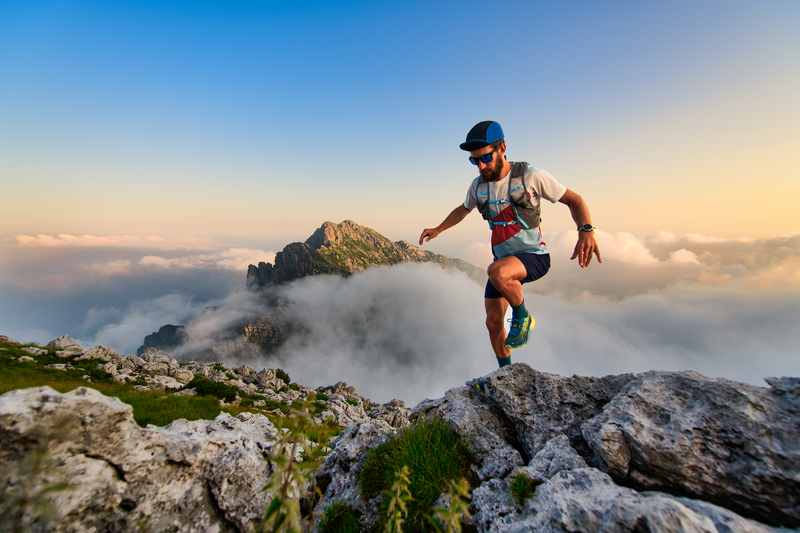
95% of researchers rate our articles as excellent or good
Learn more about the work of our research integrity team to safeguard the quality of each article we publish.
Find out more
REVIEW article
Front. Cell Dev. Biol. , 03 April 2018
Sec. Cell Adhesion and Migration
Volume 6 - 2018 | https://doi.org/10.3389/fcell.2018.00034
This article is part of the Research Topic Cell adhesion and migration in the development of multicellular organisms View all 11 articles
Most macromolecules found in cells are chiral, meaning that they cannot be superimposed onto their mirror image. However, cells themselves can also be chiral, a subject that has received little attention until very recently. In our studies on the mechanisms of left-right (LR) asymmetric development in Drosophila, we discovered that cells can have an intrinsic chirality to their structure, and that this “cell chirality” is generally responsible for the LR asymmetric development of certain organs in this species. The actin cytoskeleton plays important roles in the formation of cell chirality. In addition, Myosin31DF (Myo31DF), which encodes Drosophila Myosin ID, was identified as a molecular switch for cell chirality. In other invertebrate species, including snails and Caenorhabditis elegans, chirality of the blastomeres, another type of cell chirality, determines the LR asymmetry of structures in the body. Thus, chirality at the cellular level may broadly contribute to LR asymmetric development in various invertebrate species. Recently, cell chirality was also reported for various vertebrate cultured cells, and studies suggested that cell chirality is evolutionarily conserved, including the essential role of the actin cytoskeleton. Although the biological roles of cell chirality in vertebrates remain unknown, it may control LR asymmetric development or other morphogenetic events. The investigation of cell chirality has just begun, and this new field should provide valuable new insights in biology and medicine.
The directional left-right (LR) asymmetry of body structures and functions is found in animals across phyla. In this review, directional LR asymmetry is called “LR asymmetry.” LR asymmetry is a fundamental property of animal development, and the mechanisms of LR asymmetric development are a topic of strong interest in various biological and medical fields. To explain the molecular basis of LR asymmetric development, Walport proposed the “F molecule” hypothesis (Brown and Wolpert, 1990). In this hypothesis, the F molecule is chiral and can be arranged along the anterior-posterior and dorsal-ventral axes. An object is chiral if it cannot be superposed onto its mirror image. By virtue of these properties, the F molecule can direct the LR axis based on its chirality. This idea is supported by findings on the molecular mechanisms of LR asymmetric development in mouse.
In mouse, motile cilia in the node, which is a small pit located in the ventral side of the early embryo, rotate clockwise and induce a leftward flow of extra-embryonic fluid, named the “nodal flow” (Nonaka et al., 1998). The nodal flow is the first cue that breaks the LR symmetry of the mouse embryo. The nodal flow induces left-side-specific gene expression, which leads to subsequent LR asymmetric development (Nonaka et al., 1998, 2002; Okada and Hirokawa, 1999). In addition to their ventral location, the nodal cilia slant posteriorly, which helps them to generate the leftward fluid flow (Nonaka et al., 2005). Thus, the nodal cilia are arranged in a polar manner along both the anterior-posterior and dorsal-ventral axes. The nodal cilia also have intrinsic chirality, because they rotate clockwise (Nonaka et al., 1998). Therefore, the nodal cilia satisfy the requirements of the F molecule (Brown and Wolpert, 1990). In this case, the molecular origin of LR asymmetric development is traced back to the chiral structure and motion of cilia, which is still a subject of active investigation (Okada and Hirokawa, 2009; Nonaka, 2013).
Drosophila melanogaster is another organism in which the mechanisms of LR asymmetric development have been extensively studied (Hozumi et al., 2006; Spéder et al., 2006; González-Morales et al., 2015; Inaki et al., 2016). In this organism, chirality at the level of cells, rather than molecules, was found to contribute to LR asymmetric development for the first time (Taniguchi et al., 2011; Inaki et al., 2016). The embryonic hindgut first forms LR symmetrically along the midline, then rotates counterclockwise 90° as viewed from the posterior, and eventually exhibits a LR asymmetric morphology (Figure 1). Before the rotation, the hindgut has a hook-like shape that points ventrally, and its most posterior part is stably connected to the anus (Figure 1). The hindgut then twists, causing the hook-like shape to point rightward (Figure 1).
Figure 1. The Drosophila embryonic hindgut rotates 90° counterclockwise. (Left) The embryonic hindgut first forms as a bilaterally symmetric structure that curves ventrally (Left). It rotates 90° counterclockwise from the posterior view (Middle), and consequently curves to the right (Right). (Right) The embryonic gut curves rightward at stage 12 in wild-type Drosophila. This figure is partly adapted from Inaki et al. (2016) with permission.
Before the rotation, the anterior-posterior axis direction of the hindgut epithelial tube can be readily defined and used as a reference in analyzing the LR asymmetry of each cell. Taniguchi et al. discovered that before rotation onset, the hindgut epithelial cells show LR asymmetry in their apical surface, which faces the lumen of the hindgut tube (Figure 2; Taniguchi et al., 2011). In these cells, leftward-tilting cell boundaries are found more frequently than rightward-tilting ones (Figure 2). In addition, the hindgut epithelial cells have apical-basal polarity, like other epithelial cells. Therefore, the three-dimensional hindgut epithelial cells are chiral, because their shape cannot be superimposed onto their mirror image (Figure 3). This property of cells was called “cell chirality” (Taniguchi et al., 2011; Inaki et al., 2016). The chirality of these cells eventually disappears as the rotation progresses, and the cell shape becomes achiral (LR symmetric) when the rotation is completed (Taniguchi et al., 2011).
Figure 2. Myosin31DF mutation reverses the cell chirality and hindgut rotation. (Top) A wild-type Drosophila embryo shows normal cell chirality and a rightward-pointing hindgut. (Bottom) In the Myosin31DF mutant, the cell chirality and hindgut laterality are the mirror images of those in its wild-type counterpart. This figure is partly adapted from Inaki et al. (2016) with permission.
Figure 3. A computer simulation recapitulates the cell-chirality-driven counterclockwise rotation of the hindgut. (Top) The shape of the apical surface of hindgut epithelial cells is LR-asymmetric (Left). Considering that these cells also have apical-basal polarity, they show chirality. This property is illustrated by left-handed and right-handed chiral amino acids (Right). (Bottom) To test whether cell chirality alone could induce the axial rotation of the hindgut epithelial tube, a computer simulation based on a vertex model was performed. The introduction of LR bias to the contraction of the cell boundary was sufficient to recapitulate the cell chirality found in vivo. The introduction and subsequent release of cell chirality were sufficient to induce the LR-asymmetric rotation of the model epithelial tube (Taniguchi et al., 2011). These figures are partly adapted from Inaki et al. (2016) and Taniguchi et al. (2011) with permissions.
A computer simulation demonstrated that the introduction and subsequent dissolution of cell chirality is sufficient to induce the counterclockwise rotation of a model epithelial tube (Taniguchi et al., 2011). The results of this model study were also consistent with previous observations that cell proliferation and cell death do not occur during hindgut rotation, suggesting that cell rearrangement and/or deformation alone is responsible for the hindgut rotation (Lengyel and Iwaki, 2002; Wells et al., 2013).
Although the mechanisms of cell-chirality formation remain unclear, important clues emerged from the genetic identification of Drosophila Myosin31DF (Myo31DF) mutants, in which the LR asymmetry of various organs is reversed (Hozumi et al., 2006; Spéder et al., 2006). Myo31DF is the Drosophila ortholog of Myosin1D, which encodes an evolutionarily conserved class I myosin (Hozumi et al., 2006; Spéder et al., 2006). In loss-of-function Myo31DF mutants, the hindgut rotates 90° in the opposite direction to that of wild type in more than 80% of the flies, resulting in a hindgut in which the hook-like shape points leftward (Figure 2; Hozumi et al., 2006). Myo31DF mutants are homozygous viable and fertile, suggesting that the role of Myo31DF is highly specific for LR asymmetric development (Hozumi et al., 2006; Spéder et al., 2006). In addition, the chiral hindgut epithelial cells in these mutants are the mirror image of their wild-type counterparts (Taniguchi et al., 2011). The forced expression of wild-type Myo31DF in the hindgut epithelium of these mutants rescues both the reversed hindgut rotation and the reversed cell chirality. These observations indicated that the default states of LR asymmetry and cell chirality are the mirror image of wild type, and that Myo31DF acts to reverse them to the wild-type direction (Hozumi et al., 2006; Taniguchi et al., 2011).
A genetic mosaic analysis of Myo31DF suggested that cell chirality is generated intrinsically in each cell, but is also under some influence of neighboring cells, probably through mechanical force (Hatori et al., 2014). These findings collectively suggest that Myo31DF acts as a switch for cell chirality and determines the direction of Drosophila hindgut rotation.
In addition to the embryonic hindgut, Myo31DF mutants in Drosophila show LR inversion in various other organs, including the male genitalia, testes, and adult gut, indicating that Myo31DF determines the LR asymmetry in these organs, as well (Hozumi et al., 2006; Spéder et al., 2006). Among these organs, cell chirality is known to contribute to the LR asymmetric development of the male genitalia and adult gut (González-Morales et al., 2015; Sato et al., 2015a). Thus, cell chirality appears to be a common strategy for driving the LR asymmetric morphogenesis of tissues in Drosophila.
The male genitalia in Drosophila undergo a 360° clockwise rotation as viewed from the posterior during the pupal stage, achieved by a combination of 180° rotations in two adjacent segments, A8a and A8p (Suzanne et al., 2010; Kuranaga et al., 2011). Just before the rotation begins, A8a epithelial cells show cell chirality (Figure 4; Sato et al., 2015a). In this tissue, Myosin II (MyoII) is concentrated along the rightward tilting cell boundaries (Figure 4; Sato et al., 2015a). This chiral distribution of MyoII is maintained during the rotation and appears to drive LR asymmetric cell intercalation (Sato et al., 2015a). A computer simulation suggested that these LR asymmetric cell interactions give rise to the directional rotation of the male genitalia (Sato et al., 2015a). The cell chirality in the male genitalia is also inverted in Myo31DF mutants, consistent with the idea that cell chirality drives the LR directional rotation of this organ (Sato et al., 2015a).
Figure 4. Cell chirality drives LR asymmetric morphogenesis in various Drosophila organs. (Top) In wild-type Drosophila males, the genitalia rotate clockwise as viewed from the posterior. Just before rotation begins, genital epithelial cells in the A8 segment show a chiral cell shape and an asymmetric Myosin II (MyoII) distribution (Middle). This cell chirality drives the 360° rotation of the genitalia (Right). (Bottom) The wild-type adult Drosophila gut develops from the H2 segment during the pupal stage, while gut laterality is determined by Myo31DF expressed in the H1 segment during the larval stage (Middle). Cell chirality is observed only in the H2 segment after the H1 segment is eliminated during metamorphosis. The handedness determined by Myosin31DF in the H1 segment is propagated to the H2 segment, leading to the LR-directional looping of the adult hindgut (Right). The atypical cadherins Dachsous (Ds) and Fat (Ft) are thought to be involved in this process. Dachsous is reported to bind Myo31DF (Middle). These figures are partly adapted from Inaki et al. (2016) with permission.
Although cell chirality is also responsible for the LR asymmetric development of the adult gut, the mechanism by which Myo31DF and cell chirality contribute to the LR asymmetric morphogenesis of this organ may be different from that of the embryonic hindgut and male genitalia. Drosophila undergoes complete metamorphosis, and the adult gut develops from its primordium during the pupal stage (Robertson, 1936; Fox and Spradling, 2009). The adult gut primordium consists of two segments, H1 and H2 (Murakami and Shiotsuki, 2001; González-Morales et al., 2015). The epithelial cells in H2 proliferate to become the adult gut, while the H1 segment is eliminated during the pupal stage. Myo31DF is required only in H1 during late larval stage, and cell chirality is observed only in H2 after H1 is eliminated (Figure 4; González-Morales et al., 2015). To explain these observations, it was proposed that polarity determined by Myo31DF in H1 is propagated to H2 as cell chirality (Figure 4; González-Morales et al., 2015). In addition, evidence suggested that this propagation of cell chirality is mediated by the unconventional cadherins, Dachsous and Fat (Figure 4; González-Morales et al., 2015). Dachsous and Fat are components of the planer cell polarity (PCP) pathway (Yang et al., 2002). A connection between MyoID, which is an ortholog of Myo31DF, and the PCP pathway was also found in rat (Hegan et al., 2015). The MyoID mutation in rat results in the elimination of PCP in multi-ciliated tracheal and brain-ependymal epithelial cells (Hegan et al., 2015). Therefore, some aspects of the relationship between MyoID and PCP may be conserved between Drosophila and mammals.
The biochemical functions of the Myosin I family have been studied extensively, and their roles in membrane tension generation, membrane dynamics, and mechanosignal transduction are well-documented (McConnell and Tyska, 2010). Myo31DF is an F-actin-based motor protein, and therefore is likely to exert its function through the actin cytoskeleton; however, the molecular links between cell chirality and Myo31DF remain largely unknown (Hozumi et al., 2006; Taniguchi et al., 2011). To date, a chiral distribution of Myo31DF has not been observed in the Drosophila hindgut epithelial cells (Hatori et al., 2014). It may be necessary to observe the dynamics of Myo31DF's localization or activity to understand the biochemical basis of chirality formation.
Potential insight into the connection between the Myosin I family and cell chirality was obtained from an elegant biochemical study of a mammalian Myosin I family protein (Pyrpassopoulos et al., 2012). In this experiment, Myosin1c, a mouse Myosin I family protein (also known as Myosin IC), powered actin motility on fluid-supported lipid bilayers, and this motility was observed to occur along curved paths in a counterclockwise direction (Pyrpassopoulos et al., 2012). Thus, Myosin I family proteins may have an intrinsic chiral property that causes actin to move in a chiral manner. In addition, the actin cytoskeleton has important roles in the chirality of the hindgut epithelial cells in Drosophila. Overexpressing a dominant-negative form of Rho1 (Rho1 N19) or Rac1 (Rac1 N17), which is known to regulate F-actin, causes these epithelial cells to become achiral (Taniguchi et al., 2011). Therefore, F-actin dynamics may be important for the formation of cell chirality though Myo31DF.
The Drosophila E-Cadherin (DE-cad) may also functionally interact with Myo31DF. In DE-cad mutants, epithelial cells of the embryonic hindgut become achiral, and the embryonic hindgut rotates in a random direction or does not rotate (Taniguchi et al., 2011). DE-cad is also required for the male genitalia rotation (Petzoldt et al., 2012). Genetic analyses suggested that DE-cad functions downstream of Myo31DF in both organs (Taniguchi et al., 2011; Petzoldt et al., 2012). Myo31DF physically interacts with β-catenin and forms a complex with DE-cad, indicating that Myo31DF might regulate DE-cad through a β-catenin/cadherin complex (Petzoldt et al., 2012). In the embryonic hindgut, DE-cad chirally localizes to adherens junctions, and in Myo31DF mutants, this chiral localization of DE-cad is the mirror image of that in wild-type (Taniguchi et al., 2011). However, it is not known how Myo31DF, which does not show a chiral distribution in hindgut epithelial cells, controls the chiral localization of DE-cad.
By a genetic screen, the Abd-B gene was identified as a dominant enhancer of the Myo31DF mutation in Drosophila (Coutelis et al., 2013). The knockdown of Abd-B by RNA interference in the male genital discs stops the rotation of genitalia (Coutelis et al., 2013). The Myo31DF expression in the male genital discs is almost abolished under this condition, and the no-rotation phenotype is rescued by overexpressing Myo31DF (Coutelis et al., 2013). The knockdown of Abd-B in the embryonic hindgut also stops the hindgut rotation (Coutelis et al., 2013). These results indicate that Abd-B acts upstream of Myo31DF.
In Myo31DF mutants, the embryonic gut and male genitalia still rotate in the reverse direction, suggesting that a “default” pathway exists that drives the inverse rotation of these organs (Hozumi et al., 2006; Spéder et al., 2006). In contrast, Abd-B knockdown results in no-rotation phenotypes in these organs. Therefore, the default pathway of LR asymmetric development is also under the control of Abd-B (Coutelis et al., 2013). Further experiments are needed to uncover this default pathway. In any case, these results indicate that Abd-B may be a master controller of LR asymmetric development in Drosophila.
Drosophila has three type I myosin family proteins: Myo31DF (also known as MyoID), Myo61F (also known as MyoIC), and Myo95E (most similar to MyoIB in vertebrates) (Tzolovsky et al., 2002; Okumura et al., 2015). In addition to Myo31DF, potential roles of the other two Myosin I family proteins in LR asymmetric development have been investigated in Drosophila. Myo61F was proposed to have antagonistic functions to Myo31DF, because overexpressing Myo61F causes LR inversion of the embryonic gut and male genitalia, reminiscent of the Myo31DF loss-of-function phenotypes (Hozumi et al., 2006). Furthermore, these inversion phenotypes induced by Myo61F overexpression are suppressed by additionally overexpressing Myo31DF (Hozumi et al., 2006; Petzoldt et al., 2012). Myo61F inhibits the physical interaction between Myo31DF and the β-catenin/DE-cadherin complex, suggesting how Myo61F may antagonize Myo31DF (Petzoldt et al., 2012). However, the null Myo61F mutant alone is homozygous viable and does not show LR defects in the embryonic hindgut or genital disc (Okumura et al., 2015). Thus, the LR-reversing activity of Myo61F overexpression may not reflect its physiological function (Okumura et al., 2015). On the other hand, the Myo61F null mutant recessively enhances the clockwise (wild type) genitalia rotation of the Myo31DF mutant, suggesting that Myo61F and Myo31DF have a redundant function in promoting the dextral LR rotation of the male genitalia (Okumura et al., 2015).
Regarding Myo95E, a null mutant of Myo95E is homozygous viable and does not show any detectable LR defects (Okumura et al., 2015). In addition, Myo95E overexpression results in no obvious LR defects (Okumura et al., 2015). Myo95E does not enhance the phenotype of Myo31DF, suggesting that Myo95E is not involved in LR asymmetric development (Okumura et al., 2015). Unexpectedly, triple homozygotes for Myo31DF, Myo61F, and Myo95E lacking their maternal contributions are viable and fertile, and show no obvious developmental defects, except for the LR defects due to the Myo31DF mutation (Okumura et al., 2015). Therefore, none of the Myosin I family genes are essential for viability in Drosophila (Okumura et al., 2015).
In addition to Drosophila, the mechanisms of LR asymmetric development have been well studied in other invertebrate species, including air-breathing snails (Pulmonata) and Caenorhabditis elegans (C. elegans), which are model organisms used to study developmental biology in Ecdysozoa and Lophotrochozoa, respectively (Inaki et al., 2016). In Pulmonata and C. elegans, the LR asymmetry of blastomeres at the very early stages of embryogenesis plays central roles in the subsequent LR asymmetric development (Wood, 1991; Bergmann et al., 2003; Shibazaki et al., 2004). These LR asymmetric blastomeres are chiral, given that their shape cannot be superimposed onto its mirror image. In these cases, the chirality of the blastomeres defines the LR asymmetric axis of the next blastomere cleavage, which consequently introduces LR asymmetry into the arrangement of blastomeres (Wood, 1991; Bergmann et al., 2003; Shibazaki et al., 2004). Strikingly, in Lymnaea stagnalis (a Pulmonata species) and C. elegans, reversing the LR asymmetry in the arrangement of blastomeres by artificial manipulations inverses the entire subsequent LR asymmetric development (Wood, 1991; Kuroda et al., 2009). These results suggest that LR asymmetry in the relative position of blastomeres contains all of the information for the LR axis polarity in the subsequent development.
In Pulmonata, the initial LR asymmetry is observed as cell chirality of the blastomeres at the four-cell stage (Shibazaki et al., 2004). This chirality leads to LR slanting of the cleavage axes of the four blastomeres, which results in a helical shift of the micromeres that are generated by the next cleavage, in a particular direction (Shibazaki et al., 2004). This LR asymmetric rearrangement of macromeres is fully responsible for the entire LR asymmetric development that subsequently occurs, including the coiling direction of the shell and the laterality of the visceral organs (Shibazaki et al., 2004). Notably, in L. stagnalis, a maternal D mutant that dominantly determines the clockwise (wild type) coiling of the shell in the offspring was identified in a natural population (Pelseneer, 1920; Rolan-Alvarez and Rolan, 1995). In this mutant embryo, the chirality of blastomeres at the four-cell stage disappears, and the blastomeres become radially symmetric, which subsequently leads to the reversed LR asymmetry in the configuration of the eight-cell-stage blastomeres (Shibazaki et al., 2004). Recently, the D gene of L. stagnalis was found to be a homolog of formin (Davison et al., 2016). Formin belongs to a group of proteins that associate with the barbed end of actin filaments and are involved in actin polymerization (Pruyne et al., 2002). These results demonstrate that the actin cytoskeleton is important in the formation of blastomere chirality in this snail.
Actin also plays an important role in the blastomere chirality in C. elegans. In this organism, the initial LR asymmetry is detected as a chiral rotation of the actomyosin cortex in the one-cell-stage embryo (Naganathan et al., 2014). This chiral flow is created by turning forces that are generated by myosin under the control of Rho GTPase signaling (Naganathan et al., 2014). In the C. elegans embryo, handedness is determined at the four-cell stage (Wood, 1991). The chiral turning force in these blastomeres probably causes a LR asymmetric skewing of the mitotic spindle direction at the four-cell stage, which is responsible for the subsequent LR asymmetric development of the entire body (Wood, 1991; Bergmann et al., 2003).
In summary, the chirality of blastomeres plays critical roles in the LR asymmetric development of these two representative model organisms of Ecdysozoa and Lophotrochozoa. Given that the chirality of blastomeres is a specific version of cell chirality found during early cleavage stages, cell chirality may have general and evolutionarily conserved roles in LR asymmetric development.
Although cell chirality and blastomere chirality have been found in vivo only in invertebrates, many vertebrate cells demonstrate various indications of cell chirality under certain culture conditions (Figure 5; Xu et al., 2007; Wan et al., 2011; Chen et al., 2012; Tee et al., 2015; Worley et al., 2015; Yamanaka and Kondo, 2015; Raymond et al., 2016). Cell lines including mouse myoblasts (C2C12), human umbilical vein endothelial cells (hUVEC), and canine kidney epithelial cells (MDCK) exhibit a chiral cell shape when cultivated on ring or stripe micro-patterns of adhesive substrates (Figure 5; Wan et al., 2011; Chen et al., 2012; Worley et al., 2015; Raymond et al., 2016). Cell chirality is also found in cellular behaviors under certain culture conditions. For example, human blood cells (dHL60) show leftward biased directional movement in the absence of directional chemotactic cues (Xu et al., 2007). Cell chirality is also observed in the dynamics of intracellular structures. Cultured zebrafish melanophores show a counterclockwise rotation of their dinuclei and cytoplasm (Figure 5), and the actin cytoskeleton plays a critical role in this chiral behavior (Tee et al., 2015; Yamanaka and Kondo, 2015). Human foreskin fibroblasts seeded on a circle micro-pattern show a chiral swirling of actin fibers (Figure 5; Tee et al., 2015). Intriguingly, in addition to the appropriate actin cytoskeleton structure, Formin activity appears to be required to generate this chiral swirling of actin fibers; similarly, the formin gene has an essential role in creating blastomere chirality in a snail (Davison et al., 2016). Although the biological relevance of the vertebrate cell chirality observed in vitro remains elusive, many cell lines from various organs show distinct chirality (Wan et al., 2011), suggesting that cell chirality might drive the LR asymmetric morphogenesis of these organs, as in invertebrates.
Figure 5. Vertebrate cultured cells exhibit intrinsic cell chirality. (Top) Cultured human endothelial (hUVEC) and mouse myoblast (C2C12) cells are arranged in clockwise and counterclockwise spiral patterns on a substrate (Fibronectin) with a ring micropattern (Wan et al., 2011). (Middle) Vascular mesenchymal cells exhibit intrinsic chirality when plated on a substrate with a stripe micropattern (Fibronectin and polyethylene glycol) (Chen et al., 2012). (Bottom) A counterclockwise rotation of the nucleus is observed in cultured zebrafish melanophores (Left) (Yamanaka and Kondo, 2015). Human fibroblasts cultured on a micropattern substrate show chiral swirling (Right) (Tee et al., 2015). These figures are partly adapted from Inaki et al. (2016) with permission.
In addition to these vertebrate cells, chiral cellular behaver was recently reported in Dictyostelium discoideum (Tamada and Igarashi, 2017). During their ameboid movement, Dictyostelium cells extend filopodia that rotate in a rightward screwlike manner (Figure 6; Tamada and Igarashi, 2017). This right-screw rotation is very similar to the movement of filopodia on the growth cone of mouse neurons (Figure 6; Tamada et al., 2010; Tamada and Igarashi, 2017). It was proposed that the right-screw rotation of filopodia introduces the observed clockwise tracks in neurite growth and in cell migration on two-dimensional substrates (Figure 6; Tamada and Igarashi, 2017). These findings suggest that cell chirality can be traced back to single-celled eukaryotic organisms.
Figure 6. The chiral behavior of filopodia is evolutionarily conserved from single-celled eukaryotic organisms to mammals. (Top) Filopodia of the growth cone on mouse neurites rotate in a rightward screwlike manner (Left) (Tamada and Igarashi, 2017). The right-screw rotation of filopodia introduces a clockwise growth of neurites (Right) (Tamada and Igarashi, 2017). (Bottom) Filopodia of Dictyostelium cells also show a rightward-screwlike rotation (Left) (Tamada and Igarashi, 2017). The right-screw rotation of filopodia leads the cells to migrate in a clockwise direction (Right) (Tamada and Igarashi, 2017).
Today, it is well-accepted that many animal cells show intrinsic cell chirality. Although the molecular mechanisms of cell chirality formation are still largely unknown, F-actin appears to play critical roles in this process, as revealed in Drosophila, snails, C. elegans, and vertebrates. In particular, the essential role of Formin family proteins in the cell chirality formation of snail blastomeres and mammalian cells suggests that the axial rotation of F-actin may be one of the origins of chirality (Tee et al., 2015; Davison et al., 2016). In Drosophila, Myo31DF may switch the chiral status of the F-actin structure or dynamics, although this possibility remains to be proven.
In Drosophila, several lines of evidence show that cell chirality is a common mechanism in this organism for developing LR asymmetric structures. On the other hand, in vertebrates, although cell chirality is broadly found, its functions remain a mystery. A computer simulation predicted that a model cell with cell chirality may have an advantage for passing through a population of cells (Sato et al., 2015b). In addition, it is possible that cell chirality interferes or collaborates with PCP, thereby disrupting or modifying the architecture of the planarly polarized cells. This could occur in two ways: cell chirality itself may be superimposed onto the PCP, or chiral cells may intermingle with PCP-exhibiting cells. By such processes, cell chirality may control various morphogenetic events, not only LR asymmetric development. The investigation of cell chirality is still in its infancy, and many questions, especially about the molecular mechanisms of its formation and biological functions, are still open. Answering these questions will add valuable insight for studies in biology and medicine in the near future.
MI, TS, and KM wrote the review; MI and TS made figures.
This work was supported by JSPS KAKENHI Grant Number 15H05863, 16K07349, and 15K07077.
The authors declare that the research was conducted in the absence of any commercial or financial relationships that could be construed as a potential conflict of interest.
Bergmann, D. C., Lee, M., Robertson, B., Tsou, M. F., Rose, L. S., and Wood, W. B. (2003). Embryonic handedness choice in C. elegans involves the Galpha protein GPA-16. Development 130, 5731–5740. doi: 10.1242/dev.00839
Brown, N. A., and Wolpert, L. (1990). The development of handedness in left/right asymmetry. Development 109, 1–9.
Chen, T.-H., Hsu, J. J., Zhao, X., Guo, C., Wong, M. N., Huang, Y., et al. (2012). Left-right symmetry breaking in tissue morphogenesis via cytoskeletal mechanics novelty and significance. Circ. Res. 110, 551–559. doi: 10.1161/CIRCRESAHA.111.255927
Coutelis, J. B., Géminard, C., Spéder, P., Suzanne, M., Petzoldt, A. G., and Noselli, S. (2013). Drosophila left/right asymmetry establishment is controlled by the Hox gene abdominal-B. Dev. Cell 24, 89–97. doi: 10.1016/j.devcel.2012.11.013
Davison, A., McDowell, G. S., Holden, J. M., Johnson, H. F., Koutsovoulos, G. D., Liu, M. M., et al. (2016). Formin is associated with left-right asymmetry in the pond snail and the frog. Curr. Biol. 26, 654–660. doi: 10.1016/j.cub.2015.12.071
Fox, D. T., and Spradling, A. C. (2009). The Drosophila hindgut lacks constitutively active adult stem cells but proliferates in response to tissue damage. Cell Stem Cell 5, 290–297. doi: 10.1016/j.stem.2009.06.003
González-Morales, N., Géminard, C., Lebreton, G., Cerezo, D., Coutelis, J.-B., and Noselli, S. (2015). The atypical cadherin dachsous controls left-right asymmetry in Drosophila. Dev. Cell 33, 675–689. doi: 10.1016/j.devcel.2015.04.026
Hatori, R., Ando, T., Sasamura, T., Nakazawa, N., Nakamura, M., Taniguchi, K., et al. (2014). Left-right asymmetry is formed in individual cells by intrinsic cell chirality. Mech. Dev. 133, 146–162. doi: 10.1016/j.mod.2014.04.002
Hegan, P. S., Ostertag, E., Geurts, A. M., and Mooseker, M. S. (2015). Myosin Id is required for planar cell polarity in ciliated tracheal and ependymal epithelial cells. Cytoskeleton 72, 503–516. doi: 10.1002/cm.21259
Hozumi, S., Maeda, R., Taniguchi, K., Kanai, M., Shirakabe, S., Sasamura, T., et al. (2006). An unconventional myosin in Drosophila reverses the default handedness in visceral organs. Nature 440, 798–802. doi: 10.1038/nature04625
Inaki, M., Liu, J., and Matsuno, K. (2016). Cell chirality: its origin and roles in left-right asymmetric development. Philos. Trans. R. Soc. Lond. B Biol. Sci. 371:20150403. doi: 10.1098/rstb.2015.0403
Kuranaga, E., Matsunuma, T., Kanuka, H., Takemoto, K., Koto, A., Kimura, K., et al. (2011). Apoptosis controls the speed of looping morphogenesis in Drosophila male terminalia. Development 138, 1493–1499. doi: 10.1242/dev.058958
Kuroda, R., Endo, B., Abe, M., and Shimizu, M. (2009). Chiral blastomere arrangement dictates zygotic left-right asymmetry pathway in snails. Nature 462, 790–794. doi: 10.1038/nature08597
Lengyel, J. A., and Iwaki, D. D. (2002). It takes guts: the Drosophila hindgut as a model system for organogenesis. Dev. Biol. 243, 1–19. doi: 10.1006/dbio.2002.0577
McConnell, R. E., and Tyska, M. J. (2010). Leveraging the membrane - cytoskeleton interface with myosin-1. Trends Cell Biol. 20, 418–426. doi: 10.1016/j.tcb.2010.04.004
Murakami, R., and Shiotsuki, Y. (2001). Ultrastructure of the hindgut of Drosophila larvae, with special reference to the domains identified by specific gene expression patterns. J. Morphol. 248, 144–150. doi: 10.1002/jmor.1025
Naganathan, S. R., Fürthauer, S., Nishikawa, M., Jülicher, F., and Grill, S. W. (2014). Active torque generation by the actomyosin cell cortex drives left–right symmetry breaking. Elife 3:e04165. doi: 10.7554/eLife.04165
Nonaka, S. (2013). Visualization of mouse nodal cilia and nodal flow. Methods Enzymol. 525, 149–157. doi: 10.1016/B978-0-12-397944-5.00008-0
Nonaka, S., Shiratori, H., Saijoh, Y., and Hamada, H. (2002). Determination of left-right patterning of the mouse embryo by artificial nodal flow. Nature 418, 96–99. doi: 10.1038/nature00849
Nonaka, S., Tanaka, Y., Okada, Y., Takeda, S., Harada, A., Kanai, Y., et al. (1998). Randomization of left-right asymmetry due to loss of nodal cilia generating leftward flow of extraembryonic fluid in mice lacking KIF3B motor protein. Cell 95, 829–837. doi: 10.1016/S0092-8674(00)81705-5
Nonaka, S., Yoshiba, S., Watanabe, D., Ikeuchi, S., Goto, T., Marshall, W. F., et al. (2005). De novo formation of left-right asymmetry by posterior tilt of nodal cilia. PLoS Biol. 3:e268. doi: 10.1371/journal.pbio.0030268
Okada, Y., and Hirokawa, N. (1999). A processive single-headed motor: kinesin superfamily protein KIF1A. Science 283, 1152–1157. doi: 10.1126/science.283.5405.1152
Okada, Y., and Hirokawa, N. (2009). Observation of nodal cilia movement and measurement of nodal flow. Methods Cell Biol. 91, 265–285. doi: 10.1016/S0091-679X(08)91014-1
Okumura, T., Sasamura, T., Inatomi, M., Hozumi, S., Nakamura, M., Hatori, R., et al. (2015). Class I myosins have overlapping and specialized functions in left-right asymmetric development in Drosophila. Genetics 199, U1183–U1493. doi: 10.1534/genetics.115.174698
Petzoldt, A. G., Coutelis, J. B., Géminard, C., Spéder, P., Suzanne, M., Cerezo, D., et al. (2012). DE-Cadherin regulates unconventional Myosin, ID and Myosin IC in Drosophila left-right asymmetry establishment. Development 139, 1874–1884. doi: 10.1242/dev.047589
Pruyne, D., Evangelista, M., Yang, C., Bi, E., Zigmond, S., Bretscher, A., et al. (2002). Role of formins in actin assembly: nucleation and barbed-end association. Science 297, 612–615. doi: 10.1126/science.1072309
Pyrpassopoulos, S., Feeser, E. A., Mazerik, J. N., Tyska, M. J., and Ostap, E. M. (2012). Membrane-bound myo1c powers asymmetric motility of actin filaments. Curr. Biol. 22, 1688–1692. doi: 10.1016/j.cub.2012.06.069
Raymond, M. J., Ray, P., Kaur, G., Singh, A. V., and Wan, L. Q. (2016). Cellular and nuclear alignment analysis for determining epithelial cell chirality. Ann. Biomed. Eng. 44, 1475–1486. doi: 10.1007/s10439-015-1431-3
Robertson, C. W. (1936). The metamorphosis of Drosophila melanogaster, including an accurately timed account of the principal morphological changes. J. Morphol. 59, 351–399.
Rolan-Alvarez, E., and Rolan, E. (1995). Occurrence true symmetry inversion in gastropods: an evolutiony perspective. Thalassas 11, 93–104.
Sato, K., Hiraiwa, T., Maekawa, E., Isomura, A., Shibata, T., and Kuranaga, E. (2015a). Left-right asymmetric cell intercalation drives directional collective cell movement in epithelial morphogenesis. Nat. Commun. 6:10074. doi: 10.1038/ncomms10074
Sato, K., Hiraiwa, T., and Shibata, T. (2015b). Cell chirality induces collective cell migration in epithelial sheets. Phys. Rev. Lett. 115:188102. doi: 10.1103/PhysRevLett.115.188102
Shibazaki, Y., Shimizu, M., and Kuroda, R. (2004). Body handedness is directed by genetically determined cytoskeletal dynamics in the early embryo. Curr. Biol. 14, 1462–1467. doi: 10.1016/j.cub.2004.08.018
Spéder, P., Adám, G., and Noselli, S. (2006). Type ID unconventional myosin controls left-right asymmetry in Drosophila. Nature 440, 803–807. doi: 10.1038/nature04623
Suzanne, M., Petzoldt, A. G., Spéder, P., Coutelis, J. B., Steller, H., and Noselli, S. (2010). Coupling of apoptosis and L/R patterning controls stepwise organ looping. Curr. Biol. 20, 1773–1778. doi: 10.1016/j.cub.2010.08.056
Tamada, A., and Igarashi, M. (2017). Revealing chiral cell motility by 3D Riesz transform-differential interference contrast microscopy and computational kinematic analysis. Nat. Commun. 8:2194. doi: 10.1038/s41467-017-02193-w
Tamada, A., Kawase, S., Murakami, F., and Kamiguchi, H. (2010). Autonomous right-screw rotation of growth cone filopodia drives neurite turning. J. Cell Biol. 188, 429–441. doi: 10.1083/jcb.200906043
Taniguchi, K., Maeda, R., Ando, T., Okumura, T., Nakazawa, N., Hatori, R., et al. (2011). Chirality in planar cell shape contributes to left-right asymmetric epithelial morphogenesis. Science 333, 339–341. doi: 10.1126/science.1200940
Tee, Y. H., Shemesh, T., Thiagarajan, V., Hariadi, R. F., Anderson, K. L., Page, C., et al. (2015). Cellular chirality arising from the self-organization of the actin cytoskeleton. Nat. Cell Biol. 17, 445–457. doi: 10.1038/ncb3137
Tzolovsky, G., Millo, H., Pathirana, S., Wood, T., and Bownes, M. (2002). Identification and phylogenetic analysis of Drosophila melanogaster myosins. Mol. Biol. Evol. 19, 1041–1052. doi: 10.1093/oxfordjournals.molbev.a004163
Wan, L. Q., Ronaldson, K., Park, M., Taylor, G., Zhang, Y., Gimble, J. M., et al. (2011). Micropatterned mammalian cells exhibit phenotype-specific left-right asymmetry. Proc. Natl. Acad. Sci. U.S.A. 108, 12295–12300. doi: 10.1073/pnas.1103834108
Wells, R. E., Barry, J. D., Warrington, S. J., Cuhlmann, S., Evans, P., Huber, W., et al. (2013). Control of tissue morphology by Fasciclin III-mediated intercellular adhesion. Development 140, 3858–3868. doi: 10.1242/dev.096214
Wood, W. B. (1991). Evidence from reversal of handedness in C. elegans embryos for early cell interactions determining cell fates. Nature 349, 536–538. doi: 10.1038/349536a0
Worley, K. E., Shieh, D., and Wan, L. Q. (2015). Inhibition of cell-cell adhesion impairs directional epithelial migration on micropatterned surfaces. Integr. Biol. 7, 580–590. doi: 10.1039/C5IB00073D
Xu, J., Van Keymeulen, A., Wakida, N. M., Carlton, P., Berns, M. W., and Bourne, H. R. (2007). Polarity reveals intrinsic cell chirality. Proc. Natl. Acad. Sci. U.S.A. 104, 9296–9300. doi: 10.1073/pnas.0703153104
Yamanaka, H., and Kondo, S. (2015). Rotating pigment cells exhibit an intrinsic chirality. Genes Cells 20, 29–35. doi: 10.1111/gtc.12194
Keywords: cell chirality, left-right asymmetry, F-actin, Myosin I, Drosophila
Citation: Inaki M, Sasamura T and Matsuno K (2018) Cell Chirality Drives Left-Right Asymmetric Morphogenesis. Front. Cell Dev. Biol. 6:34. doi: 10.3389/fcell.2018.00034
Received: 17 November 2017; Accepted: 14 March 2018;
Published: 03 April 2018.
Edited by:
Takaaki Matsui, Nara Institute of Science and Technology (NAIST), JapanReviewed by:
Hidetaka Shiratori, Kyoto Sangyo University, JapanCopyright © 2018 Inaki, Sasamura and Matsuno. This is an open-access article distributed under the terms of the Creative Commons Attribution License (CC BY). The use, distribution or reproduction in other forums is permitted, provided the original author(s) and the copyright owner are credited and that the original publication in this journal is cited, in accordance with accepted academic practice. No use, distribution or reproduction is permitted which does not comply with these terms.
*Correspondence: Kenji Matsuno, a21hdHN1bm9AYmlvLnNjaS5vc2FrYS11LmFjLmpw
Disclaimer: All claims expressed in this article are solely those of the authors and do not necessarily represent those of their affiliated organizations, or those of the publisher, the editors and the reviewers. Any product that may be evaluated in this article or claim that may be made by its manufacturer is not guaranteed or endorsed by the publisher.
Research integrity at Frontiers
Learn more about the work of our research integrity team to safeguard the quality of each article we publish.