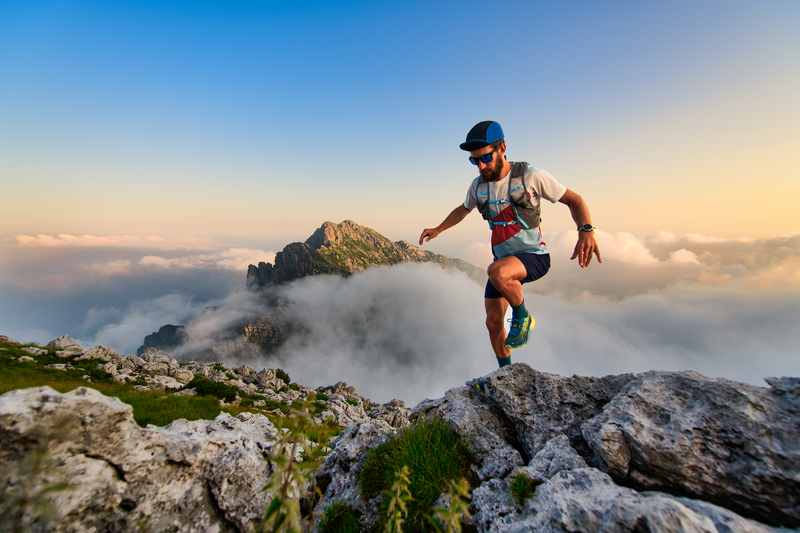
94% of researchers rate our articles as excellent or good
Learn more about the work of our research integrity team to safeguard the quality of each article we publish.
Find out more
REVIEW article
Front. Cell Dev. Biol. , 15 March 2019
Sec. Cell Adhesion and Migration
Volume 7 - 2019 | https://doi.org/10.3389/fcell.2019.00031
This article is part of the Research Topic Extracellular Matrix Dynamics in Biology, Bioengineering, and Pathology View all 12 articles
The extracellular matrix (ECM) is a biological substrate composed of collagens, proteoglycans and glycoproteins that ensures proper cell migration and adhesion and keeps the cell architecture intact. The regulation of the ECM composition is a vital process strictly controlled by, among others, proteases, growth factors and adhesion receptors. As it appears, ECM remodeling is also essential for proper neuronal and glial development and the establishment of adequate synaptic signaling. Hence, disturbances in ECM functioning are often present in neurodegenerative diseases like Alzheimer’s disease. Moreover, mutations in ECM molecules are found in some forms of epilepsy and malfunctioning of ECM-related genes and pathways can be seen in, for example, cancer or ischemic injury. Low density lipoprotein receptor-related protein 1 (Lrp1) is a member of the low density lipoprotein receptor family. Lrp1 is involved not only in ligand uptake, receptor mediated endocytosis and lipoprotein transport—functions shared by low density lipoprotein receptor family members—but also regulates cell surface protease activity, controls cellular entry and binding of toxins and viruses, protects against atherosclerosis and acts on many cell signaling pathways. Given the plethora of functions, it is not surprising that Lrp1 also impacts the ECM and is involved in its remodeling. This review focuses on the role of Lrp1 and some of its major ligands on ECM function. Specifically, interactions with two Lrp1 ligands, integrins and tissue plasminogen activator are described in more detail.
Low density lipoprotein receptor-related protein-1 (Lrp1), also known as CD91 or α-2-macroglobulin (α-2-M) receptor, is a member of the low density lipoprotein receptor family and is expressed in various tissues including liver, adipose tissue, lungs and brain. The receptor, with a mass of 600 kDa, during its biosynthesis, undergoes a furin-mediated proteolytical cleavage in the Golgi apparatus. This cleavage results in two, non-covalently bound polypeptide subunits—an 85 kDa membrane-bound C-terminal fragment (the light β chain) and a 515 kDa N-terminal fragment located extracellularly (the heavy α chain)—that form the mature Lrp1 (Figure 1). The Lrp1 α chain contains 4 ligand binding complement-like repeat clusters separated by epidermal growth factor (EGF) repeats. Clusters II and IV are considered to be responsible for the majority of ligand binding (Herz, 2001; Herz and Strickland, 2001; Croy et al., 2003; Meijer et al., 2007) and have previously been shown to display minor differences regarding the kinetics of the interactions but to be also highly similar in their ligand binding properties (duplication of the domains) (Neels et al., 1999). As suggested by Huang et al. (1999), each ligand-binding domain of Lrp1 presents very different charge densities and hydrophobic patches. These differences in turn lead to varying receptor-ligand interactions and are responsible for a distinct ligand specificity of each cluster, despite similar backbone folds. Because Lrp1 interacts with a wide variety of protein ligands (Table 1), it is necessary to study each domain individually to elucidate its exact ligand-binding capacities. Interestingly, receptor-associated protein (RAP) binds to the ligand-binding clusters and completely blocks interactions with all known Lrp1 ligands (Bu et al., 1994).
Figure 1. The structure of Lrp1. Lrp1 is cleaved by furin (red arrow) in the Golgi complex and afterwards is transported to the cell membrane. The resulting mature Lrp1 receptor consists of a 515 kDa alpha chain and an 85 kDa beta chain bound to each other non-covalently. The alpha chain consists of ligand-binding-type repeats forming four clusters (I–IV) that are rich in cysteine. The clusters contain 2, 8, 10, and 11 repeats, respectively. The clusters II and IV are responsible for the majority of ligand binding to Lrp1. The ligand-binding clusters are separated by 1–4 EGF homology domains, with cysteine-rich EGF repeats. The intracellular beta chain consists of two NPxY motifs, one YxxL motif and two di-leucine motifs that have been associated with the endocytotic functions of Lrp1. The beta chain also interacts with scaffolding proteins like PSD-95, Dab-1, and FE-65. Both the extra- and intracellular chain can act independently of each other, when the alpha chain is shed as a soluble Lrp1 and the beta chain translocates to the nucleus and activates gene transcription and signaling cascades. α-2-M, α-2-macroglobulin; ApoE, apolipoprotein E; COOH, carboxy terminal; Dab-1, disabled 1; EGF, epidermal growth factor; NH2, amino terminal; PAI-1, plasminogen activator inhibitor 1; PSD-95, postsynaptic density protein 95; tPA, tissue plasminogen activator.
As shown by Li et al. (2000, 2001), the Lrp1 β chain C-terminus contains motifs, proposed later by Deane et al. (2008) to be involved in generating the rapid endocytotic rate of Lrp1: two NPxY motifs, one YxxL motif and two di-leucine motifs. The C-terminus of Lrp1 interacts with many intracellular ligands (Betts et al., 2008; Guttman et al., 2009) and binds to endocytic and scaffold adaptors like disabled-1, FE-65 and postsynaptic density protein 95 (PSD-95) that link the Lrp1 receptor to membrane-bound proteins such as amyloid precursor protein (APP) (Herz and Chen, 2006; Waldron et al., 2008) and are involved in many cell signaling pathways (Trommsdorff et al., 1998; Gotthardt et al., 2000; May et al., 2004; Pietrzik et al., 2004; Herz et al., 2009; Klug et al., 2011). Lrp1 can additionally undergo an intramembrane proteolysis that results in a shed extracellular Lrp1 fragment and a γ-secretase cleaved intracellular Lrp1 domain. Upon cleavage, the intracellular Lrp1 domain translocates to the cell nucleus and modulates gene expression (May et al., 2002; Zurhove et al., 2008).
Lrp1 is nowadays considered to be a multifunctional receptor: it is involved not only in ligand uptake, receptor-mediated endocytosis, cellular signaling and lipoprotein transport (Herz and Bock, 2002) but also regulates cell surface protease activity (Makarova et al., 2003), controls cellular entry and binding of toxins and viruses (Kounnas et al., 1992b; Hofer et al., 1994; Liu et al., 2000), participates in dendritic cell efferocytosis (Subramanian et al., 2014), protects against atherosclerosis (Boucher and Herz, 2011), is critical for angiogenesis and the maintenance of the blood–brain barrier (BBB) (Polavarapu et al., 2007; Pi et al., 2012; Strickland et al., 2014) and acts on many signaling cascades including the Wnt and Notch pathways (Zilberberg et al., 2004; Lillis et al., 2008; Meng et al., 2010).
In the central nervous system (CNS), Lrp1 is highly expressed not only in neurons, astrocytes and microglia (Bu et al., 1994; Ishiguro et al., 1995; Rebeck et al., 1995; Marzolo et al., 2000; Makarova et al., 2003; Andersen and Willnow, 2006; Kanekiyo et al., 2011; Auderset et al., 2016) but also in brain endothelial cells, vascular smooth muscle cells, pericytes and the choroid plexus (Herz and Bock, 2002).
In the mouse, the complete knock-out of Lrp1 is lethal for the embryos (Herz et al., 1992, 1993), making it challenging to study the role and function of Lrp1 in embryonic as well as adult brain in vivo. However, various mutant mouse models generated in the last decades shed more light onto the possible function of Lrp1 in the CNS.
With their studies, May et al. (2004); Liu et al. (2010) and Nakajima et al. (2013), highlight and support earlier findings showing that Lrp1 regulates postsynaptic signaling via interactions with PSD-95 and N-methyl-D-aspartate receptor (NMDAR) and is crucial for synaptic transmission (Bacskai et al., 2000; Qiu et al., 2002). The studies of Liu et al. (2010) and Liu et al. (2011) additionally provide evidence for the importance of Lrp1 in maintaining proper brain lipid metabolism and leptin signaling and define Lrp1 as a major apolipoprotein E (ApoE) transport receptor, strengthening the role of Lrp1 in Alzheimer’s disease pathogenesis.
Knock-in mutations in the NPxY2 region lead to a reduced Lrp1 internalization rate (Roebroek et al., 2006; Reekmans et al., 2010; Gordts et al., 2012) and interfere with NMDAR recycling and NMDAR-mediated activation of the ERK1/2 pathway (Martin et al., 2008; Reekmans et al., 2010). Alterations in NMDAR subunits on the cell surface can lead to alterations and deficits in memory processes. Animals harboring the knock-in mutation in the NPxY2 motif display hyperactivity, impaired learning as well as deficits in spatial and reversal learning, similarly to animals with impaired NMDAR signaling (Bannerman et al., 1995; Sakimura et al., 1995).
A recent study from our laboratory discovered that Lrp1 is a novel carrier protein for Lewis X glycans expressed by mouse radial glial cells [neural stem precursor cells (NSPCs)] in the developing and adult CNS (Hennen et al., 2013). With this and a follow-up study we showed that Lrp1 plays a role in the differentiation of NSPCs (Hennen et al., 2013; Safina et al., 2016). The impaired differentiation of Lrp1-lacking NSPCs toward oligodendrocytes is supported by the work of Lin et al. (2017) where deficits in myelination and oligodendrocyte precursor differentiation were observed upon Lrp1 deletion specifically from the oligodendrocyte lineage. According to this study, these impairments are a combined result of altered AKT, sterol regulatory element-binding protein 2 and peroxisome proliferation-associated receptor γ pathways in oligodendrocytes.
The extracellular matrix (ECM) is a biological substrate that is composed of collagens, proteoglycans and glycoproteins. The highly organized honeycomb-like structures of the ECM were first described by Camillo Golgi over a century ago and, since then, the ECM has been found to be essential not only for cell migration, adhesion and structural support but also for proper neuronal and glial development, BBB maturation and function, synaptogenesis and synaptic signaling in the CNS (Faissner et al., 2010; Menezes et al., 2014; Song and Dityatev, 2018). The composition of the ECM is heterogeneous and tissue-specific. In the CNS, the ECM is formed by proteoglycans like heparan sulfate proteoglycans (HSPGs), chondroitin sulfate proteoglycans and glycoproteins including tenascins, laminins, and thrombospondins. During embryonic and early postnatal development, the ECM provides an environment supporting cell migration, differentiation and synapse formation. Here, the ECM forms a loose structure consisting of neuronal neurocan and matures simultaneously with synapses (Pyka et al., 2011). In adult mice, neurocan expression becomes decreased while brevican, aggrecan, and tenascin R become upregulated.
Both ECM components released by neurons and astrocytes are essential for ECM generation (Geissler et al., 2013). Such molecules tend to accumulate especially in ECM structures termed perineuronal nets (PNNs). These consist of chondroitin sulfate proteoglycans, tenascin-R, hyaluronic acid and link proteins. PNNs are found predominantly on the soma and dendrites of parvalbumin-expressing GABAergic neurons (Hartig et al., 1992), however, excitatory pyramidal neurons have also been shown to bear PNNs (Carstens et al., 2016; Lensjø et al., 2017; Morikawa et al., 2017). Both PNN and ECM formation impairments are associated with neurodegenerative and psychiatric disorders including epilepsy, Alzheimer’s disease and schizophrenia (Heck et al., 2004; Pitkänen et al., 2014; Dzyubenko et al., 2016; Song and Dityatev, 2018). Hence, it is of importance to study not only how factors released by cells impact the ECM, but also how molecules and receptors located at the plasma membrane, Lrp1 included, interact with the ECM and modify its composition.
Lipid rafts are regions of the plasma membrane enriched in cholesterol and sphingolipids that are involved in assembling protein complexes for cell signaling events (Brown and London, 1998, 2000; Simons and Toomre, 2000). In the CNS, lipid rafts are essential for synaptic integrity and they are implicated in the pathogenesis of neurodegenerative diseases (Hicks et al., 2012; Rushworth et al., 2013). Although Lrp1 has been firstly found to localize nearly exclusively to coated pits in, for example, vascular smooth muscle cells (Weaver et al., 1996), Lrp1 localization to caveolae, a specialized type of lipid rafts, was later shown by Boucher et al. (2002) on the example of human fibroblasts. In neurons and neuronal-like PC12 and N2a cells, Lrp1 localizes, at least partially, to lipid rafts (Laudati et al., 2016). Upon the disruption of lipid rafts by methyl-β-cyclodextrin, Lrp1-mediated signaling is impaired, while ligand binding and endocytosis capacities remain intact (Laudati et al., 2016). The authors suggest that the presence of Lrp1 in lipid rafts in neuronal cells is due to the scaffolding activity of PSD-95. PSD-95 is a constituent protein of the post-synaptic complex in excitatory synapses (El-Husseini et al., 2000) and functions in stabilization of dendritic spines (Ehrlich et al., 2007). Lrp1, similarly, is present at the synapse and is known to interact with PSD-95 and to affect NMDAR functioning, long-term potentiation (LTP) and synaptic signaling (May et al., 2004; Liu et al., 2010; Nakajima et al., 2013). A decrease in PSD-95 levels upon Lrp1 deletion has been previously reported for the hippocampi of the α CAMKII-Cre-Lrp1 KO mice (Liu et al., 2010). Simultaneous application of methyl-β-cyclodextrin with either enzymatically inactive tissue plasminogen activator (tPA) or active α-2-M to both PC12 cells and cultured cerebellar granule neurons, blocks neurite outgrowth, as does RAP application (Laudati et al., 2016). The mechanism behind this effect has been traced to the fact that, in PC12 and N2a cells, upon treatment with these ligands, Lrp1 forms a complex with PSD-95 and NMDARs that activates tyrosine receptor kinase receptors, stimulates ERK1/2 activity and leads to various cellular responses, including neurite outgrowth (Bacskai et al., 2000; Mantuano et al., 2013). Interestingly, upon Lrp1 loss or blockage, a reduction in the formation, length, branching and outgrowth of neurites is found (Qiu et al., 2004; Nakajima et al., 2013; Safina et al., 2016). Lrp1 is found essential also for neurite growth inhibition mediated by myelin associated-glycoprotein and CNS myelin (Stiles et al., 2013) and has been found to mediate myelin phagocytosis itself (Gaultier et al., 2009).
By a comparison of various cell types, Wu and Gonias (2005) proposed that the presence of Lrp1 in different plasma membrane compartments depends on the cell type analyzed, as does Lrp1-mediated signaling. For example, although in neuronal and neuronal-like cells Lrp1 is found both in lipid rafts and lipid raft-free membrane compartments, in vascular smooth muscle cells and CHO-K1 cells Lrp1 is present mostly in lipid raft-free membrane compartments (Wu and Gonias, 2005). Lrp1 distribution in the cell membrane remains nevertheless dynamic, as the receptor can translocate from lipid-rafts to clathrin-coated pits where it undergoes endocytosis (Boucher et al., 2002; Wu and Gonias, 2005) (Figure 2). The capacity of Lrp1 to shift between membrane compartments is influenced by plasma membrane microdomains and depends on available extracellular ligands. For example, insulin promotes Lrp1 localization to caveolae in mouse fibroblasts and adipocytes while platelet-derived growth factor (PDGF)-BB decreases it (Zhang et al., 2004). As shown in the example of cultured hepatic cells and mouse embryonic fibroblasts (MEFs), endocytosis of Lrp1 can also occur in lipid rafts. In this case, the kinesin-3 family motor protein, KIF3B, promotes caveolin-dependent endocytosis of Lrp1 by forming a complex with Lrp1 and utrophin, a cytoskeleton protein vital for adequate muscle functioning (Zhang et al., 2004; Kanai et al., 2014).
Figure 2. Lrp1 interacts with ligands located in lipid rafts and clathrin-coated pits. Lrp1 distribution in the cell membrane is dynamic: Lrp1 can be found in both lipid raft-containing and lipid raft-free membrane compartments (1). Lrp1-mediated endocytosis requires clathrin and is restricted to lipid raft-free regions (2). Lrp1 can interact with lipid raft-associated receptors and proteins, translocate back to lipid raft-free compartments and mediate the endocytosis of the bound ligand (3). The ligand undergoes either lysosomal or proteasomal degradation (4) while Lrp1 is recycled back to the membrane (5).
In the CNS, the localization of Lrp1 to both lipid rafts and clathrin-coated pits has been proposed to be one of the mechanisms enabling a separation of Lrp1 endocytic and signaling activities and their independent regulation (Laudati et al., 2016). For example, PDGF-BB via the PDGF receptor (PDGFR) β and the Src is required for mediating the phosphorylation of tyrosine residues in the cytoplasmic tail of Lrp1 (Boucher et al., 2002; Loukinova et al., 2002). This interaction occurs only when Lrp1 is located to lipid rafts and therefore modulates Lrp1-dependent cell signaling in specific cell compartments (Boucher et al., 2002).
Taken together, interactions between Lrp1, the plasma membrane and ECM molecules highlight the presence of a heterogeneous modulation of Lrp1-dependent cell signaling in specific cell compartments and cell types.
Given the multitude of Lrp1 ligands, it is not surprising that ECM molecules also interact with Lrp1. Lrp1 is primarily responsible for the endocytosis and subsequent transport of extracellular proteins to lysosomes (Herz and Strickland, 2001). Although Lrp1-mediated endocytosis occurs in non-lipid raft areas via clathrin-coated pits, due to the receptors’ mobility, GPI proteins like the prion protein and other proteins associated with lipid rafts can also undergo Lrp1-clathrin-dependent endocytosis (Czekay et al., 2001; Wu and Gonias, 2005; Taylor and Hooper, 2007; Jen et al., 2010). Protein complexes and membrane-associated receptors are also Lrp1 ligands. With their endocytosis, Lrp1 further impacts on the protein composition of the plasma membrane and the ECM (Strickland et al., 2002; Gonias et al., 2004).
Although Lrp1’s main function remains the endocytosis of extracellular ligands, it regulates the composition of the ECM also by controlling messenger ribonucleic acid (mRNA) expression and stability (Gaultier et al., 2006). In this way Lrp1 balances the protein levels of, for example, type III collagen, pigment epithelium-derived factor and urokinase-type plasminogen activator (uPA) receptor (uPAR)/Endo-180 in MEFs (Gaultier et al., 2006).
Heparan sulfate proteoglycans are core constituents of the ECM implemented in ECM integrity and in facilitating the entry of molecules including morphogens, growth factors and viruses. Some protein ligands of Lrp1 are shown to bind with low affinity to glycosaminoglycan chains of surface HSPGs that in turn facilitate binding to and Lrp1-mediated endocytosis of these proteins (Wilsie and Orlando, 2003; Kanekiyo et al., 2011). Consistent with such a mechanism, the endocytosis of such proteins is blocked by heparin and heparitinase and does not occur in cells lacking HSPGs (Kanekiyo et al., 2011). HSPGs can be cleaved by heparanase-1, an enzyme that requires a proteolytic cleavage to become active. This process is partly dependent on Lrp1-mediated internalization of the inactive pro-enzyme (Vreys et al., 2005). Interestingly, both Lrp1 and HSPGs are required for the endocytosis of mature heparanase-1 (Vreys and David, 2007).
A summary of Lrp1 interactions with the HSPGs described in this section is presented in Figures 3, 4.
Figure 3. Lrp1 interacts with heparan sulfate proteoglycans. (A) Lrp1 and heparan sulfate proteoglycans (HSPGs) are responsible for the independent endocytosis of distinct trigliceride-rich lipoproteins (TRLs) in the liver. (B) Lrp1 association with HSPGs determines the availability of lipoprotein binding sites, such that upon the dissociation of Lrp1 from HSPGs, more binding sites appear.
Figure 4. Heparan sulfate proteoglycans act as bridging molecules. Various ligands, including connective tissue growth factor (CTGF) and the prion protein, have been shown to bind to heparan sulfate proteoglycans (HSPGs) (1). After association with HSPGs, the binding of the ligand to Lrp1 is facilitated and Lrp1-mediated endocytosis is triggered (2). Upon endocytosis, the molecules are transported to lysosomes/proteasomes for degradation (3) and Lrp1 is recycled back to the membrane (4). The depicted processes are inhibited both by blockage of Lrp1 and HSPGs.
In the liver, Lrp1 was previously shown to clear trigliceride-rich lipoproteins either independently or as a coreceptor with HSPGs (Mahley and Huang, 2007). A recent study questioned this idea and suggested instead that Lrp1 and HSPGs are rather responsible for independent clearing of distinct trigliceride-rich lipoproteins (Foley et al., 2013).
Lrp1 has been proposed to regulate the availability of lipoprotein binding sites by associating with HSPGs. Upon Lrp1 dissociation from HSPGs, more lipoprotein binding sites are revealed, and lipoprotein particle clearance becomes enhanced (Wilsie and Orlando, 2003). These findings were among the first to imply a role for Lrp1 firstly as a mediator of various signaling pathways, independent of its endocytic function and secondly as a regulator of HSPG function. HSPGs were also proposed by the same study to play an active role in lipoprotein uptake (Wilsie and Orlando, 2003). So far, Lrp1 and HSPGs have been shown to mediate the uptake of a complement inhibitor, the C4b-binding protein (Spijkers et al., 2008), the coagulation factor VIII (Sarafanov et al., 2001), cellular prion protein (Sunyach et al., 2003; Taylor and Hooper, 2007), connective tissue growth factor (CTGF) (Gao and Brigstock, 2003) and thrombospondin (Mikhailenko et al., 1995). HSPGs are furthermore found essential for the binding of protease Nexin-1 to Lrp1 (Li X. et al., 2006). Although the internalization of complexed (e.g., with tPA and uPA) and free Nexin-1 is mediated by Lrp1 (Knauer et al., 1997; Crisp et al., 2000), it can be partially substituted by an Lrp1-independent pathway. In Lrp1 deficient cells, the HSPG syndecan-1 takes over the internalization of free Nexin-1 and results in the activation of the Ras-ERK pathway instead of the PKA pathway that becomes active upon Lrp1-mediated internalization (Li X. et al., 2006). Given that Nexin-1 is involved in the regulation of extracellular proteolytic activity, the efficiency of its uptake and cellular responses triggered upon its internalization are crucial, especially for tumor cell invasiveness studies (Li X. et al., 2006).
Another HSPG member involved in Lrp1-mediated endocytosis is glypican-3. Glypican-3 has been shown to regulate embryonic growth by inhibiting the Hedgehog signaling pathway (Capurro et al., 2008). The binding of Hedgehog to glypican-3 triggers Hedgehog:glypican-3 complex endocytosis and degradation, impacting Hedgehog availability for binding with Patched (Capurro et al., 2008). Mechanistically, although glypican-3 and Sonic Hedgehog can directly bind to Lrp1, endocytosis is suggested to occur only upon simultaneous glypican-3 and Sonic Hedgehog interaction with Lrp1 (Capurro et al., 2012). This mechanism is found in MEFs and breast cancer cells, indicating a general role for Lrp1 and HSPGs in Hedgehog signaling (Capurro et al., 2012).
In neuronal cells, APP processing pathways are heterogeneous. In the non-amyloidogenic pathway, APP undergoes an α-and γ-secretase-mediated cleavage that leads to the generation of the soluble α APP fragment and APP C-terminal fragments. When APP is processed in this way, no amyloid β is generated. In contrast, in the amyloidogenic pathway, upon APP cleavage by the β-secretase BACE1 and γ-secretases, amyloid β is produced together with the soluble β APP and APP C-terminal fragments (Chow et al., 2010). Depending on the position of the cleavage, two principal forms of amyloid β can be generated: amyloid β with 40 amino acid residues and amyloid β with 42 amino acid residues. Both forms of amyloid β are released outside the cell in response to normal synaptic signaling and are incorporated into the ECM (Kamenetz et al., 2003; Cirrito et al., 2005). The majority of produced amyloid β is the shorter form. If the longer amyloid β form is produced, it exhibits a higher tendency to form fibrils and later on amyloid plaques that fail to be cleared and therefore accumulate in the brain parenchyma. Amyloid β overproduction, increased processing, aggregation and deposition in the form of plaques leads to neurotoxicity and neurodegeneration and is considered central for Alzheimer’s disease development. It should be noted that, together with amyloid β, various ECM proteins are found in plaques and neurofibrillary tangles, for example ECM-located and cell-surface HSPGs (van Horssen et al., 2003). In this respect, ECM molecules have been analyzed and grouped with regard to the amyloid β form they interact with by Salza et al. (2017). The proteins investigated in this study included reelin, integrins, laminins and collagens. These authors and others showed that fibrillar and oligomeric forms of amyloid β can diversely interact with ECM and membrane proteins, influencing cell homeostasis (Genedani et al., 2010; de Jager et al., 2013; Salza et al., 2017).
For the CNS, the importance of amyloid β generation and its multiplex interactions with the ECM are highlighted by studies showing that the ECM regulates APP levels in fibroblasts and neuronal cells (Bronfman et al., 1996), amyloid β induces ECM degradation in rat astrocytes (Deb et al., 2003) and that both fibrillar and oligomeric forms of amyloid β associate with proteins present at synapses, a characteristic closely related to synapse loss and neurodegeneration observed in Alzheimer’s disease (Selkoe, 2000; Spires-Jones and Hyman, 2014).
In the CNS, Lrp1 interacts with soluble and transmembrane forms of APP and impacts their internalization, processing and transactivation (Kounnas et al., 1995a; Knauer et al., 1996; Pietrzik et al., 2002) (Figure 5). Upon Lrp1 loss in fibroblasts and CHO cells, APP degradation and trafficking is impaired (Pietrzik et al., 2002). An introduction of a truncated, intracellular domain of Lrp1 to these cells is sufficient to restore APP processing and amyloid β production (Pietrzik et al., 2002). Furthermore, the presence of a functional Lrp1 on the cell surface was shown to reduce the amount of soluble α APP produced and to favor amyloidogenic processing of APP (Ulery et al., 2000).
Figure 5. Lrp1 is crucial for the uptake and processing of amyloid precursor protein. The cytoplasmic tail of Lrp1 interacts with the cytoplasmic tail of amyloid precursor protein (APP) via the bridging molecule FE-65. This results in the endocytosis of the Lrp1:APP:FE-65 complex (1) and subsequent proteolysis of APP by the enzyme BACE1 and proteinase presenilin-1 (PS1), which is part of the γ-secretase complex (2). The proteolysis results in the generation of soluble β APP and amyloid β peptides that are released outside the cell in recycling vesicles, like Lrp1 (3). The cytoplasmic tail of APP, after γ-secretase cleavage, forms a complex with FE-65 that interacts with the transcriptional modulator Tip60 (4). This complex translocates to the nucleus where it suppresses the transcription of the Lrp1 promoter, influencing Lrp1-mediated processes (5). Upon γ-secretase-mediated cleavage of Lrp1 (6), its cytoplasmic tail can influence APP processing by competing with the intracellular domain of APP complexed with FE-65 for binding with Tip60 in the nucleus (7). After β-secretase cleavage, the shed extracellular domain of Lrp1 can bind to amyloid β peptides and enhance their clearance (8).
As Lrp1 and APP are both substrates for γ- and β-secretases, Lrp1 not only stimulates but also interferes with the processing of APP (May et al., 2002; Lleo et al., 2005; von Arnim et al., 2005). APP cleavage is favored when both Lrp1 and APP are present; however, upon Lrp1 overexpression, the cleavage of APP becomes impaired because Lrp1 competes with APP for binding to BACE1 (Lleo et al., 2005; von Arnim et al., 2005; von Einem et al., 2010). Upon γ-secretase-mediated cleavage of Lrp1 (May et al., 2002), the intracellular domain translocates to the nucleus and impacts APP-mediated signaling by interacting with the transcriptional modulator, Tip60 (May et al., 2002; Kinoshita et al., 2003). Lrp1 expression is regulated by APP itself as the APP:FE-65:Tip60 complex is able to suppress the transcription of the Lrp1 promoter (Liu et al., 2007). As a consequence, in fibroblasts of APP knock-out mice Lrp1 expression is increased (Liu et al., 2007). Interestingly, increased levels of APP can lead to a decreased production of shed Lrp1, impairing amyloid β clearance (von Einem et al., 2010).
Fibrils of amyloid β do not accumulate in excessive amounts in healthy individuals due to several efficient clearance mechanisms: extracellular proteolysis, BBB transport, efflux of soluble amyloid β to the peripheral circulation and receptor-mediated endocytosis (Deane et al., 2009; Ramanathan et al., 2015). Lrp1 is vital for the production, internalization and catabolism of amyloid β (Kounnas et al., 1995a; Knauer et al., 1996; Pietrzik et al., 2002; Deane et al., 2004, 2008; Lillis et al., 2008) (Figures 5, 6), and in the brains of Alzheimer’s disease patients, according to some studies, Lrp1 levels are significantly reduced (Kang et al., 1997, 2000; Jaeger and Pietrzik, 2008; Shinohara et al., 2017).
Figure 6. Lrp1 is a receptor mediating amyloid β uptake. Lrp1 can regulate the internalization of amyloid β in several ways. Amyloid β can directly interact with ligand-binding domains of Lrp1 (1). Amyloid β can interact with several molecules including α-2-macroglobulin (α-2-M), apolipoprotein E (ApoE) and heparan sulfate proteoglycans (HSPGs), which bridge it to Lrp1 and facilitate its endocytosis (2). Upon endocytosis, amyloid β can either undergo degradation (3) or, to a smaller extent, be recycled back to the extracellular space (4). Lrp1 can also influence signaling pathways, especially the RhoA pathway that can facilitate amyloid β clearance by stimulating actin polymerization (5). The shed extracellular domain of Lrp1 additionally sequesters soluble amyloid β present at the blood-brain barrier and in the circulation (6).
Amyloid β has so far been shown to form complexes with ApoE, lactoferrin, prion protein and activated α-2-M that undergo Lrp1-mediated endocytosis (Qiu et al., 1999; Yang et al., 1999; Kang et al., 2000; Laurén et al., 2009; Rushworth et al., 2013). Monomeric forms of amyloid β can also bind directly to the extracellular ligand-binding domains of Lrp1 and undergo endocytosis (Deane et al., 2004). Microglia were shown to migrate to plaques and engulf amyloid β predominantly via micropinocytosis (Frackowiak et al., 1992; Mandrekar et al., 2009; Lee and Landreth, 2010). Lrp1-mediated amyloid β clearance in pericytes helps prevent amyloid β deposition in the cerebrovasculature (Sagare et al., 2013). Lrp1 expressed on the surface of endothelial cells at the BBB mediates rapid clearance of amyloid β from the brain parenchyma via transcytosis and degradation (Nazer et al., 2008; Yamada et al., 2008; Pflanzner et al., 2011). In the periphery, β-secretase cleaved, shed, soluble Lrp1 can bind amyloid β, further enhancing its clearance from the brain (Sagare et al., 2007). Supporting amyloid β removal via Lrp1 from the system are the liver, spleen, and kidney (Ramanathan et al., 2015). Lrp1 impacts amyloid β metabolism also by activating signaling pathways. By modulating RhoA signaling in Schwann cells, Lrp1 influences cell adhesion and migration (Mantuano et al., 2010). RhoA has been shown to facilitate dynamin-dependent amyloid β endocytosis in neuronal cells (Yu et al., 2010). It is thereby proposed that Lrp1 could activate RhoA and facilitate RhoA-dependent endocytosis of amyloid β (Kanekiyo and Bu, 2014). HSPGs have been identified to be required for the binding of amyloid β and act as a coreceptor for Lrp1 in the process of neuronal amyloid β uptake (Kanekiyo et al., 2011, 2013; Kanekiyo and Bu, 2014). Astrocytic Lrp1 has also been shown to be essential for the uptake and degradation of amyloid β (Kanekiyo and Bu, 2014; Liu et al., 2017). Whether Lrp1 requires HSPGs for amyloid β uptake also in this cell type remains to be elucidated, however, it is suggested that astrocytic Lrp1 affects the degradation of amyloid β in the ECM by modulating the levels of matrix metalloprotease (MMP)-2 and MMP-9 (Yin et al., 2006; Liu et al., 2017).
In summary, although the involvement of Lrp1 in APP and amyloid β processing and Alzheimer’s disease pathogenesis is undeniable, given the complexity of interactions, their true nature may be hidden by the variability in cell lines and the systems used, and is still yet to be fully unraveled.
The ECM is vital for many cellular processes including cell proliferation, survival, differentiation and migration. In order for the cell to migrate, the ECM surrounding it needs to be degraded. This is performed by a variety of proteases including MMPs and serine proteases and tightly controlled by their inhibitors. Both uPA and tPA proteases mediate for example the activation of plasminogen to plasmin that leads to the digestion of the ECM and MMP activation (Werb et al., 1977; Mignatti and Rifkin, 1993; Sappino et al., 1993). One of the first reports showing Lrp1 involvement in ECM turnover and cell migration was published by Okada et al. (1996). Here, by using a Transwell filter migration assay, the authors discovered that the presence of Lrp1 facilitates the migration of smooth muscle cells on fibronectin-coated filters. These observations were reproduced by Wijnberg et al. (1997). The stimulating effect of Lrp1 was not apparent when smooth muscle cells were tested on a collagen gel invasion assay, indicating that the effects of Lrp1 depend on the composition of the matrix (Okada et al., 1996). The addition of uPA, tPA or α -2-M—potent Lrp1 ligands—to the cells is found to be stimulatory for cell migration, neurite outgrowth and axon elongation (Friedman and Seeds, 1995; Holtzman et al., 1995; Okada et al., 1996; Seeds et al., 1996, 1999; Qiu et al., 2004; Lillis et al., 2005).
Lrp1 directly interacts with uPA and the plasminogen activator inhibitor-1 (PAI-1). As the binding affinity of PAI-1 and uPA alone is lower than when in a complex with the uPAR, Lrp1-mediated endocytosis occurs only after PAI-1 binds to the uPA:uPAR complex (Herz et al., 1992; Nykjaer et al., 1994; Nykjaer et al., 1997; Czekay et al., 2001). By the binding and subsequent endocytosis of such complexes, Lrp1 reduces uPAR levels on the cell surface. In this manner, Lrp1 regulates uPAR-mediated proteolysis of the ECM (Conese et al., 1995; Weaver et al., 1996; Nykjaer et al., 1997; Degryse et al., 2001) and impacts plasminogen conversion to plasmin (Weaver et al., 1997). By regulating uPAR levels on the cell surface and decreasing uPA-mediated plasminogen activation, Lrp1 inhibits fibronectin remodeling (Gaultier et al., 2010). By regulating uPAR levels, Lrp1 also influences plasmin-mediated cleavage of ECM glycoproteins lacking collagen in their composition, as well as plasmin-dependent activation of MMP-2 and MMP-9 that degrade the ECM by cleaving collagens (Mignatti and Rifkin, 1993; Brinckerhoff and Matrisian, 2002).
Upon Lrp1 silencing from MEFs, collagen remodeling is increased (Gaultier et al., 2010). This occurs independently of the membrane type-1 matrix metalloprotease (MT1-MMP), a metalloprotease previously shown to impact collagen remodeling and Lrp1 cleavage in malignant cells (Rozanov et al., 2004; Sabeh et al., 2004; Lehti et al., 2009; Sabeh et al., 2009). As upon treatment of MT1-MMP-deficient and wild type skin fibroblasts with RAP collagen remodeling becomes enhanced in both cell types, it hints at the presence of an MT1-MMP-independent pathway that becomes active upon Lrp1 signal blockade.
PAI-1 facilitated migration in rat smooth muscle cells is Lrp1-dependent (Degryse et al., 2004). Interaction of the extracellular heat shock protein 90 with Lrp1 activates Akt 1/2 kinases that facilitate the migration of skin cells to the wound and promote its closure (Tsen et al., 2013). Lrp1 was shown to interact with PDGFR β and regulate PDGFR β cell surface levels and PDGFR β signaling which is of importance, among others, for the integrity of vascular walls and cell chemotaxis (Boucher et al., 2002, 2003; May et al., 2005; Craig et al., 2013; Strickland et al., 2014).
Actin organization and cell migration in smooth muscle cells are, for example, controlled by PDGFR β phosphatidylinositol 3-kinase (PI3K) activation that is Lrp1-dependent (Zhou et al., 2009). The interaction of PDGF β with Lrp1 can also result in the activation of the mitogen-activated protein kinase (MAPK) signaling cascade—a major player in cell survival and proliferation (Takayama et al., 2005).
Mouse embryonic fibroblasts respond with migratory behavior on fibronectin and vitronectin matrixes upon interaction of thrombospondin 1 and the calreticulin:Lrp1 complex (Orr et al., 2003a). The impaired cell migration observed upon Lrp1 loss in MEFs was suggested by Orr et al. (2003a) to be a result of improper lamellipodia generation in Lrp1-deficient cells.
Cell migration is also regulated by Lrp1 via blockage of the effect of stimulatory ligands. This is the case for ApoE, which has been shown to inhibit PDGF-B-dependent smooth muscle cell migration by binding to Lrp1 (Swertfeger et al., 2002). This interaction results in the activation of protein kinase A and increase in intracellular cAMP levels (Swertfeger et al., 2002; Zhu and Hui, 2003) and is of particular importance for protection against vascular disease. ApoE-deficient mice exhibit atherosclerosis (Plump et al., 1992; Zhang et al., 1994), while Lrp1 loss from smooth muscle cells enhances vascular cell activation and also leads to atherosclerosis (Boucher et al., 2003).
Weaver et al. (1997) detected moreover that MEFs that lacked Lrp1 and were plated on serum-, vitronectin-, and fibronectin-coated plates migrated faster than wild type MEFs upon subjection to a scratch assay in the presence of PDGF-BB. This effect was not detectable on Matrigel- and type I collagen-plated cells, highlighting the varying effects of Lrp1 in different matrices. The increased migration capacities of MEFs lacking Lrp1 were suggested by the study to be a result of increased surface levels of the uPAR in these cells (Weaver et al., 1997). As mentioned earlier, Lrp1 can bind PAI-1:uPA:uPAR complexes and facilitate their endocytosis (Nykjaer et al., 1997). This in turn leads to altered levels of the uPAR on the cell surface (Weaver et al., 1997). When cultured under high serum conditions, fibroblasts lacking Lrp1 show increased levels of Rac, a GTPase vital for lamellipodia formation and cell spreading (Ma et al., 2002). In the Lrp1-deficient MEFs, analyzed in the study of Weaver et al. (1997), elevated migration is proposed to be a result of persistent Rac activity and/or increased proteolysis. This reasoning is in agreement with studies showing that, by interacting with proteases, Lrp1 protects ECM proteins from degradation (Herz and Strickland, 2001; Strickland et al., 2002).
A requirement enabling the cell to migrate is the restructuring or disassembly of integrin-linked focal adhesion complexes. Focal adhesion disassembly is mediated by ECM proteins including thrombospondin 1 and 2. Thrombospondins are large oligomeric ECM proteins that participate in cell-cell and cell-matrix interactions by binding to other ECM molecules, cytokines and cell surface receptors. Thrombospondins are considered vital for the development of the CNS, as a lack of thrombospondins 1/2 impairs astrocyte-mediated synaptogenesis and a lack of thrombospondin 1 impairs neural progenitor proliferation and neuronal differentiation in vivo and in vitro (Christopherson et al., 2005; Lu and Kipnis, 2010). Thrombospondin 1 has been shown to interact with Lrp1, HSPGs, calreticulin and integrins in various cell types (McKeown-Longo et al., 1984; Mikhailenko et al., 1995, 1997; Merle et al., 1997; Li S. S. et al., 2006; Staniszewska et al., 2007).
Thrombospondins favor cell migration by disassembling and detaching focal adhesions from the ECM—processes dependent on calreticulin and Lrp1 and requiring intact lipid rafts (Orr et al., 2003a,b; Barker et al., 2004; Talme et al., 2013). Both the intact thrombospondin 1 and its cleaved N-terminal domain mediate focal adhesion disassembly (Murphy-Ullrich et al., 1993). The sequence responsible for this effect and binding to calreticulin is located in the N-terminal domain of thrombospondin 1, and a peptide mimetic termed hep I was developed to specifically study interactions of this thrombospondin 1 domain (Murphy-Ullrich et al., 1993). The signaling mediated by thrombospondin 1 via the calreticulin-Lrp1 complex is a process independent of Lrp1-mediated thrombospondin 1 endocytosis (Mikhailenko et al., 1995, 1997) (Figure 7A). Although the sequence responsible for the binding of thrombospondin 1 to Lrp1 and subsequent endocytosis is also located to the N-terminal domain, it does not include the sequence mimicked by hep I, as hep I lacks Lrp1 binding capacity (Orr et al., 2003b; Wang et al., 2004). Interactions of the calreticulin:Lrp1 complex with thrombospondin 1 have been evidenced to result in a temporary association of the G protein α i-2 subunit with Lrp1. This interaction results in FAK and Src phosphorylation (Thy-1-dependent) and activation of ERK, PI3K, and RhoA inactivation and favors cell migration. These events do not occur upon either loss of calreticulin or Lrp1 (Orr et al., 2002, 2003a,b, 2004; Barker et al., 2004).
Figure 7. Lrp1 interacts with thrombospondins. (A) Upon binding of thrombospondin 1 to calreticulin, its binding to Lrp1 is facilitated. The Lrp1:calreticulin complex leads to the association of the G protein αi2 that in turn phosphorylates FAK and Src. Required for the effect of thrombospondin on Src activation is additionally the GPI-linked protein Thy-1. The activation of Src and FAK further activates the ERK and phosphatidylinositol 3-kinase (PI3K) pathways and leads to the downregulation of RhoA, focal adhesion disassembly and cell migration. (B) Thrombospondins can function as bridging molecules, enabling Lrp1-mediated endocytosis of various molecules, including Notch, vascular endothelial growth factor (VEGF) and matrix metalloproteinases (MMPs).
Thrombospondins also function as bridging molecules between Lrp1 and its extracellular ligands that facilitate their clearance (Figure 7B). Thrombospondin 1 was found to participate in the clearance of vascular endothelial growth factor via Lrp1 in the ovary (Greenaway et al., 2007).
Notch signaling is crucial for proper development, hair pigmentation and homeostasis, and mediates short-range, direct communications between neighboring cells (Schouwey et al., 2007). Lrp1 facilitates Notch 3 trans-endocytosis and thrombospondin 2-mediated potentiation of Notch 3 signaling (Meng et al., 2009, 2010), highlighting that the ECM is involved in the modulation of Notch function, and Lrp1 and thrombospondins support non-cell autonomous short-range signaling (Meng et al., 2010).
The activity of MMPs, adamalysin-like metalloproteinases with thrombospondin domains and membrane-anchored adamalysins, is tightly regulated by four members of the tissue inhibitors of the MMPs (TIMPs) family. In the TIMP family, TIMP3 is unique as it controls the activity of all three metalloproteinase classes. The lack of TIMP3 leads to excessive ECM turnover caused by uncontrolled proteinase activity. TIMP3 extracellular levels are regulated by Lrp1-mediated endocytosis (Scilabra et al., 2013; Thevenard et al., 2014). The shed, soluble Lrp1 competes with cell-surface Lrp1 for TIMP binding and results in increased extracellular levels of TIMP, promoting its inhibitory action (Scilabra et al., 2013, 2017).
In addition, Lrp1 mediates the endocytosis of MMP-2, MMP-9, and MMP-13 and adamalysin-like metalloproteinases with thrombospondin domains 4 and 5, preventing their proteolytic and signaling functions (Emonard et al., 2005; Yamamoto et al., 2014). Thrombospondin 1/2 was also evidenced to interact with MMP-2 and MMP-9 (Bein and Simons, 2000). Upon binding of pro-MMP-2 to thrombospondin 2, the complex associates with Lrp1 and undergoes endocytosis, which can be blocked by an anti-thrombospondin 1 antibody (Yang et al., 2001; Emonard et al., 2004).
MMP-2 activity favors angiogenesis and endothelial cell invasion in malignant gliomas. In thrombospondin 2 knock-out mice, microvessel density is enhanced and MMP-2 levels are significantly upregulated (Fears et al., 2005). MMP-2 levels and invasion capabilities of microvessel endothelial cells in these mice are reduced upon addition of thrombospondin 2. This effect is blocked by downregulating Lrp1. Curiously, the endocytosis of pro-MMP-2 can also take place by formation of complexes with TIMP-2 and is Lrp1-dependent. This interaction, however, does not require thrombospondin (Emonard et al., 2004).
Vital for the normal function of elastic arteries are collagens and elastins, prominent members of the ECM. It is appreciated that excess protease activity can contribute to the elastic fiber degradation in these vessels. Smooth muscle cells are essential for establishment of proper vessel diameter, correct deposition of the ECM and assembly of elastic fibers. The deletion of Lrp1 from the embryo proper results in severe impairments in investment of vessels with pericytes and vascular smooth muscle cells. The deletion of Lrp1 from vascular smooth muscle cells leads to increased proliferation and aneurysms (Boucher et al., 2003; Nakajima et al., 2014). The deletion of Lrp1 from smooth muscle cells also impacts the integrity of the vasculature by altering PDGFR β signaling and levels of various ECM molecules pivotal for proper vessel development, contributing to the development of hypertension, atherosclerosis and other cardiovascular diseases (Zhou et al., 2009; Strickland et al., 2014). Alongside a lack of Lrp1 in smooth muscle cells, matrix decomposition becomes dysregulated due to CTGF protein level upregulation (Muratoglu et al., 2013). CTGF accumulation is traced to the missing Lrp1-mediated clearance of CTGF (Segarini et al., 2001). Lrp1-mediated endocytosis of CTGF occurs tissue-independently as it has also been shown to take place in the liver (Gao and Brigstock, 2003; Gerritsen et al., 2016). The disrupted elastin fiber architecture and ECM disorganization upon Lrp1 deletion from smooth muscle cells was found by Muratoglu et al. (2013) to increase protein levels of MMP-2, MMP-9, MT1-MMP, and serine protease high-temperature requirement factor A1 (HtrA1). HtrA1 is abundant in smooth muscle cells, degrades many ECM proteins including aggrecan, fibulin-5 and collagens and has been previously associated with disorganized elastic fibers (Hadfield et al., 2008; Vierkotten et al., 2011). Lrp1 was found by Muratoglu et al. (2013) to regulate the levels of HtrA1 in the vessel wall by rapid endocytosis of this protease, thereby impacting vessel wall integrity.
In conclusion, the presence of different tissue-dependent Lrp1-mediated mechanisms for serine protease and MMP
clearance highlights that Lrp1-mediated uptake and degradation provides a vital mechanism for limiting excessive extracellular proteolytic activity (Yang et al., 2001). By eliciting control over MMP extracellular levels, Lrp1 additionally modifies cell adhesion and migration capabilities that are crucial not only for processes like wound healing and angiogenesis but also for tumor growth (Fears et al., 2005; Van Gool et al., 2015). For example, EGF-mediated downregulation of Lrp1 activity in astrocytic tumors impacts ECM composition and may contribute to tumor invasiveness (Hussaini et al., 1999).
Integrins are transmembrane glycoprotein receptors consisting of α and β heterodimers. Integrins have been implemented in molecular adhesion, cell survival and migration (Pan et al., 2016).
The process of binding of integrins to the ECM provokes a change in conformation of integrins that connects the ECM with the cell and leads to adhesion. An integrin that is especially crucial for triggering the cell-matrix interactions is β1 integrin. β1 integrin outside-in signaling leads to integrin recruitment and clustering, phosphorylation of focal adhesion kinase and the assembly of focal adhesions, promoting cell spreading and adhesion.
Fibronectin is a ubiquitous and multifunctional ECM protein vital for cell adhesion, migration and differentiation. Lrp1 participates in the catabolism of fibronectin by mediating fibronectin endocytosis (Salicioni et al., 2002). In particular, Lrp1 prevents fibronectin upregulation on the cell surface and in the cell-secreted medium, as shown for MEFs and Chinese Hamster ovary cells (Salicioni et al., 2002).
Tissue transglutaminase is a ubiquitously expressed Ca2+-dependent protein crosslinking enzyme present in the cytoplasm, at the cell surface and in the ECM. Fibronectin and β integrins are major interaction partners of tissue transglutaminase that impact on cell adhesion and migration (Turner and Lorand, 1989; Akimov et al., 2000) (Figure 8). Fibronectin, aside from being internalized in an Lrp1-dependent manner, also acts as a bridging molecule and enhances Lrp1-mediated surface tissue transglutaminase endocytosis (Zemskov et al., 2007).
Figure 8. Lrp1 is vital for integrin signaling and function. Integrins are formed by α and β heterodimers. Fibronectin and integrins can interact with tissue transglutaminase (tTG) and impact on cell migration and adhesion. Upon fibronectin binding, surface tTG is endocytosed by Lrp1 (1). tTG mediates the formation of Lrp1:integrin complexes that facilitate integrin endocytosis and cell migration (2). Interaction of tTG with integrins stabilizes fibronectin:integrin complexes and stimulates RhoA activation and cell adhesion (3).
Interaction of tissue transglutaminase with β integrins results in the formation of fibronectin-tissue transglutaminase-integrin complexes that stabilize the interactions of fibronectin with integrins (Akimov et al., 2000). The interaction of tissue transglutaminase with integrins facilitates their clustering and activates RhoA (Janiak et al., 2006).
Surface tissue transglutaminase has been recently shown to associate with Lrp1 and promote the formation of β1 integrin-Lrp1 complexes (Zemskov et al., 2007). As tissue transglutaminase associates with integrins, they are internalized by Lrp1 as a complex, resulting in a modification of cell-matrix interactions. Upon Lrp1 loss, transglutaminase activity of surface tissue transglutaminase is upregulated and cell adhesion enhanced (Zemskov et al., 2007).
Fibulin-5 is an ECM integrin-binding protein that is involved in elastic fiber formation (Yanagisawa et al., 2002). The binding of fibulin-5 to uPA stimulates plasminogen activation. This leads to the proteolysis of fibulin-5, its dissociation from integrins and stimulation of β1 integrin-mediated migration of MEFs, independently of the uPAR (Kapustin et al., 2012). Czekay et al. (2003) found, however, that if uPARs are associated with integrins and the uPA:PAI-1 complex binds to such an uPAR, integrins are internalized by Lrp1 together with the uPA:PAI-1:uPAR complex, influencing integrin functioning.
Lrp1 promotes β1 integrin activation via kindlin 2 and is considered to be a driver for the trafficking and proteasomal and lysosomal degradation of endocytosed activated β1 integrin via the protein kinase C (Wujak et al., 2017) (Figure 9A). Knock-in mutations in the intracellular NPxY2 motif of Lrp1, similarly to the knock-out of Lrp1, lead to elevated levels of immature β1 integrin on the cell surface, disrupting β1 integrin functionality (Salicioni et al., 2004; Rabiej et al., 2015; Wujak et al., 2017). Immature β1 integrin lacks the full glycosylation pattern that is acquired in the endoplasmic apparatus and the Golgi complex. Changes in glycosylation patterns are known to disturb transit through the Golgi apparatus, formation of complexes with α integrin subunits and ligand-binding affinities (Bellis, 2004). Knock-in mutations in the intracellular NPxY2 motif of Lrp1 lead moreover to reduced β1 integrin recycling and cause increased cell adhesion to collagen and fibronectin matrices and reduced cell migration of MEFs (Rabiej et al., 2015). This effect is due to the fact that the impaired Lrp1 endocytosis of β1 integrin leads to increased numbers of focal adhesions, increased focal adhesion kinase phosphorylation status and decreased MMP-2 and MMP-9 activity in MEFs and neurons (Rabiej et al., 2015) (Figure 9B).
Figure 9. Lrp1 mediates β1 integrin endocytosis. (A) The NPxY2 motif located in the cytoplasmic domain of Lrp1 is responsible for the endocytosis of β1 integrins via kindlin 2 that enhances cell migration. (B) Upon mutations in the NPxY2 motif of Lrp1, interactions with kindlin 2 do not occur, resulting in reduced β1 integrin recycling and facilitation of cell adhesion.
Interactions of Lrp1 with β1 integrin are proposed to be similar to Lrp1 interactions with APP. The knock-in mutation in the intracellular NPxY2 motif of Lrp1 leads to surface accumulation of not only β1 integrin but also APP (Pietrzik et al., 2002; Rabiej et al., 2015). β1 integrin and APP are also endocytosed together by Lrp1 upon complex formation with the scaffolding protein Ran-binding protein 9, an interaction of importance for Alzheimer’s disease pathogenesis and synaptic plasticity (Woo et al., 2012a,b).
In the CNS, β1 integrin is known for participating in neuronal migration and neurite outgrowth (Belvindrah et al., 2007b; Ribeiro et al., 2013; Rabiej et al., 2015), however, its function is not limited to cell adhesion and migration. β1 integrin is expressed by NSPCs and is vital for the formation of cell layers in the cerebral cortex (Belvindrah et al., 2007a). An in vivo conditional deletion of β1 integrin in radial glia cells leads to spontaneous seizures and reactive astrogliosis (Robel et al., 2009, 2015). β1 integrin presence is put forward as being inhibitory for astrocyte differentiation in adult hippocampal neural stem cells as well as in embryonic neural stem cells (Pan et al., 2014; Brooker et al., 2016). This inhibitory effect is mediated via the integrin-linked kinase (Pan et al., 2014; Brooker et al., 2016) and appears to be more prominent in female mice, as shown by Brooker et al. (2016). Interestingly, integrin-linked kinase, as a major downstream effector kinase of β1 integrin signaling, is vital for ECM deposition and controls its expression.
In a different β1 integrin mutant, β1 integrin loss restricted to astrocytes has been found to impact endothelial cell number at the neurovascular unit, blood vessel branching and aquaporin 4 levels (Venkatesan et al., 2015). Given that Lrp1 is found on astrocytes and contributes to vessel development as well as BBB integrity (Boucher et al., 2003; Nakajima et al., 2014; Fredriksson et al., 2015; Auderset et al., 2016) it is of interest for future studies to elucidate to what extent Lrp1 participates in β1 integrin-mediated effects in the developing CNS.
As Lrp1 was recently identified as a receptor for β1 integrins also in thyroid cancer cells (Theret et al., 2017), Lrp1 and integrins emerge as potential candidate genes for preventing cancer invasiveness.
Lrp1 has also been shown to bind β2 integrins located on leukocytes and monocytes (Spijkers et al., 2005; Cao et al., 2006). The interactions of Lrp1 with β2 integrins are suggested to modulate cell adhesion and migration by regulating integrin recycling, as shown in the example of macrophages (Cao et al., 2006). Hypercholesterolemia is proposed to induce hematopoietic stem/progenitor cell (HSPC) proliferation and their migration into lesioned sites, where they are suggested to undergo differentiation into leukocytes and form plaques found in arteriosclerosis patients (Wang et al., 2014). Vital for HSPC adhesion, migration and homing is β2 integrin (Wang et al., 2014). Loss of Lrp1 does not influence expression levels of β2 integrin, but leads to inhibition of HSPC adhesion, suggesting Lrp1 in this context impacts β2 integrin functioning (Wang et al., 2014).
Tissue plasminogen activator is a serine protease that belongs to the chymotrypsin family, mostly known for its role in degrading the extracellular matrix components via activating plasmin. This glycoprotein is built up from the heavy A-chain and the light B-chain (Figure 10). The heavy A-chain is composed of the finger domain, EGF-like domain and Kringle 1 and 2 domains. The finger domain is responsible for binding to fibrin and the membrane receptors Annexin II and Lrp1 (Kagitani et al., 1985; Bu et al., 1992; Hajjar et al., 1994). The EGF-like domain is crucial for binding to the EGF receptor (Correa et al., 2011). The Kringle 1 domain is involved in the uptake of tPA in the liver and the Kringle 2 domain is vital for interactions with NMDARs and PDGFs (Kuiper et al., 1988; Fredriksson et al., 2004; Lesept et al., 2016). The light B-chain comprises the serine protease domain with the catalytic triad (His322, Asp371, and Ser478), responsible for the proteolytic activity of tPA.
Figure 10. The structure of tissue plasminogen activator. Tissue plasminogen activator (tPA), like all serine proteases, exists in two forms: the single-chain and the two-chain form. The conformation of tPA is maintained by 17 disulfide bridges (green double lines). The structure of both tPA forms consists of two chains: the A-chain and the B-chain. The A-chain consists of four domains that are color-coded on the scheme. The finger domain (red) is responsible for interacting with Lrp1, fibrin and annexin II. The EGF-like domain (blue) binds the EGF receptor. The kringle 1 domain (green) mediates tPA uptake in the liver. The kringle 2 domain (violet) is vital for interactions with NMDARs and PDGFs. The B-chain (black) consists of the catalytic triad that mediates the proteolytic activity of tPA. The single-chain form of tPA is catalytically active and can be further cleaved by kallikrein and plasmin (red double lines), resulting in the two-chain form of tPA. The two-chain tPA is also catalytically active.
Unlike other members of the chymotrypsin family, tPA is secreted as a proteolytically active single chain form that can be subsequently processed into the equally proteolytically active two-chain tPA by plasmin or kallikrein. Given its prominent matrix degradation capacities, tPA levels and activity must be strictly regulated. In the CNS, Lrp1 together with protease inhibitors PAI-1 and neuroserpin is especially crucial for controlling the effect of tPA on ECM remodeling (Orth et al., 1992; Makarova et al., 2003; Yepes and Lawrence, 2004; Czekay et al., 2011; Hébert et al., 2015).
For many years, tPA has been mostly appreciated for its fibrinolytic activity, however, it possesses a plethora of other functions vital for CNS homeostasis, some of which are summarized in Figure 11 (Sappino et al., 1993; Briens et al., 2017; Hébert et al., 2015).
Figure 11. Representative functions of tissue plasminogen activator in the CNS. (A) At the synapse, upon binding of tissue plasminogen activator (tPA), Lrp1 recruits postsynaptic density protein 95 (PSD-95). PSD-95 bridges the NPxY2 motif of Lrp1 and N-methyl-D-aspartate receptor (NMDAR). NMDAR allows Ca2+ influx, leading to an activation of signaling cascades (1). TPA can directly interact with the NMDAR, enhancing its extrasynaptic surface diffusion and resulting in excitotoxicity (2). Interactions of low levels of single-chain tPA (sc-tPA) and two-chain tPA (tc-tPA) with epidermal growth factor receptor (EGFR) result in neuroprotection and inhibition of NMDAR neurotoxicity (3). Interactions of tPA with synaptic GluN2A-NMDARs are neuroprotective, while with extrasynaptic GluN2D-NMDARs, neurotoxic (4). (B) During corticogenesis, tPA released by migrating neuroblasts clusters NMDARs on radial glia cells and ensures proper cortical migration and maturation of neurons. (C) TPA enhances blood-brain barrier permeability by interacting with astrocytic Lrp1 and platelet-derived growth factor (PDGF)-CC. (D) Both sc-tPA and tc-tPA are proteolytically active and convert plasminogen to plasmin. Plasmin digests fibrin, a process especially vital for renewing blood flow after ischemia (1). Plasmin cleaves GluN2A and GluN2B-containing NMDARs, impacting NMDAR functionality (2).
TPA, alike Lrp1, is implicated in the pathology of neurodegenerative diseases, including Alzheimer’s disease, psychotic disorders like schizophrenia (Fabbro and Seeds, 2009; Hoirisch-Clapauch and Nardi, 2015) and is recognized for supporting BBB integrity (Polavarapu et al., 2007; Fredriksson et al., 2015; Stefanitsch et al., 2015).
The tPA has been found to facilitate neurite outgrowth by promoting local proteolysis (Seeds et al., 1996) and to favor neuronal (synaptic) maturation after differentiation from cortical neural progenitor cells (Lee et al., 2014). TPA is highly expressed in brain regions undergoing cellular migration. TPA gene expression is found most prominently in the cerebellum in the period of neuronal migration and is induced in granule neurons leaving the external granule layer of the cerebellum (Friedman and Seeds, 1995). Mice that lack tPA show an increased amount of granule neurons migrating in the molecular layer of the cerebellum (Seeds et al., 1999). The mechanism behind enhanced tPA expression in granule cells is suggested to be related to NMDAR signaling as these receptors are crucial for granule cell migration (Komuro and Rakic, 1992, 1993; Friedman and Seeds, 1995). TPA-mediated signaling is also supportive for Schwann cell migration via direct interaction with NMDARs and is present even upon blockage of Lrp1 (Mantuano et al., 2015). Recently, tPA has been implicated in the control of proper radial glial cell organization and differentiation. The interaction between neuronal tPA and NMDAR on radial glia cells was found to be crucial for proper cortical migration and maturation (Pasquet et al., 2018). In a mouse model of Fragile X syndrome, a stimulating effect of tPA on Fmr1-deficient cells migrating out of neurospheres was also apparent (Achuta et al., 2014).
TPA and plasmin have been shown to regulate mossy fiber outgrowth in the hippocampus via the proteolysis of the proteoglycan DSD-1/phosphacan (Wu et al., 2000).
In murine renal and non-renal myofibroblasts, tPA promotes transforming growth factor (TGF)-β1-mediated activation and ECM production which is independent of its proteolytic activity. TPA exerts this action by directly activating Lrp1 by a phosphorylation of tyrosine residues located in the C-terminus of Lrp1. This process results in Lrp1-mediated β1 integrin recruitment and integrin-linked kinase signaling (Hu et al., 2007). Upon Lrp1 loss, tPA fails to induce myofibroblast activation and ECM overproduction as evidenced by type I collagen expression (Hu et al., 2007). TGF-β1 fibrogenic activity is not disturbed in Lrp1-deficient cells, suggesting Lrp1 loss affects only tPA-mediated signaling (Hu et al., 2007). In other cell types, however, Lrp1 was shown to impact TGF-β expression and signaling, for example during vessel wall remodeling (Muratoglu et al., 2011).
TPA is not only supportive of cell migration, but, under certain conditions, it also favors cell adhesion. This effect is reversed upon binding of tPA to PAI-1, which induces an Lrp1-mediated internalization of the adhesion complex and cell detachment (Cao et al., 2006; Kozlova et al., 2015).
Extracellular matrix remodeling by tPA and other proteases is not only involved in axonal growth and cell migration but also activates cellular signaling cascades. For example, degraded fibronectin particles interact with integrin receptors and trigger cell signaling that further remodels the ECM by regulating mRNA levels of metalloproteases and other factors (Werb et al., 1989). TPA also acts as a cytokine by upregulating MMP-9 gene expression in an Lrp1-dependent manner (Wang et al., 2003; Yepes et al., 2003; Hu et al., 2006). Lrp1 expression is increased upon ischemic stroke, and treatment with RAP or antibodies against Lrp1 decreases the degree of tissue edema after middle cerebral artery occlusion (Polavarapu et al., 2007). Vasocontraction of smooth muscle cells is facilitated by tPA and requires a functional interaction between Lrp1 and α and β integrins (Akkawi et al., 2006). Astrocytic Lrp1 and tPA have been directly shown to regulate BBB permeability (Polavarapu et al., 2007; Su et al., 2008; Niego et al., 2012). Improper tPA-Lrp1 and tPA-Mac-1-Lrp1-PDGFR α interactions at the BBB are known to influence the permeability of the neurovascular unit in relation to seizures and ischemia (Polavarapu et al., 2007; Zhang et al., 2007, 2009; Su et al., 2008; Nakajima et al., 2014; Fredriksson et al., 2015).
In the CNS, tPA is synthesized and released by various cell types including neurons and astrocytes (Hébert et al., 2015). The majority of neuronal tPA is present in dendrites and axons and is released following depolarization of presynaptic terminals. tPA liberation impacts the release of glutamate, thereby exerting a modulatory function on synaptic activity (Wu et al., 2015; Yepes et al., 2016). Simultaneously, tPA release is proposed to exhibit an independent, homeostatic effect on the cortical glutamatergic postsynapse, which is dependent on the degree of neuronal activation and extracellular Ca2+ concentrations (Jeanneret et al., 2016).
The tPA interacts with proteins and receptors located at the synapse, among them Lrp1 and NMDAR (Nicole et al., 2001; Pawlak et al., 2005; Benchenane et al., 2007; Macrez et al., 2010; Jullienne et al., 2011; Ng et al., 2012; Obiang et al., 2012; Parcq et al., 2012; Mantuano et al., 2013; Lesept et al., 2016). Both single- and two-chain tPA at low concentrations can activate neuronal EGF receptors, which decrease NMDAR signaling and result in neurotrophic effects. High concentrations of single-chain tPA lead to neurotoxic effects by activating NMDAR signaling (Bertrand et al., 2015; Chevilley et al., 2015). Neuronal survival is enhanced via astrocytic TGF-α induction of PAI-1. PAI-1 inhibits tPA and thereby prevents NMDAR-mediated neurotoxicity (Gabriel et al., 2002). Additionally, tPA exerts neuroprotective effects by activating the annexin II and mTOR pathways (Chevilley et al., 2015; Grummisch et al., 2016; Lemarchand et al., 2016).
The interaction of tPA with Lrp1 is vital for the generation of hippocampal late LTP and enhancement of PKA activity (Zhuo et al., 2000; Makarova et al., 2003).
The tPA is tightly connected with LTP induction and synaptic signaling also by its ability to degrade the ECM (Qian et al., 1993; Huang et al., 1996; Baranes et al., 1998; Madani et al., 1999; Trotter et al., 2014). TPA-mediated proteolytic cleavage of plasminogen results in the generation of plasmin that enhances the NMDAR-mediated increase in Ca2+ influx upon glutamate application to cultured hippocampal neurons (Inoue et al., 1994). TPA and plasmin have also been shown to convert pro brain-derived neurotrophic factor to mature brain-derived neurotrophic factor, a protein critical for LTP generation (Pang and Lu, 2004; Pang et al., 2004).
Healthy astrocytes possess the ability to uptake tPA, via Lrp1, from the synaptic cleft and modulate the efficacy of synaptic responses (Makarova et al., 2003; Fernández-Monreal et al., 2004; Cassé et al., 2012). Meanwhile, in vitro cultured astrocytes with reduced Lrp1 levels do not uptake neuron-derived tPA efficiently and as a result cause elevated levels of tPA in the synaptic cleft (Cassé et al., 2012). Astrocytic Lrp1 uptake of tPA is thereby crucial in preventing surplus tPA-mediated activation of NMDARs and neuronal cell death (Cassé et al., 2012) and is a promising target for epilepsy research.
The tPA is an immediate early gene expressed not only upon LTP but also early after seizures (Qian et al., 1993; Carroll et al., 1994). The exact impact of tPA on seizure generation and epilepsy progression is, however, complex (Tan et al., 2012). TPA-deficient mice are resistant to excitotoxin-induced neuronal death, but mice overexpressing tPA in adult neurons do not show neurodegeneration, only a selective enhancement of hippocampal LTP and memory (Tsirka et al., 1997; Madani et al., 1999). Involvement of tPA in activity-dependent synaptic plasticity has also been hypothesized to occur in the cerebellum upon motor learning. Here, tPA secreted at either Purkinje cell dendrites or granule neurons and parallel fibers, could eliminate synapses by degrading cell surface receptors and adhesion molecules, or facilitate new synapse formation by degrading the ECM (Seeds et al., 1995, 1996).
Lrp1 and the extracellular protease tPA impact cell migration, adhesion and NMDAR-mediated signaling. TPA exerts its effects not only via its proteolytic activity but also via activating signaling cascades (Nicole et al., 2001; Yuan et al., 2009; Parcq et al., 2012; Chevilley et al., 2015). Interactions of Lrp1 with tPA have been shown so far to depend on the animal age, the cell type, the exact co-factors available, the extracellular/intracellular tPA ratio and the form of tPA. These myriad parameters need consideration when studying the intriguing relationship between Lrp1 and tPA and partially explains contradictory findings in the literature regarding the function of this ECM protein (Nicole et al., 2001; Fernández-Monreal et al., 2004; Benchenane et al., 2007; Jullienne et al., 2011; Robinson et al., 2015).
Lrp1 is a potent modulator of ECM function in the CNS and beyond. Lrp1 can impact on the composition of the cell plasma membrane, and thereby the ECM, by the endocytosis of a wide range of ECM proteins and protein complexes. ECM molecules themselves can facilitate Lrp1-mediated endocytosis by acting as bridging and docking molecules, as do surface-bound HSPGs. By mediating the endocytosis of a wide range of proteases and protease inhibitors, Lrp1 directly impacts ECM composition. By associating with scaffolding and adaptor proteins and forming co-receptor complexes, Lrp1 appears vital for cell signaling events and activation of many downstream signaling cascades that lead to, among others, ECM remodeling. In this context, the interactions of Lrp1 with its ligands, especially tPA and integrins, have proven critical for normal development, synaptic signaling, cell adhesion and migration. Therefore, it is not surprising that changes in Lrp1 expression and phosphorylation status are associated with neurodegenerative and cardiovascular diseases and cancer.
A general overview of how Lrp1 can interact with the ECM is depicted in Figure 12.
Figure 12. Lrp1 impact on the ECM is complex. Lrp1 can undergo β- and γ-secretase-mediated cleavages that result in two functional receptor domains that exhibit different properties (1). The shed extracellular domain of Lrp1 can interact with ligands located in the matrix and in the circulation (2). The intracellular Lrp1 domain can translocate to the nucleus and regulate the expression of many genes (3). Lrp1 has been reported to interact with more than 40 different molecules, among them matrix metalloproteinases (MMPs) and protease inhibitors, thrombospondins, N-methyl-D-aspartate receptors (NMDAR) and integrins (4). Mirroring this multitude, the binding of ligands to Lrp1 can lead to various effects. Ligands can directly bind to the extracellular domain of Lrp1, undergo endocytosis (5) and be transported to lysosomes/proteasomes for degradation (7) while Lrp1 is recycled back to the membrane (6). Some ligands require bridging molecules like thrombospondins or heparan sulfate proteoglycans (HSPGs) to undergo efficient uptake (5). Upon ligand binding to the extracellular and/or cytoplasmic domains of Lrp1, the receptor can become phosphorylated (8), result in the activation of signaling cascades (9) and/or regulate gene transcription (10). Given the above, Lrp1 impacts the ECM either directly or indirectly and influences its remodeling. Lrp1 signaling alters not only the composition of the ECM but also other homeostatic processes like synaptic signaling and angiogenesis, which impact brain functioning.
In summary, as the impact of Lrp1 on the ECM is complex and both cell type- and cofactor-dependent, an integrative approach for deciphering Lrp1 function should be implemented for each studied interaction and system.
Parts of the review content (especially Figures 1, 10, 11 and the integrin and tPA sections) first appeared in the dissertation thesis (Bres, 2018). This is the only form in which this content has appeared, is in line with the author’s university policy and will be soon available online.
EB and AF discussed the concept and content of the review. EB drafted and wrote the manuscript and designed the figures, AF read and revised the manuscript. Both authors agreed to the submitted version.
This work was supported by a graduate training grant of the International Graduate School for Neuroscience (IGSN) to EB. Grant support by the German Research Foundation (DFG, SPP 1757, Fa 159-20-1 & 2) to AF is gratefully acknowledged. We acknowledge support by the DFG Open Access Publication Funds of the Ruhr-Universität Bochum.
The authors declare that the research was conducted in the absence of any commercial or financial relationships that could be construed as a potential conflict of interest.
The reviewer DL declared a shared affiliation, with no collaboration, with the authors to the handling Editor at the time of review.
Achuta, S., Rezov, V., Uutela, M., Louhivuori, V., Louhivuori, L., and Castrén, M. (2014). Tissue plasminogen activator contributes to alterations of neuronal migration and activity-dependent responses in fragile X mice. J. Neurosci. 34, 1916–1923. doi: 10.1523/JNEUROSCI.3753-13.2014
Akimov, S. S., Krylov, D., Fleischman, L. F., and Belkin, A. M. (2000). Tissue transglutaminase is an integrin-binding adhesion coreceptor for fibronectin. J. Cell Biol. 148, 825–838. doi: 10.1083/jcb.148.4.825
Akkawi, S., Nassar, T., Tarshis, M., Cines, D. B., and Higazi, A. A. (2006). LRP and alphavbeta3 mediate tPA activation of smooth muscle cells. Am. J. Physiol. Heart Circ. Physiol. 291, H1351–H1359. doi: 10.1152/ajpheart.01042.2005
Andersen, O. M., and Willnow, T. E. (2006). Lipoprotein receptors in Alzheimer’s disease. Trends Neurosci. 29, 687–694. doi: 10.1016/j.tins.2006.09.002
Ashcom, J. D., Tiller, S. E., Dickerson, K., Cravens, J. L., Argaves, W. S., and Strickland, D. K. (1990). The human alpha 2-macroglobulin receptor: identification of a 420-kD cell surface glycoprotein specific for the activated conformation of alpha 2-macroglobulin. J. Cell Biol. 110, 1041–1048. doi: 10.1083/jcb.110.4.1041
Auderset, L., Cullen, C., and Young, K. (2016). Low density lipoprotein-receptor related protein 1 is differentially expressed by neuronal and glial populations in the developing and mature mouse central nervous system. PLoS One 11:e0155878. doi: 10.1371/journal.pone.0155878
Bacskai, B., Xia, M., Strickland, D., Rebeck, G., and Hyman, B. (2000). The endocytic receptor protein LRP also mediates neuronal calcium signalling via N -methyl-D-aspartate receptors. Proc. Natl. Acad. Sci. U.S.A. 97, 11551–11556. doi: 10.1073/pnas.200238297
Bannerman, D., Good, M., Butcher, S., Ramsay, M., and Morris, R. (1995). Distinct components of spatial learning revealed by prior training and NMDA receptor blockade. Nature 378, 182–186. doi: 10.1038/378182a0
Baranes, D., Lederfein, D., Huang, Y.-Y., Chen, M., Bailey, C., and Kandel, E. (1998). Tissue plasminogen activator contributes to the late phase of LTP and to synaptic growth in the hippocampal mossy fiber pathway. Neuron 21, 813–825. doi: 10.1016/S0896-6273(00)80597-8
Barker, T. H., Pallero, M. A., MacEwen, M. W., Tilden, S. G., Woods, A., Murphy-Ullrich, J. E., et al. (2004). Thrombospondin-1-induced focal adhesion disassembly in fibroblasts requires Thy-1 surface expression, lipid raft integrity, and Src activation. J. Biol. Chem. 279, 23510–23516. doi: 10.1074/jbc.M402169200
Barmina, O. Y., Walling, H. W., Fiacco, G. J., Freije, J. M., López-Otín, C., Jeffrey, J. J., et al. (1999). Collagenase-3 binds to a specific receptor and requires the low density lipoprotein receptor-related protein for internalization. J. Biol. Chem. 274, 30087–30093. doi: 10.1074/jbc.274.42.30087
Barnes, H., Larsen, B., Tyers, M., and van der Geer, P. (2001). Tyrosine-phosphorylated low density lipoprotein receptor-related protein 1 (LRP1) associates with the adaptor protein SHC in SRC-transformed cells. J. Biol. Chem. 276, 19119–19125. doi: 10.1074/jbc.M011437200
Basu, S., Binder, R. J., Ramalingam, T., and Srivastava, P. K. (2001). CD91 is a common receptor for heat shock proteins gp96, hsp90, hsp70, and calreticulin. Immunity 14, 303–313. doi: 10.1016/S1074-7613(01)00111-X
Bein, K., and Simons, M. (2000). Thrombospondin type 1 repeats interact with matrix metalloproteinase 2. Regulation of metalloproteinase activity. J. Biol. Chem. 275, 32167–32173. doi: 10.1074/jbc.M003834200
Beisiegel, U., Weber, W., and Bengtsson-Olivecrona, G. (1991). Lipoprotein lipase enhances the binding of chylomicrons to low density lipoprotein receptor-related protein. Proc. Natl. Acad. Sci. U.S.A. 88, 8342–8346. doi: 10.1073/pnas.88.19.8342
Beisiegel, U., Weber, W., Ihrke, G., Herz, J., and Stanley, K. K. (1989). The LDL-receptor-related protein, LRP, is an apolipoprotein E-binding protein. Nature 341, 162–164. doi: 10.1038/341162a0
Bellis, S. L. (2004). Variant glycosylation: an underappreciated regulatory mechanism for beta1 integrins. Biochim. Biophys. Acta 1663, 52–60. doi: 10.1016/j.bbamem.2004.03.012
Belvindrah, R., Graus-Porta, D., Goebbels, S., Nave, K.-A., and Müller, U. (2007a). Beta1 integrins in radial glia but not in migrating neurons are essential for the formation of cell layers in the cerebral cortex. J. Neurosci. 27, 13854–13865.
Belvindrah, R., Hankel, S., Walker, J., Patton, B. L., and Müller, U. (2007b). Beta1 integrins control the formation of cell chains in the adult rostral migratory stream. J. Neurosci. 27, 2704–2717.
Benchenane, K., Castel, H., Boulouard, M., Bluthé, R., Fernández-Monreal, M., Roussel, B., et al. (2007). Anti-NR1 N-terminal-domain vaccination unmasks the crucial action of tPA on NMDA- receptor-mediated toxicity and spatial memory. J. Cell Sci. 120, 578–585. doi: 10.1242/jcs.03354
Bertrand, T., Lesept, F., Chevilley, A., Lenoir, S., Aimable, M., Briens, A., et al. (2015). Conformations of tissue plasminogen activator (tPA) orchestrate neuronal survival by a crosstalk between EGFR and NMDAR. Cell Death Dis. 6:e1924. doi: 10.1038/cddis.2015.296
Betts, G., van der Geer, P., and Komives, E. (2008). Structural and functional consequences of tyrosine phosphorylation in the LRP1 cytoplasmic domain. J. Biol. Chem. 283, 15656–15664. doi: 10.1074/jbc.M709514200
Bilodeau, N., Fiset, A., Boulanger, M. C., Bhardwaj, S., Winstall, E., Lavoie, J. N., et al. (2010). Proteomic analysis of src family kinases signaling complexes in golgi/endosomal fractions using a site-selective anti-phosphotyrosine antibody:identification of lrp1-insulin receptor complexes. J. Proteome Res. 9, 708–717. doi: 10.1021/pr900481b
Boucher, P., Gotthardt, M., Li, W., Anderson, R., and Herz, J. (2003). LRP: role in vascular wall integrity and protection from atherosclerosis. Science 300, 329–332. doi: 10.1126/science.1082095
Boucher, P., and Herz, J. (2011). Signaling through LRP1, protection from atherosclerosis and beyond. Biochem. Pharmacol. 81, 1–5. doi: 10.1016/j.bcp.2010.09.018
Boucher, P., Liu, P., Gotthardt, M., Hiesberger, T., Anderson, R., and Herz, J. (2002). Platelet-derived growth factor mediates tyrosine phosphorylation of the cytoplasmic domain of the low density lipoprotein receptor-related protein in caveolae. J. Biol. Chem. 277, 15507–15513. doi: 10.1074/jbc.M200428200
Brandan, E., Retamal, C., Cabello-Verrugio, C., and Marzolo, M. P. (2006). The low density lipoprotein receptor-related protein functions as an endocytic receptor for decorin. J. Biol. Chem. 281, 31562–31571. doi: 10.1074/jbc.M602919200
Bres, E. E. (2018). Unravelling the Role of Low-Density Lipoprotein Receptor-Related Protein-1 in Radial Glia and Neuronal and Glial Progeny in the Developing Mouse Brain. Ph.D. thesis, Ruhr University Bochum, Bochum.
Briens, A., Bardou, I., Lebas, H., Miles, L., Parmer, R., and Vivien, D. (2017). Astrocytes regulate the balance between plasminogen activation and plasmin clearance via cell-surface actin. Cell Discov. 3, 17001–17018. doi: 10.1038/celldisc.2017.1
Brinckerhoff, C. E., and Matrisian, L. M. (2002). Matrix metalloproteinases: a tail of a frog that became a prince. Nat. Rev. Mol. Cell Biol. 3, 207–214. doi: 10.1038/nrm763
Bronfman, F. C., Soto, C., Tapia, L., Tapia, V., and Inestrosa, N. C. (1996). Extracellular matrix regulates the amount of the β-amyloid precursor protein and its amyloidogenic fragments. J. Cell. Physiol. 166, 360–369. doi: 10.1002/(SICI)1097-4652(199602)166:2<360::AID-JCP14>3.0.CO;2-F
Brooker, S. M., Bond, A. M., Peng, C.-Y., and Kessler, J. A. (2016). β1-integrin restricts astrocytic differentiation of adult hippocampal neural stem cells. Glia 64, 1235–1251. doi: 10.1002/glia.22996
Brown, D. A., and London, E. (1998). Functions of lipid rafts in biological membranes. Annu. Rev. Cell Dev. Biol. 14, 111–136. doi: 10.1146/annurev.cellbio.14.1.111
Brown, D. A., and London, E. (2000). Structure and function of sphingolipid-and-cholesterol-rich membrane rafts. J. Biol. Chem. 275, 17221–17224. doi: 10.1074/jbc.R000005200
Bu, G., Maksymovitch, E., Nerbonne, J., and Schwartz, A. (1994). Expression and function of the low density lipoprotein receptor-related protein (LRP) in mammalian central neurons. J. Biol. Chem. 269, 18521–18528.
Bu, G., Williams, S., Strickland, D., and Schwartz, A. (1992). Low density lipoprotein receptor-related protein/alpha 2-macroglobulin receptor is an hepatic receptor for tissue-type plasminogen activator. Proc. Natl. Acad. Sci. U.S.A. 89, 7427–7431. doi: 10.1073/pnas.89.16.7427
Cao, C., Lawrence, D. A., Li, Y., Von Arnim, C. A., Herz, J., Su, E. J., et al. (2006). Endocytic receptor LRP together with tPA and PAI-1 coordinates Mac-1-dependent macrophage migration. EMBO J. 25, 1860–1870. doi: 10.1038/sj.emboj.7601082
Capurro, M. I., Shi, W., and Filmus, J. (2012). LRP1 mediates Hedgehog-induced endocytosis of the GPC3-Hedgehog complex. J. Cell Sci. 125, 3380–3389. doi: 10.1242/jcs.098889
Capurro, M. I., Xu, P., Shi, W., Li, F., Jia, A., and Filmus, J. (2008). Glypican-3 inhibits Hedgehog signaling during development by competing with patched for Hedgehog binding. Dev. Cell 14, 700–711. doi: 10.1016/j.devcel.2008.03.006
Carroll, P., Tsirka, S., Richards, W., Frohman, M., and Strickland, S. (1994). The mouse tissue plasminogen activator gene 5’ flanking region directs appropriate expression in development and a seizure-enhanced response in the CNS. Development 120, 3173–3183.
Carstens, K. E., Phillips, M. L., Pozzo-Miller, L., Weinberg, R. J., and Dudek, S. M. (2016). Perineuronal nets suppress plasticity of excitatory synapses on ca2 pyramidal neurons. J. Neurosci. 36, 6312–6320. doi: 10.1523/JNEUROSCI.0245-16.2016
Cassé, F., Bardou, I., Danglot, L., Briens, A., Montagne, A., Parcq, J., et al. (2012). Glutamate controls tPA recycling by astrocytes, which in turn influences glutamatergic signals. J. Neurosci. 32, 5186–5199. doi: 10.1523/JNEUROSCI.5296-11.2012
Cavallaro, U., del Vecchio, A., Lappi, D. A., and Soria, M. R. (1993a). A conjugate between human urokinase and saporin, a type 1 ribosome-inactivating protein, is selectively cytotoxic in urokinase receptor-expressing cells. J. Biol. Chem. 268, 23186–23190.
Cavallaro, U., del Vecchio, A., Tazzari, P. L., Massazza, G., and Soria, M. R. (1993b). Targeting of cytotoxic conjugates to Kaposi’s sarcoma-derived cells. Drug Deliv. 1, 119–124. doi: 10.3109/10717549309022765
Cavallaro, U., Nykjaer, A., Nielsen, M., and Soria, M. R. (1995). Alpha 2-macroglobulin receptor mediates binding and cytotoxicity of plant ribosome-inactivating proteins. Eur. J. Biochem. 232, 165–171. doi: 10.1111/j.1432-1033.1995.tb20795.x
Chan, W. L., Shaw, P. C., Tam, S. C., Jacobsen, C., Gliemann, J., and Nielsen, M. S. (2000). Trichosanthin interacts with and enters cells via LDL receptor family members. Biochem. Biophys. Res. Commun. 270, 453–457. doi: 10.1006/bbrc.2000.2441
Chappell, D. A., Fry, G. L., Waknitz, M. A., Iverius, P. H., Williams, S. E., and Strickland, D. K. (1992). The low density lipoprotein receptor-related protein/alpha 2-macroglobulin receptor binds and mediates catabolism of bovine milk lipoprotein lipase. J. Biol. Chem. 267, 25764–25767.
Chevilley, A., Lesept, F., Lenoir, S., Ali, C., Parcq, J., and Vivien, D. (2015). Impacts of tissue-type plasminogen activator (tPA) on neuronal survival. Front. Cell. Neurosci. 9:415. doi: 10.3389/fncel.2015.00415
Chow, V. W., Mattson, M. P., Wong, P. C., and Gleichmann, M. (2010). An overview of APP processing enzymes and products. Neuromolecular Med. 12, 1–12. doi: 10.1007/s12017-009-8104-z
Christopherson, K. S., Ullian, E. M., Stokes, C. C., Mullowney, C. E., Hell, J. W., Agah, A., et al. (2005). Thrombospondins are astrocyte-secreted proteins that promote CNS synaptogenesis. Cell 120, 421–433. doi: 10.1016/j.cell.2004.12.020
Cirrito, J. R., Yamada, K. A., Finn, M. B., Sloviter, R. S., Bales, K. R., May, P. C., et al. (2005). Synaptic activity regulates interstitial fluid amyloid-beta levels in vivo. Neuron 48, 913–922. doi: 10.1016/j.neuron.2005.10.028
Conese, M., Nykjaer, A., Petersen, C. M., Cremona, O., Pardi, R., Andreasen, P. A., et al. (1995). Alpha-2 macroglobulin receptor/Ldl receptor-related protein (Lrp)-dependent internalisation of the urokinase receptor. J. Cell Biol. 131, 1609–1622. doi: 10.1083/jcb.131.6.1609
Conese, M., Olson, D., and Blasi, F. (1994). Protease nexin-1-urokinase complexes are internalized and degraded through a mechanism that requires both urokinase receptor and alpha 2-macroglobulin receptor. J. Biol. Chem. 269, 17886–17892.
Correa, F., Gauberti, M., Parcq, J., Macrez, R., Hommet, Y., Obiang, P., et al. (2011). Tissue plasminogen activator prevents white matter damage following stroke. J. Exp. Med. 208, 1229–1242. doi: 10.1084/jem.20101880
Craig, J., Mikhailenko, I., Noyes, N., Migliorini, M., and Strickland, D. K. (2013). The LDL receptor-related protein 1 (LRP1) regulates the PDGF signaling pathway by binding the protein phosphatase SHP-2 and modulating SHP-2- mediated PDGF signaling events. PLoS One 8:e70432. doi: 10.1371/journal.pone.0070432
Crisp, R. J., Knauer, D. J., and Knauer, M. F. (2000). Roles of the heparin and low density lipid receptor-related protein-binding sites of protease nexin 1 (PN1) in urokinase-PN1 complex catabolism. The PN1 heparin-binding site mediates complex retention and degradation but not cell surface binding or internalization. J. Biol. Chem. 275, 19628–19637. doi: 10.1074/jbc.M909172199
Croucher, D., Saunders, D. N., and Ranson, M. (2006). The urokinase/PAI-2 complex: a new high affinity ligand for the endocytosis receptor low density lipoprotein receptor-related protein. J. Biol. Chem. 281, 10206–10213. doi: 10.1074/jbc.M513645200
Croy, J., Shin, W., Knauer, M., Knauer, D., and Komives, E. (2003). All three LDL receptors homology regions of the LDL receptor-related protein bind multiple ligands. Biochemistry 42, 13049–13057. doi: 10.1021/bi034752s
Czekay, R. P., Aertgeerts, K., Curriden, S. A., and Loskutoff, D. J. (2003). Plasminogen activator inhibitor-1 detaches cells from extracellular matrices by inactivating integrins. J. Cell Biol. 160, 781–791. doi: 10.1083/jcb.200208117
Czekay, R. P., Kuemmel, T. A., Orlando, R. A., and Farquhar, M. G. (2001). Direct binding of occupied urokinase receptor (uPAR) to LDL receptor-related protein is required for endocytosis of uPAR and regulation of cell surface urokinase activity. Mol. Biol. Cell 12, 1467–1479. doi: 10.1091/mbc.12.5.1467
Czekay, R. P., Wilkins-Port, C. E., Higgins, S. P., Freytag, J., Overstreet, J. M., Klein, R. M., et al. (2011). PAI-1: an integrator of cell signalling and migration. Int. J. Cell Biol. 2011:562481. doi: 10.1155/2011/562481
de Jager, M., van der Wildt, B., Schul, E., Bol, J. G., van Duinen, S. G., Drukarch, B., et al. (2013). Tissue transglutaminase colocalizes with extracellular matrix proteins in cerebral amyloid angiopathy. Neurobiol. Aging 34, 1159–1169. doi: 10.1016/j.neurobiolaging.2012.10.005
Deane, R., Bell, R. D., Sagare, A., and Zlokovic, B. V. (2009). Clearance of amyloid-beta peptide across the blood-brain barrier: implication for therapies in Alzheimer’s disease. CNS Neurol. Disord. Drug Targets 8, 16–30. doi: 10.2174/187152709787601867
Deane, R., Sagare, A., Hamm, K., Parisi, M., Lane, S., Finn, M., et al. (2008). apoE isoform-specific disruption of amyloid beta peptide clearance from mouse brain. J. Clin. Invest. 118, 4002–4133. doi: 10.1172/JCI36663
Deane, R., Wu, Z., Sagare, A., Davis, J., Du, Y., Hamm, K., et al. (2004). LRP/amyloid beta-peptide interaction mediates differential brain efflux of Abeta isoforms. Neuron 43, 333–344. doi: 10.1016/j.neuron.2004.07.017
Deb, S., Wenjun Zhang, J., and Gottschall, P. E. (2003). Beta-amyloid induces the production of active, matrix-degrading proteases in cultured rat astrocytes. Brain Res. 970, 205–213. doi: 10.1016/S0006-8993(03)02344-8
Degryse, B., Neels, J. G., Czekay, R. P., Aertgeerts, K., Kamikubo, Y., and Loskutoff, D. J. (2004). The low density lipoprotein receptor-related protein is a motogenic receptor for plasminogen activator inhibitor-1. J. Biol. Chem. 279, 22595–22604. doi: 10.1074/jbc.M313004200
Degryse, B., Sier, C. F., Resnati, M., Conese, M., and Blasi, F. (2001). PAI-1 inhibits urokinase-induced chemotaxis by internalizing the urokinase receptor. FEBS Lett. 505, 249–254. doi: 10.1016/S0014-5793(01)02797-1
Derocq, D., Prébois, C., Beaujouin, M., Laurent-Matha, V., Pattingre, S., and Smith, G. K. (2012). Cathepsin D is partly endocytosed by the LRP1 receptor and inhibits LRP1-regulated intramembrane proteolysis. Oncogene 31, 3202–3212. doi: 10.1038/onc.2011.501
Dzyubenko, E., Gottschling, C., and Faissner, A. (2016). Neuron-Glia interactions in neural plasticity: contributions of neural extracellular matrix and perineuronal nets. Neural Plast. 2016:5214961. doi: 10.1155/2016/5214961
Ehrlich, I., Klein, M., Rumpel, S., and Malinow, R. (2007). PSD-95 is required for activity-driven synapse stabilisation. Proc. Natl. Acad. Sci. U.S.A. 104, 4176–4181. doi: 10.1073/pnas.0609307104
El-Husseini, A., Schnell, E., Chetkovich, D., Nicoll, R., and Bredt, D. (2000). PSD-95 involvement in the maturation of excitatory synapses. Science 290, 1364–1368.
Emonard, H., Bellon, G., de Diesbach, P., Mettlen, M., Hornebeck, W., and Courtoy, P. J. (2005). Regulation of matrix metalloproteinase (MMP) activity by the low-density lipoprotein receptor-related protein (LRP). A new function for an “old friend”. Biochimie 87, 369–376. doi: 10.1016/j.biochi.2004.11.013
Emonard, H., Bellon, G., Troeberg, L., Berton, A., Robinet, A., Henriet, P., et al. (2004). Low density lipoprotein receptor-related protein mediates endocytic clearance of pro-MMP-2. TIMP-2 complex through a thrombospondin-independent mechanism. . J. Biol. Chem. 279, 54944–54951. doi: 10.1074/jbc.M406792200
Fabbro, S., and Seeds, N. (2009). Plasminogen activator activity is inhibited while neuroserpin is up-regulated in the Alzheimer disease brain. J. Neurochem. 109, 303–315. doi: 10.1111/j.1471-4159.2009.05894.x
Faissner, A., Pyka, M., Geissler, M., Sobik, T., Frischknecht, R., Gundelfinger, E. D., et al. (2010). Contributions of astrocytes to synapse formation and maturation- potential functions of the perisynaptic extracellular matrix. Brain Res. Rev. 63, 26–38. doi: 10.1016/j.brainresrev.2010.01.001
Fears, C. Y., Grammer, J. R., Stewart, JE Jr, Annis, D. S., Mosher, D. F., Bornstein, P., et al. (2005). Low-density lipoprotein receptor-related protein contributes to the antiangiogenic activity of thrombospondin-2 in a murine glioma model. Cancer Res. 65, 9338–9346. doi: 10.1158/0008-5472.CAN-05-1560
Fernández-Monreal, M., López-Atalaya, J. P., Benchenane, K., Léveillé, F., Cacquevel, M., Plawinski, L., et al. (2004). Is tissue-type plasminogen activator a neuromodulator? Mol. Cell. Neurosci. 25, 594–601. doi: 10.1016/j.mcn.2003.11.002
Foley, E. M., Gordts, P. L. S. M., Stanford, K. I., Gonzales, J. C., Lawrence, R., Stoddard, N., et al. (2013). Hepatic remnants lipoprotein clearance by heparan sulfate proteoglycans and low-density lipoprotein receptors depend on dietary conditions in mice. Arterioscler. Thromb. Vasc. Biol. 33, 2065–2074. doi: 10.1161/ATVBAHA.113.301637
Frackowiak, J., Wisniewski, H. M., Wegiel, J., Merz, G. S., Iqbal, K., and Wang, K. C. (1992). Ultrastructure of the microglia that phagocytose amyloid and the microglia that produce beta-amyloid fibrils. Acta Neuropathol. 84, 225–233. doi: 10.1007/BF00227813
Fredriksson, L., Li, H., Fieber, C., Li, X., and Eriksson, U. (2004). Tissue plasminogen activator is a potent activator of PDGF-CC. EMBO J. 23, 3793–3802. doi: 10.1038/sj.emboj.7600397
Fredriksson, L., Stevenson, T. K., Su, E. J., Ragsdale, M., Moore, S., Craciun, S., et al. (2015). Identification of a neurovascular signaling pathway regulating seizures in mice. Ann. Clin. Transl. Neurol. 2, 722–738. doi: 10.1002/acn3.209
Friedman, G. C., and Seeds, N. W. (1995). Tissue plasminogen activator mRNA expression in granule neurons coincides with their migration in the developing cerebellum. J. Comp. Neurol. 360, 658–670. doi: 10.1002/cne.903600410
Gabriel, C., Ali, C., Lesné, S., Fernández-Monreal, M., Docagne, F., Plawinski, L., et al. (2002). Transforming growth factor alpha-induced expression of type 1 plasminogen activator inhibitor in astrocytes rescues neurons from excitotoxicity. FASEB J. 17, 277–279. doi: 10.1096/fj.02-0403fje
Gao, R., and Brigstock, D. R. (2003). Low density lipoprotein receptor-related protein (LRP) is a heparin-dependent adhesion receptor for connective tissue growth factor (CTGF) in rat activated hepatic stellate cells. Hepatol. Res. 27, 214–220. doi: 10.1016/S1386-6346(03)00241-9
Gardai, S. J., McPhillips, K. A., Frasch, S. C., Janssen, W. J., Starefeldt, A., Murphy-Ullrich, J. E., et al. (2005). Cell-surface calreticulin initiates clearance of viable or apoptotic cells through trans-activation of LRP on the phagocyte. Cell 123, 321–334. doi: 10.1016/j.cell.2005.08.032
Gaultier, A., Hollister, M., Reynolds, I., Hsieh, E., and Gonias, S. L. (2010). LRP1 regulates remodelling of the extracellular matrix by fibroblasts. Matrix Biol. 29, 22–30. doi: 10.1016/j.matbio.2009.08.003
Gaultier, A., Salicioni, A. M., Arandjelovic, S., and Gonias, S. L. (2006). Regulation of the composition of the extracellular matrix by low density lipoprotein receptor-related protein-1: activities based on regulation of mRNA expression. J. Biol. Chem. 281, 7332–7340. doi: 10.1074/jbc.M511857200
Gaultier, A., Wu, X., Le Moan, N., Takimoto, S., Mukandala, G., Akassoglou, K., et al. (2009). Low-density lipoprotein receptor-related protein 1 is an essential receptor for myelin phagocytosis. J. Cell Sci. 122, 1155–1162. doi: 10.1242/jcs.040717
Geissler, M., Gottschling, C., Aguado, A., Rauch, U., Wetzel, C. H., Hatt, H., et al. (2013). Primary hippocampal neurons, which lack four crucial extracellular matrix molecules, display abnormalities of synaptic structure and function and severe deficits in perineuronal net formation. J. Neurosci. 33, 7742–7755. doi: 10.1523/JNEUROSCI.3275-12.2013
Genedani, S., Agnati, L. F., Leo, G., Buzzega, D., Maccari, F., Carone, C., et al. (2010). β-Amyloid fibrillation and/or hyperhomocysteinemia modify striatal patterns of hyaluronic acid and dermatan sulfate: possible role in the pathogenesis of Alzheimers disease. Curr. Alzheimers Res. 7, 150–157. doi: 10.2174/156720510790691074
Gerritsen, K. G. F., Bovenschen, N., Nguyen, T. Q., Sprengers, D., Koeners, M. P., van Koppen, A. N., et al. (2016). Rapid hepatic clearance of full length CCN-2/CTGF: a putative role for LRP1-mediated endocytosis. J. Cell Commun. Signal. 10, 295–303. doi: 10.1007/s12079-016-0354-6
Godyna, S., Liau, G., Popa, I., Stefansson, S., and Argraves, W. S. (1995). Identification of the low density lipoprotein receptor-related protein (LRP) as an endocytic receptor for thrombospondin-1. J. Cell Biol. 129, 1403–1410. doi: 10.1083/jcb.129.5.1403
Gonias, S. L., Wu, L., and Salicioni, A. M. (2004). Low density lipoprotein receptor-related protein: regulation of the plasma membrane proteome. Thromb. Haemost. 91, 1056–1064. doi: 10.1160/TH04-01-0023
Gordts, P. L., Bartelt, A., Nilsson, S. K., Annaert, W., Christoffersen, C., Nielsen, L. B., et al. (2012). Impaired LDL receptor-related protein 1 translocation correlates with improved dyslipidemia and atherosclerosis in apoE-deficient mice. PLoS One 7:e38330. doi: 10.1371/journal.pone.0038330
Gotthardt, M., Trommsdorff, M., Nevitt, M., Shelton, J., Richardson, J., Stockinger, W., et al. (2000). Interactions of the low density lipoprotein receptor gene family with cytosolic adaptor and scaffold proteins suggest diverse biological functions in cellular communication and signal transduction. J. Biol. Chem. 275, 25616–25624. doi: 10.1074/jbc.M000955200
Greenaway, J., Lawler, J., Moorehead, R., Bornstein, P., LaMarre, J., and Petrik, J. (2007). Thrombospondin-1 inhibits VEGF levels in the ovary directly by binding and internalization via the low density lipoprotein receptor-related protein-1 (LRP-1). J. Cell. Physiol. 210, 807–818. doi: 10.1002/jcp.20904
Grummisch, J. A., Jadavji, N. M., and Smith, P. D. (2016). TPA promotes cortical neuron survival via mTOR-dependent mechanisms. Mol. Cell. Neurosci. 74, 25–33. doi: 10.1016/j.mcn.2016.03.005
Guttman, M., Betts, G., Barnes, H., Ghassemian, M., van der Geer, P., and Komives, E. (2009). Interactions of the NPXY microdomains of the low density lipoprotein receptor-related protein 1. Proteomics 9, 5016–5028. doi: 10.1002/pmic.200900457
Hadfield, K. D., Rock, C. F., Inkson, C. A., Dallas, S. L., Sudre, L., Wallis, G. A., et al. (2008). HtrA1 inhibits mineral deposition by osteoblasts: requirement for the protease and PDZ domains. J. Biol. Chem. 283, 5928–5938. doi: 10.1074/jbc.M709299200
Hahn-Dantona, E., Ruiz, J. F., Bornstein, P., and Strickland, D. K. (2001). The low density lipoprotein receptor-related protein modulates levels of matrix metalloproteinase 9 (MMP-9) by mediating its cellular catabolism. J. Biol. Chem. 276, 15498–15503. doi: 10.1074/jbc.M100121200
Hajjar, K., Jacovina, A., and Chacko, J. (1994). An endothelial cell receptor for plasminogen/tissue plasminogen activator. I. identity with annexin II. J. Biol. Chem. 269, 21191–21197.
Hartig, W., Brauer, K., and Bruckner, G. (1992). Wisteria floribunda agglutinin-labelled nets surround parvalbumin-containing neurons. Neuroreport 3, 869–872. doi: 10.1097/00001756-199210000-00012
Hayashi, H., Campenot, R. B., Vance, D. E., and Vance, J. E. (2007). Apolipoprotein E-containing lipoproteins protect neurons from apoptosis via a signaling pathway involving low-density lipoprotein receptor-related protein-1. J. Neurosci. 27, 1933–1941. doi: 10.1523/JNEUROSCI.5471-06.2007
Hébert, M., Lesept, F., Vivien, D., and Macrez, R. (2015). The story of an exceptional serine protease, tissue-type plasminogen activator (tPA). Rev. Neurol. 172, 186–197. doi: 10.1016/j.neurol.2015.10.002
Heck, N., Garwood, J., Loeffler, J.-P., Larmet, Y., and Faissner, A. (2004). Differential upregulation of extracellular matrix molecules associated with the appearance of granule cell dispersion and mossy fiber sprouting during epileptogenesis in a murine model of temporal lobe epilepsy. Neuroscience 129, 309–324. doi: 10.1016/j.neuroscience.2004.06.078
Hennen, E., Safina, D., Haussmann, U., Wörsdörfer, P., Edenhofer, F., Poetsch, A., et al. (2013). A LewisX glycoprotein screen identifies the low density lipoprotein receptor-related protein 1 (LRP1) as a modulator of oligodendrogenesis in mice. J. Biol. Chem. 288, 16538–16545. doi: 10.1074/jbc.M112.419812
Herz, J. (2001). The LDL receptor gene family: (un)expected signal transducers in the brain. Neuron 29, 571–581. doi: 10.1016/S0896-6273(01)00234-3
Herz, J., and Bock, H. (2002). Lipoprotein receptors in the nervous system. Annu. Rev. Biochem. 71, 405–434. doi: 10.1146/annurev.biochem.71.110601.135342
Herz, J., and Chen, Y. (2006). Reelin, lipoprotein receptors and synaptic plasticity. Nat. Rev. Neurosci. 7, 850–859. doi: 10.1038/nrn2009
Herz, J., Chen, Y., Masiulis, I., and Zhou, L. (2009). Expanding functions of lipoprotein receptors. J. Lipid Res. 50, S287–S292. doi: 10.1194/jlr.R800077-JLR200
Herz, J., Clouthier, D., and Hammer, R. (1992). LDL receptor-related protein internalizes and degrades uPA-PAI-1 complexes and is essential for embryo implantation. Cell 71, 411–421. doi: 10.1016/0092-8674(92)90511-A
Herz, J., Clouthier, D., and Hammer, R. (1993). Correction: LDL receptor-related protein internalizes and degrades uPA-PAI-1 complexes and is essential for embryo implantation. Cell 73:428. doi: 10.1016/0092-8674(93)90130-I
Herz, J., Goldstein, J. L., Strickland, D. K., Ho, Y. K., and Brown, M. S. (1991). 39-kDa protein modulates binding of ligands to low density lipoprotein receptor-related protein/alpha 2-macroglobulin receptor. J. Biol. Chem. 266, 21232–21238.
Herz, J., Hamann, U., Rogne, S., Myklebost, O., Gausepohl, H., and Stanley, K. K. (1988). Surface location and high affinity for calcium of a 500-kd liver membrane protein closely related to the LDL-receptor suggest a physiological role as lipoprotein receptor. EMBO J. 7, 4119–4127. doi: 10.1002/j.1460-2075.1988.tb03306.x
Herz, J., and Strickland, D. K. (2001). LRP: a multifunctional scavenger and signaling receptor. J. Clin. Invest. 108, 779–784. doi: 10.1172/JCI200113992
Hicks, D. A., Nalivaeva, N. N., and Turner, A. J. (2012). Lipid rafts and Alzheimer’s disease: protein-lipid interactions and perturbation of signaling. Front. Physiol. 3:189. doi: 10.3389/fphys.2012.00189
Hiesberger, T., Hüttler, S., Rohlmann, A., Schneider, W., Sandhoff, K., and Herz, J. (1998). Cellular uptake of saposin (SAP) precursor and lysosomal delivery by the low density lipoprotein receptor-related protein (LRP). EMBO J. 17, 4617–4625. doi: 10.1093/emboj/17.16.4617
Ho, G., Toomey, J. R., Broze, G. J. Jr., and Schwartz, A. L. (1996). Receptor-mediated endocytosis of coagulation factor Xa requires cell surface-bound tissue factor pathway inhibitor. J. Biol. Chem. 271, 9497–9502. doi: 10.1074/jbc.271.16.9497
Hofer, F., Gruenberger, M., Kowalski, H., Machat, H., Huettinger, M., Kuechler, E., et al. (1994). Members of the low density lipoprotein receptor family mediate cell entry of a minor- group common cold virus. Proc. Natl. Acad. Sci. U.S.A. 91, 1839–1842. doi: 10.1073/pnas.91.5.1839
Hoirisch-Clapauch, S., and Nardi, A. (2015). Improvement of psychotic symptoms and the role of tissue plasminogen activator. Int. J. Mol. Sci. 16, 27550–27560. doi: 10.3390/ijms161126053
Holtzman, D. M., Pitas, R. E., Kilbridge, J., Nathan, B., Mahley, R. W., Bu, G., et al. (1995). Low density lipoprotein receptor-related protein mediates apolipoprotein E-dependent neurite outgrowth in a central nervous system-derived neuronal cell line. Proc. Natl. Acad. Sci. U.S.A. 92, 9480–9484. doi: 10.1073/pnas.92.21.9480
Hu, K., Wu, C., Mars, W. M., and Liu, Y. (2007). Tissue-type plasminogen activator promotes murine myofibroblast activation through LDL receptor-related protein 1-mediated integrin signaling. J. Clin. Invest. 117, 3821–3832. doi: 10.1172/JCI32301
Hu, K., Yang, J., Tanaka, S., Gonias, S. L., Mars, W. M., and Liu, Y. (2006). Tissue-type plasminogen activator acts as a cytokine that triggers intracellular signal transduction and induces matrix metalloproteinase-9 gene expression. J. Biol. Chem. 281, 2120–2127. doi: 10.1074/jbc.M504988200
Huang, S. S., Ling, T. Y., Tseng, W. F., Huang, Y. H., Tang, F. M., Leal, S. M., et al. (2003). Cellular growth inhibition of IGFBP-3 and TGF-(beta)1 requires LRP-1. FASEB J. 17, 2068–2081. doi: 10.1096/fj.03-0256com
Huang, W., Dolmer, K., and Gettins, P. (1999). NMR solution structure of complement-like repeat CR8 from the low density lipoprotein receptor-related protein. J. Biol. Chem. 274, 14130–14136. doi: 10.1074/jbc.274.20.14130
Huang, Y.-Y., Bach, M., Lipp, H.-P., Zhuo, M., Wolfer, D., Hawkins, R., et al. (1996). Mice lacking the gene encoding tissue-type plasminogen activator show a selective interference with late-phase long-term potentiation in both Schaffer collateral and mossy fiber pathways. Proc. Natl. Acad. Sci. U.S.A. 93, 8699–8704. doi: 10.1073/pnas.93.16.8699
Hussain, M. M., Strickland, D. K., and Bakillah, A. (1999). The mammalian low-density lipoprotein receptor family. Annu. Rev. Nutr. 19, 141–172. doi: 10.1146/annurev.nutr.19.1.141
Hussaini, I. M., Brown, M. D., Karns, L. R., Carpenter, J., Redpath, G. T., Gonias, S. L., et al. (1999). Epidermal growth factor differentially regulates low density lipoprotein receptor-related protein gene expression in neoplastic and fetal human astrocytes. Glia 25, 71–84. doi: 10.1002/(SICI)1098-1136(19990101)25:1<71::AID-GLIA7>3.0.CO;2-0
Inoue, K., Koizumi, S., Nakajima, K., Hamanoue, M., and Kohsaka, S. (1994). Modulatory effect of plasminogen on NMDA-induced increase in intracellular free calcium concentration in rat cultured hippocampal neurons. Neurosci. Lett. 179, 87–90. doi: 10.1016/0304-3940(94)90941-5
Ishiguro, M., Imai, Y., and Kohsaka, S. (1995). Expression and distribution of low density lipoprotein receptor-related protein mRNA in the rat central nervous system. Brain Res. Mol. Brain Res. 33, 37–46. doi: 10.1016/0169-328X(95)00104-Z
Jaeger, S., and Pietrzik, C. U. (2008). Functional role of lipoprotein receptors in Alzheimer’s disease. Curr. Alzheimer Res. 5, 15–25. doi: 10.2174/156720508783884675
Janiak, A., Zemskov, E. A., and Belkin, A. M. (2006). Cell surface transglutaminase promotes RhoA activation via integrin clustering and suppression of the Src-p190RhoGAP signaling pathway. Mol. Biol. Cell 17, 1605–1619. doi: 10.1091/mbc.e05-06-0549
Jeanneret, V., Wu, F., Merino, P., Torre, E., Diaz, A., Cheng, L., et al. (2016). Tissue-type plasminogen activator (tPA) modulates the postsynaptic response of cerebral cortical neurons to the presynaptic release of glutamate. Front. Mol. Neurosci. 9:121. doi: 10.3389/fnmol.2016.00121
Jen, A., Parkyn, C. J., Mootoosamy, R. C., Ford, M. J., Warley, A., Liu, Q., et al. (2010). Neuronal low-density lipoprotein receptor-related protein 1 binds and endocytoses prion fibrils via receptor cluster 4. J. Cell Sci. 123, 246–255. doi: 10.1242/jcs.058099
Jullienne, A., Montagne, A., Orset, C., Lesept, F., Jane, D., Monaghan, D., et al. (2011). Selective inhibition of GluN2D-containing n-methyl-d-aspartate receptors prevents tissue plasminogen activator-promoted neurotoxicity both in vitro and in vivo. Mol. Neurodegener. 6, 68–78. doi: 10.1186/1750-1326-6-68
Juric, V., Chen, C.-C., and Lau, L. F. (2012). TNF α-induced apoptosis enabled by CCN1/CYR61: pathways of reactive oxygen species generation and Cytochrome C release. PLoS One 7:e31303. doi: 10.1371/journal.pone.0031303
Kagitani, H., Tagawa, M., Hatanaka, K., Ikari, T., Saito, A., Bando, H., et al. (1985). Expression of e. coli of finger-domain lacking tissue-type plasminogen activator with high fibrin activity. FEBS Lett. 189, 145–149. doi: 10.1016/0014-5793(85)80860-7
Kamenetz, F., Tomita, T., Hsieh, H., Seabrook, G., Borchelt, D., Iwatsubo, T., et al. (2003). APP processing and synaptic function. Neuron 37, 925–937. doi: 10.1016/S0896-6273(03)00124-7
Kanai, Y., Wang, D., and Hirokawa, N. (2014). KIF13B enhances the endocytosis of LRP1 by recruiting LRP1 to caveolae. J. Cell Biol. 204, 395–408. doi: 10.1083/jcb.201309066
Kanekiyo, T., and Bu, G. (2014). The low-density lipoprotein receptor-related protein 1 and amyloid-β clearance in Alzheimer’s disease. Front. Aging Neurosci. 6:93. doi: 10.3389/fnagi.2014.00093
Kanekiyo, T., Cirrito, J. R., Liu, C. C., Shinohara, M., Li, J., Schuler, D. R., et al. (2013). Neuronal clearance of amyloid-ß by endocytic receptor LRP1. J. Neurosci. 33, 19276–19283. doi: 10.1523/JNEUROSCI.3487-13.2013
Kanekiyo, T., Zhang, J., Liu, Q., Liu, C., Zhang, L., and Bu, G. (2011). Heparan sulfate proteoglycan and the low-density lipoprotein receptor-related protein 1 constitute major pathways for neuronal amyloid-beta uptake. J. Neurosci. 31, 1644–1651. doi: 10.1523/JNEUROSCI.5491-10.2011
Kang, D., Pietrzik, C., Baum, L., Chevallier, N., Merriam, D., Kounnas, M., et al. (2000). Modulation of amyloid beta-protein clearance and Alzheimer’s disease susceptibility by the LDL receptor-related protein pathway. J. Clin. Invest. 106, 1159–1166. doi: 10.1172/JCI11013
Kang, D. E., Saitoh, T., Chen, X., Xia, Y., Masliah, E., Hansen, L. A., et al. (1997). Genetic association of the low-density lipoprotein receptor-related protein gene (LRP), and apolipoprotein E receptor, with late-onset Alzheimer’s disease. Neurology 49, 56–61. doi: 10.1212/WNL.49.1.56
Kapustin, A., Stepanova, V., Aniol, N., Cines, D. B., Poliakov, A., Yarovoi, S., et al. (2012). Fibulin-5 binds urokinase-type plasminogen activator and mediates urokinase-stimulated β1-integrin-dependent cell migration. Biochem. J. 443, 491–503. doi: 10.1042/BJ20110348
Kasza, A., Petersen, H. H., Heegaard, C. W., Oka, K., Christensen, A., Dubin, A., et al. (1997). Specificity of serine proteinase/serpin complex binding to very- low-density lipoprotein receptor and alpha2-macroglobulin receptor/low-density-lipoprotein-receptor-related protein. Eur. J. Biochem. 248, 270–281. doi: 10.1111/j.1432-1033.1997.00270.x
Kinoshita, A., Shah, T., Tangredi, M. M., Strickland, D. K., and Hyman, B. T. (2003). The intracellular domain of the low density lipoprotein receptor-related protein modulates transactivation mediated by amyloid precursor protein and Fe65. J. Biol. Chem. 278, 41182–41188. doi: 10.1074/jbc.M306403200
Klug, W., Dietl, A., Simon, B., Sinning, I., and Wild, K. (2011). Phosphorylation of LRP1 regulates the interaction with Fe65. FEBS Lett. 585, 3229–3235. doi: 10.1016/j.febslet.2011.09.028
Knauer, D. J., Majumdar, D., Fong, P.-C., and Knauer, M. F. (2000). Serpin regulation of factor XIa. J. Biol. Chem. 275, 37340–37346. doi: 10.1074/jbc.M003909200
Knauer, M. F., Kridel, S. J., Hawley, S. B., and Knauer, D. J. (1997). The efficient catabolism of thrombin-protease nexin 1 complexes is a synergistic mechanism that requires both the LDL receptor-related protein and cell surface heparins. J. Biol. Chem. 272, 29039–29045. doi: 10.1074/jbc.272.46.29039
Knauer, M. F., Orlando, R. A., and Glabe, C. G. (1996). Cell surface APP751 forms complexes with protease nexin 2 ligands and is internalized via the low density lipoprotein receptor-related protein (LRP). Brain Res. 740, 6–14. doi: 10.1016/S0006-8993(96)00711-1
Komuro, H., and Rakic, P. (1992). Selective role of N-type calcium channels in neuronal migration. Science 257, 806–809. doi: 10.1126/science.1323145
Komuro, H., and Rakic, P. (1993). Modulation of neuronal migration by NMDA receptors. Science 260, 95–97. doi: 10.1126/science.8096653
Kounnas, M. Z., Argraves, W., and Strickland, D. (1992a). The 39-kDa receptor-associated protein interacts with two members of the low density lipoprotein receptor family, alpha 2-macroglobulin receptor and glycoprotein 330. J. Biol. Chem. 267, 21162–21166.
Kounnas, M. Z., Chappell, D. A., Wong, H., Argraves, W. S., and Strickland, D. K. (1995b). The cellular internalization and degradation of hepatic lipase is mediated by low density lipoprotein receptor-related protein and requires cell surface proteoglycans. J. Biol. Chem. 270, 9307–9312.
Kounnas, M. Z., Church, F. C., Argraves, W. S., and Strickland, D. K. (1996). Cellular internalization and degradation of antithrombin III-thrombin, heparin cofactor II-thrombin, and α1-antitrypsin- trypsin complexes is mediated by the low density lipoprotein receptor-related protein. J. Biol. Chem. 271, 6523–6529. doi: 10.1074/jbc.271.11.6523
Kounnas, M. Z., Henkin, J., Argraves, W. S., and Strickland, D. K. (1993). Low density lipoprotein receptor-related protein/alpha 2-macroglobulin receptor mediates cellular uptake of pro-urokinase. J. Biol. Chem. 268, 21862–21867.
Kounnas, M. Z., Moir, R. D., Rebeck, G. W., Bush, A. I., Argraves, W. S., Tanzi, R. E., et al. (1995a). LDL receptor-related protein, a multifunctional apoE receptor, binds secreted β -amyloid precursor protein and mediates its degradation. Cell 82, 331–340.
Kounnas, M. Z., Morris, R. E., Thompson, M. R., FitzGerald, D. J., Strickland, D. K., and Saelinger, C. B. (1992b). The alpha 2-macroglobulin receptor/low density lipoprotein receptor-related protein binds and internalizes Pseudomonas exotoxin A. J. Biol. Chem. 267, 12420–12423.
Kowal, R. C., Herz, J., Goldstein, J. L., Esser, V., and Brown, M. S. (1989). Low density lipoprotein receptor-related protein mediates uptake of cholesteryl esters derived from apoprotein E-enriched lipoproteins. Proc. Natl. Acad. Sci. U.S.A. 86, 5810–5814. doi: 10.1073/pnas.86.15.5810
Kozlova, N., Jensen, J., Chi, T., Samoylenko, A., and Kietzmann, T. (2015). PAI-1 modulates cell migration in a LRP1-dependent manner via β-catenin and ERK1/2. Thromb. Haemost. 113, 988–998. doi: 10.1160/TH14-08-0678
Kuiper, J., Otter, M., Rijken, D., and van Berkel, T. (1988). Characterization of the interaction in vivo of tissue-type plasminogen activator with liver cells. J. Biol. Chem. 263, 18220–18224.
Landowski, L. M., Pavez, M., Brown, L. S., Gasperini, R., Taylor, B. V., West, A. K., et al. (2016). Low-density lipoprotein receptor-related proteins in a novel mechanism of axon guidance and peripheral nerve regeneration. J. Biol. Chem. 291, 1092–1102. doi: 10.1074/jbc.M115.668996
Laudati, E., Gilder, A. S., Lam, M. S., Misasi, R., Sorice, M., Gonias, S. L., et al. (2016). The activities of LDL receptor-related protein-1 (LRP1) compartmentalize into distinct plasma membrane microdomains. Mol. Cell. Neurosci. 76, 42–51. doi: 10.1016/j.mcn.2016.08.006
Laurén, J., Gimbel, D. A., Nygaard, H. B., Gilbert, J. W., and Strittmatter, S. M. (2009). Cellular prion protein mediates impairment of synaptic plasticity by amyloid-β oligomers. Nature 457, 1128–1132. doi: 10.1038/nature07761
Lee, C. Y. D., and Landreth, G. E. (2010). The role of microglia in amyloid clearance from the AD brain. J. Neural Transm. 117, 949–960. doi: 10.1007/s00702-010-0433-4
Lee, S., Ko, H., Kwon, K., Lee, J., Han, S.-H., Han, D., et al. (2014). TPA regulates neurite outgrowth by phosphorylation of LRP5/6 in neural progenitor cells. Mol. Neurobiol. 49, 199–215. doi: 10.1007/s12035-013-8511-x
Lee, S. H., Suh, H. N., Lee, Y. J., Seo, B. N., Ha, J. W., and Han, H. J. (2012). Midkine prevented hypoxic injury of mouse embryonic stem cells through activation of Akt and HIV-1α via low-density lipoprotein receptor-related protein-1. J. Cell. Physiol. 227, 1731–1739. doi: 10.1002/jcp.22897
Lehti, K., Rose, N. F., Valavaara, S., Weiss, S. J., and Keski-Oja, J. (2009). MT1-MMP promotes vascular smooth muscle dedifferentiation through LRP1 processing. J. Cell Sci. 122, 126–135. doi: 10.1242/jcs.035279
Lemarchand, E., Maubert, E., Haelewyn, B., Ali, C., Rubio, M., and Vivien, D. (2016). Stressed neurons protect themselves by a tissue-type plasminogen activator-mediated EGFR-dependent mechanism. Cell Death Differ. 23, 123–131. doi: 10.1038/cdd.2015.76
Lensjø, K. K., Christensen, A. C., Tennøe, S., Fyhn, M., and Hafting, T. (2017). Differential expression and cell-type specificity of perineuronal nets in hippocampus, medial entorhinal cortex, and visual cortex examined in the rat and mouse. eNeuro 4:ENEURO.0379-16.2017. doi: 10.1523/ENEURO.0379-16.2017
Lenting, P. J., Neels, J. G., van den Berg, B. M., Clijsters, P. P., Meijerman, D. W., Pannekoek, H., et al. (1999). The light chain of factor VIII comprises a binding site for low density lipoprotein receptor-related protein. J. Biol. Chem. 274, 23734–23739. doi: 10.1074/jbc.274.34.23734
Lesept, F., Chevilley, A., Jezequel, J., Ladépêche, L., Macrez, R., Aimable, M., et al. (2016). Tissue-type plasminogen activator controls neuronal death by raising surface dynamics of extrasynaptic NMDA receptors. Cell Death Dis. 7:e2466. doi: 10.1038/cddis.2016.279
Li, S. S., Liu, Z., and Sundqvist, K.-G. (2006). Endogenous thrombospondin-1 is a cell-surface ligand for regulation of integrin-dependent T-lymphocyte adhesion. Blood 108, 3112–3120. doi: 10.1182/blood-2006-04-016832
Li, X., Herz, J., and Monard, D. (2006). Activation of ERK signaling upon alternative protease nexin-1 internalization mediated by syndecan-1. J. Cell. Biochem. 99, 936–951. doi: 10.1002/jcb.20881
Li, Y., Lu, W., Marzolo, M. P., and Bu, G. (2001). Differential functions of members of the low density lipoprotein receptor family suggested by their distinct endocytosis rates. J. Biol. Chem. 276, 18000–18006. doi: 10.1074/jbc.M101589200
Li, Y., Marzolo, M. P., van Kerkhof, P., Strous, G. J., and Bu, G. (2000). The YXXL motif, but not the two NPXY motifs, serves as the dominant endocytosis signal for low density lipoprotein receptor-related protein. J. Biol. Chem. 275, 17187–17194. doi: 10.1074/jbc.M000490200
Lillis, A., Mikhailenko, I., and Strickland, D. (2005). Beyond endocytosis: LRP function in cell migration, proliferation and vascular permeability. J. Thromb. Haemost. 3, 1884–1893. doi: 10.1111/j.1538-7836.2005.01371.x
Lillis, A., van Duyn, L., Murphy-Ullrich, J., and Strickland, D. (2008). LDL receptor-related protein 1: unique tissue-specific functions revealed by selective gene knockout studies. Physiol. Rev. 88, 887–918. doi: 10.1152/physrev.00033.2007
Lin, J., Mironova, Y. A., Shrager, P., and Giger, R. J. (2017). LRP1 regulates peroxisome biogenesis and cholesterol homeostasis in oligodendrocytes and is required for proper CNS myelin development and repair. eLife 6:e30498. doi: 10.7554/eLife.30498
Ling, T. Y., Chen, C. L., Huang, Y. H., Liu, I. H., Huang, S. S., and Huang, J. S. (2004). Identification and characterization of the acidic pH binding sites for growth regulatory ligands of low density lipoprotein receptor-related protein-1. J. Biol. Chem. 279, 38736–38748. doi: 10.1074/jbc.M310537200
Liu, C. C., Hu, J., Zhao, N., Wang, J., Wang, N., Cirrito, J. R., et al. (2017). Astrocytic LRP1 mediates brain Aβ clearance and impacts amyloid deposition. J. Neurosci. 37, 4023–4031. doi: 10.1523/JNEUROSCI.3442-16.2017
Liu, Q., Trotter, J., Zhang, J., Peters, M. M., Cheng, H., Bao, J., et al. (2010). Neuronal LRP1 knockout in adult mice leads to impaired brain lipid metabolism and progressive, age-dependent synapse loss and neurodegeneration. J. Neurosci. 30, 17068–17078. doi: 10.1523/JNEUROSCI.4067-10.2010
Liu, Q., Zerbinatti, C. V., Zhang, J., Hoe, H. S., Wang, B., Cole, S. L., et al. (2007). Amyloid precursor protein regulates brain apolipoprotein E and cholesterol metabolism through lipoprotein receptor LRP1. Neuron 56, 66–78. doi: 10.1016/j.neuron.2007.08.008
Liu, Q., Zhang, J., Zerbinatti, C., Zhan, Y., Kolber, B. J., Herz, J., et al. (2011). Lipoprotein receptor LRP1 regulates leptin signaling and energy homeostasis in the adult central nervous system. PLoS Biol. 9:e1000575. doi: 10.1371/journal.pbio.1000575
Liu, Y., Jones, M., Hingtgen, C. M., Bu, G., Laribee, N., Tanzi, R. E., et al. (2000). Uptake of HIV-1 tat protein mediated by low-density lipoprotein receptor-related protein disrupts the neuronal metabolic balance of the receptor ligands. Nat. Med. 6, 1380–1387. doi: 10.1038/82199
Lleo, A., Waldron, E., von Arnim, C. A., Herl, L., Tangredi, M. M., Peltan, I. D., et al. (2005). Low density lipoprotein receptor-related protein (LRP) interacts with presenilin 1 and is a competitive substrate of the amyloid precursor protein (APP) for gamma-secretase. J. Biol. Chem. 280, 27303–27309. doi: 10.1074/jbc.M413969200
Loukinova, E., Ranganathan, S., Kuznetsov, S., Gorlatova, N., Migliorini, M., Ulery, P. G., et al. (2002). Platelet-derived growth factor (PDGF)-induced tyrosine phosphorylation of the low density lipoprotein receptor-related protein (LRP). Evidence for integrated co-receptor function between LRP and the PDGF. J. Biol. Chem. 277, 15499–15506. doi: 10.1074/jbc.M200427200
Lu, Z., and Kipnis, J. (2010). Thrombospondin 1 - a key astrocyte-derived neurogenic factor. FASEB J. 24, 1925–1934. doi: 10.1096/fj.09-150573
Ma, Z., Thomas, K. S., Webb, D. J., Moravec, R., Salicioni, A. M., Mars, W. M., et al. (2002). Regulation of Rac1 activation by the low density lipoprotein receptor-related protein. J. Cell Biol. 159, 1061–1070. doi: 10.1083/jcb.200207070
Macrez, R., Bezin, L., Le Mauff, B., Ali, C., and Vivien, D. (2010). Functional occurrence of the interaction of tissue plasminogen activator with the NR1 subunit of N-methyl-D-aspartate receptors during stroke. Stroke 41, 2950–2955. doi: 10.1161/STROKEAHA.110.592360
Madani, R., Hulo, S., Toni, N., Madani, H., Steimer, T., Muller, D., et al. (1999). Enhanced hippocampal long-term potentiation and learning by increased neuronal expression of tissue-type plasminogen activator in transgenic mice. EMBO J. 18, 3007–3012. doi: 10.1093/emboj/18.11.3007
Mahley, R. W., and Huang, Y. (2007). Atherogenic remnant lipoproteins: role for proteoglycans in trapping, transferring, internalizing. J. Clin. Invest. 117, 94–98. doi: 10.1172/JCI30889
Makarova, A., Mikhailenko, I., Bugge, T. H., List, K., Lawrence, D. A., and Strickland, D. K. (2003). The low density lipoprotein receptor-related protein modulates protease activity in the brain by mediating the cellular internalization of both neuroserpin and neuroserpin-tissue-type plasminogen activator complexes. J. Biol. Chem. 278, 50250–50258. doi: 10.1074/jbc.M309150200
Mandrekar, S., Jiang, Q., Lee, C. Y., Koenigsknecht-Talboo, J., Holtzman, D. M., and Landreth, G. E. (2009). Microglia mediate the clearance of soluble Abeta through fluid phase macropinocytosis. J. Neurosci. 29, 4252–4262. doi: 10.1523/JNEUROSCI.5572-08.2009
Mantuano, E., Jo, M., Gonias, S. L., and Campana, W. M. (2010). Low density lipoprotein receptor-related protein (LRP1) regulates Rac1 and RhoA reciprocally to control Schwann cell adhesion and migration. J. Biol. Chem. 285, 14259–14266. doi: 10.1074/jbc.M109.085126
Mantuano, E., Lam, M., and Gonias, S. (2013). LRP1 assembles unique co-receptor systems to initiate cel signalling in response to tissue-type plasminogen activator and myelin-associated glycoprotein. J. Biol. Chem. 288, 34009–34018. doi: 10.1074/jbc.M113.509133
Mantuano, E., Lam, M., Shibayama, M., Campana, W., and Gonias, S. (2015). The NMDA receptor functions independently and as an LRP1 co-receptor to promote Schwann cell survival and migration. J. Cell Sci. 128, 3478–3488. doi: 10.1242/jcs.173765
Martin, A. M., Kuhlmann, C., Trossbach, S., Jaeger, S., Waldron, E., Roebroek, A., et al. (2008). The functional role of the second NPXY motif of the LRP1 β-chain in tissue-type plasminogen activator-mediated activation of N-methyl-D-aspartate receptors. J. Biol. Chem. 283, 12004–12013. doi: 10.1074/jbc.M707607200
Marzolo, M. P., von Bernhardi, R., Bu, G., and Inestrosa, N. C. (2000). Expression of alpha(2)- macroglobulin receptor/low density lipoprotein receptor-related protein (LRP) in rat microglial cells. J. Neurosci. Res. 60, 401–411. doi: 10.1002/(SICI)1097-4547(20000501)60:3<401::AID-JNR15>3.0.CO;2-L
May, P., Herz, J., and Bock, H. (2005). Molecular mechanisms of lipoprotein receptor signalling. Cell. Mol. Life Sci. 62, 2325–2338. doi: 10.1007/s00018-005-5231-z
May, P., Reddy, K., and Herz, J. (2002). Proteolytic processing of low density lipoprotein receptor-related protein mediates regulated release of its intracellular domain. J. Biol. Chem. 277, 18736–18743. doi: 10.1074/jbc.M201979200
May, P., Rohlmann, A., Bock, H. H., Zurhove, K., Marth, J. D., Schomburg, E. D., et al. (2004). Neuronal LRP1 functionally associates with postsynaptic proteins and is required for normal motor function in mice. Mol. Cell. Biol. 24, 8872–8883. doi: 10.1128/MCB.24.20.8872-8883.2004
McKeown-Longo, P. J., Hanning, R., and Mosher, D. F. (1984). Binding and degradation of platelet thrombospondin by cultured fibroblasts. J. Cell Biol. 98, 22–28. doi: 10.1083/jcb.98.1.22
Meijer, A., Rohlena, J., van der Zwaan, C., van Zonneveld, A., Boertjes, R., Lenting, P., et al. (2007). Functional duplication of ligand-binding domains within low-density lipoprotein receptor-related proten for interaction with receptor associated protein, alpha2-macroglobulin, factor IXa and factor VIII. Biochim. Biophys. Acta 1774, 714–722. doi: 10.1016/j.bbapap.2007.04.003
Meilinger, M., Gschwentner, C., Burger, I., Haumer, M., Wahrmann, M., Szollar, L., et al. (1999). Metabolism of activated complement component C3 is mediated by the low density lipoprotein receptor-related protein/alpha(2)-macroglobulin receptor. J. Biol. Chem. 274, 38091–38096. doi: 10.1074/jbc.274.53.38091
Meilinger, M., Haumer, M., Szakmary, K. A., Steinbock, F., Scheiber, B., Goldenberg, H., et al. (1995). Removal of lactoferrin from plasma is mediated by binding to low density lipoprotein receptor-related protein/α 2-macroglobulin receptor and transport to endosomes. FEBS Lett. 360, 70–74. doi: 10.1016/0014-5793(95)00082-K
Menezes, M. J., McClenahan, F. K., Leiton, C. V., Aranmolate, A., Shan, X., and Colognato, H. (2014). The extracellular matrix protein laminin α2 regulates the maturation and function of the blood-brain barrier. J. Neurosci. 34, 15260–15280. doi: 10.1523/JNEUROSCI.3678-13.2014
Meng, H., Zhang, X., Hankenson, K. D., and Wang, M. M. (2009). Thrombospondin 2 potentiates notch3/jagged1 signaling. J. Biol. Chem. 284, 7866–7874. doi: 10.1074/jbc.M803650200
Meng, H., Zhang, X., Lee, S. J., Strickland, D. K., Lawrence, D. A., and Wang, M. M. (2010). Low density lipoprotein receptor-related protein-1 (LRP-1) regulates thrombospondin-2 (TSP2) enhancement of Notch3 signaling. J. Biol. Chem. 285, 23047–23055. doi: 10.1074/jbc.M110.144634
Merle, B., Malaval, L., Lawler, J., Delmas, P., and Clezardin, P. (1997). Decorin inhibits cell attachment to thrombospondin-1 by binding to a KKTR-dependent cell adhesive site present within the N-terminal domain of thrombospondin-1. J. Cell. Biochem. 67, 75–83. doi: 10.1002/(SICI)1097-4644(19971001)67:1<75::AID-JCB8>3.0.CO;2-T
Mignatti, P., and Rifkin, D. B. (1993). Biology and biochemistry of proteinases in tumor invasion. Physiol. Rev. 73, 161–195. doi: 10.1152/physrev.1993.73.1.161
Mikhailenko, I., Kounnas, M. Z., and Strickland, D. K. (1995). Low density lipoprotein receptor-related protein/alpha 2-macroglobulin receptor mediates the cellular internalization and degradation of thrombospondin. A process facilitated by cell-surface proteoglycans. J. Biol. Chem. 270, 9543–9549. doi: 10.1074/jbc.270.16.9543
Mikhailenko, I., Krylov, D., Argraves, K. M., Roberts, D. D., Liau, G., and Strickland, D. K. (1997). Cellular internalization and degradation of thrombospondin-1 is mediated by the amino-terminal heparin binding domain (HBD): high affinity interaction of dimeric HBD with the low density lipoprotein receptor-related protein. J. Biol. Chem. 272, 6784–6791. doi: 10.1074/jbc.272.10.6784
Moestrup, S. K., Christensen, E. I., Sottrup-Jensen, L., and Gliemann, J. (1987). Binding and receptor-mediated endocytosis of pregnancy zone protein-proteinase complex in rat macrophages. Biochim. Biophys. Acta 930, 297–303. doi: 10.1016/0167-4889(87)90002-4
Moestrup, S. K., Cui, S., Vorum, H., Bregengard, C., Bjorn, S. E., Norris, K., et al. (1995). Evidence that epithelial glycoprotein 330/megalin mediates uptake of polybasic drugs. J. Clin. Invest. 96, 1404–1413. doi: 10.1172/JCI118176
Morikawa, S., Ikegaya, Y., Narita, M., and Tamura, H. (2017). Activation of perineuronal net-expressing excitatory neurons during associative memory encoding and retrieval. Sci. Rep. 7:46024. doi: 10.1038/srep46024
Muramatsu, H., Zou, K., Sakaguchi, N., Ikematsu, S., Sakuma, S., and Muramatsu, T. (2000). LDL-receptor-related protein as a component of the midkine receptor. Biochem. Biophys. Res. Commun. 270, 936–941. doi: 10.1006/bbrc.2000.2549
Muratoglu, S. C., Belgrave, S., Hampton, B., Migliorini, M., Coksaygan, T., Chen, L., et al. (2013). LRP1 protects the vasculature by regulating levels of connective tissue growth factor and HtrA1. Arterioscler. Thromb. Vasc. Biol. 33, 2137–2146. doi: 10.1161/ATVBAHA.113.301893
Muratoglu, S. C., Belgrave, S., Lillis, A. P., Migliorini, M., Robinson, S., Smith, E., et al. (2011). Macrophage LRP1 supresses neo-intima formation during vascular remodeling by modulating the TGF-β signaling pathway. PLoS One 6:e28846. doi: 10.1371/journal.pone.0028846
Murphy-Ullrich, J. E., Gurusiddappa, S., Frazier, W. A., and Hook, M. (1993). Heparin-binding peptides from thrombospondins 1 and 2 contain focal adhesion-labilizing activity. J. Biol. Chem. 268, 26784–26789.
Nakajima, C., Haffner, P., Goerke, S. M., Zurhove, K., Adelmann, G., Frotscher, M., et al. (2014). The lipoprotein receptor LRP1 modulates sphingosine-1-phosphate signaling and is essential for vascular development. Development 141, 4513–4525. doi: 10.1242/dev.109124
Nakajima, C., Kulik, A., Frotscher, M., Herz, J., Schäfer, M., Bock, H. H., et al. (2013). Low density lipoprotein receptor-related protein 1 (LRP1) modulates N-methyl-D-aspartate (n.d.) receptor-dependent intracellular signaling and NMDA-induced regulation of postsynaptic protein complexes. J. Biol. Chem. 288, 21909–21923. doi: 10.1074/jbc.M112.444364
Nazer, B., Hong, S., and Selkoe, D. J. (2008). LRP promotes endocytosis and degradation, but not transcytosis, of the amyloid-β peptide in a blood-brain barrier in vitro model. Neurobiol. Dis. 30, 94–102. doi: 10.1016/j.nbd.2007.12.005
Neels, J., van den Berg, B., Lookene, A., Olivecrona, G., Pannekoek, H., and van Zonneveld, A. (1999). The second and fourth cluster of class A cysteine-rich repeats of the low density lipoprotein receptor-related protein share ligand-binding properties. J. Biol. Chem. 274, 31305–31311. doi: 10.1074/jbc.274.44.31305
Ng, K.-S., Leung, H.-W., Wong, P. T.-H., and Low, C.-M. (2012). Cleavage of the NR2B subunit amino terminus of N-methyl-D-aspartate (n.d.) receptor by tissue plasminogen activator: identification of the cleavage site and characterization of ifenprodil and glycine affinities on truncated NMDA receptor. J. Biol. Chem. 287, 25520–25529. doi: 10.1074/jbc.M112.374397
Nicole, O., Docagne, F., Ali, C., Margaill, I., Carmeliet, P., MacKenzie, E. T., et al. (2001). The proteolytic activity of tissue-plasminogen activator enhances NMDA receptor-mediated signaling. Nat. Med. 7, 59–64. doi: 10.1038/83358
Niego, B., Freeman, R., Puschmann, T. B., Turnley, A. M., and Medcalf, R. L. (2012). T-PA-specific modulation of a human blood-brain barrier model involves plasmin-mediated activation of the Rho kinase pathway in astrocytes. Blood 119, 4752–4761. doi: 10.1182/blood-2011-07-369512
Nykjaer, A., Conese, M., Christensen, E. I., Olson, D., Cremona, O., Gliemann, J., et al. (1997). Recycling of the urokinase receptor upon internalization of the uPA:serpin complexes. EMBO J. 16, 2610–2620. doi: 10.1093/emboj/16.10.2610
Nykjaer, A., Kjoller, I., Cohen, R. I., Lawrence, D. A., Garni-Wagner, B. A., Todd, R. F., et al. (1994). Regions involved in binding of urokinase-type-1 inhibitor complex and pro-urokinase to the endocytic alpha 2-macroglobulin receptor/low density lipoprotein receptor-related protein. Evidence that the urokinase receptor protects pro-urokinase against binding to the endocytic receptor. J. Biol. Chem. 269, 25668–25676.
Nykjaer, A., Petersen, C. M., Moller, B., Jensen, P. H., Moestrup, S. K., Holtet, T. L., et al. (1992). Purified alpha 2- macroglobulin receptor/LDL receptor-related protein binds urokinase plasminogen activator inhibitor type-1 complex. Evidence that the alpha 2-macroglobulin receptor mediates cellular degradation of urokinase receptor-bound complexes. J. Biol. Chem. 267, 14543–14546.
Obiang, P., Macrez, R., Jullienne, A., Bertrand, T., Lesept, F., Ali, C., et al. (2012). GluN2D subunit-containing NMDA receptors control tissue plasminogen activator-mediated spatial memory. J. Neurosci. 32, 12726–12734. doi: 10.1523/JNEUROSCI.6202-11.2012
Okada, S. S., Grobmyer, S. R., and Barnathan, E. S. (1996). Contrasting effects of plasminogen activators, urokinase receptor, LDL receptor-related protein on smooth muscle cell migration and invasion. Arterioscler. Thromb. Vasc. Biol. 16, 1269–1276. doi: 10.1161/01.ATV.16.10.1269
Orr, A. W., Elzie, C. A., Kucik, D. F., and Murphy-Ullrich, J. E. (2003a). Thrombospondin signaling through the calreticulin/LDL receptor-related protein co-complex stimulates random and directed cell migration. J. Cell Sci. 116, 2917–2927.
Orr, A. W., Pedraza, C. E., Pallero, M. A., Elzie, C. A., Goicoechea, S., Strickland, D. K., et al. (2003b). Low density lipoprotein receptor-related protein is a calreticulin coreceptor that signals focal adhesion disassembly. J. Cell Biol. 161, 1179–1189.
Orr, A. W., Pallero, M. A., and Murphy-Ullrich, J. E. (2002). Thrombospondin stimulates focal adhesion disassembly through Gi- and phosphoinositide 3-kinase-dependent ERK activation. J. Biol. Chem. 277, 20453–20460. doi: 10.1074/jbc.M112091200
Orr, A. W., Pallero, M. A., Xiong, W. C., and Murphy-Ullrich, J. E. (2004). Thrombospondin induces RhoA inactivation through FAK-dependent signaling to stimulate focal adhesion disassembly. J. Biol. Chem. 279, 48983–48992. doi: 10.1074/jbc.M404881200
Orth, K., Madison, E. L., Gething, M. J., Sambrook, J. F., and Herz, J. (1992). Complexes of tissue-type plasminogen activator and its serpin inhibitor plasminogen-activator inhibitor type 1 are internalized by means of the low density lipoprotein receptor-related protein/alpha 2-macroglobulin receptor. Proc. Natl. Acad. Sci. U.S.A. 89, 7422–7426. doi: 10.1073/pnas.89.16.7422
Pan, L., North, H. A., Sahni, V., Jeong, S. J., Mcguire, T. L., Berns, E. J., et al. (2014). β1-integrin and integrin linked kinase regulate astroctic differentiation of neural stem cells. PLoS One 9:e104335. doi: 10.1371/journal.pone.0104335
Pan, L., Zhao, Y., Zhijie, Y., and Qin, G. (2016). Research advances on structure and biological functions of integrins. Springerplus 5:1094. doi: 10.1186/s40064-016-2502-0
Pang, P. T., and Lu, B. (2004). Regulation of late-phase LTP and long-term memory in normal and aging hippocampus: role of secreted proteins tPA and BDNF. Ageing Res. Rev. 3, 407–430. doi: 10.1016/j.arr.2004.07.002
Pang, P. T., Teng, H. K., Zaitsev, E., Woo, N. T., Sakata, K., Zhen, S., et al. (2004). Cleavage of proBDNF by tPA/plasmin is essential for long-term hippocampal plasticity. Science 306, 487–491. doi: 10.1126/science.1100135
Parcq, J., Bertrand, T., Montagne, A., Baron, A. F., Macrez, R., Billard, J. M., et al. (2012). Unveiling an exceptional zymogen: the single-chain form of tPA is a selective activator of NMDA receptor-dependent signaling and neurotoxicity. Cell Death Differ. 19, 1983–1991. doi: 10.1038/cdd.2012.86
Pasquet, N., Douceau, S., Naveau, M., Lesept, F., Louessard, M., Lebouvier, L., et al. (2018). Tissue-type plasminogen activator controlled corticogenesis through a mechanism dependent of NMDA receptors expressed on radial glial cells. Cereb. Cortex 119, 1–17. doi: 10.1093/cercor/bhy119
Pawlak, R., Melchor, J., Matys, T., Skrzypiec, A., and Strickland, S. (2005). Ethanol-withdrawal seizures are controlled by tissue plasminogen activator via modulation of NR2B-containing NMDA receptors. Proc. Natl. Acad. Sci. U.S.A. 102, 443–448. doi: 10.1073/pnas.0406454102
Pflanzner, T., Janko, M. C., André-Dohmen, B., Reuss, S., Weggen, S., Roebroek, A. J., et al. (2011). LRP1 mediates bidirectional transcytosis of amyloid-β across the blood-brain barrier. Neurobiol. Aging 32, 2323.e1–2323.e11. doi: 10.1016/j.neurobiolaging.2010.05.025
Pi, X., Schmitt, C. E., Xie, L., Portbury, A. L., Wu, Y., Lockyer, P., et al. (2012). LRP1-dependent endocytic mechanism governs the signaling output of the bmp system in endothelial cells and in angiogenesis. Circ. Res. 111, 564–574. doi: 10.1161/CIRCRESAHA.112.274597
Pietrzik, C., Busse, T., Merriam, D., Weggen, S., and Koo, E. (2002). The cytoplasmic domain of the LDL receptor-related protein regulates multiple steps in APP processing. EMBO J. 21, 5691–5700. doi: 10.1093/emboj/cdf568
Pietrzik, C., Yoon, I., Jaeger, S., Busse, T., Weggen, S., and Koo, E. (2004). FE65 constitutes the functional link between the low-density lipoprotein receptor-related protein and the amyloid precursor protein. J. Neurosci. 24, 4259–4265. doi: 10.1523/JNEUROSCI.5451-03.2004
Pitkänen, A., Ndode-Ekane, X. E., Łukasiuk, K., Wilczynski, G. M., Dityatev, A., Walker, M. C., et al. (2014). Neural ECM and epilepsy. Prog. Brain Res. 214, 229–262. doi: 10.1016/B978-0-444-63486-3.00011-6
Plump, A. S., Smith, J. D., Hayek, T., Aalto-Setäla, K., Walsh, A., Verstuyft, J. G., et al. (1992). Severe hypercholesterolemia and atherosclerosis in apolipoprotein E-deficient mice created by homologous recombination in ES cells. Cell 71, 343–353. doi: 10.1016/0092-8674(92)90362-G
Polavarapu, R., Gongora, M. C., Yi, H., Ranganthan, S., Lawrence, D. A., Strickland, D., et al. (2007). Tissue-type plasminogen activator-mediated shedding of astrocytic low-density lipoprotein receptor-related protein increases the permeability of the neurovascular unit. Blood 109, 3270–3278. doi: 10.1182/blood-2006-08-043125
Poller, W., Willnow, T. E., Hilpert, J., and Herz, J. (1995). Differential recognition of alpha 1-antitrypsin-elastase and alpha 1-antichymotrypsin-cathepsin G complexes by the low density lipoprotein receptor-related protein. J. Biol. Chem. 270, 2841–2845. doi: 10.1074/jbc.270.6.2841
Pyka, M., Wetzel, C., Aguado, A., Geissler, M., Hatt, H., and Faissner, A. (2011). Chondroitin sulfate proteoglycans regulate astrocyte-dependent synaptogenesis and modulate synaptic activity in primary embryonic hippocampal neurons. Eur. J. Neurosci. 33, 2187–2202. doi: 10.1111/j.1460-9568.2011.07690.x
Qian, Z., Gilbert, M. E., Colicos, M. A., Kandel, E. R., and Kuhl, D. (1993). Tissue-plasminogen activator is induced as an immediate-early gene during seizure, kindling and long-term potentiation. Nature 361, 453–457. doi: 10.1038/361453a0
Qiu, Z., Hyman, B. T., and Rebeck, G. W. (2004). Apolipoprotein E receptors mediate neurite outgrowth through activation of p44/42 mitogen-activated protein kinase in primary neurons. J. Biol. Chem. 279, 34948–34956. doi: 10.1074/jbc.M401055200
Qiu, Z., Strickland, D., Hyman, B., and Rebeck, G. (2002). Alpha 2-macroglobulin exposure reduces calcium responses to N -methyl-D-aspartate via low density lipoprotein receptor-related protein in cultured hippocampal neurons. J. Biol. Chem. 277, 14458–14466. doi: 10.1074/jbc.M112066200
Qiu, Z., Strickland, D. K., Hyman, B. T., and Rebeck, G. W. (1999). Alpha2-macroglogulin enhances the clearance of endogeneous soluble beta-amyloid-peptide via low-density lipoprotein receptor-related protein in cortical neurons. J. Neurochem. 73, 1393–1398. doi: 10.1046/j.1471-4159.1999.0731393.x
Rabiej, V., Pflanzner, T., Wagner, T., Goetze, K., Storck, S., Eble, J., et al. (2015). Low density lipoprotein receptor-related protein 1 mediated endocytosis of β1-integrin influences cell adhesion and cell migration. Exp. Cell Res. 340, 102–115. doi: 10.1016/j.yexcr.2015.11.020
Ramanathan, A., Nelson, A. R., Sagare, A. P., and Zlokovic, B. V. (2015). Impaired vascular-mediated clearance of brain amyloid beta in Alzheimer’s disease: the role, regulation and restoration of LRP1. Front. Aging Neurosci. 7:136. doi: 10.3389/fnagi.2015.00136
Rastegarlari, G., Pegon, J. N., Casari, C., Odouard, S., Navarrete, A. M., Saint-Lu, N., et al. (2012). Macrophage LRP1 contributes to the clearance of von Willebrand factor. Blood 119, 2126–2134. doi: 10.1182/blood-2011-08-373605
Rebeck, G. W., Harr, S. D., Strickland, D. K., and Hyman, B. T. (1995). Multiple, diverse senile plaque-associated proteins are ligands of an apolipoproteinE receptor, the alpha 2-macroglobulin receptor/low-density-lipoprotein receptor related protein. Ann. Neurol. 37, 211–217. doi: 10.1002/ana.410370212
Reekmans, S. M., Pflanzner, T., Gordts, P. L., Isbert, S., Zimmermann, P., Annaert, W., et al. (2010). Inactivation of the proximal NPXY motif impairs early steps in LRP1 biosynthesis. Cell. Mol. Life Sci. 67, 135–145. doi: 10.1007/s00018-009-0171-7
Ribeiro, A., Balasubramanian, S., Hughes, D., Vargo, S., Powell, E. M., and Leach, J. B. (2013). β1-Integrin cytoskeletal signaling regulates sensory neuron response to matrix dimensionality. Neuroscience 248, 67–78. doi: 10.1016/j.neuroscience.2013.05.057
Robel, S., Buckingham, S. C., Boni, J. L., Campbell, S. L., Danbolt, N. C., Riedemann, T., et al. (2015). Reactive astrogliosis causes the development of spontaneous seizures. J. Neurosci. 35, 3330–3345. doi: 10.1523/JNEUROSCI.1574-14.2015
Robel, S., Mori, T., Zoubaa, S., Schlegel, J., Sirko, S., Faissner, A., et al. (2009). Conditional deletion of β1-integrin in astroglia causes partial reactive gliosis. Glia 57, 1630–1647. doi: 10.1002/glia.20876
Robinson, S. D., Lee, T. W., Christie, D. L., and Birch, N. P. (2015). Tissue plasminogen activator inhibits NMDA-receptor-mediated increases in calcium levels in cultured hippocampal neurons. Front. Cell. Neurosci. 9:404. doi: 10.3389/fncel.2015.00404
Roebroek, A. J., Reekmans, S., Lauwers, A., Feyaerts, N., Smeijers, L., and Hartmann, D. (2006). Mutant Lrp1 knock-in mice generated by recombinase- mediated cassette exchange reveal differential importance of the NPXY motifs in the intracellular domain of LRP1 for normal fetal development. Mol. Cell. Biol. 26, 605–616. doi: 10.1128/MCB.26.2.605-616.2006
Rohlmann, A., Gotthardt, M., Hammer, R. E., and Herz, J. (1998). Inducible inactivation of hepatic LRP gene by cre-mediated recombination confirms role of LRP in clearance of chylomicron remnants. J. Clin. Invest. 101, 689–695. doi: 10.1172/JCI1240
Rozanov, D. V., Hahn-Dantona, E., Strickland, D. K., and Strongin, A. Y. (2004). The low-density lipoprotein receptor-related protein LRP is regulated by membrane type-1 matrix metalloproteinase (MT1-MMP) proteolysis in malignant cells. J. Biol. Chem. 279, 4260–4268. doi: 10.1074/jbc.M311569200
Rushworth, J. V., Griffiths, H. H., Watt, N. T., and Hooper, N. M. (2013). Prion protein-mediated toxicity of amyloid-β oligomers requires lipid rafts and the transmembrane LRP1. J. Biol. Chem. 288, 8935–8951. doi: 10.1074/jbc.M112.400358
Sabeh, F., Ota, I., Holmbeck, K., Birkedal-Hansen, H., Soloway, P., Balbin, M., et al. (2004). Tumor cell traffic through the extracellular matrix is controlled by the membrane-anchored collagenase MT1-MMP. J. Cel Biol. 167, 769–781. doi: 10.1083/jcb.200408028
Sabeh, F., Shimizu-Hirota, R., and Weiss, S. J. (2009). Protease-dependent versus -independent cancer cell invasion programs: three-dimensional amoeboid movement revisited. J. Cell Biol. 185, 11–19. doi: 10.1083/jcb.200807195
Saenko, E. L., Yakhyaev, A. V., Mikhailenko, I., Strickland, D. K., and Sarafanov, A. G. (1999). Role of the low density lipoprotein-related protein receptor inmediation of factor VIII catabolism. J. Biol. Chem. 274, 37685–37692. doi: 10.1074/jbc.274.53.37685
Safina, D., Schlitt, F., Romeo, R., Pflanzner, T., Pietrzik, C. U., Narayanaswami, V., et al. (2016). Low-density lipoprotein receptor-related protein 1 is a novel modulator of radial glia stem cell proliferation, survival, and differentiation. Glia 64, 1363–1380. doi: 10.1002/glia.23009
Sagare, A., Deane, R., Bell, R. D., Johnson, B., Hamm, K., Pendu, R., et al. (2007). Clearance of amyloid-beta by circulating lipoprotein receptors. Nat. Med. 13, 1029–1031. doi: 10.1038/nm1635
Sagare, A. P., Bell, R. D., Zhao, Z., Ma, Q., Winkler, E. A., Ramanathan, A., et al. (2013). Pericyte loss influences Alzheimer-like neurodegeneration in mice. Nat. Commun. 4:2932. doi: 10.1038/ncomms3932
Sakimura, K., Kutsuwada, T., Ito, I., Manabe, T., Takayama, C., Kushiya, E., et al. (1995). Reduced hippocampal LTP and spatial learning in mice lacking NMDA receptor epsilon 1 subunit. Nature 373, 151–155. doi: 10.1038/373151a0
Salicioni, A., Gaultier, A., Brownlee, C., Cheezum, M., and Gonias, S. (2004). Low density lipoprotein receptor-related protein-1 promotes beta1 integrin maturation and transport to the cell surface. J. Biol. Chem. 279, 10005–10012. doi: 10.1074/jbc.M306625200
Salicioni, A. M., Mizelle, K. S., Loukinova, E., Mikhailenko, I., Strickland, D. K., and Gonias, S. L. (2002). The low density lipoprotein receptor-related protein mediates fibronectin catabolism and inhibits fibronectin accumulation on cell surfaces. J. Biol. Chem. 277, 16160–16166. doi: 10.1074/jbc.M201401200
Salza, R., Lethias, C., and Ricard-Blum, S. (2017). The multimerization state of the amyloid-β42 (Aβ 42) peptide governs its interaction network with the extracellular matrix. J. Alzheimers Dis. 56, 991–1005. doi: 10.3233/JAD-160751
Sanchez, M. C., Chiabrando, G. A., and Vides, M. A. (2001). Pregnancy zone protein-tissue-type plasminogen activator complexes bind to low-density lipoprotein receptor-related protein (LRP). Arch. Biochem. Biophys. 389, 218–222. doi: 10.1006/abbi.2001.2329
Sappino, A.-P., Madani, R., Huarte, J., Belin, D., Kiss, J. Z., Wohlwend, A., et al. (1993). Extracellular proteolysis in the adult murine brain. J. Clin. Invest. 92, 679–685. doi: 10.1172/JCI116637
Sarafanov, A. G., Ananyeva, N. M., Shima, M., and Saenko, E. L. (2001). Cell surface heparan sulfate proteoglycans participate in factor VIII catabolism mediated by low density lipoprotein receptor-related protein. J. Biol. Chem. 276, 11970–11979. doi: 10.1074/jbc.M008046200
Schorch, B., Song, S., van Diemen, F. R., Bock, H. H., May, P., Herz, J., et al. (2014). LRP1 is a receptor for Clostridium perfringens TpeL toxin indicating a two-receptor model of clostridial glycosylating toxins. Proc Natl. Acad. Sci. U.S.A. 111, 6431–6436. doi: 10.1073/pnas.1323790111
Schouwey, K., Delmas, V., Larue, L., Zimber-Strobl, U., Strobl, L. J., Radtke, F., et al. (2007). Notch1 and Notch2 receptors influence progressive hair graying in a dose-dependent manner. Dev. Dyn. 236, 282–289. doi: 10.1002/dvdy.21000
Scilabra, S. D., Troeberg, L., Yamamoto, K., Emonard, H., Thøgersen, I., Enghild, J. J., et al. (2013). Differential regulation of extracellular tissue inhibitor of metalloproteinases-3 levels by cell membrane-bound and shed low density lipoprotein receptor-related protein 1. J. Biol. Chem. 288, 332–342. doi: 10.1074/jbc.M112.393322
Scilabra, S. D., Yamamoto, K., Pigoni, M., Sakamoto, K., Müller, S. A., Papadopoulou, A., et al. (2017). Dissecting the interaction between tissue inhibitor of metalloproteinases-3 (TIMP-3) and low density lipoprotein receptor-related protein-1 (LRP-1): development of a “TRAP” to increase levels of TIMP-3 in the tissue. Matrix Biol. 59, 69–79. doi: 10.1016/j.matbio.2016.07.004
Seeds, N. W., Basham, M., and Haffke, S. (1999). Neuronal migration is retarded in mice lacking the tissue plasminogen activator gene. Proc. Natl. Acad. Sci. U.S.A. 96, 14118–14123. doi: 10.1073/pnas.96.24.14118
Seeds, N. W., Friedman, G., Hayden, S. H., Thewke, D., Haffke, S., McGuire, P., et al. (1996). Plasminogen activators and their interaction with the extracellular matrix in neural development, plasticity and regeneration. Semin. Neurosci. 8, 405–412. doi: 10.1006/smns.1996.0049
Seeds, N. W., Williams, B. L., and Bickford, P. C. (1995). Tissue plasminogen activator induction in Purkinje neurons after cerebellar motor learning. Science 270, 1992–1994. doi: 10.1126/science.270.5244.1992
Segarini, P. R., Nesbitt, J. E., Li, D., Hays, L. G., Yates, J. R. III, and Carmichael, D. F. (2001). The low density lipoprotein receptor-related protein/alpha 2-macroglobulin receptor is a receptor for connective tissue growth factor. J. Biol. Chem. 276, 40659–40667. doi: 10.1074/jbc.M105180200
Selkoe, D. J. (2000). Toward a comprehensive theory for Alzheimer’s disease. Hypothesis: alzheimer’s disease is caused by the cerebral accumulation and cytotoxicity of amyloid beta-protein. Ann. N.Y. Acad. Sci. 924, 17–25. doi: 10.1111/j.1749-6632.2000.tb05554.x
Shakibaei, M., and Frevert, U. (1996). Dual interaction of the malaria circumsporozoite protein with the low density lipoprotein receptor-related protein (LRP) and heparan sulfate proteoglycans. J. Exp. Med. 184, 1699–1711. doi: 10.1084/jem.184.5.1699
Shibata, M., Yamada, S., Kumar, S. R., Calero, M., Bading, J., Frangione, B., et al. (2000). Clearance of Alzheimer’s amyloid-β1-40 peptide from brain by LDL receptor-related protein-1 at the blood-brain barrier. J. Clin. Invest. 106, 1489–1499. doi: 10.1172/JCI10498
Shinohara, M., Tachibana, M., Kanekiyo, T., and Bu, G. (2017). Role of LRP1 in the pathogenesis of Alzheimer’s disease: evidence from clinical and preclinical studies. J. Lipid Res. 58, 1267–1281. doi: 10.1194/jlr.R075796
Simons, K., and Toomre, D. (2000). Lipid rafts and signal transduction. Nat. Rev. Mol. Cell Biol. 1, 31–39. doi: 10.1038/35036052
Song, I., and Dityatev, A. (2018). Crosstalk between glia, extracellular matrix and neurons. Brian Res. Bull. 136, 101–108. doi: 10.1016/j.brainresbull.2017.03.003
Spijkers, P. P., Denis, C. V., Blom, A. M., and Lenting, P. J. (2008). Cellular uptake of C4b-binding protein is mediated by heparan sulfate proteoglycans and CD91/LDL receptor-related protein. Eur. J. Immunol. 38, 809–817. doi: 10.1002/eji.200737722
Spijkers, P. P. E. M., Costa Martins, P., Westein, E., Gahmberg, C. G., Zwaginga, J. J., and Lenting, P. J. (2005). LDL-receptor-related protein regulates β 2-integrin-mediated leukocyte adhesion. Blood 105, 170–177. doi: 10.1182/blood-2004-02-0498
Spires-Jones, T. L., and Hyman, B. T. (2014). The intersection of amyloid beta and tau at synapses in Alzheimer’s disease. Neuron 82, 756–771. doi: 10.1016/j.neuron.2014.05.004
Staniszewska, I., Zaveri, S., Del Valle, L., Oliva, I., Rothman, V. L., Croul, S. E., et al. (2007). Interaction of alpha9beta1 integrin with thrombospondin-1 promotes angiogenesis. Circ. Res. 100, 1308–1316. doi: 10.1161/01.RES.0000266662.98355.66
Stefanitsch, C., Lawrence, A.-L., Olverling, A., Nilsson, I., and Fredriksson, L. (2015). tPA deficiency in mice leads to rearrangement in the cerebrovascular tree and cerebroventricular malformations. Front. Cell. Neurosci. 9:456. doi: 10.3389/fncel.2015.00456
Stefansson, S., Lawrence, D. A., and Argraves, W. S. (1996). Plasminogen activator inhibitor-1 and vitronectin promote the cellular clearance of thrombin by low density lipoprotein receptor-related proteins 1 and 2. J. Biol. Chem. 271, 8215–8220. doi: 10.1074/jbc.271.14.8215
Stefansson, S., Muhammad, S., Cheng, X. F., Battey, F. D., Strickland, D. K., and Lawrence, D. A. (1998). Plasminogen activator inhibitor-1 contains a cryptic high affinity binding site for the low density lipoprotein receptor-related protein. J. Biol. Chem. 273, 6358–6366. doi: 10.1074/jbc.273.11.6358
Stiles, T. L., Dickendesher, T. L., Gaultier, A., Fernandez-Castaneda, A., Mantuano, E., Giger, R. J., et al. (2013). LDL receptor-related protein-1 is a sialic-acid-independent receptor for myelin-associated glycoprotein that functions in neurite outgrowth inhibition by MAG and CNS myelin. J. Cell Sci. 126, 209–220. doi: 10.1242/jcs.113191
Storm, D., Herz, J., Trinder, P., and Loos, M. (1997). C1 inhibitor-C1s complexes are internalized and degraded by the low density lipoprotein receptor-related protein. J. Biol. Chem. 272, 31043–31050. doi: 10.1074/jbc.272.49.31043
Strickland, D. K., Ashcom, J. D., Williams, S., Burgess, W. H., Migliorini, M., and Argraves, W. S. (1990). Sequence identity between the alpha 2-macroglobulin receptor and low density lipoprotein receptor-related protein suggests that this molecule is a multifunctional receptor. J. Biol. Chem. 265, 17401–17404.
Strickland, D. K., Au, D. T., Cunfer, P., and Muratoglu, S. C. (2014). Low-density lipoprotein receptor-related protein-1: Role in the regulation of vascular integrity. Arterioscler. Thromb. Vasc. Biol. 34, 487–498. doi: 10.1161/ATVBAHA.113.301924
Strickland, D. K., Gonias, S. L., and Agraves, W. S. (2002). Diverse roles for the LDL receptor family. Trends Endocrinol. Metab. 13, 66–74. doi: 10.1016/S1043-2760(01)00526-4
Su, E. J., Fredriksson, L., Geyer, M., Folestad, E., Cale, J., Andrae, J., et al. (2008). Activation of PDGF-CC by tissue plasminogen activator impairs blood-brain barrier integrity during ischemic stroke. Nat. Med. 14, 731–737. doi: 10.1038/nm1787
Subramanian, M., Hayes, C. D., Thome, J. J., Thorp, E., Matsushima, G. K., Herz, J., et al. (2014). An AXL/LRP-1/RANBP9 complex mediates DC efferocytosis and antigen cross-presentation in vivo. J. Clin. Invest. 124, 1296–1308. doi: 10.1172/JCI72051
Sunyach, C., Jen, A., Deng, J., Fitzgerald, K. T., Frobert, Y., Grassi, J., et al. (2003). The mechanism of internalization of glycosylphosphatidylinositol-anchored prion protein. EMBO J. 22, 3591–3601. doi: 10.1093/emboj/cdg344
Swertfeger, D. K., Bu, G., and Hui, D. Y. (2002). Low density lipoprotein receptor-related protein mediates apolipoprotein E inhibition of smooth muscle cell migration. J. Biol. Chem. 277, 4141–4146. doi: 10.1074/jbc.M109124200
Takayama, Y., May, P., Anderson, R. G., and Herz, J. (2005). Low density lipoprotein receptor-related protein 1 (LRP1) controls endocytosis and c-CBL- mediated ubiquitination of the platelet-derived growth factor receptor beta (PDGFR beta). J. Biol. Chem. 280, 18504–18510. doi: 10.1074/jbc.M410265200
Talme, T., Bergdahl, E., and Sundqvist, K.-G. (2013). Regulation of T-lymphocyte motility, adhesion and de-adhesion by a cell surface mechanism directed by low density lipoprotein receptor-related protein 1 and endogenous thrombospondin-1. Immunology 142, 176–192. doi: 10.1111/imm.12229
Tan, M.-L., Ng, A., Pandher, P. S., Sashindranath, M., Hamilton, J. A., Davis, S. M., et al. (2012). Tissue plasminogen activator does not alter development of acquired epilepsy. Epilepsia 53, 1998–2004. doi: 10.1111/j.1528-1167.2012.03635.x
Taylor, D. R., and Hooper, N. M. (2007). The low-density lipoprotein receptor-related protein 1 (LRP1) mediates the endocytosis of the cellular prion protein. Biochem. J. 402, 17–23. doi: 10.1042/BJ20061736
Theret, L., Jeanne, A., Langlois, B., Hachet, C., David, M., Khrestchatisky, M., et al. (2017). Identification of LRP-1 as an endocytosis and recycling receptor for β1-integrin in thyroid cancer cells. Oncotarget 8, 78614–78632. doi: 10.18632/oncotarget.20201
Thevenard, J., Verzeaux, L., Devy, J., Etique, N., Jeanne, A., Schneider, C., et al. (2014). Low-density lipoprotein receptor-related protein-1 mediates endocytic clearance of tissue inhibitor of metalloproteinases-1 and promotes its cytokine-like activities. PLoS One 9:e103839. doi: 10.1371/journal.pone.0103839
Trommsdorff, M., Borg, J., Margolis, B., and Herz, J. (1998). Interaction of cytosolic adaptor proteins with neuronal apolipoprotein E receptors and the amyloid precursor protein. J. Biol. Chem. 273, 33556–33560. doi: 10.1074/jbc.273.50.33556
Trotter, J. H., Lussier, A. L., Psilos, K. E., Mahoney, H. L., Sponaugle, A. E., Hoe, H.-S., et al. (2014). Extracellular proteolysis of reelin by tissue plasminogen activator following synaptic potentiation. Neuroscience 274, 299–307. doi: 10.1016/j.neuroscience.2014.05.046
Tsen, F., Bhatia, A., O’Brien, K., Cheng, C.-F., Chen, M., Hay, N., et al. (2013). Extracellular heat shock protein 90 signals through subdomain II and the NPVY motif of LRP-1 receptor to Akt1 and Akt2: a circuit essential for promoting skin cell migration in vitro and wound healing in vivo. Mol. Cell. Biol. 33, 4947–4959. doi: 10.1128/MCB.00559-13
Tsirka, S., Gualandris, A., Amaral, D., and Strickland, S. (1997). Excitotoxin-induced neuronal degeneration and seizure are mediated by tissue plasminogen activator. Nature 377, 340–344. doi: 10.1038/377340a0
Turner, P. M., and Lorand, L. (1989). Complexation of fibronectin with tissue transglutaminase. Biochemistry 28, 628–635. doi: 10.1021/bi00428a032
Ulery, P. G., Beers, J., Mikhailenko, I., Tanzi, R. E., Rebeck, G. W., Hyman, B. T., et al. (2000). Modulation of beta-amyloid precursor protein processing by the low density lipoprotein receptor-related protein (LRP). Evidence that LRP contributes to the pathogenesis of Alzheimer’s disease. J. Biol. Chem. 275, 7410–7415. doi: 10.1074/jbc.275.10.7410
Vaillant, C., Michos, O., Orolicki, S., Brellier, F., Taieb, S., Moreno, E., et al. (2007). Protease nexin 1 and its receptor LRP modulate SHH signalling during cerebellar development. Development 134, 1745–1754. doi: 10.1242/dev.02840
Van Gool, B., Dedieu, S., Emonard, H., and Roebroek, A. J. (2015). The matricellular receptor LRP1 forms and interface for signaling and endocytosis in modulation of the extracellular tumor environment. Front. Pharmacol. 6:271. doi: 10.3389/fphar.2015.00271
van Horssen, J., Wesseling, P., van den Heuvel, L. P., de Waal, R. M., and Verbeek, M. M. (2003). Heparan sulfate proteoglycans in Alzheimer’s disease and amyloid-related disorders. Lancet Neurol. 2, 482–492. doi: 10.1016/S1474-4422(03)00484-8
Venkatesan, C., Birch, D., Peng, C. Y., and Kessler, J. A. (2015). Astrocytic β1-integrin affects cellular composition of murine blood brain barrier in the cerebral cortex. Int. J. Dev. Neurosci. 44, 48–54. doi: 10.1016/j.ijdevneu.2015.05.005
Vierkotten, S., Muether, P. S., and Fauser, S. (2011). Overexpression of HTRA1 leads to ultrastructural changes in the elastic layer of Bruch’s membrane via cleavage of extracellular matrix components. PLoS One 6:e22959. doi: 10.1371/journal.pone.0022959
von Arnim, C. A., Kinoshita, A., Peltan, I. D., Tangredi, M. M., Herl, L., Lee, B. M., et al. (2005). The low density lipoprotein receptor-related protein (LRP) is a novel beta-secretase (BACE1) substrate. J. Biol. Chem. 280, 17777–17785. doi: 10.1074/jbc.M414248200
von Einem, B., Schwanzar, D., Rehn, F., Beyer, A.-S., Weber, P., Wagner, M., et al. (2010). The role of low-density receptor-related protein 1 (LRP1) as a competitive substrate of the amyloid precursor protein (APP) for BACE1. Exp. Neurol. 225, 85–93. doi: 10.1016/j.expneurol.2010.05.017
Vreys, V., and David, G. (2007). Mammalian heparanase: what is the message? J. Cell. Mol. Med. 11, 427–452. doi: 10.1111/j.1582-4934.2007.00039.x
Vreys, V., Delande, N., Zhang, Z., Coomans, C., Roebroek, A., Dürr, J., et al. (2005). Cellular uptake of mammalian heparanase precursor involves low density lipoprotein receptor-related proteins, mannose 6-phosphate receptors and heparan sulfate proteoglycans. J. Biol. Chem. 280, 33141–33148. doi: 10.1074/jbc.M503007200
Waldron, E., Heilig, C., Schweitzer, A., Nadella, N., Jaeger, S., Martin, A. M., et al. (2008). LRP1 modulates APP trafficking along early compartments of the secretory pathway. Neurobiol. Dis. 31, 188–197. doi: 10.1016/j.nbd.2008.04.006
Wang, S., Herndon, M. E., Ranganathan, S., Godyna, S., Lawler, J., Argraves, W. S., et al. (2004). Internalization but not binding of thrombospondin-1 to low density lipoprotein receptor-related protein-1 requires heparan sulfate proteoglycans. J. Cell. Biochem. 91, 766–776. doi: 10.1002/jcb.10781
Wang, X., Gao, M., Schouteden, S., Roebroek, A., Eggermont, K., van Veldhoven, P. P., et al. (2014). Hematopoietic stem/progenitor cells directly contribute to arteriosclerotic progression via integrin β2. Stem Cells 33, 1230–1240. doi: 10.1002/stem.1939
Wang, X., Lee, S. R., Arai, K., Lee, S. R., Tsuji, K., Rebeck, G. W., et al. (2003). Lipoprotein receptor-mediated induction of matrix metalloproteinase by tissue plasminogen activator. Nat. Med. 10, 1313–1317. doi: 10.1038/nm926
Warshawsky, I., Broze, G. J. Jr., and Schwartz, A. L. (1994). The low density lipoprotein receptor-related protein mediates the cellular degradation of tissue factor pathway inhibitor. Proc. Natl. Acad. Sci. U.S.A. 91, 6664–6668. doi: 10.1073/pnas.91.14.6664
Weaver, A. M., Hussaini, I. M., Mazar, A., Henkin, J., and Gonias, S. L. (1997). Embryonic fibroblasts that are genetically deficient in low density lipoprotein receptor-related protein demonstrate increased activity of the urokinase receptor system and accelerated migration on vitronectin. J. Biol. Chem. 272, 14372–14379. doi: 10.1074/jbc.272.22.14372
Weaver, A. M., McCabe, M., Kim, I., Allietta, M. M., and Gonias, S. L. (1996). Epidermal growth factor and platelet-derived growth factor-BB induce a stable increase in the activity of low density lipoprotein receptor-related protein in vascular smooth muscle cells by altering receptor distribution and recycling. J. Biol. Chem. 271, 24894–24900. doi: 10.1074/jbc.271.40.24894
Werb, Z., Mainardi, C. L., Vater, C. A., and Harris, E. D. Jr. (1977). Endogenous activiation of latent collagenase by rheumatoid synovial cells. Evidence for a role of plasminogen activator. N. Engl. J. Med. 296, 1017–1023. doi: 10.1056/NEJM197705052961801
Werb, Z., Tremble, P. M., and Damsky, C. (1989). Signal transduction through the fibronectin receptor induces collagenase and stromelysin expression. J. Cell Biol. 109, 877–889. doi: 10.1083/jcb.109.2.877
Wijnberg, M. J., Quax, P. H., Nieuwenbroek, N. M., and Verheijen, J. H. (1997). The migration of human smooth muscle cells in vitro is mediated by plasminogen activation and can be inhibited by alpha2-macroglobulin receptor associated protein. Thromb. Haemost. 78, 880–886. doi: 10.1055/s-0038-1657646
Willnow, T. E., Goldstein, J. L., Orth, K., Brown, M. S., and Herz, J. (1992). Low density lipoprotein receptor-related protein and gp330 bind similar ligands, including plasminogen activator-inhibitor complexes and lactoferrin, an inhibitor of chylomicron remnant clearance. J. Biol. Chem. 267, 26172–26180.
Wilsie, L. C., and Orlando, R. A. (2003). The low density lipoprotein receptor-related protein complexes with cell surface heparan sulfate proteoglycan-mediated lipoprotein catabolism. J. Biol. Chem. 278, 15758–15764. doi: 10.1074/jbc.M208786200
Woo, J. A., Jung, A. R., Lakshmana, M. K., Bedrossian, A., Lim, Y., Bu, J. H., et al. (2012a). Pivotal role of the RanBP9-cofilin pathway in Abeta-induced apoptosis and neurodegeneration. Cell Death Differ. 19, 1413–1423. doi: 10.1038/cdd.2012
Woo, J. A., Roh, S. E., Lakshmana, M. K., and Kang, D. E. (2012b). Pivotal role of RanBP9 in integrin-dependent focal adhesion signaling and assembly. FASEB J. 26, 1672–1681. doi: 10.1096/fj.11-194423
Wu, F., Torre, E., Cuellar-Giraldo, D., Cheng, L., Yi, H., Bichler, E. K., et al. (2015). Tissue-type plasminogen activator triggers the synaptic vesicle cycle in cerebral cortical neurons. J. Cereb. Blood Flow Metab. 35, 1966–1976. doi: 10.1038/jcbfm.2015.155
Wu, L., and Gonias, S. L. (2005). The low-density lipoprotein-related protein-1 associates transiently with lipid rafts. J. Cell. Biochem. 96, 1021–1033. doi: 10.1002/jcb.20596
Wu, Y. P., Siao, C.-J., Lu, W., Sung, T.-C., Frohman, M. A., Milev, P., et al. (2000). The tissue plasminogen activator (tPA)/plasmin extracellular proteolytic system regulates seizure-induced hippocampal mossy fiber outgrowth through a proteoglycan substrate. J. Cell Biol. 148, 1295–1304. doi: 10.1083/jcb.148.6.1295
Wujak, L., Böttcher, R. T., Pak, O., Frey, H., El Agha, E., Chen, Y., et al. (2017). Low density lipoprotein receptor-related protein 1 couples β1 integrin activation to degradation. Cell. Mol. Life Sci. 75, 1671–1685. doi: 10.1007/s00018-017-2707-6
Yamada, K., Hashimoto, T., Yabuki, C., Nagae, Y., Tachikawa, M., Strickland, D. K., et al. (2008). The low density lipoprotein receptor-related protein 1 mediates uptake of amyloid β peptides in an in vitro model of the blood-brain barrier cells. J. Biol. Chem. 283, 34554–34562. doi: 10.1074/jbc.M801487200
Yamamoto, K., Owen, K., Parker, A. E., Scilabra, S. D., Dudhia, J., Strickland, D. K., et al. (2014). Low density lipoprotein receptor-related protein 1 (LRP1)-mediated endocytic clearance of a disintegrin and metalloproteinase with thrombospondin motifs-4 (ADAMTS-4): functional differences of non-catalytic domains of ADAMTS-4 and ADAMTS-5 in LRP1 binding. J. Biol. Chem. 289, 6462–6474. doi: 10.1074/jbc.M113.545376
Yanagisawa, H., Davis, E. C., Starcher, B. C., Ouchi, T., Yanagisawa, M., Richardson, J. A., et al. (2002). Fibulin-5 is an elastin-binding protein essential for elastic fibre development in vivo. Nature 415, 168–171. doi: 10.1038/415168a
Yang, D. S., Small, D. H., Seydel, U., Smith, J. D., Hallmayer, J., Gandy, S. E., et al. (1999). Apolipoprotein E promotes the binding and uptake of beta-amyloid into Chinese hamster ovary cells in an isoform-specific manner. Neuroscience 90, 1217–1226. doi: 10.1016/S0306-4522(98)00561-2
Yang, Z., Strickland, D. K., and Bornstein, P. (2001). Extracellular matrix metalloproteinase 2 levels are regulated by the low density lipoprotein-related scavenger receptor and thrombospondin 2. J. Biol. Chem. 276, 8403–8408. doi: 10.1074/jbc.M008925200
Yepes, M., and Lawrence, D. A. (2004). Neuroserpin: a selective inhibitor of tissue-type plasminogen activator in the central nervous system. Thromb. Haemost. 91, 457–464. doi: 10.1160/TH03-12-0766
Yepes, M., Sandkvist, M., Moore, E. G., Bugge, T. H., Strickland, D. K., and Lawrence, D. A. (2003). Tissue-type plasminogen activator induces opening of the blood-brain barrier via the LDL receptor-related protein. J. Clin. Invest. 112, 1533–1540. doi: 10.1172/JCI200319212
Yepes, M., Wu, F., Torre, E., Cuellar-Giraldo, D., Jia, D., and Cheng, L. (2016). Tissue-type plasminogen activator induces synaptic vesicle endocytosis in cerebral cortical neurons. Neuroscience 319, 69–78. doi: 10.1016/j.neuroscience.2016.01.046
Yin, K. J., Cirrito, J. R., Yan, P., Hu, X., Xiao, Q., Pan, X., et al. (2006). Matrix metalloproteinases expressed by astrocytes mediate extracellular amyloid-beta peptide catabolism. J. Neurosci 26, 10939–10948. doi: 10.1523/JNEUROSCI.2085-06.2006
Yu, C., Nwabuisi-Heath, E., Laxton, K., and Ladu, M. J. (2010). Endocytic pathways mediating oligomeric Aβ42 neurotoxicity. Mol. Neurodegener. 5:19. doi: 10.1186/1750-1326-5-19
Yuan, H., Vance, K. M., Junge, C. E., Geballe, M. T., Snyder, J. P., Hepler, J. R., et al. (2009). The serine protease plasmin cleaves the amino-terminal domain of the NR2A subunit to relieve zinc inhibition of the N-methyl-D-aspartate receptors. J. Biol. Chem. 284, 12862–12873. doi: 10.1074/jbc.M805123200
Zemskov, E. A., Mikhailenko, I., Strickland, D. K., and Belkin, A. M. (2007). Cell-surface transglutaminase undergoes internalization and lysosomal degradation: an essential role for LRP1. J. Cell Sci. 120, 3188–3199. doi: 10.1242/jcs.010397
Zhang, C., An, J., Haaile, W. B., Echeverry, R., Strickland, D. K., and Yepes, M. (2009). Microglial low-density lipoprotein receptor-related protein 1 mediates the effect of tissue-type plasminogen activator on matrix metalloproteinase-9 activity in the ischemic brain. J. Cereb. Blood Flow Metab. 29, 1946–1954. doi: 10.1038/jcbfm.2009.174
Zhang, H., Links, P. H., Ngsee, J. K., Tran, K., Cui, Z., Ko, K. W. S., et al. (2004). Localization of low density lipoprotein receptor-related protein 1 to caveolae in 3T3-L1 adipocytes in response to insulin treatment. J. Biol. Chem. 279, 2221–2230. doi: 10.1074/jbc.M310679200
Zhang, S. H., Reddick, R. L., Burkey, B., and Maeda, N. (1994). Diet-induced atherosclerosis in mice heterozygous and homozygous for apolipoprotein E gene disruption. J. Clin. Invest. 94, 937–945. doi: 10.1172/JCI117460
Zhang, X., Polavarapu, R., She, H., Mao, Z., and Yepes, M. (2007). Tissue-type plasminogen activator and the low-density lipoprotein receptor-related protein mediate cerebral ischemia-induced nuclear factor-kappaB pathway activation. Am. J. Pathol. 171, 1281–1290. doi: 10.2353/ajpath.2007.070472
Zhou, L., Takayama, Y., Boucher, P., Tallquist, M. D., and Herz, J. (2009). LRP1 regulates architecture of the vascular wall by controlling PDGFRβ -dependent phosphatidylinositol 3-kinase activation. PLoS One 4:e6922. doi: 10.1371/journal.pone.0006922
Zhu, Y., and Hui, D. Y. (2003). Apolipoprotein E binding to low density lipoprotein receptor-related protein-1 inhibits cell migration via activation of cAMP-dependent protein kinase A. J. Biol. Chem. 278, 36257–36263. doi: 10.1074/jbc.M303171200
Zhuo, M., Holtzman, D. M., Li, Y., Osaka, H., DeMaro, J., Jacquin, M., et al. (2000). Role of tissue plasminogen activator receptor LRP in hippocampal long-term potentiation. J. Neurosci. 20, 542–549. doi: 10.1523/JNEUROSCI.20-02-00542.2000
Zilberberg, A., Yaniv, A., and Gazit, A. (2004). The low density lipoprotein receptor-1, LRP1, interacts with the human frizzled-1 (HFz1) and down-regulates the canonical Wnt signaling pathway. J Biol. Chem. 279, 17535–17542. doi: 10.1074/jbc.M311292200
Keywords: low density receptor-related protein 1, tissue plasminogen activator, integrins, extracellular matrix, migration, matrix remodeling
Citation: Bres EE and Faissner A (2019) Low Density Receptor-Related Protein 1 Interactions With the Extracellular Matrix: More Than Meets the Eye. Front. Cell Dev. Biol. 7:31. doi: 10.3389/fcell.2019.00031
Received: 13 November 2018; Accepted: 25 February 2019;
Published: 15 March 2019.
Edited by:
Charles D. Little, University of Kansas Medical Center, United StatesReviewed by:
Nagaraj Balasubramanian, Indian Institute of Science Education and Research Pune, IndiaCopyright © 2019 Bres and Faissner. This is an open-access article distributed under the terms of the Creative Commons Attribution License (CC BY). The use, distribution or reproduction in other forums is permitted, provided the original author(s) and the copyright owner(s) are credited and that the original publication in this journal is cited, in accordance with accepted academic practice. No use, distribution or reproduction is permitted which does not comply with these terms.
*Correspondence: Andreas Faissner, YW5kcmVhcy5mYWlzc25lckBydWIuZGU=
Disclaimer: All claims expressed in this article are solely those of the authors and do not necessarily represent those of their affiliated organizations, or those of the publisher, the editors and the reviewers. Any product that may be evaluated in this article or claim that may be made by its manufacturer is not guaranteed or endorsed by the publisher.
Research integrity at Frontiers
Learn more about the work of our research integrity team to safeguard the quality of each article we publish.