth Factor-Beta 1 Mutations in Camurati-Engelmann Disease lead to Increased Signaling by Altering Either Activation or Secretion of the Mutant Protein. J. Biol. Chem. 278, 7718–7724. doi:10.1074/jbc.M208857200
Jiao, Y., Yan, J., Jiao, F., Yang, H., Donahue, L. R., Li, X., et al. (2007). A Single Nucleotide Mutation in Nppc Is Associated with a Long Bone Abnormality in Lbab Mice. BMC Genet. 8, 16. doi:10.1186/1471-2156-8-16
Joeng, K. S., and Long, F. (2009). The Gli2 Transcriptional Activator Is a Crucial Effector for Lhh Signaling in Osteoblast Development and Cartilage Vascularization. Development 136, 4177–4185. doi:10.1242/dev.041624
Kant, S. G., Polinkovsky, A., Mundlos, S., Zabel, B., Thomeer, R. T., Zonderland, H. M., et al. (1998). Acromesomelic Dysplasia Maroteaux Type Maps to Human Chromosome 9. Am. J. Hum. Genet. 63, 155–162. doi:10.1086/301917
Kim, Y. J., Osborn, D. P., Lee, J. Y., Araki, M., Araki, K., Mohun, T., et al. (2018). WDR11-mediated Hedgehog Signalling Defects Underlie a New Ciliopathy Related to Kallmann Syndrome. EMBO Rep. 19, 269–289. doi:10.15252/embr.201744632
Kinoshita, A., Saito, T., Tomita, H., Makita, Y., Yoshida, K., Ghadami, M., et al. (2000). Domain-specific Mutations in TGFB1 Result in Camurati-Engelmann Disease. Nat. Genet. 26, 19–20. doi:10.1038/79128
Klüppel, M., Wight, T. N., Chan, C., Hinek, A., and Wrana, J. L. (2005). Maintenance of Chondroitin Sulfation Balance by Chondroitin-4-Sulfotransferase 1 Is Required for Chondrocyte Development and Growth Factor Signaling during Cartilage Morphogenesis. Development 132, 3989–4003. doi:10.1242/dev.01948
Kornak, U., Kasper, D., Bösl, M. R., Kaiser, E., Schweizer, M., Schulz, A., et al. (2001). Loss of the ClC-7 Chloride Channel Leads to Osteopetrosis in Mice and Man. Cell 104, 205–215. doi:10.1016/s0092-8674(01)00206-9
Lallemand, Y., Nicola, M. A., Ramos, C., Bach, A., Cloment, C. S., and Robert, B. (2005). Analysis of Msx1; Msx2 Double Mutants Reveals Multiple Roles for Msx Genes in Limb Development. Development 132, 3003–3014. doi:10.1242/dev.01877
Lane, P. W., and Dickie, M. M. (1968). Three Recessive Mutations Producing Disproportionate Dwarfing in Mice: Achondroplasia, Brachymorphic, and Stubby. J. Hered. 59, 300–308. doi:10.1093/oxfordjournals.jhered.a107725
Le Goff, C., Mahaut, C., Wang, L. W., Allali, S., Abhyankar, A., Jensen, S., et al. (2011). Mutations in the TGFβ Binding-protein-like Domain 5 of FBN1 Are Responsible for Acromicric and Geleophysic Dysplasias. Am. J. Hum. Genet. 89, 7–14. doi:10.1016/j.ajhg.2011.05.012
Lee, Y. C., Song, I. W., Pai, Y. J., Chen, S. D., and Chen, Y. T. (2017). Knock-in Human FGFR3 Achondroplasia Mutation as a Mouse Model for Human Skeletal Dysplasia. Sci. Rep. 7, 43220. doi:10.1038/srep43220
Lefebvre, V., Behringer, R. R., and De Crombrugghe, B. (2001). L-Sox5, Sox6 and SOx9 Control Essential Steps of the Chondrocyte Differentiation Pathway. Osteoarthritis Cartilage 9, S69–S75. doi:10.1053/joca.2001.0447
Lévesque, M., Gatien, S., Finnson, K., Desmeules, S., Villiard, E., Pilote, M., et al. (2007). Transforming Growth Factor: Beta Signaling Is Essential for Limb Regeneration in Axolotls. PLoS One 2, e1227. doi:10.1371/journal.pone.0001227
Li, Y. P., Chen, W., Liang, Y., Li, E., and Stashenko, P. (1999). Atp6i-deficient Mice Exhibit Severe Osteopetrosis Due to Loss of Osteoclast-Mediated Extracellular Acidification. Nat. Genet. 23, 447–451. doi:10.1038/70563
Liu, C. F., and Lefebvre, V. (2015). The Transcription Factors SOX9 and SOX5/SOX6 Cooperate Genome-wide through Super-enhancers to Drive Chondrogenesis. Nucleic Acids Res. 43, 8183–8203. doi:10.1093/nar/gkv688
Long, F., Zhang, X. M., Karp, S., Yang, Y., and McMahon, A. P. (2001). Genetic Manipulation of Hedgehog Signaling in the Endochondral Skeleton Reveals a Direct Role in the Regulation of Chondrocyte Proliferation. Development 128, 5099–5108. doi:10.1242/dev.128.24.5099
Long, H. K., Osterwalder, M., Welsh, I. C., Hansen, K., Davies, J. O. J., Liu, Y. E., et al. (2020). Loss of Extreme Long-Range Enhancers in Human Neural Crest Drives a Craniofacial Disorder. Cell Stem Cell 27, 765–e14. doi:10.1016/j.stem.2020.09.001
Maden, M. (1983). The Effect of Vitamin A on the Regenerating Axolotl Limb. J. Embryol. Exp. Morphol. 77, 273–295. doi:10.1242/dev.77.1.273
Makanae, A., Mitogawa, K., and Satoh, A. (2014). Co-operative Bmp- and Fgf-Signaling Inputs Convert Skin Wound Healing to Limb Formation in Urodele Amphibians. Dev. Biol. 396, 57–66. doi:10.1016/j.ydbio.2014.09.021
Mancilla, E. E., De Luca, F., Uyeda, J. A., Czerwiec, F. S., and Baron, J. (1998). Effects of Fibroblast Growth Factor-2 on Longitudinal Bone Growth. Endocrinology 139, 2900–2904. doi:10.1210/endo.139.6.6032
McCusker, C., Bryant, S. V., and Gardiner, D. M. (2015). The Axolotl Limb Blastema: Cellular and Molecular Mechanisms Driving Blastema Formation and Limb Regeneration in Tetrapods. Regeneration 2, 54–71. doi:10.1002/reg2.32
Minina, E., Kreschel, C., Naski, M. C., Ornitz, D. M., and Vortkamp, A. (2002). Interaction of FGF, Ihh/Pthlh, and BMP Signaling Integrates Chondrocyte Proliferation and Hypertrophic Differentiation. Dev. Cel 3, 439–449. doi:10.1016/s1534-5807(02)00261-7
Miura, K., Kim, O. H., Lee, H. R., Namba, N., Michigami, T., Yoo, W. J., et al. (2014). Overgrowth Syndrome Associated with a Gain-Of-Function Mutation of the Natriuretic Peptide Receptor 2 (NPR2) Gene. Am. J. Med. Genet. A. 164A, 156–163. doi:10.1002/ajmg.a.36218
Miura, K., Namba, N., Fujiwara, M., Ohata, Y., Ishida, H., Kitaoka, T., et al. (2012). An Overgrowth Disorder Associated with Excessive Production of Cgmp Due to a Gain-Of-Function Mutation of the Natriuretic Peptide Receptor 2 Gene. PLoS One 7, e42180. doi:10.1371/journal.pone.0042180
Mo, R., Freer, A. M., Zinyk, D. L., Crackower, M. A., Michaud, J., Heng, H. H. Q., et al. (1997). Specific and Redundant Functions of Gli2 and Gli3 Zinc finger Genes in Skeletal Patterning and Development. Development 124, 113–123. doi:10.1242/dev.124.1.113
Neutzsky-Wulff, A. V., Karsdal, M. A., and Henriksen, K. (2008). Characterization of the Bone Phenotype in ClC-7-Deficient Mice. Calcif. Tissue Int. 83, 425–437. doi:10.1007/s00223-008-9185-7
Niazi, I. A., Pescitelli, M. J., and Stocum, D. L. (1985). Stage-dependent Effects of Retinoic Acid on Regenerating Urodele Limbs. Wilhelm Roux’s Arch. Dev. Biol. 194, 355–363. doi:10.1007/bf00877373
Nimmo, G., Monsonego, S., Descartes, M., Franklin, J., Steinberg, S., and Braverman, N. (2010). Rhizomelic Chrondrodysplasia Punctata Type 2 Resulting from Paternal Isodisomy of Chromosome 1. Am. J. Med. Genet. A. 152A, 1812–1817. doi:10.1002/ajmg.a.33489
Norrie, J. L., Lewandowski, J. P., Bouldin, C. M., Amarnath, S., Li, Q., Vokes, M. S., et al. (2014). Dynamics of BMP Signaling in Limb Bud Mesenchyme and Polydactyly. Dev. Biol. 393, 270–281. doi:10.1016/j.ydbio.2014.07.003
Ofman, R., Hettema, E. H., Hogenhout, E. M., Caruso, U., Muijsers, A. O., and Wanders, R. J. (1998). Acyl-CoA:Dihydroxyacetonephosphate Acyltransferase: Cloning of the Human cDNA and Resolution of the Molecular Basis in Rhizomelic Chondrodysplasia Punctata Type 2. Hum. Mol. Genet. 7, 847–853. doi:10.1093/hmg/7.5.847
Olsen, B. R., Reginato, A. M., and Wang, W. (2000). Bone Development. Annu. Rev. Cel Dev. Biol. 16, 191–220. doi:10.1146/annurev.cellbio.16.1.191
Potter, L. R., Yoder, A. R., Flora, D. R., Antos, L. K., and Dickey, D. M. (2009). Natriuretic Peptides: Their Structures, Receptors, Physiologic Functions and Therapeutic Applications. Handb. Exp. Pharmacol. 191, 341–366. doi:10.1007/978-3-540-68964-5_15
Purushothaman, S., Elewa, A., and Seifert, A. W. (2019). Fgf-signaling Is Compartmentalized within the Mesenchyme and Controls Proliferation during Salamander Limb Development. Elife 8, e48507. doi:10.7554/eLife.48507
Quarto, N., Li, S., Renda, A., and Longaker, M. T. (2012). Exogenous Activation of BMP-2 Signaling Overcomes TGFβ-Mediated Inhibition of Osteogenesis in Marfan Embryonic Stem Cells and Marfan Patient-specific Induced Pluripotent Stem Cells. Stem Cells 30, 2709–2719. doi:10.1002/stem.1250
Razzaghi, A., and Anastakis, D. J. (2005). Lipofibromatous Hamartoma: Review of Early Diagnosis and Treatment. Can. J. Surg. 48, 394–399.
Razzaque, M. S., Soegiarto, D. W., Chang, D., Long, F., and Lanske, B. (2005). Conditional Deletion of Indian Hedgehog from Collagen Type 2alpha1-Expressing Cells Results in Abnormal Endochondral Bone Formation. J. Pathol. 207, 453–461. doi:10.1002/path.1870
Riquelme‐Guzmán, C., Schuez, M., Böhm, A., Knapp, D., Edwards‐Jorquera, S., Ceccarelli, A. S., et al. (2021). Postembryonic Development and Aging of the Appendicular Skeleton in Ambystoma mexicanum. Dev. Dyn, 1–20. doi:10.1002/dvdy.407
Rodemer, C., Thai, T. P., Brugger, B., Kaercher, T., Werner, H., Nave, K. A., et al. (2003). Inactivation of Ether Lipid Biosynthesis Causes Male Infertility, Defects in Eye Development and Optic Nerve Hypoplasia in Mice. Hum. Mol. Genet. 12, 1881–1895. doi:10.1093/hmg/ddg191
Ruiz-Perez, V. L., Blair, H. J., Rodrigues-Andres, M. E., Blanco, M. J., Wilson, A., Liu, Y. N., et al. (2007). Evc Is a Positive Mediator of Ihh-Regulated Bone Growth that Localises at the Base of Chondrocyte Cilia. Development 134, 2903–2912. doi:10.1242/dev.007542
Satoh, A., Makanae, A., Nishimoto, Y., and Mitogawa, K. (2016). FGF and BMP Derived from Dorsal Root Ganglia Regulate Blastema Induction in Limb Regeneration in Ambystoma mexicanum. Dev. Biol. 417, 114–125. doi:10.1016/j.ydbio.2016.07.005
Segev, O., Chumakov, I., Nevo, Z., Givol, D., Madar-Shapiro, L., Sheinin, Y., et al. (2000). Restrained Chondrocyte Proliferation and Maturation with Abnormal Growth Plate Vascularization and Ossification in Human FGFR-3(G380R) Transgenic Mice. Hum. Mol. Genet. 9, 249–258. doi:10.1093/hmg/9.2.249
Settle, S. H., Rountree, R. B., Sinha, A., Thacker, A., Higgins, K., and Kingsley, D. M. (2003). Multiple Joint and Skeletal Patterning Defects Caused by Single and Double Mutations in the Mouse Gdf6 and Gdf5 Genes. Dev. Biol. 254, 116–130. doi:10.1016/s0012-1606(02)00022-2
Smits, P., Li, P., Mandel, J., Zhang, Z., Deng, J. M., Behringer, R. R., et al. (2001). The Transcription Factors L-Sox5 and Sox6 Are Essential for Cartilage Formation. Dev. Cel 1, 277–290. doi:10.1016/s1534-5807(01)00003-x
Sohaskey, M. L., Yu, J., Diaz, M. A., Plaas, A. H., and Harland, R. M. (2008). JAWS Coordinates Chondrogenesis and Synovial Joint Positioning. Development 135, 2215–2220. doi:10.1242/dev.019950
St-Jacques, B., Hammerschmidt, M., and McMahon, A. P. (1999). Indian Hedgehog Signaling Regulates Proliferation and Differentiation of Chondrocytes and Is Essential for Bone Formation. Genes Dev. 13, 2072–2086. doi:10.1101/gad.13.16.2072
Studer, D., Millan, C., Öztürk, E., Maniura-Weber, K., and Zenobi-Wong, M. (2012). Molecular and Biophysical Mechanisms Regulating Hypertrophic Differentiation in Chondrocytes and Mesenchymal Stem Cells. Eur. Cel Mater 24, 118–135. doi:10.22203/ecm.v024a09
Tang, Y., Wu, X., Lei, W., Pang, L., Wan, C., Shi, Z., et al. (2009). TGF-beta1-induced Migration of Bone Mesenchymal Stem Cells Couples Bone Resorption with Formation. Nat. Med. 15, 757–765. doi:10.1038/nm.1979
Thai, T. P., Rodemer, C., Jauch, A., Hunziker, A., Moser, A., Gorgas, K., et al. (2001). Impaired Membrane Traffic in Defective Ether Lipid Biosynthesis. Hum. Mol. Genet. 10, 127–136. doi:10.1093/hmg/10.2.127
Toydemir, R. M., Brassington, A. E., Bayrak-Toydemir, P., Krakowiak, P. A., Jorde, L. B., Whitby, F. G., et al. (2006). A Novel Mutation in FGFR3 Causes Camptodactyly, Tall Stature, and Hearing Loss (CATSHL) Syndrome. Am. J. Hum. Genet. 79, 935–941. doi:10.1086/508433
Tseng, A.-S., Beane, W. S., Lemire, J. M., Masi, A., and Levin, M. (2010). Induction of Vertebrate Regeneration by a Transient Sodium Current. J. Neurosci. 30, 13192–13200. doi:10.1523/jneurosci.3315-10.2010
Tsuge, K., and Ikuta, Y. (1973). Macrodactyly and Fibro-Fatty Proliferation of the Median Nerve. Hiroshima J. Med. Sci. 22, 83–101.
Vernersson Lindahl, E., Garcia, E. L., and Mills, A. A. (2013). An Allelic Series of Trp63 Mutations Defines TAp63 as a Modifier of EEC Syndrome. Am. J. Med. Genet. A. 161A, 1961–1971. doi:10.1002/ajmg.a.36074
Vincent, E., Villiard, E., Sader, F., Dhakal, S., Kwok, B. H., and Roy, S. (2020). BMP Signaling Is Essential for Sustaining Proximo-Distal Progression in Regenerating Axolotl Limbs. Development 147, dev170829. doi:10.1242/dev.170829
Wanders, R. J., Schumacher, H., Heikoop, J., Schutgens, R. B., and Tager, J. M. (1992). Human Dihydroxyacetonephosphate Acyltransferase Deficiency: A New Peroxisomal Disorder. J. Inherit. Metab. Dis. 15, 389–391. doi:10.1007/BF02435984
Wei, X., Hu, M., Mishina, Y., and Liu, F. (2016). Developmental Regulation of the Growth Plate and Cranial Synchondrosis. J. Dent. Res. 95, 1221–1229. doi:10.1177/0022034516651823
Weinstein, M. M., Tompson, S. W., Chen, Y., Lee, B., and Cohn, D. H. (2014). Mice Expressing Mutant Trpv4 Recapitulate the Human TRPV4 Disorders. J. Bone Miner. Res. 29, 1815–1822. doi:10.1002/jbmr.2220
Wells, K., Kelley, K., Baumel, M., Vieira, W., and McCusker, C. (2021). Neural Control of Growth and Size in the Axolotl Limb Regenerate. Elife 10, e68584. doi:10.7554/elife.68584
Wen, X., Li, X., Tang, Y., Tang, J., Zhou, S., Xie, Y., et al. (2016). Chondrocyte FGFR3 Regulates Bone Mass by Inhibiting Osteogenesis. J. Biol. Chem. 291, 24912–24921. doi:10.1074/jbc.M116.730093
Yoon, B. S., Pogue, R., Ovchinnikov, D. A., Yoshii, I., Mishina, Y., Behringer, R. R., et al. (2006). BMPs Regulate Multiple Aspects of Growth-Plate Chondrogenesis through Opposing Actions on FGF Pathways. Development 133, 4667–4678. doi:10.1242/dev.02680
Yoshida, M., Hata, K., Takashima, R., Ono, K., Nakamura, E., Takahata, Y., et al. (2015). The Transcription Factor Foxc1 Is Necessary for Ihh-Gli2-Regulated Endochondral Ossification. Nat. Commun. 6, 6653. doi:10.1038/ncomms7653
Zakany, J., and Duboule, D. (2007). The Role of Hox Genes during Vertebrate Limb Development. Curr. Opin. Genet. Dev. 17, 359–366. doi:10.1016/j.gde.2007.05.011
Zhang, H., Takeda, H., Tsuji, T., Kamiya, N., Rajderkar, S., Louie, K., et al. (2015). Generation of Evc2/Limbin Global and Conditional KO Mice and its Roles during Mineralized Tissue Formation. Genesis 53, 612–626. doi:10.1002/dvg.22879
Keywords: limb development, limb regeneration, patterning, long bone growth, growth regulation
Citation: Wells KM, Baumel M and McCusker CD (2022) The Regulation of Growth in Developing, Homeostatic, and Regenerating Tetrapod Limbs: A Minireview. Front. Cell Dev. Biol. 9:768505. doi: 10.3389/fcell.2021.768505
Received: 31 August 2021; Accepted: 19 November 2021;
Published: 03 January 2022.
Edited by:
Jennifer R. Morgan, Marine Biological Laboratory (MBL), United StatesReviewed by:
Mimi Sammarco, Tulane University, United StatesBenedikt Hallgrimsson, University of Calgary, Canada
Copyright © 2022 Wells, Baumel and McCusker. This is an open-access article distributed under the terms of the Creative Commons Attribution License (CC BY). The use, distribution or reproduction in other forums is permitted, provided the original author(s) and the copyright owner(s) are credited and that the original publication in this journal is cited, in accordance with accepted academic practice. No use, distribution or reproduction is permitted which does not comply with these terms.
*Correspondence: Catherine D. McCusker, Y2F0aGVyaW5lLm1jY3Vza2VyQHVtYi5lZHU=