- Fels Institute for Cancer Research, Lewis Katz School of Medicine, Temple University, Philadelphia, PA, United States
In mammals, sex chromosomes start to program autosomal gene expression and epigenetic patterns very soon after fertilization. Yet whether the resulting sex differences are perpetuated throughout development and how they connect to the sex-specific expression patterns in adult tissues is not known. There is a dearth of information on the timing and continuity of sex biases during development. It is also unclear whether sex-specific selection operates during embryogenesis. On the other hand, there is mounting evidence that all adult tissues exhibit sex-specific expression patterns, some of which are independent of hormonal influence and due to intrinsic regulatory effects of the sex chromosome constitution. There are many diseases with origins during embryogenesis that also exhibit sex biases. Epigenetics has provided us with viable mechanisms to explain how the genome stores the memory of developmental events. We propose that some of these marks can be traced back to the sex chromosomes, which interact with the autosomes and establish sex-specific epigenetic features soon after fertilization. Sex-biased epigenetic marks that linger after reprograming may reveal themselves at the transcriptional level at later developmental stages and possibly, throughout the lifespan. Detailed molecular information on the ontogeny of sex biases would also elucidate the sex-specific selective pressures operating on embryos and how compensatory mechanisms evolved to resolve sexual conflict.
Introduction
In the age of genomics, it has become ever more obvious that the long-known differences between males and females in health, longevity, disease risk and presentation, and response to therapy have genetic and epigenetic foundations (Yang et al., 2006; Isensee et al., 2008; Singmann et al., 2015; Chen et al., 2016; Mayne et al., 2016; Gershoni and Pietrokovski, 2017; McCormick et al., 2017). It is now clear that every adult somatic cell, to a greater or lesser degree, exhibits sex biases in gene expression and epigenetic profile in human and non-human primates, rodents, and bovines (Yang et al., 2006; Blekhman et al., 2010). There is also a growing realization that sex differences are the result of complex interactions between the sex hormones, genetic variability, and the environment, all of which operate on the background of the intrinsic effects of the sex chromosome composition, i.e., XX for females and XY for males (Arnold, 2014; Engel, 2018; Raznahan et al., 2018; Khramtsova et al., 2019).
Although the driver of sex differences in mammals has traditionally been considered to be the so-called sex determination pathway, sex-specific transcriptional and epigenomic profiles are present in the embryo very soon after fertilization in a range of mammals, i.e., well before the development of the gonads (Burgoyne et al., 1995; Bermejo-Alvarez et al., 2010; Lowe et al., 2015; Hansen et al., 2016). Moreover, male and female embryos exhibit different susceptibilities to environmental factors during early gestation (Jenkins et al., 2007; Gabory et al., 2012; Bale, 2016; Bansal et al., 2017). These differences reflect the early sexual identity of the embryo (Hansen et al., 2016) and of the placenta (Nugent et al., 2018), contrasting sharply with the prevailing view of sex-neutral development prior to gonadogenesis (Dewing et al., 2003). Long before sex hormones appear, the primary sex-determining factor is the imbalance in sex chromosome composition (Blecher and Erickson, 2007; Arnold, 2012).
If sex differences at the molecular level begin at such an early stage, the question is, do those differences matter and what are their contributions to sex differences that become apparent later in life? Do these sex biases matter more for some tissues than for others? Do expression and epigenetic sex biases wax and wane over the course of embryogenesis? How does the sex-specific molecular skewing inform on compensatory mechanisms that operate on males and females during embryogenesis from an evolutionary standpoint? The goal of this article is to identify gaps in our knowledge that impede us from answering these questions.
Sex Biases in Pre-Implantation Embryos
The Sex-Specific Regulatory Environment in Early Embryogenesis
Two observations justify how sex-biased expression in pre-implantation embryos could establish male and female-specific transcriptional and epigenetic legacies that become apparent at later stages in development. First, a number of dosage-dependent regulatory factors are expressed in a sex-biased manner in pre-implantation embryos, some of which are encoded on the X and Y chromosomes. Many transcription factors (TFs) and epigenetic regulators must be expressed at the appropriate levels for proper activation or repression of their downstream target genes. In fact, TFs are overrepresented in haploinsufficiency disorders (McKusick, 2002; Seidman and Seidman, 2002)1, in which mutations inactivating one allele produce a reduction by half in the protein levels of the TF. Second, there are precedents for epigenetic marks present after fertilization to persist after implantation. For example, genomic imprints from each parental genome are maintained throughout the genome-wide pre- and post-implantation reprograming processes (Barlow and Bartolomei, 2014; Engel, 2015).
In principle, variations in TF levels can change the response of their target genes or alter their affinity to their cognate sites (Chen et al., 2014), because in contrast to prokaryotes, transcriptional activation in eukaryotes is not always an all-or-none response. Promoters and enhancers are more or less sensitive to TF concentrations depending on the number of binding sites for specific TFs (Badis et al., 2009; Lambert et al., 2018). In addition, TFs often act synergistically or in multimers, so higher or lower levels can result in changes in downstream effects (Jolma et al., 2015).
Dosage also affects epigenetic factors (EFs), such as DNA-methyltransferases and histone modification enzymes. Because these usually act in large complexes, changes in the levels of protein components of these complexes can alter their stoichiometry and function (Veitia and Birchler, 2015; Ori et al., 2016). In turn, some TFs are sensitive to the chromatin environment (Blattler and Farnham, 2013; Inukai et al., 2017; Yin et al., 2017), so male- and female-specific epigenetic differences in binding sites can change their availability.
Unfortunately, there is a dearth of experimental data to determine how dosage differences in TFs and EFs shift transcriptomes, much less phenotypes, in mammalian model systems. ChIP data showing that sex-biased TFs are distributed differentially across the genome or that they activate their targets differentially would go a long way toward understanding dosage effects of regulatory factors. Making ChIP more quantitative and more sensitive, and expanding the availability of ChIP-grade antibodies for regulatory factors is a pre-requisite. Perhaps technology that allows us to tag TFs by genetic engineering will solve some of these issues (Savic et al., 2015). The ability to finely tune the levels of TFs is also necessary to determine if subtle variations have downstream consequences.
X Chromosome Inactivation (XCI) in Female Embryos
One of the best-studied events distinguishing male and female pre-implantation embryos is that females undergo X chromosome inactivation (XCI). XCI in placental mammals is a dosage compensation mechanism that transcriptionally silences the majority of genes on one of the X chromosomes in females. Because males have a single X chromosome, this ensures dosage equivalence between males and females. The long non-coding RNA Xist becomes highly expressed on one X chromosome, coating the entire chromosome and triggering the accumulation of DNA methylation and condensing histone modifications, ultimately resulting in heterochromatinization (Disteche and Berletch, 2015; Sahakyan et al., 2018).
Two consequences result from this massive epigenetic overhaul of an entire chromosome. First, female embryos are developmentally delayed relative to male embryos until XCI is complete (Thornhill and Burgoyne, 1993; Schulz et al., 2014). It is well-established that XCI is intimately tied to cell differentiation, at least in the mouse (Lessing et al., 2013; Payer and Lee, 2014), and its failure is lethal (Takagi and Abe, 1990; Marahrens et al., 1997). Thus, in addition to the effects of sex-biased expression of TFs, the delay in XX embryos opens a window of opportunity for TFs and EFs to act on the female genome in a sex-specific manner, even if they are not expressed in a sex-biased manner. On the other hand, the male genome may undergo specific modifications as a consequence of not needing to inactivate an X chromosome.
Another consequence of XCI that has been hypothesized is that the inactive X is a sink for epigenetic factors, altering their relative concentrations between males and female, with possible consequences for autosomal regulation (Wijchers et al., 2010; Arnold et al., 2012). The decrease in availability of EFs in females would introduce differences in the chromatin status of regulatory sequences. In turn, this would introduce a variation in how the genome is read and regulated in the female embryo. Both of these scenarios require experimental validation with sensitive genomic and proteomic tools that allow interrogation of single sexed embryos before and after XCI to determine whether females are on a different developmental clock and whether specific epigenetic factors are indeed substantially diminished relative to male embryos.
The process of XCI is stochastic in the embryo and the choice of the X chromosome to be inactivated is heritable. This means that female placental mammals are mosaics because in some cells the paternally inherited X chromosome is inactive whereas in other cells it is the maternally inherited X which is inactive (Migeon, 2007). As a result, expression of X-linked allelic variants will vary in different cell lineages (Wu et al., 2014). If the alleles exhibit variation in their expression levels, female cells in which the maternal X is active can have expression levels of X-linked genes that differ from those in male cells.
Although the majority of genes are silenced on the inactive X chromosome, a number of genes escape XCI and remain more highly expressed in female cells after implantation, contributing to sex biases in gene expression throughout the lifespan of the organism (Disteche and Berletch, 2015; Balaton and Brown, 2016).
Post-Implantation Embryogenesis and Beyond
Implantation signals a major reprograming of the genome, concomitant with lineage determination. If sex-biased epigenetic landscapes can weather the de novo DNA methylation and chromatin re-structuring that ensues, it remains to be determined which specific epigenetic marks identify the cell as male or female. If, on the other hand, implantation erases all sex biases between XX and XY embryos, there are still genes encoded on the sex chromosomes that are differentially expressed before the appearance of sex hormones that could lead to sex-biased autosomal gene expression. Such is the case of Y-linked genes, absent in female cells, X-linked allelic variants and genes that escape XCI altogether (Disteche and Berletch, 2015). It has been reported that XCI “escapees” present some degree of tissue-specificity in adult tissues (Berletch et al., 2015; Balaton and Brown, 2016; Tukiainen et al., 2017). Therefore, we need a detailed, lineage-specific catalog of what genes escape XCI over the course of development and how they affect transcriptional outcomes.
Studies in multiple non-mammalian models have revealed sex-biased expression of many genes not necessarily related to sexual function throughout embryogenesis (Mank et al., 2010; Ma et al., 2018). Such detailed characterization of the fluctuations in transcriptional and epigenetic sex biases during development is lacking for mammals. Therefore, tissue-specific developmental time-series data are needed to begin to answer these questions experimentally.
Are Sex Differences During Embryogenesis Meaningful From an Evolutionary Standpoint?
Evolutionary conflict arises between the sexes when their fitness interests diverge. Because males and females share most of their genomes, genes common to both sexes encode many of their shared traits (Cox and Calsbeek, 2009; Hosken et al., 2019). Sexually antagonistic selection emerges when optimal fitness for traits differs, leading to intra-locus sexual conflict. For example, intra-locus conflict arises when expression of a gene is beneficial in one sex but detrimental in the other. Contradictory selection pressures can lead to sub-optimal expression levels for each sex, with subsequent regulatory mechanisms evolving to offset the less-than-optimal expression level. Thus, sex-biased gene expression can be indicative of ongoing or resolved intra-locus sexual conflict (Parsch and Ellegren, 2013; Rowe et al., 2018).
Forces generating expression differences are expected to be maximal in the adults, because this is when reproductive interests diverge. However, if we envision sexual differentiation as a progressive developmental process, with independent and combined contributions from the sex chromosomes and sex hormones, it is possible that expression patterns of early embryos are also under sex-specific selection pressures and that sex-biased expression during development indicates sexual antagonism (Ingleby et al., 2015). Because we lack detailed sex-stratified data across the whole life cycle in mammals, we do not know how sex-biased transcription contributes to the male and female phenotypes, much less all of the genes involved. For example, some sex biases may need to be expressed continuously throughout development, while others may be transient, setting the stage for later sexual dimorphism.
A different mechanism of uncoupling the genetic architecture between males and females involves gene duplication and the evolution of sex-specific regulatory mechanisms for each duplicate (Wyman et al., 2012). Especially in the case of mammals, with their greatly expanded families of TFs, it would be interesting to investigate if different paralogs enable conflict resolution by harboring divergent regulatory sequences that direct sex-specific expression.
Although the majority of genes that contribute to sexually dimorphic traits are autosomal and shared between the sexes, the sex chromosomes are a separate solution to sexual conflict, expanding the range of sexual differences at the level of expression that can exist (Hosken et al., 2019; Wright et al., 2019). The special nature of the sex chromosomes is related to evolutionary forces that have driven their differentiation and the compensatory mechanisms that allow male cells to tolerate the presence of a single X chromosome (Skaletsky et al., 2003; Graves, 2016; Arnold, 2019). These forces are independent, but can interact with those related to the divergent niches of males and females in reproduction. Offsetting the imbalance in the sex chromosomes is necessary either because genes on the sex chromosomes participate in complex regulatory networks or because they encode components of dosage-sensitive protein complexes (Bellott et al., 2014; Veitia and Potier, 2015). Compensation is partially achieved by XCI in female cells. However, very little is known about the adjustments of the autosomes to the imbalance of sex chromosomes in males and females, which in principle could give rise to sex-biased expression of autosomal genes at any point in development (Veitia et al., 2015).
Detailed molecular information across all stages of development for males and females would allow us to test the major hypotheses on the ontogeny and evolutionary significance of sex biases by integrating functional studies of individual genes with systems-level analyses and identifying similarities and differences across a range of species.
Systems Biology of Sex Biases
Some genes are expressed with less than a twofold difference between the sexes (Arnold et al., 2009; Werner et al., 2017). These differences may be considered trivial, but the systems biology revolution has highlighted that genes are interconnected in complex networks and that small differences in multiple genes can shift transcriptional and phenotypic outcomes. Considering that some sexually dimorphic traits are extremely complex, many small-effect loci are likely to underlie these traits. Expression variation quantitative trait locus (eQTL) mapping of sex-biased expression in mice support this expectation (Yang et al., 2006; van Nas et al., 2010).
A recent mandate from the NIH to include sex as a biological variable in all studies has adrenalized the interest in sex differences in disease risk and susceptibility (Clayton and Collins, 2014). Significant inroads have been made in characterizing sex biases in gene expression and epigenetic features in a variety of adult tissues. Modeling of regulatory networks in adult human and mouse tissues have shown surprising differences in regulatory architecture between males and females (Chen et al., 2016; Gershoni and Pietrokovski, 2017; Karp et al., 2017; Shen et al., 2017).
The range of continuously evolving analytical tools opens the possibility of looking at the aggregate pattern of sex-biased expression to reveal sex-specific modules within the global networks that specify cellular types. There is a high degree of plasticity in developmental pathways, with a variety of intermediate states leading to the same phenotypic space (Briggs et al., 2017; Figure 1). It is conceivable, then, that sex skews some parts of a network encoding a cellular phenotype, while not affecting others. It is also possible that some cell types may require a greater degree of molecular convergence between the sexes than others. Sex-stratified transcriptional and epigenetic data from embryos would also allow a more complete understanding of how the appearance of sex hormones affect developmental processes beyond the reproductive system.
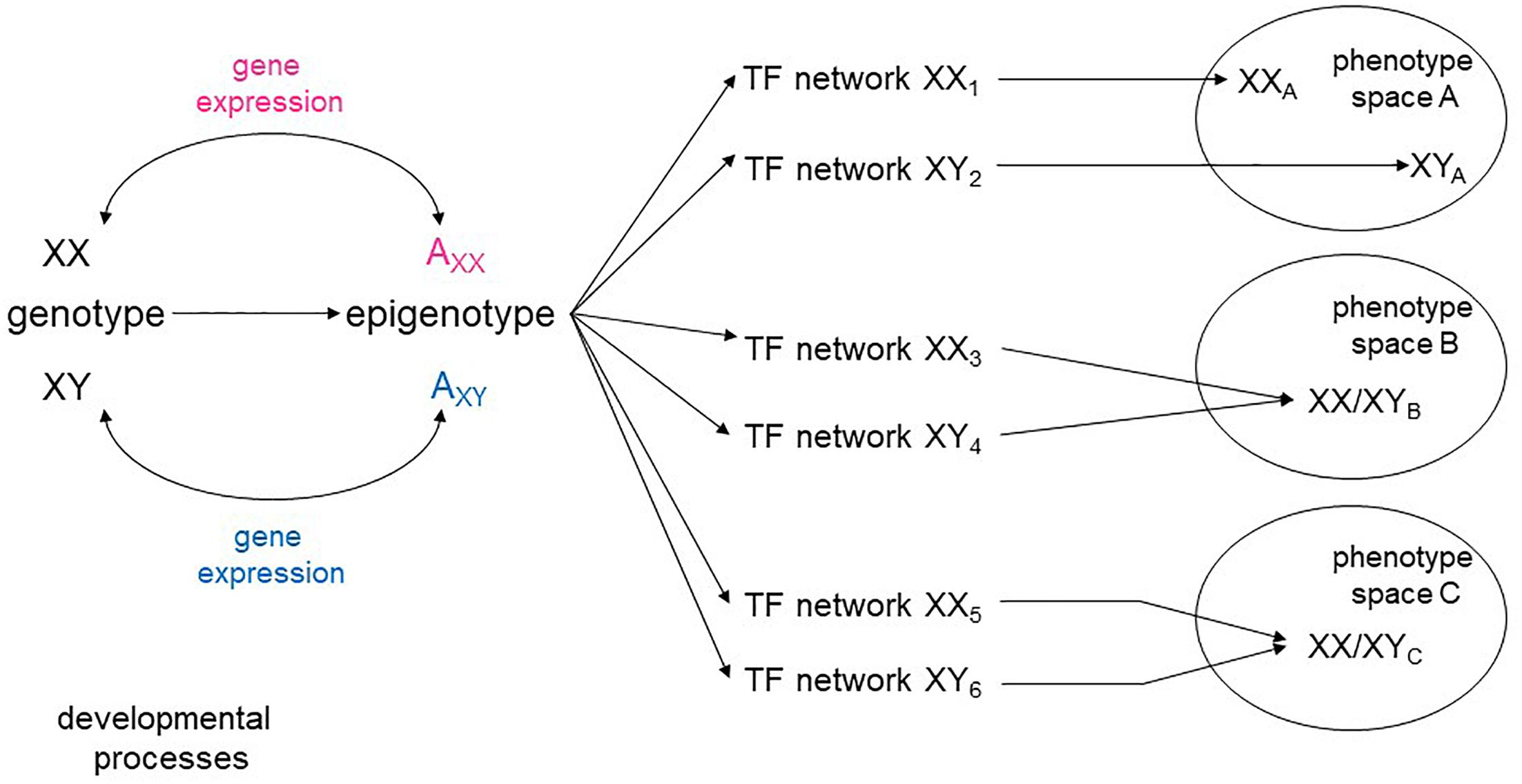
Figure 1. Sex-specific transcriptional networks and phenotype maps. Schematic representation of the relationships between genotype, transcriptional networks, and final phenotype during development. Male and female genotypes, represented as XY and XX produce distinct epigenotypes, with effects on and counter-effects from the autosomes (A). Modifications of the epigenotype on the autosomes lead to transcriptional changes that in turn influence expression from the sex chromosomes. Different transcription factor (TF) networks can either determine distinct phenotypes (space A) or converge to an equivalent phenotype (spaces B, C).
Speculations and Future Directions
We propose that genes encoded on the sex chromosomes act on autosomal genes to generate a differential regulatory and epigenetic landscape upon which later factors, such as hormones, act to counter or compound sex biases. Because the epigenome does not necessarily affect transcription until stage-specific TFs appear, epigenetic sex biases established in early development could persist and contribute to sex-specific phenotypes at later time points (Figure 2). Testing this hypothesis will first require identifying the nature of sex-biased epigenetic marks, with DNA methylation an obvious candidate. Then, we must gather and integrate dynamic, sex-stratified epigenetic, expression, and proteomic data throughout embryogenesis. The degree to which molecular sex differences are compensated for between the sexes are likely to be tissue-specific, with some cell types requiring greater molecular convergence than others for proper functionality. This can be revealed with detailed tissue-specific analyses, a time-consuming but certainly worthwhile effort. The role of sex hormones in these processes can then be inferred and validated with in vivo manipulations in animal models. This will pave the way for connecting sex biases during development to adult phenotypes.
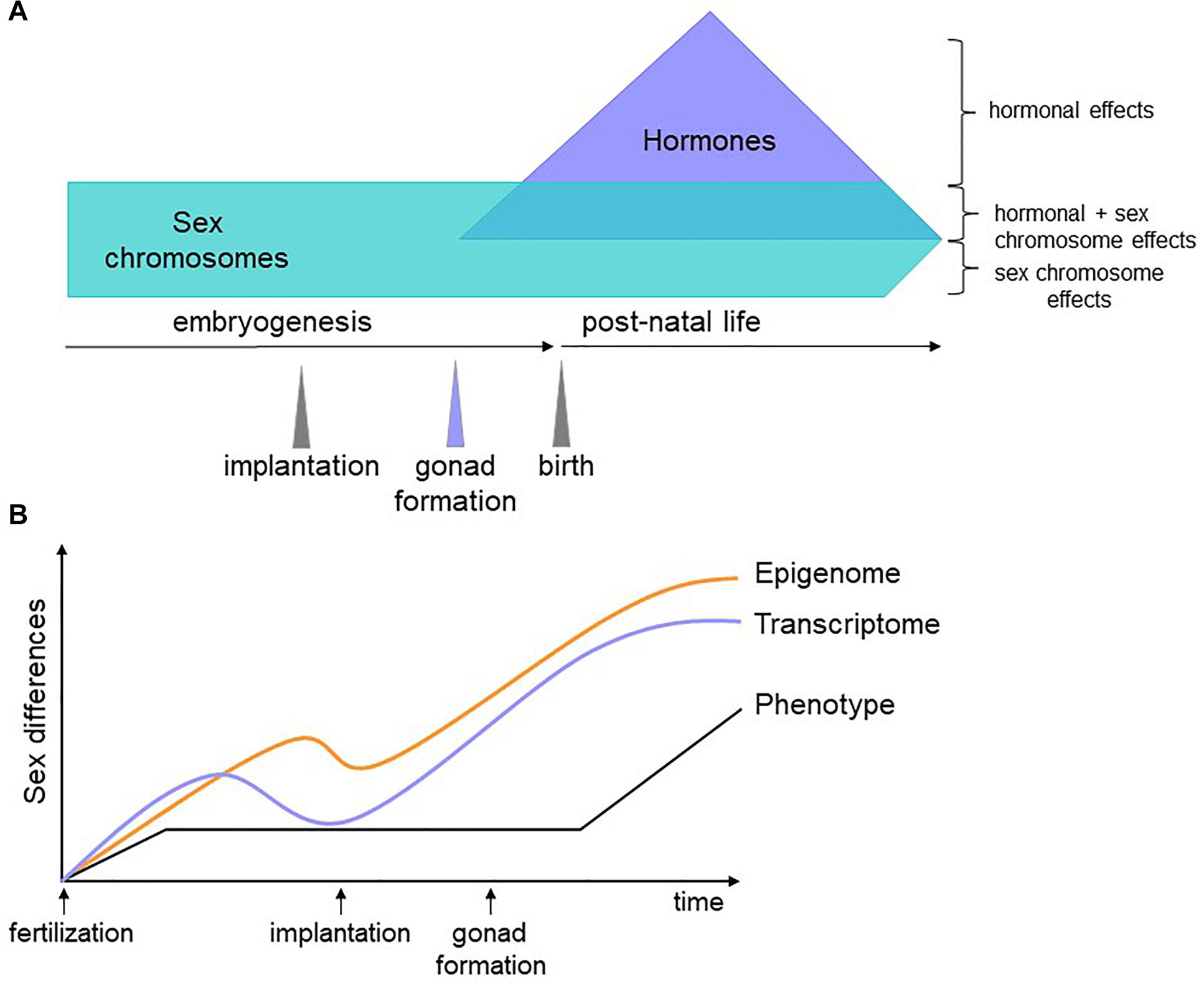
Figure 2. Schematic of our hypothesis. (A) Sex biases have different origins depending on the developmental stage of the organism. Before gonadogenesis, sex chromosomes are the primary determinants of sex differences. Sex hormones influence the transcriptome and epigenome independently of and in combination with sex chromosome effects. (B) Soon after fertilization, male and female cells have sex-specific transcriptomes, epigenomes, and phenotypes (for example, male embryos grow faster than female embryos). At implantation, lineage determination begins and gene expression differences are reduced. Epigenetic marks, however, are less constrained and some are maintained, affecting gene expression, and phenotype later in development. Once specific lineages are established, differences in gene expression increase again due to environmental, hormonal and genetic factors, some of which act on sex-specific epigenetic features established prior to differentiation.
Concluding Remarks
There are many outstanding questions on the significance and extent of sex biases in gene expression and the epigenome, especially during mammalian embryogenesis. We have evidence that the sex chromosomes and autosomes are engaged in a regulatory dialogue very soon after fertilization, but the implications for the specification of cell types and organogenesis are unclear. Stratification by sex of existing and forthcoming data, in combination with experimental validation, will allow us to determine whether early sex biases have ramifications across the lifespan. Translation of these insights into humans will require incorporating studies on how genetic background and environmental factors influence sex differences. These studies have practical value in understanding how sex functions as a variable in the developmental origins of disease (Barker, 2004). If in fact there are sex-specific evolutionary pressures acting on embryos, finding the specific genes involved and the networks they are embedded in will inform us on the compensatory mechanisms that allow males and females to develop into healthy organisms. The coming years will undoubtedly be exciting times in the field of sex differences.
Author Contributions
DD contributed to the bibliography compilation and review of the manuscript content. NE wrote and reviewed the manuscript. Both authors approved the final version of the manuscript.
Funding
This study was funded by an internal Temple University grant.
Conflict of Interest Statement
The authors declare that the research was conducted in the absence of any commercial or financial relationships that could be construed as a potential conflict of interest.
Footnotes
References
Arnold, A. P. (2012). The end of gonad-centric sex determination in mammals. Trends Genet. 28, 55–61. doi: 10.1016/j.tig.2011.10.004
Arnold, A. P. (2014). Conceptual frameworks and mouse models for studying sex differences in physiology and disease: why compensation changes the game. Exp. Neurol. 259, 2–9. doi: 10.1016/j.expneurol.2014.01.021
Arnold, A. P. (2019). Rethinking sex determination of non-gonadal tissues. Curr. Top. Dev. Biol. 134, 289–315. doi: 10.1016/bs.ctdb.2019.01.003
Arnold, A. P., Chen, X., and Itoh, Y. (2012). What a difference an X or Y makes: sex chromosomes, gene dose, and epigenetics in sexual differentiation. Handb. Exp. Pharmacol. 214, 67–88. doi: 10.1007/978-3-642-30726-3_4
Arnold, A. P., van Nas, A., and Lusis, A. J. (2009). Systems biology asks new questions about sex differences. Trends Endocrinol. Metab. 20, 471–476. doi: 10.1016/j.tem.2009.06.007
Badis, G., Berger, M. F., Philippakis, A. A., Talukder, S., Gehrke, A. R., Jaeger, S. A., et al. (2009). Diversity and complexity in DNA recognition by transcription factors. Science 324, 1720–1723. doi: 10.1126/science.1162327
Balaton, B. P., and Brown, C. J. (2016). Escape Artists of the X Chromosome. Trends Genet. 32, 348–359. doi: 10.1016/j.tig.2016.03.007
Bale, T. L. (2016). The placenta and neurodevelopment: sex differences in prenatal vulnerability. Dialogues Clin. Neurosci. 18, 459–464.
Bansal, A., Rashid, C., Xin, F., Li, C., Polyak, E., Duemler, A., et al. (2017). Sex- and dose-specific effects of maternal bisphenol a exposure on pancreatic islets of first- and second-generation adult mice offspring. Environ. Health Perspect. 125:097022. doi: 10.1289/EHP1674
Barlow, D. P., and Bartolomei, M. S. (2014). Genomic imprinting in mammals. Cold Spring Harb. Perspect. Biol. 6:a018382. doi: 10.1101/cshperspect.a018382
Bellott, D. W., Hughes, J. F., Skaletsky, H., Brown, L. G., Pyntikova, T., Cho, T. J., et al. (2014). Mammalian Y chromosomes retain widely expressed dosage-sensitive regulators. Nature 508, 494–499. doi: 10.1038/nature13206
Berletch, J. B., Ma, W., Yang, F., Shendure, J., Noble, W. S., Disteche, C. M., et al. (2015). Escape from X inactivation varies in mouse tissues. PLoS Genet 11:e1005079. doi: 10.1371/journal.pgen.1005079
Bermejo-Alvarez, P., Rizos, D., Rath, D., Lonergan, P., and Gutierrez-Adan, A. (2010). Sex determines the expression level of one third of the actively expressed genes in bovine blastocysts. Proc. Natl. Acad. Sci. U.S.A. 107, 3394–3399. doi: 10.1073/pnas.0913843107
Blattler, A., and Farnham, P. J. (2013). Cross-talk between site-specific transcription factors and DNA methylation states. J. Biol. Chem. 288, 34287–34294. doi: 10.1074/jbc.r113.512517
Blecher, S. R., and Erickson, R. P. (2007). Genetics of sexual development: a new paradigm. Am. J. Med. Genet. A 143A, 3054–3068. doi: 10.1002/ajmg.a.32037
Blekhman, R., Marioni, J. C., Zumbo, P., Stephens, M., and Gilad, Y. (2010). Sex-specific and lineage-specific alternative splicing in primates. Genome Res. 20, 180–189. doi: 10.1101/gr.099226.109
Briggs, J. A., Li, V. C., Lee, S., Woolf, C. J., Klein, A., and Kirschner, M. W. (2017). Mouse embryonic stem cells can differentiate via multiple paths to the same state. eLife 6:e26945. doi: 10.7554/eLife.26945
Burgoyne, P. S., Thornhill, A. R., Boudrean, S. K., Darling, S. M., Bishop, C. E., and Evans, E. P. (1995). The genetic basis of XX-XY differences present before gonadal sex differentiation in the mouse. Philos. Trans. R. Soc. Lond. B Biol. Sci. 350, 253–260.
Chen, C.-Y., Lopes Ramos, C., Kuijjer, M. L., Paulson, J. N., Sonawane, A. R., Fagny, M., et al. (2016). Sexual dimorphism in gene expression and regulatory networks across human tissues. bioRxiv
Chen, Z. X., Golovnina, K., Sultana, H., Kumar, S., and Oliver, B. (2014). Transcriptional effects of gene dose reduction. Biol. Sex Differ. 5:5. doi: 10.1186/2042-6410-5-5
Clayton, J. A., and Collins, F. S. (2014). Policy: NIH to balance sex in cell and animal studies. Nature 509, 282–283. doi: 10.1038/509282a
Cox, R. M., and Calsbeek, R. (2009). Sexually antagonistic selection, sexual dimorphism, and the resolution of intralocus sexual conflict. Am. Nat. 173, 176–187. doi: 10.1086/595841
Dewing, P., Shi, T., Horvath, S., and Vilain, E. (2003). Sexually dimorphic gene expression in mouse brain precedes gonadal differentiation. Brain Res. Mol. Brain Res. 118, 82–90. doi: 10.1016/s0169-328x(03)00339-5
Disteche, C. M., and Berletch, J. B. (2015). X-chromosome inactivation and escape. J. Genet. 94, 591–599. doi: 10.1007/s12041-015-0574-1
Engel, N. (2015). “Genomic imprinting in mammals: memories of generations past,” in Epigenetic Gene Expression and Regulation, eds S. Huang, M. D. Litt, and C. L. Blakely (Cambridge, MA: Academic Press).
Engel, N. (2018). Sex differences in early embryogenesis: inter-chromosomal regulation sets the stage for sex-biased gene networks: the dialogue between the sex chromosomes and autosomes imposes sexual identity soon after fertilization. Bioessays 40:e1800073. doi: 10.1002/bies.201800073
Gabory, A., Ferry, L., Fajardy, I., Jouneau, L., Gothie, J. D., Vige, A., et al. (2012). Maternal diets trigger sex-specific divergent trajectories of gene expression and epigenetic systems in mouse placenta. PLoS One 7:e47986. doi: 10.1371/journal.pone.0047986
Gershoni, M., and Pietrokovski, S. (2017). The landscape of sex-differential transcriptome and its consequent selection in human adults. BMC Biol. 15:7. doi: 10.1186/s12915-017-0352-z
Graves, J. A. (2016). Evolution of vertebrate sex chromosomes and dosage compensation. Nat. Rev. Genet. 17, 33–46. doi: 10.1038/nrg.2015.2
Hansen, P. J., Dobbs, K. B., Denicol, A. C., and Siqueira, L. G. (2016). Sex and the preimplantation embryo: implications of sexual dimorphism in the preimplantation period for maternal programming of embryonic development. Cell Tissue Res. 363, 237–247. doi: 10.1007/s00441-015-2287-4
Hosken, D. J., Archer, C. R., and Mank, J. E. (2019). Sexual conflict. Curr. Biol. 29, R451–R455. doi: 10.1016/j.cub.2019.03.052
Ingleby, F. C., Flis, I., and Morrow, E. H. (2015). Sex-biased gene expression and sexual conflict throughout development. Cold Spring Harb. Perspect. Biol. 7:a017632. doi: 10.1101/cshperspect.a017632
Inukai, S., Kock, K. H., and Bulyk, M. L. (2017). Transcription factor-DNA binding: beyond binding site motifs. Curr. Opin. Genet. Dev. 43, 110–119. doi: 10.1016/j.gde.2017.02.007
Isensee, J., Witt, H., Pregla, R., Hetzer, R., Regitz-Zagrosek, V., and Noppinger, P. R. (2008). Sexually dimorphic gene expression in the heart of mice and men. J. Mol. Med. 86, 61–74. doi: 10.1007/s00109-007-0240-z
Jenkins, K. J., Correa, A., Feinstein, J. A., Botto, L., Britt, A. E., Daniels, S. R., et al. (2007). Noninherited risk factors and congenital cardiovascular defects: current knowledge: a scientific statement from the american heart association council on cardiovascular disease in the young: endorsed by the american academy of pediatrics. Circulation 115, 2995–3014. doi: 10.1161/circulationaha.106.183216
Jolma, A., Yin, Y., Nitta, K. R., Dave, K., Popov, A., Taipale, M., et al. (2015). DNA-dependent formation of transcription factor pairs alters their binding specificity. Nature 527, 384–388. doi: 10.1038/nature15518
Karp, N. A., Mason, J., Beaudet, A. L., Benjamini, Y., Bower, L., Braun, R. E., et al. (2017). Prevalence of sexual dimorphism in mammalian phenotypic traits. Nat. Commun. 8:15475. doi: 10.1038/ncomms15475
Khramtsova, E. A., Davis, L. K., and Stranger, B. E. (2019). The role of sex in the genomics of human complex traits. Nat. Rev. Genet. 20, 173–190. doi: 10.1038/s41576-018-0083-1
Lambert, S. A., Jolma, A., Campitelli, L. F., Das, P. K., Yin, Y., Albu, M., et al. (2018). The human transcription factors. Cell 175, 598–599.
Lessing, D., Anguera, M. C., and Lee, J. T. (2013). X chromosome inactivation and epigenetic responses to cellular reprogramming. Annu. Rev. Genomics Hum. Genet. 14, 85–110. doi: 10.1146/annurev-genom-091212-153530
Lowe, R., Gemma, C., Rakyan, V. K., and Holland, M. L. (2015). Sexually dimorphic gene expression emerges with embryonic genome activation and is dynamic throughout development. BMC Genomics 16:295. doi: 10.1186/s12864-015-1506-4
Ma, W. J., Veltsos, P., Sermier, R., Parker, D. J., and Perrin, N. (2018). Evolutionary and developmental dynamics of sex-biased gene expression in common frogs with proto-Y chromosomes. Genome Biol. 19:156. doi: 10.1186/s13059-018-1548-4
Mank, J. E., Nam, K., Brunstrom, B., and Ellegren, H. (2010). Ontogenetic complexity of sexual dimorphism and sex-specific selection. Mol. Biol. Evol. 27, 1570–1578. doi: 10.1093/molbev/msq042
Marahrens, Y., Panning, B., Dausman, J., Strauss, W., and Jaenisch, R. (1997). Xist-deficient mice are defective in dosage compensation but not spermatogenesis. Genes Dev. 11, 156–166. doi: 10.1101/gad.11.2.156
Mayne, B. T., Miotto Bianco, T., Buckberry, S., Breen, J., Clifton, V., Shoubridge, C., et al. (2016). large scale gene expression meta-analysis reveals tissue-specific, sex-biased gene expression in humans. Front. Genet. 7:183. doi: 10.3389/fgene.2016.00183
McCormick, H., Young, P. E., Hur, S. S. J., Booher, K., Chung, H., Cropley, J. E., et al. (2017). Isogenic mice exhibit sexually-dimorphic DNA methylation patterns across multiple tissues. BMC Genomics 18:966. doi: 10.1186/s12864-017-4350-x
McKusick, V. A. (2002). Online Mendelian Inheritance in Man (OMIM). Baltimore, MD: McKusick-Nathans Institute of Genetic Medicine.
Migeon, B. R. (2007). Why females are mosaics, X-chromosome inactivation, and sex differences in disease. Gend. Med. 4, 97–105. doi: 10.1016/s1550-8579(07)80024-6
Nugent, B. M., O’Donnell, C. M., Epperson, C. N., and Bale, T. L. (2018). Placental H3K27me3 establishes female resilience to prenatal insults. Nat. Commun. 9:2555. doi: 10.1038/s41467-018-04992-1
Ori, A., Iskar, M., Buczak, K., Kastritis, P., Parca, L., Andres-Pons, A., et al. (2016). Spatiotemporal variation of mammalian protein complex stoichiometries. Genome Biol. 17:47. doi: 10.1186/s13059-016-0912-5
Parsch, J., and Ellegren, H. (2013). The evolutionary causes and consequences of sex-biased gene expression. Nat. Rev. Genet. 14, 83–87. doi: 10.1038/nrg3376
Payer, B., and Lee, J. T. (2014). Coupling of X-chromosome reactivation with the pluripotent stem cell state. RNA Biol. 11, 798–807. doi: 10.4161/rna.29779
Raznahan, A., Parikshak, N. N., Chandran, V., Blumenthal, J. D., Clasen, L. S., Alexander, A. F., et al. (2018). Sex-chromosome dosage effects on gene expression in humans. Proc. Natl. Acad. Sci. U.S.A. 115, 7398–7403. doi: 10.1073/pnas.1802889115
Rowe, L., Chenoweth, S. F., and Agrawal, A. F. (2018). The genomics of sexual conflict. Am. Nat. 192, 274–286. doi: 10.1086/698198
Sahakyan, A., Yang, Y., and Plath, K. (2018). The role of xist in X-chromosome dosage compensation. Trends Cell Biol. 28, 999–1013. doi: 10.1016/j.tcb.2018.05.005
Savic, D., Partridge, E. C., Newberry, K. M., Smith, S. B., Meadows, S. K., Roberts, B. S., et al. (2015). CETCh-seq: CRISPR epitope tagging ChIP-seq of DNA-binding proteins. Genome Res. 25, 1581–1589. doi: 10.1101/gr.193540.115
Schulz, E. G., Meisig, J., Nakamura, T., Okamoto, I., Sieber, A., Picard, C., et al. (2014). The two active X chromosomes in female ESCs block exit from the pluripotent state by modulating the ESC signaling network. Cell Stem Cell 14, 203–216. doi: 10.1016/j.stem.2013.11.022
Seidman, J. G., and Seidman, C. (2002). Transcription factor haploinsufficiency: when half a loaf is not enough. J. Clin. Invest. 109, 451–455. doi: 10.1172/jci15043
Shen, J. J., Wang, T. Y., and Yang, W. (2017). Regulatory and evolutionary signatures of sex-biased genes on both the X chromosome and the autosomes. Biol. Sex Differ. 8: 35. doi: 10.1186/s13293-017-0156-4
Singmann, P., Shem-Tov, D., Wahl, S., Grallert, H., Fiorito, G., Shin, S. Y., et al. (2015). Characterization of whole-genome autosomal differences of DNA methylation between men and women. Epigenetics Chromatin 8:43. doi: 10.1186/s13072-015-0035-3
Skaletsky, H., Kuroda-Kawaguchi, T., Minx, P. J., Cordum, H. S., Hillier, L., Brown, L. G., et al. (2003). The male-specific region of the human Y chromosome is a mosaic of discrete sequence classes. Nature 423, 825–837.
Takagi, N., and Abe, K. (1990). Detrimental effects of two active X chromosomes on early mouse development. Development 109, 189–201.
Thornhill, A. R., and Burgoyne, P. S. (1993). A paternally imprinted X chromosome retards the development of the early mouse embryo. Development 118, 171–174.
Tukiainen, T., Villani, A. C., Yen, A., Rivas, M. A., Marshall, J. L., Satija, R., et al. (2017). Landscape of X chromosome inactivation across human tissues. Nature 550, 244–248. doi: 10.1038/nature24265
van Nas, A., Ingram-Drake, L., Sinsheimer, J. S., Wang, S. S., Schadt, E. E., Drake, T., et al. (2010). Expression quantitative trait loci: replication, tissue- and sex-specificity in mice. Genetics 185, 1059–1068. doi: 10.1534/genetics.110.116087
Veitia, R. A., and Birchler, J. A. (2015). Models of buffering of dosage imbalances in protein complexes. Biol. Direct. 10:42. doi: 10.1186/s13062-015-0063-8
Veitia, R. A., and Potier, M. C. (2015). Gene dosage imbalances: action, reaction, and models. Trends Biochem. Sci. 40, 309–317. doi: 10.1016/j.tibs.2015.03.011
Veitia, R. A., Veyrunes, F., Bottani, S., and Birchler, J. A. (2015). X chromosome inactivation and active X upregulation in therian mammals: facts, questions, and hypotheses. J. Mol. Cell Biol. 7, 2–11. doi: 10.1093/jmcb/mjv001
Werner, R., Schultz, B., Huhn, J., Jelinek, J., Madzo, J., and Engel, N. (2017). Sex chromosomes drive gene expression and regulatory dimorphisms in mouse embryonic stem cells. Biol. Sex Diff. 8:28. doi: 10.1186/s13293-017-0150-x
Wijchers, P. J., Yandim, C., Panousopoulou, E., Ahmad, M., Harker, N., Saveliev, A., et al. (2010). Sexual dimorphism in mammalian autosomal gene regulation is determined not only by Sry but by sex chromosome complement as well. Dev. Cell 19, 477–484. doi: 10.1016/j.devcel.2010.08.005
Wright, A. E., Rogers, T. F., Fumagalli, M., Cooney, C. R., and Mank, J. E. (2019). Phenotypic sexual dimorphism is associated with genomic signatures of resolved sexual conflict. Mol. Ecol. 28, 2860–2871. doi: 10.1111/mec.15115
Wu, H., Luo, J., Yu, H., Rattner, A., Mo, A., Wang, Y., et al. (2014). Cellular resolution maps of X chromosome inactivation: implications for neural development, function, and disease. Neuron 81, 103–119. doi: 10.1016/j.neuron.2013.10.051
Wyman, M. J., Cutter, A. D., and Rowe, L. (2012). Gene duplication in the evolution of sexual dimorphism. Evolution 66, 1556–1566. doi: 10.1111/j.1558-5646.2011.01525.x
Yang, X., Schadt, E. E., Wang, S., Wang, H., Arnold, A. P., Ingram-Drake, L., et al. (2006). Tissue-specific expression and regulation of sexually dimorphic genes in mice. Genome Res. 16, 995–1004. doi: 10.1101/gr.5217506
Keywords: sexual dimorphism, sex-biased expression, transcriptional regulation, epigenetics, embryogenesis
Citation: Deegan DF and Engel N (2019) Sexual Dimorphism in the Age of Genomics: How, When, Where. Front. Cell Dev. Biol. 7:186. doi: 10.3389/fcell.2019.00186
Received: 02 July 2019; Accepted: 22 August 2019;
Published: 06 September 2019.
Edited by:
Montserrat Cecilia Anguera, University of Pennsylvania, United StatesReviewed by:
Heini Maaret Natri, Arizona State University, United StatesArthur P. Arnold, University of California, Los Angeles, United States
Copyright © 2019 Deegan and Engel. This is an open-access article distributed under the terms of the Creative Commons Attribution License (CC BY). The use, distribution or reproduction in other forums is permitted, provided the original author(s) and the copyright owner(s) are credited and that the original publication in this journal is cited, in accordance with accepted academic practice. No use, distribution or reproduction is permitted which does not comply with these terms.
*Correspondence: Nora Engel, bm9yYWVuZ2VsQHRlbXBsZS5lZHU=