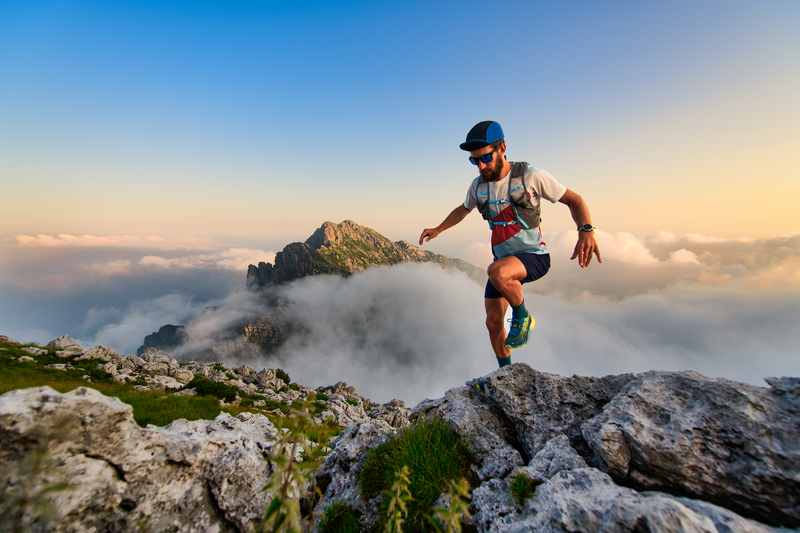
95% of researchers rate our articles as excellent or good
Learn more about the work of our research integrity team to safeguard the quality of each article we publish.
Find out more
MINI REVIEW article
Front. Cell Dev. Biol. , 13 March 2020
Sec. Molecular and Cellular Pathology
Volume 8 - 2020 | https://doi.org/10.3389/fcell.2020.00116
This article is part of the Research Topic Mechanisms of Neuronal Recovery in the Central Nervous System View all 18 articles
Neural repair in injury and disease presents a pressing unmet need in regenerative medicine. Due to the intrinsically reduced ability of the brain to replace lost and damaged neurons, reversing long-term cognitive and functional impairments poses a unique problem. Over the years, advancements in cellular and molecular understanding of neurogenesis mechanisms coupled with sophistication of biotechnology tools have transformed neural repair into a cross-disciplinary field that integrates discoveries from developmental neurobiology, transplantation and tissue engineering to design disease- and patient-specific remedies aimed at boosting either native rehabilitation or delivering exogenous hypoimmunogenic interventions. Advances in deciphering the blueprint of neural ontogenesis and annotation of the human genome has led to the development of targeted therapeutic opportunities that have the potential of treating the most vulnerable patient populations and whose findings from benchside suggest looming clinical translation. This review discusses how findings from studies of adult neurogenesis have informed development of interventions that target endogenous neural regenerative machineries and how advances in biotechnology, including the use of new gene-editing tools, have made possible the development of promising, complex neural transplant-based strategies. Adopting a multi-pronged strategy that is tailored to underlying neural pathology and that encompasses facilitation of endogenous regeneration, correction of patient’s genomic mutations and delivery of transformed neural precursors and mature disease-relevant neuronal populations to replace injured or lost neural tissue remains no longer a fantasy.
Contrary to long held belief, adult neurogenesis (AN) is now a well-recognized phenomenon in mammals. While constitutively active AN is restricted to two forebrain regions, the subgranular zone (SGZ) of the dentate gyrus (DG) of the hippocampus and the subventricular zone (SVZ) lining the ventricles, variable degrees of reactive neurogenesis is present in several other brain regions that is activated in response to injury or disease onset (Jin et al., 2006). Importantly, impairments in AN have been linked to a number of neurological and psychiatric diseases (Hoglinger et al., 2004; Reif et al., 2006), indicating the value of developing therapies that boost AN potential of cell replacement in tackling brain dysfunction.
In contrast to mammals, lower vertebrates such as teleost fish and amphibians (such as axolotl and salamander) have expanded neurogenic capacities. Unsurprisingly, most of our understanding of human AN processes continues to be informed by findings in lower vertebrate models that also display comparable expression patterns and functions of neurogenic molecular controls. Intriguingly, some amphibian species display life-long de-differentiation and trans-differentiation processes during repair (as seen during retinal and lens regeneration in newts), phenomenon absent in mammals. Using recently developed gene-editing toolkits, studies in these species can provide mechanistic insights into why such phenomenon are scaled down in mammals and be informative for developing novel disease relevant cell-replacement strategies.
The remarkable ability of the adult mammalian brain to functionally integrate new neurons into existing circuitries (Falkner et al., 2016) combined with the progress in our understanding of these phenomenon in non-mammalian and mammalian models has now made possible the development of therapies that can awaken latent neurogenic programs, direct resident neuronal fates into cell types of need and replace injured or diseased neurons in brain regions within and outside that of DG and SVZ. This review discusses AN in mammalian and non-mammalian brains, the reparative potential of cell-based transplant therapy in neurodegenerative diseases and the therapeutic application of novel gene-editing approaches in the framework of designing disease- and patient-specific curative strategies (Figure 1).
Figure 1. Adult neurogenesis in mammals and non-mammals. Lessons from non-vertebrate neurogenesis and implications for designing cell-based transplant therapies. Mammalian SVZ Neurogenesis: Characterization of neurogenesis in the rodent SVZ has revealed the existence of quiescent and active populations of proliferating cells. While heterogenous populations of NSCs have been described, “type-B” cells maintaining close proximity with the ependymal cell layer include nestin-expressing populations that asymmetrically divide to form Ascli and Dlx2 expressing “type-C” cells, also called transit-amplifying cells, which then symmetrically divide to form Dcx-positive type-A neuroblasts. These neuroblasts constitute the rostral migratory stream (RMS) that eventually contributes to OB peri-glomerular and granule cells. Recent studies have suggested that “type-B” cells are derived from embryonic NSCs that also generate striatal, septal or cortical neurons and become quiescent between E13.5-15.5 until their activation in adulthood (Fuentealba et al., 2015). Within the OB, granule cells form >95% of the adult-born population (Winner et al., 2002; Naritsuka et al., 2009; Merkle et al., 2014). Optogenetic activation paired with odor stimulation of adult-born neurons has been shown to facilitate difficult olfactory discrimination learning, an effect that is absent following photoactivation of early postnatal born neurons (Alonso et al., 2012). In addition, two photon-targeted recordings from peri-glomerular neurons have revealed that adult-born cells functionally integrate in the OB circuitry and whose activity is regulated by experience-dependent plasticity (Livneh et al., 2014). Mammalian DG Neurogenesis: Whether the adult mammalian hippocampus contains self-renewing NSCs or whether the neighboring lateral ventricular niche containing true NSCs maintains neurogenesis in the hippocampus has been contested (Seaberg and van der Kooy, 2002; Bull and Bartlett, 2005) but several recent studies have documented the presence of self-renewing, multipotent cells expressing embryonic NSC markers in the DG with fate mapping analysis confirming their stem cell behavior in vivo (Seri et al., 2001; Lagace et al., 2007; Imayoshi et al., 2008; Lugert et al., 2010; Bonaguidi et al., 2011; Encinas et al., 2011; Urban et al., 2016; Pilz et al., 2018). In the adult hippocampus (DG), SGZ radial glia-like cells give rise to proliferative non-radial-like populations that differentiate into neuroblasts. Upon differentiation, these neuroblasts develop into granule cells (Pilz et al., 2018). The embryonic origin of adult precursors in the DG in rodents was recently traced to a common Hopx-positive precursor population that is responsible for generating both developmental and AN, with the precursors not undergoing a lineage specification change during any stage of development (Berg et al., 2019). Non-mammalian Vertebrates: In contrast to mammals, non-mammalian vertebrates including certain fish and salamander species display a remarkable amount of neurogenic capacity in the adult. More than a dozen constitutive neurogenic zones have been described in the adult teleost (Grandel et al., 2006; Maruska et al., 2012), and while constitutive neurogenesis is limited to the forebrain in the newt (Berg et al., 2010) and the forebrain and ventricular zone in the axolotl (Maden et al., 2013), neuronal loss in these species following injury leads to complete regeneration (Bernardos et al., 2007; Parish et al., 2007; Berg et al., 2010; Skaggs et al., 2014). This latter capacity is reminiscent of, albeit less efficacious, reparative recruitment of quiescent progenitors found in rodents (Barnabe-Heider et al., 2010; Sirko et al., 2013; Magnusson et al., 2014). Implications for Designing Brain Repair Strategies: The limited ability of AN in mammals to enable neural repair could be due to a presence of reduced number of NSCs, available progenitors that maybe fate-restricted or strong inhibitory cues from glia. As mentioned, studies focused on naturally occurring neurogenic process that are present in lower vertebrates can yield important insights into why those mechanisms are restricted in mammals (Bhattarai et al., 2016, 2020). Evidence for the presence of evolutionary conserved genes related to regeneration as revealed by recent genome sequencing of species with enhanced regenerative capacities and the development of techniques that make those species amenable to gene-editing (Howe et al., 2013; Irion et al., 2014; Elewa et al., 2017; Nowoshilow et al., 2018) has paved the way for in-depth investigation of neurogenic molecular controls and their comparison across mammals and non-mammalian species to design novel therapies. Created with Biorender.com.
Initial anatomical evidence of the existence of AN in mammals did not arrive until the 1960s when, using thymidine-H3 autoradiographic techniques, Joseph Altman reported evidence of the formation of newborn neurons in the adult brain (Altman, 1962, 1963; Altman and Das, 1965, 1967). Follow-up electron microscopic studies extended support to the claim of radio-labeled cells as being neuronal (Kaplan and Hinds, 1977), still, a lack of use of definitive neuronal markers to co-label radio-labeled cells and a failure to replicate this in non-human primates (Rakic, 1985; Eckenhoff and Rakic, 1988) prevented the field from reaching consensus.
Almost a decade after Altman’s initial findings, Fernando Nottebohm, following up on his discovery of sexually dimorphic and seasonally regulated song-control system in the songbird (Nottebohm and Arnold, 1976; Nottebohm, 1981), crucially demonstrated adult-born cells’ neuronal identity and functional integration into adult circuits (Goldman and Nottebohm, 1983; Paton and Nottebohm, 1984; Alvarez-Buylla et al., 1990a). Soon after, the glial nature of NSCs in songbirds was described (Alvarez-Buylla et al., 1990b), which was followed by similar observations in the mammalian SVZ (Doetsch et al., 1999) and hippocampus (Seri et al., 2001, 2004). Later, the isolation of mitogen-responsive multipotent cells and successful induction of reactive neurogenesis suggested continuance of prenatal permissive regenerative programs into the adult mammalian brain (Reynolds and Weiss, 1992; Lois and Alvarez-Buylla, 1993; Luskin, 1993; Palmer et al., 1995). Finally, AN in the SVZ and hippocampus was shown to exist in humans (Eriksson et al., 1998; Spalding et al., 2013; Ernst et al., 2014).
Initial characterization of the adult human SVZ by Alvarez-Buylla et al., reported a ribbon of astrocytes lining the lateral ventricles with few surrounding proliferating cells (Sanai et al., 2004). In particular, the human SVZ was reported to lack the cellular organizational structure and rostral migratory stream (RMS) characteristics of its rodent counterpart (Figure 1, legend). A follow-up study by the same group reported strong expression of immature cell markers and RMS in the infant human SVZ, both of which sharply declined after birth (Sanai et al., 2011). In addition, a novel migratory stream to the cortex was described in the infant brain (Sanai et al., 2011). In contrast, another group found robust SVZ proliferation and a RMS containing glial cells, proliferating cells and neuroblasts organized around a lateral ventricular extension reaching the olfactory bulb (OB) in the adult (Curtis et al., 2007; Kam et al., 2009). These disparate adult human data underscore the importance of applying more reliable, species-specific progenitor/immature cell markers and optimizing methodological approaches for identifying NSCs (see also below). From a therapeutic perspective, the existence of SVZ neurogenesis in humans could have important implications for neurodegenerative disorders as neuroblasts were recently shown to migrate to and differentiate into interneurons in the striatum (Ernst et al., 2014) [but also see Dennis et al. (2016)] and carbon-14 birth-dating studies have suggested that adult-born striatal neurons might be preferentially depleted in Huntington’s disease (HD) (Ernst et al., 2014). Moreover, in animals models of PD, stimulation of SVZ precursors has been shown to rescue dopamine (DA) mediated behaviors (Spalding et al., 2005; Androutsellis-Theotokis et al., 2009).
Whether the adult human hippocampus contains self-renewing NSCs is a topic of much interest. Eriksson et al. (1998) initially reported newly generated BrdU-labeled cells in the human DG that co-expressed neuronal markers. This finding was corroborated by studies demonstrating the presence of neural progenitors in surgically excised brain specimens (Roy et al., 2000; Palmer et al., 2001) and carbon-14 birth-dating studies that revealed substantial DG neuronal turnover (Spalding et al., 2013). Recently, Boldrini et al. (2018) also found preserved DG neurogenesis in individuals 14–79 years of age, with older individuals having a smaller quiescent progenitor pool. The existence of lifelong hippocampal AN was further confirmed in two very recent studies that examined neurogenesis throughout normal and pathological aging in AD patients (Moreno-Jimenez et al., 2019) and in individuals with mild cognitive impairment (Tobin et al., 2019), with findings suggesting impaired neurogenesis in both conditions. This is not surprising since an enhanced DG neurogenic capacity has been linked to reduced susceptibility to cognitive impairments in patients with AD pathology (Briley et al., 2016) and enhancing hippocampal neurogenesis improved cognition in an AD mouse model (Choi et al., 2018). In stark contrast, other groups have observed a sharp decline in proliferating cells in childhood, observing only sparse number of proliferating cells in the adult hippocampus that were of microglia lineage (Dennis et al., 2016). In addition, Sorrells et al. (2018), using DCX and PSA-NCAM to label immature neurons, found a sharp reduction in hippocampal neurogenesis in childhood observing no new neurons in the adult. Intriguingly, the authors found that both these markers, which are widely used to identify neurogenesis in other species, can label mature neurons and glial cells in humans. Marker expression can depend on tissue preservation techniques and DCX is known to be sensitive to postmortem breakdown (Boekhoorn et al., 2006) posing significant challenges to cross-study comparisons. Future studies could benefit by allocating efforts to documenting the clinical profile and mode of death of patients as both these factors can drastically influence neurogenesis rates. In addition, applying quality control procedures, including minimizing postmortem delay, optimizing brain fixation methods to preserve neuronal morphology and marker expression, standardization of protocols for identification of neurogenic neurons and examination of gene expression patterns, along with development of more specific markers of neurogenic neurons in humans may be beneficial.
Several similarities exist between mammalian and non-mammalian species regarding AN. These include the sites of neurogenesis [the ventricular lining within the telencephalon appears to be the site of origin of new neurons in many mammalian and non-mammalian species (Goldman and Nottebohm, 1983; Doetsch et al., 1999; Ganz et al., 2010; Rothenaigner et al., 2011)], identity of neurogenic progenitors as well as the molecular machineries that regulate proliferation of NSCs. Like in mammals (Doetsch et al., 1999), some progenitor populations in birds, fishes and amphibians also have glial characteristics (Alvarez-Buylla et al., 1990b; Rothenaigner et al., 2011; Kirkham et al., 2014). Moreover, molecular controls governing quiescence of NSCs show remarkable conservation between vertebrate species: e.g., Notch signaling has been shown to regulate radial glial quiescence in zebrafish (Alunni et al., 2013), newt (Kirkham et al., 2014) and adult mouse (Imayoshi et al., 2010) and transcription factors Id1 and Fezf2 that are associated with increased quiescence in adult mouse NSCs (Nam and Benezra, 2009) have also been identified in zebrafish (Berberoglu et al., 2014; Rodriguez Viales et al., 2015). Intriguingly, Ascl1 upregulation has been linked to the activation of the retinal latent progenitors, Muller glia, in zebrafish during retinal regeneration following lesions (Ramachandran et al., 2011) and forced overexpression of Ascl1 in Muller glia in young mice coaxes these progenitors toward a neurogenic fate (Ueki et al., 2015) rather than the gliogenic fate that is normally seen in older mice (Dyer and Cepko, 2000). Comparative studies of species can thus yield important insights into the biology of AN and be informative for therapeutic strategies that harness AN potential of tissue repair (Kizil and Bhattarai, 2018; Figure 1).
On the other hand, transplantation of NSCs or progenitors to replace diseased neurons is an alternate strategy that comes with the opportunity of directing therapy to brain regions where it is most needed (Figure 1 and Table 1). Also, the self-renewing attribute and migratory nature of NSCs/progenitors make them attractive therapeutic candidates in the setting of diffuse damage to the brain.
Table 1. Summary of select studies involving cell-transplant based repair strategies in in vivo pre-clinical neurodegenerative disease models.
Initial proof-of-concept transplantation studies carried out during 70 and 80s demonstrated long-term viability of grafted neural tissue and evidence for functional replacement for missing neurons. In animal models of PD and HD, transplanted fetal rat substantia nigra tissue established connections with host striatum and reversed motor and metabolic deficits (Perlow et al., 1979; Isacson et al., 1984). Additional support came from the long-term survival of human fetal neural tissue in monkeys (Redmond et al., 1988), that led to the commencement of transplantation of fetal tissue into the striatum of PD patients (Lindvall et al., 1990; Spencer et al., 1992). Intriguingly, the initial open-label clinical trials demonstrated restoration of DA synthesis resulting in significant and long-lasting clinical improvements of motor function (Hauser et al., 1999; Piccini et al., 1999). Though variable clinical efficacy was observed, the ability of allografts to survive, integrate and function in diseased host environment, in some cases up to 20 years (Bega and Krainc, 2014), was in itself an exciting finding. Subsequent double-blinded, placebo controlled clinical trials, however, failed to reveal definitive results and long-term follow up studies revealed the development of α-synuclein aggregates in grafted fetal neurons (Kordower et al., 2008) and graft-induced dyskinesias (Freed et al., 2001; Hagell et al., 2002; Olanow et al., 2003), effects later attributed to heterogenous spread of transplanted cells and/or graft contamination by serotonergic cells (Politis et al., 2010). In addition, early withdrawal of immunosuppression, severe phenotype of transplanted patients and grafts’ non-innervation of the ventral striatum were identified as some of the limiting factors. Based on these findings, a new multi-center trial with fetal-based transplantation to PD patients is currently underway that hopes to address these concerns (Moore et al., 2014; Kirkeby et al., 2017). Therapeutic potential of neural transplantation was similarly first explored for HD in rodent and non-human primate models (Wictorin et al., 1989; Peschanski et al., 1995; Kendall et al., 1998), the positive results of which led to fetal striatal implantation in patients in the mid- to late-90s (Bachoud-Levi et al., 2000, 2006; Hauser et al., 2002). However, disease-like neuronal degeneration of grafted tissue upon postmortem analysis raised uncertainty about this approach (Cicchetti et al., 2009; Table 1).
It is worth mentioning here that both PD and HD, at least in their earlier stages, involve relatively specific cellular and regional pathologies: nigrostriatal DA neurons in PD and striatal medium spiny neurons in HD, making them suitable to tissue grafts dissected from fetal regions that contain the target cell population. However, it should also be kept in mind that pathologies in these conditions are not region-specific. For instance, in PD, non-DA and non-motor symptoms can cause significant disability, especially in patients with advanced pathology (Chaudhuri et al., 2006) and while striatal pathology dominates initial stages of HD, cell loss in the motor and cingulate cortices correlates with the degree of motor and mood dysfunction characteristic of the disease in later stages (Thu et al., 2010). Therefore, to alleviate the full repertoire of advanced disease symptomatology, adjuvant therapy for secondary disease processes maybe required. Indeed, widespread brain degenerative changes have been proposed to offset therapeutic efficacy of an otherwise viable graft (Li et al., 2016). Such a strategy may also not be applicable to disorders of diffuse pathology such as stroke, AD, SCI, and ALS, that involve pathological alterations in multiple neuronal and glial cellular phenotypes and brain regions. Lastly, the most obvious limitation to fetal transplants is the limited availability of graft sources which have traditionally been derived from human embryos causing significant logistical and ethical concerns.
Given the variable clinical efficacy, risk of contamination by non-specific cell types and ethical concerns associated with fetal grafts, alternative transplant sources have been sought to boost endogenous reparative mechanisms. Recently, pluripotent stem cells (PSCs) (Gurdon, 1962; Evans and Kaufman, 1981; Martin, 1981) including hESCs (Thomson et al., 1998; Reubinoff et al., 2000) derived from pre-implantation embryos, and hiPSCs (Takahashi and Yamanaka, 2006; Yu et al., 2007; Park et al., 2008) that are reprogrammed from somatic cells using a defined cocktail of transcription factors, have attracted tremendous interest in the field of regenerative medicine. Use of autologous hiPSCs can also overcome immune mismatch-mediated graft rejection and circumvent ethical and logistical issues associated with the use of hESCs. While hiPSC technology is by design usable to treat donor patients limiting its cost-effectiveness and scalability (Nakatsuji et al., 2008), hypo-immunogenicity in hiPSCs was recently achieved via inactivation of the major histocompatibility complex (MHC) and overexpression of the transmembrane protein CD47 (Deuse et al., 2019), potentially conferring a remarkable universal donor capability to hiPSCs (Figure 1 and Table 1).
The development of biotechnology allowing generation of hiPSCs from non-viral techniques and gene-editing tools that enable site-specific corrections of disease-causing gene mutations have made genome-edited hiPSC-based cell therapy an ideal choice in the field of precision therapeutics. Using genome-editing tools, such as zinc finger nucleases (ZFNs) (Bibikova et al., 2003), TAL effector nucleases (Boch et al., 2009) and the more specific clustered regulatory interspaced short palindromic repeats (CRISPR)/CRISPR-associated (Cas) system (Mali et al., 2013), that enable precise corrections of deleterious mutations, genome corrected-iPSCs are now being generated. Already, advances have led to generation of engineered cells that are able to deliver therapeutic factors and that carry minimal risks of tumorigenicity in preclinical models of PD (Kriks et al., 2011; Grealish et al., 2014; Hallett et al., 2015; Steinbeck et al., 2015; Kikuchi et al., 2017a; Shi et al., 2017). Using ZFNs, generation of isogenic disease and control hiPSCs from PD patients’ cortical neurons carrying the α-synuclein mutations A53T (Chung et al., 2013) and LRRK2 (Reinhardt et al., 2013) and their insertion into hESCs (Soldner et al., 2011) have been described. Lately, CRISPR/Cas system has been used to correct α-synuclein mutations in PD hiPSCs (Heman-Ackah et al., 2016; Soldner et al., 2016), remove HTT-repeat expansion mutation in HD hiPSCs (Xu et al., 2017) and correct mutations in presenilin (PSEN) in AD basal forebrain cholinergic neurons (Ortiz-Virumbrales et al., 2017). While majority of these studies have employed ex vivo manipulation of hiPSCs, successful in vivo gene editing of post mitotic cells, as has been shown in five-familial AD and amyloid precursor protein (APP) knock-in mice (Park et al., 2019), has opened up the tantalizing prospect of eliminating the need for cell transplants for brain repair. In theory, in vivo gene editing is a similar concept to the one used for in vivo direct reprogramming in which non-neuronal cells are converted into disease-specific neurons directly in situ with preclinical studies showing successful integration of reprogrammed cells into host circuits within the cerebral cortex (Guo et al., 2014), striatum (Torper et al., 2015), midbrain (Torper et al., 2013) and the spinal cord (Su et al., 2014) in heterogenous disease contexts (Figure 1 and Table 1).
Alterations in AN appear to be a common feature in many neurological and psychiatric disorders (Sahay and Hen, 2007; Winner et al., 2011; Niv et al., 2012; Pun et al., 2012). Studies in experimental models can yield important insights into AN biology including its potential for cell replacement in disease and injury contexts (van Tijn et al., 2011; Newman et al., 2014). Yet to achieve successful tissue restoration, a combinatorial therapeutic strategy might be required that includes boosting of endogenous neurogenic processes, in vivo genome-correction of endogenously generated NSCs/progenitors/mature neurons, transplantation of ex vivo gene-corrected neurons and the delivery of neurotherapeutic factors. Transplantation of fate-restricted progenitors that retain the capability to migrate and differentiate into mature neurons and functionally integrate into existing circuits has the benefit of decreasing the number of transplants and chances of developing non-specificity issues. Still, multiple cellular phenotypes might be required to construct a therapeutically efficacious graft, whose composition must be geared toward correcting primary genetic deficits and secondary phenotypes. Transplant composition for chronic neurodegenerative diseases, especially in advanced stages, might require a greater dependence on exogenous cells due to the extensive damage to host tissue, while also attempting to correct mutations in remaining diseased neuronal populations. Acute injuries, on the other hand, would require a greater role for neurotrophic factors and modification of extracellular matrix to facilitate regeneration of existing tissue, while at the same time replacing lost or damaged neural populations by promoting endogenous neurogenesis and transplanting fate-restricted precursors and/or reprogrammed mature neurons. Given the above, perhaps the most effective approach while designing cell-based therapy might be to adopt a multi-pronged strategy that cumulatively addresses the shortcomings of the above-mentioned tissue restoration strategies and that is tailored to the brain disease in hand.
MC developed the concepts and wrote the manuscript.
This work was supported by the New York State Office of Mental Health.
The author declares that the research was conducted in the absence of any commercial or financial relationships that could be construed as a potential conflict of interest.
Alonso, M., Lepousez, G., Sebastien, W., Bardy, C., Gabellec, M. M., Torquet, N., et al. (2012). Activation of adult-born neurons facilitates learning and memory. Nat. Neurosci. 15, 897–904. doi: 10.1038/nn.3108
Altman, J. (1962). Are new neurons formed in the brains of adult mammals? Science 135, 1127–1128. doi: 10.1126/science.135.3509.1127
Altman, J. (1963). Autoradiographic investigation of cell proliferation in the brains of rats and cats. Anat. Rec. 145, 573–591. doi: 10.1002/ar.1091450409
Altman, J., and Das, G. D. (1965). Post-natal origin of microneurones in the rat brain. Nature 207, 953–956. doi: 10.1038/207953a0
Altman, J., and Das, G. D. (1967). Postnatal neurogenesis in the guinea-pig. Nature 214, 1098–1101. doi: 10.1038/2141098a0
Alunni, A., Krecsmarik, M., Bosco, A., Galant, S., Pan, L., Moens, C. B., et al. (2013). Notch3 signaling gates cell cycle entry and limits neural stem cell amplification in the adult pallium. Development 140, 3335–3347. doi: 10.1242/dev.095018
Alvarez-Buylla, A., Kirn, J. R., and Nottebohm, F. (1990a). Birth of projection neurons in adult avian brain may be related to perceptual or motor learning. Science 249, 1444–1446. doi: 10.1126/science.1698312
Alvarez-Buylla, A., Theelen, M., and Nottebohm, F. (1990b). Proliferation “hot spots” in adult avian ventricular zone reveal radial cell division. Neuron 5, 101–109. doi: 10.1016/0896-6273(90)90038-h
An, M. C., Zhang, N., Scott, G., Montoro, D., Wittkop, T., Mooney, S., et al. (2012). Genetic correction of Huntington’s disease phenotypes in induced pluripotent stem cells. Cell Stem Cell 11, 253–263. doi: 10.1016/j.stem.2012.04.026
Androutsellis-Theotokis, A., Rueger, M. A., Park, D. M., Mkhikian, H., Korb, E., Poser, S. W., et al. (2009). Targeting neural precursors in the adult brain rescues injured dopamine neurons. Proc. Natl. Acad. Sci. U.S.A. 106, 13570–13575. doi: 10.1073/pnas.0905125106
Aubry, L., Bugi, A., Lefort, N., Rousseau, F., Peschanski, M., and Perrier, A. L. (2008). Striatal progenitors derived from human ES cells mature into DARPP32 neurons in vitro and in quinolinic acid-lesioned rats. Proc. Natl. Acad. Sci. U.S.A. 105, 16707–16712. doi: 10.1073/pnas.0808488105
Bachoud-Levi, A. C., Gaura, V., Brugieres, P., Lefaucheur, J. P., Boisse, M. F., Maison, P., et al. (2006). Effect of fetal neural transplants in patients with Huntington’s disease 6 years after surgery: a long-term follow-up study. Lancet Neurol. 5, 303–309. doi: 10.1016/s1474-4422(06)70381-7
Bachoud-Levi, A. C., Remy, P., Nguyen, J. P., Brugieres, P., Lefaucheur, J. P., Bourdet, C., et al. (2000). Motor and cognitive improvements in patients with Huntington’s disease after neural transplantation. Lancet 356, 1975–1979. doi: 10.1016/s0140-6736(00)03310-9
Bahat-Stroomza, M., Barhum, Y., Levy, Y. S., Karpov, O., Bulvik, S., Melamed, E., et al. (2009). Induction of adult human bone marrow mesenchymal stromal cells into functional astrocyte-like cells: potential for restorative treatment in Parkinson’s disease. J. Mol. Neurosci. 39, 199–210. doi: 10.1007/s12031-008-9166-3
Barnabe-Heider, F., Goritz, C., Sabelstrom, H., Takebayashi, H., Pfrieger, F. W., Meletis, K., et al. (2010). Origin of new glial cells in intact and injured adult spinal cord. Cell Stem Cell 7, 470–482. doi: 10.1016/j.stem.2010.07.014
Bega, D., and Krainc, D. (2014). Long-term clinical outcomes after fetal cell transplantation in parkinson disease: implications for the future of cell therapy. JAMA 311, 617–618.
Berberoglu, M. A., Dong, Z., Li, G., Zheng, J., Trejo, M., Ldel, C., et al. (2014). Heterogeneously expressed fezf2 patterns gradient Notch activity in balancing the quiescence, proliferation, and differentiation of adult neural stem cells. J. Neurosci. 34, 13911–13923. doi: 10.1523/JNEUROSCI.1976-14.2014
Berg, D. A., Kirkham, M., Beljajeva, A., Knapp, D., Habermann, B., Ryge, J., et al. (2010). Efficient regeneration by activation of neurogenesis in homeostatically quiescent regions of the adult vertebrate brain. Development 137, 4127–4134. doi: 10.1242/dev.055541
Berg, D. A., Su, Y., Jimenez-Cyrus, D., Patel, A., Huang, N., Morizet, D., et al. (2019). A common embryonic origin of stem cells drives developmental and adult neurogenesis. Cell 177, 654.e15–668.e15. doi: 10.1016/j.cell.2019.02.010
Bernardos, R. L., Barthel, L. K., Meyers, J. R., and Raymond, P. A. (2007). Late-stage neuronal progenitors in the retina are radial Muller glia that function as retinal stem cells. J. Neurosci. 27, 7028–7040. doi: 10.1523/jneurosci.1624-07.2007
Bhattarai, P., Cosacak, M. I., Mashkaryan, V., Demir, S., Popova, S. D., Govindarajan, N., et al. (2020). Neuron-glia interaction through Serotonin-BDNF-NGFR axis enables regenerative neurogenesis in Alzheimer’s model of adult zebrafish brain. PLoS Biol. 18:e3000585. doi: 10.1371/journal.pbio.3000585
Bhattarai, P., Hickman, L., and Phillips, J. L. (2016). Pain among hospitalized older people with heart failure and their preparation to manage this symptom on discharge: a descriptive-observational study. Contemp. Nurs. 52, 204–215. doi: 10.1080/10376178.2016.1175311
Bibikova, M., Beumer, K., Trautman, J. K., and Carroll, D. (2003). Enhancing gene targeting with designed zinc finger nucleases. Science 300:764. doi: 10.1126/science.1079512
Bjorklund, A., and Stenevi, U. (1979). Reconstruction of the nigrostriatal dopamine pathway by intracerebral nigral transplants. Brain Res. 177, 555–560. doi: 10.1016/0006-8993(79)90472-4
Boch, J., Scholze, H., Schornack, S., Landgraf, A., Hahn, S., Kay, S., et al. (2009). Breaking the code of DNA binding specificity of TAL-type III effectors. Science 326, 1509–1512. doi: 10.1126/science.1178811
Boekhoorn, K., Joels, M., and Lucassen, P. J. (2006). Increased proliferation reflects glial and vascular-associated changes, but not neurogenesis in the presenile Alzheimer hippocampus. Neurobiol. Dis. 24, 1–14. doi: 10.1016/j.nbd.2006.04.017
Boldrini, M., Fulmore, C. A., Tartt, A. N., Simeon, L. R., Pavlova, I., Poposka, V., et al. (2018). Human hippocampal neurogenesis persists throughout aging. Cell Stem Cell 22, 589.e5–599.e5.
Bonaguidi, M. A., Wheeler, M. A., Shapiro, J. S., Stadel, R. P., Sun, G. J., Ming, G. L., et al. (2011). In vivo clonal analysis reveals self-renewing and multipotent adult neural stem cell characteristics. Cell 145, 1142–1155. doi: 10.1016/j.cell.2011.05.024
Briley, D., Ghirardi, V., Woltjer, R., Renck, A., Zolochevska, O., Taglialatela, G., et al. (2016). Preserved neurogenesis in non-demented individuals with AD neuropathology. Sci. Rep. 6:27812. doi: 10.1038/srep27812
Bull, N. D., and Bartlett, P. F. (2005). The adult mouse hippocampal progenitor is neurogenic but not a stem cell. J. Neurosci. 25, 10815–10821. doi: 10.1523/jneurosci.3249-05.2005
Cha, M. Y., Kwon, Y. W., Ahn, H. S., Jeong, H., Lee, Y. Y., Moon, M., et al. (2017). Protein-induced pluripotent stem cells ameliorate cognitive dysfunction and reduce abeta deposition in a mouse model of Alzheimer’s disease. Stem Cells Transl. Med. 6, 293–305. doi: 10.5966/sctm.2016-0081
Chaudhuri, K. R., Healy, D. G., and Schapira, A. H. National Institute for Clinical Excellence (2006). Non-motor symptoms of Parkinson’s disease: diagnosis and management. Lancet Neurol. 5, 235–245.
Chen, Y., Xiong, M., Dong, Y., Haberman, A., Cao, J., Liu, H., et al. (2016). Chemical control of grafted human PSC-derived neurons in a mouse model of Parkinson’s disease. Cell Stem Cell. 18, 817–826. doi: 10.1016/j.stem.2016.03.014
Choi, S. H., Bylykbashi, E., Chatila, Z. K., Lee, S. W., Pulli, B., Clemenson, G. D., et al. (2018). Combined adult neurogenesis and BDNF mimic exercise effects on cognition in an Alzheimer’s mouse model. Science 361:eaan8821. doi: 10.1126/science.aan8821
Chung, C. Y., Khurana, V., Auluck, P. K., Tardiff, D. F., Mazzulli, J. R., Soldner, F., et al. (2013). Identification and rescue of alpha-synuclein toxicity in Parkinson patient-derived neurons. Science 342, 983–987. doi: 10.1126/science.1245296
Cicchetti, F., Saporta, S., Hauser, R. A., Parent, M., Saint-Pierre, M., Sanberg, P. R., et al. (2009). Neural transplants in patients with Huntington’s disease undergo disease-like neuronal degeneration. Proc. Natl. Acad. Sci. U.S.A. 106, 12483–12488. doi: 10.1073/pnas.0904239106
Curtis, M. A., Kam, M., Nannmark, U., Anderson, M. F., Axell, M. Z., Wikkelso, C., et al. (2007). Human neuroblasts migrate to the olfactory bulb via a lateral ventricular extension. Science 315, 1243–1249. doi: 10.1126/science.1136281
Dennis, C. V., Suh, L. S., Rodriguez, M. L., Kril, J. J., and Sutherland, G. T. (2016). Human adult neurogenesis across the ages: an immunohistochemical study. Neuropathol. Appl. Neurobiol. 42, 621–638. doi: 10.1111/nan.12337
Deuse, T., Hu, X., Gravina, A., Wang, D., Tediashvili, G., De, C., et al. (2019). Hypoimmunogenic derivatives of induced pluripotent stem cells evade immune rejection in fully immunocompetent allogeneic recipients. Nat. Biotechnol. 37, 252–258. doi: 10.1038/s41587-019-0016-3
Doetsch, F., Caille, I., Lim, D. A., Garcia-Verdugo, J. M., and Alvarez-Buylla, A. (1999). Subventricular zone astrocytes are neural stem cells in the adult mammalian brain. Cell 97, 703–716. doi: 10.1016/s0092-8674(00)80783-7
Dyer, M. A., and Cepko, C. L. (2000). Control of Muller glial cell proliferation and activation following retinal injury. Nat. Neurosci. 3, 873–880. doi: 10.1038/78774
Eckenhoff, M. F., and Rakic, P. (1988). Nature and fate of proliferative cells in the hippocampal dentate gyrus during the life span of the rhesus monkey. J. Neurosci. 8, 2729–2747. doi: 10.1523/jneurosci.08-08-02729.1988
El Waly, B., Cayre, M., and Durbec, P. (2018). Promoting myelin repair through in vivo neuroblast reprogramming. Stem Cell Rep. 10, 1492–1504. doi: 10.1016/j.stemcr.2018.02.015
Elewa, A., Wang, H., Talavera-Lopez, C., Joven, A., Brito, G., Kumar, A., et al. (2017). Reading and editing the Pleurodeles waltl genome reveals novel features of tetrapod regeneration. Nat. Commun. 8:2286. doi: 10.1038/s41467-017-01964-9
Encinas, J. M., Michurina, T. V., Peunova, N., Park, J. H., Tordo, J., Peterson, D. A., et al. (2011). Division-coupled astrocytic differentiation and age-related depletion of neural stem cells in the adult hippocampus. Cell Stem Cell 8, 566–579. doi: 10.1016/j.stem.2011.03.010
Eriksson, P. S., Perfilieva, E., Bjork-Eriksson, T., Alborn, A. M., Nordborg, C., Peterson, D. A., et al. (1998). Neurogenesis in the adult human hippocampus. Nat. Med. 4, 1313–1317.
Ernst, A., Alkass, K., Bernard, S., Salehpour, M., Perl, S., Tisdale, J., et al. (2014). Neurogenesis in the striatum of the adult human brain. Cell 156, 1072–1083. doi: 10.1016/j.cell.2014.01.044
Espuny-Camacho, I., Arranz, A. M., Fiers, M., Snellinx, A., Ando, K., Munck, S., et al. (2017). Hallmarks of Alzheimer’s disease in stem-cell-derived human neurons transplanted into mouse brain. Neuron 93, 1066.e8–1081.e8. doi: 10.1016/j.neuron.2017.02.001
Evans, M. J., and Kaufman, M. H. (1981). Establishment in culture of pluripotential cells from mouse embryos. Nature 292, 154–156. doi: 10.1038/292154a0
Faedo, A., Laporta, A., Segnali, A., Galimberti, M., Besusso, D., Cesana, E., et al. (2017). Differentiation of human telencephalic progenitor cells into MSNs by inducible expression of Gsx2 and Ebf1. Proc. Natl. Acad. Sci. U.S.A. 114, E1234–E1242. doi: 10.1073/pnas.1611473114
Falkner, S., Grade, S., Dimou, L., Conzelmann, K. K., Bonhoeffer, T., Gotz, M., et al. (2016). Transplanted embryonic neurons integrate into adult neocortical circuits. Nature 539, 248–253. doi: 10.1038/nature20113
Freed, C. R., Greene, P. E., Breeze, R. E., Tsai, W. Y., DuMouchel, W., Kao, R., et al. (2001). Transplantation of embryonic dopamine neurons for severe Parkinson’s disease. N. Engl. J. Med. 344, 710–719. doi: 10.1056/nejm200103083441002
Fuentealba, L. C., Rompani, S. B., Parraguez, J. I., Obernier, K., Romero, R., Cepko, C. L., et al. (2015). Embryonic origin of postnatal neural stem cells. Cell 161, 1644–1655. doi: 10.1016/j.cell.2015.05.041
Fujiwara, N., Shimizu, J., Takai, K., Arimitsu, N., Saito, A., Kono, T., et al. (2013). Restoration of spatial memory dysfunction of human APP transgenic mice by transplantation of neuronal precursors derived from human iPS cells. Neurosci. Lett. 557(Pt B), 129–134. doi: 10.1016/j.neulet.2013.10.043
Gage, F. H., Bjorklund, A., Stenevi, U., Dunnett, S. B., and Kelly, P. A. (1984). Intrahippocampal septal grafts ameliorate learning impairments in aged rats. Science 225, 533–536. doi: 10.1126/science.6539949
Gaillard, A., Prestoz, L., Dumartin, B., Cantereau, A., Morel, F., Roger, M., et al. (2007). Reestablishment of damaged adult motor pathways by grafted embryonic cortical neurons. Nat. Neurosci. 10, 1294–1299. doi: 10.1038/nn1970
Ganz, J., Kaslin, J., Hochmann, S., Freudenreich, D., and Brand, M. (2010). Heterogeneity and Fgf dependence of adult neural progenitors in the zebrafish telencephalon. Glia 58, 1345–1363. doi: 10.1002/glia.21012
Garcia, K. O., Ornellas, F. L., Martin, P. K., Patti, C. L., Mello, L. E., Frussa-Filho, R., et al. (2014). Therapeutic effects of the transplantation of VEGF overexpressing bone marrow mesenchymal stem cells in the hippocampus of murine model of Alzheimer’s disease. Front. Aging Neurosci. 6:30. doi: 10.3389/fnagi.2014.00030
Goldman, S. A., and Nottebohm, F. (1983). Neuronal production, migration, and differentiation in a vocal control nucleus of the adult female canary brain. Proc. Natl. Acad. Sci. U.S.A. 80, 2390–2394. doi: 10.1073/pnas.80.8.2390
Grandel, H., Kaslin, J., Ganz, J., Wenzel, I., and Brand, M. (2006). Neural stem cells and neurogenesis in the adult zebrafish brain: origin, proliferation dynamics, migration and cell fate. Dev. Biol. 295, 263–277. doi: 10.1016/j.ydbio.2006.03.040
Grealish, S., Diguet, E., Kirkeby, A., Mattsson, B., Heuer, A., Bramoulle, Y., et al. (2014). Human ESC-derived dopamine neurons show similar preclinical efficacy and potency to fetal neurons when grafted in a rat model of Parkinson’s disease. Cell Stem Cell 15, 653–665. doi: 10.1016/j.stem.2014.09.017
Guo, Z., Zhang, L., Wu, Z., Chen, Y., Wang, F., and Chen, G. (2014). In vivo direct reprogramming of reactive glial cells into functional neurons after brain injury and in an Alzheimer’s disease model. Cell Stem Cell 14, 188–202. doi: 10.1016/j.stem.2013.12.001
Gurdon, J. B. (1962). The developmental capacity of nuclei taken from intestinal epithelium cells of feeding tadpoles. J. Embryol. Exp. Morphol. 10, 622–640.
Hagell, P., Piccini, P., Bjorklund, A., Brundin, P., Rehncrona, S., Widner, H., et al. (2002). Dyskinesias following neural transplantation in Parkinson’s disease. Nat. Neurosci. 5, 627–628.
Hallett, P. J., Deleidi, M., Astradsson, A., Smith, G. A., Cooper, O., Osborn, T. M., et al. (2015). Successful function of autologous iPSC-derived dopamine neurons following transplantation in a non-human primate model of Parkinson’s disease. Cell Stem Cell 16, 269–274. doi: 10.1016/j.stem.2015.01.018
Hargus, G., Cooper, O., Deleidi, M., Levy, A., Lee, K., Marlow, E., et al. (2010). Differentiated Parkinson patient-derived induced pluripotent stem cells grow in the adult rodent brain and reduce motor asymmetry in Parkinsonian rats. Proc. Natl. Acad. Sci. U.S.A. 107, 15921–15926. doi: 10.1073/pnas.1010209107
Hauser, R. A., Freeman, T. B., Snow, B. J., Nauert, M., Gauger, L., Kordower, J. H., et al. (1999). Long-term evaluation of bilateral fetal nigral transplantation in Parkinson disease. Arch. Neurol. 56, 179–187.
Hauser, R. A., Sandberg, P. R., Freeman, T. B., and Stoessl, A. J. (2002). Bilateral human fetal striatal transplantation in Huntington’s disease. Neurology 58, 687–695. doi: 10.1212/wnl.58.5.687
Hellmann, M. A., Panet, H., Barhum, Y., Melamed, E., and Offen, D. (2006). Increased survival and migration of engrafted mesenchymal bone marrow stem cells in 6-hydroxydopamine-lesioned rodents. Neurosci. Lett. 395, 124–128. doi: 10.1016/j.neulet.2005.10.097
Heman-Ackah, S. M., Bassett, A. R., and Wood, M. J. (2016). Precision modulation of neurodegenerative disease-related gene expression in human iPSC-derived neurons. Sci. Rep. 6:28420. doi: 10.1038/srep28420
Hoglinger, G. U., Rizk, P., Muriel, M. P., Duyckaerts, C., Oertel, W. H., Caille, I., et al. (2004). Dopamine depletion impairs precursor cell proliferation in Parkinson disease. Nat. Neurosci. 7, 726–735. doi: 10.1038/nn1265
Howe, K., Clark, M. D., Torroja, C. F., Torrance, J., Berthelot, C., Muffato, M., et al. (2013). The zebrafish reference genome sequence and its relationship to the human genome. Nature 496, 498–503.
Imayoshi, I., Sakamoto, M., Ohtsuka, T., Takao, K., Miyakawa, T., Yamaguchi, M., et al. (2008). Roles of continuous neurogenesis in the structural and functional integrity of the adult forebrain. Nat. Neurosci. 11, 1153–1161. doi: 10.1038/nn.2185
Imayoshi, I., Sakamoto, M., Yamaguchi, M., Mori, K., and Kageyama, R. (2010). Essential roles of Notch signaling in maintenance of neural stem cells in developing and adult brains. J. Neurosci. 30, 3489–3498. doi: 10.1523/JNEUROSCI.4987-09.2010
Irion, U., Krauss, J., and Nusslein-Volhard, C. (2014). Precise and efficient genome editing in zebrafish using the CRISPR/Cas9 system. Development 141, 4827–4830. doi: 10.1242/dev.115584
Isacson, O., Brundin, P., Kelly, P. A., Gage, F. H., and Bjorklund, A. (1984). Functional neuronal replacement by grafted striatal neurones in the ibotenic acid-lesioned rat striatum. Nature 311, 458–460. doi: 10.1038/311458a0
Jeon, I., Lee, N., Li, J. Y., Park, I. H., Park, K. S., Moon, J., et al. (2012). Neuronal properties, in vivo effects, and pathology of a Huntington’s disease patient-derived induced pluripotent stem cells. Stem Cells 30, 2054–2062. doi: 10.1002/stem.1135
Jin, K., Wang, X., Xie, L., Mao, X. O., Zhu, W., Wang, Y., et al. (2006). Evidence for stroke-induced neurogenesis in the human brain. Proc. Natl. Acad. Sci. U.S.A. 103, 13198–13202. doi: 10.1073/pnas.0603512103
Kam, M., Curtis, M. A., McGlashan, S. R., Connor, B., Nannmark, U., and Faull, R. L. (2009). The cellular composition and morphological organization of the rostral migratory stream in the adult human brain. J. Chem. Neuroanat. 37, 196–205. doi: 10.1016/j.jchemneu.2008.12.009
Kaplan, M. S., and Hinds, J. W. (1977). Neurogenesis in the adult rat: electron microscopic analysis of light radioautographs. Science 197, 1092–1094. doi: 10.1126/science.887941
Kendall, A. L., Rayment, F. D., Torres, E. M., Baker, H. F., Ridley, R. M., and Dunnett, S. B. (1998). Functional integration of striatal allografts in a primate model of Huntington’s disease. Nat. Med. 4, 727–729. doi: 10.1038/nm0698-727
Kikuchi, T., Morizane, A., Doi, D., Magotani, H., Onoe, H., Hayashi, T., et al. (2017a). Human iPS cell-derived dopaminergic neurons function in a primate Parkinson’s disease model. Nature 548, 592–596. doi: 10.1038/nature23664
Kikuchi, T., Morizane, A., Doi, D., Okita, K., Nakagawa, M., Yamakado, H., et al. (2017b). Idiopathic Parkinson’s disease patient-derived induced pluripotent stem cells function as midbrain dopaminergic neurons in rodent brains. J. Neurosci. Res. 95, 1829–1837. doi: 10.1002/jnr.24014
Kim, H., Kim, H. Y., Choi, M. R., Hwang, S., Nam, K. H., Kim, H. C., et al. (2010). Dose-dependent efficacy of ALS-human mesenchymal stem cells transplantation into cisterna magna in SOD1-G93A ALS mice. Neurosci. Lett. 468, 190–194. doi: 10.1016/j.neulet.2009.10.074
Kim, K. S., Lee, H. J., An, J., Kim, Y. B., Ra, J. C., Lim, I., et al. (2014). Transplantation of human adipose tissue-derived stem cells delays clinical onset and prolongs life span in ALS mouse model. Cell Transplant. 23, 1585–1597. doi: 10.3727/096368913X673450
Kirkeby, A., Parmar, M., and Barker, R. A. (2017). Strategies for bringing stem cell-derived dopamine neurons to the clinic: a European approach (STEM-PD). Prog. Brain Res. 230, 165–190. doi: 10.1016/bs.pbr.2016.11.011
Kirkham, M., Hameed, L. S., Berg, D. A., Wang, H., and Simon, A. (2014). Progenitor cell dynamics in the Newt Telencephalon during homeostasis and neuronal regeneration. Stem Cell Rep. 2, 507–519. doi: 10.1016/j.stemcr.2014.01.018
Kizil, C., and Bhattarai, P. (2018). Is Alzheimer’s also a stem cell disease? - The zebrafish perspective. Front. Cell Dev. Biol. 6:159. doi: 10.3389/fcell.2018.00159
Kordower, J. H., Chu, Y., Hauser, R. A., Freeman, T. B., and Olanow, C. W. (2008). Lewy body-like pathology in long-term embryonic nigral transplants in Parkinson’s disease. Nat. Med. 14, 504–506. doi: 10.1038/nm1747
Kriks, S., Shim, J. W., Piao, J., Ganat, Y. M., Wakeman, D. R., Xie, Z., et al. (2011). Dopamine neurons derived from human ES cells efficiently engraft in animal models of Parkinson’s disease. Nature 480, 547–551. doi: 10.1038/nature10648
Lagace, D. C., Whitman, M. C., Noonan, M. A., Ables, J. L., DeCarolis, N. A., Arguello, A. A., et al. (2007). Dynamic contribution of nestin-expressing stem cells to adult neurogenesis. J. Neurosci. 27, 12623–12629. doi: 10.1523/jneurosci.3812-07.2007
Lee, S. T., Chu, K., Jung, K. H., Im, W. S., Park, J. E., Lim, H. C., et al. (2009). Slowed progression in models of Huntington disease by adipose stem cell transplantation. Ann. Neurol. 66, 671–681. doi: 10.1002/ana.21788
Li, W., Englund, E., Widner, H., Mattsson, B., van Westen, D., Latt, J., et al. (2016). Extensive graft-derived dopaminergic innervation is maintained 24 years after transplantation in the degenerating parkinsonian brain. Proc. Natl. Acad. Sci. U.S.A. 113, 6544–6549. doi: 10.1073/pnas.1605245113
Lin, Y. T., Chern, Y., Shen, C. K., Wen, H. L., Chang, Y. C., Li, H., et al. (2011). Human mesenchymal stem cells prolong survival and ameliorate motor deficit through trophic support in Huntington’s disease mouse models. PLoS One 6:e22924. doi: 10.1371/journal.pone.0022924
Lindvall, O., Brundin, P., Widner, H., Rehncrona, S., Gustavii, B., Frackowiak, R., et al. (1990). Grafts of fetal dopamine neurons survive and improve motor function in Parkinson’s disease. Science 247, 574–577. doi: 10.1126/science.2105529
Livneh, Y., Adam, Y., and Mizrahi, A. (2014). Odor processing by adult-born neurons. Neuron 81, 1097–1110. doi: 10.1016/j.neuron.2014.01.007
Lois, C., and Alvarez-Buylla, A. (1993). Proliferating subventricular zone cells in the adult mammalian forebrain can differentiate into neurons and glia. Proc. Natl. Acad. Sci. U.S.A. 90, 2074–2077. doi: 10.1073/pnas.90.5.2074
Low, W. C., Lewis, P. R., Bunch, S. T., Dunnett, S. B., Thomas, S. R., Iversen, S. D., et al. (1982). Function recovery following neural transplantation of embryonic septal nuclei in adult rats with septohippocampal lesions. Nature 300, 260–262. doi: 10.1038/300260a0
Lugert, S., Basak, O., Knuckles, P., Haussler, U., Fabel, K., Gotz, M., et al. (2010). Quiescent and active hippocampal neural stem cells with distinct morphologies respond selectively to physiological and pathological stimuli and aging. Cell Stem Cell 6, 445–456. doi: 10.1016/j.stem.2010.03.017
Luskin, M. B. (1993). Restricted proliferation and migration of postnatally generated neurons derived from the forebrain subventricular zone. Neuron 11, 173–189. doi: 10.1016/0896-6273(93)90281-u
Ma, L., Hu, B., Liu, Y., Vermilyea, S. C., Liu, H., Gao, L., et al. (2012). Human embryonic stem cell-derived GABA neurons correct locomotion deficits in quinolinic acid-lesioned mice. Cell Stem Cell 10, 455–464. doi: 10.1016/j.stem.2012.01.021
Maden, M., Manwell, L. A., and Ormerod, B. K. (2013). Proliferation zones in the axolotl brain and regeneration of the telencephalon. Neural Dev. 8:1. doi: 10.1186/1749-8104-8-1
Magnusson, J. P., Goritz, C., Tatarishvili, J., Dias, D. O., Smith, E. M., Lindvall, O., et al. (2014). A latent neurogenic program in astrocytes regulated by Notch signaling in the mouse. Science 346, 237–241. doi: 10.1126/science.346.6206.237
Mali, P., Yang, L., Esvelt, K. M., Aach, J., Guell, M., DiCarlo, J. E., et al. (2013). RNA-guided human genome engineering via Cas9. Science 339, 823–826. doi: 10.1126/science.1232033
Martin, G. R. (1981). Isolation of a pluripotent cell line from early mouse embryos cultured in medium conditioned by teratocarcinoma stem cells. Proc. Natl. Acad. Sci. U.S.A. 78, 7634–7638. doi: 10.1073/pnas.78.12.7634
Maruska, K. P., Carpenter, R. E., and Fernald, R. D. (2012). Characterization of cell proliferation throughout the brain of the African cichlid fish Astatotilapia burtoni and its regulation by social status. J. Comp. Neurol. 520, 3471–3491. doi: 10.1002/cne.23100
Merkle, F. T., Fuentealba, L. C., Sanders, T. A., Magno, L., Kessaris, N., and Alvarez-Buylla, A. (2014). Adult neural stem cells in distinct microdomains generate previously unknown interneuron types. Nat. Neurosci. 17, 207–214. doi: 10.1038/nn.3610
Moore, S. F., Guzman, N. V., Mason, S. L., Williams-Gray, C. H., and Barker, R. A. (2014). Which patients with Parkinson’s disease participate in clinical trials? One centre’s experiences with a new cell based therapy trial (TRANSEURO). J. Parkinsons Dis. 4, 671–676. doi: 10.3233/JPD-140432
Moreno-Jimenez, E. P., Flor-Garcia, M., Terreros-Roncal, J., Rabano, A., Cafini, F., Pallas-Bazarra, N., et al. (2019). Adult hippocampal neurogenesis is abundant in neurologically healthy subjects and drops sharply in patients with Alzheimer’s disease. Nat. Med. 25, 554–560. doi: 10.1038/s41591-019-0375-9
Mu, S., Wang, J., Zhou, G., Peng, W., He, Z., Zhao, Z., et al. (2014). Transplantation of induced pluripotent stem cells improves functional recovery in Huntington’s disease rat model. PLoS One 9:e101185. doi: 10.1371/journal.pone.0101185
Nakatsuji, N., Nakajima, F., and Tokunaga, K. (2008). HLA-haplotype banking and iPS cells. Nat. Biotechnol. 26, 739–740. doi: 10.1038/nbt0708-739
Nam, H. S., and Benezra, R. (2009). High levels of Id1 expression define B1 type adult neural stem cells. Cell Stem Cell 5, 515–526. doi: 10.1016/j.stem.2009.08.017
Naritsuka, H., Sakai, K., Hashikawa, T., Mori, K., and Yamaguchi, M. (2009). Perisomatic-targeting granule cells in the mouse olfactory bulb. J. Comp. Neurol. 515, 409–426. doi: 10.1002/cne.22063
Newman, M., Ebrahimie, E., and Lardelli, M. (2014). Using the zebrafish model for Alzheimer’s disease research. Front. Genet. 5:189. doi: 10.3389/fgene.2014.00189
Niu, W., Zang, T., Zou, Y., Fang, S., Smith, D. K., Bachoo, R., et al. (2013). In vivo reprogramming of astrocytes to neuroblasts in the adult brain. Nat. Cell Biol. 15, 1164–1175. doi: 10.1038/ncb2843
Niv, F., Keiner, S., Krishna, Witte, O. W., Lie, D. C., and Redecker, C. (2012). Aberrant neurogenesis after stroke: a retroviral cell labeling study. Stroke 43, 2468–2475. doi: 10.1161/STROKEAHA.112.660977
Nizzardo, M., Simone, C., Rizzo, F., Ruggieri, M., Salani, S., Riboldi, G., et al. (2014). Minimally invasive transplantation of iPSC-derived ALDHhiSSCloVLA4+ neural stem cells effectively improves the phenotype of an amyotrophic lateral sclerosis model. Hum. Mol. Genet. 23, 342–354. doi: 10.1093/hmg/ddt425
Nottebohm, F. (1981). A brain for all seasons: cyclical anatomical changes in song control nuclei of the canary brain. Science 214, 1368–1370. doi: 10.1126/science.7313697
Nottebohm, F., and Arnold, A. P. (1976). Sexual dimorphism in vocal control areas of the songbird brain. Science 194, 211–213. doi: 10.1126/science.959852
Nowoshilow, S., Schloissnig, S., Fei, J. F., Dahl, A., Pang, A. W. C., Pippel, M., et al. (2018). The axolotl genome and the evolution of key tissue formation regulators. Nature 554, 50–55. doi: 10.1038/nature25458
Olanow, C. W., Goetz, C. G., Kordower, J. H., Stoessl, A. J., Sossi, V., Brin, M. F., et al. (2003). A double-blind controlled trial of bilateral fetal nigral transplantation in Parkinson’s disease. Ann. Neurol. 54, 403–414. doi: 10.1002/ana.10720
Ortiz-Virumbrales, M., Moreno, C. L., Kruglikov, I., Marazuela, P., Sproul, A., Jacob, S., et al. (2017). CRISPR/Cas9-Correctable mutation-related molecular and physiological phenotypes in iPSC-derived Alzheimer’s PSEN2 (N141I) neurons. Acta Neuropathol. Commun. 5:77. doi: 10.1186/s40478-017-0475-z
Palmer, T. D., Ray, J., and Gage, F. H. (1995). FGF-2-responsive neuronal progenitors reside in proliferative and quiescent regions of the adult rodent brain. Mol. Cell. Neurosci. 6, 474–486. doi: 10.1006/mcne.1995.1035
Palmer, T. D., Schwartz, P. H., Taupin, P., Kaspar, B., Stein, S. A., and Gage, F. H. (2001). Cell culture. Progenitor cells from human brain after death. Nature 411, 42–43. doi: 10.1038/35075141
Parish, C. L., Beljajeva, A., Arenas, E., and Simon, A. (2007). Midbrain dopaminergic neurogenesis and behavioural recovery in a salamander lesion-induced regeneration model. Development 134, 2881–2887. doi: 10.1242/dev.002329
Park, H., Oh, J., Shim, G., Cho, B., Chang, Y., Kim, S., et al. (2019). In vivo neuronal gene editing via CRISPR-Cas9 amphiphilic nanocomplexes alleviates deficits in mouse models of Alzheimer’s disease. Nat. Neurosci. 22, 524–528. doi: 10.1038/s41593-019-0352-0
Park, I. H., Zhao, R., West, J. A., Yabuuchi, A., Huo, H., Ince, T. A., et al. (2008). Reprogramming of human somatic cells to pluripotency with defined factors. Nature 451, 141–146. doi: 10.1038/nature06534
Paton, J. A., and Nottebohm, F. N. (1984). Neurons generated in the adult brain are recruited into functional circuits. Science 225, 1046–1048. doi: 10.1126/science.6474166
Pereira, M., Birtele, M., Shrigley, S., Benitez, J. A., Hedlund, E., Parmar, M., et al. (2017). Direct Reprogramming of Resident NG2 Glia into Neurons with Properties of Fast-Spiking Parvalbumin-Containing Interneurons. Stem Cell Rep. 9, 742–751. doi: 10.1016/j.stemcr.2017.07.023
Perlow, M. J., Freed, W. J., Hoffer, B. J., Seiger, A., Olson, L., and Wyatt, R. J. (1979). Brain grafts reduce motor abnormalities produced by destruction of nigrostriatal dopamine system. Science 204, 643–647. doi: 10.1126/science.571147
Peschanski, M., Cesaro, P., and Hantraye, P. (1995). Rationale for intrastriatal grafting of striatal neuroblasts in patients with Huntington’s disease. Neuroscience 68, 273–285. doi: 10.1016/0306-4522(95)00162-c
Piccini, P., Brooks, D. J., Bjorklund, A., Gunn, R. N., Grasby, P. M., Rimoldi, O., et al. (1999). Dopamine release from nigral transplants visualized in vivo in a Parkinson’s patient. Nat. Neurosci. 2, 1137–1140. doi: 10.1038/16060
Pilz, G. A., Bottes, S., Betizeau, M., Jorg, D. J., Carta, S., Simons, B. D., et al. (2018). Live imaging of neurogenesis in the adult mouse hippocampus. Science 359, 658–662. doi: 10.1126/science.aao5056
Politis, M., Wu, K., Loane, C., Quinn, N. P., Brooks, D. J., Rehncrona, S., et al. (2010). Serotonergic neurons mediate dyskinesia side effects in Parkinson’s patients with neural transplants. Sci. Transl. Med. 2:38ra46. doi: 10.1126/scitranslmed.3000976
Popescu, I. R., Nicaise, C., Liu, S., Bisch, G., Knippenberg, S., Daubie, V., et al. (2013). Neural progenitors derived from human induced pluripotent stem cells survive and differentiate upon transplantation into a rat model of amyotrophic lateral sclerosis. Stem Cells Transl. Med. 2, 167–174. doi: 10.5966/sctm.2012-0042
Pun, R. Y., Rolle, I. J., Lasarge, C. L., Hosford, B. E., Rosen, J. M., Uhl, J. D., et al. (2012). Excessive activation of mTOR in postnatally generated granule cells is sufficient to cause epilepsy. Neuron 75, 1022–1034. doi: 10.1016/j.neuron.2012.08.002
Rakic, P. (1985). Limits of neurogenesis in primates. Science 227, 1054–1056. doi: 10.1126/science.3975601
Ramachandran, R., Zhao, X. F., and Goldman, D. (2011). Ascl1a/Dkk/beta-catenin signaling pathway is necessary and glycogen synthase kinase-3beta inhibition is sufficient for zebrafish retina regeneration. Proc. Natl. Acad. Sci. U.S.A. 108, 15858–15863. doi: 10.1073/pnas.1107220108
Redmond, D. E. Jr., Naftolin, F., Collier, T. J., Leranth, C., Robbins, R. J., Sladek, C. D., et al. (1988). Cryopreservation, culture, and transplantation of human fetal mesencephalic tissue into monkeys. Science 242, 768–771. doi: 10.1126/science.2903552
Reif, A., Fritzen, S., Finger, M., Strobel, A., Lauer, M., Schmitt, A., et al. (2006). Neural stem cell proliferation is decreased in schizophrenia, but not in depression. Mol. Psychiatry 11, 514–522. doi: 10.1038/sj.mp.4001791
Reinhardt, P., Schmid, B., Burbulla, L. F., Schondorf, D. C., Wagner, L., Glatza, M., et al. (2013). Genetic correction of a LRRK2 mutation in human iPSCs links parkinsonian neurodegeneration to ERK-dependent changes in gene expression. Cell Stem Cell 12, 354–367. doi: 10.1016/j.stem.2013.01.008
Reubinoff, B. E., Pera, M. F., Fong, C. Y., Trounson, A., and Bongso, A. (2000). Embryonic stem cell lines from human blastocysts: somatic differentiation in vitro. Nat. Biotechnol. 18, 399–404. doi: 10.1038/74447
Reynolds, B. A., and Weiss, S. (1992). Generation of neurons and astrocytes from isolated cells of the adult mammalian central nervous system. Science 255, 1707–1710. doi: 10.1126/science.1553558
Rivetti di Val Cervo, P., Romanov, R. A., Spigolon, G., Masini, D., et al. (2017). Induction of functional dopamine neurons from human astrocytes in vitro and mouse astrocytes in a Parkinson’s disease model. Nat. Biotechnol. 35, 444–452. doi: 10.1038/nbt.3835
Rodriguez Viales, R., Diotel, N., Ferg, M., Armant, O., Eich, J., Alunni, A., et al. (2015). The helix-loop-helix protein id1 controls stem cell proliferation during regenerative neurogenesis in the adult zebrafish telencephalon. Stem Cells 33, 892–903. doi: 10.1002/stem.1883
Rossi, S. L., Nistor, G., Wyatt, T., Yin, H. Z., Poole, A. J., Weiss, J. H., et al. (2010). Histological and functional benefit following transplantation of motor neuron progenitors to the injured rat spinal cord. PLoS One 5:e11852. doi: 10.1371/journal.pone.0011852
Rothenaigner, I., Krecsmarik, M., Hayes, J. A., Bahn, B., Lepier, A., Fortin, G., et al. (2011). Clonal analysis by distinct viral vectors identifies bona fide neural stem cells in the adult zebrafish telencephalon and characterizes their division properties and fate. Development 138, 1459–1469. doi: 10.1242/dev.058156
Roy, N. S., Wang, S., Jiang, L., Kang, J., Benraiss, A., Harrison-Restelli, C., et al. (2000). In vitro neurogenesis by progenitor cells isolated from the adult human hippocampus. Nat. Med. 6, 271–277. doi: 10.1038/73119
Sahay, A., and Hen, R. (2007). Adult hippocampal neurogenesis in depression. Nat. Neurosci. 10, 1110–1115. doi: 10.1038/nn1969
Samata, B., Doi, D., Nishimura, K., Kikuchi, T., Watanabe, A., Sakamoto, Y., et al. (2016). Purification of functional human ES and iPSC-derived midbrain dopaminergic progenitors using LRTM1. Nat. Commun. 7:13097. doi: 10.1038/ncomms13097
Sanai, N., Nguyen, T., Ihrie, R. A., Mirzadeh, Z., Tsai, H. H., Wong, M., et al. (2011). Corridors of migrating neurons in the human brain and their decline during infancy. Nature 478, 382–386. doi: 10.1038/nature10487
Sanai, N., Tramontin, A. D., Quinones-Hinojosa, A., Barbaro, N. M., Gupta, N., Kunwar, S., et al. (2004). Unique astrocyte ribbon in adult human brain contains neural stem cells but lacks chain migration. Nature 427, 740–744. doi: 10.1038/nature02301
Seaberg, R. M., and van der Kooy, D. (2002). Adult rodent neurogenic regions: the ventricular subependyma contains neural stem cells, but the dentate gyrus contains restricted progenitors. J. Neurosci. 22, 1784–1793. doi: 10.1523/jneurosci.22-05-01784.2002
Seri, B., Garcia-Verdugo, J. M., Collado-Morente, L., McEwen, B. S., and Alvarez-Buylla, A. (2004). Cell types, lineage, and architecture of the germinal zone in the adult dentate gyrus. J. Comp. Neurol. 478, 359–378. doi: 10.1002/cne.20288
Seri, B., Garcia-Verdugo, J. M., McEwen, B. S., and Alvarez-Buylla, A. (2001). Astrocytes give rise to new neurons in the adult mammalian hippocampus. J. Neurosci. 21, 7153–7160. doi: 10.1523/jneurosci.21-18-07153.2001
Shi, Y., Inoue, H., Wu, J. C., and Yamanaka, S. (2017). Induced pluripotent stem cell technology: a decade of progress. Nat. Rev. Drug Discov. 16, 115–130. doi: 10.1038/nrd.2016.245
Shin, J. Y., Park, H. J., Kim, H. N., Oh, S. H., Bae, J. S., Ha, H. J., et al. (2014). Mesenchymal stem cells enhance autophagy and increase beta-amyloid clearance in Alzheimer disease models. Autophagy 10, 32–44. doi: 10.4161/auto.26508
Sirko, S., Behrendt, G., Johansson, P. A., Tripathi, P., Costa, M., Bek, S., et al. (2013). Reactive glia in the injured brain acquire stem cell properties in response to sonic hedgehog. [corrected]. Cell Stem Cell 12, 426–439. doi: 10.1016/j.stem.2013.01.019
Skaggs, K., Goldman, D., and Parent, J. M. (2014). Excitotoxic brain injury in adult zebrafish stimulates neurogenesis and long-distance neuronal integration. Glia 62, 2061–2079. doi: 10.1002/glia.22726
Soldner, F., Laganiere, J., Cheng, A. W., Hockemeyer, D., Gao, Q., Alagappan, R., et al. (2011). Generation of isogenic pluripotent stem cells differing exclusively at two early onset Parkinson point mutations. Cell 146, 318–331. doi: 10.1016/j.cell.2011.06.019
Soldner, F., Stelzer, Y., Shivalila, C. S., Abraham, B. J., Latourelle, J. C., Barrasa, M. I., et al. (2016). Parkinson-associated risk variant in distal enhancer of alpha-synuclein modulates target gene expression. Nature 533, 95–99. doi: 10.1038/nature17939
Sorrells, S. F., Paredes, M. F., Cebrian-Silla, A., Sandoval, K., Qi, D., Kelley, K. W., et al. (2018). Human hippocampal neurogenesis drops sharply in children to undetectable levels in adults. Nature 555, 377–381. doi: 10.1038/nature25975
Spalding, K. L., Bergmann, O., Alkass, K., Bernard, S., Salehpour, M., Huttner, H. B., et al. (2013). Dynamics of hippocampal neurogenesis in adult humans. Cell 153, 1219–1227. doi: 10.1016/j.cell.2013.05.002
Spalding, K. L., Bhardwaj, R. D., Buchholz, B. A., Druid, H., and Frisen, J. (2005). Retrospective birth dating of cells in humans. Cell 122, 133–143. doi: 10.1016/j.cell.2005.04.028
Spencer, D. D., Robbins, R. J., Naftolin, F., Marek, K. L., Vollmer, T., Leranth, C., et al. (1992). Unilateral transplantation of human fetal mesencephalic tissue into the caudate nucleus of patients with Parkinson’s disease. N. Engl. J. Med. 327, 1541–1548. doi: 10.1056/nejm199211263272201
Steinbeck, J. A., Choi, S. J., Mrejeru, A., Ganat, Y., Deisseroth, K., Sulzer, D., et al. (2015). Optogenetics enables functional analysis of human embryonic stem cell-derived grafts in a Parkinson’s disease model. Nat. Biotechnol. 33, 204–209. doi: 10.1038/nbt.3124
Su, Z., Niu, W., Liu, M. L., Zou, Y., and Zhang, C. L. (2014). In vivo conversion of astrocytes to neurons in the injured adult spinal cord. Nat. Commun. 5:3338. doi: 10.1038/ncomms4338
Takahashi, K., and Yamanaka, S. (2006). Induction of pluripotent stem cells from mouse embryonic and adult fibroblast cultures by defined factors. Cell 126, 663–676. doi: 10.1016/j.cell.2006.07.024
Thomson, J. A., Itskovitz-Eldor, J., Shapiro, S. S., Waknitz, M. A., Swiergiel, J. J., Marshall, V. S., et al. (1998). Embryonic stem cell lines derived from human blastocysts. Science 282, 1145–1147. doi: 10.1126/science.282.5391.1145
Thu, D. C., Oorschot, D. E., Tippett, L. J., Nana, A. L., Hogg, V. M., Synek, B. J., et al. (2010). Cell loss in the motor and cingulate cortex correlates with symptomatology in Huntington’s disease. Brain 133(Pt 4), 1094–1110. doi: 10.1093/brain/awq047
Tobin, M. K., Musaraca, K., Disouky, A., Shetti, A., Bheri, A., Honer, W. G., et al. (2019). Human hippocampal neurogenesis persists in aged adults and Alzheimer’s disease patients. Cell Stem Cell 24, 974.e3–982.e3.
Torper, O., Ottosson, D. R., Pereira, M., Lau, S., Cardoso, T., Grealish, S., et al. (2015). In vivo reprogramming of striatal NG2 glia into functional neurons that integrate into local host circuitry. Cell Rep. 12, 474–481. doi: 10.1016/j.celrep.2015.06.040
Torper, O., Pfisterer, U., Wolf, D. A., Pereira, M., Lau, S., Jakobsson, J., et al. (2013). Generation of induced neurons via direct conversion in vivo. Proc. Natl. Acad. Sci. U.S.A. 110, 7038–7043. doi: 10.1073/pnas.1303829110
Ueki, Y., Wilken, M. S., Cox, K. E., Chipman, L., Jorstad, N., Sternhagen, K., et al. (2015). Transgenic expression of the proneural transcription factor Ascl1 in Muller glia stimulates retinal regeneration in young mice. Proc. Natl. Acad. Sci. U.S.A. 112, 13717–13722. doi: 10.1073/pnas.1510595112
Urban, N., van den Berg, D. L., Forget, A., Andersen, J., Demmers, J. A., Hunt, C., et al. (2016). Return to quiescence of mouse neural stem cells by degradation of a proactivation protein. Science 353, 292–295. doi: 10.1126/science.aaf4802
van Tijn, P., Kamphuis, W., Marlatt, M. W., Hol, E. M., and Lucassen, P. J. (2011). Presenilin mouse and zebrafish models for dementia: focus on neurogenesis. Prog. Neurobiol. 93, 149–164. doi: 10.1016/j.pneurobio.2010.10.008
Wictorin, K., Ouimet, C. C., and Bjorklund, A. (1989). Intrinsic organization and connectivity of intrastriatal striatal transplants in rats as revealed by DARPP-32 immunohistochemistry: specificity of connections with the lesioned host brain. Eur. J. Neurosci. 1, 690–701. doi: 10.1111/j.1460-9568.1989.tb00375.x
Winner, B., Cooper-Kuhn, C. M., Aigner, R., Winkler, J., and Kuhn, H. G. (2002). Long-term survival and cell death of newly generated neurons in the adult rat olfactory bulb. Eur. J. Neurosci. 16, 1681–1689. doi: 10.1046/j.1460-9568.2002.02238.x
Winner, B., Kohl, Z., and Gage, F. H. (2011). Neurodegenerative disease and adult neurogenesis. Eur. J. Neurosci. 33, 1139–1151. doi: 10.1111/j.1460-9568.2011.07613.x
Wyatt, T. J., Rossi, S. L., Siegenthaler, M. M., Frame, J., Robles, R., Nistor, G., et al. (2011). Human motor neuron progenitor transplantation leads to endogenous neuronal sparing in 3 models of motor neuron loss. Stem Cells Int. 2011, 207230. doi: 10.4061/2011/207230
Xu, X., Tay, Y., Sim, B., Yoon, S. I., Huang, Y., Ooi, J., et al. (2017). Reversal of phenotypic abnormalities by CRISPR/Cas9-mediated gene correction in huntington disease patient-derived induced pluripotent stem cells. Stem Cell Rep. 8, 619–633. doi: 10.1016/j.stemcr.2017.01.022
Yu, J., Vodyanik, M. A., Smuga-Otto, K., Antosiewicz-Bourget, J., Frane, J. L., Tian, S., et al. (2007). Induced pluripotent stem cell lines derived from human somatic cells. Science 318, 1917–1920.
Keywords: neural stem cells, transplantation, adult neurogenesis, neurological disorders, genome-editing
Citation: Chohan MO (2020) Deconstructing Neurogenesis, Transplantation and Genome-Editing as Neural Repair Strategies in Brain Disease. Front. Cell Dev. Biol. 8:116. doi: 10.3389/fcell.2020.00116
Received: 02 December 2019; Accepted: 11 February 2020;
Published: 13 March 2020.
Edited by:
Luis B. Tovar-y-Romo, National Autonomous University of Mexico, MexicoReviewed by:
Caghan Kizil, German Center for Neurodegenerative Diseases (DZNE), GermanyCopyright © 2020 Chohan. This is an open-access article distributed under the terms of the Creative Commons Attribution License (CC BY). The use, distribution or reproduction in other forums is permitted, provided the original author(s) and the copyright owner(s) are credited and that the original publication in this journal is cited, in accordance with accepted academic practice. No use, distribution or reproduction is permitted which does not comply with these terms.
*Correspondence: Muhammad O. Chohan, bXVoYW1tYWQuY2hvaGFuQG55c3BpLmNvbHVtYmlhLmVkdQ==
Disclaimer: All claims expressed in this article are solely those of the authors and do not necessarily represent those of their affiliated organizations, or those of the publisher, the editors and the reviewers. Any product that may be evaluated in this article or claim that may be made by its manufacturer is not guaranteed or endorsed by the publisher.
Research integrity at Frontiers
Learn more about the work of our research integrity team to safeguard the quality of each article we publish.