- Department of Biology and Biotechnology, Worcester Polytechnic Institute, Worcester, MA, United States
Primary cilia are specialized cellular structures that project from the surface of most cell types in metazoans and mediate transduction of major signaling pathways. The ciliary membrane is contiguous with the plasma membrane, yet it exhibits distinct protein and lipid composition, which is essential for ciliary function. Diffusion barriers at the base of a cilium are responsible for establishing unique molecular composition of this organelle. Although considerable progress has been made in identifying mechanisms of ciliary protein trafficking in and out of cilia, it remains largely unknown how the distinct lipid identity of the ciliary membrane is achieved. In this mini review, I summarize recent developments in characterizing lipid composition and organization of the ciliary membrane and discuss the emerging roles of lipids in modulating activity of ciliary signaling components including ion channels and G protein-coupled receptors.
Introduction
Cilia (or flagella) are hair-like cellular projections that are highly conserved across eukaryotes (Carvalho-Santos et al., 2011). Based on their structural features, cilia are classified into motile and non-motile subtypes. Non-motile cilia, also known as primary cilia, are present on nearly all vertebrate cell types and function as signaling hubs during development and in differentiated tissues. In fact, components of all major signaling pathways including Hedgehog (Hh), Wnt, Notch, transforming growth factor β, G protein-coupled receptors (GPCRs), receptor tyrosine kinases, and extracellular matrix receptors localize to cilia and require these organelles for proper transduction (Mykytyn and Askwith, 2017; Anvarian et al., 2019). While most cells in the human body possess a solitary primary cilium, motile cilia are also present on the surface of some specialized cells in the airway, oviduct, and brain ventricles (Brooks and Wallingford, 2014). Like their non-motile counterparts, motile cilia can detect and transmit diverse sensory cues in addition to beating and propelling fluids (Bloodgood, 2010). Due to the central role of cilia in signaling and their nearly ubiquitous distribution across human tissues, perturbations in cilia structure and/or function manifest in a spectrum of genetic disorders called ciliopathies (Reiter and Leroux, 2017). These diseases affect most human organ systems and present with pleiotropic developmental and adult phenotypes that include blindness, kidney and heart disease, obesity, and cognitive deficits (Badano et al., 2006).
Since the discovery of motile cilia in the 17th century by Antonie van Leeuwenhoek until the early 2000s, cilia research was rather scarce and focused primarily on the axoneme – the microtubule backbone of the organelle (Bloodgood, 2009). It was at the dawn of the 21st century, when the sensory functions and clinical relevance of cilia were broadly demonstrated, that an interest in cilia surged, and attention of the scientific community shifted to the ciliary membrane. Unlike other cellular organelles, cilia are not fully enclosed by membrane. Instead, the ciliary membrane is continuous with the plasma membrane, and at their base, cilia are exposed to the cytosol. Despite continuity with the plasma membrane, the ciliary membrane exhibits a unique protein and lipid composition that is maintained, at least in part, by multiple diffusion barriers at the cilia base (Verhey and Yang, 2016). During the last two decades, much progress has been made in identifying the protein constituents of the ciliary membrane and molecular mechanisms of their trafficking in and out of cilia (Nachury and Mick, 2019). In contrast, the ciliary lipidome or mechanisms controlling its establishment are only starting to come to light.
This mini-review briefly summarizes current knowledge about ciliary membrane lipid composition and the molecular mechanisms that regulate ciliary lipid content. I also discuss the emerging roles of lipids in cilia signaling and outline major outstanding questions regarding the roles of lipids in modulating cilia-based pathways and shaping ciliary membrane morphology. Addressing these questions in the future may provide insight into human pathological conditions linked to altered membrane lipid constitution.
Cilia Architecture
The cilium is comprised of a core microtubule-based structure called the axoneme ensheathed by a specialized membrane. Nine radially symmetric microtubule doublets (A- and B-tubules) of the axoneme extend from the basal body – a modified mother centriole, which nucleates the axoneme and anchors the cilium at the cell surface (Ishikawa and Marshall, 2011; Figure 1A). In addition to the nine peripheral microtubule doublets, the axoneme of the motile cilium typically contains a central pair of singlet microtubules required for ciliary beating (9 + 2 arrangement), while the axoneme of the primary cilium lacks it (9 + 0 arrangement) (Satir and Christensen, 2007; Figures 1B,C). Most motile cilia also have radial spokes and inner and outer dynein arms attached to the microtubule doublets of the axoneme to drive motility (Ishikawa, 2017; Figure 1B).
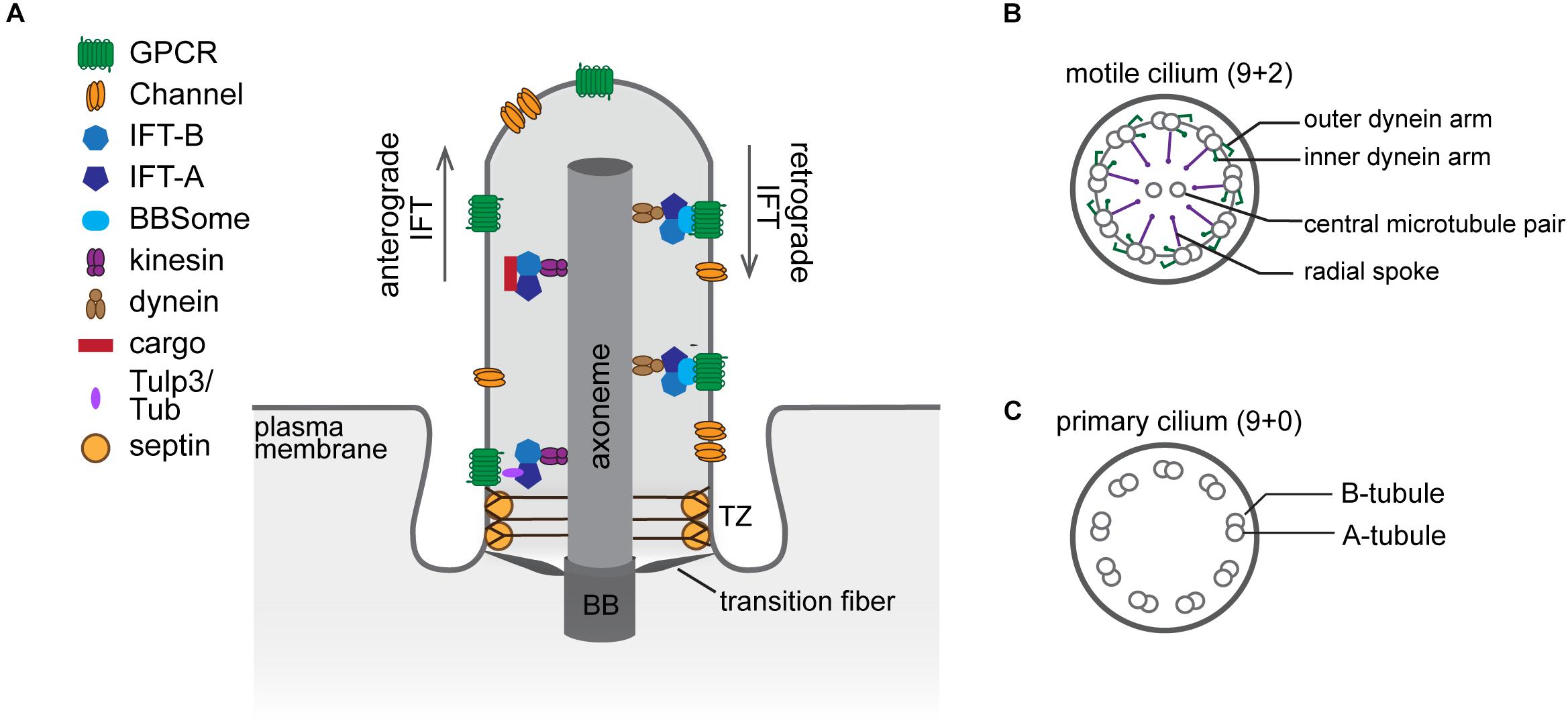
Figure 1. The structural organization of the cilium. (A) Diagram of a cilium depicting major structural components and ciliary sub-compartments. (B,C) Cross-section schematics of a typical motile (B) and primary (C) cilium. BB, basal body; TZ, transition zone.
Since there are no ribosomes inside the cilium, all ciliary proteins are imported from the cytosol. The transition zone (TZ), which constitutes the proximal 0.5–1.0 μm of the axoneme, is comprised of several macromolecular complexes that serve as a gate controlling selective entry and exit of ciliary cargoes. At the ultrastructural level, the TZ is characterized by Y-shaped fibers (Y-links) connecting the microtubule doublets of the axoneme to the ciliary membrane (Blacque and Sanders, 2014; Garcia-Gonzalo and Reiter, 2017; Figure 1A). Together with the transition fibers, which anchor the basal body to the membrane, Y-links provide a physical barrier that separates the cilium proper from the cytoplasm, and the membrane attachment points of the transition fibers demarcate the boundary between the plasma and ciliary membranes. Cilia assembly and maintenance are mediated by a bi-directional transport system called intraflagellar transport (IFT). Microtubule motors in conjunction with three multi-subunit complexes – IFT-A, IFT-B, and the Bardet–Biedl syndrome (BBS)ome – traffic proteins along the axoneme between the ciliary base and tip (reviewed in Taschner and Lorentzen, 2016; Wingfield et al., 2018; Figure 1A). Notably, mutations in genes encoding components of the basal body, TZ, and IFT are associated with ciliopathies including Meckel-Gruber and Joubert syndromes, nephronophthisis, polycystic kidney disease, and Bardet–Biedl syndrome, underscoring the importance of cilia in human health (Reiter and Leroux, 2017).
Lipid Composition of the Ciliary Membrane
Polyphosphoinositide Distribution and Roles in Ciliary Protein Trafficking
Considerable progress has been made in understanding how cilia establish their unique protein content (reviewed in Garcia-Gonzalo and Reiter, 2017; Mukhopadhyay et al., 2017; Morthorst et al., 2018; Nachury and Mick, 2019). On the other hand, much remains to be discovered about how cells maintain the ciliary membrane lipid identity. Some lipid biosynthetic enzymes localize to distinct sub-ciliary compartments and locally modulate membrane lipid composition. Conversion of polyphosphoinositides (PPIs) by multiple kinases and phosphatases provides the best-known example of lipid generation at local sites in the ciliary membrane. Polyphosphoinositides are signaling lipids generated by reversible phosphorylation of phosphatidylinositol (PI) at positions 3, 4, and 5 of its inositol ring (Balla, 2013). These phosphorylation derivatives of PI populate distinct membrane domains within cells, where they regulate many aspects of cellular physiology (Di Paolo and De Camilli, 2006). The ciliary membrane in mammals and sea urchin contains high levels of phosphatidylinositol-4-phosphate [PI(4)P] relative to the adjacent plasma membrane (Chávez et al., 2015; Garcia-Gonzalo et al., 2015). In contrast, phosphatidylinositol-4,5-bisphosphate [PI(4,5)P2] is largely depleted from the ciliary membrane in mammals, Caenorhabditis elegans, Drosophila melanogaster, and Trypanosoma brucei. Instead, PI(4,5)P2 localizes to distinct membrane domains at the cilia base creating a sharp boundary in PPI composition (Chávez et al., 2015; Garcia-Gonzalo et al., 2015; Jensen et al., 2015; Park et al., 2015; DiTirro et al., 2019; Dyson et al., 2017; Figure 2A). In retinal pigmented epithelial cells and primary mouse embryonic fibroblasts, PI(4,5)P2 is concentrated at the TZ, which also contains phosphatidylinositol-3,4,5-trisphosphate [PI(3,4,5)P3] (Dyson et al., 2017). Conversely, in C. elegans neurons and T. brucei, PI(4,5)P2 is enriched in an endocytic membrane domain (periciliary membrane compartment/ciliary pocket), which lies proximal to the TZ (Demmel et al., 2014; Jensen et al., 2015; DiTirro et al., 2019). In photoreceptors, while PI(4,5)P2 is also largely excluded from the outer segment (OS), which is a modified cilium, PI(4)P localizes to the OS as well as inner segment and perinuclear regions (Nasuhoglu et al., 2002; Finkelstein et al., 2020; Figure 2B). More studies are needed to systematically evaluate PPI composition across all ciliated cell types and to better understand the physiological significance of cell-specific differences in PPI composition and sub-ciliary distribution.
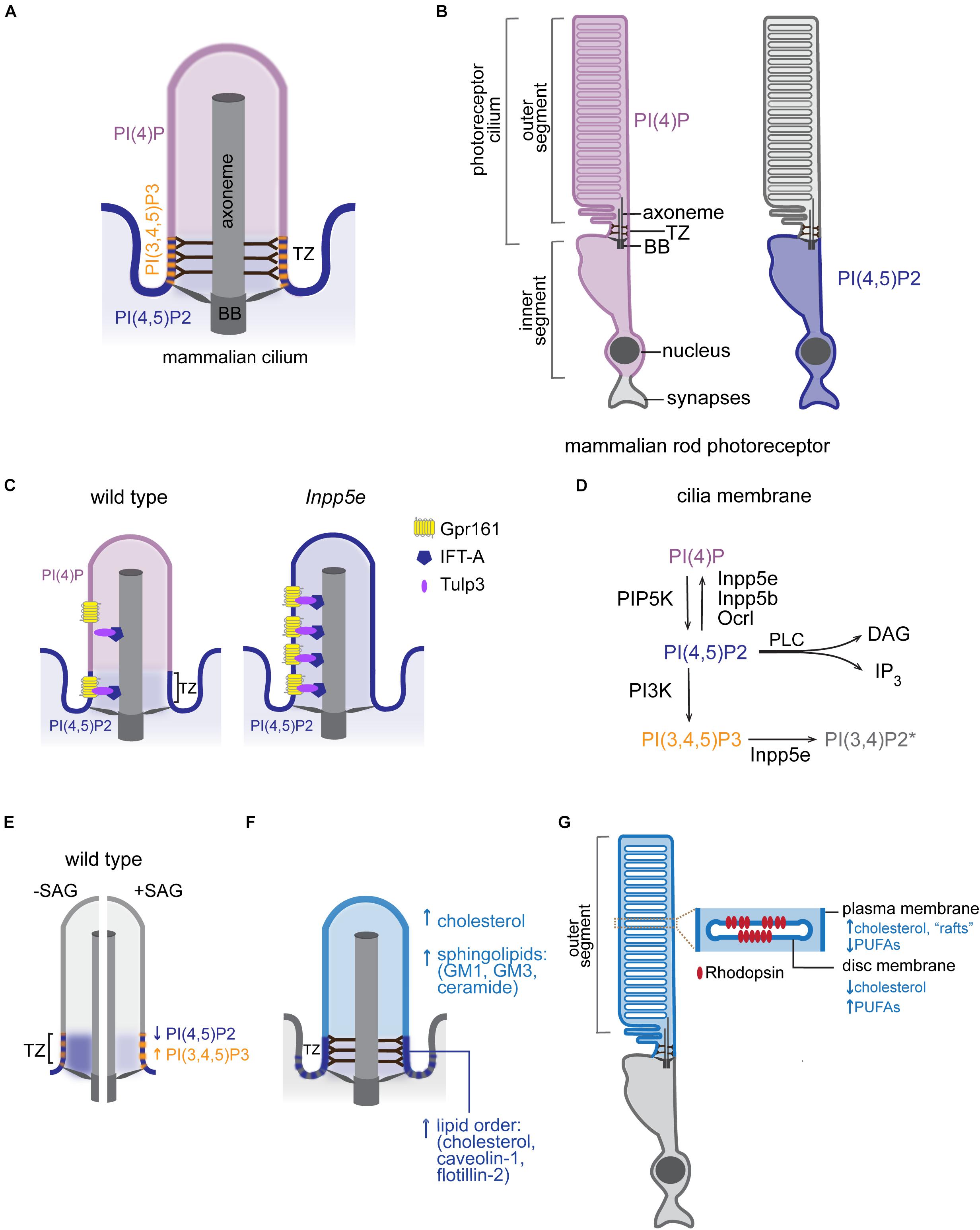
Figure 2. Lipid composition of the ciliary membrane. (A) Schematic representation of PPI distribution in the ciliary membrane of mammalian cells. BB, basal body; TZ, transition zone. (B) Distribution of the indicated PPI species in rod photoreceptors of mammalian retina. (C) Intraciliary localization of PI(4)P and PI(4,5)P2 in mammalian wild-type and Inpp5e mutant cells. Distribution of Tulp3/IFT-A trafficking complex and its GPCR cargo in control and Inpp5e mutant cells is also shown. (D) PPI species and a subset of PPI metabolizing enzymes that have been reported inside cilia. The presence of PI(3,4)P2 in the ciliary membrane is inferred based on intraciliary localization of Inpp5e and its substrate PI(3,4,5)P3 (Moore et al., 2016; Dyson et al., 2017). DAG, diacylglycerol, IP3, inositol 1,4,5-trisphosphate, PLC, phospholipase C. (E) Diagrammatic representation of changes in PPI composition at the TZ in response to Hh pathway activation. Blue and orange arrows mark direction of the observed changes in PI(4,5)P2 and PI(3,4,5)P3 levels, respectively, in the wild-type SAG-treated or untreated control cells. (F) A schematic representation of major raft-associated components (proteins and lipids) known to be enriched in the ciliary membrane. Dashed lines at cilia base represent condensed lipid microdomains detected by Laurdan microscopy in some cell types. (G) A schematic diagram of the mammalian rod photoreceptor. Inset shows an enlarged view of the disc and surrounding OS plasma membrane highlighting their distinct lipid content. PUFAs, polyunsaturated fatty acids.
How does the cilium maintain a unique PPI distribution? Inpp5e inositol polyphosphate-5-phosphatase, which converts PI(3,4,5)P3 and PI(4,5)P2 into PI(3,4)P2 and PI(4)P, respectively, localizes to mammalian cilia (Bielas et al., 2009; Jacoby et al., 2009; Luo et al., 2012, 2013). Mutations in INPP5E cause Joubert and MORM (mental retardation, truncal obesity, retinal dystrophy, and micropenis) syndromes in humans, and Inpp5e knockout mice display phenotypes consistent with ciliopathies (Jacoby et al., 2009). In the absence of Inpp5e, PI(4,5)P2 accumulates in the cilium, while ciliary PI(4)P levels drop (Chávez et al., 2015; Garcia-Gonzalo et al., 2015; Figure 2C). Similarly, in fly and worm sensory neurons, loss of INPP5E orthologs leads to increased PI(4,5)P2 levels in cilia (Park et al., 2015; DiTirro et al., 2019). Together, these findings are consistent with the model that PI(4,5)P2 diffuses laterally from the plasma to the ciliary membrane, where it is converted to PI(4)P by Inpp5e. Two other inositol polyphosphate-5-phosphatases (Inpp5b and Ocrl) have been reported to localize inside cilia of mammalian cells (Bielas et al., 2009; Jacoby et al., 2009; Luo et al., 2012, 2013). Mutations in human OCRL cause Lowe syndrome, a multisystemic disorder with characteristics of a ciliopathy, and cilia from Lowe syndrome patient fibroblasts contain high levels of PI(4,5)P2 and low levels of PI(4)P similarly to Inpp5e mutant cilia (Coon et al., 2012; Prosseda et al., 2017). Therefore, it is likely that several inositol polyphosphate-5-phosphatases contribute to the ciliary membrane PPI composition (Figure 2D). It is tempting to speculate that differences in cell and tissue distribution of PPIs and their metabolizing enzymes might contribute to symptomatic variability observed in patients carrying mutations in inositol polyphosphate-5-phosphatases (e.g., cataracts in Lowe and MORM patients versus retinitis pigmentosa in Joubert patients) (Hampshire et al., 2006; Madhivanan et al., 2012; Wang et al., 2018).
Beside inositol polyphosphate-5-phosphatases, several other phospholipid-metabolizing enzymes have been reported in the photoreceptor OS, where lipid metabolism has been extensively studied (reviewed in Giusto et al., 2000; Rajala, 2020; Wensel, 2020). Among these enzymes are phosphatidylinositol 3-kinase, which converts PI, PI(4)P, and PI(4,5)P2 into PI(3)P, PI(3,4)P2, and PI(3,4,5)P3, respectively, and phospholipase C, which cleaves PI(4,5)P2 to generate second messengers inositol 1,4,5-trisphosphate and diacylglycerol (Figure 2D). More studies are needed, however, to fully understand how ciliary phospholipid composition is modulated by these enzymes in different contexts, and how it contributes to cilia-mediated cellular functions. Since PPIs constitute <1% of total phospholipid mass in eukaryotic cells, with PI(4)P and PI(4,5)P2 being most abundant (∼0.05% each) (Fliesler and Anderson, 1983; Lemmon, 2008), PPI detectability in ciliary membranes presents a technical challenge. Development of more sensitive tools such as a recently reported ELISA-based method (He et al., 2016) is necessary to accurately measure these low-abundance lipids.
Although it remains to be determined whether diffusion barriers at the cilia base directly influence PPI distribution, the intact TZ is required for ciliary localization of Inpp5e. Mutations in TZ genes Tctn1, Tmem231, B9d1, and Mks1 disrupt Inpp5e ciliary localization (Garcia-Gonzalo et al., 2015; Roberson et al., 2015; Slaats et al., 2016; Goetz et al., 2017). The same genes are also necessary for ciliary localization of a small GTPase Arl13b, which regulates trafficking of several ciliary proteins, including Inpp5e. Thus, it is conceivable that the Tmem231/B9d1/Mks1/Tctn1 TZ complex localizes Inpp5e to the cilium via Arl13b, thereby indirectly regulating ciliary PPI distribution (Garcia-Gonzalo et al., 2011; Humbert et al., 2012).
PPIs can also directly bind to transmembrane proteins (Balla, 2013). Interestingly, the TZ levels of Mks1/Tctn1/Tmem231/B9d1 following SAG (Smoothened receptor agonist) treatment are lower in Inpp5e null compared to wild-type embryonic fibroblasts. Additionally, cilia base localization of the oligomeric GTPase Septin2 was similarly reduced under these conditions (Dyson et al., 2017). Septins interact with phospholipids including PI(4,5)P2, which in turn facilitate septin filament polymerization (Mostowy and Cossart, 2012). Like TZ proteins, septins localize to the cilia base, where they are proposed to form a diffusion barrier between the plasma and ciliary membranes and regulate localization of select TZ proteins including Tmem231 and B9d1 (Hu et al., 2010; Chih et al., 2012). Catalytic activity of Inpp5e is required for proper localization of TZ proteins and Septin2; therefore, it is likely that Inpp5e-modulated PPI composition at the cilia base can dynamically regulate TZ assembly. Future studies are needed to determine whether PPIs regulate TZ composition via direct binding to TZ proteins, indirectly by controlling Septin2 localization, or through other mechanisms.
In addition to regulating the TZ, PPIs play a key role in ciliary import of channels and GPCRs (Badgandi et al., 2017). The tubby family proteins TUB and TULP3 bind membrane PI(4,5)P2 and IFT-A and thereby serve as adaptors for delivery of transmembrane proteins into the cilium (Mukhopadhyay et al., 2010). The current model posits that the interaction of TUB/TULP3 with PI(4,5)P2 in the plasma membrane facilitates association of TUB/TULP3 with transmembrane proteins that are subsequently transported into the cilium via the IFT-A complex. Since the TUB/TULP3 interaction with protein cargoes is PI(4,5)P2-dependent, absence of PI(4,5)P2 in the ciliary membrane causes TUB/TULP3 cargoes to be released inside the cilium after traversing the TZ (Badgandi et al., 2017). Consistent with this model, depletion of Inpp5e and subsequent intraciliary accumulation of PI(4,5)P2 results in increased levels of Tulp3/IFT-A proteins and their transmembrane cargoes such as GPCR Gpr161 – a negative regulator of Shh signaling – inside cilia (Mukhopadhyay et al., 2013; Chávez et al., 2015; Garcia-Gonzalo et al., 2015; Figure 2C). Other ciliary proteins including components of the BBSome (e.g., BBS5) and the exocyst can bind PPIs in vitro suggesting a broad role for phospholipids in mediating ciliary protein trafficking (Liu et al., 2007; Nachury et al., 2007; Jin et al., 2010). Notably, recent cryo-electron microscopy structures of the native BBSome from bovine retina suggested that BBS5 may not bind PPIs in vivo or may do so via an unknown motif or after a conformational change (Singh et al., 2020).
Recent studies in mammals and C. elegans demonstrated that, similar to ciliary protein composition, PPI content of the ciliary membrane is dynamic and can change in response to signaling. For example, C. elegans mutants in odr-1, which encodes a receptor guanylyl cyclase, display elevated levels of intraciliary PI(4,5)P2 relative to wild type in a specialized sensory neuron type (DiTirro et al., 2019). In mammals, activation of Hh signaling with SAG increases PI(3,4,5)P3 while decreasing PI(4,5)P2 levels at the TZ (Dyson et al., 2017; Figure 2E). The latter study also showed that TZ levels of both PPI species were higher in Inpp5e null compared to wild-type cells upon SAG treatment, suggesting that Inpp5e is responsible, at least in part, for signaling-dependent modulation of PPI composition at the TZ. In the rod OS, several studies reported activation of PI-metabolizing enzymes in response to light as well as light-dependent changes in PI(4)P and PI(4,5)P2 levels (reviewed in (Giusto et al., 2000; Wensel, 2020). However, the direction of change in PPI composition differed among studies, and the physiological significance of these effects requires further investigation. It will be interesting to examine whether levels of other ciliary lipids are also modulated by signaling across cell types.
Microdomains of High Lipid Order
Early studies in diverse biological systems detected high levels of sterols and sphingolipids in the ciliary membrane, suggesting the presence of ordered lipid domains (i.e., “lipid rafts”) (Montesano, 1979; Souto-Padron and De Souza, 1983; Kaneshiro et al., 1984; Chailley and Boisvieux-Ulrich, 1985). More recently, sphingolipids including ceramide and raft-associated gangliosides GM1 and GM3 have been identified in primary cilia of Madin–Darby Canine Kidney (MDCK) epithelial cells by immunofluorescence (Janich and Corbeil, 2007; He et al., 2012; Figure 2F). Sphingolipids have also been detected in pure intact flagella of T. brucei using reverse-phase liquid chromatography high resolution tandem mass spectrometry (Serricchio et al., 2015). Membrane microdomains enriched in cholesterol and sphingolipids are resistant to detergent solubilization, and detergent-resistant membranes have been used as a proxy for rafts in studies probing lipid-raft composition (Farnoud et al., 2015). Caveolin-1 – an intra-membranous protein that stabilizes cholesterol-rich raft domains – localizes to the TZ in a cholesterol-dependent manner in mammalian cells and is present in the detergent resistant membranes of the photoreceptor OS (Nair et al., 2002; Lajoie et al., 2009; Schou et al., 2017). Similarly, another lipid raft scaffold flotillin-2 was detected at the TZ in epithelial cells (Schou et al., 2017; Figure 2F). In further support of the raft-like composition of ciliary membranes, the TZ membrane in Chlamydomonas reinhardtii is exceptionally resistant to detergent extraction, and Laurdan microscopy of T. brucei and MDCK cells showed condensed lipid microdomains in the trypanosome flagella and at the base of primary cilia (Kamiya and Witman, 1984; Vieira et al., 2006; Tyler et al., 2009). Collectively, these studies suggest that the ciliary membrane has unique lipid composition with distinct membrane microdomains. More research is needed, however, to determine how distinct membrane lipid domains form and contribute to cilia function.
While select phosphoinositide-metabolizing enzymes localize to cilia and directly modify intraciliary PPI content, none of the enzymes involved in sphingolipid or cholesterol metabolism have been identified inside the cilium to date. The “picket fence model” of membrane compartmentalization may provide one possible mechanism for ciliary lipid organization. This model posits that transmembrane proteins anchored to the actin network act as a “picket fence” impeding diffusion of the adjacent lipid molecules via steric hinderance and hydrodynamic slowing effects (Kusumi et al., 2012). In fact, entire raft assemblies can be confined to distinct membrane compartments by the “picket fence” according to this model. Many ciliary proteins are transmembrane, and therefore may form “pickets” to restrict diffusion of membrane molecules. Furthermore, using cryo-electron tomography, a recent study demonstrated that actin filaments surround and are intertwined with microtubules of the axoneme inside the cilia of MDCKII cells, adding further credence to the “picket fence” model as a possible mechanism of the ciliary membrane compartmentalization (Kiesel et al., 2020). Future work will need to experimentally test this model of ciliary membrane organization and determine whether same or different mechanisms regulate compartmentalization of ciliary membranes across cell types.
Lipids in Cilia-Based Signaling
PPI-Dependent Transmembrane Signaling
PPIs are key mediators of cell signaling in eukaryotes. At the plasma membrane, phospholipase C-dependent hydrolysis of PI(4,5)P2 downstream of growth factor receptors and GPCRs generates second messengers that amplify and transmit signaling from the cell surface downstream (Falkenburger et al., 2010). Furthermore, PI(4,5)P2 and PI(3,4,5)P3 facilitate assembly of signalosomes by recruiting different classes of proteins with lipid-binding domains (reviewed in Prestwich, 2004; Rajala, 2010; Hammond and Burke, 2020). Among PI(3,4,5)P3 interacting proteins are guanine nucleotide exchange factors and GTPase activating proteins for small GTPases as well as kinases and signaling scaffold proteins (Balla, 2013). In photoreceptor cilia, light stimulates PI(3,4,5)P3 binding and subsequent activation of the kinase Akt1 - a major signaling protein downstream of receptor tyrosine kinases (Li et al., 2008). Growth factor-dependent activation of Akt has also been reported at the cilia base in other cellular contexts (Zhu et al., 2009; Wang et al., 2015; Suizu et al., 2016; Walia et al., 2019). More studies are needed, however, to address the contribution of PPIs and their metabolites to cilia-based signaling in different cellular contexts.
Lipid-Dependent Regulation of Ion Channels
Membrane lipids, including phospholipids and cholesterol, can also directly modulate ion channels. For example, PI(4,5)P2 binds to and regulates the activity of voltage- and ligand-gated ion channels, inward rectifier channels, and transporters (reviewed in Suh and Hille, 2008; Duncan et al., 2020). Transient receptor potential (TRP) channels (e.g., PKD2, TRPM4, and TRPC1), voltage-gated potassium channels, cyclic nucleotide-gated channels, and epithelial sodium channels are all targets of PI(4,5)P2-dependent modulation and localize to cilia (Womack et al., 2000; Raychowdhury et al., 2005; Suh and Hille, 2008; Enuka et al., 2012; Flannery et al., 2015; Sanchez et al., 2016). The ciliary channels TRPM4 and PKD2, the latter of which is mutated in autosomal dominant polycystic kidney disease, can also bind cholesterol, suggesting that both lipids may regulate these channels’ activity (Autzen et al., 2018; Wang et al., 2019). Another ciliary channel TRPV4 possesses cholesterol recognition motifs, and both TRPV4 and TRPC1 depend on caveolin-1 and cholesterol for proper positioning in the plasma membrane (Bergdahl et al., 2003; Brazer et al., 2003; Gradilone et al., 2007; Kumari et al., 2015). Since both caveolin-1 and cholesterol have been detected in the ciliary membrane, it is possible that similar mechanisms contribute to TRPV4 and TRPC1 ciliary localization. Function of olfactory cyclic nucleotide-gated channels is also altered by cholesterol depletion (Brady et al., 2004), and both olfactory and cone cyclic nucleotide-gated channels are inhibited by PI(3,4,5)P3 (Zhainazarov et al., 2004; Brady et al., 2006; Bright et al., 2007). Taken together, these studies suggest that PPI and cholesterol compartmentalization of the ciliary membrane may be of major significance for proper function of cilia-localized ion channels. In C. elegans, polyunsaturated fatty acids also modulate function of TRPV ciliary channels, although it remains to be tested whether they do so via direct interactions (Kahn-Kirby et al., 2004). More work is needed to address the contribution of specific lipids to localization and function of different ciliary channels.
Lipid-Mediated Regulation of GPCRs
In addition to regulating channels, membrane lipids interact with and modulate multiple aspects of protein receptor physiology including oligomerization and signaling dynamics. For example, PI(4,5)P2 can bind and stabilize the active conformation of several class A GPCRs (Yen et al., 2018). Many class A GPCRs are present in cilia, where they may be similarly regulated by PPIs (Anvarian et al., 2019). Some ciliary GPCRs transiently pool in the “intermediate compartment” demarcated by the TZ distally and the transition fibers proximally before exiting or re-entering the cilium. This region is enriched in PI(4,5)P2 and may function as a distinct GPCR signaling domain (Ye et al., 2018).
The prototypical GPCR rhodopsin is enriched in the disc membrane of the photoreceptor OS. The rod OS contains a stack of closed membranous compartments (discs) encased by the OS plasma membrane (Figure 2G). Although discs form by evagination of the plasma membrane at the base of the OS followed by apical displacement, disc and OS membrane display distinct lipid composition (Boesze-Battaglia et al., 1994; Ding et al., 2015). For example, the disc membrane is enriched in polyunsaturated fatty acids and low in cholesterol relative to the surrounding OS membrane, suggesting an elaborate lipid sorting mechanism at the base of photoreceptor cilia (Aveldano and Bazan, 1983; Boesze-Battaglia and Schimmel, 1997; Nair et al., 2002; Figure 2G). Unique lipid content of disc and OS plasma membrane is critical for photoreceptor function, as aberrant distribution of cholesterol in the OS membranes is associated with photoreceptor degeneration in rats (Boesze-Battaglia et al., 1994). Both cholesterol and polyunsaturated docosahexaenoic acid interact with rhodopsin but have opposite effects on photocycle kinetics, further highlighting the significance of the lipid environment for receptor and cell function (Albert et al., 1996; Mitchell et al., 2001; Niu et al., 2002; Soubias and Gawrisch, 2005; Grossfield et al., 2006). Membrane cholesterol can also modulate ligand affinity, G protein coupling, and receptor oligomerization in select GPCRs, and membrane docosahexaenoic acid content was suggested to alter receptor oligomerization kinetics (Pucadyil and Chattopadhyay, 2004; Gahbauer and Böckmann, 2016). Cholesterol and endogenous ciliary oxysterols also bind to Smoothened and activate the Hh pathway (Luchetti et al., 2016; Raleigh et al., 2018). Cholesterol accessibility (or chemical activity) is further modulated by sphingolipids, which sequester cholesterol in complexes thereby blocking Hh transduction (Kinnebrew et al., 2019). Besides Hh, sphingolipids regulate several other cilia-based pathways including GPCRs (reviewed in Kaiser et al., 2020). More studies are needed to further evaluate the effects of lipid dynamics on ciliary signaling.
Conclusion and Future Perspectives
Lipids have recently emerged as critical regulators of cilia function. The distinct lipid composition and compartmentalization of the ciliary membrane are essential for ciliary protein trafficking and transduction of cilia-based signaling cascades. The importance of lipids in cilia biology is further underscored by the fact that many ciliopathies display defects in the membrane lipid organization. Despite the key importance of lipids in cilia biology, our knowledge about cell-specific differences in the ciliary lipid composition, dynamics, and organization in distinct microdomains remains fragmented, as does our understanding of the roles that lipids play in cilia signaling. To bridge this gap in our understanding of cilia biology, systematic analysis of the lipid composition and lipid structure of sub-ciliary compartments in different cellular contexts in vivo is necessary. Ciliary membranes across and within organisms exhibit remarkably diverse morphologies, which are important for cell-specific cilia functions and can be modulated in response to signaling. It will be important to examine whether cell-specific differences in the ciliary lipid composition and/or dynamics also contribute to the morphological diversity of ciliary membranes. Single molecule tracking of specific lipids and mass spectrometry imaging may provide some insight into these outstanding questions and advance our understanding of the repertoire of lipid-mediated physiological functions.
Author Contributions
The author wrote the manuscript and generated the figures.
Funding
This work was supported by Worcester Polytechnic Institute.
Conflict of Interest
The authors declare that the research was conducted in the absence of any commercial or financial relationships that could be construed as a potential conflict of interest.
Acknowledgments
I thank Michael P. O’Donnell and Alison Philbrook for comments on the manuscript.
References
Albert, A. D., Young, J. E., and Yeagle, P. L. (1996). Rhodopsin-cholesterol interactions in bovine rod outer segment disk membranes. Biochim. Biophys. Acta 1285, 47–55. doi: 10.1016/s0005-2736(96)00145-9
Anvarian, Z., Mykytyn, K., Mukhopadhyay, S., Pedersen, L. B., and Christensen, S. T. (2019). Cellular signalling by primary cilia in development, organ function and disease. Nat. Rev. Nephrol. 15, 199–219. doi: 10.1038/s41581-019-0116-9
Autzen, H. E., Myasnikov, A. G., Campbell, M. G., Asarnow, D., Julius, D., and Cheng, Y. (2018). Structure of the human Trpm4 ion channel in a lipid nanodisc. Science 359, 228–232. doi: 10.1126/science.aar4510
Aveldano, M. I., and Bazan, N. G. (1983). Molecular species of phosphatidylcholine, -ethanolamine, -serine, and -inositol in microsomal and photoreceptor membranes of bovine retina. J. Lipid Res. 24, 620–627.
Badano, J. L., Mitsuma, N., Beales, P. L., and Katsanis, N. (2006). The Ciliopathies: an emerging class of human genetic disorders. Annu. Rev. Genomics Hum. Genet. 7, 125–148. doi: 10.1146/annurev.genom.7.080505.115610
Badgandi, H. B., Hwang, S.-H., Shimada, I. S., Loriot, E., and Mukhopadhyay, S. (2017). Tubby family proteins are adapters for ciliary trafficking of integral membrane proteins. J. Cell Biol. 216, 743–760. doi: 10.1083/jcb.201607095
Balla, T. (2013). Phosphoinositides: tiny lipids with giant impact on cell regulation. Physiol. Rev. 93, 1019–1137. doi: 10.1152/physrev.00028.2012
Bergdahl, A., Gomez, M. F., Dreja, K., Xu, S. Z., Adner, M., Beech, D. J., et al. (2003). Cholesterol depletion impairs vascular reactivity to endothelin-1 by reducing store-operated Ca2+ entry dependent on Trpc1. Circ. Res. 93, 839–847. doi: 10.1161/01.res.0000100367.45446.a3
Bielas, S. L., Silhavy, J. L., Brancati, F., Kisseleva, M. V., Al-Gazali, L., Sztriha, L., et al. (2009). Mutations in Inpp5E, encoding inositol polyphosphate-5-phosphatase E, link phosphatidyl inositol signaling to the ciliopathies. Nat. Genet. 41, 1032–1036. doi: 10.1038/ng.423
Blacque, O. E., and Sanders, A. A. (2014). Compartments within a compartment: what C. elegans can tell us about ciliary subdomain composition, biogenesis, function, and disease. Organogenesis 10, 126–137. doi: 10.4161/org.28830
Bloodgood, R. A. (2009). From central to rudimentary to primary: the history of an underappreciated organelle whose time has come. The primary cilium. Methods Cell Biol. 94, 3–52.
Bloodgood, R. A. (2010). Sensory reception is an attribute of both primary cilia and motile cilia. J. Cell Sci. 123, 505–509. doi: 10.1242/jcs.066308
Boesze-Battaglia, K., Organisciak, D. T., and Albert, A. D. (1994). Rcs rat retinal rod outer segment membranes exhibit different cholesterol distributions than those of normal rats. Exp. Eye Res. 58, 293–300. doi: 10.1006/exer.1994.1020
Boesze-Battaglia, K., and Schimmel, R. (1997). Cell membrane lipid composition and distribution: implications for cell function and lessons learned from photoreceptors and platelets. J. Exp. Biol. 200, 2927–2936.
Brady, J. D., Rich, E. D., Martens, J. R., Karpen, J. W., Varnum, M. D., and Brown, R. L. (2006). Interplay between Pip3 and calmodulin regulation of olfactory cyclic nucleotide-gated channels. Proc. Natl. Acad. Sci. U.S.A. 103, 15635–15640. doi: 10.1073/pnas.0603344103
Brady, J. D., Rich, T. C., Le, X., Stafford, K., Fowler, C. J., Lynch, L., et al. (2004). Functional role of lipid raft microdomains in cyclic nucleotide-gated channel activation. Mol. Pharmacol. 65, 503–511. doi: 10.1124/mol.65.3.503
Brazer, S. C., Singh, B. B., Liu, X., Swaim, W., and Ambudkar, I. S. (2003). Caveolin-1 contributes to assembly of store-operated Ca2+ influx channels by regulating plasma membrane localization of Trpc1. J. Biol. Chem. 278, 27208–27215. doi: 10.1074/jbc.m301118200
Bright, S. R., Rich, E. D., and Varnum, M. D. (2007). Regulation of human cone cyclic nucleotide-gated channels by endogenous phospholipids and exogenously applied phosphatidylinositol 3,4,5-trisphosphate. Mol. Pharmacol. 71, 176–183. doi: 10.1124/mol.106.026401
Carvalho-Santos, Z., Azimzadeh, J., Pereira-Leal, J. B., and Bettencourt-Dias, M. (2011). Tracing the origins of centrioles, cilia, and flagella. J. Cell Biol. 194, 165–175. doi: 10.1083/jcb.201011152
Chailley, B., and Boisvieux-Ulrich, E. (1985). Detection of plasma membrane cholesterol by filipin during microvillogenesis and ciliogenesis in quail oviduct. J. Histochem. Cytochem. 33, 1–10. doi: 10.1177/33.1.3965567
Chávez, M., Ena, S., Van Sande, J., de Kerchove d’Exaerde, A., Schurmans, S., and Schiffmann, S. N. (2015). Modulation of ciliary phosphoinositide content regulates trafficking and sonic hedgehog signaling output. Dev. Cell 34, 338–350. doi: 10.1016/j.devcel.2015.06.016
Chih, B., Liu, P., Chinn, Y., Chalouni, C., Komuves, L. G., Hass, P. E., et al. (2012). A ciliopathy complex at the transition zone protects the cilia as a privileged membrane domain. Nat. Cell Biol. 14, 61–72. doi: 10.1038/ncb2410
Coon, B. G., Hernandez, V., Madhivanan, K., Mukherjee, D., Hanna, C. B., Barinaga-Rementeria Ramirez, I., et al. (2012). The Lowe syndrome protein Ocrl1 is involved in primary cilia assembly. Hum. Mol. Genet. 21, 1835–1847. doi: 10.1093/hmg/ddr615
Demmel, L., Schmidt, K., Lucast, L., Havlicek, K., Zankel, A., Koestler, T., et al. (2014). The endocytic activity of the flagellar pocket in Trypanosoma brucei is regulated by an adjacent phosphatidylinositol phosphate kinase. J. Cell Sci. 127, 2351–2364. doi: 10.1242/jcs.146894
Di Paolo, G., and De Camilli, P. (2006). Phosphoinositides in cell regulation and membrane dynamics. Nature 443, 651–657. doi: 10.1038/nature05185
Ding, J. D., Salinas, R. Y., and Arshavsky, V. Y. (2015). Discs of mammalian rod photoreceptors form through the membrane evagination mechanism. J. Cell Biol. 211, 495–502. doi: 10.1083/jcb.201508093
DiTirro, D., Philbrook, A., Rubino, K., and Sengupta, P. (2019). The Caenorhabditis elegans Tubby homolog dynamically modulates olfactory cilia membrane morphogenesis and phospholipid composition. eLife 8:e48789.
Duncan, A. L., Song, W., and Sansom, M. S. P. (2020). Lipid-dependent regulation of ion channels and g protein–coupled receptors: insights from structures and simulations. Annu. Rev. Pharmacol. Toxicol. 60, 31–50. doi: 10.1146/annurev-pharmtox-010919-023411
Dyson, J. M., Conduit, S. E., Feeney, S. J., Hakim, S., Ditommaso, T., Fulcher, A. J., et al. (2017). Inpp5E regulates phosphoinositide-dependent cilia transition zone function. J. Cell Biol. 216, 247–263. doi: 10.1083/jcb.201511055
Enuka, Y., Hanukoglu, I., Edelheit, O., Vaknine, H., and Hanukoglu, A. (2012). Epithelial sodium channels (EnaC) are uniformly distributed on motile cilia in the oviduct and the respiratory airways. Histochem. Cell Biol. 137, 339–353. doi: 10.1007/s00418-011-0904-1
Falkenburger, B. H., Jensen, J. B., Dickson, E. J., Suh, B.-C., and Hille, B. (2010). Symposium Review: Phosphoinositides: lipid regulators of membrane proteins. J. Physiol. 588, 3179–3185. doi: 10.1113/jphysiol.2010.192153
Farnoud, A. M., Toledo, A. M., Konopka, J. B., Del Poeta, M., and London, E. (2015). Raft-like membrane domains in pathogenic microorganisms. Curr. Top. Membr. 75, 233–268. doi: 10.1016/bs.ctm.2015.03.005
Finkelstein, S., Gospe, S. M. III, Schuhmann, K., Shevchenko, A., Arshavsky, V. Y., and Lobanova, E. S. (2020). Phosphoinositide profile of the mouse retina. Cells 9:1417. doi: 10.3390/cells9061417
Flannery, R. J., Kleene, N. K., and Kleene, S. J. (2015). A Trpm4-dependent current in murine renal primary cilia. Am. J. Physiol. Renal Physiol. 309, F697–F707.
Fliesler, S. J., and Anderson, R. E. (1983). Chemistry and metabolism of lipids in the vertebrate retina. Prog. Lipid Res. 22, 79–131. doi: 10.1016/0163-7827(83)90004-8
Gahbauer, S., and Böckmann, R. A. (2016). Membrane-mediated oligomerization of G protein coupled receptors and its implications for Gpcr function. Front. Physiol. 7:494. doi: 10.3389/fphys.2016.00494
Garcia-Gonzalo, F. R., Corbit, K. C., Sirerol-Piquer, M. S., Ramaswami, G., Otto, E. A., Noriega, T. R., et al. (2011). A transition zone complex regulates mammalian ciliogenesis and ciliary membrane composition. Nat. Genet. 43, 776–784. doi: 10.1038/ng.891
Garcia-Gonzalo, F. R., Phua, S. C., Roberson, E. C., Garcia, G. III, Abedin, M., Schurmans, S., et al. (2015). Phosphoinositides regulate ciliary protein trafficking to modulate hedgehog signaling. Dev. Cell 34, 400–409. doi: 10.1016/j.devcel.2015.08.001
Garcia-Gonzalo, F. R., and Reiter, J. F. (2017). Open sesame: how transition fibers and the transition zone control ciliary composition. Cold Spring Harb. Perspect. Biol. 9:a028134. doi: 10.1101/cshperspect.a028134
Giusto, N. M., Pasquare, S. J., Salvador, G. A., Castagnet, P. I., Roque, M. E., and Ilincheta De Boschero, M. G. (2000). Lipid metabolism in vertebrate retinal rod outer segments. Prog. Lipid Res. 39, 315–391. doi: 10.1016/s0163-7827(00)00009-6
Goetz, S. C., Bangs, F., Barrington, C. L., Katsanis, N., and Anderson, K. V. (2017). The Meckel syndrome- associated protein Mks1 functionally interacts with components of the Bbsome and Ift complexes to mediate ciliary trafficking and hedgehog signaling. PLoS One 12:e0173399. doi: 10.1371/journal.pone.0173399
Gradilone, S. A., Masyuk, A. I., Splinter, P. L., Banales, J. M., Huang, B. Q., Tietz, P. S., et al. (2007). Cholangiocyte cilia express Trpv4 and detect changes in luminal tonicity inducing bicarbonate secretion. Proc. Natl. Acad. Sci. U.S.A. 104, 19138–19143. doi: 10.1073/pnas.0705964104
Grossfield, A., Feller, S. E., and Pitman, M. C. (2006). A role for direct interactions in the modulation of rhodopsin by -3 polyunsaturated lipids. Proc. Natl. Acad. Sci. U.S.A. 103, 4888–4893. doi: 10.1073/pnas.0508352103
Hammond, G. R. V., and Burke, J. E. (2020). Novel roles of phosphoinositides in signaling, lipid transport, and disease. Curr. Opin. Cell Biol. 63, 57–67. doi: 10.1016/j.ceb.2019.12.007
Hampshire, D. J., Ayub, M., Springell, K., Roberts, E., Jafri, H., Rashid, Y., et al. (2006). Morm syndrome (mental retardation, truncal obesity, retinal dystrophy and micropenis), a new autosomal recessive disorder, links to 9q34. Eur. J. Hum. Genet. 14, 543–548. doi: 10.1038/sj.ejhg.5201577
He, F., Agosto, M. A., Anastassov, I. A., Tse, D. Y., Wu, S. M., and Wensel, T. G. (2016). Phosphatidylinositol-3-phosphate is light-regulated and essential for survival in retinal rods. Sci. Rep. 6:26978.
He, Q., Wang, G., Dasgupta, S., Dinkins, M., Zhu, G., and Bieberich, E. (2012). Characterization of an apical ceramide-enriched compartment regulating ciliogenesis. Mol. Biol. Cell 23, 3156–3166. doi: 10.1091/mbc.e12-02-0079
Hu, Q., Milenkovic, L., Jin, H., Scott, M. P., Nachury, M. V., Spiliotis, E. T., et al. (2010). A septin diffusion barrier at the base of the primary cilium maintains ciliary membrane protein distribution. Science 329, 436–439. doi: 10.1126/science.1191054
Humbert, M. C., Weihbrecht, K., Searby, C. C., Li, Y., Pope, R. M., Sheffield, V. C., et al. (2012). Arl13B, Pde6D, and Cep164 form a functional network for Inpp5E ciliary targeting. Proc. Natl. Acad. Sci. U.S.A. 109, 19691–19696. doi: 10.1073/pnas.1210916109
Ishikawa, H., and Marshall, W. F. (2011). Ciliogenesis: building the cell’s antenna. Nat. Rev. Mol. Cell Biol. 12, 222–234. doi: 10.1038/nrm3085
Ishikawa, T. (2017). Axoneme structure from motile cilia. Cold Spring Harb. Perspect. Biol. 9:a028076. doi: 10.1101/cshperspect.a028076
Jacoby, M., Cox, J. J., Gayral, S., Hampshire, D. J., Ayub, M., Blockmans, M., et al. (2009). Inpp5E mutations cause primary cilium signaling defects, ciliary instability and ciliopathies in human and mouse. Nat. Genet. 41, 1027–1031. doi: 10.1038/ng.427
Janich, P., and Corbeil, D. (2007). Gm 1 and Gm 3 gangliosides highlight distinct lipid microdomains within the apical domain of epithelial cells. FEBS Lett. 581, 1783–1787. doi: 10.1016/j.febslet.2007.03.065
Jensen, V. L., Li, C., Bowie, R. V., Clarke, L., Mohan, S., Blacque, O. E., et al. (2015). Formation of the transition zone by Mks5/Rpgrip1L establishes a ciliary zone of exclusion (Cize) that compartmentalises ciliary signalling proteins and controls Pip2 ciliary abundance. EMBO J. 34, 2537–2556. doi: 10.15252/embj.201488044
Jin, H., White, S. R., Shida, T., Schulz, S., Aguiar, M., Gygi, S. P., et al. (2010). The conserved Bardet-Biedl syndrome proteins assemble a coat that traffics membrane proteins to cilia. Cell 141, 1208–1219. doi: 10.1016/j.cell.2010.05.015
Kahn-Kirby, A. H., Dantzker, J. L., Apicella, A. J., Schafer, W. R., Browse, J., Bargmann, C. I., et al. (2004). Specific polyunsaturated fatty acids drive Trpv-dependent sensory signaling in vivo. Cell 119, 889–900. doi: 10.1016/j.cell.2004.11.005
Kaiser, F., Huebecker, M., and Wachten, D. (2020). Sphingolipids controlling cilia and microvilli function. FEBS Lett. doi: 10.1002/1873-3468.13816 [Epub ahead of print].
Kamiya, R., and Witman, G. B. (1984). Submicromolar levels of calcium control the balance of beating between the two flagella in demembranated models of Chlamydomonas. J. Cell Biol. 98, 97–107. doi: 10.1083/jcb.98.1.97
Kaneshiro, E. S., Matesic, D. F., and Jayasimhulu, K. (1984). Characterizations of six ethanolamine sphingophospholipids from Paramecium cells and cilia. J. Lipid Res. 25, 369–377.
Kiesel, P., Viar, G. A., Tsoy, N., Maraspini, R., Honigmann, A., and Pigino, G. (2020). The molecular structure of primary cilia revealed by cryo-electron tomography. bioRxiv [Preprint]. doi: 10.1101/2020.03.20.000505
Kinnebrew, M., Iverson, E. J., Patel, B. B., Pusapati, G. V., Kong, J. H., Johnson, K. A., et al. (2019). Cholesterol accessibility at the ciliary membrane controls hedgehog signaling. eLife 8:e50051.
Kumari, S., Kumar, A., Sardar, P., Yadav, M., Majhi, R. K., Kumar, A., et al. (2015). Influence of membrane cholesterol in the molecular evolution and functional regulation of Trpv4. Biochem. Biophys. Res. Commun. 456, 312–319. doi: 10.1016/j.bbrc.2014.11.077
Kusumi, A., Fujiwara, T. K., Chadda, R., Xie, M., Tsunoyama, T. A., Kalay, Z., et al. (2012). Dynamic organizing principles of the plasma membrane that regulate signal transduction: commemorating the fortieth anniversary of Singer and Nicolson’s fluid-mosaic model. Annu. Rev. Cell Dev. Biol. 28, 215–250. doi: 10.1146/annurev-cellbio-100809-151736
Lajoie, P., Goetz, J. G., Dennis, J. W., and Nabi, I. R. (2009). Lattices, rafts, and scaffolds: domain regulation of receptor signaling at the plasma membrane. J. Cell Biol. 185, 381–385. doi: 10.1083/jcb.200811059
Lemmon, M. A. (2008). Membrane recognition by phospholipid-binding domains. Nat. Rev. Mol. Cell Biol. 9, 99–111. doi: 10.1038/nrm2328
Li, G., Rajala, A., Wiechmann, A. F., Anderson, R. E., and Rajala, R. V. S. (2008). Activation and membrane binding of retinal protein kinase Bα/Akt1 is regulated through light-dependent generation of phosphoinositides. J. Neurochem. 107, 1382–1397. doi: 10.1111/j.1471-4159.2008.05707.x
Liu, J., Zuo, X., Yue, P., and Guo, W. (2007). Phosphatidylinositol 4,5-bisphosphate mediates the targeting of the exocyst to the plasma membrane for exocytosis in mammalian cells. Mol. Biol. Cell 18, 4483–4492. doi: 10.1091/mbc.e07-05-0461
Luchetti, G., Sircar, R., Kong, J. H., Nachtergaele, S., Sagner, A., Byrne, E. F., et al. (2016). Cholesterol activates the G-protein coupled receptor Smoothened to promote Hedgehog signaling. eLife 5:e20304.
Luo, N., Kumar, A., Conwell, M., Weinreb, R. N., Anderson, R., and Sun, Y. (2013). Compensatory role of inositol 5-phosphatase Inpp5B to Ocrl in primary cilia formation in Oculocerebrorenal syndrome of lowe. PLoS One 8:e66727. doi: 10.1371/journal.pone.0066727
Luo, N., West, C. C., Murga-Zamalloa, C. A., Sun, L., Anderson, R. M., Wells, C. D., et al. (2012). Ocrl localizes to the primary cilium: a new role for cilia in Lowe syndrome. Hum. Mol. Genet. 21, 3333–3344. doi: 10.1093/hmg/dds163
Madhivanan, K., Mukherjee, D., and Aguilar, R. C. (2012). Lowe syndrome. Commun. Integr. Biol. 5, 641–644.
Mitchell, D. C., Niu, S. L., and Litman, B. J. (2001). Optimization of receptor-G protein coupling by bilayer lipid composition I: kinetics of rhodopsin-transducin binding. J. Biol. Chem. 276, 42801–42806. doi: 10.1074/jbc.m105772200
Montesano, R. (1979). Inhomogeneous distribution of filipin-sterol complexes in the ciliary membrane of rat tracheal epithelium. Am. J. Anat. 156, 139–145. doi: 10.1002/aja.1001560115
Moore, B. S., Stepanchick, A. N., Tewson, P. H., Hartle, C. M., Zhang, J., Quinn, A. M., et al. (2016). Cilia have high camp levels that are inhibited by Sonic Hedgehog-regulated calcium dynamics. Proc. Natl. Acad. Sci. U.S.A. 113, 13069–13074. doi: 10.1073/pnas.1602393113
Morthorst, S. K., Christensen, S. T., and Pedersen, L. B. (2018). Regulation of ciliary membrane protein trafficking and signalling by kinesin motor proteins. FEBS J. 285, 4535–4564. doi: 10.1111/febs.14583
Mostowy, S., and Cossart, P. (2012). Septins: the fourth component of the cytoskeleton. Nat. Rev. Mol. Cell Biol. 13, 183–194. doi: 10.1038/nrm3284
Mukhopadhyay, S., Badgandi, H. B., Hwang, S. H., Somatilaka, B., Shimada, I. S., and Pal, K. (2017). Trafficking to the primary cilium membrane. Mol. Biol. Cell 28, 233–239. doi: 10.1091/mbc.e16-07-0505
Mukhopadhyay, S., Wen, X., Chih, B., Nelson, C. D., Lane, W. S., Scales, S. J., et al. (2010). Tulp3 bridges the Ift-A complex and membrane phosphoinositides to promote trafficking of G protein-coupled receptors into primary cilia. Genes Dev. 24, 2180–2193. doi: 10.1101/gad.1966210
Mukhopadhyay, S., Wen, X., Ratti, N., Loktev, A., Rangell, L., Scales, S. J., et al. (2013). The ciliary G-protein-coupled receptor Gpr161 negatively regulates the Sonic hedgehog pathway via camp signaling. Cell 152, 210–223. doi: 10.1016/j.cell.2012.12.026
Mykytyn, K., and Askwith, C. (2017). G-protein-coupled receptor signaling in cilia. Cold Spring Harb. Perspect. Biol. 9:a028183. doi: 10.1101/cshperspect.a028183
Nachury, M. V., Loktev, A. V., Zhang, Q., Westlake, C. J., Peranen, J., Merdes, A., et al. (2007). A core complex of Bbs proteins cooperates with the Gtpase Rab8 to promote ciliary membrane biogenesis. Cell 129, 1201–1213. doi: 10.1016/j.cell.2007.03.053
Nachury, M. V., and Mick, D. U. (2019). Establishing and regulating the composition of cilia for signal transduction. Nat. Rev. Mol. Cell Biol. 20, 389–405. doi: 10.1038/s41580-019-0116-4
Nair, K. S., Balasubramanian, N., and Slepak, V. Z. (2002). Signal-dependent translocation of transducin, Rgs9-1-Gβ5L complex, and arrestin to detergent-resistant membrane rafts in photoreceptors. Curr. Biol. 12, 421–425. doi: 10.1016/s0960-9822(02)00691-7
Nasuhoglu, C., Feng, S., Mao, J., Yamamoto, M., Yin, H. L., Earnest, S., et al. (2002). Nonradioactive analysis of phosphatidylinositides and other anionic phospholipids by anion-exchange high-performance liquid chromatography with suppressed conductivity detection. Anal. Biochem. 301, 243–254. doi: 10.1006/abio.2001.5489
Niu, S. L., Mitchell, D. C., and Litman, B. J. (2002). Manipulation of cholesterol levels in rod disk membranes by methyl-beta-cyclodextrin: effects on receptor activation. J. Biol. Chem. 277, 20139–20145. doi: 10.1074/jbc.m200594200
Park, J., Lee, N., Kavoussi, A., Seo, J. T., Kim, C. H., and Moon, S. J. (2015). Ciliary phosphoinositide regulates ciliary protein trafficking in Drosophila. Cell Rep. 13, 2808–2816. doi: 10.1016/j.celrep.2015.12.009
Prosseda, P. P., Luo, N., Wang, B., Alvarado, J. A., Hu, Y., and Sun, Y. (2017). Loss of Ocrl increases ciliary Pi(4,5)P2in Lowe oculocerebrorenal syndrome. J. Cell Sci. 130, 3447–3454. doi: 10.1242/jcs.200857
Pucadyil, T. J., and Chattopadhyay, A. (2004). Cholesterol modulates ligand binding and G-protein coupling to serotonin(1A) receptors from bovine hippocampus. Biochim. Biophys. Acta 1663, 188–200. doi: 10.1016/j.bbamem.2004.03.010
Rajala, R. V. (2010). Phosphoinositide 3-kinase signaling in the vertebrate retina. J. Lipid Res. 51, 4–22. doi: 10.1194/jlr.r000232
Rajala, R. V. S. (2020). Signaling roles of phosphoinositides in the retina. J. Lipid Res. doi: 10.1194/jlr.TR120000806 [Epub ahead of print].
Raleigh, D. R., Sever, N., Choksi, P. K., Sigg, M. A., Hines, K. M., Thompson, B. M., et al. (2018). Cilia-associated oxysterols activate smoothened. Mol. Cell 72, 316–327.e5. doi: 10.1016/j.molcel.2018.08.034
Raychowdhury, M. K., Mclaughlin, M., Ramos, A. J., Montalbetti, N., Bouley, R., Ausiello, D. A., et al. (2005). Characterization of single channel currents from primary cilia of renal epithelial cells. J. Biol. Chem. 280, 34718–34722. doi: 10.1074/jbc.m507793200
Reiter, J. F., and Leroux, M. R. (2017). Genes and molecular pathways underpinning ciliopathies. Nat. Rev. Mol. Cell Biol. 18, 533–547. doi: 10.1038/nrm.2017.60
Roberson, E. C., Dowdle, W. E., Ozanturk, A., Garcia-Gonzalo, F. R., Li, C., Halbritter, J., et al. (2015). Tmem231, mutated in orofaciodigital and Meckel syndromes, organizes the ciliary transition zone. J. Cell Biol. 209, 129–142. doi: 10.1083/jcb.201411087
Sanchez, A., Urrego, D., and Pardo, L. A. (2016). Cyclic expression of the voltage-gated potassium channel Kv10.1 promotes disassembly of the primary cilium. EMBO Rep. 17, 708–723. doi: 10.15252/embr.201541082
Satir, P., and Christensen, S. T. (2007). Overview of structure and function of mammalian cilia. Annu. Rev. Physiol. 69, 377–400. doi: 10.1146/annurev.physiol.69.040705.141236
Schou, K. B., Mogensen, J. B., Morthorst, S. K., Nielsen, B. S., Aleliunaite, A., Serra-Marques, A., et al. (2017). Kif13B establishes a Cav1-enriched microdomain at the ciliary transition zone to promote Sonic hedgehog signalling. Nat. Commun. 8:14177.
Serricchio, M., Schmid, A. W., Steinmann, M. E., Sigel, E., Rauch, M., Julkowska, D., et al. (2015). Flagellar membranes are rich in raft-forming phospholipids. Biol. Open 4, 1143–1153. doi: 10.1242/bio.011957
Singh, S. K., Gui, M., Koh, F., Yip, M. C., and Brown, A. (2020). Structure and activation mechanism of the Bbsome membrane protein trafficking complex. eLife 9:e53322.
Slaats, G. G., Isabella, C. R., Kroes, H. Y., Dempsey, J. C., Gremmels, H., Monroe, G. R., et al. (2016). Mks1 regulates ciliary Inpp5E levels in Joubert syndrome. J. Med. Genet. 53, 62–72. doi: 10.1136/jmedgenet-2015-103250
Soubias, O., and Gawrisch, K. (2005). Probing specific lipid-protein interaction by saturation transfer difference Nmr spectroscopy. J. Am. Chem. Soc. 127, 13110–13111. doi: 10.1021/ja0538942
Souto-Padron, T., and De Souza, W. (1983). Freeze-fracture localization of filipin-cholesterol complexes in the plasma membrane of Trypanosoma cruzi. J. Parasitol. 69, 129–137.
Suh, B.-C., and Hille, B. (2008). Pip2Is a necessary cofactor for ion channel function: Howand Why? Annu. Rev. Biophys. 37, 175–195. doi: 10.1146/annurev.biophys.37.032807.125859
Suizu, F., Hirata, N., Kimura, K., Edamura, T., Tanaka, T., Ishigaki, S., et al. (2016). Phosphorylation-dependent Akt-Inversin interaction at the basal body of primary cilia. EMBO J. 35, 1346–1363. doi: 10.15252/embj.201593003
Taschner, M., and Lorentzen, E. (2016). The intraflagellar transport machinery. Cold Spring Harb. Perspect. Biol. 8:a028092. doi: 10.1101/cshperspect.a028092
Tyler, K. M., Fridberg, A., Toriello, K. M., Olson, C. L., Cieslak, J. A., Hazlett, T. L., et al. (2009). Flagellar membrane localization via association with lipid rafts. J. Cell Sci. 122, 859–866. doi: 10.1242/jcs.037721
Verhey, K. J., and Yang, W. (2016). Permeability barriers for generating a unique ciliary protein and lipid composition. Curr. Opin. Cell Biol. 41, 109–116. doi: 10.1016/j.ceb.2016.05.004
Vieira, O. V., Gaus, K., Verkade, P., Fullekrug, J., Vaz, W. L. C., and Simons, K. (2006). Fapp2, cilium formation, and compartmentalization of the apical membrane in polarized Madin-Darby canine kidney (Mdck) cells. Proc. Natl. Acad. Sci. U.S.A. 103, 18556–18561. doi: 10.1073/pnas.0608291103
Walia, V., Cuenca, A., Vetter, M., Insinna, C., Perera, S., Lu, Q., et al. (2019). Akt Regulates a Rab11-effector switch required for ciliogenesis. Dev. Cell 50, 229–246.e7. doi: 10.1016/j.devcel.2019.05.022
Wang, H., Zou, X., Wei, Z., Wu, Y., Li, R., Zeng, R., et al. (2015). Hsp90 forms a stable complex at the cilium neck for the interaction of signalling molecules in Igf-1 receptor signalling. J. Cell Sci. 128, 100–108. doi: 10.1242/jcs.155101
Wang, Q., Corey, R. A., Hedger, G., Aryal, P., Grieben, M., Nasrallah, C., et al. (2019). Lipid Interactions of a Ciliary Membrane Trp channel: simulation and structural studies of polycystin-2. Structure 28, 169–184.e5.
Wang, S. F., Kowal, T. J., Ning, K., Koo, E. B., Wu, A. Y., Mahajan, V. B., et al. (2018). Review of ocular manifestations of Joubert Syndrome. Genes 9:605. doi: 10.3390/genes9120605
Wensel, T. G. (2020). Phosphoinositides in retinal function and disease. Cells 9:866. doi: 10.3390/cells9040866
Wingfield, J. L., Lechtreck, K. F., and Lorentzen, E. (2018). Trafficking of ciliary membrane proteins by the intraflagellar transport/Bbsome machinery. Essays Biochem. 62, 753–763. doi: 10.1042/ebc20180030
Womack, K. B., Gordon, S. E., He, F., Wensel, T. G., Lu, C.-C., and Hilgemann, D. W. (2000). Do phosphatidylinositides modulate vertebrate phototransduction? J. Neurosci. 20, 2792–2799. doi: 10.1523/jneurosci.20-08-02792.2000
Ye, F., Nager, A. R., and Nachury, M. V. (2018). Bbsome trains remove activated Gpcrs from cilia by enabling passage through the transition zone. J. Cell Biol. 217, 1847–1868. doi: 10.1083/jcb.201709041
Yen, H.-Y., Hoi, K. K., Liko, I., Hedger, G., Horrell, M. R., Song, W., et al. (2018). PtdIns(4,5)P2 stabilizes active states of Gpcrs and enhances selectivity of G-protein coupling. Nature 559, 423–427. doi: 10.1038/s41586-018-0325-6
Zhainazarov, A. B., Spehr, M., Wetzel, C. H., Hatt, H., and Ache, B. W. (2004). Modulation of the olfactory Cng channel by Ptdlns(3,4,5)P3. J. Membr. Biol. 201, 51–57. doi: 10.1007/s00232-004-0707-4
Keywords: cilia, flagella, lipids, polyphosphoinositides, cholesterol
Citation: Nechipurenko IV (2020) The Enigmatic Role of Lipids in Cilia Signaling. Front. Cell Dev. Biol. 8:777. doi: 10.3389/fcell.2020.00777
Received: 02 June 2020; Accepted: 24 July 2020;
Published: 11 August 2020.
Edited by:
Roberto Botelho, Ryerson University, CanadaReviewed by:
Richard A. Kahn, Emory University School of Medicine, United StatesHemant Khanna, University of Massachusetts Medical School, United States
Copyright © 2020 Nechipurenko. This is an open-access article distributed under the terms of the Creative Commons Attribution License (CC BY). The use, distribution or reproduction in other forums is permitted, provided the original author(s) and the copyright owner(s) are credited and that the original publication in this journal is cited, in accordance with accepted academic practice. No use, distribution or reproduction is permitted which does not comply with these terms.
*Correspondence: Inna V. Nechipurenko, aW5lY2hpcHVyZW5rb0B3cGkuZWR1