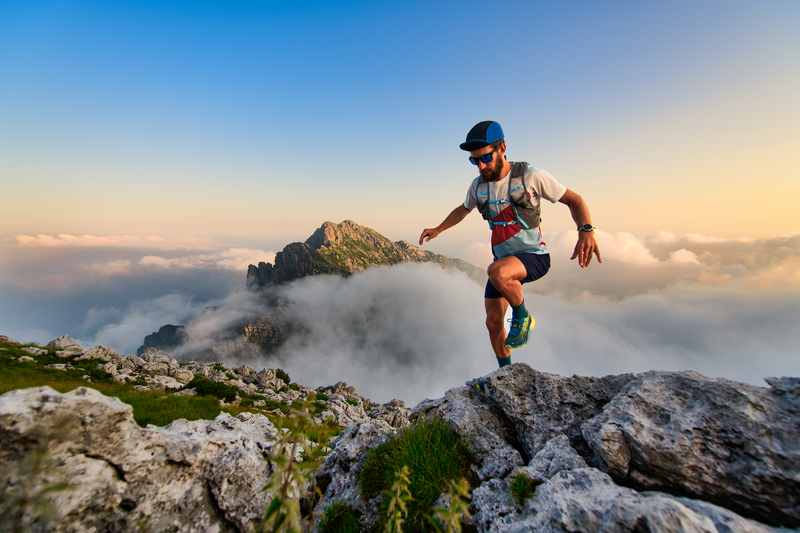
94% of researchers rate our articles as excellent or good
Learn more about the work of our research integrity team to safeguard the quality of each article we publish.
Find out more
REVIEW article
Front. Cell Dev. Biol. , 10 August 2021
Sec. Molecular and Cellular Oncology
Volume 9 - 2021 | https://doi.org/10.3389/fcell.2021.705280
This article is part of the Research Topic Unveiling the Impact of Local or Systemic Therapeutic Strategies on the Tumor Microenvironment View all 19 articles
A tumor microenvironment (TME) is composed of various cell types and extracellular components. It contains tumor cells and is nourished by a network of blood vessels. The TME not only plays a significant role in the occurrence, development, and metastasis of tumors but also has a far-reaching impact on the effect of therapeutics. Continuous interaction between tumor cells and the environment, which is mediated by their environment, may lead to drug resistance. In this review, we focus on the key cellular components of the TME and the potential strategies to improve the effectiveness of drug therapy by changing their related factors.
Tumor microenvironment (TME) refers to the cellular environment in which tumor cells and cancer stem cells (CSCs) exist. It can directly promote angiogenesis, invasion, metastasis, and chronic inflammation, and help maintain the stemness of the tumor (Denton et al., 2018). Different TMEs have not only adverse effects on the occurrence of tumors but also favorable consequences for patients. The composition of TME includes local stromal cells (such as resident fibroblasts and macrophages), remotely recruited cells (such as endothelial cells), immune cells (including myeloid cells and lymphoid cells), bone marrow-derived inflammatory cells, extracellular matrix (ECM), blood vessels, and signal molecules (Del Prete et al., 2017). Among them, tumor-associated myeloid cells (TAMCs) also include five different myeloid cell groups: tumor-associated macrophages (TAMs), monocytes expressing angiopoietin-2 receptor Tie2 (Tie2 expressing monocytes or TEM), myeloid suppressor cells (MDSCs), and tumor-associated dendritic cells (Kim and Bae, 2016). Together, they surround tumor cells while being nourished by a network of blood vessels. The TME plays a key role in the occurrence, development, and metastasis of tumors. It also has a far-reaching impact on the effect of therapeutics, and recent studies have shown that targeted the TME is clinically feasible (Table 1). Non-malignant cells in the TME usually stimulate uncontrolled proliferation of cells and play a tumor-promoting function in the overall processes of carcinogenesis. In contrast, malignant cells can metastasize to healthy tissues in other parts of the body through the lymph or circulatory system (Tu et al., 2014). As TME plays a decisive role in the progress of tumor treatment, it is essential to further understand the components associated with TME in order to provide more precise treatment for different types of cancer.
Bonnet and Dick (1997) first confirmed the existence of CSCs in patients with acute myeloid leukemia and subsequently detected CSCs in other primary tumor tissues and cell lines (Kinugasa et al., 2014; Lau et al., 2017). CSCs refer to the subpopulations of tumor cells present in tumor masses, which are characterized by tumorigenicity and self-renewal properties (Magee et al., 2012). There is increasing evidence that CSCs play a key role in tumor recurrence, metastasis, and therapeutic resistance (Najafi et al., 2019a). TME induces the interaction between cancer cells and a variety of tissue cells. The functional characteristics of CSCs are affected by differentiated cancer cells and activated extracellular signals mediated by fibroblasts, macrophages, epithelial cells, endothelial cells, and blood cells, which provide the necessary growth elements for tumor cells and play an important role in promoting and maintaining the stemness of CSCs (Rafii et al., 2002; Byrne et al., 2005; Kopp et al., 2006; Huang et al., 2010). Recent studies have shown that in addition to changes in proto-oncogenes, the occurrence and metastasis of tumors are closely related to their microenvironment.
In the TME, cancer-associated fibroblasts (CAFs) can promote and maintain the stem cell-like properties of liver cancer cells through the IL-6/STAT3/Notch signaling pathway (Xiong et al., 2018). In contrast, TAMs activate STAT3 and the hedgehog signaling pathway by secreting milk fat globule surface growth factor 8 and IL-6, thereby affecting the self-renewal and chemotherapy resistance of CSCs (Jinushi et al., 2011). Fan et al. (2014) also found that TAMs in liver cancer promote CSC phenotypes through the induction of epithelial–mesenchymal transition (EMT) by transforming growth factor β1 (TGF-β1). Moreover, IL-6 and NO secreted by MDSCs can activate STAT3 and NOTCH signaling pathways, stimulate the expression of microRNA101 in CSCs, and promote the expression of C-terminal binding protein-2 (CtBP2). The CtBP2 protein acts as a transcriptional auxiliary inhibitor factor that can directly target the core genes of stem cells Nanog and Sox2, and ultimately lead to the enhancement of the stemness of CSCs (Cui et al., 2013; Peng et al., 2016). Remarkably, these microenvironmental factors can also maintain the dryness of CSCs through Wntβ-catenin, FGFR, and MEK signaling pathways (Borah et al., 2015; Krishnamurthy and Kurzrock, 2018; Jin, 2020). CSCs can also regulate the expression and/or secretion of cytokines such as NFAT, NF-κB, and STAT signaling pathways through SOX2 and other genes, thereby regulating TME and recruiting TAMs to create an environment for the further development of tumors (Mou et al., 2015; Zeng et al., 2018). This undoubtedly supports the close connection between CSCs and TME. Considering that CSCs play a key role in the process of tumor occurrence, development, and recurrence, the microenvironment regulation strategy for the growth of CSCs is expected to become an effective means of tumor-targeted therapy.
Cancer-associated fibroblasts are the most common type of host cells in the TME. It is now generally accepted that CAFs are a heterogeneous population with distinct functions which can serve as positive and negative regulators of tumor progression (Kalluri, 2016). Under the influence of the microenvironment, CAFs obtain an activated phenotype that is different from that of normal fibroblasts. It can promote tumor progression and regulate the composition of ECM by secreting soluble factors and interacting with other types of cells (Piccard et al., 2012). In patients with prostate cancer, CAF in the TME can promote cell proliferation and sphere formation through paracrine signals, thus promoting the growth of tumor stem cells. Studies have confirmed that the presence of a large amount of CAF in the tumor stroma is associated with poor prognosis in lung, breast, and pancreatic cancer (Räsänen and Vaheri, 2010). CAF can promote tumor progression by maintaining the continuous proliferation and growth of tumor cells at the metastatic site (Li and Wang, 2011).
Most activated CAFs originate from resident fibroblasts, which can recruit and activate many growth factors and cytokines, such as transforming growth factor β, fibroblast growth factor-2, and platelet-derived growth factor (PDGF). It has been found that these growth factors and cytokines are abundant in TME (Räsänen and Vaheri, 2010). CAFs can also be derived from bone marrow mesenchymal stem cells (Figure 1), transforming from resident epithelium or endothelial cells in the tumor stroma via EMT or endothelial–mesenchymal transition (EndMT), respectively (Kidd et al., 2012). The functions of activated CAFs include the synthesis and secretion of ECM and the release of proteolytic enzymes, such as heparanase and matrix metalloproteinases (MMPs), leading to ECM remodeling (Kessenbrock et al., 2010; Wu and Dai, 2017).
Figure 1. Major cellular constituents and matrix component of the TME, including cancer cells, immune cells (T-cells, B-cells, NK cells, dendritic cells, MDSCs, TAMs), CAFs and ECM. CAF derived from bone marrow mesenchymal stem cells and transform through epithelial–mesenchymal transition (EMT) or endothelial mesenchymal transition (EndMT) from resident epithelium or endothelial cells (A). When macrophages are exposed to LPS, MAMPs, IL12, TNF, IFNG, or another TLR agonists, they will transition to M1-like. When exposed to IL4, IL5, IL10, IL13, CSF1, TGFβ1, and PGE2, it will transition to M2-like state (B).
Cancer-associated fibroblasts can interact with tumor cells through direct contact and can also secrete a variety of cytokines through paracrine methods to promote the occurrence and development of cancer (Kalluri, 2016; Salimifard et al., 2020). Orimo et al. (2005) have shown that CXCL12 (stromal cell-derived factor-1, SDF-1) secreted by CAFs directly stimulates tumor growth by acting through the cognate receptor, CXCR4, which is expressed by carcinoma cells. In addition, CAF-secreted vascular cell adhesion molecule-1 (VCAM-1) also promotes the proliferation, migration, and invasion of tumor cells by activating the AKT and MAPK signals of lung cancer cells (Zhou et al., 2020). Recently, Seino et al. (2018) found that CAFs can provide a Wnt-producing niche to support the in vivo growth of the Wnt-deficient pancreatic ductal adenocarcinoma (PDAC) organoid mode. CAFs are also an important source of growth factors and cytokines [including hepatocyte growth factor (HGF), vascular endothelial growth factor (VEGF), PDGF, etc.], which can stimulate the growth of tumor cells in vitro and lead to therapeutic drug resistance (Straussman et al., 2012; Erez et al., 2013; Paraiso and Smalley, 2013).
Angiogenesis in tumor tissues can provide oxygen and nutrients for tumor cell metabolism and promote tumor growth and metastasis. Many studies have shown that CAFs can release a variety of stimulating factors that promote angiogenesis and play an important role in the recruitment and proliferation of tumor vascular endothelial cells and the formation of vascular sprouts (Benyahia et al., 2017). CAFs promote angiogenesis by recruiting endothelial progenitor cells (EPCs) into carcinomas, an effect mediated in part by CXCL12 (Orimo et al., 2005). CXCL12 can activate the PI3K/AKT signaling pathway in tumor cells, upregulate the expression of VEGF in tumor tissues, and promote angiogenesis (Wen et al., 2019). VEGF activates the main signaling pathway in tumor angiogenesis by binding to its cognate receptor, VEGFR (Claesson-Welsh and Welsh, 2013). Mirkeshavarz et al. (2017) found that CAFs can secrete interleukin-6 (IL-6) and VEGF to induce angiogenesis in oral cancer, and that IL-6 can induce the secretion of VEGF in CAF cell lines. CAF can also release active growth factors from the ECM by expressing MMPs, which indirectly promotes angiogenesis (Najafi et al., 2019b) and serves as one of the sources of MMP9 (Boire et al., 2005) and MMP13 (Vosseler et al., 2009). Both these substances have been shown to release VEGF from the ECM to increase angiogenesis in tumors (Lederle et al., 2010).
Cancer-associated fibroblasts interact with tumor cells through inflammatory signals, thereby affecting tumor cell migration and invasion. The CAF-mediated CXCL12/CXCR4 axis plays a key role in tumor cell proliferation, invasion, and migration. The CXCL12/CXCR4 axis can activate the MEK/ERK, PI3K/AKT, and Wnt/β-catenin pathways to promote EMT, thereby promoting tumor invasion and metastasis (Guo et al., 2016; Zhou et al., 2019; Mortezaee, 2020). It also activates the PI3K, MAPK, and ERK1/2 signaling pathways, promotes the secretion of MMPs, reduces the adhesion of tumor cells, and increases their invasion and metastasis ability (Wu and Dai, 2017). In addition, a recent study found that CAF-secreted CXCL-1 can stimulate the migration and invasion of oral cancer cells, that there is an interdependent relationship between CAFs and cancer cells in the oral squamous carcinoma microenvironment, and that CXCL-1 can upregulate MMP-1 in CAF expression and activity (Wei et al., 2019). In addition, CAFs can change the structure and physical properties of the ECM, thereby affecting tumor cell migration and invasion (Egeblad et al., 2010).
The fight against drug resistance remains a major challenge in tumor treatment. CAFs mediate a variety of tumor resistance to chemotherapeutic drugs. CAFs can act on tumor cells by secreting cytokines, activating downstream signaling pathways in tumor cells, and promoting tumor resistance (Chen and Song, 2019). Studies have shown that CAFs can enhance EMT and cisplatin resistance in non-small cell lung cancer induced by transforming growth factor β by releasing high levels of IL-6, while cisplatin, in turn, promotes cancer cells to produce transforming growth factor β, resulting in CAF activation (Figure 1). CAFs can also promote chemotherapy resistance in tumor cells by secreting exosomes. Gemcitabine (GEM) is currently a chemotherapy drug that is commonly used in the treatment of pancreatic cancer. Fang et al. (2019) found that exosomal miR-106b derived from CAFs plays an important role in GEM resistance in pancreatic cancer. Recently, Zhang et al. (2020) showed that exosomal miR-522 secreted by CAFs prevents the death of cancer cells by targeting ALOX15 and blocking the accumulation of lipid-ROS. In addition, a new mechanism for obtaining gastric cancer drug resistance through the intercellular signaling pathways of USP7, hnRNPA1, exo-miR-522, and ALOX15 has been observed.
Direct ablation of CAF can promote the regression of immunogenic tumors (Feig et al., 2013), which has been explored in several recent studies, where these cells are cleared by injection of diphtheria toxin or targeting FAP-specific chimeric antigen receptor T cells; direct ablation of CAF, however, can lead to significant side effects due to lack of specificity, such as cachexia and anemia (Roberts et al., 2013; Tran et al., 2013). Because of the lack of specific markers for CAF, this method is not feasible at present, so the need to know more about the mechanism by which CAF works remains important for the development of more targeted treatments.
In a parallel study, pharmacological stimulation of the VDR was successfully performed in activated pancreatic stellate cells (PSCs). VDR is the main genomic inhibitor that is activated by PSCs. In addition, treatment with the VDR ligand calcipotriol induced matrix remodeling, which can inhibit tumor-related inflammation and fibrosis, and also improves the transport of gemcitabine to the tumor area, thus reversing chemotherapy resistance in the pancreatic ductal adenocarcinoma model (Sherman et al., 2014). Due to the complex interaction between CAF and other cells in the tumor environment, targeting some CAF subsets may cause multiple responses in the TME, which may have multiple effects depending on the individual. To eradicate cancer, the synergistic combination of CAF-targeted therapy and other effective treatments (such as immunotherapy) should also be considered.
Furthermore, the CXCL12/CXCR4 axis activates multiple signaling pathways to promote tumor cell proliferation, invasion, distant metastasis, and inhibit apoptosis. Therefore, the screening of antagonists targeting the CXCL12/CXCR4 signaling pathway is a promising target for tumor therapy. Lecavalier-Barsoum et al. (2018) found that the CXCR4 inhibitor AMD3100 can inhibit the CXCL12/CXCR4 axis in the treatment of patients with advanced disseminated high-grade serous ovarian cancer, and the combination of AMD3100 and low-dose paclitaxel can inhibit the growth of ovarian cancer cells. In osteosarcoma, AMD3100 blocks the invasion and metastasis of osteosarcoma to the lung by inhibiting the JNK and AKT pathways (Liao et al., 2015). Another CXCR4 antagonist, AMD3465, can inhibit the proliferation, colony formation, invasion, and migration of bladder cancer cells through the CXCL12/CXCR4/β-catenin axis (Zhang et al., 2018).
Micro RNA and siRNA can silence gene expression through post-transcriptional regulatory mechanisms, which may be another viable way to inhibit CXCR4 expression. In breast cancer cells, siRNA targeting CXCR4 inhibited the migration of breast cancer cells in vitro (Burger et al., 2011). miR-126 can also inactivate the RhoA signaling pathway in colon cancer by reducing the expression of CXCR4 and inducing a tumor suppressor effect (Yuan et al., 2016). These studies show that miRNA or siRNA targeting CXCR4 is of great significance in tumor treatment research. CTCE-9908 is composed of dimers of CXCL12, which is a competitive inhibitor of CXCL12 targeting CXCR4 and can inhibit the secretion of CXCL12 (Guo et al., 2016). Huang et al. (2009) reported that CTCE-9908 can target the CXCL12/CXCR4 axis and inhibit primary tumor growth and metastasis of breast cancer. Hassan et al. (2011) also found that CTCE-9908 combined with the anti-angiogenic agent DC101 also reduced the volume of the primary tumor and distant metastasis compared with DC101 alone. Moreover, an in vitro experiment proved that CTCE-9908 can inhibit the growth, invasion, and metastasis of prostate cancer (Wong et al., 2014). This evidence supports CTCE-9908 as an efficacious novel agent to prevent and treat the spread of metastatic cancer. At present, cancer treatment methods targeting CAFs and the CXCL12/CXCR4 axis are being explored and developed rapidly.
Tumor-associated macrophages account for a large proportion of most malignant tumors. They promote tumor progression at different levels by promoting genetic instability, cultivating CSCs, supporting metastasis, and taming protective adaptive immunity (Mantovani et al., 2017). TAMs can be divided into M1-like and M2-like types. When macrophages are exposed to cytokines such as bacterial lipopolysaccharide (LPS), microbe-associated molecular patterns (MAMPs), IL12, TNF, interferon-γ (IFNG), or other Toll-like receptor (TLR) agonists, they will be in a pro-inflammatory and anti-tumor state, hence M1-like. When exposed to IL4, IL5, IL10, IL13, CSF1, TFGB1, and prostaglandin E2 (PGE2), it transitions from a pro-inflammatory state to an anti-inflammatory and pro-tumor state, that is, to an M2-like state (Murray et al., 2014). TAMs have a high degree of functional plasticity and can quickly adapt to changing microenvironment (Gubin et al., 2018). The necrotic and anoxic regions of the TME contain M2-like TAMs, with low fluidity, limited antigen presentation ability, and secrete a large number of tumor support factors (Wenes et al., 2016). The metabolic spectrum of TAMs is in a dynamic model, which can change with the nutritional needs of malignant tumor cells and changes in TME. It also has a far-reaching impact on the survival of TAMs, cancer progression, and tumor-targeted immune response.
The most abundant inflammatory or immune cell type is near the CAF-populated areas in the tumor stroma, indicating a close interaction between TAMs and CAF. In prostate cancer, CAF-mediated CXCL12/CXCR4 axis induces the differentiation of monocytes and possibly M1 cells into pro-tumor M2 cells. Conversely, TAMs with the M2 phenotype activate CAFs, thereby promoting tumor malignancy (Augsten et al., 2014; Comito et al., 2014). In vitro co-culture experiments showed that CAF-like BM-MSCs enhanced the invasiveness of TAM-like macrophages. These macrophages strongly stimulate the proliferation and invasion of CAFs, thereby synergistically promoting the development of neuroblastoma (Hashimoto et al., 2016).
Tumor-associated macrophages release TNF-α to increase MMPs secreted by tumor cells and tumor stromal cells, destroy basement membrane tissue, and promote tumor metastasis (Shuman Moss et al., 2012). TAMs also stimulate vascular endothelium to secrete VEGF by synthesizing and secreting the Wnt7b protein to regulate angiogenesis (Yeo et al., 2014). TNF-α binds to tumor necrosis factor receptor 1 (TNFR-1), activates the VEGFC/VEGFR3 pathway, and promotes lymphangiogenesis (Ran and Montgomery, 2012). In addition, transforming (TGF-β) secreted by TAMs can induce EMT of colorectal cancer cells, thereby promoting the invasion and metastasis of colorectal cancer cells (Yang et al., 2019). Notably, exosomes are one of the components in TME, which carry a variety of active substances and are the mediator of information transmission between cells (Sun et al., 2018). The exosomes of tumor cells can stimulate TAMs to secrete cytokines and enhance tumor invasion and metastasis (Trivedi et al., 2016).
Tumor-associated macrophages can promote tumor repair response by coordinating tissue damage and limit the anti-tumor activity of conventional chemotherapy and radiotherapy by providing a protective niche for CSCs (Mantovani et al., 2017). There is increasing evidence that macrophages play a central role in both normal and diseased tissue remodeling, including angiogenesis, basement membrane rupture, leukocyte infiltration, and immunosuppression. Therefore, TAMs have become a promising target for the development of new anticancer treatments. These methods are mainly focused on the depletion of M2-like TAMs and/or promotion of their transformation to M1-like phenotype (Cassetta and Pollard, 2018; Pradel et al., 2018). However, the effectiveness of this method may be limited by a variety of factors, such as alternative immunosuppressive cells that can compensate for TAMs, the existence of innate and acquired drug resistance mechanisms, and the emergence of strong immunosuppression after cessation of treatment (Quail and Joyce, 2017). PLX-3397 is a small-molecule inhibitor of the CSF-1 pathway. It is not only an effective tyrosine kinase inhibitor of CSF-1R, but also targeted at cKit and FLT3. Blocking CSF-1/CSF-1R can reduce TAMS and reprogramming TAMS in the TME and enhance the activation of T cells in the TME by enhancing antigen presentation. The downstream effect blocked by CSF-1/CSF-1R hinders the growth of the tumor (Zhu et al., 2014). In a mouse model of preclinical lung adenocarcinoma, PLX-3397 has been shown to change the distribution of TAMs in the TME and reduce tumor load (Cuccarese et al., 2017). In the syngeneic mouse model of BRAFV600E mutant melanoma, PLX-3397 combined with adoptive cell metastasis immunotherapy showed a decrease in TAMs (Mok et al., 2014). In similar melanoma mouse models, PLX-3397 combined with BRAF inhibitor PLX4032 significantly reduced M2 phenotypic macrophage recruitment, resulting in significant tumor growth inhibition (Ngiow et al., 2016). In addition, recent studies have shown that M2-like TAMs, which seem to be regulators of lysosomal pH, express high levels of vacuolar ATP enzymes and are expected to become a new drug target (Kuchuk et al., 2018; Liu et al., 2019). Targeting TAMs has proven to be a promising strategy, and with the deepening of preclinical development of TAM-targeted drugs and the new progress in the study of TAM mechanism, TAM-targeted therapy will become an important supplement to anticancer drugs.
Myelogenous suppressor cells (MDSCs) are a heterogeneous population composed of bone marrow progenitor cells and immature bone marrow cells (IMCs) (Gabrilovich et al., 2012). Under normal physiological conditions, IMCs produced in the bone marrow can rapidly differentiate into mature granulocytes, macrophages, or dendritic cells. In tumors and other pathological conditions, IMCs cannot normally differentiate into mature bone marrow cells under the action of cytokines, thus forming MDSCs with immunosuppressive functions, including T cell suppression and innate immune regulation (Kumar et al., 2016). In the TME, immunosuppressive cytokines such as IL-10 and TGF-β secreted by MDSCs are important factors that inhibit the anti-tumor immune response and promote tumor progression (Yaseen et al., 2020; Salminen, 2021). Studies have shown that TGF-β can inhibit the cytotoxic activity of cytotoxic T and NK cells by reducing the production of interferon-γ (IFN-γ). On the other hand, TGF-β can also inhibit the proliferation of anti-tumor immune active cells and inhibit anti-tumor immunity from the root (Salminen et al., 2018). Bone marrow mesenchymal stem cells play a role in inducing proliferation in the TME due to the interaction between cytokines and chemokines in the tumor inflammatory environment. Conversely, MDSCs can stimulate angiogenesis by producing matrix metalloproteinase 9, pro-factor 2, and VEGF, which further induces the migration of cancer cells to endothelial cells and promotes the metastasis of cancer cells (Lee et al., 2018; Yang et al., 2020).
Myelogenous suppressor cells produce high levels of inhibitory molecules, such as Arg1, reactive oxygen species (ROS), inducible nitric oxide synthase (iNOS), and prostaglandin E2 (PGE2), to directly inhibit the anti-tumor immune response induced by effector T cells (Kusmartsev et al., 2004; Gabrilovich and Nagaraj, 2009; Condamine et al., 2015; He et al., 2018). MDSCs can also inhibit the immune response by inducing regulatory T cells (Tregs), promoting the development of macrophages into M2 phenotypes, and differentiating into TAMs (Huang et al., 2006; Weber et al., 2018). Deng et al. (2017) found that MDSC-exosomes can directly accelerate the proliferation and metastasis of tumor cells by delivering miR-126a, which indicates that MDSCs have a new regulatory mechanism on tumor cells. MDSC-induced immunosuppression promotes tumor progression by promoting EMT, accelerating immune escape, and enhancing the formation of metastatic lesions (Veglia et al., 2018). Additionally, MDSCs enhance the stemness of tumor cells, promote angiogenesis by secreting IL6 and NO, and promote tumor growth, invasion, and metastasis directly or indirectly by inhibiting T cells or natural killer cells (Condamine et al., 2015, 2016).
The key roles played by MDSCs in the TME show that it is necessary to target them effectively by blocking or deleting them. Although they play a key role in tumor progression, there are no FDA-approved drugs or treatments that directly target MDSCs. At present, clinical trials are underway to target the activities of iNOS Arg1 and STAT3, metabolism through CD36, transport through CXCR2, and other mechanisms for different types of cancer (Fleming et al., 2018). The antisense oligonucleotide STAT3 inhibitor AZD9150 has been used in phase 1b clinical trials of diffuse large B-cell lymphoma in combination with immune checkpoint inhibitors. Systemic administration of AZD9150 significantly decreased granulocyte MDSCs in peripheral blood mononuclear cells (PBMCs) (Reilley et al., 2018). Current targeting strategies mainly include induction of differentiation into mature cells, inhibiting its expansion and recruitment, and blocking its immune characteristics. Studies have shown that some neutralizing antibodies or inhibitors targeting chemokine systems (CXCR4, CXCR2, and CCL2) and tumor-derived factors (CSF1, GM-CSF, and IL-6) can inhibit the expansion or recruitment of MDSC (Bayne et al., 2012; Sumida et al., 2012; Highfill et al., 2014). For example, the chemokine receptor CCR5 plays a key role in the chemotaxis of MDSCs to TME (Weber et al., 2018). However, not all MDSCs express CCR5. In melanoma mice, MDSCs expressing CCR5 have stronger immunosuppressive ability than MDSCs that do not express CCR5. Blocking CCR5 can inhibit the recruitment and immunosuppressive activity of MDSCs and improve the survival rate of melanoma patients (Blattner et al., 2018).
It has been found that some drugs, such as phosphodiesterase-5 inhibitors (sildenafil, cyclooxygenase-2 inhibitors (acetylsalicylic acid and celecoxib), vardenafil and tadalafil and bardoxolone methyl, can directly block the immunosuppressive activity of MDSCs and restore T cell response (Serafini et al., 2006; Nagaraj et al., 2010; Fujita et al., 2011; Obermajer et al., 2011). Recent studies have found that MDSC-specific peptide-Fc fusion protein therapy can completely deplete MDSCs in the blood, spleen, and tumor without affecting other immune cells, and inhibit tumor growth process (Qin et al., 2014), which provides a new idea for inhibiting tumor growth in vivo. In patients and animal models, the failure of anti-angiogenic therapy based on inhibition of the VEGF pathway is often concomitant with an increase in the number of MDSCs or TAMs infiltrating tumor tissues (Lu-Emerson et al., 2013; Gabrusiewicz et al., 2014). Along this line of thinking, anti-VEGF therapy is thought to upregulate alternative angiogenic factors (prokinin-1 and proagonin-2) produced by myeloid cells, which may accidentally produce anti-angiogenic effects and limit tumor recurrence.
Recent studies have shown that the accumulation of MDSCs in tumors limits the effect of anti-programmed death 1 (PD1) in the treatment of rhabdomyosarcoma checkpoint blockage. Inhibition of MDSC metastasis with an anti-CXCR2 antibody can enhance the efficacy of anti-PD1 (Highfill et al., 2014). In a tumor model of tolerant mice, the removal of MDSCs with gemcitabine combined with immunotherapy can effectively break the self-tolerance and induce strong anti-tumor immunity (Ko et al., 2007). Several chemotherapeutic drugs, such as anthracyclines, platinum derivatives, and doxorubicin, can induce immunogenic cell death, thus activating an effective anti-tumor adaptive response (Kroemer et al., 2013). The chemical process for enhancing the anticancer effect of these drugs includes increasing the antigen presentation ability of dendritic cells and the subsequent CD8+ T cell response (Bracci et al., 2014). Although the current targeted therapy targeting only MDSCs does not strengthen clinical outcomes, it may play an important role in anticancer immunotherapy in the future.
Most of the treatments are focused on a certain aspect of the TME. Although some of these therapeutic responses have produced positive results, a more effective way is to promote inflammatory innate immune cells, such as CD8+ T cells, and to alter many aspects of TME through a strong inflammatory response. Breakthrough drug resistance remains a major clinical challenge. The response of tumor cells to treatment depends not only on the regulation of the TME but also on the aberration of its genome. Targeted therapy cannot focus on the complete depletion of all inherent cells in the TME, as this may cause severe complications in the patient. The solution must be a complex combination, with focus on developing multidrug management that targets both tumor cells and TME to overcome resistance and improve prognosis as much as possible.
DC, XN, JL, YH, ZC, BC, JL, and JG: wrote the review article prepared and assembled the figure and table. QD, JA, LL, and QW: critically organized and revised the manuscript by incorporating significant reports. All authors contributed to the article and approved the submitted version.
This work was funded by the National Natural Science Foundation of China (Grant No. 82000721), Post-Doctor Research Project, West China Hospital, Sichuan University (Grant No. 2019HXBH089), Health commission of Sichuan province (Grant No. 20PJ036), and Programs from the Department of Science and Technology of Sichuan Province (Grant No. 2020YJ0054).
The authors declare that the research was conducted in the absence of any commercial or financial relationships that could be construed as a potential conflict of interest.
All claims expressed in this article are solely those of the authors and do not necessarily represent those of their affiliated organizations, or those of the publisher, the editors and the reviewers. Any product that may be evaluated in this article, or claim that may be made by its manufacturer, is not guaranteed or endorsed by the publisher.
Augsten, M., Sjöberg, E., Frings, O., Vorrink, S. U., Frijhoff, J., and Olsson, E. (2014). Borg Å, Östman A: cancer-associated fibroblasts expressing CXCL14 rely upon NOS1-derived nitric oxide signaling for their tumor-supporting properties. Cancer Res. 74, 2999–3010. doi: 10.1158/0008-5472.can-13-2740
Bayne, L. J., Beatty, G. L., Jhala, N., Clark, C. E., Rhim, A. D., Stanger, B. Z., et al. (2012). Tumor-derived granulocyte-macrophage colony-stimulating factor regulates myeloid inflammation and T cell immunity in pancreatic cancer. Cancer Cell 21, 822–835. doi: 10.1016/j.ccr.2012.04.025
Benyahia, Z., Dussault, N., Cayol, M., Sigaud, R., Berenguer-Daizé, C., Delfino, C., et al. (2017). Stromal fibroblasts present in breast carcinomas promote tumor growth and angiogenesis through adrenomedullin secretion. Oncotarget 8, 15744–15762. doi: 10.18632/oncotarget.14999
Blattner, C., Fleming, V., Weber, R., Himmelhan, B., Altevogt, P., Gebhardt, C., et al. (2018). CCR5(+) Myeloid-Derived Suppressor Cells Are Enriched and Activated in Melanoma Lesions. Cancer Res. 78, 157–167. doi: 10.1158/0008-5472.can-17-0348
Boire, A., Covic, L., Agarwal, A., Jacques, S., Sherifi, S., and Kuliopulos, A. (2005). PAR1 is a matrix metalloprotease-1 receptor that promotes invasion and tumorigenesis of breast cancer cells. Cell 120, 303–313. doi: 10.1016/j.cell.2004.12.018
Bonnet, D., and Dick, J. E. (1997). Human acute myeloid leukemia is organized as a hierarchy that originates from a primitive hematopoietic cell. Nat. Med. 3, 730–737. doi: 10.1038/nm0797-730
Borah, A., Raveendran, S., Rochani, A., Maekawa, T., and Kumar, D. S. (2015). Targeting self-renewal pathways in cancer stem cells: clinical implications for cancer therapy. Oncogenesis 4:e177. doi: 10.1038/oncsis.2015.35
Bracci, L., Schiavoni, G., Sistigu, A., and Belardelli, F. (2014). Immune-based mechanisms of cytotoxic chemotherapy: implications for the design of novel and rationale-based combined treatments against cancer. Cell Death Differ. 21, 15–25. doi: 10.1038/cdd.2013.67
Burger, J. A., Stewart, D. J., Wald, O., and Peled, A. (2011). Potential of CXCR4 antagonists for the treatment of metastatic lung cancer. Expert Rev. Anticancer Ther. 11, 621–630. doi: 10.1586/era.11.11
Byrne, A. M., Bouchier-Hayes, D. J., and Harmey, J. H. (2005). Angiogenic and cell survival functions of vascular endothelial growth factor (VEGF). J. Cell. Mol. Med. 9, 777–794. doi: 10.1111/j.1582-4934.2005.tb00379.x
Cassetta, L., and Pollard, J. W. (2018). Targeting macrophages: therapeutic approaches in cancer. Nat. Rev. Drug Discov. 17, 887–904. doi: 10.1038/nrd.2018.169
Chen, X., and Song, E. (2019). Turning foes to friends: targeting cancer-associated fibroblasts. Nat. Rev. Drug Discov. 18, 99–115. doi: 10.1038/s41573-018-0004-1
Claesson-Welsh, L., and Welsh, M. (2013). VEGFA and tumour angiogenesis. J. Intern. Med. 273, 114–127. doi: 10.1111/joim.12019
Comito, G., Giannoni, E., Segura, C. P., Barcellos-de-Souza, P., Raspollini, M. R., Baroni, G., et al. (2014). Cancer-associated fibroblasts and M2-polarized macrophages synergize during prostate carcinoma progression. Oncogene 33, 2423–2431. doi: 10.1038/onc.2013.191
Condamine, T., Dominguez, G. A., Youn, J. I., Kossenkov, A. V., Mony, S., Alicea-Torres, K., et al. (2016). Lectin-type oxidized LDL receptor-1 distinguishes population of human polymorphonuclear myeloid-derived suppressor cells in cancer patients. Sci. Immunol. 1:aaf8943. doi: 10.1126/sciimmunol.aaf8943
Condamine, T., Ramachandran, I., Youn, J. I., and Gabrilovich, D. I. (2015). Regulation of tumor metastasis by myeloid-derived suppressor cells. Annu. Rev. Med. 66, 97–110. doi: 10.1146/annurev-med-051013-052304
Cuccarese, M. F., Dubach, J. M., Pfirschke, C., Engblom, C., Garris, C., Miller, M. A., et al. (2017). Heterogeneity of macrophage infiltration and therapeutic response in lung carcinoma revealed by 3D organ imaging. Nat. Commun. 8:14293.
Cui, T. X., Kryczek, I., Zhao, L., Zhao, E., Kuick, R., Roh, M. H., et al. (2013). Myeloid-derived suppressor cells enhance stemness of cancer cells by inducing microRNA101 and suppressing the corepressor CtBP2. Immunity 39, 611–621. doi: 10.1016/j.immuni.2013.08.025
Del Prete, A., Schioppa, T., Tiberio, L., Stabile, H., and Sozzani, S. (2017). Leukocyte trafficking in tumor microenvironment. Curr. Opin. Pharmacol. 35, 40–47. doi: 10.1016/j.coph.2017.05.004
Deng, Z., Rong, Y., Teng, Y., Zhuang, X., Samykutty, A., Mu, J., et al. (2017). Exosomes miR-126a released from MDSC induced by DOX treatment promotes lung metastasis. Oncogene 36, 639–651. doi: 10.1038/onc.2016.229
Denton, A. E., Roberts, E. W., and Fearon, D. T. (2018). Stromal Cells in the Tumor Microenvironment. Adv. Exp. Med. Biol. 1060, 99–114.
Egeblad, M., Rasch, M. G., and Weaver, V. M. (2010). Dynamic interplay between the collagen scaffold and tumor evolution. Curr. Opin. Cell Biol. 22, 697–706. doi: 10.1016/j.ceb.2010.08.015
Erez, N., Glanz, S., Raz, Y., Avivi, C., and Barshack, I. (2013). Cancer associated fibroblasts express pro-inflammatory factors in human breast and ovarian tumors. Biochem. Biophys. Res. Commun. 437, 397–402. doi: 10.1016/j.bbrc.2013.06.089
Fan, Q. M., Jing, Y. Y., Yu, G. F., Kou, X. R., Ye, F., Gao, L., et al. (2014). Tumor-associated macrophages promote cancer stem cell-like properties via transforming growth factor-beta1-induced epithelial-mesenchymal transition in hepatocellular carcinoma. Cancer Lett. 352, 160–168. doi: 10.1016/j.canlet.2014.05.008
Fang, Y., Zhou, W., Rong, Y., Kuang, T., Xu, X., Wu, W., et al. (2019). Exosomal miRNA-106b from cancer-associated fibroblast promotes gemcitabine resistance in pancreatic cancer. Exp. Cell Res. 383:111543. doi: 10.1016/j.yexcr.2019.111543
Feig, C., Jones, J. O., Kraman, M., Wells, R. J., Deonarine, A., Chan, D. S., et al. (2013). Targeting CXCL12 from FAP-expressing carcinoma-associated fibroblasts synergizes with anti-PD-L1 immunotherapy in pancreatic cancer. Proc. Natl. Acad. Sci. U. S. A. 110, 20212–20217. doi: 10.1073/pnas.1320318110
Fleming, V., Hu, X., Weber, R., Nagibin, V., Groth, C., Altevogt, P., et al. (2018). Targeting Myeloid-Derived Suppressor Cells to Bypass Tumor-Induced Immunosuppression. Front. Immunol. 9:398. doi: 10.3389/fimmu.2018.00398
Fujita, M., Kohanbash, G., Fellows-Mayle, W., Hamilton, R. L., Komohara, Y., Decker, S. A., et al. (2011). COX-2 blockade suppresses gliomagenesis by inhibiting myeloid-derived suppressor cells. Cancer Res. 71, 2664–2674. doi: 10.1158/0008-5472.can-10-3055
Gabrilovich, D. I., and Nagaraj, S. (2009). Myeloid-derived suppressor cells as regulators of the immune system. Nat. Rev. Immunol. 9, 162–174. doi: 10.1038/nri2506
Gabrilovich, D. I., Ostrand-Rosenberg, S., and Bronte, V. (2012). Coordinated regulation of myeloid cells by tumours. Nat. Rev. Immunol. 12, 253–268. doi: 10.1038/nri3175
Gabrusiewicz, K., Liu, D., Cortes-Santiago, N., Hossain, M. B., Conrad, C. A., Aldape, K. D., et al. (2014). Anti-vascular endothelial growth factor therapy-induced glioma invasion is associated with accumulation of Tie2-expressing monocytes. Oncotarget 5, 2208–2220. doi: 10.18632/oncotarget.1893
Gubin, M. M., Esaulova, E., Ward, J. P., Malkova, O. N., Runci, D., Wong, P., et al. (2018). High-Dimensional Analysis Delineates Myeloid and Lymphoid Compartment Remodeling during Successful Immune-Checkpoint Cancer Therapy. Cell 175, 1014–1030.e19.
Guo, F., Wang, Y., Liu, J., Mok, S. C., Xue, F., and Zhang, W. (2016). CXCL12/CXCR4: a symbiotic bridge linking cancer cells and their stromal neighbors in oncogenic communication networks. Oncogene 35, 816–826. doi: 10.1038/onc.2015.139
Hashimoto, O., Yoshida, M., Koma, Y., Yanai, T., Hasegawa, D., Kosaka, Y., et al. (2016). Collaboration of cancer-associated fibroblasts and tumour-associated macrophages for neuroblastoma development. J. Pathol. 240, 211–223. doi: 10.1002/path.4769
Hassan, S., Buchanan, M., Jahan, K., Aguilar-Mahecha, A., Gaboury, L., Muller, W. J., et al. (2011). CXCR4 peptide antagonist inhibits primary breast tumor growth, metastasis and enhances the efficacy of anti-VEGF treatment or docetaxel in a transgenic mouse model. Int. J. Cancer 129, 225–232. doi: 10.1002/ijc.25665
He, Y. M., Li, X., Perego, M., Nefedova, Y., Kossenkov, A. V., Jensen, E. A., et al. (2018). Transitory presence of myeloid-derived suppressor cells in neonates is critical for control of inflammation. Nat. Med. 24, 224–231. doi: 10.1038/nm.4467
Highfill, S. L., Cui, Y., Giles, A. J., Smith, J. P., Zhang, H., Morse, E., et al. (2014). Disruption of CXCR2-mediated MDSC tumor trafficking enhances anti-PD1 efficacy. Sci. Transl. Med. 6:237ra267.
Huang, B., Pan, P. Y., Li, Q., Sato, A. I., Levy, D. E., Bromberg, J., et al. (2006). Gr-1+CD115+ immature myeloid suppressor cells mediate the development of tumor-induced T regulatory cells and T-cell anergy in tumor-bearing host. Cancer Res. 66, 1123–1131. doi: 10.1158/0008-5472.can-05-1299
Huang, E. H., Singh, B., Cristofanilli, M., Gelovani, J., Wei, C., Vincent, L., et al. (2009). A CXCR4 antagonist CTCE-9908 inhibits primary tumor growth and metastasis of breast cancer. J. Surg. Res. 155, 231–236. doi: 10.1016/j.jss.2008.06.044
Huang, M., Li, Y., Zhang, H., and Nan, F. (2010). Breast cancer stromal fibroblasts promote the generation of CD44+CD24- cells through SDF-1/CXCR4 interaction. J. Exp. Clin. Cancer Res. 29:80.
Jin, W. (2020). Role of JAK/STAT3 Signaling in the Regulation of Metastasis, the Transition of Cancer Stem Cells, and Chemoresistance of Cancer by Epithelial-Mesenchymal Transition. Cells 9:217. doi: 10.3390/cells9010217
Jinushi, M., Chiba, S., Yoshiyama, H., Masutomi, K., Kinoshita, I., Dosaka-Akita, H., et al. (2011). Tumor-associated macrophages regulate tumorigenicity and anticancer drug responses of cancer stem/initiating cells. Proc. Natl. Acad. Sci. U. S. A. 108, 12425–12430. doi: 10.1073/pnas.1106645108
Kalluri, R. (2016). The biology and function of fibroblasts in cancer. Nat. Rev. Cancer 16, 582–598. doi: 10.1038/nrc.2016.73
Kessenbrock, K., Plaks, V., and Werb, Z. (2010). Matrix metalloproteinases: regulators of the tumor microenvironment. Cell 141, 52–67. doi: 10.1016/j.cell.2010.03.015
Kidd, S., Spaeth, E., Watson, K., Burks, J., Lu, H., Klopp, A., et al. (2012). Origins of the tumor microenvironment: quantitative assessment of adipose-derived and bone marrow-derived stroma. PLoS One 7:e30563. doi: 10.1371/journal.pone.0030563
Kim, J., and Bae, J. S. (2016). Tumor-Associated Macrophages and Neutrophils in Tumor Microenvironment. Mediators Inflamm. 2016:6058147.
Kinugasa, Y., Matsui, T., and Takakura, N. (2014). CD44 expressed on cancer-associated fibroblasts is a functional molecule supporting the stemness and drug resistance of malignant cancer cells in the tumor microenvironment. Stem Cells 32, 145–156. doi: 10.1002/stem.1556
Ko, H. J., Kim, Y. J., Kim, Y. S., Chang, W. S., Ko, S. Y., Chang, S. Y., et al. (2007). A combination of chemoimmunotherapies can efficiently break self-tolerance and induce antitumor immunity in a tolerogenic murine tumor model. Cancer Res. 67, 7477–7486. doi: 10.1158/0008-5472.can-06-4639
Kopp, H. G., Ramos, C. A., and Rafii, S. (2006). Contribution of endothelial progenitors and proangiogenic hematopoietic cells to vascularization of tumor and ischemic tissue. Curr. Opin. Hematol. 13, 175–181. doi: 10.1097/01.moh.0000219664.26528.da
Krishnamurthy, N., and Kurzrock, R. (2018). Targeting the Wnt/beta-catenin pathway in cancer: update on effectors and inhibitors. Cancer Treat. Rev. 62, 50–60. doi: 10.1016/j.ctrv.2017.11.002
Kroemer, G., Galluzzi, L., Kepp, O., and Zitvogel, L. (2013). Immunogenic cell death in cancer therapy. Annu. Rev. Immunol. 31, 51–72.
Kuchuk, O., Tuccitto, A., Citterio, D., Huber, V., Camisaschi, C., Milione, M., et al. (2018). pH regulators to target the tumor immune microenvironment in human hepatocellular carcinoma. Oncoimmunology 7:e1445452. doi: 10.1080/2162402x.2018.1445452
Kumar, V., Patel, S., Tcyganov, E., and Gabrilovich, D. I. (2016). The Nature of Myeloid-Derived Suppressor Cells in the Tumor Microenvironment. Trends Immunol. 37, 208–220.
Kusmartsev, S., Nefedova, Y., Yoder, D., and Gabrilovich, D. I. (2004). Antigen-specific inhibition of CD8+ T cell response by immature myeloid cells in cancer is mediated by reactive oxygen species. J. Immunol. 172, 989–999. doi: 10.4049/jimmunol.172.2.989
Lau, E. Y., Ho, N. P., and Lee, T. K. (2017). Cancer Stem Cells and Their Microenvironment: biology and Therapeutic Implications. Stem Cells Int. 2017:3714190.
Lecavalier-Barsoum, M., Chaudary, N., Han, K., Koritzinsky, M., Hill, R., and Milosevic, M. (2018). Targeting the CXCL12/CXCR4 pathway and myeloid cells to improve radiation treatment of locally advanced cervical cancer. Int. J. Cancer 143, 1017–1028. doi: 10.1002/ijc.31297
Lederle, W., Hartenstein, B., Meides, A., Kunzelmann, H., Werb, Z., Angel, P., et al. (2010). MMP13 as a stromal mediator in controlling persistent angiogenesis in skin carcinoma. Carcinogenesis 31, 1175–1184. doi: 10.1093/carcin/bgp248
Lee, S. E., Lim, J. Y., Kim, T. W., Jeon, Y. W., Yoon, J. H., Cho, B. S., et al. (2018). Matrix Metalloproteinase-9 in Monocytic Myeloid-Derived Suppressor Cells Correlate with Early Infections and Clinical Outcomes in Allogeneic Hematopoietic Stem Cell Transplantation. Biol. Blood Marrow Transplant. 24, 32–42. doi: 10.1016/j.bbmt.2017.08.017
Li, B., and Wang, J. H. (2011). Fibroblasts and myofibroblasts in wound healing: force generation and measurement. J. Tissue Viability 20, 108–120. doi: 10.1016/j.jtv.2009.11.004
Liao, Y. X., Fu, Z. Z., Zhou, C. H., Shan, L. C., Wang, Z. Y., Yin, F., et al. (2015). AMD3100 reduces CXCR4-mediated survival and metastasis of osteosarcoma by inhibiting JNK and Akt, but not p38 or Erk1/2, pathways in in vitro and mouse experiments. Oncol. Rep. 34, 33–42. doi: 10.3892/or.2015.3992
Liu, N., Luo, J., Kuang, D., Xu, S., Duan, Y., Xia, Y., et al. (2019). Lactate inhibits ATP6V0d2 expression in tumor-associated macrophages to promote HIF-2α-mediated tumor progression. J. Clin. Invest. 129, 631–646. doi: 10.1172/jci123027
Lu-Emerson, C., Snuderl, M., Kirkpatrick, N. D., Goveia, J., Davidson, C., Huang, Y., et al. (2013). Increase in tumor-associated macrophages after antiangiogenic therapy is associated with poor survival among patients with recurrent glioblastoma. Neuro Oncol. 15, 1079–1087. doi: 10.1093/neuonc/not082
Magee, J. A., Piskounova, E., and Morrison, S. J. (2012). Cancer stem cells: impact, heterogeneity, and uncertainty. Cancer Cell 21, 283–296. doi: 10.1016/j.ccr.2012.03.003
Mantovani, A., Marchesi, F., Malesci, A., Laghi, L., and Allavena, P. (2017). Tumour-associated macrophages as treatment targets in oncology. Nat. Rev. Clin. Oncol. 14, 399–416. doi: 10.1038/nrclinonc.2016.217
Mirkeshavarz, M., Ganjibakhsh, M., Aminishakib, P., Farzaneh, P., Mahdavi, N., Vakhshiteh, F., et al. (2017). Interleukin-6 secreted by oral cancer- associated fibroblast accelerated VEGF expression in tumor and stroma cells. Cell. Mol. Biol. 63, 131–136. doi: 10.14715/cmb/2017.63.10.21
Mok, S., Koya, R. C., Tsui, C., Xu, J., Robert, L., Wu, L., et al. (2014). Inhibition of CSF-1 receptor improves the antitumor efficacy of adoptive cell transfer immunotherapy. Cancer Res. 74, 153–161. doi: 10.1158/0008-5472.can-13-1816
Mortezaee, K. (2020). CXCL12/CXCR4 axis in the microenvironment of solid tumors: a critical mediator of metastasis. Life Sci. 249:117534. doi: 10.1016/j.lfs.2020.117534
Mou, W., Xu, Y., Ye, Y., Chen, S., Li, X., Gong, K., et al. (2015). Expression of Sox2 in breast cancer cells promotes the recruitment of M2 macrophages to tumor microenvironment. Cancer Lett. 358, 115–123. doi: 10.1016/j.canlet.2014.11.004
Murray, P. J., Allen, J. E., Biswas, S. K., Fisher, E. A., Gilroy, D. W., Goerdt, S., et al. (2014). Macrophage activation and polarization: nomenclature and experimental guidelines. Immunity 41, 14–20. doi: 10.1016/j.immuni.2014.06.008
Nagaraj, S., Youn, J. I., Weber, H., Iclozan, C., Lu, L., Cotter, M. J., et al. (2010). Anti-inflammatory triterpenoid blocks immune suppressive function of MDSCs and improves immune response in cancer. Clin. Cancer Res. 16, 1812–1823. doi: 10.1158/1078-0432.ccr-09-3272
Najafi, M., Farhood, B., and Mortezaee, K. (2019a). Cancer stem cells (CSCs) in cancer progression and therapy. J. Cell. Physiol 234, 8381–8395. doi: 10.1002/jcp.27740
Najafi, M., Farhood, B., and Mortezaee, K. (2019b). Extracellular matrix (ECM) stiffness and degradation as cancer drivers. J. Cell. Biochem. 120, 2782–2790. doi: 10.1002/jcb.27681
Ngiow, S. F., Meeth, K. M., Stannard, K., Barkauskas, D. S., Bollag, G., Bosenberg, M., et al. (2016). Co-inhibition of colony stimulating factor-1 receptor and BRAF oncogene in mouse models of BRAF(V600E) melanoma. Oncoimmunology 5:e1089381. doi: 10.1080/2162402x.2015.1089381
Obermajer, N., Muthuswamy, R., Odunsi, K., Edwards, R. P., and Kalinski, P. (2011). PGE(2)-induced CXCL12 production and CXCR4 expression controls the accumulation of human MDSCs in ovarian cancer environment. Cancer Res. 71, 7463–7470. doi: 10.1158/0008-5472.can-11-2449
Orimo, A., Gupta, P. B., Sgroi, D. C., Arenzana-Seisdedos, F., Delaunay, T., Naeem, R., et al. (2005). Stromal fibroblasts present in invasive human breast carcinomas promote tumor growth and angiogenesis through elevated SDF-1/CXCL12 secretion. Cell 121, 335–348. doi: 10.1016/j.cell.2005.02.034
Paraiso, K. H., and Smalley, K. S. (2013). Fibroblast-mediated drug resistance in cancer. Biochem. Pharmacol. 85, 1033–1041. doi: 10.1016/j.bcp.2013.01.018
Peng, D., Tanikawa, T., Li, W., Zhao, L., Vatan, L., Szeliga, W., et al. (2016). Myeloid-Derived Suppressor Cells Endow Stem-like Qualities to Breast Cancer Cells through IL6/STAT3 and NO/NOTCH Cross-talk Signaling. Cancer Res. 76, 3156–3165. doi: 10.1158/0008-5472.can-15-2528
Piccard, H., Muschel, R. J., and Opdenakker, G. (2012). On the dual roles and polarized phenotypes of neutrophils in tumor development and progression. Crit. Rev. Oncol. Hematol. 82, 296–309. doi: 10.1016/j.critrevonc.2011.06.004
Pradel, L. P., Franke, A., and Ries, C. H. (2018). Effects of IL-10 and T(h) 2 cytokines on human Mφ phenotype and response to CSF1R inhibitor. J. Leukoc. Biol. 103, 545–558. doi: 10.1002/jlb.5ma0717-282r
Qin, H., Lerman, B., Sakamaki, I., Wei, G., Cha, S. C., Rao, S. S., et al. (2014). Generation of a new therapeutic peptide that depletes myeloid-derived suppressor cells in tumor-bearing mice. Nat. Med. 20, 676–681. doi: 10.1038/nm.3560
Quail, D. F., and Joyce, J. A. (2017). Molecular Pathways: deciphering Mechanisms of Resistance to Macrophage-Targeted Therapies. Clin. Cancer Res. 23, 876–884. doi: 10.1158/1078-0432.ccr-16-0133
Rafii, S., Lyden, D., Benezra, R., Hattori, K., and Heissig, B. (2002). Vascular and haematopoietic stem cells: novel targets for anti-angiogenesis therapy? Nat. Rev. Cancer 2, 826–835. doi: 10.1038/nrc925
Ran, S., and Montgomery, K. E. (2012). Macrophage-mediated lymphangiogenesis: the emerging role of macrophages as lymphatic endothelial progenitors. Cancers 4, 618–657. doi: 10.3390/cancers4030618
Räsänen, K., and Vaheri, A. (2010). Activation of fibroblasts in cancer stroma. Exp. Cell Res. 316, 2713–2722. doi: 10.1016/j.yexcr.2010.04.032
Reilley, M. J., McCoon, P., Cook, C., Lyne, P., Kurzrock, R., Kim, Y., et al. (2018). STAT3 antisense oligonucleotide AZD9150 in a subset of patients with heavily pretreated lymphoma: results of a phase 1b trial. J. Immunother. Cancer 6:119.
Roberts, E. W., Deonarine, A., Jones, J. O., Denton, A. E., Feig, C., Lyons, S. K., et al. (2013). Depletion of stromal cells expressing fibroblast activation protein-α from skeletal muscle and bone marrow results in cachexia and anemia. J. Exp. Med. 210, 1137–1151. doi: 10.1084/jem.20122344
Salimifard, S., Masjedi, A., Hojjat-Farsangi, M., Ghalamfarsa, G., Irandoust, M., Azizi, G., et al. (2020). Cancer associated fibroblasts as novel promising therapeutic targets in breast cancer. Pathol. Res. Pract. 216:152915. doi: 10.1016/j.prp.2020.152915
Salminen, A. (2021). Increased immunosuppression impairs tissue homeostasis with aging and age-related diseases. J. Mol. Med. 99, 1–20. doi: 10.1007/s00109-020-01988-7
Salminen, A., Kauppinen, A., and Kaarniranta, K. (2018). Myeloid-derived suppressor cells (MDSC): an important partner in cellular/tissue senescence. Biogerontology 19, 325–339. doi: 10.1007/s10522-018-9762-8
Seino, T., Kawasaki, S., Shimokawa, M., Tamagawa, H., Toshimitsu, K., Fujii, M., et al. (2018). Human Pancreatic Tumor Organoids Reveal Loss of Stem Cell Niche Factor Dependence during Disease Progression. Cell Stem Cell 22, 454–467.e6.
Serafini, P., Meckel, K., Kelso, M., Noonan, K., Califano, J., Koch, W., et al. (2006). Phosphodiesterase-5 inhibition augments endogenous antitumor immunity by reducing myeloid-derived suppressor cell function. J. Exp. Med. 203, 2691–2702. doi: 10.1084/jem.20061104
Sherman, M. H., Yu, R. T., Engle, D. D., Ding, N., Atkins, A. R., Tiriac, H., et al. (2014). Vitamin D receptor-mediated stromal reprogramming suppresses pancreatitis and enhances pancreatic cancer therapy. Cell 159, 80–93. doi: 10.1016/j.cell.2014.08.007
Shuman Moss, L. A., Jensen-Taubman, S., and Stetler-Stevenson, W. G. (2012). Matrix metalloproteinases: changing roles in tumor progression and metastasis. Am. J. Pathol. 181, 1895–1899.
Straussman, R., Morikawa, T., Shee, K., Barzily-Rokni, M., Qian, Z. R., Du, J., et al. (2012). Tumour micro-environment elicits innate resistance to RAF inhibitors through HGF secretion. Nature 487, 500–504. doi: 10.1038/nature11183
Sumida, K., Wakita, D., Narita, Y., Masuko, K., Terada, S., Watanabe, K., et al. (2012). Anti-IL-6 receptor mAb eliminates myeloid-derived suppressor cells and inhibits tumor growth by enhancing T-cell responses. Eur. J. Immunol. 42, 2060–2072. doi: 10.1002/eji.201142335
Sun, W., Luo, J. D., Jiang, H., and Duan, D. D. (2018). Tumor exosomes: a double-edged sword in cancer therapy. Acta Pharmacol. Sin. 39, 534–541. doi: 10.1038/aps.2018.17
Tran, E., Chinnasamy, D., Yu, Z., Morgan, R. A., Lee, C. C., Restifo, N. P., et al. (2013). Immune targeting of fibroblast activation protein triggers recognition of multipotent bone marrow stromal cells and cachexia. J. Exp. Med. 210, 1125–1135. doi: 10.1084/jem.20130110
Trivedi, M., Talekar, M., Shah, P., Ouyang, Q., and Amiji, M. (2016). Modification of tumor cell exosome content by transfection with wt-p53 and microRNA-125b expressing plasmid DNA and its effect on macrophage polarization. Oncogenesis 5:e250. doi: 10.1038/oncsis.2016.52
Tu, E., Chia, P. Z., and Chen, W. (2014). TGFβ in T cell biology and tumor immunity: angel or devil? Cytokine Growth Factor Rev. 25, 423–435. doi: 10.1016/j.cytogfr.2014.07.014
Veglia, F., Perego, M., and Gabrilovich, D. (2018). Myeloid-derived suppressor cells coming of age. Nat. Immunol. 19, 108–119. doi: 10.1038/s41590-017-0022-x
Vosseler, S., Lederle, W., Airola, K., Obermueller, E., Fusenig, N. E., and Mueller, M. M. (2009). Distinct progression-associated expression of tumor and stromal MMPs in HaCaT skin SCCs correlates with onset of invasion. Int. J. Cancer 125, 2296–2306. doi: 10.1002/ijc.24589
Weber, R., Fleming, V., Hu, X., Nagibin, V., Groth, C., Altevogt, P., et al. (2018). Myeloid-Derived Suppressor Cells Hinder the Anti-Cancer Activity of Immune Checkpoint Inhibitors. Front. Immunol. 9:1310. doi: 10.3389/fimmu.2018.01310
Wei, L. Y., Lee, J. J., Yeh, C. Y., Yang, C. J., Kok, S. H., Ko, J. Y., et al. (2019). Reciprocal activation of cancer-associated fibroblasts and oral squamous carcinoma cells through CXCL1. Oral Oncol. 88, 115–123. doi: 10.1016/j.oraloncology.2018.11.002
Wen, N., Guo, B., Zheng, H., Xu, L., Liang, H., Wang, Q., et al. (2019). Bromodomain inhibitor jq1 induces cell cycle arrest and apoptosis of glioma stem cells through the VEGF/PI3K/AKT signaling pathway. Int. J. Oncol. 55, 879–895.
Wenes, M., Shang, M., Di Matteo, M., Goveia, J., Martín-Pérez, R., Serneels, J., et al. (2016). Macrophage Metabolism Controls Tumor Blood Vessel Morphogenesis and Metastasis. Cell Metab. 24, 701–715. doi: 10.1016/j.cmet.2016.09.008
Wong, D., Kandagatla, P., Korz, W., and Chinni, S. R. (2014). Targeting CXCR4 with CTCE-9908 inhibits prostate tumor metastasis. BMC Urol. 14:12. doi: 10.1186/1471-2490-14-12
Wu, T., and Dai, Y. (2017). Tumor microenvironment and therapeutic response. Cancer Lett. 387, 61–68. doi: 10.1016/j.canlet.2016.01.043
Xiong, S., Wang, R., Chen, Q., Luo, J., Wang, J., Zhao, Z., et al. (2018). Cancer-associated fibroblasts promote stem cell-like properties of hepatocellular carcinoma cells through IL-6/STAT3/Notch signaling. Am. J. Cancer Res. 8, 302–316.
Yang, C., Wei, C., Wang, S., Shi, D., Zhang, C., Lin, X., et al. (2019). Elevated CD163(+)/CD68(+) Ratio at Tumor Invasive Front is Closely Associated with Aggressive Phenotype and Poor Prognosis in Colorectal Cancer. Int. J. Biol. Sci. 15, 984–998. doi: 10.7150/ijbs.29836
Yang, Z., Guo, J., Cui, K., Du, Y., Zhao, H., Zhu, L., et al. (2020). Thymosin alpha-1 blocks the accumulation of myeloid suppressor cells in NSCLC by inhibiting VEGF production. Biomed. Pharmacother. 131:110740. doi: 10.1016/j.biopha.2020.110740
Yaseen, M. M., Abuharfeil, N. M., Darmani, H., and Daoud, A. (2020). Mechanisms of immune suppression by myeloid-derived suppressor cells: the role of interleukin-10 as a key immunoregulatory cytokine. Open Biol. 10:200111. doi: 10.1098/rsob.200111
Yeo, E. J., Cassetta, L., Qian, B. Z., Lewkowich, I., Li, J. F., Stefater, J. A. III, et al. (2014). Myeloid WNT7b mediates the angiogenic switch and metastasis in breast cancer. Cancer Res. 74, 2962–2973. doi: 10.1158/0008-5472.can-13-2421
Yuan, W., Guo, Y. Q., Li, X. Y., Deng, M. Z., Shen, Z. H., Bo, C. B., et al. (2016). MicroRNA-126 inhibits colon cancer cell proliferation and invasion by targeting the chemokine (C-X-C motif) receptor 4 and Ras homolog gene family, member A, signaling pathway. Oncotarget 7, 60230–60244. doi: 10.18632/oncotarget.11176
Zeng, J., Liu, Z., Sun, S., Xie, J., Cao, L., Lv, P., et al. (2018). Tumor-associated macrophages recruited by periostin in intrahepatic cholangiocarcinoma stem cells. Oncol. Lett. 15, 8681–8686.
Zhang, H., Deng, T., Liu, R., Ning, T., Yang, H., Liu, D., et al. (2020). CAF secreted miR-522 suppresses ferroptosis and promotes acquired chemo-resistance in gastric cancer. Mol. Cancer 19:43.
Zhang, T., Yang, F., Li, W., Liu, B., Li, W., Chen, Z., et al. (2018). Suppression of the SDF-1/CXCR4/β−catenin axis contributes to bladder cancer cell growth inhibition in vitro and in vivo. Oncol. Rep. 40, 1666–1674.
Zhou, W., Guo, S., Liu, M., Burow, M. E., and Wang, G. (2019). Targeting CXCL12/CXCR4 Axis in Tumor Immunotherapy. Curr. Med. Chem. 26, 3026–3041. doi: 10.2174/0929867324666170830111531
Zhou, Z., Zhou, Q., Wu, X., Xu, S., Hu, X., Tao, X., et al. (2020). VCAM-1 secreted from cancer-associated fibroblasts enhances the growth and invasion of lung cancer cells through AKT and MAPK signaling. Cancer Lett. 473, 62–73. doi: 10.1016/j.canlet.2019.12.039
Zhu, Y., Knolhoff, B. L., Meyer, M. A., Nywening, T. M., West, B. L., Luo, J., et al. (2014). CSF1/CSF1R blockade reprograms tumor-infiltrating macrophages and improves response to T-cell checkpoint immunotherapy in pancreatic cancer models. Cancer Res. 74, 5057–5069. doi: 10.1158/0008-5472.can-13-3723
Keywords: tumor microenvironment, cancer-associated fibroblasts, tumor-associated macrophages, drug therapy, targeted therapy
Citation: Cao D, Naiyila X, Li J, Huang Y, Chen Z, Chen B, Li J, Guo J, Dong Q, Ai J, Yang L, Liu L and Wei Q (2021) Potential Strategies to Improve the Effectiveness of Drug Therapy by Changing Factors Related to Tumor Microenvironment. Front. Cell Dev. Biol. 9:705280. doi: 10.3389/fcell.2021.705280
Received: 05 May 2021; Accepted: 13 July 2021;
Published: 10 August 2021.
Edited by:
Kevin J. Ni, St George Hospital, AustraliaReviewed by:
Tianyi Liu, University of California, San Francisco, United StatesCopyright © 2021 Cao, Naiyila, Li, Huang, Chen, Chen, Li, Guo, Dong, Ai, Yang, Liu and Wei. This is an open-access article distributed under the terms of the Creative Commons Attribution License (CC BY). The use, distribution or reproduction in other forums is permitted, provided the original author(s) and the copyright owner(s) are credited and that the original publication in this journal is cited, in accordance with accepted academic practice. No use, distribution or reproduction is permitted which does not comply with these terms.
*Correspondence: Liangren Liu, bGl1bGlhbmdyZW5Ac2N1LmVkdS5jbg==; Qiang Wei, d2VpcWlhbmczMzlAMTI2LmNvbQ==
†These authors have contributed equally to this work
Disclaimer: All claims expressed in this article are solely those of the authors and do not necessarily represent those of their affiliated organizations, or those of the publisher, the editors and the reviewers. Any product that may be evaluated in this article or claim that may be made by its manufacturer is not guaranteed or endorsed by the publisher.
Research integrity at Frontiers
Learn more about the work of our research integrity team to safeguard the quality of each article we publish.