- 1Instituto de Investigaciones Biomédicas Alberto Sols CSIC/UAM, Madrid, Spain
- 2Departamento de Bioquímica y Biología Molecular, Facultad de Medicina, Universidad Complutense de Madrid, Madrid, Spain
WIPIs are a conserved family of proteins with a characteristic 7-bladed β-propeller structure. They play a prominent role in autophagy, but also in other membrane trafficking processes. Mutations in human WIPI4 cause several neurodegenerative diseases. One of them is BPAN, a rare disease characterized by developmental delay, motor disorders, and seizures. Autophagy dysfunction is thought to play an important role in this disease but the precise pathological consequences of the mutations are not well established. The use of simple models such as the yeast Saccharomyces cerevisiae and the social amoeba Dictyostelium discoideum provides valuable information on the molecular and cellular function of these proteins, but also sheds light on possible pathways that may be relevant in the search for potential therapies. Here, we review the function of WIPIs as well as disease-causing mutations with a special focus on the information provided by these simple models.
Introduction
Degradation and recycling of cellular components are essential for cell homeostasis. Autophagy (from the Greek, “self-eating”) includes several pathways that deliver different types of cargos to lysosomes for degradation. Macroautophagy (referred to as autophagy hereafter) is characterized by the formation of double-membrane vesicles, known as autophagosomes, which can engulf non-specific cellular material (bulk autophagy) or specific cargoes, such as protein aggregates or defective organelles (selective autophagy). After the fusion of autophagosomes with the lysosomes, the simple biochemical compounds generated during the degradation process are transported to the cytosol and reused for energy production or recycling (see recent reviews Lahiri et al., 2019; Melia et al., 2020; Gubas and Dikic, 2021). Autophagosome biogenesis is a highly regulated process involving different stages: induction, lipidation, and elongation of the autophagosome membrane (also known as the isolation membrane or phagophore), vesicle closure, and fusion with lysosomes. These steps are controlled by protein complexes formed mainly by the so-called Atg proteins. Figure 1 summarizes schematically the essential components of the autophagy machinery and their function in the different steps of autophagosome formation.
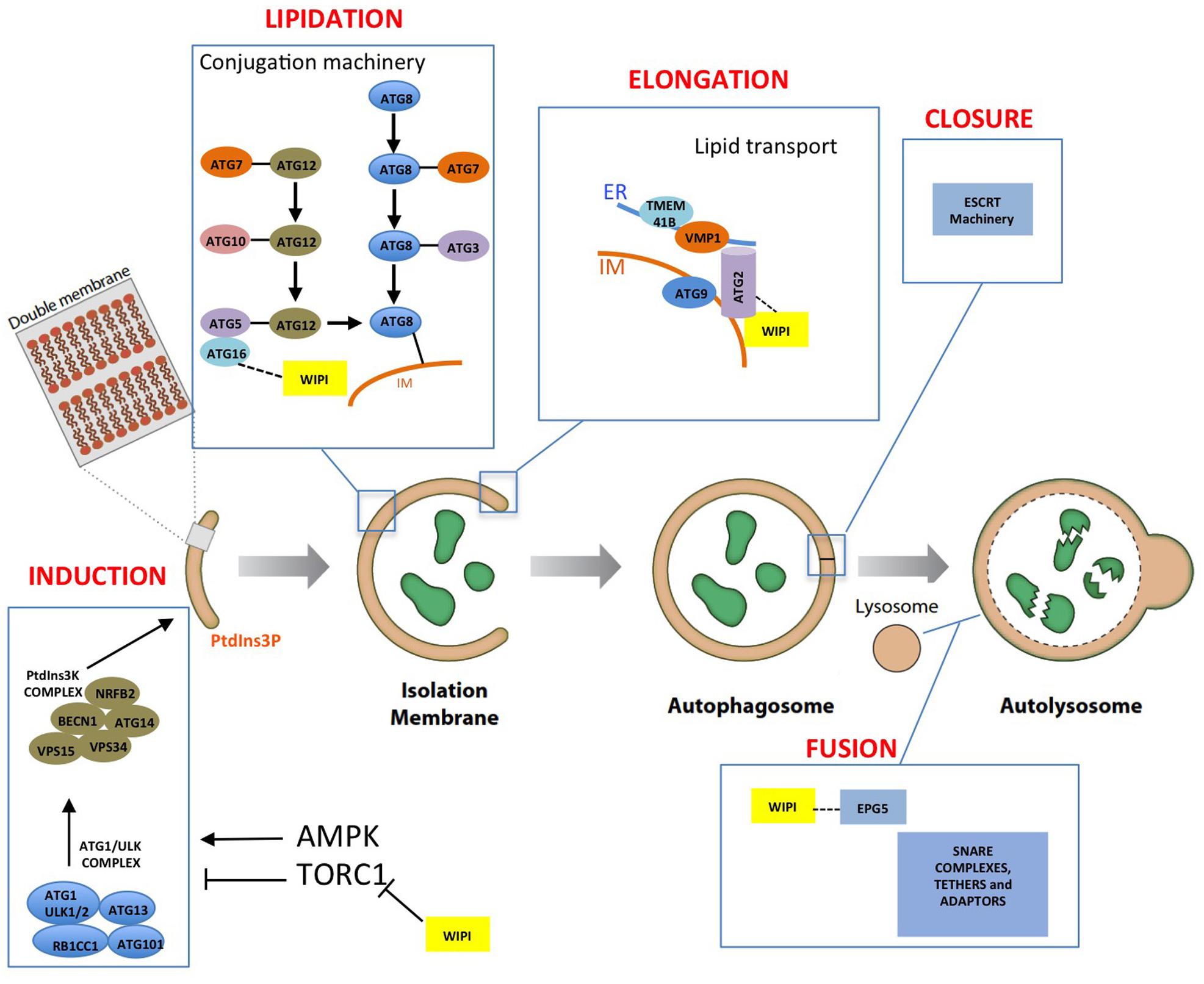
Figure 1. Autophagosome formation and the autophagic machinery. The different steps of autophagosome formation are shown together with the proteins and protein complexes involved in autophagy regulation. WIPIs (yellow boxes) are involved in different steps of autophagosome formation (see the text for a detailed explanation of the specific WIPIs involved in each step).
The nutritional and energetic status of cells regulates autophagy through two master regulators, TORC1 and AMPK (Hindupur et al., 2015). These kinases oppositely control autophagy through phosphorylation of key subunits of two essential complexes, Atg1/ULK and PI3KC3. Atg1/ULK kinase complex composed of Atg1, Atg13, Atg17, Atg29, and Atg31 in Saccharomyces cerevisiae (yeast hereafter), and by ULK1/2, ATG13, ATG101, and FIP200 in mammalian cells (Hurley and Young, 2017). Atg1, Atg13, and Atg101 have also been identified in Dictyostelium discoideum (Otto et al., 2004; Mesquita et al., 2015). During nucleation the Atg1/ULK complex is activated and recruited to specialized regions of the endoplasmic reticulum (ER) through interaction with VAMP-associated proteins (VAPs) (Zhao et al., 2018). The Atg1/ULK complex subsequently activates the PI3KC3 complex composed of Vps34, Vps15, Atg6, and Atg14 in yeast and VPS34, VPS15, BECN1, NRFB2, and ATG14 in mammals (Hurley and Young, 2017). The homologues of all these components can also be recognized in Dictyostelium (Calvo-Garrido et al., 2010; Fischer and Eichinger, 2019). The phosphoinositide 3-kinase Vps34/VPS34 generates PtdIns3P by phosphorylation of phosphatidylinositol in the nascent autophagosome membrane and in the specialized ER-derived structure known as omegasome (Axe et al., 2008; Nishimura et al., 2017). The incorporation of Atg9 containing vesicles, which seed the initial autophagosome membrane, is also under the control of the Atg1/ULK complex (Papinski et al., 2014; Karanasios et al., 2016; Nishimura et al., 2017; Sawa-Makarska et al., 2020).
The PtdIns3P generated at the autophagosome assembly site recruits a family of proteins called WIPIs (WD-repeat protein Interacting with phosphoinositides), also known as PROPPINs (beta-propellers that bind polyphosphoinositides), which are necessary for the subsequent recruitment of other autophagic proteins. Their structure, function and role in disease are the focus of this review. One of the proteins recruited by WIPIs is Atg2 in yeast (ATG2A/ATG2B in mammalian cells), a lipid transport protein that appears to be responsible for autophagosome membrane elongation through lipid transport from the ER (Maeda et al., 2019; Osawa et al., 2019, 2020; Valverde et al., 2019). Autophagosome membrane elongation also requires the function of several transmembrane proteins, ATG9 in the phagophore membrane, and VMP1 and TMEM41B in the ER membrane, which have recently been reported to have scramblase activity in vitro (Valverde et al., 2019; Matoba et al., 2020; Li et al., 2021). Since lipids are extracted by Atg2 from the outer leaflet of the ER membrane and delivered to the outer leaflet of the autophagosome membrane, lipid transport from one leaflet to the other that is accomplished by these scramblases is necessary to equilibrate the lipid composition on both sides of the bilayer of the donor and acceptor membranes.
Atg8 lipidation, a ubiquitin-like mechanism that covalently attaches the protein Atg8 to the autophagosome membrane, is also required for elongation and closure of the autophagosome membrane. In mammalian cells, Atg8 proteins are classified into three groups: LC3, GABARAP, and GABARAPL (Slobodkin and Elazar, 2013). In this process, the Atg12 protein is first conjugated to Atg5 and this complex interacts non-covalently with Atg16. The WIPI-mediated recruitment of the Atg12-Atg5/Atg16 complex to the autophagosome membrane promotes the conjugation of Atg8 to phosphatidyl-ethanolamine (PE) in a process that also involves the E1-like and E2-like enzymes Atg7 and Atg3 (Geng and Klionsky, 2008). The proteins involved in this ubiquitin-like mechanism are highly conserved in all eukaryotes, including Dictyostelium (Calvo-Garrido et al., 2010; Mesquita et al., 2017; Fischer and Eichinger, 2019). Closure of the autophagosome membrane and fusion with lysosomes requires the function of the ESCRT machinery and SNARE complexes, respectively (Lőrincz and Juhász, 2019).
Autophagy influences virtually all cellular processes, as it regulates the cellular response to the immediate metabolic demands and also plays a direct role in the quality control of protein and organelles. Consequently, autophagy has a major impact at the organismal level and regulates many physiological functions in mammalian cells, including differentiation and development, tissue and organ homeostasis, immunity and aging. Mutations in autophagy-related genes have been associated with different diseases (see a recent review for an overview; Mizushima and Levine, 2020). Mutations in WIPI genes lead to severe neurological disorders, including neurodegeneration, intellectual disability, epilepsy, developmental abnormalities, etc. Specifically, mutations in the WIPI4/WDR45 gene lead to BPAN (beta propeller-associated neurodegeneration), an ultra-rare disease with no cure that affects children. The use of simple model organisms such as Yeast and Dictyostelium can help to understand the molecular basis of these diseases.
The Yeast and Dictyostelium Models in Autophagy and Disease
Yeast has been the pioneer model in which most of the components of the autophagic machinery have been discovered and characterized. The lack of autophagy in this organism leads to reduced survival under conditions of nitrogen starvation. Nobel laureate Yoshinory Ohsumi took advantage of the powerful genetics and manipulability of yeast to screen for genes required for autophagy, whose encoded proteins were named Atg proteins (Tsukada and Ohsumi, 1993; Scott et al., 1996; Matsuura et al., 1997). Although the simplicity of the yeast model has allowed the fundamental leap of identifying the key components of the autophagic machinery, this model lacks the complexity of phenotypes usually associated with autophagy dysfunction in multicellular organisms. Dictyostelium can therefore complement the use of yeast, primarily for certain genes or pathways that are not found in yeast, while conserved between Dictyostelium and animal cells.
The species Dictyostelium discoideum was discovered in 1935 by Kenneth Raper in Western North Carolina (United States) (Raper, 1935), and the potential of this organism as an experimental model became apparent very early on. Dictyostelium belongs to the amoebozoa group, and although this group of organisms diverged before the opistokonta (fungi and animals), it retains many features of animal cells that have been lost during the evolution of fungi. Cell motility and chemotaxis, phagocytosis and macropynocytosis are very similar to those observed in animal cells and Dictyostelium presents a multicellular stage that allows the study of cell differentiation and morphogenesis (see this series of reviews collected in a special issue dedicated to Dictyostelium in IJDB (Araki and Saito, 2019; Batsios et al., 2019; Bloomfield, 2019; Bozzaro, 2019; Consalvo et al., 2019; Escalante and Cardenal-Muñoz, 2019; Farinholt et al., 2019; Fey et al., 2019; Fischer and Eichinger, 2019; Ishikawa-Ankerhold and Müller-Taubenberger, 2019; Jaiswal et al., 2019; Kawabe et al., 2019; Kay et al., 2019; Knecht et al., 2019; Kundert and Shaulsky, 2019; Kuspa and Shaulsky, 2019; Medina et al., 2019; Nanjundiah, 2019; Pal et al., 2019; Pearce et al., 2019; Pergolizzi et al., 2019; Schaf et al., 2019; Vines and King, 2019). Individual Dictyostelium cells ingest bacteria and yeasts in soil and the transition to a multicellular state, triggered when the food source is depleted, is accomplished by aggregation of preexisting cells. This developmental program culminates in the formation of a fruiting body containing spores (Figure 2). As this process takes place in the absence of nutrients, autophagy is essential to sustain the energy-demanding processes of chemotaxis, morphogenesis and cell differentiation. Therefore, autophagy dysfunction results in distinct phenotypes that vary in severity from the complete absence of development to the formation of aberrant, multi-tipped mounds that may eventually form abnormal fruiting bodies containing non-viable spores (Otto et al., 2003, 2004; Calvo-Garrido and Escalante, 2010; Tung et al., 2010; Mesquita et al., 2015; Muñoz-Braceras et al., 2015; Xiong et al., 2015, 2018; Fischer et al., 2019; Sharma et al., 2019; Yamada and Schaap, 2019, 2020; Karow et al., 2020; Figure 2). Dictyostelium has become a useful model for studying autophagy. The function of many autophagy genes have been analyzed in this organism and diverse techniques have been fine-tuned to precisely study this process (Calvo-Garrido et al., 2010; Mesquita et al., 2017; Fischer and Eichinger, 2019). In addition, Dictyostelium expresses some key autophagic proteins absent in yeast but present in animal cells. Notable examples are the Atg1/ULK complex protein Atg101 (Mesquita et al., 2015), the ER protein Vmp1 (Calvo-Garrido et al., 2008, 2014; Calvo-Garrido and Escalante, 2010), the Atg16 protein containing a C-terminal WD-repeat domain also present in mammalian ATG16L (Xiong et al., 2018) but absent in the yeast homolog, the autophagy regulators KinkyA and Bcas3 (Yamada and Schaap, 2020), and orthologues of the Gamma-secretase PSEN1 (psenA and psenB), NCSTN (nicastrin), and APH1 (gamma-secretase subunit Aph-1) (Sharma et al., 2019).
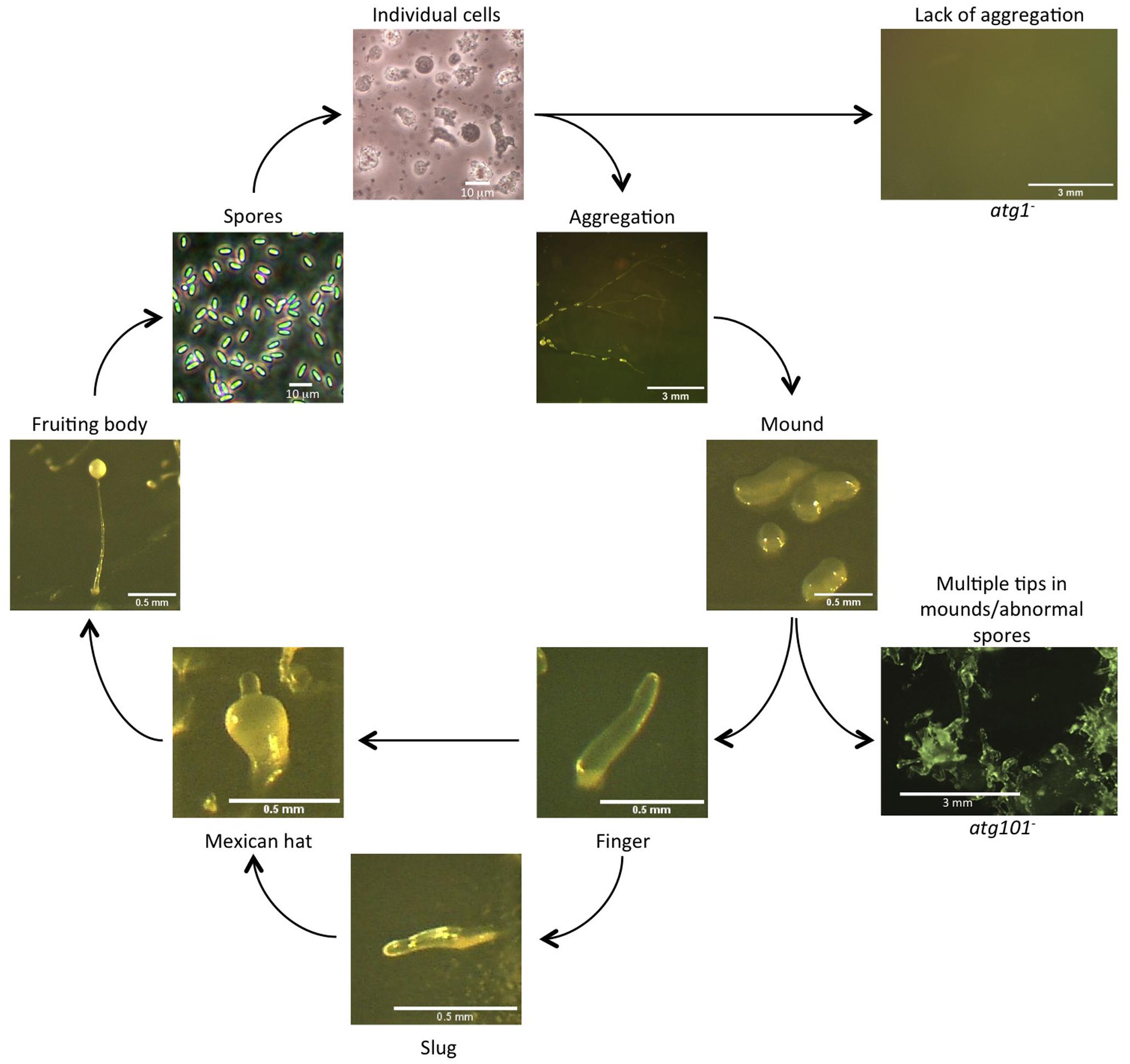
Figure 2. Autophagy is required for Dictyostelium development. Representative pictures of the Dictyostelium life cycle are shown. Strains defective in autophagy (right panels) show distinct phenotypes, such as lack of aggregation (Atg1 mutant is shown as an example) or abnormal development with a lack of spores (Atg101 mutant is shown as an example). Scale bars are shown in each photograph.
The use of simple model organisms, such as yeast and Dictyostelium, has allowed fundamental advances to be made in the basic principles of many diseases, contributing to the 3R principle (replacing, reducing and refining the use of animals in research, directive 2010/63/EU). The high conservation of protein and signaling pathways during evolution makes it possible to study diseases at different levels: (i) At the molecular level, for example by analyzing the biochemical function, structure and interactions of proteins involved in the disease; (ii) At the cellular and organism level, by analyzing cell physiology, affected pathways, differentiation and development of diseased models; and (iii) At the pharmacological levels by studying the mechanism of action of drugs or even the search for new therapeutic compounds for well-conserved pathways (see some recent works as examples of point iii; Cocorocchio et al., 2017; Schaf et al., 2019; Perry et al., 2020; Warren et al., 2020).
The Function of WIPIs in the Different Steps of Autophagosome Formation
The number of members of this protein family varies among organisms. In mammalian cells there are four members (WIPI1, WIPI2, WDR45B/WIPI3, and WDR45/WIPI4), three in yeast (Atg18, Atg21, and Hsv2) and two in Dictyostelium (Atg18 and Wdr45l). A phylogenetic analysis based on sequence similarity clusters WIPI sequences into two groups (Figure 3). One contains the highly similar WIPI1 and WIPI2 along with Dictyostelium Atg18 and yeast Atg18 and Atg21. In the other branch, human WIPI3 and WIPI4 are found together with Dictyostelium Wdr45l and yeast Hsv2 (Figure 3). Despite the apparent clarity in their distribution, the functions attributed to each member do not always correspond to this position based on sequence similarity. The most striking example is that of Hsv2, which is the closest sequence homologue in yeast of human WIPI3/4 and Dictyostelium Wdr45l, proteins that play a prominent role in autophagy, whereas Hsv2 does not appear to play a role in bulk autophagy in yeast (Krick et al., 2008). Next, we will describe in detail and in a comparative way the functions attributed to the human, yeast and Dictyostelium WIPI proteins in the different steps of autophagosome formation (Figure 1 shows the WIPIs in their proposed functions throughout the different steps of autophagosome formation).
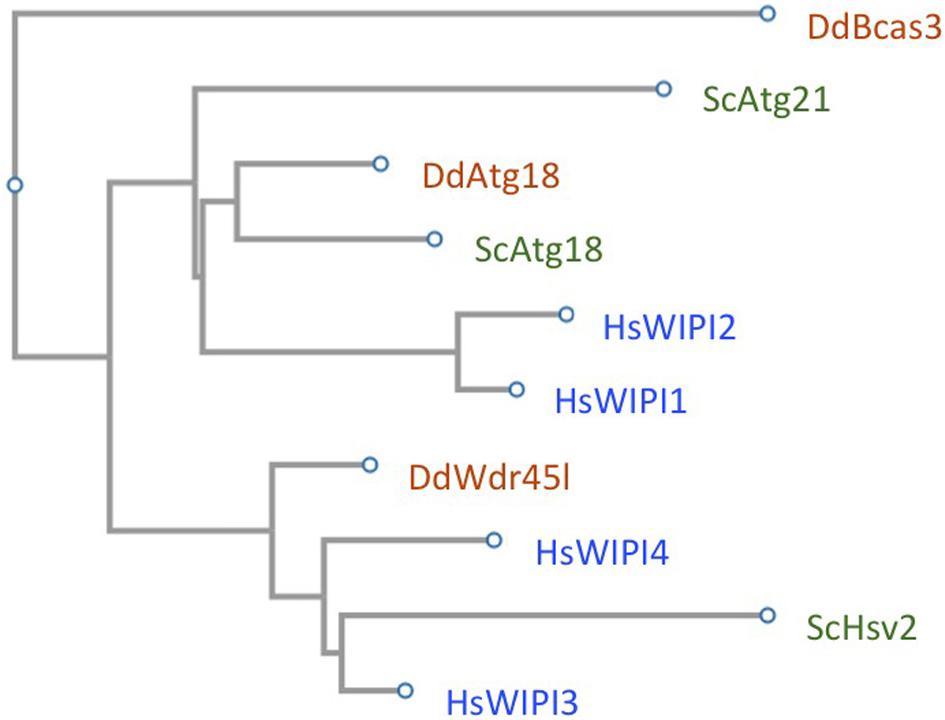
Figure 3. The WIPI family in yeast, Dictyostelium and human. The amino acid sequences of yeast Atg18, Atg21, and Hsv2 (UniProt entry: P43601; Q02887; P50079), Dictyostelium Atg18 and Wdr45l (UniProt entry: Q54NA2; Q54SA0), and the human WIPI1, WIPI2, WDR45B/WIPI3, and WDR45/WIPI4 (UniProt entry: Q5MNZ9; Q9Y4P8; Q5MNZ6; Q9Y484), were used for a phylogenetic analysis using Fast Tree (https://www.genome.jp/tools-bin/clustalw). The Dictyostelium proppin Bcas3 (UniProt entry: Q559F5) was used as an outgroup.
Induction Step
The induction of autophagy is regulated by the interplay between AMPK and TORC1. AMPK activates and TORC1 inhibits autophagy. TORC1 is activated by growth factor through RHEB, and aminoacids through RRAG/Rag GTPases that activate TORC1 on the surface of lysosomes, leading to phosphorylation of the ATG1/ULK complex and inhibition of autophagy. AMPK activates autophagy through phosphorylation and activation of the TORC1 inhibitor TSC1/2. In addition, AMPK activates autophagy directly through phosphorylation and inhibition of TORC1, and also through activation by phosphorylation of the ATG1/ULK complex. Analysis of the WIPI3 interactome suggests a regulatory role of this protein in TORC1 inhibition through the TSC1/2 complex. Specifically, WIPI3 interacts with TSC1 when the latter is phosphorylated by AMPK. The complex formed by WIPI3/TSC1/2/FIP200 localizes to the lysosome when TORC1 is absent, suggesting a role in inhibiting and releasing TORC1 from lysosomes to activate autophagy (Bakula et al., 2017). Upon starvation, WIPI3 also translocates to the nascent autophagosome colocalizing with FIP200 and other ATG proteins (Bakula et al., 2017). This possible role of human WIPI3 in the initiation step contrasts with the phenotype associated to human WIPI3 or WIPI4 knock-down (KD) (Bakula et al., 2017) and to Wdr45l knock-out (KO) in Dictyostelium (Tornero-Écija et al., 2021), as autophagy is blocked at a later step, following recruitment of the conjugation machinery and lipidation of Atg8/LC3.
Elongation and Atg8/LC3 Lipidation
WIPI proteins play an essential role in the recruitment of the conjugation machinery responsible for the lipidation of Atg8/LC3 protein at the phagophore membrane. In particular, the interaction of human WIPI2 with ATG16L1 mediates the recruitment of the ATG12-ATG5/ATG16L1 complex (Dooley et al., 2014; Fracchiolla et al., 2020). Accordingly, WIPI2 KD results in a large reduction in LC3 lipidation and autophagosome formation (Polson et al., 2010; Dooley et al., 2014; Bakula et al., 2017). There is also an accumulation of PtdIns3P and DFCP1 (double FYVE-domain containing protein 1) positive structures in WIPI2-depleted HEK293A cells, suggesting a defect in the omegasome structure (Polson et al., 2010).
WIPI1 has been proposed to assist WIPI2 in the recruitment of the conjugation machinery although its role is not essential (Bakula et al., 2017, 2018). Accordingly, WIPI1 KD in G361 cells does not prevent the formation of autophagosomes although a slight decrease in the number of autophagosomal structures is observed (Bakula et al., 2017). However, there is no detectable effect on LC3 lipidation (Proikas-Cezanne et al., 2015), indicating that WIPI1 is partially dispensable for the recruitment of the conjugation machinery.
In yeast, Atg21, like WIPI2 in humans, interacts with Atg16 and is essential for effective recruitment and lipidation of Atg8 (Juris et al., 2015; Krick and Thumm, 2016; Sawa-Makarska et al., 2020). Interestingly, the closest sequence homolog of Atg21 and WIPI2 in Dictyostelium, termed Atg18, is largely dispensable for autophagy, as Atg8-containing autophagosomes are formed in Atg18 KO cells, although a slight reduction in autophagy flux can be detected (Tornero-Écija et al., 2021). The other WIPI protein in Dictyostelium, Wdr45l, does not appear to be required for lipidation despite the complete block in autophagy, as abnormal Atg8-containing structures are detected in the Wdr45l KO strain (Tornero-Écija et al., 2021). This raises the possibility that in Dictyostelium, recruitment of the conjugation machinery is largely independent of WIPI proteins. The existence of alternative mechanisms to WIPIs in this process is consistent with the observation that LC3 lipidation is not completely blocked in mammalian cells depleted of WIPI1 or WIPI2 (Polson et al., 2010), and that a C-terminal region of ATG16L1 can bind to membranes on its own and sustain LC3 lipidation in the absence of WIPI2 (Lystad et al., 2019). Interestingly, this C-terminal extension is absent in yeast Atg16 but present in the Dictyostelium Atg16 homolog (Xiong et al., 2018), which could explain the apparently minor role of WIPIs in the conjugation process in this organism, although no direct study has been performed on the ability of this protein region in Dictyostelium to bind to the autophagosome membrane.
Elongation and Lipid Transport Mediated by ATG2
Phagophore elongation requires lipid transport from the ER by the lipid transfer protein ATG2, which forms a complex with WIPI4 in mammalian cells (Bakula et al., 2017, 2018; Chowdhury et al., 2018; Maeda et al., 2019; Osawa et al., 2020; Sawa-Makarska et al., 2020) and Atg18 in yeast (Obara et al., 2008; Gómez-Sánchez et al., 2018; Kotani et al., 2018). A proteomic analysis suggests that WIPI4 forms a complex with AMPK and ULK1 under fed conditions and is released upon starvation to localize together with ATG2 at the phagophore (Bakula et al., 2017, 2018).
Functionally, both WIPI3 and WIPI4 are downstream of LC3 lipidation as their depletion in G316 cell lines leads to abnormal accumulation of other autophagic proteins such as WIPI1 and LC3 (Bakula et al., 2017). In addition, mice with double KO of WIPI3 and 4 show a more severe autophagy defect than single KO, suggesting that these two proteins act cooperatively in autophagy (Ji et al., 2020). In Dictyostelium, accumulation of Atg18 and Atg8 is also observed in cells lacking Wdr45l, suggesting that this protein is the functional counterpart of WIPI3 and 4 (Tornero-Écija et al., 2021). Thus, mammalian WIPI3 and 4, Dictyostelium Wdr45l and yeast Atg18 appear to be functionally equivalent, even though the yeast Atg18 belongs to the other phylogenetic group formed by WIPI1 and 2, Dictyostelium Atg18 and yeast Atg21. This is another clear example of the lack of correlation between sequence similarity and functional conservation.
Fusion of Autophagosomes With Lysosomes
Defects in lipid transport during autophagosome formation resulting from lack of ATG2 or WIPI3/4 are expected to cause abnormal small phagophores to accumulate. However, a recent report showed that WIPI3/4 double KO mouse neuroblastome cells (N2a) can form autophagosomes that appear to be closed but are very small (Ji et al., 2021). The small size of these autophagosomes is overcome by overexpression of ATG2A, which is consistent with the involvement of ATG2/WIPI3/4 in lipid transfer and autophagosome membrane growth. Interestingly, WIPI3/4 appears to be required for a later stage of the autophagy pathway, as the fusion of these small autophagosomes with lysosomes is impaired in WIPI3/4 double KO (Ji et al., 2021). In the proposed model, WIPI3 or WIPI4 binds to EPG5, a protein required for the tethering of autophagosomes to lysosomes and the assembly of the SNARE complex necessary for fusion. As previously observed in the mouse model, autophagy defects are observed in WIPI3/4 double KO in N2a cells but not in single KOs of WIPI3 or WIPI4, again suggesting redundancy of function in this neural cell line.
Non-autophagic Functions of WIPIs
The versatility and complexity of WIPI proteins are also evidenced by their function in pathways other than canonical autophagy. Indeed, WIPI1 (also known as WIPI49 for 49 KD) was initially characterized for its function in endosome trafficking. It localizes to the trans-Golgi and endosomes (in COS-7 cells) and siRNA-mediated KD in COS7 leads to impaired CI-MPR (mannose 6-P receptor) trafficking (Jeffries et al., 2004). Inactivation of WIPI1 leads to enlargement of endosomes and accumulation of endosomal membrane tubules. In addition, WIPI1 (but not the other WIPIs) has recently been shown to be required for transferrin receptor recycling to the plasma membrane in HK2 (human kidney) cells (De Leo et al., 2021). It is required for the formation of tubulo-vesicular endosomal transport carriers. The binding of the two phosphatidyl inositol binding sites to PtdIns3P or PI(3, 5)P2 appear to determine WIPI1 function in autophagy or endosomal trafficking. Interestingly, yeast Atg18 (formerly known as Svp1) is required for protein recycling from the vacuole to the Golgi and its inactivation leads to vacuole enlargement and accumulation of PtdIns(3, 5)P2 (Dove et al., 2004). PI(3, 5)P2 recruits Atg18 to the vacuole where it regulates retrograde transport of proteins from the vacuole to Golgi. Its function in autophagy is independent of binding to this lipid (Dove et al., 2004).
Human WIPI3 has been implicated in an alternative form of autophagy known as GOMED (Golgi-membrane-associated degradation) that is independent of the conjugation machinery and generates autophagic structures from Golgi membranes (Yamaguchi et al., 2020).
Structural and Functional Features of Wipi Proteins: Interactions With Phosphoinositides and the Proteins Atg16 and Atg2
The crystal structure of Hsv2 from Kluyveromyces lactis revealed a characteristic structure shared by the entire WIPI protein family (Krick et al., 2012), corresponding to a seven-bladed β-propeller, each blade being formed by four antiparallel β-strands. This structure is reminiscent of a barrel-like structure and has a non-velcro closure, whereby the N-terminus is not part of the last C-terminal blade. Interaction with lipids and with proteins such as Atg2 and Atg16 involve different regions of the protein.
WIPIs bind to the membrane through two lipid-binding sites that require the conserved motif L/FRRG, which can be considered a signature of this family of proteins. Each R residue is in opposite directions, located in the two pockets (site1 and site 2) that are involved in phosphoinositide binding. Site 1 is located in blade 5 and is associated with one of the conserved R and the other site is located in blade 6 and is associated with the second R (Baskaran et al., 2012; Krick et al., 2012; Watanabe et al., 2012). In vitro analyses showed that WIPI1 (formerly known as WIPI49) binds preferentially to PtdIns3P and to a lesser extent to PtdIns5P and PtdIns(3, 5)P2 (Jeffries et al., 2004), while yeast Atg18 (Svp1) binds to both PtdIns3P and PI(3, 5)P2 (Dove et al., 2004; Strømhaug et al., 2004).
Site-directed mutagenesis analysis of the interaction between yeast Atg2 and Atg18, combined with yeast-two hybrid (Y2H) and co-immunoprecipitation studies, identified blade 2 and the region between blade 2 and 3 (loop 2) as the regions required for the interaction (Watanabe et al., 2012; Rieter et al., 2013). The interaction between mammalian ATG2 and WIPI4 proteins has been investigated by cross-linking mass spectrometry analysis and several lysine residues in blade 1 and blade 3 were found to cross-link to ATG2. In addition, alanine scanning mutagenesis located additional residues important for the interaction in loop 3 (the region between blade 3 and blade 4) (Zheng et al., 2017). These results suggest that the surface of interaction is extensive, which is consistent with results obtained from studies of the interaction between WIPI3 and the WIR (WIPI-interacting region), an ATG2A peptide that interacts with WIPI3. Structural analyses showed that the WIR peptide wraps around WIPI3 and binds to three sites in blades 1, 2, and 3 (Ren et al., 2020). Recently, a different site located on blade 7 has been proposed to be involved in the interaction between yeast Atg18 and Atg2 (Lei et al., 2021).
The interaction of the WIPI protein Atg21 with Atg16 has been characterized by the crystal structure of the yeast Atg16-Atg21 complex (using K. lactis Atg21 and A. gossypii Atg16). Three consecutive alpha-helical turns in Atg16 form a hydrophobic patch that inserts into a hydrophobic cleft of KIAtg21 between blades 2 and 3 (Munzel et al., 2020).
WIPIs and Disease
Giving the important role of WIPI proteins in autophagy and other membrane-trafficking processes, it is not surprising that they play prominent roles in human health and disease. Indeed, mutations in any of the four WIPI proteins are associated with a variety of diseases affecting the nervous system (Table 1). Among these, mutations in WIPI4 have been extensively characterized concerning β-proppeler associated neurodegeneration (BPAN), a disease also referred to as SENDA (static encephalopathy of childhood with neurodegeneration in adulthood) or NBIA5 (neurodegeneration with brain iron accumulation-5). BPAN is an X-linked neurodegenerative disease characterized by developmental delay in early childhood that later progresses to other symptoms such as dystonia, parkinsonism and psychiatric disorders. BPAN is more common in females than in males, who tend to have more severe forms of the disease. The wide spectrum of disease phenotypes associated with WIPI4 mutations probably results from a combination of different factors, including mutation severity, somatic mosaicism and skewed X-chromosome inactivation in females.
Most of the mutations reported in WIPI4 are truncations, internal deletions and nonsense mutations that profoundly alter the structure of the protein, probably resulting in loss of function. However, some are missense mutations that result in the substitution of a single amino acid (Table 1). These types of mutations can be very informative, as they can point to key residues for the function of specific domains of the protein. This is the case of the BPAN mutations N202K, G205D, A209D, and S210P in WIPI4, which are all located in the PtdIns3P binding domain (Baskaran et al., 2012; Krick et al., 2012; Watanabe et al., 2012), and therefore could potentially alter the correct subcellular localization of the protein (Figure 4). In agreement with this hypothesis, the introduction of three of these mutations in the conserved residues of the WIPI4 homolog in Dictyostelium, Wdr45l, prevents its localization in autophagic structures. In addition, these mutant proteins do not complement the phenotypic defects of the KO mutant. These findings confirm the effect of these mutations on the localization of the protein during autophagy and the relevance of this localization in vivo (Tornero-Écija et al., 2021). Notably, a pathogenic mutation in WIPI2 (V249M) is also located in the PtdIns3P binding domain (Jelani et al., 2019), and is also likely to alter the localization of the protein. In the same line, the pathogenic mutations N61K, D84G, L98P, and F100S are all located in the Atg2 binding region (Figure 4) and the introduction of two of these mutations in the conserved residues of WIPI3 prevent binding to the ATG2A WIR sequence (Ren et al., 2020). Consistent with these findings, the introduction of three of these mutations in Dictyostelium Wdr45l inactivate the protein but do not alter the normal localization of the protein in autophagosomes (Tornero-Écija et al., 2021). Moreover, a pathogenic mutation in WIPI3 (R109Q) is also located in the ATG2 binding region and this mutation has been shown to impair ATG2A WIR binding (Ren et al., 2020). Finally, in addition to mutations in the PtdIns3P and ATG2A binding domains, other pathogenic missense mutations have been identified in WIPI4 (Figure 4). Notably, unlike the mutations described above, these mutations are not associated with BPAN but with other disorders such as developmental and epileptic encephalopathy (DEE) and early onset epileptic encephalopathy (EOEE) (Table 1).
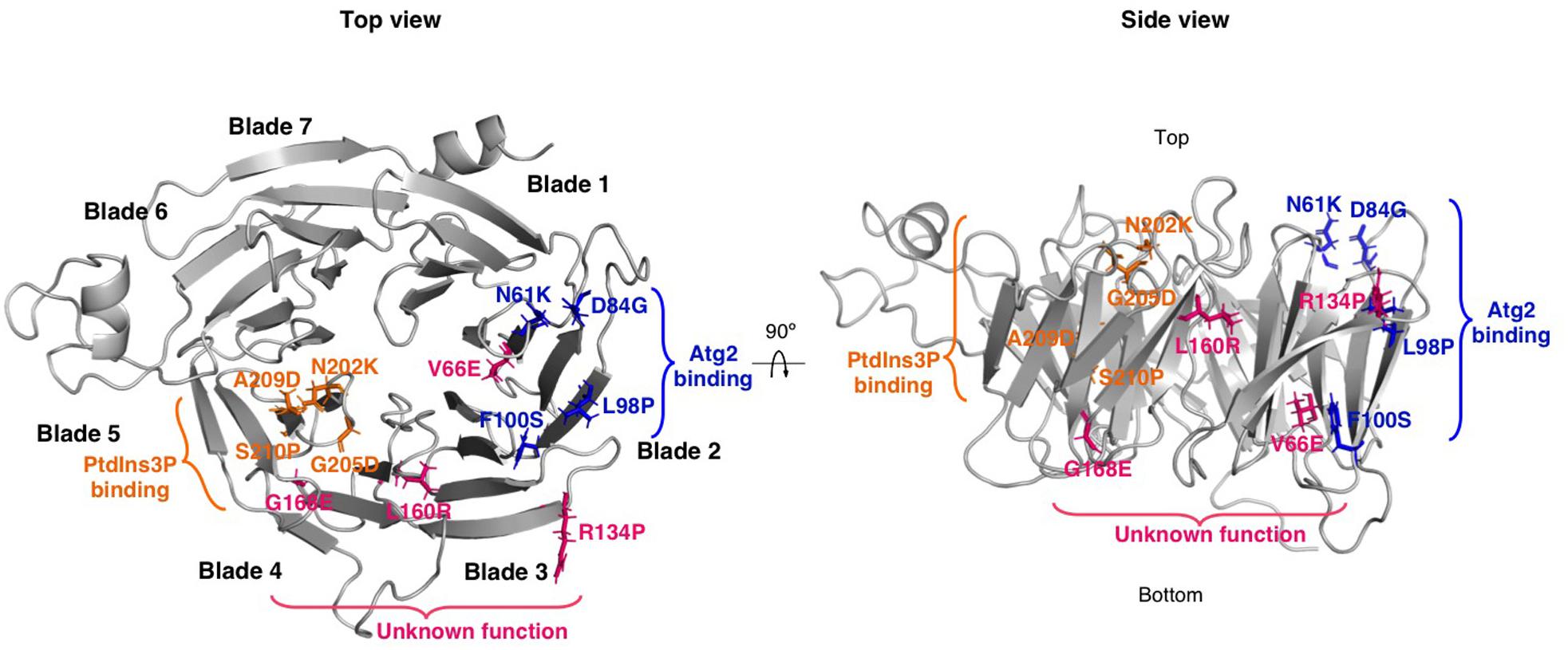
Figure 4. Localization of BPAN missense mutations in the 3D structure of WIPI4. WIPI4 structure was predicted using Robetta (http://robetta.bakerlab.org) based on the structure of WIPI3 (PDB: 6IYY). Top and side views of WIPI4 structure were generated with PyMOL software (The PyMOL Molecular Graphics System, Version 2.4.0 Schrödinger, LLC). Mutated residues localized in the PtdIns3P and ATG2 binding domains are shown as orange and blue sticks, respectively. Other mutated residues are shown as pink sticks.
The reason for the variety of clinical manifestations associated to WIPI4 mutations (see Cong et al., 2021 for a comprehensive review of the clinical symptoms) is not yet clear and could be due to the influence of genetic and environmental factors.
The severity of the phenotype of the Wdr45l KO in Dictyostelium is similar to that observed after expressing Wdr45l with BPAN mutations in the KO strain, suggesting that all these pathogenic mutations greatly affect protein function to the same extent as lack of the protein. This is consistent with the severity of the symptoms of BPAN patients, as no clear difference in severity is observed between missense mutations and those causing truncations or deletions of the protein. One exception is the mutation D84G that has been reported to cause milder symptoms in one patient (Stige et al., 2018). Notably, this mutation, as well as the pathogenic N61K mutation, is located in the ATG2 binding site. However, unlike N61K, D84G leads only to a partial defect in the interaction with ATG2, which could explain the unexpectedly mild phenotype (Bueno-Arribas et al., 2021).
Insights From the Dictyostelium Model in WIPI4-Related Diseases
KO of Wdr45l, the functional homolog of human WIPI4 in Dictyostelium, impairs growth and development in this organism, a phenotype that is remarkably similar to the KO of Vmp1 (Tornero-Écija et al., 2021). Vmp1 is an essential protein in autophagy that is conserved from Dictyostelium to humans but is absent in yeast (Calvo-Garrido et al., 2008). Mammalian VMP1 plays an essential role in organellar communication by regulating the function of membrane contact sites (MCS) between the ER and other organelles, including mitochondria, endosomes, peroxisomes and also the phagophore membrane during elongation (Tábara and Escalante, 2016; Zhao et al., 2017). Consistent with this variety of localizations, Dictyostelium Vmp1 KO exhibits pleiotropic defects affecting organellar morphology, protein sorting, and autophagy (Calvo-Garrido et al., 2008; Calvo-Garrido and Escalante, 2010). It has been recently proposed that the scramblase activity of VMP1 is essential for lipid trafficking between the ER membrane and the phagophore (Ghanbarpour et al., 2021; Li et al., 2021). The current model predicts that the lack of any of the components involved in lipid trafficking, such as the WIPI-ATG2 complex and the VMP1 and ATG9 scramblases, may lead to similar or related phenotypes. This appears to be the case for Dictyostelium Vmp1 and Wdr45l, the absence of which leads to similar phenotypes, including a block in autophagy downstream of Atg8 lipidation and accumulation of PtdIns3P at the autophagosome assembly site. This accumulation appears to be responsible for additional phenotypes, including, interestingly, chronic activation of the endoplasmic reticulum (ER) stress response (also known as UPR, unfolded protein response) (Figure 5). The UPR is a complex pathway triggered by the accumulation of unfolded proteins that regulates many aspects of cellular physiology and is closely related to neurodegenerative diseases (van Ziel and Scheper, 2020). In Dictyostelium, the UPR regulates a gene expression program that inhibits cell growth and global protein synthesis, while activating specific genes encoding proteins that will increase the folding and degradation capacity of cells (Domínguez-Martín et al., 2018a,b). Notably, mutation of the key upstream regulator Atg1 suppresses growth and UPR activation phenotypes by preventing PtdIns3P formation (Tornero-Écija et al., 2021). The possible role of the UPR pathway in BPAN is reinforced by the observation of WIPI4-deficient neurons from a KO mouse showing accumulation of misfolded proteins and activation of the ER-stress response (Wan et al., 2019). If a similar relationship between UPR and abnormal autophagy-associated PtdIns3P occurs in human cells, attenuation of PtdIns3P signaling could be a therapeutic target (Figure 5). The Dictyostelium model also suggests that mutations in any other component of the lipid transport machinery such as VMP1 and ATG2 could also lead to neurological diseases similar to BPAN.
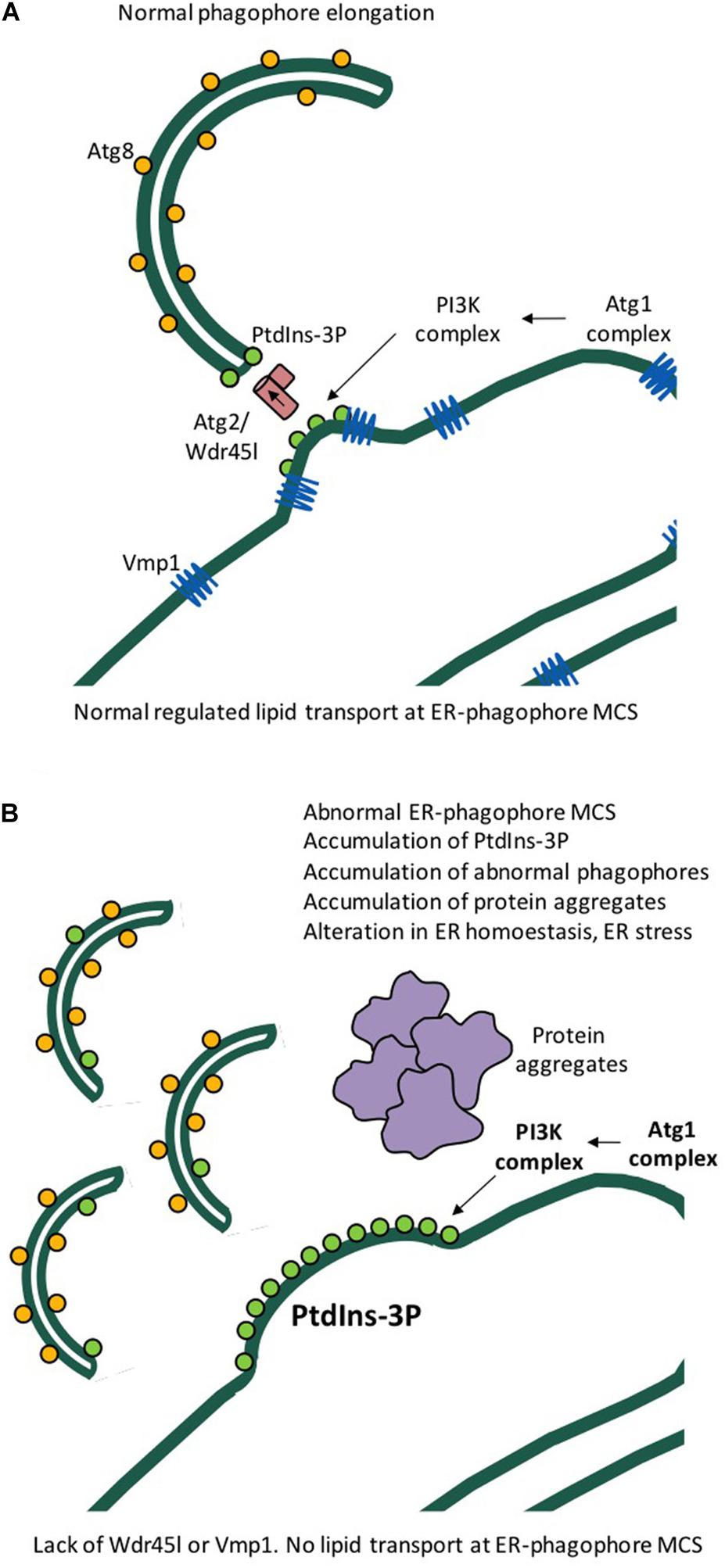
Figure 5. Model of phagophore expansion and the function of Dictyostelium Wdr45l and Vmp1. (A) Lipid transport and phagophore elongation from the ER occur at membrane contact sites (ER-phagophore MCS) and require Atg2, Wdr45l, and Vmp1. (B) Cells deficient in Vmp1 or Wdr45l have similar phenotypes, including the accumulation of PtdIns3P, abnormal autophagic structures, and formation of protein aggregates. Abnormal accumulation of PtdIns3P leads to chronic ER stress and cell growth arrest. These defects are rescued by inhibition of the upstream signaling protein Atg1.
Open Questions and Future Directions
The function of WIPI proteins is complex and not yet clearly established. Although they are phosphatidylinositol effectors that recognize at least PtdIns3P and PtdIns(3, 5)P2, their interaction with membranes is dictated not only by the presence of the signaling lipid. For example, Dictyostelium Atg18 and human WIPI1 are used as autophagic markers because of their localization at autophagic structures, as they seem to preferentially recognize autophagy-associated PtdIns3P despite the abundant presence of PtdIns3P in other cellular locations (Domínguez-Martín et al., 2017; Klionsky et al., 2021). Therefore, it is important to further investigate where and how the different WIPIs localize, and what determinants regulate their localizations. WIPIs interact with many other proteins and it is often unclear whether they act simply to recruit other proteins to particular locations or have a regulatory role as well (Bakula et al., 2017). An example of this second function has been described for WIPI2, which not only recruits but also activates the lipidation machinery (Fracchiolla et al., 2020).
WIPIs appear to have both specific and redundant functions, which further complicates their characterization and the study of their role in disease. Although autophagy appears to play a prominent role in many neurodegenerative diseases, it is unclear whether the cytopathological alterations in WIPI-related diseases are caused by defects in other autophagy-independent processes, such as the ER-stress response pathway. Many basic questions remain to be answered before effective knowledge-based treatments for these incurable diseases are feasible. In this complex scenario, the use of simple models such as yeast and Dictyostelium may lead to new avenues of discovery and understanding the function of WIPI proteins in the cell.
Author Contributions
OV: bibliographic study and manuscript writing. LA-E, MB-A, and AT-É: revision of the manuscript and figure preparation. M-ÁN: revision of manuscript. RE: coordination, bibliographic study, and manuscript writing. All authors contributed to the article and approved the submitted version.
Funding
This work has been supported by the “Ministerio de Ciencia e Innovación,” grant no. PGC2018-093604-B-I00 (MCIU/AEI/FEDER,UE). MB-A has been supported by a fellowship from the Spanish “Ministerio de Ciencia, Innovación y Universidades”.
Conflict of Interest
The authors declare that the research was conducted in the absence of any commercial or financial relationships that could be construed as a potential conflict of interest.
Publisher’s Note
All claims expressed in this article are solely those of the authors and do not necessarily represent those of their affiliated organizations, or those of the publisher, the editors and the reviewers. Any product that may be evaluated in this article, or claim that may be made by its manufacturer, is not guaranteed or endorsed by the publisher.
References
Adang, L. A., Pizzino, A., Malhotra, A., Dubbs, H., Williams, C., Sherbini, O., et al. (2020). Phenotypic and imaging spectrum associated with WDR45. Pediatric Neurol. 109, 56–62. doi: 10.1016/j.pediatrneurol.2020.03.005
Araki, T., and Saito, T. (2019). Small molecules and cell differentiation in Dictyostelium discoideum. Int. J. Dev. Biol. 63, 429–438. doi: 10.1387/ijdb.190192ts
Axe, E. L., Walker, S. A., Manifava, M., Chandra, P., Roderick, H. L., Habermann, A., et al. (2008). Autophagosome formation from membrane compartments enriched in phosphatidylinositol 3-phosphate and dynamically connected to the endoplasmic reticulum. J. Cell Biol. 182, 685–701. doi: 10.1083/jcb.200803137
Bakula, D., Mueller, A. J., and Proikas-Cezanne, T. (2018). WIPI β-propellers function as scaffolds for STK11/LKB1-AMPK and AMPK-related kinase signaling in autophagy. Autophagy 14, 1082–1083. doi: 10.1080/15548627.2017.1382784
Bakula, D., Müller, A. J., Zuleger, T., Takacs, Z., Franz-Wachtel, M., Thost, A.-K., et al. (2017). WIPI3 and WIPI4 β-propellers are scaffolds for LKB1-AMPK-TSC signalling circuits in the control of autophagy. Nat. Commun. 8:15637. doi: 10.1038/ncomms15637
Baskaran, S., Ragusa, M. J., Boura, E., and Hurley, J. H. (2012). Two-site recognition of phosphatidylinositol 3-phosphate by PROPPINs in autophagy. Mol. Cell 47, 339–348. doi: 10.1016/j.molcel.2012.05.027
Batsios, P., Gräf, R., Koonce, M. P., Larochelle, D. A., and Meyer, I. (2019). Nuclear envelope organization in Dictyostelium discoideum. Int. J. Dev. Biol. 63, 509–519. doi: 10.1387/ijdb.190184rg
Bloomfield, G. (2019). Sex and macrocyst formation in Dictyostelium. Int. J. Dev. Biol. 63, 439–446. doi: 10.1387/ijdb.190183gb
Bozzaro, S. (2019). The past, present and future of Dictyostelium as a model system. Int. J. Dev. Biol. 63, 321–331. doi: 10.1387/ijdb.190128sb
Bueno-Arribas, M., Blanca, I., Cruz-Cuevas, C., Escalante, R., Navas, M. A., and Vincent, O. (2021). A conserved ATG2 binding site WIPI4 and yeast Hsv2 is disrupted by mutations causing beta-propeller protein-associated neurodegeneration. Hum. Mol. Genet. doi: 10.1093/hmg/ddab227 Epub ahead of print.
Calvo-Garrido, J., Carilla-Latorre, S., Kubohara, Y., Santos-Rodrigo, N., Mesquita, A., Soldati, T., et al. (2010). Autophagy in Dictyostelium: genes and pathways, cell death and infection. Autophagy 6, 686–701. doi: 10.4161/auto.6.6.12513
Calvo-Garrido, J., Carilla-Latorre, S., Lázaro-Diéguez, F., Egea, G., and Escalante, R. (2008). Vacuole membrane protein 1 is an endoplasmic reticulum protein required for organelle biogenesis, protein secretion, and development. Mol. Biol. Cell 19, 3442–3453. doi: 10.1091/mbc.e08-01-0075
Calvo-Garrido, J., and Escalante, R. (2010). Autophagy dysfunction and ubiquitin-positive protein aggregates in Dictyostelium cells lacking Vmp1. Autophagy 6, 100–109. doi: 10.4161/auto.6.1.10697
Calvo-Garrido, J., King, J. S., Muñoz-Braceras, S., and Escalante, R. (2014). Vmp1 regulates PtdIns3P signaling during autophagosome formation in Dictyostelium discoideum. Traffic 15, 1235–1246. doi: 10.1111/tra.12210
Carvill, G. L., Liu, A., Mandelstam, S., Schneider, A., Lacroix, A., Zemel, M., et al. (2018). Severe infantile onset developmental and epileptic encephalopathy caused by mutations in autophagy gene WDR45. Epilepsia 59, e5–e13. doi: 10.1111/epi.13957
Chard, M., Appendino, J. P., Bello-Espinosa, L. E., Curtis, C., Rho, J. M., Wei, X.-C., et al. (2019). Single-center experience with Beta-propeller protein-associated neurodegeneration (BPAN); expanding the phenotypic spectrum. Mol. Genet. Metab. Rep. 20:100483. doi: 10.1016/j.ymgmr.2019.100483
Chen, H., Qian, Y., Yu, S., Xiao, D., Guo, X., Wang, Q., et al. (2019). Early onset developmental delay and epilepsy in pediatric patients with WDR45 variants. Eur. J. Med. Genet. 62, 149–160. doi: 10.1016/j.ejmg.2018.07.002
Chowdhury, S., Otomo, C., Leitner, A., Ohashi, K., Aebersold, R., Lander, G. C., et al. (2018). Insights into autophagosome biogenesis from structural and biochemical analyses of the ATG2A-WIPI4 complex. Proc. Natl. Acad. Sci. U.S.A. 115, E9792–E9801. doi: 10.1073/pnas.1811874115
Cocorocchio, M., Baldwin, A. J., Stewart, B., Kim, L., Harwood, A. J., Thompson, C. R. L., et al. (2017). Curcumin and derivatives function through protein phosphatase 2A and presenilin orthologues in Dictyostelium discoideum. Dis. Model. Mech. 11:dmm032375. doi: 10.1242/dmm.032375
Cong, Y., So, V., Tijssen, M. A. J., Verbeek, D. S., Reggiori, F., and Mauthe, M. (2021). WDR45, one gene associated with multiple neurodevelopmental disorders. Autophagy 1–16. doi: 10.1080/15548627.2021.1899669 Epub ahead of print.
Consalvo, K. M., Rijal, R., Tang, Y., Kirolos, S. A., Smith, M. R., and Gomer, R. H. (2019). Extracellular signaling in Dictyostelium. Int. J. Dev. Biol. 63, 395–405. doi: 10.1387/ijdb.190259rg
De Leo, M. G., Berger, P., and Mayer, A. (2021). WIPI1 promotes fission of endosomal transport carriers and formation of autophagosomes through distinct mechanisms. Autophagy 1–27. doi: 10.1080/15548627.2021.1886830 Epub ahead of print.
Domínguez-Martín, E., Cardenal-Muñoz, E., King, J., Soldati, T., Coria, R., and Escalante, R. (2017). Methods to monitor and quantify autophagy in the social amoeba Dictyostelium discoideum. Cells 6:18. doi: 10.3390/cells6030018
Domínguez-Martín, E., Hernández-Elvira, M., Vincent, O., Coria, R., and Escalante, R. (2018a). Unfolding the endoplasmic reticulum of a social amoeba: dictyostelium discoideum as a new model for the study of endoplasmic reticulum stress. Cells 7:56. doi: 10.3390/cells7060056
Domínguez-Martín, E., Ongay-Larios, L., Kawasaki, L., Vincent, O., Coello, G., Coria, R., et al. (2018b). IreA controls endoplasmic reticulum stress-induced autophagy and survival through homeostasis recovery. Mol. Cell Biol. 38, e00054–18. doi: 10.1128/MCB.00054-18
Dooley, H. C., Razi, M., Polson, H. E. J., Girardin, S. E., Wilson, M. I., and Tooze, S. A. (2014). WIPI2 Links LC3 conjugation with PI3P, Autophagosome formation, and pathogen clearance by recruiting Atg12–5-16L1. Mol. Cell 55, 238–252. doi: 10.1016/j.molcel.2014.05.021
Dove, S. K., Piper, R. C., McEwen, R. K., Yu, J. W., King, M. C., Hughes, D. C., et al. (2004). Svp1p defines a family of phosphatidylinositol 3,5-bisphosphate effectors. EMBO J 23, 1922–1933. doi: 10.1038/sj.emboj.7600203
Escalante, R., and Cardenal-Muñoz, E. (2019). The Dictyostelium discoideum model system. Int. J. Dev. Biol. 63, 317–320. doi: 10.1387/ijdb.190275re
Farinholt, T., Dinh, C., and Kuspa, A. (2019). Microbiome management in the social amoeba Dictyostelium discoideum compared to humans. Int. J. Dev. Biol. 63, 447–450. doi: 10.1387/ijdb.190240ak
Fey, P., Dodson, R. J., Basu, S., Hartline, E. C., and Chisholm, R. L. (2019). dictyBase and the dicty stock center (version 2.0) - a progress report. Int. J. Dev. Biol. 63, 563–572. doi: 10.1387/ijdb.190226pf
Fischer, S., and Eichinger, L. (2019). Dictyostelium discoideum and autophagy – a perfect pair. Int. J. Dev. Biol. 63, 485–495. doi: 10.1387/ijdb.190186LE
Fischer, S., Rijal, R., Frommolt, P., Wagle, P., Konertz, R., Faix, J., et al. (2019). Functional characterization of Ubiquitin-like core autophagy protein ATG12 in Dictyostelium discoideum. Cells 8:72. doi: 10.3390/cells8010072
Fracchiolla, D., Chang, C., Hurley, J. H., and Martens, S. (2020). A PI3K-WIPI2 positive feedback loop allosterically activates LC3 lipidation in autophagy. J. Cell Biol. 219:e201912098. doi: 10.1083/jcb.201912098
Geng, J., and Klionsky, D. J. (2008). The Atg8 and Atg12 ubiquitin-like conjugation systems in macroautophagy. EMBO Rep. 9, 859–864. doi: 10.1038/embor.2008.163
Ghanbarpour, A., Valverde, D. P., Melia, T. J., and Reinisch, K. M. (2021). A model for a partnership of lipid transfer proteins and scramblases in membrane expansion and organelle biogenesis. Proc. Natl. Acad. Sci. U.S.A. 118:e2101562118. doi: 10.1073/pnas.2101562118
Gómez-Sánchez, R., Rose, J., Guimarães, R., Mari, M., Papinski, D., Rieter, E., et al. (2018). Atg9 establishes Atg2-dependent contact sites between the endoplasmic reticulum and phagophores. J. Cell Biol. 217, 2743–2763. doi: 10.1083/jcb.201710116
Gubas, A., and Dikic, I. (2021). A guide to the regulation of selective autophagy receptors. FEBS J. doi: 10.1111/febs.15824 [Epub ahead of print].
Haack, T. B., Hogarth, P., Kruer, M. C., Gregory, A., Wieland, T., Schwarzmayr, T., et al. (2012). Exome sequencing reveals De Novo WDR45 mutations causing a phenotypically distinct, X-Linked Dominant Form of NBIA. Am. J. Hum. Genet. 91, 1144–1149. doi: 10.1016/j.ajhg.2012.10.019
Hindupur, S. K., González, A., and Hall, M. N. (2015). The opposing actions of target of rapamycin and AMP-Activated protein kinase in cell growth control. Cold Spring Harb. Perspect. Biol. 7:a019141. doi: 10.1101/cshperspect.a019141
Hurley, J. H., and Young, L. N. (2017). Mechanisms of autophagy initiation. Annu. Rev. Biochem. 86, 225–244. doi: 10.1146/annurev-biochem-061516-044820
Ishikawa-Ankerhold, H. C., and Müller-Taubenberger, A. (2019). Actin assembly states in Dictyostelium discoideum at different stages of development and during cellular stress. Int. J. Dev. Biol. 63, 417–427. doi: 10.1387/ijdb.190256am
Jaiswal, P., Majithia, A. R., Rosel, D., Liao, X.-H., Khurana, T., and Kimmel, A. R. (2019). Integrated actions of mTOR complexes 1 and 2 for growth and development of Dictyostelium. Int. J. Dev. Biol. 63, 521–527. doi: 10.1387/ijdb.190245ak
Jeffries, T. R., Dove, S. K., Michell, R. H., and Parker, P. J. (2004). PtdIns-specific MPR pathway association of a novel WD40 repeat protein. WIPI49. MBoC 15, 2652–2663. doi: 10.1091/mbc.e03-10-0732
Jelani, M., Dooley, H. C., Gubas, A., Mohamoud, H. S. A., Khan, M. T. M., Ali, Z., et al. (2019). A mutation in the major autophagy gene, WIPI2, associated with global developmental abnormalities. Brain 142, 1242–1254. doi: 10.1093/brain/awz075
Ji, C., Zhao, H., Chen, D., Zhang, H., and Zhao, Y. G. (2021). β-propeller proteins WDR45 and WDR45B regulate autophagosome maturation into autolysosomes in neural cells. Curr. Biol. 31, 1666–1677. doi: 10.1016/j.cub.2021.01.081
Ji, C., Zhao, H., Li, D., Sun, H., Hao, J., Chen, R., et al. (2020). Role of Wdr45b in maintaining neural autophagy and cognitive function. Autophagy 16, 615–625. doi: 10.1080/15548627.2019.1632621
Juris, L., Montino, M., Rube, P., Schlotterhose, P., Thumm, M., and Krick, R. (2015). PI3P binding by Atg21 organises Atg8 lipidation. EMBO J. 34, 955–973. doi: 10.15252/embj.201488957
Karanasios, E., Walker, S. A., Okkenhaug, H., Manifava, M., Hummel, E., Zimmermann, H., et al. (2016). Autophagy initiation by ULK complex assembly on ER tubulovesicular regions marked by ATG9 vesicles. Nat. Commun. 7:12420. doi: 10.1038/ncomms12420
Karow, M., Fischer, S., Meßling, S., Konertz, R., Riehl, J., Xiong, Q., et al. (2020). Functional characterisation of the autophagy ATG12~5/16 complex in Dictyostelium discoideum. Cells 9:1179. doi: 10.3390/cells9051179
Kawabe, Y., Du, Q., Schilde, C., and Schaap, P. (2019). Evolution of multicellularity in Dictyostelia. Int. J. Dev. Biol. 63, 359–369. doi: 10.1387/ijdb.190108ps
Kay, R. R., Williams, T. D., Manton, J. D., Traynor, D., and Paschke, P. (2019). Living on soup: macropinocytic feeding in amoebae. Int. J. Dev. Biol. 63, 473–483. doi: 10.1387/ijdb.190220rk
Khoury, J., Kotagal, P., and Moosa, A. N. V. (2019). Epileptic encephalopathy and brain iron accumulation due to WDR45 mutation. Seizure 71, 245–246. doi: 10.1016/j.seizure.2019.08.002
Klionsky, D. J., Abdel-Aziz, A. K., Abdelfatah, S., Abdellatif, M., Abdoli, A., Abel, S., et al. (2021). Guidelines for the use and interpretation of assays for monitoring autophagy (4th edition) 1. Autophagy 17, 1–382. doi: 10.1080/15548627.2020.1797280
Knecht, D. A., Cooper, K. M., and Moore, J. E. (2019). Teaching a biology laboratory course using Dictyostelium. Int. J. Dev. Biol. 63, 551–561. doi: 10.1387/ijdb.190249dk
Kotani, T., Kirisako, H., Koizumi, M., Ohsumi, Y., and Nakatogawa, H. (2018). The Atg2-Atg18 complex tethers pre-autophagosomal membranes to the endoplasmic reticulum for autophagosome formation. Proc. Natl. Acad. Sci. U.S.A. 115, 10363–10368. doi: 10.1073/pnas.1806727115
Krick, R., Busse, R. A., Scacioc, A., Stephan, M., Janshoff, A., Thumm, M., et al. (2012). Structural and functional characterization of the two phosphoinositide binding sites of PROPPINs, a β-propeller protein family. Proc. Natl. Acad. Sci. U.S.A. 109, E2042–E2049. doi: 10.1073/pnas.1205128109
Krick, R., Henke, S., Tolstrup, J., and Thumm, M. (2008). Dissecting the localization and function of Atg18, Atg21 and Ygr223c. Autophagy 4, 896–910. doi: 10.4161/auto.6801
Krick, R., and Thumm, M. (2016). Atg8 lipidation is coordinated in a PtdIns3P-dependent manner by the PROPPIN Atg21. Autophagy 12, 2260–2261. doi: 10.1080/15548627.2016.1221564
Kundert, P., and Shaulsky, G. (2019). Cellular allorecognition and its roles in Dictyostelium development and social evolution. Int. J. Dev. Biol. 63, 383–393. doi: 10.1387/ijdb.190239gs
Kuspa, A., and Shaulsky, G. (2019). (Auto)Biographical reflections on the contributions of William F. Loomis (1940-2016) to Dictyostelium biology. Int. J. Dev. Biol. 63, 343–357. doi: 10.1387/ijdb.190224ak
Lahiri, V., Hawkins, W. D., and Klionsky, D. J. (2019). Watch what you (Self-) Eat: autophagic mechanisms that modulate metabolism. Cell Metab. 29, 803–826. doi: 10.1016/j.cmet.2019.03.003
Lei, Y., Tang, D., Liao, G., Xu, L., Liu, S., Chen, Q., et al. (2021). The crystal structure of Atg18 reveals a new binding site for Atg2 in Saccharomyces cerevisiae. Cell. Mol. Life Sci. 78, 2131–2143. doi: 10.1007/s00018-020-03621-9
Li, Y. E., Wang, Y., Du, X., Zhang, T., Mak, H. Y., Hancock, S. E., et al. (2021). TMEM41B and VMP1 are scramblases and regulate the distribution of cholesterol and phosphatidylserine. J. Cell Biol. 220:e202103105. doi: 10.1083/jcb.202103105
Long, M., Abdeen, N., Geraghty, M. T., Hogarth, P., Hayflick, S., and Venkateswaran, S. (2015). Novel WDR45 mutation and pathognomonic BPAN imaging in a young female with mild cognitive delay. Pediatrics 136, e714–e717. doi: 10.1542/peds.2015-0750
Lőrincz, P., and Juhász, G. (2019). Autophagosome-Lysosome fusion. J. Mol. Biol. 432, 2462–2482. doi: 10.1016/j.jmb.2019.10.028
Lystad, A. H., Carlsson, S. R., de la Ballina, L. R., Kauffman, K. J., Nag, S., Yoshimori, T., et al. (2019). Distinct functions of ATG16L1 isoforms in membrane binding and LC3B lipidation in autophagy-related processes. Nat. Cell Biol. 21, 372–383. doi: 10.1038/s41556-019-0274-9
Maeda, S., Otomo, C., and Otomo, T. (2019). The autophagic membrane tether ATG2A transfers lipids between membranes. Elife 8:e45777. doi: 10.7554/eLife.45777
Matoba, K., Kotani, T., Tsutsumi, A., Tsuji, T., Mori, T., Noshiro, D., et al. (2020). Atg9 is a lipid scramblase that mediates autophagosomal membrane expansion. Nat. Struct. Mol. Biol. 27, 1185–1193. doi: 10.1038/s41594-020-00518-w
Matsuura, A., Tsukada, M., Wada, Y., and Ohsumi, Y. (1997). Apg1p, a novel protein kinase required for the autophagic process in Saccharomyces cerevisiae. Gene 192, 245–250. doi: 10.1016/S0378-1119(97)00084-X
Medina, J. M., Shreenidhi, P. M., Larsen, T. J., Queller, D. C., and Strassmann, J. E. (2019). Cooperation and conflict in the social amoeba Dictyostelium discoideum. Int. J. Dev. Biol. 63, 371–382. doi: 10.1387/ijdb.190158jm
Melia, T. J., Lystad, A. H., and Simonsen, A. (2020). Autophagosome biogenesis: from membrane growth to closure. J. Cell Biol. 219:e202002085. doi: 10.1083/jcb.202002085
Mesquita, A., Cardenal-Muñoz, E., Dominguez, E., Muñoz-Braceras, S., Nuñez-Corcuera, B., Phillips, B. A., et al. (2017). Autophagy in Dictyostelium?: mechanisms, regulation and disease in a simple biomedical model. Autophagy 13, 24–40. doi: 10.1080/15548627.2016.1226737
Mesquita, A., Tábara, L. C., Martinez-Costa, O., Santos-Rodrigo, N., Vincent, O., and Escalante, R. (2015). Dissecting the function of Atg1 complex in Dictyostelium autophagy reveals a connection with the pentose phosphate pathway enzyme transketolase. Open Biol. 5:150088. doi: 10.1098/rsob.150088
Mizushima, N., and Levine, B. (2020). Autophagy in human diseases. N. Engl. J. Med. 383, 1564–1576. doi: 10.1056/NEJMra2022774
Muñoz-Braceras, S., Calvo, R., and Escalante, R. (2015). TipC and the chorea-acanthocytosis protein VPS13A regulate autophagy in Dictyostelium and human HeLa cells. Autophagy 11, 918–927. doi: 10.1080/15548627.2015.1034413
Munzel, L., Neumann, P., Otto, F. B., Krick, R., Metje-Sprink, J., Kroppen, B., et al. (2020). Atg21 organizes Atg8 lipidation at the contact of the vacuole with the phagophore. Autophagy 17, 1458–1478. doi: 10.1080/15548627.2020.1766332
Najmabadi, H., Hu, H., Garshasbi, M., Zemojtel, T., Abedini, S. S., Chen, W., et al. (2011). Deep sequencing reveals 50 novel genes for recessive cognitive disorders. Nature 478, 57–63. doi: 10.1038/nature10423
Nanjundiah, V. (2019). Individual and collective behaviour in cellular slime mould development: contributions of John Bonner (1920-2019). Int. J. Dev. Biol. 63, 333–342. doi: 10.1387/ijdb.190272vn
Nishimura, T., Tamura, N., Kono, N., Shimanaka, Y., Arai, H., Yamamoto, H., et al. (2017). Autophagosome formation is initiated at phosphatidylinositol synthase-enriched ER subdomains. EMBO J. 36, 1719–1735. doi: 10.15252/embj.201695189
Nishioka, K., Oyama, G., Yoshino, H., Li, Y., Matsushima, T., Takeuchi, C., et al. (2015). High frequency of beta-propeller protein-associated neurodegeneration (BPAN) among patients with intellectual disability and young-onset parkinsonism. Neurobiol. Aging 36, 2004.e9–e2004.e15. doi: 10.1016/j.neurobiolaging.2015.01.020
Obara, K., Sekito, T., Niimi, K., and Ohsumi, Y. (2008). The Atg18-Atg2 complex is recruited to autophagic membranes via phosphatidylinositol 3-phosphate and exerts an essential function. J. Biol. Chem. 283, 23972–23980. doi: 10.1074/jbc.M803180200
Osawa, T., Ishii, Y., and Noda, N. N. (2020). Human ATG2B possesses a lipid transfer activity which is accelerated by negatively charged lipids and WIPI4. Genes Cells 25, 65–70. doi: 10.1111/gtc.12733
Osawa, T., Kotani, T., Kawaoka, T., Hirata, E., Suzuki, K., Nakatogawa, H., et al. (2019). Atg2 mediates direct lipid transfer between membranes for autophagosome formation. Nat. Struct. Mol. Biol. 26, 281–288. doi: 10.1038/s41594-019-0203-4
Otto, G. P., Wu, M. Y., Kazgan, N., Anderson, O. R., and Kessin, R. H. (2003). Macroautophagy is required for multicellular development of the social amoeba Dictyostelium discoideum. J. Biol. Chem. 278, 17636–17645. doi: 10.1074/jbc.M212467200
Otto, G. P., Wu, M. Y., Kazgan, N., Anderson, O. R., and Kessin, R. H. (2004). Dictyostelium macroautophagy mutants vary in the severity of their developmental defects. J. Biol. Chem. 279, 15621–15629. doi: 10.1074/jbc.M311139200
Pal, D. S., Li, X., Banerjee, T., Miao, Y., and Devreotes, P. N. (2019). The excitable signal transduction networks: movers and shapers of eukaryotic cell migration. Int. J. Dev. Biol. 63, 407–416. doi: 10.1387/ijdb.190265pd
Papinski, D., Schuschnig, M., Reiter, W., Wilhelm, L., Barnes, C. A., Maiolica, A., et al. (2014). Early steps in autophagy depend on direct phosphorylation of Atg9 by the Atg1 Kinase. Mol. Cell 53, 471–483. doi: 10.1016/j.molcel.2013.12.011
Pearce, X. G., Annesley, S. J., and Fisher, P. R. (2019). The Dictyostelium model for mitochondrial biology and disease. Int. J. Dev. Biol. 63, 497–508. doi: 10.1387/ijdb.190233pf
Pergolizzi, B., Bozzaro, S., and Bracco, E. (2019). Dictyostelium as model for studying ubiquitination and deubiquitination. Int. J. Dev. Biol. 63, 529–539. doi: 10.1387/ijdb.190260eb
Perry, C. J., Finch, P., Müller-Taubenberger, A., Leung, K., Warren, E. C., Damstra-Oddy, J., et al. (2020). A new mechanism for cannabidiol in regulating the one-carbon cycle and methionine levels in DICTYOSTELIUM and in mammalian epilepsy models. Br. J. Pharmacol. 177, 912–928. doi: 10.1111/bph.14892
Polson, H. E. J., de Lartigue, J., Rigden, D. J., Reedijk, M., Urbé, S., Clague, M. J., et al. (2010). Mammalian Atg18 (WIPI2) localizes to omegasome-anchored phagophores and positively regulates LC3 lipidation. Autophagy 6, 506–522. doi: 10.4161/auto.6.4.11863
Proikas-Cezanne, T., Takacs, Z., Donnes, P., and Kohlbacher, O. (2015). WIPI proteins: essential PtdIns3P effectors at the nascent autophagosome. J. Cell Sci. 128, 207–217. doi: 10.1242/jcs.146258
Raper, K. B. (1935). Dictyostelium discoideum, a new species of slime mold from decaying forest leaves. J. Agric. Res. 50, 135–147.
Ren, J., Liang, R., Wang, W., Zhang, D., Yu, L., and Feng, W. (2020). Multi-site-mediated entwining of the linear WIR-motif around WIPI β-propellers for autophagy. Nat. Commun. 11:2702. doi: 10.1038/s41467-020-16523-y
Rieter, E., Vinke, F., Bakula, D., Cebollero, E., Ungermann, C., Proikas-Cezanne, T., et al. (2013). Atg18 function in autophagy is regulated by specific sites within its β-propeller. J. Cell. Sci. 126, 593–604. doi: 10.1242/jcs.115725
Russo, C., Ardissone, A., Freri, E., Gasperini, S., Moscatelli, M., Zorzi, G., et al. (2019). Substantia Nigra swelling and dentate nucleus T2 hyperintensity may be early magnetic resonance imaging signs of β-propeller protein-associated neurodegeneration: early MRI Features in 4 Cases of BPAN. Mov. Disord. Clin. Pract. 6, 51–56. doi: 10.1002/mdc3.12693
Sawa-Makarska, J., Baumann, V., Coudevylle, N., von Bülow, S., Nogellova, V., Abert, C., et al. (2020). Reconstitution of autophagosome nucleation defines Atg9 vesicles as seeds for membrane formation. Science 369:eaaz7714. doi: 10.1126/science.aaz7714
Schaf, J., Damstra-Oddy, J., and Williams, R. S. B. (2019). Dictyostelium discoideum as a pharmacological model system to study the mechanisms of medicinal drugs and natural products. Int. J. Dev. Biol. 63, 541–550. doi: 10.1387/ijdb.190228rw
Scott, S. V., Hefner-Gravink, A., Morano, K. A., Noda, T., Ohsumi, Y., and Klionsky, D. J. (1996). Cytoplasm-to-vacuole targeting and autophagy employ the same machinery to deliver proteins to the yeast vacuole. Proc. Natl. Acad. Sci. U.S.A. 93, 12304–12308. doi: 10.1073/pnas.93.22.12304
Sharma, D., Otto, G., Warren, E. C., Beesley, P., King, J. S., and Williams, R. S. B. (2019). Gamma secretase orthologs are required for lysosomal activity and autophagic degradation in Dictyostelium discoideum, independent of PSEN (presenilin) proteolytic function. Autophagy 15, 1407–1418. doi: 10.1080/15548627.2019.1586245
Slobodkin, M. R., and Elazar, Z. (2013). The Atg8 family: multifunctional ubiquitin-like key regulators of autophagy. Essays Biochem. 55, 51–64. doi: 10.1042/bse0550051
Stige, K. E., Gjerde, I. O., Houge, G., Knappskog, P. M., and Tzoulis, C. (2018). Beta-propeller protein-associated neurodegeneration: a case report and review of the literature. Clin. Case Rep. 6, 353–362. doi: 10.1002/ccr3.1358
Strømhaug, P. E., Reggiori, F., Guan, J., Wang, C.-W., and Klionsky, D. J. (2004). Atg21 is a phosphoinositide binding protein required for efficient lipidation and localization of Atg8 during uptake of aminopeptidase I by selective autophagy. Mol. Biol. Cell 15, 3553–3566. doi: 10.1091/mbc.e04-02-0147
Tábara, L.-C., and Escalante, R. (2016). VMP1 establishes ER-Microdomains that regulate membrane contact sites and autophagy. PLoS One 11:e0166499. doi: 10.1371/journal.pone.0166499
Tornero-Écija, A., Tábara, L., Bueno-Arribas, M., Antón-Esteban, L., Navarro-Gómez, C., Sánchez, I., et al. (2021). A Dictyostelium model for BPAN disease reveals a functional relationship between the WDR45/WIPI4 homolog Wdr45l and Vmp1 in the regulation of autophagy-associated PtdIns3P and ER stress. Autophagy doi: 10.1080/15548627.2021.1953262 Epub ahead of print.
Tschentscher, A., Dekomien, G., Ross, S., Cremer, K., Kukuk, G. M., Epplen, J. T., et al. (2015). Analysis of the C19orf12 and WDR45 genes in patients with neurodegeneration with brain iron accumulation. J. Neurol. Sci. 349, 105–109. doi: 10.1016/j.jns.2014.12.036
Tsukada, M., and Ohsumi, Y. (1993). Isolation and characterization of autophagy-defective mutants of Saccharomyces cerevisiae. FEBS Lett. 333, 169–174. doi: 10.1016/0014-5793(93)80398-E
Tung, S. M., Unal, C., Ley, A., Peña, C., Tunggal, B., Noegel, A. A., et al. (2010). Loss of Dictyostelium ATG9 results in a pleiotropic phenotype affecting growth, development, phagocytosis and clearance and replication of Legionella pneumophila. Cell. Microbiol. 12, 765–780. doi: 10.1111/j.1462-5822.2010.01432.x
Valverde, D. P., Yu, S., Boggavarapu, V., Kumar, N., Lees, J. A., Walz, T., et al. (2019). ATG2 transports lipids to promote autophagosome biogenesis. J. Cell Biol. 218, 1787–1798. doi: 10.1083/jcb.201811139
van Ziel, A. M., and Scheper, W. (2020). The UPR in neurodegenerative disease: not just an inside job. Biomolecules 10:1090. doi: 10.3390/biom10081090
Vines, J. H., and King, J. S. (2019). The endocytic pathways of Dictyostelium discoideum. Int. J. Dev. Biol. 63, 461–471. doi: 10.1387/ijdb.190236jk
Wan, H., Wang, Q., Chen, X., Zeng, Q., Shao, Y., Fang, H., et al. (2019). WDR45 contributes to neurodegeneration through regulation of ER homeostasis and neuronal death. Autophagy 16, 531–547. doi: 10.1080/15548627.2019.1630224
Wang, L., Ren, A., Tian, T., Li, N., Cao, X., Zhang, P., et al. (2019). Whole-exome sequencing identifies damaging de novo variants in anencephalic cases. Front. Neurosci. 13:1285. doi: 10.3389/fnins.2019.01285
Warren, E. C., Dooves, S., Lugarà, E., Damstra-Oddy, J., Schaf, J., Heine, V. M., et al. (2020). Decanoic acid inhibits mTORC1 activity independent of glucose and insulin signaling. Proc. Natl. Acad. Sci. U.S.A. 117, 23617–23625. doi: 10.1073/pnas.2008980117
Watanabe, Y., Kobayashi, T., Yamamoto, H., Hoshida, H., Akada, R., Inagaki, F., et al. (2012). Structure-based analyses reveal distinct binding sites for Atg2 and phosphoinositides in Atg18. J. Biol. Chem. 287, 31681–31690. doi: 10.1074/jbc.M112.397570
Xiong, Q., Li, W., Li, P., Yang, M., Wu, C., and Eichinger, L. (2018). The role of ATG16 in autophagy and the ubiquitin proteasome system. Cells 8:2. doi: 10.3390/cells8010002
Xiong, Q., Ünal, C., Matthias, J., Steinert, M., and Eichinger, L. (2015). The phenotypes of ATG9, ATG16 and ATG9/16 knock-out mutants imply autophagy-dependent and -independent functions. Open Biol. 5:150008. doi: 10.1098/rsob.150008
Yamada, Y., and Schaap, P. (2019). Cyclic AMP induction of Dictyostelium prespore gene expression requires autophagy. Dev. Biol. 452, 114–126. doi: 10.1016/j.ydbio.2019.04.017
Yamada, Y., and Schaap, P. (2020). The proppin Bcas3 and its interactor KinkyA localize to the early phagophore and regulate autophagy. Autophagy 17, 640–655. doi: 10.1080/15548627.2020.1725403
Yamaguchi, H., Honda, S., Torii, S., Shimizu, K., Katoh, K., Miyake, K., et al. (2020). Wipi3 is essential for alternative autophagy and its loss causes neurodegeneration. Nat. Commun. 11:5311. doi: 10.1038/s41467-020-18892-w
Zhao, Y. G., Chen, Y., Miao, G., Zhao, H., Qu, W., Li, D., et al. (2017). The ER-Localized Transmembrane protein EPG-3/VMP1 regulates SERCA activity to control ER-Isolation membrane contacts for autophagosome formation. Mol. Cell 67, 974–989.e6. doi: 10.1016/j.molcel.2017.08.005
Zhao, Y. G., Liu, N., Miao, G., Chen, Y., Zhao, H., and Zhang, H. (2018). The ER contact proteins VAPA/B interact with multiple autophagy proteins to modulate autophagosome biogenesis. Curr. Biol. 28, 1234.e–1245.e. doi: 10.1016/j.cub.2018.03.002
Keywords: autophagy, BPAN, Dictyostelium discoideum, Saccharomyces cerevisiae, PROPPIN, Vmp1, WDR45, WIPI
Citation: Vincent O, Antón-Esteban L, Bueno-Arribas M, Tornero-Écija A, Navas M-Á and Escalante R (2021) The WIPI Gene Family and Neurodegenerative Diseases: Insights From Yeast and Dictyostelium Models. Front. Cell Dev. Biol. 9:737071. doi: 10.3389/fcell.2021.737071
Received: 06 July 2021; Accepted: 12 August 2021;
Published: 01 September 2021.
Edited by:
Annette Müller-Taubenberger, Ludwig Maximilian University of Munich, GermanyReviewed by:
Ludwig Eichinger, University of Cologne, GermanyMatt Scaglione, Duke University, United States
Copyright © 2021 Vincent, Antón-Esteban, Bueno-Arribas, Tornero-Écija, Navas and Escalante. This is an open-access article distributed under the terms of the Creative Commons Attribution License (CC BY). The use, distribution or reproduction in other forums is permitted, provided the original author(s) and the copyright owner(s) are credited and that the original publication in this journal is cited, in accordance with accepted academic practice. No use, distribution or reproduction is permitted which does not comply with these terms.
*Correspondence: Ricardo Escalante, ci5lc2NhbGFudGVAY3NpYy5lcw==