- Louvain Institute of Biomolecular Science and Technology, Université catholique de Louvain, Louvain-La-Neuve, Belgium
Developmentally programmed formation of DNA double-strand breaks (DSBs) by Spo11 initiates a recombination mechanism that promotes synapsis and the subsequent segregation of homologous chromosomes during meiosis. Although DSBs are induced to high levels in meiosis, their formation and repair are tightly regulated to minimize potentially dangerous consequences for genomic integrity. In S. cerevisiae, nine proteins participate with Spo11 in DSB formation, but their molecular functions have been challenging to define. Here, we describe our current view of the mechanism of meiotic DSB formation based on recent advances in the characterization of the structure and function of DSB proteins and discuss regulatory pathways in the light of recent models.
Introduction
Genomes are continuously damaged by endogenous and exogenous factors and must be accurately repaired to maintain genome integrity and function (Ceccaldi et al., 2016; Kim et al., 2016). Homologous recombination is an ancient and universal mechanism that achieves accurate repair of DNA double-strand breaks (DSBs) by copying information from an intact template (Symington, 2016; Wright et al., 2018). This repair mechanism was hijacked early during eukaryotic evolution to achieve two key goals in meiosis. First, to exchange genetic material between chromosomes, thereby breaking up allelic linkage groups and promoting genetic diversity. Second, to provide physical connections between homologous chromosomes that allow their alignment along the meiotic spindle and their accurate segregation, thereby producing chromosomally balanced haploid gametes and maintain stable genomic contents between generations (Page and Hawley, 2003; Petronczki et al., 2003; Wilkins and Holliday, 2009; Hunter, 2015; Figure 1A). Meiotic cells trigger recombination by deliberately damaging their DNA, producing hundreds of DSBs per meiosis in yeast or mice (Sun et al., 1989; Keeney, 2008; Pan et al., 2011; Kauppi et al., 2013).
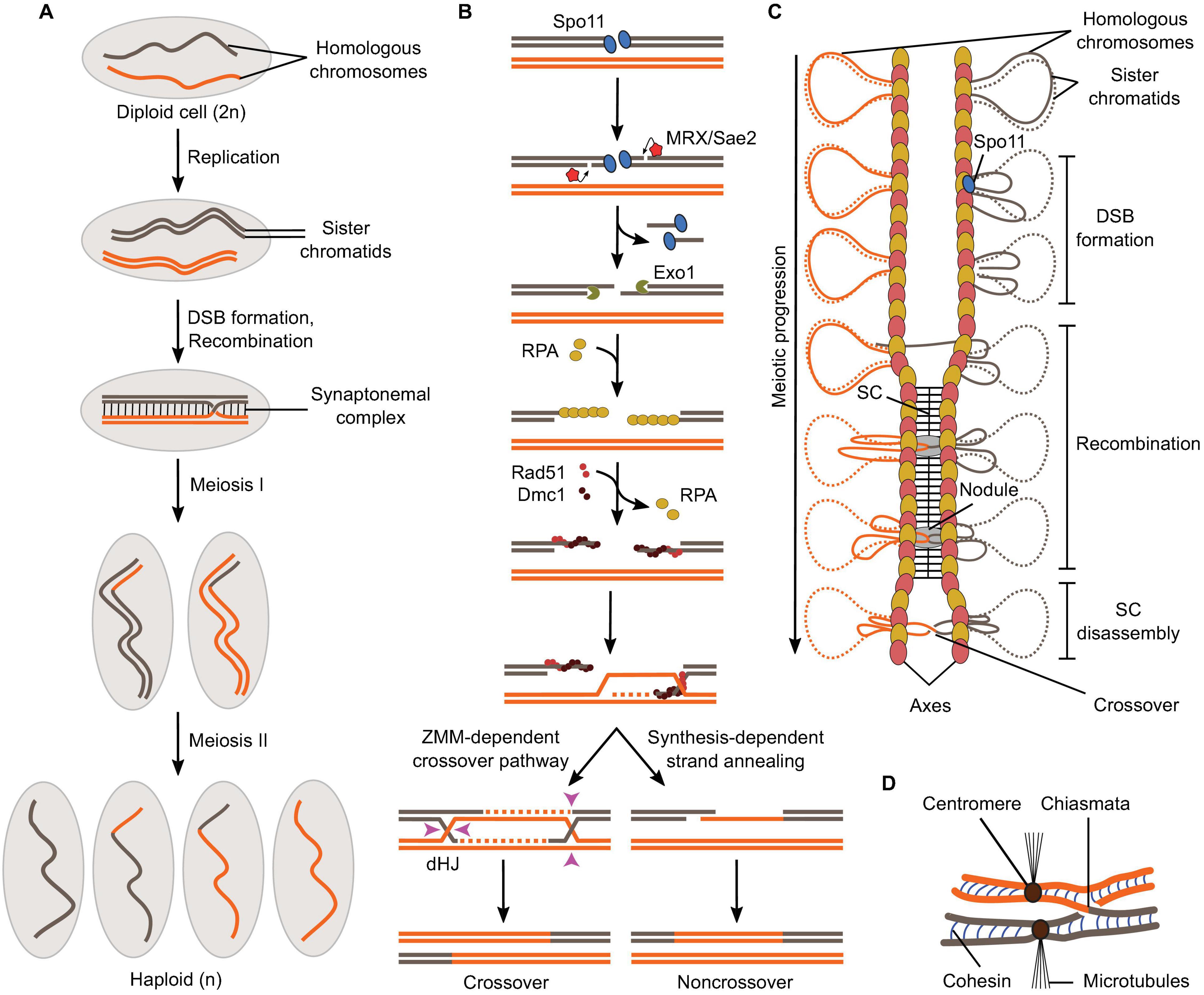
Figure 1. Overview of meiosis and meiotic recombination. (A) Schematic of the formation of haploid gametes from a diploid cell with a single pair of homologous chromosomes. DSB formation and recombination promote homolog pairing and lead to the exchange of chromosomal fragments (crossovers) in the context of synapsed chromosomes. (B) Meiotic recombination is initiated by Spo11-mediated DSB formation and leads to the formation of crossovers via a ZMM-dependent double Holliday Junction (dHJ) resolution pathway or non-crossovers by synthesis-dependent strand annealing. (C) Relationships between meiotic recombination and higher-order chromosome structure. DSB formation happens in the context of the loop-axis structure. As recombination progresses, the SC polymerizes between the axes and is disassembled prior to chromosome segregation. Axis proteins Red1 (red ovals) and Hop1 (yellow ovals) are shown. (D) In metaphase I, homologs are held together through chiasmata and sister chromatid cohesion.
Meiotic DSBs are produced by the evolutionarily conserved topoisomerase-derived protein, Spo11, along with a cohort of partner subunits (Bergerat et al., 1997; Keeney et al., 1997; Keeney, 2008; Lam and Keeney, 2015). Following break formation, Spo11 remains covalently attached to the 5′-strands at both DNA ends and is released by an endonucleolytic cleavage reaction mediated by MRX (Mre11, Rad50, and Xrs2) and Sae2, which liberates Spo11 attached to a short oligonucleotide (Neale et al., 2005; Figure 1B). The 5′-strands are further resected by 5′-3′ exonucleases (Exo1 in yeast) to produce long single-stranded tails, which are coated with ssDNA-binding protein RPA (Sun et al., 1991; Zakharyevich et al., 2010; Garcia et al., 2011; Schiller et al., 2014; Symington, 2016; Mimitou et al., 2017). RPA is then replaced by recombinases Rad51 and Dmc1 that form a nucleoprotein filament and search for sequence similarity preferentially located on the homologous chromosome, producing D-loop structures (Hong et al., 2001; San Filippo et al., 2008; Brown and Bishop, 2015). Following DNA synthesis using the homolog as a repair template, the recombination structures experience one of two main outcomes (Allers and Lichten, 2001; Hunter and Kleckner, 2001; Bishop and Zickler, 2004; De Muyt et al., 2012; Pyatnitskaya et al., 2019; Figure 1B). The invading strand can be ejected from the donor by action of helicases, which provides an opportunity for the DNA ends to re-anneal. This process is referred to as synthesis-dependent strand annealing and produces non-crossovers, that is, products not associated with reciprocal exchanges of chromosome fragments, but with local transfer of genetic information from the repair template to the broken molecule (gene conversion) (Palmer et al., 2003; Martini et al., 2011). Alternatively, recombination structures are stabilized by the “ZMM” family of proteins and channeled through a pathway that produces mostly crossovers (Börner et al., 2004; Lynn et al., 2007; Pyatnitskaya et al., 2019). Here, both ends of the break engage the donor to form a double Holliday Junction intermediate, which is resolved through a crossover-specific pathway that involves MutLγ and Exo1 (Schwacha and Kleckner, 1995; Zakharyevich et al., 2012; Gray and Cohen, 2016; Pyatnitskaya et al., 2019).
Every aspect of meiotic recombination is tied to the structural organization of the chromosomes (Figure 1C). Early in meiotic prophase, chromosomes organize as series of DNA loops that are anchored along a nucleoprotein axis. DSB formation happens in the context of this loop-axis structure. As recombination progresses, polymerization of a proteinaceous structure called the synaptonemal complex (SC) initiates between the two axes and elongates along their entire length (Kleckner, 2006; Zickler and Kleckner, 2015; Figure 1C). Recombination proceeds within the SC, inside a nodule embedded between the axes (Zickler and Kleckner, 1999). After recombination is completed, the SC disassembles and crossovers, now cytologically visible as chiasmata, provide physical connections between the homologs until their segregation at anaphase (Figure 1D).
Here, we discuss current models for meiotic DSB formation, focusing on the molecular mechanisms in S. cerevisiae. We present recent advances in deciphering the structure and function of proteins required for DSB formation, their interactions and relationships with chromosome organization, and discuss the mechanisms that regulate DSB formation in the light of these new models.
Meiotic DSB Formation in S. cerevisiae
Meiotic DSBs are distributed non-randomly throughout the genome and concentrated within distinct regions of the chromosomes called hotspots, typically ∼50–300 base-pairs wide (Baudat and Nicolas, 1997; Petes, 2001; Buhler et al., 2007; Pan et al., 2011). The primary factor determining hotspot locations in yeast is chromatin accessibility (Baudat and Nicolas, 1997; Berchowitz et al., 2009; Pan et al., 2011). Indeed, the vast majority of the ∼3,600 S. cerevisiae hotspots localize within nucleosome-depleted regions at promoters (Pan et al., 2011). However, non-randomness, in terms of break distribution and intensity, can also be observed at the chromosomal scale and at the sequence level (Wu and Lichten, 1994; Lichten and Goldman, 1995; Berchowitz et al., 2009; Pan et al., 2011; Figure 2A). Indeed, chromosome size impacts DSB formation, with smaller chromosomes experiencing higher DSB densities (Pan et al., 2011; Murakami et al., 2020). DSBs are suppressed near telomeres and centromeres, and chromosomal domains with higher or lower DSB frequency alternate, correlating positively with GC content (Baudat and Nicolas, 1997; Borde et al., 1999; Gerton et al., 2000; Petes, 2001; Blat et al., 2002; Blitzblau et al., 2007; Buhler et al., 2007; Pan et al., 2011). Hotpots themselves tend to be AT-rich and are flanked by sequences enriched for the histone H3 lysine 4 trimethylation (H3K4me3) mark (Borde et al., 2009; Pan et al., 2011; Tischfield and Keeney, 2012). In addition, break formation displays sequence bias within and around the footprint of Spo11 and at the cleavage site, with a preference for cleavage 3′ of a C (Murakami and Nicolas, 2009; Pan et al., 2011; Figure 2A).
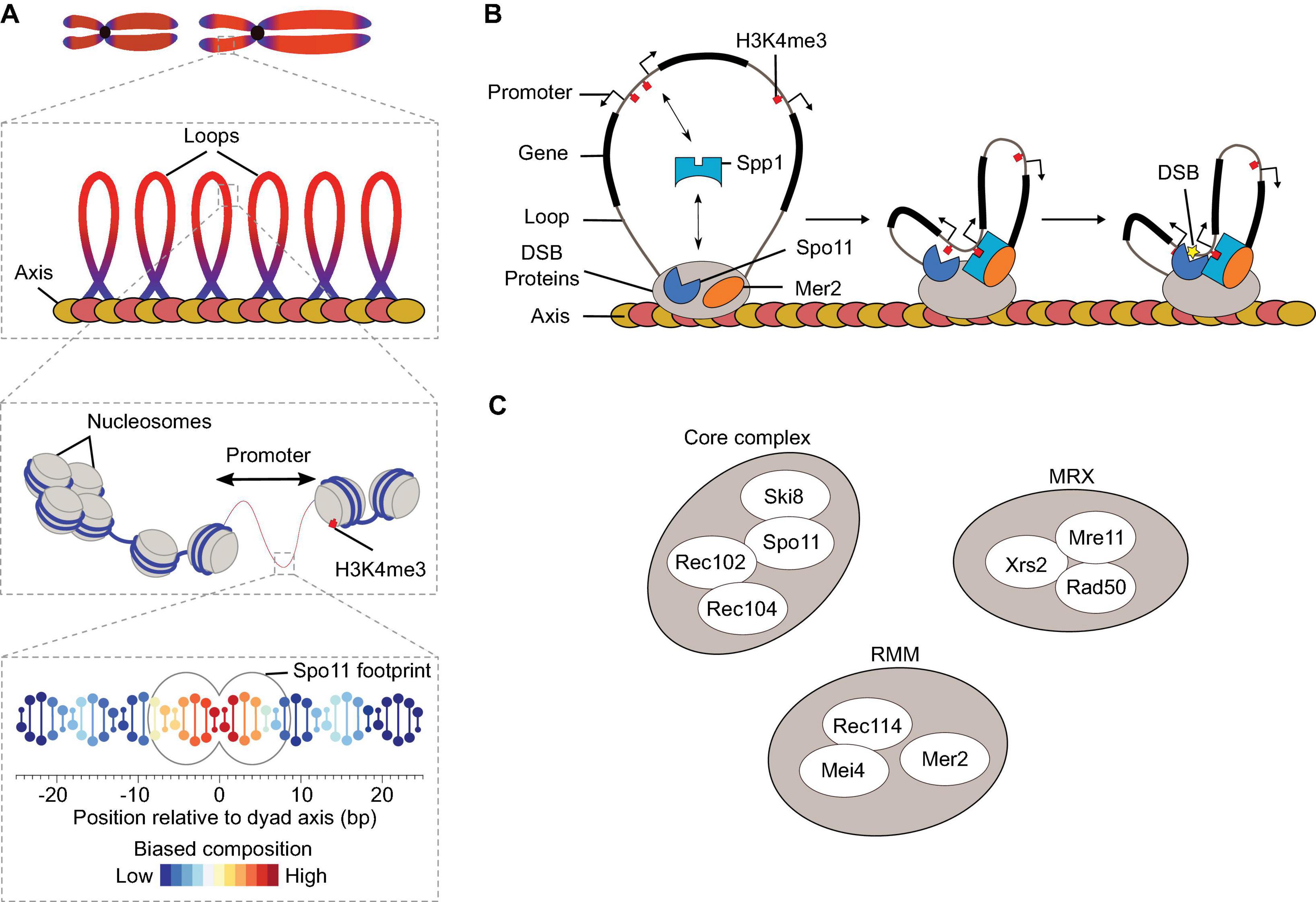
Figure 2. DSB formation in S. cerevisiae. (A) The distribution of meiotic DSBs is influenced by a combination of factors that operates at various size scales (Pan et al., 2011). Spo11 footprint indicates the expected occupancy of Spo11 on DNA based on structural modeling. (B) The tethered loop-axis model for DSB formation. Spp1 binds to H3K4me2/3 enriched around DSB hotspots and connects it to the chromosome axis through interaction with Mer2. Axis proteins Red1 (red ovals) and Hop1 (yellow ovals) are shown. (C) Ten DSB proteins in S. cerevisiae.
Although DSB formation happens primarily within chromatin loops, most of the DSB proteins are enriched along the chromosome axis (Kugou et al., 2009; Pan et al., 2011; Panizza et al., 2011). The tethered loop-axis model reconciles these findings by suggesting that DSB formation involves the capture of a DNA loop by axis-bound DSB proteins, allowing Spo11 to cleave the loop (Blat et al., 2002; Kleckner, 2006; Kim et al., 2010; Panizza et al., 2011; Figure 2B). The COMPASS subunit Spp1 was identified as a key player that connects the loops to the axis via interactions with H3K4me3 marks located at gene promoters and the axis-bound DSB protein, Mer2 (Acquaviva et al., 2013; Sommermeyer et al., 2013).
The Meiotic DSB Proteins
In S. cerevisiae, ten proteins collaborate to form DSBs, and they can be separated into three sub-groups (Figure 2C): the core complex (Spo11, Ski8, Rec102, and Rec104), the MRX complex, and the RMM proteins (Rec114, Mei4, and Mer2) (Lam and Keeney, 2015). All ten proteins are essential for DSB formation and their deletion lead to a similar meiotic phenotype in yeast: reduced homolog pairing, loss of meiotic recombination, failure to form the SC, and abnormal chromosomal segregation ultimately resulting in inviable spores (Game et al., 1980; Malone and Esposito, 1981; Klapholz et al., 1985; Menees and Roeder, 1989; Roeder et al., 1989; Malone et al., 1991; Engebrecht et al., 1991; Cool and Malone, 1992; Galbraith and Malone, 1992; Ivanov et al., 1992; Ajimura et al., 1993; Pittman et al., 1993; Rockmill et al., 1995; Gardiner et al., 1997; Lam and Keeney, 2015). Although the molecular mechanisms whereby DSB proteins collaborate during meiosis remain unclear, recent progress has been made to understand their structure, biochemical activities and regulation. Below, we provide an overview of meiotic DSB formation emphasizing some of these recent advances.
Spo11 and Topo VI
Spo11 evolved from the catalytic subunits of a type IIB topoisomerase, Topo VI (Bergerat et al., 1997; Keeney et al., 1997). Like other type II topoisomerases, Topo VI uses ATP binding and hydrolysis to coordinate the formation of a transient DSB to the passage of an intact duplex through the break, thereby modulating DNA topology (Corbett et al., 2007; Graille et al., 2008). Cleavage involves the coordinated action of two active-site tyrosines that attack opposite strands of the phosphoribose DNA backbone and produce 5′-phosphotyrosyl intermediates (Figure 3A). Both Topo VI and Spo11 produce staggered DSBs with 2-nucleotide 5′-overhangs (Liu et al., 1995; Buhler et al., 2001; Murakami and Nicolas, 2009). Spo11 can be thought of as a crippled topoisomerase in that it catalyzes break formation but is likely unable to perform strand passage and break re-sealing.
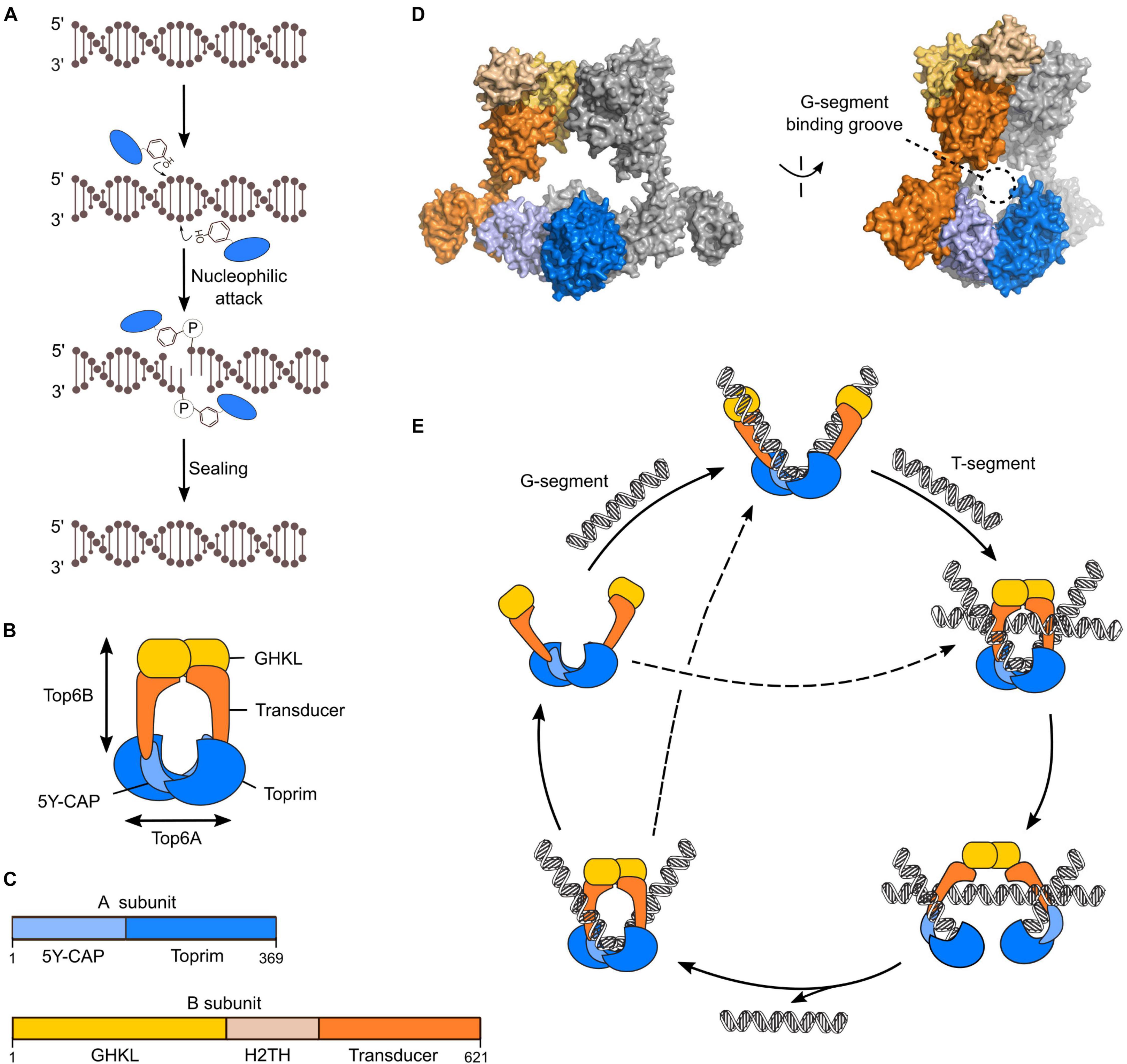
Figure 3. Mechanism of Topo VI. (A) Chemistry of strand cleavage and re-sealing in Topo VI. (B) Cartoon of the Topo VI heterotetramer. (C) Domain structure of the A and B subunits of Topo VI. (D) Structure of Topo VI (PDB: 2Q2E) showing the expected position of the G-segment within the groove formed by the A subunits (Corbett et al., 2007). (E) Catalytic cycle of Topo VI through a two-gate mechanism. ATP-dependent dimerization of the GHKL domain upon sequential or simultaneous binding to gate (G) and transfer (T) DNA duplexes is communicated to the A subunit to activate DSB formation. Topo VI can undergo multiple catalytic cycles without dissociation from the G-segment.
Topo VI has an A2B2 stoichiometry, where the A subunits perform DNA cleavage and the B subunits have ATP-binding and hydrolysis activities (Buhler et al., 2001; Corbett et al., 2007; Graille et al., 2008; Figure 3B). Although the relationship between Spo11 and Topo VIA has been recognized for over 20 years, whether Spo11 requires a B-type subunit for catalysis remained long a matter of conjecture (Bergerat et al., 1997; Buhler et al., 1998; Keeney, 2001). A few years ago, two studies eventually identified a B-type subunit in mice and plants and showed that they were essential for DSB formation (Robert et al., 2016; Vrielynck et al., 2016). This suggested that the meiotic DSB machinery is more similar to the ancestral topoisomerase than was previously appreciated. Nevertheless, while Spo11 is well-conserved and shares high sequence similarity with Topo VIA, the B-type subunits are very diverse between species and evolved almost beyond recognition from Topo VIB. Indeed, pairwise combinations of Spo11 and Topo VIA show typically 20–30% overall sequence identity with blocks that are much more conserved (Bergerat et al., 1997; Keeney et al., 1997). In contrast, conservation between the mouse Topo VIB-type subunit and S. shibatae Topo VIB is at best 11% identity over the most conserved 140 amino-acid block (Robert et al., 2016).
Topo VIA is composed of a 5Y-CAP domain (related to the DNA-binding domain of the catabolite activator protein) and a Toprim domain (also found in type IIA topoisomerases and in primase) (Bergerat et al., 1997; Nichols et al., 1999; Corbett et al., 2007; Graille et al., 2008; Figure 3C). Both domains participate in DNA binding and together form a groove that intimately engages the double helix (Figure 3D). The catalytic tyrosine is located in the 5Y-CAP domain and the Toprim domain coordinates Mg2+ ions important for catalysis. A composite active site is formed with the catalytic tyrosine and metal-ion binding pockets contributed by different subunits. Therefore, DNA cleavage necessarily requires dimerization of the A subunits. Topo VIB has an N-terminal GHKL-fold ATPase domain (found in DNA gyrase, Hsp90, Histidine Kinase, and MutL) responsible for nucleotide binding and ATP hydrolysis, a central helix two-turn helix (H2TH) fold and a C-terminal transducer domain with an extended α-helix that connects the B subunit to the 5Y-CAP domain of the A subunit (Corbett and Berger, 2003, 2005; Corbett et al., 2007; Graille et al., 2008; Figures 3C,D).
Topo VI functions through a two-gate mechanism (Corbett et al., 2007; Wendorff and Berger, 2018; Figure 3E). In its apo state, Topo VI dimerizes through the A subunits to form a U-shaped complex that can engage DNA. Topo VI binds two DNA segments, either sequentially or simultaneously (Wendorff and Berger, 2018). The G-segment (gate) binds within the DNA-binding grove formed by the A subunits and interactions between the B subunit and DNA facilitate G-segment bending (Wendorff and Berger, 2018). Engagement of the second duplex activates ATP-dependent dimerization of the GHKL domain, thereby trapping the T-segment (transfer) (Corbett et al., 2007). Dimerization of the GHKL domain is communicated to the A subunit by the transducer domain to activate DNA cleavage, whereupon ATP hydrolysis induces a conformational change that opens the DNA gate and allows strand passage (Figure 3E). Finally, the DSB is resealed, ADP in released, the ATP gate reverts to its open state, and the enzyme can dissociate from the substrate or directly engage in another round of catalysis without dissociation (Wendorff and Berger, 2018).
The Spo11 Core Complex
S. cerevisiae Spo11 has long been known to closely associate with Ski8, Rec102, and Rec104 based on genetic and cytological evidence. Indeed, yeast-two-hybrid (Y2H) experiments showed strong interactions between Spo11 and Ski8, and between Rec102 and Rec104 (Arora et al., 2004; Kee et al., 2004; Maleki et al., 2007). Spo11 and Ski8 interaction is required for chromosomal localization of Rec102 and Rec104 (Arora et al., 2004; Kee et al., 2004). In addition, Rec102 and Rec104 are essential for the association of Spo11 to DSB hotspots and for Spo11 self-interaction (Prieler et al., 2005; Sasanuma et al., 2007). Furthermore, Y2H interactions with Rec114 suggested that Rec102/Rec104 may have a role to connect Spo11 with the RMM sub-group (Maleki et al., 2007).
Recent biochemical work has shown that Spo11 indeed interacts with Ski8, Rec102, and Rec104 to form a stoichiometric complex (Claeys Bouuaert et al., 2021; Figure 4A). This complex displays structural and functional similarities expected from its relationship with Topo VI, although with differences that presumably reflect their distinct biological functions (discussed below). Since DSB formation requires two Spo11 subunits and Topo VI has an A2B2 stoichiometry, the core complex was anticipated to form a dimer of tetramers. However, purified complexes turned out to have a 1:1:1:1 stoichiometry and are catalytically inactive in vitro (Claeys Bouuaert et al., 2021). Hence, Spo11 dimerization could be an important control mechanism for DSB formation. However, what triggers Spo11 dimerization and catalysis remains unclear.
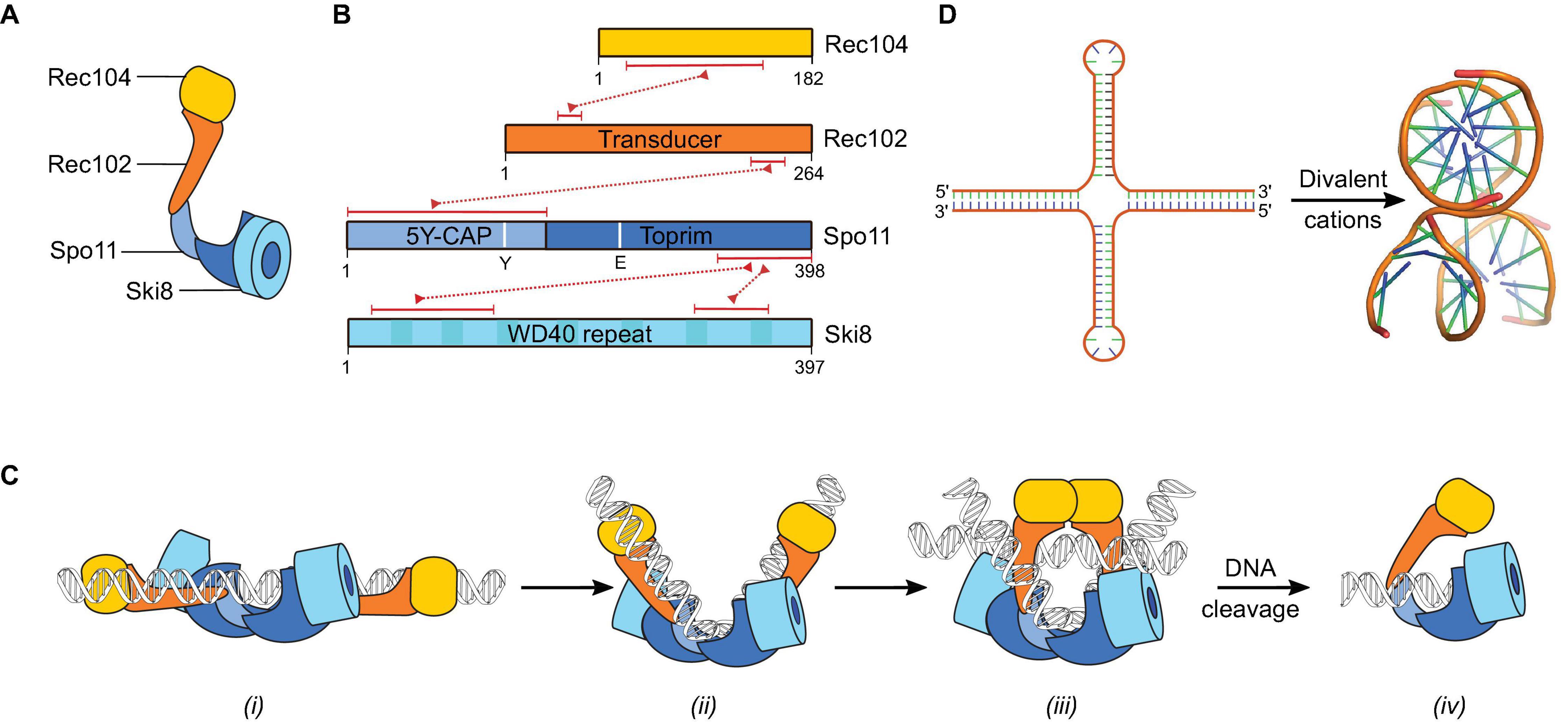
Figure 4. The Spo11 core complex. (A) Cartoon illustrating the arrangement of the different subunits in the core complex. (B) Domain structure of Rec104, Rec102, Spo11, and Ski8. The red dotted lines connecting two proteins represent their respective interaction domains. The region of Rec104 that interacts with Rec102 is predicted based on crosslinking-mass spectrometry, other interaction regions were validated by mutagenesis (Arora et al., 2004; Cheng et al., 2009; Claeys Bouuaert et al., 2021). The position in Spo11 of the catalytic tyrosine Y135 and metal-ion coordinating residue E233 are shown. (C) Proposed dynamics of the interaction between the core complex and DNA based on in vitro binding activities and analogy with Topo VI (Claeys Bouuaert et al., 2021). After DSB formation, Spo11 remains bound to the DSB through covalent and non-covalent interactions. (D) Inverted repeat sequences form cruciforms that fold into three-dimensional structures that are similar to two overlapping DNA duplexes (PDB: 1DCW) (Eichman et al., 2000).
Remote homology search had previously identified Rec102 as the Topo VIB-like subunit in S. cerevisiae (Robert et al., 2016). However, in contrast to the B-type subunit in mice and plants, Rec102 lacks the GHKL domain essential for ATP-dependent dimerization in Topo VI (Figure 4B). Crosslinking coupled to mass spectrometry and mutagenesis provided evidence that Rec104 occupies the position of the GHKL domain in the core complex (Claeys Bouuaert et al., 2021). Structural predictions were consistent with the possibility that Rec104 adopts a cryptic GHKL-like fold, but whether this is indeed the case needs to be confirmed. Rec104 lacks recognizable ATP-binding and hydrolysis motifs, while the B-type subunit in mice and plants retained some, but not all, the sequences thought to be important for ATP binding and hydrolysis (Robert et al., 2016; Vrielynck et al., 2016). Whether ATP is involved in meiotic break formation remains therefore unclear and it is possible that the answer differs between organisms.
In addition to Spo11 and Rec102/Rec104 that jointly form the A and B subunits derived from Topo VI, the S. cerevisiae core complex has an additional subunit, Ski8, with as yet unknown functions (Figure 4B). In contrast to the other core complex proteins, Ski8 is not meiosis-specific. Indeed, Ski8 has a second, independent, function as part of the Ski complex, which is involved in mRNA decay via the exosome (Anderson and Parker, 1998; Halbach et al., 2013). In vegetative cells, Ski8 localizes to the cytoplasm, but in meiotic cells it localizes to the nucleus where it interacts with Spo11 and mediates its chromosomal localization (Arora et al., 2004; Claeys Bouuaert et al., 2021). Although the meiotic function of Ski8 is conserved in S. pombe (Evans et al., 1997) and Sordaria (Tessé et al., 2003), it is not conserved in Arabidopsis (Jolivet et al., 2006).
Ski8 contains tandem copies of WD repeats folded into a seven-bladed β-propeller (Madrona and Wilson, 2004; Cheng et al., 2009; Figure 4B). A conserved patch of hydrophobic residues located on the top surface of the β-propeller was implicated in the interactions with Ski3 and Spo11 (Cheng et al., 2009). Indeed, the crystal structure of the Ski complex showed that Ski3 interacts with two Ski8 subunits through a sequence motif (Q–R–x–x–Φ) also found in Spo11 (Halbach et al., 2013). Mutations within this motif abolish the Y2H interaction with Spo11 and meiotic recombination and compromises the integrity of the core complex in vitro (Arora et al., 2004; Claeys Bouuaert et al., 2021).
Analysis of the DNA-binding properties of the S. cerevisiae core complex showed that the presence of divalent metal ions and the metal-ion binding residues (E233) stabilize the interactions with DNA, but the catalytic tyrosine (Y135) does not impact DNA binding (Claeys Bouuaert et al., 2021). Binding specificities directed toward different DNA structures were observed and suggested that DSB formation may be preceded by a series of conformational transitions, similar to the mechanism of Topo VI (Figure 4C). The core complex binds with low-nanomolar affinity to DNA duplexes, its anticipated DNA substrate (Figure 4C, i). Bound duplexes usually showed sharp ∼60° or ∼120° bends, and binding affinity was higher to pre-bent substrates than relaxed substrates, suggesting that Spo11 may bend its substrate prior to catalysis and/or bind preferentially to bendable sequences (Figure 4C, ii). Core complexes had particular affinity for positions where two DNA duplexes cross each other, such as plectonemic intertwinings of supercoiled DNA (Claeys Bouuaert et al., 2021). Binding to DNA junctions are reminiscent of other topoisomerases, including Topo VI (Corbett and Berger, 2005; Alonso-Sarduy et al., 2011; Wendorff and Berger, 2018), and suggest that core complexes dimerize in order to trap two duplexes (Figure 4C, iii). However, the stoichiometry of this intermediate was not determined and alternative interpretations remain plausible, including that monomeric core complexes have two independent duplex-binding sites. Either way, the junction-binding activity of the core complex to DNA junctions is intriguing. If the complex has more than one duplex binding site, where is the second one located? If the complex traps two duplexes like Topo VI, what is the physiological relevance of this activity, since Spo11 activity presumably does not require strand passage?
An independent line of evidence provides potential support to the hypothesis that DSB formation happens in the context of trapped DNA junctions. Insertion of long palindromes (>50 bp) within the S. cerevisiae genome generate meiotic DSB hotspots (Nasar et al., 2000). Palindromic sequences can extrude as cruciform structures (Benham, 1982), which are structurally similar to two duplexes crossing each other (Figure 4D). Hence, perhaps palindromes generate DSB hotspots by providing a preferred binding substrate to Spo11 and/or by inducing Spo11 catalysis through signaling that two duplexes have been captured. Similarly, human topoisomerase IIβ recognizes and cleaves DNA substrates that form four-way junctions (West and Austin, 1999).
Finally, the core complex binds with high affinity to the ends of DNA duplexes in vitro (Claeys Bouuaert et al., 2021; Figure 4C, iv). The end-binding activity was tightest with substrates that had a 2-nucleotide 5′-overhang identical to Spo11 cleavage products, suggesting that the core complex has intrinsic affinity for its product. Binding of Topo VI to the DSB intermediate has not been directly investigated, but in order for a topoisomerase to perform controlled strand passage, it must prevent swiveling of the DSB around the phosphotyrosyl bond and therefore hold on to both strands at both ends. Nevertheless, it is possible that Spo11 binds to DSB ends with much greater affinity than Topo VI. Indeed, since Spo11 does not turn over, increasing the stability of the complex from one intermediate to the next would help drive the reaction forward.
The significance of the end-binding activity is unclear, but it highlights the possibility that Spo11 binds strongly to DSBs after catalysis through covalent and non-covalent interactions. This may have implications regarding the first steps of DSB processing, since Spo11 could cap the DNA ends during resection and perhaps after strand invasion has initiated. Indeed, a recombination intermediate with Spo11-oligonucleotides capping the 3′-ends has been proposed to explain unanticipated patterns in genome-wide sequencing methods designed to map resection endpoints during meiosis in mice (Paiano et al., 2020; Yamada et al., 2020). In addition, scar-less repair by non-homologous end joining of meiotic DSBs that have undergone resection in a Drosophila strain with homolog pairing defects (Mcm5A7) provided further support for end-capping by Spo11-oligonucleotides after resection had initiated (Hatkevich et al., 2020). Nevertheless, end-capping by Spo11-oligonucleotide complexes has not been formally demonstrated.
The MRX Complex
MRX is an evolutionarily-conserved complex that plays key functions in the maintenance of genomic integrity in somatic cells, including the recognition of DSBs, activation of the DNA-damage checkpoint, initiation of DSB resection, and telomere maintenance, in addition to essential roles during meiosis (Symington, 2016; Gnügge and Symington, 2017). In S. cerevisiae, MRX is essential for both the formation and processing of meiotic DSBs (Alani et al., 1990; Ivanov et al., 1992; Nairz and Klein, 1997; Keeney, 2001). The DSB-processing function of MRX depends on a single-strand endonuclease activity and a 3′-5′ exonuclease activity of Mre11 directed to the 5′-strand (Figure 1B; Paull and Gellert, 1998; Neale et al., 2005; Cannavo and Cejka, 2014). The endonuclease activity is controlled by phosphorylation of Sae2, which promotes its interaction with Rad50 (Cannavo et al., 2018). Indeed, a separation-of-function mutation of Rad50 (K81I) that supports DSB formation but blocks DSB processing abolishes the interaction with phosphorylated Sae2 (Alani et al., 1990; Cannavo et al., 2018).
While the function of MRX in processing DSBs is widely conserved, its role in promoting DSB formation has only been reported in budding yeast and C. elegans (Chin and Villeneuve, 2001). Indeed, MRX orthologs are not required for DSB formation in A. thaliana (Puizina et al., 2004) and S. pombe (Young et al., 2004), and whether they are required in mice remains unknown (Lam and Keeney, 2015). In C. elegans, MRE-11 and RAD-50 are important for DSB formation (Chin and Villeneuve, 2001; Hayashi et al., 2007), but NBS-1, the ortholog of Xrs2, is not (Girard et al., 2018).
In S. cerevisiae, MRX is thought to be recruited to the DSB machinery in part through interactions between Xrs2 and Mer2, based on Y2H experiments (Arora et al., 2004; Henderson et al., 2006). In addition, chromatin immunoprecipitation (ChIP) analyses show that Mre11 associates transiently to DSB sites independently of the catalytic activity of Spo11 (Borde et al., 2004). Mre11 binding to DSB hotspots requires all other DSB proteins, except Rad50, suggesting that MRX is the last component of the DSB machinery to be recruited. Perhaps MRX recruitment activates Spo11 catalysis, but how this may be achieved is unknown. It has been proposed that the requirement of the MRX complex prior to DSB formation ensures the coordination between DSB formation and subsequent repair to limit potential impacts on genomic instability (Borde et al., 2004). Indeed, breaks detected in wild-type cells are usually fully resected, indicating that they are processed faster than they accumulate, consistent with a coordination between DSB formation and repair (Bishop et al., 1992; Tran et al., 2002; Joshi et al., 2015; Mimitou et al., 2017).
Mre11 has an N-terminal nuclease domain containing five conserved phosphoesterase motifs that form the active site (Figure 5A; Arthur et al., 2004; Williams et al., 2008). Mre11 dimerizes via its phosphodiesterase domain, which is flanked by a capping domain and creates a U-shaped structure with a cleft that binds DNA and Rad50 (Figure 5B). The capping domain is followed by a Rad50-interaction domain and a C-terminal domain with DNA-binding activity (Schiller et al., 2012). The C-terminal DNA-binding domain is dispensable for mitotic DNA repair but important for meiotic DSB formation (Furuse et al., 1998; Usui et al., 1998).
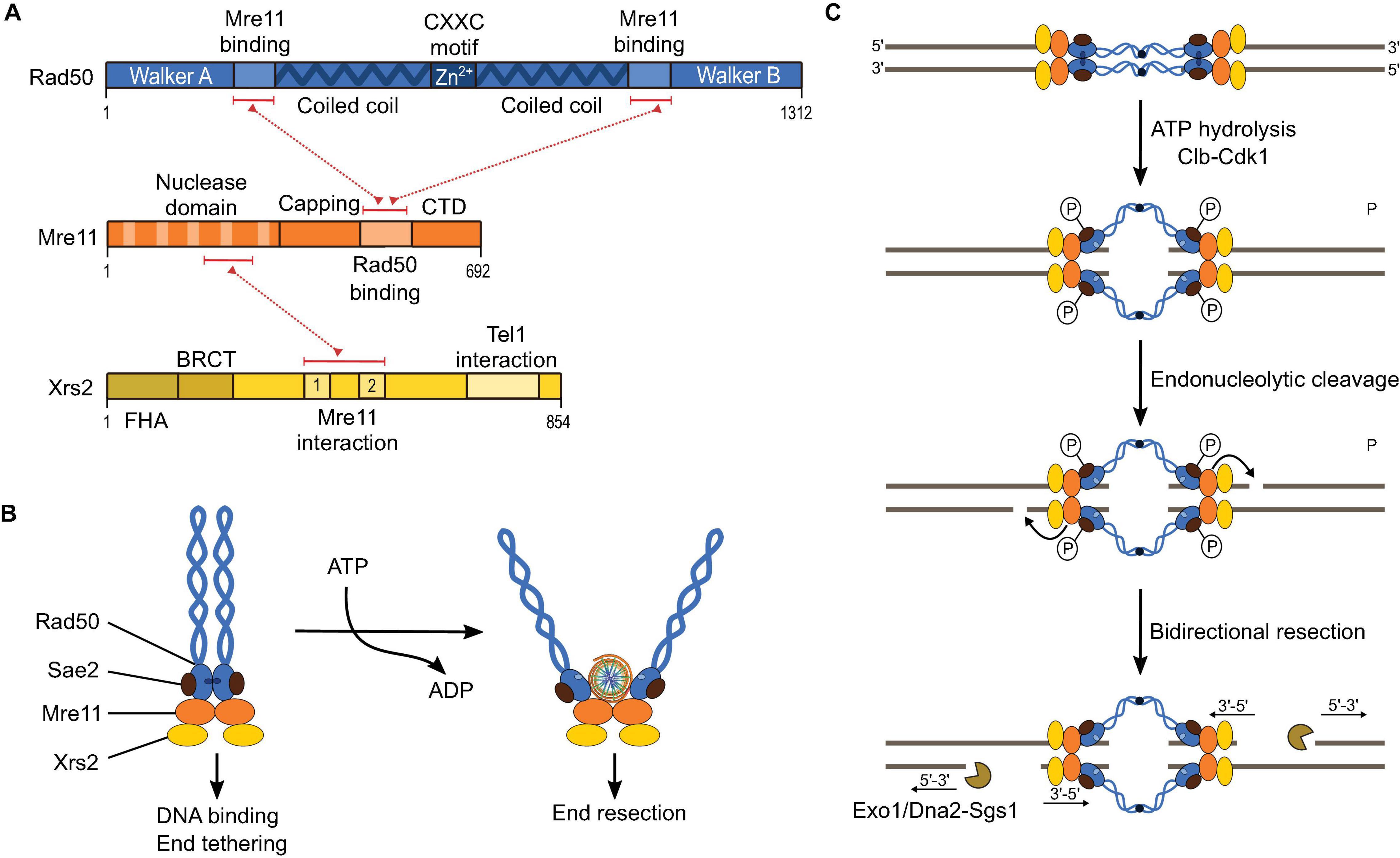
Figure 5. The MRX complex. (A) Domain structure of Mre11, Rad50, and Xrs2, and their protein-protein interacting regions (red dotted lines). (B) Cartoon illustrating the structural arrangement of the MRX complex and the conformational dynamics upon ATP hydrolysis. In an ATP-bound state, the nuclease domain of Mre11 does not access DNA. However, after ATP hydrolysis by Rad50, a conformational change exposes the nuclease domain of Mre11 to DNA. Sae2 is illustrated here as interacting with Rad50 based on Cannavo et al. (2018), but interactions with Xrs2 have also been demonstrated (Liang et al., 2015). (C) Model for DSB resection by MRX. Endonuclease activity of Mre11 directed on the 5′-strand is followed by bi-directional resection through the 3′-5′ exonuclease activity of Mre11 and the 5′-3′ exonuclease activity of ExoI or Dna2-Sgs1 in vegetative conditions or ExoI in meiosis.
Rad50 is an ATPase with Walker A and B motifs located at its N- and C-termini, respectively (Hopfner et al., 2001; Gobbini et al., 2016; Figure 5A). These are separated by a long linker that folds into a dimeric coiled-coil with the ATP-binding domain at one end and a zinc-hook domain at the other (Figure 5B; Hopfner et al., 2002; Wiltzius et al., 2005). MRX complexes can tether the two ends of a DSB via Zn2+-dependent dimerization of their hook domain (Hopfner et al., 2002; Hohl et al., 2010; Seifert et al., 2016; Figure 5C). Conformational changes within Rad50 upon ATP binding and hydrolysis control MRX function (Hopfner et al., 2001; Gobbini et al., 2016; Liu et al., 2016; Casari et al., 2019). In the presence of ATP, Rad50 adopts a closed dimeric conformation that occludes the nuclease domain of Mre11. Upon ATP hydrolysis, the Rad50 dimer dissociates, allowing the active site of Mre11 to access DNA (Hopfner et al., 2001; Liu et al., 2016; Casari et al., 2019; Figures 5B,C).
Xrs2 is thought to act as a molecular chaperone that connects Mre11 to other repair proteins, including Sae2 and the DNA-damage response kinase Tel1 (Oh et al., 2016). Xrs2 contains a fork-head associated (FHA) domain, a pair of BRCA1 C-terminus (BRCT) or BRCT-like domains, an Mre11-binding domain, and a Tel1-binding domain (Shima et al., 2005; Figure 5A). Xrs2 is essential for the nuclear localization of Mre11 (Tsukamoto et al., 2005). A mutation in Xrs2 that disrupts the interaction with Mre11 (K641E) abolishes its meiotic and vegetative functions (Tsukamoto et al., 2005). However, the Mre11-interaction domain alone (residues 630–662) is sufficient for Mre11 nuclear import and the DNA damage response but does not support meiotic recombination and telomere elongation. Although the FHA domain of Xrs2 was proposed to recruit Sae2 to the site of DNA damage (Liang et al., 2015), end resection remains Sae2-dependent in the absence of Xrs2 (Oh et al., 2016) and depends on interactions with Rad50 (Cannavo et al., 2018).
In vegetative cells, localization of Tel1 to the site of DNA damage is mediated by interactions between Tel1 and Xrs2 (Nakada et al., 2003; Iwasaki et al., 2016). Mutations in the Tel1-interaction motif of Xrs2 leads to DNA-damage signaling defects and short telomeres, similar to tel1Δ (Nakada et al., 2003). The FHA domain of Xrs2 has been shown to mediate robust Tel1 activation and to inhibit inaccurate DSB repair (Iwasaki et al., 2016). However, artificially tethering the Tel1-interaction domain of Xrs2 to an Mre11 construct containing a nuclear localization signal was sufficient for Tel1 activation (Oh et al., 2018), showing that the FHA domain was dispensable in that context.
The RMM Proteins
Rec114, Mei4, and Mer2 (RMM) form another sub-group of functionally conserved DSB proteins with enigmatic roles at the molecular level. Although they have long been recognized as meiotic DSB proteins in yeast, the identification of their homologs across the eukaryotic kingdom has been challenging because of sequence divergence. Nevertheless, RMM homologs have now been identified in many species, including mice and humans (Kumar et al., 2010, 2018; Stanzione et al., 2016; Tessé et al., 2017; Wang et al., 2019). While Rec114 and Mei4 are meiosis-specific, Mer2 is also expressed at low levels in vegetative S. cerevisiae cells and shows a unique regulation. The MER2 transcript has an intron that is only spliced efficiently during meiosis in the presence of a meiosis-specific splicing factor, Mer1 (Engebrecht et al., 1991; Nandabalan and Roeder, 1995).
Rec114, Mei4, and Mer2 localize to chromosomes in leptonema prior to DSB formation and were proposed to act as a complex based on Y2H interactions, coimmunoprecipitation, and partial foci overlap and co-dependencies (Henderson et al., 2006; Li et al., 2006; Maleki et al., 2007; Steiner et al., 2010; Miyoshi et al., 2012). Nevertheless, the existence of a stoichiometric RMM complex has never been demonstrated. In fact, their mutual dependencies are not complete, suggesting that they could exist independently. For example, chromatin binding of Rec114 and Mei4 depend on Mer2, but Mer2 foci do not depend on Rec114 and Mei4 (Maleki et al., 2007; Panizza et al., 2011).
Recent biochemical data revealed that the RMM proteins form two sub-complexes (Claeys Bouuaert et al., 2021). Rec114—Mei4 forms a complex with a 2:1 stoichiometry where the C-terminus of Rec114 homodimerizes and interacts with the N-terminus of Mei4 (Figures 6A,B). These sequences are amongst the most conserved regions of the proteins, suggesting that the interactions are also conserved (Kumar et al., 2010, 2018). In addition, the C-terminal domain of Rec114 is important for DNA binding by Rec114—Mei4 (Claeys Bouuaert et al., 2021). The N-terminus of Mus musculus REC114 was crystallized and revealed a Pleckstrin Homology (PH)-like fold with an α-helix sandwiched between two anti-parallel β-sheets (Figure 6C; Kumar et al., 2018; Boekhout et al., 2019). Blocks of amino acids previously shown to share sequence similarities across kingdoms make up the core of the domain, providing a rationale for their conservation (Maleki et al., 2007; Kumar et al., 2010). Mer2 forms a homotetramer with a predicted coiled coil thought to arrange as pairs of parallel α-helices arranged in an anti-parallel configuration (Claeys Bouuaert et al., 2021; Figure 6A). The C-terminal domain of Mer2 contains residues important for DNA binding and DSB formation (Claeys Bouuaert et al., 2021).
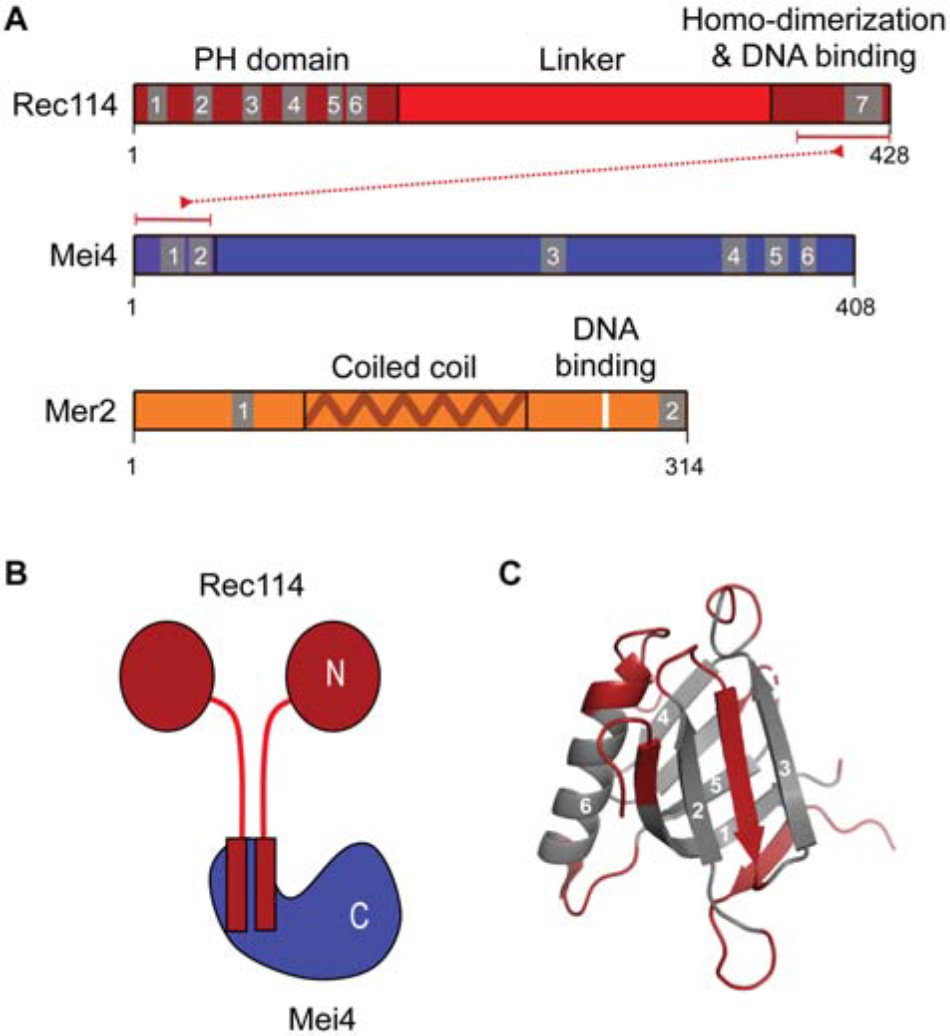
Figure 6. The RMM proteins. (A) Domain structure of Rec114, Mei4, and Mer2 with regions involved in protein-protein and protein-DNA interactions (Claeys Bouuaert et al., 2021). Numbered blocks indicate conserved sequence motifs (Kumar et al., 2010; Tessé et al., 2017). (B) Schematic of the Rec114—Mei4 complex. (C) Structure of the Pleckstrin-homology domain of mouse REC114 (PDB: 6HFG) (Kumar et al., 2018). Residues in gray are the conserved motifs highlighted in (A).
Organization of the Meiotic DSB Machinery
DSB Formation and the Chromosome Axis
It has long been appreciated that DSB formation is tied to chromosome organization (Keeney, 2001), but the relationships between local DNA-cleavage activity and higher-order structural assemblies remain poorly understood. A haploid S. cerevisiae genome contains approximately 700 loops, averaging about 15 kb each, with AT-rich sites that physically anchor a proteinaceous axis (Blat et al., 2002; Kleckner, 2006; Ito et al., 2014; Muller et al., 2018; Schalbetter et al., 2019). The loop-axis structure establishes in early prophase and plays important roles in DSB formation and inter-homolog repair (Carballo et al., 2008; Kim et al., 2010; Panizza et al., 2011; Zickler and Kleckner, 2015).
The chromosome axis in yeast includes a cohesin complex with the meiosis-specific kleisin subunit Rec8 (Klein et al., 1999), the HORMA-domain protein Hop1 (Hollingsworth et al., 1990), and the core axial protein Red1 (Smith and Roeder, 1997; Figure 7A). Axis sites are largely determined by Rec8, which localizes Red1 and Hop1 to gene ends (Panizza et al., 2011; Sun et al., 2015).
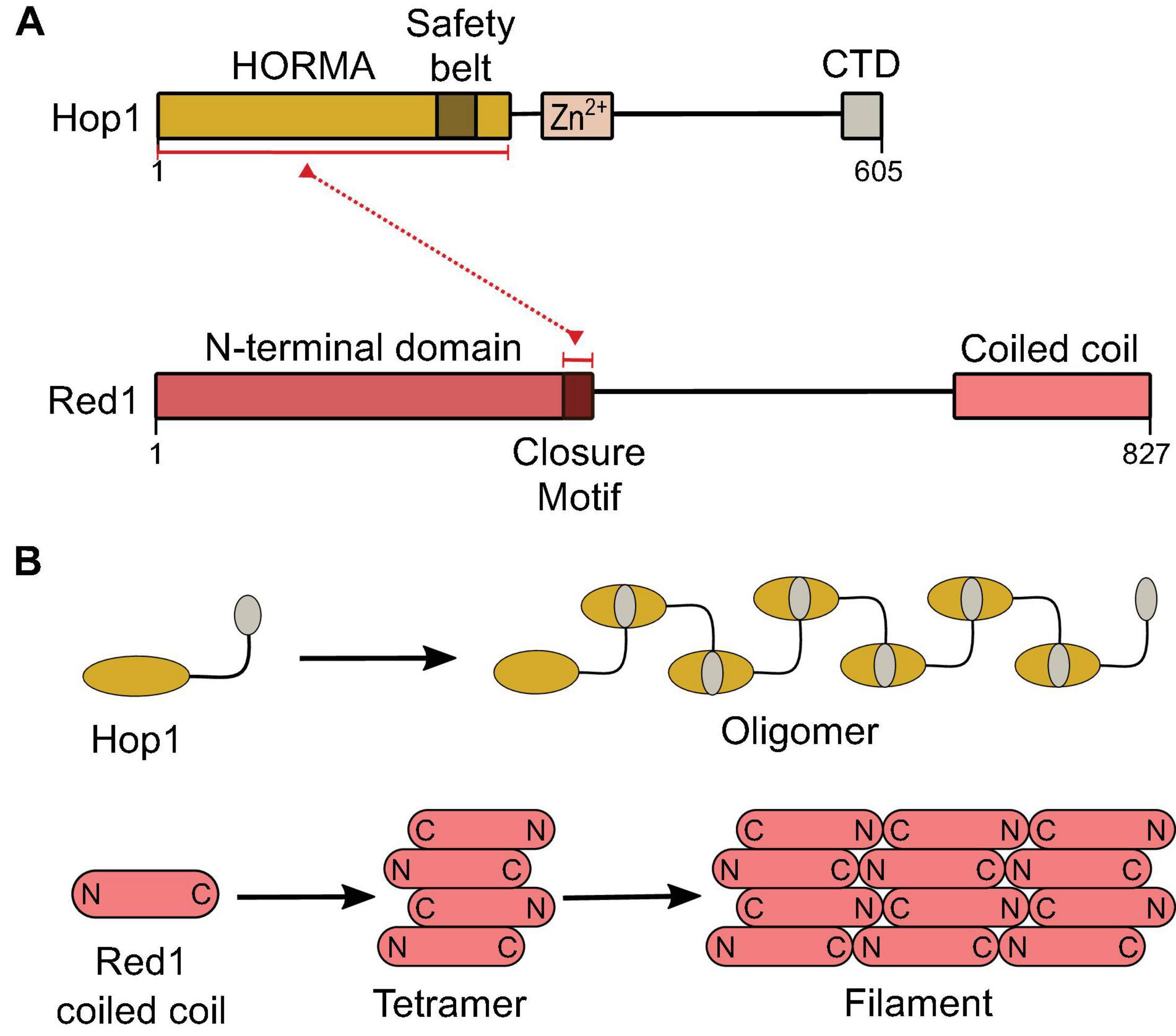
Figure 7. Structural components of the meiotic chromosome axis. (A) Domain structure of Hop1 and Red1. The C-terminal-domain (CTD) of Hop1 contains a closure motif. (B) Hop1 forms an oligomer through intermolecular interactions between the HORMA domain and the closure motif (West et al., 2018). The Red1 coiled-coil domain forms a parallel-antiparallel tetramer that can form a filament structure by end-to-end polymerization (West et al., 2019).
The C-terminal coiled-coil domain of Red1 forms a tetrameric parallel-antiparallel α-helical bundle (West et al., 2019). End-to-end polymerization of the coiled-coil is thought to underlie axis assembly (West et al., 2019). Red1 is thought to recruit Hop1 via its closure motif located in its central region (West et al., 2018) and Hop1 may also multimerize on the chromosome axis through head-to-tail self-assembly between the N-terminal HORMA domain and a closure motif located at its C-terminus (Kim et al., 2014; West et al., 2019; Figure 7B).
The DSB machinery is recruited to the chromosome axis prior to DSB formation. ChIP-seq experiments reveal similar DNA-binding distributions between RMM proteins and axis proteins, and chromatin association of RMM depends on axis proteins (Panizza et al., 2011; Murakami et al., 2020). Consistently, deletion of Red1 causes a 2.5- to 5-fold reduction in DSB formation and deletion of Hop1 decreases DSB levels by at least 10-fold (Woltering et al., 2000; Blat et al., 2002; Niu et al., 2005; Kugou et al., 2009). Axis proteins are therefore important for DSB formation, but their relationships with DSB proteins remain poorly understood at the molecular level.
DNA-Dependent Condensation of RMM
Recent characterizations of the biochemical properties of S. cerevisiae RMM brought new insights into the relationship between DSB formation and higher-order chromatin organization. In vitro, Rec114—Mei4 and Mer2 complexes bind DNA with extremely high cooperativity and lead to the assembly of large nucleoprotein structures that contain hundreds or thousands of proteins, referred to as condensates (Claeys Bouuaert et al., 2021; Figures 8A, 10A). DNA-dependent clustering is therefore an intrinsic property of Rec114—Mei4 and Mer2, suggesting that it may be important for their function. Accordingly, RMM foci are cytologically visible in vivo, implying the local accumulation of many proteins (Claeys Bouuaert et al., 2021). Nevertheless, the biophysical nature and the composition of the foci, or their relationship with break formation, remained unclear. Evidence for a direct link between foci assembly in vivo and DNA-driven condensation in vitro came from mutagenesis approaches. Mutations within Rec114 and Mer2 with mild effects on DNA binding strongly compromised DNA-driven condensation in vitro and foci formation in vivo and abolished Spo11-dependent break formation (Claeys Bouuaert et al., 2021).
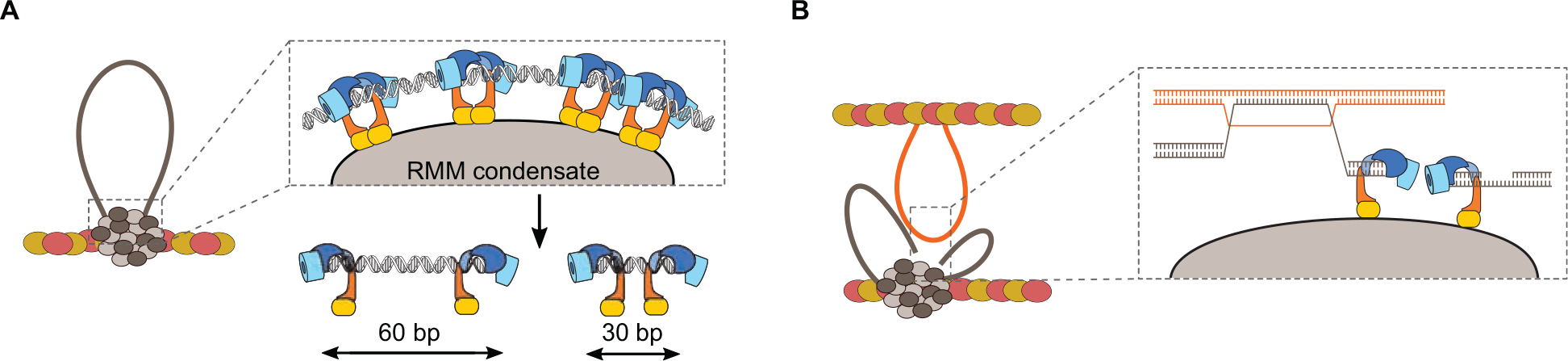
Figure 8. Model for the assembly of the meiotic DSB machinery. (A) DNA-dependent condensation of Rec114—Mei4 and Mer2 leads to the formation of large mixed nucleoprotein structures along the chromosome axis. These condensates act as a platform to recruit the Spo11 core complex, MRX, and perhaps other regulatory proteins (Claeys Bouuaert et al., 2021). This model explains the observation that Spo11 often makes closely spaced double DSBs separated with a 10-bp periodicity (Johnson et al., 2021). (B) Condensate-embedded core complexes may assist DNA repair by holding broken ends in the vicinity of one another. The condensates could also hold the broken chromatids through association with the base of the loops, independently of whether the DNA ends themselves are embedded. Axis proteins Red1 (red ovals) and Hop1 (yellow ovals) are shown.
Rec114—Mei4 and Mer2 nucleoprotein condensates share properties with systems that undergo phase-separation, including the capacity to fuse upon contact and reversibility (Claeys Bouuaert et al., 2021). In the past few years, phase separation has emerged as an important mechanism that promotes self-assembly of membrane-less intracellular compartments and exerts a variety of biological functions through local enrichment of specific biomolecules (Li et al., 2012; Banani et al., 2017; Boeynaems et al., 2018). Phase separation is often driven by weak multivalent interactions involving intrinsically disordered proteins and/or RNA. In the nucleus, chromatin sub-compartments have been proposed to assemble through one of two potential mechanisms, through the self-association of a chromatin binder, or through chromatin scaffolding by a multivalent chromatin binder (Erdel and Rippe, 2018). Condensate assembly by Rec114—Mei4 and Mer2 is driven by electrostatic interactions between the negatively charged DNA and positively charged residues within RMM proteins and appears to involve a hybrid mechanism where complexes bind multiple sites simultaneously and also engage in protein-protein interactions (Claeys Bouuaert et al., 2021).
A recent study independently reported phase separation by Mer2 and its mouse homolog IHO1 (Tsai et al., 2020). In addition, Mer2 was shown to bind directly to histone octamers, suggesting the possibility that the condensates may involve chromatinized templates, not only naked DNA (Rousova et al., 2020).
Phase separation has previously been implicated in meiosis in the assembly of the SC in C. elegans and during homolog pairing in S. pombe (Rog et al., 2017; Ding et al., 2019). In C. elegans, interactions between SC proteins are promoted by weak hydrophobic interactions (Rog et al., 2017). This creates a SC structure with mobile constituents, which is thought to allow signal transmission at the interface between pairs of homologs and to regulate crossover distribution along chromosomes (Rog et al., 2017). In fission yeast, meiosis-specific lncRNAs-protein complexes with phase-separation properties promote robust pairing of homologous chromosomes at specific loci (Ding et al., 2019).
The biochemical properties of S. cerevisiae RMM suggest a model where condensates recruit Spo11 and other regulatory proteins to provide a coherent cluster for controlled DSB formation (Figure 8A). Indeed, in vitro, the core complex can be recruited to RMM condensates via at least two sets of interactions, one dependent on Mer2, the other dependent on contacts between the PH-fold domain of Rec114 and the Rec102—Rec104 subunits of the core complex (Claeys Bouuaert et al., 2021).
The coherence provided by the condensate could provide a mechanism to keep the broken chromatids in the vicinity of each other during repair, which may reduce the risks of gross chromosomal rearrangements. Indeed, the base of the cleaved loop would remain associated with the condensate after cleavage, and one or both ends of the DSB, capped by Spo11-oligonucleotide complexes (above), could also remain embedded within the condensate (Claeys Bouuaert et al., 2021; Figure 8B).
Hyperlocalized Formation of Coincident DSBs
Independent evidence providing strong support for a higher-order assembly model of the DSB machinery came from the analysis of break patterning in S. cerevisiae (Johnson et al., 2021). Sequencing of covalently bound Spo11-DNA complexes revealed short DNA molecules (ranging from 33 to >100 bp) that are independent of MRX/Sae2-mediated nuclease activity (sae2Δ, mre11nd (nuclease dead), or rad50S). These arise from situations where two Spo11 complexes catalyze break formation in close proximity from one another. Double-cuts account for ∼5–20% of total Spo11 activity in wild-type cells, much higher than expected if the DSBs were independent from one another. Therefore, a mechanism must explain the formation of hyper-localized DSBs.
An important clue came from their spatial patterning, which shows a periodicity of ∼10.5 bp corresponding to the helical pitch of DNA (Johnson et al., 2021). Therefore, Spo11 complexes cutting adjacent to one another must attack the same side of the double helix. This could arise if Spo11 complexes were immobilized on a surface, prior to engaging the DNA substrate (Figure 8A). Given the DNA-dependent condensation property of the RMM proteins, axis-embedded RMM condensates are a good candidate to provide this surface (Claeys Bouuaert et al., 2021). However, whether the core complex is only recruited to the surface, or only active at the surface, remains unknown.
Regulation of DSB Formation
Since DSB formation is potentially dangerous, the activity of Spo11 is controlled to ensure appropriate timing, number, and distribution of breaks (Figure 9A). Complementary mechanisms overlap to achieve controlled DSB formation: (i) Activation of DSB formation is controlled temporally by protein expression and by coordination with the cell cycle and DNA replication through the reliance on post-translational modifications; (ii) Positive and negative feedback loops provide homeostatic control of DSB levels; (iii) Locally, DSBs distribution is controlled by a pro-active mechanism of hotspot competition and a reactive mechanism of DSB interference; (iv) Finally, the window of opportunity of DSB formation is controlled at the chromosomal scale through a recombination-dependent feedback mechanism, and globally through pachytene exit.
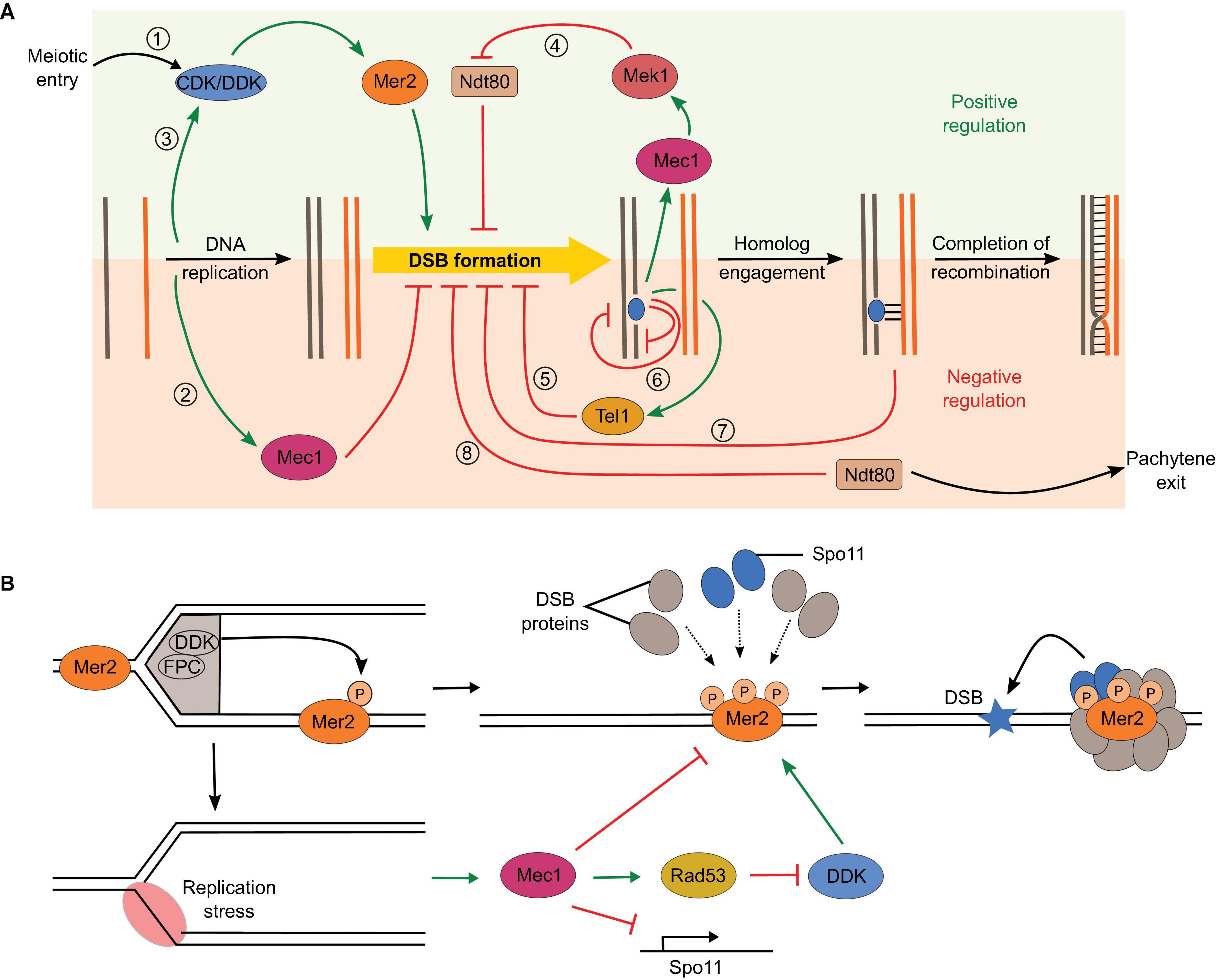
Figure 9. Overlapping regulatory circuits control DSB formation. (A) (1) DSB formation is tied to cell cycle control through dependence on CDK and DDK phosphorylation of Mer2. (2) Replication stress inhibits DSB formation by different mechanisms through activation of the Mec1 checkpoint kinase. (3) Replication also positively impacts DSB formation by promoting Mer2 phosphorylation. (4) Recombination defects activate Mec1, which extends prophase by preventing Ndt80 activation, thereby producing a positive feedback loop. (5) Activation of the DNA-damage response kinase Tel1 inhibits further DSB formation, thereby creating a negative feedback loop. (6) Hotspot competition (Tel1-independent) and DSB interference (Tel1-dependent) impact spatial distribution of DSB formation, which limits the coincident formation of two DSBs in cis within a 100-kb range or in trans between allelic regions of sister chromatids or homologs. (7) Homolog engagement shuts down DSB formation through SC-dependent removal of DSB proteins. (8) Exit of pachytene following Ndt80 activation ends the DSB-permissive period. (B) Positive and negative impacts of DNA replication on DSB formation. DDK is bound to the replisome via interactions with the fork protection complex (FPC). Phosphorylation of Mer2 in regions that have undergone replication promotes the assembly of the DSB machinery and DSB formation (Murakami and Keeney, 2014). However, replication stress activates Mec1 and inhibits DSB formation by reducing Spo11 transcription, inhibiting DDK via Rad53, and independently inhibiting chromatin association of several DSB proteins (Blitzblau and Hochwagen, 2013).
Temporo-Spatial Regulation
Meiotic DSBs occur in a narrow window of time during early prophase I. This temporal regulation is achieved by a series of factors. One level of activation is implemented by meiosis-specific transcription of genes encoding DSB proteins (SPO11, REC102, REC104, REC114, and MEI4) and meiosis-specific splicing of MER2 (Keeney, 2001, 2008). A second level is implemented through dependence of DSB formation on cell cycle progression and on coordination with DNA replication (Borde et al., 2000; Henderson et al., 2006; Wan et al., 2008; Murakami and Keeney, 2014).
S-phase cyclin-dependent kinase (CDK-S) and Dbf4-dependent kinase Cdc7 (DDK) are both essential for replication origin firing and later for DSB formation (Masai and Arai, 2002; Benjamin et al., 2003; Henderson et al., 2006; Matos et al., 2008; Wan et al., 2008). CDK-S and DDK sequentially phosphorylate Mer2 at S30 and S29, respectively, and this is important for the chromatin association of Rec114 and Mei4, and the interaction between Mer2 and Xrs2 (Henderson et al., 2006; Wan et al., 2008; Panizza et al., 2011; Figure 9A, circuit 1).
Phosphorylation of Mer2 by DDK is temporally coordinated to DNA replication by tethering of DDK to the replisome component Tof1 (Matsumoto et al., 2005; Murakami and Keeney, 2014). Mer2 phosphorylation by DDK in the wake of the replication fork therefore serves as a mark to assemble the DSB machinery in chromatin regions that have completed DNA replication (Figure 9A, circuit 3 and Figure 9B, top). However, there is a lag of about 90 min between DNA replication and DSB formation (Borde et al., 2000; Murakami and Keeney, 2014). The events that must take place between Mer2 phosphorylation and DSB formation are unclear, but in the light of the DNA-driven condensation properties of Rec114—Mei4 and Mer2, this delay could be explained by the time required to assemble the condensates and recruit the core complex and MRX.
Replication stress downregulates DSB formation through Mec1 via three complementary mechanisms: (1) partial inhibition of Spo11 transcription, (2) inhibition of DDK via Rad53 leading to hypophosphorylation of Mer2, and (3) inhibition of chromatin loading of Rec114 and Mre11 (Blitzblau and Hochwagen, 2013; Keeney et al., 2014; Figure 9A, circuit 2 and Figure 9B, bottom).
In S. pombe, blocking DNA replication also abolishes meiotic DSB formation (Ogino and Masai, 2006). In addition, early replicating regions are associated with higher DSB levels in S. pombe and in mice (Wu and Nurse, 2014; Pratto et al., 2020).
Hotspot Competition and DSB Interference
DSB formation is controlled to ensure non-random distribution of recombination events along the chromosomes (Figure 9A, circuits 5 and 6). The presence of a strong hotspot suppresses the DSB activity of an adjacent hotspot (Wu and Lichten, 1994; Xu et al., 1995; Keeney et al., 2014). This phenomenon, termed hotspot competition, is observed at a population level and can be explained by a competition between hotspots for a slowly diffusing factor that is limiting for DSB formation. Hotspot competition can therefore be implemented prior to DSB formation, and RMM proteins have been suggested to constitute this limiting factor based on the fact that they are bound to the chromosome axis, which would constrain their diffusion (Panizza et al., 2011). The condensation properties of RMM proteins provide a molecular framework to understand how this may be achieved. Partitioning of Rec114—Mei4 and Mer2 complexes within condensates lead to a local depletion of free proteins, which would reduce the probability of nucleation of other condensates nearby, leading to a non-random distribution of DSB-competent zones along the chromosomes (Claeys Bouuaert et al., 2021; Figure 10A). Consistently, in Sordaria macrospora, the Mer2 homolog Asy2 form regularly spaced foci along the chromosome axis throughout leptotene and zygotene (Tessé et al., 2017).
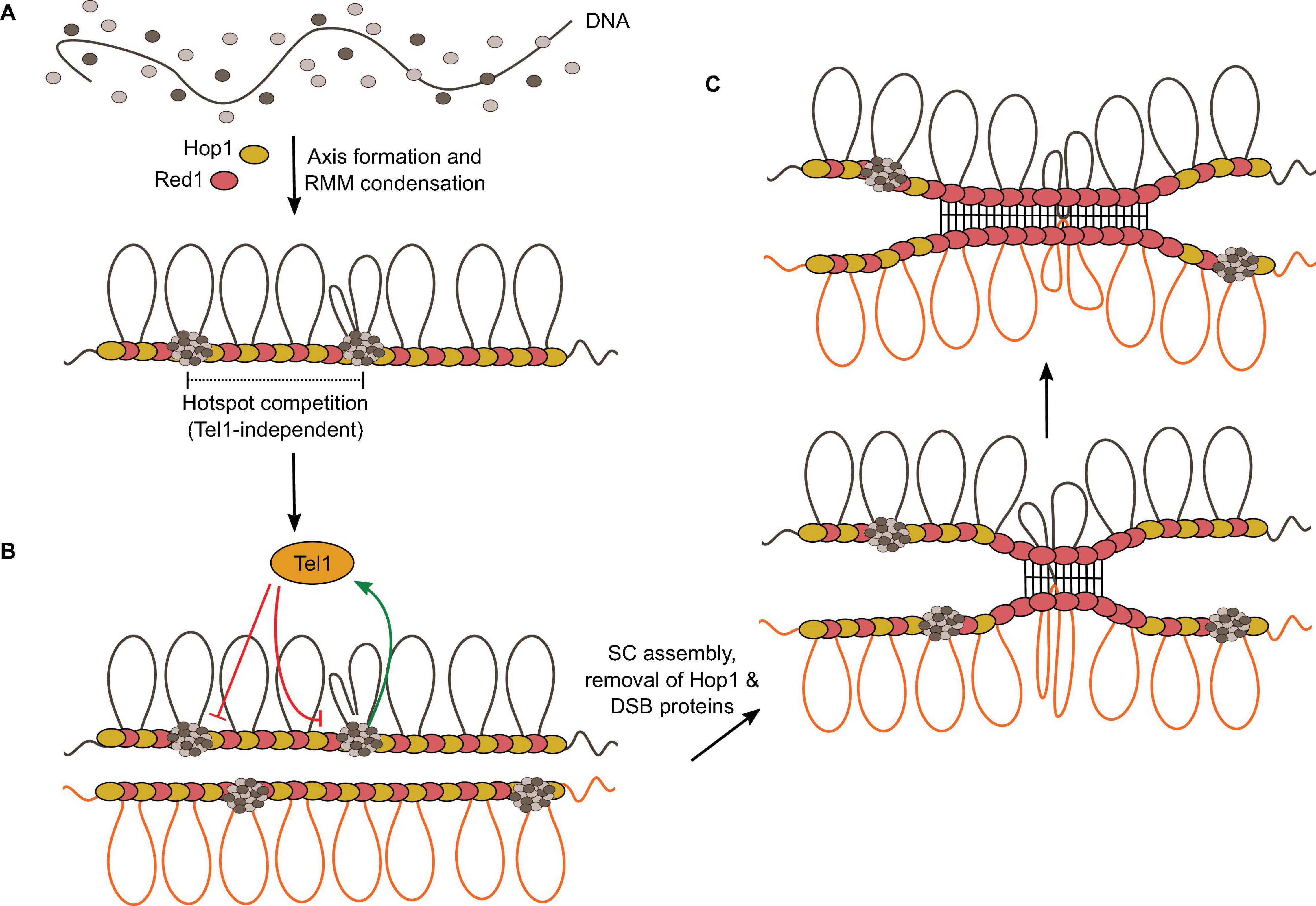
Figure 10. The condensate model for hotspot competition, DSB interference, and homolog engagement. (A) The model suggests that hotspot competition is mediated prior to DSB formation through partitioning of RMM proteins into condensates, locally depleting pools of free DSB proteins. (B) DSB formation activates Tel1, which inhibits local DSB formation. (C) SC assembly leads to the removal of Hop1 and DSB proteins from the axis, thereby shutting down further DSB formation.
Hotspot competition is genetically separable from DSB interference, the phenomenon whereby the formation of a DSB at one locus reduces the chances of another break in its vicinity. Interference is observed at the level of individual chromatids and depends on the DNA-damage response kinase Tel1, but hotspot competition does not (Mohibullah and Keeney, 2017). Upon DSB formation, Tel1 suppresses further DSB formation via a negative feedback loop thought to be implemented in part through phosphorylation of Rec114 (Zhang et al., 2011; Carballo et al., 2013; Figure 10B). Indeed, Rec114 subunit has eight [S/T]Q motifs, the known target of signal transduction kinases Tel1 and/or Mec1 (Sasanuma et al., 2007; Carballo et al., 2013), and mutation of all potential phosphorylation sites to alanine leads to elevated DSB formation, consistent with phosphorylation-dependent regulation of Rec114 (Carballo et al., 2013).
Tel1 and Mec1 mediate DSB interference in cis between different regions of the same molecule within about 100-kb range, and in trans, at allelic loci between sister chromatids (Zhang et al., 2011; Garcia et al., 2015; Fowler et al., 2018). In addition, DSB interference also occurs in trans between homologs, which must therefore depend on interhomolog contacts. Indeed, trans interference between homologs is reduced in the absence of Dmc1 (Zhang et al., 2011).
In the absence of Tel1, not only is cis interference abolished, but DSB formation shows negative interference within about a 10-kb range, meaning that coincident DSBs happen more often than predicted by chance (Garcia et al., 2015). Negative interference in the absence of Tel1 is explained by the condensate model of DSB formation since multiple Spo11 complexes are recruited within condensates, creating zones of high potential DSB activity that must be kept in check by Tel1 (Figure 8).
Hotspot competition and Tel1-dependent interference have been demonstrated in S. pombe (Fowler et al., 2018). In addition, ATM–/– mice show a high elevation of Spo11 breaks (Lange et al., 2011) and compromising ATM in Drosophila oocytes leads to increased levels of DNA damage (Joyce et al., 2011), showing that the Tel1/ATM-mediated negative feedback loop is conserved in mice and flies.
Homolog Engagement
In yeast, ZMM mutants defective for synapsis and crossing over experience persistent DSB formation (Thacker et al., 2014). This revealed that excessive DSB formation is controlled by a regulatory feedback mechanism that depends on interhomolog interactions (Figure 9A, circuit 7). Yeast strains with karyotype abnormalities show accumulation of DSBs specifically on the chromosomes that experience homolog engagement defects, showing that the feedback control operates in a chromosome-autonomous fashion (Mu et al., 2020). Smaller chromosomes also experience higher DSB levels, in part because they take more time to find each other, and as a consequence remain longer in a DSB-competent state due to the persistence of DSB proteins (Murakami et al., 2020).
Mutations in components of the SC central region (Gmc2 and Ecm11) that abolish SC elongation but not crossover formation show elevated DSBs (Humphryes and Hochwagen, 2014; Voelkel-Meiman et al., 2016; Lee et al., 2020; Mu et al., 2020). This indicates that homolog engagement feedback control operates at the level of SC assembly rather than recombination.
SC assembly removes Hop1 from the chromosome axis (Börner et al., 2008; Chen et al., 2014). This is thought to close the window of opportunity for DSB formation by triggering the dissociation of DSB proteins (Mu et al., 2020; Figure 10C). Indeed, many DSB proteins (Rec102, Rec104, Rec114, and Mei4) are removed from synapsed chromosomes (Kee et al., 2004; Li et al., 2006; Maleki et al., 2007; Panizza et al., 2011; Carballo et al., 2013). In addition, chromosomal regions ∼100 kb adjacent to telomeres retain Hop1 after synapsis and experience DSB formation in pachynema (Subramanian et al., 2019). Hop1 is removed from the axis by Pch2 that probably disrupts the interaction between Hop1 and the closure motif of Red1 (Chen et al., 2014; Kim et al., 2014; West et al., 2018).
In mice, reduced SPO11 dosage leads to synaptic defects, and unsynapsed regions display elevated DSB markers (Kauppi et al., 2013). In addition, the unsynapsed portion of the X chromosome also accumulates DSBs in wild-type male mice. Similar to yeast, synapsis leads to the removal by TRIP13 of HORMAD1 and HORMAD2, and of DSB proteins REC114 and MEI4 (Wojtasz et al., 2009; Acquaviva et al., 2020). Homolog engagement feedback control therefore appears to be conserved.
Pachytene Exit
In S. cerevisiae, exit from pachytene is controlled by the Ndt80 transcription factor (Xu et al., 1995). NDT80 activation leads to the disassembly of the SC and the removal of DSB proteins, which ends the window of opportunity for DSB formation (Figure 9A, circuit 8). As a result, ndt80 mutants accumulate more DSBs (Xu et al., 1995; Allers and Lichten, 2001; Keeney, 2001). In mutants with recombination or synapsis defects, checkpoint activation via Mec1 activates Mek1, which inhibits Ndt80 activity and leads to the extension of prophase (Figure 9A, circuit 4; Acosta et al., 2011; Gray et al., 2013; Prugar et al., 2017). Therefore, mutants that decrease Spo11 activity experience an extended window of time for DSB formation, effectively obscuring their catalytic defects. This is thought to provide homeostatic control of DSB formation.
While the negative feedback loop dependent on homolog engagement is chromosome autonomous, the Ndt80 feedback loop is nucleus-wide. The distinction was demonstrated by epistasis analysis showing that deletion of ZMM proteins in an ndt80 mutant leads to a further increase in DSB levels (Thacker et al., 2014). Therefore, the extension of prophase and synaptic defects contribute independently to persistent DSB formation.
In C. elegans and Drosophila oocytes, suppression of crossing over on a single pair of chromosomes lead to nucleus-wide increase in the retention of DSB proteins (Carlton et al., 2006; Stamper et al., 2013) or crossover frequency (Joyce and Mckim, 2010), respectively, suggesting that recombination defects extends the DSB-permissive period, leading to global increase in DSB formation.
Perspectives
To conclude, recent studies have brought new insights into the mechanism and regulation of meiotic DSB formation. However, our understanding of the structure, biochemical properties, and regulation of DSB proteins remains limited, and many important questions are yet to be addressed. Why DSB formation requires the collaborative action of so many proteins has been enigmatic for a long time. Our current model provides a tentative and partial response to this question by highlighting the organizational role of Rec114—Mei4 and Mer2 in the assembly of DSB-competent sites along chromosomes. As we have seen, the phase-separation model is consistent with, and explains, many long-standing observations regarding the behavior of DSB proteins. However, it also raises new questions regarding the biophysical properties of the condensates, their assembly and disassembly mechanisms, and how these might be controlled, perhaps through post-translational modifications. What are the minimal components required for DSB formation? In addition to known DSB proteins and essential phosphorylations, is something else needed to trigger Spo11 activity? What is the role of MRX? The rationale that its presence prior to break formation allows coordination with DSB repair is straightforward, but how is it recruited and how does it impact Spo11 activity? What is the relationship between DSB proteins and axis proteins? How are their spatial distributions controlled? Since Rec114—Mei4 and Mer2 bind DNA independently of axis proteins in vitro, why do their chromatin-association depend on the axis in vivo? Current models provide a molecular framework that will guide future experiments to better understand the mechanism of DSB formation.
Author Contributions
VKY and CCB wrote the manuscript. Both authors approved the submitted version.
Funding
This work was supported by the European Research Council under the European Union’s Horizon 2020 Research and Innovation Program (ERC Grant Agreement 802525) and by the Fonds National de la Recherche Scientifique (FNRS MIS-Ulysse Grant F.6002.20).
Conflict of Interest
The authors declare that the research was conducted in the absence of any commercial or financial relationships that could be construed as a potential conflict of interest.
Acknowledgments
We thank CCB lab members for feedback on the manuscript and Hajar Aït-Bella for help with figures.
References
Acosta, I., Ontoso, D., and San-Segundo, P. A. (2011). The budding yeast polo-like kinase Cdc5 regulates the Ndt80 branch of the meiotic recombination checkpoint pathway. Mol. Biol. Cell 22, 3478–3490. doi: 10.1091/mbc.E11-06-0482
Acquaviva, L., Boekhout, M., Karasu, M. E., Brick, K., Pratto, F., Li, T., et al. (2020). Ensuring meiotic DNA break formation in the mouse pseudoautosomal region. Nature 582, 426–431. doi: 10.1038/s41586-020-2327-4
Acquaviva, L., Székvölgyi, L., Dichtl, B., Dichtl, B. S., Saint André, C. D. L. R., Nicolas, A., et al. (2013). The COMPASS subunit Spp1 links histone methylation to initiation of meiotic recombination. Science 339, 215–218. doi: 10.1126/science.1225739
Ajimura, M., Leem, S. H., and Ogawa, H. (1993). Identification of new genes required for meiotic recombination in Saccharomyces cerevisiae. Genetics 133, 51–66.
Alani, E., Padmore, R., and Kleckner, N. (1990). Analysis of wild-type and rad50 mutants of yeast suggests an intimate relationship between meiotic chromosome synapsis and recombination. Cell 61, 419–436. doi: 10.1016/0092-8674(90)90524-I
Allers, T., and Lichten, M. (2001). Differential timing and control of noncrossover and crossover recombination during meiosis. Cell 106, 47–57. doi: 10.1016/S0092-8674(01)00416-0
Alonso-Sarduy, L., Roduit, C., Dietler, G., and Kasas, S. (2011). Human topoisomerase II-DNA interaction study by using atomic force microscopy. FEBS Lett. 585, 3139–3145. doi: 10.1016/j.febslet.2011.08.051
Anderson, J. S. J., and Parker, R. (1998). The 3′ to 5′ degradation of yeast mRNAs is a general mechanism for mRNA turnover that requires the SK12 DEVH box protein and 3′ to 5′ exonucleases of the exosome complex. EMBO J. 17, 1497–1506. doi: 10.1093/emboj/17.5.1497
Arora, C., Kee, K., Maleki, S., and Keeney, S. (2004). Antiviral protein Ski8 is a direct partner of Spo11 in meiotic DNA break formation, independent of its cytoplasmic role in RNA metabolism. Mol. Cell 13, 549–559. doi: 10.1016/S1097-2765(04)00063-2
Arthur, L. M., Gustausson, K., Hopfner, K. P., Carson, C. T., Stracker, T. H., Karcher, A., et al. (2004). Structural and functional analysis of Mre11-3. Nucleic Acids Res. 32, 1886–1893. doi: 10.1093/nar/gkh343
Banani, S. F., Lee, H. O., Hyman, A. A., and Rosen, M. K. (2017). Biomolecular condensates: organizers of cellular biochemistry. Nat. Rev. Mol. Cell Biol. 18, 285–298. doi: 10.1038/nrm.2017.7
Baudat, F., and Nicolas, A. (1997). Clustering of meiotic double-strand breaks on yeast chromosome III. Proc. Natl. Acad. Sci. U.S.A. 94, 5213–5218. doi: 10.1073/pnas.94.10.5213
Benham, C. J. (1982). Stable cruciform formation at inverted repeat sequences in supercoiled DNA. Biopolymers 21, 679–696. doi: 10.1002/bip.360210314
Benjamin, K. R., Zhang, C., Shokat, K. M., and Herskowitz, I. (2003). Control of landmark events in meiosis by the CDK Cdc28 and the meiosis-specific kinase Ime2. Genes Dev. 17, 1524–1539. doi: 10.1101/gad.1101503
Berchowitz, L. E., Hanlon, S. E., Lieb, J. D., and Copenhaver, G. P. (2009). A positive but complex association between meiotic double-strand break hotspots and open chromatin in Saccharomyces cerevisiae. Genome Res. 19, 2245–2257. doi: 10.1101/gr.096297.109
Bergerat, A., De Massy, B., Gadelle, D., Varoutas, P. C., Nicolas, A., and Forterre, P. (1997). An atypical topoisomerase II from archaea with implications for meiotic recombination. Nature 386, 414–417. doi: 10.1038/386414a0
Bishop, D. K., Park, D., Xu, L., and Kleckner, N. (1992). DMC1: a meiosis-specific yeast homolog of E. coli recA required for recombination, synaptonemal complex formation, and cell cycle progression. Cell 69, 439–456. doi: 10.1016/0092-8674(92)90446-J
Bishop, D. K., and Zickler, D. (2004). Early decision: meiotic crossover interference prior to stable strand exchange and synapsis. Cell 117, 9–15. doi: 10.1016/S0092-8674(04)00297-1
Blat, Y., Protacio, R. U., Hunter, N., and Kleckner, N. (2002). Physical and functional interactions among basic chromosome organizational features govern early steps of meiotic chiasma formation. Cell 111, 791–802. doi: 10.1016/S0092-8674(02)01167-4
Blitzblau, H. G., Bell, G. W., Rodriguez, J., Bell, S. P., and Hochwagen, A. (2007). Mapping of meiotic single-stranded DNA reveals double-strand-break hotspots near centromeres and telomeres. Curr. Biol. 17, 2003–2012. doi: 10.1016/j.cub.2007.10.066
Blitzblau, H. G., and Hochwagen, A. (2013). ATR/Mec1 prevents lethal meiotic recombination initiation on partially replicated chromosomes in budding yeast. Elife 2:e00844. doi: 10.7554/eLife.00844.001
Boekhout, M., Karasu, M. E., Wang, J., Acquaviva, L., Pratto, F., Brick, K., et al. (2019). REC114 partner ANKRD31 controls number, timing, and location of meiotic DNA breaks. Mol. Cell 74, 1053.e8–1068.e8. doi: 10.1016/j.molcel.2019.03.023
Boeynaems, S., Alberti, S., Fawzi, N. L., Mittag, T., Polymenidou, M., Rousseau, F., et al. (2018). Protein phase separation: a new phase in cell biology. Trends Cell Biol. 28, 420–435. doi: 10.1016/j.tcb.2018.02.004
Borde, V., Goldman, A. S. H., and Lichten, M. (2000). Direct coupling between meiotic DNA replication and recombination initiation. Science 290, 806–809. doi: 10.1126/science.290.5492.806
Borde, V., Lin, W., Novikov, E., Petrini, J. H., Lichten, M., and Nicolas, A. (2004). Association of Mre11p with double-strand break sites during yeast meiosis. Mol. Cell 13, 389–401. doi: 10.1016/S1097-2765(04)00034-6
Borde, V., Robine, N., Lin, W., Bonfils, S., Géli, V., and Nicolas, A. (2009). Histone H3 lysine 4 trimethylation marks meiotic recombination initiation sites. EMBO J. 28, 99–111. doi: 10.1038/emboj.2008.257
Borde, V., Wu, T.-C., and Lichten, M. (1999). Use of a recombination reporter insert to define meiotic recombination domains on chromosome III of Saccharomyces cerevisiae. Mol. Cell. Biol. 19, 4832–4842. doi: 10.1128/mcb.19.7.4832
Börner, G. V., Barot, A., and Kleckner, N. (2008). Yeast Pch2 promotes domainal axis organization, timely recombination progression, and arrest of defective recombinosomes during meiosis. Proc. Natl. Acad. Sci. U.S.A. 105, 3327–3332. doi: 10.1073/pnas.0711864105
Börner, G. V., Kleckner, N., and Hunter, N. (2004). Crossover/noncrossover differentiation, synaptonemal complex formation, and regulatory surveillance at the leptotene/zygotene transition of meiosis. Cell 117, 29–45. doi: 10.1016/S0092-8674(04)00292-2
Brown, M. S., and Bishop, D. K. (2015). DNA strand exchange and RecA homologs in meiosis. Cold Spring Harb. Perspect. Biol. 7:a016659. doi: 10.1101/cshperspect.a016659
Buhler, C., Borde, V., and Lichten, M. (2007). Mapping meiotic single-strand DNA reveals a new landscape of DNA double-strand breaks in Saccharomyces cerevisiae. PLoS Biol. 5:e324. doi: 10.1371/journal.pbio.0050324
Buhler, C., Gadelle, D., Forterre, P., Wang, J. C., and Bergerat, A. (1998). Reconstitution of DNA topoisomerase VI of the thermophilic archaeon Sulfolobus shibatae from subunits separately overexpressed in Escherichia coli. Nucleic Acids Res. 26, 5157–5162. doi: 10.1093/nar/26.22.5157
Buhler, C., Lebbink, J. H. G., Bocs, C., Ladenstein, R., and Forterre, P. (2001). DNA topoisomerase VI generates ATP-dependent double-strand breaks with two-nucleotide overhangs. J. Biol. Chem. 276, 37215–37222. doi: 10.1074/jbc.M101823200
Cannavo, E., and Cejka, P. (2014). Sae2 promotes dsDNA endonuclease activity within Mre11-Rad50-Xrs2 to resect DNA breaks. Nature 514, 122–125. doi: 10.1038/nature13771
Cannavo, E., Johnson, D., Andres, S. N., Kissling, V. M., Reinert, J. K., Garcia, V., et al. (2018). Regulatory control of DNA end resection by Sae2 phosphorylation. Nat. Commun. 9:4016. doi: 10.1038/s41467-018-06417-5
Carballo, J. A., Johnson, A. L., Sedgwick, S. G., and Cha, R. S. (2008). Phosphorylation of the axial element protein Hop1 by Mec1/Tel1 ensures meiotic interhomolog recombination. Cell 132, 758–770. doi: 10.1016/j.cell.2008.01.035
Carballo, J. A., Panizza, S., Serrentino, M. E., Johnson, A. L., Geymonat, M., Borde, V., et al. (2013). Budding yeast ATM/ATR control meiotic double-strand break (DSB) levels by down-regulating Rec114, an essential component of the DSB-machinery. PLoS Genet. 9:e1003545. doi: 10.1371/journal.pgen.1003545
Carlton, P. M., Farruggio, A. P., and Dernburg, A. F. (2006). A link between meiotic prophase progression and crossover control. PLoS Genet. 2:e12. doi: 10.1371/journal.pgen.0020012
Casari, E., Rinaldi, C., Marsella, A., Gnugnoli, M., Colombo, C. V., Bonetti, D., et al. (2019). Processing of DNA double-strand breaks by the MRX complex in a chromatin context. Front. Mol. Biosci. 6:43. doi: 10.3389/fmolb.2019.00043
Ceccaldi, R., Rondinelli, B., and D’Andrea, A. D. (2016). Repair pathway choices and consequences at the double-strand break. Trends Cell Biol. 26, 52–64. doi: 10.1016/j.tcb.2015.07.009
Chen, C., Jomaa, A., Ortega, J., and Alani, E. E. (2014). Pch2 is a hexameric ring ATPase that remodels the chromosome axis protein Hop1. Proc. Natl. Acad. Sci. U.S.A. 111, E44–E53. doi: 10.1073/pnas.1310755111
Cheng, Z., Liu, Y., Wang, C., Parker, R., and Song, H. (2009). Crystal structure of Ski8p, a WD-repeat protein with dual roles in mRNA metabolism and meiotic recombination. Protein Sci. 13, 2673–2684. doi: 10.1110/ps.04856504
Chin, G. M., and Villeneuve, A. M. (2001). C. elegans mre-11 is required for meiotic recombination and DNA repair but is dispensable for the meiotic G2 DNA damage checkpoint. Genes Dev. 15, 522–534. doi: 10.1101/gad.864101
Claeys Bouuaert, C., Pu, S., Wang, J., Oger, C., Daccache, D., Xie, W., et al. (2021). DNA-driven condensation assembles the meiotic DNA break machinery. Nature (in press).
Claeys Bouuaert, C., Tischfield, S. E., Pu, S., Mimitou, E. P., Arias-Palomo, E., Berger, J. M., et al. (2021). Structural and functional characterization of the Spo11 core complex. Nat. Struct. Mol. Biol. 28, 92–102. doi: 10.1038/s41594-020-00534-w
Cool, M., and Malone, R. E. (1992). Molecular and genetic analysis of the yeast early meiotic recombination genes REC102 and REC107/MER2. Mol. Cell. Biol. 12, 1248–1256. doi: 10.1128/mcb.12.3.1248
Corbett, K. D., Benedetti, P., and Berger, J. M. (2007). Holoenzyme assembly and ATP-mediated conformational dynamics of topoisomerase VI. Nat. Struct. Mol. Biol. 14, 611–619. doi: 10.1038/nsmb1264
Corbett, K. D., and Berger, J. M. (2003). Structure of the topoisomerase VI-B subunit: implications for type II topoisomerase mechanism and evolution. EMBO J. 22, 151–163. doi: 10.1093/emboj/cdg008
Corbett, K. D., and Berger, J. M. (2005). Structural dissection of ATP turnover in the prototypical GHL ATPase TopoVI. Structure 13, 873–882. doi: 10.1016/j.str.2005.03.013
De Muyt, A., Jessop, L., Kolar, E., Sourirajan, A., Chen, J., Dayani, Y., et al. (2012). BLM helicase ortholog Sgs1 Is a central regulator of meiotic recombination intermediate metabolism. Mol. Cell 46, 43–53. doi: 10.1016/j.molcel.2012.02.020
Ding, D. Q., Okamasa, K., Katou, Y., Oya, E., Nakayama, J. I., Chikashige, Y., et al. (2019). Chromosome-associated RNA–protein complexes promote pairing of homologous chromosomes during meiosis in Schizosaccharomyces pombe. Nat. Commun. 10:5598. doi: 10.1038/s41467-019-13609-0
Eichman, B. F., Vargason, J. M., Mooers, B. H. M., and Ho, P. S. (2000). The Holliday junction in an inverted repeat DNA sequence: sequence effects on the structure of four-way junctions. Proc. Natl. Acad. Sci. U.S.A. 97, 3971–3976. doi: 10.1073/pnas.97.8.3971
Engebrecht, J. A., Voelkel-Meiman, K., and Roeder, G. S. (1991). Meiosis-specific RNA splicing in yeast. Cell 66, 1257–1268. doi: 10.1016/0092-8674(91)90047-3
Erdel, F., and Rippe, K. (2018). Formation of chromatin subcompartments by phase separation. Biophys. J. 114, 2262–2270. doi: 10.1016/j.bpj.2018.03.011
Evans, D. H., Li, Y. F., Fox, M. E., and Smith, G. R. (1997). A WD repeat protein, Rec14, essential for meiotic recombination in Schizosaccharomyces pombe. Genetics 146, 1253–1264.
Fowler, K. R., Hyppa, R. W., Cromie, G. A., and Smith, G. R. (2018). Physical basis for long-distance communication along meiotic chromosomes. Proc. Natl. Acad. Sci. U.S.A. 115, E9333–E9342. doi: 10.1073/pnas.1801920115
Furuse, M., Nagase, Y., Tsubouchi, H., Murakami-Murofushi, K., Shibata, T., and Ohta, K. (1998). Distinct roles of two separable in vitro activities of yeast Mre11 in mitotic and meiotic recombination. EMBO J. 17, 6412–6425. doi: 10.1093/emboj/17.21.6412
Galbraith, A. M., and Malone, R. E. (1992). Characterization ofREC104, a gene required for early meiotic recombination in the yeast Saccharomyces cerevisiae. Dev. Genet. 13, 392–402. doi: 10.1002/dvg.1020130603
Game, J. C., Zamb, T. J., Braun, R. J., Resnick, M., and Roth, R. M. (1980). The role of radiation (rad) genes in meiotic recombination in yeast. Genetics 94, 51–68.
Garcia, V., Gray, S., Allison, R. M., Cooper, T. J., and Neale, M. J. (2015). Tel1ATM-mediated interference suppresses clustered meiotic double-strand-break formation. Nature 520, 114–118. doi: 10.1038/nature13993
Garcia, V., Phelps, S. E. L., Gray, S., and Neale, M. J. (2011). Bidirectional resection of DNA double-strand breaks by Mre11 and Exo1. Nature 479, 241–244. doi: 10.1038/nature10515
Gardiner, J. M., Bullard, S. A., Chrome, C., and Malone, R. E. (1997). Molecular and genetic analysis of REC103, an early meiotic recombination gene in yeast. Genetics 146, 1265–1274.
Gerton, J. L., DeRisi, J., Shroff, R., Lichten, M., Brown, P. O., and Petes, T. D. (2000). Global mapping of meiotic recombination hotspots and coldspots in the yeast Saccharomyces cerevisiae. Proc. Natl. Acad. Sci. U.S.A. 97, 11383–11390. doi: 10.1073/pnas.97.21.11383
Girard, C., Roelens, B., Zawadzki, K. A., and Villeneuve, A. M. (2018). Interdependent and separable functions of Caenorhabditis elegans MRN-C complex members couple formation and repair of meiotic DSBs. Proc. Natl. Acad. Sci. U.S.A. 115, E4443–E4452. doi: 10.1073/pnas.1719029115
Gnügge, R., and Symington, L. S. (2017). Keeping it real: MRX-Sae2 clipping of natural substrates. Genes Dev. 31, 2311–2312. doi: 10.1101/gad.310771.117
Gobbini, E., Cassani, C., Villa, M., Bonetti, D., and Longhese, M. P. (2016). Functions and regulation of the MRX complex at DNA double-strand breaks. Microb. Cell 3, 329–337. doi: 10.15698/mic2016.08.517
Graille, M., Cladière, L., Durand, D., Lecointe, F., Gadelle, D., Quevillon-Cheruel, S., et al. (2008). Crystal structure of an intact type II DNA topoisomerase: insights into DNA transfer mechanisms. Structure 16, 360–370. doi: 10.1016/j.str.2007.12.020
Gray, S., Allison, R. M., Garcia, V., Goldman, A. S. H., and Neale, M. J. (2013). Positive regulation of meiotic DNA double-strand break formation by activation of the DNA damage checkpoint kinase Mec1(ATR). Open Biol. 3:130019. doi: 10.1098/rsob.130019
Gray, S., and Cohen, P. E. (2016). Control of meiotic crossovers: from double-strand break formation to designation. Annu. Rev. Genet. 50, 175–210. doi: 10.1146/annurev-genet-120215-035111
Halbach, F., Reichelt, P., Rode, M., and Conti, E. (2013). The yeast ski complex: crystal structure and rna channeling to the exosome complex. Cell 154, 814–826. doi: 10.1016/j.cell.2013.07.017
Hatkevich, T., Miller, D. E., Turcotte, C. A., Miller, M. C., and Sekelsky, J. (2020). A pathway for error-free non-homologous end joining of resected meiotic double-strand breaks. bioRxiv [Preprint] doi: 10.1101/2020.06.17.157313
Hayashi, M., Chin, G. M., and Villeneuve, A. M. (2007). C. elegans germ cells switch between distinct modes of double-strand break repair during meiotic prophase progression. PLoS Genet. 3:e191. doi: 10.1371/journal.pgen.0030191
Henderson, K. A., Kee, K., Maleki, S., Santini, P. A., and Keeney, S. (2006). Cyclin-dependent kinase directly regulates initiation of meiotic recombination. Cell 125, 1321–1332. doi: 10.1016/j.cell.2006.04.039
Hohl, M., Kwon, Y., Galván, S. M., Xue, X., Tous, C., Aguilera, A., et al. (2010). The Rad50 coiled-coil domain is indispensable for Mre11 complex functions. Nat. Struct. Mol. Biol. 18, 1124–1131. doi: 10.1038/nsmb.2116
Hollingsworth, N. M., Goetsch, L., and Byers, B. (1990). The HOP1 gene encodes a meiosis-specific component of yeast chromosomes. Cell 61, 73–84. doi: 10.1016/0092-8674(90)90216-2
Hong, E. L., Shinohara, A., and Bishop, D. K. (2001). Saccharomyces cerevisiae Dmc1 protein promotes renaturation of single-strand DNA (ssDNA) and assimilation of ssDNA into homologous super-coiled duplex DNA. J. Biol. Chem. 276, 41906–41912. doi: 10.1074/jbc.M105563200
Hopfner, K. P., Craig, L., Moncalian, G., Zinkel, R. A., Usui, T., Owen, B. A. L., et al. (2002). The Rad50 zinc-hook is a structure joining Mre11 complexes in DNA recombination and repair. Nature 418, 562–566. doi: 10.1038/nature00922
Hopfner, K. P., Karcher, A., Craig, L., Woo, T. T., Carney, J. P., and Tainer, J. A. (2001). Structural biochemistry and interaction architecture of the DNA double-strand break repair Mre11 nuclease and Rad50-ATPase. Cell 105, 473–485. doi: 10.1016/S0092-8674(01)00335-X
Humphryes, N., and Hochwagen, A. (2014). A non-sister act: recombination template choice during meiosis. Exp. Cell Res. 329, 53–60. doi: 10.1016/j.yexcr.2014.08.024
Hunter, N. (2015). Meiotic recombination: the essence of heredity. Cold Spring Harb. Perspect. Biol. 7:a016618. doi: 10.1101/cshperspect.a016618
Hunter, N., and Kleckner, N. (2001). The single-end invasion: an asymmetric intermediate at the double-strand break to double-holliday junction transition of meiotic recombination. Cell 106, 59–70. doi: 10.1016/S0092-8674(01)00430-5
Ito, M., Kugou, K., Fawcett, J. A., Mura, S., Ikeda, S., Innan, H., et al. (2014). Meiotic recombination cold spots in chromosomal cohesion sites. Genes Cells 19, 359–373. doi: 10.1111/gtc.12138
Ivanov, E. L., Korolev, V. G., and Fabre, F. (1992). XRS2, a DNA repair gene of Saccharomyces cerevisiae, is needed for meiotic recombination. Genetics 132, 651–664.
Iwasaki, D., Hayashihara, K., Shima, H., Higashide, M., Terasawa, M., Gasser, S. M., et al. (2016). The MRX complex ensures NHEJ fidelity through multiple pathways including Xrs2-FHA–dependent tel1 activation. PLoS Genet. 12:e1005942. doi: 10.1371/journal.pgen.1005942
Johnson, D., Crawford, M., Cooper, T., Claeys Bouuaert, C., Keeney, S., Llorente, B., et al. (2021). Concerted cutting by Spo11 illuminates the mechanism of meiotic DNA break formation. Nature (in press).
Jolivet, S., Vezon, D., Froger, N., and Mercier, R. (2006). Non conservation of the meiotic function of the Ski8/Rec103 homolog in Arabidopsis. Genes Cells 11, 615–622. doi: 10.1111/j.1365-2443.2006.00972.x
Joshi, N., Brown, M. S., Bishop, D. K., and Börner, G. V. (2015). Gradual implementation of the meiotic recombination program via checkpoint pathways controlled by global DSB levels. Mol. Cell 57, 797–811. doi: 10.1016/j.molcel.2014.12.027
Joyce, E. F., and Mckim, K. S. (2010). Chromosome axis defects induce a checkpoint-mediated delay and interchromosomal effect on crossing over during drosophila meiosis. PLoS Genet. 6:e1001059. doi: 10.1371/journal.pgen.1001059
Joyce, E. F., Pedersen, M., Tiong, S., White-Brown, S. K., Paul, A., Campbell, S. D., et al. (2011). Drosophila ATM and ATR have distinct activities in the regulation of meiotic DNA damage and repair. J. Cell Biol. 195, 359–367.
Kauppi, L., Barchi, M., Lange, J., Baudat, F., Jasin, M., and Keeney, S. (2013). Numerical constraints and feedback control of double-strand breaks in mouse meiosis. Genes Dev. 27, 873–886. doi: 10.1101/gad.213652.113
Kee, K., Protacio, R. U., Arora, C., and Keeney, S. (2004). Spatial organization and dynamics of the association of Rec102 and Rec104 with meiotic chromosomes. EMBO J. 23, 1815–1824. doi: 10.1038/sj.emboj.7600184
Keeney, S. (2001). Mechanism and control of meiotic recombination initiation. Curr. Top. Dev. Biol. 52, 1–53. doi: 10.1016/s0070-2153(01)52008-6
Keeney, S. (2008). Spo11 and the formation of DNA double-strand breaks in meiosis. Genome Dyn. Stab. 2, 81–123. doi: 10.1007/7050_2007_026
Keeney, S., Giroux, C. N., and Kleckner, N. (1997). Meiosis-specific DNA double-strand breaks are catalyzed by Spo11, a member of a widely conserved protein family. Cell 88, 375–384. doi: 10.1016/S0092-8674(00)81876-0
Keeney, S., Lange, J., and Mohibullah, N. (2014). Self-organization of meiotic recombination initiation: general principles and molecular pathways. Annu. Rev. Genet. 48, 187–214. doi: 10.1146/annurev-genet-120213-092304
Kim, K. P., Weiner, B. M., Zhang, L., Jordan, A., Dekker, J., and Kleckner, N. (2010). Sister cohesion and structural axis components mediate homolog bias of meiotic recombination. Cell 143, 924–937. doi: 10.1016/j.cell.2010.11.015
Kim, S., Peterson, S. E., Jasin, M., and Keeney, S. (2016). Mechanisms of germ line genome instability. Semin. Cell Dev. Biol. 54, 177–187. doi: 10.1016/j.semcdb.2016.02.019
Kim, Y., Rosenberg, S. C., Kugel, C. L., Kostow, N., Rog, O., Davydov, V., et al. (2014). The chromosome axis controls meiotic events through a hierarchical assembly of HORMA domain proteins. Dev. Cell 31, 487–502. doi: 10.1016/j.devcel.2014.09.013
Klapholz, S., Waddell, C. S., and Esposito, R. E. (1985). The role of the Spo11 gene in meiotic recombination in yeast. Genetics 110, 187–216.
Kleckner, N. (2006). Chiasma formation: chromatin/axis interplay and the role(s) of the synaptonemal complex. Chromosoma 115, 175–194. doi: 10.1007/s00412-006-0055-7
Klein, F., Mahr, P., Galova, M., Buonomo, S. B. C., Michaelis, C., Nairz, K., et al. (1999). A central role for cohesins in sister chromatid cohesion, formation of axial elements, and recombination during yeast meiosis. Cell 98, 91–103. doi: 10.1016/S0092-8674(00)80609-1
Kugou, K., Fukuda, T., Yamada, S., Ito, M., Sasanuma, H., Mori, S., et al. (2009). Rec8 guides canonical Spo11 distribution along yeast meiotic chromosomes. Mol. Biol. Cell 20, 3064–3076. doi: 10.1091/mbc.E08-12-1223
Kumar, R., Bourbon, H. M., and De Massy, B. (2010). Functional conservation of Mei4 for meiotic DNA double-strand break formation from yeasts to mice. Genes Dev. 24, 1266–1280. doi: 10.1101/gad.571710
Kumar, R., Oliver, C., Brun, C., Juarez-Martinez, A. B., Tarabay, Y., Kadlec, J., et al. (2018). Mouse REC114 is essential for meiotic DNA double-strand break formation and forms a complex with MEI4. Life Sci. Alliance 1:e201800259. doi: 10.26508/lsa.201800259
Lam, I., and Keeney, S. (2015). Mechanism and regulation of meiotic recombination initiation. Cold Spring Harb. Perspect. Biol. 7:a016634. doi: 10.1101/cshperspect.a016634
Lange, J., Pan, J., Cole, F., Thelen, M. P., Jasin, M., and Keeney, S. (2011). ATM controls meiotic double-strand-break formation. Nature 479, 237–240. doi: 10.1038/nature10508
Lee, M.-S., Higashide, M. T., Choi, H., Li, K., Hong, S., Lee, K., et al. (2020). The synaptonemal complex central region modulates 2 crossover pathways and feedback control of meiotic double-strand break formation 4 5. bioRxiv [Preprint] doi: 10.1101/2020.07.10.198168
Li, J., Hooker, G. W., and Roeder, G. S. (2006). Saccharomyces cerevisiae Mer2, Mei4 and Rec114 form a complex required for meiotic double-strand break formation. Genetics 173, 1969–1981. doi: 10.1534/genetics.106.058768
Li, P., Banjade, S., Cheng, H. C., Kim, S., Chen, B., Guo, L., et al. (2012). Phase transitions in the assembly of multivalent signalling proteins. Nature 483, 336–340. doi: 10.1038/nature10879
Liang, J., Suhandynata, R. T., and Zhou, H. (2015). Phosphorylation of Sae2 mediates Forkhead-associated (FHA) domain-specific interaction and regulates its DNA repair function. J. Biol. Chem. 290, 10751–10763. doi: 10.1074/jbc.M114.625293
Lichten, M., and Goldman, A. S. H. (1995). Meiotic recombination hotspots. Annu. Rev. Genet. 29, 423–444. doi: 10.1146/annurev.ge.29.120195.002231
Liu, J., Wu, T. C., and Lichten, M. (1995). The location and structure of double-strand DNA breaks induced during yeast meiosis: evidence for a covalently linked DNA-protein intermediate. EMBO J. 14, 4599–4608. doi: 10.1002/j.1460-2075.1995.tb00139.x
Liu, Y., Sung, S., Kim, Y., Li, F., Gwon, G., Jo, A., et al. (2016). ATP -dependent DNA binding, unwinding, and resection by the Mre11/Rad50 complex. EMBO J. 35, 743–758. doi: 10.15252/embj.201592462
Lynn, A., Soucek, R., and Börner, G. V. (2007). ZMM proteins during meiosis: crossover artists at work. Chromosom. Res. 15, 591–605. doi: 10.1007/s10577-007-1150-1
Madrona, A. Y., and Wilson, D. K. (2004). The structure of Ski8p, a protein regulating mRNA degradation: implications for WD protein structure. Protein Sci. 13, 1557–1565. doi: 10.1110/ps.04704704
Maleki, S., Neale, M. J., Arora, C., Henderson, K. A., and Keeney, S. (2007). Interactions between Mei4, Rec114, and other proteins required for meiotic DNA double-strand break formation in Saccharomyces cerevisiae. Chromosoma 116, 471–486. doi: 10.1007/s00412-007-0111-y
Malone, R. E., Bullard, S., Hermiston, M., Rieger, R., Cool, M., and Galbraith, A. (1991). Isolation of mutants defective in early steps of meiotic recombination in the yeast Saccharomyces cerevisiae. Genetics 128, 79–88.
Malone, R. E., and Esposito, R. E. (1981). Recombinationless meiosis in Saccharomyces cerevisiae. Mol. Cell. Biol. 1, 891–901. doi: 10.1128/mcb.1.10.891
Martini, E., Borde, V., Legendre, M., Audic, S., Regnault, B., Soubigou, G., et al. (2011). Genome-wide analysis of heteroduplex DNA in mismatch repair–deficient yeast cells reveals novel properties of meiotic recombination pathways. PLoS Genet. 7:e1002305. doi: 10.1371/journal.pgen.1002305
Masai, H., and Arai, K. I. (2002). Cdc7 kinase complex: a key regulator in the initiation of DNA replication. J. Cell. Physiol. 190, 287–296. doi: 10.1002/jcp.10070
Matos, J., Lipp, J. J., Bogdanova, A., Guillot, S., Okaz, E., Junqueira, M., et al. (2008). Dbf4-dependent Cdc7 kinase links DNA replication to the segregation of homologous chromosomes in meiosis I. Cell 135, 662–678. doi: 10.1016/j.cell.2008.10.026
Matsumoto, S., Ogino, K., Noguchi, E., Russell, P., and Masai, H. (2005). Hsk1-Dfp1/Him1, the Cdc7-Dbf4 kinase in Schizosaccharomyces pombe, associates with Swi1, a component of the replication fork protection complex. J. Biol. Chem. 280, 42536–42542. doi: 10.1074/jbc.M510575200
Menees, T. M., and Roeder, G. S. (1989). MEI4, a yeast gene required for meiotic recombination. Genetics 123, 675–682.
Mimitou, E. P., Yamada, S., and Keeney, S. (2017). A global view of meiotic double-strand break end resection. Science 355, 40–45. doi: 10.1126/science.aak9704
Miyoshi, T., Ito, M., Kugou, K., Yamada, S., Furuichi, M., Oda, A., et al. (2012). A central coupler for recombination initiation linking chromosome architecture to s phase checkpoint. Mol. Cell 47, 722–733. doi: 10.1016/j.molcel.2012.06.023
Mohibullah, N., and Keeney, S. (2017). Numerical and spatial patterning of yeast meiotic DNA breaks by Tel1. Genome Res. 27, 278–288. doi: 10.1101/gr.213587.116
Mu, X., Murakami, H., Mohibullah, N., and Keeney, S. (2020). Chromosome-autonomous feedback down-regulates meiotic DNA break competence upon synaptonemal complex formation. Genes Dev. 34, 1605–1618. doi: 10.1101/gad.342873.120
Muller, H., Scolari, V. F., Agier, N., Piazza, A., Thierry, A., Mercy, G., et al. (2018). Characterizing meiotic chromosomes’ structure and pairing using a designer sequence optimized for Hi-C. Mol. Syst. Biol. 14:e8293. doi: 10.15252/msb.20188293
Murakami, H., and Keeney, S. (2014). Temporospatial coordination of meiotic dna replication and recombination via DDK recruitment to replisomes. Cell 158, 861–873. doi: 10.1016/j.cell.2014.06.028
Murakami, H., Lam, I., Huang, P.-C., Song, J., van Overbeek, M., and Keeney, S. (2020). Multilayered mechanisms ensure that short chromosomes recombine in meiosis. Nature 582, 124–128. doi: 10.1038/s41586-020-2248-2
Murakami, H., and Nicolas, A. (2009). Locally, meiotic double-strand breaks targeted by Gal4BD-Spo11 occur at discrete sites with a sequence preference. Mol. Cell. Biol. 29, 3500–3516. doi: 10.1128/mcb.00088-09
Nairz, K., and Klein, F. (1997). mre11S - a yeast mutation that blocks double-strand-break processing and permits nonhomologous synapsis in meiosis. Genes Dev. 11, 2272–2290. doi: 10.1101/gad.11.17.2272
Nakada, D., Matsumoto, K., and Sugimoto, K. (2003). ATM-related Tel1 associates with double-strand breaks through an Xrs2-dependent mechanism. Genes Dev. 17, 1957–1962. doi: 10.1101/gad.1099003
Nandabalan, K., and Roeder, G. S. (1995). Binding of a cell-type-specific RNA splicing factor to its target regulatory sequence. Mol. Cell. Biol. 15, 1953–1960. doi: 10.1128/mcb.15.4.1953
Nasar, F., Jankowski, C., and Nag, D. K. (2000). Long palindromic sequences induce double-strand breaks during meiosis in yeast. Mol. Cell. Biol. 20, 3449–3458. doi: 10.1128/mcb.20.10.3449-3458.2000
Neale, M. J., Pan, J., and Keeney, S. (2005). Endonucleolytic processing of covalent protein-linked DNA double-strand breaks. Nature 436, 1053–1057. doi: 10.1038/nature03872
Nichols, M. D., DeAngelis, K., Keck, J. L., and Berger, J. M. (1999). Structure and function of an archaeal topoisomerase VI subunit with homology to the meiotic recombination factor Spo11. EMBO J. 18, 6177–6188. doi: 10.1093/emboj/18.21.6177
Niu, H., Wan, L., Baumgartner, B., Schaefer, D., Loidl, J., and Hollingsworth, N. M. (2005). Partner choice during meiosis is regulated by Hop1-promoted dimerization of Mek1. Mol. Biol. Cell 16, 5804–5818.
Ogino, K., and Masai, H. (2006). Rad3-Cds1 mediates coupling of initiation of meiotic recombination with DNA replication: Mei4-dependent transcription as a potential target of meiotic checkpoint. J. Biol. Chem. 281, 1338–1344. doi: 10.1074/jbc.M505767200
Oh, J., Al-Zain, A., Cannavo, E., Cejka, P., and Symington, L. S. (2016). Xrs2 dependent and independent functions of the Mre11-Rad50 complex. Mol. Cell 64, 405–415. doi: 10.1016/j.molcel.2016.09.011
Oh, J., Lee, S. J., Rothstein, R., and Symington, L. S. (2018). Xrs2 and tel1 independently contribute to MR-mediated DNA tethering and replisome stability. Cell Rep. 25, 1681.e4–1692.e4. doi: 10.1016/j.celrep.2018.10.030
Page, S. L., and Hawley, R. S. (2003). Chromosome choreography: the meiotic ballet. Science 301, 785–789. doi: 10.1126/science.1086605
Paiano, J., Wu, W., Yamada, S., Sciascia, N., Callen, E., Paola Cotrim, A., et al. (2020). ATM and PRDM9 regulate SPO11-bound recombination intermediates during meiosis. Nat. Commun. 11:857. doi: 10.1038/s41467-020-14654-w
Palmer, S., Schildkraut, E., Lazarin, R., Nguyen, J., and Nickoloff, J. A. (2003). Gene conversion tracts in Saccharomyces cerevisiae can be extremely short and highly directional. Nucleic Acids Res. 31, 1164–1173. doi: 10.1093/nar/gkg219
Pan, J., Sasaki, M., Kniewel, R., Murakami, H., Blitzblau, H. G., Tischfield, S. E., et al. (2011). A hierarchical combination of factors shapes the genome-wide topography of yeast meiotic recombination initiation. Cell 144, 719–731. doi: 10.1016/j.cell.2011.02.009
Panizza, S., Mendoza, M. A., Berlinger, M., Huang, L., Nicolas, A., Shirahige, K., et al. (2011). Spo11-accessory proteins link double-strand break sites to the chromosome axis in early meiotic recombination. Cell 146, 372–383. doi: 10.1016/j.cell.2011.07.003
Paull, T. T., and Gellert, M. (1998). The 3′ to 5′ exonuclease activity of Mre11 facilitates repair of DNA double-strand breaks. Mol. Cell 1, 969–979. doi: 10.1016/S1097-2765(00)80097-0
Petes, T. D. (2001). Meiotic recombination hot spots and cold spots. Nat. Rev. Genet. 2, 360–369. doi: 10.1038/35072078
Petronczki, M., Siomos, M. F., and Nasmyth, K. (2003). Un ménage à quatre: the molecular biology of chromosome segregation in meiosis. Cell 112, 423–440. doi: 10.1016/S0092-8674(03)00083-7
Pittman, D., Lu, W., and Malone, R. E. (1993). Genetic and molecular analysis of REC114, an early meiotic recombination gene in yeast. Curr. Genet. 23, 295–304. doi: 10.1007/BF00310890
Pratto, F., Brick, K., Cheng, G., Lam, G., Cloutier, J. M., Dahiya, D., et al. (2020). Germline DNA replication shapes the recombination landscape in mammals. bioRxiv [Preprint] doi: 10.1101/2020.09.23.308874
Prieler, S., Penkner, A., Borde, V., and Klein, F. (2005). The control of Spo11’s interaction with meiotic recombination hotspots. Genes Dev. 19, 255–269. doi: 10.1101/gad.321105
Prugar, E., Burnett, C., Chen, X., and Hollingsworth, N. M. (2017). Coordination of double strand break repair and meiotic progression in yeast by a Mek1-Ndt80 negative feedback loop. Genetics 206, 497–512. doi: 10.1534/genetics.117.199703
Puizina, J., Siroky, J., Mokros, P., Schweizer, D., and Riha, K. (2004). Mre11 deficiency in Arabidopsis is associated with chromosomal instability in somatic cells and Spo11-dependent genome fragmentation during meiosis. Plant Cell 16, 1968–1978. doi: 10.1105/tpc.104.022749
Pyatnitskaya, A., Borde, V., and De Muyt, A. (2019). Crossing and zipping: molecular duties of the ZMM proteins in meiosis. Chromosoma 128, 181–198. doi: 10.1007/s00412-019-00714-8
Robert, T., Nore, A., Brun, C., Maffre, C., Crimi, B., Bourbon, H. M., et al. (2016). The topo VIB-Like protein family is required for meiotic DNA double-strand break formation. Science 351, 943–949. doi: 10.1126/science.aad5309
Rockmill, B., Engebrecht, J. A., Scherthan, H., Loidl, J., and Roeder, G. S. (1995). The yeast MER2 gene is required for chromosome synapsis and the initiation of meiotic recombination. Genetics 141, 49–59.
Roeder, G. S., Rockmill, B. M., Engebrecht, J., Thompson, E. A., and Menees, T. M. (1989). Isolation and characterization of yeast mutants defective in meiotic chromosome segregation. Prog. Clin. Biol. Res. 311, 303–326.
Rog, O., Köhler, S., and Dernburg, A. F. (2017). The synaptonemal complex has liquid crystalline properties and spatially regulates meiotic recombination factors. Elife 6:e21455. doi: 10.7554/eLife.21455
Rousova, D., Funk, S. K., Reichle, H., and Weir, J. R. (2020). Mer2 binds directly to both nucleosomes and axial proteins as the keystone of meiotic recombination. bioRxiv [Preprint] doi: 10.1101/2020.07.30.228908
San Filippo, J., Sung, P., and Klein, H. (2008). Mechanism of eukaryotic homologous recombination. Annu. Rev. Biochem. 77, 229–257. doi: 10.1146/annurev.biochem.77.061306.125255
Sasanuma, H., Murakami, H., Fukuda, T., Shibata, T., Nicolas, A., and Ohta, K. (2007). Meiotic association between Spo11 regulated by Rec102, Rec104 and Rec114. Nucleic Acids Res. 35, 1119–1133. doi: 10.1093/nar/gkl1162
Schalbetter, S. A., Fudenberg, G., Baxter, J., Pollard, K. S., and Neale, M. J. (2019). Principles of meiotic chromosome assembly revealed in S. cerevisiae. Nat. Commun. 10:4795. doi: 10.1038/s41467-019-12629-0
Schiller, C. B., Lammens, K., Guerini, I., Coordes, B., Feldmann, H., Schlauderer, F., et al. (2012). Structure of Mre11-Nbs1 complex yields insights into ataxia-telangiectasia- like disease mutations and DNA damage signaling. Nat. Struct. Mol. Biol. 19, 693–700. doi: 10.1038/nsmb.2323
Schiller, C. B., Seifert, F. U., Linke-Winnebeck, C., and Hopfner, K. P. (2014). Structural studies of DNA end detection and resection in homologous recombination. Cold Spring Harb. Perspect. Biol. 6:a017962. doi: 10.1101/cshperspect.a017962
Schwacha, A., and Kleckner, N. (1995). Identification of double holliday junctions as intermediates in meiotic recombination. Cell 83, 783–791
Seifert, F. U., Lammens, K., Stoehr, G., Kessler, B., and Hopfner, K. (2016). Structural mechanism of ATP -dependent DNA binding and DNA end bridging by eukaryotic Rad50. EMBO J. 35, 759–772. doi: 10.15252/embj.201592934
Shima, H., Suzuki, M., and Shinohara, M. (2005). Isolation and characterization of novel xrs2 mutations in Saccharomyces cerevisiae. Genetics 170, 71–85. doi: 10.1534/genetics.104.037580
Smith, A. V., and Roeder, G. S. (1997). The yeast Red1 protein localizes to the cores of meiotic chromosomes. J. Cell Biol. 136, 957–967. doi: 10.1083/jcb.136.5.957
Sommermeyer, V., Béneut, C., Chaplais, E., Serrentino, M. E., and Borde, V. (2013). Spp1, a member of the Set1 complex, promotes meiotic DSB formation in promoters by tethering histone H3K4 methylation sites to chromosome axes. Mol. Cell 49, 43–54. doi: 10.1016/j.molcel.2012.11.008
Stamper, E. L., Rodenbusch, S. E., Rosu, S., Ahringer, J., Villeneuve, A. M., and Dernburg, A. F. (2013). Identification of DSB-1, a protein required for initiation of meiotic recombination in Caenorhabditis elegans, illuminates a crossover assurance checkpoint. PLoS Genet. 9:e1003679. doi: 10.1371/journal.pgen.1003679
Stanzione, M., Baumann, M., Papanikos, F., Dereli, I., Lange, J., Ramlal, A., et al. (2016). Meiotic DNA break formation requires the unsynapsed chromosome axis-binding protein IHO1 (CCDC36) in mice. Nat. Cell Biol. 18, 1208–1220. doi: 10.1038/ncb3417
Steiner, S., Kohli, J., and Ludin, K. (2010). Functional interactions among members of the meiotic initiation complex in fission yeast. Curr. Genet. 56, 237–249. doi: 10.1007/s00294-010-0296-0
Subramanian, V. V., Zhu, X., Markowitz, T. E., Vale-Silva, L. A., San-Segundo, P. A., Hollingsworth, N. M., et al. (2019). Persistent DNA-break potential near telomeres increases initiation of meiotic recombination on short chromosomes. Nat. Commun. 10:970. doi: 10.1038/s41467-019-08875-x
Sun, H., Treco, D., Schultes, N. P., and Szostak, J. W. (1989). Double-strand breaks at an initiation site for meiotic gene conversion. Nature 338, 87–90. doi: 10.1038/338087a0
Sun, H., Treco, D., and Szostak, J. W. (1991). Extensive 3′-overhanging, single-stranded DNA associated with the meiosis-specific double-strand breaks at the ARG4 recombination initiation site. Cell 64, 1155–1161. doi: 10.1016/0092-8674(91)90270-9
Sun, X., Huang, L., Markowitz, T. E., Blitzblau, H. G., Chen, D., Klein, F., et al. (2015). Transcription dynamically patterns the meiotic chromosome-axis interface. Elife 4:e07424. doi: 10.7554/eLife.07424
Symington, L. S. (2016). Mechanism and regulation of DNA end resection in eukaryotes. Crit. Rev. Biochem. Mol. Biol. 51, 195–212. doi: 10.3109/10409238.2016.1172552
Tessé, S., Bourbon, H. M., Debuchy, R., Budin, K., Dubois, E., Liangran, Z., et al. (2017). Asy2/Mer2: an evolutionarily conserved mediator of meiotic recombination, pairing, and global chromosome compaction. Genes Dev. 31, 1880–1893. doi: 10.1101/gad.304543.117
Tessé, S., Storlazzi, A., Kleckner, N., Gargano, S., and Zickler, D. (2003). Localization and roles of Ski8p protein in Sordaria meiosis and delineation of three mechanistically distinct steps of meiotic homolog juxtaposition. Proc. Natl. Acad. Sci. U.S.A. 100, 12865–12870. doi: 10.1073/pnas.2034282100
Thacker, D., Mohibullah, N., Zhu, X., and Keeney, S. (2014). Homologue engagement controls meiotic DNA break number and distribution. Nature 510, 241–246. doi: 10.1038/nature13120
Tischfield, S. E., and Keeney, S. (2012). Scale matters. Cell Cycle 11, 1496–1503. doi: 10.4161/cc.19733
Tran, P. T., Erdeniz, N., Dudley, S., and Liskay, R. M. (2002). Characterization of nuclease-dependent functions of Exo1p in Saccharomyces cerevisiae. DNA Repair. 1, 895–912. doi: 10.1016/S1568-7864(02)00114-3
Tsai, B., Liu, W., Dong, D., Shi, K., Chen, L., and Gao, N. (2020). Phase separation of Mer2 organizes the meiotic loop-axis structure of chromatin during meiosis I. bioRxiv [Preprint] doi: 10.1101/2020.12.15.422856
Tsukamoto, Y., Mitsuoka, C., Terasawa, M., Ogawa, H., and Ogawa, T. (2005). Xrs2p regulates Mre11p translocation to the nucleus and plays a role in telomere elongation and meiotic recombination. Mol. Biol. Cell 16, 597–608. doi: 10.1091/mbc.E04-09-0782
Usui, T., Ohta, T., Oshiumi, H., Tomizawa, J. I., Ogawa, H., and Ogawa, T. (1998). Complex formation and functional versatility of Mre11 of budding yeast in recombination. Cell 95, 705–716. doi: 10.1016/S0092-8674(00)81640-2
Voelkel-Meiman, K., Cheng, S. Y., Morehouse, S. J., and Macqueen, A. J. (2016). Synaptonemal complex proteins of budding yeast define reciprocal roles in MutSγ-mediated crossover formation. Genetics 203, 1091–1103. doi: 10.1534/genetics.115.182923
Vrielynck, N., Chambon, A., Vezon, D., Pereira, L., Chelysheva, L., De Muyt, A., et al. (2016). A DNA topoisomerase VI-like complex initiates meiotic recombination. Science 351, 939–943. doi: 10.1126/science.aad5196
Wan, L., Niu, H., Futcher, B., Zhang, C., Shokat, K. M., Boulton, S. J., et al. (2008). Cdc28-Clb5 (CDK-S) and Cdc7-Dbf4 (DDK) collaborate to initiate meiotic recombination in yeast. Genes Dev. 22, 386–397. doi: 10.1101/gad.1626408
Wang, W., Dong, J., Chen, B., Du, J., Kuang, Y., Sun, X., et al. (2019). Homozygous mutations in REC114 cause female infertility characterised by multiple pronuclei formation and early embryonic arrest. J. Med. Genet. 57, 187–194. doi: 10.1136/jmedgenet-2019-106379
Wendorff, T. J., and Berger, J. M. (2018). Topoisomerase VI senses and exploits both DNA crossings and bends to facilitate strand passage. Elife 7:e31724. doi: 10.7554/eLife.31724
West, A. M., Rosenberg, S. C., Ur, S. N., Lehmer, M. K., Ye, Q., Hagemann, G., et al. (2019). A conserved filamentous assembly underlies the structure of the meiotic chromosome axis. Elife 8:e40372. doi: 10.7554/eLife.40372
West, A. M. V., Komives, E. A., and Corbett, K. D. (2018). Conformational dynamics of the Hop1 HORMA domain reveal a common mechanism with the spindle checkpoint protein Mad2. Nucleic Acids Res. 46, 279–292. doi: 10.1093/nar/gkx1196
West, K. L., and Austin, C. A. (1999). Human DNA topoisomerase IIβ binds and cleaves four-way junction DNA in vitro. Nucleic Acids Res. 27, 984–992. doi: 10.1093/nar/27.4.984
Wilkins, A. S., and Holliday, R. (2009). The evolution of meiosis from mitosis. Genetics 181, 3–12. doi: 10.1534/genetics.108.099762
Williams, R. S., Moncalian, G., Williams, J. S., Yamada, Y., Limbo, O., Shin, D. S., et al. (2008). Mre11 dimers coordinate DNA end bridging and nuclease processing in double-strand-break repair. Cell 135, 97–109. doi: 10.1016/j.cell.2008.08.017
Wiltzius, J. J. W., Hohl, M., Fleming, J. C., and Petrini, J. H. J. (2005). The Rad50 hook domain is a critical determinant of Mre11 complex functions. Nat. Struct. Mol. Biol. 12, 403–407. doi: 10.1038/nsmb928
Wojtasz, L., Daniel, K., Roig, I., Bolcun-Filas, E., Xu, H., Boonsanay, V., et al. (2009). Mouse HORMAD1 and HORMAD2, two conserved meiotic chromosomal proteins, are depleted from synapsed chromosome axes with the help of TRIP13 AAA-ATPase. PLoS Genet. 5:e1000702. doi: 10.1371/journal.pgen.1000702
Woltering, D., Baumgartner, B., Bagchi, S., Larkin, B., Loidl, J., de los Santos, T., et al. (2000). Meiotic segregation, synapsis, and recombination checkpoint functions require physical interaction between the chromosomal proteins Red1p and Hop1p. Mol. Cell. Biol. 20, 6646–6658. doi: 10.1128/mcb.20.18.6646-6658.2000
Wright, W. D., Shah, S. S., and Heyer, W. D. (2018). Homologous recombination and the repair of DNA double-strand breaks. J. Biol. Chem. 293, 10524–10535. doi: 10.1074/jbc.TM118.000372
Wu, P. Y. J., and Nurse, P. (2014). Replication origin selection regulates the distribution of meiotic recombination. Mol. Cell 53, 655–662. doi: 10.1016/j.molcel.2014.01.022
Wu, T. C., and Lichten, M. (1994). Meiosis-induced double-strand break sites determined by yeast chromatin structure. Science 263, 515–518. doi: 10.1126/science.8290959
Xu, L., Ajimura, M., Padmore, R., Klein, C., and Kleckner, N. (1995). NDT80, a meiosis-specific gene required for exit from pachytene in Saccharomyces cerevisiae. Mol. Cell. Biol. 15, 6572–6581. doi: 10.1128/mcb.15.12.6572
Yamada, S., Hinch, A. G., Kamido, H., Zhang, Y., Edelmann, W., and Keeney, S. (2020). Molecular structures and mechanisms of DNA break processing in mouse meiosis. Genes Dev. 34, 806–818. doi: 10.1101/gad.336032.119
Young, J. A., Hyppa, R. W., and Smith, G. R. (2004). Conserved and nonconserved proteins for meiotic DNA breakage and repair in yeasts. Genetics 167, 593–605. doi: 10.1534/genetics.103.023762
Zakharyevich, K., Ma, Y., Tang, S., Hwang, P. Y. H., Boiteux, S., and Hunter, N. (2010). Temporally and biochemically distinct activities of exo1 during meiosis: double-strand break resection and resolution of double holliday junctions. Mol. Cell 40, 1001–1015. doi: 10.1016/j.molcel.2010.11.032
Zakharyevich, K., Tang, S., Ma, Y., and Hunter, N. (2012). Delineation of joint molecule resolution pathways in meiosis identifies a crossover-specific resolvase. Cell 149, 334–347. doi: 10.1016/j.cell.2012.03.023
Zhang, L., Kleckner, N. E., Storlazzi, A., and Kim, K. P. (2011). Meiotic double-strand breaks occur once per pair of (sister) chromatids and, via Mec1/ATR and Tel1/ATM, once per quartet of chromatids. Proc. Natl. Acad. Sci. U. S. A. 108, 20036–20041. doi: 10.1073/pnas.1117937108
Zickler, D., and Kleckner, N. (1999). Meiotic chromosomes: integrating structure and function. Annu. Rev. Genet. 33, 603–754. doi: 10.1146/annurev.genet.33.1.603
Keywords: double-strand break, DNA recombination, meiosis, Spo11, phase separation
Citation: Yadav VK and Claeys Bouuaert C (2021) Mechanism and Control of Meiotic DNA Double-Strand Break Formation in S. cerevisiae. Front. Cell Dev. Biol. 9:642737. doi: 10.3389/fcell.2021.642737
Received: 16 December 2020; Accepted: 01 February 2021;
Published: 02 March 2021.
Edited by:
Akira Shinohara, Osaka University, JapanReviewed by:
Bernard De Massy, Université de Montpellier, FranceValérie Borde, Centre National de la Recherche Scientifique (CNRS), France
Copyright © 2021 Yadav and Claeys Bouuaert. This is an open-access article distributed under the terms of the Creative Commons Attribution License (CC BY). The use, distribution or reproduction in other forums is permitted, provided the original author(s) and the copyright owner(s) are credited and that the original publication in this journal is cited, in accordance with accepted academic practice. No use, distribution or reproduction is permitted which does not comply with these terms.
*Correspondence: Corentin Claeys Bouuaert, Y29yZW50aW4uY2xhZXlzQHVjbG91dmFpbi5iZQ==