- 1Instituto de Neurobiología, Universidad Nacional Autónoma de México, Juriquilla, Mexico
- 2Instituto Nacional de Perinatología, Mexico City, Mexico
- 3Biotecnología Médica y Farmacéutica, Centro de Investigación y Asistencia en Tecnología y Diseño del Estado de Jalisco, Guadalajara, Mexico
There have been significant advances in understanding human embryogenesis using human pluripotent stem cells (hPSCs) in conventional monolayer and 3D self-organized cultures. Thus, in vitro models have contributed to elucidate the molecular mechanisms for specification and differentiation during development. However, the molecular and functional spectrum of human pluripotency (i.e., intermediate states, pluripotency subtypes and regionalization) is still not fully understood. This review describes the mechanisms that establish and maintain pluripotency in human embryos and their differences with mouse embryos. Further, it describes a new pluripotent state representing a transition between naïve and primed pluripotency. This review also presents the data that divide pluripotency into substates expressing epiblast regionalization and amnion specification as well as primordial germ cells in primates. Finally, this work analyzes the amnion’s relevance as an “signaling center” for regionalization before the onset of gastrulation.
Introduction
Contemporary developmental biology has the challenge of deciphering the molecular bases that regulate processes during development and how these elements interact to establish patterns and construct a functional organism. Before gastrulation, embryonic cells are in a pluripotent state, which confers them the ability to differentiate into all the cells derived from the germ layers. Although pluripotency is ephemeral during development, it also constitutes a dynamic phase with molecular changes representing the epiblast’s transition before, during and after implantation (Nakamura et al., 2016). Hence, the derivation and continuous maintenance of embryonic stem cell (ESC) lines derived from preimplanted embryos of different species, but especially of the mouse, open a new window to identify distinct pluripotent states in vitro with specific molecular profiles and development potentials.
Pluripotency can also be spatially regionalized, suggesting that it is more than a transitory identity to maintain the undifferentiated epiblast before the onset of morphogenesis. Thus, there may also be substrates in the same period, which probably depend on intrinsic and extrinsic signals—depending on their position—to acquire the competence to differentiate in the anterior-posterior structures (Peng et al., 2019). Although the knowledge on pluripotency focuses on the mouse embryo and its in vitro counterparts, the human pluripotency spectrum could be more “heterogenous” due to differences with murine development. For example, in primates the epiblast coexists with extraembryonic lineages (the amniotic epithelium and extraembryonic mesoderm (EXM) before the gastrulation stage (Boroviak and Nichols, 2017). Thence, these cellular interactions could imply pluripotency states still not characterized or present in the murine model compared with the human model. Analyzing the molecular and functional spectrum of human pluripotency (intermediate states, pluripotency subtypes, and regionalization) is essential to understand the initiation of human gastrulation and morphogenesis.
Specification of the Human Epiblast and Its Differences With the Mouse
The endoderm, ectoderm and mesoderm arise from the epiblast, whereas the placenta differentiates from the trophectoderm (TE), and the primitive endoderm (PE) generates the yolk sac. Later, the epiblast contains the necessary information to initiate morphogenesis and embryogenesis once implantation occurs (Hermitte and Chazaud, 2014). The epiblast and PE originate from the inner cell mass (ICM) during the mouse’s second lineage specification.
At a stage of 3.5 days post-fertilization (E3.5), the ICM coexpresses NANOG and GATA6, and, at E4.5, NANOG+ (epiblast) and GATA6+ (PE) populations are segregated by FGF4 signaling (Yamanaka et al., 2010). Thus, the FGF receptor 1 (FGFR1)+ cells together with the autocrine FGF4 signaling commit to the epiblast, while paracrine FGF4 signaling through FGFR1 and FGFR2 derive the PE lineage. The relatively low ERK levels in FGFR1+ cells trigger ETV and SPRYs (FGF targets), which induce negative feedback with ERK, increasing the NANOG expression and subsequent epiblast identity. In contrast, FGF-ERK signaling in FGFR1+/FGFR2+ cells activates alternative targets, such as DUSP, to reduce NANOG expression and GATA6 is maintained in the prospective PE (Kang et al., 2017). FGF signaling suppression through MEK inhibitor PD0325901 (PD032) or FGFR inhibitor PD173074 induces conversion into epiblast and no specification into the PE at the morula in murine (Figure 1A) and bovine cells (McLean et al., 2014; Bessonnard et al., 2017). Consistent with reducing MEK activity to establish naïve pluripotency (see later) in the preimplantanted epiblast, PD032 is an essential component of the cocktail to derive and maintain naïve mouse embryonic stem cells (mESCs) (Nichols et al., 2009). Like in the mouse, the ICM in human blastocyst at 6 days after in vitro fertilization (IVF), showed GATA6 and NANOG colocalization before the appearance of PE and epiblast at IVF day 7 (Roode et al., 2012). However, MEK or FGFR inhibitor treatment does not change NANOG, OCT4, or GATA4+ cell numbers, suggesting that FGF-MEK signaling is not required in human embryos for the second lineage specification (Roode et al., 2012). Therefore, although the segregation of the ICM into the PE and epiblast is similar in both species, their signaling pathways contrast. These data suggest that specific molecular and cellular mechanisms induce the initiation and maintenance of epiblast pluripotency in humans.
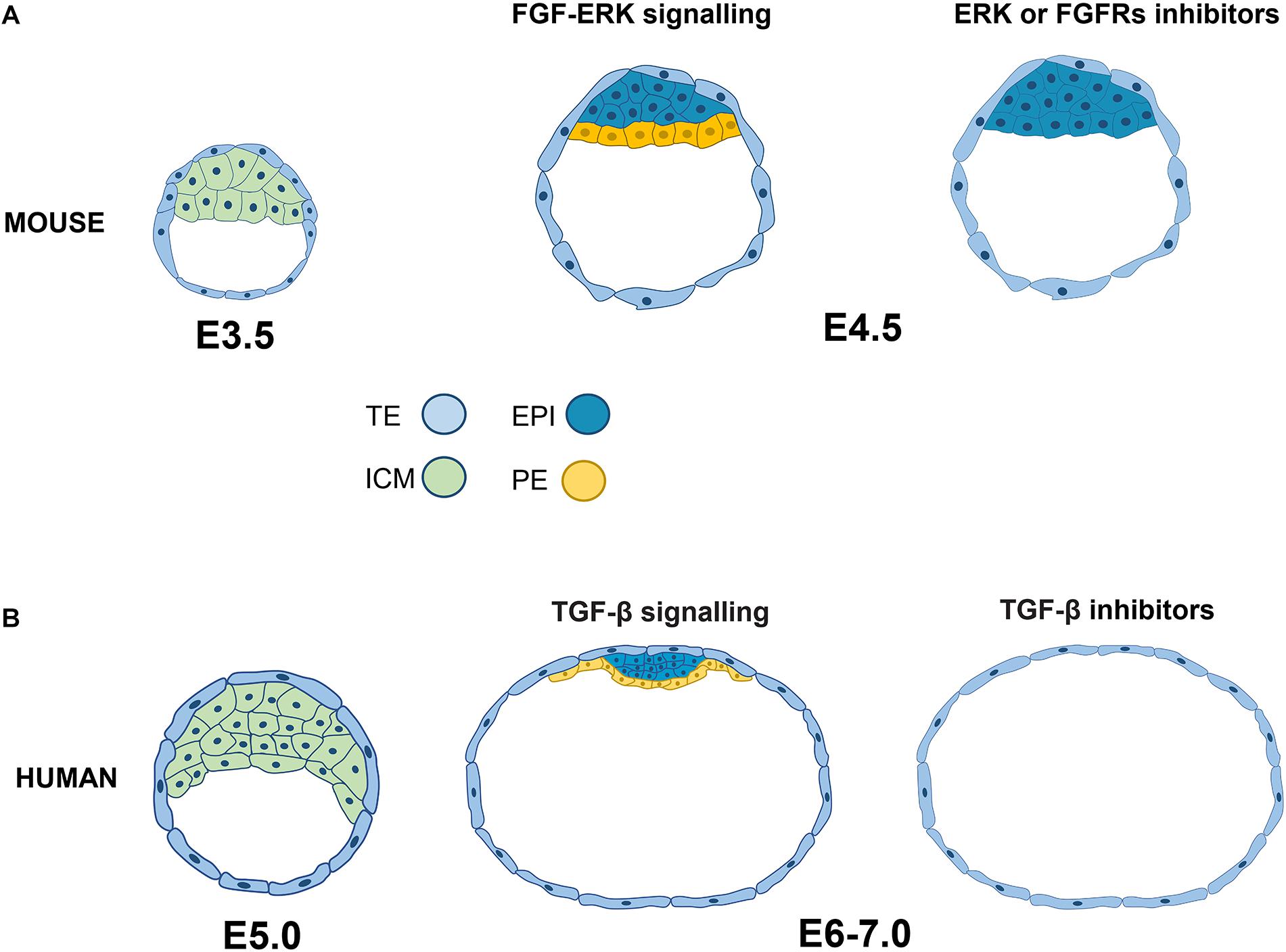
Figure 1. Signaling pathways related to the specification and segregation of pluripotent epiblast lineages in mouse and human. (A) In the mouse embryo at 3.5 days post-fertilization (E3.5), the ICM coexpresses NANOG and GATA6. By day 4.5, the ICM segregates into NANOG+ EPI and GATA6+ PE. EPI specification occurs in cells with low levels or suppression of the FGF-ERK pathway. (B) In human embryos, there is also colocalization of NANOG and GATA before lineage segregation, but the inhibition of FGF-ERK does not induce alterations in the specification of EPI (NANOG+, OCT4+) or PE (GATA4+). In contrast, blocking TGF-β signaling promotes the deficiency of both NANOG and SOX17, so this pathway is essential for the segregation of EPI and EP in the human embryo. ICM, inner cell mass; EPI, epiblast; PE, primitive endoderm; TE, trophoectoderm.
The Human Pluripotency Signaling Pathways
TGF-B Signaling in the Epiblast and Human Pluripotent Stem Cells (hPSC)
Unlike FGF signaling, the TGF-β pathway is crucial to defining and maintaining the human epiblast’s identity. TDGF1, TGFBR1, and ALK1 receptors, NODAL and GDF3 ligands and SMAD2/SMAD4 transcription factors are expressed in the human epiblast. Interestingly, SB431542 (SB) treatment disrupts the TGF-β/Activin/Nodal pathway through inhibition of ALK5, ALK4, and ALK7 receptors, which induces NANOG and SOX17 downregulation in human embryos at days 6–7, indicating that the TGF-β pathway is relevant for epiblast and PE segregation (Figure 1B). Moreover, SMAD2/SMAD5, TGFB1 and the negative regulator TGFBR3 are highly enriched in the trophectoderm (TE), suggesting the importance of this pathway in the first lineage specification (Blakeley et al., 2015).
Accordingly, the TGF-β/Activin/Nodal pathway is essential to maintain in vitro human pluripotency. hESCs cultured with iMEF conditioned medium (hESC-iMEFCM) or medium supplemented with Activin A (10 ng/mL) display SMAD 2/3 nuclear phosphorylation and have high OCT4 levels; while in differentiating cells growing in non-conditioned and non-supplemented media, the SMAD proteins decrease and their location is cytoplasmic (James et al., 2005; Vallier et al., 2005). hESCs treated with Activin A inhibitor (follistatin) lack the surface antigen TRA-1-60 expression, whereas the ALK4/5/7 receptors’ competitive inhibition by SB compromises their undifferentiated state and decreases NANOG and OCT4 levels as well as TRA-1-60 expression (James et al., 2005; Vallier et al., 2005). In contrast, SB treatment in mESCs induces low phospho-SMAD2 levels but does not affect the OCT4 level (James et al., 2005). Also, SB treatment does not affect NANOG+, OCT4+, or even SOX17+ cells in mouse blastocyst, contrasting with its human counterpart (Blakeley et al., 2015). Hence, the TGF-β/Activin/Nodal pathway activated through ALK receptors and mediated by SMAD2/3 is essential for hESCs and human pluripotency during development compared with preimplantation mouse embryo and mESCs. However, this pathway is not enough to support human pluripotency. Indeed, Activin or Nodal with FGF2 can maintain the pluripotent markers’ expression for a long time (more than ten passages) in the absence of feeders (Vallier et al., 2005).
A modified culture media supplemented with Activin A and small molecules (modulators of WNT activity) enhance the hESC derivation and single-cell clonal expansion (Ai et al., 2020). These data suggest that the TGF-β/Activin/Nodal pathway is essential in human pluripotency during development, but in hESCs its activation is not enough, as it requires synergy with other pathways like FGF2 and WNT.
FGF Pathway, the Dispensable Signaling for hPSCs
Exogenous FGF2 is enough to maintain pluripotency gene expression, promoting cell-cell adhesion and increasing survival (although not proliferation) in human pluripotent stem cells (hPSCs). The culture of hPSC (either on feeder layer or feeder-free conditions) dependent on FGF2 is known as the conventional condition. However, FGFR1, FGFR2, FGFR3, and FGFR4 expression has not been detected in epiblast; in contrast, FGFR1, FGFR2 and FGFR4 were enriched in PE (Niakan and Eggan, 2013; Kunath et al., 2014; Wamaitha et al., 2020). Furthermore, FGF2 is downregulated in epiblast compared with undifferentiated hESCs, which express the four FGF receptors and even FGF2 receptor isoforms, suggesting that the FGF2 dependence to support pluripotency is an aberrant condition in human pluripotency in vitro. However, exogenous FGF2 increases the phosphorylation of ERK1/2, AKT1/2/3, and GSK3α/β, suggesting downstream activation of MAPK and PI3K-Akt pathways and Wnt signaling regulation in hPSCs (Dvorak et al., 2005; Eiselleova et al., 2009; Ding et al., 2010). FGF receptor blockage with SU5402 or shRNA-mediated knockdown induces pluripotency marker downregulation and hESC differentiation, supporting the relevance of the FGF2 pathway to maintain the in vitro human pluripotency. LY294002 (inhibitor PI3K-Akt) administration significantly affects the hESC undifferentiated state compared with the ERK inhibition, suggesting that the principal downstream signaling cascade of FGF2 is PI3K/Akt rather than the MAPK pathway. Intriguingly, the EGF receptors (EGFR, ErbB2, and ErbB3), insulin receptor (INSR) and IGF receptor 1 (IGFR1) belong to the tyrosine kinase (RTK) family, which is highly phosphorylated in hESCs (Eiselleova et al., 2009). All these data suggest that alternative molecular pathways to FGFR activation can support human pluripotency and exist in the human epiblast.
IGF Pathways and Crosstalk With AKT/mTOR
INSR and IGFR1 are highly expressed in the epiblast as compared with TE and PE in human embryos. As previously mentioned, hESCs have these receptors, and the addition of IGF1 increases their survival and growth, suggesting a novel role for the IGF pathway to regulate human pluripotency. Hence, a defined culture medium with only Activin and IGF1 is enough to derive hESCs (AI hESC) and reprogram hiPSC, maintain a similar expression of pluripotency-associated genes compared to the FGF2-dependent condition and retain their pluripotent potential to generate derivatives of the three germ layers, demonstrated through directed and spontaneous differentiation protocols (Wamaitha et al., 2020).
In the human blastocyst, treatment with IGF1 induces NANOG+ cell proliferation but does not induce changes in the number of SOX17+ cells, indicating an IGF1 effect exclusively on the epiblast lineage (Wamaitha et al., 2020). Interestingly, FGF2 treatment does not have any impact on the human embryo. Thus, the FGF2 exogenous dependence to maintain pluripotency in hPSCs could be an artifice condition, where the role of IGF signaling in in vivo human pluripotency is supplanted. Indeed, phosphorylated AKT-S473 and p70S6 kinase detection in human blastocysts (7 days IVF) would suggest activation of PI3K-AKT and mTOR (mammalian target of rapamycin) pathways as the IGF1 downstream effectors, which are also activated in hPSCs and are essential for their survival and pluripotency (Wamaitha et al., 2020). mTOR selective inhibition with rapamycin induces death cells in hESCs, while blocking PI3K through LY294002 decreases the expression of pluripotent markers such as OCT4 and TRA-1-60 (Ding et al., 2010; Yilmaz et al., 2018). These data suggest that, unlike the FGF pathway, TGF-β signaling and the crosstalk between IGF/PI3K-AKT/mTOR pathways are indispensable during early development and maintain hPSC identity.
Modulation of the WNT Pathway in Human Pluripotency
The Wnt/ß-catenin pathway in synergy with LIF maintains mESCs self-renewal (Ogawa et al., 2006). However, the possible role of Wnt/ß-catenin in human pluripotency is contradictory. Its activation through GSK3 inhibition promotes self-renewal in hESC (Sato et al., 2004). Nonetheless, WNT signaling is not necessary for self-renewal and its activation induces differentiation (Dravid et al., 2005; Davidson et al., 2012). Thus, this suggests that the Wnt pathway promotes hESCs self-renewal or differentiation depending in the cellular location of ß-catenin: its retention in the cytoplasm by stabilizing AXIN2 supports the identity of the hESCs, but their nuclear translocation and interaction with TCF induces differentiation (Kim et al., 2013). ß-catenin also interacts with E-cadherin, α-catenin and actin in the cytoplasm, which would affect cell adhesion processes and signal transduction (Yamada et al., 2005).
This functional duality of the WNT signaling in human pluripotency could be explained by studies carried out under culture conditions where the hESCs are composed of heterogeneous populations. Indeed, the WNT pathway is decisive to the transition of pluripotent states. The diverse states of pluripotency that would reflect the progress of the epiblast during development will be discussed in the following sections.
HPSC, Artifice or In Vitro Counterpart of the Human Epiblast?
There are at least two types of pluripotency in the mouse (naïve and primed), which represent the pre- and postimplantation epiblast (preEPI and postEPI), respectively (Davidson et al., 2015; Avila-Gonzalez et al., 2016; Figure 2 and Table 1). Both states can be captured in vitro when the embryonic cells are adapted to grow in specific culture conditions. A defined medium supplemented with LIF, GSK3 inhibitor CHIR99021, and MEK inhibitor PD032 (2iLIF) is necessary to maintain the cells similar to those of the preEPI (Boroviak et al., 2014). On the other hand, the epiblast stem cells (EpiSCs) incubated with Activin A and FGF2 correspond to the in vitro counterpart of the postEPI (Brons et al., 2007). In a development context, the essence that distinguishes 2iL mESC from EpiSCs is that the first condition represents the “naïve ground state” of the preimplanted embryo, where embryonic cells do not have predefined programming but are rather a “blank slate” receiving the appropriate signals to start the specification and formation of lineage cells (Hackett and Surani, 2014). Therefore, 2iL mESC integrates efficiently into a preimplantation blastocyst, generating a chimera, while EpiSCs fail to integrate (Brons et al., 2007; Alexandrova et al., 2016).
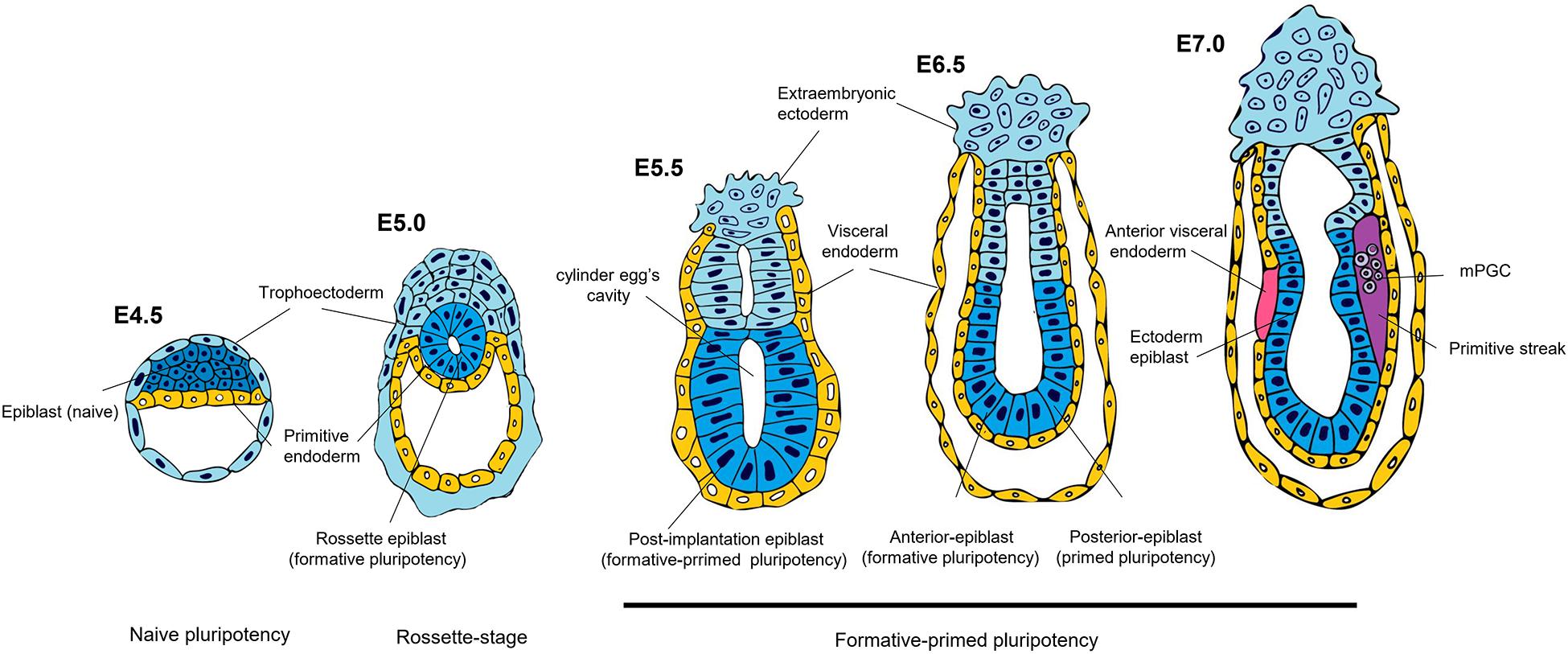
Figure 2. Spectrum of pluripotency in the mouse embryo. At 4.5 days post-fertilization (E4.5), the mouse epiblast has acquired a naïve state, the most sublime state of pluripotency. On E5.0 the blastocyst is implanted, where the epiblast forms a rosette structure. It has been proposed that this stage represents an intermediate pluripotency between the pre-implanted naïve and post-implanted primed states. In in vitro studies, this intermediate state has been described as formative pluripotency. On day 5.5 post-implantation, the formative-primed epiblast has a molecular and potential configuration different from that of the naïve state. On day 6.5, pluripotency can be divided into anterior and posterior regionalization of the epiblast. The anterior epiblast maintains a formative pluripotency, while the posterior epiblast acquires a primed pluripotency. The hypothesis proposes that on day 7.5, the posterior epiblast has a potential bias toward mesodermal precursors of the primitive streak, and the anterior epiblast acquires an ectoderm identity; its posteriorization is avoided by signals from the anterior visceral endoderm. Furthermore, mouse primordial germ cells (mPGCs) are specified in the posterior proximal epiblast from a pre-gastrulation subpopulation.
Regarding hESC, single-cell RNA sequencing demonstrated that conventional hESC and human epiblast have different transcriptomes with 1498 genes showing differential expression. Interestingly, the cells during the derivation method drastically change their gene expression; there was a downregulation in genes related to human epiblast such as NANOG, GDF3, ESRRB, DPPA5, IGF1, KLF4, and KLF17, with increased pluripotency-related (ZIC2, ZIC3, ID1, ID2, and ID3) and members of FGF and WNT signaling pathway genes. Since genes involved in the FGF pathway (FGF2, FGF5, FGFR1, and FGFR4) are also enriched in EpiSC, this was one of the first studies to suggest that conventional hESC are more similar to EpiSC than mESC, and therefore, to primed pluripotency (Yan et al., 2013).
Similarly, another single-cell RNA sequencing revealed that hESCs retain human-specific epiblast-enriched genes (KLF17 and the Nodal pathway) as well as a high expression of genes related to the FGF and WNT pathways (Blakeley et al., 2015). Additionally, principal component analysis has revealed that the presumptive transcriptional state of conventional hESCs, which do not cluster with the human blastocyst ICM and E5.0-E7.0 human epiblast, significantly resemble primed EpiSCs and the E5.5 mouse postEPI (Yang et al., 2019).
On the other hand, regarding the capture of the naïve state in human, diverse groups have reported obtaining this presumptive state using different cocktails of growth factors and small molecules (Avila-Gonzalez et al., 2016); but there is no consensus to establish the conditions to capture the in vitro human pluripotency that represents the counterpart of the preimplantation embryo. Several protocols report obtaining hPSC that express components of the circuitry which supports the naïve pluripotency of mouse epiblast and mESCs (Gafni et al., 2013; Takashima et al., 2014; Theunissen et al., 2014; Guo et al., 2016). However, naïve hPSC expressed human epiblast-enriched genes (DPPA3, DPPA5), which makes it more similar to the human epiblast than the primed conditions. They also overexpress pathways (FGF4) that are not represented in the human epiblast (Blakeley et al., 2015). According to the mESC 2iL potential, human naïve lines should be more reliable to recapitulate a differentiation process than conventional hESCs. However, presumptive naïve hESCs are deficient in differentiating at least into functional cells of cardiac and neuronal lineages, contrasting with primed hESCs (Warrier et al., 2017). Therefore, although conventional and naïve hPSCs share human epiblast molecular characteristics, the bizarre enrichment of signaling pathways and pluripotency-related genes might be an artifice due to the “unnatural” adaptation to proliferate in culture. Another possibility is that the current conditions to maintain hESCs derive a heterogeneous population mixing several “subtypes” of pluripotency.
Indeed, the failure to capture the human primed and naïve pluripotency could be explained by the broad pluripotency spectrum in primates. Delineation of the early developmental stages in the cynomolgus (cyno) monkeys by single-cell RNA seq, demonstrated that the epiblast segregates into preEPI, as well as early and late postimplantations (postE-EPI and postL-EPI). Thus, compared with cyno embryo cells, conventional hESCs have a higher correlation with the postL-EPI, compared to the naïve conditions with the preEPI (Nakamura et al., 2016; Table 2). Another series of studies in in vitro cyno embryo confirms that the later-stage cyno epiblast cells express genes related to primed pluripotency (ZIC2, ID1, and FGFR1) through single-cell seq (Niu et al., 2019), just like conventional hESC.
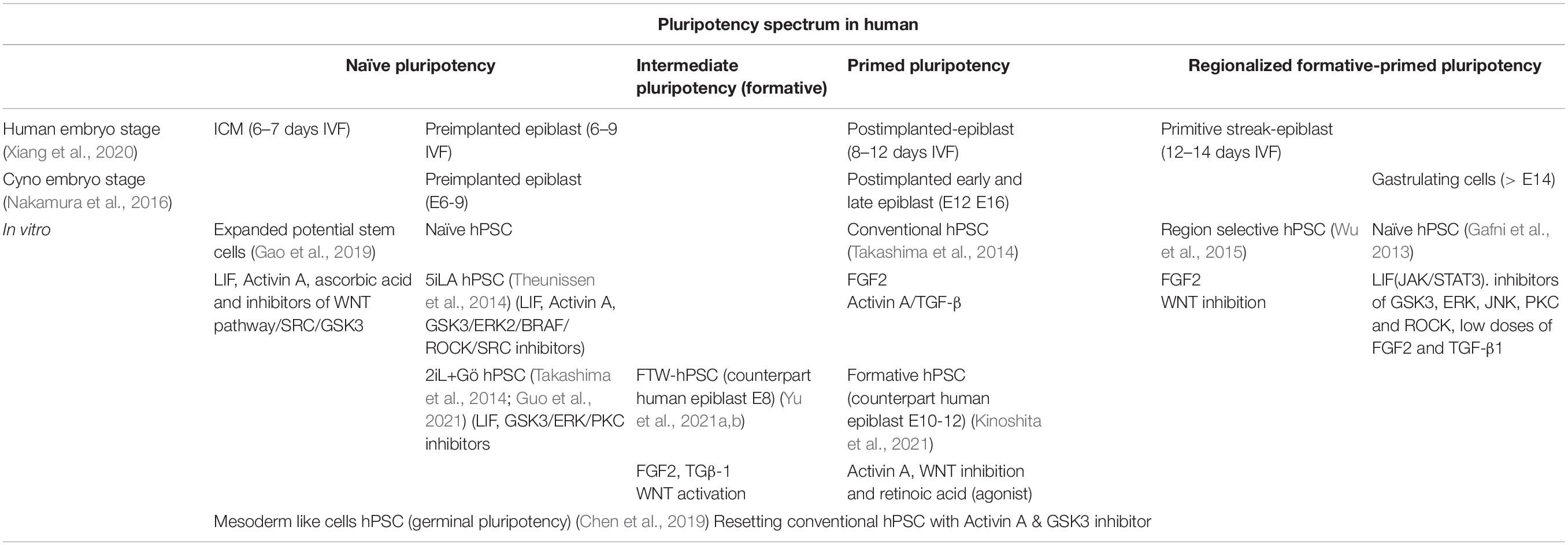
Table 2. Presumptive human pluripotent spectrum during the human development and its analogy with primate cyno.
Human epiblast embryos can be classified based on their transcriptomic profile into four stages: ICM (6–7 days IVF), preEPI (6–9 days IVF), postEPI (8–12 days IVF), and primitive streak-epiblast (PS-Epi) (12–14 days IVF) (Xiang et al., 2020). When hPSCs are compared to these transcriptomic profiles, the principal component analysis indicates that the naïve lines are clustered with PreEPI, while the primed lines are adjacent to postEPI, however, distributed toward the PS-Epi (Kinoshita et al., 2021). Therefore, conditions alternative to naïve and primed have been described in the literature where hESCs can recapitulate some fraction of this broad pluripotent spectrum of the human epiblast.
Finally, hPSC cultured with XAV939 (WNT inhibitor), Activin A and FGF2 (XAF) acquire the capacitance to substantial multi-differentiation through a transition period that might represent the epiblast progression during implantation, being a defined state different from naïve and primed (Rostovskaya et al., 2019). This intermediate phase, as well as other pluripotent substates, are described in the next section.
The Spectrum of Pluripotency in Mammals
Rossette-Stage Pluripotency in Mouse
A recent study has characterized an intermediate stage in mouse epiblast different from the naïve and primed stages. Before implantation (E4.5), the epiblast is identified by expressing naïve associated genes such as Klf4. Around E5.0, it develops a “rosette,” displaying nascent lumen at E5.5 (lumenogenesis), which will be the egg cylinder cavity (Figure 2). At the “rosette” stage, the cells are still Klf4+; however, they express Otx2 (primed pluripotency-associated gene), absent at E4.5. During the “lumen” stage, the epiblast maintains the Otx2 expression and colocalizes with Oct6 (post-implanted epiblast marker). There are defined signaling pathways to regulate this naïve-rosette-lumen transition in the mouse embryos. Treatment with IWP2, a Wnt inhibitor, prevents rosette formation. In contrast, an ERK inhibitor arrests the rosette cells and avoids lumenogenesis, demonstrating that downregulation of the Wnt pathway is necessary for the epiblast’s transition from the naïve stage into the rosette stage, while ERK signaling activation enhances the progression into the lumen stage. Therefore, cells in the rosette stage, named rosette-like stem cells (RSCs), can be captured in vitro with a defined cocktail (LIF/Wnt inhibitor IWP-2/MEK inhibitor PD032) (Table 1). They are KLF4+ /OTX2+ /OCT6- similar to the epiblast at E5.0 (rosette stage) and have a molecular profile different from 2iL mESCs (naïve) and primed epiblast-like cells (EpiLCs) and display an epigenetic landscape with a distinctive feature that possesses twice the number of bivalent genes. Also, they do not have a downregulation in naïve genes or present inactivation of the X chromosome. These data demonstrate the capture of a transition between naïve and primed states that depends on WNT and MEK/ERK signaling pathway inhibition for maintenance (Neagu et al., 2020).
Formative Pluripotency in Mouse and Human
Before the epiblast rosette stage was characterized, it had been suggested that pluripotency could have an intermediate state in addition to naïve and primed. Instead of being static and rigidly delimited, the intermediate state would be a continuum where the embryonic cells dynamically change their molecular, transcriptional and epigenetic profiles in response to autocrine signals coming from the cell-cell interaction or the extracellular environment to progress through development. According to this hypothesis, naïve cells transition into a formative phase and acquire competence for germ lineage induction and somatic lineage specification before entering primed pluripotency (Smith, 2017). The first evidence that pointed out the difference of formative from naïve and primed stages were carried out in mESCs. Thus, 2i medium was withdrawn and mESCs were monitored as they exited the naïve state. When the naïve factors disappeared, the expression of post-implantation molecules [Pou3f1 (Oct6), Otx2, Sox3, Sall2, and Fgf5] signaling pathways only present in the primed post-implantation period (ERK pathway), were observed. Also, de novo methylation levels associated with the expression of Dnmt3a/3b increased, mitochondrial respiration decreased and JAK/STAT, WNT, and mTOR signaling were downregulated (Kalkan et al., 2017).
Because WNT inhibition is important for the transition from the naïve to the rosette stage in mice, similar strategies have been designed to block this path to capture both mouse and human formative pluripotency in vitro. Activin A in a low concentration, XAV939 (WNT inhibitor) and an inverse agonist of the retinoic acid receptor (AlowXR) can derive mouse lines showing a correlation with pre-PS-Epi at stage E5.5-6.0 (Table 1). The embryonic cells cultured in AlowXR medium were designed as formative stem (FS) cells because they are more competitive for primordial germ cell (PGC) induction than EpiSC and naïve mESC; corresponding to a pluripotent formative state according to Austin Smith’s hypothesis. Similarly, naïve hPSC treated with AlowXR medium to obtain FS-like cells have a molecular profile similar to the human epiblast at 10–12 days IVF embryo (Kinoshita et al., 2021; Table 2).
Another study derived mouse and human intermediate PSC with activated FGF2, TGF-β, and WNT pathways (FTW-mESC and FTW-hPSC, respectively, Tables 1, 2). This experimental condition activates the WNT pathway (using high concentrations of WNT3A or GSK3 inhibitors such as SB216763 and CHIR99021). Intriguingly, comparable to FS cells, the FTW-mESC expression profile clusters are close to the mouse epiblast E5.5-6.0 and the cells are highly competent to form PGCs. At the same time, the FTW-hPSC transcriptome correlates to the human epiblast 8 days IVF embryo (Yu et al., 2021b).
On the other hand, after 2i withdrawal, a gene-specific network (including Klf5, Nr0b1, Nr5a2, and Pdgfa) related to naïve pluripotency was detected in mESCs, which is necessary for exiting from the naïve state and transitioning to the formative stage. Interestingly, this network was found not only in mouse embryos in the implantation stage (E5.5) but also in macaque postEPI and capacitance hPSCs (Lackner et al., 2021). These data suggest that conversion from the naïve to the formative phase is conserved in primates through a gene network mechanism.
Diversification of Postimplantation Pluripotency in Primates
In the mouse, the first pluripotent cells (Nanog+) appear at E3.5 in the ICM, differentiate into the epiblast and remain pluripotent after implantation at E5.5. However, the molecular network that maintains their identity is lost at around E7.5-8.0, once gastrulation begins at E6.5. In contrast with the mouse, whose pluripotency declines approximately 2 days after implantation, transcriptomic studies for in vivo and in vitro embryos in cyno monkeys have suggested that pluripotency extends far beyond post implantation until E16 or 16–20 IVF days (Nakamura et al., 2016; Ma et al., 2019; Niu et al., 2019). Thus, the temporal period of pluripotency is more extended in primates.
During post implantation, several critical outcomes take place: the yolk sac cavity develops, the amniotic epithelium arises (and lumenogenesis of amnion cavity occurs) and the EXM as well embryonic lineages (PGCs) and the primitive streak (PS) precursor specification take place (Niu et al., 2019). Moreover, the emergence of EXM and amniotic epithelium specification during implantation is a punctual difference that primates exhibit compared to murine (Boroviak and Nichols, 2017; Ross and Boroviak, 2020).
The amniotic epithelium is the innermost membrane that surrounds and protects the embryo during development, while the chorion and allantoides support the transport of nutrients and vascularization derived from EXM. Intriguingly, the amniotic epithelium derives from a subpopulation of the epiblast. In contrast, the origin of the EXM still is unknown, although it has been suggested that it may arise from the PE (hypoblast) or epiblast (Niu et al., 2019; Ross and Boroviak, 2020). It is unknown whether PGCs and precursors of PS derive from primed substates or an intermediate phase between naïve and primed pluripotency, although it has been proposed that they emerge from the epiblast and/or amnion (Sasaki et al., 2016; Ma et al., 2019; Niu et al., 2019) or from an intermediate pluripotent subpopulation (named germinal pluripotency; Chen et al., 2019). The cellular interactions between epiblast and the other extraembryonic lineages (at least the TE, PE and EXM) may be predicted through receptor-ligand database analyses (Niu et al., 2019). However, this has not been demonstrated experimentally in primate embryos. Thus, the multiple lineage interactions with the epiblast could influence its progression until the disappearance of its pluripotency, which would delimit other pluripotency subtypes still not characterized in primate embryos.
Germinal Pluripotency’ in Primates
One of the substates could be the putative “germline pluripotency” to specify germ cells. PGCs present pluripotency-related molecules and arise from a pluripotent source prior to gastrulation. The consensus to differentiate human PGCs (hPGCs) from conventional primed hPSCs involves Activin A and CHIR99021 exposure to induce incipient mesoderm-like cells (iMeLCs). In this transitory phase, embryonic cells form and then become hPGCs (Sasaki et al., 2015). Surprisingly, iMeLC express naïve and primed markers besides presenting an intermediate state. Upon identifying the cyno embryonic counterpart of the iMeLCs, researchers found that conventional primed hESCs were more similar to the postE-EPI and postL-EPI, whereas iMeLCs were consistent with ICM and preEPI as well as with postE-EPI and postL-EPI from cyno embryos. These data suggest that iMeLCs might recapitulate a transitory phase during implantation, where germline specification occurs, so it would be named “germinal pluripotency” (Chen et al., 2019; Table 2).
Interestingly, the signaling and molecular mechanisms that orchestrate germline specification are different in humans and mouse models (Irie et al., 2015; Kojima et al., 2017). Therefore, this “germinal pluripotency” would be primate-spectrum exclusive. Its signature gene expression (TFAP2A, EOMES GATA3, and BRACHYURY) is related to gastrulation and the amniotic epithelium, which is also derived from the human epiblast in the peri-implantation stage (Chen et al., 2019). Hence, once it leaves the naïve stage, does the human epiblast diverge into different postimplantation pluripotent subpopulations? Or is it competent for other lineages in addition to the three embryonic layers, such as the amnion and the germline?
Pluripotency Biased Toward Embryonic Lineages in hPSCs
hPSCs have subpopulations with a bias toward a particular lineage differentiation. Although they retain their high capacity for self-renewal and pluripotent potential, these subpopulations express specific lineage markers that allow them to have a strong differentiation bias. The GATA6+ hPSC subpopulation has a greater predisposition to differentiate into the endoderm lineage than GATA6– hPSCs in conventional conditions (KOSR/FGF on feeder layer, or E8 medium on feeder-free culture; Allison et al., 2018). On the other hand, E8 medium supplemented with BSA, cholesterol, CHIRON, IWP-2, and lysophophatidic acid promotes an enrichment of MIXL1+ hPSCs, which exhibit a mesodermal lineage-bias (Stavish et al., 2020). Besides, the culture medium may do more than induce heterogeneity in the hPSC differentiation potential. The change of feeder-free substrate from Vitronectin to Matrigel may influence two substates, identified by the VIMENTIN− or VIMENTIN+ population. The first type on Matrigel has an expression profile of genes involved in neuronal function (COL9A2, DGKI, GBX2, KIF26B, MARCH1, PLXNA4, SLC24A4, TLR4, and ZHX3) and a potential bias toward ectoderm lineage, in contrast with the second type cultured on Vitronectin (Mawaribuchi et al., 2019).
In light of the hPSC substrates in vitro, there would be primed subpopulations biased toward a specific embryonic layer in the post implanted human embryo. These substrates have not been characterized to complete the landscape of primed pluripotency, considering notable differences in human and other primates vs. mice in the context of their extraembryonic lineage interactions. In summary, the rosette stage in the mouse embryo, transitory in vitro iMeLCs (germinal pluripotency) in humans, and formative pluripotency in both species are clues to elucidate the transition between naïve and primed pluripotency.
Extra-Embryonic Potential of Naïve hPSC
It has recently been reported that hPSC can differentiate into both embryonic (epiblast) and extraembryonic (trophoblast and hypoblast) lineages. Therefore, it is possible to establish human pluripotent cells with the ability to differentiate into TE-like cells (named human expanded potential stem cells, hEPSC) with LIF, Activin A, ascorbic acid and inhibitors of both WNT pathway and SRCs and GSK3 kinases (Gao et al., 2019; Table 2).
Naïve hPSC-derived cells may express trophoblast markers (KRT7, TFAP2C, TEAD4, and GATA3) through spontaneous differentiation (embryonic body formation), directed differentiation to generate TE-like cells and derivation of human trophoblast stem cells (hTSC) lines (naïve-hTSC). Indeed, both TE-like cells and naïve-hTSC committed to extravillous trophoblast (EVT) and syncytiotrophoblast (STB) cells. Intriguingly, 2iL mESC cannot differentiate into TE lineages without genetic and epigenetic manipulations, while for primed hESC, its yield is meager as compared to the naïve condition to generate the trophoblast cells (KRT7, TFAP2C, TEAD4, and GATA3) (Dong et al., 2020; Guo et al., 2021; Io et al., 2021).
On the other hand, in naïve hPSC, Nodal, Wnt, and LIF activation pathways induce PE markers such as PDGFRA, GATA6, and NID2. These PE-like cells can be expanded in vitro for several passages (Linneberg-Agerholm et al., 2019). Hence naïve hPSC, unlike naïve mESC, and primed hPSC, are competent to differentiate into extraembryonic tissues, so the restriction of lineage paradigm would not be implemented in human pluripotency.
Indeed, the exceptional plasticity of human pluripotency is illustrated by recent reports, which generates blastocyst-like structures (blastoids) (Liu et al., 2021; Yu et al., 2021a). The blastoid-derived hPSC model would allow to study the dynamics of the pluripotent spectrum from the preimplantation period (specification of the epiblast, the plasticity of the naïve pluripotency, and changes in the epiblast during the implantation process, among others) and recognize their differences and similarities vs. the most studied pluripotency model of the mouse epiblast.
Spatial Regionalization of Pluripotency
Pluripotency not only progresses through a time window but is also regionalized. Its transcriptome’s dynamic expression throughout pre- and post-implanted mouse embryo showed that naïve pluripotency-related genes disappeared upon epiblast implantation, giving rise to a set of genes related to formative pluripotency at E5.5. As development continues, the first epiblast regionalization occurs, dividing into anterior and posterior regions at E6.5. Interestingly, the transcription of the formative genes is retained in the anterior epiblast, where the ectoderm specification begins at E7.0-7.5. In contrast, the posterior epiblast will produce a PS with a transcriptome profile related to primed pluripotency at E6.5 and decline its expression until E7.5 (Figure 2; Peng et al., 2019). These data suggest that pluripotency has not only a temporal progression component but also a spatial division. Indeed, embryonic cells acquire a regional identity before inducing their differentiation into either anterior or posterior structures (Metzis et al., 2018).
Interestingly, this spatial pluripotency has been described in humans. Belmonte’s laboratory discovered that hPSCs derived or maintained with FGF2 plus IWR1 displayed an alternative molecular profile different from conventional primed pluripotency (Table 2). According to their functional potential, these cells were denominated region-selective PSC (rsPSC) because they contribute to chimera formation in non-intact E7.5 mouse embryos only in the posterior region (Wu et al., 2015). rsPSC simulates in vitro features of the posterior epiblast and supports the existence of regional pluripotency in humans.
On the other hand, the blastoids which are composed of cells similar in morphology and transcriptomic profile to the three blastocyst lineages (TE, epiblast, and hypoblast), as well as with a specific spatial positioning that allowed the formation of a blastocele-like cavity (Liu et al., 2021; Yu et al., 2021a); corroborate the plasticity of hPSC to differentiate into extra-embryonic lineages, both from the first and second lineage specification of the blastocyst (Gao et al., 2019; Linneberg-Agerholm et al., 2019; Dong et al., 2020; Guo et al., 2021; Io et al., 2021). This expanded or bipotent potential of hPSCs reflects that the regional spectrum of human pluripotency might not only diverge during the post implantation period. Also, in the preimplantation stage there would be pluripotent subpopulations, one already committed to the epiblast lineage, and others with plastic capacity to give rise to the hypoblast or to retain the potential toward the TE.
Regarding the post implantation period pioneering studies demonstrated that hPSC self-organization resembles that of the embryonic sac, consisting of a cyst of asymmetric cells separated by a lumen that corresponds to the amniotic cavity. hPSCs are subjected to BMP4 signaling through a dorsal-ventral gradient and differentiate into amniotic ectoderm-like cells (AELCs) from the dorsal pole to achieve this lumenogenesis. Depending on the signals that hPSCs receive on the ventral side, embryo-like cysts acquire an anterior or posterior identity. In the posteriorized sacs, precursor cells of the PS and germline marker expression are observed at the ventral pole.
The anteriorized structure consists of AELCs on the dorsal side, separated by the lumen, and epiblast-like cells (EPILC) on the ventral part. EPILC transition to gastruloid-like cells is suppressed with inhibitors of the WNT and BMP pathways (Zheng et al., 2019). This suggests that once the amniotic cavity is formed, pluripotency is restricted in the anterior part of the embryo sac, since the PS and germ cell specification begins in the posterior embryo. Furthermore, this primed pluripotency subpopulation sheltered in the anterior region of the embryo coexists and interacts with the amniotic epithelium. Therefore, amniogenesis could be relevant for the developing embryo and notably for anteriorized structures.
The Amnion Arises From the Epiblast
Decades ago, in the absence of experimental evidence, the amnion’s origin from the epiblast was deduced from observations of histological sections of primate embryos. At around E7.0-9.0 (Carnegie stage 5a and 5b), amniogenesis begins in the human embryo, with a distinguished amniotic cavity at E10.0 (Carnegie stage 5c) (Figure 3; Hertig et al., 1956). Similarly, in the macaque embryo, the amniotic cavity lumen appears at E10.0 and is fully defined at E10.5-11.0 (Enders et al., 1986).
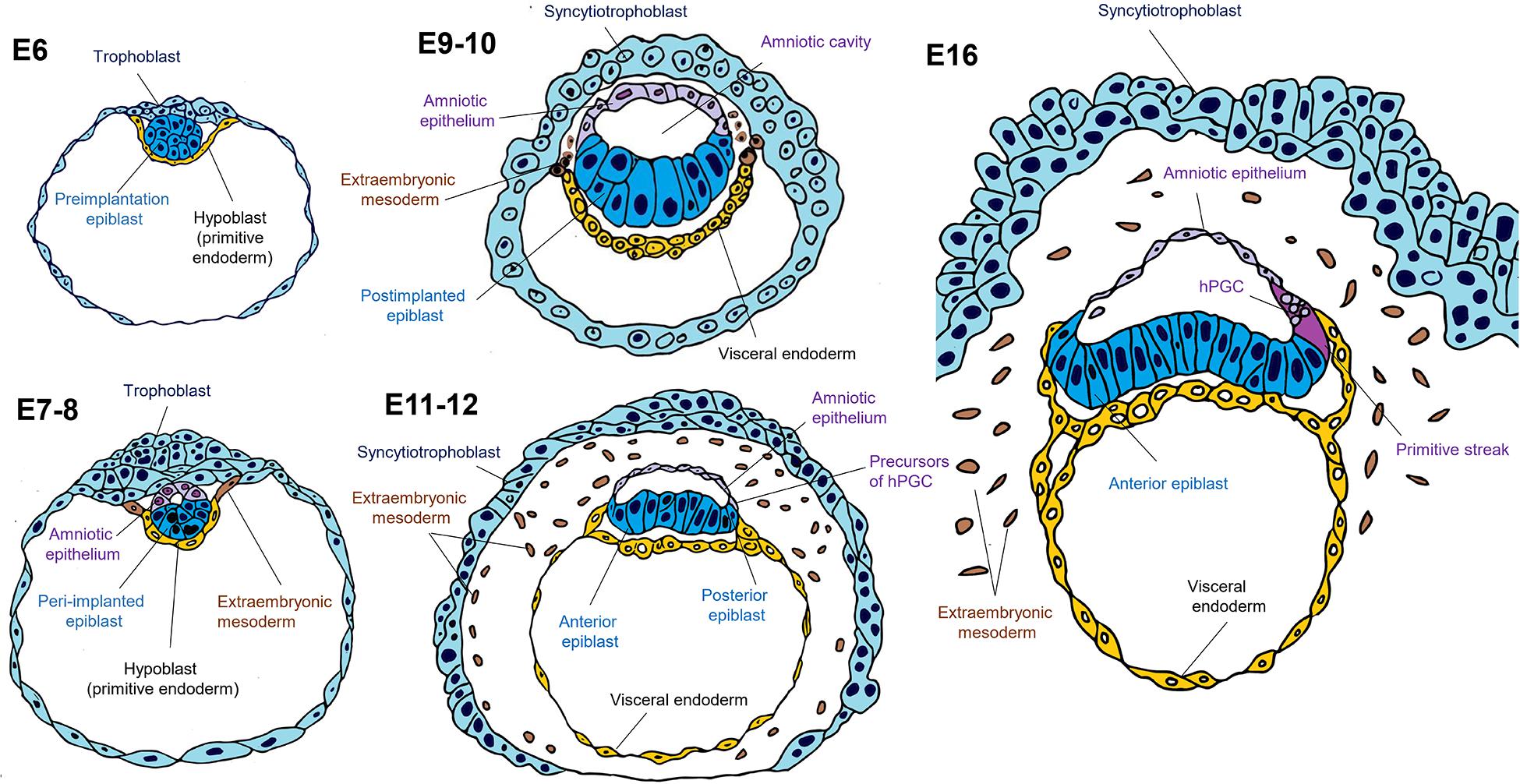
Figure 3. Spectrum of pluripotency in the human embryo. On embryonic day 6 (E6), the human blastocyst is pre-implanted, with probable naïve pluripotency. During implantation (E7-8), two lineages emerge in human embryos: the extra-embryonic mesoderm from the visceral endoderm (hypoblast) and the amniotic epithelium from the epiblast. On days 9–10, the amniotic cavity arises, while the post-implanted epiblast still expresses genes related to pluripotency but is no longer in the naïve state. It has been suggested that human primordial germ cell (hPGC) specification occurs at around E11-12, but also both lineages can arise from the same population that possesses germinal pluripotency. It is likely that the human epiblast, similar to the mouse, acquires an anterior-posterior regionalization with different subtypes of pluripotency biased toward specific lineages. On day 16, the onset of human gastrulation is preceded by the appearance of the primitive streak in the posterior region of the epiblast.
In 2016, two research groups independently reported for the first time the in vitro culture of human embryos to study their postimplantation development. Between days 7 and 8 after IVF, the embryos were adhered to a plate and implantation was simulated for up to 14 days. The research groups observed at 9–10 days that a subset of epiblast cells acquired an apical-basal polarization and organized radially around a lumen that corresponds to the nascent amniotic cavity (Deglincerti et al., 2016; Shahbazi et al., 2016).
On the other hand, the emergence of the amniotic cavity and the dissection of two OCT4+ populations, one columnar near the anterior visceral endoderm (AVE) and the other squamous on the TE side has been reported during in vitro culture of macaque embryos at 11–12 days IVF (Ma et al., 2019; Niu et al., 2019). Indeed, the single cell RNA-seq analysis carried out from recent 3D culture human embryo experiments confirmed that on 12–14 days IVF the amniotic epithelium and the epiblast are already two separate populations, each with a unique transcriptomic profile (Xiang et al., 2020).
On the other hand, it has been demonstrated that human amnion derives from the epiblast using a model of 3D self-organization of hPSCs. Thus, hPSCs were grown in a biomaterial system to mimic the implantation niche and formed a cyst constituted by a columnar epithelium (the presumptive epiblast) and squamous cells, which were considered AELCs (Shao et al., 2017; Zheng et al., 2019).
These elegant experiments confirm the hypothesis that the amniotic epithelium is derived from the human and cyno epiblast. However, the mechanisms involved in specifying the epiblast toward the amnion are still unknown.
Messmer et al. detected a subpopulation with specific genes (such as VGLL1, XAGE2, GATA3, ERP27, NR2F2, VTCN1, ODAM, HSD3B1, P2RY6, and EGFR) not expressed in naïve or primed hPSCs (Messmer et al., 2019). Besides, several of these genes are enriched in extraembryonic tissues (trophoblastic and endothelial cells from placenta) and upregulated in the amnion-like cells derived from hPSCs (VGLL1, XAGE2, GATA3, HSD3B1, and P2RY6) (Shao et al., 2017). Therefore, these “intermediate genes” sign a formative or primed pluripotent subpopulation that will originate the amnion.
On the other hand, experiments with cyno monkey embryos indicate that PGC specification is at around E11-12 days when the amnion is a separate lineage from the epiblast. Thus, it has been suggested that primate PGCs are specified from the nascent amnion (Sasaki et al., 2016; Niu et al., 2019). However, it is also possible that both amnion and PGCs derive from the same pluripotent population that later diverges (Figure 3; for PGCs, this subpopulation is described as “germinal pluripotency” in Chen et al., 2019). These data suggest that the epiblast subpopulation where amniogenesis occurs would be an intermediate state between naïve or primed pluripotency or part of the spectrum of primed epiblast amnion that precedes the PGCs or both share a common pluripotent lineage.
Possible Role of the Amnion in the Anterior-Posterior Regionalization
During cyno development, a disc-shaped embryo, composed of the columnar epithelium (epiblast), amniotic membrane, and yolk sac, and surrounded by the extraembryonic mesenchyme, is settled at E14. In this stage, the epiblast maintains the expression of a cluster of factors related to “pluripotent stemness” (POU5F1, NANOG, SOX2, and PRDM14) before gastrulation at E16. Still, the postL-EPI express genes associated with “neuron development” (Nakamura et al., 2016; Ma et al., 2019). In human embryo culture, it was also detected that the population considered postimplantation epiblast, apart from still expressing the transcriptional program related to pluripotency, begins to express genes involved with neural development (Xiang et al., 2020).
Therefore, in primates, neural induction occurs in the anterior region, as described in mice and chick embryos. The AVE is in contact with the most anterior part of epiblasts in mouse, chick and non-human primate. DKK1 and CER1, antagonists of WNT and BMP/TGF-β signaling pathways, respectively, are expressed in AVE at E11 during the development of cyno (Sasaki et al., 2016). Interestingly, WNT and BMP pathways are required to induce the formation of the PS in the posterior region of the epiblast in several species.
Thus, in human and non-human primates, the AVE would avoid the “posterization” of the anterior epiblast through WNT and BMP/TGF-β path inhibitors (Figure 4).
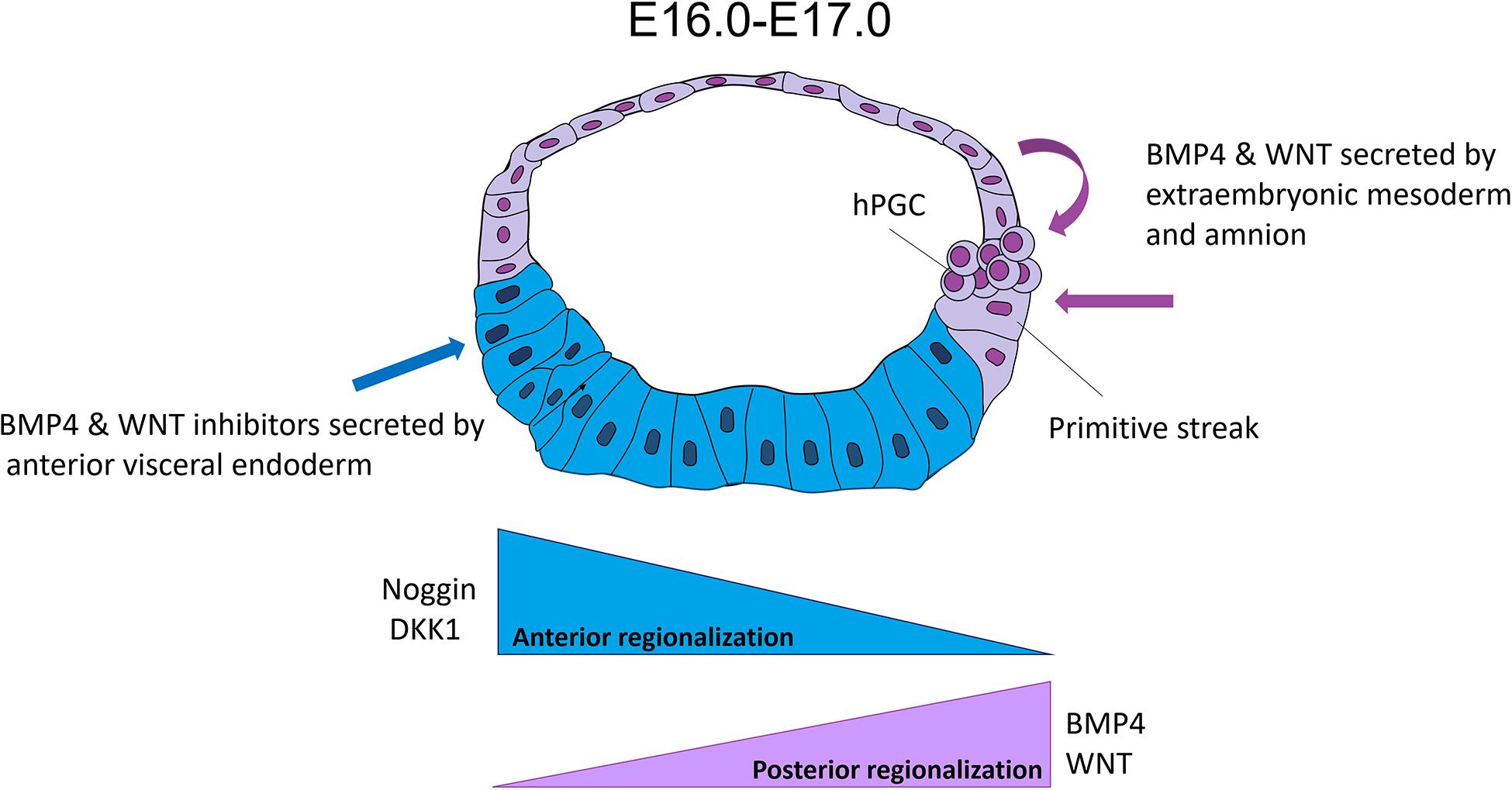
Figure 4. Hypothesis of the human epiblast regionalization. This hypothesis suggested that human pluripotency is regionalized into anterior and posterior. The posterior subpopulation has a differentiation bias toward mesoderm precursors to initiate gastrulation at E16, probably because of signals (WNT and BMP4) from the extraembryonic mesoderm and amniotic epithelium. On the other hand, the anterior region is still pluripotent and prevents its posteriorization by signals (negative regulators of BMP4 and WNT such as CER1 and DKK1, respectively) from the anterior visceral endoderm (AVE).
The amnion express components of WNT (WNT3, WNT6, and AXIN), BMP4/TGF-β (BMP4, ID2, and MSX2) and PI3K/Akt signaling pathways (Sasaki et al., 2016; Niu et al., 2019; Yang et al., 2020) during the cyno embryo development. The BMP4 decreased by the loss of ISL1 in the amnion induces a failure in the mesoderm formation of the posterior epiblast in this species (Yang et al., 2020). The amnion may also have an essential role in the PGC specification via BMP4 and WNT3 (Sasaki et al., 2016). All these data suggest that the amnion would have a role as an extraembryonic lineage and promotes the pattern of the anterior-posterior regionalization in epiblasts, in addition to the AVE at least in human and non-human primates (Figure 4).
Elucidating amnion ontogeny is relevant for embryonic development to complete the pluripotent spectrum of epiblast and its possible participation in the specification of lineages, such as PGC and the posterior mesoderm, at least in primates. As mentioned, hPSCs can self-organize into two embryonic like-sacs (ELS) with anterior (anterior-ELS) and posterior identity (posterior-ELS). Interestingly, AELCs are present in both cysts after an exposure to BMP4. The posterior-ELS comprises AELC in the dorsal part, whereas PS-like cells are found in the ventral zone. In accordance, hPSC co-culture with amniotic-like cells promotes their exit from pluripotency and begins to express PS markers (Zheng et al., 2019).
Likewise, in the anterior-ELS model, inhibition of WNT and BMP4 signaling paths avoid hPSC differentiation into posterior PS-like, maintained EPILC, where they coexist with the AELC. In addition, hESCs can be derived on a feeder layer of human amniotic epithelial cells, maintaining their expression of pluripotency-related markers and its functional potential demonstrated by the formation of embryoid bodies and teratomas (Avila-Gonzalez et al., 2015). Therefore, co-culture of isolated amniotic epithelial cells or AELC derived from hPSCs with embryonic cells is the experimental counterpart evidencing that there is a period in which the amniotic epithelium interacts with the epiblast, so it could have a role in the anterior-posterior regionalization such as the AVE.
The “default neural” model in developmental biology states that epiblast cells, in the absence of signaling for their fate, derivate ectoderm tissue (Munoz-Sanjuan and Brivanlou, 2002). However, before neural induction, the cells acquire a regional identity, which would provide the instructions and signals to establish the anterior-posterior pattern of the neuroectoderm derivatives (Metzis et al., 2018). We propose that the amniotic epithelium’s interaction is relevant for the differentiation of epiblast toward the anterior neuroectoderm fate. Indeed, the neuroectoderm is exposed to the amniotic fluid at E8.5 in the mouse embryo. Once the neural tube is closed, the amniotic fluid that remains inside the lumen would be the original cerebrospinal fluid (Chau et al., 2015). Therefore, amniotic fluid directly connects with the neuroepithelium, probably regulating embryonic neurogenesis and neural tube patterning.
As recent evidence supports the importance of the amnion and other extraembryonic lineages in human embryogenesis are two aspects where the interaction of the amnion with embryonic cells needed be studied. First, to analyze the co-culture of the amniotic-like cells with the hPSCs to simulate the peri-implantation period. With this model, to learn whether amnion specification is derived from an intermediate state between naïve and primed or shares the same origin subpopulation as the germline. In this way, studying amniogenesis would complete the spatiotemporal spectrum of human pluripotency. Second, to establish a model based on hPSC self-organization that contains two pluripotent subpopulations coexisting in the same ELS: one subpopulation with anterior fate starts neural induction, while the posterior subpopulation specifies into the mesoderm-PS. This model would also include a third group, AELC, to study their secreted factors or physical interaction with the subpopulations to promote neural induction and gastrulation.
Therefore, it can be inferred that state-of-the-art synthetic biology including the study of other non-embryonic lineages is relevant for the biology of human development and reproduction, fetal reprogramming and regenerative medicine.
Author Contributions
DÁ-G, WP, GG-L, AM-H, ND-M and ND wrote sections of the manuscript. All authors contributed to manuscript revision, read, and approved the submitted version.
Funding
This research was supported by grants from Instituto Nacional de Perinatologia (570) 212250-3230-21214-01-16 and CONACYT (A1-S-8450).
Conflict of Interest
The authors declare that the research was conducted in the absence of any commercial or financial relationships that could be construed as a potential conflict of interest.
Acknowledgments
We thank Jessica González and Susana Castro for their excellent technical assistance.
References
Ai, Z., Niu, B., Duan, K., Si, C., Wang, S., Xiang, L., et al. (2020). Modulation of Wnt and Activin/Nodal supports efficient derivation, cloning and suspension expansion of human pluripotent stem cells. Biomaterials 249:120015. doi: 10.1016/j.biomaterials.2020.120015
Alexandrova, S., Kalkan, T., Humphreys, P., Riddell, A., Scognamiglio, R., Trumpp, A., et al. (2016). Selection and dynamics of embryonic stem cell integration into early mouse embryos. Development 143, 24–34. doi: 10.1242/dev.124602
Allison, T. F., Smith, A. J. H., Anastassiadis, K., Sloane-Stanley, J., Biga, V., Stavish, D., et al. (2018). Identification and single-cell functional characterization of an endodermally biased pluripotent substate in human embryonic stem cells. Stem Cell Rep. 10, 1895–1907. doi: 10.1016/j.stemcr.2018.04.015
Avila-Gonzalez, D., Garcia-Lopez, G., Garcia-Castro, I. L., Flores-Herrera, H., Molina-Hernandez, A., Portillo, W., et al. (2016). Capturing the ephemeral human pluripotent state. Dev. Dyn. 245, 762–773. doi: 10.1002/dvdy.24405
Avila-Gonzalez, D., Vega-Hernandez, E., Regalado-Hernandez, J. C., De la Jara-Diaz, J. F., Garcia-Castro, I. L., Molina-Hernandez, A., et al. (2015). Human amniotic epithelial cells as feeder layer to derive and maintain human embryonic stem cells from poor-quality embryos. Stem Cell Res. 15, 322–324. doi: 10.1016/j.scr.2015.07.006
Bessonnard, S., Coqueran, S., Vandormael-Pournin, S., Dufour, A., Artus, J., and Cohen-Tannoudji, M. (2017). ICM conversion to epiblast by FGF/ERK inhibition is limited in time and requires transcription and protein degradation. Sci. Rep. 7:12285. doi: 10.1038/s41598-017-12120-0
Blakeley, P., Fogarty, N. M., del Valle, I., Wamaitha, S. E., Hu, T. X., Elder, K., et al. (2015). Defining the three cell lineages of the human blastocyst by single-cell RNA-seq. Development 142, 3151–3165. doi: 10.1242/dev.123547
Boroviak, T., and Nichols, J. (2017). Primate embryogenesis predicts the hallmarks of human naive pluripotency. Development 144, 175–186. doi: 10.1242/dev.145177
Boroviak, T., Loos, R., Bertone, P., Smith, A., and Nichols, J. (2014). The ability of inner-cell-mass cells to self-renew as embryonic stem cells is acquired following epiblast specification. Nat. Cell Biol. 16, 516–528. doi: 10.1038/ncb2965
Brons, I. G., Smithers, L. E., Trotter, M. W., Rugg-Gunn, P., Sun, B., Chuva de Sousa Lopes, S. M., et al. (2007). Derivation of pluripotent epiblast stem cells from mammalian embryos. Nature 448, 191–195. doi: 10.1038/nature05950
Chau, K. F., Springel, M. W., Broadbelt, K. G., Park, H. Y., Topal, S., Lun, M. P., et al. (2015). Progressive differentiation and instructive capacities of amniotic fluid and cerebrospinal fluid proteomes following neural tube closure. Dev. Cell 35, 789–802. doi: 10.1016/j.devcel.2015.11.015
Chen, D., Sun, N., Hou, L., Kim, R., Faith, J., Aslanyan, M., et al. (2019). Human primordial germ cells are specified from lineage-primed progenitors. Cell Rep 29, 4568–4582.e4565. doi: 10.1016/j.celrep.2019.11.083
Davidson, K. C., Adams, A. M., Goodson, J. M., McDonald, C. E., Potter, J. C., Berndt, J. D., et al. (2012). Wnt/beta-catenin signaling promotes differentiation, not self-renewal, of human embryonic stem cells and is repressed by Oct4. Proc. Natl. Acad. Sci. U.S.A. 109, 4485–4490. doi: 10.1073/pnas.1118777109
Davidson, K. C., Mason, E. A., and Pera, M. F. (2015). The pluripotent state in mouse and human. Development 142, 3090–3099. doi: 10.1242/dev.116061
Deglincerti, A., Croft, G. F., Pietila, L. N., Zernicka-Goetz, M., Siggia, E. D., and Brivanlou, A. H. (2016). Self-organization of the in vitro attached human embryo. Nature 533, 251–254. doi: 10.1038/nature17948
Ding, V. M., Ling, L., Natarajan, S., Yap, M. G., Cool, S. M., and Choo, A. B. (2010). FGF-2 modulates Wnt signaling in undifferentiated hESC and iPS cells through activated PI3-K/GSK3beta signaling. J. Cell Physiol. 225, 417–428. doi: 10.1002/jcp.22214
Dong, C., Beltcheva, M., Gontarz, P., Zhang, B., Popli, P., Fischer, L. A., et al. (2020). Derivation of trophoblast stem cells from naive human pluripotent stem cells. Elife 9:52504. doi: 10.7554/eLife.52504
Dravid, G., Ye, Z., Hammond, H., Chen, G., Pyle, A., Donovan, P., et al. (2005). Defining the role of Wnt/beta-catenin signaling in the survival, proliferation, and self-renewal of human embryonic stem cells. Stem Cells 23, 1489–1501. doi: 10.1634/stemcells.2005-0034
Dvorak, P., Dvorakova, D., Koskova, S., Vodinska, M., Najvirtova, M., Krekac, D., et al. (2005). Expression and potential role of fibroblast growth factor 2 and its receptors in human embryonic stem cells. Stem Cells 23, 1200–1211. doi: 10.1634/stemcells.2004-0303
Eiselleova, L., Matulka, K., Kriz, V., Kunova, M., Schmidtova, Z., Neradil, J., et al. (2009). A complex role for FGF-2 in self-renewal, survival, and adhesion of human embryonic stem cells. Stem Cells 27, 1847–1857. doi: 10.1002/stem.128
Enders, A. C., Schlafke, S., and Hendrickx, A. G. (1986). Differentiation of the embryonic disc, amnion, and yolk sac in the rhesus monkey. Am. J. Anat. 177, 161–185. doi: 10.1002/aja.1001770205
Gafni, O., Weinberger, L., Mansour, A. A., Manor, Y. S., Chomsky, E., Ben-Yosef, D., et al. (2013). Derivation of novel human ground state naive pluripotent stem cells. Nature 504, 282–286. doi: 10.1038/nature12745
Gao, X., Nowak-Imialek, M., Chen, X., Chen, D., Herrmann, D., Ruan, D., et al. (2019). Establishment of porcine and human expanded potential stem cells. Nat. Cell Biol. 21, 687–699. doi: 10.1038/s41556-019-0333-2
Guo, G., Stirparo, G. G., Strawbridge, S. E., Spindlow, D., Yang, J., Clarke, J., et al. (2021). Human naive epiblast cells possess unrestricted lineage potential. Cell Stem Cell 2:25. doi: 10.1016/j.stem.2021.02.025
Guo, G., von Meyenn, F., Santos, F., Chen, Y., Reik, W., Bertone, P., et al. (2016). Naive pluripotent stem cells derived directly from isolated cells of the human inner cell mass. Stem. Cell Rep. 6, 437–446. doi: 10.1016/j.stemcr.2016.02.005
Hackett, J. A., and Surani, M. A. (2014). Regulatory principles of pluripotency: from the ground state up. Cell Stem Cell 15, 416–430. doi: 10.1016/j.stem.2014.09.015
Hermitte, S., and Chazaud, C. (2014). Primitive endoderm differentiation: from specification to epithelium formation. Philos. Trans. R. Soc. Lond. B Biol. Sci. 369:537. doi: 10.1098/rstb.2013.0537
Hertig, A. T., Rock, J., and Adams, E. C. (1956). A description of 34 human ova within the first 17 days of development. Am. J. Anat. 98, 435–493. doi: 10.1002/aja.1000980306
Io, S., Kabata, M., Iemura, Y., Semi, K., Morone, N., Minagawa, A., et al. (2021). Capturing human trophoblast development with naive pluripotent stem cells in vitro. Cell Stem Cell 2:13. doi: 10.1016/j.stem.2021.03.013
Irie, N., Weinberger, L., Tang, W. W., Kobayashi, T., Viukov, S., Manor, Y. S., et al. (2015). SOX17 is a critical specifier of human primordial germ cell fate. Cell 160, 253–268. doi: 10.1016/j.cell.2014.12.013
James, D., Levine, A. J., Besser, D., and Hemmati-Brivanlou, A. (2005). TGFbeta/activin/nodal signaling is necessary for the maintenance of pluripotency in human embryonic stem cells. Development 132, 1273–1282. doi: 10.1242/dev.01706
Kalkan, T., Olova, N., Roode, M., Mulas, C., Lee, H. J., Nett, I., et al. (2017). Tracking the embryonic stem cell transition from ground state pluripotency. Development 144, 1221–1234. doi: 10.1242/dev.142711
Kang, M., Garg, V., and Hadjantonakis, A. K. (2017). Lineage Establishment and Progression within the Inner Cell Mass of the Mouse Blastocyst Requires FGFR1 and FGFR2. Dev. Cell 41, 8496–510.e495. doi: 10.1016/j.devcel.2017.05.003
Kim, H., Wu, J., Ye, S., Tai, C. I., Zhou, X., Yan, H., et al. (2013). Modulation of beta-catenin function maintains mouse epiblast stem cell and human embryonic stem cell self-renewal. Nat. Commun. 4:2403. doi: 10.1038/ncomms3403
Kinoshita, M., Barber, M., Mansfield, W., Cui, Y., Spindlow, D., Stirparo, G. G., et al. (2021). Capture of mouse and human stem cells with features of formative pluripotency. Cell Stem Cell 28, 453–471.e458. doi: 10.1016/j.stem.2020.11.005
Kojima, Y., Sasaki, K., Yokobayashi, S., Sakai, Y., Nakamura, T., Yabuta, Y., et al. (2017). Evolutionarily distinctive transcriptional and signaling programs drive human germ cell lineage specification from pluripotent stem cells. Cell Stem Cell 21, 517–532.e515. doi: 10.1016/j.stem.2017.09.005
Kunath, T., Yamanaka, Y., Detmar, J., MacPhee, D., Caniggia, I., Rossant, J., et al. (2014). Developmental differences in the expression of FGF receptors between human and mouse embryos. Placenta 35, 1079–1088. doi: 10.1016/j.placenta.2014.09.008
Lackner, A., Sehlke, R., Garmhausen, M., Giuseppe Stirparo, G., Huth, M., Titz-Teixeira, F., et al. (2021). Cooperative genetic networks drive embryonic stem cell transition from naive to formative pluripotency. EMBO J. 40:e105776. doi: 10.15252/embj.2020105776
Linneberg-Agerholm, M., Wong, Y. F., Romero Herrera, J. A., Monteiro, R. S., Anderson, K. G. V., and Brickman, J. M. (2019). Naive human pluripotent stem cells respond to Wnt, Nodal and LIF signalling to produce expandable naive extra-embryonic endoderm. Development 146:180620. doi: 10.1242/dev.180620
Liu, X., Tan, J. P., Schroder, J., Aberkane, A., Ouyang, J. F., Mohenska, M., et al. (2021). Modelling human blastocysts by reprogramming fibroblasts into iBlastoids. Nature 591, 627–632. doi: 10.1038/s41586-021-03372-y
Ma, H., Zhai, J., Wan, H., Jiang, X., Wang, X., Wang, L., et al. (2019). In vitro culture of cynomolgus monkey embryos beyond early gastrulation. Science 366:7890. doi: 10.1126/science.aax7890
Mawaribuchi, S., Aiki, Y., Ikeda, N., and Ito, Y. (2019). mRNA and miRNA expression profiles in an ectoderm-biased substate of human pluripotent stem cells. Sci. Rep. 9:11910. doi: 10.1038/s41598-019-48447-z
McLean, Z., Meng, F., Henderson, H., Turner, P., and Oback, B. (2014). Increased MAP kinase inhibition enhances epiblast-specific gene expression in bovine blastocysts. Biol. Reprod. 91:49. doi: 10.1095/biolreprod.114.120832
Messmer, T., von Meyenn, F., Savino, A., Santos, F., Mohammed, H., Lun, A. T. L., et al. (2019). Transcriptional heterogeneity in naive and primed human pluripotent stem cells at single-cell resolution. Cell Rep. 26, 815–824.e814. doi: 10.1016/j.celrep.2018.12.099
Metzis, V., Steinhauser, S., Pakanavicius, E., Gouti, M., Stamataki, D., Ivanovitch, K., et al. (2018). Nervous system regionalization entails axial allocation before neural differentiation. Cell 175, 1105–1118.e1117. doi: 10.1016/j.cell.2018.09.040
Munoz-Sanjuan, I., and Brivanlou, A. H. (2002). Neural induction, the default model and embryonic stem cells. Nat. Rev. Neurosci. 3, 271–280. doi: 10.1038/nrn786
Nakamura, T., Okamoto, I., Sasaki, K., Yabuta, Y., Iwatani, C., Tsuchiya, H., et al. (2016). A developmental coordinate of pluripotency among mice, monkeys and humans. Nature 537, 57–62. doi: 10.1038/nature19096
Neagu, A., van Genderen, E., Escudero, I., Verwegen, L., Kurek, D., Lehmann, J., et al. (2020). In vitro capture and characterization of embryonic rosette-stage pluripotency between naive and primed states. Nat. Cell Biol. 22, 534–545. doi: 10.1038/s41556-020-0508-x
Niakan, K. K., and Eggan, K. (2013). Analysis of human embryos from zygote to blastocyst reveals distinct gene expression patterns relative to the mouse. Dev. Biol. 375, 54–64. doi: 10.1016/j.ydbio.2012.12.008
Nichols, J., Silva, J., Roode, M., and Smith, A. (2009). Suppression of Erk signalling promotes ground state pluripotency in the mouse embryo. Development 136, 3215–3222. doi: 10.1242/dev.038893
Niu, Y., Sun, N., Li, C., Lei, Y., Huang, Z., Wu, J., et al. (2019). Dissecting primate early post-implantation development using long-term in vitro embryo culture. Science 366, 5754. doi: 10.1126/science.aaw5754
Ogawa, K., Nishinakamura, R., Iwamatsu, Y., Shimosato, D., and Niwa, H. (2006). Synergistic action of Wnt and LIF in maintaining pluripotency of mouse ES cells. Biochem. Biophys. Res. Commun. 343, 159–166. doi: 10.1016/j.bbrc.2006.02.127
Peng, G., Suo, S., Cui, G., Yu, F., Wang, R., Chen, J., et al. (2019). Molecular architecture of lineage allocation and tissue organization in early mouse embryo. Nature 572, 528–532. doi: 10.1038/s41586-019-1469-8
Roode, M., Blair, K., Snell, P., Elder, K., Marchant, S., Smith, A., et al. (2012). Human hypoblast formation is not dependent on FGF signalling. Dev. Biol. 361, 358–363. doi: 10.1016/j.ydbio.2011.10.030
Ross, C., and Boroviak, T. E. (2020). Origin and function of the yolk sac in primate embryogenesis. Nat. Commun. 11:3760. doi: 10.1038/s41467-020-17575-w
Rostovskaya, M., Stirparo, G. G., and Smith, A. (2019). Capacitation of human naive pluripotent stem cells for multi-lineage differentiation. Development 146:172916. doi: 10.1242/dev.172916
Sasaki, K., Nakamura, T., Okamoto, I., Yabuta, Y., Iwatani, C., Tsuchiya, H., et al. (2016). The germ cell fate of cynomolgus monkeys is specified in the nascent amnion. Dev. Cell 39, 169–185. doi: 10.1016/j.devcel.2016.09.007
Sasaki, K., Yokobayashi, S., Nakamura, T., Okamoto, I., Yabuta, Y., Kurimoto, K., et al. (2015). Robust in vitro induction of human germ cell fate from pluripotent stem cells. Cell Stem. Cell 17, 178–194. doi: 10.1016/j.stem.2015.06.014
Sato, N., Meijer, L., Skaltsounis, L., Greengard, P., and Brivanlou, A. H. (2004). Maintenance of pluripotency in human and mouse embryonic stem cells through activation of Wnt signaling by a pharmacological GSK-3-specific inhibitor. Nat. Med. 10, 55–63. doi: 10.1038/nm979
Shahbazi, M. N., Jedrusik, A., Vuoristo, S., Recher, G., Hupalowska, A., Bolton, V., et al. (2016). Self-organization of the human embryo in the absence of maternal tissues. Nat. Cell Biol. 18, 700–708. doi: 10.1038/ncb3347
Shao, Y., Taniguchi, K., Gurdziel, K., Townshend, R. F., Xue, X., Yong, K. M. A., et al. (2017). Self-organized amniogenesis by human pluripotent stem cells in a biomimetic implantation-like niche. Nat. Mater. 16, 419–425. doi: 10.1038/nmat4829
Smith, A. (2017). Formative pluripotency: the executive phase in a developmental continuum. Development 144, 365–373. doi: 10.1242/dev.142679
Stavish, D., Boiers, C., Price, C., Frith, T. J. R., Halliwell, J., Saldana-Guerrero, I., et al. (2020). Generation and trapping of a mesoderm biased state of human pluripotency. Nat. Commun. 11:4989. doi: 10.1038/s41467-020-18727-8
Takashima, Y., Guo, G., Loos, R., Nichols, J., Ficz, G., Krueger, F., et al. (2014). Resetting transcription factor control circuitry toward ground-state pluripotency in human. Cell 158, 1254–1269. doi: 10.1016/j.cell.2014.08.029
Theunissen, T. W., Powell, B. E., Wang, H., Mitalipova, M., Faddah, D. A., Reddy, J., et al. (2014). Systematic identification of culture conditions for induction and maintenance of naive human pluripotency. Cell Stem. Cell 15, 471–487. doi: 10.1016/j.stem.2014.07.002
Vallier, L., Alexander, M., and Pedersen, R. A. (2005). Activin/Nodal and FGF pathways cooperate to maintain pluripotency of human embryonic stem cells. J. Cell Sci. 118(Pt 19), 4495–4509. doi: 10.1242/jcs.02553
Wamaitha, S. E., Grybel, K. J., Alanis-Lobato, G., Gerri, C., Ogushi, S., McCarthy, A., et al. (2020). IGF1-mediated human embryonic stem cell self-renewal recapitulates the embryonic niche. Nat. Commun. 11:764. doi: 10.1038/s41467-020-14629-x
Warrier, S., Van der Jeught, M., Duggal, G., Tilleman, L., Sutherland, E., Taelman, J., et al. (2017). Direct comparison of distinct naive pluripotent states in human embryonic stem cells. Nat. Commun. 8:15055. doi: 10.1038/ncomms15055
Wu, J., Okamura, D., Li, M., Suzuki, K., Luo, C., Ma, L., et al. (2015). An alternative pluripotent state confers interspecies chimaeric competency. Nature 521, 316–321. doi: 10.1038/nature14413
Xiang, L., Yin, Y., Zheng, Y., Ma, Y., Li, Y., Zhao, Z., et al. (2020). A developmental landscape of 3D-cultured human pre-gastrulation embryos. Nature 577, 537–542. doi: 10.1038/s41586-019-1875-y
Yamada, S., Pokutta, S., Drees, F., Weis, W. I., and Nelson, W. J. (2005). Deconstructing the cadherin-catenin-actin complex. Cell 123, 889–901. doi: 10.1016/j.cell.2005.09.020
Yamanaka, Y., Lanner, F., and Rossant, J. (2010). FGF signal-dependent segregation of primitive endoderm and epiblast in the mouse blastocyst. Development 137, 715–724. doi: 10.1242/dev.043471
Yan, L., Yang, M., Guo, H., Yang, L., Wu, J., Li, R., et al. (2013). Single-cell RNA-Seq profiling of human preimplantation embryos and embryonic stem cells. Nat. Struct. Mol. Biol. 20, 1131–1139. doi: 10.1038/nsmb.2660
Yang, P., Humphrey, S. J., Cinghu, S., Pathania, R., Oldfield, A. J., Kumar, D., et al. (2019). Multi-omic profiling reveals dynamics of the phased progression of pluripotency. Cell Syst 8, 427–445.e410. doi: 10.1016/j.cels.2019.03.012
Yang, R. G. A., Kang, Y., Si, C., Chu, C., Zheng, Y., Chen, Z., et al. (2020). Amnion signals are essential for mesoderm formation in primates. bioRxiv [Preprint]. doi: 10.1101/2020.05.28.118703
Yilmaz, A., Peretz, M., Aharony, A., Sagi, I., and Benvenisty, N. (2018). Defining essential genes for human pluripotent stem cells by CRISPR-Cas9 screening in haploid cells. Nat. Cell Biol. 20, 610–619. doi: 10.1038/s41556-018-0088-1
Yu, L., Wei, Y., Duan, J., Schmitz, D. A., Sakurai, M., Wang, L., et al. (2021a). Blastocyst-like structures generated from human pluripotent stem cells. Nature 591, 620–626. doi: 10.1038/s41586-021-03356-y
Yu, L., Wei, Y., Sun, H. X., Mahdi, A. K., Pinzon Arteaga, C. A., Sakurai, M., et al. (2021b). Derivation of intermediate pluripotent stem cells amenable to primordial germ cell specification. Cell Stem. Cell 28, 550–567.e512. doi: 10.1016/j.stem.2020.11.003
Keywords: naïve, primed, amnion, formative, synthetic biology
Citation: Ávila-González D, Portillo W, García-López G, Molina-Hernández A, Díaz-Martínez NE and Díaz NF (2021) Unraveling the Spatiotemporal Human Pluripotency in Embryonic Development. Front. Cell Dev. Biol. 9:676998. doi: 10.3389/fcell.2021.676998
Received: 06 March 2021; Accepted: 21 May 2021;
Published: 23 June 2021.
Edited by:
Wei Jiang, Wuhan University, ChinaReviewed by:
Xudong Fu, Zhejiang University, ChinaGuangdun Peng, Chinese Academy of Sciences (CAS), China
Tianqing Li, Kunming University of Science and Technology, China
Copyright © 2021 Ávila-González, Portillo, García-López, Molina-Hernández, Díaz-Martínez and Díaz. This is an open-access article distributed under the terms of the Creative Commons Attribution License (CC BY). The use, distribution or reproduction in other forums is permitted, provided the original author(s) and the copyright owner(s) are credited and that the original publication in this journal is cited, in accordance with accepted academic practice. No use, distribution or reproduction is permitted which does not comply with these terms.
*Correspondence: Daniela Ávila-González, avila.dela@gmail.com; Néstor F. Díaz, nfdiaz00@yahoo.com.mx