- Laboratory of Cellular and Molecular Biology, Center for Cancer Research, National Cancer Institute, Bethesda, MD, United States
Transforming Growth Factor-β (TGF-β) is a key regulator of embryonic development, adult tissue homeostasis, and lesion repair. In tumors, TGF-β is a potent inhibitor of early stage tumorigenesis and promotes late stage tumor progression and metastasis. Here, we review the roles of TGF-β as well as components of its signaling pathways in tumorigenesis. We will discuss how a core property of TGF-β, namely its ability to change cell differentiation, leads to the transition of epithelial cells, endothelial cells and fibroblasts to a myofibroblastoid phenotype, changes differentiation and polarization of immune cells, and induces metabolic reprogramming of cells, all of which contribute to the progression of epithelial tumors.
Introduction
Cellular communication is crucial during development, tissue maintenance and tissue repair, and miscommunication can result in loss of organismic integrity, disease and death of the organism. During tumorigenesis, cells start to proliferate uncontrollably and invade the surrounding tissues to the detriment of the organism. Although altered behavior of tumor cells is a major contributor to tumor growth, it is understood that the surrounding stroma not only tolerates but supports tumor growth. The stroma of solid tumors provides structural support and supplies nutrients to tumor cells, and when tumor cells metastasize to distant organs they might more easily grow in locations that provide suitable conditions. This seed-and-soil theory of metastatic growth was first coined by Paget (Paget, 1889). Fidler and Hart (Hart and Fidler, 1980) showed a century later that melanoma cells spread to lung or ovarian tissue but not to renal tissue independent of the primary tumor site and concluded that tumor growth indeed depends on properties of the tumor cells (seeds) and host (soil). We now understand that tumor cells affect stromal cells and vice versa, and that the crosstalk between different tumor compartments contributes to tumor progression (Bhowmick et al., 2004a,b; Kaplan et al., 2005; Stuelten et al., 2008; Van Hove et al., 2021).
Cells interact with each other and the surrounding acellular matrix by releasing and sensing regulatory molecules. One of the master regulators of tumor-stromal crosstalk is TGF-β. TGF-β instructs cell proliferation and death, cell metabolism, cell motility and migration, tissue repair, and organ development (Morikawa et al., 2016). In tumors, TGF-β acts as a tumor suppressor during early stages of tumorigenesis by inhibiting cell proliferation and promoting cell death. As tumors progress, TGF-β promotes tumor growth and metastasis by inducing a mesenchymal transition of epithelial and endothelial cells, inducing myofibroblastoid differentiation, altering differentiation and proliferation of immune cells, modulating matrix composition, and reprogramming cell metabolism (Roberts and Wakefield, 2003; Seoane and Gomis, 2017; Hua et al., 2020; Derynck et al., 2021). Through highly regulated, local activation, TGF-β has varied and context-dependent effects including the activation of specific Smad signaling cascades and alternative signaling pathways like PI3K/AKT or MAPK signaling; in addition, cross-talking with a multitude of signaling networks such as SDF1-, FGF- HGF-, EGF- or Hippo-, Wnt-, or Rho-signaling occurs (Mu et al., 2012; Luo, 2017; Zhang, 2017; Kim et al., 2018; Miyazono et al., 2018).
Transforming Growth Factor-β Signaling: Pathways and Mechanisms
TGF-β, which exists in three isoforms, is synthesized as a propeptide consisting of the active TGF-β and the latency associated protein (LAP). The propeptide is cleaved by furin or furin-like protease during maturation, but LAP and TGF-β remain strongly associated via non-covalent interactions. LAP is tethered to latent TGF-β binding protein (LTBP) or glycoprotein-A repetitions predominant proteins (GARPs) to form latent complexes that shield the active TGF-β and prevent it from binding to receptors (Robertson and Rifkin, 2016). As such, most of the TGF-β deposited in the extracellular space is inactive, although active TGF-β is observed in specific locations (Barcellos-Hoff et al., 1994). Bioavailability of TGF-β is additionally regulated by TGF-β-binding proteins like fibromodulin and decorin which sequester TGF-β and prevent it from binding to specific TGF-β receptors (Hinz, 2015; Khan and Marshall, 2016; Nastase et al., 2018; Aubert et al., 2021). Activation of latent TGF-β is a key step in the regulation of TGF-β-signaling activity. During activation, active TGF-β is released from the latent complex by local changes in pH or shear stress, TSP1-, tenascin- or integrin binding, or by proteolytic cleavage by matrix metallo- and other proteases. Of those, integrin-mediated TGF-β activation is of particular importance, and loss of integrin-mediated TGF-β1 activation mimics the phenotype of TGF-β1-null mice (Yang et al., 2007). Likewise, mice lacking αvβ6- and αvβ8-integrins mimic the abnormalities of TGF-β1- and TGF-β3-null mice (Aluwihare et al., 2009). Integrin-mediated TGF-β activation depends on the recognition and binding of LAP’s RGD motif by integrin αv. Two mechanisms of integrin-mediated TGF-β activation are known: traction force mediated release of active TGF-β, typically seen for αvβ6 integrin (Figure 1-1), and release of TGF-β by proteolytic cleavage of LAP, observed for αvβ8 integrin (Figure 1-2; Nolte and Margadant, 2020). Integrin αvβ6 is tethered to the actomyosin cytoskeleton. After binding LAP, αvβ6 integrins link the latent complex to the actomyosin cytoskeleton. Because the latent TGF-β complex is also connected to the extracellular matrix, actomyosin generated traction forces pull on and lead to conformational changes of the latent complex and release of active TGF-β (Buscemi et al., 2011; Klingberg et al., 2014; Hinz, 2015). Notably, in this model of traction force-mediated TGF-β activation the extracellular matrix provides the counterforce for actomyosin contraction; therefore, changes in matrix stiffness should affect the traction-force mediated release of TGF-β. Indeed, integrin-mediated TGF-β activation is more efficient in stiff matrix with an elastic modulus > 10 kPa than in soft matrix (Klingberg et al., 2014; Hinz, 2015; Hiepen et al., 2020). In contrast, integrin αvβ8 does not interact with the cytoskeleton and thus cannot release active TGF-β by mechanical force transduction. It instead requires a chaperone, GARP or LRRC33, and proteases such as MT1-MMP (MMP14) to proteolytically cleave LAP and release active TGF-β (Mu et al., 2002; Liénart et al., 2018).
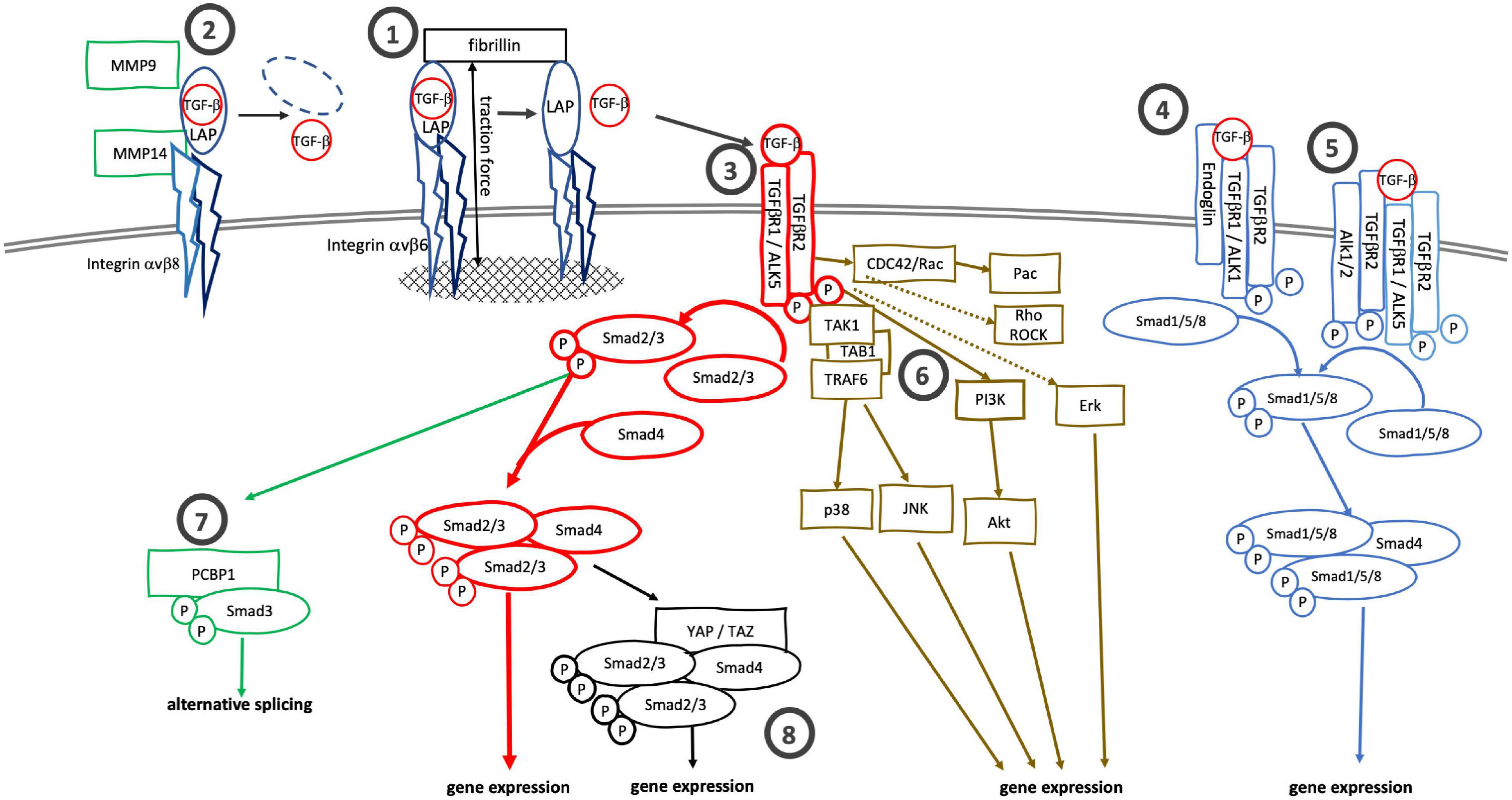
Figure 1. Canonical and alternative TGF-β signaling cascades. TGF-β can be activated by (1) traction force mediated release from the latent complex after binding to integrin αvβ6 or by (2) proteolysis after binding of the latent complex to integrin αvβ8. (3) TGF-β binds to specific receptors, TGFβR2 and TGFβR1 (ALK5), to initiate canonical Smad2/3 signaling. Alternatively, TGF-β can bind to (4) TGFβR2/ALK1/endoglin complexes or (5) TGFβR2/ALK5—TGFβR2/ALK1/2 complexes to activate Smad1/5/8 signaling. Alternatively to the Smad2/3 signaling cascade TGFβR2/ALK5 complexes can also activate MAPK-, Rho/ROCK- and cdc42/Rac/Pac signaling cascaded. pSmad3 itself can bind to (7) PCBP1 to support alternative mRNA splicing. (8) The binding of Smad2/3/Smad4 complexes to YAP/TAZ also alters gene expression profiles.
Once activated, TGF-β binds to TGF-β receptor type 2 (TGFβR2) to initiate signaling downstream. Upon binding TGF-β, TGFβR2 heterodimerizes with and phosphorylates TGF-β receptor type 1 (TGFβR1, ALK5) (Figure 1-3). In the canonical pathway, the activated receptor complex binds and phosphorylates receptor-regulated Smads (R-Smads), Smad2 and Smad3, which in turn heterotrimerize with the common Smad (Co-Smad), Smad4, to regulate TGF-β dependent gene expression (Shi and Massagué, 2003). The activity of the Smad signaling cascade is modulated by inhibitory Smads (I-Smad), Smad6 and Smad7, and Smurf1 and Smurf2, E3 ligases which ubiquitinylate TGF-β receptors and Smad proteins (Kavsak et al., 2000; Zhang et al., 2001; Tang et al., 2011; Nicklas and Saiz, 2013; Miyazawa and Miyazono, 2017; Yan et al., 2018; Sinha et al., 2021).
Several alternative TGF-β signaling cascades branch off the canonical signaling pathway beginning at the receptor level. Endoglin (CD105) is an accessory TGF-β receptor expressed in endothelial cells (Gougos and Letarte, 1988). Endoglin cannot bind TGF-β itself but does bind TGF-β1 and TGF-β3 when these interact with TGFβR3. Endoglin does not bind to TGF-β2 in any form (Barbara et al., 1999; Pawlak and Blobe, 2021). It facilitates the interaction of TGF-β and TGFβR2 with the non-classic type-1 receptor, ACVRL1/ALK1 (Nogués et al., 2020) and leads to a shift from TGF-β/TGFβR1/R2/Smad2/3 to TGF-β/ACVRL1/Smad1/5/8 signaling (Figure 1-4). Similarly, TGF-β can induce Smad1/5/8-signaling via formation of mixed TGFβR1/ALK5/ALK2 complexes (Ramachandran et al., 2018; Figure 1-5). In addition to Smad-signaling cascades, the activated TGFβR2/ALK5 receptor complex can activate TRAF6-TAB1-TAK1 and downstream p38 and JNK signaling (Sorrentino et al., 2008; Yamashita et al., 2008). The receptor complex can also activate PI3K/AKT signaling and feed into Ras/MEK/Erk, Rho/Rock, CDC42/Rac/Pac and Jak/Stat signaling cascades (Lee et al., 2007; Mu et al., 2012; Zhang et al., 2013; Tang L.-Y. et al., 2017; Zhang, 2017; Figure 1-6). Further downstream, activated Smad3 in the presence of CDK-, MAPK-, AKT- or PAK1-signaling can bind to PCBP1 and mediate alternative splicing (Tripathi et al., 2016; Figure 1-7).
Transforming Growth Factor-β Signaling in Tumors
Transforming Growth Factor-β, an Agent of Change
TGF-β is known as a potent growth inhibitor of cells of epithelial orgin, but it was first described and isolated based on its ability to transform cells and found expressed in different tumors and cell types (de Larco and Todaro, 1978; Roberts et al., 1980). In fact, TGF-β is secreted by and can act on most cells. The effects of active TGF-β are context specific (Guido et al., 2012). During development, TGF-β induces epithelial-mesenchymal transition (EMT) and facilitates gastrulation and organismic development as well as tissue repair (Thiery et al., 2009). Similarly, endothelial-mesenchymal transition (EndMT) and fibroblast-myofibroblasts transition is observed during development and tissue repair. Dysregulated EMT, EndMT and myofibroblastoid differentiation are seen in fibrotic diseases, vascular malformations, epithelial dedifferentiation and tumor growth; in advanced stages of cancers, TGF-β-induced EMT promotes tumor invasion, metastasis, and chemo-resistance (Tsubakihara and Moustakas, 2018; Katsuno and Derynck, 2021).
Cancer-Associated Fibroblasts—Bystanders Turned Culprit
Originally considered a mere presence in tumors, CAFs are now appreciated as active partners in tumor development. CAFs can modulate stemness, proliferation, invasion and dissemination of tumor cells, ECM composition, inflammatory infiltration, angiogenesis and drug resistance. They are derived from various progenitors including resident fibroblasts, mesenchymal stem cells, adipose tissue derived stem cells, and endothelial cells. Such diverse origins confer a marked heterogeneity of CAF gene expression profiles (Calon et al., 2014; Mezawa and Orimo, 2021). Nevertheless, a core signature of TGF-β regulated ECM genes has been identified in many CAFs and goes along with poor prognosis (Navab et al., 2011; Calon et al., 2015; Chakravarthy et al., 2018).
The roles of TGF-β signaling in CAFs have been demonstrated in more detail in breast cancer models, in which TGF-β and SDF1 are part of two autocrine and cross-talking signaling loops that drive myofibroblast/CAF development at the invasive front (Kojima et al., 2010; Yu et al., 2014). Increased expression of the TGF-β target SNAI1 in fibroblasts leads to increased SDF-1 secretion (Blanco-Gómez et al., 2020). The CAF-secreted TGF-β and SDF-1 promote angiogenesis by recruiting endothelial progenitor cells, and increase growth and EMT of tumor cells (Orimo et al., 2005; Yu et al., 2014; Matsumura et al., 2019). At the same time, autocrine myofibroblast TGF-β/SDF-1 signaling attenuates expression of CD26 (Dpp4), which can cleave SDF-1, such further increasing SDF-1 signaling (Mezawa et al., 2019). Thus, once triggered, this positive feedback loop maintains myofibroblast differentiation and supports tumor progression by targeting endothelial and tumor cells.
A consequence of fibroblast-myofibroblast transition, ECM secretion by CAFs changes such that matrix stiffness and density increases. This not only impacts migration of tumor cells through the matrix, immune infiltration, vascularization and drug delivery, it also affects cell differentiation and integrin signaling. Increased ECM stiffness directly impacts epithelial differentiation via increasing integrin clustering and Erk and Rho-signaling, and promoting a malignant phenotype (Wozniak et al., 2003; Paszek et al., 2005; Lu et al., 2012). In vivo, the elastic modulus of tumors increases as the tumor grows, and can reach 40–50 kPa (Samani et al., 2007; Kawano et al., 2015; Wang et al., 2017), making integrin-mediated TGF-β activation more effective (Klingberg et al., 2014; Hinz, 2015; Hiepen et al., 2020) and impacting tumor progression. Indeed, high αvβ6 expression correlates with worse prognosis in breast cancer, and integrin β6 neutralizing antibody decreased tumor growth in xenograft models of breast cancer (Moore et al., 2014). On a cellular level, integrin β1 signaling is necessary for TGF-β mediated p38-signaling and EMT in mammary epithelial cells (Bhowmick et al., 2001), and in basal carcinoma, αvβ6-mediated TGF-β activation in epithelial cells leads to fibroblast-myofibroblasts transition and secretion of HGF by myofibroblasts; HGF in turn promotes invasiveness of tumor cell (Marsh et al., 2008).
Cross-talk between activated TGF-β- and YAP/TAZ-signaling can further increase matrix stiffness via alternative signaling cascades (Figure 1–8). To this end, YAP associates with Smad7 to increase its affinity to the TGFβR1 and to increase its inhibitory effect on TGF-β signaling (Ferrigno et al., 2002). Further downstream, YAP can bind Smad3 to form a YAP-TEAD4–Smad3-p300 complex on the promotor of CTGF, a cytokine involved in EMT and tumor progression (Fujii et al., 2012; Sonnylal et al., 2013; Zhu et al., 2015). TAZ controls the nucleocytoplasmic localization of the Smad2/3-Smad4 complex by binding to Smad2/3-Smad4 and increasing nuclear accumulation of Smad2/3-Smad4 (Varelas et al., 2008). In both cases, the YAP/TAZ-Smad complexes increase the fibrotic response (Piersma et al., 2015). Matrix stiffness itself can increase YAP/TAZ activation (Dupont et al., 2011) as well as TGF-β activation, forming another positive feedback circle to drive tissue fibrosis and tumor progression.
Proteolytic degradation of the ECM, for example by MMPs, is also important for tumor progression. TGF-β regulates MMP expression and MMPs proteolytically activate TGF-β. For example, tumor cell derived TGF-β can increase MMP9-secretion by fibroblasts (Stuelten et al., 2005). MMP9 in turn can bind to CD44, and then proteolytically cleave LAP and release TGF-β in addition to remodeling the extracellular matrix (Yu and Stamenkovic, 2000). As MMPs are released into the extracellular space, activation of TGF-β by this mechanism is likely less localized than traction-force dependent αvβ6-mediated activation. Other differences between these two types of TGF-β activation are that αvbβ6-mediated activation is effective in ECM stiffness, while proteolytic activation might function in soft matrix and concurs with softening of the matrix as proteins like collagens are degraded. In turn, the degradation of ECM proteins by MMPs “opens” the matrix and might allow for smoother travel of tumor cells through the extracellular space.
In summary, CAFs contribute to tumor progression by changing ECM composition and stiffness as well as the cytokine microenvironment in the tumor. As CAF-mediated changes in matrix composition spread through the environment, one might hypothesize that the resulting changes in matrix stiffness and TGF-β activation contribute to the spread of malignant cell phenotypes through the surrounding environment.
Endothelia—More Than the Coating of the Vascular Wall
Tumors depend on blood supply for nutrients, and thus need to co-opt vessels in order to travel to distant sites. TGF-β can modulate neoangiogenesis and induce EndMT. TGF-β stimulates neoangiogenesis by inducing VEGF expression in tumor and stromal cells like macrophages in a Smad3-dependent manner (Donovan et al., 1997; Benckert et al., 2003; Kaminska et al., 2005; Sun et al., 2018). Further effects of TGF-β on endothelial cells are due the presence of the TGF-β Coreceptor Endoglin.
Endoglin has an important role in regulating angiogenesis and endothelial function (Cheifetz et al., 1992; Düwel et al., 2007; Albiñana et al., 2017). Endoglin is found to be overexpressed in the tumor neovasculature of brain, lung, breast, stomach and colon (Minhajat et al., 2006). In animal models, endoglin overexpression in tumor vasculature leads to leaky vessels with an incomplete mural coverage (Nogués et al., 2020; Ollauri-Ibáñez et al., 2020); on the other hand, haplo-insufficiency reduces the neovascularization and growth of Lewis lung tumors (Düwel et al., 2007). Mechanistically, endoglin shifts TGF-β signaling from canonical TGFβR2/ALK5-Smad2/3-signaling to the alternative TGFβR2/ALK1-Smad1/5/8 signaling cascade. While TGF-β/ALK5 signaling blocks cell proliferation, TGF-β/ALK1 signaling increases cell proliferation and motility (Lebrin et al., 2004). In addition, endoglin interacts with VEGFR2 in a VEGF-dependent manner to prevent its degradation to support tip cell formation (Tian et al., 2018). These observations support a general notion that increased endoglin expression shifts TGF-β signaling toward supporting tumor growth.
TGF-β-induced EndMT, similar to EMT, is characterized by upregulation of mesenchymal markers like α-SMA, FSP-1, vimentin and N-cadherin, by upregulation of transcription factors like Snail, Slug, Twist, and by downregulation of adhesion proteins like VE cadherin, CD31/PECAM-1 (Platel et al., 2019; Ma et al., 2020). This shift in gene expression results in endothelial cells undergoing EndMT. The loss of cell-cell contacts in the endothelial sheet during early EndMT facilitates the passing of tumor cells through the endothelial layer (Gasparics et al., 2016); later, endothelial cells acquire a pro-fibrotic phenotype with increased motility and a pro-inflammatory secretory profile, and finally convert into CAFs. Indeed, up to 40% of total CAFs in a tumor can be derived from endothelial cells (Zeisberg et al., 2007).
Mechanistically, EndMT is triggered by canonical TGF-β-signaling via ALK5/Smad2/3 or alternative signaling via TGF-β/ALK5/PI3K/Ras/TAK1 (Platel et al., 2019; Ma et al., 2020). The three TGF-β isoforms play different roles in EndMT. In colon cancer, TGF-β2 is the most important TGF-β isoform to induce EndMT (Wawro et al., 2018). Effects of TGF-β1 and TGF-β3 on EndMT are mediated by increased TGF-β2 secretion in immortalized human dermal endothelial cells, and knockdown of TGF-β2 blocks TGF-β1/2-induced EndMT (Sabbineni et al., 2018). Interestingly, the affinity of TGF-β1 and TGF-β3 to TGFβR2 is about 200–300-fold higher than that of TGF-β2 (Pawlak and Blobe, 2021). Thus, TGF-β1/3 induced ALK5 signaling might be active at low TGF-β concentrations and drive neoangiogenesis in the presence of endoglin, while TGF-β2 signaling is activate when high concentrations of TGF-β2 out-compete TGF-β1/3-binding to TGFβR2.
Transforming Growth Factor-β and the Immune System—Suppression and Polarization
TGF-β affects the immune response to tumors on several levels: it modulates accessibility of tumors for immune cells by increasing matrix density and regulating neoangiogenesis, and it regulates proliferation, differentiation and migration of immune cells.
Generally, tumor-derived TGF-β can attract myeloid and lymphoid cells, but it also leads to immunosuppression and immune evasion of tumors by changing proliferation and differentiation of residential T cells, neutrophils and macrophages, dendritic cells and NK cells (Batlle and Massagué, 2019; Brown and Marshall, 2019). Specifically, TGF-β inhibits T-cell proliferation as well as Th1 differentiation by inhibiting IL-2 expression, and together with other cytokines promotes Treg and Th17 differentiation (Zhang, 2018). Smad3/E4BP4 signaling inhibits NK cell development and reduces immune surveillance of melanoma and lung tumors (Tang P. M.-K. et al., 2017). Furthermore, tumor derived TGF-β together with other cytokines shifts the balance of tumor associated macrophages (TAM) and neutrophils (TAN) from TAM1 toward pro-tumorigenic TAM2 (Gong et al., 2012) and from TAN1 toward pro-tumorigenic TAN2 (Fridlender et al., 2009). Together, the shift toward Treg, Th17, M2 and N2 differentiation lead to a tolerogenic immune response to tumors.
The polarization of immune cells can increase their capacity to activate TGF-β. It is worth noting that immune cells, which have high motility and are not well anchored into the extracellular matrix, often employ αvbβ8-mediated TGF-β activation which relies on proteolytic TGF-β activation, rather than αvβ6-mediated activation which relies on traction forces and requires robust cell-matrix contacts. Integrin αvb8 is found on monocytes, macrophages, dendritic cells and Tregs (Fenton et al., 2017; Nolte and Margadant, 2020). Tregs, in contrast to naïve T cells, express high levels of αvβ8 and require it to release active TGF-β from the LAP/GARP complex, which in turn leads to Treg-mediated immunosuppression (Edwards et al., 2014; Stockis et al., 2017). αvβ8-activated TGF-β is necessary to quench inflammation and auto-immunity, but also to prevent anti-tumor immunity through increased Treg activity (Brown and Marshall, 2019). Likewise, αvβ8 expression on dendritic cells leads to immunosuppression (Travis et al., 2007; Fenton et al., 2017). Furthermore, αvβ8 is upregulated on M2- and downregulated on M1-macrophages (Kelly et al., 2018). In mouse models blocking of αvβ8 by monoclonal antibodies suppresses growth of squamous cell carcinoma, mammary cancer, colon cancer and prostate cancer, emphasizing the role αvβ8/TGF-β mediated immune tolerance of tumors (Dodagatta-Marri et al., 2020).
Changes of TGF-β expression and signaling in immune cells can also contribute to tumor progression. CD2-driven overexpression of TGF-β in T lymphocytes leads to delayed tumor development in dextran sodium sulfate/azoxymethane-induced colonic tumorigenesis (Becker et al., 2004). Smad3 null mice show a variety of abnormalities of the immune system, including an activated phenotype of T-lymphocytes, impaired chemotactic response of neutrophils to TGF-β, and chronic intestinal inflammation which can concur with colon tumors in aging mice (Yang et al., 1999). Loss of Smad4 in T lymphocytes increases pro-inflammatory cytokine expression and leads to increased development of epithelial tumors (Hahn et al., 2011).
Although high TGF-β-signaling in tumors leads to immune tolerance, loss of epithelial or fibroblast TGF-β signaling increases inflammation and promotes tumorigenesis: Epithelial loss of Smad4 increases inflammatory infiltration and development of dextran-sulfate-induced colon tumors; and loss of fibroblast TGF-βRII has been associated with increased inflammation, DNA damage in epithelial cells, and tumor formation in the forestomach (Achyut et al., 2013; Means et al., 2018). Thus, dysregulation of TGF-β signaling in different tumor compartments can modulate the immune response to promote tumorigenesis.
In tumor immune microenvironment, upregulated immune checkpoints protect cancer cells from immune killing (Munn and Bronte, 2016). PD-1/PD-L1 is the currently most studied immune checkpoint pathway. TGF-β has been shown to increase PD-1 expression on immune cells, while anti-PD-1 increases tumor cell pSMAD3 and can induce immunosuppression (Baas et al., 2016; Park et al., 2016; Dodagatta-Marri et al., 2019; Wu et al., 2020). Thus, blockade of TGF-β signaling enhances the effects of PD-1 inhibitors or overcomes primary resistance to PD-1 blockade in silico and in vivo (Terabe et al., 2017; Strauss et al., 2018; Chen et al., 2021; Siewe and Friedman, 2021).
Tumor Metabolism—A Symbiotic Relationship of Parenchymal and Mesenchymal Cells
To compensate for restricted blood and nutrient supply in tumors, another property of TGF-β comes in handy: it can shift the metabolism of cells in the tumor environment such that a symbiotic relationship between tumor cells and stromal cells results (Yoshida et al., 2019; Angioni et al., 2021).
Early on, it was observed that TGF-β increases glucose uptake and lactate secretion of cells (Inman and Colowick, 1985; Esposito et al., 1991). TGF-β signaling is now known to affect oxidative phosphorylation, the pentose phosphate pathway, glycolysis, fatty acid oxidation, and amino acid metabolism (Yadav et al., 2011; Angioni et al., 2021). In general, TGF-β shifts metabolism from mitochondrial oxidative phosphorylation toward a ketogenic metabolism, and EMT and EndMT, which are induced by TGF-β, can shift tumor and endothelial cell metabolism from oxidative phosphorylation toward anaerobic glycolysis (Angioni et al., 2021). Such a switching of the tumor metabolism from oxidative phosphorylation to anerobic glycolysis and lactate production was first described by Warburg (Warburg et al., 1927; Kim and Baek, 2021).
Mechanistically, auto- or paracrine TGF-β signaling reduces Cav-1 expression and concomitantly CD36 expression which leads to increased ROS production and HIF-1α stabilization. HIF-1α in turn increases glycolysis and increased lactate production (Guido et al., 2012; Heinzelmann et al., 2018; Yoshida et al., 2019). In tumor cells, TGF-β upregulates MCT1, increasing their capacity to uptake metabolites like lactate (Uddin et al., 2020).
The byproducts of anerobic glycolysis themselves have effects on cells and can further disturb cell and tissue physiology (Angioni et al., 2021). Specifically, lactate, which in tumors can be as high as 40 mM (Walenta et al., 2000), increases collagen production by fibroblasts and endothelial cells, endothelial cell migration and stimulates IL-8-dependent angiogenesis (Beckert et al., 2006; Végran et al., 2011). Lactate also has many effects on immune cells: it inhibits proliferation, cytokine production and cytotoxic activity of cytotoxic CD8 cells; increases ARG-1 expression in macrophages, such reducing T-cell activation and proliferation; and leads to differentiation of tolerogenic dendritic cells (Fischer et al., 2007; Nasi et al., 2013; Peter et al., 2015; Romero-Garcia et al., 2016).
In addition to its effects on energy metabolism, TGF-β-induced metabolic reprogramming of CAFs leads to increased reactive oxygen species (ROS) production and ROS accumulation by inactivation of CSK3 and the mitochondrial complex IV (Byun et al., 2012). The increased ROS levels in the tumor increase inflammation and DNA damage in tumor cells, and such further advance tumor progression.
TGF-β-mediated metabolic reprogramming of CAFs can spread to neighboring cells (Guido et al., 2012). Conceivably, once triggered, large parts of the tumor stroma might convert to a “Warburg-like” cancer metabolism. This metabolic flexibility would allow CAFs and other cells to better adapt to the changing demands of the tumor microenvironment to hypoxic and aerobic zones: in the fibrotic and hypoxic tumor core, tumor cells, fibroblasts and endothelial cells can utilize glucose by anaerobic glycolysis and secrete lactate and pyruvate, while at the oxygen-rich edges of the tumor lactate and pyruvate can be taken up by tumor cells, fibroblasts and endothelial cells and entered into the citrate cycle. In summary, TGF-β induces a metabolic plasticity that allows cells to successfully adapt to and thrive in the challenging and ever-changing tumor environment.
Conclusion
From its discovery 40 years ago to today, TGF-β has proven to be a major player in cell biology. The tightly regulated temporospatial activation of TGF-β as well as its wide network of canonical and alternative signaling cascades and cross-talking with other signaling networks lead to cell- and compartment specific effects. Aside from the suppression of tumor cell proliferation during the early phases of tumorigenesis, the effects of the universally present TGF-β on cells are many; in their core, they relate to cell metabolism and differentiation (Figure 2). It is these effects that explain TGF-β’s unique and multifaceted role in tumor progression, from stiffening of the tumor matrix, to neoangiogenesis, to immune tolerance, and to metabolic changes throughout the varying tumor areas. As a consequence, tumor and other cells acquire increased adaptability that enables them to thrive in hypoxic, nutrient poor and stiff tumor areas as well as in the more pliable, well vascularized marginal areas, and to contribute to tumor progression.
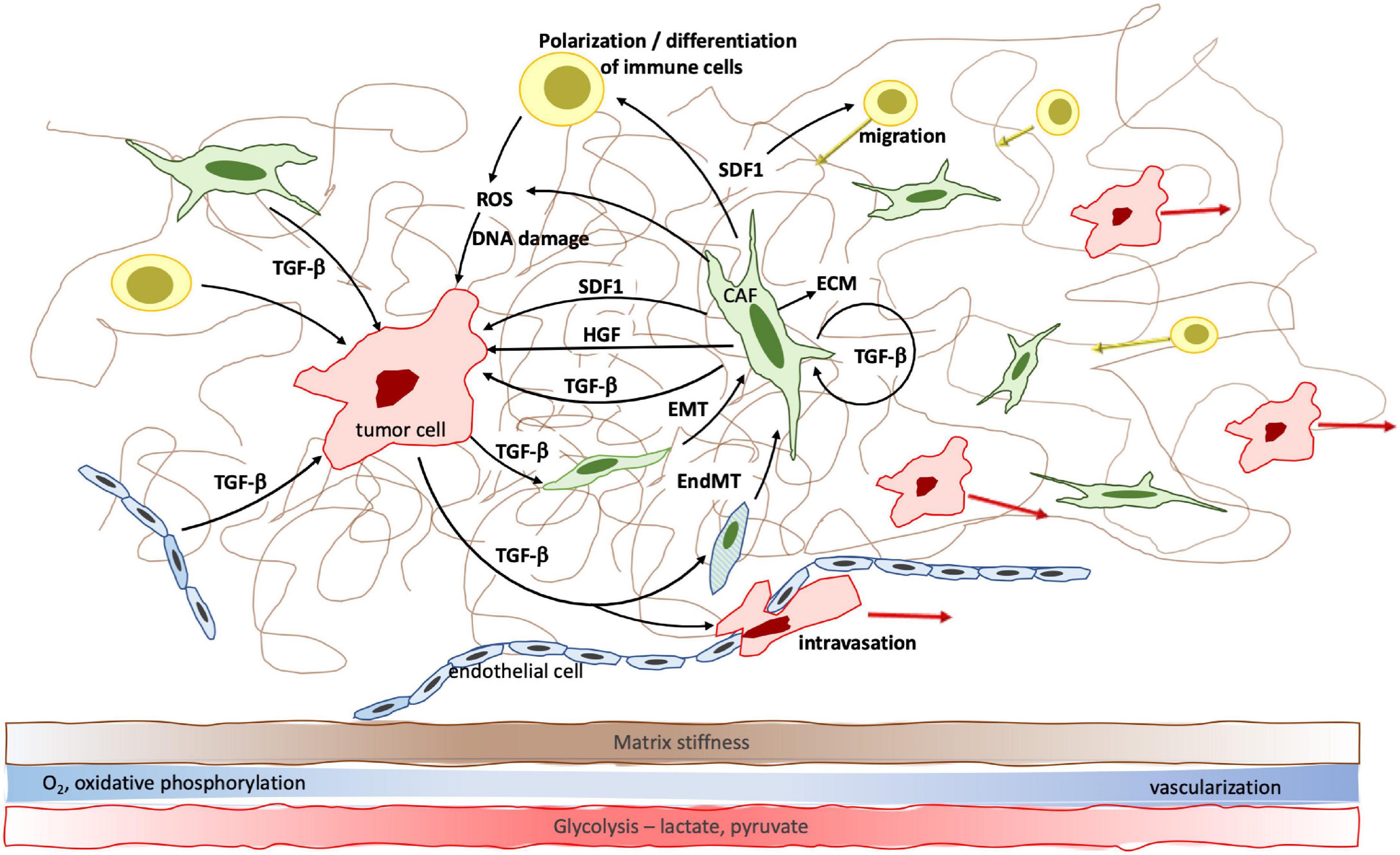
Figure 2. TGF-β facilitates cell-cell communication within the tumor microenvironment and changes cell differentiation, polarization and metabolism to promote tumor growth. Specifically, TGF-β induces fibroblast—myofibroblast transition, epithelial—mesenchymal transition (EMT) and endothelial—mesenchymal transition (EndMT) which result in increased cancer associated fibroblast (CAF) density. CAFs significantly contribute to increased matrix stiffness. EndMT furthermore leads to reduced endothelial cell-cell contacts which facilitates transmigration of tumor cells and metastatic spread. The effect of TGF-β on cell metabolism leads to a shift from oxidative phosphorylation to anaerobic glycolysis and accumulation of lactate, pyruvate and genome-damaging ROS in the hypoxic tumor center.
While the mechanisms by which TGF-β exerts its functions are increasingly unraveled, many questions still remain. How are some of the effects of TGF-β compartment specific when cells are exposed to TGF-β from different sources, that is, when fibroblasts respond to tumor cell derived TGF-β but not their own, how do they sense the difference? And regarding the activation of TGF-β one wonders: Does integrin-binding of LAP merely serve the release of active TGF-β, or also lead to active integrin signaling? Does LAP have additional functions once TGF-β is released?
As research into the mechanism of TGF-β signaling is ongoing, several clinical studies exploring the effect of modifying TGF-β signaling on tumor growth have been launched in the past two decades, starting with the pan-TGF-β binding antibody ID11. Other strategies employed in modifying TGF-β signaling for therapeutic purposes include antisense oligonucleotides, small molecule receptor kinase inhibitors, and peptide aptamers (Xie et al., 2018; Liu et al., 2021). With targeting immune checkpoints as a major focus of current cancer therapies, several clinical trials with combined inhibition of PD1/PD-L1 and TGF-β are ungoing. In addition, bifunctional fusion proteins targeting PD-L1 or CTLA-4 and the TGFβR2 to inhibit TGF-β pathway and immune checkpoint simultaneously, were shown to be superior to PD-1 or CTLA-4 inhibitors in controlling tumor growth in vitro and in vivo (David et al., 2017; Lan et al., 2018; Ravi et al., 2018).
Future clarification of the cell- and context specific effects of TGF-β will help to further harness its signaling network for tumor therapy.
Author Contributions
CS and YZ conceived and wrote the manuscript. Both authors contributed to the article and approved the submitted version.
Funding
Research in YZ’s lab was supported by intramural program of the US National Institutes of Health, National Cancer Institute, Center for Cancer Research.
Conflict of Interest
The authors declare that the research was conducted in the absence of any commercial or financial relationships that could be construed as a potential conflict of interest.
Publisher’s Note
All claims expressed in this article are solely those of the authors and do not necessarily represent those of their affiliated organizations, or those of the publisher, the editors and the reviewers. Any product that may be evaluated in this article, or claim that may be made by its manufacturer, is not guaranteed or endorsed by the publisher.
Acknowledgments
We thank Paul Randazzo and Lalage Wakefield for critically reading the manuscript.
References
Achyut, B. R., Bader, D. A., Robles, A. I., Wangsa, D., Harris, C. C., Ried, T., et al. (2013). Inflammation-mediated genetic and epigenetic alterations drive cancer development in the neighboring epithelium upon stromal abrogation of TGF-β signaling. PLoS Genet. 9:e1003251. doi: 10.1371/journal.pgen.1003251
Albiñana, V., Zafra, M. P., Colau, J., Zarrabeitia, R., Recio-Poveda, L., Olavarrieta, L., et al. (2017). Mutation affecting the proximal promoter of Endoglin as the origin of hereditary hemorrhagic telangiectasia type 1. BMC Med. Genet. 18:20. doi: 10.1186/s12881-017-0380-0
Aluwihare, P., Mu, Z., Zhao, Z., Yu, D., Weinreb, P. H., Horan, G. S., et al. (2009). Mice that lack activity of αvβ6- and αvβ8-integrins reproduce the abnormalities of Tgfb1- and Tgfb3-null mice. J. Cell Sci. 122, 227–232. doi: 10.1242/jcs.035246
Angioni, R., Sánchez-Rodríguez, R., Viola, A., and Molon, B. (2021). TGF-β in Cancer: Metabolic Driver of the Tolerogenic Crosstalk in the Tumor Microenvironment. Cancers 13:cancers13030401. doi: 10.3390/cancers13030401
Aubert, A., Mercier-Gouy, P., Aguero, S., Berthier, L., Liot, S., Prigent, L., et al. (2021). Latent TGF-β Activation Is a Hallmark of the Tenascin Family. Front. Immunol. 12:613438. doi: 10.3389/fimmu.2021.613438
Baas, M., Besançon, A., Goncalves, T., Valette, F., Yagita, H., Sawitzki, B., et al. (2016). TGFβ-dependent expression of PD-1 and PD-L1 controls CD8(+) T cell anergy in transplant tolerance. Elife 5:e08133. doi: 10.7554/eLife.08133
Barbara, N. P., Wrana, J. L., and Letarte, M. (1999). Endoglin is an accessory protein that interacts with the signaling receptor complex of multiple members of the transforming growth factor-β superfamily. J. Biol. Chem. 274, 584–594. doi: 10.1074/jbc.274.2.584
Barcellos-Hoff, M. H., Derynck, R., Tsang, M. L., and Weatherbee, J. A. (1994). Transforming growth factor-β activation in irradiated murine mammary gland. J. Clin. Invest. 93, 892–899. doi: 10.1172/JCI117045
Batlle, E., and Massagué, J. (2019). Transforming Growth Factor-β Signaling in Immunity and Cancer. Immunity 50, 924–940. doi: 10.1016/j.immuni.2019.03.024
Becker, C., Fantini, M. C., Schramm, C., Lehr, H. A., Wirtz, S., Nikolaev, A., et al. (2004). TGF-β suppresses tumor progression in colon cancer by inhibition of IL-6 trans-signaling. Immunity 21, 491–501. doi: 10.1016/j.immuni.2004.07.020
Beckert, S., Farrahi, F., Aslam, R. S., Scheuenstuhl, H., Königsrainer, A., Hussain, M. Z., et al. (2006). Lactate stimulates endothelial cell migration. Wound Repair Regen. 14, 321–324. doi: 10.1111/j.1743-6109.2006.00127.x
Benckert, C., Jonas, S., Cramer, T., Von Marschall, Z., Schäfer, G., Peters, M., et al. (2003). Transforming growth factor β 1 stimulates vascular endothelial growth factor gene transcription in human cholangiocellular carcinoma cells. Cancer Res. 63, 1083–1092.
Bhowmick, N. A., Chytil, A., Plieth, D., Gorska, A. E., Dumont, N., Shappell, S., et al. (2004a). TGF-β signaling in fibroblasts modulates the oncogenic potential of adjacent epithelia. Science 303, 848–851. doi: 10.1126/science.1090922
Bhowmick, N. A., Neilson, E. G., and Moses, H. L. (2004b). Stromal fibroblasts in cancer initiation and progression. Nature 432, 332–337. doi: 10.1038/nature03096
Bhowmick, N. A., Zent, R., Ghiassi, M., McDonnell, M., and Moses, H. L. (2001). Integrin β1 signaling is necessary for transforming growth factor-β activation of p38MAPK and epithelial plasticity. J. Biol. Chem. 276, 46707–46713. doi: 10.1074/jbc.M106176200
Blanco-Gómez, A., Hontecillas-Prieto, L., Corchado-Cobos, R., García-Sancha, N., Salvador, N., Castellanos-Martín, A., et al. (2020). Stromal SNAI2 is required for ERBB2 breast cancer progression. Cancer Res. 80, 5216–5230. doi: 10.1158/0008-5472.CAN-20-0278
Brown, N. F., and Marshall, J. F. (2019). Integrin-Mediated TGFβ Activation Modulates the Tumour Microenvironment. Cancers 11:cancers11091221. doi: 10.3390/cancers11091221
Buscemi, L., Ramonet, D., Klingberg, F., Formey, A., Smith-Clerc, J., Meister, J.-J., et al. (2011). The single-molecule mechanics of the latent TGF-β1 complex. Curr. Biol. 21, 2046–2054. doi: 10.1016/j.cub.2011.11.037
Byun, H.-O., Jung, H.-J., Seo, Y.-H., Lee, Y.-K., Hwang, S.-C., Hwang, E. S., et al. (2012). GSK3 inactivation is involved in mitochondrial complex IV defect in transforming growth factor (TGF) β1-induced senescence. Exp. Cell Res. 318, 1808–1819. doi: 10.1016/j.yexcr.2012.04.012
Calon, A., Lonardo, E., Berenguer-Llergo, A., Espinet, E., Hernando-Momblona, X., Iglesias, M., et al. (2015). Stromal gene expression defines poor-prognosis subtypes in colorectal cancer. Nat. Genet. 47, 320–329. doi: 10.1038/ng.3225
Calon, A., Tauriello, D. V. F., and Batlle, E. (2014). TGF-β in CAF-mediated tumor growth and metastasis. Semin. Cancer Biol. 25, 15–22. doi: 10.1016/j.semcancer.2013.12.008
Chakravarthy, A., Khan, L., Bensler, N. P., Bose, P., and De Carvalho, D. D. (2018). TGF-β-associated extracellular matrix genes link cancer-associated fibroblasts to immune evasion and immunotherapy failure. Nat. Commun. 9:4692. doi: 10.1038/s41467-018-06654-8
Cheifetz, S., Bellón, T., Calés, C., Vera, S., Bernabeu, C., Massagué, J., et al. (1992). Endoglin is a component of the transforming growth factor-β receptor system in human endothelial cells. J. Biol. Chem. 267, 19027–19030.
Chen, X., Yang, S., Li, S., Qu, Y., Wang, H.-Y., Liu, J., et al. (2021). Secretion of bispecific protein of anti-PD-1 fused with TGF-β trap enhances antitumor efficacy of CAR-T cell therapy. Mol. Ther. Oncolytics 21, 144–157. doi: 10.1016/j.omto.2021.03.014
David, J. M., Dominguez, C., McCampbell, K. K., Gulley, J. L., Schlom, J., and Palena, C. (2017). A novel bifunctional anti-PD-L1/TGF-β Trap fusion protein (M7824) efficiently reverts mesenchymalization of human lung cancer cells. Oncoimmunology 6:e1349589. doi: 10.1080/2162402X.2017.1349589
de Larco, J. E., and Todaro, G. J. (1978). Growth factors from murine sarcoma virus-transformed cells. Proc. Natl. Acad. Sci. U S A. 75, 4001–4005. doi: 10.1073/pnas.75.8.4001
Derynck, R., Turley, S. J., and Akhurst, R. J. (2021). TGFβ biology in cancer progression and immunotherapy. Nat. Rev. Clin. Oncol. 18, 9–34. doi: 10.1038/s41571-020-0403-1
Dodagatta-Marri, E., Ma, H.-Y., Liang, B., Li, J., Meyer, D. S., Sun, K.-H., et al. (2020). Integrin αvβ8 on T cells is responsible for suppression of anti-tumor immunity in multiple syngeneic models and is a promising target for tumor immunotherapy. BioRxiv [Preprint]. doi: 10.1101/2020.05.14.084913
Dodagatta-Marri, E., Meyer, D. S., Reeves, M. Q., Paniagua, R., To, M. D., Binnewies, M., et al. (2019). α-PD-1 therapy elevates Treg/Th balance and increases tumor cell pSmad3 that are both targeted by α-TGFβ antibody to promote durable rejection and immunity in squamous cell carcinomas. J. Immunother. Cancer 7:62. doi: 10.1186/s40425-018-0493-9
Donovan, D., Harmey, J. H., Toomey, D., Osborne, D. H., Redmond, H. P., and Bouchier-Hayes, D. J. (1997). TGF β-1 regulation of VEGF production by breast cancer cells. Ann. Surg. Oncol. 4, 621–627. doi: 10.1007/BF02303745
Dupont, S., Morsut, L., Aragona, M., Enzo, E., Giulitti, S., Cordenonsi, M., et al. (2011). Role of YAP/TAZ in mechanotransduction. Nature 474, 179–183. doi: 10.1038/nature10137
Düwel, A., Eleno, N., Jerkic, M., Arevalo, M., Bolaños, J. P., Bernabeu, C., et al. (2007). Reduced tumor growth and angiogenesis in endoglin-haploinsufficient mice. Tumour Biol. 28, 1–8. doi: 10.1159/000097040
Edwards, J. P., Thornton, A. M., and Shevach, E. M. (2014). Release of active TGF-β1 from the latent TGF-β1/GARP complex on T regulatory cells is mediated by integrin β8. J. Immunol. 193, 2843–2849. doi: 10.4049/jimmunol.1401102
Esposito, G., Keramidas, M., Mauduit, C., Feige, J. J., Morera, A. M., and Benahmed, M. (1991). Direct regulating effects of transforming growth factor-β 1 on lactate production in cultured porcine Sertoli cells. Endocrinology 128, 1441–1449. doi: 10.1210/endo-128-3-1441
Fenton, T. M., Kelly, A., Shuttleworth, E. E., Smedley, C., Atakilit, A., Powrie, F., et al. (2017). Inflammatory cues enhance TGFβ activation by distinct subsets of human intestinal dendritic cells via integrin αvβ8. Mucosal Immunol. 10, 624–634. doi: 10.1038/mi.2016.94
Ferrigno, O., Lallemand, F., Verrecchia, F., L’Hoste, S., Camonis, J., Atfi, A., et al. (2002). Yes-associated protein (YAP65) interacts with Smad7 and potentiates its inhibitory activity against TGF-β/Smad signaling. Oncogene 21, 4879–4884. doi: 10.1038/sj.onc.1205623
Fischer, K., Hoffmann, P., Voelkl, S., Meidenbauer, N., Ammer, J., Edinger, M., et al. (2007). Inhibitory effect of tumor cell-derived lactic acid on human T cells. Blood 109, 3812–3819. doi: 10.1182/blood-2006-07-035972
Fridlender, Z. G., Sun, J., Kim, S., Kapoor, V., Cheng, G., Ling, L., et al. (2009). Polarization of tumor-associated neutrophil phenotype by TGF-β: “N1” versus “N2” TAN. Cancer Cell 16, 183–194. doi: 10.1016/j.ccr.2009.06.017
Fujii, M., Nakanishi, H., Toyoda, T., Tanaka, I., Kondo, Y., Osada, H., et al. (2012). Convergent signaling in the regulation of connective tissue growth factor in malignant mesothelioma: TGFβ signaling and defects in the Hippo signaling cascade. Cell Cycle 11, 3373–3379. doi: 10.4161/cc.21397
Gasparics, Á, Rosivall, L., Krizbai, I. A., and Sebe, A. (2016). When the endothelium scores an own goal: endothelial cells actively augment metastatic extravasation through endothelial-mesenchymal transition. Am. J. Physiol. Heart Circ. Physiol. 310, H1055–H1063. doi: 10.1152/ajpheart.00042.2016
Gong, D., Shi, W., Yi, S., Chen, H., Groffen, J., and Heisterkamp, N. (2012). TGFβ signaling plays a critical role in promoting alternative macrophage activation. BMC Immunol. 13:31. doi: 10.1186/1471-2172-13-31
Gougos, A., and Letarte, M. (1988). Identification of a human endothelial cell antigen with monoclonal antibody 44G4 produced against a pre-B leukemic cell line. J. Immunol. 141, 1925–1933.
Guido, C., Whitaker-Menezes, D., Capparelli, C., Balliet, R., Lin, Z., Pestell, R. G., et al. (2012). Metabolic reprogramming of cancer-associated fibroblasts by TGF-β drives tumor growth: connecting TGF-β signaling with “Warburg-like” cancer metabolism and L-lactate production. Cell Cycle 11, 3019–3035. doi: 10.4161/cc.21384
Hahn, J. N., Falck, V. G., and Jirik, F. R. (2011). Smad4 deficiency in T cells leads to the Th17-associated development of premalignant gastroduodenal lesions in mice. J. Clin. Invest. 121, 4030–4042. doi: 10.1172/JCI45114
Hart, I. R., and Fidler, I. J. (1980). Role of organ selectivity in the determination of metastatic patterns of B16 melanoma. Cancer Res. 40, 2281–2287.
Heinzelmann, K., Lehmann, M., Gerckens, M., Noskovičová, N., Frankenberger, M., Lindner, M., et al. (2018). Cell-surface phenotyping identifies CD36 and CD97 as novel markers of fibroblast quiescence in lung fibrosis. Am. J. Physiol. Lung Cell Mol. Physiol. 315, L682–L696. doi: 10.1152/ajplung.00439.2017
Hiepen, C., Mendez, P.-L., and Knaus, P. (2020). It Takes Two to Tango: Endothelial TGFβ/BMP Signaling Crosstalk with Mechanobiology. Cells 9:cells9091965. doi: 10.3390/cells9091965
Hinz, B. (2015). The extracellular matrix and transforming growth factor-β1: Tale of a strained relationship. Matrix Biol 47, 54–65. doi: 10.1016/j.matbio.2015.05.006
Hua, W., Ten Dijke, P., Kostidis, S., Giera, M., and Hornsveld, M. (2020). TGFβ-induced metabolic reprogramming during epithelial-to-mesenchymal transition in cancer. Cell Mol. Life Sci. 77, 2103–2123. doi: 10.1007/s00018-019-03398-6
Inman, W. H., and Colowick, S. P. (1985). Stimulation of glucose uptake by transforming growth factor beta: evidence for the requirement of epidermal growth factor-receptor activation. Proc. Natl. Acad. Sci. USA 82, 1346–1349. doi: 10.1073/pnas.82.5.1346
Kaminska, B., Wesolowska, A., and Danilkiewicz, M. (2005). TGF β signalling and its role in tumour pathogenesis. Acta Biochim. Pol. 52, 329–337.
Kaplan, R. N., Riba, R. D., Zacharoulis, S., Bramley, A. H., Vincent, L., Costa, C., et al. (2005). VEGFR1-positive haematopoietic bone marrow progenitors initiate the pre-metastatic niche. Nature 438, 820–827. doi: 10.1038/nature04186
Katsuno, Y., and Derynck, R. (2021). Epithelial plasticity, epithelial-mesenchymal transition, and the TGF-β family. Dev. Cell 56, 726–746. doi: 10.1016/j.devcel.2021.02.028
Kavsak, P., Rasmussen, R. K., Causing, C. G., Bonni, S., Zhu, H., Thomsen, G. H., et al. (2000). Smad7 binds to Smurf2 to form an E3 ubiquitin ligase that targets the TGF β receptor for degradation. Mol. Cell 6, 1365–1375. doi: 10.1016/s1097-2765(00)00134-9
Kawano, S., Kojima, M., Higuchi, Y., Sugimoto, M., Ikeda, K., Sakuyama, N., et al. (2015). Assessment of elasticity of colorectal cancer tissue, clinical utility, pathological and phenotypical relevance. Cancer Sci. 106, 1232–1239. doi: 10.1111/cas.12720
Kelly, A., Gunaltay, S., McEntee, C. P., Shuttleworth, E. E., Smedley, C., Houston, S. A., et al. (2018). Human monocytes and macrophages regulate immune tolerance via integrin αvβ8-mediated TGFβ activation. J. Exp. Med. 215, 2725–2736. doi: 10.1084/jem.20171491
Khan, Z., and Marshall, J. F. (2016). The role of integrins in TGFβ activation in the tumour stroma. Cell Tissue Res. 365, 657–673. doi: 10.1007/s00441-016-2474-y
Kim, K. K., Sheppard, D., and Chapman, H. A. (2018). TGF-β1 Signaling and Tissue Fibrosis. Cold Spring Harb. Perspect. Biol. 10:cshersect.a022293. doi: 10.1101/cshperspect.a022293
Kim, S.-H., and Baek, K.-H. (2021). Regulation of cancer metabolism by deubiquitinating enzymes: the warburg effect. Int. J. Mol. Sci. 22:ijms22126173. doi: 10.3390/ijms22126173
Klingberg, F., Chow, M. L., Koehler, A., Boo, S., Buscemi, L., Quinn, T. M., et al. (2014). Prestress in the extracellular matrix sensitizes latent TGF-β1 for activation. J. Cell Biol. 207, 283–297. doi: 10.1083/jcb.201402006
Kojima, Y., Acar, A., Eaton, E. N., Mellody, K. T., Scheel, C., Ben-Porath, I., et al. (2010). Autocrine TGF-β and stromal cell-derived factor-1 (SDF-1) signaling drives the evolution of tumor-promoting mammary stromal myofibroblasts. Proc. Natl. Acad. Sci. U S A. 107, 20009–20014. doi: 10.1073/pnas.1013805107
Lan, Y., Zhang, D., Xu, C., Hance, K. W., Marelli, B., Qi, J., et al. (2018). Enhanced preclinical antitumor activity of M7824, a bifunctional fusion protein simultaneously targeting PD-L1 and TGF-β. Sci. Transl. Med. 10:scitranslmed.aan5488. doi: 10.1126/scitranslmed.aan5488
Lebrin, F., Goumans, M.-J., Jonker, L., Carvalho, R. L. C., Valdimarsdottir, G., Thorikay, M., et al. (2004). Endoglin promotes endothelial cell proliferation and TGF-β/ALK1 signal transduction. EMBO J. 23, 4018–4028. doi: 10.1038/sj.emboj.7600386
Lee, M. K., Pardoux, C., Hall, M. C., Lee, P. S., Warburton, D., Qing, J., et al. (2007). TGF-β activates Erk MAP kinase signalling through direct phosphorylation of ShcA. EMBO J. 26, 3957–3967. doi: 10.1038/sj.emboj.7601818
Liénart, S., Merceron, R., Vanderaa, C., Lambert, F., Colau, D., Stockis, J., et al. (2018). Structural basis of latent TGF-β1 presentation and activation by GARP on human regulatory T cells. Science 362, 952–956. doi: 10.1126/science.aau2909
Liu, S., Ren, J., and Ten Dijke, P. (2021). Targeting TGFβ signal transduction for cancer therapy. Signal Transduct. Target. Ther. 6:8. doi: 10.1038/s41392-020-00436-9
Lu, P., Weaver, V. M., and Werb, Z. (2012). The extracellular matrix: a dynamic niche in cancer progression. J. Cell Biol. 196, 395–406. doi: 10.1083/jcb.201102147
Luo, K. (2017). Signaling Cross Talk between TGF-β/Smad and Other Signaling Pathways. Cold Spring Harb. Perspect. Biol. 9:a022137. doi: 10.1101/cshperspect.a022137
Ma, J., Sanchez-Duffhues, G., Goumans, M.-J., and Ten Dijke, P. (2020). TGF-β-Induced Endothelial to Mesenchymal Transition in Disease and Tissue Engineering. Front. Cell Dev. Biol. 8:260. doi: 10.3389/fcell.2020.00260
Marsh, D., Dickinson, S., Neill, G. W., Marshall, J. F., Hart, I. R., and Thomas, G. J. (2008). αvβ6 Integrin promotes the invasion of morphoeic basal cell carcinoma through stromal modulation. Cancer Res. 68, 3295–3303. doi: 10.1158/0008-5472.CAN-08-0174
Matsumura, Y., Ito, Y., Mezawa, Y., Sulidan, K., Daigo, Y., Hiraga, T., et al. (2019). Stromal fibroblasts induce metastatic tumor cell clusters via epithelial-mesenchymal plasticity. Life Sci. Alliance 2:201900425. doi: 10.26508/lsa.201900425
Means, A. L., Freeman, T. J., Zhu, J., Woodbury, L. G., Marincola-Smith, P., Wu, C., et al. (2018). Epithelial Smad4 Deletion Up-Regulates Inflammation and Promotes Inflammation-Associated Cancer. Cell. Mol. Gastroenterol. Hepatol. 6, 257–276. doi: 10.1016/j.jcmgh.2018.05.006
Mezawa, Y., and Orimo, A. (2021). Phenotypic heterogeneity, stability and plasticity in tumor-promoting carcinoma-associated fibroblasts. FEBS J. [Preprint]. doi: 10.1111/febs.15851
Mezawa, Y., Daigo, Y., Takano, A., Miyagi, Y., Yokose, T., Yamashita, T., et al. (2019). CD26 expression is attenuated by TGF-β and SDF-1 autocrine signaling on stromal myofibroblasts in human breast cancers. Cancer Med. 8, 3936–3948. doi: 10.1002/cam4.2249
Minhajat, R., Mori, D., Yamasaki, F., Sugita, Y., Satoh, T., and Tokunaga, O. (2006). Organ-specific endoglin (CD105) expression in the angiogenesis of human cancers. Pathol. Int. 56, 717–723. doi: 10.1111/j.1440-1827.2006.02037.x
Miyazawa, K., and Miyazono, K. (2017). Regulation of TGF-β Family Signaling by Inhibitory Smads. Cold Spring Harb. Perspect. Biol. 9:a022095. doi: 10.1101/cshperspect.a022095
Miyazono, K., Katsuno, Y., Koinuma, D., Ehata, S., and Morikawa, M. (2018). Intracellular and extracellular TGF-β signaling in cancer: some recent topics. Front. Med. 12:387–411. doi: 10.1007/s11684-018-0646-8
Moore, K. M., Thomas, G. J., Duffy, S. W., Warwick, J., Gabe, R., Chou, P., et al. (2014). Therapeutic targeting of integrin αvβ6 in breast cancer. J. Natl. Cancer Inst. 106:dju169. doi: 10.1093/jnci/dju169
Morikawa, M., Derynck, R., and Miyazono, K. (2016). TGF-β and the TGF-β Family: Context-Dependent Roles in Cell and Tissue Physiology. Cold Spring Harb. Perspect. Biol. 8:a021873. doi: 10.1101/cshperspect.a021873
Mu, D., Cambier, S., Fjellbirkeland, L., Baron, J. L., Munger, J. S., Kawakatsu, H., et al. (2002). The integrin αvβ8 mediates epithelial homeostasis through MT1-MMP-dependent activation of TGF-β. J. Cell Biol. 157, 493–507. doi: 10.1083/jcb.200109100
Mu, Y., Gudey, S. K., and Landström, M. (2012). Non-Smad signaling pathways. Cell Tissue Res. 347, 11–20. doi: 10.1007/s00441-011-1201-y
Munn, D. H., and Bronte, V. (2016). Immune suppressive mechanisms in the tumor microenvironment. Curr. Opin. Immunol. 39, 1–6. doi: 10.1016/j.coi.2015.10.009
Nasi, A., Fekete, T., Krishnamurthy, A., Snowden, S., Rajnavölgyi, E., Catrina, A. I., et al. (2013). Dendritic cell reprogramming by endogenously produced lactic acid. J. Immunol. 191, 3090–3099. doi: 10.4049/jimmunol.1300772
Nastase, M. V., Janicova, A., Roedig, H., Hsieh, L. T.-H., Wygrecka, M., and Schaefer, L. (2018). Small Leucine-Rich Proteoglycans in Renal Inflammation: Two Sides of the Coin. J. Histochem. Cytochem. 66, 261–272. doi: 10.1369/0022155417738752
Navab, R., Strumpf, D., Bandarchi, B., Zhu, C.-Q., Pintilie, M., Ramnarine, V. R., et al. (2011). Prognostic gene-expression signature of carcinoma-associated fibroblasts in non-small cell lung cancer. Proc. Natl. Acad. Sci. U S A. 108, 7160–7165. doi: 10.1073/pnas.1014506108
Nicklas, D., and Saiz, L. (2013). Characterization of negative feedback network motifs in the TGF-β signaling pathway. PLoS One 8:e83531. doi: 10.1371/journal.pone.0083531
Nogués, A., Gallardo-Vara, E., Zafra, M. P., Mate, P., Marijuan, J. L., Alonso, A., et al. (2020). Endoglin (CD105) and VEGF as potential angiogenic and dissemination markers for colorectal cancer. World J. Surg. Oncol. 18:99. doi: 10.1186/s12957-020-01871-2
Nolte, M., and Margadant, C. (2020). Controlling Immunity and Inflammation through Integrin-Dependent Regulation of TGF-β. Trends Cell Biol. 30, 49–59. doi: 10.1016/j.tcb.2019.10.002
Ollauri-Ibáñez, C., Núñez-Gómez, E., Egido-Turrión, C., Silva-Sousa, L., Díaz-Rodríguez, E., Rodríguez-Barbero, A., et al. (2020). Continuous endoglin (CD105) overexpression disrupts angiogenesis and facilitates tumor cell metastasis. Angiogenesis 23, 231–247. doi: 10.1007/s10456-019-09703-y
Orimo, A., Gupta, P. B., Sgroi, D. C., Arenzana-Seisdedos, F., Delaunay, T., Naeem, R., et al. (2005). Stromal fibroblasts present in invasive human breast carcinomas promote tumor growth and angiogenesis through elevated SDF-1/CXCL12 secretion. Cell 121, 335–348. doi: 10.1016/j.cell.2005.02.034
Paget, S. (1889). THE DISTRIBUTION OF SECONDARY GROWTHS IN CANCER OF THE BREAST. Lancet 133, 571–573. doi: 10.1016/S0140-6736(00)49915-0
Park, B. V., Freeman, Z. T., Ghasemzadeh, A., Chattergoon, M. A., Rutebemberwa, A., Steigner, J., et al. (2016). TGFβ1-Mediated SMAD3 Enhances PD-1 Expression on Antigen-Specific T Cells in Cancer. Cancer Discov. 6, 1366–1381. doi: 10.1158/2159-8290.CD-15-1347
Paszek, M. J., Zahir, N., Johnson, K. R., Lakins, J. N., Rozenberg, G. I., Gefen, A., et al. (2005). Tensional homeostasis and the malignant phenotype. Cancer Cell 8, 241–254. doi: 10.1016/j.ccr.2005.08.010
Pawlak, J. B., and Blobe, G. C. (2021). TGF-β superfamily co-receptors in cancer. Dev. Dyn. [Preprint]. doi: 10.1002/dvdy.338
Peter, K., Rehli, M., Singer, K., Renner-Sattler, K., and Kreutz, M. (2015). Lactic acid delays the inflammatory response of human monocytes. Biochem. Biophys. Res. Commun. 457, 412–418. doi: 10.1016/j.bbrc.2015.01.005
Piersma, B., Bank, R. A., and Boersema, M. (2015). Signaling in Fibrosis: TGF-β, WNT, and YAP/TAZ Converge. Front. Med. 2:59. doi: 10.3389/fmed.2015.00059
Platel, V., Faure, S., Corre, I., and Clere, N. (2019). Endothelial-to-Mesenchymal Transition (EndoMT): Roles in Tumorigenesis, Metastatic Extravasation and Therapy Resistance. J. Oncol. 2019:8361945. doi: 10.1155/2019/8361945
Ramachandran, A., Vizán, P., Das, D., Chakravarty, P., Vogt, J., Rogers, K. W., et al. (2018). TGF-β uses a novel mode of receptor activation to phosphorylate SMAD1/5 and induce epithelial-to-mesenchymal transition. Elife 7:31756. doi: 10.7554/eLife.31756
Ravi, R., Noonan, K. A., Pham, V., Bedi, R., Zhavoronkov, A., Ozerov, I. V., et al. (2018). Bifunctional immune checkpoint-targeted antibody-ligand traps that simultaneously disable TGFβ enhance the efficacy of cancer immunotherapy. Nat. Commun. 9:741. doi: 10.1038/s41467-017-02696-6
Roberts, A. B., and Wakefield, L. M. (2003). The two faces of transforming growth factor beta in carcinogenesis. Proc. Natl. Acad. Sci. U S A. 100, 8621–8623. doi: 10.1073/pnas.1633291100
Roberts, A. B., Lamb, L. C., Newton, D. L., Sporn, M. B., De Larco, J. E., and Todaro, G. J. (1980). Transforming growth factors: isolation of polypeptides from virally and chemically transformed cells by acid/ethanol extraction. Proc. Natl. Acad. Sci. U S A. 77, 3494–3498.
Robertson, I. B., and Rifkin, D. B. (2016). Regulation of the Bioavailability of TGF-β and TGF-β-Related Proteins. Cold Spring Harb. Perspect. Biol. 8:a021907. doi: 10.1101/cshperspect.a021907
Romero-Garcia, S., Moreno-Altamirano, M. M. B., Prado-Garcia, H., and Sánchez-García, F. J. (2016). Lactate contribution to the tumor microenvironment: mechanisms, effects on immune cells and therapeutic relevance. Front. Immunol. 7:52. doi: 10.3389/fimmu.2016.00052
Sabbineni, H., Verma, A., and Somanath, P. R. (2018). Isoform-specific effects of transforming growth factor β on endothelial-to-mesenchymal transition. J. Cell Physiol. 233, 8418–8428. doi: 10.1002/jcp.26801
Samani, A., Zubovits, J., and Plewes, D. (2007). Elastic moduli of normal and pathological human breast tissues: an inversion-technique-based investigation of 169 samples. Phys. Med. Biol. 52, 1565–1576. doi: 10.1088/0031-9155/52/6/002
Seoane, J., and Gomis, R. R. (2017). TGF-β Family Signaling in Tumor Suppression and Cancer Progression. Cold Spring Harb. Perspect. Biol. 9:a022277. doi: 10.1101/cshperspect.a022277
Shi, Y., and Massagué, J. (2003). Mechanisms of TGF-β signaling from cell membrane to the nucleus. Cell 113, 685–700. doi: 10.1016/s0092-8674(03)00432-x
Siewe, N., and Friedman, A. (2021). TGF-β inhibition can overcome cancer primary resistance to PD-1 blockade: A mathematical model. PLoS One 16:e0252620. doi: 10.1371/journal.pone.0252620
Sinha, A., Iyengar, P. V., and Ten Dijke, P. (2021). E3 ubiquitin ligases: key regulators of tgfβ signaling in cancer progression. Int. J. Mol. Sci. 22:ijms22020476. doi: 10.3390/ijms22020476
Sonnylal, S., Xu, S., Jones, H., Tam, A., Sreeram, V. R., Ponticos, M., et al. (2013). Connective tissue growth factor causes EMT-like cell fate changes in vivo and in vitro. J. Cell Sci. 126, 2164–2175. doi: 10.1242/jcs.111302
Sorrentino, A., Thakur, N., Grimsby, S., Marcusson, A., von Bulow, V., Schuster, N., et al. (2008). The type I TGF-β receptor engages TRAF6 to activate TAK1 in a receptor kinase-independent manner. Nat. Cell Biol. 10, 1199–1207. doi: 10.1038/ncb1780
Stockis, J., Liénart, S., Colau, D., Collignon, A., Nishimura, S. L., Sheppard, D., et al. (2017). Blocking immunosuppression by human Tregs in vivo with antibodies targeting integrin αVβ8. Proc. Natl. Acad. Sci. U S A. 114, E10161–E10168. doi: 10.1073/pnas.1710680114
Strauss, J., Heery, C. R., Schlom, J., Madan, R. A., Cao, L., Kang, Z., et al. (2018). Phase I Trial of M7824 (MSB0011359C), a Bifunctional Fusion Protein Targeting PD-L1 and TGFβ, in Advanced Solid Tumors. Clin. Cancer Res. 24, 1287–1295. doi: 10.1158/1078-0432.CCR-17-2653
Stuelten, C. H., Barbul, A., Busch, J. I., Sutton, E., Katz, R., Sato, M., et al. (2008). Acute wounds accelerate tumorigenesis by a T cell-dependent mechanism. Cancer Res. 68, 7278–7282. doi: 10.1158/0008-5472.CAN-08-1842
Stuelten, C. H., DaCosta Byfield, S., Arany, P. R., Karpova, T. S., Stetler-Stevenson, W. G., and Roberts, A. B. (2005). Breast cancer cells induce stromal fibroblasts to express MMP-9 via secretion of TNF-α and TGF-β. J. Cell Sci. 118, 2143–2153. doi: 10.1242/jcs.02334
Sun, H., Miao, C., Liu, W., Qiao, X., Yang, W., Li, L., et al. (2018). TGF-β1/TβRII/Smad3 signaling pathway promotes VEGF expression in oral squamous cell carcinoma tumor-associated macrophages. Biochem. Biophys. Res. Commun. 497, 583–590. doi: 10.1016/j.bbrc.2018.02.104
Tang, L.-Y., Heller, M., Meng, Z., Yu, L.-R., Tang, Y., Zhou, M., et al. (2017). Transforming Growth Factor-β (TGF-β) Directly Activates the JAK1-STAT3 Axis to Induce Hepatic Fibrosis in Coordination with the SMAD Pathway. J. Biol. Chem. 292, 4302–4312. doi: 10.1074/jbc.M116.773085
Tang, L.-Y., Yamashita, M., Coussens, N. P., Tang, Y., Wang, X., Li, C., et al. (2011). Ablation of Smurf2 reveals an inhibition in TGF-β signalling through multiple mono-ubiquitination of Smad3. EMBO J. 30, 4777–4789. doi: 10.1038/emboj.2011.393
Tang, P. M.-K., Zhou, S., Meng, X.-M., Wang, Q.-M., Li, C.-J., Lian, G.-Y., et al. (2017). Smad3 promotes cancer progression by inhibiting E4BP4-mediated NK cell development. Nat. Commun. 8:14677. doi: 10.1038/ncomms14677
Terabe, M., Robertson, F. C., Clark, K., De Ravin, E., Bloom, A., Venzon, D. J., et al. (2017). Blockade of only TGF-β 1 and 2 is sufficient to enhance the efficacy of vaccine and PD-1 checkpoint blockade immunotherapy. Oncoimmunology 6:e1308616. doi: 10.1080/2162402X.2017.1308616
Thiery, J. P., Acloque, H., Huang, R. Y. J., and Nieto, M. A. (2009). Epithelial-mesenchymal transitions in development and disease. Cell 139, 871–890. doi: 10.1016/j.cell.2009.11.007
Tian, H., Huang, J. J., Golzio, C., Gao, X., Hector-Greene, M., Katsanis, N., et al. (2018). Endoglin interacts with VEGFR2 to promote angiogenesis. FASEB J. 32, 2934–2949. doi: 10.1096/fj.201700867RR
Travis, M. A., Reizis, B., Melton, A. C., Masteller, E., Tang, Q., Proctor, J. M., et al. (2007). Loss of integrin αvβ8 on dendritic cells causes autoimmunity and colitis in mice. Nature 449, 361–365. doi: 10.1038/nature06110
Tripathi, V., Sixt, K. M., Gao, S., Xu, X., Huang, J., Weigert, R., et al. (2016). Direct Regulation of Alternative Splicing by SMAD3 through PCBP1 Is Essential to the Tumor-Promoting Role of TGF-β. Mol. Cell 64, 549–564. doi: 10.1016/j.molcel.2016.09.013
Tsubakihara, Y., and Moustakas, A. (2018). Epithelial-Mesenchymal Transition and Metastasis under the Control of Transforming Growth Factor β. Int. J. Mol. Sci. 19:ijms19113672. doi: 10.3390/ijms19113672
Uddin, M., Kawami, M., Yumoto, R., and Takano, M. (2020). Effect of transforming growth factor-β1 on functional expression of monocarboxylate transporter 1 in alveolar epithelial A549 cells. Naunyn Schmiedebergs Arch. Pharmacol. 393, 889–896. doi: 10.1007/s00210-019-01802-3
Van Hove, L., Lecomte, K., Roels, J., Vandamme, N., Vikkula, H.-K., Hoorens, I., et al. (2021). Fibrotic enzymes modulate wound-induced skin tumorigenesis. EMBO Rep. 22:e51573. doi: 10.15252/embr.202051573
Varelas, X., Sakuma, R., Samavarchi-Tehrani, P., Peerani, R., Rao, B. M., Dembowy, J., et al. (2008). TAZ controls Smad nucleocytoplasmic shuttling and regulates human embryonic stem-cell self-renewal. Nat. Cell Biol. 10, 837–848. doi: 10.1038/ncb1748
Végran, F., Boidot, R., Michiels, C., Sonveaux, P., and Feron, O. (2011). Lactate influx through the endothelial cell monocarboxylate transporter MCT1 supports an NF-κB/IL-8 pathway that drives tumor angiogenesis. Cancer Res. 71, 2550–2560. doi: 10.1158/0008-5472.CAN-10-2828
Walenta, S., Wetterling, M., Lehrke, M., Schwickert, G., Sundfør, K., Rofstad, E. K., et al. (2000). High lactate levels predict likelihood of metastases, tumor recurrence, and restricted patient survival in human cervical cancers. Cancer Res. 60, 916–921.
Wang, Y., Yao, B., Li, H., Zhang, Y., Gao, H., Gao, Y., et al. (2017). Assessment of tumor stiffness with shear wave elastography in a human prostate cancer xenograft implantation model. J. Ultrasound Med. 36, 955–963. doi: 10.7863/ultra.16.03066
Warburg, O., Wind, F., and Negelein, E. (1927). The metabolism of tumors in the body. J. Gen. Physiol. 8, 519–530. doi: 10.1085/jgp.8.6.519
Wawro, M. E., Chojnacka, K., Wieczorek-Szukała, K., Sobierajska, K., and Niewiarowska, J. (2018). Invasive Colon Cancer Cells Induce Transdifferentiation of Endothelium to Cancer-Associated Fibroblasts through Microtubules Enriched in Tubulin-β3. Int. J. Mol. Sci. 20:ijms20010053. doi: 10.3390/ijms20010053
Wozniak, M. A., Desai, R., Solski, P. A., Der, C. J., and Keely, P. J. (2003). ROCK-generated contractility regulates breast epithelial cell differentiation in response to the physical properties of a three-dimensional collagen matrix. J. Cell Biol. 163, 583–595. doi: 10.1083/jcb.200305010
Wu, P., Geng, B., Chen, Q., Zhao, E., Liu, J., Sun, C., et al. (2020). Tumor Cell-Derived TGFβ1 Attenuates Antitumor Immune Activity of T Cells via Regulation of PD-1 mRNA. Cancer Immunol. Res. 8, 1470–1484. doi: 10.1158/2326-6066.CIR-20-0113
Xie, F., Ling, L., van Dam, H., Zhou, F., and Zhang, L. (2018). TGF-β signaling in cancer metastasis. Acta Biochim. Biophys. Sin. 50, 121–132. doi: 10.1093/abbs/gmx123
Yadav, H., Quijano, C., Kamaraju, A. K., Gavrilova, O., Malek, R., Chen, W., et al. (2011). Protection from obesity and diabetes by blockade of TGF-β/Smad3 signaling. Cell Metab. 14, 67–79. doi: 10.1016/j.cmet.2011.04.013
Yamashita, M., Fatyol, K., Jin, C., Wang, X., Liu, Z., and Zhang, Y. E. (2008). TRAF6 mediates Smad-independent activation of JNK and p38 by TGF-β. Mol. Cell 31, 918–924. doi: 10.1016/j.molcel.2008.09.002
Yan, X., Xiong, X., and Chen, Y.-G. (2018). Feedback regulation of TGF-β signaling. Acta Biochim. Biophys. Sin. 50, 37–50. doi: 10.1093/abbs/gmx129
Yang, X., Letterio, J. J., Lechleider, R. J., Chen, L., Hayman, R., Gu, H., et al. (1999). Targeted disruption of SMAD3 results in impaired mucosal immunity and diminished T cell responsiveness to TGF-β. EMBO J. 18, 1280–1291. doi: 10.1093/emboj/18.5.1280
Yang, Z., Mu, Z., Dabovic, B., Jurukovski, V., Yu, D., Sung, J., et al. (2007). Absence of integrin-mediated TGFβ1 activation in vivo recapitulates the phenotype of TGFβ1-null mice. J. Cell Biol. 176, 787–793. doi: 10.1083/jcb.200611044
Yoshida, G. J., Azuma, A., Miura, Y., and Orimo, A. (2019). Activated fibroblast program orchestrates tumor initiation and progression; molecular mechanisms and the associated therapeutic strategies. Int. J. Mol. Sci. 20:ijms20092256. doi: 10.3390/ijms20092256
Yu, Q., and Stamenkovic, I. (2000). Cell surface-localized matrix metalloproteinase-9 proteolytically activates TGF-β and promotes tumor invasion and angiogenesis. Genes Dev. 14, 163–176.
Yu, Y., Xiao, C. H., Tan, L. D., Wang, Q. S., Li, X. Q., and Feng, Y. M. (2014). Cancer-associated fibroblasts induce epithelial-mesenchymal transition of breast cancer cells through paracrine TGF-β signalling. Br. J. Cancer 110, 724–732. doi: 10.1038/bjc.2013.768
Zeisberg, E. M., Potenta, S., Xie, L., Zeisberg, M., and Kalluri, R. (2007). Discovery of endothelial to mesenchymal transition as a source for carcinoma-associated fibroblasts. Cancer Res. 67, 10123–10128. doi: 10.1158/0008-5472.CAN-07-3127
Zhang, L., Zhou, F., and ten Dijke, P. (2013). Signaling interplay between transforming growth factor-β receptor and PI3K/AKT pathways in cancer. Trends Biochem. Sci. 38, 612–620. doi: 10.1016/j.tibs.2013.10.001
Zhang, S. (2018). The role of transforming growth factor β in T helper 17 differentiation. Immunology 155, 24–35. doi: 10.1111/imm.12938
Zhang, Y. E. (2017). Non-Smad Signaling Pathways of the TGF-β Family. Cold Spring Harb. Perspect. Biol. 9:a022129. doi: 10.1101/cshperspect.a022129
Zhang, Y., Chang, C., Gehling, D. J., Hemmati-Brivanlou, A., and Derynck, R. (2001). Regulation of Smad degradation and activity by Smurf2, an E3 ubiquitin ligase. Proc. Natl. Acad. Sci. U S A. 98, 974–979. doi: 10.1073/pnas.98.3.974
Keywords: TGF-β, tumor-stromal crosstalk, cancer associated fibroblast (CAF), EMT—epithelial to mesenchymal transition, EndMT—endothelial to mesenchymal transition, tolerogenic differentiation, metabolic reprogramming
Citation: Stuelten CH and Zhang YE (2021) Transforming Growth Factor-β: An Agent of Change in the Tumor Microenvironment. Front. Cell Dev. Biol. 9:764727. doi: 10.3389/fcell.2021.764727
Received: 25 August 2021; Accepted: 23 September 2021;
Published: 12 October 2021.
Edited by:
Xiaohua Yan, Nanchang University, ChinaReviewed by:
Teng Fei, Northeastern University, ChinaYan Teng, Academy of Military Medical Sciences (AMMS), China
Copyright © 2021 Stuelten and Zhang. This is an open-access article distributed under the terms of the Creative Commons Attribution License (CC BY). The use, distribution or reproduction in other forums is permitted, provided the original author(s) and the copyright owner(s) are credited and that the original publication in this journal is cited, in accordance with accepted academic practice. No use, distribution or reproduction is permitted which does not comply with these terms.
*Correspondence: Christina H. Stuelten, Y2hyaXNzdHVAbWFpbC5uaWguZ292; Ying E. Zhang, emhhbmd5aW5AbWFpbC5uaWguZ292