- 1Department of Cell Pharmacology, Nagoya University Graduate School of Medicine, Nagoya, Japan
- 2Department of Cell Biology, Duke University Medical School, Durham, NC, United States
Establishment and maintenance of neuronal polarity are critical for neuronal development and function. One of the fundamental questions in neurodevelopment is how neurons generate only one axon and several dendrites from multiple minor neurites. Over the past few decades, molecular and cell biological approaches have unveiled a large number of signaling networks regulating neuronal polarity in cultured hippocampal neurons and the developing cortex. Emerging evidence reveals that positive and negative feedback signals play a crucial role in axon and dendrite specification. Positive feedback signals are continuously activated in one of minor neurites and result in axon specification and elongation, whereas negative feedback signals are propagated from a nascent axon terminal to all minor neurites and inhibit the formation of multiple axon, thereby leading to dendrite specification, and maintaining neuronal polarity. This current insight provides a holistic picture of the signaling mechanisms underlying neuronal polarization during neuronal development. Here, our review highlights recent advancements in this fascinating field, with a focus on the positive, and negative feedback signals as key regulatory mechanisms underlying neuronal polarization.
Introduction
Cell polarization is a crucial step in multiple cellular aspects such as differentiation, morphogenesis, and migration. Among the various cell types, neurons are highly polarized cells that have a long axon, and several short dendrites. These two different processes generate the information flow that is essential for brain functions such as memory, learning, and emotion. An axon is a single long process that transmits the information to other neurons by the release of neurotransmitters. Dendrites have several branched processes and dendritic spines, which contain neurotransmitter receptors to receive information from other neurons (Arimura and Kaibuchi, 2007; Takano et al., 2015). Recently, several excellent reviews have described remarkable advancements in understanding the molecular mechanisms regulating neuronal polarization, especially focusing on axon formation, and elongation in vitro and in vivo (Namba et al., 2015; Takano et al., 2015; Bentley and Banker, 2016; Schelski and Bradke, 2017; Yogev and Shen, 2017; Tortosa and Hoogenraad, 2018). In addition to these exciting topics, a major goal of neuronal development is to uncover the molecular mechanisms on how neurons stochastically determine axonal and dendritic fates to establish proper brain circuitry. Accumulating evidence has demonstrated that positive and negative feedback signals play a pivotal role in the establishment and maintenance of neuronal polarity (Arimura and Kaibuchi, 2007; Takano et al., 2015). These fascinating concepts can greatly improve the current understanding of signaling mechanisms regulating neuronal polarization. Moreover, recent studies suggest that both neuronal polarization and neuronal migration share common molecular mechanisms during neuronal development. Indeed, defects in neuronal polarization are closely tied to neuronal migration deficits in the developing cortex that result in neurodevelopmental disorders (Reiner and Sapir, 2009; Namba et al., 2015). In this brief review, we summarize the positive and negative feedback signals that are responsible for determining axonal and dendritic fates during neuronal development.
Neuronal Polarization Processes
Cultured hippocampal neurons have been an important experimental model for study neuronal polarity (Dotti et al., 1988; Banker, 2018). The neuronal morphological changes are classified into five stages (Figure 1A). Newly plated spherical hippocampal neurons extend filopodia (stage 1; shortly after plating). These neurons extend multiple minor neurites (stage 2; day 0.5–1.5), which are initially equivalent and undergo elongation and retraction. One of these equivalent minor neurites rapidly grows to become the axon (stage 3; day 1.5–3), and these neurons establish their polarity. The remaining short minor neurites continue to undergo growth and retraction, and these minor neurites subsequently develop into dendrites (stage 4; day 4–7). These neurons finally form dendritic spines and establish synaptic contacts (stage 5; >7 days in culture). Since axonal fate is stochastically determined in cultured hippocampal neurons, this process is called “the stochastic model” of neuronal polarization.
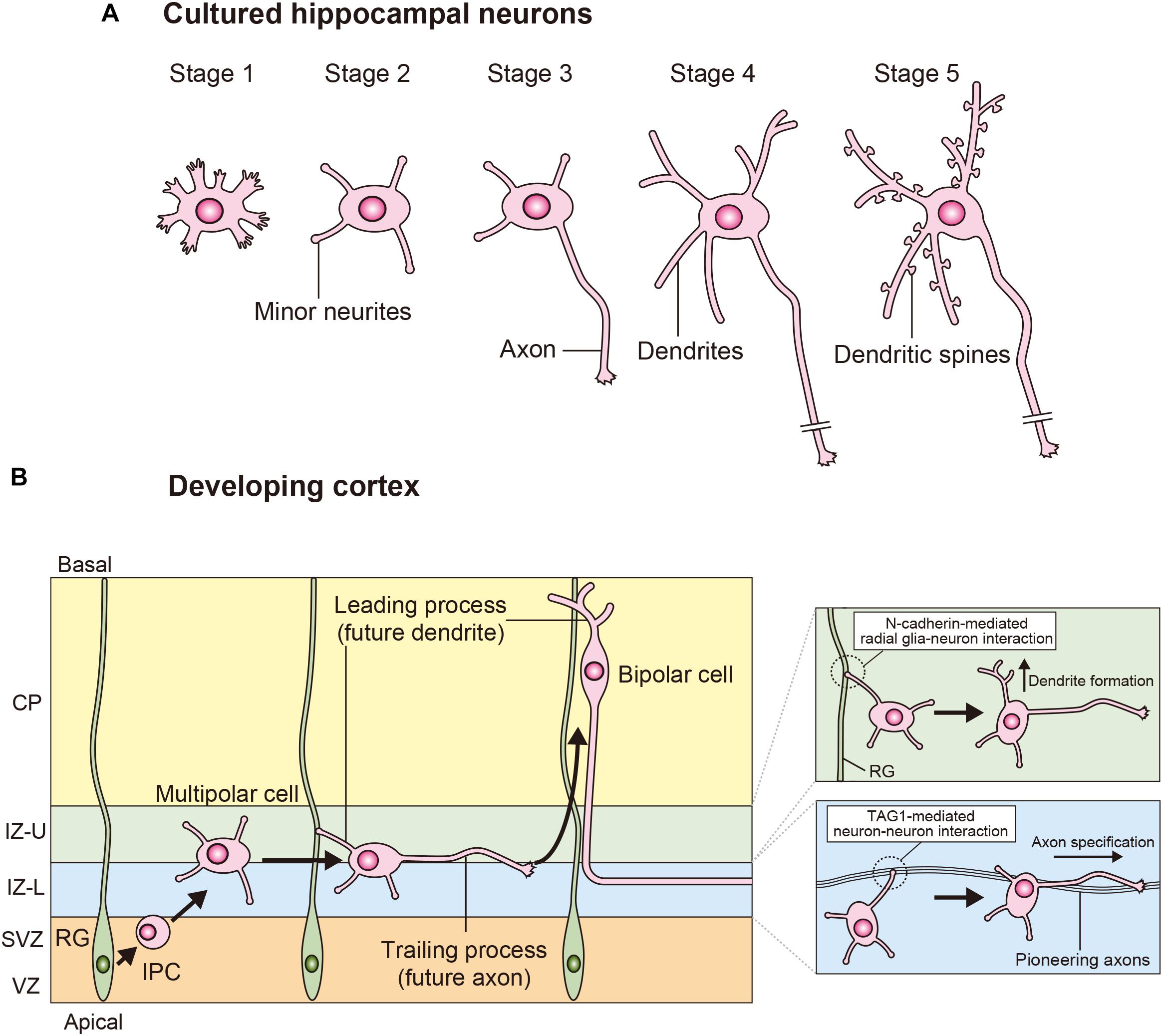
Figure 1. Processes of neuronal polarization in vitro and in vivo. (A) Upon isolation, hippocampal neurons form filopodia (stage 1). These neurons subsequently extend several minor neurites (stage 2), which show characteristic alternations of growth, and retraction. The major polarity event occurs when one of these equivalent minor neurites grows rapidly to become the axon (stage 3). The next steps are the morphological development of the remaining short minor neurites into dendrites (stage 4) and the functional polarization of axons and dendrites, including dendritic spine formation (stage 5). (B) In the developing neocortex, cortical neurons are generated from the radial glia in the VZ. These neurons extend multiple minor neurites and migrate toward the IZ through the SVZ. The multipolar (MP) cells generate a trailing process (future axon) and a leading process (future dendrite), and then these MP cells transform into bipolar (BP) cells and migrate toward the cortical plate (CP). A TAG-mediated interaction between a minor neurite of an MP cell and pioneering axons determines axon specification in the lower part of the IZ (IZ-L). N-cadherin-mediated interactions between the radial glia and neuron interactions determines dendrite specification in the upper part of the IZ (IZ-U).
In the developing cortex, cortical neurons are generated in the ventricular zone (VZ) and subsequently form multiple minor neurites. These multipolar (MP) cells migrate toward the intermediate zone (IZ) through the subventricular zone (SVZ) (Miyata et al., 2004; Noctor et al., 2004). MP cells form a trailing process (future axon) and a leading process (future dendrite), and transform into bipolar (BP) cells in the IZ (Miyata et al., 2004; Noctor et al., 2004). BP cells migrate toward the cortical plate (CP) along radial glias. Since the formation of leading process is necessary for neuronal migration, the MP-to-BP transition is a crucial step not only in the establishment of neuronal polarity but also in neuronal migration in developing cortex (Figure 1B).
Extrinsic Signaling in Axon and Dendrite Specification
In the developing cortex, neurons are surrounded by specific microenvironments containing extracellular molecules such as neurotrophins [brain-derived neurotrophic factor (BDNF) and neurotrophin 3 (NT3)], insulin-like growth factor 1 (IGF1), Wnt5A, transforming growth factor-β (TGF-β) and Semaphorin 3A, and cell adhesion molecules such as TAG1 and N-cadherin, which provide cues for neuronal polarization (Figure 2A; Funahashi et al., 2014; Namba et al., 2015; Takano et al., 2015; Rozes-Salvador et al., 2018). Indeed, inhibition of neurotrophin receptors, TrkB and TrkC, block the MP-to-BP transition in vivo (Nakamuta et al., 2011). Knockdown of TrkB also shows impairment of neuronal migration (Cheng et al., 2011). Recently, it has been shown that knockdown of the IGF-1 receptor impairs the MP-to-BP transition and neuronal migration (Nieto Guil et al., 2017). The expression level of Wnt5A is increased during the MP-to-BP transition in IZ and inhibition of Wnt5A blocks the MP-to-BP transition and neuronal migration (Boitard et al., 2015). Wnt5A activates atypical PKC (aPKC) in complex with Par3 and Par6 through Disheveled (Dvl) and promotes axon specification (Zhang et al., 2007). Because TGF-β is highly expressed in the VZ compared to that of CP in the developing cortex, the expression pattern of TGF-β is graded along the VZ-to-CP axis. TGF-β receptor (TβR2) conditional knockout mice fail to form axons of pyramidal neurons in vivo (Yi et al., 2010). TβR2 induces axon formation through phosphorylation of Par6 (Yi et al., 2010). In contrast, Semaphorin 3A is predominantly expressed in the CP and its expression decreases in the VZ (Polleux et al., 2000). Semaphorin 3A suppresses axon formation and promotes dendrite formation in vitro, and regulates the MP-to-BP transition and neuronal migration in vivo (Shelly et al., 2011). The gradient of these secreted factors was initially thought to determine axon or dendrite specification (Polleux et al., 2000; Yi et al., 2010). However, recent studies have shown that MP cells form the trailing process in any direction and subsequently migrate toward the CP, leaving behind the trailing process, which results in axonal elongation toward the VZ (Nakamuta et al., 2011; Namba et al., 2014). A gradient of extracellular molecules might be responsible for the axon formation and directional migration, but not for the determination of axon specification.
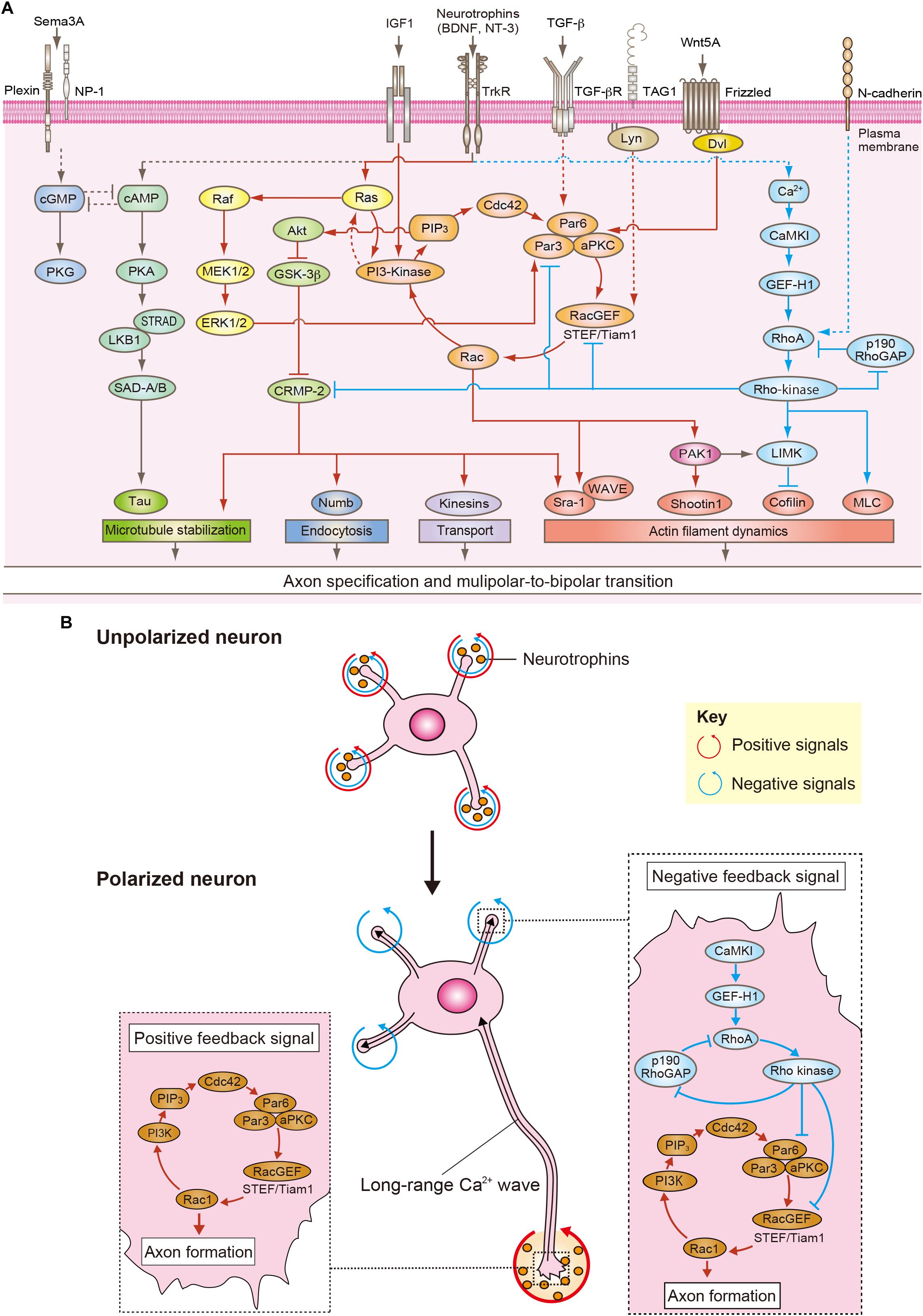
Figure 2. Positive and negative feedback signals for neuronal polarization. (A) Overview of positive (red arrows) and negative (blue arrows) feedback signaling that is responsible for neuronal polarization. Neurotrophins, TGF-β, Wnt5A, and TAG1 activate the Rac1/PI3-kinase/PIP3/Cdc42/Par complex/RacGEFs (STEF/Tiam) pathway that acts as a positive feedback loop underlying axon specification and MP-to-BP transition (red arrows). Neurotrophins induce Ras activation and thereby activate PI3-kinase. Ras also activates ERK1/2 through Raf/MEK pathway, and ERK1/2 regulates the selective transport of the Par complex to the nascent axon. TGF-β induces the phosphorylation of Par6, which is required for axon formation. Wnt5A activates aPKC through Dvl and promotes axon specification. TAG1 induces Rac1 activation through the Src family kinase Lyn. Rac1 regulates actin polymerization through PAK1-mediated phosphorylation of Shootin 1 and the Sra-1/WAVE complex during neuronal polarization. Rac1/PI3-kinase/PIP3/Cdc42/Par complex/RacGEFs (STEF/Tiam) pathway also activates CRMP-2 through Akt/GSK-3β pathway. CRMP-2 modulates microtubule stabilization, endocytosis, selective transport and actin filament dynamics through its binding to tubulin, Numb, the kinesin light chain subunit of kinesin-1, and Sra-1. Neurotrophins activate cAMP/PKA/LKB1/SAD-A/B kinases pathway, which regulates microtubule dynamics through the phosphorylation of Tau. Neurotrophins also induce Ca2+/CaMKK/CaMKI/GEF-H1/RhoA/Rho-kinase pathway that acts as a negative feedback signaling for preventing multiple axonal formation (blue arrows). N-cadherin activates RhoA/Rho-kinase. Rho-kinase maintains RhoA activation through inactivation of p190RhoGAP. Rho-kinase regulates actin filament dynamics and microtubule stabilization through MLC, LIM-kinase, and CRMP-2. Rho-kinase can inactivate Rac1 through the disruption of the Par complex and inactivation of Rac GEF (STEF). Semaphorin3A (Sema3A) suppresses axon formation through cGMP/PKG pathway. (B) In unpolarized neurons, the positive, and negative feedback signals are balanced in all minor neurites (red and blue arrows). When the balance is upset by the local amplification of neurotrophins, the Rac1/PI3-kinase/PIP3/Cdc42/Par complex/RacGEFs pathway is continuously activated within one minor neurite and thereby induces axon specification and elongation (red arrows). Concurrently, the long-range Ca2+ waves are propagated from the nascent axon to the cell body. The Ca2+ waves activate the CaMKI/GEF-H1/RhoA/Rho-kinase pathway, which represses the positive feedback loop in all minor neurites to prevent the formation of multiple axons and determine dendritic specification (blue arrows).
Given that MP cells constantly establish neuronal polarity within the IZ, cell-to-cell interactions should be noted. In the lower part of the IZ, approximately 60% of the MP cells first generate a trailing process and subsequently form a leading process (Hatanaka and Yamauchi, 2013; Takano et al., 2015). In this region. pioneering axons of cortical neurons are enriched (Namba et al., 2014). When a minor neurite of an MP cell “touches” a pioneering axon, it rapidly extends (“goes”) and becomes an axon. This process has been proposed the “Touch & Go model” (Namba et al., 2014, 2015; Takano et al., 2015). The cell adhesion molecule TAG1 is highly expressed in the pioneering axons and regulates this process. The TAG1-mediated neuron-neuron interaction activates Rac1 at the minor neurite of the MP cell through the Src family kinase Lyn and induces axon initiation (Namba et al., 2014; Figure 2A). Thus, TAG1-mediated neuron-neuron interaction in the lower part of IZ is essential for axon specification in the developing cortex (Namba et al., 2014; Figure 1B). The remaining approximately 40% of MP cells first form the leading process and then generate the trailing process in the upper part of IZ (Namba et al., 2014; Takano et al., 2015). In this region, a minor neurite of an MP cell becomes the leading process by making contact with a radial glia (Xu et al., 2015). N-cadherin accumulates in the upper part of IZ and regulates the radial glia-neuron interactions that determine neuronal polarity (Xu et al., 2015; Figure 1B). Inhibition of N-cadherin by the expression of dominant-negative N-cadherin or knockdown disrupts axon-dendrite polarity and neuronal migration in vivo (Kawauchi et al., 2010; Jossin and Cooper, 2011; Gartner et al., 2012; Xu et al., 2015). N-cadherin activates RhoA at the contacting neurite and then induces the formation of a leading process (Xu et al., 2015; Figure 2A). Thus, the TAG1-mediated neuron-neuron interaction and the N-cadherin-mediated radial glia–neuron interaction are essential for neuronal polarization in vivo as complementary mechanisms (Figure 1B).
Positive Feedback Signals
The involvement of “positive and negative feedback signals” play a crucial role in neuronal polarization (Arimura and Kaibuchi, 2007; Inagaki et al., 2011; Takano et al., 2015; Schelski and Bradke, 2017). “Positive feedback signals” induce axon specification and elongation. “Negative feedback signals” determine dendrite specification and maintain neuronal polarity by preventing the formation of multiple axons.
In the developing cortex, neuronal polarization is established in response to environmental cues (Funahashi et al., 2014; Namba et al., 2015). In contrast, cultured neurons stochastically generate a single axon and multiple dendrites that never develop into axons without the need for exogenous factors (Arimura and Kaibuchi, 2007; Takano et al., 2015). How do neurons on the stochastic model establish their polarity without these environmental cues? Recent results showed that inhibition of neurotrophins, such as BDNF and NT-3, or their receptors through the use of neutralizing antibodies and inhibitors prevents axon specification. These findings indicate that cultured hippocampal neurons secrete these neurotrophins for establishing neuronal polarity (Cheng et al., 2011; Nakamuta et al., 2011). Neurotrophin receptors are predominantly transported toward the tip of the nascent axon by kinesin-1 (Arimura et al., 2009). Furthermore, BDNF induces the production of cAMP and activation of PKA, which leads to further BDNF secretion and membrane insertion of TrkB, indicating that local amplification of neurotrophin signals are essential for neuronal polarity (Arimura et al., 2009; Cheng et al., 2011; Nakamuta et al., 2011).
The local amplification of neurotrophins induces PKA-dependent phophsoryaltion of the serine/threonine kinase LKB1, which promotes axon specification (Barnes et al., 2007; Shelly et al., 2007). LKB1 interacts with the STE20-related pseudokinase (STRAD) and the LKB1/STRAD complex is enriched in the growing axon (Shelly et al., 2007). Overexpression of LKB1 induces the formation of multiple axons, whereas inhibition of LKB1 attenuates axon specification in cultured hippocampal neurons (Barnes et al., 2007; Shelly et al., 2007). LKB1 conditional knockout mice fail to form axons of pyramidal neurons in the cortex, but neuronal migration is unaffected (Barnes et al., 2007). LKB1 phosphorylates and activates SAD-A/-B kinases, which regulate microtubule dynamics through the phosphorylation of Tau during neuronal polarization (Barnes et al., 2007; Shelly and Poo, 2011). SAD-A/-B kinases-deficient neurons show a mixed axon/dendrite identity in the developing neocortex (Kishi et al., 2005).
Neurotrophins also activate phosphoinositide 3-kinase (PI3-kinase) by Ras activation, thereby leading to axon specification in vitro (Shi et al., 2003; Menager et al., 2004; Yoshimura et al., 2006). Ras activation is abolished by the action of the PI3-kinase inhibitor in the tip of the axon, indicating a positive feedback loop between Ras, and PI3-kinase appears to be involved in neuronal polarization (Fivaz et al., 2008; Figure 2A). However, the molecular mechanism of Ras activation by PI3-kinase that is responsible for axon specification remains unknown. PI3-kinase induces activation of Cdc42 by phosphatidylinositol 3,4,5-trisphosphate (PIP3) (Menager et al., 2004; Nishimura et al., 2005; Reichardt, 2006). Cdc42 interacts with the Par complex and then induces Rac1 activation through Rac-specific GEFs (STEF/Tiam1). The active Rac1 leads to further activation of PI3-kinase (Nishimura et al., 2005). Thus, the PI3-kinase/Cdc42/Par complex/RacGEF/Rac1 pathway represents a positive-feedback loop that responsible for axon specification (Arimura and Kaibuchi, 2007; Takano et al., 2015; Figure 2B). The Par complex is selectively transported into the nascent axon through the interaction of KIF3A (Nishimura et al., 2004). A recent study showed that this interaction is regulated by extracellular signal-regulated kinase 2 (ERK2)-mediated phosphorylation of Par3 and is important for neuronal polarization in vivo (Funahashi et al., 2013). In the developing cortex, the constitutively active or dominant-negative form of Rac1 or STEF/Tiam1 shows a loss of leading and trailing processes and neuronal migration defects, indicating that the appropriate activity of Rac1 is essential for MP-to-BP transition and neuronal migration (Kawauchi et al., 2003). Moreover, knockdown of Par3 disrupts the MP-to-BP transition and neuronal migration (Funahashi et al., 2013). These findings suggest that the PI3-kinase/Cdc42/Par complex/RacGEF/Rac1 pathway has a vital role in the establishment of neuronal polarization as a positive feedback signal.
Downstream Targets of Positive Feedback Signals
How do positive feedback signals regulate axon specification and elongation? Positive feedback signals lead to a local accumulation of additional effectors such as CRMP-2, Par complex, and Shootin1 in the nascent axon during neuronal polarization (Toriyama et al., 2010; Inagaki et al., 2011; Naoki et al., 2011; Naoki and Ishii, 2014). These accumulated molecules govern multiple cellular events including microtubule stabilization, endocytosis, selective transport, and actin filament dynamics to induce axon specification and elongation (Conde and Caceres, 2009; Namba et al., 2015; Schelski and Bradke, 2017; Figure 2A).
The PI3-kinase/Cdc42/Par complex/RacGEF/Rac1 pathway phosphorylates and inactivates glycogen synthase kinase-3β (GSK-3β) through interleukin-like kinase (ILK) and Akt. Phosphorylated GSK-3β is enriched in the nascent axon (Jiang et al., 2005; Yoshimura et al., 2005). The expression of a constitutive active mutant form of GSK-3β inhibits axon specification, whereas inhibition of GSK-3β induces multiple axonal formation (Jiang et al., 2005; Yoshimura et al., 2005). GSK-3β phosphorylates CRMP-2 at Thr514 and suppresses its activity (Yoshimura et al., 2005). The expression of CRMP-2 induces the formation of multiple axons in culred hippocampal neurons (Inagaki et al., 2001). Consistently, inhibition of CRMP-2 suppresses axon formation in vitro and MP-to-BP transition in vivo (Inagaki et al., 2001; Sun et al., 2010). CRMP-2 regulates microtubule dynamics that are essential for axon specification (Arimura and Kaibuchi, 2007; Witte and Bradke, 2008; Takano et al., 2015; Schelski and Bradke, 2017). Indeed, the microtubule stabilizing agent Taxol induces the formation of multiple axons in vitro, indicating that the microtubule stabilization is required for axon specification (Witte and Bradke, 2008; Schelski and Bradke, 2017). CRMP-2 also modulates endocytosis, selective transport and actin filament dynamics through its binding to Numb, the kinesin light chain subunit of kinesin-1 and Sra-1 (Arimura and Kaibuchi, 2007; Takano et al., 2015; Figure 2A).
The Par complex (Par3/Par6/aPKC) was identified as a key regulator of cell polarity by a genetic screen for mutaions that disturb asymmetric cell divisions (Kemphues et al., 1988; Rodriguez-Boulan and Macara, 2014). The Par complex is enriched in the growing axon by KIF3A-dependent transport (Shi et al., 2003; Nishimura et al., 2004; Funahashi et al., 2013). The phosphorylation of Par3 at Ser1116 by ERK2 disrupts this Par3-KIF3A interaction and thereby leads to the accumulation of the Par complex at the nascent axon that results in axon specification (Funahashi et al., 2014). Knockdown of Par3 disrupts the MP-to-BP transition in vivo (Chen et al., 2013; Funahashi et al., 2013). The phospho-mimic mutant of Par3, which cannot bind to KIF3A, failed to rescue the knockdown phenotype, indicating that the accumulation of Par3 in the nascent axon is essential for MP-to-BP transition in vivo (Funahashi et al., 2013). Par6 also colocalizes with the TGF-β receptor (TβR2) and the phosphorylation of Par6 at Ser345 by the TGF-β receptor regulates axon formation and neuronal migration (Ozdamar et al., 2005; Yi et al., 2010; Rozes-Salvador et al., 2018).
Rac1 is a key regulator of actin polymerization during neuronal polarization through p21 activated kinase (PAK) and the Sra/Wiskott–Aldrich syndrome protein (WASP)-family verprolin-homologs protein (WAVE) complex (Kawano et al., 2005; Tahirovic et al., 2010; Schelski and Bradke, 2017). PAK1 phosphorylates Shootin1, which is selectively transported in a neurite-length-dependent manner and leads to axon specification in cultured hippocampal neurons (Toriyama et al., 2006; Toriyama et al., 2010). Phosphorylation of Shootin1 mediates binding between L1 cell adhesion molecule (L1-CAM) and F-actin retrograde flow as a molecular clutch (Toriyama et al., 2013; Kubo et al., 2015). This interaction generates a traction force in the growth cone of the nascent axon through decreasing retrograde actin flow, thereby increasing actin polymerization for pushing against the membrane of the nascent axon. In the developing cortex, knockdown of Shootin1 impairs the MP-to-BP transition, and neuronal migration (Sapir et al., 2013). Rac1 also recruits the WAVE complex to the plasma membrane of the nascent axon, and the WAVE complex regulates actin filament dynamics through actin related protein 2/3 (Arp2/3) to induce axon elongation (Tahirovic et al., 2010; San Miguel-Ruiz and Letourneau, 2014). Thus, actin filaments are more dynamic in the nascent axon than in those of other minor neurites (Witte and Bradke, 2008; Schelski and Bradke, 2017). Indeed, actin destabilizing drugs induce the formation of multiple axons, and local application of the actin destabilizing drugs to one minor neurite leads to axon specification (Bradke and Dotti, 1999; Witte and Bradke, 2008). These results indicate that actin filament dynamics are required for determining axonal specification. Altogether, positive feedback signals are continuously activated within one minor neurite and result in axon specification through many downstream pathways such as cytoskeletal organization and intracellular trafficking (Arimura and Kaibuchi, 2007; Takano et al., 2015; Figure 2A).
Negative Feedback Signals
Since neurons constantly generate only one axon and other minor neurites that never become axons, negative feedback signals play an important role in the formation of a single axon, and multiple dendrites during neuronal development (Arimura and Kaibuchi, 2007; Takano et al., 2015). Several models of negative feedback signals have been proposed (Inagaki et al., 2011; Takano et al., 2015; Schelski and Bradke, 2017; Yogev and Shen, 2017). cAMP and cGMP show antagonistic actions on each other during neuronal polarization (Shelly et al., 2010). Local elevation of cAMP level induces axon specification through PKA activation, whereas the cGMP level is increased by reducing the amount of cAMP in other minor neurites and then leads to dendritic specification (Shelly et al., 2010). However, it remains unclear how the cAMP elevation in the nascent axon regulates the cGMP level in all of the other minor neurites. It has also been proposed that there is a winner-take-all model for the establishment of neuronal polarity (Inagaki et al., 2011; Schelski and Bradke, 2017). As the amount of growth-relating factors are limited, local accumulation of these factors in the nascent axon deplete them in all of the other minor neurites. In turn, all of the other minor neurites could not become axons (Inagaki et al., 2011; Schelski and Bradke, 2017). More recently, a spatiotemporal long-range negative feedback signal for guaranteeing the proper neuronal polarization has been identified (Takano et al., 2017). The negative feedback signal is mediated by unique long-range Ca2+ waves, which are generated by NT-3 and propagate from the growing nascent axon to the cell body (Takano et al., 2017). The long-range Ca2+ waves subsequently activate calmodulin-dependent protein kinase I (CaMKI) at the cell body (Takano et al., 2017). CaMKI induces phosphorylation and activation of a RhoA-specific GEF, GEF-H1, and thereby activates RhoA and its effector Rho-kinase at the cell body (Takano et al., 2017). RhoA and Rho-kinase are well known as key negative regulators of neurogenesis through modulating the actin cytoskeleton and myosin-based contractility in several cell lines (Da Silva et al., 2003; Conde et al., 2010). Interestingly, photoactivation of RhoA or Rho-kinase by an optogenetic approach, LOV2 trap and release of the protein (LOVTRAP), in the cell body specifically inhibits minor neurite elongation in polarized neurons (Takano et al., 2017). Additionally, computational modeling has shown that active Rho-kinase spreads from the cell body into the minor neurites but not into the axons for preventing multiple axonal formation (Takano et al., 2017). Consistently, inhibition of Rho-kinase induces minor neurite elongation that develops into multiple axons (Takano et al., 2017). These results indicate the polarized activation of RhoA/Rho-kinase is necessary for generation of the single axon and multiple dendrites during neuronal development (Gonzalez-Billault et al., 2012; Takano et al., 2017). In the developing cortex, inhibition of RhoA or Rho-kinase by the expression of the dominant negative mutant impairs the MP-to-BP transition, and neuronal migration (Xu et al., 2015). The expression of a phospho-mimic mutant of GEF-H1, which leads to RhoA activation, also disrupts the MP-to-BP transition and neuronal migration, indicating the polarized RhoA/Rho-kinase activity is essential for neuronal polarization and neuronal migration in vivo (Xu et al., 2015; Takano et al., 2017). Rho-kinase maintains RhoA activation through phosphorylation and inactivation of p190RhoGAP (Mori et al., 2009). Importantly, Rho-kinase can inactivate Rac1 through the disruption of Par complex and inactivation of STEF in a phosphorylation-dependent manner (Takefuji et al., 2007; Nakayama et al., 2008). Thus, the long-range Ca2+ waves/CaMKI/GEF-H1/RhoA/Rho-kinase pathway represents a negative feedback signal that functions to repress the positive feedback loop in all minor neurites to inhibit multiple axonal formation and determine dendritic specification (Arimura and Kaibuchi, 2007; Takano et al., 2015, 2017; Figure 2). Together, Rac1-dependent positive feedback signals in the nascent axon and RhoA/Rho-kinase-dependent negative feedback signals in all other minor neurites guarantee proper neuronal polarization (Figure 2B).
Concluding Remarks
Banker and colleagues discovered neuronal morphological changes using cultured hippocampal neurons and reported it as “neuronal polarity” (Dotti et al., 1988; Banker, 2018). Over the past three decades, this new research field has been growing and revealed a large number of signaling networks regulating neuronal polarity (Arimura and Kaibuchi, 2007; Barnes and Polleux, 2009; Takano et al., 2015; Schelski and Bradke, 2017). Particularly important in this regard are most of the environmental polarity cues being tightly connected to Rac1-dependent positive feedback signals and RhoA/Rho-kinase-dependent negative feedback signlas (Figure 2). The positive and negative feedback signals remodel the actin and microtubule cytoskeleton underlying axon and dendrite specification during neuronal development. Rac1-depedent positive feedback signals induce dynamics of actin filaments and stabilization of microtubules in one of minor neurites through several downstream molecules including PAK1, the Sra-1/WAVE complex and CRMP-2, thereby leading to axon specification and elongation (Kawano et al., 2005; Tahirovic et al., 2010; Schelski and Bradke, 2017; Figure 2). In contrast, RhoA/Rho-kinase-dependent negative feedback signal stabilizes actin filaments in the minor neurites through myosin light chain (MLC) and LIM-kinase to prevent the formation of multiple axons and determine dendritic specification (Tahirovic and Bradke, 2009; Amano et al., 2010; Takano et al., 2017; Figure 2). Although negative feedback signals that are delivered from the nascent axon induce dendritic specification in cultured hippocampal neurons, some neurons that do not have axons by knockout of TGF-β receptor or LKB1 show dendrite formation in the developing cortex (Barnes et al., 2007; Yi et al., 2010). These findings suggest that additional molecular mechanisms might be involved in dendritic specification in vivo. Recently, it has been shown that N-cadherin-mediated glia-neuron interactions determine dendritic specification before axon formation (Takano et al., 2015; Xu et al., 2015). Interestingly, N-cadherin also activates RhoA/Rho-kinase in this process, indicating that RhoA/Rho-kinase plays critical role in dendritic specification during neuronal development. Thus, Rac1-dependent positive feedback signals and RhoA/Rho-kinase-dependent negative feedback signals are major key regulatory systems underlying neuronal polarization. However, our current knowledge of the signaling networks regulating neuronal polarization in vivo remains underdeveloped. In recent years, in utero electroporation approaches have become very useful for analyzing the role of various molecules on neuronal polarity in the mammalian cerebral cortex (Saito and Nakatsuji, 2001; Namba et al., 2014; Xu et al., 2015; Takano et al., 2017). However, it is difficult to distinguish the neuronal polarity defects and the neuronal migration deficits because neuronal polarization occurs during neuronal migration in the neocortex. Furthermore, these defects have a tremendous effect on the synaptic connections, which result in neurodevelopmental disorders such as schizophrenia, autism, lissencephaly, and microcephaly (Francis et al., 2006; Manzini and Walsh, 2011; Ishii et al., 2016; Reiner et al., 2016). How an altered neuronal polarization impacts these diseases has remained an open question. Future studies are necessary to uncover not only molecular mechanisms underlying neuronal polarization in vivo but also how these mechanisms are coordinated with other neuronal events including neuronal migration and synaptic functions to establish proper brain circuits. These broad approaches to study neuronal polarization would provide new insight into neuronal development and shed light on therapeutic approaches for neurodevelopmental disorders.
Author Contributions
TT and KK wrote the manuscript. YF contributed to the discussion about the manuscript.
Funding
This work was supported by Japan Society for the Promotion of Science (JSPS) KAKENHI (25251021 to KK), Grant-in-Aid for Young Scientists (B) (26830045 to TT), Grant-in-Aid for JSPS Fellows (PD) (20153173 to TT), Ministry of Education, Culture, Sports, Science and Technology (MEXT) KAKENHI on Innovative Areas “Neural Diversity and Neocortical Organization” (23123507 to KK), and “Bioinformatics of Brain Sciences” conducted under the Strategic Research Program for Brain Science (SRPBS) by MEXT (to KK).
Conflict of Interest Statement
The authors declare that the research was conducted in the absence of any commercial or financial relationships that could be construed as a potential conflict of interest.
Acknowledgments
We thank T. Ishii for secretarial and technical assistance. We also thank J. Wallace at Duke University for reading and discussing the manuscript.
References
Amano, M., Nakayama, M., and Kaibuchi, K. (2010). Rho-kinase/ROCK: a key regulator of the cytoskeleton and cell polarity. Cytoskeleton 67, 545–554. doi: 10.1002/cm.20472
Arimura, N., and Kaibuchi, K. (2007). Neuronal polarity: from extracellular signals to intracellular mechanisms. Nat. Rev. Neurosci. 8, 194–205. doi: 10.1038/nrn2056
Arimura, N., Kimura, T., Nakamuta, S., Taya, S., Funahashi, Y., Hattori, A., et al. (2009). Anterograde transport of TrkB in axons is mediated by direct interaction with Slp1 and Rab27. Dev. Cell. 16, 675–686. doi: 10.1016/j.devcel.2009.03.005
Banker, G. (2018). The development of neuronal polarity: a retrospective view. J. Neurosci. 38, 1867–1873. doi: 10.1523/JNEUROSCI.1372-16.2018
Barnes, A. P., Lilley, B. N., Pan, Y. A., Plummer, L. J., Powell, A. W., Raines, A. N., et al. (2007). LKB1 and SAD kinases define a pathway required for the polarization of cortical neurons. Cell 129, 549–563. doi: 10.1016/j.cell.2007.03.025
Barnes, A. P., and Polleux, F. (2009). Establishment of axon-dendrite polarity in developing neurons. Annu. Rev. Neurosci. 32, 347–381. doi: 10.1146/annurev.neuro.31.060407.125536
Bentley, M., and Banker, G. (2016). The cellular mechanisms that maintain neuronal polarity. Nat. Rev. Neurosci. 17, 611–622. doi: 10.1038/nrn.2016.100
Boitard, M., Bocchi, R., Egervari, K., Petrenko, V., Viale, B., Gremaud, S., et al. (2015). Wnt signaling regulates multipolar-to-bipolar transition of migrating neurons in the cerebral cortex. Cell Rep. 10, 1349–1361. doi: 10.1016/j.celrep.2015.01.061
Bradke, F., and Dotti, C. G. (1999). The role of local actin instability in axon formation. Science 283, 1931–1934. doi: 10.1126/science.283.5409.1931
Chen, S., Chen, J., Shi, H., Wei, M., Castaneda-Castellanos, D. R., Bultje, R. S., et al. (2013). Regulation of microtubule stability and organization by mammalian Par3 in specifying neuronal polarity. Dev. Cell. 24, 26–40. doi: 10.1016/j.devcel.2012.11.014
Cheng, P. L., Song, A. H., Wong, Y. H., Wang, S., Zhang, X., and Poo, M. M. (2011). Self-amplifying autocrine actions of BDNF in axon development. Proc. Natl. Acad. Sci. U.S.A. 108, 18430–18435. doi: 10.1073/pnas.1115907108
Conde, C., Arias, C., Robin, M., Li, A., Saito, M., Chuang, J.-Z., et al. (2010). Evidence for the involvement of Lfc and Tctex-1 in axon formation. J. Neurosci. 30, 6793–6800. doi: 10.1523/JNEUROSCI.5420-09.2010
Conde C., and Caceres, A. (2009). Microtubule assembly, organization and dynamics in axons and dendrites. Nat. Rev. Neurosci. 10, 319–332. doi: 10.1038/nrn2631
Da Silva, J. S., Medina, M., Zuliani, C., Di Nardo, A., Witke, W., and Dotti, C. G. (2003). RhoA/ROCK regulation of neuritogenesis via profilin IIa-mediated control of actin stability. J. Cell. Biol. 162, 1267–1279. doi: 10.1083/jcb.200304021
Dotti, C. G., Sullivan, C. A., and Banker, G. A. (1988). The establishment of polarity by hippocampal neurons in culture. J. Neurosci. 8, 1454–1468. doi: 10.1523/jneurosci.08-04-01454.1988
Fivaz, M., Bandara, S., Inoue, T., and Meyer, T. (2008). Robust neuronal symmetry breaking by Ras-triggered local positive feedback. Curr. Biol. 18, 44–50. doi: 10.1016/j.cub.2007.11.051
Francis, F., Meyer, G., Fallet-Bianco, C., Moreno, S., Kappeler, C., Socorro, A. C., et al. (2006). Human disorders of cortical development: from past to present. Eur. J. Neurosci. 23, 877–893. doi: 10.1111/j.1460-9568.2006.04649.x
Funahashi, Y., Namba, T., Fujisue, S., Itoh, N., Nakamuta, S., Kato, K., et al. (2013). ERK2-mediated phosphorylation of Par3 regulates neuronal polarization. J. Neurosci. 33, 13270–13285. doi: 10.1523/JNEUROSCI.4210-12.2013
Funahashi, Y., Namba, T., Nakamuta, S., and Kaibuchi, K. (2014). Neuronal polarization in vivo: growing in a complex environment. Curr. Opin. Neurobiol. 27, 215–223. doi: 10.1016/j.conb.2014.04.009
Gartner, A., Fornasiero, E. F., Munck, S., Vennekens, K., Seuntjens, E., Huttner, W. B., et al. (2012). N-cadherin specifies first asymmetry in developing neurons. EMBO J. 31, 1893–1903. doi: 10.1038/emboj.2012.41
Gonzalez-Billault, C., Munoz-Llancao, P., Henriquez, D. R., Wojnacki, J., Conde, C., and Caceres, A. (2012). The role of small GTPases in neuronal morphogenesis and polarity. Cytoskeleton 69, 464–485. doi: 10.1002/cm.21034
Hatanaka, Y., and Yamauchi, K. (2013). Excitatory cortical neurons with multipolar shape establish neuronal polarity by forming a tangentially oriented axon in the intermediate zone. Cereb. Cortex 23, 105–113. doi: 10.1093/cercor/bhr383
Inagaki, N., Chihara, K., Arimura, N., Menager, C., Kawano, Y., Matsuo, N., et al. (2001). CRMP-2 induces axons in cultured hippocampal neurons. Nat. Neurosci. 4, 781–782. doi: 10.1038/90476
Inagaki, N., Toriyama, M., and Sakumura, Y. (2011). Systems biology of symmetry breaking during neuronal polarity formation. Dev. Neurobiol. 71, 584–593. doi: 10.1002/dneu.20837
Ishii, K., Kubo, K. I., and Nakajima, K. (2016). Reelin and neuropsychiatric disorders. Front. Cell. Neurosci. 10:229. doi: 10.3389/fncel.2016.00229
Jiang, H., Guo, W., Liang, X., and Rao, Y. (2005). Both the establishment and the maintenance of neuronal polarity require active mechanisms: critical roles of GSK-3beta and its upstream regulators. Cell 120, 123–135. doi: 10.1016/j.cell.2004.12.033
Jossin, Y., and Cooper, J. A. (2011). Reelin, Rap1 and N-cadherin orient the migration of multipolar neurons in the developing neocortex. Nat. Neurosci. 14, 697–703. doi: 10.1038/nn.2816
Kawano, Y., Yoshimura, T., Tsuboi, D., Kawabata, S., Kaneko-Kawano, T., Shirataki, H., et al. (2005). CRMP-2 is involved in kinesin-1-dependent transport of the Sra-1/WAVE1 complex and axon formation. Mol. Cell. Biol. 25, 9920–9935. doi: 10.1128/MCB.25.22.9920-9935.2005
Kawauchi, T., Chihama, K., Nabeshima, Y., and Hoshino, M. (2003). The in vivo roles of STEF/Tiam1, Rac1 and JNK in cortical neuronal migration. EMBO J. 22, 4190–4201. doi: 10.1093/emboj/cdg413
Kawauchi, T., Sekine, K., Shikanai, M., Chihama, K., Tomita, K., Kubo, K., et al. (2010). Rab GTPases-dependent endocytic pathways regulate neuronal migration and maturation through N-cadherin trafficking. Neuron 67, 588–602. doi: 10.1016/j.neuron.2010.07.007
Kemphues, K. J., Priess, J. R., Morton, D. G., and Cheng, N. S. (1988). Identification of genes required for cytoplasmic localization in early C. elegans embryos. Cell 52, 311–320. doi: 10.1016/s0092-8674(88)80024-2
Kishi, M., Pan, Y. A., Crump, J. G., and Sanes, J. R. (2005). Mammalian SAD kinases are required for neuronal polarization. Science 307, 929–932. doi: 10.1126/science.1107403
Kubo, Y., Baba, K., Toriyama, M., Minegishi, T., Sugiura, T., Kozawa, S., et al. (2015). Shootin1-cortactin interaction mediates signal-force transduction for axon outgrowth. J. Cel. Biol. 210, 663–676. doi: 10.1083/jcb.201505011
Manzini, M. C., and Walsh, C. A. (2011). What disorders of cortical development tell us about the cortex: one plus one does not always make two. Curr. Opin. Genet. Dev. 21, 333–339. doi: 10.1016/j.gde.2011.01.006
Menager, C., Arimura, N., Fukata, Y., and Kaibuchi, K. (2004). PIP3 is involved in neuronal polarization and axon formation. J. Neurosci. 89, 109–118. doi: 10.1046/j.1471-4159.2004.02302.x
Miyata, T., Kawaguchi, A., Saito, K., Kawano, M., Muto, T., and Ogawa, M. (2004). Asymmetric production of surface-dividing and non-surface-dividing cortical progenitor cells. Development 131, 3133–3145. doi: 10.1242/dev.01173
Mori, K., Amano, M., Takefuji, M., Kato, K., Morita, Y., Nishioka, T., et al. (2009). Rho-kinase contributes to sustained RhoA activation through phosphorylation of p190A RhoGAP. J. Biol. Chem. 284, 5067–5076. doi: 10.1074/jbc.M806853200
Nakamuta, S., Funahashi, Y., Namba, T., Arimura, N., Picciotto, M. R., Tokumitsu, H., et al. (2011). Local application of neurotrophins specifies axons through inositol 1,4,5-trisphosphate, calcium, and Ca2+/calmodulin-dependent protein kinases. Sci. Signal. 4:ra76. doi: 10.1126/scisignal.2002011
Nakayama, M., Goto, T. M., Sugimoto, M., Nishimura, T., Shinagawa, T., Ohno, S., et al. (2008). Rho-kinase phosphorylates PAR-3 and disrupts PAR complex formation. Dev. Cell 14, 205–215. doi: 10.1016/j.devcel.2007.11.021
Namba, T., Funahashi, Y., Nakamuta, S., Xu, C., Takano, T., and Kaibuchi, K. (2015). Extracellular and intracellular signaling for neuronal polarity. Physiol. Rev. 95, 995–1024. doi: 10.1152/physrev.00025.2014
Namba, T., Kibe, Y., Funahashi, Y., Nakamuta, S., Takano, T., Ueno, T., et al. (2014). Pioneering axons regulate neuronal polarization in the developing cerebral cortex. Neuron 81, 814–829. doi: 10.1016/j.neuron.2013.12.015
Naoki, H., and Ishii, S. (2014). Mathematical modeling of neuronal polarization during development. Prog. Mol. Biol. Transl. Sci. 123, 127–141. doi: 10.1016/B978-0-12-397897-4.00003-6
Naoki, H., Nakamuta, S., Kaibuchi, K., and Ishii, S. (2011). Flexible search for single-axon morphology during neuronal spontaneous polarization. PLoS One 6:e19034. doi: 10.1371/journal.pone.0019034
Nieto Guil, A. F., Oksdath, M., Weiss, L. A., Grassi, D. J., Sosa, L. J., Nieto, M., et al. (2017). IGF-1 receptor regulates dynamic changes in neuronal polarity during cerebral cortical migration. Sci. Rep. 7:7703. doi: 10.1038/s41598-017-08140-5
Nishimura, T., Kato, K., Yamaguchi, T., Fukata, Y., Ohno, S., and Kaibuchi, K. (2004). Role of the PAR-3-KIF3 complex in the establishment of neuronal polarity. Nat. Cell Biol. 6, 328–334. doi: 10.1038/ncb1118
Nishimura, T., Yamaguchi, T., Kato, K., Yoshizawa, M., Nabeshima, Y., Ohno, S., et al. (2005). PAR-6-PAR-3 mediates Cdc42-induced Rac activation through the Rac GEFs STEF/Tiam1. Nat. Cell Biol. 7, 270–277. doi: 10.1038/ncb1227
Noctor, S. C., Martinez-Cerdeno, V., Ivic, L., and Kriegstein, A. R. (2004). Cortical neurons arise in symmetric and asymmetric division zones and migrate through specific phases. Nat. Neurosci. 7, 136–144. doi: 10.1038/nn1172
Ozdamar, B., Bose, R., Barrios-Rodiles, M., Wang, H. R., Zhang, Y., and Wrana, J. L. (2005). Regulation of the polarity protein Par6 by TGFbeta receptors controls epithelial cell plasticity. Science 307, 1603–1609. doi: 10.1126/science.1105718
Polleux, F., Morrow, T., and Ghosh, A. (2000). Semaphorin 3A is a chemoattractant for cortical apical dendrites. Nature 404, 567–573. doi: 10.1038/35007001
Reichardt, L. F. (2006). Neurotrophin-regulated signalling pathways. Philos. Trans. R. Soc. Lond. B. Biol. Sci. 361, 1545–1564. doi: 10.1098/rstb.2006.1894
Reiner, O., Karzbrun, E., Kshirsagar, A., and Kaibuchi, K. (2016). Regulation of neuronal migration, an emerging topic in autism spectrum disorders. J. Neurochem. 136, 440–456. doi: 10.1111/jnc.13403
Reiner, O., and Sapir, T. (2009). Polarity regulation in migrating neurons in the cortex. Mol. Neurobiol. 40, 1–14. doi: 10.1007/s12035-009-8065-0
Rodriguez-Boulan, E., and Macara, I. G. (2014). Organization and execution of the epithelial polarity programme. Nat. Rev. Mol. Cell. Biol. 15, 225–242. doi: 10.1038/nrm3775
Rozes-Salvador, V., Siri, S. O., Musri, M. M., and Conde, C. (2018). New player in endosomal trafficking: differential roles of smad anchor for receptor activation (SARA) protein. Mol. Cell. Biol. 38:e00446-18. doi: 10.1128/MCB.00446-18
Saito, T., and Nakatsuji, N. (2001). Efficient gene transfer into the embryonic mouse brain using in vivo electroporation. Dev. Biol. 240, 237–246. doi: 10.1006/dbio.2001.0439
San Miguel-Ruiz, J. E., and Letourneau, P. C. (2014). The role of Arp2/3 in growth cone actin dynamics and guidance is substrate dependent. J. Neurosci. 34, 5895–5908. doi: 10.1523/JNEUROSCI.0672-14.2014
Sapir, T., Levy, T., Sakakibara, A., Rabinkov, A., Miyata, T., and Reiner, O. (2013). Shootin1 acts in concert with KIF20B to promote polarization of migrating neurons. J. Neurosci. 33, 11932–11948. doi: 10.1523/JNEUROSCI.5425-12.2013
Schelski, M., and Bradke, F. (2017). Neuronal polarization: from spatiotemporal signaling to cytoskeletal dynamics. Mol. Cell. Neurosci. 84, 11–28. doi: 10.1016/j.mcn.2017.03.008
Shelly, M., Cancedda, L., Heilshorn, S., Sumbre, G., and Poo, M. M. (2007). LKB1/STRAD promotes axon initiation during neuronal polarization. Cell 129, 565–577. doi: 10.1016/j.cell.2007.04.012
Shelly, M., Cancedda, L., Lim, B. K., Popescu, A. T., Cheng, P. L., Gao, H., et al. (2011). Semaphorin3A regulates neuronal polarization by suppressing axon formation and promoting dendrite growth. Neuron 71, 433–446. doi: 10.1016/j.neuron.2011.06.041
Shelly, M., Lim, B. K., Cancedda, L., Heilshorn, S. C., Gao, H., and Poo, M. M. (2010). Local and long-range reciprocal regulation of cAMP and cGMP in axon/dendrite formation. Science 327, 547–552. doi: 10.1126/science.1179735
Shelly, M., and Poo, M. M. (2011). Role of LKB1-SAD/MARK pathway in neuronal polarization. Dev. Neurobiol. 71, 508–527. doi: 10.1002/dneu.20884
Shi, S. H., Jan, L. Y., and Jan, Y. N. (2003). Hippocampal neuronal polarity specified by spatially localized mPar3/mPar6 and PI 3-kinase activity. Cell 112, 63–75. doi: 10.1016/s0092-8674(02)01249-7
Sun, Y., Fei, T., Yang, T., Zhang, F., Chen, Y. G., Li, H., et al. (2010). The suppression of CRMP2 expression by bone morphogenetic protein (BMP)-SMAD gradient signaling controls multiple stages of neuronal development. J. Biol. Chem. 285, 39039–39050. doi: 10.1074/jbc.M110.168351
Tahirovic, S., and Bradke, F. (2009). Neuronal polarity. Cold Spring Harb. Perspect. Biol. 1:a001644. doi: 10.1101/cshperspect.a001644
Tahirovic, S., Hellal, F., Neukirchen, D., Hindges, R., Garvalov, B. K., Flynn, K. C., et al. (2010). Rac1 regulates neuronal polarization through the WAVE complex. J. Neurosci. 30, 6930–6943. doi: 10.1523/JNEUROSCI.5395-09.2010
Takano, T., Wu, M., Nakamuta, S., Naoki, H., Ishizawa, N., Namba, T., et al. (2017). Discovery of long-range inhibitory signaling to ensure single axon formation. Nat. Commun. 8:33. doi: 10.1038/s41467-017-00044-2
Takano, T., Xu, C., Funahashi, Y., Namba, T., and Kaibuchi, K. (2015). Neuronal polarization. Development 142, 2088–2093. doi: 10.1242/dev.114454
Takefuji, M., Mori, K., Morita, Y., Arimura, N., Nishimura, T., Nakayama, M., et al. (2007). Rho-kinase modulates the function of STEF, a Rac GEF, through its phosphorylation. Biochem. Biophys. Res. Commun. 355, 788–794. doi: 10.1016/j.bbrc.2007.02.028
Toriyama, M., Kozawa, S., Sakumura, Y., and Inagaki, N. (2013). Conversion of a signal into forces for axon outgrowth through Pak1-mediated shootin1 phosphorylation. Curr. Biol. 23, 529–534. doi: 10.1016/j.cub.2013.02.017
Toriyama, M., Sakumura, Y., Shimada, T., Ishii, S., and Inagaki, N. (2010). A diffusion-based neurite length-sensing mechanism involved in neuronal symmetry breaking. Mol. Syst. Biol. 6:394. doi: 10.1038/msb.2010.51
Toriyama, M., Shimada, T., Kim, K. B., Mitsuba, M., Nomura, E., Katsuta, K., et al. (2006). Shootin1: a protein involved in the organization of an asymmetric signal for neuronal polarization. J. Cell. Biol. 175, 147–157. doi: 10.1083/jcb.200604160
Tortosa, E., and Hoogenraad, C. C. (2018). Polarized trafficking: the palmitoylation cycle distributes cytoplasmic proteins to distinct neuronal compartments. Curr. Opin. Cell. Biol. 50, 64–71. doi: 10.1016/j.ceb.2018.02.004
Witte, H., and Bradke, F. (2008). The role of the cytoskeleton during neuronal polarization. Curr. Opin Neurobiol. 18, 479–487. doi: 10.1016/j.conb.2008.09.019
Xu, C., Funahashi, Y., Watanabe, T., Takano, T., Nakamuta, S., Namba, T., et al. (2015). Radial glial cell-neuron interaction directs axon formation at the opposite side of the neuron from the contact site. J. Neurosci. 35, 14517–14532. doi: 10.1523/JNEUROSCI.1266-15.2015
Yi, J. J., Barnes, A. P., Hand, R., Polleux, F., and Ehlers, M. D. (2010). TGF-beta signaling specifies axons during brain development. Cell 142, 144–157. doi: 10.1016/j.cell.2010.06.010
Yogev, S., and Shen, K. (2017). Establishing neuronal polarity with environmental and intrinsic mechanisms. Neuron 96, 638–650. doi: 10.1016/j.neuron.2017.10.021
Yoshimura, T., Arimura, N., Kawano, Y., Kawabata, S., Wang, S., and Kaibuchi, K. (2006). Ras regulates neuronal polarity via the PI3-kinase/Akt/GSK-3beta/CRMP-2 pathway. Biochem. Biophys Res. Commun. 340, 62–68. doi: 10.1016/j.bbrc.2005.11.147
Yoshimura, T., Kawano, Y., Arimura, N., Kawabata, S., Kikuchi, A., and Kaibuchi, K. (2005). GSK-3beta regulates phosphorylation of CRMP-2 and neuronal polarity. Cell 120, 137–149. doi: 10.1016/j.cell.2004.11.012
Keywords: neuronal polarity, axon specification, dendrite specification, positive feedback loop, negative feedback signal
Citation: Takano T, Funahashi Y and Kaibuchi K (2019) Neuronal Polarity: Positive and Negative Feedback Signals. Front. Cell Dev. Biol. 7:69. doi: 10.3389/fcell.2019.00069
Received: 21 January 2019; Accepted: 09 April 2019;
Published: 24 April 2019.
Edited by:
Kazuhito Toyo-oka, Drexel University, United StatesReviewed by:
Maya Shelly, Stony Brook University, United StatesIryna Leshchyns’Ka, University of New South Wales, Australia
Copyright © 2019 Takano, Funahashi and Kaibuchi. This is an open-access article distributed under the terms of the Creative Commons Attribution License (CC BY). The use, distribution or reproduction in other forums is permitted, provided the original author(s) and the copyright owner(s) are credited and that the original publication in this journal is cited, in accordance with accepted academic practice. No use, distribution or reproduction is permitted which does not comply with these terms.
*Correspondence: Kozo Kaibuchi, a2FpYnVjaGlAbWVkLm5hZ295YS11LmFjLmpw