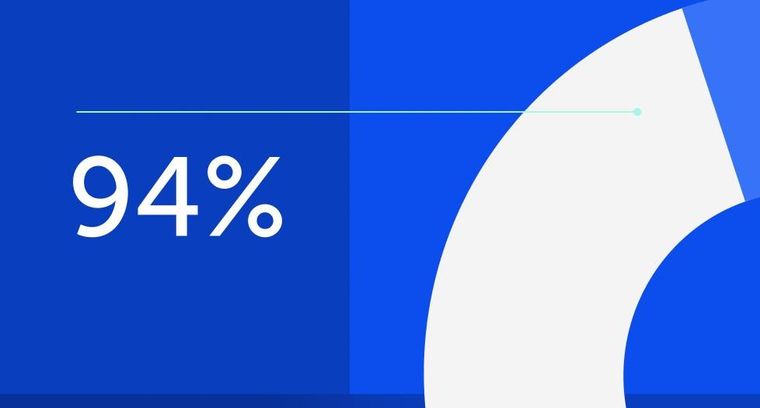
94% of researchers rate our articles as excellent or good
Learn more about the work of our research integrity team to safeguard the quality of each article we publish.
Find out more
ORIGINAL RESEARCH article
Front. Cell Dev. Biol., 30 April 2021
Sec. Cell Growth and Division
Volume 9 - 2021 | https://doi.org/10.3389/fcell.2021.669182
This article is part of the Research TopicMolecular and Cellular Physiology of Gametes in Domestic and Wild Animal ModelsView all 17 articles
Sperm present a highly particular DNA condensation that is acquired during their differentiation. Protamines are key elements for DNA condensation. However, whereas the presence of protamine 1 (P1) is conserved across mammalian species, that of protamine 2 (P2) has evolved differentially, existing only few species that use both protamines for sperm DNA condensation. In addition, altered P1/P2 ratios and alterations in the expression of P1 have previously been associated to infertility and DNA damage disorders. On the other hand, different methods evaluating DNA integrity, such as Sperm Chromatin Dispersion (SCD) and Comet tests, need a previous complete DNA decondensation to properly assess DNA breaks. Related with this, the present study aims to analyze the resilience of sperm DNA to decodensation in different eutherian mammals. Sperm samples from humans, horses, cattle, pigs and donkeys were used. Samples were embedded in low melting point agarose and treated with lysis solutions to induce DNA decondensation and formation of sperm haloes. The treatment consisted of three steps: (1) incubation in SDS + DTT for 30 min; (2) incubation in DTT + NaCl for 30 min; and (3) incubation in DTT + NaCl with or without proteinase K for a variable time of 0, 30, or 180 min. How incubation with the third lysis solution (with or without proteinase K) for 0, 30, and 180 min affected DNA decondensation was tested through analyzing core and halo diameters in 50 sperm per sample. Halo/core length ratio was used as an indicator of complete chromatin decondensation. While incubation time with the third lysis solution had no impact on halo/core length ratios in species having P1 and P2 (human, equine and donkey), DNA decondensation of pig and cattle sperm, which only present P1, significantly (P < 0.05) increased following incubation with the third lysis solution for 180 min. In addition, the inclusion of proteinase K was found to accelerate DNA decondensation. In conclusion, longer incubations in lysis solution including proteinase K lead to higher DNA decondensation in porcine and bovine sperm. This suggests that tests intended to analyze DNA damage, such as halo or Comet assays, require complete chromatin deprotamination to achieve high sensitivity in the detection of DNA breaks.
Different proteins encoded in nuclear and mitochondrial DNA allow cells to accomplish multiple functions to survive and proliferate. Nuclear chromatin consists of a complex of DNA and histone octamers. Histone octamers are formed by histones 2A, 2B, 3, and 4 (H2A, H2B, H3, and H4) and are separated with linker DNA of about 20–60 base pairs; each octamer condenses around 146 base pairs of DNA. The DNA-histone complex is highly supercoiled, forms chromatin fibers of 100–400 Kb in length and is organized in loop domains (Luger et al., 1997; Rao et al., 2014; Gibson et al., 2019). According to the models of chromatin organization, in each of these loops can one find a matrix attachment region (MAR) whose function is attaching chromatin to the nuclear matrix, a protein scaffold that supports nuclear organization (Ward, 2010) and contributes to the regulation of DNA replication and transcription in somatic cells, providing epigenetic plasticity for gene expression (Getzenberg, 1994; Linnemann et al., 2009; Ji et al., 2013; Narwade et al., 2019).
Spermatozoa are highly differentiated cells whose main function is the transport of genetic material to the oocyte through the female reproductive tract. To achieve this, millions of years of evolution have conferred mammalian sperm with highly specialized characteristics, such as the presence of flagellum, organization of mid-piece, and a very particular chromatin condensation and organization, as DNA is mainly condensed in protamines rather than in histones (Ward and Coffey, 1991; Wykes and Krawetz, 2003; Oliva, 2006). Organization of sperm chromatin changes during spermatogenesis, as histones are replaced by protamines through a series of events that, in some species such as humans, mouse, rat or sheep, involve transition nuclear proteins (Fuentes-Mascorro et al., 2000; Kierszenbaum, 2001; Hao et al., 2019). Condensation of sperm DNA through protamines protects genetic information from damaging agents (Zini and Libman, 2006), facilitating the delivery of an intact genetic material to the future embryo. This is very relevant as sperm DNA damage has been proposed as an important affectation that may underlie fertilization failure, poor embryo development, miscarriage and the production of unhealthy offspring (Makker et al., 2009; Lewis and Simon, 2010). These effects, however, are subject to the DNA damage repair capacity provided by the oocyte and, in this regard, different works described the activation of embryo checkpoints upon fertilization with damaged sperm (Adiga et al., 2007; Toyoshima, 2009). Also, a recent study in humans showed that ICSI cycles performed with sperm containing DNA damage depict lower embryo quality, pregnancy and implantation rates and higher miscarriage rates in older than in younger women (<36 years old) (Setti et al., 2021).
Protamines are arginine-rich proteins sized about half of the size of a typical histone and with highly positive charge, features that result in a tight binding to the nuclear sperm DNA, neutralizing the phosphodiester backbone (Balhorn, 1982). Protamine-DNA complex is responsible for the higher level of DNA condensation of spermatozoa compared to somatic cells; nuclear sperm DNA is known to be about six times more condensed than mitotic chromosomes, resulting in a nucleus with about 44 times less volume than that of a mouse liver cell (Ward and Coffey, 1991). Due to this DNA condensation, sperm chromatin is transcriptionally silent and, after fertilization, the replacement of protamines by histones is one of the main processes happening in the male pronuclear stage (Ajduk et al., 2006; Ward, 2010). In mammals, two different types of protamines (protamine 1 and protamine 2 family) are encoded in the genome. While protamine 1 is present in sperm from all species, protamine 2 family, formed by P2, P3, and P4 components, is only found in species such as humans, mice and horses (Fuentes-Mascorro et al., 2000; Corzett et al., 2002; Oliva, 2006; Gosálvez et al., 2011). Whereas protamine 1 is synthesized as a mature protein, those belonging to protamine 2 family (mainly P2, P3, and P4 are present in a minor percentage) need post-translational proteolysis prior to conversion into the mature form (Oliva, 2006). As Protamine 2 family proteins have never been found to compact sperm DNA alone, they appear not to be capable of condensing sperm chromatin without protamine 1 (Corzett et al., 2002). Alterations in the expression of protamine 1 have been shown to be linked to sperm morphology anomalies and premature DNA condensation during sperm differentiation (Peschon et al., 1989; Lee et al., 1995); alterations in protamine 2 content lead to male infertility and DNA damage (Aoki et al., 2005; Nanassy et al., 2011), as confirmed by Prm2± knockouts (Oliva and Dixon, 1991; Cho et al., 2001).
The currently accepted model for chromatin organization in mammalian sperm purports that protamine-DNA complex forms toroidal structures, which condense about 48 kb of DNA, and are estimated to measure between 60 and 100 nm in diameter and 20 nm in thickness (Brewer et al., 1999, 2003; Miller et al., 2010). These toroidal structures are stabilized by disulfide covalent bonds between neighbor protamines, and are linked through uncoiled linker DNA in toroid linker regions (TLR). The function of TLR is to attach the chromatin to the nuclear matrix and is thus supposed to contain matrix attachment regions (MAR; Ward, 2010). Toroid linker regions are assumed to be condensed in histones containing complex epigenetic information that may play a crucial role in the very early zygote by influencing gene expression after fertilization and during early embryo development (Jung et al., 2017; Yoshida et al., 2018). In the context of sperm chromatin, localization of histone regions in gene-rich or gene-poor DNA is a subject of much debate among scientists, as different methodologies have been shown to lead to opposite results (Yamaguchi et al., 2018). Moreover, it has been demonstrated that different species may have different percentages of histone-condensed DNA, ranging between 3 and 15% in humans, between 1 and 15% in mouse or even at higher rates (like 50%) in some marsupials (Fuentes-Mascorro et al., 2000; Hammoud et al., 2009; Miller et al., 2010; Samans et al., 2014). Despite the fact that the toroidal DNA and the linker DNA coupled to the nuclear matrix constitute the main unit of chromatin structure, evidences obtained through freeze-fracture, circular dichroism and atomic force microscopy support the presence of a higher superstructure of sperm chromatin, composed by parallel stacks of lamellar sheets oriented to the nuclear long axis in bulls, bucks and men (Koehler et al., 1983; Fuentes-Mascorro et al., 2000). Also, other evidences support a nodular chromatin sub-organization, sized between 100 and 300 nm, in mouse, human, cattle and rat sperm (Allen et al., 1993; Haaf and Ward, 1995). However, the presence of smaller nucleosome-like particles is not discarded, especially in the nuclear periphery (Allen et al., 1996). Regardless of chromatin superorganization, different studies also reported a chromosomal region-specific organization, since centromeres were found to be positioned in the most internal parts of the sperm nucleus, whereas telomeres that remain condensed in histones appeared to attach to the internal nuclear membrane, positioned in the external parts of the nucleus (Zalensky et al., 1997; Mudrak et al., 2005; Zalensky and Zalenskaya, 2007). Moreover, other authors showed that chromosomal territories exist in the sperm nucleus, leading to a precise organization of chromosomal DNA (Luetjens et al., 1999; Zalenskaya and Zalensky, 2004; Foster et al., 2005).
While the high degree of sperm DNA condensation requires protamines to be replaced by histones upon fertilization, it also represents a challenge for the in vitro study of sperm chromatin. In this sense, previous studies tested the in vivo sperm DNA decondensation of different animal species after microinjection of hamster oocytes; whereas nuclei of human, mouse, chinchilla and hamster sperm were properly decondensed, this was not the case of cattle or rat sperm (Perreault et al., 1988). The same study showed that, while DTT and SDS led to sperm DNA decondensation, incubation with these two agents needed to be 10 times longer in cattle than in human sperm to achieve similar levels of decondensation (Perreault et al., 1988). Nowadays, NaCl is known to be strictly necessary to remove protamines from DNA, a required step to achieve complete DNA decondensation and form sperm haloes of decondensed chromatin, leaving TLR attached to the nuclear matrix (Mohar et al., 2002; Sotolongo et al., 2003).
Different methods require complete sperm DNA decondensation to evaluate DNA breaks. DNA fragmentation assays, such as the SCD test, rely on the differential chromatin decondensation of spermatozoa containing DNA breaks compared to those with non-damaged DNA (Fernández et al., 2003; Cortés-Gutiérrez et al., 2009). Likewise, the Comet assay requires complete DNA decondensation for migration of fragments and the subsequent formation of the Comet tail, and some protocols include incubations with proteinase K overnight (Simon et al., 2014; Ribas-Maynou and Benet, 2019). Even the TUNEL assay has been proposed to display higher sensitivity following mild decondensation with DTT (Mitchell et al., 2011). Decondensation of sperm chromatin is therefore applied to multiple species, with several studies linking DNA breaks to fertility disorders (Pérez-Llano et al., 2010; Gosálvez et al., 2011; Esteves et al., 2020; Ribas-Maynou et al., 2020).
Against this background, this study aimed at comparing the decondensation capacity of sperm DNA between different species, some described to contain both protamine 1 and protamine 2, and others known to have protamine 1 only.
Unless stated, all reagents used for this study were purchased from Sigma-Aldrich (St. Louis, MO, United States).
Five human semen samples were obtained from healthy volunteers that were recruited by University of Girona, Spain. All donors signed an informed consent before providing their biological samples, and the study was approved by the Ethics Committee, Josep Trueta Hospital, Spain (Reference PTI-HUMA 10012018) thus complying with the Helsinki declaration for the research with human specimens. All men provided a semen sample through ejaculation in a sterile cup. This ejaculation was maintained at 37°C for 30 min and was subsequently cryopreserved.
Cryopreservation was performed using Test Yolk Buffer (TYB, 14% glycerol, 30% egg yolk, 1.98% glucose, 1.72% sodium citrate, and 2% glycine, pH 7.4). Briefly, each semen sample was gently mixed 1:1 (v:v) with TYB, poured into a 0.5-mL cryotube, and cooled down in an isopropyl alcohol bath placed within a −80°C freezer for 12 h. Afterward, cryotubes were transferred to liquid nitrogen. Samples were thawed by submerging cryotubes in a water bath at 37°C, and then diluted with PBS (1:3 dilution), added drop-by-drop in order to avoid osmotic shock.
Researchers did not manipulate animals, but semen samples were rather purchased from a local farm (Servicios Genéticos Porcinos, S.L.; Roda de Ter, Spain), which provided commercial, standard doses (90 mL) intended to artificial insemination (AI). A total of five semen samples were obtained from five sexually mature Piétrain boars of proven fertility. Ejaculates were collected using the gloved-hand method, and the sperm rich fraction was diluted to 3.3 × 107 spermatozoa/mL with a long-term commercial extender (Vitasem LD; Magapor SL, Zaragoza, Spain).
Cryopreservation was performed using the following protocol. First, 50-mL aliquots were centrifuged at 2,400 × g and 15°C for 3 min, and sperm suspensions were diluted in β-lactose-egg yolk (LEY) medium (80% v:v lactose, 20% egg yolk) to a final concentration of 1.5 × 109 sperm/mL. Thereafter, all aliquots were cooled down to a final temperature of 5°C, at a rate of −0.1°C/min. Then, samples were diluted to a final concentration of 1 × 109 sperm/mL in LEYGO medium, which consisted of LEY medium supplemented with 6% v:v glycerol and 1.5% Orvus ES Paste (Equex STM; Nova Chemical Sales Inc., Scituate, MA, United States). Finally, samples were packed into 0.5-mL plastic straws (Minitüb, Tiefenbach, Germany), and a programmable controlled-rate freezer (Ice-Cube, Minitüb) was used to cryopreserve samples at the following cooling rates: 6°C/min from 5 to –5°C (100 s); –39.82°C/min from –5 to –80°C (113 s); holt at –80°C for 30 s; and cooled at –60°C/min from –80 to –150°C (70 s). Samples were finally plunged into liquid nitrogen.
Samples were thawed through submerging a 0.5-mL straw in a 38 °C water bath for 15 s, with shaking. The entire volume of the straw was diluted in three volumes (1:3, v:v) of pre-warmed Beltsville Thawing Solution (BTS).
Five cryopreserved straws from five separate animals were used. All stallions were donors of proven fertility, and were housed at the Equine Reproduction Service, Autonomous University of Barcelona (Spain), which is a European Union approved equine semen collection center (Authorization code: ES09RS01E). Semen samples were obtained using a Hannover-type artificial vagina (MiniTüb, Tiefenbach, Germany) that contains a nylon mesh filter to separate the ejaculate gel fraction. The obtained ejaculates were directly diluted (1:5, v:v) in previously warmed (37°C) Kenney buffer (49% glucose, w:v; 24% powdered milk, w:v).
Cryopreservation was performed according to the following protocol: first, samples were centrifuged at 600 × g and 20°C for 15 min. Then, pellets were resuspended in BotuCRYO commercial extender (Botupharma, Botucatu, Brazil) to reach a concentration of 2 × 106 viable sperm/mL. Samples were then packaged into 0.5-mL straws and frozen in a programmable freezer (Ice-Cube) as follows: (i) −0.25°C/min from 20 to 5°C (60 min); (ii) −4.75°C/min from 5 to −90°C (20 min); and (iii) −11.11°C/min from −90 to −120°C (2.7 min). Straws were finally plunged into liquid nitrogen for storage.
Thawing was performed by submerging straws in a water bath at 38°C for 20 s. The volume of each straw was diluted in three volumes of pre-warmed Kenney medium (1:3, v:v).
Five semen samples coming from five different jackasses of proven fertility were used. Animals were located at the Equine Reproduction Service, Autonomous University of Barcelona (Bellaterra, Spain). Semen samples were obtained using a Hannover artificial vagina (Minitüb) with a nylon mesh filter to remove the gel fraction. Immediately after collection, semen was mixed with Kenney extender (1:5, v:v) at 37°C.
For cryopreservation, the following protocol was used: first, diluted semen was centrifuged at 600 × g and 20°C for 15 min, and pellets were resuspended in 2 mL of INRA-freeze freezing extender (INRA, Paris, France). Then, concentration and motility were evaluated, and samples were diluted again to reach a final concentration of 2 × 106 viable sperm/mL. Following this, sperm were packaged into 0.5-mL straws and frozen with a programmable freezer (Ice-Cube, Minitüb) with the following cooling rates: (i) from 20 to 5°C at a rate of −0.25°C/min; (ii) from 5 to −90°C at a rate of −4.75°C/min; and (iii) from −90 to −120°C at a rate of −11.11°C/min. Finally, straws were plunged into liquid nitrogen and stored.
For thawing, straws were submerged in a water bath at 38°C for 20 s, and the content was diluted with three volumes of in 1.5 mL of Kenney medium at 37°C per straw.
Five separate samples from five healthy and sexually mature Holstein bulls were used. All animals were kept in an artificial insemination center (Cenero-Asturias, Gijón, Spain), and ejaculates were collected through an artificial vagina. Collected semen was diluted in Bioxcell extender reaching a concentration of 92 × 106 sperm/mL. Then, samples were cooled down to 4°C at a rate of −0.2°C/min, and after 3 h of equilibration, extended semen was packaged into 0.25-mL straws containing 23 × 106 sperm/straw.
Cryopreservation was performed in a programmable freezer (Digit-cool; IMV Technologies, L’Aigle, France) following the standard curve for bovine sperm: −5°C/min from 4 to −10°C, −40°C/min from −10 to −100°C, and −20°C/min from −100 to −140°C. Thawing was performed by submerging straws in a water bath at 38°C for 40 s, with shaking.
Halo formation was induced following the same protocol for all species. First, samples were diluted in phosphate-buffered saline (PBS) to reach a final concentration of 1 × 106 sperm/mL. Meanwhile, an aliquot of low melting point agarose at 1% was melted at 70°C for 10 min and warmed to 37.5°C for further 10 min. Next, the sample and low melting point agarose were mixed at a 1:2 (v:v) ratio, obtaining a final agarose concentration of 0.66%. A drop of 6 μL of the mixture was then dispensed onto an agarose pre-treated slide, covered with a 8-mm circular coverslip, and allowed to jellify on a metal cold plate at 4°C for 5 min. Afterward, the coverslip was gently removed and the slide was incubated in a first lysis solution (0.8 M Tris-HCl, 0.8 M DTT, 1% SDS; pH = 7.5) at room temperature for 30 min. Following this, slides were incubated in a second lysis solution (0.4 M Tris-HCl, 0.4 M DTT, 50 mM EDTA, 2 M NaCl, 1% Tween20; pH = 7.5) at room temperature for 30 min. At this point, slides were differentially incubated with a third lysis solution that contained proteinase K (0.4 M Tris-HCl, 0.4 M DTT, 50 mM EDTA, 2 M NaCl, 1% Tween20, 100 μg/mL proteinase K; pH = 7.5). Incubation with this third lysis solution was also performed at room temperature for 0, 30, or 180 min. To assess the effect of incubating pig and cattle sperm with proteinase K, decondensation with a third lysis solution that did not contain proteinase K (0.4 M Tris-HCl, 0.4 M DTT, 50 mM EDTA, 2 M NaCl, 1% Tween20, 100 μg/mL proteinase K; pH = 7.5) was also performed for 0, 30, or 180 min. After all lysis steps, slides were washed with a neutral pH solution (0.4 M Tris-HCl, pH 7.5) for 5 min, and dehydrated in ethanol series (70, 90, and 100%; 2 min/step).
Halo and core diameters are used as DNA decondensation indicators. In order to obtain both measures, samples were labeled with Safeview DNA stain (NBS Biologicals, Huntingdon, United Kingdom) prior to evaluation under a Zeiss Imager Z1 epifluorescence microscope (Carl Zeiss AG, Oberkochen, Germany). Captures at 1,000× magnification of at least 50 cells per experimental condition were obtained using Axiovision 4.6 software (Carl Zeiss AG, Oberkochen, Germany). In these captures, exposure time was adapted to correctly differentiate haloes from core sperm heads. From these pictures, diameters of the core and the halo were measured as indicated in Figure 1, using the Image J measurement tool. These diameters were obtained in duplicate, and mean values were calculated for each sperm cell. In order to normalize data, a scale bar was used to obtain the correspondence of pixels to μm at the resolution used (1,388 × 1,040 pixels).
In order to measure the size of nuclear DNA, a sperm sample from each species was smeared onto a glass slide, permeabilized with PBS containing 0.25% Triton X-100 for 2 min, and stained with Safeview DNA stain. Measures of nuclear core were made following the same procedure depicted in Figure 1.
Data were analyzed using the Statistics Package for the Social Sciences software (IBM SPSS ver. 25; IBM Corp., Armonk, NY, United States). Graphs were drawn using GraphPad Prism Software (GraphPad; San Diego, CA, United States). Once data were recorded and compiled, normal distribution and homogeneity of variances were checked through Shapiro-Wilk and Levene tests. Since, even after linear transformation [log(x), arcsine √x, √x], data did not fit with parametric assumptions, Scheirer-Ray-Hare and Mann-Whitney tests were run. A significance level of 95% for the confidence interval was established to consider statistically significant differences.
Figure 2 shows the formation of sperm haloes in different species, following different incubation times with proteinase K (third lysis step). The application of the third lysis step containing proteinase K had a differential effect on the separate species studied. Whilst human, horse and donkey sperm did not present higher decondensation when incubated with proteinase K for a longer period (third lysis step), pig and cattle sperm showed higher DNA decondensation after incubation with proteinase K and exhibited larger sperm haloes.
Figure 2. Decondensation of sperm DNA through the formation of sperm haloes. It is worth noting that porcine and bovine sperm presented higher DNA decondensation with increasing incubation times over the third lysis step containing proteinase K. In contrast, no significant changes in human, horse and donkey sperm were observed. Scale bar: 5 μm.
Following DNA staining of sperm spreads/smears, dimensions of nuclei were found to be: 4.14 μm ± 0.41 μm (mean ± standard deviation) for human sperm, 3.62 μm ± 0.55 μm for horse sperm, 3.89 μm ± 0.52 μm for donkey sperm, 4.43 μm ± 0.34 μm for pig sperm, and 4.80 μm ± 0.34 μm for cattle sperm. While treatment with lysis solutions that did not contain proteinase K led to chromatin decondensation of sperm core, the extent of that decondensation differed between species. In effect, while, after this step, the cores of human, horse and donkey sperm were 7.11 μm ± 0.96 μm, 5.67 μm ± 0.55 μm, and 5.83 ± 0.35, respectively, they were found to measure 4.98 μm ± 0.50 μm in pig sperm and 4.34 μm ± 0.79 μm in cattle sperm. In spite of this, this increase in the diameter of the core, which indicated DNA decondensation, was statistically significant in all cases (human, P < 0.001; horse, P < 0.001; donkey, P < 0.001; pig, P < 0.001; and cattle, P = 0.004).
Moreover, nuclear core also showed an increase in decondensation associated to the additional treatment of sperm cells with a lysis solution containing proteinase K (Table 1). This increase was statistically significant in horse, donkey, porcine and bovine sperm when comparing 180 min of incubation with 0 min of incubation (P < 0.05); and in human, porcine and bovine sperm when comparing 180 min of incubation with 30 min of incubation (P < 0.05).
Table 1. Effect of the third lysis solution containing proteinase K on halo and core diameter in different species.
Table 1 shows the effect of incubation in the third lysis solution containing proteinase K on halo diameter. We observed that exposure to proteinase K led to higher halo diameters in all species. In effect, there was a significant (P < 0.05) increase in halo diameters of human, horse, pig and cattle sperm, when 0 and 180 min of incubation were compared. Furthermore, 180-min incubations compared to their 30-min counterparts also showed a significant increase in halo diameters of human, donkey, pig and cattle sperm (P < 0.05). However, and in spite of these significant differences, the extent of the increase of halo diameter in porcine and bovine sperm was much higher (Table 1).
Since both sperm haloes and cores showed an increased size associated to proteinase K lysis treatment, the relationship between halo size and core size appeared to be an indicator of sperm DNA decondensation. Figure 3 and Table 1 show the halo/core ratio for all species involved in this study. Interestingly, while human, horse and donkey sperm did not exhibit an increase in halo/core ratios associated to longer proteinase k treatments (P > 0.05), these ratios augmented in porcine and bovine sperm (0 min vs. 30 min; 30 min vs. 180 min; P < 0.05).
Figure 3. Halo/core ratio in relation to proteinase K treatment (0, 30, or 180 min). ∗∗Statistically significant differences (P < 0.05).
To determine the importance of including proteinase K in the third lysis solution, we tested DNA decondensation in pig and cattle sperm after incubation with the same lysis solution without proteinase K for 30 and 180 min. Table 2 shows core diameter, halo diameter and halo/core ratio after such incubations. Figure 4 depicts halo/core ratio as a measure of DNA decondensation. When proteinase K was included, halo diameter significantly increased both in pig and cattle sperm after 30 and 180 min of incubation (P < 0.001). Similarly, all tested conditions also augmented halo/core ratios in both species (P < 0.001).
Table 2. DNA decondensation of pig and cattle sperm after incubation in the third lysis solution with or without proteinase K.
Figure 4. Halo/core ratios for incubations (0, 30, or 180 min) of pig and cattle sperm in the third lysis solution without (empty dots) or with proteinase K (filled dots). ∗∗Statistically significant differences (P < 0.001).
In the present study, we observed complete decondensation of sperm DNA when samples were exposed to a lysis solution containing proteinase K for a long period (up to 180 min). We found inter-specific differences regarding sperm chromatin decondensation following that lysis treatment since, while human, horse and donkey sperm did not effectively augment their DNA decondensation, pig and cattle sperm achieved a 2.3- and 1.75-fold increase, respectively (based on halo/core ratios). Moreover, in an additional experiment, we could establish that proteinase K accelerates DNA decondensation in pig and cattle sperm.
A high DNA condensation up to almost crystalline state is a key feature that is only present in paternal germ cells and allows them to transport the male genome through the female tract to reach the oocyte. This highly condensed state helps in the protection of sperm cells from genotoxic damage to which they are exposed (Adiga et al., 2007; Marchetti et al., 2015). Due to their transcriptional silence and the loss of most of their cytoplasmic proteins, sperm cells are devoid of DNA repair systems; however, they are continuously exposed to exogenous and endogenous DNA damage, caused mainly through oxidative stress (Aitken and De Iuliis, 2007). Not only are reactive oxygen species produced by sperm mitochondria through oxidative phosphorylation, which is essential for ATP generation (Camello-Almaraz et al., 2006), but can also result from the presence of immature sperm, leucocytes, and other exogenous effectors, such as pollutants, heat stress, infections, radiations, or other toxicants (Agarwal et al., 2008; Ribas-Maynou et al., 2020).
Different methods that indirectly evaluate DNA integrity are based on the differential decondensation of sperm cells that contain DNA breaks. In this context, in vitro DNA decondensation is required in order for external and internal chromatin to be accessible. As previous studies showed, methods evaluating sperm DNA integrity differ in their sensitivity to detect the actual DNA damage (Ribas-Maynou et al., 2013; Simon et al., 2014). In this regard, achieving complete in vitro DNA decondensation is critical to increase the sensitivity of methods evaluating its integrity, thus allowing researchers to obtain reliable values of DNA damage. In fact, some authors explained the low sensitivity of techniques assessing DNA integrity through the hypothesis of “the iceberg tip,” which surmises that, while some sperm detected as “normal” actually have DNA breaks, they are out of the method threshold (Gosálvez et al., 2013; Cho et al., 2017).
In the present study, we found that pig and cattle sperm present higher grade of DNA condensation, which is related to an increased difficulty to achieve its complete decondensation in vitro and form a sperm halo in an agarose matrix (Figure 2). In pigs, previous studies using the SCD test considered that sperm with non-fragmented DNA lacked a halo, whereas those with fragmented DNA showed a large halo (Enciso et al., 2006; Alkmin et al., 2013; Batista et al., 2016). As shown for pig sperm after 0 min of incubation in the third lysis solution containing proteinase K (Figure 2), the lack of halo identified in other studies (Enciso et al., 2006) could be due to an incomplete DNA decondensation. Therefore, when complete DNA decondensation was achieved following incubation in the third lysis solution containing proteinase K for 180 min, sperm with non-fragmented DNA showed large halos, as occurs when the halo assay is used with human sperm (Fernández et al., 2005). Remarkably, our results showed that complete sperm DNA decondensation is more easily achieved in the human than in the pig. In effect, in order for similar levels of decondensed DNA to be obtained, pig sperm were observed to need a longer lysis treatment with proteinase K. In fact, proteinase K was found to act as a DNA decondensation accelerator, probably because it degrades protamines extracted by the lysis buffer (Figure 4). Previous studies using the SCD test in pig sperm lysed for 5 min, regardless of the composition of solutions (Enciso et al., 2006; Alkmin et al., 2013; Yeste et al., 2013a, b; Batista et al., 2016), are in agreement with our results. In a similar way, using the Comet assay in pig sperm without a long lysis containing proteinase K has also been suggested to lead to sensitivity-compromised results (Pérez-Llano et al., 2010; Enciso et al., 2011). Also matching with our data, a previous study aimed at elucidating alkaline labile sites in pig sperm showed the need of incubating these cells in a lysis solution containing SDS, DTT and proteinase K for 3.5 h at 37°C to observe the same amount of those sites in leukocytes from the same species (Cortés-Gutiérrez et al., 2009). Furthermore, other authors incubated pig sperm with lysis solutions containing proteinase K and found that Comet images depicted complete DNA decondensation (Fraser and Strzezek, 2005).
Regarding the analysis of sperm DNA integrity in cattle, previous studies showed that sperm with non-fragmented DNA depicted small halos, whereas those with fragmented DNA presented big halos, which was in agreement with what found in pigs (García-Macías et al., 2007; Dogan et al., 2015). Our results also indicated that bovine sperm exhibited a small halo when either not exposed or shortly exposed to proteinase K for 30 min. However, when the time of incubation with proteinase K increased, sperm chromatin of bovine sperm completely decondensed, in a similar fashion to what observed in other species (Figure 3). Thus, our results confirm that proteinase K acts as an accelerator of DNA decondensation, and suggest that previous studies evaluating DNA fragmentation in bovine sperm could not have decondensed DNA enough, since the lysis period was, regardless of the solutions used, short (10 min) (García-Macías et al., 2007; Dogan et al., 2015). The use of the Comet assay in cattle sperm also demonstrated that longer lysis treatments increased the degree of DNA decondensation (Villani et al., 2010). However, it remains unclear whether exposure to lysis solutions overnight in Fraser and Strzezek (2005) could have induced artifactual DNA breaks, thus misestimating the actual results.
It is widely known that sperm DNA in humans and horses is condensed around both protamine 1 and protamine 2, whereas only protamine 1 but not protamine 2 is present in the chromatin of pig and cattle sperm (Corzett et al., 2002). Moreover, morphometric studies indicated that pig and cattle sperm display a bigger disk-shaped nucleus, whereas human and horse sperm are smaller and more oval-shaped (Gu et al., 2019). Our results on the resilience of sperm DNA to decondensation in the aforementioned species suggest that while sperm containing only protamine 1 display less efficiency in condensing their DNA, they have stronger protamine-protamine junctions. In fact, protamine 1 in pig sperm displays the highest number of cysteine residues (10 residues) compared to other species, such as donkey (8 residues), horse (7 residues) or human (6 residues), which would support the relevance of disulfide bonds between protamines in the pig (Gosálvez et al., 2011). Furthermore, bovine protamine 1 has less cysteine residues (7 residues) than its pig counterpart, which could explain the less degree of DNA decondensation observed at 0 min of incubation with the third lysis solution (Gosálvez et al., 2011). Alternatively, sperm from species containing both P1 and P2 appear to exhibit higher DNA condensation, with the contraindication of having less protamine-protamine unions, as different protamines from P2 family have less cysteine residues than P1 (Corzett et al., 2002; Oliva, 2006). Moreover, when the number cysteine residues of P1 is related to the P1/P2 ratio, the amount of cysteine residues in sperm chromatin (cysteine residues of P1 × %P1) in each species appears to be linked to the degree of DNA condensation (halo/core ratio) after 0 min of incubation with the third lysis solution (Supplementary Table 1). All the aforementioned could also explain why alterations in the relative amounts of protamine 2 lead to higher sperm DNA decondensation, and underlie DNA damage and infertility (Aoki et al., 2005; García-Peiró et al., 2011; Nanassy et al., 2011).
In conclusion, long lysis treatment of porcine and bovine sperm is required for complete sperm DNA decondensation in these species. This is not necessary for human, horse and donkey sperm, as lysis solutions used in previous studies led to complete DNA decondensation without any additional treatment. Moreover, we observed that the inclusion of proteinase K accelerates DNA decondensation. Thus, we hypothesize that the species-specific differences in the resilience of sperm DNA to decondensation rely upon whether sperm DNA is condensed around protamine 1 and protamine 2, or around protamine 1 only.
The original contributions presented in the study are included in the article/Supplementary Material, further inquiries can be directed to the corresponding author/s.
The studies involving human participants were reviewed and approved by the Josep Trueta Hospital Ethics Committee (Reference PTI-HUMA 10012018). The patients/participants provided their written informed consent to participate in this study.
JR-M conceived the study, performed the experiments, analyzed the data, run statistics, wrote the manuscript, and approved the final version. EG-B performed the experiments, critically revised the document, and approved the final version. CH, JC, and JM cryopreserved semen samples, critically revised the manuscript, and approved the final document. MY contributed to the experimental design, coordinated the work, discussed results, critically revised the manuscript, and approved the final document. All authors contributed to the article and approved the submitted version.
The authors acknowledge the support from the Ministry of Science and Innovation, Spain (Grant No. AGL2017-88329-R), the Regional Government of Catalonia, Spain (Grant No. 2017-SGR-1229), la Marató de TV3 (Grant No. 214/857-202039), and University of Girona (Postdoc UdG-2020, to JR-M).
The authors declare that the research was conducted in the absence of any commercial or financial relationships that could be construed as a potential conflict of interest.
The Supplementary Material for this article can be found online at: https://www.frontiersin.org/articles/10.3389/fcell.2021.669182/full#supplementary-material
Adiga, S. K., Toyoshima, M., Shiraishi, K., Shimura, T., Takeda, J., Taga, M., et al. (2007). p21 provides stage specific DNA damage control to preimplantation embryos. Oncogene 26, 6141–6149. doi: 10.1038/sj.onc.1210444
Agarwal, A., Makker, K., and Sharma, R. (2008). Clinical relevance of oxidative stress in male factor infertility: an update. Am. J. Reprod. Immunol. 59, 2–11. doi: 10.1111/j.1600-0897.2007.00559.x
Aitken, R. J., and De Iuliis, G. N. (2007). Origins and consequences of DNA damage in male germ cells. Reprod. Biomed. Online 14, 727–733.
Ajduk, A., Yamauchi, Y., and Ward, M. A. (2006). Sperm chromatin remodeling after intracytoplasmic sperm injection differs from that of in vitro fertilization. Biol. Reprod. 75, 442–451. doi: 10.1095/biolreprod.106.053223
Alkmin, D. V., Martinez-Alborcia, M. J., Parrilla, I., Vazquez, J. M., Martinez, E. A., and Roca, J. (2013). The nuclear DNA longevity in cryopreserved boar spermatozoa assessed using the Sperm-Sus-Halomax. Theriogenology 79, 1294–1300. doi: 10.1016/j.theriogenology.2013.02.026
Allen, M. J., Bradbury, E. M., and Balhorn, R. (1996). The chromatin structure of well-spread demembranated sperm nuclei revealed by atomic force microscopy. Scanning Microsc. 10, 989–996.
Allen, M. J., Lee, C., Lee, J. D., Pogany, G. C., Balooch, M., Siekhaus, W. J., et al. (1993). Atomic force microscopy of mammalian sperm chromatin. Chromosoma 102, 623–630. doi: 10.1007/BF00352310
Aoki, V. W., Moskovtsev, S. I., Willis, J., Liu, L., Mullen, J. B. M., and Carrell, D. T. (2005). DNA integrity is compromised in protamine-deficient human sperm. J. Androl. 26, 741–748. doi: 10.2164/jandrol.05063
Balhorn, R. (1982). A model for the structure of chromatin in mammalian sperm. J. Cell Biol. 93, 298–305.
Batista, C., van Lier, E., and Petrocelli, H. (2016). Dynamics of sperm DNA fragmentation in raw boar semen and fertility. Reprod. Domest. Anim. 51, 774–780. doi: 10.1111/rda.12749
Brewer, L., Corzett, M., Lau, E. Y., and Balhorn, R. (2003). Dynamics of protamine 1 binding to single DNA molecules. J. Biol. Chem. 278, 42403–42408. doi: 10.1074/jbc.M303610200
Brewer, L. R., Corzett, M., and Balhorn, R. (1999). Protamine-induced condensation and decondensation of the same DNA molecule. Science 286, 120–123.
Camello-Almaraz, C., Gomez-Pinilla, P. J., Pozo, M. J., and Camello, P. J. (2006). Mitochondrial reactive oxygen species and Ca2+ signaling. Am. J. Physiol. Cell Physiol. 291, C1082–C1088. doi: 10.1152/ajpcell.00217.2006
Cho, C., Willis, W. D., Goulding, E. H., Jung-Ha, H., Choi, Y. C., Hecht, N. B., et al. (2001). Haploinsufficiency of protamine-1 or -2 causes infertility in mice. Nat. Genet. 28, 82–86. doi: 10.1038/ng0501-82
Cho, C.-L., Agarwal, A., Majzoub, A., and Esteves, S. C. (2017). The correct interpretation of sperm DNA fragmentation test. Transl. Androl. Urol. 6, S621–S623. doi: 10.21037/tau.2017.06.25
Cortés-Gutiérrez, E. I., Crespo, F., Serres-Dalmau, C., Gutiérrez de las Rozas, A. L., Dávila-Rodríguez, M. I., López-Fernández, C., et al. (2009). Assessment of sperm DNA fragmentation in stallion (Equus caballus) and donkey (Equus asinus) using the sperm chromatin dispersion test. Reprod. Domest. Anim. 44, 823–828. doi: 10.1111/j.1439-0531.2008.01091.x
Corzett, M., Mazrimas, J., and Balhorn, R. (2002). Protamine 1: protamine 2 stoichiometry in the sperm of eutherian mammals. Mol. Reprod. Dev. 61, 519–527. doi: 10.1002/mrd.10105
Dogan, S., Vargovic, P., Oliveira, R., Belser, L. E., Kaya, A., Moura, A., et al. (2015). Sperm protamine-status correlates to the fertility of breeding bulls. Biol. Reprod. 92:92. doi: 10.1095/biolreprod.114.124255
Enciso, M., Johnston, S. D., and Gosálvez, J. (2011). Differential resistance of mammalian sperm chromatin to oxidative stress as assessed by a two-tailed comet assay. Reprod. Fertil. Dev. 23, 633–637. doi: 10.1071/RD10269
Enciso, M., López-Fernández, C., Fernández, J. L., García, P., Gosálbez, A., and Gosálvez, J. (2006). A new method to analyze boar sperm DNA fragmentation under bright-field or fluorescence microscopy. Theriogenology 65, 308–316. doi: 10.1016/j.theriogenology.2005.05.044
Esteves, S. C., Zini, A., Coward, R. M., Evenson, D. P., Gosálvez, J., Lewis, S. E. M., et al. (2020). Sperm DNA fragmentation testing: summary evidence and clinical practice recommendations. Andrologia 53:e13874. doi: 10.1111/and.13874
Fernández, J. L., Muriel, L., Goyanes, V., Segrelles, E., Gosálvez, J., Enciso, M., et al. (2005). Simple determination of human sperm DNA fragmentation with an improved sperm chromatin dispersion test. Fertil. Steril. 84, 833–842. doi: 10.1016/j.fertnstert.2004.11.089
Fernández, J. L., Muriel, L., Rivero, M. T., Goyanes, V., Vazquez, R., and Alvarez, J. G. (2003). The sperm chromatin dispersion test: a simple method for the determination of sperm DNA fragmentation. J. Androl. 24, 59–66. doi: 10.1002/j.1939-4640.2003.tb02641.x
Foster, H. A., Abeydeera, L. R., Griffin, D. K., and Bridger, J. M. (2005). Non-random chromosome positioning in mammalian sperm nuclei, with migration of the sex chromosomes during late spermatogenesis. J. Cell Sci. 118, 1811–1820. doi: 10.1242/jcs.02301
Fraser, L., and Strzezek, J. (2005). Effects of freezing-thawing on DNA integrity of boar spermatozoa assessed by the neutral comet assay. Reprod. Domest. Anim. 40, 530–536. doi: 10.1111/j.1439-0531.2005.00626.x
Fuentes-Mascorro, G., Serrano, H., and Rosado, A. (2000). Sperm chromatin. Arch. Androl. 45, 215–225.
García-Macías, V., de Paz, P., Martinez-Pastor, F., Alvarez, M., Gomes-Alves, S., Bernardo, J., et al. (2007). DNA fragmentation assessment by flow cytometry and sperm-bos-halomax (bright-field microscopy and fluorescence microscopy) in bull sperm. Int. J. Androl. 30, 88–98. doi: 10.1111/j.1365-2605.2006.00723.x
García-Peiró, A., Martínez-Heredia, J., Oliver-Bonet, M., Abad, C., Amengual, M. J., Navarro, J., et al. (2011). Protamine 1 to protamine 2 ratio correlates with dynamic aspects of DNA fragmentation in human sperm. Fertil. Steril. 95, 105–109. doi: 10.1016/j.fertnstert.2010.06.053
Getzenberg, R. H. (1994). Nuclear matrix and the regulation of gene expression: tissue specificity. J. Cell. Biochem. 55, 22–31. doi: 10.1002/jcb.240550105
Gibson, B. A., Doolittle, L. K., Schneider, M. W. G., Jensen, L. E., Gamarra, N., Henry, L., et al. (2019). Organization of chromatin by intrinsic and regulated phase separation. Cell 179, 470–484. doi: 10.1016/j.cell.2019.08.037
Gosálvez, J., Caballero, P., López-Fernández, C., Ortega, L., Guijarro, J. A., Fernández, J. L., et al. (2013). Can DNA fragmentation of neat or swim-up spermatozoa be used to predict pregnancy following ICSI of fertile oocyte donors? Asian J. Androl. 15, 812–818. doi: 10.1038/aja.2013.74
Gosálvez, J., López-Fernández, C., Fernández, J. L., Gouraud, A., and Holt, W. V. (2011). Relationships between the dynamics of iatrogenic DNA damage and genomic design in mammalian spermatozoa from eleven species. Mol. Reprod. Dev. 78, 951–961. doi: 10.1002/mrd.21394
Gu, N.-H., Zhao, W.-L., Wang, G.-S., and Sun, F. (2019). Comparative analysis of mammalian sperm ultrastructure reveals relationships between sperm morphology, mitochondrial functions and motility. Reprod. Biol. Endocrinol. 17:66. doi: 10.1186/s12958-019-0510-y
Haaf, T., and Ward, D. (1995). Higher order nuclear structure in mammalian sperm revealed by in situ hybridization and extended chromatin fibers. Exp. Cell Res. 219, 604–611. doi: 10.1006/EXCR.1995.1270
Hammoud, S. S., Nix, D. A., Zhang, H., Purwar, J., Carrell, D. T., and Cairns, B. R. (2009). Distinctive chromatin in human sperm packages genes for embryo development. Nature 460, 473–478. doi: 10.1038/nature08162
Hao, S.-L., Ni, F.-D., and Yang, W.-X. (2019). The dynamics and regulation of chromatin remodeling during spermiogenesis. Gene 706, 201–210. doi: 10.1016/j.gene.2019.05.027
Ji, L., Xu, R., Lu, L., Zhang, J., Yang, G., Huang, J., et al. (2013). TM6, a novel nuclear matrix attachment region, enhances its flanking gene expression through influencing their chromatin structure. Mol. Cells 36, 127–137. doi: 10.1007/s10059-013-0092-z
Jung, Y. H., Sauria, M. E. G., Lyu, X., Cheema, M. S., Ausio, J., Taylor, J., et al. (2017). Chromatin states in mouse sperm correlate with embryonic and adult regulatory landscapes. Cell Rep. 18, 1366–1382. doi: 10.1016/j.celrep.2017.01.034
Kierszenbaum, A. L. (2001). Transition nuclear proteins during spermiogenesis: unrepaired DNA breaks not allowed. Mol. Reprod. Dev. 58, 357–358.
Koehler, J. K., Würschmidt, U., and Larsen, M. P. (1983). Nuclear and chromatin structure in rat spermatozoa. Gamete Res. 8, 357–370. doi: 10.1002/mrd.1120080406
Lee, K., Haugen, H. S., Clegg, C. H., and Braun, R. E. (1995). Premature translation of protamine 1 mRNA causes precocious nuclear condensation and arrests spermatid differentiation in mice. Proc. Natl. Acad. Sci. U. S. A. 92, 12451–12455. doi: 10.1073/pnas.92.26.12451
Lewis, S. E. M., and Simon, L. (2010). Clinical implications of sperm DNA damage. Hum. Fertil. 13, 201–207. doi: 10.3109/14647273.2010.528823
Linnemann, A. K., Platts, A. E., and Krawetz, S. A. (2009). Differential nuclear scaffold/matrix attachment marks expressed genes. Hum. Mol. Genet. 18, 645–654. doi: 10.1093/hmg/ddn394
Luetjens, C. M., Payne, C., and Schatten, G. (1999). Non-random chromosome positioning in human sperm and sex chromosome anomalies following intracytoplasmic sperm injection. Lancet 353:1240. doi: 10.1016/S0140-6736(99)80059-2
Luger, K., Mäder, A. W., Richmond, R. K., Sargent, D. F., and Richmond, T. J. (1997). Crystal structure of the nucleosome core particle at 2.8 A resolution. Nature 389, 251–260. doi: 10.1038/38444
Makker, K., Agarwal, A., and Sharma, R. (2009). Oxidative stress & male infertility. Indian J. Med. Res. 129, 357–367.
Marchetti, F., Bishop, J., Gingerich, J., and Wyrobek, A. J. (2015). Meiotic interstrand DNA damage escapes paternal repair and causes chromosomal aberrations in the zygote by maternal misrepair. Sci. Rep. 5:7689. doi: 10.1038/srep07689
Miller, D., Brinkworth, M., and Iles, D. (2010). Paternal DNA packaging in spermatozoa: more than the sum of its parts? DNA, histones, protamines and epigenetics. Reproduction 139, 287–301. doi: 10.1530/REP-09-0281
Mitchell, L. A., De Iuliis, G. N., and Aitken, R. J. (2011). The TUNEL assay consistently underestimates DNA damage in human spermatozoa and is influenced by DNA compaction and cell vitality: development of an improved methodology. Int. J. Androl. 34, 2–13. doi: 10.1111/j.1365-2605.2009.01042.x
Mohar, I., Szczygiel, M. A., Yanagimachi, R., and Ward, W. S. (2002). Sperm nuclear halos can transform into normal chromosomes after injection into oocytes. Mol. Reprod. Dev. 62, 416–420. doi: 10.1002/mrd.10147
Mudrak, O., Tomilin, N., and Zalensky, A. (2005). Chromosome architecture in the decondensing human sperm nucleus. J. Cell Sci. 118, 4541–4550. doi: 10.1242/jcs.02581
Nanassy, L., Liu, L., Griffin, J., and Carrell, D. T. (2011). The clinical utility of the protamine 1/protamine 2 ratio in sperm. Protein Pept. Lett. 18, 772–777.
Narwade, N., Patel, S., Alam, A., Chattopadhyay, S., Mittal, S., and Kulkarni, A. (2019). Mapping of scaffold/matrix attachment regions in human genome: a data mining exercise. Nucleic Acids Res. 47, 7247–7261. doi: 10.1093/nar/gkz562
Oliva, R. (2006). Protamines and male infertility. Hum. Reprod. Update 12, 417–435. doi: 10.1093/humupd/dml009
Oliva, R., and Dixon, G. H. (1991). Vertebrate protamine genes and the histone-to-protamine replacement reaction. Prog. Nucleic Acid Res. Mol. Biol. 40, 25–94. doi: 10.1016/s0079-6603(08)60839-9
Pérez-Llano, B., López-Fernández, C., García-Casado, P., Arroyo, F., Gosalbez, A., Sala, R., et al. (2010). Dynamics of sperm DNA fragmentation in the swine: ejaculate and temperature effects. Anim. Reprod. Sci. 119, 235–243. doi: 10.1016/j.anireprosci.2010.01.002
Perreault, S. D., Barbee, R. R., Elstein, K. H., Zucker, R. M., and Keefer, C. L. (1988). Interspecies differences in the stability of mammalian sperm nuclei assessed in vivo by sperm microinjection and in vitro by flow cytometry. Biol. Reprod. 39, 157–167. doi: 10.1095/biolreprod39.1.157
Peschon, J. J., Behringer, R. R., Palmiter, R. D., and Brinster, R. L. (1989). Expression of mouse protamine 1 genes in transgenic mice. Ann. N. Y. Acad. Sci. 564, 186–197. doi: 10.1111/j.1749-6632.1989.tb25897.x
Rao, S. S. P., Huntley, M. H., Durand, N. C., Stamenova, E. K., Bochkov, I. D., Robinson, J. T., et al. (2014). A 3D map of the human genome at kilobase resolution reveals principles of chromatin looping. Cell 159, 1665–1680. doi: 10.1016/J.CELL.2014.11.021
Ribas-Maynou, J., and Benet, J. (2019). Single and double strand sperm DNA damage: different reproductive effects on male fertility. Genes 10:105. doi: 10.3390/genes10020105
Ribas-Maynou, J., García-Peiró, A., Fernández-Encinas, A., Abad, C., Amengual, M. J. J., Prada, E., et al. (2013). Comprehensive analysis of sperm DNA fragmentation by five different assays: TUNEL assay, SCSA, SCD test and alkaline and neutral comet assay. Andrology 1, 715–722. doi: 10.1111/j.2047-2927.2013.00111.x
Ribas-Maynou, J., Yeste, M., and Salas-Huetos, A. (2020). The relationship between sperm oxidative stress alterations and IVF/ICSI outcomes: a systematic review from nonhuman mammals. Biology 9, 1–18. doi: 10.3390/biology9070178
Samans, B., Yang, Y., Krebs, S., Sarode, G. V., Blum, H., Reichenbach, M., et al. (2014). Uniformity of nucleosome preservation pattern in mammalian sperm and its connection to repetitive DNA elements. Dev. Cell 30, 23–35. doi: 10.1016/j.devcel.2014.05.023
Setti, A. S., Braga, D. P., de, A. F., Provenza, R. R., Iaconelli, A., and Borges, E. (2021). Oocyte ability to repair sperm DNA fragmentation: the impact of maternal age on intracytoplasmic sperm injection outcomes. Fertil. Steril. S0015-0282(20)32617-0. doi: 10.1016/j.fertnstert.2020.10.045 [Epub ahead of print].
Simon, L., Liu, L., Murphy, K., Ge, S., Hotaling, J., Aston, K. I., et al. (2014). Comparative analysis of three sperm DNA damage assays and sperm nuclear protein content in couples undergoing assisted reproduction treatment. Hum. Reprod. 29, 904–917. doi: 10.1093/humrep/deu040
Sotolongo, B., Lino, E., and Ward, W. S. (2003). Ability of hamster spermatozoa to digest their own DNA. Biol. Reprod. 69, 2029–2035. doi: 10.1095/biolreprod.103.020594
Toyoshima, M. (2009). Analysis of p53 dependent damage response in sperm-irradiated mouse embryos. J. Radiat. Res. 50, 11–17.
Villani, P., Eleuteri, P., Grollino, M. G., Rescia, M., Altavista, P., Spanò, M., et al. (2010). Sperm DNA fragmentation induced by DNAse I and hydrogen peroxide: an in vitro comparative study among different mammalian species. Reproduction 140, 445–452. doi: 10.1530/REP-10-0176
Ward, W. S. (2010). Function of sperm chromatin structural elements in fertilization and development. Mol. Hum. Reprod. 16, 30–36. doi: 10.1093/molehr/gap080
Ward, W. S., and Coffey, D. S. (1991). DNA packaging and organization in mammalian spermatozoa: comparison with somatic cells. Biol. Reprod. 44, 569–574.
Wykes, S. M., and Krawetz, S. A. (2003). The structural organization of sperm chromatin. J. Biol. Chem. 278, 29471–29477. doi: 10.1074/jbc.M304545200
Yamaguchi, K., Hada, M., Fukuda, Y., Inoue, E., Makino, Y., Katou, Y., et al. (2018). Re-evaluating the localization of sperm-retained histones revealed the modification-dependent accumulation in specific genome regions. Cell Rep. 23, 3920–3932. doi: 10.1016/j.celrep.2018.05.094
Yeste, M., Estrada, E., Casas, I., Bonet, S., and Rodríguez-Gil, J. E. (2013a). Good and bad freezability boar ejaculates differ in the integrity of nucleoprotein structure after freeze-thawing but not in ROS levels. Theriogenology 79, 929–939. doi: 10.1016/j.theriogenology.2013.01.008
Yeste, M., Flores, E., Estrada, E., Bonet, S., Rigau, T., and Rodríguez-Gil, J. E. (2013b). Reduced glutathione and procaine hydrochloride protect the nucleoprotein structure of boar spermatozoa during freeze-thawing by stabilising disulfide bonds. Reprod. Fertil. Dev. 25, 1036–1050. doi: 10.1071/RD12230
Yoshida, K., Muratani, M., Araki, H., Miura, F., Suzuki, T., Dohmae, N., et al. (2018). Mapping of histone-binding sites in histone replacement-completed spermatozoa. Nat. Commun. 9:3885. doi: 10.1038/s41467-018-06243-9
Zalenskaya, I. A., and Zalensky, A. O. (2004). Non-random positioning of chromosomes in human sperm nuclei. Chromosome Res. 12, 163–173.
Zalensky, A., and Zalenskaya, I. (2007). Organization of chromosomes in spermatozoa: an additional layer of epigenetic information? Biochem. Soc. Trans. 35, 609–611. doi: 10.1042/BST0350609
Zalensky, A. O., Tomilin, N. V., Zalenskaya, I. A., Teplitz, R. L., and Bradbury, E. M. (1997). Telomere-telomere interactions and candidate telomere binding protein(s) in mammalian sperm cells. Exp. Cell Res. 232, 29–41. doi: 10.1006/excr.1997.3482
Keywords: sperm, DNA condensation, DNA damage, protamine, mammals
Citation: Ribas-Maynou J, Garcia-Bonavila E, Hidalgo CO, Catalán J, Miró J and Yeste M (2021) Species-Specific Differences in Sperm Chromatin Decondensation Between Eutherian Mammals Underlie Distinct Lysis Requirements. Front. Cell Dev. Biol. 9:669182. doi: 10.3389/fcell.2021.669182
Received: 18 February 2021; Accepted: 12 April 2021;
Published: 30 April 2021.
Edited by:
Silvina Perez-Martinez, CONICET Centro de Estudios Farmacológicos y Botánicos (CEFYBO), ArgentinaReviewed by:
Juan Carlos Calvo, CONICET Instituto de Biología y Medicina Experimental (IBYME), ArgentinaCopyright © 2021 Ribas-Maynou, Garcia-Bonavila, Hidalgo, Catalán, Miró and Yeste. This is an open-access article distributed under the terms of the Creative Commons Attribution License (CC BY). The use, distribution or reproduction in other forums is permitted, provided the original author(s) and the copyright owner(s) are credited and that the original publication in this journal is cited, in accordance with accepted academic practice. No use, distribution or reproduction is permitted which does not comply with these terms.
*Correspondence: Jordi Ribas-Maynou, am9yZGkucmliYXNtYXlub3VAdWRnLmVkdQ==; orcid.org/0000-0002-9101-2044
Disclaimer: All claims expressed in this article are solely those of the authors and do not necessarily represent those of their affiliated organizations, or those of the publisher, the editors and the reviewers. Any product that may be evaluated in this article or claim that may be made by its manufacturer is not guaranteed or endorsed by the publisher.
Research integrity at Frontiers
Learn more about the work of our research integrity team to safeguard the quality of each article we publish.