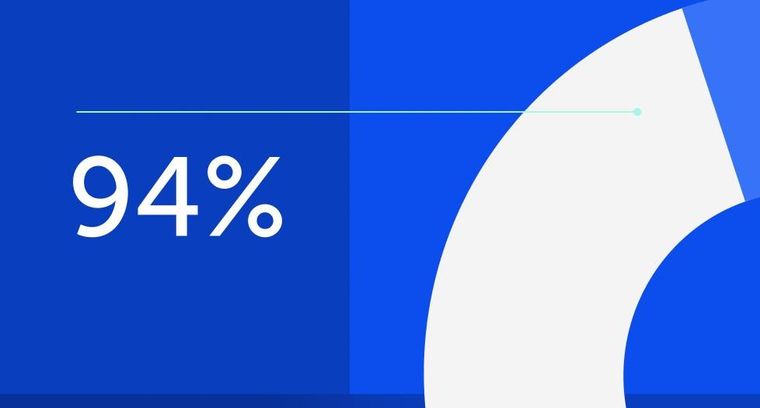
94% of researchers rate our articles as excellent or good
Learn more about the work of our research integrity team to safeguard the quality of each article we publish.
Find out more
SPECIALTY GRAND CHALLENGE article
Front. Cell Dev. Biol., 13 October 2021
Sec. Cell Adhesion and Migration
Volume 9 - 2021 | https://doi.org/10.3389/fcell.2021.720494
The processes of cell adhesion and migration fulfill crucial tasks during physiological developmental processes, immune responses, angiogenesis, or tissue injury and pathological diseased states including the cancer, cancer angiogenesis and its malignant progression, referred to as metastasis. Thereby, the cells undergo tremendous alterations that obviously lead to pronounced morphological alterations that are closely coupled to intracellular structural changes and mechanical perturbations (Humphrey et al., 2002; Guck et al., 2005; Mierke et al., 2008a, 2011; Kumar and Weaver, 2009; Menon and Beningo, 2011; Lekka et al., 2012; Humphries et al., 2019; Mierke, 2019a). These alterations occur on different cellular length scales, such as bulk alterations, compartmental alterations, structural compositional changes, molecular alterations down to gene expression regulatory events. All of these types of changes cannot be treated as purely separate events that can be fully deciphered in an independent manner. Hence, the specific microenvironmental constraints play a prominent role in unraveling the impact of the intricate interplay. Due to the vastly high number of molecules that function in cell adhesion under physiological and pathological processes, the agglomeration of proteins within focal adhesion has been termed cancer cellular adhesome to discriminate them from randomly distributed surrounding proteins (Maziveyi and Alahari, 2017). The contributing proteins of the adhesome can be divided into four different branches of the basic adhesion system which includes the Talin-Vinculin (Mierke et al., 2008a, 2010; Golji et al., 2011; Wang et al., 2019; Boujemaa-Paterski et al., 2020), FAK-Paxillin (Hu et al., 2015; Mierke et al., 2017; Ripamonti et al., 2021), α-Actinin-Zyxin-VASP (Oldenburg et al., 2015), and ILK-PINCH-Kindlin biochemical signal transduction pathways (Honda et al., 2013; Horton et al., 2015; Kunschmann et al., 2017). All of them represent critical pathways or mechanosensory systems to respond to changes in the mechanical homoestatic stage of cells. There are also additional organizational structures such as protrusions, podosomes, invadosomes, and similar structures for the motile function or migratory capacity of cells. When these structures, such as invadopodia are altered, the process of cancer metastasis can be impaired, such as for melanoma cells (Karamanou et al., 2021). Cancer cells act in various types of directional cell migration compromising chemotaxis (chemoattractant gradient), haptotaxis (environmental gradient), electrotaxis (ionic flux), galvanotaxis (electrical attractant), pilotaxis, and durotaxis (rigidity attractant) (Roussos et al., 2011; Allen et al., 2013; Mierke, 2021). Recognition of the microenvironment by cancer cells allows them to translate diverse signaling conveyed through focal adhesions. The stiffness produced by the extracellular matrix initiates and synergizes with the cell-matrix forces imposed by the cells (Krieg et al., 2008). Cells are able to capture multiple properties of the extracellular matrix in terms of stiffness and analysis of anisotropy (Geiger et al., 2009).
In the field of cell adhesion and migration, the impact of the extracellular matrix structure on the motility of single or grouped cells has been investigated quite well-during the last decade. However, the mechanical cues of the microenvironment on the adhesive, migratory, and functional tasks of cells or tissues has not yet been addressed on a broad scale (Mierke, 2020). Instead, it has been largely explored how alterations in cellular metabolism influence cell adhesion and migration. Recently, it has been stated that cellular metabolism and matrix mechanics are coupled (Levental et al., 2009; Tilghman et al., 2012; Chaudhuri et al., 2014; Ge et al., 2021). Moreover, it needs to be revealed whether there exist even similarities between the migration and invasion of individual or grouped cells in terms of the impact of mechanical characteristics. It has turned out that the cell mechanical characteristics can either foster or impair the adhesion and migration processes, what can be considered as one of the biggest grand challenges in this field (Bergert et al., 2012; Mierke, 2013; De Pascalis and Etienne-Manneville, 2017). The same holds true for the matrix mechanics or confinement on cell migration and invasion (Mierke et al., 2011; Mierke, 2014; Winkler et al., 2020; Kiran et al., 2021). In specific detail, the viscoelasticity has emerged as a key characteristic feature of cellular behavior, overall cellular shape, morphology and function of whole tissues (Clément et al., 2017; Tan et al., 2018; Barriga and Mayor, 2019; Chaudhuri et al., 2020). Thus, the field of cell adhesion and migration needs to cover also cell mechanical aspects as well as matrix mechanical aspects.
Besides the migration and invasion of individual cells, the collective cell migration plays a prominent role in a number of physiological processes, including wound healing and embryogenesis, and in several pathological processes, such as cancer metastasis. Although there is abundant experimental and theoretical evidence (Haeger et al., 2014; Pajic-Lijakovic and Milivojevic, 2019; Lohmann et al., 2020; Mitchel et al., 2020), the unifying mechanism governing collective cell migration is not best identified. However, there may also remain differences in the collective motion of cells, such as the tricellular junctions, which appear and mature at vertexes where three cells come together. Moreover, they are an ideal place to guide and govern the shape of cells and coordination of the multicellular migration (Lohmann et al., 2020). However, their function in epithelial tissue dynamic remains poorly defined. Most investigations have examined engineered model wounds to analyze collective cell migration in an epithelial solitary layer. These synthetic model wounds have a high-density of cells relative to physiological scenes such as a wound healing setting, where cell damage occurred from applied incision, and cancer metastasis settings, where smaller cell clusters are commonly involved. Based on these findings, the two systems may not be fully related, and further studies are necessary to better comprehend collective cell migration patterns in physiological scenes, which turns out to be a new frontier in cell migratory research. In specific, even the expansion of tissues can be restricted by strong confinements (Kiran et al., 2021). In addition, the migration of a collection of cells needs to be subdivided in areas of high, intermediate or low migration can be related to mechanical cues. Despite confinement, collective cell migration is more efficacious than individual cell migration by virtue of its intercellular forces (De Pascalis and Etienne-Manneville, 2017).
Apart from the pure cell mechanics, the environment is a prominent regulator of cell and tissue mechanics that needs to be regarded as well (Pokki et al., 2021). Thereby the interaction between cells can take place on different levels, such as the macroscopic level, including the bulk cell and tissue mechanical level, the mesoscopic level, encompassing structural alterations of extracellular matrices through degradation (Kessenbrock et al., 2010; Slattery et al., 2013; Grolman et al., 2020; Winkler et al., 2020; Curtis et al., 2021) or cross-linking processes (Levental et al., 2009) and the storage of growth factors, cytokines, chemokines, cell adhesion receptor ligands or enzyme-inhibiting molecules and the microscopic level, including fiber mechanics and cross-linker protein mechanics. On the microscopic scale, the organelles of cells, such as the nucleus, nucleolus, mitochondria, endoplasmic reticulum, Golgi apparatus and lysosomes have an additional effect on cell adhesion and migration, since these compartments may represent major steric obstacles for cell migration (Friedl et al., 2011; Janel et al., 2019; Krause et al., 2019; Efremov et al., 2020; Fischer et al., 2020; Zuela-Sopilniak et al., 2020), which can be broken down by rupture or elevated by fusion among organelles, such as mitochondria. The connection between cell, matrix and tissue mechanics on different length scales and dynamically on different time scales seems to be an additional new frontier in cell adhesion and cell migratory research (Figure 1).
Figure 1. Existing interactions between cells or a collection of cells and the microenvironment during the adhesion and migration of cells. The current gaps represent new frontiers for cell adhesion and migration research. There is a mutual interplay between cells and their surroundings that takes place at vastly different length scales and depends dynamically on time (different time scales).
Besides cell mechanical cues, the release of exosomes, microvesicles and apoptotic bodies from cancer cells can impact their migratory and invasive capacity and phenotype, as they have been shown to regulate cell adhesion and migration (Antonyak et al., 2011; Yoon et al., 2014; Sedgwick et al., 2015; Ståhl et al., 2019). Consequently, their effect on matrix mechanics, and in turn on their own regulation by matrix mechanical cues, appears to be evolving and forming a potential new frontier for cell adhesion and migration. Delivery of specific substances to recipient cells, such as matrix embedded cells or neighboring cancer cell subpopulations, generally occurs through the uptake of exosomes or microvesicles and may alter the mechanical and structural phenotype of the target cell. Finally, the intercellular communication has been altered as a result.
Apart from the mechanics of these extracellular elements, matrix embedded living cells, such as stroma fibroblasts or vessel-lining endothelial cells with or without pericytes can modify the whole scene of cell adhesion and migration on all length scales in terms of mechanical cues. In specific, these cells can alter their own mechanical characteristics and functions to either indirectly affect the adhesion and migration properties of targeted cells through changes of the local microenvironment or directedly release substances that then stimulate a structural or mechanical response of these cells. The connection to other embedded cells and their impact on cell mechanical properties represents another new frontier in this field.
It can be asserted that the interaction of various cell types or the interference of cells with their close microenvironment depends on the mechanical phenotype of each associated element. Thus, the focus demands to be shifted toward the mechanics of cells, nearby cells and extracellular matrix. The investigation of the mechanical cues will encourage the sharpening of the focus spot and provide a clear character.
Apart from stroma cells, such as tissue fibroblasts, immune cells can affect the mechanical properties of the extracellular matrix microenvironment (Hynes and Naba, 2012; Cox et al., 2013; Gonzalez et al., 2018; Pakshir et al., 2019; D'Urso and Kurniawan, 2020) and other nearby cells, such as cancer cells (Fiegl et al., 2006; Zhan et al., 2017; Zheng and Li, 2020). Thus, these immune cell types, encompassing γδT-cells, B-lymphocytes, tumor-associated macrophages, CD8+ and CD4+ lymphocytes, natural killer cells, dendritic cells, T-helper-1 (Th1) cells, Th9 cells and M1 macrophages, T-regulatory (Treg) cells, N1 and N2 neutrophils and cancer associated eosinophiles need to be included in this scenario of cell adhesion and migration (Stankovic et al., 2019; Grisaru-Tal et al., 2020). Thereby it requires the discrimination between the various types of immune cells and their individual impact on matrix and neighboring cell mechanics. The interplay of these different subsets of immune cells are crucial in challenging the overall mechanical phenotypes of cells and tissues. There may also be raised the question whether specific mechanical cues can induce an adherent state of distinct immune cells on their way through tissue microenvironment.
Since the impact of embedded cells on 3D migration and invasion, the role of the mechanical characteristics of cells and their microenvironment including its bi-directional interplay have been recognized, they all can be determined as new frontiers that have been identified on individual and collective migration. Consequently, the goals for future cell adhesion and migration efforts are the knowledge of the role of embedded cells, such as stroma cells and immune cells, the effect of mechanics on cell adhesion and motility and its mutual interplay including the release of exosomes and microvesicles. However, it is not yet entirely obvious how viscoelasticity alters the functional phenotype of cells, but it is definitely an encouraging new frontier. In particular, the biophysical technologies are yet to be improved and advanced. Hence, this specialty grand challenge article focusses in the following section on some crucial technologies employed to analyze the viscoelasticity of cells and extracellular matrices. It also illustrates how the cellular mechanophenotype is essential to identify, characterize, and therapeutically treat a variety of diseases such as cancer, tissue injury, acute or chronic inflammation, or fibrotic diseases. Finally, a future perspective on the importance of viscoelasticity of cells and matrices is presented.
Firstly, there is still an urgent need for the development of novel biophysical techniques and analytical procedures to measure the mechanical properties of individual cells, collection of cells and tissues. Thereby, statistical analyses need to be involved due to the broad heterogeneity of cell populations. Secondly, the further development of existing biophysical techniques, such as super-resolution microscopy or microrheological techniques or microfluidic approaches seems to be highly promising in addressing the mechanical feature of cell migration. Thirdly, the assessment of the microenvironmental physical deformation of cells or collections of cells seems to be key for revealing the interplay between specific cell types and their extracellular matrix environment. Thereby, the dynamically evolving matrix displacement, referred to as flow displacements are highly required.
Super-resolution microscopy, a technique that relies on single-molecule spatial information localization, comprises photoactivation localization microscopy (PALM), stochastic optical reconstruction microscopy (STORM), ground state depletion microscopy followed by individual molecular return (GSDIM) and universal point accumulation imaging in the nanoscale topography (uPAINT) (Godin et al., 2014; Paszek et al., 2014). iPALM represents a truly singular type of super-resolution microscopy that couples PALM with concurrent multiphase photon interferometry of single fluorescent molecules (Betzig et al., 2006). This type of imaging technology can be applied to visualize fluorescently labeled focal adhesion proteins with three-dimensional nanoscale spatial resolution (Kanchanawong et al., 2010). The improvement of these techniques can help to address the intracellular organelle level including the fission and fusion of organelles that can alter cell mechanical properties and subsequently cell adhesion and migration behavior.
Microrheological approaches, such as Magnetic tweezers (Kollmannsberger and Fabry, 2007; Sarkar and Rybenkov, 2016), Magnetic twisting cytometry, and Single article tracking approaches are both still state-of-the-art biophysical techniques that can be refined by including temperature issues (Aermes et al., 2021), cytoskeletal coupling or membrane receptor strengths to characterize the mechanophenotype of cells.
When addressing the mechanical properties of cells without the impact of cell adhesion, improved cell deformation/stretching biophysical techniques can be used, compromising an optical cell stretcher (Guck et al., 2005; Mierke et al., 2017, 2020; Mierke, 2019b) and microfluidics-based cell stretcher that represents a channel confinement for directional flowing or migrating cells (Huang et al., 2020; Yao et al., 2020).
Other even more famous biophysical techniques are atomic force microscopy (AFM) (Fischer et al., 2020), Micropipettes or Dual Micropipettes (González-Bermúdez et al., 2019), which the latter to be employed for intercellular adhesion force analyses. Last, but not least, the analysis of matrix displacement by cellular forces, such as Flow displacement technique in 3D, and traction force techniques in 2D, 2.5 D and 3D require to be further developed and combined with biological techniques (Mierke et al., 2008b; Legant et al., 2013; Cóndor et al., 2017; Hazlett et al., 2020). These dimensions can be expanded to 4D by adding time as fourth dimension. These forces may help to enlighten the impact of internal mechanotransduction events on cell adhesion and migration and vice versa the external mechanotransduction through other neighboring cells.
There is no other way in the future then that of combined cell biological and biophysical research in the broad field of cell adhesion and migration. The coupling of cell adhesion and migration to specific diseased states and non-equilibrium states seems to be required and promising. The transition of cellular states, such as individual cells from epithelial to mesenchymal or intermediate states, or such of a collection of cells from jamming to unjamming including intermediate states, are crucial feature for understanding under which structural or mechanical constraints cells or collections of cells start to move. Thereby the formation of adhesomes compromising all four branches plays a critical role and requires the connection of cellular and developmental biophysics to systems biological or genetic approaches. All the gained knowledge on the impact of the 3D cellular microenvironment and in specific the extracellular matrix scaffold can be employed to develop beyond state-of-the art theranostic approaches and propose new smart biomaterials.
CM contributed conception, design, wrote the manuscript, and prepared the figure herself.
The author declares that the research was conducted in the absence of any commercial or financial relationships that could be construed as a potential conflict of interest.
All claims expressed in this article are solely those of the authors and do not necessarily represent those of their affiliated organizations, or those of the publisher, the editors and the reviewers. Any product that may be evaluated in this article, or claim that may be made by its manufacturer, is not guaranteed or endorsed by the publisher.
Aermes, C., Hayn, A., Fischer, T., and Mierke, C. T. (2021). Cell mechanical properties of human breast carcinoma cells depend on temperature. Sci. Rep. 11:10771. doi: 10.1038/s41598-021-90173-y
Allen, G. M., Mogilner, A., and Theriot, J. A. (2013). Electrophoresis of cellular membrane components creates the directional cue guiding keratocyte galvanotaxis. Curr. Biol. 23, 560–568. doi: 10.1016/j.cub.2013.02.047
Antonyak, M. A., Li, B., Boroughs, L. K., Johnson, J. L., Druso, J. E., Bryant, K. L., et al. (2011). Cancer cell-derived microvesicles induce transformation by transferring tissue transglutaminase and fibronectin to recipient cells. Proc. Natl. Acad. Sci. U. S. A. 108, 4852–4857. doi: 10.1073/pnas.1017667108
Barriga, E. H., and Mayor, R. (2019). Adjustable viscoelasticity allows for efficient collective cell migration. Semin. Cell Dev. Biol. 93, 55–68. doi: 10.1016/j.semcdb.2018.05.027
Bergert, M., Chandradoss, S. D., Desai, R. A., and Paluch, E. (2012). Cell mechanics control rapid transitions between blebs and lamellipodia during migration. Proc. Natl. Acad. Sci. U. S. A. 109, 14434–14439. doi: 10.1073/pnas.1207968109
Betzig, E., Patterson, G. H., Sougrat, R., Lindwasser, O. W., Olenych, S., Bonifacino, J. S., et al. (2006). Imaging intracellular fluorescent proteins at nanometer resolution. Science 313, 1642–1645. doi: 10.1126/science.1127344
Boujemaa-Paterski, R., Martins, B., Eibauer, M., Beales, C. T., Geiger, B., and Medalia, O. (2020). Talin-activated vinculin interacts with branched actin networks to initiate bundles. ELife 9:e53990. doi: 10.7554/eLife.53990.sa2
Chaudhuri, O., Cooper-White, J., Janmey, P. A., Mooney, D. J., and Shenoy, V. B. (2020). Effects of extracellular matrix viscoelasticity on cellular behaviour. Nature 584, 535–546. doi: 10.1038/s41586-020-2612-2
Chaudhuri, O., Koshy, S. T., Branco da Cunha, C., Shin, J.-W., Verbeke, C. S., Allison, K. H., et al. (2014). Extracellular matrix stiffness and composition jointly regulate the induction of malignant phenotypes in mammary epithelium. Nat. Mater. 13, 970–978. doi: 10.1038/nmat4009
Clément, R., Dehapiot, B., Collinet, C., Lecuit, T., and Lenne, P.-F. (2017). Viscoelastic dissipation stabilizes cell shape changes during tissue morphogenesis. Curr. Biol. 27, 3132–3142.e4. doi: 10.1016/j.cub.2017.09.005
Cóndor, M., Steinwachs, J., Mark, C., García-Aznar, J. M., and Fabry, B. (2017). Traction force microscopy in 3-dimensional extracellular matrix networks. Curr. Protoc. Cell Biol. 75:10.22.1–10.22.20. doi: 10.1002/cpcb.24
Cox, T. R., Bird, D., Baker, A.-M., Barker, H. E., Ho, M. W.-Y., Lang, G., et al. (2013). LOX-mediated collagen crosslinking is responsible for fibrosis-enhanced metastasis. Cancer Res. 73, 1721–1732. doi: 10.1158/0008-5472.CAN-12-2233
Curtis, K. J., Mai, C., Martin, H., Oberman, A. G., Alderfer, L., Romero-Moreno, R., et al. (2021). The effect of marrow secretome and culture environment on the rate of metastatic breast cancer cell migration in two and three dimensions. Mol. Biol. Cell 32, 1009–1019. doi: 10.1091/mbc.E19-12-0682
De Pascalis, C., and Etienne-Manneville, S. (2017). Single and collective cell migration: the mechanics of adhesions. Mol. Biol. Cell 28, 1833–1846. doi: 10.1091/mbc.e17-03-0134
D'Urso, M., and Kurniawan, N. A. (2020). Mechanical and physical regulation of fibroblast-myofibroblast transition: from cellular mechanoresponse to tissue pathology. Front. Bioeng. Biotechnol. 8:609653. doi: 10.3389/fbioe.2020.609653
Efremov, Y. M., Kotova, S. L., Akovantseva, A. A., and Timashev, P. S. (2020). Nanomechanical properties of enucleated cells: contribution of the nucleus to the passive cell mechanics. J. Nanobiotechnol. 18:134. doi: 10.1186/s12951-020-00696-1
Fiegl, H., Millinger, S., Goebel, G., Müller-Holzner, E., Marth, C., Laird, P. W., et al. (2006). Breast cancer DNA methylation profiles in cancer cells and tumor stroma: association with HER-2/neu status in primary breast cancer. Cancer Res. 66, 29–33. doi: 10.1158/0008-5472.CAN-05-2508
Fischer, T., Hayn, A., and Mierke, C. T. (2020). Effect of nuclear stiffness on cell mechanics and migration of human breast cancer cells. Front. Cell Dev. Biol. 8:393. doi: 10.3389/fcell.2020.00393
Friedl, P., Wolf, K., and Lammerding, J. (2011). Nuclear mechanics during cell migration. Curr. Opin. Cell Biol. 23, 55–64. doi: 10.1016/j.ceb.2010.10.015
Ge, H., Tian, M., Pei, Q., Tan, F., and Pei, H. (2021). Extracellular matrix stiffness: new areas affecting cell metabolism. Front. Oncol. 11:631991. doi: 10.3389/fonc.2021.631991
Geiger, B., Spatz, J. P., and Bershadsky, A. D. (2009). Environmental sensing through focal adhesions. Nat. Rev. Mol. Cell Biol. 10, 21–33. doi: 10.1038/nrm2593
Godin, A. G., Lounis, B., and Cognet, L. (2014). Super-resolution microscopy approaches for live cell imaging. Biophys. J. 107, 1777–1784. doi: 10.1016/j.bpj.2014.08.028
Golji, J., Lam, J., and Mofrad, M. R. K. (2011). Vinculin activation is necessary for complete talin binding. Biophys. J. 100, 332–340. doi: 10.1016/j.bpj.2010.11.024
Gonzalez, H., Hagerling, C., and Werb, Z. (2018). Roles of the immune system in cancer: from tumor initiation to metastatic progression. Genes Dev. 32, 1267–1284. doi: 10.1101/gad.314617.118
González-Bermúdez, B., Guinea, G. V., and Plaza, G. R. (2019). Advances in micropipette aspiration: applications in cell biomechanics, models, and extended studies. Biophys. J. 116, 587–594. doi: 10.1016/j.bpj.2019.01.004
Grisaru-Tal, S., Itan, M., Klion, A. D., and Munitz, A. (2020). A new dawn for eosinophils in the tumour microenvironment. Nat. Rev. Cancer 20, 594–607. doi: 10.1038/s41568-020-0283-9
Grolman, J. M., Weinand, P., and Mooney, D. J. (2020). Extracellular matrix plasticity as a driver of cell spreading. Proc. Natl. Acad. Sci. U. S. A. 117, 25999–26007. doi: 10.1073/pnas.2008801117
Guck, J., Schinkinger, S., Lincoln, B., Wottawah, F., Ebert, S., Romeyke, M., et al. (2005). Optical deformability as an inherent cell marker for testing malignant transformation and metastatic competence. Biophys. J. 88, 3689–3698. doi: 10.1529/biophysj.104.045476
Haeger, A., Krause, M., Wolf, K., and Friedl, P. (2014). Cell jamming: collective invasion of mesenchymal tumor cells imposed by tissue confinement. Biochim. Biophys. Acta 1840, 2386–2395. doi: 10.1016/j.bbagen.2014.03.020
Hazlett, L., Landauer, A. K., Patel, M., Witt, H. A., Yang, J., Reichner, J. S., et al. (2020). Epifluorescence-based three-dimensional traction force microscopy. Sci. Rep. 10:16599. doi: 10.1038/s41598-020-72931-6
Honda, S., Shirotani-Ikejima, H., Tadokoro, S., Tomiyama, Y., and Miyata, T. (2013). The integrin-linked kinase-PINCH-parvin complex supports integrin αIIbβ3 activation. PLoS One 8:e85498. doi: 10.1371/journal.pone.0085498
Horton, E. R., Byron, A., Askari, J. A., Ng, D. H. J., Millon-Frémillon, A., Robertson, J., et al. (2015). Definition of a consensus integrin adhesome and its dynamics during adhesion complex assembly and disassembly. Nat. Cell Biol. 17, 1577–1587. doi: 10.1038/ncb3257
Hu, Y.-L., Lu, S., Szeto, K. W., Sun, J., Wang, Y., Lasheras, J. C., et al. (2015). FAK and paxillin dynamics at focal adhesions in the protrusions of migrating cells. Sci. Rep. 4:6024. doi: 10.1038/srep06024
Huang, L., Liang, F., Feng, Y., Zhao, P., and Wang, W. (2020). On-chip integrated optical stretching and electrorotation enabling single-cell biophysical analysis. Microsyst. Nanoeng. 6:57. doi: 10.1038/s41378-020-0162-2
Humphrey, D., Duggan, C., Saha, D., Smith, D., and Käs, J. (2002). Active fluidization of polymer networks through molecular motors. Nature 416, 413–416. doi: 10.1038/416413a
Humphries, J. D., Chastney, M. R., Askari, J. A., and Humphries, M. J. (2019). Signal transduction via integrin adhesion complexes. Curr. Opin. Cell Biol. 56, 14–21. doi: 10.1016/j.ceb.2018.08.004
Hynes, R. O., and Naba, A. (2012). Overview of the matrisome-an inventory of extracellular matrix constituents and functions. Cold Spring Harbor Perspect. Biol. 4:a004903. doi: 10.1101/cshperspect.a004903
Janel, S., Popoff, M., Barois, N., Werkmeister, E., Divoux, S., Perez, F., et al. (2019). Stiffness tomography of eukaryotic intracellular compartments by atomic force microscopy. Nanoscale 11, 10320–10328. doi: 10.1039/C8NR08955H
Kanchanawong, P., Shtengel, G., Pasapera, A. M., Ramko, E. B., Davidson, M. W., Hess, H. F., et al. (2010). Nanoscale architecture of integrin-based cell adhesions. Nature 468, 580–584. doi: 10.1038/nature09621
Karamanou, K., Franchi, M., Proult, I., Rivet, R., Vynios, D., and Brézillon, S. (2021). Lumican inhibits in vivo melanoma metastasis by altering matrix-effectors and invadopodia markers. Cells 10:841. doi: 10.3390/cells10040841
Kessenbrock, K., Plaks, V., and Werb, Z. (2010). Matrix metalloproteinases: regulators of the tumor microenvironment. Cell 141, 52–67. doi: 10.1016/j.cell.2010.03.015
Kiran, A., Kumar, N., and Mehandia, V. (2021). Distinct modes of tissue expansion in free versus earlier-confined boundaries for more physiological modeling of wound healing, cancer metastasis, and tissue formation. ACS Omega 6, 11209–11222. doi: 10.1021/acsomega.0c06232
Kollmannsberger, P., and Fabry, B. (2007). BaHigh-force magnetic tweezers with force feedback for biological applications. Rev. Sci. Instrum. 78:114301. doi: 10.1063/1.2804771
Krause, M., Yang, F. W., te Lindert, M., Isermann, P., Schepens, J., Maas, R. J. A., et al. (2019). Cell migration through three-dimensional confining pores: speed accelerations by deformation and recoil of the nucleus. Philos. Trans. R. Soc. B Biol. Sci. 374:20180225. doi: 10.1098/rstb.2018.0225
Krieg, M., Arboleda-Estudillo, Y., Puech, P.-H., Käfer, J., Graner, F., Müller, D. J., et al. (2008). Tensile forces govern germ-layer organization in zebrafish. Nat. Cell Biol. 10, 429–436. doi: 10.1038/ncb1705
Kumar, S., and Weaver, V. M. (2009). Mechanics, malignancy, and metastasis: the force journey of a tumor cell. Cancer Metastasis Rev. 28, 113–127. doi: 10.1007/s10555-008-9173-4
Kunschmann, T., Puder, S., Fischer, T., Perez, J., Wilharm, N., and Mierke, C. T. (2017). Integrin-linked kinase regulates cellular mechanics facilitating the motility in 3D extracellular matrices. Biochim. Biophys. Acta 1864, 580–593. doi: 10.1016/j.bbamcr.2016.12.019
Legant, W. R., Choi, C. K., Miller, J. S., Shao, L., Gao, L., Betzig, E., et al. (2013). Multidimensional traction force microscopy reveals out-of-plane rotational moments about focal adhesions. Proc. Natl. Acad. Sci. U. S. A. 110, 881–886. doi: 10.1073/pnas.1207997110
Lekka, M., Pogoda, K., Gostek, J., Klymenko, O., Prauzner-Bechcicki, S., Wiltowska-Zuber, J., et al. (2012). Cancer cell recognition - mechanical phenotype. Micron 43, 1259–1266. doi: 10.1016/j.micron.2012.01.019
Levental, K. R., Yu, H., Kass, L., Lakins, J. N., Egeblad, M., Erler, J. T., et al. (2009). Matrix crosslinking forces tumor progression by enhancing integrin signaling. Cell 139, 891–906. doi: 10.1016/j.cell.2009.10.027
Lohmann, S., Giampietro, C., Pramotton, F. M., Al-Nuaimi, D., Poli, A., Maiuri, P., et al. (2020). The role of tricellulin in epithelial jamming and unjamming via segmentation of tricellular junctions. Adv. Sci. 7:2001213. doi: 10.1002/advs.202001213
Maziveyi, M., and Alahari, S. K. (2017). Cell matrix adhesions in cancer: the proteins that form the glue. Oncotarget 8, 48471–48487. doi: 10.18632/oncotarget.17265
Menon, S., and Beningo, K. A. (2011). Cancer cell invasion is enhanced by applied mechanical stimulation. PLoS One 6:e17277. doi: 10.1371/journal.pone.0017277
Mierke, C. T. (2013). Phagocytized beads reduce the α5β1 integrin facilitated invasiveness of cancer cells by regulating cellular stiffness. Cell Biochem. Biophys. 66, 599–622. doi: 10.1007/s12013-012-9506-3
Mierke, C. T. (2014). The fundamental role of mechanical properties in the progression of cancer disease and inflammation. Rep. Prog. Phys. 77:076602. doi: 10.1088/0034-4885/77/7/076602
Mierke, C. T. (2019a). The matrix environmental and cell mechanical properties regulate cell migration and contribute to the invasive phenotype of cancer cells. Rep. Prog. Phys. 82:064602. doi: 10.1088/1361-6633/ab1628
Mierke, C. T. (2019b). The role of the optical stretcher is crucial in the investigation of cell mechanics regulating cell adhesion and motility. Front. Cell Dev. Biol. 7:184. doi: 10.3389/fcell.2019.00184
Mierke, C. T. (2020). Mechanical cues affect migration and invasion of cells from three different directions. Front. Cell Dev. Biol. 8:583226. doi: 10.3389/fcell.2020.583226
Mierke, C. T. (2021). Physics of Cancer, Volume 3 (Second Edition): Experimental Biophysical Techniques in Cancer Research. Bristol: IOP Publishing.
Mierke, C. T., Fischer, T., Puder, S., Kunschmann, T., Soetje, B., and Ziegler, W. H. (2017). Focal adhesion kinase activity is required for actomyosin contractility-based invasion of cells into dense 3D matrices. Sci. Rep. 7:42780. doi: 10.1038/srep46435
Mierke, C. T., Frey, B., Fellner, M., Herrmann, M., and Fabry, B. (2011). Integrin α5β1 facilitates cancer cell invasion through enhanced contractile forces. J. Cell Sci. 124, 369–383. doi: 10.1242/jcs.071985
Mierke, C. T., Kollmannsberger, P., Paranhos Zitterbart, D., Smith, J., Fabry, B., and Goldmann, W. H. (2008a). Mechano-coupling and regulation of contractility by the vinculin tail domain. Biophys. J. 94, 661–670. doi: 10.1529/biophysj.107.108472
Mierke, C. T., Kollmannsberger, P., Zitterbart, D. P., Diez, G., Koch, T. M., Marg, S., et al. (2010). Vinculin facilitates cell invasion into three-dimensional collagen matrices. J. Biol. Chem. 285, 13121–13130. doi: 10.1074/jbc.M109.087171
Mierke, C. T., Puder, S., Aermes, C., Fischer, T., and Kunschmann, T. (2020). Effect of PAK inhibition on cell mechanics depends on Rac1. Front. Cell Dev. Biol. 8:13. doi: 10.3389/fcell.2020.00013
Mierke, C. T., Zitterbart, D. P., Kollmannsberger, P., Raupach, C., Schlötzer-Schrehardt, U., Goecke, T. W., et al. (2008b). Breakdown of the endothelial barrier function in tumor cell transmigration. Biophys. J. 94, 2832–2846. doi: 10.1529/biophysj.107.113613
Mitchel, J. A., Das, A., O'Sullivan, M. J., Stancil, I. T., DeCamp, S. J., Koehler, S., et al. (2020). In primary airway epithelial cells, the unjamming transition is distinct from the epithelial-to-mesenchymal transition. Nat. Commun. 11:5053. doi: 10.1038/s41467-020-18841-7
Oldenburg, J., van der Krogt, G., Twiss, F., Bongaarts, A., Habani, Y., Slotman, J. A., et al. (2015). VASP, zyxin and TES are tension-dependent members of Focal Adherens Junctions independent of the α-catenin-vinculin module. Sci. Rep. 5:17225. doi: 10.1038/srep17225
Pajic-Lijakovic, I., and Milivojevic, M. (2019). Long-time viscoelasticity of multicellular surfaces caused by collective cell migration - Multi-scale modeling considerations. Semin. Cell Dev. Biol. 93, 87–96. doi: 10.1016/j.semcdb.2018.08.002
Pakshir, P., Alizadehgiashi, M., Wong, B., Coelho, N. M., Chen, X., Gong, Z., et al. (2019). Dynamic fibroblast contractions attract remote macrophages in fibrillar collagen matrix. Nat. Commun. 10:1850. doi: 10.1038/s41467-019-10344-4
Paszek, M. J., DuFort, C. C., Rossier, O., Bainer, R., Mouw, J. K., Godula, K., et al. (2014). The cancer glycocalyx mechanically primes integrin-mediated growth and survival. Nature 511, 319–325. doi: 10.1038/nature13535
Pokki, J., Zisi, I., Schulman, E., Indana, D., and Chaudhuri, O. (2021). Magnetic probe-based microrheology reveals local softening and stiffening of 3D collagen matrices by fibroblasts. Biomed. Microdevices 23:27. doi: 10.1007/s10544-021-00547-2
Ripamonti, M., Liaudet, N., Azizi, L., Bouvard, D., Hytönen, V. P., and Wehrle-Haller, B. (2021). Structural and functional analysis of LIM domain-dependent recruitment of paxillin to αvβ3 integrin-positive focal adhesions. Commun. Biol. 4:380. doi: 10.1038/s42003-021-01886-9
Roussos, E. T., Condeelis, J. S., and Patsialou, A. (2011). Chemotaxis in cancer. Nat. Rev. Cancer 11, 573–587. doi: 10.1038/nrc3078
Sarkar, R., and Rybenkov, V. V. (2016). A guide to magnetic tweezers and their applications. Front. Phys. 4:48. doi: 10.3389/fphy.2016.00048
Sedgwick, A. E., Clancy, J. W., Olivia Balmert, M., and D'Souza-Schorey, C. (2015). Extracellular microvesicles and invadopodia mediate non-overlapping modes of tumor cell invasion. Sci. Rep. 5:14748. doi: 10.1038/srep14748
Slattery, M. L., John, E., Torres-Mejia, G., Stern, M., Lundgreen, A., Hines, L., et al. (2013). Matrix metalloproteinase genes are associated with breast cancer risk and survival: the Breast Cancer Health Disparities Study. PLoS One 8:e63165. doi: 10.1371/journal.pone.0063165
Ståhl, A., Johansson, K., Mossberg, M., Kahn, R., and Karpman, D. (2019). Exosomes and microvesicles in normal physiology, pathophysiology, and renal diseases. Pediatr. Nephrol. 34, 11–30. doi: 10.1007/s00467-017-3816-z
Stankovic, B., Bjørhovde, H. A. K., Skarshaug, R., Aamodt, H., Frafjord, A., Müller, E., et al. (2019). Immune cell composition in human non-small cell lung cancer. Front. Immunol. 9:3101. doi: 10.3389/fimmu.2018.03101
Tan, Y., Huang, H., Ayers, D. C., and Song, J. (2018). Modulating viscoelasticity, stiffness, and degradation of synthetic cellular niches via stoichiometric tuning of covalent versus dynamic noncovalent cross-linking. ACS Cent. Sci. 4, 971–981. doi: 10.1021/acscentsci.8b00170
Tilghman, R. W., Blais, E. M., Cowan, C. R., Sherman, N. E., Grigera, P. R., Jeffery, E. D., et al. (2012). Matrix rigidity regulates cancer cell growth by modulating cellular metabolism and protein synthesis. PLoS One 7:e37231. doi: 10.1371/journal.pone.0037231
Wang, P., Wu, J., Wood, A., Jones, M., Pedley, R., Li, W., et al. (2019). Vinculins interaction with talin is essential for mammary epithelial differentiation. Sci. Rep. 9:18400. doi: 10.1038/s41598-019-54784-w
Winkler, J., Abisoye-Ogunniyan, A., Metcalf, K. J., and Werb, Z. (2020). Concepts of extracellular matrix remodelling in tumour progression and metastasis. Nat. Commun. 11:5120. doi: 10.1038/s41467-020-18794-x
Yao, Z., Kwan, C. C., and Poon, A. W. (2020). An optofluidic “tweeze-and-drag” cell stretcher in a microfluidic channel. Lab Chip 20, 601–613. doi: 10.1039/C9LC01026B
Yoon, Y. J., Kim, O. Y., and Gho, Y. S. (2014). Extracellular vesicles as emerging intercellular communicasomes. BMB Rep. 47, 531–539. doi: 10.5483/BMBRep.2014.47.10.164
Zhan, H., Zhou, B., Cheng, Y., Xu, J., Wang, L., Zhang, G., et al. (2017). Crosstalk between stromal cells and cancer cells in pancreatic cancer: New insights into stromal biology. Cancer Lett. 392, 83–93. doi: 10.1016/j.canlet.2017.01.041
Zheng, P., and Li, W. (2020). Crosstalk between mesenchymal stromal cells and tumor-associated macrophages in gastric cancer. Front. Oncol. 10:571516. doi: 10.3389/fonc.2020.571516
Zuela-Sopilniak, N., Bar-Sela, D., Charar, C., Wintner, O., Gruenbaum, Y., and Buxboim, A. (2020). Measuring nucleus mechanics within a living multicellular organism: physical decoupling and attenuated recovery rate are physiological protective mechanisms of the cell nucleus under high mechanical load. Mol. Biol. Cell 31, 1943–1950. doi: 10.1091/mbc.E20-01-0085
Keywords: cell and matrix mechanics, constraints, extracellular matrix, alignment, viscoelasticity, stiffness, endothelium, immune cells
Citation: Mierke CT (2021) The Pertinent Role of Cell and Matrix Mechanics in Cell Adhesion and Migration. Front. Cell Dev. Biol. 9:720494. doi: 10.3389/fcell.2021.720494
Received: 04 June 2021; Accepted: 20 September 2021;
Published: 13 October 2021.
Approved by:
Shamik Sen, Indian Institute of Technology Bombay, IndiaCopyright © 2021 Mierke. This is an open-access article distributed under the terms of the Creative Commons Attribution License (CC BY). The use, distribution or reproduction in other forums is permitted, provided the original author(s) and the copyright owner(s) are credited and that the original publication in this journal is cited, in accordance with accepted academic practice. No use, distribution or reproduction is permitted which does not comply with these terms.
*Correspondence: Claudia Tanja Mierke, Y2xhdWRpYS5taWVya2VAdW5pLWxlaXB6aWcuZGU=
Disclaimer: All claims expressed in this article are solely those of the authors and do not necessarily represent those of their affiliated organizations, or those of the publisher, the editors and the reviewers. Any product that may be evaluated in this article or claim that may be made by its manufacturer is not guaranteed or endorsed by the publisher.
Research integrity at Frontiers
Learn more about the work of our research integrity team to safeguard the quality of each article we publish.