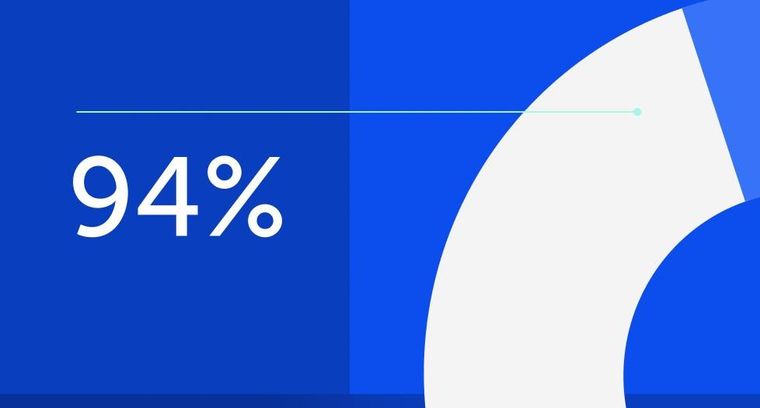
94% of researchers rate our articles as excellent or good
Learn more about the work of our research integrity team to safeguard the quality of each article we publish.
Find out more
REVIEW article
Front. Cell Dev. Biol., 09 February 2021
Sec. Stem Cell Research
Volume 9 - 2021 | https://doi.org/10.3389/fcell.2021.627429
This article is part of the Research TopicMechanisms of Cellular Differentiation, Organ Development, and Novel Model SystemsView all 25 articles
Mesenchymal stem cells (MSCs) give rise to adipocytes, osteocytes, and chondrocytes and reside in various tissues, including bone marrow and adipose tissue. The differentiation choices of MSCs are controlled by several signaling pathways, including the Wnt/β-catenin signaling. When MSCs undergo adipogenesis, they first differentiate into preadipocytes, a proliferative adipocyte precursor cell, after which they undergo terminal differentiation into mature adipocytes. These two steps are controlled by the Wnt/β-catenin pathway, in such a way that when signaling is abrogated, the next step in adipocyte differentiation can start. This sequence suggests that the main role of Wnt/β-catenin signaling is to suppress differentiation while increasing MSC and preadipocytes cell mass. During later steps of MSC differentiation, however, active Wnt signaling can promote osteogenesis instead of keeping the MSCs undifferentiated and proliferative. The exact mechanisms behind the various functions of Wnt signaling remain elusive, although recent research has revealed that during lineage commitment of MSCs into preadipocytes, Wnt signaling is inactivated by endogenous Wnt inhibitors. In part, this process is regulated by histone-modifying enzymes, which can lead to increased or decreased Wnt gene expression. The role of Wnt in adipogenesis, as well as in osteogenesis, has implications for metabolic diseases since Wnt signaling may serve as a therapeutic target.
Decades of research has established a main role of Wnt signaling in stem cell fate and control (Nusse, 2008; Van Camp et al., 2014). For example, Wnt/β-catenin signaling is among the main cell communication pathways in the intestinal stem cell niche in the crypts (Gehart and Clevers, 2019). Wnt signaling is also involved in differentiation and lineage fate decisions, as evinced in embryonic hematopoietic stem cell development (Richter et al., 2017). In mesenchymal stem cell (MSC) biology, activation of the β-catenin-dependent canonical Wnt pathway is essential to induce osteogenic differentiation (Kang et al., 2007). Over recent years, it has become clear that Wnt signaling also plays a major role in the process of adipogenesis, the differentiation of MSCs into mature fat cells, or adipocytes. Studies from Ross et al. and Bennet et al. demonstrated for the first time that autocrine Wnt expression suppresses terminal differentiation into mature adipocytes from the precursor preadipocytes (Ross et al., 2000; Bennett et al., 2002). Moreover, Wnt is also involved during the first stages of adipogenesis, when adipocyte commitment is at the expense of osteogenesis, the formation of bone cells (Han et al., 2019).
Adipose tissue is a potential source of easily accessible MSCs (Tsuji et al., 2014). Applications include the development of replacement therapies for bone, cartilage, and adipose tissues (Lazar et al., 2018). Likewise, MSCs are also used to treat other diseases unrelated to connective tissue dysfunction, due to their signaling and inflammatory mediating properties (Alfaro et al., 2011). Wnt effectors may be used as pharmaceutical targets for the treatment of Wnt-related metabolic diseases such as obesity, type 2 diabetes (T2D), and osteoporosis (Baron and Gori, 2018; Chen et al., 2018). A better understanding of the signaling routes during stem cell maintenance and differentiation is essential for utilization in tissue engineering and safe applications in patients. In this review, we aim at clarifying the role of Wnt signaling from MSCs until adipogenesis.
MSCs are multipotent adult stem cells and are the progenitors of many connective tissue types, including bone, fat, cartilage, and muscle. Thus, MSCs have the ability to differentiate into adipocytes, osteocytes, chondrocytes, as well as myocytes and fibroblasts (Figure 1). The cells can be found in many adult tissues, including bone marrow (BM), and adipose tissue (Alfaro et al., 2011). MSCs cell populations are quite heterogeneous but they can be distinguished from each other by a set of positive and negative surface markers. The markers that multipotent MSCs typically express are CD13, CD29, CD44, CD63, CD73, CD90, and CD105 and they are negative for hematopoietic antigens CD14, CD31, and CD45 (Alfaro et al., 2011; Tsuji et al., 2014). MSCs are identified as having the ability to differentiate into bone, cartilage, and fat lineages both in vitro and in vivo. Primary MSCs can be isolated from tissues and can be cultured in vitro. Typically, the multipotency of primary isolated MSCs is tested by differentiation into the individual descendants, confirmed by Oil Red-O staining of neutral lipids for adipocytes, staining for mineralization for osteocytes, and staining for glycosaminoglycans for chondrocytes (Alfaro et al., 2011). After a number of passages cultured MSCs generally become senescent (Bork et al., 2010). Consequently, permanent MSC lines like the murine C3H10T1/2, are used experimentally (Tang et al., 2004). The heterogeneous nature of MSCs is also true for resident populations in tissues: while adipose-residing cells possess multi-lineage potential, they have greater adipogenic potential (Tsuji et al., 2014). Likewise, BM-MSCs are more biased to differentiate into an osteogenic lineage, in addition to forming BM-adipocytes (Tsuji et al., 2014; Bukowska et al., 2018).
Figure 1. Differentiation lineage potential of MSCs into bone (osteogenesis), adipose tissue (adipogenesis), cartilage (chondrogenesis), and muscle (myogenesis).
The Wnt signaling pathways are a collective of signaling pathways activated by a family of Wnt proteins. The most commonly implicated Wnt pathway is through β-catenin, also termed the canonical Wnt pathway. Non-canonical systems include the planar cell polarity (PCP) and Wnt/Calcium pathways. Wnt signaling plays important roles during embryonic development as well as in adults (Nusse, 2008). When Wnt is not present, β-catenin, localized in the cytoplasm, is continuously degraded through the action of the “destruction complex.” This multiprotein complex consisting of scaffold protein axis inhibition protein (Axin), adenomatous polyposis coli (APC), casein kinase 1 (CK1), and glycogen synthase kinase 3 (GSK3), phosphorylates the N-terminus of β-catenin which is then recognized by β-Trcp, an E3 ubiquitin ligase subunit, followed by ubiquitination and subsequent proteasome degradation. Destruction of β-catenin prevents its nuclear localization. The signaling cascade of the Wnt pathway begins when one of the Wnt ligands binds to the receptor Frizzled (Fz) and its co-receptor low-density lipoprotein receptor-related protein 5 and 6 (LRP5/6). Once bound, this can recruit the scaffolding protein Disheveled (Dvl) to the receptor complex and after phosphorylation of LRP5/6, this recruits the destruction complex to the receptors, inhibiting the β-catenin phosphorylating activity. If β-catenin is active and stable, it translocates to the nucleus where it forms complexes with co-activators, such as transcription factor family DNA-bound T cell factor/lymphoid enhancer factor (TCF/LEF) (MacDonald et al., 2009). This transcription factor complex starts the expression of downstream targets genes, including Axin2, a Wnt signaling repressor (Leung et al., 2002). Several mechanisms are involved in endogenous inhibition of Wnt signaling.
Secreted Wnt inhibitors factors include Wnt inhibitor protein (WIF) and secreted Frizzled-related proteins (sFRPs). Dickkopf (DKK) is a secreted factor that binds and inhibits the LRP5/6 co-receptors (MacDonald et al., 2009). Other endogenous inhibitors, such as Axin2, act downstream of the Wnt signaling pathway (Leung et al., 2002).
Adipose tissues fall into two distinct groups: the more common white adipose tissue (WAT; e.g., subcutaneous WAT and visceral WAT) and brown adipose tissue (BAT). These are both derived from adipose-residing MSCs and are generated in a stepwise differentiation program. MSCs first differentiate into an intermediate cell type called preadipocytes, during which the cells undergo growth arrest and mitotic clonal expansion (Lazar et al., 2018). Preadipocytes are proliferative, are presumably located near the vasculature in adipose tissues (Tsuji et al., 2014; Zhang et al., 2018) and have fibroblast-like morphology. Preadipocytes express the adipogenic transcription factors CCAAT-enhancer-binding protein β and δ (C/EBPβ/δ) and low but detectable levels of Peroxisome proliferator-activated receptor γ (PPARγ) (Park et al., 2004). In preadipocytes C/EBPβ is present in an inactive state and unable to start transcription of genes that initiate terminal differentiation (Park et al., 2004). PPARγ is an indispensable master regulator of the adipocyte transcriptional program. PPARγ expression is also required during the maintenance of mature adipocytes (Lee and Ge, 2014).
Terminal differentiation of preadipocytes into mature adipocytes can be triggered by pro-adipogenic signals (e.g., insulin; dexamethasone; 3-isobutyl-1-methylxanthine, IBMX; or bone morphogenetic proteins, BMPs), resulting in the upregulation of the adipogenic transcription factors PPARγ and C/EBPα (Tang et al., 2004; Wang et al., 2014). The differentiating cells morphologically change from a fibroblast-like morphology into a spherical shape with a single large lipid droplet (Lazar et al., 2018). Mature adipocytes are cells with metabolic and endocrine properties. These characteristics are initiated after activation of metabolic and adipogenic-specific genes, like fatty acid-binding protein 4 (FABP4), glucose transporter type 4 (GLUT4), leptin, and adiponectin. The differentiating cells assemble the machinery that mediates lipogenesis, the metabolic pathway that converts carbohydrates into triacylglycerols for storage. In addition, the cells become insulin sensitive, which is responsible for carbohydrate uptake (Cristancho and Lazar, 2011). Although the scope of this review is to discuss Wnt during adipogenesis, Wnt signaling also has notable roles in mature adipocytes, such as lipogenesis (Bagchi et al., 2020). A commonly used cell line for adipogenesis research is the mouse-derived permanent 3T3-L1 preadipocyte line. The cells can easily be grown in vitro and can be differentiated into a mature adipocyte phenotype (Bennett et al., 2002).
In general, Wnt signaling is often associated with stem cell control and maintenance. Stem cells receive signaling cues from themselves or neighboring cells and can respond with the appropriate manner; self-renewal or differentiation (Nusse, 2008). Two decades ago Wnt10b was identified in preadipocytes as an important factor in cell maintenance and proliferation. Wnt10b is highly expressed in preadipocytes of both human and mouse origins (Ross et al., 2000; Bennett et al., 2002; Christodoulides et al., 2006). Later, Wnt10a and Wnt6 were also found to have similar control on stem cell maintenance (Cawthorn et al., 2012). In vitro studies have shown that blocking the Wnt pathway downstream of Wnt10b/10a/6 leads to spontaneous differentiation into mature adipocytes, and accordingly, overexpression of Wnt10b/10a/6 prevented adipogenesis (Ross et al., 2000; Bennett et al., 2002; Cawthorn et al., 2012). Genetically engineered mice that miss both alleles of Wnt10b have adipogenic as well as osteogenic abnormalities (Ross et al., 2000; Bennett et al., 2002; Stevens et al., 2010; Cawthorn et al., 2012). Perhaps the most compelling evidence that Wnt10b controls MSC and preadipocyte cell maintenance in mice is the progressive absence of adipogenic and osteogenic progenitors and premature adipogenesis/osteogenesis in Wnt10b-null mice (Stevens et al., 2010) Moreover, there is evidence that the expression of endogenous Wnt inhibitors (e.g., WT1 and sFRP1) in preadipocytes is significantly lower compared to mature adipocytes (Cho et al., 2019).
Wnt signaling impacts the expression of key transcription factors important in adipocyte differentiation, as has been shown by the MacDougald lab: active Wnt signaling in preadipocytes lowers RNA and protein levels of transcription factors C/EBPα and PPARγ, both needed for terminal differentiation. This suggests that Wnt signaling actively inhibits the expression of C/EBPα and PPARγ in preadipocytes (Ross et al., 2000; Bennett et al., 2002; Longo et al., 2004). The exact underlying mechanism of these effects was, until recently, poorly defined. However, a study by Xie et al. found that Wnt signaling through Axin2 directly inhibited the transcriptional activity of C/EBPβ (Figure 2) (Ross et al., 2000; Bennett et al., 2002; Longo et al., 2004; Xie et al., 2018). Active Wnt signaling in preadipocytes maintains the expression of Axin2. In the cytoplasm, Axin2 binds with the Wnt-related kinase GSK3β, which prevents GSK3β localization in the nucleus. GSK3β is mainly present in the cytoplasm, but can also be localized into the nucleus where it possesses kinase activity (Ross et al., 2000; Bennett et al., 2002; Longo et al., 2004; Shin et al., 2012; Xie et al., 2018). Given the fact that Axin1 and 2 are largely functional and structural similar (Chia and Costantini, 2005), it remains to be investigated why GSK3β is not retained in the cytoplasm by Axin1 during the Wnt off state. Without GSK3β in the nucleus C/EBPβ and Snail remain unphosphorylated, the absence of phosphorylation decreases the DNA binding activity of C/EBPβ and increases the stability of Snail (Park et al., 2004; Tang et al., 2005). Unphosphorylated C/EBPβ cannot start the expression of C/EBPα and PPARγ and stable Snail blocks PPARγ expression. Disruption of Wnt signaling leads to nuclear localization and phosphorylation activity of GSK3β, followed by the expression of C/EBPα and PPARγ mediated by active C/EBPβ (Xie et al., 2018). In turn, PPARγ binds to the Axin2 promoter and activates its transcription, leading to continuous canonical Wnt inhibition (Jho et al., 2002; Hu et al., 2015). Although Axin2 blocks PPARγ expression, PPARγ does not inhibit its own expression in a negative feedback loop. In 3T3-L1 cells, the expression of PPARγ peaks at day 6 of adipocyte differentiation and remains expressed even in mature adipocytes (Jitrapakdee et al., 2005). This could be explained by the fact that several transcription factors (e.g., C/EBPs, KLFs, etc.), induced at various time points of differentiation can directly activate gene expression of PPARγ and hereby maintaining its expression pattern (Jitrapakdee et al., 2005; Lee and Ge, 2014).
Figure 2. The mechanism of the inhibitory role of Wnt/β-catenin pathway in preadipocytes during adipogenesis. (Left) When in preadipocytes the canonical Wnt pathway is turned on by autocrine Wnt10b, this leads to nuclear localization of β-catenin in the nucleus, where it can bind with LEF/TCF. This transcription factor complex starts the expression of Axin2 and other genes related to stem cell maintenance and proliferation. Axin2 binds with GSK3β in the cytoplasm, thereby preventing migration into the nucleus. In this state, inactive C/EBPβ prevents the expression of transcription factors C/EBPα and PPARγ. Additionally, Snail inhibits the expression of C/EBPα. (Right) If the Wnt pathway is turned off this results in the start of terminal differentiation into mature adipocytes. Immediately preceding Wnt inactivation in preadipocytes, destruction of β-catenin in the cytoplasm by the destruction complex results in an absence of nuclear β-catenin. Without Axin2, GSK3β localizes into the nucleus where it phosphorylates Snail and C/EBPβ. Phosphorylation of Snail is accompanied by its degradation while phosphorylation of C/EBPβ renders its activation. These events start the expression of PPARγ C/EBPα, both crucial for initiating the final stage of adipogenesis. Changes in gene expression and epigenetic regulators prevent the production and secretion of endogenous Wnt ligands. Moreover, PPARγ starts the expression of Axin2, a Wnt signaling inhibiter.
Once Wnt signaling is inactivate in preadipocytes, the persistent Wnt expression has to be shut off. Recent research has shown that the control of enzymes involved in epigenetic regulation plays a role in this process. Histone acetyltransferases and histone methyltransferases are enzymes that modify lysine and arginine residues on histone proteins, by doing that they change the DNA accessibility, making genes more or less likely to be transcribed (Jing et al., 2018). Several studies suggest that this epigenetic regulation is involved in the way cells respond to Wnt signaling during adipogenesis. After activation of C/EBPβ during terminal differentiation, this transcription factor is able to start the transcription of lysine demethylase 5A (KDM5A), a transcriptional repressor. KDM5A knockdown by siRNAs resulted in the upregulation of anti-adipogenic Wnt6, so it is believed that the epigenetic changes caused by KDM5A after induction of adipogenesis inhibit the Wnt signaling pathway by preventing the expression of Wnt ligands (Guo et al., 2019).
Enhancer of zeste homolog 2 (EZH2) marks the promoters of Wnt1/6/10a/10b during adipocyte differentiation with the repressive epigenetic marker H3K27me3, thus reducing Wnt expression. EZH2 knockout blocks adipogenesis of brown preadipocytes, accompanied by significant upregulation of the Wnt genes. Moreover, these studies reported on a decrease of H3K27me3 on Wnt promoters and a global increase of the activating marker H3K27ac levels (Wang et al., 2010). Due to the changes of H3K27ac in EZH2 knockout cells, which can alter overall gene expression, the precise role of EZH2 remains unknown.
Additionally, the activity of epigenetic enzymes also affects how MSCs and preadipocytes respond to Wnt signaling. Histone Acetyltransferase GCN5 (GCN5), an enzyme that acetylates histone 3 on lysine 9 (H3K9ac), is known to prime the activation of Wnt genes, resulting in reduced adipogenesis (Jing et al., 2018). In BM-MSCs, GCN5 is able to prime transition of cells into an osteogenic lineage and to alter osteogenic activating signaling pathways (Zhang et al., 2016). A recent study by Sen et al. found that the histone methyltransferase activity of EZH2 activity could be responsible for keeping MSC in an undifferentiated state during Wnt signaling activation, since EZH2 knockdown in BM-MSCs resulted in osteogenesis (Sen et al., 2020). These findings emphasize that the epigenetic landscape of MSCs and preadipocytes has a regulatory role in the precise effect of Wnt signaling. The epigenetic enzymes change the likelihood of gene transcription, which is cell type-dependent. Therefore, in order to describe the role of Wnt signaling on heterogeneous cell populations such as MSCs and preadipocytes, it is required to have a better understanding of the underlying epigenetics.
Since inhibition of the autocrine effect of Wnt10b leads to spontaneous adipocyte differentiation, it is widely believed that Wnt signaling inactivation is needed for differentiation (Figure 3). In fact, when 3T3-L1 cells are treated with the common terminal adipocyte differentiation protocol (methyl-isobutylxanthine, dexamethasone, and insulin) the cells have elevated levels of intracellular cAMP which leads to subsequent downregulation of Wnt10b expression (Bennett et al., 2002; Prestwich and MacDougald, 2007). Groucho family member transducin-like enhancer of split 3 (TLE3), a transcriptional coregulator, antagonizes TCF4 during terminal differentiation. This mechanism is speculated to prevent the expression of β-catenin-dependent Wnt target gene expression during differentiation (Villanueva et al., 2011). The antagonistic effect of pro-adipogenic factors on Wnt signaling is also seen in other signaling factors, including BMPs. These are members of the transforming growth factor β (TGF-β) signaling family and are essential molecules for triggering of differentiation of MSCs (Wang et al., 2014). BMP2 and BMP4 are known for their importance in the first step of adipogenesis. BMP2 mainly possesses pro-osteogenic properties but was also shown to be adipogenic. BMP4 is a known pro-adipogenic factor. BMP4 or 2 treatment in cultured murine C3H10T1/2 MSC cells differentiates these cells into a proliferating preadipocyte phenotype. Applying the terminal adipocyte differentiation protocol on BMP4-induced preadipocytes successfully generated mature adipocytes (Tang et al., 2004). Mechanistically BMPs act via SMAD1/5/8 proteins or an alternative pathway, via p38 mitogen-activated protein kinase (p38MAPK) (Wang et al., 2014). Lysyl oxidase (Lox) is a protein expressed downstream of p38MAPK and stimulates adipogenesis. Inhibition of Lox not only results in an absence of adipogenesis but also leads to an elevated activation of the Wnt signaling pathway and BMP4-induced osteogenesis (Jiang et al., 2018). These findings suggest that the on or off state of the canonical Wnt pathway determines the lineage potential during BMP-induced differentiation of MSCs. This is in line with the well-established required role of Wnt signaling activation during osteogenesis (Day et al., 2005; Hill et al., 2005).
Figure 3. The effect of canonical Wnt during the several stages of adipogenesis and early osteogenesis from MSCs.
Wnt5a and b, two Wnt proteins responsible for turning on non-canonical signaling have been described to have pro-adipogenic properties. Importantly, Wnt5a/b have a direct effect on the adipose transcription factors, since they are able to activate PPARγ (van Tienen et al., 2009). However, the effects of Wnt5a/b are not always consistent and unlike the canonical Wnt signaling pathway, the importance of non-canonical Wnts during adipogenesis in vivo is less established (Fuster et al., 2015). For instance, Wnt5a/b have a completely opposite effect in BM-MSCs compared to preadipocytes. In BM-MSCs they are anti-adipogenic and have the potential to induce osteogenesis by upregulation of osteogenic master regulator Runt-related transcription factor 2 (Runx2) (Kitagawa et al., 2007). In addition, the role of Wnt5a is dose-dependent in rat MSCs and preadipocytes, lower concentrations have been shown to prevent adipogenesis and higher concentrations are pro-adipogenic, via an anti-β-catenin and Wnt/PCP-independent manner (Tang et al., 2004; Kitagawa et al., 2007). The multifactorial role is related to which type of signaling pathways are activated by Wnt5a/b proteins. For instance, Park and colleagues found an alternative Wnt signaling mechanism independent of the canonical Wnt pathway. This signaling pathway, named Wnt-YAP/TAZ, interacts with the non-canonical PCP/Wnt pathway and have Yes-associated proteins (YAP) and transcriptional coactivator with PDZ-binding motif (TAZ) as downstream effectors (Park et al., 2015). Wnt5a/b are agonists of Wnt-YAP/TAZ signaling and there is evidence that it can initiate terminal adipocyte differentiation (Park et al., 2015). To activate this pathway, Wnt5a/b bind to the Fz receptor, and the tyrosine kinase-like orphan receptor 1 and 2 (ROR1/2) have to act as co-receptors (Ackers and Malgor, 2018). MSCs from adipose tissue have been shown to contain a significantly higher population of ROR2 positive cells compared to BM-MSCs (Dickinson et al., 2017). Consequently, this could point out that the effect of non-canonical Wnt is cell type-dependent.
The formation of bone cells (or osteogenesis), happens at the expense of adipocyte differentiation, and this bifurcation is regulated by Wnt signaling. Two distinct mechanisms are involved in skeletal bone development, intramembranous and endochondral ossification. The first is characterized by direct formation of osteoblasts while the latter proceeds through an intermediate step of chondrocytes (Day et al., 2005). During the formation of intramembranous bone, MSCs directly differentiate into heterogenous osteoblast precursors which can later develop into osteocytes (Franz-Odendaal et al., 2006). The differentiation program is launched when the cells start expressing the osteogenic transcription factors Runx2 and SP7/Osterix (Komori, 2011). Runx2 is for example responsible for the expression of bone matrix proteins and Osterix for the upregulation of proteins initiating mineralization (Renn and Winkler, 2009; Komori, 2010). It is well-established that Wnt signaling can directly promote this differentiation process. Activated β-catenin starts the expression and activation of Runx2 which then results in the expression of transcription factors Osterix and TCF7. Noteworthy, Runx2 expression can also be induced by other signals such as fibroblast growth factor or Hedgehog (Komori, 2011). Mice with a conditional deletion of β-catenin in preosteoblasts exhibit a complete block of osteoblast differentiation, but these cells still expressed Runx2 (Day et al., 2005; Hill et al., 2005). This means that the induction of Runx2 expression does not depend on Wnt signaling, but that the signaling cascade is vital to proceed osteogenesis. A recent study found that a novel regulator of the Wnt pathway, Z-DNA-binding protein 1 (ZBP1), formed a positive feedback loop with the Wnt signaling pathway that could inhibit adipogenesis and promote osteogenesis. Moreover, ZBP1 was required for Wnt signaling in MSCs, indicating that ZBP1 could play a role in lineage specification of MSCs (Zhao et al., 2020).
MSCs can truly be beneficial for use in RM since there are many clinical applications for the cells, including bone, fat, and cartilage graft development for (auto)transplantation. Although isolated human MSCs are self-renewing stem cells, they have limited doubling potential when cultured in vitro. Long term culture of MSCs renders the cells senescent, and the cells stop proliferating (Liu et al., 2020). This is a major hurdle, since expansion might be needed to collect enough cells to create an MSC-derived graft (Liu et al., 2020). Signaling factors such as FGF, have the potential to stimulate MSC expansion and prevent them from becoming senescent (Tsutsumi et al., 2001; Bork et al., 2010). Likewise, active β-catenin in MSCs has been associated with increased proliferation without altering its potential to differentiate into adipocytes, osteocytes, or chondrocytes. Treatment of murine C3H10T1/2 cells with β-catenin agonist, 6-bromoindirubin-3′-oxime (BIO), showed that besides significant increase of proliferation, the cells exhibited higher levels of expression of pluripotency genes SRY-box 2 (Sox2), Nanog, octamer-binding transcription factor 4 (Oct4), and Cyclin D1 (Hoffman and Benoit, 2015). In MSCs, these markers have been demonstrated to have a direct effect on maintaining the undifferentiated state as well as promoting proliferation (Tsai and Hung, 2012). MSCs extracted from different locations express several Wnt ligands, and endogenous Wnt signaling is required for stem cell maintenance (Etheridge et al., 2004; Tantrawatpan et al., 2013; Jothimani et al., 2020). Thus, this suggests that the canonical Wnt pathway mediates the stemness as well as osteogenesis in an intricate balance. However, more research has to be carried out to establish this underlying mechanism and how to apply this in vitro.
Impaired Wnt signaling can lead to disease, and this is also true for mesenchymal tissues. Since mesenchymal tissue types are constantly renewed (Alfaro et al., 2011), impaired signaling can have significant effects on metabolism, as seen in osteoporosis, T2D, and obesity (Baron and Kneissel, 2013; Chen et al., 2018). One example of overly active Wnt signaling in adipose tissue is seen in T2D patients with a mutation in the transcription factor 7-like 2 gene (TCF7L2) (Chen et al., 2018), a transcriptional repressor of the Wnt signaling pathway (Mandal et al., 2017). In vivo experiments with inactive mutations of TCF7L2 results in impaired adipogenesis characterized by increased subcutaneous adipose tissue mass, adipocyte hypertrophy, and inflammation. All mechanisms that are manifested when there is an excess of lipids. Moreover, this was accompanied by whole-body glucose intolerance and hepatic insulin resistance, a T2D hallmark (Kahn et al., 2006; Jo et al., 2009; Chen et al., 2018). On the other hand, a decrease in Wnt activity can also be detrimental, as seen in osteoporosis. Osteoporosis is a bone disease characterized by lowered bone density and increased bone fragility. Patients suffering from this disease have diminished osteogenesis and the Wnt signaling pathways are shown to be responsible. More specifically, loss-of-function mutations of the co-receptors LRP5/6 were identified as the cause (Baron and Kneissel, 2013). Increased BM adipose tissue (BMAT), a special type of adipose tissue distinct from WAT or BAT naturally residing in BM (Bukowska et al., 2018), is also related to osteoporosis. The lineage potential of MSCs from patients with osteoporosis was more biased toward differentiation into adipocytes and signaling from BMAT was shown to promote adipogenesis of BM-MSCs (Astudillo et al., 2008). Wnt and its downstream effectors could serve as potential therapeutic targets (Hoeppner et al., 2009).
Adipogenesis, the process of the formation of adipocytes from MSCs, is tightly regulated by signaling pathways. The Wnt signaling pathways are among these and have been proven to be crucial for MSC lineage specification. It is widely documented that canonical Wnt signaling regulates several steps in this differentiation process, but the precise underlying mechanisms remain poorly understood. In recent years, MSCs have been shown to be promising for use in regenerative medicine (Hoeppner et al., 2009; Alfaro et al., 2011).
In adipose tissue, Wnt signaling promotes stem cell maintenance of preadipocytes. The stem cell niche of preadipocytes, however, does not solely consist of preadipocytes and adipocytes. Other cell types that reside in the cell type niche are fibroblasts, immune cells, endothelial cells, and a small fraction of MSCs. Preadipocytes can be found in the stromal vascular fraction of adipose tissue and this is believed to be in close proximity of the blood vessels (Tsuji et al., 2014; Zhang et al., 2018). Secretion of signaling factors by some of these neighboring cells was shown to be essential in the mediation of adipogenesis (Zhang et al., 2018; Sebo and Rodeheffer, 2019). It remains unclear if these other cell types also express Wnt ligands, but autocrine action of Wnt10b/10a/6 by preadipocytes is believed to be the main source (Ross et al., 2000; Bennett et al., 2002; Cawthorn et al., 2012).
A limitation of many studies related to adipogenesis and MSCs biology is the use of non-human permanent cell lines 3T3-L1 and C3H10T1/2 (Ross et al., 2000; Longo et al., 2004; Tang et al., 2004), although they provide consistency across experiments, which would be difficult with isolated human MSCs and preadipocytes. These permanent cell line do not faithfully represent the highly heterogeneous nature of MSCs, however. Primary cultures of MSCs should be included as well, but culturing these cells has been proven difficult (Bork et al., 2010).
In conclusion, Wnt signaling mainly plays a role in suppressing adipogenesis during several stages of differentiation. It seems that the main role of suppression is to increase the MSC and preadipocytes cell mass. Epigenetic and transcriptional regulators have a significant impact on this process, but it is still poorly understood how transcriptional regulation and Wnt signaling interact during adipogenesis.
TW performed the literature review, analyzed and interpreted the findings, and wrote the manuscript. RN supervised the writing process and edited the manuscript. All authors contributed to the article and approved the submitted version.
The authors declare that the research was conducted in the absence of any commercial or financial relationships that could be construed as a potential conflict of interest.
We thank Catriona Logan for comments on the manuscript. Work in the laboratory of RN was supported by the Howard Hughes Medical Institute. Figures were created with BioRender.com.
Ackers, I., and Malgor, R. (2018). Interrelationship of canonical and non-canonical wnt signalling pathways in chronic metabolic diseases. Diabet. Vasc. Dis. Res. 15, 3–13. doi: 10.1177/1479164117738442
Alfaro, M. P., Saraswati, S., and Young, P. P. (2011). Molecular mediators of mesenchymal stem cell biology. Vitam. Horm. 87, 39–59. doi: 10.1016/B978-0-12-386015-6.00023-8
Astudillo, P., Ríos, S., Pastenes, L., Pino, A. M., and Rodríguez, J. P. (2008). Increased adipogenesis of osteoporotic human-mesenchymal stem cells (MSCs) characterizes by impaired leptin action. J. Cell. Biochem. 103, 1054–1065. doi: 10.1002/jcb.21516
Bagchi, D. P., Nishii, A., Li, Z., DelProposto, J. B., Corsa, C. A., Mori, H., et al. (2020). Wnt/β-catenin signaling regulates adipose tissue lipogenesis and adipocyte-specific loss is rigorously defended by neighboring stromal-vascular cells. Mol. Metab. 42:101078. doi: 10.1016/j.molmet.2020.101078
Baron, R., and Gori, F. (2018). Targeting WNT signaling in the treatment of osteoporosis. Curr. Opin. Pharmacol. 40, 134–141. doi: 10.1016/j.coph.2018.04.011
Baron, R., and Kneissel, M. (2013). WNT signaling in bone homeostasis and disease: from human mutations to treatments. Nat. Med. 19, 179–192. doi: 10.1038/nm.3074
Bennett, C. N., Ross, S. E., Longo, K. A., Bajnok, L., Hemati, N., Johnson, K. W., et al. (2002). Regulation of wnt signaling during adipogenesis. J. Biol. Chem. 277, 30998–31004. doi: 10.1074/jbc.M204527200
Bork, S., Pfister, S., Witt, H., Horn, P., Korn, B., Ho, A. D., et al. (2010). DNA methylation pattern changes upon long-term culture and aging of human mesenchymal stromal cells. Aging Cell 9, 54–63. doi: 10.1111/j.1474-9726.2009.00535.x
Bukowska, J., Frazier, T., Smith, S., Brown, T., Bender, R., McCarthy, M., et al. (2018). Bone marrow adipocyte developmental origin and biology. Curr. Osteoporos. Rep. 16, 312–319. doi: 10.1007/s11914-018-0442-z
Cawthorn, W. P., Bree, A. J., Yao, Y., Du, B., Hemati, N., Martinez-Santibañez, G., et al. (2012). Wnt6, Wnt10a and Wnt10b inhibit adipogenesis and stimulate osteoblastogenesis through a β-catenin-dependent mechanism. Bone 50, 477–489. doi: 10.1016/j.bone.2011.08.010
Chen, X., Ayala, I., Shannon, C., Fourcaudot, M., Acharya, N. K., Jenkinson, C. P., et al. (2018). The diabetes gene and wnt pathway effector TCF7L2 regulates adipocyte development and function. Diabetes 67, 554–568. doi: 10.2337/db17-0318
Chia, I. V., and Costantini, F. (2005). Mouse axin and axin2/conductin proteins are functionally equivalent in vivo. Mol. Cell. Biol. 25, 4371–4376. doi: 10.1128/MCB.25.11.4371-4376.2005
Cho, D. S., Lee, B., and Doles, J. D. (2019). Refining the adipose progenitor cell landscape in healthy and obese visceral adipose tissue using single-cell gene expression profiling. Life Sci. Allian. 2:6. doi: 10.26508/lsa.201900561
Christodoulides, C., Scarda, A., Granzotto, M., Milan, G., Dalla Nora, E., Keogh, J., et al. (2006). WNT10B mutations in human obesity. Diabetologia 49, 678–684. doi: 10.1007/s00125-006-0144-4
Cristancho, A. G., and Lazar, M. A. (2011). Forming functional fat: a growing understanding of adipocyte differentiation. Nat. Rev. 12, 722–734. doi: 10.1038/nrm3198
Day, T. F., Guo, X., Garrett-Beal, L., and Yang, Y. (2005). Wnt/β-catenin signaling in mesenchymal progenitors controls osteoblast and chondrocyte differentiation during vertebrate skeletogenesis. Dev. Cell. 8, 739–750. doi: 10.1016/j.devcel.2005.03.016
Dickinson, S. C., Sutton, C. A., Brady, K., Salerno, A., Katopodi, T., Williams, R. L., et al. (2017). The Wnt5a receptor, receptor tyrosine Kinase-Like orphan receptor 2, is a predictive cell surface marker of human mesenchymal stem cells with an enhanced capacity for chondrogenic differentiation. Stem Cells 35, 2280–2291. doi: 10.1002/stem.2691
Etheridge, S. L., Spencer, G. J., Heath, D. J., and Genever, P. G. (2004). Expression profiling and functional analysis of wnt signaling mechanisms in mesenchymal stem cells. Stem Cells 22, 849–860. doi: 10.1634/stemcells.22-5-849
Franz-Odendaal, T. A., Hall, B. K., and Witten, P. E. (2006). Buried alive: how osteoblasts become osteocytes. Dev. Dynam. 235, 176–190. doi: 10.1002/dvdy.20603
Fuster, J. J., Zuriaga, M. A., Ngo, D. T., Farb, M. G., Aprahamian, T., Yamaguchi, T. P., et al. (2015). Noncanonical wnt signaling promotes obesity-induced adipose tissue inflammation and metabolic dysfunction independent of adipose tissue expansion. Diabetes 64, 1235–1248. doi: 10.2337/db14-1164
Gehart, H., and Clevers, H. (2019). Tales from the crypt: new insights into intestinal stem cells. Nat. Rev. 16, 19–34. doi: 10.1038/s41575-018-0081-y
Guo, L., Guo, Y., Li, B., Peng, W., and Tang, Q. (2019). Histone demethylase KDM5A is transactivated by the transcription factor C/EBPβ and promotes preadipocyte differentiation by inhibiting wnt/β-catenin signaling. J. Biol. Chem. 294, 9642–9654. doi: 10.1074/jbc.RA119.008419
Han, L., Wang, B., Wang, R., Gong, S., Chen, G., and Xu, W. (2019). The shift in the balance between osteoblastogenesis and adipogenesis of mesenchymal stem cells mediated by glucocorticoid receptor. Stem Cell Res. Ther. 10:377. doi: 10.1186/s13287-019-1498-0
Hill, T. P., Später, D., Taketo, M. M., Birchmeier, W., and Hartmann, C. (2005). Canonical wnt/beta-catenin signaling prevents osteoblasts from differentiating into chondrocytes. Dev. Cell 8, 727–738. doi: 10.1016/j.devcel.2005.02.013
Hoeppner, L. H., Secreto, F. J., and Westendorf, J. J. (2009). Wnt signaling as a therapeutic target for bone diseases. Expert Opin. Ther. Targets 13, 485–496. doi: 10.1517/14728220902841961
Hoffman, M. D., and Benoit, D. S. W. (2015). Agonism of wnt/β-catenin signaling promotes mesenchymal stem cell (MSC) expansion. J. Tissue Eng. Regen. Med. 9, E13–E26. doi: 10.1002/term.1736
Hu, L., Yang, G., Hägg, D., Sun, G., Ahn, J. M., Jiang, N., et al. (2015). IGF1 promotes adipogenesis by a lineage bias of endogenous adipose stem/progenitor cells. Stem Cells 33, 2483–2495. doi: 10.1002/stem.2052
Jho, E., Zhang, T., Domon, C., Joo, C., Freund, J., and Costantini, F. (2002). Wnt/beta-catenin/tcf signaling induces the transcription of Axin2, a negative regulator of the signaling pathway. Mol. Cell. Biol. 22, 1172–1183. doi: 10.1128/MCB.22.4.1172-1183.2002
Jiang, W., Xing, C., Wang, H., Wang, W., Chen, S., Ning, L., et al. (2018). A lox/CHOP-10 crosstalk governs osteogenic and adipogenic cell fate by MSCs. J. Cell. Mol. Med. 22, 5097–5108. doi: 10.1111/jcmm.13798
Jing, H., Su, X., Gao, B., Shuai, Y., Chen, J., Deng, Z., et al. (2018). Epigenetic inhibition of wnt pathway suppresses osteogenic differentiation of BMSCs during osteoporosis. Cell Death Dis. 9:176. doi: 10.1038/s41419-017-0231-0
Jitrapakdee, S., Slawik, M., Medina-Gomez, G., Campbell, M., Wallace, J. C., Sethi, J. K., et al. (2005). The peroxisome proliferator-activated receptor-gamma regulates murine pyruvate carboxylase gene expression in vivo and in vitro. J. Biol. Chem. 280, 27466–27476. doi: 10.1074/jbc.M503836200
Jo, J., Gavrilova, O., Pack, S., Jou, W., Mullen, S., Sumner, A. E., et al. (2009). Hypertrophy and/or hyperplasia: dynamics of adipose tissue growth. PLoS Comput. Biol. 5:e1000324. doi: 10.1371/journal.pcbi.1000324
Jothimani, G., Liddo, R. D., Pathak, S., Piccione, M., Sriramulu, S., and Banerjee, A. (2020). Wnt signaling regulates the proliferation potential and lineage commitment of human umbilical cord derived mesenchymal stem cells. Mol. Biol. Rep. 47, 1293–1308. doi: 10.1007/s11033-019-05232-5
Kahn, S. E., Hul, R. L., and Utzschneider, K. M. (2006). Mechanisms linking obesity to insulin resistance and type 2 diabetes. Nature 444, 840–846. doi: 10.1038/nature05482
Kang, S., Bennett, C. N., Gerin, I., Rapp, L. A., Hankenson, K. D., and Macdougald, O. A. (2007). Wnt signaling stimulates osteoblastogenesis of mesenchymal precursors by suppressing CCAAT/enhancer-binding protein alpha and peroxisome proliferator-activated receptor gamma. J. Biol. Chem. 282, 14515–14524. doi: 10.1074/jbc.M700030200
Kitagawa, H., Igarashi, M., Takeyama, K., Shibuya, H., Suzawa, M., Mezaki, Y., et al. (2007). A histone lysine methyltransferase activated by non-canonical wnt signalling suppresses PPAR-γ transactivation. Nat. Cell Biol. 9, 1273–1285. doi: 10.1038/ncb1647
Komori, T. (2010). Regulation of bone development and extracellular matrix protein genes by RUNX2. Cell Tissue Res. 339, 189–195. doi: 10.1007/s00441-009-0832-8
Komori, T. (2011). Signaling networks in RUNX2-dependent bone development. J. Cell. Biochem. 112, 750–755. doi: 10.1002/jcb.22994
Lazar, A., Dinescu, S., and Costache, M. (2018). Adipose tissue engineering and adipogenesis – a review. Rev. Biol. Biomed. Sci. 1, 17–26. doi: 10.31178/rbbs.2018.1.1.3
Lee, J., and Ge, K. (2014). Transcriptional and epigenetic regulation of PPARγ expression during adipogenesis. Cell Biosci. 4:29. doi: 10.1186/2045-3701-4-29
Leung, J. Y., Kolligs, F. T., Wu, R., Zhai, Y., Kuick, R., Hanash, S., et al. (2002). Activation of AXIN2 expression by beta-catenin-T cell factor. A feedback repressor pathway regulating wnt signaling. J. Biol. Chem. 277, 21657–21665. doi: 10.1074/jbc.M200139200
Liu, J., Ding, Y., Liu, Z., and Liang, X. (2020). Senescence in mesenchymal stem cells: functional alterations, molecular mechanisms, and rejuvenation strategies. Front. Cell Dev. Biol. 8:258. doi: 10.3389/fcell.2020.00258
Longo, K. A., Wright, W. S., Kang, S., Gerin, I., Chiang, S., Lucas, P. C., et al. (2004). Wnt10b inhibits development of white and brown adipose tissues. J. Biol. Chem. 279, 35503–35509. doi: 10.1074/jbc.M402937200
MacDonald, B. T., Tamai, K., and He, X. (2009). Wnt/β-catenin signaling: components, mechanisms, and diseases. Dev. Cell. 17, 9–26. doi: 10.1016/j.devcel.2009.06.016
Mandal, A., Holowiecki, A., Song, Y. C., and Waxman, J. S. (2017). Wnt signaling balances specification of the cardiac and pharyngeal muscle fields. Mech. Dev. 143, 32–41. doi: 10.1016/j.mod.2017.01.003
Nusse, R. (2008). Wnt signaling and stem cell control. Cell Res. 18, 523–527. doi: 10.1038/cr.2008.47
Park, B., Qiang, L., and Farmer, S. R. (2004). Phosphorylation of C/EBPbeta at a consensus extracellular signal-regulated kinase/glycogen synthase kinase 3 site is required for the induction of adiponectin gene expression during the differentiation of mouse fibroblasts into adipocytes. Mol. Cell. Biol. 24, 8671–8680. doi: 10.1128/MCB.24.19.8671-8680.2004
Park, H. W., Kim, Y. C., Yu, B., Moroishi, T., Mo, J., Plouffe, S. W., et al. (2015). Alternative wnt signaling activates YAP/TAZ. Cell 162, 780–794. doi: 10.1016/j.cell.2015.07.013
Prestwich, T. C., and MacDougald, O. A. (2007). Wnt/β-catenin signaling in adipogenesis and metabolism. Curr. Opin. Cell Biol. 19, 612–617. doi: 10.1016/j.ceb.2007.09.014
Renn, J., and Winkler, C. (2009). Osterix-mCherry transgenic medaka for in vivo imaging of bone formation. Dev. Dynam. 238, 241–248. doi: 10.1002/dvdy.21836
Richter, J., Traver, D., and Willert, K. (2017). The role of wnt signaling in hematopoietic stem cell development. Crit. Rev. Biochem. Mol. Biol. 52, 414–424. doi: 10.1080/10409238.2017.1325828
Ross, S., Hemati, N., Longo, K., Bennett, C., Lucas, P., Erickson, R., et al. (2000). Inhibition of adipogenesis by wnt signaling. Science 289, 950–953. doi: 10.1126/science.289.5481.950
Sebo, Z. L., and Rodeheffer, M. S. (2019). Assembling the adipose organ: adipocyte lineage segregation and adipogenesis in vivo. Development 146:172098. doi: 10.1242/dev.172098
Sen, B., Paradise, C. R., Xie, Z., Sankaran, J., Uzer, G., Styner, M., et al. (2020). B-catenin preserves the stem state of murine bone marrow stromal cells through activation of EZH2. J. Bone Mineral Res. doi: 10.1002/jbmr.3975
Shin, S., Lee, E., Chun, J., Hyun, S., Kim, Y., and Kang, S. (2012). The nuclear localization of glycogen synthase kinase 3β is required its putative PY-nuclear localization sequences. Mol. Cells 34, 375–382. doi: 10.1007/s10059-012-0167-2
Stevens, J. R., Miranda-Carboni, G. A., Singer, M. A., Brugger, S. M., Lyons, K. M., and Lane, T. F. (2010). Wnt10b deficiency results in age-dependent loss of bone mass and progressive reduction of mesenchymal progenitor cells. J. Bone Mineral Res. 25, 2138–2147. doi: 10.1002/jbmr.118
Tang, Q., Grønborg, M., Huang, H., Kim, J., Otto, T. C., Pandey, A., et al. (2005). Sequential phosphorylation of CCAAT enhancer-binding protein beta by MAPK and glycogen synthase kinase 3beta is required for adipogenesis. Proc. Natl. Acad. Sci. U. S. A. 102, 9766–9771. doi: 10.1073/pnas.0503891102
Tang, Q., Otto, T. C., and Lane, M. D. (2004). Commitment of C3H10T1/2 pluripotent stem cells to the adipocyte lineage. Proc. Natl. Acad. Sci. U. S. A. 101, 9607–9611. doi: 10.1073/pnas.0403100101
Tantrawatpan, C., Manochantr, S., Kheolamai, P.-U., Pratya, Y., Supokawej, A., and Issaragrisil, S. (2013). Pluripotent gene expression in mesenchymal stem cells from human umbilical cord wharton's jelly and their differentiation potential to neural-like cells. J. Med. Assoc. Thai 96, 1208–1217.
Tsai, C., and Hung, S. (2012). Functional roles of pluripotency transcription factors in mesenchymal stem cells. Cell Cycle 11, 3711–3712. doi: 10.4161/cc.22048
Tsuji, W., Rubin, J. P., and Marra, K. G. (2014). Adipose-derived stem cells: implications in tissue regeneration. World J. Stem Cells 6, 312–321. doi: 10.4252/wjsc.v6.i3.312
Tsutsumi, S., Shimazu, A., Miyazaki, K., Pan, H., Koike, C., Yoshida, E., et al. (2001). Retention of multilineage differentiation potential of mesenchymal cells during proliferation in response to FGF. Biochem. Biophys. Res. Commun. 288, 413–419. doi: 10.1006/bbrc.2001.5777
Van Camp, J. K., Beckers, S., Zegers, D., and Van Hul, W. (2014). Wnt signaling and the control of human stem cell fate. Stem Cell Rev. Rep. 10, 207–229. doi: 10.1007/s12015-013-9486-8
van Tienen, F. H. J., Laeremans, H., van der Kallen, C. J. H., and Smeets, H. J. M. (2009). Wnt5b stimulates adipogenesis by activating PPARgamma, and inhibiting the beta-catenin dependent wnt signaling pathway together with Wnt5a. Biochem. Biophys. Res. Commun. 387, 207–211. doi: 10.1016/j.bbrc.2009.07.004
Villanueva, C. J., Waki, H., Godio, C., Nielsen, R., Chou, W., Vargas, L., et al. (2011). TLE3 is a dual-function transcriptional coregulator of adipogenesis. Cell Metab. 13, 413–427. doi: 10.1016/j.cmet.2011.02.014
Wang, L., Jin, Q., Lee, J., Su, I., and Ge, K. (2010). Histone H3K27 methyltransferase Ezh2 represses wnt genes to facilitate adipogenesis. Proc. Natl. Acad. Sci. U. S. A. 107, 7317–7322. doi: 10.1073/pnas.1000031107
Wang, R. N., Green, J., Wang, Z., Deng, Y., Qiao, M., Peabody, M., et al. (2014). Bone morphogenetic protein (BMP) signaling in development and human diseases. Genes Dis. 1, 87–105. doi: 10.1016/j.gendis.2014.07.005
Xie, Y., Mo, C., Cai, Y., Wang, W., Hong, X., Zhang, K., et al. (2018). Pygo2 regulates adiposity and glucose homeostasis via β-catenin-Axin2-GSK3β signaling pathway. Diabetes 67, 2569–2584. doi: 10.2337/db18-0311
Zhang, P., Liu, Y., Jin, C., Zhang, M., Tang, F., and Zhou, Y. (2016). Histone acetyltransferase GCN5 regulates osteogenic differentiation of mesenchymal stem cells by inhibiting NF-κB. J. Bone Mineral Res. 31, 391–402. doi: 10.1002/jbmr.2704
Zhang, R., Gao, Y., Zhao, X., Gao, M., Wu, Y., Han, Y., et al. (2018). FSP1-positive fibroblasts are adipogenic niche and regulate adipose homeostasis. PLoS Biol. 16:e2001493. doi: 10.1371/journal.pbio.2001493
Zhao, X., Xie, L., Wang, Z., Wang, J., Xu, H., Han, X., et al. (2020). ZBP1 (DAI/DLM-1) promotes osteogenic differentiation while inhibiting adipogenic differentiation in mesenchymal stem cells through a positive feedback loop of wnt/β-catenin signaling. Bone Res. 8, 1–10. doi: 10.1038/s41413-020-0085-4
Keywords: adipogenesis, Wnt signaling, mesenchymal stem cells, preadipocyte, osteogenesis, C/EBP, PPARγ
Citation: de Winter TJJ and Nusse R (2021) Running Against the Wnt: How Wnt/β-Catenin Suppresses Adipogenesis. Front. Cell Dev. Biol. 9:627429. doi: 10.3389/fcell.2021.627429
Received: 09 November 2020; Accepted: 14 January 2021;
Published: 09 February 2021.
Edited by:
Delilah Hendriks, Hubrecht Institute (KNAW), NetherlandsReviewed by:
Ormond MacDougald, University of Michigan, United StatesCopyright © 2021 de Winter and Nusse. This is an open-access article distributed under the terms of the Creative Commons Attribution License (CC BY). The use, distribution or reproduction in other forums is permitted, provided the original author(s) and the copyright owner(s) are credited and that the original publication in this journal is cited, in accordance with accepted academic practice. No use, distribution or reproduction is permitted which does not comply with these terms.
*Correspondence: Roeland Nusse, cm51c3NlQHN0YW5mb3JkLmVkdQ==
Disclaimer: All claims expressed in this article are solely those of the authors and do not necessarily represent those of their affiliated organizations, or those of the publisher, the editors and the reviewers. Any product that may be evaluated in this article or claim that may be made by its manufacturer is not guaranteed or endorsed by the publisher.
Research integrity at Frontiers
Learn more about the work of our research integrity team to safeguard the quality of each article we publish.