- 1Glycotherapeutics Group, Institute of Medical Biology, Agency for Science, Technology and Research (A∗STAR), Singapore, Singapore
- 2Department of Orthopaedic Surgery, Yong Loo Lin School of Medicine, National University of Singapore, Singapore, Singapore
- 3Lee Kong Chian School of Medicine, Nanyang Technological University–Imperial College London, Singapore, Singapore
Heparan sulfate proteoglycans (HSPGs) are an evolutionarily ancient subclass of glycoproteins with exquisite structural complexity. They are ubiquitously expressed across tissues and have been found to exert a multitude of effects on cell behavior and the surrounding microenvironment. Evidence has shown that heterogeneity in HSPG composition is crucial to its functions as an essential scaffolding component in the extracellular matrix as well as a vital cell surface signaling co-receptor. Here, we provide an overview of the significance of HSPGs as essential regulators of stem cell function. We discuss the various roles of HSPGs in distinct stem cell types during key physiological events, from development through to tissue homeostasis and regeneration. The contribution of aberrant HSPG production to altered stem cell properties and dysregulated cellular homeostasis characteristic of cancer is also reviewed. Finally, we consider approaches to better understand and exploit the multifaceted functions of HSPGs in influencing stem cell characteristics for cell therapy and associated culture expansion strategies.
Introduction
The glycocalyx is a dense layer of glycoproteins and glycolipids that coats the exterior of the cell membrane. It is comprised of a variety of polysaccharides covalently attached to lipids or proteins, and functions as an essential interface between external and internal cellular environments. Heparan sulfate (HS) is one of the many constituent polysaccharides, and is long (40–300 residues), linear and highly charged (Sarrazin et al., 2011). HS belongs to the glycosaminoglycan (GAG) family of carbohydrates, which also includes the closely related heparin, hyaluronan (HA), chondroitin and dermatan sulfate (CS/DS), and keratan sulfate (KS) (Esko and Lindahl, 2001; Gallagher, 2001). The base structure of HS is highly conserved throughout multicellular organisms, including mammals, fruit flies (Drosophila melanogaster) (Nakato et al., 1995), nematodes (Caenorhabditis elegans) (Townley and Bülow, 2011) and hydra (Hydra Magnipapillata and Hydra vulgaris) (Sarras et al., 1991; Yamada et al., 2007), indicating an ancient evolutionary origin.
HS is present in the extracellular matrix (ECM) of every tissue and on the surface of virtually every cell within an organism. HS chains are covalently attached to core proteins belonging to multiple families, and are collectively known as heparan sulfate proteoglycans (HSPGs). Mammalian HSPGs may be present as transmembrane proteins (syndecan 1–4, CD44, neuropilin-1, betaglycan) (Rapraeger et al., 1985; Sanderson and Bernfield, 1988; Andres et al., 1989; Brown et al., 1991; Kojima et al., 1992; Shintani et al., 2006; Multhaupt et al., 2009; Couchman, 2010; Gopal et al., 2010), GPI-anchored membrane proteins (glypican 1–6) (David et al., 1990; Filmus and Selleck, 2001; Filmus et al., 2008; Feil et al., 2009), secreted proteins retained within the ECM (perlecan, agrin, and collagen XVIII) (Noonan et al., 1991; Tsen et al., 1995; Halfter et al., 1998; Knox et al., 2002; Knox and Whitelock, 2006) or in secretory granules (serglycin) (Tantravahi et al., 1986; Stevens et al., 1988; Kolset and Tveit, 2008).
The ubiquitous presence of HSPGs is indicative of the multitude of important biological roles they play within an organism. For example, HS-rich perlecan is an integral basement membrane constituent, along with agrin and collagen XVIII, and a critical scaffolding component of the ECM (Hassell et al., 1980; Costell et al., 1999; Farach-Carson and Carson, 2007). In this regard, HSPGs provide biomechanical support and help maintain integrity of the extracellular microenvironment. Apart from their structural functions, HS chains can bind to a large number of proteins, of which at least 437 have been identified, and serve as low-affinity co-receptors during signaling events (Xu and Esko, 2014; Rudd et al., 2017; Dubey et al., 2020). Additionally, the protein-binding properties of HS afford it the ability to arrange morphogens into spatial and temporal gradients, which is vital for orderly development and tissue repair (Bernfield et al., 1999). HS can also bind and sequester various proteins, protecting them from thermal or proteolytic degradation (Sadir et al., 2004; Makarenkova et al., 2009; Xu and Esko, 2014; Kjellén and Lindahl, 2018; Poon et al., 2018; Sun C. et al., 2019). The distinct combinations of functional groups and chain modifications laid down during biosynthesis confer unique structural conformations and binding properties to an HS chain, underpinning its propensity to interact with a wide array of ligands and cognate receptors (Kreuger et al., 2006; Lindahl and Li, 2009).
HSPGs may also interact with proteins via the cytoplasmic domains of their core proteins. The syndecans are especially known to engage components of the cytoskeleton and focal adhesions, such as α-actinin and integrins, despite an absence of inherent kinase activity (Rapraeger et al., 1986; Saoncella et al., 1999; Greene et al., 2003). Such interactions implicate HSPGs as crucial components of protein binding events not only at the cell surface, but also those within adjacent intracellular regions that may influence cytoskeletal organization and downstream signaling cascades. All of these functional features confer an indispensability of HSPGs in cellular responses that influence cell morphology, adhesion, migration, and fate decisions (Longley et al., 1999; Cool and Nurcombe, 2006; Gopal et al., 2010; Bass et al., 2011; Okina et al., 2012; Vuong et al., 2015; Mitsou et al., 2017). In this review, we highlight some of the intricate structural features of HS and their proteoglycans (PGs) that underpin such functionality, and discuss the importance of specific HSPG signatures in mediating the behavior of different stem cell types.
HS Structure and Biosynthesis
The basic structural unit of the HS polysaccharide is that of a repeating disaccharide composed of uronic acid (either glucuronic acid or its C5-epimer, iduronic acid) β1,4 linked to N-glucosamine, which may be N-acetylated, N-sulfated or in rare circumstances, N-unsubstituted (free amine) (Figure 1; Esko and Lindahl, 2001). A wide range of structural diversity and functional complexity is conferred upon modification of the constituent monosaccharides—the uronic acid may be sulfated at the C2 position and the glucosamine at the N, C6 and rarely, C3 positions. The synthesis of HS is carried out within the Golgi apparatus by a series of biosynthetic enzymes (Figure 2), through the catalysis of nucleotide sugars (such as UDP-glucosamine) and the nucleotide sulfate donor 3′-phosphoadenosine-5′-phosphosulfate (PAPS) (Esko and Lindahl, 2001). The majority of HS biosynthetic enzymes are type II integral Golgi membrane proteins except for 3-O-sulphotransferase 1 (3OST1), which possesses an intraluminal resident form (Shworak et al., 1997).
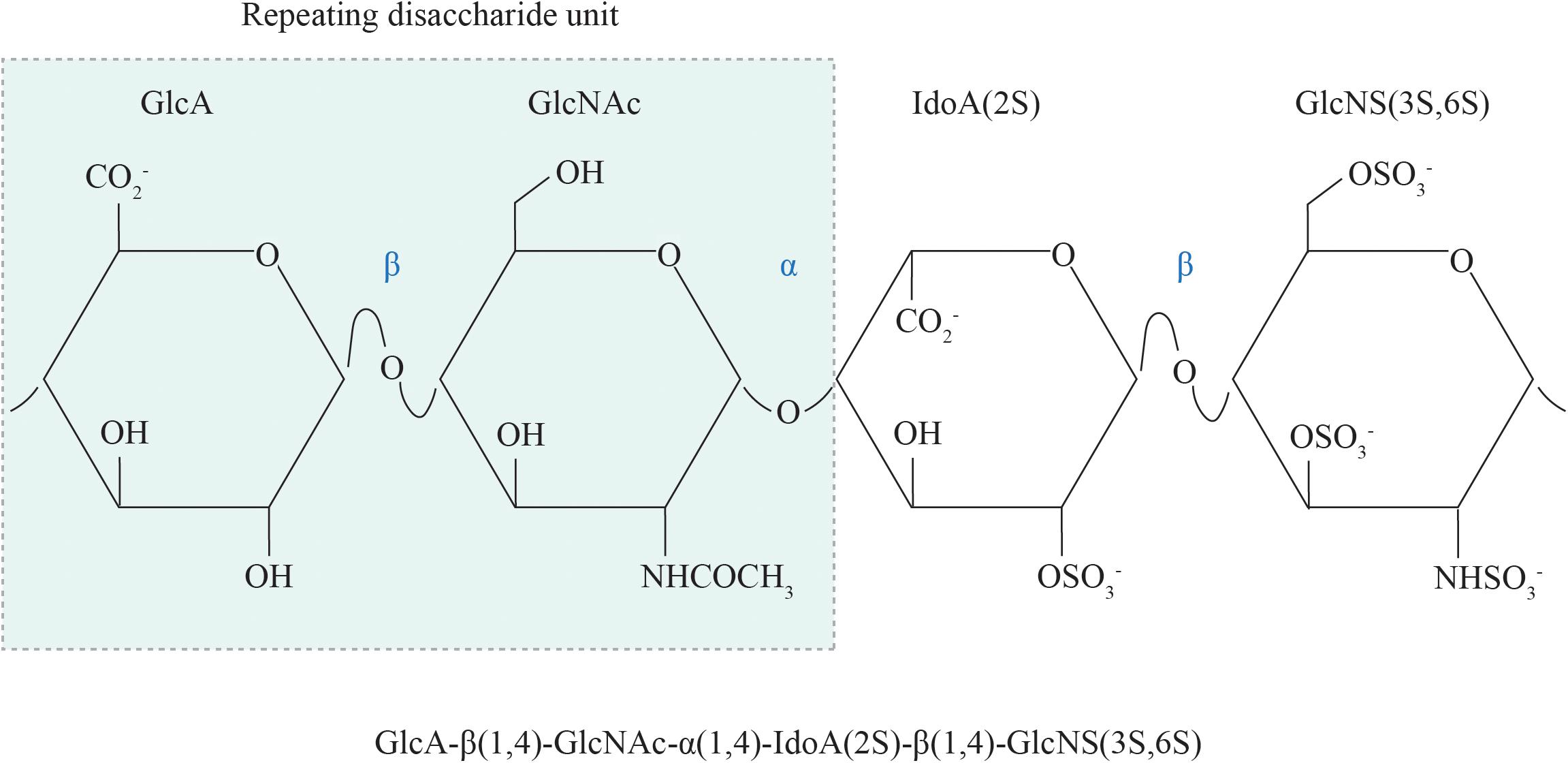
Figure 1. The chemical structure of HS. The repeating disaccharide unit of HS comprises a GlcA residue linked to GlcNAc residue via a β1,4 glycosidic bond. Selective GlcA monosaccharides undergo epimerization to IdoA, which is particularly susceptible to sulfation at the C2 position. Sulfate moieties may also be added to the N, C6 and rarely, C3 positions of the glucosamine residue. GlcA, glucuronic acid; GlcNAc, N-acetyl glucosamine; IdoA, iduronic acid; GlcNS, N-sulfated glucosamine; 2S, 2-O-sulfation; 6S, 6-O-sulfation; 3S, 3-O-sulfation.
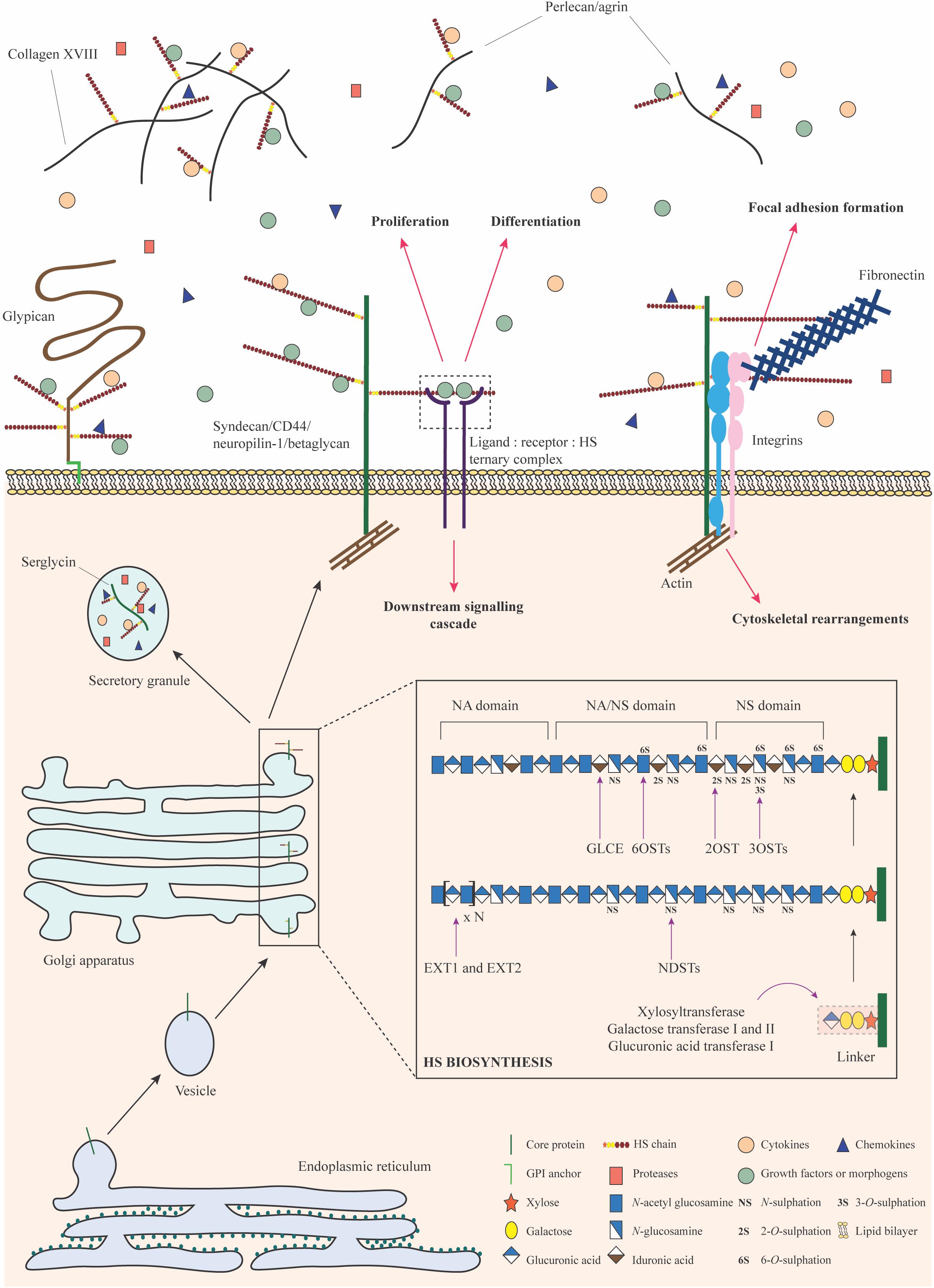
Figure 2. HSPG biosynthesis and functions. HS biosynthesis is a sequential process that occurs in the Golgi apparatus. It is initiated by the formation and attachment of a tetrasaccharide linker to a serine residue on the core protein. Subsequently, chain polymerization is carried out by EXT1 and EXT2, where N-acetylglucosamine and glucuronic acid residues are added in succession. The HS chain is then modified by a collection of enzymes including the NDSTs, GLCE, 2OST, 6OSTs, and 3OSTs. Selective sulfation and epimerization confer intricate structural nuances to the HS chain, underpinning the formation of domains with distinct functional features. Upon exit from the Golgi apparatus, HSPGs may be stored in secretory granules, transported to the plasma membrane or secreted into the ECM. They bind to a variety of signaling factors and function as co-receptors to mediate signaling cascades important for stem cell proliferation and differentiation. HSPGs may engage with integrins, fibronectin, and actin filaments to bring about cytoskeletal reorganization for focal adhesion formation or migration. HSPGs can also sequester signaling molecules in the ECM and regulate their bioavailability. Monosaccharides in this figure have been represented in accordance with the symbol nomenclature for glycans (SNFG) (Varki et al., 2015).
HS chains are attached to PG core proteins as post-translational modifications via a linkage tetrasaccharide. This “linker” sequence is composed of a glucuronic acid residue, two galactose residues and a xylose residue covalently bound to a serine hydroxyl group through a xylosidic bond (Lindahl and Rodén, 1965; Lindahl, 1966). A heterodimeric complex of the HS co-polymerases exostosin 1 and 2 (EXT1 and EXT2) alternatively adds N-glucosamine and glucuronic acid residues, resulting in the basic structure and length of the chain. Following this, N-deacetylase/N-sulphotransferase (NDST; 4 isoforms) removes acetyl groups and adds a sulfate moiety to the N position of select glucosamine residues. This process yields short N-sulfated domains that serve as substrates for further modification. On rare occasion, deacetylated glucosamine residues may be left as free amine groups (Sheng et al., 2011; Dou et al., 2015). Next, glucuronyl C5-epimerase (GLCE) selectively converts glucuronic acid residues into iduronic acid, a process that requires an adjacent N-sulphoglucosamine at the non-reducing end (Hagner-McWhirter et al., 2004). This is followed by 2-O-sulfation at the C2 position of the uronic acid, brought about by 2-O-sulphotransferase (2OST); this modification occurs predominantly in iduronic acid residues rather than glucuronic acid (Smeds et al., 2010). Subsequently, the C6 positions of select glucosamine residues are sulfated by 6-O-sulphotransferase (6OST; 3 isoforms) (Xu et al., 2017). In rare cases, the C3 position may also be sulfated by 3-O-sulphotransferase (3OST; 7 isoforms) (Shworak et al., 1999). At this stage, HS is transported to the cell surface where a final modification involving the selective removal of 6-O-sulfate groups from glucosamine residues may occur, through the action of extracellular 6-O-endosulphatase (SULF; 2 isoforms collectively referred to as “sulfatases”) (Ai et al., 2003).
The Intricate Structure of HS Imparts Essential Functions
A complete HS chain possesses complex structural heterogeneity seldom observed in most biomolecules. Short, highly sulfated, and heterogeneous regions (NS domains) are flanked by short transition domains of variable sulfation (NA/NS domains) (Figure 2). The NA/NS domains are interconnected by long, flexible regions of little to no modification (NA domains) (Turnbull and Gallagher, 1990, 1991; Maccarana et al., 1996; Gallagher, 2001). HS functionality is imparted predominantly by the highly negatively charged NS domains. The disaccharide residues in these regions are capable of interacting with small hydrophilic clusters of positively charged amino acid residues, such as lysine and arginine, common characteristics observed within the three-dimensional structure of HS-binding proteins (Cardin and Weintraub, 1989; Faham et al., 1996; Rudd et al., 2017). Interactions of HS with the fibroblast growth factor (FGF) family and their cognate receptors (FGFRs) serve as quintessential examples of this feature, which underpins the role of HSPGs as low-affinity co-receptors (Rapraeger et al., 1991; Yayon et al., 1991; Nurcombe et al., 1993).
A high affinity binding site for FGF2 in fibroblast-derived HS was discovered to be an oligosaccharide that is 7 disaccharides in length or has a degree of polymerization of 7 (dp7) (Turnbull et al., 1992). This dp7 fragment and other longer heparin/HS-derived oligosaccharides containing the dp7 sequence were characterized by an enrichment of 2-O-sulfated iduronic acid (IdoA2S) and N-sulfated glucosamine (GlcNS) residues (Turnbull et al., 1992; Ishihara et al., 1994; Kreuger et al., 1999). The necessity of these IdoA2S and GlcNS residues for FGF2 binding was evidenced when treatment of the dp7 fragment with heparinase led to an abolishment of FGF2 binding. Moreover, other similarly sized oligosaccharides with reduced 2-O-sulfation exhibited lower affinities for FGF2, highlighting the requirement of contiguous stretches of highly sulfated disaccharides for strong FGF2 binding (Turnbull et al., 1992). Further enquiry into the compositional features of HS and heparin oligosaccharides that are required for FGF interactions has shown that the FGFs bind to certain shared HS epitopes with low affinity and selectivity (Kreuger et al., 2005). However, distinct sulfation patterns and chain lengths allow for the formation of specialized NS domains in the GAG chain, which preferentially bind specific FGFs with a higher affinity than others (Guimond and Turnbull, 1999; Schultz et al., 2017). For example, while IdoA2S and GlcNS residues are essential for FGF2 binding, additional 6-O-sulfation of GlcNS residues (GlcNS6S) is required for high affinity binding to FGF1 and FGF4 (Ishihara, 1994; Kreuger et al., 1999; Ashikari-Hada et al., 2004). The binding affinity of HS/heparin oligosaccharides to FGF1 and FGF4 is also reported to correlate with chain length. Kreuger et al. (1999) have shown that the minimum length required for a heparin oligosaccharide to exhibit binding affinity to FGF1 is dp6. However, dp10 fragments and larger are needed for tight binding with both FGF1 and FGF4 (Ishihara, 1994; Kreuger et al., 1999).
In addition to FGF binding, HS chains bind to corresponding FGFRs to enhance ligand–receptor interactions and promote receptor dimerization for ternary complex formation (Ornitz et al., 1992; Spivak-Kroizman et al., 1994; Pellegrini et al., 2000; Schlessinger et al., 2000). While 6-O-sulfate moieties on glucosamine residues of HS/heparin are dispensable for FGF2 binding (Guimond et al., 1993; Maccarana et al., 1993; Ishihara et al., 1994), they are required for engaging FGFR, facilitating ternary complex formation and subsequently, activating the mitogenic effect of FGF2–FGFR interactions (Ostrovsky et al., 2002). In a study by Guimond and colleagues, exogenous full-length heparin was capable of rescuing the mitogenic effect of FGF2 in fibroblasts devoid of HS. However, selective 6-O-desulfation of heparin inactivated FGF2-induced mitogenesis, even though the oligosaccharide was able to bind FGF2 and compete with endogenous heparin for FGF2 binding (Guimond et al., 1993). The requirement of 6-O-sulfate moieties for activating FGF2-dependent mitogenesis upon HS/heparin binding was further established when disaccharide analysis of a range of different HS oligosaccharides clearly showed that the major distinguishing factor between activating and inhibitory fragments was the extent of 6-O-sulfation (Pye et al., 1998). Apart from appropriately 6-O-sulfated residues, chain length was also found to be an important factor for determining the ability of a HS/heparin oligosaccharide to support FGF2 mitogenic activity. An exogenous heparin-derived dodecasaccharide (dp12) was comparable to full-length heparin in rescuing the mitogenic effect of FGF2 in fibroblasts devoid of HS, whereas HS/heparin fragments lower than dp10 lacked the ability to support this activity (Guimond et al., 1993; Ishihara, 1994; Pye et al., 1998). Therefore, the structural and compositional features of HS heavily influence the functional properties of the chain, and consequently, the ability of HSPGs to operate effectively as signaling co-receptors (Goodger et al., 2008; Jastrebova et al., 2010).
Heterogeneity in HSPG Composition and Localization Can Influence Stem Cell Fate
Structural and functional heterogeneity in HSPGs has been observed between different cell types and within particular cell populations over time (Turnbull and Gallagher, 1988; Allen et al., 2001). The HSPGs produced by a cell (heparanome) can be rapidly altered with changes in cell state. Such alterations can influence cell activity in distinct ways depending on the compositional complexity of the heparanome (Turnbull et al., 2001).
HSPG heterogeneity can be seen at both the GAG chain and core protein levels. Heterogeneity at the HS chain level has largely been attributed to the non-template and incomplete nature of the HS biosynthetic process, as well as the activity of multiple biosynthetic enzyme isoforms that may differ in their substrate specificities and the products they generate (Aikawa and Esko, 1999; Liu et al., 1999; Habuchi et al., 2000; Turnbull et al., 2001). Post-biosynthetic alterations to sulfation and chain length are also known to contribute to the structural diversity and associated functional variability in HS. For example, selective removal of 6-O-sulfate groups by the SULF enzymes, SULF2 in particular, may lead to domain reorganization within the HS chain, prevent the binding of HS to signaling factors and consequently, alter ligand–receptor interactions that influence stem cell or progenitor cell fate decisions (Ai et al., 2003; Lai et al., 2003; Hammond et al., 2014). The drastic effects that a lack of appropriate HS moieties can have on signaling and progenitor cell function was examined in MM14 myoblast cultures in vitro—desulfation of cell surface HS by heparinase or chlorate treatment prevented FGF2 binding to cognate cell surface receptors and removed the suppressive effect of FGF2 on myoblast differentiation (Rapraeger et al., 1991). A similar trend was observed with the addition of selectively 6-O-desulfated heparin to MM14 cultures. The heparin oligosaccharides competed with endogenous HS for FGF2 binding and precluded FGF2–FGFR interactions that normally suppress cell cycle exit and myogenic activation (Guimond et al., 1993). The cleavage of 6-O-sulfates from cell surface and secreted HSPGs can also lead to the release of previously bound signaling factors and an increase in the bioavailability of these factors within the ECM (Uchimura et al., 2006; Rosen and Lemjabbar-Alaoui, 2010). Alternatively, the biodistribution of signaling molecules in the ECM may be altered as a result of HS chain cleavage by the single copy heparanase enzyme (HPSE), since cleavage releases biologically active HS fragments that are capable of binding and sequestering signaling factors within the extracellular space (Kussie et al., 1999; Hulett et al., 2000; Vlodavsky et al., 2002).
In the case of core proteins, heterogeneity can result from the differential expression of encoding genes. Spatiotemporal variability in the expression of core proteins, especially during development and wound healing, can influence signaling events at the cell surface and affect a stem cell’s responsiveness to its surroundings (Bernfield et al., 1993; Olczyk et al., 2015). The collection of core proteins present at the cell surface and their localization may also be altered upon cleavage of PG ectodomains in a process known as “shedding” (Nam and Park, 2012). Studies have shown that shedding is mediated by a variety of proteases and phospholipases, collectively known as “sheddases,” which can exhibit specificity toward particular core proteins. For example, the syndecans are known targets of a number of matrix metalloproteinases (MMPs), such as MMP7, MMP9, and ADAM17 (Ding et al., 2005; Brule et al., 2006; Pruessmeyer et al., 2010). Recently, ADAM17 has also been reported as a glypican sheddase, in addition to conventional phospholipases, such as Notum, that cleave at the GPI anchor (Traister et al., 2008; Kawahara et al., 2017). The shedding process yields bioactive soluble forms of HSPGs in the ECM that act as paracrine or autocrine effectors, and compete against cell-surface/matrix-bound HS (Nam and Park, 2012). The intact HS chains attached to the cleaved PG ectodomains can also operate as reservoirs that bind signaling molecules and regulate their diffusion, aiding in the maintenance of morphogen gradients that direct stem cell responses (Yan and Lin, 2009).
Heterogeneity in chain composition, core protein expression and PG localization underpin a highly diverse heparanome, which may directly influence the repertoire of molecular cues within a cell’s microenvironment as well as the cell’s ability to respond to them. However, there are several important questions yet to be answered regarding this diversity, especially pertaining to potential differences in functionality between cell surface HSPGs, cleaved or shed HSPGs, and secreted HSPGs. It remains to be understood whether HSPGs vary in the HS chains they carry depending on the core protein type and positional status. Current experimental methods to examine HSPG composition and function involve the extraction of PG pools from cell cultures or tissues and therefore, lack the resolution to interrogate distinct HSPG fractions or individual HSPGs. Such an inability to probe potentially unique compositional characteristics and mechanisms of action of specific HSPGs presents a major challenge in the field, and has hindered an understanding of fine functional nuances that may exist between HSPGs.
HSPGs as Orchestrators of Development and Differentiation
Lessons From Knockout Mouse Models
Over the past two decades, genetic modification has been one of the primary methods used for investigating the functions of HS modifications in mediating stem cell fate during development and differentiation. The knockout (KO) of individual genes from the HS biosynthetic pathway has revealed essential roles played by distinct modifications in tuning cellular responses to GFs, cytokines, chemokines, and morphogens.
Ext1 Knockout
In 2000, Lin and colleagues first developed the Ext1–/– mouse, which was devoid of HS due to the loss of the HS co-polymerase EXT1 (Lin et al., 2000). Ext1–/– embryos failed to develop beyond the blastocyst stage, clearly indicating that HS is an essential regulator of gastrulation and embryogenesis. Analysis of Ext1–/– embryonic stem cell (ESC) behavior in vitro has been carried out extensively by the Merry lab, specifically with regards to the development of the neurectoderm and mesoderm (Johnson et al., 2007; Baldwin et al., 2008; Holley et al., 2011; Pickford et al., 2011; Meade et al., 2013). Over the course of several studies, the essential regulatory role of HS in the exit of murine ESCs (mESCs) from pluripotency was discovered; Ext1–/– ESCs were incapable of exiting the pluripotent state under neural (Johnson et al., 2007; Pickford et al., 2011; Meade et al., 2013) or mesodermal (Holley et al., 2011) differentiation conditions, instead retaining characteristics of pluripotent cells, such as high levels of OCT4 expression. Interestingly, the addition of exogenous heparin or HS was able to rescue differentiation in these studies, further indicating that HS is a crucial regulator of stem cell function.
These findings were further validated in two studies by Kraushaar et al. (2010, 2012), who revealed that Ext1–/– mESCs were capable of maintaining pluripotency despite the removal of leukemia inhibitory factor. The absence of HS resulted in defective FGF and BMP signaling, such that the KO mESCs were unable to respond to pro-differentiation factors. Additional studies have revealed potential compensatory mechanisms by which the levels of other sulfated GAGs (such as CS and DS) appear to be upregulated in Ext1- null cells. This idea was initially proposed in a study by Le Jan and colleagues, wherein Ext1–/– embryoid bodies (EBs; spheroid cell aggregates capable of crudely recapitulating early embryonic development) displayed increased levels of CS/DS, and were able to respond to VEGF as well as undergo sprouting angiogenesis despite the loss of HS (Le Jan et al., 2012). The existence of putative compensatory mechanisms between different GAG species and consequent effects on signaling cascades suggests yet another layer of complexity in the GAG-dependent modulation of stem cell behavior, which is yet to be fully explored.
Ndst1/2 Knockout
Whilst the loss of Ext1–/– highlights the absolute requirement of HS in development and differentiation, it does little to reveal how changes in specific modifications within the HS chain, such as N-sulfation, within the HS chain could affect the behavior of cells. As described previously, NDST1 and 2 are primarily responsible for laying down N-sulfate modifications and forming NS domains within HS (NDST1) and heparin (NDST2) (Forsberg and Kjellén, 2001). In 2004, Holmborn and colleagues derived Ndst1/2–/– mESCs; analysis of the HS produced in these cells indicated a total loss of N- and 2-O-sulfation. Interestingly, 6-O-sulfation was still present and transcripts for all three 6OSTs were expressed, with 6OST1 displaying the greatest transcript abundance (Holmborn et al., 2004). Although functional assays were not performed, it was revealed that the presence of NS domains was not a pre-requisite for 6-O-sulfation. This important observation may be attributed to the unique orientation in which the 6OSTs engage with the HS chain, which is markedly different to that adopted by other sulphotransferases (Xu et al., 2017).
A number of other studies have sought to better elucidate the role of HS N-sulfation in early differentiation events and maintenance of pluripotency through use of the Ndst1/2–/– mESC line. The loss of N-sulfation results in perturbed FGF4 signaling, which in turn manifests as a similar phenotype to Ext1–/– mESCs: an inability to exit the pluripotent state (Lanner et al., 2010). Subsequent investigation indicated that despite the loss of N-sulfation, Ndst1/2–/– mESCs were still able to respond to stimulation by BMP4 (Forsberg et al., 2012). This finding clearly reflected the differential nature and context-dependent role of HS in FGF and BMP signaling. HS is not essential for BMP:BMPRI complex formation, as is the case for FGF:FGFR. Instead, HS binds to BMPRII and enhances its recruitment to BMP:BMPRI complexes, facilitating receptor hetero-oligomerisation for signaling (Kuo et al., 2010). Recent studies have suggested that HS is also able to antagonize BMP signaling (Mundy et al., 2018), offering an insight into why Ndst1/2–/– mESCs are still capable of responding to BMP4 and differentiating down an osteoblastic lineage.
Further studies have revealed an essential requirement for N-sulfation in angiogenic sprouting and the response to VEGF (Jakobsson et al., 2006). Lack of N-sulfation prevented Ndst1/2–/– EBs from undergoing angiogenesis. However, chimeric EBs formed with Vegfr2–/– mESCs were able to effectively respond to VEGF. This indicated that HS in trans was capable of potentiating VEGF signaling and was sufficient to rescue the differentiation capacity of cells devoid of N-sulfated residues.
Embryonic Stem Cells
Preliminary studies on HS expression and function in ESCs focused on mESCs. Immortal, easy to culture and rapidly proliferating, these cells were ideal for obtaining sufficient quantities of biological material to conduct detailed analyses of HS and its biosynthetic pathway. Nairn et al. (2007) used mESCs to study changes in GAG content and composition during differentiation to EBs and extra embryonic endoderm. Findings indicated that HS synthesis was upregulated, together with that of HA and CS/DS, during differentiation. Overall GAG sulfation increased as cells differentiated, with particularly high levels of N-sulfated HS disaccharides and di-sulfated CS type E disaccharides (Nairn et al., 2007).
Further studies from the Merry lab focused on early lineage commitment through the use of well-defined differentiation protocols aimed at recapitulating early germ layer specification (Smith et al., 2011). In 2007, an mESC Sox1-green fluorescence protein (GFP) reporter line, 46C, was used to analyze changes in HS sulfation through the course of differentiation to neural progenitor cells (NPCs) (Johnson et al., 2007). The study reported a clear rise in HS sulfation as cells exited a pluripotent state and differentiated into NPCs. Increases in HS biosynthetic enzyme transcripts, FGF2 cell surface binding and N, 6- and 2-O-sulfated disaccharides were also observed. A similar study in 2008 assessed the changes in HS expression through the course of early mesoderm commitment in mESCs by using an epitope-specific single chain fragment-variable antibody (HS4C3) (Baldwin et al., 2008). During mesodermal differentiation toward the haemangioblast lineage, cells upregulated the HS4C3 epitope [previously identified as containing 3-O-sulfation (ten Dam et al., 2006)], coinciding with an upregulation in the transcription factor brachyury. Isolation and reaggregation of cells with high expression of the HS4C3 epitope and brachyury yielded a higher efficiency of differentiation into haemangioblasts, indicating that specific changes in HS fine structure are correlated with enhanced commitment to a particular mature lineage (Baldwin et al., 2008). This was further confirmed via immunocytochemical staining of sectioned primitive streak stage mouse embryos, which displayed high expression of the HS4C3 epitope in the developing mesoderm during gastrulation (Baldwin et al., 2008).
Hirano et al. (2012) proposed that the HS4C3 epitope influenced Fas signaling during mESC differentiation, with overexpression of 3-O-sulfation leading to enhanced Fas signaling and differentiation. Conversely, removal of 3-O-sulfation reduced Fas signaling and the differentiation potential of mESCs. A follow-up study showed that Fas signaling via the 3-O-sulfated epitope recognized by HS4C3 was indeed required for the transition between naïve and primed states, as mESCs differentiated into murine epiblast-like cells (Hirano et al., 2013). Such observations confirmed the importance of understanding rapid and subtle changes in HS fine structure during early differentiation events and tissue specification. Temporal and lineage-specific alterations in mESC HS structure can influence signaling pathways that mediate exit from pluripotency, highlighting HS as a key orchestrator of early developmental events.
Whilst many studies investigating the role of HS in pluripotent stem cell function have utilized mESCs due to their relative ease of culture and ability to readily produce genetic mutants, focus has begun to shift onto more relevant human ESCs (hESCs) and induced pluripotent stem cells (iPSCs). Gasimli and colleagues investigated changes in the structure of various GAGs, including HS, during the differentiation of hESCs toward mesodermal and endodermal lineages (Gasimli et al., 2014). Upon differentiation toward splanchnic mesoderm, several PG transcripts were markedly upregulated and an increase in HS sulfation was observed (predominantly N-sulfation). Differentiation toward an immature hepatocyte lineage yielded similar increases in HS sulfation, with an accentuation of N-sulfated, 6-O-sulfated HS (Gasimli et al., 2014). Such evidence highlights the potential importance of regulated variation in HSPG expression and HS fine structure during specification toward different germ layers and cell lineages. It also suggests a possibility that particular HSPG signatures, which predominate over others at distinct stages of lineage commitment, may be used to develop potential markers of differentiation. However, more evidence detailing chronological changes in HSPG composition and associated sequence motifs during lineage commitment is needed to improve an understanding of the relationship between the heparanome and differentiation status.
The use of hESCs has also expanded knowledge regarding the requirement of HS within the extracellular environment for guiding stem cell fate. A 2012 study indicated that matrix-bound HS is essential for the effective culture expansion and maintenance of pluripotency of hESCs (Stelling et al., 2012). Cells were cultured on a variety of substrates, including mouse embryonic fibroblasts (MEFs), ethanol-fixed MEFs, and MEFs devoid of HS. It was observed that hESCs maintained pluripotency marker gene expression when cultured on feeder layers presenting HS (both live and fixed). In contrast, HS-deficient layers were unable to support hESC attachment and growth (Stelling et al., 2012). Another group demonstrated that decellularized organ matrices were capable of influencing differentiation and lineage commitment of pluripotent stem cell-derived mesoderm progenitors through HSPG-bound tissue-specific factors (Ullah et al., 2020). Together, these data highlight that HS in trans within the surrounding matrix or culture substrata is crucial for the maintenance of homeostasis, as well as the regulation of ESC function.
Neuroepithelial Cells
The significance of HSPGs in early central nervous system (CNS) development was established from studies where the absence of key biosynthetic enzymes resulted in pronounced developmental abnormalities (Poulain and Yost, 2015). Conditional knockout of Ext1 in the nervous system of murine embryos gave rise to severe midbrain and cerebellar deformities, underdeveloped cerebral cortices and a lack of key neuronal tracts, leading to postnatal lethality (Inatani et al., 2003). Defects in forebrain development, cerebral hypoplasia as well as craniofacial malformations, such as hydrocephalus, have also been described with deficiencies in Ndst1, Hs2st1, and Sulf2, respectively (McLaughlin et al., 2003; Grobe et al., 2005; Kalus et al., 2009). Notably, these developmental phenotypes were observed to overlap extensively with those obtained by knocking out genes encoding key morphogens, such as Fgf8, Wnt1, and Shh, during embryogenesis (Thomas et al., 1991; Chiang et al., 1996; Meyers et al., 1998). This suggested an HS-dependency of these signaling factors in directing stem cell function for neural tube patterning.
Further inquiry revealed that HSPGs are essential prior to and during neurogenesis (Yamaguchi, 2001). Distinct spatiotemporal expression patterns of HS-binding FGFs have been observed to mediate the proliferation, survival and differentiation of neuroepithelial cells (NECs) as well as their radial glial cell (RGC) progeny (Murphy et al., 1990; Guillemot and Zimmer, 2011). For example, FGF2 and FGF8 support the expansion of NECs soon after specification in the neural tube, while the onset of FGF10 expression promotes the asymmetric division of mature NECs to form RGCs (Raballo et al., 2000; Storm et al., 2006; Sahara and O’Leary, 2009). Such nuanced FGF expression, FGF2 in particular, is accompanied by the presence of HSPGs as early as embryonic day 9 (E9) in murine neuroepithelial tissue (Nurcombe et al., 1993). Importantly, the functional properties of neuroepithelial HS were observed to change as embryogenesis progressed, in synchrony with changes in FGF expression. While HS derived from E9 tissue was able to bind FGF2 effectively in comparison to FGF1, a shift in binding affinity toward FGF1, rather than FGF2, was found in HS from E11. Such an alteration in binding affinity at E11 was attributed to compositional variations of HS, including longer chain length, greater number of sulfated domains and increased 6-O-sulfation in comparison to E9 chains (Brickman et al., 1998). Furthermore, a single core protein species was detected in both E9 and E11 neuroepithelial cultures, suggesting that HS chains on an individual PG may be rapidly modulated to complement, or even augment, the action of signaling molecules that regulate distinct NEC phenotypes (Nurcombe et al., 1993).
Several aspects of NEC behavior, including quiescence, responses to morphogen gradients, migration, self-renewal, and the generation of RGCs capable of differentiating, are regulated by HS-binding signaling factors. Moreover, distinct HSPGs appear to mediate such cellular events within the developing CNS. Perlecan has been identified to promote NSC proliferation by providing HS chains that function as co-receptors for FGF2 signaling, and also operate as an important component of the neural tube basement membrane (Joseph et al., 1996; Haubst et al., 2006; Girós et al., 2007). The presence of several membrane-bound syndecans has also been determined as important for neurogenesis. At E10, all 4 syndecans were found on the surface of NECs, with syndecan-1 mRNA expressed at the highest level (Ford-Perriss et al., 2003). Knockdown of syndecan-1 has been observed to attenuate canonical WNT signaling in NECs and RGCs, and severely diminish the proliferative capacity of these cells (Wang et al., 2012). This suggests that syndecan-1 normally functions to modulate proliferation-associated signaling cascades in early CNS development, much like syndecan-4, which has also been described to regulate proliferation during zebrafish neurogenesis (Luo et al., 2016). Additionally, syndecan-3 has been reported to facilitate neuroblast migration by triggering actin cytoskeletal changes. HS-dependent interactions with pleiotrophin and GDNF activate key intracellular signaling proteins, such as SRC kinase, and induce morphological changes required for cell motility (Raulo et al., 1994; Bespalov et al., 2011).
In addition to the syndecans, Ford-Perriss and colleagues also reported mRNA expression of most of the glypicans in E10 murine neuroepithelial tissue, with the exception of glypican-5 (Ford-Perriss et al., 2003). While knowledge regarding the exact functions of glypicans during neurogenesis remains limited, some studies have suggested roles in regulating stem cell proliferation, presumably via associations with FGFs. A loss of glypican-1 and corresponding FGF17 interactions in homozygous-null murine mutant embryos has been observed to hamper the proliferation of neuronal precursors and induce premature differentiation around E9, leading to a reduction in brain size (Jen et al., 2009). Maintenance of a proliferative stem cell phenotype has also been shown to depend on appropriate glypican-4 expression and putative FGF-binding. Glypican-4 has been observed to be persistently present in NECs within the ventricular zone but downregulated in their post-mitotic progeny (Hagihara et al., 2000). Although the mechanisms regulating such spatiotemporal variations in HSPG expression are yet to be elucidated, dynamic PG patterns seem to be hallmarks of neurogenesis, where distinct PG combinations function to fine-tune NEC responses at each stage of the developmental process. Such findings have led to the identification of particular HSPGs as potential biomarkers for neural lineage commitment, and have also suggested therapeutic targeting of these HSPGs for regulating neural stem cell function (Oikari et al., 2016; Yu et al., 2017).
HSPGs as Regulators of Adult Stem Cell and Progenitor Functions
The role of HSPGs in adult stem cell (AdSC) populations is less well understood compared to ESCs and their progeny, primarily because such analysis is dependent on the ability to identify and purify tissue-specific stem cells. While some AdSC populations have been relatively easy to access for cell isolation, others remain elusive.
Haematopoietic Stem Cells and Progenitors
Studies investigating the presence and role of polysaccharides in the bone marrow stroma gained momentum during the 1970s; a 1978 study demonstrated that GAGs or mucopolysaccharides could influence in vitro erythrocytic proliferation and differentiation (Ploemacher et al., 1978). Other investigations detected the presence of sulfated GAGs in the bone marrow stroma and suggested a role for stromal cells in producing them to support haematopoiesis (McCuskey and Meineke, 1973; Del Rosso et al., 1981). Simultaneous inquiry into the conditions required for long-term culture of HSCs and their progenitors (HPCs) revealed the dependence of these cells on adhesion with mesenchymal stromal cell feeder layers (Dexter et al., 1977; Reimann and Burger, 1979; Gartner and Kaplan, 1980; Bentley and Tralka, 1983), further endorsing the idea of stroma-derived HS as a putative mediator of cell–cell and cell–matrix interactions required for HSC survival and function.
The significance of stromal cell HS for in vitro haematopoiesis was confirmed when HS was found to be enriched in extracts of stromal feeder cells of long-term haematopoietic cultures and haematopoiesis-supportive bone marrow stromal cell lines (Gallagher et al., 1983; Wight et al., 1986; Kirby and Bentley, 1987). In a study by Gallagher et al. (1983), high proportions of disaccharide and tetrasaccharide species obtained by nitrous acid digestion of stromal HS demonstrated the presence of N-sulfate rich domains. Oligosaccharide mapping revealed that HS produced by cultured bone marrow stromal cells is enriched in highly sulfated disaccharides, indicating extensive protein-binding properties (Turnbull and Gallagher, 1988). Furthermore, work from our group has shown that in the presence of pro-haematopoietic cytokines, an exogenous 6-O-sulfate-rich bone marrow stromal cell-derived HS variant is capable of maintaining a subset of primitive HSCs during ex vivo expansion. These cells exhibit improved clonogenicity and an increased propensity to form erythroid and granulocyte progenitors, highlighting the capacity of 6-O-sulfate-rich HS fragments to enhance HSC properties (Bramono et al., 2011). Other studies have detailed the role of extracellular HS in inducing differentiation of naïve HPC-like cells. For example, HL-60 promyelocytic leukemia cells were observed to acquire a mature phenotype when grown on marrow stroma-derived matrix, which was abrogated upon heparinase treatment (Luikart et al., 1990). Moreover, the pro-differentiation effect was recapitulated when exogenous heparin was added to the cultures. The ability of HS/heparin in trans to guide HPC fate was similarly exhibited, when addition of HS to megakaryocyte progenitor cultures augmented megakaryocytopoiesis (Han et al., 1996).
To better understand the function of stromal HS during haematopoiesis, several studies investigated HS binding to bone marrow-relevant signaling molecules. Gordon and colleagues found that stroma-derived GAGs, of which HS was a major constituent, were capable of binding to granulocyte-macrophage colony stimulating factor (GM-CSF), while negligible binding was observed between liver-derived GAGs and GM-CSF (Gordon et al., 1987). They posited that this selective binding was due to stroma-specific structural features of GAGs that enabled compartmentalization and appropriate presentation of signaling molecules important for HPC function. In addition to GM-CSF, stroma-derived HS was also capable of binding interleukin (IL)-3, a key multilineage haematopoietic signaling factor (Roberts et al., 1988). Subsequently, various heparin/HS-binding proteins were found in the bone marrow stroma including GFs, chemokines as well as morphogenetic proteins such as BMPs, WNT, and SHH (reviewed in Papy-Garcia and Albanese, 2017).
In addition to its involvement in signaling events, HS produced by the bone marrow stroma was also found to be involved in the adhesion of HPCs and HSCs to stromal cells. Treating HPC-stromal cell cultures with sodium borohydride was observed to strip HS from cell-surface protein cores and destroy cell–cell binding activity (Siczkowski et al., 1992). De-sulfation of HSPGs as a result of sodium chlorate treatment was also found to decrease HPC adhesion to stromal feeder cells (Zweegman et al., 2004). Apart from adhesion, HSPGs in the marrow stroma are important participants in CXCL12/CXCR4-dependent cascades that orchestrate HPC migration, homing and retention, especially after transplantation. Netelenbos and colleagues conducted a study where subendothelial matrices produced by bone marrow endothelial cells were used to coat filters in a transwell migration assay using HPCs (Netelenbos et al., 2002). Cells were observed to migrate through the filters toward CXCL12 (stromal cell-derived factor-1; SDF-1)-enriched media, which did not occur in the absence of CXCL12. Importantly, HPC migration was significantly reduced when the matrix filter was treated with heparinases. This indicated that subendothelial ECM-associated HS was essential for trans-matrix migration in response to CXCL12 and is likely involved in CXCL12 presentation. A follow-up paper by the same group demonstrated that HS produced by bone marrow endothelial cells binds to cell surface CXCL12 in an autocrine manner, and assists in its interactions with CXCR4 on migrating HPCs (Netelenbos et al., 2003).
While the requirement of stromal HS in mediating HSC and HPC function has been well established, the significance of specific sequence motifs and particular PG core proteins remains to be understood. In-depth compositional analysis of stroma-derived HS coupled with gene expression profiling of HSPG core proteins in stromal cells may provide preliminary insights. Nonetheless, studies exploring the significance of HS in HSC and HPC function has progressed at a rapid pace, whereas evidence of the roles of HS in other marrow resident progenitor functions has unfolded more slowly. An important factor that has facilitated thorough investigations of HSC properties is a well-defined, robust in vitro culture system. This highlights the need for similar approaches to be adopted to further our understanding of other tissue-specific stem cell types that may be less amenable to ex vivo expansion.
Mesenchymal Stem Cells
Mesenchymal stem cells (MSCs) were initially described as marrow-derived proliferative, colony-forming and plastic adherent cells (Friedenstein et al., 1974). Subsequent studies led by Caplan’s group to better characterize these cells led to the discovery of their multilineage potency: they were able to form osteoblasts, chondrocytes and adipocytes in vitro and when transplanted in vivo (Osdoby and Caplan, 1976; Caplan, 1991). Although the in situ identity and function of MSCs has remained controversial, these cells continue to be widely studied and are valuable tools for use in cell therapy applications, especially by virtue of their immunomodulatory secretome (Weiss and Dahlke, 2019).
An important challenge for the successful clinical use of MSCs is their inherent heterogeneity, particularly after culture expansion. MSCs have been reported to show variability in both surface marker expression and function, including biased differentiation potential and clonogenic capacity, depending on their tissue of origin and/or culture conditions (da Silva Meirelles et al., 2006; Traktuev et al., 2008; Hagmann et al., 2013; Holley et al., 2015; Elabd et al., 2018). While transcriptomic approaches have dominated the toolkit for MSC analysis, glycomic analysis and GAG profiling of MSCs are yet to be adopted for more comprehensive characterization. As discussed earlier (see section “Heterogeneity in HSPG Composition and Localization Can Influence Stem Cell Fate”), heterogeneity in HS composition can influence stem cell fate decisions. Owing to the significant tissue-dependent differences observed in MSC functional properties, it is highly likely that these are accompanied by distinct tissue-specific HS signatures. Therefore, we deem it prudent to investigate the status of HSPGs, as well as other GAGs/PGs, in MSCs.
Together with transcriptomic analysis, glycomic data will allow for a better understanding of the mechanisms related to the functional heterogeneity of these cells. Furthermore, knowledge regarding the roles of particular HS signatures in mediating MSC properties will allow for the synthesis of desirable HS adjuvants to culture media (see section “Exploiting the HSPG-Stem Cell Relationship for Therapeutic Use: HS as an Adjuvant for the Expansion of Potent MSCs”). This approach may permit the development of improved expansion regimens, wherein tailored HS oligosaccharides are capable of maintaining reservoirs of appropriate GFs and other signaling molecules in the ECM for better control of MSC fate in vitro.
Osteogenic and Chondrogenic Progenitors
Osteogenic and chondrogenic differentiation have been widely studied in vitro. Both trajectories involve drastic changes in the composition of the ECM, with the laying down of a mineralized matrix being a hallmark of osteogenesis (Assis-Ribas et al., 2018). Importantly, alterations in the expression of genes encoding PG core proteins and HS biosynthetic machinery have been reported during osteogenesis (Haupt et al., 2009). Pre-osteoblasts cultured in osteogenic media were observed to increase the mRNA and protein levels of several PG core proteins as they matured. While glypican-3 displayed the most significant increase, followed by all 4 syndecans, glypican-2 and glypican-4 showed moderate increases. There were also variations in gene expression levels of some biosynthetic enzymes: a decrease in Ext1/2 gene expression was noted, with contrasting increases in Ndst1/2, Hs2st1, and Hs6st1 as differentiation progressed.
A study from Zhao and colleagues also reported an upregulation of 6OST3 at the mRNA and protein levels as MSCs underwent calcification (Zhao et al., 2015). Collectively, data from these studies indicate that as pre-osteoblasts or MSCs progress through osteogenesis, HS chain initiation may decrease while overall chain sulfation may be increased. Moreover, siRNA knockdown of glypican-3 or 6OST3 was found to decrease differentiation down the osteogenic lineage (Haupt et al., 2009; Zhao et al., 2015), suggesting the necessity of unique HSPG motifs for progression through the lineage commitment process. Further investigations into the functional features of unique HS/heparin signatures in osteogenesis have demonstrated distinct binding properties for proteins that mediate important pro-differentiation signaling cascades. For example, bone marrow-derived HS is able to bind with high affinity to, and enhance the osteoinductivity of BMP2 (Bramono et al., 2012). In another study, highly sulfated heparin was found to bind WNT3A with high affinity and promote the osteogenic activity of pre-osteoblasts (Ling et al., 2010). To improve our understanding of how the heparanome may be altered during osteogenesis and the resulting functional effects this may have on stem cell differentiation, additional studies may be undertaken where HS pools are extracted from cultures at various stages of the osteogenic process. Subsequent HS disaccharide isolation and characterization would provide information regarding the compositional features of HS during differentiation, and also offer evidence to complement existing gene expression data for biosynthetic enzymes.
The role of HS as an important regulator of chondrogenesis was first demonstrated in 1987 using micromass cultures of chick limb bud mesenchyme (San Antonio et al., 1987). Addition of either exogenous HS or heparin was found to enhance chondrogenesis and cartilage nodule formation in a dose-dependent manner. The involvement of HSPG core proteins in chondrogenesis has also been demonstrated in vitro (Gomes et al., 2004). The ability of perlecan to induce chondrogenesis was tested by plating MSCs on surfaces coated with intact perlecan or recombinant perlecan domain 1. Plated cells were found to attach and aggregate into dense cell condensations, and proceed to express chondrogenic differentiation markers such as collagen type II and aggrecan. This phenotype was not obtained with cells plated with other PGs or perlecan domains, suggesting that only certain HSPGs possess structural features that support chondrogenesis. Other groups have further explored the role of HS in chondrogenesis primarily by assessing the effects of exogenous HS on chick limb mesenchymal cells (LMCs) or MSC cultures. Addition of HS or BMP2 alone to LMC cultures led to only a moderate improvement of Alcian blue staining (Fisher et al., 2006). However, the addition of HS and BMP2 together significantly accentuated staining compared to individual treatments. The presence of HS was able to potentiate the activity of BMP2, as indicated by increased phosphorylation of SMAD proteins, and lowered the concentration required to stimulate chondrogenesis to an equal or even greater extent as compared to high concentrations of BMP2 alone. Similarly, combined exposure of HS and TGF-β3 resulted in higher cartilage-specific gene expression levels and even induced remodeling of the surrounding microenvironment by increasing cartilage matrix protein production compared to TGF-β3-only or HS-only treatments (Chen et al., 2016).
While the significance of HSPGs in mediating chondrogenesis has been documented mainly through examining the effects of exogenous HSPGs on differentiation, the heparanome status of the stem cells themselves during chondrogenic lineage commitment is unknown. Gene expression analysis of biosynthetic enzymes coupled with glycomic profiling of cultures undergoing chondrogenesis would strengthen our knowledge of how HSPGs may influence this differentiation process. Furthermore, such data would provide a basis for subsequent mechanistic studies that probe into the functional attributes of the heparanome as chondrogenesis ensues.
Muscle Satellite Cells and Myoblasts
Satellite cells (MuSCs) are stem cells within skeletal muscle that are normally quiescent within a specialized niche located between the plasma membrane and basement membrane of myofibres (Mauro, 1961). They exit quiescence, resume cell cycle activity and acquire a myoblast identity for muscle regeneration, in response to triggers such as exercise or injury. A fine balance of signaling events is essential for an appropriate MuSC response. Of the many families of signaling factors, FGFs emerged as key participants in mediating MuSC activity (Pawlikowski et al., 2017). Studies in the 1980s showed that exposure of muscle progenitors to FGF1 or FGF2 resulted in strong mitogenic effects and concomitant repression of terminal differentiation (Lathrop et al., 1985; Clegg et al., 1987). FGF deprivation of cells that were initially exposed resulted in withdrawal from the cell cycle and simultaneous expression of myosin heavy chain. The indication of FGFs as inhibitors of myogenic differentiation was further corroborated when it was shown that exposure of myoblasts to FGF also inhibited myogenin expression (Brunetti and Goldfine, 1990). Not long after, the integral role of HS, and their 6-O-sulfated residues in particular, in facilitating FGF signaling in MuSCs became evident. Rapraeger and colleagues demonstrated that treatment of MM14 cultures with either sodium chlorate or heparinase abolished FGF signaling (Rapraeger et al., 1991). Cells ceased proliferation and stained positive for myosin heavy chain, recapitulating results obtained upon FGF deprivation (Lathrop et al., 1985; Clegg et al., 1987). In a follow up study, the group reported that the addition of heparin to chlorate-treated cultures rescued FGF signaling, further highlighting the role of HS in mediating FGF-dependent repression of myogenic differentiation (Olwin and Rapraeger, 1992).
The use of the MM14 culture system has provided information on another key feature of myogenesis, which is a change in the expression of myoblast HSPG core proteins. Analysis of MM14 PGs showed a decrease in syndecan expression in differentiated cells compared to proliferative counterparts (Olwin and Rapraeger, 1992). A decrease in syndecan-3 expression along with perlecan, at both protein and mRNA levels, was also observed as C2C12 myoblasts differentiated into myotubes (Larraín et al., 1997; Fuentealba et al., 1999). Inhibition or genetic ablation of syndecan-3 expression in myoblasts or MuSCs drastically decreases sensitivity to FGF2-dependent inhibition of myogenesis, and also precludes signaling via the Notch pathway (Fuentealba et al., 1999; Pisconti et al., 2010). As a result, cells undergo reduced self-renewal and precocious acquisition of a differentiated phenotype, which can be reversed by exogenous heparin. In contrast to syndecan and perlecan, glypican-1 expression has been observed to be maintained both on the cell surface and as a secreted form in the ECM throughout the myogenic process (Campos et al., 1993; Brandan et al., 1996). Localization of glypican-1 in lipid rafts at the cell membrane was found to be an important mechanism that sequesters FGF2, prevents interactions with FGFRs and inhibits myoblast differentiation. A deficiency in glypican-1 was unable to restrict FGF:FGFR interactions and led to defective differentiation of C2C12 cells (Gutierrez and Brandan, 2010). Collectively, these results indicate that intricate spatiotemporal variation and control of HSPG expression in vitro is pivotal in modulating FGF signaling for myogenic activity.
Further investigations of the roles of HSPGs in myogenesis have extended such in vitro findings and shown that heterogeneity in core protein expression in vivo is crucial during muscle development and in adult skeletal muscle. While syndecans-1, -3, and -4 were found to be enriched in developing mouse skeletal muscle tissue, only syndecan-3 and syndecan-4 expression persisted in adult muscle, colocalized with FGFR1 (Cornelison et al., 2001; Olguin and Brandan, 2001). Cornelison and colleagues observed that the expression of both these FGFR-associated syndecans overlapped with c-MET, a key MuSC marker, suggesting a role for HSPG-mediated signaling in MuSC function in vivo. Moreover, treatment of adult myofibre explant cultures with sodium chlorate led to a significant decrease in MuSC numbers, and an associated increase in the percentage of cells expressing MyoD (Cornelison et al., 2001). Thus, a switch from MuSC proliferation to differentiation could be triggered in response to disruption of HS-dependent FGF signaling in MuSCs. The significance of specific patterns of HSPG expression in adult skeletal muscle was further supported by a study conducted by Casar and colleagues. They detected widespread HSPG expression by immunostaining [using the 3G10 monoclonal antibody (David et al., 1992)] in the myofibres of healthy mouse muscle (Casar et al., 2004). Following injury, an increased 3G10 signal was detected in the regenerating myotubes. Subsequent analysis of upregulated HSPGs identified syndecan-3, syndecan-4, glypican, and perlecan, with syndecan-3 upregulated the earliest in regenerating structures. In comparison, dysregulated HSPG expression, as in the case of syndecan-3–/– and syndecan-4–/– mice, severely impacted the ability to maintain homeostasis in the myogenic tissue and activate regenerative programs upon injury (Cornelison et al., 2004).
Apart from dynamic PG core protein expression patterns, variability in HS biosynthetic enzyme expression has also been identified in MuSCs. Langsdorf and colleagues found that only SULF1 was expressed, both at protein and mRNA levels, in quiescent mouse MuSCs. However, following muscle injury, a shift in Sulf expression was observed such that both SULF1 and SULF2 were detected in Pax7-positive MuSCs. To investigate the role of the SULF enzymes in myofibre regeneration, the group went on to characterize Sulf double mutant mice (Langsdorf et al., 2007; Tran et al., 2012). In the absence of Sulf1 and Sulf2, the animals displayed an acutely delayed and compromised ability to regenerate muscle. GAG disaccharide analysis revealed that the lack of SULF enzymes led to a significant increase in 6-O-sulfation, with a notable rise in trisulfated disaccharide levels. Such an overtly sulfated HS signature was associated with an overactivation of the FGF pathway as well as an elevation in non-canonical WNT signaling, leading to an inhibition of differentiation and myoblast fusion (Langsdorf et al., 2007; Tran et al., 2012). These results identified SULF proteins as repressors of inhibitory FGF signaling in activated MuSCs and important gatekeepers of HS composition.
Although specific patterns of PG and biosynthetic enzyme expression seem to be important for myogenic activity during development and regeneration, the significance of specific HS domain structures and sulfation patterns in mediating these processes is largely unknown. Future studies focused on glycomic characterization are required for a better mechanistic understanding of the functions of HS in regulating myoblast or MuSC fate.
Skin Stem Cells and Progenitors
Homeostasis within the skin layers and its appendages is maintained by several distinct stem cell and progenitor populations. The epidermal basal layer consists of rapidly diving stem cell progenitors (transit-amplifying cells) that work to continually replenish the epidermal layers (Potten and Morris, 1988; Fuchs and Raghavan, 2002). In response to appropriate triggers, these cells lose their attachment, both to one another as well as the underlying basement membrane (BM), and differentiate into keratinocytes (KCs) destined for stratification (Barrandon and Green, 1987; Blanpain and Fuchs, 2009). The involvement of HS and its PGs in the functional fidelity of KCs and the structural integrity of the skin BM has been an important area of research.
The role of HSPGs as key regulators of signaling is conserved in epidermal progenitors, as in the case of other previously discussed AdSC types. Several studies have highlighted the necessity of HS-binding GFs, including FGFs, GM-CSF and TGF-β, for the control of basal layer progenitor proliferation as well as differentiation (Mansbridge and Hanawalt, 1988; Finch et al., 1989; Szabowski et al., 2000; Zhu et al., 2014). The expression of HSPG core proteins has also been well established within the epidermis as well as along the entire length of the dermo-epidermal junction in the BM (Hassell et al., 1980; Caughman et al., 1987; Horiguchi et al., 1989). Importantly, the patterns of core protein expression within the epidermis are dynamic and have been found to change as KCs progress through terminal differentiation. Syndecan-1 expression was reported as modest in the basal layer, enriched in the suprabasal layer, and absent in superficial layers (Sanderson et al., 1992). This trend in syndecan-1 expression was recapitulated when stratification of KC monolayers was induced in vitro. The purified syndecan-1 from stratified samples was found to be more abundant than in monolayer controls and also had a lower molecular mass, which was attributed to differences in the composition of HS chains (Sanderson et al., 1992).
Unlike the syndecans, the expression patterns of glypicans are less well known in the epidermis. A study from 2006 utilized an immunohistochemical staining approach and detected diffused glypican-1 expression throughout the epidermis, while glypican-3 appeared restricted to the basal layer (Patterson et al., 2008). These results collectively indicate that unique profiles of HSPGs at different stages of KC differentiation serve specific functions in mediating cell fate decisions. In this regard, Sanderson et al. (1992) suggested that changes in HSPG composition are essential to facilitate the drastic alterations in KC adhesive properties required for detachment from the basal layer and subsequent migration. Likewise, the presence of HSPGs, such as perlecan, within the ECM has been found to heavily influence KC migration and organization. In a study by Sher et al. (2006), perlecan was detected in all epidermal layers apart from the stratum corneum when KCs were seeded on dermal equivalents. However, upon transfecting a perlecan antisense construct into KCs, differentiation was significantly altered. The cells formed an aberrantly organized epidermis that was only 1–2 layers thick. Notably, exogenous perlecan addition rescued the ability of KCs to form a well-differentiation, stratified epidermis.
Apart from the epidermis, HSPGs, syndecans in particular, have been identified in stem cell-rich hair follicles (HFs) (Couchman, 1993). To elucidate the role of HS in the HF, Coulson-Thomas et al. (2014) characterized a transgenic mouse model that was devoid of Ext1 only in the outer root sheath (ORS), inner root sheath (IRS) and hair shaft. They observed a significantly increased number of HFs in transgenic mice compared to littermate controls. These HFs were observed to be arrested at anagen, unable to transition into catagen and telogen. Subsequent analysis revealed that cells within these HFs had impaired differentiation potential, due to uncontrolled signaling involving SHH. Thus, in the absence of HS, control of the intricate molecular networks that preside over stem cell fate decisions during HF cycling and homeostasis is severely perturbed. Widespread HSPG expression has also been observed in dermal papillae (DP), important stem cell-containing signaling centers at the base of HFs that regulate epithelial stem cells and keratinocytes for HF cycling (Driskell et al., 2011; Morgan, 2014). Notably, HSPG expression patterns have been found to be spatiotemporally variable in the DP matrix and the adjacent ORS (contiguous with the epidermal BM) throughout HF cycle stages, except for telogen (Couchman, 1986; Westgate et al., 1991; Couchman, 1993). While perlecan expression persisted in both the BM and DP, syndecans localized at different positions during the HF cycle (Kaplan and Holbrook, 1994). During anagen, syndecan-1 is strongly detected in the ORS of the HF and to a lesser extent in DP (Malgouries et al., 2008). As follicles proceed through catagen, syndecan-1 levels diminish in the ORS, below that within the DP (Bayer-Garner et al., 2002).
Stem cell populations within the epidermis, HF and DP appear to share a commonality in that distinct PG core protein expression patterns appear to correlate with specific stem cell responses and fate decisions. However, the role of HS chains attached to these proteins and their functional significance is currently unknown. The extraction of HS from epidermal tissue and subsequent structural analysis may provide preliminary insights into the overall status of the epidermal heparanome, and may also help identify sequence motifs that impart protein-binding properties.
Cancer Stem Cells
Dysregulated HSPG biosynthesis and aberrant chain modifications have been documented in several solid tumors and hematological malignancies. Changes in HS sulfation patterns due to alterations in expression of the sulphotransferases and SULF enzymes, coupled with increased cleavage by HPSE, have long been implicated in key tumorigenic events (Vlodavsky et al., 2007; Rosen and Lemjabbar-Alaoui, 2010; Hammond et al., 2014; Nagarajan et al., 2018). Increased localization of HS binding signaling factors and ECM remodeling events (such as breakdown of HSPGs in the basement membrane) are known to underpin tumor proliferation, angiogenesis and metastasis (Knelson et al., 2014). Research over the last decade has also uncovered vital roles for HSPGs in mediating cancer stem cell (CSC) function.
CSCs have been described to fuel tumor growth and heterogeneity due to their highly proliferative, phenotypically plastic, and treatment-resistant nature (Plaks et al., 2015; Batlle and Clevers, 2017). Several HSPG signatures have been identified to promote CSC survival and oncogenic properties. For example, upon profiling triple negative inflammatory breast CSCs, Ibrahim, and colleagues found significantly high levels of syndecan-1 mRNA and protein levels (Ibrahim et al., 2017). Notably, siRNA knockdown of syndecan-1 was observed to directly affect CSC survival and decrease the pool of available cells. These knockdown cells were unable to efficiently form colonies and spheroids, highlighting a perturbation in their self-renewal capacity. Such a dependency on syndecan-1 for maintaining oncogenic CSC activity was also observed in murine mammary glands (Liu et al., 2004). Overexpression of Wnt1 failed to trigger the accumulation of mammary CSCs and progenitors for oncogenic transformation in syndecan-1-null mice, whereas an enrichment in the progenitor population and tumor initiation were induced in syndecan-1 expressing counterparts. A follow up study showed that the tumor resistance conferred by the absence of syndecan-1 extended beyond the mammary glands in null mice and was a multi-organ effect (McDermott et al., 2007). While this evidence clearly indicates an involvement of syndecan-1 in augmenting the tumorigenic properties of CSCs, it is unclear whether this phenomenon relates to the properties of the HS chains they carry. Further investigation is required to establish if syndecan-1-dependent enhancement of CSC activity is an HS-independent feature or not.
Although syndecan-1 may be important for tumorigenesis in certain tumor types, patterns of HSPG expression in CSC populations appear to be contextual and tissue-specific. For example, decreases in syndecan-1 expression have been observed to correlate with epithelial-mesenchymal transitions (EMT) and poorly differentiated phenotypes in colon cancer (Hashimoto et al., 2008). To understand the significance of syndecan-1 in colon CSCs, Kumar Katakam et al. (2020) performed an siRNA knockdown study. Silenced cells showed enhanced expression of stemness markers, such as SOX2 and NANOG, an accentuated ability to self-renew and a greater tendency to undergo EMT compared to control cells. Furthermore, it was observed that dampened syndecan-1 levels in the knockdown cells were accompanied by a concomitant rise in WNT signaling and expression of downstream transcription factors of the TCF/LEF family, boosting the CSC phenotype. Attenuated HSPG expression in colon CSCs was thus sufficient to induce pro-oncogenic features and trigger associated signaling events. In a follow-up study, the group also found that HPSE expression was elevated in syndecan-1 depleted colon cancer cells (Katakam et al., 2020). These cells exhibited enhanced stem cell properties including sphere formation capacity and strong expression of stemness markers. While such observations suggest the involvement of HS remodeling mechanisms in tumor progression, accompanying analyses at the level of HS compositional characterization and associated bioactivity will be essential to confirm this, and to discern whether particular glycan signatures are associated with enhanced CSC function. Apart from the syndecans, variations in CSC glypican expression have also been reported. Enriched glypican-4 expression has been identified in chemotherapy-resistant pancreatic CSCs (Cao et al., 2018). Knockdown of glypican-4 resulted in a dramatic decrease in stemness marker expression (such as OCT4, SOX2, and NANOG) in these cells and increased their vulnerability to 5-fluorouracil, a chemotherapeutic agent. Moreover, glypican-4 knockdown cells exhibited suppressed WNT signaling and a decreased level of nuclear β-catenin. This highlights a dependence on the expression of particular HSPGs for the progression of important signaling cascades that accentuate stemness features in CSCs.
In addition to the HSPG core proteins, the significance of unique HS fragments in mediating CSC responses has also emerged as an important area of research, mainly through investigating the effects of exogenous HS on CSC properties. Patel et al. (2016) adopted a screening strategy to test the effects of treating CSCs with a library of heparin/HS oligosaccharides of varying chain lengths. These oligosaccharides shared a common repeating disaccharide structure comprising a 2-O-sulfated iduronic acid residue linked to a glucosamine residue with sulfate moieties at the C6 and N positions (i.e., IdoA2S-GlcNS6S). The group discovered a chain length-dependent inhibition of self-renewal in CSCs across a variety of tumor cell lines. Spheroid growth was inhibited upon exposure to HS chains from dp6 to dp12, while longer and shorter chains were ineffective. Importantly, the dp6 oligosaccharide was the most potent and treatment was found to induce a decrease in the expression of CSC markers CD44 and LGRF5. The group found that these effects on CSCs were brought about by a dp6-dependent activation of a specific isoform of p38, a stress-activated mitogen activated protein kinase (MAPK) with tumor suppressive functions. Although the mechanistic details of this interaction are yet to be fully elucidated and the functional properties of the HS fragments require validation, the results from this study offer important insights into the roles of HS oligosaccharides as anti-cancer therapy agents, depending on their compositional signatures.
Examining how chain attributes, such as length, domain organization, sulfate modifications and protein binding sites, may be tailored for the synthesis of HS oligosaccharides with desirable therapeutic properties holds immense promise in expanding the scope of glycotherapeutics. Moreover, modulating the expression levels and localization of HSPG core proteins, and utilizing methods to enhance or inhibit HS function may be adopted as potential approaches to regulate the heparanome of CSCs and consequently, ameliorate their aberrant behavior.
Experimental Methods to Strengthen Our Understanding of the HSPG-Stem Cell Relationship
Current knowledge regarding the specific compositional and associated functional features of HSPGs in mediating AdSC fate is limited, with unaddressed gaps in mechanistic understanding and restricted clinical relevance. To acquire a holistic appreciation of the HSPG-stem cell relationship, future studies may adopt a multi-omics approach that examines the heparanome of different stem cell types using a combination of glycomic, genomic, chemical, and proteomic methods. Thorough HS compositional profiling would be useful for uncovering distinct glycomic signatures that may predominate during differentiation down a particular lineage. Employing liquid chromatography-tandem mass spectrometry (LC-MS/MS) would allow for the necessary identification of fine structural features of HS and associated temporal changes, with a high level of sensitivity and accuracy (Zaia, 2013). Additionally, perturbation of HS structure and subsequent loss-of-function effects in differentiating stem cells may be investigated to identify the required structural nuances and roles of HSPGs at distinct time-points during lineage commitment.
Apart from traditional genetic manipulation approaches, gene editing through drug-inducible CRISPR/Cas9 systems may be utilized to knockout key HS biosynthetic and regulatory enzymes or PG core proteins (Cao et al., 2016; Sun N. et al., 2019). The loss of such key HSPG-related proteins at specific stages of differentiation will provide insights into the relationship between the HSPG profiles of progenitors or stem cells and their differentiation status. A range of chemical methods have also been developed to inhibit HS function in vitro, including the use of sodium chlorate, xylosides, and surfen (Humphries and Silbert, 1988; Garud et al., 2008; Weiss et al., 2015). Xylosides serve as primers for HS chain synthesis, while fluoroxylosides prevent chain elongation and effectively inhibit HS and CS/DS biosynthesis (Garud et al., 2008). In a 2018 study, Huang and colleagues investigated effects of the heparin/heparan sulfate antagonist surfen (bis-2-methyl-4-amino-quinolyl-6-carbamide) (Weiss et al., 2015) on HS function in Oct4-GFP and Sox1-GFP mESC reporter lines (Huang et al., 2018). Treated cells showed persistent pluripotency and an inability to differentiate, iterating a requirement of HS in mediating lineage commitment decisions. Moreover, this effect was reversible through the removal of surfen, suggesting that this molecule could prove useful for the temporal inhibition of HS activity throughout various stages of differentiation in vitro (Huang et al., 2018).
Other than chemical inhibition, the most widespread method to perturb HS function is the use of heparinases isolated from bacteria (Flavobacterium heparinum). When all three enzymes are used together, HS chains are cleaved into constituent disaccharides. Whilst this approach is simple to execute in cell culture models, the rapid turnover of cell surface HS allows only for a limited period to conduct subsequent live cell experiments. Alternatively, heparinase digestion may be carried out prior to fixation of cells, followed by immunostaining and flow cytometry analysis (Ayerst et al., 2017). HS participation as a signaling co-receptor can also be inhibited through a variety of peptide or protein-based methods. The HS/heparin-binding domain of HS binding proteins may be engineered, such that GAG-protein interactions are precluded. A non-HS binding variant of BMP2 has been generated in this manner, through the replacement of the first 12 of the 17 N-terminal amino acids which constitute the heparin-binding domain (Ruppert et al., 1996; Kuo et al., 2010). Alternatively, HS-protein interactions can be hindered by ligand binding competition. For example, synthetic heparin-binding peptides competed with and prevented the attachment of cytomegalovirus envelop proteins to cell surface HS in fibroblasts (Dogra et al., 2015). Another approach involves the use of soluble decoy FGFR fusion proteins, which compete with cell surface FGFR for FGF binding and affect ternary complex formation with HS (Harding et al., 2013; Li et al., 2014). HS/heparin binding to protein partners and signaling complex formation may also be intercepted by the use of custom designed antibodies that mask the GAG binding site on the protein. Our lab has previously developed an antibody (IMB-R1) that was able to bind to FGFR1 and prevent FGFR1-heparin interactions (Ling et al., 2015).
Exploiting the HSPG-Stem Cell Relationship for Therapeutic Use: HS as an Adjuvant for the Expansion of Potent MSCs
The critical roles HS plays in mediating stem cell function in vitro and in vivo makes it an ideal candidate for use as an adjuvant in cell therapy and associated culture expansion strategies. Our own work has focused on enhancing the expansion of potent human MSCs (hMSCs) by using exogenous HS as a supplement to culture media throughout expansion. Initial studies using rat MSCs revealed that the addition of exogenous HS resulted in enhanced proliferation and enhanced osteogenic differentiation (Dombrowski et al., 2009). Importantly, this effect was potentiated through FGFR1, a mechanism that has since become an extensive focus in our studies. In 2012, we sought to understand the effects of adding exogenous HS (HS2, a murine embryonic forebrain-derived variant that displays enhanced binding affinity toward FGF2) to hMSCs during in vitro culture (Helledie et al., 2012). As with rat MSCs, addition of exogenous HS augmented hMSC proliferation, especially of subpopulations with long telomeres and high expression of multipotency markers as well as the cell surface marker STRO-1. When HS2-treated hMSCs were assessed in an animal model of a critical-sized bone defect, bone regeneration was enhanced over hMSCs which were not pre-cultured with HS2. This gave us some indication that HS, specifically the HS2 variant, could contribute to the selection and expansion of a more potent sub-population of hMSCs.
Due to the difficulties of isolating sufficient HS2 from developing mouse brains for extensive analyses, we went on to develop an affinity isolation platform that utilizes the heparin-binding domain (HBD) of proteins. HBDs were peptide synthesized and bound to streptavidin-functionalised resin through a terminal biotin molecule (Murali et al., 2013; Wang et al., 2014; Wijesinghe et al., 2017). Using such methods, we developed a peptide modeled on an HBD from within FGF2. We then fractionated commercial porcine intestinal mucosal HS over the column. The retained material, termed “HS8,” was subjected to comprehensive testing against hMSCs (Wijesinghe et al., 2017). Addition of HS8 to culture media enhanced the proliferation and colony-forming efficiency of hMSCs, much like the effects observed after the addition of HS2. HS8 enhanced the stability of FGF2 in media and led to an accumulation over time, resulting in prolonged FGFR1 and ERK1/2 phosphorylation in hMSCs treated with sub-optimal concentrations of FGF2. The affinity isolation methodology demonstrated our ability to effectively scale production of a desired HS variant, yielding larger quantities suitable for more extensive biochemical and cellular analysis, including larger animal studies. HS variants isolated in this manner proved to be amiable to gamma irradiation, without a loss of structure or function (Smith et al., 2018). This technique could be used to sterilize potential HS-based cell media supplements prior to use in stem cell expansion. We next employed a microbioreactor array to further elucidate the mechanism by which HS8 functions in the in vitro hMSC microenvironment (Titmarsh et al., 2017). Using microfluidics, various concentrations of HS8 and FGF2 were mixed and applied to hMSCs under constant flow across sequential flow cells. The addition of HS8 not only increased production of endogenous FGF2, but also facilitated release of FGF2 from the cell surface (and likely ECM), resulting in perfusion of released FGF2 into downstream flow cells. HS8 also sustained FGF2 availability over time, an effect we have observed in previous studies.
Our most recent work explored the therapeutic potential of clinically sourced hMSCs isolated from fresh bone marrow aspirates (Ling et al., 2020). Extensive analysis revealed that HS supplementation of culture media increased hMSC proliferation, telomere length, expression of desirable cell surface markers and multipotency. We hypothesize that this is a result of the expansion of a sub-population of MSCs that display a more “naïve” phenotype. MSCs were subsequently expanded in media with or without HS8 and used in two animal models of a knee osteochondral defect (rat and pig). In the rat model, MSCs cultured with HS8 demonstrated enhanced defect healing over the control groups (empty, carrier, MSCs cultured without HS8), including increased type II collagen and GAG deposition within the wound site. Using a large animal model of an osteochondral defect, the micro pig, we found that animals treated with cells cultured in HS8 showed a marked improvement in wound healing, increased collagen II and GAG deposition, and improved mechanical properties at 4 and 8 months post-surgery. Throughout these studies, there was clear indication that supplementation of HS to the in vitro MSC microenvironment leads to an expansion of an MSC subpopulation with increased potency. HS supports this potent MSC cell type at least in part through its influence as a co-receptor in the FGF2:FGFR1 signaling cascade. Indeed, the rapid expansion of MSCs to desirable quantities for cell therapies often utilizes large quantities of FGF2 and other recombinant factors, many of which are heparin-binding. For this reason, the development of HS variants for the purpose of stem cell expansion could yield greater numbers of more potent cells by promoting the secretion of autocrine factors, or by increasing the stability of decreased concentrations of the exogenous factors ordinarily supplemented into the culture media.
Apart from media supplementation, HS may be introduced into cell cultures in other formats. In a 2019 study, Treiger and colleagues developed a novel heparinoid-bovine serum albumin (BSA) bioconjugate, wherein a copper-free click reaction was used to conjugate various heparin derivatives to cyclooctyne-functionalized BSA (Trieger et al., 2019). These constructs were passively adsorbed onto tissue culture plastic surfaces and served as ECM PGs. They were found to variably sequester FGF2 based on their sulfation patterns, resulting in increased proliferation of cultured hMSCs. One benefit of such reactions and methodologies is the ability to use a generic anchoring molecule (in this case, BSA) for surface coating, whilst a library of different functional moieties may be generated and employed depending upon the required use. Surface coatings offer potential advantages over traditional supplementation in solution: firstly, a much lower quantity of HS is required to achieve a maximum surface coating. Secondly, surface coatings more effectively recapitulate the extracellular environment of a typical stem cell, as HSPGs are either found contained within the ECM, on the cell surface or immediately adjacent to it, not as free-floating HS chains in solution. Finally, the large-scale culture of hMSCs requires the use of large bioreactors, utilizing microcarriers to which hMSCs may bind and proliferate in suspension. The use of HS-coated microcarriers should greatly reduce the amounts of HS required for such culture systems over HS supplementation into the bulk media. The last of these points is yet to be investigated but could provide useful insights into the importance of HS localization during stem cell expansion in artificial environments.
Concluding Remarks
HSPGs have emerged as key mediators of stem cell function, essential for the regulation of development, homeostasis and regeneration. Early evidence from ESC studies highlighted the significance of HSPGs and their biosynthetic machinery in mediating key lineage commitment and cell fate decisions. Recent observations have indicated conservation in HSPG function and compositional regulation across AdSC types, as well as perturbation in analogous mechanisms in CSC populations. The use of HS variants as media adjuvants offers an encouraging avenue for the development of customized media formulations for bioprocessing cells suitable for clinical application. However, further investigations are required to unravel the importance of particular HSPG compositional characteristics and associated functional features in guiding the behavior of various stem cell types, especially prior to clinical adoption.
It is worth noting that in our own experience, the structure and composition of HS varies widely depending upon the source, with highly sulfated HS variants more akin to heparin. We and others have previously discussed the negative impact of long-term culture supplementation with heparin has on the molecular phenotype of MSCs (Hemeda et al., 2013; Ling et al., 2016), in addition to the negative impact heparin has on GDF5 signaling (Ayerst et al., 2017). Yet, we do not observe such phenomena with HS, a molecule which relies on smaller but distinctly heparin-like NS domains to facilitate interactions with ligands and receptors. These subtle distinctions in structure, which yield significant differences in subsequent biological events, require more exhaustive examination. Rigorous inquiry into the biochemistry of HSPGs will accelerate their development as tools and pharmacological agents to modulate stem cell responses in vitro or in vivo, and is a necessary step to be undertaken if HS is to see widespread therapeutic application. Despite this, the amalgamation of glycotherapeutics and stem cell therapy holds considerable promise in bringing forth novel strategies for regenerative medicine.
Author Contributions
MR and RS conceptualized, drafted and edited the manuscript. VN contributed to editing the manuscript. SC was invited by the journal to provide the review, revised the manuscript and approved the final version for submission. All authors contributed to the article and approved the submitted version.
Funding
This work was supported by the Industry Alignment Fund Pre-Positioning (IAF-PP) funding (H18/01/a0/021) from the Agency for Science, Technology and Research (A∗STAR), Singapore.
Conflict of Interest
The authors declare that the research was conducted in the absence of any commercial or financial relationships that could be construed as a potential conflict of interest.
Acknowledgments
We also thank A∗STAR for providing MR with support for her graduate studies through a Singapore International Graduate Award (SINGA). The authors further acknowledge support from the Institute of Medical Biology (IMB) and the Biomedical Research Council (BMRC), A∗STAR.
References
Ai, X., Do, A.-T., Lozynska, O., Kusche-Gullberg, M., Lindahl, U., and Emerson, C. P. Jr. (2003). QSulf1 remodels the 6-O sulfation states of cell surface heparan sulfate proteoglycans to promote Wnt signaling. J. Cell Biol. 162, 341–351. doi: 10.1083/jcb.200212083
Aikawa, J., and Esko, J. D. (1999). Molecular cloning and expression of a third member of the heparan sulfate/heparin GlcNAc N-deacetylase/N-sulfotransferase family. J. Biol. Chem. 274, 2690–2695. doi: 10.1074/jbc.274.5.2690
Allen, B. L., Filla, M. S., and Rapraeger, A. C. (2001). Role of heparan sulfate as a tissue-specific regulator of FGF-4 and FGF receptor recognition. J. Cell Biol. 155, 845–858. doi: 10.1083/jcb.200106075
Andres, J. L., Stanley, K., Cheifetz, S., and Massagué, J. (1989). Membrane-anchored and soluble forms of betaglycan, a polymorphic proteoglycan that binds transforming growth factor-beta. J. Cell Biol. 109, 3137–3145. doi: 10.1083/jcb.109.6.3137
Ashikari-Hada, S., Habuchi, H., Kariya, Y., Itoh, N., Reddi, A. H., and Kimata, K. (2004). Characterization of growth factor-binding structures in heparin/heparan sulfate using an octasaccharide library. J. Biol. Chem. 279, 12346–12354. doi: 10.1074/jbc.M313523200
Assis-Ribas, T., Forni, M. F., Winnischofer, S. M. B., Sogayar, M. C., and Trombetta-Lima, M. (2018). Extracellular matrix dynamics during mesenchymal stem cells differentiation. Dev. Biol. 437, 63–74. doi: 10.1016/j.ydbio.2018.03.002
Ayerst, B. I., Smith, R. A., Nurcombe, V., Day, A. J., Merry, C. L., and Cool, S. M. (2017). Growth differentiation factor 5-mediated enhancement of chondrocyte phenotype is inhibited by heparin: implications for the use of heparin in the clinic and in tissue engineering applications. Tissue Eng. Part A 23, 275–292. doi: 10.1089/ten.TEA.2016.0364
Baldwin, R. J., ten Dam, G. B., van Kuppevelt, T. H., Lacaud, G., Gallagher, J. T., Kouskoff, V., et al. (2008). A developmentally regulated heparan sulfate epitope defines a subpopulation with increased blood potential during mesodermal differentiation. Stem Cells 26, 3108–3118. doi: 10.1634/stemcells.2008-0311
Barrandon, Y., and Green, H. (1987). Three clonal types of keratinocyte with different capacities for multiplication. Proc. Natl. Acad. Sci. U.S.A. 84, 2302–2306. doi: 10.1073/pnas.84.8.2302
Bass, M. D., Williamson, Rosalind, C., Nunan, Robert, D., and Humphries, et al. (2011). A Syndecan-4 hair trigger initiates wound healing through caveolin- and RhoG-regulated integrin endocytosis. Dev. Cell 21, 681–693. doi: 10.1016/j.devcel.2011.08.007
Batlle, E., and Clevers, H. (2017). Cancer stem cells revisited. Nat. Med. 23, 1124–1134. doi: 10.1038/nm.4409
Bayer-Garner, I. B., Sanderson, R. D., and Smoller, B. R. (2002). Syndecan-1 is strongly expressed in the anagen hair follicle outer root sheath and in the dermal papilla but expression diminishes with involution of the hair follicle. Am. J. Dermatopathol. 24, 484–489. doi: 10.1097/00000372-200212000-00005
Bentley, S. A., and Tralka, T. S. (1983). Fibronectin-mediated attachment of hematopoietic cells to stromal elements in continuous bone marrow culture. Exp. Hematol. 11, 129–138.
Bernfield, M., Götte, M., Park, P. W., Reizes, O., Fitzgerald, M. L., Lincecum, J., et al. (1999). Functions of cell surface heparan sulfate proteoglycans. Annu. Rev. Biochem. 68, 729–777. doi: 10.1146/annurev.biochem.68.1.729
Bernfield, M., Hinkes, M. T., and Gallo, R. L. (1993). Developmental expression of the syndecans: possible function and regulation. Development 119, 205–212. doi: 10.1006/dbio.1997.8824
Bespalov, M. M., Sidorova, Y. A., Tumova, S., Ahonen-Bishopp, A., Magalhães, A. C., Kulesskiy, E., et al. (2011). Heparan sulfate proteoglycan syndecan-3 is a novel receptor for GDNF, neurturin, and artemin. J. Cell Biol. 192, 153–169. doi: 10.1083/jcb.201009136
Blanpain, C., and Fuchs, E. (2009). Epidermal homeostasis: a balancing act of stem cells in the skin. Nat. Rev. Mol. Cell Biol. 10, 207–217. doi: 10.1038/nrm2636
Bramono, D. S., Murali, S., Rai, B., Ling, L., Poh, W. T., Lim, Z. X., et al. (2012). Bone marrow-derived heparan sulfate potentiates the osteogenic activity of bone morphogenetic protein-2 (BMP-2). Bone 50, 954–964. doi: 10.1016/j.bone.2011.12.013
Bramono, D. S., Rider, D. A., Murali, S., Nurcombe, V., and Cool, S. M. (2011). The effect of human bone marrow stroma-derived heparan sulfate on the ex vivo expansion of human cord blood hematopoietic stem cells. Pharm. Res. 28, 1385–1394. doi: 10.1007/s11095-010-0352-y
Brandan, E., Carey, D. J., Larrain, J., Melo, F., and Campos, A. (1996). Synthesis and processing of glypican during differentiation of skeletal muscle cells. Eur. J. Cell Biol. 71, 170–176.
Brickman, Y. G., Ford, M. D., Gallagher, J. T., Nurcombe, V., Bartlett, P. F., and Turnbull, J. E. (1998). Structural modification of fibroblast growth factor-binding heparan sulfate at a determinative stage of neural development. J. Biol. Chem. 273, 4350–4359. doi: 10.1074/jbc.273.8.4350
Brown, T. A., Bouchard, T., St John, T., Wayner, E., and Carter, W. G. (1991). Human keratinocytes express a new CD44 core protein (CD44E) as a heparan-sulfate intrinsic membrane proteoglycan with additional exons. J. Cell Biol. 113, 207–221. doi: 10.1083/jcb.113.1.207
Brule, S., Charnaux, N., Sutton, A., Ledoux, D., Chaigneau, T., Saffar, L., et al. (2006). The shedding of syndecan-4 and syndecan-1 from HeLa cells and human primary macrophages is accelerated by SDF-1/CXCL12 and mediated by the matrix metalloproteinase-9. Glycobiology 16, 488–501. doi: 10.1093/glycob/cwj098
Brunetti, A., and Goldfine, I. D. (1990). Role of myogenin in myoblast differentiation and its regulation by fibroblast growth factor. J. Biol. Chem. 265, 5960–5963.
Campos, A., Nùñez, R., Koenig, C. S., Carey, D. J., and Brandan, E. (1993). A lipid-anchored heparan sulfate proteoglycan is present in the surface of differentiated skeletal muscle cells. Eur. J. Biochem. 216, 587–595. doi: 10.1111/j.1432-1033.1993.tb18178.x
Cao, J., Ma, J., Sun, L., Li, J., Qin, T., Zhou, C., et al. (2018). Targeting glypican-4 overcomes 5-FU resistance and attenuates stem cell-like properties via suppression of Wnt/beta-catenin pathway in pancreatic cancer cells. J. Cell. Biochem. 119, 9498–9512. doi: 10.1002/jcb.27266
Cao, J., Wu, L., Zhang, S. M., Lu, M., Cheung, W. K., Cai, W., et al. (2016). An easy and efficient inducible CRISPR/Cas9 platform with improved specificity for multiple gene targeting. Nucleic Acids Res. 44, e149. doi: 10.1093/nar/gkw660
Caplan, A. I. (1991). Mesenchymal stem cells. J. Orthop. Res. 9, 641–650. doi: 10.1002/jor.1100090504
Cardin, A. D., and Weintraub, H. J. (1989). Molecular modeling of protein-glycosaminoglycan interactions. Arteriosclerosis 9, 21–32. doi: 10.1161/01.ATV.9.1.21
Casar, J. C., Cabello-Verrugio, C., Olguin, H., Aldunate, R., Inestrosa, N. C., and Brandan, E. (2004). Heparan sulfate proteoglycans are increased during skeletal muscle regeneration: requirement of syndecan-3 for successful fiber formation. J. Cell Sci. 117(Pt 1), 73–84. doi: 10.1242/jcs.00828
Caughman, S. W., Krieg, T., Timpl, R., Hintner, H., and Katz, S. I. (1987). Nidogen and heparan sulfate proteoglycan: detection of newly isolated basement membrane components in normal and epidermolysis bullosa skin. J. Invest. Dermatol. 89, 547–550. doi: 10.1111/1523-1747.ep12461192
Chen, J., Wang, Y., Chen, C., Lian, C., Zhou, T., Gao, B., et al. (2016). Exogenous heparan sulfate enhances the TGF-beta3-induced chondrogenesis in human mesenchymal stem cells by activating TGF-beta/Smad signaling. Stem Cells Int. 2016:1520136. doi: 10.1155/2016/1520136
Chiang, C., Litingtung, Y., Lee, E., Young, K. E., Corden, J. L., Westphal, H., et al. (1996). Cyclopia and defective axial patterning in mice lacking Sonic hedgehog gene function. Nature 383, 407–413. doi: 10.1038/383407a0
Clegg, C. H., Linkhart, T. A., Olwin, B. B., and Hauschka, S. D. (1987). Growth factor control of skeletal muscle differentiation: commitment to terminal differentiation occurs in G1 phase and is repressed by fibroblast growth factor. J. Cell Biol. 105, 949–956. doi: 10.1083/jcb.105.2.949
Cool, S. M., and Nurcombe, V. (2006). Heparan sulfate regulation of progenitor cell fate. J. Cell. Biochem. 99, 1040–1051. doi: 10.1002/jcb.20936
Cornelison, D. D., Filla, M. S., Stanley, H. M., Rapraeger, A. C., and Olwin, B. B. (2001). Syndecan-3 and syndecan-4 specifically mark skeletal muscle satellite cells and are implicated in satellite cell maintenance and muscle regeneration. Dev. Biol. 239, 79–94. doi: 10.1006/dbio.2001.0416
Cornelison, D. D., Wilcox-Adelman, S. A., Goetinck, P. F., Rauvala, H., Rapraeger, A. C., and Olwin, B. B. (2004). Essential and separable roles for Syndecan-3 and Syndecan-4 in skeletal muscle development and regeneration. Genes Dev. 18, 2231–2236. doi: 10.1101/gad.1214204
Costell, M., Gustafsson, E., Aszódi, A., Mörgelin, M., Bloch, W., Hunziker, E., et al. (1999). Perlecan maintains the integrity of cartilage and some basement membranes. J. Cell Biol. 147, 1109–1122. doi: 10.1083/jcb.147.5.1109
Couchman, J. R. (1986). Rat hair follicle dermal papillae have an extracellular matrix containing basement membrane components. J. Invest. Dermatol. 87, 762–767. doi: 10.1111/1523-1747.ep12456955
Couchman, J. R. (1993). Hair follicle proteoglycans. J. Invest. Dermatol. 101, S60–S64. doi: 10.1016/0022-202X(93)90502-9
Couchman, J. R. (2010). Transmembrane signaling proteoglycans. Annu. Rev. Cell Dev. Biol. 26, 89–114. doi: 10.1146/annurev-cellbio-100109-104126
Coulson-Thomas, V. J., Gesteira, T. F., Esko, J., and Kao, W. (2014). Heparan sulfate regulates hair follicle and sebaceous gland morphogenesis and homeostasis. J. Biol. Chem. 289, 25211–25226. doi: 10.1074/jbc.M114.572511
da Silva, Meirelles, L., Chagastelles, P. C., and Nardi, N. B. (2006). Mesenchymal stem cells reside in virtually all post-natal organs and tissues. J. Cell Sci. 119(Pt 11), 2204–2213. doi: 10.1242/jcs.02932
David, G., Bai, X. M., Van der Schueren, B., Cassiman, J. J., and Van den Berghe, H. (1992). Developmental changes in heparan sulfate expression: in situ detection with mAbs. J. Cell Biol. 119, 961–975. doi: 10.1083/jcb.119.4.961
David, G., Lories, V., Decock, B., Marynen, P., Cassiman, J. J., and Van den Berghe, H. (1990). Molecular cloning of a phosphatidylinositol-anchored membrane heparan sulfate proteoglycan from human lung fibroblasts. J. Cell Biol. 111, 3165–3176. doi: 10.1083/jcb.111.6.3165
Del Rosso, M., Cappelletti, R., Dini, G., Fibbi, G., Vannucchi, S., Chiarugi, V., et al. (1981). Involvement of glycosaminoglycans in detachment of early myeloid precursors from bone-marrow stromal cells. Biochim. Biophys. Acta 676, 129–136. doi: 10.1016/0304-4165(81)90180-x
Dexter, T. M., Allen, T. D., and Lajtha, L. G. (1977). Conditions controlling the proliferation of haemopoietic stem cells in vitro. J. Cell. Physiol. 91, 335–344. doi: 10.1002/jcp.1040910303
Ding, K., Lopez-Burks, M., Sanchez-Duran, J. A., Korc, M., and Lander, A. D. (2005). Growth factor-induced shedding of syndecan-1 confers glypican-1 dependence on mitogenic responses of cancer cells. J. Cell Biol. 171, 729–738. doi: 10.1083/jcb.200508010
Dogra, P., Martin, E. B., Williams, A., Richardson, R. L., Foster, J. S., Hackenback, N., et al. (2015). Novel heparan sulfate-binding peptides for blocking herpesvirus entry. PLoS One 10:e0126239. doi: 10.1371/journal.pone.0126239
Dombrowski, C., Song, S. J., Chuan, P., Lim, X., Susanto, E., Sawyer, A. A., et al. (2009). Heparan sulfate mediates the proliferation and differentiation of rat mesenchymal stem cells. Stem Cells Dev. 18, 661–670. doi: 10.1089/scd.2008.0157
Dou, W., Xu, Y., Pagadala, V., Pedersen, L. C., and Liu, J. (2015). Role of deacetylase activity of N-Deacetylase/N-Sulfotransferase 1 in forming n-sulfated domain in heparan sulfate. J. Biol. Chem. 290, 20427–20437. doi: 10.1074/jbc.m115.664409
Driskell, R. R., Clavel, C., Rendl, M., and Watt, F. M. (2011). Hair follicle dermal papilla cells at a glance. J. Cell Sci. 124(Pt 8), 1179–1182. doi: 10.1242/jcs.082446
Dubey, R., van Kerkhof, P., Jordens, I., Malinauskas, T., Pusapati, G. V., McKenna, J. K., et al. (2020). R-spondins engage heparan sulfate proteoglycans to potentiate WNT signaling. eLife 9:e54469. doi: 10.7554/eLife.54469
Elabd, C., Ichim, T. E., Miller, K., Anneling, A., Grinstein, V., Vargas, V., et al. (2018). Comparing atmospheric and hypoxic cultured mesenchymal stem cell transcriptome: implication for stem cell therapies targeting intervertebral discs. J. Transl. Med. 16:222. doi: 10.1186/s12967-018-1601-9
Esko, J. D., and Lindahl, U. (2001). Molecular diversity of heparan sulfate. J. Clin. Invest. 108, 169–173. doi: 10.1172/jci200113530
Faham, S., Hileman, R. E., Fromm, J. R., Linhardt, R. J., and Rees, D. C. (1996). Heparin structure and interactions with basic fibroblast growth factor. Science 271, 1116–1120. doi: 10.1126/science.271.5252.1116
Farach-Carson, M. C., and Carson, D. D. (2007). Perlecan–a multifunctional extracellular proteoglycan scaffold. Glycobiology 17, 897–905. doi: 10.1093/glycob/cwm043
Feil, S., Valtcheva, N., and Feil, R. (2009). Inducible cre mice. Methods Mol. Biol. 530, 343–363. doi: 10.1007/978-1-59745-471-1_18
Filmus, J., and Selleck, S. B. (2001). Glypican: proteoglycans with a suprise. J. Clin. Invest. 108, 497–501.
Finch, P. W., Rubin, J. S., Miki, T., Ron, D., and Aaronson, S. A. (1989). Human KGF is FGF-related with properties of a paracrine effector of epithelial cell growth. Science 245, 752–755. doi: 10.1126/science.2475908
Fisher, M. C., Li, Y., Seghatoleslami, M. R., Dealy, C. N., and Kosher, R. A. (2006). Heparan sulfate proteoglycans including syndecan-3 modulate BMP activity during limb cartilage differentiation. Matrix Biol. 25, 27–39. doi: 10.1016/j.matbio.2005.07.008
Ford-Perriss, M., Turner, K., Guimond, S., Apedaile, A., Haubeck, H. D., Turnbull, J., et al. (2003). Localisation of specific heparan sulfate proteoglycans during the proliferative phase of brain development. Dev. Dyn. 227, 170–184. doi: 10.1002/dvdy.10298
Forsberg, E., and Kjellén, L. (2001). Heparan sulfate: lessons from knockout mice. J. Clin. Invest. 108, 175–180. doi: 10.1172/JCI13561
Forsberg, M., Holmborn, K., Kundu, S., Dagalv, A., Kjellen, L., and Forsberg-Nilsson, K. (2012). Undersulfation of heparan sulfate restricts differentiation potential of mouse embryonic stem cells. J. Biol. Chem. 287, 10853–10862. doi: 10.1074/jbc.M111.337030
Friedenstein, A. J., Chailakhyan, R. K., Latsinik, N. V., Panasyuk, A. F., and Keiliss-Borok, I. V. (1974). Stromal cells responsible for transferring the microenvironment of the hemopoietic tissues. Cloning in vitro and retransplantation in vivo. Transplantation 17, 331–340. doi: 10.1097/00007890-197404000-00001
Fuchs, E., and Raghavan, S. (2002). Getting under the skin of epidermal morphogenesis. Nat. Rev. Genet. 3, 199–209. doi: 10.1038/nrg758
Fuentealba, L., Carey, D. J., and Brandan, E. (1999). Antisense inhibition of syndecan-3 expression during skeletal muscle differentiation accelerates myogenesis through a basic fibroblast growth factor-dependent mechanism. J. Biol. Chem. 274, 37876–37884. doi: 10.1074/jbc.274.53.37876
Gallagher, J. T. (2001). Heparan sulfate: growth control with a restricted sequence menu. J. Clin. Invest. 108, 357–361. doi: 10.1172/jci13713
Gallagher, J. T., Spooncer, E., and Dexter, T. M. (1983). Role of the cellular matrix in haemopoiesis. I. Synthesis of glycosaminoglycans by mouse bone marrow cell cultures. J. Cell Sci. 63, 155–171.
Gartner, S., and Kaplan, H. S. (1980). Long-term culture of human bone marrow cells. Proc. Natl. Acad. Sci. U.S.A. 77, 4756–4759. doi: 10.1073/pnas.77.8.4756
Garud, D. R., Tran, V. M., Victor, X. V., Koketsu, M., and Kuberan, B. (2008). Inhibition of heparan sulfate and chondroitin sulfate proteoglycan biosynthesis. J. Biol. Chem. 283, 28881–28887. doi: 10.1074/jbc.m805939200
Gasimli, L., Hickey, A. M., Yang, B., Li, G., dela Rosa, M., Nairn, A. V., et al. (2014). Changes in glycosaminoglycan structure on differentiation of human embryonic stem cells towards mesoderm and endoderm lineages. Biochim. Biophys. Acta 1840, 1993–2003. doi: 10.1016/j.bbagen.2014.01.007
Girós, A., Morante, J., Gil-Sanz, C., Fairén, A., and Costell, M. (2007). Perlecan controls neurogenesis in the developing telencephalon. BMC Dev. Biol. 7:29. doi: 10.1186/1471-213x-7-29
Gomes, R. R. Jr., Farach-Carson, M. C., and Carson, D. D. (2004). Perlecan functions in chondrogenesis: insights from in vitro and in vivo models. Cells Tissues Organs 176, 79–86. doi: 10.1159/000075029
Goodger, S. J., Robinson, C. J., Murphy, K. J., Gasiunas, N., Harmer, N. J., Blundell, T. L., et al. (2008). Evidence that heparin saccharides promote FGF2 mitogenesis through two distinct mechanisms. J. Biol. Chem. 283, 13001–13008. doi: 10.1074/jbc.M704531200
Gopal, S., Bober, A., Whiteford, J. R., Multhaupt, H. A. B., Yoneda, A., and Couchman, J. R. (2010). Heparan sulfate chain valency controls syndecan-4 function in cell adhesion. J. Biol. Chem. 285, 14247–14258. doi: 10.1074/jbc.m109.056945
Gordon, M. Y., Riley, G. P., Watt, S. M., and Greaves, M. F. (1987). Compartmentalization of a haematopoietic growth factor (GM-CSF) by glycosaminoglycans in the bone marrow microenvironment. Nature 326, 403–405. doi: 10.1038/326403a0
Greene, D. K., Tumova, S., Couchman, J. R., and Woods, A. (2003). Syndecan-4 associates with alpha-actinin. J. Biol. Chem. 278, 7617–7623. doi: 10.1074/jbc.M207123200
Grobe, K., Inatani, M., Pallerla, S. R., Castagnola, J., Yamaguchi, Y., and Esko, J. D. (2005). Cerebral hypoplasia and craniofacial defects in mice lacking heparan sulfate Ndst1 gene function. Development 132, 3777–3786. doi: 10.1242/dev.01935
Guillemot, F., and Zimmer, C. (2011). From cradle to grave: the multiple roles of fibroblast growth factors in neural development. Neuron 71, 574–588. doi: 10.1016/j.neuron.2011.08.002
Guimond, S., Maccarana, M., Olwin, B. B., Lindahl, U., and Rapraeger, A. C. (1993). Activating and inhibitory heparin sequences for FGF-2 (basic FGF). Distinct requirements for FGF-1, FGF-2, and FGF-4. J. Biol. Chem. 268, 23906–23914.
Guimond, S. E., and Turnbull, J. E. (1999). Fibroblast growth factor receptor signalling is dictated by specific heparan sulphate saccharides. Curr. Biol. 9, 1343–1346. doi: 10.1016/s0960-9822(00)80060-3
Gutierrez, J., and Brandan, E. (2010). A novel mechanism of sequestering fibroblast growth factor 2 by glypican in lipid rafts, allowing skeletal muscle differentiation. Mol. Cell. Biol. 30, 1634–1649. doi: 10.1128/MCB.01164-09
Habuchi, H., Tanaka, M., Habuchi, O., Yoshida, K., Suzuki, H., Ban, K., et al. (2000). The occurrence of three isoforms of heparan sulfate 6-O-sulfotransferase having different specificities for hexuronic acid adjacent to the targeted N-sulfoglucosamine. J. Biol. Chem. 275, 2859–2868. doi: 10.1074/jbc.275.4.2859
Hagihara, K., Watanabe, K., Chun, J., and Yamaguchi, Y. (2000). Glypican-4 is an FGF2-binding heparan sulfate proteoglycan expressed in neural precursor cells. Dev. Dyn. 219, 353–367. doi: 10.1002/1097-017720009999:9999<::AID-DVDY1059>3.0.CO;2-\#
Hagmann, S., Moradi, B., Frank, S., Dreher, T., Kämmerer, P. W., Richter, W., et al. (2013). Different culture media affect growth characteristics, surface marker distribution and chondrogenic differentiation of human bone marrow-derived mesenchymal stromal cells. BMC Musculoskelet. Disord. 14:223. doi: 10.1186/1471-2474-14-223
Hagner-McWhirter, Å, Li, J.-P., Oscarson, S., and Lindahl, U. (2004). Irreversible Glucuronyl C5-epimerization in the biosynthesis of heparan sulfate. J. Biol. Chem. 279, 14631–14638. doi: 10.1074/jbc.m313760200
Halfter, W., Dong, S., Schurer, B., and Cole, G. J. (1998). Collagen XVIII is a basement membrane heparan sulfate proteoglycan. J. Biol. Chem. 273, 25404–25412. doi: 10.1074/jbc.273.39.25404
Hammond, E., Khurana, A., Shridhar, V., and Dredge, K. (2014). The role of heparanase and sulfatases in the modification of heparan sulfate proteoglycans within the tumor microenvironment and opportunities for novel cancer therapeutics. Front. Oncol. 4:195. doi: 10.3389/fonc.2014.00195
Han, Z. C., Bellucci, S., Shen, Z. X., Maffrand, J. P., Pascal, M., Petitou, M., et al. (1996). Glycosaminoglycans enhance megakaryocytopoiesis by modifying the activities of hematopoietic growth regulators. J. Cell. Physiol. 168, 97–104. doi: 10.1002/(sici)1097-4652(199607)168:1<97::Aid-jcp12>3.0.Co;2-m
Harding, T. C., Long, L., Palencia, S., Zhang, H., Sadra, A., Hestir, K., et al. (2013). Blockade of nonhormonal fibroblast growth factors by FP-1039 inhibits growth of multiple types of cancer. Sci. Transl. Med. 5:178ra139. doi: 10.1126/scitranslmed.3005414
Hashimoto, Y., Skacel, M., and Adams, J. C. (2008). Association of loss of epithelial syndecan-1 with stage and local metastasis of colorectal adenocarcinomas: an immunohistochemical study of clinically annotated tumors. BMC Cancer 8:185. doi: 10.1186/1471-2407-8-185
Hassell, J. R., Robey, P. G., Barrach, H. J., Wilczek, J., Rennard, S. I., and Martin, G. R. (1980). Isolation of a heparan sulfate-containing proteoglycan from basement membrane. Proc. Natl. Acad. Sci. U.S.A. 77, 4494–4498. doi: 10.1073/pnas.77.8.4494
Haubst, N., Georges-Labouesse, E., De Arcangelis, A., Mayer, U., and Götz, M. (2006). Basement membrane attachment is dispensable for radial glial cell fate and for proliferation, but affects positioning of neuronal subtypes. Development 133, 3245–3254. doi: 10.1242/dev.02486
Haupt, L. M., Murali, S., Mun, F. K., Teplyuk, N., Mei, L. F., Stein, G. S., et al. (2009). The heparan sulfate proteoglycan (HSPG) glypican-3 mediates commitment of MC3T3-E1 cells toward osteogenesis. J. Cell. Physiol. 220, 780–791. doi: 10.1002/jcp.21825
Helledie, T., Dombrowski, C., Rai, B., Lim, Z. X., Hin, I. L., Rider, D. A., et al. (2012). Heparan sulfate enhances the self-renewal and therapeutic potential of mesenchymal stem cells from human adult bone marrow. Stem Cells Dev. 21, 1897–1910. doi: 10.1089/scd.2011.0367
Hemeda, H., Kalz, J., Walenda, G., Lohmann, M., and Wagner, W. (2013). Heparin concentration is critical for cell culture with human platelet lysate. Cytotherapy 15, 1174–1181. doi: 10.1016/j.jcyt.2013.05.006
Hirano, K., Sasaki, N., Ichimiya, T., Miura, T., Van Kuppevelt, T. H., and Nishihara, S. (2012). 3-O-Sulfated heparan sulfate recognized by the antibody HS4C3 contribute to the differentiation of mouse embryonic stem cells via fas signaling. PLoS One 7:e43440. doi: 10.1371/journal.pone.0043440
Hirano, K., Van Kuppevelt, T. H., and Nishihara, S. (2013). The transition of mouse pluripotent stem cells from the naïve to the primed state requires Fas signaling through 3-O sulfated heparan sulfate structures recognized by the HS4C3 antibody. Biochem. Biophys. Res. Commun. 430, 1175–1181. doi: 10.1016/j.bbrc.2012.12.005
Holley, R. J., Pickford, C. E., Rushton, G., Lacaud, G., Gallagher, J. T., Kouskoff, V., et al. (2011). Influencing hematopoietic differentiation of mouse embryonic stem cells using soluble heparin and heparan sulfate saccharides. J. Biol. Chem. 286, 6241–6252. doi: 10.1074/jbc.m110.178483
Holley, R. J., Tai, G., Williamson, A. J., Taylor, S., Cain, S. A., Richardson, S. M., et al. (2015). Comparative quantification of the surfaceome of human multipotent mesenchymal progenitor cells. Stem Cell Rep. 4, 473–488. doi: 10.1016/j.stemcr.2015.01.007
Holmborn, K., Ledin, J., Smeds, E., Eriksson, I., Kusche-Gullberg, M., and Kjellen, L. (2004). Heparan sulfate synthesized by mouse embryonic stem cells deficient in NDST1 and NDST2 is 6-O-sulfated but contains no N-sulfate groups. J. Biol. Chem. 279, 42355–42358. doi: 10.1074/jbc.C400373200
Horiguchi, Y., Couchman, J. R., Ljubimov, A. V., Yamasaki, H., and Fine, J. D. (1989). Distribution, ultrastructural localization, and ontogeny of the core protein of a heparan sulfate proteoglycan in human skin and other basement membranes. J. Histochem. Cytochem. 37, 961–970. doi: 10.1177/37.7.2659664
Huang, M. L., Michalak, A. L., Fisher, C. J., Christy, M., Smith, R. A. A., and Godula, K. (2018). Small molecule antagonist of cell surface glycosaminoglycans restricts mouse embryonic stem cells in a pluripotent state. Stem Cells 36, 45–54. doi: 10.1002/stem.2714
Hulett, M. D., Hornby, J. R., Ohms, S. J., Zuegg, J., Freeman, C., Gready, J. E., et al. (2000). Identification of active-site residues of the pro-metastatic endoglycosidase heparanase. Biochemistry 39, 15659–15667. doi: 10.1021/bi002080p
Humphries, D. E., and Silbert, J. E. (1988). Chlorate: a reversible inhibitor of proteoglycan sulfation. Biochem. Biophys. Res. Commun. 154, 365–371. doi: 10.1016/0006-291X(88)90694-8
Ibrahim, S. A., Gadalla, R., El-Ghonaimy, E. A., Samir, O., Mohamed, H. T., Hassan, H., et al. (2017). Syndecan-1 is a novel molecular marker for triple negative inflammatory breast cancer and modulates the cancer stem cell phenotype via the IL-6/STAT3, Notch and EGFR signaling pathways. Mol. Cancer 16:57. doi: 10.1186/s12943-017-0621-z
Inatani, M., Irie, F., Plump, A. S., Tessier-Lavigne, M., and Yamaguchi, Y. (2003). Mammalian brain morphogenesis and midline axon guidance require heparan sulfate. Science 302, 1044–1046. doi: 10.1126/science.1090497
Ishihara, M. (1994). Structural requirements in heparin for binding and activation of FGF-1 and FGF-4 are different from that for FGF-2. Glycobiology 4, 817–824. doi: 10.1093/glycob/4.6.817
Ishihara, M., Shaklee, P. N., Yang, Z., Liang, W., Wei, Z., Stack, R. J., et al. (1994). Structural features in heparin which modulate specific biological activities mediated by basic fibroblast growth factor. Glycobiology 4, 451–458. doi: 10.1093/glycob/4.4.451
Jakobsson, L., Kreuger, J., Holmborn, K., Lundin, L., Eriksson, I., Kjellen, L., et al. (2006). Heparan sulfate in trans potentiates VEGFR-mediated angiogenesis. Dev. Cell 10, 625–634. doi: 10.1016/j.devcel.2006.03.009
Jastrebova, N., Vanwildemeersch, M., Lindahl, U., and Spillmann, D. (2010). Heparan sulfate domain organization and sulfation modulate FGF-induced cell signaling. J. Biol. Chem. 285, 26842–26851. doi: 10.1074/jbc.M109.093542
Jen, Y. H., Musacchio, M., and Lander, A. D. (2009). Glypican-1 controls brain size through regulation of fibroblast growth factor signaling in early neurogenesis. Neural Dev. 4:33. doi: 10.1186/1749-8104-4-33
Johnson, C. E., Ward, C. M., Wilson, V., van Kuppevelt, T. H., Esko, J. D., Smith, A., et al. (2007). Essential alterations of heparan sulfate during the differentiation of embryonic stem cells to Sox1-enhanced green fluorescent protein-expressing neural progenitor cells. Stem Cells 25, 1913–1923. doi: 10.1634/stemcells.2006-0445
Joseph, S. J., Ford, M. D., Barth, C., Portbury, S., Bartlett, P. F., Nurcombe, V., et al. (1996). A proteoglycan that activates fibroblast growth factors during early neuronal development is a perlecan variant. Development 122, 3443–3452.
Kalus, I., Salmen, B., Viebahn, C., Figura, K. V., Schmitz, D., D’Hooge, R., et al. (2009). Differential involvement of the extracellular 6-O-endosulfatases Sulf1 and Sulf2 in brain development and neuronal and behavioural plasticity. J. Cell Mol. Med. 13, 4505–4521. doi: 10.1111/j.1582-4934.2008.00558.x
Kaplan, E. D., and Holbrook, K. A. (1994). Dynamic expression patterns of tenascin, proteoglycans, and cell adhesion molecules during human hair follicle morphogenesis. Dev. Dyn. 199, 141–155. doi: 10.1002/aja.1001990207
Katakam, S. K., Pelucchi, P., Cocola, C., Reinbold, R., Vlodavsky, I., Greve, B., et al. (2020). Syndecan-1-dependent regulation of heparanase affects invasiveness, stem cell properties, and therapeutic resistance of Caco2 colon cancer cells. Front. Oncol. 10:774. doi: 10.3389/fonc.2020.00774
Kawahara, R., Granato, D. C., Yokoo, S., Domingues, R. R., Trindade, D. M., and Paes Leme, A. F. (2017). Mass spectrometry-based proteomics revealed Glypican-1 as a novel ADAM17 substrate. J. Proteomics 151, 53–65. doi: 10.1016/j.jprot.2016.08.017
Kirby, S. L., and Bentley, S. A. (1987). Proteoglycan synthesis in two murine bone marrow stromal cell lines. Blood 70, 1777–1783. doi: 10.1182/blood.v70.6.1777.bloodjournal7061777
Kjellén, L., and Lindahl, U. (2018). Specificity of glycosaminoglycan–protein interactions. Curr. Opin. Struct. Biol. 50, 101–108. doi: 10.1016/j.sbi.2017.12.011
Knelson, E. H., Nee, J. C., and Blobe, G. C. (2014). Heparan sulfate signaling in cancer. Trends Biochem. Sci. 39, 277–288. doi: 10.1016/j.tibs.2014.03.001
Knox, S., Merry, C., Stringer, S., Melrose, J., and Whitelock, J. (2002). Not all perlecans are created equal: interactions with fibroblast growth factor (FGF) 2 and FGF receptors. J. Biol. Chem. 277, 14657–14665. doi: 10.1074/jbc.M111826200
Knox, S. M., and Whitelock, J. M. (2006). Perlecan: how does one molecule do so many things? Cell. Mol. Life Sci. 63, 2435–2445. doi: 10.1007/s00018-006-6162-z
Kojima, T., Shworak, N. W., and Rosenberg, R. D. (1992). Molecular cloning and expression of two distinct cDNA-encoding heparan sulfate proteoglycan core proteins from a rat endothelial cell line. J. Biol. Chem. 267, 4870–4877.
Kolset, S. O., and Tveit, H. (2008). Serglycin–structure and biology. Cell Mol. Life. Sci. 65, 1073–1085. doi: 10.1007/s00018-007-7455-6
Kraushaar, D. C., Rai, S., Condac, E., Nairn, A., Zhang, S., Yamaguchi, Y., et al. (2012). Heparan sulfare facilitates FGF and BMP signaling to drive mesoderm differentiation of mouse embryonic stem cells. J. Biol. Chem. 287, 22691–22700. doi: 10.1074/jbc.m112.368241
Kraushaar, D. C., Yamaguchi, Y., and Wang, L. (2010). Heparan sulfate is required for embryonic stem cells to exit from self-renewal. J. Biol. Chem. 285, 5907–5916. doi: 10.1074/jbc.m109.066837
Kreuger, J., Jemth, P., Sanders-Lindberg, E., Eliahu, L., Ron, D., Basilico, C., et al. (2005). Fibroblast growth factors share binding sites in heparan sulphate. Biochem. J. 389(Pt 1), 145–150. doi: 10.1042/bj20042129
Kreuger, J., Prydz, K., Pettersson, R. F., Lindahl, U., and Salmivirta, M. (1999). Characterization of fibroblast growth factor 1 binding heparan sulfate domain. Glycobiology 9, 723–729. doi: 10.1093/glycob/9.7.723
Kreuger, J., Spillmann, D., Li, J. P., and Lindahl, U. (2006). Interactions between heparan sulfate and proteins: the concept of specificity. J. Cell Biol. 174, 323–327. doi: 10.1083/jcb.200604035
Kumar Katakam, S., Tria, V., Sim, W. C., Yip, G. W., Molgora, S., Karnavas, T., et al. (2020). The heparan sulfate proteoglycan Syndecan-1 regulates colon cancer stem cell function via a focal adhesion kinase - Wnt signaling axis. FEBS J. [Epub ahead of print]. doi: 10.1111/febs.15356
Kuo, W. J., Digman, M. A., and Lander, A. D. (2010). Heparan sulfate acts as a bone morphogenetic protein coreceptor by facilitating ligand-induced receptor hetero-oligomerization. Mol. Biol. Cell 21, 4028–4041. doi: 10.1091/mbc.E10-04-0348
Kussie, P. H., Hulmes, J. D., Ludwig, D. L., Patel, S., Navarro, E. C., Seddon, A. P., et al. (1999). Cloning and functional expression of a human heparanase gene. Biochem. Biophys. Res. Commun. 261, 183–187. doi: 10.1006/bbrc.1999.0962
Lai, J., Chien, J., Staub, J., Avula, R., Greene, E. L., Matthews, T. A., et al. (2003). Loss of HSulf-1 up-regulates heparin-binding growth factor signaling in cancer. J. Biol. Chem. 278, 23107–23117. doi: 10.1074/jbc.M302203200
Langsdorf, A., Do, A. T., Kusche-Gullberg, M., Emerson, C. P. Jr., and Ai, X. (2007). Sulfs are regulators of growth factor signaling for satellite cell differentiation and muscle regeneration. Dev. Biol. 311, 464–477. doi: 10.1016/j.ydbio.2007.08.053
Lanner, F., Lee, K. L., Sohl, M., Holmborn, K., Yang, H., Wilbertz, J., et al. (2010). Heparan sulfation–dependent fibroblast growth factor signaling maintains embryonic stem cells primed for differentiation in a heterogeneous state. Stem Cells 28, 191–200. doi: 10.1002/stem.265
Larraín, J., Alvarez, J., Hassell, J. R., and Brandan, E. (1997). Expression of perlecan, a proteoglycan that binds myogenic inhibitory basic fibroblast growth factor, is down regulated during skeletal muscle differentiation. Exp. Cell Res. 234, 405–412. doi: 10.1006/excr.1997.3648
Lathrop, B., Olson, E., and Glaser, L. (1985). Control by fibroblast growth factor of differentiation in the BC3H1 muscle cell line. J. Cell Biol. 100, 1540–1547. doi: 10.1083/jcb.100.5.1540
Le Jan, S., Hayashi, M., Kasza, Z., Eriksson, I., Bishop, J. R., Weibrecht, I., et al. (2012). Functional overlap between chondroitin and heparan sulfate proteoglycans during VEGF-induced sprouting angiogenesis. Arterioscler. Thromb. Vasc. Biol. 32, 1255–1263. doi: 10.1161/atvbaha.111.240622
Li, D., Wei, X., Xie, K., Chen, K., Li, J., and Fang, J. (2014). A novel decoy receptor fusion protein for FGF-2 potently inhibits tumour growth. Br. J. Cancer 111, 68–77. doi: 10.1038/bjc.2014.282
Lin, X., Wei, G., Shi, Z., Dryer, L., Esko, J. D., Wells, D. E., et al. (2000). Disruption of gastrulation and heparan sulfate biosynthesis in EXT1-deficient mice. Dev. Biol. 224, 299–311. doi: 10.1006/dbio.2000.9798
Lindahl, U. (1966). The structures of xylosylserine and galactosylxylosylserine from heparin. Biochim. Biophys. Acta 130, 361–367. doi: 10.1016/0304-4165(66)90232-7
Lindahl, U., and Li, J. P. (2009). Interactions between heparan sulfate and proteins - design and functional implications. Int. Rev. Cell Mol. Biol. 276, 105–159. doi: 10.1016/s1937-6448(09)76003-4
Lindahl, U., and Rodén, L. (1965). The role of galactose and xylose in the linkage of heparin to protein. J. Biol. Chem. 240, 2821–2826.
Ling, L., Camilleri, E. T., Helledie, T., Samsonraj, R. M., Titmarsh, D. M., Chua, R. J., et al. (2016). Effect of heparin on the biological properties and molecular signature of human mesenchymal stem cells. Gene 576(1 Pt 2), 292–303. doi: 10.1016/j.gene.2015.10.039
Ling, L., Dombrowski, C., Foong, K. M., Haupt, L. M., Stein, G. S., Nurcombe, V., et al. (2010). Synergism between Wnt3a and heparin enhances osteogenesis via a phosphoinositide 3-kinase/Akt/RUNX2 pathway. J. Biol. Chem. 285, 26233–26244. doi: 10.1074/jbc.M110.122069
Ling, L., Ren, X., Cao, X., Hassan, A. B. M., Mah, S., Sathiyanathan, P., et al. (2020). Enhancing the efficacy of stem cell therapy with glycosaminoglycans. Stem Cell Rep. 14, 105–121. doi: 10.1016/j.stemcr.2019.12.003
Ling, L., Tan, S. K., Goh, T. H., Cheung, E., Nurcombe, V., van Wijnen, A. J., et al. (2015). Targeting the heparin-binding domain of fibroblast growth factor receptor 1 as a potential cancer therapy. Mol. Cancer 14:136. doi: 10.1186/s12943-015-0391-4
Liu, B. Y., McDermott, S. P., Khwaja, S. S., and Alexander, C. M. (2004). The transforming activity of Wnt effectors correlates with their ability to induce the accumulation of mammary progenitor cells. Proc. Natl. Acad. Sci. U.S.A. 101, 4158–4163. doi: 10.1073/pnas.0400699101
Liu, J., Shworak, N. W., Sinay, P., Schwartz, J. J., Zhang, L., Fritze, L. M. S., et al. (1999). Expression of heparan Sulfate d-Glucosaminyl 3-O-Sulfotransferase isoforms reveals novel substrate specificities. J. Biol. Chem. 274, 5185–5192. doi: 10.1074/jbc.274.8.5185
Longley, R. L., Woods, A., Fleetwood, A., Cowling, G. J., Gallagher, J. T., and Couchman, J. R. (1999). Control of morphology, cytoskeleton and migration by syndecan-4. J. Cell Sci. 112, 3421.
Luikart, S. D., Maniglia, C. A., Furcht, L. T., McCarthy, J. B., et al. (1990). A heparan sulfate-containing fraction of bone marrow stroma induces maturation of HL-60 cells in vitro. Cancer Res. 50, 3781–3785.
Luo, N., Li, H., Xiang, B., Qiao, L., He, J., Ji, Y., et al. (2016). Syndecan-4 modulates the proliferation of neural cells and the formation of CaP axons during zebrafish embryonic neurogenesis. Sci. Rep. 6, 25300–25300. doi: 10.1038/srep25300
Maccarana, M., Casu, B., and Lindahl, U. (1993). Minimal sequence in heparin/heparan sulfate required for binding of basic fibroblast growth factor. J. Biol. Chem. 268, 23898–23905.
Maccarana, M., Sakura, Y., Tawada, A., Yoshida, K., and Lindahl, U. (1996). Domain structure of heparan sulfates from bovine organs. J. Biol. Chem. 271, 17804–17810. doi: 10.1074/jbc.271.30.17804
Makarenkova, H. P., Hoffman, M. P., Beenken, A., Eliseenkova, A. V., Meech, R., Tsau, C., et al. (2009). Differential interactions of FGFs with heparan sulfate control gradient formation and branching morphogenesis. Sci. Signal. 2:ra55. doi: 10.1126/scisignal.2000304
Malgouries, S., Thibaut, S., and Bernard, B. A. (2008). Proteoglycan expression patterns in human hair follicle. Br. J. Dermatol. 158, 234–242. doi: 10.1111/j.1365-2133.2007.08339.x
Mansbridge, J. N., and Hanawalt, P. C. (1988). Role of transforming growth factor beta in the maturation of human epidermal keratinocytes. J. Invest. Dermatol. 90, 336–341. doi: 10.1111/1523-1747.ep12456286
Mauro, A. (1961). Satellite cell of skeletal muscle fibers. J. Biophys. Biochem. Cytol. 9, 493–495. doi: 10.1083/jcb.9.2.493
McCuskey, R. S., and Meineke, H. A. (1973). Studies of the hemopoietic microenvironment. 3. Differences in the splenic microvascular system and stroma between SL-SL d and W-W v anemic mice. Am. J. Anat. 137, 187–197. doi: 10.1002/aja.1001370205
McDermott, S. P., Ranheim, E. A., Leatherberry, V. S., Khwaja, S. S., Klos, K. S., and Alexander, C. M. (2007). Juvenile syndecan-1 null mice are protected from carcinogen-induced tumor development. Oncogene 26, 1407–1416. doi: 10.1038/sj.onc.1209930
McLaughlin, D., Karlsson, F., Tian, N., Pratt, T., Bullock, S. L., Wilson, V. A., et al. (2003). Specific modification of heparan sulphate is required for normal cerebral cortical development. Mech. Dev. 120, 1481–1488. doi: 10.1016/j.mod.2003.08.008
Meade, K. A., White, K. J., Pickford, C. E., Holley, R. J., Marson, A., Tillotson, D., et al. (2013). Immobilization of heparan sulfate on electrospun meshes to support embryonic stem cell culture and differentiation. J. Biol. Chem. 288, 5530–5538. doi: 10.1074/jbc.M112.423012
Meyers, E. N., Lewandoski, M., and Martin, G. R. (1998). An Fgf8 mutant allelic series generated by Cre- and Flp-mediated recombination. Nat. Genet. 18, 136–141. doi: 10.1038/ng0298-136
Mitsou, I., Multhaupt, H. A. B., and Couchman, J. R. (2017). Proteoglycans, ion channels and cell-matrix adhesion. Biochem. J. 474, 1965–1979. doi: 10.1042/BCJ20160747
Morgan, B. A. (2014). The dermal papilla: an instructive niche for epithelial stem and progenitor cells in development and regeneration of the hair follicle. Cold Spring Harb. Perspect. Med. 4:a015180. doi: 10.1101/cshperspect.a015180
Multhaupt, H. A. B., Yoneda, A., Whiteford, J. R., Oh, E. S., Lee, W., and Couchman, J. R. (2009). Syndecan signalling: when, where and why? J. Physiol. Pharmacol. 60(Suppl. 4), 31–38.
Mundy, C., Yang, E., Takano, H., Billings, P. C., and Pacifici, M. (2018). Heparan sulfate antagonism alters bone morphogenetic protein signaling and receptor dynamics, suggesting a mechanism in hereditary multiple exostoses. J. Biol. Chem. 293, 7703–7716. doi: 10.1074/jbc.ra117.000264
Murali, S., Rai, B., Dombrowski, C., Lee, J. L., Lim, Z. X., Bramono, D. S., et al. (2013). Affinity-selected heparan sulfate for bone repair. Biomaterials 34, 5594–5605. doi: 10.1016/j.biomaterials.2013.04.017
Murphy, M., Drago, J., and Bartlett, P. F. (1990). Fibroblast growth factor stimulates the proliferation and differentiation of neural precursor cells in vitro. J. Neurosci. Res. 25, 463–475. doi: 10.1002/jnr.490250404
Nagarajan, A., Malvi, P., and Wajapeyee, N. (2018). Heparan sulfate and heparan sulfate proteoglycans in cancer initiation and progression. Front. Endocrinol. 9:483. doi: 10.3389/fendo.2018.00483
Nairn, A. V., Kinoshita-Toyoda, A., Toyoda, H., Xie, J., Harris, K., Dalton, S., et al. (2007). Glycomics of proteoglycan biosynthesis in murine embryonic stem cell differentiation. J. Proteome Res. 6, 4374–4387. doi: 10.1021/pr070446f
Nakato, H., Futch, T. A., and Selleck, S. B. (1995). The division abnormally delayed (dally) gene: a putative integral membrane proteoglycan required for cell division patterning during postembryonic development of the nervous system in Drosophila. Development 121:3687.
Nam, E. J., and Park, P. W. (2012). Shedding of cell membrane-bound proteoglycans. Methods Mol. Biol. 836, 291–305. doi: 10.1007/978-1-61779-498-8_19
Netelenbos, T., van den Born, J., Kessler, F. L., Zweegman, S., Merle, P. A., van Oostveen, J. W., et al. (2003). Proteoglycans on bone marrow endothelial cells bind and present SDF-1 towards hematopoietic progenitor cells. Leukemia 17, 175–184. doi: 10.1038/sj.leu.2402738
Netelenbos, T., Zuijderduijn, S., Van Den Born, J., Kessler, F. L., Zweegman, S., Huijgens, P. C., et al. (2002). Proteoglycans guide SDF-1-induced migration of hematopoietic progenitor cells. J. Leukoc. Biol. 72, 353–362.
Noonan, D. M., Fulle, A., Valente, P., Cai, S., Horigan, E., Sasaki, M., et al. (1991). The complete sequence of perlecan, a basement membrane heparan sulfate proteoglycan, reveals extensive similarity with laminin A chain, low density lipoprotein-receptor, and the neural cell adhesion molecule. J. Biol. Chem. 266, 22939–22947.
Nurcombe, V., Ford, M. D., Wildschut, J. A., and Bartlett, P. F. (1993). Developmental regulation of neural response to FGF-1 and FGF-2 by heparan sulfate proteoglycan. Science 260:103. doi: 10.1126/science.7682010
Oikari, L. E., Okolicsanyi, R. K., Qin, A., Yu, C., Griffiths, L. R., and Haupt, L. M. (2016). Cell surface heparan sulfate proteoglycans as novel markers of human neural stem cell fate determination. Stem Cell Res. 16, 92–104. doi: 10.1016/j.scr.2015.12.011
Okina, E., Grossi, A., Gopal, S., Multhaupt, H. A. B., and Couchman, J. R. (2012). Alpha-actinin interactions with syndecan-4 are integral to fibroblast–matrix adhesion and regulate cytoskeletal architecture. Int. J. Biochem. Cell Biol. 44, 2161–2174. doi: 10.1016/j.biocel.2012.08.017
Olczyk, P., Mencner, Ł, and Komosinska-Vassev, K. (2015). Diverse roles of heparan sulfate and heparin in wound repair. BioMed Res. Int. 2015, 549417. doi: 10.1155/2015/549417
Olguin, H., and Brandan, E. (2001). Expression and localization of proteoglycans during limb myogenic activation. Dev. Dyn. 221, 106–115. doi: 10.1002/dvdy.1129
Olwin, B. B., and Rapraeger, A. (1992). Repression of myogenic differentiation by aFGF, bFGF, and K-FGF is dependent on cellular heparan sulfate. J. Cell Biol. 118, 631–639. doi: 10.1083/jcb.118.3.631
Ornitz, D. M., Yayon, A., Flanagan, J. G., Svahn, C. M., Levi, E., and Leder, P. (1992). Heparin is required for cell-free binding of basic fibroblast growth factor to a soluble receptor and for mitogenesis in whole cells. Mol. Cell. Biol. 12, 240–247. doi: 10.1128/mcb.12.1.240
Osdoby, P., and Caplan, A. I. (1976). The possible differentiation of osteogenic elements in vitro from chick limb mesodermal cells. I. Morphological evidence. Dev. Biol. 52, 283–299. doi: 10.1016/0012-1606(76)90246-3
Ostrovsky, O., Berman, B., Gallagher, J., Mulloy, B., Fernig, D. G., Delehedde, M., et al. (2002). Differential effects of heparin saccharides on the formation of specific fibroblast growth factor (FGF) and FGF receptor complexes. J. Biol. Chem. 277, 2444–2453. doi: 10.1074/jbc.M108540200
Papy-Garcia, D., and Albanese, P. (2017). Heparan sulfate proteoglycans as key regulators of the mesenchymal niche of hematopoietic stem cells. Glycoconj. J. 34, 377–391. doi: 10.1007/s10719-017-9773-8
Patel, N. J., Sharon, C., Baranwal, S., Boothello, R. S., Desai, U. R., and Patel, B. B. (2016). Heparan sulfate hexasaccharide selectively inhibits cancer stem cells self-renewal by activating p38 MAP kinase. Oncotarget 7, 84608–84622. doi: 10.18632/oncotarget.12358
Patterson, A. M., Cartwright, A., David, G., Fitzgerald, O., Bresnihan, B., Ashton, B. A., et al. (2008). Differential expression of syndecans and glypicans in chronically inflamed synovium. Ann. Rheum. Dis. 67, 592–601. doi: 10.1136/ard.2006.063875
Pawlikowski, B., Vogler, T. O., Gadek, K., and Olwin, B. B. (2017). Regulation of skeletal muscle stem cells by fibroblast growth factors. Dev. Dyn. 246, 359–367. doi: 10.1002/dvdy.24495
Pellegrini, L., Burke, D. F., von Delft, F., Mulloy, B., and Blundell, T. L. (2000). Crystal structure of fibroblast growth factor receptor ectodomain bound to ligand and heparin. Nature 407, 1029–1034. doi: 10.1038/35039551
Pickford, C. E., Holley, R. J., Rushton, G., Stavridis, M., Ward, C. M., and Merry, C. L. R. (2011). Specific glycosaminoglycans modulate neural specification of mouse embryonic stem cells. Stem Cells 29, 629–640. doi: 10.1002/stem.610
Pisconti, A., Cornelison, D. D., Olguin, H. C., Antwine, T. L., and Olwin, B. B. (2010). Syndecan-3 and Notch cooperate in regulating adult myogenesis. J. Cell Biol. 190, 427–441. doi: 10.1083/jcb.201003081
Plaks, V., Kong, N., and Werb, Z. (2015). The cancer stem cell niche: how essential is the niche in regulating stemness of tumor cells? Cell Stem Cell 16, 225–238. doi: 10.1016/j.stem.2015.02.015
Ploemacher, R. E., van’t Hull, E., and van Soest, P. L. (1978). Studies of the hemopoietic microenvironments: effects of acid mucopolysaccharides and dextran sulphate on erythroid and granuloid differentiation in vitro. Exp. Hematol. 6, 311–320.
Poon, S., Lu, X., Smith, R. A. A., Ho, P., Bhakoo, K., Nurcombe, V., et al. (2018). Improved recovery from limb ischaemia by delivery of an affinity-isolated heparan sulphate. Angiogenesis 21, 777–791. doi: 10.1007/s10456-018-9622-9
Potten, C. S., and Morris, R. J. (1988). Epithelial stem cells in vivo. J. Cell Sci. Suppl. 10, 45–62. doi: 10.1242/jcs.1988.supplement_10.4
Poulain, F. E., and Yost, H. J. (2015). Heparan sulfate proteoglycans: a sugar code for vertebrate development? Development 142, 3456–3467. doi: 10.1242/dev.098178
Pruessmeyer, J., Martin, C., Hess, F. M., Schwarz, N., Schmidt, S., Kogel, T., et al. (2010). A disintegrin and metalloproteinase 17 (ADAM17) mediates inflammation-induced shedding of syndecan-1 and -4 by lung epithelial cells. J. Biol. Chem. 285, 555–564. doi: 10.1074/jbc.M109.059394
Pye, D. A., Vives, R. R., Turnbull, J. E., Hyde, P., and Gallagher, J. T. (1998). Heparan sulfate oligosaccharides require 6-O-sulfation for promotion of basic fibroblast growth factor mitogenic activity. J. Biol. Chem. 273, 22936–22942. doi: 10.1074/jbc.273.36.22936
Raballo, R., Rhee, J., Lyn-Cook, R., Leckman, J. F., Schwartz, M. L., and Vaccarino, F. M. (2000). Basic fibroblast growth factor (Fgf2) is necessary for cell proliferation and neurogenesis in the developing cerebral cortex. J. Neurosci. 20:5012. doi: 10.1523/JNEUROSCI.20-13-05012.2000
Rapraeger, A., Jalkanen, M., and Bernfield, M. (1986). Cell surface proteoglycan associates with the cytoskeleton at the basolateral cell surface of mouse mammary epithelial cells. J. Cell Biol. 103, 2683–2696. doi: 10.1083/jcb.103.6.2683
Rapraeger, A., Jalkanen, M., Endo, E., Koda, J., and Bernfield, M. (1985). The cell surface proteoglycan from mouse mammary epithelial cells bears chondroitin sulfate and heparan sulfate glycosaminoglycans. J. Biol. Chem. 260, 11046–11052.
Rapraeger, A. C., Krufka, A., and Olwin, B. B. (1991). Requirement of heparan sulfate for bFGF-mediated fibroblast growth and myoblast differentiation. Science 252:1705. doi: 10.1126/science.1646484
Raulo, E., Chernousov, M. A., Carey, D. J., Nolo, R., and Rauvala, H. (1994). Isolation of a neuronal cell surface receptor of heparin binding growth-associated molecule (HB-GAM). Identification as N-syndecan (syndecan-3). J. Biol. Chem. 269, 12999–13004.
Reimann, J., and Burger, H. (1979). In vitro proliferation of haemopoietic cells in the presence of adherent cell layers. II. Differential effect of adherent cell layers derived from various organs. Exp. Hematol. 7, 52–58.
Roberts, R., Gallagher, J., Spooncer, E., Alien, T. D., Bloomfield, F., and Dexter, T. M. (1988). Heparan sulphate bound growth factors: a mechanism for stromal cell mediated haemopoiesis. Nature 332, 376–378. doi: 10.1038/332376a0
Rosen, S. D., and Lemjabbar-Alaoui, H. (2010). Sulf-2: an extracellular modulator of cell signaling and a cancer target candidate. Expert Opin. Ther. Targets 14, 935–949. doi: 10.1517/14728222.2010.504718
Rudd, T. R., Preston, M. D., and Yates, E. A. (2017). The nature of the conserved basic amino acid sequences found among 437 heparin binding proteins determined by network analysis. Mol. BioSyst. 13, 852–865. doi: 10.1039/c6mb00857g
Ruppert, R., Hoffmann, E., and Sebald, W. (1996). Human bone morphogenetic protein 2 contains a heparin-binding site which modifies its biological activity. Eur. J. Biochem. 237, 295–302. doi: 10.1111/j.1432-1033.1996.0295n.x
Sadir, R., Imberty, A., Baleux, F., and Lortat-Jacob, H. (2004). Heparan sulfate/heparin oligosaccharides protect stromal cell-derived Factor-1 (SDF-1)/CXCL12 against proteolysis induced by CD26/Dipeptidyl Peptidase IV. J. Biol. Chem. 279, 43854–43860. doi: 10.1074/jbc.m405392200
Sahara, S., and O’Leary, D. D. M. (2009). Fgf10 regulates transition period of cortical stem cell differentiation to radial glia controlling generation of neurons and basal progenitors. Neuron 63, 48–62. doi: 10.1016/j.neuron.2009.06.006
San Antonio, J. D., Winston, B. M., and Tuan, R. S. (1987). Regulation of chondrogenesis by heparan sulfate and structurally related glycosaminoglycans. Dev. Biol. 123, 17–24. doi: 10.1016/0012-1606(87)90422-2
Sanderson, R. D., and Bernfield, M. (1988). Molecular polymorphism of a cell surface proteoglycan: distinct structures on simple and stratified epithelia. Proc. Natl. Acad. Sci. U.S.A. 85:9562. doi: 10.1073/pnas.85.24.9562
Sanderson, R. D., Hinkes, M. T., and Bernfield, M. (1992). Syndecan-1, a cell-surface proteoglycan, changes in size and abundance when keratinocytes stratify. J. Invest. Dermatol. 99, 390–396. doi: 10.1111/1523-1747.ep12616103
Saoncella, S., Echtermeyer, F., Denhez, F., Nowlen, J. K., Mosher, D. F., Robinson, S. D., et al. (1999). Syndecan-4 signals cooperatively with integrins in a Rho-dependent manner in the assembly of focal adhesions and actin stress fibers. Proc. Natl. Acad. Sci. U.S.A. 96, 2805–2810. doi: 10.1073/pnas.96.6.2805
Sarras, M. P., Madden, M. E., Zhang, X., Gunwar, S., Huff, J. K., and Hudson, B. G. (1991). Extracellular matrix (mesoglea) of Hydra vulgaris: I. Isolation and characterization. Dev. Biol. 148, 481–494. doi: 10.1016/0012-1606(91)90266-6
Sarrazin, S., Lamanna, W. C., and Esko, J. D. (2011). Heparan sulfate proteoglycans. Cold Spring Harb. Perspect. Biol. 3:a004952. doi: 10.1101/cshperspect.a004952
Schlessinger, J., Plotnikov, A. N., Ibrahimi, O. A., Eliseenkova, A. V., Yeh, B. K., Yayon, A., et al. (2000). Crystal structure of a ternary FGF-FGFR-Heparin complex reveals a dual role for heparin in FGFR binding and dimerization. Mol. Cell. 6, 743–750. doi: 10.1016/S1097-2765(00)00073-3
Schultz, V., Suflita, M., Liu, X., Zhang, X., Yu, Y., Li, L., et al. (2017). Heparan sulfate domains required for fibroblast growth factor 1 and 2 signaling through fibroblast growth factor receptor 1c. J. Biol. Chem. 292, 2495–2509. doi: 10.1074/jbc.M116.761585
Sheng, J., Liu, R., Xu, Y., and Liu, J. (2011). The dominating role of N-Deacetylase/N-Sulfotransferase 1 in forming domain structures in heparan sulfate. J. Biol. Chem. 286, 19768–19776. doi: 10.1074/jbc.m111.224311
Sher, I., Zisman-Rozen, S., Eliahu, L., Whitelock, J. M., Maas-Szabowski, N., Yamada, Y., et al. (2006). Targeting perlecan in human keratinocytes reveals novel roles for perlecan in epidermal formation. J. Biol. Chem. 281, 5178–5187. doi: 10.1074/jbc.M509500200
Shintani, Y., Takashima, S., Asano, Y., Kato, H., Liao, Y., Yamazaki, S., et al. (2006). Glycosaminoglycan modification of neuropilin-1 modulates VEGFR2 signaling. Embo J. 25, 3045–3055. doi: 10.1038/sj.emboj.7601188
Shworak, N. W., Liu, J., Fritze, L. M. S., Schwartz, J. J., Zhang, L., Logeart, D., et al. (1997). Molecular cloning and expression of mouse and human cDNAs encoding heparan sulfate d-Glucosaminyl 3-O-Sulfotransferase. J. Biol. Chem. 272, 28008–28019. doi: 10.1074/jbc.272.44.28008
Shworak, N. W., Liu, J., Petros, L. M., Zhang, L., Kobayashi, M., Copeland, N. G., et al. (1999). Multiple isoforms of heparan Sulfate d-Glucosaminyl 3-O-Sulfotransferase: isolation, characterization, and expression of human cdnas and identification of distinct genomic loci. J. Biol. Chem. 274, 5170–5184. doi: 10.1074/jbc.274.8.5170
Siczkowski, M., Clarke, D., and Gordon, M. Y. (1992). Binding of primitive hematopoietic progenitor cells to marrow stromal cells involves heparan sulfate. Blood 80, 912–919. doi: 10.1182/blood.v80.4.912.912
Smeds, E., Feta, A., and Kusche-Gullberg, M. (2010). Target selection of heparan sulfate hexuronic acid 2-O-sulfotransferase. Glycobiology 20, 1274–1282. doi: 10.1093/glycob/cwq089
Smith, R. A. A., Chua, R. J. E., Carnachan, S. M., Tan, C. L. L., Sims, I. M., Hinkley, S. F. R., et al. (2018). Retention of the structure and function of heparan sulfate biomaterials after gamma irradiation. Tissue Eng. Part A 24, 729–739. doi: 10.1089/ten.TEA.2017.0263
Smith, R. A. A., Meade, K., Pickford, C. E., Holley, R. J., and Merry, C. L. (2011). Glycosaminoglycans as regulators of stem cell differentiation. Biochem. Soc. Trans. 39, 383–387. doi: 10.1042/BST0390383
Spivak-Kroizman, T., Lemmon, M. A., Dikic, I., Ladbury, J. E., Pinchasi, D., Huang, J., et al. (1994). Heparin-induced oligomerization of FGF molecules is responsible for FGF receptor dimerization, activation, and cell proliferation. Cell 79, 1015–1024. doi: 10.1016/0092-8674(94)90032-9
Stelling, M. P., Lages, Y. M. V., Tovar, A. M. F., Mourão, P. A. S., and Rehen, S. K. (2012). Matrix-bound heparan sulfate is essential for the growth and pluripotency of human embryonic stem cells. Glycobiology 23, 337–345. doi: 10.1093/glycob/cws133
Stevens, R. L., Fox, C. C., Lichtenstein, L. M., and Austen, K. F. (1988). Identification of chondroitin sulfate E proteoglycans and heparin proteoglycans in the secretory granules of human lung mast cells. Proc. Natl. Acad. Sci. U.S.A. 85, 2284. doi: 10.1073/pnas.85.7.2284
Storm, E. E., Garel, S., Borello, U., Hebert, J. M., Martinez, S., McConnell, S. K., et al. (2006). Dose-dependent functions of Fgf8 in regulating telencephalic patterning centers. Development 133, 1831–1844. doi: 10.1242/dev.02324
Sun, C., Liu, M., Sun, P., Yang, M., Yates, E. A., Guo, Z., et al. (2019). Sulfated polysaccharides interact with fibroblast growth factors and protect from denaturation. FEBS Open Biol. 9, 1477–1487. doi: 10.1002/2211-5463.12696
Sun, N., Petiwala, S., Wang, R., Lu, C., Hu, M., Ghosh, S., et al. (2019). Development of drug-inducible CRISPR-Cas9 systems for large-scale functional screening. BMC Genomics 20:225. doi: 10.1186/s12864-019-5601-9
Szabowski, A., Maas-Szabowski, N., Andrecht, S., Kolbus, A., Schorpp-Kistner, M., Fusenig, N. E., et al. (2000). c-Jun and JunB antagonistically control cytokine-regulated mesenchymal-epidermal interaction in skin. Cell 103, 745–755. doi: 10.1016/s0092-8674(00)00178-1
Tantravahi, R. V., Stevens, R. L., Austen, K. F., and Weis, J. H. (1986). A single gene in mast cells encodes the core peptides of heparin and chondroitin sulfate proteoglycans. Proc. Natl. Acad. Sci. U.S.A. 83, 9207–9210. doi: 10.1073/pnas.83.23.9207
ten Dam, G. B., Kurup, S., van de Westerlo, E. M. A., Versteeg, E. M. M., Lindahl, U., Spillmann, D., et al. (2006). 3-O-Sulfated Oligosaccharide Structures Are Recgonized by Anti-heparan Sulfate Antibody HS4C3. J. Biol. Chem. 281, 4654–4662. doi: 10.1074/jbc.m506357200
Thomas, K. R., Musci, T. S., Neumann, P. E., and Capecchi, M. R. (1991). Swaying is a mutant allele of the proto-oncogene Wnt-1. Cell 67, 969–976. doi: 10.1016/0092-8674(91)90369-a
Titmarsh, D. M., Tan, C. L. L., Glass, N. R., Nurcombe, V., Cooper-White, J. J., and Cool, S. M. (2017). Microfluidic screening reveals heparan sulfate enhances human mesenchymal stem cell growth by modulating fibroblast growth factor-2 transport. Stem Cells Transl. Med. 6, 1178–1190. doi: 10.1002/sctm.16-0343
Townley, R. A., and Bülow, H. E. (2011). Genetic analysis of the heparan modification network in Caenorhabditis elegans. J. Biol. Chem. 286, 16824–16831. doi: 10.1074/jbc.m111.227926
Traister, A., Shi, W., and Filmus, J. (2008). Mammalian Notum induces the release of glypicans and other GPI-anchored proteins from the cell surface. Biochem. J. 410, 503–511. doi: 10.1042/BJ20070511
Traktuev, D. O., Merfeld-Clauss, S., Li, J., Kolonin, M., Arap, W., Pasqualini, R., et al. (2008). A population of multipotent CD34-positive adipose stromal cells share pericyte and mesenchymal surface markers, reside in a periendothelial location, and stabilize endothelial networks. Circ. Res. 102, 77–85. doi: 10.1161/CIRCRESAHA.107.159475
Tran, T. H., Shi, X., Zaia, J., and Ai, X. (2012). Heparan sulfate 6-O-endosulfatases (Sulfs) coordinate the Wnt signaling pathways to regulate myoblast fusion during skeletal muscle regeneration. J. Biol. Chem. 287, 32651–32664. doi: 10.1074/jbc.M112.353243
Trieger, G. W., Verespy, S. III, Gordts, P., and Godula, K. (2019). Efficient synthesis of heparinoid bioconjugates for tailoring FGF2 activity at the stem cell-matrix interface. Bioconjug. Chem. 30, 833–840. doi: 10.1021/acs.bioconjchem.8b00921
Tsen, G., Halfer, W., Kroger, S., and Cole, G. J. (1995). Agrin is a heparan sulfate proteoglycan. J. Biol. Chem. 270, 3392–3399. doi: 10.1074/jbc.270.7.3392
Turnbull, J., Powell, A., and Guimond, S. (2001). Heparan sulfate: decoding a dynamic multifunctional cell regulator. Trends Cell Biol. 11, 75–82. doi: 10.1016/S0962-8924(00)01897-3
Turnbull, J. E., Fernig, D. G., Ke, Y., Wilkinson, M. C., and Gallagher, J. T. (1992). Identification of the basic fibroblast growth factor binding sequence in fibroblast heparan sulfate. J. Biol. Chem. 267, 10337–10341.
Turnbull, J. E., and Gallagher, J. T. (1988). Oligosaccharide mapping of heparan sulphate by polyacrylamide-gradient-gel electrophoresis and electrotransfer to nylon membrane. Biochem. J. 251, 597–608. doi: 10.1042/bj2510597
Turnbull, J. E., and Gallagher, J. T. (1990). Molecular organization of heparan sulphate from human skin fibroblasts. Biochem. J. 265, 715–724. doi: 10.1042/bj2650715
Turnbull, J. E., and Gallagher, J. T. (1991). Distribution of iduronate 2-sulphate residues in heparan sulphate. Evidence for an ordered polymeric structure. Biochem. J. 273, 553–559. doi: 10.1042/bj2730553
Uchimura, K., Morimoto-Tomita, M., Bistrup, A., Li, J., Lyon, M., Gallagher, J., et al. (2006). HSulf-2, an extracellular endoglucosamine-6-sulfatase, selectively mobilizes heparin-bound growth factors and chemokines: effects on VEGF, FGF-1, and SDF-1. BMC Biochem. 7:2. doi: 10.1186/1471-2091-7-2
Ullah, I., Busch, J. F., Rabien, A., Ergün, B., Stamm, C., Knosalla, C., et al. (2020). Adult tissue extracellular matrix determines tissue specification of human iPSC-derived embryonic stage mesodermal precursor cells. Adv. Sci. 7:1901198. doi: 10.1002/advs.201901198
Varki, A., Cummings, R. D., Aebi, M., Packer, N. H., Seeberger, P. H., Esko, J. D., et al. (2015). Symbol nomenclature for graphical representations of glycans. Glycobiology 25, 1323–1324. doi: 10.1093/glycob/cwv091
Vlodavsky, I., Goldshmidt, O., Zcharia, E., Atzmon, R., Rangini-Guatta, Z., Elkin, M., et al. (2002). Mammalian heparanase: involvement in cancer metastasis, angiogenesis and normal development. Semin Cancer Biol. 12, 121–129. doi: 10.1006/scbi.2001.0420
Vlodavsky, I., Ilan, N., Naggi, A., and Casu, B. (2007). Heparanase: structure, biological functions, and inhibition by heparin-derived mimetics of heparan sulfate. Curr. Pharm. Des. 13, 2057–2073. doi: 10.2174/138161207781039742
Vuong, T. T., Reine, T. M., Sudworth, A., Jenssen, T. G., and Kolset, S. O. (2015). Syndecan-4 is a major syndecan in primary human endothelial cells in vitro, modulated by inflammatory stimuli and involved in wound healing. J. Histochem. Cytochem. 63, 280–292. doi: 10.1369/0022155415568995
Wang, C., Poon, S., Murali, S., Koo, C. Y., Bell, T. J., Hinkley, S. F., et al. (2014). Engineering a vascular endothelial growth factor 165-binding heparan sulfate for vascular therapy. Biomaterials 35, 6776–6786. doi: 10.1016/j.biomaterials.2014.04.084
Wang, Q., Yang, L., Alexander, C., and Temple, S. (2012). The niche factor syndecan-1 regulates the maintenance and proliferation of neural progenitor cells during mammalian cortical development. PLoS One 7:e42883. doi: 10.1371/journal.pone.0042883
Weiss, A. R. R., and Dahlke, M. H. (2019). Immunomodulation by mesenchymal stem cells (MSCs): mechanisms of action of living, apoptotic, and dead MSCs. Front. Immunol. 10:1191. doi: 10.3389/fimmu.2019.01191
Weiss, R. J., Gordts, P. L. S. M., Le, D., Xu, D., Esko, J. D., and Tor, Y. (2015). Small molecule antagonists of cell-surface heparan sulfate and heparin–protein interactions. Chem. Sci. 6, 5984–5993. doi: 10.1039/C5SC01208B
Westgate, G. E., Messenger, A. G., Watson, L. P., and Gibson, W. T. (1991). Distribution of proteoglycans during the hair growth cycle in human skin. J. Invest. Dermatol. 96, 191–195. doi: 10.1111/1523-1747.ep12461019
Wight, T. N., Kinsella, M. G., Keating, A., and Singer, J. W. (1986). Proteoglycans in human long-term bone marrow cultures: biochemical and ultrastructural analyses. Blood 67, 1333–1343. doi: 10.1182/blood.v67.5.1333.bloodjournal6751333
Wijesinghe, S. J., Ling, L., Murali, S., Qing, Y. H., Hinkley, S. F., Carnachan, S. M., et al. (2017). Affinity selection of FGF2-binding heparan sulfates for ex vivo expansion of human mesenchymal stem cells. J. Cell. Physiol. 232, 566–575. doi: 10.1002/jcp.25454
Xu, D., and Esko, J. D. (2014). Demystifying heparan sulfate-protein interactions. Annu. Rev. Biochem. 83, 129–157. doi: 10.1146/annurev-biochem-060713-035314
Xu, Y., Moon, A. F., Xu, S., Krahn, J. M., Liu, J., and Pedersen, L. C. (2017). Structure based substrate specificity analysis of heparan sulfate 6-O-Sulfotransferases. ACS Chem. Biol. 12, 73–82. doi: 10.1021/acschembio.6b00841
Yamada, S., Morimoto, H., Fujisawa, T., and Sugahara, K. (2007). Glycosaminoglycans in Hydra magnipapillata (Hydrozoa, Cnidaria): demonstration of chondroitin in the developing nematocyst, the sting organelle, and structural characterization of glycosaminoglycans. Glycobiology 17, 886–894. doi: 10.1093/glycob/cwm051
Yamaguchi, Y. (2001). Heparan sulfate proteoglycans in the nervous system: their diverse roles in neurogenesis, axon guidance, and synaptogenesis. Semin. Cell Dev. Biol. 12, 99–106. doi: 10.1006/scdb.2000.0238
Yan, D., and Lin, X. (2009). Shaping morphogen gradients by proteoglycans. Cold Spring Harb. Perspect. Biol. 1:a002493. doi: 10.1101/cshperspect.a002493
Yayon, A., Klagsbrun, M., Esko, J. D., Leder, P., and Ornitz, D. M. (1991). Cell surface, heparin-like molecules are required for binding of basic fibroblast growth factor to its high affinity receptor. Cell 64, 841–848. doi: 10.1016/0092-8674(91)90512-W
Yu, C., Griffiths, L. R., and Haupt, L. M. (2017). Exploiting heparan sulfate proteoglycans in human neurogenesis-controlling lineage specification and fate. Front. Integr. Neurosci. 11:28. doi: 10.3389/fnint.2017.00028
Zaia, J. (2013). Glycosaminoglycan glycomics using mass spectrometry. Mol. Cell. Proteomics 12, 885–892. doi: 10.1074/mcp.R112.026294
Zhao, S., Deng, C., Wang, Z., Teng, L., and Chen, J. (2015). Heparan sulfate 6-O-sulfotransferase 3 is involved in bone marrow mesenchymal stromal cell osteogenic differentiation. Biochemistry 80, 379–389. doi: 10.1134/s000629791503013x
Zhu, X. J., Liu, Y., Dai, Z. M., Zhang, X., Yang, X., Li, Y., et al. (2014). BMP-FGF signaling axis mediates Wnt-induced epidermal stratification in developing mammalian skin. PLoS Genet. 10:e1004687. doi: 10.1371/journal.pgen.1004687
Keywords: proteoglycans, GAGs, stem cells, embryonic stem cells, adult stem cells, extracellular matrix, glycosaminoglycan, heparan sulfate
Citation: Ravikumar M, Smith RAA, Nurcombe V and Cool SM (2020) Heparan Sulfate Proteoglycans: Key Mediators of Stem Cell Function. Front. Cell Dev. Biol. 8:581213. doi: 10.3389/fcell.2020.581213
Received: 08 July 2020; Accepted: 29 October 2020;
Published: 19 November 2020.
Edited by:
Jeremy Turnbull, University of Liverpool, United KingdomReviewed by:
Martin Götte, University of Münster, GermanyDeirdre R. Coombe, Curtin University, Australia
Copyright © 2020 Ravikumar, Smith, Nurcombe and Cool. This is an open-access article distributed under the terms of the Creative Commons Attribution License (CC BY). The use, distribution or reproduction in other forums is permitted, provided the original author(s) and the copyright owner(s) are credited and that the original publication in this journal is cited, in accordance with accepted academic practice. No use, distribution or reproduction is permitted which does not comply with these terms.
*Correspondence: Simon M. Cool, U2ltb24uQ09PTEBpbWIuYS1zdGFyLmVkdS5zZw==