- Department of Veterinary and Animal Sciences, Animal Models Core Facility, Institute for Applied Life Sciences (IALS), University of Massachusetts Amherst, Amherst, MA, United States
In mammals, including humans, mature oocytes are ovulated into the oviduct for fertilization. Normally, these oocytes are arrested at metaphase of the second meiosis (MII), and this arrest can be maintained for a certain period, which is essential for fertilization in vivo and oocyte manipulations in vitro, such as assisted reproduction in clinics and nuclear/spindle transfer in laboratories. However, in some species and under certain circumstances, exit from MII occurs spontaneously without any obvious stimulation or morphological signs, which is so-called oocyte spontaneous activation (OSA). This mini-review summarizes two types of OSA. In the first type (e.g., most rat strains), oocytes can maintain MII arrest in vivo, but once removed out, oocytes undergo OSA with sister chromatids separated and eventually scattered in the cytoplasm. Because the stimulation is minimal (oocyte collection itself), this OSA is incomplete and cannot force oocytes into interphase. Notably, once re-activated by sperm or chemicals, those scattered chromatids will form multiple pronuclei (MPN), which may recapitulate certain MPN and aneuploidy cases observed in fertility clinics. The second type of OSA occurs in ovarian oocytes (e.g., certain mouse strains and dromedary camel). Without ovulation or fertilization, these OSA-oocytes can initiate intrafollicular development, but these parthenotes cannot develop to term due to aberrant genomic imprinting. Instead, they either degrade or give rise to ovarian teratomas, which have also been reported in female patients. Last but not the least, genetic models displaying OSA phenotypes and the lessons we can learn from animal OSA for human reproduction are also discussed.
Introduction
Except some species (e.g., canine), mammalian females ovulate mature metaphase-II (MII) oocytes into the oviduct following luteinizing hormone (LH)-triggered oocyte maturation and follicular rupture (Cui and Kim, 2007; Duan and Sun, 2019). Normally, these ovulated oocytes can maintain MII arrest for a certain period until fertilization occurs (Fissore et al., 2002; Yin et al., 2008). Maintaining at MII stage is essential for not only fertilization in vivo but also oocyte manipulations in vitro, such as assisted reproduction, nuclear transfer cloning, and other therapeutic approaches (Sun et al., 2014; Herbert and Turnbull, 2018; Matoba and Zhang, 2018). However, in some species and under certain circumstances, exit from MII occurs spontaneously without any obvious stimulation or morphological signs, which is so-called oocyte spontaneous activation (OSA). In this mini-review, we highlight insights gained on two types of OSA through various animal models and discuss the effects of OSA on human fertility and reproductive health.
First Type of OSA
In the first type of OSA (type-1 OSA) (Figure 1A), ovulated mature oocytes can maintain MII arrest in vivo (in the oviduct); however, once collected out without any obvious or artificial stimulation, oocytes undergo OSA. In other words, these oocytes have very limited ability to maintain the MII arrest, and only oocyte recovery procedure itself can trigger parthenogenetic activation in these oocytes. This type of OSA has been known and studied in multiple species, mainly on rat (Keefer and Schuetz, 1982; Zernicka-Goetz, 1991) and golden hamster (Goud et al., 1998; Sun et al., 2002), together with case reports from human in vitro fertilization (IVF) clinics (Van Blerkom et al., 1994; Osman et al., 2019; Ye et al., 2020).
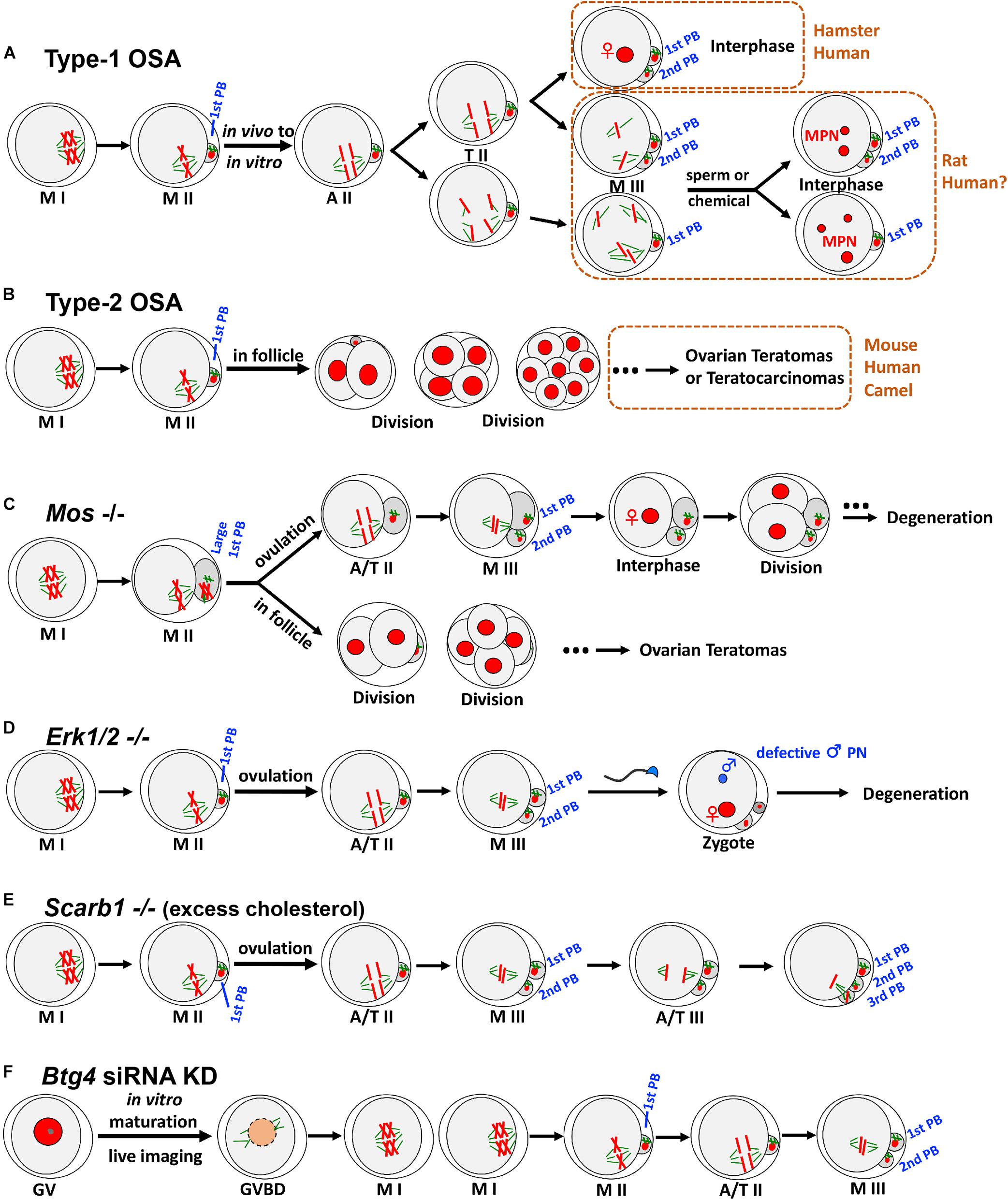
Figure 1. Two types of OSA and representative animal models exhibiting OSA phenotypes. (A) In type-1 OSA, ovulated mature oocytes can maintain MII arrest in vivo; however, once collected out without any obvious or artificial stimulation, oocytes undergo OSA. In hamster and some human IVF cases, OSA-oocytes can extrude 2nd PB and reach interphase with visible pronucleus. In rat, OSA-oocytes either extrude 2nd PB or not, depending on the oocyte postovulatory age, rat strain, external environment, and microtubule integrity. When OSA is finished, rat OSA-oocytes reach M-III arrest, with chromatids scattered around but no pronucleus formation. Once re-activated, those scattered chromatids will form multiple pronuclei (MPN), which may recapitulate certain MPN and aneuploidy cases observed in human fertility clinics. Notably, due to the nature of IVF lab protocol, only the consequence (3PN or MPN) has been reported, but information about the process prior to MPN formation was not available. We speculate that certain cases of human 3PN and MPN, especially after ICSI, are due to OSA. (B) In certain mouse strains, such as LT/Sv, some oocytes that have completed the first meiotic division can undergo type-2 OSA. Intrafollicular development of these parthenotes can cause ovarian teratomas (occasionally to teratocarcinomas). Similar phenotypes have also been reported in human and dromedary camel. (C) Mos– oocytes frequently produce large 1st PBs due to the failure of metaphase spindle movement. Ovulated MII oocytes undergo OSA to extrude 2nd PB and reach M-III, followed by pronucleus formation and cell divisions. Unovulated OSA-oocytes can initiate intrafollicular development to form ovarian teratomas. (D) Soon after ovulation, Erk1/2– oocytes undergo OSA and exit MII arrest with 2nd PB extruded spontaneously, reaching M-III stage but not into interphase. After fertilization, male pronucleus formation shows severe defects. (E) Scarb1 knockout causes excess cholesterol deposition in oocytes, which does not affect oocyte maturation significantly. However, excess cholesterol in MII oocytes can induce an elevation of (Ca2+)i, leading to OSA and extrusion of 2nd PB to M-III after ovulation. Furthermore, this cholesterol-induced OSA can result in a further round of meiosis with extrusion of 3rd PB. (F) Btg4 knockdown immature GV oocytes under live imaging can resume meiosis and reach MII. BTG4 deficiency causes a global delay in maternal mRNA degradation, and excess polyadenylated mRNA would occupy the translational machinery, which then leads to an insufficient capacity of the oocyte to translate the mRNAs that are essential for MII arrest, leading to OSA and reaching M-III. MI, metaphase of the first meiosis; MII, metaphase of the second meiosis; A/T, anaphase/telophase; PB, polar body; GV, germinal vesicle; GVBD, GV breakdown; KD, knockdown. For clarity, only four of 40 chromatids at MII are illustrated.
Morphological and cytoskeletal changes during type-1 OSA have been relatively well studied in rats. The initial separation of sister chromatids is similar to normal MII-to-AII (anaphase II) transition as seen in sperm or chemical-induced meiotic resumption and oocyte activation (Ross et al., 2006; Cui et al., 2012). Following this, oocytes either extrude the second polar body (2nd PB) or just exhibit a protrusion without 2nd PB extrusion or even no obvious change at all, depending on the oocyte postovulatory age, rat strain, external environment, and microtubule integrity (Zernicka-Goetz, 1991; Ross et al., 2006; Chaube et al., 2007; Cui et al., 2012). When OSA is done (normally around 6 h post in vitro culture), scattered chromatids and surrounding microtubules form multiple small spindle-like structures, reaching a new metaphase-like arrest (Zernicka-Goetz et al., 1993; Tomioka et al., 2007; Cui et al., 2012). Because the stimulation is minimal (oocyte collection itself and the following in vitro culture), this OSA is incomplete/abortive and cannot force oocytes into interphase, and therefore, no pronucleus formation occurs after OSA. Instead, OSA-oocytes enter a so-called “metaphase III-like” (M-III) arrest (Zernicka-Goetz, 1991). Notably, these OSA-oocytes can be re-activated by sperm or chemicals, and once re-activated, those scattered chromatids will form multiple pronuclei (MPN), which may recapitulate certain MPN and aneuploidy cases observed in human fertility clinics (Van Blerkom et al., 1984; Dozortsev et al., 1998; Hayes et al., 2001; Dai et al., 2017; Grigoryan et al., 2019). Different from rat, some hamster and human OSA-oocytes can reach interphase with visible pronuclei (Longo, 1974; Van Blerkom et al., 1994; Sun et al., 2002; Jiang et al., 2015; Osman et al., 2019; Ye et al., 2020).
Mechanism and Control of Type-1 OSA
Although no obvious or artificial stimulation is applied on OSA-oocytes, substantial subtle changes could happen during oocyte collection and in vitro culture. Among all factors, exposure to cold and prolonged retention in the oviduct after animal sacrifice can significantly increase rat OSA (Keefer and Schuetz, 1982; Zernicka-Goetz, 1991; Kito et al., 2010). Another widely recognized factor is postovulatory aging, which has been shown in rat (Ben-Yosef et al., 1995; Chaube et al., 2007; Cui et al., 2012), golden hamster (Sun et al., 2002; Jiang et al., 2015), and human (Santos et al., 2003) to facilitate OSA (Table 1). Same as other vertebrates, the two most critical kinases of cytostatic factor (CSF), maturation-promoting factor (MPF), and mitogen-activated protein kinase (MAPK), are also involved in OSA (Tiwari et al., 2018). Previous studies revealed that rat oocytes carry only 40% MPF kinase activity of that in mouse oocytes (Ito et al., 2005), which could explain the susceptibility of rat oocytes to OSA, although variation in MPF activity has been detected among different rat strains (Hirabayashi et al., 2003; Ross et al., 2006; Sterthaus et al., 2009). As a master regulator of microtubule organization and spindle assembly during oocyte meiosis, MAPK, the other pivotal CSF, has also been studied in rat OSA. Different from sperm or chemical-induced oocyte activation where high MAPK activity still lasts several hours after the stimulation (Fan and Sun, 2004), rat OSA exhibits a quick decrease in both Mos and MAPK kinase (MEK)/MAPK (Ito et al., 2007; Table 1), which could explain the reason underlying the disintegrated microtubules and failure of 2nd PB extrusion during OSA in some rat strains (Cui et al., 2012; Prasad et al., 2015). Regarding the mechanism of re-entering the so-called M-III arrest after OSA, a plausible scenario is that defects in attachment of kinetochores and/or spindle assembly caused by premature MAPK decline could activate the spindle assembly checkpoint (SAC) proteins, which then mobilize cyclin B protein and actuate MPF activity (Cui et al., 2012).
Similar to sperm and chemical-induced mammalian oocyte activation (Miao and Williams, 2012; Parrington et al., 2019), Ca2+ and calmodulin-dependent protein kinase II (CaMKII) are also involved in OSA attributing to MPF inactivation and probably a premature decline in Mos/MEK/MAPK (Ito et al., 2006; Ito et al., 2007; Yoo and Smith, 2007; Table 1). It is noteworthy that increase of intracellular free Ca2+ [(Ca2+)i] during OSA is insufficient (Cui et al., 2012; Premkumar and Chaube, 2013) compared with the pattern caused by sperm or chemical, which could explain why OSA is incomplete and abortive that cannot force oocytes into interphase to form pronucleus. To block increase of (Ca2+)i and its cascade during OSA, Ca2+-free medium (Hayes et al., 2001; Sun et al., 2002; Premkumar and Chaube, 2013), Ca2+ chelator (Ito et al., 2007), multiple Ca2+ channel blockers (Chaube et al., 2007; Yoo and Smith, 2007), and CaMKII inhibitors (Ito et al., 2006; Yoo and Smith, 2007) have been applied (Table 1), but these methods cannot fully block OSA or can cause obvious side effects [reviewed in Chebotareva et al. (2011)]. A novel physiological method focusing on sodium/calcium exchanger-mediated Ca2+ efflux has been demonstrated effective to block OSA, but this method cannot override the stimulation caused by enucleation during somatic cell nuclear transfer (SCNT) (Cui et al., 2013). To inhibit cyclin-B degradation and MPF inactivation, proteasome inhibitor MG132 was also widely applied (Zhou et al., 2003; Ito et al., 2007; Popova et al., 2009; Sterthaus et al., 2009; Mizumoto et al., 2010; Cui et al., 2013), but caution should be exercised due to its profound side effects [reviewed in Chebotareva et al. (2011)]. Furthermore, other factors have also been evaluated to better elucidate type-1 OSA, such as intracellular nitric oxide (Premkumar and Chaube, 2015; Prasad and Chaube, 2016), cyclin-dependent kinase 1 (Prasad et al., 2016a,b), ubiquitin-proteasome pathway (Tan et al., 2005), and reactive oxygen species (Premkumar and Chaube, 2016). To summarize, although more factors and pathways involved in type-1 OSA are emerging (Table 1), the nature of trigger and the best way to prevent the onset of OSA are still unclear. With advancement in rat and hamster genome editing especially under CRISPR/Cas9 system (Meek et al., 2017; Li et al., 2018), we hope a clear picture of type-1 OSA could be achieved soon.
“Confusing” Terms
Given the theme of this mini-review and the following content (type-2 OSA) to discuss, it seems helpful to clarify some “confusing” terms here.
Spontaneous Meiotic Resumption
Once mammalian oocytes are separated from the antral follicles and cultured under appropriate conditions, they can resume meiosis spontaneously from the diplotene stage of the first meiotic prophase to MII, which is also called spontaneous maturation (Liang et al., 2007; Pan and Li, 2019).
Spontaneous Ovulation
Most mammals including women display a continuous cycling of reproductive hormones with ovulation occurring at regular intervals, which is different from those induced ovulators (e.g., rabbits, cats, and camelids) that copulation is responsible for hormone regulation and ovulation (Ratto et al., 2019).
Postovulatory Oocyte Aging vs. OSA
Normally, mature oocytes can maintain MII arrest for a certain period in vivo or in vitro. If fertilization does not occur, oocytes undergo postovulatory oocyte aging, and too “aged” oocytes may GRADUALLY exit MII arrest with some initiating OSA (Pickering et al., 1988; Xu et al., 1997; Gordo et al., 2002; Miao et al., 2009). In short, long-time postovulatory oocyte aging may facilitate OSA both in vivo and in vitro, but OSA can also occur in very “young and fresh” oocytes, and OSA process is relatively much faster and uncontrollable (discussed in section “First Type Of OSA”).
Type-2 OSA and Mechanism
Although oocytes spend majority of their life in the ovary and follicles, to become an embryo, it has to be ovulated from the follicle into oviduct for fertilization with sperm. However, OSA-induced embryogenesis is an exception. The second type of OSA (Figure 1B) occurs in ovarian oocytes within the follicles and it can initiate intrafollicular development to a certain stage. For example, in LT/Sv mice, a substantial portion of oocytes that have completed the first meiotic division can undergo OSA (Eppig et al., 1977). Although these ovarian OSA-embryos resemble normal until the blastocyst or even primitive streak stage, later on, most of them become disorganized and form ovarian teratomas (Stevens and Varnum, 1974). Usually, these teratomas are benign, but occasionally, they grow progressively and are malignant, containing multiple types of tissue and proliferating pluripotent stem cells (embryonal carcinoma cells that are called teratocarcinomas) (Stevens, 1980). Notably, these phenotypes were also reported in humans, including ovarian zygotes (Combelles et al., 2011), two-cell embryo (Padilla et al., 1987), four-cell embryo (Oliveira et al., 2004), and teratomas (Linder et al., 1975). To clarify, the existence of nuclei had been confirmed in all blastomeres of the above-mentioned human ovarian OSA-embryos, ruling out the possibly of cytoplasmic fragmentation, which is a relatively common phenomenon in aged unfertilized oocytes or during human preimplantation development (Alikani et al., 1999; Lord and Aitken, 2013).
In addition to spontaneous ovulators, type-2 OSA and ovarian teratoma have also been detected in induced ovulators, such as dromedary camel (Camelus dromedarius) (Mesbah et al., 2002, 2004). Similarly, OSA-oocytes can initiate intrafollicular development to blastocyst stage with clear inner cell mass and trophectoderm (Abdoon et al., 2007, 2020), suggesting the occurrence of the first cell lineage specification in these parthenotes (Cui and Mager, 2018; Ho et al., 2019). Although the underlying molecular mechanism that causes type-2 OSA is not fully understood yet, current knowledge from mouse models suggests that type-2 OSA and teratoma formation are multigenic traits (Eppig et al., 1996), involving genetic background (Lee et al., 1997; Ciemerych and Kubiak, 1998; Cheng et al., 2012; Abdoon et al., 2020), cytoskeletal arrangement and SAC (Albertini and Eppig, 1995; Maciejewska et al., 2009), companion somatic cells (Eppig et al., 2000), AMPK signaling (Downs et al., 2010; Ya and Downs, 2013), and hormonal regulation (Speirs and Kaufman, 1988).
Genetic Models Displaying OSA Phenotypes
With success in embryonic stem cell (ESC)-mediated gene targeting and CRISPR/Cas9-mediated genome editing, more engineered animal models have been generated for studying mammalian oocyte meiosis. Here, we briefly review some examples.
Mos knockout female mice can produce MII oocytes; however, these oocytes cannot arrest at MII stage (Figure 1C). For those ovulated MII oocytes, they will spontaneously extrude 2nd PB and reach M-III, followed by pronucleus formation and cell divisions. Meanwhile, unovulated OSA-oocytes can initiate intrafollicular development, which then causes ovarian teratomas (Colledge et al., 1994; Hashimoto et al., 1994; Araki et al., 1996). In addition, Mos– oocytes frequently produce large first polar bodies (1st PBs) due to the failure of metaphase spindle movement (Choi et al., 1996; Verlhac et al., 1996). Given the phenotypes detected from the knockout mice, these models are valuable for studying human ovarian pathology and teratogenesis.
Although MOS/MEK/ERK cascade has been relatively well studied in oocyte meiosis, the explicit role of extracellular signal-regulated kinase (ERK) in vivo was not clear. Through the knockout of both Erk1 and Erk2 in mouse oocytes (Figure 1D), data indicates that Erk1/2– oocytes exit MII arrest and extrude 2nd PB spontaneously, reaching M-III stage. Different from Mos– oocytes, Erk1/2– MII oocytes do not exhibit large 1st PBs, and subsequent M-III oocytes display low frequency of pronucleus formation, explaining why ovarian teratomas were not detected in the females. Interestingly, ERK1/2 deletion also severely prevents male pronucleus formation after fertilization (Zhang et al., 2015), representing another major contributing cause of female infertility.
Female fertility can be affected by many factors, including diet and nutrient metabolism. Two recent studies using genetically modified mice revealed that maintenance of cholesterol within a physiological range during oocyte development and maturation is essential for female fertility. Excess cholesterol deposition in MII oocytes can induce an elevation of (Ca2+)i, which then triggers reduction in both MPF and MAPK, leading to OSA and extrusion of 2nd PB to M-III (Figure 1E). Different from all the above-mentioned OSAs, this cholesterol-induced OSA can result in multiple cell cycles, including execution of the third meiosis with extrusion of 3rd PB (Yesilaltay et al., 2014; Quiroz et al., 2020), which was found in partially activated oocytes (Kubiak, 1989). Importantly, this excess-cholesterol-induced OSA can be reversed both in vivo and in vitro (Yesilaltay et al., 2014; Quiroz et al., 2020), highlighting the possibility that cholesterol metabolism may underlie some woman infertility of unknown etiology and this could be cured with appropriate treatments.
In mammals, oocyte meiotic maturation not only produces a haploid gamete but also initiates maternal mRNA transition from stable to unstable (Wu and Dean, 2016; Sha et al., 2019), serving as a prolog to maternal-zygotic transition (MZT) which involves maternal mRNA destabilization and degradation. Recently, three laboratories independently identified BTG4 as a key mediator that links mRNA decay machinery and meiotic cell cycle progression, and loss of BTG4 causes a global delay in maternal mRNA degradation (Liu et al., 2016; Pasternak et al., 2016; Yu et al., 2016). In addition, BTG4 was also identified as essential for MII arrest (Figure 1F), because excess polyadenylated mRNA caused by Btg4 knockdown could occupy the translational machinery, which then leads to an insufficient capacity of the oocyte to translate the mRNAs that are essential for MII arrest (e.g., mRNAs encoding EMI2), and all of this finally resulted in OSA to M-III (Pasternak et al., 2016). Interestingly, this OSA phenotype was not detected in knockout models, which could be due to the environment (in vitro live imaging vs. in vivo) and/or methodologies (difference in genetic compensation and specificity between knockdown and knockout).
Discussion
We reviewed two types of OSA and representative animal models exhibiting OSA phenotypes due to genetic defects. Regarding type-1 OSA (in vivo to in vitro), we propose that more caution should be exercised during assisted human reproduction, as many steps could trigger OSA, such as oocyte retrieval (Muechler et al., 1989), cryopreservation (Gook et al., 1995), and intracytoplasmic sperm injection (ICSI) (Sultan et al., 1995). Furthermore, as learned from rat OSA, certain OSA-oocytes could show minimal morphological signs (e.g., sister chromatids separated or even scattered in cytoplasm but without 2nd PB or pronucleus formation). Therefore, we propose OSA should be considered for those unexplained abnormal fertilization with repeated triploid pronuclei (3PN) (Grigoryan et al., 2019) or even more pronuclei (e.g., up to 8PN) (Dai et al., 2017) after ICSI. Other lessons we can learn from animal models and issues that should be addressed are as follows: (1) time interval between oocyte pickup and IVF/ICSI. Currently, there is no consensus among clinics about this interval, and 2–6 h are widely accepted for a better cytoplasmic maturity but without aging (Van de Velde et al., 1998; Garor et al., 2015). This routine interval seems fine for most patients; however, for those that cannot achieve pregnancy after multiple cycles and especially with repeated 3PN or MPN, this interval probably needs to be avoided as OSA could be the reason that is much faster than natural aging. (2) Make everything ready for a rapid ICSI. For oocytes that are susceptible to OSA (Dozortsev et al., 1998; Morishita et al., 2019), all things should be well prepared before oocyte retrieval; right after which, a careful and rapid oocyte denudation and ICSI should follow to mitigate OSA-induced abnormal fertilization and possible aneuploidy.
A substantial number of patients cannot achieve successful pregnancy after multiple IVF cycles, and it is generally believed that genetic defects underlie many of these unrecognized pathologies (Conti and Franciosi, 2018; Cui, 2020). Dissecting the association between genetic variants and human OSA is challenging because the etiology is highly heterogeneous and patients have different genetic predispositions and epigenetic modifications (Marshall and Rivera, 2018; Ou et al., 2019), ages and lifestyles (Qiao et al., 2014; Grondahl et al., 2017), and exposures to diverse environments and pollutants (Peretz et al., 2014). To gain a better understanding of human oocyte meiosis and idiopathic infertility, animal models have been generated to define key factors and pathways involved in meiotic cell cycle regulation. Although more than 400 mutant mouse models with reproductive phenotypes have been established (Matzuk and Lamb, 2008), many genes and pathways regulating oocyte meiosis and OSA are still not fully delineated due to insufficient models and possible limitations when translating the information from mice to humans. With more mouse models being generated by the Knockout Mouse Program and the International Mouse Phenotyping Consortium1 and recent application of CRISPR/Cas9 in other species that can bypass barriers of ESCs and SCNT, we believe more essential genes will be screened out and more appropriate animal models (e.g., point mutation by knock-in) will be generated. We hope, with more precise animal models available, more sophisticated clinical protocols (Sachs et al., 2000; Socolov et al., 2015), faster genetic tests in clinics, more advanced assisted reproductive technologies (Smith and Takayama, 2017; Belli et al., 2019; Hawkins et al., 2021), and genetic diagnosis in preimplantation embryos, we will fully understand the underpinnings of human OSA, an overlooked meiotic instability problem that requires global attention (Premkumar et al., 2020).
Author Contributions
WC conceived the study, prepared the figures, and wrote the manuscript.
Funding
This work was supported by grants from the National Institutes of Health/National Institute of Child Health and Human Development (NIH/NICHD R21HD098686) and USDA National Institute of Food and Agriculture/Hatch (NIFA/Hatch #1024792) to WC. The contents are solely the responsibility of the author and do not necessarily represent the official views of the funding agencies.
Conflict of Interest
The author declares that the research was conducted in the absence of any commercial or financial relationships that could be construed as a potential conflict of interest.
Footnotes
References
Abdoon, A. S., Kandil, O. M., Berisha, B., Kliem, H., and Schams, D. (2007). Morphology of dromedary camel oocytes and their ability to spontaneous and chemical parthenogenetic activation. Reprod. Domest. Anim. 42, 88–93. doi: 10.1111/j.1439-0531.2006.00737.x
Abdoon, A. S. S., Kandil, O. M., and Zeng, S. M. (2020). Intrafollicular spontaneous parthenogenetic development of dromedary camel oocytes. Mol. Reprod. Dev. 87, 704–710. doi: 10.1002/mrd.23350
Albertini, D. F., and Eppig, J. J. (1995). Unusual cytoskeletal and chromatin configurations in mouse oocytes that are atypical in meiotic progression. Dev. Genet. 16, 13–19. doi: 10.1002/dvg.1020160105
Alikani, M., Cohen, J., Tomkin, G., Garrisi, G. J., Mack, C., and Scott, R. T. (1999). Human embryo fragmentation in vitro and its implications for pregnancy and implantation. Fertil. Steril. 71, 836–842. doi: 10.1016/S0015-0282(99)00092-8
Araki, K., Naito, K., Haraguchi, S., Suzuki, R., Yokoyama, M., Inoue, M., et al. (1996). Meiotic abnormalities of c-mos knockout mouse oocytes: activation after first meiosis or entrance into third meiotic metaphase. Biol. Reprod. 55, 1315–1324. doi: 10.1095/biolreprod55.6.1315
Belli, M., Zhang, L., Liu, X., Donjacour, A., Ruggeri, E., Palmerini, M. G., et al. (2019). Oxygen concentration alters mitochondrial structure and function in in vitro fertilized preimplantation mouse embryos. Hum. Reprod. 34, 601–611. doi: 10.1093/humrep/dez011
Ben-Yosef, D., Oron, Y., and Shalgi, R. (1995). Low temperature and fertilization-induced Ca2+ changes in rat eggs. Mol. Reprod. Dev. 42, 122–129. doi: 10.1002/mrd.1080420116
Chaube, S. K., Dubey, P. K., Mishra, S. K., and Shrivastav, T. G. (2007). Verapamil reversibly inhibits spontaneous parthenogenetic activation in aged rat eggs cultured in vitro. Cloning Stem Cells 9, 608–617. doi: 10.1089/clo.2007.0001
Chebotareva, T., Taylor, J., Mullins, J. J., and Wilmut, I. (2011). Rat eggs cannot wait: spontaneous exit from meiotic metaphase-II arrest. Mol. Reprod. Dev. 78, 795–807. doi: 10.1002/mrd.21385
Cheng, Y., Zhong, Z., and Latham, K. E. (2012). Strain-specific spontaneous activation during mouse oocyte maturation. Fertil. Steril. 98, 200–206. doi: 10.1016/j.fertnstert.2012.03.060
Choi, T., Fukasawa, K., Zhou, R., Tessarollo, L., Borror, K., Resau, J., et al. (1996). The Mos/mitogen-activated protein kinase (MAPK) pathway regulates the size and degradation of the first polar body in maturing mouse oocytes. Proc. Natl. Acad. Sci. U.S.A. 93, 7032–7035. doi: 10.1073/pnas.93.14.7032
Ciemerych, M. A., and Kubiak, J. Z. (1998). Cytostatic activity develops during meiosis I in oocytes of LT/Sv mice. Dev. Biol. 200, 198–211. doi: 10.1006/dbio.1998.8930
Colledge, W. H., Carlton, M. B., Udy, G. B., and Evans, M. J. (1994). Disruption of c-mos causes parthenogenetic development of unfertilized mouse eggs. Nature 370, 65–68. doi: 10.1038/370065a0
Combelles, C. M., Kearns, W. G., Fox, J. H., and Racowsky, C. (2011). Cellular and genetic analysis of oocytes and embryos in a human case of spontaneous oocyte activation. Hum. Reprod. 26, 545–552. doi: 10.1093/humrep/deq363
Conti, M., and Franciosi, F. (2018). Acquisition of oocyte competence to develop as an embryo: integrated nuclear and cytoplasmic events. Hum. Reprod. Update 24, 245–266. doi: 10.1093/humupd/dmx040
Cui, W. (2020). SOHLHs are essential for fertility regardless of gender or population. Fertil. Steril. 114, 283–284. doi: 10.1016/j.fertnstert.2020.06.010
Cui, W., and Mager, J. (2018). Transcriptional regulation and genes involved in first lineage specification during preimplantation development. Adv. Anat. Embryol. Cell Biol. 229, 31–46. doi: 10.1007/978-3-319-63187-5_4
Cui, W., Zhang, J., Lian, H. Y., Wang, H. L., Miao, D. Q., Zhang, C. X., et al. (2012). Roles of MAPK and spindle assembly checkpoint in spontaneous activation and MIII arrest of rat oocytes. PLoS One 7:e32044. doi: 10.1371/journal.pone.0032044
Cui, W., Zhang, J., Zhang, C. X., Jiao, G. Z., Zhang, M., Wang, T. Y., et al. (2013). Control of spontaneous activation of rat oocytes by regulating plasma membrane Na+/Ca2+ exchanger activities. Biol. Reprod. 88:160. doi: 10.1095/biolreprod.113.108266
Cui, X. S., and Kim, N. H. (2007). Maternally derived transcripts: identification and characterisation during oocyte maturation and early cleavage. Reprod. Fertil. Dev. 19, 25–34. doi: 10.1071/RD06128
Dai, J., Leng, L. Z., Lu, C. F., Gong, F., Zhang, S. P., Zheng, W., et al. (2017). Time-lapse observation and transcriptome analysis of a case with repeated multiple pronuclei after IVF/ICSI. J. Assist. Reprod. Genet. 34, 1189–1197. doi: 10.1007/s10815-017-0972-9
Downs, S. M., Ya, R., and Davis, C. C. (2010). Role of AMPK throughout meiotic maturation in the mouse oocyte: evidence for promotion of polar body formation and suppression of premature activation. Mol. Reprod. Dev. 77, 888–899. doi: 10.1002/mrd.21229
Dozortsev, D., Wakaiama, T., Ermilov, A., and Yanagimachi, R. (1998). Intracytoplasmic sperm injection in the rat. Zygote 6, 143–147. doi: 10.1017/S0967199498000069
Duan, X., and Sun, S. C. (2019). Actin cytoskeleton dynamics in mammalian oocyte meiosis. Biol. Reprod. 100, 15–24. doi: 10.1093/biolre/ioy163
Eppig, J. J., Kozak, L. P., Eicher, E. M., and Stevens, L. C. (1977). Ovarian teratomas in mice are derived from oocytes that have completed the first meiotic division. Nature 269, 517–518. doi: 10.1038/269517a0
Eppig, J. J., Wigglesworth, K., and Hirao, Y. (2000). Metaphase I arrest and spontaneous parthenogenetic activation of strain LTXBO oocytes: chimeric reaggregated ovaries establish primary lesion in oocytes. Dev. Biol. 224, 60–68. doi: 10.1006/dbio.2000.9764
Eppig, J. J., Wigglesworth, K., Varnum, D. S., and Nadeau, J. H. (1996). Genetic regulation of traits essential for spontaneous ovarian teratocarcinogenesis in strain LT/Sv mice: aberrant meiotic cell cycle, oocyte activation, and parthenogenetic development. Cancer Res. 56, 5047–5054.
Fan, H. Y., and Sun, Q. Y. (2004). Involvement of mitogen-activated protein kinase cascade during oocyte maturation and fertilization in mammals. Biol. Reprod. 70, 535–547. doi: 10.1095/biolreprod.103.022830
Fissore, R. A., Kurokawa, M., Knott, J., Zhang, M., and Smyth, J. (2002). Mechanisms underlying oocyte activation and postovulatory ageing. Reproduction 124, 745–754. doi: 10.1530/rep.0.1240745
Garor, R., Shufaro, Y., Kotler, N., Shefer, D., Krasilnikov, N., Ben-Haroush, A., et al. (2015). Prolonging oocyte in vitro culture and handling time does not compensate for a shorter interval from human chorionic gonadotropin administration to oocyte pickup. Fertil. Steril. 103, 72–75. doi: 10.1016/j.fertnstert.2014.09.022
Gook, D. A., Osborn, S. M., and Johnston, W. I. (1995). Parthenogenetic activation of human oocytes following cryopreservation using 1,2-propanediol. Hum. Reprod. 10, 654–658. doi: 10.1093/oxfordjournals.humrep.a136005
Gordo, A. C., Rodrigues, P., Kurokawa, M., Jellerette, T., Exley, G. E., Warner, C., et al. (2002). Intracellular calcium oscillations signal apoptosis rather than activation in in vitro aged mouse eggs. Biol. Reprod. 66, 1828–1837. doi: 10.1095/biolreprod66.6.1828
Goud, P. T., Goud, A. P., Rybouchkin, A. V., De Sutter, P., and Dhont, M. (1998). Chromatin decondensation, pronucleus formation, metaphase entry and chromosome complements of human spermatozoa after intracytoplasmic sperm injection into hamster oocytes. Hum. Reprod. 13, 1336–1345. doi: 10.1093/humrep/13.5.1336
Grigoryan, H., Levkov, L., Sciorio, R., and Hambartsoumian, E. (2019). Unexplained total abnormal fertilization of donor oocytes in ICSI with using spermatozoa from different patients. Gynecol. Endocrinol. 35, 60–62. doi: 10.1080/09513590.2019.1632086
Grondahl, M. L., Christiansen, S. L., Kesmodel, U. S., Agerholm, I. E., Lemmen, J. G., Lundstrom, P., et al. (2017). Effect of women’s age on embryo morphology, cleavage rate and competence-a multicenter cohort study. PLoS One 12:e0172456. doi: 10.1371/journal.pone.0172456
Hashimoto, N., Watanabe, N., Furuta, Y., Tamemoto, H., Sagata, N., Yokoyama, M., et al. (1994). Parthenogenetic activation of oocytes in c-mos-deficient mice. Nature 370, 68–71. doi: 10.1038/370068a0
Hawkins, J., Miao, X., Cui, W., and Sun, Y. (2021). Biophysical optimization of preimplantation embryo culture: what mechanics can offer ART. Mol. Hum. Reprod. 27:gaaa087. doi: 10.1093/molehr/gaaa087
Hayes, E., Galea, S., Verkuylen, A., Pera, M., Morrison, J., Lacham-Kaplan, O., et al. (2001). Nuclear transfer of adult and genetically modified fetal cells of the rat. Physiol. Genomics 5, 193–204. doi: 10.1152/physiolgenomics.2001.5.4.193
Herbert, M., and Turnbull, D. (2018). Progress in mitochondrial replacement therapies. Nat. Rev. Mol. Cell Biol. 19, 71–72. doi: 10.1038/nrm.2018.3
Hirabayashi, M., Kato, M., Ishikawa, A., and Hochi, S. (2003). Factors influencing chromosome condensation and development of cloned rat embryos. Cloning Stem Cells 5, 35–42. doi: 10.1089/153623003321512148
Ho, N. T. K., Nguyen, T. V. T., Nguyen, T. V., and Bui, H. T. (2019). Epigenetic impairments in development of parthenogenetic preimplantation mouse embryos. J. Reprod. Dev. 65, 83–90. doi: 10.1262/jrd.2018-028
Ito, J., Hirabayashi, M., Kato, M., Takeuchi, A., Ito, M., Shimada, M., et al. (2005). Contribution of high p34cdc2 kinase activity to premature chromosome condensation of injected somatic cell nuclei in rat oocytes. Reproduction 129, 171–180. doi: 10.1530/rep.1.00431
Ito, J., Kaneko, R., and Hirabayashi, M. (2006). The regulation of calcium/calmodulin-dependent protein kinase II during oocyte activation in the rat. J. Reprod. Dev. 52, 439–447. doi: 10.1262/jrd.17047
Ito, J., Shimada, M., Hochi, S., and Hirabayashi, M. (2007). Involvement of Ca2+-dependent proteasome in the degradation of both cyclin B1 and Mos during spontaneous activation of matured rat oocytes. Theriogenology 67, 475–485. doi: 10.1016/j.theriogenology.2006.08.012
Jiang, H., Wang, C., Guan, J., Wang, L., and Li, Z. (2015). Changes of spontaneous parthenogenetic activation and development potential of golden hamster oocytes during the aging process. Acta Histochem. 117, 104–110. doi: 10.1016/j.acthis.2014.11.008
Keefer, C. L., and Schuetz, A. W. (1982). Spontaneous activation of ovulated rat oocytes during in vitro culture. J. Exp. Zool. 224, 371–377. doi: 10.1002/jez.1402240310
Kito, S., Yano, H., Ohta, Y., and Tsukamoto, S. (2010). Superovulatory response, oocyte spontaneous activation, and embryo development in WMN/Nrs inbred rats. Exp. Anim. 59, 35–45. doi: 10.1538/expanim.59.35
Kubiak, J. Z. (1989). Mouse oocytes gradually develop the capacity for activation during the metaphase II arrest. Dev. Biol. 136, 537–545. doi: 10.1016/0012-1606(89)90279-0
Lee, G. H., Bugni, J. M., Obata, M., Nishimori, H., Ogawa, K., and Drinkwater, N. R. (1997). Genetic dissection of susceptibility to murine ovarian teratomas that originate from parthenogenetic oocytes. Cancer Res. 57, 590–593.
Li, R., Miao, J., Fan, Z., Song, S., Kong, I. K., Wang, Y., et al. (2018). Production of genetically engineered golden Syrian hamsters by pronuclear injection of the CRISPR/Cas9 complex. J. Vis. Exp. 131:56263. doi: 10.3791/56263
Liang, C. G., Su, Y. Q., Fan, H. Y., Schatten, H., and Sun, Q. Y. (2007). Mechanisms regulating oocyte meiotic resumption: roles of mitogen-activated protein kinase. Mol. Endocrinol. 21, 2037–2055. doi: 10.1210/me.2006-0408
Linder, D., Mccaw, B. K., and Hecht, F. (1975). Parthenogenic origin of benign ovarian teratomas. N. Engl. J. Med. 292, 63–66. doi: 10.1056/NEJM197501092920202
Liu, Y., Lu, X., Shi, J., Yu, X., Zhang, X., Zhu, K., et al. (2016). BTG4 is a key regulator for maternal mRNA clearance during mouse early embryogenesis. J. Mol. Cell Biol. 8, 366–368. doi: 10.1093/jmcb/mjw023
Longo, F. J. (1974). An ultrastructural analysis of spontaneous activation of hamster eggs aged in vivo. Anat. Rec. 179, 27–55. doi: 10.1002/ar.1091790104
Lord, T., and Aitken, R. J. (2013). Oxidative stress and ageing of the post-ovulatory oocyte. Reproduction 146, R217–R227. doi: 10.1530/REP-13-0111
Maciejewska, Z., Polanski, Z., Kisiel, K., Kubiak, J. Z., and Ciemerych, M. A. (2009). Spindle assembly checkpoint-related failure perturbs early embryonic divisions and reduces reproductive performance of LT/Sv mice. Reproduction 137, 931–942. doi: 10.1530/REP-09-0011
Marshall, K. L., and Rivera, R. M. (2018). The effects of superovulation and reproductive aging on the epigenome of the oocyte and embryo. Mol. Reprod. Dev. 85, 90–105. doi: 10.1002/mrd.22951
Matoba, S., and Zhang, Y. (2018). Somatic cell nuclear transfer reprogramming: mechanisms and applications. Cell Stem Cell 23, 471–485. doi: 10.1016/j.stem.2018.06.018
Matzuk, M. M., and Lamb, D. J. (2008). The biology of infertility: research advances and clinical challenges. Nat. Med. 14, 1197–1213. doi: 10.1038/nm.f.1895
Meek, S., Mashimo, T., and Burdon, T. (2017). From engineering to editing the rat genome. Mamm. Genome 28, 302–314. doi: 10.1007/s00335-017-9705-8
Mesbah, S. F., Kafi, I. M., and Nili, H. (2002). Ovarian teratoma in a camel (Camelus dromedarius). Vet. Rec. 151:776.
Mesbah, S. F., Kafi, M., Nili, H., and Nasr-Esfahani, M. H. (2004). Spontaneous parthenogenesis and development of camel (Camelus dromedarius) oocytes. Vet. Rec. 155, 498–500. doi: 10.1136/vr.155.16.498
Miao, Y. L., Kikuchi, K., Sun, Q. Y., and Schatten, H. (2009). Oocyte aging: cellular and molecular changes, developmental potential and reversal possibility. Hum. Reprod. Update 15, 573–585. doi: 10.1093/humupd/dmp014
Miao, Y. L., and Williams, C. J. (2012). Calcium signaling in mammalian egg activation and embryo development: the influence of subcellular localization. Mol. Reprod. Dev. 79, 742–756. doi: 10.1002/mrd.22078
Mizumoto, S., Kato, Y., and Tsunoda, Y. (2010). The effect of the time interval between injection and parthenogenetic activation on the spindle formation and the in vitro developmental potential of somatic cell nuclear-transferred rat oocytes. Zygote 18, 9–15. doi: 10.1017/S0967199409990025
Morishita, N., Ochi, M., and Horiuchi, T. (2019). Development of golden hamster embryos effectively produced by injection of sperm heads sonicated in Tris-HCl buffer with EGTA. Reprod. Med. Biol. 18, 83–90. doi: 10.1002/rmb2.12253
Muechler, E. K., Graham, M. C., Huang, K. E., Partridge, A. B., and Jones, K. (1989). Parthenogenesis of human oocytes as a function of vacuum pressure. J. In Vitro Fert. Embryo Transf. 6, 335–337. doi: 10.1007/BF01138772
Oliveira, F. G., Dozortsev, D., Diamond, M. P., Fracasso, A., Abdelmassih, S., Abdelmassih, V., et al. (2004). Evidence of parthenogenetic origin of ovarian teratoma: case report. Hum. Reprod. 19, 1867–1870. doi: 10.1093/humrep/deh345
Osman, E. K., Hong, K. H., and Scott, R. T. (2019). A case of recurrent spontaneous parthenogenetic oocyte activation. Reprod. Biomed. Online 39(Suppl. 2) e7–e8. doi: 10.1016/j.rbmo.2019.07.018
Ou, X. H., Zhu, C. C., and Sun, S. C. (2019). Effects of obesity and diabetes on the epigenetic modification of mammalian gametes. J. Cell. Physiol. 234, 7847–7855. doi: 10.1002/jcp.27847
Padilla, S. L., Boldt, J. P., and Mcdonough, P. G. (1987). Possible parthenogenesis with in vitro fertilization subsequent to ovarian cystic teratomas. Am. J. Obstet. Gynecol. 156, 1127–1129. doi: 10.1016/0002-9378(87)90124-4
Pan, B., and Li, J. (2019). The art of oocyte meiotic arrest regulation. Reprod. Biol. Endocrinol. 17:8. doi: 10.1186/s12958-018-0445-8
Parrington, J., Arnoult, C., and Fissore, R. A. (2019). The eggstraordinary story of how life begins. Mol. Reprod. Dev. 86, 4–19. doi: 10.1002/mrd.23083
Pasternak, M., Pfender, S., Santhanam, B., and Schuh, M. (2016). The BTG4 and CAF1 complex prevents the spontaneous activation of eggs by deadenylating maternal mRNAs. Open Biol. 6:160184. doi: 10.1098/rsob.160184
Peretz, J., Vrooman, L., Ricke, W. A., Hunt, P. A., Ehrlich, S., Hauser, R., et al. (2014). Bisphenol a and reproductive health: update of experimental and human evidence, 2007-2013. Environ. Health Perspect. 122, 775–786. doi: 10.1289/ehp.1307728
Pickering, S. J., Johnson, M. H., Braude, P. R., and Houliston, E. (1988). Cytoskeletal organization in fresh, aged and spontaneously activated human oocytes. Hum. Reprod. 3, 978–989. doi: 10.1093/oxfordjournals.humrep.a136828
Popova, E., Bader, M., and Krivokharchenko, A. (2009). Efficient production of nuclear transferred rat embryos by modified methods of reconstruction. Mol. Reprod. Dev. 76, 208–216. doi: 10.1002/mrd.20944
Prasad, S., and Chaube, S. K. (2016). S-nitroso-N-acetyl penicillamine inhibits spontaneous exit from metaphase-II arrest in rat eggs cultured in vitro. Biomed. Pharmacother. 84, 680–686. doi: 10.1016/j.biopha.2016.09.059
Prasad, S., Koch, B., and Chaube, S. K. (2016a). Involvement of cyclin-dependent kinase 1 during postovulatory aging-mediated abortive spontaneous egg activation in rat eggs cultured in vitro. Cell Reprogram 18, 96–107. doi: 10.1089/cell.2015.0068
Prasad, S., Koch, B., and Chaube, S. K. (2016b). RO-3306 prevents postovulatory aging-mediated spontaneous exit from M-II arrest in rat eggs cultured in vitro. Biomed. Pharmacother. 78, 216–225. doi: 10.1016/j.biopha.2016.01.013
Prasad, S., Tiwari, M., Koch, B., and Chaube, S. K. (2015). Morphological, cellular and molecular changes during postovulatory egg aging in mammals. J. Biomed. Sci. 22:36. doi: 10.1186/s12929-015-0143-1
Premkumar, K. V., and Chaube, S. K. (2013). An insufficient increase of cytosolic free calcium level results postovulatory aging-induced abortive spontaneous egg activation in rat. J. Assist. Reprod. Genet. 30, 117–123. doi: 10.1007/s10815-012-9908-6
Premkumar, K. V., and Chaube, S. K. (2015). Nitric oxide signals postovulatory aging-induced abortive spontaneous egg activation in rats. Redox Rep. 20, 184–192. doi: 10.1179/1351000215Y.0000000003
Premkumar, K. V., and Chaube, S. K. (2016). Increased level of reactive oxygen species persuades postovulatory aging-mediated spontaneous egg activation in rat eggs cultured in vitro. In Vitro Cell. Dev. Biol. Anim. 52, 576–588. doi: 10.1007/s11626-016-0007-3
Premkumar, K. V., Prasad, S., Tiwari, M., Pandey, A. N., Gupta, A., Sharma, A., et al. (2020). Meiotic instability generates a pathological condition in mammalian ovum. Stem Cell Rev. Rep. doi: 10.1007/s12015-020-10072-z [Epub ahead of print].
Qiao, J., Wang, Z. B., Feng, H. L., Miao, Y. L., Wang, Q., Yu, Y., et al. (2014). The root of reduced fertility in aged women and possible therapentic options: current status and future perspects. Mol. Aspects Med. 38, 54–85. doi: 10.1016/j.mam.2013.06.001
Quiroz, A., Molina, P., Santander, N., Gallardo, D., Rigotti, A., and Busso, D. (2020). Ovarian cholesterol efflux: ATP-binding cassette transporters and follicular fluid HDL regulate cholesterol content in mouse oocytesdagger. Biol. Reprod. 102, 348–361. doi: 10.1093/biolre/ioz159
Ratto, M. H., Berland, M., Silva, M. E., and Adams, G. P. (2019). New insights of the role of beta-NGF in the ovulation mechanism of induced ovulating species. Reproduction 157, R199–R207. doi: 10.1530/REP-18-0305
Ross, P. J., Yabuuchi, A., and Cibelli, J. B. (2006). Oocyte spontaneous activation in different rat strains. Cloning Stem Cells 8, 275–282. doi: 10.1089/clo.2006.8.275
Sachs, A. R., Politch, J. A., Jackson, K. V., Racowsky, C., Hornstein, M. D., and Ginsburg, E. S. (2000). Factors associated with the formation of triploid zygotes after intracytoplasmic sperm injection. Fertil. Steril. 73, 1109–1114. doi: 10.1016/S0015-0282(00)00521-5
Santos, T. A., Dias, C., Henriques, P., Brito, R., Barbosa, A., Regateiro, F., et al. (2003). Cytogenetic analysis of spontaneously activated noninseminated oocytes and parthenogenetically activated failed fertilized human oocytes–implications for the use of primate parthenotes for stem cell production. J. Assist. Reprod. Genet. 20, 122–130. doi: 10.1023/A:1022630924236
Sha, Q. Q., Zhang, J., and Fan, H. Y. (2019). A story of birth and death: mRNA translation and clearance at the onset of maternal-to-zygotic transition in mammalsdagger. Biol. Reprod. 101, 579–590. doi: 10.1093/biolre/ioz012
Smith, G. D., and Takayama, S. (2017). Application of microfluidic technologies to human assisted reproduction. Mol. Hum. Reprod. 23, 257–268. doi: 10.1093/molehr/gaw076
Socolov, R., Ebner, T., Gorduza, V., Martiniuc, V., Angioni, S., and Socolov, D. (2015). Self-oocyte activation and parthenogenesis: an unusual outcome of a misconducted IVF cycle. Gynecol. Endocrinol. 31, 529–530. doi: 10.3109/09513590.2015.1062861
Speirs, S., and Kaufman, M. H. (1988). Effect of exogenous hormones on the ovulation of primary and secondary oocytes in LT/Sv strain mice. Gamete Res. 21, 179–184. doi: 10.1002/mrd.1120210208
Sterthaus, O., Skoczylas, E., De Geyter, C., Burki, K., and Ledermann, B. (2009). Evaluation of in vitro cultured rat oocytes, from different strains, by spindle morphology and maturation-promoting-factor activity combined with nuclear-transfer experiments. Cloning Stem Cells 11, 463–472. doi: 10.1089/clo.2009.0014
Stevens, L. C. (1980). Teratocarcinogenesis and spontaneous parthenogenesis in mice. Results Probl. Cell Differ. 11, 265–274. doi: 10.1007/978-3-540-38267-6_34
Stevens, L. C., and Varnum, D. S. (1974). The development of teratomas from parthenogenetically activated ovarian mouse eggs. Dev. Biol. 37, 369–380. doi: 10.1016/0012-1606(74)90155-9
Sultan, K. M., Munne, S., Palermo, G. D., Alikani, M., and Cohen, J. (1995). Chromosomal status of uni-pronuclear human zygotes following in-vitro fertilization and intracytoplasmic sperm injection. Hum. Reprod. 10, 132–136. doi: 10.1093/humrep/10.1.132
Sun, X. S., Yue, K. Z., Zhou, J. B., Chen, Q. X., and Tan, J. H. (2002). In vitro spontaneous parthenogenetic activation of golden hamster oocytes. Theriogenology 57, 845–851. doi: 10.1016/S0093-691X(01)00680-X
Sun, Y. C., Cheng, S. F., Sun, R., Zhao, Y., and Shen, W. (2014). Reconstitution of gametogenesis in vitro: meiosis is the biggest obstacle. J. Genet. Genomics 41, 87–95. doi: 10.1016/j.jgg.2013.12.008
Tan, X., Peng, A., Wang, Y. C., Wang, Y., and Sun, Q. Y. (2005). Participation of the ubiquitin-proteasome pathway in rat oocyte activation. Zygote 13, 87–95. doi: 10.1017/S0967199405003114
Tiwari, M., Gupta, A., Sharma, A., Prasad, S., Pandey, A. N., Yadav, P. K., et al. (2018). Role of mitogen activated protein kinase and maturation promoting factor during the achievement of meiotic competency in mammalian oocytes. J. Cell. Biochem. 119, 123–129. doi: 10.1002/jcb.26184
Tomioka, I., Mizutani, E., Yoshida, T., Sugawara, A., Inai, K., Sasada, H., et al. (2007). Spindle formation and microtubule organization during first division in reconstructed rat embryos produced by somatic cell nuclear transfer. J. Reprod. Dev. 53, 835–842. doi: 10.1262/jrd.18144
Van Blerkom, J., Davis, P. W., and Merriam, J. (1994). A retrospective analysis of unfertilized and presumed parthenogentically activated human oocytes demonstrates a high frequency of sperm penetration. Hum. Reprod. 9, 2381–2388. doi: 10.1093/oxfordjournals.humrep.a138456
Van Blerkom, J., Henry, G., and Porreco, R. (1984). Preimplantation human embryonic development from polypronuclear eggs after in vitro fertilization. Fertil. Steril. 41, 686–696. doi: 10.1016/S0015-0282(16)47833-7
Van de Velde, H., De Vos, A., Joris, H., Nagy, Z. P., and Van Steirteghem, A. C. (1998). Effect of timing of oocyte denudation and micro-injection on survival, fertilization and embryo quality after intracytoplasmic sperm injection. Hum. Reprod. 13, 3160–3164. doi: 10.1093/humrep/13.11.3160
Verlhac, M. H., Kubiak, J. Z., Weber, M., Geraud, G., Colledge, W. H., Evans, M. J., et al. (1996). Mos is required for MAP kinase activation and is involved in microtubule organization during meiotic maturation in the mouse. Development 122, 815–822.
Wu, D., and Dean, J. (2016). BTG4, a maternal mRNA cleaner. J. Mol. Cell Biol. 8, 369–370. doi: 10.1093/jmcb/mjw031
Xu, Z., Abbott, A., Kopf, G. S., Schultz, R. M., and Ducibella, T. (1997). Spontaneous activation of ovulated mouse eggs: time-dependent effects on M-phase exit, cortical granule exocytosis, maternal messenger ribonucleic acid recruitment, and inositol 1,4,5-trisphosphate sensitivity. Biol. Reprod. 57, 743–750. doi: 10.1095/biolreprod57.4.743
Ya, R., and Downs, S. M. (2013). Suppression of chemically induced and spontaneous mouse oocyte activation by AMP-activated protein kinase. Biol. Reprod. 88:70. doi: 10.1095/biolreprod.112.106120
Ye, Y., Li, N., Yan, X., Wu, R., Zhou, W., Cheng, L., et al. (2020). Genetic analysis of embryo in a human case of spontaneous oocyte activation: a case report. Gynecol. Endocrinol. 36, 294–296. doi: 10.1080/09513590.2019.1687671
Yesilaltay, A., Dokshin, G. A., Busso, D., Wang, L., Galiani, D., Chavarria, T., et al. (2014). Excess cholesterol induces mouse egg activation and may cause female infertility. Proc. Natl. Acad. Sci. U.S.A. 111, E4972–E4980. doi: 10.1073/pnas.1418954111
Yin, S., Sun, X. F., Schatten, H., and Sun, Q. Y. (2008). Molecular insights into mechanisms regulating faithful chromosome separation in female meiosis. Cell Cycle 7, 2997–3005. doi: 10.4161/cc.7.19.6809
Yoo, J. G., and Smith, L. C. (2007). Extracellular calcium induces activation of Ca(2+)/calmodulin-dependent protein kinase II and mediates spontaneous activation in rat oocytes. Biochem. Biophys. Res. Commun. 359, 854–859. doi: 10.1016/j.bbrc.2007.05.181
Yu, C., Ji, S. Y., Sha, Q. Q., Dang, Y., Zhou, J. J., Zhang, Y. L., et al. (2016). BTG4 is a meiotic cell cycle-coupled maternal-zygotic-transition licensing factor in oocytes. Nat. Struct. Mol. Biol. 23, 387–394. doi: 10.1038/nsmb.3204
Zernicka-Goetz, M. (1991). Spontaneous and induced activation of rat oocytes. Mol. Reprod. Dev. 28, 169–176. doi: 10.1002/mrd.1080280210
Zernicka-Goetz, M., Kubiak, J. Z., Antony, C., and Maro, B. (1993). Cytoskeletal organization of rat oocytes during metaphase II arrest and following abortive activation: a study by confocal laser scanning microscopy. Mol. Reprod. Dev. 35, 165–175. doi: 10.1002/mrd.1080350210
Zhang, Y. L., Liu, X. M., Ji, S. Y., Sha, Q. Q., Zhang, J., and Fan, H. Y. (2015). ERK1/2 activities are dispensable for oocyte growth but are required for meiotic maturation and pronuclear formation in mouse. J. Genet. Genomics 42, 477–485. doi: 10.1016/j.jgg.2015.07.004
Keywords: meiosis, cell cycle, assisted reproduction, ovarian teratoma, multiple pronuclei, aneuploidy, metaphase arrest, triploid pronuclei
Citation: Cui W (2021) Oocyte Spontaneous Activation: An Overlooked Cellular Event That Impairs Female Fertility in Mammals. Front. Cell Dev. Biol. 9:648057. doi: 10.3389/fcell.2021.648057
Received: 31 December 2020; Accepted: 08 February 2021;
Published: 08 March 2021.
Edited by:
Shao-Chen Sun, Nanjing Agricultural University, ChinaReviewed by:
Hai-Long Wang, Xiamen University, ChinaJunya Ito, Azabu University, Japan
Chuan Zhou, University of California, Davis, United States
Copyright © 2021 Cui. This is an open-access article distributed under the terms of the Creative Commons Attribution License (CC BY). The use, distribution or reproduction in other forums is permitted, provided the original author(s) and the copyright owner(s) are credited and that the original publication in this journal is cited, in accordance with accepted academic practice. No use, distribution or reproduction is permitted which does not comply with these terms.
*Correspondence: Wei Cui, d2N1aUB1bWFzcy5lZHU=