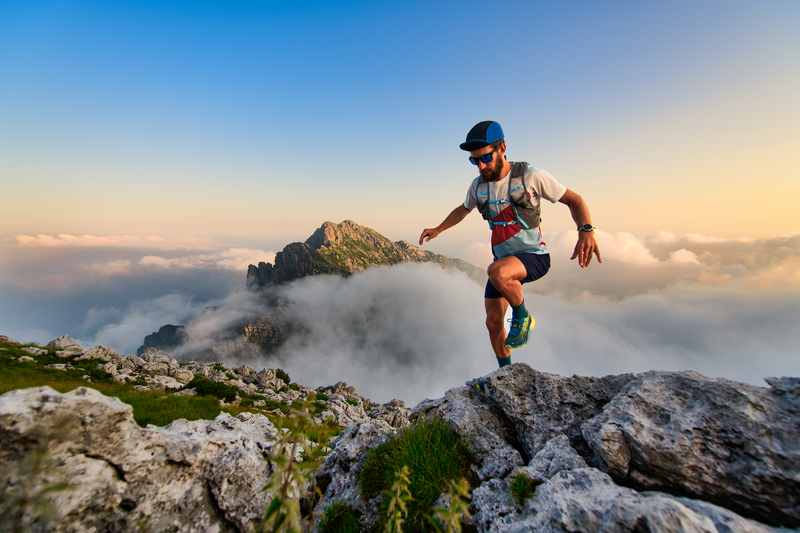
94% of researchers rate our articles as excellent or good
Learn more about the work of our research integrity team to safeguard the quality of each article we publish.
Find out more
REVIEW article
Front. Cell Dev. Biol. , 06 September 2021
Sec. Molecular and Cellular Oncology
Volume 9 - 2021 | https://doi.org/10.3389/fcell.2021.728759
This article is part of the Research Topic Metabolite and Nutrient Transporters in Cancer-Cell Metabolism: Role in Cancer Progression and Metastasis View all 12 articles
Breast cancer is the most common malignancy in women worldwide and is associated with high mortality rates despite the continuously advancing treatment strategies. Glucose is essential for cancer cell metabolism owing to the Warburg effect. During the process of glucose metabolism, various glycolytic metabolites, such as serine and glycine metabolites, are produced and other metabolic pathways, such as the pentose phosphate pathway (PPP), are associated with the process. Glucose is transported into the cell by glucose transporters, such as GLUT. Breast cancer shows high expressions of glucose metabolism-related enzymes and GLUT, which are also related to breast cancer prognosis. Triple negative breast cancer (TNBC), which is a high-grade breast cancer, is especially dependent on glucose metabolism. Breast cancer also harbors various stromal cells such as cancer-associated fibroblasts and immune cells as tumor microenvironment, and there exists a metabolic interaction between these stromal cells and breast cancer cells as explained by the reverse Warburg effect. Breast cancer is heterogeneous, and, consequently, its metabolic status is also diverse, which is especially affected by the molecular subtype, progression stage, and metastatic site. In this review, we will focus on glucose metabolism and glucose transporters in breast cancer, and we will additionally discuss their potential applications as cancer imaging tracers and treatment targets.
Breast cancer is the most common malignancy in women worldwide, and ranks top in the cause of death in female cancers worldwide (Bray et al., 2018). A total of 2.1 million women were newly diagnosed with breast cancer in 2018, and 627,000 women died of breast cancer (Bray et al., 2018). Breast cancer is increasing in underdeveloped and developing countries, and it is decreasing in developed countries since the early 2000s (Rossouw et al., 2002; Bray et al., 2004; DeSantis et al., 2015). Breast cancer presents with diverse characteristics. To categorize such diverse features of breast cancer, molecular subtypes have been developed: luminal A, luminal B, HER-2, and basal-like type. Moreover, estrogen receptor (ER), progesterone receptor (PR), and HER-2 are the main targets for targeted therapy in breast cancer, and samples/cases that are negative for these three receptors are defined as triple negative breast cancer (TNBC), which comprises about 15% of breast cancer cases. Each of the molecular subtypes of breast cancer and TNBC shows distinct clinical and molecular features in treatment response. Generally, breast cancer is treated with surgery, chemo-radiotherapy, and targeted therapy for biomarkers. Breast cancer showing hormone receptor expressions is treated with hormonal therapy such as tamoxifen, and breast cancer showing HER-2 amplification is treated with targeted therapy such as trastuzumab. Those that do not have any treatment targets are treated with a non-specific chemotherapy.
One of the fundamental characteristics of cancer cells that differs from normal cells is metabolic reprogramming—producing energy through glycolysis rather than mitochondrial oxidative phosphorylation, which is known as the Warburg effect after the German scientist Otto Warburg who first described it in the 1950s. The Warburg effect was first described in the 1950s by Otto Warburg, a German scientist, who stated that cancer cells secrete high levels of lactate because of an increase in glycolysis (Warburg, 1956). In the process of glycolysis, which is one of the main processes of glucose metabolism, glucose can enter cancer cells by glucose transporters. As a result, various glucose metabolites are produced that are related to diverse metabolic pathways, such as the serine/glycine metabolic pathway and pentose phosphate pathway (PPP). These glucose metabolic pathways and glucose transporters have pivotal roles in cancer metabolism as well as in cancer progression and metastasis, and such metabolic characteristics can be used in imaging diagnosis and targeted therapies. This review will focus on the glucose metabolic pathways, such as glycolysis, serine/glycine pathway, and PPP, in breast cancer and glucose transporters used in glycolysis and their potential implications in clinical practice.
Glucose metabolism consists of glycolysis and PPP, and glycolysis-related metabolic pathways consist of serine and glycine metabolism (Figure 1). A major pathway in the glucose metabolism of cancer cells is aerobic glycolysis, in the process of which glucose is first transported into the cancer cells by glucose transporters and then metabolized to pyruvate by various enzymes. Many enzymes are involved in this process, of which, the key enzymes are hexokinase II (HKII), phosphofructokinase (PFK), and pyruvate kinase (PK) (Li et al., 2015). Pyruvates produced in glycolysis are then moved into the mitochondria by mitochondrial pyruvate carriers 1 and 2, where they are turned into acetyl-CoA and oxaloacetate by pyruvate dehydrogenase and pyruvate carboxylase, respectively, to enter the TCA cycle for oxidative phosphorylation (OXPHOS) (Corbet and Feron, 2017). With one of the intermediate metabolites produced during the process of glycolysis, 3-phosphoglycerate (3PG), starts the serine pathway, in which 3-phosphoglycerate (3PG) is oxidized to 3-phosphohydroxypyruvate (pPYR) by phosphoglycerate dehydrogenase (PHGDH) and pPYR is transaminated to phosphoserine (pSER) by phosphoserine aminotransferase (PSAT). pSER is dephosphorylated to serine by phosphoserine phosphatase. In glycine metabolism, glycine is metabolized to H-protein-S-aminomethyldihydrolipoyllysine by glycine decarboxylase (GLDC), an important component of the glycine cleavage system. This serine metabolism and glycine metabolism are linked by serine hydroxymethyltransferse (SHMT), which causes a reversible conversion of serine and glycine (Locasale, 2013). Lastly, PPP is a metabolic pathway that occurs with glycolysis (Ramos-Martinez, 2017), playing a pivotal role in cell survival and growth by providing pentose phosphate for nucleic acid synthesis and also nicotinamide adenine dinucleotide phosphate (NADPH) for fatty acid synthesis and cell survival (Patra and Hay, 2014). PPP is comprised of two branches, the oxidative branch and non-oxidative branch. The oxidative branch converts glucose 6-phosphate (G6P) to ribulose-5-phosphate, CO2, and NADPH (Kruger and von Schaewen, 2003), and the non-oxidative branch produces glycolytic intermediates, such as fructose 6-phosphate (F6P), glyceraldehyde 3-phosphate (G3P), and sedoheptulose. These glycolytic intermediates are important for amino acid synthesis and produce ribose-5-phosphate (R5P) that is also important for nucleic acid synthesis (Stincone et al., 2015). Enzymes that are involved in the oxidative branch are 6-phosphogluconate dehydrogenase (6PGD) and glucose 6-phosphate dehydrogenase (G6PD), and those that are involved in the non-oxidative branch are ribulose-5-phosphate epimerase (RPE), ribose 5-phosphate isomerase (RPI), transaldolase (TALDO), and transketolase (TKT).
Figure 1. Overview of glucose metabolism in cancer cells. Glucose metabolism in tumor cells consists of three main types: glycolysis, the pentose phosphate pathway (PPP), and the serine/glycine pathway. First, in glycolysis, glucose influx occurs in the cell by glucose transporter GLUT1. Using HK2, PFK, and PKM2, glucose becomes pyruvate and is eventually converted to lactate by LDHA. PPP comprises an oxidative branch and a non-oxidative branch, where glucose 6-phosphate is converted to 6-phosphogluconolactone and then ribulose-5-phosphate by 6PGD and G6PD. The non-oxidative branch produces xylulose-5-phosphate by RPE and ribose-5-phosphate by RPI, and then produces fructose 6-phosphate, glyceraldehyde 3-phosphate, sedoheptulose- 7-phosphate, and erythrose-4-phosphate by TKT and TALDO through complex interchangeable reactions. The serine pathway starts with 3-phosphoglycerate, which is converted to phosphohydroxypyruvate by PHGDH, which is converted to 3-phosphoserine by PSAT1, and 3-phosphoserine is converted to serine by PSPH. In addition, glycine is converted by GLDC to H-protein-S-aminomethyldihydrolipoyllysine in glycine metabolism, which is linked to serine metabolism by SHMT in the form of reversible conversion. HK2, hexokinase II; PFK, phosphofructokinase; PKM2, pyruvate kinase isozymes M2; LDHA, lactate dehydrogenase A; G6PD, glucose 6-phosphate dehydrogenase; 6PGD, 6-phosphogluconate dehydrogenase; RPE, ribulose-5-phosphate epimerase; RPI, ribose 5-phosphate isomerase; TKT, transketolase; TALDO, transaldolase; PHGDH, phosphoglycerate dehydrogenase; PSAT1, phosphohydroxythreonine aminotransferase; PSPH, phosphoserine phosphatase; SHMT, serine hydroxymethyltransferase; GLDC, glycine decarboxylase.
Cancer cells produce a high level of reactive oxygen species (ROS) compared to normal cells due to the increased activation of various metabolic pathways (Ahmad et al., 2005). Cancer cell metabolism is closely related to ROS homeostasis; they cause ROS detoxifications by using various substrates and metabolic intermediates in metabolic pathways, the most representative of which are glycolysis by the Warburg effect and PPP (Aykin-Burns et al., 2009). Glycolysis by the Warburg effect maintains redox homeostasis by being independent of mitochondrial OXPHOS that produces a large amount of ROS (Lee and Yoon, 2015), and PPP by producing ROS-detoxifying molecule, NADPH, by G6PD and 6-Phosphogluconate dehydrogenase (6PGDH) (Salazar, 2018).
Molecules involved in the regulation of glucose metabolism in cancer in general are oncogenes such as Ras, Src, and MYC, transcription factors such as hypoxia-inducible factor-1 (HIF-1), signaling pathway such as phosphoinositide 3-kinase (PI3K)/Akt/mammalian target of rapamycin (mTOR), and tumor suppressor such as p53. Oncogenes such as Ras, Src, and MYC increase the expression of HIF-1 that increases the expression of various glycolytic enzymes, and HIF-1, MYC, and KRAS increase glucose uptake by inducing GLUT expression. In addition, the PI3K/Akt/mTOR pathway induces glycolytic enzymes and GLUT expression, and p53 regulates glycolysis and GLUT through mTOR and AMP-activated protein kinase (AMPK) (Abdel-Wahab et al., 2019; Ghanavat et al., 2021).
There are two families of glucose transporters: facilitative sugar transporters (GLUT, gene family name SLC2A) and Na+/glucose co-transporters (SGLT, gene family name solute carrier SLC5A). Additionally found families of glucose transporters are the Sugars Will Eventually be Exported Transporters (SWEET; SLC50) family and the Spinter protein (SLC63) family. SLC50 is a Na (+)/substrate co-transporter involved in the transport of glucose, myoinositol, and anions and located in the plasma membrane. SGLT1 (SLC5A1) and SGLT2 (SLC5A2) are important in glucose uptake with the former expressed mainly in the intestine and the latter in the kidney (Wright, 2013). GLUT has 14 isoforms that share structural features, such as 12 transmembrane domains, amino terminus, carboxy-terminus, and an N-glycosylation site. GLUTs can be subgrouped into three classes: class I (GLUT1–4 and GLUT14), class II (GLUT5, 7, 9, and 11), and class III (GLUT6, 8, 10, 12, and 13). Class I and class II GLUTs are called odd transporters, whereas class III GLUTs are called even transporters (Mueckler and Thorens, 2013). Except for GLUT13, which is a proton-driven myoinositol transporter, all GLUTs are facilitative transporters. These GLUT isoforms differ in the tissue type in which they are present, their location within the cells, cohesiveness with substrates, and control mechanism (Mueckler and Thorens, 2013). For instance, GLUT1 and GLUT3 are found in the brain, where they function mainly in glucose transport (Leino et al., 1997; Yeh et al., 2008), whereas GLUT3–5 and GLUT10–11 are found in the muscle (Bilan et al., 1992; McVie-Wylie et al., 2001; Rogers et al., 2002; Douard and Ferraris, 2008). Glucose is an important substrate for GLUT, but GLUT can also transport other substrates such as galactose, mannose, glucosamine, dehydroacetic acid, fructose, urate, and myo-inositol (Barron et al., 2016; Holman, 2020).
Cancer cells harbor a metabolic shift to aerobic glycolysis that plays an important role in tumor growth, progression, and metastasis; therefore, glucose metabolism and glycolysis-related metabolic pathways can have a diverse impact on cancer cells in breast cancer.
Breast cancer shows an increased expression of glycolysis-related enzymes, namely, HKII (Brown et al., 2002; Yang T. et al., 2018), 6-phosphofructo-2-kinase/fructose-2, 6-biphosphatase 3 (PFKFB3) (O’Neal et al., 2016), and pyruvate kinase M2 (PKM2) (Lin et al., 2015). In primary breast cancer, HKII is overexpressed in about 79% of tumors (Brown et al., 2002), which has been correlated with an increased histologic grade and proliferative activity (Sato-Tadano et al., 2013). The expression of 6-phosphofructo-2-kinase/fructose-2, 6-biphosphatase 3 activates PFK-1, a key enzyme in glycolysis (Okar et al., 2001), and is correlated with HER-2 expression and poor prognosis (O’Neal et al., 2016; Peng et al., 2018). Additionally, 6-phosphofructo-2-kinase/fructose-2, 6-biphosphatase 3 expression is related to the expression of vascular endothelial growth factor (VEGF)-α in breast cancer, which contributes to angiogenesis and distant metastasis (Peng et al., 2018). PFK-2 is a muscle isoform M2 of PK, a key enzyme in glycolysis, and its expression is correlated with a poor prognosis in breast cancer (Lin et al., 2015). Lactates produced by glycolysis are transported in and out of cells by monocarboxylate transporter (MCT) (Wilde et al., 2017). MCT1 overexpression in breast cancer is correlated with ER negativity, PR negativity, high Ki-67 labeling index (Li et al., 2018), basal-like type (Pinheiro et al., 2010), high grade, high stage, increased recurrence, and poor prognosis (Johnson et al., 2017). As for MCT4, tumoral MCT4 expression (Li et al., 2018) and stromal MCT4 expression (Baenke et al., 2015) are associated with poor prognosis.
Breast cancer has been reported to have an increased expression of GLUT1–6 and 12 (Table 1; Barron et al., 2016), and the most important glucose transporter for glucose uptake in breast cancer is GLUT1 (Grover-McKay et al., 1998; López-Lázaro, 2008; Furuta et al., 2010; Wuest et al., 2018). Glucose uptake by GLUT1 is important in the carcinomatous transformation and carcinogenesis of breast cancer, and it plays an important role in the early phase of breast cancer development (Young et al., 2011; Wellberg et al., 2016). GLUT1 overexpression in breast cancer is correlated with high histologic grade, high proliferative activity, poor differentiation, and poor prognosis (Pinheiro et al., 2011; Krzeslak et al., 2012). GLUT4 is an insulin-stimulated glucose transporter (Vargas et al., 2021), and glucose uptake is dependent on insulin stimulation in cancer cell lines (Harmon and Patel, 2004; Moreira et al., 2013; Guedes et al., 2016). It has also been reported that hyperinsulinemia increases the risk of breast cancer irrespective of the body mass index (BMI) (Lawlor et al., 2004; Kabat et al., 2009; Gunter et al., 2015), and so it can be postulated that insulin is associated with breast cancer. Cross-talks between signaling pathways regulated by 17 beta-estradiol (E2) and insulin-like growth factor (IGF) (Bruning et al., 1992; Conover et al., 1992), strong mitogen for cancer cells (Beckwith and Yee, 2014), and actions through ER-signaling (Katzenellenbogen and Norman, 1990) are some possible mechanisms associated with the insulin effect on breast cancer.
Overexpression of glycolysis-related enzymes and GLUTs in breast cancer is due to the activation of the signaling pathways controlling the enzyme expression in breast cancer (Figure 2). The main molecular pathways involved in the control of aerobic glycolysis are the PI3K/AKT, AMP-activated protein kinase (AMPK), mitogen-activated protein kinase, Wnt, and mTOR pathways (Engelman et al., 2006; Han et al., 2015; Cai et al., 2018; Hibdon et al., 2019; Irey et al., 2019). Among these, the PI3K/AKT, AMPK, and mTOR pathways are activated in breast cancer. PI3K/AKT activates phosphofructokinase-2 (PFK-2) by phosphorylation (Novellasdemunt et al., 2013; Lee et al., 2018). PI3K/AKT pathway activation leads to GLUT1 overexpression, which is then translocated from the cytoplasm to the plasma membrane (Samih et al., 2000). AKT is activated by E2, thus increasing the glucose uptake in MCF-7 breast cancer cell line through translocation of GLUT4 to the plasma membrane (Garrido et al., 2013). PIK3CA and AKT1 gene mutations are common in breast cancer (Castaneda et al., 2010; Koboldt et al., 2012), and PIK3CA mutation is usually found in ER-positive and HER-2 positive breast cancer. AMPK translocates GLUT4 to the cytoplasmic membrane by activating PFK-2 (Marsin et al., 2000) and increases GLUT1 expression (Barnes et al., 2002). AMPK is highly expressed in TNBC and known to be associated with poor prognosis (Huang et al., 2016). mTOR is a downstream effector of AKT, comprising mTOR complex 1 (mTORC1) and mTOR complex 2 (mTORC2) (Hara et al., 2002; Vivanco and Sawyers, 2002; Baretić and Williams, 2014). mTORC1 promotes the transition from OXPHOS to glycolysis and increases the expression of HIF-1α, which in turn increases the expression of glycolysis-related enzymes such as PFK (Düvel et al., 2010). mTORC2 promotes glycolysis by activating AKT (García-Martínez and Alessi, 2008; Ikenoue et al., 2008; Cybulski and Hall, 2009) and GLUT1-related glucose uptake (Beg et al., 2017). mTOR is activated in breast cancer through HER-2 overexpression, PI3K pathway alteration, and mTOR mutation (Hare and Harvey, 2017). Second, the increased expression of glycolysis-related enzymes in breast cancer is because of the activation of transcription factors (Figure 2). The transcription factors associated with glycolysis are c-myc, p53, and HIF-1. c-myc is responsible for increasing the gene expression of glycolysis-related genes and, consequently, glycolysis-related enzymes, such as GLUT, HK, and PFK (Hsieh et al., 2015). Moreover, estrogen is responsible for the increased expression of c-myc, and about 80% of breast cancers are ER-positive (Butt et al., 2008). p53 is a well-known tumor suppressor, gene mutations of which are found in most cancers including breast cancer. p53 mutation is found in about 20%–30% of breast cancers and more often in ER-negative breast cancer. p53 suppresses phosphoglycerate mutase (PGM), GLUT1, GLUT3, and GLUT4 expression (Kawauchi et al., 2008; Vousden and Ryan, 2009); hence, p53 mutation leads to an increased glycolysis in breast cancer. Lastly, the transcription factor HIF-1α, which is activated by hypoxia, is an important regulator in glycolysis and increases the expression of glycolysis-related molecules, such as HKII, PFK-1, lactate dehydrogenase (LDH) A, GLUT-1, and GLUT-3. HIF-1α promotes the metabolic shift to glycolysis by suppressing the mitochondrial function through the activation of pyruvate dehydrogenase kinase 1 (PKD1) and MAX interactor 1 (MXI1) (Denko, 2008). HIF-1α overexpression has been reported in breast cancer (Zhong et al., 1999), and it is attributed to the increased expression of glycolysis-related proteins in breast cancer because HIF-1α overexpression is related to HER-2 positivity (Giatromanolaki et al., 2004) and TNBC (Jin et al., 2016).
Figure 2. Regulation of glycolysis and glucose transporters in breast cancer. Important signaling pathways regulating glycolysis and glucose transporters in breast cancer are the PI3K/AKT, AMPK, and mTOR pathways. PI3K/AKT pathway activated by 17-estradiol (E2) or genetic mutations increases expression of PFK2 and GLUT. AMPK pathway activated in breast cancer transports GLUT4 to cell membrane through activation of PFK-2 and increases GLUT expression. mTORC1 among the mTOR complex increases the expression of PFK by activating HIF-1α. mTORC2 either activates AKT or increases GLUT1 expression. Transcription factors regulating glucose metabolism in breast cancer are c-myc, p53, and HIF-1α. As such, breast cancer with p53 mutation shows increased expression of GLUT because c-myc induces increased expression of GLUT, HK, and PFK, and p53 suppresses expression of GLUT. Lastly, activated HIF-1α increases expressions of HK, PFK, LDHA, and GLUT, and suppresses mitochondrial function by activating PKD1 and MXII. PI3K, phosphoinositide 3-kinase; AKT, Ak strain transforming protein kinase B; AMPK, AMP-activated protein kinase; mTOR, mechanistic target of rapamycins; PFK, phosphofructokinase; HIF, hypoxia-inducible factor; HK, hexokinase; LDHA, lactate dehydrogenase A; PKD1, pyruvate dehydrogenase kinase 1; MXI1, MAX interactor 1.
Breast cancer is susceptible to sex hormones such as estrogen, which may have an effect on the regulation of glucose metabolism. E2 and ERα stimulation activates the MAPK pathway (Ronda et al., 2010a, b), regulates expression of GLUT4 (Barros et al., 2006, 2008), and increases glucose uptake (Niu et al., 2003; Gorres et al., 2011). Furthermore, E2 activates the PI3K pathway that is involved in glucose metabolism in breast cancer cells (Simoncini et al., 2000; Lee et al., 2005), and suppresses phosphatase and tensin homolog (PTEN), a phosphatidylinositol-3 kinase inhibitory protein (Noh et al., 2011).
Triple negative breast cancer is defined as breast cancer that is negative for ER, PR, and HER-2 and accounts for about 15% of breast cancer cases. Basal-like breast cancer (BLBC) is defined as those that have high expressions of basal genes in gene expression studies such as DNA microarray. Therefore, TNBC and BLBC are not the same in the strict sense of definitions (Carey et al., 2010), although they can overlap in many instances. TNBC is a heterogeneous group, and many researches have focused on the subgrouping of TNBC. Lehmann et al. (2011) have grouped TNBC further into basal-like1, basal-like2, mesenchymal, and luminal androgen receptor, and Burstein et al. (2015) have grouped TNBC further into basal-like immune-activated, basal-like immune suppressed, mesenchymal, and luminal androgen receptor. The general characteristics of TNBC include the histological characteristics of high grade, high proposition index, and tumor necrosis, and clinical characteristics of higher rate of metastasis and poor prognosis (Kumar and Aggarwal, 2016; Borri and Granaglia, 2020). With these histological and clinical features, TNBC can be postulated to be of high metabolic status. One of the important metabolic features of TNBC is high glucose uptake, and GLUT1 overexpression is seen in TNBC (Hussein et al., 2011; Oh et al., 2017). High expression of glycolysis-related enzymes, such as HK2 (Jiang S. et al., 2012), PKM2 (Christofk et al., 2008; Ma et al., 2019), and LDH (McCleland et al., 2012; Huang et al., 2016; Dong et al., 2017), and that of lactate transporters MCT1 and MCT4 have also been reported in TNBC (Pinheiro et al., 2010; McCleland et al., 2012; Doyen et al., 2014). The high expression of glycolysis-related proteins in TNBC is owing to the fact that the glycolysis regulatory factors, such as HIF-1 (Lin et al., 2016; De Blasio et al., 2020), c-myc (Palaskas et al., 2011; Shen et al., 2015), and EGF signaling (Avanzato et al., 2018), are promoted in TNBC. Therefore, TNBC cells are much more dependent on glucose metabolism than non-TNBC cells (MCF-7) (Robey et al., 2005), and GLUT1 inhibition shows a more anti-proliferative effect for TNBC cells than non-TNBC cells (MCF-7) (Yang et al., 2021).
In glucose metabolism, non-glycolysis metabolic pathways, such as the serine/glycine metabolic pathway and PPP, play important roles in breast cancer. The expression of serine/glycine metabolic pathway-related proteins in breast cancer differs depending on the breast cancer molecular subtype. Serine metabolic pathway-related proteins were highly expressed in TNBC (Labuschagne et al., 2014), and glycine metabolic pathway-related proteins were highly expressed in HER-2 type breast cancer (Kim S. K. et al., 2014). The basal-like type also showed a higher expression of serine/glycine metabolic pathway-related proteins among the TNBC subtypes (Noh et al., 2014). Analysis using the cBioPortal TCGA Pan-Cancer Atlas shows PHGDH amplification in approximately 2.2% of breast cancers (Geeraerts et al., 2021a). PHGDH expression is observed frequently in ER-negative breast cancer (Possemato et al., 2011), and increased PHGDH expression in breast cancer is associated with poor prognosis (Locasale et al., 2011; Possemato et al., 2011). Similarly, phosphoserine aminotransferase 1 (PSAT1) is more frequently expressed in ER-negative breast cancer and is associated with poor prognosis (Gao et al., 2017). Serine hydroxymethyltransferase 2 expression level is associated with the histologic grade of breast cancer (Yin, 2015).
High expression of PPP-related enzymes, such as 6PGD (Yang X. et al., 2018) and TKT (Benito et al., 2017; Yang X. et al., 2018), is reported in breast cancer. G6PD, one of the PPP-related enzymes, is associated with the molecular subtype of breast cancer, and G6PD overexpression is associated with poor prognosis of breast cancer (Pu et al., 2015; Dong et al., 2016). 6PGDH expression is high in TNBC, and the expression of G6PDH and 6PGL are high in HER-2 type (Choi et al., 2018b). The expression of G6PDH is also the highest in brain metastasis among metastatic breast cancers (Cha et al., 2017). The expression of TKT is associated with tumor size and high TKT expression is associated with poor prognosis in a mouse model of breast cancer (Tseng et al., 2018). Increased PPP flux by G6PD and HK2 enhancement induces tamoxifen resistance in breast cancer (Wang et al., 2016). An increase in HK2 transcription by the yes-associated protein (YAP) axis also promotes the migration of breast cancer cells (Tseng et al., 2018).
Breast cancer is one of those tumors that harbors tumor stroma, the main cell components of which include cancer-associated fibroblasts (CAFs), cancer-associated adipocytes (CAAs), and immune cells. These stromal cells affect the development, progression, and metastasis of breast cancer through various interactions with breast cancer cells (Mao et al., 2013; Soysal et al., 2015; Choi et al., 2018a; Mittal et al., 2018; Wu et al., 2019b). Thus, metabolic interactions are present between breast cancer cells and stromal cells (Figure 3), and glucose metabolism in tumor stromal cells is suggested in the reverse Warburg effect. According to the reverse Warburg effect, aerobic glycolysis occurs in CAFs that are present in the breast cancer stroma. In brief, the reverse Warburg theory describes the glycolysis that occurs in CAFs by ROS, HIF1A, and nuclear factor-κB (NF-κB), resulting in lactate being released from CAFs by MCT4, which is then transported into the tumor cells by MCT1 in breast cancer, creating energy by mitochondrial OXPHOS (Pavlides et al., 2009; Fu et al., 2017; Wilde et al., 2017). Lactate produced by CAFs is transported into the tumor cells as potent nutrients for the TCA cycle, and this lactate can be an important source of energy for cancer cells because lactate is the primary source of carbon for the TCA cycle among circulating metabolites (Hui et al., 2017; Martínez-Reyes and Chandel, 2017). In co-cultural studies of breast cancer cell lines and fibroblasts and studies of human breast cancer tissue, MCT4 was expressed in CAFs, whereas MCT1 was expressed in tumor cells (Whitaker-Menezes et al., 2011; Witkiewicz et al., 2012; Johnson et al., 2017). In a co-cultural study of MCF7 breast cancer cells and normal fibroblasts, culture of MCF7 breast cancer cells alone or fibroblasts alone did not exhibit MCT4 expression, whereas co-culture of MCF7 breast cancer cells and fibroblasts showed MCT4 expression in CAFs. The co-culture with fibroblasts showed MCT1 upregulation in MCF7 breast cancer cells (Whitaker-Menezes et al., 2011). Breast CAFs showed higher expressions of GLUT1 and PDK1 than normal fibroblasts (Pasanen et al., 2016), and the co-cultural study of breast cancer cells and fibroblasts showed an increase in glycolysis and glucose transporter-related genes in CAFs (Ueno et al., 2015). The reverse Warburg effect is not only observed between cancer cells and CAFs but also between hypoxic and oxygenated cancer cells (Sonveaux et al., 2008; Doherty and Cleveland, 2013).
Figure 3. Glucose metabolic interaction between breast cancer cells and stromal cells. The glucose metabolic interaction between the breast cancer cell and CAF is presented as the reverse Warburg effect, where mitochondrial dysfunction results in a decrease in caveolin-1 levels because of increased autophagy, and an increase in glycolysis occurs by enhanced HIF-1α and NF-κB in CAF. Lactate produced by glycolysis is transferred to cancer cells by MCT4 in CAF and MCT1 in cancer cells, which is converted to pyruvate and used as a material for mitochondrial OXPHOS. ROS produced by the OXPHOS process cause an increase in HIF-1α and NF-κB in CAF. TAM, one of the immune cells of breast cancer stroma, shows increased glycolysis because of the increased GLUT1 and HK2 activity by enhanced HIF-1α expression; therefore, TAM can compete with cancer cells for glucose. G protein-coupled receptor 132 (Gpr132) senses the lactate produced by glycolysis to convert the macrophage to an M2-like phenotype, which promotes cancer cell adaptation, migration, and invasion. HIF-1α-stabilizing long non-coding RNA (HISLA) is transferred from TAM to breast cancer cells through extracellular vesicle transmission, and then, HISLA promotes glycolysis in breast cancer cells. Breast cancer cells have a metabolic switch that controls glycolysis and OXPHOS depending on the circumstances. CAF, cancer-associated fibroblast; HIF, hypoxia-inducible factor; MCT, monocarboxylate transporter; OXPHOS, oxidative phosphorylation; HK, hexokinase; ROS, reactive oxygen species; TAM, tumor-associated macrophage.
One type of immune cells in the tumor stroma is tumor-associated macrophages (TAMs) that inhibit antitumor immunity in breast cancer, resulting in tumor progression. In general, TAMs exhibit properties of M2 macrophages (Mantovani et al., 2002; Hollmén et al., 2015), and TAMs in hypoxic tumor regions express HIF-1 (Burke et al., 2003), which controls the expression of glycolysis-related genes, including GLUT1, HK2, PFFB3, and PGK1 (Semenza et al., 1994). Therefore, TAMs in hypoxic tumor environments may utilize glycolysis. In addition, lactate generated in the glycolysis process is an important metabolite, which activates M2 macrophages (Colegio et al., 2014; Chen P. et al., 2017; Mu et al., 2018). In a co-culture study of breast cancer cells and macrophages, G protein-coupled receptor 132 (Gpr132) senses lactate in the tumor environment to transform macrophages into M2-like phenotypes to promote cancer cell adherence, migration, and invasion (Chen P. et al., 2017). In addition, HIF-1α-stabilizing long non-coding RNA (HISLA) is transferred from TAMs to breast cancer cells via extracurricular vessel transmission, which increases glycolysis in breast cancer cells (Chen et al., 2019).
First, the proliferation of tumor cells requires a lot of energy and a variety of materials are needed to create new tumor cells, which is also true for breast cancer cells. Therefore, glucose metabolism and glucose transporters, which provide energy sources for breast cancer, and PPP, which provides the materials needed for the synthesis of nucleotides, lipids, and non-essential amino acids, play important roles in breast cancer proliferation. Second, glucose metabolism affects the maintenance of epithelial-mesenchymal transition (EMT) and cancer stem cell (CSC) phenotype in breast cancer. Increased glycolysis and PPP by epigenetic silencing of fructose-1,6-biphosphatase can increase NADPH and reduce ROS levels, which enhance EMT and CSC phenotype in basal-like breast cancer (Dong et al., 2013; Schieber and Chandel, 2013). In a breast cancer cell line study, high glucose levels increased glycolytic enzyme, motor protein, and NF-κB levels and glucose uptake, and reduced actin, resulting in EMT phenotype activation (Santos and Hussain, 2020). In addition, HIF-1 activation by hypoxia maintains ROS homeostasis through the glycolytic pathway and serine synthesis pathway, which is important for breast CSC induction (Semenza, 2017). Moreover, glucose metabolism is associated with treatment resistance in breast cancer, where induced glycolysis is observed by AKT/mTOR/HIF-1α axis activation in tamoxifen resistant breast cancer cells, and when HKII is inhibited, tamoxifen sensitivity is recovered (Woo et al., 2015). Increased glycolysis is observed in trastuzumab resistant breast cancer cells, and glycolytic inhibition reduces trastuzumab resistance (Zhao et al., 2011). The expression of PFK-2 is linked to the responsiveness of anticancer drugs such as epirubicin and 5-fluorouacil in breast cancer cells (Benesch et al., 2010; Lin et al., 2015). Chemoresistant TNBC cells exhibit increased glycolysis and lactate permutation (Zhou et al., 2010), and PHGDH expression correlates with the responsiveness of chemotherapy in TNBC cells (Samanta et al., 2016). GLUT is associated with breast cancer metastasis; a proteomic analysis of MDA-MB-231 (metastatic breast cancer cell line) and MCF-10A (normal breast epithelial cell line) showed that one of the three strongest breast cancer-related proteins was GLUT1 (Risha et al., 2020). The GLUT expression showed a difference according to the metastatic sites, and the expression of GLUT1 was the highest in brain metastasis (Kim H. M. et al., 2014). Additionally, GLUT12 plays an important role in tumor growth and metastasis through aerobic glycolysis in TNBC (Shi et al., 2020).
As we have seen earlier, glucose transporter expression is high in breast cancer, and glucose metabolism is carried through the glycolytic, serine/glycine, and PPPs that play important roles in tumor growth and progression. Therefore, they may have a variety of clinical applications, especially in imaging diagnosis and targeted therapy.
Positron emission tomography (PET) using 18F-fluorodeoxy glucose (FDG), a radioactive analog of glucose, is the representative functional imaging technique based on the principle that tumor cells uptake large amounts of glucose by GLUT via the Warburg effect. These PETs are used for tumor staging and treatment response monitoring (Bohndiek and Brindle, 2010). These FDG-PET/CTs are also useful for diagnosis, staging, and treatment evaluation in breast cancer (Groheux et al., 2016; Caresia Aroztegui et al., 2017; Paydary et al., 2019). In addition to FDG-PET/CT, functional imaging based on glucose metabolism can be performed using magnetic resistance spectroscopy (MRS). Multiple metabolites can be simultaneously identified in tumor tissues using MRS, which can analyze labeling patterns using stable isotopic traces, and glucose metabolites can be analyzed using 13C-MRS and [13C]-labeled glucose to image the glycolysis status. MRS can perform effective metabolic monitoring in breast cancer (Rivenzon-Segal et al., 2002). Breast cancer with different 13C-MRS expression patterns show a different glucose metabolism (Grinde et al., 2011). A high-resolution magic angle spinning MRS analysis of metabolites in breast cancer, such as β-glucose, lactate, and glycine, shows good prognosis with reduced concentrations of glycine. The concentration of β-glucose shows a negative correlation with proliferation index (MIB-1), indicating that MR metabolite analysis is valuable in breast cancer prognostication (Sitter et al., 2010).
The expression of glucose transporters and glucose metabolic enzymes in breast cancer is high; thus, their inhibition can serve as an effective treatment strategy against breast cancer (Figure 4). Various preclinical and clinical studies have been conducted to investigate this implication.
Figure 4. Candidate drugs for the inhibition of glucose metabolism and glucose transporters in breast cancer. Inhibitors for GLUT1 involved in glucose influx in glycolysis include WZB117, SFT-31, BAY-876, anti-GLUT1 antibody, and polyphenols such as resveratrol, hesperetin, quercetin, glabridin, EGCG, cantharidin, kudingcha, and vitamin D3. 2-DG competes with glucose for binding GLUT1. Enzyme inhibitors for HK2 involved in glycolysis include 3-BrPA and methyl jasmonate, and resveratrol as PKF inhibitors; cyclosporine A as PKM2 inhibitor; and oxamate, gossypol, AT-101, and galloflavin as LDHA inhibitors. Enzyme inhibitors for G6PD involved in PPP include DHEA, 6-aminonicotinamide, and CB83 and oxythiamine as TKT inhibitor. In the serine and glycine pathway, PHGDH inhibitors include NCT-503, CBR-5884, PKUMDL-WQ-2101, PKUMDL-WQ-2201, and 15 fragments, and sertraline as SHMT inhibitors. HK, hexokinase; PKF, phosphofructokinase; PKM2, pyruvate kinase isozymes M2; LDHA, lactate dehydrogenase A; G6PD, glucose 6-phosphate dehydrogenase; PPP, pentose phosphate pathway; DHEA, dehydroepiandrosterone; TKT, transketolase; PHGDH, phosphoglycerate dehydrogenase; SHMT, serine hydroxymethyltransferase.
GLUT1 inhibitors—WZB117 and SFT-31—inhibit cell proliferation and promote apoptosis in breast cancer cell lines (Xintaropoulou et al., 2015). WZB117 increases the effectiveness of radiation (Zhao et al., 2016) and anticancer drugs in breast cancer cell lines (Liu et al., 2012; Chen Q. et al., 2017). BAY-876, a selective GLUT1 inhibitor, decreases glucose uptake in TNBC cell lines (Wu et al., 2019a) and 2-deoxy-D-glucose (2-DG), a synthetic non-metabolizable glucose analog, competes with glucose for binding GLUT, which reduces glucose uptake in the MDA-MB-231 TNBC cell line (Amaral et al., 2018). As for 2-DG, there are two different phenomena resulting from the suppression of glycolysis: first, glucose can be deviated to PPP because 2-DG is not metabolized any further after phosphorylation into 2-deoxy-D-glucose-6-phosphate (2-DG-6-P) by HKII (Ralser et al., 2008); and second, 2-DG induces autophagy due to endoplasmic reticulum (ER) stress. Suppression of glycolysis leads to a decreased ATP, by which N-linked glycosylation is suppressed and AMPK is activated. AMPK activation and N-linked glycosylation lead to ER stress (Xi et al., 2011, 2013). Autophagy promotes tumor growth in the early stage of cancer (Cheong, 2015), maintains tumor survival, and increases metastasis in the advanced stage (Yang et al., 2011). Anti-GLUT1 monoclonal antibody decreases glucose uptake in the MDA-MB-231 TNBC cell line, and decreases cancer cell proliferation and promotes apoptosis in MCF-1 and T47D breast cancer cell lines (Rastogi et al., 2007). Polyphenols, a huge family of natural compounds found in plants or food, is one category of the GLUT1 inhibitors (Williamson, 2017) that shows an anti-tumoral effect against various cancers including breast cancer. The anti-tumoral mechanism of polyphenols against breast cancer includes increased apoptosis, cell cycle arrest, enhanced autophagy, decreased angiogenesis, anti-inflammatory effect, blockade for estrogen, aromatase modulation, altered redox balance, and inhibition of the HER-2 pathway (Mocanu et al., 2015; Losada-Echeberría et al., 2017). Polyphenols inhibiting GLUT1 in breast cancer are as follows: Resveratol suppresses glucose uptake in T-47D cell line by reducing GLUT1 protein level (Jung et al., 2013), and hesperetin suppresses glucose uptake by decreasing GLUT 1 mRNA and protein levels (Yang et al., 2013). Quercetin decreases the glucose uptake in MCF-7 and MDA-MB-231 by reducing GLUT1 protein level (Jia L. et al., 2018), as does glabridin in MDA-MB-231 (Li et al., 2019). Epigallocatechin-3-gallate (EGCG) decreases the glucose and lactate levels in cancer cells by reducing GLUT1 mRNA levels in 4T1 cell line (Wei et al., 2018), and cantharidin suppresses metastasis by inhibiting glucose uptake and lactate production through decreasing GLUT1 protein level in MCF-7 and MDA-MB-231 (Pan et al., 2019). Kudingcha, one of the Ligustrum robustum species, inhibit cancer proliferation through decreasing GLUT1 protein level in MDA-MB-231 and HCC1806 (Zhu et al., 2020). Vitamin D3 decreases glucose uptake by decreasing GLUT1 mRNA and protein levels in MCF-7 and MDA-MB-231 (Santos et al., 2018).
First, 3-bromopyruvate (3-BrPA), an inhibitor of hexokinase, causes apoptosis in MDA-MB-231 breast cancer cell line (Liu et al., 2014; Chen et al., 2018) and increases the response to daunorubicin (Liu et al., 2015) and tamoxifen (Attia et al., 2015) in breast cancer. Methyl jasmonate, another hexokinase inhibitor, caused a decrease in tumor volume in mice bearing 4T1 breast cancer cell line (Yousefi et al., 2020). Resveratrol, an inhibitor of PFK, decreases the cell viability and glucose consumption in MCF-7 breast cancer cell line (Gomez et al., 2013). Cyclosporin A, an immunosuppressive agent, inhibits the expression and activity of PKM2 in breast cancer cell lines (MCF-7, MDA-MB-435, and MDA-MB-231) and causes tumor cell death by reducing cell viability (Jiang K. et al., 2012). Cyclosporin A also maintains mitochondrial function by suppressing mitochondrial permeability transition pore (Halestrap et al., 1997; Mishra et al., 2019). When oxamate, an LDH inhibitor, is administered in conjunction with doxorubicin and metformin, it causes a rapid tumor growth inhibition in the xenograft model using human MDA-MB-231 TNBC cell line (García-Castillo et al., 2017). When paclitaxel and oxamate are administered together, they induce an effective killing of paclitaxel-resident TNBC cells (Zhou et al., 2010). Gossypol, a lipid soluble polyphenolic compound, exhibits antitumor effects by inhibiting glycolysis through LDH isoenzyme type 5 inhibition (Coyle et al., 1994). Gossypol causes anti-proliferative activity and apoptosis in breast cancer cells (Gilbert et al., 1995; Ye et al., 2010; Messeha et al., 2019), and when R-(-)-gossypol (AT-101) is administered in conjunction with trastuzumab in HER-2 positive breast cancer cell line, it causes synergistic cytotoxicity and apoptosis (Bulut et al., 2020). Galloflavin, an LDHA inhibitor, induces cell death in MDA-MB-231 cell lines and acquired tamoxifen resistance MCF-7 breast cancer cell lines (Farabegoli et al., 2012).
Serine and glycine pathway inhibitors can be used for the management of tumors that use serine and glycine metabolism and for treatment of tumors showing recurrence and treatment resistance. PHGDH inhibitors—NCT-503 and CBR-5884—are both allosteric PHGDH inhibitors; NCT-503 binds to the near substrate-binding pockets; and CBR-5884 hinders PHGD holigomerization (Mullarky et al., 2016; Pacold et al., 2016). NCT-503 inhibits tumor growth in PHGDH-amplified breast cancer xenografts (Pacold et al., 2016), and CBR-5884 inhibits tumor cell proliferation in high PHGDH-expressing breast cancer cell lines (MDA-MB-468, MDA-MB-436, HCC70, and Hs578T) (Mullarky et al., 2016). PKUMDL-WQ-2101 and PKUMDL-WQ-2201, which are allosteric PHGDH inhibitors, show an antitumor activity in PHGDH-amplified breast cancer cell lines (MDA-MB-468 and HCC70) (Wang et al., 2017). An NAD-competitive PHGDH inhibitor, 15 fragments, reduces cell proliferation in PHGDH-amplified breast cancer cell line (MDA-MB-468) (Unterlass et al., 2018). Sertraline, an antidepressant, is a selective serotonin reuptake inhibitor (SSRI) class (MacQueen et al., 2001), but it also works as a competitive dual SHMT1/2 inhibitor, reducing the cell growth in serine/glycine synthesis-addicted breast cancer cell line (MDA-MB-468) and decreasing the tumor growth in a mouse xenograft study (Geeraerts et al., 2021b).
G6PD, one of the important enzymes in PPP, has a potent non-competitive inhibitor, dehydroepiandrosterone (DHEA), which is an adrenal cortical steroid. DHEA inhibits the growth and migration of breast cancer cell lines (MCF-7, MDA-MB-231, and Hs578T) (López-Marure et al., 2011). DHEA can bind estrogen or androgen receptors because it is metabolized to estrogen or androgen (Labrie et al., 2001), however, the suppression of MCT-7 cell line growth by DHEA is reported to be independent of estrogen or androgen receptors (Gayosso et al., 2006). 6-aminonicotinamide, a G6PD inhibitor, can decrease mammosphere formation and aldehyde dehydrogenase (ALDH) activity when given with DHEA in breast cancer stem-like cells that show high PPP activity (Debeb et al., 2016). CB83, another G6PD inhibitor, can inhibit growth of MCF10-AT1 breast cancer cell line (Preuss et al., 2013). Oxythiamine, an inhibitor of TKT, also increases the response of breast cancer cells to doxorubicin or docetaxel (Tseng et al., 2018).
Because of the high expressions of GLUT-1 and the enzymes involved in glucose metabolism, tumor cells in breast cancer, as in other tumors, are provided with energy through glucose metabolism. There are several characteristic factors to consider in the glucose metabolism of breast cancer. Because breast cancer is heterogeneous, inter- and intratumoral heterogeneity is also seen in glucose metabolism. First, glucose metabolic activity is different among the molecular subtypes, especially in TNBC, which shows an increased glycolytic phenotype (Wang et al., 2020). According to the traditional Warburg theory, tumors showing aerobic glycolysis are suggested to exhibit a decreased mitochondrial OXPHOS; however, TNBC with a high metabolic activity shows both enhanced glycolysis and sustained mitochondrial OXPHOS (Park et al., 2016; Lanning et al., 2017; Luo et al., 2018; Jia et al., 2019). Luminal type breast cancer rely more on OXPHOS than glycolysis compared to TNBC (Pelicano et al., 2014). It also presents metabolic switches between glycolysis and OXPHOS during cancer progression (Levine and Puzio-Kuter, 2010; Jia D. et al., 2018; Lai et al., 2020; Moldogazieva et al., 2020). Therefore, metabolic intratumoral heterogeneity is exhibited in breast cancer, showing different glycolytic activities depending on the tumor cell type. Second, there is a metabolic interaction between tumor cells and the surrounding stromal cells in breast cancer. Breast cancer is a typical tumor that contains various stromal cells, the main components of which are CAFs, CAAs, and immune cells. Metabolic interactions exist between breast cancer cells and stromal cells; especially according to the reverse Warburg theory, lactates produced by glycolysis in CAFs enter tumor cells and produce energy through OXPHOS. Among the immune cells, B-cells and NK cells use glycolysis, and tumor-associated neutrophils use glycolysis and PPP, allowing a metabolic competition with the tumor cells. Third, unlike in other tumors, CAAs are stromal cells that are specifically present in breast cancer, and previous studies suggest that β-oxidation in tumor cells is primarily studied through the lipid transfer between CAAs and tumor cells. As the glucose metabolic interaction between CAAs and tumor cells is rarely studied in breast cancer, it requires further study. Metabolic interactions between tumor cells and stromal cells in these breast cancer cases are also reported to be affected by cancer phenotypes (Brauer et al., 2013), which may require further research on the metabolic cross-talk between the cancer cells and stromal cells according to the molecular subtype of breast cancer. Fourth, breast cancer shows differential metabolic features depending on the stage and metastatic site. In order for the tumor to progress into distant metastasis, multiple and complex processes, such as intravasation, survival in blood stream, and extravasation, must be accomplished during this process, and the hurdles, such as anchorage independent survival and tumor cell proliferation in foreign microenvironment, should be overcome. One way to overcome this challenge is metabolic reprogramming. Breast cancer shows metabolic differences between the primary and metastatic tumors (Chen et al., 2007; LeBleu et al., 2014; Dupuy et al., 2015; Simões et al., 2015; Andrzejewski et al., 2017), and breast cancer does not rely on a single metabolic pathway, but uses multiple metabolic pathways. Highly metastatic 4T1 cells show increased glycolysis and OXPHOS compared to non-metastatic 67NR breast cancer cells (Simões et al., 2015). The most common metastatic sites are the brain, bone, lung, and liver, which exhibit differential metabolic features owing to different microenvironments. Liver metastatic breast cancer demonstrates increased glycolytic pathways compared to bone and lung metastatic breast cancer (Dupuy et al., 2015), whereas brain metastatic breast cancer shows increased glycolysis and PPP compared to bone metastatic breast cancer (Chen et al., 2007). As a result of the above characteristics of glucose metabolism in breast cancer, further studies are needed to consider tumor imaging using glucose metabolism and glucose metabolic markers as treatment targets. In addition, because glucose metabolism is associated with resistance to anticancer drugs or targeted treatments in breast cancer, glucose metabolic inhibitors can also be considered for a combined therapy with conventional treatments.
ES and JK: writing—original draft, investigation, and writing—review and editing. Both authors contributed to the article and approved the submitted version.
The authors declare that the research was conducted in the absence of any commercial or financial relationships that could be construed as a potential conflict of interest.
All claims expressed in this article are solely those of the authors and do not necessarily represent those of their affiliated organizations, or those of the publisher, the editors and the reviewers. Any product that may be evaluated in this article, or claim that may be made by its manufacturer, is not guaranteed or endorsed by the publisher.
The authors would like to thank Dong-Su Jang, MFA (Medical Illustrator), for his help with the illustrations.
HK2, hexokinase II; PFK, phosphofructokinase; PKM2, pyruvate kinase isozymes M2; LDHA, lactate dehydrogenase A; G6PD, glucose 6-phosphate dehydrogenase; 6PGD, 6-phosphogluconate dehydrogenase; RPE, ribulose-5-phosphate epimerase; RPI, ribose 5-phosphate isomerase; TKT, transketolase; TALDO, transaldolase; PHGDH, phosphoglycerate dehydrogenase; PSAT1, phosphohydroxythreonine aminotransferase; PSPH, phosphoserine phosphatase; SHMT, serine hydroxymethyltransferase; GLDC, glycine decarboxylase; CAF, cancer-associated fibroblast; HIF, hypoxia-inducible factor; MCT, Monocarboxylate transporter; OXPHOS, oxidative phosphorylation; TAM, tumor-associated macrophage; PPP, pentose phosphate pathway; DHEA, dehydroepiandrosterone; PHGDH, phosphoglycerate dehydrogenase; ROS, reactive oxygen species; EMT, epithelial-mesenchymal transition; CSC, cancer stem cell; NADPH, nicotinamide adenine dinucleotide phosphate; 6PGDH, 6-phosphogluconate dehydrogenase; PI3K, phosphoinositide 3-kinase; mTOR, mammalian target of rapamycin; AMPK, AMP-activated protein kinase; VEGF, vascular endothelial growth factor; BMI, body mass index; E2, 17 beta-estradiol; IGF, insulin-like growth factor.
Abdel-Wahab, A. F., Mahmoud, W., and Al-Harizy, R. M. (2019). Targeting glucose metabolism to suppress cancer progression: prospective of anti-glycolytic cancer therapy. Pharmacol. Res. 150:104511.
Ahmad, I. M., Aykin-Burns, N., Sim, J. E., Walsh, S. A., Higashikubo, R., Buettner, G. R., et al. (2005). Mitochondrial O2∗- and H2O2 mediate glucose deprivation-induced stress in human cancer cells. J. Biol. Chem. 280, 4254–4263.
Alò, P. L., Visca, P., Botti, C., Galati, G. M., Sebastiani, V., Andreano, T., et al. (2001). Immunohistochemical expression of human erythrocyte glucose transporter and fatty acid synthase in infiltrating breast carcinomas and adjacent typical/atypical hyperplastic or normal breast tissue. Am. J. Clin. Pathol. 116, 129–134. doi: 10.1309/5y2l-cdck-yb55-kdk6
Amaral, I., Silva, C., Correia-Branco, A., and Martel, F. (2018). Effect of metformin on estrogen and progesterone receptor-positive (MCF-7) and triple-negative (MDA-MB-231) breast cancer cells. Biomed. Pharmacother. 102, 94–101. doi: 10.1016/j.biopha.2018.03.008
Andrzejewski, S., Klimcakova, E., Johnson, R. M., Tabariès, S., Annis, M. G., McGuirk, S., et al. (2017). PGC-1α Promotes Breast Cancer Metastasis and Confers Bioenergetic Flexibility against Metabolic Drugs. Cell Metab. 26, 778–787.e5. doi: 10.1016/j.cmet.2017.09.006
Attia, Y. M., El-Abhar, H. S., Al Marzabani, M. M., and Shouman, S. A. (2015). Targeting glycolysis by 3-bromopyruvate improves tamoxifen cytotoxicity of breast cancer cell lines. BMC Cancer 15:838. doi: 10.1186/s12885-015-1850-4
Avanzato, D., Pupo, E., Ducano, N., Isella, C., Bertalot, G., Luise, C., et al. (2018). High USP6NL Levels in Breast Cancer Sustain Chronic AKT Phosphorylation and GLUT1 Stability Fueling Aerobic Glycolysis. Cancer Res. 78, 3432–3444. doi: 10.1158/0008-5472.Can-17-3018
Aykin-Burns, N., Ahmad, I. M., Zhu, Y., Oberley, L. W., and Spitz, D. R. (2009). Increased levels of superoxide and H2O2 mediate the differential susceptibility of cancer cells versus normal cells to glucose deprivation. Biochem. J. 418, 29–37. doi: 10.1042/bj20081258
Baenke, F., Dubuis, S., Brault, C., Weigelt, B., Dankworth, B., Griffiths, B., et al. (2015). Functional screening identifies MCT4 as a key regulator of breast cancer cell metabolism and survival. J. Pathol. 237, 152–165. doi: 10.1002/path.4562
Baretić, D., and Williams, R. L. (2014). The structural basis for mTOR function. Semin. Cell Dev. Biol. 36, 91–101. doi: 10.1016/j.semcdb.2014.09.024
Barnes, K., Ingram, J. C., Porras, O. H., Barros, L. F., Hudson, E. R., Fryer, L. G., et al. (2002). Activation of GLUT1 by metabolic and osmotic stress: potential involvement of AMP-activated protein kinase (AMPK). J. Cell Sci. 115, 2433–2442. doi: 10.1242/jcs.115.11.2433
Barron, C. C., Bilan, P. J., Tsakiridis, T., and Tsiani, E. (2016). Facilitative glucose transporters: implications for cancer detection, prognosis and treatment. Metabolism 65, 124–139. doi: 10.1016/j.metabol.2015.10.007
Barros, R. P., Machado, U. F., Warner, M., and Gustafsson, J. A. (2006). Muscle GLUT4 regulation by estrogen receptors ERbeta and ERalpha. Proc. Natl. Acad. Sci. U. S. A. 103, 1605–1608. doi: 10.1073/pnas.0510391103
Barros, R. P., Morani, A., Moriscot, A., and Machado, U. F. (2008). Insulin resistance of pregnancy involves estrogen-induced repression of muscle GLUT4. Mol. Cell Endocrinol. 295, 24–31. doi: 10.1016/j.mce.2008.07.008
Beckwith, H., and Yee, D. (2014). Insulin-like growth factors, insulin, and growth hormone signaling in breast cancer: implications for targeted therapy. Endocr. Pract. 20, 1214–1221. doi: 10.4158/ep14208.Ra
Beg, M., Abdullah, N., Thowfeik, F. S., Altorki, N. K., and McGraw, T. E. (2017). Distinct Akt phosphorylation states are required for insulin regulated Glut4 and Glut1-mediated glucose uptake. Elife 6:e26896. doi: 10.7554/eLife.26896
Benesch, C., Schneider, C., Voelker, H. U., Kapp, M., Caffier, H., Krockenberger, M., et al. (2010). The clinicopathological and prognostic relevance of pyruvate kinase M2 and pAkt expression in breast cancer. Anticancer Res. 30, 1689–1694.
Benito, A., Polat, I. H., Noé, V., Ciudad, C. J., Marin, S., and Cascante, M. (2017). Glucose-6-phosphate dehydrogenase and transketolase modulate breast cancer cell metabolic reprogramming and correlate with poor patient outcome. Oncotarget 8, 106693–106706. doi: 10.18632/oncotarget.21601
Bilan, P. J., Mitsumoto, Y., Maher, F., Simpson, I. A., and Klip, A. (1992). Detection of the GLUT3 facilitative glucose transporter in rat L6 muscle cells: regulation by cellular differentiation, insulin and insulin-like growth factor-I. Biochem. Biophys. Res. Commun. 186, 1129–1137. doi: 10.1016/0006-291x(92)90864-h
Binder, C., Binder, L., Marx, D., Schauer, A., and Hiddemann, W. (1997). Deregulated simultaneous expression of multiple glucose transporter isoforms in malignant cells and tissues. Anticancer Res. 17, 4299–4304.
Bohndiek, S. E., and Brindle, K. M. (2010). Imaging and ‘omic’ methods for the molecular diagnosis of cancer. Expert. Rev. Mol. Diagn. 10, 417–434. doi: 10.1586/erm.10.20
Borri, F., and Granaglia, A. (2020). Pathology of triple negative breast cancer. Semin. Cancer Biol. 72, 136–145. doi: 10.1016/j.semcancer.2020.06.005
Brauer, H. A., Makowski, L., Hoadley, K. A., Casbas-Hernandez, P., Lang, L. J., Romàn-Pèrez, E., et al. (2013). Impact of tumor microenvironment and epithelial phenotypes on metabolism in breast cancer. Clin. Cancer Res. 19, 571–585. doi: 10.1158/1078-0432.Ccr-12-2123
Bray, F., Ferlay, J., Soerjomataram, I., Siegel, R. L., Torre, L. A., and Jemal, A. (2018). Global cancer statistics 2018: GLOBOCAN estimates of incidence and mortality worldwide for 36 cancers in 185 countries. CA Cancer J. Clin. 68, 394–424. doi: 10.3322/caac.21492
Bray, F., McCarron, P., and Parkin, D. M. (2004). The changing global patterns of female breast cancer incidence and mortality. Breast Cancer Res. 6, 229–239. doi: 10.1186/bcr932
Brown, R. S., Goodman, T. M., Zasadny, K. R., Greenson, J. K., and Wahl, R. L. (2002). Expression of hexokinase II and Glut-1 in untreated human breast cancer. Nucl. Med. Biol. 29, 443–453. doi: 10.1016/s0969-8051(02)00288-3
Brown, R. S., and Wahl, R. L. (1993). Overexpression of Glut-1 glucose transporter in human breast cancer. An immunohistochemical study. Cancer 72, 2979–2985. doi: 10.1002/1097-0142(19931115)72:10<2979::aid-cncr2820721020<3.0.co;2-x
Bruning, P. F., Bonfrèr, J. M., van Noord, P. A., Hart, A. A., de Jong-Bakker, M., and Nooijen, W. J. (1992). Insulin resistance and breast-cancer risk. Int. J. Cancer 52, 511–516. doi: 10.1002/ijc.2910520402
Bulut, G., Atmaca, H., and Karaca, B. (2020). Trastuzumab in combination with AT-101 induces cytotoxicity and apoptosis in Her2 positive breast cancer cells. Future Oncol. 16, 4485–4495. doi: 10.2217/fon-2019-0521
Burke, B., Giannoudis, A., Corke, K. P., Gill, D., Wells, M., Ziegler-Heitbrock, L., et al. (2003). Hypoxia-induced gene expression in human macrophages: implications for ischemic tissues and hypoxia-regulated gene therapy. Am. J. Pathol. 163, 1233–1243. doi: 10.1016/s0002-9440(10)63483-9
Burstein, M. D., Tsimelzon, A., Poage, G. M., Covington, K. R., Contreras, A., Fuqua, S. A., et al. (2015). Comprehensive genomic analysis identifies novel subtypes and targets of triple-negative breast cancer. Clin. Cancer Res. 21, 1688–1698. doi: 10.1158/1078-0432.Ccr-14-0432
Butt, A. J., Sergio, C. M., Inman, C. K., Anderson, L. R., McNeil, C. M., Russell, A. J., et al. (2008). The estrogen and c-Myc target gene HSPC111 is over-expressed in breast cancer and associated with poor patient outcome. Breast Cancer Res. 10:R28. doi: 10.1186/bcr1985
Cai, C. F., Ye, G. D., Shen, D. Y., Zhang, W., Chen, M. L., Chen, X. X., et al. (2018). Chibby suppresses aerobic glycolysis and proliferation of nasopharyngeal carcinoma via the Wnt/β-catenin-Lin28/let7-PDK1 cascade. J. Exp. Clin. Cancer Res. 37:104. doi: 10.1186/s13046-018-0769-4
Caresia Aroztegui, A. P., García Vicente, A. M., Alvarez Ruiz, S., Delgado Bolton, R. C., Orcajo Rincon, J., Garcia Garzon, J. R., et al. (2017). 18F-FDG PET/CT in breast cancer: evidence-based recommendations in initial staging. Tumour Biol. 39:1010428317728285. doi: 10.1177/1010428317728285
Carey, L., Winer, E., Viale, G., Cameron, D., and Gianni, L. (2010). Triple-negative breast cancer: disease entity or title of convenience? Nat. Rev. Clin. Oncol. 7, 683–692. doi: 10.1038/nrclinonc.2010.154
Castaneda, C. A., Cortes-Funes, H., Gomez, H. L., and Ciruelos, E. M. (2010). The phosphatidyl inositol 3-kinase/AKT signaling pathway in breast cancer. Cancer Metastasis Rev. 29, 751–759. doi: 10.1007/s10555-010-9261-0
Cha, Y. J., Jung, W. H., and Koo, J. S. (2017). Differential Site-Based Expression of Pentose Phosphate Pathway-Related Proteins among Breast Cancer Metastases. Dis. Markers 2017:7062517. doi: 10.1155/2017/7062517
Chen, E. I., Hewel, J., Krueger, J. S., Tiraby, C., Weber, M. R., Kralli, A., et al. (2007). Adaptation of energy metabolism in breast cancer brain metastases. Cancer Res. 67, 1472–1486. doi: 10.1158/0008-5472.Can-06-3137
Chen, F., Chen, J., Yang, L., Liu, J., Zhang, X., Zhang, Y., et al. (2019). Extracellular vesicle-packaged HIF-1α-stabilizing lncRNA from tumour-associated macrophages regulates aerobic glycolysis of breast cancer cells. Nat. Cell Biol. 21, 498–510. doi: 10.1038/s41556-019-0299-0
Chen, P., Zuo, H., Xiong, H., Kolar, M. J., Chu, Q., Saghatelian, A., et al. (2017). Gpr132 sensing of lactate mediates tumor-macrophage interplay to promote breast cancer metastasis. Proc. Natl. Acad. Sci. U. S. A. 114, 580–585. doi: 10.1073/pnas.1614035114
Chen, Q., Meng, Y. Q., Xu, X. F., and Gu, J. (2017). Blockade of GLUT1 by WZB117 resensitizes breast cancer cells to adriamycin. Anticancer Drugs 28, 880–887. doi: 10.1097/cad.0000000000000529
Chen, Y., Wei, L., Zhang, X., Liu, X., Chen, Y., Zhang, S., et al. (2018). 3-Bromopyruvate sensitizes human breast cancer cells to TRAIL-induced apoptosis via the phosphorylated AMPK-mediated upregulation of DR5. Oncol. Rep. 40, 2435–2444. doi: 10.3892/or.2018.6644
Cheong, H. (2015). Integrating autophagy and metabolism in cancer. Arch. Pharm. Res. 38, 358–371. doi: 10.1007/s12272-015-0562-2
Choi, J., Gyamfi, J., Jang, H., and Koo, J. S. (2018a). The role of tumor-associated macrophage in breast cancer biology. Histol. Histopathol. 33, 133–145. doi: 10.14670/hh-11-916
Choi, J., Kim, E. S., and Koo, J. S. (2018b). Expression of Pentose Phosphate Pathway-Related Proteins in Breast Cancer. Dis. Markers 2018:9369358. doi: 10.1155/2018/9369358
Choi, J., Jung, W. H., and Koo, J. S. (2013). Metabolism-related proteins are differentially expressed according to the molecular subtype of invasive breast cancer defined by surrogate immunohistochemistry. Pathobiology 80, 41–52. doi: 10.1159/000339513
Christofk, H. R., Vander Heiden, M. G., Harris, M. H., Ramanathan, A., Gerszten, R. E., Wei, R., et al. (2008). The M2 splice isoform of pyruvate kinase is important for cancer metabolism and tumour growth. Nature 452, 230–233. doi: 10.1038/nature06734
Colegio, O. R., Chu, N. Q., Szabo, A. L., Chu, T., Rhebergen, A. M., Jairam, V., et al. (2014). Functional polarization of tumour-associated macrophages by tumour-derived lactic acid. Nature 513, 559–563. doi: 10.1038/nature13490
Conover, C. A., Lee, P. D., Kanaley, J. A., Clarkson, J. T., and Jensen, M. D. (1992). Insulin regulation of insulin-like growth factor binding protein-1 in obese and nonobese humans. J. Clin. Endocrinol. Metab. 74, 1355–1360. doi: 10.1210/jcem.74.6.1375600
Corbet, C., and Feron, O. (2017). Cancer cell metabolism and mitochondria: nutrient plasticity for TCA cycle fueling. Biochim. Biophys. Acta Rev. Cancer 1868, 7–15. doi: 10.1016/j.bbcan.2017.01.002
Coyle, T., Levante, S., Shetler, M., and Winfield, J. (1994). In vitro and in vivo cytotoxicity of gossypol against central nervous system tumor cell lines. J. Neurooncol. 19, 25–35. doi: 10.1007/bf01051046
Cybulski, N., and Hall, M. N. (2009). TOR complex 2: a signaling pathway of its own. Trends Biochem. Sci. 34, 620–627. doi: 10.1016/j.tibs.2009.09.004
De Blasio, A., Di Fiore, R., Pratelli, G., Drago-Ferrante, R., Saliba, C., Baldacchino, S., et al. (2020). A loop involving NRF2, miR-29b-1-5p and AKT, regulates cell fate of MDA-MB-231 triple-negative breast cancer cells. J. Cell Physiol. 235, 629–637. doi: 10.1002/jcp.29062
Debeb, B. G., Lacerda, L., Larson, R., Wolfe, A. R., Krishnamurthy, S., Reuben, J. M., et al. (2016). Histone deacetylase inhibitor-induced cancer stem cells exhibit high pentose phosphate pathway metabolism. Oncotarget 7, 28329–28339. doi: 10.18632/oncotarget.8631
Denko, N. C. (2008). Hypoxia, HIF1 and glucose metabolism in the solid tumour. Nat. Rev. Cancer 8, 705–713. doi: 10.1038/nrc2468
DeSantis, C. E., Bray, F., Ferlay, J., Lortet-Tieulent, J., Anderson, B. O., and Jemal, A. (2015). International Variation in Female Breast Cancer Incidence and Mortality Rates. Cancer Epidemiol. Biomarkers Prev. 24, 1495–1506. doi: 10.1158/1055-9965.Epi-15-0535
Doherty, J. R., and Cleveland, J. L. (2013). Targeting lactate metabolism for cancer therapeutics. J. Clin. Invest. 123, 3685–3692. doi: 10.1172/jci69741
Dong, C., Yuan, T., Wu, Y., Wang, Y., Fan, T. W., Miriyala, S., et al. (2013). Loss of FBP1 by Snail-mediated repression provides metabolic advantages in basal-like breast cancer. Cancer Cell 23, 316–331. doi: 10.1016/j.ccr.2013.01.022
Dong, T., Kang, X., Liu, Z., Zhao, S., Ma, W., Xuan, Q., et al. (2016). Altered glycometabolism affects both clinical features and prognosis of triple-negative and neoadjuvant chemotherapy-treated breast cancer. Tumour Biol. 37, 8159–8168. doi: 10.1007/s13277-015-4729-8
Dong, T., Liu, Z., Xuan, Q., Wang, Z., Ma, W., and Zhang, Q. (2017). Tumor LDH-A expression and serum LDH status are two metabolic predictors for triple negative breast cancer brain metastasis. Sci. Rep. 7:6069. doi: 10.1038/s41598-017-06378-7
Douard, V., and Ferraris, R. P. (2008). Regulation of the fructose transporter GLUT5 in health and disease. Am. J. Physiol. Endocrinol. Metab. 295, E227–E237. doi: 10.1152/ajpendo.90245.2008
Doyen, J., Trastour, C., Ettore, F., Peyrottes, I., Toussant, N., Gal, J., et al. (2014). Expression of the hypoxia-inducible monocarboxylate transporter MCT4 is increased in triple negative breast cancer and correlates independently with clinical outcome. Biochem. Biophys. Res. Commun. 451, 54–61. doi: 10.1016/j.bbrc.2014.07.050
Dupuy, F., Tabariès, S., Andrzejewski, S., Dong, Z., Blagih, J., Annis, M. G., et al. (2015). PDK1-Dependent Metabolic Reprogramming Dictates Metastatic Potential in Breast Cancer. Cell Metab. 22, 577–589. doi: 10.1016/j.cmet.2015.08.007
Düvel, K., Yecies, J. L., Menon, S., Raman, P., Lipovsky, A. I., Souza, A. L., et al. (2010). Activation of a metabolic gene regulatory network downstream of mTOR complex 1. Mol. Cell 39, 171–183. doi: 10.1016/j.molcel.2010.06.022
Engelman, J. A., Luo, J., and Cantley, L. C. (2006). The evolution of phosphatidylinositol 3-kinases as regulators of growth and metabolism. Nat. Rev. Genet. 7, 606–619. doi: 10.1038/nrg1879
Farabegoli, F., Vettraino, M., Manerba, M., Fiume, L., Roberti, M., and Di Stefano, G. (2012). Galloflavin, a new lactate dehydrogenase inhibitor, induces the death of human breast cancer cells with different glycolytic attitude by affecting distinct signaling pathways. Eur. J. Pharm. Sci. 47, 729–738. doi: 10.1016/j.ejps.2012.08.012
Fu, Y., Liu, S., Yin, S., Niu, W., Xiong, W., Tan, M., et al. (2017). The reverse Warburg effect is likely to be an Achilles’ heel of cancer that can be exploited for cancer therapy. Oncotarget 8, 57813–57825. doi: 10.18632/oncotarget.18175
Furuta, E., Okuda, H., Kobayashi, A., and Watabe, K. (2010). Metabolic genes in cancer: their roles in tumor progression and clinical implications. Biochim. Biophys. Acta 1805, 141–152. doi: 10.1016/j.bbcan.2010.01.005
Gao, S., Ge, A., Xu, S., You, Z., Ning, S., Zhao, Y., et al. (2017). PSAT1 is regulated by ATF4 and enhances cell proliferation via the GSK3β/β-catenin/cyclin D1 signaling pathway in ER-negative breast cancer. J. Exp. Clin. Cancer Res. 36:179. doi: 10.1186/s13046-017-0648-4
García-Castillo, V., López-Urrutia, E., Villanueva-Sánchez, O., Ávila-Rodríguez, M., Zentella-Dehesa, A., Cortés-González, C., et al. (2017). Targeting Metabolic Remodeling in Triple Negative Breast Cancer in a Murine Model. J. Cancer 8, 178–189. doi: 10.7150/jca.16387
García-Martínez, J. M., and Alessi, D. R. (2008). mTOR complex 2 (mTORC2) controls hydrophobic motif phosphorylation and activation of serum- and glucocorticoid-induced protein kinase 1 (SGK1). Biochem. J. 416, 375–385. doi: 10.1042/bj20081668
Garrido, P., Morán, J., Alonso, A., González, S., and González, C. (2013). 17β-estradiol activates glucose uptake via GLUT4 translocation and PI3K/Akt signaling pathway in MCF-7 cells. Endocrinology 154, 1979–1989. doi: 10.1210/en.2012-1558
Gayosso, V., Montano, L. F., and López-Marure, R. (2006). DHEA-induced antiproliferative effect in MCF-7 cells is androgen- and estrogen receptor-independent. Cancer J. 12, 160–165.
Geeraerts, S. L., Heylen, E., De Keersmaecker, K., and Kampen, K. R. (2021a). The ins and outs of serine and glycine metabolism in cancer. Nat. Metab. 3, 131–141. doi: 10.1038/s42255-020-00329-9
Geeraerts, S. L., Kampen, K. R., Rinaldi, G., Gupta, P., Planque, M., Louros, N., et al. (2021b). Repurposing the Antidepressant Sertraline as SHMT Inhibitor to Suppress Serine/Glycine Synthesis-Addicted Breast Tumor Growth. Mol. Cancer Ther. 20, 50–63. doi: 10.1158/1535-7163.Mct-20-0480
Ghanavat, M., Shahrouzian, M., Deris Zayeri, Z., Banihashemi, S., Kazemi, S. M., and Saki, N. (2021). Digging deeper through glucose metabolism and its regulators in cancer and metastasis. Life Sci. 264:118603. doi: 10.1016/j.lfs.2020.118603
Giatromanolaki, A., Koukourakis, M. I., Simopoulos, C., Polychronidis, A., Gatter, K. C., Harris, A. L., et al. (2004). c-erbB-2 related aggressiveness in breast cancer is hypoxia inducible factor-1alpha dependent. Clin. Cancer Res. 10, 7972–7977. doi: 10.1158/1078-0432.Ccr-04-1068
Gilbert, N. E., O’Reilly, J. E., Chang, C. J., Lin, Y. C., and Brueggemeier, R. W. (1995). Antiproliferative activity of gossypol and gossypolone on human breast cancer cells. Life Sci. 57, 61–67. doi: 10.1016/0024-3205(95)00243-y
Godoy, A., Ulloa, V., Rodríguez, F., Reinicke, K., Yañez, A. J., García Mde, L., et al. (2006). Differential subcellular distribution of glucose transporters GLUT1-6 and GLUT9 in human cancer: ultrastructural localization of GLUT1 and GLUT5 in breast tumor tissues. J. Cell Physiol. 207, 614–627. doi: 10.1002/jcp.20606
Gomez, L. S., Zancan, P., Marcondes, M. C., Ramos-Santos, L., Meyer-Fernandes, J. R., Sola-Penna, M., et al. (2013). Resveratrol decreases breast cancer cell viability and glucose metabolism by inhibiting 6-phosphofructo-1-kinase. Biochimie 95, 1336–1343. doi: 10.1016/j.biochi.2013.02.013
Gorres, B. K., Bomhoff, G. L., Morris, J. K., and Geiger, P. C. (2011). In vivo stimulation of oestrogen receptor α increases insulin-stimulated skeletal muscle glucose uptake. J. Physiol. 589, 2041–2054. doi: 10.1113/jphysiol.2010.199018
Grinde, M. T., Moestue, S. A., Borgan, E., Risa, Ø, Engebraaten, O., and Gribbestad, I. S. (2011). 13C high-resolution-magic angle spinning MRS reveals differences in glucose metabolism between two breast cancer xenograft models with different gene expression patterns. NMR Biomed. 24, 1243–1252. doi: 10.1002/nbm.1683
Groheux, D., Cochet, A., Humbert, O., Alberini, J. L., Hindié, E., and Mankoff, D. (2016). 18F-FDG PET/CT for Staging and Restaging of Breast Cancer. J. Nucl. Med. 57, 17s–26s. doi: 10.2967/jnumed.115.157859
Grover-McKay, M., Walsh, S. A., Seftor, E. A., Thomas, P. A., and Hendrix, M. J. (1998). Role for glucose transporter 1 protein in human breast cancer. Pathol. Oncol. Res. 4, 115–120. doi: 10.1007/bf02904704
Guedes, M., Araújo, J. R., Correia-Branco, A., Gregório, I., Martel, F., and Keating, E. (2016). Modulation of the uptake of critical nutrients by breast cancer cells by lactate: impact on cell survival, proliferation and migration. Exp. Cell Res. 341, 111–122. doi: 10.1016/j.yexcr.2016.01.008
Gunter, M. J., Xie, X., Xue, X., Kabat, G. C., Rohan, T. E., Wassertheil-Smoller, S., et al. (2015). Breast cancer risk in metabolically healthy but overweight postmenopausal women. Cancer Res. 75, 270–274. doi: 10.1158/0008-5472.Can-14-2317
Halestrap, A. P., Connern, C. P., Griffiths, E. J., and Kerr, P. M. (1997). Cyclosporin A binding to mitochondrial cyclophilin inhibits the permeability transition pore and protects hearts from ischaemia/reperfusion injury. Mol. Cell Biochem. 174, 167–172. doi: 10.1007/978-1-4615-6111-8_25
Han, J., Zhang, L., Guo, H., Wysham, W. Z., Roque, D. R., Willson, A. K., et al. (2015). Glucose promotes cell proliferation, glucose uptake and invasion in endometrial cancer cells via AMPK/mTOR/S6 and MAPK signaling. Gynecol. Oncol. 138, 668–675. doi: 10.1016/j.ygyno.2015.06.036
Hara, K., Maruki, Y., Long, X., Yoshino, K., Oshiro, N., Hidayat, S., et al. (2002). Raptor, a binding partner of target of rapamycin (TOR), mediates TOR action. Cell 110, 177–189. doi: 10.1016/s0092-8674(02)00833-4
Hare, S. H., and Harvey, A. J. (2017). mTOR function and therapeutic targeting in breast cancer. Am. J. Cancer Res. 7, 383–404.
Harmon, A. W., and Patel, Y. M. (2004). Naringenin inhibits glucose uptake in MCF-7 breast cancer cells: a mechanism for impaired cellular proliferation. Breast Cancer Res. Treat 85, 103–110. doi: 10.1023/B:BREA.0000025397.56192.e2
Hibdon, E. S., Razumilava, N., Keeley, T. M., Wong, G., Solanki, S., Shah, Y. M., et al. (2019). Notch and mTOR Signaling Pathways Promote Human Gastric Cancer Cell Proliferation. Neoplasia 21, 702–712. doi: 10.1016/j.neo.2019.05.002
Hollmén, M., Roudnicky, F., Karaman, S., and Detmar, M. (2015). Characterization of macrophage–cancer cell crosstalk in estrogen receptor positive and triple-negative breast cancer. Sci. Rep. 5:9188. doi: 10.1038/srep09188
Holman, G. D. (2020). Structure, function and regulation of mammalian glucose transporters of the SLC2 family. Pflugers Arch. 472, 1155–1175. doi: 10.1007/s00424-020-02411-3
Hsieh, A. L., Walton, Z. E., Altman, B. J., Stine, Z. E., and Dang, C. V. (2015). MYC and metabolism on the path to cancer. Semin. Cell Dev. Biol. 43, 11–21. doi: 10.1016/j.semcdb.2015.08.003
Huang, X., Li, X., Xie, X., Ye, F., Chen, B., Song, C., et al. (2016). High expressions of LDHA and AMPK as prognostic biomarkers for breast cancer. Breast 30, 39–46. doi: 10.1016/j.breast.2016.08.014
Hui, S., Ghergurovich, J. M., Morscher, R. J., Jang, C., Teng, X., Lu, W., et al. (2017). Glucose feeds the TCA cycle via circulating lactate. Nature 551, 115–118. doi: 10.1038/nature24057
Hussein, Y. R., Bandyopadhyay, S., Semaan, A., Ahmed, Q., Albashiti, B., Jazaerly, T., et al. (2011). Glut-1 Expression Correlates with Basal-like Breast Cancer. Transl. Oncol. 4, 321–327. doi: 10.1593/tlo.11256
Ikenoue, T., Inoki, K., Yang, Q., Zhou, X., and Guan, K. L. (2008). Essential function of TORC2 in PKC and Akt turn motif phosphorylation, maturation and signalling. EMBO J. 27, 1919–1931. doi: 10.1038/emboj.2008.119
Irey, E. A., Lassiter, C. M., Brady, N. J., Chuntova, P., Wang, Y., Knutson, T. P., et al. (2019). JAK/STAT inhibition in macrophages promotes therapeutic resistance by inducing expression of protumorigenic factors. Proc. Natl. Acad. Sci. U. S. A. 116, 12442–12451. doi: 10.1073/pnas.1816410116
Jia, D., Lu, M., Jung, K. H., Park, J. H., Yu, L., Onuchic, J. N., et al. (2019). Elucidating cancer metabolic plasticity by coupling gene regulation with metabolic pathways. Proc. Natl. Acad. Sci. U. S. A. 116, 3909–3918. doi: 10.1073/pnas.1816391116
Jia, D., Park, J. H., Jung, K. H., Levine, H., and Kaipparettu, B. A. (2018). Elucidating the Metabolic Plasticity of Cancer: mitochondrial Reprogramming and Hybrid Metabolic States. Cells 7:21. doi: 10.3390/cells7030021
Jia, L., Huang, S., Yin, X., Zan, Y., Guo, Y., and Han, L. (2018). Quercetin suppresses the mobility of breast cancer by suppressing glycolysis through Akt-mTOR pathway mediated autophagy induction. Life Sci. 208, 123–130. doi: 10.1016/j.lfs.2018.07.027
Jiang, K., He, B., Lai, L., Chen, Q., Liu, Y., Guo, Q., et al. (2012). Cyclosporine A inhibits breast cancer cell growth by downregulating the expression of pyruvate kinase subtype M2. Int. J. Mol. Med. 30, 302–308. doi: 10.3892/ijmm.2012.989
Jiang, S., Zhang, L. F., Zhang, H. W., Hu, S., Lu, M. H., Liang, S., et al. (2012). A novel miR-155/miR-143 cascade controls glycolysis by regulating hexokinase 2 in breast cancer cells. EMBO J. 31, 1985–1998. doi: 10.1038/emboj.2012.45
Jin, M. S., Lee, H., Park, I. A., Chung, Y. R., Im, S. A., Lee, K. H., et al. (2016). Overexpression of HIF1α and CAXI predicts poor outcome in early-stage triple negative breast cancer. Virchows Arch. 469, 183–190. doi: 10.1007/s00428-016-1953-6
Johnson, J. M., Cotzia, P., Fratamico, R., Mikkilineni, L., Chen, J., Colombo, D., et al. (2017). MCT1 in Invasive Ductal Carcinoma: monocarboxylate Metabolism and Aggressive Breast Cancer. Front. Cell Dev. Biol. 5:27. doi: 10.3389/fcell.2017.00027
Jung, K. H., Lee, J. H., Thien Quach, C. H., Paik, J. Y., Oh, H., Park, J. W., et al. (2013). Resveratrol suppresses cancer cell glucose uptake by targeting reactive oxygen species-mediated hypoxia-inducible factor-1α activation. J. Nucl. Med. 54, 2161–2167. doi: 10.2967/jnumed.112.115436
Kabat, G. C., Kim, M., Caan, B. J., Chlebowski, R. T., Gunter, M. J., Ho, G. Y., et al. (2009). Repeated measures of serum glucose and insulin in relation to postmenopausal breast cancer. Int. J. Cancer 125, 2704–2710. doi: 10.1002/ijc.24609
Kang, S. S., Chun, Y. K., Hur, M. H., Lee, H. K., Kim, Y. J., Hong, S. R., et al. (2002). Clinical significance of glucose transporter 1 (GLUT1) expression in human breast carcinoma. Jpn J. Cancer Res. 93, 1123–1128. doi: 10.1111/j.1349-7006.2002.tb01214.x
Katzenellenbogen, B. S., and Norman, M. J. (1990). Multihormonal regulation of the progesterone receptor in MCF-7 human breast cancer cells: interrelationships among insulin/insulin-like growth factor-I, serum, and estrogen. Endocrinology 126, 891–898. doi: 10.1210/endo-126-2-891
Kawauchi, K., Araki, K., Tobiume, K., and Tanaka, N. (2008). p53 regulates glucose metabolism through an IKK-NF-kappaB pathway and inhibits cell transformation. Nat. Cell Biol. 10, 611–618. doi: 10.1038/ncb1724
Kim, H. M., Jung, W. H., and Koo, J. S. (2014). Site-specific metabolic phenotypes in metastatic breast cancer. J. Transl. Med. 12:354. doi: 10.1186/s12967-014-0354-3
Kim, S. K., Jung, W. H., and Koo, J. S. (2014). Differential expression of enzymes associated with serine/glycine metabolism in different breast cancer subtypes. PLoS One 9:e101004. doi: 10.1371/journal.pone.0101004
Kim, Y. H., Jung, W. H., and Koo, J. S. (2014). Expression of metabolism-related proteins in invasive lobular carcinoma: comparison to invasive ductal carcinoma. Tumour Biol. 35, 10381–10393. doi: 10.1007/s13277-014-2345-7
Kim, S., Kim, D. H., Jung, W. H., and Koo, J. S. (2013). Metabolic phenotypes in triple-negative breast cancer. Tumour Biol. 34, 1699–1712. doi: 10.1007/s13277-013-0707-1
Koboldt, D. C., Fulton, R. S., McLellan, M. D., Schmidt, H., Kalicki-Veizer, J., McMichael, J. F., et al. (2012). Comprehensive molecular portraits of human breast tumours. Nature 490, 61–70. doi: 10.1038/nature11412
Kruger, N. J., and von Schaewen, A. (2003). The oxidative pentose phosphate pathway: structure and organisation. Curr. Opin. Plant Biol. 6, 236–246. doi: 10.1016/s1369-5266(03)00039-6
Krzeslak, A., Wojcik-Krowiranda, K., Forma, E., Jozwiak, P., Romanowicz, H., Bienkiewicz, A., et al. (2012). Expression of GLUT1 and GLUT3 glucose transporters in endometrial and breast cancers. Pathol. Oncol. Res. 18, 721–728. doi: 10.1007/s12253-012-9500-5
Kumar, P., and Aggarwal, R. (2016). An overview of triple-negative breast cancer. Arch. Gynecol. Obstet. 293, 247–269. doi: 10.1007/s00404-015-3859-y
Labrie, F., Luu-The, V., Labrie, C., and Simard, J. (2001). DHEA and its transformation into androgens and estrogens in peripheral target tissues: intracrinology. Front. Neuroendocrinol. 22, 185–212. doi: 10.1006/frne.2001.0216
Labuschagne, C. F., van den Broek, N. J., Mackay, G. M., Vousden, K. H., and Maddocks, O. D. (2014). Serine, but not glycine, supports one-carbon metabolism and proliferation of cancer cells. Cell Rep. 7, 1248–1258. doi: 10.1016/j.celrep.2014.04.045
Lai, X., Li, Q., Wu, F., Lin, J., Chen, J., Zheng, H., et al. (2020). Epithelial-Mesenchymal Transition and Metabolic Switching in Cancer: lessons From Somatic Cell Reprogramming. Front. Cell Dev. Biol. 8:760. doi: 10.3389/fcell.2020.00760
Lanning, N. J., Castle, J. P., Singh, S. J., Leon, A. N., Tovar, E. A., Sanghera, A., et al. (2017). Metabolic profiling of triple-negative breast cancer cells reveals metabolic vulnerabilities. Cancer Metab. 5:6. doi: 10.1186/s40170-017-0168-x
Lawlor, D. A., Smith, G. D., and Ebrahim, S. (2004). Hyperinsulinaemia and increased risk of breast cancer: findings from the British Women’s Heart and Health Study. Cancer Causes Control 15, 267–275. doi: 10.1023/B:CACO.0000024225.14618.a8
LeBleu, V. S., O’Connell, J. T., Gonzalez Herrera, K. N., Wikman, H., Pantel, K., Haigis, M. C., et al. (2014). PGC-1α mediates mitochondrial biogenesis and oxidative phosphorylation in cancer cells to promote metastasis. Nat. Cell Biol. 16, 992–1003. doi: 10.1038/ncb3039
Lee, J. H., Liu, R., Li, J., Wang, Y., Tan, L., Li, X. J., et al. (2018). EGFR-Phosphorylated Platelet Isoform of Phosphofructokinase 1 Promotes PI3K Activation. Mol. Cell 70, 197–210.e7. doi: 10.1016/j.molcel.2018.03.018
Lee, M., and Yoon, J. H. (2015). Metabolic interplay between glycolysis and mitochondrial oxidation: the reverse Warburg effect and its therapeutic implication. World J. Biol. Chem. 6, 148–161. doi: 10.4331/wjbc.v6.i3.148
Lee, Y. R., Park, J., Yu, H. N., Kim, J. S., Youn, H. J., and Jung, S. H. (2005). Up-regulation of PI3K/Akt signaling by 17beta-estradiol through activation of estrogen receptor-alpha, but not estrogen receptor-beta, and stimulates cell growth in breast cancer cells. Biochem. Biophys. Res. Commun. 336, 1221–1226. doi: 10.1016/j.bbrc.2005.08.256
Lehmann, B. D., Bauer, J. A., Chen, X., Sanders, M. E., Chakravarthy, A. B., Shyr, Y., et al. (2011). Identification of human triple-negative breast cancer subtypes and preclinical models for selection of targeted therapies. J. Clin. Invest. 121, 2750–2767. doi: 10.1172/jci45014
Leino, R. L., Gerhart, D. Z., van Bueren, A. M., McCall, A. L., and Drewes, L. R. (1997). Ultrastructural localization of GLUT 1 and GLUT 3 glucose transporters in rat brain. J. Neurosci. Res. 49, 617–626. doi: 10.1002/(sici)1097-4547(19970901)49:5<617::Aid-jnr12<3.0.Co;2-s
Levine, A. J., and Puzio-Kuter, A. M. (2010). The control of the metabolic switch in cancers by oncogenes and tumor suppressor genes. Science 330, 1340–1344. doi: 10.1126/science.1193494
Li, L. J., Li, G. W., and Xie, Y. (2019). [Regulatory effects of glabridin and quercetin on energy metabolism of breast cancer cells]. Zhongguo Zhong Yao Za Zhi 44, 3786–3791. doi: 10.19540/j.cnki.cjcmm.20190505.401
Li, X. B., Gu, J. D., and Zhou, Q. H. (2015). Review of aerobic glycolysis and its key enzymes - new targets for lung cancer therapy. Thorac Cancer 6, 17–24. doi: 10.1111/1759-7714.12148
Li, Z., Wu, Q., Sun, S., Wu, J., Li, J., Zhang, Y., et al. (2018). Monocarboxylate transporters in breast cancer and adipose tissue are novel biomarkers and potential therapeutic targets. Biochem. Biophys. Res. Commun. 501, 962–967. doi: 10.1016/j.bbrc.2018.05.091
Lin, A., Li, C., Xing, Z., Hu, Q., Liang, K., Han, L., et al. (2016). The LINK-A lncRNA activates normoxic HIF1α signalling in triple-negative breast cancer. Nat. Cell Biol. 18, 213–224. doi: 10.1038/ncb3295
Lin, Y., Lv, F., Liu, F., Guo, X., Fan, Y., Gu, F., et al. (2015). High Expression of Pyruvate Kinase M2 is Associated with Chemosensitivity to Epirubicin and 5-Fluorouracil in Breast Cancer. J. Cancer 6, 1130–1139. doi: 10.7150/jca.12719
Liu, Y., Cao, Y., Zhang, W., Bergmeier, S., Qian, Y., Akbar, H., et al. (2012). A small-molecule inhibitor of glucose transporter 1 downregulates glycolysis, induces cell-cycle arrest, and inhibits cancer cell growth in vitro and in vivo. Mol. Cancer Ther. 11, 1672–1682. doi: 10.1158/1535-7163.Mct-12-0131
Liu, Z., Sun, Y., Hong, H., Zhao, S., Zou, X., Ma, R., et al. (2015). 3-bromopyruvate enhanced daunorubicin-induced cytotoxicity involved in monocarboxylate transporter 1 in breast cancer cells. Am. J. Cancer Res. 5, 2673–2685.
Liu, Z., Zhang, Y. Y., Zhang, Q. W., Zhao, S. R., Wu, C. Z., Cheng, X., et al. (2014). 3-Bromopyruvate induces apoptosis in breast cancer cells by downregulating Mcl-1 through the PI3K/Akt signaling pathway. Anticancer Drugs 25, 447–455. doi: 10.1097/cad.0000000000000081
Locasale, J. W. (2013). Serine, glycine and one-carbon units: cancer metabolism in full circle. Nat. Rev. Cancer 13, 572–583. doi: 10.1038/nrc3557
Locasale, J. W., Grassian, A. R., Melman, T., Lyssiotis, C. A., Mattaini, K. R., Bass, A. J., et al. (2011). Phosphoglycerate dehydrogenase diverts glycolytic flux and contributes to oncogenesis. Nat. Genet. 43, 869–874. doi: 10.1038/ng.890
López-Lázaro, M. (2008). The warburg effect: why and how do cancer cells activate glycolysis in the presence of oxygen? Anticancer Agents Med. Chem. 8, 305–312. doi: 10.2174/187152008783961932
López-Marure, R., Contreras, P. G., and Dillon, J. S. (2011). Effects of dehydroepiandrosterone on proliferation, migration, and death of breast cancer cells. Eur. J. Pharmacol. 660, 268–274. doi: 10.1016/j.ejphar.2011.03.040
Losada-Echeberría, M., Herranz-López, M., Micol, V., and Barrajón-Catalán, E. (2017). Polyphenols as Promising Drugs against Main Breast Cancer Signatures. Antioxidants (Basel) 6:88. doi: 10.3390/antiox6040088
Luo, M., Shang, L., Brooks, M. D., Jiagge, E., Zhu, Y., Buschhaus, J. M., et al. (2018). Targeting Breast Cancer Stem Cell State Equilibrium through Modulation of Redox Signaling. Cell Metab. 28, 69–86.e6. doi: 10.1016/j.cmet.2018.06.006
Ma, C., Zu, X., Liu, K., Bode, A. M., Dong, Z., Liu, Z., et al. (2019). Knockdown of Pyruvate Kinase M Inhibits Cell Growth and Migration by Reducing NF-kB Activity in Triple-Negative Breast Cancer Cells. Mol. Cells 42, 628–636. doi: 10.14348/molcells.2019.0038
MacQueen, G., Born, L., and Steiner, M. (2001). The selective serotonin reuptake inhibitor sertraline: its profile and use in psychiatric disorders. CNS Drug Rev. 7, 1–24. doi: 10.1111/j.1527-3458.2001.tb00188.x
Mantovani, A., Sozzani, S., Locati, M., Allavena, P., and Sica, A. (2002). Macrophage polarization: tumor-associated macrophages as a paradigm for polarized M2 mononuclear phagocytes. Trends Immunol. 23, 549–555. doi: 10.1016/s1471-4906(02)02302-5
Mao, Y., Keller, E. T., Garfield, D. H., Shen, K., and Wang, J. (2013). Stromal cells in tumor microenvironment and breast cancer. Cancer Metastasis Rev. 32, 303–315. doi: 10.1007/s10555-012-9415-3
Marsin, A. S., Bertrand, L., Rider, M. H., Deprez, J., Beauloye, C., Vincent, M. F., et al. (2000). Phosphorylation and activation of heart PFK-2 by AMPK has a role in the stimulation of glycolysis during ischaemia. Curr. Biol. 10, 1247–1255. doi: 10.1016/s0960-9822(00)00742-9
Martínez-Reyes, I., and Chandel, N. S. (2017). Waste Not, Want Not: lactate Oxidation Fuels the TCA Cycle. Cell Metab. 26, 803–804. doi: 10.1016/j.cmet.2017.11.005
McCleland, M. L., Adler, A. S., Shang, Y., Hunsaker, T., Truong, T., Peterson, D., et al. (2012). An integrated genomic screen identifies LDHB as an essential gene for triple-negative breast cancer. Cancer Res. 72, 5812–5823. doi: 10.1158/0008-5472.Can-12-1098
McVie-Wylie, A. J., Lamson, D. R., and Chen, Y. T. (2001). Molecular cloning of a novel member of the GLUT family of transporters, SLC2a10 (GLUT10), localized on chromosome 20q13.1: a candidate gene for NIDDM susceptibility. Genomics 72, 113–117. doi: 10.1006/geno.2000.6457
Messeha, S. S., Zarmouh, N. O., Mendonca, P., Alwagdani, H., Cotton, C., and Soliman, K. F. A. (2019). Effects of gossypol on apoptosis-related gene expression in racially distinct triple-negative breast cancer cells. Oncol. Rep. 42, 467–478. doi: 10.3892/or.2019.7179
Mishra, J., Davani, A. J., Natarajan, G. K., Kwok, W. M., Stowe, D. F., and Camara, A. K. S. (2019). Cyclosporin A Increases Mitochondrial Buffering of Calcium: an Additional Mechanism in Delaying Mitochondrial Permeability Transition Pore Opening. Cells 8:1052. doi: 10.3390/cells8091052
Mittal, S., Brown, N. J., and Holen, I. (2018). The breast tumor microenvironment: role in cancer development, progression and response to therapy. Expert. Rev. Mol. Diagn. 18, 227–243. doi: 10.1080/14737159.2018.1439382
Mocanu, M. M., Nagy, P., and Szöllõsi, J. (2015). Chemoprevention of Breast Cancer by Dietary Polyphenols. Molecules 20, 22578–22620. doi: 10.3390/molecules201219864
Moldogazieva, N. T., Mokhosoev, I. M., and Terentiev, A. A. (2020). Metabolic Heterogeneity of Cancer Cells: an Interplay between HIF-1, GLUTs, and AMPK. Cancers (Basel) 12:862. doi: 10.3390/cancers12040862
Moreira, L., Araújo, I., Costa, T., Correia-Branco, A., Faria, A., Martel, F., et al. (2013). Quercetin and epigallocatechin gallate inhibit glucose uptake and metabolism by breast cancer cells by an estrogen receptor-independent mechanism. Exp. Cell Res. 319, 1784–1795. doi: 10.1016/j.yexcr.2013.05.001
Mu, X., Shi, W., Xu, Y., Xu, C., Zhao, T., Geng, B., et al. (2018). Tumor-derived lactate induces M2 macrophage polarization via the activation of the ERK/STAT3 signaling pathway in breast cancer. Cell Cycle 17, 428–438. doi: 10.1080/15384101.2018.1444305
Mueckler, M., and Thorens, B. (2013). The SLC2 (GLUT) family of membrane transporters. Mol. Aspects Med. 34, 121–138. doi: 10.1016/j.mam.2012.07.001
Mullarky, E., Lucki, N. C., Beheshti Zavareh, R., Anglin, J. L., Gomes, A. P., Nicolay, B. N., et al. (2016). Identification of a small molecule inhibitor of 3-phosphoglycerate dehydrogenase to target serine biosynthesis in cancers. Proc. Natl. Acad. Sci. U. S. A. 113, 1778–1783. doi: 10.1073/pnas.1521548113
Niu, W., Huang, C., Nawaz, Z., Levy, M., Somwar, R., Li, D., et al. (2003). Maturation of the regulation of GLUT4 activity by p38 MAPK during L6 cell myogenesis. J. Biol. Chem. 278, 17953–17962. doi: 10.1074/jbc.M211136200
Noh, E. M., Lee, Y. R., Chay, K. O., Chung, E. Y., Jung, S. H., Kim, J. S., et al. (2011). Estrogen receptor α induces down-regulation of PTEN through PI3-kinase activation in breast cancer cells. Mol. Med Rep. 4, 215–219. doi: 10.3892/mmr.2011.412
Noh, S., Kim, D. H., Jung, W. H., and Koo, J. S. (2014). Expression levels of serine/glycine metabolism-related proteins in triple negative breast cancer tissues. Tumour Biol. 35, 4457–4468. doi: 10.1007/s13277-013-1588-z
Novellasdemunt, L., Tato, I., Navarro-Sabate, A., Ruiz-Meana, M., Méndez-Lucas, A., Perales, J. C., et al. (2013). Akt-dependent activation of the heart 6-phosphofructo-2-kinase/fructose-2,6-bisphosphatase (PFKFB2) isoenzyme by amino acids. J. Biol. Chem. 288, 10640–10651. doi: 10.1074/jbc.M113.455998
Oh, S., Kim, H., Nam, K., and Shin, I. (2017). Glut1 promotes cell proliferation, migration and invasion by regulating epidermal growth factor receptor and integrin signaling in triple-negative breast cancer cells. BMB Rep. 50, 132–137. doi: 10.5483/bmbrep.2017.50.3.189
Okar, D. A., Manzano, A., Navarro-Sabatè, A., Riera, L., Bartrons, R., and Lange, A. J. (2001). PFK-2/FBPase-2: maker and breaker of the essential biofactor fructose-2,6-bisphosphate. Trends Biochem. Sci. 26, 30–35. doi: 10.1016/s0968-0004(00)01699-6
O’Neal, J., Clem, A., Reynolds, L., Dougherty, S., Imbert-Fernandez, Y., Telang, S., et al. (2016). Inhibition of 6-phosphofructo-2-kinase (PFKFB3) suppresses glucose metabolism and the growth of HER2+ breast cancer. Breast Cancer Res. Treat. 160, 29–40. doi: 10.1007/s10549-016-3968-8
Pacold, M. E., Brimacombe, K. R., Chan, S. H., Rohde, J. M., Lewis, C. A., Swier, L. J., et al. (2016). A PHGDH inhibitor reveals coordination of serine synthesis and one-carbon unit fate. Nat. Chem. Biol. 12, 452–458. doi: 10.1038/nchembio.2070
Palaskas, N., Larson, S. M., Schultz, N., Komisopoulou, E., Wong, J., Rohle, D., et al. (2011). 18F-fluorodeoxy-glucose positron emission tomography marks MYC-overexpressing human basal-like breast cancers. Cancer Res. 71, 5164–5174. doi: 10.1158/0008-5472.Can-10-4633
Pan, Y., Zheng, Q., Ni, W., Wei, Z., Yu, S., Jia, Q., et al. (2019). Breaking Glucose Transporter 1/Pyruvate Kinase M2 Glycolytic Loop Is Required for Cantharidin Inhibition of Metastasis in Highly Metastatic Breast Cancer. Front. Pharmacol. 10:590. doi: 10.3389/fphar.2019.00590
Park, J. H., Vithayathil, S., Kumar, S., Sung, P. L., Dobrolecki, L. E., Putluri, V., et al. (2016). Fatty Acid Oxidation-Driven Src Links Mitochondrial Energy Reprogramming and Oncogenic Properties in Triple-Negative Breast Cancer. Cell Rep. 14, 2154–2165. doi: 10.1016/j.celrep.2016.02.004
Pasanen, I., Lehtonen, S., Sormunen, R., Skarp, S., Lehtilahti, E., Pietilä, M., et al. (2016). Breast cancer carcinoma-associated fibroblasts differ from breast fibroblasts in immunological and extracellular matrix regulating pathways. Exp. Cell Res. 344, 53–66. doi: 10.1016/j.yexcr.2016.04.016
Patra, K. C., and Hay, N. (2014). The pentose phosphate pathway and cancer. Trends Biochem. Sci. 39, 347–354. doi: 10.1016/j.tibs.2014.06.005
Pavlides, S., Whitaker-Menezes, D., Castello-Cros, R., Flomenberg, N., Witkiewicz, A. K., Frank, P. G., et al. (2009). The reverse Warburg effect: aerobic glycolysis in cancer associated fibroblasts and the tumor stroma. Cell Cycle 8, 3984–4001. doi: 10.4161/cc.8.23.10238
Paydary, K., Seraj, S. M., Zadeh, M. Z., Emamzadehfard, S., Shamchi, S. P., Gholami, S., et al. (2019). The Evolving Role of FDG-PET/CT in the Diagnosis, Staging, and Treatment of Breast Cancer. Mol. Imaging Biol. 21, 1–10. doi: 10.1007/s11307-018-1181-3
Pelicano, H., Zhang, W., Liu, J., Hammoudi, N., Dai, J., Xu, R. H., et al. (2014). Mitochondrial dysfunction in some triple-negative breast cancer cell lines: role of mTOR pathway and therapeutic potential. Breast Cancer Res. 16:434. doi: 10.1186/s13058-014-0434-6
Peng, F., Li, Q., Sun, J. Y., Luo, Y., Chen, M., and Bao, Y. (2018). PFKFB3 is involved in breast cancer proliferation, migration, invasion and angiogenesis. Int. J. Oncol. 52, 945–954. doi: 10.3892/ijo.2018.4257
Pinheiro, C., Albergaria, A., Paredes, J., Sousa, B., Dufloth, R., Vieira, D., et al. (2010). Monocarboxylate transporter 1 is up-regulated in basal-like breast carcinoma. Histopathology 56, 860–867. doi: 10.1111/j.1365-2559.2010.03560.x
Pinheiro, C., Sousa, B., Albergaria, A., Paredes, J., Dufloth, R., Vieira, D., et al. (2011). GLUT1 and CAIX expression profiles in breast cancer correlate with adverse prognostic factors and MCT1 overexpression. Histol. Histopathol. 26, 1279–1286. doi: 10.14670/hh-26.1279
Possemato, R., Marks, K. M., Shaul, Y. D., Pacold, M. E., Kim, D., Birsoy, K., et al. (2011). Functional genomics reveal that the serine synthesis pathway is essential in breast cancer. Nature 476, 346–350. doi: 10.1038/nature10350
Preuss, J., Richardson, A. D., Pinkerton, A., Hedrick, M., Sergienko, E., Rahlfs, S., et al. (2013). Identification and characterization of novel human glucose-6-phosphate dehydrogenase inhibitors. J. Biomol. Screen 18, 286–297. doi: 10.1177/1087057112462131
Pu, H., Zhang, Q., Zhao, C., Shi, L., Wang, Y., Wang, J., et al. (2015). Overexpression of G6PD is associated with high risks of recurrent metastasis and poor progression-free survival in primary breast carcinoma. World J. Surg. Oncol. 13:323. doi: 10.1186/s12957-015-0733-0
Ralser, M., Wamelink, M. M., Struys, E. A., Joppich, C., Krobitsch, S., Jakobs, C., et al. (2008). A catabolic block does not sufficiently explain how 2-deoxy-D-glucose inhibits cell growth. Proc. Natl. Acad. Sci. U. S. A. 105, 17807–17811. doi: 10.1073/pnas.0803090105
Ramos-Martinez, J. I. (2017). The regulation of the pentose phosphate pathway: remember Krebs. Arch. Biochem. Biophys. 614, 50–52. doi: 10.1016/j.abb.2016.12.012
Rastogi, S., Banerjee, S., Chellappan, S., and Simon, G. R. (2007). Glut-1 antibodies induce growth arrest and apoptosis in human cancer cell lines. Cancer Lett. 257, 244–251. doi: 10.1016/j.canlet.2007.07.021
Ravazoula, P., Batistatou, A., Aletra, C., Ladopoulos, J., Kourounis, G., and Tzigounis, B. (2003). Immunohistochemical expression of glucose transporter Glut1 and cyclin D1 in breast carcinomas with negative lymph nodes. Eur. J. Gynaecol. Oncol. 24, 544–546.
Risha, Y., Minic, Z., Ghobadloo, S. M., and Berezovski, M. V. (2020). The proteomic analysis of breast cell line exosomes reveals disease patterns and potential biomarkers. Sci. Rep. 10:13572. doi: 10.1038/s41598-020-70393-4
Rivenzon-Segal, D., Margalit, R., and Degani, H. (2002). Glycolysis as a metabolic marker in orthotopic breast cancer, monitored by in vivo (13)C MRS. Am. J. Physiol. Endocrinol. Metab. 283, E623–E630. doi: 10.1152/ajpendo.00050.2002
Robey, I. F., Lien, A. D., Welsh, S. J., Baggett, B. K., and Gillies, R. J. (2005). Hypoxia-inducible factor-1alpha and the glycolytic phenotype in tumors. Neoplasia 7, 324–330. doi: 10.1593/neo.04430
Rogers, S., Docherty, S. E., Slavin, J. L., Henderson, M. A., and Best, J. D. (2003). Differential expression of GLUT12 in breast cancer and normal breast tissue. Cancer Lett. 193, 225–233. doi: 10.1016/s0304-3835(03)00010-7
Rogers, S., Macheda, M. L., Docherty, S. E., Carty, M. D., Henderson, M. A., Soeller, W. C., et al. (2002). Identification of a novel glucose transporter-like protein-GLUT-12. Am. J. Physiol. Endocrinol. Metab. 282, E733–E738. doi: 10.1152/ajpendo.2002.282.3.E733
Ronda, A. C., Buitrago, C., and Boland, R. (2010a). Role of estrogen receptors, PKC and Src in ERK2 and p38 MAPK signaling triggered by 17β-estradiol in skeletal muscle cells. J. Steroid Biochem. Mol. Biol. 122, 287–294. doi: 10.1016/j.jsbmb.2010.05.002
Ronda, A. C., Vasconsuelo, A., and Boland, R. (2010b). Extracellular-regulated kinase and p38 mitogen-activated protein kinases are involved in the antiapoptotic action of 17beta-estradiol in skeletal muscle cells. J. Endocrinol. 206, 235–246. doi: 10.1677/joe-09-0429
Rossouw, J. E., Anderson, G. L., Prentice, R. L., LaCroix, A. Z., Kooperberg, C., Stefanick, M. L., et al. (2002). Risks and benefits of estrogen plus progestin in healthy postmenopausal women: principal results From the Women’s Health Initiative randomized controlled trial. JAMA 288, 321–333. doi: 10.1001/jama.288.3.321
Salazar, G. (2018). NADPH Oxidases and Mitochondria in Vascular Senescence. Int. J. Mol. Sci. 19:1327. doi: 10.3390/ijms19051327
Samanta, D., Park, Y., Andrabi, S. A., Shelton, L. M., Gilkes, D. M., and Semenza, G. L. (2016). PHGDH Expression Is Required for Mitochondrial Redox Homeostasis, Breast Cancer Stem Cell Maintenance, and Lung Metastasis. Cancer Res. 76, 4430–4442. doi: 10.1158/0008-5472.Can-16-0530
Samih, N., Hovsepian, S., Aouani, A., Lombardo, D., and Fayet, G. (2000). Glut-1 translocation in FRTL-5 thyroid cells: role of phosphatidylinositol 3-kinase and N-glycosylation. Endocrinology 141, 4146–4155. doi: 10.1210/endo.141.11.7793
Santos, J. M., and Hussain, F. (2020). Higher Glucose Enhances Breast Cancer Cell Aggressiveness. Nutr. Cancer 72, 734–746. doi: 10.1080/01635581.2019.1654527
Santos, J. M., Khan, Z. S., Munir, M. T., Tarafdar, K., Rahman, S. M., and Hussain, F. (2018). Vitamin D(3) decreases glycolysis and invasiveness, and increases cellular stiffness in breast cancer cells. J. Nutr. Biochem. 53, 111–120. doi: 10.1016/j.jnutbio.2017.10.013
Sato-Tadano, A., Suzuki, T., Amari, M., Takagi, K., Miki, Y., Tamaki, K., et al. (2013). Hexokinase II in breast carcinoma: a potent prognostic factor associated with hypoxia-inducible factor-1α and Ki-67. Cancer Sci. 104, 1380–1388. doi: 10.1111/cas.12238
Schieber, M. S., and Chandel, N. S. (2013). ROS links glucose metabolism to breast cancer stem cell and EMT phenotype. Cancer Cell 23, 265–267. doi: 10.1016/j.ccr.2013.02.021
Semenza, G. L. (2017). Hypoxia-inducible factors: coupling glucose metabolism and redox regulation with induction of the breast cancer stem cell phenotype. EMBO J. 36, 252–259. doi: 10.15252/embj.201695204
Semenza, G. L., Roth, P. H., Fang, H. M., and Wang, G. L. (1994). Transcriptional regulation of genes encoding glycolytic enzymes by hypoxia-inducible factor 1. J. Biol. Chem. 269, 23757–23763. doi: 10.1016/s0021-9258(17)31580-6
Shen, L., O’Shea, J. M., Kaadige, M. R., Cunha, S., Wilde, B. R., Cohen, A. L., et al. (2015). Metabolic reprogramming in triple-negative breast cancer through Myc suppression of TXNIP. Proc. Natl. Acad. Sci. U. S. A. 112, 5425–5430. doi: 10.1073/pnas.1501555112
Shi, Y., Zhang, Y., Ran, F., Liu, J., Lin, J., Hao, X., et al. (2020). Let-7a-5p inhibits triple-negative breast tumor growth and metastasis through GLUT12-mediated warburg effect. Cancer Lett. 495, 53–65. doi: 10.1016/j.canlet.2020.09.012
Simões, R. V., Serganova, I. S., Kruchevsky, N., Leftin, A., Shestov, A. A., Thaler, H. T., et al. (2015). Metabolic plasticity of metastatic breast cancer cells: adaptation to changes in the microenvironment. Neoplasia 17, 671–684. doi: 10.1016/j.neo.2015.08.005
Simoncini, T., Hafezi-Moghadam, A., Brazil, D. P., Ley, K., Chin, W. W., and Liao, J. K. (2000). Interaction of oestrogen receptor with the regulatory subunit of phosphatidylinositol-3-OH kinase. Nature 407, 538–541. doi: 10.1038/35035131
Sitter, B., Bathen, T. F., Singstad, T. E., Fjøsne, H. E., Lundgren, S., Halgunset, J., et al. (2010). Quantification of metabolites in breast cancer patients with different clinical prognosis using HR MAS MR spectroscopy. NMR Biomed. 23, 424–431. doi: 10.1002/nbm.1478
Sonveaux, P., Végran, F., Schroeder, T., Wergin, M. C., Verrax, J., Rabbani, Z. N., et al. (2008). Targeting lactate-fueled respiration selectively kills hypoxic tumor cells in mice. J. Clin. Invest. 118, 3930–3942. doi: 10.1172/jci36843
Soysal, S. D., Tzankov, A., and Muenst, S. E. (2015). Role of the Tumor Microenvironment in Breast Cancer. Pathobiology 82, 142–152. doi: 10.1159/000430499
Stincone, A., Prigione, A., Cramer, T., Wamelink, M. M., Campbell, K., Cheung, E., et al. (2015). The return of metabolism: biochemistry and physiology of the pentose phosphate pathway. Biol. Rev. Camb. Philos. Soc. 90, 927–963. doi: 10.1111/brv.12140
Tseng, C. W., Kuo, W. H., Chan, S. H., Chan, H. L., Chang, K. J., and Wang, L. H. (2018). Transketolase Regulates the Metabolic Switch to Control Breast Cancer Cell Metastasis via the α-Ketoglutarate Signaling Pathway. Cancer Res. 78, 2799–2812. doi: 10.1158/0008-5472.Can-17-2906
Ueno, T., Utsumi, J., Toi, M., and Shimizu, K. (2015). Characteristic Gene Expression Profiles of Human Fibroblasts and Breast Cancer Cells in a Newly Developed Bilateral Coculture System. Biomed. Res. Int. 2015:960840. doi: 10.1155/2015/960840
Unterlass, J. E., Baslé, A., Blackburn, T. J., Tucker, J., Cano, C., Noble, M. E. M., et al. (2018). Validating and enabling phosphoglycerate dehydrogenase (PHGDH) as a target for fragment-based drug discovery in PHGDH-amplified breast cancer. Oncotarget 9, 13139–13153. doi: 10.18632/oncotarget.11487
Vargas, E., Podder, V., and Carrillo Sepulveda, M. A. (2021). Physiology, Glucose Transporter Type 4. Treasure Island: StatPearls Publishing.
Vivanco, I., and Sawyers, C. L. (2002). The phosphatidylinositol 3-Kinase AKT pathway in human cancer. Nat. Rev. Cancer 2, 489–501. doi: 10.1038/nrc839
Vousden, K. H., and Ryan, K. M. (2009). p53 and metabolism. Nat. Rev. Cancer 9, 691–700. doi: 10.1038/nrc2715
Wang, J., Duan, Z., Nugent, Z., Zou, J. X., Borowsky, A. D., Zhang, Y., et al. (2016). Reprogramming metabolism by histone methyltransferase NSD2 drives endocrine resistance via coordinated activation of pentose phosphate pathway enzymes. Cancer Lett. 378, 69–79. doi: 10.1016/j.canlet.2016.05.004
Wang, Q., Liberti, M. V., Liu, P., Deng, X., Liu, Y., Locasale, J. W., et al. (2017). Rational Design of Selective Allosteric Inhibitors of PHGDH and Serine Synthesis with Anti-tumor Activity. Cell Chem. Biol. 24, 55–65. doi: 10.1016/j.chembiol.2016.11.013
Wang, Z., Jiang, Q., and Dong, C. (2020). Metabolic reprogramming in triple-negative breast cancer. Cancer Biol. Med. 17, 44–59. doi: 10.20892/j.issn.2095-3941.2019.0210
Warburg, O. (1956). On the origin of cancer cells. Science 123, 309–314. doi: 10.1126/science.123.3191.309
Wei, R., Mao, L., Xu, P., Zheng, X., Hackman, R. M., Mackenzie, G. G., et al. (2018). Suppressing glucose metabolism with epigallocatechin-3-gallate (EGCG) reduces breast cancer cell growth in preclinical models. Food Funct. 9, 5682–5696. doi: 10.1039/c8fo01397g
Wellberg, E. A., Johnson, S., Finlay-Schultz, J., Lewis, A. S., Terrell, K. L., Sartorius, C. A., et al. (2016). The glucose transporter GLUT1 is required for ErbB2-induced mammary tumorigenesis. Breast Cancer Res. 18:131. doi: 10.1186/s13058-016-0795-0
Whitaker-Menezes, D., Martinez-Outschoorn, U. E., Lin, Z., Ertel, A., Flomenberg, N., Witkiewicz, A. K., et al. (2011). Evidence for a stromal-epithelial “lactate shuttle” in human tumors: MCT4 is a marker of oxidative stress in cancer-associated fibroblasts. Cell Cycle 10, 1772–1783. doi: 10.4161/cc.10.11.15659
Wilde, L., Roche, M., Domingo-Vidal, M., Tanson, K., Philp, N., Curry, J., et al. (2017). Metabolic coupling and the Reverse Warburg Effect in cancer: implications for novel biomarker and anticancer agent development. Semin. Oncol. 44, 198–203. doi: 10.1053/j.seminoncol.2017.10.004
Williamson, G. (2017). The role of polyphenols in modern nutrition. Nutr. Bull. 42, 226–235. doi: 10.1111/nbu.12278
Witkiewicz, A. K., Whitaker-Menezes, D., Dasgupta, A., Philp, N. J., Lin, Z., Gandara, R., et al. (2012). Using the “reverse Warburg effect” to identify high-risk breast cancer patients: stromal MCT4 predicts poor clinical outcome in triple-negative breast cancers. Cell Cycle 11, 1108–1117. doi: 10.4161/cc.11.6.19530
Woo, Y. M., Shin, Y., Lee, E. J., Lee, S., Jeong, S. H., Kong, H. K., et al. (2015). Inhibition of Aerobic Glycolysis Represses Akt/mTOR/HIF-1α Axis and Restores Tamoxifen Sensitivity in Antiestrogen-Resistant Breast Cancer Cells. PLoS One 10:e0132285. doi: 10.1371/journal.pone.0132285
Wright, E. M. (2013). Glucose transport families SLC5 and SLC50. Mol Aspects Med. 34, 183–196. doi: 10.1016/j.mam.2012.11.002
Wu, Q., Heidenreich, D., Zhou, S., Ackloo, S., Krämer, A., Nakka, K., et al. (2019a). A chemical toolbox for the study of bromodomains and epigenetic signaling. Nat. Commun. 10:1915. doi: 10.1038/s41467-019-09672-2
Wu, Q., Li, B., Li, Z., Li, J., Sun, S., and Sun, S. (2019b). Cancer-associated adipocytes: key players in breast cancer progression. J. Hematol. Oncol. 12:95. doi: 10.1186/s13045-019-0778-6
Wuest, M., Hamann, I., Bouvet, V., Glubrecht, D., Marshall, A., Trayner, B., et al. (2018). Molecular Imaging of GLUT1 and GLUT5 in Breast Cancer: a Multitracer Positron Emission Tomography Imaging Study in Mice. Mol. Pharmacol. 93, 79–89. doi: 10.1124/mol.117.110007
Xi, H., Barredo, J. C., Merchan, J. R., and Lampidis, T. J. (2013). Endoplasmic reticulum stress induced by 2-deoxyglucose but not glucose starvation activates AMPK through CaMKKβ leading to autophagy. Biochem. Pharmacol. 85, 1463–1477. doi: 10.1016/j.bcp.2013.02.037
Xi, H., Kurtoglu, M., Liu, H., Wangpaichitr, M., You, M., Liu, X., et al. (2011). 2-Deoxy-D-glucose activates autophagy via endoplasmic reticulum stress rather than ATP depletion. Cancer Chemother. Pharmacol. 67, 899–910. doi: 10.1007/s00280-010-1391-0
Xintaropoulou, C., Ward, C., Wise, A., Marston, H., Turnbull, A., and Langdon, S. P. (2015). A comparative analysis of inhibitors of the glycolysis pathway in breast and ovarian cancer cell line models. Oncotarget 6, 25677–25695. doi: 10.18632/oncotarget.4499
Yang, L., Li, J., Li, Y., Zhou, Y., Wang, Z., Zhang, D., et al. (2021). Diclofenac impairs the proliferation and glucose metabolism of triple-negative breast cancer cells by targeting the c-Myc pathway. Exp. Ther. Med. 21:584. doi: 10.3892/etm.2021.10016
Yang, T., Ren, C., Qiao, P., Han, X., Wang, L., Lv, S., et al. (2018). PIM2-mediated phosphorylation of hexokinase 2 is critical for tumor growth and paclitaxel resistance in breast cancer. Oncogene 37, 5997–6009. doi: 10.1038/s41388-018-0386-x
Yang, X., Peng, X., and Huang, J. (2018). Inhibiting 6-phosphogluconate dehydrogenase selectively targets breast cancer through AMPK activation. Clin. Transl. Oncol. 20, 1145–1152. doi: 10.1007/s12094-018-1833-4
Yang, Y., Wolfram, J., Boom, K., Fang, X., Shen, H., and Ferrari, M. (2013). Hesperetin impairs glucose uptake and inhibits proliferation of breast cancer cells. Cell Biochem. Funct. 31, 374–379. doi: 10.1002/cbf.2905
Yang, Z. J., Chee, C. E., Huang, S., and Sinicrope, F. A. (2011). The role of autophagy in cancer: therapeutic implications. Mol. Cancer Ther. 10, 1533–1541. doi: 10.1158/1535-7163.Mct-11-0047
Ye, W., Chang, H. L., Wang, L. S., Huang, Y. W., Shu, S., Sugimoto, Y., et al. (2010). Induction of apoptosis by (-)-gossypol-enriched cottonseed oil in human breast cancer cells. Int. J. Mol. Med. 26, 113–119.
Yeh, W. L., Lin, C. J., and Fu, W. M. (2008). Enhancement of glucose transporter expression of brain endothelial cells by vascular endothelial growth factor derived from glioma exposed to hypoxia. Mol. Pharmacol. 73, 170–177. doi: 10.1124/mol.107.038851
Yin, K. (2015). Positive correlation between expression level of mitochondrial serine hydroxymethyltransferase and breast cancer grade. Onco. Targets Ther. 8, 1069–1074. doi: 10.2147/ott.S82433
Younes, M., Brown, R. W., Mody, D. R., Fernandez, L., and Laucirica, R. (1995). GLUT1 expression in human breast carcinoma: correlation with known prognostic markers. Anticancer Res. 15, 2895–2898.
Young, C. D., Lewis, A. S., Rudolph, M. C., Ruehle, M. D., Jackman, M. R., Yun, U. J., et al. (2011). Modulation of glucose transporter 1 (GLUT1) expression levels alters mouse mammary tumor cell growth in vitro and in vivo. PLoS One 6:e23205. doi: 10.1371/journal.pone.0023205
Yousefi, S., Darvishi, P., Yousefi, Z., and Pourfathollah, A. A. (2020). Effect of methyl jasmonate and 3-bromopyruvate combination therapy on mice bearing the 4 T1 breast cancer cell line. J. Bioenerg. Biomembr. 52, 103–111. doi: 10.1007/s10863-019-09811-w
Zamora-León, S. P., Golde, D. W., Concha, I. I., Rivas, C. I., Delgado-López, F., Baselga, J., et al. (1996). Expression of the fructose transporter GLUT5 in human breast cancer. Proc. Natl. Acad. Sci. U. S. A. 93, 1847–1852. doi: 10.1073/pnas.93.5.1847
Zhao, F., Ming, J., Zhou, Y., and Fan, L. (2016). Inhibition of Glut1 by WZB117 sensitizes radioresistant breast cancer cells to irradiation. Cancer Chemother. Pharmacol. 77, 963–972. doi: 10.1007/s00280-016-3007-9
Zhao, Y., Liu, H., Liu, Z., Ding, Y., Ledoux, S. P., Wilson, G. L., et al. (2011). Overcoming trastuzumab resistance in breast cancer by targeting dysregulated glucose metabolism. Cancer Res. 71, 4585–4597. doi: 10.1158/0008-5472.Can-11-0127
Zhong, H., De Marzo, A. M., Laughner, E., Lim, M., Hilton, D. A., Zagzag, D., et al. (1999). Overexpression of hypoxia-inducible factor 1alpha in common human cancers and their metastases. Cancer Res. 59, 5830–5835.
Zhou, M., Zhao, Y., Ding, Y., Liu, H., Liu, Z., Fodstad, O., et al. (2010). Warburg effect in chemosensitivity: targeting lactate dehydrogenase-A re-sensitizes taxol-resistant cancer cells to taxol. Mol. Cancer 9:33. doi: 10.1186/1476-4598-9-33
Keywords: breast cancer, glucose metabolism, glucose transporter, pentose phosphate pathway, serine/glycine pathway
Citation: Shin E and Koo JS (2021) Glucose Metabolism and Glucose Transporters in Breast Cancer. Front. Cell Dev. Biol. 9:728759. doi: 10.3389/fcell.2021.728759
Received: 22 June 2021; Accepted: 10 August 2021;
Published: 06 September 2021.
Edited by:
Maria E. Mycielska, University Medical Center Regensburg, GermanyReviewed by:
Fátima Martel, Universidade do Porto, PortugalCopyright © 2021 Shin and Koo. This is an open-access article distributed under the terms of the Creative Commons Attribution License (CC BY). The use, distribution or reproduction in other forums is permitted, provided the original author(s) and the copyright owner(s) are credited and that the original publication in this journal is cited, in accordance with accepted academic practice. No use, distribution or reproduction is permitted which does not comply with these terms.
*Correspondence: Ja Seung Koo, a2pzMTk3NkB5dWhzLmFj
Disclaimer: All claims expressed in this article are solely those of the authors and do not necessarily represent those of their affiliated organizations, or those of the publisher, the editors and the reviewers. Any product that may be evaluated in this article or claim that may be made by its manufacturer is not guaranteed or endorsed by the publisher.
Research integrity at Frontiers
Learn more about the work of our research integrity team to safeguard the quality of each article we publish.