- 1Central Research Laboratory, Bashkir State Medical University, Ufa, Russia
- 2Department of Neurosurgery, The First Affiliated Hospital of Harbin Medical University, Harbin, China
- 3Institute of Brain Science, Harbin Medical University, Harbin, China
- 4Interim Translational Research Institute, Academic Health System, Hamad Medical Corporation, Doha, Qatar
Brain tumors in children and adults are challenging tumors to treat. Malignant primary brain tumors (MPBTs) such as glioblastoma have very poor outcomes, emphasizing the need to better understand their pathogenesis. Developing novel strategies to slow down or even stop the growth of brain tumors remains one of the major clinical challenges. Modern treatment strategies for MPBTs are based on open surgery, chemotherapy, and radiation therapy. However, none of these treatments, alone or in combination, are considered effective in controlling tumor progression. MicroRNAs (miRNAs) are 18–22 nucleotide long endogenous non-coding RNAs that regulate gene expression at the post-transcriptional level by interacting with 3′-untranslated regions (3′-UTR) of mRNA-targets. It has been proven that miRNAs play a significant role in various biological processes, including the cell cycle, apoptosis, proliferation, differentiation, etc. Over the last decade, there has been an emergence of a large number of studies devoted to the role of miRNAs in the oncogenesis of brain tumors and the development of resistance to radio- and chemotherapy. Wherein, among the variety of molecules secreted by tumor cells into the external environment, extracellular vesicles (EVs) (exosomes and microvesicles) play a special role. Various elements were found in the EVs, including miRNAs, which can be transported as part of these EVs both between neighboring cells and between remotely located cells of different tissues using biological fluids. Some of these miRNAs in EVs can contribute to the development of resistance to radio- and chemotherapy in MPBTs, including multidrug resistance (MDR). This comprehensive review examines the role of miRNAs in the resistance of MPBTs (e.g., high-grade meningiomas, medulloblastoma (MB), pituitary adenomas (PAs) with aggressive behavior, and glioblastoma) to chemoradiotherapy and pharmacological treatment. It is believed that miRNAs are future therapeutic targets in MPBTs and such the role of miRNAs needs to be critically evaluated to focus on solving the problems of resistance to therapy this kind of human tumors.
Introduction
Malignant primary brain tumors (MPBTs) are one of the most difficult to treat types of tumors, resulting in significant morbidity and mortality in both children and adults. The most common MPBTs are glioblastomas, high-grade meningiomas, medulloblastoma (MB), and pituitary adenomas (PAs) with aggressive behavior, as aggressive prolactin PAs (Schiff and Alyahya, 2020; Thakkar et al., 2021). For example, for patients with glioblastoma or MB, the overall survival remains is poor, with conventional therapies such as radio- and chemotherapy only providing marginal benefits to patient survival. Therefore, new strategies are needed to overcome the barriers to successful treatment (Thakkar et al., 2021).
Over the past decades, significant progress has been achieved in the study of tumor biology, the study of the mechanisms of control of tumor metastasis, apoptosis, invasion, angiogenesis, and proliferation of tumor cells. These data were obtained by studying the cellular composition and microenvironment of tumors, various intracellular signaling pathways, and molecular processes of oncogenesis (Richardson et al., 2020). MicroRNAs (miRNAs) are 18–22 nucleotide endogenous non-coding RNAs that regulate gene expression at the post-transcriptional level by interacting with 3′-untranslated regions (3′-UTR) of mRNA-targets (Lu and Rothenberg, 2018; Figure 1). It is estimated that more than 60% of all human protein-coding genes are directly regulated by miRNAs (Ha and Kim, 2014; Dexheimer and Cochella, 2020). It has been proven that miRNAs are involved in various biological processes, including the cell cycle, apoptosis, cell proliferation, and differentiation (Rupaimoole and Slack, 2017; Saliminejad et al., 2019). In addition, miRNAs play a role in the oncogenesis of various human tumors, including brain tumors (Bertoli et al., 2015; Qadir and Faheem, 2017; Ali Syeda et al., 2020; Balachandran et al., 2020). Recently, most research has focused on the role of miRNAs in resistance to malignant human tumors therapy. In MPBTs, the role of miRNAs in radio- and chemotherapy resistance is an attractive area of research and is expected to lead to the development of novel treatment strategies. This review will focus on differential expression of miRNAs in MPBTs (e.g., high-grade meningiomas, MB, PAs with aggressive behavior, and glioblastoma) with their gene-targets and their potential role in resistance to radio- and chemotherapy, and to pharmacological treatment.
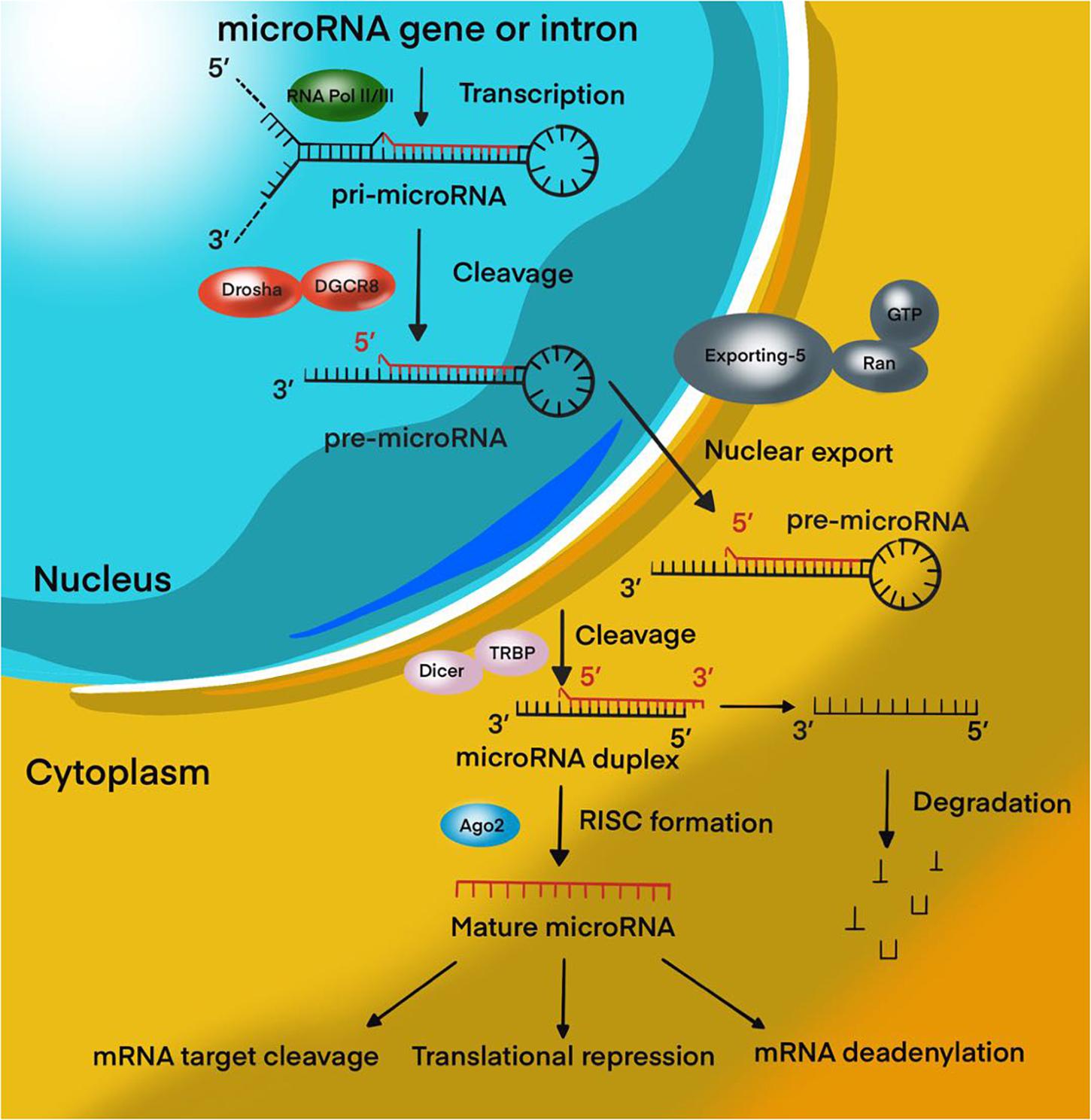
Figure 1. MiRNA biogenesis pathway. Overview schematic representation of canonical miRNA biogenesis pathway.
Micrornas Dysregulation in Malignant Primary Brain Tumors
MicroRNAs perform an important function in the complex mechanism of regulation of gene activity, since they determine the qualitative and quantitative composition of transcripts and proteins necessary for the development of individual tissues, organs and the whole organism. A growing body of evidence points to the importance of miRNAs deregulation in the initiation and progression of tumors, where they can act as oncogenic miRNAs (oncomiRs) or tumor-suppressor miRNAs, depending on the cellular function of their gene-targets (Liu et al., 2014). Moreover, the activation or suppression of specific miRNA families is the mechanism by which oncogenes such as epidermal growth factor receptor (EGFR) and MET or tumor suppressor genes such as phosphatase and tensin homolog deleted on chromosome 10 (PTEN), adenomatous polyposis coli (APC), and breast cancer type 1/2 (BRCA1/2) induce or inhibit oncogenesis (Zhang et al., 2007; Lee and Muller, 2010).
To date, a lot of evidence has been collected about the aberrant expression of miRNAs in various tumors, particular, malignant (Van Roosbroeck and Calin, 2017). It was shown that miRNAs control the expression of genes of regulatory pathways that play a key role in tumor development, control apoptosis and proliferation of tumor cells, tumor growth in response to DNA damage and repair, angiogenesis and response to hypoxia, the interaction of tumor cells with the microenvironment (Rupaimoole and Slack, 2017; Van Roosbroeck and Calin, 2017). A number of researchers isolate miRNAs directly related to individual processes in a tumor, or stages of development, of a disease (Vishnoi and Rani, 2017). Many miRNAs are involved in key signaling pathways associated with the regulation of the cell cycle and apoptosis. In one of the latest studies, Allahverdi et al. (2020) found that adipose-derived mesenchymal stem cells (AD-MSCs) delivering miR-4731 induces apoptosis and cell cycle arrest in the glioblastoma cell line. Another study provided evidence that miR-221-3p reduces MB cell proliferation by inducing apoptosis and G0/G1 arrest by suppressing eukaryotic translation initiation factor 5A-2 (EIF5A2) (Yang et al., 2019).
There is also a separate group of miRNAs associated with the metastatic activity of tumors – metastamiRs. Moreover, among such miRNAs, some promote (miR-9, miR-210, miR-21, miR-218, etc.) tumor metastasis, while others (miR-145, miR-7, miR-146-a, etc.), on the contrary, suppress it (Alsidawi et al., 2014; Lu et al., 2015; Lima et al., 2017; Ji et al., 2018; Maryam et al., 2021).
In recent years, thanks to advances in molecular oncology, it has been possible to decipher some of the mechanisms of oncogenesis and to determine the signs of a malignant phenotype, one of which is angiogenesis. Malignant tumors requires more oxygen and nutrients to grow (Viallard and Larrivée, 2017). The solution to this issue is to trigger the mechanism of angiogenesis in the tumor. Vascular endothelial growth factor (VEGF) is extremely important for the formation of an adequate functioning vascular system during embryogenesis and in the early postnatal period, but it also plays an important role in pathological angiogenesis. In many types of tumors, increased VEGF expression correlates with poor prognosis, including aggressive tumor growth, recurrence, metastasis, and decreased survival (Melincovici et al., 2018). In addition, VEGF expression correlates with a decrease in the density of the microvascular network in the malignant brain tumors, which in itself serves as an indicator of the prognosis of vascular rupture, followed by hemorrhage in the tumor bed (Apte et al., 2019). To date, a number of miRNAs have been identified that are highly expressed in endothelial cells (ECs) and/or are activated under hypoxic conditions. Among these miRNAs, it is worth noting miR-126, which is specifically expressed in the ECs and is a key regulator of the integrity of the vascular wall and angiogenesis in various tumors, including brain tumors (Fish et al., 2008). Smits et al. (2012) showed there is significant low-expression of miR-125b in ECs co-cultured with U87 glioblastoma line cells. Moreover, the authors demonstrated that miRNA-125b inhibits angiogenic processes by directly regulating Myc-associated zinc finger protein (MAZ)/VEGF signaling pathway expression. It is known that, MAZ-binding sites are located in the promoter regions of angiogenic factor VEGF.
Xiao et al. (2016) demonstrated that miR-566 was overexpressed in glioblastoma in vitro and in vivo, and inhibition of miR-566 was able to suppress the invasion and migration of glioblastoma cells, and angiogenesis via the VEGF/Von Hippel–Lindau tumor suppressor (VHL) pathway. This suggests that miR-566 may function as an oncogene, and therefore, miR-566 may be considered a novel therapeutic target of glioblastoma (Xiao et al., 2016).
There is evidence that some miRNAs can participate in the processes of malignant transformation in benign brain tumors. Brain tumors of different histology are characterized by specific miRNAs expression profiles associated with the clinical and pathological properties of the tumor (Van Roosbroeck and Calin, 2017). For instance, miR-21 makes it possible to distinguish between the main histological types of meningioma, for which miR-21 expression showed a significant increase in World Health Organization (WHO) grade 2 and 3 lesions as compared to WHO grade 1 lesions (Katar et al., 2017).
Currently, there is an active search for new miRNAs and their target genes involved in other important processes associated with oncogenesis (and not only), for example, the control of the balance of self-renewal and differentiation of stem cells, epithelial-mesenchymal transition (EMT), regulation of the immune response, the relationship of the tumor with the microenvironment, etc.
It should also be borne in mind that the reason for the change in the expression of miRNA may be a violation of the expression of proteins involved in miRNA biogenesis. It is known that the loss or insufficient expression of Drosha, Dicer, and TRBP can lead to the development of a tumor process (Olejniczak et al., 2018). Several groups showed impaired expression of Drosha and Dicer in glioblastoma, pineoblastoma, and neuroblastoma, all of which correlated with a poor prognosis of survival (Lin et al., 2010; Mansouri et al., 2016; de Kock et al., 2020). Decreased expression of these proteins can be mediated by mutations or epigenetic inactivation of their genes. In addition, mutations in Dicer can lead to impaired recognition of miRNA precursors and a change in the balance of strands. Argonaute 2 (Ago2) and GW proteins that act as direct partners of miRNAs are often susceptible to somatic mutations in glioblastoma, which are accompanied by a high level of instability of microsatellite tumor DNA (Li S. et al., 2014; Li Z. et al., 2019). Disruption of the transport of pre-miRNAs into the cytoplasm can also lead to a decrease in their expression. A mutation in the exportin-5 gene, leading to the synthesis of a truncated protein that is unable to recognize pre-miRNAs, causes a decrease in the level of mature miRNAs in a number of tumors (Wu et al., 2018).
A number of oncogenic proteins can directly interfere with miRNA biogenesis in tumors. Thus, wild and mutant forms of p53 are involved in the biogenesis of a number of miRNAs, primarily miR-34 (Zhang et al., 2019). It is known that mutant forms of p53 can inhibit Drosha activity and prevent the formation of pre-miRNA (Garibaldi et al., 2016). Transforming growth factor beta (TGF-β) affects the processing of miRNA through the binding of effector proteins to the microprocessor complex and pri-miRNA. YES-associated protein 1 (YAP1), one of the components of the Hippo pathway, regulates the activity of the microprocessor complex depending on the density of cells in culture (Ruan et al., 2016). Tumor suppressor protein BRCA1 also stimulates the activity of the microprocessor complex (Lee and Muller, 2010). Under hypoxic conditions, activated EGFR can phosphorylate Ago2, inhibiting its interaction with Dicer and decreasing the level of activity of miRNA effector systems (Shen et al., 2013). In addition, the interaction of p53 with Ago2 leads to a change in the spectrum of miRNAs associated with it, leading to the formation of complexes carrying tumor suppressor miRNAs, for example, let-7 (Krell et al., 2016).
Thus, miRNAs are one of the key factors in the development of malignant forms of brain tumors, both as drivers of malignant transformation and as a victim of the deregulation of cellular regulatory systems. The close relationship of miRNAs with MPBTs has led to the fact that they are currently being actively studied (Table 1; Grunder et al., 2011; Kliese et al., 2013; Asuthkar et al., 2014; Wang et al., 2015; Wei et al., 2015; Yu et al., 2016; Zheng et al., 2017; Xu et al., 2018; Xue et al., 2019; Hou et al., 2020; Muñoz-Hidalgo et al., 2020; Negroni et al., 2020; Song et al., 2020), including with the aim of creating diagnostic and therapeutic systems designed to increase the effectiveness of treatment of this disease.
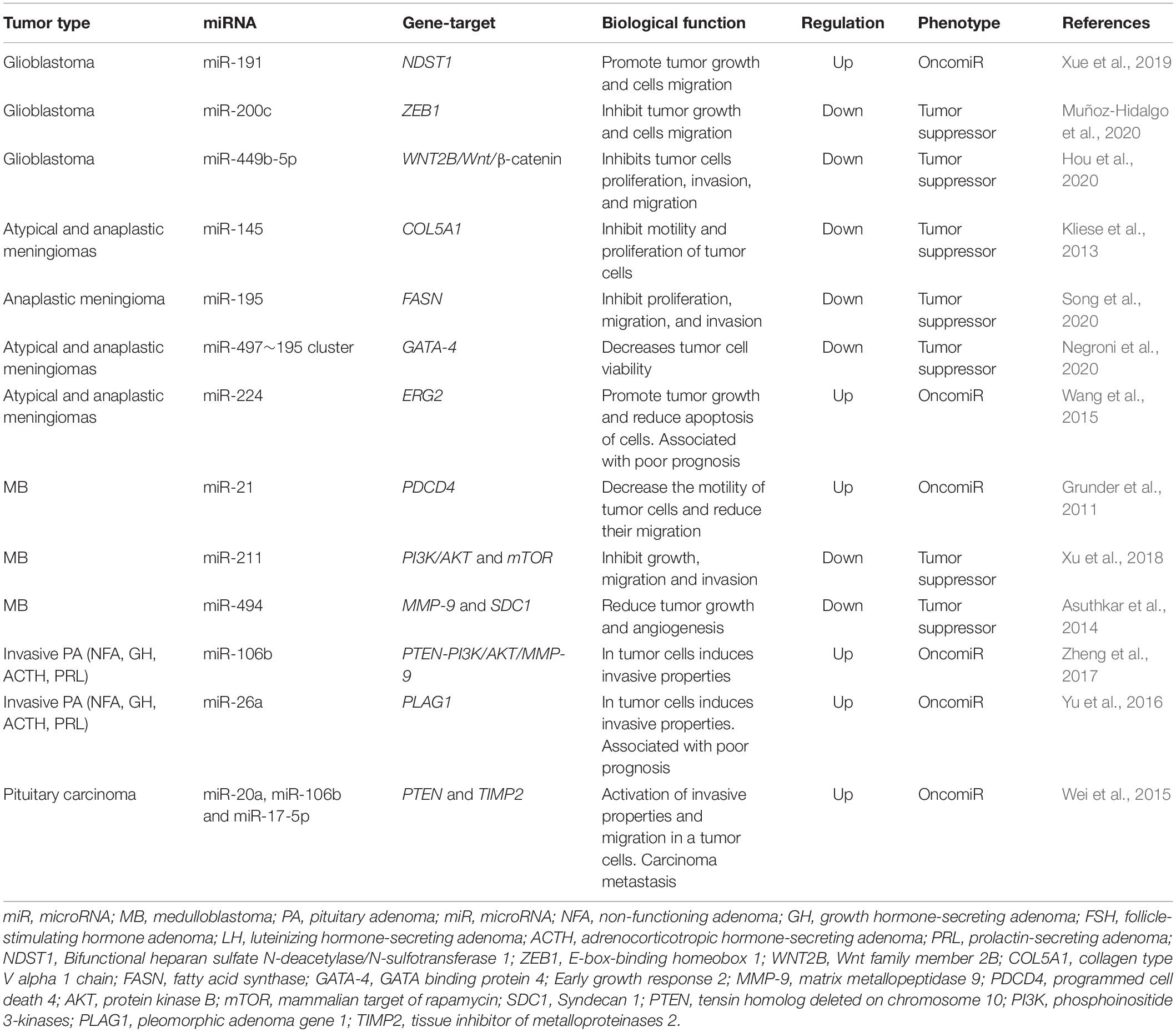
Table 1. The most relevant studies to study the role of miRNAs in the oncogenesis of malignant primary brain tumors (MPBTs).
Micrornas in Resistance to Chemoradiotherapy and Pharmacological Treatment
Resistance to therapy of brain tumors is an important problem in modern neurosurgery. There are two main mechanisms for the emergence of radio- and chemotherapy resistance: (1) activation of specific signaling pathways responsible for the “neutralization” of the chemotherapy drug and ionizing radiation in the tumor cell. Such these signaling pathways include phosphoinositide 3-kinases/protein kinase B (PI3K/AKT) è mitogen-activated protein kinase (MAPK)/ERK; and 2) violation of the mechanism of cell death under the influence of chemotherapy and ionizing radiation (Cao et al., 2019; Kapoor et al., 2020). This mechanism includes the blocking of apoptosis with p53 mutations, overexpression of B-cell lymphoma 2 (Bcl-2), a decrease in the expression of cluster of differentiation 95 (CD95) (Kapoor et al., 2020). It is now known that miRNAs can control the regulation of target genes or signaling pathways involved in malignant tumor resistance to therapy, including MPBTs. Among these miRNAs, miR-21 is the most studied in glioblastoma. MiR-21 is one of the important miRNAs involved in glioblastoma oncogenesis. A large number of studies indicated that miR-21 could affect a variety of cellular and molecular pathways. It has been showing that deregulation of miR-21 could be associated with resistance to radio- and chemotherapy of glioblastoma (Figure 2; Lan et al., 2015; Masoudi et al., 2018; Buruiană et al., 2020). In this article, we will consider current knowledge about the role of miRNAs in the mechanisms of resistance to therapy in MPBTs. In addition, a summary of the role of some miRNAs in therapy resistance by targeting their gene targets is shown in Table 2 (Pannuru et al., 2014; Dénes et al., 2015; Abdelfattah et al., 2018; Chen et al., 2018; Li J. et al., 2019; Bogner et al., 2020; Hu et al., 2020; Sun et al., 2020; Zhao C. et al., 2020; Cardoso et al., 2021) and Table 3 (Lee et al., 2014; Li W. et al., 2014; Huynh et al., 2015; Wu et al., 2015; Yang F. et al., 2017; Zhang Q. et al., 2020).
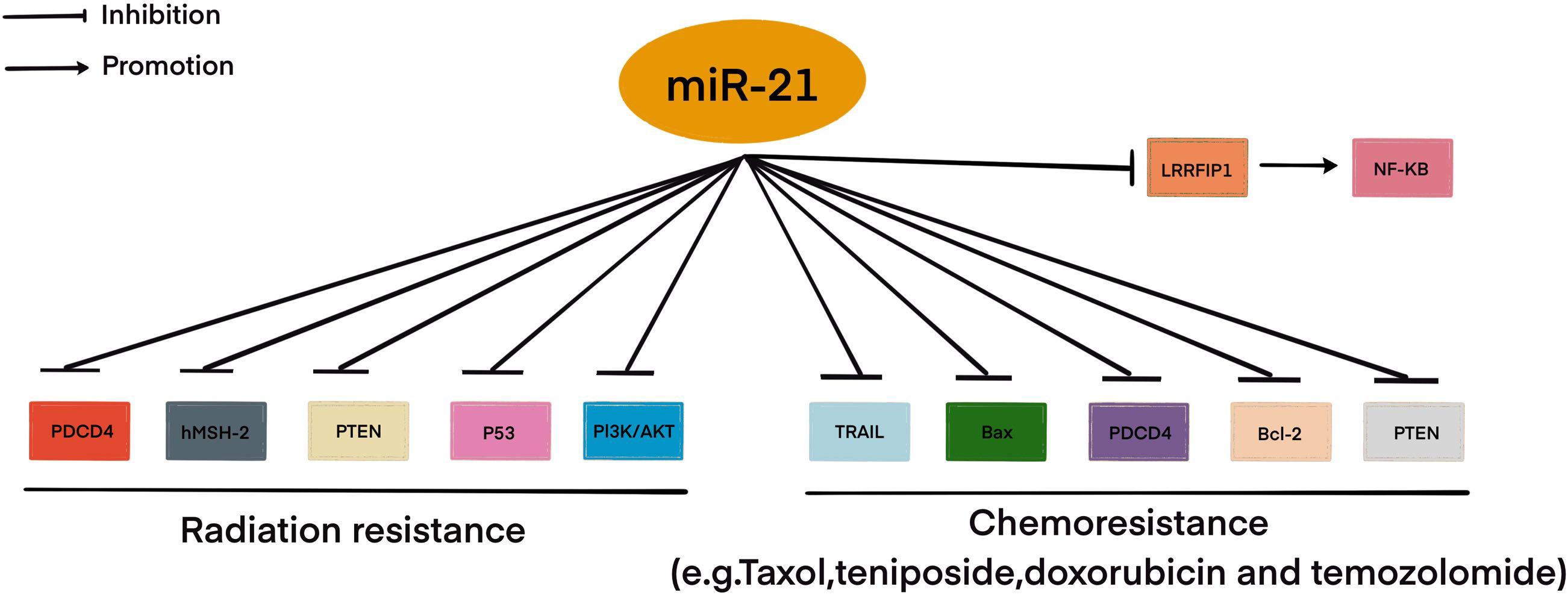
Figure 2. MiR-21 involved in radio- and chemoresistance in glioblastoma. This figure shows through which signaling pathways miR-21 may be involved in resistance to therapy in glioblastoma.
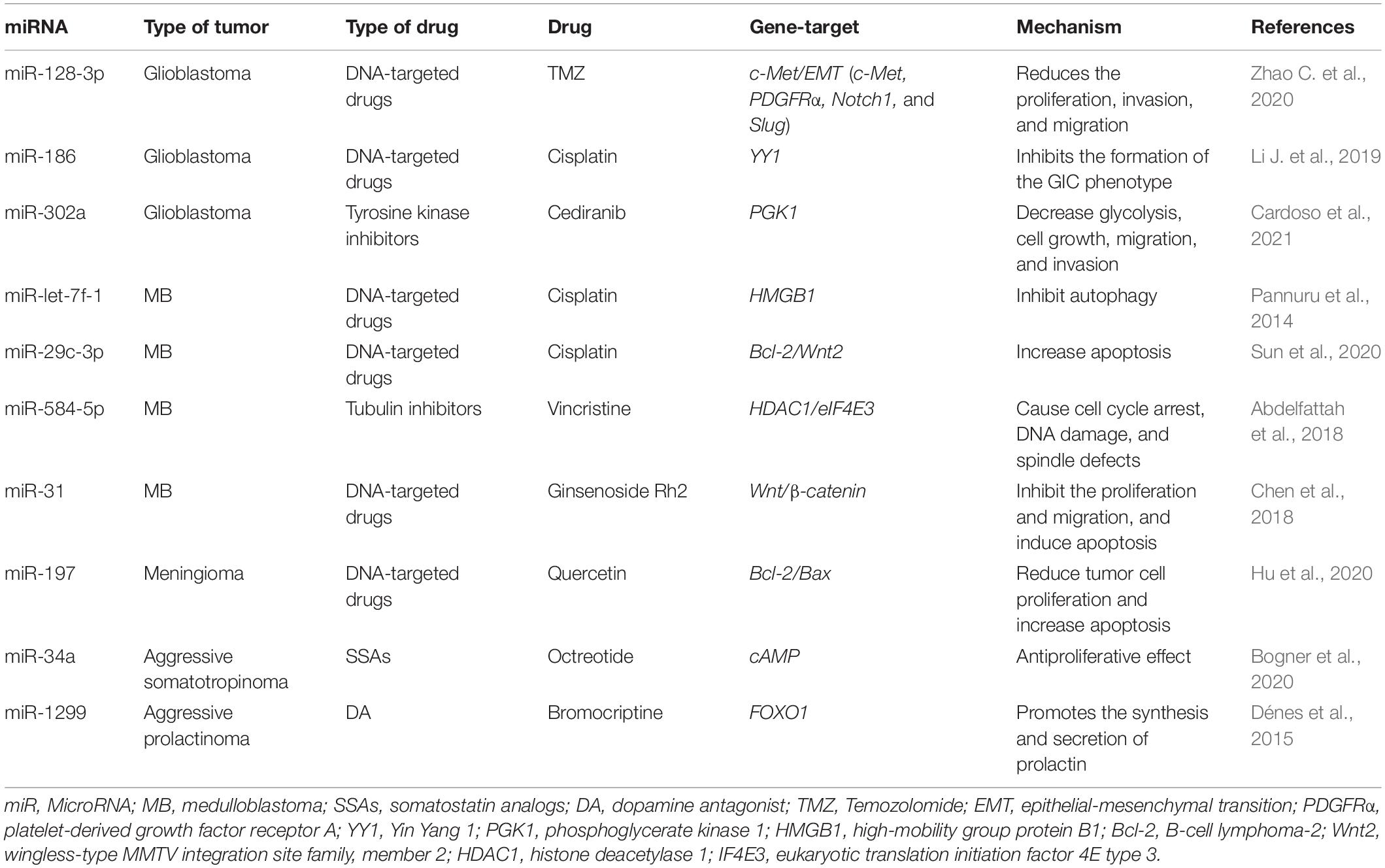
Table 2. MiRNAs involved in the regulation of chemotherapeutic and pharmacological drug treatment resistance in malignant primary brain tumors (MPBTs).
Glioblastoma
Glioblastoma is the most aggressive primary brain tumor and usually has a poor prognosis. Thus, the median survival rate of patients with glioblastoma after surgical resection and standard radio- and chemotherapy is no more than 12–15 months, while the 2-year survival rate for this group of patients varies from 26 to 33% (Wirsching et al., 2016; Schiff and Alyahya, 2020). Angiogenesis is the most important pathophysiological mechanism for the growth and progression of glioblastoma due to the active development of the microvascular network. The accelerated development of the microvascular network in glioblastoma occurs due to the synthesis of a large number of growth factors by tumor cells, including the VEGF family, placental growth factor (PLGF), platelet-derived growth factor (PDGF), and fibroblast growth factor (FGF) (Le Rhun et al., 2019). It should be noted that the microvascular network of glioblastoma is characterized by a high degree of tortuosity, increased permeability, as well as an increased diameter of the vascular lumen, and a thickened basement membrane (Ahir et al., 2020). It is believed that these features of the microvascular network of glioblastoma increase the hypoxia of the tumor tissue, thereby reducing the effectiveness of the use of cytotoxic drugs. It is for this reason that the development and use of anti-angiogenic drugs seem to be one of the most promising methods of targeted treatment of glioblastoma patients. The effectiveness of the use of anti-angiogenic drugs in the treatment of glioblastoma has been clearly demonstrated in a number of clinical studies (Sousa et al., 2019; Schulte et al., 2020). However, the widespread use of anti-angiogenic drugs in clinical practice has led to the development of glioblastoma resistance to drugs of this group. The formation of drug resistance of glioblastoma to anti-angiogenic drugs is associated with molecular and cellular features of the behavior of tumor cells, including with the participation of certain miRNAs (Zeng et al., 2018b).
Autophagy is one of the main mechanisms of tumor resistance. It should be noted that autophagy is the main cellular defense mechanism in the development of hypoxic conditions, which does not require tissue and extracellular matrix remodeling (Kimmelman and White, 2017). As is already known, the use combination of anti-angiogenic therapy with radiotherapy leads to the development of tumor tissue hypoxia due to the disturbance of the angiogenesis process necessary for the growth and progression of the tumor (Le Rhun et al., 2019). It is generally accepted that the process of autophagy in tumor cells is aimed at the destruction of proteins and signaling molecules formed during hypoxic conditions, which leads to the preservation of intracellular structures and the leveling of the secondary effects of anti-angiogenic drugs. It is assumed that in glioblastoma, drug resistance to bevacizumab is associated with non-selective hypoxia-induced factor (HIF)-dependent autophagy-mediated through the activity of the protein-interacting protein 3 (BNIP 3) and hypoxia-inducible factor 1-alpha (HIF1-α) protein (Hu et al., 2012). It is known that HIF-1α can cause cell cycle arrest, initiating angiogenesis, and regulating cellular metabolism (Gabriely et al., 2017; Huang et al., 2019). Huang et al. identified that the expression of HIF-1α was increased in glioblastoma in vitro and in vivo under hypoxia (Cardoso et al., 2021). Moreover, the longer the duration of hypoxia, the higher was the expression of HIF-1α. However, the expression of miR-224-3p was decreased under hypoxia conditions in a time-dependent manner. Their data showed that the miR-224-3p mimic significantly suppressed the expression HIF-1α and inhibited cell mobility while increased chemosensitivity to Temozolomide (TMZ) of glioblastoma. In addition, the miR-224-3p mimic suppressed the expression of VEGF with an increased cell apoptosis rate. In another study, miR-203 could be a useful target for overcoming the radioresistance of glioblastoma by suppressing HIF-1α expression in vitro (Chang et al., 2016). However, further experiments are needed to understand the complex link between miR-203 and HIF-1α expression.
Medulloblastoma
Medulloblastoma is a malignant primary tumor of the posterior fossa (WHO grade 4), mainly manifested in children. MB arises in the posterior fossa, usually from the cerebellar vermis and in the roof of the fourth ventricle (Schiff and Alyahya, 2020). MBs tend to metastasize along with the cerebrospinal fluid (CSF) pathways, which is detected in 35% of cases at the time of diagnosis. MBs are the most common malignant neoplasms of the brain in childhood and account for 15 to 30% of all primary CNS tumors in children, and about 70% of all cases are diagnosed in children under 15 years of age (Quinlan and Rizzolo, 2017). The age peak of diagnosis is between 3 and 5 years, and only 25% are patients between the ages of 20–44 (Millard and De Braganca, 2016; Quinlan and Rizzolo, 2017). Patients with MB have a poor outcome despite surgical, radio- and chemotherapy. However, the molecular mechanisms that confer sensitivity or resistance of MB to chemoradiation therapy are still unclear.
Recent evidence has implicated miRNAs in modulating chemo- and radiosensitivity in MBs (Joshi et al., 2019). It has been shown that in MB therapy there is a balance between cell cycle arrest and cell death (Kasuga et al., 2008). Melanoma-associated antigen-A (MAGE-A) family acts as a cell cycle regulatory protein and plays a key role in the oncogenesis and therapy resistance in MB. Kasuga et al. (2008) demonstrate that knockdown of MAGE-A increases apoptosis and sensitizes MB cells to chemotherapeutic agents such as cisplatin and etoposide. This finding supports the hypothesis that knockdown of MAGE-A genes increases the susceptibility of MB cells to cisplatin and etoposide, potentially by accumulating cells in the S phase. In contrast, Sheamal et al. showed that miR-34a directly targets the MAGE-A family (MAGE-A2, MAGE-A3, MAGE-A6, and MAGE-A12), disengaging p53 from MAGE-A–mediated repression (Weeraratne et al., 2011). Moreover, an important consequence of this is a positive feedback loop that sensitizes MB cells to cisplatin and etoposide via delayed G2/M progression and increased tumor cells apoptosis.
There is evidence that phosphatase and tensin homolog deleted on chromosome 10 (PTEN) dysfunction plays a crucial role in the development and progression MB. PTEN plays important roles in many cellular processes, including cell-cycle progression and apoptosis (Tolonen et al., 2020). Li et al. (2015) reported the upregulation of miR-106b in MB. In their study, the suppression of miR-106b inhibited cell proliferation, migration and invasion, and anchorage-independent growth, tumorsphere formation. In addition, downregulation of miR-106b suppressed the tumor growth by promoting G1 arrest and apoptosis. Besides, PTEN can be modulated by miR-106 family in various human cancers. For instance, miR-106b caused cell radio resistance in colorectal cancer via the PTEN/PI3K/AKT pathways (Zheng et al., 2015). MiR-106a induced cisplatin resistance via the PTEN/AKT pathway in gastric cancer cells (Fang et al., 2013). However, the role of miR-106b in therapy resistance of MB is still largely unknown. Nevertheless, this is an excellent opportunity to continue research on the role of miR-106b directly targeted PTEN in therapy resistance of MB.
Pituitary Adenomas With Aggressive Behavior
Among the tumors of the chiasmatic-sellar region, the most common are PAs, accounting for about 18% of all tumors of this localization. In the overwhelming majority of cases, these are benign neoplasms, characterized by slow growth rates and progression (Schiff and Alyahya, 2020). However, among them, there are PAs with aggressive behavior, which exhibits the properties of resistance to traditional treatment methods (Lake et al., 2020). Among PAs, the first place is occupied by tumors accompanied by the syndrome of hyperprolactinemia – prolactinomas, as well as non-functioning (hormonally inactive) PAs, each approximately 40%. The next most frequent is somatotropinomas, about 13–15%, accompanied by symptoms of acromegaly. Gonadotropin-secreting PAs, ACTH-secreting PAs, GH-secreting PAs, mixed forms are less common. In the age range, PAs occupy a period from 30 to 50 years, which is the working age (Elsarrag et al., 2020; Lake et al., 2020). In connection with all of the above, PAs, their diagnosis, and especially, treatment are important medical and social problems.
The main methods of treatment for PAs are surgical removal of the tumor and pharmacological treatment and their combinations. Radio- and chemotherapy are used, as a rule, when it is impossible to perform a surgical intervention or when it is at high risk, and when the tumor is highly aggressive (Fleseriu and Popovic, 2020). Since there is no clear definition and availability of reliable prognostic markers, PAs with aggressive behavior are difficult to identify at initial presentation, and therefore the primary therapeutic approach is no different from other PAs depending on the type of tumor (Mete and Lopes, 2017). Resistance to drugs presenting as escalating hormone levels and/or tumor growth where can be an early indicator of aggressiveness. There are many studies examining changes in miRNA expression in PAs. Among them are studies on their role in drug resistance in various types of PAs (Ciato and Albani, 2020). However, there is no evidence base on their potential role in resistance to chemo- and radiotherapy in patients with PAs with aggressive behavior and pituitary carcinomas. It is possible to suggest from previous studies which miRNAs and through which signaling pathways can participate in the mechanisms of resistance to chemo- and radiotherapy. For instance, in a recent study, Wang Z. et al. (2019) successfully identified one key target gene, EGFR, and two crucial miRNAs, miR-489 and miR-520b, associated with aggressiveness of prolactinomas based on bioinformatics analysis. It is also known that EGFR is one of the most frequently altered oncogenes in tumors, which important role in therapy resistance and is often associated with a negative prognosis (Lee and Muller, 2010).
Prolactinoma is the most commonly seen secretory tumor of pituitary glands. More than 90% of prolactinomas are microprolactinomas (<1.0 cm), while the rest are macroprolactinomas (≥1.0 cm). Macroprolactinomas account for approximately half of all functioning pituitary macroadenomas (Huynh et al., 2021). Without a doubt, prolactinoma is an innocent tumor of its kind. Prolactinomas are more aggressive and are characterized by increased proliferative ability; they can turn into recurrent, invasive giant prolactinomas (Castinetti et al., 2021). Most patients with prolactinomas respond to standard doses of dopamine agonists (DA), while the rest of the patients remain resistant to therapy. In this case, the term is used – resistance to DA, the inability to achieve normalization of prolactin levels, and tumor reduction by 50%, while taking the maximum tolerated dosage of the drug (Giraldi and Ioachimescu, 2020). To overcome resistance, it is necessary to increase the dose of drugs, in this regard, the risk of developing side effects, such as liquorrhea, headaches, acute psychosis, etc., (Molitch, 2020; Vermeulen et al., 2020; Castinetti et al., 2021). Surgical intervention is indicated in case of intolerance or resistance to DA, or with a persistent increase in tumor size with the development of neuro-ophthalmic symptoms, or if there is a rapid loss of vision or cranial nerve paralysis due to intratumoral hemorrhage (Panigrahi et al., 2020). However, complete surgical removal of a giant tumor is rarely performed due to the technical complexity and the greater risk of side effects. Radio- and chemotherapy have a limited role in the treatment of giant prolactinomas; on the one hand, because of its dubious chemotherapy effectiveness and, on the other hand, because of the complications that appear during tumor irradiation (Iglesias et al., 2018). Therefore, a thorough and deeper understanding of the molecular mechanisms underlying drug resistance of PAs with aggressive behavior like aggressive prolactinomas are urgently needed to find potential new targets for improving therapeutic efficacy. For instance, Jian et al. (2019) demonstrated that miR-145-5p was greatly downregulated in bromocriptine-resistant prolactinoma cell lines and tissues in vitro and in vivo. In addition, transfer miR-145 mimic into tumor cells and revealed that overexpression of miR-145-5p increased sensitivity for bromocriptine markedly. In conclusion, identification of tumor protein, translationally controlled 1 (TPT1) as a direct target gene of miR-145-5p. In another study, miR-93-5p was related to fibrosis and was involved in the bromocriptine -resistance mechanisms in prolactinoma by regulating the transforming growth factor beta 1/mothers against decapentaplegic homolog 3 (TGF-β1/Smad3) signaling pathway (Hu et al., 2019). Interesting that previous studies showed that TGF-β1 promotes the synthesis and secretion of collagen fibers in fibroblasts and that the TGF-β1/Smad3 signaling pathway is involved in the drug-resistance mechanism of prolactinoma by increasing fibrosis through interactions with fibroblasts (Hu et al., 2018).
High-Grade Meningiomas
Meningiomas are common tumors of the CNS, originating from the meninges of the brain or spinal cord. Most meningiomas are benign tumors characterized by slow growth and are histologically WHO Grade 1 (Schiff and Alyahya, 2020). However, high-grade meningiomas [atypical (WHO Grade 2) and anaplastic (WHO Grade 3)] exhibit more aggressive biological behavior and are clinically associated with a high risk of recurrence and a less favorable prognosis (Maier et al., 2020). Atypical and anaplastic meningiomas account for about 30% of the total number of intracranial meningiomas (Maier et al., 2020). Atypical and anaplastic meningiomas are often accompanied by invasive growth into the surrounding anatomical structures, which is the main factor limiting the radical nature of the surgery and increasing the frequency of recurrence. The problem of diagnosis and treatment, including resistance to radio- and chemotherapy, of patients with high-grade meningiomas, is still far from its final solution, which cannot but affect the long-term results and the level of mortality and mortality (Zhao L. et al., 2020). A better understanding of the molecular mechanisms involved in meningioma oncogenesis may lead to the identification of new therapeutic targets responsible for therapeutic resistance. Numerous studies have identified multiple signaling pathways involved in the therapeutic resistance of high-grade meningiomas and have suggested many important molecular targets for the development of new drugs for the treatment of high-grade meningiomas that are resistant to radio- and chemotherapy. In particular, growth factors, such as PDGF, epidermal growth factor (EGF) and their receptors, and cytokines, such as TGF-β, serve as major factors in high-grade meningiomas leading to therapeutic resistance (Birzu et al., 2020; Shao et al., 2020).
Unfortunately, studies on the role of miRNAs in the mechanisms of resistance to therapy in meningiomas are limited. For instance, microarray analysis, using the atypical meningioma tissue samples of 55 patients (43 from the radiosensitive and 12 from the radioresistant group), indicated that 14 miRNAs were significantly dysregulated in tumor tissue (Zhang X. et al., 2020). Among them 7 significantly upregulated miRNAs (miR-4286, miR-4695-5p, miR-6732-5p, miR-6855-5p, miR-7977, miR-6765-3p, miR-6787-5p) and 7 significantly downregulated miRNAs (miR-1275, miR-30c-1-3p, miR-4449, miR-4539, miR-4684-3p, miR-6129, miR-6891-5p) in patients resistant to radiotherapy. Furthermore, in order to investigate the signaling pathways affected by the differentially expressed 14 miRNAs between radiosensitive and radioresistant atypical meningioma, the authors used the DIANA-miRPath software and found three enriched pathways: two pathways were fatty acid biosynthesis and metabolism, and TGF-β signaling pathway. The role of TGF-β and these miRNAs in the oncogenesis, particularly radiosensitivity, of meningiomas remains to be established.
Extracellular Micrornas in Tumor Resistance
The main part of miRNAs is localized inside the cell. However, a certain proportion of miRNAs are present outside the cells and they are called extracellular or circulating miRNAs. A series of studies are devoted to the detection of extracellular miRNAs in various human fluids including whole blood, plasma/serum, saliva, urine, cerebrospinal fluid (Valihrach et al., 2020). In these biological fluids, the total concentration of miRNAs and their ratio varies considerably, which may be due to the peculiarities of the pathological or physiological status of the organism. The discovery of significant changes in the expression level of extracellular miRNAs in various diseases promoted the positioning of these molecules as promising non-invasive biomarkers (Sohel, 2020). MiRNAs can be secreted by the cell as part of extracellular vesicles (EVs) (exosomes and microvesicles) or apoptotic bodies; they can be found in the form of high-density lipoprotein (HDL) bound and mostly in the form of Argonaute2-containing ribonucleoprotein complexes (miRNA-Ago2) (Figure 3; Valihrach et al., 2020). Then, regardless of the forms, miRNAs pass from the extracellular space into the biological fluid (for example, the general blood flow).
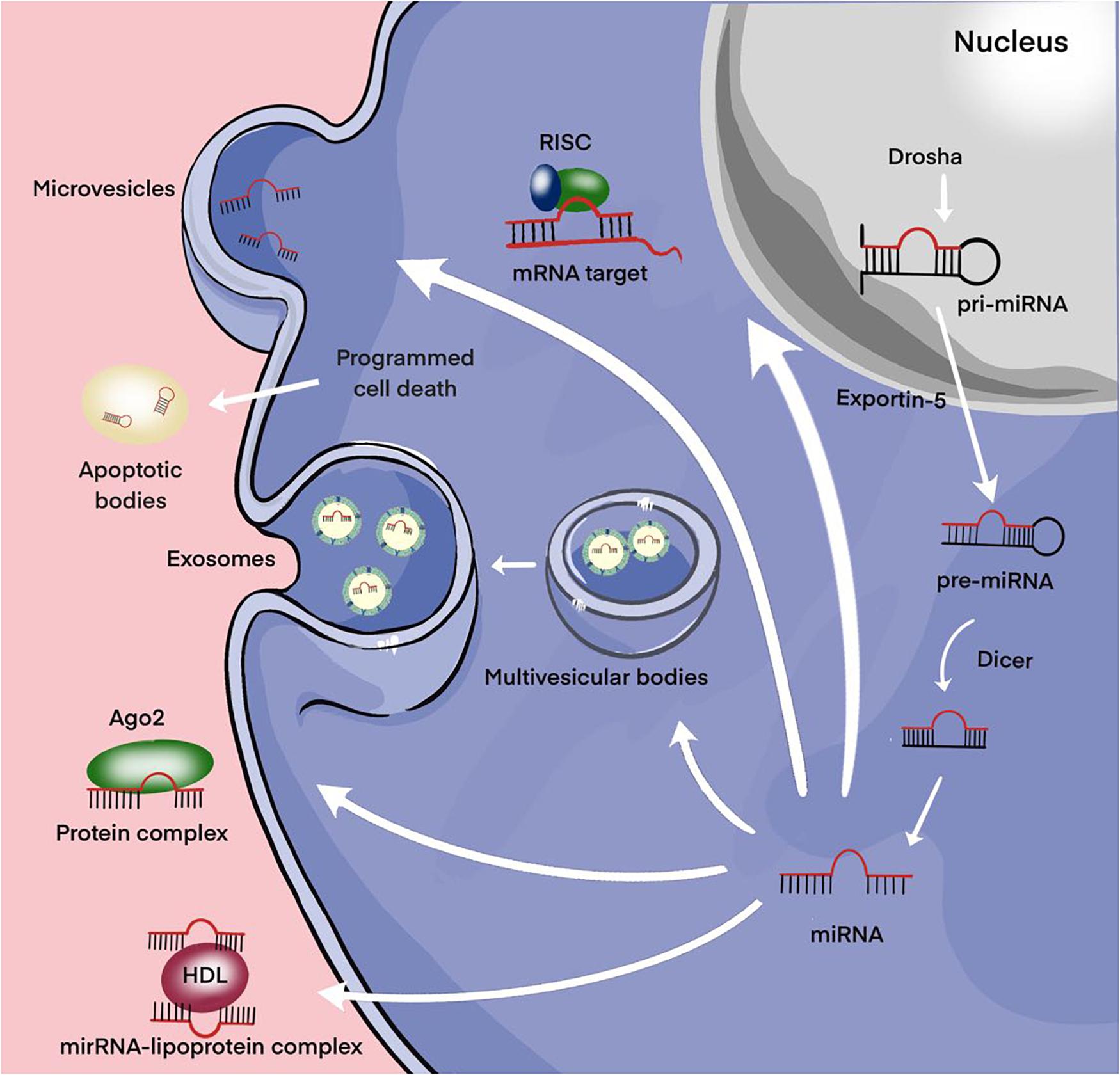
Figure 3. Secretion of miRNAs into the extracellular environment. Primary microRNA (pri-miRNAs) are transcribed by RNA polymerase II and then processed by Drosha into precursor-miRNAs (pre-miRNAs). Exportin5 transfers these pre-miRNAs from the nucleus to the cytoplasm, where Dicer converts them into mature miRNAs. Mature miRNAs can be selectively incorporated into extracellular vesicles (EVs) (exosomes and microvesicles) or linked to the Argonaute 2 (Ago2) protein and released into the extracellular environment. Alternatively, they can be attached to high-density lipoprotein (HDL) or contained in apoptotic bodies and then released into the extracellular environment.
Moreover, extracellular miRNAs, as exosomal miRNAs, play an important role in intracellular communication and the signaling system of cells. Over the past two decades, a broad evidence base has been obtained regarding the role of extracellular miRNAs in maintaining cellular homeostasis. Exosomes are a new form of intercellular communication (Pegtel and Gould, 2019). These are small membrane vesicles of endosomal origin with a diameter of 30 to 100 nm, which are secreted by various types of cells, normal or abnormal (Pegtel and Gould, 2019). Tumor cells play a particularly important role in the production of exosomes (Kalluri and LeBleu, 2020). In addition to miRNAs, exosomes contain a diverse set of molecules such as DNA, proteins, other non-coding RNAs, translation factors, metabolic enzymes, etc. There is evidence that exosomal miRNAs are actively involved in the oncogenesis of MPBTs, including resistance to therapy (Zhang and Yu, 2019). The presence of surface protein markers and adhesion molecules allows exosomes to bind to cells (including tumor cells) that exhibit the corresponding receptors, via micropinocytosis or endocytosis, to be transported into these cells. All this suggests that miRNAs carried by exosomes can enter recipient cells and regulate the expression of their target genes (Figure 4; Pegtel and Gould, 2019; Kalluri and LeBleu, 2020).
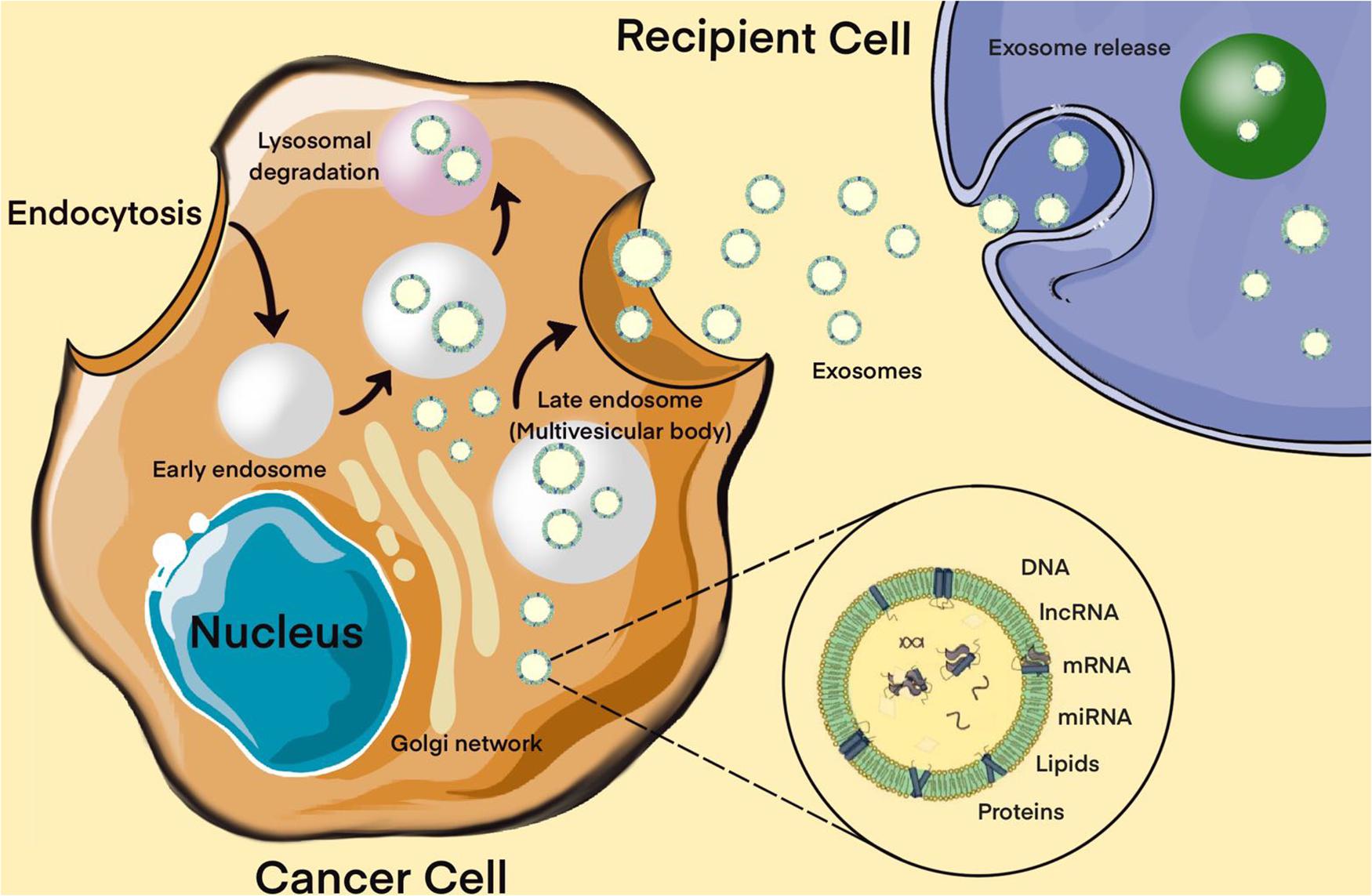
Figure 4. Biogenesis of exosomes. The exosome membrane is formed as a result of the invagination of the early endosome into the membrane. Proteins, lipids, RNA, DNA enter the exosome from the cell cytoplasm. The fate of an endosome depends on the marking of its membrane with certain lipids: if it is labeled with lysobisphosphatidic acid, then its contents will be destroyed, and if ceramides, it will be pushed out of the cell. These processes are controlled by the GTPases of the Rab (G-protein) family, whose various members perform different functions: Rab5 directs the formation of the endosome, Rab7 organizes the degradation of the contents of the multivesicular body (late endosome) in the lysosome, and Rab11, Rab27, and Rab35 are necessary for the secretion of exosomes into the extracellular space. It has been shown that exosomes contain about 4,000 different proteins, more than 1,500 different miRNAs and mRNAs, as well as DNA. Bottom right – enlarged “generalized” exosome.
Glioblastoma
If we talk about the role of EVs in the formation of a resistant phenotype of tumor cells, then, of course, one of the most studied areas is the participation of exosomes in the development of multiple drug resistance (MDR) (Zhang and Yu, 2019). Further studies made it possible to significantly expand the list of biomolecules that are part of resistant tumor cell EVs and are capable of inducing MDR in recipient cells. This primarily refers to miRNAs that regulate the expression level of a number of genes. For instance, Munoz et al. demonstrated that anti-miR-9 delivered via exosomes from MSCs to the TMZ-resistant glioblastoma cells was able to reduce the endogenous upregulation of miR-9 in response to TMZ (Koritzinsky et al., 2017). Moreover, to determine whether multidrug resistance gene 1(MDR1) expression is miR-9 dependent for sensitivity to TMZ, the authors knocked down MDR1 with short hairpin RNA (shRNA), and then examined whether this affects the sensitivity of glioblastoma cells to TMZ. The results indicated that anti-miR-9 increased active caspase by decreased the expression of MDR1 and concomitantly caused enhanced glioblastoma cell death in response to TMZ treatment. At the same time, in the protocol using manumycin A, which prevented the release of vesicles, it was indicated that the transfer of anti-miR-9 occurs by vesicular transfer, particularly via exosomes.
It should be noted that not only miRNAs but also mRNAs could be transported by EVs. Therefore, exosomes secreted by glioblastoma cells are enriched in mRNA, which enzymes of DNA repair as methylation of the O(6)-Methylguanine-DNA methyltransferase (MGMT) promoter and alkylpurine–DNA–N-glycosylase (APNG), and the transfer of these mRNAs into recipient cells can significantly increase the level of chemoresistance (Shao et al., 2015).
While the participation of EVs in the formation of chemoresistance of tumor cells is not in doubt today, the role of EVs in the regulation of the response of cells to irradiation has been studied to a much lesser extent. Only a few studies are known in which the participation of EVs in the development of the tumor response to radiation has been demonstrated, and the results of these studies are rather contradictory. This is primarily due to the bystander effect, a well-known biological process, radio-induced changes transmitted from irradiated cells to non-irradiated ones (Li and Nabet, 2019). It is assumed that this effect is based on the transmission of induced or radiation-modified biometabolites (reactive oxygen species, cytokines, growth factors, nucleic acid fragments, etc.) non-irradiated cells either by paracrine pathway or through intercellular gap junctions (Ni et al., 2019). In recent years, studies have appeared in which, in on both normal and tumor cells, direct evidence has been obtained for the participation of EVs of irradiated cells in the induction of radiation changes (primarily, genetic instability, telomere contraction) in non-irradiated cells. At the same time, EVs of irradiated cells can also have protective properties, in particular, in experiments on glioblastoma cells, the ability of exosomes from irradiated cells has been described to promotes a migratory phenotype of non-irradiated cells, as the authors believe, as a result of the active accumulation of DNA repair enzymes in the exosomes of irradiated cells (Arscott et al., 2013).
Pituitary Adenomas With Aggressive Behavior
Hormone therapy is one of the most common types of treatment for hormone-dependent malignant neoplasms, primarily PAs with aggressive behavior. Hormone therapy is based on the principle of creating an artificial deficiency of hormones necessary for the growth of hormone-dependent tumors, which is achieved mainly in two ways: (1) by reducing the concentration of endogenous hormones and (2) by suppressing their synthesis or replacing hormones with their inactive analogs (Iglesias et al., 2020; van Bunderen and Olsson, 2021). Despite the unconditional effectiveness, the use of hormonal therapy is limited by the development of tumor resistance to hormones. The mechanism of hormonal resistance is well understood. Much less is known about the role of intercellular interactions in the development of hormonal resistance of tumors and, in particular, PAs. For instance, Zhao et al. (2021) demonstrated that 20 differentially expressed miRNAs were identified in human invasive and non-invasive PAs tissue, and rat PA cells, where among them, the expression level of miR-99a-3p and mir-149 was significantly reduced. Furthermore, it was shown that overexpression of miR-149 and miR-99a-3p inhibits the growth and metastasis of PA cells and the formation of EC tubes. Interestingly, delivery of miR-149 mimic and miR-99a-3p mimic via exosomes showed similar suppressive effects on cell viability, metastasis, tube formation ability, tumor growth in vivo, and expression of markers associated with angiogenesis as VEGF. In additional, the authors showed that NOVA1, denticleless E3 ubiquitin protein ligase homolog (DTL), and RAB27B were targeted by miR-99a-3p. It is known that this group of genes is directly promoted EMT in various human tumors (Aleksakhina et al., 2019). Finally, EMT has been shown to contribute to drug resistance in tumors (Du and Shim, 2016; Aleksakhina et al., 2019). Therefore, we can suggest that these miRNAs can be involved in the oncogenesis of PAs, and in particular, be responsible for drug resistance through intercellular communications. In another pilot study to discover that the expression levels of circulating miR-200a in plasma of patients with invasive PAs were significantly higher than that in plasma of patients with non-invasive PAs. Moreover, invasive PA patients with residual after surgery had lower expression levels of circulating miR-200a. Therefore, miR-200 was a potential influencing factor for invasiveness in PAs patients. However, further research is required for exploring the relationships between circulating miR-200a expression and tumor size, clinical characteristics, and molecular mechanism for packaging and secretion to biofluids of miR-200a (Beylerli et al., 2021).
High-Grade Meningiomas and Medulloblastoma
Aberrant expression of extracellular miRNA circulating in biofluids of certain brain tumor patients has recently been reported to be non-invasive biomarkers and potential regulators of the disease (Sohel, 2020; Valihrach et al., 2020). However, the existence and role of miRNAs in MB and malignant meningioma extracellular environment are unknown. Therefore, better understanding of extracellular miRNA secretion and function in MB and malignant meningioma seems crucial for the development of novel insights for its regulation of oncogenesis including resistance to therapy. For instance, Choi et al. (2020) investigated whether secreted exosomal miR-135b and miR-135a function at the microenvironment level by possibly impacting the stemness of brain tumor spheroid-forming cells (BTSCs). The authors suggest that the inhibition of miR-135b or miR-135a can suppress the self-renewal capacity and expression of stem cell-related markers of BTSCs. In additional, they demonstrated that miR-135b, miR-135a targeted angiomotin-like2 (AMOTL2), and the expression of AMOTL2 can be increased through miR-135b and miR-135a inhibition. This result might be a clue that exosomal miR-135b and miR-135a derived from BTSCs may be able to regulate the Hippo pathway via AMOTL2, which plays a significant role in chemoresistance (Mohajan et al., 2021; Zeng and Dong, 2021).
Negroni et al. (2020) using reverse transcriptase real-time quantitative polymerase chain reaction (qRT-PCR) assay demonstrated that exosomal miR-497 and miR-195 are downregulated in serum of patients with high-grade meningioma (WHO grade 2 and 3) compared to benign meningioma (WHO grade 1). However, receiver operating characteristic (ROC) curve analysis showed that exosomal miR-497 has better sensitivity and specificity than exosomal miR-195 in distinguishing between low-grade (WHO grade 1) and higher-grade (WHO grade 2 and 3) meningioma patients, where area under the curve (AUC) was 0.89 and 0.78, respectively. Furthermore, the authors demonstrated that the transcription factor GATA binding protein 4 (GATA-4) is overexpressed in malignant meningioma, which in turn regulates Cyclin D1, and it negatively regulates the miR-497 expression with an increase in cell viability in vitro. Importantly, that a cell cycle protein cyclin D1 is an established cancer-driving protein (Montalto and De Amicis, 2020). In recent years, studies have reported that the high expression of Cyclin D1 is involved in drug resistance processes such as chemo- and radiation treatment, and targeted therapy in various human tumors (Liu et al., 2020; Zuo et al., 2021). Therefore, the clinical values of exosomal miR-497 in the therapy resistance of high-grade meningioma must investigate in the future.
Discussion and Implications
The introduction of extracellular miRNAs into clinical practice is quite active. The search results of the clinical trial database clinicaltrials.gov for the keywords “extracellular,” “vesicle,” “exosomes,” “miRNA,” and “tumor” contain more than 50 projects, some of which are in the recruitment phase. A number of projects have been launched to assess the effectiveness of tumor therapy. In previous studies, it was shown that metformin has cytotoxicity and decreases the viability of glioblastoma cells, a promising biguanide with pronounced antitumor activity (Al Hassan et al., 2018). Furthermore, Soraya et al. (2021) demonstrated that metformin significantly decreased the expression of miR-21, miR-155, and miR-182, indicating suppression of oncogenesis in glioblastoma cells. In addition, confirm metformin increased the exosome biogenesis and secretion in glioblastoma cells. Their result also showed that the expression level of Rab27a, Rab27b, and Rab11 upregulated in treated cells with metformin than that of control cells. In the author’s opinion, the decreased expression levels of miR-21 and miR-182 may be responsible for Rab genes upregulation, which may correlate with increased exosomes secretion.
It turned out that not only exosomes of resistant cells, but also exosomes produced by cells of the tumor stroma can induce drug resistance in tumor cells. In experiments on head and neck cancer, it was found that exosomes produced by tumor-associated fibroblasts are able to induce cisplatin resistance in nearby tumor cells by transferring miR-196a and through targeting cyclin-dependent kinase inhibitor 1B (CDKN1B) and inhibitor of growth protein 5 (ING5) (Qin et al., 2019).
Another, no less important question is to what extent EVs can affect the initial level of tumor radiosensitivity and, given their protective properties, contribute to the spread of radioresistance to the brain tumors population. Research in this direction is just beginning, and we can expect that soon it will be possible to get answers to this and other questions concerning the role of EVs in the tumor response to radiation.
Thus, in recent years, extensive information has been accumulated on the correlations of miRNA profiles of EV s and the development of a resistant phenotype of tumor cells. Despite significant advances in research on the role of extracellular miRNAs in therapeutic resistance in MPBTs, studies besides glioblastoma with other tumors remains to be seen. In conclusion, the most common miRNAs in EVs that have been reported to be involved in glioblastoma therapeutic resistance are shown in Table 4 (Zeng et al., 2018a).
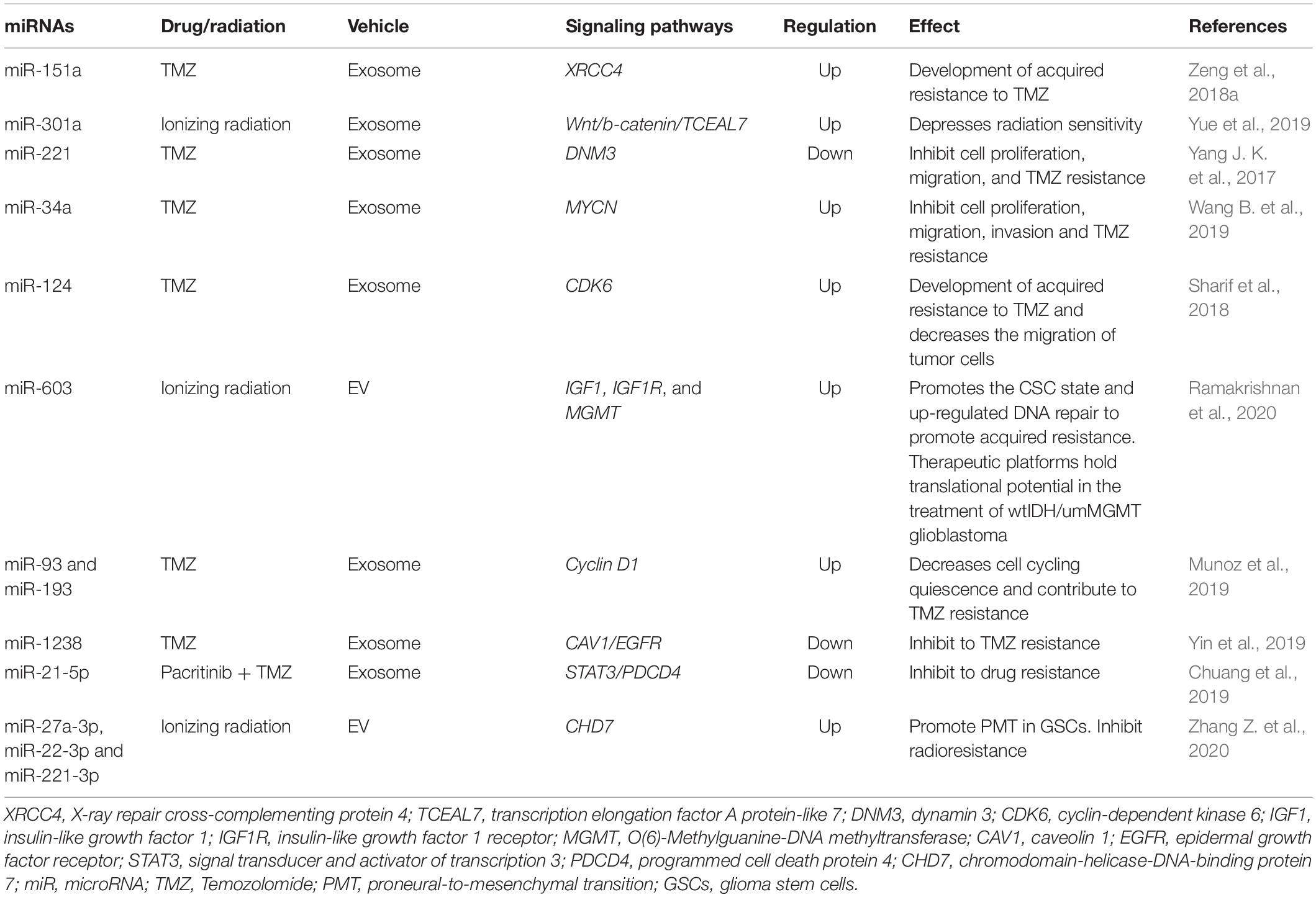
Table 4. MiRNAs in extracellular vesicles (EVs) and their signaling pathways through which they may be responsible for glioblastoma therapeutic resistance.
Conclusion
The last decade has been accompanied by the emergence of a large number of studies devoted to the role of miRNAs in oncogenesis and the development of resistance to antitumor therapy. The discovery of EVs and, most importantly, their ability to transfer biological material as miRNAs from cell to cell has largely changed the understanding of the mechanism of tumor development and progression. First, this is the revealed ability of EVs to induce tumor transformation and/or induce a tumor-like phenotype in the cells of the surrounding tissue. Moreover, of course, one of the most significant achievements in this reign – the influence of EVs on the formation of a resistant phenotype of tumor cells. EVs can indeed provide the spread of resistance from resistant to sensitive cells through various mechanisms based on the transfer of specific regulatory molecules into cells including proteins, miRNAs, mRNA, etc. The induction of resistance to chemoradiotherapy and pharmacological treatment of MPBTs by miRNAs has been convincingly demonstrated in in vitro and in vivo experiments. However, there are a number of questions. And one of them in which To the extent that miRNAs are involved in the development of tumor resistance to antitumor therapy, can miRNAs actually participate in the development of resistance across the entire pool of tumor cells under in vivo conditions, and, most importantly, how important this process is in the development of acquired tumor resistance. Today these issues are being actively investigated, and, of course, their solution will make it possible to make significant progress in solving such an important problem in neurosurgery as the resistance of MPBTs to antitumor therapy.
Author Contributions
IG: conceptualization, writing – original draft, and supervision. OB: writing – review and editing, investigation, project administration, and resources. AA: formal analysis, methodology, and original draft. YL, HX, CL, XX, and CY: data curation. GY: validation, visualization, and funding acquisition. All authors have read and agreed to the published version of the manuscript.
Funding
This work was supported by the National Natural Science Foundation of China (81971135); Natural Science Foundation of Heilongjiang (YQ2020H014); “Chunhui Plan” of Ministry of Education (HLJ2019009); Distinguished Young Foundations of the First Affiliated Hospital of Harbin Medical University (HYD2020JQ0014); and the reported study was funded by RFBR and NSFC, project number 21-515-53017.
Conflict of Interest
The authors declare that the research was conducted in the absence of any commercial or financial relationships that could be construed as a potential conflict of interest.
Publisher’s Note
All claims expressed in this article are solely those of the authors and do not necessarily represent those of their affiliated organizations, or those of the publisher, the editors and the reviewers. Any product that may be evaluated in this article, or claim that may be made by its manufacturer, is not guaranteed or endorsed by the publisher.
References
Abdelfattah, N., Rajamanickam, S., Panneerdoss, S., Timilsina, S., Yadav, P., Onyeagucha, B. C., et al. (2018). MiR-584-5p potentiates vincristine and radiation response by inducing spindle defects and DNA damage in medulloblastoma. Nat. Commun. 9:4541. doi: 10.1038/s41467-018-06808-8
Ahir, B. K., Engelhard, H. H., and Lakka, S. S. (2020). Tumor development and angiogenesis in adult brain tumor: glioblastoma. Mol. Neurobiol. 57, 2461–2478. doi: 10.1007/s12035-020-01892-8
Al Hassan, M., Fakhoury, I., El Masri, Z., Ghazale, N., Dennaoui, R., El Atat, O., et al. (2018). Metformin treatment inhibits motility and invasion of glioblastoma cancer cells. Anal. Cell. Pathol. (Amst.) 2018:5917470. doi: 10.1155/2018/5917470
Aleksakhina, S. N., Kashyap, A., and Imyanitov, E. N. (2019). Mechanisms of acquired tumor drug resistance. Biochim. Biophys. Acta Rev. Cancer 1872:188310. doi: 10.1016/j.bbcan.2019.188310
Ali Syeda, Z., Langden, S. S. S., Munkhzul, C., Lee, M., and Song, S. J. (2020). Regulatory mechanism of MicroRNA expression in cancer. Int. J. Mol. Sci. 21:1723. doi: 10.3390/ijms21051723
Allahverdi, A., Arefian, E., Soleimani, M., Ai, J., Nahanmoghaddam, N., Yousefi-Ahmadipour, A., et al. (2020). MicroRNA-4731-5p delivered by AD-mesenchymal stem cells induces cell cycle arrest and apoptosis in glioblastoma. J. Cell. Physiol. 235, 8167–8175. doi: 10.1002/jcp.29472
Alsidawi, S., Malek, E., and Driscoll, J. J. (2014). MicroRNAs in brain metastases: potential role as diagnostics and therapeutics. Int. J. Mol. Sci. 15, 10508–10526. doi: 10.3390/ijms150610508
Apte, R. S., Chen, D. S., and Ferrara, N. (2019). VEGF in signaling and disease: beyond discovery and development. Cell 176, 1248–1264. doi: 10.1016/j.cell.2019.01.021
Arscott, W. T., Tandle, A. T., Zhao, S., Shabason, J. E., Gordon, I. K., Schlaff, C. D., et al. (2013). Ionizing radiation and glioblastoma exosomes: implications in tumor biology and cell migration. Transl. Oncol. 6, 638–648. doi: 10.1593/tlo.13640
Asuthkar, S., Velpula, K. K., Nalla, A. K., Gogineni, V., Gondi, C., and Rao, J. S. (2014). Irradiation-induced angiogenesis is associated with an MMP-9-miR-494-syndecan-1 regulatory loop in medulloblastoma cells. Oncogene 33, 1922–1933. doi: 10.1038/onc.2013.151
Balachandran, A. A., Larcher, L. M., Chen, S., and Veedu, R. N. (2020). Therapeutically significant MicroRNAs in primary and metastatic brain malignancies. Cancers (Basel) 12:2534. doi: 10.3390/cancers12092534
Bertoli, G., Cava, C., and Castiglioni, I. (2015). MicroRNAs: new biomarkers for diagnosis, prognosis, therapy prediction and therapeutic tools for breast cancer. Theranostics 5, 1122–1143. doi: 10.7150/thno.11543
Beylerli, O., Khasanov, D., Gareev, I., Valitov, E., Sokhatskii, A., Wang, C., et al. (2021). Differential non-coding RNAs expression profiles of invasive and non-invasive pituitary adenomas. Noncoding RNA Res. 6, 115–122. doi: 10.1016/j.ncrna.2021.06.004
Birzu, C., Peyre, M., and Sahm, F. (2020). Molecular alterations in meningioma: prognostic and therapeutic perspectives. Curr. Opin. Oncol. 32, 613–622. doi: 10.1097/CCO.0000000000000687
Bogner, E. M., Daly, A. F., Gulde, S., Karhu, A., Irmler, M., Beckers, J., et al. (2020). miR-34a is upregulated in AIP-mutated somatotropinomas and promotes octreotide resistance. Int. J. Cancer. 147, 3523–3538. doi: 10.1002/ijc.33268
Buruiană, A., Florian, ŞI., Florian, A. I., Timiş, T. L., Mihu, C. M., Miclăuş, M., et al. (2020). The roles of miRNA in glioblastoma tumor cell communication: diplomatic and aggressive negotiations. Int. J. Mol. Sci. 21:1950. doi: 10.3390/ijms21061950
Cao, Z., Liao, Q., Su, M., Huang, K., Jin, J., and Cao, D. (2019). AKT and ERK dual inhibitors: the way forward? Cancer Lett. 459, 30–40. doi: 10.1016/j.canlet.2019.05.025
Cardoso, A. M., Morais, C. M., Rebelo, O., Tão, H., Barbosa, M., de Lima, M. C. P., et al. (2021). Downregulation of long non-protein coding RNA MVIH impairs glioblastoma cell proliferation and invasion through a miR-302a-dependent mechanism. Hum. Mol. Genet. 30, 46–64.
Castinetti, F., Albarel, F., Amodru, V., Cuny, T., Dufour, H., Graillon, T., et al. (2021). The risks of medical treatment of prolactinoma. Ann. Endocrinol. (Paris) 82, 15–19. doi: 10.1016/j.ando.2020.12.008
Chang, J. H., Hwang, Y. H., Lee, D. J., Kim, D. H., Park, J. M., Wu, H. G., et al. (2016). MicroRNA-203 modulates the radiosensitivity of human malignant glioma cells. Int. J. Radiat. Oncol. Biol. Phys. 94, 412–420. doi: 10.1016/j.ijrobp.2015.10.001
Chen, Y., Shang, H., Zhang, S., and Zhang, X. (2018). Ginsenoside Rh2 inhibits proliferation and migration of medulloblastoma Daoy by down-regulation of microRNA-31. J. Cell. Biochem. 119, 6527–6534. doi: 10.1002/jcb.26716
Choi, S. A., Koh, E. J., Kim, R. N., Byun, J. W., Phi, J. H., Yang, J., et al. (2020). Extracellular vesicle-associated miR-135b and -135a regulate stemness in Group 4 medulloblastoma cells by targeting angiomotin-like 2. Cancer Cell Int. 20:558. doi: 10.1186/s12935-020-01645-6
Chuang, H. Y., Su, Y. K., Liu, H. W., Chen, C. H., Chiu, S. C., Cho, D. Y., et al. (2019). Preclinical evidence of STAT3 inhibitor pacritinib overcoming temozolomide resistance via downregulating miR-21-Enriched exosomes from M2 glioblastoma-associated macrophages. J. Clin. Med. 8:959. doi: 10.3390/jcm8070959
Ciato, D., and Albani, A. (2020). Molecular mechanisms of glucocorticoid resistance in corticotropinomas: new developments and drug targets. Front. Endocrinol. (Lausanne) 11:21. doi: 10.3389/fendo.2020.00021
de Kock, L., Rivera, B., and Foulkes, W. D. (2020). Pineoblastoma is uniquely tolerant of mutually exclusive loss of DICER1, DROSHA or DGCR8. Acta Neuropathol. 139, 1115–1118. doi: 10.1007/s00401-020-02139-5
Dénes, J., Kasuki, L., Trivellin, G., Colli, L. M., Takiya, C. M., Stiles, C. E., et al. (2015). Regulation of aryl hydrocarbon receptor interacting protein (AIP) protein expression by MiR-34a in sporadic somatotropinomas. PLoS One 10:e0117107. doi: 10.1371/journal.pone.0117107
Dexheimer, P. J., and Cochella, L. (2020). MicroRNAs: from mechanism to organism. Front. Cell Dev. Biol. 8:409. doi: 10.3389/fcell.2020.00409
Du, B., and Shim, J. S. (2016). Targeting epithelial-mesenchymal transition (EMT) to overcome drug resistance in cancer. Molecules 21:965. doi: 10.3390/molecules21070965
Elsarrag, M., Patel, P. D., Chatrath, A., Taylor, D., and Jane, J. A. (2020). Genomic and molecular characterization of pituitary adenoma pathogenesis: review and translational opportunities. Neurosurg. Focus 48:E11. doi: 10.3171/2020.3.FOCUS20104
Fang, Y., Shen, H., Li, H., Cao, Y., Qin, R., Long, L., et al. (2013). miR-106a confers cisplatin resistance by regulating PTEN/Akt pathway in gastric cancer cells. Acta Biochim. Biophys. Sin. (Shanghai) 45, 963–972. doi: 10.1093/abbs/gmt106
Fish, J. E., Santoro, M. M., Morton, S. U., Yu, S., Yeh, R. F., Wythe, J. D., et al. (2008). miR-126 regulates angiogenic signaling and vascular integrity. Dev. Cell 15, 272–284. doi: 10.1016/j.devcel.2008.07.008
Fleseriu, M., and Popovic, V. (2020). The journey in diagnosis and treatment, from pituitary adenoma to aggressive pituitary tumors. Rev. Endocr. Metab. Disord. 21, 201–202. doi: 10.1007/s11154-020-09561-w
Gabriely, G., Wheeler, M. A., Takenaka, M. C., and Quintana, F. J. (2017). Role of AHR and HIF-1alpha in glioblastoma metabolism. Trends Endocrinol. Metab. 28, 428–436. doi: 10.1016/j.tem.2017.02.009
Garibaldi, F., Falcone, E., Trisciuoglio, D., Colombo, T., Lisek, K., Walerych, D., et al. (2016). Mutant p53 inhibits miRNA biogenesis by interfering with the microprocessor complex. Oncogene 35, 3760–3770. doi: 10.1038/onc.2016.51
Giraldi, E. A., and Ioachimescu, A. G. (2020). The role of dopamine agonists in pituitary adenomas. Endocrinol. Metab. Clin. North Am. 49, 453–474. doi: 10.1016/j.ecl.2020.05.006
Grunder, E., D’Ambrosio, R., Fiaschetti, G., Abela, L., Arcaro, A., Zuzak, T., et al. (2011). MicroRNA-21 suppression impedes medulloblastoma cell migration. Eur. J. Cancer 47, 2479–2490. doi: 10.1016/j.ejca.2011.06.041
Ha, M., and Kim, V. N. (2014). Regulation of microRNA biogenesis. Nat. Rev. Mol. Cell Biol. 15, 509–524. doi: 10.1038/nrm3838
Hou, W. Z., Chen, X. L., Qin, L. S., Xu, Z. J., Liao, G. M., Chen, D., et al. (2020). MiR-449b-5p inhibits human glioblastoma cell proliferation by inactivating WNT2B/Wnt/β-catenin signaling pathway. Eur. Rev. Med. Pharmacol. Sci. 24, 5549–5557. doi: 10.26355/eurrev_202005_21340
Hu, B., Mao, Z., Du, Q., Jiang, X., Wang, Z., Xiao, Z., et al. (2019). miR-93-5p targets Smad7 to regulate the transforming growth factor-β1/Smad3 pathway and mediate fibrosis in drug-resistant prolactinoma. Brain Res. Bull. 149, 21–31. doi: 10.1016/j.brainresbull.2019.03.013
Hu, B., Mao, Z., Jiang, X., He, D., Wang, Z., Wang, X., et al. (2018). Role of TGF-beta1/Smad3-mediated fibrosis in drug resistance mechanism of prolactinoma. Brain Res. 1698, 204–212. doi: 10.1016/j.brainres.2018.07.024
Hu, S. A., Cheng, J., Zhao, W. H., and Zhao, H. Y. (2020). Quercetin induces apoptosis in meningioma cells through the miR-197/IGFBP5 cascade. Environ. Toxicol. Pharmacol. 80:103439. doi: 10.1016/j.etap.2020.103439
Hu, Y. L., DeLay, M., Jahangiri, A., Molinaro, A. M., Rose, S. D., Carbonell, W. S., et al. (2012). Hypoxia-induced autophagy promotes tumor cell survival and adaptation to antiangiogenic treatment in glioblastoma. Cancer Res. 72, 1773–1783. doi: 10.1158/0008-5472.CAN-11-3831
Huang, S., Qi, P., Zhang, T., Li, F., and He, X. (2019). The HIF-1α/miR-224-3p/ATG5 axis affects cell mobility and chemosensitivity by regulating hypoxia-induced protective autophagy in glioblastoma and astrocytoma. Oncol. Rep. 41, 1759–1768. doi: 10.3892/or.2018.6929
Huynh, P. P., Ishii, L. E., and Ishii, M. (2021). Prolactinomas. JAMA 325:195. doi: 10.1001/jama.2020.3744
Huynh, T. T., Lin, C. M., Lee, W. H., Wu, A. T., Lin, Y. K., Lin, Y. F., et al. (2015). Pterostilbene suppressed irradiation-resistant glioma stem cells by modulating GRP78/miR-205 axis. J. Nutr. Biochem. 26, 466–475. doi: 10.1016/j.jnutbio.2014.11.015
Iglesias, P., Magallón, R., Mitjavila, M., Rodríguez Berrocal, V., Pian, H., and Díez, J. J. (2020). Multimodal therapy in aggressive pituitary tumors. Endocrinol. Diabetes Nutr. 67, 469–485. doi: 10.1016/j.endinu.2019.08.004
Iglesias, P., Rodríguez Berrocal, V., and Díez, J. J. (2018). Giant pituitary adenoma: histological types, clinical features and therapeutic approaches. Endocrine 61, 407–421. doi: 10.1007/s12020-018-1645-x
Ji, J., Rong, Y., Luo, C. L., Li, S., Jiang, X., Weng, H., et al. (2018). Up-Regulation of hsa-miR-210 promotes venous metastasis and predicts poor prognosis in hepatocellular carcinoma. Front. Oncol. 8:569. doi: 10.3389/fonc.2018.00569
Jian, M., Du, Q., Zhu, D., Mao, Z., Wang, X., Feng, Y., et al. (2019). Tumor suppressor miR-145-5p sensitizes prolactinoma to bromocriptine by downregulating TPT1. J. Endocrinol. Invest. 42, 639–652. doi: 10.1007/s40618-018-0963-4
Joshi, P., Katsushima, K., Zhou, R., Meoded, A., Stapleton, S., Jallo, G., et al. (2019). The therapeutic and diagnostic potential of regulatory noncoding RNAs in medulloblastoma. Neurooncol. Adv. 1:vdz023. doi: 10.1093/noajnl/vdz023
Kalluri, R., and LeBleu, V. S. (2020). The biology, function, and biomedical applications of exosomes. Science 367:eaau6977. doi: 10.1126/science.aau6977
Kapoor, I., Bodo, J., Hill, B. T., Hsi, E. D., and Almasan, A. (2020). Targeting BCL-2 in B-cell malignancies and overcoming therapeutic resistance. Cell Death Dis. 11:941. doi: 10.1038/s41419-020-03144-y
Kasuga, C., Nakahara, Y., Ueda, S., Hawkins, C., Taylor, M. D., Smith, C. A., et al. (2008). Expression of MAGE and GAGE genes in medulloblastoma and modulation of resistance to chemotherapy. Laboratory investigation. J. Neurosurg. Pediatr. 1, 305–313. doi: 10.3171/PED/2008/1/4/305
Katar, S., Baran, O., Evran, S., Cevik, S., Akkaya, E., Baran, G., et al. (2017). Expression of miRNA-21, miRNA-107, miRNA-137 and miRNA-29b in meningioma. Clin. Neurol. Neurosurg. 156, 66–70. doi: 10.1016/j.clineuro.2017.03.016
Kimmelman, A. C., and White, E. (2017). Autophagy and tumor metabolism. Cell Metab. 25, 1037–1043. doi: 10.1016/j.cmet.2017.04.004
Kliese, N., Gobrecht, P., Pachow, D., Andrae, N., Wilisch-Neumann, A., Kirches, E., et al. (2013). miRNA-145 is downregulated in atypical and anaplastic meningiomas and negatively regulates motility and proliferation of meningioma cells. Oncogene 32, 4712–4720. doi: 10.1038/onc.2012.468
Koritzinsky, E. H., Street, J. M., Star, R. A., and Yuen, P. S. (2017). Quantification of exosomes. J. Cell. Physiol. 232, 1587–1590. doi: 10.1002/jcp.25387
Krell, J., Stebbing, J., Carissimi, C., Dabrowska, A. F., de Giorgio, A., Frampton, A. E., et al. (2016). TP53 regulates miRNA association with AGO2 to remodel the miRNA-mRNA interaction network. Genome Res. 26, 331–341. doi: 10.1101/gr.191759.115
Lake, M. G., Krook, L. S., and Cruz, S. V. (2020). Pituitary adenomas: an overview. Am. Fam. Physician 88, 319–327. Rev Endocr Metab Disord. 21, 201–202.
Lan, F., Pan, Q., Yu, H., and Yue, X. (2015). Sulforaphane enhances temozolomide-induced apoptosis because of down-regulation of miR-21 via Wnt/beta-catenin signaling in glioblastoma. J. Neurochem. 134, 811–818. doi: 10.1111/jnc.13174
Le Rhun, E., Preusser, M., Roth, P., Reardon, D. A., van den Bent, M., Wen, P., et al. (2019). Molecular targeted therapy of glioblastoma. Cancer Treat. Rev. 80:101896. doi: 10.1016/j.ctrv.2019.101896
Lee, E. Y., and Muller, W. J. (2010). Oncogenes and tumor suppressor genes. Cold Spring Harb. Perspect. Biol. 2:a003236. doi: 10.1101/cshperspect.a003236
Lee, Y. Y., Yang, Y. P., Huang, M. C., Wang, M. L., Yen, S. H., Huang, P. I., et al. (2014). MicroRNA142-3p promotes tumor-initiating and radioresistant properties in malignant pediatric brain tumors. Cell Transplant. 23, 669–690. doi: 10.3727/096368914X678364
Li, I., and Nabet, B. Y. (2019). Exosomes in the tumor microenvironment as mediators of cancer therapy resistance. Mol. Cancer 18:32. doi: 10.1186/s12943-019-0975-5
Li, J., Song, J., and Guo, F. (2019). miR-186 reverses cisplatin resistance and inhibits the formation of the glioblastoma-initiating cell phenotype by degrading Yin Yang 1 in glioblastoma. Int. J. Mol. Med. 43, 517–524. doi: 10.3892/ijmm.2018.3940
Li, K. K., Xia, T., Ma, F. M., Zhang, R., Mao, Y., Wang, Y., et al. (2015). miR-106b is overexpressed in medulloblastomas and interacts directly with PTEN. Neuropathol. Appl. Neurobiol. 41, 145–164. doi: 10.1111/nan.12169
Li, S., Wang, L., Fu, B., Berman, M. A., Diallo, A., and Dorf, M. E. (2014). TRIM65 regulates microRNA activity by ubiquitination of TNRC6. Proc. Natl. Acad. Sci. U.S.A. 111, 6970–6975. doi: 10.1073/pnas.1322545111
Li, W., Guo, F., Wang, P., Hong, S., and Zhang, C. (2014). miR-221/222 confers radioresistance in glioblastoma cells through activating Akt independent of PTEN status. Curr. Mol. Med. 14, 185–195. doi: 10.2174/1566524013666131203103147
Li, Z., Hu, C., Zhen, Y., Pang, B., Yi, H., and Chen, X. (2019). Pristimerin inhibits glioma progression by targeting AGO2 and PTPN1 expression via miR-542-5p. Biosci. Rep. 39:BSR20182389. doi: 10.1042/BSR20182389
Lima, C. R., Gomes, C. C., and Santos, M. F. (2017). Role of microRNAs in endocrine cancer metastasis. Mol. Cell. Endocrinol. 456, 62–75. doi: 10.1016/j.mce.2017.03.015
Lin, R. J., Lin, Y. C., Chen, J., Kuo, H. H., Chen, Y. Y., Diccianni, M. B., et al. (2010). microRNA signature and expression of Dicer and Drosha can predict prognosis and delineate risk groups in neuroblastoma. Cancer Res. 70, 7841–7850. doi: 10.1158/0008-5472.CAN-10-0970
Liu, B., Chen, D., Chen, S., Saber, A., and Haisma, H. (2020). Transcriptional activation of cyclin D1 via HER2/HER3 contributes to EGFR-TKI resistance in lung cancer. Biochem. Pharmacol. 178:114095. doi: 10.1016/j.bcp.2020.114095
Liu, B., Li, J., and Cairns, M. J. (2014). Identifying miRNAs, targets and functions. Brief. Bioinform. 15, 1–19. doi: 10.1093/bib/bbs075
Lu, T. X., and Rothenberg, M. E. (2018). MicroRNA. J. Allergy Clin. Immunol. 141, 1202–1207. doi: 10.1016/j.jaci.2017.08.034
Lu, Y. F., Zhang, L., Waye, M. M., Fu, W. M., and Zhang, J. F. (2015). MiR-218 mediates tumorigenesis and metastasis: perspectives and implications. Exp. Cell Res. 334, 173–182. doi: 10.1016/j.yexcr.2015.03.027
Maier, A. D., Bartek, J. Jr., Eriksson, F., Ugleholdt, H., Juhler, M., Broholm, H., et al. (2020). Clinical and histopathological predictors of outcome in malignant meningioma. Neurosurg. Rev. 43, 643–653. doi: 10.1007/s10143-019-01093-5
Mansouri, S., Singh, S., Alamsahebpour, A., Burrell, K., Li, M., Karabork, M., et al. (2016). DICER governs characteristics of glioma stem cells and the resulting tumors in xenograft mouse models of glioblastoma. Oncotarget 7, 56431–56446. doi: 10.18632/oncotarget.10570
Maryam, M., Naemi, M., and Hasani, S. S. (2021). A comprehensive review on oncogenic miRNAs in breast cancer. J. Genet. 100:15.
Masoudi, M. S., Mehrabian, E., and Mirzaei, H. (2018). MiR-21: a key player in glioblastoma pathogenesis. J. Cell. Biochem. 119, 1285–1290. doi: 10.1002/jcb.26300
Melincovici, C. S., Boşca, A. B., Şuşman, S., Mărginean, M., Mihu, C., Istrate, M., et al. (2018). Vascular endothelial growth factor (VEGF) - key factor in normal and pathological angiogenesis. Rom. J. Morphol. Embryol. 59, 455–467.
Mete, O., and Lopes, M. B. (2017). Overview of the 2017 WHO classification of pituitary tumors. Endocr. Pathol. 28, 228–243. doi: 10.1007/s12022-017-9498-z
Millard, N. E., and De Braganca, K. C. (2016). Medulloblastoma. J. Child Neurol. 31, 1341–1353. doi: 10.1177/0883073815600866
Mohajan, S., Jaiswal, P. K., Vatanmakarian, M., Yousefi, H., Sankaralingam, S., Alahari, S. K., et al. (2021). Hippo pathway: regulation, deregulation and potential therapeutic targets in cancer. Cancer Lett. 507, 112–123. doi: 10.1016/j.canlet.2021.03.006
Molitch, M. E. (2020). Dopamine agonists and antipsychotics. Eur. J. Endocrinol. 183, C11–C13. doi: 10.1530/EJE-20-0607
Montalto, F. I., and De Amicis, F. (2020). Cyclin D1 in cancer: a molecular connection for cell cycle control, adhesion and invasion in tumor and stroma. Cells 9:2648. doi: 10.3390/cells9122648
Munoz, J. L., Walker, N. D., Mareedu, S., Pamarthi, S. H., Sinha, G., Greco, S. J., et al. (2019). Cycling quiescence in temozolomide resistant glioblastoma cells is partly explained by microRNA-93 and -193-mediated decrease of cyclin D. Front. Pharmacol. 10:134. doi: 10.3389/fphar.2019.00134
Muñoz-Hidalgo, L., San-Miguel, T., Megías, J., Serna, E., Calabuig-Fariñas, S., Monleón, D., et al. (2020). The status of EGFR modulates the effect of miRNA-200c on ZEB1 expression and cell migration in glioblastoma cells. Int. J. Mol. Sci. 22:368. doi: 10.3390/ijms22010368
Negroni, C., Hilton, D. A., Ercolano, E., Adams, C. L., Kurian, K. M., Baiz, D., et al. (2020). GATA-4, a potential novel therapeutic target for high-grade meningioma, regulates miR-497, a potential novel circulating biomarker for high-grade meningioma. EBioMedicine 59:102941. doi: 10.1016/j.ebiom.2020.102941
Ni, J., Bucci, J., Malouf, D., Knox, M., Graham, P., and Li, Y. (2019). Exosomes in cancer radioresistance. Front. Oncol. 6:869. doi: 10.3389/fonc.2019.00869
Olejniczak, M., Kotowska-Zimmer, A., and Krzyzosiak, W. (2018). Stress-induced changes in miRNA biogenesis and functioning. Cell. Mol. Life Sci. 75, 177–191. doi: 10.1007/s00018-017-2591-0
Panigrahi, M. K., Chandrasekhar, Y. B. V. K., and Vooturi, S. (2020). Current status of surgery in management of prolactinomas. Neurol. India 68(Suppl.), S39–S43. doi: 10.4103/0028-3886.287668
Pannuru, P., Dontula, R., Khan, A. A., Herbert, E., Ozer, H., Chetty, C., et al. (2014). miR-let-7f-1 regulates SPARC mediated cisplatin resistance in medulloblastoma cells. Cell. Signal. 26, 2193–2201. doi: 10.1016/j.cellsig.2014.06.014
Pegtel, D. M., and Gould, S. J. (2019). Exosomes. Annu. Rev. Biochem. 88, 487–514. doi: 10.1146/annurev-biochem-013118-111902
Qadir, M. I., and Faheem, A. (2017). miRNA: a diagnostic and therapeutic tool for pancreatic cancer. Crit. Rev. Eukaryot. Gene Expr. 27, 197–204. doi: 10.1615/CritRevEukaryotGeneExpr.2017019494
Qin, X., Guo, H., Wang, X., Zhu, X., Yan, M., Wang, X., et al. (2019). Exosomal miR-196a derived from cancer-associated fibroblasts confers cisplatin resistance in head and neck cancer through targeting CDKN1B and ING5. Genome Biol. 20:12. doi: 10.1186/s13059-018-1604-0
Quinlan, A., and Rizzolo, D. (2017). Understanding medulloblastoma. JAAPA 30, 30–36. doi: 10.1097/01.JAA.0000524717.71084.50
Ramakrishnan, V., Xu, B., Akers, J., Nguyen, T., Ma, J., Dhawan, S., et al. (2020). Radiation-induced extracellular vesicle (EV) release of miR-603 promotes IGF1-mediated stem cell state in glioblastomas. EBioMedicine 55:102736. doi: 10.1016/j.ebiom.2020.102736
Richardson, H. E., Cordero, J. B., and Grifoni, D. (2020). Basic and translational models of cooperative oncogenesis. Int. J. Mol. Sci. 21:5919. doi: 10.3390/ijms21165919
Ruan, T., He, X., Yu, J., and Hang, Z. (2016). MicroRNA-186 targets Yes-associated protein 1 to inhibit Hippo signaling and tumorigenesis in hepatocellular carcinoma. Oncol. Lett. 11, 2941–2945. doi: 10.3892/ol.2016.4312
Rupaimoole, R., and Slack, F. J. (2017). MicroRNA therapeutics: towards a new era for the management of cancer and other diseases. Nat. Rev. Drug Discov. 16, 203–222. doi: 10.1038/nrd.2016.246
Saliminejad, K., Khorram Khorshid, H. R., Soleymani Fard, S., and Ghaffari, S. H. (2019). An overview of microRNAs: biology, functions, therapeutics, and analysis methods. J. Cell. Physiol. 234, 5451–5465. doi: 10.1002/jcp.27486
Schiff, D., and Alyahya, M. (2020). Neurological and medical complications in brain tumor patients. Curr. Neurol. Neurosci. Rep. 20:33. doi: 10.1007/s11910-020-01054-2
Schulte, J. D., Aghi, M. K., and Taylor, J. W. (2020). Anti-angiogenic therapies in the management of glioblastoma. Chin. Clin. Oncol. 10:37. doi: 10.21037/cco.2020.03.06
Shao, H., Chung, J., Lee, K., Balaj, L., Min, C., Carter, B. S., et al. (2015). Chip-based analysis of exosomal mRNA mediating drug resistance in glioblastoma. Nat. Commun. 6:6999. doi: 10.1038/ncomms7999
Shao, Z., Liu, L., Zheng, Y., Tu, S., Pan, Y., Yan, S., et al. (2020). Molecular mechanism and approach in progression of meningioma. Front. Oncol. 10:538845. doi: 10.3389/fonc.2020.538845
Sharif, S., Ghahremani, M. H., and Soleimani, M. (2018). Delivery of exogenous miR-124 to glioblastoma multiform cells by Wharton’s jelly mesenchymal stem cells decreases cell proliferation and migration, and confers chemosensitivity. Stem Cell Rev. Rep. 14, 236–246. doi: 10.1007/s12015-017-9788-3
Shen, J., Xia, W., Khotskaya, Y. B., Huo, L., Nakanishi, K., Lim, S. O., et al. (2013). EGFR modulates microRNA maturation in response to hypoxia through phosphorylation of AGO2. Nature 497, 383–387. doi: 10.1038/nature12080
Smits, M., Wurdinger, T., van het Hof, B., Drexhage, J. A., Geerts, D., Wesseling, P., et al. (2012). Myc-associated zinc finger protein (MAZ) is regulated by miR-125b and mediates VEGF-induced angiogenesis in glioblastoma. FASEB J. 26, 2639–2647. doi: 10.1096/fj.11-202820
Sohel, M. M. H. (2020). Circulating microRNAs as biomarkers in cancer diagnosis. Life Sci. 248:117473. doi: 10.1016/j.lfs.2020.117473
Song, L. R., Li, D., Weng, J. C., Li, C. B., Wang, L., Wu, Z., et al. (2020). MicroRNA-195 functions as a tumor suppressor by directly targeting fatty acid synthase in malignant meningioma. World Neurosurg. 136, e355–e364. doi: 10.1016/j.wneu.2019.12.182
Soraya, H., Sani, N. A., Jabbari, N., and Rezaie, J. (2021). Metformin increases exosome biogenesis and secretion in U87 MG human glioblastoma cells: a possible mechanism of therapeutic resistance. Arch. Med. Res. 52, 151–162. doi: 10.1016/j.arcmed.2020.10.007
Sousa, F., Dhaliwal, H. K., Gattacceca, F., Sarmento, B., and Amiji, M. M. (2019). Enhanced anti-angiogenic effects of bevacizumab in glioblastoma treatment upon intranasal administration in polymeric nanoparticles. J. Control. Release. 309, 37–47. doi: 10.1016/j.jconrel.2019.07.033
Sun, X. H., Fan, W. J., An, Z. J., and Sun, Y. (2020). Inhibition of long noncoding RNA CRNDE increases chemosensitivity of medulloblastoma cells by targeting miR-29c-3p. Oncol. Res. 28, 95–102. doi: 10.3727/096504019X15742472027401
Thakkar, J. P., Prabhu, V. C., Peters, K. B., and Lukas, R. V. (2021). What is new in neuro-oncology? Neurol. Clin. 39, 163–179. doi: 10.1016/j.ncl.2020.09.009
Tolonen, J. P., Hekkala, A., Kuismin, O., Tuominen, H., Suo-Palosaari, M., Tynninen, O., et al. (2020). Medulloblastoma, macrocephaly, and a pathogenic germline PTEN variant: cause or coincidence? Mol. Genet. Genomic Med. 8:e1302. doi: 10.1002/mgg3.1302
Valihrach, L., Androvic, P., and Kubista, M. (2020). Circulating miRNA analysis for cancer diagnostics and therapy. Mol. Aspects Med. 72:100825. doi: 10.1016/j.mam.2019.10.002
van Bunderen, C. C., and Olsson, D. S. (2021). Growth hormone deficiency and replacement therapy in adults: impact on survival. Rev. Endocr. Metab. Disord. 22, 125–133. doi: 10.1007/s11154-020-09599-w
Van Roosbroeck, K., and Calin, G. A. (2017). Cancer hallmarks and MicroRNAs: the therapeutic connection. Adv. Cancer Res. 135, 119–149. doi: 10.1016/bs.acr.2017.06.002
Vermeulen, E., D’Haens, J., Stadnik, T., Unuane, D., Barbe, K., Van Velthoven, V., et al. (2020). Predictors of dopamine agonist resistance in prolactinoma patients. BMC Endocr. Disord. 20:68. doi: 10.1186/s12902-020-0543-4
Viallard, C., and Larrivée, B. (2017). Tumor angiogenesis and vascular normalization: alternative therapeutic targets. Angiogenesis 20, 409–426. doi: 10.1007/s10456-017-9562-9
Vishnoi, A., and Rani, S. (2017). MiRNA biogenesis and regulation of diseases: an overview. Methods Mol. Biol. 1509, 1–10. doi: 10.1007/978-1-4939-6524-3_1
Wang, B., Wu, Z. H., Lou, P. Y., Chai, C., Han, S. Y., Ning, J. F., et al. (2019). Human bone marrow-derived mesenchymal stem cell-secreted exosomes overexpressing microRNA-34a ameliorate glioblastoma development via down-regulating MYCN. Cell. Oncol. (Dordr.) 42, 783–799. doi: 10.1007/s13402-019-00461-z
Wang, M., Deng, X., Ying, Q., Jin, T., Li, M., and Liang, C. (2015). MicroRNA-224 targets ERG2 and contributes to malignant progressions of meningioma. Biochem. Biophys. Res. Commun. 460, 354–361. doi: 10.1016/j.bbrc.2015.03.038
Wang, Z., Gao, L., Guo, X., Feng, C., Deng, K., Lian, W., et al. (2019). Identification of microRNAs associated with the aggressiveness of prolactin pituitary tumors using bioinformatic analysis. Oncol. Rep. 42, 533–548. doi: 10.3892/or.2019.7173
Weeraratne, S. D., Amani, V., Neiss, A., Teider, N., Scott, D. K., Pomeroy, S. L., et al. (2011). miR-34a confers chemosensitivity through modulation of MAGE-A and p53 in medulloblastoma. Neuro Oncol. 13, 165–175. doi: 10.1093/neuonc/noq179
Wei, Z., Zhou, C., Liu, M., Yao, Y., Sun, J., Xiao, J., et al. (2015). MicroRNA involvement in a metastatic non-functioning pituitary carcinoma. Pituitary 18, 710–721. doi: 10.1007/s11102-015-0648-3
Wirsching, H. G., Galanis, E., and Weller, M. (2016). Glioblastoma. Handb. Clin. Neurol. 134, 381–397. doi: 10.1016/B978-0-12-802997-8.00023-2
Wu, H. M., Wang, H. D., Tang, Y., Fan, Y. W., Hu, Y. B., Tohti, M., et al. (2015). Differential expression of microRNAs in postoperative radiotherapy sensitive and resistant patients with glioblastoma multiforme. Tumour Biol. 36, 4723–4730. doi: 10.1007/s13277-015-3121-z
Wu, K., He, J., Pu, W., and Peng, Y. (2018). The role of exportin-5 in MicroRNA biogenesis and cancer. Genomics Proteomics Bioinformatics 16, 120–126. doi: 10.1016/j.gpb.2017.09.004
Xiao, B., Zhou, X., Ye, M., Lv, S., Wu, M., Liao, C., et al. (2016). MicroRNA-566 modulates vascular endothelial growth factor by targeting Von Hippel-Landau in human glioblastoma in vitro and in vivo. Mol. Med. Rep. 13, 379–385. doi: 10.3892/mmr.2015.4537
Xu, D., Chi, G., Zhao, C., and Li, D. (2018). Ligustrazine inhibits growth, migration and invasion of medulloblastoma daoy cells by up-regulation of miR-211. Cell. Physiol. Biochem. 49, 2012–2021. doi: 10.1159/000493712
Xue, J., Yang, M., Hua, L. H., and Wang, Z. P. (2019). MiRNA-191 functions as an oncogene in primary glioblastoma by directly targeting NDST1. Eur. Rev. Med. Pharmacol. Sci. 23, 6242–6249. doi: 10.26355/eurrev_201907_18443
Yang, F., Liu, X., Liu, Y., Liu, Y., Zhang, C., Wang, Z., et al. (2017). miR-181d/MALT1 regulatory axis attenuates mesenchymal phenotype through NF-kappaB pathways in glioblastoma. Cancer Lett. 396, 1–9. doi: 10.1016/j.canlet.2017.03.002
Yang, J. K., Yang, J. P., Tong, J., Jing, S. Y., Fan, B., Wang, F., et al. (2017). Exosomal miR-221 targets DNM3 to induce tumor progression and temozolomide resistance in glioma. J. Neurooncol. 131, 255–265. doi: 10.1007/s11060-016-2308-5
Yang, Y., Cui, H., and Wang, X. (2019). Downregulation of EIF5A2 by miR-221-3p inhibits cell proliferation, promotes cell cycle arrest and apoptosis in medulloblastoma cells. Biosci. Biotechnol. Biochem. 83, 400–408. doi: 10.1080/09168451.2018.1553604
Yin, J., Zeng, A., Zhang, Z., Shi, Z., Yan, W., and You, Y. (2019). Exosomal transfer of miR-1238 contributes to temozolomide-resistance in glioblastoma. EBioMedicine 42, 238–251. doi: 10.1016/j.ebiom.2019.03.016
Yu, C., Li, J., Sun, F., Cui, J., Fang, H., and Sui, G. (2016). Expression and clinical significance of miR-26a and pleomorphic adenoma gene 1 (PLAG1) in invasive pituitary adenoma. Med. Sci. Monit. 22, 5101–5108. doi: 10.12659/msm.898908
Yue, X., Lan, F., and Xia, T. (2019). Hypoxic glioma cell-secreted exosomal miR-301a activates Wnt/b-catenin signaling and promotes radiation resistance by targeting TCEAL7. Mol. Ther. 27, 1939–1949. doi: 10.1016/j.ymthe.2019.07.011
Zeng, A., Yin, J., Li, Y., Li, R., Wang, Z., Zhou, X., et al. (2018b). miR-129-5p targets Wnt5a to block PKC/ERK/NF-kappaB and JNK pathways in glioblastoma. Cell Death Dis. 9:394. doi: 10.1038/s41419-018-0343-1
Zeng, A., Wei, Z., Yan, W., Yin, J., Huang, X., Zhou, X., et al. (2018a). Exosomal transfer of miR-151a enhances chemosensitivity to temozolomide in drug-resistant glioblastoma. Cancer Lett. 436, 10–21. doi: 10.1016/j.canlet.2018.08.004
Zeng, R., and Dong, J. (2021). The hippo signaling pathway in drug resistance in cancer. Cancers (Basel) 13:318. doi: 10.3390/cancers13020318
Zhang, B., Pan, X., Cobb, G. P., and Anderson, T. A. (2007). microRNAs as oncogenes and tumor suppressors. Dev. Biol. 302, 1–12. doi: 10.1016/j.ydbio.2006.08.028
Zhang, L., and Yu, D. (2019). Exosomes in cancer development, metastasis, and immunity. Biochim. Biophys. Acta Rev. Cancer 1871, 455–468. doi: 10.1016/j.bbcan.2019.04.004
Zhang, L., Liao, Y., and Tang, L. (2019). MicroRNA-34 family: a potential tumor suppressor and therapeutic candidate in cancer. J. Exp. Clin. Cancer Res. 38:53. doi: 10.1186/s13046-019-1059-5
Zhang, Q., Song, L. R., Huo, X. L., Wang, L., Zhang, G. B., Hao, S. Y., et al. (2020). MicroRNA-221/222 inhibits the radiation-induced invasiveness and promotes the radiosensitivity of malignant meningioma cells. Front. Oncol. 10:1441. doi: 10.3389/fonc.2020.01441
Zhang, X., Zhang, G., Huang, H., Li, H., Lin, S., and Wang, Y. (2020). Differentially expressed MicroRNAs in radioresistant and radiosensitive atypical meningioma: a clinical study in Chinese patients. Front. Oncol. 10:501. doi: 10.3389/fonc.2020.00501
Zhang, Z., Xu, J., Chen, Z., Wang, H., Xue, H., Yang, C., et al. (2020). Transfer of microRNA via macrophage-derived extracellular vesicles promotes proneural-to-mesenchymal transition in glioma stem cells. Cancer Immunol. Res. 8, 966–981. doi: 10.1158/2326-6066.CIR-19-0759
Zhao, C., Guo, R., Guan, F., Ma, S., Li, M., Wu, J., et al. (2020). MicroRNA-128-3p enhances the chemosensitivity of temozolomide in glioblastoma by targeting c-Met and EMT. Sci. Rep. 10:9471. doi: 10.1038/s41598-020-65331-3
Zhao, L., Zhao, W., Hou, Y., Wen, C., Wang, J., Wu, P., et al. (2020). An overview of managements in meningiomas. Front. Oncol. 10:1523. doi: 10.3389/fonc.2020.01523
Zhao, P., Cheng, J., Li, B., Nie, D., Li, C., Gui, S., et al. (2021). Up-regulation of the expressions of MiR-149-5p and MiR-99a-3p in exosome inhibits the progress of pituitary adenomas. Cell Biol. Toxicol. 37, 633–651. doi: 10.1007/s10565-020-09570-0
Zheng, L., Zhang, Y., Liu, Y., Zhou, M., Lu, Y., Yuan, L., et al. (2015). MiR-106b induces cell radioresistance via the PTEN/PI3K/AKT pathways and p21 in colorectal cancer. J. Transl. Med. 13:252. doi: 10.1186/s12967-015-0592-z
Zheng, Z., Zhang, Y., Zhang, Z., Yang, Y., and Song, T. (2017). Effect of miR-106b on Invasiveness of Pituitary Adenoma via PTEN-PI3K/AKT. Med. Sci. Monit. 23, 1277–1285. doi: 10.12659/msm.900092
Keywords: malignant primary brain tumors, miRNAs, resistance, therapy, oncogenesis, exosomes
Citation: Gareev I, Beylerli O, Liang Y, Xiang H, Liu C, Xu X, Yuan C, Ahmad A and Yang G (2021) The Role of MicroRNAs in Therapeutic Resistance of Malignant Primary Brain Tumors. Front. Cell Dev. Biol. 9:740303. doi: 10.3389/fcell.2021.740303
Received: 12 July 2021; Accepted: 17 September 2021;
Published: 07 October 2021.
Edited by:
Ata Abbas, Case Western Reserve University, United StatesReviewed by:
Mohd Wasim Nasser, University of Nebraska Medical Center, United StatesMaria Braoudaki, University of Hertfordshire, United Kingdom
Copyright © 2021 Gareev, Beylerli, Liang, Xiang, Liu, Xu, Yuan, Ahmad and Yang. This is an open-access article distributed under the terms of the Creative Commons Attribution License (CC BY). The use, distribution or reproduction in other forums is permitted, provided the original author(s) and the copyright owner(s) are credited and that the original publication in this journal is cited, in accordance with accepted academic practice. No use, distribution or reproduction is permitted which does not comply with these terms.
*Correspondence: Guang Yang, eWFuZ2d1YW5nMTIyN0AxNjMuY29t; Aamir Ahmad, YWFobWFkOUBoYW1hZC5xYQ==
†These authors have contributed equally to this work