- 1Department of Clinical Microbiology, Rigshospitalet, Copenhagen University Hospital, Copenhagen, Denmark
- 2Department of Cardiology, Rigshospitalet, Copenhagen University Hospital, Copenhagen, Denmark
- 3Department of Cardiology, Herlev and Gentofte Hospital, University of Copenhagen, Herlev, Denmark
- 4Department of Emergency Medicine, Herlev and Gentofte Hospital, University of Copenhagen, Herlev, Denmark
- 5Costerton Biofilm Center, Department of Immunology and Microbiology, University of Copenhagen, Copenhagen, Denmark
Infective endocarditis (IE) is a life-threatening infective disease with increasing incidence worldwide. From early on, in the antibiotic era, it was recognized that high-dose and long-term antibiotic therapy was correlated to improved outcome. In addition, for several of the common microbial IE etiologies, the use of combination antibiotic therapy further improves outcome. IE vegetations on affected heart valves from patients and experimental animal models resemble biofilm infections. Besides the recalcitrant nature of IE, the microorganisms often present in an aggregated form, and gradients of bacterial activity in the vegetations can be observed. Even after appropriate antibiotic therapy, such microbial formations can often be identified in surgically removed, infected heart valves. Therefore, persistent or recurrent cases of IE, after apparent initial infection control, can be related to biofilm formation in the heart valve vegetations. On this background, the present review will describe potentially novel non-antibiotic, antimicrobial approaches in IE, with special focus on anti-thrombotic strategies and hyperbaric oxygen therapy targeting the biofilm formation of the infected heart valves caused by Staphylococcus aureus. The format is translational from preclinical models to actual clinical treatment strategies.
Introduction
Infective endocarditis (IE) is defined as an infection of the inner surface of the heart, the endocardium, most prevalent on the heart valves, or on implanted cardiac devices (Moreillon and Que, 2004). In most cases, left-sided IE is considered a more complicated and severe infection compared with patients affected by right-sided IE. Although IE is relatively rare, it is increasing in incidence based on quality reports mainly from high income countries (Holland et al., 2017; Jensen et al., 2020). IE is one of the most serious infections with 100% mortality if untreated and a high rate of complications. Under modern treatment, especially if infectious control is not obtained and septic shock develops, a mortality rate of 20–25% (Murdoch et al., 2009) and a 1-year mortality rate of up to 40% are reported (Alestig et al., 2000; Cabell et al., 2002; Que and Moreillon, 2011). The highly critical appearance of the infections indicates a need for improvement in diagnosis and treatment strategies (Fowler et al., 2005; Que and Moreillon, 2011; Werdan et al., 2014). Rheumatic heart disease and IE in younger persons are still dominant features in low-income countries (Werdan et al., 2014). In contrast, an increase in the population of elderly people is reported from most high-income countries, resulting in further predisposed persons for IE (Werdan et al., 2014). Modern medical treatment also seems to predispose for IE, not the least cardiac implants, hemodialysis, and additional reasons for intravascular catheters (i.e., of cancer and short bowel disease patients), including other patients in risk of healthcare-associated infections due to invasive procedures (Tong et al., 2015). Especially cardiac implants together with improved diagnosis and awareness of IE seem to drive this development. Importantly, an increasing age of the affected population also leads to challenges in therapy due to reduced organ functions, increased susceptibility to potential antibiotic toxicity, and comorbidities.
In geographical areas with quality data, often high-income countries, Staphylococcus aureus on native heart valves and coagulase negative staphylococci (CoNS) on implants, is referred to as the dominant IE etiology, closely followed by viridans streptococci (i.e., Streptococcus mutans, S. sanguis, S. sanguinis, S. mitis, S. salivarius, and S. bovis) (Lalani et al., 2006; Murdoch et al., 2009; Østergaard et al., 2019). The third most common bacterial etiology causing IE on native heart valves is Enterococcus faecalis, especially in patients above 70 years of age (Hoen and Duval, 2013; Østergaard et al., 2019). These three groups of Gram-positive bacteria constitute approximately 80% of all microbial etiologies of IE (Que and Moreillon, 2011; Østergaard et al., 2019). Substantially less frequent are species of the HACEK group [Aggregatibacter (formerly Haemophilus) aphrophilus/paraaphrophis, Aggregatibacter actinomycetemcomitans, Cardiobacterium hominis, Eikenella corrodens, and Kingella spp.] identified as the fourth most common cause of IE on native heart valves (Que and Moreillon, 2011). Indeed, the intact endocardium, including the heart valves, is resistant to microbial colonization (Werdan et al., 2014). However, if micro-lesions of the endocardium appear, a healing process involving fibrin formation and thrombocyte aggregation is initiated with the formation of non-bacterial thrombotic endocarditis (NBTE) (Figure 1; Werdan et al., 2014). The healing process of the endothelium is estimated to last for 2 weeks, and in this period, the breakage of the endothelial barrier is highly susceptible to bacterial colonization (Werdan et al., 2014). The bacteria will adhere to the damaged heart valve by means of surface adhesins, consisting of proteins and polysaccharides (reviewed by Que and Moreillon, 2011). Such colonization can occur during temporary bacteremia of less than 10 min duration due to activities potentially resulting in minor lesions in the oral cavity, like tooth brushing, additional mucosal surfaces lesions, or from the skin microbiota (Hall et al., 1999; Werdan et al., 2014). An established infection subsequently induces an inflammatory response, with recruitment of polymorphonuclear neutrophils (PMNs), resulting in further damages of the heart valve, thus, worsening the process (Werdan et al., 2014).
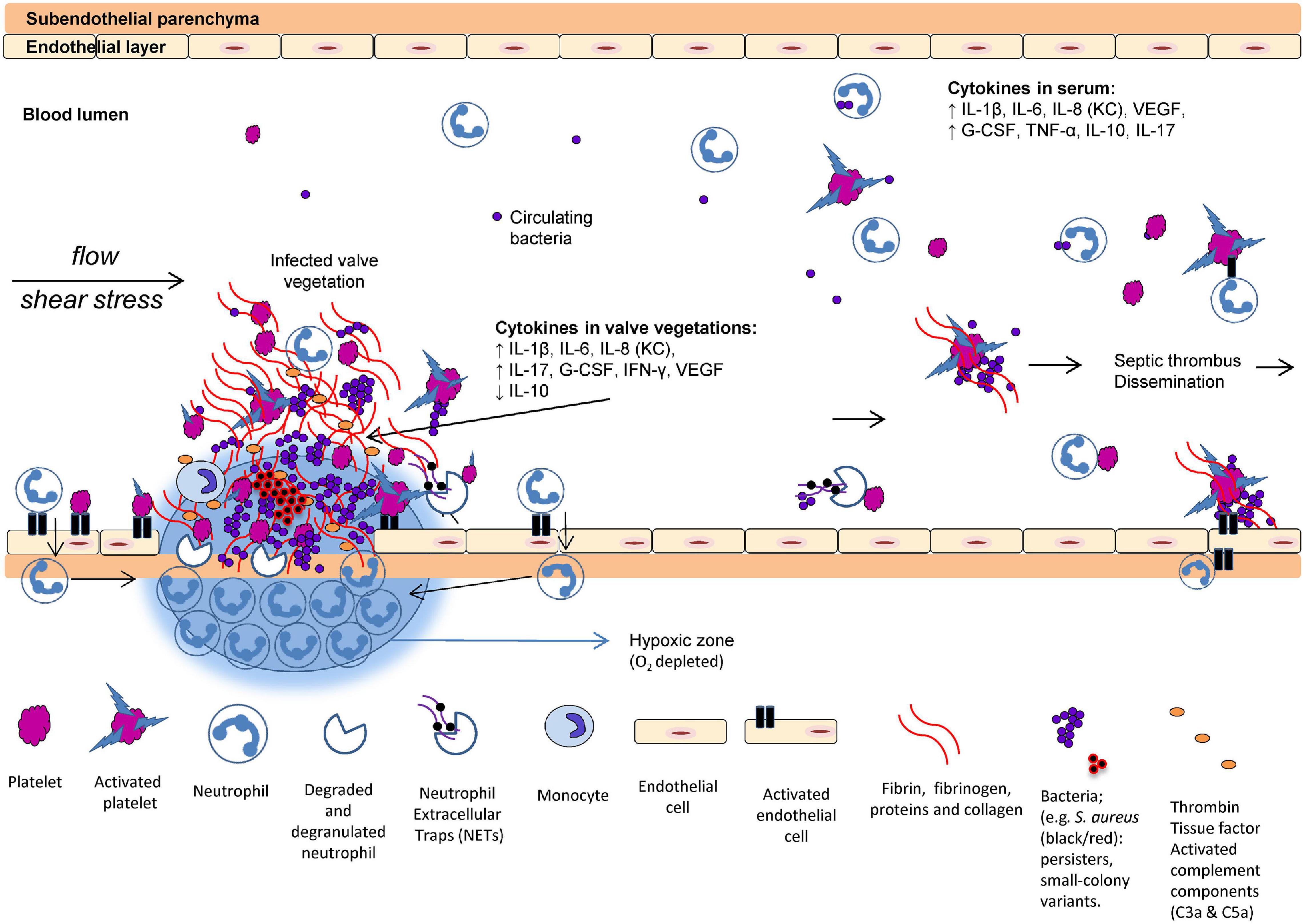
Figure 1. Proposed scenario of interaction between the host and pathogens in infective endocarditis (IE). The formation of valve vegetations is induced by the damaged and infected endothelium. Platelets aggregate to the injured endothelial cells followed by accumulation of innate immune cells and upregulation of tissue factor, fibrinogen, fibrin, and cytokines. O2 consumption in the valve vegetations (biofilm) and by the activated neutrophils may prevent appropriate O2 in the tissue. Increased IL-8, interaction between activated platelets and neutrophils trigger the formation of neutrophil extracellular traps (NETs). Many bacteria exhibit virulence mechanisms to survive the NET formation. Deep-seated bacteria are less metabolically active, consequently reducing the efficacy of oxygen-dependent antibiotics. In the local tissue of the valve vegetations, key inflammatory markers of progression are elevated, for example, G-CSF, IL-1β, IL-6, IL-8 (analog to KC in rodents), IL-17, IFN-γ, and VEGF (Moser et al., 2017) (with permission from the editor of APMIS and authors).
The following biofilm IE review is divided into sections on Early host–pathogen interactions, Clinical biofilms, IE biofilm characteristics and appearance, Antibiotic tolerance, Small colony variants, In vitro and in vivo model systems, and Potential novel IE treatments.
Early Host–Pathogen Interactions in Infective Endocarditis
The first essential step in the development of IE is the interaction of the pathogen and the activated endothelial layer and platelets (Figure 1). Several adhesive molecules are expressed on the cell wall of Gram-positive bacteria and facilitate the bridging and adherence to the endothelial cells, platelets, and the extracellular matrix [fibrin(ogen) and collagen]. These adhesins can either be covalently bound to the cell wall [microbial surface components reorganizing adhesive matrix aolecules (MSCRAMMs)] (Foster and Höök, 1998) or secreted [secretable expanded repertoire adhesive molecules (SERAMs)] (Chavakis et al., 2005). It is well known that these adhesins play an important role in the development and pathogenesis of IE. Most Gram-positive bacteria can bind directly to fibrin(ogen) (Que et al., 2001, 2005; Entenza et al., 2005) as a bridging molecule (Cheung et al., 1991), fibronectin (Que et al., 2005), collagen (Hienz et al., 1996), and integrins of platelets (Siboo et al., 2001; Niemann et al., 2004; Loughman et al., 2005; Veloso et al., 2013) and endothelial cells (Edwards et al., 2012; Pappelbaum et al., 2013; Liesenborghs et al., 2016). These important host–pathogen interactions are thoroughly reviewed elsewhere (Moreillon et al., 2002; Moreillon and Que, 2004; Que and Moreillon, 2011; Werdan et al., 2014).
Platelets are short-lived cells—they die after approximately 10 days—and is the smallest of the formed blood elements. Platelets were generally thought to be important in hemostatic functions, but are also crucial innate effector cells cross-talking with other innate immune cells and enhancing their functions and recruitment (Yeaman, 1997; Zarbock et al., 2007; Maugeri et al., 2014). Platelets are circulating sensor cells and respond to the activated or damaged endothelium by accumulation of facilitating tissue factor and cytokine production as an initial inflammatory response. Tissue factors are essential for the development of thrombi (von Brühl et al., 2012) and stimulate the thrombin generation, which facilitates the fibrin formation (Panizzi et al., 2011). Activated platelets also release procoagulant molecules facilitating additional platelet aggregation (Rivera et al., 2009). However, these essential and protective properties of the hemostatic system are dysregulated in severe infections and further enhanced by virulent Gram-positive bacteria colonizing the valve endothelium facilitating hyperactivation of complex pathways, resulting in vegetational growth and biofilm formation in cardiac valves (Figure 2).
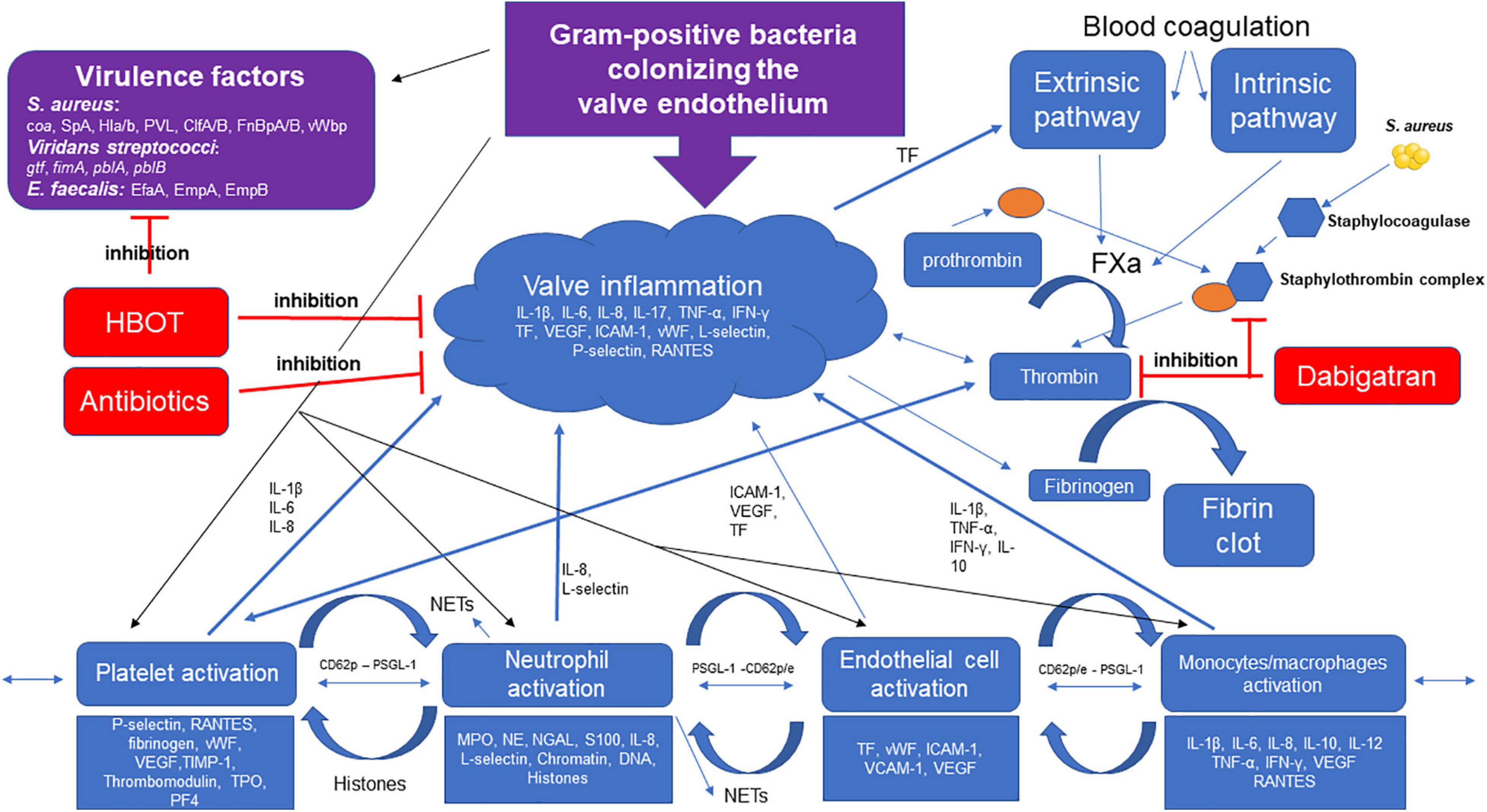
Figure 2. Gram-positive bacteria colonizing the valve endothelium trigger multiple pathways of inflammation. S. aureus, viridans streptococci, and E. faecalis express important adhesion surface proteins/glucans, i.e., clumping factor A and B (ClfA/B), fibrinogen binding protein A and B (FnBpA/B), Coagulase (coa) von willebrand binding protein (vWbp), and glucosyltransferase (gtf), binding to the activated endothelial cell and extracellular matrix proteins in the vegetation. Host cells (platelets, neutrophils, and monocytes/macrophages) are recruited to the activated endothelium promoted by the pathogen’s increasing production of inflammatory markers. Tissue factor (TF) stimulates the extrinsic pathway increasing thrombin generation converting fibrinogen to fibrin inducing clot formation. Dabigatran is a direct thrombin inhibitor, limiting fibrin formation, HBOT potentiates the effect of antibiotics by decreasing inflammation and decreasing virulence of S. aureus. S. aureus and thrombin are potent activators of platelets, facilitating recruitment of neutrophil-binding to the surface of the vegetation, stimulating degranulation and neutrophil extracellular traps (NETs) formation, and further enhancing the clot and fibrin formation. The activated endothelial cells also stimulate neutrophil-adhesion and NET release. S. aureus produces Staphylocoagulase-forming Staphylothrombin complexes with prothrombin facilitating fibrin formation, dabigatran inhibits the formation of Staphylothrombin complex and fibrin. Abbreviations: S. aureus, Staphylococcus aureus; Enterococcus faecalis, E. faecalis; SpA, Staphylococcus aureus protein A; Hl,a/b, alpha and beta hemolysis; PVL, Panton–Valentine leucocidin; E. faecalis antigen A, EfA; Interleukin-1beta (-1β, 6, 8, 10, 17); tumor necrosis factor alpha (TNF-α); interferon gamma (IFN-γ); vascular endothelial growth factor, VEGF; tissue factor, TF; intercellular adhesion molecule 1 (ICAM-1); vascular cell adhesion molecule 1 VCAM-1; von Willebrand factor, vWF; Thrombopoietin, TPO; platelet factor 4, PF4; myeloperoxidase, MPO; neutrophil elastase, NE; neutrophil gelatinase-associated lipocalin, NGAL; P-selectin high-affinity ligand, PSGL-1.
Clinical Biofilms
Microbial biofilms can be defined as bacterial aggregates embedded in a self-produced extracellular polymeric matrix constituted of extracellular polysaccharides, proteins, extracellular DNA (eDNA), LPS, and other bacterial products and occasionally also host factors and elements from the surroundings (Høiby et al., 2015). Biofilm research is dominated by thorough investigations on only a limited number of pathogens and clinical syndromes. Therefore, precautions should be taken when transferring results of biofilm infections in general to a specific syndrome, even though there are several common biofilm features. The clinical consequences of biofilm infections are frequent antibiotic treatment failure and relapse of infection when antibiotic therapy is terminated, which cannot be attributed to development of antibiotic resistance per se (Høiby et al., 2015). The recalcitrant nature of the biofilm infections has been supported by observations using in vitro and in vivo animal model systems revealing tolerance of the biofilm-associated microorganisms to both, antimicrobials and the immune system (Høiby et al., 2015). Biofilm growth is probably the preferred lifestyle of extracellular bacteria and yeast in nature and can be considered an ancient survival mechanism (Allen, 2016; Moser et al., 2017). The aggregating microorganisms in the matrix also allow for interbacterial communication, known as quorum sensing—a chemical and bacterial density-dependent mutual impact of the microorganisms on the expression of virulence factors in the microenvironment of the biofilms (Høiby et al., 2010).
Biofilm Formation in Infective Endocarditis
Whereas it seems well-accepted that device-associated IE involves microbial biofilm formation on the implanted foreign body, biofilm involvement in IE on native valves is more debatable (Marrie et al., 1982). From early observations of penicillin therapy against IE, it became evident that increased and frequent dosing for long treatment periods were necessary for successful outcome, compared with acute infections, indicating a bacterial mode of growth that resulted in a certain tolerance toward the antibiotic provided (Degraff, 1941; Bloomfield et al., 1945; Christie, 1946; Tan et al., 1971). When the bacteria were cultured from the blood or the heart valves at relapse, they still appeared fully penicillin susceptible, when tested in vitro, and a second treatment with penicillin could be successful (Christie, 1946; Tan et al., 1971). Relatively early on in the antibiotic era of IE treatments, a synergistic effect of additional streptomycin (and later newer aminoglycosides) to the penicillin therapy against enterococci IE was observed (Hunter, 1947). This approach successfully expanded to the treatment of less penicillin susceptible streptococci. It was also revealed that the synergistic effect was due to inhibition of the cell wall synthesis, allowing intra-bacterial entry of the aminoglycosides (Moellering and Weinberg, 1971).
The recalcitrant nature of IE which necessitates involvement of heart valve surgery in almost 50% of all IE cases, strongly supports the biofilm behavior of IE (Elgharably et al., 2016, 2018). Although, a proportion of surgery in IE is due to valve damage, a substantial fraction of all heart valve surgery during IE is due to insufficient antibiotic effect and lack of infection control (Elgharably et al., 2016, 2018).
Quantification of the bacterial level during IE has revealed a relatively constant level of bacteria in the peripheral blood stream during the natural course of IE (Werner et al., 1967). Performing consecutive blood cultures of patients with streptococcal or staphylococcal IE revealed a substantial varied interpatient level of quantitative bacteriology from less than 10 CFU/ml to above 300 CFU/ml peripheral blood, with a median around 30 CFU/ml (approximately a total of 150,000 bacteria in a person with 5 L of blood) (Werner et al., 1967). However, the individual patient showed a steady level of bacteremia over 2–9 days of observation (Werner et al., 1967). From the onset of appropriate antibiotic treatment, it is reported that the planktonic bacteria of the peripheral blood stream are normally cleared within 48 h, paralleled by a reduction in the valve bacteriology (Bloomfield et al., 1945). However, the free-floating bacteria in the blood stream only constitute an extreme minority of the total IE bacteriology, since the number of CFUs in heart valve vegetations has been reported to be as high as 109–1011 CFU per gram tissue (Bennett et al., 2014). This means that the planktonic peripheral blood bacteria at an average only constitute 0.15‰ of the total bacteriology of an IE patient, if we estimate the weight of a vegetation to 0.1 g, and even 0.015‰ in case of a more realistic 1 g vegetation. The majority of the microorganisms are not immediately available for investigations like antibiotic susceptibility testing.
The pronounced chronicity of biofilm infections results in a special immunological dynamic with ongoing activation of both the innate and the adaptive immune responses (Jensen et al., 2010; Moser et al., 2017). This has been investigated thoroughly in patients with the inherited disease cystic fibrosis (CF), suffering from chronic Pseudomonas aeruginosa biofilm lung infections (Høiby et al., 1977; Bjarnsholt et al., 2009). Instead of clearing the chronic lung infection, activation of the adaptive immune response contributes to the pathology by means of forming immune complexes, inducing PMN-dominated inflammation and activating the complement system resulting in collateral tissue damage (Høiby et al., 1977; Jensen et al., 2010; Moser et al., 2017). A parallel can be drawn to patients with IE. Indeed, a specific adaptive humoral immune response has been described in subacute IE by means of precipitating antibodies (Kjerulf et al., 1993). Several immunological phenomena during IE have been recognized, including erythema nodosum, glomerulonephritis, and vasculitis, like Osler’s nodes. During IE, hyperglobulinemia has been described (Laxdal et al., 1968). The presence of IgM anti-IgG rheumatoid factor has been reported in half the patients if IE lasts for more than 6 weeks (Sheagren et al., 1976). Additionally, antinuclear antibodies have also been reported during IE (Bacon et al., 1974). How these auto-antibodies contribute to pathology has not been clarified. Circulating immune complexes have been observed in almost all IE patients (Bayer et al., 1976; Holland et al., 2017). Circulating immune complexes are believed to be involved in the peripheral manifestations of IE, like Osler’s nodes and Roth spots resembling Arthus reaction. Whether the humoral response directly contributes to the pathology is not clarified, although antibodies probably both bind and neutralize bacterial virulence factors beneficially, but also precipitate inflammation and thereby contribute to the pathology. However, therapeutic failure has been demonstrated to correlate with a rise in circulating immune complexes, similar to CF (Kauffmann et al., 1981).
Infective Endocarditis and Biofilms—Valve Vegetation Appearance
Histopathological evaluations of surgically removed heart valves from IE patients, as well as of heart valves from experimental IE animal studies, has shown that the bacteria are organized in biofilm characteristic microcolony-aggregates within the vegetations (Allen, 1939, 1941; Angrist et al., 1960). In a study on the ultrastructure of six aortic valves, myriads of bacteria were observed in areas where the surface of the vegetation was broken (Marrie et al., 1987). Transmission electron microscopy revealed that the bacteria were embedded in an electron dense matrix, even with negative culture results, and cell wall and cell division appeared abnormal (Marrie et al., 1987). Masses of bacteria seen in the vegetations, usually clustered in discrete colonies, were reported many years ago by Allen and colleagues (Allen, 1939). In addition, Hobby et al. (1942), based on histopathological examination of vegetations, suggested that a proportion of the bacteria in the vegetation, though alive and capable of multiplication, are in a resting phase, and hence not subject to rapid killing when exposed to penicillin. Based on observations made in a streptococcal IE rabbit model, Durack and Beeson (1972b) reported gradients of bacterial activity in vegetations, since surface colonies incorporated a Tritium-labeled L-alanin, while deeply seated colonies remained unlabeled, and furthermore, they described that the colonies were surrounded by an amorphous debris (Table 1; Durack and Beeson, 1972b). In a large retrospective study using Gram staining, culture, and histopathology on 506 heart valves removed from patients with IE, a microscopic positive Gram staining of visual bacteria was reported in the majority of the samples (Morris et al., 2003). Even after completed antibiotic treatment, more than 60% of the valves were microscopy positive, increasing to 88% if less than 25% of the treatment was completed (Morris et al., 2003). However, although the majority of the removed heart valves were microscopy positive by means of Gram stain, only 5% were culture positive at completion of antibiotic therapy (Morris et al., 2003). The findings were supported by revealing signs of acute inflammation in approximately one-third of the samples after completion of antibiotic therapy (Morris et al., 2003). By means of fluorescence in situ hybridization (FISH) with bacteria-specific probes, Mallmann et al. (2010) revealed causative pathogens in heart valves from patients with culture-negative IE. The authors demonstrated large colonies of streptococci, organized in typical dense bacterial clusters (Mallmann et al., 2010). Additional IE pathogens have also been demonstrated using the FISH technique (Frickmann et al., 2017). These early microscopic descriptions resemble modern definitions and descriptions of biofilms, in accordance with our own clinical and preclinical observations (Table 1; Høiby et al., 2015).
Infective Endocarditis and Biofilms—Antibiotic Tolerance
It is known for investigated bacteria and fungi that the MIC—or rather, the minimum biofilm inhibitory concentration (MBIC)—is increased 10- to 1,000-fold for microorganisms in the biofilm mode compared with the exact same microorganism growing planktonically (Anwar et al., 1990; Moskowitz et al., 2004; Hengzhuang et al., 2011). This difference is accentuated if the minimum bactericidal concentration (MBC) is compared with the minimum biofilm eradication concentration (MBEC) (Hengzhuang et al., 2011). In the latter case, it is almost impossible to obtain sufficient antibiotic levels by systemic administration of antibiotics (Hengzhuang et al., 2011). The observed phenomenon is due to the biofilm mode of growth and not mutational antibiotic resistance per se (Fisher et al., 2017). Recently, a study investigating bacterial response to biofilm growing bacteria from a smaller number of IE patients demonstrated how the normal antibiotic susceptibility testing differed from the susceptibility of the bacteria growing as biofilms in an in vitro biofilm setup (Di Domenico et al., 2019). The explanation for this biofilm antibiotic tolerance phenomenon is multifactorial (Ciofu et al., 2017). A key reason is believed to be the significantly changed physiology of the biofilms, with gradients from the biofilm surface to the center (Jensen et al., 2017). Inside the biofilm there can be limited access to nutrients and not the least, oxygen (Zhang et al., 2013; Jensen et al., 2017). Indeed, hypoxic conditions just 50 μm inside biofilms have been described (Zhang et al., 2013; Jensen et al., 2017). The result is an adaptation of the bacterial metabolism to the biofilm environment, leading to a significantly reduced activity and prolonged generation time (Kragh et al., 2016). These observations are in concordance with the described microscopic observations of heart valves from patients with IE and from animal models (Allen, 1939, 1941; Angrist and Oka, 1963; Durack and Beeson, 1972a, b; Durack et al., 1973; Durack, 1974). Prolonged division times result in reduced activity of antibiotics, which are dependent on division of the bacterial cells (Tuomanen et al., 1986). The biofilm-generated hypoxia may be adding to the heart valve disease progression as indicated in a study on effect of hypoxia on pathological changes of porcine aortic and mitral valves using an oxygen diffusion chamber (Sapp et al., 2016). Interestingly, vascular endothelial growth factor (VEGF) receptor 2 seemed to be involved in this effect, and we have shown that HBOT was able to reduce VEGF expression levels in the infected aortic valves using a rat model of left-sided S. aureus IE (Lerche et al., 2017).
A further consequence of the limited oxygen below the biofilm surface is a reduction in the oxygen-dependent effect of bactericidal antibiotic drugs (Kohanski et al., 2007). Antibiotic exposure to bacteria results in metabolic changes of the bacteria as a stress response, resulting in intra-bacterial accumulation of toxic oxygen radicals, which will cause damage to the DNA/RNA, proteins, cell membranes, and other essential macro-molecules and subsequentially death of the bacteria (Dwyer et al., 2015). This mechanism adds to the target-specific effect of the bactericidal antibiotics, including interactions with cell wall synthesis, DNA replication, or protein synthesis (Jensen et al., 2014). This important oxygen-dependent potentiation of bactericidal antibiotics can be a target to augment the antibiotic efficacy in IE by supplying excessive oxygen under pressure (more details in the Adjunctive treatment with dabigatran section).
The efficiency of antibiotic penetration into deep-seeded biofilms and into bacterial vegetations in IE is a topic of extensive research. It may be argued that the antibiotic penetration is lowered by the limited direct blood supply of the valves, the dense valve vegetations, mainly constructed of fibrin and platelets, as well as the bacterial aggregates with their polysaccharide matrix (Elgharably et al., 2018). However, for the heart valve vegetations, antibiotic penetration is probably more refined and individual, and due to the limited access to samples, not thoroughly examined. However, using autoradiographic distribution techniques with labeled antibiotic compounds and experimental animal models, three different diffusion patterns have been described and reviewed by Cremieux and Carbon (1992). The first pattern was observed for teicoplanin with a high concentration of the antibiotic in the periphery of the vegetation, but a limited penetration into the vegetation resulting in decreased anti-bacterial activity (Cremieux and Carbon, 1992). The second pattern was observed for ceftriaxone, where a progressive diffusion, but also a substantial gradient from the vegetation surface to the center was reported (Cremieux and Carbon, 1992). The same pattern, although to a lesser degree, was observed for penicillin. In contrast, a third pattern of diffusion was observed for some fluoroquinolones and tobramycin which provided a homogenous distribution throughout the vegetation (Cremieux and Carbon, 1992). In support, aminoglycosides have, in an experimental study by means of autoradiography, been shown to distribute homogeneously inside the vegetations (Table 1; Cremieux et al., 1989).
The limited antibiotic penetration into the bacterial vegetation in IE can, at least partially, be translated to other well-known biofilm infections characterized by bacterial aggregates surrounded by a polysaccharide matrix. It has been suggested and shown that bacterial biofilms can be considered as independent pharmacokinetic microcompartments (Zimmerli and Moser, 2012; Cao et al., 2015). It was revealed that tobramycin was bound to substances of the biofilm matrix and, thus, not free for bacterial killing (Cao et al., 2015, 2016). The substantial matrix binding resulted in a delayed diffusion of tobramycin following a power law, instead of a First-order kinetic (Cao et al., 2016). Recently, this phenomenon was also shown in vivo (Christophersen et al., 2020). Finally, it has been shown that the pharmacokinetics and pharmacodynamics of antibiotics in biofilms follow the same rules (time-, concentration-, or dose-dependent killing) as for planktonically growing bacteria, although significantly higher antibiotic concentrations are needed (Hengzhuang et al., 2011).
The density of the bacterial aggregates and the exceptionally high number of bacteria inside the vegetations also lead to an inoculum effect of the bacteria against different antibiotics (Song et al., 2018). The inoculum effect describes the phenomenon that a high number of bacteria (high inoculum) leads to an increase in MICs, to values even above the breakpoint. This has been reported for β-lactam antibiotics like piperacillin/tazobactam, ampicillin/sulbactam, and, to some degree, oxacillin, whereas cephalosporins and meropenem were less influenced by the inoculum effect (Song et al., 2019). The inoculum effect is likely to also contribute to the reduced antibiotic effect in case of IE.
Infective Endocarditis and Biofilms—Small Colony Variants
Chronic antibiotic-refractory infections can lead to the appearance of small colony variants (SCV) (Proctor et al., 2006). SCVs obtain a survival advantage by slow and/or intracellular replication and, thereby, reduced susceptibility to antibiotics (Vulin et al., 2018). As the SCVs phenotype is infrequent and most of the times not a consequence of mutations, and therefore not stable, SCVs are often missed in the standard clinical laboratory testing unless there is specific focus on the variants (Table 1; Tuchscherr et al., 2011). For S. aureus and IE, SCVs have been intensively studied and reviewed elsewhere, but are characterized as non-hemolytic and non-pigmented colony morphologies 5–10 times smaller than the most common colony morphologies, due to auxotrophy (Proctor et al., 2006; Garcia et al., 2013). On animal experiments, S. aureus SCVs appeared from the heart valves of rats with aortic IE treated with tobramycin (Lerche et al., 2017). Interestingly, hyperbaric oxygen therapy (HBOT) significantly increased the appearance of SCVs (Lerche et al., 2017). This could be explained by the increased oxidative stress from HBOT and aminoglycoside treatment, highlighting the need to treat IE with antibiotics, which do not have an oxygen-dependent killing mechanism (i.e., rifampicin and linezolid) to prevent SCVs within the biofilm vegetations. For E. faecalis, SCVs have only been sparsely and casuistically described, although in relation to IE (Wellinghausen et al., 2009; Ogihara et al., 2016). However, for E. faecalis SCV, reduced sugar fermentation, changed ultrastructural morphology, and growth behavior, as well as changed antimicrobial susceptibility were observed (Wellinghausen et al., 2009). Especially, a reduced aminoglycoside susceptibility was reported (Wellinghausen et al., 2009; Ogihara et al., 2016). Our own observation is that E. faecalis SCVs are only identified from surgically removed heart valves of IE patients, and not from the corresponding blood cultures, even though we have had focus on this issue. For streptococci, SCVs have only been sparsely investigated and almost exclusively for S. pneumoniae.
Model Systems of Infective Endocarditis
Experimental representative model systems of IE are essential to identify potentially novel treatment strategies. The recently published POET study is a rare exception of a large randomized controlled trial within IE (Iversen et al., 2019). The conduction of clinical trials in IE is especially challenging because of factors like co-morbidities, different microbiological etiologies, difficulties and delays of diagnosis, the admission of the patients to many different hospitals, as well as surgery in almost half the patients (Bundgaard et al., 2019; Iversen et al., 2019). Therefore, we are dependent on clinically relevant animal models to provide solid preclinical testing of potential improvements for IE. By regarding IE as a biofilm-related infection, new in vitro model systems could be developed taking these important aspects into consideration. The benefit of such design could improve screening of new treatment options before heading to preclinical studies and helps in reducing the substantial gap from in vitro to in vivo and lastly into clinical studies.
In vitro and ex vivo Model Systems of Infective Endocarditis
Even though biofilms can have a heterogenic and complex appearance, they share certain characteristics, such as the extracellular polymeric matrix, high cell densities, and decreased susceptibility toward antimicrobial treatments. It is therefore possible to mimic biofilm environments in vitro to a certain degree, to improve our understanding of biofilms, and gain insight into possible new treatment options. Multiple different model systems have been developed to study different angles of biofilms. For initial screening or high throughput experiments, in vitro models can be exceptionally helpful in identifying lead candidates for novel treatment strategies to be tested in more clinically relevant model systems. However, the majority of those in vitro model systems are not specifically orientated toward IE. Different biofilm models with their advantages and limitations have been described intensively elsewhere (Coenye and Nelis, 2010; Lebeaux et al., 2013; Brackman and Coenye, 2015; Roberts et al., 2015; Maske et al., 2017) and will not be included in the present review. They cover both various biofilm physiological, metabolic, genetical behavior, and appearance, as well as methods for preliminary antibiotic susceptibility testing. Considering that the present review is especially focusing on native valve IE, it is also a drawback that most of the biofilm in vitro models are based on material adherent biofilms. However, a few specific in vitro IE models and an ex vivo model have been published (Table 2).
One of the first in vitro model of IE was performed from ex vivo human and canine aortic valves by Gould et al. (1975) to investigate the ability of 14 strains of both Gram-positive cocci and Gram-negative rods to adhere to heart valves and confirmed the superiority of the most frequent IE Gram-positive cocci to adhere to the endothelium compared with the Gram-negative rods. This was followed by an investigation of adherence of Streptococcus sanguis to fibrin and platelets in an in vitro assay system showing that dextran-producing streptococci increased the ability to adhere to fibrin–platelet matrix. This observation was reconfirmed in the in vivo rabbit IE model increasing the probability of IE in the rabbits compared with rabbits inoculated with pretreated bacteria with dextranase (an enzyme that removes dextran from the bacterial cell surface) (Scheld et al., 1978).
Simulated Endocardial Vegetations
Later, in 1994, a group around M. J. Rybak (McGrath et al., 1994) published a novel in vitro model simulating infective endocarditis (Table 2). They produced human fibrin clots, called simulated endocardial vegetations (SEVs), on monofilament lines by adding S. aureus to human cryoprecipitate. A sterile monofilament line was introduced, and bovine thrombin added. The resulting fibrin clot was removed and introduced into ports in a one-compartment model, previously developed for pharmacodynamic studies (Garrison et al., 1990). The model consists of a main chamber that is provided with a flow of fresh medium. Antibiotics or other drugs of interest can be administered directly into the main chamber. To assess the pharmacokinetics and pharmacodynamics of the chosen drugs, McGrath et al. removed the fibrin clots or medium at different points in time and analyzed the bacterial killing and the half-lives of the drugs (McGrath et al., 1994).
Several findings with relevance for IE have been made utilizing this model. The authors have shown that combination treatment of the SEV with humanized doses of different combinations of vancomycin, teicoplanin, and gentamicin could reduce the CFU of methicillin-susceptible S. aureus vegetations, while monotreatment with either of the antibiotics had limited effect (McGrath et al., 1994). Those results were comparable with previous observations in rabbit IE models (McGrath et al., 1994). Similar results have been obtained for the combination of vancomycin and quinupristin/dalfopristin against a methicillin-susceptible S. aureus (MSSA) and a methicillin-resistant S. aureus (MRSA) strain (Kang and Rybak, 1995). While quinupristin/dalfopristin alone was more effective than vancomycin, the combination treatment achieved the highest CFU reductions. At the same time, Kang and Rybak (1995) observed that quinupristin/dalfopristin monotherapy in both bacterial strains led to resistance development toward the compound, as well as to an increase in the erythromycin MIC for the MRSA strain. In the same model, dosing strategies for daptomycin against different MRSA isolates have been evaluated (Vidaillac et al., 2011). By exposing the SEVs to either high (10 mg/kg of body weight/day) or low (6 mg/kg/day) daptomycin doses for 4 days and afterward increasing or decreasing the dose with respect to concentration. The use of high initial antibiotic concentrations followed by a concentration decrease after 4 days was shown to be the most effective strategy. Likewise, combinations with dalbavancin and ceftaroline or telavancin combined with ceftaroline or rifampicin, as well as tedizolid and daptomycin have been tested in the setup against different MRSA or MSSA S. aureus isolates (Jahanbakhsh et al., 2018; Smith et al., 2018; Kebriaei et al., 2019a). Additional combinations with daptomycin have been tested against daptomycin non-susceptible MRSA isolates for identification of most optimal antibiotic combinations (Steed et al., 2010). The before-mentioned IE-characteristic inoculum effect was investigated with daptomycin in combination with β-lactams against E. faecium predisposed for daptomycin resistance, harboring the LiaFSR substitutions (Kebriaei et al., 2018). Surprisingly, a beneficial effect of combining daptomycin with ampicillin, ceftaroline, or ertapenem was observed (Kebriaei et al., 2018). Daptomycin β-lactam combinations were also tested against the vancomycin-resistant E. faecium and E. faecalis using SEVs simulating PK/PD, and especially the combination with ceftaroline was promising (Snyder et al., 2014; Werth and Shireman, 2017; Jahanbakhsh et al., 2020).
The effect of daptomycin against Streptococcus mitis and Streptococcus oralis was evaluated in other recent studies using the SEV model (Yim et al., 2017; Kebriaei et al., 2019b). Here, it was shown that monotreatment with daptomycin was ineffective, with high development of resistance. When daptomycin was combined with ceftriaxone, significant bacterial CFU reductions were observed without formation of daptomycin resistance. On the basis of these observations, the authors suggest further studies on the clinical relevance for the treatment of IE patients (Kebriaei et al., 2019b). The SEV model has also been utilized to study the role of platelets in S. aureus IE. The results indicate that platelets can inhibit the bacterial proliferation inside the vegetations dependent on the bacterial susceptibility toward the thrombin-induced platelet microbicidal protein-1 (tPMP) (Mercier et al., 2000).
The SEV in vitro model has been validated by comparison with four experimental rabbit models of IE (Hershberger et al., 2000). The study found that the effects of various fluroquinolone treatments against MRSA and MSSA as well as on E. faecalis and E. faecium were comparable with the results obtained in rabbit vegetations.
Shear Flow Model
In an attempt to study the adhesion of bacteria to vessel walls under flow conditions, several groups attempted to model the shear conditions with in vitro models. The idea of such flow chambers is to immobilize either bacteria and/or different host components on a surface and then model a flow by leading fluid at the desired flow rate past that surface and the possibility of adjusting the flow rates seen in human vessels.
Such in vitro flow models are valuable to identify components involved in the bacterial adhesion to tissues under shear stress. In the context of IE, this can help to understand the process of bacterial adhesion to the endothelial cells during shear stress under the constant flow of fluid.
Using this model, Claes et al. (2015) found that the adhesion of S. aureus to endothelial cells under shear conditions was dependent on the presence of von Willebrand factor (vWF) on the endothelium. Interestingly, bacterial adhesion was increased with increasing shear rates (Claes et al., 2015). In vivo experiments could confirm the dependence of adhesion on vWF (Claes et al., 2015). The study concludes that shear stress plays an important role in the initial adhesion mediated by vWF of bacteria to the endothelium. Similar observations in vitro and in vivo have been made for Staphylococcus lugdunensis (Liesenborghs et al., 2016). For S. aureus, it has also been found that platelet aggregation is dependent on the bacterial clumping factor A and the platelet immunoglobulin receptor FcyRIIa (Kerrigan et al., 2008).
Using a similar in vitro technique, Yakovenko et al. (2018) investigated the shear-enhanced binding of streptococci to platelets and found that three streptococcal serine-rich repeat glycoproteins were able to mediate the adhesion to the endothelium under shear conditions. Another publication reports that the glycoproteins Iba and FcyRIIa were important for platelet activation by Streptococcus oralis (Tilley et al., 2013). A similar model has also been developed to study the bacterial adhesion to components of graft tissue (Ditkowski et al., 2018).
Two-Chamber Compartment Model With Porcine Valves
A recent publication by Lauten et al. (2020) presents a novel IE model, using a pulsatile two-chamber compartment mimicking the heart (Table 2). In this device, the group created monospecies S. epidermidis biofilms on native porcine aortic valves under physiologic hemodynamic and temperature conditions. A piston pump establishes pulsatile flow conditions in the chamber that are comparable to the human in vivo situation. Bacterial growth in IE can therefore be mimicked taking hydrodynamic factors and physical shear stress into account. The chamber system, as well as the porcine aortic valves were tested to be sterile before inoculation with bacteria. The results indicate that this model can reproduce the pathogenesis and pathophysiology of the biofilm formation on native valves in IE under physiologic flow conditions. However, the model is limited by the lack of host defense factors, such as platelets, fibrin, and leukocytes.
In vivo Infective Endocarditis Model Systems
The history of the experimental model of endocarditis dates to 1878 where Dr. Ottomar Rosenbach was the pioneer to establish the principal of mechanically induced lesions of the heart valves in rabbits (Rosenbach, 1878; Jarcho, 1967) and was followed by a range of other investigators (Jarcho, 1972). The real breakthrough was made in the 1970s with the introduction of polyethylene catheter models, first used in a rabbit right-sided endocarditis model by Garrison and Freeman (Garrison and Freedman, 1970), shortly after, followed by a left-side IE model (Perlman and Freedman, 1971). Durack and Beeson modified the model into streptococcal IE and described the important findings in detail, including the colonization of the sterile lesions, the limited number of leukocytes inside the vegetations, the composition of degraded platelets, and the high grade of bacterial aggregation with low metabolic activity of the deep-seated bacteria compared with the active surface bacteria (Durack and Beeson, 1972a, b; Durack et al., 1973; Durack, 1974). Important experiments of E. faecalis IE in rabbits have been performed by E. Gutschik (Gutschik and Christensen, 1978a, b; Gutschik et al., 1979, 1982; Gutschik, 1982). Santoro and Levison (1978) and Kjerulf et al. (1998) reproduced the model in rats, which is now the most used model of experimental IE. Most of the experimental work has been performed on left-sided endocarditis, not only because it is the more clinically relevant for the disease but also due to technical aspects. The in vivo model of IE is therefore one of the most investigated infection models known.
The Principle of the in vivo Model of Infective Endocarditis
Independent of the model animal, the most common way to induce IE is by introducing mechanical damage to the heart valve. The following part briefly describes the most common method for the induction of IE.
The surgery is performed on fully anaesthetized animals (Figure 3). The neck area is disinfected, and a sterile incision of the skin is applied vertically above the sternum. By separating the underlying fascia, the carotid artery is exposed (either left or right). The artery is carefully lifted out and tied off using two sutures. A small hole is introduced into the artery through which a catheter is inserted. The suture toward the heart is removed and the catheter can be pushed down until resistance and pulsation of the catheter is obtained. The catheter is then fixated by caudal suture around the right carotid artery. Excess sutures and catheters are cut, and the skin is closed over the catheter using clips or suture. The catheter can then be left in place for the whole duration of experiments, or it can be removed after 24 h making sufficient mechanical damage to the endothelium of the aortic valves. There are several limitations to leaving the catheter in situ during the experiment. First, it increases the mortality and risk of septic and thrombotic embolism; second, it resembles a foreign body infection/IE; and third, it makes long-term observation studies impossible (Kjerulf et al., 1998). After inducing catheter damage of the endothelium of the heart valves, the pathogen of interest is injected in a peripheral vein inducing IE and complications as seen in human IE, here illustrated by aortic valve IE in rats and kidney infarction (Figure 4).
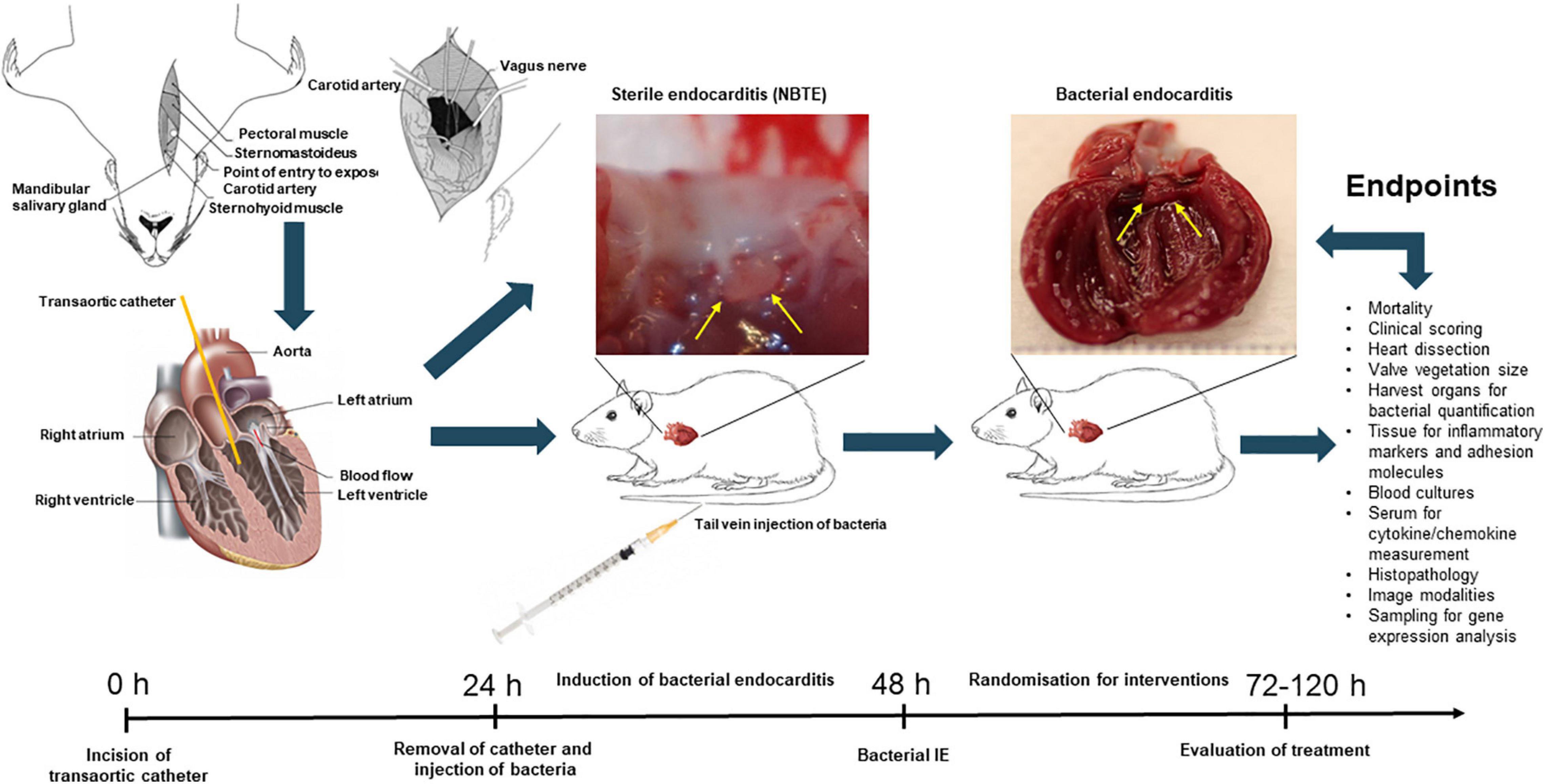
Figure 3. Schematic overview of in vivo experimental endocarditis, here illustrated by the experimental model of rat endocarditis. Abbrevations: non-bacterial thrombotic endocarditis, NBTE; infective endocarditis, IE.
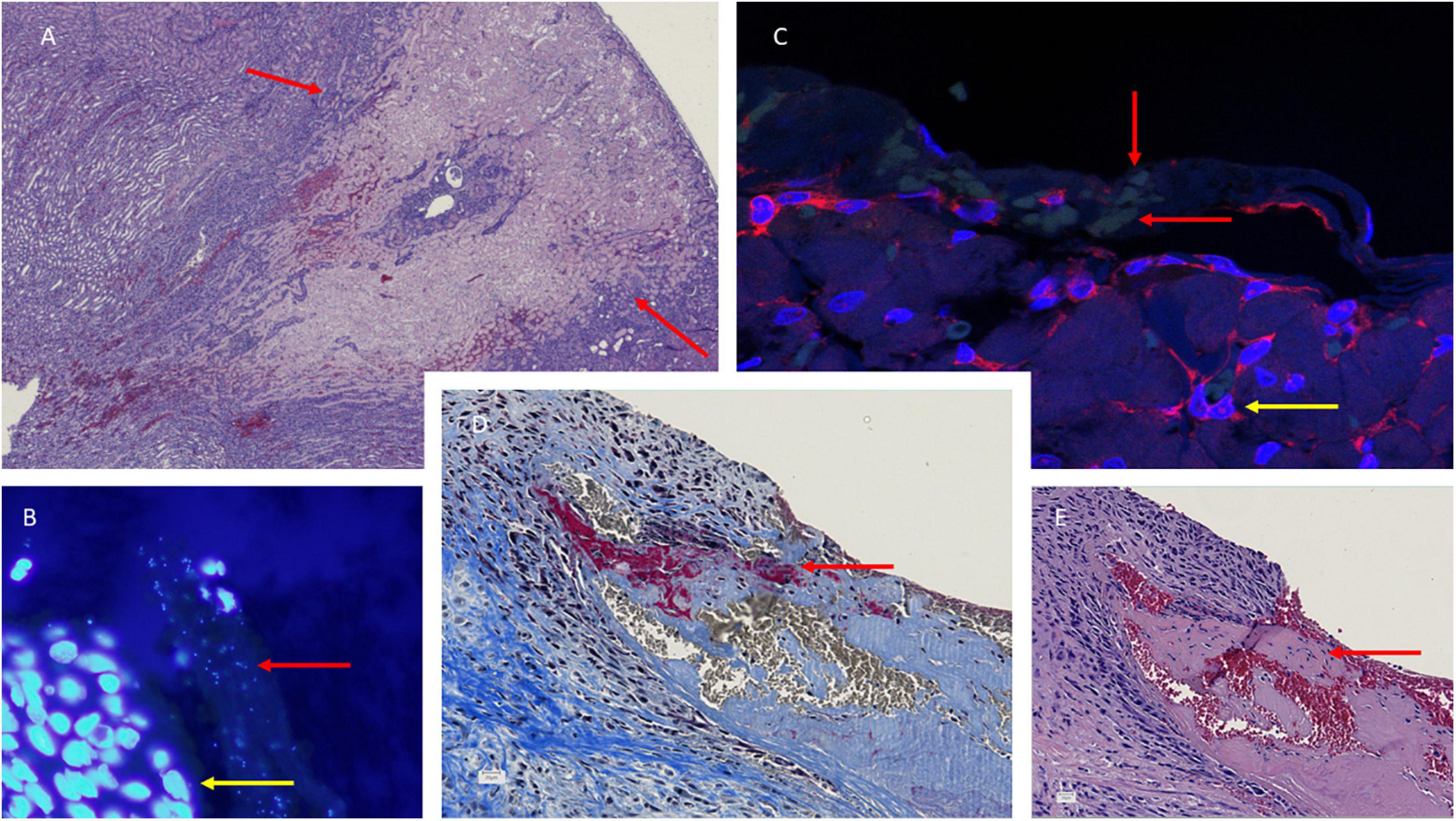
Figure 4. Histology of the aortic valves (B–E) and kidney necrosis (A) in S. aureus infective endocarditis (IE) experimental rat model. Hematoxylin-eosin (HE) staining reveals the large necrosis in the cortex of the kidney in a rat with IE. Representative sections of S. aureus infected valve [(B), fluorescens microscope ×100 and (C), confocal microscope, ×62] with DAPI/PNA-flourescence in situ hybridization (FISH) stain showing S. aureus microcolonies in valve vegetations, red arrow indicating the microcolonies and yellow arrow indicating recruited polymorphonuclear neutrophils. Martius, Scarlet, and Blue (MSB) and hematoxylin-eosin (HE) (E) reveals fibrin in red indicated (D) and amorphous structures (E) in valve vegetations indicated by red arrow, respectively.
Mouse Models of Infective Endocarditis
Even though rabbit and rat models are more prevalent in studying IE, some research has been performed in mice, mostly by induction of endothelial damage on the heart valves through the carotid artery with a catheter (Ring et al., 2014; Gibson et al., 2015). Mice have the advantage over bigger animals to be less expensive, also in housing costs, and that less compounds for treatments are needed. For the induction of endocarditis, microsurgery has to be performed. However, the resulting vegetations cannot be observed macroscopically or isolated individually.
Gibson et al. (2015) developed an MSSA (SA-3529) and MRSA (SA-2015) IE in CD1 mice by inducing aortic valve damage through the left carotid artery. The catheters were kept in place throughout the study duration. The group also attempted an approach through the right carotid artery, which presented more difficulty and was less consistent and was therefore not further used. Bacterial infection was performed by injecting bacteria into the lateral tail vein 18–24 h after placing the catheter. They found that 70% of the mice survive the surgical procedure. Furthermore, they determined the optimal infection rate to be 10E+6 CFU/mouse, as this led to maximal survival compared with higher concentrations, while still showing sufficient infection of the heart. Using histopathology, they confirmed the formation of IE pathology that could be alleviated by vancomycin treatment. They also characterized the time course of their mouse model and found the CFU to increase until 48 h after infection, while more than 80% of the mice did not survive 72 h after infection.
Other groups have contributed to further characterize this mouse model. Ring et al. (2014) performed MRI screening on mouse hearts at 48 h after induction of valve damage via the right carotid artery, corresponding to 24 h after the infection with 10E+5 CFU S. aureus (ATCC 53657). Their experiments included a sham control group, a group that received unlabeled bacteria, and one group receiving bacteria labeled with iron oxide nano particles. Subsets of the groups receiving labeled or unlabeled bacteria additionally were intravenously injected with an iron oxide nano particle suspension. The iron particles are partly taken up by macrophages and are therefore a way to separate the magnetic resonance imaging (MRI) signal changes resulting from bacteria from the changes resulting from immune infiltration. They achieved comparable surgery survival rates with those of Gibson et al. and found their MRI results to be highly comparable with the standard microbiologically technique, quantitative bacteriology. By comparing the different groups, they confirmed that the changes observed in the MRI resulted from bacterial vegetations and not from macrophage infiltration. Labeling of S. aureus was shown to not be required for detection. They concluded that MRI is a promising strategy for precisely and time efficiently monitoring the development of IE in mice; however, the use of MRI to evaluate treatment efficacy remains to be studied. MRI has also been used by other researchers to evaluate a different setup of inducing S. aureus IE in mice (Schwarz et al., 2020).
Liesenborghs et al. (2019) compared three different methods of establishing S. aureus IE in mice (C57Bl/6). Endocarditis was induced by intravenous injection of bacteria, either in healthy mice (bacteremia group), or by catheter-induced endothelial damage of the heart valves. A new technique applied was a third group, receiving locally infused histamine to activate the endothelium prior to bacterial challenge though a catheter placed at the aortic valves. They found that bacterial adhesion in the bacteremia control group only occurred in mice above the age of 1.5 years, which is a very high age for mice. In the group that received endothelial damage prior to bacterial challenge, the bacterial adhesion was significantly increased compared with the bacteremia group. The highest bacterial adhesion was observed in the histamine-pretreated group.
In a 3-day study, they observed a development of endocarditis in some, but not all, of the mice in the damage-induced model as well as in the histamine-induced model. Their results show that cardiac valve damage, as well as valve inflammation both play a role in the development of endocarditis. Furthermore, they found that the initial bacterial adhesion is mediated by different mechanisms. While S. aureus adhesion following heart valve damage is dependent on fibrinogen and Sortase A, the adhesion following cardiac valve inflammation is mediated by platelets.
Rat Models of Infective Endocarditis
IE has extensively been studied in rats as described previously. Santoro and Levison (1978) published the first experimental rat IE model of left-sided IE, based on existing rabbit models of right- and left-sided IE. They introduced a polyethylene tubing through the right carotid artery into the left ventricle, leaving it in place for the duration of the experiment. The bacterial challenge was performed 24–48 h after surgery, with 1 ml of overnight cultures of either Streptococcus mitis, S. aureus, or E. faecalis. They could confirm the formation of valve vegetations that were comparable with results obtained from studies performed on rabbits.
Several studies have been performed in experimental rat IE models, which contributed to the understanding of the pathogenesis of the disease and were used for evaluation of numerous therapeutic studies. One study demonstrated that the removal of the catheter before bacterial injection could reduce the efficiency of the infection and that S. aureus was superior in establishing IE, compared with Streptococcus intermedius and E. coli (Heraief et al., 1982). Xiong et al. found that real-time in vivo bioluminescent imaging is a valid tool for evaluating antibiotic efficacies (Xiong et al., 2005). However, bioluminescent imaging has limitations because it is less sensitive than conventional bacterial culture, and it might not reveal bacteria, which are less active due to metabolic downregulation (biofilm formation). Furthermore, the stability of the different bioluminescent-modified bacteria varies greatly especially for S. aureus compared with other Gram-negative strains. The advances with this method are the reduction in animals needed and the possibility to follow each animal until the evaluation point (Xiong et al., 2005). The plasma and extracellular matrix protein gC1qR/p33 and S. aureus clumping factors, as well as coagulase have been found to be important factors in the pathogenesis of S. aureus IE (Moreillon et al., 1995; Peerschke et al., 2006). The gene expression of S. aureus during IE has been evaluated by Hanses et al. (2014), who found a higher degree of bacteremia and increased vegetation size in diabetic rats compared with non-diabetic rats, underlining diabetes as a risk factor for IE. Microarray analysis revealed an upregulation of 61 S. aureus genes, predominantly involved in amino acid and carbohydrate metabolism in the diabetic rats (Smith et al., 2018). In vivo gene expression revealed an upregulation of toxins and proteases, although, in general, similar in diabetic compared with non-diabetic rats (Smith et al., 2018).
The experimental model of IE has also been used to investigate the host response of antibody production in rats exposed to the infection (Kjerulf et al., 1998). In this study, Kjerulf et al. observed that a significant specific anti-E. faecalis response was not seen until 3 weeks after establishment of the infection, which corresponds to the clinical observation, that antibody measurements are mainly useful in cases of subacute and chronic IE (Kjerulf et al., 1993).
Several experimental rat IE studies have also unraveled new promising treatment strategies for IE. Results from different in vivo studies suggest daptomycin and daptomycin combinations with β-lactams as a promising treatment option for recalcitrant E. faecium infections (Pericàs et al., 2017; Kebriaei et al., 2018). Recently, animal experiments have suggested that tedizolid is an effective step-down therapy following daptomycin against enterococcal and S. aureus endocarditis (Singh et al., 2020).
Other aspects of in vivo IE research are focused on finding non-antibiotic adjunctive strategies to supplement antibiotic treatment of IE. Previously, we showed that tobramycin treatment alone was inefficient (Lerche et al., 2015), but could be significantly augmented by supplementing with hyperbaric oxygen therapy (Lerche et al., 2017). We further found the thrombin inhibitor, dabigatran, to be an effective adjunctive treatment strategy to antibiotic (gentamicin) treatment in vivo (Lerche et al., 2019) (for details, see the Antithrombotic treatment in infective endocarditis section).
Rabbit Models of Infective Endocarditis
Experimental rabbit models for IE have been used extensively to understand the disease and find promising new treatment strategies.
Within the last 5 years, the model has mostly been used to investigate bacterial virulence factors and their contribution to the infection of the endocardium and heart valves (Crosby et al., 2016; Herrera et al., 2016, 2017; King et al., 2016; Colomer-Winter et al., 2018a, b; Baker and Nulton, 2019; Reed et al., 2019; Martini et al., 2020). Using rabbit models for IE rather than mice or rats, bears the advantage that although they are still relatively small animals, the rabbit carotid artery is substantially easier to work with due to its size. Furthermore, the immune and cardiovascular system of rabbits is thought to be more comparable with humans (Salgado-Pabón and Schlievert, 2014) and human platelet physiology (Yeaman et al., 1996).
Other Species Models of Infective Endocarditis
Apart from the most used models, experimental endocarditis models have also been established in pigs, dogs, and opossums. In pigs, models with (Johnson et al., 1986; Christiansen et al., 2013) and without (Jones, 1972, 1981, 1982) afflicting damage to the heart prior to infection have been published. More recent IE-related research involving pigs is focused on utilizing biological porcine xenografts as valve replacements for elderly patients with aortic valve stenosis and mitral valve regurgitation (Jawad et al., 2020).
Captive opossums have been shown to be susceptible to spontaneously occurring bacteria endocarditis (Sherwood et al., 1968). Sherwood et al. (1971) described an opossum IE model, in which, by giving single i.v. injections of either Streptococcus viridans or S. aureus, 58% of the S. viridans or 100% of the S. aureus group of animals developed bacterial endocarditis. Streptococcal endocarditis was mostly located on the left side of the heart, while S. aureus-induced endocarditis was seen in both sides of the heart. Injection of three different fungi did not lead to IE induction. Even though the spontaneous development of bacterial endocarditis in opossum points at its usefulness in clinical research, the opossum model has not been used in IE research after 1972. This is mostly due to difficulties in housing and handling the animals, and because the animals were obtained from the wilderness. Also, non-infected opossums in the control groups acquired different infections over the course of experiments.
Potential Novel Therapies
In the present review, we describe new treatment targets and strategies based on the findings of preclinical studies with the potential to be used in patients with IE.
Antithrombotic Treatment in Infective Endocarditis
IE is a prothrombic condition and subclinical or silent embolic phenomena that occur in a high number of patients (Hess et al., 2013; Monteiro et al., 2017), especially in S. aureus IE as cerebral ischemic strokes (Rasmussen et al., 2009). Embolism is one of the most frequent and serious complications in IE and is primarily related to the size of the vegetations (Røder et al., 1997; Heiro et al., 2000; Di Salvo et al., 2001; Vilacosta et al., 2002; Thuny et al., 2005; Snygg-Martin et al., 2008; García-Cabrera et al., 2013). Since the early antibiotic era, experimental treatment of patients with IE with antithrombotic medication has been tested with unsuccessful outcome (Cooke and Taylor, 1943). Since then, IE treatment with antibiotic and antithrombic therapy has changed significantly. The knowledge of treatment and safety margins for antithrombotic therapy is widely used in the clinical treatment of patients with beneficial outcomes. Later studies have investigated the effect of antiplatelet therapy preclinically, which has shown a beneficial effect (Kupferwasser et al., 1999, 2003).
The basis of anticoagulant treatment of IE dates back to the 1940s where heparin and dicoumarol were used as adjunctive treatment to penicillin therapy (Loewe, 1945; Priest et al., 1946; Thill and Meyer, 1947). In a clinical study from Tornos et al. (1999), examined 56 cases of left-sided native and prosthetic valve S. aureus IE in a 12-year period and reported the deleterious effect of antiplatelet therapy with aspirin. Ninety percent (n = 21) of patients with prosthetic valve IE receiving anticoagulant treatment displayed a higher mortality due to CNS complications compared with native IE patients. The authors concluded that left-sided prosthetic IE caused by S. aureus is a contraindication for anticoagulant treatment.
This study has been the primary reason that the ESC guidelines in 2009 do not recommend anticoagulation (Tornos et al., 1999; Habib et al., 2009). This recommendation was kept in the 2015 guidelines (Habib et al., 2015) based on Chan et al. (2003). However, in a prospective study by Rasmussen et al. (2009), the frequencies of ischemic stroke and intracranial hemorrhage were reported to be 34 and 3%, respectively. Patients who were on anticoagulation therapy prior to diagnosis showed reduced numbers of detectable vegetations, they were smaller, and resulted in an almost fourfold reduction in the number of strokes (OR: 0.27; 95% CI: 0.076–0.96; p = 0.04), strongly indicating that anticoagulation reduces the vegetation size. Furthermore, the study did not find any increase in hemorrhagic events in patients receiving anticoagulation therapy, neither in patients with native nor in patients with prosthetic IE. There are several important differences between Tornos’ and Rasmussen’s studies. A much larger number of patients is included in Rasmussen et al. (2009), and the patients were recruited during a considerably shorter time period than in the Tornos et al. (1999) study. The Rasmussen et al. (2009) study also better reflects the current IE management and the higher rate of surgical intervention. However, most studies include prosthetic IE patients already receiving anticoagulation treatment, whereas the majority of patients with native IE in the clinical setting do not receive anticoagulation therapy.
Another prospective study by Snygg–Martin and colleagues studied the role of anticoagulation in 587 episodes of left-sided native IE. The majority of patients were not on anticoagulation treatments, but 8% of the patients received warfarin for indications other than IE. No increase in hemorrhage lesions was seen in the subgroup of anticoagulated patients compared with the group not receiving anticoagulation medicine. Symptomatic cerebrovascular complications were reduced (OR: 0.26; 95% CI: 0.07–0.94) in patients receiving warfarin at admission and were especially prevalent in S. aureus IE (Snygg-Martin et al., 2011). A small prospective observational study by Taha et al. (1992) evaluated the effect of low-dose aspirin (75 mg/day) on the incidence of stroke in IE patients and measured the change of the vegetation size by means of echocardiography. Patients receiving aspirin (n = 4) did not have embolic complications, while two patients in the control group (n = 5) developed focal neurological deficits with cerebral infarction and one of them additionally developed myocardial infarction (Taha et al., 1992).
Adjunctive Treatment With Dabigatran
Dabigatran is a direct inhibitor of thrombin (FIIa), which is the last serine protease in the clotting cascade converting fibrinogen to fibrin. Thrombin is the most potent platelet activator, further contributing to hemostatic clot formation or thrombus formation in pathologic conditions. Virulence factors of S. aureus enhance the clotting cascade and platelet activation leading to a large burst of thrombin and the coagulase-prothrombin complex facilitating the fibrin formation. Dabigatran could therefore be an important treatment strategy by dampening the excess fibrin and clot formation on cardiac valves, resulting in reduced size of the heart valve vegetation and, presumably, a reduced risk of embolization.
Staphylocoagulase is also an important virulence factor and expressed in all S. aureus isolates. Coagulase has been known for over 100 years and is important in abscess formation and the pathogenesis of sepsis and IE (Moreillon et al., 1995). Secreted coagulase is central in activation of the coagulation cascade promoting thrombin generation and activating prothrombin without proteolysis (Panizzi et al., 2006). Staphylocoagulase can form a complex with thrombin (Staphylothrombin complex) using fibrinogen as substrate for the conversion of fibrinogen to fibrin (Panizzi et al., 2006) and is important for the expansion of cardiac vegetations (Panizzi et al., 2011). Another coagulase produced by S. aureus is vWb protein, also assisting coagulation and clot formation by activation of prothrombin (Kroh et al., 2009). vWb also has adhesion properties resulting in firm adhesion and biofilm formation in valve vegetations (Claes et al., 2014).
Interestingly, it has been shown that dabigatran inhibits the formation of Staphylothrombin complex and reduces the virulence of S. aureus in an in vivo abscess model in mice (Vanassche et al., 2011). Dabigatran also prevents clotting in a dose-dependent fashion (Vanassche et al., 2010), increasing the time to death in septic mice in combination with anti-ClfA (McAdow et al., 2011), and reduces the fibrin and clot formation (Panizzi et al., 2011; Vanassche et al., 2012, 2013). Furthermore, dabigatran has been effective as a prophylactic treatment in a low-grade S. aureus induced model of experimental IE (Veloso et al., 2015).
Several other preclinical studies have also shown dose-dependent beneficial effects of dabigatran by inhibition of the thrombus formation in a venous model of thrombosis (Wienen et al., 2007), and by attenuating inflammation and infarction size in pre-treated rats in a cerebral stroke model (Dittmeier et al., 2016).
These beneficial indications of dabigatran against S. aureus led to a recent study by Lerche et al. evaluating dabigatran as adjunctive treatment in an experimental rat model of S. aureus IE (Lerche et al., 2019). Here, animals received adjunctive treatment with dabigatran together with gentamicin compared with the control group, receiving gentamicin alone. The dabigatran treated group showed a significantly reduced valve vegetations size compared with the control valves. Furthermore, the bacterial load in valve vegetations was significantly reduced in dabigatran-treated animals, in parallel with key proinflammatory markers of IE (i.e., IL-8 and IL-6). These findings indicate that dabigatran could be a beneficial non-antibiotic treatment option in acute and severe S. aureus IE. The authors demonstrated that adjunctive dabigatran treatment reduced the valve vegetation size, platelet aggregation, bacterial load, inflammation, and bacterial dissemination (Lerche et al., 2019). However, these findings need to be evaluated and confirmed in future clinical studies of S. aureus IE.
Recently, a single-center, randomized (Peetermans et al., 2018), controlled feasibility, and safety trial of staphylothrombin inhibition with direct thrombin inhibitors in patients with S. aureus bacteremia has been performed. Eligible patients were randomized 1:1 to oral dabigatran (110 mg twice a day) or i.v. argatroban for 7–10 days or subcutaneous enoxaparin 40 mg once daily (standard care). Primary outcomes were the feasibility and safety of thrombin inhibitors in patients with S. aureus bacteremia. Secondary outcomes included D-dimer evolution (days 0–4) as a marker of coagulation activation, inflammatory and microbiological parameters, and clinical outcomes including metastatic infections. Thirty-one percent (94/303) of screened patients were enrolled. The study showed that similar frequencies of thrombotic and bleeding events occurred in both thromboprophylactic treatment groups, suggesting a similar safety profile of thrombin inhibitors compared with standard thromboprophylaxis based on low molecular weight heparins (LMWH). The secondary outcome parameter D-dimers, which reflects degradation of fibrin, regardless of whether this fibrin is generated by host thrombin or by staphylothrombin, showed a faster resolution in the direct thrombin inhibitor groups compared with the standard LMWH group. In this selected patient group with S. aureus bacteremia, direct thrombin inhibitors showed a comparable safety profile to standard care with similar rates of bleeding and thrombotic events.
In a recent Danish nationwide cohort study (Butt et al., 2020), 112,537 patients with atrial fibrillation receiving direct oral anticoagulants were identified from nationwide registries. A median follow-up of 2.0 years was found and 186 patients in the dabigatran group and 356 patients in the factor Xa-inhibitor group were admitted with S. aureus bacteremia. The crude incidence rate of S. aureus bacteremia was lower in the dabigatran group compared with the factor Xa-inhibitor group {22.8 [95% confidence interval (CI), 19.7–26.3] and 33.8 (95% CI, 30.5–37.6) events per 10,000 person-years, respectively}. In adjusted analyses, dabigatran was associated with a significantly lower incidence of S. aureus bacteremia compared with factor Xa-inhibitors (incidence rate ratio, 0.76; 95% CI, 0.63–0.93). These findings, both preclinical and clinical, indicate that adjunctive treatment with dabigatran might have a place in the treatment of S. aureus IE. However, as invasive S. aureus causes complicated infections with multiple factors influencing the course of disease, future prospective studies in S. aureus IE should be made with precautions and selective candidates, with specific relevant end points. A further question is, of course, whether a similar beneficial effect of anti-thrombotic therapy could be expected in IE caused by the other frequent bacterial etiologies since they do not produce coagulase-like molecules.
Clinical Application of Hyperbaric Oxygen Therapy in Infective Endocarditis
Hyperbaric oxygen therapy (HBOT) is an ancient treatment modality, first documented in 1662 (Henshaw, 1664; Henshaw and Simpson, 1857), where Henshaw built a chamber called “domicilium,” followed by Beddoes in 1795 (Beddoes and Watt) and the first described adjunctive treatment performed by Fontaine (1879).
HBOT is based on the administration of 100% oxygen in a treatment chamber that is pressurized greater than sea level (1 ATA). HBOT enhances the amount of dissolved oxygen in the plasma and increases the supplied oxygen in tissues, independently of hemoglobin (Gill and Bell, 2004). The treatment is commonly applied for 60–90 min at a pressure of 2.5–2.8 ATA corresponding to diving at 15–18 m below sea level. During treatment, the arterial oxygen tension often exceeds 2,000 mmHg (6.8 ml O2/100 ml of blood) and levels of 200–400 mmHg in tissues (Thom, 1989). In comparison, the normal oxygen tension in arterial blood is 80–100 mmHg, in venous blood 30–40 mmHg and in a healthy human kidney ∼16 mmHg (Collins et al., 2015). The oxygen concentration in air is 160 mmHg, and in normal human tissue, it is about 60 mmHg (Kanick et al., 2019).
Hypoxia is a hallmark of infectious diseases, and HBOT is approved and indicated in severe bacterial infections such as necrotizing soft tissue infection (Wilkinson and Doolette, 2004), chronic wounds (Sanford et al., 2018), refractory osteomyelitis (Mader et al., 1978, 1990), and intracranial abscesses (Bilic et al., 2012). Although HBOT is a well-established treatment used both for infectious and non-infectious diseases, there is a need for improved clinical documentation. Hopefully, more randomized control trials are on the way in this area (Hansen et al., 2015). The mechanisms of action of HBOT are numerous and far from all have been completely understood (Figure 5). HBOT potentially affects all cells in the mammalian body due to the fundamental need for oxygen in maintaining cellular functions and survival. In inflammatory diseases, the need for oxygen is increased significantly and HBOT is an important strategy to obtain and maintain cellular function in inflamed hypoxic tissue.
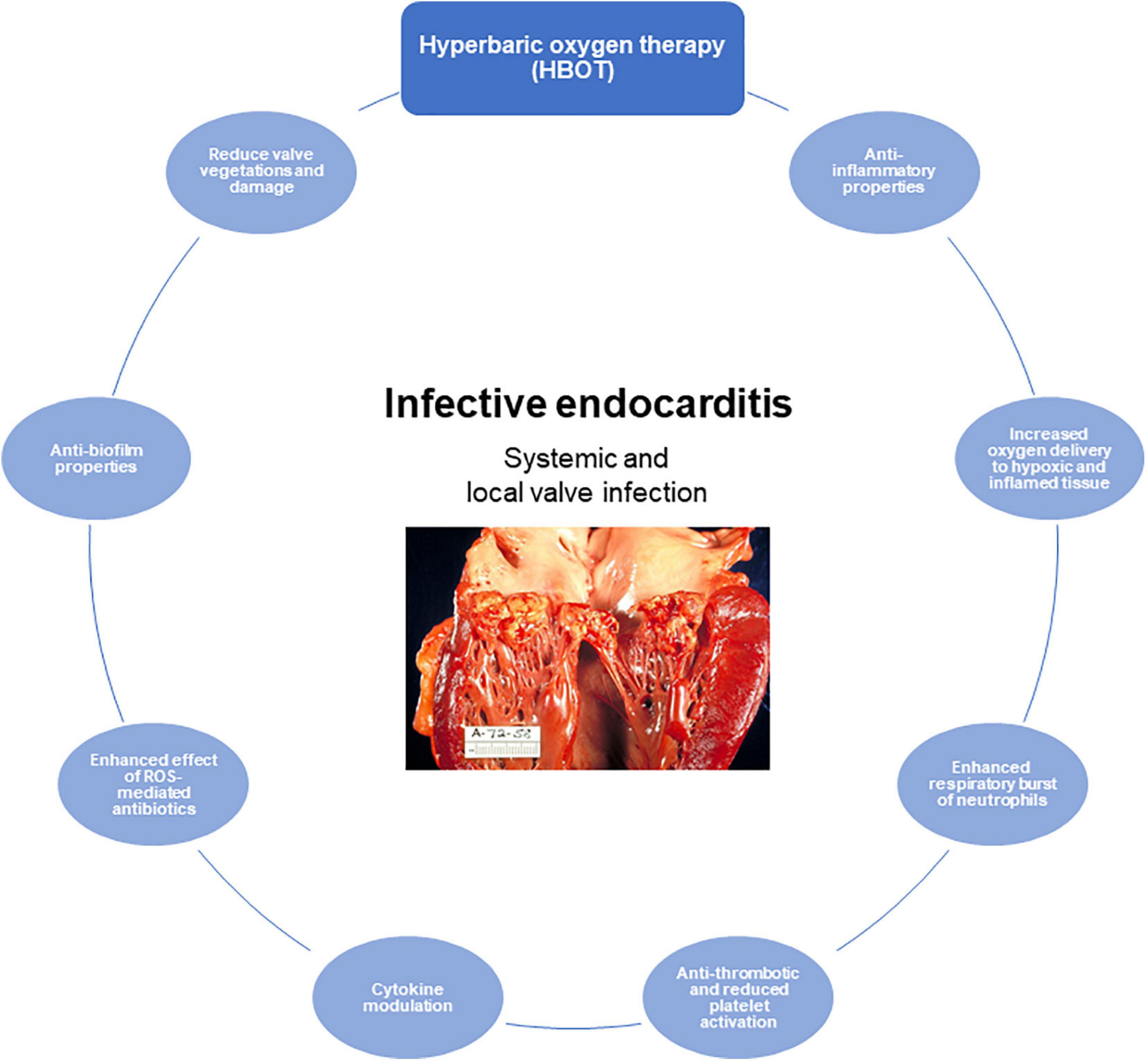
Figure 5. The role of hyperbaric oxygen treatment (HBOT) of infective endocarditis (IE). Proposed and important effects of HBOT in IE. Illustration of human IE reused with permission from Wikimedia Commons, the free media repository.
Severe and complicated infections, i.e., S. aureus IE, may result in abscess formation and biofilm formation, resulting in low oxygen tension in the inflamed tissue, compromising host responses, and antibiotic effect. In vitro studies applying HBOT to treat bacterial biofilms have shown to increase the antimicrobial effect of ciprofloxacin (Kolpen et al., 2016, 2017). Furthermore, by knockout of the catalase gene in Pseudomonas aeruginosa (ΔkatA), the oxygen-dependent antibiotic killing is further enhanced (Kolpen et al., 2016). Moreover, HBOT increases the oxygen penetration into the biofilm by a factor of four, increasing the metabolic activity and growth rate of the bacteria, thus, making them more susceptible to antibiotic treatment, within the biofilms (Gade et al., 2018).
A limited number of experimental studies have shown a beneficial effect of HBOT in different S. aureus infections (Mader et al., 1978; Turhan et al., 2009; Oguz et al., 2011; Bilic et al., 2012), but in IE, evidence for the benefits of HBOT are sparse (Özkan et al., 2016; Lerche et al., 2017). HBOT might potentially be used as adjunctive treatments for the following reasons: first, to reduce the tissue hypoxia induced by inflammation (Proctor et al., 2014). Second, to enhance the oxygen-dependent killing mechanism by the leukocytes (respiratory burst) (Mader et al., 1978; Jüttner et al., 2003; Almzaiel et al., 2013), decreasing the β2 integrin expression of leukocytes thereby reducing the adherence and cytotoxicity (Thom et al., 1997), amelioration of ischemia–reperfusion injury (Thom, 2009), reduce neutrophil extracellular trap (NET)-induced inflammation by neutrophils (Grimberg-Peters et al., 2016), and increase intracellular ROS generation. Third, effects of β-lactam antibiotics, fluoroquinolones, and aminoglycosides are potentiated by oxygen, which leads to the formation of intrabacterial reactive oxygen species, which participate in the toxic killing of bacteria, thereby increasing the antibiotic efficacy (Verklin and Mandell, 1977; Jensen et al., 2014; Kolpen et al., 2016, 2017).
In experimental IE, hyperbaric oxygen has shown similar effects on S. aureus biofilms treated with tobramycin and HBOT. Yet, we still need to explore other groups of antibiotics, which are common in the standard treatment of IE, in combination with HBOT Furthermore, we need to evaluate which antibiotics are most efficient together with HBOT for different pathogens. However, two experimental studies have shown augmented effects of HBOT together with linezolid, teicoplanin, and vancomycin in an experimental model of S. aureus IE and mediastinitis (Turhan et al., 2009; Özkan et al., 2016).
A preclinical study of adjunctive HBOT in S. aureus IE has shown a beneficial effect by improving clinical scores and reducing the bacterial load in the aortic valves, myocardium, and spleen compared with the non-HBOT group (Lerche et al., 2017). Furthermore, the valve vegetation size, weight of the vegetations, and key pro-inflammatory markers was significantly reduced in the HBOT group compared with the control group. These key findings indicate that HBOT has a potential use as adjuvant treatment of IE. These results have also led to the conduction of a clinical feasibility study (currently recruiting) in patients with Gram-positive IE.
Together with the documented positive effects of HBOT in clinical studies of other infectious diseases (Korhonen, 2000; Escobar et al., 2005; Kaur et al., 2012; Skeik et al., 2015; Sanford et al., 2018), this supports the concept of using HBOT in biofilm infections and the rationality of setting up a clinical feasibility study in patients with IE. The benefit of HBOT in IE can most likely be extended to most courses, although the effect in patients that do not respond to conservative antibiotic therapy might be significantly limited. However, IE patients, that are not candidates for invasive cardiac surgery or are in the acute state of IE with severe infections, would probably be likely candidates where HBOT could be highly beneficial.
Bacteriophage Therapy
With the increase of antibiotic resistance worldwide, there is an increasing interest in developing novel treatments against resistant bacteria. Considering the challenges of antibiotic tolerance of biofilm growing bacteria, some of these novel treatments are also directed against biofilm related infections. Such an approach is the bacteriophage therapy, either alone or in combination with antibiotic drugs. There are limited studies of phage therapy in IE. Oechslin et al. (2017) recently performed an experimental study using a phage cocktail alone or in combination with ciprofloxacin against Pseudomonas aeruginosa IE, a very rare pathogen to cause IE. However, the study revealed a 7-log reduction in P. aeruginosa in the clots by a single-dose phage (Oechslin et al., 2017). In the rat model, a 2.5-log reduction was observed by both phage and ciprofloxacin monotherapy, whereas the combination revealed a highly synergistic effect with > 6-log killing (Oechslin et al., 2017). In vitro, but not in vivo, phage-resistant mutants were observed after 24 h, however, this could be prevented by combining the phages with ciprofloxacin (Oechslin et al., 2017). In a case report, anti-S. aureus phage therapy with AB-SA01, in a patient with severe S. aureus IE on a mechanical aortic valve, native mitral valve, and a possible paravalvular root abscess who could not undergo heart valve surgery, was reported (Gilbey et al., 2019). Although the bacterial strain was antibiotic-susceptible and the patient received treatment with a high dose of flucloxacillin, ciprofloxacin, and rifampicin, blood cultures were repeatedly positive. After the onset of phage therapy, the perpetuating bacteremia vanished, the patient became afebrile, the inflammatory markers were reduced, and the patient recovered after 40 days (Gilbey et al., 2019). Unfortunately, the patient died at day 103 from a new culture negative IE. Although only a case study, the potential of adjunctive bacteriophage therapy in IE remains to be evaluated in randomized controlled trials. The safety of bacteriophage therapy in 13 patients with severe S. aureus infection (including IE and septic shock) has been conducted in a single-arm non-comparative trial with no adverse reactions that indicates this as a promising treatment candidate (Petrovic Fabijan et al., 2020). However, bacteriophage therapy as a monotherapy at the current state seems obsolete and should at least be in combination therapy with an efficient antibiotic for a successful outcome (Kebriaei et al., 2021).
Cyclic Diguanylate(c-di-GMP)[Bis(3′,5′)- Cyclic Diguanyllic Acid]—Disruption of Biofilms
The mechanisms, which lead to biofilm formation per se, are essential for the characteristics and functions of the biofilm-related chronic infections. Therefore, disruption of the biofilm to render it vulnerable to antibiotic treatments or other anti-biofilm strategies have gained much attention. One can argue that the surgical approach is a mechanical disruption of the biofilm, in concordance with the mechanical removal of dental plaques and wound debridement of non-healing wounds. Indeed, uncontrolled infections, in the form of large or enlarging valve vegetations despite appropriate antibiotic therapy, are indications of early heart valve surgery (Habib et al., 2015). A non-surgical manner to disrupt the biofilm structure could be to interfere with the gene regulation of the bacteria during biofilm formation. C-di-GMP is a prokaryotic second messenger implicated in complex biological processes including biofilm formation (Yan et al., 2010; Nair et al., 2017). Adding C-di-GMP to S. mutans significantly impaired biofilm formation and tooth surface adhesion, compared with untreated controls (Yan et al., 2010). Likewise C-di-GMP exposure to S. aureus has shown reduced intercellular adhesive interaction, as well as reduced biofilm formation and adhesion to the human epithelial cell line HeLa cells (Karaolis et al., 2005). Treatment of mice in a mouse model of biofilm-related mastitis significantly reduced the quantitative bacteriology in a dose-dependent manner (Brouillette et al., 2005). Obviously, this kind of interference with biofilm formation remains to be studied in IE models.
Antibiotic Combinations
For several biofilm infections, it is well established that combinations of antibiotics, and even combinations of antibiotic drugs with non-antibiotic strategies as discussed above, are essential for optimal outcome (Høiby et al., 2015). Most of these observations have been established in CF and in patients with orthopedic implant infections (Zimmerli and Moser, 2012; Høiby et al., 2015). For antibiotic combinations, in vitro experiments have shown how antibiotics affect different niches of the biofilms (Pamp et al., 2008; Herrmann et al., 2010; Siala et al., 2018). Using the flow chamber system and live/dead staining, Pamp and colleagues showed how ciprofloxacin mainly killed active P. aeruginosa in the periphery of in vitro biofilms, whereas colistin mainly killed the centrally living, but slowly dividing, P. aeruginosa (Pamp et al., 2008). A similar finding was obtained combining tobramycin and colistin (Herrmann et al., 2010). For orthopedic implant infections, there are both animal experimental studies as well as clinical observations that show a beneficial effect of adding rifampicin to the antibiotic therapy, if the planktonic pathogens are susceptible (Sendi and Zimmerli, 2017; Zimmerli and Sendi, 2019). Likewise, the addition of rifampicin improves outcome in case of infection on cardiac implants or vascular grafts. For IE on native valves, studies have already stated the beneficial outcome of IE by adding aminoglycoside to β-lactam antibiotics (or glycopeptide) in cases of E. faecalis IE or cases with less susceptible streptococci. In the POET trial, shifting to oral antibiotic IE therapy was designed not only as a combination therapy to compensate for variability in pharmacokinetics but also to compensate for biofilm formation on the heart valves (Bundgaard et al., 2019). However, for the remaining cases of IE, it is still debatable whether combination antibiotic therapy is beneficial. If the IE pathogen is highly susceptible to the provided antibiotic drug, and this drug is known to have an acceptable penetration into the vegetation, and the patient is not critically ill, antibiotic monotherapy in correct dosages is probably sufficient. Although, as mentioned previously, resistant SCVs may be selected. However, in cases of prostheses, large vegetations, abscess formation, recurrent infection, enlargement of vegetation, unsecure pharmacokinetic, or critical illness, using two bactericidal antibiotic drugs with different microbe killing mechanisms in combination is a recommended strategy in our opinion.
Conclusion
In the present review, we describe how the observed characteristics of some of the most prevalent and important pathogens causing IE, meet and fulfill the criteria for IE being considered a biofilm infection. Within the vegetations, microorganisms present as biofilm-like aggregates that are responsible for the recurrent and chronic nature of biofilm infections. They show the typical extensive tolerance of biofilms to antibiotic therapy and the host response.
In all cases, IE is a serious fatal infection if not treated. Even following the guidelines for IE treatment, there is a high mortality rate of 20–25%, and a 1-year mortality rate of up to 40% in some reports. However, this represents a wide spectrum of courses of left-sided IE from relatively mild cases responding very well to a 2-week antibiotic course using the IE dosages (and combinations), to serious infections with septic shock, heart valve destruction, and severe embolization. This calls for an optimization of therapy to improve the clinical outcome. Accepting IE as a biofilm infection can be an inspiration for novel treatment candidates, as elaborated in the present review.
One can argue that if early infection control is achieved in IE, there is a substantial likelihood of treatment success. Therefore, in contrast to the chronic pulmonary biofilm infection in CF and orthopedic alloplastic-related infections, where it is almost impossible to eliminate an already established infection. IE is a curable biofilm infection for several reasons (Høiby et al., 2015). It is important to recognize that almost 50% of all IE patients in specialized referral centers and approximately one-third are undergoing valve replacement surgery for various indications, one of them being lack of infection control (Iversen et al., 2019). Additional indications include abscess formation, extended valve destruction leading to heart failure, and embolization leaving a rest vegetation. The beneficial outcome of conservative antibiotic treatments may also be due to the location of the infection, centrally in the blood stream with optimal antibiotic concentrations. It probably also can be of significance that the valves are living tissue, in contrast to prostheses.
In IE, model systems are essential, since it is difficult to perform controlled and randomized clinical trials. However, in the present review, we have covered the major model systems available for research in IE. From the observations, it can be seen that the in vitro models, although helpful, may not sufficiently mirror the ongoing IE in the patients. The animal models are all reflective of the clinical IE when focusing on the valve pathology and suitable for initial investigation of new treatment strategies, both for antibiotic as non-antibiotics. However, animal models are technically difficult and may not lead to the clinical impact they may deserve—since there is a substantial gap to the clinical trials. However, preclinical studies are important for testing hypotheses that could be evaluated in clinical trials.
Author Contributions
CL and FS contributed equally to the literature collection and the writing of the manuscript. MT, EF, KI, and HB edited and commented on the final manuscript. NH contributed with invaluable knowledge and comments to the manuscript and edited the final manuscript. CM was the senior and corresponding author, wrote the draft, provided the ideas, supervised the entire process from inception to the final submission, and edited the final manuscript. All authors contributed to the article and approved the submitted version.
Funding
CM was supported by the Novo Nordisk Foundation-“Borregaard Clinical Scientist Grant” (grant no. NNF17OC0025074).
Conflict of Interest
The authors declare that the research was conducted in the absence of any commercial or financial relationships that could be construed as a potential conflict of interest.
References
Alestig, K., Hogevik, H., and Olaison, L. (2000). Infective endocarditis: a diagnostic and therapeutic challenge for the new millennium. Scand. J. Infect. Dis. 32, 343–356. doi: 10.1080/003655400750044908
Allen, A. C. (1941). A case of bacterial endocarditis illustrating the mechanism of localization and the nature of vegetations. Am. Heart J. 21, 667–675. doi: 10.1016/s0002-8703(41)90730-5
Allen, J. F. (2016). A proposal for formation of Archaean stromatolites before the advent of oxygenic photosynthesis. Front. Microbiol. 7:1784. doi: 10.3389/fmicb.2016.01784
Almzaiel, A. J., Billington, R., Smerdon, G., and Moody, A. J. (2013). Effects of hyperbaric oxygen treatment on antimicrobial function and apoptosis of differentiated HL-60 (neutrophil-like) cells. Life Sci. 93, 125–131. doi: 10.1016/j.lfs.2013.06.003
Angrist, A. A., and Oka, M. (1963). Pathogenesis of bacterial endocarditis. JAMA 183, 249–252. doi: 10.1007/978-94-011-0746-4_18
Angrist, A. A., Oka, M., Nakao, K., and Marquiss, J. (1960). Studies in experimental endocarditis. I. Production of valvular lesions by mechanisms not involving infection or sensitivity factors. Am. J. Pathol. 36, 181–199.
Anwar, H., Dasgupta, M. K., and Costerton, J. W. (1990). Testing the susceptibility of bacteria in biofilms to antibacterial agents. Antimicrob. Agents Chemother. 34, 2043–2046. doi: 10.1128/aac.34.11.2043
Bacon, P. A., Davidson, C., and Smith, B. (1974). Antibodies to candida and autoantibodies in sub-acute bacterial endocarditis. Q. J. Med. 43, 537–550.
Baddour, L. M., Simpson, W. A., Weems, J. J., Hill, M. M., Christensen, G. D., and Baddour, L. M. (1988). Phenotypic selection of small-colony variant forms of Staphylococcus epidermidis in the rat model of endocarditis. J. Infect. Dis. 157, 757–763. doi: 10.1093/infdis/157.4.757
Baker, S. P., and Nulton, T. J. (2019). Genomic, Phenotypic, and virulence analysis of Streptococcus sanguinis oral and infective-endocarditis isolates. Infect. Immun. 87:e00703-18.
Bayer, A. S., Theofilopoulos, A. N., Eisenberg, E., Dixon, F. J., and Guze, L. B. (1976). Circulating Immune Complexes in Infective Endocarditis. N. Engl. J. Med. 295, 1500–1505. doi: 10.1056/nejm197612302952703
Beddoes, T., and Watt, J. (0000). Medicinal Uses of Factitious Airs, and on the Manner of Obtaining Them in Large Quantities, Vol. 2. Bristol: Bulgin and Rosser, 15.
Bennett, J. E., Dolin, R., and Blaser, M. J. (2014). Mandell, Douglas, and Bennett’s Principles and Practice of Infectious Diseases. Philadelphia, PA: Elsevier Saunders.
Bhattacharyya, S., Roy, S., Pk, M., Rit, K., Jb, D., Ganguly, U., et al. (2012). Small colony variants of Staphylococcus aureus isolated from a patient with infective endocarditis: a case report and review of the literature. Iran. J. Microbiol. 4, 98–99.
Bilic, I., Petri, N. M., Krstulja, M., Vuckovic, M., Salamunic, I., Kraljevic, K. S., et al. (2012). Hyperbaric oxygen is effective in early stage of healing of experimental brain abscess in rats. Neurol. Res. 34, 931–936. doi: 10.1179/1743132812y.0000000091
Bjarnsholt, T., Jensen, P. Ø, Fiandaca, M. J., Pedersen, J., Hansen, C. R., Andersen, C. B., et al. (2009). Pseudomonas aeruginosa biofilms in the respiratory tract of cystic fibrosis patients. Pediatr. Pulmonol. 44, 547–558.
Bloomfield, A. L., Armstrong, C. D., and Kirby, W. M. (1945). The treatment of subacute bacterial endocarditis with penicillin. J. Clin. Invest. 24, 251–267.
Brackman, G., and Coenye, T. (2015). In vitro and in vivo biofilm wound models and their application. Adv. Exp. Med. Biol. 897, 15–32. doi: 10.1007/5584_2015_5002
Brouillette, E., Hyodo, M., Hayakawa, Y., Karaolis, D. K. R., and Malouin, F. (2005). 3′,5′-cyclic diguanylic acid reduces the virulence of biofilm-forming Staphylococcus aureus strains in a mouse model of mastitis infection. Antimicrob. Agents Chemother. 49, 3109–3113. doi: 10.1128/aac.49.8.3109-3113.2005
Bundgaard, H., Ihlemann, N., Gill, S. U., Bruun, N. E., Elming, H., Madsen, T., et al. (2019). Long-term outcomes of partial oral treatment of endocarditis. N. Engl. J. Med. 380, 1373–1374.
Butt, J. H., Fosbøl, E. L., Verhamme, P., Gerds, T. A., Iversen, K., Bundgaard, H., et al. (2020). Dabigatran and the risk of Staphylococcus aureus bacteremia: a nationwide cohort study. Clin. Infect. Dis. [Epub ahead of print].
Cabell, C. H., Jollis, J. G., Peterson, G. E., Corey, G. R., Anderson, D. J., Sexton, D. J., et al. (2002). Changing patient characteristics and the effect on mortality in endocarditis. Arch. Intern. Med. 162, 90–94. doi: 10.1097/00019048-200202000-00017
Cao, B., Christophersen, L., Kolpen, M., Jensen, P. Ø, Sneppen, K., Høiby, N., et al. (2016). Diffusion retardation by binding of tobramycin in an alginate biofilm model. PLoS One 11:e0153616. doi: 10.1371/journal.pone.0153616
Cao, B., Christophersen, L., Thomsen, K., Sønderholm, M., Bjarnsholt, T., and Jensen, P. Ø, et al. (2015). Antibiotic penetration and bacterial killing in a Pseudomonas aeruginosa biofilm model. J. Antimicrob. Chemother. 70, 2057–2063.
Chan, K. L., Dumesnil, J. G., Cujec, B., Sanfilippo, A. J., Jue, J., Turek, M. A., et al. (2003). A randomized trial of aspirin on the risk of embolic events in patients with infective endocarditis. J. Am. Coll. Cardiol. 42, 775–780. doi: 10.1016/s0735-1097(03)00829-5
Chauhan, A., Lebeaux, D., Decante, B., Kriegel, I., Escande, M.-C., Ghigo, J.-M., et al. (2012). A rat model of central venous catheter to study establishment of long-term bacterial biofilm and related acute and chronic infections. PLoS One 7:e37281. doi: 10.1371/journal.pone.0037281
Chavakis, T., Wiechmann, K., Preissner, K. T., and Herrmann, M. (2005). Staphylococcus aureus interactions with the endothelium. The role of bacterial “secretable expanded repertoire adhesive molecules” (SERAM) in disturbing host defense systems. Thrombosis Haemostasis 94, 278–285.
Cheung, A. L., Krishnan, M., Jaffe, E. A., and Fischetti, V. A. (1991). Fibrinogen acts as a bridging molecule in the adherence of Staphylococcus aureus to cultured human endothelial cells. J. Clin. Invest. 87, 2236–2245. doi: 10.1172/jci115259
Christiansen, J. G., Jensen, H. E., Johansen, L. K., Kochl, J., Koch, J., Aalbaek, B., et al. (2013). Porcine models of non-bacterial thrombotic endocarditis (NBTE) and infective endocarditis (IE) caused by Staphylococcus aureus: a preliminary study. J. Heart Valve Dis. 22, 368–376.
Christophersen, L., Schwartz, F. A., Lerche, C. J., Svanekjær, T., Kragh, K. N., Laulund, A. S., et al. (2020). In vivo demonstration of Pseudomonas aeruginosa biofilms as independent pharmacological microcompartments. J. Cystic Fibrosis 19, 996–1003. doi: 10.1016/j.jcf.2020.01.009
Ciofu, O., Rojo-Molinero, E., Macià, M. D., and Oliver, A. (2017). Antibiotic treatment of biofilm infections. APMIS 125, 304–319. doi: 10.1111/apm.12673
Claes, J., Liesenborghs, L., Lox, M., Verhamme, P., Vanassche, T., and Peetermans, M. (2015). In vitro and in vivo model to study bacterial adhesion to the vessel wall under flow conditions. J. Vis. Exp. 100:e52862.
Claes, J., Vanassche, T., Peetermans, M., Liesenborghs, L., Vandenbriele, C., Vanhoorelbeke, K., et al. (2014). Adhesion of Staphylococcus aureus to the vessel wall under flow is mediated by von Willebrand factor-binding protein. Blood 124, 1669–1676. doi: 10.1182/blood-2014-02-558890
Coenye, T., and Nelis, H. J. (2010). In vitro and in vivo model systems to study microbial biofilm formation. J. Microbiol. Methods 83, 89–105. doi: 10.1016/j.mimet.2010.08.018
Collins, J.-A., Rudenski, A., Gibson, J., Howard, L., and O’Driscoll, R. (2015). Relating oxygen partial pressure, saturation and content: the haemoglobin-oxygen dissociation curve. Breathe (Sheff.) 11, 194–201. doi: 10.1183/20734735.001415
Colomer-Winter, C., Flores-Mireles, A. L., Baker, S. P., Frank, K. L., Lynch, A. J. L., Hultgren, S. J., et al. (2018a). Manganese acquisition is essential for virulence of Enterococcus faecalis. PLoS Pathog. 14:e1007102. doi: 10.1371/journal.ppat.1007102
Colomer-Winter, C., Gaca, A. O., Chuang-Smith, O. N., Lemos, J. A., and Frank, K. L. (2018b). Basal levels of (p)ppgpp differentially affect the pathogenesis of infective endocarditis in enterococcus faecalis. Microbiology (United Kingdom) 164, 1254–1265. doi: 10.1099/mic.0.000703
Cooke, W. T., and Taylor, A. B. (1943). Treatment of subacute infective endocarditis with heparin and chemotherapy. Br. Heart J. 5, 229–237. doi: 10.1136/hrt.5.4.229
Cremieux, A. C., and Carbon, C. (1992). Pharmacokinetic and pharmacodynamic requirements for antibiotic therapy of experimental endocarditis. Antimicrob. Agents Chemother. 36, 2069–2074. doi: 10.1128/aac.36.10.2069
Cremieux, A. C., Maziere, B., Vallois, J. M., Ottaviani, M., Azancot, A., Raffoul, H., et al. (1989). Evaluation of antibiotic diffusion into cardiac vegetations by quantitative autoradiography. J. Infect. Dis. 159, 938–944. doi: 10.1093/infdis/159.5.938
Crosby, H. A., Schlievert, P. M., Merriman, J. A., King, J. M., Salgado-Pabón, W., and Horswill, A. R. (2016). The Staphylococcus aureus global regulator MgrA modulates clumping and virulence by controlling surface protein expression. PLoS Pathog. 12:e1005604. doi: 10.1371/journal.ppat.1005604
Dahl, A., Rasmussen, R. V., Bundgaard, H., Hassager, C., Bruun, L. E., Lauridsen, T. K., et al. (2013). Enterococcus faecalis infective endocarditis: a pilot study of the relationship between duration of gentamicin treatment and outcome. Circulation 127, 1810–1817. doi: 10.1161/circulationaha.112.001170
Degraff, A. C. (1941). The present status of the treatment of subacute bacterial endocarditis. Bull. N. Y. Acad. Med. 17, 423–433.
Di Domenico, E. G., Rimoldi, S. G., Cavallo, I., D’Agosto, G., Trento, E., Cagnoni, G., et al. (2019). Microbial biofilm correlates with an increased antibiotic tolerance and poor therapeutic outcome in infective endocarditis. BMC Microbiol. 19:228. doi: 10.1186/s12866-019-1596-2
Di Salvo, G., Habib, G., Pergola, V., Avierinos, J.-F., Philip, E., Casalta, J.-P., et al. (2001). Echocardiography predicts embolic events in infective endocarditis. J. Am. Coll. Cardiol. 37, 1069–1076. doi: 10.1016/s0735-1097(00)01206-7
Ditkowski, B., Veloso, T. R., Bezulska-Ditkowska, M., Lubig, A., Jockenhoevel, S., Mela, P., et al. (2018). An In Vitro model of a parallel-plate perfusion system to study bacterial adherence to graft tissues. J. Vis. Exp. 143:e58476.
Dittmeier, M., Wassmuth, K., Schuhmann, M. K., Kraft, P., Kleinschnitz, C., and Fluri, F. (2016). Dabigatran etexilate reduces thrombin-induced inflammation and thrombus formation in experimental ischemic stroke. Curr. Neurovasc. Res. 13, 199–206. doi: 10.2174/1567202613666160517122605
Durack, D. T. (1974). Experimental bacterial endocarditis - IV. Structure and evolution of very early lesions. J. Pathol. 115, 81–89. doi: 10.1002/path.1711150204
Durack, D. T., and Beeson, P. B. (1972a). Experimental bacterial endocarditis. I. Colonization of a sterile vegetation. Br. J. Exp. Pathol. 53, 44–49.
Durack, D. T., and Beeson, P. B. (1972b). Experimental bacterial endocarditis. II. Survival of a bacteria in endocardial vegetations. Br. J. Exp. Pathol. 53, 50–53.
Durack, D. T., Beeson, P. B., and Petersdorf, R. G. (1973). Experimental bacterial endocarditis. 3. Production and progress of the disease in rabbits. Br. J. Exp. Pathol. 54, 142–151.
Dwyer, D. J., Collins, J. J., and Walker, G. C. (2015). Unraveling the physiological complexities of antibiotic lethality. Ann. Rev. Pharmacol. Toxicol. 55, 313–332. doi: 10.1146/annurev-pharmtox-010814-124712
Edwards, A. M., Bowden, M. G., Brown, E. L., Laabei, M., and Massey, R. C. (2012). Staphylococcus aureus extracellular adherence protein triggers TNFα release, promoting attachment to endothelial cells via protein A. PLoS One 7:e43046. doi: 10.1371/journal.pone.0043046
Elgharably, H., Hussain, S. T., Shrestha, N. K., Blackstone, E. H., and Pettersson, G. B. (2016). Current hypotheses in cardiac surgery: biofilm in infective endocarditis. Semin. Thorac. Cardiovasc. Surg. 28, 56–59. doi: 10.1053/j.semtcvs.2015.12.005
Elgharably, H., Hussain, S. T., Shrestha, N. K., and Pettersson, G. B. (2018). “Biofilm in infective endocarditis and clinical implications,” in Biofilm, Pilonidal Cysts and Sinuses. Recent Clinical Techniques, Results, and Research in Wounds, Vol. 1, eds M. Shiffman and M. Low (Cham: Springer), 109–120. doi: 10.1007/15695_2018_109
Entenza, J.-M., Moreillon, P., Senn, M. M., Kormanec, J., Dunman, P. M., Berger-Bächi, B., et al. (2005). Role of sigmaB in the expression of Staphylococcus aureus cell wall adhesins ClfA and FnbA and contribution to infectivity in a rat model of experimental endocarditis. Infect. Immun. 73, 990–998. doi: 10.1128/iai.73.2.990-998.2005
Escobar, S. J., Slade, J. B., Hunt, T. K., and Cianci, P. (2005). Adjuvant hyperbaric oxygen therapy (HBO2)for treatment of necrotizing fasciitis reduces mortality and amputation rate. Undersea Hyperb. Med. 32, 437–443.
Fernández Guerrero, M. L., Álvarez, B., Manzarbeitia, F., and Renedo, G. (2012). Infective endocarditis at autopsy: a review of pathologic manifestations and clinical correlates. Medicine (Baltimore) 91, 152–164. doi: 10.1097/md.0b013e31825631ea
Fernández-Hidalgo, N., Almirante, B., Gavaldà, J., Gurgui, M., Peña, C., De Alarcón, A., et al. (2013). Ampicillin plus ceftriaxone is as effective as ampicillin plus gentamicin for treating enterococcus faecalis infective endocarditis. Clin. Infect. Dis. 56, 1261–1268.
Fisher, R. A., Gollan, B., and Helaine, S. (2017). Persistent bacterial infections and persister cells. Nat. Rev. Microbiol. 15, 453–464. doi: 10.1038/nrmicro.2017.42
Foster, T. J., and Höök, M. (1998). Surface protein adhesins of Staphylococcus aureus. Trends Microbiol. 6, 484–488. doi: 10.1016/s0966-842x(98)01400-0
Fowler, V. G., Miro, J. M., Hoen, B., Cabell, C. H., Abrutyn, E., Rubinstein, E., et al. (2005). Staphylococcus aureus endocarditis: a consequence of medical progress. JAMA 293, 3012–3021.
Francioli, P., Ruch, W., and Stamboulian, D. (1995). Treatment of streptococcal endocarditis with a single daily dose of ceftriaxone and netilmicin for 14 Days: a prospective multicenter study. Clin. Infect. Dis. 21, 1406–1410. doi: 10.1093/clinids/21.6.1406
Frickmann, H., Zautner, A. E., Moter, A., Kikhney, J., Hagen, R. M., Stender, H., et al. (2017). Fluorescence in situ hybridization (FISH) in the microbiological diagnostic routine laboratory: a review. Crit. Rev. Microbiol. 43, 263–293.
Gade, P. A. V., Olsen, T. B., Jensen, P. Ø, Kolpen, M., Høiby, N., and Henneberg, K. -Å, et al. (2018). Modelling of ciprofloxacin killing enhanced by hyperbaric oxygen treatment in Pseudomonas aeruginosa PAO1 biofilms. Omri A, editor. PLoS One 13:e0198909. doi: 10.1371/journal.pone.0198909
Garcia, L. G., Lemaire, S., Kahl, B. C., Becker, K., Proctor, R. A., Denis, O., et al. (2013). Antibiotic activity against small-colony variants of Staphylococcus aureus: review of in vitro, animal and clinical data. J. Antimicrob. Chemother. 68, 1455–1464. doi: 10.1093/jac/dkt072
García-Cabrera, E., Fernández-Hidalgo, N., Almirante, B., Ivanova-Georgieva, R., Noureddine, M., Plata, A., et al. (2013). Neurological complications of infective endocarditis: risk factors, outcome, and impact of cardiac surgery: a multicenter observational study. Circulation 127, 2272–2284. doi: 10.1161/circulationaha.112.000813
Garrison, M. W., Vance-Bryan, K., Larson, T. A., Toscano, J. P., and Rotschafer, J. C. (1990). Assessments of effects of protein binding on daptomycin and vancomycin killing of Staphylococcus aureus by using an in vitro pharmacodynamic model. Antimicrob. Agents Chemother. 34, 1925–1931. doi: 10.1128/aac.34.10.1925
Garrison, P. K., and Freedman, L. R. (1970). Experimental endocarditis I. Staphylococcal endocarditis in rabbits resulting from placement of a polyethylene catheter in the right side of the heart. Yale J. Biol. Med. 42, 394–410.
Gavaldá, J., Onrubia, P. L., Gómez, M. T. M., Gomis, X., Ramírez, J. L., Len, O., et al. (2003). Efficacy of ampicillin combined with ceftriaxone and gentamicin in the treatment of experimental endocarditis due to Enterococcus faecalis with no high-level resistance to aminoglycosides. J. Antimicrob. Chemother. 52, 514–517. doi: 10.1093/jac/dkg360
Gibson, G. W., Kreuser, S. C., Riley, J. M., Rosebury-smith, W. S., Courtney, C. L., Juneau, P. L., et al. (2015). Development of a Mouse model of induced Staphylococcus aureus infective endocarditis. Comp. Med. 57, 563–569.
Gilbey, T., Ho, J., Cooley, L. A., Petrovic Fabijan, A., and Iredell, J. R. (2019). Adjunctive bacteriophage therapy for prosthetic valve endocarditis due to Staphylococcus aureus. Med. J. Aust. 211, 142–143.e1.
Gill, A. L., and Bell, C. N. A. (2004). Hyperbaric oxygen: its uses, mechanisms of action and outcomes. QJM 97, 385–395. doi: 10.1093/qjmed/hch074
Gomes, A., van Oosten, M., Bijker, K. L. B., Boiten, K. E., Salomon, E. N., Rosema, S., et al. (2018). Sonication of heart valves detects more bacteria in infective endocarditis. Sci. Rep. 8:12967.
Gould, K., Ramirez-Ronda, C. H., Holmes, R. K., and Sanford, J. P. (1975). Adherence of bacteria to heart valves in vitro. J. Clin. Invest.. 56, 1364–1370. doi: 10.1172/jci108216
Grimberg-Peters, D., Büren, C., Windolf, J., Wahlers, T., and Paunel-Görgülü, A. (2016). Hyperbaric oxygen reduces production of reactive oxygen species in neutrophils from polytraumatized patients yielding in the inhibition of p38 MAP kinase and downstream pathways. van Griensven M, editor. PLoS One 11:e0161343. doi: 10.1371/journal.pone.0161343
Gutschik, E. (1982). Experimental endocarditis in rabbits. 7. Results of long-term combined therapy of Streptococcus faecalis endocarditis with penicillin and gentamicin. Acta Pathol. Microbiol. Immunol. Scand. B 90, 295–302. doi: 10.1111/j.1699-0463.1982.tb00121.x
Gutschik, E., and Christensen, N. (1978a). Experimental endocarditis in rabbits. I. Technique and spontaneous course of non-bacterial thrombotic endocarditis. Acta Pathol. Microbiol. Immunol. Scand. B 86, 215–221. doi: 10.1111/j.1699-0463.1978.tb00034.x
Gutschik, E., and Christensen, N. (1978b). Experimental endocarditis in rabbits. 2. Course of untreated Streptococcus faecalis infection. Acta Pathol. Microbiol. Immunol. Scand. B 86, 223–228. doi: 10.1111/j.1699-0463.1978.tb00035.x
Gutschik, E., Møller, S., and Christensen, N. (1979). Experimental endocarditis in rabbits. 3. Significance of the proteolytic capacity of the infecting strains of Streptococcus faecalis. Acta Pathol. Microbiol. Immunol. Scand. B 87, 353–362. doi: 10.1111/j.1699-0463.1979.tb02450.x
Gutschik, E., Mortensen, I., and Møller, S. (1982). Experimental endocarditis in rabbits. 5. Results of long-term penicillin or streptomycin treatment of Streptococcus faecalis endocarditis and the effect of long-term exposure of healthy rabbits to the same drugs. Acta Pathol. Microbiol. Immunol. Scand. B 90, 25–35. doi: 10.1111/j.1699-0463.1982.tb00078.x
Habib, G., Hoen, B., Tornos, P., Thuny, F., Prendergast, B., Vilacosta, I., et al. (2009). Guidelines on the prevention, diagnosis, and treatment of infective endocarditis (new version 2009): the task force on the prevention, diagnosis, and treatment of infective endocarditis of the European society of cardiology (ESC). Endorsed by the European. Eur. Heart J. 30, 2369–2413.
Habib, G., Lancellotti, P., Antunes, M. J., Bongiorni, M. G., Casalta, J.-P., Del Zotti, F., et al. (2015). 2015 ESC guidelines for the management of infective endocarditis. Eur. Heart J. 36, 3075–3128.
Hall, G., Heimdahl, A., and Nord, C. E. (1999). Bacteremia after oral surgery and antibiotic prophylaxis for endocarditis. Clin. Infect. Dis. 29, 1–10. doi: 10.1086/520134
Haller, C., Berthold, M., Wobser, D., Kropec, A., Lauriola, M., Schlensak, C., et al. (2014). Cell-Wall glycolipid mutations and their effects on virulence of E. faecalis in a rat model of infective endocarditis. PLoS One 9:e91863. doi: 10.1371/journal.pone.0091863
Hansen, M. B., Simonsen, U., Garred, P., Hyldegaard, O., and Norrby-Teglund, A. (2015). Biomarkers of necrotising soft tissue infections: aspects of the innate immune response and effects of hyperbaric oxygenation–the protocol of the prospective cohort BIONEC study. BMJ Open 5:e006995. doi: 10.1136/bmjopen-2014-006995
Hanses, F., Roux, C., Dunman, P. M., Salzberger, B., and Lee, J. C. (2014). Staphylococcus aureus gene expression in a rat model of infective endocarditis. Genome Med. 6:93. doi: 10.1186/preaccept-4819325051343079
Heiro, M., Nikoskelainen, J., Engblom, E., Kotilainen, E., Marttila, R., and Kotilainen, P. (2000). Neurologic manifestations of infective endocarditis: a 17-year experience in a teaching hospital in Finland. Arch. Intern. Med. 160, 2781–2787. doi: 10.1001/archinte.160.18.2781
Hengzhuang, W., Wu, H., Ciofu, O., Song, Z., and Høiby, N. (2011). Pharmacokinetics/pharmacodynamics of colistin and imipenem on mucoid and nonmucoid Pseudomonas aeruginosa biofilms. Antimicrob. Agents Chemother. 55, 4469–4474. doi: 10.1128/aac.00126-11
Henshaw, I. N., and Simpson, A. (1857). Compressed air as a Therapeutic Agent in the Treatment of Consumption, Asthma, Chronic Bronchitis and Other Diseases. Edinburgh: Sutherland and Knox.
Heraief, E., Glauser, M. P., and Freedman, L. R. (1982). Natural history of aortic valve endocarditis in rats. Infect. Immun. 37, 127–131. doi: 10.1128/iai.37.1.127-131.1982
Herrera, A., Kulhankova, K., Sonkar, V. K., Dayal, S., Klingelhutz, A. J., Salgado-Pabon, W., et al. (2017). Staphylococcal b -Toxin modulates human aortic endothelial cell and platelet function through sphingomyelinase and biofilm ligase activities. mBio 8:e00273-17.
Herrera, A., Stach, C. S., Merriman, J. A., Horswill, A. R., Salgado-Pabon, W., and Schlievert, P. M. (2016). Staphylococcus aureus β-Toxin mutants are defective in biofilm ligase and sphingomyelinase activity, and causation of infective endocarditis/sepsis. Biochemistry 55, 2510–2517. doi: 10.1021/acs.biochem.6b00083
Herrmann, G., Yang, L., Wu, H., Song, Z., Wang, H., Høiby, N., et al. (2010). Colistin-tobramycin combinations are superior to monotherapy concerning the killing of biofilm Pseudomonas aeruginosa. J. Infect. Dis. 202, 1585–1592. doi: 10.1086/656788
Hershberger, E., Coyle, E. A., Kaatz, G. W., Zervos, M. J., and Rybak, M. J. (2000). Comparison of a rabbit model of bacterial endocarditis and an in vitro infection model with simulated endocardial vegetations. Antimicrob. Agents Chemother. 44, 1921–1924. doi: 10.1128/aac.44.7.1921-1924.2000
Hess, A., Klein, I., Iung, B., Lavallée, P., Ilic-Habensus, E., Dornic, Q., et al. (2013). Brain MRI findings in neurologically asymptomatic patients with infective endocarditis. Am. J. Neuroradiol. 34, 1579–1584. doi: 10.3174/ajnr.a3582
Hienz, S. A., Schennings, T., Heimdahl, A., and Flock, J.-I. (1996). Collagen binding of Staphylococcus aureus is a virulence factor in experimental endocarditis. J. Infect. Dis. 174, 83–88. doi: 10.1093/infdis/174.1.83
Hobby, G. L., Meyer, K., and Chaffee, E. (1942). Observations on the mechanism of action of penicillin. Exp. Biol. Med. 50, 281–285. doi: 10.3181/00379727-50-13773
Hoen, B., and Duval, X. (2013). Clinical practice. Infective endocarditis. N. Engl. J. Med. 368, 1425–1433.
Høiby, N., Bjarnsholt, T., Givskov, M., Molin, S., and Ciofu, O. (2010). Antibiotic resistance of bacterial biofilms. Int. J. Antimicrob. Agents 35, 322–332.
Høiby, N., Bjarnsholt, T., Moser, C., Bassi, G. L. L., Coenye, T., Donelli, G., et al. (2015). ESCMID guideline for the diagnosis and treatment of biofilm infections 2014. Clin. Microbiol. Infect. 21, S1–S25.
Høiby, N., Flensborg, E. W., Friis, B., Jacobsen, S. V., and Jacobsen, L. (1977). Pseudomonas aeruginosa infection in cystic fibrosis. Diagnostic and prognostic significance of Pseudomonas aeruginosa precipitins determined by means of crossed immunoelectrophoresis. Scand. J. Respir. Dis. 58, 65–79.
Holland, T., Baddour, L., Bayer, A., Hoen, B., Miro, J., and Fowler, V. G. (2017). Infective endocarditis. Nat. Rev. Dis. Primers 2:16059.
Hsu, C.-C., Hsu, R.-B., Ohniwa, R. L., Chen, J.-W., Yuan, C.-T., Chia, J.-S., et al. (2019). Neutrophil extracellular traps enhance Staphylococcus Aureus vegetation formation through interaction with platelets in infective endocarditis. Thromb Haemost 119, 786–796. doi: 10.1055/s-0039-1678665
Hunter, T. H. (1947). Use of streptomycin in the treatment of bacterial endocarditis. Am. J. Med. 2, 436–442. doi: 10.1016/0002-9343(47)90088-0
Illingworth, B. L., Tweden, K., Schroeder, R. F., and Cameron, J. D. (1998). In vivo efficacy of silver-coated (Silzone(TM)) infection-resistant polyester fabric against a biofilm-producing bacteria, Staphylococcus epidermidis. J. Heart Valve Dis. 7, 524–530.
Iversen, K., Ihlemann, N., Gill, S. U., Madsen, T., Elming, H., Jensen, K. T., et al. (2019). Partial oral versus intravenous antibiotic treatment of endocarditis. N. Engl. J. Med. 380, 415–424. doi: 10.1056/nejmoa1808312
Jahanbakhsh, S., Singh, N. B., Yim, J., Kebriaei, R., Smith, J. R., Lev, K., et al. (2020). Impact of daptomycin dose exposure alone or in combination with β-lactams or rifampin against vancomycin-resistant enterococci in an in Vitro biofilm model. Antimicrob. Agents Chemother. 64:e02074-19.
Jahanbakhsh, S., Singh, N. B., Yim, J., Rose, W. E., and Rybak, M. J. (2018). Evaluation of telavancin alone and combined with ceftaroline or rifampin against methicillin-resistant Staphylococcus aureus in an in vitro biofilm model. Antimicrob. Agents Chemother. 62:e00567-18.
Jarcho, S. (1967). Artificial insufficiency of heart valves (Rosenbach, 1878). Am. J. Cardiol. 19, 850–853. doi: 10.1016/0002-9149(67)90507-3
Jarcho, S. (1972). Experimental myocarditis and endocarditis (Ribbert, 1886). Am. J. Cardiol. 30, 865–867. doi: 10.1016/0002-9149(72)90011-2
Jawad, K., Lehmann, S., Koziarz, A., Dieterlen, M., Feder, S., Misfeld, M., et al. (2020). Midterm results after St Jude medical epic porcine xenograft for aortic, mitral, and double valve replacement. J. Card. Surg. 35, 1769–1777. doi: 10.1111/jocs.14554
Jensen, A. D., Bundgaard, H., Butt, J. H., Bruun, N. E., Voldstedlund, M., Torp-Pedersen, C., et al. (2020). Temporal changes in the incidence of infective endocarditis in Denmark 1997–2017: a nationwide study. Int. J. Cardiol. 326, 145–152. doi: 10.1016/j.ijcard.2020.10.029
Jensen, P., Briales, A., Brochmann, R. P., Wang, H., Kragh, K. N., Kolpen, M., et al. (2014). Formation of hydroxyl radicals contributes to the bactericidal activity of ciprofloxacin against Pseudomonas aeruginosa biofilms. Pathog. Dis. 70, 440–443. doi: 10.1111/2049-632x.12120
Jensen, P. Ø, Givskov, M., Bjarnsholt, T., and Moser, C. (2010). The immune system vs. Pseudomonas aeruginosa biofilms. FEMS Immunol. Med. Microbiol. 59, 292–305.
Jensen, P. Ø, Kolpen, M., Kragh, K. N., and Kühl, M. (2017). Microenvironmental characteristics and physiology of biofilms in chronic infections of CF patients are strongly affected by the host immune response. APMIS 125, 276–288. doi: 10.1111/apm.12668
Johnson, C. M., Bahn, R. C., and Fass, D. N. (1986). Experimental porcine infective endocarditis: description of a clinical model. Vet. Pathol. 23, 780–782. doi: 10.1177/030098588602300620
Jones, J. E. T. (1972). Experimental bacterial endocarditis in the pig. Proc. R. Soc. Med. 65, 990–994. doi: 10.1177/003591577206501138
Jones, J. E. T. (1981). Experimental streptococcal endocarditis in the pig: the development of lesions 3 to 14 days after inoculation. J. Comp. Pathol. 91, 51–62. doi: 10.1016/0021-9975(81)90044-x
Jones, J. E. T. (1982). Experimental streptococcal endocarditis in the pig: the development of lesions 18 to 48 hours after inoculation. J. Comp. Pathol. 92, 301–308. doi: 10.1016/0021-9975(82)90089-5
Jung, C.-J., Yeh, C.-Y., Shun, C.-T., Hsu, R.-B., Cheng, H.-W., Lin, C.-S., et al. (2012). Platelets enhance biofilm formation and resistance of endocarditis-inducing streptococci on the injured heart valve. J. Infect. Dis. 205, 1066–1075. doi: 10.1093/infdis/jis021
Jüttner, B., Scheinichen, D., Bartsch, S., Heine, J., Ruschulte, H., Elsner, H. A., et al. (2003). Lack of toxic side effects in neutrophils following hyperbaric oxygen. Undersea Hyperb. Med. 30, 305–311.
Kang, S. L., and Rybak, M. J. (1995). Pharmacodynamics of RP 59500 alone and in combination with vancomycin against Staphylococcus aureus in an in vitro-infected fibrin clot model. Antimicrob. Agents Chemother. 39, 1505–1511. doi: 10.1128/aac.39.7.1505
Kanick, S. C., Schneider, P. A., Klitzman, B., Wisniewski, N. A., and Rebrin, K. (2019). Continuous monitoring of interstitial tissue oxygen using subcutaneous oxygen microsensors: in vivo characterization in healthy volunteers. Microvasc. Res. 124, 6–18. doi: 10.1016/j.mvr.2019.02.002
Karaolis, D. K. R., Rashid, M. H., Chythanya, R., Luo, W., Hyodo, M., and Hayakawa, Y. (2005). c-di-GMP (3′-5′-cyclic diguanylic acid) inhibits Staphylococcus aureus cell-cell interactions and biofilm formation. Antimicrob. Agents Chemother. 49, 1029–1038. doi: 10.1128/aac.49.3.1029-1038.2005
Kauffmann, R. H., Thompson, J., Valentijn, R. M., Daha, M. R., and Van Es, L. A. (1981). The clinical implications and the pathogenetic significance of circulating immune complexes in infective endocarditis. Am. J. Med. 71, 17–25. doi: 10.1016/0002-9343(81)90253-9
Kaur, S., Pawar, M., Banerjee, N., and Garg, R. (2012). Evaluation of the efficacy of hyperbaric oxygen therapy in the management of chronic nonhealing ulcer and role of periwound transcutaneous oximetry as a predictor of wound healing response: a randomized prospective controlled trial. J. Anaesthesiol. Clin. Pharmacol. 28, 70–75. doi: 10.4103/0970-9185.92444
Kebriaei, R., Lev, K. L., Stamper, K. C., Lehman, S. M., Morales, S., and Rybak, M. J. (2021). Bacteriophage AB-SA01 cocktail in combination with antibiotics against mrsa-visa strain in an in vitro pharmacokinetic/pharmacodynamic model. Antimicrob. Agents Chemother. 65:e01863-20.
Kebriaei, R., Rice, S. A., Singh, K. V., Stamper, K. C., Dinh, A. Q., Rios, R., et al. (2018). Influence of inoculum effect on the efficacy of daptomycin monotherapy and in combination with b-lactams against daptomycin-susceptible Enterococcus faecium harboring LiaSR substitutions. Antimicrob. Agents Chemother. 62:e00315-18.
Kebriaei, R., Rice, S. A., Stamper, K. C., and Rybak, M. J. (2019a). Dalbavancin alone and in combination with ceftaroline against four different phenotypes of Staphylococcus aureus in a simulated pharmacodynamic/pharmacokinetic model. Antimicrob. Agents Chemother. 63:e01743-18.
Kebriaei, R., Rice, S. A., Stamper, K. C., Seepersaud, R., Garcia-De-la-Maria, C., Mishra, N. N., et al. (2019b). Daptomycin dose-ranging evaluation with single-dose versus multidose ceftriaxone combinations against streptococcus mitis/oralis in an ex vivo simulated endocarditis vegetation model. Antimicrob. Agents Chemother. 63, 1–11.
Kerrigan, S. W., Clarke, N., Loughman, A., Meade, G., Foster, T. J., and Cox, D. (2008). Molecular basis for Staphylococcus aureus-mediated platelet aggregate formation under arterial shear in vitro. Arterioscler. Thromb. Vasc. Biol. 28, 335–340. doi: 10.1161/atvbaha.107.152058
King, J. M., Kulhankova, K., Stach, C. S., Vu, B. G., and Salgado-pabón, W. (2016). Phenotypes and virulence among Staphylococcus aureus USA100, USA200, USA300, USA400, and USA600 clonal lineages. mSphere 1:e00071-16.
Kjerulf, A., Espersen, F., Gutschik, E., Majcherczyk, P. A., Hougen, H. P., Rygaard, J., et al. (1998). Serological diagnosis of experimental Enterococcus faecalis endocarditis. APMIS 106, 997–1008.
Kjerulf, A., Tvede, M., and Høiby, N. (1993). Crossed immunoelectrophoresis used for bacteriological diagnosis in patients with endocarditis. APMIS 101, 746–752. doi: 10.1111/j.1699-0463.1993.tb00175.x
Kohanski, M. A., Dwyer, D. J., Hayete, B., Lawrence, C. A., and Collins, J. J. A. (2007). Common mechanism of cellular death induced by bactericidal antibiotics. Cell 130, 797–810. doi: 10.1016/j.cell.2007.06.049
Kolpen, M., Lerche, C. J., Kragh, K. N., Sams, T., Koren, K., Jensen, A. S., et al. (2017). Hyperbaric oxygen sensitizes anoxic Pseudomonas aeruginosa biofilm to ciprofloxacin. Antimicrob. Agents Chemother. 61:e01024-17.
Kolpen, M., Mousavi, N., Sams, T., Bjarnsholt, T., Ciofu, O., Moser, C., et al. (2016). Reinforcement of the bactericidal effect of ciprofloxacin on Pseudomonas aeruginosa biofilm by hyperbaric oxygen treatment. Int. J. Antimicrob. Agents 47, 163–167. doi: 10.1016/j.ijantimicag.2015.12.005
Korhonen, K. (2000). Hyperbaric oxygen therapy in acute necrotizing infections. With a special reference to the effects on tissue gas tensions. Ann. Chir. Gynaecol. 214, 7–36.
Kragh, K. N., Hutchison, J. B., Melaugh, G., Rodesney, C., Roberts, A. E. L., Irie, Y., et al. (2016). Role of multicellular aggregates in biofilm formation. mBio 7:e00237.
Kroh, H. K., Panizzi, P., and Bock, P. E. (2009). Von Willebrand factor-binding protein is a hysteretic conformational activator of prothrombin. Proc. Natl. Acad. Sci. U.S.A. 106, 7786–7791. doi: 10.1073/pnas.0811750106
Kupferwasser, L. I., Yeaman, M. R., Nast, C. C., Kupferwasser, D., Xiong, Y.-Q., Palma, M., et al. (2003). Salicylic acid attenuates virulence in endovascular infections by targeting global regulatory pathways in Staphylococcus aureus. J. Clin. Invest. 112, 222–233. doi: 10.1172/jci200316876
Kupferwasser, L. I., Yeaman, M. R., Shapiro, S. M., Nast, C. C., Sullam, P. M., Filler, S. G., et al. (1999). Acetylsalicylic acid reduces vegetation bacterial density, hematogenous bacterial dissemination, and frequency of embolic events in experimental Staphylococcus aureus endocarditis through antiplatelet and antibacterial effects. Circulation 99, 2791–2797. doi: 10.1161/01.cir.99.21.2791
Lalani, T., Kanafani, Z. A., Chu, V. H., Moore, L., Corey, G. R., Pappas, P., et al. (2006). Prosthetic valve endocarditis due to coagulase-negative staphylococci: findings from the international collaboration on endocarditis merged database. Eur. J. Clin. Microbiol. Infect. Dis. 25, 365–368. doi: 10.1007/s10096-006-0141-z
LaPlante, K. L., and Rybak, M. J. (2004). Impact of high-inoculum Staphylococcus aureus on the activities of nafcillin, vancomycin, linezolid, and daptomycin, alone and in combination with gentamicin, in an in vitro pharmacodynamic model. Antimicrob. Agents Chemother. 48, 4665–4672. doi: 10.1128/aac.48.12.4665-4672.2004
Lauten, A., Martinović, M., Kursawe, L., Kikhney, J., Affeld, K., Kertzscher, U., et al. (2020). Bacterial biofilms in infective endocarditis: an in vitro model to investigate emerging technologies of antimicrobial cardiovascular device coatings. Clin. Res. Cardiol. 110, 323–331. doi: 10.1007/s00392-020-01669-y
Laxdal, T., Messner, R. P., Williams, R. C. Jr., and Quie, P. G. (1968). Opsonic, agglutinating, and complement-fixing antibodies in patients with subacute bacterial endocarditis. J. Lab. Clin. Med. 71, 638–653.
Lebeaux, D., Chauhan, A., Rendueles, O., and Beloin, C. (2013). From in vitro to in vivo models of bacterial biofilm-related infections. Pathogens 2, 288–356. doi: 10.3390/pathogens2020288
Lerche, C. J., Christophersen, L. J., Goetze, J. P., Nielsen, P. R., Thomsen, K., Enevold, C., et al. (2019). Adjunctive dabigatran therapy improves outcome of experimental left-sided Staphylococcus aureus endocarditis. Garcia de Frutos P, editor. PLoS One 14:e0215333. doi: 10.1371/journal.pone.0215333
Lerche, C. J., Christophersen, L. J., Kolpen, M., Nielsen, P. R., Trøstrup, H., Thomsen, K., et al. (2017). Hyperbaric oxygen therapy augments tobramycin efficacy in experimental Staphylococcus aureus endocarditis. Int. J. Antimicrob. Agents 50, 406–412. doi: 10.1016/j.ijantimicag.2017.04.025
Lerche, C. J., Christophersen, L. J., Trøstrup, H., Thomsen, K., Jensen, P., Hougen, H. P., et al. (2015). Low efficacy of tobramycin in experimental Staphylococcus aureus endocarditis. Eur. J. Clin. Microbiol. Infect. Dis. 34, 2349–2357. doi: 10.1007/s10096-015-2488-5
Liesenborghs, L., Meyers, S., Lox, M., Criel, M., Claes, J., Peetermans, M., et al. (2019). Staphylococcus aureus endocarditis: distinct mechanisms of bacterial adhesion to damaged and inflamed heart valves. Eur. Heart J. 40, 3248–3259. doi: 10.1093/eurheartj/ehz175
Liesenborghs, L., Peetermans, M., Claes, J., Veloso, T. R., Vandenbriele, C., Criel, M., et al. (2016). Shear-resistant binding to von willebrand factor allows Staphylococcus lugdunensis to adhere to the cardiac valves and initiate endocarditis. J. Infect. Dis. 213, 1148–1156. doi: 10.1093/infdis/jiv773
Loewe, L. (1945). The combined use of anti-infectives and anticoagulants in the treatment of subacute bacterial endocarditis. Bull. N. Y. Acad. Med. 21, 59–86.
Loughman, A., Fitzgerald, J. R., Brennan, M. P., Higgins, J., Downer, R., Cox, D., et al. (2005). Roles for fibrinogen, immunoglobulin and complement in platelet activation promoted by Staphylococcus aureus clumping factor A. Mol. Microbiol. 57, 804–818. doi: 10.1111/j.1365-2958.2005.04731.x
Mader, J. T., Adams, K. R., Wallace, W. R., and Calhoun, J. H. (1990). Hyperbaric oxygen as adjunctive therapy for osteomyelitis. Infect. Dis. Clin. North Am. 4, 433–440. doi: 10.1016/s0891-5520(20)30355-x
Mader, J. T., Guckian, J. C., Glass, D. L., and Reinarz, J. A. (1978). Therapy with hyperbaric oxygen for experimental osteomyelitis due to Staphylococcus aureus in rabbits. J. Infect. Dis. 138, 312–318. doi: 10.1093/infdis/138.3.312
Mallmann, C., Siemoneit, S., Schmiedel, D., Petrich, A., Gescher, D. M., Halle, E., et al. (2010). Fluorescence in situ hybridization to improve the diagnosis of endocarditis: a pilot study. Clin. Microbiol. Infect. 16, 767–773. doi: 10.1111/j.1469-0691.2009.02936.x
Marrie, T. J., Cooper, J. H., and Costerton, J. W. (1987). Ultrastructure of cardiac bacterial vegetations on native valves with emphasis on alterations in bacterial morphology following antibiotic treatment. Can. J. Cardiol. 3, 275–280.
Marrie, T. J., Nelligan, J., and Costerton, J. W. (1982). A scanning and transmission electron microscopic study of human nodular goitre. Circulation 66, 65–76. doi: 10.1111/j.1365-2559.1983.tb02216.x
Martini, A. M., Moricz, B. S., Ripperger, A. K., Tran, P. M., Sharp, M. E., Forsythe, A. N., et al. (2020). Association of novel Streptococcus sanguinis virulence factors with pathogenesis in a native valve infective endocarditis model. Front. Microbiol. 11:10. doi: 10.3389/fmicb.2020.00010
Maske, T. T., van de Sande, F. H., Arthur, R. A., Huysmans, M. C. D. N. J. M., and Cenci, M. S. (2017). In vitro biofilm models to study dental caries: a systematic review. Biofouling 33, 661–675. doi: 10.1080/08927014.2017.1354248
Maugeri, N., Campana, L., Gavina, M., Covino, C., De Metrio, M., Panciroli, C., et al. (2014). Activated platelets present high mobility group box 1 to neutrophils, inducing autophagy and promoting the extrusion of neutrophil extracellular traps. J. Thromb. Haemost. 12, 2074–2088. doi: 10.1111/jth.12710
McAdow, M., Kim, H. K., DeDent, A. C., Hendrickx, A. P. A., Schneewind, O., and Missiakas, D. M. (2011). Preventing Staphylococcus aureus sepsis through the inhibition of its agglutination in blood. Sullam PM, editor. PLoS Pathog. 7:e1002307. doi: 10.1371/journal.ppat.1002307
McGrath, B. J., Kang, S. L., Kaatz, G. W., and Rybak, M. J. (1994). Bactericidal activities of teicoplanin, vancomycin, and gentamicin alone and in combination against Staphylococcus aureus in an in vitro pharmacodynamic model of endocarditis. Antimicrob. Agents Chemother. 38, 2034–2040. doi: 10.1128/aac.38.9.2034
Mercier, R. C., Rybak, M. J., Bayer, A. S., and Yeaman, M. R. (2000). Influence of platelets and platelet microbicidal protein susceptibility on the fate of Staphylococcus aureus in an in vitro model of infective endocarditis. Infect. Drug Resist. 48, 2551–2557.
Moellering, R. C., and Weinberg, A. N. (1971). Studies on antibiotic synergism against enterococci. J. Clin. Invest. 50, 2580–2584. doi: 10.1172/jci106758
Monteiro, T. S., Correia, M. G., Golebiovski, W. F., Barbosa, G. I. F., Weksler, C., and Lamas, C. C. (2017). Asymptomatic and symptomatic embolic events in infective endocarditis: associated factors and clinical impact. Braz. J. Infect. Dis. 21, 240–247. doi: 10.1016/j.bjid.2017.01.006
Moreillon, P., Entenza, J. M., Francioli, P., McDevitt, D., Foster, T. J., François, P., et al. (1995). Role of Staphylococcus aureus coagulase and clumping factor in pathogenesis of experimental endocarditis. Infect. Immun. 63, 4738–4743. doi: 10.1128/iai.63.12.4738-4743.1995
Moreillon, P., Que, Y. A., and Bayer, A. S. (2002). Pathogenesis of streptococcal and staphylococcal endocarditis. Infect. Dis. Clin. North Am. 16, 297–318. doi: 10.1016/s0891-5520(01)00009-5
Morris, A. J., Drinkovic, D., Pottumarthy, S., Strickett, M. G., MacCulloch, D., Lambie, N., et al. (2003). Gram stain, culture, and histopathological examination findings for heart valves removed because of infective endocarditis. Clin. Infect. Dis. 36, 697–704. doi: 10.1086/367842
Moser, C., Pedersen, H. T., Lerche, C. J., Kolpen, M., Line, L., Thomsen, K., et al. (2017). Biofilms and host response–helpful or harmful. APMIS 125, 320–338. doi: 10.1111/apm.12674
Moskowitz, S. M., Foster, J. M., Emerson, J., and Burns, J. L. (2004). Clinically feasible biofilm susceptibility assay for isolates of Pseudomonas aeruginosa from patients with cystic fibrosis. J. Clin. Microbiol. 42, 1915–1922. doi: 10.1128/jcm.42.5.1915-1922.2004
Murdoch, D. R., Corey, G. R., Hoen, B., Miró, J. M., Fowler, V. G., Bayer, A. S., et al. (2009). Clinical presentation, etiology, and outcome of infective endocarditis in the 21st century: the international collaboration on endocarditis-prospective cohort study. Arch. Intern. Med. 169, 463–473. doi: 10.1001/archinternmed.2008.603
Nair, H. A. S., Periasamy, S., Yang, L., Kjelleberg, S., and Rice, S. A. (2017). Real time, spatial, and temporal mapping of the distribution of c-di-GMP during biofilm development. J. Biol. Chem. 292, 477–487. doi: 10.1074/jbc.m116.746743
Niemann, S., Spehr, N., Van Aken, H., Morgenstern, E., Peters, G., Herrmann, M., et al. (2004). Soluble fibrin is the main mediator of Staphylococcus aureus adhesion to platelets. Circulation 110, 193–200. doi: 10.1161/01.cir.0000134486.93030.e7
Oechslin, F., Piccardi, P., Mancini, S., Gabard, J., Moreillon, P., Entenza, J. M., et al. (2017). Synergistic interaction between phage therapy and antibiotics clears Pseudomonas Aeruginosa infection in endocarditis and reduces virulence. J. Infect. Dis. 215, 703–712.
Ogihara, S., Saito, R., Sawabe, E., Hagihara, M., and Tohda, S. (2016). First Japanese case of infectious endocarditis due to Enterococcus faecalis small-colony variants. J. Infect. Chemother. 22, 716–719. doi: 10.1016/j.jiac.2016.03.010
Oguz, E., Ekinci, S., Eroglu, M., Bilgic, S., Koca, K., Durusu, M., et al. (2011). Evaluation and comparison of the effects of hyperbaric oxygen and ozonized oxygen as adjuvant treatments in an experimental osteomyelitis model. J. Surg. Res. 171, e61–e68.
Olaison, L., and Schadewitz, K. (2002). Enterococcal endocarditis in Sweden, 1995-1999: can shorter therapy with aminoglycosides be used? Clin. Infect. Dis. 34, 159–166. doi: 10.1086/338233
Østergaard, L., Mogensen, U. M., Bundgaard, J. S., Dahl, A., Wang, A., Torp-Pedersen, C., et al. (2019). Duration and complications of diabetes mellitus and the associated risk of infective endocarditis. Int. J. Cardiol. 278, 280–284. doi: 10.1016/j.ijcard.2018.09.106
Özkan, M. T. A., Vural, A., Çiçek, ÖF., Yener, A. Ü, Özcan, S., Toman, H., et al. (2016). Is hyperbaric oxygen or ozone effective in experimental endocarditis? J. Surg. Res. 202, 66–70. doi: 10.1016/j.jss.2015.12.006
Pamp, S. J., Gjermansen, M., Johansen, H. K., and Tolker-Nielsen, T. (2008). Tolerance to the antimicrobial peptide colistin in Pseudomonas aeruginosa biofilms is linked to metabolically active cells, and depends on the pmr and mexAB-oprM genes. Mol. Microbiol. 68, 223–240. doi: 10.1111/j.1365-2958.2008.06152.x
Panizzi, P., Friedrich, R., Fuentes-Prior, P., Richter, K., Bock, P. E., and Bode, W. (2006). Fibrinogen substrate recognition by staphylocoagulase(Pro)thrombin complexes. J. Biol. Chem. 281, 1179–1187. doi: 10.1074/jbc.m507956200
Panizzi, P., Nahrendorf, M., Figueiredo, J.-L., Panizzi, J., Marinelli, B., Iwamoto, Y., et al. (2011). In vivo detection of Staphylococcus aureus endocarditis by targeting pathogen-specific prothrombin activation. Nat. Med. 17, 1142–1146. doi: 10.1038/nm.2423
Pappelbaum, K. I., Gorzelanny, C., Grässle, S., Suckau, J., Laschke, M. W., Bischoff, M., et al. (2013). Ultralarge von Willebrand factor fibers mediate luminal Staphylococcus aureus adhesion to an intact endothelial cell layer under shear stress. Circulation 128, 50–59. doi: 10.1161/circulationaha.113.002008
Peerschke, E. I. B., Bayer, A. S., Ghebrehiwet, B., and Xiong, Y. Q. (2006). gC1qR/p33 blockade reduces Staphylococcus aureus colonization of target tissues in an animal model of infective endocarditis. Infect. Immun. 74, 4418–4423. doi: 10.1128/iai.01794-05
Peetermans, M., Liesenborghs, L., Peerlinck, K., Van Wijngaerden, E., Gheysens, O., Goffin, K. E., et al. (2018). Targeting coagulase activity in Staphylococcus aureus bacteraemia: a randomized controlled single-centre trial of staphylothrombin inhibition. Thromb. Haemost. 118, 818–829. doi: 10.1055/s-0038-1639586
Pericàs, J. M., García-de-la-Mària, C., Brunet, M., Armero, Y., García-González, J., Casals, G., et al. (2017). Early in vitro development of daptomycin non-susceptibility in high-level aminoglycoside-resistant Enterococcus faecalis predicts the efficacy of the combination of high-dose daptomycin plus ampicillin in an in vivo model of experimental endocarditis. J. Antimicrob. Chemother. 72, 1714–1722. doi: 10.1093/jac/dkx016
Perlman, B. B., and Freedman, L. R. (1971). Experimental endocarditis. II. Staphylococcal infection of the aortic valve following placement of a polyethylene catheter in the left side of the heart. Yale J. Biol. Med. 44, 206–213.
Petrovic Fabijan, A., Lin, R. C. Y., Ho, J., Maddocks, S., Ben Zakour, N. L., Iredell, J. R., et al. (2020). Safety of bacteriophage therapy in severe Staphylococcus aureus infection. Nat. Microbiol. 5, 465–472. doi: 10.1038/s41564-019-0634-z
Priest, W. S., Smith, J. M., and McGee, C. J. (1946). The effect of anticoagulants on the penicillin therapy and the pathologic lesion of subacute bacterial endocarditis. N. Engl. J. Med. 235, 699–706. doi: 10.1056/nejm194611142352001
Proctor, R. A., Kriegeskorte, A. A., Kahl, B. C., Becker, K., Löffler, B., Peters, G., et al. (2014). Staphylococcus aureus small colony variants (SCVs): a road map for the metabolic pathways involved in persistent infections. Front. Cell. Infect. Microbiol. 4:99. doi: 10.3389/fcimb.2014.00099
Proctor, R. A., von Eiff, C., Kahl, B. C., Becker, K., McNamara, P., Herrmann, M., et al. (2006). Small colony variants: a pathogenic form of bacteria that facilitates persistent and recurrent infections. Nat. Rev. Microbiol. 4, 295–305. doi: 10.1038/nrmicro1384
Que, Y. A., Francois, P., Haefliger, J. A., Entenza, J. M., Vaudaux, P., Moreillon, P., et al. (2001). Reassessing the role of Staphylococcus aureus clumping factor and fibronectin-binding protein by expression in Lactococcus lactis. Infect. Immun. 69, 6296–6302. doi: 10.1128/iai.69.10.6296-6302.2001
Que, Y.-A., Haefliger, J.-A., Piroth, L., François, P., Widmer, E., Entenza, J. M., et al. (2005). Fibrinogen and fibronectin binding cooperate for valve infection and invasion in Staphylococcus aureus experimental endocarditis. J. Exp. Med. 201, 1627–1635. doi: 10.1084/jem.20050125
Rasmussen, R. V., Snygg-Martin, U., Olaison, L., Buchholtz, K., Larsen, C. T., Hassager, C., et al. (2009). Major cerebral events in Staphylococcus aureus infective endocarditis: is anticoagulant therapy safe? Cardiology 114, 284–291. doi: 10.1159/000235579
Reed, J. M., Gardner, S. G., Mishra, N. N., Bayer, A. S., and Somerville, G. A. (2019). Metabolic interventions for the prevention and treatment of daptomycin non-susceptibility in Staphylococcus aureus. J. Antimicrob. Chemother. 74, 2274–2283. doi: 10.1093/jac/dkz194
Ring, J., Hoerr, V., Tuchscherr, L., Kuhlmann, M. T., Löffler, B., and Faber, C. (2014). MRI visualization of Staphyloccocus aureus-induced infective endocarditis in mice. PLoS One 9:e107179. doi: 10.1371/journal.pone.0107179
Rivera, J., Lozano, M. L., Navarro-Núñez, L., and Vicente, V. (2009). Platelet receptors and signaling in the dynamics of thrombus formation. Haematologica 94, 700–711. doi: 10.3324/haematol.2008.003178
Roberts, A. E. L., Kragh, K. N., Bjarnsholt, T., and Diggle, S. P. (2015). The limitations of in vitro experimentation in understanding biofilms and chronic infection. J. Mol. Biol. 427, 3646–3661. doi: 10.1016/j.jmb.2015.09.002
Røder, B. L., Wandall, D. A., Espersen, F., Frimodt-Møller, N., Skinhøj, P., and Rosdahl, V. T. (1997). Neurologic manifestations in Staphylococcus aureus endocarditis: a review of 260 bacteremic cases in nondrug addicts. Am. J. Med. 102, 379–386. doi: 10.1016/s0002-9343(97)00090-9
Rosenbach, O. (1878). Ueber artificielle herzklappenfehler. Arch. Exp. Pathol. Pharmakol. 9, 1–30. doi: 10.1007/bf02125952
Rowe, S. E., Wagner, N. J., Li, L., Beam, J. E., Wilkinson, A. D., Radlinski, L. C., et al. (2020). Reactive oxygen species induce antibiotic tolerance during systemic Staphylococcus aureus infection. Nat. Microbiol. Nat. Res. 5, 282–290. doi: 10.1038/s41564-019-0627-y
Salgado-Pabón, W., and Schlievert, P. M. (2014). Models matter: the search for an effective Staphylococcus aureus vaccine. Nat. Rev. Microbiol. 12, 585–591. doi: 10.1038/nrmicro3308
Sande, M. A., and Courtney, K. B. (1976). Nafcillin gentamicin synergism in experimental staphylococcal endocarditis. J. Lab. Clin. Med. 88, 118–124.
Sanford, N. E., Wilkinson, J. E., Nguyen, H., Diaz, G., and Wolcott, R. (2018). Efficacy of hyperbaric oxygen therapy in bacterial biofilm eradication. J. Wound Care 27(Suppl. 1), S20–S28.
Santoro, J., and Levison, M. E. (1978). Rat model of experimental endocarditis. Infect. Immun. 19, 915–918. doi: 10.1128/iai.19.3.915-918.1978
Sapp, M. C., Krishnamurthy, V. K., Puperi, B. S., Bhatnagar, S., Fatora, G., Mutyala, N., et al. (2016). Differential cell-matrix responses in hypoxia-stimulated aortic versus mitral valves. J. R. Soc. Interface 13:20160449. doi: 10.1098/rsif.2016.0449
Scheld, W. M., Valone, J. A., and Sande, M. A. (1978). Bacterial adherence in the pathogenesis of endocarditis. Interaction of bacterial dextran, platelets, and fibrin. J. Clin. Invest. 61, 1394–1404. doi: 10.1172/jci109057
Schwarz, C., Hoerr, V., Töre, Y., Hösker, V., Hansen, U., Van de Vyver, H., et al. (2020). Isolating crucial steps in induction of infective endocarditis with preclinical modeling of host pathogen interaction. Front. Microbiol. 11:1325. doi: 10.3389/fmicb.2020.01325
Sendi, P., and Zimmerli, W. (2017). The use of rifampin in staphylococcal orthopaedic-device-related infections. Clin. Microbiol. Infect. 23, 349–350. doi: 10.1016/j.cmi.2016.10.002
Sheagren, J. N., Tuazon, C. U., Griffin, C., and Padmore, N. (1976). Rheumatoid factor in acute bacterial endocarditis. Arthritis Rheum. 19, 887–890. doi: 10.1002/art.1780190509
Sherwood, B. F., Rowlands, D. T., Hackel, D. B., and LeMay, J. C. (1968). Bacterial endocarditis, glomerulonephritis and amyloidosis in the opossum (Didelphis virginiana). Am. J. Pathol. 53, 115–126.
Sherwood, B. F., Rowlands, D. T., Vakilzadeh, J., and LeMay, J. C. (1971). Experimental bacterial endocarditis in the opossum (Didelphis virginiana). Am. J. Pathol. 122, 89–92. doi: 10.1093/infdis/122.1-2.89
Siala, W., Rodriguez-Villalobos, H., Fernandes, P., Tulkens, P. M., and Van Bambeke, F. (2018). Activities of combinations of antistaphylococcal antibiotics with fusidic acid against staphylococcal biofilms in in vitro static and dynamic models. Antimicrob. Agents Chemother. 62:e00598-18.
Siboo, I. R., Cheung, A. L., Bayer, A. S., and Sullam, P. M. (2001). Clumping factor A mediates binding of Staphylococcus aureus to human platelets. Infect. Immun. 69, 3120–3127. doi: 10.1128/iai.69.5.3120-3127.2001
Singh, K. V., Arias, C. A., and Murray, B. E. (2020). Tedizolid as step-down therapy following daptomycin versus continuation of daptomycin against enterococci and methicillin- and vancomycin-resistant Staphylococcus aureus in a rat endocarditis model. Antimicrob. Agents Chemother. 64:e02303-19.
Skeik, N., Porten, B. R., Isaacson, E., Seong, J., Klosterman, D. L., Garberich, R. F., et al. (2015). Hyperbaric oxygen treatment outcome for different indications from a single center. Ann. Vasc. Surg. 29, 206–214. doi: 10.1016/j.avsg.2014.07.034
Smith, J. R., Yim, J., Rice, S., Stamper, K., Kebriaei, R., and Rybak, J. (2018). Combination of tedizolid and daptomycin against methicillin- resistant Staphylococcus aureus in an in vitro model of simulated endocardial vegetations. Antimicrob. Agents Chemother. 62:e00101-18.
Snyder, A. H., Werth, B. J., Barber, K. E., Sakoulas, G., and Rybak, M. J. (2014). Evaluation of the novel combination of daptomycin plus ceftriaxone against vancomycin-resistant enterococci in an in vitro pharmacokinetic/pharmacodynamic simulated endocardial vegetation model. J. Antimicrob. Chemother. 69, 2148–2154. doi: 10.1093/jac/dku113
Snygg-Martin, U., Gustafsson, L., Rosengren, L., Alsiö, Å, Ackerholm, P., Andersson, R., et al. (2008). Cerebrovascular complications in patients with left-sided infective endocarditis are common: a prospective study using magnetic resonance imaging and neurochemical brain damage markers. Clin. Infect. Dis. 47, 23–30. doi: 10.1086/588663
Snygg-Martin, U., Rasmussen, R. V., Hassager, C., Bruun, N. E., Andersson, R., and Olaison, L. (2011). Warfarin therapy and incidence of cerebrovascular complications in left-sided native valve endocarditis. Eur. J. Clin. Microbiol. Infect. Dis. 30, 151–157. doi: 10.1007/s10096-010-1063-3
Song, K. H., Jung, S. I., Lee, S., Park, S., Kim, E. S., Park, K. H., et al. (2018). Inoculum effect of methicillin-susceptible Staphylococcus aureus against broad-spectrum beta-lactam antibiotics. Eur. J. Clin. Microbiol. Infect. Dis. 38, 67–74. doi: 10.1007/s10096-018-3392-6
Song, K.-H., Jung, S.-I., Lee, S., Park, S., Kim, E. S., Park, K.-H., et al. (2019). Inoculum effect of methicillin-susceptible Staphylococcus aureus against broad-spectrum beta-lactam antibiotics. Eur. J. Clin. Microbiol. Infect. Dis. 38, 67–74.
Steed, M. E., Vidaillac, C., and Rybak, M. J. (2010). Novel daptomycin combinations against daptomycin-nonsusceptible methicillin-resistant Staphylococcus aureus in an in vitro model of simulated endocardial vegetations. Antimicrob. Agents Chemother. 54, 5187–5192. doi: 10.1128/aac.00536-10
Taha, T. H., Durrant, S. S., Mazeika, P. K., Nihoyannopoulos, P., and Oakley, C. M. (1992). Aspirin to prevent growth of vegetations and cerebral emboli in infective endocarditis. J. Intern. Med. 231, 543–546. doi: 10.1111/j.1365-2796.1992.tb00971.x
Tan, J. S., Terhune, C. A., Kaplan, S., and Hamburger, M. (1971). Successful two-week treatment schedule for penicillin-susceptible Streptococcus viridans endocarditis. Lancet 2, 1340–1343. doi: 10.1016/s0140-6736(71)92360-9
Thill, C. J., and Meyer, O. O. (1947). Experiences with penicillin and dicumarol in the treatment of subacute bacterial endocarditis. Am. J. Med. Sci. 213, 300–307. doi: 10.1016/0002-8703(47)90329-3
Thom, S. R. (1989). Analytic reviews: hyperbaric oxygen therapy. J. Intensive Care Med. 4, 58–74. doi: 10.1177/088506668900400204
Thom, S. R. (2009). Oxidative stress is fundamental to hyperbaric oxygen therapy. J. Appl. Physiol. 106, 988–995. doi: 10.1152/japplphysiol.91004.2008
Thom, S. R., Mendiguren, I., Hardy, K., Bolotin, T., Fisher, D., Nebolon, M., et al. (1997). Inhibition of human neutrophil beta2-integrin-dependent adherence by hyperbaric O2. Am. J. Physiol. 272(Pt. 1), C770–C777.
Thuny, F., Disalvo, G., Belliard, O., Avierinos, J.-F. F., Pergola, V., Rosenberg, V., et al. (2005). Risk of embolism and death in infective endocarditis: prognostic value of echocardiography–a prospective multicenter study. Circulation 112, 69–75. doi: 10.1161/circulationaha.104.493155
Tilley, D. O., Arman, M., Smolenski, A., Cox, D., O’Donnell, J. S., Douglas, C. W. I., et al. (2013). Glycoprotein Ibα and FcγRIIa play key roles in platelet activation by the colonizing bacterium, Streptococcus oralis. J. Thromb. Haemost. 11, 941–950. doi: 10.1111/jth.12175
Tissot-Dupont, H., Gouriet, F., Oliver, L., Jamme, M., Casalta, J. P., Jimeno, M. T., et al. (2019). High-dose trimethoprim-sulfamethoxazole and clindamycin for Staphylococcus aureus endocarditis. Int. J. Antimicrob. Agents 54, 143–148. doi: 10.1016/j.ijantimicag.2019.06.006
Tong, S. Y. C., Davis, J. S., Eichenberger, E., Holland, T. L., and Fowler, V. G. Jr. (2015). Staphylococcus aureus infections: epidemiology, pathophysiology, clinical manifestations, and management. Clin. Microbiol. rev. 28, 603–661. doi: 10.1128/cmr.00134-14
Tornos, P., Almirante, B., Mirabet, S., Permanyer, G., Pahissa, A., and Soler-Soler, J. (1999). Infective endocarditis due to Staphylococcus aureus: deleterious effect of anticoagulant therapy. Arch. Intern. Med. 159, 473–475. doi: 10.1001/archinte.159.5.473
Tuchscherr, L., Medina, E., Hussain, M., Völker, W., Heitmann, V., Niemann, S., et al. (2011). Staphylococcus aureus phenotype switching: an effective bacterial strategy to escape host immune response and establish a chronic infection. EMBO Mol. Med. 3, 129–141. doi: 10.1002/emmm.201000115
Tuomanen, E., Cozens, R., Tosch, W., Zak, O., and Tomasz, A. (1986). The rate of killing of Escherichia coli by beta-lactam antibiotics is strictly proportional to the rate of bacterial growth. J. Gen. Microbiol. 132, 1297–1304. doi: 10.1099/00221287-132-5-1297
Turhan, V., Sacar, S., Uzun, G., Sacar, M., Yildiz, S., Ceran, N., et al. (2009). Hyperbaric oxygen as adjunctive therapy in experimental mediastinitis. J. Surg. Res. 155, 111–115. doi: 10.1016/j.jss.2008.08.031
Vanassche, T., Kauskot, A., Verhaegen, J., Peetermans, W. E., van Ryn, J., Schneewind, O., et al. (2012). Fibrin formation by staphylothrombin facilitates Staphylococcus aureus-induced platelet aggregation. Thromb. Haemost. 107, 1107–1121. doi: 10.1160/th11-12-0891
Vanassche, T., Peetermans, M., Van Aelst, L. N. L., Peetermans, W. E., Verhaegen, J., Missiakas, D. M., et al. (2013). The role of staphylothrombin-mediated fibrin deposition in catheter-related Staphylococcus aureus infections. J. Infect. Dis. 208, 92–100. doi: 10.1093/infdis/jit130
Vanassche, T., Verhaegen, J., Peetermans, W. E., Hoylaerts, M. F., and Verhamme, P. (2010). Dabigatran inhibits Staphylococcus aureus coagulase activity. J. Clin. Microbiol. 48, 4248–4250. doi: 10.1128/jcm.00896-10
Vanassche, T., Verhaegen, J., Peetermans, W. E., Van Ryn, J., Cheng, A., Schneewind, O., et al. (2011). Inhibition of staphylothrombin by dabigatran reduces Staphylococcus aureus virulence. J. Thromb. Haemost JTH 9, 2436–2446. doi: 10.1111/j.1538-7836.2011.04529.x
Veloso, T. R., Chaouch, A., Roger, T., Giddey, M., Vouillamoz, J., Majcherczyk, P., et al. (2013). Use of a human-like low-grade bacteremia model of experimental endocarditis to study the role of Staphylococcus aureus adhesins and platelet aggregation in early endocarditis. Infect. Immun. 81, 697–703. doi: 10.1128/iai.01030-12
Veloso, T. R., Que, Y.-A., Chaouch, A., Giddey, M., Vouillamoz, J., Rousson, V., et al. (2015). Prophylaxis of experimental endocarditis with antiplatelet and antithrombin agents: a role for long-term prevention of infective endocarditis in humans? J. Infect. Dis. 211, 72–79. doi: 10.1093/infdis/jiu426
Verklin, R. M., and Mandell, G. L. (1977). Alteration of effectiveness of antibiotics by anaerobiosis. J. Lab. Clin. med. 89, 65–71.
Vidaillac, C., Steed, M. E., and Rybak, M. J. (2011). Impact of dose de-escalation and escalation on daptomycin’s pharmacodynamics against clinical methicillin-resistant Staphylococcus aureus isolates in an in vitro model. Antimicrob. Agents Chemother. 55, 2160–2165. doi: 10.1128/aac.01291-10
Vilacosta, I., Graupner, C., San Román, J. A., Sarriá, C., Ronderos, R., Fernández, C., et al. (2002). Risk of embolization after institution of antibiotic therapy for infective endocarditis. J. Am. Coll. Cardiol. 39, 1489–1495. doi: 10.1016/s0735-1097(02)01790-4
von Brühl, M.-L., Stark, K., Steinhart, A., Chandraratne, S., Konrad, I., Lorenz, M., et al. (2012). Monocytes, neutrophils, and platelets cooperate to initiate and propagate venous thrombosis in mice in vivo. J. Exp. Med. 209, 819–835. doi: 10.1084/jem.20112322
Vulin, C., Leimer, N., Huemer, M., Ackermann, M., and Zinkernagel, A. S. (2018). Prolonged bacterial lag time results in small colony variants that represent a sub-population of persisters. Nat. Commun. 9:4074.
Wellinghausen, N., Chatterjee, I., Berger, A., Niederfuehr, A., Proctor, R. A., and Kahl, B. C. (2009). Characterization of clinical Enterococcus faecalis small-colony variants. J. Clin. Microbiol. 47, 2802–2811. doi: 10.1128/jcm.00485-09
Werdan, K., Dietz, S., Löffler, B., Niemann, S., Bushnaq, H., Silber, R.-E., et al. (2014). Mechanisms of infective endocarditis: pathogen-host interaction and risk states. Nat. Rev. Cardiol. 11, 35–50. doi: 10.1038/nrcardio.2013.174
Werner, A. S., Cobbs, G., and Kaye, D. (1967). Studies on the bacteremia of bacterial endocarditis. JAMA 202, 199–203. doi: 10.1001/jama.202.3.199
Werth, B. J., and Shireman, L. M. (2017). Pharmacodynamics of ceftaroline plus ampicillin against Enterococcus faecalis in an in vitro pharmacokinetic/pharmacodynamic model of simulated endocardial vegetations. Antimicrob. Agents Chemother. 61:e02235-16.
Wienen, W., Stassen, J.-M., Priepke, H., Ries, U. J., and Hauel, N. (2007). Antithrombotic and anticoagulant effects of the direct thrombin inhibitor dabigatran, and its oral prodrug, dabigatran etexilate, in a rabbit model of venous thrombosis. J. Thromb. Haemost. 5, 1237–1242. doi: 10.1111/j.1538-7836.2007.02526.x
Wilkinson, D., and Doolette, D. (2004). Hyperbaric oxygen treatment and survival from necrotizing soft tissue infection. Arch. Surg. 139, 1339–1345. doi: 10.1001/archsurg.139.12.1339
Xiong, Y. Q., Willard, J., Kadurugamuwa, J. L., Yu, J., Francis, K. P., and Bayer, A. S. (2005). Real-time in vivo bioluminescent imaging for evaluating the efficacy of antibiotics in a rat Staphylococcus aureus endocarditis model. Antimicrob. Agents Chemother. 49, 380–387. doi: 10.1128/aac.49.1.380-387.2005
Yakovenko, O., Nunez, J., Bensing, B., Yu, H., Mount, J., Zeng, J., et al. (2018). Serine-rich repeat adhesins mediate shear-enhanced streptococcal binding to platelets. Infect. Immun. 86, e160–e118.
Yan, W., Qu, T., Zhao, H., Su, L., Yu, Q., Gao, J., et al. (2010). The effect of c-di-GMP (3′-5′-cyclic diguanylic acid) on the biofilm formation and adherence of Streptococcus mutans. Microbiol. Res. 165, 87–96. doi: 10.1016/j.micres.2008.10.001
Yeaman, M. R. (1997). State-of-the-art clinical article the role of platelets in antimicrobial host defense. Clin. Infect. Dis. 25, 951–968. doi: 10.1086/516120
Yeaman, M. R., Soldan, S. S., Ghannoum, M. A., Edwards, J. E., Filler, S. G., Bayer, A. S., et al. (1996). Resistance to platelet microbicidal protein results in increased severity of experimental Candida albicans endocarditis. Infect. Immun. 64, 1379–1384. doi: 10.1128/iai.64.4.1379-1384.1996
Yim, J., Smith, J. R., Singh, N. B., Rice, S., Stamper, K., Garcia de la Maria, C., et al. (2017). Evaluation of daptomycin combinations with cephalosporins or gentamicin against Streptococcus mitis group strains in an in vitro model of simulated endocardial vegetations (SEVs). J. Antimicrob. Chemother. 56, 6192–6200.
Zarbock, A., Polanowska-Grabowska, R. K., and Ley, K. (2007). Platelet-neutrophil-interactions: linking hemostasis and inflammation. Blood Rev. 21, 99–111. doi: 10.1016/j.blre.2006.06.001
Zhang, T., Pabst, B., Klapper, I., and Stewart, P. S. (2013). General theory for integrated analysis of growth, gene, and protein expression in biofilms. PLoS One 8:e83626. doi: 10.1371/journal.pone.0083626
Zimmerli, W., and Moser, C. (2012). Pathogenesis and treatment concepts of orthopaedic biofilm infections. FEMS Immun. Med. Microbiol. 65, 158–168. doi: 10.1111/j.1574-695x.2012.00938.x
Keywords: biofilm, infective endocarditis, innate immunity, Staphylococcus aureus, dabigatran, hyperbaric oxygen therapy, in vivo, in vitro
Citation: Lerche CJ, Schwartz F, Theut M, Fosbøl EL, Iversen K, Bundgaard H, Høiby N and Moser C (2021) Anti-biofilm Approach in Infective Endocarditis Exposes New Treatment Strategies for Improved Outcome. Front. Cell Dev. Biol. 9:643335. doi: 10.3389/fcell.2021.643335
Received: 17 December 2020; Accepted: 04 May 2021;
Published: 18 June 2021.
Edited by:
Wiilem Jan Bastiaan Van Wamel, Erasmus Medical Center, NetherlandsReviewed by:
Yan Q Xiong, UCLA David Geffen School of Medicine, United StatesBenito Almirante, Vall d’Hebron University Hospital, Spain
Copyright © 2021 Lerche, Schwartz, Theut, Fosbøl, Iversen, Bundgaard, Høiby and Moser. This is an open-access article distributed under the terms of the Creative Commons Attribution License (CC BY). The use, distribution or reproduction in other forums is permitted, provided the original author(s) and the copyright owner(s) are credited and that the original publication in this journal is cited, in accordance with accepted academic practice. No use, distribution or reproduction is permitted which does not comply with these terms.
*Correspondence: Claus Moser, bW9zZXJAZGFkbG5ldC5kaw==