- 1Vascular Biology Program, Boston Children’s Hospital, Boston, MA, United States
- 2Department of Surgery, Harvard Medical School, Boston, MA, United States
- 3Department of Cardiology, Boston Children’s Hospital, Boston, MA, United States
- 4Division of Cardiology, Department of Medicine, University of California, San Diego, San Diego, CA, United States
Endocytosis is the process of actively transporting materials into a cell by membrane engulfment. Traditionally, endocytosis was divided into three forms: phagocytosis (cell eating), pinocytosis (cell drinking), and the more selective receptor-mediated endocytosis (clathrin-mediated endocytosis); however, other important endocytic pathways (e.g., caveolin-dependent endocytosis) contribute to the uptake of extracellular substances. In each, the plasma membrane changes shape to allow the ingestion and internalization of materials, resulting in the formation of an intracellular vesicle. While receptor-mediated endocytosis remains the best understood pathway, mammalian cells utilize each form of endocytosis to respond to their environment. Receptor-mediated endocytosis permits the internalization of cell surface receptors and their ligands through a complex membrane invagination process that is facilitated by clathrin and adaptor proteins. Internalized vesicles containing these receptor-ligand cargoes fuse with early endosomes, which can then be recycled back to the plasma membrane, delivered to other cellular compartments, or destined for degradation by fusing with lysosomes. These intracellular fates are largely determined by the interaction of specific cargoes with adaptor proteins, such as the epsins, disabled-homolog 2 (Dab2), the stonin proteins, epidermal growth factor receptor substrate 15, and adaptor protein 2 (AP-2). In this review, we focus on the role of epsins and Dab2 in controlling these sorting processes in the context of cardiovascular disease. In particular, we will focus on the function of epsins and Dab2 in inflammation, cholesterol metabolism, and their fundamental contribution to atherogenicity.
Endocytosis is the method that cells utilize to uptake material from outside of the membrane to inside of the cells. There are three major forms of endocytosis, phagocytosis, pinocytosis, and clathrin-mediated endocytosis, each involving its own specific cell machinery.
Clathrin-Mediated Endocytosis
Receptor-mediated endocytosis (i.e., clathrin-mediated endocytosis) is a process by which cells internalize metabolites, hormones, and proteins to allow them to respond to their local environment. This form of endocytosis typically consists of the following steps: (1) extracellular ligand binding to cell surface receptors, (2) the formation of a clathrin cage around the receptor-ligand complex resulting from the interaction with a multitude of molecules and proteins, such as phosphatidylinositol 4,5-bisphosphate (PIP2), adaptor protein 2 (AP-2), clathrin-coat assembly protein 180 (AP180), and epsin proteins, (3) lipid bilayer invagination with the aid of membrane curvature promoting proteins, such as members of the epsin family, (4) vesicle formation and release from the plasma membrane, and (5) sorting of the vesicle and receptor-ligand cargo within the cell (Figure 1). Each of these steps requires a variety of endocytic adaptor proteins that include the epsins, epidermal growth factor receptor substrate 15 (Eps15), disabled homolog 2 (Dab2), AP-2, and PIP2 (Eberhard et al., 1990; Chen et al., 1998; Pearse et al., 2000; Ford et al., 2002; Polo et al., 2002; Maurer and Cooper, 2005; Bhattacharjee et al., 2020). In this review we focus on the epsin and Dab2 proteins, which play crucial roles in clathrin-mediated endocytosis and are implicated as important modulators of cardiovascular diseases.
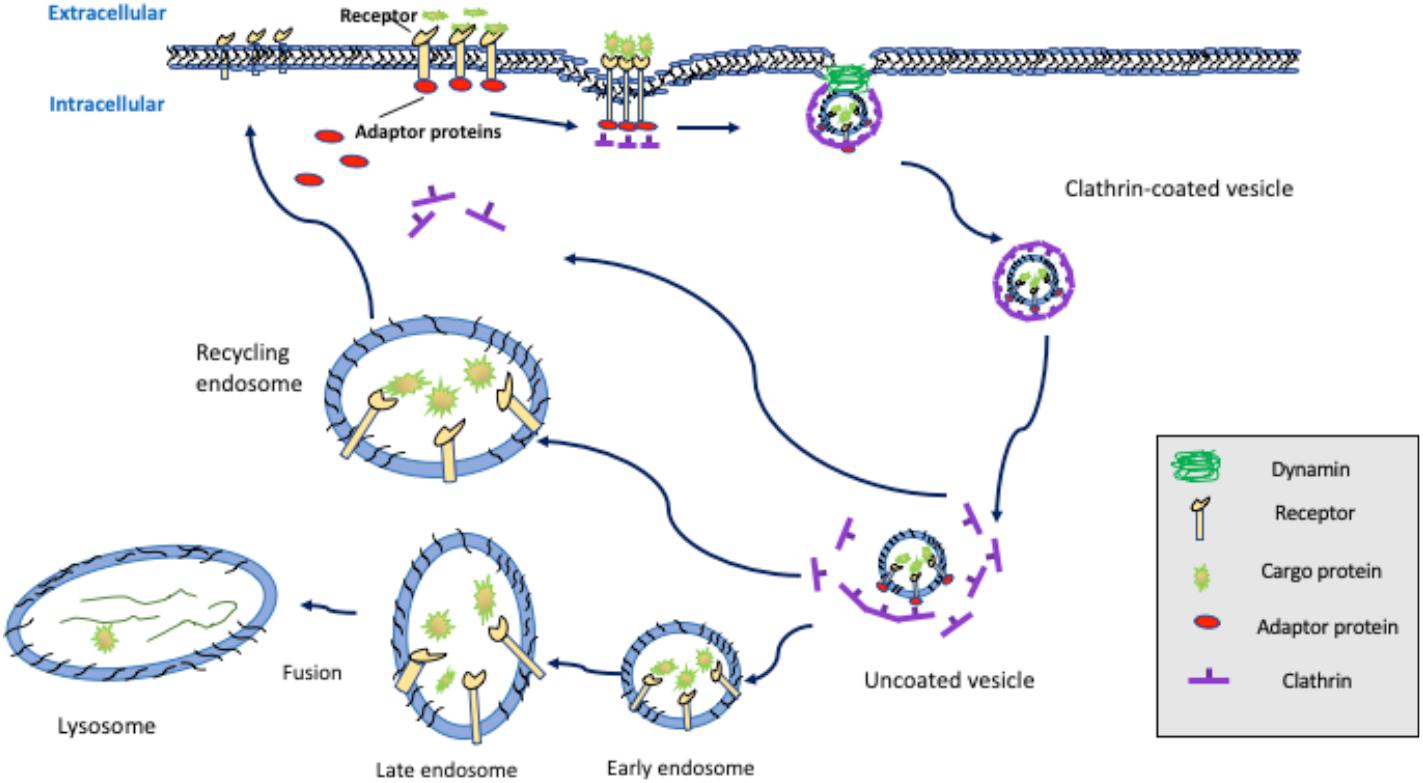
Figure 1. Clathrin-mediated endocytosis. Extracellular ligands binding to cell surface receptors triggers the recruitment of the adaptor proteins, such as AP-2, Dab2, and/or members of the epsin family, which induces plasma membrane invagination and formation of clathrin-coated pits that are subsequently released via dynamin-mediated scission. Clathrin-coated vesicles undergo de-coating and sorting through fusion with early endosomes, late endosomes, and ultimately, lysosomes, leading to receptor degradation, or sorting back to the cell surface by recycling endosomes.
Phagocytosis
Phagocytosis, also called “cell eating,” is used by the cells, such as neutrophils, macrophages and other white blood cells, to engulf debris, bacteria, or other solid objects through the produced pseudopodia (Jaggi et al., 2020; Joffe et al., 2020; Reine et al., 2020; Smirnov et al., 2020). The invagination of cell membrane produces phagosomes, which later fuse with lysosomes containing enzymes. Materials in the phagosome are broken down into simpler substance by these enzymes and degraded.
Pinocytosis
Pinocytosis is called “cell drinking” that cells, such as hepatocytes, kidney cells and epithelial cells, engulf extracellular fluid, including various solutes, such as sugars, ions, amino acids, and proteins. The cell membrane folds inward to form invagination, which takes up the extracellular fluid and releases it inside the cells. Kidney cell can utilize pinocytosis to separate nutrients and fluid from the urine (Bode et al., 1975). Capillary epithelial cells can use pinocytosis to engulf the liquid part of blood at its surface (Orlov, 1988).
Epsins
Epsins are a family of adaptor proteins associated with clathrin-coated pits that support lipid bilayer curvature and coordinate the recruitment of ubiquitinated cargo proteins (Ko et al., 2010). The first member of this family to be isolated was epsin 1, which was identified through its interaction with the clathrin-associated protein Eps15 (Chen et al., 1998). Subsequent investigations established that there are three classic members of this family (epsins 1, 2, and 3) in addition to a non-classic isoform named either epsin 4 or epsin R, which is now more commonly referred to as clathrin interactor 1 (CLINT1). In mammals, epsins 1 and 2 are ubiquitously expressed and particularly enriched in the brain (Rosenthal et al., 1999). While epsins 1 and 2 are functionally redundant, epsin 3 is predominantly expressed in the gastric parietal cells of the stomach (Spradling et al., 2001).
Epsins 1, 2, and 3 share a structure termed the Epsin N-terminal Homology (ENTH) domain, which interacts with PIP2 at the plasma membrane (Chen et al., 1999; De Camilli et al., 2002; Chen and De Camilli, 2005). Ubiquitin-interacting motifs (UIMs) that recognize and recruit ubiquitinated surface receptors to clathrin-coated pits for internalization are situated next to the ENTH domain (Oldham et al., 2002; Sakamoto et al., 2004; Chen and De Camilli, 2005). An adjacent region, characterized by DPW (Asp-Pro-Trp)-rich amino acid motifs flanked by a clathrin-binding domain, is responsible for binding to AP-2 and clathrin, respectively (Figure 2). In the COOH-terminal region, NPF (Asn-Pro-Phe) motifs function to bind Eps15-homology (EH) domain-containing proteins, such as Eps15 and the BTB/POZ domain-containing protein POB1 (Salcini et al., 1999; De Camilli et al., 2002).

Figure 2. Epsin domain structure. Epsin interacting regions for binding partners. PIP2, phosphatidylinositol-4,5-bisphosphate; ENTH, epsin N-terminal homology; UIM, Ubiquitin-interacting motifs; AP-2, Adaptor protein 2; EH, Eps 15 homology domain.
Disabled Homolog 2 (Dab2)
Dab2 is another clathrin- and cargo-binding endocytic adaptor protein that was first isolated as a mitogen-responsive phosphoprotein called p96 (Xu et al., 1995). Although the messenger RNA transcript encoding this protein was initially named DOC2 (for Differentially expressed in Ovarian Cancer) because it was differentially expressed in ovarian cancer, the protein is now referred to as Disabled homolog-2 or Dab2 as it is transcribed from an ortholog of the Drosophila Dab gene (Gertler et al., 1989). At the same time, a neuronal-specific isoform named Dab1 represents an additional protein produced from a mammalian ortholog of this Drosophila gene (Howell et al., 1997). Interestingly, several spliced isoforms of Dab2 have been identified, including p96 and p67 (Xu et al., 1995; Sheng et al., 2000, 2001) and mammalian Dab2 is expressed in a wide variety of cells and tissues including macrophages, kidney, white adipose tissues, the placenta, and the adrenal gland (Xu et al., 1995; Fazili et al., 1999; Moore R. et al., 2013; Hocevar, 2019). Aside from Dab1, Dab2 shares sequence similarity to other endocytic adaptors, such as Numb, Numbl, and Arh.
Dab2, Numb, Numblike, and Arh are similar in structure and share an N-terminal phosphotyrosine-binding domain (PTB) or phosphotyrosine-interacting domain (PID) for cargo recognition (Bork and Margolis, 1995; Howell et al., 1999). The PID/PTB domain of Dab2 can interact with transmembrane proteins, such as the LDL and EGF receptors as well as integrins through a NPXY (Asn-Pro-x-Tyr) motif (Morris and Cooper, 2001; Tao et al., 2016a). The interaction between Dab2 and the endocytic proteins clathrin and α-adaptin is mediated by the middle and C-terminal portions of this motif (Traub, 2003). The C-terminus also binds to the motor protein myosin IV (Inoue et al., 2002; Morris et al., 2002a), which facilitates its role in clathrin-mediated endocytosis and trafficking (Figure 3).
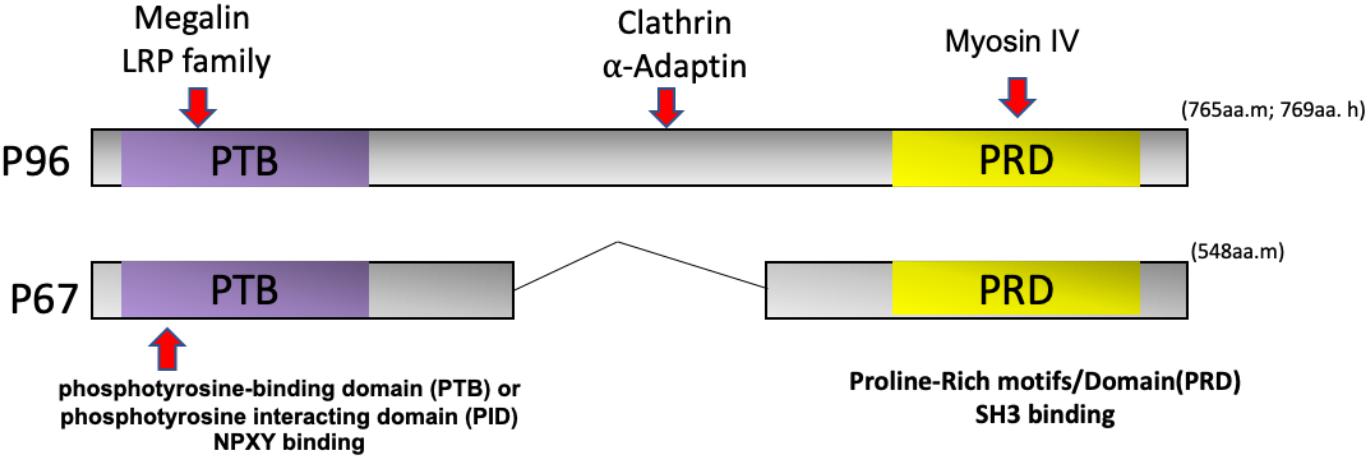
Figure 3. Dab2 domain structure and isoforms. Dab2 has two splice variants called p96 and p67. The NH2-terminal phosphotyrosine-binding domain (PTB) or phosphotyrosine-interacting domain (PID) interacts with receptors, such as the LDL receptor or LDL receptor-related protein (LRP) via the NPXY (Asn-Pro-x-Tyr) motif. The middle region of p96 interacts with endocytic proteins clathrin and α-Adaptin. The COOH-terminal of Dab2 contains a Proline-Rich Domain (PRD) that can bind to Src homology 3 (SH3)-containing proteins. The Dab2 COOH-terminus can also bind myosin VI to mediate endocytic trafficking.
Epsin-Deficient Animal Models
Epsins 1 and 2 are expressed in most vertebrates, contribute to clathrin-mediated endocytosis, and are located near the plasma membrane. Given their ubiquitous expression, their function is strictly cell-type and tissue dependent. Epsins 1 and 2 are widely expressed (Rosenthal et al., 1999), whereas epsin 3 is predominantly expressed in the gastric parietal cells of the stomach (Spradling et al., 2001). As discussed above, epsins are multi-functional proteins that act as endocytic adapters and sort ubiquitinated cargoes. Several studies show that epsins sort ubiquitinated proteins, such as vascular endothelial growth factor receptor-2 (VEGFR2) (Pasula et al., 2012; Dong et al., 2015, 2017), the linear ubiquitin chain assembly complex (LUBAC) (Song et al., 2020), the receptor tyrosine-protein kinase ErbB3 (Szymanska et al., 2016), Notch ligands (Tian et al., 2004; Wang and Struhl, 2004; Chen et al., 2009; Xie et al., 2012; Langridge and Struhl, 2017), and low-density lipoprotein receptor-related protein 1 (LRP1) (Brophy et al., 2019), in addition to playing a role in establishing cell polarity (Li L. et al., 2016). Because epsins are involved in the Notch signaling pathway, which is essential for normal embryonic development, the deficiency of both epsins 1 and 2 results in embryonic lethality. As a result, the study of these proteins in adulthood has relied on inducible knock-out models (Chen and De Camilli, 2005; Chen et al., 2009).
Inducible deficiency of epsins 1 and 2 in endothelial cells in a tumor angiogenesis mouse model revealed the regulatory role of these proteins in vascular development (Song et al., 2017; Dong et al., 2018). More recent studies showed that epsins are involved in regulating Notch signaling to modulate murine embryonic stem cells exit from pluripotency (Cardano et al., 2019). In addition, epsin-mediated Notch signaling has also been reported in another study where the lethality of epsins 1 and 2 double knockout mice die at embryonic day 9.5–10 (E9.5-10), owing to defects in organogenesis, which included failure of blood vessel and heart tube formation and insufficient yolk sac circulation (Chen et al., 2009). Using cell-type and tissue-specific epsin mutant mouse models, the regulatory role of these proteins in development (Chen et al., 2009), tumor angiogenesis (Pasula et al., 2012; Dong et al., 2015), developmental and physiological angiogenesis (Tessneer et al., 2014; Rahman et al., 2016), lymph angiogenesis (Liu et al., 2014; Wu et al., 2018), atherosclerosis (Brophy et al., 2019; Dong et al., 2020), diabetes (Wu et al., 2018), and cancer progression (Pasula et al., 2012; Tessneer et al., 2013a,b; Chang et al., 2015; Dong et al., 2015; Song et al., 2017) have become better understood.
Epsins in Cardiovascular Disease
Atherosclerosis, a leading cause of morbidity and mortality in cardiovascular diseases, is a multi-factorial and chronic inflammatory disease (Lusis, 2000; Falk, 2006; Yin et al., 2013; Fang et al., 2014). It is characterized by low-density lipoprotein (LDL) cholesterol deposition and macrophage accumulation in the arterial wall (Charo and Taubman, 2004; Zhang et al., 2012; Hilgendorf et al., 2015; Bobryshev et al., 2016). Excessive oxidized LDL (oxLDL) and cholesterol esterification result in the formation of foam cells, which subsequently generate atheromatous plaques (Moore K. J. et al., 2013; Yu et al., 2013; Chistiakov et al., 2016). These plaques can rupture and hemorrhage, which leads to severe conditions including myocardial infarction, peripheral artery disease, stroke, and kidney dysfunction (Swirski and Nahrendorf, 2013). Further, this complex process involves the interaction of pathological mediators (e.g., oxLDL) with arterial wall constituents, such as endothelial cells (Mai et al., 2013; Yin et al., 2015; Li X. et al., 2016; Xi et al., 2016; Li et al., 2017, 2018a; Shao et al., 2020), monocyte-derived macrophages, immune cells (including T cells) (Pastrana et al., 2012; Li et al., 2018b), and vascular smooth muscle cells (Galkina and Ley, 2009). Consequently, atherosclerosis has been defined as a metabolic and immune disease that involves multiple cell types (Paoletti et al., 2006; Galkina and Ley, 2009; Sun et al., 2020; Zhong et al., 2020), as well as the liver (Liu and Czaja, 2013; Fargion et al., 2014; Palmal et al., 2014).
Numerous studies indicate that macrophages are crucial for the development of atherosclerotic lesions because they participate in all stages of plaque formation and progression (Yang et al., 2014; Bories and Leitinger, 2017; Groh et al., 2018). In the early stages, circulating monocytes migrate from the blood stream to the arterial intima. Locally polarized macrophage subsets engulf accumulated oxidized lipids, and become foam cells, which accumulate at the lesion sites and, eventually, cause the failure of plaque resolution. Therefore, monocytes/macrophages are central to lesion formation and the progression of atherosclerosis (Shapiro and Fazio, 2017).
Genome wide-association studies have reported several genes related to cancer biology that are also associated with cardiovascular diseases—suggesting the involvement of epsins in both cancer and atherosclerosis (Holdt and Teupser, 2012). In addition, it has been reported that epsin 1 binds to LDLR to facilitate LDLR internalization through an FxNPxY-independent mechanism in Caenorhabditis elegans (Kang et al., 2013). Studies from our laboratory show that epsins are upregulated in lesional macrophages. Using engineered myeloid cell-specific epsin 1 and 2 knock-out mice (LysM-DKO) on an ApoE–/– background and fed a “Western Diet,” these mice display a dramatic reduction in atherosclerotic plaque size and lesion number as well as decreased immune cell infiltration in the aorta and reduced necrotic core formation with an increase in smooth muscle cell number in the aortic root (Brophy et al., 2019). In vitro studies demonstrate the absence of epsins inhibited foam cell formation and reduced M1 phenotype macrophages, but increased M2 phenotype macrophages. We also observed a pro-atherogeneic role for myeloid-specific epsins that was resulted from a downregulation of LRP-1. LRP-1 is known to have anti-atherosclerotic and anti-inflammatory functions, which is mediated by the interaction of the epsin UIM domain with LRP-1. With the treatment of oxLDL, the ubiquitination of LRP-1 was markedly increased, resulting in the enhanced interaction between LRP-1 and epsins 1/2. Genetic reduction of LRP-1 in ApoE–/–/LysM-DKO-LRP1fl/+ mice restored atherosclerosis and confirmed the interaction between LRP1 and epsins in atherosclerosis (Brophy et al., 2019). These findings suggest that myeloid-epsin-mediated LRP-1 downregulation plays a vital role in promoting atherogenesis.
In another study, we found that the inducible deletion of epsins 1 and 2 in endothelial cells significantly attenuated atherosclerosis (Dong et al., 2020). Using cultured aortic endothelial cells from double knock-out (DKO) mice treated with atherogenic cholesterol, we discovered that epsins interact with ubiquitinated inositol 1,4,5-trisphosphate receptor type 1 (IP3R1) to promote the degradation of this calcium release channel (Dong et al., 2020). Furthermore, we confirmed that the binding of epsin to IP3R1 in atherogenic conditions occurred through the UIM and N-terminal suppressor domain (SD) of these proteins, respectively. These findings established the role of epsins in endothelial cell dysfunction and the initiation and progression of atherosclerosis.
Dab2-Deficient Animal Models
Dab2 is a multi-functional adaptor protein and plays roles in many cell functions, including endocytosis (Morris and Cooper, 2001), cell signaling (Drahos et al., 2009; Schutte-Nutgen et al., 2019), lipid uptake (Morris and Cooper, 2001), cholesterol homeostasis (Eden et al., 2007), and cell adhesion (Rosenbauer et al., 2002). Dab2 is also important in embryonic development as deletion of the Dab2 gene in mice leads to early embryonic lethality prior to gastrulation (Morris et al., 2002b). Interestingly, conditional deletion of Dab2 in embryonic stem cells did not affect the development of embryos, but showed reduced clathrin-coated pits, decreased transport mediated by the lipoprotein receptor in kidney proximal tubule, and increased serum cholesterol levels, which suggest a regulatory role for Dab2 in embryonic development and lipoprotein receptor trafficking (Morris et al., 2002b). In addition, the endocytosis of megalin (also known as low density lipoprotein-related protein 2) is mediated by Dab2 by binding to NPXY motifs on the receptor (Maurer and Cooper, 2005). Studies showing the rescue of embryonic viability also indicates that Dab2-mediated endocytosis is critical for embryonic development (Maurer and Cooper, 2005).
Dab2 in Inflammation and Cholesterol Metabolism
Recent studies from Norbert Leitinger’s laboratory found that the expression of Dab2 was increased in M2 macrophages and suppressed in M1 macrophages in both mice and humans (Adamson et al., 2016). Deletion of Dab2 results in a pro-inflammatory M1 phenotype, which suggests that Dab2 regulates macrophage phenotypic polarization and inflammatory signaling by inhibiting the NF-κB pathway by binding to TNF receptor associated factor (TRAF) 6 (Adamson et al., 2016). In other studies, analysis of Dab2-deficient bone marrow revealed increased systemic inflammation and cytokine expression, which led to liver injury; however, the effect of Dab2 deletion in atherosclerosis has yet to be determined (Adamson et al., 2018). Moreover, whether the decreased serum lipids as a result of liver injury could counter the elevated inflammation resulted in pro-inflammatory macrophage accumulation in atherogenesis and whether atherosclerotic lesion formation is impacted because of a myeloid deficiency in Dab2 are poorly understood. Consistent with its role in regulating inflammation, Dab2 was also markedly reduced by toll like receptor (TLR) ligands in a TRIF- and MyD88-dependent manner, resulting in a switch in mucosal dendritic cells from a tolerogenic to a pro-inflammatory phenotype (Figliuolo da Paz et al., 2019). Nevertheless, whether Dab2-mediated modulation of aforesaid inflammation by regulating endocytosis of the plasma membrane cargo is unclear.
On the contrary, Dab2 has been shown to potentially regulate LDL receptor (LDLR) endocytosis, and consequently, LDL uptake and cholesterol metabolism as the PTB/PID domain of Dab2 can bind to the NPXY (Asn-Pro-X-Tyr) motif expressed on LDLR. Lipid uptake is mediated by the LDLR through clathrin-dependent endocytosis and the adaptor proteins Arh and Dab2 can specifically interact with the NPXF motif of LDLR and recruit clathrin/AP-2 to facilitate internalization (Tao et al., 2016b). The latter studies suggest that the deletion of both Dab2 and Arh in liver endothelial cells dramatically elevates serum LDL and cholesterol levels, which is different from a single knockout of either Dab2 or Arh. The authors conclude that Arh and Dab2 work together to regulate hepatic cholesterol synthesis through LDLR endocytosis (Tao et al., 2016b).
It is notable that despite the multiple physiological roles of endocytic adaptor proteins in governing cell signaling, adhesion, nutrient uptake, and synaptic transmission, malfunction of these proteins cause a suite of endocytic defects that perturb cholesterol homeostasis and inaugurate cardiovascular disease, and produce developmental defects, cancer and neurological disorders (Figure 4). There are numerous molecular mechanisms that remain to be uncovered to determine how these adaptor proteins function. In particular, more work is required to understand how epsins and Dab2 act in a cell context-dependent manner as well as elucidate their interacting partners, post-translational modifications, and their role in the development and progression of diseases.
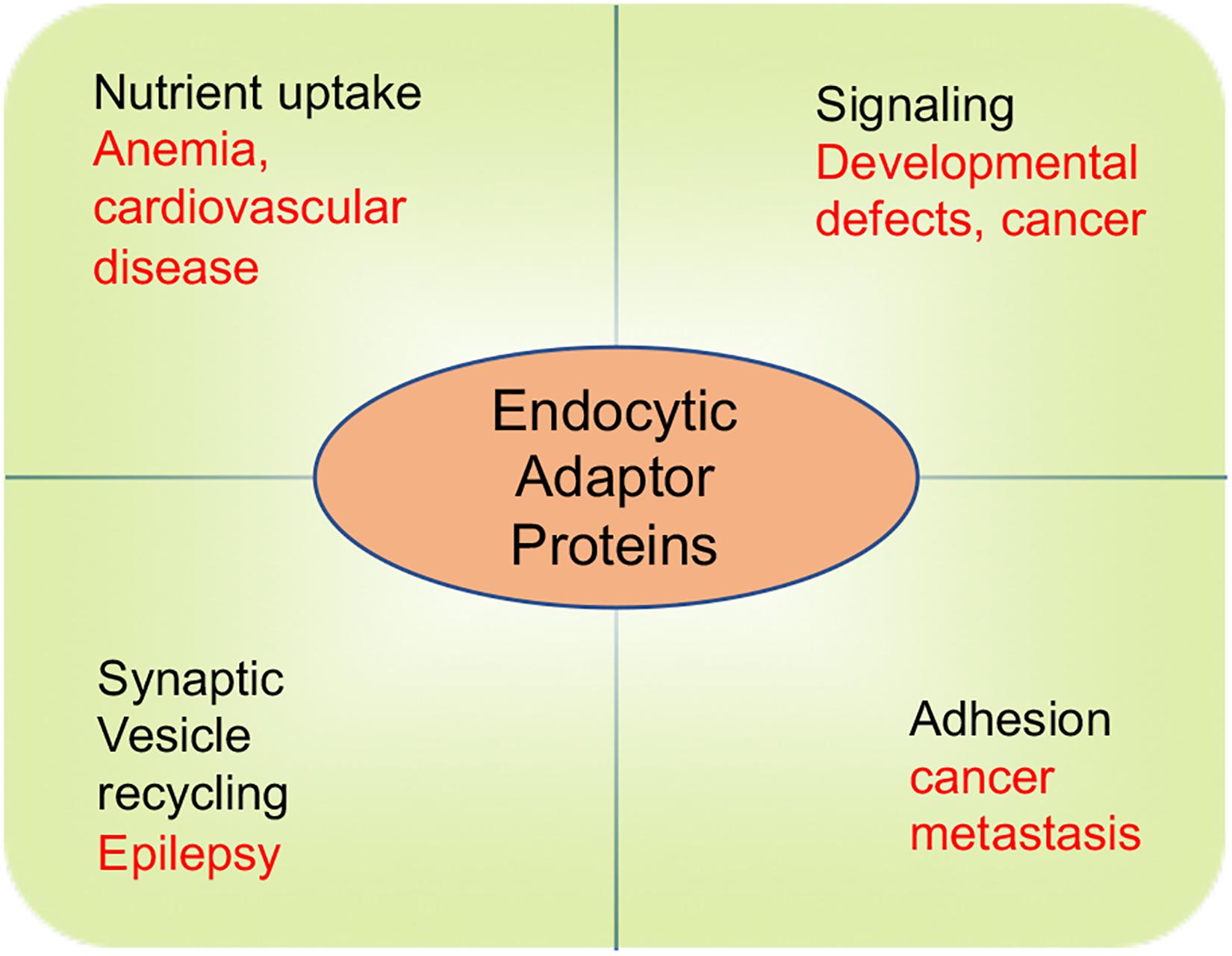
Figure 4. The role of endocytic adaptor proteins in physiological and pathophysiological processes. Physiological processes are depicted by words in black color. Pathological consequences of endocytic defects are depicted by words in red color.
Targeting Epsins and Dab2 to Treat Atherosclerosis
Statins remain the most commonly used drugs to treat or prevent atherosclerosis by lowering cholesterol levels in the circulation (Endo et al., 1976; Goldstein and Brown, 2015); although, anti-hypertensive drugs, such as anti-platelet medications, beta blockers, angiotensin-converting enzyme (ACE) inhibitors, and calcium channel blockers are also used to reduce the symptoms of this disease. Unfortunately, due to their potential side effects in multiple organ systems combined with an increased risk for developing diabetes and cancer (Ramkumar et al., 2016), the safety of wide-spread use of statins remains questionable. Consequently, the identification of new therapeutic targets is warranted.
Promising alternatives to reduce atherosclerosis include targeted therapies for the renin-angiotensin system (RAS) and administration of CD47 blocking antibodies to target macrophages (Lu et al., 2008; Kojima et al., 2016). Targeted therapy of PCSK9 in the liver to manage cholesterol levels is another recent alternative (Rader and Daugherty, 2008; Goldstein and Brown, 2015) and Amgen has recently been marketing an antibody to this protein. In addition, anti-inflammatory therapies are being developed to combat atherosclerosis (Rader and Daugherty, 2008; Tabas and Glass, 2013; Li et al., 2019, 2020). In particular, the CANTOS trial clearly suggests that reducing inflammation in patients with prior cardiovascular events using anti-Interleukin-1β antibodies (i.e., Canakinumab) significantly diminishes the risk of recurrent myocardial infarction (Ridker et al., 2017). At the same time, selectively targeting the activated aortic endothelium has proven more difficult despite its obvious potential for treating atherosclerosis.
In our studies, we have shown that endothelial and macrophage epsins are possible therapeutic targets for the treatment of atherosclerosis (Brophy et al., 2019; Dong et al., 2020). Based on the molecular mechanism that we have uncovered, it may be possible to treat this disease by: (1) targeting atheroma-specific epsins using lipid nanoparticle-based delivery of siRNAs to mitigate inflammatory signaling, (2) blocking epsin binding of IP3R1 or LRP1 using UIM-containing peptides in both the endothelium and macrophages, and (3) using an adeno-associated virus gene therapy approach to downregulate epsin expression in lesions.
While several studies show that Dab2 plays an important role in cellular trafficking of LDLR, the relationship between Dab2 and the development of atherosclerosis remains somewhat obscure. A recent study shows that a Dab2 gene variant is associated with increased coronary artery disease risk (Wang et al., 2020). In another study, quercetin attenuated the progression of atherosclerosis by regulating dendritic cell maturation by upregulating Dab2 expression (Lin et al., 2017). Giving the important role of epsins and Dab2 in endocytosis and the regulation of LDLR and LRP trafficking, fully uncovering the regulatory roles of these proteins in different cells and tissues may result in the development of new anti-atherosclerotic therapeutics.
Perspective
Because of the complexity of atherosclerosis, there have been few effective drugs developed to treat this disease despite decades-long relentless efforts (Weber and Noels, 2011; Libby, 2012; Ridker et al., 2017). As epsins are ubiquitin-binding proteins that play important roles in the vascular system as well as the initiation and progression of atherosclerosis, these endocytic adaptors could represent an important therapeutic target for this disease. Using genetically modified mice, we have demonstrated that the loss of epsins in the aortic endothelium or macrophages inhibits atherosclerosis (Brophy et al., 2019; Dong et al., 2020). Mechanistically, epsins bind IP3R1 in the endothelium and LRP-1 in macrophages via their UIM domain to potentiate atherosclerosis. Targeting epsins in atherosclerotic plaques using liposome nanoparticles containing epsins siRNAs, UIM-containing peptides as competitive inhibitors, or by using a gene therapy approach, may open new avenues to treat atherosclerosis. At the same time, a detailed knowledge of Dab2-receptor interactions in atherosclerosis may identify additional, therapeutically relevant avenues of investigation. In addition, as other endocytic adaptor proteins, such as Numb and Arh, have been implicated in regulating the uptake of cholesterol or cholesterol synthesis (Tao et al., 2016a; Azarnia Tehran et al., 2019). Modulating levels of these endocytic adaptor proteins in cell-restrictive manner would offer equally inspiring opportunity to intervene diseased conditions including dyslipidemia. Thus, developing novel class of drugs that potentially target these endocytic adaptors should offer great promise to circumvent the challenge for the management of dyslipidemia and to reduce cardiovascular diseases.
Author Contributions
KC, YD, BW, DC, S-LC, JS, and HC wrote the manuscript. KC and HC created the figures. All authors contributed to the article and approved the submitted version.
Funding
This work was supported in part by the NIH Grants R01HL093242, R01HL130845, R01HL133216, R01HL137229, R01HL141858, R01HL146134, and R01HL141853 to HC, as well as an Established Investigator Award and a Transformational Project Award from American Heart Association to HC.
Conflict of Interest
The authors declare that the research was conducted in the absence of any commercial or financial relationships that could be construed as a potential conflict of interest.
References
Adamson, S. E., Griffiths, R., Moravec, R., Senthivinayagam, S., Montgomery, G., Chen, W., et al. (2016). Disabled homolog 2 controls macrophage phenotypic polarization and adipose tissue inflammation. J. Clin. Invest. 126, 1311–1322. doi: 10.1172/JCI79590
Adamson, S. E., Polanowska-Grabowska, R., Marqueen, K., Griffiths, R., Angdisen, J., Breevoort, S. R., et al. (2018). Deficiency of Dab2 (Disabled Homolog 2) in myeloid cells exacerbates inflammation in liver and atherosclerotic plaques in LDLR (low-density lipoprotein receptor)-null mice-brief report. Arterioscler Thromb. Vasc. Biol. 38, 1020–1029. doi: 10.1161/ATVBAHA.117.310467
Azarnia Tehran, D., Lopez-Hernandez, T., and Maritzen, T. (2019). Endocytic adaptor proteins in health and disease: lessons from model organisms and human mutations. Cells 8:1345. doi: 10.3390/cells8111345
Bhattacharjee, S., Lee, Y., Zhu, B., Wu, H., Chen, Y., and Chen, H. (2020). Epsins in vascular development, function and disease. Cell Mol. Life Sci. doi: 10.1007/s00018-020-03642-4 [Epub ahead of print]
Bobryshev, Y. V., Ivanova, E. A., Chistiakov, D. A., Nikiforov, N. G., and Orekhov, A. N. (2016). Macrophages and their role in atherosclerosis: pathophysiology and transcriptome analysis. Biomed. Res. Int. 2016:9582430. doi: 10.1155/2016/9582430
Bode, F., Baumann, K., and Kinne, R. (1975). Biochemical aspects of pinocytosis in kidney. Contrib. Nephrol. 1, 21–27. doi: 10.1159/000398225
Bories, G. F. P., and Leitinger, N. (2017). Macrophage metabolism in atherosclerosis. FEBS Lett. 591, 3042–3060. doi: 10.1002/1873-3468.12786
Bork, P., and Margolis, B. (1995). A phosphotyrosine interaction domain. Cell 80, 693–694. doi: 10.1016/0092-8674(95)90347-x
Brophy, M. L., Dong, Y., Tao, H., Yancey, P. G., Song, K., Zhang, K., et al. (2019). Myeloid-specific deletion of epsins 1 and 2 reduces atherosclerosis by preventing LRP-1 downregulation. Circ. Res. 124, e6–e19. doi: 10.1161/CIRCRESAHA.118.313028
Cardano, M., Zasso, J., Ruggiero, L., Di Giacomo, G., Marcatili, M., Cremona, O., et al. (2019). Epsins regulate mouse embryonic stem cell exit from pluripotency and neural commitment by controlling notch activation. Stem Cells Int. 2019:4084351. doi: 10.1155/2019/4084351
Chang, B., Tessneer, K. L., McManus, J., Liu, X., Hahn, S., Pasula, S., et al. (2015). Epsin is required for Dishevelled stability and Wnt signalling activation in colon cancer development. Nat. Commun. 6:6380. doi: 10.1038/ncomms7380
Charo, I. F., and Taubman, M. B. (2004). Chemokines in the pathogenesis of vascular disease. Circ. Res. 95, 858–866. doi: 10.1161/01.RES.0000146672.10582.17
Chen, H., and De Camilli, P. (2005). The association of epsin with ubiquitinated cargo along the endocytic pathway is negatively regulated by its interaction with clathrin. Proc. Natl. Acad. Sci. U.S.A. 102, 2766–2771. doi: 10.1073/pnas.0409719102
Chen, H., Fre, S., Slepnev, V. I., Capua, M. R., Takei, K., Butler, M. H., et al. (1998). Epsin is an EH-domain-binding protein implicated in clathrin-mediated endocytosis. Nature 394, 793–797. doi: 10.1038/29555
Chen, H., Ko, G., Zatti, A., Di Giacomo, G., Liu, L., Raiteri, E., et al. (2009). Embryonic arrest at midgestation and disruption of Notch signaling produced by the absence of both epsin 1 and epsin 2 in mice. Proc. Natl. Acad. Sci. U.S.A. 106, 13838–13843. doi: 10.1073/pnas.0907008106
Chen, H., Slepnev, V. I., Di Fiore, P. P., and De Camilli, P. (1999). The interaction of epsin and Eps15 with the clathrin adaptor AP-2 is inhibited by mitotic phosphorylation and enhanced by stimulation-dependent dephosphorylation in nerve terminals. J. Biol. Chem. 274, 3257–3260. doi: 10.1074/jbc.274.6.3257
Chistiakov, D. A., Bobryshev, Y. V., and Orekhov, A. N. (2016). Macrophage-mediated cholesterol handling in atherosclerosis. J. Cell Mol. Med. 20, 17–28. doi: 10.1111/jcmm.12689
De Camilli, P., Chen, H., Hyman, J., Panepucci, E., Bateman, A., and Brunger, A. T. (2002). The ENTH domain. FEBS Lett. 513, 11–18. doi: 10.1016/s0014-5793(01)03306-3
Dong, J., Saunders, D., Silasi-Mansat, R., Yu, L., Zhu, H., Lupu, F., et al. (2018). Therapeutic efficacy of a synthetic epsin mimetic peptide in glioma tumor model: uncovering multiple mechanisms beyond the VEGF-associated tumor angiogenesis. J. Neurooncol. 138, 17–27. doi: 10.1007/s11060-018-2766-z
Dong, Y., Lee, Y., Cui, K., He, M., Wang, B., Bhattacharjee, S., et al. (2020). Epsin-mediated degradation of IP3R1 fuels atherosclerosis. Nat. Commun. 11:3984. doi: 10.1038/s41467-020-17848-4
Dong, Y., Wu, H., Dong, J., Song, K., Rahman, H. A., Towner, R., et al. (2017). Mimetic peptide of ubiquitin-interacting motif of epsin as a cancer therapeutic-perspective in brain tumor therapy through regulating VEGFR2 signaling. Vessel Plus 1, 3–11. doi: 10.20517/2574-1209.2016.01
Dong, Y., Wu, H., Rahman, H. N., Liu, Y., Pasula, S., Tessneer, K. L., et al. (2015). Motif mimetic of epsin perturbs tumor growth and metastasis. J. Clin. Invest. 125, 4349–4364. doi: 10.1172/JCI80349
Drahos, K. E., Welsh, J. D., Finkielstein, C. V., and Capelluto, D. G. (2009). Sulfatides partition disabled-2 in response to platelet activation. PLoS One 4:e8007. doi: 10.1371/journal.pone.0008007
Eberhard, D. A., Cooper, C. L., Low, M. G., and Holz, R. W. (1990). Evidence that the inositol phospholipids are necessary for exocytosis. Loss of inositol phospholipids and inhibition of secretion in permeabilized cells caused by a bacterial phospholipase C and removal of ATP. Biochem. J. 268, 15–25. doi: 10.1042/bj2680015
Eden, E. R., Sun, X. M., Patel, D. D., and Soutar, A. K. (2007). Adaptor protein disabled-2 modulates low density lipoprotein receptor synthesis in fibroblasts from patients with autosomal recessive hypercholesterolaemia. Hum. Mol. Genet. 16, 2751–2759. doi: 10.1093/hmg/ddm232
Endo, A., Kuroda, M., and Tanzawa, K. (1976). Competitive inhibition of 3-hydroxy-3-methylglutaryl coenzyme A reductase by ML-236A and ML-236B fungal metabolites, having hypocholesterolemic activity. FEBS Lett. 72, 323–326. doi: 10.1016/0014-5793(76)80996-9
Falk, E. (2006). Pathogenesis of atherosclerosis. J. Am. Coll. Cardiol. 47, C7–C12. doi: 10.1016/j.jacc.2005.09.068
Fang, P., Zhang, D., Cheng, Z., Yan, C., Jiang, X., Kruger, W. D., et al. (2014). Hyperhomocysteinemia potentiates hyperglycemia-induced inflammatory monocyte differentiation and atherosclerosis. Diabetes 63, 4275–4290. doi: 10.2337/db14-0809
Fargion, S., Porzio, M., and Fracanzani, A. L. (2014). Nonalcoholic fatty liver disease and vascular disease: state-of-the-art. World J. Gastroenterol. 20, 13306–13324. doi: 10.3748/wjg.v20.i37.13306
Fazili, Z., Sun, W., Mittelstaedt, S., Cohen, C., and Xu, X. X. (1999). Disabled-2 inactivation is an early step in ovarian tumorigenicity. Oncogene 18, 3104–3113. doi: 10.1038/sj.onc.1202649
Figliuolo da Paz, V., Jamwal, D. R., Gurney, M., Midura-Kiela, M., Harrison, C. A., Cox, C., et al. (2019). Rapid downregulation of DAB2 by toll-like receptor activation contributes to a pro-inflammatory switch in activated dendritic cells. Front. Immunol. 10:304. doi: 10.3389/fimmu.2019.00304
Ford, M. G., Mills, I. G., Peter, B. J., Vallis, Y., Praefcke, G. J., Evans, P. R., et al. (2002). Curvature of clathrin-coated pits driven by epsin. Nature 419, 361–366. doi: 10.1038/nature01020
Galkina, E., and Ley, K. (2009). Immune and inflammatory mechanisms of atherosclerosis (∗). Annu. Rev. Immunol. 27, 165–197. doi: 10.1146/annurev.immunol.021908.132620
Gertler, F. B., Bennett, R. L., Clark, M. J., and Hoffmann, F. M. (1989). Drosophila abl tyrosine kinase in embryonic CNS axons: a role in axonogenesis is revealed through dosage-sensitive interactions with disabled. Cell 58, 103–113. doi: 10.1016/0092-8674(89)90407-8
Goldstein, J. L., and Brown, M. S. (2015). A century of cholesterol and coronaries: from plaques to genes to statins. Cell 161, 161–172. doi: 10.1016/j.cell.2015.01.036
Groh, L., Keating, S. T., Joosten, L. A. B., Netea, M. G., and Riksen, N. P. (2018). Monocyte and macrophage immunometabolism in atherosclerosis. Semin. Immunopathol. 40, 203–214. doi: 10.1007/s00281-017-0656-7
Hilgendorf, I., Swirski, F. K., and Robbins, C. S. (2015). Monocyte fate in atherosclerosis. Arterioscler Thromb. Vasc. Biol. 35, 272–279. doi: 10.1161/ATVBAHA.114.303565
Hocevar, B. A. (2019). Loss of disabled-2 expression in pancreatic cancer progression. Sci. Rep. 9:7532. doi: 10.1038/s41598-019-43992-z
Holdt, L. M., and Teupser, D. (2012). Recent studies of the human chromosome 9p21 locus, which is associated with atherosclerosis in human populations. Arterioscler Thromb. Vasc. Biol 32, 196–206. doi: 10.1161/ATVBAHA.111.232678
Howell, B. W., Hawkes, R., Soriano, P., and Cooper, J. A. (1997). Neuronal position in the developing brain is regulated by mouse disabled-1. Nature 389, 733–737. doi: 10.1038/39607
Howell, B. W., Lanier, L. M., Frank, R., Gertler, F. B., and Cooper, J. A. (1999). The disabled 1 phosphotyrosine-binding domain binds to the internalization signals of transmembrane glycoproteins and to phospholipids. Mol. Cell Biol. 19, 5179–5188. doi: 10.1128/mcb.19.7.5179
Inoue, A., Sato, O., Homma, K., and Ikebe, M. (2002). DOC-2/DAB2 is the binding partner of myosin VI. Biochem. Biophys. Res. Commun. 292, 300–307. doi: 10.1006/bbrc.2002.6636
Jaggi, U., Yang, M., Matundan, H. H., Hirose, S., Shah, P. K., Sharifi, B. G., et al. (2020). Increased phagocytosis in the presence of enhanced M2-like macrophage responses correlates with increased primary and latent HSV-1 infection. PLoS Pathog. 16:e1008971. doi: 10.1371/journal.ppat.1008971
Joffe, A. M., Bakalar, M. H., and Fletcher, D. A. (2020). Macrophage phagocytosis assay with reconstituted target particles. Nat. Protoc. 15, 2230–2246. doi: 10.1038/s41596-020-0330-8
Kang, Y. L., Yochem, J., Bell, L., Sorensen, E. B., Chen, L., and Conner, S. D. (2013). Caenorhabditis elegans reveals a FxNPxY-independent low-density lipoprotein receptor internalization mechanism mediated by epsin1. Mol. Biol. Cell 24, 308–318. doi: 10.1091/mbc.E12-02-0163
Ko, G., Paradise, S., Chen, H., Graham, M., Vecchi, M., Bianchi, F., et al. (2010). Selective high-level expression of epsin 3 in gastric parietal cells, where it is localized at endocytic sites of apical canaliculi. Proc. Natl. Acad. Sci. U.S.A. 107, 21511–21516. doi: 10.1073/pnas.1016390107
Kojima, Y., Volkmer, J. P., McKenna, K., Civelek, M., Lusis, A. J., Miller, C. L., et al. (2016). CD47-blocking antibodies restore phagocytosis and prevent atherosclerosis. Nature 536, 86–90. doi: 10.1038/nature18935
Langridge, P. D., and Struhl, G. (2017). Epsin-dependent ligand endocytosis activates notch by force. Cell 171, 1383–1396.e12. doi: 10.1016/j.cell.2017.10.048
Li, L., Han, L., Zhang, J., Liu, X., Ma, R., Hou, X., et al. (2016). Epsin2 promotes polarity establishment and meiotic division through activating Cdc42 in mouse oocyte. Oncotarget 7, 50927–50936. doi: 10.18632/oncotarget.10815
Li, X., Fang, P., Li, Y., Kuo, Y. M., Andrews, A. J., Nanayakkara, G., et al. (2016). Mitochondrial reactive oxygen species mediate lysophosphatidylcholine-induced endothelial cell activation. Arterioscler Thromb. Vasc. Biol. 36, 1090–1100. doi: 10.1161/ATVBAHA.115.306964
Li, X., Fang, P., Sun, Y., Shao, Y., Yang, W. Y., Jiang, X., et al. (2020). Anti-inflammatory cytokines IL-35 and IL-10 block atherogenic lysophosphatidylcholine-induced, mitochondrial ROS-mediated innate immune activation, but spare innate immune memory signature in endothelial cells. Redox Biol. 28:101373. doi: 10.1016/j.redox.2019.101373
Li, X., Fang, P., Yang, W. Y., Chan, K., Lavallee, M., Xu, K., et al. (2017). Mitochondrial ROS, uncoupled from ATP synthesis, determine endothelial activation for both physiological recruitment of patrolling cells and pathological recruitment of inflammatory cells. Can. J. Physiol. Pharmacol. 95, 247–252. doi: 10.1139/cjpp-2016-0515
Li, X., Fang, P., Yang, W. Y., Wang, H., and Yang, X. (2019). IL-35, as a newly proposed homeostasis-associated molecular pattern, plays three major functions including anti-inflammatory initiator, effector, and blocker in cardiovascular diseases. Cytokine 122:154076. doi: 10.1016/j.cyto.2017.06.003
Li, X., Shao, Y., Sha, X., Fang, P., Kuo, Y. M., Andrews, A. J., et al. (2018a). IL-35 (Interleukin-35) suppresses endothelial cell activation by inhibiting mitochondrial reactive oxygen species-mediated site-specific acetylation of H3K14 (Histone 3 Lysine 14). Arterioscler Thromb. Vasc. Biol. 38, 599–609. doi: 10.1161/ATVBAHA.117.310626
Li, X., Wang, L., Fang, P., Sun, Y., Jiang, X., Wang, H., et al. (2018b). Lysophospholipids induce innate immune transdifferentiation of endothelial cells, resulting in prolonged endothelial activation. J. Biol. Chem. 293, 11033–11045. doi: 10.1074/jbc.RA118.002752
Libby, P. (2012). Inflammation in atherosclerosis. Arterioscler Thromb. Vasc. Biol. 32, 2045–2051. doi: 10.1161/ATVBAHA.108.179705
Lin, W., Wang, W., Wang, D., and Ling, W. (2017). Quercetin protects against atherosclerosis by inhibiting dendritic cell activation. Mol. Nutr. Food Res. 61:1700031. doi: 10.1002/mnfr.201700031
Liu, K., and Czaja, M. J. (2013). Regulation of lipid stores and metabolism by lipophagy. Cell Death Differ. 20, 3–11. doi: 10.1038/cdd.2012.63
Liu, X., Pasula, S., Song, H., Tessneer, K. L., Dong, Y., Hahn, S., et al. (2014). Temporal and spatial regulation of epsin abundance and VEGFR3 signaling are required for lymphatic valve formation and function. Sci. Signal. 7:ra97. doi: 10.1126/scisignal.2005413
Lu, H., Rateri, D. L., Feldman, D. L., Charnigo, R. J. Jr., Fukamizu, A., Ishida, J., et al. (2008). Renin inhibition reduces hypercholesterolemia-induced atherosclerosis in mice. J. Clin. Invest. 118, 984–993. doi: 10.1172/JCI32970
Mai, J., Virtue, A., Shen, J., Wang, H., and Yang, X. F. (2013). An evolving new paradigm: endothelial cells–conditional innate immune cells. J. Hematol. Oncol. 6:61. doi: 10.1186/1756-8722-6-61
Maurer, M. E., and Cooper, J. A. (2005). Endocytosis of megalin by visceral endoderm cells requires the Dab2 adaptor protein. J. Cell Sci. 118(Pt 22), 5345–5355. doi: 10.1242/jcs.02650
Moore, K. J., Sheedy, F. J., and Fisher, E. A. (2013). Macrophages in atherosclerosis: a dynamic balance. Nat. Rev. Immunol. 13, 709–721. doi: 10.1038/nri3520
Moore, R., Cai, K. Q., Tao, W., Smith, E. R., and Xu, X. X. (2013). Differential requirement for Dab2 in the development of embryonic and extra-embryonic tissues. BMC Dev. Biol. 13:39. doi: 10.1186/1471-213X-13-39
Morris, S. M., Arden, S. D., Roberts, R. C., Kendrick-Jones, J., Cooper, J. A., Luzio, J. P., et al. (2002a). Myosin VI binds to and localises with Dab2, potentially linking receptor-mediated endocytosis and the actin cytoskeleton. Traffic 3, 331–341. doi: 10.1034/j.1600-0854.2002.30503.x
Morris, S. M., and Cooper, J. A. (2001). Disabled-2 colocalizes with the LDLR in clathrin-coated pits and interacts with AP-2. Traffic 2, 111–123. doi: 10.1034/j.1600-0854.2001.020206.x
Morris, S. M., Tallquist, M. D., Rock, C. O., and Cooper, J. A. (2002b). Dual roles for the Dab2 adaptor protein in embryonic development and kidney transport. EMBO J. 21, 1555–1564. doi: 10.1093/emboj/21.7.1555
Oldham, C. E., Mohney, R. P., Miller, S. L., Hanes, R. N., and O’Bryan, J. P. (2002). The ubiquitin-interacting motifs target the endocytic adaptor protein epsin for ubiquitination. Curr. Biol. 12, 1112–1116. doi: 10.1016/s0960-9822(02)00900-4
Orlov, V. S. (1988). The theory of pinocytosis. Factors determining the dynamics of pinocytosis in capillary endothelium. Biofizika 33, 117–120.
Palmal, S., Maity, A. R., Singh, B. K., Basu, S., Jana, N. R., and Jana, N. R. (2014). Inhibition of amyloid fibril growth and dissolution of amyloid fibrils by curcumin-gold nanoparticles. Chemistry 20, 6184–6191. doi: 10.1002/chem.201400079
Paoletti, R., Bolego, C., Poli, A., and Cignarella, A. (2006). Metabolic syndrome, inflammation and atherosclerosis. Vasc. Health Risk Manag. 2, 145–152. doi: 10.2147/vhrm.2006.2.2.145
Pastrana, J. L., Sha, X., Virtue, A., Mai, J., Cueto, R., Lee, I. A., et al. (2012). Regulatory T cells and Atherosclerosis. J. Clin. Exp. Cardiolog. 2012(Suppl. 12), 2. doi: 10.4172/2155-9880.S12-002
Pasula, S., Cai, X., Dong, Y., Messa, M., McManus, J., Chang, B., et al. (2012). Endothelial epsin deficiency decreases tumor growth by enhancing VEGF signaling. J. Clin. Invest. 122, 4424–4438. doi: 10.1172/JCI64537
Pearse, B. M., Smith, C. J., and Owen, D. J. (2000). Clathrin coat construction in endocytosis. Curr. Opin. Struct. Biol. 10, 220–228. doi: 10.1016/s0959-440x(00)00071-3
Polo, S., Sigismund, S., Faretta, M., Guidi, M., Capua, M. R., Bossi, G., et al. (2002). A single motif responsible for ubiquitin recognition and monoubiquitination in endocytic proteins. Nature 416, 451–455. doi: 10.1038/416451a
Rader, D. J., and Daugherty, A. (2008). Translating molecular discoveries into new therapies for atherosclerosis. Nature 451, 904–913. doi: 10.1038/nature06796
Rahman, H. A., Wu, H., Dong, Y., Pasula, S., Wen, A., Sun, Y., et al. (2016). Selective targeting of a novel epsin-VEGFR2 interaction promotes VEGF-mediated angiogenesis. Circ. Res. 118, 957–969. doi: 10.1161/CIRCRESAHA.115.307679
Ramkumar, S., Raghunath, A., and Raghunath, S. (2016). Statin therapy: review of safety and potential side effects. Acta Cardiol. Sin. 32, 631–639. doi: 10.6515/acs20160611a
Reine, J., Rylance, J., Ferreira, D. M., Pennington, S. H., Welters, I. D., Parker, R., et al. (2020). The whole blood phagocytosis assay: a clinically relevant test of neutrophil function and dysfunction in community-acquired pneumonia. BMC Res. Notes 13:203. doi: 10.1186/s13104-020-05034-0
Ridker, P. M., Everett, B. M., Thuren, T., MacFadyen, J. G., Chang, W. H., Ballantyne, C., et al. (2017). Antiinflammatory therapy with canakinumab for atherosclerotic disease. N. Engl. J. Med. 377, 1119–1131. doi: 10.1056/NEJMoa1707914
Rosenbauer, F., Kallies, A., Scheller, M., Knobeloch, K. P., Rock, C. O., Schwieger, M., et al. (2002). Disabled-2 is transcriptionally regulated by ICSBP and augments macrophage spreading and adhesion. EMBO J. 21, 211–220. doi: 10.1093/emboj/21.3.211
Rosenthal, J. A., Chen, H., Slepnev, V. I., Pellegrini, L., Salcini, A. E., Di Fiore, P. P., et al. (1999). The epsins define a family of proteins that interact with components of the clathrin coat and contain a new protein module. J. Biol. Chem. 274, 33959–33965. doi: 10.1074/jbc.274.48.33959
Sakamoto, C., Kawamoto, C., Takeuchi, K., Miyamoto, I., and Shuntoh, H. (2004). Fission yeast epsin, Ent1p is required for endocytosis and involved in actin organization. Kobe J. Med. Sci. 50, 47–57.
Salcini, A. E., Chen, H., Iannolo, G., De Camilli, P., and Di Fiore, P. P. (1999). Epidermal growth factor pathway substrate 15, Eps15. Int. J. Biochem. Cell Biol. 31, 805–809. doi: 10.1016/s1357-2725(99)00042-4
Schutte-Nutgen, K., Edeling, M., Mendl, G., Krahn, M. P., Edemir, B., Weide, T., et al. (2019). Getting a Notch closer to renal dysfunction: activated Notch suppresses expression of the adaptor protein Disabled-2 in tubular epithelial cells. FASEB J. 33, 821–832. doi: 10.1096/fj.201800392RR
Shao, Y., Saredy, J., Yang, W. Y., Sun, Y., Lu, Y., Saaoud, F., et al. (2020). Vascular endothelial cells and innate immunity. Arterioscler Thromb. Vasc. Biol 40, e138–e152. doi: 10.1161/ATVBAHA.120.314330
Shapiro, M. D., and Fazio, S. (2017). Apolipoprotein B-containing lipoproteins and atherosclerotic cardiovascular disease. F1000Res. 6:134. doi: 10.12688/f1000research.9845.1
Sheng, Z., He, J., Tuppen, J. A., Sun, W., Fazili, Z., Smith, E. R., et al. (2000). Structure, sequence, and promoter analysis of human disabled-2 gene (DAB2). Genomics 70, 381–386. doi: 10.1006/geno.2000.6383
Sheng, Z., Smith, E. R., He, J., Tuppen, J. A., Martin, W. D., Dong, F. B., et al. (2001). Chromosomal location of murine disabled-2 gene and structural comparison with its human ortholog. Gene 268, 31–39. doi: 10.1016/s0378-1119(01)00401-2
Smirnov, A., Solga, M. D., Lannigan, J., and Criss, A. K. (2020). Using imaging flow cytometry to quantify neutrophil phagocytosis. Methods Mol. Biol. 2087, 127–140. doi: 10.1007/978-1-0716-0154-9_10
Song, K., Cai, X., Dong, Y., Wu, H., Wei, Y., Shankavaram, U., et al. (2020). Epsins 1 and 2 promote NEMO linear ubiquitination via LUBAC to drive breast cancer development. J. Clin. Invest. doi: 10.1172/JCI129374 [Epub ahead of print]
Song, K., Wu, H., Rahman, H. N., Dong, Y., Wen, A., Brophy, M. L., et al. (2017). Endothelial epsins as regulators and potential therapeutic targets of tumor angiogenesis. Cell Mol. Life Sci. 74, 393–398. doi: 10.1007/s00018-016-2347-2
Spradling, K. D., McDaniel, A. E., Lohi, J., and Pilcher, B. K. (2001). Epsin 3 is a novel extracellular matrix-induced transcript specific to wounded epithelia. J. Biol. Chem. 276, 29257–29267. doi: 10.1074/jbc.M101663200
Sun, L., Yang, X., Yuan, Z., and Wang, H. (2020). Metabolic reprogramming in immune response and tissue inflammation. Arterioscler Thromb. Vasc. Biol. 40, 1990–2001. doi: 10.1161/ATVBAHA.120.314037
Swirski, F. K., and Nahrendorf, M. (2013). Leukocyte behavior in atherosclerosis, myocardial infarction, and heart failure. Science 339, 161–166. doi: 10.1126/science.1230719
Szymanska, M., Fosdahl, A. M., Raiborg, C., Dietrich, M., Liestol, K., Stang, E., et al. (2016). Interaction with epsin 1 regulates the constitutive clathrin-dependent internalization of ErbB3. Biochim. Biophys. Acta 1863, 1179–1188. doi: 10.1016/j.bbamcr.2016.03.011
Tabas, I., and Glass, C. K. (2013). Anti-inflammatory therapy in chronic disease: challenges and opportunities. Science 339, 166–172. doi: 10.1126/science.1230720
Tao, W., Moore, R., Meng, Y., Smith, E. R., and Xu, X. X. (2016a). Endocytic adaptors Arh and Dab2 control homeostasis of circulatory cholesterol. J. Lipid Res. 57, 809–817. doi: 10.1194/jlr.M063065
Tao, W., Moore, R., Smith, E. R., and Xu, X. X. (2016b). Endocytosis and physiology: insights from disabled-2 deficient mice. Front. Cell Dev. Biol. 4:129. doi: 10.3389/fcell.2016.00129
Tessneer, K. L., Cai, X., Pasula, S., Dong, Y., Liu, X., Chang, B., et al. (2013a). Epsin family of endocytic adaptor proteins as oncogenic regulators of cancer progression. J. Can. Res. Updates 2, 144–150. doi: 10.6000/1929-2279.2013.02.03.2
Tessneer, K. L., Pasula, S., Cai, X., Dong, Y., Liu, X., Yu, L., et al. (2013b). Endocytic adaptor protein epsin is elevated in prostate cancer and required for cancer progression. ISRN Oncol. 2013:420597. doi: 10.1155/2013/420597
Tessneer, K. L., Pasula, S., Cai, X., Dong, Y., McManus, J., Liu, X., et al. (2014). Genetic reduction of vascular endothelial growth factor receptor 2 rescues aberrant angiogenesis caused by epsin deficiency. Arterioscler Thromb. Vasc. Biol. 34, 331–337. doi: 10.1161/ATVBAHA.113.302586
Tian, X., Hansen, D., Schedl, T., and Skeath, J. B. (2004). Epsin potentiates Notch pathway activity in Drosophila and C. elegans. Development 131, 5807–5815. doi: 10.1242/dev.01459
Traub, L. M. (2003). Sorting it out: AP-2 and alternate clathrin adaptors in endocytic cargo selection. J. Cell Biol. 163, 203–208. doi: 10.1083/jcb.200309175
Wang, W., and Struhl, G. (2004). Drosophila Epsin mediates a select endocytic pathway that DSL ligands must enter to activate Notch. Development 131, 5367–5380. doi: 10.1242/dev.01413
Wang, Y., Wang, Y., Adi, D., He, X., Liu, F., Abudesimu, A., et al. (2020). Dab2 gene variant is associated with increased coronary artery disease risk in Chinese Han population. Medicine (Baltimore) 99:e20924. doi: 10.1097/MD.0000000000020924
Weber, C., and Noels, H. (2011). Atherosclerosis: current pathogenesis and therapeutic options. Nat. Med. 17, 1410–1422. doi: 10.1038/nm.2538
Wu, H., Rahman, H. N. A., Dong, Y., Liu, X., Lee, Y., Wen, A., et al. (2018). Epsin deficiency promotes lymphangiogenesis through regulation of VEGFR3 degradation in diabetes. J. Clin. Invest. 128, 4025–4043. doi: 10.1172/JCI96063
Xi, H., Zhang, Y., Xu, Y., Yang, W. Y., Jiang, X., Sha, X., et al. (2016). Caspase-1 inflammasome activation mediates homocysteine-induced pyrop-apoptosis in endothelial cells. Circ. Res. 118, 1525–1539. doi: 10.1161/CIRCRESAHA.116.308501
Xie, X., Cho, B., and Fischer, J. A. (2012). Drosophila Epsin’s role in Notch ligand cells requires three Epsin protein functions: the lipid binding function of the ENTH domain, a single Ubiquitin interaction motif, and a subset of the C-terminal protein binding modules. Dev. Biol. 363, 399–412. doi: 10.1016/j.ydbio.2012.01.004
Xu, X. X., Yang, W., Jackowski, S., and Rock, C. O. (1995). Cloning of a novel phosphoprotein regulated by colony-stimulating factor 1 shares a domain with the Drosophila disabled gene product. J. Biol. Chem. 270, 14184–14191. doi: 10.1074/jbc.270.23.14184
Yang, J., Zhang, L., Yu, C., Yang, X. F., and Wang, H. (2014). Monocyte and macrophage differentiation: circulation inflammatory monocyte as biomarker for inflammatory diseases. Biomark. Res. 2:1. doi: 10.1186/2050-7771-2-1
Yin, Y., Li, X., Sha, X., Xi, H., Li, Y. F., Shao, Y., et al. (2015). Early hyperlipidemia promotes endothelial activation via a caspase-1-sirtuin 1 pathway. Arterioscler Thromb. Vasc. Biol. 35, 804–816. doi: 10.1161/ATVBAHA.115.305282
Yin, Y., Pastrana, J. L., Li, X., Huang, X., Mallilankaraman, K., Choi, E. T., et al. (2013). Inflammasomes: sensors of metabolic stresses for vascular inflammation. Front. Biosci. (Landmark Ed) 18:638–649.
Yu, X. H., Fu, Y. C., Zhang, D. W., Yin, K., and Tang, C. K. (2013). Foam cells in atherosclerosis. Clin. Chim. Acta 424, 245–252. doi: 10.1016/j.cca.2013.06.006
Zhang, D., Fang, P., Jiang, X., Nelson, J., Moore, J. K., Kruger, W. D., et al. (2012). Severe hyperhomocysteinemia promotes bone marrow-derived and resident inflammatory monocyte differentiation and atherosclerosis in LDLr/CBS-deficient mice. Circ. Res. 111, 37–49. doi: 10.1161/CIRCRESAHA.112.269472
Keywords: epsin, disabled-homolog 2, endocytic adaptor proteins, atherosclerosis, diabetes, inflammation, receptor-mediated endocytosis, clathrin
Citation: Cui K, Dong Y, Wang B, Cowan DB, Chan S-L, Shyy J and Chen H (2020) Endocytic Adaptors in Cardiovascular Disease. Front. Cell Dev. Biol. 8:624159. doi: 10.3389/fcell.2020.624159
Received: 30 October 2020; Accepted: 23 November 2020;
Published: 11 December 2020.
Edited by:
Xiaofeng Yang, Temple University, United StatesReviewed by:
Jun Yu, Temple University, United StatesTohru Fukai, Augusta University, United States
Copyright © 2020 Cui, Dong, Wang, Cowan, Chan, Shyy and Chen. This is an open-access article distributed under the terms of the Creative Commons Attribution License (CC BY). The use, distribution or reproduction in other forums is permitted, provided the original author(s) and the copyright owner(s) are credited and that the original publication in this journal is cited, in accordance with accepted academic practice. No use, distribution or reproduction is permitted which does not comply with these terms.
*Correspondence: Hong Chen, aG9uZy5jaGVuQGNoaWxkcmVucy5oYXJ2YXJkLmVkdQ==