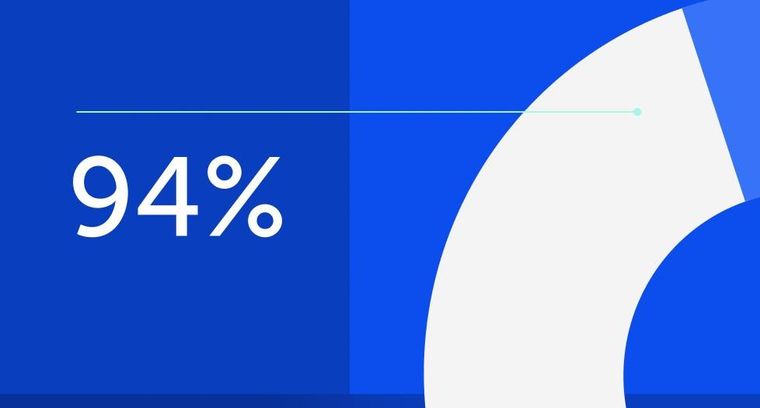
94% of researchers rate our articles as excellent or good
Learn more about the work of our research integrity team to safeguard the quality of each article we publish.
Find out more
REVIEW article
Front. Cell Dev. Biol., 11 February 2021
Sec. Membrane Traffic and Organelle Dynamics
Volume 9 - 2021 | https://doi.org/10.3389/fcell.2021.635518
This article is part of the Research TopicCoupling and Uncoupling: Dynamic Control of Membrane ContactsView all 15 articles
The compartmentalisation achieved by confining cytoplasm into membrane-enclosed organelles in eukaryotic cells is essential for maintaining vital functions including ATP production, synthetic and degradative pathways. While intracellular organelles are highly specialised in these functions, the restricting membranes also impede exchange of molecules responsible for the synchronised and responsive cellular activities. The initial identification of contact sites between the ER and plasma membrane (PM) provided a potential candidate structure for communication between organelles without mixing by fusion. Over the past decades, research has revealed a far broader picture of the events. Membrane contact sites (MCSs) have been recognized as increasingly important actors in cell differentiation, plasticity and maintenance, and, upon dysfunction, responsible for pathological conditions such as cancer and neurodegenerative diseases. Present in multiple organelles and cell types, MCSs promote transport of lipids and Ca2+ homoeostasis, with a range of associated protein families. Interestingly, each MCS displays a unique molecular signature, adapted to organelle functions. This review will explore the literature describing the molecular components and interactions taking place at ER-PM contact sites, their functions, and implications in eukaryotic cells, particularly neurons, with emphasis on lipid transfer proteins and emerging function of SNAREs.
A distinctive property of eukaryotic cells is the compartmentaliasation of the cytoplasm by intracellular membranes. In addition to the plasma membrane (PM), phospholipid bilayers and monolayers form complex internal networks, defining individual organelles specialised for specific functions. While this is useful for separating incompatible biochemical reactions and restricting specific conditions such as low pH, and/or redox potential, compartmentalisation can impede the transfer of molecules which instead relies on vesicular trafficking (Helle et al., 2013; Scorrano et al., 2019). Recent studies however have shown that long known specialised regions of membrane in close proximity to each other, called membrane contact sites (MCSs), could also be involved in communication (Gallo et al., 2016; Kang et al., 2019; Bohnert, 2020). Early research identified closely apposed domains of the endoplasmic reticulum (ER) and mitochondria in hepatocytes (Bernhard and Rouiller, 1956) and of ER with T-tubule invaginations of the PM in muscle cells (Porter and Palade, 1957). However, the physiological significance of many MCSs was poorly understood. The first insights only arrived in the 1990s, with observations suggesting that phosphoserine-derivatives in the mitochondrial membranes are synthesised by the ER, and that contact between the organelles was involved in lipid transfer (Vance, 1990; Stefan et al., 2017). Since, MCSs have been identified in a range of organisms, cell types and between different organelles, the structure and function of many are still yet to be fully understood. MCSs are defined as domains of organelles in close apposition to each other, in general via biochemically defined interactions. They involve most organelles and can be homotypic (contacts between distal regions of an organelle or organelles of the same type) or heterotypic (between organelles of different nature) (Gatta and Levine, 2017; Scorrano et al., 2019). Due to its extensive and dynamic network throughout the cell (Nixon-Abell et al., 2016), the ER is engaged as a partner in almost all heterotypic connections and mitochondria have also been shown to form contacts with multiple organelles (Gatta and Levine, 2017).
The diversity of interactions observed suggests a variety of functions and processes that MCSs are involved in. For example, ER contacts with mitochondria are important in lipid transport and metabolism (Dimmer and Rapaport, 2017). Additionally, the ER can wrap around mitochondrial tubules and facilitate fission (Tilokani et al., 2018). Remarkably, numerous cellular processes depend on ER contacts beside organelle dynamics. ER-mediated contacts sites have been shown to facilitate Ca2+ homoeostasis (Wu et al., 2006) lipid transport (Tavassoli et al., 2013), modulate autophagic biogenesis (Nascimbeni et al., 2017), organelle morphology (Manford et al., 2012), and excitability in neurons and muscle cells (Ito et al., 2001; Sahu et al., 2019). Incidentally, several protein families of no obvious similarity have been identified at MCSs. Specific isoforms or even entirely separate families are allocated to MCSs depending on the membranes involved and the cell type, raising questions regarding their functionality and expression patterns. This review will summarize the literature describing the nature and molecular composition of ER-PM MCSs, presenting the roles of various populating protein families, emphasising MCSs in neurons and among related functions, the emerging role of SNAREs at MCSs.
The ER forms an extensive network of tubules and cisternae throughout the cell and has a range of roles integral to cell survival and function including lipid synthesis, protein synthesis, and Ca2+ regulation. The network projects to all parts of the cell and is functionally connected to other organelles (Nixon-Abell et al., 2016). ER-mediated contact sites typically present the following characteristics: 1) the two membranes involved are tethered within 7 – 30 nm of each other, 2) despite this close proximity, membrane composition is maintained and there are no reports of fusion between organelles, and 3) specific proteins and lipids are enriched at MCSs, creating microdomains (Prinz, 2014; Eisenberg-Bord et al., 2016; Gallo et al., 2016). Depending on their type, MCSs can be transient or stable over time, according to their role in cellular structure and physiology, as noted in Orai–Stim (Helle et al., 2013) and Kv2.1 (Fox et al., 2015) induced tethering respectively. Importantly, the interactions at MCSs influence the function of either or both the participating organelles.
Endoplasmic reticulum-mediated MCSs also show specific characteristics. Studies in Saccharomyces cerevisiae indicate that tethering another membrane excludes ribosomes from the cytoplasmic face of the ER (Wolf et al., 2012) and controls membrane curvature, vital for maintaining membrane integrity (Collado et al., 2019). The ER MCSs with other organelles, including mitochondria and peroxisomes (Elbaz and Schuldiner, 2011), are some of the best characterized examples of MCSs and have been described in multiple cell types and organisms, mediating additional processes at each contact site.
Initial evidence for ER-PM MCSs in neurons and muscle cells came from observation by electron microscopy, identifying subsurface cisternae “closely opposed” to the PM, maintaining a separation of 5–8 nm between the membranes (Porter and Palade, 1957; Rosenbluth, 1962). The high concentration of proteins in the membrane at these sites, as observed by freeze-fracture techniques, opened the possibility that these junctions had functions related to cell excitability (Henkart et al., 1976). Much later, this hypothesis was reinforced in rat hippocampal neurons (Spacek and Harris, 1997) with subsequent characterisation of novel tethers such as Kv2.1 K+ channels (Fox et al., 2015; Kirmiz et al., 2018) along with several SNARE proteins (Petkovic et al., 2014). 3D reconstructions show that the percentage of cell body PM engaged in MCSs with the ER as approximately 12.5% in the Nucleus accumbens, but varies in other brain regions (Wu et al., 2017). In agreement with earlier studies, the authors also found that, while the ER extends throughout neuronal cells, ER-PM MCSs are far less frequent in dendrites, only at the periphery of the post-synaptic densities and not at all in spines (Spacek and Harris, 1997; Wu et al., 2017). In contrast, yeast ER MCSs can cover 25–40% of the PM while separation reports have varied between studies, averaging 33 nm (West et al., 2011) but also reported lower at 21 nm (Collado et al., 2019). Several studies have also distinguished the morphology of ER associated with the PM in various models (West et al., 2011; Manford et al., 2012; Nixon-Abell et al., 2016; Wu et al., 2017). While the ER network surrounds the nucleus to form the nuclear envelope (Helle et al., 2013), tubules extend throughout the cell into the periphery, referred to as cortical ER (cER). Here the ER presents highly variable morphology, with “flattened sheets” with lumen width >25 nm (in neurons) (Orci et al., 2009; Wu et al., 2017) separated by thin tubules (Fernández-Busnadiego et al., 2015; Wu et al., 2017; Collado et al., 2019). Consistent with the dynamic nature of the ER, the larger sheets observed can quickly assemble and disassemble, likely due to interactions with motor proteins on microtubules (Nixon-Abell et al., 2016), which are frequently found in alignment with the cER (Orci et al., 2009). Extending into dendrites, the ER predominantly forms tubules and is less frequently in contact with the PM (Wu et al., 2017). ER-PM MCSs in the axons and dendrites of neurons are smaller and less frequent than in the cell body, and while the ER can enter the necks of large spines, they do not seem to form contacts (Spacek and Harris, 1997; Wu et al., 2017).
Membrane contact sites could be viewed as microenvironments where selected proteins, namely tethering proteins, first establish physical bridge(s) between membranes, then recruit partner proteins which perform contact specific functions (Helle et al., 2013). In MCSs, membrane-associated tethering proteins maintain two distinct membranes in close apposition, under conditions where no membrane fusion occurs. Tethering proteins are often ER integral membrane proteins able to directly bind to either PM lipids or soluble lipid-binding proteins (Prinz, 2014; Eisenberg-Bord et al., 2016). Alternatively, trans-interactions involve integral membrane proteins that can project from each membrane into the cytoplasm. Broadly, tethers are defined as proteins or protein complexes that a) are physically present at the MCS, b) contribute a tethering force between the participating membranes, c) affect the processes taking place at the MCS, and d) modulate the degree of proximity and number of MCSs (Helle et al., 2013; Eisenberg-Bord et al., 2016).
Multiple protein families have been indicated at ER-PM junctions, hosting unusual molecular compositions including selected proteomes and potentially lipidomes (Phillips and Voeltz, 2016). No single-family appears to solely perform as a tether. More commonly, tethers are involved in additional functions either by themselves or together with binding partners, identified in model systems and translated to human homologues by sequencing and proteomics. Accordingly, studies presenting ablation of a tethering family generally show reduced numbers of contact sites rather than complete abolition, as exemplified in ER-endolysosomal contacts (Henne et al., 2015) and ER-PM contacts (Manford et al., 2012), further indicating functional redundancy. Loss of all the aforementioned proteins leads to ER-PM dissociation, together with the accumulation of cytoplasmic ER, resulting in misregulation of phosphoinositide (at PM) and Ca2+ signalling; compromising cellular integrity, and activating ER unfolded response (Manford et al., 2012; Stefan, 2018; Wang and Dehesh, 2018). The role played by ER-PM MCS in maintaining membrane homoeostasis remains to be investigated. So far, several families of proteins have been identified for their functions in lipid modification and/or transfer between membranes; the TMEM16 family, E-Syt (Extended Synaptotagmins), VAPs (VAMP-associated proteins), Junctophilins, and more recently, SNAREs (Figure 1), as described below.
Figure 1. Schematic illustration of the organization and topology of ER-PM tethering proteins. Shown are the so-far identified protein complexes acting in ER to PM membrane recruitment, in yeast and mammals: Sec22b-Syntaxin1/3 (Sso1/2p in yeast) complex, TMEM16 (Ist2 in Yeast), TMEM24 (C2CD2L in Yeast), Kv Channels, Junctophillins, VAPs (Sc2-22 in Yeast), Extended Synaptotagmin (E-Syt). SNARE complexes generate short-range (∼10 nm) MCS whereas other tethers generate longer range (∼20–30 nm). Created with BioRender.com.
Ist2 is an integral membrane protein predominantly found in the cER in yeast. Studies of the Ist2 family have shown its members can bind to phosphatidylinositol-4,5-bisphosphate (PI(4,5)P2) in the PM, via a C-terminal basic stretch of 69 amino acids (Ercan et al., 2009; Fischer et al., 2009; Maass et al., 2009). This interaction not only contributes to the localisation of Ist2 to cER but also recruits ER to the PM, creating a compartment devoid of ribosomes (Wolf et al., 2012). Furthermore, deletion of Ist2 increased the distance between ER-PM associations, while overexpression dramatically increased the percentage of cER associated with the yeast PM (Wolf et al., 2012).
The first identified mammalian orthologues, TMEM16A/Ano1 and TMEM16B/Ano2 are Ca2+ activated Cl– channels (Yang et al., 2008; Caputo et al., 2008). Other Anoctamins of the 10-member family include TMEM16C (ANO3), TMEM16D (ANO4), TMEM16F (ANO6), TMEM16G (ANO7), and TMEM16J (ANO9) and although some of which can be found localised to ER-PM MCSs (Duran et al., 2012; Bushell et al., 2019), their functions are not fully understood (Picollo et al., 2015). The family members display substantial functional diversity (Milenkovic et al., 2010) and different tissue localisation (Schreiber et al., 2010), though their structural analysis shows a conserved topology including eight N-terminal transmembrane domains (TMDs) and stretches of basic amino acids at the C-terminus (Pedemonte and Galietta, 2014). As TMEM family members are tethers with an intrinsic activity in lipid transfer (for example, that of phosphatidylserine, i.e., PS) (Lees et al., 2017), it was unexpected to discover that, in yeast, efficient transport of PS to the PM by the cytosolic lipid transfer protein (LTP) Osh6, requires its association with Ist2 (D’Ambrosio et al., 2020). This functionally distinct contribution to PS homoeostasis illustrates the complexity of TMEM16 biology at MCSs. Besides delineating the regulatory role of Ca2+ channels, the structural bases of the family members’ functional diversity remain to be elucidated (Picollo et al., 2015). The TMEM16F interactome includes Munc18-1, a partner of the PM SNARE Syntaxin1 (Stx1) (Brooks et al., 2015). TMEM16K, an ER lipid scramblase involved in spinocerebellar ataxia, was found at ER-endosome MCSs further emphasising the importance of this family of proteins. Several ER and endosomal SNAREs were found in close proximity to TMEM16K (Petkovic et al., 2020) but the potential functional relationship is unknown.
VAMP-associated proteins or VAPs are another family suggested to act as tethers at MCSs, with orthologues identified in plants (Siao et al., 2016) and yeast (Loewen et al., 2003). VAP’s acronym refer to the initial interaction of VAP-A with the SNARE VAMP in Aplysia (Skehel et al., 1995) but the functional relevance of VAP-SNARE interactions is not yet well defined (Weir et al., 2001). VAPs are highly conserved type II integral ER membrane proteins and interact with a wide variety of intracellular proteins (Nishimura et al., 1999; Murphy and Levine, 2016), regulating several cellular processes (Soussan et al., 1999; Kagiwada and Zen, 2003). Structurally, VAPs consist of an N-terminal MSP (major sperm protein) domain, a coiled-coil domain involved in dimerisation (Kim et al., 2010) and finally a C-terminal TMD, responsible for anchoring in the ER membrane (Murphy and Levine, 2016; Sun and De Camilli, 2018). VAPs are prominently present at several ER-mediated contact sites including with the PM, Golgi, and mitochondria (Eisenberg-Bord et al., 2016). Deletion of the yeast homologues Scs2 and Scs22 result in a loss of cER associated with the PM (Manford et al., 2012). VAPs can also recruit proteins containing an FFAT motif, which consists of two phenylalanine residues flanked by an acidic tract which binds to a positively charged binding motif on the face of the MSP domain (Murphy and Levine, 2016). FFAT motifs have been found in the oxysterol-binding protein (OSBP) related protein family (ORPs), Ceramide transfer protein and N-terminal domain-interacting receptor 1–3 (Loewen et al., 2003; Murphy and Levine, 2016). The versatility of this structure gives VAP an opening to interact with multiple proteins, divided into three categories, 1) SNAREs, 2) Viral proteins, and 3) FFAT-motif-containing proteins (Loewen et al., 2003). VAPs, therefore, have a diverse catalogue of binding partners, including LTPs such as oxysterol-binding homology (Osh) proteins and Nir2, located at ER-PM contact sites. Accordingly, the presence of VAP-A and VAP-B is paramount for lipid transport at ER-Golgi contact sites, with knockdown experiments showing detrimental effects on Golgi conformation and distorted composition of phosphatidylinositol-4-phosphate (PI4P), sphingomyelin, and diacylglycerol (DAG) in the Golgi membrane (Peretti et al., 2008).
Two groups independently identified an unexpected VAP binding partner in hippocampal neurons. Despite lacking a typical FFAT motif, PM-localised Kv2 channels form ER-PM MCSs via the recruitment of the ER-resident VAPs. This interaction is thought to be regulated by Kv2 phosphorylation (Kirmiz et al., 2018; Johnson et al., 2019), thus involving negative charges acting like the acidic amino acid residues of the FFAT motif of ORPs/Oshs (Sun and De Camilli, 2018) (see below). Incidentally, VAP-A and VAP-B knockdown reduces Kv2 clustering (Kirmiz et al., 2018; Johnson et al., 2019). Finally, VAP-B in particular is associated with the progressive neurodegenerative diseases Amyotrophic Lateral Sclerosis and Parkinson’s disease. Mutations in the MSP domain result in conformational changes that promote oligomerisation and degradation (Kim et al., 2010; Murphy and Levine, 2016), altering ER morphology (Yamanaka et al., 2020). This leads to a range of mechanistic dysfunctions including the different extent of MCS tethering, cytoskeletal coordination, and lipid homoeostasis (Murphy and Levine, 2016) as shown in Drosophila (Forrest et al., 2013), highlighting the role of VAPs as an important mediator in ER-PM contact formation (Figures 1, 2).
Figure 2. SNAREs and lipid transfer proteins at the MCS. Using varying mechanisms such as FFAT to bridge SNAP25-OSH/ORP-VAP-A, and non-fusogenic Sec22b-Stx1- E-Syt complex, LTPs promote MCS tethering and mediate lipid transfer [PI4P, sterol, glycerolipids, and diacylglycerol (DAG)]. PI4P phosphatase at the ER membrane Sac1 was not represented for simplicity. Created with BioRender.com.
Junctophilins were originally identified as junction components in muscle cells, with JPH-2 knockouts presenting reduced associations between the sarcoplasmic reticulum (SR) and PM (Takeshima et al., 2000). While invertebrates have one genomic copy, mammals have four, all identified in excitable cells (Landstrom et al., 2014). Subtypes 1 and 2 are expressed in skeletal and cardiac muscle respectively, while 3 and 4 have been shown to be expressed in hippocampal pyramidal neurons (Nishi et al., 2003). JPHs are embedded in the ER/SR via a C-terminal TMD (Nishi et al., 2000; Takeshima et al., 2000), following a defining feature of the family; eight N-terminal MORN (membrane and recognition nexus) motifs, the final two of which are separated by a joining region (Landstrom et al., 2014). These MORN motifs are hypothesised to bind phospholipids, specifically phosphatidylinositol phosphates (PIPs) such as PI4P and PIP2 (Landstrom et al., 2014), mediating attachment to the PM according to the number and sequence present, as removal of specific MORN motifs in JPH-1 leads to localisation in the nucleus and cytoplasm rather than PM (Takeshima et al., 2000). There is evidence that the N-terminal region (containing the MORN repeats) mediates PM targeting, though the direct involvement of MORN repeats has not been demonstrated (Nakada et al., 2018; Rossi et al., 2019). Between the MORN motifs and C-terminal TMD sits an alpha helix, which modulates the distance between the ER/SR and PM, followed by a divergent region of unknown function, so named due to limited sequence conservation between isoforms (Garbino et al., 2009) (Figure 1).
Initially thought to be primarily structural components, Ito et al. (2001) highlights roles for JPHs mediating Ca2+ regulation in muscle cells by stabilising a complex of Ryanodine receptor and DHPR Ca2+ channels in the SR and PM, respectively. More recently, this has also been observed in T cells (Woo et al., 2016) and several neuronal populations. For example, JPH-3 and -4 double knockouts result in behavioural and physiological defects in mice in addition to dysfunctional Ca2+ release and impaired plasticity in hippocampal neurons (Moriguchi et al., 2006). Loss of JPH isoforms prevents the after hyperpolarisation necessary to form Ca2+ microdomains (Kakizawa et al., 2008; Takeshima et al., 2015) as JPH-3 and/or -4 are needed to tether and stabilise a Cav1-RyR2-KCa3.1 Tripartite Complex necessary for neuronal excitability regulation (Sahu et al., 2019). Aside from functional studies, JPH-3 has been implicated in neurodegenerative disorders, notably in Huntington’s disease-like (HDL) syndrome. Up to 15% of patients lacking pathological mutations in Huntingtin, the canonical cause of Huntington’s disease, show CAG/CTG repeats in JPH-3 (Holmes et al., 2001) which generate toxic RNA foci and protein aggregation. Loss of JPH-3 in mouse models presents progressive motor abnormalities, consistent with JPH-3 as an, albeit rare, cause of HDL syndrome (Seixas et al., 2012). JPHs have not been connected to SNAREs yet.
Extended synaptogamins (E-Syts) are evolutionarily conserved proteins shown to function at ER-PM membrane contact sites in multicellular organisms. Sequence and domain analysis revealed homology with the yeast Tricalbins family, also identified at ER-PM MCSs. The deletion of all three known Tricalbin isoforms in combination with deletion of other associated tethers reduces the number of contact sites (Manford et al., 2012). Tricalbins consist of an N-terminal TMD, followed by a synaptotagmin-like mitochondrial lipid-binding protein (SMP) domain and three to five C2 domains (Toulmay and Prinz, 2012), which bind to membranes in a Ca2+-dependent manner (Schulz and Creutz, 2004). Indeed, of the three mammalian E-Syt isoforms, E-Syts differs from the yeast orthologues in the number of C2 domains; E-Syt1 has five, while E-Syts2/3 have three C2 domains (Saheki and De Camilli, 2017), enabling recruitment to ER-PM interfaces by membrane-binding of C2 domains upon an increase of cytosolic Ca2+ level (Chang et al., 2013; Giordano et al., 2013; Saheki et al., 2016).
In yeast, the Tricalbins are involved in lipid transfer and maintenance of ER curvature, specifically in the formation of cER peaks which are essential for lipid transfer to maintain PM integrity (Collado et al., 2019; Hoffmann et al., 2019). As such, all E-Syts have been shown to perform similar roles under Ca2+ regulation. E-Syts2/3 bind PI(4,5)P2 at the PM via the C2C domain (Figure 1), but E-Syt1, which is distributed over the whole ER surface area under resting conditions, binds at low Ca2+ concentrations via C2E (Giordano et al., 2013). Upon micromolar changes in cytosolic Ca2+, E-Syt1 is recruited to ER-PM sites where it can control the distance between the membranes, after Ca2+-binding to the C2C domain (Fernández-Busnadiego et al., 2015; Idevall-Hagren et al., 2015). Additionally, hetero- or homodimerisation of SMP domains forms a hydrophobic channel that can facilitate lipid transfer in vitro (Schauder et al., 2014; Yu et al., 2016) (Figures 1, 2). Finally, the single E-Syt orthologue in Drosophila, catalysing lipid transfer in photoreceptors at the submicrovillar cisternae and the growth of the organism (Nath et al., 2020), was also suggested to modulate synaptogenesis (Kikuma et al., 2017). E-Syts have since been identified in mammalian neuronal cells but, while it is plausible that they can perform these functions (Giordano et al., 2013; Fernández-Busnadiego et al., 2015) their physiological significance in neurons is so far unclear and may only be noticeable under specific conditions. Indeed, hippocampal neurons from E-Syt triple knockout mice exhibited no change in ER morphology or protein composition, and neuronal survival under stress was not impaired, suggesting that other tethers may provide compensation for their loss (Sclip et al., 2016). ESyts have recently been connected to SNAREs as discussed below.
Soluble N-ethylmaleimide-sensitive factor attachment protein receptors (SNAREs) constitute the basic molecular machinery of intracellular membrane fusion. Positioned on opposite membranes, vesicular SNAREs on one side and target SNAREs on the other assemble in a ternary complex which allows for close docking and subsequent fusion as exemplified in the case of synaptic vesicle fusion with the presynaptic PM (Galli and Haucke, 2004). ER-localised vesicular SNARE Sec22b contains an N-terminal Longin domain, followed by the characteristic SNARE domain and finally a C-terminal TMD (Petkovic et al., 2014) (Figure 1). Previously shown to be involved in trafficking between the ER and Golgi via its interaction with t-SNAREs Syntaxin 5, membrin, and rbet1 (Williams et al., 2004), Sec22b was shown to associate with Stx1 at ER-PM contact sites in the growth cones of developing neurons (Petkovic et al., 2014). This complex does not include SNAP23/25/29/47. However, liposome assays, while confirming a fusogenic activity of Sec22b in a tripartite assembly with Stx1 and SNAP-25, did not elicit the fusion of membranes in the absence of SNAP25. Additionally, Sec22b was not found at the cell surface. Altogether, this evidence suggest that Sec22b/Stx1 association does not lead to membrane fusion. This assembly represents a new short range (∼10 nm) tether between ER and the PM (Petkovic et al., 2014) able to determine MCS distance, as elongating the linker between the SNARE and TMD domains of Sec22b with polyproline motifs increased the distance between the ER and PM.
Meanwhile, Sec22b was also essential for neuronal development, as RNAi-mediated knockdown of Sec22b inhibited axonal and dendritic growth. Altogether this supports the notion that the complex is not involved in membrane fusion but instead acts as a tether and facilitates additional functions such as lipid transfer. Indeed, in the brain, E-Syts were recently found in complexes containing Stx1 or Stx3 and Sec22b but not SNAP25 (Gallo et al., 2020). Notably, the interaction between the ER-resident ESyts and Sec22b depends on the latter’s Longin domain. Removal of the Longin domain induces recovery of SNAP25 in Sec22b immunoprecipitates. Therefore, Sec22b Longin domain likely excludes SNAP25 allowing the occurrence of a non-fusogenic Sec22b-Stx1 complex. Super-resolution imaging of growth cones in developing neurons demonstrated the very close proximity of E-Syt2 and Sec22b beneath the PM, plausibly populating ER-PM contact sites. Moreover, the interaction between E-Syt and Sec22b seems to promote and/or stabilize the association of Sec22b with Stx1/3. E-Syt overexpression is responsible for neuronal membrane expansion in the form of filopodia leading to protuberant hyper-ramified axons, an effect which depends on Stx1 and Sec22b as shown using clostridial toxins and expression of Sec22b extended by a polyproline spacer and the Longin domain. Brought together, these observations allow postulating E-Syt engagement in a tripartite complex with Sec22b and Stx1/3, regulating lipid transfer to the PM within ER-PM contact sites (Gallo et al., 2020). This situation provides the first example of a SNARE-containing MCS where an aborted formation of a fusogenic SNARE complex contributes to the function of an LTP, namely E-Syts. It further emphasises the importance of Longin domains conserved molecular functions in SNARE regulation via promoting and inhibiting membrane fusion by folding back onto the fusion-inducing SNARE coiled-coil domain, along with managing Longin SNAREs interactions with proteins controlling intracellular sorting (Daste et al., 2015; Gallo et al., 2020; Singh et al., 2020).
In addition to the above tethering proteins, which appear to be ubiquitously expressed among mammalian cells, there is recent evidence for tethering candidates exclusive to neuronal populations. For example, TMEM24/C2CD2L is an ER-localised protein shown to transport phosphatidylinositol between the ER and the PM via its SMP domain, binding to the PM in a Ca2+-dependent manner (Lees et al., 2017). TMEM24 is also enriched at ER-PM MCSs in neurons and acts as a tether. TMEM24 is redistributed throughout the ER at high cytosolic Ca2+ levels and may interact with other potential tethers including VAPs and Kv2 channels, indicating a regulatory loop related to neuronal activity (Sun et al., 2019) (Table 1).
Widely expressed throughout mammalian brain Kv2 K+ channels are distinguished for their neuronal surface localisation pattern divided into a) freely diffusive channels in PM and b) micron-sized clusters present on the soma, dendrites, and axon initial segment (Johnson et al., 2019). Kv2 voltage-gated K+ channels are frequently identified as interactor partners with known tethers. However, several lines of evidence have recently implicated Kv2 channels as tethers in their own right. Multiple studies identified Kv2 (Kv2.1 and Kv2.2) channel clustering at the PM in hippocampal neurons (Antonucci et al., 2001), cerebellar Purkinje cells (Kaufmann et al., 2009), and even Aplysia neurons (Zhang et al., 2008) due to a 26-amino acid long targeting sequence called the Proximal Restriction and Clustering domain (Lim et al., 2000). While early studies recognised clusters at close appositions, more recent studies have shown that Kv2 clustering remodels the ER, initiating the formation of ER-PM contact sites (Fox et al., 2015; Kirmiz et al., 2018), where L-type Ca2+ channels and Ryanodine receptors are recruited to mediate burst firing (Mandikian et al., 2014; Irie and Trussell, 2017; Kirmiz et al., 2018). Further investigation conducted by Kirmiz et al. (2019) has shown that Kv2.1 knockout mice have significantly reduced PI4P and PI(4,5)P2, suggesting their integral involvement in PI4P and PI(4,5)P2 regulation; components crucial for generating secondary messengers inositol trisphosphate (IP3) and DAG (Balla et al., 2020; Jing et al., 2020). However, clustering at close appositions was not equal in all cells; Kv2.1 (also called BK channels) expression differed between pyramidal neurons and interneurons of the hippocampus, potentially in reference to their different functions (Antonucci et al., 2001). Furthermore, Kv2.1 channels in the soma are commonly found clustering opposite to astrocytic processes, so may mediate a method of intercellular communication with non-neuronal cells, not just by regulating synaptic firing (Du et al., 1998).
Interestingly, disrupting the phosphorylation-dependent interaction between Kv2.1 and VAP-A (Kirmiz et al., 2019) is neuroprotective and prevents pro-apoptotic K+ entry upon ischaemic injury (Schulien et al., 2020). It is particularly interesting that Kv1.1 and Kv2.1 channels were also found to interact with Stx1 (Fili et al., 2001) and SNAP-25 (MacDonald et al., 2002; Leung et al., 2003). These interactions appear conserved in plants where they were shown to regulate membrane expansion for cell growth independently of vesicle traffic (Honsbein et al., 2009). Future studies should address the potential role of Kv-SNARE interactions in regulating MCSs’ protein complexes and lipid transfer.
The characterisation of the molecular composition of MCSs has aided the evaluation of their physiological significance, with most tethering proteins seemingly mediating functions such as Ca2+ homoeostasis or lipid transport. We will thereafter go on to review the recognised functions occurring at MCSs and their significance in neurons.
The translocation of lipids between membranes has been shown since some of the earliest studies (Vance, 1990). More recent evidence has begun to shed light on the potential mechanisms and the components involved. LTPs are able to bind lipids in one membrane and deliver them to another, closely situated membrane. Consequently, it is logical that LTPs function at MCSs (Toulmay and Prinz, 2012). While the mechanisms of translocation are still contested, there are two prevailing theories related to their mechanism of action. The tunnel model proposes that lipid-binding domains form a tunnel bridging the intermembrane space, with a small channel open along the length of the domain, such that the polar head group is exposed to the hydrophilic environment as it traverses the channel (Schauder et al., 2014). Hydrophobic residues line the inside of the tunnel stabilising the tails, with conformational changes revealing lipid-binding sites at the tunnel entrance, permitting lipid binding and translocation to the opposing membrane (Schauder et al., 2014; Lees and Reinisch, 2020). Alternatively, the shuttle model suggests that LTPs specifically deliver lipids from the donor membrane, particularly across short distances (under ∼200Å). A series of conformational changes extract the lipid from the single entry point along the closed hydrophobic environment and insert the lipid into the acceptor membrane (Schauder et al., 2014; Wong et al., 2019). Some LTPs have been shown to dimerise to form the tunnel, while others utilise β-barrels (Im et al., 2005), α-helices (Tong et al., 2018), or a mix to form the bridge (Wong et al., 2019). In concert with this, it has been suggested some LTPs (e.g., E-Syt2) may necessarily interact with partners, including other LTPs, to discriminate and transport specific lipids (Schauder et al., 2014). The channel model of E-Syts action clearly fits well with the notion that lipid transfer would work best at the closest contacts such as those involving SNAREs (<10 nm) (Gallo et al., 2020) (Figure 2).
Sequence analysis of SMP domains, identified in proteins of the ERMES complex in yeast ER-mitochondrial MCSs (Lee and Hong, 2006), showed homology with the TULIP superfamily, confirming lipid-binding interactions (Kopec et al., 2010; Alva and Lupas, 2016). SMP domains have been shown to dimerise in order to facilitate lipid transport. A shuttle mechanism, as described above, likely operates in E-Syt as the 90Å-long SMP dimer is too short to span the distance between membranes (Schauder et al., 2014). E-Syts were found to mediate the transport of glycerophospholipids in a bidirectional manner using in vitro assays (Saheki et al., 2016; Yu et al., 2016; Bian et al., 2018; Bian and De Camilli, 2019). E-Syts might also participate in mechanisms altering lipid composition. For example, PI(4,5)P2 hydrolysis following phospholipase C (PLC) activation by mediated via G-protein coupled receptor (GPCR) (Figure 3) led to the release and accumulation of DAG in E-Syt knockout cells (Saheki et al., 2016). In addition, Ca2+ influx is needed to relieve autoinhibition of the SMP domain in E-Syt1 by the C2A domain (Yu et al., 2016; Bian et al., 2018). Furthermore, a study in human embryonic kidney cells and rat superior cervical ganglia neurons has shown that E-Syt2 stabilises Sac1, an ER-localised lipid phosphatase, at ER-PM MCSs (Dickson et al., 2016). Here, Sac1 dephosphorylates phosphatidylinositol monophosphates; overexpression of Sac1 increased PI levels approximately two-fold, while PI4P and PIP2 levels decreased. In accordance with this role, Sac1 and E-Syt2 were shown to co-localise while E-Syt2 knockdown significantly reduced Sac1 puncta at MCSs coinciding with accumulation of PI4P and PIP2 (Dickson et al., 2016). A role of E-Syt2 in PM PI regulation fits well with the observation that E-Syts regulates growth as shown in Drosophila (Nath et al., 2020) and mammalian neurons (Gallo et al., 2020) because of the role of PIP2 in PM dynamics (Scholze et al., 2018). Furthermore, E-Syts were found to interact with FGFR1 (Tremblay et al., 2015), a receptor that regulates PM PIP2 (Herdman and Moss, 2016).
Figure 3. Ca2+ influx at the ER-PM contact site. Tethering proteins involved in the control of the Ca2+ ions fluxes between the ER lumen, the cytosol and the extracellular medium: ORA1, STIM1, SERCA, and IP3 Receptor (IP3R). IP3R is activated by IP3 generated by Phospholipase C (PLC) upon activation of G-protein coupled receptor (GPCR). The G protein subunits ware not represented for simplicity. Created with BioRender.com.
Another major class of LTPs are ORPs (Table 1). There are 12 members of the ORP family in humans and seven in yeast (homologue Osh proteins), each containing a conserved OSBP-related domain (ORD). In addition, members usually contain ER-targeting sequences (e.g., FFAT motif or C-terminal TMD) and membrane-binding domains, most commonly a Pleckstrin homology (PH) domain (Lehto et al., 2001). Recently, studies have shown that ORPs facilitate lipid transport at several MCSs, including ER-lipid droplets (Du et al., 2020) and ER-PM MCSs (Chung et al., 2015; Mochizuki et al., 2018). The function of ORPs (and Oshs) relies on their binding to the ER transmembrane protein, VAP (Scs2p in yeast) via the FFAT motif (Loewen et al., 2003). In yeast, Osh2/3p-Scs2p complexes are located at ER-PM MCSs (Weber-Boyvat et al., 2015) where they are thought to be responsible for the extensive tethering of the cortical ER and PM (Figure 2). Although being topologically adapted to this function through selective Scs2p binding, Osh were also shown to be highly integrated in an intriguing interplay with SNARE proteins (Weber-Boyvat et al., 2020). This later study demonstrated the direct interaction of Osh and the SNARE domain of Sec9p (SNAP25 homologue), along with the previously defined Sec9p interaction with Sso1 (Stx homologue). Furthermore, this crosstalk was conserved in hippocampal neurons. Knocking down ORP2 in neurons reduced both neurite growth and synaptogenesis, further emphasising the importance of lipid transfer. In this situation, a plausible hypothesis is the recruitment of Sec9p (SNAP25) by an Osh-Scs2p complex reinforcing tethering between ER and PM (Figure 2). In the absence of an ER-resident v-SNARE and thus membrane fusion, negative regulation of exocytosis by Osh overexpression (Weber-Boyvat et al., 2020) could be due to a re-routing of Sec9p (SNAP25) by Osh towards a ternary Osh-Scs2p-Sec9p complex, allowing only lipid transfer/modification.
Additional sterol transporters were identified as StARt (steroidogenic acute regulatory protein-related lipid transfer) domain (StARD) proteins (Table 1), also known as the StARkin superfamily (Jentsch et al., 2018). Following, LAM/Ltc proteins were identified in yeast to contain StART-like domains, structurally similar to the StART domains (Gatta et al., 2015). LAM/Ltcs are localised to the ER via a C-terminal TMD and can be found at ER-PM MCSs. A hydrophobic cavity the StARt domain accommodates sterols, supported by slowed sterol transport upon deletion (Horenkamp et al., 2018; Tong et al., 2018). LAM isoforms also contain a PH-like domain, N-terminal to the StARt domain, thought to be involved in targeting, although unable to bind lipids (Jentsch et al., 2018; Tong et al., 2018). In mammals, several StART (e.g., StARDs) and StART-like domain-containing proteins have been suggested to transport cholesterol to the PM and between intracellular organelles (Horenkamp et al., 2018; Clark, 2020). For example, mammalian homologues of yeast LAM proteins GRAMD1s/ASTERs contain a C-terminal TMD localising the protein at the ER, an N-terminal GRAM domain associating with the PM and a StART-like domain (also called VaST domain) to facilitate lipid transfer (Sandhu et al., 2018; Naito et al., 2019). While consistent with a role in sterol homoeostasis at MCSs, the potential physiological function in neurons remains to be investigated.
While much of the mechanistic detail describing lipid transfer remains elusive, the diversity among the identified molecular components (and potential for unidentified members) indicates extensive functional redundancy, highlighting the significance of lipid homoeostasis in biological membranes. Enhancement of ER-PM MCSs by E-Syt recruitment also induces translocation of Nir2 to ER-PM MCSs, potentially via interactions with VAP isoforms (Amarilio et al., 2005). Here, Nir2, a phosphatidylinositol transfer protein, replenishes PIP2 in the PM by promoting transfer from the ER, offering a feedback loop for receptor-induced Ca2+ signalling (Chang et al., 2013). Abnormal localisation and disproportionate lipid concentrations can have dire effects on organelle function and downstream signalling pathways. In this manner, it has been suggested LTPs do not simply move lipids between compartments, but they also present them to metabolising enzymes to maintain specific compositions (e.g., Sac1 and E-Syt2 localisation). For example, studies in yeast show that the lipid transporter Osh3 is needed not only for PI4P transport, but is independently necessary for the localisation of Opi3, a phosphatidylethanolamine N-methyltransferase involved in phosphatidylcholine synthesis (Tavassoli et al., 2013). In accordance with roles in signalling pathways, temperature stress in yeast induces recruitment of phosphoinositide-dependent kinase orthologues by PI4P and PIP2, modulating the PDK-TORC2-Akt cascade thus activating sphingolipid synthesis in the ER. A key component of the PM, sphingolipid transfer from the ER is essential in maintaining PM integrity under stress (Omnus et al., 2016). Following, the same study showed that deletion of ER-PM tethers resulting in dysfunctional Ca2+ signalling and subsequent impaired sphingolipid synthesis, manifesting in PM integrity defects.
Ca2+ is an essential second messenger utilised ubiquitously in eukaryotes. Early work by Michell (1975) suggested an integral role played by ER-PM contacts in Ca2+ signaling. A prerequisite for Ca2+ signaling is a low baseline Ca2+ concentration in the cytosol; in resting cells, Ca2+ levels are actively maintained in the nanomolar range, several orders of magnitude below typical extracellular concentrations (Carrasco and Meyer, 2011). With low intracellular concentrations, Ca2+ is acquired from the extracellular milieu and/or from specific stores in ER, Golgi, and mitochondria (Giorgi et al., 2018). When low, these stocks must be replenished from the extracellular milieu, requiring signalling between organelles, thus MCSs are obvious candidate hubs for signal transduction (Wu et al., 2006). Post ER Ca2+ depletion, mediated by GPCR, PLC and IP3, Ca2+ is replenished through a process called store-operated Ca2+ entry (SOCE) (Figure 3), directly mediated by ER-PM junctions through ER Ca2+ sensor STIM1 and PM Ca2+ channel Orai1 (Michell, 1975; Chang et al., 2013). These form an elementary unit upon Ca2+ depletion, sensed by the lumenal EF domain of STIM1 protein, generating cytoplasmic Ca2+ signals and refilling ER Ca2+ (Balla et al., 2020; Stefan, 2020) (Figure 3). Defects in this mechanism could contribute to degenerative diseases such as Alzheimer’s disease (Popugaeva and Bezprozvanny, 2014).
At rest, STIM1 and Orai1 are evenly distributed across ER/PM, however, post ER Ca2+ depletion, STIM1 is activated and releases Ca2+ from the lumenal EF-hand, eventually enabling oligomerization and translocation. STIM1 in activated state directly interacts and gates Orai1 Ca2+ channel (Jing et al., 2020). Orai1 permits the entry of Ca2+ into the cytosol, where repletion of the ER as a Ca2+ store is facilitated by SERCA channels (Luik et al., 2008; Chang and Liou, 2016). Mammals express two STIM isoforms, which share high sequence homology (Berna-Erro et al., 2009). Both isoforms are widely expressed in the brain, although show differential patterns with STIM1 more common in Purkinje neurons of the cerebellar cortex (Hartmann et al., 2014) while STIM2 is the predominant isoform expressed in the hippocampus (Berna-Erro et al., 2009) and cortex (Gruszczynska-Biegala et al., 2011). Further research has revealed additional regulatory and stabilising partners. Receptor-induced PIP2 hydrolysis results in Ca2+ influx due to the generation of IP3 and subsequent opening of IP3 receptors, Ca2+ channels in the ER. Additionally, E-Syts are Ca2+-activated, transferring PLC generated DAG from the PM to ER (Saheki et al., 2016). Recruitment of E-Syt1 to ER-PM junctions (Chang et al., 2013; Idevall-Hagren et al., 2015) modifies ER which form a ring around the site of Ca2+ entry, thereby stabilising MCSs and accelerating Ca2+ replenishment (Kang et al., 2019). Furthermore, localisation at PI(4,5)P2-rich microdomains tethered by E-Syt1 seems to be necessary for binding and inactivation of STIM by SARAF (Maléth et al., 2014). Interestingly, knockdown of Sec22b does not impair SOCE but increasing the length of Sec22b strongly affects SOCE (Petkovic et al., 2014), suggesting that different tethering mechanisms could operate in an independent manner at ER-PM MCSs to a limited extent. In addition, an essential role of α-SNAP, a SNARE interactor, was found in binding to and mediating functional coupling of Stim1 and Orai1. This function for α-SNAP is direct and independent of its known activity in NSF dependent SNARE complex disassembly (Miao et al., 2013).
Another intriguing protein family playing a critical role in modulating Ca2+ at ER-PM MCSs is GRAMD1/Aster. GRAMD1 and GRAMD2 localise to separate MCSs, suggesting different physiological functions. For example, GRAMD2 localisation at ER-PM MCSs is PI(4,5)P2-dependent, highlighting the MCS for recruitment and translocation of STIM1 post depletion of Ca2+ (Besprozvannaya et al., 2018).
Lipid transfer is essential to maintain cell homoeostasis and simultaneously mediate dynamic changes upon stimulus. Indeed, transfer can modulate lipid synthesis, cell growth and is also vital for organelle biogenesis and function, as each membrane possesses specific lipid compositions, including asymmetric distributions between leaflets. This requires targeted and often large-scale transport of lipids throughout the cell (Prinz, 2014). Lipid transfer operates complementarily to vesicular transport and membrane fusion. Contrary to the latter, lipid transfer does not imply full lipid mixing between organelles, nor co-transfer of proteins and can operate for amounts of molecules not defined by quantal size of organelles. This complementarity between vesicular transport and lipid transfer at contact sites is illustrated by the formation of the phagophore, the initial step of autophagy. For instance, macroautophagy is dependent on the formation of the phosphatidylinositol-3,4,5-trisphosphate (PIP3)-enriched phagophore, which requires progressive vesicular fusion to form the maturing structure (Rubinsztein et al., 2012; Wang et al., 2016) and also lipid transfer by ATG2 (Maeda et al., 2019; Sawa-Makarska et al., 2020). Further evidence suggests that ER-PM contact sites can be directly involved in autophagy. Induction of autophagy by nutrient deprivation results in upregulated and stabilised E-Syt-mediated MCSs in mammalian cells. Co-localisation of phagophore markers such as LC3 and DCFP1 with E-Syt2 or 3 suggests that about 30% of all autophagosome assembly occurs at ER-PM MCSs (Nascimbeni et al., 2017). SiRNA-mediated knockdown of E-Syt2 and 3 resulted in decreased expression of some members of the PI3KC3 complex, such as Beclin1 and VMP1. Both precipitated with E-Syt2, suggesting E-Syts stabilise the PI3KC3 complex, needed for local PI3P synthesis, therefore making E-Syt-mediated ER-PM MCSs ideal locations for autophagosome assembly. The extent of this mechanism in neurons and how this may contribute to a disease characterised by deficiencies in the autophagy-lysosomal pathway has yet to be investigated. Sec22b was suggested to mediate the fusion of autophagosome with the PM via interaction with syntaxin 3/4 and SNAP-23/29 in on neuronal cells (Kimura et al., 2017). It is tempting to speculate that autophagosome Sec22b might differ from ER Sec22b in a regulatory process, maybe related to the Longin domain, which would allow for fusion with the PM in the case of the autophagosome but not the ER.
The study of MCSs has grown tremendously in the last 20 years, however, our understanding of their structure and functions still shows many gaps. Much of this difficulty arises from the inherent features of MCSs; they are minute, dynamic structures with diverse molecular compositions between organelles and organisms and thus can be difficult to study. ER-PM MCSs, while relatively well characterised, exemplify the complexity surrounding MCSs, with multiple tethering complexes and binding partners defining the structure’s performance as a signalling hub and as key homeostatic regulators, responsive to physiological and stressful conditions. What remains a major question in the field is the relationship between MCS and particularly their lipid transfer activity with membrane fusion events. The fact that SNAREs, which constitute the basic membrane fusion machinery, would interact with LTPs and be found both at MCSs suggest that their regulation might be key to either generate a contact or fuse. In such scenario, we propose an important function for the Longin domain of Sec22b. In other words, could membrane contact correspond to a membrane docking with frustrated fusion? An evolutionary view of these processes would certainly solve this question.
BH wrote initial version and revised last version. NS and CV extensively rewrote and completed. NS designed the figures using BioRender. TG supervised and wrote final draft.
Work in our group was funded by grants from the French National Research Agency (MetDePaDi ANR-16-CE16-0012), the Institut National du Cancer (PLBIO 2018-149), the Fondation pour la Recherche Médicale (FRM), and Fondation Bettencourt-Schueller to TG. NS is recipient of a fellowship from the University de Paris – CD4Impact international program (ANR-18-IDEX-0001).
The authors declare that the research was conducted in the absence of any commercial or financial relationships that could be construed as a potential conflict of interest.
Alva, V., and Lupas, A. N. (2016). The TULIP superfamily of eukaryotic lipid-binding proteins as a mediator of lipid sensing and transport. Biochim. Biophys. Acta 1861, 913–923. doi: 10.1016/j.bbalip.2016.01.016
Amarilio, R., Ramachandran, S., Sabanay, H., and Lev, S. (2005). Differential regulation of endoplasmic reticulum structure through VAP-Nir interactions. J. Bio. Chem. 280, 5934–5944. doi: 10.1074/jbc.m409566200
Antonucci, D. E., Lim, S. T., Vassanelli, S., and Trimmer, J. S. (2001). Dynamic localization and clustering of dendritic Kv2.1 voltage-dependent potassium channels in developing hippocampal neurons. Neuroscience 108, 69–81. doi: 10.1016/s0306-4522(01)00476-476
Balla, T., Gulyas, G., Kim, Y. J., and Pemberton, J. (2020). Phosphoinositides and calcium signaling. a marriage arranged in er-pm contact sites. Curr. Opin. Physiol. 17, 149–157. doi: 10.1016/j.cophys.2020.08.007
Berna-Erro, A., Braun, A., Kraft, R., Kleinschnitz, C., Schuhmann, M. K., Stegner, D., et al. (2009). STIM2 regulates capacitive Ca2+ entry in neurons and plays a key role in hypoxic neuronal cell death. Sci. Signal. 2:ra67. doi: 10.1126/scisignal.2000522
Bernhard, W., and Rouiller, C. (1956). Close topographical relationship between mitochondria and ergastoplasm of liver cells in a definite phase of cellular activity. J. Biophys. Biochem. Cytol. 2, 73–78. doi: 10.1083/jcb.2.4.73
Besprozvannaya, M., Dickson, E., Li, H., Ginburg, K. S., Bers, D. M., Auwerx, J., et al. (2018). GRAM domain proteins specialize functionally distinct ER-PM contact sites in human cells. eLife 7:e31019. doi: 10.7554/eLife.31019
Bian, X., and De Camilli, P. (2019). In vitro assays to measure the membrane tethering and lipid transport activities of the extended synaptotagmins. Methods Mol. Biol. 1949, 201–212. doi: 10.1007/978-1-4939-9136-5_15
Bian, X., Saheki, Y., and De Camilli, P. (2018). Ca 2+ releases E-Syt1 autoinhibition to couple ER -plasma membrane tethering with lipid transport. EMBO J. 37, 219–234. doi: 10.15252/embj.201797359
Bohnert, M. (2020). Tether me, tether me not-dynamic organelle contact sites in metabolic rewiring. Dev. Cell 54, 212–225. doi: 10.1016/j.devcel.2020.06.026
Brooks, M. B., Catalfamo, J. L., MacNguyen, R., Tim, D., Fancher, S., and McCardle, J. A. (2015). A TMEM16F point mutation causes an absence of canine platelet TMEM16F and ineffective activation and death-induced phospholipid scrambling. J. Thromb. Haemost. 13, 2240–2252. doi: 10.1111/jth.13157
Bushell, S. R., Pike, A. C. W., Falzone, M. E., Rorsman, N. J. G., Ta, C. M., Corey, R. A., et al. (2019). The structural basis of lipid scrambling and inactivation in the endoplasmic reticulum scramblase TMEM16K. Nat. Commun. 10:3956. doi: 10.1038/s41467-019-11753-11751
Caputo, A., Caci, E., Ferrera, L., Pedemonte, N., Barsanti, C., Sondo, E., et al. (2008). TMEM16A, a membrane protein associated with calcium-dependent chloride channel activity. Science 322, 590–594. doi: 10.1126/science.1163518
Carrasco, S., and Meyer, T. (2011). STIM proteins and the endoplasmic reticulum-plasma membrane junctions. Annu. Rev. Biochem. 80, 973–1000. doi: 10.1146/annurev-biochem-061609-165311
Chang, C.-L., Hsieh, T.-S., Yang, T. T., Rothberg, K. G., Azizoglu, D. B., Volk, E., et al. (2013). Feedback regulation of receptor-induced Ca2+ signaling mediated by E-Syt1 and Nir2 at endoplasmic reticulum-plasma membrane junctions. Cell Rep. 5, 813–825. doi: 10.1016/j.celrep.2013.09.038
Chang, C.-L., and Liou, J. (2016). Homeostatic regulation of the PI(4,5)P2-Ca(2+) signaling system at ER-PM junctions. Biochim. Biophys. Acta 1861, 862–873. doi: 10.1016/j.bbalip.2016.02.015
Chung, J., Torta, F., Masai, K., Lucast, L., Czapla, H., Tanner, L. B., et al. (2015). Intracellular transport. PI4P/phosphatidylserine countertransport at ORP5- and ORP8-mediated ER-plasma membrane contacts. Science 349, 428–432. doi: 10.1126/science.aab1370
Clark, B. J. (2020). The START-domain proteins in intracellular lipid transport and beyond. Mol. Cell. Endocrinol. 54:110704. doi: 10.1016/j.mce.2020.110704
Collado, J., Kalemanov, M., Campelo, F., Bourgoint, C., Thomas, F., Loewith, R., et al. (2019). Tricalbin-Mediated contact sites control ER curvature to maintain plasma membrane integrity. Dev. Cell 51, 476–487.e7. doi: 10.1016/j.devcel.2019.10.018
D’Ambrosio, J. M., Albanèse, V., Lipp, N.-F., Fleuriot, L., Debayle, D., Drin, G., et al. (2020). Osh6 requires Ist2 for localization to ER-PM contacts and efficient phosphatidylserine transport in budding yeast. J. Cell Sci. 133:jcs243733. doi: 10.1242/jcs.243733
Daste, F., Galli, T., and Tareste, D. (2015). Structure and function of longin SNAREs. J. Cell Sci. 128, 4263–4272. doi: 10.1242/jcs.178574
Dickson, E. J., Jensen, J. B., Vivas, O., Kruse, M., Traynor-Kaplan, A. E., and Hille, B. (2016). Dynamic formation of ER-PM junctions presents a lipid phosphatase to regulate phosphoinositides. J. Cell Biol. 213, 33–48. doi: 10.1083/jcb.201508106
Dimmer, K. S., and Rapaport, D. (2017). Mitochondrial contact sites as platforms for phospholipid exchange. Biochim. Biophys. Acta Mol. Cell Biol. Lipids 1862, 69–80. doi: 10.1016/j.bbalip.2016.07.010
Du, J., Tao-Cheng, J. H., Zerfas, P., and McBain, C. J. (1998). The K+ channel, Kv2.1, is apposed to astrocytic processes and is associated with inhibitory postsynaptic membranes in hippocampal and cortical principal neurons and inhibitory interneurons. Neuroscience 84, 37–48. doi: 10.1016/s0306-4522(97)00519-518
Du, X., Zhou, L., Aw, Y. C., Mak, H. Y., Xu, Y., Rae, J., et al. (2020). ORP5 localizes to ER-lipid droplet contacts and regulates the level of PI(4)P on lipid droplets. J. Cell Biol. 219:e201905162. doi: 10.1083/jcb.201905162
Duran, C., Qu, Z., Osunkoya, A. O., Cui, Y., and Hartzell, H. C. (2012). ANOs 3-7 in the anoctamin/Tmem16 Cl- channel family are intracellular proteins. Am. J. Physiol. Cell Physiol. 302, C482–C493. doi: 10.1152/ajpcell.00140.2011
Eisenberg-Bord, M., Shai, N., Schuldiner, M., and Bohnert, M. (2016). A tether is a tether is a tether: tethering at membrane contact sites. Dev. Cell 39, 395–409. doi: 10.1016/j.devcel.2016.10.022
Elbaz, Y., and Schuldiner, M. (2011). Staying in touch: the molecular era of organelle contact sites. Trends Biochem. Sci. 36, 616–623. doi: 10.1016/j.tibs.2011.08.004
Ercan, E., Momburg, F., Engel, U., Temmerman, K., Nickel, W., and Seedorf, M. (2009). A conserved, lipid-mediated sorting mechanism of yeast Ist2 and mammalian STIM proteins to the peripheral ER. Traffic 10, 1802–1818. doi: 10.1111/j.1600-0854.2009.00995
Fernández-Busnadiego, R., Saheki, Y., and De Camilli, P. (2015). Three-dimensional architecture of extended synaptotagmin-mediated endoplasmic reticulum-plasma membrane contact sites. Proc. Natl. Acad. Sci. U S A. 112, E2004–E2013. doi: 10.1073/pnas.1503191112
Fili, O., Michaelevski, I., Bledi, Y., Chikvashvili, D., Singer-Lahat, D., Boshwitz, H., et al. (2001). Direct interaction of a brain voltage-gated K+ channel with syntaxin 1A: functional impact on channel gating. J. Neurosci. 21, 1964–1974. doi: 10.1523/jneurosci.21-06-01964.2001
Fischer, M. A., Temmerman, K., Ercan, E., Nickel, W., and Seedorf, M. (2009). Binding of plasma membrane lipids recruits the yeast integral membrane protein Ist2 to the cortical ER. Traffic 10, 1084–1097. doi: 10.1111/j.1600-0854.2009.00926.x
Forrest, S., Chai, A., Sanhueza, M., Marescotti, M., Parry, K., Georgiev, A., et al. (2013). Increased levels of phosphoinositides cause neurodegeneration in a Drosophila model of amyotrophic lateral sclerosis. Hum. Mol. Genet. 22, 2689–2704. doi: 10.1093/hmg/ddt118
Fox, P. D., Haberkorn, C. J., Akin, E. J., Seel, P. J., Krapf, D., and Tamkun, M. M. (2015). Induction of stable ER-plasma-membrane junctions by Kv2.1 potassium channels. J. Cell Sci. 128, 2096–2105. doi: 10.1242/jcs.166009
Galli, T., and Haucke, V. (2004). Cycling of synaptic vesicles: how far? How fast! Sci. STKE 2004:re19. doi: 10.1126/stke.2642004re19
Gallo, A., Danglot, L., Giordano, F., Hewlett, B., Binz, T., Vannier, C., et al. (2020). Role of the Sec22b-E-Syt complex in neurite growth and ramification. J. Cell Sci. 133:jcs247148. doi: 10.1242/jcs.247148
Gallo, A., Vannier, C., and Galli, T. (2016). Endoplasmic reticulum-plasma membrane associations:structures and functions. Annu. Rev. Cell Dev. Biol. 32, 279–301. doi: 10.1146/annurev-cellbio-111315-125024
Garbino, A., van Oort, R. J., Dixit, S. S., Landstrom, A. P., Ackerman, M. J., and Wehrens, X. H. T. (2009). Molecular evolution of the junctophilin gene family. Physiol. Genom. 37, 175–186. doi: 10.1152/physiolgenomics.00017.2009
Gatta, A. T., and Levine, T. P. (2017). Piecing together the patchwork of contact sites. Trends Cell Biol. 27, 214–229. doi: 10.1016/j.tcb.2016.08.010
Gatta, A. T., Wong, L. H., Sere, Y. Y., Calderón-Noreña, D. M., Cockcroft, S., Menon, A. K., et al. (2015). A new family of StART domain proteins at membrane contact sites has a role in ER-PM sterol transport. eLife 4:e07253. doi: 10.7554/eLife.07253
Giordano, F., Saheki, Y., Idevall-hagren, O., Colombo, S. F., Pirruccello, M., Milosevic, I., et al. (2013). PI(4,5)P2-Dependent and Ca2+-Regulated ER-PM interactions mediated by the extended synaptotagmins. Cell 153, 1494–1509. doi: 10.1016/j.cell.2013.05.026
Giorgi, C., Danese, A., Missiroli, S., Patergnani, S., and Pinton, P. (2018). Calcium dynamics as a machine for decoding signals. Trends Cell Biol. 28, 258–273. doi: 10.1016/j.tcb.2018.01.002
Gruszczynska-Biegala, J., Pomorski, P., Wisniewska, M. B., and Kuznicki, J. (2011). Differential roles for STIM1 and STIM2 in store-operated calcium entry in rat neurons. PLoS One 6:e19285. doi: 10.1371/journal.pone.0019285
Hartmann, J., Karl, R. M., Alexander, R. P. D., Adelsberger, H., Brill, M. S., Rühlmann, C., et al. (2014). STIM1 controls neuronal Ca2+ signaling, mGluR1-dependent synaptic transmission, and cerebellar motor behavior. Neuron 82, 635–644. doi: 10.1016/j.neuron.2014.03.027
Helle, S. C. J., Kanfer, G., Kolar, K., Lang, A., Michel, A. H., and Kornmann, B. (2013). Organization and function of membrane contact sites. Biochim. Biophys. Acta 1833, 2526–2541. doi: 10.1016/j.bbamcr.2013.01.028
Henkart, M., Landis, D. M., and Reese, T. S. (1976). Similarity of junctions between plasma membranes and endoplasmic reticulum in muscle and neurons. J. Cell Biol. 70, 338–347. doi: 10.1083/jcb.70.2.338
Henne, W. M., Zhu, L., Balogi, Z., Stefan, C., Pleiss, J. A., and Emr, S. D. (2015). Mdm1/Snx13 is a novel ER-endolysosomal interorganelle tethering protein. J. Cell Biol. 210, 541–551. doi: 10.1083/jcb.201503088
Herdman, C., and Moss, T. (2016). Extended-Synaptotagmins (E-Syts); the extended story. Pharmacol. Res. 107, 48–56. doi: 10.1016/j.phrs.2016.01.034
Hoffmann, P. C., Bharat, T. A. M., Wozny, M. R., Boulanger, J., Miller, E. A., and Kukulski, W. (2019). Tricalbins contribute to cellular lipid flux and form curved ER-PM contacts that are bridged by rod-shaped structures. Dev. Cell 51, 488–502.e8. doi: 10.1016/j.devcel.2019.09.019
Holmes, S. E., O’Hearn, E., Rosenblatt, A., Callahan, C., Hwang, H. S., Ingersoll-Ashworth, R. G., et al. (2001). A repeat expansion in the gene encoding junctophilin-3 is associated with Huntington disease-like 2. Nat. Genet. 29, 377–378. doi: 10.1038/ng760
Honsbein, A., Sokolovski, S., Grefen, C., Campanoni, P., Pratelli, R., Paneque, M., et al. (2009). A tripartite SNARE-K+ channel complex mediates in channel-dependent K+ nutrition in Arabidopsis. Plant Cell 21, 2859–2877. doi: 10.1105/tpc.109.066118
Horenkamp, F. A., Valverde, D. P., Nunnari, J., and Reinisch, K. M. (2018). Molecular basis for sterol transport by StART-like lipid transfer domains. EMBO J. 37:e98002. doi: 10.15252/embj.201798002
Idevall-Hagren, O., Lü, A., Xie, B., and De Camilli, P. (2015). Triggered Ca2+ influx is required for extended synaptotagmin 1-induced ER-plasma membrane tethering. EMBO J. 34, 2291–2305. doi: 10.15252/embj.201591565
Im, Y. J., Raychaudhuri, S., Prinz, W. A., and Hurley, J. H. (2005). Structural mechanism for sterol sensing and transport by OSBP-related proteins. Nature 437, 154–158. doi: 10.1038/nature03923
Irie, T., and Trussell, L. O. (2017). Double-Nanodomain coupling of calcium channels, ryanodine receptors, and BK channels controls the generation of burst firing. Neuron 96, 856–870.e4. doi: 10.1016/j.neuron.2017.10.014
Ito, K., Komazaki, S., Sasamoto, K., Yoshida, M., Nishi, M., Kitamura, K., et al. (2001). Deficiency of triad junction and contraction in mutant skeletal muscle lacking junctophilin type 1. J. Cell Biol. 154, 1059–1067. doi: 10.1083/jcb.200105040
Jentsch, J.-A., Kiburu, I., Pandey, K., Timme, M., Ramlall, T., Levkau, B., et al. (2018). Structural basis of sterol binding and transport by a yeast StARkin domain. J. Biol. Chem. 293, 5522–5531. doi: 10.1074/jbc.RA118.001881
Jing, J., Liu, G., Huang, Y., and Zhou, Y. (2020). A molecular toolbox for interrogation of membrane contact sites. J. Physiol. (Lond.) 598, 1725–1739. doi: 10.1113/JP277761
Johnson, B., Leek, A. N., and Tamkun, M. M. (2019). Kv2 channels create endoplasmic reticulum/plasma membrane junctions: a brief history of Kv2 channel subcellular localization. Channels 13, 88–101. doi: 10.1080/19336950.2019.1568824
Kagiwada, S., and Zen, R. (2003). Role of the yeast VAP homolog, Scs2p, in INO1 expression and phospholipid metabolism. J. Biochem. 133, 515–522. doi: 10.1093/jb/mvg068
Kakizawa, S., Moriguchi, S., Ikeda, A., Iino, M., and Takeshima, H. (2008). Functional crosstalk between cell-surface and intracellular channels mediated by junctophilins essential for neuronal functions. Cerebellum 7, 385–391. doi: 10.1007/s12311-008-0040-41
Kang, F., Zhou, M., Huang, X., Fan, J., Wei, L., Boulanger, J., et al. (2019). E-syt1 Re-arranges STIM1 Clusters to stabilize ring-shaped ER-PM contact sites and accelerate Ca2+ store replenishment. Sci. Rep. 9:3975. doi: 10.1038/s41598-019-40331-40330
Kaufmann, W. A., Ferraguti, F., Fukazawa, Y., Kasugai, Y., Shigemoto, R., Laake, P., et al. (2009). Large-conductance calcium-activated potassium channels in purkinje cell plasma membranes are clustered at sites of hypolemmal microdomains. J. Comp. Neurol. 515, 215–230. doi: 10.1002/cne.22066
Kikuma, K., Li, X., Kim, D., Sutter, D., and Dickman, D. K. (2017). Extended synaptotagmin localizes to presynaptic er and promotes neurotransmission and synaptic growth in Drosophila. Genetics 207, 993–1006. doi: 10.1534/genetics.117.300261
Kim, S., Leal, S. S., Ben Halevy, D., Gomes, C. M., and Lev, S. (2010). Structural requirements for VAP-B oligomerization and their implication in amyotrophic lateral sclerosis-associated VAP-B(P56S) neurotoxicity. J. Biol. Chem. 285, 13839–13849. doi: 10.1074/jbc.M109.097345
Kimura, T., Jia, J., Kumar, S., Choi, S. W., Gu, Y., Mudd, M., et al. (2017). Dedicated SNAREs and specialized TRIM cargo receptors mediate secretory autophagy. EMBO J. 36, 42–60. doi: 10.15252/embj.201695081
Kirmiz, M., Gillies, T. E., Dickson, E. J., and Trimmer, J. S. (2019). Neuronal ER-plasma membrane junctions organized by Kv2-VAP pairing recruit Nir proteins and affect phosphoinositide homeostasis. J. Biol. Chem. 294, 17735–17757. doi: 10.1074/jbc.RA119.007635
Kirmiz, M., Palacio, S., Thapa, P., King, A. N., Sack, J. T., and Trimmer, J. S. (2018). Remodeling neuronal ER-PM junctions is a conserved nonconducting function of Kv2 plasma membrane ion channels. Mol. Biol. Cell 29, 2410–2432. doi: 10.1091/mbc.E18-05-0337
Kopec, K. O., Alva, V., and Lupas, A. N. (2010). Homology of SMP domains to the TULIP superfamily of lipid-binding proteins provides a structural basis for lipid exchange between ER and mitochondria. Bioinformatics 26, 1927–1931. doi: 10.1093/bioinformatics/btq326
Landstrom, A. P., Beavers, D. L., and Wehrens, X. H. T. (2014). The junctophilin family of proteins: from bench to bedside. Trends Mol. Med. 20, 353–362. doi: 10.1016/j.molmed.2014.02.004
Lee, I., and Hong, W. (2006). Diverse membrane-associated proteins contain a novel SMP domain. FASEB J. 20, 202–206. doi: 10.1096/fj.05-4581hyp
Lees, J. A., Messa, M., Sun, E. W., Wheeler, H., Torta, F., Wenk, M. R., et al. (2017). Lipid transport by TMEM24 at ER-plasma membrane contacts regulates pulsatile insulin secretion. Science 355:eaah6171. doi: 10.1126/science.aah6171
Lees, J. A., and Reinisch, K. M. (2020). Inter-organelle lipid transfer: a channel model for Vps13 and chorein-N motif proteins. Curr. Opin. Cell Biol. 65, 66–71. doi: 10.1016/j.ceb.2020.02.008
Lehto, M., Laitinen, S., Chinetti, G., Johansson, M., Ehnholm, C., Staels, B., et al. (2001). The OSBP-related protein family in humans. J. Lipid Res. 42, 1203–1213. doi: 10.1016/s0022-2275(20)31570-4
Leung, Y. M., Kang, Y., Gao, X., Xia, F., Xie, H., Sheu, L., et al. (2003). Syntaxin 1A binds to the cytoplasmic C terminus of Kv2.1 to regulate channel gating and trafficking. J. Biol. Chem. 278, 17532–17538. doi: 10.1074/jbc.M213088200
Lim, S. T., Antonucci, D. E., Scannevin, R. H., and Trimmer, J. S. (2000). A novel targeting signal for proximal clustering of the Kv2.1 K+ channel in hippocampal neurons. Neuron 25, 385–397. doi: 10.1016/s0896-6273(00)80902-80902
Loewen, C. J. R., Roy, A., and Levine, T. P. (2003). A conserved ER targeting motif in three families of lipid binding proteins and in Opi1p binds VAP. EMBO J. 22, 2025–2035. doi: 10.1093/emboj/cdg201
Luik, R. M., Wang, B., Prakriya, M., Wu, M. M., and Lewis, R. S. (2008). Oligomerization of STIM1 couples ER calcium depletion to CRAC channel activation. Nature 454, 538–542. doi: 10.1038/nature07065
Maass, K., Fischer, M. A., Seiler, M., Temmerman, K., Nickel, W., and Seedorf, M. (2009). A signal comprising a basic cluster and an amphipathic alpha-helix interacts with lipids and is required for the transport of Ist2 to the yeast cortical ER. J. Cell Sci. 122, 625–635. doi: 10.1242/jcs.036012
MacDonald, P. E., Wang, G., Tsuk, S., Dodo, C., Kang, Y., Tang, L., et al. (2002). Synaptosome-associated protein of 25 kilodaltons modulates Kv2.1 voltage-dependent K(+) channels in neuroendocrine islet beta-cells through an interaction with the channel N terminus. Mol. Endocrinol. 16, 2452–2461. doi: 10.1210/me.2002-2058
Maeda, S., Otomo, C., and Otomo, T. (2019). The autophagic membrane tether ATG2A transfers lipids between membranes. eLife 8:e45777. doi: 10.7554/eLife.45777
Maléth, J., Choi, S., Muallem, S., and Ahuja, M. (2014). Translocation between PI(4,5)P2-poor and PI(4,5)P2-rich microdomains during store depletion determines STIM1 conformation and Orai1 gating. Nat. Commun. 5:5843. doi: 10.1038/ncomms6843
Mandikian, D., Bocksteins, E., Parajuli, L. K., Bishop, H. I., Cerda, O., Shigemoto, R., et al. (2014). Cell type-specific spatial and functional coupling between mammalian brain Kv2.1 K+ channels and ryanodine receptors. J. Comp. Neurol. 522, 3555–3574. doi: 10.1002/cne.23641
Manford, A. G., Stefan, C. J., Yuan, H. L., Macgurn, J. A., and Emr, S. D. (2012). ER-to-plasma membrane tethering proteins regulate cell signaling and ER morphology. Dev. Cell 23, 1129–1140. doi: 10.1016/j.devcel.2012.11.004
Miao, Y., Miner, C., Zhang, L., Hanson, P. I., Dani, A., and Vig, M. (2013). An essential and NSF independent role for α-SNAP in store-operated calcium entry. eLife 2:e00802. doi: 10.7554/eLife.00802
Michell, R. H. (1975). Inositol phospholipids and cell surface receptor function. Biochim. Biophys. Acta 415, 81–47. doi: 10.1016/0304-4157(75)90017-90019
Milenkovic, V. M., Brockmann, M., Stöhr, H., Weber, B. H., and Strauss, O. (2010). Evolution and functional divergence of the anoctamin family of membrane proteins. BMC Evol. Biol. 10:319. doi: 10.1186/1471-2148-10-319
Mochizuki, S., Miki, H., Zhou, R., Kido, Y., Nishimura, W., Kikuchi, M., et al. (2018). Oxysterol-binding protein-related protein (ORP) 6 localizes to the ER and ER-plasma membrane contact sites and is involved in the turnover of PI4P in cerebellar granule neurons. Exp. Cell Res. 370, 601–612. doi: 10.1016/j.yexcr.2018.07.025
Moriguchi, S., Nishi, M., Komazaki, S., Sakagami, H., Miyazaki, T., Masumiya, H., et al. (2006). Functional uncoupling between Ca2+ release and afterhyperpolarization in mutant hippocampal neurons lacking junctophilins. Proc. Natl. Acad. Sci. U S A. 103, 10811–10816. doi: 10.1073/pnas.0509863103
Murphy, S. E., and Levine, T. P. (2016). VAP, a versatile access point for the endoplasmic reticulum: review and analysis of FFAT-like motifs in the VAPome. Biochim. Biophys. Acta 1861, 952–961. doi: 10.1016/j.bbalip.2016.02.009
Naito, T., Ercan, B., Krshnan, L., Triebl, A., Koh, D. H. Z., Wei, F. Y., et al. (2019). Movement of accessible plasma membrane cholesterol by GRAMD1 lipid transfer protein complex. eLife 8:e51401. doi: 10.7554/eLife.51401
Nakada, T., Kashihara, T., Komatsu, M., Kojima, K., Takeshita, T., and Yamada, M. (2018). Physical interaction of junctophilin and the CaV1.1 C terminus is crucial for skeletal muscle contraction. Proc. Natl. Acad. Sci. U S A. 115, 4507–4512. doi: 10.1073/pnas.1716649115
Nascimbeni, A. C., Giordano, F., Dupont, N., Grasso, D., Vaccaro, M. I., Codogno, P., et al. (2017). ER-plasma membrane contact sites contribute to autophagosome biogenesis by regulation of local PI3P synthesis. EMBO J. 36, 2018–2033. doi: 10.15252/embj.201797006
Nath, V. R., Mishra, S., Basak, B., Trivedi, D., and Raghu, P. (2020). Extended synaptotagmin regulates membrane contact site structure and lipid transfer function in vivo. EMBO Rep. 21:e50264. doi: 10.15252/embr.202050264
Nishi, M., Mizushima, A., Nakagawara, K. I., and Takeshima, H. (2000). Characterization of human junctophilin subtype genes. Biochem. Biophys. Res. Commun. 273, 920–927. doi: 10.1006/bbrc.2000.3011
Nishi, M., Sakagami, H., Komazaki, S., Kondo, H., and Takeshima, H. (2003). Coexpression of junctophilin type 3 and type 4 in brain. Brain Res. Mol. Brain Res. 118, 102–110. doi: 10.1016/s0169-328x(03)00341-343
Nishimura, Y., Hayashi, M., Inada, H., and Tanaka, T. (1999). Molecular cloning and characterization of mammalian homologues of vesicle-associated membrane protein-associated (VAMP-associated) proteins. Biochem. Biophys. Res. Commun. 254, 21–26. doi: 10.1006/bbrc.1998.9876
Nixon-Abell, J., Obara, C. J., Weigel, A. V., Li, D., Legant, W. R., Xu, C. S., et al. (2016). Increased spatiotemporal resolution reveals highly dynamic dense tubular matrices in the peripheral ER. Science 354:aaf3928. doi: 10.1126/science.aaf3928
Omnus, D. J., Manford, A. G., Bader, J. M., Emr, S. D., and Stefan, C. J. (2016). Phosphoinositide kinase signaling controls ER-PM cross-talk. Mol. Biol. Cell 27, 1170–1180. doi: 10.1091/mbc.E16-01-0002
Orci, L., Ravazzola, M., Le Coadic, M., Shen, W.-W., Demaurex, N., and Cosson, P. (2009). From the cover: STIM1-induced precortical and cortical subdomains of the endoplasmic reticulum. Proc. Natl. Acad. Sci. U S A. 106, 19358–19362. doi: 10.1073/pnas.0911280106
Pedemonte, N., and Galietta, L. J. V. (2014). Structure and function of tmem16 proteins (anoctamins). Physiol. Rev. 94, 419–459. doi: 10.1152/physrev.00039.2011
Peretti, D., Dahan, N., Shimoni, E., Hirschberg, K., and Lev, S. (2008). Coordinated lipid transfer between the endoplasmic reticulum and the golgi complex requires the VAP proteins and is essential for Golgi-mediated transport. Mol. Biol. Cell 19, 3871–3884. doi: 10.1091/mbc.E08-05-0498
Petkovic, M., Jemaiel, A., Daste, F., Specht, C. G., Izeddin, I., Vorkel, D., et al. (2014). The SNARE Sec22b has a non-fusogenic function in plasma membrane expansion. Nat. Cell Biol. 16, 434–444. doi: 10.1038/ncb2937
Petkovic, M., Oses-Prieto, J., Burlingame, A., Jan, L. Y., and Jan, Y. N. (2020). TMEM16K is an interorganelle regulator of endosomal sorting. Nat. Commun. 11, 3298. doi: 10.1038/s41467-020-17016-17018
Phillips, M. J., and Voeltz, G. K. (2016). Structure and function of ER membrane contact sites with other organelles. Nat. Rev. Mol. Cell Biol. 17, 69–82. doi: 10.1038/nrm.2015.8
Picollo, A., Malvezzi, M., and Accardi, A. (2015). TMEM16 proteins: unknown structure and confusing functions. J. Mol. Biol. 427, 94–105. doi: 10.1016/j.jmb.2014.09.028
Popugaeva, E., and Bezprozvanny, I. (2014). Can the calcium hypothesis explain synaptic loss in Alzheimer’s disease? Neurodegener. Dis. 13, 139–141. doi: 10.1159/000354778
Porter, K. R., and Palade, G. E. (1957). Studies on the endoplasmic reticulum. III. Its form and distribution in striated muscle cells. J. Biophys. Biochem. Cytol. 3, 269–300.
Prinz, W. A. (2014). Bridging the gap: membrane contact sites in signaling, metabolism, and organelle dynamics. J. Cell Biol. 205, 759–769. doi: 10.1083/jcb.201401126
Rosenbluth, J. (1962). Subsurface cisterns and their relationship to the neuronal plasma membrane. J. Cell Biol. 13, 405–421. doi: 10.1083/jcb.13.3.405
Rossi, D., Scarcella, A. M., Liguori, E., Lorenzini, S., Pierantozzi, E., Kutchukian, C., et al. (2019). Molecular determinants of homo- and heteromeric interactions of Junctophilin-1 at triads in adult skeletal muscle fibers. Proc. Natl. Acad. Sci. U S A. 116, 15716–15724. doi: 10.1073/pnas.1820980116
Rubinsztein, D. C., Shpilka, T., and Elazar, Z. (2012). Mechanisms of autophagosome biogenesis. Curr. Biol. 22, R29–R34. doi: 10.1016/j.cub.2011.11.034
Saheki, Y., Bian, X., Schauder, C. M., Sawaki, Y., Surma, M. A., Klose, C., et al. (2016). Control of plasma membrane lipid homeostasis by the extended synaptotagmins. Nat. Cell Biol. 18, 504–515. doi: 10.1038/ncb3339
Saheki, Y., and De Camilli, P. (2017). The Extended-Synaptotagmins. Biochim. et Biophys. Acta - Mol. Cell Res. 1864, 1490–1493. doi: 10.1016/j.bbamcr.2017.03.013
Sahu, G., Wazen, R.-M., Colarusso, P., Chen, S. R. W., Zamponi, G. W., and Turner, R. W. (2019). Junctophilin proteins tether a Cav1-RyR2-KCa3.1 tripartite complex to regulate neuronal excitability. Cell Rep. 28, 2427–2442.e6. doi: 10.1016/j.celrep.2019.07.075
Sandhu, J., Li, S., Fairall, L., Pfisterer, S. G., Gurnett, J. E., Xiao, X., et al. (2018). Aster proteins facilitate nonvesicular plasma membrane to ER cholesterol transport in mammalian cells. Cell 175, 514–529.e20. doi: 10.1016/j.cell.2018.08.033
Sawa-Makarska, J., Baumann, V., Coudevylle, N., von Bülow, S., Nogellova, V., Abert, C., et al. (2020). Reconstitution of autophagosome nucleation defines Atg9 vesicles as seeds for membrane formation. Science 369:eaaz7714. doi: 10.1126/science.aaz7714
Schauder, C. M., Wu, X., Saheki, Y., Narayanaswamy, P., Torta, F., Wenk, M. R., et al. (2014). Structure of a lipid-bound extended synaptotagmin indicates a role in lipid transfer. Nature 510, 552–555. doi: 10.1038/nature13269
Scholze, M. J., Barbieux, K. S., De Simone, A., Boumasmoud, M., Süess, C. C. N., Wang, R., et al. (2018). PI(4,5)P2 forms dynamic cortical structures and directs actin distribution as well as polarity in Caenorhabditis elegans embryos. Development 145:dev164988. doi: 10.1242/dev.164988
Schreiber, R., Uliyakina, I., Kongsuphol, P., Warth, R., Mirza, M., Martins, J. R., et al. (2010). Expression and function of epithelial anoctamins. J. Biol. Chem. 285, 7838–7845. doi: 10.1074/jbc.M109.065367
Schulien, A. J., Yeh, C., Orange, B. N., Pav, O. J., Hopkins, M. P., Moutal, A., et al. (2020). Targeted disruption of Kv2. 1-VAPA association provides neuroprotection against ischemic stroke in mice by declustering Kv2. 1 channels. Cell. Neurosci. 6:eaaz8110. doi: 10.1126/sciadv.aaz8110
Schulz, T. A., and Creutz, C. E. (2004). The tricalbin C2 domains: lipid-binding properties of a novel, synaptotagmin-like yeast protein family. Biochemistry 43, 3987–3995. doi: 10.1021/bi036082w
Sclip, A., Bacaj, T., Giam, L. R., and Südhof, T. C. (2016). Extended Synaptotagmin (ESyt) triple knock-out mice are viable and fertile without obvious endoplasmic reticulum dysfunction. PLoS One 11:e0158295. doi: 10.1371/journal.pone.0158295
Scorrano, L., De Matteis, M. A., Emr, S., Giordano, F., Hajnóczky, G., Kornmann, B., et al. (2019). Coming together to define membrane contact sites. Nat. Commun. 10:1287. doi: 10.1038/s41467-019-09253-9253
Seixas, A. I., Holmes, S. E., Takeshima, H., Pavlovich, A., Sachs, N., Pruitt, J. L., et al. (2012). Loss of junctophilin-3 contributes to Huntington disease-like 2 pathogenesis. Ann. Neurol. 71, 245–257. doi: 10.1002/ana.22598
Siao, W., Wang, P., Voigt, B., Hussey, P. J., and Baluska, F. (2016). Arabidopsis SYT1 maintains stability of cortical endoplasmic reticulum networks and VAP27-1-enriched endoplasmic reticulum-plasma membrane contact sites. J. Exp. Bot. 67, 6161–6171. doi: 10.1093/jxb/erw381
Singh, N. P., Vannier, C., and Galli, T. (2020). SNAP iN, SNAP oUT—SNAREs at ER-PM contact sites. Contact 3:251525642097958. doi: 10.1177/2515256420979586
Skehel, P. A., Martin, K. C., Kandel, E. R., and Bartsch, D. (1995). A VAMP-binding protein from Aplysia required for neurotransmitter release. Science 269, 1580–1583. doi: 10.1126/science.7667638
Soussan, L., Burakov, D., Daniels, M. P., Toister-Achituv, M., Porat, A., Yarden, Y., et al. (1999). ERG30, a VAP-33-related protein, functions in protein transport mediated by COPI vesicles. J. Cell Biol. 146, 301–311. doi: 10.1083/jcb.146.2.301
Spacek, J., and Harris, K. M. (1997). Three-dimensional organization of smooth endoplasmic reticulum in hippocampal CA1 dendrites and dendritic spines of the immature and mature rat. J. Neurosci. 17, 190–203. doi: 10.1523/jneurosci.17-01-00190.1997
Stefan, C. J. (2018). Building ER-PM contacts: keeping calm and ready on alarm. Curr. Opin. Cell Biol. 53, 1–8. doi: 10.1016/j.ceb.2018.03.008
Stefan, C. J. (2020). Endoplasmic reticulum-plasma membrane contacts: principals of phosphoinositide and calcium signaling. Curr. Opin. Cell Biol. 63, 125–134. doi: 10.1016/j.ceb.2020.01.010
Stefan, C. J., Trimble, W. S., Grinstein, S., Drin, G., Reinisch, K., De Camilli, P., et al. (2017). Membrane dynamics and organelle biogenesis-lipid pipelines and vesicular carriers. BMC Biol. 15:102. doi: 10.1186/s12915-017-0432-430
Sun, E. W., and De Camilli, P. (2018). Kv2 potassium channels meet VAP. Proc. Natl. Acad. Sci. U S A. 115, 7849–7851. doi: 10.1073/pnas.1810059115
Sun, E. W., Guillén-Samander, A., Bian, X., Wu, Y., Cai, Y., Messa, M., et al. (2019). Lipid transporter TMEM24/C2CD2L is a Ca2+-regulated component of ER-plasma membrane contacts in mammalian neurons. Proc. Natl. Acad. Sci. U S A. 116, 5775–5784. doi: 10.1073/pnas.1820156116
Takeshima, H., Hoshijima, M., and Song, L.-S. (2015). Ca2+ microdomains organized by junctophilins. Cell Calcium 58, 349–356. doi: 10.1016/j.ceca.2015.01.007
Takeshima, H., Komazaki, S., Nishi, M., Iino, M., and Kangawa, K. (2000). Junctophilins: a novel family of junctional membrane complex proteins. Mol. Cell 6, 11–22. doi: 10.1016/s1097-2765(00)00003-4
Tavassoli, S., Chao, J. T., Young, B. P., Cox, R. C., Prinz, W. A., de Kroon, A. I. P. M., et al. (2013). Plasma membrane–endoplasmic reticulum contact sites regulate phosphatidylcholine synthesis. EMBO Rep. 14, 434–440. doi: 10.1038/embor.2013.36
Tilokani, L., Nagashima, S., Paupe, V., and Prudent, J. (2018). Mitochondrial dynamics: overview of molecular mechanisms. Essays Biochem. 62, 341–360. doi: 10.1042/EBC20170104
Tong, J., Manik, M. K., and Im, Y. J. (2018). Structural basis of sterol recognition and nonvesicular transport by lipid transfer proteins anchored at membrane contact sites. Proc. Natl. Acad. Sci. U S A. 115, E856–E865. doi: 10.1073/pnas.1719709115
Toulmay, A., and Prinz, W. A. (2012). A conserved membrane-binding domain targets proteins to organelle contact sites. J. Cell Sci. 125, 49–58. doi: 10.1242/jcs.085118
Tremblay, M. G., Herdman, C., Guillou, F., Mishra, P. K., Baril, J., Bellenfant, S., et al. (2015). Extended synaptotagmin interaction with the fibroblast growth factor receptor depends on receptor conformation, not catalytic activity. J. Biol. Chem. 290, 16142–16156. doi: 10.1074/jbc.M115.656918
Vance, J. E. (1990). Phospholipid synthesis in a membrane fraction associated with mitochondria. J. Biol. Chem. 265, 7248–7256. doi: 10.1016/s0021-9258(19)39106-9
Wang, J.-Z., and Dehesh, K. (2018). ER: the silk road of interorganellar communication. Curr. Opin. Plant Biol. 45, 171–177. doi: 10.1016/j.pbi.2018.07.012
Wang, Y., Li, L., Hou, C., Lai, Y., Long, J., Liu, J., et al. (2016). SNARE-mediated membrane fusion in autophagy. Semin. Cell Dev. Biol. 60, 97–104. doi: 10.1016/j.semcdb.2016.07.009
Weber-Boyvat, M., Kentala, H., Peränen, J., and Olkkonen, V. M. (2015). Ligand-dependent localization and function of ORP-VAP complexes at membrane contact sites. Cell Mol. Life Sci. 72, 1967–1987. doi: 10.1007/s00018-014-1786-x
Weber-Boyvat, M., Trimbuch, T., Shah, S., Jäntti, J., Olkkonen, V. M., and Rosenmund, C. (2020). ORP/Osh mediate cross-talk between ER-plasma membrane contact site components and plasma membrane SNAREs. Cell Mol. Life Sci. doi: 10.1007/s00018-020-03604-w Online ahead of print.
Weir, M. L., Xie, H., Klip, A., and Trimble, W. S. (2001). VAP-A binds promiscuously to both v- and tSNAREs. Biochem. Biophys. Res. Commun. 286, 616–621. doi: 10.1006/bbrc.2001.5437
West, M., Zurek, N., Hoenger, A., and Voeltz, G. K. (2011). A 3D analysis of yeast ER structure reveals how ER domains are organized by membrane curvature. J. Cell Biol. 193, 333–346. doi: 10.1083/jcb.201011039
Williams, A. L., Ehm, S., Jacobson, N. C., Xu, D., and Hay, J. C. (2004). rsly1 binding to syntaxin 5 is required for endoplasmic reticulum-to-golgi transport but does not promote SNARE motif accessibility. Mol. Biol. Cell. 15, 162–175. doi: 10.1091/mbc.E03-07-0535
Wolf, W., Kilic, A., Schrul, B., Lorenz, H., Schwappach, B., and Seedorf, M. (2012). Yeast Ist2 recruits the endoplasmic reticulum to the plasma membrane and creates a ribosome-free membrane microcompartment. PLoS One 7:e39703. doi: 10.1371/journal.pone.0039703
Wong, L. H., Gatta, A. T., and Levine, T. P. (2019). Lipid transfer proteins: the lipid commute via shuttles, bridges and tubes. Nat. Rev. Mol. Cell Biol. 20, 85–101. doi: 10.1038/s41580-018-0071-75
Woo, J. S., Srikanth, S., Nishi, M., Ping, P., Takeshima, H., and Gwack, Y. (2016). Junctophilin-4, a component of the endoplasmic reticulum-plasma membrane junctions, regulates Ca2+ dynamics in T cells. Proc. Natl. Acad. Sci. U S A. 113, 2762–2767. doi: 10.1073/pnas.1524229113
Wu, M. M., Buchanan, J., Luik, R. M., and Lewis, R. S. (2006). Ca2+ store depletion causes STIM1 to accumulate in ER regions closely associated with the plasma membrane. J. Cell Biol. 174, 803–813. doi: 10.1083/jcb.200604014
Wu, Y., Whiteus, C., Xu, C. S., Hayworth, K. J., Weinberg, R. J., Hess, H. F., et al. (2017). Contacts between the endoplasmic reticulum and other membranes in neurons. Proc. Natl. Acad. Sci. U S A. 114, E4859–E4867. doi: 10.1073/pnas.1701078114
Yamanaka, T., Nishiyama, R., Shimogori, T., and Nukina, N. (2020). Proteomics-Based approach identifies altered ER domain properties by ALS-linked VAPB mutation. Sci. Rep. 10:7610. doi: 10.1038/s41598-020-64517-z
Yang, Y. D., Cho, H., Koo, J. Y., Tak, M. H., Cho, Y., Shim, W.-S., et al. (2008). TMEM16A confers receptor-activated calcium-dependent chloride conductance. Nature 455, 1210–1215. doi: 10.1038/nature07313
Yu, H., Liu, Y., Gulbranson, D. R., Paine, A., Rathore, S. S., and Shen, J. (2016). Extended synaptotagmins are Ca2+-dependent lipid transfer proteins at membrane contact sites. Proc. Natl. Acad. Sci. U S A. 113, 4362–4367. doi: 10.1073/pnas.1517259113
Keywords: membrane contact sites, tethers, lipid transfer, SNAREs, neurons
Citation: Hewlett B, Singh NP, Vannier C and Galli T (2021) ER-PM Contact Sites – SNARING Actors in Emerging Functions. Front. Cell Dev. Biol. 9:635518. doi: 10.3389/fcell.2021.635518
Received: 30 November 2020; Accepted: 21 January 2021;
Published: 11 February 2021.
Edited by:
Yasunori Saheki, Lee Kong Chian School of Medicine, Nanyang Technological University, SingaporeReviewed by:
Raghu Padinjat, National Centre for Biological Sciences, IndiaCopyright © 2021 Hewlett, Singh, Vannier and Galli. This is an open-access article distributed under the terms of the Creative Commons Attribution License (CC BY). The use, distribution or reproduction in other forums is permitted, provided the original author(s) and the copyright owner(s) are credited and that the original publication in this journal is cited, in accordance with accepted academic practice. No use, distribution or reproduction is permitted which does not comply with these terms.
*Correspondence: Thierry Galli, dGhpZXJyeS5nYWxsaUBpbnNlcm0uZnI=
†Present address: Bailey Hewlett, MRC-LMCB, University College London, London, United Kingdom
Disclaimer: All claims expressed in this article are solely those of the authors and do not necessarily represent those of their affiliated organizations, or those of the publisher, the editors and the reviewers. Any product that may be evaluated in this article or claim that may be made by its manufacturer is not guaranteed or endorsed by the publisher.
Research integrity at Frontiers
Learn more about the work of our research integrity team to safeguard the quality of each article we publish.