- Department of Biology, Texas A&M University, College Station, TX, United States
Acute respiratory distress syndrome (ARDS) involves damage to lungs causing an influx of neutrophils from the blood into the lung airspaces, and the neutrophils causing further damage, which attracts more neutrophils in a vicious cycle. There are ∼190,000 cases of ARDS per year in the US, and because of the lack of therapeutics, the mortality rate is ∼40%. Repelling neutrophils out of the lung airspaces, or simply preventing neutrophil entry, is a potential therapeutic. In this minireview, we discuss how our lab noticed that a protein called AprA secreted by growing Dictyostelium cells functions as a repellent for Dictyostelium cells, causing cells to move away from a source of AprA. We then found that AprA has structural similarity to a human secreted protein called dipeptidyl peptidase IV (DPPIV), and that DPPIV is a repellent for human neutrophils. In animal models of ARDS, inhalation of DPPIV or DPPIV mimetics blocks neutrophil influx into the lungs. To move DPPIV or DPPIV mimetics into the clinic, we need to know how this repulsion works to understand possible drug interactions and side effects. Combining biochemistry and genetics in Dictyostelium to elucidate the AprA signal transduction pathway, followed by drug studies in human neutrophils to determine similarities and differences between neutrophil and Dictyostelium chemorepulsion, will hopefully lead to the safe use of DPPIV or DPPIV mimetics in the clinic.
Acute Respiratory Distress Syndrome (Ards)
Acute respiratory distress syndrome (ARDS) is an acute onset of low blood oxygen levels due to abnormal accumulation of multiple cell types in the lungs, including immune cells (Ashbaugh et al., 1967). The cells can damage the lungs, as well as clog airspaces, leading to lung dysfunction and thus low blood oxygen levels. The abnormal accumulation of cells is caused by either direct or indirect lung injury; direct lung injury can be from pneumonia caused by viruses, bacteria, or fungi, trauma from mechanical ventilation, or injury caused by inhaling harmful substances, while indirect lung injury is primarily from inflammation or trauma to other organ systems (Shaver and Bastarache, 2014; Cochi et al., 2016; Lynn et al., 2019). There are approximately 190,000 cases of ARDS in the United States each year (Rubenfeld et al., 2005). ARDS can have a rapid progression, with patients advancing from breathing normally despite lung damage (mild ARDS) to requiring a ventilator (moderate or severe ARDS) within a week (Ranieri et al., 2012). The mortality rate for ARDS is 27% for mild, 32% for moderate, and 45% for severe ARDS (Ranieri et al., 2012) and the 3-year mortality rate is 44, 47, and 71% respectively (Parhar et al., 2019).
In ARDS patients, neutrophils migrate from the blood into the airspaces of the lungs (Steinberg et al., 1994; Zemans et al., 2009) in response to increased levels of inflammatory mediators, chemokines, and cell damage (Figures 1A–C; Thompson et al., 2017; Lin and Fessler, 2021). Once in the lungs, the neutrophils target pathogens for phagocytosis and release proteases, reactive oxidants, and neutrophil extracellular traps (Zemans et al., 2009). In ARDS, some neutrophils in the lungs release proteases and reactive oxygen species even if there is no pathogen present, causing lung damage, and this then recruits more neutrophils in a vicious cycle (Figures 1D,E; Weiss, 1989). The inflammation and damage reduce gas exchange, promotes vascular permeability, and increases fluid in the lung tissues and air spaces (Matthay et al., 2012; Kao et al., 2015; Lynn et al., 2019). The only effective management for ARDS is protection of the lungs, putting the patient on oxygen and a ventilator with low tidal volume ventilation to reduce stretching of the lung tissue (Acute Respiratory Distress Syndrome Network et al., 2000). There are currently no therapeutics for ARDS (Chen et al., 2020).
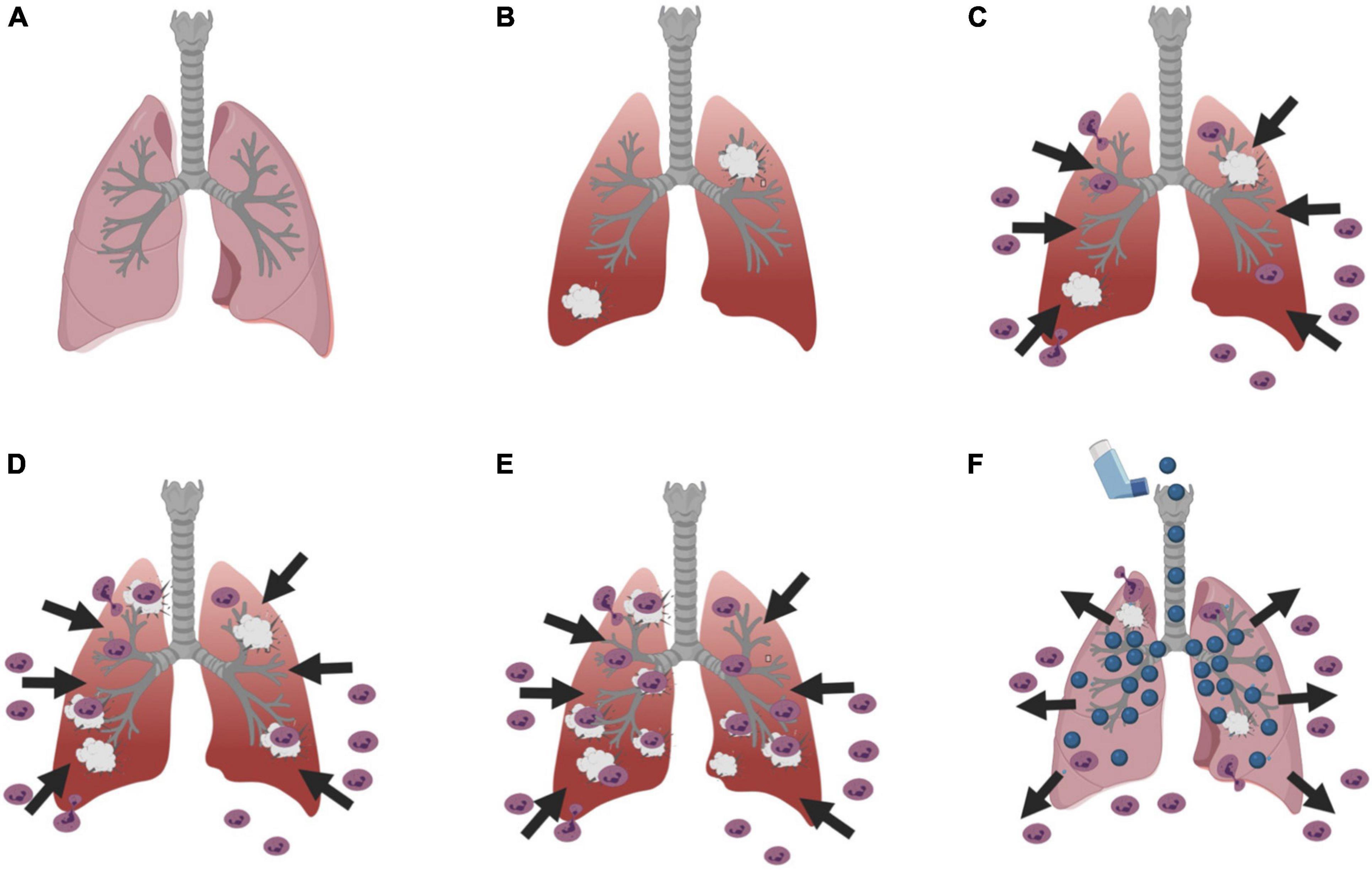
Figure 1. Diagram of the basic process of ARDS and a potential therapeutic mechanism. (A) A cartoon of a normal lung, and (B) a lung with damage. (C) Neutrophils leave the blood and enter the lung tissue and airspaces in response to the damage. (D) In ARDS, this can result in further lung damage, which (E) results in even more neutrophils attracted to the lungs causing more damage in a vicious cycle. (F) An intriguing possibility is that inhalation of a nebulized neutrophil chemorepellent could drive neutrophils out of the lungs and/or prevent neutrophils from entering the lungs, and thus break the vicious cycle and reduce neutrophil-induced lung damage. Figures were created using BioRender.com.
As described above, neutrophils entering the lungs appear to cause most of the damage in ARDS. An intriguing possible therapy for ARDS is to prevent neutrophils from entering the lungs, or to repel neutrophils that have entered the lungs back out of the lungs (Figure 1F). Some prokaryotes can sense repellent chemicals (chemorepellents) and move away from the source of the chemorepellent, and this process is fairly well understood (Pandey and Jain, 2002; Anderson et al., 2015). As described below, there are a few examples of chemorepellents in eukaryotic cells, including neutrophils, but how these affect cells is poorly understood. To move a neutrophil chemorepellent into the clinic, we need to know how this repulsion works to understand possible drug interactions and side effects.
Chemorepulsion Can Cause Cells to Move Away From a Signal
Chemotaxis allows migratory cells to either move toward (chemoattract) or move away (chemorepel) from an external chemotactic stimulus (Figure 2; Vianello et al., 2005). In eukaryotes, chemoattraction plays important roles during development and morphogenesis, and in immune responses (Sadik and Luster, 2012; Kolaczkowska and Kubes, 2013; Theveneau and Mayor, 2013). Directed migration of a cell toward a chemoattractant involves chemoattractant gradient sensing through a receptor-mediated signal transduction processes to induce rearrangement of cytoskeletal proteins at the front and rear of a cell, a conserved mechanism used by migrating cells (Dandekar et al., 2013; Fukujin et al., 2016). Similar to chemoattraction, chemorepulsion also plays an important role in development and immune responses (Mark et al., 1997; Vianello et al., 2005; Levine et al., 2006; Clark et al., 2014a; Guidobaldi et al., 2017; Tang, 2017). However, the mechanisms of eukaryotic chemorepulsion is still being elucidated.
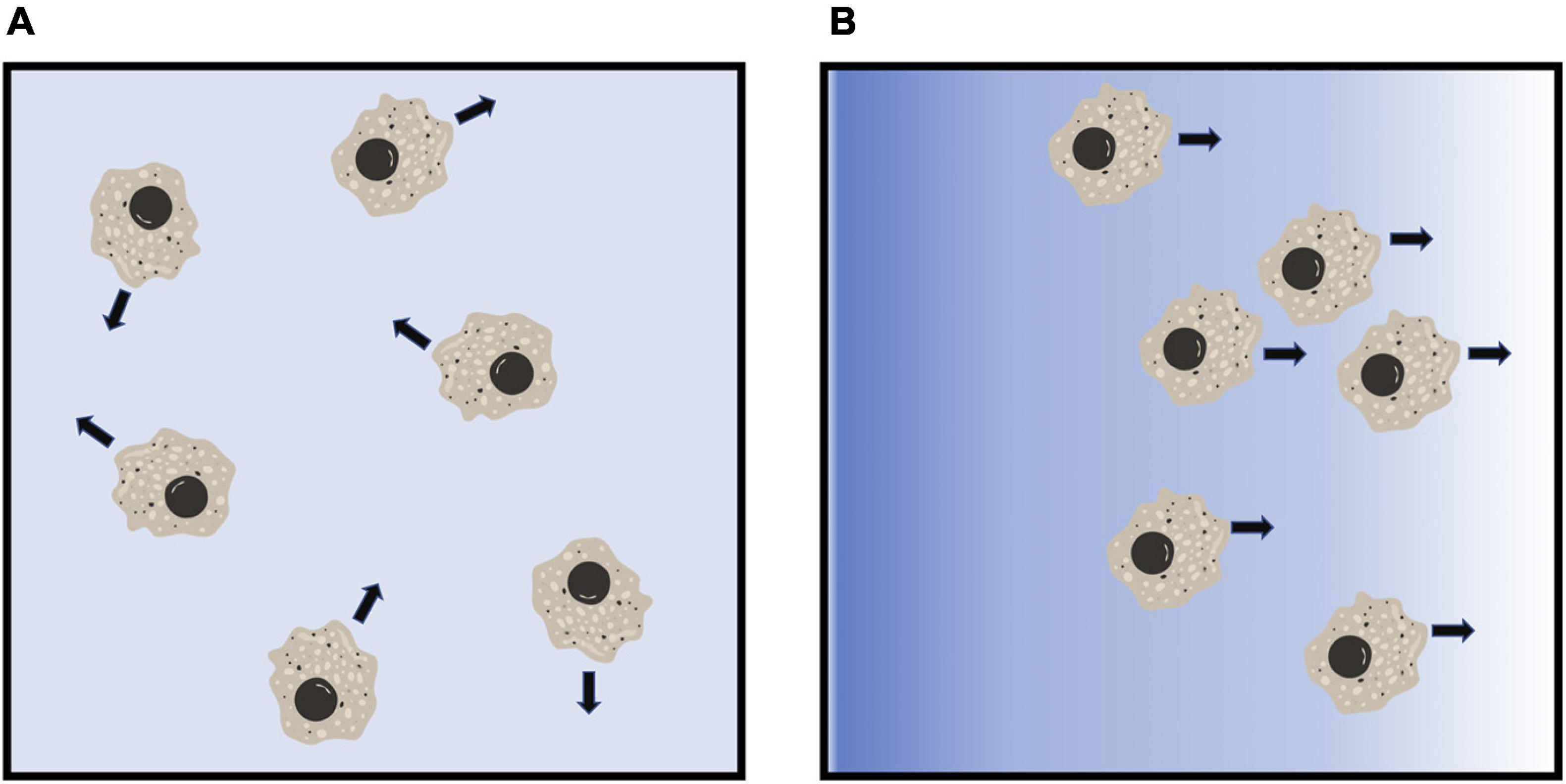
Figure 2. Diagram of eukaryotic chemorepulsion. (A) In the absence of a gradient, cells extend pseudopods, and move, in random directions. (B) In a gradient of a chemorepellent (blue shading), cells move away from the higher concentration of the chemorepellent. Figures were created using BioRender.com.
The bacterium Escherichia coli (E. coli) responds to both chemoattractants and chemorepellents (Adler and Templeton, 1967; Berg and Brown, 1972; Berg and Tedesco, 1975). E. coli cells that sense decreasing concentrations of an attractant, or increasing concentrations of a repellent, causes the cells to tumble induced by clockwise flagellar rotation instead of smooth swimming induced by counter clockwise flagellar rotation, and the cells thus change direction to increase the probability that they will be moving in the right direction (Larsen et al., 1974). In Helicobacter pylori, chemorepulsion from the autocrine quorum sensing signal autoinducer-2 determines spatial organization and dispersal of biofilms (Anderson et al., 2015). The unicellular eukaryote Trichomonas vaginalis chemorepels from toxic agents such as metronidazole, ketoconazole, and miconazole (Sugarman and Mummaw, 1988). In the presence of a chemorepellent, such as conditioned supernatant factor (CSF), unicellular eukaryotes such as Tetrahymena and Paramecium swim backward (Rodgers et al., 2008; Plattner, 2015; Zou and Hennessey, 2017). In fungi, chemorepulsion can direct filament growth and mat formation (Karunanithi et al., 2012).
Several Proteins Act as Neuronal Chemorepellents
In higher eukaryotes, during neuronal development, neurons extend axons to reach specific targets (Kalil et al., 2011; Santos et al., 2020). For axons to correctly navigate to their targets, attractive and repulsive factors guide neuronal growth, regeneration, and collapse (Cregg et al., 2014; Thiede-Stan and Schwab, 2015). Many of the repulsive factors inhibit growth cone outgrowth and promote their collapse (Liu et al., 2006). Some of these repulsive molecules are proteins such as Nogo, Ephrins, Semaphorins, Draxin and Netrins. Nogo-A (the active form is called Nogo-66) is expressed by many projection neurons in the central and peripheral nervous systems (Nash et al., 2009; Schwab, 2010). Ephrins are negative guidance molecules for axons (Kolpak et al., 2009; Chatzizacharias et al., 2014; Savino et al., 2015). Ephrins signal through neuronal Eph receptors including EphA5 (Wahl et al., 2000). Other repulsive molecules such as the semaphorins Sema3A and 3F bind to plexin and neuropilin co-receptors to induce the repulsion of axons (Liu et al., 2006; Andrews et al., 2013; Vo et al., 2013).
Draxin is an axon guidance cue that is vital for the development of the thick bundle of nerve fibers, called the corpus collosum, between the two hemispheres of the brain (Islam et al., 2009) and signals through the “deleted in colorectal cancer (DCC)” receptor (Islam et al., 2009; Meli et al., 2015). Although DCC is vital for axonal repulsive behavior, the binding of Netrin-1 to neuronal DCC induces attraction (Guijarro et al., 2006). Netrin-1 has both chemoattractive and chemorepulsive effects on many migratory axons during development and injury repair (Colamarino and Tessierlavigne, 1995; Furne et al., 2008). The binding of Netrin-1 to uncoordinated family member 5 (UNC-5) (Bashaw and Goodman, 1999; Hong et al., 1999) induces chemorepulsion in axons and immune cells (Hedgecock et al., 1990; Tadagavadi et al., 2010).
In mammals, multiple pathways are required for chemorepulsion (Riches et al., 2013; Holt et al., 2021). The Wnt signaling pathway is a highly conserved pathway involved in multiple cell processes such as fate determination, polarity, migration, and neural patterning (Komiya and Habas, 2008). Wnt5a activation of the non- canonical Wnt receptor RYK on specific neurons induces chemorepulsion (Clark et al., 2014b). Secreted Wnt protein also bind to Frizzled receptors, a family of integral membrane protein receptors, to repel axon growth (Huang and Klein, 2004; Freese et al., 2010). Slit guidance ligand 2 (Slit2), a chemoattractant or chemorepellent dependent on the isoform, and activation of Roundabout receptor (Robo1), a coreceptor of Slit2, induces chemorepulsion of axons as well as neutrophils (Hu, 1999; Nguyen Ba-Charvet et al., 1999; Park et al., 2016). Slit1 and Slit3 also induce chemorepulsion of olfactory tract and spinal motor axons during development (Patel et al., 2001).
Several Proteins Act as Immune Cell Chemorepellents
In addition to Slit2, protein signals such as eotaxin-3, CXCL10, IL-8 and stromal cell derived factor-1 induce dendritic cell, monocyte, leukocyte, and/or neutrophil chemorepulsion (Ogilvie et al., 2003; Kohrgruber et al., 2004; Tharp et al., 2006). The chemokine stromal derived factor-1/CXCL12 is involved in tumor growth, metastasis and promotion of tumor immunity (Chen et al., 1994; Jager et al., 1996), possibly because CXCL12 secreted by tumors decreases T-cell infiltration (Vianello et al., 2006; d’Onofrio, 2012). In neutrophils, Slit2 plays a role in neutrophil migration toward and away from regions of inflammation (Tole et al., 2009; Ye B. Q. et al., 2010; Pilling et al., 2019). A ∼110- kDa N-terminal fragment of Slit2 induces chemorepulsion in neutrophils, and inhibiting Slit2 receptors Robo1 and syndecan-4 diminishes neutrophil chemorepulsion (Pilling et al., 2019).
Eukaryotic Chemorepulsion Involves Regulation of the Cytoskeleton
Downstream of their receptors, many axon growth chemorepellents induce axonal repulsion by activating Rho GTPases, small signaling G protein molecular switches that play a role in cytoskeletal organization, cell movement, and cell polarity (Hall, 1998; Bustelo et al., 2007; Ridley, 2015). The activation of Rho GTPases in turn activates Rho-associated protein kinases (ROCKs), effector molecules downstream of Rho GTPases that play a role in cell shape and movement by regulating cytoskeletal elements (Leung et al., 1996; Maekawa et al., 1999). The signal transduction pathways then diverge to rearrange essential cytoskeletal proteins necessary for directed cell migration (Schmandke et al., 2007; Gelfand et al., 2009; Schwab, 2010).
Dictyostelium discoideum Secretes An Endogenous Chemorepellent Called AprA
The simple eukaryote Dictyostelium discoideum is an excellent model system to study chemotaxis. In a nutrient-rich environment, D. discoideum cells grow and proliferate as single cells. When the nutrients become depleted, D. discoideum cells aggregate and form multicellular structures bearing spores that can survive harsh conditions (Bonner and Scharf, 1978; Kessin, 2001). The aggregation is mediated by cells secreting, and moving toward, relayed pulses of 3′, 5′-cyclic adenosine monophosphate (cAMP) (Bonner, 1970; Tomchik and Devreotes, 1981).
During their growth phase, D. discoideum cells secrete a protein called autocrine proliferation repressor A (AprA). AprA acts as a signal that partially inhibits cell division without inhibiting cell growth (the increase in accumulated mass) (Brock and Gomer, 2005). AprA is a 60 kDa protein which forms a ∼150 kDa complex with a protein called CfaD (Brock and Gomer, 2005; Bakthavatsalam et al., 2008).
How cells regulate the accumulation of AprA is not fully understood. Eukaryotic initiation factor 2 (eIF2) (Dever, 2002; Ye J. et al., 2010), initiation factor kinases, IfkA, IfkB, and IfkC (Fang et al., 2003; Rai et al., 2006), and Ceroid lipofuscinosis neuronal 3 (Cln3) and Cln5 that are associated with a childhood onset neurological disorder called Batten disease or neuronal ceroid lipofuscinosis (NCL) (Santavuori, 1988), are important for extracellular accumulation of AprA and CfaD (Bowman et al., 2011; Huber et al., 2014; Huber and Mathavarajah, 2018; Figure 3).
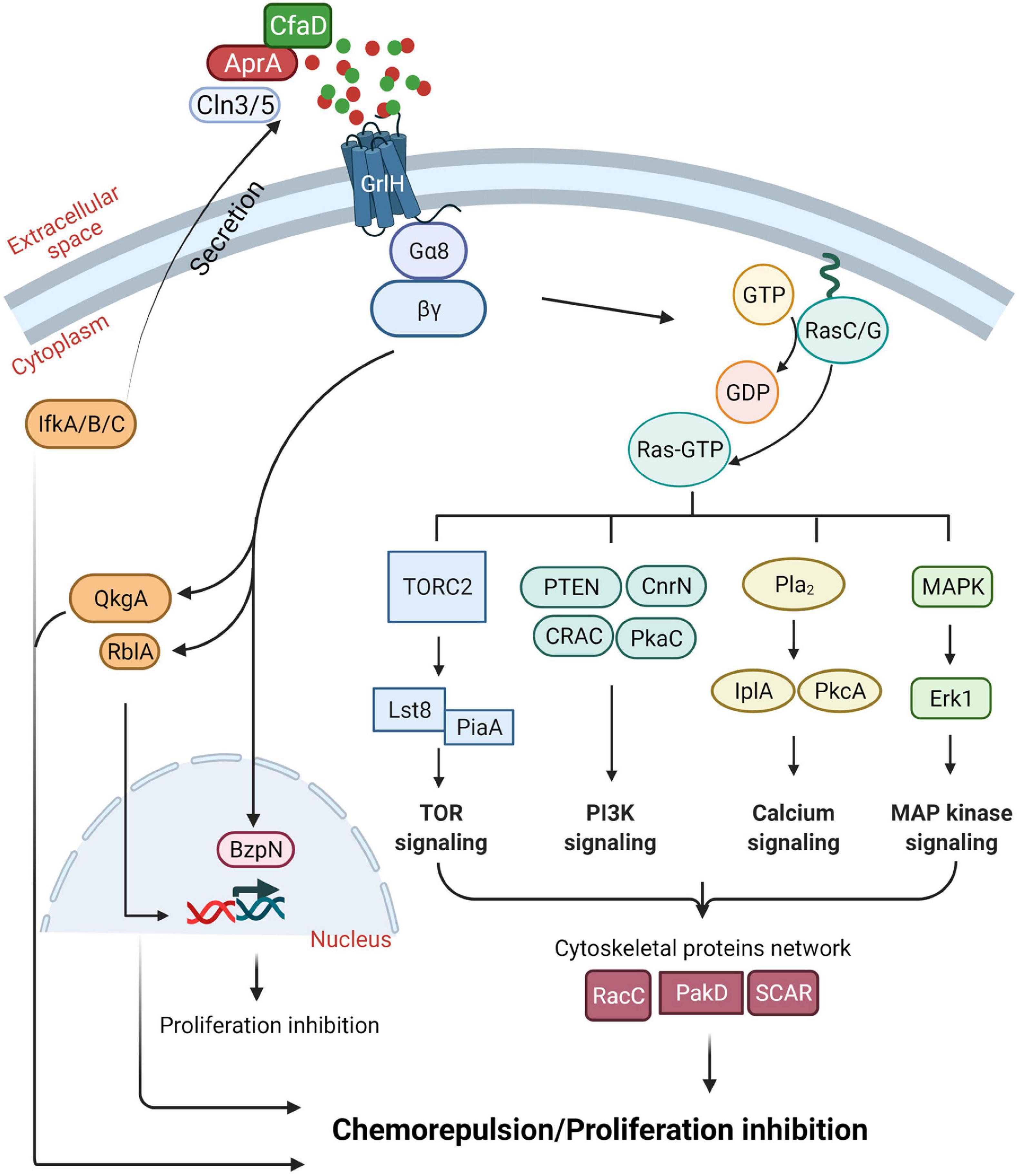
Figure 3. Summary of our current understanding of the AprA signal transduction pathway. See text for details. Figure created with BioRender.com.
Dictyostelium discoideum cells lacking AprA are able to aggregate, but form abnormal structures with fewer and less viable spores, suggesting that cells lacking AprA can still migrate toward cAMP and aggregate (Brock and Gomer, 2005). In colonies of growing cells, wild type cells show directed movement away from the edge of the colony while cells lacking AprA do not move away from the edge of the colony (Phillips and Gomer, 2010). This suggested that AprA acts as a chemorepellent (Phillips and Gomer, 2010). In artificial gradients, D. discoideum cells move away from physiological concentrations of AprA, indicating that AprA is indeed a chemorepellent (Phillips and Gomer, 2010).
AprA Activates a Complex Signal Transduction Pathway to Induce Chemorepulsion
Dictyostelium discoideum cells migrating toward cAMP and folic acid (a waste product released by bacteria, which D. discoideum cells uses as a chemoattractant to find and eat bacteria) require G protein-coupled receptors (GPCRs) and multiple conserved signal transduction pathways (De Wit and Bulgakov, 1985; Hadwiger et al., 1994; Ma et al., 1997; Lim et al., 2005; Kortholt et al., 2011; Yan et al., 2012). AprA activates G-proteins Gα8 and Gβ through the GPCR glutamate receptor-like H (GrlH) to induce chemorepulsion (Bakthavatsalam et al., 2009; Phillips and Gomer, 2012; Tang et al., 2018; Rijal et al., 2019; Figure 3). To decrease cell proliferation and induce chemorepulsion, AprA requires QkgA, a ROCO family kinase (Gotthardt et al., 2008; Phillips and Gomer, 2010), PakD, a member of a conserved family of p-21 activated kinases (PAKs) (Bokoch, 2003), CnrN, a phosphatase and tensin homolog (PTEN)-like phosphatase (Tang and Gomer, 2008), RblA, a homolog of a human Retinoblastoma (Rb) protein (MacWilliams et al., 2006), protein kinase A, components of the target of rapamycin (TOR) complex 2, phospholipase A, extracellular signal-regulated protein kinase (Erk1), and the Ras proteins RasC and RasG (Bakthavatsalam et al., 2009; Garcia et al., 2014; Phillips and Gomer, 2014; Tang et al., 2018; Rijal et al., 2019). In addition, AprA requires the putative bZIP transcription factor BzpN for its proliferation-inhibiting activity but not for chemorepulsion activity (Phillips et al., 2011), suggesting that AprA uses partially overlapping pathways to mediate proliferation inhibition and chemorepulsion.
The AprA Chemorepulsion Mechanism Has a Partial but Not Complete Overlap With the camp Chemoattraction Mechanism
For chemorepulsion, in addition to the components mentioned above, AprA also uses some but not all components of the cAMP chemoattraction signal transduction pathway (Rijal et al., 2019). Similar to chemoattraction to cAMP, cells in a rAprA gradient show actin-rich protrusions at the leading edge of the cell and myosin II mediated contraction at the trailing edge of the cell, allowing the cells to move in a biased direction away from the chemorepellent (Phillips and Gomer, 2012). Although phospholipase C (PLC) and PI3 kinases 1 and 2 are important for chemorepulsion of starved D. discoideum cells by the synthetic cAMP analog 8-CPT-cAMP, and for cAMP chemoattraction (Keizer-Gunnink et al., 2007), PLC and PI3 kinases are not necessary for AprA chemorepulsion (Phillips and Gomer, 2012). In addition, unlike chemoattraction toward cAMP, AprA does not require Akt and PKB, guanylyl cyclases, and the cytosolic regulator of adenylate cyclase (CRAC) (Parent et al., 1998; Rijal et al., 2019).
Some of the key regulators of the AprA-induced chemorepulsion pathway are Ras GTPases. AprA induces translocation of RBDRaf1-GFP, an active Ras binding protein that when translocated to the cell cortex indicates Ras activation (Rijal et al., 2019). Although PakD is necessary for AprA mediated chemorepulsion, PakD is not necessary for AprA induced translocation of RBDRaf1-GFP to the cell cortex (Rijal et al., 2019). While PakD localizes at the rear of a migrating cell (Phillips and Gomer, 2014), PakD may negatively regulate Ras activation at the side of the cells facing away from AprA during chemorepulsion (Rijal et al., 2019). Several other proteins can negatively regulate Ras local activity at the membrane including Rho GTPases and ERK1/2 (Wang et al., 2013; Lake et al., 2016). AprA does not cause translocation of RBDRaf1-GFP in cells lacking a WASP-related cytoskeletal protein suppressor of cAR (SCAR), suggesting that AprA requires cytoskeletal proteins to activate Ras (Rijal et al., 2019).
AprA Induces Chemorepulsion by Inhibiting Pseudopod Formation at the Side of the Cell Closest to the Source of AprA
When cells such as D. discoideum and neutrophils move, they induce a localized polymerization of actin to form a pseudopod that protrudes from the cell, and when this attaches to a substrate, the cells can then move following their pseudopod (King and Insall, 2009). In the absence of a chemoattractant or chemorepellent gradient, cells form pseudopods at random locations, and thus move in random directions (Sasaki et al., 2004; Rijal et al., 2019). During chemoattraction, cells polymerize actin and extend a pseudopod toward the source of the attractant (Sasaki et al., 2004). During chemorepulsion, AprA inhibits filamentous actin (F-actin) polymerization at the region of the cell closest to the source of AprA, inhibiting pseudopod formation in the sector of the cell closest to the source of AprA (Rijal et al., 2019). This then allows the cells to move in any direction except the direction toward the source of AprA, resulting in chemorepulsion. This then indicates a fundamental difference between chemoattraction and chemorepulsion in D. discoideum: if a chemoattractant is coming from the West, a cell will tend to extend a pseudopod and move toward the West; to a first approximation, if a chemorepellent is coming from the West, cells will extend pseudopods randomly, and move toward, the East, North, or South.
The Identification of AprA Led to the Identification of a Human Neutrophil Chemorepellent
Dictyostelium discoideum and neutrophils share chemotaxis properties (Wang, 2009; Wang et al., 2011). Although AprA has little similarity to mammalian proteins, a predicted tertiary structure of AprA showed similarity to the structure of human dipeptidyl peptidase IV (DPPIV) (Zhang, 2008; Roy et al., 2010, 2012; Herlihy et al., 2013). DPPIV is a 110 kDa serine protease that localizes on the extracellular surface of some lymphocytes, endothelial cells, and is also present in plasma, serum, cerebrospinal fluid, synovial fluid, semen, and urine in a soluble form, and cleaves peptides with a proline or alanine in the second position at the N-terminus end (Walborg et al., 1985; Thoma et al., 2003; Cordero et al., 2009; Kotacková et al., 2009; Nauck et al., 2011; Pan et al., 2021). In addition to the predicted structural similarity, AprA has a DPPIV -like protease activity (Herlihy et al., 2013, 2017). Although AprA is not able to repel human neutrophils, DPPIV induces chemorepulsion of D. discoideum cells, suggesting a conserved mechanism of action (Herlihy et al., 2017). In a range of concentrations encompassing its concentration in human plasma, DPPIV acts as a chemorepellent for human and mouse neutrophils, but does not affect the motility of macrophages and lymphocytes (Herlihy et al., 2013). In the presence of the DPPIV inhibitors Diprotin A and DPPP 1c hydrochloride, the chemorepellent activity of DPPIV was significantly reduced, and suggesting that the protease activity of DPPIV mediates its ability to induce neutrophil chemorepulsion (Herlihy et al., 2013).
Dppiv, the AprA-Like the Neutrophil Chemorepellent, Shows Efficacy in a Mouse Model of Ards as Well as a Mouse Model of Rheumatoid Arthritis
A standard mouse model of ARDS is to damage the lungs with aspiration of a drug called bleomycin (Walters and Kleeberger, 2008). The mouse is lightly anesthetized, and 50 μl of saline with bleomycin is quickly pipetted through the mouth into the trachea as the mouse is inhaling. A gentle tailward shake of the mouse then disperses the bleomycin into the lungs. One day later, activated neutrophils start accumulating in the lungs (Walters and Kleeberger, 2008). Using this model, recombinant DPPIV or an equal volume of saline was introduced into the airspaces of the lungs of bleomycin-treated mice on day 1 and day 2 after bleomycin treatment by a similar aspiration procedure (Herlihy et al., 2013). Three days after bleomycin treatment, mice were euthanized. Phosphate buffered saline was put into the lungs through the trachea and then removed along with cells and other material in the airspaces in a procedure called bronchoalveolar lavage (BAL) (Herlihy et al., 2013). At day 3, the DPPIV treatments reduced both neutrophils in the BAL fluid as well as neutrophils remaining in the lungs (as determined by immunostaining of sections of the post-BAL lungs), but did not affect the numbers of macrophages and lymphocytes, suggesting that the effect of DPPIV is specific to neutrophils (Herlihy et al., 2013). Inhalation of DPPIV thus showed efficacy in a mouse model of ARDS.
Inflammatory arthritis, also known as rheumatoid arthritis (RA), is an autoimmune disorder with characteristic chronic inflammation due to neutrophil accumulation in the joints causing destruction of the joints (Kaplan, 2013). RA patients have lower level of DPPIV in plasma compared to non-inflammatory osteoarthritis (Busso et al., 2005). Injection of DPPIV directly into the joint in a mouse model of arthritis reduced the accumulation of neutrophils in the joint, and reduced the severity of arthritis and synovial inflammation (Herlihy et al., 2015). Together with the ARDS model results, this suggests that DPPIV can reduce inflammation by causing neutrophils to move away from a site of accumulation.
Agonists of the Dppiv Receptor Par2 Induce Neutrophil Chemorepulsion and Show Efficacy in a Mouse Model of Ards
As mentioned above, soluble DPPIV acts as a human and mouse neutrophil chemorepellent (Herlihy et al., 2013; White et al., 2018) via the activation of protease activated receptor 2 (PAR2) (White et al., 2018). PAR2 is a member of the PAR family of GPCRs, which consists of PAR1/2/3/4 (Ossovskaya and Bunnett, 2004). PAR2 is activated by the proteolytic cleavage of the extracellular N-terminus domain causing the remaining tethered N-terminus to act as a ligand that binds to extracellular loop 2 of the receptor thereby activating it (Vu et al., 1991; Gardell et al., 2008) DPPIV requires protease activated receptor (PAR2) to induce chemorepulsion of human neutrophils (White et al., 2018). Similar to DPPIV, the PAR2 agonists 2f-LIGRL-amide (a small peptide with modifications at the N and C termini), SLIGKVNH2 (a small peptide with a modifications at the C terminus), and AC55541 (a small molecule) induce chemorepulsion of human and mouse neutrophils (White et al., 2018). Although DPPIV induces stronger chemorepulsion of male neutrophils than female neutrophils, PAR2 agonists induce chemorepulsion of neutrophils from both male and female mice (White et al., 2018). In the mouse ARDS model described above, aspiration of SLIGKVNH2, starting 24 h after oropharyngeal aspiration of bleomycin, reduced neutrophil accumulation in lungs at day 3, similar to the effects of DPPIV described above (White et al., 2018), suggesting that aspiration of PAR2 agonists could be used to treat ARDS in human patients (Herlihy et al., 2013; White et al., 2018). Since DPPIV is a protease that cleaves many target molecules in addition to PAR2 (Zhu et al., 2003; Mulvihill and Drucker, 2014; Wronkowitz et al., 2014; White et al., 2018; Deacon, 2019; Trzaskalski et al., 2020), in terms of potential therapeutics, exogenous delivery of PAR2 agonists may have fewer side effects than exogenous delivery of DPPIV.
Conclusion
Chemorepulsion is an essential process for development, morphogenesis, and immune responses in eukaryotes. The identification of AprA, an endogenous chemorepellent in D. discoideum led to the identification of DPPIV, a chemorepellent that acts on human (and mouse) neutrophils. This in turn led to the identification of small-molecule PAR2 agonists as neutrophil chemorepellents. Given by aspiration into the lungs, both DPPIV and a PAR2 agonist showed efficacy in a mouse model of ARDS. Although the activation of PAR2 can reduce damage caused by arthritis, ARDS, and ischemia (McLean et al., 2002; McCulloch et al., 2018; White et al., 2018), the activation of PAR2 can lead to unwanted effects such as fetal injury, fibrosis, and inflammatory, metabolic and cardiovascular disorders (Cicala, 2002; Redecha et al., 2008; Grimsey et al., 2011; Kagota et al., 2016; Shearer et al., 2016; Heuberger and Schuepbach, 2019). Although intriguing possibilities are that inhalation of a nebulized mist containing DPPIV and/or PAR2 agonists might be useful as therapeutics for ARDS, and localized delivery of these neutrophil chemorepellents might be useful in other neutrophil-driven diseases, caution will be needed to limit dosing to prevent systemic toxicity.
Author Contributions
All authors listed have made a substantial, direct and intellectual contribution to the work, and approved it for publication.
Conflict of Interest
The authors declare that the research was conducted in the absence of any commercial or financial relationships that could be construed as a potential conflict of interest.
Funding
This work was supported by NIH grants GM118355 and GM139486 to RG. The figures were created using BioRender.com.
References
Acute Respiratory Distress Syndrome Network, Brower, R. G., Matthay, M. A., Morris, A., Schoenfeld, D., Thompson, B. T., et al. (2000). Ventilation with lower tidal volumes as compared with traditional tidal volumes for acute lung injury and the acute respiratory distress syndrome. N. Engl. J. Med. 342, 1301–1308. doi: 10.1056/nejm200005043421801
Adler, J., and Templeton, B. (1967). The effect of environmental conditions on the motility of Escherichia coli. J. Gen. Microbiol. 46, 175–184. doi: 10.1099/00221287-46-2-175
Anderson, J. K., Huang, J. Y., Wreden, C., Sweeney, E. G., Goers, J., Remington, S. J., et al. (2015). Chemorepulsion from the quorum signal autoinducer-2 promotes Helicobacter pylori biofilm dispersal. mBio 6:e00379.
Andrews, W. D., Zito, A., Memi, F., Jones, G., Tamamaki, N., and Parnavelas, J. G. (2013). Limk2 mediates semaphorin signalling in cortical interneurons migrating through the subpallium. Biol. Open 2, 277–282. doi: 10.1242/bio.20133202
Ashbaugh, D. G., Bigelow, D. B., Petty, T. L., and Levine, B. E. (1967). Acute respiratory distress in adults. Lancet 290, 319–323.
Bakthavatsalam, D., Brock, D. A., Nikravan, N. N., Houston, K. D., Hatton, R. D., and Gomer, R. H. (2008). The secreted Dictyostelium protein CfaD is a chalone. J. Cell Sci. 121, 2473–2480. doi: 10.1242/jcs.026682
Bakthavatsalam, D., Choe, J. M., Hanson, N. E., and Gomer, R. H. (2009). A Dictyostelium chalone uses G proteins to regulate proliferation. BMC Biol. 7:44. doi: 10.1186/1741-7007-7-44
Bashaw, G. J., and Goodman, C. S. (1999). Chimeric axon guidance receptors: the cytoplasmic domains of slit and netrin receptors specify attraction versus repulsion. Cell 97, 917–926. doi: 10.1016/s0092-8674(00)80803-x
Berg, H. C., and Brown, D. A. (1972). Chemotaxis in Escherichia coli analysed by three-dimensional tracking. Nature 239, 500–504. doi: 10.1038/239500a0
Berg, H. C., and Tedesco, P. M. (1975). Transient response to chemotactic stimuli in Escherichia coli. Proc. Natl. Acad. Sci. U.S.A. 72, 3235–3239. doi: 10.1073/pnas.72.8.3235
Bokoch, G. M. (2003). Biology of the p21-activated kinases. Annu. Rev. Biochem. 72, 743–781. doi: 10.1146/annurev.biochem.72.121801.161742
Bonner, J. T. (1970). Induction of stalk cell differentiation by cyclic AMP in the cellular slime mold Dictyostelium discoideum. Proc. Natl. Acad. Sci. U.S.A. 65, 110–113. doi: 10.1073/pnas.65.1.110
Bowman, R. L., Xiong, Y., Kirsten, J. H., and Singleton, C. K. (2011). eIF2α kinases control chalone production in Dictyostelium discoideum. Eukaryot. Cell 10, 494–501. doi: 10.1128/ec.00270-10
Brock, D. A., and Gomer, R. H. (2005). A secreted factor represses cell proliferation in Dictyostelium. Development 132, 4553–4562. doi: 10.1242/dev.02032
Busso, N., Wagtmann, N., Herling, C., Chobaz-Péclat, V., Bischof-Delaloye, A., So, A., et al. (2005). Circulating CD26 is negatively associated with inflammation in human and experimental arthritis. Am. J. Pathol. 166, 433–442. doi: 10.1016/s0002-9440(10)62266-3
Bustelo, X. R., Sauzeau, V., and Berenjeno, I. M. (2007). GTP-binding proteins of the Rho/Rac family: regulation, effectors and functions in vivo. BioEssays 29, 356–370. doi: 10.1002/bies.20558
Chatzizacharias, N. A., Giaginis, C. T., Agapitos, E., and Theocharis, S. E. (2014). The role of ephrins’ receptors and ephrins’ ligands in normal placental development and disease. Expert. Opin. Ther. Targets 18, 269–275. doi: 10.1517/14728222.2014.864638
Chen, C. M., Lu, H. C., Tung, Y. T., and Chen, W. (2020). Antiplatelet therapy for acute respiratory distress syndrome. Biomedicines 8:230. doi: 10.3390/biomedicines8070230
Chen, L., McGowan, P., Ashe, S., Johnston, J., Li, Y., Hellstrom, I., et al. (1994). Tumor immunogenicity determines the effect of B7 costimulation on T cell-mediated tumor immunity. J. Exp. Med. 179, 523–532. doi: 10.1084/jem.179.2.523
Cicala, C. (2002). Protease activated receptor 2 and the cardiovascular system. Br. J. Pharmacol. 135, 14–20. doi: 10.1038/sj.bjp.0704438
Clark, C. E., Liu, Y., and Cooper, H. M. (2014a). The Yin and Yang of Wnt/Ryk axon guidance in development and regeneration. Sci. China Life Sci. 57, 366–371. doi: 10.1007/s11427-014-4640-3
Clark, C. E., Richards, L. J., Stacker, S. A., and Cooper, H. M. (2014b). Wnt5a induces Ryk-dependent and -independent effects on callosal axon and dendrite growth. Growth Factors 32, 11–17. doi: 10.3109/08977194.2013.875544
Cochi, S. E., Kempker, J. A., Annangi, S., Kramer, M. R., and Martin, G. S. (2016). Mortality trends of acute respiratory distress syndrome in the United States from 1999 to 2013. Ann. Am. Thorac. Soc. 13, 1742–1751.
Colamarino, S. A., and Tessierlavigne, M. (1995). The axonal chemoattractant netrin-1 is also a chemorepellent for trochlear motor axons. Cell 81, 621–629. doi: 10.1016/0092-8674(95)90083-7
Cordero, O. J., Salgado, F. J., and Nogueira, M. (2009). On the origin of serum CD26 and its altered concentration in cancer patients. Cancer Immunol. Immunother. 58, 1723–1747. doi: 10.1007/s00262-009-0728-1
Cregg, J. M., DePaul, M. A., Filous, A. R., Lang, B. T., Tran, A., and Silver, J. (2014). Functional regeneration beyond the glial scar. Exp. Neurol. 253, 197–207. doi: 10.1016/j.expneurol.2013.12.024
Dandekar, S. N., Park, J. S., Peng, G. E., Onuffer, J. J., Lim, W. A., and Weiner, O. D. (2013). Actin dynamics rapidly reset chemoattractant receptor sensitivity following adaptation in neutrophils. Philos. Trans. R. Soc. Lond. B Biol. Sci. 368:20130008. doi: 10.1098/rstb.2013.0008
De Wit, R. J., and Bulgakov, R. (1985). Guanine nucleotides modulate the ligand binding properties of cell surface folate receptors in Dictyostelium discoideum. FEBS Lett. 179, 257–261. doi: 10.1016/0014-5793(85)80530-5
Deacon, C. F. (2019). Physiology and pharmacology of DPP-4 in glucose homeostasis and the treatment of type 2 diabetes. Front. Endocrinol. 10:80. doi: 10.3389/fendo.2019.00080
Dever, T. E. (2002). Gene-specific regulation by general translation factors. Cell 108, 545–556. doi: 10.1016/s0092-8674(02)00642-6
d’Onofrio, A. (2012). Spatiotemporal effects of a possible chemorepulsion of tumor cells by immune system effectors. J. Theor. Biol. 296, 41–48. doi: 10.1016/j.jtbi.2011.11.013
Fang, R., Xiong, Y., and Singleton, C. K. (2003). IfkA, a presumptive eIF2 alpha kinase of Dictyostelium, is required for proper timing of aggregation and regulation of mound size. BMC Dev. Biol. 3:3. doi: 10.1186/1471-213x-3-3
Freese, J. L., Pino, D., and Pleasure, S. J. (2010). Wnt signaling in development and disease. Neurobiol. Dis. 38, 148–153.
Fukujin, F., Nakajima, A., Shimada, N., and Sawai, S. (2016). Self-organization of chemoattractant waves in dictyostelium depends on F-actin and cell-substrate adhesion. J. R. Soc. Interface 13:20160233. doi: 10.1098/rsif.2016.0233
Furne, C., Rama, N., Corset, V., Chedotal, A., and Mehlen, P. (2008). Netrin-1 is a survival factor during commissural neuron navigation. Proc. Natl. Acad. Sci. U.S.A. 105, 14465–14470. doi: 10.1073/pnas.0803645105
Garcia, M., Ray, S., Brown, I., Irom, J., and Brazill, D. (2014). PakD, a putative p21-activated protein kinase in Dictyostelium discoideum, regulates actin. Eukaryot. Cell 13, 119–126. doi: 10.1128/ec.00216-13
Gardell, L. R., Ma, J. N., Seitzberg, J. G., Knapp, A. E., Schiffer, H. H., Tabatabaei, A., et al. (2008). Identification and characterization of novel small-molecule protease-activated receptor 2 agonists. J. Pharmacol. Exp. Ther. 327, 799–808. doi: 10.1124/jpet.108.142570
Gelfand, M. V., Hong, S., and Gu, C. (2009). Guidance from above: common cues direct distinct signaling outcomes in vascular and neural patterning. Trends Cell Biol. 19, 99–110. doi: 10.1016/j.tcb.2009.01.001
Gotthardt, K., Weyand, M., Kortholt, A., Van Haastert, P. J., and Wittinghofer, A. (2008). Structure of the Roc-COR domain tandem of C. tepidum. a prokaryotic homologue of the human LRRK2 Parkinson kinase. Embo J. 27, 2239–2249. doi: 10.1038/emboj.2008.150
Grimsey, N., Soto, A. G., and Trejo, J. (2011). Regulation of protease-activated receptor signaling by post-translational modifications. IUBMB Life 63, 403–411. doi: 10.1002/iub.442
Guidobaldi, H. A., Cubilla, M., Moreno, A., Molino, M. V., Bahamondes, L., and Giojalas, L. C. (2017). Sperm chemorepulsion, a supplementary mechanism to regulate fertilization. Hum. Reprod. 32, 1560–1573. doi: 10.1093/humrep/dex232
Guijarro, P., Simo, S., Pascual, M., Abasolo, I., Del Rio, J. A., and Soriano, E. (2006). Netrin1 exerts a chemorepulsive effect on migrating cerebellar interneurons in a Dcc-independent way. Mol. Cell Neurosci. 33, 389–400. doi: 10.1016/j.mcn.2006.08.010
Hadwiger, J. A., Lee, S., and Firtel, R. A. (1994). The G alpha subunit G alpha 4 couples to pterin receptors and identifies a signaling pathway that is essential for multicellular development in Dictyostelium. Proc. Natl. Acad. Sci. U.S.A. 91:10566. doi: 10.1073/pnas.91.22.10566
Hall, A. (1998). Rho GTPases and the actin cytoskeleton. Science 279, 509–514. doi: 10.1126/science.279.5350.509
Hedgecock, E. M., Culotti, J. G., and Hall, D. H. (1990). The unc-5, unc-6, and unc-40 genes guide circumferential migrations of pioneer axons and mesodermal cells on the epidermis in C. elegans. Neuron 4, 61–85. doi: 10.1016/0896-6273(90)90444-k
Herlihy, S. E., Brown, M. L., Pilling, D., Weeks, B. R., Myers, L. K., and Gomer, R. H. (2015). Role of the neutrophil chemorepellent soluble dipeptidyl peptidase IV in decreasing inflammation in a murine model of arthritis. Arthritis Rheumatol. 67, 2634–2638. doi: 10.1002/art.39250
Herlihy, S. E., Pilling, D., Maharjan, A. S., and Gomer, R. H. (2013). Dipeptidyl peptidase IV is a human and murine neutrophil chemorepellent. J. immunol. 190, 6468–6477. doi: 10.4049/jimmunol.1202583
Herlihy, S. E., Tang, Y., Phillips, J. E., and Gomer, R. H. (2017). Functional similarities between the Dictyostelium protein AprA and the human protein dipeptidyl-peptidase IV. Protein Sci. 26, 578–585. doi: 10.1002/pro.3107
Heuberger, D. M., and Schuepbach, R. A. (2019). Protease-activated receptors (PARs): mechanisms of action and potential therapeutic modulators in PAR-driven inflammatory diseases. Thromb. J. 17:4.
Holt, E., Stanton-Turcotte, D., and Iulianella, A. (2021). Development of the vertebrate trunk sensory system: origins, specification, axon guidance, and central connectivity. Neuroscience 458, 229–243. doi: 10.1016/j.neuroscience.2020.12.037
Hong, K. S., Hinck, L., Nishiyama, M., Poo, M. M., Tessier-Lavigne, M., and Stein, E. (1999). A ligand-gated association between cytoplasmic domains of UNC5 and DCC family receptors converts netrin-induced growth cone attraction to repulsion. Cell 97, 927–941. doi: 10.1016/s0092-8674(00)80804-1
Hu, H. (1999). Chemorepulsion of neuronal migration by Slit2 in the developing mammalian forebrain. Neuron 23, 703–711. doi: 10.1016/s0896-6273(01)80029-5
Huang, H. C., and Klein, P. S. (2004). The Frizzled family: receptors for multiple signal transduction pathways. Genome Biol. 5:234.
Huber, R. J., and Mathavarajah, S. (2018). Cln5 is secreted and functions as a glycoside hydrolase in Dictyostelium. Cell Signal 42, 236–248. doi: 10.1016/j.cellsig.2017.11.001
Huber, R. J., Myre, M. A., and Cotman, S. L. (2014). Loss of Cln3 function in the social amoeba Dictyostelium discoideum causes pleiotropic effects that are rescued by human CLN3. PLoS One 9:e110544. doi: 10.1371/journal.pone.0110544
Islam, S. M., Shinmyo, Y., Okafuji, T., Su, Y., Naser, I. B., Ahmed, G., et al. (2009). Draxin, a repulsive guidance protein for spinal cord and forebrain commissures. Science 323, 388–393. doi: 10.1126/science.1165187
Jager, E., Ringhoffer, M., Karbach, J., Arand, M., Oesch, F., and Knuth, A. (1996). Inverse relationship of melanocyte differentiation antigen expression in melanoma tissues and CD8+ cytotoxic-T-cell responses: evidence for immunoselection of antigen-loss variants in vivo. Int. J. Cancer 66, 470–476. doi: 10.1002/(sici)1097-0215(19960516)66:4<470::aid-ijc10>3.0.co;2-c
Kagota, S., Maruyama, K., and McGuire, J. J. (2016). Characterization and functions of protease-activated receptor 2 in obesity, diabetes, and metabolic syndrome: a systematic review. Biomed. Res. Int. 2016:3130496.
Kalil, K., Li, L., and Hutchins, B. I. (2011). Signaling mechanisms in cortical axon growth, guidance, and branching. Front. Neuroanat. 5:62. doi: 10.3389/fnana.2011.00062
Kao, K. C., Hu, H. C., Chang, C. H., Hung, C. Y., Chiu, L. C., Li, S. H., et al. (2015). Diffuse alveolar damage associated mortality in selected acute respiratory distress syndrome patients with open lung biopsy. Crit. Care 19:228.
Kaplan, M. J. (2013). Role of neutrophils in systemic autoimmune diseases. Arthritis Res. Ther. 15:219. doi: 10.1186/ar4325
Karunanithi, S., Joshi, J., Chavel, C., Birkaya, B., Grell, L., and Cullen, P. J. (2012). Regulation of mat responses by a differentiation MAPK pathway in Saccharomyces cerevisiae. PLoS One 7:e32294. doi: 10.1371/journal.pone.0032294
Keizer-Gunnink, I., Kortholt, A., and Van Haastert, P. J. (2007). Chemoattractants and chemorepellents act by inducing opposite polarity in phospholipase C and PI3-kinase signaling. J. Cell Biol. 177, 579–585. doi: 10.1083/jcb.200611046
Kessin, R. H. (2001). Dictyostelium: Evolution, Cell Biology, and the Development of Multicellularity. Cambridge: Cambridge University Press.
King, J. S., and Insall, R. H. (2009). Chemotaxis: finding the way forward with Dictyostelium. Trends Cell Biol. 19, 523–530. doi: 10.1016/j.tcb.2009.07.004
Kohrgruber, N., Groger, M., Meraner, P., Kriehuber, E., Petzelbauer, P., Brandt, S., et al. (2004). Plasmacytoid dendritic cell recruitment by immobilized CXCR3 ligands. J. Immunol. 173, 6592–6602. doi: 10.4049/jimmunol.173.11.6592
Kolaczkowska, E., and Kubes, P. (2013). Neutrophil recruitment and function in health and inflammation. Nat. Rev. Immunol. 13, 159–175. doi: 10.1038/nri3399
Kolpak, A. L., Jiang, J., Guo, D., Standley, C., Bellve, K., Fogarty, K., et al. (2009). Negative guidance factor-induced macropinocytosis in the growth cone plays a critical role in repulsive axon turning. J. Neurosci. 29, 10488–10498. doi: 10.1523/jneurosci.2355-09.2009
Komiya, Y., and Habas, R. (2008). Wnt signal transduction pathways. Organogenesis 4, 68–75. doi: 10.4161/org.4.2.5851
Kortholt, A., Kataria, R., Keizer-Gunnink, I., Van Egmond, W. N., Khanna, A., and Van Haastert, P. J. (2011). Dictyostelium chemotaxis: essential Ras activation and accessory signalling pathways for amplification. EMBO Rep. 12, 1273–1279. doi: 10.1038/embor.2011.210
Kotacková, L., Baláziová, E., and Sedo, A. (2009). Expression pattern of dipeptidyl peptidase IV activity and/or structure homologues in cancer. Folia Biol. 55, 77–84.
Lake, D., Correa, S. A., and Muller, J. (2016). Negative feedback regulation of the ERK1/2 MAPK pathway. Cell Mol. Life Sci. 73, 4397–4413. doi: 10.1007/s00018-016-2297-8
Larsen, S. H., Reader, R. W., Kort, E. N., Tso, W. W., and Adler, J. (1974). Change in direction of flagellar rotation is the basis of the chemotactic response in Escherichia coli. Nature 249, 74–77. doi: 10.1038/249074a0
Leung, T., Chen, X. Q., Manser, E., and Lim, L. (1996). The p160 RhoA-binding kinase ROK alpha is a member of a kinase family and is involved in the reorganization of the cytoskeleton. Mol. Cell. Biol. 16, 5313–5327. doi: 10.1128/mcb.16.10.5313
Levine, H., Kessler, D. A., and Rappel, W. J. (2006). Directional sensing in eukaryotic chemotaxis: a balanced inactivation model. Proc. Natl. Acad. Sci. U.S.A. 103, 9761–9766. doi: 10.1073/pnas.0601302103
Lim, C. J., Zawadzki, K. A., Khosla, M., Secko, D. M., Spiegelman, G. B., and Weeks, G. (2005). Loss of the Dictyostelium RasC protein alters vegetative cell size, motility and endocytosis. Exp. Cell Res. 306, 47–55. doi: 10.1016/j.yexcr.2005.02.002
Lin, W. C., and Fessler, M. B. (2021). Regulatory mechanisms of neutrophil migration from the circulation to the airspace. Cell Mol. Life Sci. 78, 4095–4124. doi: 10.1007/s00018-021-03768-z
Liu, B. P., Cafferty, W. B., Budel, S. O., and Strittmatter, S. M. (2006). Extracellular regulators of axonal growth in the adult central nervous system. Philos. Trans. R. Soc. Lond. B Biol. Sci. 361, 1593–1610. doi: 10.1098/rstb.2006.1891
Lynn, H., Sun, X., Casanova, N., Gonzales-Garay, M., Bime, C., and Garcia, J. G. N. (2019). Genomic and genetic approaches to deciphering acute respiratory distress syndrome risk and mortality. Antioxid. Redox Signal. 31, 1027–1052. doi: 10.1089/ars.2018.7701
Ma, H., Gamper, M., Parent, C., and Firtel, R. A. (1997). The Dictyostelium MAP kinase kinase DdMEK1 regulates chemotaxis and is essential for chemoattractant-mediated activation of guanylyl cyclase. Embo. J. 16, 4317–4332. doi: 10.1093/emboj/16.14.4317
MacWilliams, H., Doquang, K., Pedrola, R., Dollman, G., Grassi, D., Peis, T., et al. (2006). A retinoblastoma ortholog controls stalk/spore preference in Dictyostelium. Development 133, 1287–1297. doi: 10.1242/dev.02287
Maekawa, M., Ishizaki, T., Boku, S., Watanabe, N., Fujita, A., Iwamatsu, A., et al. (1999). Signaling from Rho to the actin cytoskeleton through protein kinases ROCK and LIM-kinase. Science 285, 895–898. doi: 10.1126/science.285.5429.895
Mark, M. D., Lohrum, M., and Puschel, A. W. (1997). Patterning neuronal connections by chemorepulsion: the semaphorins. Cell Tissue Res. 290, 299–306. doi: 10.1007/978-3-642-60905-3_15
Matthay, M. A., Ware, L. B., and Zimmerman, G. A. (2012). The acute respiratory distress syndrome. J. Clin. Invest. 122, 2731–2740.
McCulloch, K., McGrath, S., Huesa, C., Dunning, L., Litherland, G., Crilly, A., et al. (2018). Rheumatic disease: protease-activated receptor-2 in synovial joint pathobiology. Front. Endocrinol. 9:257. doi: 10.3389/fendo.2018.00257
McLean, P. G., Aston, D., Sarkar, D., and Ahluwalia, A. (2002). Protease-activated receptor-2 activation causes EDHF-like coronary vasodilation: selective preservation in ischemia/reperfusion injury: involvement of lipoxygenase products, VR1 receptors, and C-fibers. Circ Res. 90, 465–472. doi: 10.1161/hh0402.105372
Meli, R., Weisova, P., and Propst, F. (2015). Repulsive axon guidance by Draxin is mediated by protein Kinase B (Akt), glycogen synthase kinase-3beta (GSK-3beta) and microtubule-associated protein 1B. PLoS One 10:e0119524. doi: 10.1371/journal.pone.0119524
Mulvihill, E. E., and Drucker, D. J. (2014). Pharmacology, physiology, and mechanisms of action of dipeptidyl peptidase-4 inhibitors. Endocr. Rev. 35, 992–1019. doi: 10.1210/er.2014-1035
Nash, M., Pribiag, H., Fournier, A. E., and Jacobson, C. (2009). Central nervous system regeneration inhibitors and their intracellular substrates. Mol. Neurobiol. 40, 224–235. doi: 10.1007/s12035-009-8083-y
Nauck, M. A., Vardarli, I., Deacon, C. F., Holst, J. J., and Meier, J. J. (2011). Secretion of glucagon-like peptide-1 (GLP-1) in type 2 diabetes: what is up, what is down? Diabetologia 54, 10–18. doi: 10.1007/s00125-010-1896-4
Nguyen Ba-Charvet, K. T., Brose, K., Marillat, V., Kidd, T., Goodman, C. S., Tessier-Lavigne, M., et al. (1999). Slit2-Mediated chemorepulsion and collapse of developing forebrain axons. Neuron 22, 463–473. doi: 10.1016/s0896-6273(00)80702-3
Ogilvie, P., Paoletti, S., Clark-Lewis, I., and Uguccioni, M. (2003). Eotaxin-3 is a natural antagonist for CCR2 and exerts a repulsive effect on human monocytes. Blood 102, 789–794. doi: 10.1182/blood-2002-09-2773
Ossovskaya, V. S., and Bunnett, N. W. (2004). Protease-activated receptors: contribution to physiology and disease. Physiol Rev. 84, 579–621. doi: 10.1152/physrev.00028.2003
Pan, K., Ohnuma, K., Morimoto, C., and Dang, N. H. (2021). CD26/Dipeptidyl peptidase IV and its multiple biological functions. Cureus 13, e13495.
Pandey, G., and Jain, R. K. (2002). Bacterial chemotaxis toward environmental pollutants: role in bioremediation. Appl. Environ. Microbiol. 68, 5789–5795. doi: 10.1128/aem.68.12.5789-5795.2002
Parent, C. A., Blacklock, B. J., Froehlich, W. M., Murphy, D. B., and Devreotes, P. N. (1998). G protein signaling events are activated at the leading edge of chemotactic cells. Cell 95, 81–91. doi: 10.1016/s0092-8674(00)81784-5
Parhar, K. K. S., Zjadewicz, K., Soo, A., Sutton, A., Zjadewicz, M., Doig, L., et al. (2019). Epidemiology, mechanical power, and 3-Year outcomes in acute respiratory distress syndrome patients using standardized screening. An observational cohort study. Ann. Am. Thorac. Soc. 16, 1263–1272. doi: 10.1513/annalsats.201812-910oc
Park, J. H., Pak, H. J., Riew, T. R., Shin, Y. J., and Lee, M. Y. (2016). Increased expression of Slit2 and its receptors Robo1 and Robo4 in reactive astrocytes of the rat hippocampus after transient forebrain ischemia. Brain Res. 1634, 45–56. doi: 10.1016/j.brainres.2015.12.056
Patel, K., Nash, J. A., Itoh, A., Liu, Z., Sundaresan, V., and Pini, A. (2001). Slit proteins are not dominant chemorepellents for olfactory tract and spinal motor axons. Development 128, 5031–5037. doi: 10.1242/dev.128.24.5031
Phillips, J. E., and Gomer, R. H. (2010). The ROCO kinase QkgA is necessary for proliferation inhibition by autocrine signals in Dictyostelium discoideum. Eukaryot. Cell 9, 1557–1565. doi: 10.1128/ec.00121-10
Phillips, J. E., and Gomer, R. H. (2012). A secreted protein is an endogenous chemorepellant in Dictyostelium discoideum. Proc. Natl. Acad. Sci. U.S.A. 109, 10990–10995. doi: 10.1073/pnas.1206350109
Phillips, J. E., and Gomer, R. H. (2014). The p21-activated kinase (PAK) family member PakD is required for chemorepulsion and proliferation inhibition by autocrine signals in Dictyostelium discoideum. PLoS One 9:e96633. doi: 10.1371/journal.pone.0096633
Phillips, J. E., Huang, E., Shaulsky, G., and Gomer, R. H. (2011). The putative bZIP transcription factor BzpN slows proliferation and functions in the regulation of cell density by autocrine signals in Dictyostelium. PLoS One 6:e21765. doi: 10.1371/journal.pone.0021765
Pilling, D., Chinea, L. E., Consalvo, K. M., and Gomer, R. H. (2019). Different isoforms of the neuronal guidance molecule Slit2 directly cause chemoattraction or chemorepulsion of human neutrophils. J. Immunol. 202, 239–248. doi: 10.4049/jimmunol.1800681
Plattner, H. (2015). Molecular aspects of calcium signalling at the crossroads of unikont and bikont eukaryote evolution–the ciliated protozoan Paramecium in focus. Cell Calcium 57, 174–185. doi: 10.1016/j.ceca.2014.12.002
Rai, M., Xiong, Y., and Singleton, C. K. (2006). Disruption of the ifkA and ifkB genes results in altered cell adhesion, morphological defects and a propensity to form pre-stalk O cells during development of Dictyostelium. Differentiation 74, 583–595. doi: 10.1111/j.1432-0436.2006.00085.x
Ranieri, V. M., Rubenfeld, G. D., Thompson, B. T., Ferguson, N. D., Caldwell, E., Fan, E., et al. (2012). Acute respiratory distress syndrome: the Berlin Definition. JAMA 307, 2526–2533.
Redecha, P., Franzke, C. W., Ruf, W., Mackman, N., and Girardi, G. (2008). Neutrophil activation by the tissue factor/Factor VIIa/PAR2 axis mediates fetal death in a mouse model of antiphospholipid syndrome. J. Clin. Invest. 118, 3453–3461.
Riches, K., Franklin, L., Maqbool, A., Peckham, M., Adams, M., Bond, J., et al. (2013). Apolipoprotein(a) acts as a chemorepellent to human vascular smooth muscle cells via integrin alphaVbeta3 and RhoA/ROCK-mediated mechanisms. Int. J. Biochem. Cell Biol. 45, 1776–1783. doi: 10.1016/j.biocel.2013.05.021
Ridley, A. J. (2015). Rho GTPase signalling in cell migration. Curr. Opin. Cell Biol. 36, 103–112. doi: 10.1016/j.ceb.2015.08.005
Rijal, R., Consalvo, K. M., Lindsey, C. K., and Gomer, R. H. (2019). An endogenous chemorepellent directs cell movement by inhibiting pseudopods at one side of cells. Mol. Biol. Cell 30, 242–255. doi: 10.1091/mbc.e18-09-0562
Rodgers, L. F., Markle, K. L., and Hennessey, T. M. (2008). Responses of the ciliates tetrahymena and paramecium to vertebrate odorants and tastants. J. Eukaryot. Microbiol. 55, 27–33. doi: 10.1111/j.1550-7408.2007.00300.x
Roy, A., Kucukural, A., and Zhang, Y. (2010). I-TASSER: a unified platform for automated protein structure and function prediction. Nat. Protoc. 5, 725–738. doi: 10.1038/nprot.2010.5
Roy, A., Yang, J., and Zhang, Y. (2012). COFACTOR: an accurate comparative algorithm for structure-based protein function annotation. Nucleic Acids Res. 40, W471–W477.
Rubenfeld, G. D., Caldwell, E., Peabody, E., Weaver, J., Martin, D. P., Neff, M., et al. (2005). Incidence and outcomes of acute lung injury. N. Engl. J. Med. 353, 1685–1693.
Sadik, C. D., and Luster, A. D. (2012). Lipid-cytokine-chemokine cascades orchestrate leukocyte recruitment in inflammation. J. Leukoc. Biol. 91, 207–215. doi: 10.1189/jlb.0811402
Santavuori, P. (1988). Neuronal ceroid-lipofuscinoses in childhood. Brain Dev. 10, 80–83. doi: 10.1016/s0387-7604(88)80075-5
Santos, T. E., Schaffran, B., Broguiere, N., Meyn, L., Zenobi-Wong, M., and Bradke, F. (2020). Axon growth of CNS neurons in three dimensions is amoeboid and independent of adhesions. Cell Rep. 32:107907. doi: 10.1016/j.celrep.2020.107907
Sasaki, A. T., Chun, C., Takeda, K., and Firtel, R. A. (2004). Localized Ras signaling at the leading edge regulates PI3K, cell polarity, and directional cell movement. J. Cell Biol. 167, 505–518. doi: 10.1083/jcb.200406177
Savino, W., Mendes-da-Cruz, D. A., Golbert, D. C., Riederer, I., and Cotta-de-Almeida, V. (2015). Laminin-mediated interactions in thymocyte migration and development. Front. Immunol. 6:579. doi: 10.3389/fimmu.2015.00579
Schmandke, A., Schmandke, A., and Strittmatter, S. M. (2007). ROCK and Rho: biochemistry and neuronal functions of Rho-associated protein kinases. Neuroscientist 13, 454–469. doi: 10.1177/1073858407303611
Schwab, M. E. (2010). Functions of Nogo proteins and their receptors in the nervous system. Nat. Rev. Neurosci. 11, 799–811. doi: 10.1038/nrn2936
Shaver, C. M., and Bastarache, J. A. (2014). Clinical and biological heterogeneity in acute respiratory distress syndrome: direct versus indirect lung injury. Clin. Chest Med. 35, 639–653.
Shearer, A. M., Rana, R., Austin, K., Baleja, J. D., Nguyen, N., Bohm, A., et al. (2016). Targeting liver fibrosis with a cell-penetrating protease-activated receptor-2 (PAR2) pepducin. J. Biol. Chem. 291, 23188–23198. doi: 10.1074/jbc.m116.732743
Steinberg, K. P., Milberg, J. A., Martin, T. R., Maunder, R. J., Cockrill, B. A., and Hudson, L. D. (1994). Evolution of bronchoalveolar cell populations in the adult respiratory distress syndrome. Am. J. Respir. Crit. Care Med. 150, 113–122. doi: 10.1164/ajrccm.150.1.8025736
Sugarman, B., and Mummaw, N. (1988). Effects of antimicrobial agents on growth and chemotaxis of Trichomonas vaginalis. Antimicrob. Agents Chemother. 32, 1323–1326. doi: 10.1128/aac.32.9.1323
Tadagavadi, R. K., Wang, W., and Ramesh, G. (2010). Netrin-1 regulates Th1/Th2/Th17 cytokine production and inflammation through UNC5B receptor and protects kidney against ischemia-reperfusion injury. J. Immunol. 185, 3750–3758. doi: 10.4049/jimmunol.1000435
Tang, V. (2017). Cell-cell adhesion interface: rise of the lateral membrane. F1000Res. 6:276. doi: 10.12688/f1000research.10680.1
Tang, Y., and Gomer, R. H. (2008). A protein with similarity to PTEN regulates aggregation territory size by decreasing cyclic AMP pulse size during Dictyostelium discoideum development. Eukaryot. Cell 7, 1758–1770. doi: 10.1128/ec.00210-08
Tang, Y., Wu, Y., Herlihy, S. E., Brito-Aleman, F. J., Ting, J. H., Janetopoulos, C., et al. (2018). An autocrine proliferation repressor regulates Dictyostelium discoideum proliferation and chemorepulsion using the G protein-coupled receptor GrlH. mBio 9:e2443-17.
Tharp, W. G., Yadav, R., Irimia, D., Upadhyaya, A., Samadani, A., Hurtado, O., et al. (2006). Neutrophil chemorepulsion in defined interleukin-8 gradients in vitro and in vivo. J. Leukoc. Biol. 79, 539–554. doi: 10.1189/jlb.0905516
Theveneau, E., and Mayor, R. (2013). Collective cell migration of epithelial and mesenchymal cells. Cell Mol. Life Sci. 70, 3481–3492. doi: 10.1007/s00018-012-1251-7
Thiede-Stan, N. K., and Schwab, M. E. (2015). Attractive and repulsive factors act through multi-subunit receptor complexes to regulate nerve fiber growth. J. Cell Sci. 128, 2403–2414.
Thoma, R., Löffler, B., Stihle, M., Huber, W., Ruf, A., and Hennig, M. (2003). Structural basis of proline-specific exopeptidase activity as observed in human dipeptidyl peptidase-IV. Structure 11, 947–959. doi: 10.1016/s0969-2126(03)00160-6
Thompson, B. T., Chambers, R. C., and Liu, K. D. (2017). Acute respiratory distress syndrome. N. Engl. J. Med. 377, 562–572.
Tole, S., Mukovozov, I. M., Huang, Y. W., Magalhaes, M. A., Yan, M., Crow, M. R., et al. (2009). The axonal repellent, Slit2, inhibits directional migration of circulating neutrophils. J. Leukoc. Biol. 86, 1403–1415. doi: 10.1189/jlb.0609391
Tomchik, K. J., and Devreotes, P. N. (1981). Adenosine 3.5′-monophosphate waves in Dictyostelium discoideum: a demonstration by isotope dilution–fluorography. Science 212, 443–446. doi: 10.1126/science.6259734
Trzaskalski, N. A., Fadzeyeva, E., and Mulvihill, E. E. (2020). Dipeptidyl Peptidase-4 at the Interface Between Inflammation and Metabolism. Clin. Med. Insights Endocrinol. Diabetes 13:1179551420912972.
Vianello, F., Olszak, I. T., and Poznansky, M. C. (2005). Fugetaxis: active movement of leukocytes away from a chemokinetic agent. J. Mol. Med. 83, 752–763. doi: 10.1007/s00109-005-0675-z
Vianello, F., Papeta, N., Chen, T., Kraft, P., White, N., Hart, W. K., et al. (2006). Murine B16 melanomas expressing high levels of the chemokine stromal-derived factor-1/CXCL12 induce tumor-specific T cell chemorepulsion and escape from immune control. J. Immunol. 176, 2902–2914. doi: 10.4049/jimmunol.176.5.2902
Vo, T., Carulli, D., Ehlert, E. M., Kwok, J. C., Dick, G., Mecollari, V., et al. (2013). The chemorepulsive axon guidance protein semaphorin3A is a constituent of perineuronal nets in the adult rodent brain. Mol. Cell Neurosci. 56, 186–200. doi: 10.1016/j.mcn.2013.04.009
Vu, T. K., Hung, D. T., Wheaton, V. I., and Coughlin, S. R. (1991). Molecular cloning of a functional thrombin receptor reveals a novel proteolytic mechanism of receptor activation. Cell 64, 1057–1068. doi: 10.1016/0092-8674(91)90261-v
Wahl, S., Barth, H., Ciossek, T., Aktories, K., and Mueller, B. K. (2000). Ephrin-A5 induces collapse of growth cones by activating Rho and Rho kinase. J. Cell Biol. 149, 263–270. doi: 10.1083/jcb.149.2.263
Walborg, E. F. Jr., Tsuchida, S., Weeden, D. S., Thomas, M. W., Barrick, A., McEntire, K. D., et al. (1985). Identification of dipeptidyl peptidase IV as a protein shared by the plasma membrane of hepatocytes and liver biomatrix. Exp. Cell Res. 158, 509–518. doi: 10.1016/0014-4827(85)90474-4
Walters, D. M., and Kleeberger, S. R. (2008). Mouse models of bleomycin-induced pulmonary fibrosis. Curr. Protoc. Pharmacol. 40, 5.46.1–5.46.17.
Wang, F. (2009). The signaling mechanisms underlying cell polarity and chemotaxis. Cold Spring Harb. Perspect Biol. 1:a002980. doi: 10.1101/cshperspect.a002980
Wang, Y., Chen, C. L., and Iijima, M. (2011). Signaling mechanisms for chemotaxis. Dev. Growth Differ. 53, 495–502. doi: 10.1111/j.1440-169x.2011.01265.x
Wang, Y., Senoo, H., Sesaki, H., and Iijima, M. (2013). Rho GTPases orient directional sensing in chemotaxis. Proc. Natl. Acad. Sci. U.S.A. 110, E4723–E4732.
Weiss, S. J. (1989). Tissue destruction by neutrophils. N. Engl. J. Med. 320, 365–376. doi: 10.1056/nejm198902093200606
White, M. J. V., Chinea, L. E., Pilling, D., and Gomer, R. H. (2018). Protease activated-receptor 2 is necessary for neutrophil chemorepulsion induced by trypsin, tryptase, or dipeptidyl peptidase IV. J. Leukoc. Biol. 103, 119–128. doi: 10.1002/jlb.3a0717-308r
Wronkowitz, N., Görgens, S. W., Romacho, T., Villalobos, L. A., Sánchez-Ferrer, C. F., Peiró, C., et al. (2014). Soluble DPP4 induces inflammation and proliferation of human smooth muscle cells via protease-activated receptor 2. Biochim. Biophys. Acta 1842, 1613–1621. doi: 10.1016/j.bbadis.2014.06.004
Yan, J., Mihaylov, V., Xu, X., Brzostowski, J. A., Li, H., and Liu, L. (2012). A Gβγ Effector, ElmoE, transduces GPCR signaling to the actin network during chemotaxis. Dev. Cell 22, 92–103. doi: 10.1016/j.devcel.2011.11.007
Ye, B. Q., Geng, Z. H., Ma, L., and Geng, J. G. (2010). Slit2 regulates attractive eosinophil and repulsive neutrophil chemotaxis through differential srGAP1 expression during lung inflammation. J. Immunol. 185, 6294–6305. doi: 10.4049/jimmunol.1001648
Ye, J., Kumanova, M., Hart, L. S., Sloane, K., Zhang, H., De Panis, D. N., et al. (2010). The GCN2-ATF4 pathway is critical for tumour cell survival and proliferation in response to nutrient deprivation. Embo J. 29, 2082–2096. doi: 10.1038/emboj.2010.81
Zemans, R. L., Colgan, S. P., and Downey, G. P. (2009). Transepithelial migration of neutrophils: mechanisms and implications for acute lung injury. Am. J. Respir. Cell Mol. Biol. 40, 519–535. doi: 10.1165/rcmb.2008-0348tr
Zhang, Y. (2008). I-TASSER server for protein 3D structure prediction. BMC Bioinformatics 9:40. doi: 10.1186/1471-2105-9-40
Zhu, L., Tamvakopoulos, C., Xie, D., Dragovic, J., Shen, X., Fenyk-Melody, J. E., et al. (2003). The role of dipeptidyl peptidase IV in the cleavage of glucagon family peptides: in vivo metabolism of pituitary adenylate cyclase activating polypeptide-(1-38). J. Biol. Chem. 278, 22418–22423.
Keywords: Dictyostelium discoideum, chemorepulsion, acute respiratory disease syndrome, neutrophil (PMN), DPPIV, PAR2
Citation: Kirolos SA, Rijal R, Consalvo KM and Gomer RH (2021) Using Dictyostelium to Develop Therapeutics for Acute Respiratory Distress Syndrome. Front. Cell Dev. Biol. 9:710005. doi: 10.3389/fcell.2021.710005
Received: 14 May 2021; Accepted: 22 June 2021;
Published: 19 July 2021.
Edited by:
Annette Müller-Taubenberger, Ludwig Maximilian University of Munich, GermanyReviewed by:
Salvatore Bozzaro, University of Turin, ItalyJeffrey Hadiwger, Oklahoma State University, United States
Shigehiko Yumura, Yamaguchi University, Japan
Copyright © 2021 Kirolos, Rijal, Consalvo and Gomer. This is an open-access article distributed under the terms of the Creative Commons Attribution License (CC BY). The use, distribution or reproduction in other forums is permitted, provided the original author(s) and the copyright owner(s) are credited and that the original publication in this journal is cited, in accordance with accepted academic practice. No use, distribution or reproduction is permitted which does not comply with these terms.
*Correspondence: Richard H. Gomer, cmdvbWVyQHRhbXUuZWR1