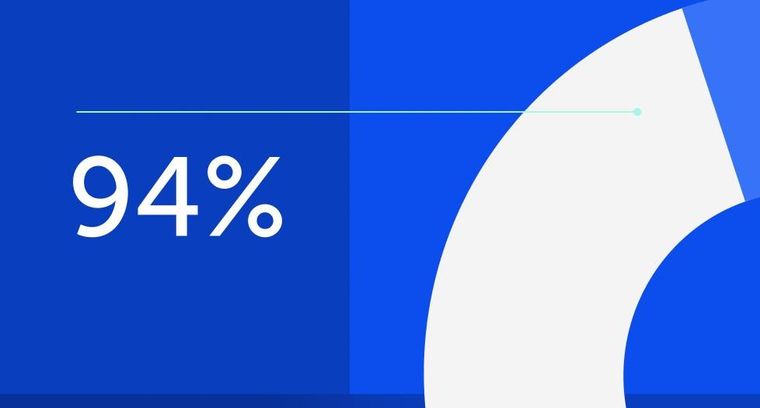
94% of researchers rate our articles as excellent or good
Learn more about the work of our research integrity team to safeguard the quality of each article we publish.
Find out more
ORIGINAL RESEARCH article
Front. Cell Dev. Biol., 26 February 2021
Sec. Cell Growth and Division
Volume 9 - 2021 | https://doi.org/10.3389/fcell.2021.638115
This article is part of the Research TopicBiomimetic Materials for Tissue RegenerationsView all 25 articles
Scaffold-free cartilage-sheet technology can stably regenerate high-quality cartilage tissue in vivo. However, uncontrolled shape maintenance and mechanical strength greatly hinder its clinical translation. Decalcified bone matrix (DBM) has high porosity, a suitable pore structure, and good biocompatibility, as well as controlled shape and mechanical strength. In this study, cartilage sheet was prepared into engineered cartilage gel (ECG) and combined with DBM to explore the feasibility of regenerating 3D cartilage with controlled shape and mechanical strength. The results indicated that ECG cultured in vitro for 3 days (3 d) and 15 days (15 d) showed good biocompatibility with DBM, and the ECG–DBM constructs successfully regenerated viable 3D cartilage with typical mature cartilage features in both nude mice and autologous goats. Additionally, the regenerated cartilage had comparable mechanical properties to native cartilage and maintained its original shape. To further determine the optimal seeding parameters for ECG, the 3 d ECG regenerated using human chondrocytes was diluted in different concentrations (1:3, 1:2, and 1:1) for seeding and in vivo implantation. The results showed that the regenerated cartilage in the 1:2 group exhibited better shape maintenance and homogeneity than the other groups. The current study established a novel mode of 3D cartilage regeneration based on the design concept of steel (DBM)-reinforced concrete (ECG) and successfully regenerated homogenous and mature 3D cartilage with controlled shape and mechanical strength, which hopefully provides an ideal cartilage graft for the repair of various cartilage defects.
The clinical treatment of cartilage defects is a significant challenge (Gomez et al., 2020; Mardones et al., 2020; Pattappa et al., 2020), as current methods do not provide an ideal graft for repairing the cartilage defects. Autologous cartilage transplantation is currently the most effective method for treating cartilage defects; however, there is limited source tissue, and the potential exists for irreversible damage to the donor area (Schinhan et al., 2013; Ruta et al., 2016). Tissue engineering provides a new approach for treating various cartilage defects and can be used to regenerate sufficient autologous viable cartilage tissue during in vitro proliferation with a small number of chondrocytes and minimal trauma (Zheng et al., 2014; He et al., 2018; Zhou et al., 2018).
Various animal and clinical experiments have demonstrated that scaffold-free cartilage-sheet technologies can stably regenerate high-quality cartilage tissue in vivo (Li et al., 2017, 2019; He et al., 2018). Our latest studies demonstrated that engineered cartilage gel (ECG) produced by cartilage-sheet technology have excellent ability to regenerate cartilage (unpublished data). However, the application of ECG is mainly limited to minimally invasive fillings, which are not suitable for repairing cartilage defects with specific shape and strength requirements because ECG cannot be shaped, ECG does not provide adequate mechanical strength, and ECG particles are too large to seed in conventional tissue regeneration scaffolds that have a relatively small pore size.
The above limitations greatly hinder the clinical application of ECG. A suitable framework with controlled shape, mechanical strength, and fine pore size is required to address these problems. Current scaffolds suitable for cell seeding cannot meet these requirements; therefore, here, we introduce DBM as a framework for ECG.
DBM is an ideal natural tissue regeneration scaffold with biocompatibility and immunogenicity, controllable shape, and mechanical strength that can be controlled by the degree of decalcification (Zheng et al., 2004; Liese et al., 2013; Meng et al., 2015; Xie et al., 2017). However, because of its large pore size and the difficulty in controlling its uniformity, DBM readily loses cells after seeding and cannot be directly used for cartilage regeneration (Man et al., 2016; Xu B. et al., 2017). Additionally, because of its rapid degradation (ten Koppel et al., 1998; van Osch et al., 1999), DBM cannot be used alone as a tissue regeneration scaffold, which greatly limits its application in the field of cartilage regeneration. However, the large pore size and controllable mechanical strength of DBM are precisely suitable for seeding ECG, which has a large particle size. Therefore, the current study investigated a novel mode of 3D cartilage regeneration based on the design concept of steel (DBM)-reinforced concrete (ECG). Although the combination of ECG and DBM can overcome their individual limitations and take full advantage of both materials, there are no reports on whether the steel (DBM)-reinforced concrete (ECG) concept can achieve 3D cartilage regeneration with controlled shape and mechanical strength. To prove the steel (DBM)-reinforced concrete (ECG) concept, the following scientific questions must be answered. (1) Can cartilage regeneration be realized using this concept? (2) Which mature stage of ECG is better for seeding DBM for cartilage regeneration? (3) Does the ECG need to be diluted, and if so, what proportion is optimal? These parameters not only affect the feasibility of the future clinical translation of ECG–DBM constructs and but also play an important role in the efficiency of cartilage regeneration in vivo.
To address these questions, goat chondrocyte suspensions with a high density were seeded in six-well cell culture plates and cultured for 3 days (3 d) to produce early ECG or 15 days (15 d) for mature ECG. The ECG–DBM constructs were formed by direct seeding (early ECG) or centrifuging (mature ECG) and implanted into nude mice and autologous goats to verify the feasibility and the proper parameter of cartilage regeneration. Furthermore, human chondrocyte ECG was suspended in gradient dilutions, seeded in DBM, and implanted into nude mice to determine whether the steel (DBM)-reinforced concrete (ECG) concept is suitable for human cartilage regeneration. The optimal ECG dilution for cartilage regeneration efficiency in future clinical applications was also evaluated.
Three 6-month-old goats (Shanghai Jiagan Biological Technology Co., Shanghai, China) were used in this study. All animal experiments were approved by the Weifang Medical College Ethics Committee.
Auricular cartilage was obtained from autologous goat and cut into 1.0 mm pieces, washed using phosphate-buffered solution (PBS), and digested using 0.15% collagenase (Worthington Biochemical Corp., NJ, United States) for isolating chondrocytes, as previously reported (Rodriguez et al., 1999). Isolated chondrocytes were cultured in Dulbecco’s modified Eagle’s medium (DMEM) medium (Gibco BRL, Grand Island, NY, United States) containing 10% fetal bovine serum (Gibco BRL) and 1% antibiotic-antimycotic (Gibco BRL). Passage 2 chondrocytes were used to form cartilage sheets (Li et al., 2019).
Cartilage sheets were prepared as previously established (Xue et al., 2018). Goat chondrocytes in the second passage were suspended and seeded in six-well cell culture plates at a density of 1.5 × 107 cells/well. The chondrocytes were then cultured in chondrogenic medium comprising DMEM, 10 ng/ml of TGF-β1 (R&D Systems Inc. Minneapolis, MN, United States), 40 ng/ml of dexamethasone (Sigma-Aldrich, St. Louis, MO, United States), 100 ng/ml of IGF-I (R&D Systems Inc. Minneapolis, MN, United States), 1% insulin-transferrin-selenium-linoleic acid (ITS, ScienCell, CA, United States), and 1% antibiotic-antimycotic (Gibco BRL) for 3 and 15 days. The 3 d ECG was prepared from 3 days cartilage sheets that were collected directly using a 5 ml syringe and mixed well with the 1.5-fold chondrogenic medium. The 15 d ECG was prepared from 15 days cartilage sheets that were minced into pieces and collected in a centrifuge tube without any further attenuation.
The protocols for the use of human cells were approved by the Weifang Medical College Ethics Committee. Abandoned cartilage tissue was donated by patients. To isolate the chondrocytes, the cartilage pieces were washed by PBS and digested by 0.15% collagenase (Worthington Biochemical Corp., Freehold, NJ, United States) as previously described (Deng et al., 2009). The cells were then harvested and cultured as previously reported (Deng et al., 2009). Chondrocytes from passage 2 were used to form human cartilage sheets (Li et al., 2019).
Human cartilage sheets were prepared using a process that was similar to that used with the goat chondrocytes. Human chondrocytes from passage 2 were seeded in six-well cell culture plates at a density of 1.5 × 107 cells/well. The chondrocytes were cultured in chondrogenic medium for 3 days, and the 3 days cartilage sheets were collected using a 5 ml syringe and mixed with onefold, twofold, or threefold chondrogenic medium.
DBM frameworks (Daqing Bio Co. LTD, Chongqing, China) were cut into cuboid constructs 7 mm long, 5 mm wide, and 2.5 mm thick. Different methods were used to form the chondrocyte ECG–DBM constructs. The 3 d ECG was directly seeded into the DBM and incubated for 2 h. The 15 d ECG and DBM were combined by centrifuging for 2 min at 500 rpm and then incubated for 2 h. The ECG with different dilution ratios were directly seeded into DBM and incubated for 2 h. All ECG–DBM constructs were then gently transferred to new six-well plates. After 3 days, the constructs were subcutaneously implanted into autologous goats and nude mice.
After in vitro culture for 24 and 72 h, the ECG–DBM constructs were washed by PBS and fixed overnight at 4°C in 0.05% glutaraldehyde. Both the DBM framework and ECG–DBM constructs were critical point dried and examined by scanning electron microscopy (SEM; Philips XL-30, Amsterdam, Netherlands) to observe the pore size distribution of the DBM framework and attachment and distribution of ECG and to assess the ECG synthesis on the DBM (Xu Y. et al., 2017). The ECG adhesion rate was determined by the ratio of the DNA content of ECG that was seeded into the DBM and the DNA content of ECG–DBM constructs that were cultured for 24 h. The DNA content of the samples (n = 5 per group) was quantified by a Quant-iT PicoGreen dsDNA assay (Invitrogen, Carlsbad, CA, United States) as previously described (Schagemann et al., 2010).
The Young modulus of the regenerated cartilage was tested using a mechanical analyzer (Instron-5542, Instron, Canton, MA). As previously described (Liu et al., 2008), samples from different groups (n = 6 per group) were cut into 4 mm-diameter cylinders. A constant compressive strain at a speed of 0.5 mm/min was applied until 80% of the maximal deformation. The stress–strain curves were obtained from the first 40%. The Young modulus was calculated according to the stress–strain curves.
To evaluate the structure and extracellular matrix (ECM) deposition of the regenerated cartilage, hematoxylin, and eosin (HE), Safranin-O, and type II collagen (mouse anti-human type II collagen monoclonal antibody, 1:100, Santa Cruz Biotechnology, Dallas, TX), staining was performed (Liu et al., 2008). The terminal deoxynucleotidyl transferase biotin dUTP nick end labeling assay (TUNEL) for apoptosis was analyzed using a TUNEL kit (Roche, Indianapolis, IN). CD3 was analyzed using mouse anti-human CD3 monoclonal antibody (1:200 in PBS, Santa Cruz Biotechnology, Santa Cruz, CA, United States). CD68 was analyzed using mouse anti-human CD68 monoclonal antibody (1:200 in PBS, Santa Cruz). Horseradish peroxidase (HRP)-conjugated anti-mouse antibody (1:200 in PBS, Santa Cruz) was then applied as a secondary antibody.
Quantitative analysis was performed as previously described (Enobakhare et al., 1996; Reddy and Enwemeka, 1996; Schagemann et al., 2010). Briefly, all samples (n = 5 per group) were weighed using an electronic balance. The volume of each sample (n = 5 per group) was measured using a water displacement method. The volume change rate was determined by the ratio of the volume of the samples that were cultured in vitro for 72 h and the volume of the samples after 12 weeks in vivo The total glycosaminoglycan (GAG) content of the samples (n = 5 per group) was quantified using the Alcian blue method. The total collagen content of the samples (n = 5 per group) was detected using a hydroxyproline assay.
The total RNA was extracted from 3 d and 15 d ECGs (n = 3 per group), and cDNA was obtained by reverse transcription (RT) according to a previously described method (Jiang et al., 2010). RT-qPCR was performed according to the manufacturer’s protocol (Thermo Fisher Scientific, Waltham, MA, United States). The expression levels of the genes Aggrecan, COLIIA1, and Sox9 were analyzed. The housekeeping gene encoding β-actin was quantified as an internal control. Forward and reverse primer sequences are listed in Table 1. Expression levels were analyzed using the 2–ΔΔCT method, as previously described (Livak and Schmittgen, 2001).
All values were expressed as mean ± standard. A t-test analysis of the variance was used to determine the statistical between groups using SPSS 23 software, and a value of ∗P < 0.05 was considered statistically significant.
As shown in Figure 1, both types of 3 days cartilage sheet—goat chondrocyte and human chondrocyte—had liquid properties and could be collected using a 5 ml syringe (Figures 1B1,B3 and Supplementary Videos 1, 2), and the 15 days cartilage sheets were relatively tough sheets that could not be directly collected (Figure 1B2). The 3 d ECG was successfully prepared by mixing 3 days cartilage sheets with 1.5-fold medium (Figure 1C1). The 15 d ECG was prepared from 15 days cartilage sheets that were minced into pieces (Figure 1C2). And as for the expression of cartilage-related genes, 15 d ECG was higher than 3 d ECG (Supplementary Figure 1). Different dilution ECGs exhibited different behaviors: the ECG in 1:1 and 1:2 groups stably maintained a suspension, while the ECG in the 1:3 group rapidly sedimented and presented obvious stratification (Figure 1C3).
Figure 1. Formation of engineered cartilage gel. Gross (A1) and mature status (B1) images of 3 d goat cartilage sheet and gross images (C1) of 3 d engineered cartilage gel (ECG). Gross (A2) and mature status (B2) images of 15 d goat cartilage sheet and gross images (C2) of 15 d ECG. Gross (A3) and mature status (B3) images of a 3 d human cartilage sheet and 3 d cartilage-gel in different dilution ratios (C3).
The biocompatibility between ECG and DBM was evaluated using gross imaging and SEM. As shown in Figure 2, the pore size of DBM was too large and unsuitable for cell seeding (Figures 2A1,B1,C). However, both 3 d and 15 d ECGs showed biocompatibility with DBM, and the ECGs stably adhered to DBM. In the 3 days in vitro culture, the DBM pores of both groups were filled with ECG (Figures 2A2–A5,B2–B5). Both 3 d and 15 d ECGs had fine ECG adhesion efficiency (Figures 2D,E).
Figure 2. Evaluation of the biocompatibility of engineered cartilage gel (ECG) and decalcified bone matrix (DBM), and the ECG adhesion efficiency. Gross (A1) and SEM (B1) images of the DBM framework. Gross (A2) and SEM (B2) images of the 3 d ECG–DBM constructs after culture in vitro for 24 h. Gross (A3) and SEM (B3) images of the 3 d ECG–DBM constructs after culture in vitro for 72 h. Gross (A4) and SEM (B4) images of the 15 d ECG–DBM constructs after in vitro culture for 24 h. Gross (A5) and SEM (B5) images of the 15 d ECG–DBM constructs after in vitro culture for 72 h. The pore size distribution of the DBM framework (C). The ECG adhesion rate of ECG (D). The DNA content of the ECG–DBM framework at different times (E).
The feasibility of cartilage regeneration using ECG–DBM constructs was the first key point in the current study. After 12 weeks, both 3 d and 15 d ECG–DBMs successfully regenerated ivory white, cartilage-like tissues that maintained their shape in both nude mouse (Figures 3A1,B1) and autologous goat models (Figures 4A1,B1). Histological analysis performed at 12 weeks showed that both the 3 d and 15 d ECM–DBM constructs formed mature cartilage-like tissue in the outer areas of the sample with abundant lacuna structure and homogenous cartilage ECM distribution in both nude mouse (Figures 3A2–A8,B2–B8) and autologous goat models (Figures 4A2–A8,B2–B8). Additionally, some immature cartilage-like tissue (15 d) and fibrous-like tissue (3 d) were observed in the inner areas of all the samples (likely because of nutrient deficiency). Consistent with the histological staining, the quantitative analysis showed that the GAG content and total collagen content and the mechanical strength of the regenerated cartilage reached favorable levels at 12 weeks for both 3 d ECG–DBM and 15 d ECG–DBM (Figure 5).
Figure 3. Gross view and histological examination of the cartilage regenerated by goat engineered cartilage gel (ECG) after 12 weeks in vivo in nude mice. Gross view, HE, Safranin-O, and collagen II immunohistochemical staining of 3 d ECG–decalcified bone matrix (DBM) (A1–A8) and 15 d ECG–DBM constructs (B1–B8). The red box represents the mature cartilage-like tissue areas, and the orange box represents the immature cartilage-like tissue.
Figure 4. Gross view and histological examination of the cartilage regenerated by goat engineered cartilage gel (ECG) after 12 weeks in vivo in autologous goat. Gross view, HE, Safranin-O, and collagen II immunohistochemical staining of 3 d ECG–decalcified bone matrix (DBM) (A1–A8) and 15 d ECG–DBM (B1–B8) constructs. The red box represents the mature cartilage-like tissue areas, and the orange box represents immature cartilage-like tissue.
Figure 5. Quantitative evaluations of the cartilage regenerated by goat engineered cartilage gel (ECG) after 12 weeks in vivo. Quantitative analysis of the wet weight (A), volume (B), Young’s modulus (C), total glycosaminoglycan (GAG) (D), and total collagen (E) in different groups. N-3-12 = the cartilage regenerated by 3 d ECG–decalcified bone matrix (DBM) constructs after 12 weeks in vivo in nude mice; N-15-12 = the cartilage regenerated by 15 d ECG–DBM constructs after 12 weeks in vivo in nude mice; G-3-12 = the cartilage regenerated by 3 d ECG–DBM constructs after 12 weeks in vivo in autologous goats; G-15-12 = the cartilage regenerated by 15 d ECG–DBM constructs after 12 weeks in vivo in autologous goats. *P < 0.05.
To further clarify the reasons for the formation of the immature cartilage/fibrous-like tissue, immunohistological examinations of CD3 (T-cell marker), CD68 (monocyte/macrophage marker), and the TUNEL assay for apoptosis were conducted. As shown in Figure 6, both the outer cartilage-like tissue and inner immature cartilage/fibrous tissue showed negative CD3 (Figures 6A1–A3,D1–D3) and CD68 expression (Figures 6B1–B3,E1–E3), which indicates that no host inflammatory cells participated in the cartilage regeneration. TUNEL staining confirmed that a large number of apoptotic cells were detected in the inner areas but not in the outer areas (Figures 6C1–C3,F1–F3), which implies that the immature cartilage/fibrous-like tissue in the inner areas probably derived from implanted ECG, whose cells were dying because of nutrient deficiency caused by the continuous cartilage-like tissue on the periphery of the implant blocking nutrient transport.
Figure 6. Inflammatory reactions characterized by CD3, CD68, and terminal deoxynucleotidyl transferase biotin dUTP nick end labeling assay (TUNEL) staining. CD3 staining of 3 d engineered cartilage gel–decalcified bone matrix (ECG–DBM) (A1–A3) and 15 d ECG–DBM constructs (D1–D3) after 12 weeks in vivo in autologous goat. CD68 staining of 3 d ECG–DBM (B1–B3) and 15 d ECG–DBM (E1–E3) constructs after 12 weeks in vivo in autologous goat. TUNEL staining of 3 d ECG–DBM (C1–C3) and 15 d ECG–DBM (F1–F3) constructs after 12 weeks in vivo in autologous goat. The red box represents the mature cartilage-like tissue areas, and the orange box represents immature cartilage-like tissue.
Enhancing cartilage regeneration efficiency is vital for the clinical translation of ECG. To explore the feasibility of enhancing cartilage regeneration efficiency, 3 d ECG was diluted at different ratios (1:3, 1:2, and 1:1) and combined with DBM followed by in vivo implantation. After 12 weeks, gross views showed that the samples in all groups formed mature cartilage-like tissues. However, the samples in the 1:1 group exhibited visible excessive proliferation and deformation compared with before implantation, while the samples in the 1:2 and 1:3 groups had relatively satisfactory shape maintenance (volume change rate < 30%, Figures 8A–C) and homogeneity of the regenerated cartilage (Figures 7A1,B1,C1, 8A–C). Histological examinations showed that the samples in the 1:2 and 1:1 groups regenerated continuous and mature cartilage-like tissue (Figures 7B2–B8,C2–C8), while only a small amount of mature cartilage-like tissue was observed in the inner areas in 1:3 group (Figures 7A2–A8). Quantitative analyses showed that all quantitative indexes, including the wet weight, volume, and cartilage ECM contents, increased significantly with decreasing dilution (Figure 8). These results indicate that an appropriate dilution is important for enhancing the cartilage regeneration efficiency and preventing excessive proliferation of the ECG–DBM constructs. In terms of efficiency, quality, homogeneity, and shape maintenance, a 1:2 dilution of ECG is appropriate for cartilage regeneration of the ECG–DBM constructs.
Figure 7. Gross view and histological examination of the cartilage regenerated by human engineered cartilage gel (ECG) after 12 weeks in vivo implantation in nude mouse. Gross view, HE, Safranin-O, and collagen II immunohistochemical staining of 3 d ECG–decalcified bone matrix (DBM) constructs in 1:1 (A1–A8), 1:2 (B1–B8), and 1:3 (C1–C8) dilution ratios. The red box represents the mature cartilage-like tissue areas, and the orange box represents immature cartilage-like tissue.
Figure 8. Quantitative evaluations of human engineered cartilage gel–decalcified bone matrix (ECG–DBM) constructs. Quantitative analysis of the wet weight (A), volume (B), and volume change rate (C) in different groups after 3-day culture in vitro (in vitro) and 12 weeks in vivo (in vivo). Total glycosaminoglycan (GAG) (D) and total collagen (E) in different groups after 12 weeks in vivo implantation (in vivo). 1:1 = ECG mixed well with onefold medium; 1:2 = ECG mixed well with twofold medium; 1:3 = ECG mixed well with threefold medium. *P < 0.05.
Although cartilage-sheet technology is a promising strategy for cartilage regeneration, an uncontrolled shape and poor mechanical strength greatly hinder further clinical application (Li et al., 2017, 2019). The results demonstrated that ECG produced by cartilage-sheet technology combined with DBM can regenerate 3D cartilage tissue with controlled shape and mechanical strength in both nude mice and autologous goats. Additionally, both 3 d and 15 d ECGs combined with DBM regenerated favorable cartilage tissue in vivo. More importantly, ECG–DBM constructed from human chondrocytes also successfully regenerated mature cartilage tissue in nude mice, and 3 days ECG at a 1:2 dilution provided the optimal conditions of those tested. The current study provides a new cartilage regeneration mode and predicts its potential in future clinical translation.
Whether ECG combined with DBM can successfully regenerate viable cartilage with a certain shape and mechanical strength is the first important issue. The current study showed that ECG of goat chondrocytes could regenerate fine cartilage tissue when combined with DBM, in both nude mice and autologous goats. Additionally, histological staining, immunohistochemical staining, and related quantification analyses confirmed that the regenerated cartilage had typical cartilage lacunae and cartilage specific ECM deposition. More importantly, these regenerated cartilages maintained their original shape and presented satisfactory mechanical strength (even greater than normal levels). These findings strongly support the concept of feasibility of steel (DBM)-reinforced concrete (ECG) to regenerate homogenous and mature 3D cartilage with controlled shape and mechanical strength. The regeneration process and principle should be as follows: At an early stage, DBM provides shape maintenance and mechanical support for cartilage regeneration. At a later stage, following DBM degradation, ECG-regenerated cartilage gradually matures and finally forms intact and continuous cartilaginous tissue, which contributes to stable shape maintenance and mechanical support. Importantly, the same results were verified using human ECG and demonstrated a high potential for future clinical applications.
After verifying the feasibility of the concept, identifying if the mature status of ECG was conducive to cartilage regeneration became the key step for future clinical application. Therefore, the cartilage tissues regenerated by short-term (3 days) and long-term (15 days) ECG–DBM constructs were systematically compared. The chondrocyte sheets cultured for 3 days were only preliminarily formed and were relatively immature. Consequently, the sheets could be directly collected to form ECG and seeded into DBM. After culture for 15 days, the formed chondrocyte sheets were mature, tough cartilage tissues could be picked up with tweezers, and they required being cut into pieces to form ECG for seeding into DBM by centrifugation. The in vivo results confirmed that both 3 d ECG–DBM and 15 d ECG–DBM constructs could regenerate mature cartilage tissue, and there was no significant difference, although 15 d ECG had higher expression of cartilage-related genes before seeding into the DBM construct. Nevertheless, compared with 15 d ECG, 3 d ECG had obvious advantages, including a shorter culture period, less medium requirements, easier seeding operation, higher ECG seeding efficiency, and lower contamination risk. Therefore, 3 d ECG was more suitable for the current mode of 3D cartilage regeneration.
After confirming the suitable mature status of ECG, the optimal ratio of ECG dilution was the next key parameter. To determine the effect of ECG dilution on cartilage regeneration, 3 d human ECG was diluted by gradient, and the differences in cartilage regeneration among the various dilution ratios were compared. According to the results, the samples in the 1:2 dilution group showed relatively satisfactory cartilage regeneration in terms of homogeneity, shape maintenance, and efficiency of the regenerated cartilage. In the 1:1 dilution group, although relatively mature and homogenous cartilage was successfully regenerated, the samples presented visible excessive proliferation and deformation on the surface. ECG at this dilution ratio had a high viscosity, which led to local accumulation on the surface of the construct liquidity that caused excessive proliferation and deformation on the surface. Alternatively, in the 1:3 dilution group, the samples showed heterogeneous cartilage regeneration, and mature cartilage was mainly observed in the inner areas. ECG at this dilution ratio had a relatively low viscosity, which led to ECG loss in the outer areas of the construct because of the low viscosity and low adhesion efficiency after seeding, which resulted in inferior cartilage regeneration in the outer areas. These findings suggested that appropriate ECG dilution is vital for satisfactory cartilage regeneration using ECG–DBM constructs.
Although the feasibility of the cartilage regeneration was verified using the current mode, most of samples showed inferior cartilage formation inside, which led to an obviously heterogeneous structure of the sample. The inferior cartilage formation inside was possibly caused by nutrient deficiency. After implantation in vivo, ECG in the outer areas of the samples easily obtained sufficient nutrients and rapidly formed a relatively mature and continuous cartilage layer, which severely blocked nutrient diffusion that led to nutrient deficiency in the inner areas and caused cell apoptosis and inferior cartilage formation (Wu et al., 2010). The results of CD3, CD68, and TUNEL staining further verified that the immature cartilage/fibrous-like tissue in the inner areas was mainly composed of apoptotic chondrocytes rather than host inflammatory cells. Alternatively, when the outer layer cartilage was discontinuous (nutrients could freely diffuse into inner areas), as shown in the 1:3 dilution group, mature cartilage was also observed in the inner areas. It is accepted that cartilage regeneration has a thickness limitation because of its avascular feature (Bonzani et al., 2006; Ge et al., 2012). Therefore, to realize homogeneity of a whole regenerated cartilage, multiple module cartilage regenerations, and a multi-layer assembly might be a feasible strategy for future clinical applications.
This study established a new concept for cartilage regeneration—analogous to steel-reinforced concrete—based on ECG and DBM and demonstrated its feasibility. Additionally, the formation method and relative parameters were investigated and optimized. However, some important issues, including how to precisely control the shape and realize homogeneity of the whole regenerated cartilage, still need to be investigated. The presented results provide a new cartilage regeneration strategy with potential for future clinical translation.
The original contributions presented in the study are included in the article/Supplementary Material, further inquiries can be directed to the corresponding author/s.
The studies involving human participants were reviewed and approved by the Weifang Medical College Ethics Committee. The patients/participants provided their written informed consent to participate in this study. The animal study was reviewed and approved by the Weifang Medical College Ethics Committee.
ZC, YZ, and YW: scaffolds design, culture cells, and animal operation. GW, MH, PZ, LJ, and BB: relative data analyses. YC and YL: revision. GZ: funding acquisition, review, and editing. All authors contributed to the article and approved the submitted version.
This research was supported by the National Key Research and Development Program of China (2017YFC1103900), the National Natural Science Foundation of China (81871502, 81701843, and 81671837), the Shanghai Excellent Technical Leader (18XD1421500), the Program of Shanghai Academic/Technology Research Leader (19XD1431100), and the Shanghai Collaborative Innovation Program on Regenerative Medicine and Stem Cell Research (2019CXJQ01).
The authors declare that the research was conducted in the absence of any commercial or financial relationships that could be construed as a potential conflict of interest.
The Supplementary Material for this article can be found online at: https://www.frontiersin.org/articles/10.3389/fcell.2021.638115/full#supplementary-material
Bonzani, I. C., George, J. H., and Stevens, M. M. (2006). Novel materials for bone and cartilage regeneration. Curr. Opin. Chem. Biol. 10, 568–575. doi: 10.1016/j.cbpa.2006.09.009
Deng, D., Liu, W., Xu, F., Yang, Y., Zhou, G., Zhang, W. J., et al. (2009). Engineering human neo-tendon tissue in vitro with human dermal fibroblasts under static mechanical strain. Biomaterials 30, 6724–6730. doi: 10.1016/j.biomaterials.2009.08.054
Enobakhare, B. O., Bader, D. L., and Lee, D. A. (1996). Quantification of sulfated glycosaminoglycans in chondrocyte/alginate cultures, by use of 1,9-dimethylmethylene blue. Anal. Biochem. 243, 189–191. doi: 10.1006/abio.1996.0502
Ge, Z., Li, C., Heng, B. C., Cao, G., and Yang, Z. (2012). Functional biomaterials for cartilage regeneration. J. Biomed. Mater. Res. A 100, 2526–2536.
Gomez, M., Wittig, O., Diaz-Solano, D., and Cardier, J. E. (2020). Mesenchymal stromal cell transplantation induces regeneration of large and full-thickness cartilage defect of the temporomandibular joint. Cartilage doi: 10.1177/1947603520926711 Online ahead of print.
He, A., Xia, H., Xiao, K., Wang, T., Liu, Y., Xue, J., et al. (2018). Cell yield, chondrogenic potential, and regenerated cartilage type of chondrocytes derived from ear, nasoseptal, and costal cartilage. J. Tissue Eng. Regen. Med. 12, 1123–1132. doi: 10.1002/term.2613
Jiang, T., Liu, W., Lv, X., Sun, H., Zhang, L., Liu, Y., et al. (2010). Potent in vitro chondrogenesis of CD105 enriched human adipose-derived stem cells. Biomaterials 31, 3564–3571. doi: 10.1016/j.biomaterials.2010.01.050
Li, D., Yin, Z., Liu, Y., Feng, S., Liu, Y., Lu, F., et al. (2019). Regeneration of trachea graft with cartilage support, vascularization, and epithelization. Acta Biomater. 89, 206–216. doi: 10.1016/j.actbio.2019.03.003
Li, D., Zhu, L., Liu, Y., Yin, Z., Liu, Y., Liu, F., et al. (2017). Stable subcutaneous cartilage regeneration of bone marrow stromal cells directed by chondrocyte sheet. Acta Biomater. 54, 321–332. doi: 10.1016/j.actbio.2017.03.031
Liese, J., Marzahn, U., El Sayed, K., Pruss, A., Haisch, A., and Stoelzel, K. (2013). Cartilage tissue engineering of nasal septal chondrocyte-macroaggregates in human demineralized bone matrix. Cell Tissue Bank 14, 255–266. doi: 10.1007/s10561-012-9322-4
Liu, K., Zhou, G. D., Liu, W., Zhang, W. J., Cui, L., Liu, X., et al. (2008). The dependence of in vivo stable ectopic chondrogenesis by human mesenchymal stem cells on chondrogenic differentiation in vitro. Biomaterials 29, 2183–2192. doi: 10.1016/j.biomaterials.2008.01.021
Livak, K. J., and Schmittgen, T. D. (2001). Analysis of relative gene expression data using real-time quantitative PCR and the 2(-Delta Delta C(T)) Method. Methods 25, 402–408. doi: 10.1006/meth.2001.1262
Man, Z., Hu, X., Liu, Z., Huang, H., Meng, Q., Zhang, X., et al. (2016). Transplantation of allogenic chondrocytes with chitosan hydrogel-demineralized bone matrix hybrid scaffold to repair rabbit cartilage injury. Biomaterials 108, 157–167. doi: 10.1016/j.biomaterials.2016.09.002
Mardones, R., Giai Via, A., Pipino, G., Jofre, C. M., Munoz, S., Narvaez, E., et al. (2020). BM-MSCs differentiated to chondrocytes for treatment of full-thickness cartilage defect of the knee. J. Orthop. Surg. Res. 15:455.
Meng, Q., Man, Z., Dai, L., Huang, H., Zhang, X., Hu, X., et al. (2015). A composite scaffold of MSC affinity peptide-modified demineralized bone matrix particles and chitosan hydrogel for cartilage regeneration. Sci. Rep. 5:17802.
Pattappa, G., Krueckel, J., Schewior, R., Franke, D., Mench, A., Koch, M., et al. (2020). Physioxia expanded bone marrow derived mesenchymal stem cells have improved cartilage repair in an early osteoarthritic focal defect model. Biology 9:230. doi: 10.3390/biology9080230
Reddy, G. K., and Enwemeka, C. S. (1996). A simplified method for the analysis of hydroxyproline in biological tissues. Clin. Biochem. 29, 225–229. doi: 10.1016/0009-9120(96)00003-6
Rodriguez, A., Cao, Y. L., Ibarra, C., Pap, S., Vacanti, M., Eavey, R. D., et al. (1999). Characteristics of cartilage engineered from human pediatric auricular cartilage. Plast. Reconstr. Surg. 103, 1111–1119. doi: 10.1097/00006534-199904040-00001
Ruta, D. J., Villarreal, A. D., and Richardson, D. R. (2016). Orthopedic surgical options for joint cartilage repair and restoration. Phys. Med. Rehabil. Clin. N. Am. 27, 1019–1042. doi: 10.1016/j.pmr.2016.06.007
Schagemann, J. C., Chung, H. W., Mrosek, E. H., Stone, J. J., Fitzsimmons, J. S., O’Driscoll, S. W., et al. (2010). Poly-epsilon-caprolactone/gel hybrid scaffolds for cartilage tissue engineering. J. Biomed. Mater. Res. A 93, 454–463.
Schinhan, M., Gruber, M., Dorotka, R., Pilz, M., Stelzeneder, D., Chiari, C., et al. (2013). Matrix-associated autologous chondrocyte transplantation in a compartmentalized early stage of osteoarthritis. Osteoarthritis Cartilage 21, 217–225. doi: 10.1016/j.joca.2012.10.006
ten Koppel, P. G., van Osch, G. J., Verwoerd, C. D., and Verwoerd-Verhoef, H. L. (1998). Efficacy of perichondrium and a trabecular demineralized bone matrix for generating cartilage. Plast. Reconstr. Surg. 102, 2012–2020. doi: 10.1097/00006534-199811000-00031
van Osch, G. J., ten Koppel, P. G., van der Veen, S. W., Poppe, P., Burger, E. H., and Verwoerd-Verhoef, H. L. (1999). The role of trabecular demineralized bone in combination with perichondrium in the generation of cartilage grafts. Biomaterials 20, 233–240. doi: 10.1016/s0142-9612(98)00165-3
Wu, Y., Zhu, L., Jiang, H., Liu, W., Liu, Y., Cao, Y., et al. (2010). Engineering cartilage substitute with a specific size and shape using porous high-density polyethylene (HDPE) as internal support. J. Plast. Reconstr. Aesthet. Surg. 63, e370–e375.
Xie, H., Wang, Z., Zhang, L., Lei, Q., Zhao, A., Wang, H., et al. (2017). Extracellular vesicle-functionalized decalcified bone matrix scaffolds with enhanced pro-angiogenic and pro-bone regeneration activities. Sci. Rep. 7:45622.
Xu, B., Wang, R., Wang, H., and Xu, H. G. (2017). Coculture of allogenic DBM and BMSCs in the knee joint cavity of rabbits for cartilage tissue engineering. Biosci. Rep. 37:BSR20170804.
Xu, Y., Li, D., Yin, Z., He, A., Lin, M., Jiang, G., et al. (2017). Tissue-engineered trachea regeneration using decellularized trachea matrix treated with laser micropore technique. Acta Biomater. 58, 113–121. doi: 10.1016/j.actbio.2017.05.010
Xue, J., He, A., Zhu, Y., Liu, Y., Li, D., Yin, Z., et al. (2018). Repair of articular cartilage defects with acellular cartilage sheets in a swine model. Biomed. Mater. 13:025016. doi: 10.1088/1748-605x/aa99a4
Zheng, D., Yang, S., Li, J., Xu, W., Yang, C., Liu, Y., et al. (2004). Experimental study on allogenic decalcified bone matrix as carrier for bone tissue engineering. J. Hua. Univ. Sci. Technolog. Med. Sci. 24, 147–150.
Zheng, R., Duan, H., Xue, J., Liu, Y., Feng, B., Zhao, S., et al. (2014). The influence of Gelatin/PCL ratio and 3-D construct shape of electrospun membranes on cartilage regeneration. Biomaterials 35, 152–164.
Keywords: 3D cartilage regeneration, engineered cartilage gel, decalcified bone matrix, tissue engineering, nutrient efficiency
Citation: Ci Z, Zhang Y, Wang Y, Wu G, Hou M, Zhang P, Jia L, Bai B, Cao Y, Liu Y and Zhou G (2021) 3D Cartilage Regeneration With Certain Shape and Mechanical Strength Based on Engineered Cartilage Gel and Decalcified Bone Matrix. Front. Cell Dev. Biol. 9:638115. doi: 10.3389/fcell.2021.638115
Received: 05 December 2020; Accepted: 26 January 2021;
Published: 26 February 2021.
Edited by:
Naresh Kumar Rajendran, University of Rochester, United StatesReviewed by:
Li Zheng, Guangxi Medical University, ChinaCopyright © 2021 Ci, Zhang, Wang, Wu, Hou, Zhang, Jia, Bai, Cao, Liu and Zhou. This is an open-access article distributed under the terms of the Creative Commons Attribution License (CC BY). The use, distribution or reproduction in other forums is permitted, provided the original author(s) and the copyright owner(s) are credited and that the original publication in this journal is cited, in accordance with accepted academic practice. No use, distribution or reproduction is permitted which does not comply with these terms.
*Correspondence: Yu Liu, eXVsaXUxMjExQDE2My5jb20=; Guangdong Zhou, Z3Vhbmdkb25nemhvdUAxMjYuY29t
†These authors have contributed equally to this work
Disclaimer: All claims expressed in this article are solely those of the authors and do not necessarily represent those of their affiliated organizations, or those of the publisher, the editors and the reviewers. Any product that may be evaluated in this article or claim that may be made by its manufacturer is not guaranteed or endorsed by the publisher.
Research integrity at Frontiers
Learn more about the work of our research integrity team to safeguard the quality of each article we publish.