- Department of Biochemistry, School of Medicine, University of Puerto Rico Medical Sciences Campus, San Juan, Puerto Rico
The Rho family GTPases Rho, Rac, and Cdc42 have emerged as key players in cancer metastasis, due to their essential roles in regulating cell division and actin cytoskeletal rearrangements; and thus, cell growth, migration/invasion, polarity, and adhesion. This review will focus on the close homologs Rac and Cdc42, which have been established as drivers of metastasis and therapy resistance in multiple cancer types. Rac and Cdc42 are often dysregulated in cancer due to hyperactivation by guanine nucleotide exchange factors (GEFs), belonging to both the diffuse B-cell lymphoma (Dbl) and dedicator of cytokinesis (DOCK) families. Rac/Cdc42 GEFs are activated by a myriad of oncogenic cell surface receptors, such as growth factor receptors, G-protein coupled receptors, cytokine receptors, and integrins; consequently, a number of Rac/Cdc42 GEFs have been implicated in metastatic cancer. Hence, inhibiting GEF-mediated Rac/Cdc42 activation represents a promising strategy for targeted metastatic cancer therapy. Herein, we focus on the role of oncogenic Rac/Cdc42 GEFs and discuss the recent advancements in the development of Rac and Cdc42 GEF-interacting inhibitors as targeted therapy for metastatic cancer, as well as their potential for overcoming cancer therapy resistance.
Introduction
Rho GTPases have emerged as pivotal drivers of cancer metastasis in multiple cancer types. Of the Rho GTPases, Rac (1,2,3) and the close homolog Cdc42 are central regulators of cancer cell migration and invasion, and thus, metastasis. Therefore, recent studies have focused on demonstrating the dysregulation of Rac and Cdc42 in cancer, understanding their contribution to the hallmarks of metastasis: cancer cell growth, cell cycle progression, survival, epithelial to mesenchymal transition (EMT), cell-cell and cell-substrate adhesion, cell polarization, vesicle trafficking, angiogenesis, immune function, and migration/invasion, and consequently, therapy resistance. Even though oncogenic point mutations and splice variants have been reported from a number of cancers, Rac and Cdc42 do not have to be mutated in cancer to drive cancer progression. This is due to their activation by a number of oncogenic cell surface receptors, including receptor tyrosine kinases (RTKs), G protein coupled receptors (GPCR), cytokine receptors, and integrin receptors, as well as Wnt and Notch signaling, which converge on Rac and Cdc42 by activating specific guanine nucleotide exchange factors (GEFs) (Figure 1). As culled from the Cancer Genome Atlas (TCGA) data, many GEFs themselves are oncogenic and are overexpressed or mutated in a number of cancer types (Table 1). Therefore, Rac and Cdc42 activation, even in the absence of oncogenic mutations or dysregulated expression, can play a critical role in cancer progression to metastasis.
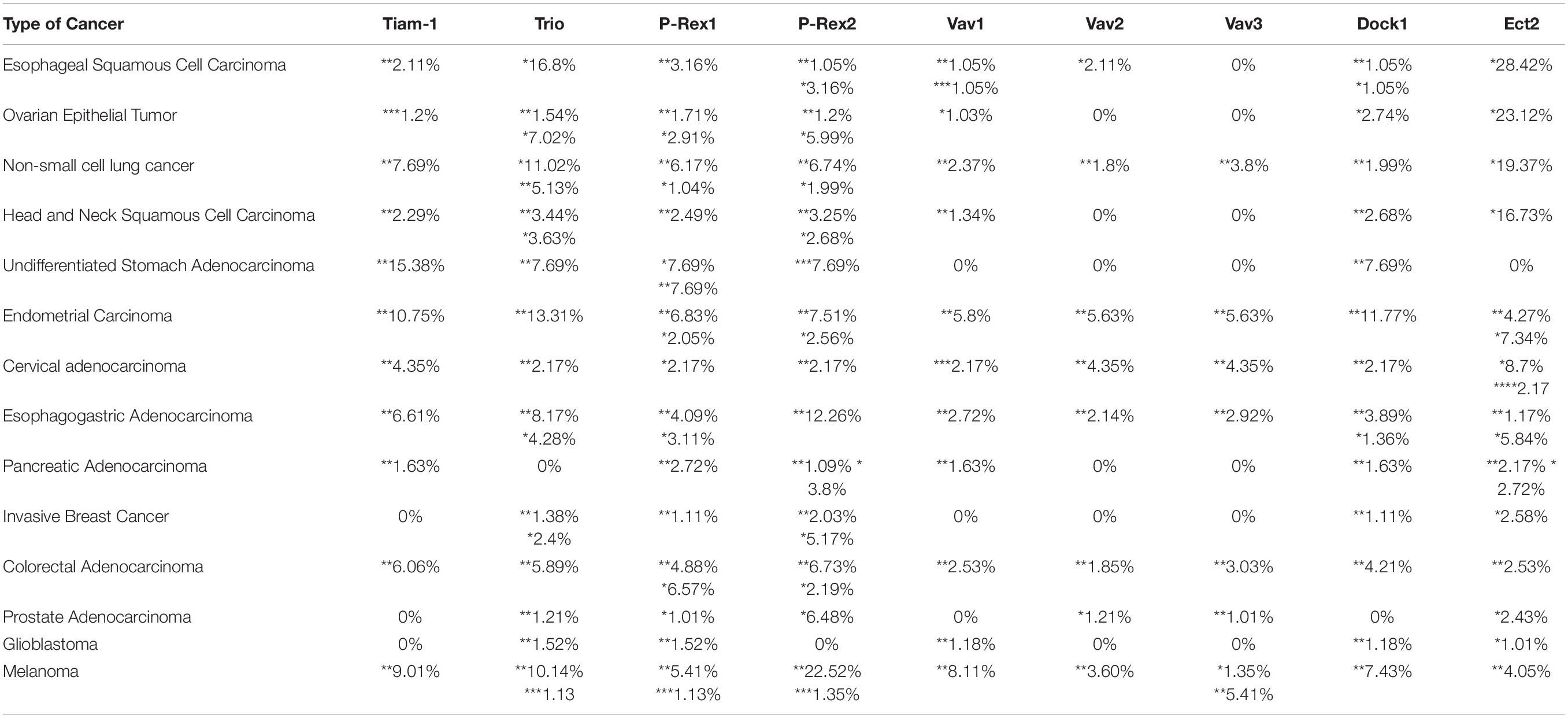
Table 1. Percentage (%) alterations (*amplification, **mutations, ***deep deletion, ****multiple alterations) in GEFs of multiple cancers from the TCGA PanCancer Atlas (Cerami et al., 2012; Gao et al., 2013).
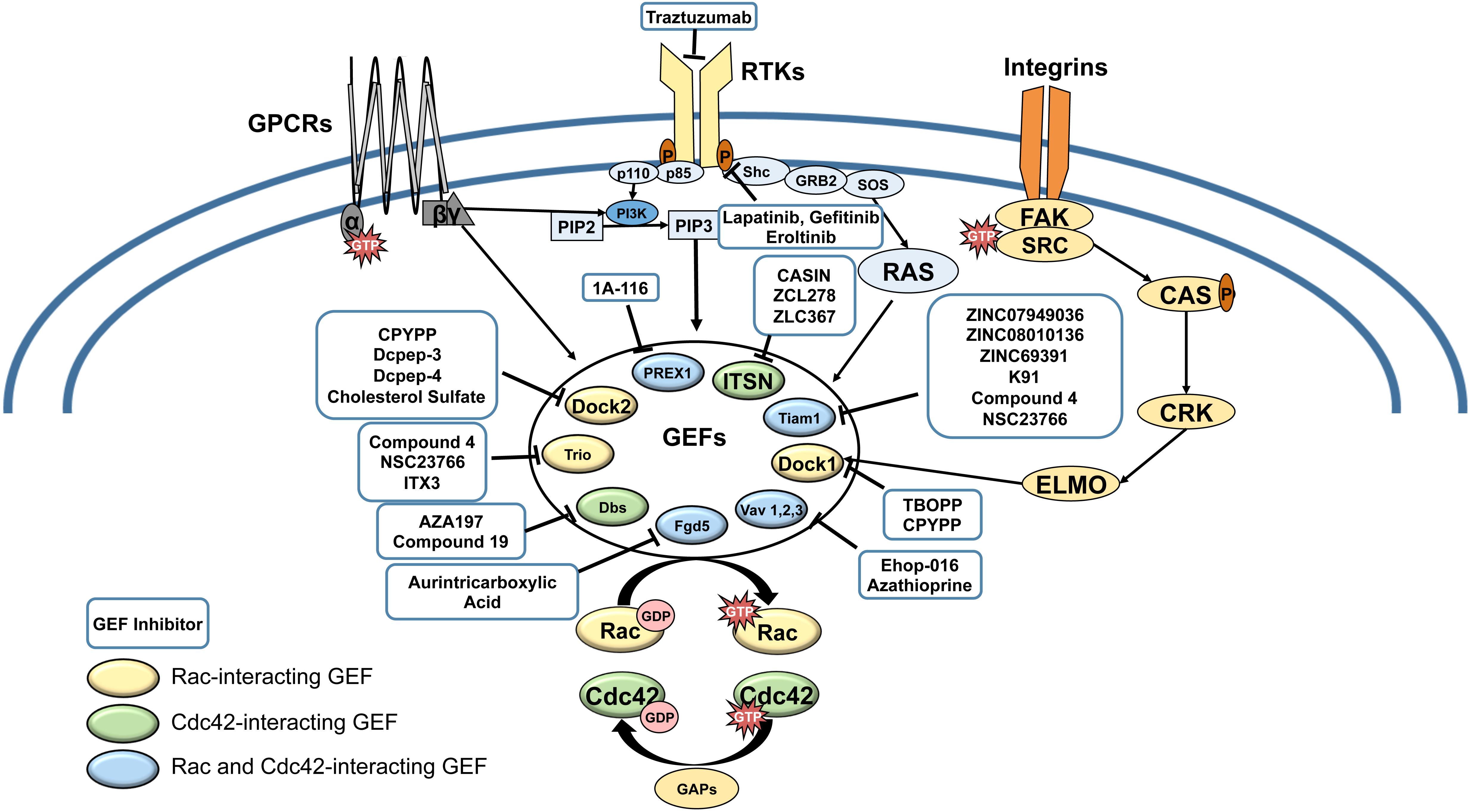
Figure 1. Receptor tyrosine kinases (RTKs), G-protein coupled receptors (GPCRs) and Integrin pathways converge in the activation of Rac and Cdc42 GEFs. Cell surface receptors activate GEFs by a variety of mechanisms, involving phosphoinside 3-kinase (PI3-K)-mediated production of phosphatidyl-inositol (3,4,5) tris phosphate (PIP3), which activates GEFs such as P-Rex1, DOCKs, and Vavs; Ras, which activates Tiam-1; and Focal adhesion kinase (FAK)/ signaling to activate ELMO a partner for DOCK1. Upregulation of Rac and Cdc42 can lead to resistance to cell surface receptor targeted therapies (eg. Trastuzumab, Lapatinib, Gefitinib, Eroltinib). Rac/Cdc42-interaction inhibitors are shown in blue boxes. Color-coding of GEFs represent Rac-exclusive GEFs, Cdc42-exclusive GEFs, and GEFs that interact with both Rac and Cdc42.
The pivotal role of Rac and Cdc42 in multiple cancers has been extensively reviewed by us and others (Pai et al., 2010; Mack et al., 2011; Stengel and Zheng, 2011; Wertheimer et al., 2012; Bid et al., 2013; Kazanietz and Caloca, 2017; Casado-Medrano et al., 2018; Maldonado and Dharmawardhane, 2018; De et al., 2019). In addition, the related Rho GTPase Rho has also been implicated in tumorigenesis and cancer progression. Multiple recent reviews have discussed the relevance, especially of RhoA and RhoC signaling in cancer, and thus, all three GTPases Rho, Rac, and Cdc42 are potential targets for anticancer drug development (Orgaz et al., 2014; Aspenström, 2018; Svensmark and Brakebusch, 2019; Porazinski et al., 2020). However, Rac and Cdc42 proteins share 72% sequence identity, and therefore, have a number of common upstream and downstream effectors such as p21-activated kinase (PAK), while Rho proteins activate different effectors such as Rho kinase (ROCK) and thus, activate distinct signaling cascades (Mott et al., 1999; Schmidt and Hall, 2002; Bustelo et al., 2007; Prudnikova et al., 2015). Therefore, even though all Rho family GTPases play a role in cancer malignancy, this review will focus on new strategies to target Rac and Cdc42 activation via blocking the exchange of GDP to GTP. We will also briefly discuss the burgeoning relevance of blocking Rac and Cdc42 to overcome cancer therapy resistance.
Targeting Rac and Cdc42/GEF Interaction
The Rho GTPases have been considered “undruggable” for many years due to their limited availability of binding pockets and globular structure. The fact that they bind GTP with high affinity, and the micromolar concentrations of intracellular GTP, further complicates the development of GTP-competitive inhibitors. However, elucidation of the molecular basis for Rho GTPase activation by GEFs using crystal structure analysis coupled to functional mutant analysis of specific residues in Rac and Cdc42 that interact with different GEFs have enabled a deeper understanding of Rho GTPase/GEF molecular interactions. This knowledge in turn have fueled efforts to develop inhibitors that block the molecular interaction between GEFs and Rac and Cdc42. Herein, we discuss the relevance of Rac/Cdc42 GEFs in metastatic cancer cell signaling in the context of the GEF interaction inhibitors that target Rac and Cdc42 activation (Table 2).
Rac and Cdc42 are structural homologs of the Rho GTPase family that are activated by common and distinct GEFs. The ∼80 different Rac/Cdc42 GEFs, can be divided into two groups depending on their conserved active domains: the diffuse B cell lymphoma (Dbl) Homology (DH)/Pleckstrin Homology (PH) proteins and Dedicator of Cytokinesis (DOCK) proteins. Approximately 70 GEFs share DH/PH domains, while 11 GEFs belong to the DOCK family (Rossman et al., 2005). The DH domain is required for GEFs to interact with Rho GTPases and catalyze GDP/GTP exchange, while the PH domain is used to stabilize or anchor with lipids and proteins (Harian et al., 1994; Sondek et al., 2000; Hoffman and Cerione, 2002; Cook et al., 2014). The DOCK family GEFs share the DOCK Homology Region 2 (DHR2), and a phospholipid-binding domain (DHR1) (Meller et al., 2005). DOCK proteins catalyze their GEF activity through the DHR2 domain, while the DHR1 is used to localize to the membrane by interacting with phosphoinositides, such as phosphatidyl-inositol (3,4,5) tris phosphate (PIP3). DOCK family GEFs interact only with Rac and Cdc42 and not RhoA.
The expression of GEFs are dependent on different tissues and cellular functions (Rossman et al., 2005). In addition, GEFs are activated by a myriad of cell surface receptors and cytosolic proteins, thus, adding to the complexity of their regulation. In cancer, Rac1 and Cdc42 are mutated, overexpressed and (or) hyperactivated. Hyperactivation of Rac and Cdc42 is primarily due to signaling via oncogenic upstream receptors, which in turn stimulate GEF activity (Arias-Romero and Chernoff, 2013; Kazanietz and Caloca, 2017; Maldonado and Dharmawardhane, 2018; De et al., 2019). However, GEFs themselves can become oncogenic by mutation or dysregulated expression (Table 1). Overexpressed GEFs have been detected in several types of cancer and correlated with clinicopathological features like poor prognosis and low survival rates. The most common GEFs implicated in cancer are T-cell lymphoma invasion and metastasis 1 (Tiam1), Trio, Vav1/2/3, Ect2, Phosphatidylinositol 3,4,5-trisphosphate (PIP3)-dependent Rac exchanger 1 (P-Rex1), and Dock1 (Wertheimer et al., 2012; Porter et al., 2016; Kazanietz and Caloca, 2017). This review will summarize the current knowledge on the GEFs that contribute to cancer malignancy and discuss therapies targeting the interaction of oncogenic GEFs with Rac and Cdc42.
Targeting Tiam1
Tiam1 is a ∼177 kD protein that was first identified from invasive variants of murine leukemia cells (Habets et al., 1994). Tiam1 has affinity for both Rac1 and Cdc42, but is specific for Rac1 and Rac2 activation (Jaiswal et al., 2013). Structurally, Tiam-1 is composed of a myristoylation sequence, coiled-coil region, PEST, N-terminal and C-terminal PH, extended, Ras binding, PSD-95/DlgA/ZO-1, and DH domains. Similar to other GEFs, Tiam1 is auto-inhibited by its N-terminal PH domain coiled-coiled extension, blocking its GEF activity (Xu Z. et al., 2017). Tiam1 is regulated through phosphorylation, which localizes Tiam1 to the membrane to interact with cell surface receptors (Boissier, 2013). The myristoylation sequence, coiled-coil region and extended domain of Tiam1 interacts with membrane associated proteins, such as Par3, which controls polarization via Rac1 activation (Chen and Macara, 2005; Nishimura et al., 2005; Boissier, 2013; Matsuzawa et al., 2016).
Several studies have identified TIAM1 as an oncogene due to its overexpression or mutation in epithelial cancers with poor prognosis, such as non-small cell lung cancer (NSCLC), stomach, endometrial, colorectal, pancreatic, and cervical cancer (Table 1). For instance, in colorectal cancer, Tiam1 overexpression was associated with resistance to late stage therapeutics FOLFOX (5-fluorouracil and oxaliplatin) and FOLFIRI (folfinic acid, fluorouracil and irinotecan) (Izumi et al., 2019). Moreover, in papillary thyroid cancer, esophageal squamous cell carcinoma, metastatic hepatocellular carcinoma, and cervical cancer, Tiam1 overexpression was implicated with poor prognosis and a low survival rate (Hsueh et al., 2011; Liu et al., 2011; Huang et al., 2013; Yang et al., 2018). In addition to overexpression, Tiam1 was shown to be mutated in neuroblastoma where certain point mutations were associated with a better outcome in patients with neuroblastoma, and thus, implicated with dysregulation in Tiam1 signaling (Sanmartín et al., 2017). In breast cancer, Tiam1 has been implicated with invasion and metastasis, where Tiam1 overexpression was associated with high-grade breast cancer (Li et al., 2016). Therefore, blocking the Tiam1/Rac1 interaction has the potential to reduce metastasis from multiple cancer types.
Resolution of the crystal structure of the DH/PH domain of Tiam1 with Rac1 facilitated the rational identification of specific inhibitors of this interaction Worthylake et al., 2000). Structure-based mutagenesis of specific residues in the β2 and β3 strands in the switch 1 (residues 30–48) and Switch II (residues 59–75) regions of Rac1 identified Trp56 as a residue that is critical for GEF binding to Rac (Gao et al., 2001; Karnoub et al., 2001). Based on this premise, the small molecule NSC23766 was identified through a structure-based virtual screen as an inhibitor of the Tiam1 and Trio GEF interactions with Rac1 (Gao et al., 2004). Even though its high IC50 (∼50 μM) limits its therapeutic use, NSC23766 reduces invasion and growth of multiple cancer types, including prostate, breast, gastric, leukemia, lymphoma, and glioblastoma (Gao et al., 2004; Thomas et al., 2007; Yoshida et al., 2010; Colomba et al., 2011; Karpel-Massler et al., 2013; Lee et al., 2015). Recent studies have also demonstrated the potential of NSC23766 in combination therapy to overcome resistance to targeted as well as cytotoxic therapies (Karpel-Massler et al., 2017; Tian et al., 2018). Nonetheless, although the use of NSC23766 may look promising in some instances, the high effective concentrations (μM range) and the reported off-target effects in platelets, on alfa-1-adrenergic receptors and CXCR4 chemokine receptors, limits its therapeutic use (Dutting et al., 2015; Mills et al., 2018; Yu et al., 2019).
Other small molecule inhibitors of the Tiam-1/Rac1 interaction were developed by the Ferri et al. (2009, 2013a,b) and Ruffoni et al. (2014) group through a docking-based virtual library screening, also using the Trp56 residue as a target. Compounds ZINC08010136 (IC50, 12.2 μM) and ZINC07949036 (IC50, 24.1 μM), and ZINC69391 (IC50 of 61 μM) are some of the molecules identified by this group as Rac1/Tiam1 inhibitors. In the case of ZINC69391, this molecule was able to inhibit cell cycle progression, cell proliferation, migration, and lung metastasis in highly invasive breast cancer models (Cardama et al., 2014a). Using the structure of ZINC69391 as a template, a more potent Rac inhibitor, named 1A-116, was produced, but this molecule has a different mechanism of action that does not involve Tiam-1.
Besides chemical compounds, nucleic acid aptamers can also target Tiam1-mediated activation of Rac. K91 is an RNA aptamer inhibitor of the Rac-Tiam1 interaction. This RNA inhibitor consists of a full-length sequence of 94 nucleotides, corresponding to a molecular mass of 31 kDa, which specifically binds to the DH/PH domain of Tiam-1 in vitro (KD = 209 ± 45 nM) (Niebel et al., 2013). However, the efficacy of K91 in cell-based and in vivo models remains unknown. Potential challenges of this therapeutic strategy include difficulties in targeting the delivery to cancer cells and instability in vivo.
Targeting Trio
Trio is a related ∼350 kD protein of the Dbl family of GEFs that contain two GEF domains: the first N-terminal domain contains the catalytic activity for Rac1 and RhoG and the second domain for RhoA (Debant et al., 1996). Additionally, it contains a CRAL-TRIO/SEC14 domain, two SH3 domains, a kinase domain, immunoglobulin motif and several spectrum repeats (Cannet et al., 2014). Moreover, Trio contains approximately five isoforms, Trio A-E, as a result of alternative splicing and differing in the C-terminus. One of the oncogenic isoforms, Tgat, is detected in adult leukemia and is specific for RhoA (Yoshizuka et al., 2004). Trio is activated by oncogenic upstream receptors such as RTKs and GPCRs. For example, in uveal melanoma, prolonged signaling via GPRCs activated Rac1 through Trio to signal to the transcriptional co-activator YAP and induce tumorigenesis (Feng et al., 2014).
The extension of lamellipodia by Trio requires the N-terminal GEF domain to interact with the C-terminus of Rac1 (Rijssel et al., 2012). During cytokinesis, Trio controls F-actin remodeling via the Rac1-actin related protein (Arp)2/3 pathway (Cannet et al., 2014). Phosphorylation of Trio at tyrosine 2681 (Y2681) leads to the activation of Rac1 and promotes colorectal cancer invasion. Trio pY1990 and Trio pY2681 phosphorylations were also correlated with clinicopathological parameters, where patients with high levels of Trio pY2681 demonstrated reduced survival (Sonoshita et al., 2015). Studies have also reported Trio to regulate invadopodia via Rac1/PAK signaling, specifically in metastatic breast carcinoma (Moshfegh et al., 2014). Therefore, in breast cancer tissues, Trio levels, but not Tiam1 or Vav1, were high and correlated with a poor outcome (Lane et al., 2008). Studies have shown Trio overexpression and (or) mutations in esophageal squamous cell carcinoma, NSCLC, stomach cancer, endometrial cancer, colorectal cancer, and glioblastoma (Table 1) (Schmidt et al., 2014). In glioblastoma, patients with a low survival rate had higher expression of Trio, Ect2 and Vav3, as well as high Rac1 expression (Salhia et al., 2008).
The compounds NSC23766 and CPYPP also inhibit Trio and have been tested in a variety of cancer models. Another Trio inhibitor is the small molecule ITX3, which blocks Trio’s binding to Rac with high specificity; however, its low potency (IC50 ∼50–100 μM) limits its therapeutic potential (Bouquier et al., 2009). Lastly, compound 4, one of the molecules identified by the Ferri et al. (2013a) group, also inhibits Trio-mediated activation of Rac in a concentration-dependent manner; nonetheless its action is not-specific to Trio, since it also inhibits the GEFs Vav and Tiam 1.
Targeting Vav1/2/3
Vav family proteins Vav1, Vav2, and Vav3 are composed of zinc-finger, proline rich motif, calponin-homology, Acidic, DH, PH, SH2, and two SH3 domains. Vav1 and 2 have broad specificity and act as GEFs for Rho, Rac, and Cdc42 (Movilla et al., 2001); however, studies have shown that Vav2, which is expressed in non-hematopoietic tissues is specific for Rac1 activation (Jaiswal et al., 2013; Cook et al., 2014). The GEF activity of Vav proteins are activated through phosphorylation of the c-terminal tyrosine residues via kinases activated in response to GPCRs, RTKs, integrin, and T-cell and B-cell receptors (Aghazadeh et al., 2000; Moores et al., 2000; Bartolomé et al., 2007).
Vav1 is exclusively expressed in hematopoietic tissues but not non-hematopoietic normal tissues. However, studies have detected Vav1 expression in several types of cancer cells and tumor tissue, including neuroblastoma, ovarian, lung, pancreatic, head and neck, gastric, endometrial, cervical, and colorectal cancer, metastatic melanoma, and B-cell chronic lymphocytic leukemia (Cook et al., 2014; Farago et al., 2020) (Table 1). Vav1 knockdown reduced tumor growth in nude mice from NSCLC cells expressing oncogenic K-Ras (Lazer et al., 2009). Vav1 also regulated pancreatic cancer cell migration via Rac1 (Razidlo et al., 2013), and its expression was correlated with pancreatic cancer cell lines but not in the normal human pancreas. Moreover, demethylation of VAV1 in the promoter region was shown to increase Vav1 expression in pancreatic cancer, and targeting Vav1 inhibited pancreatic cancer metastasis (Fernandez-zapico et al., 2005; Razidlo et al., 2015). Similarly, VAV1 gene is hypomethylated in medulloblastoma, leading to increased expression of Vav1 protein, which was correlated with the proto-oncogene MYCN amplification (Lindsey et al., 2014). Therefore, there is a need to elucidate epigenetic mechanisms regulating Vav expression, which can lead to novel therapeutic strategies.
Vav1 is expressed in a majority of breast tumors; however, Vav1 may induce apoptosis in certain breast cancer cell lines, in a p53 dependent manner (Sebban et al., 2013). This effect may explain the observation that in early invasive breast cancer, high nuclear expression of Vav1 was correlated with a lower incidence of disease relapse (Grassilli et al., 2014). The E59K and D51E oncogenic Vav1 point mutations identified from human lung adenocarcinoma resulted in truncation and overexpression of Vav1 with increased catalytic GEF activity for Rac1 activation (Shalom et al., 2018). D797N, another Vav1 mutation, described from NIH-3T3 fibroblasts, also demonstrated enhanced activation of Rac1 and upregulated the downstream effector c-Jun, thereby confirming transformation properties to Vav1 (Razanadrakoto et al., 2015). Therefore, Vav1 may play opposing roles depending on the type of cancer, and further studies are needed to determine and differentiate the signaling axis by which Vav1 is expressed and activated in malignant tumors.
Vav2 and Vav3 homologs are expressed in non-hematopoietic tissues and have been implicated with cardiovascular diseases and cancer (Perretta-Tejedor et al., 2017). Vav2/3 has been shown to specifically activate Rac1 and Rac3, the isoforms expressed in non-hematopoietic cells (Jaiswal et al., 2013). Moreover, Vav2/3 overexpression and activation has been reported from multiple cancers, including metastatic melanoma, endometrial, cervical, breast, and prostate cancer (Cook et al., 2014) (Table 1). In gastric cancer, Vav2/3 expression and activities were related to the degree of tumor differentiation, lymph node metastasis, and higher grade invasive and metastatic tumors (Tan et al., 2017a). Vav2 is also activated in papillary thyroid cancer, being phosphorylated through EphB3 tyrosine kinase receptors (Li et al., 2017).
In breast cancer, Vav2 activates Rac1/3 to regulate actin polymerization, invadopodia extension, matrix degradation, vesicular trafficking, and invasion (Donnelly et al., 2017; Rosenberg et al., 2017). In triple negative breast cancer cell lines, Vav2 was shown to interact with NEDD9, a scaffolding protein involved in cell migration and thus, activate Rac1 in breast cancer cell lines (Jones et al., 2017). Moreover, Vav2 was also shown to activate Rac1 and cell proliferation via its interaction with Mammalian STE20-like kinase 3, which is overexpressed in breast tumors (Cho et al., 2016). In breast cancer specimens, Vav2 expression was significantly higher in invasive cancer tissues compared to ductal carcinoma in situ or normal breast tissue (Jiang et al., 2014). Therefore, Vav2 is a central regulator of breast cancer metastasis.
Similar to Vav2, Vav3 expression has been associated with multiple epithelial cancers (Table 1). Vav3 expression in gastric cancer tissues was related to tumor differentiation, tumor invasion, lymphatic metastasis, neurovascular invasion and clinicopathological stage (Lin et al., 2012b; Tan, 2014; Tan et al., 2017b). Vav3 is also present in breast cancer, associating with poorly differentiated lesions. In breast cancer cells, Vav3 activated estrogen receptor (ER) α partially via PI3K-Akt signaling and promoted cell growth (Chen et al., 2015). However, Vav3 expression was higher in ER negative tissue, and high levels of nuclear Vav3 was associated with poorer endocrine therapy response (Aguilar et al., 2014). Similarly, in colorectal cancer, Vav3 expression was higher in malignant tissue compared to normal tissue, correlating with invasion and proliferation, and thus an advanced stage with poorer prognosis (Uen et al., 2015). Vav3 has been extensively studied in prostate cancer, being detected in prostate and androgen-independent prostate cancer cells. During androgen deprivation, Vav3 expression was induced and increased in LNCaP prostate cancer cells activating PI3K-Akt signaling, similar to its action in breast cancer (Hirai et al., 2014). Therefore, Vav3 overexpression may contribute to androgen receptor (AR) signaling through the PI3K-Akt pathway to stimulate cancer cell growth (Dong et al., 2006). Consequently, higher Vav3 expression was correlated with prostate cancer metastasis and recurrence. Several studies have also shown that Vav3 is activated by the tyrosine kinase EphA2 receptor to activate Rac and thus, increase cancer cell migration and proliferation (Lin et al., 2012a). Therefore, Vav3 appears to be activated by multiple signaling mechanisms to converge on Rac activation in metastatic cancer.
Structural and mutational analysis of the Vav1 DH-PH-CRD domain interaction with Rac1 revealed unique interactions of the Vav1 DH domain with the switch I and II regions of Rac1 (Chrencik et al., 2008). Accordingly, EHop-016, a Rac inhibitor developed by our group as a structural derivative of NSC23766, unlike NSC23766, which interacts with Tryp56 in the Switch II region and inhibits Tiam-1 and Trio, interacts with Val36 in the Switch I region and Asn 39 in the Switch II region of Rac, in silico (Montalvo-Ortiz et al., 2012). These residues have been shown to interact tightly with Glu201 and Gln331 in the DH domain of Vav (Chrencik et al., 2008). Accordingly, EHop-016 blocked the interaction between Vav1/2 and Rac with an IC50 of ∼1 μM in metastatic breast cancer cells. EHop-016 also reduced mammary tumor growth, angiogenesis, and metastasis in vivo (Hernández et al., 2010; Montalvo-Ortiz et al., 2012; Dharmawardhane et al., 2013; Castillo-Pichardo et al., 2014). Subsequently, EHop-016 was demonstrated to be effective in other cancer types, such as prostate, leukemia, melanoma, T lymphocytes, and fibrosarcoma (Montalvo-Ortiz et al., 2012; Manes and Pober, 2013; Martin et al., 2013; Maes et al., 2014; Okada et al., 2017; Chen et al., 2019). Moreover, EHop-016 was recently shown to revert cisplatin resistance in esophageal squamous cell carcinoma in vivo and in vitro, further validating its therapeutic applicability (Schmit et al., 2019). Vlaar et al. (2018) recently synthesized a series of EHop-016 derivatives in an attempt to improve its potency further. Specifically, the compounds 3b and 11b were described, where compound 11b was four times more potent than EHop-016 in inhibiting metastasis. Another derivative of EHop-016 that was recently described by us, is the small molecule MBQ-167, a dual Rac/Cdc42 inhibitor, which is ten times more potent than its predecessor (Humphries-Bickley et al., 2017). Nonetheless, the specific mechanism by which this compound interacts with Rac and Cdc42 GTPases has yet to be described in detail.
Another VAV family targeting drug is Azathioprine, an immunosuppressive drug that has been shown to inhibit Vav1 signaling in immune cells. In a recent study, azathioprine also blocked Vav1 signaling in pancreatic tumor cells, inhibiting cell migration, and decreasing metastasis in mouse models (Razidlo et al., 2015). The metabolite of azathioprine, 6-Thio-GTP, also inhibits Vav-mediated activation of Rac1, as reported from breast cancer cells and lymphocytes (Poppe et al., 2006; Menna et al., 2009).
Targeting P-Rex
P-Rex1 was first identified in neutrophils as a GEF unique for Rac1, even though it can activate Rac1,2,3, RhoG, Cdc42, and TC10 (Jaiswal et al., 2013). P-Rex1 is composed of a DH, PH, 2 DEP, PDZ, and an inositol polyphosphate 4-phosphatase homology domain, and is thus, activated by phosphatidylinositol 3,4,5 trisphosphate (PIP3) through the PH domain, and by Gβγ via the DH domain. P-Rex activity is also regulated by a negative phosphorylation of the DEP domain by protein kinase A (PKA), which results in an autoinhibitory interaction with the DH/PH domains (Kazanietz et al., 2018). PAK has also been shown to phosphorylate P-Rex1 and P-Rex2, decreasing its GEF activity in a feedback inhibition manner (Barrows et al., 2015; Barrows et al., 2016). Thus, phosphorylation patterns in both P-Rex1 and 2 regulate Rac1 activation and has potential for designing novel targeted therapies (Mayeenuddin and Garrison, 2006; Montero et al., 2016).
Both P-Rex1 and P-Rex2, possess oncogenic activities, and are expressed and mutated in multiple cancer types (Srijakotre et al., 2017) (Table 1). P-Rex1 is activated by human epidermal growth factor receptor (HER2) and GPCRs, such as the chemokine receptor CXCR4 in breast cancer, and overexpressed in ER and HER2 positive luminal A and B breast cancer tissues compared to normal breast tissue. P-Rex1 expression was actually low in triple-negative primary tumors but was upregulated in distant metastases (Marotti et al., 2017; Casado-Medrano et al., 2018; Kazanietz et al., 2018). Furthermore, demethylation in the PREX promoter has been associated with breast cancer mortality (Montero et al., 2011). However, recent data using MMTV-P-Rex1 transgenic mice have shown that P-Rex1 is not necessary for mitogenesis or survival in breast cancer, thus indicting that it may be active in late stage metastases, as has been shown by microarray analysis of breast cancer cells where P-Rex1 expression was associated with matrix metalloproteinase (MMP-10) expression, and thus, a more invasive phenotype (Barrio-Real et al., 2016).
The P-Rex2 isoform P-Rex2a, is an inhibitor of the PTEN tumor suppressor, consequently activating the PI3-Kinase pathway, and thus cancer malignancy (Leslie, 2009). In endometrial tumors tissues, P-Rex2a was associated with poor prognosis independent of PTEN expression status (Takeshita et al., 2018). P-Rex2 mutations are also reported from metastatic melanoma, which results in a truncated protein, losing its ability to bind to PTEN and increasing its GEF activity (Berger et al., 2012; Deribe, 2016; Lissanu et al., 2016).
Therefore, inhibiting P-Rex may block breast cancer metastasis, and the compound 1A-116, an analog of the previously discussed ZINC69391, was shown to inhibit the interaction of the GEF P-Rex1 with Rac1 with an IC50 = 4 μM. IA-116 demonstrated higher antiproliferative potency than its parental compound in metastatic breast cancer cells, and decreased metastasis to the lung by 60% in an in vivo model of experimental metastasis (Cardama et al., 2014a). Also, IA-116 reduced cell proliferation and invasion in other cancer types, such as leukemia and glioma (Bouquier et al., 2009; Cardama et al., 2014b). In another study, treatment with 1A-116 reverted therapy resistance to tamoxifen in breast cancer cells (Gonzalez et al., 2017), demonstrating the therapeutic potential of Rac inhibitors in overcoming therapy resistance.
Targeting FYVE, RhoGEF and PH Domain-Containing Protein or Faciogenital Dysplasia Protein (Fgd)
From the human FYVE domain containing proteins, those within the Fgd subfamily act as Rac/Cdc42 GEFs due to their DH domain and two PH domains (Eitzen et al., 2019). Recently, biochemical analysis of Fgd5, a member of the Fgd family of proteins, showed specificity for Rac1 activation (Park et al., 2019), even though FGD1 has been described as a GEF for Cdc42 (Egorov et al., 2009). Fgd5 has a DH domain similar to Trio with preferential activation of Rac1, and has been related to poorer prognosis in breast cancer patients (Valla et al., 2017). Park et al. (2019) identified the small molecule aurintricarboxylic acid through a surface plasmon resonance screening as a compound that binds to Fgd5, thus inactivating Rac1 with an IC50 of 157 nM. Further studies are needed to validate these results in vivo and to delineate the utility of targeting Fgd5 to block cancer progression.
Targeting DOCKs
One of the most commonly known oncogenic GEFs from the DOCK family is DOCK1 (also known as DOCK180), a Rac specific GEF. In addition to DHR1 lipid binding and catalytic DHR2 domains, DOCK1 also contains a SH3 domain and a proline rich motif. The GEF function of DOCK1 is activated by binding to engulfment and cell motility protein 1 (ELMO) through its SH3 domain, as well as phosphorylation. DOCKs are activated by oncogenic RTKs, where in glioblastoma, the oncogenic epidermal growth factor receptor (EGFRvIII) and platelet derived growth factor receptor (PDGFR) induced Src-mediated tyrosine phosphorylation of DOCK1 to activate Rac1 and promote cell migration and invasion (Feng et al., 2011, 2012). PDGFRα mediated protein kinase A action was shown to activate DOCK1 via serine phosphorylation (Feng et al., 2011, 2015). Moreover, in a HER2+ breast cancer mouse model, DOCK1 promoted invasion to the lungs, where the lung metastases overexpressed DOCK1 compared to primary tumors (Laurin et al., 2013). DOCK1 expression and mutations have been reported from a number of cancers (Table 1).
Such evidence of an oncogenic and metastasis promoting role for DOCK family members has led to an interest in the development of DOCK inhibitors. TBOPP, a DOCK1 selective inhibitor, inhibited tumor growth and metastasis in mice with lung carcinoma tumors (Tajiri et al., 2017). This compound also targeted DOCK1 in melanoma cells with the oncogenic Rac1 P29S mutation (Tomino et al., 2018). Hence, DOCK1 inhibition could be an approach for the treatment of cancers associated with the Rac1 P29S oncogenic mutation.
DOCK2, is expressed in hematopoietic cells and has been implicated in a number of inflammatory diseases and cancer (Chen et al., 2018). Therefore, DOCK2 is considered to be a viable drug target for leukemia, where DOCK2 is overexpressed in chronic lymphocytic leukemia (Wu et al., 2017; Hasan et al., 2018).
The small molecule CPYPP was identified as a DOCK2-inhibitor that binds to its DHR-2 domain (Nishikimi et al., 2012). Nonetheless, the utility of this compound is limited by its weak potency (IC50 = 23 μM for DOCK2) and non-specificity, since it also inhibits the GEFs DOCK5 and Trio (Ferrandez et al., 2017), which could lead to unwanted side effects.
On the other hand, a naturally occurring DOCK2 inhibitor is cholesterol sulfate, which also binds to the catalytic domain of DOCK2 but at a higher potency in vitro (IC50 = 2 μM). Cholesterol sulfate limited inflammation by inhibiting leukocyte migration and Rac activation in murine T-cells, an effect not observed with other cholesterol derivatives (Sakurai et al., 2018). However, the therapeutic efficacy and specificity of this compound in cancer models remains to be elucidated.
Recent efforts have been directed toward the development of DOCK2 inhibitory peptides conjugated with different moieties to allow cell permeability. Adachi et al. (2017) produced various cell-penetrating peptides (CPP) that were conjugated with a partial sequence of influenza A viral protein (PB1-F2) to increase cell permeability. Inhibitory activity of these peptides to DOCK2 was in the nanomolar range, and conjugation with PB1-F2 increased cellular uptake of these peptides (Adachi et al., 2017). Other DOCK2-selective inhibitory peptides are Dcpep-3 and Dcpep-4 (Sakamoto et al., 2017). Dcep-4 has an IC50 of 6 nM for Rac1 and could be valuable in the treatment of leukemia and autoimmune diseases. Nonetheless, variable absorption and lack of specific localization could prove to be a challenge in the development of these peptides as therapeutics. Additionally, in vivo efficacy of cell-penetrating peptides remains to be demonstrated.
Another activator of Rac1 that is also an attractive target of the DOCK family is DOCK5. DOCK5 has also being implicated in acute myeloid leukemia (Biswas et al., 2019, 30668141), and recently, an oncogenic variant of DOCK5 was described from head and neck squamous cell carcinoma (Liu et al., 2018).
Vives et al. (2011) discovered the small molecule, C21, as an inhibitor of DOCK5 through screening, using a yeast-based assay. This molecule targets the DHR2 domain of DOCK5, causing an abortive conformation that results in non-competitive inhibition (Ferrandez et al., 2017). In models of bone metastasis, C21 was able to protect mice against bone degradation without causing side effects, which makes it a potential therapeutic against osteolytic diseases (Vives et al., 2015).
Inhibitors of the Cdc42-GEF Interaction
Targeting [Dbl’s Big Sister (Dbs)]
Dbs is another DH family member that is ubiquitously expressed and was identified as an oncogene by its ability to transform NIH3T3 fibroblasts (Whitehead et al., 1995). Full-length Dbs encompasses 1149 amino acids and contains, in addition to the DH and PH domains, a putative N-terminal Sec14 domain, two spectrin-like repeats and a C-terminal SH3 domain. Structural studies of the DH/PH domains of Dbs complexed with Cdc42 revealed that the binding interactions were unique and distinct from those described for Tiam-1 and Trio (Rossman et al., 2002). Even though Dbs was first described as an activator of RhoA and Cdc42, but not Rac1, in the activation of multiple signaling pathways using specific inhibitors (Whitehead et al., 1999; Snyder et al., 2002), a subsequent study using siRNA directed at Cdc42 and Rac1 demonstrated a role for both Cdc42 and Rac1 in activating breast cancer cell migration. However, in this study, oncogenic Dbs demonstrated increased Rho activation, and thus, even though Rho activity may not be relevant for the demonstrated Rac/Cdc42 mediated breast cancer cell migration via downstream activation of focal adhesion kinase/p130Crk associated substrate, the enhanced Rho activity in oncogenic Dbs expressing breast cancer cells may still contribute to other cancer promoting signaling pathways in cancer (Liu et al., 2009).
AZA197 is a small-molecule inhibitor of the Cdc42-Dbs interaction that was shown to inhibit cancer cell proliferation and invasion with an IC50 of 1–10 μM. AZA197 also reduced tumor growth and extended survival in a mouse model of colon cancer (Zins et al., 2013). This molecule was identified through an in vitro screen of compounds similar to the previously discussed Rac-inhibitor NSC23766. Nonetheless, AZA197 showed toxicity in concentrations higher than 20 μM in NIH3T3 fibroblasts and colon cancer cells, and may not prove to be pharmacologically useful.
Another molecule that inhibits Cdc42 activation through Dbs is Compound 19, an α-helix mimetic that targets the K774, L777, and Q770 residues of Dbs that mediate the interaction between Dbs and Cdc42 (Cummings et al., 2009). However, the therapeutic applicability of Compound 19 in cancer remains to be tested. Additionally, its high IC50 (IC50 = 67 μM) limits its therapeutic potential.
Targeting Intersectin (ITSN)
ITSN is another DH family member, which primarily activates Cdc42 to regulate the actin cytoskeleton (Humphries et al., 2014). ITSNs are multidomain adaptor proteins regulating endocytosis and cell signaling via RTK/PI3-K pathways, and have been implicated in neurodegenerative diseases and cancer. Structurally, N-terminal Eps15 homology (EH1 and EH2) domains, a coiled coil region, and five SH3 domains are present in the short isoform (ITSN-S), while the long isoform of ITSN (ITSN-L) has an additional C-terminal DH/PH domain, which confers GEF activity (Hunter et al., 2013). Even though low expression of ITSN1 was recently correlated with higher grade in breast and lung cancer (Xie et al., 2019; Zhang and Zhang, 2019), A role for ITSN in neuroblastoma has been shown by a study where knockdown of ITSN decreased orthotopic neuroblastoma growth in mice (Harris et al., 2017).
So far, four small molecules have shown to block the interaction of Cdc42 with ITSN; these are Pirl1, CASIN, ZCL278, and ZCL367. The compound Pirl1 was developed to target the Phe56 residue in Cdc42, which has been demonstrated to be crucial for GEF binding. This molecule inhibits the PIP2-mediated GEF activity of the Cdc42/RhoGDI complex (Peterson et al., 2006). A derivative of Pirl1, CASIN (Cdc42 activity-specific inhibitor), demonstrated in vitro and in vivo efficacy in colorectal cancer models with an IC50 of 2 μM (Sakamori et al., 2014). CASIN could also prove to be useful in improving hematopoietic stem cell mobilization and benefiting bone marrow transplant recipients (Liu et al., 2019). ZCL278 is another ITSN inhibitor, identified through virtual screening to directly bind Cdc42 by interacting with several residues of its Switch I region, essential for the interaction with ITSN. ZCL278 blocked prostate cancer cell migration and may have potential as a metastatic prostate cancer therapeutic (Friesland et al., 2013).
Recently, Aguilar et al., 2019 developed a potent inhibitor of the Cdc42-ITSN interaction, named ZCL367. With an IC50 of 0.098 nM, ZCL367 demonstrated beneficial effects in lung and prostate cancer cells by inhibiting migration, proliferation, and cell cycle progression. Its anticancer potential was further validated in a xenograft mouse model of lung cancer, where it decreased tumor growth. This molecule also inhibits Rac1 but, to a lesser degree (IC50 = 0.19 nM) (Aguilar et al., 2019). Hence, ZCL367’s efficacy and low IC50 makes it a promising anti metastatic cancer therapeutic for further development.
Rac/Cdc42 Inhibitors in Therapy Resistance
So far, no Rac/Cdc42 inhibitors have received FDA approval for clinical trials; however, burgeoning evidence has positioned Rac and Cdc42 as ideal targets for anti-metastatic cancer therapy. Rac1 and Cdc42 are unique in that they act as molecular switches that do not have to be mutated to drive cancer progression but are activated by oncogenic cell surface receptors. These receptors include RTKs, GPCRs, and integrins, which signal to other oncogenes such as PI3-K and Ras that can in turn activate Rac/Cdc42 GEFs, which themselves can be oncogenic (Figure 1). Therefore, upregulated Rac and Cdc42 can drive malignant signaling during acquisition of resistance to cancer therapies targeting cell surface receptors, by acting downstream of therapy resistance mechanisms such as Ras/MAPK and PI3-K/mTOR signaling (Baker et al., 2014; Cardama et al., 2018; Maldonado and Dharmawardhane, 2018; De et al., 2019).
Numerous studies have implicated Rac/PAK activities with the maintenance of mesenchymal stem cell-like populations in epithelial cancers; and thus, therapy resistance (Zhao et al., 2011; Ong et al., 2013; Zhu et al., 2015; Goel et al., 2016; Huynh et al., 2016; Aboukameel et al., 2017; Lai et al., 2017; Morrison Joly et al., 2017; Cardama et al., 2018; Goka et al., 2019). Specifically in breast cancer, Rac/Cdc42/PAK signaling is implicated with therapy resistance of HER2-type (Wang et al., 2006; Ebi et al., 2013; Laurin et al., 2013; Dokmanovic et al., 2014; Desai et al., 2016; Hampsch et al., 2017), triple negative (De et al., 2017), and ER(+) cancers (Cai et al., 2003; Gonzalez et al., 2017). TCGA data show that Rac1 or PAK1 overexpression is associated with malignant breast cancer and significantly diminishes HER2 type patient survival within 10 years following diagnosis (Fang et al., 2016). Accordingly, Rac/PAK inhibitors have the potential to overcome resistance to HER2/EGFR targeted therapies in epithelial cancers that overexpress these receptors.
The success of current oncogenic receptor targeted therapies for high grade cancers continue to be marred by acquired and intrinsic therapy resistance due to activation of downstream signaling. Therefore, a viable strategy to mitigate HER2/EGFR therapy resistance is combination therapy with other therapeutics targeting the downstream signaling pathways (Vrbic et al., 2013). Of the 20% of invasive HER2 type breast cancers that are treated with HER2 targeted therapeutics, such as trastuzumab (Herceptin), the monoclonal antibody targeting HER2, ∼25% of patients eventually develop resistance. Trastuzumab resistant breast cancers circumvent HER2 inhibition via bypass signaling and activation of downstream pathways independent of HER2 (Normanno et al., 2006; Bender and Nahta, 2008; Hynes and MacDonald, 2009; Weigelt et al., 2010; Wilken and Maihle, 2010; Brünner-Kubath et al., 2011; Nahta, 2012; Vu and Claret, 2012; Wong and Lee, 2012). Notably, a number of HER2 therapy resistance signaling pathways (Vu and Claret, 2012) are regulated by Rac signaling and targeting Rac has been shown to block breast cancer metastasis (Bracho-Valdés et al., 2011; Saci et al., 2011; Katz et al., 2012). Studies have shown that Rac1 activation is critical for resistance to trastuzumab in HER2+ breast cancer cells with upregulated insulin growth factor receptor (IGFR) (Zhao et al., 2011). Our data using a trastuzumab resistant HER2 type metastatic cancer have demonstrated the efficacy of Rac/Cdc42 inhibitors to block metastatic cancer cell functions, tumor growth, and metastasis (Montalvo-Ortiz et al., 2012; Dharmawardhane et al., 2013; Castillo-Pichardo et al., 2014); thus highlighting the utility of Rac inhibitors to overcome trastuzumab resistance.
Therapy with the next generation small molecule EGFR/HER2 inhibitor lapatinib also results in resistance. Similar to trastuzumab, lapatinib resistance circumvents its kinase inhibitory function by acquiring point mutations in HER2 and EGFR, as well as via elevated downstream signaling (Weigelt et al., 2010; Wilken and Maihle, 2010; Zuo et al., 2016; Xu X. et al., 2017). Rac1 has been shown to be overexpressed in lapatinib-resistant HER2 type breast cancer and is considered to be a viable therapeutic for sensitization of lapatinib resistant tumors (Wetterskog et al., 2014). In addition, Rac1 inhibition has been shown to overcome gefitinib (EGFR-targeted therapeutic) resistance in NSCLC (Kaneto et al., 2014). Recent studies with glioblastoma cells also demonstrate the potential of the Tiam-1/Trio/Rac inhibitor NSC23766 in combination therapy with eroltinib, another tyrosine kinase inhibitor (Karpel-Massler et al., 2017). Moreover, EHop-016, a Vav/Rac inhibitor developed by us, was recently shown to overcome resistance by combined cancer therapy with Akt/mTOR inhibitors (Okada et al., 2017). Thus, Rac inhibitors are poised for dual therapy with HER2/EGFR or Akt/mTOR targeting therapies.
In addition, Rac/Cdc42 inhibition has the potential to sensitize cancers to current chemotherapies such as taxanes and anthracyclines. Since cytotoxic cancer therapeutics are designed to kill actively dividing cells, this can lead to selection of non-dividing stem cells that still have the capacity to migrate and establish tumors at distant sites. Several studies have implicated Rac and Cdc42 signaling in stem cell maintenance through activation of Wnt, Notch, and YAP signaling in breast cancer, NSCLC, and lymphomas (De et al., 2017; Hicks-Berthet and Varelas, 2017; Lai et al., 2017; Olabi et al., 2018; Katoh and Katoh, 2020). Therefore, as has been shown by us using mammosphere assays, Rac/Cdc42 inhibition can reduce stem cell-like growth in breast cancer (Humphries-Bickley et al., 2017).
Recent studies on metastasis show that chemotherapy may also affect multicellular interactions in the tumor microenvironment for metastasis (TMEM) by recruiting macrophages, mesenchymal stem cells, platelets, etc., which increases cancer cell survival, invasion, angiogenesis, and metastasis (Karagiannis et al., 2018). Given the central role of Rac and Cdc42 in immune cell function, Rac/Cdc42 inhibitors also have potential for targeting immune cell migration and inflammation, including infiltration of perivascular macrophages, increased vascular permeability, and lymphatic penetration (Infante and Ridley, 2013; Biro et al., 2014; Durand-Onaylı et al., 2018). Indeed, we have shown that Rac inhibition decreases macrophage and neutrophil penetration of the TMEM (Castillo-Pichardo et al., 2014; Humphries-Bickley et al., 2017). Therefore, an additional benefit of Rac/Cdc42 inhibition is the reduction in bone marrow-derived cells that promote the metastatic dissemination of cancer cells in the TMEM.
Accordingly, recent studies have also implicated a role for Rac/Cdc42 inhibitors in combination with cytotoxic therapies for maximum efficacy. In esophageal squamous cell carcinoma, Rac1 expression was associated with poor prognosis and therapy resistance. Use of the Vav/Rac inhibitor EHop-016 was shown to overcome cisplatin resistance in esophageal squamous cell carcinoma in vitro and in vivo and by decreasing Akt/FOXO3a signaling and glycolysis (Schmit et al., 2019; Zeng et al., 2019), further validating its therapeutic applicability. Moreover, Tiam1 and Rac1 were overexpressed in a 3-D model of lymphoma, where treatment with Tiam1/Rac1 inhibitor NSC23766 overcame chemoresistance to doxorubicin (Ikram et al., 2018). NSC23766 has also been shown to overcome resistance to fludarabine in chronic lymphocytic leukemia where Tiam1/Rac1 is upregulated (Hofbauer et al., 2014). Also, Rac1 inhibition with NSC23766 increases the cytotoxic effect of Adriamycin, in the treatment of mantle cell lymphoma, an aggressive B-cell lymphoma (Tian et al., 2018). Therefore, combination therapy studies with standard cytotoxic agents further supports the clinical significance of targeting Rac and Cdc42.
Conclusion
Current studies on targeting Rac and Cdc42 have identified compounds that are poised to emerge as leading antimetastatic drugs for combination therapy with cytotoxic cancer therapies and to overcome resistance to current therapies targeting RTKs and PI3-K/Akt/mTOR signaling (Figure 1). Due to the specificity of their interaction with different oncogenic GEFs, drugs that block the Rac/Cdc42-GEF interaction need to be prudently targeted to different tissues and types of cancer according to their expression and activation of specific GEFs. However, there is a pressing need to improve the efficacy of the currently available GEF targeting drugs and demonstrate in vivo activity at physiologically relevant concentrations, prior to their translational development.
In addition, there is also a need to target multiple GEFs, such as the DH family GEF Ect2. Ect2 is a proto-oncogene with GEF activity toward Rho, Rac, and Cdc42, and thus regulates cell division, polarity, and invasion/migration (Tatsumoto et al., 1999). Ect2 has been shown to be overexpressed in multiple cancers and associated with poor survival (Saito et al., 2004; Justilien and Fields, 2009; Fields and Justilien, 2010; Iyoda et al., 2010; Huff et al., 2013; Wang et al., 2016, 2018; Guo et al., 2017; Bai et al., 2018) (Table 1). Inhibitors targeting Ect2 have yet to be identified, but it is imperative to develop specific inhibitors to block the activation of Rho GTPases by Ect2 in cancer cells.
Overall, this review provides ample evidence for the utility of Rac/Cdc42 inhibitors both individually, and in combination with current targeted and cytotoxic therapeutics to overcome therapy resistance and augment established cancer therapies to specifically block metastasis. In addition to combined therapy with existing cancer therapeutics, cocktails of Rac/Cdc42 GEF targeting compounds may also be developed for maximum therapeutic efficacy. In conclusion, the plethora of compelling data discussed in this review realizes the promise of Rac/Cdc42 inhibitors as viable therapeutics for metastatic cancer, and we look forward to their routine use in standard cancer care.
Author Contributions
MM, JM, and SD wrote the manuscript. MM prepared the Table 2. JM prepared the Table 1. LV prepared the Figure 1.
Funding
MM and JM were supported by NIH/NIGMS R25GM061838 to UPR MSC. SD was supported by the Puerto Rico Science, Technology and Research Trust; NIH/NIGMS SC3 GM084824; and DoD/US Army W81XWH2010041.
Conflict of Interest
The authors declare that the research was conducted in the absence of any commercial or financial relationships that could be construed as a potential conflict of interest.
References
Aboukameel, A., Muqbil, I., Senapedis, W., Baloglu, E., Landesman, Y., Shacham, S., et al. (2017). Novel p21-activated kinase 4 (PAK4) allosteric modulators overcome drug resistance and stemness in pancreatic ductal adenocarcinoma. Mol. Cancer Ther. 16, 76–87. doi: 10.1158/1535-7163.MCT-16-0205
Adachi, Y., Sakamoto, K., Umemoto, T., Fukuda, Y., Tani, A., and Asami, T. (2017). Investigation on cellular uptake and pharmacodynamics of DOCK2-inhibitory peptides conjugated with cell-penetrating peptides. Bioorganic Med. Chem. 25, 2148–2155. doi: 10.1016/j.bmc.2017.02.022
Aghazadeh, B., Lowry, W. E., Huang, X., and Rosen, M. K. (2000). Structural basis for relief of autoinhibition of the Dbl homology domain of proto-oncogene Vav by tyrosine phosphorylation. Cell 102, 625–633. doi: 10.1016/s0092-8674(00)00085-4
Aguilar, B. J., Zhao, Y., Zhou, H., Huo, S., Chen, Y. H., and Lu, Q. (2019). Inhibition of Cdc42–intersectin interaction by small molecule ZCL367 impedes cancer cell cycle progression, proliferation, migration, and tumor growth. Cancer Biol. Ther. 20, 740–749. doi: 10.1080/15384047.2018.1564559
Aguilar, H., Urruticoechea, A., Halonen, P., Kiyotani, K., Mushiroda, T., Barril, X., et al. (2014). VAV3 mediates resistance to breast cancer endocrine therapy. Breast Cancer Res. 16:R53. doi: 10.1186/bcr3664
Arias-Romero, L. E., and Chernoff, J. (2013). Targeting Cdc42 in cancer. Expert Opin. Ther. Targets 17, 1263–1273. doi: 10.1517/14728222.2013.828037
Aspenström, P. (2018). Activated Rho GTPases in cancer — the beginning of a new paradigm. Int. J. Mol. Sci. 19:3949. doi: 10.3390/ijms19123949
Bai, X., Yi, M., Xia, X., Yu, S., Zheng, X., and Wu, K. (2018). Progression and prognostic value of ECT2 in non-small-cell lung cancer and its correlation with PCNA. Cancer Manag. Res. 10, 4039–4050. doi: 10.2147/CMAR.S170033
Baker, N. M., Chow, H. Y., Chernoff, J., and Der, C. J. (2014). Molecular pathways: targeting RAC-p21-activated serine-threonine kinase signaling in RAS-driven cancers. Clin. Cancer Res. 20, 4740–4746. doi: 10.1158/1078-0432.CCR-13-1727
Barrio-Real, L., Wertheimer, E., Garg, R., Abba, M. C., and Kazanietz, M. G. (2016). Characterization of a P-Rex1 gene signature in breast cancer cells. Oncotarget 7, 51335–51348. doi: 10.18632/oncotarget.10285
Barrows, D., He, J. Z., and Parsons, R. (2016). PREX1 protein function is negatively regulated downstream of receptor tyrosine kinase activation by p21-activated kinases (PAKs). J. Biol. Chem. 291, 20042–20054. doi: 10.1074/jbc.M116.723882
Barrows, D., Schoenfeld, S. M., Hodakoski, C., Silkov, A., Honig, B., Couvillon, A., et al. (2015). p21-activated kinases (PAKs) mediate the phosphorylation of PREX2 protein to initiate feedback inhibition of Rac1 GTPase. J. Biol. Chem. 290, 28915–28931. doi: 10.1074/jbc.M115.668244
Bartolomé, R. A., Molina-Ortiz, I., Samaniego, R., Sánchez-Mateos, P., Bustelo, X. R., and Teixidó, J. (2007). Activation of Vav/Rho GTPase signaling by CXCL12 controls membrane-type matrix metalloproteinase–dependent melanoma cell invasion. Cancer Res. 66, 248–258. doi: 10.1158/0008-5472.CAN-05-2489
Bender, L. M., and Nahta, R. (2008). Her2 cross talk and therapeutic resistance in breast cancer. Front. Biosci. 13, 3906–3912. doi: 10.2741/2978
Berger, M. F., Heffernan, T. P., Deribe, Y. L., Lawrence, M. S., Protopopov, A., Ivanova, E., et al. (2012). Melanoma genome sequencing reveals frequent PREX2 mutations. Nature 485, 502–506. doi: 10.1038/nature11071
Bid, H. K., Roberts, R. D., Manchanda, P. K., and Houghton, P. J. (2013). RAC1: an emerging therapeutic option for targeting cancer angiogenesis and metastasis. Mol. Cancer Ther. 12, 1925–1934. doi: 10.1158/1535-7163.MCT-13-0164
Biro, M., Munoz, M. A., and Weninger, W. (2014). Targeting Rho-GTPases in immune cell migration and inflammation. Br. J. Pharmacol. 171, 5491–5506. doi: 10.1111/bph.12658
Biswas, M., Chatterjee, S. S., Boila, L. D., Chakraborty, S., Banerjee, D., and Sengupta, A. (2019). MBBD3/NuRD loss participates with KDM6A program to promote DOCK5/8 expression and Rac GTPase activation in human acute myeloid leukemia. FASEB J. 33, 5268–5286. doi: 10.1096/fj201801035R
Boissier, P. (2013). The guanine nucleotide exchange factor Tiam1: a janus-faced molecule in cellular signaling. Cell. Signal. 26, 483–491. doi: 10.1016/j.cellsig.2013.11.034
Bouquier, N., Vignal, E., Charrasse, S., Weill, M., Schmidt, S., Léonetti, J. P., et al. (2009). A cell active chemical GEF inhibitor selectively targets the Trio/RhoG/Rac1 signaling pathway. Chem. Biol. 16, 657–666. doi: 10.1016/j.chembiol.2009.04.012
Bracho-Valdés, I., Moreno-Alvarez, P., Valencia-Martínez, I., Robles-Molina, E., Chávez-Vargas, L., and Vázquez-Prado, J. (2011). MTORC1- and mTORC2-interacting proteins keep their multifunctional partners focused. IUBMB Life 63, 896–914. doi: 10.1002/iub.558
Brünner-Kubath, C., Shabbir, W., Saferding, V., Wagner, R., Singer, C. F., Valent, P., et al. (2011). The PI3 kinase/mTOR blocker NVP-BEZ235 overrides resistance against irreversible ErbB inhibitors in breast cancer cells. Breast Cancer Res. Treat. 129, 387–400. doi: 10.1007/s10549-010-1232-1
Bustelo, X. R., Sauzeau, V., and Berenjeno, I. M. (2007). GTP-binding proteins of the Rho/Rac family: regulation, effectors and functions in vivo. BioEssays 29, 356–370. doi: 10.1002/bies.20558
Cai, D., Iyer, A., Felekkis, K. N., Near, R. I., Luo, Z., Chernoff, J., et al. (2003). AND-34/BCAR3, a GDP exchange factor whose overexpression confers antiestrogen resistance. Activates Rac, PAK1, and the Cyclin D1 promoter. Cancer Res. 63, 6802–6808.
Cannet, A., Schmidt, S., Delaval, B., Debant, A., and Chang, F. (2014). Identification of a mitotic Rac-GEF, Trio, that counteracts MgcRacGAP function during cytokinesis. Mol. Biol. Cell 25, 4063–4071. doi: 10.1091/mbc.E14-06-1153
Cardama, G. A., Alonso, D. F., Gonzalez, N., Maggio, J., Gomez, D. E., Rolfo, C., et al. (2018). Relevance of small GTPase Rac1 pathway in drug and radio-resistance mechanisms: opportunities in cancer therapeutics. Crit. Rev. Oncol. Hematol. 124, 29–36. doi: 10.1016/j.critrevonc.2018.01.012
Cardama, G. A., Comin, M. J., Hornos, L., Gonzalez, N., Defelipe, L., Turjanski, A. G., et al. (2014a). Preclinical development of novel Rac1-GEF signaling inhibitors using a rational design approach in highly aggressive breast cancer cell lines. Anticancer. Agents Med. Chem. 14, 840–851. doi: 10.2174/18715206113136660334
Cardama, G. A., Gonzalez, N., Ciarlantini, M., Gandolfi Donadío, L., Comin, M. J., Alonso, D. F., et al. (2014b). Proapoptotic and antiinvasive activity of Rac1 small molecule inhibitors on malignant glioma cells. Onco. Targets. Ther. 7, 2021–2033. doi: 10.2147/OTT.S67998
Casado-Medrano, V., Baker, M. J., Lopez-Haber, C., Cooke, M., Wang, S., Caloca, M. J., et al. (2018). The role of Rac in tumor susceptibility and disease progression: from biochemistry to the clinic. Biochem. Soc. Trans. 46, 1003–1012. doi: 10.1042/bst20170519
Castillo-Pichardo, L., Humphries-Bickley, T., De La Parra, C., Forestier-Roman, I., Martinez-Ferrer, M., Hernandez, E., et al. (2014). The Rac inhibitor EHop-016 inhibits mammary tumor growth and metastasis in a nude mouse model. Transl. Oncol. 7, 546–555. doi: 10.1016/j.tranon.2014.07.004
Cerami, E., Gao, J., Dogrusoz, U., Gross, B. E., Sumer, S. O., Arman, B., et al. (2012). The cBio cancer genomics portal: an open platform for exploring multidimensional cancer genomics data. Cancer Discov. 2, 401–404. doi: 10.1158/2159-8290.CD-12-0095
Chen, B., Zhang, C., Wang, Z., Chen, Y., Xie, H., Li, S., et al. (2019). Mechanistic insights into Nav1.7-dependent regulation of rat prostate cancer cell invasiveness revealed by toxin probes and proteomic analysis. FEBS J. 286, 2549–2561. doi: 10.1111/febs.14823
Chen, X., Chen, S., Liu, X.-A., Zhou, W., Ma, R.-R., and Chen, L. (2015). Vav3 oncogene is upregulated and a poor prognostic factor in breast cancer patients. Oncol. Lett. 9, 2143–2148. doi: 10.3892/ol.2015.3004
Chen, X., and Macara, I. G. (2005). Par-3 controls tight junction assembly through the Rac exchange factor Tiam1. Nat. Cell Biol. 7, 262–269. doi: 10.1038/ncb1226
Chen, Y., Meng, F., Wang, B., He, L., Liu, Y., and Liu, Z. (2018). Dock2 in the development of inflammation and cancer. Eur. J. Immunol. 48, 915–922. doi: 10.1002/eji.201747157
Cho, C., Lee, K., Chen, W., and Wang, C. (2016). MST3 promotes proliferation and tumorigenicity through the VAV2/Rac1 signal axis in breast cancer. Oncotarget 7, 14586–14604. doi: 10.18632/oncotarget.7542
Chrencik, J. E., Brooun, A., Zhang, H., Mathews, I. I., Hura, G. L., Foster, S. A., et al. (2008). Structural basis of guanine nucleotide exchange mediated by the T-Cell essential Vav1. J. Mol. Biol. 380, 828–843. doi: 10.1016/j.jmb.2008.05.024
Colomba, A., Giuriato, S., Dejean, E., Thornber, K., Delsol, G., Tronchère, H., et al. (2011). Inhibition of Rac controls NPM–ALK-dependent lymphoma development and dissemination. Blood Cancer J. 1:e21. doi: 10.1038/bcj.2011.19
Cook, D. R., Rossman, K. L., and Der, C. J. (2014). Rho guanine nucleotide exchange factors: regulators of Rho GTPase activity in development and disease. Oncogene 33, 4021–4035. doi: 10.1038/onc.2013.362
Cummings, C. G., Ross, N. T., Katt, W. P., and Hamilton, A. D. (2009). Synthesis and biological evaluation of a 5-6-5 imidazole-phenyl-thiazole based a-helix mimetic. Org. Lett. 11, 25–28. doi: 10.1021/ol8022962
De, P., Aske, J. C., and Dey, N. (2019). RAC1 takes the lead in solid tumors. Cells 8:382. doi: 10.3390/cells8050382
De, P., Carlson, J. H., Jepperson, T., Willis, S., Leyland-Jones, B., and Dey, N. (2017). RAC1 GTP-ase signals wnt-beta-catenin pathway mediated integrin-directed metastasis-associated tumor cell phenotypes in triple negative breast cancers. Oncotarget 8, 3072–3103. doi: 10.18632/oncotarget.13618
Debant, A., Serra-PagèS, C., Seipel, K., O’Brien, S., Tang, M., Park, S. H., et al. (1996). The multidomain protein Trio binds the LAR transmembrane tyrosine phosphatase, contains a protein kinase domain, and has separate rac-specific and rho-specific guanine nucleotide exchange factor domains. Proc. Natl. Acad. Sci. U.S.A. 93, 5466–5471. doi: 10.1073/pnas.93.11.5466
Deribe, Y. L. (2016). Interplay between PREX2 mutations and the PI3K pathway and its effect on epigenetic regulation of gene expression in NRAS-mutant melanoma. Small GTPases 7, 178–185. doi: 10.1080/21541248.2016.1178366
Desai, K., Nair, M. G., Prabhu, J. S., Vinod, A., Korlimarla, A., Rajarajan, S., et al. (2016). High expression of integrin β6 in association with the Rho–Rac pathway identifies a poor prognostic subgroup within HER2 amplified breast cancers. Cancer Med. 5, 2000–2011. doi: 10.1002/cam4.756
Dharmawardhane, S., Hernandez, E., and Vlaar, C. (2013). Development of EHop-016: a small molecule inhibitor of Rac. Enzym 33(Pt A), 117–146. doi: 10.1016/B978-0-12-416749-0.00006-3
Dokmanovic, M., Wu, Y., Shen, Y., Chen, J., Hirsch, D. S., and Wu, W. J. (2014). Trastuzumab-induced recruitment of Csk-homologous kinase (CHK) to ErbB2 receptor is associated with ErbB2-Y1248 phosphorylation and ErbB2 degradation to mediate cell growth inhibition. Cancer Biol. Ther. 15, 1029–1041. doi: 10.4161/cbt.29171
Dong, Z., Liu, Y., Lu, S., Wang, A., Lee, K., Wang, L., et al. (2006). Vav3 oncogene is overexpressed and regulates cell growth and androgen receptor activity in human prostate cancer. Mol. Endocrinol. 20, 2315–2325. doi: 10.1210/me.2006-2048
Donnelly, S. K., Cabrera, R., Mao, S. P. H., Christin, J. R., Wu, B., Guo, W., et al. (2017). Rac3 regulates breast cancer invasion and metastasis by controlling adhesion and matrix degradation. J. Biol. Chem. 216, 4331–4349. doi: 10.1083/jcb.201704048
Durand-Onaylı, V., Haslauer, T., Härzschel, A., and Hartmann, T. N. (2018). Rac GTPases in hematological malignancies. Int. J. Mol. Sci. 19:4041. doi: 10.3390/ijms19124041
Dutting, S., Heidenreich, J., Cherpokova, D., Amin, E., Zhang, S. C., Ahmadian, M. R., et al. (2015). Critical off-target effects of the widely used Rac1 inhibitors NSC23766 and EHT1864 in mouse platelets. J. Thromb. Haemost. 13, 827–838. doi: 10.1111/jth.12861
Ebi, H., Costa, C., Faber, A. C., Nishtala, M., Kotani, H., Juric, D., et al. (2013). PI3K regulates MEK/ERK signaling in breast cancer via the Rac-GEF, P-Rex1. Proc. Natl. Acad. Sci. U.S.A. 110, 21124–21129. doi: 10.1073/pnas.1314124110
Egorov, M. V., Capestrano, M., Vorontsova, O. A., Di Pentima, A., Egorova, A. V., Mariggiò, S., et al. (2009). Faciogenital dysplasia protein (FGD1) Regulates export of cargo proteins from the golgi complex via Cdc42 activation. Mol. Biol. Cell 20, 2413–2427. doi: 10.1091/mbc.E08-11-1136
Eitzen, G., Smithers, C. C., Murray, A. G., and Overduin, M. (2019). Structure and function of the Fgd family of divergent FYVE domain proteins. Biochem. Cell Biol. 97, 257–264. doi: 10.1139/bcb-2018-2185
Fang, F., Pan, J., Li, Y. P., Li, G., Xu, L. X., Su, G. H., et al. (2016). p21-activated kinase 1 (PAK1) expression correlates with prognosis in solid tumors: a systematic review and metaanalysis. Oncotarget 7, 27422–27429. doi: 10.18632/oncotarget.8320
Farago, M., Yarnitzky, T., Shalom, B., and Katzav, S. (2020). Vav1 mutations: what makes them oncogenic? Cell. Signal. 65:109438. doi: 10.1016/j.cellsig.2019.109438
Feng, H., Hu, B., Jarzynka, M. J., Li, Y., Keezer, S., Johns, T. G., et al. (2012). Phosphorylation of dedicator of cytokinesis 1 (Dock180) at tyrosine residue Y722 by Src family kinases mediates EGFRvIII-driven glioblastoma tumorigenesis. Proc. Natl. Acad. Sci. U.S.A. 109, 3018–3023. doi: 10.1073/pnas.1121457109
Feng, H., Hu, B., Liu, K., Li, Y., Lu, X., Cheng, T., et al. (2011). Activation of Rac1 by Src-dependent phosphorylation of Dock180 Y1811 mediates PDGFR α -stimulated glioma tumorigenesis in mice and humans. J. Clin. Investig. 121, 4670–4684. doi: 10.1172/JCI58559DS1
Feng, H., Li, Y., Yin, Y., Zhang, W., Hou, Y., Zhang, L., et al. (2015). Protein kinase A–dependent phosphorylation of Dock180 at serine residue 1250 is important for glioma growth and invasion stimulated by platelet derived-growth factor receptor a. Neuro Oncol. 17, 832–842. doi: 10.1093/neuonc/nou323
Feng, X., Degese, M. S., Iglesias-bartolome, R., Vaque, J. P., Molinolo, A. A., Rodrigues, M., et al. (2014). Hippo-independent activation of YAP by the GNAQ uveal melanoma oncogene through a trio-regulated Rho GTPase signaling circuitry. Cancer Cell 25, 831–845. doi: 10.1016/j.ccr.2014.04.016
Fernandez-zapico, M. E., Gonzalez-paz, N. C., Weiss, E., Savoy, D. N., Molina, J. R., Fonseca, R., et al. (2005). Ectopic expression of VAV1 reveals an unexpected role in pancreatic cancer tumorigenesis. Cancer Cell 7, 39–49. doi: 10.1016/j.ccr.2004.11.024
Ferrandez, Y., Zhang, W., Peurois, F., Akendengué, L., Blangy, A., Zeghouf, M., et al. (2017). Allosteric inhibition of the guanine nucleotide exchange factor DOCK5 by a small molecule. Sci. Rep. 7:14409. doi: 10.1038/s41598-017-13619-2
Ferri, N., Bernini, S. K., Corsini, A., Clerici, F., Erba, E., Stragliotto, S., et al. (2013a). 3-Aryl-N-aminoylsulfonylphenyl-1H-pyrazole-5-carboxamides: a new class of selective Rac inhibitors. Medchemcomm 4, 537–541. doi: 10.1039/c2md20328f
Ferri, N., Contini, A., Bernini, S. K., and Corsini, A. (2013b). Role of small GTPase protein Rac1 in cardiovascular diseases: development of new selective pharmacological inhibitors. J. Cardiovasc. Pharmacol. 62, 425–435. doi: 10.1097/FJC.0b013e3182a18bcc
Ferri, N., Corsini, A., Bottino, P., Clerici, F., and Contini, A. (2009). Virtual screening approach for the identification of new Rac1 inhibitors. J. Med. Chem. 52, 4087–4090. doi: 10.1021/jm8015987
Fields, A. P., and Justilien, V. (2010). The guanine nucleotide exchange factor (GEF) Ect2 is an oncogene in human cancer. Adv. Enzyme Regul. 50, 190–200. doi: 10.1016/j.advenzreg.2009.10.010
Friesland, A., Zhao, Y., Chen, Y.-H., Wang, L., Zhou, H., and Lu, Q. (2013). Small molecule targeting Cdc42-intersectin interaction disrupts Golgi organization and suppresses cell motility. Proc. Natl. Acad. Sci. U.S.A. 110, 1261–1266. doi: 10.1073/pnas.1116051110
Gao, J., Aksoy, B. A., Dogrusoz, U., Dresdner, G., Gross, B., Onur Sumer, S., et al. (2013). Integrative analysis of complex cancer genomics and clinical profiles using the cBioPortal. Sci. Signal. 6:269. doi: 10.1126/scisignal.2004088.Integrative
Gao, Y., Dickerson, J. B., Guo, F., Zheng, J., and Zheng, Y. (2004). Rational design and characterization of a Rac GTPase-specific small molecule inhibitor. Proc. Natl. Acad. Sci. U.SA. 101, 7618–7623. doi: 10.1073/pnas.0307512101
Gao, Y., Xing, J., Streuli, M., Leto, T. L., and Zheng, Y. (2001). Trp56 of Rac1 specifies interaction with a subset of guanine nucleotide exchange factors. J. Biol. Chem. 276, 47530–47541. doi: 10.1074/jbc.M108865200
Goel, H. L., Pursell, B., Shultz, L. D., Greiner, D. L., Brekken, R. A., Vander Kooi, C. W., et al. (2016). P-Rex1 promotes resistance to VEGF/VEGFR-targeted therapy in prostate cancer. Cell Rep. 14, 2193–2208. doi: 10.1016/j.celrep.2016.02.016
Goka, E. T., Chaturvedi, P., Lopez, D. T. M., De La Garza, A., and Lippman, M. E. (2019). RAC1b overexpression confers resistance to chemotherapy treatment in colorectal cancer. Mol. Cancer Ther. 18, 957–968. doi: 10.1158/1535-7163.MCT-18-0955
Gonzalez, N., Cardama, G. A., Comin, M. J., Segatori, V. I., Pifano, M., Alonso, D. F., et al. (2017). Pharmacological inhibition of Rac1-PAK1 axis restores tamoxifen sensitivity in human resistant breast cancer cells. Cell. Signal. 30, 154–161. doi: 10.1016/j.cellsig.2016.12.002
Grassilli, S., Brugnoli, F., Lattanzio, R., Rossi, C., Mottolese, M., Marchisio, M., et al. (2014). High nuclear level of Vav1 is a positive prognostic factor in early invasive breast tumors: a role in modulating genes related to the efficiency of metastatic process. Oncotarget 5, 4320–4336. doi: 10.18632/oncotarget.2011
Guo, Z., Chen, X., Du, T., Zhu, D., Lai, Y., Dong, W., et al. (2017). Elevated levels of epithelial cell transforming sequence 2 predicts poor prognosis for prostate cancer. Med. Oncol. 34:13. doi: 10.1007/s12032-016-0872-3
Habets, G. G. M., Scholtes, E. H. M., Zuydgeest, D., Van Der Kammen, R. A., Stam, J. C., Berns, A., et al. (1994). Identification of an invasion-inducing Tiam-7 Gen, that encodes a protein with homology to GDP-GTP exchangers for Rho-like proteins. Cell 77, 537–549
Hampsch, R. A., Shee, K., Bates, D., Lewis, L. D., Désiré, L., Leblond, B., et al. (2017). Therapeutic sensitivity to Rac GTPase inhibition requires consequential suppression of mTORC1, AKT, and MEK signaling in breast cancer. Oncotarget 8, 21806–21817. doi: 10.18632/oncotarget.15586
Harian, J. E., Hajduk, P. J., Yoon, H. S., and Fesik, S. W. (1994). Pleckstrin homology domains bind to phosphatidylinositol-4,5-biphosphate. Nature 371, 168–170. doi: 10.1038/371168a0
Harris, J., Herrero-Garcia, E., Russo, A., Kajdacsy-Balla, A., O’Bryan, J. P., and Chiu, B. (2017). Silencing intersectin 1 slows orthotopic neuroblastoma growth in mice. J. Pediatr. Hematol. Oncol. 39, 413–418. doi: 10.1097/MPH.0000000000000931
Hasan, K., Yu, J., Widhopf, G. F., Rassenti, L. Z., Chen, L., Shen, Z., et al. (2018). Wnt5a induces ROR1 to recruit DOCK2 to activate Rac1/2 in chronic lymphocytic leukemia. Blood 132, 170–178. doi: 10.1182/blood-2017-12-819383
Hernández, E., De La Mota-Peynado, A., Dharmawardhane, S., and Vlaar, C. P. (2010). Novel inhibitors of Rac1 in metastatic breast cancer. P. R. Health Sci. J. 29, 348–356.
Hicks-Berthet, J., and Varelas, X. (2017). Integrin-FAK-CDC42-PP1A signaling gnaws at YAP/TAZ activity to control incisor stem cells. BioEssays 39:1700116. doi: 10.1002/bies.201700116
Hirai, K., Nomura, T., Yamasaki, M., Inoue, T., Narimatsu, T., Nakada, C., et al. (2014). The Vav3 oncogene enhances the malignant potential of prostate cancer cells under chronic hypoxia. Urol. Oncol. 32, 101–109. doi: 10.1016/j.urolonc.2012.09.005
Hofbauer, S. W., Krenn, P. W., Ganghammer, S., Asslaber, D., Pichler, U., Oberascher, K., et al. (2014). Tiam1/Rac1 signals contribute to the proliferation and chemoresistance, but not motility, of chronic lymphocytic leukemia cells. Blood 123, 2181–2188. doi: 10.1182/blood-2013-08-523563
Hoffman, G. R., and Cerione, R. A. (2002). Signaling to the Rho GTPases: networking with the DH domain. FEBS Lett. 513, 85–91. doi: 10.1016/S0014-5793(01)03310-5
Hsueh, C., Lin, J., and Yang, C. (2011). Prognostic significance of Tiam1 expression in papillary thyroid carcinoma. Virchows Arch. 459, 587–593. doi: 10.1007/s00428-011-1164-0
Huang, J., Ye, X., Guan, J., Chen, B., Li, Q., Zheng, X., et al. (2013). Tiam1 is associated with hepatocellular carcinoma metastasis. Int. J. Cancer 100, 90–100. doi: 10.1002/ijc.27627
Huff, L. P., Decristo, M. J., Trembath, D., Kuan, P. F., Yim, M., Liu, J., et al. (2013). The role of Ect2 nuclear RhoGEF activity in ovarian cancer cell transformation. Genes Cancer 4, 460–475. doi: 10.1177/1947601913514851
Humphries, A. C., Donnelly, S. K., and Way, M. (2014). Cdc42 and the Rho GEF intersectin-1 collaborate with Nck to promote N-WASP-dependent actin polymerisation. J. Cell Sci. 127, 673–685. doi: 10.1242/jcs.141366
Humphries-Bickley, T., Castillo-Pichardo, L., Hernandez-O-Farrill, E., Borrero-Garcia, L. D., Forestier-Roman, I., Gerena, Y., et al. (2017). Characterization of a dual Rac/Cdc42 inhibitor MBQ-167 in metastatic cancer. Mol. Cancer Ther. 16, 805–818. doi: 10.1158/1535-7163.mct-16-0442
Hunter, M. P., Russo, A., and O’Bryan, J. P. (2013). Emerging roles for intersectin (ITSN) in regulating signaling and disease pathways. Int. J. Mol. Sci. 14, 7829–7852. doi: 10.3390/ijms14047829
Huynh, N., Shulkes, A., Baldwin, G., and He, H. (2016). Up-regulation of stem cell markers by P21-activated kinase 1 contributes to 5-fluorouracil resistance of colorectal cancer. Cancer Biol. Ther. 17, 813–823. doi: 10.1080/15384047.2016.1195045
Hynes, N. E., and MacDonald, G. (2009). ErbB receptors and signaling pathways in cancer. Curr. Opin. Cell Biol. 21, 177–184. doi: 10.1016/j.ceb.2008.12.010
Ikram, M., Lim, Y., Baek, S. Y., Jin, S., Jeong, Y. H., Kwak, J. Y., et al. (2018). Co-targeting of Tiam1/Rac1 and Notch ameliorates chemoresistance against doxorubicin in a biomimetic 3D lymphoma model. Oncotarget 9, 2058–2075. doi: 10.18632/oncotarget.23156
Infante, E., and Ridley, A. J. (2013). Roles of Rho GTPases in leucocyte and leukaemia cell transendothelial migration. Philos. Trans. R. Soc. B Biol. Sci. 368:1629. doi: 10.1098/rstb.2013.0013
Iyoda, M., Kasamatsu, A., Ishigami, T., Nakashima, D., Endo-Sakamoto, Y., Ogawara, K., et al. (2010). Epithelial cell transforming sequence 2 in human oral cancer. PLoS One 5:e14082. doi: 10.1371/journal.pone.0014082
Izumi, D., Toden, S., Ureta, E., and Ishimoto, T. (2019). TIAM1 promotes chemoresistance and tumor invasiveness in colorectal cancer. Cell Death Dis. 10:267. doi: 10.1038/s41419-019-1493-5
Jaiswal, M., Dvorsky, R., and Ahmadian, M. R. (2013). Deciphering the molecular and functional basis of Dbl family proteins: a novel systematic approach toward classification of selective activation of the Rho family proteins. J. Biol. Chem. 288, 4486–4500. doi: 10.1074/jbc.m112.429746
Jiang, Y., Prabakaran, I., Wan, F., Mitra, N., Furstenau, D. K., Hung, R. K., et al. (2014). Vav2 protein overexpression marks and may predict the aggressive subtype of ductal carcinoma in situ. Biomark. Res. 2:22. doi: 10.1186/2050-7771-2-22
Jones, B. C., Kelley, L. C., Loskutov, Y. V., Marinak, K. M., Kozyreva, V. K., Smolkin, M. B., et al. (2017). Dual targeting of mesenchymal and amoeboid motility hinders metastatic behavior. Mol. Cancer Res. 15, 670–683. doi: 10.1158/1541-7786.MCR-16-0411
Justilien, V., and Fields, A. P. (2009). Ect2 links the PKCι-Par6α complex to Rac1 activation and cellular transformation. Oncogene 28, 3597–3607. doi: 10.1038/onc.2009.217
Kaneto, N., Yokoyama, S., Hayakawa, Y., Kato, S., Sakurai, H., and Saiki, I. (2014). RAC1 inhibition as a therapeutic target for gefitinib-resistant non-small-cell lung cancer. Cancer Sci. 105, 788–794. doi: 10.1111/cas.12425
Karagiannis, G. S., Condeelis, J. S., and Oktay, M. H. (2018). Chemotherapy-induced metastasis: mechanisms and translational opportunities. Clin. Exp. Metastasis 35, 269–284. doi: 10.1007/s10585-017-9870-x
Karnoub, A. E., Worthylake, D. K., Rossman, K. L., Pruitt, W. M., Campbell, S. L., Sondek, J., et al. (2001). Molecular basis for Rac1 recognition by guanine nucleotide exchange factors. Nat. Struct. Biol. 8, 1037–1041. doi: 10.1038/nsb719
Karpel-Massler, G., Westhoff, M. A., Kast, R. E., Dwucet, A., Karpel-Massler, S., Nonnenmacher, L., et al. (2017). Simultaneous interference with HER1/EGFR and RAC1 signaling drives cytostasis and suppression of survivin in human glioma cells in vitro. Neurochem. Res. 42, 1543–1554. doi: 10.1007/s11064-017-2213-0
Karpel-Massler, G., Westhoff, M.-A., Zhou, S., Nonnenmacher, L., Dwucet, A., Kast, R. E., et al. (2013). Combined inhibition of HER1/EGFR and RAC1 results in a synergistic antiproliferative effect on established and primary cultured human glioblastoma cells. Mol. Cancer Ther. 12, 1783–1795. doi: 10.1158/1535-7163.MCT-13-0052
Katoh, M., and Katoh, M. (2020). Precision medicine for human cancers with Notch signaling dysregulation (Review). Int. J. Mol. Med. 45, 279–297. doi: 10.3892/ijmm.2019.4418
Katz, E., Sims, A. H., Sproul, D., Caldwell, H., Michael Dixon, J., Meehan, R. R., et al. (2012). Targeting of Rac GTPases blocks the spread of intact human breast cancer. Oncotarget 3, 608–619. doi: 10.18632/oncotarget.520
Kazanietz, M. G., Barrio-real, L., Casado-medrano, V., Martin, J., Lopez-haber, C., Kazanietz, M. G., et al. (2018). The P-Rex1 / Rac signaling pathway as a point of convergence for HER / ErbB receptor and GPCR responses. Small GTPases 9, 297–303. doi: 10.1080/21541248.2016.1221273
Kazanietz, M. G., and Caloca, M. J. (2017). The Rac GTPase in cancer: from old concepts to new paradigms. Cancer Res. 77, 5445–5451. doi: 10.1158/0008-5472.CAN-17-1456
Lai, Y. J., Tsai, J. C., Tseng, Y. T., Wu, M. S., Liu, W. S., Lam, H. I., et al. (2017). Small G protein Rac GTPases regulate the maintenance of glioblastoma stem-like cells in vitro and in vivo. Oncotarget 8, 18031–18049. doi: 10.18632/oncotarget.14949
Lane, J., Martin, T. A., Mansel, R. E., and Jiang, W. G. (2008). The expression and prognostic value of the guanine nucleotide exchange factors (GEFs) Trio, Vav1 and TIAM-1 in human breast cancer. Int. Semin. Surg. Oncol. 5:23. doi: 10.1186/1477-7800-5-23
Laurin, M., Huber, J., Pelletier, A., Houalla, T., Park, M., Fukui, Y., et al. (2013). Rac-specific guanine nucleotide exchange factor DOCK1 is a critical regulator of HER2-mediated breast cancer metastasis. Proc. Natl. Acad. Sci. U.S.A. 110, 7434–7439. doi: 10.1073/pnas.1213050110
Lazer, G., Idelchuk, Y., Schapira, V., Pikarsky, E., and Katzav, S. (2009). The haematopoietic specific signal transducer Vav1 is aberrantly expressed in lung cancer and plays a role in tumourigenesis. J. Pathol. 219, 25–34. doi: 10.1002/path.2579
Lee, J. W., Ryu, Y. K., Ji, Y. H., Kang, J. H., and Moon, E. Y. (2015). Hypoxia/reoxygenation-experienced cancer cell migration and metastasis are regulated by Rap1-and Rac1-GTPase activation via the expression of thymosin beta-4. Oncotarget 6, 9820–9833. doi: 10.18632/oncotarget.3218
Leslie, N. R. (2009). P-REX2a driving tumorigenesis by PTEN inhibition. Cancer Biol. 2:e68. doi: 10.1126/scisignal.294pe68
Li, J., Sun, Z., Yuan, Y., Yin, F., Bian, Y., Long, L., et al. (2017). EphB3 stimulates cell migration and metastasis in a kinase-dependent manner through Vav2-Rho GTPase axis in papillary thyroid cancer. J. Biol. Chem. 292, 1112–1121. doi: 10.1074/jbc.M116.750349
Li, Z., Liu, Q., Piao, J., Hua, F., Wang, J., Jin, G., et al. (2016). Clinicopathological implications of Tiam1 overexpression in invasive ductal carcinoma of the breast. BMC Cancer 16:681. doi: 10.1186/s12885-016-2724-0
Lin, K., Gong, J., Li, C., Jang, T., Chen, W., Chen, H., et al. (2012a). Vav3-Rac1 signaling regulates prostate cancer metastasis with elevated Vav3 expression correlating with prostate cancer progression and posttreatment recurrence. Cancer Res. 72, 3000–3010. doi: 10.1158/0008-5472.CAN-11-2502
Lin, K., Wang, L., Hseu, Y., Fang, C., Yang, H., and Kumar, K. J. S. (2012b). Clinical significance of increased guanine nucleotide exchange factor Vav3 expression in human gastric cancer. Mol. Cancer Res. 10, 750–760. doi: 10.1158/1541-7786.MCR-11-0598-T
Lindsey, J. C., Kawauchi, D., Schwalbe, E. C., Solecki, D. J., Selby, M. P., Mckinnon, P. J., et al. (2014). Cross-species epigenetics identifies a critical role for VAV1 in SHH subgroup medulloblastoma maintenance. Oncogene 34, 4746–4757. doi: 10.1038/onc.2014.405
Lissanu, Y., Shi, Y., Rai, K., Nezi, L., Amin, S. B., Wu, C., et al. (2016). Truncating PREX2 mutations activate its GEF activity and alter gene expression regulation in NRAS-mutant melanoma. Proc. Natl. Acad. Sci. U.S.A. 113, 1296–1305. doi: 10.1073/pnas.1513801113
Liu, C., Guo, T., Xu, G., Sakai, A., Ren, S., Fukusumi, T., et al. (2018). Characterization of alternative splicing events in HPV-negative head and neck squamous cell carcinoma identifies an oncogenic DOCK5 variant. Clin. Cancer Res. 24, 5123–5132. doi: 10.1158/1078-0432.CCR-18-0752
Liu, H., Shi, G., Liu, X., Wu, H. U. I., Fan, Q., and Wang, X. I. N. (2011). Overexpression of Tiam1 predicts poor prognosis in patients with esophageal squamous cell carcinoma. Oncol. Rep. 25, 841–848. doi: 10.3892/or.2010.1122
Liu, W., Du, W., Shang, X., Wang, L., Evelyn, C., Florian, M. C., et al. (2019). Rational identification of a Cdc42 inhibitor presents a new regimen for long-term hematopoietic stem cell mobilization. Leukemia 33, 749–761. doi: 10.1038/s41375-018-0251-5
Liu, Z., Adams, H. C., and Whitehead, I. P. (2009). The rho-specific guanine nucleotide exchange factor Dbs regulates breast cancer cell migration. J. Biol. Chem. 284, 15771–15780. doi: 10.1074/jbc.M901853200
Mack, N. A., Whalley, H. J., Castillo-Lluva, S., and Malliri, A. (2011). The diverse roles of Rac signaling in tumorigenesis. Cell Cycle 10, 1571–1581. doi: 10.4161/cc.10.10.15612
Maes, H., Van Eygen, S., Krysko, D. V., Vandenabeele, P., Nys, K., Rillaerts, K., et al. (2014). BNIP3 supports melanoma cell migration and vasculogenic mimicry by orchestrating the actin cytoskeleton. Cell Death Dis. 5:e1127. doi: 10.1038/cddis.2014.94
Maldonado, M. D. M., and Dharmawardhane, S. (2018). Targeting Rac and Cdc42 GTpases in cancer. Cancer Res. 78, 3101–3111. doi: 10.1158/0008-5472.CAN-18-0619
Manes, T. D., and Pober, J. S. (2013). TCR-driven transendothelial migration of human effector memory CD4 T cells involves Vav, Rac, and myosin IIA. J. Immunol. 190, 3079–3088. doi: 10.4049/jimmunol.1201817
Marotti, J. D., Muller, K. E., Tafe, L. J., Demidenko, E., and Miller, T. W. (2017). P-Rex1 expression in invasive breast cancer in relation to receptor status and distant metastatic site. Int. J. Breast Cancer 2017:4537532. doi: 10.1155/2017/4537532
Martin, H., Mali, R. S., Ma, P., Chatterjee, A., Ramdas, B., Sims, E., et al. (2013). Pak and Rac GTPases promote oncogenic KIT-induced neoplasms. J. Clin. Invest. 123, 4449–4463. doi: 10.1172/JCI67509
Matsuzawa, K., Akita, H., Watanabe, T., Kakeno, M., Matsui, T., and Chernoff, J. (2016). PAR3-aPKC regulates Tiam1 by modulating suppressive internal interactions. Mol. Biol. Cell 27, 1511–1523. doi: 10.1091/mbc.E15-09-0670
Mayeenuddin, L. H., and Garrison, J. C. (2006). Phosphorylation of P-Rex1 by the cyclic AMP-dependent protein kinase inhibits the phosphatidylinositiol (3, 4, 5)-Trisphosphate and Gβγ-mediated regulation of its activity. J. Biol. Chem. 281, 1921–1928. doi: 10.1074/jbc.M506035200
Meller, N., Merlot, S., and Guda, C. (2005). CZH proteins: a new family of Rho-GEFs. J. Cell Sci. 118, 4937–4946. doi: 10.1242/jcs.02671
Menna, P. L., Parera, R. L., Cardama, G. A., Alonso, D. F., Gomez, D. E., and Farina, H. G. (2009). Enhanced cytostatic activity of statins in mouse mammary carcinoma cells overexpressing beta2-chimaerin. Mol. Med. Rep. 2, 97–102. doi: 10.3892/mmr_00000068
Mills, S. C., Howell, L., Beekman, A., Stokes, L., and Mueller, A. (2018). Rac1 plays a role in CXCL12 but not CCL3-induced chemotaxis and Rac1 GEF inhibitor NSC23766 has off target effects on CXCR4. Cell. Signal. 42, 88–96. doi: 10.1016/j.cellsig.2017.10.006
Montalvo-Ortiz, B. L., Castillo-Pichardo, L., Hernández, E., Humphries-Bickley, T., De La Mota-Peynado, A., Cubano, L. A., et al. (2012). Characterization of EHop-016, novel small molecule inhibitor of Rac GTPase. J. Biol. Chem. 287, 13228–13238. doi: 10.1074/jbc.M111.334524
Montero, J. C., Seoane, S., and Ocan, A. (2011). P-Rex1 participates in Neuregulin-ErbB signal transduction and its expression correlates with patient outcome in breast cancer. Oncogene 30, 1059–1071. doi: 10.1038/onc.2010.489
Montero, J. C., Seoane, S., and Pandiella, A. (2016). Multisite phosphorylation of P-Rex1 by protein kinase C. Oncotarget 7, 77937–77949. doi: 10.18632/oncotarget.12846
Moores, S. L., Selfors, L. M., Fredericks, J., Breit, T., Fujikawa, K., Alt, F. W., et al. (2000). Vav family proteins couple to diverse cell surface receptors. Mol. Cell. Biol. 20, 6364–6373. doi: 10.1128/mcb.20.17.6364-6373.2000
Morrison Joly, M., Williams, M. M., Hicks, D. J., Jones, B., Sanchez, V., Young, C. D., et al. (2017). Two distinct mTORC2-dependent pathways converge on Rac1 to drive breast cancer metastasis. Breast Cancer Res. 19:74. doi: 10.1186/s13058-017-0868-8
Moshfegh, Y., Bravo-cordero, J. J., Miskolci, V., Condeelis, J., and Hodgson, L. (2014). A Trio–Rac1–Pak1 signalling axis drives invadopodia disassembly. Nat. Cell Biol. 16, 574–586. doi: 10.1038/ncb2972
Mott, H. R., Owen, D., Nietlispach, D., Lowe, P. N., Manser, E., Lim, L., et al. (1999). Structure of the small G protein Cdc42 bound to the GTPase- binding domain of ACK. Nature 399:6734. doi: 10.1038/20732
Movilla, N., Dosil, M., Zheng, Y., and Bustelo, X. R. (2001). How Vav proteins discriminate the GTPases Rac1 and Rhoa from Cdc42. Oncogene 20, 8057–8065. doi: 10.1038/sj.onc.1205000
Nahta, R. (2012). Molecular mechanisms of trastuzumab-based treatment in HER2-overexpressing breast cancer. ISRN Oncol. 2012:42806. doi: 10.5402/2012/428062
Niebel, B., Wosnitza, C. I., and Famulok, M. (2013). RNA-aptamers that modulate the RhoGEF activity of Tiam1. Bioorganic Med. Chem. 21, 6239–6246. doi: 10.1016/j.bmc.2013.05.021
Nishikimi, A., Uruno, T., Duan, X., Cao, Q., Okamura, Y., Saitoh, T., et al. (2012). Blockade of inflammatory responses by a small-molecule inhibitor of the Rac activator DOCK2. Chem. Biol. 19, 488–497. doi: 10.1016/j.chembiol.2012.03.008
Nishimura, T., Yamaguchi, T., Kato, K., Yoshizawa, M., and Nabeshima, Y. (2005). PAR-6 – PAR-3 mediates Cdc42-induced Rac activation through the Rac GEFs STEF / Tiam1. Nat. Cell Biol. 7, 270–277. doi: 10.1038/ncb1227
Normanno, N., Antonella, A. L., Maiello, M. R., Campiglio, M., Napolitano, M., Mancino, M., et al. (2006). The MEK/MAPK pathway is involved in the resistance of breast cancer cells to the EGFR tyrosine kinase inhibitor gefitinib. J. Cell. Physiol. 207, 420–427. doi: 10.1002/JCP
Okada, T., Lee, A. Y., Qin, L. X., Agaram, N., Mimae, T., Shen, Y., et al. (2017). Integrin-alpha 10 dependency identifies RAC and RICTOR as therapeutic targets in high-grade myxofibrosarcoma. Cancer Discov. 6, 1148–1165. doi: 10.1158/2159-8290.CD-15-1481.Integrin-
Olabi, S., Ucar, A., Brennan, K., and Streuli, C. H. (2018). Integrin-Rac signalling for mammary epithelial stem cell self-renewal. Breast Cancer Res. 20:128. doi: 10.1186/s13058-018-1048-1
Ong, C. C., Jubb, A. M., Jakubiak, D., Zhou, W., Rudolph, J., Haverty, P. M., et al. (2013). P21-activated kinase 1 (PAK1) as a therapeutic target in BRAF wild-type melanoma. J. Natl. Cancer Inst. 105, 606–615. doi: 10.1093/jnci/djt054
Orgaz, J. L., Herraiz, C., Sanz-moreno, V., Orgaz, J. L., Herraiz, C., and Sanz-moreno, V. (2014). Rho GTPases modulate malignant transformation of tumor cells Rho GTPases modulate malignant transformation of tumor cells. Small GTPases 5:e29019. doi: 10.4161/sgtp.29019
Pai, S. Y., Kim, C., and Williams, D. A. (2010). Rac GTPases in human diseases. Dis. Markers 29, 177–187. doi: 10.3233/DMA-2010-2738
Park, S., Guo, Y., Negre, J., Preto, J., Smithers, C. C., Azad, A. K., et al. (2019). Fgd5 is a Rac1-specific Rho GEF that is selectively inhibited by aurintricarboxylic acid. Small GTPases doi: 10.1080/21541248.2019.1674765 [Epub ahead of print].
Perretta-Tejedor, N., Fernández-Mateos, J., García-Ortiz, L., Gómez-Marcos, M. A., Recio-Rodríguez, J. I., Agudo-Conde, C., et al. (2017). Association of VAV2 and VAV3 polymorphisms with cardiovascular risk factors. Sci. Rep. 7:41875. doi: 10.1038/srep41875
Peterson, J. R., Lebensohn, A. M., Pelish, H. E., and Kirschner, M. W. (2006). Biochemical suppression of small molecule inhibitors: a new strategy to identify inhibitor targets and signaling pathway components. Chem. Biol. 13, 443–452. doi: 10.1016/j.chembiol.2006.02.009
Poppe, D., Tiede, I., Fritz, G., Becker, C., Bartsch, B., Wirtz, S., et al. (2006). Azathioprine suppresses ezrin-radixin-moesin-dependent T cell-APC conjugation through inhibition of Vav guanosine exchange activity on Rac proteins. J. Immunol. 176, 640–651. doi: 10.4049/jimmunol.176.1.640
Porazinski, S., Parkin, A., and Pajic, M. (2020). “Rho-ROCK signaling in normal physiology and as a key player in shaping the tumor microenvironment,” in Advances in Experimental Medicine and Biology, ed. A. Birbrair (Cham: Springer), 99–127.
Porter, A. P., Papaioannou, A., and Malliri, A. (2016). Deregulation of Rho GTPases in cancer. Small GTPases 7, 123–138. doi: 10.1080/21541248.2016.1173767
Prudnikova, T. Y., Rawat, S. J., and Chernoff, J. (2015). Molecular pathways: targeting the kinase effectors of RHO-family GTPases. Clin. Cancer Res. 21, 24–29. doi: 10.1158/1078-0432.CCR-14-0827
Razanadrakoto, L., Cormier, F., and Laurienté, V. (2015). Mutation of Vav1 adaptor region reveals a new oncogenic activation. Oncotarget 6, 2524–2538. doi: 10.18632/oncotarget.2629
Razidlo, G. L., Magnine, C., Sletten, A. C., Hurley, R. M., Almada, L. L., Fernandez-zapico, M. E., et al. (2015). Targeting pancreatic cancer metastasis by inhibition of Vav1, a driver of tumor cell invasion. Cancer Res. 75, 2907–2916. doi: 10.1158/0008-5472.CAN-14-3103
Razidlo, G. L., Wang, Y., Chen, J., Krueger, E. W., Billadeau, D. D., and Mcniven, M. A. (2013). Dynamin 2 potentiates invasive migration of pancreatic tumor cells through stabilization of the Rac1 GEF Vav1. Cell 24, 573–585. doi: 10.1016/j.devcel.2013.02.010
Rijssel, J., Van Hoogenboezem, M., Wester, L., Hordijk, P. L., and Buul, J. D. (2012). The N-terminal DH-PH domain of trio induces cell spreading and migration by regulating lamellipodia dynamics in a Rac1-dependent fashion. PLoS One 7:e29912. doi: 10.1371/journal.pone.0029912
Rosenberg, B. J., Gil-henn, H., Mader, C. C., Halo, T., and Yin, T. (2017). Phosphorylated cortactin recruits Vav2 guanine nucleotide exchange factor to activate Rac3 and promote invadopodial function in invasive breast cancer cells. Mol. Biol. Cell 28, 1347–1360. doi: 10.1091/mbc.E16-12-0885
Rossman, K. L., Der, C. J., and Sondek, J. (2005). GEF means go: turning on Rho GTPases with guanine nucleotide-exchange factors. Nat. Rev. Mol. Cell Biol. 6, 167–180. doi: 10.1038/nrm1587
Rossman, K. L., Worthylake, D. K., Snyder, J. T., Siderovski, D. P., Campbell, S. L., and Sondek, J. (2002). A crystallographic view of interactions between Dbs and Cdc42: PH domain-assisted guanine nucleotide exchange. EMBO J. 21, 1315–1326. doi: 10.1093/emboj/21.6.1315
Ruffoni, A., Ferri, N., Bernini, S. K., Ricci, C., Corsini, A., Maffuci, I., et al. (2014). 2-Amino-3-(phenylsulfanyl)norbornane-2-carboxylate: an appealing scaffold for the design of Rac1-Tiam1 protein-protein interaction inhibitors. J. Med. Chem. 57, 2953–2962. doi: 10.1021/jm401924s
Saci, A., Cantley, L. C., and Carpenter, C. L. (2011). Rac1 regulates the activity of mTORC1 and mTORC2 and controls cellular size. Mol. Cell 42, 50–61. doi: 10.1016/j.molcel.2011.03.017
Saito, S., Liu, X. F., Kamijo, K., Raziuddin, R., Tatsumoto, T., Okamoto, I., et al. (2004). Deregulation and mislocalization of the cytokinesis regulator ECT2 activate the Rho signaling pathways leading to malignant transformation. J. Biol. Chem. 279, 7169–7179. doi: 10:1075/jbc.M306725200
Sakamori, R., Yu, S., Zhang, X., Hoffman, A., Sun, J., Das, S., et al. (2014). CDC42 inhibition suppresses progression of incipient intestinal tumors. Cancer Res. 74, 5480–5492. doi: 10.1158/0008-5472.CAN-14-0267
Sakamoto, K., Adachi, Y., Komoike, Y., Kamada, Y., Koyama, R., Fukuda, Y., et al. (2017). Novel DOCK2-selective inhibitory peptide that suppresses B-cell line migration. Biochem. Biophys. Res. Commun. 483, 183–190. doi: 10.1016/j.bbrc.2016.12.170
Sakurai, T., Uruno, T., Sugiura, Y., Tatsuguchi, T., Yamamura, K., Ushijima, M., et al. (2018). Cholesterol sulfate is a DOCK2 inhibitor that mediates tissue-specific immune evasion in the eye. Sci. Signal. 11:541. doi: 10.1126/scisignal.aao4874
Salhia, B., Tran, N. L., Chan, A., Wolf, A., Nakada, M., Rutka, F., et al. (2008). The guanine nucleotide exchange factors trio, Ect2, and Vav3 mediate the invasive behavior of glioblastoma. Tumorigenes. Neoplast. Progress. 173, 1828–1838. doi: 10.2353/ajpath.2008.080043
Sanmartín, E., Yáñez, Y., Fornés-Ferrer, V., and Zugaza, J. L. (2017). TIAM1 variants improve clinical outcome in neuroblastoma. Oncotarget 8, 45286–45297. doi: 10.18632/oncotarget.16787
Schmidt, A., and Hall, A. (2002). Guanine nucleotide exchange factors for Rho GTPases: turning on the switch. Genes Dev. 16, 1587–1609. doi: 10.1101/gad.1003302
Schmidt, S., Debant, A., Schmidt, S., and Debant, A. (2014). Function and regulation of the Rho guanine nucleotide exchange factor Trio. Small GTPases 5:4. doi: 10.4161/sgtp.29769
Schmit, S. L., Edlund, C. K., Schumacher, F. R., Gong, J., Harrison, T. A., Huyghe, J. R., et al. (2019). Novel common genetic susceptibility loci for colorectal cancer. J. Natl. Cancer Inst. 111, 146–157. doi: 10.1093/jnci/djy099
Sebban, S., Farago, M., Gashai, D., Ilan, L., Pikarsky, E., Ben-porath, I., et al. (2013). Vav1 fine tunes p53 control of apoptosis versus proliferation in breast cancer. PLoS One 8:e54321. doi: 10.1371/journal.pone.0054321
Shalom, B., Farago, M., Pikarsky, E., and Katzav, S. (2018). Vav1 mutations identified in human cancers give rise to different oncogenic phenotypes. Oncogenesis 7:80. doi: 10.1038/s41389-018-0091-1
Snyder, J. T., Worthylake, D. K., Rossman, K. L., Betts, L., Pruitt, W. M., Siderovski, D. P., et al. (2002). Structural basis for the selective activation of Rho GTPases by Dbl exchange factors. Nat. Struct. Biol. 9, 468–473. doi: 10.1038/nsb796
Sondek, J., Worthylake, D. K., and Rossman, K. L. (2000). Crystal structure of Rac1 in complex with the guanine nucleotide exchange region of Tiam1. Nature 408, 682–688. doi: 10.1038/35047014
Sonoshita, M., Itatani, Y., Kakizaki, F., Sakimura, K., and Terashima, T. (2015). Promotion of colorectal cancer invasion and metastasis through activation of NOTCH–DAB1– ABL–RHOGEF protein TRIO. Cancer Discov. 5, 198–211. doi: 10.1158/2159-8290.CD-14-0595
Srijakotre, N., Man, J., Ooms, L. M., Lucato, C. M., Ellisdon, A. M., and Mitchell, C. A. (2017). P-Rex1 and P-Rex2 RacGEFs and cancer. Biochem. Soc. Trans. 45, 963–977. doi: 10.1042/BST20160269
Stengel, K., and Zheng, Y. (2011). Cdc42 in oncogenic transformation, invasion, and tumorigenesis. Bone 23, 1415–1423. doi: 10.1038/jid.2014.371
Svensmark, J. H., and Brakebusch, C. (2019). Rho GTPases in cancer: friend or foe? Oncogene 38, 7447–7456. doi: 10.1038/s41388-019-0963-7
Tajiri, H., Uruno, T., Shirai, T., Takaya, D., Matsunaga, S., Setoyama, D., et al. (2017). Targeting ras-driven cancer cell survival and invasion through selective inhibition of DOCK1. Cell Rep. 19, 969–980. doi: 10.1016/j.celrep.2017.04.016
Takeshita, S., Yamashita, Y., Shiomi, K., Suzuki, N., and Yoshida, J. (2018). Expression of P-REX2a is associated with poor prognosis in endometrial malignancies. Oncotarget 9, 24778–24786. doi: 10.18632/oncotarget.25349
Tan, B. (2014). Inhibition of gastric cancer cell growth and invasion through siRNA-mediated knockdown of guanine nucleotide exchange factor Vav3. Tumor Biol. 35, 1481–1488. doi: 10.1007/s13277-013-1204-2
Tan, B., Li, Y., Fan, L., Zhao, Q., Liu, Q., Liu, Y., et al. (2017a). Upregulated Vav2 in gastric cancer tissues promotes tumor invasion and metastasis. Tumor Biol. 39:1010428317698392. doi: 10.1177/1010428317698392
Tan, B., Li, Y., Shi, X., Fan, L., Zhao, Q., Liu, Y., et al. (2017b). Expression of Vav3 protein and its prognostic value in patients with gastric cancer. Pathol. Pract. 213, 435–440. doi: 10.1016/j.prp.2017.01.028
Tatsumoto, T., Xie, X., Blumenthal, R., Okamoto, I., and Miki, T. (1999). Human ECT2 is an exchange factor for Rho GTPases, phosphorylated in G2/M phases, and involved in cytokinesis. J. Cell Biol. 147, 921–928. doi: 10.1083/jcb.147.5.921
Thomas, E. K., Cancelas, J. A., Chae, H. D., Cox, A. D., Keller, P. J., Perrotti, D., et al. (2007). Rac guanosine triphosphatases represent integrating molecular therapeutic targets for BCR-ABL-induced myeloproliferative disease. Cancer Cell 12, 467–478. doi: 10.1016/j.ccr.2007.10.015
Tian, T., Bi, C., Hein, A. L., Zhang, X., Wang, C., Shen, S., et al. (2018). Rac1 is a novel therapeutic target in mantle cell lymphoma. Blood Cancer J. 8:17. doi: 10.1038/s41408-018-0052-0
Tomino, T., Tajiri, H., Tatsuguchi, T., Shirai, T., Oisaki, K., Matsunaga, S., et al. (2018). DOCK1 inhibition suppresses cancer cell invasion and macropinocytosis induced by self-activating Rac1P29S mutation. Biochem. Biophys. Res. Commun. 497, 298–304. doi: 10.1016/j.bbrc.2018.02.073
Uen, Y., Fang, C., Hseu, Y., Shen, P., and Yang, H. (2015). VAV3 oncogene expression in colorectal cancer: clinical aspects and functional characterization. Sci. Rep. 5:9360. doi: 10.1038/srep09360
Valla, M., Engstrøm, M. J., Ytterhus, B., Hansen, Å. K. S., Akslen, L. A., Vatten, L. J., et al. (2017). FGD5 amplification in breast cancer patients is associated with tumour proliferation and a poorer prognosis. Breast Cancer Res. Treat. 162, 243–253. doi: 10.1007/s10549-017-4125-8
Vives, V., Cres, G., Richard, C., Busson, M., Ferrandez, Y., Planson, A. G., et al. (2015). Pharmacological inhibition of Dock5 prevents osteolysis by affecting osteoclast podosome organization while preserving bone formation. Nat. Commun. 6:6218. doi: 10.1038/ncomms7218
Vives, V., Laurin, M., Cres, G., Larrousse, P., Morichaud, Z., Noel, D., et al. (2011). The Rac1 exchange factor Dock5 is essential for bone resorption by osteoclasts. J. Bone Miner. Res. 26, 1099–1110. doi: 10.1002/jbmr.282
Vlaar, C. P., Castillo-Pichardo, L., Medina, J. I., Marrero-Serra, C. M., Velez, E., Ramos, Z., et al. (2018). Design, synthesis and biological evaluation of new carbazole derivatives as anti-cancer and anti-migratory agents. Bioorg. Med. Chem. 26, 884–890. doi: 10.1016/j.bmc.2018.01.003
Vrbic, S., Pejcic, I., Filipovic, S., Kocic, B., and Vrbic, M. (2013). Current and future anti-HER2 therapy in breast cancer. J. BUON 18, 4–16.
Vu, T., and Claret, F. X. (2012). Trastuzumab: updated mechanisms of action and resistance in breast cancer. Front. Oncol. 2:62. doi: 10.3389/fonc.2012.00062
Wang, H.-B., Yan, H.-C., and Liu, Y. (2016). Clinical significance of ECT2 expression in tissue and serum of gastric cancer patients. Clin. Transl. Oncol. 18, 735–742. doi: 10.1007/s12094-015-1428-2
Wang, H.-K., Liang, J.-F., Zheng, H.-X., and Xiao, H. (2018). Expression and prognostic significance of ECT2 in invasive breast cancer. J. Clin. Pathol. 71, 442–445. doi: 10.1136/jclinpath-2017-204569
Wang, S. E., Shin, I., Wu, F. Y., Friedman, D. B., and Arteaga, C. L. (2006). HER2/Neu (ErbB2) signaling to Rac1-Pak1 is temporally and spatially modulated by transforming growth factor β. Cancer Res. 66, 9591–9600. doi: 10.1158/0008-5472.CAN-06-2071
Weigelt, B., Lo, A. T., Park, C. C., Gray, J. W., and Bissell, M. J. (2010). HER2 signaling pathway activation and response of breast cancer cells to HER2-targeting agents is dependent strongly on the 3D microenvironment. Breast Cancer Res. Treat. 122, 35–43. doi: 10.1007/s10549-009-0502-2
Wertheimer, E., Gutierrez-Uzquiza, A., Rosemblit, C., Lopez-Haber, C., Sosa, M. S., and Kazanietz, M. G. (2012). Rac signaling in breast cancer: a tale of GEFs and GAPs. Cell. Signal. 24, 353–362. doi: 10.1016/j.cellsig.2011.08.011
Wetterskog, D., Shiu, K. K., Chong, I., Meijer, T., Mackay, A., Lambros, M., et al. (2014). Identification of novel determinants of resistance to lapatinib in ERBB2-amplified cancers. Oncogene 33, 966–976. doi: 10.1038/onc.2013.41
Whitehead, I., Kirk, H., and Kay, R. (1995). Retroviral transduction and oncogenic selection of a cDNA encoding Dbs, a homolog of the Dbl guanine nucleotide exchange factor. Oncogene 10, 713–271.
Whitehead, I. P., Lambert, Q. T., Glaven, J. A., Abe, K., Rossman, K. L., Mahon, G. M., et al. (1999). Dependence of Dbl and Dbs transformation on MEK and NF-κB Activation. Mol. Cell. Biol. 19, 7759–7770. doi: 10.1128/mcb.19.11.7759
Wilken, J. A., and Maihle, N. J. (2010). Primary trastuzumab resistance: new tricks for an old drug. Ann. N. Y. Acad. Sci. 1210, 53–65. doi: 10.1111/j.1749-6632.2010.05782.x
Wong, A. L. A., and Lee, S.-C. (2012). Mechanisms of resistance to trastuzumab and novel therapeutic strategies in HER2-positive breast cancer. Int. J. Breast Cancer 2012:41517. doi: 10.1155/2012/415170
Worthylake, D. K., Rossman, K. L., and Sondek, J. (2000). Crystal structure of Rac1 in complex with the guanine nucleotide exchange region of Tiam1. Nature 408, 682–688. doi: 10.1038/35047014
Wu, M., Small, D., and Duffield, A. S. (2017). DOCK2: a novel FLT3/ITD leukemia drug target. Oncotarget 8, 88253–88254. doi: 10.18632/oncotarget.21390
Xie, C., Xiong, W., Li, J., Wang, X., Xu, C., and Yang, L. (2019). Intersectin 1 (ITSN1) identified by comprehensive bioinformatic analysis and experimental validation as a key candidate biological target in breast cancer. Onco. Targets. Ther. 2019, 7079–7093. doi: 10.2147/OTT.S216286
Xu, X., De Angelis, C., Burke, K. A., Nardone, A., Hu, H., Qin, L., et al. (2017). HER2 reactivation through acquisition of the HER2 L755S mutation as a mechanism of acquired resistance to HER2-targeted therapy in HER2+ breast cancer. Clin. Cancer Res. 23, 5123–5134. doi: 10.1158/1078-0432.CCR-16-2191
Xu, Z., Gakhar, L., Bain, F. E., and Spies, M. (2017). The Tiam1 guanine nucleotide exchange factor is auto- inhibited by its pleckstrin homology coiled-coil extension. J. Biol. Chem. 292, 17777–17793. doi: 10.1074/jbc.M117.799114
Yang, Y., Wu, Q., Li, N., Che, S., Jin, T., Nan, Y., et al. (2018). Upregulation of Tiam1 contributes to cervical cancer disease progression and indicates poor. Hum. Pathol. 75, 179–188. doi: 10.1016/j.humpath.2018.02.006
Yoshida, T., Zhang, Y., Rivera Rosado, L. A., Chen, J., Khan, T., Moon, S. Y., et al. (2010). Blockade of Rac1 activity induces G1 cell cycle arrest or apoptosis in breast cancer cells through downregulation of cyclin D1, survivin, and X-linked inhibitor of apoptosis protein. Mol. Cancer Ther. 9, 1657–1668. doi: 10.1158/1535-7163.MCT-09-0906
Yoshizuka, N., Moriuchi, R., Mori, T., Yamada, K., Hasegawa, S., Maeda, T., et al. (2004). An alternative transcript derived from the trio locus encodes a guanosine nucleotide exchange factor with mouse cell-transforming potential. J. Biol. Chem. 279, 43998–44004. doi: 10.1074/jbc.M406082200
Yu, Q., Gratzke, C., Wang, Y., Wang, X., Li, B., Strittmatter, F., et al. (2019). New strategies for inhibition of non-adrenergic prostate smooth muscle contraction by pharmacologic intervention. Prostate 79, 746–756. doi: 10.1002/pros.23780
Zeng, R. J., Zheng, C. W., Gu, J. E., Zhang, H. X., Xie, L., Xu, L. Y., et al. (2019). RAC1 inhibition reverses cisplatin resistance in esophageal squamous cell carcinoma and induces downregulation of glycolytic enzymes. Mol. Oncol. 13, 2010–2030. doi: 10.1002/1878-0261.12548
Zhang, N., and Zhang, S. W. (2019). Identification of differentially expressed genes between primary lung cancer and lymph node metastasis via bioinformatic analysis. Oncol. Lett. 18, 3754–3768. doi: 10.3892/ol.2019.10723
Zhao, Y., Wang, Z., Jiang, Y., and Yang, C. (2011). Inactivation of Rac1 reduces Trastuzumab resistance in PTEN deficient and insulin-like growth factor I receptor overexpressing human breast cancer SKBR3 cells. Cancer Lett. 313, 54–63. doi: 10.1016/j.canlet.2011.08.023
Zhu, Y., Liu, H., Xu, L., An, H., Liu, W., Liu, Y., et al. (2015). P21-activated kinase 1 determines stem-like phenotype and sunitinib resistance via NF-κB/IL-6 activation in renal cell carcinoma. Cell Death Dis. 6:e1637. doi: 10.1038/cddis.2015.2
Zins, K., Gunawardhana, S., Lucas, T., Abraham, D., and Aharinejad, S. (2013). Targeting Cdc42 with the small molecule drug AZA197 suppresses primary colon cancer growth and prolongs survival in a preclinical mouse xenograft model by downregulation of PAK1 activity. J. Transl. Med. 11:295. doi: 10.1186/1479-5876-11-295
Keywords: Rac, Cdc42, guanine nucleotide exchange factors, metastasis therapy, guanine nucleotide exchange factor (GEF), metastasis, targeted (selective) treatment
Citation: Maldonado MdM, Medina JI, Velazquez L and Dharmawardhane S (2020) Targeting Rac and Cdc42 GEFs in Metastatic Cancer. Front. Cell Dev. Biol. 8:201. doi: 10.3389/fcell.2020.00201
Received: 03 February 2020; Accepted: 09 March 2020;
Published: 08 April 2020.
Edited by:
Peter J. Parker, King’s College London, United KingdomReviewed by:
Jose Orgaz, Queen Mary University of London, United KingdomPiero Crespo, Instituto de Biomedicina y Biotecnología de Cantabria, Consejo Superior de Investigaciones Científicas, Spain
Copyright © 2020 Maldonado, Medina, Velazquez and Dharmawardhane. This is an open-access article distributed under the terms of the Creative Commons Attribution License (CC BY). The use, distribution or reproduction in other forums is permitted, provided the original author(s) and the copyright owner(s) are credited and that the original publication in this journal is cited, in accordance with accepted academic practice. No use, distribution or reproduction is permitted which does not comply with these terms.
*Correspondence: Suranganie Dharmawardhane, c3UuZEB1cHIuZWR1