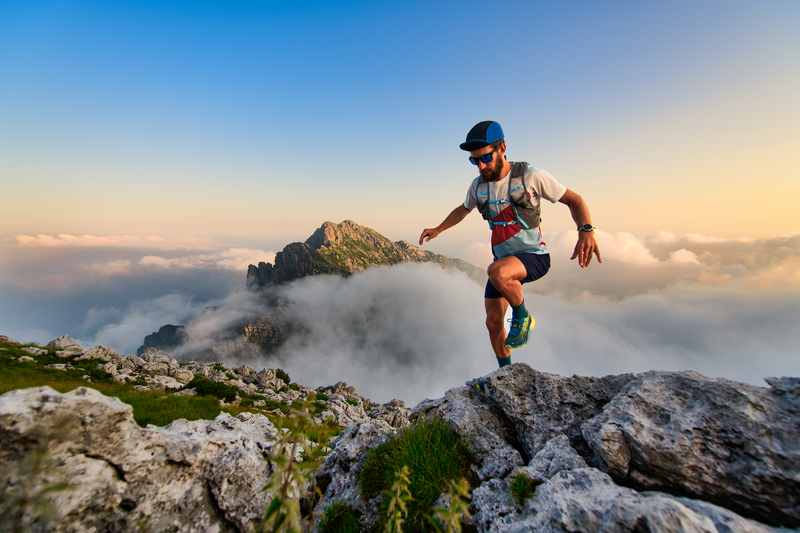
95% of researchers rate our articles as excellent or good
Learn more about the work of our research integrity team to safeguard the quality of each article we publish.
Find out more
REVIEW article
Front. Cell Dev. Biol. , 02 August 2021
Sec. Molecular and Cellular Reproduction
Volume 9 - 2021 | https://doi.org/10.3389/fcell.2021.710033
This article is part of the Research Topic Germ Cell Development and Reproductive Aging View all 21 articles
Successful human reproduction relies on the well-orchestrated development of competent gametes through the process of meiosis. The loading of cohesin, a multi-protein complex, is a key event in the initiation of mammalian meiosis. Establishment of sister chromatid cohesion via cohesin rings is essential for ensuring homologous recombination-mediated DNA repair and future proper chromosome segregation. Cohesin proteins loaded during female fetal life are not replenished over time, and therefore are a potential etiology of age-related aneuploidy in oocytes resulting in decreased fecundity and increased infertility and miscarriage rates with advancing maternal age. Herein, we provide a brief overview of meiotic cohesin and summarize the human genetic studies which have identified genetic variants of cohesin proteins and the associated reproductive phenotypes including primary ovarian insufficiency, trisomy in offspring, and non-obstructive azoospermia. The association of cohesion defects with cancer predisposition and potential impact on aging are also described. Expansion of genetic testing within clinical medicine, with a focus on cohesin protein-related genes, may provide additional insight to previously unknown etiologies of disorders contributing to gamete exhaustion in females, and infertility and reproductive aging in both men and women.
Infertility, a disease defined by the failure to achieve a successful pregnancy after 12 or more months of regular, unprotected intercourse or due to an impairment of a person’s capacity to reproduce (Practice Committee of the American Society for Reproductive Medicine, 2020), is estimated to affect approximately 15% of the reproductive age population. A woman’s age is the single most important factor determining her ability to conceive as the quantity and quality of oocytes decrease progressively with advancing age (American College of Obstetricians and Gynecologists Committee on Gynecologic Practice and Practice Committee, 2014; Steiner and Jukic, 2016). The number of primordial germ cells, or oogonia, peaks at approximately 6–7 million during mid-gestation, followed by progressive atresia with approximately 1–2 million oocytes present at birth (Motta et al., 1997; Pereda et al., 2006; Mamsen et al., 2012). By puberty, there is estimated to be 300,000–500,000 oocytes remaining, and approximately 25,000 at age 37 years (Forabosco and Sforza, 2007; Albamonte et al., 2019). Menopause occurs when the number of remaining oocytes falls below a critical threshold of about 1000, regardless of age (American College of Obstetricians and Gynecologists Committee on Gynecologic Practice and Practice Committee, 2014).
Intentional delays in childbearing until later maternal age has been an increasing phenomenon over several decades. In the United States, between 1970 and 2006, the proportion of first births to women age 35 or over increased nearly eight times (Matthews and Hamilton, 2014). This trend has been attributed to multiple factors including women’s educational and professional pursuits as well as increasing availability of contraception (Simoni et al., 2017; Kavanaugh et al., 2021). Additionally, surveys of older women have demonstrated misperceptions about the magnitude of fertility decline with age. Reasons for this include recollections of messaging about pregnancy prevention starting in adolescence, healthy lifestyle and family history of normal fertility, as well as incorrect information from media reports of pregnancies in older celebrity women (Mac Dougall et al., 2013).
With later maternal age, women experience a gradual decrease in fecundity and increased rates of miscarriage (American College of Obstetricians and Gynecologists Committee on Gynecologic Practice and Practice Committee, 2014). These poor outcomes with advancing age reflect a decline in oocyte quality which can be associated with no or abnormal fertilization, no implantation, or aneuploidy (notably trisomy) of conceived embryos (Balasch and Gratacos, 2012). This manifests clinically as a higher incidence of infertility, early pregnancy loss, or developmental defects in ongoing pregnancies with increasing maternal age.
Throughout a woman’s reproductive life, successful meiosis requires homologous recombination-mediated DNA repair and proper segregation of chromosomes and sister chromatids to yield haploid oocytes. Sister chromatid cohesion, mediated by cohesin rings which tether the two sister chromatids of replicated chromosomes, is essential to ensure that these processes are carried out correctly. Loss or weakening of chromosome cohesion with advancing maternal age has been proposed as a leading cause of age-related aneuploidy in oocytes (Chiang et al., 2010, 2011; Jessberger, 2012; Herbert et al., 2015; Gruhn et al., 2019). Therefore, it is likely that deterioration of the complex of proteins involved in chromosome cohesion, referred to as cohesin proteins, or genetic variants affecting these proteins are potential drivers of age-related infertility and aneuploidy. This review provides an overview of meiotic cohesin and summarizes the human genetic studies that have identified genetic variants of cohesin proteins and the associated reproductive phenotypes.
Understanding the etiology of aneuploidy with advancing maternal age requires an understanding of female mammalian meiosis. Mammalian meiosis is a specialized form of cell division characterized by a single round of DNA replication, followed by two rounds of chromosome segregation resulting in haploid gametes. Segregation of homologous chromosomes occurs during meiosis I (MI) and segregation of sister chromatids occurs during meiosis II (MII) (Zickler and Kleckner, 2015; Bolcun-Filas and Handel, 2018). Female mammalian meiosis commences during fetal life. Chromosomes within oocytes undergo replication and subsequently enter meiotic prophase I, and arrest in this stage for decades until future ovulation or atresia.
Meiotic prophase is the first and longest stage of mammalian meiosis in which the homologous chromosomes must pair, synapse, and undergo meiotic recombination to generate a crossover event. Meiotic prophase I include unique processes which are distinct from mitotic prophase. Following pre-meiotic DNA replication, meiotic prophase I initiates and progresses through various stages including leptonema, zygonema, pachynema, diplonema, and diakinesis (Zickler and Kleckner, 1999, 2015; Figure 1). Pairing-synapsis and recombination are hallmarks of prophase I, and are both essential for ensuring homolog interactions leading to the formation of at least one crossover event per chromosome pair. In fact, the number of crossovers and their location is critical for ensuring appropriate disjunction at metaphase I and for maintaining genomic stability. Pairing of homologous chromosomes occurs through the formation of a proteinaceous structure called the synaptonemal complex that forms between homologous chromosomes, while crossover recombination occurs at the DNA level, between two non-sister chromatids (Svetlanov and Cohen, 2004; Holloway and Cohen, 2015; Gray and Cohen, 2016). During leptonema, synaptonemal complex proteins (e.g., SYCP2/3) begin to form a proteinaceous scaffold (axial elements) along each homologous chromosome (Dietrich et al., 1992; Page and Hawley, 2004; Fraune et al., 2012; Cahoon et al., 2019). During zygonema, central element proteins (e.g., SYCP1, TEX12), begin to localize between the lateral elements allowing for continued pairing and synapsis between homologous chromosomes, essentially providing a proteinaceous structure physically tethering them by the end of this stage. At pachynema, lateral elements are completely formed, and the homologous chromosomes are completely synapsed (Zickler and Kleckner, 2015; Cahoon and Hawley, 2016; Gao and Colaiacovo, 2018). During diplonema, the central element of the synaptonemal complex breaks down and the chromosomes begin to repel each other. By diakinesis, homologous chromosomes are only tethered at the sites of crossovers and sister chromatids at sites of centromeres (Figure 1).
Figure 1. Meiotic Prophase I. Following DNA replication, meiotic prophase initiates. DNA double strand breaks (DSBs) are created during leptonema and the chromosomes initiate the processes of pairing and recombination. These processes progress into zygonema with DSB repair mechanisms ensuring proper homologous recombination. By pachynema, the chromosomes are fully synapsed and sites of crossovers (CO) are apparent. Sites of CO tether the homologous chromosomes together until anaphase I, allowing for proper segregation of homologous chromosomes and reducing the risk of aneuploidy in future gametes. Diplonema is marked by breakdown of the central element of the synaptonemal complex and chromosomes begin to repel each other. By diakinesis, homologous chromosomes are only tethered at the sites of crossovers and sister chromatids at the centromeres.
Recombination during prophase I occurs at the DNA level, between two non-sister chromatids (Svetlanov and Cohen, 2004; Holloway and Cohen, 2015; Gray and Cohen, 2016). Programmed double strand breaks (DSBs) are generated by the protein SPO11 throughout the genome in a very controlled and specific fashion. After creating hundreds of DSBs, they are repaired either as crossovers (COs) or non-crossovers (NCO) (Keeney and Kleckner, 1995; Keeney, 2008; Massy, 2016; Robert et al., 2016) or can undergo inter-sister repair as well (Garcia-Muse et al., 2019; Almanzar et al., 2021; Toraason et al., 2021). Generation of COs of homologs is required for proper segregation of homologous chromosomes during MI. The resulting bivalent chromosomes are linked at chiasmata which correspond to sites of crossovers. COs are stabilized by cohesin rings encompassing the sister chromatids distal to these sites on the chromosome arms (Petronczki et al., 2003). Full literature reviews about these processes have been described (Handel and Schimenti, 2010; Hunter, 2015; Gray and Cohen, 2016; Pereira et al., 2020).
Chromosome cohesion is established during pre-meiotic S phase and early prophase I via loading of cohesin rings/complexes. While mitotic and meiotic cohesin subunits are widely conserved in diverse species (Ishiguro, 2019), three types of meiotic cohesin complexes exist in mammalian cells. The subunits of STAG3, SMC1β, and SMC3 are shared in most of these complexes while the kleisin component (REC8, RAD21L, or RAD21) can vary (Herran et al., 2011; McNicoll et al., 2013; Ishiguro, 2019; Figure 2A). REC8 and RAD21L are meiosis-specific cohesin components while RAD21 is seen in both mitosis and meiosis. During meiotic prophase, REC8-containing cohesin begins to localize along the chromosomes prior to and during replication of DNA. Alternatively, RAD21L-containing cohesin rings are localized on chromosomes during leptonema and zygonema stages of prophase I and dissociates following late pachynema. The early loading of cohesin during meiosis provides a cohesin axis which serves as a structural core for chromosome organization during meiosis (Ishiguro, 2019). Thus, the cohesin complex is essential for maintaining sister chromatid cohesion and for ensuring correct chromosome segregation (Nasmyth and Haering, 2009). While mitotic and meiotic cohesion events are largely conserved, there are specific differences that exist, specifically, the unique and sequential chromosome segregation profiles during meiosis.
Figure 2. Mitotic vs. Meiotic Cohesin ring components and associated proteins. (A) Schematic representation of mitotic and meiotic cohesin complexes. The meiosis-specific cohesin ring proteins are SMC1β, RAD21L, REC8, and STAG3. (B) PDS5B, WAPL, and Sororin are associated with the cohesin complex and regulate the dynamic interaction of cohesin with chromatin. Phosphorylation of Sororin dissociates it from the cohesin ring permitting interaction of WAPL with PDS5B to open the of the cohesin ring via the prophase pathway.
The most well-defined mechanism of cohesin removal during meiosis involves Separase-induced proteolytic cleavage (Verni et al., 2000; Waizenegger et al., 2000; Losada et al., 2002; Buheitel and Stemmann, 2013; Wassmann, 2013; Haarhuis et al., 2014; Hirano, 2015). During meiosis, cleavage of REC8 by Separase is initially restricted to the chromosome arms, and it is not until anaphase II that centromeric REC8 cleavage is initiated (Buonomo et al., 2000; Kitajima et al., 2003; Kudo et al., 2009; Ishiguro et al., 2010; Katis et al., 2010; Figure 3A). Centromeric cohesin is essential to maintain sister chromatid cohesion until the second meiotic division. These functions of cohesin ensure future proper chromosome segregation upon resumption of meiosis in sexually mature females at the time of ovulation and later during fertilization. The cohesin rings at the centromeric region are protected by the interaction of Shugoshin 2 (SGO2) and protein phosphatase 2A (PP2A) which keeps REC8 in a hypo-phosphorylated state (Kitajima et al., 2006; Riedel et al., 2006). Maintenance of centromeric cohesin rings during MI protects from premature segregation of sister chromatids (PSSC). During MII, dissociation of SGO2/PP2A at the centromeres results in phosphorylation of REC8, thus allowing the action of Separase to cleave centromeric cohesin rings which permits segregation of sister chromatids (Lee et al., 2008; Xu et al., 2009; Figure 3A).
Figure 3. Sequential removal of cohesin ensures proper chromosome segregation and completion of meiosis. (A) Removal of cohesin rings from the chromosome arms during MI permits segregation of homologous chromosomes during anaphase I. Cleavage of centromeric cohesin during MII allows for segregation of sister chromatids and formation of haploid gametes. (B) The LH surge induces the transition of the oocyte from meiotic prophase I to complete the first meiotic division of homologous chromosomes resulting the extrusion of the first polar body and arrest at metaphase II. Fertilization is the subsequent trigger for the second meiotic division with segregation of sister chromatids and extrusion of the second polar body.
Cohesin removal also depends on a non-proteolytic mechanism known as the prophase pathway, which precedes the action of Separase. The prophase pathway is orchestrated by an interaction between PDS5B, Sororin and wings apart-like (WAPL) protein (Verni et al., 2000; Nishiyama et al., 2013; Tedeschi et al., 2013). WAPL facilitates unloading of cohesin during prophase through an antagonistic mechanism mediated by competition between WAPL and Sororin for binding to PDS5B (Figure 2B; Kitajima et al., 2003; Kudo et al., 2009; Shintomi and Hirano, 2009; Ishiguro et al., 2010; Katis et al., 2010; Nishiyama et al., 2010; Carretero et al., 2013). In meiosis, this pathway has been described in yeast, worms, and mice (Brieno-Enriquez et al., 2016; Crawley et al., 2016; Wolf et al., 2018; Challa et al., 2019).
The proper step-wise removal of the cohesin rings is essential to ensure correct chromosome segregation during MI and MII. In sexually mature females, follicle-stimulating hormone (FSH) promotes growth of a dominant follicle in the ovary. Proliferation of granulosa cells surrounding the follicle leads to estrogen production which ultimately triggers the pre-ovulatory luteinizing hormone (LH) surge (McGee and Hsueh, 2000; Edson et al., 2009; Grive, 2020). The LH surge induces a decline in oocyte cAMP levels which then activates cyclin-dependent kinase 1 (CDK1) driving the transition of the oocyte out of prophase I arrest (Mehlmann et al., 2002; Mehlmann, 2005; Norris et al., 2010; Li et al., 2019). Removal of cohesin from the chromosome arms leads to the resolution of chiasmata and allows homologous chromosomes to segregate during anaphase I. If the first chromosome segregation commences correctly, the daughter cell receives a complete set of chromatids and the other set of chromatids are encompassed in the first polar body. Sister chromatids are maintained together via cohesin rings located at the centromere region. Mammalian oocyte meiosis then arrests at metaphase II until fertilization, as this event is the trigger for resumption and completion of MII. Resolution of centromeric cohesin rings during MII then allows for the segregation of sister chromatids. These events culminate in the formation of the haploid gamete and the second polar body (Figure 3B).
Cohesin established in females during fetal life is not replenished over time during postnatal development. Oocyte cohesin expression is restricted to pre- and early meiotic prophase stages and allows for complete fertility and prevents aneuploidy (Revenkova et al., 2010). Additionally, mouse models have shown that no or very little cohesin loading occurs postnatally (Burkhardt et al., 2016). Therefore, it appears that the cohesin established during fetal life provides chromosome cohesion throughout the reproductive lifespan and the loss or weakening of chromosome cohesin may underpin the issue of declining oocyte quality and aneuploidy of aging females. Specifically, cohesin loss appears to occur while oocytes age during the time they are arrested in prophase I (Lister et al., 2010). Long-lived mouse strains as well as humans show an increased interkinetochore distance (IKD) with increasing female age (Chiang et al., 2010; Lister et al., 2010; Gruhn et al., 2019) which is thought to be a surrogate marker for loss of centromeric cohesion. Studies in human females have demonstrated that PSSC is the most common segregation error leading to aneuploidy (Pellestor et al., 2003; Garcia-Cruz et al., 2010; Ottolini et al., 2015; Gruhn et al., 2019). Therefore, weakening of centromeric cohesion is likely a major contributor to aneuploidy seen in women of advanced age. Additionally, studies of human oocytes and embryos have found higher rates of recombination and therefore sites of crossovers in euploid as opposed to aneuploid oocytes (Ottolini et al., 2015; Wang et al., 2017; Gruhn et al., 2019; Hassold et al., 2021). Given the presence of cohesin rings along the chromosome arms adjacent to the sites of crossovers, this data provides another link for the lack or loss of cohesin being a driver of aneuploidy in older women.
Primary ovarian insufficiency (POI) is defined by oligomenorrhea or amenorrhea prior to age 40 in conjunction with elevated serum FSH levels in the menopausal range, as defined by the reporting laboratory, drawn on two separate occasions at least 1 month apart (Nelson, 2009). Affecting approximately 1% of reproductive-aged women, POI is a heterogeneous disorder with a spectrum of etiologies including cytogenetic abnormalities, autoimmune factors, or various genetic causes. Additionally, POI may result from iatrogenic factors such as gonadotoxic cancer treatments (Nelson, 2009; Kort et al., 2014; Anderson et al., 2015; Hoekman et al., 2018) or ovarian surgeries. More recent advancements in clinical genetics have uncovered significant genetic contributions to POI, though the etiology in most spontaneous cases remains to be elucidated. Additionally, POI exhibits a spectrum of patient presentations including young girls who present with primary amenorrhea already qualifying for the diagnosis, compared to women in their thirties with subfertility/infertility and/or diminished ovarian reserve who subsequently go on to be formally diagnosed with POI once criteria are met.
Recent studies of families with POI from various ethnic backgrounds have identified several variants in the gene encoding the meiosis-specific cohesin protein STAG3 as a potential etiology for their diagnosis (Table 1; Caburet et al., 2014; Le Quesne Stabej et al., 2016; Colombo et al., 2017; He et al., 2018; Franca et al., 2019; Heddar et al., 2019). Interestingly, in all studies which have identified variants in STAG3, the index patient case(s) have presented with primary amenorrhea, elevated FSH levels in menopausal range, and streak gonads on ultrasound. These findings are consistent with early gonadal dysgenesis in young girls with otherwise normal 46XX karyotypes.
The first report of mutant cohesin leading to POI was reported by Caburet et al. (2014), in a Palestinian family with four affected sisters and one affected maternal aunt. Whole exome sequencing (WES) was performed on one affected sister and one unaffected sister which identified a deleterious 1 bp deletion (c.968delC) in STAG3 resulting in a frameshift mutation and subsequent premature stop codon (Caburet et al., 2014). Ultimately, the four affected sisters were found to be homozygous for the mutation while unaffected family members were either heterozygous or homozygous for the non-mutant allele. These authors created a Stag3–/– mouse model which had no overt phenotype beyond sterility in both male and female mice. Histologic analysis of ovaries from these mice revealed a lack of oocytes and ovarian follicles as well as dense stroma indicating severe and early ovarian dysgenesis. Fetal oocyte meiotic chromosome spreads also demonstrated that oocytes from the Stag3–/– mouse model had loss of centromeric sister chromatid cohesion and axial elements did not progress beyond the leptonema stage of meiotic prophase. Additionally, findings from a different Stag3–/– mouse model demonstrated disruption in other meiosis-specific cohesin localization to the chromosome cores which led to disruption in DNA repair processes, synapsis of chromosomes, and pericentromeric heterochromatin clustering. This culminated in disruption of centromeric cohesion during meiotic prophase I with early prophase I arrest and apoptosis of both male and female germ cells (Hopkins et al., 2014). Therefore, studies in mice support the finding that this family (and others in which STAG3 variants have been identified) has POI on the more severe end of the spectrum with diagnosis at a young age with primary amenorrhea, elevated FSH levels, and streak gonads on ultrasound consistent with a clinical picture of gonadal dysgenesis.
The second report of a STAG3 variant identified in a family with POI was by Le Quesne Stabej et al. (2016) in a consanguineous Lebanese family with two affected sisters who presented with primary amenorrhea and absent pubertal development. Linkage analysis and WES found a homozygous 2 bp duplication (c.1947_48dupCT) which resulted in a transcript encoding a truncated protein in the STAG3 gene. Furthermore, Colombo et al. (2017) reported on a consanguineous family of Asian origin with two sisters affected by POI. WES identified a C to G transversion at nucleotide c.677 (c.677C > G) in STAG3–both affected sisters were homozygous for this mutation which resulted in a premature stop codon and thus, predicted a truncated protein (p.[Ser227∗]). Interestingly, the 87-year-old paternal grandmother and 48-year-old maternal aunt of the sisters were both carriers of this mutation and did not suffer from amenorrhea or infertility, though they did go through premature menopause at age 40 and 37 years old, respectively.
In addition, two sisters affected with POI from a Han Chinese family were found to have a homozygous donor splice site mutation in STAG3 (c.1573 + 5G > A) which was predicted to result in a frameshift mutation and premature stop codon. The unaffected parents and brother in this family were heterozygous carriers for the mutation (He et al., 2018). Additionally, more recent reports have shown the impact of compound heterozygous STAG3 variants on families with POI. Heddar et al. (2019) reported on two Caucasian sisters with non-syndromic POI. WES of the proband and unaffected mother identified two novel pathogenic variants including a 1 bp deletion in exon 28 of STAG3 (c.305delC) which yielded a premature stop codon predicting a truncated protein. The second variant was a T to G substitution in exon 7 (c.659T > G) which led to a missense mutation. Interestingly, this variant was also identified in the unaffected mother who proceeded through menopause at age 51. Franca et al. (2019) identified two rare loss-of function variants in STAG3 (c.291dupC and c1950C > A) leading to POI in a 21-year-old Brazilian woman. Compound heterozygosity for these variants was felt to be the mode of inheritance given the rarity of both variants and their impact on the transcript and protein; however, the affected patient was adopted and therefore parental DNA was not available to confirm this assumption.
Additional cohesin-ring components have also been implicated in human POI, specifically, SMC1β and REC8 (Bouilly et al., 2016). Bouilly et al. (2016) used multiplex sequencing technology to screen 100 patients with unexplained POI for 19 different candidate genes either known or suspected to play a role in POI pathogenesis. Four patients were identified to harbor variants in genes encoding cohesin-associated proteins. Three of these patients presented with primary amenorrhea and two of them were also siblings. These sisters were diagnosed with POI at age 14 and 17, respectively. They had inherited a REC8 variant (c.641A > G; Q154R) from their mother and a variant in GDF9 (c.1360C > T; R54C) from their father. Both variants were predicted to be damaging by the Polyphen2 database (prediction of functional effects of human nsSNPs), a tool which predicts the potential impact of amino acid changes on the structure and function of a protein. The other patient who presented with primary amenorrhea was diagnosed with POI at age 13 and was found to have a variant in SMC1β (c.3530A > T; Q1177L) as well as BMP15 (c.13A > C; S5R), both of which were predicted to be damaging by the Polyphen2 database. An additional SMC1β variant (c.662T > C; I221T) was identified in one patient with secondary amenorrhea and POI diagnosed at age 22, yet she was also found to have two variants in the oocyte-specific gene, NOBOX. The findings of these studies demonstrate that variants in genes encoding cohesin-associated proteins may play a role in POI pathogenesis. Moreover, digenic and perhaps polygenic inheritance likely plays a role in the timing of onset of POI as well. Future studies of patients with POI, and their evaluation for potential etiologies, may need to consider these candidate genes, and specifically STAG3, given multiple affected families across various ethnic backgrounds have been identified to harbor these variants.
The vast majority of meiotic errors detected in human pregnancies result from errors in female meiosis and are a leading cause of pregnancy loss (Hassold and Hunt, 2001; Nagaoka et al., 2012). Meiosis in females is prone to segregation errors such as non-disjunction of homologous chromosomes as well as PSSC resulting in aneuploidy, with trisomy being the most common aneuploidy. While all autosomal chromosomes are susceptible to missegregation (Handyside et al., 2012), most autosomal aneuploidy is not compatible with embryogenesis or implantation. Therefore, autosomal aneuploidy manifests clinically as infertility or subfertility, especially in women at advanced ages. Some pregnancies affected by trisomy can progress to later stages, but most commonly result in miscarriage. Trisomy 13, 18, or 21 can result in live births with children being affected by Patau Syndrome, Edwards Syndrome, or Down Syndrome, respectively. Of these, Trisomy 21 is the most common and the incidence has been increasing in recent decades, which is likely related to women having children later in the reproductive lifespan (Morris and Alberman, 2009; Loane et al., 2013). Given that Trisomy 21 is one of the few chromosomal aneuploidies which may survive to a live birth, studying families (parents and affected child) is a resource to further understand mechanisms of meiotic segregation errors in humans.
Regarding trisomy 21, both increased maternal age as well as altered recombination events, regardless of age, have been associated with meiotic errors (Lamb et al., 1997; Oliver et al., 2008; Ray et al., 2018). The absence of recombination or the presence of a single peri-telomeric recombination event on the long arm of chromosome 21 have been associated with errors during MI and appear to be age-independent, and thus, the more common etiology of trisomy 21 in younger women (Lamb et al., 1997; Oliver et al., 2008; Ray et al., 2018). Errors during MII are associated with increasing maternal age and a peri-centromeric recombinant event on chromosome 21 (Lamb et al., 1997; Oliver et al., 2008, 2012; Ray et al., 2018). This peri-centromeric recombination pattern may lead to a suboptimal configuration which could compromise proteins involved in centromeric cohesin, exacerbating the normal degradation of this complex with age. Alternatively, the peri-centromeric recombinant event may in fact stabilize the tetrad through MI, leading to enrichment in errors during MII in older oocytes (Oliver et al., 2008).
A recent study by Chernus et al. (2019) was undertaken to discover genetic variants which may increase the risk for maternal non-disjunction of chromosome 21 using a candidate gene approach as well as a genome-wide association study (GWAS). The study sample included 749 liveborn offspring with a free, maternally derived, trisomy 21, as well as their available biological parents (n = 1,437 parents). DNA samples were genotyped on the HumanOmniExpressExome-8v1-2 array. These genotypes were then used to determine (1) if the non-disjunction error was maternally derived and (2) if this occurred during MI or MII. Subgroup analyses of mothers vs. fathers, MI mothers vs. fathers, MII mothers vs. fathers, and MI mothers vs. MII mothers were performed. Given that defects in cohesin proteins may play a role in PSSC and aneuploidy, the authors chose to assess candidate genes for meiosis-specific cohesin [SMC1β, REC8, RAD21L (SNP rs450739), and STAG3] within the subgroup analyses. Of the analyzed genes involved in the meiotic cohesin complex in this GWAS, there was a statistically significant association with a missense variant in RAD21L in the all mothers vs. fathers (OR 0.67) and MI mothers vs. fathers (OR 0.67) subgroup analyses, suggesting variants in this gene may be a risk factor for non-disjunction, more commonly in MI. None of the other candidate genes for cohesin-associated proteins demonstrated significance.
RAD21L is a part of the cohesin complex, which is an important regulator of chromosome dynamics and structure during mitosis and meiosis. Gene disruption of RAD21L in male mice leads to infertility whereas in female mice there is apparent age-related infertility (Herran et al., 2011). Additionally, RAD21L has been associated with recombination in males, but less so in females (Kong et al., 2014). Therefore, disruption of RAD21L seems to have sexually dimorphic phenotypes–possibly playing a larger role in recombination events in males and potentially a role related to chromosome segregation in females. The RAD21L variant association with trisomy 21 seen by Chernus et al. (2019) was more commonly seen in maternal MI errors which would be consistent with prior studies relating errors during MI to aberrations of recombination (Lamb et al., 1997; Oliver et al., 2008; Ray et al., 2018). Interestingly, the RAD21L variant association was not significant for MII errors despite RAD21L being a member of the cohesin complex. We would have hypothesized seeing an association with MII errors given their association with maternal age. The authors noted, however, that their sample size was limited and they did not see any unique age-associated variants. Therefore, further studies are needed to investigate alternative genetic variants which may be associated with trisomy 21 as well as how they relate to the timing of the meiotic error and maternal age at conception.
The most severe form of male factor infertility is non-obstructive azoospermia (NOA). This occurs in approximately 1% of men of reproductive age (Tournaye et al., 2017). Approximately, 14% of those patients are found to have karyotype abnormalities (Van Assche et al., 1996) while approximately 8–10% have identified Y chromosome microdeletions [azoospermia factor (AZF) deletions] (Ferlin et al., 2007). When NOA is “idiopathic” the assumption is that there is likely a genetic factor involved that is yet to be determined. In recent years, sequencing approaches in families with members affected by azoospermia or severe oligospermia have identified candidate genes using a discovery-oriented approach. This method has not yet identified a cohesin-associated variant.
An alternative approach, utilizing information known from mouse models, has recently identified STAG3 variants in a cohort of men with azoospermia. Riera-Escamilla et al. (2019) designed a “mouse azoospermia” gene panel consisting of 175 genes and subsequently analyzed those candidate genes via next-generation sequencing in a highly selected cohort of men with idiopathic NOA (n = 33). This cohort included 31 unrelated men and two brothers from a consanguineous family. After sequencing data was filtered, one patient was found to harbor two variants in STAG3 (compound heterozygote). The STAG3 variants included a frameshift insertion which generated a premature stop gain in the 597 amino acid; this is predicted to result in a protein lacking the entire armadillo-type domain of STAG3 which is involved in DNA and protein interactions. The other STAG3 variant identified was a novel splicing variant located on exon 23, an indispensable site for normal splicing. The patient’s unaffected fertile brother was only a heterozygous carrier for the frameshift mutation. Upon histologic analysis of testis biopsies including immunohistochemistry, the authors concluded that meiotic entry occurred with normal frequency, however, meiosis was noted to arrest as there was evidence of DSB formation, but failure to complete chromosome pairing. Therefore, it appears that STAG3 mutations in this patient resulted in complete bilateral meiotic arrest (MA) of spermatogenesis resulting in NOA.
In similar fashion, van der Bijl et al. (2019) took a candidate gene approach to assessing sequence variants in STAG3 in a different cohort of men with idiopathic NOA with MA (n = 28). In this analysis, the full coding region of STAG3 was sequenced directly. These authors identified two compound heterozygous variants in STAG3 (c.1262T > G; 1312C > T) in exon 13 leading to complete bilateral MA in an otherwise healthy human male. The c.1262T > G variant was paternally inherited and is a missense variant that changes a highly conserved neutral amino acid (Leucine) to a basic amino acid (Arginine) likely affecting protein folding and post-translational modifications. This variant is predicted to be disease-causing by all utilized prediction programs. The c.1312C > T variant was maternally inherited and is a nonsense substitution which introduces a premature stop codon (p.Arg438Ter). The index patient had bilateral small testis volumes (12–13 mL) and normal testosterone levels. The most developed germ cell type in any seminiferous tubule was the primary spermatocyte and a microdissection testicular sperm extraction (microTESE) procedure did not yield any sperm from this patient. Analysis of meiotic chromosome spreads from the spermatocytes of the affected patient found that no meiotic cells progressed through the zygonema stage of meiotic prophase I, which is consistent with MA. Additionally, compound heterozygosity was confirmed by Sanger sequencing of the patient’s parents. His brother inherited the c.1262T > G variant and spontaneously conceived two children.
In another recent study by Krausz et al. (2020) performed exome sequencing on 17 men with NOA due to MA who had a negative microTESE procedure. Interestingly, a plausible genetic cause of NOA was identified in 14 of these 17 patients. One patient with complete MA and normal sized testis was found to carry two novel heterozygous loss of function variants in STAG3–one splicing variant and one frameshift deletion in exon 16 which led to a premature stop codon at the 558th amino acid affecting the armadillo-type domain.
These studies are the first to demonstrate STAG3 variants negatively impact protein function and lead to human male infertility due to MA. Similar to observations in females harboring variants in STAG3 have been shown to be associated with POI as previously described. With further evidence of the important role of cohesin-associated STAG3 in meiotic events in both males and females, consideration can be made for analysis of STAG3 in men and women with otherwise “idiopathic” NOA or POI, respectively.
RAD21L is another meiosis-specific cohesin protein which interacts with structural maintenance of chromosome proteins SMC3 and SMC1α/β as well as STAG3 (Gutierrez-Caballero et al., 2011; Ishiguro et al., 2011; Lee and Hirano, 2011). RAD21L is transcribed abundantly in testis and localized to the lateral and axial elements of the synaptonemal complex playing an essential role in homologous chromosome synapsis during meiotic prophase I. Male mice deficient in RAD21L are defective in homologous chromosome synapsis which in turn leads to zygonema arrest and subsequent azoospermia with male sterility in mice (Herran et al., 2011). Interestingly, age-dependent sterility is seen in female mice lacking RAD21L (Gutierrez-Caballero et al., 2011; Herran et al., 2011).
In the study by Krausz et al. (2020) described above, one patient with NOA was noted to have a homozygous stop gain variant in RAD21L (c.1543C > T; p.Arg515Ter), which resulted in the removal of the final 41 amino acids of the protein. His fertile brother was found to be a heterozygous carrier. The affected patient had bilaterally small testis and histology demonstrated complete MA. In a targeted gene approach, Minase et al. (2017) evaluated the RAD21L coding region in a Japanese cohort with 38 men with NOA due to MA and 140 men with NOA due to Sertoli cell-only syndrome (SCOS) and compared these men to 200 fertile controls. The RAD21L coding region was sequenced, and three variants were found (c.454C > A; c.1268A > C; and c.1610G > A). The distribution of two of the variants (c.1268A > C, His423Pro; and c.1610G > A, Ser537Asn) was significantly different between the NOA patients with either MA or SCOS compared with the fertile controls. The authors postulated that these amino acid substitutions may play a role in disruption of spermatogenesis in Japanese patients, however, the function of the SNPs in those positions were predicted to be benign via the PolyPhen2 database. Therefore, the relationship between these identified SNPs and a mechanistic cause of NOA has yet to be determined.
In addition to its role in sister chromatid cohesion and chromosome segregation, cohesin has been implicated in genome stability owing to its role in DNA repair and recombination. DNA damage arises continuously by various endogenous and exogenous factors and cells must possess mechanisms to repair these lesions. One mechanism is via homologous recombination (HR). In this process, BRCA2 (BRCA2 DNA repair associated) and PALB2 (Partner and Localizer of BRCA2) are key regulators and promote RAD51 activity which is essential for HR. Failure to repair DNA properly may lead to cell apoptosis or cancer (Hoeijmakers, 2001; Prakash et al., 2015). Cohesin subunits have been demonstrated to undergo various mutations in cancer (Losada, 2014; Waldman, 2020). Somatic mutations in cohesin ring components have been observed in colorectal cancer (Sasaki et al., 2021), bladder cancer (Aquila et al., 2018), and hematologic malignancies and germline mutations in cohesin or its regulators are found in cohesinopathies (Horsfield et al., 2012; Cancer Genome Atlas Research Network et al., 2013).
The cohesin-associated protein PDS5B has been shown to be a mediator of homologous recombination in vitro. PDS5B associates with BRCA2 in early S-phase and depletion of PDS5B compromises the localization of both RAD51 and BRCA2 to the nucleus essentially disturbing HR and leading to increased cell sensitivity to DNA damaging agents (Brough et al., 2012). Based upon these findings, Couturier et al. (2016) sought to elucidate the role of PDS5B in HR and ovarian cancer prediction. In their analysis, tumor samples were obtained from chemotherapy naïve patients undergoing surgery for ovarian cancer between 1992 and 2012. The authors found that low levels of PDS5B expression correlated with improved survival in these patients. Similarly, Brough et al. (2012) assessed the expression of PDS5B in a panel of 160 invasive breast tumors and found that levels were associated with both histological grade of the breast cancer as well as outcome in patients treated with chemotherapy. In general, lower expression of PDS5B was associated with higher grade tumors and triple negative (estrogen receptor, progesterone receptor, Her2/Neu negative) tumors. Additionally, tumors with lower expression of PDS5B had a favorable response to chemotherapy.
Regarding the meiosis-specific cohesin components, loss of heterozygosity in STAG3 has been noted in epithelial ovarian carcinomas (Notaridou et al., 2011). In the review of the literature outlined above for women with POI and associated STAG3 variants, there was one patient noted to have bilateral ovarian tumors diagnosed at age 19 which consisted of a gonadoblastoma on the right ovary and a complex tumor of the left ovary consisting of embryonal carcinoma, choriocarcinoma, and dysgerminoma (Caburet et al., 2014). With this finding and the knowledge that cohesin plays a role in DNA repair and genome stability, additional investigation is needed to determine if women with POI related to cohesin defects are at risk for ovarian cancer and specifically, germ cell tumors.
As described above, loss or weakening of cohesin has been implicated in reproductive aging and aneuploidy. The end result of reproductive aging is POI/menopause in females or NOA in males and human genetic variants in cohesin-protein components have been implicated in both diagnoses. It is well characterized that POI/early menopause is associated with age-related diseases such as osteoporosis, cardiovascular disease (CVD), and all-cause mortality (Qiu et al., 2013; Tao et al., 2016; Quinn and Cedars, 2018). Regarding CVD pathogenesis, estrogen is felt to be cardioprotective due to effects on vascular endothelium (Mori et al., 2000; Chandrasekar et al., 2001), hence the increase in CVD risk in patients with early menopause. An alternative hypothesis is that premenopausal CVD may have effects on ovarian microvasculature predisposing to ovarian aging (Kok et al., 2006). A recent study by Wang et al. (2021) utilizing prospective data from the Nurses Health Study II found that spontaneous abortion (SAB) was associated with a greater risk of premature death, particularly from CVD. Whether or not SAB was related to microvascular changes or genetic changes was not determined, however, the authors postulated that SAB could be an early marker for future health risk in women, though the underlying mechanisms linking SAB with premature death from CVD still need to be elucidated. Therefore, the question remains if ovarian aging leads to CVD or if general aging in the individual patient is a common underlying risk factor for both CVD and ovarian aging (Cedars, 2013). For example, Hanson et al. (2020) studied young women with poor ovarian response to stimulation in assisted reproductive technology cycles and correlated oocyte yield with DNA methylation profiles in white blood cell (WBC) samples according to the “epigenetic clock” age prediction model (Horvath, 2013, 2015). Interestingly, the authors found that poor ovarian response was associated with epigenetic age acceleration in patient WBC samples.
More recent reports have also implicated male factor infertility in diseases of aging and mortality (Glazer et al., 2017; Francesco et al., 2021). Eisenberg (2016) and Eisenberg et al. (2016) reported that men with oligospermia and azoospermia have higher risks of incident diabetes, kidney disease, and ischemic heart disease in the years following their infertility evaluation. Additionally, men with infertility are at increased risk of various malignancies including testicular, prostate, bladder, and thyroid cancers; melanoma; and hematologic malignancies (Eisenberg et al., 2015). A recent systematic review by Francesco et al. (2021), found that infertile men (as compared to fertile men) have an increased risk of death, with the highest risk being associated with azoospermia.
An underlying link connecting male and female infertility with overall health and lifespan remains uncertain due to the multifactorial pathogenesis of infertility and aging as well as heterogeneity of the literature. However, the use of infertility, specifically the most extreme versions including POI in females and NOA in males, may provide a biomarker for further counseling on genetic implications for current attempts at conception as well as the future health of patients and their offspring.
Expansion of genetic testing within clinical medicine, and specifically reproductive medicine, may offer additional insight into previously unknown etiologies of disease. Specifically focusing on cohesin protein-related genes may inform the diagnosis of disorders contributing to gamete exhaustion in females, as well as infertility and reproductive aging in both men and women. The regulation of cohesin loading and removal requires the coordination of various kinases and phosphatases and further studies are needed to gain a better understanding of these mechanisms. Given that protein kinases are an emerging class of drug targets, identification of genetic defects in cohesin-related genes or regulators may serve as potential targets for future therapeutic studies of reproductive aging.
RB, MS, and MB-E collected the references and wrote the manuscript. All authors contributed to the article and approved the submitted version.
This study was supported by Eunice Kennedy Shriver National Institute of Child Health and Human Development (R00HD090289) and the Magee Auxiliary Research Scholar (MARS) endowment.
The authors declare that the research was conducted in the absence of any commercial or financial relationships that could be construed as a potential conflict of interest.
All claims expressed in this article are solely those of the authors and do not necessarily represent those of their affiliated organizations, or those of the publisher, the editors and the reviewers. Any product that may be evaluated in this article, or claim that may be made by its manufacturer, is not guaranteed or endorsed by the publisher.
Albamonte, M. I., Albamonte, M. S., Bou-Khair, R. M., Zuccardi, L., and Vitullo, A. D. (2019). The ovarian germinal reserve and apoptosis-related proteins in the infant and adolescent human ovary. J. Ovarian Res. 12:22.
Almanzar, D. E., Gordon, S. G., and Rog, O. (2021). Meiotic sister chromatid exchanges are rare in C. elegans. Curr. Biol. 31, 1499–1507.e3.
American College of Obstetricians and Gynecologists Committee on Gynecologic Practice and Practice Committee (2014). Female age-related fertility decline. Committee Opinion No. 589. Fertil. Steril. 101, 633–634. doi: 10.1016/j.fertnstert.2013.12.032
Anderson, R. A., Mitchell, R. T., Kelsey, T. W., Spears, N., Telfer, E. E., and Wallace, W. H. (2015). Cancer treatment and gonadal function: experimental and established strategies for fertility preservation in children and young adults. Lancet Diabetes Endocrinol. 3, 556–567. doi: 10.1016/s2213-8587(15)00039-x
Aquila, L., Ohm, J., and Woloszynska-Read, A. (2018). The role of STAG2 in bladder cancer. Pharmacol. Res. 131, 143–149. doi: 10.1016/j.phrs.2018.02.025
Balasch, J., and Gratacos, E. (2012). Delayed childbearing: effects on fertility and the outcome of pregnancy. Curr. Opin. Obstet. Gynecol. 24, 187–193. doi: 10.1097/gco.0b013e3283517908
Bolcun-Filas, E., and Handel, M. A. (2018). Meiosis: the chromosomal foundation of reproduction. Biol. Reprod. 99, 112–126. doi: 10.1093/biolre/ioy021
Bouilly, J., Beau, I., Barraud, S., Bernard, V., Azibi, K., Fagart, J., et al. (2016). Identification of multiple gene mutations accounts for a new genetic architecture of primary ovarian insufficiency. J. Clin. Endocrinol. Metab. 101, 4541–4550. doi: 10.1210/jc.2016-2152
Brieno-Enriquez, M. A., Moak, S. L., Toledo, M., Filter, J. J., Gray, S., Barbero, J. L., et al. (2016). Cohesin removal along the chromosome arms during the first meiotic division depends on a NEK1-PP1gamma-WAPL axis in the mouse. Cell Rep. 17, 977–986. doi: 10.1016/j.celrep.2016.09.059
Brough, R., Bajrami, I., Vatcheva, R., Natrajan, R., Reis-Filho, J. S., Lord, C. J., et al. (2012). APRIN is a cell cycle specific BRCA2-interacting protein required for genome integrity and a predictor of outcome after chemotherapy in breast cancer. EMBO J. 31, 1160–1176. doi: 10.1038/emboj.2011.490
Buheitel, J., and Stemmann, O. (2013). Prophase pathway-dependent removal of cohesin from human chromosomes requires opening of the Smc3-Scc1 gate. EMBO J. 32, 666–676. doi: 10.1038/emboj.2013.7
Buonomo, S. B., Clyne, R. K., Fuchs, J., Loidl, J., Uhlmann, F., and Nasmyth, K. (2000). Disjunction of homologous chromosomes in meiosis I depends on proteolytic cleavage of the meiotic cohesin Rec8 by separin. Cell 103, 387–398. doi: 10.1016/s0092-8674(00)00131-8
Burkhardt, S., Borsos, M., Szydlowska, A., Godwin, J., Williams, S. A., Cohen, P. E., et al. (2016). Chromosome cohesion established by Rec8-cohesin in fetal oocytes is maintained without detectable turnover in oocytes arrested for months in mice. Curr. Biol. 26, 678–685. doi: 10.1016/j.cub.2015.12.073
Caburet, S., Arboleda, V. A., Llano, E., Overbeek, P. A., Barbero, J. L., Oka, K., et al. (2014). Mutant cohesin in premature ovarian failure. N. Engl. J. Med. 370, 943–949. doi: 10.1056/nejmoa1309635
Cahoon, C. K., and Hawley, R. S. (2016). Regulating the construction and demolition of the synaptonemal complex. Nat. Struct. Mol. Biol. 23, 369–377. doi: 10.1038/nsmb.3208
Cahoon, C. K., Helm, J. M., and Libuda, D. E. (2019). Synaptonemal complex central region proteins promote localization of pro-crossover factors to recombination events during Caenorhabditis elegans Meiosis. Genetics 213, 395–409. doi: 10.1534/genetics.119.302625
Cancer Genome Atlas Research Network, Ley, T. J., Miller, C., Ding, L., Raphael, B. J., Mungall, A. J., et al. (2013). Genomic and epigenomic landscapes of adult de novo acute myeloid leukemia. N. Engl. J. Med. 368, 2059–2074. doi: 10.1056/nejmoa1301689
Carretero, M., Ruiz-Torres, M., Rodriguez-Corsino, M., Barthelemy, I., and Losada, A. (2013). Pds5B is required for cohesion establishment and Aurora B accumulation at centromeres. EMBO J. 32, 2938–2949. doi: 10.1038/emboj.2013.230
Cedars, M. I. (2013). Biomarkers of ovarian reserve–do they predict somatic aging? Semin. Reprod. Med. 31, 443–451. doi: 10.1055/s-0033-1356480
Challa, K., Shinohara, M., and Shinohara, A. (2019). Meiotic prophase-like pathway for cleavage-independent removal of cohesin for chromosome morphogenesis. Curr. Genet. 65, 817–827. doi: 10.1007/s00294-019-00959-x
Chandrasekar, B., Nattel, S., and Tanguay, J. F. (2001). Coronary artery endothelial protection after local delivery of 17beta-estradiol during balloon angioplasty in a porcine model: a potential new pharmacologic approach to improve endothelial function. J. Am. Coll. Cardiol. 38, 1570–1576. doi: 10.1016/s0735-1097(01)01552-2
Chernus, J. M., Allen, E. G., Zeng, Z., Hoffman, E. R., Hassold, T. J., Feingold, E., et al. (2019). A candidate gene analysis and GWAS for genes associated with maternal nondisjunction of chromosome 21. PLoS Genet. 15:e1008414. doi: 10.1371/journal.pgen.1008414
Chiang, T., Duncan, F. E., Schindler, K., Schultz, R. M., and Lampson, M. A. (2010). Evidence that weakened centromere cohesion is a leading cause of age-related aneuploidy in oocytes. Curr. Biol. 20, 1522–1528. doi: 10.1016/j.cub.2010.06.069
Chiang, T., Schultz, R. M., and Lampson, M. A. (2011). Age-dependent susceptibility of chromosome cohesion to premature separase activation in mouse oocytes. Biol. Reprod. 85, 1279–1283. doi: 10.1095/biolreprod.111.094094
Colombo, R., Pontoglio, A., and Bini, M. (2017). A STAG3 missense mutation in two sisters with primary ovarian insufficiency. Eur. J. Obstet. Gynecol. Reprod. Biol. 216, 269–271. doi: 10.1016/j.ejogrb.2017.08.005
Couturier, A. M., Fleury, H., Patenaude, A. M., Bentley, V. L., Rodrigue, A., Coulombe, Y., et al. (2016). Roles for APRIN (PDS5B) in homologous recombination and in ovarian cancer prediction. Nucleic Acids Res. 44, 10879–10897. doi: 10.1093/nar/gkw921
Crawley, O., Barroso, C., Testori, S., Ferrandiz, N., Silva, N., Castellano-Pozo, M., et al. (2016). Cohesin-interacting protein WAPL-1 regulates meiotic chromosome structure and cohesion by antagonizing specific cohesin complexes. Elife 5:e10851.
Dietrich, A. J., van Marle, J., Heyting, C., and Vink, A. C. (1992). Ultrastructural evidence for a triple structure of the lateral element of the synaptonemal complex. J. Struct. Biol. 109, 196–200. doi: 10.1016/1047-8477(92)90031-5
Edson, M. A., Nagaraja, A. K., and Matzuk, M. M. (2009). The mammalian ovary from genesis to revelation. Endocr. Rev. 30, 624–712. doi: 10.1210/er.2009-0012
Eisenberg, M. L. (2016). Risks beyond reproduction for infertile men. Fertil. Steril. 105, 300–301. doi: 10.1016/j.fertnstert.2015.11.040
Eisenberg, M. L., Li, S., Brooks, J. D., Cullen, M. R., and Baker, L. C. (2015). Increased risk of cancer in infertile men: analysis of U.S. claims data. J. Urol. 193, 1596–1601. doi: 10.1016/j.juro.2014.11.080
Eisenberg, M. L., Li, S., Cullen, M. R., and Baker, L. C. (2016). Increased risk of incident chronic medical conditions in infertile men: analysis of United States claims data. Fertil. Steril. 105, 629–636. doi: 10.1016/j.fertnstert.2015.11.011
Ferlin, A., Arredi, B., Speltra, E., Cazzadore, C., Selice, R., Garolla, A., et al. (2007). Molecular and clinical characterization of Y chromosome microdeletions in infertile men: a 10-year experience in Italy. J. Clin. Endocrinol. Metab. 92, 762–770. doi: 10.1210/jc.2006-1981
Forabosco, A., and Sforza, C. (2007). Establishment of ovarian reserve: a quantitative morphometric study of the developing human ovary. Fertil. Steril. 88, 675–683. doi: 10.1016/j.fertnstert.2006.11.191
Franca, M. M., Nishi, M. Y., Funari, M. F. A., Lerario, A. M., Baracat, E. C., Hayashida, S. A. Y., et al. (2019). Two rare loss-of-function variants in the STAG3 gene leading to primary ovarian insufficiency. Eur. J. Med. Genet. 62, 186–189. doi: 10.1016/j.ejmg.2018.07.008
Francesco, D. G., Alex, M. K., Tony, C., Ettore, B., Maria, B. G., Alessandro, S., et al. (2021). The association between mortality and male infertility: systematic review and meta-analysis. Urology S0090-4295(21)00287-9. doi: 10.1016/j.urology.2021.02.041
Fraune, J., Schramm, S., Alsheimer, M., and Benavente, R. (2012). The mammalian synaptonemal complex: protein components, assembly and role in meiotic recombination. Exp. Cell Res. 318, 1340–1346. doi: 10.1016/j.yexcr.2012.02.018
Gao, J., and Colaiacovo, M. P. (2018). Zipping and unzipping: protein modifications regulating synaptonemal complex dynamics. Trends Genet. 34, 232–245. doi: 10.1016/j.tig.2017.12.001
Garcia-Cruz, R., Casanovas, A., Brieno-Enriquez, M., Robles, P., Roig, I., Pujol, A., et al. (2010). Cytogenetic analyses of human oocytes provide new data on non-disjunction mechanisms and the origin of trisomy 16. Hum. Reprod. 25, 179–191. doi: 10.1093/humrep/dep347
Garcia-Muse, T., Galindo-Diaz, U., Garcia-Rubio, M., Martin, J. S., Polanowska, J., O’Reilly, N., et al. (2019). A meiotic checkpoint alters repair partner bias to permit inter-sister repair of persistent DSBs. Cell Rep. 26, 775–787.e5.
Glazer, C. H., Bonde, J. P., Eisenberg, M. L., Giwercman, A., Haervig, K. K., Rimborg, S., et al. (2017). Male infertility and risk of nonmalignant chronic diseases: a systematic review of the epidemiological evidence. Semin. Reprod. Med. 35, 282–290. doi: 10.1055/s-0037-1603568
Gray, S., and Cohen, P. E. (2016). Control of meiotic crossovers: from double-strand break formation to designation. Annu. Rev. Genet. 50, 175–210. doi: 10.1146/annurev-genet-120215-035111
Grive, K. J. (2020). Pathways coordinating oocyte attrition and abundance during mammalian ovarian reserve establishment. Mol. Reprod. Dev. 87, 843–856. doi: 10.1002/mrd.23401
Gruhn, J. R., Zielinska, A. P., Shukla, V., Blanshard, R., Capalbo, A., Cimadomo, D., et al. (2019). Chromosome errors in human eggs shape natural fertility over reproductive life span. Science 365, 1466–1469. doi: 10.1126/science.aav7321
Gutierrez-Caballero, C., Herran, Y., Sanchez-Martin, M., Suja, J. A., Barbero, J. L., Llano, E., et al. (2011). Identification and molecular characterization of the mammalian alpha-kleisin RAD21L. Cell Cycle 10, 1477–1487. doi: 10.4161/cc.10.9.15515
Haarhuis, J. H., Elbatsh, A. M., and Rowland, B. D. (2014). Cohesin and its regulation: on the logic of X-shaped chromosomes. Dev Cell 31, 7–18. doi: 10.1016/j.devcel.2014.09.010
Handel, M. A., and Schimenti, J. C. (2010). Genetics of mammalian meiosis: regulation, dynamics and impact on fertility. Nat. Rev. Genet. 11, 124–136. doi: 10.1038/nrg2723
Handyside, A. H., Montag, M., Magli, M. C., Repping, S., Harper, J., Schmutzler, A., et al. (2012). Multiple meiotic errors caused by predivision of chromatids in women of advanced maternal age undergoing in vitro fertilisation. Eur. J. Hum. Genet. 20, 742–747. doi: 10.1038/ejhg.2011.272
Hanson, B. M., Tao, X., Zhan, Y., Jenkins, T. G., Morin, S. J., Scott, R. T., et al. (2020). Young women with poor ovarian response exhibit epigenetic age acceleration based on evaluation of white blood cells using a DNA methylation-derived age prediction model. Hum. Reprod. 35, 2579–2588. doi: 10.1093/humrep/deaa206
Hassold, T., and Hunt, P. (2001). To err (meiotically) is human: the genesis of human aneuploidy. Nat. Rev. Genet. 2, 280–291. doi: 10.1038/35066065
Hassold, T., Maylor-Hagen, H., Wood, A., Gruhn, J., Hoffmann, E., Broman, K. W., et al. (2021). Failure to recombine is a common feature of human oogenesis. Am. J. Hum. Genet. 108, 16–24. doi: 10.1016/j.ajhg.2020.11.010
He, W. B., Banerjee, S., Meng, L. L., Du, J., Gong, F., Huang, H., et al. (2018). Whole-exome sequencing identifies a homozygous donor splice-site mutation in STAG3 that causes primary ovarian insufficiency. Clin. Genet. 93, 340–344. doi: 10.1111/cge.13034
Heddar, A., Dessen, P., Flatters, D., and Misrahi, M. (2019). Novel STAG3 mutations in a Caucasian family with primary ovarian insufficiency. Mol. Genet. Genomics 294, 1527–1534. doi: 10.1007/s00438-019-01594-4
Herbert, M., Kalleas, D., Cooney, D., Lamb, M., and Lister, L. (2015). Meiosis and maternal aging: insights from aneuploid oocytes and trisomy births. Cold Spring Harb. Perspect. Biol. 7:a017970. doi: 10.1101/cshperspect.a017970
Herran, Y., Gutierrez-Caballero, C., Sanchez-Martin, M., Hernandez, T., Viera, A., Barbero, J. L., et al. (2011). The cohesin subunit RAD21L functions in meiotic synapsis and exhibits sexual dimorphism in fertility. EMBO J. 30, 3091–3105. doi: 10.1038/emboj.2011.222
Hirano, T. (2015). Chromosome dynamics during mitosis. Cold Spring Harb. Perspect. Biol. 7:a015792. doi: 10.1101/cshperspect.a015792
Hoeijmakers, J. H. (2001). Genome maintenance mechanisms for preventing cancer. Nature 411, 366–374. doi: 10.1038/35077232
Hoekman, E. J., Knoester, D., Peters, A. A. W., Jansen, F. W., de Kroon, C. D., and Hilders, C. (2018). Ovarian survival after pelvic radiation: transposition until the age of 35 years. Arch. Gynecol. Obstet. 298, 1001–1007. doi: 10.1007/s00404-018-4883-5
Holloway, J. K., and Cohen, P. E. (2015). “Mammalian meiosis,” in Knobil and Neill’s Physiology of Reproduction, Vol. 1, eds T. M. Plant and A. J. Zeleznik (Boston, MA: Academic Press), 5–57. doi: 10.1016/b978-0-12-397175-3.00001-6
Hopkins, J., Hwang, G., Jacob, J., Sapp, N., Bedigian, R., Oka, K., et al. (2014). Meiosis-specific cohesin component, Stag3 is essential for maintaining centromere chromatid cohesion, and required for DNA repair and synapsis between homologous chromosomes. PLoS Genet. 10:e1004413. doi: 10.1371/journal.pgen.1004413
Horsfield, J. A., Print, C. G., and Monnich, M. (2012). Diverse developmental disorders from the one ring: distinct molecular pathways underlie the cohesinopathies. Front. Genet. 3:171. doi: 10.3389/fgene.2012.00171
Horvath, S. (2015). Erratum to: DNA methylation age of human tissues and cell types. Genome Biol. 16, 96.
Hunter, N. (2015). Meiotic recombination: the essence of heredity. Cold Spring Harb. Perspect. Biol. 7:a016618. doi: 10.1101/cshperspect.a016618
Ishiguro, K. I. (2019). The cohesin complex in mammalian meiosis. Genes Cells 24, 6–30. doi: 10.1111/gtc.12652
Ishiguro, K., Kim, J., Fujiyama-Nakamura, S., Kato, S., and Watanabe, Y. (2011). A new meiosis-specific cohesin complex implicated in the cohesin code for homologous pairing. EMBO Rep. 12, 267–275. doi: 10.1038/embor.2011.2
Ishiguro, T., Tanaka, K., Sakuno, T., and Watanabe, Y. (2010). Shugoshin-PP2A counteracts casein-kinase-1-dependent cleavage of Rec8 by separase. Nat. Cell Biol. 12, 500–U201.
Jessberger, R. (2012). Age-related aneuploidy through cohesion exhaustion. EMBO Rep. 13, 539–546. doi: 10.1038/embor.2012.54
Katis, V. L., Lipp, J. J., Imre, R., Bogdanova, A., Okaz, E., Habermann, B., et al. (2010). Rec8 Phosphorylation by casein kinase 1 and Cdc7-Dbf4 kinase regulates cohesin cleavage by separase during meiosis. Dev. Cell 18, 397–409. doi: 10.1016/j.devcel.2010.01.014
Kavanaugh, M. L., Pliskin, E., and Jerman, J. (2021). Use of concurrent multiple methods of contraception in the United States, 2008 to 2015. Contracept X 3:100060. doi: 10.1016/j.conx.2021.100060
Keeney, S. (2008). Spo11 and the formation of DNA double-strand breaks in meiosis. Genome Dyn. Stab. 2, 81–123. doi: 10.1007/7050_2007_026
Keeney, S., and Kleckner, N. (1995). Covalent protein-DNA complexes at the 5’ strand termini of meiosis-specific double-strand breaks in yeast. Proc. Natl. Acad. Sci. U.S.A. 92, 11274–11278. doi: 10.1073/pnas.92.24.11274
Kitajima, T. S., Miyazaki, Y., Yamamoto, M., and Watanabe, Y. (2003). Rec8 cleavage by separase is required for meiotic nuclear divisions in fission yeast. EMBO J. 22, 5643–5653. doi: 10.1093/emboj/cdg527
Kitajima, T. S., Sakuno, T., Ishiguro, K., Iemura, S., Natsume, T., Kawashima, S. A., et al. (2006). Shugoshin collaborates with protein phosphatase 2A to protect cohesin. Nature 441, 46–52. doi: 10.1038/nature04663
Kok, H. S., van Asselt, K. M., van der Schouw, Y. T., van der Tweel, I., Peeters, P. H., Wilson, P. W., et al. (2006). Heart disease risk determines menopausal age rather than the reverse. J. Am. Coll. Cardiol. 47, 1976–1983. doi: 10.1016/j.jacc.2005.12.066
Kong, A., Thorleifsson, G., Frigge, M. L., Masson, G., Gudbjartsson, D. F., Villemoes, R., et al. (2014). Common and low-frequency variants associated with genome-wide recombination rate. Nat. Genet. 46, 11–16. doi: 10.1038/ng.2833
Kort, J. D., Eisenberg, M. L., Millheiser, L. S., and Westphal, L. M. (2014). Fertility issues in cancer survivorship. CA Cancer J. Clin. 64, 118–134. doi: 10.3322/caac.21205
Krausz, C., Riera-Escamilla, A., Moreno-Mendoza, D., Holleman, K., Cioppi, F., Algaba, F., et al. (2020). Genetic dissection of spermatogenic arrest through exome analysis: clinical implications for the management of azoospermic men. Genet. Med. 22, 1956–1966. doi: 10.1038/s41436-020-0907-1
Kudo, N. R., Anger, M., Peters, A. H. F. M., Stemmann, O., Theussl, H. C., Helmhart, W., et al. (2009). Role of cleavage by separase of the Rec8 kleisin subunit of cohesin during mammalian meiosis I. J. Cell Sci. 122, 2686–2698. doi: 10.1242/jcs.035287
Lamb, N. E., Feingold, E., Savage, A., Avramopoulos, D., Freeman, S., Gu, Y., et al. (1997). Characterization of susceptible chiasma configurations that increase the risk for maternal nondisjunction of chromosome 21. Hum. Mol. Genet. 6, 1391–1399. doi: 10.1093/hmg/6.9.1391
Le Quesne Stabej, P., Williams, H. J., James, C., Tekman, M., Stanescu, H. C., Kleta, R., et al. (2016). STAG3 truncating variant as the cause of primary ovarian insufficiency. Eur. J. Hum. Genet. 24, 135–138. doi: 10.1038/ejhg.2015.107
Lee, J., and Hirano, T. (2011). RAD21L, a novel cohesin subunit implicated in linking homologous chromosomes in mammalian meiosis. J. Cell Biol. 192, 263–276. doi: 10.1083/jcb.201008005
Lee, J., Kitajima, T. S., Tanno, Y., Yoshida, K., Morita, T., Miyano, T., et al. (2008). Unified mode of centromeric protection by shugoshin in mammalian oocytes and somatic cells. Nat. Cell Biol. 10, 42–52. doi: 10.1038/ncb1667
Li, J., Qian, W. P., and Sun, Q. Y. (2019). Cyclins regulating oocyte meiotic cell cycle progressiondagger. Biol. Reprod. 101, 878–881. doi: 10.1093/biolre/ioz143
Lister, L. M., Kouznetsova, A., Hyslop, L. A., Kalleas, D., Pace, S. L., Barel, J. C., et al. (2010). Age-related meiotic segregation errors in mammalian oocytes are preceded by depletion of cohesin and Sgo2. Curr. Biol. 20, 1511–1521. doi: 10.1016/j.cub.2010.08.023
Loane, M., Morris, J. K., Addor, M. C., Arriola, L., Budd, J., Doray, B., et al. (2013). Twenty-year trends in the prevalence of Down syndrome and other trisomies in Europe: impact of maternal age and prenatal screening. Eur. J. Hum. Genet. 21, 27–33. doi: 10.1038/ejhg.2012.94
Losada, A. (2014). Cohesin in cancer: chromosome segregation and beyond. Nat. Rev. Cancer 14, 389–393. doi: 10.1038/nrc3743
Losada, A., Hirano, M., and Hirano, T. (2002). Cohesin release is required for sister chromatid resolution, but not for condensin-mediated compaction, at the onset of mitosis. Genes Dev. 16, 3004–3016. doi: 10.1101/gad.249202
Mac Dougall, K., Beyene, Y., and Nachtigall, R. D. (2013). Age shock: misperceptions of the impact of age on fertility before and after IVF in women who conceived after age 40. Hum. Reprod. 28, 350–356. doi: 10.1093/humrep/des409
Mamsen, L. S., Brochner, C. B., Byskov, A. G., and Mollgard, K. (2012). The migration and loss of human primordial germ stem cells from the hind gut epithelium towards the gonadal ridge. Int. J. Dev. Biol. 56, 771–778. doi: 10.1387/ijdb.120202lm
Massy, B. (2016). Meiosis: to pair and recombine, a sophisticated chromosome dance. Semin. Cell Dev. Biol. 54, 104–105. doi: 10.1016/j.semcdb.2016.04.016
Matthews, T. J., and Hamilton, B. E. (2014). First births to older women continue to rise. NCHS Data Brief. 152, 1–8.
McGee, E. A., and Hsueh, A. J. (2000). Initial and cyclic recruitment of ovarian follicles. Endocr. Rev. 21, 200–214. doi: 10.1210/er.21.2.200
McNicoll, F., Stevense, M., and Jessberger, R. (2013). Cohesin in gametogenesis. Curr. Top. Dev. Biol. 102, 1–34. doi: 10.1016/b978-0-12-416024-8.00001-5
Mehlmann, L. M. (2005). Stops and starts in mammalian oocytes: recent advances in understanding the regulation of meiotic arrest and oocyte maturation. Reproduction 130, 791–799. doi: 10.1530/rep.1.00793
Mehlmann, L. M., Jones, T. L., and Jaffe, L. A. (2002). Meiotic arrest in the mouse follicle maintained by a Gs protein in the oocyte. Science 297, 1343–1345. doi: 10.1126/science.1073978
Minase, G., Miyamoto, T., Miyagawa, Y., Iijima, M., Ueda, H., Saijo, Y., et al. (2017). Single-nucleotide polymorphisms in the human RAD21L gene may be a genetic risk factor for Japanese patients with azoospermia caused by meiotic arrest and Sertoli cell-only syndrome. Hum. Fertil. (Camb.) 20, 217–220. doi: 10.1080/14647273.2017.1292004
Mori, T., Durand, J., Chen, Y., Thompson, J. A., Bakir, S., and Oparil, S. (2000). Effects of short-term estrogen treatment on the neointimal response to balloon injury of rat carotid artery. Am. J. Cardiol. 85, 1276–1279. doi: 10.1016/s0002-9149(00)00748-7
Morris, J. K., and Alberman, E. (2009). Trends in Down’s syndrome live births and antenatal diagnoses in England and Wales from 1989 to 2008: analysis of data from the National Down syndrome cytogenetic register. BMJ 339:b3794. doi: 10.1136/bmj.b3794
Motta, P. M., Makabe, S., and Nottola, S. A. (1997). The ultrastructure of human reproduction. I. The natural history of the female germ cell: origin, migration and differentiation inside the developing ovary. Hum. Reprod. Update 3, 281–295. doi: 10.1093/humupd/3.3.281
Nagaoka, S. I., Hassold, T. J., and Hunt, P. A. (2012). Human aneuploidy: mechanisms and new insights into an age-old problem. Nat. Rev. Genet. 13, 493–504. doi: 10.1038/nrg3245
Nasmyth, K., and Haering, C. H. (2009). Cohesin: its roles and mechanisms. Annu. Rev. Genet. 43, 525–558. doi: 10.1146/annurev-genet-102108-134233
Nelson, L. M. (2009). Clinical practice. Primary ovarian insufficiency. N. Engl. J. Med. 360, 606–614.
Nishiyama, T., Ladurner, R., Schmitz, J., Kreidl, E., Schleiffer, A., Bhaskara, V., et al. (2010). Sororin mediates sister chromatid cohesion by antagonizing Wapl. Cell 143, 737–749. doi: 10.1016/j.cell.2010.10.031
Nishiyama, T., Sykora, M. M., Huis in ’t Veld, P. J., Mechtler, K., and Peters, J. M. (2013). Aurora B and Cdk1 mediate Wapl activation and release of acetylated cohesin from chromosomes by phosphorylating Sororin. Proc. Natl. Acad. Sci. U.S.A. 110, 13404–13409. doi: 10.1073/pnas.1305020110
Norris, R. P., Freudzon, M., Nikolaev, V. O., and Jaffe, L. A. (2010). Epidermal growth factor receptor kinase activity is required for gap junction closure and for part of the decrease in ovarian follicle cGMP in response to LH. Reproduction 140, 655–662. doi: 10.1530/rep-10-0288
Notaridou, M., Quaye, L., Dafou, D., Jones, C., Song, H., Hogdall, E., et al. (2011). Common alleles in candidate susceptibility genes associated with risk and development of epithelial ovarian cancer. Int. J. Cancer 128, 2063–2074.
Oliver, T. R., Feingold, E., Yu, K., Cheung, V., Tinker, S., Yadav-Shah, M., et al. (2008). New insights into human nondisjunction of chromosome 21 in oocytes. PLoS Genet. 4:e1000033. doi: 10.1371/journal.pgen.1000033
Oliver, T. R., Tinker, S. W., Allen, E. G., Hollis, N., Locke, A. E., Bean, L. J., et al. (2012). Altered patterns of multiple recombinant events are associated with nondisjunction of chromosome 21. Hum Genet 131, 1039–1046. doi: 10.1007/s00439-011-1121-7
Ottolini, C. S., Newnham, L., Capalbo, A., Natesan, S. A., Joshi, H. A., Cimadomo, D., et al. (2015). Genome-wide maps of recombination and chromosome segregation in human oocytes and embryos show selection for maternal recombination rates. Nat. Genet. 47, 727–735. doi: 10.1038/ng.3306
Page, S. L., and Hawley, R. S. (2004). The genetics and molecular biology of the synaptonemal complex. Annu. Rev. Cell Dev. Biol. 20, 525–558. doi: 10.1146/annurev.cellbio.19.111301.155141
Pellestor, F., Andreo, B., Arnal, F., Humeau, C., and Demaille, J. (2003). Maternal aging and chromosomal abnormalities: new data drawn from in vitro unfertilized human oocytes. Hum. Genet. 112, 195–203. doi: 10.1007/s00439-002-0852-x
Pereda, J., Zorn, T., and Soto-Suazo, M. (2006). Migration of human and mouse primordial germ cells and colonization of the developing ovary: an ultrastructural and cytochemical study. Microsc. Res. Tech. 69, 386–395. doi: 10.1002/jemt.20298
Pereira, C., Smolka, M. B., Weiss, R. S., and Brieno-Enriquez, M. A. (2020). ATR signaling in mammalian meiosis: from upstream scaffolds to downstream signaling. Environ. Mol. Mutagen. 61, 752–766. doi: 10.1002/em.22401
Petronczki, M., Siomos, M. F., and Nasmyth, K. (2003). Un menage a quatre: the molecular biology of chromosome segregation in meiosis. Cell 112, 423–440. doi: 10.1016/s0092-8674(03)00083-7
Practice Committee of the American Society for Reproductive Medicine (2020). Definitions of infertility and recurrent pregnancy loss: a committee opinion. Fertil. Steril. 113, 533–535. doi: 10.1016/j.fertnstert.2019.11.025
Prakash, R., Zhang, Y., Feng, W., and Jasin, M. (2015). Homologous recombination and human health: the roles of BRCA1, BRCA2, and associated proteins. Cold Spring Harb. Perspect. Biol. 7:a016600. doi: 10.1101/cshperspect.a016600
Qiu, C., Chen, H., Wen, J., Zhu, P., Lin, F., Huang, B., et al. (2013). Associations between age at menarche and menopause with cardiovascular disease, diabetes, and osteoporosis in Chinese women. J. Clin. Endocrinol. Metab. 98, 1612–1621. doi: 10.1210/jc.2012-2919
Quinn, M. M., and Cedars, M. I. (2018). Cardiovascular health and ovarian aging. Fertil. Steril. 110, 790–793. doi: 10.1016/j.fertnstert.2018.07.1152
Ray, A., Oliver, T. R., Halder, P., Pal, U., Sarkar, S., Dutta, S., et al. (2018). Risk of Down syndrome birth: consanguineous marriage is associated with maternal meiosis-II nondisjunction at younger age and without any detectable recombination error. Am. J. Med. Genet. A 176, 2342–2349. doi: 10.1002/ajmg.a.40511
Revenkova, E., Herrmann, K., Adelfalk, C., and Jessberger, R. (2010). Oocyte cohesin expression restricted to predictyate stages provides full fertility and prevents aneuploidy. Curr. Biol. 20, 1529–1533. doi: 10.1016/j.cub.2010.08.024
Riedel, C. G., Katis, V. L., Katou, Y., Mori, S., Itoh, T., Helmhart, W., et al. (2006). Protein phosphatase 2A protects centromeric sister chromatid cohesion during meiosis I. Nature 441, 53–61. doi: 10.1038/nature04664
Riera-Escamilla, A., Enguita-Marruedo, A., Moreno-Mendoza, D., Chianese, C., Sleddens-Linkels, E., Contini, E., et al. (2019). Sequencing of a ‘mouse azoospermia’ gene panel in azoospermic men: identification of RNF212 and STAG3 mutations as novel genetic causes of meiotic arrest. Hum. Reprod. 34, 978–988. doi: 10.1093/humrep/dez042
Robert, T., Nore, A., Brun, C., Maffre, C., Crimi, B., Bourbon, H. M., et al. (2016). The TopoVIB-like protein family is required for meiotic DNA double-strand break formation. Science 351, 943–949. doi: 10.1126/science.aad5309
Sasaki, M., Miyoshi, N., Fujino, S., Saso, K., Ogino, T., Takahashi, H., et al. (2021). The meiosis-specific cohesin component stromal antigen 3 promotes cell migration and chemotherapeutic resistance in colorectal cancer. Cancer Lett. 497, 112–122. doi: 10.1016/j.canlet.2020.10.006
Shintomi, K., and Hirano, T. (2009). Releasing cohesin from chromosome arms in early mitosis: opposing actions of Wapl-Pds5 and Sgo1. Genes Dev. 23, 2224–2236. doi: 10.1101/gad.1844309
Simoni, M. K., Mu, L., and Collins, S. C. (2017). Women’s career priority is associated with attitudes towards family planning and ethical acceptance of reproductive technologies. Hum. Reprod. 32, 2069–2075. doi: 10.1093/humrep/dex275
Steiner, A. Z., and Jukic, A. M. (2016). Impact of female age and nulligravidity on fecundity in an older reproductive age cohort. Fertil. Steril. 105, 1584–1588.e1.
Svetlanov, A., and Cohen, P. E. (2004). Mismatch repair proteins, meiosis, and mice: understanding the complexities of mammalian meiosis. Exp. Cell Res. 296, 71–79. doi: 10.1016/j.yexcr.2004.03.020
Tao, X. Y., Zuo, A. Z., Wang, J. Q., and Tao, F. B. (2016). Effect of primary ovarian insufficiency and early natural menopause on mortality: a meta-analysis. Climacteric 19, 27–36. doi: 10.3109/13697137.2015.1094784
Tedeschi, A., Wutz, G., Huet, S., Jaritz, M., Wuensche, A., and Schirghuber, E. I., et al. (2013). Wapl is an essential regulator of chromatin structure and chromosome segregation. Nature 501, 564–568. doi: 10.1038/nature12471
Toraason, E., Horacek, A., Clark, C., Glover, M. L., Adler, V. L., Premkumar, T., et al. (2021). Meiotic DNA break repair can utilize homolog-independent chromatid templates in C. elegans. Curr. Biol. 31, 1508–1514.e5.
Tournaye, H., Krausz, C., and Oates, R. D. (2017). Novel concepts in the aetiology of male reproductive impairment. Lancet Diabetes Endocrinol. 5, 544–553. doi: 10.1016/s2213-8587(16)30040-7
Van Assche, E., Bonduelle, M., Tournaye, H., Joris, H., Verheyen, G., Devroey, P., et al. (1996). Cytogenetics of infertile men. Hum. Reprod. 11(Suppl 4), 1–24; discussion 25–26.
van der Bijl, N., Ropke, A., Biswas, U., Woste, M., Jessberger, R., Kliesch, S., et al. (2019). Mutations in the stromal antigen 3 (STAG3) gene cause male infertility due to meiotic arrest. Hum. Reprod. 34, 2112–2119.
Verni, F., Gandhi, R., Goldberg, M. L., and Gatti, M. (2000). Genetic and molecular analysis of wings apart-like (wapl), a gene controlling heterochromatin organization in Drosophila melanogaster. Genetics 154, 1693–1710. doi: 10.1093/genetics/154.4.1693
Waizenegger, I. C., Hauf, S., Meinke, A., and Peters, J. M. (2000). Two distinct pathways remove mammalian cohesin from chromosome arms in prophase and from centromeres in anaphase. Cell 103, 399–410. doi: 10.1016/s0092-8674(00)00132-x
Waldman, T. (2020). Emerging themes in cohesin cancer biology. Nat. Rev. Cancer 20, 504–515. doi: 10.1038/s41568-020-0270-1
Wang, S., Hassold, T., Hunt, P., White, M. A., Zickler, D., Kleckner, N., et al. (2017). Inefficient crossover maturation underlies elevated aneuploidy in human female meiosis. Cell 168, 977–989.e17.
Wang, Y. X., Minguez-Alarcon, L., Gaskins, A. J., Missmer, S. A., Rich-Edwards, J. W., Manson, J. E., et al. (2021). Association of spontaneous abortion with all cause and cause specific premature mortality: prospective cohort study. BMJ 372:n530. doi: 10.1136/bmj.n530
Wassmann, K. (2013). Sister chromatid segregation in meiosis II: deprotection through phosphorylation. Cell Cycle 12, 1352–1359. doi: 10.4161/cc.24600
Wolf, P. G., Cuba Ramos, A., Kenzel, J., Neumann, B., and Stemmann, O. (2018). Studying meiotic cohesin in somatic cells reveals that Rec8-containing cohesin requires Stag3 to function and is regulated by Wapl and sororin. J. Cell Sci. 131:jcs212100.
Xu, Z., Cetin, B., Anger, M., Cho, U. S., Helmhart, W., Nasmyth, K., et al. (2009). Structure and function of the PP2A-shugoshin interaction. Mol. Cell 35, 426–441. doi: 10.1016/j.molcel.2009.06.031
Zickler, D., and Kleckner, N. (1999). Meiotic chromosomes: integrating structure and function. Annu. Rev. Genet. 33, 603–754. doi: 10.1146/annurev.genet.33.1.603
Keywords: cohesin, aging, reproduction, human, primary ovarian insufficiency, meiosis
Citation: Beverley R, Snook ML and Brieño-Enríquez MA (2021) Meiotic Cohesin and Variants Associated With Human Reproductive Aging and Disease. Front. Cell Dev. Biol. 9:710033. doi: 10.3389/fcell.2021.710033
Received: 17 May 2021; Accepted: 13 July 2021;
Published: 02 August 2021.
Edited by:
Karen Schindler, Rutgers, The State University of New Jersey, United StatesReviewed by:
Diana Libuda, University of Oregon, United StatesCopyright © 2021 Beverley, Snook and Brieño-Enríquez. This is an open-access article distributed under the terms of the Creative Commons Attribution License (CC BY). The use, distribution or reproduction in other forums is permitted, provided the original author(s) and the copyright owner(s) are credited and that the original publication in this journal is cited, in accordance with accepted academic practice. No use, distribution or reproduction is permitted which does not comply with these terms.
*Correspondence: Miguel Angel Brieño-Enríquez, YnJpZW5vZW5yaXF1ZXptYUBtd3JpLm1hZ2VlLmVkdQ==
Disclaimer: All claims expressed in this article are solely those of the authors and do not necessarily represent those of their affiliated organizations, or those of the publisher, the editors and the reviewers. Any product that may be evaluated in this article or claim that may be made by its manufacturer is not guaranteed or endorsed by the publisher.
Research integrity at Frontiers
Learn more about the work of our research integrity team to safeguard the quality of each article we publish.