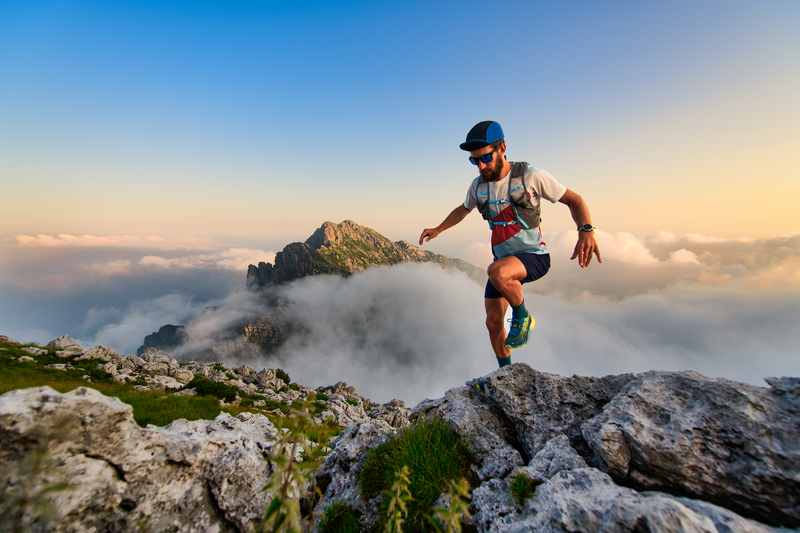
94% of researchers rate our articles as excellent or good
Learn more about the work of our research integrity team to safeguard the quality of each article we publish.
Find out more
REVIEW article
Front. Cell Dev. Biol. , 19 June 2020
Sec. Molecular and Cellular Oncology
Volume 8 - 2020 | https://doi.org/10.3389/fcell.2020.00507
This article is part of the Research Topic Bone Metastases View all 11 articles
Osteoclasts are myeloid lineage-derived bone-resorbing cells of hematopoietic origin. They differentiate from myeloid precursors through a complex regulation process where the differentiation of preosteoclasts is followed by intercellular fusion to generate large multinucleated cells. Under physiological conditions, osteoclastogenesis is primarily directed by interactions between CSF-1R and macrophage colony-stimulating factor (M-CSF, CSF-1), receptor activator of nuclear factor NF-κB (RANK) and RANK ligand (RANKL), as well as adhesion receptors (e.g., integrins) and their ligands. Osteoclasts play a central role in physiological and pathological bone resorption and are also required for excessive bone loss during osteoporosis, inflammatory bone and joint diseases (such as rheumatoid arthritis) and cancer cell-induced osteolysis. Due to the major role of osteoclasts in these diseases the better understanding of their intracellular signaling pathways can lead to the identification of potential novel therapeutic targets. Non-receptor tyrosine kinases and lipid kinases play major roles in osteoclasts and small-molecule kinase inhibitors are emerging new therapeutics in diseases with pathological bone loss. During the last few years, we and others have shown that certain lipid (such as phosphoinositide 3-kinases PI3Kβ and PI3Kδ) and tyrosine (Src−family and Syk) kinases play a critical role in osteoclast differentiation and function in humans and mice. Some of these signaling pathways shows similarity to immunoreceptor-like receptor signaling and involves important other enzymes (e.g., PLCγ2) and adapter proteins (such as the ITAM−bearing adapters DAP12 and the Fc-receptor γ-chain). Here, we review recently identified osteoclast signaling pathways and their role in osteoclast differentiation and function as well as pathological bone loss associated with osteolytic tumors of the bone. A better understanding of osteoclast signaling may facilitate the design of novel and more efficient therapies for pathological bone resorption and osteolytic skeletal metastasis formation.
Bone tissue plays a crucial role in structural support and movement of the body as well as it stores minerals. It also hosts the bone marrow, which is the major site of postnatal hematopoiesis (Zaidi, 2007). Bone matrix is an essential component of the bone and it is built up from inorganic salts and organic matrix. Besides providing structural support, bone matrix also stores a wide range of growth factors capable of regulating normal bone homeostasis. Bone microenvironment contains a wide repertoire of cellular elements: hematopoietic and mesenchymal stem cells, chondrocytes, fibroblasts, adipocytes, endothelial and nerve cells as well as the bone cells themselves (Arron and Choi, 2000). The most well-known of these latter ones are bone-resorbing osteoclasts, bone-forming osteoblasts and osteocytes regulating the bone remodeling process (Bonewald, 2011).
Osteoclasts are derived from myeloid precursors, which express several cytokine receptors. Osteoclast differentiation is mainly governed by receptor activator of NF-κB ligand (RANKL) and macrophage colony-stimulating factor (M-CSF or CSF-1), as well as integrin and immunoreceptor-like adhesion signals and interactions, which are provided by osteoclastogenesis-supporting cells, such as osteoblasts, osteocytes and other stromal cells under physiological conditions (Boyle et al., 2003). The early phase of osteoclast differentiation is characterized by the expression of osteoclast-specific genes, such as tartrate-resistant acidic phosphatase (TRAP) in committed precursors (preosteoclasts). Fusion of these preosteoclasts will then lead to formation of large, multinucleated osteoclasts. These giant polykarions spread over the bone surface and digest the underlying bone tissue through the simultaneous release of hydrochloric acid and digestive enzymes onto the bone (Teitelbaum, 2000).
The tightly regulated balance between bone resorption and bone formation can be altered under pathological conditions. Enhanced maturation and activation of osteoclasts leads to pathological bone resorption as seen in osteoporosis and inflammatory bone diseases (Győri and Mócsai, 2015). During the pathogenesis of inflammatory bone diseases such as rheumatoid arthritis, gout and periodontitis, the chronic inflammation irreversibly affects the surrounding bone tissue. Bone degradation in inflammatory arthritis is best characterized in human rheumatoid arthritis (Győri and Mócsai, 2015). Rheumatoid arthritis is a chronic autoimmune disease, eventually leading to the destruction of surface cartilage and subchondral bone primarily in the small synovial joints of the hands and feet (Firestein, 2003). Osteoclasts play a key role in the pathogenesis of rheumatoid arthritis (McInnes and Schett, 2007) and numerous studies focused on the crosstalk between osteoclast and the immune system in rheumatoid arthritis. Mature osteoclasts are present at the sites of bone destruction and osteoclastogenesis is enhanced in the close proximity of the inflamed joints (Schett and Teitelbaum, 2009). This pronounced osteoclast formation is due to the accumulation of osteoclast precursors at the sites of erosion and enhanced maturation of these preosteoclasts to bone-resorbing polykarions in the presence of osteoclastogenic cytokines derived from the immune and stromal cells (Schett, 2009). Besides inflammatory bone diseases and osteoporosis, the third major disease where excessive bone loss occurs due to hyperactivation of osteoclasts is tumor-induced osteolysis and the formation of bone metastases.
Bone tissue is one of the most common sites for metastasis formation by a large number of solid tumors including lung, prostate, breast, thyroid, colorectal, ovarian cancers, and malignant melanoma. Further, two-third of patients with stage II/III prostate and breast cancers develop bone metastasis (Hernandez et al., 2018). Bone metastases are classified as osteolytic, osteoblastic and mixed lesions. The role of osteoclasts and induction of osteoclastogenesis is best described in the process of osteolytic bone metastasis formation. The presence of osteolytic bone lesions are associated with a set of different morbidities including pathological fractures, pain and hypercalcemia (Weilbaecher et al., 2011), seriously affecting the patient’s wellbeing and life expectancy (Coleman and Rubens, 1987).
Although solid tumors capable of forming osteolytic lesions have proteolytic activity, the extent of this is far from being able to break down the bone matrix. Degradation of both the organic and inorganic components of the bone is therefore carried out by osteoclasts, the unique bone-resorbing cells, accumulating in the vicinity of tumor cells forming osteolytic metastases (Croucher et al., 2016). Tumor cells can promote osteoclast-mediated osteolysis via several mechanisms. Either tumor cells can induce osteoclast differentiation directly via the expression of RANKL or they can stimulate osteoclastogenesis indirectly via the activation of osteoblasts (Mundy, 2002). During this latter process, a wide range of tumor cell-derived growth factors such as parathyroid hormone related peptide – (PTHrP) can induce the expression of RANKL on osteoblasts, which in turn drives the differentiation of multinucleated osteoclasts from myeloid precursors (Suva et al., 1987). Mature osteoclasts then resorb the bone matrix and allow tumor cells to grow and spread within the tissue.
Skeletal metastases formation is a self-perpetuating cycle where tumor cells and bone-resorbing osteoclasts are enrolled in a “vicious” cycle characterized by the release of bone-stored growth factors by osteoclast-mediated bone resorption, which further stimulates cancer cell survival and proliferation (Faccio, 2011). Malignant cells express a wide range of growth factors and cytokines, which can directly or indirectly activate osteoclasts. On the other hand, osteoclast-mediated bone resorption can lead to the release of bone-stored cytokines including TGFβ, which are able to promote cancer cell survival and growth (Kakonen et al., 2002). Bone matrix-derived cytokines can also provide a chemotactic stimulus for directed cancer cell migration (Orr et al., 1979). Further, RANKL itself via a paracrine mechanism can serve as a chemoattractant and increase migration of RANK-positive cancer cells (Jones et al., 2006). As a consequence, osteoclast-mediated osteolysis results in an altered bone microenvironment, which facilitates cancer growth and metastasis formation. Later during the disease, these interactions between tumor and bone cells result in a locked cycle of tissue destruction and cancer growth (“vicious cycle” of bone metastasis formation) (Roodman and Dougall, 2008). In line with this, it has been found in mice, that cancer cells, which are more closely located to the bone surface showed increased proliferation compared to the ones distant from the bone (Kostenuik et al., 1992). Underlying the role of osteoclasts in the process of bone metastasis formation, bisphosphonate pyrophosphate analogs that target osteoclasts are used to prevent bone destruction and modify progression of skeletal metastasis in cancer (Choi et al., 2009).
Bone remodeling is also closely coupled with the lympho-hematopoietic system. The similarity between the signaling mechanism of the bone and immune systems in this shared microenvironment indicate that cancer cell growth associated with osteolytic bone degradation can also drive local immunosuppression and accumulation of metastasis-promoting immune cell populations (Lorenzo et al., 2007). A large body of experimental evidence has implicated the role of the immune system in the regulation of bone homeostasis both in humans and mice (Lorenzo et al., 2010).
Tumor development can alter both the skeletal and immune homeostasis (Nakashima and Takayanagi, 2009). Malignant cells are able to suppresses certain effector immune cells subsets, such as conventional CD8+ T cells, which can recognize and kill cancer cells (Schreiber et al., 2011). Other immune cells, such as regulatory T cells (Tregs), myeloid-derived suppressor cells (MDSC) and tumor-associated macrophages (TAMs) also play important roles in promoting cancer growth and metastasis formation. On the other hand, the cellular elements and humoral factors of the innate and adaptive immune systems can affect osteoclastogenesis as well (Takayanagi, 2010). Macrophages and dendritic cells also share common precursors with osteoclasts, which underline the importance of the field of osteoimmunology (Takayanagi, 2007).
While Th1 and Th2 cytokines exert an inhibitory effect on osteoclastogenesis, the IL-17 producing T helper type 17 (Th17) cells have been described to be highly osteoclastogenic (Sato et al., 2006b). Th17 cells can express high levels of RANKL and as a consequence directly promote osteoclastogenesis. Moreover, they may activate inflammation locally, leading to the release of proinflammatory mediators (e.g., TNF-α, IL-1, and IL-6), which can potentiate RANKL expression on osteoclastogenesis-supporting cells (Sato et al., 2006b). Further, Th17 cells were described to activate osteoclastogenesis-driven osteolysis through RANKL production during inflammatory arthritis (Okamoto and Takayanagi, 2011). However, no direct role for Th17 cells in cancer induced bone disease has been reported so far. In line with this, IL-17F, which shows 50% homology with IL-17A and shares its receptor, was produced in high levels in the 4T1 preclinical tumor model, but found not to be necessary for the development of pre-metastatic bone disease (Monteiro et al., 2013).
The effects of conventional T cells on osteoclastogenesis are normally suppressed by regulatory T (Treg) cells. These cells are able to inhibit osteoclast development and function via the release of tumor growth factor-β (TGF-β), IL-10 (Kim et al., 2007b; Kelchtermans et al., 2009) and expression of CTLA-4 (Zaiss et al., 2007). In addition to their suppressive capabilities, tumor-infiltrating Treg cells have also been described to express RANKL (Tan et al., 2011). As a consequence, the effect of Treg cells on osteoclastogenesis depends on the balance between positive and negative factors within the tumor microenvironment.
Myeloid-derived suppressor cells (MDSCs) are a heterogeneous population of immature myeloid cells, which are capable of potently suppressing the anti-tumor functions of conventional T lymphocytes (Gabrilovich and Nagaraj, 2009). It has been shown that MDSCs derived from the tumor microenvironment can differentiate into bone-resorbing osteoclasts under tissue culture conditions (Sawant et al., 2013) and MDSCs from tumor-bearing mice have increased osteoclastogenic potential (Zhuang et al., 2012).
Tumor-associated macrophages (TAMs) are a predominant white blood cell subset both in the bone and tumor microenvironment, which can influence tumor development, proliferation, growth, survival, and metastasis formation. Macrophages classically have been divided into proinflammatory M1 and anti-inflammatory M2 subsets (Mosser and Edwards, 2008). When activated, M1 macrophages secrete high levels of proinflammatory cytokines and participate in the elimination of tumor cells (Gabrilovich et al., 2012). However, M2 macrophages are characterized by high expression of mannose receptors, scavenger receptors and IL-1Ra (Zhang et al., 2012), and are also often found in human solid tumors. Activated M2 macrophages generate high levels of IL-10 and TGF-β, which can suppress CD4+ and CD8+ conventional T cells (Biswas and Mantovani, 2010). In preclinical studies, where tumor-associated macrophages were depleted using clodronate liposomes, reduced number of bone metastatic lesions were detected (Hiraoka et al., 2008).
Receptor activator of NF-κB ligand (RANKL) belongs to the tumor necrosis factor (TNF) superfamily of cytokines and it is expressed by monocytes, T and B cells, dendritic cells and osteoclastogenesis-supporting cells, such as osteoblasts and synovial fibroblasts (Caetano-Lopes et al., 2009). Parathyroid hormone, 1,25-dihydroxy-cholecalciferol (active vitamin D3) and prostaglandins can promote the secretion of RANKL by osteoblasts and other stromal cellular elements (Takayanagi, 2007). CD4+ conventional and regulatory T lymphocytes are also able to provide RANKL in membrane-bound form as well as release it in a soluble form (Wong et al., 1997). TNF-α, IL-1, IL-6 and IL-17 cytokines can increase RANKL expression on osteoclastogenesis-supporting cells, thereby stimulating RANKL signaling (Takayanagi, 2007). Osteoprotegerin (OPG), expressed by osteoblasts and other stromal cells, is a soluble decoy receptor for RANKL capable of inhibiting RANK signaling (Simonet et al., 1997).
The RANKL receptor, RANK, is highly expressed by preosteoclast. RANKL binding to RANK leads to receptor trimerization and activation of the adapter protein TRAF6, which further stimulates transcription factor NF-κB and members of the mitogen-activated protein kinase (MAPK) family (Takayanagi, 2010) as shown on Figure 1. Nuclear factor of activated T-cell cytoplasmic 1 (NFATc1), the master regulator of osteoclast differentiation, is also activated by RANK receptor signaling (Takayanagi, 2010). NFATc1 translocates to the nucleus and then amplifies its own expression resulting in strong induction of NFATc1 expression (Asagiri et al., 2005). Generation of calcium signal and calcineurin activation are also important for NFATc1 induction. NFATc1 together with activator protein 1 (AP-1) and microphthalmia-associated transcription factor (MITF) induce then the expression of osteoclast-specific genes encoding for tartrate-resistant acid phosphatase (TRAP), cathepsin K (CTSK) and the β3 integrin (Takayanagi, 2007).
Figure 1. Summary of osteoclast differentiation induced by integration of CSF-1R, RANK, immunoreceptor-like and integrin receptor signaling. CSF-1 and its receptor CSF-1R activate the MAPK cascade pathway leading to the survival and proliferation of preosteoclasts. RANKL and its receptor RANK transduce signals via the adaptor molecule TRAF6, which activates the NF-κB and MAPK pathways leading to the differentiation of osteoclasts. The expression of the master regulator of osteoclastogenesis, NFATc1, is driven by NF-κB and NFATc1. The activation of NFATc1 is also regulated by the costimulatory signaling pathways, where FcRγ, DAP12 and their associating partners (OSCAR and TREM2, respectively) recruit Syk, which further activates PLCγ2, resulting in the activation of calcium signaling. The calcium signaling activates then calcineurin, which in turn promotes NFATc1 expression. The calcium signal also induces Vav3 activation involved in αvβ3 integrin signaling, which leads to cytoskeletal reorganization and osteoclastic bone resorption.
The expression of NFATc1 in osteoclasts and their precursors is also regulated on the epigenetic level. An important step in the differentiation of osteoclasts occurs at the NFATc1 promoter when the histone methylation changes from H3K4me3/H3K27me3 to H3K4me3 (Yasui et al., 2011). A histone demethylase, Jmjd3 converts the bivalent H3K4/H3K27 trimethylation to monovalent H3K4me3 in preosteoclasts following RANKL stimulation, leading to increased osteoclast differentiation as well (Yasui et al., 2011). On the other hand, NFATc1 expression can also be inhibited by other transcription factors such as Interferon regulatory factor 8 (IRF-8) (Zhao et al., 2009), bZIP motif containing transcription factor MafB (Kim et al., 2007a), B-cell lymphoma 6 (Bcl-6) (Miyauchi et al., 2010), and Leukemia/lymphoma related factor (LRF) (Tsuji-Takechi et al., 2012). The expression of those transcription factors decrease during osteoclast differentiation, which is mediated by the DNA methyl-transferase 3A (Dnmt3A) (Nishikawa et al., 2015). Furthermore, the B lymphocyte-induced maturation protein (Blimp) was shown to be able to inhibit IRF-8, MafB, Bcl-6 and LRZ transcription factors, leading to upregulated NFATc1 expression and enhanced osteoclastogenesis (Nishikawa et al., 2010). In line with this, Blimp1-deficient mice exhibit increased bone mass and osteopetrotic disease (Nishikawa et al., 2010).
Cancer cells enhance osteoclast-driven osteolysis via several different mechanisms. Upregulation of the expression of RANKL on osteoclastogenesis-supporting cells, downregulation of OPG expression or increased secretion of factors activating RANK receptor signaling have all been described in the context of breast cancer bone metastases (Kearns et al., 2008). Prostate tumor cells can even express RANKL themselves (Brown et al., 2001). Further, secretion of RANKL has also been described by multiple myeloma cells (Farrugia et al., 2003; Sezer et al., 2003). While breast tumors do not upregulate RANKL (Thomas et al., 1999), those cells can eventually induce the expression of RANKL on osteoblasts (Kitazawa and Kitazawa, 2002) and other osteoclastogenesis-supporting cells via the production and release of PTHrP (Mancino et al., 2001). RANK receptor expression by melanoma, breast and prostate cancer cell lines has also been described (Jones et al., 2006), and involved in the autocrine effect of tumor cell-derived RANKL on promoting cancer cell migration. Downregulation of OPG secretion has been found to be characteristic for breast cancer and multiple myeloma cells (Thomas et al., 1999; Giuliani et al., 2001). As a consequence, the RANKL-OPG balance is disturbed within the bone microenvironment in favor of supporting osteoclast-induced osteolysis and bone metastasis formation (Grimaud et al., 2003). Denosumab, a monoclonal antibody raised against RANKL, demonstrated efficacy in preventing tumor-induced bone loss in patients with skeletal metastasis (Choi et al., 2009).
It has also been reported that certain tumor cell-derived soluble factors are able to induce osteoclastogenesis independent of RANKL. Secretion of lysyl oxidase (LOX) from primary breast carcinoma cells induced osteoclast differentiation and osteolytic skeletal lesion formation in animal tumor models (Cox et al., 2015). However, LOX failed to substitute for RANKL when Tnfrsf11a (RANK)-deficient bone marrow cells were treated with recombinant LOX protein in subsequent experiments (Tsukasaki et al., 2017). Similarly, initial data indicated that Tnfrsf11a–/– primary bone marrow cells were capable to differentiate into osteoclasts under TNFα stimulation, when RBP-J transcription factor was deleted in the progenitors (Kim et al., 2005; Zhao et al., 2012). However, RBP-J deletion in mice did not exhibit obvious defects in bone phenotype, suggesting that RBP-J plays a minor role in osteoclast development under physiological conditions (Zhao et al., 2012). Although there have been other factors indicated to induce RANKL-independent osteoclastogenesis, they may be able to promote osteoclast differentiation only under certain conditions and cannot completely substitute for RANK ligand (Tanaka, 2017). Identifying the precise role of these factors in osteoclasts within the solid tumor microenvironment requires further investigation.
Another key osteoclastogenic cytokine that governs osteoclastogenesis is CSF-1 (or macrophage-colony stimulating factor, M-CSF). CSF-1 is a polypeptide growth factor that binds to its plasmamembrane receptor (CSF-1R) encoded by the c-fms gene (Stanley et al., 1983). CSF-1 is essential for the development and survival of preosteoclasts (Ross and Teitelbaum, 2005). CSF-1 was originally identified as a regulator of macrophages and their bone marrow precursors (Stanley et al., 1983). As shown on Figure 1, once CSF-1 binds to its transmembrane receptor CSF-1R, it induces tyrosine phosphorylation of the cytoplasmic domain of the receptor (Pixley and Stanley, 2004). Then Src homology 2 domain (SH2)-bearing adapter molecules (such as Grb2) are recruited to the phosphorylated tyrosine residues and initiate different signaling cascades (including the MAP-kinase cascade) that lead to cell proliferation and differentiation (Pixley and Stanley, 2004). CSF-1 is also known to induce cytoskeletal rearrangement in osteoclasts by activation of the c-Src and phosphoinositide 3-kinases (Nakamura et al., 1995; Insogna et al., 1997; Pilkington et al., 1998). CSF-1 deficient mice are osteopetrotic (Wiktor-Jedrzejczak et al., 1990).
When considering CSF-1R and RANK-driven non-pathological osteoclastogenesis, it is important to note, that apart from TGF-β, the cytokine IL-1 is supposed to be released from the bone as an autocrine factor. It has been described, that osteoclast precursor interaction with bone matrix (but not plastic surface) can induce osteoclast formation directly by an interleukin-1-mediated autocrine mechanism in the presence of CSF-1 (Yao et al., 2008; De Vries et al., 2015).
In addition to its physiological role in myeloid cells, increased expression of CSF-1 has been detected in breast, colorectal, ovarian and uterine cancers, where the extent of its expression correlates with poor prognosis (Kacinski, 1995; Smith et al., 1995). Furthermore, in human breast carcinomas, overexpression of CSF-1 and its receptor positively correlates with high grade invasiveness (Kacinski, 1995; Smith et al., 1995). Importantly, a strong correlation of CSF-1 expression with CSF-1R-positive tumor-associated macrophage infiltration has also been detected in human carcinomas (Tang et al., 1990; Scholl et al., 1994). We recently demonstrated that pharmacological inhibition or genetic ablation of CSF1 in cancer cells reduces the accumulation of immunosuppressive CSF-1R+ tumor-associated macrophages and increases CD8+ T cell attack on tumors (Győri et al., 2018).
In line with this, it is highly likely that certain tumor cells capable of forming osteolytic bone metastasis recruit not only tumor-associated macrophages, but monocytes/osteoclast precursors via the secretion of CSF-1 into the tumor microenvironment. Furthermore, tumor cell-derived CSF-1 can also enhance the maturation of those precursors to bone-resorbing osteoclasts in the presence of an osteoclastogenic milieu. As a consequence, pharmacological blockade of CSF-1 might offer benefits for patients with osteolytic bone metastases and CSF-1 inhibitors are being evaluated in clinical trials. PLX3397, an orally available CSF-1R inhibitor is currently being administered in clinical trials and early data suggested good tolerability and beneficial effects (Ries et al., 2015). It has also been described, that CSF-1R and αvβ3 integrins collaborate during osteoclast differentiation via shared activation of downstream signaling pathways (Faccio et al., 2003; Ross and Teitelbaum, 2005).
Integrins are heterodimeric transmembrane proteins that facilitate cell-cell and cell-matrix interactions (Schwartz et al., 1995). Integrins can activate many intracellular signaling pathways and induce the proliferation, survival, and cytoskeletal rearrangements of the target cells (Hynes, 1992). So far 8 beta and 18 alpha integrin subunits have been described, which can combine into 24 unique heterodimers within the different cell types, each of those characterized by different ligand binding characteristics, signaling and regulatory mechanisms (Hynes, 2002). Integrin heterodimers can be activated by conformational changes in their extracellular domains. When inactive, integrin dimers are found in a closed conformation within the cell membrane. Upon activation through the cytoplasmic domains, the α and β cytoplasmic and transmembrane regions become separated resulting in the unfolding of the extracellular ligand binding domain (inside-out signaling) (Shattil et al., 2010). This open conformation then promotes extracellular ligand binding and initiates integrin-driven intracellular signaling pathways (outside-in signaling) (Qin et al., 2004).
Integrins play critical roles on both tumor cells and osteoclasts in promoting bone metastasis formation. During the process of bone resorption, mature osteoclasts attach to the bone surface, generate an actin ring mediated sealing zone and then secrete hydrochloric acid and enzymes to lyse the underlying bone matrix. All of these steps at least in part are regulated by integrin heterodimers located on the surface of osteoclasts (Teitelbaum and Ross, 2003). Different integrins are involved in the binding of osteoclasts to the bone, including αvβ3, αvβ5, and α2β1, of which, αvβ3 is the dominant integrin on osteoclasts (Ross and Teitelbaum, 2005; Novack and Teitelbaum, 2008; Ross et al., 1993). αvβ3 integrins are responsible for mediating osteoclast-bone matrix recognition and subsequent attachment to the bone surface (Ross et al., 1993). αvβ3 integrin signaling is essential for osteoclast spreading and to create the characteristic ruffled border of the plasmamembrane for subsequent resorption (Faccio et al., 2003; McHugh, 2000). Furthermore, osteopontin binding to αvβ3 induce podosome formation and cytoskeletal rearrangement (Miyauchi et al., 1991). Additional studies identified further downstream signaling effector molecules such as Vav, a critical regulator of osteoclast differentiation and actin ring formation (Faccio et al., 2005). As a consequence, mice with genetic inactivation of β3 integrin (β3–/–) have defective osteoclast function (McHugh, 2000) and are protected from cancer cell-induced bone loss (Bakewell et al., 2003). αvβ3 integrins are also critical for the activation of c-Src which is a key signaling molecule in osteoclast spreading and cytoskeletal reorganization (Zhang et al., 2000; Faccio et al., 2003).
Cancer cells display altered integrin expression and signaling, allowing them to colonize new tissues by escaping from cell–cell and cell–matrix connections. Maintaining adhesion to the extracellular matrix via integrins is key to cell survival (Frisch and Screaton, 2001). Disruption of cell–cell or cell–matrix interactions lead to loss of survival signals and non-transformed cells which are anchorage-dependent undergo a form of programmed cell death called anoikis (Frisch and Screaton, 2001). Under physiological conditions, this form of apoptosis assures that isolated cells are not able to migrate to inappropriate locations (Frisch and Screaton, 2001). Metastatic cancer cells that can resist anoikis utilize several different mechanisms in order to be able to settle in a novel microenvironment. These mechanisms include altered integrin expression (Bissell and Radisky, 2001), activation of integrin signaling cascade downstream molecules such as focal adhesion (Frisch et al., 1996) and c-Src kinases (Shain et al., 2002), EGFR (Demers et al., 2009), as well as suppression of apoptotic pathways (Simpson et al., 2008). Expression of αvβ3 integrin is elevated on human breast cancer bone metastases (Zhao et al., 2007), and ectopic overexpression of the β3 subunit on breast cancer cells has been demonstrated to enhance tumor establishment in bone (Sloan et al., 2006).
The non-receptor tyrosine kinase, c-Src is a key signaling molecule in bone metabolism and plays an important role in the regulation of growth, survival, proliferation, adhesion and motility (Brunton and Frame, 2008). Preclinical studies demonstrated an important role for Src-family kinases in osteoclast-mediated bone resorption (Horne et al., 1992). Mature osteoclasts express high levels of c-Src (Miyazaki et al., 2004). Pharmacological inhibition of c-Src kinase-activity decreased preosteoclast migration and inhibited the subsequent formation of resorption pits in in vitro studies (de Vries et al., 2009). Mice with genetic inactivation of the c-Src gene exhibit osteopetrosis, a severe disease that makes bones abnormally dense and prone to fractures (Soriano et al., 1991). Since c-Src-deficient mice had normal osteoclast numbers, the osteopetrotic phenotype is rather due to a failure in osteoclast function (Soriano et al., 1991). Further, Src-deficient mature osteoclasts fail to form actin rings and sealing zones (Boyce et al., 1992).
Besides regulating cytoskeletal rearrangement and ruffled border formation, Src-family kinases are also present at vesicular membranes, where they are required for the secretion of hydrochloric acid and bone-degrading enzymes (Furuyama and Fujisawa, 2000; Edwards et al., 2006). Among Src-family kinase binding partners, Tks5 has been shown to mediate podosome formation and cell-cell fusion in osteoclasts (Oikawa et al., 2012). Tks5, which has been originally described as a regulator of invadopodia formation in tumor cells, was reported to be phosphorylated on tyrosine residues in a c-Src-dependent manner within osteoclasts (Oikawa et al., 2012). Furthermore, it was also shown that co-culturing malignant melanoma cells with osteoclasts promoted the formation of melanoma-osteoclast hybrid cells (Oikawa et al., 2012). Fusion of osteoclasts with cancer cells can contribute to increased bone resorption activity, secretion of chemokines promoting osteolytic bone metastasis formation and even the evasion of immune surveillance (Oikawa et al., 2012).
An elevated level of activity of c-Src is suggested to be linked to cancer progression and a large body of evidence suggests that Src-family kinase has a critical role in cancer growth and invasion (Thomas and Brugge, 1997). Further, Src expression positively correlates with the metastatic spread of cancer cells (Boyer and Poupon, 2002). Importantly, correlation was also observed between tumor cell colonization in bone and Src kinase activity (Myoui et al., 2003). Increased Src family kinase activity fueled tumor cell growth and enhanced parathyroid hormone related peptide (PTHrP) release within bone metastases (Myoui et al., 2003). Small-molecule kinase inhibitors are emerging new therapeutics in diseases with pathological bone loss and have potential for the treatment of bone metastases as well. Preclinical studies with therapeutic Src inhibitors (dasatinib, saracatinib, and bosutinib) demonstrated anti-tumor and anti-osteoclast effects, as well as clinical studies provided evidence that Src-family kinase inhibitors might be beneficial for patients with refractory disease (Boyce and Xing, 2011).
Classical immunoreceptors, such as T and B cell receptors (TCR, BCR, respectively) as well as Fc-receptors (FcR) use a common signaling machinery within the innate and adaptive immune systems. Firstly, when ligand binds to the classical immunoreceptor, tyrosine residues within the immunoreceptor tyrosine-based activation motifs (ITAMs) are phosphorylated by Src kinases as shown on Figure 1. This then results in the SH2-domain dependent recruitment of the spleen tyrosine kinase (Syk) (Fodor et al., 2006). Src and Syk non-receptor tyrosine kinases then activate downstream effector molecules such as the phospholipase Cγ2 (PLCγ2) and phosphoinositide 3-kinase (PI3K) isoforms. We and others recently recognized that this classical immunoreceptor-like signaling mechanism is present in a range of non-lymphoid cell types (e.g., osteoclasts) too (Koga et al., 2004; Mócsai et al., 2004).
Osteoclasts carry at least two ITAM sequence-containing adapter molecules, namely the DAP12 and the FcR γ-chain (FcRγ). These proteins likely work together with adhesion receptors OSCAR and TREM2 on osteoclasts (Koga et al., 2004). Deletion of DAP12 and TREM2 in mice results in failure of osteoclast differentiation and function (Paloneva et al., 2003; Humphrey et al., 2004). On the other hand, TREM2 and DAP12 deficient mice are not osteopetrotic, which indicates that osteoclastogenesis can proceed through a mechanism that requires the other ITAM-bearing molecule FcR γ-chain. FcRγ, may be able to compensate for the lack of DAP12, since double mutant mice for FcRγ and DAP12 are severely osteopetrotic (Koga et al., 2004; Mócsai et al., 2004). These ITAM-bearing co-receptors most likely mediate osteoclast-osteoblast and osteoclast-bone matrix interactions together with integrin adhesion receptors (Koga et al., 2004; Mócsai et al., 2004). More recently, paired immunoglobulin-like receptor-A (PIR-A) and osteoclast-associated receptor (OSCAR) has been shown to associate with the FcRγ-chain (FcRγ), while DAP12 interaction with signal regulatory protein β1 (SIRPβ1), sialic acid-binding immunoglobulin-like lectin 15 (Siglec-15) and myeloid DAP12-associating lectin (MDL)-1 besides TREM2 has also been reported (Koga et al., 2004; Mócsai et al., 2004; Joyce-Shaikh et al., 2010; Kameda et al., 2013; Negishi-Koga et al., 2015).
As discussed before, β3 integrin ligation leads to activation of Src-family kinases and a non-receptor kinase, Syk. The Syk tyrosine kinase is required for the differentiation and function of osteoclasts as well (Mócsai et al., 2004). Activation of Syk requires the presence of ITAM-bearing adapters, DAP12 and FcRγ-chain in the osteoclast (Mócsai et al., 2004). We recently showed, that osteoclast-specific genetic inactivation of Syk also leads to elevated bone volume in mice (Csete et al., 2019). Syk inhibitors (like fostamatinib) has shown promising effects in rheumatoid arthritis and may also provide significant bone protection in a tumor-associated environment (Mócsai et al., 2010). The osteopetrotic phenotype (Soriano et al., 1991) and defective osteoclastogenesis in the Src-deficient mice indicates that Src-family kinases might be also required for the phosphorylation of DAP12 and FcRγ in osteoclasts (Lowe et al., 1993). Syk then promotes the formation of the Bruton’s tyrosine kinase (Btk)/B cell linker protein (BLNK)/SH2 domain-containing leukocyte protein of 76 kDa (SLP76) complex, which activates the phospholipase Cγ2 (PLCγ2) enzyme. PLCγ2 cleaves the plasmamembrane phosphatidylinositol 4,5-bisphosphate (PIP2) into inositol 1,4,5-triphosphate (IP3) and diacylglycerol (DAG) (Feng et al., 2012). We and others showed that PLCγ2 is needed for the differentiation and function of osteoclasts and PLCγ2–/– mice have increased bone mass (Mao et al., 2006; Chen et al., 2008; Epple et al., 2008; Kertész et al., 2012). IP3 generated by PLCγ2 then binds to its receptor, which in turn induces the release of calcium ions from the sarco/endoplasmic reticulum (SR/ER). Sarco/endoplasmic reticulum calcium ATPase 2 (SERCA2) re-uptakes the calcium into SR/ER (Yang et al., 2009). Repetitive influx and efflux of calcium ions results in calcium oscillations within the cytoplasm of the osteoclast. Calcium oscillations activate calcineurin, which leads to the dephosphorylation of the master regulator of osteoclastogenesis, NFATc1, enabling its autoamplification and entry to the nucleus. Calcium signaling can also induce c-Fos transcription factor via Calcium/calmodulin dependent kinase (CAMK) IV and cAMP response-element binding protein (CREB) pathways (Sato et al., 2006a). Further, transmembrane protein 64 (Tmem64) has been reported to be essential for the function of SERCA2 (Kim et al., 2013), while the transmembrane protein 178 (Tmem178) was shown to be required to suppress the excessive efflux of calcium in a PLCγ2-dependent manner (Decker et al., 2015). Accordingly, mice deficient in DAP12/FcRγ, SERCA2 and Tmem64 are osteopetrotic and exhibit defective calcium oscillations (Yang et al., 2009; Kim et al., 2013). On the other hand, lack of Tmem178 in mice results in an osteopenic phenotype with increased amplitude of calcium oscillations (Decker et al., 2015).
Immunoreceptor-like signaling has been implicated in metastatic spread and homing of cancer cells to distant organs such as the bone. TREM2 was found to be upregulated on peripheral blood monocytes in tumor-bearing hosts and elevated expression levels in pulmonary macrophages correlated with high pathological stage of lung adenocarcinomas (Yao et al., 2016). Further, DAP12 expression in colorectal cancer cells was associated with higher tumor grade (Shabo et al., 2013) and Syk is also frequently upregulated in recurrent ovarian carcinomas metastasizing to the bone (Yu et al., 2019). On the other hand, while PLCγ2 has been implicated in the proliferation and migration of several human cancers (Feng et al., 2012), its genetic deletion in mice resulted in increased tumor growth (Zhang et al., 2011). Interestingly, it has been shown that PLCγ2–/– mice exhibit increased tumor mass in the bone, despite the decreased osteoclast numbers. Although PLCγ2 is not needed for T cell receptor signaling, altered CD8+ T cell activation was observed in PLCγ2–/– tumor-bearing mice (Zhang et al., 2011). Recent evidence indicated that downregulation of PLCγ2 signaling results in the accumulation of immunosuppressive MDSCs in tumor-bearing mice instead (Capietto et al., 2013). This study highlights the role of a permissive immune phenotype and microenvironment as the requirement for osteoclast-mediated promotion of tumor growth and bone metastasis.
Cell–cell interactions are critical for normal bone homeostasis. Ephrins and Semaphorins are known to be expressed in several cell types and regulate intercellular communication. Eph tyrosine kinase receptors and Ephrin ligands mediate interactions between bone cells through a bidirectional signaling. These membrane-bound proteins have been demonstrated to play important roles in osteoblasts and osteoclasts (Edwards and Mundy, 2008). EphrinB2 has been shown to be regulated by PTH and PTHrP in osteoblasts, while the role of EphrinA4 in modulating osteoclast activity via β3-integrin signaling has recently been identified (Stiffel et al., 2014). The altered expression of Eph tyrosine kinase receptors and ephrins has also been demonstrated in several human cancers, e.g., EphrinA2 levels were detected to be elevated in late-stage and bone metastatic prostate cancers (Lin et al., 2012).
Semaphorins play critical roles in the immune system, organ and tumor development. It has been shown that Semaphorin 3A (Sema3A) and its receptor neuropilin-1 (Nrp1) suppress osteoclast differentiation and protect mice from excessive bone loss (Hayashi et al., 2012). The Semaphorin 6D (Sema6D), which associate with TREM2/DAP12 via its receptor plexin-A1 (PlxnA1), on the other hand, promoted osteoclast differentiation and function (Takegahara et al., 2006). Semaphorins were also shown to contribute to the pathogenesis of cancer, including the regulation of epithelial-mesenchymal transitions and stem cell properties (Lontos et al., 2018). In the process of bone metastasis formation Semaphorin 4D, a coupling factor expressed on osteoclasts that inhibits osteoblast differentiation, has recently been implicated (Lontos et al., 2018).
Phosphoinositide 3-kinases (PI3Ks) generate 3-phosphoinositide lipids in cell membranes and play important roles in key biological functions including cellular proliferation, survival, cytoskeletal reorganization, migration, metabolism and vesicular trafficking (Vanhaesebroeck et al., 2012; Hawkins and Stephens, 2015). PI3Ks are subgrouped into three unique classes of which the Class I. PI3K family comprise of PI3Kα, PI3Kβ, PI3Kγ, and PI3Kδ (Hawkins et al., 2006; Vanhaesebroeck et al., 2010). PI3Kα and PI3Kβ are ubiquitously expressed, while the PI3Kγ and PI3Kδ isoforms are mainly restricted to hematopoietic lineages, e.g., white blood cells (Okkenhaug, 2013). 3-phosphoinositide lipids such as PIP3 can initiate a number of downstream signaling pathways and activate effector molecules, like the 3-phosphoinositide dependent protein kinase-1 (PDK1), Ser/Thr kinase Akt (or protein kinase B) and the mammalian target of rapamycin complex 1 (mTORC1) (Hawkins et al., 2006).
PI3-kinases are central downstream effectors of the RANK, CSF-1 and αvβ3 integrin receptors in osteoclasts. Activation of Class I. PI3Ks downstream of those receptors results in the generation of PIP3 from PIP2. Class I.A PI3Ks (PI3Kα, PI3Kβ, PI3Kδ) are designated based on catalytic subunits p110α, p110β, and p110δ, which associate with regulatory subunits p85α, p85β, and p55γ. The sole Class I.B member PI3Kγ consists of catalytic subunit p110γ and regulatory subunit p101 or p84. Mice lacking p110α exhibit embryonic lethality (Bi et al., 1999), while p110γ mutant mice are viable but show defects in immune cell proliferation and function (Sasaki et al., 2000). Mice knockout for the p85α/β subunit showed impaired osteoclast differentiation and ruffled border formation (Munugalavadla et al., 2008; Shinohara et al., 2012). These data indicated that PIP3 is a strong inducer of osteoclast differentiation and PI3-kinases might play an important role in regulating osteoclastogenesis.
We and others have shown that Class I. PI3Ks play a critical role in osteoclasts and could be potential therapeutic targets in bone metastases (Shinohara et al., 2012; Shugg et al., 2013; Győri et al., 2014). Previous studies using non-selective PI3K inhibitors (such as wortmannin) indicated a role for PI3-kinases in osteoclastogenesis (Hall et al., 1995; Nakamura et al., 1995; Sato et al., 1996). Using pharmacological and genetic approaches we demonstrated that PI3Kβ is important for normal osteoclast differentiation and function (Győri et al., 2014). PI3Kβ–/– mice had increased bone mass and PI3Kβ–/– osteoclasts displayed altered morphology in vivo (Győri et al., 2014). Further, PI3Kβ–/– osteoclasts were unable to form actin rings and to release intracellular vesicles and cathepsin K (Győri et al., 2014). In addition to this, the PI3Kδ isoform, which is mainly expressed in hematopoietic cells, has been found to be an attractive target for anti-resorptive agents since recent data indicated that PI3Kδ regulates osteoclast cytoskeleton and resorptive activity (Shugg et al., 2013).
Class I. PI3Ks are often mutated in human cancers (Fruman and Rommel, 2014). Aberrant activation and amplification of PI3Kα is one of the most frequently observed mutations associated with malignant transformation (Samuels et al., 2005; Thorpe et al., 2015). Oncogenic mutations have also been detected in PI3Kβ, but rarely in PI3Kγ and PI3Kδ (Hill et al., 2010; Dbouk et al., 2013). An elevated level of activity of PI3Kδ was found in non-hematopoietic cell types, including those of breast and melanocytic origin (Sawyer et al., 2003). Further, PI3K downstream targets are also often seen abnormally activated in human malignancies. Overexpression of Akt/PKB has been observed in a wide range of solid tumors, including breast, ovarian and prostate carcinomas (Scheid and Woodgett, 2001; Vivanco and Sawyers, 2002). The recognition of the tumor suppressor PTEN, a phosphatase for 3-phosphoinositide lipids, proved to be an indicator of aberrant PI3K signal transduction in cancer (Cantley and Neel, 1999). Deletions and mutations in the PTEN gene also lead to the accumulation of PI3K lipid products, which is observed in many human tumors (Di Cristofano and Pandolfi, 2000).
There is substantial interest in the pharmaceutical industry to develop PI3K inhibitors for the treatment of human cancers. To avoid the serious side effects of pan–Class (I) PI3K drugs affecting glucose homeostasis (Busaidy et al., 2012), administration of isoform-selective PI3K inhibitors below their maximum tolerated dose, most likely in combination may be beneficial. Based on its role in the tumor microenvironment PI3Kβ and/or PI3Kδ may be suitable therapeutic targets for bone metastases with tumor-induced osteolysis.
MicroRNAs are non-coding RNAs of approximately 20–22 nucleotides in length which regulate critical cellular processes including proliferation, differentiation, and survival. Recent data indicated that microRNAs are involved in tumorigenesis and osteolytic bone metastasis formation as well. Various tumor-cell derived mature microRNAs were implicated in regulating osteoclast differentiation. miR-223 was shown to regulate the level of the CSF1 receptor in preosteoclasts (Sugatani and Hruska, 2007), while miR-503 targets RANK (Chen et al., 2014). Further, miR-29 promotes murine osteoclastogenesis by regulating osteoclast commitment and migration (Franceschetti et al., 2013). On the other hand, miR-124 was detected to suppress transcription factor NFATc1 during osteoclastogenesis (Lee et al., 2013), while miR-155 represses MITF and PU.1 transcription factors (Mann et al., 2010). It has been reported that administration of miR-141 and miR-219 results in a significant drop in the number of bone-resorbing osteoclasts in vivo and tumor burden in breast carcinoma-bearing mice (Ell et al., 2013). Similarly, miR-33a suppressed bone metastasis formation by targeting PTHrP and altering the RANKL/OPG ratios in osteoblasts (Kuo et al., 2013).
MicroRNAs were recently revealed to be present in extracellular vesicles (Valadi et al., 2007). Extracellular vesicles are lipid bilayer coated vesicles ranging from approximately 50 to 5000 nm in diameter. Three main types of extracellular vesicles exist: microvesicles, exosomes and apoptotic bodies (Marton et al., 2017). MicroRNA-containing extracellular vesicle secretion depends on various different factors. Numerous studies suggested the involvement of extracellular vesicles secreted by tumor cells in the development of osteolytic bone metastases (Kagiya, 2015). Exosomal miR-21, miR-210 and miR-378 were found to be important for regulating osteoclast differentiation (Kagiya, 2015). Further, miR-16 and miR-378 were detected to be higher in the serum of metastatic breast cancer patients (Arroyo et al., 2011; Ell et al., 2013). Accordingly, extracellular vesicles play an important role in intercellular communication via the transfer of not only microRNAs and proteins, but also bioactive lipids such as PIP3 generated by phosphoinositide 3-kinases.
Tight coupling between bone formation and resorption is essential for bone remodeling. Disruption of this balance can lead to skeletal disorders. Skeletal metastatic disease is a severe consequence of tumor cell dissemination from primary cancer sites, and significantly decreases wellbeing and life expectancy in patients. The bone is a major target for tumor metastasis as it provides a unique environment, which promotes solid cancer cell dissemination (Weilbaecher et al., 2011). The balance between bone-forming osteoblasts and bone-resorbing osteoclasts is altered by bone metastasis formation (Mundy, 2002). Cancer cell invasion into the bone is associated with the activation of osteoclasts. Osteoclast-mediated osteolysis then leads to the release of cytokines and growth factors deposited in the bone matrix. This further fuels tumor growth and dissemination within the tissue (“vicious cycle” of bone metastasis formation) (Faccio, 2011).
RANK ligand, an important osteoclastogenic factor, is expressed by osteoblasts and cancer cells (Kearns et al., 2008). PTHrP secreted by tumor cells indirectly promotes osteoclastogenesis-supporting cells to express RANK ligand (Suva et al., 1987; Mancino et al., 2001). CSF-1, necessary for the development of preosteoclast to osteoclasts, is also derived from cancer cells (Stanley et al., 1983; Smith et al., 1995). Once bone metastasis is established, cancer cells recruit other tumor-associated cells, including fibroblasts and immune cells which secrete cytokines (such as IL-17, IL-1, IL-6, and TNF-α) that increase RANK ligand expression (Mancino et al., 2001; Kitazawa and Kitazawa, 2002). Integrin and immunoreceptor-like signaling leads to the activation of key effector molecules, such as Src-family kinases (Myoui et al., 2003), Syk non-receptor tyrosine kinase (Mócsai et al., 2010) and phosphoinositide 3-kinases (PI3Ks), which further enhance osteoclast activity and subsequent bone destruction (Di Cristofano and Pandolfi, 2000). Summary of the mechanisms of osteoclast activation during cancer cell-induced osteolysis as well as the key osteoclast signaling molecules are shown on Figure 2.
Figure 2. Schematic representation of physiological and pathological osteoclast activation during bone metastasis formation and key signaling molecules within the solid tumor microenvironment from a therapeutic aspect. Osteoblast-derived cytokines and interactions with the bone matrix lead to physiological osteoclast activation via CSF1, RANK, costimulatory and β3 integrin receptors. “Osteotropic” tumor cell recruited regulatory T cells (Tregs) and myeloid derived suppressor cells (MDSC) inhibit conventional T cells (Tconv) and promote cancer cell-induced pathological osteoclast activation via PI3Kβ, PI3Kδ, c-Src, and Syk.
Better understanding of the molecular mechanisms governing osteoclast activation in tumor induced-osteolysis and bone metastasis formation may result in the development of novel therapeutic approaches. As several of the osteoclast signal transduction pathways are also activated in cancer cells, they might be tackled synergistically within the solid tumor microenvironment resulting in a parallel reduction in cancer growth and osteoclast-mediated bone loss. On the other hand, a precision medicine-based approach is required to overcome cancer cell resistance mechanisms and to minimize side effects and interference with the mechanisms of physiological bone homeostasis. Taken together, pharmacological targeting of osteoclast and tumor cell signaling pathways may provide novel approaches to improve the long-term survival of cancer patients with bone metastases.
All authors listed have made a substantial, direct and intellectual contribution to the work, and approved it for publication.
This work was supported by the Hungarian National Scientific Research Fund (NKFIH-OTKA Grant No. FK132971 to DG and NKFIH-OTKA Grant No. K119653 to AM).
The authors declare that the research was conducted in the absence of any commercial or financial relationships that could be construed as a potential conflict of interest.
Arron, J. R., and Choi, Y. (2000). Bone versus immune system. Nature 408, 535–536. doi: 10.1038/35046196
Arroyo, J. D., Chevillet, J. R., Kroh, E. M., Ruf, I. K., Pritchard, C. C., Gibson, D. F., et al. (2011). Argonaute2 complexes carry a population of circulating microRNAs independent of vesicles in human plasma. Proc. Natl. Acad. Sci. U.S.A. 108, 5003–5008. doi: 10.1073/pnas.1019055108
Asagiri, M., Sato, K., Usami, T., Ochi, S., Nishina, H., Yoshida, H., et al. (2005). Autoamplification of NFATc1 expression determines its essential role in bone homeostasis. J. Exp. Med. 202, 1261–1269. doi: 10.1084/jem.20051150
Bakewell, S. J., Nestor, P., Prasad, S., Tomasson, M. H., Dowland, N., Mehrotra, M., et al. (2003). Platelet and osteoclast beta3 integrins are critical for bone metastasis. Proc. Natl. Acad. Sci. U.S.A. 100, 14205–14210. doi: 10.1073/pnas.2234372100
Bi, L., Okabe, I., Bernard, D. J., Wynshaw-Boris, A., and Nussbaum, R. L. (1999). Proliferative defect and embryonic lethality in mice homozygous for a deletion in the p110alpha subunit of phosphoinositide 3-kinase. J. Biol. Chem. 274, 10963–10968. doi: 10.1074/jbc.274.16.10963
Bissell, M. J., and Radisky, D. (2001). Putting tumours in context. Nat. Rev. Cancer 1, 46–54. doi: 10.1038/35094059
Biswas, S. K., and Mantovani, A. (2010). Macrophage plasticity and interaction with lymphocyte subsets: cancer as a paradigm. Nat. Immunol. 11, 889–896. doi: 10.1038/ni.1937
Bonewald, L. F. (2011). The amazing osteocyte. J. Bone Miner. Res. 26, 229–238. doi: 10.1002/jbmr.320
Boyce, B., and Xing, L. (2011). Src inhibitors in the treatment of metastatic bone disease: rationale and clinical data. Clin. Investig. (Lond.) 1, 1695–1706. doi: 10.4155/cli.11.150
Boyce, B. F., Yoneda, T., Lowe, C., Soriano, P., and Mundy, G. R. (1992). Requirement of pp60c-src expression for osteoclasts to form ruffled borders and resorb bone in mice. J. Clin. Invest. 90, 1622–1627. doi: 10.1172/jci116032
Boyer, B. Y., and Poupon, M. F. B. (2002). Src kinase contributes to the metastatic spread of carcinoma cells. Oncogene 21, 2347–2356. doi: 10.1038/sj.onc.1205298
Boyle, W. J., Simonet, W. S., and Lacey, D. L. (2003). Osteoclast differentiation and activation. Nature 423, 337–342. doi: 10.1038/nature01658
Brown, J. M., Corey, E., Lee, Z. D., True, L. D., Yun, T. J., Tondravi, M., et al. (2001). Osteoprotegerin and rank ligand expression in prostate cancer. Urology 57, 611–616. doi: 10.1016/s0090-4295(00)01122-5
Brunton, V. G., and Frame, M. C. (2008). Src and focal adhesion kinase as therapeutic targets in cancer. Curr. Opin. Pharmacol. 8, 427–432. doi: 10.1016/j.coph.2008.06.012
Busaidy, N., Farooki, A., Dowlati, A., Perentesis, J. P., Dancey, J. E., Doyle, L. A., et al. (2012). Management of metabolic effects associated with anticancer agents targeting the PI3K-Akt-mTOR pathway. J. Clin. Oncol. 30, 2919–2928. doi: 10.1200/jco.2011.39.7356
Caetano-Lopes, J., Canhao, H., and Fonseca, J. E. (2009). Osteoimmunology-the hidden immune regulation of bone. Autoimmun. Rev. 8, 250–255. doi: 10.1016/j.autrev.2008.07.038
Cantley, L. C., and Neel, B. G. (1999). New insights into tumor suppression: PTEN suppresses tumor formation by restraining the phosphoinositide 3-kinase/AKT pathway. Proc. Natl. Acad. Sci. U.S.A. 96, 4240–4245. doi: 10.1073/pnas.96.8.4240
Capietto, A. H., Kim, S., Sanford, D. E., Linehan, D. C., Hikida, M., Kumosaki, T., et al. (2013). Down-regulation of PLCγ2-β-catenin pathway promotes activation and expansion of myeloid-derived suppressor cells in cancer. J. Exp. Med. 210, 2257–2271. doi: 10.1084/jem.20130281
Chen, C., Cheng, P., Xie, H., Zhou, H. D., Wu, X. P., Liao, E. Y., et al. (2014). MiR-503 regulates osteoclastogenesis via targeting RANK. J. Bone Miner. Res. 29, 338–347. doi: 10.1002/jbmr.2032
Chen, Y., Wang, X., Di, L., Fu, G., Bai, L., Liu, J., et al. (2008). Phospholipase Cγ2 mediates RANKLstimulated lymph node organogenesis and osteoclastogenesis. J. Biol. Chem. 283, 29593–29601. doi: 10.1074/jbc.m802493200
Choi, Y., Arron, J. R., and Townsend, M. J. (2009). Promising bone-related therapeutic targets for rheumatoid arthritis. Nat. Rev. Rheumatol. 5, 543–548. doi: 10.1038/nrrheum.2009.175
Coleman, R., and Rubens, R. D. (1987). The clinical course of bone metastases from breast cancer. Br. J. Cancer 55, 61–66. doi: 10.1038/bjc.1987.13
Cox, T. R., Rumney, R. M. H., Schoof, E. M., Perryman, L., Høye, A. M., Agrawal, A., et al. (2015). The hypoxic cancer secretome induces pre-metastatic bone lesions through lysyl oxidase. Nature 522, 106–110. doi: 10.1038/nature14492
Croucher, P. I., McDonald, M. M., and Martin, T. J. (2016). Bone metastasis: the importance of the neighbourhood. Nat. Rev. Cancer 16, 373–386. doi: 10.1038/nrc.2016.44
Csete, D., Simon, E., Alatshan, A., Aradi, P., Dobó-Nagy, C., Jakus, Z., et al. (2019). Hematopoietic or osteoclast-specific deletion of Syk leads to increased bone mass in experimental mice. Front. Immunol. 10:937. doi: 10.3389/fimmu.2019.00937
Dbouk, H., Khalil, B. D., Wu, H., Shymanets, A., Nürnberg, B., and Backer, J. M. (2013). Characterization of a tumor-associated activating mutation of the p110β PI 3-kinase. PLoS One 8:e63833. doi: 10.1371/journal.pone.0063833
De Vries, T., Schoenmaker, T., Aerts, D., Grevers, L. C., Souza, P. P., Nazmi, K., et al. (2015). M-CSF priming of osteoclast precursors can cause osteoclastogenesis-insensitivity, which can be prevented and overcome on bone. J. Cell Physiol. 230, 210–225. doi: 10.1002/jcp.24702
de Vries, T. J., Mullender, M. G., van Duin, M. A., Semeins, C. M., James, N., Green, T. P., et al. (2009). The Src inhibitor AZD0530 reversibly inhibits the formation and activity of human osteoclasts. Mol. Cancer Res. 7, 476–488. doi: 10.1158/1541-7786.mcr-08-0219
Decker, C. E., Yang, Z., Rimer, R., Park-Min, K. H., Macaubas, C., Mellins, E. D., et al. (2015). Tmem178 acts in a novel negative feedback loop targeting NFATc1 to regulate bone mass. Proc. Natl. Acad. Sci. U.S.A. 112, 15654–15659. doi: 10.1073/pnas.1511285112
Demers, M. J., Thibodeau, S., Noel, D., Fujita, N., Tsuruo, T., Gauthier, R., et al. (2009). Intestinal epithelial cancer cell anoikis resistance: EGFR-mediated sustained activation of Src overrides Fak-dependent signaling to MEK/Erk and/or PI3-K/Akt-1. J. Cell Biochem. 107, 639–654. doi: 10.1002/jcb.22131
Di Cristofano, A., and Pandolfi, P. P. (2000). The multiple roles of PTEN in tumor suppression. Cell 100, 387–390. doi: 10.1016/s0092-8674(00)80674-1
Edwards, C., and Mundy, G. R. (2008). Eph receptors and ephrin signaling pathways: a role in bone homeostasis. Int. J. Med. Sci. 5, 263–272. doi: 10.7150/ijms.5.263
Edwards, J. C., Cohen, C., Xu, W., and Schlesinger, P. H. (2006). c-Src control of chloride channel support for osteoclast HCl transport and bone resorption. J. Biol. Chem. 281, 28011–28022. doi: 10.1074/jbc.m605865200
Ell, B., Mercatali, L., Ibrahim, T., Campbell, N., Schwarzenbach, H., Pantel, K., et al. (2013). Tumor-induced osteoclast miRNA changes as regulators and biomarkers of osteolytic bone metastasis. Cancer Cell 24, 542–556. doi: 10.1016/j.ccr.2013.09.008
Epple, H., Cremasco, V., Zhang, K., Mao, D., Longmore, G. D., and Faccio, R. (2008). Phospholipase Cγ2 modulates integrin signaling in the osteoclast by affecting the localization and activation of Src kinase. Mol. Cell Biol. 28, 3610–3622. doi: 10.1128/mcb.00259-08
Faccio, R. (2011). Immune regulation of the tumor/bone vicious cycle. Ann. N. Y. Acad. Sci. 1237, 71–78. doi: 10.1111/j.1749-6632.2011.06244.x
Faccio, R., Takeshita, S., Zallone, A., Ross, F. P., and Teitelbaum, S. L. (2003). c-Fms and the alphavbeta3 integrin collaborate during osteoclast differentiation. J. Clin. Invest. 115, 749–758. doi: 10.1172/jci200316924
Faccio, R., Teitelbaum, S. L., Fujikawa, K., Chappel, J., Zallone, A., Tybulewicz, V. L., et al. (2005). Vav3 regulates osteoclast function and bone mass. Nat. Med. 11, 284–290. doi: 10.1038/nm1194
Farrugia, A. N., Atkins, G. J., To, L. B., Pan, B., Horvath, N., Kostakis, P., et al. (2003). Receptor activator of nuclear factor-kappaB ligand expression by human myeloma cells mediates osteoclast formation in vitro and correlates with bone destruction in vivo. Cancer Res. 63, 5438–5445.
Feng, L., Reynisdóttir, I., and Reynisson, J. (2012). The effect of PLC-γ2 inhibitors on the growth of human tumour cells. Eur. J. Med. Chem. 54, 463–469. doi: 10.1016/j.ejmech.2012.05.029
Firestein, G. S. (2003). Evolving concepts of rheumatoid arthritis. Nature 423, 356–361. doi: 10.1038/nature01661
Fodor, S., Jakus, Z., and Mócsai, A. (2006). ITAM-based signaling beyond the adaptive immune response. Immunol. Lett. 104, 29–37. doi: 10.1016/j.imlet.2005.11.001
Franceschetti, T., Kessler, C. B., Lee, S. K., and Delany, A. M. (2013). miR-29 promotes murine osteoclastogenesis by regulating osteoclast commitment and migration. J. Biol. Chem. 288, 33347–33360. doi: 10.1074/jbc.m113.484568
Frisch, S. M., and Screaton, R. A. (2001). Anoikis mechanisms. Curr. Opin. Cell Biol. 13, 555–562. doi: 10.1016/s0955-0674(00)00251-9
Frisch, S. M., Vuori, K., Ruoslahti, E., and Chan-Hui, P. Y. (1996). Control of adhesion-dependent cell survival by focal adhesion kinase. J. Cell Biol. 134, 793–799. doi: 10.1083/jcb.134.3.793
Fruman, D., and Rommel, C. (2014). PI3K and cancer: lessons, challenges and opportunities. Nat. Rev. Drug Discov. 13, 140–156. doi: 10.1038/nrd4204
Furuyama, N., and Fujisawa, Y. (2000). Regulation of collagenolytic protease secretion through c-Src in osteoclasts. Biochem. Biophys. Res. Commun. 272, 116–124. doi: 10.1006/bbrc.2000.2698
Gabrilovich, D. I., and Nagaraj, S. (2009). Myeloid-derived suppressor cells as regulators of the immune system. Nat. Rev. Immunol. 9, 162–174. doi: 10.1038/nri2506
Gabrilovich, D. I., Ostrand-Rosenberg, S., and Bronte, V. (2012). Coordinated regulation of myeloid cells by tumours. Nat. Rev. Immunol. 12, 253–268. doi: 10.1038/nri3175
Giuliani, N., Bataille, R., Mancini, C., Lazzaretti, M., and Barille, S. (2001). Myeloma cells induce imbalance in the osteoprotegerin/osteoprotegerin ligand system in the human bone marrow environment. Blood 98, 3527–3533. doi: 10.1182/blood.v98.13.3527
Grimaud, E., Soubigou, L., Couillaud, S., Coipeau, P., Moreau, A., Passuti, N., et al. (2003). Receptor activator of nuclear factor kappaB ligand (RANKL)/osteoprotegerin (OPG) ratio is increased in severe osteolysis. Am. J. Pathol. 163, 2021–2031. doi: 10.1016/s0002-9440(10)63560-2
Győri, D., Csete, D., Benkő, S., Kulkarni, S., Mandl, P., Dobó-Nagy, C., et al. (2014). The phosphoinositide 3-kinase isoform PI3Kβ regulates osteoclast-mediated bone resorption in humans and mice. Arthritis Rheumatol. 66, 2210–2221. doi: 10.1002/art.38660
Győri, D., Lim, E. L., Grant, F. M., Spensberger, D., Roychoudhuri, R., Shuttleworth, S. J., et al. (2018). Compensation between CSF1R+ macrophages and Foxp3+ Treg cells drives resistance to tumor immunotherapy. JCI Insight. 3:120631.
Hall, T. J., Jeker, H., and Schaueblin, M. (1995). Wortmannin, a potent inhibitor of phosphatidylinositol 3-kinase, inhibits osteoclastic bone resorption in vitro. Calcif. Tissue Int. 56, 336–338. doi: 10.1007/bf00318056
Hawkins, P. T., Anderson, K. E., Davidson, K., and Stephens, L. R. (2006). Signalling through class I PI3Ks in mammalian cells. Biochem. Soc. Trans. 34, 647–662. doi: 10.1042/bst0340647
Hawkins, P. T., and Stephens, L. R. (2015). PI3K signalling in inflammation. Biochim. Biophys. Acta 1851, 882–897. doi: 10.1016/j.bbalip.2014.12.006
Hayashi, M., Nakashima, T., Taniguchi, M., Kodama, T., Kumanogoh, A., and Takayanagi, H. (2012). Osteoprotection by semaphorin 3A. Nature 485, 69–74. doi: 10.1038/nature11000
Hernandez, R. K., Wade, S. W., Reich, A., Pirolli, M., Liede, A., and Lyman, G. H. (2018). Incidence of bone metastases in patients with solid tumors: analysis of oncology electronic medical records in the United States. BMC Cancer 18:44. doi: 10.1186/s12885-017-3922-0
Hill, K., Kalifa, S., Das, J. R., Bhatti, T., Gay, M., Williams, D., et al. (2010). The role of PI 3-kinase p110beta in AKT signally, cell survival, and proliferation in human prostate cancer cells. Prostate 70, 755–764.
Hiraoka, K., Zenmyo, M., Watari, K., Iguchi, H., Fotovati, A., Kimura, Y. N., et al. (2008). Inhibition of bone and muscle metastases of lung cancer cells by a decrease in the number of monocytes/macrophages. Cancer Sci. 99, 1595–1602. doi: 10.1111/j.1349-7006.2008.00880.x
Horne, W. C., Neff, L., Chatterjee, D., Lomri, A., Levy, J. B., and Baron, R. (1992). Osteoclasts express high levels of pp60c-src in association with intracellular membranes. J. Cell Biol. 119, 1003–1013. doi: 10.1083/jcb.119.4.1003
Humphrey, M. B., Ogasawara, K., Yao, W., Spusta, S. C., Daws, M. R., Lane, N. E., et al. (2004). The signaling adapter protein DAP12 regulates multinucleation during osteoclast development. J. Bone Miner. Res. 19, 224–234. doi: 10.1359/jbmr.0301234
Hynes, R. O. (1992). Integrins: versatility, modulation, and signaling in cell adhesion. Cell 69, 11–25. doi: 10.1016/0092-8674(92)90115-s
Insogna, K. L., Sahni, M., Grey, A. B., Tanaka, S., Horne, W. C., Neff, L., et al. (1997). Colony-stimulating factor-1 induces cytoskeletal reorganization and c-src-dependent tyrosine phosphorylation of selected cellular proteins in rodent osteoclasts. J. Clin. Invest. 100, 2476–2485. doi: 10.1172/jci119790
Jones, D. H., Nakashima, T., Sanchez, O. H., Kozieradzki, I., Komarova, S. V., Sarosi, I., et al. (2006). Regulation of cancer cell migration and bone metastasis by RANKL. Nature 440, 692–696.
Joyce-Shaikh, B., Bigler, M. E., Chao, C. C., Murphy, E. E., Blumenschein, W. M., Adamopoulos, I. E., et al. (2010). Myeloid DAP12-associating lectin (MDL)-1 regulates synovial inflammation and bone erosion associated with autoimmune arthritis. J. Exp. Med. 207, 579–589. doi: 10.1084/jem.20090516
Kacinski, B. M. (1995). CSF-1 and its receptor in ovarian, endometrial and breast cancer. Ann. Med. 27, 79–85. doi: 10.3109/07853899509031941
Kagiya, T. (2015). MicroRNAs and osteolytic bone metastasis: the roles of microRNAs in tumor-induced osteoclast differentiation. J. Clin. Med. 4, 1741–1752. doi: 10.3390/jcm4091741
Kakonen, S. M., Selander, K. S., Chirgwin, J. M., Yin, J. J., Burns, S., Rankin, W. A., et al. (2002). Transforming growth factor-beta stimulates parathyroid hormone-related protein and osteolytic metastases via Smad and mitogen-activated protein kinase signaling pathways. J. Biol. Chem. 277, 24571–24578. doi: 10.1074/jbc.m202561200
Kameda, Y., Takahata, M., Komatsu, M., Mikuni, S., Hatakeyama, S., Shimizu, T., et al. (2013). Siglec-15 regulates osteoclast differentiation by modulating RANKL-induced phosphatidylinositol 3-kinase/Akt and Erk pathways in association with signaling Adaptor DAP12. J. Bone Miner. Res. 28, 2463–2475. doi: 10.1002/jbmr.1989
Kearns, A., Khosla, S., and Kostenuik, P. J. (2008). Receptor activator of nuclear factor kappaB ligand and osteoprotegerin regulation of bone remodeling in health and disease. Endocr. Rev. 29, 155–192. doi: 10.1210/er.2007-0014
Kelchtermans, H., Geboes, L., Mitera, T., Huskens, D., Leclercq, G., and Matthys, P. (2009). Activated CD4+CD25+ regulatory T cells inhibit osteoclastogenesis and collageninduced arthritis. Ann. Rheum. Dis. 68, 744–750. doi: 10.1136/ard.2007.086066
Kertész, Z., Győri, D., Körmendi, S., Fekete, T., Kis-Tóth, K., Jakus, Z., et al. (2012). Phospholipase Cγ2 is required for basal but not oestrogen deficiency-induced bone resorption. Eur. J. Clin. Invest. 42, 49–60. doi: 10.1111/j.1365-2362.2011.02556.x
Kim, H., Kim, T., Jeong, B. C., Cho, I. T., Han, D., Takegahara, N., et al. (2013). Tmem64 modulates calcium signaling during RANKL-mediated osteoclast differentiation. Cell Metab. 17, 249–260. doi: 10.1016/j.cmet.2013.01.002
Kim, K., Kim, J. H., Lee, J., Jin, H. M., Kook, H., Kim, K. K., et al. (2007a). MafB negatively regulates RANKL-mediated osteoclast differentiation. Blood 109, 3253–3259. doi: 10.1182/blood-2006-09-048249
Kim, N., Kadono, Y., Takami, M., Lee, J., Lee, S. H., Okada, F., et al. (2005). Osteoclast differentiation independent of the TRANCE-RANK-TRAF6 axis. J. Exp. Med. 202, 589–595. doi: 10.1084/jem.20050978
Kim, Y., Lee, C. K., Nah, S. S., Mun, S. H., Yoo, B., and Moon, H. B. (2007b). Human CD4+CD25+ regulatory T cells inhibit the differentiation of osteoclasts from peripheral blood mononuclear cells. Biochem. Biophys. Res. Commun. 357, 1046–1052. doi: 10.1016/j.bbrc.2007.04.042
Kitazawa, S., and Kitazawa, R. (2002). RANK ligand is a prerequisite for cancer-associated osteolytic lesions. J. Pathol. 198, 228–236. doi: 10.1002/path.1199
Koga, T., Inui, M., Inoue, K., Kim, S., Suematsu, A., Kobayashi, E., et al. (2004). Costimulatory signals mediated by the ITAM motif cooperate with RANKL for bone homeostasis. Nature 428, 758–763. doi: 10.1038/nature02444
Kostenuik, P. J., Singh, G., Suyama, K. L., and Orr, F. W. (1992). A quantitative model for spontaneous bone metastasis: evidence for a mitogenic effect of bone on Walker 256 cancer cells. Clin. Exp. Metastasis 10, 403–410. doi: 10.1007/bf00133469
Kuo, P. L., Liao, S. H., Hung, J. Y., Huang, M. S., and Hsu, Y. L. (2013). MicroRNA-33a functions as a bone metastasis suppressor in lung cancer by targeting parathyroid hormone related protein. Biochim. Biophys. Acta 1830, 3756–3766. doi: 10.1016/j.bbagen.2013.02.022
Lee, Y., Kim, H. J., Park, C. K., Kim, Y. G., Lee, H. J., Kim, J. Y., et al. (2013). MicroRNA-124 regulates osteoclast differentiation. Bone 56, 383–389. doi: 10.1016/j.bone.2013.07.007
Lin, K., Gong, J., Li, C. F., Jang, T. H., Chen, W. L., Chen, H. J., et al. (2012). Vav3-rac1 signaling regulates prostate cancer metastasis with elevated Vav3 expression correlating with prostate cancer progression and posttreatment recurrence. Cancer Res. 72, 3000–3009. doi: 10.1158/0008-5472.can-11-2502
Lontos, K., Adamik, J., Tsagianni, A., Galson, D. L., Chirgwin, J. M., and Suvannasankha, A. (2018). The Role of semaphorin 4D in bone remodeling and cancer metastasis. Front. Endocrinol. 9:322. doi: 10.3389/fendo.2018.00322
Lorenzo, J., Horowitz, M., and Choi, Y. (2007). Osteoimmunology: interaction of the bone and immune systems. End. Rev. 29, 403–440.
Lorenzo, J., Horowitz, M., Choi, Y., and Takayanagi, H. (2010). Osteoimmunology: Interactions of the Immune and Skeletal Systems. Cambridge, MA: Academic Press.
Lowe, C., Yoneda, T., Boyce, B. F., Chen, H., Mundy, G. R., and Soriano, P. (1993). Osteopetrosis in Src-deficient mice is due to an autonomous defect of osteoclasts. Proc. Natl. Acad. Sci. U.S.A. 90, 4485–4489. doi: 10.1073/pnas.90.10.4485
Mancino, A. T., Klimberg, V. S., Yamamoto, M., Manolagas, S. C., and Abe, E. (2001). Breast cancer increases osteoclastogenesis by secreting M-CSF and upregulating RANKL in stromal cells. J. Surg. Res. 100, 18–24. doi: 10.1006/jsre.2001.6204
Mann, M., Barad, O., Agami, R., Geiger, B., and Hornstein, E. (2010). miRNA-based mechanism for the commitment of multipotent progenitors to a single cellular fate. Proc. Natl. Acad. Sci. U.S.A. 107, 15804–15809. doi: 10.1073/pnas.0915022107
Mao, D., Epple, H., Uthgenannt, B., Novack, D. V., and Faccio, R. (2006). PLCγ2 regulates osteoclastogenesis via its interaction with ITAM proteins and GAB2. J. Clin. Invest. 116, 2869–2879. doi: 10.1172/jci28775
Marton, N., Kovacs, O. T., Baricza, E., Kittel, A., Győri, D., Mocsai, A., et al. (2017). Extracellular vesicles regulate the human osteoclastogenesis: divergent roles in discrete inflammatory arthropathies. Cell Mol. Life Sci. 74, 3599–3611. doi: 10.1007/s00018-017-2535-8
McHugh, K. P. (2000). Mice lacking b3 integrins are osteosclerotic because of dysfunctional osteoclasts. J. Clin. Invest. 105, 433–440. doi: 10.1172/jci8905
McInnes, I. B., and Schett, G. (2007). Cytokines in the pathogenesis of rheumatoid arthritis. Nat. Rev. Immunol. 7, 429–442.
Miyauchi, A., Alvarez, J., Greenfield, E. M., Teti, A., Grano, M., Colucci, S., et al. (1991). Recognition of osteopontin and related peptides by an alpha v beta 3 integrin stimulates immediate cell signals in osteoclasts. J. Biol. Chem. 266, 20369–20374.
Miyauchi, Y., Ninomiya, K., Miyamoto, H., Sakamoto, A., Iwasaki, R., Hoshi, H., et al. (2010). The Blimp1-Bcl6 axis is critical to regulate osteoclast differentiation and bone homeostasis. J. Exp. Med. 207, 751–762. doi: 10.1084/jem.20091957
Miyazaki, T., Sanjay, A., Neff, L., Tanaka, S., Horne, W. C., and Baron, R. (2004). Src kinase activity is essential for osteoclast function. J. Biol. Chem. 279, 17660–17666. doi: 10.1074/jbc.m311032200
Mócsai, A., Humphrey, M. B., Ziffle, J.A. Van, Hu, Y., Burghardt, A., Spusta, S. C., et al. (2004). The immunomodulatory adapter proteins DAP12 and Fc receptor gamma-chain (FcRγ) regulate development of functional osteoclasts through the Syk tyrosine kinase. Proc. Natl. Acad. Sci. U.S.A. 101, 6158–6163. doi: 10.1073/pnas.0401602101
Mócsai, A., Ruland, J., and Tybulewicz, V. L. (2010). The SYK tyrosine kinase: a crucial player in diverse biological functions. Nat. Rev. Immunol. 10, 387–402. doi: 10.1038/nri2765
Monteiro, A. C., Leal, A. C., Goncalves-Silva, T., Mercadante, A. C., Kestelman, F., Chaves, S. B., et al. (2013). T cells induce pre-metastatic osteolytic disease and help bone metastases establishment in a mouse model of metastatic breast cancer. PLoS One 8:e68171. doi: 10.1371/journal.pone.0068171
Mosser, D. M., and Edwards, J. P. (2008). Exploring the full spectrum of macrophage activation. Nat. Rev. Immunol. 8, 958–969. doi: 10.1038/nri2448
Mundy, G. R. (2002). Metastasis: metastasis to bone: causes, consequences and therapeutic opportunities. Nat. Rev. Cancer 2, 584–593. doi: 10.1038/nrc867
Munugalavadla, V., Vemula, S., Sims, E. C., Krishnan, S., Chen, S., Yan, J., et al. (2008). The p85alpha subunit of class IA phosphatidylinositol 3-kinase regulates the expression of multiple genes involved in osteoclast maturation and migration. Mol. Cell Biol. 28, 7182–7198. doi: 10.1128/mcb.00920-08
Myoui, A., Nishimura, R., Williams, P. J., Hiraga, T., Tamura, D., Michigami, T., et al. (2003). C-SRC tyrosine kinase activity is associated with tumor colonization in bone and lung in an animal model of human breast cancer metastasis. Cancer Res. 63, 5028–5033.
Nakamura, I., Takahashi, N., Sasaki, T., Tanaka, S., Udagawa, N., and Murakami, H. (1995). Wortmannin, a specific inhibitor of phosphatidylinositol-3 kinase, blocks osteoclastic bone resorption. FEBS Lett. 361, 79–84. doi: 10.1016/0014-5793(95)00153-z
Nakashima, T., and Takayanagi, H. (2009). Osteoimmunology: crosstalk between the immune and bone systems. J. Clin. Immunol. 29, 555–567. doi: 10.1007/s10875-009-9316-6
Negishi-Koga, T., Gober, H. J., Sumiya, E., Komatsu, N., Okamoto, K., Sawa, S., et al. (2015). Immune complexes regulate bone metabolism through FcRγ signalling. Nat. Commun. 6:6637.
Nishikawa, K., Iwamoto, Y., Kobayashi, Y., Katsuoka, F., Kawaguchi, S., Tsujita, T., et al. (2015). DNA methyltransferase 3a regulates osteoclast differentiation by coupling to an S-adenosylmethionine-producing metabolic pathway. Nat. Med. 21, 281–287. doi: 10.1038/nm.3774
Nishikawa, K., Nakashima, T., Hayashi, M., Fukunaga, T., Kato, S., Kodama, T., et al. (2010). Blimp1-mediated repression of negative regulators is required for osteoclast differentiation. Proc. Natl. Acad. Sci. U.S.A. 107, 3117–3122. doi: 10.1073/pnas.0912779107
Novack, D. V., and Teitelbaum, S. L. (2008). The osteoclast: friend or foe? Annu. Rev. Pathol. 3, 457–484.
Oikawa, T., Oyama, M., Kozuka-Hata, H., Uehara, S., Udagawa, N., Saya, H., et al. (2012). Tks5-dependent formation of circumferential podosomes/invadopodia mediates cell-cell fusion. J. Cell Biol. 197, 553–568. doi: 10.1083/jcb.201111116
Okamoto, K., and Takayanagi, H. (2011). Regulation of bone by the adaptive immune system in arthritis. Arthritis Res. Ther. 13, 219. doi: 10.1186/ar3323
Okkenhaug, K. (2013). Signaling by the phosphoinositide 3-kinase family in immune cells. Annu. Rev. Immunol. 31, 675–704. doi: 10.1146/annurev-immunol-032712-095946
Orr, W., Varani, J., Gondex, M. K., Ward, P. A., and Mundy, G. R. (1979). Chemotactic responses of tumor cells to products of resorbing bone. Science 203, 176–179. doi: 10.1126/science.569363
Paloneva, J., Mandelin, J., Kiialainen, A., Bohling, T., Prudlo, J., Hakola, P., et al. (2003). DAP12/TREM2 deficiency results in impaired osteoclast differentiation and osteoporotic features. J. Exp. Med. 198, 669–675. doi: 10.1084/jem.20030027
Pilkington, M. F., Sims, S. M., and Dixon, S. J. (1998). Wortmannin inhibits spreading and chemotaxis of rat osteoclasts in vitro. J. Bone Miner. Res. 13, 688–694. doi: 10.1359/jbmr.1998.13.4.688
Pixley, F. J., and Stanley, E. R. (2004). CSF-1 regulation of the wandering macrophage: complexity in action. Trends Cell Biol. 14, 628–638. doi: 10.1016/j.tcb.2004.09.016
Qin, J., Vinogradova, O., and Plow, E. F. (2004). Integrin bidirectional signaling: a molecular view. PLoS Biol. 2:e169. doi: 10.1371/journal.pbio.0020169
Ries, C., Hoves, S., Cannarile, M. A., and Rüttinger, D. (2015). CSF-1/CSF-1R targeting agents in clinical development for cancer therapy. Curr. Opin. Pharmacol. 23, 45–51. doi: 10.1016/j.coph.2015.05.008
Roodman, G. D., and Dougall, W. C. (2008). RANK ligand as a therapeutic target for bone metastases and multiple myeloma. Cancer Treat. Rev. 34, 92–101. doi: 10.1016/j.ctrv.2007.09.002
Ross, F. P., Chappel, J., Alvarez, J. I., Sander, D., Butler, W. T., Farach-Carson, M. C., et al. (1993). Interactions between the bone matrix proteins osteopontin and bone sialoprotein and the osteoclast integrin alpha v beta 3 potentiate bone resorption. J. Biol. Chem. 268, 9901–9907.
Ross, F. P., and Teitelbaum, S. L. (2005). αvβ3 and macrophage colony-stimulating factor: partners in osteoclast biology. Immunol. Rev. 208, 88–105. doi: 10.1111/j.0105-2896.2005.00331.x
Samuels, Y., Diaz, L. A., Schmidt-Kittler, O., Cummins, J. M., Delong, L., Cheong, I., et al. (2005). Mutant PIK3CA promotes cell growth and invasion of human cancer cells. Cancer Cell 7, 561–573. doi: 10.1016/j.ccr.2005.05.014
Sasaki, T., Irie-Sasaki, J., Jones, R. G., Oliveira-dos-Santos, A. J., Stanford, W. L., Bolon, B., et al. (2000). Function of PI3Kgamma in thymocyte development, T cell activation, and neutrophil migration. Science 287, 1040–1046. doi: 10.1126/science.287.5455.1040
Sato, K., Suematsu, A., Nakashima, T., Takemoto-Kimura, S., Aoki, K., Morishita, Y., et al. (2006a). Regulation of osteoclast differentiation and function by the CaMK-CREB pathway. Nat. Med. 12, 1410–1416. doi: 10.1038/nm1515
Sato, K., Suematsu, A., Okamoto, K., Yamaguchi, A., Morishita, Y., Kadono, Y., et al. (2006b). Th17 functions as an osteoclastogenic helper T cell subset that links T cell activation and bone destruction. J. Exp. Med. 203, 2673–2682. doi: 10.1084/jem.20061775
Sato, M., Bryant, H. U., Dodge, J. A., Davis, H., Matter, W. F., and Vlahos, C. J. (1996). Effects of wortmannin analogs on bone in vitro and in vivo. J. Pharmacol. Exp. Ther. 277, 543–550.
Sawant, A., Deshane, J., Jules, J., Lee, C. M., Harris, B. A., Feng, X., et al. (2013). Myeloid-derived suppressor cells function as novel osteoclast progenitors enhancing bone loss in breast cancer. Cancer Res. 73, 672–682. doi: 10.1158/0008-5472.can-12-2202
Sawyer, C., Sturge, J., Bennett, D. C., O’Hare, M. J., Allen, W. E., Bain, J., et al. (2003). Regulation of breast cancer cell chemotaxis by the phosphoinositide 3-kinase p110delta. Cancer Res. 63, 1667–1675.
Scheid, M. P., and Woodgett, J. R. (2001). PKB/Akt: functional insights from genetic models. Nat. Rev. Mol. Cell Biol. 2, 760–768. doi: 10.1038/35096067
Schett, G. (2009). Osteoimmunology in rheumatic diseases. Arth. Res. Ther. 11:210. doi: 10.1186/ar2571
Schett, G., and Teitelbaum, S. L. (2009). Osteoclasts and arthritis. J. Bone Miner. Res. 24, 1142–1146.
Scholl, S. M., Pallud, C., Beuvon, F., Hacene, K., Stanley, E. R., Rohrschneider, L. R., et al. (1994). Anti-colony-stimulating factor-1 antibody staining in primary breast adenocarcinomas correlates with marked inflammatory cell infiltrates and prognosis. J. Natl. Cancer Inst. 86, 120–126. doi: 10.1093/jnci/86.2.120
Schreiber, R. D., Old, L. J., and Smyth, M. J. (2011). Cancer immunoediting: integrating immunity’s roles in cancer suppression and promotion. Science 331, 1565–1570. doi: 10.1126/science.1203486
Schwartz, M. A., Schaller, M. D., and Ginsberg, M. H. (1995). Integrins: emerging paradigms of signal transduction. Annu. Rev. Cell Dev. Biol. 11, 549–599. doi: 10.1146/annurev.cb.11.110195.003001
Sezer, O., Heider, U., Zavrski, I., Kuhne, C. A., and Hofbauer, L. C. (2003). RANK ligand and osteoprotegerin in myeloma bone disease. Blood 101, 2094–2098. doi: 10.1182/blood-2002-09-2684
Shabo, I., Olsson, H., Stål, O., and Svanvik, J. (2013). Breast cancer expression of DAP12 is associated with skeletal and liver metastases and poor survival. Clin. Breast. Cancer 13, 371–377. doi: 10.1016/j.clbc.2013.05.003
Shain, K. H., Landowski, T. H., and Dalton, W. S. (2002). Adhesion-mediated intracellular redistribution of c-Fas-associated death domain-like IL-1-converting enzyme-like inhibitory protein-long confers resistance to CD95-induced apoptosis in hematopoietic cancer cell lines. J. Immunol. 168, 2544–2553. doi: 10.4049/jimmunol.168.5.2544
Shattil, S. J., Kim, C., and Ginsberg, M. H. (2010). The final steps of integrin activation: the end game. Nat. Rev. Mol. Cell Biol. 11, 288–300. doi: 10.1038/nrm2871
Shinohara, M., Nakamura, M., Masuda, H., Hirose, J., Kadono, Y., Iwasawa, M., et al. (2012). Class IA phosphatidylinositol 3-kinase regulates osteoclastic bone resorption through protein kinase B-mediated vesicle transport. J. Bone Miner. Res. 27, 2464–2475. doi: 10.1002/jbmr.1703
Shugg, R. P., Thomson, A., Tanabe, N., Kashishian, A., Steiner, B. H., Puri, K. D., et al. (2013). Effects of isoform-selective phosphatidylinositol 3-kinase inhibitors on osteoclasts: actions on cytoskeletal organization, survival, and resorption. J. Biol. Chem. 288, 35346–35357. doi: 10.1074/jbc.m113.507525
Simonet, W. S., Lacey, D. L., Dunstan, C. R., Kelley, M., Chang, M. S., Luthy, R., et al. (1997). Osteoprotegerin: a novel secreted protein involved in the regulation of bone density. Cell 89, 309–319. doi: 10.1016/s0092-8674(00)80209-3
Simpson, K. J., Selfors, L. M., Bui, J., Reynolds, A., Leake, D., Khvorova, A., et al. (2008). Identification of genes that regulate epithelial cell migration using an siRNA screening approach. Nat. Cell. Biol. 10, 1027–1038. doi: 10.1038/ncb1762
Sloan, E., Pouliot, N., Stanley, K. L., Chia, J., Moseley, J. M., Hards, D. K., et al. (2006). Tumor-specific expression of alphavbeta3 integrin promotes spontaneous metastasis of breast cancer to bone. Breast Cancer Res. 8:R20.
Smith, H. O., Anderson, P. S., Kuo, D. Y. K., Goldberg, G. L., DeVictoria, C. L., Boocock, C. A., et al. (1995). The role of colony-stimulating factor 1 and its receptor in the etiopathogenesis of endometrial adenocarcinoma. Clin. Cancer Res. 1, 313–325.
Soriano, P., Montgomery, C., Geske, R., and Bradley, A. (1991). Targeted disruption of the c-src proto-oncogene leads to osteopetrosis in mice. Cell 64, 693–702. doi: 10.1016/0092-8674(91)90499-o
Stanley, E. R., Guilbert, L. T., Tushinski, R. J., and Bartelmez, S. H. (1983). CSF-1 A mononuclear phagocyte lineage-specific hemopoietic growth factor. J. Cell Biochem. 21, 151–159. doi: 10.1002/jcb.240210206
Stiffel, V., Amoui, M., Sheng, M. H., Mohan, S., and Lau, K. H. (2014). EphA4 receptor is a novel negative regulator of osteoclast activity. J. Bone Miner. Res. 29, 804–819. doi: 10.1002/jbmr.2084
Sugatani, T., and Hruska, K. A. (2007). MicroRNA-223 is a key factor in osteoclast differentiation. J. Cell Biochem. 101, 996–999. doi: 10.1002/jcb.21335
Suva, L. J., Winslow, G. A., Wettenhall, R. E., Hammonds, R. G., Moseley, J. M., Diefenbach-Jagger, H., et al. (1987). A parathyroid hormone-related protein implicated in malignant hypercalcemia: cloning and expression. Science 237, 893–896. doi: 10.1126/science.3616618
Takayanagi, H. (2007). Osteoimmunology: shared mechanisms and crosstalk between the immune and bone systems. Nat. Rev. Immunol. 7, 292–304. doi: 10.1038/nri2062
Takayanagi, H. (2010). New immune connections in osteoclast formation. Ann. N. Y. Acad. Sci. 1192, 117–123. doi: 10.1111/j.1749-6632.2009.05303.x
Takegahara, N., Takamatsu, H., Toyofuku, T., Tsujimura, T., Okuno, T., Yukawa, K., et al. (2006). Plexin-A1 and its interaction with DAP12 in immune responses and bone homeostasis. Nat. Cell Biol. 8, 615–622. doi: 10.1038/ncb1416
Tan, W., Zhang, W., Strasner, A., Grivennikov, S., Cheng, J. Q., Hoffman, R. M., et al. (2011). Tumour-infiltrating regulatory T cells stimulate mammary cancer metastasis through RANKL–RANK signalling. Nature 470, 548–553. doi: 10.1038/nature09707
Tanaka, S. (2017). RANKL-independent osteoclastogenesis: a long-standing controversy. J. Bone Miner. Res. 32, 431–433. doi: 10.1002/jbmr.3092
Tang, R. P., Kackinski, B., Validire, P., Beuvon, F., Sastre, X., Benoit, P., et al. (1990). Oncogene amplification correlates with dense lymphocyte infiltration in human breast cancers: a role for hematopoietic growth factor release by tumor cells? J. Cell Biochem. 44, 189–198. doi: 10.1002/jcb.240440307
Teitelbaum, S. L. (2000). Bone resorption by osteoclasts. Science 289, 1504–1508. doi: 10.1126/science.289.5484.1504
Teitelbaum, S. L., and Ross, F. P. (2003). Genetic regulation of osteoclast development and function. Nat. Rev. Genet. 4, 638–649. doi: 10.1038/nrg1122
Thomas, R. J., Guise, T. A., Yin, J. J., Elliott, J., Horwood, N. J., Martin, T. J., et al. (1999). Breast cancer cells interact with osteoblasts to support osteoclast formation. Endocrinology 140, 4451–4458. doi: 10.1210/endo.140.10.7037
Thomas, S. M., and Brugge, J. S. (1997). Cellular functions regulated by Src family kinases. Annu. Rev. Cell Dev. Biol. 13, 513–609. doi: 10.1146/annurev.cellbio.13.1.513
Thorpe, L., Yuzugullu, H., and Zhao, J. J. (2015). PI3K in cancer: divergent roles of isoforms, modes of activation and therapeutic targeting. Nat. Rev. Cancer 15, 7–24. doi: 10.1038/nrc3860
Tsuji-Takechi, K., Negishi-Koga, T., Sumiya, E., Kukita, A., Kato, S., Maeda, T., et al. (2012). Stage-specific functions of leukemia/lymphoma-related factor (LRF) in the transcriptional control of osteoclast development. Proc. Natl. Acad. Sci. U.S.A. 109, 2561–2566. doi: 10.1073/pnas.1116042109
Tsukasaki, M., Hamada, K., Okamoto, K., Nagashima, K., Terashima, A., Komatsu, N., et al. (2017). LOX fails to substitute for RANKL in osteoclastogenesis. J. Bone Miner. Res. 32, 434–439. doi: 10.1002/jbmr.2990
Valadi, H., Ekstrom, K., Bossios, A., Sjostrand, M., Lee, J. J., and Lotvall, J. O. (2007). Exosome-mediated transfer of mRNAs and microRNAs is a novel mechanism of genetic exchange between cells. Nat. Cell Biol. 9, 654–659. doi: 10.1038/ncb1596
Vanhaesebroeck, B., Guillermet-Guibert, J., Graupera, M., and Bilanges, B. (2010). The emerging mechanisms of isoform-specific PI3K signalling. Nat. Rev. Mol. Cell Biol. 11, 329–341. doi: 10.1038/nrm2882
Vanhaesebroeck, B., Stephens, L. R., and Hawkins, P. T. (2012). PI3K signalling: the path to discovery and understanding. Nat. Rev. Mol. Cell Biol. 13, 195–203. doi: 10.1038/nrm3290
Vivanco, I., and Sawyers, C. L. (2002). The phosphatidylinositol 3-Kinase AKT pathway in human cancer. Nat. Rev. Cancer 2, 489–501. doi: 10.1038/nrc839
Weilbaecher, K. N., Guise, T. A., and McCauley, L. K. (2011). Cancer to bone: a fatal attraction. Nat. Rev. Cancer 11, 411–425. doi: 10.1038/nrc3055
Wiktor-Jedrzejczak, W., Bartocci, A., Ferrante, A. W. Jr., Ahmed-Ansari, A., Sell, K. W., Pollard, J. W., et al. (1990). Total absence of colony-stimulating factor 1 in the macrophage-deficient osteopetrotic (op/op) mouse. Proc. Natl. Acad. Sci. U.S.A. 87, 4828–4832. doi: 10.1073/pnas.87.12.4828
Wong, B., Rho, J., Arron, J., Robinson, E., Orlinick, J., Chao, M., et al. (1997). TRANCE is a novel ligand of the tumor necrosis factor receptor family that activates c-Jun N-terminal kinase in T cells. J. Biol. Chem. 272, 25190–25194. doi: 10.1074/jbc.272.40.25190
Yang, Y. M., Kim, M. S., Son, A., Hong, J. H., Kim, K. H., Seo, J. T., et al. (2009). Alteration of RANKL-induced osteoclastogenesis in primary cultured osteoclasts from SERCA2+/- mice. J. Bone Miner. Res. 24, 1763–1769. doi: 10.1359/jbmr.090420
Yao, Y., Li, H., Chen, J., Xu, W., Yang, G., Bao, Z., et al. (2016). TREM-2 serves as a negative immune regulator through Syk pathway in an IL-10 dependent manner in lung cancer. Oncotarget 7, 29620–29634. doi: 10.18632/oncotarget.8813
Yao, Z., Xing, L., Qin, C., Schwarz, E. M., and Boyce, B. F. (2008). Osteoclast precursor interaction with bone matrix induces osteoclast formation directly by an interleukin-1-mediated autocrine mechanism. J. Biol. Chem. 283, 9917–9924. doi: 10.1074/jbc.m706415200
Yasui, T., Hirose, J., Tsutsumi, S., Nakamura, K., Aburatani, H., and Tanaka, S. (2011). Epigenetic regulation of osteoclast differentiation: possible involvement of Jmjd3 in the histone demethylation of Nfatc1. J. Bone. Miner. Res. 26, 2665–2671. doi: 10.1002/jbmr.464
Yu, Y., Suryo Rahmanto, Y., Shen, Y. A., Ardighieri, L., Davidson, B., Gaillard, S., et al. (2019). Spleen tyrosine kinase activity regulates epidermal growth factor receptor signaling pathway in ovarian cancer. EBioMedicine 47, 184–194. doi: 10.1016/j.ebiom.2019.08.055
Zaidi, M. (2007). Skeletal remodeling in health and disease. Nat. Med. 13, 791–801. doi: 10.1038/nm1593
Zaiss, M., Axmann, R., Zwerina, J., Polzer, K., Gückel, E., Skapenko, A., et al. (2007). Treg cells suppress osteoclast formation: a new link between the immune system and bone. Arthritis Rheum. 56, 4104–4112. doi: 10.1002/art.23138
Zhang, K., Kim, S., Cremasco, V., Hirbe, A. C., Collins, L., Piwnica-Worms, D., et al. (2011). CD8+ T cells regulate bone tumor burden independent of osteoclast resorption. Cancer Res. 71, 4799–4808. doi: 10.1158/0008-5472.can-10-3922
Zhang, Q. W., Liu, L., Gong, C. Y., Shi, H. S., Zeng, Y. H., Wang, X. Z., et al. (2012). Prognostic significance of tumor-associated macrophages in solid tumor: a meta-analysis of the literature. PLoS One 7:e50946. doi: 10.1371/journal.pone.0050946
Zhang, Z., Baron, R., and Horne, W. C. (2000). Integrin engagement, the actin cytoskeleton, and c-Src are required for the calcitonin-induced tyrosine phosphorylation of paxillin and HEF1, but not for calcitonin-induced Erk1/2 phosphorylation. J. Biol. Chem. 275, 37219–37223. doi: 10.1074/jbc.m001818200
Zhao, B., Grimes, S. N., Li, S., Hu, X., and Ivashkiv, L. B. (2012). TNF-induced osteoclastogenesis and inflammatory bone resorption are inhibited by transcription factor RBP-J. J. Exp. Med. 209, 319–334. doi: 10.1084/jem.20111566
Zhao, B., Takami, M., Yamada, A., Wang, X., Koga, T., Hu, X., et al. (2009). Interferon regulatory factor-8 regulates bone metabolism by suppressing osteoclastogenesis. Nat. Med. 15, 1066–1071.
Zhao, Y., Bachelier, R., Treilleux, I., Pujuguet, P., Peyruchaud, O., Baron, R., et al. (2007). Tumor alphavbeta3 integrin is a therapeutic target for breast cancer bone metastases. Cancer Res. 67, 5821–5830.
Keywords: osteoclast (OC), signaling/signaling pathways, tumor, bone metastases (BM), osteolysis
Citation: Győri DS and Mócsai A (2020) Osteoclast Signal Transduction During Bone Metastasis Formation. Front. Cell Dev. Biol. 8:507. doi: 10.3389/fcell.2020.00507
Received: 18 November 2019; Accepted: 27 May 2020;
Published: 19 June 2020.
Edited by:
Maria Teresa Valenti, University of Verona, ItalyReviewed by:
Teun J. De Vries, VU University Amsterdam, NetherlandsCopyright © 2020 Győri and Mócsai. This is an open-access article distributed under the terms of the Creative Commons Attribution License (CC BY). The use, distribution or reproduction in other forums is permitted, provided the original author(s) and the copyright owner(s) are credited and that the original publication in this journal is cited, in accordance with accepted academic practice. No use, distribution or reproduction is permitted which does not comply with these terms.
*Correspondence: Dávid S. Győri, Z3lvcmkuZGF2aWRAbWVkLnNlbW1lbHdlaXMtdW5pdi5odQ==
Disclaimer: All claims expressed in this article are solely those of the authors and do not necessarily represent those of their affiliated organizations, or those of the publisher, the editors and the reviewers. Any product that may be evaluated in this article or claim that may be made by its manufacturer is not guaranteed or endorsed by the publisher.
Research integrity at Frontiers
Learn more about the work of our research integrity team to safeguard the quality of each article we publish.