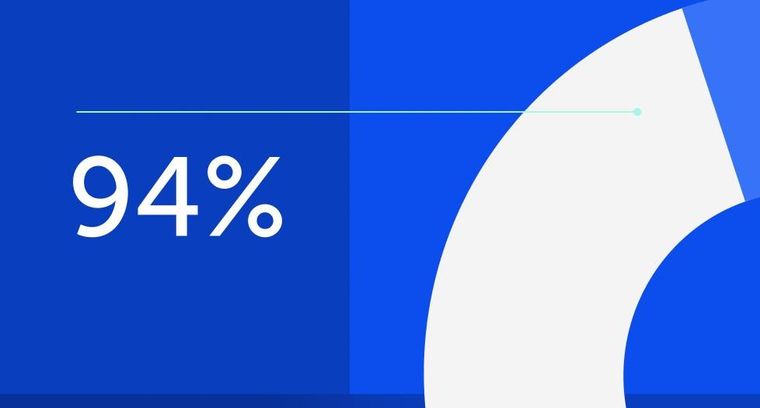
94% of researchers rate our articles as excellent or good
Learn more about the work of our research integrity team to safeguard the quality of each article we publish.
Find out more
REVIEW article
Front. Cell Dev. Biol., 26 February 2020
Sec. Cell Growth and Division
Volume 8 - 2020 | https://doi.org/10.3389/fcell.2020.00061
This article is part of the Research TopicSperm Differentiation and Spermatozoa Function: Mechanisms, Diagnostics, and TreatmentView all 21 articles
With the continued steep rise of the global human population, and the paucity of safe and practical contraceptive options available to men, the need for development of effective and reversible non-hormonal methods of male fertility control is widely recognized. Currently there are several contraceptive options available to men, however, none of the non-hormonal alternatives have been clinically approved. To advance progress in the development of a safe and reversible contraceptive for men, further identification of novel reproductive tract-specific druggable protein targets is required. Here we provide an overview of genes/proteins identified in the last decade as specific or highly expressed in the male reproductive tract, with deletion phenotypes leading to complete male infertility in mice. These phenotypes include arrest of spermatogenesis and/or spermiogenesis, abnormal spermiation, abnormal spermatid morphology, abnormal sperm motility, azoospermia, globozoospermia, asthenozoospermia, and/or teratozoospermia, which are all desirable outcomes for a novel male contraceptive. We also consider other associated deletion phenotypes that could impact the desirability of a potential contraceptive. We further discuss novel contraceptive targets underscoring promising leads with the objective of presenting data for potential druggability and whether collateral effects may exist from paralogs with close sequence similarity.
Currently, fertility control approaches for men fall into one of two categories: hormonal and non-hormonal. Several approaches have been investigated involving injectable or transdermal regimes using testosterone alone or combined with other molecules (Bagatell et al., 1993; Ilani et al., 2012). Although claims of total reversibility and full recovery to fertility have been made with hormonal contraception in males (Pasztor et al., 2017), prolonged use of exogenous hormones is associated with off-target effects, such as decreased high density lipoprotein cholesterol levels and potential cardiovascular risk in otherwise healthy men (Meriggiola et al., 1995). Therefore, there has been considerable interest in alternative methods for safe and reversible fertility control. Several non-hormonal methods are currently under development including gel-based obstruction of vas deferens, contraceptive vaccines, sperm-specific calcium ion channel blockers, and anti-spermatogenic indenopyridines with varied effectiveness and risks involved (Aitken, 2002; Hild et al., 2007; Morakinyo et al., 2009; Baggelaar et al., 2019).
Past efforts on the development of a safe non-hormonal contraceptive for men has resided on a diverse array of targets identified through both forward and reverse approaches. Forward approaches include identification of a target protein prior to drug development, while reverse approaches identify a drug with contraceptive effect prior to identification of the target protein. Often reverse approaches fail to identify specific drug targets with minimal side effects as discussed below.
Adjudin, a derivative of 1H-indazole-3-carboxylic acid, was shown to have potent anti-spermatogenic activity in rats, rabbits, and dogs prior to identification of its targets (Mok et al., 2011). Through further studies, it was later found to disrupt the Sertoli-germ cell junction proteins ACTB, ITGA6, ITGB1, MYH11, and OCLN (Mok et al., 2011, 2012; Mruk and Cheng, 2011). Unsurprisingly, administration of this drug resulted in significant toxicity, including effects on liver and skeletal muscle (Mruk et al., 2006) necessitating efforts to lower systemic toxicity by developing a conjugate capable of delivering Adjudin directly to the testis (Mruk et al., 2006), and by utilizing different formulations of the drug for its oral use to reduce the effective dose (Chen et al., 2016a). However, the efficacy of these approaches has yet to be determined.
H2-Gamendazole, an additional indazole carboxylic acid analog, blocks spermatogenesis by inhibiting production of inhibin B by primary Sertoli cells (Tash et al., 2008a), inhibiting HSP90AB1 and EEF1A1, and increasing Interleukin 1 Alpha expression in Sertoli cells (Tash et al., 2008b), which is a known disruptor of Sertoli cell-spermatid junction integrity (Nilsson et al., 1998). Although Gamendazole was shown to induce significant loss of spermatids (Tash et al., 2008b) and cause complete infertility after just a single dose, the damages to the seminiferous epithelium were not fully reversible (Tash et al., 2008a). The failure of some animals to regain fertility may have resulted from the combined effects of this drug on EEF1A1 and HSP90 in less differentiated spermatogenic cells. Therefore, further progress on this drug has been halted until these issues can be addressed. Generation of potentially safer analogs of gamendazole are currently under development (Kanishchev and Dolbier, 2018).
One major obstacle in the development of a safe, reversible, and non-hormonal male contraceptive is identifying small molecule inhibitors that can penetrate the highly selective blood–testis barrier (BTB), or Sertoli cell barrier (SCB). Established through a strong network of tight junction proteins between adjacent Sertoli cells, the BTB/SCB partitions the seminiferous epithelium into two compartments: basal and adluminal (lumen-facing) (Franca et al., 2016). The basal compartment contains the undifferentiated spermatogonia, differentiating spermatogonia, and early differentiating spermatocytes. Meanwhile, the lumen-facing compartment contains differentiated spermatocytes, and round and elongated spermatids that—due to homologous recombination during meiosis—are no longer recognized by the immune system and thus require an immune privileged location. Many molecules have been shown to not cross the BTB/SCB and are thus used as molecular tools for measuring an intact BTB/SCB in manipulated animal models (Chen et al., 2018).
To the best of our knowledge, the small-molecule inhibitor JQ1, which inhibits the bromodomain-containing, testis-specific protein, BRDT, is currently the only molecule that has been shown to be present beyond the BTB/SCB (Matzuk et al., 2012). By binding to the BRDT acetyl-lysine binding pocket, JQ1 disrupts spermatogenesis at the spermatocyte and round spermatid stages, producing a reversible contraceptive effect in mice (Matzuk et al., 2012) that phenocopies the male infertility phenotype of BRDT knockout mice (Shang et al., 2007). Development of JQ1 was done in a target-based drug discovery approach focused on finding inhibitors of BRD4, a close paralog of BRDT. JQ1 blocks the production of sperm in the testes by inhibiting BRDT, however, it also acts on other bromodomains (BRD) of the bromo-and-extra-terminal (BET) proteins known to regulate transcription and DNA repair, which include important BRDT paralogs with oncogenic effects (French et al., 2008). BRD4, has become known as an important therapeutic target in several types of cancers, including breast and prostate cancers, as well as glioblastoma multiforme (GMB) (Shi et al., 2014; Zhou et al., 2016; Wen et al., 2019). JQ1 possesses anti-tumor effects (Shao et al., 2014; Das et al., 2015) and has been identified as a promising inhibitor for treating GBM (Wen et al., 2019). Along with other related pan-BET BRD compounds JQ1 was also associated with improvement of associated memory and enhancement of special memory precision in mice, showing potential for treatment of dementia (Benito et al., 2017). Given the therapeutic potential of JQ1 significant efforts have been made to understand the mechanisms of its action on the BET BRD family, giving insight into the intricate protein–protein interactions (PPI) with transcriptional complexes, and most importantly, providing means for enhancing inhibition specificity for a single BET (Lambert et al., 2019).
The epididymis is a prime target for the development of a male contraceptive. This is because sperm leaving the testis are neither motile nor able to recognize or fertilize an egg; they must transit through the epididymis to acquire these abilities (Sullivan and Mieusset, 2016). Knockout mice of several epididymis-specific proteins have confirmed that the epididymis is essential for sperm maturation in the mouse (Davies et al., 2004; Roberts et al., 2006; Sipila et al., 2009). To the best of our knowledge, inhibitors that target an epididymis-specific protein do not yet exist. However, one of the most promising reversible, non-hormonal male contraceptives developed thus far has been against EPPIN (epididymal protease inhibitor; SPINLW1), which was developed through a reverse approach. EPPIN is a protein secreted by Sertoli cells and epithelial cells of the epididymis that gets deposited on the surface of maturing spermatozoa (Silva et al., 2012). The small molecule inhibitor EP055, which targets EPPIN, causes reversible infertility in primates (Silva et al., 2012) most likely by targeting the testes and epididymis where the drug can be found (O’Rand et al., 2018). In fact, deposition of EPPIN on the sperm surface appears to be greatest in the epididymis, indicating that the drug primarily acts in the epididymis (O’Rand et al., 2018). Current efforts are focused on increasing the half-life of the drug molecule (O’Rand et al., 2018).
Additionally, the inhibitors cyclosporine A and FK506, which are used as immunosuppressant drugs, target the sperm calcineurin subunits PPP3CC and PPP3R2 in the epididymis causing reversible effects on sperm morphology and motility (Miyata et al., 2015). Treatment of mice with cyclosporine A or FK506 creates phenocopies of the sperm motility and morphological defects apparent in knockout mice, which appear within 4 to 5 days of treatment and are reversed a week after discontinuation (Miyata et al., 2015). Unfortunately, cyclosporine A and FK506 are undesirable candidates due to their immunosuppressive effect (Stucker and Ackermann, 2011).
When evaluating potential druggability in a target-based drug discovery process, one must consider the protein properties that are required for safe and effective inhibition. Among the most significant is tissue expression specificity to minimize potential adverse effects; protein function and whether protein activity or interaction with other proteins is potentially druggable; sequence similarity to closely related paralogs that may be ubiquitously expressed; whether genetically manipulated animal models demonstrate a functional requirement for the target of interest; and other considerations as discussed below.
By ensuring in the first steps of development that candidate drug targets are near exclusively expressed in the male reproductive tract, the potential for off-target effects in humans is minimized. Gamendazole, for example, targets HSP90AB1 and EEF1A1, which are highly expressed in non-reproductive tissues (Djureinovic et al., 2014; Uhlen et al., 2015). Therefore, significant toxicity as evidenced through previous studies (Tash et al., 2008a, b) could have been predicted based on target gene expression analysis. Likewise, the toxicity of Adjudin has resulted in attempts to target the drug specifically to the testes through conjugation with FSH (Chen et al., 2016a), a complicated approach which may minimize, but not completely remove, off-target effects. Since the identified molecular targets of Adjudin are ACTB, ITGA6, ITGB1, MYH11, and OCLN (Mruk and Cheng, 2011; Mok et al., 2012), which are all widely expressed in non-reproductive organs (Djureinovic et al., 2014; Uhlen et al., 2015), likewise, the toxicity of Adjudin could have been predicted and avoided had the drug’s development began with a target-based discovery approach.
Protein druggability is often based on the protein family for which other members are known drug targets (Hopkins and Groom, 2002). For instance, an enzyme with a known binding site might be an easier target when compared to a novel protein that has not already been categorized. The development of an inhibiting drug for an uncategorized protein might seem challenging, however, disrupting PPI has recently been gaining attention as one of the possible methods. Generally, PPI are considered to be more challenging than traditional drug targets due to the smaller protein interfaces and difficulty with finding a sufficiently binding ligand capable of interrupting the interaction site at a suitable concentration (Whitty and Kumaravel, 2006). However, PPI targets are not deemed undruggable, based on the discovery of small molecules capable of deeper and higher affinity binding within the contact surfaces of the target protein (Wells and McClendon, 2007). Therefore, although not initially compelling, uncategorized genes have the likelihood of becoming potential drug targets by using druglike compounds that can modulate the PPI at multiple interface sites—increasing the ligand binding affinity—and consequently lowering the necessary drug dose administered (Fuller et al., 2009). Table 1 presents the list of male reproductive tract-specific genes expressed at various stages of spermatogenesis with assigned categories of protein families. Besides a handful of enzymes (HFM1, MOV10L1, PGK2, PRSS37, and LRGUK), two transcription factors (SOX30 and TERB1), a few epigenetically active proteins (SCML2 and TDRD5) and a sperm specific ion channel KCNU1, most of the proteins discussed in this review belong to an unknown category. However, the contraceptive potential of these genes should not be overseen, but rather investigated for the identification of a high-affinity small molecule that can either (1) interfere with PPI, or (2) target the protein specifically for degradation as discussed below.
Table 1. Recently identified reproductive tract-specific genes with male infertility phenotypes in mice: potential non-hormonal male contraceptive drug targets.
Many of the potential drug targets, such as non-enzymatic proteins, are uncategorized and identified as “undruggable” due to various challenges with existing targeting approaches. However, an emerging targeted protein degradation method called Proteolysis Targeting Chimeras (PROTACs) is a promising technique that can address these issues. The traditional approach to most enzymatic proteins serves to interfere with the functional aspect of the protein target, whereas PROTACs eliminate the protein through utilization of the ubiquitin-proteasome system to promote selective degradation (Bondeson et al., 2015; Lu et al., 2015; Olson et al., 2018). Thus, PROTACs do not require that an identified small molecule both bind with high affinity and reduce activity of the target protein (through interference of the binding pocket, etc.). PROTACs only requires that the identified small molecule bind with high affinity to the protein target. Additional design and chemistry are conducted to conjugate the small molecule to a high affinity E3 ubiquitin ligase ligand that results in target protein ubiquitination. There are currently various combinations of PROTACs developed to overcome the limitations of cell permeability, stability, solubility, selectivity, and tissue distribution (Brooks et al., 2005; Bechara and Sagan, 2013; Ottis et al., 2017; Bondeson et al., 2018). Therefore, PROTACs technology provides the potential to greatly promote the development of contraceptive drugs against the “undruggable” non-enzymatic protein targets.
The probability of two or more paralogs sharing a specific function increases with the percentage of sequence similarity (Zallot et al., 2016). If a reproductive tract-specific target is found to have high sequence similarity to a ubiquitously expressed protein or paralog, especially in the potentially druggable domain, this would make the target a poor choice for contraceptive development due to potential off-target effects. However, if a reproductive tract-specific protein has one or more ubiquitously expressed paralogs with low sequence similarity, this indicates that the function of the expressed proteins in the reproductive tract could be disrupted without significant off-target risks. Considerably high contraceptive potential resides in genes without a confirmed paralog; however, one must remain cautious in assuming a complete lack of paralogs as the druggable domain may be present in completely unrelated proteins, and further investigation should be conducted before proceeding to contraceptive drug development.
Mice serve as one of the most efficient and effective models to understanding human physiology for a variety of reasons. The mouse genome is very well-characterized with almost all genes sharing similar functions to human orthologs (Cheng et al., 2014; Lin et al., 2014). Mice are biologically very similar, yet because they are small and have very short lifespans compared to humans, developmental processes can be studied economically and at an accelerated rate. Genetically manipulated knockout mouse models have significantly advanced our understanding of male gamete differentiation and the molecular mechanisms underlying male fertility. Nevertheless, there is still much to be learned about these intricate processes considering only approximately half of the protein coding genes have been individually ablated in mice (Dickinson et al., 2016; Smith et al., 2018). By ensuring that a knockout animal model of an individual protein target of interest leads to a complete male infertility phenotype, not subfertility, confidence in potential drug efficacy is established.
The current popularity of CRISPR-generated knockout mouse models as a method to study the function of a gene in vivo has dominated over other approaches. While knockout methods lead to a complete depletion of gene expression, or complete elimination of gene function, methods involving gene knockdowns lead to reduction in protein expression, the results of which may yield valuable information. RNA interference (RNAi) was found to be useful for silencing gene expression in mammals and employed to study the functional relevance of genes in a relatively fast and easy way. Past reproductive studies have utilized short interfering RNA (siRNA) to determine functional significance of genes in male mouse fertility and has, in some cases, resulted in impaired or complete infertility phenotype in viable mice (Nagai et al., 2011; Welborn et al., 2015). Several interesting experiments that compared the phenotypes resulting from knocking-out and knocking-down mouse genes have revealed that the two methods are complimentary in conducting gene functional studies (De Souza et al., 2006). The focus of this review, however, lies in male reproductive genes whose functional relevance was determined through knockout mouse models, since incisive genetic approaches provide a more definitive foundation for establishing the contraceptive potential of a novel reproductive tract-specific gene. A variable and indeterminate level of off-target effects introduced from transfection or lentivirus-mediated infection cannot be excluded as confounding variables in knockdown experiments.
Many reproductive tract-specific genes have been identified as dispensable for male fertility when individually ablated in mice (Miyata et al., 2016; Holcomb et al., 2020; Lu et al., 2019). Functional compensation through upregulation of other functionally similar genes or molecular pathways may explain the lack of phenotype (Marschang et al., 2004; Hanada et al., 2009; Kashiwabara et al., 2016). Therefore, identifying a small molecule inhibitor against an individually dispensable target would yield no contraceptive effect.
As previously discussed, BRDT is a testis-specific gene that when individually ablated in mice results in arrest of male meiosis, azoospermia, and complete male infertility (Barda et al., 2016). Since BRDT has enough sequence dissimilarity— >55% sequence dissimilarity with its closest paralogs that are ubiquitously expressed, BRD4 and BRD2, development of BRDT inhibitors with enhanced specificity and drug selectivity is feasible and currently underway (Matzuk et al., 2012; Miller et al., 2016). On the other end of the spectrum, GJA1 is an example of a gene that when conditionally ablated in the Sertoli cells of male mice leads to male infertility (Brehm et al., 2007). However, because the gene is not exclusively expressed in the reproductive tract and (Djureinovic et al., 2014; Uhlen et al., 2015) other knockout animal models of this gene display a variety of non-reproductive phenotypes including severe heart abnormalities (Gutstein et al., 2001; Liao et al., 2001), thus demonstrating that this target is not suitable for the development of a safe male contraceptive.
Mutations causing male infertility in humans might also be informative in the context of contraception and should be taken into consideration in the search for a potential non-hormonal target. There are 1,517 human genes associated with male infertility and/or either azoospermia, globozospermia, or oligospermia as reported by GeneCards and MalaCards (Stelzer et al., 2016; Rappaport et al., 2017). Of these 1,517 genes, 202 genes are reproductive tract-specific in humans as reported through the CITDBase Contraceptive Target Database (GTEx Consortium, 2013; Djureinovic et al., 2014; Uhlen et al., 2015; Schmidt et al., 2017; Lee, 2019; Samaras et al., 2019) and/or Djureinovic et al. (2014) and Uhlen et al. (2015) (Figure 1). Of these 202 genes, 21 genes do not have a corresponding mouse ortholog and 3 of these genes (KLK2, CDY2A, and RHOXF2) may be of potential interest for further study as they encode an enzyme, an epigenetic protein, and a transcription factor, respectively; all proteins with potential druggable activity (Supplementary Table S1). The remaining 18 genes without a corresponding mouse ortholog encode proteins of unknown drug target type (Supplementary Table S1). Of the 202 reproductive tract-specific human genes associated with human infertility, 78 genes have a corresponding mouse ortholog displaying male infertility in the mouse (Figure 1 and Supplementary Table S1). The remaining 124 genes either (1) have a single mouse ortholog each and none have been knocked-out in the mouse, (2) have two or more mouse ortholog genes, whereby none or an incomplete number of orthologs have been knocked-out, and of those that have been knocked-out, none display a male infertility phenotype, (3) have a single mouse ortholog or multiple mouse orthologs that when individually ablated all lack a male infertility phenotype, or (4) have multiple mouse orthologs that have all been knocked out and that individually do not display a male infertility phenotype (Figure 1 and Supplementary Table S1). While the first two categories (categories 1 and 2) require further study for functional validation of the contraceptive potential of these genes, the latter categories (categories 3 and 4) do not require any further study. Genes listed in the first two categories (47 genes total) encode 2 enzymes (KLK3 and SPAM1), 1 kinase (TSSK2), 3 transcription factors (HSFY1, TGIF2LX, and TGIF2LY), and 41 proteins of unknown drug target type (Supplementary Table S1). Of the 202 reproductive tract-specific human genes associated with human infertility, 32 genes are mentioned in Figure 2, and four genes (BOLL, KCNU, SPATA22, and TEX101) are discussed in further detail within this review.
Figure 1. Knockout mouse model availability and phenotype outcome of single and multiple mouse ortholog genes corresponding to 202 reproductive tract-specific human genes associated with male infertility and/or either azoospermia, globozospermia, or oligospermia as reported by GeneCards and MalaCards (Stelzer et al., 2016; Rappaport et al., 2017).
Figure 2. A review of reviews from the past decade. Targets identified by the listed reviews are color-coded based on reproductive tract-specificity and fertility phenotype as follows: red = reproductive tract-specific displaying male infertility phenotype; green = non-reproductive tract-expressed and male infertility phenotype; blue = reproductive tract-specific displaying fertile or subfertile phenotype; orange = reproductive tract-specific with unknown fertility phenotype; black = non-reproductive tract-expressed displaying fertile or subfertile phenotype; gray = non-reproductive tract-expressed with unknown fertility phenotype. Expression and phenotype data obtained from Contraceptive Infertility Target DataBase (CITDBase), Human Protein Atlas (HPA), Ensembl Biomart, Mouse Genome Informatics (MGI), and the International Mouse Phenotyping Consortium (IMPC). *denotes genes implicated in human male infertility according to GeneCards and MalaCards.
Potential contraceptives could hit targets expressed along various stages of sperm development in the testis and epididymis, including targets active during the maintenance of the progenitor spermatogonia pool, entry into and passage through the various stages of meiosis, spermatid development and release, and sperm maturation through the epididymis. The contraceptive potential at these various stages varies in advantages and disadvantages. Targeting genes involved in early sperm development, for instance, could potentially be more effective, as suggested by several previous reviews identifying groups of male reproductive tract-expressed genes as promising drug targets (Schultz et al., 2003; Archambeault and Matzuk, 2014; Payne and Goldberg, 2014; O’Rand et al., 2016). However, disrupting the early stages of spermatogenesis poses the risk of testicular atrophy, longer recovery, and an increased possibility of irreversibility. Genes involved in later phases of spermatogenesis, namely spermiogenesis, acrosome and flagella formation, spermiation, and sperm maturation, would be more desirable contraceptive targets because testicular size would most likely remain unaffected, with a quicker, more reliable return to full fertility. Functional analysis of the expression patterns and specificity of male reproductive tract genes is imperative as it often provides additional insight into the molecular mechanisms of various stages of spermatogenesis, understanding of which is necessary in the process of developing a safe and effective non-hormonal male contraceptive.
Identification of a drug-like small molecule that can effectively modulate the activity of a given target should also be assessed based on protein properties such as structure, size, and complexity (Kozakov et al., 2015). Targets that contain transmembrane helices could increase the difficulty of obtaining a properly folded and soluble protein for drug selection purposes. Likewise, due to glycosylation and processing through the secretory pathway, secreted proteins require protein production in eukaryotic expression systems, which could increase the difficulty of obtaining suitable quantities of purified protein. Therefore, whether drug development is feasible resides on careful consideration of the biophysical properties of the protein.
Several notable reviews published in the last decade have mentioned promising, non-hormonal, contraceptive leads that include both meiotically and post-meiotically expressed genes that are testis-specific or epididymis-specific genes required for sperm maturation (O’Rand et al., 2011; Alves et al., 2014; Archambeault and Matzuk, 2014; Murdoch and Goldberg, 2014; Payne and Goldberg, 2014; Chen et al., 2016b; O’Rand et al., 2016; Drevet, 2018) (Figure 2). Some are, in fact, not reproductive tract-specific, but are still required for fertility, while others that are indeed reproductive tract-specific, lead to subfertility, not infertility, which is an ineffective and highly undesirable outcome for a contraceptive. Thus, the genes in Figure 2 are color-coded according to reproductive tract-specificity and infertility phenotype in the mouse. It is worth noting that while the reviews were restricted to the past decade, the reproductive tract-specific genes mentioned in these reviews were first identified beyond the past decade including some that were reported in 2006 and earlier, such as TNP1 (Yu et al., 2000), CATSPER1 (Ren et al., 2001), and TEX14 (Greenbaum et al., 2006).
In this review, 45 genes are discussed in further detail as they were identified in the last decade as required for male fertility through knockout mouse studies, whereby many of these genes were not discussed or discussed minimally in any previous male contraceptive drug target review. Henceforth, these 45 genes will be listed according to their published expression patterns (Figure 3), which is an important criterium that should be considered in the selection of a safe and/or desirable contraceptive target. It is worth noting that nearly all of the genes mentioned below are male reproductive tract-specific or highly enriched as reported in the literature and with additional confirmation through the published expression data from CITDBase (GTEx Consortium, 2013; Uhlen et al., 2015; Schmidt et al., 2017; Lee, 2019; Samaras et al., 2019) and/or the Human Protein Atlas (Djureinovic et al., 2014; Uhlen et al., 2015). A graphical summary of the RNAseq-based expression data for these 45 genes is depicted in Figure 4. Those that are not reproductive tract-specific are identified as such through the level of non-reproductive tissue expression. The following discussion provides detailed information about individual genes with focus on determining the contraceptive potential of each. Additional relevant information for these genes is listed in Table 1.
Figure 3. Diagrammatic representation of germ cells along the spermatogenic pathway showing the cell types displaying expression of the potential targets. Targets in red display decreased testes size in mice.
Figure 4. Digital PCR (heatmap) depicting the average transcripts per million (TPM) value per tissue per gene from human RNAseq data published by the Human Protein Atlas (Djureinovic et al., 2014; Uhlen et al., 2015). White = 0 TPM, Black ≥ 30 TPM. The genes are ordered from most reproductive tract-specific to least based on the level of non-reproductive tissue expression. “Fold” = testis/max normal. The data was obtained from a tab-separated file including Ensembl gene identifier, analyzed sample, and TPM value per gene that was downloaded from the Human Protein Atlas website. The expression profile of the housekeeping genes, GAPDH, is included.
In the last decade, only four reproductive tract-specific genes with infertile mouse models have been identified that fit the criteria of being expressed as early as the spermatogonia stage: ASZ1 (Ma et al., 2009), MOV10L1 (Frost et al., 2010; Zheng et al., 2010), SCML2 (Hasegawa et al., 2015), and TEX101 (Fujihara et al., 2013; Li et al., 2013) (Table 1). With expression as early as the spermatogonia stage (Teng et al., 2006; Ma et al., 2009; Frost et al., 2010; Maezawa et al., 2018), a deficiency of these genes’ functions will likely require at least 2 months prior to a contraceptive effect, at least 2 months for recovery, and in some cases, depending on the gene, the potential for irreversibility of the contraceptive effect. Nevertheless, since this form of contraceptive may be considered desirable due to the permanence of the effect, and the need for a compound that can traverse the BTB is not required, these genes are worthy of consideration. Since the conserved domains of human ASZ1, MOV10L1, and TEX101 show sequence similarity below 40% to their respective, non-reproductive tract expressed paralogs, ANKRD34C, CT55, and CD177, then these protein targets have a reasonable potential for drug specificity with a low risk of collateral effects. However, as it has been previously reported that human SCML2 is expressed ubiquitously at low levels (Bonasio et al., 2014) (Figure 4), low but potentially physiologically relevant expression of SCML2 across many non-reproductive tissues may therefore be of concern in the process of drug development. Additionally, the closest paralog to SCML2, SCMH1, is ubiquitously expressed and shows 60% sequence similarity in its conserved domain. Based on this information, human SCML2 may not be an ideal contraceptive target.
The genes discussed here are expressed in spermatocytes during preleptotene, leptotene, zygotene, pachytene, and/or diplotene stages and typically serve an essential role in meiosis I or meiosis II. A deficiency of these early stage genes results in male infertility typically due to meiotic arrest, which indicates a strong contraceptive potential. Important to note: although these genes may not directly impact spermatogonial stem cell self-renewal, and a healthy pool of spermatogonial stem cells may still remain for the regeneration of spermatogenesis, recovery time following cessation of targeting these genes may still take considerably longer than drugs targeting later stages of spermatogenesis or sperm. Consistently, the onset of a reliable contraceptive effect could take weeks, which may be an undesirable timeframe for drug action.
In the last decade, at least twenty-five reproductive tract-specific genes with infertile mouse models have been identified that fit the criteria of being expressed in spermatocytes (Table 1). Of these, seven genes—MEIOB, MEIOC, PIH1D3, SPATA22, SYCE3, TERB1, and TOPAZ1—encode the most promising target candidates as they do not have an associated paralog, or conserved domain with sequence similarity to any known protein, and thus these proteins bear the lowest risks of generating off-target effects from inhibiting compounds. Additionally, HORMAD2 is also an attractive target considering it only has one paralog, HORMAD1, which is also highly enriched in the male reproductive tract and leads to male infertility phenotype when ablated in mice (Shin et al., 2010; Daniel et al., 2011; Kogo et al., 2012b). Although HORMAD1 shows low, but appreciable gene expression in two non-reproductive tissues (Figure 4) this fact may be offset by the sequence dissimilarity between HORMAD1 and HORMAD2 (50% whole protein and 40% conserved domain) which can aid in the identification of a selective inhibitor with careful drug selection and design. C14orf39 and CNBD2 would both be attractive candidates because they also lack paralogs, however both have tissue specificity issues of concern (Figure 4), and Cnbd2 null mice display incomplete penetrance (Krahling et al., 2013), which may translate to potential ineffectiveness in humans. Therefore, while MEIOB, MEIOC, PIH1D3, SPATA22, SYCE3, TERB1, and TOPAZ1 are ideal candidates, C14orf39 and CNBD2 are not.
The next most promising candidates (another six genes)—BTBD18, CCDC63, CCDC155, HFM1, MCMDC2, and TDRD5—encode proteins that show sequence similarity below 40% to their respective, non-reproductive tract expressed paralogs, and within the conserved domains of their paralogs, KLHL26, SSH2, CCDC114, SNRNP200, MCM3, and TDRD1; thus, these potential targets have a reasonable potential for drug specificity with a low risk of collateral effects. However, the following six candidates—BOLL, FBXO43, INSL6, NUP210L, SOX30, and SPDYA—may require careful drug selection and design since the conserved domains of these proteins are 48–60% similar to the conserved domains of their respective, non-reproductive tract expressed paralogs, DAZ4, FBXO5, RLN1, NUP210, SOX7, and SPDYC. With respect to NUP210L, spermatid-Sertoli cell interaction was severely impaired in the knockout mouse model, resulting in Sertoli cell degeneration (Walters et al., 2009). Since this may cause irreversible disruption of spermatogenesis, this candidate may pose potential irreversibility issues. LY6K would be an attractive candidate because its closest ubiquitously expressed paralog, GML, has only 28% sequence similarity to LY6K; however, low, but appreciable expression of LY6K in non-reproductive tissues is of concern (Figure 4).
The most difficult spermatocyte-expressed candidates to target specifically are RAD21L1 and PGK2, which have ubiquitously expressed paralogs, RAD21 and PGK1, that have 83 and 87% similarity in their respective conserved domains. Since human mutations in RAD21 and PGK1 are characterized by significant disorders affecting numerous organ systems—Cornelia de Lange syndrome 4 (Deardorff et al., 2012), Mungan syndrome (Bonora et al., 2015), and phosphoglycerate kinase 1 deficiency (Fermo et al., 2012)—extraordinary effort would need to be made to generate specific drug molecules against RAD21L1 and PGK2.
The proteins discussed here are expressed during the later stages of spermatogenesis and sperm maturation (Table 1). These proteins are found in round or elongating spermatids, either in the acrosome, acroplaxome, basal body, manchette, or flagellum during spermiogenesis, and these proteins function in either proper sperm head formation and attachment, midpiece formation, acrosome formation and attachment, generating sperm with normal motility, or generating sperm capable of normal sperm-zona binding. Targeting genes at these post-meiotic stages is more likely to act within a faster timeframe and lead to better and potentially faster recovery of fertility upon cessation of drug.
In the last decade, at least nine reproductive tract-specific genes with infertile mouse models have been identified that fit the criteria of being expressed in spermatids (Table 1). Again, the most attractive candidates are those that show excellent reproductive tract-specificity and that do not have any ubiquitously expressed paralogs, or that have ubiquitously expressed paralogs with low sequence similarity. CCDC62, SPACA1, and TCTE1 do not have any paralogs and TDRD12, CCDC42, PRSS37, and LRGUK have ubiquitously expressed paralogs, TDRD15, CFAP73, KLK15, and PPP1R42, that share less than 40% sequence similarity at the whole protein and conserved domain level. CALR3 has a ubiquitously expressed paralog, CALR, that shows 51% and 55% sequence similarity at the whole protein and conserved domain level, respectively, which may necessitate careful drug selection and design to ensure off-target effects are minimized. RIMBP3, RIMBP3B, and RIMBP3C in humans are all testis-specific and RIMBP3B and RIMBP3C share conserved domains with identical protein sequences, indicating a strong evolutionary requirement for the function of these proteins and high likelihood of targeting more than one isoform with one small molecule inhibitor. However, the closest ubiquitously expressed paralog to these proteins is RIMBP2, which shares 76% sequence similarity in its conserved domain, which may be of concern in finding a specific drug molecule that targets this region.
Genes in this group are highly expressed in spermatozoa and disrupting their function would most likely affect spermatozoa maturation as they pass through the epididymis, while still maintaining the pool of testicular spermatogonia and spermatocytes, or inhibit proper spermatozoa function after sperm maturation. These targets are typically localized to the neck, principal piece, flagellum, or the central microtubule apparatus of mature spermatozoa. Since these targets act late, it is important to note that some—depending on the binding kinetics of the drugs and if the drugs are reversible or irreversible inhibitors—may only provide a momentary decrease in sperm function while in the male reproductive tract, and shortly thereafter, but not indefinitely in the female reproductive tract after ejaculation as the drug concentration invariably decreases over time. Thus, although this category of drug may be the most desirable due to having fastest onset of drug action, fastest recovery after cessation of drug, and no effect on testicular size, there may be additional challenges to address during drug development to ensure contraceptive efficacy.
In the last decade, at least seven reproductive tract-specific genes with infertile mouse models have been identified that fit the criteria of being present in mature sperm (Table 1). CATSPERD, KCNU1, PMIS2, and SUN5 show the lowest potentials for off-target effects as their closest ubiquitously expressed paralogs, CASC4, KCNMA1, SYNDIG1L, and SUN1, have less than 50% similarity at the whole protein and conserved domain level. After that, RSPH6A, has 52% (whole) and 63% (CD) similarity to its closest ubiquitously expressed paralog, RSPH4A, which may make it a promising lead with additional attention during drug selection and design to minimize off-target effects. Although, CFAP54 would be an excellent candidate with less than 22% similarity (whole and CD) to its closest ubiquitously expressed paralog, NPBWR2, expression in cilia of the respiratory epithelium (Djureinovic et al., 2014; Uhlen et al., 2015) and additional low but potentially physiologically relevant expression across many non-reproductive tissues (Figure 4) indicate targeting this candidate may yield side effects. Likewise, ATP1A4 is not an ideal candidate due to having high sequence similarity—81% similarity (whole and CD)—to its closest ubiquitously expressed paralog, ATP1A2, and also having low but potentially physiologically relevant expression levels across many non-reproductive tissues (Figure 4).
Not discussed in terms of potential drug target specificity, however of additional potential interest are the following genes that are also reproductive tract-specific in humans according to CITDBase (GTEx Consortium, 2013; Uhlen et al., 2015; Schmidt et al., 2017; Lee, 2019; Samaras et al., 2019) with mouse models displaying male infertility phenotype published in peer-reviewed journals in the last 10 years: 3 genes encoding enzymes [ENO4 (Nakamura et al., 2013), PNLDC1 (Nishimura et al., 2018), and SPINK2 (Lee et al., 2011)] and 8 genes encoding proteins of unknown drug target type [M1AP (Arango et al., 2013), MEIG1 (Zhang et al., 2009), MEIKIN (Kim et al., 2015), NXF2 (Pan et al., 2009), ODF1 (Yang et al., 2012), PPP3R2 (Miyata et al., 2015), SMC1B (Revenkova et al., 2010), and SPATA16 (Fujihara et al., 2017)]. Furthermore, the following genes are reproductive tract-specific in humans according to CITDBase (GTEx Consortium, 2013; Uhlen et al., 2015; Schmidt et al., 2017; Lee, 2019; Samaras et al., 2019) with mouse models displaying male infertility phenotypes as reported by the International Mouse Phenotyping Consortium (IMPC) (Munoz-Fuentes et al., 2018): 1 gene encoding an enzyme (ADAD2); 1 gene encoding an epigenetic-related protein (PHF7); and 11 genes encoding proteins of unknown drug target type (ACTL7B, ADGB, ARRDC5, C11orf94, C16orf92, C3orf20, DNAH17, FBXO47, NUTM1, ODF4, TEX38). The expression pattern of these additional individually published and IMPC-reported genes are listed in Figure 4.
Global demand for the development of a “male pill” is at an all-time high with the exponential growth of the human population. Efforts to reduce unplanned pregnancies are recognized as the search for a safe and effective method of male contraception has been a decades-long quest (Prasad and Rajalakshmi, 1976; Frick and Aulitzky, 1988; Herndon, 1992; Waites, 1993; Gottwald et al., 2006; Mruk, 2008). Identification of reproductive tract-specific targets through transcriptomic and proteomic approaches followed by validation of their functional requirement using knockout mice models has helped advance this quest. In this review, we discuss novel reproductive tract-specific protein targets that have been identified in the past 10 years, their potential druggability, the factors that contribute to their druggability and why they should be taken into consideration when selecting male contraceptive targets. We direct the reader to consider that optimal gene targets are those that contribute to the later phases of spermatogenesis as disrupting genes in the earlier phases could potentially lead to permanent infertility. Additionally, the reader is directed to consider the potential adverse effects that may exist when targets whose protein sequences bear high sequence similarity to other ubiquitously expressed proteins. This information can be valuable in future studies since off-target effects can considerably hamper development of a safe, non-hormonal male contraceptive. Continued persistence in the search for an optimal protein target should lead to a clinically approved, affordable product.
KK and TG designed the research and wrote the manuscript. KK, MJ, NS, and TG performed the research and analyzed the data.
This research was supported by the Eunice Kennedy Shriver National Institute of Child Health and Human Development (R01HD095341 to TG).
The authors declare that the research was conducted in the absence of any commercial or financial relationships that could be construed as a potential conflict of interest.
The Supplementary Material for this article can be found online at: https://www.frontiersin.org/articles/10.3389/fcell.2020.00061/full#supplementary-material
TABLE S1 | Orthologous mouse genes and mouse model information of 202 reproductive tract-specific human genes associated with male infertility in humans. Mouse ortholog gene symbols and phenotype information collected from Ensembl BioMart and MGI. For publication references refer to MGI. “Incomplete mouse data available” means not all orthologs have been knocked-out. ‡Genes that have been knocked-out are indicated in bold. *In instances where more than one mouse ortholog exists, the male infertile mouse ortholog is indicated in bold.
Abbasi, F., Miyata, H., Shimada, K., Morohoshi, A., Nozawa, K., Matsumura, T., et al. (2018). RSPH6A is required for sperm flagellum formation and male fertility in mice. J. Cell Sci. 131:jcs221648. doi: 10.1242/jcs.221648
Abby, E., Tourpin, S., Ribeiro, J., Daniel, K., Messiaen, S., Moison, D., et al. (2016). Implementation of meiosis prophase I programme requires a conserved retinoid-independent stabilizer of meiotic transcripts. Nat. Commun. 7:10324. doi: 10.1038/ncomms10324
Aitken, R. J. (2002). Immunocontraceptive vaccines for human use. J. Reprod. Immunol. 57, 273–287. doi: 10.1016/s0165-0378(02)00010-4
Alves, M. G., Dias, T. R., Silva, B. M., and Oliveira, P. F. (2014). Metabolic cooperation in testis as a pharmacological target: from disease to contraception. Curr. Mol. Pharmacol. 7, 83–95. doi: 10.2174/1874467208666150126153830
Arango, N. A., Li, L., Dabir, D., Nicolau, F., Pieretti-Vanmarcke, R., Koehler, C., et al. (2013). Meiosis I arrest abnormalities lead to severe oligozoospermia in meiosis 1 arresting protein (M1ap)-deficient mice. Biol. Reprod. 88:76. doi: 10.1095/biolreprod.111.098673
Archambeault, D. R., and Matzuk, M. M. (2014). Disrupting the male germ line to find infertility and contraception targets. Ann. Endocrinol. 75, 101–108. doi: 10.1016/j.ando.2014.04.006
Asano, Y., Akiyama, K., Tsuji, T., Takahashi, S., Noguchi, J., and Kunieda, T. (2009). Characterization and linkage mapping of an ENU-induced mutant mouse with defective spermatogenesis. Exp. Anim. 58, 525–532. doi: 10.1538/expanim.58.525
Bagatell, C. J., Matsumoto, A. M., Christensen, R. B., Rivier, J. E., and Bremner, W. J. (1993). Comparison of a gonadotropin releasing-hormone antagonist plus testosterone (T) versus T alone as potential male contraceptive regimens. J. Clin. Endocrinol. Metab. 77, 427–432. doi: 10.1210/jcem.77.2.8345047
Baggelaar, M. P., den Dulk, H., Florea, B. I., Fazio, D., Bernabo, N., Raspa, M., et al. (2019). ABHD2 inhibitor identified by activity-based protein profiling reduces acrosome reaction. ACS Chem. Biol. 14, 2295–2304. doi: 10.1021/acschembio.9b00640
Barda, S., Yogev, L., Paz, G., Yavetz, H., Hauser, R., Breitbart, H., et al. (2016). New insights into the role of the Brdt protein in the regulation of development and spermatogenesis in the mouse. Gene Expr. Patterns 20, 130–137. doi: 10.1016/j.gep.2016.03.003
Bechara, C., and Sagan, S. (2013). Cell-penetrating peptides: 20 years later, where do we stand? FEBS Lett. 587, 1693–1702. doi: 10.1016/j.febslet.2013.04.031
Benito, E., Ramachandran, B., Schroeder, H., Schmidt, G., Urbanke, H., Burkhardt, S., et al. (2017). The BET/BRD inhibitor JQ1 improves brain plasticity in WT and APP mice. Transl. Psychiatry 7:e1239. doi: 10.1038/tp.2017.202
Bonasio, R., Lecona, E., Narendra, V., Voigt, P., Parisi, F., Kluger, Y., et al. (2014). Interactions with RNA direct the Polycomb group protein SCML2 to chromatin where it represses target genes. eLife 3:e02637. doi: 10.7554/eLife.02637
Bondeson, D. P., Mares, A., Smith, I. E., Ko, E., Campos, S., Miah, A. H., et al. (2015). Catalytic in vivo protein knockdown by small-molecule PROTACs. Nat. Chem. Biol. 11, 611–617. doi: 10.1038/nchembio.1858
Bondeson, D. P., Smith, B. E., Burslem, G. M., Buhimschi, A. D., Hines, J., Jaime-Figueroa, S., et al. (2018). Lessons in PROTAC design from selective degradation with a promiscuous warhead. Cell Chem. Biol. 25, 78.e5–87.e5. doi: 10.1016/j.chembiol.2017.09.010
Bonora, E., Bianco, F., Cordeddu, L., Bamshad, M., Francescatto, L., Dowless, D., et al. (2015). Mutations in RAD21 disrupt regulation of APOB in patients with chronic intestinal pseudo-obstruction. Gastroenterology 148, 771.e11–782.e11. doi: 10.1053/j.gastro.2014.12.034
Brehm, R., Zeiler, M., Ruttinger, C., Herde, K., Kibschull, M., Winterhager, E., et al. (2007). A sertoli cell-specific knockout of connexin43 prevents initiation of spermatogenesis. Am. J. Pathol. 171, 19–31. doi: 10.2353/ajpath.2007.061171
Brooks, H., Lebleu, B., and Vives, E. (2005). Tat peptide-mediated cellular delivery: back to basics. Adv. Drug Deliv. Rev. 57, 559–577. doi: 10.1016/j.addr.2004.12.001
Burnicka-Turek, O., Shirneshan, K., Paprotta, I., Grzmil, P., Meinhardt, A., Engel, W., et al. (2009). Inactivation of insulin-like factor 6 disrupts the progression of spermatogenesis at late meiotic prophase. Endocrinology 150, 4348–4357. doi: 10.1210/en.2009-0201
Castaneda, J. M., Hua, R., Miyata, H., Oji, A., Guo, Y., Cheng, Y., et al. (2017). TCTE1 is a conserved component of the dynein regulatory complex and is required for motility and metabolism in mouse spermatozoa. Proc. Natl. Acad. Sci. U.S.A. 114, E5370–E5378. doi: 10.1073/pnas.1621279114
Chen, H., Lui, W. Y., Mruk, D. D., Xiao, X., Ge, R., Lian, Q., et al. (2018). Monitoring the integrity of the blood-testis barrier (BTB): an in vivo assay. Methods Mol. Biol. 1748, 245–252. doi: 10.1007/978-1-4939-7698-0_17
Chen, H., Mruk, D. D., Xia, W., Bonanomi, M., Silvestrini, B., and Cheng, C. Y. (2016a). Effective delivery of male contraceptives behind the blood-testis barrier (BTB) - lesson from adjudin. Curr. Med. Chem. 23, 701–713. doi: 10.2174/0929867323666160112122724
Chen, S. R., Batool, A., Wang, Y. Q., Hao, X. X., Chang, C. S., Cheng, C. Y., et al. (2016b). The control of male fertility by spermatid-specific factors: searching for contraceptive targets from spermatozoon’s head to tail. Cell Death Dis. 7:e2472. doi: 10.1038/cddis.2016.344
Cheng, Y., Ma, Z., Kim, B. H., Wu, W., Cayting, P., Boyle, A. P., et al. (2014). Principles of regulatory information conservation between mouse and human. Nature 515, 371–375. doi: 10.1038/nature13985
Chung, J. J., Navarro, B., Krapivinsky, G., Krapivinsky, L., and Clapham, D. E. (2011). A novel gene required for male fertility and functional CATSPER channel formation in spermatozoa. Nat. Commun. 2:153. doi: 10.1038/ncomms1153
Daniel, K., Lange, J., Hached, K., Fu, J., Anastassiadis, K., Roig, I., et al. (2011). Meiotic homologue alignment and its quality surveillance are controlled by mouse HORMAD1. Nat. Cell Biol. 13, 599–610. doi: 10.1038/ncb2213
Danshina, P. V., Geyer, C. B., Dai, Q., Goulding, E. H., Willis, W. D., Kitto, G. B., et al. (2010). Phosphoglycerate kinase 2 (PGK2) is essential for sperm function and male fertility in mice. Biol. Reprod. 82, 136–145. doi: 10.1095/biolreprod.109.079699
Das, A., Chai, J. C., Yang, C. S., Lee, Y. S., Das, N. D., Jung, K. H., et al. (2015). Dual transcriptome sequencing reveals resistance of TLR4 ligand-activated bone marrow-derived macrophages to inflammation mediated by the BET inhibitor JQ1. Sci. Rep. 5:16932. doi: 10.1038/srep16932
Davies, B., Baumann, C., Kirchhoff, C., Ivell, R., Nubbemeyer, R., Habenicht, U. F., et al. (2004). Targeted deletion of the epididymal receptor HE6 results in fluid dysregulation and male infertility. Mol. Cell Biol. 24, 8642–8648. doi: 10.1128/MCB.24.19.8642-8648.2004
De Souza, A. T., Dai, X., Spencer, A. G., Reppen, T., Menzie, A., Roesch, P. L., et al. (2006). Transcriptional and phenotypic comparisons of Ppara knockout and siRNA knockdown mice. Nucleic Acids Res. 34, 4486–4494. doi: 10.1093/nar/gkl609
Deardorff, M. A., Wilde, J. J., Albrecht, M., Dickinson, E., Tennstedt, S., Braunholz, D., et al. (2012). RAD21 mutations cause a human cohesinopathy. Am. J. Hum. Genet. 90, 1014–1027. doi: 10.1016/j.ajhg.2012.04.019
Dickinson, M. E., Flenniken, A. M., Ji, X., Teboul, L., Wong, M. D., White, J. K., et al. (2016). High-throughput discovery of novel developmental phenotypes. Nature 537, 508–514. doi: 10.1038/nature19356
Djureinovic, D., Fagerberg, L., Hallstrom, B., Danielsson, A., Lindskog, C., Uhlen, M., et al. (2014). The human testis-specific proteome defined by transcriptomics and antibody-based profiling. Mol. Hum. Reprod. 20, 476–488. doi: 10.1093/molehr/gau018
Dong, F., Shinohara, K., Botilde, Y., Nabeshima, R., Asai, Y., Fukumoto, A., et al. (2014). Pih1d3 is required for cytoplasmic preassembly of axonemal dynein in mouse sperm. J. Cell Biol. 204, 203–213. doi: 10.1083/jcb.201304076
Drevet, J. R. (2018). Epididymal approaches to male contraception. Basic Clin. Androl. 28:12. doi: 10.1186/s12610-018-0078-y
Feng, C. A., Spiller, C., Merriner, D. J., O’Bryan, M. K., Bowles, J., and Koopman, P. (2017). SOX30 is required for male fertility in mice. Sci. Rep. 7:17619. doi: 10.1038/s41598-017-17854-5
Fermo, E., Bianchi, P., Chiarelli, L. R., Maggi, M., Mandara, G. M., Vercellati, C., et al. (2012). A new variant of phosphoglycerate kinase deficiency (p.I371K) with multiple tissue involvement: molecular and functional characterization. Mol. Genet. Metab. 106, 455–461. doi: 10.1016/j.ymgme.2012.05.015
Finsterbusch, F., Ravindranathan, R., Dereli, I., Stanzione, M., Trankner, D., and Toth, A. (2016). Alignment of homologous chromosomes and effective repair of programmed DNA double-strand breaks during mouse meiosis require the minichromosome maintenance domain containing 2 (MCMDC2) protein. PLoS Genet. 12:e1006393. doi: 10.1371/journal.pgen.1006393
Franca, L. R., Hess, R. A., Dufour, J. M., Hofmann, M. C., and Griswold, M. D. (2016). The Sertoli cell: one hundred fifty years of beauty and plasticity. Andrology 4, 189–212. doi: 10.1111/andr.12165
French, C. A., Ramirez, C. L., Kolmakova, J., Hickman, T. T., Cameron, M. J., Thyne, M. E., et al. (2008). BRD-NUT oncoproteins: a family of closely related nuclear proteins that block epithelial differentiation and maintain the growth of carcinoma cells. Oncogene 27, 2237–2242. doi: 10.1038/sj.onc.1210852
Frick, J., and Aulitzky, W. (1988). Male contraception. Hum. Reprod. 3, 147–151. doi: 10.1093/oxfordjournals.humrep.a136664
Frost, R. J., Hamra, F. K., Richardson, J. A., Qi, X., Bassel-Duby, R., and Olson, E. N. (2010). MOV10L1 is necessary for protection of spermatocytes against retrotransposons by Piwi-interacting RNAs. Proc. Natl. Acad. Sci. U.S.A. 107, 11847–11852. doi: 10.1073/pnas.1007158107
Fujihara, Y., Oji, A., Larasati, T., Kojima-Kita, K., and Ikawa, M. (2017). Human globozoospermia-related gene Spata16 is required for sperm formation revealed by CRISPR/Cas9-mediated mouse models. Int. J. Mol. Sci. 18:2208. doi: 10.3390/ijms18102208
Fujihara, Y., Okabe, M., and Ikawa, M. (2014). GPI-anchored protein complex, LY6K/TEX101, is required for sperm migration into the oviduct and male fertility in mice. Biol. Reprod. 90:60. doi: 10.1095/biolreprod.113.112888
Fujihara, Y., Satouh, Y., Inoue, N., Isotani, A., Ikawa, M., and Okabe, M. (2012). SPACA1-deficient male mice are infertile with abnormally shaped sperm heads reminiscent of globozoospermia. Development 139, 3583–3589. doi: 10.1242/dev.081778
Fujihara, Y., Tokuhiro, K., Muro, Y., Kondoh, G., Araki, Y., Ikawa, M., et al. (2013). Expression of TEX101, regulated by ACE, is essential for the production of fertile mouse spermatozoa. Proc. Natl. Acad. Sci. U.S.A. 110, 8111–8116. doi: 10.1073/pnas.1222166110
Fuller, J. C., Burgoyne, N. J., and Jackson, R. M. (2009). Predicting druggable binding sites at the protein-protein interface. Drug Discov. Today 14, 155–161. doi: 10.1016/j.drudis.2008.10.009
Gomez, H. L., Felipe-Medina, N., Sanchez-Martin, M., Davies, O. R., Ramos, I., Garcia-Tunon, I., et al. (2016). C14ORF39/SIX6OS1 is a constituent of the synaptonemal complex and is essential for mouse fertility. Nat. Commun. 7:13298. doi: 10.1038/ncomms13298
Gopinathan, L., Szmyd, R., Low, D., Diril, M. K., Chang, H. Y., Coppola, V., et al. (2017). Emi2 is essential for mouse spermatogenesis. Cell Rep. 20, 697–708. doi: 10.1016/j.celrep.2017.06.033
Gottwald, U., Davies, B., Fritsch, M., and Habenicht, U. F. (2006). New approaches for male fertility control: HE6 as an example of a putative target. Mol. Cell. Endocrinol. 250, 49–57. doi: 10.1016/j.mce.2005.12.024
Greenbaum, M. P., Yan, W., Wu, M. H., Lin, Y. N., Agno, J. E., Sharma, M., et al. (2006). TEX14 is essential for intercellular bridges and fertility in male mice. Proc. Natl. Acad. Sci. U.S.A. 103, 4982–4987. doi: 10.1073/pnas.0505123103
GTEx Consortium (2013). The genotype-tissue expression (GTEx) project. Nat. Genet. 45, 580–585. doi: 10.1038/ng.2653
Guiraldelli, M. F., Eyster, C., Wilkerson, J. L., Dresser, M. E., and Pezza, R. J. (2013). Mouse HFM1/Mer3 is required for crossover formation and complete synapsis of homologous chromosomes during meiosis. PLoS Genet. 9:e1003383. doi: 10.1371/journal.pgen.1003383
Gutstein, D. E., Morley, G. E., Tamaddon, H., Vaidya, D., Schneider, M. D., Chen, J., et al. (2001). Conduction slowing and sudden arrhythmic death in mice with cardiac-restricted inactivation of connexin43. Circ. Res. 88, 333–339. doi: 10.1161/01.res.88.3.333
Hanada, K., Kuromori, T., Myouga, F., Toyoda, T., Li, W. H., and Shinozaki, K. (2009). Evolutionary persistence of functional compensation by duplicate genes in Arabidopsis. Genome Biol. Evol. 1, 409–414. doi: 10.1093/gbe/evp043
Hasegawa, K., Sin, H. S., Maezawa, S., Broering, T. J., Kartashov, A. V., Alavattam, K. G., et al. (2015). SCML2 establishes the male germline epigenome through regulation of histone H2A ubiquitination. Dev. Cell 32, 574–588. doi: 10.1016/j.devcel.2015.01.014
Herran, Y., Gutierrez-Caballero, C., Sanchez-Martin, M., Hernandez, T., Viera, A., Barbero, J. L., et al. (2011). The cohesin subunit RAD21L functions in meiotic synapsis and exhibits sexual dimorphism in fertility. EMBO J. 30, 3091–3105. doi: 10.1038/emboj.2011.222
Hild, S. A., Reel, J. R., Dykstra, M. J., Mann, P. C., and Marshall, G. R. (2007). Acute adverse effects of the indenopyridine CDB-4022 on the ultrastructure of sertoli cells, spermatocytes, and spermatids in rat testes: comparison to the known sertoli cell toxicant Di-n-pentylphthalate (DPP). J. Androl. 28, 621–629. doi: 10.2164/jandrol.106.002295
Holcomb, R. J., Oura, S., Nozawa, K., Kent, K., Yu, Z., Robertson, M. J., et al. (2020). The testis-specific serine proteases Prss44, Prss46, and Prss54 are dispensable for male mouse fertility†. Biol. Reprod. 102, 84–91. doi: 10.1093/biolre/ioz158
Hopkins, A. L., and Groom, C. R. (2002). The druggable genome. Nat. Rev. Drug Discov. 1, 727–730. doi: 10.1038/nrd892
Horn, H. F., Kim, D. I., Wright, G. D., Wong, E. S., Stewart, C. L., Burke, B., et al. (2013). A mammalian KASH domain protein coupling meiotic chromosomes to the cytoskeleton. J. Cell Biol. 202, 1023–1039. doi: 10.1083/jcb.201304004
Ikawa, M., Tokuhiro, K., Yamaguchi, R., Benham, A. M., Tamura, T., Wada, I., et al. (2011). Calsperin is a testis-specific chaperone required for sperm fertility. J. Biol. Chem. 286, 5639–5646. doi: 10.1074/jbc.M110.140152
Ilani, N., Roth, M. Y., Amory, J. K., Swerdloff, R. S., Dart, C., Page, S. T., et al. (2012). A new combination of testosterone and nestorone transdermal gels for male hormonal contraception. J. Clin. Endocrinol. Metab. 97, 3476–3486. doi: 10.1210/jc.2012-1384
Jimenez, T., McDermott, J. P., Sanchez, G., and Blanco, G. (2011). Na,K-ATPase alpha4 isoform is essential for sperm fertility. Proc. Natl. Acad. Sci. U.S.A. 108, 644–649. doi: 10.1073/pnas.1016902108
Kanishchev, O. S., and Dolbier, W. R. (2018). Synthesis of 6-SF5-indazoles and an SF5-analog of gamendazole. Org. Biomol. Chem. 16, 5793–5799. doi: 10.1039/c8ob01460d
Kashiwabara, S., Tsuruta, S., Okada, K., Saegusa, A., Miyagaki, Y., and Baba, T. (2016). Functional compensation for the loss of testis-specific poly(A)-binding protein, PABPC2, during mouse spermatogenesis. J. Reprod. Dev. 62, 305–310. doi: 10.1262/jrd.2016-023
Kim, J., Ishiguro, K., Nambu, A., Akiyoshi, B., Yokobayashi, S., Kagami, A., et al. (2015). Meikin is a conserved regulator of meiosis-I-specific kinetochore function. Nature 517, 466–471. doi: 10.1038/nature14097
Kogo, H., Tsutsumi, M., Inagaki, H., Ohye, T., Kiyonari, H., and Kurahashi, H. (2012a). HORMAD2 is essential for synapsis surveillance during meiotic prophase via the recruitment of ATR activity. Genes Cells 17, 897–912. doi: 10.1111/gtc.12005
Kogo, H., Tsutsumi, M., Ohye, T., Inagaki, H., Abe, T., and Kurahashi, H. (2012b). HORMAD1-dependent checkpoint/surveillance mechanism eliminates asynaptic oocytes. Genes Cells 17, 439–454. doi: 10.1111/j.1365-2443.2012.01600.x
Kozakov, D., Hall, D. R., Napoleon, R. L., Yueh, C., Whitty, A., and Vajda, S. (2015). New frontiers in druggability. J. Med. Chem. 58, 9063–9088. doi: 10.1021/acs.jmedchem.5b00586
Krahling, A. M., Alvarez, L., Debowski, K., Van, Q., Gunkel, M., Irsen, S., et al. (2013). CRIS-a novel cAMP-binding protein controlling spermiogenesis and the development of flagellar bending. PLoS Genet. 9:e1003960. doi: 10.1371/journal.pgen.1003960
La Salle, S., Palmer, K., O’Brien, M., Schimenti, J. C., Eppig, J., and Handel, M. A. (2012). Spata22, a novel vertebrate-specific gene, is required for meiotic progress in mouse germ cells. Biol. Reprod. 86:45. doi: 10.1095/biolreprod.111.095752
Lambert, J. P., Picaud, S., Fujisawa, T., Hou, H., Savitsky, P., Uuskula-Reimand, L., et al. (2019). Interactome rewiring following pharmacological targeting of BET bromodomains. Mol. Cell 73, 621.e17–638.e17. doi: 10.1016/j.molcel.2018.11.006
Lee, B., Park, I., Jin, S., Choi, H., Kwon, J. T., Kim, J., et al. (2011). Impaired spermatogenesis and fertility in mice carrying a mutation in the Spink2 gene expressed predominantly in testes. J. Biol. Chem. 286, 29108–29117. doi: 10.1074/jbc.M111.244905
Lee, M. S. (2019). CITDBase: Contraceptive Infertility Target DataBase. Available: https://www.citdbase.org/ (accessed October, 2019).
Li, W., Guo, X. J., Teng, F., Hou, X. J., Lv, Z., Zhou, S. Y., et al. (2013). Tex101 is essential for male fertility by affecting sperm migration into the oviduct in mice. J. Mol. Cell Biol. 5, 345–347. doi: 10.1093/jmcb/mjt031
Li, Y., Li, C., Lin, S., Yang, B., Huang, W., Wu, H., et al. (2017). A nonsense mutation in Ccdc62 gene is responsible for spermiogenesis defects and male infertility in repro29/repro29 mice. Biol. Reprod. 96, 587–597. doi: 10.1095/biolreprod.116.141408
Liao, Y., Day, K. H., Damon, D. N., and Duling, B. R. (2001). Endothelial cell-specific knockout of connexin 43 causes hypotension and bradycardia in mice. Proc. Natl. Acad. Sci. U.S.A. 98, 9989–9994. doi: 10.1073/pnas.171305298
Lin, S., Lin, Y., Nery, J. R., Urich, M. A., Breschi, A., Davis, C. A., et al. (2014). Comparison of the transcriptional landscapes between human and mouse tissues. Proc. Natl. Acad. Sci. U.S.A. 111, 17224–17229. doi: 10.1073/pnas.1413624111
Liu, Y., DeBoer, K., de Kretser, D. M., O’Donnell, L., O’Connor, A. E., Merriner, D. J., et al. (2015). LRGUK-1 is required for basal body and manchette function during spermatogenesis and male fertility. PLoS Genet. 11:e1005090. doi: 10.1371/journal.pgen.1005090
Lu, J., Qian, Y., Altieri, M., Dong, H., Wang, J., Raina, K., et al. (2015). Hijacking the E3 ubiquitin ligase cereblon to efficiently target BRD4. Chem. Biol. 22, 755–763. doi: 10.1016/j.chembiol.2015.05.009
Lu, Y., Oura, S., Matsumura, T., Oji, A., Sakurai, N., Fujihara, Y., et al. (2019). CRISPR/Cas9-mediated genome editing reveals 30 testis-enriched genes dispensable for male fertility in mice. Biol. Reprod. 101, 501–511. doi: 10.1093/biolre/ioz103
Luangpraseuth-Prosper, A., Lesueur, E., Jouneau, L., Pailhoux, E., Cotinot, C., and Mandon-Pepin, B. (2015). TOPAZ1, a germ cell specific factor, is essential for male meiotic progression. Dev. Biol. 406, 158–171. doi: 10.1016/j.ydbio.2015.09.002
Luo, M., Yang, F., Leu, N. A., Landaiche, J., Handel, M. A., Benavente, R., et al. (2013). MEIOB exhibits single-stranded DNA-binding and exonuclease activities and is essential for meiotic recombination. Nat. Commun. 4:2788. doi: 10.1038/ncomms3788
Ma, L., Buchold, G. M., Greenbaum, M. P., Roy, A., Burns, K. H., Zhu, H., et al. (2009). GASZ is essential for male meiosis and suppression of retrotransposon expression in the male germline. PLoS Genet. 5:e1000635. doi: 10.1371/journal.pgen.1000635
Maezawa, S., Hasegawa, K., Yukawa, M., Kubo, N., Sakashita, A., Alavattam, K. G., et al. (2018). Polycomb protein SCML2 facilitates H3K27me3 to establish bivalent domains in the male germline. Proc. Natl. Acad. Sci. U.S.A. 115, 4957–4962. doi: 10.1073/pnas.1804512115
Marschang, P., Brich, J., Weeber, E. J., Sweatt, J. D., Shelton, J. M., Richardson, J. A., et al. (2004). Normal development and fertility of knockout mice lacking the tumor suppressor gene LRP1b suggest functional compensation by LRP1. Mol. Cell Biol. 24, 3782–3793. doi: 10.1128/mcb.24.9.3782-3793.2004
Matzuk, M. M., McKeown, M. R., Filippakopoulos, P., Li, Q., Ma, L., Agno, J. E., et al. (2012). Small-molecule inhibition of BRDT for male contraception. Cell 150, 673–684. doi: 10.1016/j.cell.2012.06.045
McKenzie, C. W., Craige, B., Kroeger, T. V., Finn, R., Wyatt, T. A., Sisson, J. H., et al. (2015). CFAP54 is required for proper ciliary motility and assembly of the central pair apparatus in mice. Mol. Biol. Cell 26, 3140–3149. doi: 10.1091/mbc.E15-02-0121
Meriggiola, M. C., Marcovina, S., Paulsen, C. A., and Bremner, W. J. (1995). Testosterone enanthate at a dose of 200 mg/week decreases HDL-cholesterol levels in healthy men. Int. J. Androl. 18, 237–242. doi: 10.1111/ijan.1995.18.5.237
Miller, T. C., Simon, B., Rybin, V., Grotsch, H., Curtet, S., Khochbin, S., et al. (2016). A bromodomain-DNA interaction facilitates acetylation-dependent bivalent nucleosome recognition by the BET protein BRDT. Nat. Commun. 7:13855. doi: 10.1038/ncomms13855
Miyata, H., Castaneda, J. M., Fujihara, Y., Yu, Z., Archambeault, D. R., Isotani, A., et al. (2016). Genome engineering uncovers 54 evolutionarily conserved and testis-enriched genes that are not required for male fertility in mice. Proc. Natl. Acad. Sci. U.S.A. 113, 7704–7710. doi: 10.1073/pnas.1608458113
Miyata, H., Satouh, Y., Mashiko, D., Muto, M., Nozawa, K., Shiba, K., et al. (2015). Sperm calcineurin inhibition prevents mouse fertility with implications for male contraceptive. Science 350, 442–445. doi: 10.1126/science.aad0836
Mok, K. W., Lie, P. P. Y., Mruk, D. D., Mannu, J., Mathur, P. P., Silvestrini, B., et al. (2012). The apical ectoplasmic specialization-blood-testis barrier functional axis is a novel target for male contraception. Adv. Exp. Med. Biol. 763, 334–355. doi: 10.1007/978-1-4614-4711-5_17
Mok, K. W., Mruk, D. D., Lie, P. P., Lui, W. Y., and Cheng, C. Y. (2011). Adjudin, a potential male contraceptive, exerts its effects locally in the seminiferous epithelium of mammalian testes. Reproduction 141, 571–580. doi: 10.1530/REP-10-0464
Morakinyo, A. O., Iranloye, B. O., and Adegoke, O. A. (2009). Antireproductive effect of calcium channel blockers on male rats. Reprod. Med. Biol. 8, 97–102. doi: 10.1007/s12522-009-0018-9
Mruk, D. D. (2008). New perspectives in non-hormonal male contraception. Trends Endocrinol. Metab. 19, 57–64. doi: 10.1016/j.tem.2007.11.002
Mruk, D. D., and Cheng, C. Y. (2011). Testin and actin are key molecular targets of adjudin, an anti-spermatogenic agent, in the testis. Spermatogenesis 1, 137–146. doi: 10.4161/spmg.1.2.16449
Mruk, D. D., Wong, C. H., Silvestrini, B., and Cheng, C. Y. (2006). A male contraceptive targeting germ cell adhesion. Nat. Med. 12, 1323–1328. doi: 10.1038/nm1420
Munoz-Fuentes, V., Cacheiro, P., Meehan, T. F., Aguilar-Pimentel, J. A., Brown, S. D. M., Flenniken, A. M., et al. (2018). The international mouse phenotyping consortium (IMPC): a functional catalogue of the mammalian genome that informs conservation. Conserv. Genet. 19, 995–1005. doi: 10.1007/s10592-018-1072-9
Murdoch, F. E., and Goldberg, E. (2014). Male contraception: another Holy Grail. Bioorg. Med. Chem. Lett. 24, 419–424. doi: 10.1016/j.bmcl.2013.12.004
Nagai, M., Moriyama, T., Mehmood, R., Tokuhiro, K., Ikawa, M., Okabe, M., et al. (2011). Mice lacking Ran binding protein 1 are viable and show male infertility. FEBS Lett. 585, 791–796. doi: 10.1016/j.febslet.2011.02.002
Nakamura, N., Dai, Q., Williams, J., Goulding, E. H., Willis, W. D., Brown, P. R., et al. (2013). Disruption of a spermatogenic cell-specific mouse enolase 4 (eno4) gene causes sperm structural defects and male infertility. Biol. Reprod. 88:90. doi: 10.1095/biolreprod.112.107128
Nilsson, M., Husmark, J., Bjorkman, U., and Ericson, L. E. (1998). Cytokines and thyroid epithelial integrity: interleukin-1alpha induces dissociation of the junctional complex and paracellular leakage in filter-cultured human thyrocytes. J. Clin. Endocrinol. Metab. 83, 945–952. doi: 10.1210/jcem.83.3.4626
Nishimura, T., Nagamori, I., Nakatani, T., Izumi, N., Tomari, Y., Kuramochi-Miyagawa, S., et al. (2018). PNLDC1, mouse pre-piRNA Trimmer, is required for meiotic and post-meiotic male germ cell development. EMBO Rep. 19:e44957. doi: 10.15252/embr.201744957
Olson, C. M., Jiang, B., Erb, M. A., Liang, Y., Doctor, Z. M., Zhang, Z., et al. (2018). Pharmacological perturbation of CDK9 using selective CDK9 inhibition or degradation. Nat. Chem. Biol. 14, 163–170. doi: 10.1038/nchembio.2538
O’Rand, M. G., Hamil, K. G., Adevai, T., and Zelinski, M. (2018). Inhibition of sperm motility in male macaques with EP055, a potential non-hormonal male contraceptive. PLoS One 13:e0195953. doi: 10.1371/journal.pone.0195953
O’Rand, M. G., Silva, E. J., and Hamil, K. G. (2016). Non-hormonal male contraception: a review and development of an Eppin based contraceptive. Pharmacol. Ther. 157, 105–111. doi: 10.1016/j.pharmthera.2015.11.004
O’Rand, M. G., Widgren, E. E., Hamil, K. G., Silva, E. J., and Richardson, R. T. (2011). Epididymal protein targets: a brief history of the development of epididymal protease inhibitor as a contraceptive. J. Androl. 32, 698–704. doi: 10.2164/jandrol.110.012781
Ottis, P., Toure, M., Cromm, P. M., Ko, E., Gustafson, J. L., and Crews, C. M. (2017). Assessing different E3 ligases for small molecule induced protein ubiquitination and degradation. ACS Chem. Biol. 12, 2570–2578. doi: 10.1021/acschembio.7b00485
Pan, J., Eckardt, S., Leu, N. A., Buffone, M. G., Zhou, J., Gerton, G. L., et al. (2009). Inactivation of Nxf2 causes defects in male meiosis and age-dependent depletion of spermatogonia. Dev. Biol. 330, 167–174. doi: 10.1016/j.ydbio.2009.03.022
Pandey, R. R., Tokuzawa, Y., Yang, Z., Hayashi, E., Ichisaka, T., Kajita, S., et al. (2013). Tudor domain containing 12 (TDRD12) is essential for secondary PIWI interacting RNA biogenesis in mice. Proc. Natl. Acad. Sci. U.S.A. 110, 16492–16497. doi: 10.1073/pnas.1316316110
Pasek, R. C., Malarkey, E., Berbari, N. F., Sharma, N., Kesterson, R. A., Tres, L. L., et al. (2016). Coiled-coil domain containing 42 (Ccdc42) is necessary for proper sperm development and male fertility in the mouse. Dev. Biol. 412, 208–218. doi: 10.1016/j.ydbio.2016.01.042
Pasztor, N., Hegyi, B. E., Bado, A., and Nemeth, G. (2017). [Male hormonal contraception: past, present, future]. Orv. Hetil. 158, 1819–1830. doi: 10.1556/650.2017.30876
Payne, C., and Goldberg, E. (2014). Male contraception: past, present and future. Curr. Mol. Pharmacol. 7, 175–181.
Prasad, M. R., and Rajalakshmi, M. (1976). Target sites for suppressing fertility in the male. Adv. Sex. Horm. Res. 2, 263–287.
Rappaport, N., Twik, M., Plaschkes, I., Nudel, R., Iny Stein, T., Levitt, J., et al. (2017). MalaCards: an amalgamated human disease compendium with diverse clinical and genetic annotation and structured search. Nucleic Acids Res. 45, D877–D887. doi: 10.1093/nar/gkw1012
Ren, D., Navarro, B., Perez, G., Jackson, A. C., Hsu, S., Shi, Q., et al. (2001). A sperm ion channel required for sperm motility and male fertility. Nature 413, 603–609. doi: 10.1038/35098027
Revenkova, E., Herrmann, K., Adelfalk, C., and Jessberger, R. (2010). Oocyte cohesin expression restricted to predictyate stages provides full fertility and prevents aneuploidy. Curr. Biol. 20, 1529–1533. doi: 10.1016/j.cub.2010.08.024
Roberts, K. P., Ensrud, K. M., Wooters, J. L., Nolan, M. A., Johnston, D. S., and Hamilton, D. W. (2006). Epididymal secreted protein Crisp-1 and sperm function. Mol. Cell. Endocrinol. 250, 122–127. doi: 10.1016/j.mce.2005.12.034
Samaras, P., Schmidt, T., Frejno, M., Gessulat, S., Reinecke, M., Jarzab, A., et al. (2019). ProteomicsDB: a multi-omics and multi-organism resource for life science research. Nucleic Acids Res. 48, D1153–D1163. doi: 10.1093/nar/gkz974
Santi, C. M., Martinez-Lopez, P., de la Vega-Beltran, J. L., Butler, A., Alisio, A., and Darszon, A. (2010). The SLO3 sperm-specific potassium channel plays a vital role in male fertility. FEBS Lett. 584, 1041–1046. doi: 10.1016/j.febslet.2010.02.005
Schmidt, T., Samaras, P., Frejno, M., Gessulat, S., Barnert, M., Kienegger, H., et al. (2017). ProteomicsDB. Nucleic Acids Res. 46, D1271–D1281. doi: 10.1093/nar/gkx1029
Schramm, S., Fraune, J., Naumann, R., Hernandez-Hernandez, A., Hoog, C., Cooke, H. J., et al. (2011). A novel mouse synaptonemal complex protein is essential for loading of central element proteins, recombination, and fertility. PLoS Genet. 7:e1002088. doi: 10.1371/journal.pgen.1002088
Schultz, N., Hamra, F. K., and Garbers, D. L. (2003). A multitude of genes expressed solely in meiotic or postmeiotic spermatogenic cells offers a myriad of contraceptive targets. Proc. Natl. Acad. Sci. U.S.A. 100, 12201–12206. doi: 10.1073/pnas.1635054100
Shang, E., Nickerson, H. D., Wen, D., Wang, X., and Wolgemuth, D. J. (2007). The first bromodomain of Brdt, a testis-specific member of the BET sub-family of double-bromodomain-containing proteins, is essential for male germ cell differentiation. Development 134, 3507–3515. doi: 10.1242/dev.004481
Shang, Y., Zhu, F., Wang, L., Ouyang, Y. C., Dong, M. Z., Liu, C., et al. (2017). Essential role for SUN5 in anchoring sperm head to the tail. eLife 6:e28199. doi: 10.7554/eLife.28199
Shao, Q., Kannan, A., Lin, Z., Stack, B. C. Jr., Suen, J. Y., and Gao, L. (2014). BET protein inhibitor JQ1 attenuates Myc-amplified MCC tumor growth in vivo. Cancer Res. 74, 7090–7102. doi: 10.1158/0008-5472.CAN-14-0305
Shen, C., Kuang, Y., Liu, J., Feng, J., Chen, X., Wu, W., et al. (2013). Prss37 is required for male fertility in the mouse. Biol. Reprod. 88:123. doi: 10.1095/biolreprod.112.107086
Shi, J., Wang, Y., Zeng, L., Wu, Y., Deng, J., Zhang, Q., et al. (2014). Disrupting the interaction of BRD4 with diacetylated Twist suppresses tumorigenesis in basal-like breast cancer. Cancer Cell 25, 210–225. doi: 10.1016/j.ccr.2014.01.028
Shibuya, H., Ishiguro, K., and Watanabe, Y. (2014). The TRF1-binding protein TERB1 promotes chromosome movement and telomere rigidity in meiosis. Nat. Cell Biol. 16, 145–156. doi: 10.1038/ncb2896
Shin, Y. H., Choi, Y., Erdin, S. U., Yatsenko, S. A., Kloc, M., Yang, F., et al. (2010). Hormad1 mutation disrupts synaptonemal complex formation, recombination, and chromosome segregation in mammalian meiosis. PLoS Genet. 6:e1001190. doi: 10.1371/journal.pgen.1001190
Silva, E. J., Patrao, M. T., Tsuruta, J. K., O’Rand, M. G., and Avellar, M. C. (2012). Epididymal protease inhibitor (EPPIN) is differentially expressed in the male rat reproductive tract and immunolocalized in maturing spermatozoa. Mol. Reprod. Dev. 79, 832–842. doi: 10.1002/mrd.22119
Sipila, P., Jalkanen, J., Huhtaniemi, I. T., and Poutanen, M. (2009). Novel epididymal proteins as targets for the development of post-testicular male contraception. Reproduction 137, 379–389. doi: 10.1530/REP-08-0132
Smith, C. L., Blake, J. A., Kadin, J. A., Richardson, J. E., and Bult, C. J., and Mouse Genome Database Group, (2018). Mouse genome database (MGD)-2018: knowledgebase for the laboratory mouse. Nucleic Acids Res. 46, D836–D842. doi: 10.1093/nar/gkx1006
Souquet, B., Abby, E., Herve, R., Finsterbusch, F., Tourpin, S., Le Bouffant, R., et al. (2013). MEIOB targets single-strand DNA and is necessary for meiotic recombination. PLoS Genet. 9:e1003784. doi: 10.1371/journal.pgen.1003784
Stelzer, G., Rosen, N., Plaschkes, I., Zimmerman, S., Twik, M., Fishilevich, S., et al. (2016). The GeneCards suite: from gene data mining to disease genome sequence analyses. Curr. Protoc. Bioinform. 54, 1.30.1–1.30.33. doi: 10.1002/cpbi.5
Stucker, F., and Ackermann, D. (2011). [Immunosuppressive drugs - how they work, their side effects and interactions]. Ther. Umsch. 68, 679–686. doi: 10.1024/0040-5930/a000230
Sullivan, R., and Mieusset, R. (2016). The human epididymis: its function in sperm maturation. Hum. Reprod. Update 22, 574–587. doi: 10.1093/humupd/dmw015
Tash, J. S., Attardi, B., Hild, S. A., Chakrasali, R., Jakkaraj, S. R., and Georg, G. I. (2008a). A novel potent indazole carboxylic acid derivative blocks spermatogenesis and is contraceptive in rats after a single oral dose. Biol. Reprod. 78, 1127–1138. doi: 10.1095/biolreprod.106.057810
Tash, J. S., Chakrasali, R., Jakkaraj, S. R., Hughes, J., Smith, S. K., Hornbaker, K., et al. (2008b). Gamendazole, an orally active indazole carboxylic acid male contraceptive agent, targets HSP90AB1 (HSP90BETA) and EEF1A1 (eEF1A), and stimulates Il1a transcription in rat Sertoli cells. Biol. Reprod. 78, 1139–1152. doi: 10.1095/biolreprod.107.062679
Teng, X., Yang, J., Xie, Y., Ni, Z., Hu, R., Shi, L., et al. (2006). A novel spermatogenesis-specific uPAR gene expressed in human and mouse testis. Biochem. Biophys. Res. Commun. 342, 1223–1227. doi: 10.1016/j.bbrc.2006.01.175
Tu, Z., Bayazit, M. B., Liu, H., Zhang, J., Busayavalasa, K., Risal, S., et al. (2017). Speedy A-Cdk2 binding mediates initial telomere-nuclear envelope attachment during meiotic prophase I independent of Cdk2 activation. Proc. Natl. Acad. Sci. U.S.A. 114, 592–597. doi: 10.1073/pnas.1618465114
Uhlen, M., Fagerberg, L., Hallstrom, B. M., Lindskog, C., Oksvold, P., Mardinoglu, A., et al. (2015). Tissue-based map of the human proteome. Science 347:1260419. doi: 10.1126/science.1260419
VanGompel, M. J., and Xu, E. Y. (2010). A novel requirement in mammalian spermatid differentiation for the DAZ-family protein Boule. Hum. Mol. Genet. 19, 2360–2369. doi: 10.1093/hmg/ddq109
Waites, G. M. (1993). Male fertility regulation: the challenges for the year 2000. Br. Med. Bull. 49, 210–221. doi: 10.1093/oxfordjournals.bmb.a072599
Walters, E. M., Bauer, B. A., Franklin, C. L., Evans, T. J., Bryda, E. C., Riley, L. K., et al. (2009). Mutational insertion of a ROSA26-EGFP transgene leads to defects in spermiogenesis and male infertility in mice. Comp. Med. 59, 545–552.
Welborn, J. P., Davis, M. G., Ebers, S. D., Stodden, G. R., Hayashi, K., Cheatwood, J. L., et al. (2015). Rhox8 ablation in the sertoli cells using a tissue-specific RNAi approach results in impaired male fertility in mice. Biol. Reprod. 93:8. doi: 10.1095/biolreprod.114.124834
Wells, J. A., and McClendon, C. L. (2007). Reaching for high-hanging fruit in drug discovery at protein-protein interfaces. Nature 450, 1001–1009. doi: 10.1038/nature06526
Wen, N., Guo, B., Zheng, H., Xu, L., Liang, H., Wang, Q., et al. (2019). Bromodomain inhibitor jq1 induces cell cycle arrest and apoptosis of glioma stem cells through the VEGF/PI3K/AKT signaling pathway. Int. J. Oncol. 55, 879–895. doi: 10.3892/ijo.2019.4863
Whitty, A., and Kumaravel, G. (2006). Between a rock and a hard place? Nat. Chem. Biol. 2, 112–118. doi: 10.1038/nchembio0306-112
Yabuta, Y., Ohta, H., Abe, T., Kurimoto, K., Chuma, S., and Saitou, M. (2011). TDRD5 is required for retrotransposon silencing, chromatoid body assembly, and spermiogenesis in mice. J. Cell Biol. 192, 781–795. doi: 10.1083/jcb.201009043
Yamaguchi, R., Fujihara, Y., Ikawa, M., and Okabe, M. (2012). Mice expressing aberrant sperm-specific protein PMIS2 produce normal-looking but fertilization-incompetent spermatozoa. Mol. Biol. Cell 23, 2671–2679. doi: 10.1091/mbc.E11-12-1025
Yang, K., Meinhardt, A., Zhang, B., Grzmil, P., Adham, I. M., and Hoyer-Fender, S. (2012). The small heat shock protein ODF1/HSPB10 is essential for tight linkage of sperm head to tail and male fertility in mice. Mol. Cell Biol. 32, 216–225. doi: 10.1128/MCB.06158-11
Young, S. A., Miyata, H., Satouh, Y., Kato, H., Nozawa, K., Isotani, A., et al. (2015). CRISPR/Cas9-mediated rapid generation of multiple mouse lines identified Ccdc63 as essential for spermiogenesis. Int. J. Mol. Sci. 16, 24732–24750. doi: 10.3390/ijms161024732
Yu, Y. E., Zhang, Y., Unni, E., Shirley, C. R., Deng, J. M., Russell, L. D., et al. (2000). Abnormal spermatogenesis and reduced fertility in transition nuclear protein 1-deficient mice. Proc. Natl. Acad. Sci. U.S.A. 97, 4683–4688. doi: 10.1073/pnas.97.9.4683
Zallot, R., Harrison, K. J., Kolaczkowski, B., and de Crecy-Lagard, V. (2016). Functional annotations of paralogs: a blessing and a curse. Life 6:39. doi: 10.3390/life6030039
Zeng, X. H., Yang, C., Kim, S. T., Lingle, C. J., and Xia, X. M. (2011). Deletion of the Slo3 gene abolishes alkalization-activated K+ current in mouse spermatozoa. Proc. Natl. Acad. Sci. U.S.A. 108, 5879–5884. doi: 10.1073/pnas.1100240108
Zhang, Z., Shen, X., Gude, D. R., Wilkinson, B. M., Justice, M. J., Flickinger, C. J., et al. (2009). MEIG1 is essential for spermiogenesis in mice. Proc. Natl. Acad. Sci. U.S.A. 106, 17055–17060. doi: 10.1073/pnas.0906414106
Zheng, K., Xiol, J., Reuter, M., Eckardt, S., Leu, N. A., McLaughlin, K. J., et al. (2010). Mouse MOV10L1 associates with Piwi proteins and is an essential component of the Piwi-interacting RNA (piRNA) pathway. Proc. Natl. Acad. Sci. U.S.A. 107, 11841–11846. doi: 10.1073/pnas.1003953107
Zhou, J., Du, Y. R., Qin, W. H., Hu, Y. G., Huang, Y. N., Bao, L., et al. (2009). RIM-BP3 is a manchette-associated protein essential for spermiogenesis. Development 136, 373–382. doi: 10.1242/dev.030858
Zhou, J., Li, W., Guo, J., Li, G., Chen, F., and Zhou, J. (2016). Downregulation of miR-329 promotes cell invasion by regulating BRD4 and predicts poor prognosis in hepatocellular carcinoma. Tumour Biol. 37, 3561–3569. doi: 10.1007/s13277-015-4109-4
Keywords: contraception, drug target, knockout mouse, spermatozoa, druggability
Citation: Kent K, Johnston M, Strump N and Garcia TX (2020) Toward Development of the Male Pill: A Decade of Potential Non-hormonal Contraceptive Targets. Front. Cell Dev. Biol. 8:61. doi: 10.3389/fcell.2020.00061
Received: 23 July 2019; Accepted: 22 January 2020;
Published: 26 February 2020.
Edited by:
Zhibing Zhang, Virginia Commonwealth University, United StatesReviewed by:
C. Yan Cheng, Population Council, United StatesCopyright © 2020 Kent, Johnston, Strump and Garcia. This is an open-access article distributed under the terms of the Creative Commons Attribution License (CC BY). The use, distribution or reproduction in other forums is permitted, provided the original author(s) and the copyright owner(s) are credited and that the original publication in this journal is cited, in accordance with accepted academic practice. No use, distribution or reproduction is permitted which does not comply with these terms.
*Correspondence: Thomas X. Garcia, dGhvbWFzLmdhcmNpYUBiY20uZWR1
Disclaimer: All claims expressed in this article are solely those of the authors and do not necessarily represent those of their affiliated organizations, or those of the publisher, the editors and the reviewers. Any product that may be evaluated in this article or claim that may be made by its manufacturer is not guaranteed or endorsed by the publisher.
Research integrity at Frontiers
Learn more about the work of our research integrity team to safeguard the quality of each article we publish.