- Department of Biochemistry and Molecular Biology, The Uniformed Services University of the Health Sciences - F. Edward Hébert School of Medicine, Bethesda, MD, United States
Cytoplasmic dynein-1 is a minus-end-directed microtubule motor that transports a variety of cargoes including early endosomes, late endosomes and other organelles. In many cell types, dynein accumulates at the microtubule plus end, where it interacts with its cargo to be moved toward the minus end. Dynein binds to its various cargoes via the dynactin complex and specific cargo adapters. Dynactin and some of the coiled-coil-domain-containing cargo adapters not only link dynein to cargo but also activate dynein motility, which implies that dynein is activated by its cellular cargo. Structural studies indicate that a dynein dimer switches between the autoinhibited phi state and an open state; and the binding of dynactin and a cargo adapter to the dynein tails causes the dynein motor domains to have a parallel configuration, allowing dynein to walk processively along a microtubule. Recently, the dynein regulator LIS1 has been shown to be required for dynein activation in vivo, and its mechanism of action involves preventing dynein from switching back to the autoinhibited state. In this review, we will discuss our current understanding of dynein activation and point out the gaps of knowledge on the spatial regulation of dynein in live cells. In addition, we will emphasize the importance of studying a complete set of dynein regulators for a better understanding of dynein regulation in vivo.
Introduction
In eukaryotic cells, motor proteins such as dyneins, kinesins and myosins are ATPases, and they use the energy from ATP hydrolysis to drive intracellular motility (Hirokawa et al., 2009; Verhey and Hammond, 2009; Dodding and Way, 2011; Hammer and Sellers, 2011; Reck-Peterson et al., 2018; Olenick and Holzbaur, 2019; Scherer et al., 2020). In most mammalian cells, polarized microtubules serve as tracks for long-distance transport: while the plus-end-directed kinesins transport cargoes toward the microtubule plus ends near the cell periphery, the minus end-directed cytoplasmic dynein transports cargoes inward from the cell periphery (Reck-Peterson et al., 2018). Cytoplasmic dynein-1 (called “dynein” hereafter for simplicity) powers the intracellular transport of nuclei/mitotic spindles, Golgi, mitochondria, early endosomes, late endosomes, autophagosomes, proteins, mRNAs and/or virus particles (Dodding and Way, 2011; Reck-Peterson et al., 2018; Olenick and Holzbaur, 2019; Scherer et al., 2020). Deficiencies in dynein and its regulators such as dynactin and LIS1 (Lissencephaly-1) cause devastating neurodegenerative diseases and brain developmental disorders (Wynshaw-Boris, 2007; Maday et al., 2014; Guedes-Dias and Holzbaur, 2019; Markus et al., 2020).
Studies have shown that dynein binds to its various cargoes via the dynactin complex and cargo adapters. Importantly, some cargo adapters not only link dynein to cargos but also activate dynein motility, which implies that the dynein motor is activated by its cargo (Reck-Peterson et al., 2018; Olenick and Holzbaur, 2019; Canty and Yildiz, 2020). While some kinesin and myosin motors are also known to be activated by cargo or specific adapters/scaffolding proteins (Sellers and Knight, 2007; Trybus, 2008; Hirokawa et al., 2009; Verhey and Hammond, 2009; Fu and Holzbaur, 2014; Sweeney and Holzbaur, 2018), the mechanism of dynein activation is distinct due to the unique structure of the dynein motor. In this review, we will cover our current understanding on the mechanism of dynein activation. We will mainly use early endosome transport in filamentous fungi as an example to discuss how dynein activation is spatially regulated in vivo and point out unresolved issues that need to be addressed in the future.
Dynactin and Cargo Adapter Proteins Mediate the Dynein-Cargo Interaction
Compared to kinesins or myosins, dynein is extremely huge and complex (Höök and Vallee, 2006; Sweeney and Holzbaur, 2018). It is a multi-protein complex of ∼1.4 MDa containing two dynein heavy chains (HCs) as well as other subunits such as intermediate chains (ICs), light intermediate chains (LICs), and light chains (LCs) (Pfister et al., 2005; Reck-Peterson et al., 2018). The HCs form a homodimer, and each HC monomer contains the C-terminal motor head and the N-terminal tail. The motor head is responsible for motility, while the tail is responsible for HC-HC dimerization and also binds other dynein subunits as well as the dynactin complex (King, 2000; Carter et al., 2016; Schmidt and Carter, 2016). The motor head of the dynein HC consists a motor ring with six AAA (ATPases Associated with diverse cellular Activities) domains, a linker (∼10 nm) connecting the motor ring with the tail (Burgess et al., 2003), and a microtubule-binding domain that is connected to the motor ring via a coiled-coil stalk extending out between AAA4 and AAA5 (Gee et al., 1997; Gibbons et al., 2005; Cianfrocco et al., 2015; Figure 1A). ATP binding and hydrolysis at AAA1 cause conformational changes in the ring, which can be transmitted via the coiled-coil stalk to the microtubule binding site, driving dynein movement along a microtubule (Roberts et al., 2013; Carter et al., 2016). ATP hydrolysis at AAA3 allows the proper transmission of conformational changes around the ring, allowing dynein to be released from the microtubule when ATP is bound to AAA1 (Bhabha et al., 2014; Dewitt et al., 2015; Nicholas et al., 2015).
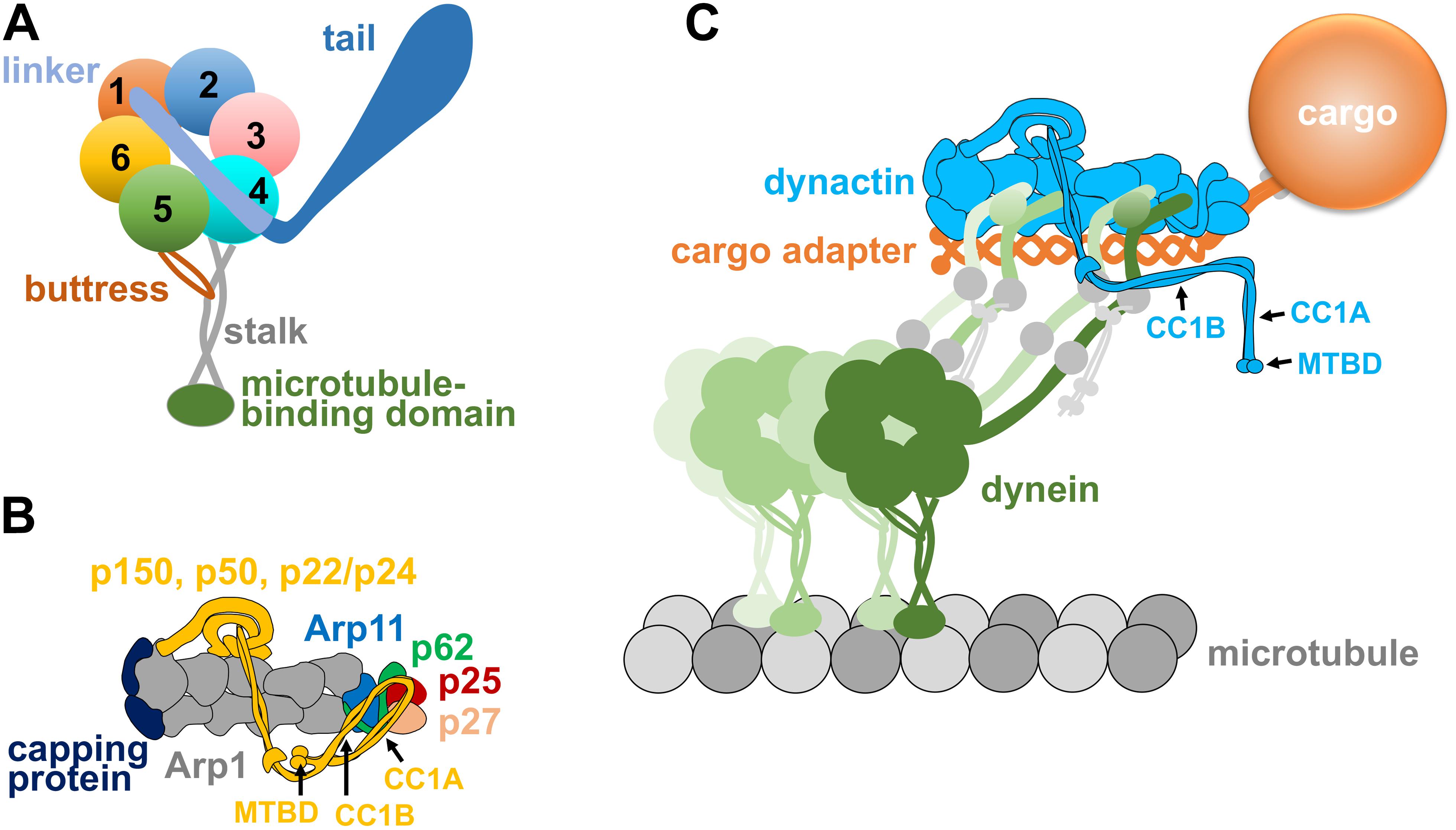
Figure 1. (A) A diagram of the dynein heavy chain with a motor ring containing six AAA domains (1–6), a linker domain connected to the beginning of AAA1 and the N-terminal tail domain connected to the linker. A microtubule-binding domain is connected to the coiled-coil stalk that emerges from a location between AAA4 and AAA5, and the buttress coming from AAA5 supports the stalk. All these domains work together to ensure minus-end-directed motility of dynein as well as other properties of the dynein motor such as tension sensing (Roberts et al., 2013; Carter et al., 2016; Can et al., 2019; Rao et al., 2019). (B) A diagram of the dynactin complex with its Arp1 mini-filament, barbed-end capping protein, pointed-end proteins Arp11, p62, p25, and p27, as well as the shoulder/sidearm proteins p150, p22/p24, and p50 (Schroer, 2004; Urnavicius et al., 2015). Note that p150 is depicted as a folded molecule, and its CC1A, CC1B, and microtubule-binding domain (MTBD) are indicated by arrows. (C) A diagram of the dynein-dynactin-cargo adapter complex and the cargo linked to the cargo adapter. The cargo adapter, depicted as a homodimer, contains coiled-coil domains. Note that the dynein tails bind to the Arp1 mini-filament of dynactin, as shown by EM structural analyses (Schlager et al., 2014; Chowdhury et al., 2015; Urnavicius et al., 2015). An extended form of p150 is depicted, and its CC1A, CC1B, and microtubule-binding domain (MTBD) are indicated by arrows. Two dynein dimers are depicted, since a fraction of the dynein-dynactin-cargo adapter complexes could contain a second dynein dimer along the Arp1 mini-filament (Grotjahn et al., 2018; Urnavicius et al., 2018). Dynein intermediate chains and dynein light intermediate chains are depicted as gray circles on the dynein tails, and dynein light chains are depicted as small gray circles connected to the dynein intermediate chains.
The dynactin complex of ∼1 MDa is involved in almost all functions of cytoplasmic dynein (Schroer, 2004). The backbone of the dynactin complex is an Arp1 mini-filament of ∼37 nm (Schafer et al., 1994), which provides the binding sites for dynein tails and cargo adapters (Chowdhury et al., 2015; Urnavicius et al., 2015). The pointed end of the Arp1 mini-filament is occupied by the pointed-end subcomplex containing p25, p27, p62 and Arp11 (Eckley et al., 1999; Figure 1B). The barbed end of the Arp1 mini-filament is occupied by the actin-capping protein, which also caps the barbed ends of conventional actin filaments (Schafer et al., 1994; Wear and Cooper, 2004). The largest subunit of the dynactin complex is p150Glued (or p150 for simplicity), which contains a microtubule-binding domain (MTBD) at its N-terminus (Holzbaur et al., 1991; Waterman-Storer et al., 1995). Following the MT-binding domain are the coiled-coil domains CC1 and CC2, and CC1 interacts with the N-terminus of dynein IC in biochemical assays (Karki and Holzbaur, 1995; Vaughan and Vallee, 1995; King et al., 2003). The CC1 domain of p150 can be further divided into CC1A and CC1B (Figures 1B,C), and CC1B contains a dynein-IC-binding domain (McKenney et al., 2011; Loening et al., 2020) whose function may be modulated by the binding of CC1A (Tripathy et al., 2014; Saito et al., 2020). Two other subunit of the dynactin complex, p22/p24 and p50 dynamitin that forms an oligomer (Echeverri et al., 1996; Karki et al., 1998; Melkonian et al., 2007), together with part of the p150 subunit, form a “shoulder/side arm” adjacent to the Arp1 mini-filament, and the p50 oligomer was proposed to function as a template for Arp1 mini-filament assembly (Urnavicius et al., 2015). Interestingly, in the dynein-dynactin cargo adapter tripartite complex, it is the Arp1 mini-filament rather than the p150 subunit that interacts with the tails of dynein (Schlager et al., 2014; Chowdhury et al., 2015; Urnavicius et al., 2015, 2018; Grotjahn et al., 2018; Figure 1C). Future work will be needed to address whether the dynein IC-p150 interaction found by biochemical assays is important for initiating the dynein-dynactin interaction before cargo adapter binding.
The dynactin complex is needed for the dynein-cargo interaction. This function of dynactin was proposed many years ago (Schroer and Sheetz, 1991; Waterman-Storer et al., 1997), but this idea had remained controversial (Haghnia et al., 2007). This subject was revisited in two independent studies on the pointed-end proteins of the Arp1-minifilament, in the filamentous fungus Aspergillus nidulans and mammalian cells (Zhang et al., 2011; Yeh et al., 2012). These studies have found that the pointed-end proteins p25 in A. nidulans and the p25/p27 heterodimer in mammalian cells play a critical role in the interaction of dynein with its early endosome cargo (Zhang et al., 2011; Yeh et al., 2012). Both p25 and p27 adopt a left-handed beta-helix structure (Parisi et al., 2004; Yeh et al., 2013). Because p25 has many hydrophobic residues, it seemed plausible that p25 may contact membrane directly (Yeh et al., 2012). However, follow-up genetic screens in A. nidulans as well as another filamentous fungus Ustilago maydis led to the discovery that the FTS-Hook-FHIP (FHF) complex (Xu et al., 2008) functions as an adapter allowing dynein-dynactin to link with early endosomes (Bielska et al., 2014b; Yao et al., 2014; Zhang et al., 2014).
The Hook proteins (three in mammalian cells: Hook1, Hook2 and Hook3) and the FHF complex were initially discovered in higher eukaryotic cells (Krämer and Phistry, 1996; Walenta et al., 2001; Xu et al., 2008). Within the fungal FHF complex, HookA in A. nidulans and Hok1 in U. maydis use their N-terminal Hook domain (Schroeder and Vale, 2016) and the coiled-coil domains to interact with dynein-dynactin (Bielska et al., 2014b; Zhang et al., 2014; Qiu et al., 2018), and this interaction depends on Arp1 and p25 (Zhang et al., 2014). Structural studies on the mammalian Hook3 protein further demonstrate that a Hook protein binds dynein-dynactin directly via dynein light intermediate chain (Schroeder and Vale, 2016; Lee et al., 2018) and the Arp1 filament (Urnavicius et al., 2018). The interaction of fungal hook proteins with the early endosome depends on FTS and FHIP (Yao et al., 2014; Guo et al., 2016); FHIP makes the closest contact with early endosome (Yao et al., 2014), most likely via its direct interaction with Rab5 (Guo et al., 2016). It is unclear whether FTS and FHIP are involved in targeting mammalian Hook1, Hook2, or Hook3 onto other cargoes or cellular structures, such as the TrkB–BDNF-signaling endosome (Olenick et al., 2019), nuclear envelope (Dwivedi et al., 2019b), centrosome (Szebenyi et al., 2007a), aggresome (Szebenyi et al., 2007b), and the Golgi apparatus (Walenta et al., 2001). It should be pointed out that Hook1 interacts directly with cargo proteins of the recycling endosomes (Maldonado-Báez et al., 2013), and Hook1 and Hook2 bind directly with AP4 (adaptor protein complex 4 of the trans-Golgi network), which is responsible for trafficking of the autophagy protein ATG9A (Mattera et al., 2020).
There are several other important dynein adapters (Reck-Peterson et al., 2018; Olenick and Holzbaur, 2019), such as proteins of the Bicaudal D (BICD) family including BicD2 (Hoogenraad and Akhmanova, 2016), Rab11-FIP3 (Horgan et al., 2010) and Spindly (Griffis et al., 2007). The domain organization of these dynein adapter proteins are similar in that they all contain an N-terminal portion including the coiled-coil domains important for binding dynein-dynactin and a C-terminus required for cargo binding (Reck-Peterson et al., 2018; Dwivedi et al., 2019a; Olenick and Holzbaur, 2019). In vitro experiments show that the N-terminal portion of the cargo adapters can enhance the interaction between the dynein complex and the dynactin complex (Splinter et al., 2012; McKenney et al., 2014; Olenick et al., 2016). Such an effect was first shown for the N-terminal part of the BicD2 protein (Splinter et al., 2012), a result highly instrumental to the ground-breaking experiments revealing cargo adapters being critical for dynein activation (McKenney et al., 2014; Schlager et al., 2014).
Dynactin and Specific Cargo Adapter Proteins Activate Dynein
Mammalian dynein by itself is incapable of moving along the microtubule processively, although the dynein is active in a microtubule-gliding assay (Trokter et al., 2012; McKenney et al., 2014; Schlager et al., 2014). Adding dynactin alone does not seem to help even when dynactin is added in large excess (McKenney et al., 2014; Schlager et al., 2014). Astonishingly, addition of both BicD2 and dynactin enhances the processivity of dynein dramatically (McKenney et al., 2014; Schlager et al., 2014). Importantly, not only the BicD2 N-terminus but also the dynein-dynactin-binding portion of Hook3 and other cargo adapters with a similar domain organization, including Rab11-FIP3 that targets dynein to Rab11-positive vesicles (Horgan et al., 2010) and Spindly that targets dynein to kinetochores (Griffis et al., 2007; Gama et al., 2017), all stimulated dynein processivity via an enhancement of the dynein-dynactin interaction (McKenney et al., 2014). The processivity and velocity of dynein motility activated by the N-terminal Hook1 and Hook3 are even higher than that by the N-terminal BicD2 (Olenick et al., 2016). These coiled-coil domain-containing dynein adapters are considered as “activating adapters,” and new dynein activators with this domain signature have continuously been discovered (Redwine et al., 2017; Reck-Peterson et al., 2018; Olenick and Holzbaur, 2019; Wang et al., 2019).
As revealed by a cryo-EM study, dynein activation results from a conformational change of dynein upon binding to dynactin and a cargo adapter (Zhang K. et al., 2017). Specifically, the two dynein heavy chains within the dimer are initially held in an auto-inhibited “phi” conformation with the two heavy chains positioned very close to each other (Amos, 1989; Torisawa et al., 2014; Zhang K. et al., 2017). This conformation can be switched to an “open” state with the two dynein heavy chains separated from each other. Although the “open dynein” has a higher affinity for microtubules, it is still not configured properly to move along a microtubule (Zhang K. et al., 2017). Only after the dynein tails bind dynactin and a cargo adapter, the two motor domains with the microtubule-binding stalks become parallel, which allows processive movement (Zhang K. et al., 2017). It is important to point out that since dynactin and cargo adapters bind dynein tails (Schlager et al., 2014; Chowdhury et al., 2015; Urnavicius et al., 2015; Zhang K. et al., 2017), the conformational change in the dynein motor domains must be transmitted through the dynein tails. This may explain why changes in the dynein tail, especially subtle mutations that do not affect the dynein-dynactin interaction, can lead to a severe defect in dynein function (Ori-McKenney et al., 2010; Sivagurunathan et al., 2012; Qiu et al., 2013; Hoang et al., 2017; Marzo et al., 2019).
EM-based structural studies have shown that in some dynein-dynactin-cargo adapter complexes, there are two dynein dimers instead of one, and both dimers have their tails positioned along the Arp1 mini-filament (Grotjahn et al., 2018; Urnavicius et al., 2018). Some cargo adapters including Hook3 and BicDR1 have a much stronger tendency to form this type of complexes with an extra dynein compared to other cargo adapters such as BicD2, and the presence of two dynein dimers enhances dynein’s speed and force output (Urnavicius et al., 2018). This may be part of the reason why some of the dynein-dynactin-Hook3 complexes move with a higher velocity compared to the dynein-dynactin-BicD2 complexes (Olenick et al., 2016). Recently, the dynein regulator LIS1 has also been shown to enhance the recruitment of the second dynein dimer to the dynein-dynactin-cargo adapter complex in vitro (Elshenawy et al., 2020; Htet et al., 2020), further suggesting the importance of the second dynein dimer. However, it still remains to be determined what proportion of cargo-bound dynactin in live cells contain two dynein dimers associated with the Arp1 mini-filament and how different regulators change this proportion.
While the in vitro motility studies and structural analysis provided significant insights into the mechanism of dynein activation, knowledges gained from in vivo studies further shed light on the spatial regulation of dynein activity. In filamentous fungi including Aspergillus nidulans and Ustilago maydis, the dynamic microtubule plus ends face the hyphal tip, and both dynein and dynactin are strongly enriched at the microtubule plus ends (Han et al., 2001; Zhang et al., 2003; Lenz et al., 2006). Fungal dynein transports many cargoes and its major cargo is the early endosome, which undergo rapid bi-directional movements (Wedlich-Soldner et al., 2002; Lenz et al., 2006; Abenza et al., 2009; Zekert and Fischer, 2009; Penalva et al., 2017; Hernández-González et al., 2018; Otamendi et al., 2019; Bieger et al., 2020). Early endosome motility not only is coupled to endosome maturation (Abenza et al., 2010, 2012), it also helps distribute hitchhiking cargoes including peroxisomes, ribosomes and RNAs (Baumann et al., 2012; Bielska et al., 2014a; Higuchi et al., 2014; Guimaraes et al., 2015; Pohlmann et al., 2015; Lin et al., 2016; Salogiannis et al., 2016). Early endosomes are moved by kinesin-3 toward the plus ends near the hyphal tip and then delivered to dynein to be moved away from the hyphal tip (Lenz et al., 2006). The accumulation of dynein at the microtubule plus end depends on kinesin-1 and dynactin (especially the microtubule-binding domain of p150) (Xiang et al., 2000; Zhang et al., 2003; Lenz et al., 2006; Egan et al., 2012; Yao et al., 2012), and dynein-mediated early endosome transport depends on kinesin-1, most likely because the accumulation of dynein at the plus end enhances the chance for the dynein-early endosome interaction (Lenz et al., 2006; Zhang et al., 2010).
Given the current understanding of dynein’s structural change during its activation (Zhang K. et al., 2017), we speculate that fungal dynein is in the autoinhibited phi conformation while being transported by kinesin-1 toward the microtubule plus end. This would prevent a tug-of-war between kinesin-1 and dynein. Conceptually, this is similar to the regulatory mechanism of dynein-2 in intraflagellar transport (IFT) as revealed by cryo-electron tomography: dynein-2 is in an autoinhibited conformation when it is being transported to the plus end by kinesin-2 (Jordan et al., 2018). In A. nidulans and U. maydis, the microtubule plus end-localized dynein-dynactin interact with early endosomes on which the activating cargo adapter HookA or Hok1 is bound (Bielska et al., 2014b; Zhang et al., 2014). In A. nidulans, overexpression of the cytosolic ΔC-HookA drives dynein departure from the microtubule plus ends and causes dynein to accumulate at the minus ends (Qiu et al., 2019; Figure 2). This supports not only the idea of cargo adapter-mediated dynein activation emerged from in vitro studies (McKenney et al., 2014; Schlager et al., 2014) but also the postulation that the plus-end dynein is activated by its early endosome cargo (Lenz et al., 2006).
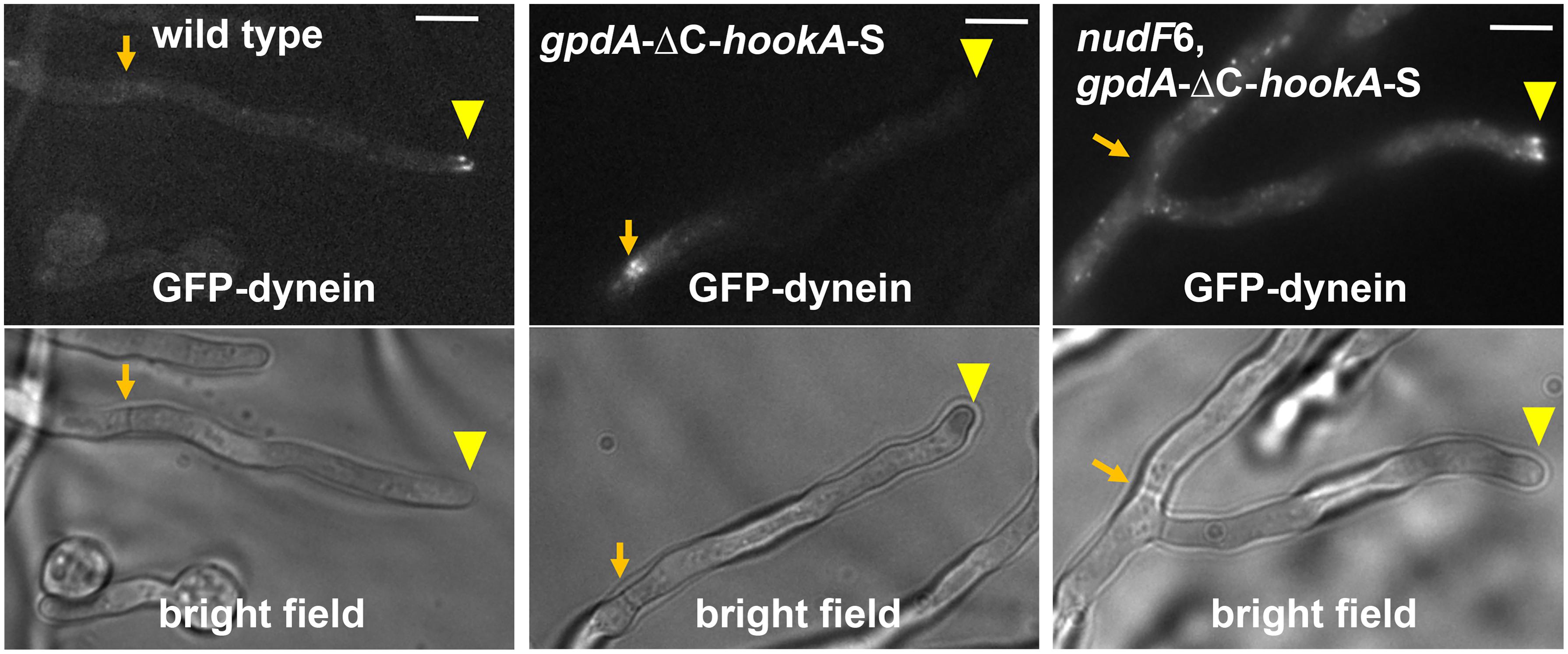
Figure 2. Dynein activation in A. nidulans depends on NudF/LIS1. In wild-type cells, dynein is accumulated at the microtubule plus ends, and this accumulation is represented by the comet-like structures formed by GFP-labeled dynein near the hyphal tip (Xiang et al., 2000; Han et al., 2001). Dynein activation, as judged by dynein relocation from the microtubule plus ends at hyphal tip (yellow arrowhead) to the minus ends at septum (Zhang Y. et al., 2017) (brown arrow), is driven by the dynein-dynactin-binding portion of the cargo adapter HookA, ΔC-HookA, overexpressed under the gpdA promoter (gpdA-ΔC-hookA-S, note that “S” indicates S-tag, an affinity tag for biochemical studies) (Qiu et al., 2019). In the nudF6 mutant, a NudF/LIS1 loss-of-function mutant, dynein is retained at the microtubule plus ends. Bright-field images are shown below to indicate hyphal shape and septal position. Bars, 5 μm. These images have been published previously in the Journal of Cell Biology (Qiu et al., 2019).
The activating function of the cargo adapters has been thought to be involved in the enhancement of the dynein-dynactin interaction. Interestingly, the dynein-dynactin interaction must have occurred to a certain extent before cargo binding in cells. In filamentous fungi, dynactin is required for the plus-end localization of dynein (Xiang et al., 2000; Zhang et al., 2003, 2008; Lenz et al., 2006; Egan et al., 2012; Yao et al., 2012), suggesting that either the two complexes are transported together by kinesin-1 to the plus end or they associate at the plus end after being transported separately. It seems likely that they are transported together because loss of Arp1 reduced the interaction between dynein and kinesin-1 (Qiu et al., 2018). HookA is able to interact with dynein or dynactin only when both complexes are present (Zhang et al., 2014), although dynein LIC binds the Hook domain directly (Malone et al., 2003; Schroeder and Vale, 2016; Lee et al., 2018). Thus, it is most likely that an early endosome interacts with a plus end dynein-dynactin complex and changes the configuration of the dynein-dynactin interaction to make it productive for minus-end-directed movement. This notion is consistent with previous findings that while the dynein IC in the dynein tail interacts with the p150 subunit of dynactin without cargo adapter (Karki and Holzbaur, 1995; Vaughan and Vallee, 1995; King et al., 2003), the dynein tails bind the Arp1 filament in the presence of cargo adapters (Schlager et al., 2014; Chowdhury et al., 2015; Urnavicius et al., 2015, 2018; Zhang K. et al., 2017; Grotjahn et al., 2018).
The spatial regulation of dynein activation appears to be evolutionarily conserved. In Drosophila oocyte, and in Caenorhabditis elegans and mammalian neurons, kinesin-1 has been implicated in transporting dynein toward the microtubule plus ends for function (Brendza et al., 2002; Duncan and Warrior, 2002; Januschke et al., 2002; Palacios and St Johnston, 2002; Yamada et al., 2008, 2010; Arimoto et al., 2011; Twelvetrees et al., 2016). The kinesin-1-dynein interaction has been dissected in detail, and it was found that the dynein intermediate chain interacts directly with the light chains of kinesin-1 in mammalian hippocampal neurons (Ligon et al., 2004; Twelvetrees et al., 2016). In C. elegans, however, the interaction between kinesin-1 and dynein is mediated by UNC-16 that binds to the dynein light intermediate chain (Arimoto et al., 2011). In mouse DRG neurons, dynactin and dynein are transported separately by kinesin-1 via mNudC (Yamada et al., 2010). Thus, how kinesin-1 transports dynein may differ in different cell types. What appears to be conserved is the need to get dynein-dynactin to the microtubule plus end using the plus-end-directed kinesin-1, which could enhance the chance of dynein-cargo interaction. In mammalian and Drosophila neurons, the microtubule-binding domain of p150 dynactin is required for enriching dynactin at the distal end of an axon, thereby facilitating the initiation of retrograde transport from the neurite tip or synaptic termini (Lloyd et al., 2012; Moughamian and Holzbaur, 2012). Hook1, which is able to activate dynein (Olenick et al., 2016), is required for transporting signaling endosomes in axons (Olenick et al., 2019).
In the budding yeast, dynein is almost exclusively used for moving nuclei/spindles (Eshel et al., 1993; Li et al., 1993; Winey and Bloom, 2012), and both dynein and dynactin are clearly accumulated at the microtubule plus end (Lee et al., 2003; Sheeman et al., 2003; Moore et al., 2008). Although dynein can be recruited directly from a cytoplasm to the plus end via Bik1/Clip170 and Pac1/LIS1, Kip2 (kinesin-7) also plays an important role in transporting Bik1/Clip170 and dynein to the microtubule plus end (Lee et al., 2003; Sheeman et al., 2003; Carvalho et al., 2004; Markus et al., 2011; Roberts et al., 2014). Dynein accumulated at the microtubule plus end is activated by its cortical anchor Num1, which is a cargo adapter-like molecule containing coiled-coil domains (Farkasovsky and Küntzel, 1995; Lee et al., 2003; Sheeman et al., 2003; Markus and Lee, 2011; Tang et al., 2012; Lammers and Markus, 2015). In the fission yeast, dynein drives the oscillatory movement of meiotic prophase nucleus (Yamamoto et al., 1999), and this function of dynein depends on dynactin and the Num1-like cortical anchor (Niccoli et al., 2004; Yamashita and Yamamoto, 2006). It was found that dynein molecules along microtubules are inactive but activated by the cortical Num1 homolog (Ananthanarayanan et al., 2013). Thus, although yeast dynein is active on its own in vitro (Reck-Peterson et al., 2006), yeast dynein’s cortical anchor functions as an activating cargo adapter in vivo.
Lis1 Is a Positive Regulator for Dynein Activation
Beside dynactin and cargo adapters, another important protein involved in dynein activation is LIS1 (Lissencephaly-1) (Markus et al., 2020). The mechanism of LIS1 action in the dynein pathway has been controversial, but multiple recent studies suggest that LIS1 promotes the open dynein conformation, thereby facilitating dynein activation (Qiu et al., 2019; Elshenawy et al., 2020; Htet et al., 2020; Marzo et al., 2020; McKenney, 2020). LIS1, a WD40-repeats-containing protein, was initially identified as a causal gene for type 1 lissencephaly, a human brain developmental disorder (Reiner et al., 1993). Its functional connection to dynein was first suggested by genetic studies in fungi and further demonstrated in higher eukaryotic organisms and cell types (Xiang et al., 1995; Geiser et al., 1997; Liu et al., 1999, 2000; Faulkner et al., 2000; Lei and Warrior, 2000; Smith et al., 2000; Markus et al., 2020). In contrast to dynactin that binds to the dynein tail (Karki and Holzbaur, 1995; Vaughan and Vallee, 1995; Chowdhury et al., 2015; Urnavicius et al., 2015), LIS1 binds directly to the dynein motor ring at AAA3/AAA4 as shown by cryo-EM studies (Huang et al., 2012; Toropova et al., 2014; Desantis et al., 2017; Htet et al., 2020). LIS1’s binding to this site is not compatible with the autoinhibited phi conformation of dynein (Htet et al., 2020; Marzo et al., 2020), which supports a “check valve” (Markus et al., 2020) model of LIS1 mechanism of action: it stabilizes the open dynein conformation and prevents it from switching to the autoinhibited phi state, thereby facilitating cargo-adapter-mediated dynein activation (Qiu et al., 2019; Canty and Yildiz, 2020; Elshenawy et al., 2020; Htet et al., 2020; Markus et al., 2020; Marzo et al., 2020).
In budding yeast and mammalian cells, LIS1 is required for the microtubule plus-end accumulation of dynein and consequently dynein offloading to cortex or cargoes (Lee et al., 2003; Sheeman et al., 2003; Markus and Lee, 2011; Markus et al., 2011; Splinter et al., 2012; Tame et al., 2014). However, dynactin rather than LIS1 plays a critical role in the plus-end accumulation of dynein in filamentous fungi (Zhang et al., 2003; Lenz et al., 2006; Egan et al., 2012). This allows the role of LIS1 in cargo-adapter-mediated dynein activation in vivo to be shown clearly in A. nidulans (Qiu et al., 2019). Specifically, while overexpression of ΔC-HookA drives almost a complete relocation of dynein from the microtubule plus ends to the minus ends, dynein remains at the plus ends in the nudF (lis1) loss-of-function mutants when ΔC-HookA is overexpressed (Qiu et al., 2019; Figure 2). This requirement of LIS1 for HookA-mediated dynein activation in vivo is consistent with a role of LIS1 in enhancing the frequency of departure of the dynein-dynactin-cargo adapter complex from the microtubule plus end in vitro (Baumbach et al., 2017; Jie et al., 2017). Importantly, a phi mutation that promotes open dynein (Zhang K. et al., 2017) bypasses the requirement for LIS1 to a significant extent (Qiu et al., 2019), suggesting that LIS1 is involved in promoting open dynein, thereby facilitating cargo adapter-mediated dynein activation (Qiu et al., 2019). This main conclusion agrees with three other recent studies (Elshenawy et al., 2020; Htet et al., 2020; Marzo et al., 2020), although LIS1 in A. nidulans does not seem to significantly affect the formation of the dynein-dynactin-ΔC-Hook complex (Qiu et al., 2019), while LIS1 enhances the recruitment of the second dynein to the dynein-dynactin-BicD2N complexes in vitro (Elshenawy et al., 2020; Htet et al., 2020). Possibly, A. nidulans LIS1 still enhances the dynein-dynactin interaction as described in other systems (Dix et al., 2013; Wang et al., 2013), but this effect was not easily detected when the concentration of cytosolic cargo adapters is high enough. We should also point out that LIS1 may play roles beyond stabilizing the open dynein because constitutively opening dynein does not allow the requirement for LIS1 to be completely bypassed (Qiu et al., 2019; Elshenawy et al., 2020; Htet et al., 2020; Marzo et al., 2020).
In filamentous fungi and budding yeast, LIS1 accumulates at the microtubule plus end just like dynein (Han et al., 2001; Lee et al., 2003; Callejas-Negrete et al., 2015). LIS1’s plus-end accumulation depends partly on dynein, its binding partner NudE as well as the CLIP170 homolog CLIPA or Bik1 (Zhang et al., 2003; Li et al., 2005; Efimov et al., 2006; Markus et al., 2011). This is consistent with earlier data from mammalian cells and budding yeast indicating a direct interaction between LIS1 and CLIP170/Bik1 (Coquelle et al., 2002; Sheeman et al., 2003). In the budding yeast, the dynein-LIS1-Bik1/CLIP170 complex could be transported by the Kip2 kinesin-7 to the microtubule plus end, or, dynein and LIS1 form a complex before being directly recruited from the cytosol to the plus end via Bik1/CLIP170 (Carvalho et al., 2004; Markus et al., 2011). Thus, the plus-end dynein in yeast is most likely in the open conformation and can interact effectively with dynactin and Num1 (Markus et al., 2020; Marzo et al., 2020). In cultured cells and in reconstituted in vitro systems with dynamic microtubules, LIS1 also enhances the plus-end targeting of mammalian dynein, although the plus-end dynein localization also requires dynactin (Splinter et al., 2012; Baumbach et al., 2017; Jha et al., 2017). In the filamentous fungi such as A. nidulans and U. maydis, the plus-end accumulation of dynein requires kinesin-1 and dynactin but not LIS1 (Zhang et al., 2003; Lenz et al., 2006; Egan et al., 2012). Thus, the plus-end dynein in filamentous fungi could be in the autoinhibited phi conformation before it interacts with LIS1 (Figure 3). In A. nidulans, dynein localized along microtubules in cells lacking kinesin-1 is still able to be activated by LIS1 and ΔC-HookA (Qiu et al., 2019), suggesting that LIS1 can also bind to dynein not at the plus end. It cannot be excluded that some LIS1 molecules may bind to dynein during plus-end-directed transport mediated by kinesin-1, and in that case, dynein at the plus end is in the open conformation, waiting to be activated by the early endosome cargo.
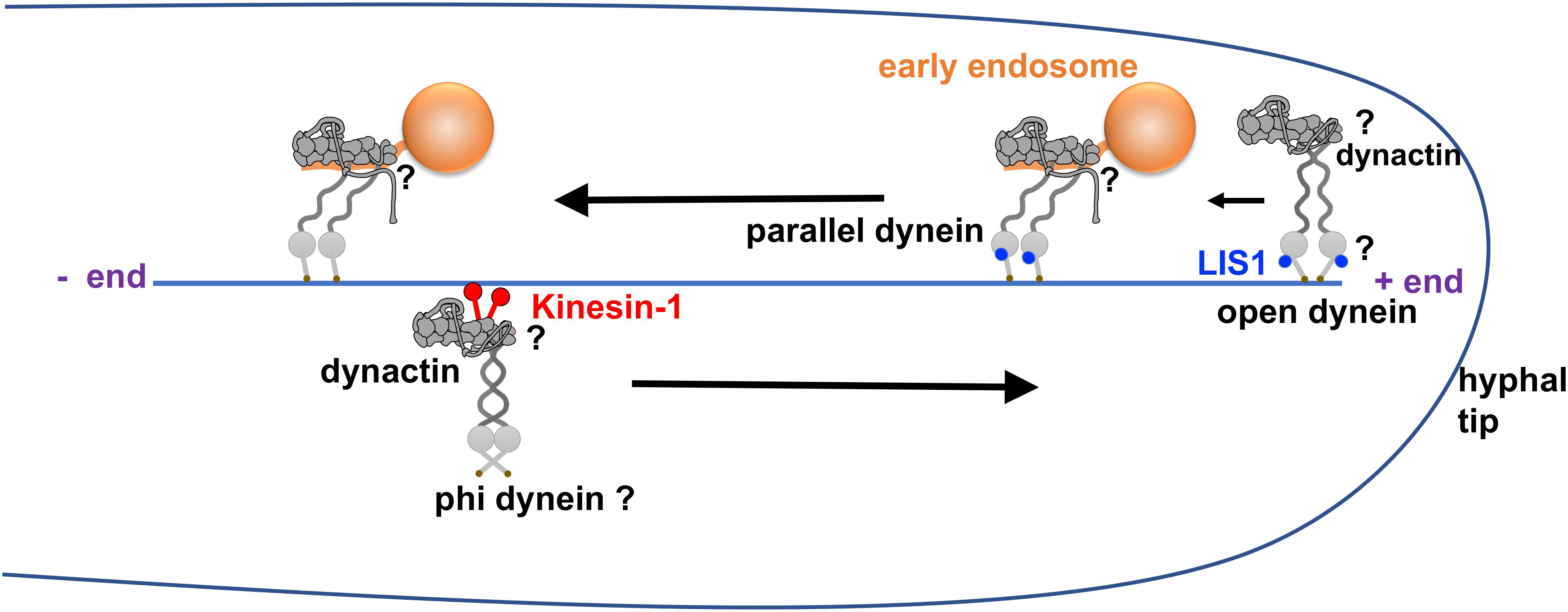
Figure 3. Model of dynein activation in filamentous fungi. This model is based on the structural data on the LIS1-dynein interaction and cargo-adapter-mediated dynein activation (Huang et al., 2012; Zhang K. et al., 2017; Htet et al., 2020), live cell imaging data on dynein, dynactin and LIS1 from filamentous fungi (Han et al., 2001; Zhang et al., 2003; Efimov et al., 2006; Lenz et al., 2006; Egan et al., 2012; Yao et al., 2012) as well as recent data on LIS1 mechanism (Qiu et al., 2019; Elshenawy et al., 2020; Htet et al., 2020; Marzo et al., 2020). In this model, dynein in the autoinhibited phi conformation is transported by kinesin-1 to the microtubule plus end together with dynactin. At the microtubule plus end, the dynein-LIS1 interaction keeps the motor domains of the dynein dimer in an open configuration but still being incapable of processive movement until dynein-dynactin interacts with an early endosome cargo. The cargo adapter (HookA or Hok1) on the early endosome interacts with dynein-dynactin-LIS1 and switches the dynein dimer into a parallel configuration, allowing it to walk toward the microtubule minus end. Note that there is also a conformational change of dynactin after cargo adapter binding so that the p150 protein is in an extended conformation (Urnavicius et al., 2015). Finally, LIS1 dissociates from dynein during the minus-end-directed movement (Lenz et al., 2006; Egan et al., 2012). The questions marks in this figure indicate the speculative nature of the dynein-dynactin configurations at these sites.
When bound to dynactin and cargo adapters, dynein carrying LIS1 at its motor ring is able to undergo processive movement toward the microtubule minus end (Baumbach et al., 2017; Gutierrez et al., 2017), which differs from the inhibitory function of LIS1 on dynein alone (Yamada et al., 2008; McKenney et al., 2010; Huang et al., 2012). However, LIS1 tends to dissociate from the motile dynein-dynactin-cargo adapter complex both in vivo and in vitro (Lenz et al., 2006; Egan et al., 2012; Lammers and Markus, 2015; Jha et al., 2017; Elshenawy et al., 2020; Htet et al., 2020). In the budding yeast, LIS1 has never been observed to co-localize with dynein-dynactin at the cell cortex (Markus et al., 2011). In filamentous fungi such as U. maydis and A. nidulans, while dynactin remains associated with a motile early endosome after its dynein-mediated movement has been initiated, LIS1 tends to fall off from it (Lenz et al., 2006; Egan et al., 2012). By observing many A. nidulans hyphal tip cells of a nudF/lis1 deletion mutant in which early endosome motility is rarely observed, it was found that loss of LIS1 does not affect the speed of occasional dynein-mediated transporting events, and thus, LIS1 was considered as an initiation factor (Egan et al., 2012). The idea that LIS1 is only important for transport initiation is consistent with the current results on LIS1 promoting the open dynein state (Qiu et al., 2019; Elshenawy et al., 2020; Htet et al., 2020; Marzo et al., 2020), thereby enhancing the formation of the dynein-dynactin-cargo-adapter complex (Zhang K. et al., 2017). However, the dynein-dynactin-ΔC-HookA complex still forms without LIS1 in A. nidulans, suggesting that dynein-dynactin at the microtubule plus end are capable of binding the HookA-linked early endosome but cannot initiate minus-end-directed movement (Qiu et al., 2019). Further studies will be needed to determine the structure of the dynein-dynactin-ΔC-HookA complex isolated from cells with or without NudF/LIS1, as it seems intriguing why such a complex is not capable of leaving the microtubule plus end in vivo in the absence of NudF/LIS1. We should also point out that in neurons, while the microtubule-binding domain of the p150 dynactin is only required for transporting initiation from the distal axon containing dynamic MT plus ends (Lloyd et al., 2012; Moughamian and Holzbaur, 2012), LIS1 is additionally required for continued transport in the mid-axon with much more stable MT (Moughamian et al., 2013). Why is LIS1 so critical in vivo while the dynein-dynactin-cargo adapter complex can move without LIS1 in vitro? We can envision two possibilities. First, the assembly of the dynein-dynactin-cargo adapter complex containing a second dynein, which is promoted by LIS1 (Elshenawy et al., 2020; Htet et al., 2020), leads to a higher force production (Urnavicius et al., 2018; Elshenawy et al., 2020), thereby facilitating the movement of dynein cargoes in a viscous cytoplasm. Second, there could be a negative regulator that keeps dynein at the phi state in vivo, which makes LIS1 absolutely necessary to work against such an inhibition. Future studies will be needed to address these possibilities.
Other Proteins Important for Dynein Activation in vivo-Nude and P150 of Dynactin
LIS1’s binding partner NudE and its homologs are also involved in dynein function (Minke et al., 1999; Efimov and Morris, 2000; Feng et al., 2000; Niethammer et al., 2000; Sasaki et al., 2000; Yan et al., 2003; Liang et al., 2004, 2007; Shu et al., 2004; Li et al., 2005; Guo et al., 2006; Stehman et al., 2007; Ma et al., 2009; Lam et al., 2010; Pandey and Smith, 2011; Wang and Zheng, 2011; Zylkiewicz et al., 2011; Wang et al., 2013; Klinman and Holzbaur, 2015; Reddy et al., 2016; Simões et al., 2018). NudE (homologous to Ro11 in Neurospora crassa) (Minke et al., 1999) and its interaction with NudF/LIS1 were first identified in A. nidulans and in higher eukaryotic model systems (Efimov and Morris, 2000; Feng et al., 2000; Niethammer et al., 2000; Sasaki et al., 2000). In both A. nidulans and budding yeast, loss of NudE causes defects in nuclear migration/spindle orientation, but the defects are much milder compared to that caused by loss of LIS1/NudF/Pac1 (Efimov and Morris, 2000; Li et al., 2005). In A. nidulans, mammalian cells, budding yeast and Xenopus egg extract, NudE becomes dispensable if LIS1 concentration is increased (Efimov, 2003; Shu et al., 2004; Li et al., 2005; Wang and Zheng, 2011), consistent with a role of NudE in recruiting LIS1 to dynein (McKenney et al., 2010). In A. nidulans, NudE is required for ΔC-HookA-mediated dynein activation, and the requirement of NudE for dynein-mediated early endosome transport can be partially bypassed by constitutively opening dynein using the phi mutations (Qiu et al., 2019). Thus, NudE supports LIS1’s function in dynein activation. NudE not only binds LIS1 but also binds dynein (Sasaki et al., 2000; Liang et al., 2004; McKenney et al., 2011; Wang and Zheng, 2011; Zylkiewicz et al., 2011; Nyarko et al., 2012), but intriguingly, NudE and dynactin p150 compete for binding to the N-terminal site of dynein IC (McKenney et al., 2011; Nyarko et al., 2012). Further studies are needed to reveal how these binding events are regulated to allow dynein activation by LIS1, dynactin and cargo adapters.
EM structural analysis suggests that cargo adapter binding to dynactin may change the conformation of p150 dynactin (Urnavicius et al., 2015). Without cargo adapters, p150 proteins can be seen under EM to exist in either folded or more extended conformations (Urnavicius et al., 2015; Saito et al., 2020). In the folded state, p150’s CC1A and CC1B domains contact the pointed-end complex of the Arp1 mini-filament (Urnavicius et al., 2015), and its microtubule-binding domain (MTBD) is most likely folded inside rather than being exposed (Figure 1B). Although only a minority of dynactin complexes contain folded p150 under EM (Urnavicius et al., 2015), the idea that p150 is mainly in a folded state in the absence of cargo adapters is consistent with the observation that isolated dynactin does not bind microtubules in vitro in the presence of dynein without cargo adapters (McKenney et al., 2014). Interestingly, the pointed end proteins including p25 interact with both the cargo adapter and p150’s CC1A and CC1B domains (Urnavicius et al., 2015; Qiu et al., 2018), and the binding sites of the dynein cargo adapters BicD2, Hook3, and BicDR1 at the pointed end overlap with the CC1A- and CC1B-binding sites (Urnavicius et al., 2015; Lau et al., 2020). Thus, it seems possible that BicD2, Hook3, BicDR1 or other similar cargo adapters may compete with p150’s CC1A and CC1B domains for binding to the pointed end, thereby forcing p150 to open up (Cianfrocco et al., 2015; Lau et al., 2020). The pointed end protein p25 is likely to be critically involved in this process as it plays a dual role in cargo adapter binding and the regulation of dynactin-microtubule interaction (Qiu et al., 2018). The most recent structural analysis shows that the whole pointed end complex including p25 acts as an interaction hub for cargo adapters and p150 (Lau et al., 2020). Future studies are needed to address whether cargo adapter binding indeed causes p150 to change its conformation, and if so, how this contributes to the process of dynein activation.
Future Directions
While the dynein field has made significant progress toward understanding how dynein is activated, some basic questions still remain to be answered. For example, what is the physiological significance of cargo adapter-mediated dynein activation? One obvious purpose of such a regulatory strategy could be to allow inactive dynein to be delivered to the microtubule plus end and stay there to receive its cargo rather than leaving prematurely toward the minus end without the cargo. However, could this regulatory strategy also help save the cellular energy currency ATP and ease the cellular burden of using metabolic pathways to generate ATP, especially when food source is limited? In A. nidulans, overexpressing ΔC-HookA in phi mutant cells where dynein is constitutively open produces colonies that are nearly inviable (much sicker than the dynein-null mutant) (Qiu et al., 2019). Could this be related to abnormal ATP consumption, which affects other ATP-utilizing processes or causes the overproduction of unhealthy metabolic products (like lactic acid in a skeletal muscle cell)? Currently, the published dynein ATPase activity (∼200 nmol/min/mg dynein) (Mesngon et al., 2006; McKenney et al., 2010) is likely from inactive dynein, and given the cellular ATP concentration of ∼2 nmol/μl, a fungal tip cell with the volume of ∼1 × 10–7 μl would need about half a million dynein molecules to consume the total cellular ATP within a minute (if ATP is not generated by metabolic pathways). It does seem unlikely to have so many dynein molecules in a fungal cell to significantly affect the cellular ATP pool. However, if a fully active dynein has a much higher ATPase activity, this number of dynein molecules will become smaller and more reasonable. It is known that the ATPase activity of an activated myosin II motor can be >100× higher than that of an inactive one (Trybus, 1989; Heissler and Sellers, 2016). In this context, it would be worthwhile to measure the ATPase activities of the inactive phi dynein, open dynein and fully activated dynein (with dynactin and cargo adapters).
There are important open questions on the spatial regulation of dynein in live cells. For example, what are the dynein-dynactin conformational states during kinesin-mediated transport to the microtubule plus end and/or at the plus end? While it will be technically challenging to apply structural analysis on live cells to reveal these states, it should be possible to use dynein-dynactin isolated from specific mutants for biochemical, single molecule and structural analyses. For example, in an A. nidulans ΔhookA mutant without any early endosomal dynein adapters, many dynein and dynactin molecules accumulate at the microtubule plus end, and it will be interesting to determine their conformational states. Similarly, it would be interesting to determine the conformation of dynein-dynactin or dynein-dynactin-cargo-adapter isolated from cells with or without LIS1. Another interesting issue is how kinesin-3 delivers an early endosome to dynein in vivo without being a competitor of dynein, given that U. maydis Hok1 affects the kinesin-3-early-endosome interaction and the mammalian Hook3 binds both dynein and kinesin-3 (Bielska et al., 2014b; Kendrick et al., 2019; Siddiqui et al., 2019)? In addition, how do the interactions between the microtubule-binding domain of p150 dynactin and differently modified tubulins affect dynein-mediated transport in vivo (Barisic and Maiato, 2016; McKenney et al., 2016; Nirschl et al., 2016; Roll-Mecak, 2020)?
One specific question that deserves to be discussed in more detail is how p150 of dynactin is involved in the dynein activation process. The microtubule-binding domain (MTBD) of p150 is needed for the plus-end accumulation of dynactin-dynein (Vaughan et al., 2002; Kim et al., 2007; Yao et al., 2012) and the initiation of minus-end-directed transport, especially in neurons (Lloyd et al., 2012; Moughamian and Holzbaur, 2012), possibly by helping dynein landing on tyrosinated microtubules (McKenney et al., 2016; Nirschl et al., 2016). However, data from multiple labs also suggest that p150 is an allosteric activator of dynein rather than simply a microtubule-tethering factor (Kim et al., 2007; Dixit et al., 2008; Kardon et al., 2009; Tripathy et al., 2014; Feng et al., 2020). The possibility that cargo binding may change p150 conformation is of great interest in this context. Before cargo binding, p150 could be in folded and more extended conformations and these states are in equilibria (Urnavicius et al., 2015; Saito et al., 2020). We speculate that in fungal cells, before the formation of the dynein-dynactin-kinesin-1 complex, the extended p150 allows its MTBD to be exposed to bind microtubule (Yao et al., 2012). This will help recruit dynein to the microtubule, possibly via the p150 (CC1B)-IC interaction (Karki and Holzbaur, 1995; Vaughan and Vallee, 1995; King et al., 2003; McKenney et al., 2011). We speculate that the subsequent binding to kinesin-1 changes this interaction mode and promotes a folded state of p150 to prevent its MTBD from interfering with kinesin-1-mediated transport, although it is unclear how dynein binds dynactin with a folded p150 (Figure 3). We also speculate that after kinesin-1 is dissociated from dynein-dynactin at the plus end, a transient NudE-IC interaction (Efimov, 2003; McKenney et al., 2011; Wang and Zheng, 2011) may prevent p150’s CC1B from binding to IC (McKenney et al., 2011), thereby stabilizing the folded state of p150. Does cargo adapter binding promote the open state of p150 at the microtubule plus end (Figure 3) and allow its CC1B domain to bind dynein IC again (Urnavicius et al., 2015)? If so, how does the p150-IC interaction in the presence of the cargo adapter change the configuration of dynein HC tails to position them along the Arp1 filament, which eventually leads to dynein activation (Zhang K. et al., 2017)?
Related to the questions on the conformational states of dynactin p150, another important question is how dynein stays at the microtubule plus end. Since the binding to a cargo adapter is a prerequisite for dynein activation, dynein is expected to remain at the plus end before cargo binding. However, we do not know exactly how dynein interacts with the microtubule plus end. In the budding yeast, the microtubule-binding domain of dynein HC is not needed for dynein’s plus-end accumulation (Lammers and Markus, 2015), and thus, yeast dynein is most likely retained at the plus end via LIS1 that binds the plus end-tracking protein Bik1/CLIP170 (Lin et al., 2001; Sheeman et al., 2003; Markus et al., 2011). However, the plus-end accumulation of dynein in filamentous fungi does not need LIS1 or CLIP170 homologs (Zhang et al., 2003; Efimov et al., 2006; Lenz et al., 2006; Egan et al., 2012), but it needs the MTBD of p150 (Yao et al., 2012). Nevertheless, if p150 is folded at the plus end before cargo binding, its MTBD is unlikely to be exposed and used for anchoring dynein at the plus end. Thus, we hypothesize that in filamentous fungi, an open dynein (with LIS1 bound) contacts the microtubule plus end directly using its own microtubule-binding domains (Figure 3), and this open dynein is primed for cargo binding and the subsequent minus-end-directed movement. More data will be needed to either support or refute this hypothesis.
Finally, to gain a full picture of dynein regulation in vivo, it will also be important to study the new regulators of dynein. A good example for illustrating this point is the recent progress toward understanding LIS1’s mechanism of action, which was possible only after specific cargo adapters were identified and shown to activate dynein. In this context, we should also point out the need of further dissecting how NudE participates in dynein activation (Qiu et al., 2019), as NudE competes with the CC1B domain of p150 for binding to dynein IC (McKenney et al., 2011; Jie et al., 2017). Moreover, is there any negative regulator that helps keep dynein in the autoinhibited conformation in vivo? While we still do not know if such a regulator exists for cytoplasmic dynein, a recent study using Tetrahymena has identified a novel axonemal dynein-binding protein, Shulin, as a regulator that keeps axonemal dynein in an inactivate conformation before it is delivered to cilia (Mali et al., 2020). While both biochemical and genetic approaches can be powerful, genetic screens in A. nidulans have been highly valuable in identifying new proteins involved in dynein-mediated intracellular transport (Osmani et al., 1990; Xiang et al., 1995; Efimov and Morris, 2000; Yao et al., 2014; Zhang et al., 2014; Salogiannis et al., 2016). Recently, two new proteins, VezA/vezatin and Prp40A/PRPF40A, have been identified in A. nidulans as important factors for dynein-mediated early endosome transport (Yao et al., 2015; Qiu et al., 2020). Vezatin was initially identified as a protein involved in stabilizing cell-cell adhesions (Kussel-Andermann et al., 2000), and PRPF40A is homologous to the yeast RNA-splicing factor Prp40 (Kao and Siliciano, 1996). Interestingly, both vezatin and PRPF40A were identified as Arp1-binding proteins in a biochemical pulldown assay (Hein et al., 2015; Qiu et al., 2020). For vezatin, the interaction with Arp1 could be direct as Arp1 (ACTR1A) was identified as a protein in close proximity to vezatin (VEZT) in human cells (Go et al., 2019)1. VezA/vezatin in A. nidulans is clearly not a cargo adapter like HookA, and intriguingly, it localizes at the hyphal tip in an actin cytoskeleton-dependent fashion (Yao et al., 2015), and how it affects the microtubule plus end-localized dynein-dynactin will need to be addressed. Recently, a forward genetic screen in Drosophila has also identified a vezatin homolog as being important for dynein-mediated axonal transport, and furthermore, a zebrafish vezatin homolog is also involved in a similar dynein-mediated process (Spinner et al., 2020). The mechanisms of actions of these proteins will need to be further studied in different experimental systems.
Author Contributions
XX and RQ: writing, editing, and making figures. Both authors contributed to the article and approved the submitted version.
Conflict of Interest
The authors declare that the research was conducted in the absence of any commercial or financial relationships that could be construed as a potential conflict of interest.
Funding
The authors’ recent work on dynein regulation in vivo was supported by the National Institutes of Health grant RO1 GM121850 (to XX).
Footnotes
References
Abenza, J. F., Galindo, A., Pantazopoulou, A., Gil, C., De Los Ríos, V., and Peñalva, M. A. (2010). Aspergillus RabB Rab5 integrates acquisition of degradative identity with the long distance movement of early endosomes. Mol. Biol. Cell. 21, 2756–2769. doi: 10.1091/mbc.e10-02-0119
Abenza, J. F., Galindo, A., Pinar, M., Pantazopoulou, A., De Los Ríos, V., and Peñalva, M. A. (2012). Endosomal maturation by Rab conversion in Aspergillus nidulans is coupled to dynein-mediated basipetal movement. Mol. Biol. Cell. 23, 1889–1901. doi: 10.1091/mbc.e11-11-0925
Abenza, J. F., Pantazopoulou, A., Rodriguez, J. M., Galindo, A., and Penalva, M. A. (2009). Long-distance movement of Aspergillus nidulans early endosomes on microtubule tracks. Traffic 10, 57–75. doi: 10.1111/j.1600-0854.2008.00848.x
Amos, L. A. (1989). Brain dynein crossbridges microtubules into bundles. J. Cell. Sci. 93(Pt 1), 19–28.
Ananthanarayanan, V., Schattat, M., Vogel, S. K., Krull, A., Pavin, N., and Toliæ-Nørrelykke, I. M. (2013). Dynein motion switches from diffusive to directed upon cortical anchoring. Cell 153, 1526–1536. doi: 10.1016/j.cell.2013.05.020
Arimoto, M., Koushika, S. P., Choudhary, B. C., Li, C., Matsumoto, K., and Hisamoto, N. (2011). The Caenorhabditis elegans JIP3 protein UNC-16 functions as an adaptor to link kinesin-1 with cytoplasmic dynein. J. Neurosci. 31, 2216–2224. doi: 10.1523/jneurosci.2653-10.2011
Barisic, M., and Maiato, H. (2016). The tubulin code: a navigation system for chromosomes during mitosis. Trends Cell. Biol. 26, 766–775. doi: 10.1016/j.tcb.2016.06.001
Baumann, S., Pohlmann, T., Jungbluth, M., Brachmann, A., and Feldbrugge, M. (2012). Kinesin-3 and dynein mediate microtubule-dependent co-transport of mRNPs and endosomes. J. Cell. Sci. 125, 2740–2752. doi: 10.1242/jcs.101212
Baumbach, J., Murthy, A., Mcclintock, M. A., Dix, C. I., Zalyte, R., Hoang, H. T., et al. (2017). Lissencephaly-1 is a context-dependent regulator of the human dynein complex. eLife 6:e21768.
Bhabha, G., Cheng, H. C., Zhang, N., Moeller, A., Liao, M., Speir, J. A., et al. (2014). Allosteric communication in the dynein motor domain. Cell 159, 857–868. doi: 10.1016/j.cell.2014.10.018
Bieger, B. D., Rogers, A. M., Bates, S., and Egan, M. J. (2020). Long-distance early endosome motility in Aspergillus fumigatus promotes normal hyphal growth behaviors in controlled microenvironments but is dispensable for virulence. Traffic 21, 479–487. doi: 10.1111/tra.12735
Bielska, E., Higuchi, Y., Schuster, M., Steinberg, N., Kilaru, S., Talbot, N. J., et al. (2014a). Long-distance endosome trafficking drives fungal effector production during plant infection. Nat. Commun. 5:5097.
Bielska, E., Schuster, M., Roger, Y., Berepiki, A., Soanes, D. M., Talbot, N. J., et al. (2014b). Hook is an adapter that coordinates kinesin-3 and dynein cargo attachment on early endosomes. J. Cell. Biol. 204, 989–1007. doi: 10.1083/jcb.201309022
Brendza, R. P., Serbus, L. R., Saxton, W. M., and Duffy, J. B. (2002). Posterior localization of dynein and dorsal-ventral axis formation depend on kinesin in Drosophila oocytes. Curr. Biol. 12, 1541–1545. doi: 10.1016/s0960-9822(02)01108-9
Burgess, S. A., Walker, M. L., Sakakibara, H., Knight, P. J., and Oiwa, K. (2003). Dynein structure and power stroke. Nature 421, 715–718. doi: 10.1038/nature01377
Callejas-Negrete, O. A., Plamann, M., Schnittker, R., Bartnicki-García, S., Roberson, R. W., Pimienta, G., et al. (2015). Two microtubule-plus-end binding proteins LIS1-1 and LIS1-2, homologues of human LIS1 in Neurospora crassa. Fungal Genet. Biol. 82, 213–227. doi: 10.1016/j.fgb.2015.07.009
Can, S., Lacey, S., Gur, M., Carter, A. P., and Yildiz, A. (2019). Directionality of dynein is controlled by the angle and length of its stalk. Nature 566, 407–410. doi: 10.1038/s41586-019-0914-z
Canty, J. T., and Yildiz, A. (2020). Activation and regulation of cytoplasmic dynein. Trends Biochem. Sci. 45, 440–453. doi: 10.1016/j.tibs.2020.02.002
Carter, A. P., Diamant, A. G., and Urnavicius, L. (2016). How dynein and dynactin transport cargos: a structural perspective. Curr. Opin. Struct. Biol. 37, 62–70. doi: 10.1016/j.sbi.2015.12.003
Carvalho, P., Gupta, M. L. Jr., Hoyt, M. A., and Pellman, D. (2004). Cell cycle control of kinesin-mediated transport of Bik1 (CLIP-170) regulates microtubule stability and dynein activation. Dev. Cell. 6, 815–829. doi: 10.1016/j.devcel.2004.05.001
Chowdhury, S., Ketcham, S. A., Schroer, T. A., and Lander, G. C. (2015). Structural organization of the dynein-dynactin complex bound to microtubules. Nat. Struct. Mol. Biol. 22, 345–347. doi: 10.1038/nsmb.2996
Cianfrocco, M. A., Desantis, M. E., Leschziner, A. E., and Reck-Peterson, S. L. (2015). Mechanism and regulation of cytoplasmic dynein. Annu. Rev. Cell. Dev. Biol. 31, 83–108.
Coquelle, F. M., Caspi, M., Cordelières, F. P., Dompierre, J. P., Dujardin, D. L., Koifman, C., et al. (2002). LIS1, CLIP-170’s key to the dynein/dynactin pathway. Mol. Cell. Biol. 22, 3089–3102. doi: 10.1128/mcb.22.9.3089-3102.2002
Desantis, M. E., Cianfrocco, M. A., Htet, Z. M., Tran, P. T., Reck-Peterson, S. L., and Leschziner, A. E. (2017). Lis1 has two opposing modes of regulating cytoplasmic dynein. Cell 170, 1197.e12–1208.e12.
Dewitt, M. A., Cypranowska, C. A., Cleary, F. B., Belyy, V., and Yildiz, A. (2015). The AAA3 domain of cytoplasmic dynein acts as a switch to facilitate microtubule release. Nat. Struct. Mol. Biol. 22, 73–80. doi: 10.1038/nsmb.2930
Dix, C. I., Soundararajan, H. C., Dzhindzhev, N. S., Begum, F., Suter, B., Ohkura, H., et al. (2013). Lissencephaly-1 promotes the recruitment of dynein and dynactin to transported mRNAs. J. Cell. Biol. 202, 479–494. doi: 10.1083/jcb.201211052
Dixit, R., Levy, J. R., Tokito, M., Ligon, L. A., and Holzbaur, E. L. (2008). Regulation of dynactin through the differential expression of p150Glued isoforms. J. Biol. Chem. 283, 33611–33619. doi: 10.1074/jbc.m804840200
Dodding, M. P., and Way, M. (2011). Coupling viruses to dynein and kinesin-1. EMBO J. 30, 3527–3539. doi: 10.1038/emboj.2011.283
Duncan, J. E., and Warrior, R. (2002). The cytoplasmic dynein and kinesin motors have interdependent roles in patterning the Drosophila oocyte. Curr. Biol. 12, 1982–1991. doi: 10.1016/s0960-9822(02)01303-9
Dwivedi, D., Chawla, P., and Sharma, M. (2019a). Incorporating motility in the motor: role of the hook protein family in regulating dynein motility. Biochemistry 58, 1026–1031. doi: 10.1021/acs.biochem.8b01065
Dwivedi, D., Kumari, A., Rathi, S., Mylavarapu, S. V. S., and Sharma, M. (2019b). The dynein adaptor Hook2 plays essential roles in mitotic progression and cytokinesis. J. Cell. Biol. 218, 871–894. doi: 10.1083/jcb.201804183
Echeverri, C. J., Paschal, B. M., Vaughan, K. T., and Vallee, R. B. (1996). Molecular characterization of the 50-kD subunit of dynactin reveals function for the complex in chromosome alignment and spindle organization during mitosis. J. Cell. Biol. 132, 617–633. doi: 10.1083/jcb.132.4.617
Eckley, D. M., Gill, S. R., Melkonian, K. A., Bingham, J. B., Goodson, H. V., Heuser, J. E., et al. (1999). Analysis of dynactin subcomplexes reveals a novel actin-related protein associated with the arp1 minifilament pointed end. J. Cell. Biol. 147, 307–320. doi: 10.1083/jcb.147.2.307
Efimov, V. P. (2003). Roles of NUDE and NUDF proteins of Aspergillus nidulans: insights from intracellular localization and overexpression effects. Mol. Biol. Cell. 14, 871–888. doi: 10.1091/mbc.e02-06-0359
Efimov, V. P., and Morris, N. R. (2000). The LIS1-related NUDF protein of Aspergillus nidulans interacts with the coiled-coil domain of the NUDE/RO11 protein. J. Cell. Biol. 150, 681–688. doi: 10.1083/jcb.150.3.681
Efimov, V. P., Zhang, J., and Xiang, X. (2006). CLIP-170 homologue and NUDE play overlapping roles in NUDF localization in Aspergillus nidulans. Mol. Biol. Cell. 17, 2021–2034. doi: 10.1091/mbc.e05-11-1084
Egan, M. J., Tan, K., and Reck-Peterson, S. L. (2012). Lis1 is an initiation factor for dynein-driven organelle transport. J. Cell. Biol. 197, 971–982. doi: 10.1083/jcb.201112101
Elshenawy, M. M., Kusakci, E., Volz, S., Baumbach, J., Bullock, S. L., and Yildiz, A. (2020). Lis1 activates dynein motility by modulating its pairing with dynactin. Nat. Cell. Biol. 22, 570–578. doi: 10.1038/s41556-020-0501-4
Eshel, D., Urrestarazu, L. A., Vissers, S., Jauniaux, J. C., Van Vliet-Reedijk, J. C., Planta, R. J., et al. (1993). Cytoplasmic dynein is required for normal nuclear segregation in yeast. Proc. Natl. Acad. Sci. U.S.A. 90, 11172–11176. doi: 10.1073/pnas.90.23.11172
Farkasovsky, M., and Küntzel, H. (1995). Yeast Num1p associates with the mother cell cortex during S/G2 phase and affects microtubular functions. J. Cell. Biol. 131, 1003–1014. doi: 10.1083/jcb.131.4.1003
Faulkner, N. E., Dujardin, D. L., Tai, C. Y., Vaughan, K. T., O’connell, C. B., Wang, Y., et al. (2000). A role for the lissencephaly gene LIS1 in mitosis and cytoplasmic dynein function. Nat. Cell. Biol. 2, 784–791. doi: 10.1038/35041020
Feng, Q., Gicking, A. M., and Hancock, W. O. (2020). Dynactin p150 promotes processive motility of DDB complexes by minimizing diffusional behavior of dynein. Mol. Biol. Cell. 31, 782–792. doi: 10.1091/mbc.e19-09-0495
Feng, Y., Olson, E. C., Stukenberg, P. T., Flanagan, L. A., Kirschner, M. W., and Walsh, C. A. (2000). LIS1 regulates CNS lamination by interacting with mNudE, a central component of the centrosome. Neuron 28, 665–679. doi: 10.1016/s0896-6273(00)00145-8
Fu, M. M., and Holzbaur, E. L. (2014). Integrated regulation of motor-driven organelle transport by scaffolding proteins. Trends Cell. Biol. 24, 564–574. doi: 10.1016/j.tcb.2014.05.002
Gama, J. B., Pereira, C., Simões, P. A., Celestino, R., Reis, R. M., Barbosa, D. J., et al. (2017). Molecular mechanism of dynein recruitment to kinetochores by the Rod-Zw10-Zwilch complex and spindly. J. Cell. Biol. 216, 943–960. doi: 10.1083/jcb.201610108
Gee, M. A., Heuser, J. E., and Vallee, R. B. (1997). An extended microtubule-binding structure within the dynein motor domain. Nature 390, 636–639. doi: 10.1038/37663
Geiser, J. R., Schott, E. J., Kingsbury, T. J., Cole, N. B., Totis, L. J., Bhattacharyya, G., et al. (1997). Saccharomyces cerevisiae genes required in the absence of the CIN8-encoded spindle motor act in functionally diverse mitotic pathways. Mol. Biol. Cell. 8, 1035–1050. doi: 10.1091/mbc.8.6.1035
Gibbons, I. R., Garbarino, J. E., Tan, C. E., Reck-Peterson, S. L., Vale, R. D., and Carter, A. P. (2005). The affinity of the dynein microtubule-binding domain is modulated by the conformation of its coiled-coil stalk. J. Biol. Chem. 280, 23960–23965. doi: 10.1074/jbc.m501636200
Go, C. D., Knight, J. D. R., Rajasekharan, A., Rathod, B., Hesketh, G. G., Abe, K. T., et al. (2019). A proximity biotinylation map of a human cell. biorxiv [Preprint] doi: 10.1101/796391
Griffis, E. R., Stuurman, N., and Vale, R. D. (2007). Spindly, a novel protein essential for silencing the spindle assembly checkpoint, recruits dynein to the kinetochore. J. Cell. Biol. 177, 1005–1015. doi: 10.1083/jcb.200702062
Grotjahn, D. A., Chowdhury, S., Xu, Y., McKenney, R. J., Schroer, T. A., and Lander, G. C. (2018). Cryo-electron tomography reveals that dynactin recruits a team of dyneins for processive motility. Nat. Struct. Mol. Biol. 25, 203–207. doi: 10.1038/s41594-018-0027-7
Guedes-Dias, P., and Holzbaur, E. L. F. (2019). Axonal transport: driving synaptic function. Science 366:eaaw9997. doi: 10.1126/science.aaw9997
Guimaraes, S. C., Schuster, M., Bielska, E., Dagdas, G., Kilaru, S., Meadows, B. R., et al. (2015). Peroxisomes, lipid droplets, and endoplasmic reticulum “hitchhike” on motile early endosomes. J. Cell. Biol. 211, 945–954. doi: 10.1083/jcb.201505086
Guo, J., Yang, Z., Song, W., Chen, Q., Wang, F., Zhang, Q., et al. (2006). Nudel contributes to microtubule anchoring at the mother centriole and is involved in both dynein-dependent and -independent centrosomal protein assembly. Mol. Biol. Cell. 17, 680–689. doi: 10.1091/mbc.e05-04-0360
Guo, X., Farias, G. G., Mattera, R., and Bonifacino, J. S. (2016). Rab5 and its effector FHF contribute to neuronal polarity through dynein-dependent retrieval of somatodendritic proteins from the axon. Proc. Natl. Acad. Sci. U.S.A. 113, E5318–E5327.
Gutierrez, P. A., Ackermann, B. E., Vershinin, M., and McKenney, R. J. (2017). Differential effects of the dynein-regulatory factor Lissencephaly-1 on processive dynein-dynactin motility. J. Biol. Chem. 292, 12245–12255. doi: 10.1074/jbc.m117.790048
Haghnia, M., Cavalli, V., Shah, S. B., Schimmelpfeng, K., Brusch, R., Yang, G., et al. (2007). Dynactin is required for coordinated bidirectional motility, but not for dynein membrane attachment. Mol. Biol. Cell. 18, 2081–2089. doi: 10.1091/mbc.e06-08-0695
Hammer, J. A. III, and Sellers, J. R. (2011). Walking to work: roles for class V myosins as cargo transporters. Nat. Rev. Mol. Cell. Biol. 13, 13–26. doi: 10.1038/nrm3248
Han, G., Liu, B., Zhang, J., Zuo, W., Morris, N. R., and Xiang, X. (2001). The Aspergillus cytoplasmic dynein heavy chain and NUDF localize to microtubule ends and affect microtubule dynamics. Curr. Biol. 11, 719–724. doi: 10.1016/s0960-9822(01)00200-7
Hein, M. Y., Hubner, N. C., Poser, I., Cox, J., Nagaraj, N., Toyoda, Y., et al. (2015). A human interactome in three quantitative dimensions organized by stoichiometries and abundances. Cell 163, 712–723. doi: 10.1016/j.cell.2015.09.053
Heissler, S. M., and Sellers, J. R. (2016). Various themes of myosin regulation. J. Mol. Biol. 428, 1927–1946. doi: 10.1016/j.jmb.2016.01.022
Hernández-González, M., Bravo-Plaza, I., Pinar, M., De Los Ríos, V., Arst, H. N. Jr., and Peñalva, M. A. (2018). Endocytic recycling via the TGN underlies the polarized hyphal mode of life. PLoS Genet. 14:e1007291. doi: 10.1371/journal.pgen.1007291
Higuchi, Y., Ashwin, P., Roger, Y., and Steinberg, G. (2014). Early endosome motility spatially organizes polysome distribution. J. Cell. Biol. 204, 343–357. doi: 10.1083/jcb.201307164
Hirokawa, N., Noda, Y., Tanaka, Y., and Niwa, S. (2009). Kinesin superfamily motor proteins and intracellular transport. Nat. Rev. Mol. Cell. Biol. 10, 682–696. doi: 10.1038/nrm2774
Hoang, H. T., Schlager, M. A., Carter, A. P., and Bullock, S. L. (2017). DYNC1H1 mutations associated with neurological diseases compromise processivity of dynein-dynactin-cargo adaptor complexes. Proc. Natl. Acad. Sci. U.S.A. 114, E1597–E1606.
Holzbaur, E. L., Hammarback, J. A., Paschal, B. M., Kravit, N. G., Pfister, K. K., and Vallee, R. B. (1991). Homology of a 150K cytoplasmic dynein-associated polypeptide with the Drosophila gene Glued. Nature 351, 579–583. doi: 10.1038/351579a0
Hoogenraad, C. C., and Akhmanova, A. (2016). Bicaudal D family of motor adaptors: linking dynein motility to cargo binding. Trends Cell Biol. 26, 327–340. doi: 10.1016/j.tcb.2016.01.001
Höök, P., and Vallee, R. B. (2006). The dynein family at a glance. J. Cell. Sci. 119, 4369–4371. doi: 10.1242/jcs.03176
Horgan, C. P., Hanscom, S. R., Jolly, R. S., Futter, C. E., and Mccaffrey, M. W. (2010). Rab11-FIP3 links the Rab11 GTPase and cytoplasmic dynein to mediate transport to the endosomal-recycling compartment. J. Cell. Sci. 123, 181–191. doi: 10.1242/jcs.052670
Htet, Z. M., Gillies, J. P., Baker, R. W., Leschziner, A. E., Desantis, M. E., and Reck-Peterson, S. L. (2020). LIS1 promotes the formation of activated cytoplasmic dynein-1 complexes. Nat. Cell. Biol. 22, 518–525. doi: 10.1038/s41556-020-0506-z
Huang, J., Roberts, A. J., Leschziner, A. E., and Reck-Peterson, S. L. (2012). Lis1 Acts as a “Clutch” between the ATPase and microtubule-binding domains of the dynein motor. Cell 150, 975–986. doi: 10.1016/j.cell.2012.07.022
Januschke, J., Gervais, L., Dass, S., Kaltschmidt, J. A., Lopez-Schier, H., St Johnston, D., et al. (2002). Polar transport in the Drosophila oocyte requires Dynein and Kinesin I cooperation. Curr. Biol. 12, 1971–1981. doi: 10.1016/s0960-9822(02)01302-7
Jha, R., Roostalu, J., Cade, N. I., Trokter, M., and Surrey, T. (2017). Combinatorial regulation of the balance between dynein microtubule end accumulation and initiation of directed motility. EMBO J. 36, 3387–3404. doi: 10.15252/embj.201797077
Jie, J., Lohr, F., and Barbar, E. (2017). Dynein binding of competitive regulators dynactin and NudE involves novel interplay between phosphorylation site and disordered spliced linkers. Structure 25, 421–433. doi: 10.1016/j.str.2017.01.003
Jordan, M. A., Diener, D. R., Stepanek, L., and Pigino, G. (2018). The cryo-EM structure of intraflagellar transport trains reveals how dynein is inactivated to ensure unidirectional anterograde movement in cilia. Nat. Cell. Biol. 20, 1250–1255. doi: 10.1038/s41556-018-0213-1
Kao, H. Y., and Siliciano, P. G. (1996). Identification of Prp40, a novel essential yeast splicing factor associated with the U1 small nuclear ribonucleoprotein particle. Mol. Cell. Biol. 16, 960–967. doi: 10.1128/mcb.16.3.960
Kardon, J. R., Reck-Peterson, S. L., and Vale, R. D. (2009). Regulation of the processivity and intracellular localization of Saccharomyces cerevisiae dynein by dynactin. Proc. Natl. Acad. Sci. U.S.A. 106, 5669–5674. doi: 10.1073/pnas.0900976106
Karki, S., and Holzbaur, E. L. (1995). Affinity chromatography demonstrates a direct binding between cytoplasmic dynein and the dynactin complex. J. Biol. Chem. 270, 28806–28811. doi: 10.1074/jbc.270.48.28806
Karki, S., Lamonte, B., and Holzbaur, E. L. (1998). Characterization of the p22 subunit of dynactin reveals the localization of cytoplasmic dynein and dynactin to the midbody of dividing cells. J. Cell. Biol. 142, 1023–1034. doi: 10.1083/jcb.142.4.1023
Kendrick, A. A., Dickey, A. M., Redwine, W. B., Tran, P. T., Vaites, L. P., Dzieciatkowska, M., et al. (2019). Hook3 is a scaffold for the opposite-polarity microtubule-based motors cytoplasmic dynein-1 and KIF1C. J. Cell. Biol. 218, 2982–3001. doi: 10.1083/jcb.201812170
Kim, H., Ling, S. C., Rogers, G. C., Kural, C., Selvin, P. R., Rogers, S. L., et al. (2007). Microtubule binding by dynactin is required for microtubule organization but not cargo transport. J. Cell. Biol. 176, 641–651. doi: 10.1083/jcb.200608128
King, S. J., Brown, C. L., Maier, K. C., Quintyne, N. J., and Schroer, T. A. (2003). Analysis of the dynein-dynactin interaction in vitro and in vivo. Mol. Biol. Cell. 14, 5089–5097. doi: 10.1091/mbc.e03-01-0025
King, S. M. (2000). AAA domains and organization of the dynein motor unit. J. Cell. Sci. 113(Pt 14), 2521–2526.
Klinman, E., and Holzbaur, E. L. (2015). Stress-induced CDK5 activation disrupts axonal transport via Lis1/Ndel1/Dynein. Cell Rep. 12, 462–473. doi: 10.1016/j.celrep.2015.06.032
Krämer, H., and Phistry, M. (1996). Mutations in the Drosophila hook gene inhibit endocytosis of the boss transmembrane ligand into multivesicular bodies. J. Cell. Biol. 133, 1205–1215. doi: 10.1083/jcb.133.6.1205
Kussel-Andermann, P., El-Amraoui, A., Safieddine, S., Nouaille, S., Perfettini, I., Lecuit, M., et al. (2000). Vezatin, a novel transmembrane protein, bridges myosin VIIA to the cadherin-catenins complex. EMBO J. 19, 6020–6029. doi: 10.1093/emboj/19.22.6020
Lam, C., Vergnolle, M. A., Thorpe, L., Woodman, P. G., and Allan, V. J. (2010). Functional interplay between LIS1, NDE1 and NDEL1 in dynein-dependent organelle positioning. J. Cell. Sci. 123, 202–212. doi: 10.1242/jcs.059337
Lammers, L. G., and Markus, S. M. (2015). The dynein cortical anchor Num1 activates dynein motility by relieving Pac1/LIS1-mediated inhibition. J. Cell. Biol. 211, 309–322. doi: 10.1083/jcb.201506119
Lau, C. K., O’Reilly, F. J., Santhanam, B., Lacey, S. E., Rappsilber, J., and Carter, A. P. (2020). Cryo-EM reveals the complex architecture of dynactin’s shoulder and pointed end. BioRxiv [Preprint]. doi: 10.1101/2020.07.16.206359
Lee, I. G., Olenick, M. A., Boczkowska, M., Franzini-Armstrong, C., Holzbaur, E. L. F., and Dominguez, R. (2018). A conserved interaction of the dynein light intermediate chain with dynein-dynactin effectors necessary for processivity. Nat. Commun. 9:986.
Lee, W. L., Oberle, J. R., and Cooper, J. A. (2003). The role of the lissencephaly protein Pac1 during nuclear migration in budding yeast. J. Cell. Biol. 160, 355–364. doi: 10.1083/jcb.200209022
Lei, Y., and Warrior, R. (2000). The Drosophila lissencephaly1 (DLis1) gene is required for nuclear migration. Dev. Biol. 226, 57–72. doi: 10.1006/dbio.2000.9848
Lenz, J. H., Schuchardt, I., Straube, A., and Steinberg, G. (2006). A dynein loading zone for retrograde endosome motility at microtubule plus-ends. EMBO J. 25, 2275–2286. doi: 10.1038/sj.emboj.7601119
Li, J., Lee, W. L., and Cooper, J. A. (2005). NudEL targets dynein to microtubule ends through LIS1. Nat. Cell. Biol. 7, 686–690. doi: 10.1038/ncb1273
Li, Y. Y., Yeh, E., Hays, T., and Bloom, K. (1993). Disruption of mitotic spindle orientation in a yeast dynein mutant. Proc. Natl. Acad. Sci. U.S.A. 90, 10096–10100. doi: 10.1073/pnas.90.21.10096
Liang, Y., Yu, W., Li, Y., Yang, Z., Yan, X., Huang, Q., et al. (2004). Nudel functions in membrane traffic mainly through association with Lis1 and cytoplasmic dynein. J. Cell. Biol. 164, 557–566. doi: 10.1083/jcb.200308058
Liang, Y., Yu, W., Li, Y., Yu, L., Zhang, Q., Wang, F., et al. (2007). Nudel modulates kinetochore association and function of cytoplasmic dynein in M phase. Mol. Biol. Cell. 18, 2656–2666. doi: 10.1091/mbc.e06-04-0345
Ligon, L. A., Tokito, M., Finklestein, J. M., Grossman, F. E., and Holzbaur, E. L. (2004). A direct interaction between cytoplasmic dynein and kinesin I may coordinate motor activity. J. Biol. Chem. 279, 19201–19208. doi: 10.1074/jbc.m313472200
Lin, C., Schuster, M., Guimaraes, S. C., Ashwin, P., Schrader, M., Metz, J., et al. (2016). Active diffusion and microtubule-based transport oppose myosin forces to position organelles in cells. Nat. Commun. 7:11814.
Lin, H., De Carvalho, P., Kho, D., Tai, C. Y., Pierre, P., Fink, G. R., et al. (2001). Polyploids require Bik1 for kinetochore-microtubule attachment. J. Cell. Biol. 155, 1173–1184. doi: 10.1083/jcb.200108119
Liu, Z., Steward, R., and Luo, L. (2000). Drosophila Lis1 is required for neuroblast proliferation, dendritic elaboration and axonal transport. Nat. Cell. Biol. 2, 776–783. doi: 10.1038/35041011
Liu, Z., Xie, T., and Steward, R. (1999). Lis1, the Drosophila homolog of a human lissencephaly disease gene, is required for germline cell division and oocyte differentiation. Development 126, 4477–4488.
Lloyd, T. E., Machamer, J., O’hara, K., Kim, J. H., Collins, S. E., Wong, M. Y., et al. (2012). The p150(Glued) CAP-Gly domain regulates initiation of retrograde transport at synaptic termini. Neuron 74, 344–360. doi: 10.1016/j.neuron.2012.02.026
Loening, N. M., Saravanan, S., Jespersen, N. E., Jara, K., and Barbar, E. (2020). Interplay of disorder and sequence specificity in the formation of stable dynein-dynactin complexes. Biophys. J. 119, 950–965. doi: 10.1016/j.bpj.2020.07.023
Ma, L., Tsai, M. Y., Wang, S., Lu, B., Chen, R., and Yates, J. R. III, et al (2009). Requirement for Nudel and dynein for assembly of the lamin B spindle matrix. Nat. Cell. Biol. 11, 247–256. doi: 10.1038/ncb1832
Maday, S., Twelvetrees, A. E., Moughamian, A. J., and Holzbaur, E. L. (2014). Axonal transport: cargo-specific mechanisms of motility and regulation. Neuron 84, 292–309. doi: 10.1016/j.neuron.2014.10.019
Maldonado-Báez, L., Cole, N. B., Krämer, H., and Donaldson, J. G. (2013). Microtubule-dependent endosomal sorting of clathrin-independent cargo by Hook1. J. Cell. Biol. 201, 233–247. doi: 10.1083/jcb.201208172
Mali, G. R., Ali, F. A., Lau, C. K., Begum, F., Skehel, M., and Carter, A. P. (2020). Shulin packages axonemal outer dynein arms for ciliary targeting. bioRxiv [Preprint]. doi: 10.1101/2020.09.04.282897
Malone, C. J., Misner, L., Le Bot, N., Tsai, M. C., Campbell, J. M., Ahringer, J., et al. (2003). The C. elegans hook protein, ZYG-12, mediates the essential attachment between the centrosome and nucleus. Cell 115, 825–836. doi: 10.1016/s0092-8674(03)00985-1
Markus, S. M., and Lee, W. L. (2011). Regulated offloading of cytoplasmic dynein from microtubule plus ends to the cortex. Dev. Cell. 20, 639–651. doi: 10.1016/j.devcel.2011.04.011
Markus, S. M., Marzo, M. G., and McKenney, R. J. (2020). New insights into the mechanism of dynein motor regulation by lissencephaly-1. eLife 9:e59737.
Markus, S. M., Plevock, K. M., St Germain, B. J., Punch, J. J., Meaden, C. W., and Lee, W. L. (2011). Quantitative analysis of Pac1/LIS1-mediated dynein targeting: implications for regulation of dynein activity in budding yeast. Cytoskeleton 68, 157–174. doi: 10.1002/cm.20502
Marzo, M. G., Griswold, J. M., and Markus, S. M. (2020). Pac1/LIS1 stabilizes an uninhibited conformation of dynein to coordinate its localization and activity. Nat. Cell. Biol. 22, 559–569. doi: 10.1038/s41556-020-0492-1
Marzo, M. G., Griswold, J. M., Ruff, K. M., Buchmeier, R. E., Fees, C. P., and Markus, S. M. (2019). Molecular basis for dyneinopathies reveals insight into dynein regulation and dysfunction. eLife 8: e47246.
Mattera, R., Williamson, C. D., Ren, X., and Bonifacino, J. S. (2020). The FTS-Hook-FHIP (FHF) complex interacts with AP-4 to mediate perinuclear distribution of AP-4 and its cargo ATG9A. Mol. Biol. Cell. 31, 963–979. doi: 10.1091/mbc.e19-11-0658
McKenney, R. J. (2020). LIS1 cracks open dynein. Nat. Cell. Biol. 22, 515–517. doi: 10.1038/s41556-020-0500-5
McKenney, R. J., Huynh, W., Tanenbaum, M. E., Bhabha, G., and Vale, R. D. (2014). Activation of cytoplasmic dynein motility by dynactin-cargo adapter complexes. Science 345, 337–341. doi: 10.1126/science.1254198
McKenney, R. J., Huynh, W., Vale, R. D., and Sirajuddin, M. (2016). Tyrosination of alpha-tubulin controls the initiation of processive dynein-dynactin motility. EMBO J. 35, 1175–1185. doi: 10.15252/embj.201593071
McKenney, R. J., Vershinin, M., Kunwar, A., Vallee, R. B., and Gross, S. P. (2010). LIS1 and NudE induce a persistent dynein force-producing state. Cell 141, 304–314. doi: 10.1016/j.cell.2010.02.035
McKenney, R. J., Weil, S. J., Scherer, J., and Vallee, R. B. (2011). Mutually exclusive cytoplasmic dynein regulation by NudE-Lis1 and dynactin. J. Biol. Chem. 286, 39615–39622. doi: 10.1074/jbc.m111.289017
Melkonian, K. A., Maier, K. C., Godfrey, J. E., Rodgers, M., and Schroer, T. A. (2007). Mechanism of dynamitin-mediated disruption of dynactin. J. Biol. Chem. 282, 19355–19364. doi: 10.1074/jbc.m700003200
Mesngon, M. T., Tarricone, C., Hebbar, S., Guillotte, A. M., Schmitt, E. W., Lanier, L., et al. (2006). Regulation of cytoplasmic dynein ATPase by Lis1. J. Neurosci. 26, 2132–2139. doi: 10.1523/jneurosci.5095-05.2006
Minke, P. F., Lee, I. H., Tinsley, J. H., Bruno, K. S., and Plamann, M. (1999). Neurospora crassa ro-10 and ro-11 genes encode novel proteins required for nuclear distribution. Mol. Microbiol. 32, 1065–1076. doi: 10.1046/j.1365-2958.1999.01421.x
Moore, J. K., Li, J., and Cooper, J. A. (2008). Dynactin function in mitotic spindle positioning. Traffic 9, 510–527. doi: 10.1111/j.1600-0854.2008.00710.x
Moughamian, A. J., and Holzbaur, E. L. (2012). Dynactin is required for transport initiation from the distal axon. Neuron 74, 331–343. doi: 10.1016/j.neuron.2012.02.025
Moughamian, A. J., Osborn, G. E., Lazarus, J. E., Maday, S., and Holzbaur, E. L. (2013). Ordered recruitment of dynactin to the microtubule plus-end is required for efficient initiation of retrograde axonal transport. J. Neurosci. 33, 13190–13203. doi: 10.1523/jneurosci.0935-13.2013
Niccoli, T., Yamashita, A., Nurse, P., and Yamamoto, M. (2004). The p150-Glued Ssm4p regulates microtubular dynamics and nuclear movement in fission yeast. J. Cell. Sci. 117, 5543–5556. doi: 10.1242/jcs.01475
Nicholas, M. P., Berger, F., Rao, L., Brenner, S., Cho, C., and Gennerich, A. (2015). Cytoplasmic dynein regulates its attachment to microtubules via nucleotide state-switched mechanosensing at multiple AAA domains. Proc. Natl. Acad. Sci. U.S.A. 112, 6371–6376. doi: 10.1073/pnas.1417422112
Niethammer, M., Smith, D. S., Ayala, R., Peng, J., Ko, J., Lee, M. S., et al. (2000). NUDEL is a novel Cdk5 substrate that associates with LIS1 and cytoplasmic dynein. Neuron 28, 697–711. doi: 10.1016/s0896-6273(00)00147-1
Nirschl, J. J., Magiera, M. M., Lazarus, J. E., Janke, C., and Holzbaur, E. L. (2016). alpha-tubulin tyrosination and CLIP-170 phosphorylation regulate the initiation of dynein-driven transport in neurons. Cell. Rep. 14, 2637–2652. doi: 10.1016/j.celrep.2016.02.046
Nyarko, A., Song, Y., and Barbar, E. (2012). Intrinsic disorder in dynein intermediate chain modulates its interactions with NudE and dynactin. J. Biol. Chem. 287, 24884–24893. doi: 10.1074/jbc.m112.376038
Olenick, M. A., Dominguez, R., and Holzbaur, E. L. F. (2019). Dynein activator Hook1 is required for trafficking of BDNF-signaling endosomes in neurons. J. Cell. Biol. 218, 220–233. doi: 10.1083/jcb.201805016
Olenick, M. A., and Holzbaur, E. L. F. (2019). Dynein activators and adaptors at a glance. J. Cell. Sci. 132:jcs227132. doi: 10.1242/jcs.227132
Olenick, M. A., Tokito, M., Boczkowska, M., Dominguez, R., and Holzbaur, E. L. (2016). Hook adaptors induce unidirectional processive motility by enhancing the dynein-dynactin interaction. J. Biol. Chem. 291, 18239–18251. doi: 10.1074/jbc.m116.738211
Ori-McKenney, K. M., Xu, J., Gross, S. P., and Vallee, R. B. (2010). A cytoplasmic dynein tail mutation impairs motor processivity. Nat. Cell. Biol. 12, 1228–1234. doi: 10.1038/ncb2127
Osmani, A. H., Osmani, S. A., and Morris, N. R. (1990). The molecular cloning and identification of a gene product specifically required for nuclear movement in Aspergillus nidulans. J. Cell. Biol. 111, 543–551. doi: 10.1083/jcb.111.2.543
Otamendi, A., Perez-De-Nanclares-Arregi, E., Oiartzabal-Arano, E., Cortese, M. S., Espeso, E. A., and Etxebeste, O. (2019). Developmental regulators FlbE/D orchestrate the polarity site-to-nucleus dynamics of the fungal bZIP transcription factor FlbB. Cell. Mol. Life Sci. 76, 4369–4390. doi: 10.1007/s00018-019-03121-5
Palacios, I. M., and St Johnston, D. (2002). Kinesin light chain-independent function of the Kinesin heavy chain in cytoplasmic streaming and posterior localisation in the Drosophila oocyte. Development 129, 5473–5485. doi: 10.1242/dev.00119
Pandey, J. P., and Smith, D. S. (2011). A Cdk5-dependent switch regulates Lis1/Ndel1/dynein-driven organelle transport in adult axons. J. Neurosci. 31, 17207–17219. doi: 10.1523/jneurosci.4108-11.2011
Parisi, G., Fornasari, M. S., and Echave, J. (2004). Dynactins p25 and p27 are predicted to adopt the LbetaH fold. FEBS Lett. 562, 1–4. doi: 10.1016/s0014-5793(04)00165-6
Penalva, M. A., Zhang, J., Xiang, X., and Pantazopoulou, A. (2017). Transport of fungal RAB11 secretory vesicles involves myosin-5, dynein/dynactin/p25, and kinesin-1 and is independent of kinesin-3. Mol. Biol. Cell. 28, 947–961. doi: 10.1091/mbc.e16-08-0566
Pfister, K. K., Fisher, E. M., Gibbons, I. R., Hays, T. S., Holzbaur, E. L., Mcintosh, J. R., et al. (2005). Cytoplasmic dynein nomenclature. J. Cell. Biol. 171, 411–413.
Pohlmann, T., Baumann, S., Haag, C., Albrecht, M., and Feldbrugge, M. (2015). A FYVE zinc finger domain protein specifically links mRNA transport to endosome trafficking. eLife 4:e06041.
Qiu, R., Zhang, J., and Xiang, X. (2013). Identification of a novel site in the tail of dynein heavy chain important for dynein function in vivo. J. Biol. Chem. 288, 2271–2280. doi: 10.1074/jbc.m112.412403
Qiu, R., Zhang, J., and Xiang, X. (2018). p25 of the dynactin complex plays a dual role in cargo binding and dynactin regulation. J. Biol. Chem. 293, 15606–15619. doi: 10.1074/jbc.ra118.004000
Qiu, R., Zhang, J., and Xiang, X. (2019). LIS1 regulates cargo-adapter-mediated activation of dynein by overcoming its autoinhibition in vivo. J. Cell. Biol. 218, 3630–3646. doi: 10.1083/jcb.201905178
Qiu, R., Zhang, J., and Xiang, X. (2020). The splicing-factor Prp40 affects dynein-dynactin function in Aspergillus nidulans. Mol. Biol. Cell. 31, 1289–1301. doi: 10.1091/mbc.e20-03-0166
Rao, L., Berger, F., Nicholas, M. P., and Gennerich, A. (2019). Molecular mechanism of cytoplasmic dynein tension sensing. Nat. Commun. 10:3332.
Reck-Peterson, S. L., Redwine, W. B., Vale, R. D., and Carter, A. P. (2018). The cytoplasmic dynein transport machinery and its many cargoes. Nat. Rev. Mol. Cell. Biol. 19, 382–398. doi: 10.1038/s41580-018-0004-3
Reck-Peterson, S. L., Yildiz, A., Carter, A. P., Gennerich, A., Zhang, N., and Vale, R. D. (2006). Single-molecule analysis of dynein processivity and stepping behavior. Cell 126, 335–348. doi: 10.1016/j.cell.2006.05.046
Reddy, B. J., Mattson, M., Wynne, C. L., Vadpey, O., Durra, A., Chapman, D., et al. (2016). Load-induced enhancement of Dynein force production by LIS1-NudE in vivo and in vitro. Nat. Commun. 7, 12259.
Redwine, W. B., Desantis, M. E., Hollyer, I., Htet, Z. M., Tran, P. T., Swanson, S. K., et al. (2017). The human cytoplasmic dynein interactome reveals novel activators of motility. eLife 6:e28257.
Reiner, O., Carrozzo, R., Shen, Y., Wehnert, M., Faustinella, F., Dobyns, W. B., et al. (1993). Isolation of a Miller-Dieker lissencephaly gene containing G protein beta-subunit-like repeats. Nature 364, 717–721. doi: 10.1038/364717a0
Roberts, A. J., Goodman, B. S., and Reck-Peterson, S. L. (2014). Reconstitution of dynein transport to the microtubule plus end by kinesin. eLife 3:e02641.
Roberts, A. J., Kon, T., Knight, P. J., Sutoh, K., and Burgess, S. A. (2013). Functions and mechanics of dynein motor proteins. Nat. Rev. Mol. Cell. Biol. 14, 713–726. doi: 10.1038/nrm3667
Roll-Mecak, A. (2020). The tubulin code in microtubule dynamics and information encoding. Dev. Cell. 54, 7–20. doi: 10.1016/j.devcel.2020.06.008
Saito, K., Murayama, T., Hata, T., Kobayashi, T., Shibata, K., Kazuno, S., et al. (2020). Conformational diversity of dynactin sidearm and domain organization of its subunit p150. Mol. Biol. Cell. 31, 1218–1231. doi: 10.1091/mbc.e20-01-0031
Salogiannis, J., Egan, M. J., and Reck-Peterson, S. L. (2016). Peroxisomes move by hitchhiking on early endosomes using the novel linker protein PxdA. J. Cell. Biol. 212, 289–296. doi: 10.1083/jcb.201512020
Sasaki, S., Shionoya, A., Ishida, M., Gambello, M. J., Yingling, J., Wynshaw-Boris, A., et al. (2000). A LIS1/NUDEL/cytoplasmic dynein heavy chain complex in the developing and adult nervous system. Neuron 28, 681–696. doi: 10.1016/s0896-6273(00)00146-x
Schafer, D. A., Gill, S. R., Cooper, J. A., Heuser, J. E., and Schroer, T. A. (1994). Ultrastructural analysis of the dynactin complex: an actin-related protein is a component of a filament that resembles F-actin. J. Cell. Biol. 126, 403–412. doi: 10.1083/jcb.126.2.403
Scherer, J., Yi, J., and Vallee, R. B. (2020). Role of cytoplasmic dynein and kinesins in adenovirus transport. FEBS Lett. 594, 1838–1847. doi: 10.1002/1873-3468.13777
Schlager, M. A., Hoang, H. T., Urnavicius, L., Bullock, S. L., and Carter, A. P. (2014). In vitro reconstitution of a highly processive recombinant human dynein complex. EMBO J. 33, 1855–1868. doi: 10.15252/embj.201488792
Schmidt, H., and Carter, A. P. (2016). Review: structure and mechanism of the dynein motor ATPase. Biopolymers 105, 557–567. doi: 10.1002/bip.22856
Schroeder, C. M., and Vale, R. D. (2016). Assembly and activation of dynein-dynactin by the cargo adaptor protein Hook3. J. Cell. Biol. 214, 309–318. doi: 10.1083/jcb.201604002
Schroer, T. A., and Sheetz, M. P. (1991). Two activators of microtubule-based vesicle transport. J. Cell. Biol. 115, 1309–1318. doi: 10.1083/jcb.115.5.1309
Sellers, J. R., and Knight, P. J. (2007). Folding and regulation in myosins II and V. J. Muscle Res. Cell. Motil. 28, 363–370. doi: 10.1007/s10974-008-9134-0
Sheeman, B., Carvalho, P., Sagot, I., Geiser, J., Kho, D., Hoyt, M. A., et al. (2003). Determinants of S. cerevisiae dynein localization and activation: implications for the mechanism of spindle positioning. Curr. Biol. 13, 364–372. doi: 10.1016/s0960-9822(03)00013-7
Shu, T., Ayala, R., Nguyen, M. D., Xie, Z., Gleeson, J. G., and Tsai, L. H. (2004). Ndel1 operates in a common pathway with LIS1 and cytoplasmic dynein to regulate cortical neuronal positioning. Neuron 44, 263–277. doi: 10.1016/j.neuron.2004.09.030
Siddiqui, N., Zwetsloot, A. J., Bachmann, A., Roth, D., Hussain, H., Brandt, J., et al. (2019). PTPN21 and Hook3 relieve KIF1C autoinhibition and activate intracellular transport. Nat. Commun. 10:2693.
Simões, P. A., Celestino, R., Carvalho, A. X., and Gassmann, R. (2018). NudE regulates dynein at kinetochores but is dispensable for other dynein functions in the C. elegans early embryo. J. Cell. Sci. 131:jcs212159. doi: 10.1242/jcs.212159
Sivagurunathan, S., Schnittker, R. R., Razafsky, D. S., Nandini, S., Plamann, M. D., and King, S. J. (2012). Analyses of dynein heavy chain mutations reveal complex interactions between dynein motor domains and cellular dynein functions. Genetics 191, 1157–1179. doi: 10.1534/genetics.112.141580
Smith, D. S., Niethammer, M., Ayala, R., Zhou, Y., Gambello, M. J., Wynshaw-Boris, A., et al. (2000). Regulation of cytoplasmic dynein behaviour and microtubule organization by mammalian Lis1. Nat. Cell. Biol. 2, 767–775. doi: 10.1038/35041000
Spinner, M. A., Pinter, K., Drerup, C. M., and Herman, T. G. (2020). A conserved role for vezatin proteins in cargo-specific regulation of retrograde axonal transport. Genetics 216, 431–445. doi: 10.1534/genetics.120.303499
Splinter, D., Razafsky, D. S., Schlager, M. A., Serra-Marques, A., Grigoriev, I., Demmers, J., et al. (2012). BICD2, dynactin, and LIS1 cooperate in regulating dynein recruitment to cellular structures. Mol. Biol. Cell. 23, 4226–4241. doi: 10.1091/mbc.e12-03-0210
Stehman, S. A., Chen, Y., McKenney, R. J., and Vallee, R. B. (2007). NudE and NudEL are required for mitotic progression and are involved in dynein recruitment to kinetochores. J. Cell. Biol. 178, 583–594. doi: 10.1083/jcb.200610112
Sweeney, H. L., and Holzbaur, E. L. F. (2018). Motor proteins. Cold Spring Harb. Perspect. Biol. 10:a021931.
Szebenyi, G., Hall, B., Yu, R., Hashim, A. I., and Krämer, H. (2007a). Hook2 localizes to the centrosome, binds directly to centriolin/CEP110 and contributes to centrosomal function. Traffic 8, 32–46. doi: 10.1111/j.1600-0854.2006.00511.x
Szebenyi, G., Wigley, W. C., Hall, B., Didier, A., Yu, M., Thomas, P., et al. (2007b). Hook2 contributes to aggresome formation. BMC Cell. Biol. 8:19. doi: 10.1186/1471-2121-8-19
Tame, M. A., Raaijmakers, J. A., Van Den Broek, B., Lindqvist, A., Jalink, K., and Medema, R. H. (2014). Astral microtubules control redistribution of dynein at the cell cortex to facilitate spindle positioning. Cell Cycle 13, 1162–1170. doi: 10.4161/cc.28031
Tang, X., Germain, B. S., and Lee, W. L. (2012). A novel patch assembly domain in Num1 mediates dynein anchoring at the cortex during spindle positioning. J. Cell. Biol. 196, 743–756. doi: 10.1083/jcb.201112017
Torisawa, T., Ichikawa, M., Furuta, A., Saito, K., Oiwa, K., Kojima, H., et al. (2014). Autoinhibition and cooperative activation mechanisms of cytoplasmic dynein. Nat. Cell. Biol. 16, 1118–1124. doi: 10.1038/ncb3048
Toropova, K., Zou, S., Roberts, A. J., Redwine, W. B., Goodman, B. S., Reck-Peterson, S. L., et al. (2014). Lis1 regulates dynein by sterically blocking its mechanochemical cycle. eLife 3:e03372.
Tripathy, S. K., Weil, S. J., Chen, C., Anand, P., Vallee, R. B., and Gross, S. P. (2014). Autoregulatory mechanism for dynactin control of processive and diffusive dynein transport. Nat. Cell. Biol. 16, 1192–1201. doi: 10.1038/ncb3063
Trokter, M., Mücke, N., and Surrey, T. (2012). Reconstitution of the human cytoplasmic dynein complex. Proc. Natl. Acad. Sci. U.S.A. 109, 20895–20900. doi: 10.1073/pnas.1210573110
Trybus, K. M. (1989). Filamentous smooth muscle myosin is regulated by phosphorylation. J. Cell. Biol. 109, 2887–2894. doi: 10.1083/jcb.109.6.2887
Trybus, K. M. (2008). Myosin V from head to tail. Cell. Mol. Life Sci. 65, 1378–1389. doi: 10.1007/s00018-008-7507-6
Twelvetrees, A. E., Pernigo, S., Sanger, A., Guedes-Dias, P., Schiavo, G., Steiner, R. A., et al. (2016). The dynamic localization of cytoplasmic dynein in neurons is driven by kinesin-1. Neuron 90, 1000–1015. doi: 10.1016/j.neuron.2016.04.046
Urnavicius, L., Lau, C. K., Elshenawy, M. M., Morales-Rios, E., Motz, C., Yildiz, A., et al. (2018). Cryo-EM shows how dynactin recruits two dyneins for faster movement. Nature 554, 202–206. doi: 10.1038/nature25462
Urnavicius, L., Zhang, K., Diamant, A. G., Motz, C., Schlager, M. A., Yu, M., et al. (2015). The structure of the dynactin complex and its interaction with dynein. Science 347, 1441–1446. doi: 10.1126/science.aaa4080
Vaughan, K. T., and Vallee, R. B. (1995). Cytoplasmic dynein binds dynactin through a direct interaction between the intermediate chains and p150Glued. J. Cell. Biol. 131, 1507–1516. doi: 10.1083/jcb.131.6.1507
Vaughan, P. S., Miura, P., Henderson, M., Byrne, B., and Vaughan, K. T. (2002). A role for regulated binding of p150(Glued) to microtubule plus ends in organelle transport. J. Cell. Biol. 158, 305–319. doi: 10.1083/jcb.200201029
Verhey, K. J., and Hammond, J. W. (2009). Traffic control: regulation of kinesin motors. Nat. Rev. Mol. Cell. Biol. 10, 765–777. doi: 10.1038/nrm2782
Walenta, J. H., Didier, A. J., Liu, X., and Kramer, H. (2001). The Golgi-associated hook3 protein is a member of a novel family of microtubule-binding proteins. J. Cell. Biol. 152, 923–934. doi: 10.1083/jcb.152.5.923
Wang, S., Ketcham, S. A., Schon, A., Goodman, B., Wang, Y., and Yates, J. III, et al (2013). Nudel/NudE and Lis1 promote dynein and dynactin interaction in the context of spindle morphogenesis. Mol. Biol. Cell. 24, 3522–3533. doi: 10.1091/mbc.e13-05-0283
Wang, S., and Zheng, Y. (2011). Identification of a novel dynein binding domain in nudel essential for spindle pole organization in Xenopus egg extract. J. Biol. Chem. 286, 587–593. doi: 10.1074/jbc.m110.181578
Wang, Y., Huynh, W., Skokan, T. D., Lu, W., Weiss, A., and Vale, R. D. (2019). CRACR2a is a calcium-activated dynein adaptor protein that regulates endocytic traffic. J. Cell. Biol. 218, 1619–1633. doi: 10.1083/jcb.201806097
Waterman-Storer, C. M., Karki, S., and Holzbaur, E. L. (1995). The p150Glued component of the dynactin complex binds to both microtubules and the actin-related protein centractin (Arp-1). Proc. Natl. Acad. Sci. U.S.A. 92, 1634–1638. doi: 10.1073/pnas.92.5.1634
Waterman-Storer, C. M., Karki, S. B., Kuznetsov, S. A., Tabb, J. S., Weiss, D. G., Langford, G. M., et al. (1997). The interaction between cytoplasmic dynein and dynactin is required for fast axonal transport. Proc. Natl. Acad. Sci. U.S.A. 94, 12180–12185. doi: 10.1073/pnas.94.22.12180
Wear, M. A., and Cooper, J. A. (2004). Capping protein: new insights into mechanism and regulation. Trends Biochem. Sci. 29, 418–428. doi: 10.1016/j.tibs.2004.06.003
Wedlich-Soldner, R., Straube, A., Friedrich, M. W., and Steinberg, G. (2002). A balance of KIF1A-like kinesin and dynein organizes early endosomes in the fungus Ustilago maydis. EMBO J. 21, 2946–2957. doi: 10.1093/emboj/cdf296
Winey, M., and Bloom, K. (2012). Mitotic spindle form and function. Genetics 190, 1197–1224. doi: 10.1534/genetics.111.128710
Wynshaw-Boris, A. (2007). Lissencephaly and LIS1: insights into the molecular mechanisms of neuronal migration and development. Clin. Genet. 72, 296–304. doi: 10.1111/j.1399-0004.2007.00888.x
Xiang, X., Han, G., Winkelmann, D. A., Zuo, W., and Morris, N. R. (2000). Dynamics of cytoplasmic dynein in living cells and the effect of a mutation in the dynactin complex actin-related protein Arp1. Curr. Biol. 10, 603–606. doi: 10.1016/s0960-9822(00)00488-7
Xiang, X., Osmani, A. H., Osmani, S. A., Xin, M., and Morris, N. R. (1995). NudF, a nuclear migration gene in Aspergillus nidulans, is similar to the human LIS-1 gene required for neuronal migration. Mol. Biol. Cell. 6, 297–310. doi: 10.1091/mbc.6.3.297
Xu, L., Sowa, M. E., Chen, J., Li, X., Gygi, S. P., and Harper, J. W. (2008). An FTS/Hook/p107(FHIP) complex interacts with and promotes endosomal clustering by the homotypic vacuolar protein sorting complex. Mol. Biol. Cell. 19, 5059–5071. doi: 10.1091/mbc.e08-05-0473
Yamada, M., Toba, S., Takitoh, T., Yoshida, Y., Mori, D., Nakamura, T., et al. (2010). mNUDC is required for plus-end-directed transport of cytoplasmic dynein and dynactins by kinesin-1. EMBO J. 29, 517–531. doi: 10.1038/emboj.2009.378
Yamada, M., Toba, S., Yoshida, Y., Haratani, K., Mori, D., Yano, Y., et al. (2008). LIS1 and NDEL1 coordinate the plus-end-directed transport of cytoplasmic dynein. EMBO J. 27, 2471–2483. doi: 10.1038/emboj.2008.182
Yamamoto, A., West, R. R., Mcintosh, J. R., and Hiraoka, Y. (1999). A cytoplasmic dynein heavy chain is required for oscillatory nuclear movement of meiotic prophase and efficient meiotic recombination in fission yeast. J. Cell. Biol. 145, 1233–1249. doi: 10.1083/jcb.145.6.1233
Yamashita, A., and Yamamoto, M. (2006). Fission yeast Num1p is a cortical factor anchoring dynein and is essential for the horse-tail nuclear movement during meiotic prophase. Genetics 173, 1187–1196. doi: 10.1534/genetics.105.050062
Yan, X., Li, F., Liang, Y., Shen, Y., Zhao, X., Huang, Q., et al. (2003). Human Nudel and NudE as regulators of cytoplasmic dynein in poleward protein transport along the mitotic spindle. Mol. Cell. Biol. 23, 1239–1250. doi: 10.1128/mcb.23.4.1239-1250.2003
Yao, X., Arst, H. N. Jr., Wang, X., and Xiang, X. (2015). Discovery of a vezatin-like protein for dynein-mediated early endosome transport. Mol. Biol. Cell. 26, 3816–3827. doi: 10.1091/mbc.e15-08-0602
Yao, X., Wang, X., and Xiang, X. (2014). FHIP and FTS proteins are critical for dynein-mediated transport of early endosomes in Aspergillus. Mol. Biol. Cell. 25, 2181–2189. doi: 10.1091/mbc.e14-04-0873
Yao, X., Zhang, J., Zhou, H., Wang, E., and Xiang, X. (2012). In vivo roles of the basic domain of dynactin p150 in microtubule plus-end tracking and dynein function. Traffic 13, 375–387. doi: 10.1111/j.1600-0854.2011.01312.x
Yeh, T. Y., Kowalska, A. K., Scipioni, B. R., Cheong, F. K., Zheng, M., Derewenda, U., et al. (2013). Dynactin helps target Polo-like kinase 1 to kinetochores via its left-handed beta-helical p27 subunit. EMBO J. 32, 1023–1035. doi: 10.1038/emboj.2013.30
Yeh, T. Y., Quintyne, N. J., Scipioni, B. R., Eckley, D. M., and Schroer, T. A. (2012). Dynactin’s pointed-end complex is a cargo-targeting module. Mol. Biol. Cell. 23, 3827–3837. doi: 10.1091/mbc.e12-07-0496
Zekert, N., and Fischer, R. (2009). The Aspergillus nidulans kinesin-3 UncA motor moves vesicles along a subpopulation of microtubules. Mol. Biol. Cell. 20, 673–684. doi: 10.1091/mbc.e08-07-0685
Zhang, J., Li, S., Fischer, R., and Xiang, X. (2003). Accumulation of cytoplasmic dynein and dynactin at microtubule plus ends in Aspergillus nidulans is kinesin dependent. Mol. Biol. Cell. 14, 1479–1488. doi: 10.1091/mbc.e02-08-0516
Zhang, J., Qiu, R., Arst, H. N. Jr., Penalva, M. A., and Xiang, X. (2014). HookA is a novel dynein-early endosome linker critical for cargo movement in vivo. J. Cell. Biol. 204, 1009–1026. doi: 10.1083/jcb.201308009
Zhang, J., Wang, L., Zhuang, L., Huo, L., Musa, S., Li, S., et al. (2008). Arp11 affects dynein-dynactin interaction and is essential for dynein function in Aspergillus nidulans. Traffic 9, 1073–1087. doi: 10.1111/j.1600-0854.2008.00748.x
Zhang, J., Yao, X., Fischer, L., Abenza, J. F., Penalva, M. A., and Xiang, X. (2011). The p25 subunit of the dynactin complex is required for dynein-early endosome interaction. J. Cell. Biol. 193, 1245–1255. doi: 10.1083/jcb.201011022
Zhang, J., Zhuang, L., Lee, Y., Abenza, J. F., Penalva, M. A., and Xiang, X. (2010). The microtubule plus-end localization of Aspergillus dynein is important for dynein-early-endosome interaction but not for dynein ATPase activation. J. Cell. Sci. 123, 3596–3604. doi: 10.1242/jcs.075259
Zhang, K., Foster, H. E., Rondelet, A., Lacey, S. E., Bahi-Buisson, N., Bird, A. W., et al. (2017). Cryo-EM reveals how human cytoplasmic dynein is auto-inhibited and activated. Cell 169, 1303.e18–1314.e18.
Zhang, Y., Gao, X., Manck, R., Schmid, M., Osmani, A. H., Osmani, S. A., et al. (2017). Microtubule-organizing centers of Aspergillus nidulans are anchored at septa by a disordered protein. Mol. Microbiol. 106, 285–303. doi: 10.1111/mmi.13763
Keywords: dynactin, cargo adapter, LIS1, early endosome, microtubule plus end, fungi, dynein
Citation: Xiang X and Qiu R (2020) Cargo-Mediated Activation of Cytoplasmic Dynein in vivo. Front. Cell Dev. Biol. 8:598952. doi: 10.3389/fcell.2020.598952
Received: 26 August 2020; Accepted: 07 October 2020;
Published: 23 October 2020.
Edited by:
Carlos M. Guardia, National Institutes of Health (NIH), United StatesReviewed by:
Steven Markus, Colorado State University, United StatesRichard McKenney, University of California, Davis, United States
Copyright © 2020 Xiang and Qiu. This is an open-access article distributed under the terms of the Creative Commons Attribution License (CC BY). The use, distribution or reproduction in other forums is permitted, provided the original author(s) and the copyright owner(s) are credited and that the original publication in this journal is cited, in accordance with accepted academic practice. No use, distribution or reproduction is permitted which does not comply with these terms.
*Correspondence: Xin Xiang, eGluLnhpYW5nQHVzdWhzLmVkdQ==