- 1Department of Orthopedic, Taizhou Hospital of Zhejiang Province Affiliated to Wenzhou Medical University, Linhai, China
- 2Department of Pathology, Taizhou Hospital of Zhejiang Province Affiliated to Wenzhou Medical University, Linhai, China
Acylglycerol kinase (AGK) is a recently discovered mitochondrial lipid kinase, and mutation of its gene is the fundamental cause of Sengers syndrome. AGK is not only involved in the stability of lipid metabolism but also closely related to mitochondrial protein transport, glycolysis, and thrombocytopoiesis. Evidence indicates that AGK is an important factor in the occurrence and development of tumors. Specifically, AGK has been identified as an oncogene that partakes in the regulation of tumor cell growth, invasion, metastasis, and drug resistance. The versatility of AGK and its unique role in different types of cancerous and normal cells greatly piqued our interest. We believe that AGK is a promising target for cancer therapy. Therefore, this review summarizes the main research advances concerning AGK, including the discovery of its physiological/pathogenic mechanisms, and provides a reference for the feasible evaluation of AGK as a therapeutic target for human diseases, particularly tumors.
Introduction
Acylglycerol kinase (AGK), also named multi-substrate lipid kinases (MULK), was initially found through its mutation in Sengers syndrome (Mayr et al., 2012). In the past 10 years, new insights into the biological function of AGK and its significant role in human diseases (Abu El-Asrar et al., 2012, 2013, 2014) (especially tumors) have been established (Figure 1). AGK is renowned as a mitochondrial lipid kinase (Bektas et al., 2005). However, in-depth studies have proclaimed that AGK has a variety of kinase-independent biological responses in multitudinous cells, including as a subunit of the mitochondrial translocase of the inner membrane 22 (TIM22) complex (Kang et al., 2017; Vukotic et al., 2017), which involves transmembrane proteins [such as mitochondrial carrier family members (SLC25A family)] entering the mitochondrial interior from the cytoplasm (Kang et al., 2018; Jackson et al., 2021). Also, AGK partakes in glycolysis in CD8 T cells (Hu et al., 2019) and thrombocytopoiesis (Jiang et al., 2020). Importantly, AGK has been identified as a key oncogene that is highly expressed in a range of tumor types, such as prostate cancer (Spiegel and Milstien, 2005; Nouh et al., 2009; Zeng et al., 2009), breast cancer (Wang et al., 2014), cervical squamous cell carcinoma (Sun et al., 2016), and esophageal squamous cell carcinoma (ESCC) (Chen et al., 2013). AGK participates in the regulation of multiple signaling pathways during tumor occurrence and progression, which makes it a potential target for tumor therapy. In this paper, we comprehensively summarize the structure, function, and regulatory mechanism of AGK, focusing on its regulatory role in the tumor signaling pathway. Also, we describe the latest research results from AGK studies and prospects for future challenges.
AGK Gene, Protein Structure, and Function
Gene
The human AGK gene is located on chromosome 7q34 and contains 15 exons and 1,269 bases, making it highly similar (90% similarity) to AGK in mice. The AGK gene in mice is located on chromosome 6, comprising 16 exons and 1,263 bases in an open reading frame.
Protein Structure
AGK is a nuclear gene-encoded protein mainly located in the mitochondrial membrane, while very recent studies suggest that a portion of AGK is localized elsewhere in the cell (Hu et al., 2019; Jiang et al., 2020). As a newly discovered member of the lipid kinase family, AGK is diffusely expressed in the heart, muscle, brain, and other tissues. AGK is a 47,137-Da protein composed of 422 amino acids. It consists of a typical two-domain fold (DGK domain 1 and DGK domain 2) that mediates the phosphorylation of monoacylglycerols or diacylglycerols (Bektas et al., 2005; Qi et al., 2021). Also, AGK has an N-terminal α1 helix which is complete across the membrane, and a C-terminal key region bound to the membrane (Figure 2).
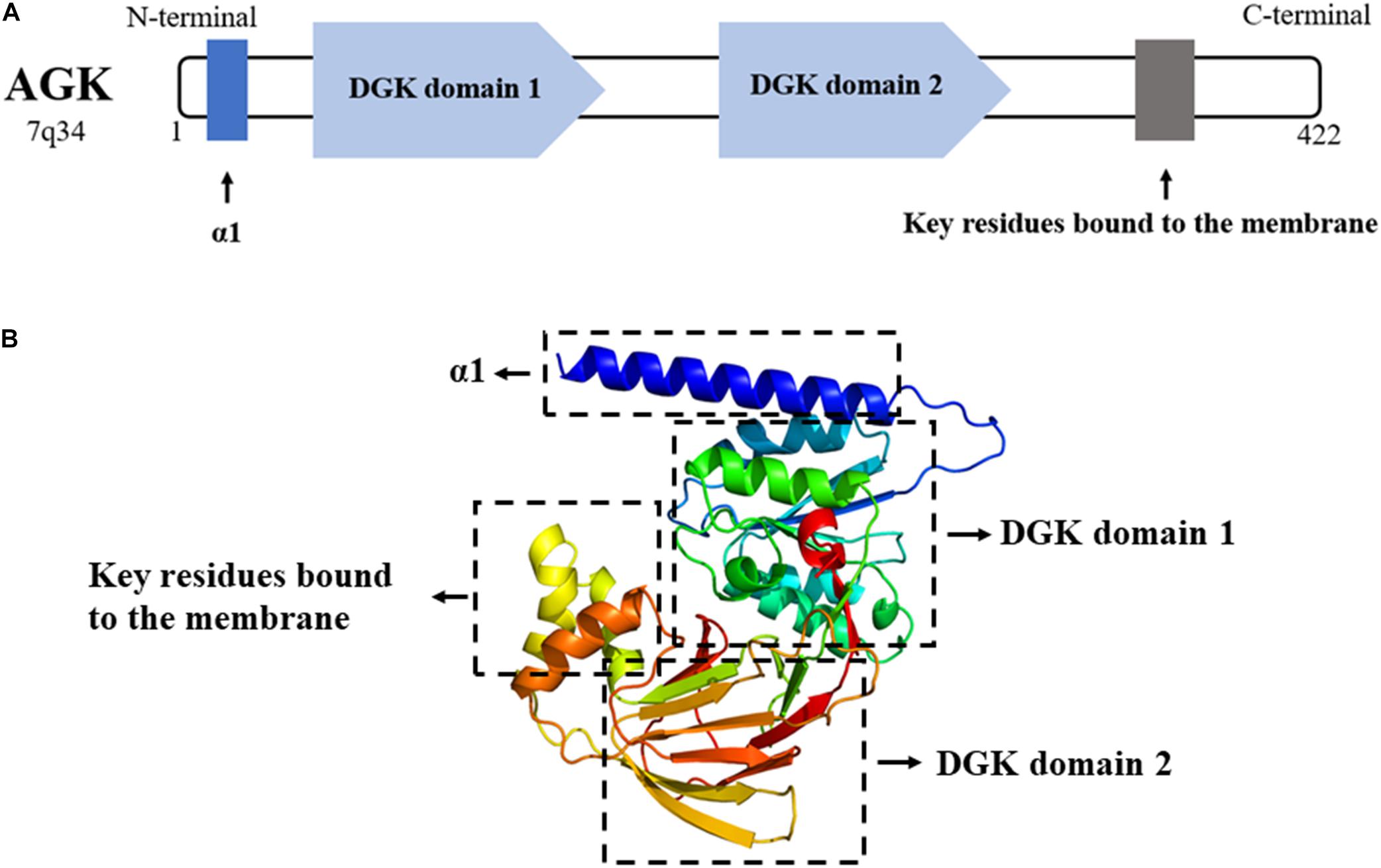
Figure 2. (A) AGK is composed of 422 amino acids and contains an α1 helix, a DGK domain 1, a DGK domain 2, and a C-terminal key residue bound to the membrane. DGK, diacylglycerol kinase. (B) 3D structure of AGK generated by X-ray crystal diffraction and visualized in Phyre2 (Kelley et al., 2015).
Protein Kinase Function of AGK
Mitochondrial lipids maintain organelle homeostasis and participate in mitophagy and cytochrome C-mediated apoptosis (Mayr, 2015). AGK is originally found to be an important mitochondrial kinase involved in lipid metabolism. Its major kinase function is to catalyze the production of lysophosphatidic acid (LPA) and phosphatidic acid (PA) from monoacylglycerol (MAG) and diacylglycerol (DAG) (Bektas et al., 2005). AGK deficiency will lead to the disorder of mitochondria lipid membrane composition and affect mitochondrial homeostasis. In addition, it is believed that the ability of AGK to promote tumorigenesis involves the production of LPA, which is an effective signaling molecule that can drive cell growth and proliferation (Geraldo et al., 2021).
A decrease in the oxygen consumption rate and severe damage of mitochondrial ultrastructure were observed in AGK–/– and AGKG126E cells (a cell line in which the AGK enzyme activity is inhibited) (Vukotic et al., 2017), enlightening that effective mitochondrial respiration and mitochondrial structural integrity depend on AGK kinase activity. However, the specific mechanism remains to be clarified.
Non-kinase Function of AGK
On the one hand, AGK participates in phospholipid homeostasis as a mitochondrial kinase; on the other hand, AGK has a kinase-independent function (Figure 3). TIM22 complex mediates the insertion of multichannel transmembrane proteins into the mitochondrial membrane (Qi et al., 2021). Kang et al. (2018) discovered that AGK, as a subunit of the human TIM22 complex, plays an important role in stabilizing the TIM22 complex and regulating the import and assembly of mitochondrial carrier proteins [including adenine nucleotide transporter 1 (ANT1, also known as SLC25A4) and TDP-43 (Yu et al., 2020a)]. Surprisingly, the transport function of AGK does not depend on its kinase domain, which opens a new area for studying AGK as a non-kinase (Vukotic et al., 2017). Recently, Qi et al. (2021) revealed the Cryo-EM structure of the TIM22 complex, which includes TIM22, TIM9, TIM10a, TIM10b, TIM29, and AGK. This greatly enriched our understanding of the structure of the TIM22 complex.
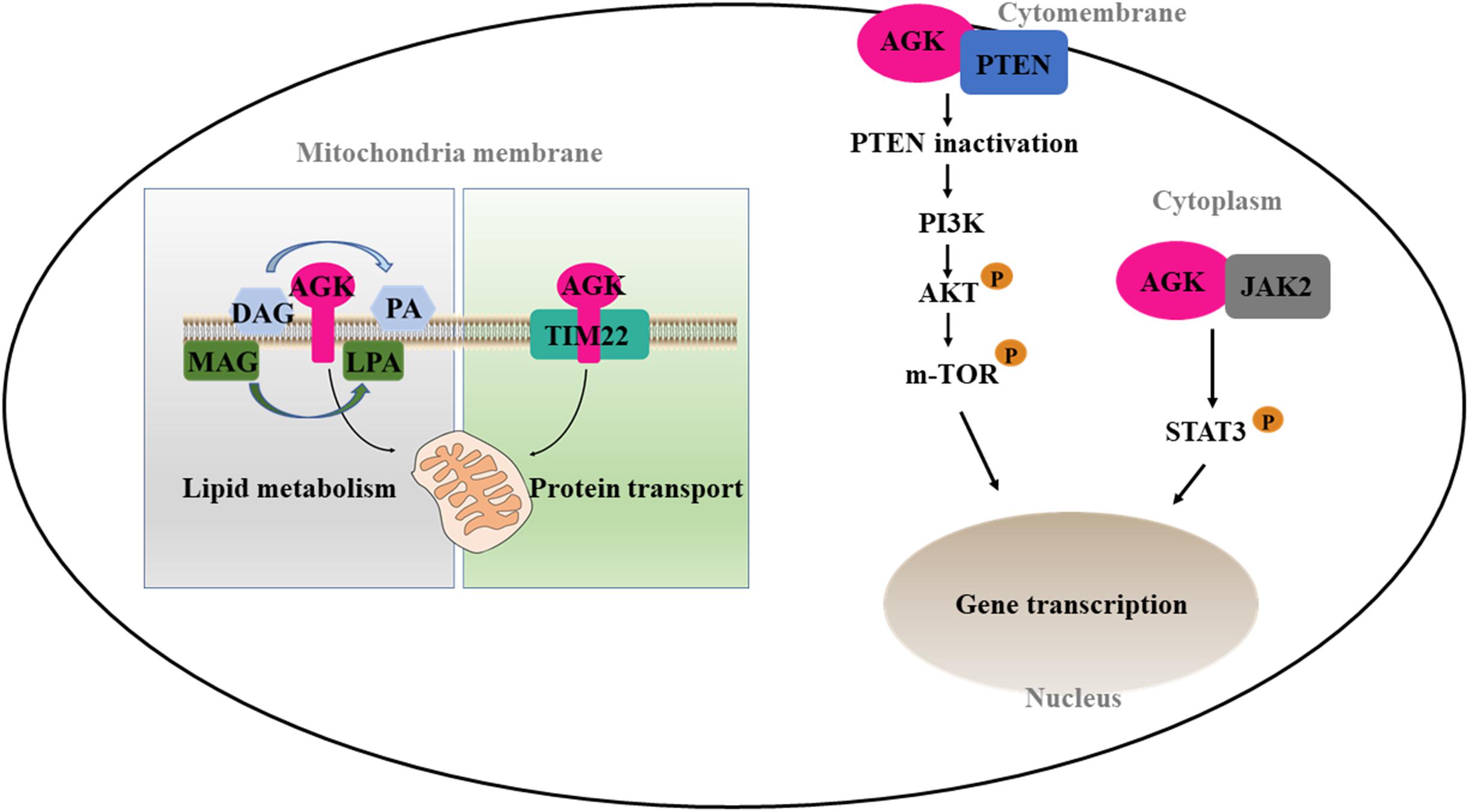
Figure 3. Kinase and non-kinase function of AGK. AGK as a kinase can phosphorylate MAG and DAG to form PLA and PA, respectively. As a non-kinase, AGK participates in mitochondrial protein transfer as a part of the TIM22 complex. Also, extramitochondrial AGK has a hand in the glycolysis of CD8 T cells and the formation of platelets by interacting with PTEN or JAK2, respectively. MAG, monoacylglycerol; DAG, diacylglycerol; LPA, lysophosphatidic acid; PA, phosphatidic acid; TIM22, translocase of the inner membrane 22; PTEN, phosphatase and tension homolog; PI3K, phosphatidylinositol 3-kinase; Akt, protein kinase B; mTOR, mammalian target of rapamycin; JAK2, Janus kinase 2; STAT3, signal transducer and activator of transcription 3.
CD8 T cells are important in adaptive immunity. Oxidative phosphorylation provides most of the energy for primitive and memory CD8 T cells (Vardhana et al., 2020). However, the increase of glycolysis is a prerequisite for the growth and activation of CD8 T cells (Varanasi et al., 2020). Loss of phosphatase and tension homolog (PTEN) in T cells leads to dysregulation of immune homeostasis (Lin et al., 2021). Moreover, PTEN is well known as a tumor inhibitor and phosphatidylinositol 3-kinase (PI3K)–mammalian target of rapamycin (mTOR) signaling inhibitor (Zheng et al., 2020). AGK on cytomembrane is involved in cell proliferation and activation by binding PTEN and activating the PI3K-mTOR pathway (Hu et al., 2019). On the contrary, AGK deficiency dramatically inhibits the antitumor function of CD8 T cells in vivo and in vitro, suggesting that targeting AGK in immune cells may be useful as an antitumor therapy.
Platelets are an important part of the blood, involved in hemostasis, tumor metastasis, and inflammation. The MPL/Janus kinase 2 (JAK2)/signal transducer and activator of transcription 3 (STAT3) signaling pathway is the most important pathway regulating megakaryocyte development and platelet formation (Plo et al., 2017). The most recent research declared that megakaryocyte/platelet-specific AGK-deficient mice show thrombocytopenia and splenomegaly. Cytoplasmic AGK promotes JAK2/STAT3 signal transduction by binding to JAK2 in megakaryocytes/platelets, ultimately inducing the formation of platelets (Jiang et al., 2020). These results suggest that AGK may be a new strategy for the treatment of thrombocytopenia and thrombocytopenia-related diseases.
Whether AGK kinase or kinase-independent functions are activated may depend on specific cell types and subcellular localization, and more wide-ranging studies are needed to explore the roles of AGK in specific organs and cell types.
Regulation of AGK Activity
MicroRNA (miRNA) are known to play important roles in cancer progression by directly downregulating multiple targets (Goodall and Wickramasinghe, 2021). MiR-194 and miR-610 inhibit the proliferation and invasion of tumor cells by downregulating the expression of AGK (Chi, 2015; Yao et al., 2019), suggesting that AGK is an important downstream target of some miRNA mediated antitumor effects.
The Hippo signaling pathway is a newly discovered signal transduction pathway of tumors targeted for inhibition that is widely involved in cellular processes (Yu et al., 2015; Kulkarni et al., 2020). In gastric cancer, AGK is involved in tumor progression through the Hippo pathway. On the one hand, AGK, as the upstream matrix of Yes-associated protein 1 (YAP1), can induce the nuclear localization of YAP1 to enhance the transcriptional activity of YAP1/transcriptional enhanced associate domain (TEAD)s, and eventually lead to the proliferation, invasion, and epithelial–mesenchymal transition of cancer cells (Huang et al., 2020). On the other hand, the activity of AGK is regulated by the transcription of the large tumor suppressor (LATS)1/2 proteins upstream in the Hippo signaling pathway as their downstream target (Huang et al., 2020).
Our understanding of the regulation of AGK is currently based on very few studies (Table 1). The mechanism by which the upstream/downstream signals of AGK are regulated to initiate specific responses remains elusive. To gain a better understanding of the regulatory mechanisms of AGK, we analyzed and predicted the possible interaction gene (Figure 4) and protein (Figure 5) networks of AGK using GeneMANIA and STRING data to provide a reference for those who are interested in AGK research in the future.
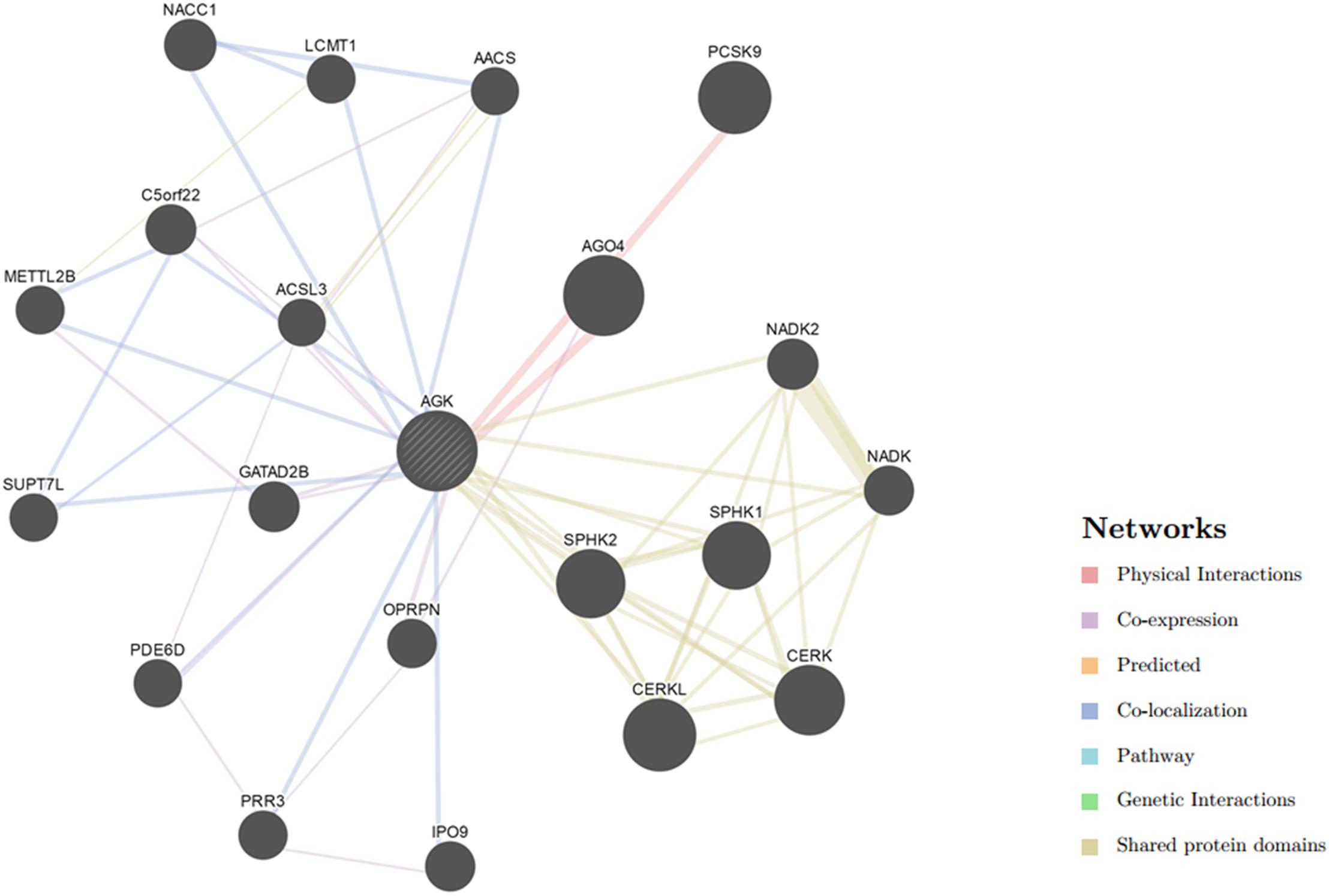
Figure 4. Gene interaction network of AGK. The gene–gene interaction network was generated by GeneMANIA (Warde-Farley et al., 2010). AGO4, argonaute 4; RISC, catalytic component; CERKL, ceramide kinase like; PCSK9, proprotein convertase subtilisin/kexin type 9; CERK, ceramide kinase; SPHK1, sphingosine kinase 1; SPHK2, sphingosine kinase 2; NACC1, nucleus accumbens associated 1; NADK2, NAD kinase 2, mitochondrial; GATAD2B, GATA zinc finger domain containing 2B; C5orf22, chromosome 5 open reading frame 22; IPO9, importin 9; NADK, NAD kinase; OPRPN, opiorphin prepropeptide; PRR3, proline rich 3; METTL2B, methyltransferase like 2B; LCMT1, leucine carboxyl methyltransferase 1; PDE6D, phosphodiesterase 6D; SUPT7L, SPT7-like STAGA complex gamma subunit; AACS, acetoacetyl-CoA synthetase; ACSL3, acyl-CoA synthetase long-chain family member 3.
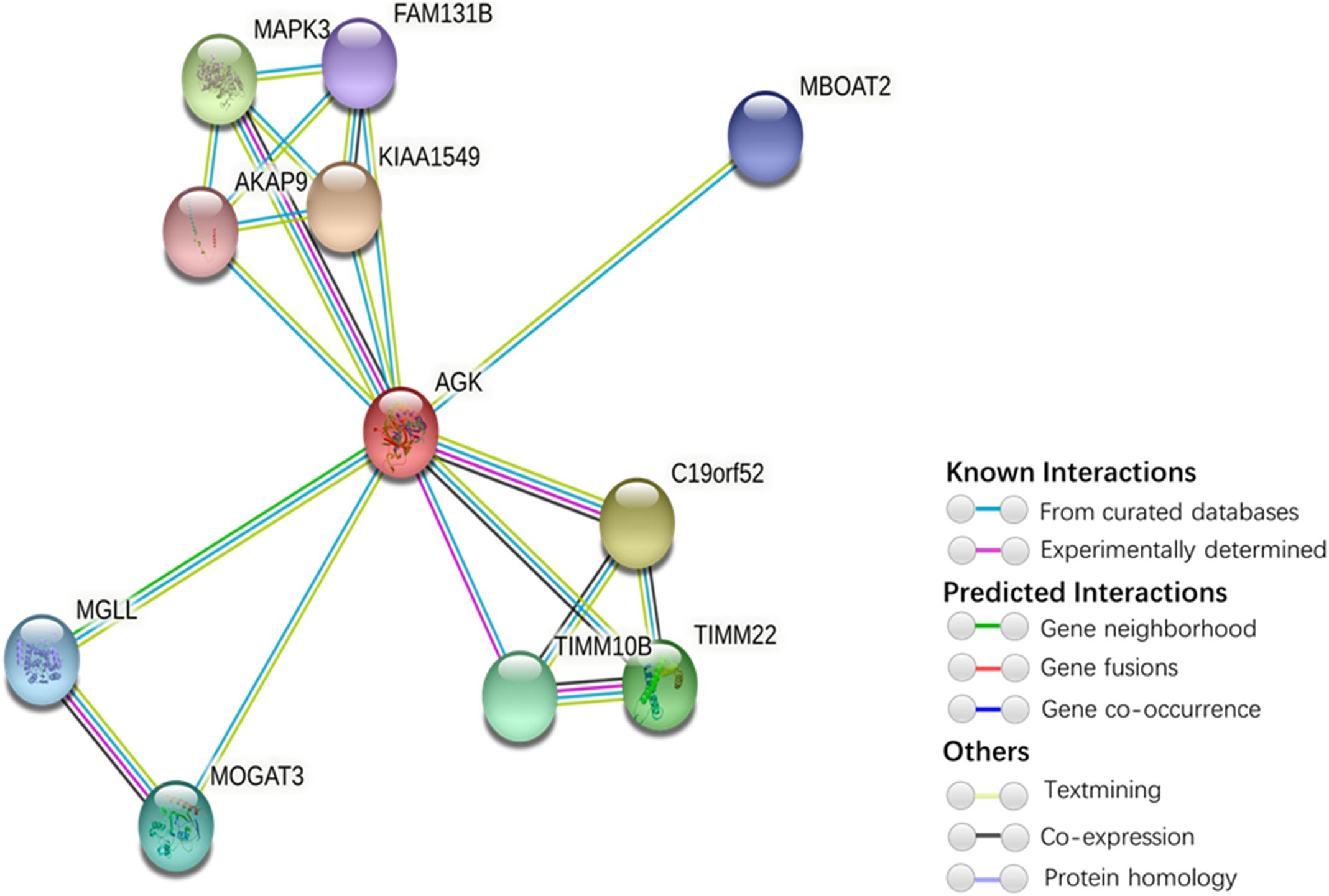
Figure 5. Protein interaction network of AGK-related proteins. The protein–protein interaction network was generated by STRING (Szklarczyk et al., 2019). Network nodes represent proteins. Edges represent protein–protein associations (associations are meant to be specific and meaningful, i.e., proteins jointly contribute to a shared function; this does not necessarily mean they are physically binding each other). Kiaa1545, UPF0606 protein KIAA1549; C19orf52, mitochondrial import inner membrane translocase subunit Tim29; MAPK3, mitogen-activated protein kinase 3; TIMM22, mitochondrial import inner membrane translocase subunit Tim22; TIMM10B, mitochondrial import inner membrane translocase subunit Tim10 B; MOGAT3, 2-acylglycerol O-acyltransferase 3; MGLL, monoglyceride lipase; MBOAT2, lysophospholipid acyltransferase 2; FAM131B, protein FAM131B; AKAP9, A-kinase anchor protein 9.
AGK and Sengers Syndrome
Sengers syndrome is an autosomal recessive disorder caused by nuclear AGK gene mutation and was firstly described by Sengers et al. (1975). The clinical manifestation of Sengers syndrome closely resembles that of mitochondrial ATP synthesis disorder and cardiolipin metabolism deficiency. The predominant symptoms of the disease are congenital cataracts, hypertrophic cardiomyopathy, skeletal myopathy, exercise intolerance, and lactic acidosis (Mayr et al., 2012; Beck et al., 2018). Patients with severe cases die in infancy, while people with mild cases can survive for decades (Haghighi et al., 2014). Most patients died of heart failure caused by hypertrophic cardiomyopathy (Haghighi et al., 2014).
An increasing number of reports have investigated families with Sengers syndrome and via whole-exome sequencing identified different types of mutations in the AGK gene, and patients with different AGK mutations show clinical heterogeneity (Mayr et al., 2012; Siriwardena et al., 2013; Haghighi et al., 2014; Beck et al., 2018; Aggarwal et al., 2021). Adenine nucleotide transporter 1 (ANT1, also known as SLC25A4) is a mitochondrial protein with dual functions. It participates not only in metabolism through the regulation of ATP/ADP release in mitochondria but also in the regulation of cell death as a part of the mitochondrial permeability transition pore (Zhao et al., 2020b). In, Johnston et al. (2002) found that SLC25A4 activity decreased in two Sengers syndrome families without SLC25A4 gene mutation. Because of the importance of phospholipid metabolism for mitochondrial function, the main function of AGK is to produce LPA and PA through phosphorylation of MAG and DAG (Bektas et al., 2005). Mayr et al. (2012) speculated that AGK affects the stability of SLC25A4 by affecting the metabolism of mitochondrial phospholipids and suggested that Sengers syndrome is a mitochondrial disease associated with the lack of mitochondrial carrier SLC25A4. Importantly, SLC25A4 knockout mice exhibit phenotypes of hypertrophic cardiomyopathy, exercise intolerance, and lactic acidemia, which are extraordinarily similar to the symptom of Sengers syndrome. In addition, we were surprised to find that a newly published study drew the mitochondrial proteome map of fibroblasts from patients with Sengers syndrome by using the quantitative proteomics method and found that the enzymes related to mitochondrial carbon metabolism were downregulated, which was also detected in AGK-deficient cell lines (Jackson et al., 2021). These results suggest that the imbalance of carbon metabolism may be involved in the mitochondrial dysfunction associated with AGK deficiency, which provides a new insight for us to understand mitochondrial diseases, although AGK mutation is the root cause of Sengers syndrome. Although impaired mitochondrial protein input, the disorder of lipid metabolism, and one-carbon metabolism may contribute to the pathogenesis of Sengers syndrome, it is unfortunate that there is no method or drug available to cure this fatal disease. Until recently, some researchers have proposed that one-carbon metabolic disorder is a new molecular feature in the biology of Sengers syndrome, and serine supplementation may be a promising treatment for AGK deficiency (Jackson et al., 2021).
AGK in Cancer
We used the cBioPortal tool (Cerami et al., 2012) to analyze the frequency of AGK mutation types in various malignant tumors (Figure 6) and found that AGK mutations are the most common, amplification and deletion mutations are found in some cancers, and fusions are found only in melanoma and prostate cancer. Moreover, the abnormal expression of AGK in various types of tumors has been reported (Table 2) and is involved in many processes of tumor biology, such as proliferation, migration, metastasis, and even drug resistance. Therefore, AGK, as an oncogene, may become a potential target for cancer treatment. Due to the heterogeneity and genetic instability of cancer, AGK seems to play a core role in cancer progression through different signaling pathways and molecules, such as the Hippo-YAP1, JAK2/STAT3, nuclear factor-kappa B (NF-κB), and PI3K/protein kinase B (Akt) signaling pathways as well as the forkhead box O1 (FOXO1) and epidermal growth factor (EGF) transcription factors (Figure 7). Next, we will introduce the specific mechanism of AGK involved in tumor progression from the perspective of the AGK-regulated signaling pathway.
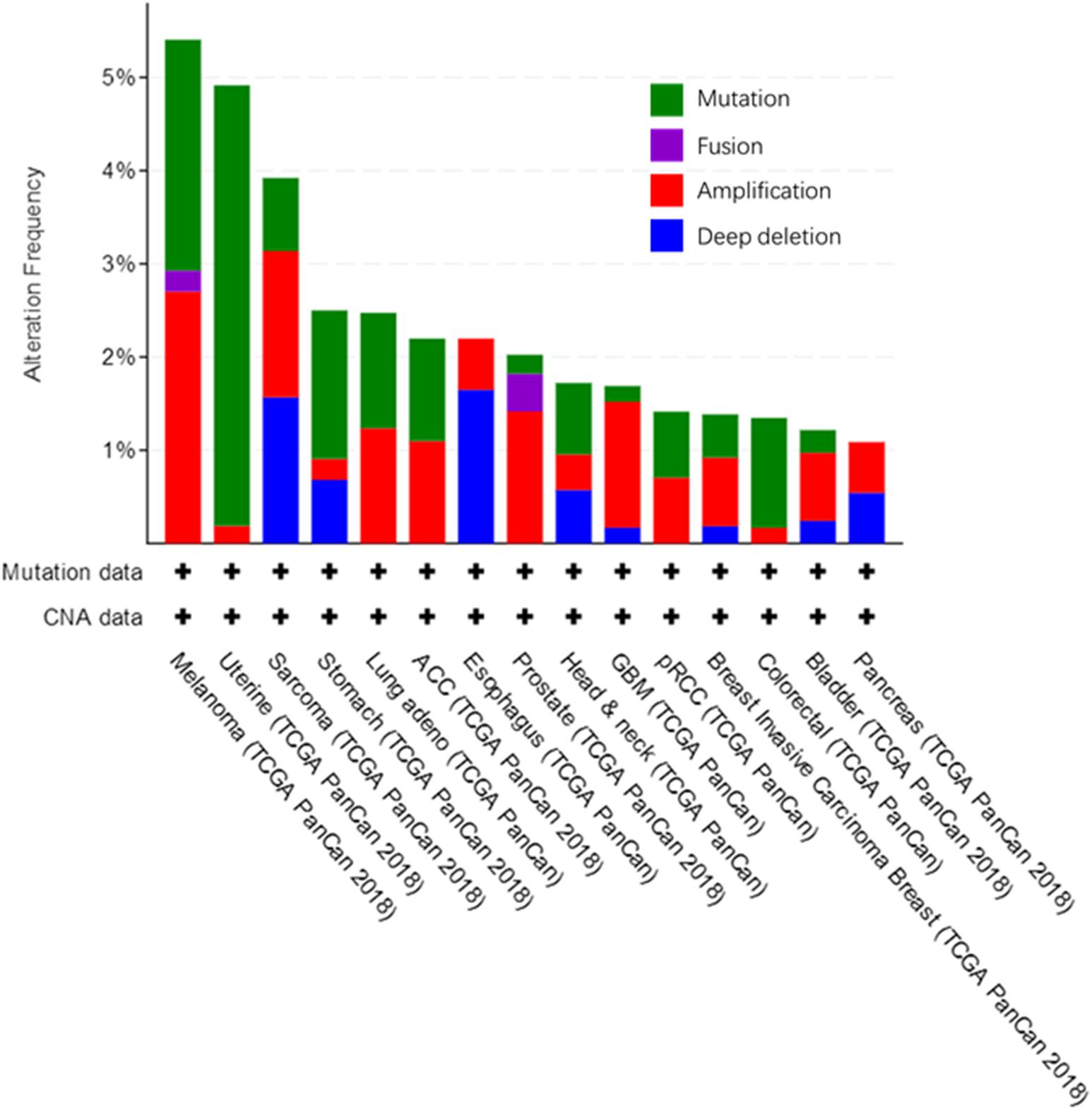
Figure 6. Alteration frequency in AGK gene across various cancers. All these data are concluded in cBioPortal, from TCGA Pan-Cancer Atlas Studies which cover 10,953 patients/10,967 samples.
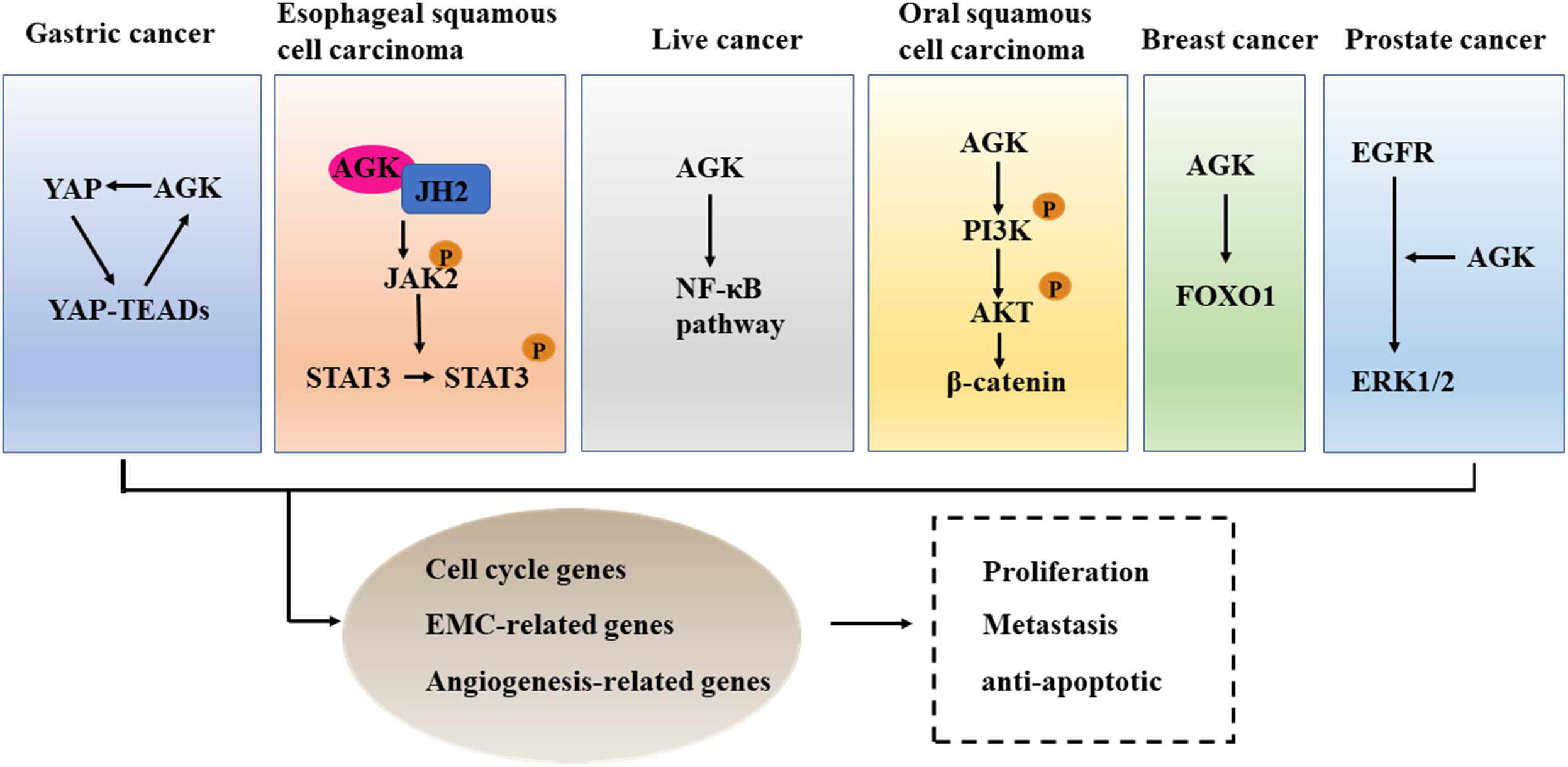
Figure 7. The cross talk between AGK and cancer-related pathways. AGK is participating in the differentiation, metastasis, and anti-apoptosis of tumor cells by adjusting Hippo-YAP1, JAK2/STAT3, NF-κB, and PI3K/Akt signaling pathways, FOXO1, and EGF transcription factor. YAP1, Yes-associated protein 1; TEAD, transcriptional enhanced associated domain; JH2, Janus kinase homology 2; JAK2, Janus kinase 2; STAT3, signal transducer and activator of transcription 3; NF-κB, nuclear factor-kappa B; PI3K, phosphatidylinositol 3-kinase; Akt, protein kinase B; FOXO1, forkhead box O1; EGFR, epidermal growth factor receptor; ERK, extracellular regulated protein kinases.
Hippo-YAP1
The overexpression of YAP/transcriptional coactivator with PDZ-binding motif (TAZ), downstream effectors in the Hippo signaling pathway, leads to uncontrolled cell proliferation and malignant transformation (Dey et al., 2020). Therefore, an anomalously high expression of YAP/TAZ has been proved to be an independent predictor of tumor prognosis and an indicator of cell proliferation, metastasis, and poor survival (Kulkarni et al., 2020). In gastric cancer, YAP1/TAZ acts as an oncogene and promotes tumor formation by co-transcription with the TEAD transcription factor (Qiao et al., 2018). AGK was able to enhance the expression of nuclear YAP1 protein and then induce the transcriptional activity of the YAP1/TEAD gene (Huang et al., 2020). Therefore, targeting the expression or activity of AGK seems to be a therapeutic target to control the progression of gastric cancer.
JAK/STAT
The JAK/STAT signaling pathway is constitutively activated in many kinds of cancer (Lin and Farooqi, 2020), and inhibition of its activation can suppress the occurrence and progression of cancer (Toh et al., 2020). In esophageal squamous cell carcinoma (ESCC), AGK directly binds to the Janus kinase homology 2 (JH2) domain of JAK2 to block the inhibition of JAK2 mediated by JH2, which leads to the activation of the JAK2/STAT3 signaling pathway and enhances the tumorigenicity of ESCC cells in vivo and in vitro (Chen et al., 2013). Additionally, AGK expression was positively correlated with STAT3 activation in lung cancer and breast cancer (Wang et al., 2014). Therefore, inhibiting the expression of AGK or using competitive AGK peptide to inhibit AGK-JAK interaction can be used as a novel and valuable method to block the activation of JAK/STAT3 in some tumors, such as ESCC (Chen et al., 2013).
NF-κB
As a double-edged sword, the role of the NF-κB signal in cancer is complex (Yu et al., 2020b). On the one hand, NF-κB activation can promote the proliferation, invasion, and angiogenesis of tumor cells. On the other hand, the activation of atypical NF-κB can promote the apoptosis of cancer cells (Taniguchi and Karin, 2018). Studies have confirmed that NF-κB is abnormally activated in hepatocellular carcinoma and plays an important role in the malignant transformation of tumors (Czauderna et al., 2019). The overexpression of AGK promotes angiogenesis and enhances the resistance of tumor cells to apoptosis by activating the NF-κB signal in hepatocellular carcinoma (Cui et al., 2014). Therefore, targeted inhibition of AGK may be a favorable strategy for anti-angiogenesis and pro-apoptotic cancer therapy.
PI3K/Akt
PI3K is a kind of lipid kinase that can transmit intracellular signal cascade and regulate a variety of cellular physiological processes (Koch et al., 2021). Protein kinase B (AKT) is an important downstream effector of PI3K signal transduction that regulates a variety of signaling pathways, including the inhibition of cell apoptosis, stimulation of cell growth, and regulation of cell metabolism (Hoxhaj and Manning, 2020). Activated PI3K/AKT can catalyze the phosphorylation of a series of proteins, ultimately promoting the growth and proliferation of tumor cells, inhibiting apoptosis, promoting invasion and metastasis, regulating the growth and angiogenesis of endothelial cells, and increasing the sensitivity to radiation (Lee et al., 2015; Alzahrani, 2019). MiRNAs are small non-coding RNAs that control the specificity of gene expression (McGeary et al., 2019). Increasing evidence shows that miRNAs regulate gene expression by targeting messenger RNAs (mRNAs) and thus participate in the process of malignant tumorigenesis (Goodall and Wickramasinghe, 2021). For example, in oral squamous cell carcinoma, miR-194 can inhibit the PI3K/Akt/FOXO3a signaling pathway by inhibiting AGK, resulting in the decreased expression of cyclin D1 and the increased expression of P21, which ultimately inhibits the proliferation and cell cycle progression of cancer cells (Chi, 2015). Additionally, a study of renal cell carcinoma and nasopharyngeal carcinoma found that AGK is involved in tumor progression and lymph node metastasis by activating the PI3K/Akt pathway (Zhu et al., 2020).
FOXO1
FOXO1 is a member of the forkhead box containing an O subfamily of transcription factors (Deng et al., 2018). It plays an important role in many biological processes, including cell cycle arrest, cell death, apoptosis, stress response, cell differentiation, and metabolism (Kim et al., 2018). FOXO1 is downregulated in a variety of cancers and is considered to be a tumor suppressor (Jiang et al., 2018). The overexpression of AGK can reduce the activation of FOXO1 activity and its downstream targets, thereby enhancing the proliferation and tumorigenicity of cancer cells (Wang et al., 2014).
EGF
The proliferation of many types of cancer cells is partly controlled by the autocrine EGF stimulation loop because epidermal growth factor receptor (EGFR) is consistently overexpressed in these cells (Sabbah et al., 2020). AGK in prostate cancer cells increases the formation and secretion of LPA, which leads to the transactivation of EGFR and the activation of the downstream MAPK signaling pathway, resulting in the growth of cancer cells. When the endogenous AGK was removed, the activation of extracellular regulated protein kinases (ERK)1/2 and cell proliferation induced by EGF was significantly inhibited (Spiegel and Milstien, 2005). Therefore, targeted inhibition of AGK may provide additional therapeutic benefits for patients with prostate cancer.
Drug Resistance
EGFR mutant non-small cell lung cancers are resistant to EGFR tyrosine kinase inhibitor (EGFR-TKI) (Westover et al., 2018), and BRAF fusion is one of the reasons for their resistance (Yu et al., 2013). Lung cancer patients show secondary resistance to EGFR TKIs due to acquired AGK/BRAF fusion (Vojnic et al., 2019; Boyle et al., 2020), which suggests that AGK is involved in some acquired tumor resistance, but the specific AGK mechanism in this context needs to be clarified.
Prognosis
Through the data analysis results of the relevant data of the protein atlas database (Table 3), we found that the high expression of AGK does not seem to predict the prognosis of tumor patients. While some studies have indicated that high AGK expression is associated with a poor prognosis for patients with certain types of cancer, AGK may be used to identify the risk of patients and guide personalized treatment. There seems to be a contradiction. However, we notice that the database takes the whole population as the research object for overall survival prognosis analysis, while the related research reports involve some specific groups and statistics of the overall survival rate, clinical stage, Fuhrman classification, recurrence with metastasis, and vital status, which may be the reason for the difference in statistical outcomes between the two. Since tumor prognosis is related to many factors, whether AGK can be used as a prognostic indicator of pan-cancer in the whole population remains to be supported by further clinical investigations and surveys.
AGK-Related Mouse Models
To date, some investigators have tried to elucidate additional features of AGK by studying AGK-related genes in mice (Table 4). Agkfl/flPf4-Cre mice (megakaryocyte/platelet specific knockout mice) showed abnormal platelet development, similar to some Sengers syndrome patients with thrombocytopenia (Jiang et al., 2020). Additionally, Agkfl/flCd4-Cre mice, Agkfl/flCd4-Cre OT-I mice, and Agkfl/flCd4-Cre OT-II mice all showed weak antitumor ability (Hu et al., 2019). AgkG126E/G126E mice and AgkG126E/G126E OT-I mice, which were generated after G126E knock-in, showed uncontrolled tumor growth and lethality (Hu et al., 2019). However, the Agkfl/flPtenfl/flCd4-Cre mice with PTEN knockout showed increased glycolysis in CD8 T cells, which led to the antitumor effect (Hu et al., 2019). AGK was necessary for the antitumor function of these CD8 T cells.
Conclusion and Prospects
AGK, as a mitochondrial lipid kinase, has multiple kinases and kinase-independent biological functions, and its mutation leads to Sengers syndrome, which is characterized by multiple-organ dysfunction. As an effective oncogene, AGK is involved in the occurrence and development of a variety of cancers. Inhibiting its expression in certain cancer cells has led to anticancer effects, indicating that AGK is a potential therapeutic target in a variety of cancers. AGK shows diverse functions in specific cell and subcellular localization through different mechanisms, which helps us to develop antitumor strategies targeting AGK from different perspectives. By targeting the subcellular localization of AGK, or destroying its kinase or non-kinase function, it can be a promising antitumor strategy. However, there is no targeted and selective inhibitor for AGK, and one needs to be further developed and verified by drug researchers. The function of AGK in physiological and pathological conditions has not been fully elucidated. First, the diverse regulation of AGK in genomics, epigenetics, and posttranslational modification is rarely studied. Second, the function of AGK as a non-kinase in different cells is worthy of attention and exploration. In addition to tumor diseases, the roles of AGK in various systemic diseases remain unclear. In the future, it will be necessary to further study the interaction pathways and precise molecular targets of AGK to further clarify its physiological and pathological mechanisms.
Author Contributions
BC, ZH, and XZ participated in designing and writing the manuscript. All authors contributed to the article and approved the submitted version.
Funding
This work was sponsored by the National Natural Science Foundation of China (80212076) and the Medical Health Science and Technology Project of Zhejiang Provincial Health Commission (2020384729).
Conflict of Interest
The authors declare that the research was conducted in the absence of any commercial or financial relationships that could be construed as a potential conflict of interest.
References
Abu El-Asrar, A. M., Missotten, L., and Geboes, K. (2012). Expression of autotaxin and acylglycerol kinase in proliferative vitreoretinal epiretinal membranes. Acta Ophthalmol. 90, e84–e89. doi: 10.1111/j.1755-3768.2011.02303.x
Abu El-Asrar, A. M., Mohammad, G., Nawaz, M. I., Siddiquei, M. M., Kangave, D., and Opdenakker, G. (2013). Expression of lysophosphatidic acid, autotaxin and acylglycerol kinase as biomarkers in diabetic retinopathy. Acta Diabetol. 50, 363–371. doi: 10.1007/s00592-012-0422-1
Abu El-Asrar, A. M., Nawaz, M. I., Mohammad, G., Siddiquei, M. M., Alam, K., Mousa, A., et al. (2014). Expression of bioactive lysophospholipids and processing enzymes in the vitreous from patients with proliferative diabetic retinopathy. Lipids Health Dis. 13:187. doi: 10.1186/1476-511X-13-187
Aggarwal, B., Kabra, M., and Gupta, N. (2021). Report of an Indian family with sengers syndrome. Indian J. Pediatr. 88:92. doi: 10.1007/s12098-020-03471-0
Alzahrani, A. S. (2019). PI3K/Akt/mTOR inhibitors in cancer: at the bench and bedside. Semin Cancer Biol. 59, 125–132. doi: 10.1016/j.semcancer.2019.07.009
Beck, D. B., Cusmano-Ozog, K., Andescavage, N., and Leon, E. (2018). Extending the phenotypic spectrum of Sengers syndrome: congenital lactic acidosis with synthetic liver dysfunction. Transl. Sci. Rare Dis. 3, 45–48. doi: 10.3233/TRD-180020
Bektas, M., Payne, S. G., Liu, H., Goparaju, S., Milstien, S., and Spiegel, S. (2005). A novel acylglycerol kinase that produces lysophosphatidic acid modulates cross talk with EGFR in prostate cancer cells. J. Cell Biol. 169, 801–811. doi: 10.1083/jcb.200407123
Boyle, T. A., Quinn, G. P., Schabath, M. B., Munoz-Antonia, T., Saller, J. J., Duarte, L. F., et al. (2020). A community-based lung cancer rapid tissue donation protocol provides high-quality drug-resistant specimens for proteogenomic analyses. Cancer Med. 9, 225–237. doi: 10.1002/cam4.2670
Cerami, E., Gao, J., Dogrusoz, U., Gross, B. E., Sumer, S. O., Aksoy, B. A., et al. (2012). The cBio cancer genomics portal: an open platform for exploring multidimensional cancer genomics data. Cancer Discov. 2, 401–404. doi: 10.1158/2159-8290.CD-12-0095
Chen, X., Ying, Z., Lin, X., Lin, H., Wu, J., Li, M., et al. (2013). Acylglycerol kinase augments JAK2/STAT3 signaling in esophageal squamous cells. J. Clin. Invest. 123, 2576–2589. doi: 10.1172/JCI68143
Chi, H. (2015). miR-194 regulated AGK and inhibited cell proliferation of oral squamous cell carcinoma by reducing PI3K-Akt-FoxO3a signaling. Biomed. Pharmacother. 71, 53–57. doi: 10.1016/j.biopha.2015.02.011
Cui, Y., Lin, C., Wu, Z., Liu, A., Zhang, X., Zhu, J., et al. (2014). AGK enhances angiogenesis and inhibits apoptosis via activation of the NF-kappaB signaling pathway in hepatocellular carcinoma. Oncotarget 5, 12057–12069. doi: 10.18632/oncotarget.2666
Czauderna, C., Castven, D., Mahn, F. L., and Marquardt, J. U. (2019). Context-dependent role of NF-kappaB signaling in primary liver cancer-from tumor development to therapeutic implications. Cancers (Basel) 11:1053. doi: 10.3390/cancers11081053
Deng, Y., Wang, F., Hughes, T., and Yu, J. (2018). FOXOs in cancer immunity: Knowns and unknowns. Semin Cancer Biol. 50, 53–64. doi: 10.1016/j.semcancer.2018.01.005
Dey, A., Varelas, X., and Guan, K. L. (2020). Targeting the hippo pathway in cancer, fibrosis, wound healing and regenerative medicine. Nat. Rev. Drug. Discov. 19, 480–494. doi: 10.1038/s41573-020-0070-z
Geraldo, L. H. M., Spohr, Tcls, Amaral, R. F. D., and Fonseca, Accd, et al. (2021). Role of lysophosphatidic acid and its receptors in health and disease: novel therapeutic strategies. Signal Transduct. Target. Ther. 6:45. doi: 10.1038/s41392-020-00367-5
Goodall, G. J., and Wickramasinghe, V. O. (2021). RNA in cancer. Nat. Rev. Cancer 21, 22–36. doi: 10.1038/s41568-020-00306-0
Haghighi, A., Haack, T. B., Atiq, M., Mottaghi, H., Haghighi-Kakhki, H., Bashir, R. A., et al. (2014). Sengers syndrome: six novel AGK mutations in seven new families and review of the phenotypic and mutational spectrum of 29 patients. Orphanet. J. Rare Dis. 9:119. doi: 10.1186/s13023-014-0119-3
Hoxhaj, G., and Manning, B. D. (2020). The PI3K-AKT network at the interface of oncogenic signalling and cancer metabolism. Nat. Rev. Cancer 20, 74–88. doi: 10.1038/s41568-019-0216-7
Hu, Z., Qu, G., Yu, X., Jiang, H., Teng, X. L., Ding, L., et al. (2019). Acylglycerol kinase maintains metabolic state and immune responses of CD8(+) T cells. Cell Metab 30, 290–302.e295. doi: 10.1016/j.cmet.2019.05.016
Huang, S., Cao, Y., Guo, H., Yao, Y., Li, L., Chen, J., et al. (2020). Up-regulated acylglycerol kinase (AGK) expression associates with gastric cancer progression through the formation of a novel YAP1-AGK-positive loop. J. Cell. Mol. Med. 24, 11133–11145. doi: 10.1111/jcmm.15613
Jackson, T. D., Hock, D. H., Fujihara, K. M., Palmer, C. S., Frazier, A. E., Low, Y. C., et al. (2021). The TIM22 complex mediates the import of Sideroflexins and is required for efficient mitochondrial one-carbon metabolism. Mol. Biol. Cell. 32, 475–491. doi: 10.1091/mbc.E20-06-0390
Jiang, H., Yu, Z., Ding, N., Yang, M., Zhang, L., Fan, X., et al. (2020). The role of AGK in thrombocytopoiesis and possible therapeutic strategies. Blood 136, 119–129. doi: 10.1182/blood.2019003851
Jiang, S., Li, T., Yang, Z., Hu, W., and Yang, Y. (2018). Deciphering the roles of FOXO1 in human neoplasms. Int. J. Cancer 143, 1560–1568. doi: 10.1002/ijc.31338
Johnston, A. J., Hoogenraad, J., Dougan, D. A., Truscott, K. N., Yano, M., Mori, M., et al. (2002). Insertion and assembly of human tom7 into the preprotein translocase complex of the outer mitochondrial membrane. J. Biol. Chem. 277, 42197–42204. doi: 10.1074/jbc.M205613200
Kang, Y., Fielden, L. F., and Stojanovski, D. (2018). Mitochondrial protein transport in health and disease. Semin Cell Dev. Biol. 76, 142–153. doi: 10.1016/j.semcdb.2017.07.028
Kang, Y., Stroud, D. A., Baker, M. J., De Souza, D. P., Frazier, A. E., Liem, M., et al. (2017). Sengers syndrome-associated mitochondrial acylglycerol kinase is a subunit of the human TIM22 protein import complex. Mol. Cell 67, 457–470.e5. doi: 10.1016/j.molcel.2017.06.014
Kelley, L. A., Mezulis, S., Yates, C. M., Wass, M. N., and Sternberg, M. J. (2015). The Phyre2 web portal for protein modeling, prediction and analysis. Nat. Protoc. 10, 845–858. doi: 10.1038/nprot.2015.053
Kim, C. G., Lee, H., Gupta, N., Ramachandran, S., Kaushik, I., Srivastava, S., et al. (2018). Role of forkhead box class O proteins in cancer progression and metastasis. Semin Cancer Biol. 50, 142–151. doi: 10.1016/j.semcancer.2017.07.007
Koch, P. A., Dornan, G. L., Hessenberger, M., and Haucke, V. (2021). The Molecular mechanisms mediating class II PI 3-kinase function in cell physiology. FEBS J. doi: 10.1111/febs.15692 [Epub ahead of print].
Kulkarni, A., Chang, M. T., Vissers, J. H. A., Dey, A., and Harvey, K. F. (2020). The hippo pathway as a driver of select human cancers. Trends Cancer 6, 781–796. doi: 10.1016/j.trecan.2020.04.004
Lee, M. S., Jeong, M. H., Lee, H. W., Han, H. J., Ko, A., Hewitt, S. M., et al. (2015). PI3K/AKT activation induces PTEN ubiquitination and destabilization accelerating tumourigenesis. Nat. Commun. 6:7769. doi: 10.1038/ncomms8769
Lin, X., and Farooqi, A. A. (2020). Cucurbitacin mediated regulation of deregulated oncogenic signaling cascades and non-coding RNAs in different cancers: Spotlight on JAK/STAT, Wnt/beta-catenin, mTOR, TRAIL-mediated pathways. Semin Cancer Biol. 73, 302–309. doi: 10.1016/j.semcancer.2020.10.012
Lin, Z., Huang, L., Li, S. L., Gu, J., Cui, X., and Zhou, Y. (2021). PTEN loss correlates with T cell exclusion across human cancers. BMC Cancer 21:429. doi: 10.1186/s12885-021-08114-x
Liu, N., Wang, Z., Cheng, Y., Zhang, P., Wang, X., Yang, H., et al. (2016). Acylglycerol kinase functions as an oncogene and an unfavorable prognostic marker of human gliomas. Hum. Pathol. 58, 105–112. doi: 10.1016/j.humpath.2016.07.034
Mayr, J. A. (2015). Lipid metabolism in mitochondrial membranes. J. Inherit. Metab Dis. 38, 137–144. doi: 10.1007/s10545-014-9748-x
Mayr, J. A., Haack, T. B., Graf, E., Zimmermann, F. A., Wieland, T., Haberberger, B., et al. (2012). Lack of the mitochondrial protein acylglycerol kinase causes Sengers syndrome. Am. J. Hum. Genet. 90, 314–320. doi: 10.1016/j.ajhg.2011.12.005
McGeary, S. E., Lin, K. S., Shi, C. Y., Pham, T. M., Bisaria, N., Kelley, G. M., et al. (2019). The biochemical basis of microRNA targeting efficacy. Science 366:eaav1741. doi: 10.1126/science.aav1741
Nouh, M. A. A. M., Wu, X.-X., Okazoe, H., Tsunemori, H., Haba, R., Abou-Zeid, A. M. M., et al. (2009). Expression of autotaxin and acylglycerol kinase in prostate cancer: association with cancer development and progression. Cancer Sci. 100, 1631–1638. doi: 10.1111/j.1349-7006.2009.01234.x
Plo, I., Bellanne-Chantelot, C., Mosca, M., Mazzi, S., Marty, C., and Vainchenker, W. (2017). Genetic alterations of the thrombopoietin/MPL/JAK2 axis impacting megakaryopoiesis. Front. Endocrinol. (Lausanne) 8:234. doi: 10.3389/fendo.2017.00234
Qi, L., Wang, Q., Guan, Z., Wu, Y., Shen, C., Hong, S., et al. (2021). Cryo-EM structure of the human mitochondrial translocase TIM22 complex. Cell Res. 31, 369–372. doi: 10.1038/s41422-020-00400-w
Qiao, Y., Li, T., Zheng, S., and Wang, H. (2018). The Hippo pathway as a drug target in gastric cancer. Cancer Lett. 420, 14–25. doi: 10.1016/j.canlet.2018.01.062
Sabbah, D. A., Hajjo, R., and Sweidan, K. (2020). Review on epidermal growth factor receptor (EGFR) structure, signaling pathways, interactions, and recent updates of EGFR inhibitors. Curr. Top. Med. Chem. 20, 815–834. doi: 10.2174/1568026620666200303123102
Sengers, R. C., Trijbels, J. M., Willems, J. L., Daniels, O., and Stadhouders, A. M. (1975). Congenital cataract and mitochondrial myopathy of skeletal and heart muscle associated with lactic acidosis after exercise. J. Pediatr. 86, 873–880. doi: 10.1016/s0022-3476(75)80217-4
Siriwardena, K., Mackay, N., Levandovskiy, V., Blaser, S., Raiman, J., Kantor, P. F., et al. (2013). Mitochondrial citrate synthase crystals: novel finding in Sengers syndrome caused by acylglycerol kinase (AGK) mutations. Mol. Genet. Metab 108, 40–50. doi: 10.1016/j.ymgme.2012.11.282
Spiegel, S., and Milstien, S. (2005). Critical role of acylglycerol kinase in epidermal growth factor-induced mitogenesis of prostate cancer cells. Biochem. Soc. Trans 33(Pt. 6), 1362–1365. doi: 10.1042/BST20051362
Sun, F., Xiong, Y., Zhou, X. H., Li, Q., Xiao, L., Long, P., et al. (2016). Acylglycerol kinase is over-expressed in early-stage cervical squamous cell cancer and predicts poor prognosis. Tumour. Biol. 37, 6729–6736. doi: 10.1007/s13277-015-4498-4
Szklarczyk, D., Gable, A. L., Lyon, D., Junge, A., Wyder, S., Huerta-Cepas, J., et al. (2019). STRING v11: protein-protein association networks with increased coverage, supporting functional discovery in genome-wide experimental datasets. Nucleic Acids Res. 47, D607–D613. doi: 10.1093/nar/gky1131
Taniguchi, K., and Karin, M. (2018). NF-kappaB, inflammation, immunity and cancer: coming of age. Nat. Rev. Immunol. 18, 309–324. doi: 10.1038/nri.2017.142
Toh, T. B., Lim, J. J., Hooi, L., and Rashid, Mbma, Chow, E. K. (2020). Targeting Jak/Stat pathway as a therapeutic strategy against SP/CD44+ tumorigenic cells in Akt/beta-catenin-driven hepatocellular carcinoma. J. Hepatol. 72, 104–118. doi: 10.1016/j.jhep.2019.08.035
Varanasi, S. K., Kumar, S. V., and Rouse, B. T. (2020). Determinants of tissue-specific metabolic adaptation of T cells. Cell Metab 32, 908–919. doi: 10.1016/j.cmet.2020.10.013
Vardhana, S. A., Hwee, M. A., Berisa, M., Wells, D. K., Yost, K. E., King, B., et al. (2020). Impaired mitochondrial oxidative phosphorylation limits the self-renewal of T cells exposed to persistent antigen. Nat. Immunol. 21, 1022–1033. doi: 10.1038/s41590-020-0725-2
Vojnic, M., Kubota, D., Kurzatkowski, C., Offin, M., Suzawa, K., Benayed, R., et al. (2019). Acquired BRAF rearrangements induce secondary resistance to EGFR therapy in EGFR-mutated lung cancers. J. Thorac. Oncol. 14, 802–815. doi: 10.1016/j.jtho.2018.12.038
Vukotic, M., Nolte, H., Konig, T., Saita, S., Ananjew, M., Kruger, M., et al. (2017). acylglycerol kinase mutated in sengers syndrome is a subunit of the TIM22 protein translocase in mitochondria. Mol. Cell 67, 471–483.e477. doi: 10.1016/j.molcel.2017.06.013
Wang, X., Lin, C., Zhao, X., Liu, A., Zhu, J., Li, X., et al. (2014). Acylglycerol kinase promotes cell proliferation and tumorigenicity in breast cancer via suppression of the FOXO1 transcription factor. Mol. Cancer 13:106. doi: 10.1186/1476-4598-13-106
Warde-Farley, D., Donaldson, S. L., Comes, O., Zuberi, K., Badrawi, R., Chao, P., et al. (2010). The GeneMANIA prediction server: biological network integration for gene prioritization and predicting gene function. Nucleic Acids Res. 38, W214–W220. doi: 10.1093/nar/gkq537
Westover, D., Zugazagoitia, J., Cho, B. C., Lovly, C. M., and Paz-Ares, L. (2018). Mechanisms of acquired resistance to first- and second-generation EGFR tyrosine kinase inhibitors. Ann. Oncol. 29(Suppl. 1), i10–i19. doi: 10.1093/annonc/mdx703
Yao, L. X., Liu, J., and Xu, L. (2019). MiR-610 functions as a tumor suppressor in oral squamous cell carcinoma by directly targeting AGK. Eur. Rev. Med. Pharmacol. Sci. 23, 187–197. doi: 10.26355/eurrev_201901_16764
Yu, C. H., Davidson, S., Harapas, C. R., Hilton, J. B., Mlodzianoski, M. J., Laohamonthonkul, P., et al. (2020a). TDP-43 triggers mitochondrial DNA release via mPTP to activate cGAS/STING in ALS. Cell 183, 636–649.e18. doi: 10.1016/j.cell.2020.09.020
Yu, F. X., Meng, Z., Plouffe, S. W., and Guan, K. L. (2015). Hippo pathway regulation of gastrointestinal tissues. Annu. Rev. Physiol. 77, 201–227. doi: 10.1146/annurev-physiol-021014-071733
Yu, H., Lin, L., Zhang, Z., Zhang, H., and Hu, H. (2020b). Targeting NF-kappaB pathway for the therapy of diseases: mechanism and clinical study. Signal Transduct. Target. Ther. 5:209. doi: 10.1038/s41392-020-00312-6
Yu, H. A., Arcila, M. E., Rekhtman, N., Sima, C. S., Zakowski, M. F., Pao, W., et al. (2013). Analysis of tumor specimens at the time of acquired resistance to EGFR-TKI therapy in 155 patients with EGFR-mutant lung cancers. Clin. Cancer Res. 19, 2240–2247. doi: 10.1158/1078-0432.CCR-12-2246
Zeng, Y., Kakehi, Y., Nouh, M. A., Tsunemori, H., Sugimoto, M., and Wu, X. X. (2009). Gene expression profiles of lysophosphatidic acid-related molecules in the prostate: relevance to prostate cancer and benign hyperplasia. Prostate 69, 283–292. doi: 10.1002/pros.20879
Zhao, Q., Sun, P., Qin, S., and Liu, J. (2020a). Acylglycerol kinase promotes the stemness of nasopharyngeal carcinoma cells by promoting beta-catenin translocation to the nucleus through activating PI3K/Akt pathway. Environ. Toxicol. 35, 1299–1307. doi: 10.1002/tox.22994
Zhao, L., Tang, M., Bode, A. M., Liao, W., and Cao, Y. (2020b). ANTs and cancer: emerging pathogenesis, mechanisms, and perspectives. Biochim. Biophys. Acta Rev. Cancer 1875:188485. doi: 10.1016/j.bbcan.2020.188485
Zheng, C., Tang, F., Min, L., Hornicek, F., Duan, Z., and Tu, C. (2020). PTEN in osteosarcoma: recent advances and the therapeutic potential. Biochim. Biophys. Acta Rev. Cancer 1874:188405. doi: 10.1016/j.bbcan.2020.188405
Keywords: acylglycerol kinase, mitochondria, Sengers syndrome, oncogenesis, metabolism
Citation: Chu B, Hong Z and Zheng X (2021) Acylglycerol Kinase-Targeted Therapies in Oncology. Front. Cell Dev. Biol. 9:659158. doi: 10.3389/fcell.2021.659158
Received: 27 January 2021; Accepted: 28 June 2021;
Published: 22 July 2021.
Edited by:
Varda Shoshan-Barmatz, Ben-Gurion University of the Negev, IsraelReviewed by:
Sotirios G. Zarogiannis, University of Thessaly, GreeceDavid Stroud, The University of Melbourne, Australia
Copyright © 2021 Chu, Hong and Zheng. This is an open-access article distributed under the terms of the Creative Commons Attribution License (CC BY). The use, distribution or reproduction in other forums is permitted, provided the original author(s) and the copyright owner(s) are credited and that the original publication in this journal is cited, in accordance with accepted academic practice. No use, distribution or reproduction is permitted which does not comply with these terms.
*Correspondence: Zhenghua Hong, aHpodHpoMDAxQDE2My5jb20=; Xiaohe Zheng, MjE2MTg0NjhAemp1LmVkdS5jbg==