- 1Department of Animal Reproduction, National Institute for Agriculture and Food Research and Technology (INIA), Madrid, Spain
- 2Servicio Regional de Investigación y Desarrollo Agroalimentario (SERIDA), Gijón, Spain
According to the World Health Organization, infertility affects up to 14% of couples under reproductive age, leading to an exponential rise in the use of assisted reproduction as a route for conceiving a baby. In the same way, thousands of embryos are produced in cattle and other farm animals annually, leading to increased numbers of individuals born. All reproductive manipulations entail deviations of natural phenotypes and genotypes, with in vitro embryo technologies perhaps showing the biggest effects, although these alterations are still emerging. Most of these indications have been provided by animal models, in particular the bovine species, due to its similarities to human early embryo development. Oocytes and embryos are highly sensitive to environmental stress in vivo and in vitro. Thus, during in vitro culture, a number of stressful conditions affect embryonic quality and viability, inducing subfertility and/or long-term consequences that may reach the offspring. A high proportion of the embryos produced in vitro are arrested at a species-specific stage of development during the first cell divisions. These arrested embryos do not show signs of programmed cell death during early cleavage stages. Instead, defective in vitro produced embryos would enter a permanent cell cycle arrest compatible with cellular senescence, in which they show active metabolism and high reactive oxygen species levels. Later in development, mainly during the morula and blastocyst stages, apoptosis would mediate the elimination of certain cells, accomplishing both a physiological role in to balancing cell proliferation and death, and a pathological role preventing the transmission of damaged cells with an altered genome. The latter would acquire relevant importance in in vitro produced embryos that are submitted to stressful environmental stimuli. In this article, we review the mechanisms mediating apoptosis and senescence during early embryo development, with a focus on in vitro produced bovine embryos. Additionally, we shed light on the protective role of senescence and apoptosis to ensure that unhealthy cells and early embryos do not progress in development, avoiding long-term detrimental effects.
Introduction
Human in vitro embryo production has been one of the most remarkable medical achievements of the past century, enabling infertile couples, persons with heritable genetic diseases, or oncology patients to conceive a baby. Since then, more than 8 million babies have been born from assisted reproductive technologies (ARTs) around the world, and it is estimated that around 2.4 million ART cycles are performed every year, leading to 500,000 babies born (Eshre, 2018). The first human baby produced by in vitro fertilization (IVF) was born 42 years ago (Steptoe and Edwards, 1978), and since then ARTs have been optimized in various mammalian species. Soon after, IVF was introduced as a very complex experimental procedure in cattle (Brackett et al., 1982), the species other than human with better-developed ARTs. Over the years, the procedures have been simplified and improved due to the development of in vitro maturation (IVM) of oocytes recovered from slaughterhouse ovaries or a living animal (transvaginal ovum pickup: OPU), followed by IVF and in vitro culture (IVC). Nowadays, in vitro embryo production in cattle is not only used to overcome infertility (due to anovulation, fertilization failure (Looney et al., 1994; Block et al., 2010; Gomez et al., 2020), or productive and heat stress (Ealy et al., 1993; Block et al., 2010; Stewart et al., 2011), but as a faster means to obtain embryos of high genetic value. Worldwide, while the number of in vivo collected embryos that are transferred seems to have stabilized, the transfer of in vitro-produced (IVP) embryos continues to grow (742,908 embryos in 2018) (Viana, 2019). Although there are important differences between cattle and humans that need to be considered, such as the techniques employed, with intracytoplasmic sperm injection (ICSI) being used only in humans, and the age and fertility status of the animals/patients, cattle are frequently used as a model to study preimplantation embryo development, as well as infertility issues in humans, since most technologies used share many similarities. In fact, much of the initial human-based ARTs were based on work completed in cattle (Sirard, 2018). In vitro studies in cattle with early IVP and in vivo developed (IVD) embryos are normally focused on the period into which the blastocyst remains enclosed in the zona pellucida (Rizos et al., 2017). These blastocysts, which are 7–8 days old, bear the best pregnancy and birth rates for embryo transfer (ET) to recipients (Ledgard et al., 2012; Randi et al., 2016). Subsequent processes (i.e., trophectoderm –TE- elongation in concurrence with gastrulation) are maternally driven in ruminants, with no in vitro studies being up to now supportive of a functionally representative elongation.
However, despite all efforts performed to optimize ARTs, gametes and embryos are still submitted to stressful conditions in vitro. IVF and intracytoplasmic sperm injection (ICSI) bypass natural selection barriers and involve IVC of gametes and embryos, where nutrients are limited and toxic substrates and end-products of metabolism can be present (Calle et al., 2012; Ramos-Ibeas et al., 2019). Embryos show outstanding plasticity during preimplantation development and can often grow under suboptimal conditions. However, severe alterations can also lead to a state of developmental arrest similar to cellular senescence or trigger apoptotic cell death (Betts and King, 2001). In this review, we shed some light on the debated aspects of embryonic senescence and apoptosis as mechanisms to impede damaged cells and compromised embryos to progress in development. Such important restrictions at peri-conception stages would avoid long-term deleterious effects on the offspring (Barker, 2007).
Impact of Assisted Reproduction on Embryo Development: Differences Between in vivo and in vitro Produced Embryos
Despite many improvements made in the field of assisted reproduction, in vitro embryo production systems are still not as efficient as in vivo development (Rizos et al., 2017). Notwithstanding, it should be emphasized that gametes and embryos are exposed to spatial and temporal unnatural conditions during ART, whose consequences are not completely known (Van Eetvelde et al., 2017). In cattle, approximately 90% of oocytes cultured in vitro undergo nuclear and cytoplasmic maturation, from which 80% are fertilized and cleave at least once (Lonergan et al., 2003). Contrary to in vivo development, whereby one or less frequently two oocytes are ovulated in the cow, in vitro procedures permit maturation of virtually all oocytes in the absence of the inhibitory effects of the dominant follicle (Labrecque et al., 2016; Nagano, 2019). Nonetheless, only between 30 and 40% of such oocytes reach the blastocyst stage (Rizos et al., 2017). While at first appearance this may appear inefficient, it is important to remember that the oocytes used for IVM come from small (2–8 mm) follicles, which would never ovulate in vivo and whose fate would be atresia. Moreover, it has been demonstrated that pregnancy rate following transfer of IVP blastocysts in heifers is approximately 40–50% compared to 60–70% for IVD embryos (Hasler et al., 1995; Hoshi, 2003; see review by Hansen, 2020). Therefore, the challenge today is to improve current IVM conditions to obtain more blastocysts and to improve IVC conditions to produce better-quality blastocysts, capable of continuing development and implantation after transfer to recipient and resulting in births of viable and healthy individuals.
Oocyte developmental competence, often defined as the ability of the oocyte to mature, be fertilized, and develop to the blastocyst stage, has been associated with the size of the antral follicle from which it is recovered, the stage of the follicular wave, and the site of maturation—in vivo or in vitro (Pavlok et al., 1992, see review by Lonergan and Fair, 2016). Oocytes matured in vivo are of better quality than those matured in vitro, and this is reflected in the rates of embryos produced subsequently. Indeed, irrespective of whether embryo development occurred in vivo or in vitro, when bovine oocytes were matured in vivo from superovulated cows, the resultant blastocyst rate was almost 80%, while when oocytes were matured in vitro, it was limited to about 35% (Rizos et al., 2002).
In mammals, the cumulus-oocyte complex (COC) provides metabolites and nutrients, like pyruvate, oxaloacetic acid, and amino acids to the oocytes during their growth, besides stimulating them to resume meiosis and progress to metaphase II (Chen et al., 1990). Several studies demonstrated that gene functions and signaling pathways interact between the oocyte and CCs (Regassa et al., 2011). Thus, gene expression patterns in CCs are currently used as indicators of oocyte quality (Tesfaye et al., 2009; Bunel et al., 2015). Besides, IVM and in vivo maturation differ in the amounts of mRNA transcripts stored in the ooplasm (Lonergan et al., 2003), with more than 100 differentially expressed genes between in vitro- and in vivo-matured bovine oocytes being responsible for depleting their developmental competence (Katz-Jaffe et al., 2009; Adona et al., 2016).
In humans, IVM can be an alternative for patients with ovarian pathologies affecting oocyte quality (Ata et al., 2010; Shalom-Paz et al., 2010) and can also reduce the risks of ovarian hyperstimulation syndrome (Sauerbrun-Cutler et al., 2015). However, despite its potential benefits, IVM is still marginally used in human ART, with applications mainly in fertility preservation (Kasum et al., 2015; Shirasawa and Terada, 2017). Therefore, a better understanding of the mechanisms involved in oocyte competence during IVM is crucial in the optimization of this technology.
The fate of an embryo is determined at fertilization. Delays in fertilization or fertilization by a damaged spermatozoon could conceivably lead to oocyte aging or to the formation of a defective embryo, respectively (Tarín et al., 2000). However, in the bovine species, IVC seems to be one of the major factors determining embryo quality. As mentioned before, IVC embryos show poorer morphology, cryotolerance, distinct transcript expression profiles, and pregnancy rates after transfer than IVD embryos (see review by Lonergan, 2007). Alternating in vitro and in vivo embryo culture demonstrated the importance of developing in vitro systems mimicking the in vivo situation. Thus, the culture of IVP bovine zygotes in vivo in the oviduct of sheep (Rizos et al., 2002), cow (Tesfaye et al., 2007), or mouse (Rizos et al., 2007), increased the quality of the resulting blastocysts. Conversely, IVC of in vivo produced bovine zygotes resulted in blastocysts of low quality (Rizos et al., 2002). However, IVC before and during embryonic genome activation (EGA) did not affect embryo development rates, irrespective of where culture took place, although IVC did affect the transcriptome of such blastocysts (Gad et al., 2012).
Negative factors within IVC include media supplementation with fetal calf serum (FCS), which reduces embryo cryotolerance, induces alterations in gene expression and leads to large offspring syndrome in cattle, a disorder caused by epigenetic alterations in the embryo that is phenotypically similar to Beckwith-Wiedemann syndrome in humans (Young et al., 1998; Lazzari et al., 2002; Rizos et al., 2003; Wrenzycki et al., 2005). Compared to in vivo derived embryos, IVP blastocysts produced with FCS had 5 times more differentially expressed genes than serum-free IVP blastocysts (1,109 vs. 207) (Heras et al., 2016). Serum derivatives added to culture can also trigger long term detrimental effects, as shown by improved survival to cryopreservation and reduced miscarriage rates observed when bovine serum albumin (BSA) was removed from the culture at the time of blastocyst formation (Murillo-Rios et al., 2017; Gomez et al., 2020). The embryonic genome is epigenetically reprogrammed after fertilization. This process involves activating or silencing specific genes by laying on appropriate methylation patterns (Reik et al., 2001). During this critical period, the embryo is especially vulnerable to epigenetic defects, which can be induced by IVC (El Hajj and Haaf, 2013). Indeed, animal studies have revealed links between different ARTs and imprinting disorders, via altered DNA methylation patterns and histone codes (Urrego et al., 2014). Such disorders are more prevalent in gametes and embryos after ARTs than in their in vivo-derived counterparts (Urrego et al., 2014). Thus, IVP embryos show culture-induced, stage-specific, and non-stage-specific, aberrant DNA methylation patterns in paternally or maternally imprinted genes and in several genomic clusters (Salilew-Wondim et al., 2015, 2018). The post-fertilization culture environment is therefore crucial for the quality of the resulting blastocysts (see review by Rizos et al., 2017; Wrenzycki, 2018). To overcome the limitations of conventional IVC and mimic in vivo conditions, different embryo culture systems have been developed such as co-culture with bovine oviduct epithelial cells (BOECs) (Schmaltz-Panneau et al., 2014) or supplementation of culture media with oviductal and uterine fluid in sequential culture, as well as the use of extracellular vesicles (EVs) (Rodriguez-Alonso et al., 2020). All these systems entail improvements in IVC for blastocyst development and their quality in terms of cryotolerance, cell counts, cell apoptosis and gene expression (Alminana et al., 2017; Lopera-Vasquez et al., 2017; Almiñana and Bauersachs, 2019). In particular, EVs secreted by donor oviductal cells increased birth rates after embryo transfer in mice due to decreased apoptosis and improved cellular differentiation in embryos (Qu et al., 2019). Furthermore, supplementation of IVC medium with uterine exosomes improved the developmental capacity of embryos generated by somatic cell nuclear transfer (SCNT) (Qiao et al., 2018). Taking together the above findings, it could be suggested that the effect of EVs on early embryonic development may be fundamental, and IVC systems containing EVs can provide new insights to improve gamete maturation, fertilization, and embryo development during ARTs.
In parallel, other research lines based on chemical modifications of simple medium, polarized and three-dimensional (3D) cell co-cultures, lead to improved embryo development and quality. These systems could allow cultured gametes and embryos to experience some of the same mechanical forces, mimicking interactions with the substratum of the oviduct and endometrium, and dynamic changes that occur in the microenvironment of the reproductive tract. The BOEC polarized system consists of growing the cells on inserts to allow media access from basolateral and apical sides, and to maintain the polarized asymmetrical structure of the oviductal epithelial cells. Epithelial cells derived from human, porcine or bovine oviduct maintained polarity and in vivo–like morphology when they were cultured for long term in a polarized air-liquid interface system (Levanon et al., 2010; Chen et al., 2013, 2017). It was evidenced that air-liquid system supports embryo development in vitro without culture medium supply in porcine, mouse and bovine species; however, the obtained blastocyst rates could not yet match the outcome of optimized standard IVP procedures, suggesting that further improvement of the model is required (Chen et al., 2017). In cattle, using the 3D printing technology in combination with microfluidics resulted in the creation of “oviduct-on-a-chip” with a U-shaped porous membrane allowing BOEC polarization that could be maintained during long-term mimicking tissue- and organ-specific micro-architecture (Ferraz et al., 2017b). It was demonstrated that culturing BOEC in 3D system improved embryo production by allowing proper sperm and oocyte interactions, fertilization, and completely abolishing polyspermic and parthenogenic activation of oocytes in the absence of added sperm activating factors (Ferraz et al., 2017a). Although technical issues contributed to lower cleavage and developmental rates than in a conventional embryo production system, the DNA methylation intensity and transcript abundance in zygotes produced in the microfluidics device were more similar to embryos produced in vivo than to embryos produced in a conventional IVP system (Ferraz et al., 2018).
Senescence During Early Embryo Development
Cellular senescence is a state of irreversible cell cycle arrest that can be induced through different mechanisms, such as genomic and telomeric damage, epigenetic perturbations, reactive oxygen species (ROS), or activation of oncogenes, and mitogenic signals (Lopez-Otin et al., 2013). This event was first noticed in primary human fibroblasts that had undergone replicative exhaustion after a finite number of passages (“Hayflick’s limit”) (Hayflick and Moorhead, 1961). Nowadays, this state is known as replicative senescence and is associated with telomeres shortening. However, stress-induced premature senescence can be also induced as a defense mechanism in damaged cells that are not able to undergo apoptosis, and programmed senescence has been reported during development (Lozono-Torres et al., 2019).
Senescent cells show specific morphological and biochemical features, often presenting a large flattened morphology with increased cytoplasmic and nuclear volume in culture (Rhinn et al., 2019). These cells remain metabolically active, resist to apoptosis-inducing stimuli (Baar et al., 2017) and show high ROS levels and β-galactosidase activity (Wang and Dreesen, 2018). At the molecular level, the senescent response is mediated by two main components: the intrinsic arm and the extrinsic arm (Rhinn et al., 2019). The intrinsic arm is mediated by proteins that inhibit cell cycle progression, including tumor protein P53 (p53), p21 (encoded by Cyclin Dependent Kinase Inhibitor 1A; Cdkn1a), p16INKA and p19ARF (both encoded by Cyclin Dependent Kinase Inhibitor 2A Cdkn2a) (Kuilman et al., 2010; Martinez-Zamudio et al., 2017), and by microRNA-mediated gene silencing (Benhamed et al., 2012). The extrinsic arm comprises the “senescence-associated secretory phenotype” (SASP), mediated by cytokines, chemokines, growth factors, extracellular matrix (ECM), and ECM-remodeling proteins (Freund et al., 2010; Acosta et al., 2013) that are transcriptionally regulated by nuclear factor kappa-light-chain-enhancer of activated B cells (Nfkb), CCAAT/enhancer-binding protein beta (Cebpβ), p38, p53, and GATA binding protein 4 (Gata4) transcription factors (Acosta et al., 2008; Kuilman et al., 2008; Kang et al., 2015). The SASP allows senescent cells to secrete signaling molecules, generating a microenvironment, and activating the immune system to remove damaged or stressed cells (Kang et al., 2011; Sagiv et al., 2016).
Although cellular senescence has been mainly associated with tumor suppression, tissue repair or aging (Baker et al., 2011; Campisi, 2013; Ritschka et al., 2017), features of senescence have been also reported in cells from different mammalian species during embryonic development (Rhinn et al., 2019). In the mouse, senescent cells have been identified by expression of β-galactosidase, p21 and SASP factors in many tissues during specific time windows of fetal development (mesonephros, neural tube, gut endoderm, heart, and the developing limbs, etc.) (Munoz-Espin et al., 2013; Storer et al., 2013; Lorda-Diez et al., 2019). These cells emerge and are removed by macrophages in a tightly controlled manner, resulting in tissue remodeling and patterning. Interestingly, senescent cells in the developing fetus are negative to certain senescence markers described in adult tissues, such as p53, p16INKA, p19ARF, and DNA damage. Instead, developmental senescence is strictly dependent on p21 and is regulated by the TGFβ/SMAD and PI3K/FOXO pathways (Munoz-Espin et al., 2013; Storer et al., 2013; Table 1). This senescence model seems to be conserved in vertebrate development, as similar patterns have been observed in chick (Storer et al., 2013) and human embryos (Munoz-Espin et al., 2013), and could have emerged initially as a basic developmental mechanism that subsequently evolved to a protective role to face aging and tumor suppression in adult tissues.
Going one more step back in development up to the preimplantational embryo, the existence of senescence was first hypothesized almost 20 years ago (Betts and King, 2001). A high proportion of the embryos produced in vitro are arrested at a species-specific stage of development during the first cell divisions, before EGA. These arrested embryos do not show signs of apoptosis or necrosis, since features of programmed cell death are not detected during the early cleavage stages (Byrne et al., 1999; Hardy, 1999; Matwee et al., 2000). Interestingly, when the apoptotic pathway is partially activated by exposing embryos at early cleavage stages to protein kinase inhibitors or mitochondria depolarizing agents, limited caspase activation, and DNA fragmentation can be observed (Matwee et al., 2000; Gjorret et al., 2007). This might suggest that components of the apoptosis machinery are available before EGA, but cannot be physiologically activated due to the absence of mature mitochondria (Plante and King, 1994; Van Blerkom, 2004) or to certain inhibition of the apoptotic pathway (Brad et al., 2007). Instead, defective IVP embryos would enter a state of permanent cell cycle arrest before EGA compatible with cellular senescence, in which they show active metabolism and high ROS levels (Betts and Madan, 2008; Figure 1).
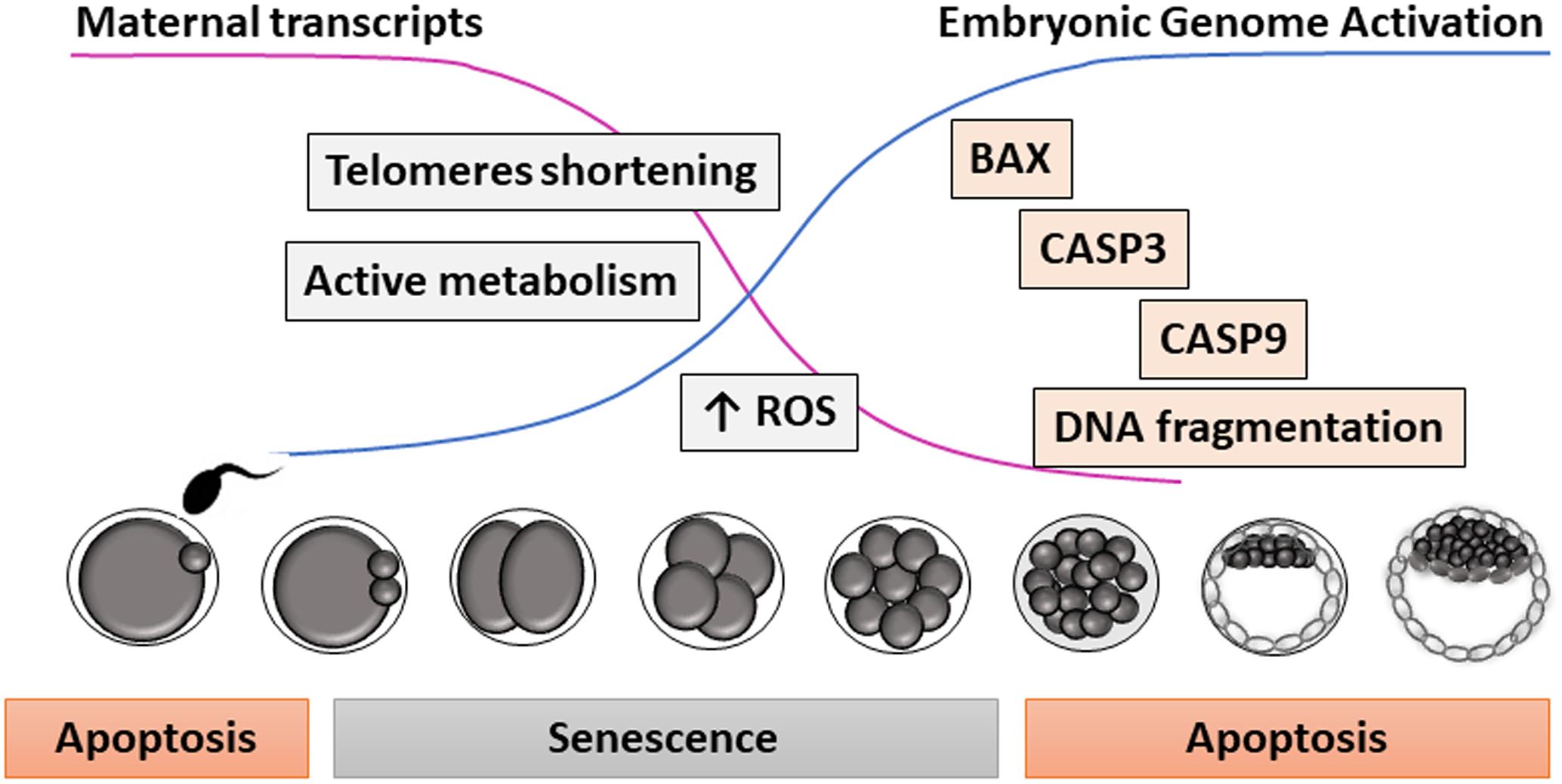
Figure 1. Proposed model for apoptosis and senescence during early embryo development. Due to the low amount of specific embryonic transcripts before embryonic genome activation (i.e., telomerase), embryos are very sensitive to environmental stressors during early cleavage stages, entering into a state of cell arrest resembling senescence. Later in development, embryos (and oocytes before fertilization) can show DNA fragmentation and other morphological signs of apoptosis.
Assisted reproductive technologies induce oxidative stress in the embryos (Ramos-Ibeas et al., 2019), which might also generate DNA and telomeric damage before EGA (Betts and Madan, 2008). At these stages of development, many transcripts are scarce, including telomerase, the enzyme responsible for telomere elongation (Betts and King, 1999). Alternatively spliced telomerase variants associated with a lack of telomerase activity have been detected in some human oocytes and poorly developing embryos (Brenner et al., 1999). Moreover, telomere length in human oocytes predicts embryo fragmentation at day 3 of development, supporting the theory of reproductive senescence in women (Keefe et al., 2005). Thus, stress-mediated telomeric damage due to sub-optimal culture conditions, shortened telomeres derived from aged oocytes, or absence of proper telomerase activity in low-quality oocytes or embryos could trigger embryo senescence before EGA (Betts and King, 2001; Figure 2).
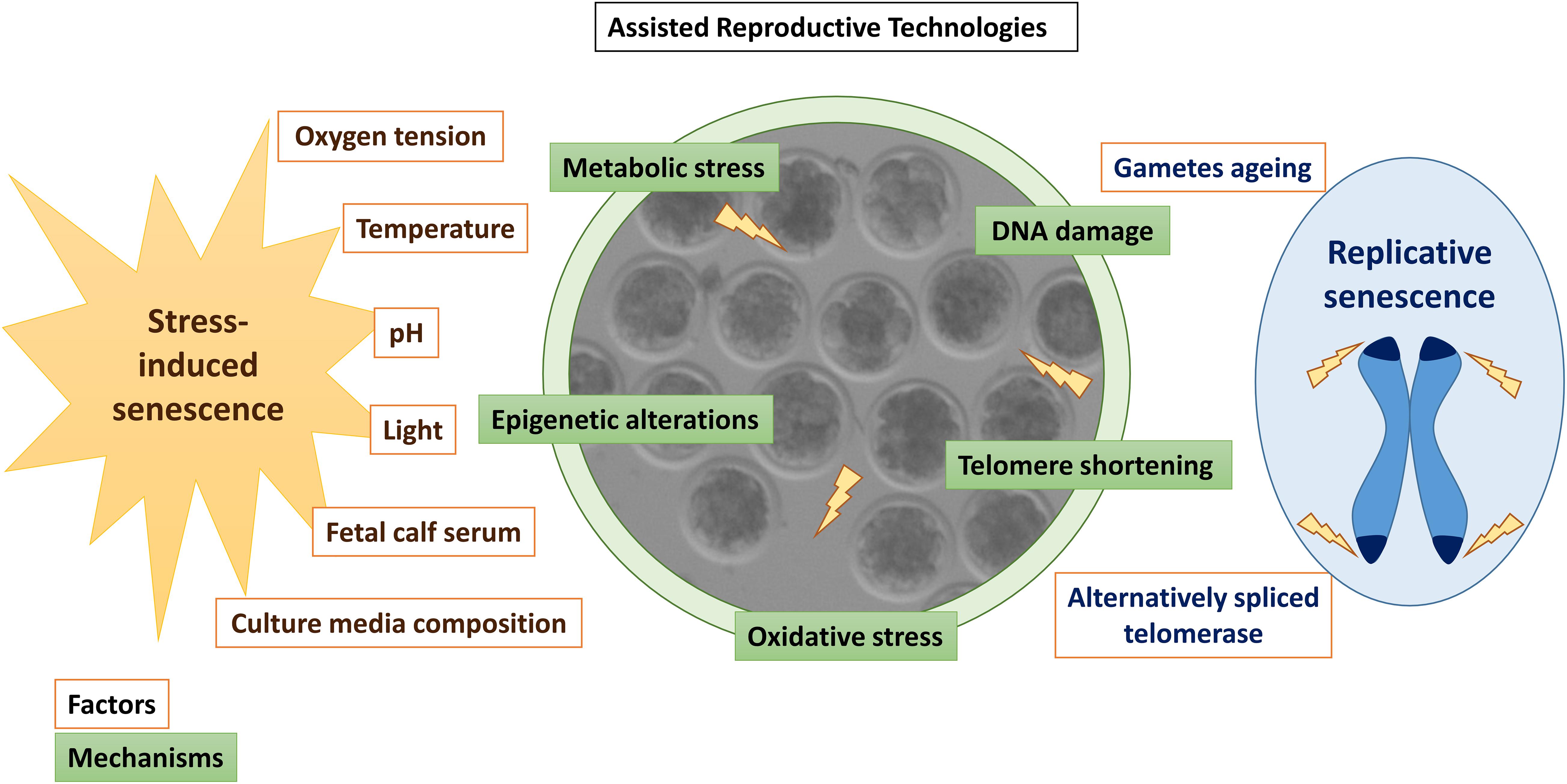
Figure 2. Summary of factors (orange) and mechanisms (green) inducing senescence in in vitro produced embryos. Stressors associated with in vitro embryo culture are written in brown letters, while factors associated with replicative senescence are written in blue.
In contrast to senescent adult cells and in the same way as in fetal cells, p53 is not involved in embryo senescence during early cleavage stages (Favetta et al., 2004; Favetta et al., 2007a; Velez-Pardo et al., 2007; Meuter et al., 2014). Furthermore, p16INKA, which is involved in the senescence response in adult tissues, has not been detected in embryos (Egashira et al., 2011; Meuter et al., 2014). However, IVP bovine embryos arrested at the 2-cell stage were positive for phosphorylated γH2AX, a senescence marker that was not detected in proliferating 2-cell embryos (Betts and Madan, 2008). Besides, a direct correlation between the oxidative stress adaptor protein p66Shc levels and the duration of the embryonic arrest was found in these relatively transcriptionally silent embryos, so p66Shc might play a key role in the senescence response induced by ROS in early embryos (Favetta et al., 2004, 2007b). Additional markers of senescence in IVP embryos are β-galactosidase and p21 expression (Meuter et al., 2014; Ock et al., 2020; Table 1). Upregulation of p21 has been reported in mouse preimplantation embryos in response to heat stress or X-ray radiation that led to developmental delay or blockage in damaged embryos (Ock et al., 2020). In the absence of p21, damaged embryos can progress to the blastocyst stage, although exhibiting increased apoptosis and chromosome instability (Adiga et al., 2007). Thus, p21 plays a protective role in the embryo, mediating a senescent phenotype in response to exogenous stressors.
Therefore, senescence seems to play a protective role during preimplantational development, acting as a checkpoint mechanism to ensure that unhealthy embryos enter into a permanently arrested state and do not progress in development, avoiding long-term effects on health and reproduction. This mechanism would become even more important in IVP embryos, which are exposed to multiple stressors (Ramos-Ibeas et al., 2019). It would be interesting to investigate whether senescent embryos secrete signaling factors that could exert a detrimental effect on the rest of the embryos in culture.
Apoptosis During Early Embryo Development
Cell death exerts an essential role in governing early mammalian embryo development (Hardy, 1997; Betts and King, 2001; Leidenfrost et al., 2011; Vandaele and Van Soom, 2011). Early embryos progress in development faster than tumor cells because of the lower embryonic demand for membranes construction and lack of new biomass synthesis (Krisher and Prather, 2012). A delicate balance between cell proliferation and death is therefore required for successful development to term. This view of cell death, as a regulatory process, alternates with its pathological role (Betts and King, 2001; Leidenfrost et al., 2011). Regulated cell death (RCD) exists mainly in multicellular organisms as a form of genetically programmed elimination of cells that are perceived as dispensable, noxious, or with irreversible damage. Physiological forms of RCD do not require intracellular or extracellular alterations (Conradt, 2009; Fuchs and Steller, 2011). On the contrary, prolonged and/or intense stimulation, insofar as they produce stress and disturb cellular homeostasis, induce pathological RCD (Galluzzi et al., 2016). Apoptosis is a form of RCD existing in multiple tissues during embryonic and fetal development in mammals. The apoptotic cell is rounded and shrunk, showing condensed, marginalized, and later fragmented chromatin, intact nuclear envelope, shrunk organelles and contained cytoplasm (Voss and Strasser, 2020). A recent hypothesis postulated that apoptosis and cell proliferation are independent processes that work together better than alone in regulating growth (Voss and Strasser, 2020). The role of apoptosis in mammalian development is not only to control cell proliferation, including the deletion of cells and portions of tissues, but also to regulate morphogenesis, including the formation of cavities, and vesicles in compact structures and modeling tissue shape (reviewed by Diamantis et al., 2008). Since the framework of early morphogenetic events is not yet started in early embryo development, the apoptosis mechanisms seem to be limited to eliminate abnormal cells, as there is no information about apoptosis regulating other essential hallmarks until the blastocyst stage. A recent study in a mouse model of chromosome mosaicism supports this hypothesis, where abnormal aneuploid cells are eliminated by autophagy and apoptosis at the peri- and post-implantation stages of development (Singla et al., 2020). In human ART, apoptosis has been postulated as a candidate mechanism to explain elevated monozygotic twining (MZT), which would be facilitated by more labile junctions between the ICM cells in IVP embryos (Hviid et al., 2018). Thus, high glucose in culture increases MZT rates, explained as increased ROS production and subsequent apoptosis that would disrupt the ICM, with the possible help of overpressure during hatching (Ménézo and Sakkas, 2002). Frequent blastocyst collapse could relocate some ICM cells on the trophectoderm wall, thereby forming a second, ectopic ICM, and subsequent MZT (Payne et al., 2007). Embryo biopsy and cryopreservation, together with specific donor conditions have also been cited as factors underlying increased MZT, always with apoptosis as a triggering factor (reviewed by Tauwinklova et al., 2010). In the cow, increased MZT has been not documented within IVP embryos.
Thus, the interest in apoptosis within in vivo and in vitro produced embryos lies in its strong consistency with a variety of stresses and poor development conditions, and this makes the apoptosis signs timely markers of an abnormal environment and likely responsible of increased MZT in humans. Hence, one of the hallmarks of IVP is an apoptosis rate generally higher than embryos naturally developing in the genital tract (Pomar et al., 2005), indicating that in vitro conditions are poorer for the embryo.
Apoptotic Pathways Within Early Embryos
Apoptosis is regulated through two convergent pathways, depending on whether the signals initiating cell death originate within or outside the cell: (1) the extrinsic or death receptor pathway, mainly activated by members of the tumor necrosis factor (TNF) family that bind to their family of cognate receptors (TNFR), and (2) the intrinsic, mitochondrial, or B-cell lymphoma 2 (BCL-2) regulated pathway. Both apoptotic pathways are active –but not completely- in early embryos under stage-specific conditions (Leidenfrost et al., 2011).
Intrinsic Apoptosis
Intrinsic apoptosis pathway proceeds at least by well-defined environmental perturbations (Galluzzi et al., 2018). The bovine embryo has been identified as responsive to some of such alterations: (1) Endoplasmic reticulum (ER) stress, which simultaneously increases expression of ER stress (Glucose-Regulated Protein, 78 kDa (GRP78), Activating Transcription Factor 4 (ATF4), Activating Transcription Factor 6 (ATF6), Regulator Of G Protein Signaling 1 (RGS1) and X-Box Binding Protein 1 (XBP1)] and pro-apoptotic [C/EBP Homologous Protein (CHOP) and BCL2 Associated X, Apoptosis Regulator (BAX)] genes, at the same time that development rates, cryotolerance, cell survival and apoptotic cell rates improve (Yoon et al., 2014; Khatun et al., 2020); (2) ROS overload can also trigger apoptosis and developmental affectance (Huang and Chan, 2017; Luo et al., 2020); (3) Irregular DNA structures, out of which only a subset can be identified by TUNEL (Gjorret et al., 2003; Leidenfrost et al., 2011); abnormal segregation of chromosomes in mitosis is a possible origin of such structures (Gjorret et al., 2003); (4) Mitotic effects, where apoptosis was observed in response to multinucleate cells (Paula and Hansen, 2008) and cytokinesis becomes blocked leading to a “mitotic catastrophe” by checkpoint activation (Huang et al., 2005); (5) Specific miRNAs that trigger apoptosis, since slow-cleaving bovine embryos in culture release miRNA-30c, which targets cyclin-dependent kinase 12 (CDK12) and lead to apoptosis (Juan et al., 2016; Lin et al., 2019), although it is unknown whether dead cells or live cells with a commitment release miR-30c; 6) Growth factors removal as shown by specific growth factors identified in the genital tract that reduce apoptosis when added to IVC (Trigal et al., 2011; Gomez et al., 2014). In pigs, vascular endothelial growth factor (VEGF) decreased in parallel to an increase in embryo development, concomitant with a reduction in mRNA abundance of caspase 3 (CASP3) and increased BCL2 Apoptosis Regulator (BCL2) and Nuclear Factor, Erythroid 2 Like 2 NRF-2 (Biswas et al., 2018).
Synergies between the above inducers may exert a more tuned regulation of apoptosis and proliferation, as shown in cancer cells, where more than 300 miRNAs respond to retinoic acid (RA) to inhibit cell invasiveness and deregulate growth (Lima et al., 2019). Interestingly, RA is a main activated pathway in the bovine uterus (Bauersachs et al., 2005). In vitro, RA regulates apoptosis in the bovine blastocyst through both retinoid receptors RXR and RAR (Rodriguez et al., 2006, 2007; Gomez et al., 2008).
Extrinsic Apoptosis
Extracellular perturbations can trigger apoptosis through the extrinsic pathway, activated by receptor-ligand interaction between extrinsic molecules and mainly two types of receptors in the plasma membrane: (1) Death receptors, activated by cognate ligands; (2) Dependence receptors, activated by falling of ligand concentrations below specific thresholds (Galluzzi et al., 2018). Death receptors include Fas cell surface receptor (FAS, also termed as APO-1 or CD95), and TNF receptor superfamily member 1A (TNFR1), 10a (DR4 or TRAILR1), and 10b (DR5 or TRAIL2) (Wajant, 2002; Aggarwal et al., 2012; Von Karstedt et al., 2017). The extrinsic apoptosis pathway could be partly inactive in bovine embryos because of the lack of convergence with the intrinsic pathway to activate the effector caspases, since caspase-8 would be absent or inactive. Together with caspase-8, FAS/FASL transcripts are at very low abundance or absent in embryos (Leidenfrost et al., 2011). Furthermore, the FAS signaling pathway is inactive in bovine, human and mouse oocytes (Sato, 1998; De Los Santos et al., 2000; Pomar et al., 2004). The alternative pathway of necroptosis, a regulated common morphology of necrotic and apoptotic cells, which in turn should become active by caspase-8 inhibition, has not been studied in bovine embryos to our knowledge. However, morphological evidence associated with this RCD type has been described (Gjorret et al., 2003; Rodriguez et al., 2006). A third signaling pathway through death receptor is represented by Nuclear factor-Kappa-B (NF-h) activation, which usually leads to a wide inflammatory response linked to cell survival (Nguyen-Chi et al., 2017; Von Karstedt et al., 2017). The bovine blastocyst is equipped with NF-
B inducers, such as TNF (Correia-Alvarez et al., 2015b) and Interleukin 1 Beta (IL1b) (Correia-Alvarez et al., 2015a). In the uterus, Day 6/Day 8 embryos need to depress a natural pro-inflammatory status to progress in development; evidence of such depression, including reduced NF-
in the uterine fluid, has been provided (Munoz et al., 2012). Interestingly, the sex of the embryo determines the extent of such a response in the uterus, with female blastocysts showing higher apoptotic rates in their ICM (Gomez et al., 2013).
Apoptosis Chronology During Early Development Stages
The timing at which the IVP embryo responds to external stimuli through apoptosis -as an endogenous mechanism of removing cells- has been defined in stage-specific studies with embryos. However, perturbations of the oocyte and spermatozoa environments can also result in an apoptotic response in the generated embryo (El Hajj and Haaf, 2013; Wang et al., 2017; Bittner et al., 2018), although this topic is out of the scope of the present review.
Apoptosis and kinetic patterns of development are related, since the incidence of embryos with dying/dead cells increases through development by stage and day. However, the most advanced embryonic stages within a day show lower apoptosis rates. Pioneering studies (Byrne et al., 1999; Matwee et al., 2000; Gjorret et al., 2003) defined the chronology of apoptosis within IVD and IVP cattle embryos. Embryos were examined by morphological apoptotic traits (M) and analysis of DNA fragmentation using terminal deoxynucleotidyl transferase-mediated dUTP nick end labeling (TUNEL) (T) (Gjorret et al., 2003). Only nuclei showing T + M were regarded as completely apoptotic. Apoptotic M was rarely detectable before the 9–16 cell stage. In contrast, significant proportions of mature and immature oocytes showed T staining (Matwee et al., 2000), indicating that apoptotic processes became repressed after fertilization, since no IVD embryos and very few IVP embryos (< 3%) showed complete apoptotic T + M up to the morula stage. The first sign of T + M was observed at the 8-cell stage (Byrne et al., 1999; Matwee et al., 2000; Paula-Lopes and Hansen, 2002; Gjorret et al., 2003), while close to 50% Day-3 IVP embryos (i.e., at different development stages) contained at least one cell showing any sign of M (Leidenfrost et al., 2011). Thus, at the blastocyst stage rarely an embryo was found without dying/dead cells, with steadily higher apoptotic rates in the ICM (usually 25–40% total cells in blastocysts) than in the trophectoderm (Gjorret et al., 2003; Rodriguez et al., 2006; Gomez et al., 2008, 2014, 2020; Leidenfrost et al., 2011; Munoz et al., 2014; Murillo et al., 2017), suggesting a more stringent regulation in the developmentally important and yet undifferentiated ICM. Such differences are not apparent in human embryos, although appropriate studies are limited and with low sample numbers (Sjöblom et al., 2002; Hardy et al., 2003).
Signaling pathways and genes involved in the apoptotic response have been analyzed during early embryo development in the bovine species. In an elegant study, Leidenfrost and co-workers analyzed genes involved in both extrinsic and intrinsic apoptosis initiation (Figure 3; Leidenfrost et al., 2011). Within IVP embryos, the copy numbers of CASP3, X-linked Inhibitor of Apoptosis (XIAP), BAX, BCL2 Like 1 (BCL2L1), Caspase 9 (CASP9), and Signal Transducer and Activator of Transcription 3 (STAT3) declined from the oocyte until day 3/4, to gradually increase thereafter. Interestingly, transcripts for the extrinsic initiator caspase-8 were undetectable from the oocyte up to the hatching blastocyst stage (Leidenfrost et al., 2011). Contrary to the caspase-9 protein from the intrinsic apoptotic pathway, caspase-8 was not induced in 2-cell embryos by the apoptotic promoter ceramide, which increases the numbers of multinucleated cells (Paula and Hansen, 2008), an effect by which cytokinesis becomes blocked leading to a “mitotic catastrophe” by checkpoint activation (Huang et al., 2005).
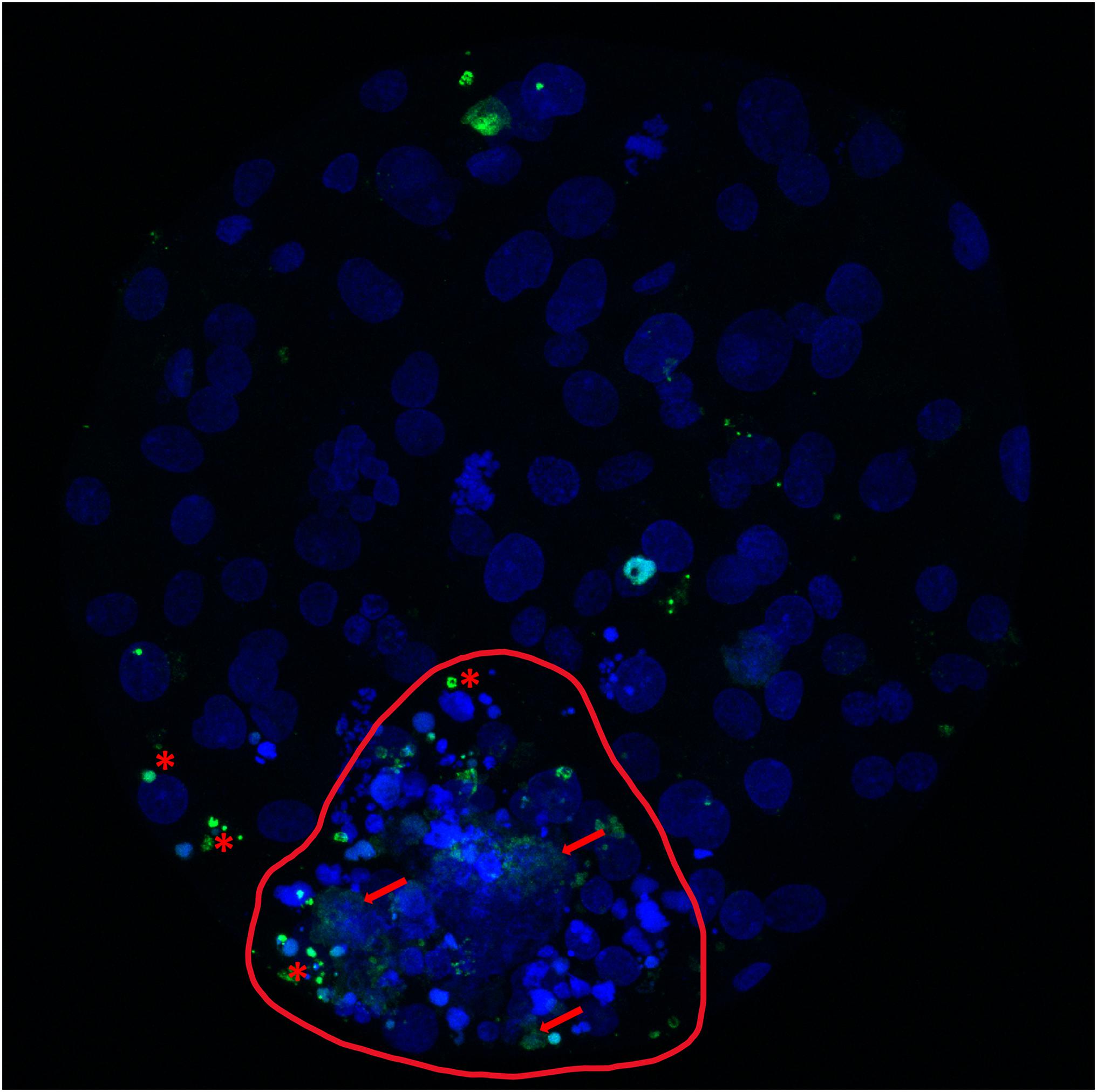
Figure 3. Cell death analysis in a bovine expanded blastocyst produced in vitro. Bovine expanded blastocyst produced in vitro and stained with bisbenzimide (blue) to visualize all nuclei and TdT-mediated dUTP nick-end labeling (TUNEL; green). Red asterisks mark TUNEL-positive nuclei undergoing the apoptotic pathway (condensed, TUNEL-positive structures that can appear scattered as smaller apoptotic bodies and correspond to chromatin and cytoplasmic condensation, with intact nuclear membrane). Red arrows mark nuclei undergoing necrosis (TUNEL-positive structures, with unclear, swollen and frayed edges; red arrows). The inner cell mass (circled, red line) shows the highest incidence of identified and non-recognizable forms of cell death, in contrast with a lower incidence in the more abundant trophectoderm cells.
Immunocytochemical staining of the effector protein CASP3, revealed also increasing apoptotic rates from cleavage through the blastocyst stages, but CASP3 positive embryos appeared before the 8-cell stage (Oliveira et al., 2016) at higher rates than those with T + M (see the above-cited works). Such differences may represent incomplete repression of apoptotic mechanisms, or activation of specific subsections, as suggested (Gjorret et al., 2003). The EGA, at the 8–16 cell stage in cattle, entails a complete re-organization of the apoptotic machinery in the embryo, which in this way would reach complete capacity to reorganize cell growth and differentiation. Altered EGA within IVP embryos would be involved in the distinct occurrence of apoptosis features between IVP embryos and their in vivo counterparts.
Embryo Apoptotic Responses to in vitro and in vivo Stress Sources
Early embryos can respond to a variety of stressors through apoptosis activation in vivo and in vitro (Figure 4). Heat-shock-induced apoptosis can occur in cattle embryos exposed to high environmental temperatures, being more acute in high-producing dairy cows due to their higher metabolic heat; 8–16 cell embryos are the first stage able to respond to heat stress (De Barros and Paula-Lopes, 2018). Nutritional stress is also harmful for embryo development and pregnancy. In a diabetic pregnancy, hyperglycemia has been related with congenital malformations in humans and animals (Eriksson and Wentzel, 2016; Shub and Lappas, 2020). Mimicking maternal diabetes with very high concentrations of glucose in culture reduces blastocyst development rates and trophectoderm cells, while increases apoptotic cell rates in bovine and mouse (Bermejo-Alvarez et al., 2012; Uhde et al., 2018). The metabolomic profile of bovine embryos produced in high-glucose medium showed an increase in glycolysis and hexosamine pathways, and a reduction in the components of the tricarboxylic acid cycle, resulting in a deficient energy production from pyruvate, and in an increase in the polyol pathway as an alternative to produce energy (Uhde et al., 2018). Also, high glucose increased the expression of pro-apoptotic genes Bax and Casp3 and decreased the mitochondrial content and expression of glucose transporters in mouse blastocysts (Shen et al., 2009). All these alterations may induce oxidative stress and the activation of the apoptosis pathway in the embryo (Jimenez et al., 2003). Moreover, negative energy balance (NEB) in cows and obesity in humans are associated with upregulated lipolysis, which increases non-esterified fatty acids (FA) concentrations in blood. Bovine blastocyst obtained from COCs matured with a high concentration of FA had an increased apoptotic incidence and lower cell numbers (Van Hoeck et al., 2011; Marei et al., 2017), caused by lipid accumulation and increased mitochondrial ROS (Prastowo et al., 2016).
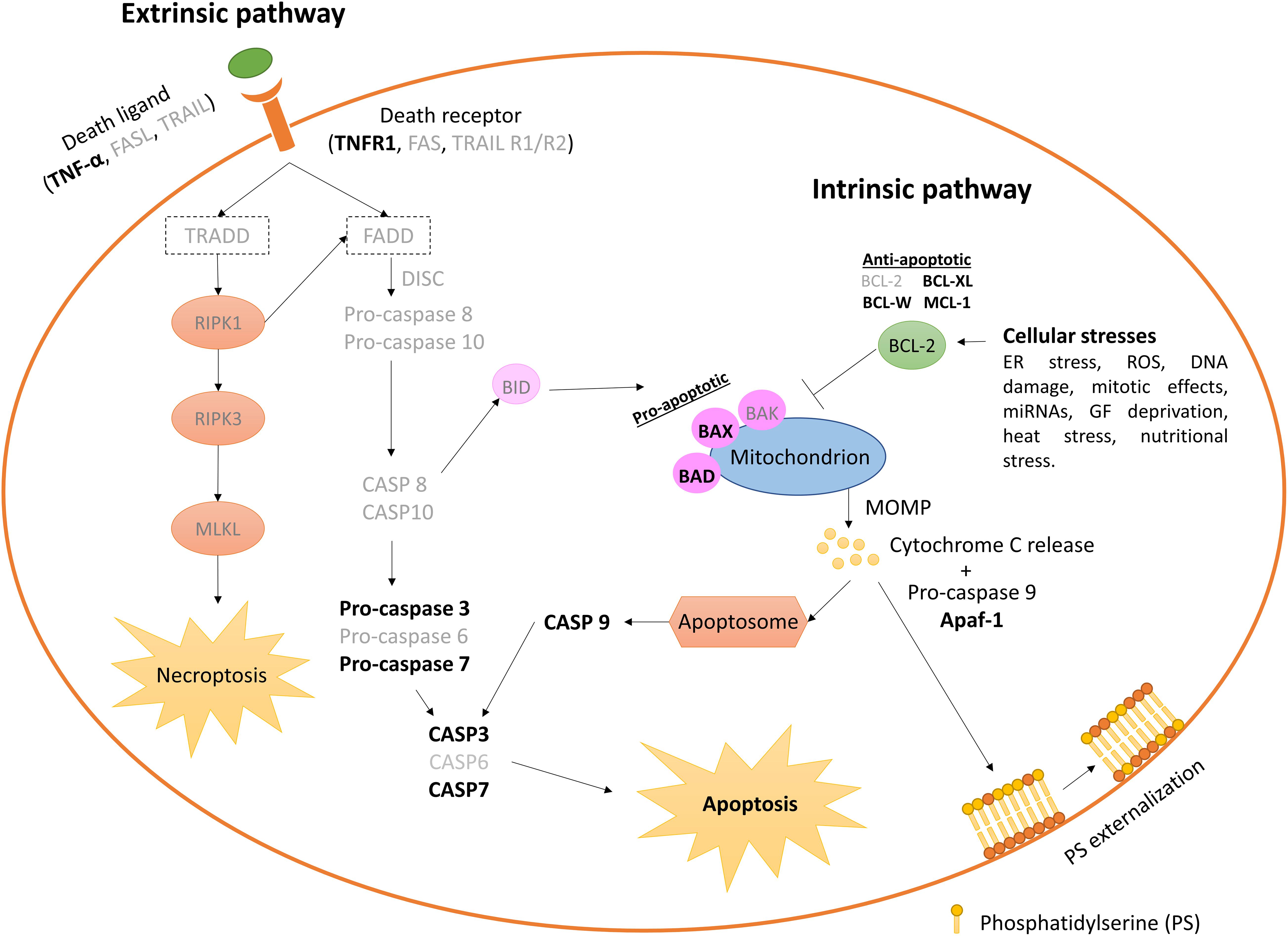
Figure 4. Extrinsic and intrinsic pathways leading apoptosis on the in vitro produced bovine embryos. Elements not yet identified in early bovine embryos are shown in lighter types. Only stress sources to which the bovine embryo responds triggering apoptosis cascade are cited. Anti-apoptotic BCL2 and BCL-W gene expression decreases between the oocyte and > 16 cell-stage embryos, while pro-apoptotic BAX increases. BAX and BCL2 are absent in > 20 cells embryos (Melka et al., 2010; Fear and Hansen, 2011), although other authors have reported absence of BCL2 during early embryo development (Leidenfrost et al., 2011). CASP3 and CASP9 are found at very low abundance, and CASP8 is undetectable neither in oocytes nor in embryos at any analyzed stage (Leidenfrost et al., 2011). DISC, death-inducing signaling complex; MOMP, mitochondrial outer membrane permeabilization.
Incidence of apoptosis within in vivo embryos is generally lower than in IVP embryos (Gjorret et al., 2003; Pomar et al., 2005; Warzych et al., 2007), although this appreciation is not unanimous (Leidenfrost et al., 2011). Thus, embryonic quality is normally associated with lower apoptotic rates. IVP blastocysts show higher transcript levels for XIAP, BAX, BCL2L1, CASP3, and CASP9 than in vivo embryos. However, neither in vivo nor IVP embryos show transcripts for BCL2, Caspase 8 (CASP8), and Fas Ligand (FASLG); and Fas Cell Surface Death Receptor (FAS) transcripts were detected only in the in vivo group at very low abundance (Leidenfrost et al., 2011).
Supplementation of in vitro culture media with serum increases embryonic Bax expression (Rizos et al., 2003), while improved formulations that increase the stability of glutamine (e.g., as glutamyl-glycine), avoids ammonia production by glutamine degradation (Zuo et al., 2020). Embryo cryopreservation, in particular vitrification and warming, enhances the expression of apoptotic genes like Forkhead Box O3A (FOXO3A), Patatin Like Phospholipase Domain Containing 2 (PNPLA2), BCL2L1, and BAX, although apoptotic phenotypes do not necessarily appear modified (Madrid Gaviria et al., 2019).
Interestingly, Day-6 IVP female embryos can show higher apoptotic rates than males (Oliveira et al., 2016). Such differences, nevertheless, can be due to culture conditions potentially inappropriate for female embryos, as there is sufficient evidence of metabolic differences between males and females (Sturmey et al., 2010; Munoz et al., 2020) and no sex-specific culture media have been presently developed. Finally, the individual bull sperm used for IVF can interact with sex factors to alter the incidence of apoptosis during embryonic development (Oliveira et al., 2016).
Assessment of Embryo Quality Through Senescence and Apoptosis Markers
Until now, the most widely used method to select IVP embryos for transfer is a morphological evaluation by standard microscopy. However, even when performed by experienced embryologists, it does not completely reflect embryo competence. Cytoplasmic fragmentation and cytokinesis failure, often observed in IVP embryos (Mateusen et al., 2005), can be estimated by morphological evaluation to assess the viability of the embryo, although the outcome can be imprecise and subjective, depending on the evaluator’s criteria. Therefore, the use of alternative markers could improve the selection of more competent embryos. Fragmentation has been associated with apoptosis induction and can lead to a reduction of embryo viability if present to a high degree (Jurisicova et al., 1996). However, although apoptosis evaluation has been suggested as an additional technique to assess embryo quality and viability (Pomar et al., 2005), activation of apoptosis might happen in the embryo as a physiological mechanism to maintain the equilibrium between cell proliferation and death, regardless of external damage or initial oocyte quality (Bakri et al., 2016). In the authors’ opinion, current systems to evaluate apoptosis are relative and invasive. Therefore, due to the large variety of embryo production systems available, measuring a critical level of apoptosis able to trigger developmental arrest is not possible yet, although it would be a highly desirable test to analyze embryonic quality. Comparison between embryo culture systems and in vivo models is the reference used in practice. Moreover, the early mammalian embryo is able to cope with a number of adverse environmental situations (Laguna-Barraza et al., 2012; Eckert et al., 2015; Fleming et al., 2015; Hansen et al., 2016) without a clear measurable evidence of the embryo resilience. Thus, appropriate analysis of the efficiency of embryonic “repair mechanisms” (i.e., confident evaluation of embryonic quality) would request pregnancy establishment and progression until birth, which makes such experiments highly expensive or, in most cases, directly unapproachable.
Nowadays, apoptosis can be analyzed by different techniques, differentiating those based on biochemical analyses, being the most used the comet and TUNEL reaction assays and annexin V staining, and those based on the analysis of genes and proteins involved in apoptosis pathways. The comet assay is a sensitive technique to detect DNA damage used in numerous eukaryotic cell types and commonly applied in genetic toxicity studies. It is based on electrophoresis migration of denatured DNA fragments and visualization by fluorescence of the comet-like shape that produces the fragmented DNA (Kucharova et al., 2019). Comet is used to detect presence of apoptosis through the visualization of the comet containing broken DNA fragments, but apoptosis level is difficult to quantify. Although in the reproduction field, this technique is mainly used to assess sperm quality and sometimes in oocytes (Chang et al., 2019; Haddock et al., 2020), it has also been adapted to study early embryos, even though the number of studies are very limited and comprise different species. One of its applications is the evaluation of the persistence of induced damage from maternal germ cells in early embryos (Rolland et al., 2017). However, an important limitation of this technique is that it cannot distinguish between DNA fragmentation coming from polar body disintegration (Tatemoto et al., 2000) and DNA fragmentation induced through apoptosis in embryonic cells (Fabian et al., 2003). Moreover, the conventional comet assay cannot distinguish between apoptosis and necrosis, requiring additional techniques to discriminate between them (Morley et al., 2006).
TUNEL assay recognizes fragmented DNA, an event that occurs at the later stage of the apoptotic process. This technique is widely used in studies for quality assessment of embryos and has revealed differences in the onset of apoptosis between in vitro- and in vivo-produced bovine embryos (Gjorret et al., 2003). Sudano et al. (2014) reported a correlation in apoptosis levels quantified by TUNEL between fresh bovine embryos and post-vitrification survival, which is also used as predictor of embryo quality. In this context, apoptosis incidence has also been analyzed to compare culture medium formulations and IVC conditions, providing information about embryo status. Thus, high oxygen tension and FCS in culture increase apoptosis rates in embryos (He et al., 2020). The effect of FCS is dose-dependent, and very low doses of serum (0.1% FCS) are not pro-apoptotic, although the embryonic quality and competence are reduced (Murillo et al., 2017; Gomez et al., 2020).
Suboptimal conditions during ARTs subject the embryos to chemical and physical stress, triggering apoptosis, and compromising the integrity of the embryo or leading to long-term deleterious effects (Ramos-Ibeas et al., 2019). IVP equine, porcine, ovine, caprine, and bovine embryos show higher incidence of TUNEL quantified apoptosis than their in vivo counterparts (Pomar et al., 2005). However, although TUNEL is a frequently used method for apoptosis detection, its specificity is low since the assay labels all free 3/hydroxyl termini in the DNA, which are also detected during necrosis, DNA repair, mechanical damage, and even active gene transcription. Thus, TUNEL staining would detect any type of DNA damage and should be used in combination with other apoptosis-specific assays (Loo, 2011) or analyzing the morphological features of apoptosis and necrosis observed in the target cells (Rodriguez et al., 2006).
Another technique commonly used for apoptosis assessment within IVP is annexin V staining, which allows the detection of phosphatidylserine (PS) in the membrane lipid bilayer surface. The externalization of PS is one of the earliest events in the apoptosis pathway. Annexin V staining has been observed in all human embryos arrested from the 2-cell stage to uncompacted morula, while only 30% of these embryos were TUNEL-positive (Levy et al., 1998), as well as in 1-cell to 9-cell fragmented embryos (Liu et al., 2000). The presence of PS on the outer leaflet of the membrane lipid bilayer of a cell is the earliest event of the apoptosis process. In healthy cells, PS is found exclusively on the inner layer of the membrane cell (Hanshaw et al., 2005). The externalization of PS had been observed by Levy et al. (1998) and Mateusen et al. (2005) in all stages of the apoptotic preimplantation embryo (2-cell stage embryo until blastocyst stage). There are three possible explanations to these results: (1) Annexin V allows the detection of apoptotic cells in arrested embryos earlier than using TUNEL; (2) Annexin V could be also labeling late-stage senescent cells in arrested embryos, which show mitochondrial depolarization by ROS, an event that may precede apoptosis (Wang et al., 2016); and (3) Necrotic cells can exhibit annexin V (Crowley et al., 2016). Therefore, as recommended for TUNEL, detection of annexin V should be combined with other apoptosis-specific assays or apoptotic morphology studies.
Increased expression of pro-apoptotic genes BAX and CASPASE-3 is associated to IVP 2–4 cell bovine embryos bearing poor morphology (Melka et al., 2010). In the same way, the anti-apoptotic gene BCL2 increases in bovine embryos with good morphological quality (Yang and Rajamahendran, 2002), and in non-fragmented mouse blastocysts (Exley et al., 1999). Therefore, the ratio between pro-apoptotic BAX and anti-apoptotic BCL2 is used to determine embryo quality and marker of differences between IVP and in vivo collected embryos. This occurs in bovine (Gutierrez-Adan et al., 2004) and humans (Metcalfe et al., 2004).
MicroRNAs (miRNAs) are key regulators of gene expression through post-transcriptional and post-translational modifications of mRNA. Mammalian blastocysts release miRNA via exosomes and apoptotic bodies in the spent embryo culture medium. Recently, a new approach to study apoptosis through miRNAs in the spent culture medium of individually cultured blastocysts has been proposed. In this study, miR-294 levels in the spent culture medium were directly correlated with apoptosis in mouse embryos. Although the biological role of miR-294 remains unknown, two hypotheses could explain these results. One is that high levels of miR-294 are involved in the suppression of the apoptosis inhibitor BCL2, which in the last term acts promoting apoptosis; although no BCL2 expression could be detected in this study, so this hypothesis could not be confirmed. The other possibility is that apoptosis triggers miR-294 release; which was confirmed by exposing the embryos to ultraviolet radiation (Makri et al., 2020). In bovine embryos, high levels of miR-30c have been associated with increased apoptosis and reduced development rates (Lin et al., 2019). miR-30c, as miR-30 family member, regulates p53 expression and cell apoptosis (Li et al., 2010), and downregulates Cyclin Dependent Kinase 12 (CDK12) and DNA damage response (DDR) genes, which may impact on cell cycle progression. miR-30c is highly conserved between species, and in humans, miR-30c levels increase in the spent culture medium of those embryos that will successfully implant (Capalbo et al., 2016). Thus, miRNAs secreted to the culture medium are potential biomarkers of embryo apoptosis and viability, although their biological functions remain unknown or poorly studied. The analysis of compounds secreted to or taken up from the culture media by embryos, such as metabolites and non-coding RNAs, is advantageous, and non-invasive. In contrast, gene expression analysis, TUNEL assay and annexin V staining are invasive techniques that require biopsy or embryo destruction. Biopsy can be detrimental for the embryo and can also lead to false negatives, discarding embryos with actual pregnancy potential.
Controversies between the association of apoptosis and embryo viability still exist (Haouzi and Hamamah, 2009), as shown by similar apoptotic indexes found by TUNEL between developmentally arrested in vivo bovine embryos, fragmented morulae and non-fragmented morulae (Ikeda et al., 2006). Similar results have been reported in the human species by TUNEL and Annexin V (Antczak and Van Blerkom, 1999). These results suggest that an alternative process might be going on in poor-quality embryos at these stages, which causes developmental arrest and cannot be detected with the TUNEL assay, such as cellular senescence.
Embryo senescence might underlie the high frequency of early developmental failure associated with sub-optimal culture conditions (Betts and King, 2001). Senescence markers β-galactosidase, phosphorylated H2A.X and p21 (Cdkn1a) mRNA are more abundant in mouse IVP than in vivo blastocysts. However, their expression was reduced when embryos were cultured in low oxygen tension (5%), pointing to a stress-induced senescence associated with in vitro conditions (Meuter et al., 2014). Another marker of embryo senescence could be telomere length in the oocyte, which is negatively correlated with Day-3 human embryos fragmentation (Keefe et al., 2005). However, the use of such senescence markers entails embryo biopsy or destruction to perform Fluorescent In Situ Hybridization (FISH), gene expression analysis or embryo staining. Therefore, novel markers and non-invasive methods to predict embryo senescence are still needed.
Conclusion and Future Directions
Early embryo development represents a very delicate developmental window in which aberrant environmental conditions can easily lead to developmental failure or to long-term negative consequences in health. Due to the increasing use of reproductive technologies in cattle and to the similarities between bovine and human pre-implantation embryos, the bovine species has allowed us to explore many mechanisms involved in developmental failure during this period. However, despite the significant progress made in the field of assisted reproduction during the last decades, still more than 50% of IVP embryos do not reach the blastocyst stage. A high proportion of these embryos enter a state of permanent cell cycle arrest compatible with cellular senescence during the first cell divisions, not showing signs of apoptosis. This protective mechanism might prevent further development of abnormal embryos showing chromosomal anomalies (Almeida and Bolton, 1998) or failure to activate the embryonic genome (Artley et al., 1992), avoiding long-term effects on health and reproduction.
Later in development, after EGA, and mainly at the morula and blastocyst stages, apoptosis would mediate a controlled elimination of certain cells. Apoptosis would then fulfill two different roles: a physiological role maintaining an equilibrium between cell proliferation and death, and a prophylactic role preventing the transmission of damaged cells with an altered genome. This last role would acquire relevant importance in IVP embryos that are submitted to environmental stress, in which apoptosis incidence increases in comparison to IVD embryos. Thus, although later in development, an individual cell might be faced with apoptosis and senescence as alternative fates (Childs et al., 2014), senescence and apoptosis during pre-implantation development would be available during restricted time windows, acting as complementary processes to ensure that damaged cells/embryos do not progress in development.
Currently, common methods to assess apoptosis and senescence in the early embryo, such as immunocytochemistry, gene expression analyses or TUNEL and annexin V staining, are not adequate to evaluate the viability of the embryo before transfer due to their invasive nature. The identification of novel non-invasive markers for apoptosis or senescence remains a crucial goal, such as the analysis of compounds secreted to or taken up from the culture media by embryos, or the possibility of using cumulus cells to analyze the quality of the future embryo, since apoptotic signaling might be already triggered in the oocyte. In parallel, there are other RCD types, such as necroptosis, ferroptosis or cell death induced by the panoptosome, which remain to be explored in early embryos. We hypothesize that such RCD types can be susceptible to alteration in the context of specific traits of early embryonic cells and/or embryo cryopreservation.
Necroptosis is a type of RCD that can be activated through the death receptor pathway via FAS receptor and TNFR1 when caspase-8 lacks or becomes inactive (Shan et al., 2018). In this situation, receptor-interacting serine/threonine-protein kinase-1 (RIPK1) and -3 (RIPK3) activate a mixed lineage kinase domain-like pseudokinase (MLKL). At least in specific circumstances, RIPK1 could also behave as an inhibitor for RIPK3-dependent necroptosis and/or CASP8-dependent extrinsic apoptosis (Orozco et al., 2014). Elucidating these roles in bovine embryo development is challenging. Although necroptosis has not been specifically studied in the early embryo, studies performed by TUNEL alone could have identified a regulated common morphology of necrotic and apoptotic cells. Combining TUNEL staining with a caspase staining may allow such distinction.
Ferroptosis is also a form of RCD driven by iron-dependent lipid peroxidation. Peroxidation of phospholipids, which are integrated into cell membranes, triggers ferroptotic death (Stockwell et al., 2017). The oocyte could be an appropriate objective for ferroptosis damage because of its largest size among the body cells and the high mitochondrial endowment (100,000–300,000 mitochondria in the human gamete (Barritt et al., 2002; Cummins, 2004), which entails a considerable amount and extent of membranes containing the ferroptosis-target phospholipid able to be peroxidized. The mitochondrial numbers decrease with cleavage stages, but they remain higher than normal cells and restart replication in the human expanded blastocyst to reach the maximal counts (Hashimoto et al., 2017) comparable to adult somatic cells (between 80 and 2000 mitochondria per cell) (Cole, 2016). The expanded IVP blastocyst is a preferential stage for cryopreservation, and a ferroptosis target because of the high amount of mitochondrial membranes that can be damaged during cryopreservation.
Panoptosis has been recently described as a multifaceted cell-death signaling platform that integrates multiple cell death types as apoptosis, pyroptosis, and necroptosis which form the collective cell death pathway or PANoptosis (Samir et al., 2020). The PANoptosome drives an inflammatory form of cell death for immune-mediated protection. Modulation of inflammation and immune responses has been described as a strong need in the bovine uterus for embryos to progress in pregnancy (Munoz et al., 2012; Gomez et al., 2013), by which PANoptosis should be considered in future studies that lead to pregnancy establishment in cattle ET.
Author Contributions
PR-I, IG, KC-B, AG-A, DR, and EG conducted literature review and wrote the manuscript. All authors contributed to the article and approved the submitted version.
Funding
This work was supported by the Spanish Ministry of Science and Innovation (AG-A: RTI2018-093548-B-I00; DR and KC-B: PID2019-111641RB-I00). PR-I was funded by a Ramón y Cajal contract from MINECO (RYC2018-025666-I). IG was supported by MINECO BES-2017-082200. EG and IG were supported by the Spanish Ministry of Economy and Competitiveness (MINECO) projects AGL2016-78597R and AGL2016-81890-REDT and Fondo Europeo de Desarrollo Regional (FEDER).
Conflict of Interest
The authors declare that the research was conducted in the absence of any commercial or financial relationships that could be construed as a potential conflict of interest.
References
Acosta, J. C., Banito, A., Wuestefeld, T., Georgilis, A., Janich, P., Morton, J. P., et al. (2013). A complex secretory program orchestrated by the inflammasome controls paracrine senescence. Nat. Cell Biol. 15, 978-990.
Acosta, J. C., O’loghlen, A., Banito, A., Guijarro, M. V., Augert, A., Raguz, S., et al. (2008). Chemokine signaling via the CXCR2 receptor reinforces senescence. Cell 133, 1006–1018. doi: 10.1016/j.cell.2008.03.038
Adiga, S. K., Toyoshima, M., Shiraishi, K., Shimura, T., Takeda, J., Taga, M., et al. (2007). p21 provides stage specific DNA damage control to preimplantation embryos. Oncogene 26, 6141–6149. doi: 10.1038/sj.onc.1210444
Adona, P.R., Leal, C.L., Biase, F.H., De Bem, T.H., Mesquita, L.G., Meirelles, F.V., et al. (2016). In vitro maturation alters gene expression in bovine oocytes. Zygote 24, 624-633.
Aggarwal, B. B., Gupta, S. C., and Kim, J. H. (2012). Historical perspectives on tumor necrosis factor and its superfamily: 25 years later, a golden journey. Blood 119, 651–665. doi: 10.1182/blood-2011-04-325225
Almeida, P. A., and Bolton, V. N. (1998). Cytogenetic analysis of human preimplantation embryos following developmental arrest in vitro. Reprod. Fertil. Dev. 10, 505–513. doi: 10.1071/rd98040
Almiñana, C., and Bauersachs, S. (2019). Extracellular Vesicles in the Oviduct: Progress, Challenges and Implications for the Reproductive Success. Bioengineering 6:32. doi: 10.3390/bioengineering6020032
Alminana, C., Corbin, E., Tsikis, G., Alcantara-Neto, A. S., Labas, V., Reynaud, K., et al. (2017). Oviduct extracellular vesicles protein content and their role during oviduct-embryo cross-talk. Reproduction 154, 253–268. doi: 10.1530/rep-17-0054
Antczak, M., and Van Blerkom, J. (1999). Temporal and spatial aspects of fragmentation in early human embryos: possible effects on developmental competence and association with the differential elimination of regulatory proteins from polarized domains. Hum. Reprod. 14, 429–447. doi: 10.1093/humrep/14.2.429
Artley, J. K., Braude, P. R., and Johnson, M. H. (1992). Gene activity and cleavage arrest in human pre-embryos. Hum. Reprod. 7, 1014–1021. doi: 10.1093/oxfordjournals.humrep.a137761
Ata, B., Shalom-Paz, E., Chian, R.C., and Tan, S.L. (2010). In vitro maturation of oocytes as a strategy for fertility preservation. Clin. Obstet Gynecol. 53, 775-786.
Baar, M. P., Brandt, R. M. C., Putavet, D. A., Klein, J. D. D., Derks, K. W. J., Bourgeois, B. R. M., et al. (2017). Targeted apoptosis of senescent cells restores tissue homeostasis in response to chemotoxicity and aging. Cell 169:132. doi: 10.1016/j.cell.2017.02.031
Baker, D. J., Wijshake, T., Tchkonia, T., Lebrasseur, N. K., Childs, B. G., Van De Sluis, B., et al. (2011). Clearance of p16(Ink4a)-positive senescent cells delays ageing-associated disorders. Nature 479, 232-236.
Bakri, N. M., Ibrahim, S. F., Osman, N. A., Hasan, N., Jaffar, F. H., Rahman, Z. A., et al. (2016). Embryo apoptosis identification: Oocyte grade or cleavage stage? Saudi J. Biol. Sci. 23, S50-S55.
Barker, D. J. (2007). The origins of the developmental origins theory. J. Intern. Med. 261, 412–417. doi: 10.1111/j.1365-2796.2007.01809.x
Barritt, J. A., Kokot, M., Cohen, J., Steuerwald, N., and Brenner, C. A. (2002). Quantification of human ooplasmic mitochondria. Reprod. Biomed. Online 4, 243–247. doi: 10.1016/s1472-6483(10)61813-5
Bauersachs, S., Ulbrich, S. E., Gross, K., Schmidt, S. E. M., Meyer, H. H. D., Einspanier, R., et al. (2005). Gene expression profiling of bovine endometrium during the oestrous cycle: detection of molecular pathways involved in functional changes. J. Mol. Endocrinol. 34, 889–908. doi: 10.1677/jme.1.01799
Benhamed, M., Herbig, U., Ye, T., Dejean, A., and Bischof, O. (2012). Senescence is an endogenous trigger for microRNA-directed transcriptional gene silencing in human cells. Nat. Cell Biol. 14:266. doi: 10.1038/ncb2443
Bermejo-Alvarez, P., Roberts, R. M., and Rosenfeld, C. S. (2012). Effect of glucose concentration during in vitro culture of mouse embryos on development to blastocyst, success of embryo transfer, and litter sex ratio. Mol. Reprod. Dev. 79, 329–336. doi: 10.1002/mrd.22028
Betts, D. H., and King, W. A. (1999). Telomerase activity and telomere detection during early bovine development. Dev. Genet. 25, 397–403. doi: 10.1002/(sici)1520-6408(1999)25:4<397::aid-dvg13>3.0.co;2-j
Betts, D. H., and King, W. A. (2001). Genetic regulation of embryo death and senescence. Theriogenology 55, 171–191. doi: 10.1016/s0093-691x(00)00453-2
Betts, D. H., and Madan, P. (2008). Permanent embryo arrest: molecular and cellular concepts. Mol. Hum. Reprod. 14, 445–453. doi: 10.1093/molehr/gan035
Biswas, D., So, K. H., Hwang, S. U., Yoon, J. D., Kim, M., Kim, D. Y., et al. (2018). Embryotropic effects of vascular endothelial growth factor on porcine embryos produced by in vitro fertilization. Theriogenology 120, 147–156. doi: 10.1016/j.theriogenology.2018.07.024
Bittner, L., Wyck, S., Herrera, C., Siuda, M., Wrenzycki, C., Van Loon, B., et al. (2018). Negative effects of oxidative stress in bovine spermatozoa on in vitro development and DNA integrity of embryos. Reprod. Fertil. Dev. 30, 1359–1368. doi: 10.1071/rd17533
Block, J., Bonilla, L., and Hansen, P. J. (2010). Efficacy of in vitro embryo transfer in lactating dairy cows using fresh or vitrified embryos produced in a novel embryo culture medium. J. Dairy Sci. 93, 5234–5242. doi: 10.3168/jds.2010-3443
Brackett, B. G., Bousquet, D., Boice, M. L., Donawick, W. J., Evans, J. F., and Dressel, M. A. (1982). Normal development following in vitro fertilization in the cow. Biol. Reprod. 27, 147–158. doi: 10.1095/biolreprod27.1.147
Brad, A. M., Hendricks, K. E. M., and Hansen, P. J. (2007). The block to apoptosis in bovine two-cell embryos involves inhibition of caspase-9 activation and caspase-mediated DNA damage. Reproduction 134, 789–797. doi: 10.1530/rep-07-0146
Brenner, C. A., Wolny, Y. M., Adler, R. R., and Cohen, J. (1999). Alternative splicing of the telomerase catalytic subunit in human oocytes and embryos. Mol. Hum. Reprod. 5, 845–850. doi: 10.1093/molehr/5.9.845
Bunel, A., Jorssen, E. P., Merckx, E., Leroy, J. L., Bols, P. E., and Sirard, M. A. (2015). Individual bovine in vitro embryo production and cumulus cell transcriptomic analysis to distinguish cumulus-oocyte complexes with high or low developmental potential. Theriogenology 83, 228–237. doi: 10.1016/j.theriogenology.2014.09.019
Byrne, A. T., Southgate, J., Brison, D. R., and Leese, H. J. (1999). Analysis of apoptosis in the preimplantation bovine embryo using TUNEL. J. Reprod. Fertil. 117, 97–105. doi: 10.1530/jrf.0.1170097
Calle, A., Fernandez-Gonzalez, R., Ramos-Ibeas, P., Laguna-Barraza, R., Perez-Cerezales, S., Bermejo-Alvarez, P., et al. (2012). Long-term and transgenerational effects of in vitro culture on mouse embryos. Theriogenology 77, 785–793. doi: 10.1016/j.theriogenology.2011.07.016
Capalbo, A., Ubaldi, F. M., Cimadomo, D., Noli, L., Khalaf, Y., Farcomeni, A., et al. (2016). MicroRNAs in spent blastocyst culture medium are derived from trophectoderm cells and can be explored for human embryo reproductive competence assessment. Fertil. Steril. 105:225. doi: 10.1016/j.fertnstert.2015.09.014
Chang, H. Y., Chen, H. H., Zhang, L., Wang, Y. L., Xie, X. G., Zhang, Y., et al. (2019). Effect of oocyte vitrification on DNA damage in metaphase II oocytes and the resulting preimplantation embryos. Mol. Reprod. Dev. 86, 1603–1614. doi: 10.1002/mrd.23247
Chen, L., Wert, S. E., Hendrix, E. M., Russell, P. T., Cannon, M., and Larsen, W. J. (1990). Hyaluronic acid synthesis and gap junction endocytosis are necessary for normal expansion of the cumulus mass. Mol. Reprod. Dev. 26, 236–247. doi: 10.1002/mrd.1080260307
Chen, S., Einspanier, R., and Schoen, J. (2013). In vitro mimicking of estrous cycle stages in porcine oviduct epithelium cells: estradiol and progesterone regulate differentiation, gene expression, and cellular function. Biol. Reprod. 89:54.
Chen, S., Palma-Vera, S. E., Langhammer, M., Galuska, S. P., Braun, B. C., Krause, E., et al. (2017). An air-liquid interphase approach for modeling the early embryo-maternal contact zone. Sci. Rep. 7:42298.
Childs, B. G., Baker, D. J., Kirkland, J. L., Campisi, J., and Van Deursen, J. M. (2014). Senescence and apoptosis: dueling or complementary cell fates? EMBO Rep. 15, 1139–1153. doi: 10.15252/embr.201439245
Cole, L. W. (2016). The evolution of per-cell organelle number. Front. Cell Dev. Biol. 4:85. doi: 10.3389/fcell.2016.00085
Conradt, B. (2009). Genetic control of programmed cell death during animal development. Annu. Rev. Genet. 43, 493–523. doi: 10.1146/annurev.genet.42.110807.091533
Correia-Alvarez, E., Gomez, E., Martin, D., Carrocera, S., Perez, S., Otero, J., et al. (2015a). Expression and localization of interleukin 1 beta and interleukin 1 receptor (type I) in the bovine endometrium and embryo. J. Reprod. Immunol. 110, 1–13. doi: 10.1016/j.jri.2015.03.006
Correia-Alvarez, E., Gomez, E., Martin, D., Carrocera, S., Perez, S., and Peynot, N. et al. (2015b). Early embryonic and endometrial regulation of tumor necrosis factor and tumor necrosis factor receptor 2 in the cattle uterus. Theriogenology 83, 1028–1037. doi: 10.1016/j.theriogenology.2014.12.007
Crowley, L. C., Marfell, B. J., Scott, A. P., and Waterhouse, N. J. (2016). Quantitation of Apoptosis and Necrosis by Annexin V Binding, Propidium Iodide Uptake, and Flow Cytometry. Cold Spring Harb Protoc. 11, 953–957.
Cummins, J. M. (2004). The role of mitochondria in the establishment of oocyte functional competence. Eur. J. Obstet. Gynecol. Reprod. Biol. 115(Suppl 1), S23-S29.
De Barros, F. R. O., and Paula-Lopes, F. F. (2018). Cellular and epigenetic changes induced by heat stress in bovine preimplantation embryos. Mol. Reprod. Dev. 85, 810–820. doi: 10.1002/mrd.23040
De Los Santos, M. J., Anderson, D. J., Racowsky, C., and Hill, J. A. (2000). Presence of Fas-Fas ligand system and bcl-2 gene products in cells and fluids from gonadotropin-stimulated human ovaries. Biol. Reprod. 63, 1811–1816. doi: 10.1095/biolreprod63.6.1811
Diamantis, A., Magiorkinis, E., Sakorafas, G. H., and Androutsos, G. (2008). A brief history of apoptosis: from ancient to modern times. Onkologie 31, 702–706. doi: 10.1159/000165071
Ealy, A. D., Drost, M., and Hansen, P. J. (1993). Developmental changes in embryonic resistance to adverse effects of maternal heat stress in cows. J. Dairy Sci. 76, 2899–2905. doi: 10.3168/jds.s0022-0302(93)77629-8
Eckert, J. J., Velazquez, M. A., and Fleming, T. P. (2015). Cell signalling during blastocyst morphogenesis. Adv. Exp. Med. Biol. 843, 1–21. doi: 10.1007/978-1-4939-2480-6_1
Egashira, A., Kano, K., and Naito, K. (2011). Preimplantation-embryo-specific cell-cycle regulation is attributable to a low expression of retinoblastoma protein rather than its phosphorylation. J. Reprod. Dev. 57, 492–499. doi: 10.1262/jrd.10-170o
El Hajj, N., and Haaf, T. (2013). Epigenetic disturbances in in vitro cultured gametes and embryos: implications for human assisted reproduction. Fertil. Sterility 99, 632–641. doi: 10.1016/j.fertnstert.2012.12.044
Eriksson, U. J., and Wentzel, P. (2016). The status of diabetic embryopathy. Upsala J. Med. Sci. 121, 96–112.
Eshre (2018). “ART Fact Sheet”. Available online at: https://www.eshre.eu/Press-Room/Resources.aspx (accessed October 7, 2020).
Exley, G. E., Tang, C. Y., Mcelhinny, A. S., and Warner, C. M. (1999). Expression of caspase and BCL-2 apoptotic family members in mouse preimplantation embryos. Biol. Reprod. 61, 231–239. doi: 10.1095/biolreprod61.1.231
Fabian, D., Rehak, P., Czikkova, S., Il’kova, G., Baran, V., and Koppel, J. (2003). Induced cell death of preimplantation mouse embryos cultured in vitro evaluated by comet assay. Theriogenology 60, 691–706. doi: 10.1016/s0093-691x(03)00087-6
Favetta, L. A., Madan, P., Mastromonaco, G. F., St John, E. J., King, A., and Betts, D. H. C. (2007a). The oxidative stress adaptor p66Shc is required for permanent embryo arrest in vitro. BMC Developmental Biology 7:132. doi: 10.1186/1471-213X-7-132
Favetta, L. A., Robert, C., St John, E. J., Betts, D. H., and King, W. A. (2004). p66(shc), but not p53, is involved in early arrest of in vitro-produced bovine embryos. Mol. Hum. Reprod. 10, 383–392. doi: 10.1093/molehr/gah057
Favetta, L. A., St John, E. J., King, W. A., and Betts, D. H. (2007b). High levels of p66(shc) and intracellular ROS in permanently arrested early embryos. Free Radic. Biol. Med. 42, 1201–1210. doi: 10.1016/j.freeradbiomed.2007.01.018
Fear, J. M., and Hansen, P. J. (2011). Developmental changes in expression of genes involved in regulation of apoptosis in the bovine preimplantation embryo. Biol. Reprod. 84, 43–51. doi: 10.1095/biolreprod.110.086249
Ferraz, M., Henning, H. H. W., Costa, P. F., Malda, J., Melchels, F. P., Wubbolts, R., et al. (2017a). Improved bovine embryo production in an oviduct-on-a-chip system: prevention of poly-spermic fertilization and parthenogenic activation. Lab. Chip 17, 905–916. doi: 10.1039/c6lc01566b
Ferraz, M., Henning, H. H. W., Stout, T. A. E., Vos, P., and Gadella, B. M. (2017b). Designing 3-dimensional in vitro oviduct culture systems to study mammalian fertilization and embryo production. Ann. Biomed. Eng 45, 1731-1744.
Ferraz, M., Rho, H. S., Hemerich, D., Henning, H. H. W., Van Tol, H. T. A., Holker, M., et al. (2018). An oviduct-on-a-chip provides an enhanced in vitro environment for zygote genome reprogramming. Nat. Commun. 9:4934.
Fleming, T. P., Watkins, A. J., Sun, C., Velazquez, M. A., Smyth, N. R., and Eckert, J. J. (2015). Do little embryos make big decisions? How maternal dietary protein restriction can permanently change an embryo’s potential, affecting adult health. Reprod. Fertil. Dev. 27, 684–692. doi: 10.1071/rd14455
Freund, A., Orjalo, A. V., Desprez, P. -Y., and Campisi, J. (2010). Inflammatory networks during cellular senescence: causes and consequences. Trends Mol. Med. 16, 238–246. doi: 10.1016/j.molmed.2010.03.003
Fuchs, Y., and Steller, H. (2011). Programmed cell death in animal development and disease. Cell 147, 742–758. doi: 10.1016/j.cell.2011.10.033
Gad, A., Hoelker, M., Besenfelder, U., Havlicek, V., Cinar, U., Rings, F., (2012). Molecular mechanisms and pathways involved in bovine embryonic genome activation and their regulation by alternative in vivo and in vitro culture conditions. Biol. Reprod. 87, 1–13. doi: 10.1095/biolreprod.112.099697
Galluzzi, L., Manuel Bravo-San Pedro, J., Kepp, O., and Kroemer, G. (2016). Regulated cell death and adaptive stress responses. Cell. Mol. Life Sci. 73, 2405–2410. doi: 10.1007/s00018-016-2209-y
Galluzzi, L., Vitale, I., Aaronson, S. A., Abrams, J. M., Adam, D., Agostinis, P., et al. (2018). Molecular mechanisms of cell death: recommendations of the nomenclature committee on cell death 2018. Cell Death Differ. 25, 486–541.
Gjorret, J. O., Fabian, D., Avery, B., and Maddox-Hyttel, P. (2007). Active caspase-3 and ultrastructural evidence of apoptosis in spontaneous and induced cell death in bovine in vitro produced pre-implantation embryos. Mol. Reprod. Dev. 74, 961–971. doi: 10.1002/mrd.20714
Gjorret, J. O., Knijn, H. M., Dieleman, S. J., Avery, B., Larsson, L. I., and Maddox-Hyttel, P. (2003). Chronology of apoptosis in bovine embryos produced in vivo and in vitro. Biol. Reprod. 69, 1193–1200. doi: 10.1095/biolreprod.102.013243
Gomez, E., Caamano, J. N., Corrales, F. J., Diez, C., Correia-Alvarez, E., Martin, D., et al. (2013). Embryonic sex induces differential expression of proteins in bovine uterine fluid. J. Prot. Res. 12, 1199–1210. doi: 10.1021/pr300845e
Gomez, E., Carrocera, S., Martin, D., Jose Perez-Janez, J., Prendes, J., Manuel Prendes, J., et al. (2020). Efficient one-step direct transfer to recipients of thawed bovine embryos cultured in vitro and frozen in chemically defined medium. Theriogenology 146, 39–47. doi: 10.1016/j.theriogenology.2020.01.056
Gomez, E., Correia-Alvarez, E., Caamano, J. N., Diez, C., Carrocera, S., Peynot, N., et al. (2014). Hepatoma-derived growth factor: from the bovine uterus to the in vitro embryo culture. Reproduction 148, 353–365. doi: 10.1530/rep-14-0304
Gomez, E., Rodriguez, A., Munoz, M., Nestor Caamano, J., Carrocera, S., Martin, D., et al. (2008). Development and quality of bovine morulae cultured in serum-free medium with specific retinoid receptor agonists. Reprod. Fertil. Dev. 20, 884–891. doi: 10.1071/rd08103
Gutierrez-Adan, A., Rizos, D., Fair, T., Moreira, P. N., Pintado, B., De La Fuente, J., et al. (2004). Effect of speed of development on mRNA expression pattern in early bovine embryos cultured in vivo or in vitro. Mol. Reprod. Dev. 68, 441–448. doi: 10.1002/mrd.20113
Haddock, L., Gordon, S., Lewis, S. E. M., Larsen, P., Shehata, A., and Shehata, H. (2020). Sperm DNA fragmentation is a novel biomarker for early pregnancy loss. Reprod. Biomed. Online.
Hansen, P. J. (2020). The incompletely fulfilled promise of embryo transfer in cattle-why aren’t pregnancy rates greater and what can we do about it? J. Anim. Sci. 98:skaa288.
Hansen, P. J., Dobbs, K. B., Denicol, A. C., and Siqueira, L. G. B. (2016). Sex and the preimplantation embryo: implications of sexual dimorphism in the preimplantation period for maternal programming of embryonic development. Cell Tissue Res. 363, 237–247. doi: 10.1007/s00441-015-2287-4
Hanshaw, R. G., Lakshmi, C., Lambert, T. N., Johnson, J. R., and Smith, B. D. (2005). Fluorescent detection of apoptotic cells by using zinc coordination complexes with a selective affinity for membrane surfaces enriched with phosphatidylserine. Chembiochem 6, 2214–2220. doi: 10.1002/cbic.200500149
Haouzi, D., and Hamamah, S. (2009). Pertinence of apoptosis markers for the improvement of in vitro fertilization (IVF). Curr. Med. Chem. 16, 1905-1916.
Hardy, K. (1997). Cell death in the mammalian blastocyst. Mol. Hum. Reprod. 3, 919–925. doi: 10.1093/molehr/3.10.919
Hardy, K. (1999). Apoptosis in the human embryo. Rev. Reprod. 4, 125–134. doi: 10.1530/revreprod/4.3.125
Hardy, K., Stark, J., and Winston, R. M. L. (2003). Maintenance of the inner cell mass in human blastocysts from fragmented embryos. Biol. Reprod. 68, 1165–1169. doi: 10.1095/biolreprod.102.010090
Hashimoto, S., Morimoto, N., Yamanaka, M., Matsumoto, H., Yamochi, T., Goto, H., et al. (2017). Quantitative and qualitative changes of mitochondria in human preimplantation embryos. J. Assist. Reprod. Genet. 34, 573–580. doi: 10.1007/s10815-017-0886-6
Hasler, J. F., Henderson, W. B., Hurtgen, P. J., Jin, Z. Q., Mccauley, A. D., Mower, S. A., et al. (1995). Production, freezing and transfer of bovine ivf embryos and subsequent calving results. Theriogenology 43, 141–152. doi: 10.1016/0093-691x(94)00020-u
Hayflick, L., and Moorhead, P. S. (1961). The serial cultivation of human diploid cell strains. Exp. Cell Res. 25, 585–621. doi: 10.1016/0014-4827(61)90192-6
He, H., Zhang, H., Li, Q., Fan, J., Pan, Y., Zhang, T., et al. (2020). Low oxygen concentrations improve yak oocyte maturation and enhance the developmental competence of preimplantation embryos. Theriogenology 156, 46–58. doi: 10.1016/j.theriogenology.2020.06.022
Heras, S., De Coninck, D. I., Van Poucke, M., Goossens, K., Bogado Pascottini, O., Van Nieuwerburgh, F., et al. (2016). Suboptimal culture conditions induce more deviations in gene expression in male than female bovine blastocysts. BMC Genomics 17:72. doi: 10.1186/s12864-016-2393-z
Hoshi, H. (2003). In vitro production of bovine embryos and their application for embryo transfer. Theriogenology 59, 675-685.
Huang, C. -H., and Chan, W. -H. (2017). Rhein induces oxidative stress and apoptosis in mouse blastocysts and has immunotoxic effects during embryonic development. Int. J. Mol. Sci. 18:2018. doi: 10.3390/ijms18092018
Huang, X. X., Tran, T., Zhang, L. N., Hatcher, R., and Zhang, P. M. (2005). DNA damage-induced mitotic catastrophe is mediated by the Chk1-dependent mitotic exit DNA damage checkpoint. Proc. Natl. Acad. Sci. U.S.A. 102, 1065–1070. doi: 10.1073/pnas.0409130102
Hviid, K. V. R., Malchau, S. S., Pinborg, A., and Nielsen, H. S. (2018). Determinants of monozygotic twinning in ART: a systematic review and a meta-analysis. Hum. Reprod. Update 24, 468–483. doi: 10.1093/humupd/dmy006
Ikeda, S., Prendes, J. M., Alonso-Montes, C., Rodriguez, A., Diez, C., Kitagawa, M., et al. (2006). Apoptosis-independent poor morphology of bovine embryos produced by multiple ovulation. Reprod. Domestic Anim. 41, 383–385. doi: 10.1111/j.1439-0531.2006.00672.x
Jimenez, A., Madrid-Bury, N., Fernandez, R., Perez-Garnelo, S., Moreira, P., Pintado, B., et al. (2003). Hyperglycemia-induced apoptosis affects sex ratio of bovine and murine preimplantation embryos. Mol. Reprod. Dev. 65, 180–187. doi: 10.1002/mrd.10286
Juan, H. C., Lin, Y., Chen, H. R., and Fann, M. J. (2016). Cdk12 is essential for embryonic development and the maintenance of genomic stability. Cell Death Differ. 23, 1038–1048. doi: 10.1038/cdd.2015.157
Jurisicova, A., Varmuza, S., and Casper, R. F. (1996). Programmed cell death and human embryo fragmentation. Mol. Hum. Reprod. 2, 93–98. doi: 10.1093/molehr/2.2.93
Kang, C., Xu, Q., Martin, T. D., Li, M. Z., Demaria, M., Aron, L., et al. (2015). The DNA damage response induces inflammation and senescence by inhibiting autophagy of GATA4. Science 349:aaa5612. doi: 10.1126/science.aaa5612
Kang, T. W., Yevsa, T., Woller, N., Hoenicke, L., Wuestefeld, T., Dauch, D., et al. (2011). Senescence surveillance of pre-malignant hepatocytes limits liver cancer development. Nature 479, 547–551. doi: 10.1038/nature10599
Kasum, M., Von Wolff, M., Franulić, D., Čehić, E., Klepac-Pulanić, T., Orešković, S., et al. (2015). Fertility preservation options in breast cancer patients. Gynecol. Endocrinol. 31, 846–851.
Katz-Jaffe, M. G., Mccallie, B. R., Preis, K. A., Filipovits, J., and Gardner, D. K. (2009). Transcriptome analysis of in vivo and in vitro matured bovine MII oocytes. Theriogenology 71, 939–946. doi: 10.1016/j.theriogenology.2008.10.024
Keefe, D. L., Franco, S., Liu, L., Trimarchi, J., Cao, B., Weitzen, S., et al. (2005). Telomere length predicts embryo fragmentation after in vitro fertilization in women - Toward a telomere theory of reproductive aging in women. Am. J. Obstetr. Gynecol. 192, 1256–1260. doi: 10.1016/j.ajog.2005.01.036
Khatun, H., Ihara, Y., Takakura, K., Egashira, J., Wada, Y., Konno, T., et al. (2020). Role of endoplasmic reticulum stress on developmental competency and cryo-tolerance in bovine embryos. Theriogenology 142, 131–137. doi: 10.1016/j.theriogenology.2019.09.042
Krisher, R. L., and Prather, R. S. (2012). A role for the Warburg effect in preimplantation embryo development: metabolic modification to support rapid cell proliferation. Mol. Reprod. Dev. 79, 311–320. doi: 10.1002/mrd.22037
Kucharova, M., Hronek, M., Rybakova, K., Zadak, Z., Stetina, R., Joskova, V., et al. (2019). Comet assay and its use for evaluating oxidative DNA damage in some pathological states. Physiol. Res. 68, 1–15. doi: 10.33549/physiolres.933901
Kuilman, T., Michaloglou, C., Mooi, W. J., and Peeper, D. S. (2010). The essence of senescence. Genes Dev. 24, 2463–2479. doi: 10.1101/gad.1971610
Kuilman, T., Michaloglou, C., Vredeveld, L. C. W., Douma, S., Van Doom, R., Desmet, C. J., et al. (2008). Oncogene-induced senescence relayed by an interleukin-dependent inflammatory network. Cell 133, 1019–1031. doi: 10.1016/j.cell.2008.03.039
Labrecque, R., Fournier, E., and Sirard, M. A. (2016). Transcriptome Analysis of Bovine Oocytes From Distinct Follicle Sizes: Insights From Correlation Network Analysis. Mol. Reprod. Dev. 83, 558–569. doi: 10.1002/mrd.22651
Laguna-Barraza, R., Bermejo-Alvarez, P., Ramos-Ibeas, P., De Frutos, C., Lopez-Cardona, A. P., Calle, A., et al. (2012). Sex-specific embryonic origin of postnatal phenotypic variability. Reprod. Fertil. Dev. 25, 38–47. doi: 10.1071/rd12262
Lazzari, G., Wrenzycki, C., Herrmann, D., Duchi, R., Kruip, T., Niemann, H., et al. (2002). Cellular and molecular deviations in bovine in vitro-produced embryos are related to the large offspring syndrome. Biol. Reprod. 67, 767–775. doi: 10.1095/biolreprod.102.004481
Ledgard, A. M., Berg, M. C., Mcmillan, W. H., Smolenski, G., and Peterson, A. J. (2012). Effect of asynchronous transfer on bovine embryonic development and relationship with early cycle uterine proteome profiles. Reprod. Fertil. Dev. 24, 962–972.
Leidenfrost, S., Boelhauve, M., Reichenbach, M., Guengoer, T., Reichenbach, H. -D., Sinowatz, F., et al. (2011). Cell arrest and cell death in mammalian preimplantation development: lessons from the bovine model. PLoS One 6:e22121. doi: 10.1371/journal.pone.0022121
Levanon, K., Ng, V., Piao, H. Y., Zhang, Y., Chang, M. C., Roh, M. H., et al. (2010). Primary ex vivo cultures of human fallopian tube epithelium as a model for serous ovarian carcinogenesis. Oncogene 29, 1103–1113. doi: 10.1038/onc.2009.402
Levy, R., Benchaib, M., Cordonier, H., Souchier, C., and Guerin, J. F. (1998). Annexin V labelling and terminal transferase-mediated DNA end labelling (TUNEL) assay in human arrested embryos. Mol. Hum. Reprod. 4, 775–783. doi: 10.1093/molehr/4.8.775
Li, J. C., Donath, S., Li, Y. R., Qin, D., Prabhakar, B. S., and Li, P. F. (2010). miR-30 Regulates mitochondrial fission through targeting p53 and the dynamin-related protein-1 pathway. PLoS Genet. 6:e1000795. doi: 10.1371/journal.pgen.1000795.
Lima, L., Tavares De Melo, T. C., Marques, D., Goes De Araujo, J. N., Fernandes Leite, I. S., Alves, C. X., et al. (2019). Modulation of all-trans retinoic acid-induced MiRNA expression in neoplastic cell lines: a systematic review. BMC Cancer 19:866. doi: 10.1186/s12885-019-6081-7.
Lin, X. Y., Beckers, E., Mc Cafferty, S., Gansemans, Y., Szymanska, K. J., Pavani, K. C., et al. (2019). Bovine embryo-secreted microRNA-30c is a potential non-invasive biomarker for hampered preimplantation developmental competence. Front. Genet. 10:315. doi: 10.3389/fgene.2019.00315
Liu, H. C., He, Z. Y., Mele, C. A., Veeck, L. L., Davis, O., and Rosenwaks, Z. C. P. (2000). Expression of apoptosis-related genes in human oocytes and embryos. J. Assist. Reprod. Genet. 17, 521–533.
Lonergan, P. (2007). State-of-the-art embryo technologies in cattle. Soc. Reprod. Fertil. Suppl. 64, 315–325. doi: 10.5661/rdr-vi-315
Lonergan, P., and Fair, T. (2016). Maturation of Oocytes in Vitro. Annu. Rev. Anim. Biosci. 4, 255–268.
Lonergan, P., Rizos, D., Gutiérrez-Adán, A., Fair, T., and Boland, M. P. (2003). Effect of culture environment on embryo quality and gene expression - experience from animal studies. Reprod. Biomed. Online 7, 657–663. doi: 10.1016/s1472-6483(10)62088-3
Loo, D.T. (2011). In situ detection of apoptosis by the TUNEL assay: an overview of techniques. Methods Prot. 682, 3-13.
Looney, C. R., Lindsey, B. R., Gonseth, C. L., and Johnson, D. L. (1994). Commercial aspects of oocyte retrieval and in-vitro fertilization (ivf) for embryo production in problem cows. Theriogenology 41, 67–72. doi: 10.1016/s0093-691x(05)80050-0
Lopera-Vasquez, R., Hamdi, M., Maillo, V., Lloreda, V., Coy, P., Gutierrez-Adan, A., et al. (2017). Effect of bovine oviductal fluid on development and quality of bovine embryos produced in vitro. Reprod. Fertil. Dev. 29, 621–629. doi: 10.1071/rd15238
Lopez-Otin, C., Blasco, M. A., Partridge, L., Serrano, M., and Kroemer, G. (2013). The hallmarks of aging. Cell 153, 1194–1217.
Lorda-Diez, C. I., Solis-Mancilla, M. E., Sanchez-Fernandez, C., Garcia-Porrero, J. A., Hurle, J. M., and Montero, J. A. (2019). Cell senescence, apoptosis and DNA damage cooperate in the remodeling processes accounting for heart morphogenesis. J.Anat. 234, 815–829. doi: 10.1111/joa.12972
Lozono-Torres, B., Estepa-Fernandez, A., Rovira, M., Orzaez, M., Serrano, M., Martinez-Manez, R., et al. (2019). The chemistry of senescence. Nat. Rev. Chem. 3, 426–441.
Luo, D., Zhang, J. -B., Liu, W., Yao, X. -R., Guo, H., Jin, Z. L., et al. (2020). Leonurine improves invitro porcine embryo development competence by reducing reactive oxygen species production and protecting mitochondrial function. Theriogenology 156, 116–123. doi: 10.1016/j.theriogenology.2020.06.038
Madrid Gaviria, S., Lopez Herrera, A., Urrego, R., Restrepo Betancur, G., and Echeverri Zuluaga, J. J. (2019). Effect of resveratrol on vitrified in vitro produced bovine embryos: recovering the initial quality. Cryobiology 89, 42–50. doi: 10.1016/j.cryobiol.2019.05.008
Makri, D., Efstathiou, P., Michailidou, E., and Maalouf, W. E. (2020). Apoptosis triggers the release of microRNA miR-294 in spent culture media of blastocysts. J. Assist. Reprod. Genet. 37, 1685–1694. doi: 10.1007/s10815-020-01796-5
Marei, W. F. A., Alvarez, M. A., Van Hoeck, V., Gutierrez-Adan, A., Bols, P. E. J., and Leroy, J. L. M. R. (2017). Effect of nutritionally induced hyperlipidaemia on in vitro bovine embryo quality depends on the type of major fatty acid in the diet. Reprod. Fertil. Dev. 29, 1856–1867. doi: 10.1071/rd16297
Martinez-Zamudio, R. I., Robinson, L., Roux, P. -F., and Bischof, O. (2017). SnapShot: cellular senescence pathways. Cell 170:816.e811.
Mateusen, B., Van Soom, A., Maes, D. G. D., Donnay, I., Duchateau, L., and Lequarre, A. S. (2005). Porcine embryo development and fragmentation and their relation to apoptotic markers: a cinematographic and confocal laser scanning microscopic study. Reproduction 129, 443–452. doi: 10.1530/rep.1.00533
Matwee, C., Betts, D. H., and King, W. A. (2000). Apoptosis in the early bovine embryo. Zygote 8, 57–68. doi: 10.1017/s0967199400000836
Melka, M.G., Rings, F., Hoelker, M., Tholen, E., Havlicek, V., Besenfelder, U., et al. (2010). Expression of apoptosis regulatory genes and incidence of apoptosis in different morphological quality groups of in vitro-produced bovine pre-implantation embryos. Reprod. Domestic Anim. 45, 915-921.
Ménézo, Y. J., and Sakkas, D. (2002). Monozygotic twinning: is it related to apoptosis in the embryo? Hum. Reprod. 17, 247–248. doi: 10.1093/humrep/17.1.247
Metcalfe, A. D., Hunter, H. R., Bloor, D. J., Lieberman, B. A., Picton, H. M., Leese, H. J., et al. (2004). Expression of 11 members of the BCL-2 family of apoptosis regulatory molecules during human preimplantation embryo development and fragmentation. Mol. Reprod. Dev. 68, 35–50. doi: 10.1002/mrd.20055
Meuter, A., Rogmann, L. M., Winterhoff, B. J., Tchkonia, T., Kirkland, J. L., and Morbeck, D. E. (2014). Markers of cellular senescence are elevated in murine blastocysts cultured in vitro: molecular consequences of culture in atmospheric oxygen. J. Assist. Reprod. Genet. 31, 1259–1267. doi: 10.1007/s10815-014-0299-8
Morley, N., Rapp, A., Dittmar, H., Salter, L., Gould, D., Greulich, K. O., et al. (2006). UVA-induced apoptosis studied by the new apo/necro-Comet-assay which distinguishes viable, apoptotic and necrotic cells. Mutagenesis 21, 105–114. doi: 10.1093/mutage/gel004
Munoz, M., Corrales, F. J., Caamano, J. N., Diez, C., Trigal, B., Mora, M. I., et al. (2012). Proteome of the Early Embryo-Maternal Dialogue in the Cattle Uterus. J. Prot. Res. 11, 751–766. doi: 10.1021/pr200969a
Munoz, M., Gatien, J., Salvetti, P., Martin-Gonzalez, D., Carrocera, S., and Gomez, E. (2020). Nuclear magnetic resonance analysis of female and male pre-hatching embryo metabolites at the embryo-maternal interface. Metabolomics 16:47
Munoz, M., Uyar, A., Correia, E., Ponsart, C., Guyader-Joly, C., Martinez-Bello, D., et al. (2014). Metabolomic Prediction of Pregnancy Viability in Superovulated Cattle Embryos and Recipients with Fourier Transform Infrared Spectroscopy. Biomed. Res. Int. 2014:608579
Munoz-Espin, D., Canamero, M., Maraver, A., Gomez-Lopez, G., Contreras, J., Murillo-Cuesta, S., et al. (2013). Programmed cell senescence during mammalian embryonic development. Cell 155, 1104–1118. doi: 10.1016/j.cell.2013.10.019
Murillo, A., Muñoz, M., Martín-González, D., Carrocera, S., Martínez-Nistal, A., and Gómez, E. (2017). Low serum concentration in bovine embryo culture enhances early blastocyst rates on Day-6 with quality traits in the expanded blastocyst stage similar to BSA-cultured embryos. Reprod. Biol. 17, 162–171. doi: 10.1016/j.repbio.2017.04.002
Murillo-Rios, A., Maillo, V., Munoz, M., Gutierrez-Adan, A., Carrocera, S., Martin-Gonzalez, D., et al. (2017). Short- and long-term outcomes of the absence of protein during bovine blastocyst formation in vitro. Reprod. Fertil. Dev. 29, 1064–1073. doi: 10.1071/rd15485
Nagano, M. (2019). Acquisition of developmental competence and in vitro growth culture of bovine oocytes. J. Reprod. Dev. 65, 195–201. doi: 10.1262/jrd.2019-022
Nguyen-Chi, M., Laplace-Builhe, B., Travnickova, J., Luz-Crawford, P., Tejedor, G., Lutfalla, G., et al. (2017). TNF signaling and macrophages govern fin regeneration in zebrafish larvae. Cell Death Dis. 8:e2979. doi: 10.1038/cddis.2017.374
Ock, S. A., Knott, J. G., and Choi, I. (2020). Involvement of CDKN1A (p21) in cellular senescence in response to heat and irradiation stress during preimplantation development. Cell Stress Chaperones 25, 503–508. doi: 10.1007/s12192-020-01090-4
Oliveira, C. S., Saraiva, N. Z., De Lima, M. R., Oliveira, L. Z., Serapiao, R. V., Garcia, J. M., et al. (2016). Cell death is involved in sexual dimorphism during preimplantation development. Mech. Dev. 139, 42–50. doi: 10.1016/j.mod.2015.12.001
Orozco, S., Yatim, N., Werner, M. R., Tran, H., Gunja, S. Y., Tait, S. W., et al. (2014). RIPK1 both positively and negatively regulates RIPK3 oligomerization and necroptosis. Cell Death Differ. 21, 1511–1521. doi: 10.1038/cdd.2014.76
Paula, L. A. D. C. E., and Hansen, P. J. (2008). Ceramide inhibits development and cytokinesis and induces apoptosis in preimplantation bovine embryos. Mol. Reprod. Dev. 75, 1063–1070. doi: 10.1002/mrd.20841
Paula-Lopes, F. F., and Hansen, P. J. (2002). Heat shock-induced apoptosis in preimplantation bovine embryos is a developmentally regulated phenomenon. Biol. Reprod. 66, 1169–1177. doi: 10.1093/biolreprod/66.4.1169
Pavlok, A., Lucas-Hahn, A., and Niemann, H. (1992). Fertilization and developmental competence of bovine oocytes derived from different categories of antral follicles. Mol. Reprod. Dev. 31, 63–67. doi: 10.1002/mrd.1080310111
Payne, D., Okuda, A., Wakatsuki, Y., Takeshita, C., Iwata, K., Shimura, T., et al. (2007). Time-lapse recording identifies human blastocysts at risk of producing monzygotic twins. Hum. Reprod. 22, I9-I10.
Plante, L., and King, W. A. (1994). Light and electron-microscopic analysis of bovine embryos derived by in-vitro and in-vivo fertilization. J. Assist. Reprod. Genet. 11, 515–529. doi: 10.1007/bf02216032
Pomar, F. J. R., Roelen, B. A. J., Slot, K. A., Van Tol, H. T. A., Colenbrander, B., and Teerds, K. J. (2004). Role of Fas-mediated apoptosis and follicle-stimulating hormone on the developmental capacity of bovine cumulus oocyte complexes in vitro. Biol. Reprod. 71, 790–796. doi: 10.1095/biolreprod.104.028613
Pomar, F. J. R., Teerds, K. J., Kidson, A., Colenbrander, B., Tharasanit, T., Aguilar, B., et al. (2005). Differences in the incidence of apoptosis between in vivo and in vitro produced blastocysts of farm animal species: a comparative study. Theriogenology 63, 2254–2268. doi: 10.1016/j.theriogenology.2004.10.015
Prastowo, S., Amin, A., Rings, F., Held, E., Wondim, D. S., Gad, A., et al. (2016). Fateful triad of reactive oxygen species, mitochondrial dysfunction and lipid accumulation is associated with expression outline of the AMP-activated protein kinase pathway in bovine blastocysts. Reprod. Fertil. Dev. doi: 10.1071/RD15319 [Epub ahead of print].
Qiao, F., Ge, H., Ma, X. N., Zhang, Y., Zuo, Z. Z., Wang, M. Y., et al. (2018). Bovine uterus-derived exosomes improve developmental competence of somatic cell nuclear transfer embryos. Theriogenology 114, 199–205. doi: 10.1016/j.theriogenology.2018.03.027
Qu, P. X., Zhao, Y. L., Wang, R., Zhang, Y. L., Li, L., Fan, J. L., et al. (2019). Extracellular vesicles derived from donor oviduct fluid improved birth rates after embryo transfer in mice. Reprod. Fertil. Dev. 31, 324–332. doi: 10.1071/rd18203
Ramos-Ibeas, P., Heras, S., Gómez-Redondo, I., Planells, B., Fernández-González, R., Pericuesta, E., et al. (2019). Embryo responses to stress induced by assisted reproductive technologies. Mol. Reprod. Dev. 86, 1292–1306.
Randi, F., Fernandez-Fuertes, B., Mcdonald, M., Forde, N., Kelly, A. K., Amorin, H. B., et al. (2016). Asynchronous embryo transfer as a tool to understand embryo-uterine interaction in cattle: is a large conceptus a good thing? Reprod. Fertil. Dev. 28, 1999–2006. doi: 10.1071/rd15195
Regassa, A., Rings, F., Hoelker, M., Cinar, U., Tholen, E., Looft, C., et al. (2011). Transcriptome dynamics and molecular cross-talk between bovine oocyte and its companion cumulus cells. BMC Genomics 12:57. doi: 10.1186/1471-2164-12-57
Reik, W., Dean, W., and Walter, J. (2001). Epigenetic reprogramming in mammalian development. Science 293, 1089–1093. doi: 10.1126/science.1063443
Rhinn, M., Ritschka, B., and Keyes, W. M. (2019). Cellular senescence in development, regeneration and disease. Development 146:dev151837. doi: 10.1242/dev.151837
Ritschka, B., Storer, M., Mas, A., Heinzmann, F., Ortells, M. C., Morton, J. P., et al. (2017). The senescence-associated secretory phenotype induces cellular plasticity and tissue regeneration. Genes Dev. 31, 172–183. doi: 10.1101/gad.290635.116
Rizos, D., Gutierrez-Adan, A., Perez-Garnelo, S., De La Fuente, J., Boland, M. P., and Lonergan, P. (2003). Bovine embryo culture in the presence or absence of serum: Implications for blastocyst development, cryotolerance, and messenger RNA expression. Biol. Reprod. 68, 236–243. doi: 10.1095/biolreprod.102.007799
Rizos, D., Maillo, V., Sanchez-Calabuig, M. J., and Lonergan, P. (2017). The Consequences of Maternal-Embryonic Cross Talk During the Periconception Period on Subsequent Embryonic Development. Adv. Exp. Med. Biol. 1014, 69–86. doi: 10.1007/978-3-319-62414-3_4
Rizos, D., Pintado, B., De La Fuente, J., Lonergan, P., and Gutiérrez-Adán, A. (2007). Development and pattern of mRNA relative abundance of bovine embryos cultured in the isolated mouse oviduct in organ culture. Mol. Reprod. Dev. 74, 716–723. doi: 10.1002/mrd.20652
Rizos, D., Ward, F., Duffy, P., Boland, M. P., and Lonergan, P. (2002). Consequences of bovine oocyte maturation, fertilization or early embryo development in vitro versus in vivo: implications for blastocyst yield and blastocyst quality. Mol. Reprod. Dev. 61, 234–248. doi: 10.1002/mrd.1153
Rodriguez, A., Diez, C., Caamano, J. N., De Frutos, C., Royo, L. J., Munoz, M., et al. (2007). Retinoid receptor-specific agonists regulate bovine in vitro early embryonic development, differentiation and expression of genes related to cell cycle arrest and apoptosis. Theriogenology 68, 1118–1127. doi: 10.1016/j.theriogenology.2007.08.007
Rodriguez, A., Diez, C., Ikeda, S., Royo, L. J., Caamano, J. N., Alonso-Montes, C., et al. (2006). Retinoids during the in vitro transition from bovine morula to blastocyst. Hum. Reprod. 21, 2149–2157. doi: 10.1093/humrep/del099
Rodriguez-Alonso, B., Sanchez, J. M., Gonzalez, E., Lonergan, P., and Rizos, D. (2020). Challenges in studying preimplantation embryo-maternal interaction in cattle. Theriogenology 150, 139–149. doi: 10.1016/j.theriogenology.2020.01.019
Rolland, L., Courbiere, B., Tassistro, V., Sansoni, A., Orsiere, T., Liu, W., et al. (2017). Comet assay on thawed embryos: an optimized technique to evaluate DNA damage in mouse embryos. Toxicol. Vitro 44, 266–272. doi: 10.1016/j.tiv.2017.07.010
Sagiv, A., Burton, D. G. A., Moshayev, Z., Vadai, E., Wensveen, F., Ben-Dor, S., et al. (2016). NKG2D ligands mediate immunosurveillance of senescent cells. Aging Us 8, 328–344. doi: 10.18632/aging.100897
Salilew-Wondim, D., Fournier, E., Hoelker, M., Saeed-Zidane, M., Tholen, E., Looft, C., et al. (2015). Genome-wide DNA methylation patterns of bovine blastocysts developed in vivo from embryos completed different stages of development in vitro. PLoS One 10:e0140467. doi: 10.1371/journal.pone.0140467
Salilew-Wondim, D., Saeed-Zidane, M., Hoelker, M., Gebremedhn, S., Poirier, M., Pandey, H. O., et al. (2018). Genome-wide DNA methylation patterns of bovine blastocysts derived from in vivo embryos subjected to in vitro culture before, during or after embryonic genome activation. BMC Genomics 19:424. doi: 10.1186/s12864-018-4826-3
Samir, P., Malireddi, R. K. S., and Kanneganti, T. D. (2020). The PANoptosome: A Deadly Protein Complex Driving Pyroptosis, Apoptosis, and Necroptosis (PANoptosis). Front. Cell Infect. Microbiol. 10:238. doi: 10.3389/fcimb.2020.00238
Sato, E. (1998). Morphological dynamics of cumulus-oocyte complex during oocyte maturation. New Trends Microanat. Reprod. 6, 103–118.
Sauerbrun-Cutler, M.T., Vega, M., Keltz, M., and Mcgovern, P.G. (2015). In vitro maturation and its role in clinical assisted reproductive technology. Obstet. Gynecol. Surv. 70, 45-57.
Schmaltz-Panneau, B., Cordova, A., Dhorne-Pollet, S., Hennequet-Antier, C., Uzbekova, S., Martinot, E., et al. (2014). Early bovine embryos regulate oviduct epithelial cell gene expression during in vitro co-culture. Anim,. Reprod. Sci. 149, 103–116. doi: 10.1016/j.anireprosci.2014.06.022
Shalom-Paz, E., Almog, B., Shehata, F., Huang, J., Holzer, H., Chian, R. C., et al. (2010). Fertility preservation for breast-cancer patients using IVM followed by oocyte or embryo vitrification. Reprod. Biomed. Online 21, 566–571. doi: 10.1016/j.rbmo.2010.05.003
Shan, B., Pan, H., Najafov, A., and Yuan, J. (2018). Necroptosis in development and diseases. Genes Dev. 32, 327–340. doi: 10.1101/gad.312561.118
Shen, X.H., Han, Y.J., Yang, B.C., Cui, X.S., and Kim, N.H. (2009). Hyperglycemia reduces mitochondrial content and glucose transporter expression in mouse embryos developing in vitro. J. Reprod. Dev. 55, 534-541.
Shirasawa, H., and Terada, Y. (2017). In vitro maturation of human immature oocytes for fertility preservation and research material. Reprod. Med. Biol. 16, 258-267.
Shub, A., and Lappas, M. (2020). Pregestational diabetes in pregnancy: Complications, management, surveillance, and mechanisms of disease-A review. Prenatal Diag. 40, 1092–1098. doi: 10.1002/pd.5718
Singla, S., Iwamoto-Stohl, L. K., Zhu, M., and Zernicka-Goetz, M. (2020). Autophagy-mediated apoptosis eliminates aneuploid cells in a mouse model of chromosome mosaicism. Nat. Commun. 11:2958.
Sirard, M. A. (2018). 40 years of bovine IVF in the new genomic selection context. Reproduction 156, R1-R7.
Sjöblom, C., Wikland, M., and Robertson, S. A. (2002). Granulocyte-macrophage colony-stimulating factor (GM-CSF) acts independently of the beta common subunit of the GM-CSF receptor to prevent inner cell mass apoptosis in human embryos. Biol. Reprod. 67, 1817–1823. doi: 10.1095/biolreprod.101.001503
Steptoe, P. C., and Edwards, R. G. (1978). Birth after the reimplantation of a human embryo. Lancet 2:366. doi: 10.1016/s0140-6736(78)92957-4
Stewart, B. M., Block, J., Morelli, P., Navarette, A. E., Amstalden, M., Bonilla, L., et al. (2011). Efficacy of embryo transfer in lactating dairy cows during summer using fresh or vitrified embryos produced in vitro with sex-sorted semen. J. Dairy Sci. 94, 3437–3445. doi: 10.3168/jds.2010-4008
Stockwell, B. R., Friedmann Angeli, J. P., Bayir, H., Bush, A. I., Conrad, M., Dixon, S. J., et al. (2017). Ferroptosis: a regulated cell death nexus linking metabolism, redox biology, and disease. Cell 171, 273–285. doi: 10.1016/j.cell.2017.09.021
Storer, M., Mas, A., Robert-Moreno, A., Pecoraro, M., Ortells, M. C., Di Giacomo, V., et al. (2013). Senescence is a developmental mechanism that contributes to embryonic growth and patterning. Cell 155, 1119–1130. doi: 10.1016/j.cell.2013.10.041
Sturmey, R. G., Bermejo-Alvarez, P., Gutierrez-Adan, A., Rizos, D., Leese, H. J., and Lonergan, P. (2010). Amino acid metabolism of bovine blastocysts: a biomarker of sex and viability. Mol. Reprod. Dev. 77, 285–296. doi: 10.1002/mrd.21145
Sudano, M. J., Paschoal, D. M., Rascado, T. D., Crocomo, L. F., Magalhaes, L. C. O., Martins, A., et al. (2014). Crucial surviving aspects for vitrified in vitro-produced bovine embryos. Zygote 22, 124–131. doi: 10.1017/s0967199412000196
Tarín, J. J., Pérez-Albalá, S., and Cano, A. (2000). Consequences on offspring of abnormal function in ageing gametes. Hum. Reprod. Update 6, 532–549. doi: 10.1093/humupd/6.6.532
Tatemoto, H., Sakurai, N., and Muto, N. (2000). Protection of porcine oocytes against apoptotic cell death caused by oxidative stress during in vitro maturation: Role of cumulus cells. Biol. Reprod. 63, 805–810. doi: 10.1095/biolreprod63.3.805
Tauwinklova, G., Gaillyova, R., Travnik, P., Oracova, E., Vesela, K., Hromadova, L., et al. (2010). Monozygotic twins with discordant karyotypes following preimplantation genetic screening and single embryo transfer: case report. J. Assist. Reprod. Genet. 27, 649–655. doi: 10.1007/s10815-010-9462-z
Tesfaye, D., Ghanem, N., Carter, F., Fair, T., Sirard, M. A., Hoelker, M., et al. (2009). Gene expression profile of cumulus cells derived from cumulus-oocyte complexes matured either in vivo or in vitro. Reprod. Fertil. Dev. 21, 451–461. doi: 10.1071/rd08190
Tesfaye, D., Lonergan, P., Hoelker, M., Rings, F., Nganvongpanit, K., Havlicek, V., et al. (2007). Suppression of connexin 43 and E-cadherin transcripts in in vitro derived bovine embryos following culture in vitro or in vivo in the homologous bovine oviduct. Mol. Reprod. Dev. 74, 978–988. doi: 10.1002/mrd.20678
Trigal, B., Gomez, E., Diez, C., Caamano, J.N., Martin, D., Carrocera, S., et al. (2011). In vitro development of bovine embryos cultured with activin A. Theriogenology 75, 584-588.
Uhde, K., Van Tol, H. T. A., Stout, T. A. E., and Roelen, B. A. J. (2018). Exposure to elevated glucose concentrations alters the metabolomic profile of bovine blastocysts. PLos One 13:e0199310. doi: 10.1371/journal.pone.0199310
Urrego, R., Rodriguez-Osorio, N., and Niemann, H. (2014). Epigenetic disorders and altered gene expression after use of Assisted Reproductive Technologies in domestic cattle. Epigenetics 9, 803–815. doi: 10.4161/epi.28711
Van Blerkom, J. (2004). Mitochondria in human oogenesis and preimplantation embryogenesis: engines of metabolism, ionic regulation and developmental competence. Reproduction 128, 269–280. doi: 10.1530/rep.1.00240
Van Eetvelde, M., Heras, S., Leroy, J. L. M. R., Van Soom, A., and Opsomer, G. (2017). The Importance of the Periconception Period: Immediate Effects in Cattle Breeding and in Assisted Reproduction Such as Artificial Insemination and Embryo Transfer. Adv. Exp. Med. Biol. 1014, 41–68. doi: 10.1007/978-3-319-62414-3_3
Van Hoeck, V., Sturmey, R. G., Bermejo-Alvarez, P., Rizos, D., Gutierrez-Adan, A., Leese, H. J., et al. (2011). Elevated non-esterified fatty acid concentrations during bovine oocyte maturation compromise early embryo physiology. PLoS One 6:e23183. doi: 10.1371/journal.pone.0023183
Vandaele, L., and Van Soom, A. (2011). Intrinsic factors affecting apoptosis in bovine in vitro produced embryos. Verhandelingen - Koninklijke Academie voor Geneeskunde van Belgie 73, 79–104.
Velez-Pardo, C., Morales, A. T., Del Rio, M. J., and Olivera-Angel, M. (2007). Endogenously generated hydrogen peroxide induces apoptosis via mitochondrial damage independent of NF-kappa B and p53 activation in bovine embryos. Theriogenology 67, 1285–1296. doi: 10.1016/j.theriogenology.2007.01.018
Von Karstedt, S., Montinaro, A., and Walczak, H. (2017). Exploring the TRAILs less travelled: TRAIL in cancer biology and therapy. Nat. Rev. Cancer 17, 352–366. doi: 10.1038/nrc.2017.28
Voss, A. K., and Strasser, A. (2020). The essentials of developmental apoptosis. F1000Research 9, 1–12.
Wajant, H. (2002). The Fas signaling pathway: more than a paradigm. Science 296, 1635–1636. doi: 10.1126/science.1071553
Wang, A. S., and Dreesen, O. (2018). Biomarkers of cellular senescence and skin aging. Front. Genet. 9:247. doi: 10.3389/fgene.2018.00247
Wang, D., Liu, Y., Zhang, R., Zhang, F., Sui, W., Chen, L., et al. (2016). Apoptotic transition of senescent cells accompanied with mitochondrial hyper-function. Oncotarget 7 28286–28300.
Wang, M., Gao, Y., Qu, P., Qing, S., Qiao, F., Zhang, Y., et al. (2017). Sperm-borne miR-449b influences cleavage, epigenetic reprogramming and apoptosis of SCNT embryos in bovine. Sci. Rep. 7:13403.
Warzych, E., Peippo, J., Szydlowski, M., and Lechniak, D. (2007). Supplements to in vitro maturation media affect the production of bovine blastocysts and their apoptotic index but not the proportions of matured and apoptotic oocytes. Anim. Reprod. Sci. 97, 334–343. doi: 10.1016/j.anireprosci.2006.01.011
Wrenzycki, C. (2018). Gene expression analysis and in vitro production procedures for bovine preimplantation embryos: Past highlights, present concepts and future prospects. Reprod. Domestic Anim. 53, 14–19. doi: 10.1111/rda.13260
Wrenzycki, C., Herrmann, D., Lucas-Hahn, A., Korsawe, K., Lemme, E., and Niemann, H. (2005). Addition to methods used already, such asMessenger RNA expression patterns in bovine embryos derived from in vitro procedures and their implications for development. Reprod. Fertil. Dev. 17, 23–35. doi: 10.1071/rd04109
Yang, M. Y., and Rajamahendran, R. C. P. S. (2002). Expression of Bcl-2 and Bax proteins in relation to quality of bovine oocytes and embryos produced in vitro. Anim. Reprod. Sci. 70, 159–169. doi: 10.1016/s0378-4320(01)00186-5
Yoon, S. B., Choi, S. A., Sim, B. W., Kim, J. S., Mun, S. E., Jeong, P. S., et al. (2014). Developmental Competence of Bovine Early Embryos Depends on the Coupled Response Between Oxidative and Endoplasmic Reticulum Stress. Biol. Reprod. 90:104.
Young, L. E., Sinclair, K. D., and Wilmut, I. (1998). Large offspring syndrome in cattle and sheep. Rev. Reprod. 3, 155–163. doi: 10.1530/revreprod/3.3.155
Keywords: assisted reproductive technologies, programmed cell death, cell cycle arrest, DNA fragmentation, TUNEL, caspase
Citation: Ramos-Ibeas P, Gimeno I, Cañón-Beltrán K, Cañón-Beltrán A, Rizos D and Gómez E (2020) Senescence and Apoptosis During in vitro Embryo Development in a Bovine Model. Front. Cell Dev. Biol. 8:619902. doi: 10.3389/fcell.2020.619902
Received: 21 October 2020; Accepted: 01 December 2020;
Published: 18 December 2020.
Edited by:
Wolfgang Knabe, Universität Münster, GermanyReviewed by:
Wilfried A. Kues, Institute of Farm Animal Genetics, Friedrich Loeffler Institute (FLI), GermanyHeiner Niemann, Hannover Medical School, Germany
Copyright © 2020 Ramos-Ibeas, Gimeno, Cañón-Beltrán, w, Rizos and Gómez. This is an open-access article distributed under the terms of the Creative Commons Attribution License (CC BY). The use, distribution or reproduction in other forums is permitted, provided the original author(s) and the copyright owner(s) are credited and that the original publication in this journal is cited, in accordance with accepted academic practice. No use, distribution or reproduction is permitted which does not comply with these terms.
*Correspondence: Enrique Gómez, ZWdvbWV6QHNlcmlkYS5vcmc=