- 1Key Laboratory of Zoonosis Research, Ministry of Education, College of Veterinary Medicine, Jilin University, Changchun, China
- 2Department of Parasite, Jilin Academy of Animal Husbandry and Veterinary Medicine, Changchun, China
Giardia duodenalis, also known as Giardia lamblia or Giardia intestinalis, is an important opportunistic, pathogenic, zoonotic, protozoan parasite that infects the small intestines of humans and animals, causing giardiasis. Several studies have demonstrated that innate immunity-associated Toll-like receptors (TLRs) are critical for the elimination of G. duodenalis; however, whether TLR9 has a role in innate immune responses against Giardia infection remains unknown. In the present study, various methods, including reverse transcriptase–quantitative polymerase chain reaction, Western blot, enzyme-linked immunosorbent assay, immunofluorescence, inhibitor assays, and small-interfering RNA interference, were utilized to probe the role of TLR9 in mouse macrophage-mediated defenses against G. lamblia virus (GLV)–free or GLV-containing Giardia trophozoites. The results revealed that in G. duodenalis–stimulated mouse macrophages, the secretion of proinflammatory cytokines, including interleukin 6 (IL-6), tumor necrosis factor α (TNF-α), and IL-12 p40, was enhanced, concomitant with the significant activation of TLR9, whereas silencing TLR9 attenuated the host inflammatory response. Notably, the presence of GLV exacerbated the secretion of host proinflammatory cytokines. Moreover, G. duodenalis stimulation activated multiple signaling pathways, including the nuclear factor κB p65 (NF-κB p65), p38, ERK, and AKT pathways, the latter three in a TLR9-dependent manner. Additionally, inhibiting the p38 or ERK pathway downregulated the G. duodenalis–induced inflammatory response, whereas AKT inhibition aggravated this process. Taken together, these results indicated that G. duodenalis may induce the secretion of proinflammatory cytokines by activating the p38 and ERK signaling pathways in a TLR9-dependent manner in mouse macrophages. Our in vitro findings on the mechanism underlying the TLR9-mediated host inflammatory response may help establish the foundation for an in-depth investigation of the role of TLR9 in the pathogenicity of G. duodenalis.
Introduction
Giardia duodenalis, also known as Giardia lamblia and Giardia intestinalis, is an opportunist protozoan parasite that predominantly parasitizes the duodenum of humans, as well as of numerous domestic and wild animals, causing giardiasis. The disease is distinguished by a broad spectrum of clinical manifestations ranging from asymptomatic infection to vomiting, abdominal pain, weight loss, severe diarrhea, and malabsorption syndrome. Giardiasis is one of the most common pathogenic parasite infections in humans, with approximately 280 million cases of symptomatic giardiasis being reported annually worldwide (Einarsson et al., 2016). Giardiasis has been included in the World Health Organization’s neglected disease initiative since 2004 (Savioli et al., 2006), owing to its severe impact on children, which includes severe malnutrition, physical retardation, and poor cognitive function (Berkman et al., 2002). In addition, giardiasis has been reported to the Centers for Disease Control and Prevention (CDC) of the United States since 1992 and became a nationally notifiable disease in 2002 (Coffey et al., 2021). According to the CDC, because of poverty, poor drinking water quality, and limited treatment options, nearly 33% of the population in developing countries is afflicted with giardiasis, as is 2% of the adult population in the developed world (Kunz et al., 2017). Giardia infection has clearly become a non-negligible problem, attracting widespread research attention from an increasing number of scientists.
Giardia lamblia virus (GLV), first identified in 1986 (Wang and Wang, 1986), is a double-stranded RNA virus in the family Totiviridae that specifically infects trophozoites of G. duodenalis (Lagunas-Rangel et al., 2021). GLV is the only one of the known protozoal dsRNA viruses that can transmit efficiently by extracellular means, although the pathway involved is not well outlined (Wang and Wang, 1986; Miller et al., 1988). Similarly, several other protozoan parasites, including Leishmania, Trichomonas, and Cryptosporidium, also harbor small dsRNA viruses, and several studies have reported that these viruses can enhance the pathogenicity of their respective protozoa and thus exacerbate the disease (Wang et al., 1987; Jenkins et al., 2008; Ives et al., 2011; Fichorova et al., 2012, 2013). Although an early review found no significant correlation between GLV and Giardia virulence (Wang and Wang, 1991), it is currently unclear whether GLV affects Giardia infectivity.
Innate immunity and adaptive immunity, two main components of the immune system, are crucial for the eradication of Giardia infection (Fink and Singer, 2017). Innate immunity acts as the first line of defense against infections by pathogenic microorganisms. The mammalian innate immune system mainly serves to recognize pathogen-associated molecular patterns (PAMPs) found in viruses, bacteria, fungi, and parasites but absent in mammalian cells, through corresponding pattern recognition receptors (PRRs) (Vadillo and Pelayo, 2012), which, in turn, initiate the relevant immune responses. Toll-like receptors (TLRs) are an ancient family of innate immune receptors and play a significant role in resisting parasite infections (Gay and Gangloff, 2007). When TLRs recognize parasite-derived PAMPs, they can activate downstream regulatory factors, such as nuclear factor κB (NF-κB) and interferon regulatory factor (IRF), which regulate immune-related signal transduction and induce the transcription and expression of proinflammatory factors, interferons, and chemokines, among other factors. They can also initiate innate and adaptive immune responses targeting parasite infection through the regulation of antigen-presenting cells (Takeda and Akira, 2004).
TLR9 is known to specifically recognize unmethylated cytosine–phosphate–guanine (CpG) motifs present in bacterial and viral DNA, thereby eliciting innate immune responses (Kumagai et al., 2008; Yasuda et al., 2009; Wu and Kuo, 2015), whereas TLR3, which also recognizes nucleic acids, chiefly recognizes the dsRNA genome from viruses (Vercammen et al., 2008; Tatematsu et al., 2014). Additionally, CpG motifs in parasites such as Leishmania have been found to act as ligands for TLR9 (Gupta et al., 2015), and dsRNA genome of Leishmaniavirus can be recognized by the host’s TLR3 (Ives et al., 2011), whereas Leishmania parasites that do not carry Leishmaniavirus cannot express dsRNA for TLR3 recognition (Franco et al., 2017). TLR9 activation has been shown to promote a host-protective response in Leishmania-infected mouse macrophages (Srivastava et al., 2013). Ligand recognition by TLR9 results in the activation of the mitogen-activated protein kinase (MAPK), AKT, and NF-κB signaling pathways (Xu et al., 2003; Das et al., 2015), which promotes the secretion of proinflammatory cytokines (Gazzinelli and Denkers, 2006; Kawai and Akira, 2009). Macrophages are a key component of the innate immune system, and many PRRs, such as TLRs, localize to either the cell membrane (TLR1, TLR2, TLR4, TLR5, and TLR6) or endosomes (TLR3, TLR4, TLR7, TLR8, and TLR9) of these cells (Park et al., 2011; Cui et al., 2014; Liu et al., 2017). Activated macrophages can produce a wide array of cytokines, including interleukin 6 (IL-6), tumor necrosis factor α (TNF-α), and IL-12 p40, which initiate the inflammatory response (Sodhi and Pandey, 2011). Furthermore, it has been demonstrated that mouse peritoneal macrophages (PMφs) infected with G. duodenalis secrete large amounts of these proinflammatory cytokines (Li et al., 2017). However, whether TLR9 plays a role in G. duodenalis–induced host macrophage-mediated inflammation and cytokine secretion, whether it plays a protective part in promoting host cytokine secretion or exacerbates disease progression or whether TLR9 has differential roles in host macrophages infected with GLV-free Giardia trophozoites and those infected with GLV-containing Giardia trophozoites remains unknown.
In the present study, we undertook an in vitro analysis of the role of TLR9 in the inflammatory response of mouse macrophages mediated by GLV-free and GLV-containing Giardia trophozoites and sought to identify the signaling pathways involved in this process. We found that, compared with GLV-free Giardia trophozoites, those containing GLV induced a stronger inflammatory response in mouse macrophages. We further found that TLR9 was significantly activated in mouse macrophages with G. duodenalis stimulation, an effect that involved proinflammatory cytokine production mediated by the TLR9–p38/ERK signaling pathways. These findings may establish the foundation for further research on the role of TLR9 in the pathogenicity of G. duodenalis.
Materials and Methods
Cultivation of G. duodenalis Trophozoites and Separation of Mouse PMφs
Giardia lamblia virus-free Giardia trophozoites were derived from the G. duodenalis WB strain (ATCC30957; American Type Culture Collection, Manassas, VA, United States), and GLV-containing Giardia trophozoites were derived from G. duodenalis Assemblage A1 preserved in the parasite laboratory of College of Veterinary Medicine, Jilin University (Gong et al., 2020). GLV-free and GLV-containing Giardia trophozoites were both cultivated for 48 h in modified TYI-S-33 medium. To enrich macrophages, wild-type (WT) female C57BL/6 mice (6–8 weeks old) were intraperitoneally injected with 3 mL of sterile 2.98% Difo fluid thioglycolate medium (Becton, Dickinson and Company, Sparks, MD, United States) (Murphy et al., 2017). Four days later, the mice were euthanized by an overdose of diethyl ether and then sterilized by immersion in 75% ethanol for 10 min (Li et al., 2017). The mouse peritoneal cavity was rinsed twice with 16 mL of sterile phosphate-buffered saline (PBS; pH 7.4), and separated PMφs were collected by centrifugation at 1,000 × g for 10 min and then washed twice with 16 mL of sterile PBS. A total of 3 × 106 cells were incubated per well of 6-well culture plates (JET BIOFIL, Guangzhou, China) in 1 mL of RPMI 1640 medium supplemented with 10% fetal bovine serum (FBS), 2 mM L-glutamine, 100 U/mL penicillin, and 100 mg/mL streptomycin at 37°C with 5% CO2. Unattached cells were removed, and fresh medium was added before stimulation. All animal experiments were performed strictly according to the Regulations for the Administration of Affairs Concerning Experimental Animals approved through the State Council of the People’s Republic of China (1988.11.1) and with the approval of the Animal Welfare and Research Ethics Committee at Jilin University (IACUC permit no. 20160612). The C57BL/6 mice were purchased from Changsheng Experimental Animal Centre (Anshan, China) and were housed in filter-top cages in an air-conditioned animal facility in the National Experimental Teaching Demonstration Center of Jilin University (Changchun, China). Water and normal mouse food were provided ad libitum.
Extraction of Genomic DNA (gDNA) From G. duodenalis Trophozoites
Giardia gDNA was extracted from GLV-free Giardia trophozoites using the TIANamp Genomic DNA Kit (Tiangen, Beijing, China). GLV-free Giardia trophozoites were collected by centrifugation at 1,000 × g for 10 min and washed three times with sterile PBS. GLV-free Giardia trophozoites (1 × 106 per sample) were digested with proteinase K for 1 h, following which gDNA was extracted according to the manufacturer’s instructions. The concentration of gDNA was measured by UV absorbance at 260 nm using a Nanodrop (Thermo Fisher Scientific, Waltham, MA, United States).
Analysis of TLR9 Gene Expression by Reverse Transcriptase-Quantitative Polymerase Chain Reaction
A total of 3 × 106 WT PMφs were stimulated with 1 × 106 G. duodenalis trophozoites for different times (2, 4, 6, 8, and 12 h). Unstimulated PMφs served as a blank control, whereas PMφs stimulated with 5 μM/mL CpG ODN 1668 (a murine TLR9 ligand; InvivoGen, San Diego, CA, United States) served as a positive control. Subsequently, 3 × 106 WT macrophages were stimulated with Giardia gDNA (3 μg of gDNA extracted from 1 × 106 GLV-free Giardia trophozoites), 1 × 106 GLV-free Giardia trophozoites, 1 × 106 GLV-containing Giardia trophozoites, or 5 μM/mL CpG ODN 1668 in 1 mL of RPMI 1640 medium containing 1% FBS, 2 mM L-glutamine, 100 U/mL penicillin, and 100 mg/mL streptomycin at 37°C with 5% CO2. After treatment, the supernatants of the cell cultures were discarded, and total RNA was extracted from mouse macrophages using TRIzol reagent (Invitrogen, Carlsbad, CA, United States). Total RNA was reverse-transcribed into cDNA with oligo(dT) primers using the PrimeScript 1st Strand cDNA Synthesis Kit (Takara, Dalian, China). The TLR9 mRNA level was normalized to that of β-actin. For polymerase chain reaction (PCR) amplification, the conditions were as follows: 95°C for 3 min, followed by 44 cycles of 95°C for 30 s, 56°C for 30 s, and 72°C for 30 s, with a final extension at 72°C for 10 min. Melting curves were analyzed following the PCR run to validate the homogeneity of PCR products. All the primers were synthesized by Sangon (Shanghai, China) and contained the following sequences: TLR9, 5′-CTGCCCAAACTCCACACTCT-3′ forward primer and 5′-ACAAGTCCACAAAGCGAAGG-3′ reverse primer (Zhao et al., 2021); and β-actin, 5′-TGCTGTCCCTGTATGCCTCT-3′ forward primer and 5′-GGTCTTTACGGATGTCAACG-3′ reverse primer (Li et al., 2017).
SiRNA-Mediated Knockdown Assay
The TLR9-specific small-interfering RNA (siTLR9) (target sequence: 1#, 5′-GGAACTGCTACTACAAGAA-3′; target sequence 2#, 5′-CCTTCGTGGTGTTCGATAA-3′; and target sequence 3#, 5′-CCTATAACCTCATTGTCAA-3′) and scramble negative control of small-interfering RNA (siRNA) (siNC) were synthesized by RiboBio (Guangzhou, China). A total of 3 × 106 WT PMφs were incubated per well of a 6-well culture plate, following which adherent cells were transfected with the indicated siRNAs using Lipofectamine 2000 (Invitrogen). Lipofectamine 2000 and siRNA were separately diluted in serum-free and antibiotic-free Opti-MEM medium, separately incubated at room temperature for 5 min, mixed and incubated for 15 min at room temperature, and finally added to the cells. After 6 h, the transfection medium was discarded; the cells were washed twice with RPMI 1640 medium and maintained in 1 mL of RPMI 1640 medium plus 2% FBS for another 24 h at 37°C with 5% CO2. The cells were incubated with 1 × 106 GLV-free Giardia trophozoites or GLV-containing Giardia trophozoites for 18 h after transfection. The medium and bottom-layer cells were harvested separately for enzyme-linked immunosorbent assay (ELISA) and Western blot, respectively.
Analysis of Cytokine Levels by ELISA
A total of 3 × 106 WT or siTLR9-treated PMφs were stimulated with 1 × 106 GLV-free Giardia trophozoites, 1 × 106 GLV-containing Giardia trophozoites, 3 μg of Giardia gDNA, 5 μM/mL CpG ODN 1668, or 100 ng/mL lipopolysaccharide (LPS) (Escherichia coli 0111: B4; Sigma-Aldrich, St. Louis, MO, United States) for 18 h in 1 mL of RPMI 1640 medium containing 1% FBS at 37°C with 5% CO2 (Murphy et al., 2017; Li et al., 2019). The collected supernatants were temporarily stored at −80°C for ELISA. Cytokine concentrations in the supernatants were determined by uncoated ELISA kits specific for mouse IL-6 (88–7064), TNF-α (88–7324), and IL-12/IL-23 (total p40) (88–7120, all from eBioscience, San Diego, CA, United States) following the manufacturer’s instructions.
Western Blotting
A total of 3 × 106 WT PMφs were stimulated with 1 × 106 G. duodenalis trophozoites for different times (0.5, 1, 2, 3, 4, 5, and 6 h) with unstimulated PMφs serving as a blank control. Following stimulation, the medium was collected, and the bottom-layer cells were scraped with a cell scraper, followed by centrifugation at 12,000 × g for 30 min at 4°C. The harvested cells were lysed with RIPA buffer (BOSTER, Wuhan, China) containing protease/phosphatase inhibitors (Sangon, dilution 1/100). Protein was quantified by bicinchoninic acid assay. Equal amounts (30 μg) of protein from the different samples were separated by 10% sodium dodecyl sulfate–polyacrylamide gel electrophoresis and then transferred to polyvinyl difluoride membranes (Millipore, Billerica, MA, United States). After blocking with 5% bovine serum albumin (BSA) in Tris-buffered saline 0.1 Tween 20 (TBST) for 2 h at room temperature, the membranes were incubated overnight at 4°C with primary antibodies targeting TLR9, p38, ERK, AKT, NF-κB p65, phospho-p38, phospho-ERK, phospho-AKT, phospho-p65, phospho-IκBα, β-actin (all rabbit), and IκBα (mouse) (all from Cell Signaling Technology, Danvers, MA, United States) diluted 1/1,000 in 5% BSA. The next day, the membranes were washed three times with TBST, incubated with secondary horseradish peroxidase–conjugated goat anti-rabbit immunoglobulin G (IgG) or anti–mouse IgG antibodies (Proteintech, Wuhan, China), diluted 1/5,000 in 5% non-fat milk for 45 min at room temperature, and then washed again three times with TBST. Protein bands were detected by enhanced chemiluminescence (Vigorous, Beijing, China). The protein expression level was densitometrically quantified using ImageJ.
Analysis of NF-κB p65 by Immunofluorescence
A total of 7.5 × 105 WT and siTLR9-treated PMφs were cultivated in 24-well culture plates on sterile glass coverslips and stimulated with 7.5 μg of Giardia gDNA, 2.5 × 105 GLV-free Giardia trophozoites, 2.5 × 105 GLV-containing Giardia trophozoites, or 5 μM/mL CpG ODN 1668 at 37°C with 5% CO2. After incubation for 60 min, the cells were washed three times with sterile PBS, fixed in 4% formaldehyde solution for 20 min at room temperature, washed three times with sterile PBS, permeabilized with 0.25% Triton X-100 for 20 min, washed three times, blocked in 3% BSA in PBST for 1 h at room temperature, and incubated overnight at 4°C with rabbit anti–NF-κB p65 antibody (Cell Signaling Technology) diluted 1/1,000 in 1% BSA. After washing three times, the cells were incubated with fluorescein isothiocyanate–conjugated goat anti–rabbit IgG (H + L) antibody (Proteintech) diluted 1/100 in 1% BSA for 1 h at room temperature, washed again three times, and then counterstained with DAPI at room temperature for 20 min. NF-κB p65 localization was visualized under a confocal microscope (LSM-710, Carl Zeiss, Oberkochen, Germany) equipped with a 63×, 1.4-NA, oil-immersion objective.
Inhibition Assay
Wild-type PMφs (3 × 106) were pretreated with the p38 inhibitor SB203580 (30 μM), ERK inhibitor PD98059 (40 μM), or AKT inhibitor MK-2206 2HCl (5 μM) (all from Selleck, Shanghai, China) for 30 min at 37°C with 5% CO2, followed by coincubation with 1 × 106 GLV-free Giardia trophozoites or 1 × 106 GLV-containing Giardia trophozoites in 1 mL of RPMI 1640 medium supplemented with 1% FBS. The medium and bottom-layer cells were separately harvested for ELISA and Western blot analysis.
Statistical Analysis
Data were expressed as means ± SD from three separate experiments. GraphPad Prism 8 (GraphPad Software Inc., La Jolla, CA, United States) was utilized for ELISA data analysis. SPSS version 19.0 (SPSS Inc., Chicago, IL, United States) was employed for statistical analysis. Unpaired t tests were used to compare data between two groups, whereas one-way analysis of variance followed by Tukey test was used to compare data between multiple groups. p < 0.05 was considered statistically significant.
Results
G. duodenalis Trophozoites Induced TLR9 Activation and Proinflammatory Cytokine Production in PMφs
To explore the role of TLR9 in Giardia infection, we first assessed TLR9 gene expression levels at different time points (0, 2, 4, 6, 8, and 12 h) using reverse transcriptase (RT)–quantitative PCR (qPCR). The results showed that PMφs incubated with G. duodenalis exhibited significantly enhanced transcription levels of TLR9 within 12 h compared to the control PMφs, peaking at 8 h and then decreasing (Figure 1A). To then address whether TLR9 activation was indeed due to stimulation by Giardia gDNA and not GLV or any other factor, we compared TLR9 mRNA levels among several stimulation groups, including a positive control group. RT-qPCR analysis indicated that TLR9 transcript levels in WT PMφs were increased to varying degrees, and no significant difference was found between PMφs stimulated with GLV-free and GLV-containing Giardia trophozoites (Figure 1B). Furthermore, to determine the effect of TLR9 on cytokine production by innate immune cells, we measured the production of IL-6, TNF-α, and IL-12 p40 in siTLR9-treated and WT PMφs. The results showed that incubation with G. duodenalis trophozoites increased the secretion levels of IL-6, TNF-α, and IL-12 p40 in WT PMφs; however, compared to WT PMφs, the secretion of these cytokines was markedly decreased in siTLR9-treated PMφs stimulated with CpG ODN 1668 or G. duodenalis trophozoites, but remained largely unchanged in the LPS-stimulated groups. In addition, WT PMφs stimulated with GLV-containing Giardia trophozoites exhibited significantly higher levels of cytokine secretion compared with stimulation with GLV-free Giardia trophozoites (Figure 1C and Supplementary Figure 1B).
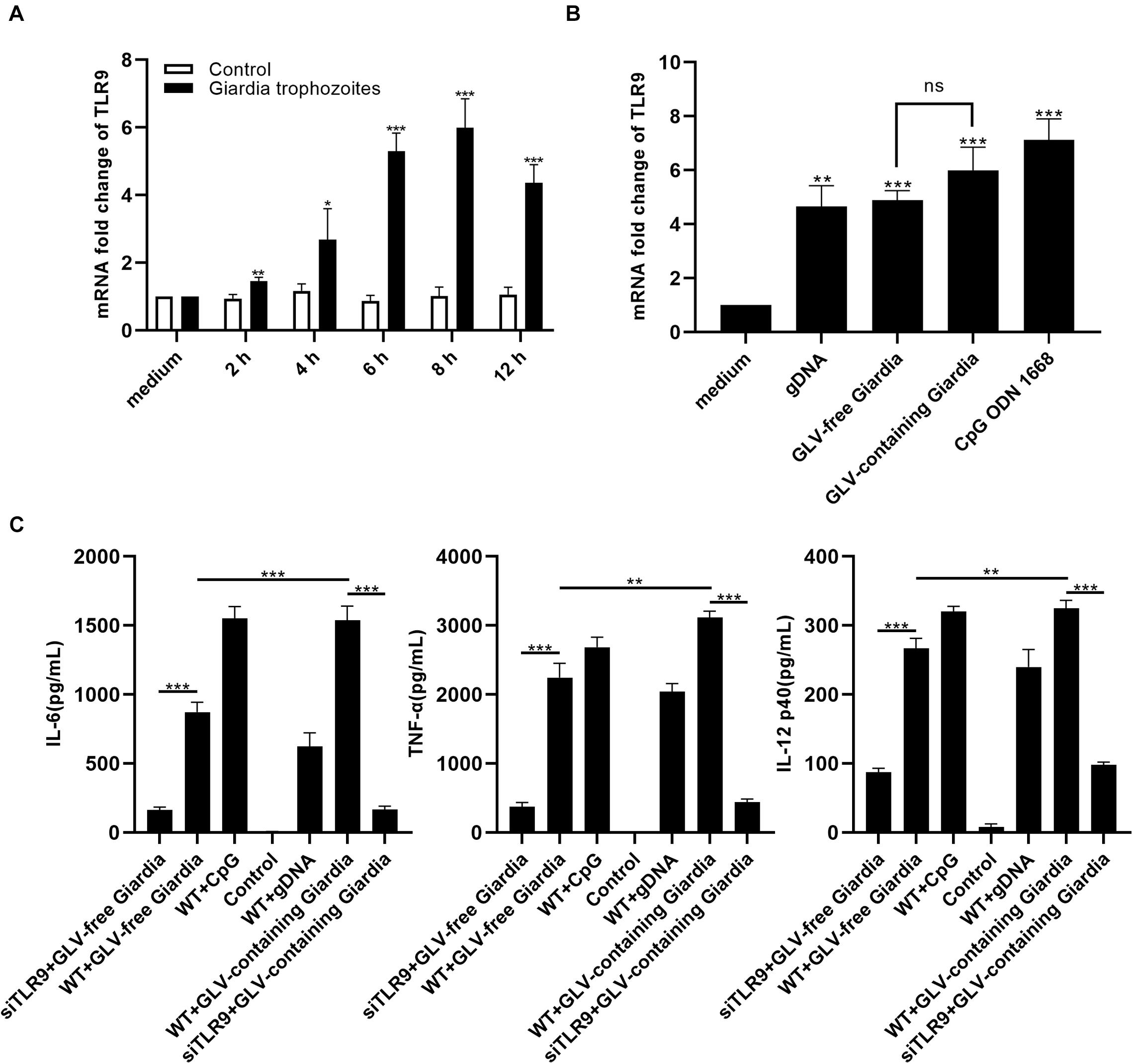
Figure 1. Giardia duodenalis trophozoites induced cytokine production in a TLR9-dependent manner. (A) RT-qPCR analysis of the relative fold change in the levels of TLR9 mRNA extracted from 3 × 106 mouse peritoneal macrophages stimulated with 1 × 106 Giardia lamblia virus (GLV)–containing Giardia trophozoites for various periods (0, 2, 4, 6, 8, and 12 h). (B) RT-qPCR analysis of the relative fold change in the levels of TLR9 mRNA extracted from 3 × 106 mouse peritoneal macrophages stimulated with gDNA (3 μg, extracted from 1 × 106 GLV-free Giardia trophozoites), 1 × 106 GLV-free Giardia trophozoites, 1 × 106 GLV-containing Giardia trophozoites, or CpG ODN 1668 (5 μM/mL). The TLR9 mRNA level was normalized to that of β-actin. (C) The secretion levels of IL-6, TNF-α, and IL-12 p40 in cell culture supernatants were measured by ELISA. Data are expressed as means ± SD from three separate experiments. ns, no significant difference, *p < 0.05, **p < 0.01, ***p < 0.001.
G. duodenalis Trophozoites Induced Cytokine Secretion in PMφs by Activating the p38 and ERK Pathways via TLR9
To examine whether stimulation with G. duodenalis trophozoites could activate the p38 and ERK pathways in mouse macrophages, the phosphorylation levels of p38 and ERK in WT PMφs were measured by Western blot after stimulation with G. duodenalis trophozoites. The phosphorylation of p38 and ERK was increased within 6 h compared with that in control PMφs, with that of p38 peaking after 3 h and then decreasing and that of ERK peaking at 4 h followed by a decrease (Figure 2A). Additionally, to evaluate cytokine levels following G. duodenalis stimulation, we assessed the levels of secreted cytokines (IL-6, TNF-α, and IL-12 p40) in cell supernatants and found that production of these cytokines by WT PMφs showed a gradual increase (Figure 2B).
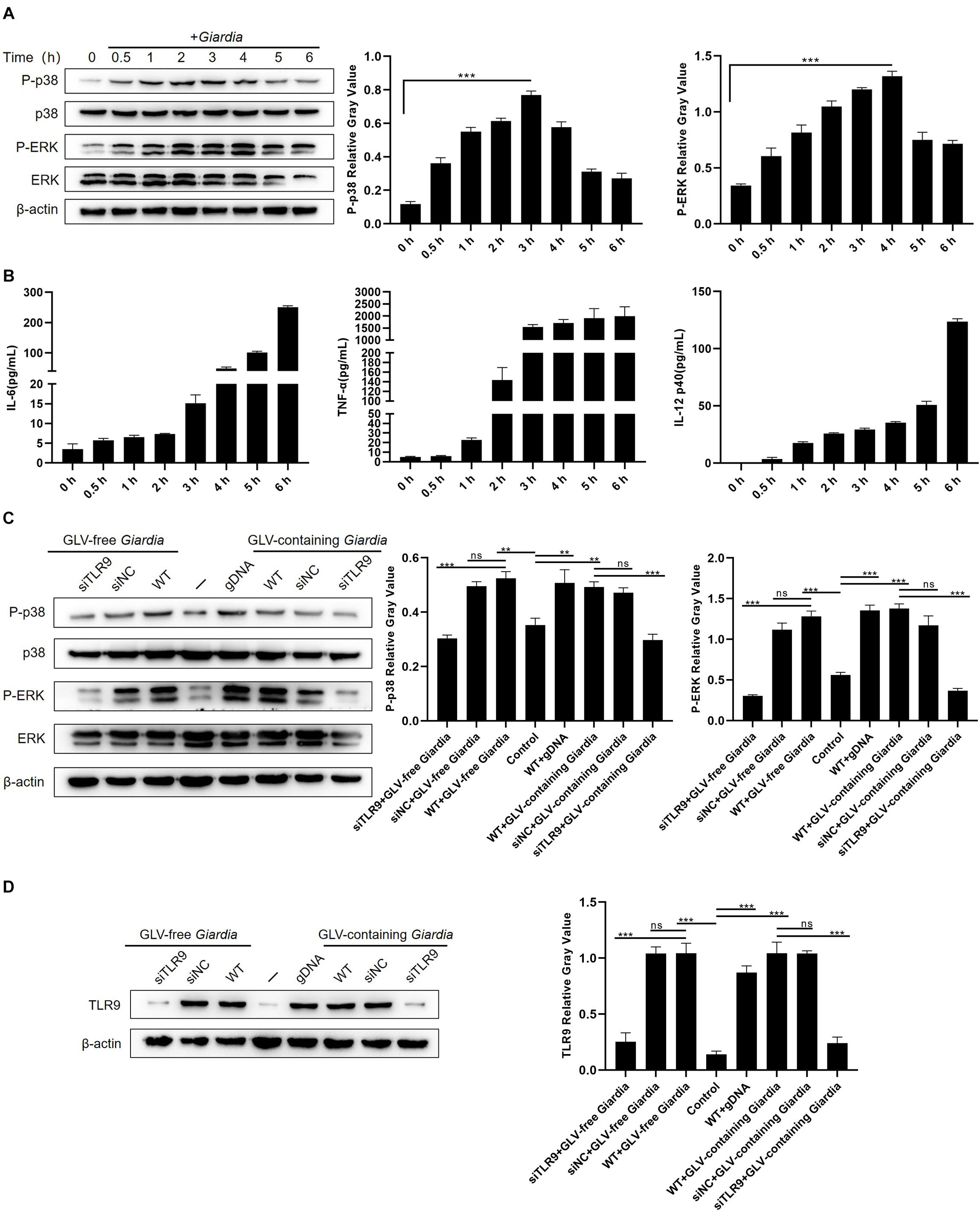
Figure 2. Giardia duodenalis trophozoites activated the p38 and ERK/MAPK signaling pathways via TLR9. (A) A total of 3 × 106 wild-type (WT) mouse peritoneal macrophages were stimulated with 1 × 106 G. duodenalis trophozoites for various periods (0–6 h) following which the phosphorylation levels of p38 and ERK were analyzed by Western blot. (B) The secretion levels of IL-6, TNF-α, and IL-12 p40 in cell culture supernatants were measured by ELISA. (C) Macrophages treated or not with small-interfering RNA (siRNA) targeting TLR9 (siTLR9) were stimulated with Giardia lamblia virus (GLV)–free or GLV-containing Giardia trophozoites for 3 h. (D) A total of 3 × 106 WT macrophages pretreated or not with siTLR9 were incubated for 3 h with 1 × 106 GLV-free Giardia trophozoites or 1 × 106 GLV-containing Giardia trophozoites, following which TLR9 expression levels were analyzed by Western blot. Relative protein expression was quantified by densitometric analysis using β-actin as an internal reference. Data are expressed as means ± SD from three separate experiments. ns, no significant difference, *p < 0.05, **p < 0.01, ***p < 0.001.
To estimate whether the G. duodenalis trophozoites–induced activation of the p38 and ERK pathways was mediated by TLR9, PMφs treated or not with siTLR9 were stimulated with GLV-free or GLV-containing Giardia trophozoites for 3 h at 37°C. Compared with WT or siNC-treated PMφs incubated with G. duodenalis trophozoites, the phosphorylation levels of p38 and ERK in siTLR9-treated PMφs incubated with G. duodenalis trophozoites were greatly decreased (Figure 2C). Furthermore, to assess the efficacy of siTLR9, we measured the TLR9 protein expression levels by Western blot in siTLR9-treated PMφs. The results showed that stimulated with LPS, CpG ODN 1668, GLV-free Giardia trophozoites, or GLV-containing Giardia trophozoites, protein expression levels of TLR9 significantly decreased in WT PMφs with TLR9-siRNA pretreatment compared with those in untreated PMφs (Figure 2D and Supplementary Figure 1A). These results suggested that G. duodenalis trophozoites–induced activation of the p38 and ERK signaling pathways was mediated via TLR9.
To evaluate the role of the p38 and ERK signaling pathways in modulating the production of IL-6, TNF-α, and IL-12 p40, PMφs were pretreated or not with the p38 inhibitor SB203580 or the ERK inhibitor PD98059 for 30 min at 37°C. Following incubation with GLV-free or GLV-containing Giardia trophozoites for 18 h, the bottom-layer cells were harvested for Western blotting, and the cell supernatants were used for the measurement of cytokine concentrations by ELISA. Western blot analysis demonstrated that p38 and ERK phosphorylation levels were markedly diminished in PMφs pretreated with the inhibitors compared with those in untreated PMφs (Figure 3A). Moreover, the G. duodenalis trophozoites–induced secretion of IL-6, TNF-α, and IL-12 p40 was significantly decreased in PMφs pretreated with the inhibitors compared with that of untreated PMφs (Figure 3B). These data demonstrated that the p38 and ERK pathways were activated via TLR9, leading to increased secretion of IL-6, TNF-α, and IL-12 p40 in PMφs exposed to G. duodenalis trophozoites.
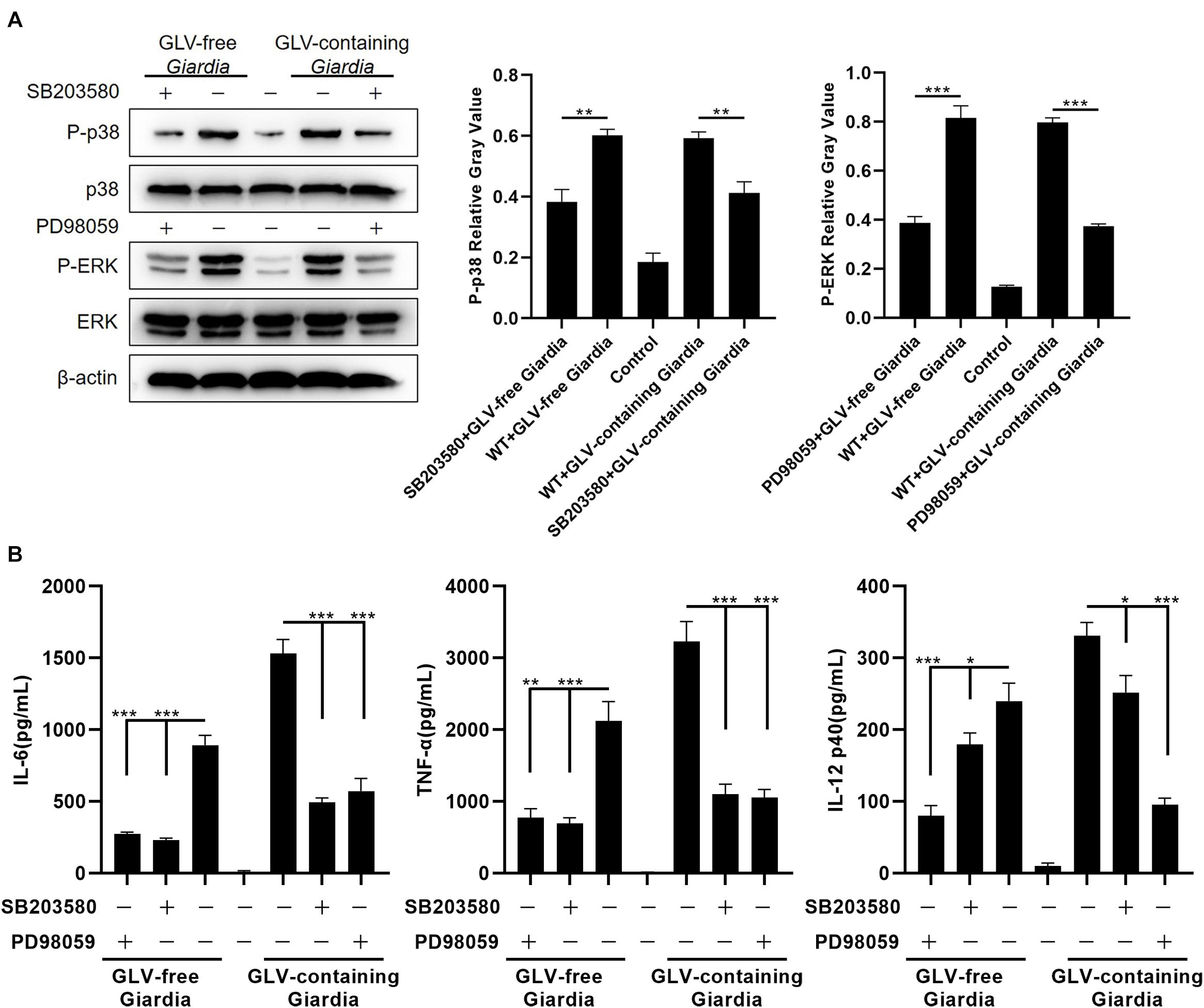
Figure 3. Giardia duodenalis trophozoites–induced cytokine production was disrupted by p38 and ERK inhibitor treatment. (A) A total of 3 × 106 wild-type (WT) mouse peritoneal macrophages were pretreated for 30 min with the p38 inhibitor SB203580 (30 μM) or the ERK inhibitor PD98059 (40 μM) before stimulation with 1 × 106 Giardia lamblia virus (GLV)–free Giardia trophozoites or 1 × 106 GLV-containing Giardia trophozoites. The phosphorylation levels of p38 and ERK were subsequently analyzed by Western blot. Relative protein expression was quantified by densitometric analysis using β-actin as an internal reference. (B) The production of IL-6, TNF-α, and IL-12 p40 in cell supernatants was measured by ELISA. Data are expressed as means ± SD from three separate experiments. *p < 0.05, **p < 0.01, ***p < 0.001.
G. duodenalis Trophozoites Reduced Cytokine Production by Activating the AKT Pathway
To investigate whether stimulation with G. duodenalis trophozoites could activate the AKT pathway in mouse macrophages, AKT phosphorylation levels in G. duodenalis trophozoites–incubated PMφs were determined by Western blot. The results showed that AKT phosphorylation levels were first increased compared with that of control PMφs, peaking at 3 h, and then decreased to baseline levels at 6 h (Figure 4A).
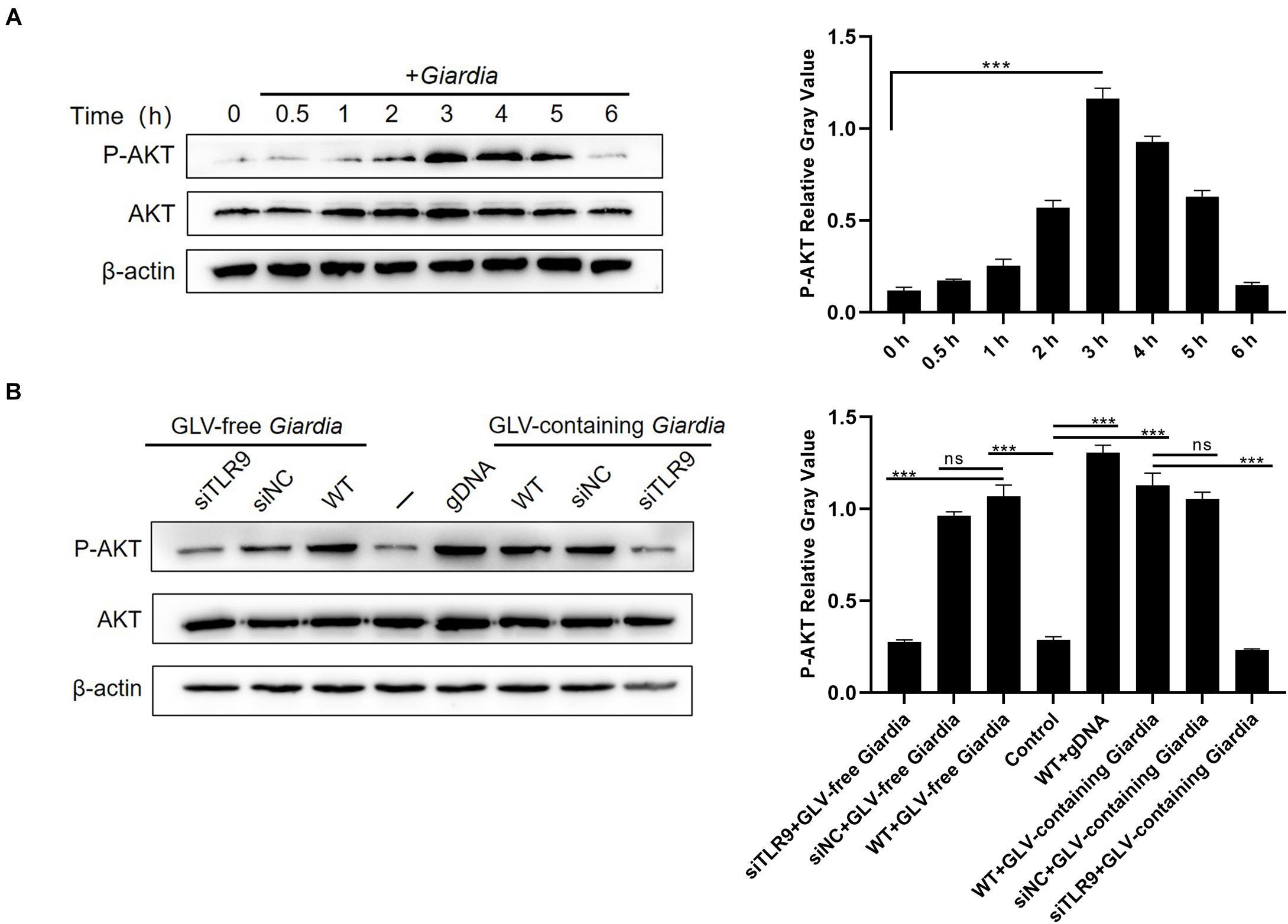
Figure 4. Giardia duodenalis trophozoites induced the phosphorylation of AKT via TLR9. (A) A total of 3 × 106 wild-type (WT) mouse peritoneal macrophages were stimulated with 1 × 106 G. duodenalis trophozoites for various periods (0–6 h) after which the phosphorylation level of AKT was analyzed by Western blot. (B) A total of 3 × 106 macrophages treated or not with siRNA targeting TLR9 were stimulated with 1 × 106 Giardia lamblia virus (GLV)–free Giardia trophozoites or 1 × 106 GLV-containing Giardia trophozoites for 3 h. Data are expressed as means ± SD from three separate experiments. ns, no significant difference, *p < 0.05, **p < 0.01, ***p < 0.001.
To assess whether the G. duodenalis trophozoites–induced activation of the AKT pathway was mediated via TLR9, AKT phosphorylation levels were measured in PMφs treated or not with siTLR9 and stimulated with GLV-free or GLV-containing Giardia trophozoites for 3 h at 37°C. AKT phosphorylation levels were significantly reduced in the siTLR9-treated groups with G. duodenalis trophozoites compared with those in stimulated WT PMφs. These results suggested that G. duodenalis trophozoites activated the AKT signaling pathway through TLR9 (Figure 4B).
To explore the role of the AKT signaling pathway in regulating the production of IL-6, TNF-α, and IL-12 p40, PMφs were pretreated or not with the AKT inhibitor MK-2206 2HCl for 30 min at 37°C. Following coincubation with GLV-free or GLV-containing Giardia trophozoites for 18 h, the bottom-layer cells were harvested for Western blotting, whereas the cell supernatants were used for the determination of cytokine concentrations by ELISA. Western blot analysis demonstrated that pretreatment with the AKT inhibitor significantly reduced AKT phosphorylation levels (Figure 5A). Furthermore, compared with untreated PMφs, the G. duodenalis trophozoites–induced secretion of IL-6, TNF-α, and IL-12 p40 was increased to varying degrees in PMφs pretreated with the AKT inhibitor (Figure 5B). These data indicated that G. duodenalis reduced the secretion of these cytokines in PMφs by activating the AKT signaling pathway.
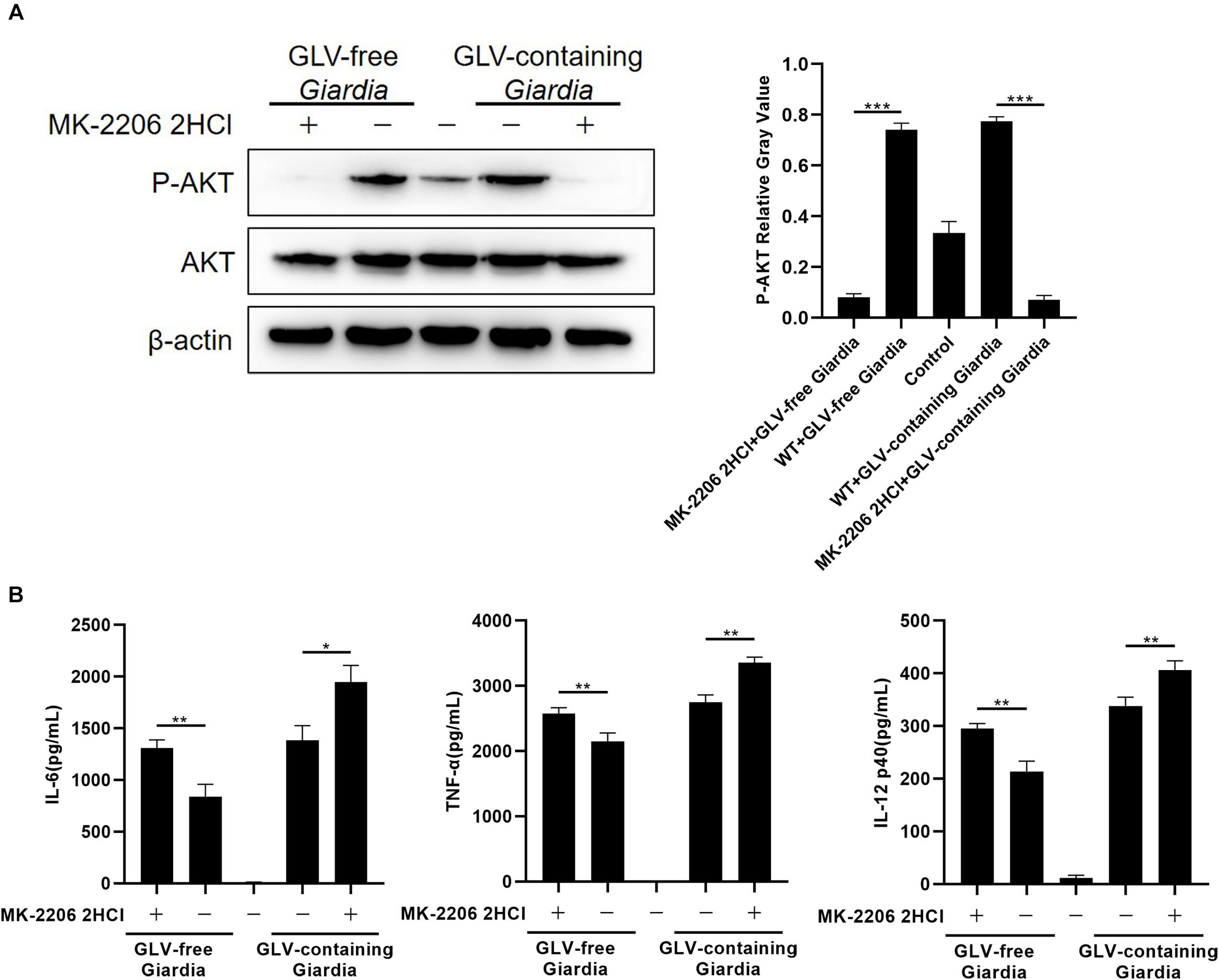
Figure 5. Giardia duodenalis trophozoites suppressed cytokine production by activating the AKT signaling pathway. (A) A total of 3 × 106 wild-type (WT) mouse peritoneal macrophages were pretreated for 30 min with the AKT inhibitor MK-2206 2HCl (5 μM) before stimulation with Giardia lamblia virus (GLV)–free or GLV-containing Giardia trophozoites. The AKT phosphorylation level was subsequently analyzed by Western blot. Relative protein expression was quantified by densitometric analysis using β-actin as an internal reference. (B) The secretion levels of IL-6, TNF-α, and IL-12 p40 in cell supernatants were measured by ELISA. Data are expressed as means ± SD from three separate experiments. *p < 0.05, **p < 0.01, ***p < 0.001.
G. duodenalis Trophozoites Induced the Nuclear Translocation of NF-κB p65 in SiTLR9-Treated and WT PMφs
To determine the effects of G. duodenalis trophozoites on NF-κB activation, immunofluorescence staining and Western blot were used to detect the localization and expression of NF-κB p65, respectively. Translocation of NF-κB p65 into the nucleus of PMφs following incubation with G. duodenalis trophozoites was visualized by laser confocal microscopy (Figure 6A). Western blot analysis showed that, in WT PMφs incubated with G. duodenalis trophozoites, NF-κB p65 phosphorylation peaked after 60 min and then gradually decreased, whereas the phosphorylation of IκBα peaked at 30 min and then gradually decreased (Figure 6B). Laser confocal microscopy revealed that after stimulation with G. duodenalis trophozoites for 60 min, NF-κB p65 could be seen in the nuclei of both siTLR9-treated and WT PMφs, whereas no nuclear translocation was detected in WT PMφs without G. duodenalis trophozoite stimulation as well as in group stimulated with CpG ODN 1668 or gDNA (Figure 6A). To further investigate whether the G. duodenalis trophozoites–induced activation of NF-κB was mediated via TLR9, PMφs treated or not with siTLR9 were stimulated with GLV-free or GLV-containing Giardia trophozoites for 60 min at 37°C. No differences in NF-κB p65 or IκBα phosphorylation levels were found between siTLR9-treated and WT PMφs (Figure 6C), confirming the results obtained by immunofluorescence staining. Combined, these results indicated that the G. duodenalis trophozoites–induced activation of NF-κB was not mediated via TLR9.
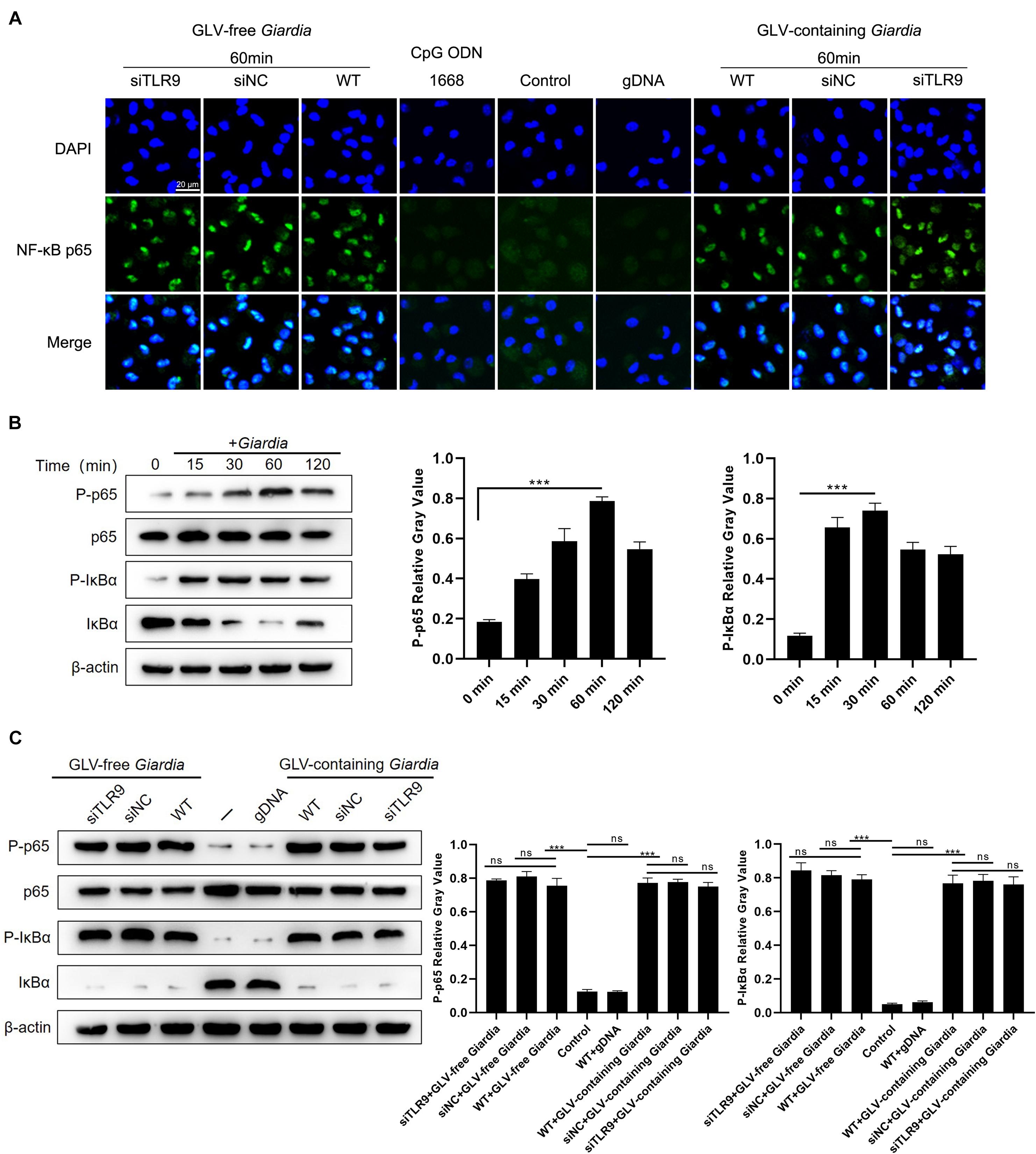
Figure 6. Giardia duodenalis trophozoites activated the NF-κB signaling pathway by inducing NF-κB p65 nuclear translocation. (A) Laser confocal microscopic images showing the facilitation of the nuclear translocation of NF-κB p65 by G. duodenalis in wild-type (WT) mouse macrophages treated or not with small-interfering RNA (siRNA) targeting TLR9 (siTLR9). (B) A total of 3 × 106 WT macrophages were stimulated with 1 × 106 G. duodenalis trophozoites for various periods (0–120 min) after which the phosphorylation levels of NF-κB p65 and IκBα were analyzed by Western blot. (C) A total of 3 × 106 WT macrophages treated or not with siTLR9 were stimulated with 1 × 106 Giardia lamblia virus (GLV)–free Giardia trophozoites or 1 × 106 GLV-containing Giardia trophozoites for 60 min. Relative protein expression was quantified by densitometric analysis using β-actin as an internal reference. Data are expressed as means ± SD from three separate experiments. ns, no significant difference, *p < 0.05, **p < 0.01, ***p < 0.001.
Discussion
Giardia duodenalis colonization of the intestinal tract of the host damages the host intestinal mucosa (Roxström-Lindquist et al., 2005; Chen et al., 2013). This leads to macrophage recruitment in the intestine, which triggers the innate immune response, followed by the secretion of proinflammatory cytokines to defend against Giardia infection (Roxström-Lindquist et al., 2005; Maloney et al., 2015). Meanwhile, adaptive immune responses are initiated, including the mobilization and recruitment of dendritic cells (DCs) for antigen processing and presentation (Rescigno et al., 2001; Roxström-Lindquist et al., 2006), thereby further enhancing the host immune response to G. duodenalis. Throughout this process, macrophages play a pivotal role. It has been demonstrated that macrophages can intake G. duodenalis trophozoites and gather them in the lamina propria of the small intestine (Belosevic and Daniels, 1992; Maloney et al., 2015). TLRs are crucial components of innate immunity, mediating inflammatory responses to defend against invading pathogens. Located on the cell membrane and in intracellular endosomes, TLRs can monitor and recognize a range of PAMPs derived from bacteria, parasites, viruses, and fungi. Importantly, studies have revealed the functions and mechanisms associated with the innate immune responses mediated by TLR2 and TLR4 during Giardia infection (Lee et al., 2014; Li et al., 2017; Serradell et al., 2019; Zhao et al., 2021). Additionally, it has been shown that the Plasmodium falciparum–derived metabolite, hemozoin, can activate TLR9 in host macrophages and DCs, leading to the massive secretion of proinflammatory factors and chemokines (Kawai and Akira, 2009; Kumar et al., 2009). Here, we found that both GLV-free and GLV-containing Giardia trophozoites could significantly enhance TLR9 gene expression in WT mouse PMφs. Notably, our study was performed on mouse macrophages infected with G. duodenalis, whereas Giardia muris might be a better option for establishing a model of Giardia infection in mice (Roberts-Thomson et al., 1976; Stevens and Roberts-Thompson, 1978); moreover, Giardia-induced activation of TLRs, including TLR9, may differ among Giardia species (Koh et al., 2013; Bhargava et al., 2015). Given that the disparities between strains were not examined in this study, further studies are warranted to investigate the differences between Giardia strains in eliciting TLR-mediated host innate immune responses. In addition, G. duodenalis is the only known zoonotic protozoan parasite among Giardia species, and studies on the involvement of immune cells in host–pathogen interactions are commonly performed using human peripheral blood mononuclear cells (Cheng et al., 2017; Cromarty et al., 2019), which may be a better alternative than mouse macrophages for investigating the mechanisms involved in immune cell defenses against G. duodenalis infection. Relevant studies involving human immune cells and various animal models are needed to further validate the current findings.
The proinflammatory cytokines TNF-α and IL-6 are essential for the elimination and early control of giardiasis in mice (Zhou et al., 2003, 2007; Li et al., 2004). Interestingly, TNF-α deficiency does not affect the mechanisms involved in host defenses against Giardia infection, which includes the generation of IgA, the proliferation of mast cells, and the secretion of IL-6 or IL-4, suggesting that TNF-α does not exert its effects through these mechanisms mentioned previously in control of Giardia, but through other effector responses downstream of it (Zhou et al., 2007). IL-6 serves a vital function in the eradication of Giardia in infected mice (Zhou et al., 2003). For example, IL-6 deficiency can significantly affect the immune response to G. duodenalis (Bienz et al., 2003), and IL-6 can regulate B-cell maturation and induce a shift of antibody type to IgA in response to Giardia infection (Ekdahl and Andersson, 2005). Additionally, mast cells can rapidly produce IL-6 to control Giardia infection in mice (Li et al., 2004). Giardia induces inflammatory responses that involve blood platelets and the release of IL-6 and TNF-α in patients infected with G. duodenalis (Matowicka-Karna et al., 2009). Additionally, bone marrow–derived DCs coincubated with Giardia extracts and TLR ligands, including CpG DNA, exhibited increased secretion of IL-10 and diminished secretion of IL-12 (Kamda and Singer, 2009). The results of the present study indicated that, following stimulation with G. duodenalis trophozoites or CpG ODN 1668, the secretion levels of IL-6, TNF-α, and IL-12 p40 were significantly higher in WT macrophages than in macrophages treated with siTLR9, but were largely unchanged in the LPS-stimulated groups, suggesting that siTLR9 treatment specifically silenced TLR9 without blocking the activation of macrophages by another pathway. These data indicated that the G. duodenalis–induced secretion of proinflammatory cytokines in macrophages was in part TLR9-dependent. Furthermore, IL-6 and TNF-α are known to be involved in the elimination and early control of Giardia infection. In the present study, we further found that, with G. duodenalis trophozoite stimulation, the secretion levels of IL-6 and TNF-α were significantly lower in siTLR9-treated mouse macrophages than in WT mouse macrophages, suggesting that activated TLR9 not only has a role in the regulation of cytokine secretion by the host in the early stages of infection but also plays a host-protective role. Importantly, GLV-containing Giardia trophozoites elicited significantly greater production of proinflammatory cytokines, including IL-6, TNF-α, and IL-12 p40, than GLV-free Giardia trophozoites, suggesting GLV may be a factor that contributed to this difference. And it has been reported that the dsRNA genome of Leishmaniavirus is recognized by the host endosomal TLR3, which triggers the secretion of proinflammatory cytokines and chemokines (Ives et al., 2011; Zangger et al., 2014). In the present study, TLR9 in mouse macrophages could recognize CpG DNA motifs, which may exist in G. duodenalis rather than the dsRNA genome of GLV. That the TLR3 of the host recognizes dsRNA viral genomes, such as that of the Leishmania RNA virus, suggests that the GLV dsRNA genome in GLV-containing Giardia trophozoites may be recognized by TLR3 in mouse macrophages, thus causing the host to initiate a more intense inflammatory response. In addition, another potential explanation for the difference in host inflammatory response induced by G. duodenalis is that GLV-free and GLV-containing Giardia trophozoites are derived from different G. duodenalis isolates, rather than the effect of GLV. And studies have found that G. duodenalis assemblage B, including GS isolate, produces more inflammatory responses both in vitro and in vivo compared to G. duodenalis assemblage A (Hanevik et al., 2007; Bénéré et al., 2012; Lee et al., 2012).
Mitogen-activated protein kinase signaling cascades are crucial for monitoring the host’s immune responses to infection, regulating the transcription of numerous proinflammatory cytokine-related genes through the phosphorylation of transcription factors and the promotion of chromatin remodeling (Kirk et al., 2020). The expression of TLR2, TLR4, and TLR9 was reported to be significantly upregulated in HeLa cells stimulated with Trichomonas vaginalis in a p38 signaling pathway-dependent manner (Chang et al., 2006). G. duodenalis GS excretory/secretory products contain factors that prompt HT-29 cells to secrete IL-8 through activating the p38 and ERK1/2 signaling pathways (Lee et al., 2012). Our findings revealed that the phosphorylation levels of p38 and ERK/MAPKs in siTLR9-treated macrophages were significantly decreased compared with those of WT macrophages. Moreover, stimulation of mouse macrophages with GLV-free and GLV-containing Giardia trophozoites, respectively, resulted in different cytokines secretion, and higher secretion levels of IL-6, TNF-α, and IL-12 p40 were observed in macrophages with GLV-containing Giardia trophozoite stimulation compared to GLV-free Giardia trophozoites; however, such secretion of G. duodenalis–induced cytokines was abolished by pretreatment with p38 and ERK inhibitors. Corroborating the findings of our present study, the G. duodenalis–induced secretion of IL-6, TNF-α, and IL-12 p40 was decreased in siTLR9-treated mouse macrophages and WT mouse macrophages pretreated with p38 and ERK inhibitors, implying that the TLR9–p38/ERK signaling pathways activated by G. duodenalis may contribute to host defenses against Giardia infection. These findings suggested that the G. duodenalis–induced secretion of proinflammatory cytokines in macrophages was achieved via TLR9-mediated activation of p38 and ERK signaling.
AKT is a serine/threonine-protein kinase with a pivotal part in the regulation of cell metabolism, survival, and proliferation. In macrophage-mediated innate immunity, AKT regulates the expression and production of proinflammatory cytokines (Lee et al., 2011). Studies have shown that, under chronic stress, the levels of phospho-AKT are lower in TLR9-deficient macrophages than in WT macrophages (Xiang et al., 2015). Additionally, G. duodenalis exposure was reported to reduce the secretion of proinflammatory cytokines in a TLR2-mediated, AKT-dependent manner (Li et al., 2017). In our study, the phosphorylation of AKT in siTLR9-treated macrophages was significantly decreased compared with that in WT macrophages. Furthermore, pretreatment with an AKT inhibitor increased the secretion levels of IL-6, TNF-α, and IL-12 p40 in mouse macrophages stimulated with GLV-free or GLV-containing Giardia trophozoites, implying that both GLV-free and GLV-containing Giardia trophozoites can attenuate the secretion levels of these cytokines in mouse macrophages via the AKT signaling pathway. These data suggested that G. duodenalis trophozoites can downregulate cytokine secretion by mouse macrophages in an AKT-dependent manner. However, the differences between these results and those obtained with the TLR9 silencing assay and p38/ERK inhibition experiments suggest that the positive regulatory effects associated with the p38 and ERK pathways were more important than the negative regulatory effects related to the AKT pathway for the TLR9-mediated innate immune response against Giardia infection in mouse macrophages.
The NF-κB transcriptional complex is known to be a key regulator of cellular stress responses and immune responses to infection (Ashall et al., 2009). NF-κB activity in the nucleus is controlled by IκB proteins, which provide transient or dynamic regulation through their stimuli-responsive degradation and resynthesis (Adelaja and Hoffmann, 2019). Studies have suggested that the transition from p50 to p65 heterodimers to p50 homodimer in NF-κB may be involved in inflammation (Lawrence et al., 2001; Ashall et al., 2009). Additionally, as a TLR9 ligand, CpG DNA triggers the production of immune mediators by immune cells through TLR9-mediated signal transduction, which, in turn, leads to NF-κB activation (Xu et al., 2003). It has been reported that G. duodenalis may target NF-κB in a differential manner to modulate the inflammatory functions of macrophages (Faria et al., 2020). In our study, the immunofluorescence assay showed that both GLV-free and GLV-containing Giardia trophozoites could induce NF-κB p65 accumulation in the nucleus in both siTLR9-treated and WT mouse macrophages. Further, Western blot analysis showed that the phosphorylation levels of p65 and IκBα in siTLR9-treated macrophages were similar to those of WT macrophages. Additionally, GLV-free and GLV-containing Giardia trophozoites exerted similar effects on activating the NF-κB signaling pathway. These results indicated that G. duodenalis can activate NF-κB p65 signaling in a TLR9-independent manner in mouse macrophages.
Collectively, our results showed that G. duodenalis can induce the production of proinflammatory cytokines through the TLR9-mediated activation of the p38 and ERK signaling pathways in mouse PMφs, whereas GLV-containing Giardia trophozoites can enhance cytokine secretion in mouse macrophages to a greater extent than GLV-free Giardia trophozoites. Furthermore, G. duodenalis–induced activation of TLR9–p38/ERK signaling enhanced immune defense responses, thus forming a host-protective barrier. Our study complements those on TLR-mediated innate immune responses of host cells against Giardia infection and provides potential molecular targets for the further development of novel strategies for the treatment of giardiasis.
Data Availability Statement
The original contributions presented in the study are included in the article/Supplementary Material, further inquiries can be directed to the corresponding author.
Ethics Statement
The animal study was reviewed and approved by the Animal Welfare and Research Ethics Committee at Jilin University (IACUC Permit Number: 20160612).
Author Contributions
XP, XL, and PZ drafted the manuscript and analyzed the data. XP, KY, LC, and PG planned and performed the experiments. XP, XL, ML, ZZ, NZ, XW, and PG designed the experiments. XZ, JL, and PG provided guidance and support and revised the manuscript. All authors contributed to the article and approved the submitted version.
Funding
This study was supported by the National Natural Science Foundation of China (Nos. 31772732, 31672288, and 31101804) and the Fundamental Research Funds for the Central Universities.
Conflict of Interest
The authors declare that the research was conducted in the absence of any commercial or financial relationships that could be construed as a potential conflict of interest.
Supplementary Material
The Supplementary Material for this article can be found online at: https://www.frontiersin.org/articles/10.3389/fcell.2021.694675/full#supplementary-material
References
Adelaja, A., and Hoffmann, A. (2019). Signaling crosstalk mechanisms that may fine-tune pathogen-responsive NFκB. Front. Immunol. 10:433. doi: 10.3389/fimmu.2019.00433
Ashall, L., Horton, C. A., Nelson, D. E., Paszek, P., Harper, C. V., Sillitoe, K., et al. (2009). Pulsatile stimulation determines timing and specificity of NF-κB-dependent transcription. Science 324, 242–246. doi: 10.1126/science.1164860
Belosevic, M., and Daniels, C. W. (1992). Phagocytosis of Giardia lamblia trophozoites by cytokine-activated macrophages. Clin. Exp. Immunol. 87, 304–309. doi: 10.1111/j.1365-2249.1992.tb02992.x
Bénéré, E., Van Assche, T., Van Ginneken, C., Peulen, O., Cos, P., and Maes, L. (2012). Intestinal growth and pathology of Giardia duodenalis assemblage subtype AI. AII, B and E in the gerbil model. Parasitology 139, 424–433. doi: 10.1017/S0031182011002137
Berkman, D. S., Lescano, A. G., Gilman, R. H., Lopez, S. L., and Black, M. M. (2002). Effects of stunting, diarrhoeal disease, and parasitic infection during infancy on cognition in late childhood: a follow-up study. Lancet 359, 564–571. doi: 10.1016/S0140-6736(02)07744-9
Bhargava, A., Cotton, J. A., Dixon, B. R., Gedamu, L., Yates, R. M., and Buret, A. G. (2015). Giardia duodenalis surface cysteine proteases induce cleavage of the intestinal epithelial cytoskeletal protein villin via myosin light chain kinase. PLoS One 10:e0136102. doi: 10.1371/journal.pone.0136102
Bienz, M., Dai, W. J., Welle, M., Gottstein, B., and Müller, N. (2003). Interleukin-6-deficient mice are highly susceptible to Giardia lamblia infection but exhibit normal intestinal immunoglobulin A responses against the parasite. Infect. Immun. 71, 1569–1573. doi: 10.1128/IAI.71.3.1569-1573.2003
Chang, J.-H., Park, J.-Y., and Kim, S.-K. (2006). Dependence on p38 MAPK signalling in the up-regulation of TLR2, TLR4 and TLR9 gene expression in Trichomonas vaginalis-treated HeLa cells. Immunology 118, 164–170. doi: 10.1111/j.1365-2567.2006.02347.x
Chen, T. L., Chen, S., Wu, H. W., Lee, T. C., Lu, Y. Z., Wu, L. L., et al. (2013). Persistent gut barrier damage and commensal bacterial influx following eradication of Giardia infection in mice. Gut Pathog. 5:26. doi: 10.1186/1757-4749-5-26
Cheng, L., Zhang, Z., Li, G., Li, F., Wang, L., Zhang, L., et al. (2017). Human innate responses and adjuvant activity of TLR ligands in vivo in mice reconstituted with a human immune system. Vaccine 35, 6143–6153. doi: 10.1016/j.vaccine.2017.09.052
Coffey, C. M., Collier, S. A., Gleason, M. E., Yoder, J. S., Kirk, M. D., Richardson, A. M., et al. (2021). Evolving Epidemiology of Reported Giardiasis Cases in the United States, 1995–2016. Clin. Infect. Dis. 72, 764–770. doi: 10.1093/cid/ciaa128
Cromarty, R., Sigal, A., Liebenberg, L. J. P., McKinnon, L. R., Abdool Karim, S. S., Passmore, J. A. S., et al. (2019). Diminished HIV Infection of Target CD4+ T Cells in a Toll-Like Receptor 4 Stimulated in vitro Model. Front. Immunol. 10:1705. doi: 10.3389/fimmu.2019.01705
Cui, J., Chen, Y., Wang, H. Y., and Wang, R. F. (2014). Mechanisms and pathways of innate immune activation and regulation in health and cancer. Hum. Vaccines Immunother. 10, 3270–3285. doi: 10.4161/21645515.2014.979640
Das, S., Ghosh, A. K., Singh, S., Saha, B., Ganguly, A., and Das, P. (2015). Unmethylated CpG motifs in the L. donovani DNA regulate TLR9-dependent delay of programmed cell death in macrophages. J. Leukoc. Biol. 97, 363–378. doi: 10.1189/jlb.4a0713-378rr
Einarsson, E., Ma’ayeh, S., and Svärd, S. G. (2016). An up-date on Giardia and giardiasis. Curr. Opin. Microbiol. 34, 47–52. doi: 10.1016/j.mib.2016.07.019
Ekdahl, K., and Andersson, Y. (2005). Imported giardiasis: impact of international travel, immigration, and adoption. Am. J. Trop. Med. Hyg. 72, 825–830. doi: 10.4269/ajtmh.2005.72.825
Faria, C. P., Neves, B. M., Lourenço, Á, Cruz, M. T., Martins, J. D., Silva, A., et al. (2020). Giardia lamblia Decreases NF-κB p65RelA Protein Levels and Modulates LPS-Induced Pro-Inflammatory Response in Macrophages. Sci. Rep. 10:6234. doi: 10.1038/s41598-020-63231-0
Fichorova, R. N., Buck, O. R., Yamamoto, H. S., Fashemi, T., Dawood, H. Y., Fashemi, B., et al. (2013). The villain team-up or how trichomonas vaginalis and bacterial vaginosis alter innate immunity in concert. Sex. Transm. Infect. 89, 460–466. doi: 10.1136/sextrans-2013-051052
Fichorova, R. N., Lee, Y., Yamamoto, H. S., Takagi, Y., Hayes, G. R., Goodman, R. P., et al. (2012). Endobiont Viruses Sensed by the Human Host-Beyond Conventional Antiparasitic Therapy. PLoS One 7:e48418. doi: 10.1371/journal.pone.0048418
Fink, M. Y., and Singer, S. M. (2017). The Intersection of Immune Responses, Microbiota, and Pathogenesis in Giardiasis. Trends Parasitol. 33, 901–913. doi: 10.1016/j.pt.2017.08.001
Franco, L. H., Fleuri, A. K. A., Pellison, N. C., Quirino, G. F. S., Horta, C. V., De Carvalho, R. V. H., et al. (2017). Autophagy downstream of endosomal Toll-like receptor signaling in macrophages is a key mechanism for resistance to Leishmania major infection. J. Biol. Chem. 292, 13087–13096. doi: 10.1074/jbc.M117.780981
Gay, N. J., and Gangloff, M. (2007). Structure and function of toll receptors and their ligands. Annu. Rev. Biochem. 76, 141–145. doi: 10.1146/annurev.biochem.76.060305.151318
Gazzinelli, R. T., and Denkers, E. Y. (2006). Protozoan encounters with Toll-like receptor signalling pathways: implications for host parasitism. Nat. Rev. Immunol. 6, 895–906. doi: 10.1038/nri1978
Gong, P., Li, X., Wu, W., Cao, L., Zhao, P., Li, X., et al. (2020). A Novel MicroRNA From the Translated Region of the Giardiavirus rdrp Gene Governs Virus Copy Number in Giardia duodenalis. Front. Microbiol. 11:569412. doi: 10.3389/fmicb.2020.569412
Gupta, C. L., Akhtar, S., Waye, A., Pandey, N. R., Pathak, N., and Bajpai, P. (2015). Cross talk between Leishmania donovani CpG DNA and Toll-like receptor 9: an immunoinformatics approach. Biochem. Biophys. Res. Commun. 459, 424–429. doi: 10.1016/j.bbrc.2015.02.121
Hanevik, K., Hausken, T., Morken, M. H., Strand, E. A., Mørch, K., Coll, P., et al. (2007). Persisting symptoms and duodenal inflammation related to Giardia duodenalis infection. J. Infect. 55, 524–530. doi: 10.1016/j.jinf.2007.09.004
Ives, A., Ronet, C., Prevel, F., Ruzzante, G., Fuertes-Marraco, S., Schutz, F., et al. (2011). Leishmania RNA virus controls the severity of mucocutaneous leishmaniasis. Science 331, 775–778. doi: 10.1126/science.1199326
Jenkins, M. C., Higgins, J., Abrahante, J. E., Kniel, K. E., O’Brien, C., Trout, J., et al. (2008). Fecundity of Cryptosporidium parvum is correlated with intracellular levels of the viral symbiont CPV. Int. J. Parasitol. 38, 1051–1055. doi: 10.1016/j.ijpara.2007.11.005
Kamda, J. D., and Singer, S. M. (2009). Phosphoinositide 3-Kinase-Dependent inhibition of dendritic cell interleukin-12 production by giardia lamblia. Infect. Immun. 77, 685–693. doi: 10.1128/IAI.00718-08
Kawai, T., and Akira, S. (2009). The roles of TLRs, RLRs and NLRs in pathogen recognition. Int. Immunol. 21, 317–337. doi: 10.1093/intimm/dxp017
Kirk, S. G., Samavati, L., and Liu, Y. (2020). MAP kinase phosphatase-1, a gatekeeper of the acute innate immune response. Life Sci. 241:117157. doi: 10.1016/j.lfs.2019.117157
Koh, W. H., Geurden, T., Paget, T., O’Handley, R., Steuart, R. F., Thompson, R. C. A., et al. (2013). Giardia duodenalis assemblage-specific induction of apoptosis and tight junction disruption in human intestinal epithelial cells: effects of mixed infections. J. Parasitol. 99, 353–358. doi: 10.1645/GE-3021.1
Kumagai, Y., Takeuchi, O., and Akira, S. (2008). TLR9 as a key receptor for the recognition of DNA. Adv. Drug Deliv. Rev. 60, 795–804. doi: 10.1016/j.addr.2007.12.004
Kumar, H., Kawai, T., and Akira, S. (2009). Pathogen recognition in the innate immune response. Biochem. J. 420, 1–16. doi: 10.1042/BJ20090272
Kunz, S., Balmer, V., Sterk, G. J., Pollastri, M. P., Leurs, R., Müller, N., et al. (2017). The single cyclic nucleotide-specific phosphodiesterase of the intestinal parasite Giardia lamblia represents a potential drug target. PLoS Negl. Trop. Dis. 11:e0005891. doi: 10.1371/journal.pntd.0005891
Lagunas-Rangel, F. A., Kameyama-Kawabe, L. Y., and Bermúdez-Cruz, R. M. (2021). Giardiavirus: an update. Parasitol. Res. 120, 1943–1948. doi: 10.1007/s00436-021-07167-y
Lawrence, T., Gilroy, D. W., Colville-Nash, P. R., and Willoughby, D. A. (2001). Possible new role for NF-κB in the resolution of inflammation. Nat. Med. 7, 1291–1297. doi: 10.1038/nm1201-1291
Lee, H. Y., Hyung, S., Lee, N. Y., Yong, T. S., Han, S. H., and Park, S. J. (2012). Excretory-secretory products of Giardia lamblia induce interleukin-8 production in human colonic cells via activation of p38, ERK1/2, NF-κB and AP-1. Parasite Immunol. 34, 183–198. doi: 10.1111/j.1365-3024.2012.01354.x
Lee, H. Y., Kim, J., Noh, H. J., Kim, H. P., and Park, S. J. (2014). Giardia lamblia binding immunoglobulin protein triggers maturation of dendritic cells via activation of TLR4-MyD88-p38 and ERK1/2 MAPKs. Parasite Immunol. 36, 627–646. doi: 10.1111/pim.12119
Lee, Y. G., Lee, J., Byeon, S. E., Yoo, D. S., Kim, M. H., Lee, S. Y., et al. (2011). Functional role of Akt in macrophage-mediated innate immunity. Front. Biosci. 16, 517–530. doi: 10.2741/3702
Li, E., Zhou, P., Petrin, Z., and Singer, S. M. (2004). Mast cell-dependent control of Giardia lamblia infections in mice. Infect. Immun. 72, 6642–6649. doi: 10.1128/IAI.72.11.6642-6649.2004
Li, P., Wu, Y. H., Zhu, Y. T., Li, M. X., and Pei, H. H. (2019). Requirement of Rab21 in LPS-induced TLR4 signaling and pro-inflammatory responses in macrophages and monocytes. Biochem. Biophys. Res. Commun. 508, 169–176. doi: 10.1016/j.bbrc.2018.11.074
Li, X., Zhang, X., Gong, P., Xia, F., Li, L., Yang, Z., et al. (2017). TLR2–/– mice display decreased severity of Giardiasis via enhanced proinflammatory cytokines production dependent on AKT signal pathway. Front. Immunol. 8:1186. doi: 10.3389/fimmu.2017.01186
Liu, S., Huang, L., Lin, Z., Hu, Y., Chen, R., Wang, L., et al. (2017). RhoB induces the production of proinflammatory cytokines in TLR-triggered macrophages. Mol. Immunol. 87, 200–206. doi: 10.1016/j.molimm.2017.04.015
Maloney, J., Keselman, A., Li, E., and Singer, S. M. (2015). Macrophages expressing arginase 1 and nitric oxide synthase 2 accumulate in the small intestine during Giardia lamblia infection. Microbes Infect. 17, 462–467. doi: 10.1016/j.micinf.2015.03.006
Matowicka-Karna, J., Dymicka-Piekarska, V., and Kemona, H. (2009). IFN-gamma, IL-5, IL-6 and IgE in patients infected with Giardia intestinalis. Folia Histochem. Cytobiol. 47, 93–97. doi: 10.2478/v10042-009-0013-3
Miller, R. L., Wang, A. L., and Wang, C. C. (1988). Identification of Giardia lamblia isolates susceptible and resistant to infection by the double-stranded RNA virus. Exp. Parasitol. 66, 118–123. doi: 10.1016/0014-4894(88)90056-2
Murphy, P. S., Wang, J., Bhagwat, S. P., Munger, J. C., Janssen, W. J., Wright, T. W., et al. (2017). CD73 regulates anti-inflammatory signaling between apoptotic cells and endotoxin-conditioned tissue macrophages. Cell Death Differ. 24, 559–570. doi: 10.1038/cdd.2016.159
Park, B., Buti, L., Lee, S., Matsuwaki, T., Spooner, E., Brinkmann, M. M., et al. (2011). Granulin Is a Soluble Cofactor for Toll-like Receptor 9 Signaling. Immunity 34, 505–513. doi: 10.1016/j.immuni.2011.01.018
Rescigno, M., Urbano, M., Valzasina, B., Francolini, M., Rotta, G., Bonasio, R., et al. (2001). Dendritic cells express tight junction proteins and penetrate gut epithelial monolayers to sample bacteria. Nat. Immunol. 2, 361–367. doi: 10.1038/86373
Roberts-Thomson, I. C., Stevens, D. P., Mahmoud, A. A., and Warren, K. S. (1976). Giardiasis in the Mouse: an Animal Model. Gastroenterology 71, 57–61. doi: 10.1016/S0016-5085(76)80097-2
Roxström-Lindquist, K., Palm, D., Reiner, D., Ringqvist, E., and Svärd, S. G. (2006). Giardia immunity-An update. Trends Parasitol. 22, 26–31. doi: 10.1016/j.pt.2005.11.005
Roxström-Lindquist, K., Ringqvist, E., Palm, D., and Svärd, S. (2005). Giardia lamblia-induced changes in gene expression in differentiated caco-2 human intestinal epithelial cells. Infect. Immun. 73, 8204–8208. doi: 10.1128/IAI.73.12.8204-8208.2005
Savioli, L., Smith, H., and Thompson, A. (2006). Giardia and Cryptosporidium join the “Neglected Diseases Initiative.”. Trends Parasitol. 22, 203–208. doi: 10.1016/j.pt.2006.02.015
Serradell, M. C., Rupil, L. L., Martino, R. A., Prucca, C. G., Carranza, P. G., Saura, A., et al. (2019). Efficient oral vaccination by bioengineering virus-like particles with protozoan surface proteins. Nat. Commun. 10:361. doi: 10.1038/s41467-018-08265-9
Sodhi, A., and Pandey, A. K. (2011). In vitro activation of murine peritoneal macrophages by recombinant YopJ: production of nitric oxide, proinflammatory cytokines and chemokines. Immunobiology 216, 358–366. doi: 10.1016/j.imbio.2010.07.002
Srivastava, S., Pandey, S. P., Jha, M. K., Chandel, H. S., and Saha, B. (2013). Leishmania expressed lipophosphoglycan interacts with Toll-like receptor (TLR)-2 to decrease TLR-9 expression and reduce anti-leishmanial responses. Clin. Exp. Immunol. 172, 403–409. doi: 10.1111/cei.12074
Stevens, D. P., and Roberts-Thompson, I. C. (1978). Animal model of human disease: giardiasis. Am. J. Pathol. 90, 529–532.
Takeda, K., and Akira, S. (2004). TLR signaling pathways. Semin. Immunol. 16, 3–9. doi: 10.1016/j.smim.2003.10.003
Tatematsu, M., Seya, T., and Matsumoto, M. (2014). Beyond dsRNA: toll-like receptor 3 signalling in RNA-induced immune responses. Biochem. J. 458, 195–201. doi: 10.1042/BJ20131492
Vadillo, E., and Pelayo, R. (2012). [Toll-like receptors in development and function of the hematopoietic system]. Rev. Invest. Clin. 64, 461–476.
Vercammen, E., Staal, J., and Beyaert, R. (2008). Sensing of viral infection and activation of innate immunity by toll-like receptor 3. Clin. Microbiol. Rev. 21, 13–25. doi: 10.1128/CMR.00022-07
Wang, A., Wang, C. C., and Alderete, J. F. (1987). Trichomonas Vaginalis phenotypic variation occurs only among trichomonads infected with the double-stranded RNA virus. J. Exp. Med. 166, 142–150. doi: 10.1084/jem.166.1.142
Wang, A. L., and Wang, C. C. (1986). Discovery of a specific double-stranded RNA virus in Giardia lamblia. Mol. Biochem. Parasitol. 21, 269–276. doi: 10.1016/0166-6851(86)90132-5
Wang, A. L., and Wang, C. C. (1991). Viruses of parasitic protozoa. Parasitol. Today 7, 76–80. doi: 10.1016/0169-4758(91)90198-W
Wu, J. Y., and Kuo, C. C. (2015). ADP-Ribosylation Factor 3 Mediates Cytidine-Phosphate-Guanosine Oligodeoxynucleotide-Induced Responses by Regulating Toll-Like Receptor 9 Trafficking. J. Innate Immun. 7, 623–636. doi: 10.1159/000430785
Xiang, Y., Yan, H., Zhou, J., Zhang, Q., Hanley, G., Caudle, Y., et al. (2015). The role of toll-like receptor 9 in chronic stress-induced apoptosis in macrophage. PLoS One 10:e0123447. doi: 10.1371/journal.pone.0123447
Xu, H., An, H., Yu, Y., Zhang, M., Qi, R., and Cao, X. (2003). Ras participates in CpG oligodeoxynucleotide signaling through association with toll-like receptor 9 and promotion of interleukin-1 receptor-associated kinase/tumor necrosis factor receptor-associated factor 6 complex formation in macrophages. J. Biol. Chem. 278, 36334–36340. doi: 10.1074/jbc.M305698200
Yasuda, K., Richez, C., Uccellini, M. B., Richards, R. J., Bonegio, R. G., Akira, S., et al. (2009). Requirement for DNA CpG Content in TLR9-Dependent Dendritic Cell Activation Induced by DNA-Containing Immune Complexes. J. Immunol. 183, 3109–3117. doi: 10.4049/jimmunol.0900399
Zangger, H., Hailu, A., Desponds, C., Lye, L. F., Akopyants, N. S., Dobson, D. E., et al. (2014). Leishmania aethiopica Field Isolates Bearing an Endosymbiontic dsRNA Virus Induce Pro-inflammatory Cytokine Response. PLoS Negl. Trop. Dis. 8:e2836. doi: 10.1371/journal.pntd.0002836
Zhao, P., Cao, L., Wang, X., Dong, J., Zhang, N., Li, X., et al. (2021). Extracellular vesicles secreted by Giardia duodenalis regulate host cell innate immunity via TLR2 and NLRP3 inflammasome signaling pathways. PLoS Negl. Trop. Dis. 15:e0009304. doi: 10.1371/journal.pntd.0009304
Zhou, P., Li, E., Shea-Donohue, T., and Singer, S. M. (2007). Tumour necrosis factor α contributes to protection against Giardia lamblia infection in mice. Parasite Immunol. 29, 367–374. doi: 10.1111/j.1365-3024.2007.00953.x
Keywords: Giardia duodenalis, TLR9, p38, ERK, cytokines
Citation: Pu X, Li X, Cao L, Yue K, Zhao P, Wang X, Li J, Zhang X, Zhang N, Zhao Z, Liang M and Gong P (2021) Giardia duodenalis Induces Proinflammatory Cytokine Production in Mouse Macrophages via TLR9-Mediated p38 and ERK Signaling Pathways. Front. Cell Dev. Biol. 9:694675. doi: 10.3389/fcell.2021.694675
Received: 13 April 2021; Accepted: 16 June 2021;
Published: 15 July 2021.
Edited by:
Gaoqian Feng, Burnet Institute, AustraliaReviewed by:
Steven Singer, Georgetown University, United StatesJean-Paul Motta, INSERM U1220 Institut de Recherche en Santé Digestive, France
Copyright © 2021 Pu, Li, Cao, Yue, Zhao, Wang, Li, Zhang, Zhang, Zhao, Liang and Gong. This is an open-access article distributed under the terms of the Creative Commons Attribution License (CC BY). The use, distribution or reproduction in other forums is permitted, provided the original author(s) and the copyright owner(s) are credited and that the original publication in this journal is cited, in accordance with accepted academic practice. No use, distribution or reproduction is permitted which does not comply with these terms.
*Correspondence: Pengtao Gong, Z29uZ3B0QGpsdS5lZHUuY24=
†These authors have contributed equally to this work