- 1Biochemistry-Proteomics Laboratory, Victor Babes National Institute of Pathology, Bucharest, Romania
- 2Cell Biology and Histology Department, Carol Davila University of Medicine and Pharmacy, Bucharest, Romania
- 3Clinical Biochemistry Department, Faculty of Medicine, Titu Maiorescu University, Bucharest, Romania
Caveolae are membrane microdomains described in many cell types involved in endocytocis, transcytosis, cell signaling, mechanotransduction, and aging. They are found at the interface with the extracellular environment and are structured by caveolin and cavin proteins. Caveolae and caveolins mediate transduction of chemical messages via signaling pathways, as well as non-chemical messages, such as stretching or shear stress. Various pathogens or signals can hijack these gates, leading to infectious, oncogenic and even caveolin-related diseases named caveolinopathies. By contrast, preclinical and clinical research have fallen behind in their attempts to hijack caveolae and caveolins for therapeutic purposes. Caveolae involvement in human disease is not yet fully explored or understood and, of all their scaffold proteins, only caveolin-1 is being considered in clinical trials as a possible biomarker of disease. This review briefly summarizes current knowledge about caveolae cell signaling and raises the hypothesis whether these microdomains could serve as hijackable “gatekeepers” or “gateways” in cell communication. Furthermore, because cell signaling is one of the most dynamic domains in translating data from basic to clinical research, we pay special attention to translation of caveolae, caveolin, and cavin research into clinical practice.
Introduction
The term “caveolae” is more than 60 years old and traces back to seminal electron microscopy studies conducted independently by G.E. Palade (Palade, 1953; Bruns and Palade, 1968) and E. Yamada of various tissues including endothelia (Yamada, 1955a,b; Smith and Ryan, 1972) and muscle (Merrillees, 1960; Zampighi et al., 1975; Sawada et al., 1978). For a long time, their function(s) remained elusive, until electron microscopy studies complemented by biochemical investigations led to in vitro functional studies and in vivo models. In 2001, Lisanti’s lab produced the first caveolin knockout (KO) mouse strain (Cav1–/–) (Razani et al., 2001). Further research revealed that caveolae and their scaffold proteins are involved in specific cellular processes, such as plasma microdomain organization and cell signaling (Boscher and Nabi, 2012)—in both normal cells (Sowa, 2012) or tumor cells (Hehlgans and Cordes, 2011)—or even in a specific kind of tumor (Parat and Riggins, 2012). Caveolae also are involved in cell migration and metastasis (Nunez-Wehinger et al., 2014), mechano-reception (Nassoy and Lamaze, 2012), and mechano-protection in certain tissues (Lo et al., 2015). Embryologic development is a less explored area in caveolin research (but for review, see Sohn et al., 2016).
Technological progress in cell imaging has improved our understanding of how biochemical components of caveolae assemble into a functional ultrastructural domain of the cell membrane. Knockout animal models have added more to a comprehensive picture of these microdomains. Tracking the literature describing all of these membrane microdomains means navigating a flood of publications on caveolae and their scaffold proteins, caveolins, and cavins. Of about 100 reviews published in the last 5 years, only a few offer an integrated view (Cheng and Nichols, 2016; Han et al., 2016; Busija et al., 2017; Lamaze et al., 2017; Bhogal et al., 2018; Filippini et al., 2018; Parton et al., 2018; Leo et al., 2020). An inventory of (un)resolved issues regarding caveolae is exquisitely summarized in a recent review unconventionally entitled “Caveolae: The FAQs” (Parton et al., 2020).
This review briefly summarizes the knowledge in caveolae cell signaling and assesses the status of these microdomains as both gatekeepers and gateways in cell communication. Furthermore, because cell signaling is one of the most dynamic domains in translating data from basic to clinical research, special attention will be paid to caveolae, caveolins, and cavins research translation into clinical practice.
Ultrastructure of Caveolae, Related to Caveolins, and Cavins Expression
Based on the first electron micrograph reports, caveolae were categorized and further evaluated as endocytotic vesicles (Gabella, 1978; Levin and Page, 1980; McGuire and Twietmeyer, 1983; Noguchi et al., 1987; Severs, 1988; Anderson, 1993). Organized as omega-shaped plasma membrane microdomains (segments of membrane with special lipid composition; Field, 2017), caveolae have been studied in experimental setups relying on cholesterol depletion (Liu and Pilch, 2008; Grundner and Zemljic Jokhadar, 2014). However, cholesterol is also enriched in other membrane microdomains, such as lipid rafts and chlatrin coated pits. Cholesterol depletion affects with variable degree a significant number of endocytotic pathways (reviewed in Thottacherry et al., 2019), thus generating results that lack specificity. Caveolae have been described in many cell types, including endothelial cells, smooth and striated muscle cells, interstitial cells of the heart (Gherghiceanu et al., 2009), adipose cells, fibroblasts, and Schwann cells of myelinated or unmyelinated peripheral nerve fibers (Figure 1). The number of caveolae varies in different cell types and has been reported to be up to 10,000/cell in endothelial cells (Couet et al., 1997c) and about 1,000,000/cell in adipocytes (Thorn et al., 2003). The number of caveolae also varies in smooth muscle cells of different tissues from Wistar rats, with 0.48 c/μm in the muscularis mucosa of stomach, 0.57 c/μm in the media of the aorta, 0.74 c/μm in the bladder, and 1.06 c/μm in the myometrium (Popescu et al., 2006). More so, the number of caveolae seems to be modified in pathological conditions, and a decreased number of caveolae in aortic smooth muscle cells has been associated with hypertension (Potje et al., 2019).
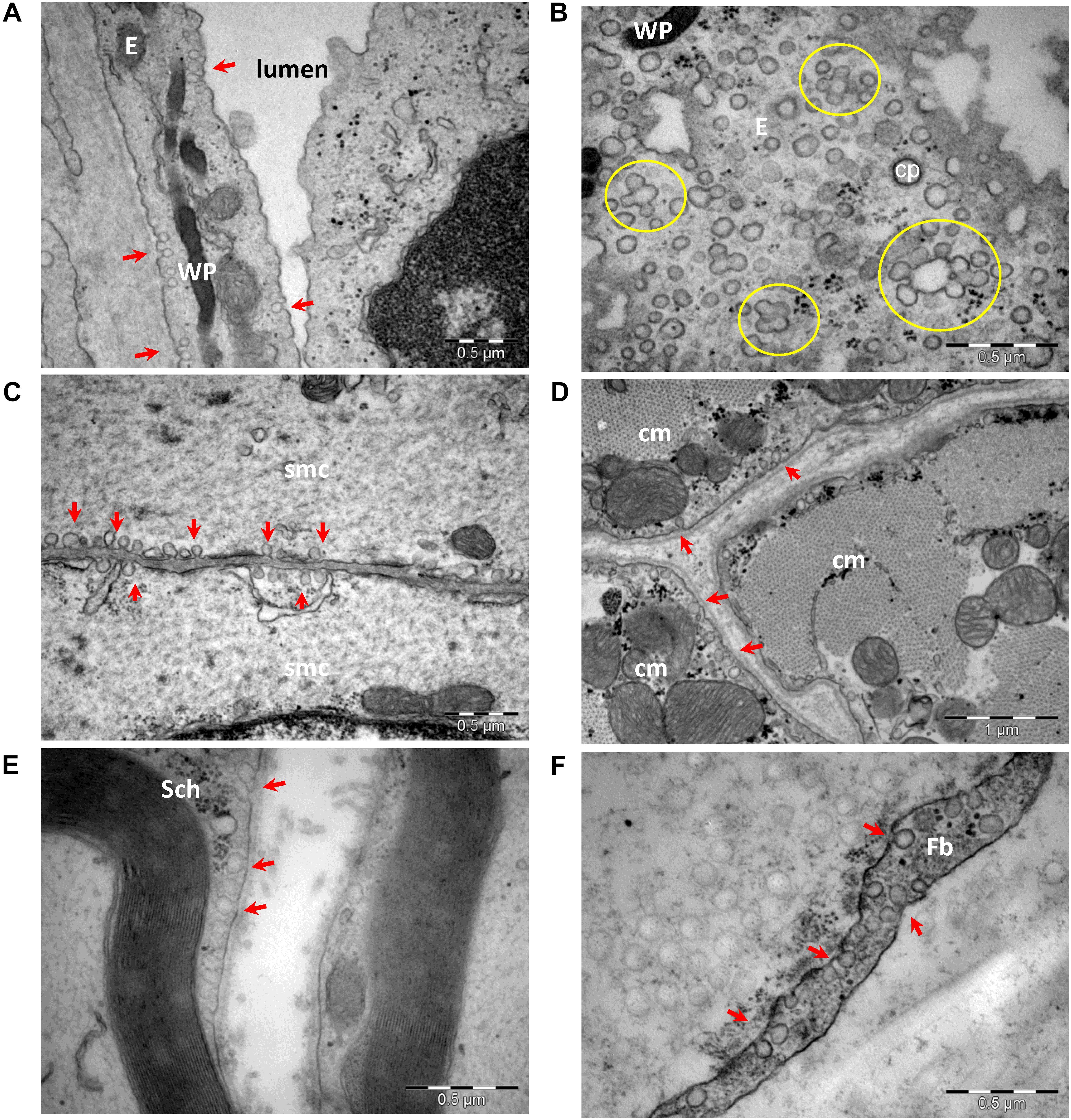
Figure 1. Transmission electron microscopy of cells with caveolae (arrows): endothelial cells (A,B), smooth (C) and striated (D) muscle cells, Schwann cell (E), and perineurial fibroblast (F). Caveolae in a section parallel to an endothelial cell plasma membrane (B) shows numerous caveolae as single units or clustered forming rosettes (encircled). E, endothelial cell; cp, clathrin-coated pit; WP, Weibel–Palade bodies in endothelial cell; smc, smooth muscle cell; cm, cardiac muscle cell; Sch, Schwann cell; Fb, fibroblast (image collection, Department of Ultrastructural Pathology, “Victor Babes” Institute of Pathology, Bucharest).
The protein scaffold composition of caveolae was resolved by mass spectrometry and cryoelectron tomography (Ludwig et al., 2013, 2016). The main scaffolding proteins are members of the caveolin family [caveolin (Cav)-1, -2, and -3], which associate as homo- or heterooligomers. The protein expression of caveolins differs in various tissues, as well as in their propensity to associate in heterooligomers. Caveolins have an even wider distribution, being detected even in cells that do not organize caveolae. Cav-1 is the main protein to form caveolae in non-muscle cells, alone or with Cav-2 (de Almeida, 2017), and its absence results in a lack of caveolae at least in endothelial and smooth muscle cells (Figure 2). Cav-2 KO mice show evidence of severe pulmonary dysfunction without disruption of caveolae (Razani et al., 2002). Cav-1/3 double-KO mice are viable but lack both muscle and non-muscle caveolae and develop a severe cardiomyopathic phenotype (Park et al., 2002). Cav-3 is the main scaffold protein of muscle cell membrane caveolae (Sohn et al., 2016). Mutations in this gene lead to skeletal muscle disease through multiple pathogenetic mechanisms, and Cav-3 deficiency has led to the recognition of a new category of pathologies labeled the caveolinopathies. These conditions include sarcolemmal membrane alterations, disorganization of the skeletal muscle T-tubule network, and disruption of distinct cell-signaling pathways. To date, 30 Cav-3 mutations have been identified in the human population. Cav-3 defects underlie four distinct skeletal muscle disease phenotypes: limb girdle muscular dystrophy, rippling muscle disease, distal myopathy, and hyperCKemia. In addition, one Cav-3 mutant has been described in a case of hypertrophic cardiomyopathy (Gazzerro et al., 2010).
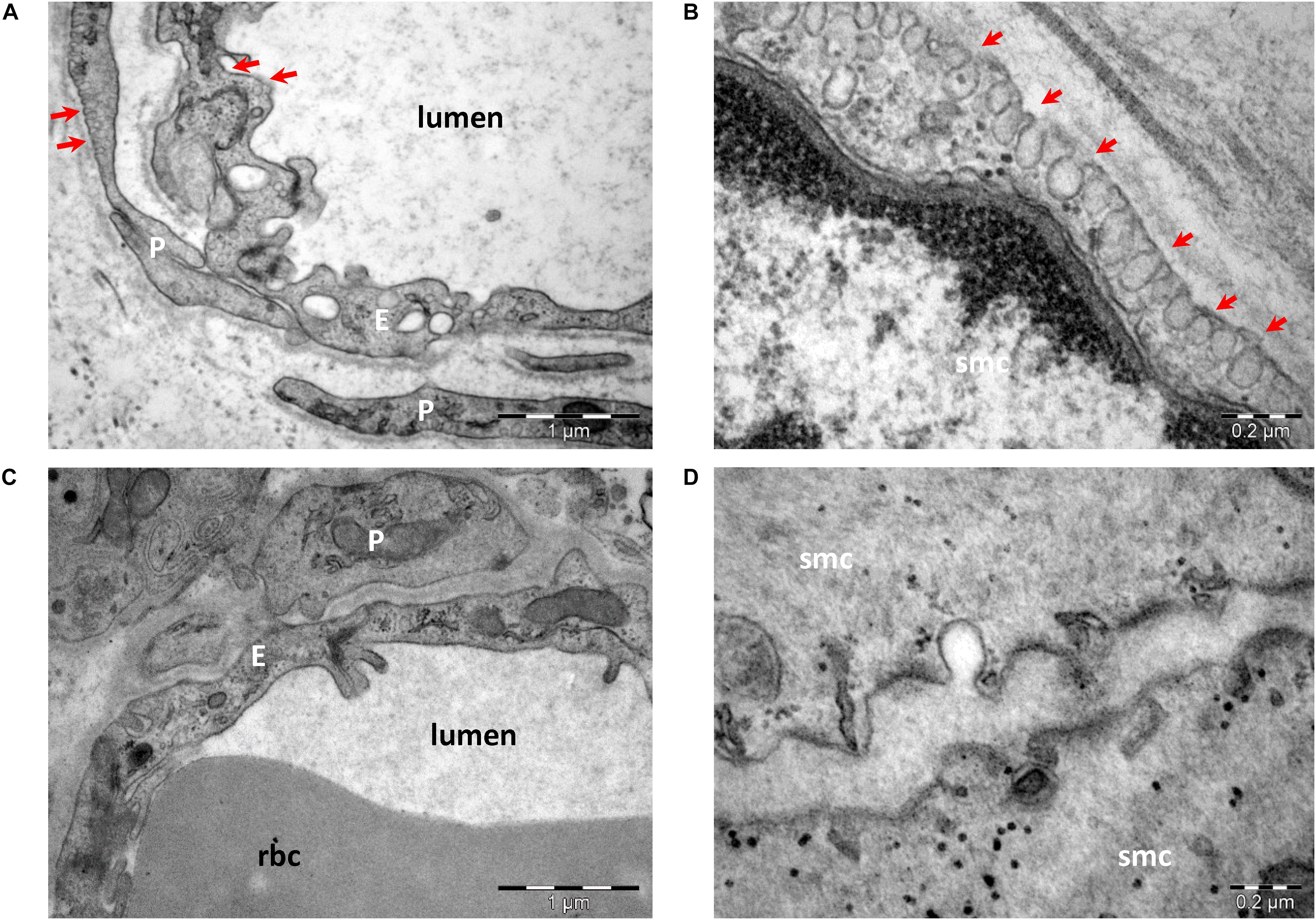
Figure 2. Transmission electron microscopy of endothelial cells (A,C) and smooth muscle cells (B,D) from wild-type (A,B) and Cav-1 KO (C,D) mice. Caveolae (arrows) are present in endothelial cells (E), pericytes (P), and smooth muscle cells (smc) in wild type (A,B). Note the lack of caveolae in cells from Cav-1 KO mice (C,D) (image collection, Department of Ultrastructural Pathology, “Victor Babes” Institute of Pathology, Bucharest).
In addition, cavin proteins (cavins 1–4) are recruited from cytosol to caveolae in the presence of caveolins and are required to stabilize the caveolar structure by multiple low-affinity interactions with caveolins and membrane lipids (Parton et al., 2018; Figure 3).
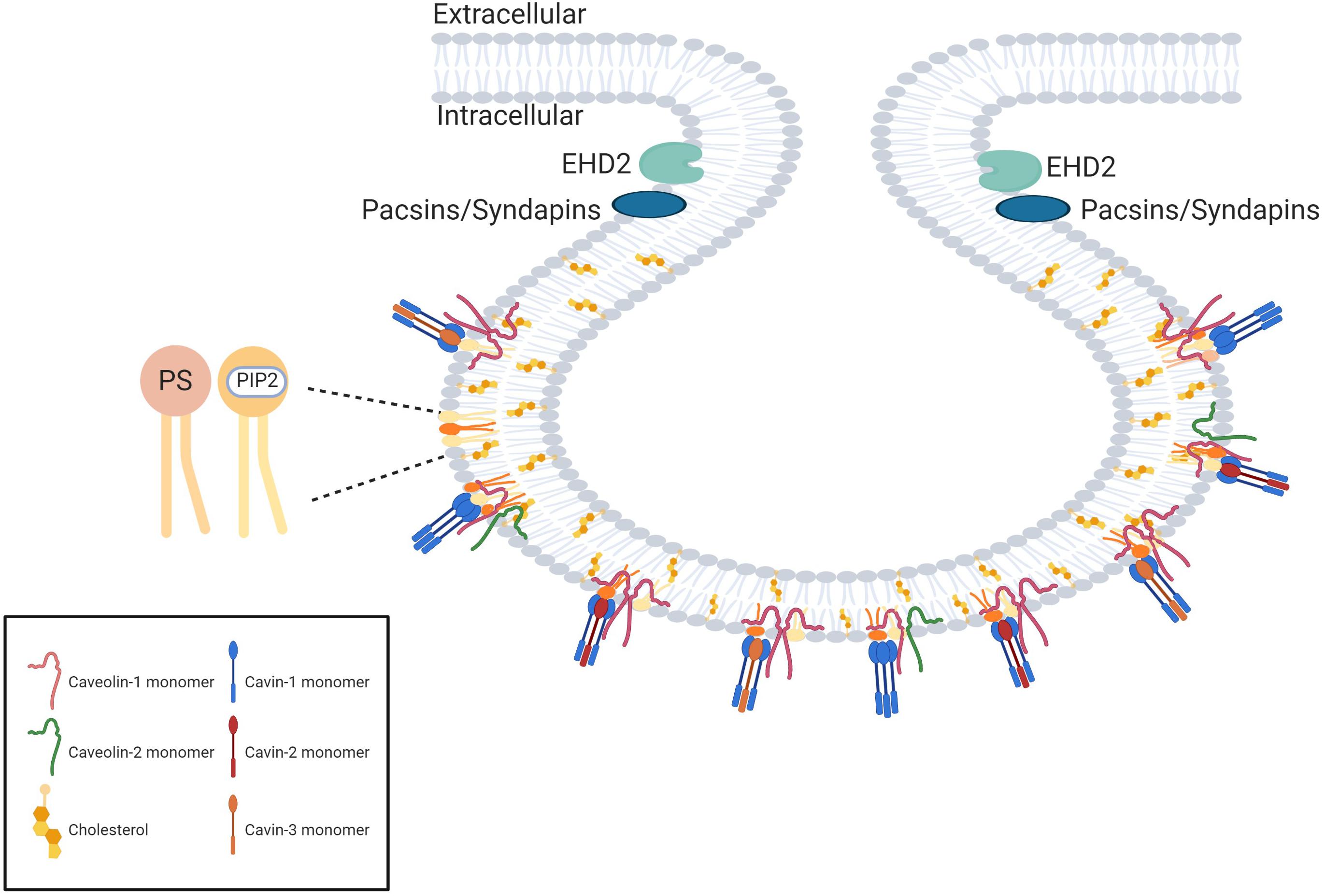
Figure 3. The protein scaffold of caveolae. The proteins forming the caveolae coat are named caveolins and cavins. The main proteins of the coat are caveolin-1 (which oligomerizes in homooligomers or heterooligomers with caveolin-2) and cavin-1 which forms homotrimers or heterotrimers with cavin-2 or cavin-3. The curvature of the neck of the caveolae is dependent on EH-domain containing protein 2 (EDH2) and pacsins. Created with BioRender.com.
Similar to Cav-1, cavin-1 is required for caveolae formation, and KO of either leads to caveolae loss (Hill et al., 2008; Liu and Pilch, 2008). Cavin-2 deficiency in mice causes tissue-specific loss of caveolae, affecting lung endothelium and adipose tissue but not the endothelium in skeletal and cardiac tissue (Hansen et al., 2013). In vitro, cavin-2 induces membrane curvature in caveolae (Hansen et al., 2009), serves as part of a cholesterol sensor, and relocalizes cavin-1 from the cytosol to the plasma membrane (Breen et al., 2012). Cavin-3 does not play a major role in caveolae formation and composition (Liu et al., 2014a). Less is known about the pathogenic impact of cavin mutations in humans. Cavin-1 mutations cause secondary deficiency of caveolins, resulting in muscular dystrophy with generalized lipodystrophy (Hayashi et al., 2009). Also, muscle hypertrophy, muscle mounding, mild metabolic complications, and elevated serum creatine kinase levels have been observed in these patients (Dwianingsih et al., 2010; Rajab et al., 2010). Cavin-1 mutations also are associated with congenital general lipodystrophy type 4 (Shastry et al., 2010; Jelani et al., 2015; Patni et al., 2019). Cavin-4 (MURC) has been associated with dilated cardiomyopathy (Rodriguez G. et al., 2011; Szabadosova et al., 2018).
Biogenesis and stabilization of caveolae are further supported by accessory proteins, such as membrane curvature–regulating protein PACSIN2/Syndapin II, Eps-15 homology domain 2 ATPase, and receptor tyrosine kinase–like orphan receptor 1 (Lamaze et al., 2017). Both syndapin II (Koch et al., 2012; Senju and Suetsugu, 2015) and III (Seemann et al., 2017) were shown to be important in membrane shaping and stabilization of caveolae.
Apart from proteins, caveolae have a high cholesterol content, sphingomyelin, and phosphatidylserine to serve as anchoring points for cavins (Lamaze et al., 2017). The reverse of this interaction—how caveolae and their protein constituents affect lipid metabolism—is less studied (Ariotti et al., 2014; Chen et al., 2014; Golani et al., 2019).
Clustering of caveolae has been observed in different cells as “caveolar cluster-rosettes” or “multiple bulbs packed in a flower-like fashion,” and mechanisms of assembling in superstructures have been examined by computational analysis (Golani et al., 2019). The assumption has been that attractive forces originating from the energy of membrane deformation generated by the bulbs are responsible for this feature. The mechano-protective role of these structures (Szabadosova et al., 2018) putatively relays on the ability to disintegrate upon cell stretching (Golani et al., 2019). Formation of clusters of caveole is promoted by caveolar neck proteins EDH 1, 2, and 4, which partially compensate for each other to protect the cell against mechanical stress (Yeow et al., 2017).
Novel imagistic methods began to call into question some of putative characteristic features of caveolae: the specific smooth omega shape was actually an artifact of glutaraldehyde fixation (Schlormann et al., 2010), high resolution scanning EM and quick-freeze deep-etch techniques detected striations or ridges on the cytoplasmic side (Izumi et al., 1991), also detectable on flattened caveolae (Rothberg et al., 1992). A 3-D electron tomographic study showed a spiral organization of the coating (Lebbink et al., 2010), adding evidence to previous suggestions that caveolae are indeed covered by a spiky coat (Richter et al., 2008). This protein complex has been designated the caveolar coat complex, consisting of Cav-1, Cav-2 and cavins 1, 2, and 3, found in a rather strict stoichiometry of cavin-1: total caveolin—1:4. Cavins 2 and 3 were detected in a ratio of 1:2 to cavin-1 (Ludwig et al., 2013). This stoichiometry was confirmed by further studies and the coatomer organization of the caveolae was unraveled. Cavin-1 homotrimers or heterotrimers of cavins (cavin 1 to either cavin 2 or 3 in a stoichiometry of 2–3:1) are the core constituent of the coat, interacting with approximately 12 caveolin molecules (Krijnse Locker and Schmid, 2013). Using single-molecule analysis of fluorescently tagged cavins, Gambin et al. (2013) showed that in cavin hetrotrimers, expression of cavin 2 and 3 was mutually exclusive and the ratio of cavin 1: cavin 2 may vary, whereas the ratio of cavin 1: cavin 3 was 3:1. A ratio of Cav-1:cavin 1 of 3–4:1 was also confirmed. Cryoelectron tomography revealed that this caveolar coat has an inner layer composed of caveolins that assemble into a polyhedral cage, and a peripheral filamentous layer composed of cavins (Ludwig et al., 2016), responsible for the spiral aspect noticed in deep-etch studies. Using mutational analysis and cryoelectron investigations, Stoeber et al. (2016) proposed a regular dodecahedron model for the cavin coat Cav-1 oligomers associate into discs that occupy the faces of the dodecahedron. Superresolution microscopy studies further proposed a modular superstructure of caveolae, constructed on smaller scaffolds of Cav-1 oligomers, which can dimerize and oligomerize into the polyhedral caveolae coat (Khater et al., 2018, 2019a,b).
From a functional point of view, caveolae initially were considered to be endocytotic vesicles, but following early ultrastructural reports, Popescu et al. (1974) proposed a specific role in calcium signaling and smooth muscle contraction. That step opened the way to the “renaissance in thinking of caveolae as organizing centers for signal transduction” (Ostrom and Insel, 1999), a shift in scientific perception supported by identification and further study of the first caveolin (Rothberg et al., 1992). Ever since, opinions about what deserves the most attention in caveolins research have been shifting, from markers for caveolae to demonstrate co-localization of other proteins to these membrane-microdomains (Bilderback et al., 1997; Lupu et al., 1997; Scherer and Lisanti, 1997; Wu et al., 1997), to active players involved in protein–protein interactions and recruitment of other proteins, to caveolin scaffolding domains (CSDs) (Couet et al., 1997a). Caveolins were found to co-purify with different kinases and to participate in signaling events (Li et al., 1995, 1996a; Couet et al., 1997b). Still, interaction between caveolins and various proteins via caveolin-binding motifs was challenged, based on the variety of the latter, their structural role and their accesibility to interacting proteins (Byrne et al., 2012; Collins et al., 2012). Nevertheless, aberrant cell signaling is a hallmark of cancer, and caveolins were reported to be deregulated in tumor pathogenesis (Razani et al., 2000; Del Pozo and Schwartz, 2007; Quest et al., 2008). An early yet continuous trend in caveolae research has been their involvement in the physiology of muscle tissue (Gabella, 1971), leading to studies of caveolins in muscle contraction (Thyberg, 2000; Betz et al., 2001; Ohsawa et al., 2004) mechanosensing (Boyd et al., 2003; Spisni et al., 2003) and muscle disease (Galbiati et al., 2001; Tanase et al., 2009). They are now also considered dynamic membrane reservoirs, providing mechanoprotection against membrane damage upon changes in membrane tensions (Sinha et al., 2011), which leads to flattening of caveolae and lateral diffusion of Cav-1, followed by slow reconstruction (Tachikawa et al., 2017).
Caveolae in Cell Communication
To date, functional studies have shown that caveolaedo not simply convey extracellular signals but also are actively involved in their modulation. Caveolin binding is reported to inhibit kinase activity for (i) heterotrimeric G proteins, with caveolin interacting directly with multiple G protein alpha subunits, including G(s), G(o), and G(i2) (Song et al., 1996); (ii) members of the Ras superfamily, such as H-Ras (Song et al., 1996) and RhoC (Lin et al., 2005); and (iii) Src tyrosine kinases (Li et al., 1996a), acting as negative regulators and sequestering them to the plasma membrane. Caveolins also are associated with endothelial nitric oxide synthase signaling (Bucci et al., 2000) through direct protein–protein interactions (Feron et al., 1996), exerting a suppressive effect (Razani et al., 2001). Protein–protein interaction has been reported for CSDs and other kinases, such as PKA (Levin et al., 2006), and co-localization with Cav-1 has been demonstrated, although without confirming a physical interaction (Stubbs et al., 2005). Caveolae also harbor key proteins involved in calcium signaling (Pani and Singh, 2009), with a functional impact on striated (Bryant et al., 2014) and smooth muscle contraction, either directly (Yang et al., 2015) or indirectly via endothelial nitric oxide synthase endothelial signaling and shear stress in endothelial cells (Yamamoto et al., 2018). In cardiac muscle, Cav-3 participates in scaffolding a molecular complex centered on calcium channels (Balijepalli et al., 2006; Harvey and Hell, 2013). In neurons, Cav-1 binds to calcium-sensing proteins and mediates photoreceptor activity (Vladimirov et al., 2018), or independently of calcium, to neurotransmitter receptors (Schmitz et al., 2010; Roh et al., 2014).
Caveolae might act as reservoirs of signaling proteins, maintained in inactive forms, until the “holders” are signaled to release them. Conformational changes in caveolins are at least in part phosphorylation-dependent (Li et al., 1996b), under the control of the very same kinases that they inactivate, acting as negative feedback signals (Chen et al., 2012).
One of the few exceptions from the caveolae inhibitory effect is insulin signaling (Yamamoto et al., 1998). Early studies have indicated that in adipocytes and pre-adipocytes, downstream insulin signaling requires intact caveolae (Parpal et al., 2001). In an embryonic kidney cell line, both Cav-1 and Cav-3 could directly stimulate insulin receptor kinase activity (Yamamoto et al., 1998). Furthermore, insulin signaling triggered relocation of Glut4 to caveolae (for review, see Cohen et al., 2003; Ishikawa et al., 2005). Recently, other caveolae-associated proteins, such as EHD2 (Moren et al., 2019) and NECC2 (Travez et al., 2018), have been identified as part of the insulin-caveolae signaling mechanism. Studies using a Cavin-1 null mouse have revealed a distinct lipodystrophic, insulin-resistant phenotype (Liu et al., 2008), which also has been subsequently documented in patients who are cavin-1 deficient (Pilch and Liu, 2011). In contrast, loss of cavin-3 does not have a significant impact on adipose tissue and glucose metabolism, as shown in cavin-3 KO mice (Liu et al., 2014a).
Of note, Cav-1 retains signaling functions in the absence of caveolae, as it continues to act as a scaffolding platform for signaling proteins. In neurons, Cav-1 was proposed to scaffold signaling components promoting neuronal survival, growth cone arborization (Head et al., 2011) and axonal growth (Wang et al., 2019). Furthermore, flattening of caveolae under membrane tension might trigger itself downstream signaling by cavin-1 release (Sinha et al., 2011), protein kinase C activation (Senju et al., 2015) or cooperation with cytoskeleton (Echarri and Del Pozo, 2015).
Oncogenic Signaling and Caveolins
A significant body of evidence involves caveolins in oncogenesis and emerged as a consequence of caveolins’ ability to suppress cellular signaling pathways. Initial reports highlighted caveolae and caveolin downregulation in transformed cells (Koleske et al., 1995; Galbiati et al., 1998; Capozza et al., 2003), and murine Cav-1 and 2 genes were mapped to a tumor suppressor locus (Engelman et al., 1998). Since then, numerous data have been collected from cell lines involving both Cav-1 and -2 (Sagara et al., 2004), [colon carcinoma (Bender et al., 2000), human breast cancer (Lee et al., 1998), and ovarian carcinoma (Miotti et al., 2005)], or only Cav-1 (Racine et al., 1999). Further data have come from studies with KO animals (Capozza et al., 2003; Williams et al., 2004) and human tissue samples of human colon carcinoma (Bender et al., 2000), pancreatic adenocarcinoma (Tanase, 2008; Tanase et al., 2009) lung neoplasia (Kato et al., 2004), breast (Chen et al., 2004) and ovarian cancer (Wiechen et al., 2001a), and malignant mesenchymal tumors (Wiechen et al., 2001b). The role for caveolins as guardians against oncogenic transformation is supported by reports that loss of Cav-1 in tumor-associated fibroblasts drives a change in phenotype from “normal” to “fuel-supplier,” modifying the stromal environment of cancer cells into a medium favoring survival (Mercier et al., 2008; Trimmer et al., 2011).
This suppressor status has been challenged over time, however, with some limited but fairly consistent evidence of Cav-1 overexpression in various types of cancer. These findings have sometimes been in contradiction with datasets from the same types of cancer in which Cav-1 overexpression was reported (Patlolla et al., 2004) and in other cases have arisen in areas of cancer study where caveolins have otherwise gone unexamined (Ito et al., 2002; Suzuoki et al., 2002; Steffens et al., 2011). Caveolin expression seems to be increased in urogenital cancers (Kasahara et al., 2002; Fong et al., 2003; Joo et al., 2004), although not exclusively so. Some results suggest involvement of Cav-1 in resistance to cancer treatments (Sekhar et al., 2013, as reviewed in Ketteler and Klein, 2018), and in tumor spreading (reviewed in Senetta et al., 2013; Campos et al., 2019).
On the other hand, involvement of Cav-2 in tumor biology was not investigated until recently. The few data reported so far converge toward a protumorigenic role for Cav-2. In a Cav-2 KO mouse model, its loss seems to favor infiltration of tumor-associated macrophages into the tumor tissue and tumor regression (Liu et al., 2019). It also reduces metastatic potential of pancreatic cell lines (Liang et al., 2018) and its serum levels are increased in patients with pancreatic cancer, a finding associated with poor prognosis (Liang et al., 2018). Similar to Cav-1, Cav-2 involvement in tumorigenesis seems to depend on the tumor type. Investigation of Cav-2 expression in lung cancer have revealed a loss of protein during metastasis in lymph nodes, which correlated with poor prognosis (Gerstenberger et al., 2018).
The role of the cavin family as an oncogene or tumor suppressor is also controversial, depending on the cell and/or tissue type.
Most reports show the same trends for cavins as observed for Cav-1. Loss of cavin-1 is correlated with poor prognosis in colorectal cancer (Wang et al., 2017) and liposarcoma (Codenotti et al., 2017). Loss of cavin-2 also is correlated with a poor prognosis in liposarcoma (Codenotti et al., 2017) and hepatocellular carcinoma (Jing et al., 2016). In glioblastoma (Huang et al., 2018) and pancreatic adenocarcinoma, cavin-1 enhances the prognostic potency of Cav-1 (Liu et al., 2014b).
Cell-to-Pathogen Interaction at Caveolar Sites
Caveolins were related to both antiviral and antibacterial defense. Cav-1 was proposed as a T cell–intrinsic orchestrator of TCR-mediated membrane polarity and signal specificity selectively employed by CD8 T cells to customize TCR responsiveness (Tomassian et al., 2011). Downregulation of Cav-1 apparently disperses clusters of antiviral defense–related receptors (Gabor et al., 2013), and Cav-1 KO cells display increased exosome uptake (Svensson et al., 2013). Overexpressing Cav-1 in macrophages seems to lower virion infectivity by altering cholesterol content (Lin et al., 2012) or cellular proteins required for viral replication (Simmons et al., 2012).
Viruses
Conversely, Cav-1 is a target of viral attacks, serving as a safe environment for viral replication (Yan et al., 2012), including of coronavirus strains (Nomura et al., 2004; Guo et al., 2017; Wang et al., 2020). Similar to other membrane microdomains, caveolae serve as entry gates for viruses, such as simian immunodeficiency virus (Neu et al., 2008), HCV (Shi et al., 2003), some species of adenoviruses (Leung and Brown, 2011), some herpesviridae (Hasebe et al., 2009; Kerur et al., 2010), group B of coxsackie viruses (Patel et al., 2009), foot-and-mouth disease virus (O’Donnell et al., 2008), some types of papilloma virus (Smith et al., 2007), echovirus-1 (Pietiainen et al., 2004), some coronaviruses (Nomura et al., 2004; Guo et al., 2017; Wang et al., 2020), and some types of flavivirus (Zhu et al., 2012). Viral hijacking of caveolae is summarized in Figure 4 (based on Xing et al., 2020).
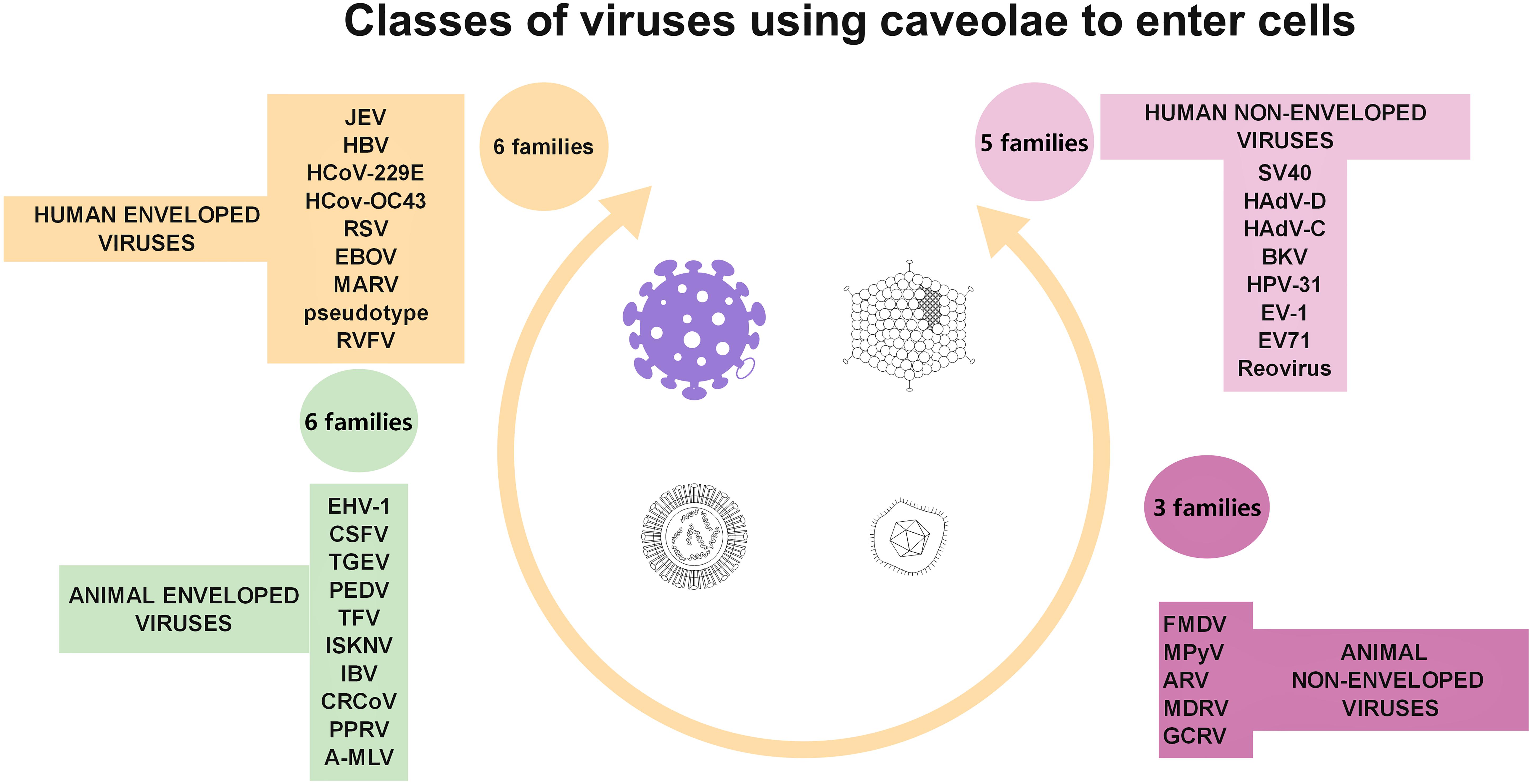
Figure 4. Diagrammatic representation of the four classes of viruses (taking into account virus structure and target cell type) documented to use caveolae as gates to entry cells.
A few viruses even seem to incorporate Cav-1 into new virions (Brown et al., 2002; Laliberte et al., 2006; Ravid et al., 2010). Whether caveolins are only part of the “vessel” or are being hijacked and used as “anchors” has been elucidated for some pathogens. Molecular modeling and simulation have suggested the existence of caveolin-binding sites for SARS-CoV proteins (Cai et al., 2003), although a Cav-independent mechanism also has been described (Wang et al., 2008), and for rotavirus endotoxin (Mir et al., 2007), HIV envelope proteins (Huang et al., 2007), human influenza A viruses (Sun et al., 2010), and murine leukemia viruses (Yu Z. et al., 2006).
Bacteria
Recent results offer growing evidence of Cav-1 acts against bacterial infections, as well (Tsai et al., 2011; Hitkova et al., 2013). It plays a protective role against fibronectin-binding pathogens, such as Staphylococcus aureus (Hoffmann et al., 2010) and Pseudomonas aeruginosa (Gadjeva et al., 2010), prevents Neisseria gonorrhoeae uptake (Boettcher et al., 2010), and is a critical protective modulator of sepsis (Feng et al., 2010).
On the other hand, some strains of streptococci (Rohde et al., 2003), chlamydia (Webley et al., 2004), Salmonella (Lim et al., 2010), Mycobacterium, Brucella species, FimH expressing E. coli (for review see Duncan et al., 2002; Medina-Kauwe, 2007), other aggressive strains of E. coli (Rogers et al., 2012), Campylobacter jejuni (Watson and Galan, 2008), Bordetella pertussis (Martin et al., 2015), Leptospira interrogans (Li et al., 2019), and Rickettsia (Chan et al., 2009) also use this endocytic pathway for host infectivity. In addition, caveolae are targeted by Gram-negative bacterial outer membrane vesicles, structures involved in secretion of virulence determinants, modulation of the host immune response, and contributions to biofilm formation and stability (Sharpe et al., 2011). Gonococci use Cav-1 phosphorylation and downstream signaling to switch from local to invasive infection (Faulstich et al., 2013). Bacterial entry into cells was divided in active and passive entry (Duncan et al., 2002). Salmonella typhimurium was identified as an example of active entry, driven by secreted proteins, while Chlamydia trachomatis as a typical example of passive entering germ (Duncan et al., 2002). Caveolae-mediated endocytosis was shown to be not involved in Clostridium difficile toxin A endocytosis (Chandrasekaran et al., 2016). This paper illustrates a thinking pattern, suggesting that clathrin-dependent and caveolae-dependent-mediated endocytosis have to be equally explored as routes for both bacteria or bacterial products. Clathrin, caveolae, macropinocytosis and secretory lysosomes were investigated as the four major points (gates) pathogens can hijack (Eto et al., 2008). Regarding mechanisms used for hijacking, data is scares as only a few articles examined specifically this subject (Eto et al., 2008; Watson and Galan, 2008; Chi et al., 2010, 2011; Lim et al., 2014; Martin et al., 2015; Chandrasekaran et al., 2016; see also Table 1).
Some other papers were mentioning bacterial entry, but in a broader context, examining, for example, clathrin-independent mechanisms of endocytosis. It is noteworthy to observe that “nomenclature is imprecise,” criteria used to classify such transport systems relying on “cargoes, or plasma membrane markers, or carrier morphologies, or speed of the process” (for details see Ferreira and Boucrot, 2018).
Cav-1–/– mice show an increased production of inflammatory cytokines, chemokines (Codrici et al., 2018), and nitric oxide and an inability to control systemic infection by Salmonella. The increased chemokine production in these mice leads to greater infiltration of neutrophils into granulomas but no changes in the number of granulomas present. Cav-1–/– macrophages show increased inflammatory responses and increased nitric oxide production in vitro in response to Salmonella lipopolysaccharide. These results show that Cav-1 plays a key role in regulating anti-inflammatory responses in macrophages. These data collectively suggest that the increased production of toxic mediators from macrophages lacking Cav-1 is likely to be responsible for the marked susceptibility of Cav-1–deficient mice to S. enterica serovar Typhimurium (Medina et al., 2006).
Prions
PrP(C) were shown to be associated in rafts with caveolin-1 and signaling molecules, including Fyn and Src tyrosine kinases (Taylor and Hooper, 2006; Toni et al., 2006). These data open the avenue for exploring conditions in which cell are handling prion-like proteins (i.e., neurodegenerative diseases; Muradashvili et al., 2016; Puangmalai et al., 2020).
Parasites
Last, but not least, parasitic pathogens such as Leishmania infantum (Leishmania chagasi) (Rodriguez et al., 2006), Plasmodium vivax (Bracho et al., 2006), and Trypanosoma cruzi (Soeiro Mde et al., 1999) also use caveolae as cellular entry point. “Leishmania spp. include the infectious promastigote and the replicative intracellular amastigote.” It was shown that caveolae are contributing to uptake and intracellular survival of virulent promastigotes by macrophages (Rodriguez N. E. et al., 2011). Parasites of the genus Plasmodium induce changes within the host cell, among which, a type of “caveola-vesicle complex” (Sherling and van Ooij, 2016), defined also as “distinctive caveolae nanostructures” (Malleret et al., 2015). Chagas disease is caused by the protozoan parasite Trypanosoma cruzi. Cardiac injury observed during chagasic cardiomyopathy imply caveolae components (reduced expression of caveolin-3) contributing to the feature of disease (Adesse et al., 2010). Caveolae/raft-mediated endocytosis was demonstrated as the main route to AgB internalization, “a major component of Echinococcus granulosus metacestode hydatid fluid” (da Silva et al., 2018).
Of note, as presented in table 1, caveolae are not exclusive entry gates for these pathogens, who “hijack” other cellular entry pathways [for an update on bacterial manipulation of clathrin see (Latomanski and Newton, 2019), for endocytotic mechanisms used by viruses, (Slonska et al., 2016)and for parasites (Horta et al., 2020)]. Furthermore, lipids enriched in caveolae could play a part in virus entry (Ewers et al., 2010)and virus replication (Favard et al., 2019), but can be found elsewhere in the plasma membrane. This may explain partly why pathogens can enter the cells via caveolae with more or less specificity.
Current data support the idea that functions of caveolae may be hijacked by different means, in different target cells, with different consequences. Diverse viruses, prions, bacteria or parasites are capable of such hijacking. From this point of view caveolae could be considered as potential hijackable cell gates. Identification of key points during the process could make more effectively the treatment of such infectious diseases. Along with these efforts, development new drug delivery techniques could become be more likely.
Gatekeepers of Aging
Cho and Park proposed Cav-1 as a “gatekeeper molecule” and a “major determinant of aging process” (Cho and Park, 2005). Cav-1 is involved in the regulation of many cellular processes relevant to stem cell biology, such as growth, control of mitochondrial antioxidant levels, migration, and senescence (Baker and Tuan, 2013). The observation that caveolae are decreased in senescent cells is now more than a decade old (Somara et al., 2007; Lowalekar et al., 2012, reviewed in Nguyen and Cho, 2017) and has been associated with an apparent paradox of increased cellular caveolins (Wheaton et al., 2001). In vitro data have confirmed that overexpression of Cav-1 induces early senescence in different cell types (Volonte et al., 2002; Cho et al., 2004; Dai et al., 2006), and Cav-1 KO animal models have a reduced lifespan (Park et al., 2003). Several reports have focused on signaling alterations in normally and induced aging cells (Castro et al., 2004; Favetta et al., 2004). A direct correlation between caveolin deficit and senescence has been reported to involve the Mdm–p53–p21 axis (Bartholomew et al., 2009). In-depth analysis of this correlation has shown that this interaction is cavin-1 dependent (Bai et al., 2011), and through inhibition of Nrf2-mediated signaling, Cav-1 links free radicals to activation of the p53/senescence pathway (Volonte et al., 2013). The same research group has argued that Cav-1 appears to play a major role in the signaling events linking oxidative stress to cellular senescence and identified an oxidant-responsive Cav-1 promoter sequence (Bartholomew and Galbiati, 2010). Furthermore, Cav-1 deficiency inhibited cardiolipin synthesis and induced mitochondrial dysfunction (Yu et al., 2017).
Different tissue types age differently in terms of caveolae and caveolins: cardiac muscle maintains the same levels of total Cav-1 and -3, but levels of Cav-1 alpha and -3 increase with aging in purified fractions of caveolae (Ratajczak et al., 2003). Other studies have reported a decrease in Cav-3 with age in ventricular myocytes (Kong et al., 2018). Aged smooth muscle has a reduced number of caveolae and reduced levels of Cav-2 and 3, but non-altered levels of Cav-1 and cavin-1 (Lowalekar et al., 2012), and aged endothelial cells have increased levels of total Cav-1 (Yoon et al., 2010). The hippocampus of aged mice shows low Cav-1 expression, and knocking it out triggers a predisposition to an early Alzheimer’s disease–like phenotype (Head et al., 2010). Total rat brain levels of Cav-1 are decreased in aged animals (Yang et al., 2018), and skin fibroblasts upregulate Cav-1 in both chronological and UV-induced skin aging (Kruglikov et al., 2019).
Transduction of Environmental, Non-chemical Signals
Of all environmental cues perceived by cells, caveolae play an important part in translating those involved in mechanotransduction (Rizzo et al., 2003; Sinha et al., 2011; Joshi et al., 2012). This role has been comprehensively reviewed (Nassoy and Lamaze, 2012; Shihata et al., 2016), including the involvement of caveolae in signaling events, but new facets are being constantly added to this particular facet of caveolae behavior. Caveolae sensitivity to environmental physical stimuli was being uncovered as researchers began to also reveal their involvement in cellular signaling (Rizzo et al., 1998, 2003; Spisni et al., 2003), and changes in caveolar morphology under muscle stretch were reported even earlier (Gabella and Blundell, 1978). Caveolae morphology and number, as well as caveolins expression and distribution, seem to depend on shear stress (Boyd et al., 2003), gravitational force (Grenon et al., 2013), and mechanical stretch (Sinha et al., 2011). Along with disappearance of caveolae following mechanical stress in muscle cells, Cav-1 and -3 are translocated to non-caveolar membrane sites (Kawabe et al., 2004) and Cav-1 showed increased phosphorylation (Zhang et al., 2007) in a context of increased kinase activity and signaling events. Cavins are released into the cytosol, where they form a pool for subsequent caveolar reconstruction (Tillu et al., 2015).
Although Cav-3 is muscle specific, its involvement in stretch-induced cell signaling has been less studied than that of Cav-1 and may not even be mandatory for some signaling pathways (Bellott et al., 1985). Cav-3 has been recently demonstrated to regulate IL6/STAT3 mechano-signaling (and mechano-protection) (Tate et al., 1987). Both the increase in shear stress and the cessation of flow trigger a mechano-signaling cascade that leads to the generation of reactive oxygen species (Noel et al., 2013). Through their structure, composition, and mechanical properties, caveolae limit activity of mechanosensitive ion channels, which seems to require Cav-3 (Huang et al., 2013). One hypothesis is that the reservoir of Cav-1 and glycosphingolipids can be released to control mechano-signaling (Nassoy and Lamaze, 2012).
Localization of small GTPases involved in cytoskeleton rearrangement within the caveolar compartments seems to be essential for stretch-signal transduction (Kawamura et al., 2003). Regulation of RhoA, for example, drives actomyosin contractility and other mechanosensitive pathways, suggesting that caveolae could couple mechanotransduction pathways to actin-controlled changes in tension through their association with stress fibers (Echarri and Del Pozo, 2015). Unfolding of caveolae under stress, followed by activation of Src and redistribution of caveolin and glycosphingolipids, might reflect mechanisms of the cellular adaptation to mechanical stresses (Gervasio et al., 2011). The role of caveolae as mechanosensors has been extensively reviewed (Nassoy and Lamaze, 2012; Echarri and Del Pozo, 2015).
The mechanosensing of caveolae is only partially supplemented by other cellular mechanisms, as demonstrated by KO animal models. Cav-1 KO mice have impaired-flow–induced vasodilation and flow-dependent arterial remodeling, effects that are rescued by re-expression of endothelial Cav-1 (Yu J. et al., 2006). Muscle fibers from cavin-1–/– mice have a prominent sarcolemmal organization, aberrant T-tubule structures, and increased sensitivity to membrane tension, effects that could be rescued by muscle-specific cavin-1 re-expression. In vivo imaging of live zebrafish embryos showed that loss of muscle-specific cavin-1 or expression of a dystrophy-associated Cav-3 mutant both led to sarcolemmal damage but only in response to vigorous muscle activity (Lo et al., 2015).
Another aspect of environmental communication is extracellular matrix sensing, which triggers cytoskeleton remodeling. Disruption of lipid rafts or knockdown of Cav-1 decreased cell spreading on a stiff matrix, and this effect was mediated by β1 integrin downregulation (Yeh et al., 2017). Effects of defective extracellular matrix stiffness sensing in Cav-1 loss can be rescued by constitutive activation of yes-associated protein (Moreno-Vicente et al., 2018).
Traffic of Macromolecules and Metabolic Regulation
Caveolae are a conserved traffic pathway for a number of proteins, including albumin transfer across the endothelium in physiological (Predescu et al., 2002) and inflammatory conditions (Hu and Minshall, 2009), matrix metalloproteinase traffic (Galvez et al., 2004), endocytosis of many surface receptors, and traffic to the Golgi apparatus and endoplasmic reticulum (Le and Nabi, 2003). Involvement of caveolin in endocytosis, as well as the dynamics of caveolae between membrane-attached and scissored state are the topics of several reviews (Parton and Richards, 2003; Lajoie and Nabi, 2010; Hubert et al., 2020).
This prompted the hypothesis that caveolae are “metabolic platforms” and “gateways for the uptake of nutrients across the plasma membrane” (Örtegren et al., 2007). Caveolin-1 has been reported to regulate metabolism of lipid droplets in adipocytes (Briand et al., 2014, reviewed in Martin, 2013) and endothelial cells (Kuo et al., 2018), including the composition in peripheral phospholipids and associated proteins (Blouin et al., 2010). CAV-1 gene polymorphisms have been reported in patients with altered lipid metabolism in adult (Mora-Garcia et al., 2017, 2018) and juvenile forms (Nizam et al., 2018). Metabolic alterations are partially mediated by impaired insulin signaling (Gonzalez-Munoz et al., 2009; Perez-Verdaguer et al., 2018; Travez et al., 2018). Conversely, it was recently shown that lifestyle interventions in patients with impaired glucose regulation changed tissular and plasma cav-1 expression (Fachim et al., 2020).
To summarize, caveolae can be viewed as “hijackable gates.” They selectively filter extracellular signals, and no general rule has yet been identified for opening or closing them. The variability of response from cell type to cell type or tumor type to tumor type can be partially explained by different interaction partners. The complete picture of caveolin and cavin alterations in any given type of tumor remains elusive. Another gap that is just gaining attention is the comparative analysis of behavior between the primary tumor and metastatic sites.
The Foreseen Trend: Caveolin Research (Not Yet) Translated Into Clinical Practice
As a consequence of cancer-related studies, Cav-1 and -2 have been investigated as tumor prognostic markers for different types of cancers, mostly carcinomas (Sloan et al., 2009; Langeberg et al., 2010; Steffens et al., 2011; Zhan et al., 2012; Lobos-Gonzalez et al., 2014). The discovery of new communication patterns between cancer and stromal cells, in which Cav-1 seems to be an important key note for both sides (Sotgia et al., 2012), prompted a new paradigm for prognosis of certain cancer types, based on evaluation of Cav-1 in stromal cells (Witkiewicz et al., 2009; Zhao et al., 2013).
In addition to cancer, caveolins are reported to be involved as gatekeepers in a wide variety of pathological processes from neurodegeneration (Head et al., 2010) to lipodystrophy (Martin et al., 2012), atherosclerosis (Pavlides et al., 2014), pulmonary fibrosis (Tourkina et al., 2008), and glaucoma (Thorleifsson et al., 2010). Genetic polymorphisms in the Cav-1 gene are associated with transplant-related renal (Moore et al., 2010) and respiratory pathologies (Kastelijn et al., 2011). Because of its tissue specificity, Cav-3 is involved in specific muscle pathologies, ranging from myodystrophies (Minetti et al., 1998) to arrhythmias (Balijepalli and Kamp, 2008).
Following the many pieces of evidence of caveolin involvement in several pathologies, attempts have been made to target them for therapeutic development in cancer (Tamaskar and Zhou, 2008), cardiovascular diseases (Sellers et al., 2012), and kidney disease (van Dokkum and Buikema, 2009). To date, only 13 clinical trials related to caveolin are listed in the U.S. National Institutes of Health database (Table 2), and only one is registered in Europe, a 2013 clinical study regarding nab-Paclitaxel treatment in HER2-negative metastatic (stage IV) breast cancer. This ongoing European study is evaluating Cav-1 expression as a secondary prognostic marker.
As a potential drug, Cav-1 is being tested in a form of a peptide-mimetic in a US randomized, double-blind, placebo-controlled trial (NCT04233814) that is starting phase 1 in 2020. The trial will assess the initial safety, tolerability, and pharmacokinetic profile of inhaled LTI-03, a Cav-1 scaffold protein–derived 7-amino acid peptide (LTI-03) in healthy participants. The results will guide any future clinical development of LTI-03 for the treatment of idiopathic pulmonary fibrosis.
Other trials are evaluating caveolin as a prognostic biomarker of therapy response in gynaecologic cancers (NCT02874430), breast cancer (NCT01878695, NCT01746225, NCT02701348 NCT00371254), and gastric adenocarcinoma (NCT01641783), and vascular response to hypertensive stimuli (NCT01426529).
No clinical trials that involve cavin are yet available.
Cell-penetrating-peptides (CPPs) are small amino acid sequences characterized by the ability to cross cellular membranes. In therapy, they can be useful for delivering bioactive molecules, such as CPP-mediated delivery of anti-tumoral proteins (Habault and Poyet, 2019).
Conclusion
Caveolae are complex membrane microdomains of (now better) known molecular composition, with wide tissue distribution. Two classes of proteins (caveolins and cavins) cooperate to generate a gateway involved in transduction of messages from the extracellular environment. Integrity of this gate is essential for cell signaling, metabolism, antibacterial defense, mechanoreception, and aging. Mutation or loss of caveolae proteins affects human health and may generate disease phenotypes called “caveolinopathies.” Caveolae research has only recently been oriented toward translational potential, with several clinical trials to investigate their role as prognostic biomarkers in tumors. Although various cell types (prokaryotic cells, parasitic cells, tumor cells) have learned how to hijack them, we still need to learn how to exploit caveolae and their proteins for our own therapeutic purposes.
Author Contributions
MD and EC collected data and drafted the manuscript. CT critically revised the manuscript. MG acquired EM images and prepared the Figures 1, 2. A-ME and MH performed study conception and design, and addressed all revisions. All authors contributed to the article and approved the submitted version.
Funding
This work was supported by the Romanian Ministry of Education and Research (MEC) under grants no. PN 1N/2019_19.29.01.04 and no. 7PFE/2018.
Conflict of Interest
The authors declare that the research was conducted in the absence of any commercial or financial relationships that could be construed as a potential conflict of interest.
Acknowledgments
We would like to acknowledge Radu Huica and Lucian Albulescu for early data gathering and valuable input.
Abbreviations
A-MLV, Murine leukemia virus (Retroviridae); ARV, Avian reovirus (Reoviridae); BKV, BK virus (Polyomaviridae); CrCov, Canine respiratory coronavirus (Coronaviridae); CSFV, Classical swine fever virus (Flaviviridae); EBOV, Ebolavirus (Filoviridae); EHV, Equine herpesviruses (Herpesviridae); EV1, Echovirus type 1 (Picornaviridae); EV-71, Enterovirus type 71 (Picornaviridae); FMDV, Foot-and-mouth disease virus (Picornaviridae); GCRV, Grass carp reovirus (Reoviridae); HadV-C, Human mastadenovirus-C (Adenoviridae); HadV-D, Human mastadenovirus-D (Adenoviridae); HBV, Hepatitis B virus (Hepadnaviridae); HCoV-229E, Human coronavirus (HCoV) 229E (Coronaviridae); HcoVO-C43, Human betacoronavirus OC43 (Coronaviridae); HPV-31, Human papillomavirus type 31 (Papillomaviridae); ISKNV, Infectious spleen and kidney necrosis virus (Iridoviridae); IVB, Infectious bronchitis virus (Coronaviridae); JEV, Japanese encephalitis virus (Flaviviridae); MARV, Marburg virus (Filoviridae); MDRV, Muscovy duck reovirus (Reoviridae); MpyV, Mouse polyomavirus (Polyomaviridae); PEDV, Porcine epidemic diarrhea virus (Coronaviridae); PPRV, Peste des petits ruminants virus (Paramyxoviridae); Reovirus, Reovirus (Reoviridae); RSV, Respiratory syncytial virus (Paramixoviridae); RVFV, Rift Valley fever virus (Phenuiviridae); SV40, simian virus 40 (Polyomaviridae); TGEV, transmissible gastroenteritis virus (Coronaviridae); TVF, tiger frog virus (Iridoviridae).
References
Adesse, D., Lisanti, M. P., Spray, D. C., Machado, F. S., Meirelles, M. D. N., Tanowitz, H. B., et al. (2010). Trypanosoma cruzi infection results in the reduced expression of caveolin-3 in the heart. Cell Cycle 9, 1639–1646. doi: 10.4161/cc.9.8.11509
Anderson, R. G. (1993). Caveolae: where incoming and outgoing messengers meet. Proc. Natl. Acad. Sci. U.S.A. 90, 10909–10913. doi: 10.1073/pnas.90.23.10909
Ariotti, N., Fernandez-Rojo, M. A., Zhou, Y., Hill, M. M., Rodkey, T. L., Inder, K. L., et al. (2014). Caveolae regulate the nanoscale organization of the plasma membrane to remotely control Ras signaling. J. Cell Biol. 204, 777–792. doi: 10.1083/jcb.201307055
Bai, L., Deng, X., Li, J., Wang, M., Li, Q., An, W., et al. (2011). Regulation of cellular senescence by the essential caveolar component PTRF/Cavin-1. Cell Res. 21, 1088–1101. doi: 10.1038/cr.2011.56
Baker, N., and Tuan, R. S. (2013). The less-often-traveled surface of stem cells: caveolin-1 and caveolae in stem cells, tissue repair and regeneration. Stem Cell Res. Ther. 4:90. doi: 10.1186/scrt276
Balijepalli, R. C., Foell, J. D., Hall, D. D., Hell, J. W., and Kamp, T. J. (2006). Localization of cardiac L-type Ca(2+) channels to a caveolar macromolecular signaling complex is required for beta(2)-adrenergic regulation. Proc. Natl. Acad. Sci. U.S.A. 103, 7500–7505. doi: 10.1073/pnas.0503465103
Balijepalli, R. C., and Kamp, T. J. (2008). Caveolae, ion channels and cardiac arrhythmias. Prog. Biophys. Mol. Biol. 98, 149–160. doi: 10.1016/j.pbiomolbio.2009.01.012
Barrias, E. S., Dutra, J. M., De Souza, W., and Carvalho, T. M. (2007). Participation of macrophage membrane rafts in Trypanosoma cruzi invasion process. Biochem. Biophys. Res. Commun. 363, 828–834. doi: 10.1016/j.bbrc.2007.09.068
Bartholomew, J. N., and Galbiati, F. (2010). Mapping of oxidative stress response elements of the caveolin-1 promoter. Methods Mol. Biol. 594, 409–423. doi: 10.1007/978-1-60761-411-1_29
Bartholomew, J. N., Volonte, D., and Galbiati, F. (2009). Caveolin-1 regulates the antagonistic pleiotropic properties of cellular senescence through a novel Mdm2/p53-mediated pathway. Cancer Res. 69, 2878–2886. doi: 10.1158/0008-5472.can-08-2857
Beer, C., Andersen, D. S., Rojek, A., and Pedersen, L. (2005). Caveola-dependent endocytic entry of amphotropic murine leukemia virus. J. Virol. 79, 10776–10787. doi: 10.1128/jvi.79.16.10776-10787.2005
Bellott, A. C., Patel, K. C., and Burkholder, T. J. (1985). Reduction of caveolin-3 expression does not inhibit stretch-induced phosphorylation of ERK2 in skeletal muscle myotubes. J. Appl. Physiol. 98, 1554–1561. doi: 10.1152/japplphysiol.01070.2004
Bender, F. C., Reymond, M. A., Bron, C., and Quest, A. F. (2000). Caveolin-1 levels are down-regulated in human colon tumors, and ectopic expression of caveolin-1 in colon carcinoma cell lines reduces cell tumorigenicity. Cancer Res. 60, 5870–5878.
Betz, R. C., Schoser, B. G., Kasper, D., Ricker, K., Ramirez, A., Stein, V., et al. (2001). Mutations in CAV3 cause mechanical hyperirritability of skeletal muscle in rippling muscle disease. Nat. Genet. 28, 218–219. doi: 10.1038/90050
Bhogal, N. K., Hasan, A., and Gorelik, J. (2018). The development of compartmentation of cAMP signaling in cardiomyocytes: the role of T-tubules and caveolae microdomains. J. Cardiovasc. Dev. Dis. 5:25. doi: 10.3390/jcdd5020025
Bilderback, T. R., Grigsby, R. J., and Dobrowsky, R. T. (1997). Association of p75(NTR) with caveolin and localization of neurotrophin-induced sphingomyelin hydrolysis to caveolae. J. Biol. Chem. 272, 10922–10927. doi: 10.1074/jbc.272.16.10922
Blouin, C. M., Le Lay, S., Eberl, A., Kofeler, H. C., Guerrera, I. C., Klein, C., et al. (2010). Lipid droplet analysis in caveolin-deficient adipocytes: alterations in surface phospholipid composition and maturation defects. J. Lipid Res. 51, 945–956. doi: 10.1194/jlr.m001016
Boettcher, J. P., Kirchner, M., Churin, Y., Kaushansky, A., Pompaiah, M., Thorn, H., et al. (2010). Tyrosine-phosphorylated caveolin-1 blocks bacterial uptake by inducing Vav2-RhoA-mediated cytoskeletal rearrangements. PLoS Biol. 8:e1000457. doi: 10.1371/journal.pbio.1000457
Boscher, C., and Nabi, I. R. (2012). Caveolin-1: role in cell signaling. Adv. Exp. Med. Biol. 729, 29–50. doi: 10.1007/978-1-4614-1222-9_3
Boyd, N. L., Park, H., Yi, H., Boo, Y. C., Sorescu, G. P., Sykes, M., et al. (2003). Chronic shear induces caveolae formation and alters ERK and Akt responses in endothelial cells. Am. J. Physiol. Heart Circ. Physiol. 285, H1113–H1122.
Bracho, C., Dunia, I., Romano, M., Raposo, G., De La Rosa, M., Benedetti, E. L., et al. (2006). Caveolins and flotillin-2 are present in the blood stages of Plasmodium vivax. Parasitol. Res. 99, 153–159. doi: 10.1007/s00436-006-0139-6
Breen, M. R., Camps, M., Carvalho-Simoes, F., Zorzano, A., and Pilch, P. F. (2012). Cholesterol depletion in adipocytes causes caveolae collapse concomitant with proteosomal degradation of cavin-2 in a switch-like fashion. PLoS One 7:e34516. doi: 10.1371/journal.pone.0034516
Briand, N., Prado, C., Mabilleau, G., Lasnier, F., Le Liepvre, X., Covington, J. D., et al. (2014). Caveolin-1 expression and cavin stability regulate caveolae dynamics in adipocyte lipid store fluctuation. Diabetes 63, 4032–4044. doi: 10.2337/db13-1961
Brown, G., Aitken, J., Rixon, H. W., and Sugrue, R. J. (2002). Caveolin-1 is incorporated into mature respiratory syncytial virus particles during virus assembly on the surface of virus-infected cells. J. Gen. Virol. 83, 611–621. doi: 10.1099/0022-1317-83-3-611
Bruns, R. R., and Palade, G. E. (1968). Studies on blood capillaries. I. General organization of blood capillaries in muscle. J. Cell Biol. 37, 244–276.
Bryant, S., Kimura, T. E., Kong, C. H., Watson, J. J., Chase, A., Suleiman, M. S., et al. (2014). Stimulation of ICa by basal PKA activity is facilitated by caveolin-3 in cardiac ventricular myocytes. J. Mol. Cell. Cardiol. 68, 47–55. doi: 10.1016/j.yjmcc.2013.12.026
Bucci, M., Gratton, J. P., Rudic, R. D., Acevedo, L., Roviezzo, F., Cirino, G., et al. (2000). In vivo delivery of the caveolin-1 scaffolding domain inhibits nitric oxide synthesis and reduces inflammation. Nat. Med. 6, 1362–1367. doi: 10.1038/82176
Busija, A. R., Patel, H. H., and Insel, P. A. (2017). Caveolins and cavins in the trafficking, maturation, and degradation of caveolae: implications for cell physiology. Am. J. Physiol. Cell Physiol. 312, C459–C477.
Byrne, D. P., Dart, C., and Rigden, D. J. (2012). Evaluating caveolin interactions: do proteins interact with the caveolin scaffolding domain through a widespread aromatic residue-rich motif? PLoS One 7:e44879. doi: 10.1371/journal.pone.0044879
Cai, Q. C., Jiang, Q. W., Zhao, G. M., Guo, Q., Cao, G. W., and Chen, T. (2003). Putative caveolin-binding sites in SARS-CoV proteins. Acta Pharmacol. Sin. 24, 1051–1059.
Campos, A., Burgos-Ravanal, R., Gonzalez, M. F., Huilcaman, R., Lobos Gonzalez, L., and Quest, A. F. G. (2019). Cell intrinsic and extrinsic mechanisms of caveolin-1-enhanced metastasis. Biomolecules 9:314. doi: 10.3390/biom9080314
Capozza, F., Williams, T. M., Schubert, W., McClain, S., Bouzahzah, B., Sotgia, F., et al. (2003). Absence of caveolin-1 sensitizes mouse skin to carcinogen-induced epidermal hyperplasia and tumor formation. Am. J. Pathol. 162, 2029–2039. doi: 10.1016/s0002-9440(10)64335-0
Castro, M. E., del Valle Guijarro, M., Moneo, V., and Carnero, A. (2004). Cellular senescence induced by p53-ras cooperation is independent of p21waf1 in murine embryo fibroblasts. J. Cell. Biochem. 92, 514–524. doi: 10.1002/jcb.20079
Chan, Y. G., Cardwell, M. M., Hermanas, T. M., Uchiyama, T., and Martinez, J. J. (2009). Rickettsial outer-membrane protein B (rOmpB) mediates bacterial invasion through Ku70 in an actin, c-Cbl, clathrin and caveolin 2-dependent manner. Cell. Microbiol. 11, 629–644. doi: 10.1111/j.1462-5822.2008.01279.x
Chandrasekaran, R., Kenworthy, A. K., and Lacy, D. B. (2016). Clostridium difficile Toxin A undergoes clathrin-independent, PACSIN2-dependent endocytosis. PLoS Pathog. 12:e1006070. doi: 10.1371/journal.ppat.1006070
Chen, Y. H., Lin, W. W., Liu, C. S., Hsu, L. S., Lin, Y. M., and Su, S. L. (2014). Caveolin-1 provides palliation for adverse hepatic reactions in hypercholesterolemic rabbits. PLoS One 9:e71862. doi: 10.1371/journal.pone.0071862
Chen, Z., Bakhshi, F. R., Shajahan, A. N., Sharma, T., Mao, M., Trane, A., et al. (2012). Nitric oxide-dependent Src activation and resultant caveolin-1 phosphorylation promote eNOS/caveolin-1 binding and eNOS inhibition. Mol. Biol. Cell 23, 1388–1398. doi: 10.1091/mbc.e11-09-0811
Chen, S. T., Lin, S. Y., Yeh, K. T., Kuo, S. J., Chan, W. L., Chu, Y. P., et al. (2004). Mutational, epigenetic and expressional analyses of caveolin-1 gene in breast cancers. Int. J. Mol. Med. 14, 577–582.
Cheng, J. P. X., and Nichols, B. J. (2016). Caveolae: One function or many? Trends Cell Biol. 26, 177–189. doi: 10.1016/j.tcb.2015.10.010
Chi, F., Jong, T. D., Wang, L., Ouyang, Y., Wu, C., Li, W., et al. (2010). Vimentin-mediated signalling is required for IbeA+ E. coli K1 invasion of human brain microvascular endothelial cells. Biochem. J. 427, 79–90. doi: 10.1042/bj20091097
Chi, F., Wang, L., Zheng, X., Jong, A., and Huang, S. H. (2011). Recruitment of alpha7 nicotinic acetylcholine receptor to caveolin-1-enriched lipid rafts is required for nicotine-enhanced Escherichia coli K1 entry into brain endothelial cells. Future Microbiol. 6, 953–966. doi: 10.2217/fmb.11.65
Cho, K. A., and Park, S. C. (2005). Caveolin-1 as a prime modulator of aging: A new modality for phenotypic restoration? Mech. Ageing Dev. 126, 105–110. doi: 10.1016/j.mad.2004.09.029
Cho, K. A., Ryu, S. J., Oh, Y. S., Park, J. H., Lee, J. W., Kim, H. P., et al. (2004). Morphological adjustment of senescent cells by modulating caveolin-1 status. J. Biol. Chem. 279, 42270–42278. doi: 10.1074/jbc.m402352200
Codenotti, S., Vezzoli, M., Poliani, P. L., Cominelli, M., Monti, E., and Fanzani, A. (2017). Cavin-2 is a specific marker for detection of well-differentiated liposarcoma. Biochem. Biophys. Res. Commun. 493, 660–665. doi: 10.1016/j.bbrc.2017.08.135
Codrici, E., Albulescu, L., Popescu, I. D., Mihai, S., Enciu, A. M., Albulescu, R., et al. (2018). Caveolin-1-knockout mouse as a model of inflammatory diseases. J. Immunol. Res. 2018:2498576.
Cohen, A. W., Combs, T. P., Scherer, P. E., and Lisanti, M. P. (2003). Role of caveolin and caveolae in insulin signaling and diabetes. Am. J. Physiol. Endocrinol. Metab. 285, E1151–E1160.
Collins, B. M., Davis, M. J., Hancock, J. F., and Parton, R. G. (2012). Structure-based reassessment of the caveolin signaling model: Do caveolae regulate signaling through caveolin-protein interactions? Dev. Cell 23, 11–20. doi: 10.1016/j.devcel.2012.06.012
Couet, J., Li, S., Okamoto, T., Ikezu, T., and Lisanti, M. P. (1997a). Identification of peptide and protein ligands for the caveolin-scaffolding domain. Implications for the interaction of caveolin with caveolae-associated proteins. J. Biol. Chem. 272, 6525–6533. doi: 10.1074/jbc.272.10.6525
Couet, J., Sargiacomo, M., and Lisanti, M. P. (1997b). Interaction of a receptor tyrosine kinase, EGF-R, with caveolins. Caveolin binding negatively regulates tyrosine and serine/threonine kinase activities. J. Biol. Chem. 272, 30429–30438. doi: 10.1074/jbc.272.48.30429
Couet, J., Shengwen, L., Okamoto, T., Scherer, P. E., and Lisanti, M. P. (1997c). Molecular and cellular biology of caveolae paradoxes and plasticities. Trends Cardiovasc. Med. 7, 103–110. doi: 10.1016/s1050-1738(97)00001-7
da Silva, E. D., Cancela, M., Monteiro, K. M., Ferreira, H. B., and Zaha, A. (2018). Antigen B from Echinococcus granulosus enters mammalian cells by endocytic pathways. PLoS Negl. Trop. Dis. 12:e0006473. doi: 10.1371/journal.pntd.0006473
Dai, S. M., Shan, Z. Z., Nakamura, H., Masuko-Hongo, K., Kato, T., Nishioka, K., et al. (2006). Catabolic stress induces features of chondrocyte senescence through overexpression of caveolin 1: possible involvement of caveolin 1-induced down-regulation of articular chondrocytes in the pathogenesis of osteoarthritis. Arthritis Rheum. 54, 818–831. doi: 10.1002/art.21639
de Almeida, C. J. G. (2017). Caveolin-1 and Caveolin-2 can be antagonistic partners in inflammation and beyond. Front. Immunol. 8:1530. doi: 10.3389/fimmu.2017.01530
Del Pozo, M. A., and Schwartz, M. A. (2007). Rac, membrane heterogeneity, caveolin and regulation of growth by integrins. Trends Cell Biol. 17, 246–250. doi: 10.1016/j.tcb.2007.03.001
Duncan, M. J., Li, G., Shin, J. S., Carson, J. L., and Abraham, S. N. (2004). Bacterial penetration of bladder epithelium through lipid rafts. J. Biol. Chem. 279, 18944–18951. doi: 10.1074/jbc.m400769200
Duncan, M. J., Shin, J. S., and Abraham, S. N. (2002). Microbial entry through caveolae: variations on a theme. Cell. Microbiol. 4, 783–791. doi: 10.1046/j.1462-5822.2002.00230.x
Dwianingsih, E. K., Takeshima, Y., Itoh, K., Yamauchi, Y., Awano, H., Malueka, R. G., et al. (2010). A Japanese child with asymptomatic elevation of serum creatine kinase shows PTRF-CAVIN mutation matching with congenital generalized lipodystrophy type 4. Mol. Genet. Metab. 101, 233–237. doi: 10.1016/j.ymgme.2010.06.016
Echarri, A., and Del Pozo, M. A. (2015). Caveolae - mechanosensitive membrane invaginations linked to actin filaments. J. Cell Sci. 128, 2747–2758. doi: 10.1242/jcs.153940
Engelman, J. A., Zhang, X. L., Galbiati, F., and Lisanti, M. P. (1998). Chromosomal localization, genomic organization, and developmental expression of the murine caveolin gene family (Cav-1, -2, and -3). Cav-1 and Cav-2 genes map to a known tumor suppressor locus (6-A2/7q31). FEBS Lett. 429, 330–336. doi: 10.1016/s0014-5793(98)00619-x
Eto, D. S., Gordon, H. B., Dhakal, B. K., Jones, T. A., and Mulvey, M. A. (2008). Clathrin, AP-2, and the NPXY-binding subset of alternate endocytic adaptors facilitate FimH-mediated bacterial invasion of host cells. Cell. Microbiol. 10, 2553–2567. doi: 10.1111/j.1462-5822.2008.01229.x
Ewers, H., Romer, W., Smith, A. E., Bacia, K., Dmitrieff, S., Chai, W., et al. (2010). GM1 structure determines SV40-induced membrane invagination and infection. Nat. Cell Biol. 12, 11–18. doi: 10.1038/ncb1999
Fachim, H. A., Siddals, K., Malipatil, N., Donn, R. P., Moreno, G. Y., Dalton, C. F., et al. (2020). Lifestyle intervention in individuals with impaired glucose regulation affects Caveolin-1 expression and DNA methylation. Adipocyte 9, 96–107. doi: 10.1080/21623945.2020.1732513
Faulstich, M., Bottcher, J. P., Meyer, T. F., Fraunholz, M., and Rudel, T. (2013). Pilus phase variation switches gonococcal adherence to invasion by caveolin-1-dependent host cell signaling. PLoS Pathog. 9:e1003373. doi: 10.1371/journal.ppat.1003373
Favard, C., Chojnacki, J., Merida, P., Yandrapalli, N., Mak, J., Eggeling, C., et al. (2019). HIV-1 Gag specifically restricts PI(4,5)P2 and cholesterol mobility in living cells creating a nanodomain platform for virus assembly. Sci. Adv. 5:eaaw8651. doi: 10.1126/sciadv.aaw8651
Favetta, L. A., Robert, C., King, W. A., and Betts, D. H. (2004). Expression profiles of p53 and p66shc during oxidative stress-induced senescence in fetal bovine fibroblasts. Exp. Cell Res. 299, 36–48. doi: 10.1016/j.yexcr.2004.05.009
Feng, H., Guo, L., Song, Z., Gao, H., Wang, D., Fu, W., et al. (2010). Caveolin-1 protects against sepsis by modulating inflammatory response, alleviating bacterial burden, and suppressing thymocyte apoptosis. J. Biol. Chem. 285, 25154–25160. doi: 10.1074/jbc.m110.116897
Feron, O., Belhassen, L., Kobzik, L., Smith, T. W., Kelly, R. A., and Michel, T. (1996). Endothelial nitric oxide synthase targeting to caveolae. Specific interactions with caveolin isoforms in cardiac myocytes and endothelial cells. J. Biol. Chem. 271, 22810–22814. doi: 10.1074/jbc.271.37.22810
Ferreira, A. P. A., and Boucrot, E. (2018). Mechanisms of carrier formation during clathrin-independent endocytosis. Trends Cell Biol. 28, 188–200. doi: 10.1016/j.tcb.2017.11.004
Field, S. J. (2017). Spelunking for lipids in caveolae. J. Biol. Chem. 292, 14308–14309. doi: 10.1074/jbc.h117.791400
Filippini, A., Sica, G., and D’Alessio, A. (2018). The caveolar membrane system in endothelium: from cell signaling to vascular pathology. J. Cell. Biochem. 119, 5060–5071. doi: 10.1002/jcb.26793
Fong, A., Garcia, E., Gwynn, L., Lisanti, M. P., Fazzari, M. J., and Li, M. (2003). Expression of caveolin-1 and caveolin-2 in urothelial carcinoma of the urinary bladder correlates with tumor grade and squamous differentiation. Am. J. Clin. Pathol. 120, 93–100. doi: 10.1309/292nhaynwavrej37
Gabella, G., and Blundell, D. (1978). Effect of stretch and contraction on caveolae of smooth muscle cells. Cell Tissue Res. 190, 255–271. doi: 10.1007/978-0-387-68234-1_9
Gabella, G. (1971). Caveolae intracellulares and sarcoplasmic reticulum in smooth muscle. J. Cell Sci. 8, 601–609.
Gabella, G. (1978). Inpocketings of the cell membrane (caveolae) in the rat myocardium. J. Ultrastruct. Res. 65, 135–147. doi: 10.1016/s0022-5320(78)90051-5
Gabor, K. A., Stevens, C. R., Pietraszewski, M. J., Gould, T. J., Shim, J., Yoder, J. A., et al. (2013). Super resolution microscopy reveals that caveolin-1 is required for spatial organization of crfb1 and subsequent antiviral signaling in Zebrafish. PLoS One 8:e68759. doi: 10.1371/journal.pone.0068759
Gadjeva, M., Paradis-Bleau, C., Priebe, G. P., Fichorova, R., and Pier, G. B. (2010). Caveolin-1 modifies the immunity to Pseudomonas aeruginosa. J. Immunol. 184, 296–302. doi: 10.4049/jimmunol.0900604
Galbiati, F., Volonte, D., Engelman, J. A., Watanabe, G., Burk, R., Pestell, R. G., et al. (1998). Targeted downregulation of caveolin-1 is sufficient to drive cell transformation and hyperactivate the p42/44 MAP kinase cascade. EMBO J. 17, 6633–6648. doi: 10.1093/emboj/17.22.6633
Galbiati, F., Razani, B., and Lisanti, M. P. 2001. Caveolae and caveolin-3 in muscular dystrophy. Trends Mol. Med. 7, 435–441. doi: 10.1016/s1471-4914(01)02105-0
Galvez, B. G., Matias-Roman, S., Yanez-Mo, M., Vicente-Manzanares, M., Sanchez-Madrid, F., and Arroyo, A. G. (2004). Caveolae are a novel pathway for membrane-type 1 matrix metalloproteinase traffic in human endothelial cells. Mol. Biol. Cell 15, 678–687. doi: 10.1091/mbc.e03-07-0516
Gambin, Y., Ariotti, N., McMahon, K. A., Bastiani, M., Sierecki, E., Kovtun, O., et al. (2013). Single-molecule analysis reveals self assembly and nanoscale segregation of two distinct cavin subcomplexes on caveolae. eLife 3:e01434.
Gazzerro, E., Sotgia, F., Bruno, C., Lisanti, M. P., and Minetti, C. (2010). Caveolinopathies: from the biology of caveolin-3 to human diseases. Eur. J. Hum. Genet. 18, 137–145. doi: 10.1038/ejhg.2009.103
Gerstenberger, W., Wrage, M., Kettunen, E., Pantel, K., Anttila, S., Steurer, S., et al. (2018). Stromal Caveolin-1 and caveolin-2 expression in primary tumors and lymph node metastases. Anal. Cell. Pathol. 2018:8651790.
Gervasio, O. L., Phillips, W. D., Cole, L., and Allen, D. G. (2011). Caveolae respond to cell stretch and contribute to stretch-induced signaling. J. Cell Sci. 124(Pt 21), 3581–3590. doi: 10.1242/jcs.084376
Gherghiceanu, M., Hinescu, M. E., and Popescu, L. M. (2009). Myocardial interstitial Cajal-like cells (ICLC) in caveolin-1 KO mice. J. Cell. Mol. Med. 13, 202–206. doi: 10.1111/j.1582-4934.2008.00615.x
Golani, G., Ariotti, N., Parton, R. G., and Kozlov, M. M. (2019). Membrane curvature and tension control the formation and collapse of caveolar superstructures. Dev. Cell 48:523. doi: 10.1016/j.devcel.2018.12.005
Gonzalez-Munoz, E., Lopez-Iglesias, C., Calvo, M., Palacin, M., Zorzano, A., and Camps, M. (2009). Caveolin-1 loss of function accelerates glucose transporter 4 and insulin receptor degradation in 3T3-L1 adipocytes. Endocrinology 150, 3493–3502. doi: 10.1210/en.2008-1520
Grenon, S. M., Jeanne, M., Aguado-Zuniga, J., Conte, M. S., and Hughes-Fulford, M. (2013). Effects of gravitational mechanical unloading in endothelial cells: association between caveolins, inflammation and adhesion molecules. Sci. Rep. 3:1494.
Grundner, M., and Zemljic Jokhadar, S. (2014). Cytoskeleton modification and cholesterol depletion affect membrane properties and caveolae positioning of CHO Cells. J. Membr. Biol. 247, 201–210. doi: 10.1007/s00232-013-9625-9
Guo, H., Huang, M., Yuan, Q., Wei, Y., Gao, Y., Mao, L., et al. (2017). The important role of lipid raft-mediated attachment in the infection of cultured cells by coronavirus infectious bronchitis virus Beaudette Strain. PLoS One 12:e0170123. doi: 10.1371/journal.pone.0170123
Habault, J., and Poyet, J. L. (2019). Recent advances in cell penetrating peptide-based anticancer therapies. Molecules 24:927. doi: 10.3390/molecules24050927
Han, B., Copeland, C. A., Tiwari, A., and Kenworthy, A. K. (2016). Assembly and turnover of caveolae: What do we really know? Front. Cell Dev. Biol. 4:68. doi: 10.3389/fcell.2016.00068
Hansen, C. G., Bright, N. A., Howard, G., and Nichols, B. J. (2009). SDPR induces membrane curvature and functions in the formation of caveolae. Nat. Cell Biol. 11, 807–814. doi: 10.1038/ncb1887
Hansen, C. G., Shvets, E., Howard, G., Riento, K., and Nichols, B. J. (2013). Deletion of cavin genes reveals tissue-specific mechanisms for morphogenesis of endothelial caveolae. Nat. Commun. 4:1831.
Harmon, B., Schudel, B. R., Maar, D., Kozina, C., Ikegami, T., Tseng, C. T., et al. (2012). Rift Valley fever virus strain MP-12 enters mammalian host cells via caveola-mediated endocytosis. J. Virol. 86, 12954–12970. doi: 10.1128/jvi.02242-12
Harvey, R. D., and Hell, J. W. (2013). CaV1.2 signaling complexes in the heart. J. Mol. Cell. Cardiol. 58, 143–152.
Hasebe, R., Sasaki, M., Sawa, H., Wada, R., Umemura, T., and Kimura, T. (2009). Infectious entry of equine herpesvirus-1 into host cells through different endocytic pathways. Virology 393, 198–209. doi: 10.1016/j.virol.2009.07.032
Hayashi, Y. K., Matsuda, C., Ogawa, M., Goto, K., Tominaga, K., Mitsuhashi, S., et al. (2009). Human PTRF mutations cause secondary deficiency of caveolins resulting in muscular dystrophy with generalized lipodystrophy. J. Clin. Invest. 119, 2623–2633. doi: 10.1172/jci38660
Head, B. P., Hu, Y., Finley, J. C., Saldana, M. D., Bonds, J. A., Miyanohara, A., et al. (2011). Neuron-targeted caveolin-1 protein enhances signaling and promotes arborization of primary neurons. J. Biol. Chem. 286, 33310–33321. doi: 10.1074/jbc.m111.255976
Head, B. P., Peart, J. N., Panneerselvam, M., Yokoyama, T., Pearn, M. L., Niesman, I. R., et al. (2010). Loss of caveolin-1 accelerates neurodegeneration and aging. PLoS One 5:e15697. doi: 10.1371/journal.pone.0015697
Hehlgans, S., and Cordes, N. (2011). Caveolin-1: an essential modulator of cancer cell radio-and chemoresistance. Am. J. Cancer Res. 1, 521–530.
Hill, M. M., Bastiani, M., Luetterforst, R., Kirkham, M., Kirkham, A., Nixon, S. J., et al. (2008). PTRF-Cavin, a conserved cytoplasmic protein required for caveola formation and function. Cell 132, 113–124. doi: 10.1016/j.cell.2007.11.042
Hitkova, I., Yuan, G., Anderl, F., Gerhard, M., Kirchner, T., Reu, S., et al. (2013). Caveolin-1 protects B6129 mice against Helicobacter pylori gastritis. PLoS Pathog. 9:e1003251. doi: 10.1371/journal.ppat.1003251
Hoffmann, C., Berking, A., Agerer, F., Buntru, A., Neske, F., Chhatwal, G. S., et al. (2010). Caveolin limits membrane microdomain mobility and integrin-mediated uptake of fibronectin-binding pathogens. J. Cell Sci. 123(Pt 24), 4280–4291. doi: 10.1242/jcs.064006
Horta, M. F., Andrade, L. O., Martins-Duarte, E. S., and Castro-Gomes, T. (2020). Cell invasion by intracellular parasites - the many roads to infection. J. Cell Sci. 133:jcs232488. doi: 10.1242/jcs.232488
Hu, G., and Minshall, R. D. (2009). Regulation of transendothelial permeability by Src kinase. Microvasc. Res. 77, 21–25. doi: 10.1016/j.mvr.2008.10.002
Hu, Q., Wang, J., Shen, J., Liu, M., Jin, X., Tang, G., et al. (2012). Intracellular pathways and nuclear localization signal peptide-mediated gene transfection by cationic polymeric nanovectors. Biomaterials 33, 1135–1145. doi: 10.1016/j.biomaterials.2011.10.023
Huang, H., Bae, C., Sachs, F., and Suchyna, T. M. (2013). Caveolae regulation of mechanosensitive channel function in myotubes. PLoS One 8:e72894. doi: 10.1371/journal.pone.0072894
Huang, J. H., Lu, L., Lu, H., Chen, X., Jiang, S., and Chen, Y. H. (2007). Identification of the HIV-1 gp41 core-binding motif in the scaffolding domain of caveolin-1. J. Biol. Chem. 282, 6143–6152. doi: 10.1074/jbc.m607701200
Huang, K., Fang, C., Yi, K., Liu, X., Qi, H., Tan, Y., et al. (2018). The role of PTRF/Cavin1 as a biomarker in both glioma and serum exosomes. Theranostics 8, 1540–1557. doi: 10.7150/thno.22952
Hubert, M., Larsson, E., and Lundmark, R. (2020). Keeping in touch with the membrane; protein- and lipid-mediated confinement of caveolae to the cell surface. Biochem. Soc. Trans. 48, 155–163. doi: 10.1042/bst20190386
Ishikawa, Y., Otsu, K., and Oshikawa, J. (2005). Caveolin; different roles for insulin signal? Cell. Signal. 17, 1175–1182.
Ito, Y., Yoshida, H., Nakano, K., Kobayashi, K., Yokozawa, T., Hirai, K., et al. (2002). Caveolin-1 overexpression is an early event in the progression of papillary carcinoma of the thyroid. Br. J. Cancer 86, 912–916. doi: 10.1038/sj.bjc.6600172
Izumi, T., Shibata, Y., and Yamamoto, T. (1991). Quick-freeze, deep-etch studies of endothelial components, with special reference to cytoskeletons and vesicle structures. J. Electron. Microsc. Tech. 19, 316–326. doi: 10.1002/jemt.1060190307
Jelani, M., Ahmed, S., Almramhi, M. M., Mohamoud, H. S., Bakur, K., Anshasi, W., et al. (2015). Novel nonsense mutation in the PTRF gene underlies congenital generalized lipodystrophy in a consanguineous Saudi family. Eur. J. Med. Genet. 58, 216–221. doi: 10.1016/j.ejmg.2015.02.002
Jing, W., Luo, P., Zhu, M., Ai, Q., Chai, H., and Tu, J. (2016). Prognostic and Diagnostic Significance of SDPR-Cavin-2 in Hepatocellular Carcinoma. Cell. Physiol. Biochem. 39, 950–960. doi: 10.1159/000447803
Joo, H. J., Oh, D. K., Kim, Y. S., Lee, K. B., and Kim, S. J. (2004). Increased expression of caveolin-1 and microvessel density correlates with metastasis and poor prognosis in clear cell renal cell carcinoma. BJU Int. 93, 291–296. doi: 10.1111/j.1464-410x.2004.04604.x
Joshi, B., Bastiani, M., Strugnell, S. S., Boscher, C., Parton, R. G., and Nabi, I. R. (2012). Phosphocaveolin-1 is a mechanotransducer that induces caveola biogenesis via Egr1 transcriptional regulation. J. Cell Biol. 199, 425–435. doi: 10.1083/jcb.201207089
Kasahara, T., Hara, N., Bilim, V., Tomita, Y., Tsutsui, T., and Takahashi, K. (2002). Immunohistochemical studies of caveolin-3 in germ cell tumors of the testis. Urol. Int. 69, 63–68. doi: 10.1159/000064363
Kastelijn, E. A., van Moorsel, C. H., Kazemier, K. M., Roothaan, S. M., Ruven, H. J., Kwakkel-van Erp, J. M., et al. (2011). A genetic polymorphism in the CAV1 gene associates with the development of bronchiolitis obliterans syndrome after lung transplantation. Fibrogenesis Tissue Repair. 4:24.
Kato, T., Miyamoto, M., Kato, K., Cho, Y., Itoh, T., Morikawa, T., et al. (2004). Difference of caveolin-1 expression pattern in human lung neoplastic tissue. Atypical adenomatous hyperplasia, adenocarcinoma and squamous cell carcinoma. Cancer Lett. 214, 121–128. doi: 10.1016/j.canlet.2004.04.017
Kawabe, J., Okumura, S., Lee, M. C., Sadoshima, J., and Ishikawa, Y. (2004). Translocation of caveolin regulates stretch-induced ERK activity in vascular smooth muscle cells. Am. J. Physiol. Heart Circ. Physiol. 286, H1845–H1852.
Kawamura, S., Miyamoto, S., and Brown, J. H. (2003). Initiation and transduction of stretch-induced RhoA and Rac1 activation through caveolae: cytoskeletal regulation of ERK translocation. J. Biol. Chem. 278, 31111–31117. doi: 10.1074/jbc.m300725200
Kerur, N., Veettil, M. V., Sharma-Walia, N., Sadagopan, S., Bottero, V., Paul, A. G., et al. (2010). Characterization of entry and infection of monocytic THP-1 cells by Kaposi’s sarcoma associated herpesvirus (KSHV): role of heparan sulfate, DC-SIGN, integrins and signaling. Virology 406, 103–116. doi: 10.1016/j.virol.2010.07.012
Ketteler, J., and Klein, D. (2018). Caveolin-1, cancer and therapy resistance. Int. J. Cancer 143, 2092–2104. doi: 10.1002/ijc.31369
Khater, I. M., Liu, Q., Chou, K. C., Hamarneh, G., and Nabi, I. R. (2019a). Super-resolution modularity analysis shows polyhedral caveolin-1 oligomers combine to form scaffolds and caveolae. Sci. Rep. 9:9888.
Khater, I. M., Meng, F., Nabi, I. R., and Hamarneh, G. (2019b). Identification of caveolin-1 domain signatures via machine learning and graphlet analysis of single-molecule super-resolution data. Bioinformatics 35, 3468–3475. doi: 10.1093/bioinformatics/btz113
Khater, I. M., Meng, F., Wong, T. H., Nabi, I. R., and Hamarneh, G. (2018). Super resolution network analysis defines the molecular architecture of caveolae and caveolin-1 scaffolds. Sci. Rep. 8:9009.
Koch, D., Westermann, M., Kessels, M. M., and Qualmann, B. (2012). Ultrastructural freeze-fracture immunolabeling identifies plasma membrane-localized syndapin II as a crucial factor in shaping caveolae. Histochem. Cell Biol. 138, 215–230. doi: 10.1007/s00418-012-0945-0
Koleske, A. J., Baltimore, D., and Lisanti, M. P. (1995). Reduction of caveolin and caveolae in oncogenically transformed cells. Proc. Natl. Acad. Sci. U.S.A. 92, 1381–1385. doi: 10.1073/pnas.92.5.1381
Kong, C. H. T., Bryant, S. M., Watson, J. J., Gadeberg, H. C., Roth, D. M., Patel, H. H., et al. (2018). The effects of aging on the regulation of T-Tubular ICa by caveolin in mouse ventricular myocytes. J. Gerontol. Ser. A Biol. Sci. Med. Sci. 73, 711–719. doi: 10.1093/gerona/glx242
Kotze, L. A., Young, C., Leukes, V. N., John, V., Fang, Z., Walzl, G., et al. (2020). Mycobacterium tuberculosis and myeloid-derived suppressor cells: insights into caveolin rich lipid rafts. EBioMedicine 53:102670. doi: 10.1016/j.ebiom.2020.102670
Krijnse Locker, J., and Schmid, S. L. (2013). Integrated electron microscopy: super-duper resolution. PLoS Biol. 11:e1001639. doi: 10.1371/journal.pbio.1001639
Kruglikov, I. L., Zhang, Z., and Scherer, P. E. (2019). Caveolin-1 in skin aging - From innocent bystander to major contributor. Ageing Res. Rev. 55:100959. doi: 10.1016/j.arr.2019.100959
Kuo, A., Lee, M. Y., Yang, K., Gross, R. W., and Sessa, W. C. (2018). Caveolin-1 regulates lipid droplet metabolism in endothelial cells via autocrine prostacyclin-stimulated, cAMP-mediated lipolysis. J. Biol. Chem. 293, 973–983. doi: 10.1074/jbc.ra117.000980
Lajoie, P., and Nabi, I. R. (2010). Lipid rafts, caveolae, and their endocytosis. Int. Rev. Cell Mol. Biol. 282, 135–163. doi: 10.1016/s1937-6448(10)82003-9
Laliberte, J. P., McGinnes, L. W., Peeples, M. E., and Morrison, T. G. (2006). Integrity of membrane lipid rafts is necessary for the ordered assembly and release of infectious Newcastle disease virus particles. J. Virol. 80, 10652–10662. doi: 10.1128/jvi.01183-06
Lamaze, C., Tardif, N., Dewulf, M., Vassilopoulos, S., and Blouin, C. M. (2017). The caveolae dress code: structure and signaling. Curr. Opin. Cell Biol. 47, 117–125. doi: 10.1016/j.ceb.2017.02.014
Langeberg, W. J., Tahir, S. A., Feng, Z., Kwon, E. M., Ostrander, E. A., Thompson, T. C., et al. (2010). Association of caveolin-1 and -2 genetic variants and post-treatment serum caveolin-1 with prostate cancer risk and outcomes. Prostate 70, 1020–1035. doi: 10.1002/pros.21137
Latomanski, E. A., and Newton, H. J. (2019). Taming the triskelion: bacterial manipulation of clathrin. Microbiol. Mol. Biol. Rev. 83:e00058-18.
Le, P. U., and Nabi, I. R. (2003). Distinct caveolae-mediated endocytic pathways target the Golgi apparatus and the endoplasmic reticulum. J. Cell Sci. 116(Pt 6):1059–1071. doi: 10.1242/jcs.00327
Lebbink, M. N., Jimenez, N., Vocking, K., Hekking, L. H., Verkleij, A. J., and Post, J. A. (2010). Spiral coating of the endothelial caveolar membranes as revealed by electron tomography and template matching. Traffic 11, 138–150. doi: 10.1111/j.1600-0854.2009.01008.x
Lee, S. W., Reimer, C. L., Oh, P., Campbell, D. B., and Schnitzer, J. E. (1998). Tumor cell growth inhibition by caveolin re-expression in human breast cancer cells. Oncogene 16, 1391–1397. doi: 10.1038/sj.onc.1201661
Leo, F., Hutzler, B., Ruddiman, C. A., Isakson, B. E., and Cortese-Krott, M. M. (2020). Cellular microdomains for nitric oxide signaling in endothelium and red blood cells. Nitric Oxide Biol. Chem. 96, 44–53. doi: 10.1016/j.niox.2020.01.002
Leung, T. K., and Brown, M. (2011). Block in entry of enteric adenovirus type 41 in HEK293 cells. Virus Res. 156, 54–63. doi: 10.1016/j.virusres.2010.12.018
Levin, A. M., Coroneus, J. G., Cocco, M. J., and Weiss, G. A. (2006). Exploring the interaction between the protein kinase A catalytic subunit and caveolin-1 scaffolding domain with shotgun scanning, oligomer complementation, NMR, and docking. Protein Sci. 15, 478–486. doi: 10.1110/ps.051911706
Levin, K. R., and Page, E. (1980). Quantitative studies on plasmalemmal folds and caveolae of rabbit ventricular myocardial cells. Circ. Res. 46, 244–255. doi: 10.1161/01.res.46.2.244
Li, S., Couet, J., and Lisanti, M. P. (1996a). Src tyrosine kinases, Galpha subunits, and H-Ras share a common membrane-anchored scaffolding protein, caveolin. Caveolin binding negatively regulates the auto-activation of Src tyrosine kinases. J. Biol. Chem. 271, 29182–29190. doi: 10.1074/jbc.271.46.29182
Li, S., Okamoto, T., Chun, M., Sargiacomo, M., Casanova, J. E., Hansen, S. H., et al. (1995). Evidence for a regulated interaction between heterotrimeric G proteins and caveolin. J. Biol. Chem. 270, 15693–15701. doi: 10.1074/jbc.270.26.15693
Li, S., Seitz, R., and Lisanti, M. P. (1996b). Phosphorylation of caveolin by src tyrosine kinases. The alpha-isoform of caveolin is selectively phosphorylated by v-Src in vivo. J. Biol. Chem. 271, 3863–3868. doi: 10.1074/jbc.271.7.3863
Li, Y., Li, K. X., Hu, W. L., Ojcius, D. M., Fang, J. Q., Li, S. J., et al. (2019). Endocytic recycling and vesicular transport systems mediate transcytosis of Leptospira interrogans across cell monolayer. eLife 8:e44594.
Liang, C., Shi, S., Meng, Q., Liang, D., Hua, J., Qin, Y., et al. (2018). MiR-29a, targeting caveolin 2 expression, is responsible for limitation of pancreatic cancer metastasis in patients with normal level of serum CA125. Int. J. Cancer 143, 2919–2931. doi: 10.1002/ijc.31654
Lim, J. S., Choy, H. E., Park, S. C., Han, J. M., Jang, I. S., and Cho, K. A. (2010). Caveolae-mediated entry of Salmonella typhimurium into senescent nonphagocytotic host cells. Aging Cell 9, 243–251. doi: 10.1111/j.1474-9726.2010.00554.x
Lim, J. S., Shin, M., Kim, H. J., Kim, K. S., Choy, H. E., and Cho, K. A. (2014). Caveolin-1 mediates Salmonella invasion via the regulation of SopE-dependent Rac1 activation and actin reorganization. J. Infect. Dis. 210, 793–802. doi: 10.1093/infdis/jiu152
Lin, M., DiVito, M. M., Merajver, S. D., Boyanapalli, M., and van Golen, K. L. (2005). Regulation of pancreatic cancer cell migration and invasion by RhoC GTPase and caveolin-1. Mol. Cancer 4:21.
Lin, M., and Rikihisa, Y. (2003). Obligatory intracellular parasitism by Ehrlichia chaffeensis and Anaplasma phagocytophilum involves caveolae and glycosylphosphatidylinositol-anchored proteins. Cell. Microbiol. 5, 809–820. doi: 10.1046/j.1462-5822.2003.00322.x
Lin, S., Nadeau, P. E., Wang, X., and Mergia, A. (2012). Caveolin-1 reduces HIV-1 infectivity by restoration of HIV Nef mediated impairment of cholesterol efflux by apoA-I. Retrovirology 9:85. doi: 10.1186/1742-4690-9-85
Liu, L., Brown, D., McKee, M., Lebrasseur, N. K., Yang, D., Albrecht, K. H., et al. (2008). Deletion of Cavin/PTRF causes global loss of caveolae, dyslipidemia, and glucose intolerance. Cell Metab. 8, 310–317. doi: 10.1016/j.cmet.2008.07.008
Liu, L., Hansen, C. G., Honeyman, B. J., Nichols, B. J., and Pilch, P. F. (2014a). Cavin-3 knockout mice show that cavin-3 is not essential for caveolae formation, for maintenance of body composition, or for glucose tolerance. PLoS One 9:e102935. doi: 10.1371/journal.pone.0102935
Liu, L., and Pilch, P. F. (2008). A critical role of cavin (polymerase I and transcript release factor) in caveolae formation and organization. J. Biol. Chem. 283, 4314–4322. doi: 10.1074/jbc.m707890200
Liu, L., Xu, H. X., Wang, W. Q., Wu, C. T., Chen, T., Qin, Y., et al. (2014b). Cavin-1 is essential for the tumor-promoting effect of caveolin-1 and enhances its prognostic potency in pancreatic cancer. Oncogene 33, 2728–2736. doi: 10.1038/onc.2013.223
Liu, Y., Qi, X., Li, G., and Sowa, G. (2019). Caveolin-2 deficiency induces a rapid anti-tumor immune response prior to regression of implanted murine lung carcinoma tumors. Sci. Rep. 9:18970.
Lo, H. P., Nixon, S. J., Hall, T. E., Cowling, B. S., Ferguson, C., Morgan, G. P., et al. (2015). The caveolin-cavin system plays a conserved and critical role in mechanoprotection of skeletal muscle. J. Cell Biol. 210, 833–849. doi: 10.1083/jcb.201501046
Lobos-Gonzalez, L., Aguilarguzman, L., Fernandez, J. G., Munoz, N., Hossain, M., Bieneck, S., et al. (2014). Caveolin-1 is a risk factor for postsurgery metastasis in preclinical melanoma models. Melanoma Res. 24, 108–119 doi: 10.1097/cmr.0000000000000046
Lowalekar, S. K., Cristofaro, V., Radisavljevic, Z. M., Yalla, S. V., and Sullivan, M. P. (2012). Loss of bladder smooth muscle caveolae in the aging bladder. Neurourol. Urodyn. 31, 586–592. doi: 10.1002/nau.21217
Ludwig, A., Howard, G., Mendoza-Topaz, C., Deerinck, T., Mackey, M., Sandin, S., et al. (2013). Molecular composition and ultrastructure of the caveolar coat complex. PLoS Biol. 11:e1001640. doi: 10.1371/journal.pbio.1001640
Ludwig, A., Nguyen, T. H., Leong, D., Ravi, L. I., Tan, B. H., Sandin, S., et al. (2017). Caveolae provide a specialized membrane environment for respiratory syncytial virus assembly. J. Cell Sci. 130, 1037–1050.
Ludwig, A., Nichols, B. J., and Sandin, S. (2016). Architecture of the caveolar coat complex. J. Cell Sci. 129, 3077–3083. doi: 10.1242/jcs.191262
Lupu, C., Goodwin, C. A., Westmuckett, A. D., Emeis, J. J., Scully, M. F., Kakkar, V. V., et al. (1997). Tissue factor pathway inhibitor in endothelial cells colocalizes with glycolipid microdomains/caveolae. Regulatory mechanism(s) of the anticoagulant properties of the endothelium. Arterioscler. Thromb. Vasc. Biol. 17, 2964–2974. doi: 10.1161/01.atv.17.11.2964
Macovei, A., Radulescu, C., Lazar, C., Petrescu, S., Durantel, D., Dwek, R. A., et al. (2010). Hepatitis B virus requires intact caveolin-1 function for productive infection in HepaRG cells. J. Virol. 84, 243–253. doi: 10.1128/jvi.01207-09
Malleret, B., Li, A., Zhang, R., Tan, K. S., Suwanarusk, R., Claser, C., et al. (2015). Plasmodium vivax: restricted tropism and rapid remodeling of CD71-positive reticulocytes. Blood 125, 1314–1324. doi: 10.1182/blood-2014-08-596015
Marjomaki, V., Pietiainen, V., Matilainen, H., Upla, P., Ivaska, J., Nissinen, L., et al. (2002). Internalization of echovirus 1 in caveolae. J. Virol. 76, 1856–1865. doi: 10.1128/jvi.76.4.1856-1865.2002
Martin, C., Etxaniz, A., Uribe, K. B., Etxebarria, A., Gonzalez-Bullon, D., Arlucea, J., et al. (2015). Adenylate Cyclase Toxin promotes bacterial internalisation into non phagocytic cells. Sci. Rep. 5:13774.
Martin, S., Fernandez-Rojo, M. A., Stanley, A. C., Bastiani, M., Okano, S., Nixon, S. J., et al. (2012). Caveolin-1 deficiency leads to increased susceptibility to cell death and fibrosis in white adipose tissue: characterization of a lipodystrophic model. PLoS One 7:e46242. doi: 10.1371/journal.pone.0046242
Martin, S. (2013). Caveolae, lipid droplets, and adipose tissue biology: pathophysiological aspects. Horm. Mol. Biol. Clin. Invest. 15, 11–18.
McGuire, P. G., and Twietmeyer, T. A. (1983). Morphology of rapidly frozen aortic endothelial cells. Glutaraldehyde fixation increases the number of caveolae. Circ. Res. 53, 424–429. doi: 10.1161/01.res.53.3.424
Medina, F. A., de Almeida, C. J., Dew, E., Li, J., Bonuccelli, G., Williams, T. M., et al. (2006). Caveolin-1-deficient mice show defects in innate immunity and inflammatory immune response during Salmonella enterica serovar Typhimurium infection. Infect. Immun. 74, 6665–6674. doi: 10.1128/iai.00949-06
Medina-Kauwe, L. K. (2007). “Alternative” endocytic mechanisms exploited by pathogens: new avenues for therapeutic delivery? Adv. Drug Deliv. Rev. 59, 798–809. doi: 10.1016/j.addr.2007.06.009
Mercier, I., Casimiro, M. C., Wang, C., Rosenberg, A. L., Quong, J., Minkeu, A., et al. (2008). Human breast cancer-associated fibroblasts (CAFs) show caveolin-1 downregulation and RB tumor suppressor functional inactivation: implications for the response to hormonal therapy. Cancer Biol. Ther. 7, 1212–1225. doi: 10.4161/cbt.7.8.6220
Mergia, A. (2017). The role of caveolin 1 in HIV infection and pathogenesis. Viruses 9:129. doi: 10.3390/v9060129
Merrillees, N. C. (1960). The fine structure of muscle spindles in the lumbrical muscles of the rat. J. Biophys. Biochem. Cytol. 7, 725–742. doi: 10.1083/jcb.7.4.725
Minetti, C., Sotgia, F., Bruno, C., Scartezzini, P., Broda, P., Bado, M., et al. (1998). Mutations in the caveolin-3 gene cause autosomal dominant limb-girdle muscular dystrophy. Nat. Genet. 18, 365–368.
Miotti, S., Tomassetti, A., Facetti, I., Sanna, E., Berno, V., and Canevari, S. (2005). Simultaneous expression of caveolin-1 and E-cadherin in ovarian carcinoma cells stabilizes adherens junctions through inhibition of src-related kinases. Am. J. Pathol. 167, 1411–1427. doi: 10.1016/s0002-9440(10)61228-x
Mir, K. D., Parr, R. D., Schroeder, F., and Ball, J. M. (2007). Rotavirus NSP4 interacts with both the amino- and carboxyl-termini of caveolin-1. Virus Res. 126, 106–115. doi: 10.1016/j.virusres.2007.02.004
Moore, J., McKnight, A. J., Simmonds, M. J., Courtney, A. E., Hanvesakul, R., Brand, O. J., et al. (2010). Association of caveolin-1 gene polymorphism with kidney transplant fibrosis and allograft failure. JAMA 303, 1282–1287. doi: 10.1001/jama.2010.356
Mora-Garcia, G., Gomez-Camargo, D., Alario, A., and Gomez-Alegria, C. (2018). A common variation in the Caveolin 1 gene is associated with high serum triglycerides and metabolic syndrome in an admixed Latin American population. Metab. Syndr. Relat. Disord. 16, 453–463. doi: 10.1089/met.2018.0004
Mora-Garcia, G. J., Ruiz-Diaz, M. S., Gomez-Camargo, D. E., and Gomez-Alegria, C. J. (2017). Frequency of common polymorphisms in Caveolin 1 (CAV1) gene in adults with high serum triglycerides from Colombian Caribbean Coast. Colomb. Med. 48, 167–173. doi: 10.25100/cm.v48i4.2625
Moren, B., Hansson, B., Negoita, F., Fryklund, C., Lundmark, R., Goransson, O., et al. (2019). EHD2 regulates adipocyte function and is enriched at cell surface-associated lipid droplets in primary human adipocytes. Mol. Biol. Cell 30, 1147–1159. doi: 10.1091/mbc.e18-10-0680
Moreno-Vicente, R., Pavon, D. M., Martin-Padura, I., Catala-Montoro, M., Diez-Sanchez, A., Quilez-Alvarez, A., et al. (2018). Caveolin-1 modulates mechanotransduction responses to substrate stiffness through actin-dependent control of YAP. Cell Rep. 25:1622. doi: 10.1016/j.celrep.2018.10.024
Muradashvili, N., Tyagi, R., Tyagi, N., Tyagi, S. C., and Lominadze, D. (2016). Cerebrovascular disorders caused by hyperfibrinogenaemia. J. Physiol. 594, 5941–5957. doi: 10.1113/jp272558
Nassoy, P., and Lamaze, C. (2012). Stressing caveolae new role in cell mechanics. Trends Cell Biol. 22, 381–389. doi: 10.1016/j.tcb.2012.04.007
Neu, U., Woellner, K., Gauglitz, G., and Stehle, T. (2008). Structural basis of GM1 ganglioside recognition by simian virus 40. Proc. Natl. Acad. Sci. U.S.A. 105, 5219–5224. doi: 10.1073/pnas.0710301105
Nguyen, K. C., and Cho, K. A. (2017). Versatile functions of Caveolin-1 in aging-related diseases. Chonnam Med. J. 53, 28–36. doi: 10.4068/cmj.2017.53.1.28
Nizam, R., Al-Ozairi, E., Goodson, J. M., Melhem, M., Davidsson, L., Alkhandari, H., et al. (2018). Caveolin-1 variant is associated with the metabolic syndrome in kuwaiti children. Front. Genet. 9:689. doi: 10.3389/fgene.2018.00689
Noel, J., Wang, H., Hong, N., Tao, J. Q., Yu, K. J., Sorokina, E. M., et al. (2013). PECAM-1 and caveolae form the mechanosensing complex necessary for NOX2 activation and angiogenic signaling with stopped flow in pulmonary endothelium. Am. J. Physiol. Lung Cell. Mol. Physiol. 305: L805–L818.
Noguchi, Y., Shibata, Y., and Yamamoto, T. (1987). Endothelial vesicular system in rapid-frozen muscle capillaries revealed by serial sectioning and deep etching. Anat. Rec. 217, 355–360. doi: 10.1002/ar.1092170406
Nomura, R., Kiyota, A., Suzaki, E., Kataoka, K., Ohe, Y., Miyamoto, K., et al. (2004). Human coronavirus 229E binds to CD13 in rafts and enters the cell through caveolae. J. Virol. 78, 8701–8708. doi: 10.1128/jvi.78.16.8701-8708.2004
Nunez-Wehinger, S., Ortiz, R. J., Diaz, N., Diaz, J., Lobos-Gonzalez, L., and Quest, A. F. (2014). Caveolin-1 in cell migration and metastasis. Curr. Mol. Med. 14, 255–274. doi: 10.2174/1566524014666140128112827
O’Donnell, V., Larocco, M., and Baxt, B. (2008). Heparan sulfate-binding foot-and-mouth disease virus enters cells via caveola-mediated endocytosis. J. Virol. 82, 9075–9085. doi: 10.1128/jvi.00732-08
Ohsawa, Y., Toko, H., Katsura, M., Morimoto, K., Yamada, H., Ichikawa, Y., et al. (2004). Overexpression of P104L mutant caveolin-3 in mice develops hypertrophic cardiomyopathy with enhanced contractility in association with increased endothelial nitric oxide synthase activity. Hum. Mol. Genet. 13, 151–157. doi: 10.1093/hmg/ddh014
Örtegren, U., Aboulaich, N., Öst, A., and Strålfors, P. (2007). A new role for caveolae as metabolic platforms. Trends Endocrinol. Metab. 18, 344–349. doi: 10.1016/j.tem.2007.08.007
Ostrom, R. S., and Insel, P. A. (1999). Caveolar microdomains of the sarcolemma: compartmentation of signaling molecules comes of age. Circ. Res. 84, 1110–1112. doi: 10.1161/01.res.84.9.1110
Owczarek, K., Szczepanski, A., Milewska, A., Baster, Z., Rajfur, Z., Sarna, M., et al. (2018). Early events during human coronavirus OC43 entry to the cell. Sci. Rep. 8:7124.
Pani, B., and Singh, B. B. (2009). Lipid rafts/caveolae as microdomains of calcium signaling. Cell Calcium 45, 625–633. doi: 10.1016/j.ceca.2009.02.009
Parat, M. O., and Riggins, G. J. (2012). Caveolin-1, caveolae, and glioblastoma. Neuro Oncol. 14, 679–688. doi: 10.1093/neuonc/nos079
Park, D. S., Cohen, A. W., Frank, P. G., Razani, B., Lee, H., Williams, T. M., et al. (2003). Caveolin-1 null (-/-) mice show dramatic reductions in life span. Biochemistry 42, 15124–15131. doi: 10.1021/bi0356348
Park, D. S., Woodman, S. E., Schubert, W., Cohen, A. W., Frank, P. G., Chandra, M., et al. (2002). Caveolin-1/3 double-knockout mice are viable, but lack both muscle and non-muscle caveolae, and develop a severe cardiomyopathic phenotype. Am. J. Pathol. 160, 2207–2217. doi: 10.1016/s0002-9440(10)61168-6
Parpal, S., Karlsson, M., Thorn, H., and Stralforsm, P. (2001). Cholesterol depletion disrupts caveolae and insulin receptor signaling for metabolic control via insulin receptor substrate-1, but not for mitogen-activated protein kinase control. J. Biol. Chem. 276, 9670–9678. doi: 10.1074/jbc.m007454200
Parton, R. G., Del Pozo, M. A., Vassilopoulos, S., Nabi, I. R., Le Lay, S., Lundmark, R., et al. (2020). Caveolae: the FAQs. Traffic 21, 181–185.
Parton, R. G., and Richards, A. A. (2003). Lipid rafts and caveolae as portals for endocytosis: new insights and common mechanisms. Traffic 4, 724–738. doi: 10.1034/j.1600-0854.2003.00128.x
Patel, K. P., Coyne, C. B., and Bergelson, J. M. (2009). Dynamin- and lipid raft-dependent entry of decay-accelerating factor (DAF)-binding and non-DAF-binding coxsackieviruses into nonpolarized cells. J. Virol. 83, 11064–11077. doi: 10.1128/jvi.01016-09
Patlolla, J. M., Swamy, M. V., Raju, J., and Rao, C. V. (2004). Overexpression of caveolin-1 in experimental colon adenocarcinomas and human colon cancer cell lines. Oncol. Rep. 11, 957–963.
Patni, N., Vuitch, F., and Garg, A. (2019). Postmortem findings in a young man with congenital generalized lipodystrophy, Type 4 Due to CAVIN1 Mutations. J. Clin. Endocrinol. Metab. 104, 957–960. doi: 10.1210/jc.2018-01331
Pavlides, S., Gutierrez-Pajares, J. L., Iturrieta, J., Lisanti, M. P., and Frank, P. G. (2014). Endothelial caveolin-1 plays a major role in the development of atherosclerosis. Cell Tissue Res. 356, 147–157. doi: 10.1007/s00441-013-1767-7
Perez-Verdaguer, M., Capera, J., Ortego-Dominguez, M., Bielanska, J., Comes, N., Montoro, R. J., et al. (2018). Caveolar targeting links Kv1.3 with the insulin-dependent adipocyte physiology. Cell. Mol. Life Sci. 75, 4059–4075. doi: 10.1007/s00018-018-2851-7
Pietiainen, V., Marjomaki, V., Upla, P., Pelkmans, L., Helenius, A., and Hyypia, T. (2004). Echovirus 1 endocytosis into caveosomes requires lipid rafts, dynamin, I. I., and signaling events. Mol. Biol. Cell 15, 4911–4925. doi: 10.1091/mbc.e04-01-0070
Pilch, P. F., and Liu, L. (2011). Fat caves: caveolae, lipid trafficking and lipid metabolism in adipocytes. Trends Endocrinol. Metab. 22, 318–324. doi: 10.1016/j.tem.2011.04.001
Popescu, L. M., Diculescu, I., Zelck, U., and Ionescu, N. (1974). Ultrastructural distribution of calcium in smooth muscle cells of guinea-pig taenia coli. A correlated electron microscopic and quantitative study. Cell Tissue Res. 154, 357–378.
Popescu, L. M., Gherghiceanu, M., Mandache, E., and Cretoiu, D. (2006). Caveolae in smooth muscles: nanocontacts. J. Cell. Mol. Med. 10, 960–990. doi: 10.1111/j.1582-4934.2006.tb00539.x
Potje, S. R., Grando, M. D., Chignalia, A. Z., Antoniali, C., and Bendhack, L. M. (2019). Reduced caveolae density in arteries of SHR contributes to endothelial dysfunction and ROS production. Sci. Rep. 9:6696.
Predescu, D., Predescu, S., and Malik, A. B. (2002). Transport of nitrated albumin across continuous vascular endothelium. Proc. Natl. Acad. Sci. U.S.A. 99, 13932–13937. doi: 10.1073/pnas.212253499
Puangmalai, N., Bhatt, N., Montalbano, M., Sengupta, U., Gaikwad, S., Ventura, F., et al. (2020). Internalization mechanisms of brain-derived tau oligomers from patients with Alzheimer’s disease, progressive supranuclear palsy and dementia with Lewy bodies. Cell Death Dis. 11:314.
Quest, A. F., Gutierrez-Pajares, J. L., and Torres, V. A. (2008). Caveolin-1: an ambiguous partner in cell signalling and cancer. J. Cell. Mol. Med. 12, 1130–1150. doi: 10.1111/j.1582-4934.2008.00331.x
Racine, C., Belanger, M., Hirabayashi, H., Boucher, M., Chakir, J., and Couet, J. (1999). Reduction of caveolin 1 gene expression in lung carcinoma cell lines. Biochem. Biophys. Res. Commun. 255, 580–586. doi: 10.1006/bbrc.1999.0236
Rajab, A., Straub, V., McCann, L. J., Seelow, D., Varon, R., Barresi, R., et al. (2010). Fatal cardiac arrhythmia and long-QT syndrome in a new form of congenital generalized lipodystrophy with muscle rippling (CGL4) due to PTRF-CAVIN mutations. PLoS Genet. 6:e1000874. doi: 10.1371/journal.pgen.1000874
Rasmussen, I., and Vilhardt, F. (2015). Macropinocytosis is the entry mechanism of amphotropic murine leukemia virus. J. Virol. 89, 1851–1866. doi: 10.1128/jvi.02343-14
Ratajczak, P., Damy, T., Heymes, C., Oliviero, P., Marotte, F., Robidel, E., et al. (2003). Caveolin-1 and -3 dissociations from caveolae to cytosol in the heart during aging and after myocardial infarction in rat. Cardiovasc. Res. 57, 358–369. doi: 10.1016/s0008-6363(02)00660-0
Ravid, D., Leser, G. P., and Lamb, R. A. (2010). A role for caveolin 1 in assembly and budding of the paramyxovirus parainfluenza virus 5. J. Virol. 84, 9749–9759. doi: 10.1128/jvi.01079-10
Razani, B., Engelman, J. A., Wang, X. B., Schubert, W., Zhang, X. L., Marks, C. B., et al. (2001). Caveolin-1 null mice are viable but show evidence of hyperproliferative and vascular abnormalities. J. Biol. Chem. 276, 38121–38138.
Razani, B., Schlegel, A., and Lisanti, M. P. (2000). Caveolin proteins in signaling, oncogenic transformation and muscular dystrophy. J. Cell Sci. 113, (Pt 12), 2103–2109.
Razani, B., Wang, X. B., Engelman, J. A., Battista, M., Lagaud, G., Zhang, X. L., et al. (2002). Caveolin-2-deficient mice show evidence of severe pulmonary dysfunction without disruption of caveolae. Mol. Cell. Biol. 22, 2329–2344. doi: 10.1128/mcb.22.7.2329-2344.2002
Richter, T., Floetenmeyer, M., Ferguson, C., Galea, J., Goh, J., Lindsay, M. R., et al. (2008). High-resolution 3D quantitative analysis of caveolar ultrastructure and caveola-cytoskeleton interactions. Traffic 9, 893–909. doi: 10.1111/j.1600-0854.2008.00733.x
Rizzo, V., Morton, C., DePaola, N., Schnitzer, J. E., and Davies, P. F. (2003). Recruitment of endothelial caveolae into mechanotransduction pathways by flow conditioning in vitro. Am. J. Physiol. Heart Circ. Physiol. 285, H1720–H1729.
Rizzo, V., McIntosh, D. P., Oh, P., and Schnitzer, J. E. (1998). In situ flow activates endothelial nitric oxide synthase in luminal caveolae of endothelium with rapid caveolin dissociation and calmodulin association. J. Biol. Chem. 273, 34724–34729. doi: 10.1074/jbc.273.52.34724
Rodriguez, G., Ueyama, T., Ogata, T., Czernuszewicz, G., Tan, Y., Dorn, G. W., et al. (2011). Molecular genetic and functional characterization implicate muscle-restricted coiled-coil gene (MURC) as a causal gene for familial dilated cardiomyopathy. Circ. Cardiovasc. Genet. 4, 349–358. doi: 10.1161/circgenetics.111.959866
Rodriguez, N. E., Gaur, U., and Wilson, M. E. (2006). Role of caveolae in Leishmania chagasi phagocytosis and intracellular survival in macrophages. Cell. Microbiol. 8, 1106–1120. doi: 10.1111/j.1462-5822.2006.00695.x
Rodriguez, N. E., Gaur Dixit, U., Allen, L. A., and Wilson, M. E. (2011). Stage-specific pathways of Leishmania infantum chagasi entry and phagosome maturation in macrophages. PLoS One 6:e19000. doi: 10.1371/journal.pone.0019000
Rogers, T. J., Thorpe, C. M., Paton, A. W., and Paton, J. C. (2012). Role of lipid rafts and flagellin in invasion of colonic epithelial cells by Shiga-toxigenic Escherichia coli O113:H21. Infect. Immun. 80, 2858–2867. doi: 10.1128/iai.00336-12
Roh, S. E., Hong, Y. H., Jang, D. C., Kim, J., and Kim, S. J. (2014). Lipid rafts serve as signaling platforms for mGlu1 receptor-mediated calcium signaling in association with caveolin. Mol. Brain 7:9. doi: 10.1186/1756-6606-7-9
Rohde, M., Muller, E., Chhatwal, G. S., and Talay, S. R. (2003). Host cell caveolae act as an entry-port for group A streptococci. Cell. Microbiol. 5, 323–342. doi: 10.1046/j.1462-5822.2003.00279.x
Rothberg, K. G., Heuser, J. E., Donzell, W. C., Ying, Y. S., Glenney, J. R., and Anderson, R. G. (1992). Caveolin, a protein component of caveolae membrane coats. Cell 68, 673–682. doi: 10.1016/0092-8674(92)90143-z
Sagara, Y., Mimori, K., Yoshinaga, K., Tanaka, F., Nishida, K., Ohno, S., et al. (2004). Clinical significance of Caveolin-1, Caveolin-2 and HER2/neu mRNA expression in human breast cancer. Br. J. Cancer 91, 959–965. doi: 10.1038/sj.bjc.6602029
Samanta, D., Mulye, M., Clemente, T. M., Justis, A. V., and Gilk, S. D. (2017). Manipulation of Host Cholesterol by Obligate Intracellular Bacteria. Front. Cell. Infect. Microbiol. 7:165. doi: 10.3389/fcimb.2017.00165
Sanderlin, A. G., Vondrak, C., Scricco, A. J., Fedrigo, I., Ahyong, V., and Lamason, R. L. (2019). RNAi screen reveals a role for PACSIN2 and caveolins during bacterial cell-to-cell spread. Mol. Biol. Cell 30, 2124–2133. doi: 10.1091/mbc.e19-04-0197
Sawada, H., Ishikawa, H., and Yamada, E. (1978). High resolution scanning electron microscopy of frog sartorius muscle. Tissue Cell 10, 179–190. doi: 10.1016/0040-8166(78)90016-2
Scherer, P. E., and Lisanti, M. P. (1997). Association of phosphofructokinase-M with caveolin-3 in differentiated skeletal myotubes. Dynamic regulation by extracellular glucose and intracellular metabolites. J. Biol. Chem. 272, 20698–20705. doi: 10.1074/jbc.272.33.20698
Schlormann, W., Steiniger, F., Richter, W., Kaufmann, R., Hause, G., Lemke, C., et al. (2010). The shape of caveolae is omega-like after glutaraldehyde fixation and cup-like after cryofixation. Histochem. Cell Biol. 133, 223–228. doi: 10.1007/s00418-009-0651-8
Schmitz, M., Kloppner, S., Klopfleisch, S., Mobius, W., Schwartz, P., Zerr, I., et al. (2010). Mutual effects of caveolin and nerve growth factor signaling in pig oligodendrocytes. J. Neurosci. Res. 88, 572–588.
Seemann, E., Sun, M., Krueger, S., Troger, J., Hou, W., Haag, N., et al. (2017). Deciphering caveolar functions by syndapin III KO-mediated impairment of caveolar invagination. eLife 6:e29854.
Sekhar, S. C., Kasai, T., Satoh, A., Shigehiro, T., Mizutani, A., Murakami, H., et al. (2013). Identification of caveolin-1 as a potential causative factor in the generation of trastuzumab resistance in breast cancer cells. J. Cancer 4, 391–401. doi: 10.7150/jca.6470
Sellers, S. L., Trane, A. E., and Bernatchez, P. N. (2012). Caveolin as a potential drug target for cardiovascular protection. Front. Physiol. 3:280. doi: 10.3389/fphys.2012.00280
Senetta, R., Stella, G., Pozzi, E., Sturli, N., Massi, D., and Cassoni, P. (2013). Caveolin-1 as a promoter of tumour spreading: when, how, where and why. J. Cell. Mol. Med. 17, 325–336. doi: 10.1111/jcmm.12030
Senju, Y., Rosenbaum, E., Shah, C., Hamada-Nakahara, S., Itoh, Y., Yamamoto, K., et al. (2015). Phosphorylation of PACSIN2 by protein kinase C triggers the removal of caveolae from the plasma membrane. J. Cell Sci. 128, 2766–2780. doi: 10.1242/jcs.167775
Senju, Y., and Suetsugu, S. (2015). Possible regulation of caveolar endocytosis and flattening by phosphorylation of F-BAR domain protein PACSIN2/Syndapin II. Bioarchitecture 5, 70–77. doi: 10.1080/19490992.2015.1128604
Severs, N. J. (1988). Caveolae: static inpocketings of the plasma membrane, dynamic vesicles or plain artifact? J. Cell Sci. 90, (Pt 3):341–348.
Sharpe, S. W., Kuehn, M. J., and Mason, K. M. (2011). Elicitation of epithelial cell-derived immune effectors by outer membrane vesicles of nontypeable Haemophilus influenzae. Infect. Immun. 79, 4361–4369. doi: 10.1128/iai.05332-11
Shastry, S., Delgado, M. R., Dirik, E., Turkmen, M., Agarwal, A. K., and Garg, A. (2010). Congenital generalized lipodystrophy, type 4 (CGL4) associated with myopathy due to novel PTRF mutations. Am. J. Med. Genet. A 152A, 2245–2253. doi: 10.1002/ajmg.a.33578
Sherling, E. S., and van Ooij, C. (2016). Host cell remodeling by pathogens: the exomembrane system in Plasmodium-infected erythrocytes. FEMS Microbiol. Rev. 40, 701–721. doi: 10.1093/femsre/fuw016
Shi, S. T., Lee, K. J., Aizaki, H., Hwang, S. B., and Lai, M. M. (2003). Hepatitis C virus RNA replication occurs on a detergent-resistant membrane that cofractionates with caveolin-2. J. Virol. 77, 4160–4168. doi: 10.1128/jvi.77.7.4160-4168.2003
Shihata, W. A., Michell, D. L., Andrews, K. L., and Chin-Dusting, J. P. (2016). Caveolae: A role in endothelial inflammation and mechanotransduction? Front. Physiol. 7:628. doi: 10.3389/fphys.2016.00628
Simmons, G. E. Jr., Taylor, H. E., and Hildreth, J. E. (2012). Caveolin-1 suppresses human immunodeficiency virus-1 replication by inhibiting acetylation of NF-kappaB. Virology 432, 110–119. doi: 10.1016/j.virol.2012.05.016
Sinha, B., Köster, D., Ruez, R., Gonnord, P., Bastiani, M., Abankwa, D., et al. (2011). Cells respond to mechanical stress by rapid disassembly of Caveolae. Cell 144, 402–413. doi: 10.1016/j.cell.2010.12.031
Sloan, E. K., Ciocca, D. R., Pouliot, N., Natoli, A., Restall, C., Henderson, M. A., et al. (2009). Stromal cell expression of caveolin-1 predicts outcome in breast cancer. Am. J. Pathol. 174, 2035–2043. doi: 10.2353/ajpath.2009.080924
Slonska, A., Cymerys, J., and Banbura, M. W. (2016). Mechanisms of endocytosis utilized by viruses during infection. Postepy Hig. Med. Dosw. 70, 572–580. doi: 10.5604/17322693.1203721
Smith, J. L., Campos, S. K., and Ozbun, M. A. (2007). Human papillomavirus type 31 uses a caveolin 1- and dynamin 2-mediated entry pathway for infection of human keratinocytes. J. Virol. 81, 9922–9931. doi: 10.1128/jvi.00988-07
Smith, U., and Ryan, J. W. (1972). Substructural features of pulmonary endothelial caveolae. Tissue Cell 4, 49–54. doi: 10.1016/s0040-8166(72)80005-3
Soeiro Mde, N., Paiva, M. M., Barbosa, H. S., Meirelles Mde, N., and Araujo-Jorge, T. C. (1999). A cardiomyocyte mannose receptor system is involved in Trypanosoma cruzi invasion and is down-modulated after infection. Cell Struct. Funct. 24, 139–149. doi: 10.1247/csf.24.139
Sohn, J., Brick, R. M., and Tuan, R. S. (2016). From embryonic development to human diseases: the functional role of caveolae/caveolin. Birth Defects Res. C Embryo Today 108, 45–64. doi: 10.1002/bdrc.21121
Somara, S., Gilmont, R. R., Martens, J. R., and Bitar, K. N. (2007). Ectopic expression of caveolin-1 restores physiological contractile response of aged colonic smooth muscle. Am. J. Physiol. Gastrointest. Liver Physiol. 293, G240–G249.
Song, K. S., Li, S., Okamoto, T., Quilliam, L. A., Sargiacomo, M., and Lisanti, M. P. (1996). Co-purification and direct interaction of Ras with caveolin, an integral membrane protein of caveolae microdomains. Detergent-free purification of caveolae microdomains. J. Biol. Chem. 271, 9690–9697. doi: 10.1074/jbc.271.16.9690
Sotgia, F., Martinez-Outschoorn, U. E., Howell, A., Pestell, R. G., Pavlides, S., and Lisanti, M. P. (2012). Caveolin-1 and cancer metabolism in the tumor microenvironment: markers, models, and mechanisms. Annu. Rev. Pathol. 7, 423–467. doi: 10.1146/annurev-pathol-011811-120856
Sowa, G. (2012). Caveolae, caveolins, cavins, and endothelial cell function: new insights. Front. Physiol. 2:120. doi: 10.3389/fphys.2011.00120
Spisni, E., Bianco, M. C., Griffoni, C., Toni, M., D’Angelo, R., Santi, S., et al. (2003). Mechanosensing role of caveolae and caveolar constituents in human endothelial cells. J. Cell. Physiol. 197, 198–204. doi: 10.1002/jcp.10344
Steffens, S., Schrader, A. J., Blasig, H., Vetter, G., Eggers, H., Trankenschuh, W., et al. (2011). Caveolin 1 protein expression in renal cell carcinoma predicts survival. BMC Urol. 11:25. doi: 10.1186/1471-2490-11-25.
Stoeber, M., Schellenberger, P., Siebert, C. A., Leyrat, C., Helenius, A., and Grunewald, K. (2016). Model for the architecture of caveolae based on a flexible, net-like assembly of Cavin1 and Caveolin discs. Proc. Natl. Acad. Sci. U.S.A. 113, E8069–E8078.
Stubbs, C. D., Botchway, S. W., Slater, S. J., and Parker, A. W. (2005). The use of time-resolved fluorescence imaging in the study of protein kinase C localisation in cells. BMC Cell Biol. 6:22. doi: 10.1186/1471-2121-6-22
Sui, Z. H., Xu, H., Wang, H., Jiang, S., Chi, H., and Sun, L. (2017). Intracellular trafficking pathways of Edwardsiella tarda: from Clathrin- and Caveolin-mediated endocytosis to endosome and lysosome. Front. Cell. Infect. Microbiol. 7:400. doi: 10.3389/fcimb.2017.00400
Sun, L., Hemgard, G. V., Susanto, S. A., and Wirth, M. (2010). Caveolin-1 influences human influenza A virus (H1N1) multiplication in cell culture. Virol. J. 7:108. doi: 10.1186/1743-422x-7-108
Suzuoki, M., Miyamoto, M., Kato, K., Hiraoka, K., Oshikiri, T., Nakakubo, Y., et al. (2002). Impact of caveolin-1 expression on prognosis of pancreatic ductal adenocarcinoma. Br. J. Cancer 87, 1140–1144. doi: 10.1038/sj.bjc.6600619
Svensson, K. J., Christianson, H. C., Wittrup, A., Bourseau-Guilmain, E., Lindqvist, E., Svensson, L. M., et al. (2013). Exosome uptake depends on ERK1/2-heat shock protein 27 signaling and lipid raft-mediated endocytosis negatively regulated by Caveolin-1. J. Biol. Chem. 288, 17713–17724. doi: 10.1074/jbc.m112.445403
Szabadosova, V., Boronova, I., Ferenc, P., Tothova, I., Bernasovska, J., Zigova, M., et al. (2018). Analysis of selected genes associated with cardiomyopathy by next-generation sequencing. J. Clin. Lab. Anal. 32:e22254. doi: 10.1002/jcla.22254
Tachikawa, M., Morone, N., Senju, Y., Sugiura, T., Hanawa-Suetsugu, K., Mochizuki, A., et al. (2017). Measurement of caveolin-1 densities in the cell membrane for quantification of caveolar deformation after exposure to hypotonic membrane tension. Sci. Rep. 7:7794.
Tamaskar, I., and Zhou, M. (2008). Clinical implications of caveolins in malignancy and their potential as therapeutic targets. Curr. Oncol. Rep. 10, 101–106. doi: 10.1007/s11912-008-0017-8
Tanase, C. P. (2008). Caveolin-1: a marker for pancreatic cancer diagnosis. Expert Rev. Mol. Diagn. 8, 395–404. doi: 10.1586/14737159.8.4.395
Tanase, C. P., Dima, S., Mihai, M., Raducan, E., Nicolescu, M. I., Albulescu, L., et al. (2009). Caveolin-1 overexpression correlates with tumour progression markers in pancreatic ductal adenocarcinoma. J. Mol. Histol. 40, 23–29. doi: 10.1007/s10735-008-9209-7
Tate, K. M., Higgins, D. L., Holmes, W. E., Winkler, M. E., Heyneker, H. L., and Vehar, G. A. (1987). Functional role of proteolytic cleavage at arginine-275 of human tissue plasminogen activator as assessed by site-directed mutagenesis. Biochemistry 26, 338–343. doi: 10.1021/bi00376a002
Taylor, D. R., and Hooper, N. M. (2006). The prion protein and lipid rafts. Mol. Membr. Biol. 23, 89–99. doi: 10.1080/09687860500449994
Thorleifsson, G., Walters, G. B., Hewitt, A. W., Masson, G., Helgason, A., DeWan, A., et al. (2010). Common variants near CAV1 and CAV2 are associated with primary open-angle glaucoma. Nat. Genet. 42, 906–909.
Thorn, H., Stenkula, K. G., Karlsson, M., Ortegren, U., Nystrom, F. H., Gustavsson, J., et al. (2003). Cell surface orifices of caveolae and localization of caveolin to the necks of caveolae in adipocytes. Mol. Biol. Cell 14, 3967–3976. doi: 10.1091/mbc.e03-01-0050
Thottacherry, J. J., Sathe, M., Prabhakara, C., and Mayor, S. (2019). Spoiled for choice: diverse endocytic pathways function at the cell surface. Annu. Rev. Cell Dev. Biol. 35, 55–84. doi: 10.1146/annurev-cellbio-100617-062710
Thyberg, J. (2000). Differences in caveolae dynamics in vascular smooth muscle cells of different phenotypes. Lab. Invest. 80, 915–929. doi: 10.1038/labinvest.3780095
Tillu, V. A., Kovtun, O., McMahon, K. A., Collins, B. M., and Parton, R. G. (2015). A phosphoinositide-binding cluster in cavin1 acts as a molecular sensor for cavin1 degradation. Mol. Biol. Cell 26, 3561–3569. doi: 10.1091/mbc.e15-06-0359
Tomassian, T., Humphries, L. A., Liu, S. D., Silva, O., Brooks, D. G., and Miceli, M. C. (2011). Caveolin-1 orchestrates TCR synaptic polarity, signal specificity, and function in CD8 T cells. J. Immunol. 187, 2993–3002. doi: 10.4049/jimmunol.1101447
Toni, M., Spisni, E., Griffoni, C., Santi, S., Riccio, M., Lenaz, P., et al. (2006). Cellular prion protein and caveolin-1 interaction in a neuronal cell line precedes Fyn/Erk 1/2 signal transduction. J. Biomed. Biotechnol. 2006:69469.
Tourkina, E., Richard, M., Gooz, P., Bonner, M., Pannu, J., Harley, R., et al. (2008). Antifibrotic properties of caveolin-1 scaffolding domain in vitro and in vivo. Am. J. Physiol. Lung Cell. Mol. Physiol. 294, L843–L8461.
Travez, A., Rabanal-Ruiz, Y., Lopez-Alcala, J., Molero-Murillo, L., Diaz-Ruiz, A., Guzman-Ruiz, R., et al. (2018). The caveolae-associated coiled-coil protein, NECC2, regulates insulin signalling in Adipocytes. J. Cell. Mol. Med. 22, 5648–5661. doi: 10.1111/jcmm.13840
Trimmer, C., Sotgia, F., Whitaker-Menezes, D., Balliet, R. M., Eaton, G., Martinez-Outschoorn, U. E., et al. (2011). Caveolin-1 and mitochondrial SOD2 (MnSOD) function as tumor suppressors in the stromal microenvironment: a new genetically tractable model for human cancer associated fibroblasts. Cancer Biol. Ther. 11, 383–394. doi: 10.4161/cbt.11.4.14101
Tsai, T. H., Chen, S. F., Huang, T. Y., Tzeng, C. F., Chiang, A. S., Kou, Y. R., et al. (2011). Impaired Cd14 and Cd36 expression, bacterial clearance, and Toll-like receptor 4-Myd88 signaling in caveolin-1-deleted macrophages and mice. Shock 35, 92–99. doi: 10.1097/shk.0b013e3181ea45ca
van Dokkum, R. P., and Buikema, H. (2009). Possible new druggable targets for the treatment of nephrosis. Perhaps we should find them in caveolea? Curr. Opin. Pharmacol. 9, 132–138. doi: 10.1016/j.coph.2008.12.008
Vladimirov, V. I., Zernii, E. Y., Baksheeva, V. E., Wimberg, H., Kazakov, A. S., Tikhomirova, N. K., et al. (2018). Photoreceptor calcium sensor proteins in detergent-resistant membrane rafts are regulated via binding to caveolin-1. Cell Calcium 73, 55–69. doi: 10.1016/j.ceca.2018.04.003
Volonte, D., Liu, Z., Musille, P. M., Stoppani, E., Wakabayashi, N., Di, Y. P., et al. (2013). Inhibition of nuclear factor-erythroid 2-related factor (Nrf2) by caveolin-1 promotes stress-induced premature senescence. Mol. Biol. Cell 24, 1852–1862. doi: 10.1091/mbc.e12-09-0666
Volonte, D., Zhang, K., Lisanti, M. P., and Galbiati, F. (2002). Expression of caveolin-1 induces premature cellular senescence in primary cultures of murine fibroblasts. Mol. Biol. Cell 13, 2502–2517. doi: 10.1091/mbc.01-11-0529
Wang, F., Zheng, Y., Orange, M., Yang, C., Yang, B., Liu, J., et al. (2017). PTRF suppresses the progression of colorectal cancers. Oncotarget 8, 48650–48659. doi: 10.18632/oncotarget.9424
Wang, H., Yang, P., Liu, K., Guo, F., Zhang, Y., Zhang, G., et al. (2008). SARS coronavirus entry into host cells through a novel clathrin- and caveolae-independent endocytic pathway. Cell Res. 18, 290–301. doi: 10.1038/cr.2008.15
Wang, J., Li, Y., Wang, S., and Liu, F. (2020). Dynamics of transmissible gastroenteritis virus internalization unraveled by single-virus tracking in live cells. FASEB J. 34, 4653–4669. doi: 10.1096/fj.201902455r
Wang, S., Zhang, Z., Almenar-Queralt, A., Leem, J., DerMardirossian, C., Roth, D. M., et al. (2019). Caveolin-1 phosphorylation is essential for axonal growth of human neurons derived from iPSCs. Front. Cell. Neurosci. 13:324. doi: 10.3389/fncel.2019.00324
Watson, R. O., and Galan, J. E. (2008). Campylobacter jejuni survives within epithelial cells by avoiding delivery to lysosomes. PLoS Pathog. 4:e14. doi: 10.1371/journal.ppat.0040014
Webley, W. C., Norkin, L. C., and Stuart, E. S. (2004). Caveolin-2 associates with intracellular chlamydial inclusions independently of caveolin-1. BMC Infect. Dis. 4:23. doi: 10.1186/1471-2334-4-23
Werling, D., Hope, J. C., Chaplin, P., Collins, R. A., Taylor, G., and Howard, C. J. (1999). Involvement of caveolae in the uptake of respiratory syncytial virus antigen by dendritic cells. J. Leukoc. Biol. 66, 50–58. doi: 10.1002/jlb.66.1.50
Wheaton, K., Sampsel, K., Boisvert, F. M., Davy, A., Robbins, S., and Riabowol, K. (2001). Loss of functional caveolae during senescence of human fibroblasts. J. Cell. Physiol. 187, 226–235. doi: 10.1002/jcp.1071
Wiechen, K., Diatchenko, L., Agoulnik, A., Scharff, K. M., Schober, H., Arlt, K., et al. (2001a). Caveolin-1 is down-regulated in human ovarian carcinoma and acts as a candidate tumor suppressor gene. Am. J. Pathol. 159, 1635–1643. doi: 10.1016/s0002-9440(10)63010-6
Wiechen, K., Sers, C., Agoulnik, A., Arlt, K., Dietel, M., Schlag, P. M., et al. (2001b). Down-regulation of caveolin-1, a candidate tumor suppressor gene, in sarcomas. Am. J. Pathol. 158, 833–839. doi: 10.1016/s0002-9440(10)64031-x
Williams, T. M., Medina, F., Badano, I., Hazan, R. B., Hutchinson, J., Muller, W. J., et al. (2004). Caveolin-1 gene disruption promotes mammary tumorigenesis and dramatically enhances lung metastasis in vivo. Role of Cav-1 in cell invasiveness and matrix metalloproteinase (MMP-2/9) secretion. J. Biol. Chem. 279, 51630–51646. doi: 10.1074/jbc.m409214200
Witkiewicz, A. K., Casimiro, M. C., Dasgupta, A., Mercier, I., Wang, C., Bonuccelli, G., et al. (2009). Towards a new “stromal-based” classification system for human breast cancer prognosis and therapy. Cell Cycle 8, 1654–1658. doi: 10.4161/cc.8.11.8544
Wu, M., Fan, J., Gunning, W., and Ratnam, M. (1997). Clustering of GPI-anchored folate receptor independent of both cross-linking and association with caveolin. J. Membr. Biol. 159, 137–147. doi: 10.1007/s002329900277
Xing, Y., Wen, Z., Gao, W., Lin, Z., Zhong, J., and Jiu, Y. (2020). Multifaceted functions of host cell Caveolae/Caveolin-1 in virus infections. Viruses 12:487. doi: 10.3390/v12050487
Xu, Q., Cao, M., Song, H., Chen, S., Qian, X., Zhao, P., et al. (2016). Caveolin-1-mediated Japanese encephalitis virus entry requires a two-step regulation of actin reorganization. Future Microbiol. 11, 1227–1248. doi: 10.2217/fmb-2016-0002
Yamada, E. (1955a). The fine structure of the gall bladder epithelium of the mouse. J. Biophys. Biochem. Cytol. 1, 445–458. doi: 10.1083/jcb.1.5.445
Yamada, E. (1955b). The fine structure of the renal glomerulus of the mouse. J. Biophys. Biochem. Cytol. 1, 551–566. doi: 10.1083/jcb.1.6.551
Yamamoto, K., Imamura, H., and Ando, J. (2018). Shear stress augments mitochondrial ATP generation that triggers ATP release and Ca(2+) signaling in vascular endothelial cells. Am. J. Physiol. Heart Circ. Physiol. 315:H1477-H85.
Yamamoto, M., Toya, Y., Schwencke, C., Lisanti, M. P., and Myers, M. G. Jr., and Ishikawa, Y. (1998). Caveolin is an activator of insulin receptor signaling. J. Biol. Chem. 273, 26962–26968. doi: 10.1074/jbc.273.41.26962
Yan, J., Lu, Q., Dong, J., Li, X., Ma, K., and Cai, L. (2012). Hepatitis B virus X protein suppresses caveolin-1 expression in hepatocellular carcinoma by regulating DNA methylation. BMC Cancer 12:353. doi: 10.1186/1471-2407-12-353
Yang, C., DeMars, K. M., and Candelario-Jalil, E. (2018). Age-dependent decrease in adropin is associated with reduced levels of endothelial nitric oxide synthase and increased oxidative stress in the rat brain. Aging Dis. 9, 322–330. doi: 10.14336/ad.2017.0523
Yang, K., Lu, W., Jiang, Q., Yun, X., Zhao, M., Jiang, H., et al. (2015). Peroxisome proliferator-activated receptor gamma-mediated inhibition on hypoxia-triggered store-operated calcium entry. A Caveolin-1-dependent mechanism. Am. J. Respir. Cell. Mol. Biol. 53, 882–892. doi: 10.1165/rcmb.2015-0002oc
Yang, S., He, M., Liu, X., Li, X., Fan, B., and Zhao, S. (2013). Japanese encephalitis virus infects porcine kidney epithelial PK15 cells via clathrin- and cholesterol-dependent endocytosis. Virol. J. 10:258. doi: 10.1186/1743-422x-10-258
Yeh, Y. C., Ling, J. Y., Chen, W. C., Lin, H. H., and Tang, M. J. (2017). Mechanotransduction of matrix stiffness in regulation of focal adhesion size and number: reciprocal regulation of caveolin-1 and beta1 integrin. Sci. Rep. 7:15008.
Yeow, I., Howard, G., Chadwick, J., Mendoza-Topaz, C., Hansen, C. G., Nichols, B. J., et al. (2017). EHD proteins cooperate to generate caveolar clusters and to maintain caveolae during repeated mechanical stress. Curr. Biol. 27, 2951–2962.e5.
Yoon, H. J., Cho, S. W., Ahn, B. W., and Yang, S. Y. (2010). Alterations in the activity and expression of endothelial NO synthase in aged human endothelial cells. Mech. Ageing Dev. 131, 119–123. doi: 10.1016/j.mad.2009.12.010
Yu, D. M., Jung, S. H., An, H. T., Lee, S., Hong, J., Park, J. S., et al. (2017). Caveolin-1 deficiency induces premature senescence with mitochondrial dysfunction. Aging Cell 16, 773–784. doi: 10.1111/acel.12606
Yu, J., Bergaya, S., Murata, T., Alp, I. F., Bauer, M. P., Lin, M. I., et al. (2006). Direct evidence for the role of caveolin-1 and caveolae in mechanotransduction and remodeling of blood vessels. J. Clin. Invest. 116, 1284–1291. doi: 10.1172/jci27100
Yu, Z., Beer, C., Koester, M., and Wirth, M. (2006). Caveolin-1 interacts with the Gag precursor of murine leukaemia virus and modulates virus production. Virol. J. 3:73.
Zaas, D. W., Swan, Z. D., Brown, B. J., Li, G., Randell, S. H., Degan, S., et al. (2009). Counteracting signaling activities in lipid rafts associated with the invasion of lung epithelial cells by Pseudomonas aeruginosa. J. Biol. Chem. 284, 9955–9964. doi: 10.1074/jbc.m808629200
Zampighi, G., Vergara, J., and Ramon, F. (1975). On the connection between the transverse tubules and the plasma membrane in frog semitendinosus skeletal muscle. Are caveolae the mouths of the transverse tubule system? J. Cell Biol. 64, 734–740. doi: 10.1083/jcb.64.3.734
Zhan, P., Shen, X. K., Qian, Q., Wang, Q., Zhu, J. P., Zhang, Y., et al. (2012). Expression of caveolin-1 is correlated with disease stage and survival in lung adenocarcinomas. Oncol. Rep. 27, 1072–1078. doi: 10.3892/or.2011.1605
Zhang, B., Peng, F., Wu, D., Ingram, A. J., Gao, B., and Krepinsky, J. C. (2007). Caveolin-1 phosphorylation is required for stretch-induced EGFR and Akt activation in mesangial cells. Cell. Signal. 19, 1690–1700. doi: 10.1016/j.cellsig.2007.03.005
Zhao, X., He, Y., Gao, J., Fan, L., Li, Z., Yang, G., et al. (2013). Caveolin-1 expression level in cancer associated fibroblasts predicts outcome in gastric cancer. PLoS One 8:e59102. doi: 10.1371/journal.pone.0059102
Keywords: caveolae, caveolins, cell communication, cell signaling, gatekeeper, hijack, oncogenic signal, clinical trials
Citation: Dudãu M, Codrici E, Tanase C, Gherghiceanu M, Enciu A-M and Hinescu ME (2020) Caveolae as Potential Hijackable Gates in Cell Communication. Front. Cell Dev. Biol. 8:581732. doi: 10.3389/fcell.2020.581732
Received: 09 July 2020; Accepted: 08 October 2020;
Published: 27 October 2020.
Edited by:
Jiri Novotny, Charles University, CzechiaReviewed by:
Cedric M. Blouin, Institut Curie, FranceBrett Collins, University of Queensland, Australia
Ivan Robert Nabi, University of British Columbia, Canada
Copyright © 2020 Dudãu, Codrici, Tanase, Gherghiceanu, Enciu and Hinescu. This is an open-access article distributed under the terms of the Creative Commons Attribution License (CC BY). The use, distribution or reproduction in other forums is permitted, provided the original author(s) and the copyright owner(s) are credited and that the original publication in this journal is cited, in accordance with accepted academic practice. No use, distribution or reproduction is permitted which does not comply with these terms.
*Correspondence: Ana-Maria Enciu, YW5hLmVuY2l1QHVtZmNkLnJv
†These authors share first authorship