- 1Guangdong Provincial Key Laboratory of Malignant Tumor Epigenetics and Gene Regulation, Guangdong-Hong Kong Joint Laboratory for RNA Medicine, Sun Yat-sen Memorial Hospital, Sun Yat-sen University, Guangzhou, China
- 2Medical Research Center, Sun Yat-sen Memorial Hospital, Sun Yat-sen University, Guangzhou, China
Pericytes (PCs), known as mural cells, play an important blood vessel (BV) supporting role in regulating vascular stabilization, permeability and blood flow in microcirculation as well as blood brain barrier. In carcinogenesis, defective interaction between PCs and endothelial cells (ECs) contributes to the formation of leaky, chaotic and dysfunctional vasculature in tumors. However, recent works from other laboratories and our own demonstrate that the direct interaction between PCs and other stromal cells/cancer cells can modulate tumor microenvironment (TME) to favor cancer growth and progression, independent of its BV supporting role. Furthermore, accumulating evidence suggests that PCs have an immunomodulatory role. In the current review, we focus on recent advancement in understanding PC’s regulatory role in the TME by communicating with ECs, immune cells, and tumor cells, and discuss how we can target PC’s functions to re-model TME for an improved cancer treatment strategy.
Introduction
Pericytes (PCs) are embedded in the basement membrane of blood microvessels (Bergers and Song, 2005), which play a vital role in regulating physiological and pathological events, including vascular development, homeostasis, fibrosis, and stroke. Generally, PCs are responsible for the regulation of vascular stabilization, vascular permeability, blood flow, and angiogenesis along with endothelial cells (ECs) in blood vessels (BVs) (Hellstrom et al., 2001; Pallone and Silldorff, 2001; Enge et al., 2002). In angiogenesis, sprouting ECs secrete platelet derived growth factor (PDGF) to recruit platelet derived growth factor receptor-beta (PDGFR-β) positive mural cells (including PCs), which then interact with ECs and stabilize the newly formed BVs (Carmeliet and Jain, 2000). Unlike other stromal cells, PCs can be distinguished by dynamic molecular marker expression pattern under different conditions, such as PDGFR-β, CD13 (alanine aminopeptidase), Cluster of differentiation 146 (CD146), alpha-smooth muscle actin (α-SMA) (Dermietzel and Krause, 1991; Lindahl et al., 1997; Ozerdem et al., 2001). In recent years, PCs are defined as heterogeneous, tissue-specific, and multipotent cell population in BVs (Ferland-McCollough et al., 2017), which are mainly due to their tissue/organ-specific roles (Shepro and Morel, 1993; Armulik et al., 2005; Corselli et al., 2013; Kitano and Bloomston, 2016) and ability to give rise to various cell populations (Dore-Duffy et al., 2006; Dellavalle et al., 2007; Crisan et al., 2008; Olson and Soriano, 2011). During tumor angiogenesis, defective EC–PC interaction is one of the major causes of the formation of dysfunctional tumor vasculature and hypoxic tumor microenvironment (TME), which favors cancer growth and metastasis (Song et al., 2005). Therefore, it is important to investigate the underlying role of PCs in modulating tumor angiogenesis and TME in order to develop an improved anti-cancer treatment.
Anti-angiogenetic therapy is recognized as a promising treatment strategy for cancer, while many anti-angiogenic drugs have been approved for certain types of cancers, including anti-vascular endothelial growth factor (VEGF) drug (i.e., Bevacizumab, Ranibizumab) and some tyrosine kinase inhibitors (i.e., Sorafenib, Sunitinib) (Meng et al., 2015; Ramjiawan et al., 2017; Li et al., 2018). However, the resistance to anti-angiogenetic therapy have jeopardized their clinical benefits in cancer patients (Ramjiawan et al., 2017; Li et al., 2018). Previous studies suggested that PCs can protect ECs from anti-angiogenic therapies probably by secreting pro-angiogenic factors (Franco et al., 2011) or soluble factors (Prete et al., 2018). In addition, PCs may increase their coverage around tumor BVs adaptively and cause resistance to anti-angiogenetic therapy in preclinical models (Benjamin et al., 1999; Bergers and Hanahan, 2008). Nevertheless, combination treatment with PDGF receptor kinase inhibitor erlotinib/imatinib and bevacizumab showed very limited benefits in the clinical trials and even displayed an additive toxicity in some cancer patients (Hainsworth et al., 2007). The failure behind these trials suggests that PC may have other potential roles in controlling tumor growth and progression. Indeed, recent work from our laboratory shows that PC can regulate tumor cell growth via paracrine signals controlled by β3-integrin (Wong et al., 2020), independent of its BV supporting function, suggesting that its regulatory role in the TME is far more complicated than we previously thought.
In this review, we will exploit the current progress of understanding the role of PC in regulating TME, and discuss its functions in regulating tumor cells and other stromal cells to dictate cancer growth and progression. For comprehensive reviews of its role in BV formation and supporting function, please see Betsholtz and Crivellato (Armulik et al., 2011; Ribatti et al., 2011).
Crosstalk Between Pericytes and Tumor/Stromal Cells in Tumor Microenvironment
Although the composition of TME varies in different cancer types, some features seem to be typical characteristics in most solid tumors. Indeed, TME consists of cancer cells and some non-malignant cells, including ECs, PCs, immune inflammatory cells, cancer-associated fibroblasts (CAFs), and also extracellular matrix (ECM) components (including cytokines, chemokines, matrix metalloproteinases, integrins, and other secreted molecules) (Hanahan and Weinberg, 2011). In this section, we review and discuss the multifaceted roles of PCs in regulating tumor cell and stromal cell’s functions in details.
Abnormal Endothelial Cell–Pericyte Interaction and Signaling in Tumor Vasculature
Endothelial cells are the fundamental cells lining the interior face of BV walls, which are surrounded by quiescent mural cells (including PCs). PCs are capable of interacting with newly proliferating ECs to form nascent BVs and secrete angiogenetic factors to stabilize the newly-formed vessels (Abramsson et al., 2003). In tumorigenesis, defective EC–PC interaction leads to the formation of disorganized tumor vasculature (Ferland-McCollough et al., 2017). This is because PC is an essential mediator to maintain the integrity of tumor BVs, while PDGF-B/PDGFR-β signaling is critical for controlling PC migration during angiogenesis. Preceding findings have suggested that PDGFR-β mediated paracrine loop activates ECs to produce PDGF-B in order to recruit PDGFR-β-positive PCs, which in return releases VEGF-A and Ang-1 to stabilize the newly formed BVs (Armulik et al., 2005). Afterward, Ang-1 regulates the maturation and integrity of BV through binding to the endothelial cell-Tie-2 receptor (Harrell et al., 2018). During sprouting angiogenesis, ECs can also secrete Ang-2 to compete with Ang-1 for the binding to endothelial cell-Tie-2 receptor, which in turn destructs EC–PC interaction and destabilizes BVs (Saharinen et al., 2017). Interestingly, overexpression of PDGF-B by ECs causes an increase in PC coverage and vascular stability as well as accelerated tumor progression (Guo et al., 2003; Furuhashi et al., 2004). Moreover, tumor-derived PDGF-B induces endothelial cell-SDF-1α secretion, which then promotes PC migration and recruitment during tumor angiogenesis (Song et al., 2009). Furthermore, EC- and PC-derived HB-EGF (heparin-binding epidermal growth factor-like growth factor) activates EGFR (epidermal growth factor receptor) specifically in tumor-associated perivascular cells, resulting in increased PC coverage and enhanced angiogenesis (Nolan-Stevaux et al., 2010). Conversely, it has been suggested that inadequate PDGF-B in the stroma results in inappropriate attachment of PCs to ECs (Raza et al., 2010). Previous works have demonstrated that the blockade of Notch signaling inhibits vascular co-option and disrupts the EC-PC interaction during tumor angiogenesis (Hernandez et al., 2013), while Jagged-1 expression and Notch signaling are shown to be important for the growth of ECs and PCs as well as the maintenance of EC–PC interaction (Tattersall et al., 2016). In the study of Meng et al. (2015), they discover that ECs and PCs can build an “EC-PC shield” to protect tumor cells from cancer-directed therapy and immune surveillance in the TME, while the maintenance of BV integrity ensures an adequate oxygen and nutrient supply to tumor cells, which in turn promotes cancer growth and progression (Ferland-McCollough et al., 2017). Indeed, clinical studies show that high BV’s PC coverage is associated with increased tumor growth and poor prognosis (Furuhashi et al., 2004), while it is correlated with reduced distant metastasis in colorectal cancer patients (Yonenaga et al., 2005). Overall, these findings suggest that PC overabundance and deficiency occur in different tumor types during vascularization with mixed clinical outcome, implying that targeting PC coverage may not be an ideal strategy for anti-cancer treatment. Instead, our recent study indicates that PC-derived paracrine signals can modulate tumor cell growth independent of its BV supporting role and coverage (Wong et al., 2020), suggesting that targeting PC derived paracrine signals could be an alternative method for cancer therapy.
Direct Paracrine Crosstalk Between Tumor Cells and Pericytes Determines Cancer Growth and Progression
Although PCs have been considered as a critical compartment of the TME, the underlying mechanism of tumor cell–PC interaction has yet to be elucidated. Recently, we have shown that high percentage of mural-β3-integrin negative BVs correlates with increased tumor size and progression in multiple cancers (Wong et al., 2020), while PC specific knock out of β3-integrin expression enhances tumor growth independent of its BV supporting role. Further mechanistic study shows that loss of PC-β3-integrin expression increases the production of paracrine factors, including CCL2, CXCL1, and TIMP1, via activation of the FAK-HGFR-Akt-NF-κB signaling pathway, while PC-derived CCL2 enhances MEK1-ERK1/2-ROCK2 mediated tumor growth, suggesting that inhibition of ROCK in tumors with low PC-β3-integrin expression could potentially control cancer growth (Wong et al., 2020). Interestingly, a recent study from Lechertier et al. (2020) show that loss of PC FAK enhances p-Pyk2-Gas6-Axl-Akt signaling and its downstream Cyr61 expression to stimulate tumor angiogenesis, while PC-derived Cyr61 is also able to enhance tissue factor expression in tumor cells and its mediated cell proliferation. This work provides first evidence that PCs can crosstalk with ECs and tumor cells via the same paracrine signal (Lechertier et al., 2020). Furthermore, Caspani et al. study shows that a pathogenic crosstalk between PCs and tumor cells determines glioblastoma progression in mouse models (Caspani et al., 2014).
Pericytes Modulate Immunosuppressive Tumor Microenvironment
Inflammatory cells, an important component in the TME, are often associated with the inflammatory and immune responses in carcinogenesis. It is known that solid tumors are infiltrated by various innate and adaptive immune cells with both pro-tumor and anti-tumor functions (Turley et al., 2015). Previous works have shown that PCs release chemokines and cytokines in response to the pro-inflammatory stimulus, such as CCL2, CCL3, CXCL1, IFN-γ, and IL-8 (Navarro et al., 2016), while they also express some functional pattern-recognition receptors (i.e., TLR4, TLR2, NOD1) and macrophage markers (i.e., ED-2), implying that they may also have a role in modulating immune response (Navarro et al., 2016). Interestingly, PCs display phagocytic and pinocytic ability and regulate different types of leukocytes trafficking (Navarro et al., 2016). Accordingly, tumor PCs have distinct effects on tumor-associated macrophages (TAMs) in TME, while IL-33 produced by PDGF-BB-stimulated PCs has been shown to recruit TAMs in order to promote cancer metastasis in several human and mouse xenograft models (Figure 1, process ➀) (Yang et al., 2016). PC-derived chemokine CXCL12 (SDF-1) can trigger the EGF/CSF-1 paracrine invasion loop to mediate the co-migration of TAM and tumor cells, after binding to its receptor CXCR4 on both TAMs and tumor cells (Figure 1, process ➁) (Qian and Pollard, 2010). Meanwhile, crosstalk between M2-like macrophages and PCs in glioblastoma (GBM) promotes PC recruitment and upregulates the expression of the proangiogenic ECM component periostin deposition in PCs through the CECR1–PDGF-B–PDGFR-β signaling pathway (Zhu et al., 2017). In the pdgfbret/ret mouse model, PCs deficiency-driven hypoxia result in IL-6 upregulation and an increased myeloid-derived suppressor cell (MDSC) transmigration in tumors, and the MDSC accumulation leads to increased tumor growth, while the amounts of circulating malignant cells can be abrogated upon the recovery of PC coverage (Figure 1, process ➂) (Hong et al., 2015).
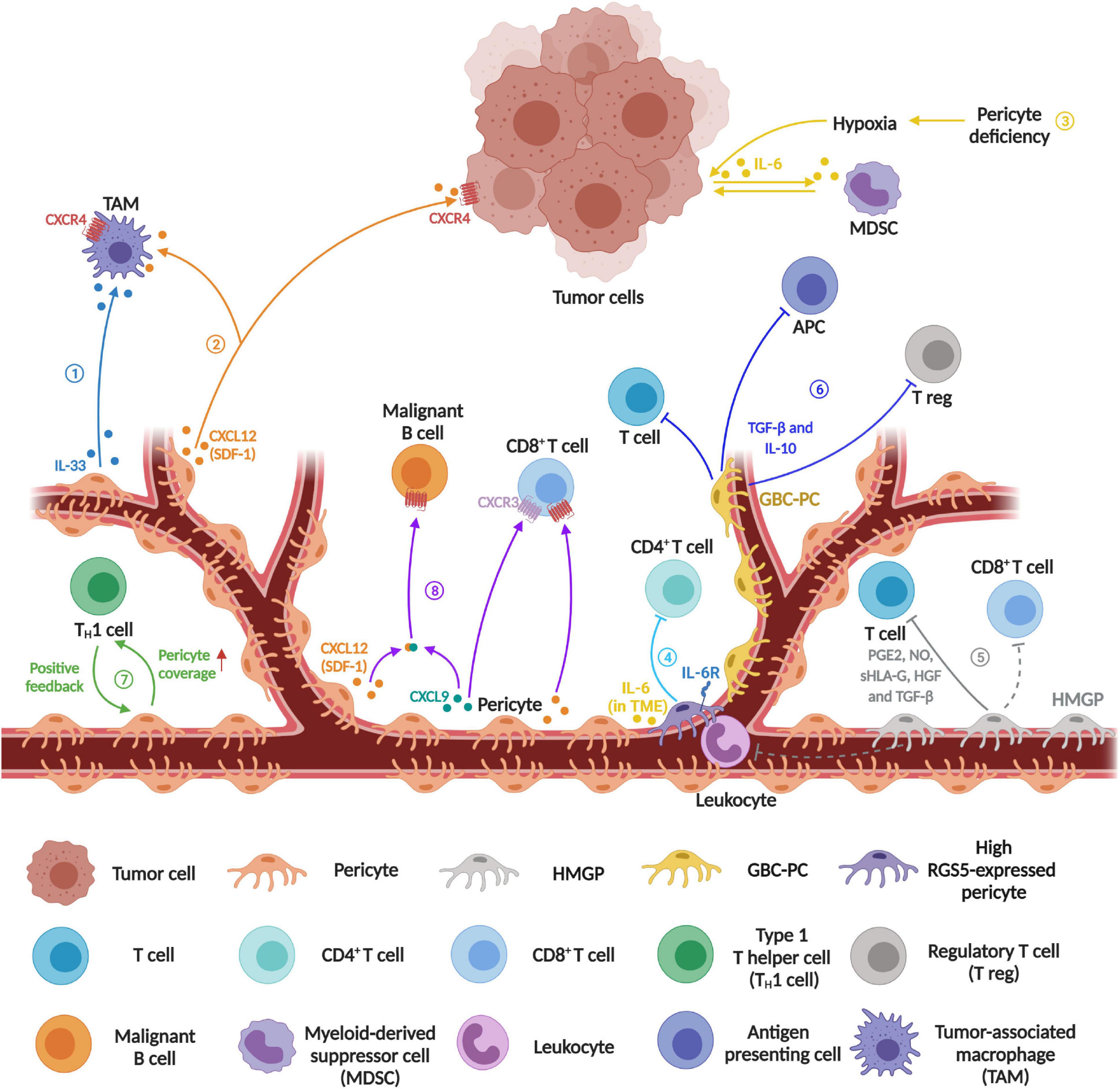
Figure 1. Schematics diagram represents the emerging immunomodulatory role of pericytes in tumor microenvironment. ➀Recruitment of tumor-associated macrophage (TAM). PDGF-BB-stimulated PCs release IL-33 to recruit more TAMs. ➁ Increased co-migration of TAMs and tumor cells. PC-derived chemokine CXCL12 (SDF-1) contributes to the co-migration of TAMs and tumor cells during innate immune response. ➂ Increased myeloid-derived suppressor cells (MDSCs) transmigration. PC loss causes leaky blood vessels and inadequate oxygen supply leading to tumor hypoxia, which then induces IL-6 expression in tumor cells to increase MDSC transmigration, resulting in suppression of the T cell-mediated anti-tumor response. ➃ Induced CD4+ T cell anergy. Tumor PCs act as negative regulators of CD4+ T cell activity. ➄ Inhibition of mitogen- and allogeneic-stimulated T cell proliferation. Human malignant glioma-derived pericyte (HMGP) releases PGE2, NO, sHLA-G, HGF, and TDF-β to suppress T cell proliferation, while CD90-positive PCs may function as suppressors of the infiltration of leukocytes and CD8+ T cells in malignant glioma. ➅ Inhibition of T cell and antigen presenting cell activity, and increased recruitment of regulatory T cells. Glioblastoma conditioned-pericyte (GBC-PC) not only negatively regulates T cell and antigen presenting cell (APC) but also recruits regulatory T cell (T reg). ➆ Regulation of blood vessel normalization and immune cell infiltration. In the positive feedback loop between type 1 T helper (TH1) and blood vessel normalization, PC coverage has a certain impact on TH1-mediated immune cell infiltration. ➇ Enhanced CD8+ T cell recruitment and malignant B cell migration. Perivascular cell derived CXCL9 and CXCL12 can recruit CD8+ T cell effectors by binding to their corresponding receptor CXCR3 and CXCR4 respectively. Besides, CXCL9 forms a heterocomplex with CXCL12, which then enhances CXCR4-dependent malignant B cell migration to accumulate on the vessel wall (Created with BioRender.com).
Newer evidence suggests that tumor-derived PCs regulate the activity and proliferation of T lymphocytes in TME (Figure 1). In a mouse spontaneous model of pancreatic cancer (RIP1-Tag5), knocking out RGS5 (regulator of G-protein signaling 5) gene results in PC maturation, vascular normalization and consequently a marked reduction in tumor hypoxia and vessel leakiness, while these changes enhance immune cell infiltration and extend the survival of tumor bearing mice (Hamzah et al., 2008). Furthermore, PC-RGS5 overexpression has been observed in several types of human tumors including kidney, liver, and head and neck cancers (Furuya et al., 2004; Hamzah et al., 2008). Coincidently, Bose et al. show that the expression of PC-RGS5 is upregulated after co-cultured with tumor-derived supernatant or established subcutaneous tumors (Bose et al., 2013). Tumor derived PCs inhibit CD4+ T cell proliferation and activation while promoting CD4+ T cell anergy in vitro, which is also regulated by RGS5- and IL-6-dependent signaling pathways (Figure 1, process ➃). In addition, the expression of PD-L1 is up-regulated in PCs after co-cultured with tumor fragments (Bose et al., 2013). These results suggest that the combined effect of PC-PD-L1 and RGS5 expression might protect tumor cells from cytotoxic T cells. In a different study, the authors show that human malignant glioma-derived pericytes (HMGP), which co-expressed CD90, CD248, and PDGFR-β, are capable of inhibiting the proliferation of mitogen- and allogeneic-stimulated T cells via the release of prostaglandin E2 (PGE2), serum human leukocyte antigen G (sHLA-G), hepatocyte growth factor (HGF), and transforming growth factor-beta (TGF-β) (Figure 1, process ➄). Clinically, the expression level of CD90 in perivascular cells positively correlates with glioma malignancy, while it is negatively associated with BV-associated leukocytes and CD8+ T cell infiltration (Ochs et al., 2013). Recently, Valdor et al. report that GBM-conditioned-pericytes (GBC-PCs) can secrete a high level of anti-inflammatory cytokines and immunosuppressive molecules while reducing their surface co-stimulatory molecule expression, which in turn suppresses CD4+ T cell response and IL-2 production in vitro (Figure 1, process ➅) (Valdor et al., 2017). Further study shows that GBC-PCs upregulate chaperone-mediated autophagy (CMA) to enhance the expression of anti-inflammatory cytokines TGF-β and IL-10, which then inhibit T cell and antigen presenting cell activity and recruit regulatory T cells (Figure 1, process ➅) (Valdor et al., 2019). Additionally, PCs contribute to the subsequent positive feedback loop of type 1 T helper cells-mediated vessel normalization and immune response (Figure 1, process ➆) (Tian et al., 2017).
Interestingly, Daniel et al., show that PCs may also possess a potential regulatory role of malignant B cell recruitment in primary central nervous system lymphoma (Figure 1, process ➇). Clinically, the localization and density of activated CD8+ T cells within tumors is correlated with the expression level of inflammatory chemokine CXC chemokine ligand 9 (CXCL9), which is an agonist of the CXC chemokine receptor 3 (CXCR3), mainly secreted by PCs and perivascular macrophages. In the perivascular TME, CXCL9 can form heterocomplex with B-cell chemoattractant CXCL12 to enhance CXCL12-induced CD8+ T cell as well as malignant B cell recruitment toward BV walls in the primary central nervous system lymphomas (Venetz et al., 2010). In addition, our recent work shows that β3-integrin controls the secretion of CCL2, CXCL1, and TIMP1 from PCs via the FAK-HGFR-Akt-NF-κB signaling (Wong et al., 2020), while these cytokines have been linked to immune cell infiltration and activity in TME (Navarro et al., 2016), suggesting that targeting PC-β3-integrin and its downstream signaling pathway can be a potential strategy to modulate immunosuppressive TME.
The Role of Pericytes-Fibroblast Transition in Tumor Microenvironment
As a fundamental component of the tumor stroma, cancer associated fibroblast (CAFs) have a role in modulating TME and changing the behavior of neoplastic cells in either a tumor-promoting or tumor-inhibiting manner (Kalluri, 2016). In the tumor-promoting property, CAFs support carcinogenesis through secretion of cytokine, growth factors and angiogenic factors, production and remodeling of the ECM, as well as suppression of immune surveillance in the TME (Matsuda and Seki, 2020). Recently, PC is considered to be one of the major sources of CAFs in tumors and fibrosis (Öhlund et al., 2014; Kalluri, 2016). A novel finding reveals that PDGF-BB-PDGFRβ signaling can induce pericytes-fibroblast transition (PFT), while the detached PCs from tumor microvasculature can transdifferentiate to fibroblasts that significantly contributed to tumor invasion and metastasis (Hosaka et al., 2016).
Targeting Pericytes as a Cancer Treatment Strategy: Challenges vs Opportunities
It has become a research hot topic for developing direct/indirect PC-targeted agents against angiogenesis and cancer growth in the last decades (Supplementary Table 1). However, majority of these agents showed modest or no effect on tumor growth and progression as a single agent in preclinical animal models, especially for PDGFR-targeted therapy. Combining anti-PDGFR agent with chemotherapy or other agent-targeted therapy displayed slightly better anti-tumor effect in mouse models of certain cancer types (Supplementary Table 1). Furthermore, the phase 3 clinical trials of PC-related antitumor therapy have so far shown modest clinical benefits in certain cancers (Table 1). Besides, the combination therapy of anti-PDGFB and anti-VEGF had very limited effect in the clinical trials and even showed additive side effects in some patients (Hainsworth et al., 2007). After interpreting these studies, we speculate that drug dosing strategy is a critical variable which may determine whether PC-targeted drugs promote vascular function and immune cell infiltration or induce tumor vasculature destruction and cancer metastasis. Therefore, it is a clinically unmet need to investigate how to target PC coverage or recondition PC functions (i.e., immunomodulatory role) for preferred immunobiology/vascular function in TME. Apart from targeting PCs directly, Cantelmo et al. show that inhibition of the glycolytic activator PFKFB3 in ECs induces tumor vessel normalization to improve PC coverage and chemotherapy delivery in the preclinical models. The authors also claimed that depletion of PFKFB3 significantly inhibits placenta derived PC proliferation, while improves PC coverage and adherence to ECs in tumor BVs (Cantelmo et al., 2016). However, the short-term effect of BV normalization raises a question about its application in the clinic (Wong et al., 2015). Recently, we discover that loss of mural-β3-integrin expression significantly enhances FAK-p-HGFR-p-Akt-p-p65 mediated CCL2 cytokine production, which in turn activates CCR2-MEK1-ROCK2 dependent tumor growth (Wong et al., 2020). These findings suggest that cancer patients with low PC-β3-integrin expression can be potentially treated with CCR2 or ROCK inhibitors.
Conclusion
As an obligatory constituent of the TME, PCs modulate the TME by interacting with tumor cells, ECs, immune cells, and CAFs, beyond their BV supporting role. Recent work supports direct cross-talk between PCs and tumor cells in the TME, which can promote tumor growth independent of tumor angiogenesis. Also, the interplay between ECs and PCs in regulating vascular formation and remodeling has been demonstrated in numerous studies. Disrupting EC-PC interactions in tumor vasculature not only affects PC coverage on tumor BVs but also alter vascular and perivascular TME to influence the efficacy of anti-tumor therapies. Indeed, new studies have highlighted that PCs protect tumor cells from immune surveillance through suppressing the proliferation or response of inflammatory cells around the tumor parenchyma, which could be a new potential target for cancer immunotherapy. Besides, the observation of PC-fibroblast transition suggests the potential progenitor cell property of PC in the TME. In this review, we provide new information to support an integral role for PCs in promoting tumor progression in part through their regulatory activities of tumor cells and dominated stromal cells, suggesting that PCs can serve as a therapeutic target for anticancer treatment in addition to anti-angiogenesis. Meanwhile, the stromal cells within TME may also provide potential therapeutic targets for intending anti-angiogenesis combination therapy since their underlying relationships with PCs. Future studies should focus on exploring the underlying mechanisms of PC-stromal cell/tumor cell interaction in the TME in order to identify new therapeutic targets for an improved cancer treatment strategy.
Author Contributions
RS wrote the manuscript and made the figure as well as the table. XK, XQ, and CH reviewed the manuscript. P-PW conceptualized, wrote, and reviewed the final version. All authors approved the submission for publication.
Funding
This work was supported by the Natural Science Foundation of China (81920108028 and 81872142), Guangzhou Science and Technology Program (201904020008), Guangdong Science and Technology Department (2020A0505100029, 2020B1212060018, and 2020B1212030004), and the Key Training Program for Young Scholars of Sun Yat-sen University (18ykzd07).
Conflict of Interest
The authors declare that the research was conducted in the absence of any commercial or financial relationships that could be construed as a potential conflict of interest.
Supplementary Material
The Supplementary Material for this article can be found online at: https://www.frontiersin.org/articles/10.3389/fcell.2021.676342/full#supplementary-material
Supplementary Table 1 | Preclinical testing of Pericyte-targeted antitumor therapy.
References
Abramsson, A., Lindblom, P., and Betsholtz, C. (2003). Endothelial and nonendothelial sources of PDGF-B regulate pericyte recruitment and influence vascular pattern formation in tumors. J. Clin. Invest. 112, 1142–1151. doi: 10.1172/jci18549
Armulik, A., Abramsson, A., and Betsholtz, C. (2005). Endothelial/pericyte interactions. Circ. Res. 97, 512–523. doi: 10.1161/01.RES.0000182903.16652.d7
Armulik, A., Genove, G., and Betsholtz, C. (2011). Pericytes: developmental, physiological, and pathological perspectives, problems, and promises. Dev. Cell 21, 193–215. doi: 10.1016/j.devcel.2011.07.001
Benjamin, L. E., Golijanin, D., Itin, A., Pode, D., and Keshet, E. (1999). Selective ablation of immature blood vessels in established human tumors follows vascular endothelial growth factor withdrawal. J. Clin. Invest. 103, 159–165. doi: 10.1172/JCI5028
Bergers, G., and Hanahan, D. (2008). Modes of resistance to anti-angiogenic therapy. Nat. Rev. Cancer 8, 592–603. doi: 10.1038/nrc2442
Bergers, G., and Song, S. (2005). The role of pericytes in blood-vessel formation and maintenance. Neuro Oncol. 7, 452–464. doi: 10.1215/S1152851705000232
Bergers, G., Song, S., Meyer-Morse, N., Bergsland, E., and Hanahan, D. (2003). Benefits of targeting both pericytes and endothelial cells in the tumor vasculature with kinase inhibitors. J. Clin. Invest. 111, 1287–1295. doi: 10.1172/JCI17929
Blay, J. Y., Shen, L., Kang, Y. K., Rutkowski, P., Qin, S., Nosov, D., et al. (2015). Nilotinib versus imatinib as first-line therapy for patients with unresectable or metastatic gastrointestinal stromal tumours (ENESTg1): a randomised phase 3 trial. Lancet Oncol. 16, 550–560. doi: 10.1016/s1470-2045(15)70105-1
Bose, A., Barik, S., Banerjee, S., Ghosh, T., Mallick, A., Bhattacharyya Majumdar, S., et al. (2013). Tumor-derived vascular pericytes anergize Th cells. J. Immunol. 191, 971–981. doi: 10.4049/jimmunol.1300280
Brand, C., Schliemann, C., Ring, J., Kessler, T., Baumer, S., Angenendt, L., et al. (2016). NG2 proteoglycan as a pericyte target for anticancer therapy by tumor vessel infarction with retargeted tissue factor. Oncotarget 7, 6774–6789. doi: 10.18632/oncotarget.6725
Brose, M. S., Nutting, C. M., Jarzab, B., Elisei, R., Siena, S., Bastholt, L., et al. (2014). Sorafenib in radioactive iodine-refractory, locally advanced or metastatic differentiated thyroid cancer: a randomised, double-blind, phase 3 trial. Lancet 384, 319–328. doi: 10.1016/s0140-6736(14)60421-9
Bruix, J., Qin, S., Merle, P., Granito, A., Huang, Y. H., Bodoky, G., et al. (2017). Regorafenib for patients with hepatocellular carcinoma who progressed on sorafenib treatment (RESORCE): a randomised, double-blind, placebo-controlled, phase 3 trial. Lancet 389, 56–66. doi: 10.1016/s0140-6736(16)32453-9
Bruix, J., Takayama, T., Mazzaferro, V., Chau, G. Y., Yang, J., Kudo, M., et al. (2015). Adjuvant sorafenib for hepatocellular carcinoma after resection or ablation (STORM): a phase 3, randomised, double-blind, placebo-controlled trial. Lancet Oncol. 16, 1344–1354. doi: 10.1016/s1470-2045(15)00198-9
Cantelmo, A. R., Conradi, L. C., Brajic, A., Goveia, J., Kalucka, J., Pircher, A., et al. (2016). Inhibition of the glycolytic activator PFKFB3 in endothelium induces tumor vessel normalization, impairs metastasis, and improves chemotherapy. Cancer Cell 30, 968–985. doi: 10.1016/j.ccell.2016.10.006
Carmeliet, P., and Jain, R. K. (2000). Angiogenesis in cancer and other diseases. Nature 407, 249–257. doi: 10.1038/35025220
Caspani, E. M., Crossley, P. H., Redondo-Garcia, C., and Martinez, S. (2014). Glioblastoma: a pathogenic crosstalk between tumor cells and pericytes. PLoS One 9:e101402. doi: 10.1371/journal.pone.0101402
Chen, M., Lei, X., Shi, C., Huang, M., Li, X., Wu, B., et al. (2017). Pericyte-targeting prodrug overcomes tumor resistance to vascular disrupting agents. J. Clin. Invest. 127, 3689–3701. doi: 10.1172/jci94258
Cheng, A. L., Kang, Y. K., Chen, Z., Tsao, C. J., Qin, S., Kim, J. S., et al. (2009). Efficacy and safety of sorafenib in patients in the Asia-Pacific region with advanced hepatocellular carcinoma: a phase III randomised, double-blind, placebo-controlled trial. Lancet Oncol. 10, 25–34. doi: 10.1016/s1470-2045(08)70285-7
Chi Sabins, N., Taylor, J. L., Fabian, K. P., Appleman, L. J., Maranchie, J. K., Stolz, D. B., et al. (2013). DLK1: a novel target for immunotherapeutic remodeling of the tumor blood vasculature. Mol. Ther. 21, 1958–1968. doi: 10.1038/mt.2013.133
Corselli, M., Chin, C. J., Parekh, C., Sahaghian, A., Wang, W., Ge, S., et al. (2013). Perivascular support of human hematopoietic stem/progenitor cells. Blood 121, 2891–2901. doi: 10.1182/blood-2012-08-451864
Crisan, M., Yap, S., Casteilla, L., Chen, C. W., Corselli, M., Park, T. S., et al. (2008). A perivascular origin for mesenchymal stem cells in multiple human organs. Cell Stem Cell 3, 301–313. doi: 10.1016/j.stem.2008.07.003
Dellavalle, A., Sampaolesi, M., Tonlorenzi, R., Tagliafico, E., Sacchetti, B., Perani, L., et al. (2007). Pericytes of human skeletal muscle are myogenic precursors distinct from satellite cells. Nat. Cell Biol. 9, 255–267. doi: 10.1038/ncb1542
Demetri, G. D., Reichardt, P., Kang, Y. K., Blay, J. Y., Rutkowski, P., Gelderblom, H., et al. (2013). Efficacy and safety of regorafenib for advanced gastrointestinal stromal tumours after failure of imatinib and sunitinib (GRID): an international, multicentre, randomised, placebo-controlled, phase 3 trial. Lancet 381, 295–302. doi: 10.1016/s0140-6736(12)61857-1
Demetri, G. D., van Oosterom, A. T., Garrett, C. R., Blackstein, M. E., Shah, M. H., Verweij, J., et al. (2006). Efficacy and safety of sunitinib in patients with advanced gastrointestinal stromal tumour after failure of imatinib: a randomised controlled trial. Lancet 368, 1329–1338. doi: 10.1016/s0140-6736(06)69446-4
Dermietzel, R., and Krause, D. (1991). Molecular anatomy of the blood-brain barrier as defined by immunocytochemistry. Intern. Rev. Cytol. 127, 57–109. doi: 10.1016/s0074-7696(08)60692-0
Dore-Duffy, P., Katychev, A., Wang, X., and Van Buren, E. (2006). CNS microvascular pericytes exhibit multipotential stem cell activity. J. Cereb. Blood Flow Metab. 26, 613–624. doi: 10.1038/sj.jcbfm.9600272
Dresemann, G., Weller, M., Rosenthal, M. A., Wedding, U., Wagner, W., Engel, E., et al. (2010). Imatinib in combination with hydroxyurea versus hydroxyurea alone as oral therapy in patients with progressive pretreated glioblastoma resistant to standard dose temozolomide. J. Neurooncol. 96, 393–402. doi: 10.1007/s11060-009-9976-3
du Bois, A., Kristensen, G., Ray-Coquard, I., Reuss, A., Pignata, S., Colombo, N., et al. (2016). Standard first-line chemotherapy with or without nintedanib for advanced ovarian cancer (AGO-OVAR 12): a randomised, double-blind, placebo-controlled phase 3 trial. Lancet Oncol. 17, 78–89. doi: 10.1016/s1470-2045(15)00366-6
Edwards, A. K., Glithero, K., Grzesik, P., Kitajewski, A. A., Munabi, N. C., Hardy, K., et al. (2017). NOTCH3 regulates stem-to-mural cell differentiation in infantile hemangioma. JCI Insight. 2, 93764. doi: 10.1172/jci.insight.93764
Enge, M., Bjarnegard, M., Gerhardt, H., Gustafsson, E., Kalen, M., Asker, N., et al. (2002). Endothelium-specific platelet-derived growth factor-B ablation mimics diabetic retinopathy. EMBO J. 21, 4307–4316. doi: 10.1093/emboj/cdf418
Fabian, K. P. L., Chi-Sabins, N., Taylor, J. L., Fecek, R., Weinstein, A., and Storkus, W. J. (2017). Therapeutic efficacy of combined vaccination against tumor pericyte-associated antigens DLK1 and DLK2 in mice. OncoImmunology 6:e1290035. doi: 10.1080/2162402X.2017.1290035
Falcon, B. L., Hashizume, H., Koumoutsakos, P., Chou, J., Bready, J. V., Coxon, A., et al. (2009). Contrasting actions of selective inhibitors of angiopoietin-1 and angiopoietin-2 on the normalization of tumor blood vessels. Am. J. Pathol. 175, 2159–2170. doi: 10.2353/ajpath.2009.090391
Falcon, B. L., Pietras, K., Chou, J., Chen, D., Sennino, B., Hanahan, D., et al. (2011). Increased vascular delivery and efficacy of chemotherapy after inhibition of platelet-derived growth factor-B. Am. J. Pathol. 178, 2920–2930. doi: 10.1016/j.ajpath.2011.02.019
Ferland-McCollough, D., Slater, S., Richard, J., Reni, C., and Mangialardi, G. (2017). Pericytes, an overlooked player in vascular pathobiology. Pharmacol. Ther. 171, 30–42. doi: 10.1016/j.pharmthera.2016.11.008
Franco, M., Roswall, P., Cortez, E., Hanahan, D., and Pietras, K. (2011). Pericytes promote endothelial cell survival through induction of autocrine VEGF-A signaling and Bcl-w expression. Blood 118, 2906–2917. doi: 10.1182/blood-2011-01-331694
Furuhashi, M., Sjöblom, T., Abramsson, A., Ellingsen, J., Micke, P., Li, H., et al. (2004). Platelet-derived growth factor production by B16 melanoma cells leads to increased pericyte abundance in tumors and an associated increase in tumor growth rate. Cancer Res. 64, 2725–2733. doi: 10.1158/0008-5472.can-03-1489
Furuya, M., Nishiyama, M., Kimura, S., Suyama, T., Naya, Y., Ito, H., et al. (2004). Expression of regulator of G protein signalling protein 5 (RGS5) in the tumour vasculature of human renal cell carcinoma. J. Pathol. 203, 551–558. doi: 10.1002/path.1543
Grothey, A., Van Cutsem, E., Sobrero, A., Siena, S., Falcone, A., Ychou, M., et al. (2013). Regorafenib monotherapy for previously treated metastatic colorectal cancer (CORRECT): an international, multicentre, randomised, placebo-controlled, phase 3 trial. Lancet 381, 303–312. doi: 10.1016/s0140-6736(12)61900-x
Guan, Y. Y., Luan, X., Xu, J. R., Liu, Y. R., Lu, Q., Wang, C., et al. (2014). Selective eradication of tumor vascular pericytes by peptide-conjugated nanoparticles for antiangiogenic therapy of melanoma lung metastasis. Biomaterials 35, 3060–3070. doi: 10.1016/j.biomaterials.2013.12.027
Guo, P., Hu, B., Gu, W., Xu, L., Wang, D., Huang, H. J., et al. (2003). Platelet-derived growth factor-B enhances glioma angiogenesis by stimulating vascular endothelial growth factor expression in tumor endothelia and by promoting pericyte recruitment. Am. J. Pathol. 162, 1083–1093. doi: 10.1016/s0002-9440(10)63905-3
Haas, N. B., Manola, J., Uzzo, R. G., Flaherty, K. T., Wood, C. G., Kane, C., et al. (2016). Adjuvant sunitinib or sorafenib for high-risk, non-metastatic renal-cell carcinoma (ECOG-ACRIN E2805): a double-blind, placebo-controlled, randomised, phase 3 trial. Lancet 387, 2008–2016. doi: 10.1016/s0140-6736(16)00559-6
Hainsworth, J. D., Spigel, D. R., Sosman, J. A., Burris, H. A. III, Farley, C., Cucullu, H., et al. (2007). Treatment of advanced renal cell carcinoma with the combination bevacizumab/erlotinib/imatinib: a phase I/II trial. Clin. Genitourin. Cancer 5, 427–432. doi: 10.3816/CGC.2007.n.030
Hamzah, J., Jugold, M., Kiessling, F., Rigby, P., Manzur, M., Marti, H. H., et al. (2008). Vascular normalization in Rgs5-deficient tumours promotes immune destruction. Nature 453, 410–414. doi: 10.1038/nature06868
Han, B., Li, K., Wang, Q., Zhang, L., Shi, J., Wang, Z., et al. (2018). Effect of anlotinib as a third-line or further treatment on overall survival of patients with advanced non-small cell lung cancer: the alter 0303 phase 3 randomized clinical trial. JAMA Oncol. 4, 1569–1575. doi: 10.1001/jamaoncol.2018.3039
Hanahan, D., and Weinberg, R. A. (2011). Hallmarks of cancer: the next generation. Cell 144, 646–674. doi: 10.1016/j.cell.2011.02.013
Harrell, C. R., Simovic Markovic, B., Fellabaum, C., Arsenijevic, A., Djonov, V., and Volarevic, V. (2018). Molecular mechanisms underlying therapeutic potential of pericytes. J. Biomed. Sci. 25:21. doi: 10.1186/s12929-018-0423-7
Hellstrom, M., Gerhardt, H., Kalen, M., Li, X., Eriksson, U., Wolburg, H., et al. (2001). Lack of pericytes leads to endothelial hyperplasia and abnormal vascular morphogenesis. J. Cell Biol. 153, 543–553. doi: 10.1083/jcb.153.3.543
Hernandez, S. L., Banerjee, D., Garcia, A., Kangsamaksin, T., Cheng, W.-Y., Anastassiou, D., et al. (2013). Notch and VEGF pathways play distinct but complementary roles in tumor angiogenesis. Vasc. Cell 5:17. doi: 10.1186/2045-824X-5-17
Hong, J., Tobin, N. P., Rundqvist, H., Li, T., Lavergne, M., García-Ibáñez, Y., et al. (2015). Role of tumor pericytes in the recruitment of myeloid-derived suppressor cells. J. Natl. Cancer Instit. 107:djv209. doi: 10.1093/jnci/djv209
Hosaka, K., Yang, Y., Seki, T., Fischer, C., Dubey, O., Fredlund, E., et al. (2016). Pericyte-fibroblast transition promotes tumor growth and metastasis. Proc. Natl. Acad. Sci. U.S.A. 113, E5618–E5627. doi: 10.1073/pnas.1608384113
Kalluri, R. (2016). The biology and function of fibroblasts in cancer. Nat. Rev. Cancer 16, 582–598. doi: 10.1038/nrc.2016.73
Kang, Y. K., Ryu, M. H., Yoo, C., Ryoo, B. Y., Kim, H. J., Lee, J. J., et al. (2013). Resumption of imatinib to control metastatic or unresectable gastrointestinal stromal tumours after failure of imatinib and sunitinib (RIGHT): a randomised, placebo-controlled, phase 3 trial. Lancet Oncol. 14, 1175–1182. doi: 10.1016/s1470-2045(13)70453-4
Kawai, A., Araki, N., Hiraga, H., Sugiura, H., Matsumine, A., Ozaki, T., et al. (2016). A randomized, double-blind, placebo-controlled, Phase III study of pazopanib in patients with soft tissue sarcoma: results from the Japanese subgroup. Jpn. J. Clin. Oncol. 46, 248–253. doi: 10.1093/jjco/hyv184
Keskin, D., Kim, J., Cooke, V. G., Wu, C. C., Sugimoto, H., Gu, C., et al. (2015). Targeting vascular pericytes in hypoxic tumors increases lung metastasis via angiopoietin-2. Cell Rep. 10, 1066–1081. doi: 10.1016/j.celrep.2015.01.035
Kitano, M., and Bloomston, P. M. (2016). Hepatic stellate cells and microRNAs in pathogenesis of liver fibrosis. J. Clin. Med. 5:38. doi: 10.3390/jcm5030038
Kłosowska-Wardega, A., Hasumi, Y., Burmakin, M., Ahgren, A., Stuhr, L., Moen, I., et al. (2009). Combined anti-angiogenic therapy targeting PDGF and VEGF receptors lowers the interstitial fluid pressure in a murine experimental carcinoma. PLoS One 4:e8149. doi: 10.1371/journal.pone.0008149
Kuhnert, F., Tam, B. Y., Sennino, B., Gray, J. T., Yuan, J., Jocson, A., et al. (2008). Soluble receptor-mediated selective inhibition of VEGFR and PDGFRbeta signaling during physiologic and tumor angiogenesis. Proc. Natl. Acad. Sci. U.S.A. 105, 10185–10190. doi: 10.1073/pnas.0803194105
Lechertier, T., Reynolds, L. E., Kim, H., Pedrosa, A. R., Gómez-Escudero, J., Muñoz-Félix, J. M., et al. (2020). Pericyte FAK negatively regulates Gas6/Axl signalling to suppress tumour angiogenesis and tumour growth. Nat. Commun. 11:2810. doi: 10.1038/s41467-020-16618-6
Lederle, W., Linde, N., Heusel, J., Bzyl, J., Woenne, E. C., Zwick, S., et al. (2010). Platelet-derived growth factor-B normalizes micromorphology and vessel function in vascular endothelial growth factor-A-induced squamous cell carcinomas. Am. J. Pathol. 176, 981–994. doi: 10.2353/ajpath.2010.080998
Leow, C. C., Coffman, K., Inigo, I., Breen, S., Czapiga, M., Soukharev, S., et al. (2012). MEDI3617, a human anti-angiopoietin 2 monoclonal antibody, inhibits angiogenesis and tumor growth in human tumor xenograft models. Int. J. Oncol. 40, 1321–1330. doi: 10.3892/ijo.2012.1366
Li, T., Kang, G., Wang, T., and Huang, H. (2018). Tumor angiogenesis and anti-angiogenic gene therapy for cancer. Oncol. Lett. 16, 687–702. doi: 10.3892/ol.2018.8733
Lindahl, P., Johansson, B. R., Leveen, P., and Betsholtz, C. (1997). Pericyte loss and microaneurysm formation in PDGF-B-deficient mice. Science 277, 242–245. doi: 10.1126/science.277.5323.242
Lu, C., Kamat, A. A., Lin, Y. G., Merritt, W. M., Landen, C. N., Kim, T. J., et al. (2007). Dual targeting of endothelial cells and pericytes in antivascular therapy for ovarian carcinoma. Clin. Cancer Res. 13, 4209–4217. doi: 10.1158/1078-0432.CCR-07-0197
Lu, C., Shahzad, M. M., Moreno-Smith, M., Lin, Y. G., Jennings, N. B., Allen, J. K., et al. (2010). Targeting pericytes with a PDGF-B aptamer in human ovarian carcinoma models. Cancer Biol. Ther. 9, 176–182. doi: 10.4161/cbt.9.3.10635
Marth, C., Vergote, I., Scambia, G., Oberaigner, W., Clamp, A., Berger, R., et al. (2017). ENGOT-ov-6/TRINOVA-2: randomised, double-blind, phase 3 study of pegylated liposomal doxorubicin plus trebananib or placebo in women with recurrent partially platinum-sensitive or resistant ovarian cancer. Eur. J. Cancer 70, 111–121. doi: 10.1016/j.ejca.2016.09.004
Matsuda, M., and Seki, E. (2020). Hepatic stellate cell-macrophage crosstalk in liver fibrosis and carcinogenesis. Semin. Liver Dis. 40, 307–320. doi: 10.1055/s-0040-1708876
McCarty, M. F., Somcio, R. J., Stoeltzing, O., Wey, J., Fan, F., Liu, W., et al. (2007). Overexpression of PDGF-BB decreases colorectal and pancreatic cancer growth by increasing tumor pericyte content. J. Clin. Invest. 117, 2114–2122. doi: 10.1172/JCI31334
Meng, M. B., Zaorsky, N. G., Deng, L., Wang, H. H., Chao, J., Zhao, L. J., et al. (2015). Pericytes: a double-edged sword in cancer therapy. Future Oncol. 11, 169–179. doi: 10.2217/fon.14.123
Miljkovic-Licina, M., Hammel, P., Garrido-Urbani, S., Lee, B. P., Meguenani, M., Chaabane, C., et al. (2012). Targeting olfactomedin-like 3 inhibits tumor growth by impairing angiogenesis and pericyte coverage. Mol. Cancer Ther. 11, 2588–2599. doi: 10.1158/1535-7163.MCT-12-0245
Monk, B. J., Poveda, A., Vergote, I., Raspagliesi, F., Fujiwara, K., Bae, D. S., et al. (2014). Anti-angiopoietin therapy with trebananib for recurrent ovarian cancer (TRINOVA-1): a randomised, multicentre, double-blind, placebo-controlled phase 3 trial. Lancet Oncol. 15, 799–808. doi: 10.1016/s1470-2045(14)70244-x
Motzer, R. J., Hutson, T. E., Tomczak, P., Michaelson, M. D., Bukowski, R. M., Rixe, O., et al. (2007). Sunitinib versus interferon alfa in metastatic renal-cell carcinoma. N. Engl. J. Med. 356, 115–124. doi: 10.1056/NEJMoa065044
Nasarre, P., Thomas, M., Kruse, K., Helfrich, I., Wolter, V., Deppermann, C., et al. (2009). Host-derived angiopoietin-2 affects early stages of tumor development and vessel maturation but is dispensable for later stages of tumor growth. Cancer Res. 69, 1324–1333. doi: 10.1158/0008-5472.CAN-08-3030
Navarro, R., Compte, M., Álvarez-Vallina, L., and Sanz, L. (2016). Immune regulation by pericytes: modulating innate and adaptive immunity. Front. Immunol. 7:480. doi: 10.3389/fimmu.2016.00480
Nisancioglu, M. H., Betsholtz, C., and Genove, G. (2010). The absence of pericytes does not increase the sensitivity of tumor vasculature to vascular endothelial growth factor-A blockade. Cancer Res. 70, 5109–5115. doi: 10.1158/0008-5472.CAN-09-4245
Nolan-Stevaux, O., Truitt, M. C., Pahler, J. C., Olson, P., Guinto, C., Lee, D. C., et al. (2010). Differential contribution to neuroendocrine tumorigenesis of parallel egfr signaling in cancer cells and pericytes. Genes Cancer 1, 125–141. doi: 10.1177/1947601909358722
Ochs, K., Sahm, F., Opitz, C. A., Lanz, T. V., Oezen, I., Couraud, P.-O., et al. (2013). Immature mesenchymal stem cell-like pericytes as mediators of immunosuppression in human malignant glioma. J. Neuroimmunol. 265, 106–116. doi: 10.1016/j.jneuroim.2013.09.011
Öhlund, D., Elyada, E., and Tuveson, D. (2014). Fibroblast heterogeneity in the cancer wound. J. Exper. Med. 211, 1503–1523. doi: 10.1084/jem.20140692
Olson, L. E., and Soriano, P. (2011). PDGFRbeta signaling regulates mural cell plasticity and inhibits fat development. Dev. Cell 20, 815–826. doi: 10.1016/j.devcel.2011.04.019
Onoyama, M., Kitadai, Y., Tanaka, Y., Yuge, R., Shinagawa, K., Tanaka, S., et al. (2013). Combining molecular targeted drugs to inhibit both cancer cells and activated stromal cells in gastric cancer. Neoplasia 15, 1391–1399. doi: 10.1593/neo.131668
Ozerdem, U., Grako, K. A., Dahlin-Huppe, K., Monosov, E., and Stallcup, W. B. (2001). NG2 proteoglycan is expressed exclusively by mural cells during vascular morphogenesis. Dev. Dynam. 222, 218–227. doi: 10.1002/dvdy.1200
Pallone, T. L., and Silldorff, E. P. (2001). Pericyte regulation of renal medullary blood flow. Exp. Nephrol. 9, 165–170. doi: 10.1159/000052608
Pietras, K., and Hanahan, D. (2005). A multitargeted, metronomic, and maximum-tolerated dose “chemo-switch” regimen is antiangiogenic, producing objective responses and survival benefit in a mouse model of cancer. J. Clin. Oncol. 23, 939–952. doi: 10.1200/jco.2005.07.093
Pietras, K., Pahler, J., Bergers, G., and Hanahan, D. (2008). Functions of paracrine PDGF signaling in the proangiogenic tumor stroma revealed by pharmacological targeting. PLoS Med. 5:e19. doi: 10.1371/journal.pmed.0050019
Prete, A., Lo, A. S., Sadow, P. M., Bhasin, S. S., Antonello, Z. A., Vodopivec, D. M., et al. (2018). Pericytes elicit resistance to Vemurafenib and Sorafenib therapy in thyroid carcinoma via the TSP-1/TGFβ1 Axis. Clin. Cancer Res. 24, 6078–6097. doi: 10.1158/1078-0432.CCR-18-0693
Qian, B. Z., and Pollard, J. W. (2010). Macrophage diversity enhances tumor progression and metastasis. Cell 141, 39–51. doi: 10.1016/j.cell.2010.03.014
Ramjiawan, R. R., Griffioen, A. W., and Duda, D. G. (2017). Anti-angiogenesis for cancer revisited: is there a role for combinations with immunotherapy? Angiogenesis 20, 185–204. doi: 10.1007/s10456-017-9552-y
Ravaud, A., Motzer, R. J., Pandha, H. S., George, D. J., Pantuck, A. J., Patel, A., et al. (2016). Adjuvant sunitinib in high-risk renal-cell carcinoma after nephrectomy. N. Engl. J. Med. 375, 2246–2254. doi: 10.1056/NEJMoa1611406
Raymond, E., Dahan, L., Raoul, J. L., Bang, Y. J., Borbath, I., Lombard-Bohas, C., et al. (2011). Sunitinib malate for the treatment of pancreatic neuroendocrine tumors. N. Engl. J. Med. 364, 501–513. doi: 10.1056/NEJMoa1003825
Raza, A., Franklin, M. J., and Dudek, A. Z. (2010). Pericytes and vessel maturation during tumor angiogenesis and metastasis. Am. J. Hematol. 85, 593–598. doi: 10.1002/ajh.21745
Ribatti, D., Nico, B., and Crivellato, E. (2011). The role of pericytes in angiogenesis. Int. J. Dev. Biol. 55, 261–268. doi: 10.1387/ijdb.103167dr
Rimassa, L., and Santoro, A. (2009). Sorafenib therapy in advanced hepatocellular carcinoma: the SHARP trial. Expert. Rev. Anticancer Ther. 9, 739–745. doi: 10.1586/era.09.41
Rini, B. I., Escudier, B., Tomczak, P., Kaprin, A., Szczylik, C., Hutson, T. E., et al. (2011). Comparative effectiveness of axitinib versus sorafenib in advanced renal cell carcinoma (AXIS): a randomised phase 3 trial. Lancet 378, 1931–1939. doi: 10.1016/s0140-6736(11)61613-9
Ruan, J., Luo, M., Wang, C., Fan, L., Yang, S. N., Cardenas, M., et al. (2013). Imatinib disrupts lymphoma angiogenesis by targeting vascular pericytes. Blood 121, 5192–5202. doi: 10.1182/blood-2013-03-490763
Rybinski, K., Imtiyaz, H. Z., Mittica, B., Drozdowski, B., Fulmer, J., Furuuchi, K., et al. (2015). Targeting endosialin/CD248 through antibody-mediated internalization results in impaired pericyte maturation and dysfunctional tumor microvasculature. Oncotarget 6, 25429–25440. doi: 10.18632/oncotarget.4559
Saharinen, P., Eklund, L., and Alitalo, K. (2017). Therapeutic targeting of the angiopoietin-TIE pathway. Nat. Rev. Drug Discov. 16, 635–661. doi: 10.1038/nrd.2016.278
Schiffmann, L. M., Brunold, M., Liwschitz, M., Goede, V., Loges, S., Wroblewski, M., et al. (2017). A combination of low-dose bevacizumab and imatinib enhances vascular normalisation without inducing extracellular matrix deposition. Br. J. Cancer 116, 600–608. doi: 10.1038/bjc.2017.13
Schlumberger, M., Tahara, M., Wirth, L. J., Robinson, B., Brose, M. S., Elisei, R., et al. (2015). Lenvatinib versus placebo in radioiodine-refractory thyroid cancer. N. Engl. J. Med. 372, 621–630. doi: 10.1056/NEJMoa1406470
Scholz, A., Harter, P. N., Cremer, S., Yalcin, B. H., Gurnik, S., Yamaji, M., et al. (2016). Endothelial cell-derived angiopoietin-2 is a therapeutic target in treatment-naive and bevacizumab-resistant glioblastoma. EMBO Mol. Med. 8, 39–57. doi: 10.15252/emmm.201505505
Sennino, B., Falcon, B. L., McCauley, D., Le, T., McCauley, T., Kurz, J. C., et al. (2007). Sequential loss of tumor vessel pericytes and endothelial cells after inhibition of platelet-derived growth factor B by selective aptamer AX102. Cancer Res. 67, 7358–7367. doi: 10.1158/0008-5472.CAN-07-0293
Shen, J., Vil, M. D., Prewett, M., Damoci, C., Zhang, H., Li, H., et al. (2009). Development of a fully human anti-PDGFRbeta antibody that suppresses growth of human tumor xenografts and enhances antitumor activity of an anti-VEGFR2 antibody. Neoplasia 11, 594–604. doi: 10.1593/neo.09278
Shepro, D., and Morel, N. M. (1993). Pericyte physiology. FASEB J. 7, 1031–1038. doi: 10.1096/fasebj.7.11.8370472
Song, N., Huang, Y., Shi, H., Yuan, S., Ding, Y., Song, X., et al. (2009). Overexpression of platelet-derived growth factor-BB increases tumor pericyte content via stromal-derived factor-1alpha/CXCR4 axis. Cancer Res. 69, 6057–6064. doi: 10.1158/0008-5472.Can-08-2007
Song, S., Ewald, A. J., Stallcup, W., Werb, Z., and Bergers, G. (2005). PDGFRbeta+ perivascular progenitor cells in tumours regulate pericyte differentiation and vascular survival. Nat. Cell Biol. 7, 870–879. doi: 10.1038/ncb1288
Sternberg, C. N., Davis, I. D., Mardiak, J., Szczylik, C., Lee, E., Wagstaff, J., et al. (2010). Pazopanib in locally advanced or metastatic renal cell carcinoma: results of a randomized phase III trial. J. Clin. Oncol. 28, 1061–1068. doi: 10.1200/jco.2009.23.9764
Taeger, J., Moser, C., Hellerbrand, C., Mycielska, M. E., Glockzin, G., Schlitt, H. J., et al. (2011). Targeting FGFR/PDGFR/VEGFR impairs tumor growth, angiogenesis, and metastasis by effects on tumor cells, endothelial cells, and pericytes in pancreatic cancer. Mol. Cancer Ther. 10, 2157–2167. doi: 10.1158/1535-7163.MCT-11-0312
Tao, Z., Yang, H., Shi, Q., Fan, Q., Wan, L., and Lu, X. (2017). Targeted delivery to tumor-associated Pericytes via an Affibody with High Affinity for PDGFRβ enhances the in vivo antitumor effects of human TRAIL. Theranostics 7, 2261–2276. doi: 10.7150/thno.19091
Tattersall, I. W., Du, J., Cong, Z., Cho, B. S., Klein, A. M., Dieck, C. L., et al. (2016). In vitro modeling of endothelial interaction with macrophages and pericytes demonstrates Notch signaling function in the vascular microenvironment. Angiogenesis 19, 201–215. doi: 10.1007/s10456-016-9501-1
Thijssen, V. L. J. L., Paulis, Y. W. J., Nowak-Sliwinska, P., Deumelandt, K. L., Hosaka, K., Soetekouw, P. M. M. B., et al. (2018). Targeting PDGF-mediated recruitment of pericytes blocks vascular mimicry and tumor growth. J. Pathol. 246, 447–458. doi: 10.1002/path.5152
Tian, L., Goldstein, A., Wang, H., Ching, L. H., Sun, K. I., Welte, T., et al. (2017). Mutual regulation of tumour vessel normalization and immunostimulatory reprogramming. Nature 544, 250–254. doi: 10.1038/nature21724
Turley, S. J., Cremasco, V., and Astarita, J. L. (2015). Immunological hallmarks of stromal cells in the tumour microenvironment. Nat. Rev. Immunol. 15, 669–682. doi: 10.1038/nri3902
Valdor, R., García-Bernal, D., Bueno, C., Ródenas, M., Moraleda, J. M., Macian, F., et al. (2017). Glioblastoma progression is assisted by induction of immunosuppressive function of pericytes through interaction with tumor cells. Oncotarget 8, 68614–68626. doi: 10.18632/oncotarget.19804
Valdor, R., García-Bernal, D., Riquelme, D., Martinez, C. M., Moraleda, J. M., Cuervo, A. M., et al. (2019). Glioblastoma ablates pericytes antitumor immune function through aberrant up-regulation of chaperone-mediated autophagy. Proc. Natl. Acad. Sci. U.S.A. 116, 20655–20665. doi: 10.1073/pnas.1903542116
van der Graaf, W. T., Blay, J. Y., Chawla, S. P., Kim, D. W., Bui-Nguyen, B., Casali, P. G., et al. (2012). Pazopanib for metastatic soft-tissue sarcoma (PALETTE): a randomised, double-blind, placebo-controlled phase 3 trial. Lancet 379, 1879–1886. doi: 10.1016/s0140-6736(12)60651-5
Venetz, D., Ponzoni, M., Schiraldi, M., Ferreri, A. J., Bertoni, F., Doglioni, C., et al. (2010). Perivascular expression of CXCL9 and CXCL12 in primary central nervous system lymphoma: T-cell infiltration and positioning of malignant B cells. Int. J. Cancer 127, 2300–2312. doi: 10.1002/ijc.25236
Vergote, I., Scambia, G., O’Malley, D. M., Van Calster, B., Park, S. Y., Del Campo, J. M., et al. (2019). Trebananib or placebo plus carboplatin and paclitaxel as first-line treatment for advanced ovarian cancer (TRINOVA-3/ENGOT-ov2/GOG-3001): a randomised, double-blind, phase 3 trial. Lancet Oncol. 20, 862–876. doi: 10.1016/s1470-2045(19)30178-0
Wong, P. P., Demircioglu, F., Ghazaly, E., Alrawashdeh, W., Stratford, M. R., Scudamore, C. L., et al. (2015). Dual-action combination therapy enhances angiogenesis while reducing tumor growth and spread. Cancer Cell 27, 123–137. doi: 10.1016/j.ccell.2014.10.015
Wong, P. P., Munoz-Felix, J. M., Hijazi, M., Kim, H., Robinson, S. D., De Luxan-Delgado, B., et al. (2020). Cancer burden is controlled by mural cell-beta3-integrin regulated crosstalk with tumor cells. Cell 181, 1346–1363.e1321. doi: 10.1016/j.cell.2020.02.003
Yang, Y., Andersson, P., Hosaka, K., Zhang, Y., Cao, R., Iwamoto, H., et al. (2016). The PDGF-BB-SOX7 axis-modulated IL-33 in pericytes and stromal cells promotes metastasis through tumour-associated macrophages. Nat. Commun. 7:11385. doi: 10.1038/ncomms11385
Yin, L., He, J., Xue, J., Na, F., Tong, R., Wang, J., et al. (2018). PDGFR-beta inhibitor slows tumor growth but increases metastasis in combined radiotherapy and Endostar therapy. Biomed. Pharmacother. 99, 615–621. doi: 10.1016/j.biopha.2018.01.095
Yonenaga, Y., Mori, A., Onodera, H., Yasuda, S., Oe, H., Fujimoto, A., et al. (2005). Absence of smooth muscle actin-positive pericyte coverage of tumor vessels correlates with hematogenous metastasis and prognosis of colorectal cancer patients. Oncology 69, 159–166. doi: 10.1159/000087840
Zhou, W., Chen, C., Shi, Y., Wu, Q., Gimple, R. C., Fang, X., et al. (2017). Targeting Glioma stem cell-derived Pericytes disrupts the blood-tumor barrier and improves chemotherapeutic efficacy. Cell Stem Cell 21, 591–603.e594. doi: 10.1016/j.stem.2017.10.002
Keywords: pericyte, mural cell, tumor microenvironment, angiogenesis, immunomodulation
Citation: Sun R, Kong X, Qiu X, Huang C and Wong P-P (2021) The Emerging Roles of Pericytes in Modulating Tumor Microenvironment. Front. Cell Dev. Biol. 9:676342. doi: 10.3389/fcell.2021.676342
Received: 05 March 2021; Accepted: 06 April 2021;
Published: 11 June 2021.
Edited by:
Liu Yang, Zhejiang Provincial People’s Hospital, ChinaReviewed by:
Walter J. Storkus, University of Pittsburgh, United StatesAnantha Koteswararao Kanugula, University of Massachusetts Medical School, United States
John Chappell, Fralin Biomedical Research Institute, Virginia Tech Carilion, United States
Copyright © 2021 Sun, Kong, Qiu, Huang and Wong. This is an open-access article distributed under the terms of the Creative Commons Attribution License (CC BY). The use, distribution or reproduction in other forums is permitted, provided the original author(s) and the copyright owner(s) are credited and that the original publication in this journal is cited, in accordance with accepted academic practice. No use, distribution or reproduction is permitted which does not comply with these terms.
*Correspondence: Ping-Pui Wong, aHVhbmdicDNAbWFpbC5zeXN1LmVkdS5jbg==