- 1Bone Marrow Transplantation Center, The First Affiliated Hospital, School of Medicine, Zhejiang University, Hangzhou, China
- 2Liangzhu Laboratory, Zhejiang University Medical Center, Hangzhou, China
- 3Zhejiang Province Engineering Laboratory for Stem Cell and Immunity Therapy, Hangzhou, China
- 4Institute of Hematology, Zhejiang University, Hangzhou, China
Myeloid-derived suppressor cells (MDSCs) represent a population of heterogeneous myeloid cells, which are characterized by their remarkable ability to suppress T cells and natural killer cells. MDSCs have been proven to play a positive role in protecting acute graft-versus-host disease (aGVHD). Here, we aimed to describe the mechanism behind how mTOR signaling regulates MDSCs’ generation and explore its prophylactic and therapeutic potential in aGVHD. Reducing mTOR expression retains myeloid cells with immature characteristics and promotes polymorphonuclear MDSC (PMN-MDSC) immunosuppressive function through STAT3-C/EBPβ pathway. Prophylactic transfusion of mTORKO PMN-MDSCs could alleviate aGVHD while maintaining the graft-versus-leukemia (GVL) effect, which could downregulate the Th1/Th2 ratio, decrease serum proinflammatory cytokines, and increase the proportion of regulatory T cells (Tregs) in aGVHD models at the early stage after transplantation. Moreover, transfusion therapy could promote the reconstruction and function of donor-derived PMN-MDSCs. Not only the percentage and the absolute number of donor-derived PMN-MDSCs significantly increased but also the immunosuppressive ability was much more robust compared to other groups. Altogether, these findings indicated that mTOR is an intrinsic regulator for PMN-MDSCs’ differentiation and immunosuppressive function. Together, mTORKO PMN-MDSC transfusion can play a protective role in alleviating cytokine storm at the initial stage and promoting the quantitative and functional recoveries of donor-derived PMN-MDSCs in aGVHD.
Introduction
Allogeneic hematopoietic stem cell transplantation (allo-HSCT) is a potentially curative therapy for different hematopoietic diseases. The graft-versus-host disease (GVHD) represents one of the major complications and causes of death after allo-HSCT, where T cells play a central role in the pathogenesis and progression of acute GVHD (aGVHD) (Korngold and Sprent 1987; Vogelsang, Lee, and Bensen-Kennedy 2003). Recently, immune-regulatory cell therapy is gaining widespread attention in GVHD control, including mesenchymal stromal cells (MSCs) (Ringden et al., 2006; Le Blanc et al., 2008), regulatory T cells (Tregs) (Hess 2006; Koreth et al., 2011), and myeloid-derived suppressor cells (MDSCs) (Messmann et al., 2015; Zhang et al., 2019).
MDSCs represent a population of heterogeneous myeloid cells, precursors of dendritic cells (DCs), macrophages, and granulocytes, which accumulate in various pathophysiological settings, such as cancers, chronic inflammations, and autoimmune diseases (Gabrilovich and Nagaraj 2009). Currently, there is a lack of specific gene signatures or surface markers to identify MDSCs among myeloid cells due to their plasticity in different microenvironments. Therefore, their immunosuppressive ability become the most unique feature of MDSCs. In mice, MDSCs are historically defined as cells expressing both Gr1 and CD11b markers. The CD11b+Ly6G−Ly6Chi subset is identified as monocytic MDSCs (M-MDSCs), while the CD11b+Ly6G+Ly6Clo subgroup is defined as polymorphonuclear MDSCs (PMN-MDSCs) (Bronte et al., 2016). MDSCs exert their suppressive functions via several mechanisms including the expression of enzymes, such as arginase (Arg), nitric oxide synthase (NOS), and indamine 2,3-dioxygenase (IDO); releasing reactive oxygen species (ROS); and inducing Tregs and secreting cytokines (e.g., IL-6, IL-10, and TGF-β) (Dugast et al., 2008; Gabrilovich and Nagaraj 2009; Garcia et al., 2010; Deng et al., 2018).
It has been observed that a higher proportion of MDSCs in grafts was correlated to a lower incidence of aGVHD (Vendramin et al., 2014; Lv et al., 2015; Fan et al., 2017; Wang et al., 2019). The granulocyte colony-stimulating factor (G-CSF) is clinically used as a mobilizer to increase the number of circulating hematopoietic stem cells. Both PMN-MDSCs and M-MDSCs induced by G-CSF could exert suppressive function to reduce aGVHD (Perobelli et al., 2016; D'Aveni et al., 2015). After allo-HSCT, MDSCs recovering at the early stage after transplantation presented immunomodulatory properties to suppress donor-derived T cell proliferation and differentiation and promote Tregs development (Mougiakakos et al., 2013; Rieber et al., 2014; Guan et al., 2015). Besides, co-transfusing MDSCs generated in vitro could alleviate aGVHD, while preserving the graft-versus-leukemia (GVL) effect in murine models (Messmann et al., 2015; Zhang et al., 2019). Hence, a better understanding of how to enhance MDSCs’ suppressive ability and what are their regulatory networks in vivo requires to be further investigated.
Suppressive MDSCs can be induced and expanded by various methods (Zhou et al., 2010; Gomez et al., 2014). Culturing bone marrow cells for 4 days with G-CSF and granulocyte-macrophage (GM)-CSF can induce functional MDSCs able to inhibit allogeneic T cell responses in vitro and in vivo (Highfill et al., 2010). MDSCs induced by progenipoietin-1 (a synthetic G-CSF/Flt-3 ligand molecule) can promote transplant tolerance by inducing MHC class II-restricted, IL-10-secreting, antigen-specific Tregs (MacDonald et al., 2005). mTOR is an evolutionary conserved serine/threonine kinase that plays key regulatory roles in several biological processes, such as cell survival, proliferation, differentiation, metabolism, and autophagy (Beauchamp and Platanias 2013). The activity of mTOR affects the differentiation and functions of various innate and adaptive immune cells, including effector T cells, Tregs, and antigen-presenting cells (Delgoffe et al., 2009; Thomson et al., 2009; Rao et al., 2010). Targeting mTOR on the recruitment, expansion, and function of MDSCs has been reported in different disease models. Rapamycin, an mTOR inhibitor, regulated the differentiation and suppressive function of MDSCs in protecting against immunological hepatic injury and aGVHD (Zhang et al., 2014; Lin et al., 2018). Additionally, rapamycin could prolong cardiac or corneal allograft survival by inducing MDSCs after transplantation (Nakamura et al., 2015; Wei et al., 2018). However, the underlying mechanisms of how mTOR signals regulate MDSCs are currently not well defined.
In our present study, we demonstrated that the mTOR signaling regulated the suppressive function of PMN-MDSCs through the STAT3-C/EBPβ pathway. Prophylactic transfusion of mTOR-deficient PMN-MDSCs could play a protective role in alleviating cytokine storm at the initial stage and promote a quantitative and functional recovery of donor-derived PMN-MDSCs in aGVHD models. We also explored the mechanism of how mTOR regulated MDSCs and demonstrated that mTOR-deficient PMN-MDSCs might become an effective approach for aGVHD prevention in allo-HSCT.
Materials and Methods
Mice
Experiments were performed with age-matched mTORfl/fl (referred to as wild-type, WT control) mice and LysM-Cre mTORfl/fl (referred as to mTOR knockout, mTOR KO) mice. LysM-Cre mTORfl/fl mice were crossed with LysM-Cre and mTORfl/fl mice, confirmed by genotyping, which were kindly provided by Prof. H Shen. BALB/c (H-2Kd) and C57BL/6 (H-2Kb) mice were directly purchased from SLAC Laboratory Supplies (China). The CD45.1 congenic mice (C57BL/6) were a gift from Prof. P Qian. Animal experiments were conducted under specific pathogen-free conditions in the Laboratory Animal Center of Zhejiang University and approved by the Institutional Animal Care and Use Committee of Zhejiang University.
Reagents and Antibodies
The antibodies used for flow cytometric analyses are listed in Supplementary Table S1. Purified Fc Block (anti-mouse FcRγII/III monoclonal antibody) and isotype control antibodies were obtained from eBioscience. Rapamycin and STAT3 inhibitor were purchased from TargetMol (China). Carboxyfluorescein diacetate succinimidyl ester (CFSE) was purchased from Invitrogen (United States). L-NG-Monomethylarginine, Acetate Salt (L-NMMA) and N(omega)-hydroxy-nor-L-arginine (nor-NOHA) were purchased from MCE (United States). Recombinant murine IL-2 was obtained from PeproTech (United States).
aGVHD and GVL Models
The MHC-mismatched aGVHD model was used as previously established (Hill et al., 1997; Lin et al., 2018). Briefly, BALB/c mice received 5 × 106 bone marrow (BM) cells (Mouse CD3 Positive Selection, Biolegend) after lethal irradiation with 8 Gy (split doses of 2 × 4.0 Gy 4 h apart) for the C57BL/6 → BALB/c combinations. To induce aGVHD, CD3+ T cells were isolated (Pan T Cell Isolation Kit, Miltenyi Biotec) from donor’s spleens and given at a dosage of 5 × 105 i.v. on day 0. Clinical and histopathological scores were assigned following the criteria described by Hill et al. (1997). In GVL models, recipients received grafts containing 2 × 104 A20 tumor cells. Representative samples of GVHD target organs were excised from recipients on day 21 post-BMT and subjected to pathology scoring as previously described (Lin et al., 2018).
Cellular Flow Cytometry
First, cells were stained with surface markers for 30 min at 4°C in stain buffer (BD Biosciences) and washed with PBS (2% FBS). Foxp3 and granzyme B analyses were performed following the manufacturer’s instructions (True-Nuclear Transcription Factor Buffer Set, BioLegend). For intracellular staining, cells were fixed and permeabilized with Cytofix/Permwash buffer (BD Biosciences) for 30 min at 4°C. Then, they were incubated with intracellular antibodies for another 30 min. Isotype controls were used for intracellular staining, whereas extracellular markers were gated according to the fluorescence minus one control. For T cell intracellular staining, cells isolated from each group were individually cultured for 6 h in the presence or absence of PMA (20 ng/ ml) and ionomycin (1 μg/ ml), with GolgiStop brefeldin A solution (BD Biosciences) during the last 4 h. The FlowJo v.10 software (BD Biosciences) was used to analyze and calculate the MFI for each sample.
Transduction of shRNA
The specific STAT3 and control shRNA were synthesized by Shanghai GenePharma Co., Ltd (Ling and Arlinghaus 2005). The control (E20150630A) and specific C/EBPβ (V3LMM_504726) shRNA were purchased from Dharmacon. Briefly, target cells were transduced with lentiviruses accompanied with polybrene 8 μg/ ml and centrifuged at 500g for 1 h. After 12 h, the serum-free culture medium was replaced by a fresh complete medium and incubated for 48 h. The transfection efficiency was analyzed by flow cytometry.
Cell Isolation and Culture
A20 (BALB/c B-cell lymphoma cell line) was bought from ATCC and cultured in RPMI-1640 (Gibco) supplemented with 10% FBS. To detect immunosuppressive function, PMN-MDSCs and M-MDSCs were sorted by MDSC Isolation Kit (Miltenyi Biotec) or flow cytometry cell sorting (BD FACSAria II). Human CD15+ cells were positively isolated by human CD15 MicroBeads (Miltenyi Biotec) from the bone marrow of healthy volunteers. All subjects signed informed consent forms. The purity was normally over 95% as assessed by flow cytometry (CytoFLEX LX, Beckman). The isolated PMN-MDSCs were cultured in RPMI-1640 (Gibco) with 2 mM l-glutamine, 10 mM HEPES, 1 mM sodium pyruvate, 50 mM 2-mercaptoethanol, 1% penicillin/streptomycin (Sigma), and 10% fetal bovine serum (Gibco) at 5% CO2 and 37°C. Media were supplemented with 20 ng/mL GM-CSF. PMN-MDSCs co-cultured with T cells activated by anti-CD3/CD28 antibodies (Invitrogen) for 3 days were harvested for further studies.
Quantitative RT-PCR
RNA was extracted using TRIzol (Invitrogen), and cDNA was synthesized using Takara. A SYBR Premix Ex Taq (Takara) Real-Time PCR system was used for quantitative PCR. The primers used are shown in Supplementary Table S2. The expression of target genes was determined using the comparative CT (ΔΔCT) method and presented as the “fold change” relative to control samples.
Functional Assay of PMN-MDSCs
The in vitro-suppressive function of MDSCs was assessed by determining their ability to inhibit T cell activation as described previously. Briefly, purified T cells from the spleen were labeled with CFSE (2.5 mM) and plated at a density of 1 × 105 cells/well in 5 μg/ml anti-CD3 antibody-coated, round-bottom, 96-well plates (4°C, overnight) and in the presence of 2 μg/ml soluble anti-CD28 antibody. Isolated MDSCs were added to the wells at the same size ratio. Cell proliferation was determined 3.5 days later by CFSE expression.
Cytokine Measurements
Serum samples were obtained from different recipients at the time specified. G-Series Mouse Inflammation Array (Ray Biotech) was used for semi-quantitative measurement of mouse cytokines from serum according to the manufacturer’s instructions.
Western Blot Analyses
Protein was extracted from cells using RIPA buffer (Beyotime Biotechnology) with protease and phosphatase inhibitors (Roche Diagnostics GmbH). Samples were electrophoresed through 10% polyacrylamide gels and immunoblotted with the relevant antibodies using standard methods. The antibodies for β-actin (4970), mTOR (2972), STAT3 (9139), p-STAT3 (9145), and C/EBPβ (3087) were purchased from CST.
RNA Sequencing
Total RNA was isolated using TRIzol (Invitrogen), and sequencing libraries were prepared from 10 to 100 ng total RNA using the TruSeq RNA Sample Preparation Kit v2 (Illumina). RNA sequencing (RNA-seq) and the subsequent analyses were completed by Huada Gene Biological Company (China). The heatmap was constructed using the “heatmap” package in R 3.4.4. The RNA sequencing data were deposited in the Sequence Read Archive (SRA) database: PRJNA726285.
Statistical Analyses
Experiments were performed with at least three independent biological replicates, and data are presented as means ± standard deviations (SD). Statistical analyses were performed by conducting an unpaired (two-sided) Student’s t-test for normally distributed data. The Mann–Whitney U (MWU) test was conducted if the data failed to meet normality criteria. Comparison of the survival curves was generated using the Kaplan–Meier method and compared by log-rank test. To obtain unbiased data, the histopathologic scoring of the aGVHD severity was performed by researchers who were blinded to the treatment groups. A p < 0.05 was considered statistically significant. All statistical analyses and graphics generation were performed using GraphPad Prism v.7.0 (GraphPad Software, United States).
Results
Reducing mTOR Expression Retains Myeloid Cells’ Immature Characteristics and Promotes PMN-MDSCs’ Immunosuppressive Function
As we previously demonstrated, the mTOR inhibitor rapamycin could induce a strong immunosuppressive function in PMN-MDSCs from murine BM in vitro, which prompted us to explore how mTOR regulates MDSCs’ generation and function. Thus, we generated mice with myeloid-specific mTOR deficiency by crossing mTORfl/fl with LysM-Cre recombinase mice. LysM-Cre mTORfl/fl (referred to as mTORKO) mice presented a similar absolute number of BM and spleen (SP) cells with mTORfl/fl (referred to as WT) control mice (Supplementary Figure S1A). The proportion of PMN-MDSCs and M-MDSCs in the BM and SP was comparable in WT and mTORKO mice (Supplementary Figure S1B). mTOR knockout increased the percentage of proliferating cells in S and G2/M phases and elevated resistance to apoptosis under active T cell stimulation (Supplementary Figures S1C, D). The expression of c-Kit and CXCR4 was higher in mTORKO PMN-MDSCs (Figure 1A), which revealed that they presented precursor myeloid cells characteristics. Additionally, mTORKO PMN-MDSCs had lower expression of MHC II and CD86 compared to WT PMN-MDSCs (Figure 1B), suggesting that loss of mTOR maintains PMN-MDSCs in an immature state.
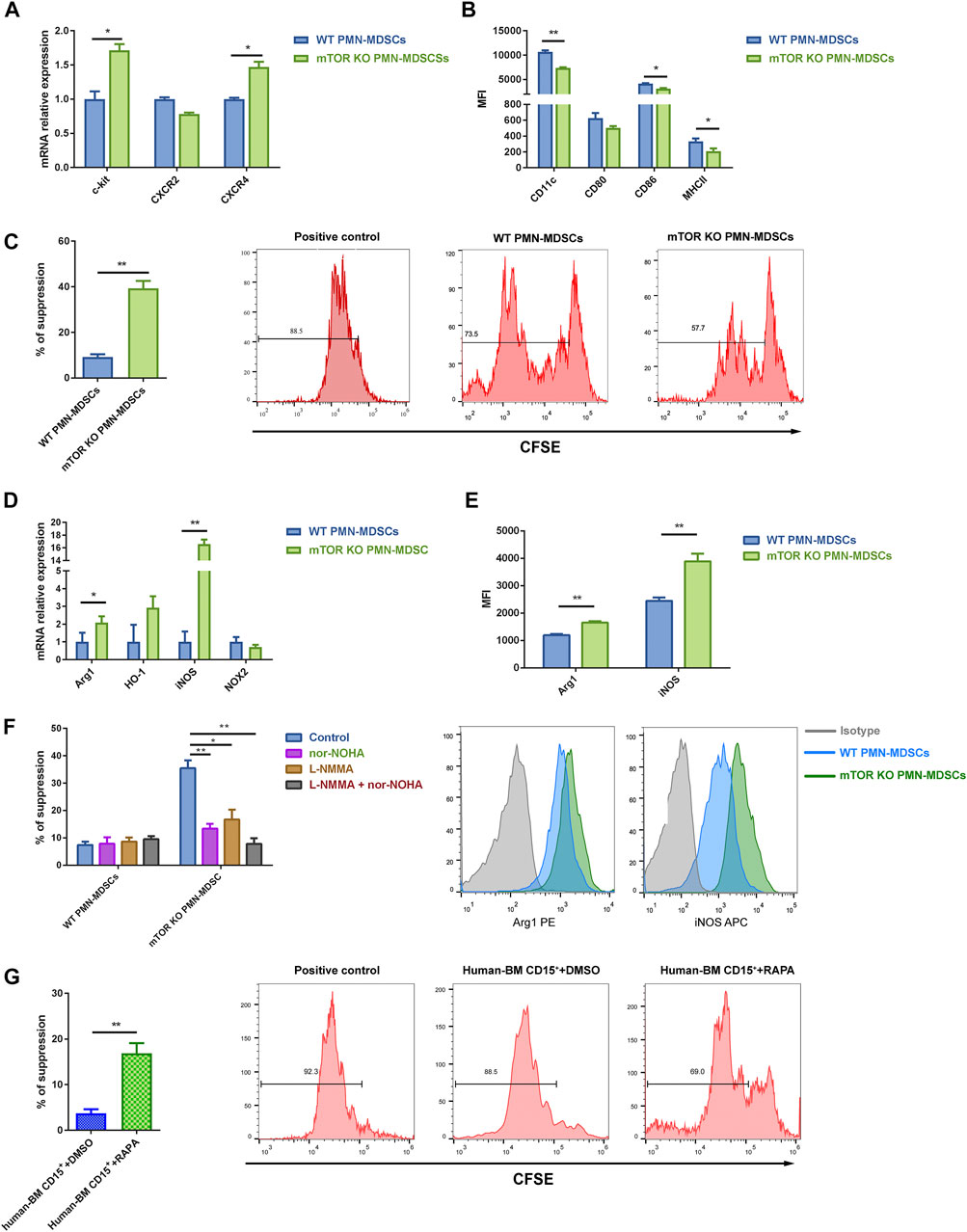
FIGURE 1. mTOR depletion induced the suppressive function of bone marrow (BM)-derived polymorphonuclear myeloid-derived suppressor cells (PMN-MDSCs). (A) PMN-MDSCs were generated in vitro from mTORfl/fl and LysM-Cre mTORfl/fl mice BM cells co-cultured with active T cells for 3 days. High transcriptional levels of neutrophil precursor markers were detected in mTORKO PMN-MDSCs. (B) mTOR depletion downregulated surface maturation markers. (C) Carboxyfluorescein diacetate succinimidyl ester (CFSE)-labeled splenic T cells were stimulated with anti-CD3/CD28 in the presence of wild-type (WT) or mTORKO PMN-MDSCs at a 1:1 ratio for 3.5 days. The suppression of splenic T cell proliferation was analyzed and calculated as PMN-MDSCs’ suppressive ability (mean ± SD; n = 8). (D) Transcript levels of the MDSC immunosuppressive markers were determined by qPCR (mean ± SEM; n = 5). (E) Flow cytometry analyses of Arg1 and iNOS expression in WT and mTORKO PMN-MDSCs after co-culturing with active T cells. Representative Arg1 and iNOS histogram showing overlays of WT PMN-MDSCs, mTORKO PMN-MDSCs, and the appropriate isotype control. (F) Arg1 inhibitor (nor-NOHA) and/or iNOS inhibitor [L-NG-Monomethylarginine, Acetate Salt (L-NMMA)] were added in PMN-MDSCs’ suppressive function assays. (G) CD15+ BM cells isolated from healthy donors were pretreated with rapamycin for 4 h, which significantly suppressed the active peripheral blood mononuclear cell (PBMC) proliferation compared to DMSO treatment (control). Data are shown as mean ± SD of one representative experiment of three–four experiments performed. **p < 0.01, *p < 0.05; p-values reflected analyses with two-tailed unpaired Student’s t-test.
Next, we determined the effect of mTOR deficiency on the suppressive function of PMN-MDSCs. T cell proliferation assays were performed by co-incubating BM-derived PMN-MDSCs with splenic T cells. The mTORKO PMN-MDSCs expressed a stronger immunosuppressive ability compared with WT PMN-MDSCs (Figure 1C), while mTOR deficiency ameliorated M-MDSCs’ immunosuppressive ability (Supplementary Figure S2A). Due to the larger amount of PMN-MDSCs compared to M-MDSCs, focusing on PMN-MDSCs as an overall treatment strategy deserves further investigation. Then, we explored the molecular mechanisms underlying their suppressive function. Arg1 and iNOS were significantly upregulated at transcriptional and protein levels in mTORKO PMN-MDSCs (Figure 1D, E). The administration of L-NMMA (an iNOS inhibitor) or nor-NOHA (an Arg1 inhibitor), either alone or combined, efficiently reduced immunosuppressive activity (Figure 1F).
Next, we hypothesized whether mTOR played the same role in human myeloid cells. After treating healthy donor BM cells with rapamycin for 4 h, the number of CD15+ cells and PMN-MDSC and M-MDSC proportions were not affected (Supplementary Figure S2B). The rapamycin pretreatment induced human BM CD15+ cells’ immunosuppressive function, but the variation degree was much smaller than that of murine cells (Figure 1G). These results showed that mTOR inhibition could promote PMN-MDSCs’ suppressive function both in mice and human BM cells.
Reducing mTOR Expression Enhances STAT3 Activity in PMN-MDSCs
Furthermore, we performed RNA-seq to uncover the molecular mechanisms underlying how mTOR deficiency rejuvenated PMN-MDSCs. A total of 2,860 differentially expressed genes were identified, 631 upregulated and 2,175 downregulated (Supplementary Figure S3A). Particularly, NOS2 showed a pronounced change in mTORKO PMN-MDSCs, which provided another evidence for the suppressive ability elevation (Supplementary Figure S3B). Through Kyoto Encyclopedia of Genes and Genomes (KEGG) analysis, many immune-related pathways were identified including TNF signaling, PI3K-Akt, and JAK-STAT (Figure 2A). STAT3 and C/EBPβ were enriched in multiple signaling pathways. Several negative regulators of STAT3 signaling including Pias, SOCS, and PTP cluster were downregulated in mTORKO PMN-MDSCs, but not in WT PMN-MDSCs (Figure 2B). The qPCR results validated significant decreases in Pias2 and Pias4 in mTORKO PMN-MDSCs; moreover, the upregulation of STAT3 and C/EBPβ was also demonstrated (Figure 2C). We verified the role of STAT3 in suppressive function using the STAT3 inhibitor, Stattic. We found that Stattic could significantly reduce the mTORKO PMN-MDSCs’ suppressive function (Figure 2D). Meanwhile, the expression of Arg1 and iNOS was downregulated (Figure 2E). Previous studies have shown that C/EBPβ is a downstream target of mTOR and STAT3 signaling pathways (Bitto et al., 2021). Western blot analyses confirmed that C/EBPβ (at 35 kDa) increased in mTORKO PMN-MDSCs and concurrently decreased with the downregulated STAT3 phosphorylation in the group treated with Stattic (Figure 2F). This indicated that STAT3 activation was required for C/EBPβ expression. To further verify the relationship between STAT3-C/EBPβ signaling and the mTORKO PMN-MDSC suppressive function, shRNA was performed to knockdown STAT3 and C/EBPβ in mTORKO PMN-MDSCs, respectively. STAT3 activation was not affected by C/EBPβ-shRNA (Supplementary Figure S4A, B), but the expression of Arg1 and iNOS decreased in both STAT3-shRNA and C/EBPβ-shRNA groups (Figure 2G). These results indicated that mTOR regulated the suppressive function of PMN-MDSCs through STAT3-C/EBPβ pathway activation.
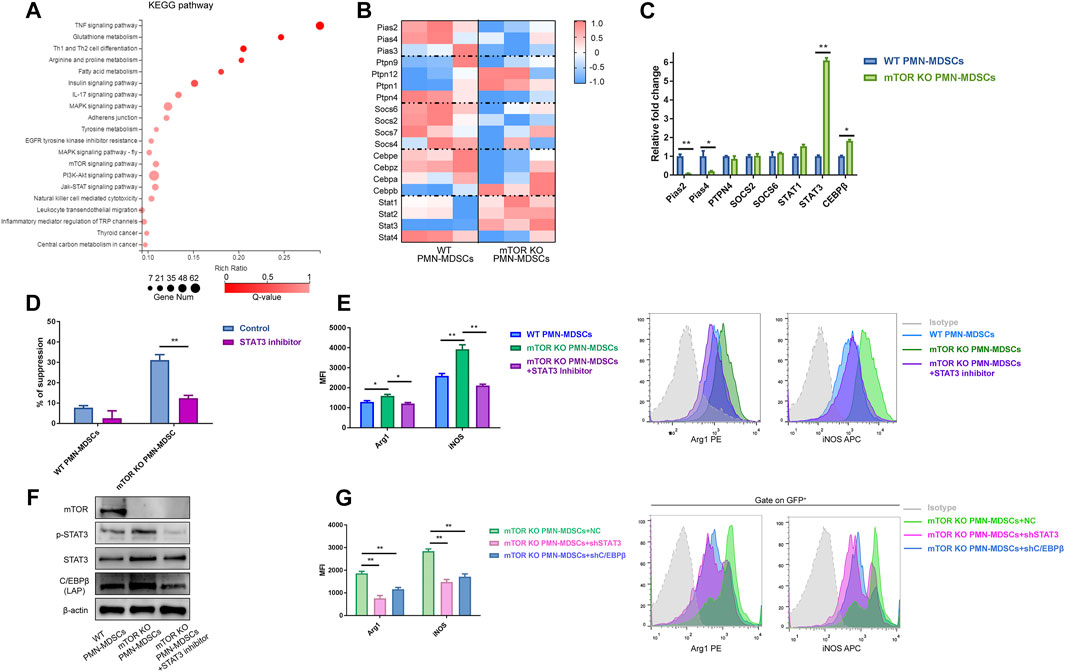
FIGURE 2. Reducing mTOR expression mainly regulated PMN-MDSCs through the STAT3-C/EBPβ pathway. (A) Kyoto Encyclopedia of Genes and Genomes (KEGG) pathway enrichment analysis of differentially expressed genes between WT and mTORKO PMN-MDSCs. (B) Heatmap showing different expressions of STAT3-related genes. (C) qPCR validations of selected genes (mean ± SEM, n = 5). (D) mTORKO PMN-MDSCs were generated with or without the STAT3 inhibitor Stattic (10 µM) for 4 h followed by co-culture with activated splenic T cells for 3.5 days at 1:1 ratios (mean ± SD, n = 6). (E) Effects of Stattic on Arg1 and iNOS expressions of mTORKO PMN-MDSCs. The representative histogram shows different expressions of suppressive markers among groups. (F) STAT3-C/EBPβ pathway western blot analyses. (G) The decline of Arg1 and iNOS expressions in mTORKO PMN-MDSCs after transfection with STAT3-shRNA or C/EBPβ-shRNA. Representative histogram of five experiments. **p < 0.01, *p < 0.05; p-values reflected analyses with the Mann–Whitney U (MWU) test.
mTORKO PMN-MDSCs Could Alleviate aGVHD While Retaining the GVL Effect
Since mTORKO PMN-MDSCs displayed a remarkable immunosuppressive function, we further explored their preventive and therapeutic capacity in murine aGVHD models. First, lethally irradiated BALB/c mice (H-2Kd) were reconstituted with T cell-depleted (TCD)-BM alone or TCD-BM plus CD3+ splenic T cells from B6 mice (H-2Kb). Then, WT PMN-MDSCs or mTORKO PMN-MDSCs were transfused with TCD-BM + T cells (Figure 3A). Mice who received TCD-BM alone achieved long-term survival within 60 days. Mice in the TCD-BM + T cells group had poor survival with a mortality rate of around 90% at 60 days and presented severe clinical aGVHD symptoms, such as weight loss, hunched posture, hair loss, and diarrhea. Histological examination of the intestine, liver, and skin showed severe tissue damage and lymphocyte infiltration. Infusion of WT PMN-MDSCs did not effectively improve the survival or alleviate the aGVHD symptoms. However, in the mTORKO PMN-MDSC infusion group, lower mortality rate and aGVHD scores were observed, and nearly 60% of the mice survived more than 60 days (Figure 3B–D). Lymphocyte infiltration and tissue damage were remarkably relieved, and pathological scores of the liver, intestine, and skin decreased in the mTORKO PMN-MDSC infusion group (Figure 3E, F). These observations suggested that mTORKO PMN-MDSC co-transfusion was an effective method to prevent aGVHD after allo-HSCT. However, when the mTORKO PMN-MDSC transfusion was performed on day 14 after aGVHD onset, the therapeutic ability was limited (Supplementary Figure S5A). The survival and aGVHD clinical and pathological scores did not differ among the TCD-BM + T cells, WT PMN-MDSC transfusion, and mTORKO PMN-MDSC transfusion groups (Supplementary Figure S5B–F).
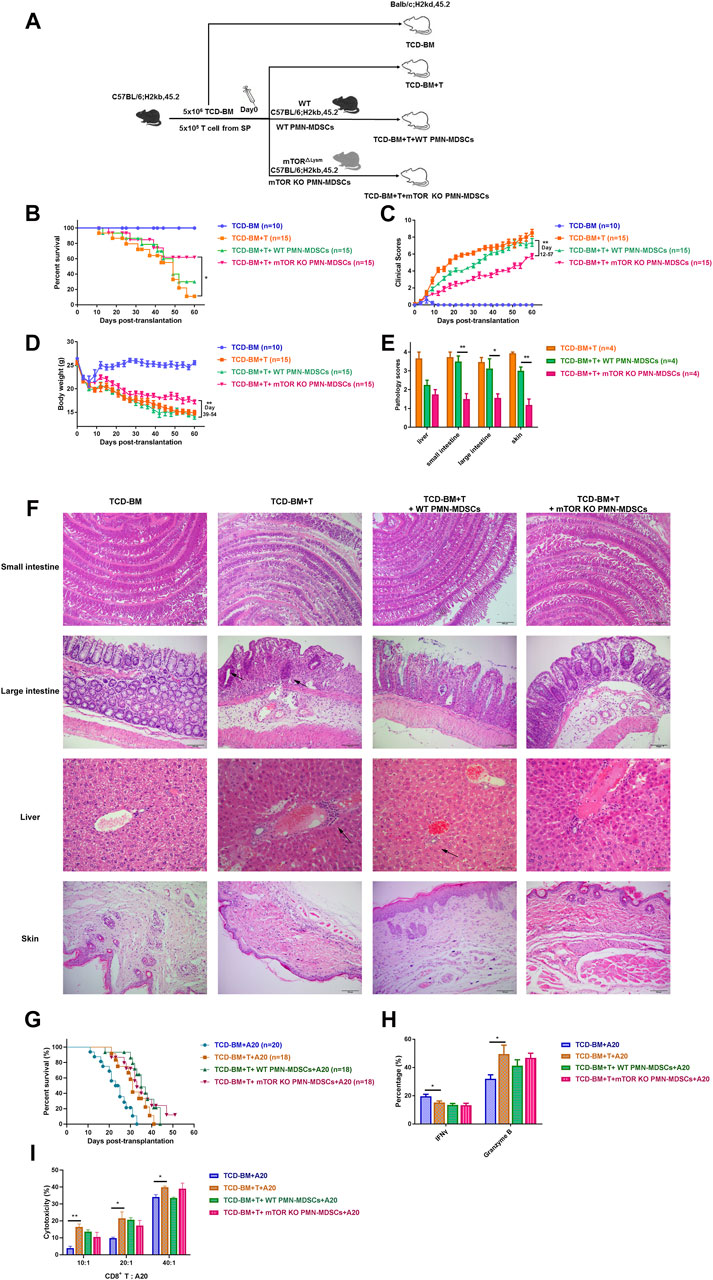
FIGURE 3. Transferring mTORKO PMN-MDSCs could alleviate acute graft-versus-host disease (aGVHD) while retaining the graft-versus-leukemia (GVL) effect. (A) Schematic diagram of the experimental design. BALB/c mice received allo-genetic C57B/L6 grafts in the absence or presence of 1 × 106 WT or mTORKO PMN-MDSCs after lethal irradiation. (B) The improvements were observed in survival time (log-rank test, p < 0.05). (C) aGVHD clinical scores (days 12–57, p < 0.01) and (D) weight restoration (days 3–27, p < 0.05; days 39–54, p < 0.01) in the TCD-BM + T cells + mTORKO PMN-MDSC group. (E) Histopathological scores of aGVHD target tissues on day 21 after allogeneic hematopoietic stem cell transplantation (allo-HSCT). (F) Representative sections of organs are shown for each group. The area indicated by the arrow shows infiltrated inflammatory cells. (G) The survival of GVL models induced by A20 leukemic cell (H-2d) injection into recipients. (H) The frequency of donor-derived interferon-γ (IFN-γ) production and granzyme B expression in CD8+ splenic T cells on day 14. (I) The cytotoxic activity of donor CD8+ T cells was analyzed on day 14 after allo-HSCT. The data above are presented as mean ± SD and was assessed by unpaired Student’s t-test (two-sided) or MWU test, pooled from two–three independent experiments, with at least five mice per group. Numerals in brackets indicate the number of mice tested.
Then, A20 cells were injected into recipients to induce tumor development to clarify the influence of mTORKO PMN-MDSCs on the GVL effect. Mice that received only TCD-BM plus A20 cells died within 32 days due to leukemia progression. Mice in the TCD-BM + T cells plus A20 cells group displayed minimal tumor infiltration but died due to aGVHD-related damage. The mTORKO PMN-MDSC transfusion group showed the best survival compared with other groups (Figure 3G). These results indicated that mTORKO PMN-MDSCs could actively suppress aGVHD after allo-HSCT while retaining GVL activity. To explore the underlying mechanisms, we examined the activation and functional activity of donor-derived CD8+ T cells, known as the main effector cells governing anti-tumor response. Intercellular staining of effective markers revealed no difference in interferon-γ (IFN-γ) or granzyme B expression in CD8+ T cells among the mTORKO PMN-MDSC treatment, WT PMN-MDSC treatment, and untreated groups (Figure 3H). Correspondingly, isolated CD8+ T cells from mTORKO PMN-MDSC-treated mice exhibited a cytotoxic activity against allogeneic A20 cells, which showed no clear difference compared with other groups (Figure 3I). These data provided further evidence that the cytotoxic capabilities of donor-derived CD8+ T cells were unaffected by mTORKO PMN-MDSCs.
mTORKO PMN-MDSCs Could Alleviate Cytokine Storm at aGVHD Initial Stage
Our previous findings confirmed that only co-transfusing mTORKO PMN-MDSCs with grafts could exert a protective effect of aGVHD development, rather than a therapeutic activity after aGVHD occurrence. Therefore, we speculated that the infused mTORKO PMN-MDSCs could restrain donor-derived T lymphocyte activation and expansion at a very early stage of aGVHD. Thus, we analyzed the proportions of several T cell subsets in each group on days 3, 7, and 14 after transplantation. In peripheral blood (PB) and SP, the T helper cell type 1 versus type 2 (Th1/Th2) ratio was remarkably elevated in TCD-BM + T cells and WT PMN-MDSC infusion groups, whereas decreased in the mTORKO PMN-MDSCs infusion group (Figure 4A). Since inducing Tregs generation is considered as an important MDSC mechanism to suppress GVHD progression (Edinger et al., 2003; Zhao et al., 2008), we further detected the percentage of Tregs in total CD4+ T cells from PB and SP. During the early period after transplantation, mice who received mTORKO PMN-MDSCs had a higher percentage of Tregs compared to other aGVHD groups (Figure 4B). Proinflammatory cytokines in serum including IL-1β, IL-6, TNF-a, and IFN-γ remarkably increased in the TCD-BM + T cells and WT PMN-MDSC infusion groups, but reduced in the mTORKO PMN-MDSC infusion group on days 7 and 14 (Figure 4C, D). These findings demonstrated that adoptive transferring of mTORKO PMN-MDSC could suppress the activation and proliferation of allo-active T cells at the beginning of aGVHD, thereby stopping cytokine storm amplification.
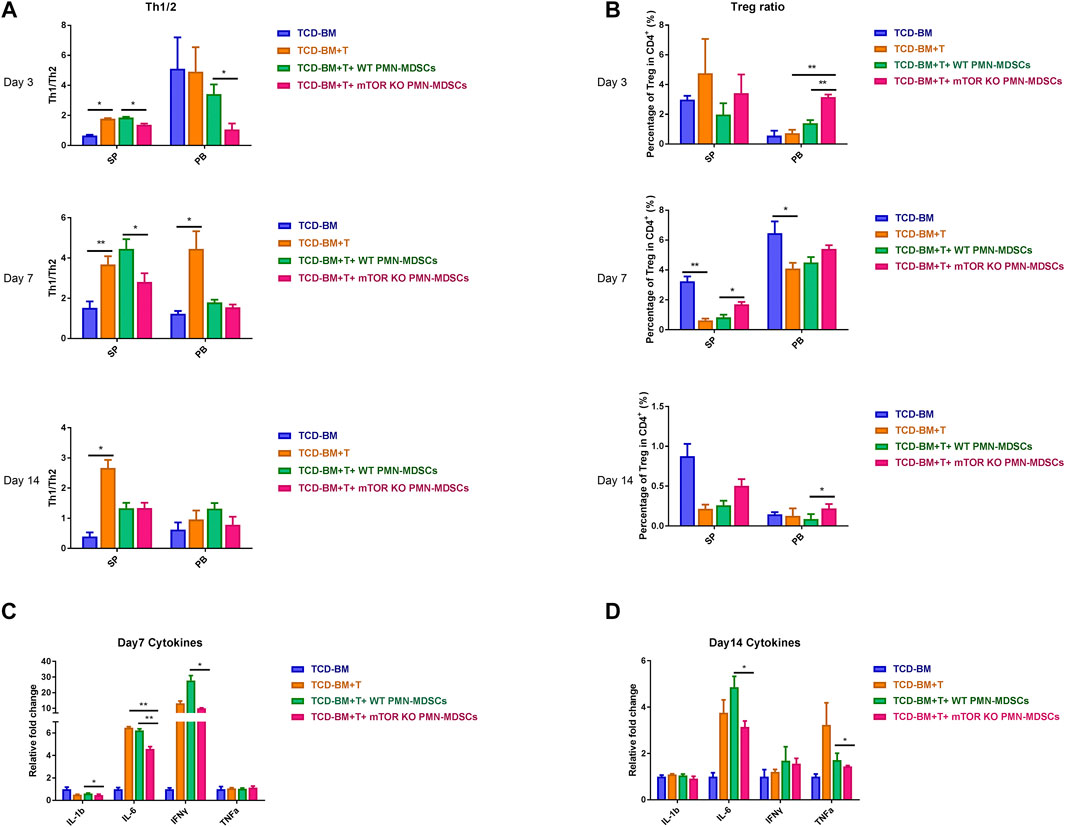
FIGURE 4. mTORKO PMN-MDSCs alleviated cytokine storm in aGVHD mice and restrained Th1/Th2 cell differentiation but promoted Tregs induction. (A–B) Th1/Th2 ratios (A) and Tregs percentage out of the CD4+ T cells total (B) detected in the spleen (SP) and peripheral blood (PB) on days 3, 7, and 14 after allo-HSCT. Data are presented as mean ± SD (n = 6). One representative experiment of three is shown. (C–D) The relative level of inflammatory cytokines in serum was measured on days 7 (C) and 14 (D). Data are presented as mean ± SD (n = 4). The p-values were determined by MWU test, *p < 0.05, **p < 0.01. One representative experiment of three is shown.
mTORKO PMN-MDSCs Could Promote a Quantitative and Functional Recovery of Donor-Derived PMN-MDSCs at aGVHD Later Stage
Next, we investigated how long the infused mTORKO PMN-MDSC could persist in vivo and whether they could play a long-term suppressive function. Thus, BALB/c mice (H2Kd, CD45.2) received B6-derived TCD-BM + T cells (H2Kb, CD45.1) with WT or mTORKO PMN-MDSCs (H2kb, CD45.2) to distinguish the host-derived or co-transfused PMN-MDSCs by CD45.1 and CD45.2 markers (Figure 5A). Both the transfused CD45.2+ WT and mTORKO PMN-MDSCs remained at a relatively high level on day 1 after transplantation, then markedly declined over time. After the transfusion, we found that either WT or mTORKO PMN-MDSCs (CD45.2, H-2Kb) persisted for no more than 10 days in BM, SP, and PB. In contrast, donor-derived PMN-MDSCs (CD45.1, H-2Kb) rapidly differentiated from progenitor cells, and the difference between the two groups gradually increased over time (Figure 5B). Therefore, these results indicated that mTORKO PMN-MDSC transfusion could promote a better recovery of donor-derived PMN-MDSCs.
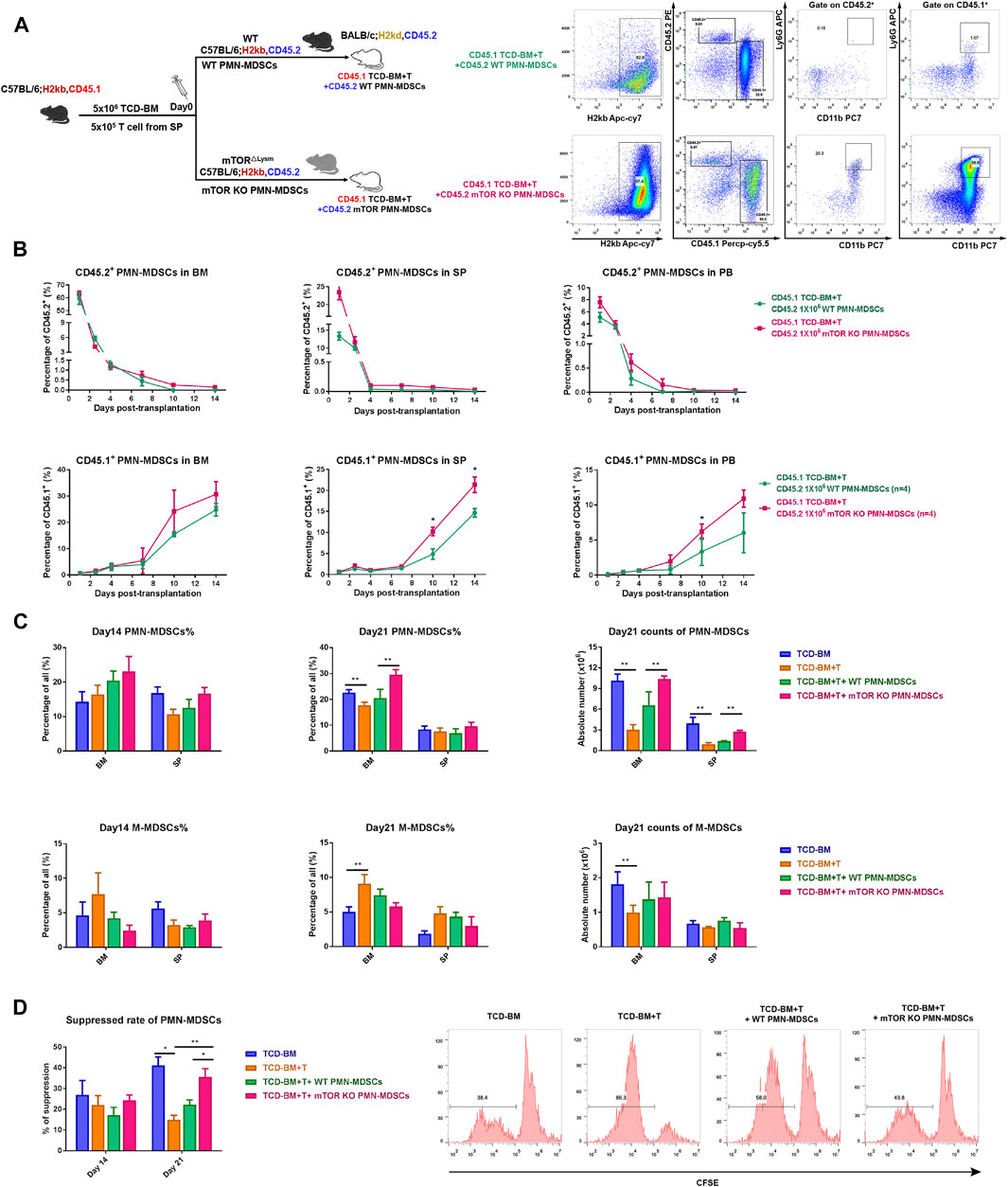
FIGURE 5. Transfused mTORKO PMN-MDSCs exerted suppressive effects during the early post-transplant period and contributed to donor-derived PMN-MDSC functional recovery. (A) Lethally irradiated CD45.2 BALB/c recipients reconstituted with CD45.1 congenic C57BL/6 mice (left). Gating strategy to discriminate the original donor (CD45.1) or host (CD45.2) PMN-MDSCs in the BM, SP, and PB, and the flow analyses of BM on day 10 was used as an example (right). (B) Transferred CD45.2+ (top row) and donor-derived CD45.1+ (bottom row) PMN-MDSCs were monitored on days 1, 3, 5, 7, 10, and 14 in the BM, SP, and PB. (C) PMN-MDSC and monocytic MDSC (M-MDSC) percentage and absolute number in the BM and SP. (D) The suppressive ability of BM PMN-MDSCs in each group on days 14 and 21. Data are presented as mean ± SD (n > 3) of one representative experiment out of three. The p-values were determined by unpaired Student’s t-test (two-sided) or MWU test, *p < 0.05, **p < 0.01.
Then, we measured donor-derived PMN-MDSCs and M-MDSCs in BM and SP on days 14 and 21 after transplantation. The percentage and absolute number of PMN-MDSCs remarkably increased in BM and SP of the mTORKO PMN-MDSC-infused group (Figure 5C). The immunosuppressive ability of PMN-MDSCs from the mTORKO PMN-MDSC infusion group was also much more robust on day 21 compared to other groups (Figure 5D). Meanwhile, M-MDSCs possessed a powerful suppressive function but with no significant difference among groups (Supplementary Figure S6A). Overall, our results showed that although transfused mTORKO PMN-MDSCs could not maintain a long-lasting persistence in BM, SP, and PB, they could promote the proliferation and functional recovery of donor-derived PMN-MDSCs.
Discussion
The sensor mTOR is important for various environmental or cellular changes, such as nutrient deprivation, energy insufficiency, and cytokine stimulation. Also, mTOR is important to control the fate of diverse immune cells (Powell et al., 2012; Yang et al., 2014). Generally, cytokines such as G-CSF, GM-CSF, IL-1β, IL-6, IL-4, and S100A8/A9 are essential to induce immunosuppressive function of BM-derived PMN-MDSCs (Talmadge and Gabrilovich 2013). In the current study, we found that isolated mTORKO PMN-MDSCs could directly exhibit a strong suppressive ability without long-term and complex cytokine induction in vitro. Consistent with previous studies, our results showed that mTOR inhibition could decrease M-MDSCs’ immunosuppressive ability (Wu et al., 2016), which revealed that the mTOR signaling might have an opposite regulatory function on these two subpopulations. Thus, we investigated how mTOR regulates PMN-MDSCs’ generation and function. According to Evrard et al. (2018), neutrophils in the BM can be identified by mass cytometry and cell cycle-based analyses as neutrophil precursors, immature neutrophils, and mature neutrophils. Among them, immature neutrophils have suppressive ability. Correspondingly, we found that the proportion of the immature subset increased in the BM of mTORKO mice (Supplementary Figure S7). These findings implied that the mTOR deficiency maintained myeloid cells in an immature state, which might be consistent with its functional properties.
The JAK-STAT signaling is considered to play a critical role in the expansion and function of MDSCs (Vasquez-Dunddel et al., 2013; Waldmann and Chen 2017). STAT3 is an essential transcription factor that activates genes involved in the differentiation and proliferation of MDSCs, such as c-myc, cyclin D1, and S100A8/A9, and induces genes associated with suppressive functions including NADPH oxidase subunits, p47phox and gp91phox, and C/EBPβ (Marigo et al., 2010). C/EBPβ is an intronless gene that generates three isoforms—liver activating protein* (LAP*, 38 kDa), liver activating protein (LAP, 35 kDa), and liver inhibitory protein (LIP, 20 kDa)—which are highly expressed in innate immune cells (Guo, Li, and Tang 2015). When mTORC1 activity is low (e.g., with the presence of rapamycin), the downstream translation of C/EBPβ-LAP is favored (Bitto et al., 2021). We observed an increased level of C/EBPβ-LAP in mTORKO PMN-MDSCs, which was STAT3 signal-dependent. The suppressive function of mTORKO PMN-MDSCs could be abolished when STAT3 activation or C/EBPβ-LAP expression was obstructed. Therefore, we concluded that mTOR regulated the suppressive function of PMN-MDSCs via STAT3-C/EBPβ pathway activation. Recent studies have demonstrated that mTOR and STAT pathways interact to control and optimize both innate and adaptive immunity regulations. T cell-specific TSC1-deficient and RICTOR-deficient mice presented increased Tregs number, which was correlated with higher STAT5 activation and proliferation rates (Chen et al., 2013; Park et al., 2013). DCs treated with Torin 1, a catalytic mTOR inhibitor, were found to express lower SOCS3 levels, resulting in increased STAT3 activation, increased B7-H1 expression, and enhanced Tregs induction (Rosborough et al., 2013). In our study, we uncovered that STAT3 was up-regulated in mTORKO PMN-MDSCs, while its negative regulator Pias was significantly decreased. However, the relationship between mTOR and Pias needs to be further studied. We will carry out further experiments to identify whether STAT3 activation is a direct result of mTOR deficiency or a subsequent signaling cascade.
Adoptive transfusion of MDSCs has been reported to be an effective strategy to control aGVHD after allo-HSCT. In vitro-generated MDSCs abrogated allogeneic antigen-specific T cell functions by skewing T cells into Th2, thereby preventing GVHD without affecting the GVL effect (Messmann et al., 2015). In our current study, we found that co-transplanting mTORKO PMN-MDSCs with grafts could alleviate aGVHD while retaining the GVL effect. In previous studies, adoptive transferred MDSCs were mainly generated in vitro by cytokines or isolated from tumor-bearing mice. As BM PMN-MDSCs freshly obtained from WT mice presented limited suppressive function, M-MDSCs with natural powerful suppressive function were considered to be a better source of therapeutic cells (Lin et al., 2018). However, M-MDSCs are present only in a small fraction in the BM. leading to an insufficient amount for treatment. In contrast, PMN-MDSCs possessing a larger proportion and quantity and can be a better choice for cellular immunotherapy. Thus, we assumed that prophylactic infusion of mTORKO PMN-MDSCs could significantly reduce mortality and alleviate aGVHD. But, it was found before that the intense inflammatory environment could directly undermine the suppressive capacity of MDSCs, which was associated with their conversion toward a mature state (Koehn et al., 2015). Transfusing on day 14 after aGVHD occurrence led to an effect that is less than satisfactory, suggesting that the therapeutic ability of these cells was limited.
In the mTORKO PMN-MDSC infusion group, the Th1/Th2 ratio was much lower and peripheral proinflammatory cytokines were maintained at a low level in serum, but the Tregs percentage was higher. Combined with the evidence that mTORKO PMN-MDSCs could only play a preventive role but not a therapeutic effect, we speculated that the adoptive transferred mTORKO PMN-MDSCs had a suppressive function at the very beginning of aGVHD and further stopped the cytokine storm amplification. Using CD45.1 and CD45.2 to distinguish adoptive transferred and donor-derived PMN-MDSCs, we found that the co-transplanted PMN-MDSCs could only persist for a short time in the BM and PB. Previous studies found that the adoptive transfused MDSCs could be detected in the lymphoid organs and liver until day 30 after transplantation (Messmann et al., 2015). We assumed that co-transplanted MDSCs might rapidly migrate to the peripheral organs or differentiate to a mature state after transfusion. On aGVHD at a later stage, we detected a better quantitative and functional recovery of donor-derived PMN-MDSCs in the mTORKO PMN-MDSC transfusion group. mTORKO PMN-MDSC transfusion plays an effective role in aGVHD prevention in a mouse model. However, the application of gene-edited MDSCs in clinical practice needs to be further explored due to the uncertain safety and technical constraints. The mTOR inhibitor rapamycin would become a potential alternative choice to regulate the differentiation and suppressive function of PMN-MDSCs. Our findings provide more evidence for the application of rapamycin for aGVHD control.
Overall, we demonstrated that mTOR is an effective intrinsic regulatory factor for PMN-MDSCs’ differentiation and immunosuppressive function. Also, mTORKO PMN-MDSC transfusion can be a therapeutic option to prevent cytokine storms at early stages and promote a quantitative and functional recovery of donor-derived PMN-MDSCs in aGVHD. Our findings indicate that mTOR manipulation of PMN-MDSCs might be an effective way in aGVHD prevention.
Data Availability Statement
The original contributions presented in the study are publicly available. This data can be found here: BioProject: PRJNA726285.
Ethics Statement
The studies involving human participants were reviewed and approved by the Committee of the First Affiliated Hospital, School of Medicine, Zhejiang University. The patients/participants provided their written informed consent to participate in this study. The animal study was reviewed and approved by the Institutional Animal Care and used Committee of Zhejiang University.
Author Contributions
XL and YL designed the study and conducted most of the experiments. QY and YL conducted some of the experiments, analyzed the data, and created some the figures. CW, SF, and LX conducted some of the experiments. YL and JS provided the research guidance. XL and YL prepared the first draft. YL, PQ, and HH provided subsequent revisions and revised the article. All authors contributed to the article and approved the submitted version.
Funding
This work was supported by grants from the National Natural Science Foundation of China (82000194, 82070179, and 81970158), the Key R and D projects of Science Technology Department of Zhejiang Province (2020C03G2013586), and the Zhejiang Provincial Natural Science Foundation (LQ19H080006 and LY19H080008).
Conflict of Interest
The authors declare that the research was conducted in the absence of any commercial or financial relationships that could be construed as a potential conflict of interest.
Publisher’s Note
All claims expressed in this article are solely those of the authors and do not necessarily represent those of their affiliated organizations, or those of the publisher, the editors, and the reviewers. Any product that may be evaluated in this article, or claim that may be made by its manufacturer, is not guaranteed or endorsed by the publisher.
Acknowledgments
We thank the support and help from the members of core facilities of the Zhejiang University School of Medicine and Laboratory Animal Center of Zhejiang University.
Supplementary Material
The Supplementary Material for this article can be found online at: https://www.frontiersin.org/articles/10.3389/fcell.2021.741911/full#supplementary-material
References
Beauchamp, E. M., and Platanias, L. C. (2013). The Evolution of the TOR Pathway and its Role in Cancer. Oncogene 32 (34), 3923–3932. doi:10.1038/onc.2012.567
Bitto, A., Tatom, N., Krivak, T., Grotz, P., and Kaeberlein, M. (2021). Evidence that C/EBP-β LAP Increases Fat Metabolism and Protects against Diet-Induced Obesity in Response to mTOR Inhibition. Front. Aging 2, 738512. doi:10.3389/fragi.2021.738512
Bronte, V., Brandau, S., Chen, S. H., Colombo, M. P., Frey, A. B., Greten, T. F., et al. (2016). Recommendations for Myeloid-Derived Suppressor Cell Nomenclature and Characterization Standards. Nat. Commun. 7, 12150. doi:10.1038/ncomms12150
Chen, H., Zhang, L., Zhang, H., Xiao, Y., Shao, L., Li, H., et al. (2013). Disruption of TSC1/2 Signaling Complex Reveals a Checkpoint Governing Thymic CD4 + CD25 + Foxp3 + Regulatory T‐cell Development in Mice. FASEB j. 27 (10), 3979–3990. doi:10.1096/fj.13-235408
D'Aveni, M., Rossignol, J., Coman, T., Sivakumaran, S., Henderson, S., Manzo, T., et al. (2015). G-CSF Mobilizes CD34+ Regulatory Monocytes that Inhibit Graft-Versus-Host Disease. Sci. Transl Med. 7 (281), 281ra42. doi:10.1126/scitranslmed.3010435
Delgoffe, G. M., Kole, T. P., Zheng, Y., Zarek, P. E., Matthews, K. L., Xiao, B., et al. (2009). The mTOR Kinase Differentially Regulates Effector and Regulatory T Cell Lineage Commitment. Immunity 30 (6), 832–844. doi:10.1016/j.immuni.2009.04.014
Deng, Y., Yang, J., Luo, F., Qian, J., Liu, R., Zhang, D., et al. (2018). mTOR-Mediated Glycolysis Contributes to the Enhanced Suppressive Function of Murine Tumor-Infiltrating Monocytic Myeloid-Derived Suppressor Cells. Cancer Immunol. Immunother. 67 (9), 1355–1364. doi:10.1007/s00262-018-2177-1
Dugast, A.-S., Haudebourg, T., Coulon, F., Heslan, M., Haspot, F., Poirier, N., et al. (2008). Myeloid-derived Suppressor Cells Accumulate in Kidney Allograft Tolerance and Specifically Suppress Effector T Cell Expansion. J. Immunol. 180 (12), 7898–7906. doi:10.4049/jimmunol.180.12.7898
Edinger, M., Hoffmann, P., Ermann, J., Drago, K., Fathman, C. G., Strober, S., et al. (2003). CD4+CD25+ Regulatory T Cells Preserve Graft-Versus-Tumor Activity while Inhibiting Graft-Versus-Host Disease after Bone Marrow Transplantation. Nat. Med. 9 (9), 1144–1150. doi:10.1038/nm915
Evrard, M., Kwok, I. W. H., Chong, S. Z., Teng, K. W. W., Becht, E., Chen, J., et al. (2018). Developmental Analysis of Bone Marrow Neutrophils Reveals Populations Specialized in Expansion, Trafficking, and Effector Functions. Immunity 48 (2), 364–379.e8. doi:10.1016/j.immuni.2018.02.002
Fan, Q., Liu, H., Liang, X., Yang, T., Fan, Z., Huang, F., et al. (2017). Superior GVHD-free, Relapse-free Survival for G-BM to G-PBSC Grafts Is Associated with Higher MDSCs Content in Allografting for Patients with Acute Leukemia. J. Hematol. Oncol. 10, 135. doi:10.1186/s13045-017-0503-2
Gabrilovich, D. I., and Nagaraj, S. (2009). Myeloid-derived Suppressor Cells as Regulators of the Immune System. Nat. Rev. Immunol. 9 (3), 162–174. doi:10.1038/nri2506
Garcia, M. R., Ledgerwood, L., Yang, Y., Xu, J., Lal, G., Burrell, B., et al. (2010). Monocytic Suppressive Cells Mediate Cardiovascular Transplantation Tolerance in Mice. J. Clin. Invest. 120 (7), 2486–2496. doi:10.1172/Jci41628
Gomez, A., Espejo, C., Eixarch, H., Casacuberta-Serra, S., Mansilla, M. J., Sanchez, R., et al. (2014). Myeloid-Derived Suppressor Cells Are Generated during Retroviral Transduction of Murine Bone Marrow. Cel Transpl. 23 (1), 73–85. doi:10.3727/096368912x658971
Guan, Q., Blankstein, A. R., Anjos, K., Synova, O., Tulloch, M., Giftakis, A., et al. (2015). Functional Myeloid-Derived Suppressor Cell Subsets Recover Rapidly after Allogeneic Hematopoietic Stem/Progenitor Cell Transplantation. Biol. Blood Marrow Transplant. 21 (7), 1205–1214. doi:10.1016/j.bbmt.2015.04.015
Guo, L., Li, X., and Tang, Q.-Q. (2015). Transcriptional Regulation of Adipocyte Differentiation: A Central Role for CCAAT/Enhancer-binding Protein (C/EBP) β. J. Biol. Chem. 290 (2), 755–761. doi:10.1074/jbc.R114.619957
Hess, A. D. (2006). Modulation of Graft-Versus-Host Disease: Role of Regulatory T Lymphocytes. Biol. Blood Marrow Transplant. 12 (1 Suppl. 2), 13–21. doi:10.1016/j.bbmt.2005.11.002
Highfill, S. L., Rodriguez, P. C., Zhou, Q., Goetz, C. A., Koehn, B. H., Veenstra, R., et al. (2010). Bone Marrow Myeloid-Derived Suppressor Cells (MDSCs) Inhibit Graft-Versus-Host Disease (GVHD) via an Arginase-1-dependent Mechanism that Is Up-Regulated by Interleukin-13. Blood 116 (25), 5738–5747. doi:10.1182/blood-2010-06-287839
Hill, G. R., Crawford, J. M., Cooke, K. R., Brinson, Y. S., Pan, L., and Ferrara, J. L. M. (1997). Total Body Irradiation and Acute Graft-Versus-Host Disease: the Role of Gastrointestinal Damage and Inflammatory Cytokines. Blood 90 (8), 3204–3213. doi:10.1182/blood.v90.8.3204
Koehn, B. H., Apostolova, P., Haverkamp, J. M., Miller, J. S., McCullar, V., Tolar, J., et al. (2015). GVHD-associated, Inflammasome-Mediated Loss of Function in Adoptively Transferred Myeloid-Derived Suppressor Cells. Blood 126 (13), 1621–1628. doi:10.1182/blood-2015-03-634691
Koreth, J., Matsuoka, K.-i., Kim, H. T., McDonough, S. M., Bindra, B., Alyea, E. P., et al. (2011). Interleukin-2 and Regulatory T Cells in Graft-Versus-Host Disease. N. Engl. J. Med. 365 (22), 2055–2066. doi:10.1056/NEJMoa1108188
Korngold, R., and Sprent, J. (1987). Variable Capacity of L3T4+ T Cells to Cause Lethal Graft-Versus-Host Disease across Minor Histocompatibility Barriers in Mice. J. Exp. Med. 165 (6), 1552–1564. doi:10.1084/jem.165.6.1552
Le Blanc, K., Frassoni, F., Ball, L., Locatelli, F., Roelofs, H., Lewis, I., et al. (2008). Blood Developmental Committee of the European Group for, and Transplantation Marrow Mesenchymal Stem Cells for Treatment of Steroid-Resistant, Severe, Acute Graft-Versus-Host Disease: a Phase II Study. The Lancet 371 (9624), 1579–1586. doi:10.1016/S0140-6736(08)60690-X
Lin, Y., Wang, B., Shan, W., Tan, Y., Feng, J., Xu, L., et al. (2018). mTOR Inhibitor Rapamycin Induce Polymorphonuclear Myeloid-Derived Suppressor Cells Mobilization and Function in Protecting against Acute Graft-Versus-Host Disease after Bone Marrow Transplantation. Clin. Immunol. 187, 122–131. doi:10.1016/j.clim.2017.11.005
Ling, X., and Arlinghaus, R. B. (2005). Knockdown of STAT3 Expression by RNA Interference Inhibits the Induction of Breast Tumors in Immunocompetent Mice. Cancer Res. 65 (11), 2532–2536. doi:10.1158/0008-5472.CAN-04-2425
Lv, M., Zhao, X.-S., Hu, Y., Chang, Y.-J., Zhao, X.-Y., Kong, Y., et al. (2015). Monocytic and Promyelocytic Myeloid-Derived Suppressor Cells May Contribute to G-CSF-Induced Immune Tolerance in Haplo-Identical Allogeneic Hematopoietic Stem Cell Transplantation. Am. J. Hematol. 90 (1), E9–E16. doi:10.1002/ajh.23865
MacDonald, K. P. A., Rowe, V., Clouston, A. D., Welply, J. K., Kuns, R. D., Ferrara, J. L. M., et al. (2005). Cytokine Expanded Myeloid Precursors Function as Regulatory Antigen-Presenting Cells and Promote Tolerance through IL-10-producing Regulatory T Cells. J. Immunol. 174 (4), 1841–1850. doi:10.4049/jimmunol.174.4.1841
Marigo, I., Bosio, E., Solito, S., Mesa, C., Fernandez, A., Dolcetti, L., et al. (2010). Tumor-Induced Tolerance and Immune Suppression Depend on the C/EBPβ Transcription Factor. Immunity 32 (6), 790–802. doi:10.1016/j.immuni.2010.05.010
Messmann, J. J., Reisser, T., Leithäuser, F., Lutz, M. B., Debatin, K.-M., and Strauss, G. (2015). In Vitro-generated MDSCs Prevent Murine GVHD by Inducing Type 2 T Cells without Disabling Antitumor Cytotoxicity. Blood 126 (9), 1138–1148. doi:10.1182/blood-2015-01-624163
Mougiakakos, D., Jitschin, R., von Bahr, L., Poschke, I., Gary, R., Sundberg, B., et al. (2013). Immunosuppressive CD14+HLA-DRlow/neg Ido+ Myeloid Cells in Patients Following Allogeneic Hematopoietic Stem Cell Transplantation. Leukemia 27 (2), 377–388. doi:10.1038/leu.2012.215
Nakamura, T., Nakao, T., Yoshimura, N., and Ashihara, E. (2015). Rapamycin Prolongs Cardiac Allograft Survival in a Mouse Model by Inducing Myeloid-Derived Suppressor Cells. Am. J. Transplant. 15 (9), 2364–2377. doi:10.1111/ajt.13276
Park, Y., Jin, H.-S., Lopez, J., Elly, C., Kim, G., Murai, M., et al. (2013). TSC1 Regulates the Balance between Effector and Regulatory T Cells. J. Clin. Invest. 123 (12), 5165–5178. doi:10.1172/Jci69751
Perobelli, S. M., Mercadante, A. C. T., Galvani, R. G., Gonçalves-Silva, T., Alves, A. P. G., Pereira-Neves, A., et al. (2016). G-CSF-Induced Suppressor IL-10+Neutrophils Promote Regulatory T Cells that Inhibit Graft-Versus-Host Disease in a Long-Lasting and Specific Way. J Immunol. 197 (9), 3725–3734. doi:10.4049/jimmunol.1502023
Powell, J. D., Pollizzi, K. N., Heikamp, E. B., and Horton, M. R. (2012). Regulation of Immune Responses by mTOR. Annu. Rev. Immunol. 30, 39–68. doi:10.1146/annurev-immunol-020711-075024
Rao, R. R., Li, Q., Odunsi, K., and Shrikant, P. A. (2010). The mTOR Kinase Determines Effector versus Memory CD8 + T Cell Fate by Regulating the Expression of Transcription Factors T-Bet and Eomesodermin. Immunity 32 (1), 67–78. doi:10.1016/j.immuni.2009.10.010
Rieber, N., Wecker, I., Neri, D., Fuchs, K., Schäfer, I., Brand, A., et al. (2014). Extracorporeal Photopheresis Increases Neutrophilic Myeloid-Derived Suppressor Cells in Patients with GvHD. Bone Marrow Transpl. 49 (4), 545–552. doi:10.1038/bmt.2013.236
Ringdén, O., Uzunel, M., Rasmusson, I., Remberger, M., Sundberg, B., Lönnies, H., et al. (2006). Mesenchymal Stem Cells for Treatment of Therapy-Resistant Graft-Versus-Host Disease. Transplantation 81 (10), 1390–1397. doi:10.1097/01.tp.0000214462.63943.14
Rosborough, B. R., Raïch-Regué, D., Matta, B. M., Lee, K., Gan, B., DePinho, R. A., et al. (2013). Murine Dendritic Cell Rapamycin-Resistant and Rictor-independent mTOR Controls IL-10, B7-H1, and Regulatory T-Cell Induction. Blood 121 (18), 3619–3630. doi:10.1182/blood-2012-08-448290
Talmadge, J. E., and Gabrilovich, D. I. (2013). History of Myeloid-Derived Suppressor Cells. Nat. Rev. Cancer 13 (10), 739–752. doi:10.1038/nrc3581
Thomson, A. W., Turnquist, H. R., and Raimondi, G. (2009). Immunoregulatory Functions of mTOR Inhibition. Nat. Rev. Immunol. 9 (5), 324–337. doi:10.1038/nri2546
Vasquez-Dunddel, D., Pan, F., Zeng, Q., Gorbounov, M., Albesiano, E., Fu, J., et al. (2013). STAT3 Regulates Arginase-I in Myeloid-Derived Suppressor Cells from Cancer Patients. J. Clin. Invest. 123 (4), 1580–1589. doi:10.1172/Jci60083
Vendramin, A., Gimondi, S., Bermema, A., Longoni, P., Rizzitano, S., Corradini, P., et al. (2014). Graft Monocytic Myeloid-Derived Suppressor Cell Content Predicts the Risk of Acute Graft-Versus-Host Disease after Allogeneic Transplantation of Granulocyte Colony-Stimulating Factor-Mobilized Peripheral Blood Stem Cells. Biol. Blood Marrow Transplant. 20 (12), 2049–2055. doi:10.1016/j.bbmt.2014.09.011
Vogelsang, G. B., Lee, L., and Bensen-Kennedy, D. M. (2003). Pathogenesis and Treatment of Graft-Versus-Host Disease after Bone Marrow Transplant. Annu. Rev. Med. 54, 29–52. doi:10.1146/annurev.med.54.101601.152339
Waldmann, T. A., and Chen, J. (2017). Disorders of the JAK/STAT Pathway in T Cell Lymphoma Pathogenesis: Implications for Immunotherapy. Annu. Rev. Immunol. 35, 533–550. doi:10.1146/annurev-immunol-110416-120628
Wang, K., Lv, M., Chang, Y.-J., Zhao, X.-Y., Zhao, X.-S., Zhang, Y.-Y., et al. (2019). Early Myeloid-Derived Suppressor Cells (HLA-DR−/lowCD33+CD16−) Expanded by Granulocyte colony-stimulating Factor Prevent Acute Graft-Versus-Host Disease (GVHD) in Humanized Mouse and Might Contribute to Lower GVHD in Patients post Allo-HSCT. J. Hematol. Oncol. 12 (1), 31. doi:10.1186/s13045-019-0710-0
Wei, C., Wang, Y. X., Ma, L., Wang, X., Chi, H., Zhang, S., et al. (2018). Rapamycin Nano-Micelle Ophthalmic Solution Reduces Corneal Allograft Rejection by Potentiating Myeloid-Derived Suppressor Cells' Function. Front. Immunol. 9, 2283. doi:10.3389/fimmu.2018.02283
Wu, T., Zhao, Y., Wang, H., Li, Y., Shao, L., Wang, R., et al. (2016). mTOR Masters Monocytic Myeloid-Derived Suppressor Cells in Mice with Allografts or Tumors. Sci. Rep. 6, 20250. doi:10.1038/srep20250
Yang, H., Wang, X., Zhang, Y., Liu, H., Liao, J., Shao, K., et al. (2013). Modulation of TSC-mTOR Signaling on Immune Cells in Immunity and Autoimmunity. J. Cel. Physiol. 229 (1), 17–26. doi:10.1002/jcp.24426
Zhang, J., Chen, H.-M., Ma, G., Zhou, Z., Raulet, D., Rivera, A. L., et al. (2019). The Mechanistic Study behind Suppression of GVHD while Retaining GVL Activities by Myeloid-Derived Suppressor Cells. Leukemia 33 (8), 2078–2089. doi:10.1038/s41375-019-0394-z
Zhang, Y., Bi, Y., Yang, H., Chen, X., Liu, H., Lu, Y., et al. (2014). mTOR Limits the Recruitment of CD11b+ Gr1+ Ly6Chigh Myeloid-Derived Suppressor Cells in Protecting against Murine Immunological Hepatic Injury. J. Leukoc. Biol. 95 (6), 961–970. doi:10.1189/jlb.0913473
Zhao, D., Zhang, C., Yi, T., Lin, C.-L., Todorov, I., Kandeel, F., et al. (2008). In Vivo-activated CD103+CD4+ Regulatory T Cells Ameliorate Ongoing Chronic Graft-Versus-Host Disease. Blood 112 (5), 2129–2138. doi:10.1182/blood-2008-02-140277
Keywords: polymorphonuclear MDSCs, mTOR, acute GVHD, GVL effect, allogeneic HSCT
Citation: Li X, Li Y, Yu Q, Xu L, Fu S, Wei C, Wang L, Luo Y, Shi J, Qian P, Huang H and Lin Y (2021) mTOR Signaling Regulates the Development and Therapeutic Efficacy of PMN-MDSCs in Acute GVHD. Front. Cell Dev. Biol. 9:741911. doi: 10.3389/fcell.2021.741911
Received: 15 July 2021; Accepted: 19 November 2021;
Published: 23 December 2021.
Edited by:
Kai Yang, Indiana University School of Medicine–Lafayette, United StatesReviewed by:
Snehalata Pawar, Upstate Medical University, United StatesNamratha Sheshadri, Rutgers University, United States
Copyright © 2021 Li, Li, Yu, Xu, Fu, Wei, Wang, Luo, Shi, Qian, Huang and Lin. This is an open-access article distributed under the terms of the Creative Commons Attribution License (CC BY). The use, distribution or reproduction in other forums is permitted, provided the original author(s) and the copyright owner(s) are credited and that the original publication in this journal is cited, in accordance with accepted academic practice. No use, distribution or reproduction is permitted which does not comply with these terms.
*Correspondence: He Huang, aHVhbmdoZUB6anUuZWR1LmNu; Yu Lin, bGlueXU1MTdAemp1LmVkdS5jbg==