- Neuroimmunology Unit, Division of Neuroscience, Institute of Experimental Neurology, San Raffaele Scientific Institute, Milan, Italy
The physiological and pathological properties of the neural germinal stem cell niche have been well-studied in the past 30 years, mainly in animals and within given limits in humans, and knowledge is available for the cyto-architectonic structure, the cellular components, the timing of development and the energetic maintenance of the niche, as well as for the therapeutic potential and the cross talk between neural and immune cells. In recent years we have gained detailed understanding of the potentiality of neural stem cells (NSCs), although we are only beginning to understand their molecular, metabolic, and epigenetic profile in physiopathology and, further, more can be invested to measure quantitatively the activity of those cells, to model in vitro their therapeutic responses or to predict interactions in silico. Information in this direction has been put forward for other organs but is still limited in the complex and very less accessible context of the brain. A comprehensive understanding of the behavior of endogenous NSCs will help to tune or model them toward a desired response in order to treat complex neurodegenerative diseases. NSCs have the ability to modulate multiple cellular functions and exploiting their plasticity might make them into potent and versatile cellular drugs.
Introduction
Although it has been thought for a long time that mammalian neurogenesis occurs only during embryonic and perinatal stages, young neurons are continuously incorporated into the adult brain as demonstrated by Altman and Das already in the early sixties (Altman, 1962; Altman and Das, 1965). Indeed, neural stem cells (NSCs), residing in the brain of most adult mammals in the so-called “neurogenic niches,” sustain neurogenesis throughout life. It is estimated that 700 new neurons are generated every day by the neuropoietic niche in an adult human hippocampus, outlining one aspect of the plasticity/renewal capacity of NSCs (Knoth et al., 2010; Spalding et al., 2013). As for other stem cells, the specialized microenvironment of the neurogenic niche ensures not only NSC self-renewal but also differentiation, mainly into neurons. However, neurogenesis is not the only activity of NSCs in the adulthood. As a matter of fact, recent studies indicate that adult NSCs residing within the sub-ventricular zone (SVZ) might physiologically exert alternative functions to cell replacement, the so-called non-neurogenic functions (Martino et al., 2014), mainly aimed at protecting CNS homeostasis, in both physiological and pathological conditions. They regulate and are regulated by several signaling pathways (Faigle and Song, 2013) that, tuning the evolution of progenitor proliferation, division, and migration, can per se also impact the composition of the niche (Preston and Sherman, 2011; Gattazzo et al., 2014). Neighboring cells, the vasculature and the cerebrospinal fluid constitute the main routes through which molecular signals reach NSCs and affect their behavior.
Overall, knowing the physiological properties of NSCs and what changes in pathological conditions opens up the possibility of exploiting NSC plasticity for preventive/therapeutic purposes.
This review will primarily focus on (i) the properties of precursors of the adult neurogenic niches of the central nervous system (CNS); (ii) the mechanisms of inter- and intra-cellular communication of NSCs and other cells, resident or not in the niche, in physio- and patho-logical conditions, with focus on multiple sclerosis (MS) and ischemic stroke, neurodegenerative disorders of the brain that unfold acute and chronic consequences.
What Defines a NSC and a NSC Niche?
At the onset of murine neurogenesis, at embryonic day 9.5, the precursors in the CNS are neuroepithelial cells (NECs) that form a tube with a central canal (Taverna et al., 2014). NECs are highly proliferative and initially divide symmetrically to expand; afterwards they convert into radial glial cells (RGCs) that divide both symmetrically and asymmetrically. Basal processes of RGCs are used by newborn neurons as guiding scaffolds while they migrate away from the germinal niche toward the pial surface.
Although most CNS regions largely extinguish their NSC pool after development, discrete areas of the adult brain retain NSCs and active neurogenesis throughout life (Ming and Song, 2005, 2011). Namely, the striatal subventricular zone (SVZ) and the hippocampal dentate gyrus (DG, subgranular zone SGZ) are the most extensively characterized adult neurogenic niches. However, according to the most recent evidences, sites of neurogenesis are present also in the ependyma (Alvarez-Buylla and Lim, 2004; Bjornsson et al., 2015), near the third and fourth ventricle, in the forebrain, in the striatum, in the amygdala, in the hypothalamus, in the substantia nigra and in the subcortical white matter or spinal cord root ganglia (Bernier et al., 2002; Lie et al., 2002; Kokoeva et al., 2005; Chang et al., 2008; Ernst et al., 2014; Muratori et al., 2015; Stolp and Molnar, 2015). Proliferating cells from those regions, namely somatic NSCs, can be isolated and established as virtually perpetual cell lines in response to fibroblast growth factor 2 (FGF-2) and epidermal growth factor (EGF) similar to their embryonic counterparts (Temple, 2001).
In the adult neural stem cell niche, NSCs, immature neurons, supporting astrocytes, blood vessels and epithelial ciliated cells are in close contact and the vasculature with “leaky” features supports adult neurogenesis (Butti et al., 2014). In the mouse, the SVZ contains slowly dividing progenitors that can be subdivided into two types: type B1 cells, in close contact with both the cerebrospinal fluid (CSF) and the blood vessels of the SVZ, and type B2 cells, closer to the striatum (Ihrie et al., 2011). B1 cells give rise to transit amplifying cells (type C cells), located in close proximity to blood vessels, and along with B2 cells, they form a glial supportive sheath around their more differentiated progeny and migrating neuroblasts, type A cells, that originate from type C cells. Type A cells migrate tangentially to form the rostral migratory stream (RMS) to the olfactory bulb for terminal differentiation. Once in the olfactory bulb, the neuroblasts defasciculate from the stream and migrate radially to their site of terminal differentiation into neurons (Alvarez-Buylla et al., 2000). SVZ-NSCs give rise also to oligodendrocyte precursors and mature oligodendrocytes, continuously replenishing cells in the corpus callosum (Menn et al., 2006).
The primary role of the neurogenic SGZ niche instead is to generate new granule cells, primary excitatory neurons that support hippocampus-dependent cognitive functions (Zhao et al., 2008). Stem cells of the SGZ give rise to radial astrocytes that convert into immature progenitors (Type 1, the counterpart of type B in the SVZ) and eventually into neuroblasts (Type 2, the counterpart of Type C-A cells in the SVZ) (Zhao et al., 2008). Complete depletion either of type 2 or type C cells, respectively in the SGZ and SVZ, (non-radial glia like cells) stops neurogenesis (Doetsch et al., 1999; Ahn and Joyner, 2005), although, but infrequently, dividing radial glia could sustain neurogenesis as well (Seri et al., 2004). NSCs of the SGZ are also in close contact with blood vessels and endothelial cells, that act as scaffolding cells for NSCs (Palmer et al., 2000) and play a major role in directing NSC specification (Shen et al., 2004, 2008; Tavazoie et al., 2008; Kokovay et al., 2010).
This is the conventional cell classification for the mouse neural germinal center, which has been better characterized.
In humans the SVZ differs from the one in rodents because it organizes into four layers instead: the ependymal layer, the hypocellular gap, the astrocytic ribbon, and the transitional zone to the parenchyma, rich in myelin and oligodendrocytes (Quinones-Hinojosa et al., 2006). Migrating neuron-like cells can occasionally be found in the layers II and III as individual cells (Sanai et al., 2004; Quinones-Hinojosa et al., 2006). In detail, layer I consists of an ependymal monolayer lining the ventricular wall with astrocytic processes contacting the ventricular wall. Layer II, also known as the gap region, is rich in GFAP+ processes, with only some neuroblasts in the anterior regions. Ependymal cells send basal processes into Layer II, making critical contacts with the underlying basal lamina. Layer II may function as the corridor for neuroblast migration. Layer III is the proliferative region of the human SVZ, with GFAP+/Ki67+ and CD133+ cells. Few neuroblasts are present in the human SVZ, compared to the rodent, and are found mainly in Layer III. Some ependymal cells, which typically comprise the epithelial barrier of the ventricles, have motile cilia and are found in small clusters (4–14 cells) in Layer III (Quinones-Hinojosa et al., 2006; Kam et al., 2009). Layer IV represents the first portion of brain parenchyma away from the parietal ventricle and where the first evidence of neurons is found.
Extracellular Cues and Intrinsic Genetic Programs Control the NSC Functions in Physiological Conditions in the Adult Brain
Main feature of NSCs is their plasticity (Suh et al., 2009; Martino et al., 2011). The two major adult stem neurogenic niches take advantage of different mechanisms to exploit this property, which manifests as self renewal capacity, quiescence, metabolic modulation, homing, differentiation capacity, cellular cross-talk, and immune surveillance (Figure 1A, Table 1).
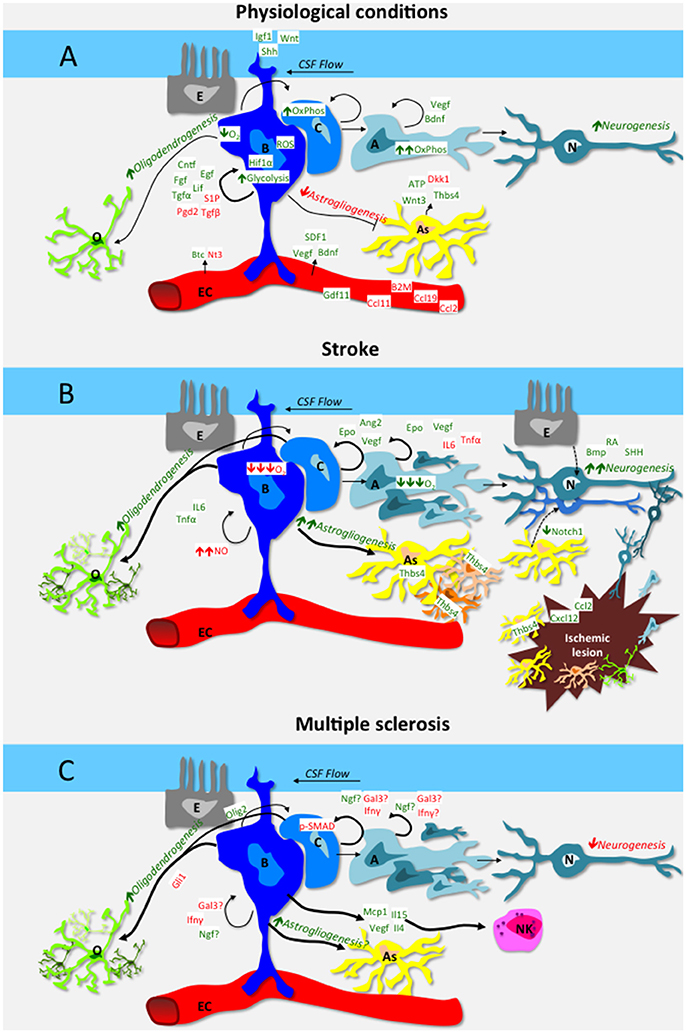
Figure 1. Schematic representation of the interplay among cells of the neural stem cell niche of the subventricular zone (A,B,C), vascular endothelial cells (EC), ependymal cells (E), differentiated oligodendrocytes (O), astrocytes (As), and neurons (N). Green is used for positive regulators of neural stem cell function, red for inhibitory regulators. Mechanisms are in italic. (A) depicts mechanisms and factors in physiological conditions: in steady-state, B cells self renewal is promoted by niche-derived factors such as CNTF, EGF, FGF2, LIF, PGD2, S1P, and TGFα, as well as by systemic-derived factors as VEGF, BDNF and SDF1. The cerebrospinal fluid also contributes actively to niche homeostasis via IGF1, Wnt and Shh that signal to B cells via their apical cilium. Aging increases neurogenesis-inhibitory factors such as B2M, CCL2, CCL11, CCL19, while pro-neurogeneic factors as GDF11 decrease. The hypoxic milieu of the niche favors B cell quiescence, while C and A precursors rely on oxidative phosphorylation. In steady-state, astrogliogenesis is generally inhibited, while growing astrocytes secrete both pro-neurogeneic and anti-neurogeneic mediators. Nonetheless, a basal level of oligodendrogenesis and in particular neurogenesis occurs also during the steady state. (B) depicts mechanisms and factors that are altered in the SVZ niche in the context of stroke. Ischemia increases Epo, Ang2 and VEGF as well as morphogens BMP, RA and SHH, which stimulate neurogenesis. Moreover, chemotactic and growth factors produced within the lesion (e.g. CXCL12, CCL2) guide newly formed glial and neuronal cells toward the ischemic area. Hypoxia and increased nitric oxide inhibit B cell cycling while low O2 promotes precursor differentiation. Direct transdifferentiation (dashed arrow lines) from ependymal cells and astrocytes to neurons might also contribute to stroke-induced neurogenesis. Stroke per se increases oligodendrogenesis and astrogliogenesis as well. In particular, SVZ-derived, Thbs4 positive astrocytes are pivotal in containing tissue damage and preventing hemorrhagic transformation. (C) depicts mechanisms and factors that are altered in the SVZ niche in the context of MS. Neurogenesis in inhibited by IFNγ, Gal3 and upregulation of phoshorylated-SMAD (pSMAD) in neurogenic precursors. IFNγ also inhibits oligodendrogenesis via upregulation of Gli1. NSCs produce a wide array of soluble mediators, including IL15 that attract NK cells, which in turn contribute to the neurogenic niche dysfunction observed in MS models.
The maintenance of the neurogenic niche itself and the renewal capacity depends on active intrinsic genetic (Tirone et al., 2013) and epigenetic (Liu et al., 2010; Yao et al., 2016) programs along with microenvironment-dependent specific properties. Autocrine regulators of NSC proliferation, such as transforming growth factor α (Tropepe et al., 1997; Guerra-Crespo et al., 2009) and β (Dias et al., 2014), amphyregulin, fibroblast growth factor-2 (FGF-2), insulin-like growth factor 2 (IGF2) (Marques et al., 2011) are released from specific subsets of NSCs along with leukemia inhibitory factor (LIF), ciliary neurotrophic factor (CNTF) that promote proliferation (Emsley and Hagg, 2003; Lee et al., 2013) and sphingosine-1-phosphate (S1P) or prostaglandin D2 (PGD2) that instead maintain quiescence (Codega et al., 2014; Chaker et al., 2016). The environment-contribution to NSCs plasticity encompasses several elements as described below.
Close anatomical association between NSCs and the vascular structure (vascular niche) is preserved both in the SVZ and SGZ and vascular endothelial cells of the niche secrete Notch ligands Jagged1, Jagged2, and Delta-like-4, crucial factors for self-renewal and neurogenesis (Shen et al., 2004; Androutsellis-Theotokis et al., 2010; Lu et al., 2011). Recent evidence supports the hypothesis that the cross-talk between blood vessels and NSCs is bi-directional: NSCs can indeed provide juxtacrine and paracrine signals to drive endothelial cells (Chou and Modo, 2016) and promote angiogenesis (Hicks et al., 2013). Moreover, vascular endothelial growth factor (VEGF) is a shared cue both for angiogenesis and neurogenesis because on one side it promotes the angiogenic development of capillaries, on the other, the secretion of neurogenic molecules by proximal endothelial cells (Jin et al., 2002; Cao et al., 2004; Kim et al., 2004; Udo et al., 2008; Ruiz de Almodovar et al., 2009). Further, other cues secreted from vascular endothelial cells such as neurotrophin 3 (NT3) or betacellulin (BTC) maintain quiescence or promote proliferation of NSCs, respectively (Gomez-Gaviro et al., 2012; Delgado et al., 2014), although there is evidence of NT3 effect on differentiation (Shimazu et al., 2006), while stromal derived factor-1 (SDF1) stimulates the motility of type A, B, and C neuroblasts (Kokovay et al., 2010).
The extracellular matrix (ECM), a dynamic and complex environmental element characterized by biophysical and biochemical properties specific for each tissue and able to regulate cell behavior, represents also an essential player in stem cell niche (Gattazzo et al., 2014). Extensions of the extracellular matrix known as fractones project from the blood vessels of the subventricular plexus as thin, highly branching ECM stalks that expand into bulbs where they contact the basal surface of the ependymal layer (Mercier et al., 2002). Fractones are enriched in laminin, heparan sulfate, perlecan, nidogen, and collagens. Those associations are able to bind several growth factors, suggesting that they may play a role in concentrating, activating, and presenting trophic factors to cells within the niche (Kerever et al., 2007).
NSCs receive inputs also from other cells such as microglia which reside in close proximity to NSCs of the niche. Indeed, resting microglia secrete factors that promote NSC niche maintenance and, at the same time, astrocyte differentiation of striatal NSCs via the Jak/Stat3 pathway (Zhu et al., 2008). Moreover, microglia contribute to the development of cytoarchitectonic and functional differences across cortical areas of the brain, secreting growth factors, and cytokines that tightly regulate the neurogenic process (Kim and de Vellis, 2005; Harry, 2013; Su et al., 2014). Conversely, in vitro, microglia promote neuronal differentiation, but not maintenance or self-renewal (Walton et al., 2006). On the other side, activated microglia inhibit neurogenesis (Sierra et al., 2014) favoring gliogenesis via tumor necrosis factor-α (TNFα) (Carpentier and Palmer, 2009), and when exposed to interleukin 4 (IL4) and interferon-γ (IFNγ), they secrete insulin-like growth factor 1 (IGF1) and promote neuronal differentiation of NSCs (Butovsky et al., 2006). Conversely, NSCs can also influence microglia via VEGF that in turn modulates microglial activation, proliferation and phagocytosis (Mosher et al., 2012).
Stem plasticity is modulated by other cell types as well, namely astrocytes, residing in close proximity with NSCs both in the SVZ and in the SGZ. Their contribution to NSC proliferation is likely exploited via ATP release (Cao et al., 2013) while Wnt3, neurogenesin-1 (NG1), thrombospondin-1 (TSP1) as well as interleukin-1β (IL1β) and interleukin-6 (IL6) promote hippocampal neurodifferentiation (Ueki et al., 2003; Lie et al., 2005; Barkho et al., 2006; Lu and Kipnis, 2010). Of note, when FGF2-producing astrocytes age, neurogenesis is impaired (Shetty et al., 2005).
Signals arising from the ependymal and meningeal cells and released in the CSF may also influence NSC activity (Lim et al., 2000; Siegenthaler et al., 2009). Indeed, NSCs possess primary cilia which sense liquor morphogens, such as FGF2, IGF2 (effective at lower level postnatally), Wnt and Sonic Hedgehog (SHH) (Corbit et al., 2005; Rohatgi et al., 2007; Breunig et al., 2008; Kim et al., 2010; Ihrie et al., 2011; Lehtinen and Walsh, 2011) and, possibly, the CSF flow itself. The latter indeed can, via mechano-sensing signaling, promote proliferation and differentiation (Li et al., 2011; Arulmoli et al., 2015; Jagielska et al., 2017).
Moreover, cytoarchitectonic innervation via GABA (γ-Aminobutyric acid)-, glutamin-, colin-, serotonin-, and dopamin-ergic neurons sustains neurogenesis in the niche (Suh et al., 2009; Song et al., 2012; Paez-Gonzalez et al., 2014; Young et al., 2014; Alunni and Bally-Cuif, 2016; Chaker et al., 2016). Conversely, it is not clear yet whether NSCs have an impact on axons and neuronal circuitry (Zhang Y. et al., 2016).
The niche is also very much dependent on and prompt to metabolic changes. While lipid metabolism maintains proliferation and neurogenesis (structural and energy support), glycolysis regulates NSCs development and differentiation (Knobloch et al., 2013). The metabolic activity strongly depends on oxidative saturation because in mammalian CNS oxygen regulates the growth and differentiation state of stem cells (De Filippis and Delia, 2011; Ivanovic and Vlaski-Lafarge, 2016; Sandvig et al., 2017). Dividing progenitor cells depend more on glycolysis, whereas differentiated progeny relies on energetically efficient oxidative phosphorylation occurring at low oxygen concentration. Apart from those, other important metabolic pathways are active in neural stem cells, such as (i) glycogen synthesis or glutamine/folate metabolism (Goodman and Hajihosseini, 2015); (ii) phosphatidylinositol 3-kinase/AKT (PI3K/AKT) growth factor pathway insulin-dependent; (iii) mTOR pathway nutrient-dependent (Rafalski et al., 2012); (iv) AMP-activated protein kinase (AMPK)/LKB1 pathway, sensor of intracellular adenosine monophosphate (AMP) to ATP ratios, and (v) the sirtuin pathway, metabolic sensors of NAD (nicotinamide adenine dinucleotide) level and epigenetic repressors (Folmes et al., 2012; Shyh-Chang et al., 2013).
Cell to cell contact has also been shown to play a role in exploiting the plasticity of NSCs. Astrocytes of the niche negatively control neuronal differentiation through astrocyte-secreted factors such as insulin like growth factor binding protein 6 (IGFBP6) and decorin (Barkho et al., 2006; Wilhelmsson et al., 2012), while astrocytic ephrin-B2 positively regulates proliferation (Ashton et al., 2012).
Another peculiar property of NSCs consists in their capacity to migrate where their replacement or bystander effect is needed. Indeed, endogenous NSCs migrate out of the niche at physiological rate to maintain brain homeostasis, either differentiating or releasing tropic factors (Shen et al., 2004; Kokaia et al., 2012). When transplanted during acute or chronic neuroinflammatory disorders, NSCs show remarkable pathotropism: they follow the molecular gradient of chemotactic inflammatory factors (Muller et al., 2006) and reach the damaged site where they start secreting a series of molecules (i.e., bone morphogenetic protein 4, noggin, Notch, Jagged, and SHH) to recapitulate the microenvironment of the SVZ niche (atypical ectopic perivascular niche) (Pluchino et al., 2003, 2010; Irvin et al., 2004; Stidworthy et al., 2004; Martino and Pluchino, 2006; Bonaguidi et al., 2008).
Of note, beside communication via soluble factors, NSCs sense and can release intracellular messengers wrapped in vesicles (Cossetti et al., 2014). Although their role in adult NSCs is almost unexplored (more is known for other types of stem cells), vesicles can transfer information in the form of mRNA, ribosomal RNA, long non-coding RNA, microRNA, DNA, protein, or lipids (Thery et al., 2002; Huang et al., 2013; Batiz et al., 2015; Kirby et al., 2015).
NSC Function in Neuroinflammation and Neurodegeneration. Focus on Multiple Sclerosis and Ischemic Stroke.
The peculiar plasticity of the CNS and of its NSCs manifests after CNS injury, when (i) proliferation and differentiation of NSCs is enhanced; (ii) striatal spiny interneurons and glutamatergic neurons are ectopically found in the injured cortex and in the striatum after stroke (Thored et al., 2007) while migrating neuroblasts become oligodendrocytes in area of demyelination after exiting the niche; (iii) ependymal cells behave as progenitors (Luo et al., 2008) and directly convert into neurons (Carlen et al., 2009); (iv) reactive astrocytes in ischemic brain injury exhibit self renewal capacity and multipotency (Buffo et al., 2008; Gabel et al., 2016).
The fact that non-neurogenic precursors can convert into neurogenic cells clearly highlights how exogenous factors can trigger plasticity within and outside of the niche (Carlen et al., 2009; Magnusson et al., 2014; Shetty and Hattiangady, 2016). Moreover, during pathology and steady-state, NSCs also exert trophic non-neurogenic functions which are crucial to maintain brain homeostasis.
In the following section we will explore how NSC niches function during CNS injury, with focus on stroke, multiple sclerosis and their animal models (Figures 1B,C, Table 1).
Effect of Oxygen Supply on Neural Stem Cell Plasticity, Connection with Stroke, and Multiple Sclerosis
In physiological conditions, neural stem cells are exposed to an oxygen concentration between 2.5 and 5.0%, which promotes NSC self-renewal via VEGF and erythropoietin (EPO) production induced by hypoxia inducible factor 1α, HIF1α (Pavlica et al., 2012; Li et al., 2014).
In the quiescent state, NSC mitochondria are quite immature with globular shape, do not depend much for energy on oxidative phosphorylation (OXPHOS), rather on glycolysis, with high lactate production (Zheng et al., 2016). Although glycolysis produces less ATP than mitochondrial OXPHOS, the pathway is very fast in NSCs (Ito and Suda, 2014). Their anaerobic metabolism is sustained mainly by mitochondria uncoupling protein 2 (UCP2), high level of hexokinase II and low pyruvate dehydrogenase to keep under control the production of reactive oxygen species (ROS) (Madhavan et al., 2006; Zheng et al., 2016). In this way, DNA and proteins of the cells are protected from ROS-dependent potential damage. Further, ROS, produced in limited amount in physiological conditions and normally neutralized, are also beneficial because they trigger self-renewal and neurogenesis (Le Belle et al., 2011). Conversely, differentiated cells present elongated, crystae-rich mitochondria, higher ratio of mitochondrial glucose oxidation (OXPHOS) over glycolysis as metabolic support (Zhang et al., 2014; Marcialis et al., 2016). Moreover, NSCs increase Krebs' cycle functionality and decrease lactate production, concurrent with increased number and total mitochondrial mass (Sola et al., 2013).
On the other side, the first consequence of the blood flow occlusion in brain ischemia is hypoxic injury that causes extensive neural cell death (Niquet et al., 2003). Nonetheless and surprisingly, hypoxia, while being detrimental in the acute phase of stroke, in a second step, contributes to promote neurogenesis (Arvidsson et al., 2002; Tonchev et al., 2003; Jin et al., 2006; Macas et al., 2006; Minger et al., 2007; Wang et al., 2011; Zhang and Chopp, 2016), to support replenishment of neurons in the RMS, migration in the region of ischemic brain injury, and growth of oligodendrocyte progenitors that disperse to the gray and white matter (Zhang et al., 2001, 2012; Parent et al., 2002; Minger et al., 2007; Li et al., 2010a; Zhang and Chopp, 2016).
Hypoxia has recently emerged as a concurrent complication of disease progression also in MS. Indeed, important lack of oxygen, occurring as a consequence of inflammation, has been measured in brain gray matter regions of MS patients, where it can per se be considered co-causative for neurodegeneration (Haider et al., 2014; Yang et al., 2015). Since hypoxia and inflammation are strictly linked, it is still difficult to tease apart their respective contributions in MS (Sun et al., 1998; Lassmann, 2003; Davies et al., 2013). It looks like hypoxia can occur in MS patients as a consequence of increased oxygen demand. When this request is not satisfied, hypoxia can be further detrimental for surrounding neural stem cells (Trapp and Stys, 2009).
Effect of Inflammation on Neural Stem Cell Plasticity, Connection with Stroke, and Multiple Sclerosis
It has been well-documented that the brain cannot be simplistically considered an immune privileged site (Kleine and Benes, 2006) and actually immune system activation in the brain exerts both damaging and beneficial effects on CNS functions (Martino, 2004), depending on the onset of the inflammation, on the cell types involved in the process and on the chronicity of the response (Crutcher et al., 2006; Kyritsis et al., 2012).
NSCs share with the immune system an array of secreted mediators and receptors, which are all relevant for the maintenance of the neurogenic niche and, at the same time, represent the prerequisite for the interaction of NPCs with the microenvironment, especially during neuroinflammation (De Feo et al., 2012; Kokaia et al., 2012).
Indeed, inflammation, via its associated cues (i.e. cytokines, chemokines, chemical species….), strongly impacts structure and function of the stem cell niche, acts directly on NSCs and affects tissue restoration/regeneration via microglia and astrocyte activation (Ekdahl et al., 2003; Pluchino et al., 2008; Pourabdolhossein et al., 2017).
In homeostatic conditions, microglia are ramified in shape to maintain surveillance. Upon pathogen invasion or insults, they retract the protrusions, become amoeboid, increase their migratory behavior, and secrete cytokines. IL6, TNFα, IL1β, and complement 1 subunit q (C1q) are among the most potent microglia–derived cytokines, able to compromise the niche environment, inhibit neurogenesis (Vallieres et al., 2002; Monje et al., 2003), induce oligodendrogenesis (Valerio et al., 2002) and a subtype of reactive astrocytes (Liddelow et al., 2017). Further, while NSC-derived nitric oxide synthase promotes release of small amounts of nitric oxide (NO) with neurogenic properties (Carreira et al., 2010; Luo et al., 2010), abundant NO released by microglia or astrocytes in inflammatory conditions (i) inhibits proliferation of NSCs acting on the EGF receptor (Carreira et al., 2014) or on the transcription factor complex Neuron-Restrictive Silencer Factor /RE1-Silencing Transcription factor, NRSF/REST (Bergsland et al., 2014) and (ii) promotes gliogenesis (Bergsland et al., 2014).
IFNγ, a pleiotropic cytokine, has a central role in regulating NSCs proliferation and quiescence (Kulkarni et al., 2016). In inflammatory conditions, such as experimental autoimmune encephalomyelitis (EAE), the preclinical model of MS, IFNγ dramatically decreases progenitor proliferation (Pluchino et al., 2008; Pereira et al., 2015) and inhibits the recruitment of newborn neurons to the olfactory bulb (OB). IFNγ might also play a crucial role in regulating glioma-associated oncogene homolog-1 (Gli1), a Shh-induced trasncription factor that drives the oligodendroglial fate of NSCs (Wang et al., 2008; Li et al., 2010b; Samanta et al., 2015).
The complement system also inhibits NSC activity as demonstrated with complement receptor 2 (Cr2) knock out mice that have increased basal neurogenesis (Moriyama et al., 2011). Moreover, lipid modifiers present in inflammation, such as leukotriene, can negatively regulate NSC proliferation likely via TNFα and IL1β (Bonizzi et al., 1999; Wu et al., 2013). Of note, montelukast, a leukotriene receptor blocker protects from this event (Marschallinger et al., 2015).
IL6 is another crucial mediator of NSC function and its induction, e.g., during maternal infection and stroke, permanently perturbs NSC proliferation and neurogenesis (Gallagher et al., 2013; Chucair-Elliott et al., 2014; Meng et al., 2015).
Conversely, the anti-inflammatory interleukin-10 (IL10) in rodents keeps NSCs in an undifferentiated proliferation state, rather than promoting differentiation (Ben-Hur et al., 2003; Perez-Asensio et al., 2013; Wang et al., 2015).
Nevertheless, as anticipated, inflammation can be also beneficial for NSCs. First, the immune system in general and T cells in particular are involved in maintaining niche neurogenesis, as indeed genetic T-cell depleted mice present compromised cognitive functions (Ziv et al., 2006). Moreover, chemokines such as monocyte chemoattractant protein 1 (MCP1) or SDF1, released in inflammatory conditions, promote migration of NSCs to site of injury and local TNFα or IL1β trigger their proliferative capacity (Widera et al., 2006). Second, injection of moderately activated microglia can regulate brain homeostasis and neurogenesis (Butovsky et al., 2006; Bachstetter et al., 2011).
Further, NSCs directly produce inflammatory chemokines (Covacu et al., 2009), promoting a feed forward loop at sites of tissue damage (Imitola et al., 2004; Belmadani et al., 2006; Widera et al., 2006; Wang et al., 2007). NSCs can also directly change inflammatory responses through immunomodulatory factors (Pluchino et al., 2005; Ben-Hur, 2008; Yong and Rivest, 2009; Butti et al., 2012) or trophic factors (Huang et al., 2014). In particular, SVZ-NSCs can protect striatal neurons from the excitotoxic damage occurring in stroke and epilepsy, releasing endogenous endocannabinoids, likely upon inflammatory stimuli (Butti et al., 2012). Endogenous NSCs also secrete MCP1, VEGF, IL4, and interleukin-15 (IL15), the latter known to retain NK cells in the chronic phase of EAE in mice and of MS in humans. Indeed, in the SVZ, NK cells contribute to the dysfunction of the neurogenic niche observed in EAE by killing stem cells (Liu et al., 2016). Moreover, inflammatory cytokines lead to metabolic reprogramming of the arginase pathway in NSCs ultimately impacting on the NSC mediated anti-proliferative effect on T cells (Drago et al., 2016). Further, it has been reported that human NSCs hinder the differentiation of myeloid precursor cells into immature dendritic cells and the final maturation into functional antigen presenting cells (Pluchino et al., 2009).
In stroke, immune cell infiltration and inflammation take place as secondary events to hypoxic injury, which is responsible of the massive neuronal death. Upon brain injury, the blood-brain barrier (BBB) is disrupted, its permeability increases, migrating immature neurons associate with the angiogenic fenestrated endothelium, thus mimicking the neurogenic niche structure (Ohab et al., 2006; Ohab and Carmichael, 2008). Neutrophils and innate immune cells are the first players, although also T and B cells cross the damaged-BBB, inducing a rapid adaptive autoimmune response to neuronal antigens (Chamorro et al., 2012). As consequence of inflammation, neurogenesis is activated in stroke (Wang et al., 2011; Katajisto et al., 2015) supported by soluble factors such as bone morphogenic protein (Forni et al., 2013), retinoic acid (Plane et al., 2008), sonic hedgehog (Cheng et al., 2015), C-C motif chemokine Ligand 2 (CCL2) (Osman et al., 2016) along with SDF1 and angiopoietin-2 (Ang2) that home NSC progenitors to the site of injury (Thored et al., 2006), where they improve functional recovery (Guzman et al., 2008) and differentiate into neurons (Darsalia et al., 2007). Nevertheless, they present only limited reparative properties because matured neurons tend to die (Arvidsson et al., 2002; Marti-Fabregas et al., 2010; Kazanis et al., 2013). Moreover, given that NSCs themselves express and release respectively C-X-C motif chemokine receptor 4 (CXCR4) and SDF1, they might play a role in regulating axonal remodeling in ischemic brain. On the other hand, SVZ-derived astrocytes have a crucial role in ischemic stroke: photothrombotic cortical ischemia induces a strong gliogenic, Notch1-dependent response in the SVZ, which generates thrombospondin-4 (Thbs4)-positive astrocytes. Thbs4 astrocytes are essential components of the glial scar and their proliferation might be favored over neurogenesis after cortical injury (Benner et al., 2013). Conversely, it has also been reported that inflammation can promote neurogenesis in dormant neural progenitors in nonconventional neurogenic regions (Jiao and Chen, 2008).
Further, stroke increases generation of oligodendrocyte precursor cells (OPCs) from NSC in the ischemic brain, although it remains to be defined whether NSC-derived OPCs, beside oligodendrogenesis, help with brain repair, likely communicating with cerebrovasculature and other brain parenchyma cells (Itoh et al., 2015).
Overall, according to several evidences, inflammation in stroke can be beneficial for NSCs, nonetheless other reports, evaluating microglia function, claim that activated ED1+ microglia impair basal neurogenesis (Ekdahl et al., 2003) likely via TNFα/TNFR1 signaling (Iosif et al., 2008; Chen and Palmer, 2013; Gebara et al., 2013). In humans, a definitive robust evidence of clinical post-stroke neurogenesis remains a matter of investigation as analysis of autopsy tissue provided positive results (Ekonomou et al., 2012) which are instead lacking when labeling newly born neurons with 14C (Huttner et al., 2014).
In MS preclinical models, it is important to distinguish among experimental conditions since they influence the experimental evidence. Nonetheless, either chemically (LPC), focal (site injection of TNFα-IFNγ combined with subclinical immunization) or immune-mediated (EAE) demyelination causes reduced neuroblast proliferation in the olfactory bulb of mice, while neutralization of immune mediators, such as IFNγ and Galectin3 inflammatory cytokines, restores neurogenesis both in vitro (Pluchino et al., 2008; Tepavcevic et al., 2011) and in vivo (Monje et al., 2003; James et al., 2016). Indeed, in human samples, the SVZ of post-mortem MS brains is altered, with reduced number of neuroblasts in the SVZ of the lateral ventricle. Conversely, neuroinflammation increases neurotrophin levels (e.g., nerve growth factor-NGF) in the SVZ (Calza et al., 1998; Triaca et al., 2005), likely counteracting the inhibitory effect of proinflammatory molecules. Further, demyelination is able to boost proliferation of NSCs in the neurogenic niches promoting their differentiation (Pluchino et al., 2005; Menn et al., 2006). Only in conditions of acute cytokine exposure, either by focal intrathecal cytokine injection or as a consequence of strong microglia activation, the proliferative capacity of the niche is inhibited. In terms of gliogenic differentiation, inflammatory conditions may increase the percentage of Olig2+ cells originating from the SVZ via modulation of bone morphogenetic protein (BMP) signaling (Tepavcevic et al., 2011). The transcription factor Gli1 seems to be a key regulator of SVZ oligodendrogenesis and may be a promising target for reparative strategies in MS, as pharmacological inhibition of Gli1 during EAE promotes remyelination and improves clinical outcome (Samanta et al., 2015).
Leveraging the regenerative potential of NSCs and their immunomodulatory and trophic functions, NSC transplant has been proposed as therapeutic strategy for inflammatory diseases of the CNS. In stroke, transplanted NSCs directly modulate neuronal circuit plasticity (Zhang et al., 2009) through the expression of developmental molecules such as guidance molecules (i.e., slit, thrombospondin-1 and -2) and trophic factor such as VEGF (Andres et al., 2011). In fact, transplanted NSCs in stroke stimulate the proliferation of endogenous neural stem cells (Zhang et al., 2011; Hassani et al., 2012; Mine et al., 2013), increase endogenous angiogenesis after stroke (Jiang et al., 2005), scavenge the neurotoxic molecules (Emsley et al., 2004) and contribute both directly and indirectly (via astrocytes) to glutamate clearance (Bacigaluppi et al., 2009, 2016).
In EAE, transplanted NSCs directly inhibit both T cell and myeloid cell immune responses. In the CNS, they block inflammatory cell recruitment, T cell proliferation and promote the apoptosis of brain-reactive T cells (Einstein et al., 2003; Pluchino et al., 2005). In peripheral secondary lymphoid organs of EAE mice, antigen-specific proliferation of T cells, dendritic cell antigen presentation and chemotactic gradients are impaired by NSCs transplanted either subcutaneously or intravenously. As an example, NSC-secreted LIF, on one hand, stimulates endogenous remyelination in the CNS (Laterza et al., 2013), on the other, inhibits Th17 cell differentiation in the periphery (Cao et al., 2011).
Overall, the current literature shows that a controlled use of inflammation in CNS injury could be of help in regenerative approaches when NSC proliferation needs to be boosted and inflammatory tweaking can have beneficial outcomes. An option could consist in reducing NSC sensitivity to inflammatory mediators and, at the same time, increasing the therapeutic efficacy of NSCs when transplanting engineered cells.
Effect of Aging on Neural Stem Cell Plasticity, Connection with Stroke, and Multiple Sclerosis
Aging is a common feature of both stroke and progressive multiple sclerosis and loss of niche integrity, depletion of the stem cell pool, cellular senescence, defect in cell-cell contact in the niche or metabolic changes are all shared characteristics that contribute to the demise of the aging neurogenic niche (Oh et al., 2014).
The most significant consequence of age in the SVZ consists in the alteration of the niche cytostructure and in reduction of the neuroblast population, with reduced proliferation and neurogenic capacity (Kerever et al., 2015). On the contrary, oligodendrogenesis is substantially not affected by aging, rather oligodendrocyte recruitment and differentiation are impaired (Sim et al., 2002; Franklin and Ffrench-Constant, 2008; Conover and Todd, 2016). The latter is indeed a causal pathogenic characteristic of chronically demyelinated MS lesions in humans (Kuhlmann et al., 2008).
Concurrently, given that senescence does not spare the vasculature (Farkas and Luiten, 2001), the environment of the niche may enrich for toxic factors which further sustain exhaustion, reduce neuroplasticity and cause cognitive decline (Villeda et al., 2011). Potential therapeutic strategies to counteract the decline of adult stem cells may involve the promotion of a rejuvenating environment (Katsimpardi et al., 2014) or the prevention of premature exhaustion of long-lived self-renewing NSC populations. Neurogenic decline in aged SVZ can occur because of the accumulation of damaged DNA and both nuclear and mitochondrial DNA are susceptible to age-related changes (Bailey et al., 2004). Upon sensing DNA damage, SVZ cells upregulate the inhibitor of cyclin kinase to post-pone cell cycle entry and reduce proliferation of NSCs (Molofsky et al., 2006).
NSC age-dependent cell plasticity is also influenced by metabolic changes (Rabie et al., 2011; Chaker et al., 2015). As far as nutrient sensing pathways, growth differentiation factor-11 (GDF11), has been identified as one of the extrinsic circulating youth signals that maintain neurogenesis (Katsimpardi et al., 2014), although those findings are currently matter of further validation (Egerman et al., 2015; Smith S. C. et al., 2015). It is still unknown whether the effect is direct onto NSCs or indirect via improved microvascular network. IGF1, another critical growth factor, per se stimulates proliferation and differentiation of NSCs (Aberg, 2010), but decreases with aging (Sonntag et al., 2005). Nonetheless, it is also life–long exposure to IGF1 that with age causes decline of neurogenesis (Chaker et al., 2015). Along the same line, NAD+ and AMP levels, which reflect cellular energy state, decline with age in the hippocampus, in parallel with reduction of differentiation and self-renewal capacity of NSCs, because their regulatory enzyme decreases (Liu et al., 2012; Stein and Imai, 2014). As far as oxygen-dependent effect, those are linked to failure in maintaining appropriate mitochondrial regulations. Indeed, accumulation of mutations in mitochondrial DNA (mtDNA) occurs during aging and comes along with abnormal accumulation of toxic by-products (Siqueira et al., 2005) because mtDNA lacks protective histones and is located close to the major source of ROS, the electron transport chain (Park and Larsson, 2011).
Inflammation is another comorbidity factor associated with aging. The immune molecules C-C motif chemokine-11 (CCL11) and β2-microglobulin (B2M), as well as CCL2, C-C motif chemokine-19 (CCL19) and haptoglobin, contribute to low-grade of inflammation in aging (inflammaging) (Franceschi and Campisi, 2014), and negatively affect neurogenesis (Villeda et al., 2011; Wyss-Coray, 2016). Indeed, heterochronic parabiosis between aged and young mice rejuvenates and reverses stem cell aging in numerous tissues (Conboy et al., 2005) or administration of plasma from young mice can ameliorate cognitive impairment in aged mice (Villeda et al., 2014).
Last, it is worth mentioning that also the cellular environment of the niche sustains aging features. Astrocytes of the niche, with age, reduce release of Wnt3 (Lie et al., 2005; Okamoto et al., 2011), that in turn down regulates Survivin expression and increases release of Dickkopf-related protein 1 (DKK1), a canonical Wnt signaling inhibitor. The final effect is induced quiescence on one side (Miranda et al., 2012) and negative regulation of NSC neurogenesis (Seib et al., 2013) on the other. This is paired with physical changes of the extracellular matrix, which also affect senescence of the niche (Gattazzo et al., 2014). Conversely, it is also possible that NSCs efficiently graft the SVZ and well differentiate in both young and aged hippocampus, suggesting that advanced age of the host at the time of grafting has no major adverse effects on engraftment, migration, and differentiation of SVZ-NSCs (Shetty and Hattiangady, 2016).
Age is an independent risk factor for brain ischemia (Marinigh et al., 2010; Roger et al., 2012) and stroke models using aged animals are clinically very relevant (Popa-Wagner et al., 2007). From work on animals, it has been reported a 50% decline in neurogenesis in the SVZ of elder compared with young–adult animals (Darsalia et al., 2005; Macas et al., 2006; Ahlenius et al., 2009). Unfortunately, although endogenous neurogenesis has been observed even in aged humans, it is still not clear to what extent newly formed neurons are functionally relevant for stroke recovery in human patients. Thus, cell therapies have been implemented to address this question. An open-label, single-site, dose-escalation study was performed on 13 aged patients after ischemic stroke, transplanting by stereotaxis the neural stem cell line CTX0E03 in the ipsilateral putamen. After 2 years, improvement in the disability score scale (NIHSS) ranging from 0 to 5 (median 2) points has been recorded (Borlongan, 2016; Kalladka et al., 2016). Similarly, studies in animal models examined whether exogenous NSC delivery might restore neurogenesis in the DG of old rodents. Both embryonic and fetal derived NSCs stimulated neurogenesis in young or old animal models. Likely the effect was exerted via suppression of microglia/macrophage activation (Jin et al., 2011). Of note, inhibition of microglial activation has also been reported in ischemic mice following systemic delivery of mouse NSCs (Bacigaluppi et al., 2009).
Those data suggest that the problem with NSCs in ischemic stroke is to some extent cell autonomous, because the environment of the aged brain does not preclude NSC engraftment.
In MS, loss of myelin, a specialized membrane produced by oligodendrocytes, essential for normal CNS function, is a characteristic feature. Aging impairs not only the neurogenic but also the oligodendrogenic properties of the brain germinal niches as a consequence of longer cell cycle length of NSCs and of their progeny, loss of growth factors and upregulation of inhibitors (Hamilton et al., 2013). Iron accumulation (Andersen et al., 2014), linked in cascade to oxidative alteration and to mitochondrial dysfunction (Mahad et al., 2015) are other recognized characteristics of neurodegeneration in progressive aged MS individuals. A direct impact also on neural stem cells has not been experimentally proven (Crabbe et al., 2010) but can reasonable be speculated.
In the context of demyelination, work by Crawford et al. demonstrated responsiveness of OPCs to demyelination, although remyelination and susceptibility to aging is regionally dependent: in chemically-induced demyelination of the corpus callosum in aged animals, dorsal SVZ-NSCs are efficiently recruited to lesions and their differentiation into OPCs is impaired, whereas ventral NSCs are recruited more slowly but differentiate rapidly into OPCs; similarly, while in aged animals the percentage of differentiated OPCs from dorsal NSCs is half of the one seen in young animals, the percentage of ventral NSCs differentiating into OPCs remained constant (Crawford et al., 2016). This evidence has significant implications for the progressive form of MS, for which age is the current best associated risk factor (Minden et al., 2004; Scalfari et al., 2011). As disease progresses with age, repair, remyelination and other physiological functions become less robust. Animal models have shown that while OPC recruitment toward lesions remains intact, differentiation into myelinating oligodendrocytes decreases with age with a slower and less effective remyelination process compared to young rats (Rist and Franklin, 2008; Ruckh et al., 2012). Similarly, elder human subjects showed reduced remyelinating capabilities. Multiple hypotheses can explain these findings, including either extrinsic factors or intrinsic characteristics such as epigenetic changes known to be modulated during aging and thus to impact OPC maturation (Rist and Franklin, 2008).
How Do Neural Precursors from Induced Pluripotent Stem Cells Stand?
The technology of somatic cell reprogramming (induced pluripotent stem cells, iPSCs) that developed in the last 10 years (Takahashi et al., 2007), represents an invaluable alternative strategy to obtain, in large scale and indefinitely, neural precursors, unfolding new research and clinical avenues. Expandable intermediate neural precursors can be obtained with published protocols (Falk et al., 2012; Reinhardt et al., 2013) and the cells resemble most of the specific properties of endogenous plastic cells. To treat neurodegenerative conditions, both iPSC-derived NSCs or terminally differentiated neurons have been transplanted in preclinical models. Early on, it has been demonstrated that those cells are able to survive and adequately integrate into the host brain (Oki et al., 2012; Tornero et al., 2013; Qi et al., 2017), and recently it was reported that transplanted human iPSC-derived cortical neurons can be incorporated also into injured cortical circuits and could contribute to functional recovery (Tornero et al., 2017). Beside reparative activity, transplanted cells can improve the brain functional outcome, directly waking up brain plasticity via their bystander effects. Indeed, transplanted cells promote the formation of new synapses among existing neurons or preserve axonal integrity releasing trophic factor for myelinating cells as described in ischemic stroke and in a preclinical model of MS (Oki et al., 2012; Espuny-Camacho et al., 2013; Laterza et al., 2013; Tornero et al., 2013).
In addition, the release of soluble factors by (neural) stem cells along with cell-cell interactions supports angiogenesis that, beside self organizing blood vessels (Kusuma et al., 2013), guarantees trophic support for proper integration of neuronal cells and sustains synaptogenesis. Further, the bystander effect is exploited also in terms of (i) inhibition of neutrophil and peripheral dendritic cell activation and recruitment, (ii) inhibition of effector T- and B-cells, (iii) attenuation of BBB damage, or (iv) stimulation of the M2 microglial phenotype (Drago et al., 2013). Similar evidence of the (neuro)-protective, regenerative, and angiogenic properties of iPSCs is not available yet from their complete secretome, nonetheless it can be reasonably predicted.
As of now, while work by Jensen et al. reported no iPSC-mediated improvement in behavioral function in stroke (Jensen et al., 2013), several others, as previously mentioned, show that also direct iPSC transplantation improves neurological score, motor, sensory and cognitive functions, already within the first week after transplantation, when migration, maturation, synaptic integration, and paracrine release into the host brain is completed (Oki et al., 2012; Polentes et al., 2012; Tornero et al., 2013, 2017; Yuan et al., 2013; Tatarishvili et al., 2014).
Similarly, transplant of iPSC-derived neural precursors in the MS context dramatically reduced T cell infiltration and ameliorated white matter damage in treated EAE mice (Laterza et al., 2013; Zhang C. et al., 2016), both via improved differentiation toward cells of the oligo-glial lineage and via soluble factor release (Laterza et al., 2013).
Experimental validation in humans, both for stroke and MS, could be useful in the future but regulatory guidelines for safe clinical trial are awaited.
Can We Guide Neural Stem Cell Plasticity Toward a Desired Response?
Taking advantage of their plasticity, stem cells can generate, repair, and change nervous system functions. Fine-tuning of the inflammatory responses or proper modulation of the hypoxic and aging conditions should provide a permissive milieu for an effective reparative process. In this sense, the perspective that aged hippocampus licenses efficient and functional engraftment (Shetty and Hattiangady, 2016) puts forward the possibility that grafted niches can continuously generate new neurons and glia which, in turn, are expected to improve the plasticity and function of aged brain tissue.
As engineering and reprogramming approaches advance, transplant of exogenous human NSCs are optimized and better understanding of NSC regulation in physio- pathological conditions is achieved, it will be possible to fully take advantage of the therapeutic potential of those cells and successfully exploit their plasticity.
So far, it is possible to (i) engineer NSCs to deliver extrinsic stem cell fate determinants or intrinsic factors that allow the development of biomimetic environmental cues to control stem cell fates (Michailidou et al., 2014; Karimi et al., 2016); (ii) culture and expand stem cells outside of their native environments (Komura et al., 2015); (iii) perform localized delivery of microspheres to specifically differentiate endogenous stem cells (Gomez et al., 2015); (iv) engineer somatic cells to obtain induced NSCs with reparative functions (Hargus et al., 2014); (v) engineer human stem cells to obtain indefinitely expandable lines for clinical purposes (Pollock et al., 2006); (vi) snapshot the metabolic profile of cells in physio-pathological conditions (Bystrom et al., 2014; Dislich et al., 2015).
Systems biology will likely represent a fundamental tool to discover novel drugs to modulate NSC plasticity, as it can integrate and analyze in a quantitative manner several aspects of NSC behavior (e.g., differentiation potential and self-renewal) (Wells et al., 2013; Hussein et al., 2014).
Conclusions and Future Outlook
Neural progenitor cells represent an underdeveloped therapeutic resource in clinical settings. Although their paracrine and endocrine bystander potential is well-known, much of their plasticity still needs to be properly manipulated for therapeutic purposes.
On one end, cells can be expanded and bioengineering approaches are becoming available to further potentiate their availability (Bressan et al., 2014; Bouzid et al., 2016; Gardin et al., 2016). For instance, in vitro preconditioning with low oxygen tension might enhance NSC survival as it mimics the oxygen supply normally found in brain tissues (Hawkins et al., 2013; Sandvig et al., 2017). Nonetheless, it is this same plasticity that could threaten their therapeutic capacity, because the manipulation of cells themselves or of the trophic microenvironment, might induce unwanted side-effects, such as senescence from over-stimulation. Thus, it might be worth increasing the knowledge on (i) the influence of cell metabolism on NSC behavior during CNS diseases; (ii) the ex-vivo maintenance of NSCs; (iii) the most suitable window time for transplant to best leverage graft survival and disease specific environmental conditions. Indeed engraftment efficiency strongly correlates with therapeutic benefits.
Furthermore, this additional knowledge should shed light on the age-related decline of the stem cell niche, which contributes to neurodegeneration in both chronic and acute diseases. Last, careful analysis of the microenvironment is warranted to overcome complications and to improve the efficacy of cell therapy approaches.
Author Contributions
LO, AM wrote and approved the manuscript. GM, developed the concept wrote and approved the manuscript.
Conflict of Interest Statement
The authors declare that the research was conducted in the absence of any commercial or financial relationships that could be construed as a potential conflict of interest.
Acknowledgments
We apologize to researchers whose studies we were unable to cite due to the space limitation of this review. We would like to thank the TargetBrain (EU Framework 7 project HEALTH-F2-2012-279017), NEUROKINE network (EU Framework 7 ITN project), and Ricerca Finalizzata GR-2011-02348160 for financial support.
References
Aberg, D. (2010). Role of the growth hormone/insulin-like growth factor 1 axis in neurogenesis. Endocr. Dev. 17, 63–76. doi: 10.1159/000262529
Ahlenius, H., Visan, V., Kokaia, M., Lindvall, O., and Kokaia, Z. (2009). Neural stem and progenitor cells retain their potential for proliferation and differentiation into functional neurons despite lower number in aged brain. J. Neurosci. 29, 4408–4419. doi: 10.1523/JNEUROSCI.6003-08.2009
Ahn, S., and Joyner, A. L. (2005). In vivo analysis of quiescent adult neural stem cells responding to Sonic hedgehog. Nature 437, 894–897. doi: 10.1038/nature03994
Altman, J., and Das, G. D. (1965). Autoradiographic and histological evidence of postnatal hippocampal neurogenesis in rats. J. Comp. Neurol. 124, 319–335.
Alunni, A., and Bally-Cuif, L. (2016). A comparative view of regenerative neurogenesis in vertebrates. Development 143, 741–753. doi: 10.1242/dev.122796
Alvarez-Buylla, A., and Lim, D. A. (2004). For the long run: maintaining germinal niches in the adult brain. Neuron 41, 683–686. doi: 10.1016/S0896-6273(04)00111-4
Alvarez-Buylla, A., Herrera, D. G., and Wichterle, H. (2000). The subventricular zone: source of neuronal precursors for brain repair. Prog. Brain Res. 127, 1–11. doi: 10.1016/S0079-6123(00)27002-7
Andersen, H. H., Johnsen, K. B., and Moos, T. (2014). Iron deposits in the chronically inflamed central nervous system and contributes to neurodegeneration. Cell. Mol. Life Sci. 71, 1607–1622. doi: 10.1007/s00018-013-1509-8
Andres, R. H., Horie, N., Slikker, W., Keren-Gill, H., Zhan, K., Sun, G., et al. (2011). Human neural stem cells enhance structural plasticity and axonal transport in the ischaemic brain. Brain 134, 1777–1789. doi: 10.1093/brain/awr094
Androutsellis-Theotokis, A., Rueger, M. A., Park, D. M., Boyd, J. D., Padmanabhan, R., Campanati, L., et al. (2010). Angiogenic factors stimulate growth of adult neural stem cells. PLoS ONE 5:e9414. doi: 10.1371/journal.pone.0009414
Arulmoli, J., Pathak, M. M., McDonnell, L. P., Nourse, J. L., Tombola, F., Earthman, J. C., et al. (2015). Static stretch affects neural stem cell differentiation in an extracellular matrix-dependent manner. Sci. Rep. 5:8499. doi: 10.1038/srep08499
Arvidsson, A., Collin, T., Kirik, D., Kokaia, Z., and Lindvall, O. (2002). Neuronal replacement from endogenous precursors in the adult brain after stroke. Nat. Med. 8, 963–970. doi: 10.1038/nm747
Ashton, R. S., Conway, A., Pangarkar, C., Bergen, J., Lim, K. I., Shah, P., et al. (2012). Astrocytes regulate adult hippocampal neurogenesis through ephrin-B signaling. Nat. Neurosci. 15, 1399–1406. doi: 10.1038/nn.3212
Bachstetter, A. D., Morganti, J. M., Jernberg, J., Schlunk, A., Mitchell, S. H., Brewster, K. W., et al. (2011). Fractalkine and CX3CR1 regulate hippocampal neurogenesis in adult and aged rats. Neurobiol. Aging 32, 2030–2044. doi: 10.1016/j.neurobiolaging.2009.11.022
Bacigaluppi, M., Pluchino, S., Peruzzotti-Jametti, L., Kilic, E., Kilic, U., Salani, G., et al. (2009). Delayed post-ischaemic neuroprotection following systemic neural stem cell transplantation involves multiple mechanisms. Brain 132(Pt 8), 2239–2251. doi: 10.1093/brain/awp174
Bacigaluppi, M., Russo, G. L., Peruzzotti-Jametti, L., Rossi, S., Sandrone, S., Butti, E., et al. (2016). Neural stem cell transplantation induces stroke recovery by upregulating glutamate transporter GLT-1 in astrocytes. J. Neurosci. 36, 10529–10544. doi: 10.1523/JNEUROSCI.1643-16.2016
Bailey, K. J., Maslov, A. Y., and Pruitt, S. C. (2004). Accumulation of mutations and somatic selection in aging neural stem/progenitor cells. Aging Cell 3, 391–397. doi: 10.1111/j.1474-9728.2004.00128.x
Barkho, B. Z., Song, H., Aimone, J. B., Smrt, R. D., Kuwabara, T., Nakashima, K., et al. (2006). Identification of astrocyte-expressed factors that modulate neural stem/progenitor cell differentiation. Stem Cells Dev. 15, 407–421. doi: 10.1089/scd.2006.15.407
Batiz, L. F., Castro, M. A., Burgos, P. V., Velasquez, Z. D., Munoz, R. I., Lafourcade, C. A., et al. (2015). Exosomes as novel regulators of adult neurogenic niches. Front. Cell. Neurosci. 9:501. doi: 10.3389/fncel.2015.00501
Belmadani, A., Tran, P. B., Ren, D., and Miller, R. J. (2006). Chemokines regulate the migration of neural progenitors to sites of neuroinflammation. J. Neurosci. 26, 3182–3191. doi: 10.1523/JNEUROSCI.0156-06.2006
Ben-Hur, T. (2008). Immunomodulation by neural stem cells. J. Neurol. Sci. 265, 102–104. doi: 10.1016/j.jns.2007.05.007
Ben-Hur, T., Ben-Menachem, O., Furer, V., Einstein, O., Mizrachi-Kol, R., and Grigoriadis, N. (2003). Effects of proinflammatory cytokines on the growth, fate, and motility of multipotential neural precursor cells. Mol. Cell. Neurosci. 24, 623–631. doi: 10.1016/S1044-7431(03)00218-5
Benner, E. J., Luciano, D., Jo, R., Abdi, K., Paez-Gonzalez, P., Sheng, H., et al. (2013). Protective astrogenesis from the SVZ niche after injury is controlled by Notch modulator Thbs4. Nature 497, 369–373. doi: 10.1038/nature12069
Bergsland, M., Covacu, R., Perez Estrada, C., Svensson, M., and Brundin, L. (2014). Nitric oxide-induced neuronal to glial lineage fate-change depends on NRSF/REST function in neural progenitor cells. Stem Cells 32, 2539–2549. doi: 10.1002/stem.1749
Bernier, P. J., Bedard, A., Vinet, J., Levesque, M., and Parent, A. (2002). Newly generated neurons in the amygdala and adjoining cortex of adult primates. Proc. Natl. Acad. Sci. U.S.A. 99, 11464–11469. doi: 10.1073/pnas.172403999
Bjornsson, C. S., Apostolopoulou, M., Tian, Y., and Temple, S. (2015). It takes a village: constructing the neurogenic niche. Dev. Cell 32, 435–446. doi: 10.1016/j.devcel.2015.01.010
Bonaguidi, M. A., McGuire, T., Hu, M., Kan, L., Samanta, J., and Kessler, J. A. (2005). LIF and BMP signaling generate separate and discrete types of GFAP-expressing cells. Development 132, 5503–5514. doi: 10.1242/dev.02166
Bonaguidi, M. A., Peng, C. Y., McGuire, T., Falciglia, G., Gobeske, K. T., Czeisler, C., et al. (2008). Noggin expands neural stem cells in the adult hippocampus. J. Neurosci. 28, 9194–9204. doi: 10.1523/JNEUROSCI.3314-07.2008
Bonizzi, G., Piette, J., Schoonbroodt, S., Greimers, R., Havard, L., Merville, M. P., et al. (1999). Reactive oxygen intermediate-dependent NF-kappaB activation by interleukin-1beta requires 5-lipoxygenase or NADPH oxidase activity. Mol. Cell. Biol. 19, 1950–1960.
Borlongan, C. V. (2016). Age of PISCES: stem-cell clinical trials in stroke. Lancet 388, 736–738. doi: 10.1016/S0140-6736(16)31259-4
Bouzid, T., Sinitskii, A., and Lim, J. Y. (2016). Graphene platform for neural regenerative medicine. Neural Regen Res. 11, 894–895. doi: 10.4103/1673-5374.184454
Bressan, E., Ferroni, L., Gardin, C., Sbricoli, L., Gobbato, L., Ludovichetti, F. S., et al. (2014). Graphene based scaffolds effects on stem cells commitment. J. Transl. Med. 12:296. doi: 10.1186/s12967-014-0296-9
Breunig, J. J., Sarkisian, M. R., Arellano, J. I., Morozov, Y. M., Ayoub, A. E., Sojitra, S., et al. (2008). Primary cilia regulate hippocampal neurogenesis by mediating sonic hedgehog signaling. Proc. Natl. Acad. Sci. U.S.A. 105, 13127–13132. doi: 10.1073/pnas.0804558105
Brezun, J. M., and Daszuta, A. (1999). Depletion in serotonin decreases neurogenesis in the dentate gyrus and the subventricular zone of adult rats. Neuroscience 89, 999–1002.
Buffo, A., Rite, I., Tripathi, P., Lepier, A., Colak, D., Horn, A. P., et al. (2008). Origin and progeny of reactive gliosis: a source of multipotent cells in the injured brain. Proc. Natl. Acad. Sci. U.S.A. 105, 3581–3586. doi: 10.1073/pnas.0709002105
Butovsky, O., Ziv, Y., Schwartz, A., Landa, G., Talpalar, A. E., Pluchino, S., et al. (2006). Microglia activated by IL-4 or IFN-gamma differentially induce neurogenesis and oligodendrogenesis from adult stem/progenitor cells. Mol. Cell. Neurosci. 31, 149–160. doi: 10.1016/j.mcn.2005.10.006
Butti, E., Bacigaluppi, M., Rossi, S., Cambiaghi, M., Bari, M., Cebrian Silla, A., et al. (2012). Subventricular zone neural progenitors protect striatal neurons from glutamatergic excitotoxicity. Brain 135(Pt 11), 3320–3335. doi: 10.1093/brain/aws194
Butti, E., Cusimano, M., Bacigaluppi, M., and Martino, G. (2014). Neurogenic and non-neurogenic functions of endogenous neural stem cells. Front. Neurosci. 8:92. doi: 10.3389/fnins.2014.00092
Bystrom, S., Ayoglu, B., Haggmark, A., Mitsios, N., Hong, M. G., Drobin, K., et al. (2014). Affinity proteomic profiling of plasma, cerebrospinal fluid, and brain tissue within multiple sclerosis. J. Proteome Res. 13, 4607–4619. doi: 10.1021/pr500609e
Calza, L., Giardino, L., Pozza, M., Bettelli, C., Micera, A., and Aloe, L. (1998). Proliferation and phenotype regulation in the subventricular zone during experimental allergic encephalomyelitis: in vivo evidence of a role for nerve growth factor. Proc. Natl. Acad. Sci. U.S.A. 95, 3209–3214.
Cao, L., Jiao, X., Zuzga, D. S., Liu, Y., Fong, D. M., Young, D., et al. (2004). VEGF links hippocampal activity with neurogenesis, learning and memory. Nat. Genet. 36, 827–835. doi: 10.1038/ng1395
Cao, W., Yang, Y., Wang, Z., Liu, A., Fang, L., Wu, F., et al. (2011). Leukemia inhibitory factor inhibits T helper 17 cell differentiation and confers treatment effects of neural progenitor cell therapy in autoimmune disease. Immunity 35, 273–284. doi: 10.1016/j.immuni.2011.06.011
Cao, X., Li, L. P., Qin, X. H., Li, S. J., Zhang, M., Wang, Q., et al. (2013). Astrocytic adenosine 5'-triphosphate release regulates the proliferation of neural stem cells in the adult hippocampus. Stem Cells 31, 1633–1643. doi: 10.1002/stem.1408
Carbajal, K. S., Schaumburg, C., Strieter, R., Kane, J., and Lane, T. E. (2010). Migration of engrafted neural stem cells is mediated by CXCL12 signaling through CXCR4 in a viral model of multiple sclerosis. Proc. Natl. Acad. Sci. U.S.A. 107, 11068–11073. doi: 10.1073/pnas.1006375107
Carlen, M., Meletis, K., Goritz, C., Darsalia, V., Evergren, E., Tanigaki, K., et al. (2009). Forebrain ependymal cells are Notch-dependent and generate neuroblasts and astrocytes after stroke. Nat. Neurosci. 12, 259–267. doi: 10.1038/nn.2268
Carpentier, P. A., and Palmer, T. D. (2009). Immune influence on adult neural stem cell regulation and function. Neuron 64, 79–92. doi: 10.1016/j.neuron.2009.08.038
Carreira, B. P., Morte, M. I., Inacio, A., Costa, G., Rosmaninho-Salgado, J., Agasse, F., et al. (2010). Nitric oxide stimulates the proliferation of neural stem cells bypassing the epidermal growth factor receptor. Stem Cells 28, 1219–1230. doi: 10.1002/stem.444
Carreira, B. P., Morte, M. I., Santos, A. I., Lourenco, A. S., Ambrosio, A. F., Carvalho, C. M., et al. (2014). Nitric oxide from inflammatory origin impairs neural stem cell proliferation by inhibiting epidermal growth factor receptor signaling. Front. Cell. Neurosci. 8:343. doi: 10.3389/fncel.2014.00343
Chaker, Z., Aid, S., Berry, H., and Holzenberger, M. (2015). Suppression of IGF-I signals in neural stem cells enhances neurogenesis and olfactory function during aging. Aging Cell 14, 847–856. doi: 10.1111/acel.12365
Chaker, Z., Codega, P., and Doetsch, F. (2016). A mosaic world: puzzles revealed by adult neural stem cell heterogeneity. Wiley Interdiscip. Rev. Dev. Biol. 5, 640–658. doi: 10.1002/wdev.248
Chamorro, A., Meisel, A., Planas, A. M., Urra, X., van de Beek, D., and Veltkamp, R. (2012). The immunology of acute stroke. Nat. Rev. Neurol. 8, 401–410. doi: 10.1038/nrneurol.2012.98
Chang, A., Smith, M. C., Yin, X., Fox, R. J., Staugaitis, S. M., and Trapp, B. D. (2008). Neurogenesis in the chronic lesions of multiple sclerosis. Brain 131(Pt 9), 2366–2375. doi: 10.1093/brain/awn157
Chen, J., Zacharek, A., Zhang, C., Jiang, H., Li, Y., Roberts, C., et al. (2005). Endothelial nitric oxide synthase regulates brain-derived neurotrophic factor expression and neurogenesis after stroke in mice. J. Neurosci. 25, 2366–2375. doi: 10.1523/JNEUROSCI.5071-04.2005
Chen, Z., and Palmer, T. D. (2013). Differential roles of TNFR1 and TNFR2 signaling in adult hippocampal neurogenesis. Brain Behav. Immun. 30, 45–53. doi: 10.1016/j.bbi.2013.01.083
Cheng, W., Yu, P., Wang, L., Shen, C., Song, X., Chen, J., et al. (2015). Sonic hedgehog signaling mediates resveratrol to increase proliferation of neural stem cells after oxygen-glucose deprivation/reoxygenation injury in vitro. Cell. Physiol. Biochem. 35, 2019–2032. doi: 10.1159/000374009
Chou, C. H., and Modo, M. (2016). Human neural stem cell-induced endothelial morphogenesis requires autocrine/paracrine and juxtacrine signaling. Sci. Rep. 6:29029. doi: 10.1038/srep29029
Chucair-Elliott, A. J., Conrady, C., Zheng, M., Kroll, C. M., Lane, T. E., and Carr, D. J. (2014). Microglia-induced IL-6 protects against neuronal loss following HSV-1 infection of neural progenitor cells. Glia 62, 1418–1434. doi: 10.1002/glia.22689
Codega, P., Silva-Vargas, V., Paul, A., Maldonado-Soto, A. R., Deleo, A. M., Pastrana, E., et al. (2014). Prospective identification and purification of quiescent adult neural stem cells from their in vivo niche. Neuron 82, 545–559. doi: 10.1016/j.neuron.2014.02.039
Colucci-D'Amato, L., Cicatiello, A. E., Reccia, M. G., Volpicelli, F., Severino, V., Russo, R., et al. (2015). A targeted secretome profiling by multiplexed immunoassay revealed that secreted chemokine ligand 2 (MCP-1/CCL2) affects neural differentiation in mesencephalic neural progenitor cells. Proteomics 15, 714–724. doi: 10.1002/pmic.201400360
Conboy, I. M., Conboy, M. J., Wagers, A. J., Girma, E. R., Weissman, I. L., and Rando, T. A. (2005). Rejuvenation of aged progenitor cells by exposure to a young systemic environment. Nature 433, 760–764. doi: 10.1038/nature03260
Conover, J. C., and Todd, K. L. (2016). Development and aging of a brain neural stem cell niche. Exp. Gerontol. doi: 10.1016/j.exger.2016.11.007. [Epub ahead of print].
Corbit, K. C., Aanstad, P., Singla, V., Norman, A. R., Stainier, D. Y., and Reiter, J. F. (2005). Vertebrate smoothened functions at the primary cilium. Nature 437, 1018–1021. doi: 10.1038/nature04117
Cossetti, C., Iraci, N., Mercer, T. R., Leonardi, T., Alpi, E., Drago, D., et al. (2014). Extracellular vesicles from neural stem cells transfer IFN-gamma via Ifngr1 to activate Stat1 signaling in target cells. Mol. Cell 56, 193–204. doi: 10.1016/j.molcel.2014.08.020
Covacu, R., Arvidsson, L., Andersson, A., Khademi, M., Erlandsson-Harris, H., Harris, R. A., et al. (2009). TLR activation induces TNF-alpha production from adult neural stem/progenitor cells. J. Immunol. 182, 6889–6895. doi: 10.4049/jimmunol.0802907
Crabbe, A., Vandeputte, C., Dresselaers, T., Sacido, A. A., Verdugo, J. M., Eyckmans, J., et al. (2010). Effects of MRI contrast agents on the stem cell phenotype. Cell Transplant. 19, 919–936. doi: 10.3727/096368910X494623
Crawford, A. H., Tripathi, R. B., Richardson, W. D., and Franklin, R. J. (2016). Developmental origin of oligodendrocyte lineage cells determines response to demyelination and susceptibility to age-associated functional decline. Cell Rep. 13, pii: S2211–1247(16)30358-8. doi: 10.1016/j.celrep.2016.03.069
Crutcher, K. A., Gendelman, H. E., Kipnis, J., Perez-Polo, J. R., Perry, V. H., Popovich, P. G., et al. (2006). Debate: “Is increasing neuroinflammation beneficial for neural repair?” J. Neuroimmune Pharmacol. 1, 195–211. doi: 10.1007/s11481-006-9021-7
Cui, X., Chen, J., Zacharek, A., Roberts, C., Yang, Y., and Chopp, M. (2009). Nitric oxide donor up-regulation of SDF1/CXCR4 and Ang1/Tie2 promotes neuroblast cell migration after stroke. J. Neurosci. Res. 87, 86–95. doi: 10.1002/jnr.21836
Darsalia, V., Heldmann, U., Lindvall, O., and Kokaia, Z. (2005). Stroke-induced neurogenesis in aged brain. Stroke 36, 1790–1795. doi: 10.1161/01.STR.0000173151.36031.be
Darsalia, V., Kallur, T., and Kokaia, Z. (2007). Survival, migration and neuronal differentiation of human fetal striatal and cortical neural stem cells grafted in stroke-damaged rat striatum. Eur. J. Neurosci. 26, 605–614. doi: 10.1111/j.1460-9568.2007.05702.x
Davies, A. L., Desai, R. A., Bloomfield, P. S., McIntosh, P. R., Chapple, K. J., Linington, C., et al. (2013). Neurological deficits caused by tissue hypoxia in neuroinflammatory disease. Ann. Neurol. 74, 815–825. doi: 10.1002/ana.24006
De Feo, D., Merlini, A., Laterza, C., and Martino, G. (2012). Neural stem cell transplantation in central nervous system disorders: from cell replacement to neuroprotection. Curr. Opin. Neurol. 25, 322–333. doi: 10.1097/WCO.0b013e328352ec45
De Filippis, L., and Delia, D. (2011). Hypoxia in the regulation of neural stem cells. Cell. Mol. Life Sci. 68, 2831–2844. doi: 10.1007/s00018-011-0723-5
Delgado, A. C., Ferron, S. R., Vicente, D., Porlan, E., Perez-Villalba, A., Trujillo, C. M., et al. (2014). Endothelial NT-3 delivered by vasculature and CSF promotes quiescence of subependymal neural stem cells through nitric oxide induction. Neuron 83, 572–585. doi: 10.1016/j.neuron.2014.06.015
Dias, J. M., Alekseenko, Z., Applequist, J. M., and Ericson, J. (2014). Tgfβ signaling regulates temporal neurogenesis and potency of neural stem cells in the CNS. Neuron 84, 927–939. doi: 10.1016/j.neuron.2014.10.033
Dislich, B., Wohlrab, F., Bachhuber, T., Muller, S. A., Kuhn, P. H., Hogl, S., et al. (2015). Label-free quantitative proteomics of mouse cerebrospinal fluid detects β-site APP cleaving enzyme (BACE1) protease substrates in vivo. Mol. Cell. Proteomics 14, 2550–2563. doi: 10.1074/mcp.M114.041533
Doetsch, F., Caille, I., Lim, D. A., Garcia-Verdugo, J. M., and Alvarez-Buylla, A. (1999). Subventricular zone astrocytes are neural stem cells in the adult mammalian brain. Cell 97, 703–716.
Drago, D., Basso, V., Gaude, E., Volpe, G., Peruzzotti-Jametti, L., Bachi, A., et al. (2016). Metabolic determinants of the immune modulatory function of neural stem cells. J. Neuroinflammation 13, 232. doi: 10.1186/s12974-016-0667-7
Drago, D., Cossetti, C., Iraci, N., Gaude, E., Musco, G., Bachi, A., et al. (2013). The stem cell secretome and its role in brain repair. Biochimie 95, 2271–2285. doi: 10.1016/j.biochi.2013.06.020
Egerman, M. A., Cadena, S. M., Gilbert, J. A., Meyer, A., Nelson, H. N., Swalley, S. E., et al. (2015). GDF11 increases with age and inhibits skeletal muscle regeneration. Cell Metab. 22, 164–174. doi: 10.1016/j.cmet.2015.05.010
Einstein, O., Karussis, D., Grigoriadis, N., Mizrachi-Kol, R., Reinhartz, E., Abramsky, O., et al. (2003). Intraventricular transplantation of neural precursor cell spheres attenuates acute experimental allergic encephalomyelitis. Mol. Cell. Neurosci. 24, 1074–1082. doi: 10.1016/j.mcn.2003.08.009
Ekdahl, C. T., Claasen, J. H., Bonde, S., Kokaia, Z., and Lindvall, O. (2003). Inflammation is detrimental for neurogenesis in adult brain. Proc. Natl. Acad. Sci. U.S.A. 100, 13632–13637. doi: 10.1073/pnas.2234031100
Ekonomou, A., Johnson, M., Perry, R. H., Perry, E. K., Kalaria, R. N., Minger, S. L., et al. (2012). Increased neural progenitors in individuals with cerebral small vessel disease. Neuropathol. Appl. Neurobiol. 38, 344–353. doi: 10.1111/j.1365-2990.2011.01224.x
Emsley, J. G., and Hagg, T. (2003). Endogenous and exogenous ciliary neurotrophic factor enhances forebrain neurogenesis in adult mice. Exp. Neurol. 183, 298–310. doi: 10.1016/S0014-4886(03)00129-8
Emsley, J. G., Mitchell, B. D., Magavi, S. S., Arlotta, P., and Macklis, J. D. (2004). The repair of complex neuronal circuitry by transplanted and endogenous precursors. NeuroRx 1, 452–471. doi: 10.1602/neurorx.1.4.452
Ernst, A., Alkass, K., Bernard, S., Salehpour, M., Perl, S., Tisdale, J., et al. (2014). Neurogenesis in the striatum of the adult human brain. Cell 156, 1072–1083. doi: 10.1016/j.cell.2014.01.044
Espuny-Camacho, I., Michelsen, K. A., Gall, D., Linaro, D., Hasche, A., Bonnefont, J., et al. (2013). Pyramidal neurons derived from human pluripotent stem cells integrate efficiently into mouse brain circuits in vivo. Neuron 77, 440–456. doi: 10.1016/j.neuron.2012.12.011
Faigle, R., and Song, H. (2013). Signaling mechanisms regulating adult neural stem cells and neurogenesis. Biochim. Biophys. Acta 1830, 2435–2448. doi: 10.1016/j.bbagen.2012.09.002
Falk, A., Koch, P., Kesavan, J., Takashima, Y., Ladewig, J., Alexander, M., et al. (2012). Capture of neuroepithelial-like stem cells from pluripotent stem cells provides a versatile system for in vitro production of human neurons. PLoS ONE 7:e29597. doi: 10.1371/journal.pone.0029597
Farkas, E., and Luiten, P. G. (2001). Cerebral microvascular pathology in aging and Alzheimer's disease. Prog. Neurobiol. 64, 575–611. doi: 10.1016/S0301-0082(00)00068-X
Felfly, H., Zambon, A. C., Xue, J., Muotri, A., Zhou, D., Snyder, E. Y., et al. (2011). Severe hypoxia: consequences to neural stem cells and neurons. J. Neurol. Res. 1, 177–189. doi: 10.4021/jnr70w
Folmes, C. D., Dzeja, P. P., Nelson, T. J., and Terzic, A. (2012). Metabolic plasticity in stem cell homeostasis and differentiation. Cell Stem Cell 11, 596–606. doi: 10.1016/j.stem.2012.10.002
Forni, P. E., Bharti, K., Flannery, E. M., Shimogori, T., and Wray, S. (2013). The indirect role of fibroblast growth factor-8 in defining neurogenic niches of the olfactory/GnRH systems. J. Neurosci. 33, 19620–19634. doi: 10.1523/JNEUROSCI.3238-13.2013
Franceschi, C., and Campisi, J. (2014). Chronic inflammation (inflammaging) and its potential contribution to age-associated diseases. J. Gerontol. A Biol. Sci. Med. Sci. 69(Suppl. 1), S4–S9. doi: 10.1093/gerona/glu057
Franklin, R. J., and Ffrench-Constant, C. (2008). Remyelination in the CNS: from biology to therapy. Nat. Rev. Neurosci. 9, 839–855. doi: 10.1038/nrn2480
Gabel, S., Koncina, E., Dorban, G., Heurtaux, T., Birck, C., Glaab, E., et al. (2016). Inflammation promotes a conversion of astrocytes into neural progenitor cells via NF-κB activation. Mol. Neurobiol. 53, 5041–5055. doi: 10.1007/s12035-015-9428-3
Gajera, C. R., Emich, H., Lioubinski, O., Christ, A., Beckervordersandforth-Bonk, R., Yoshikawa, K., et al. (2010). LRP2 in ependymal cells regulates BMP signaling in the adult neurogenic niche. J. Cell Sci. 123(Pt 11), 1922–1930. doi: 10.1242/jcs.065912
Gallagher, D., Norman, A. A., Woodard, C. L., Yang, G., Gauthier-Fisher, A., Fujitani, M., et al. (2013). Transient maternal IL-6 mediates long-lasting changes in neural stem cell pools by deregulating an endogenous self-renewal pathway. Cell Stem Cell 13, 564–576. doi: 10.1016/j.stem.2013.10.002
Gardin, C., Piattelli, A., and Zavan, B. (2016). Graphene in regenerative medicine: focus on stem cells and neuronal differentiation. Trends Biotechnol. 34, 435–437. doi: 10.1016/j.tibtech.2016.01.006
Gattazzo, F., Urciuolo, A., and Bonaldo, P. (2014). Extracellular matrix: a dynamic microenvironment for stem cell niche. Biochim. Biophys. Acta 1840, 2506–2519. doi: 10.1016/j.bbagen.2014.01.010
Gebara, E., Sultan, S., Kocher-Braissant, J., and Toni, N. (2013). Adult hippocampal neurogenesis inversely correlates with microglia in conditions of voluntary running and aging. Front. Neurosci. 7:145. doi: 10.3389/fnins.2013.00145
Ghashghaei, H. T., Weber, J., Pevny, L., Schmid, R., Schwab, M. H., Lloyd, K. C., et al. (2006). The role of neuregulin-ErbB4 interactions on the proliferation and organization of cells in the subventricular zone. Proc. Natl. Acad. Sci. U.S.A. 103, 1930–1935. doi: 10.1073/pnas.0510410103
Gomez, J. E. J., Agbay, A., Bibault, E., Montgomery, A., Mohtaram, N., and Willerth, S. (2015). Incorporation of retinoic acid releasing microspheres into pluripotent stem cell aggregates for inducing neuronal differentiation. Cell. Mol. Bioeng. 8, 307–331. doi: 10.1007/s12195-015-0401-z
Gomez-Gaviro, M. V., Scott, C. E., Sesay, A. K., Matheu, A., Booth, S., Galichet, C., et al. (2012). Betacellulin promotes cell proliferation in the neural stem cell niche and stimulates neurogenesis. Proc. Natl. Acad. Sci. U.S.A. 109, 1317–1322. doi: 10.1073/pnas.1016199109
Goodman, T., and Hajihosseini, M. K. (2015). Hypothalamic tanycytes-masters and servants of metabolic, neuroendocrine, and neurogenic functions. Front. Neurosci. 9:387. doi: 10.3389/fnins.2015.00387
Guadagno, J., Swan, P., Shaikh, R., and Cregan, S. P. (2015). Microglia-derived IL-1β triggers p53-mediated cell cycle arrest and apoptosis in neural precursor cells. Cell Death Dis. 6:e1779. doi: 10.1038/cddis.2015.151
Guadagno, J., Xu, X., Karajgikar, M., Brown, A., and Cregan, S. P. (2013). Microglia-derived TNFa induces apoptosis in neural precursor cells via transcriptional activation of the Bcl-2 family member Puma. Cell Death Dis. 4, e538. doi: 10.1038/cddis.2013.59
Guerra-Crespo, M., Gleason, D., Sistos, A., Toosky, T., Solaroglu, I., Zhang, J. H., et al. (2009). Transforming growth factor-alpha induces neurogenesis and behavioral improvement in a chronic stroke model. Neuroscience 160, 470–483. doi: 10.1016/j.neuroscience.2009.02.029
Guzman, R., De Los Angeles, A., Cheshier, S., Choi, R., Hoang, S., Liauw, J., et al. (2008). Intracarotid injection of fluorescence activated cell-sorted CD49d-positive neural stem cells improves targeted cell delivery and behavior after stroke in a mouse stroke model. Stroke 39, 1300–1306. doi: 10.1161/STROKEAHA.107.500470
Haider, L., Simeonidou, C., Steinberger, G., Hametner, S., Grigoriadis, N., Deretzi, G., et al. (2014). Multiple sclerosis deep grey matter: the relation between demyelination, neurodegeneration, inflammation and iron. J. Neurol. Neurosurg. Psychiatr. 85, 1386–1395. doi: 10.1136/jnnp-2014-307712
Hamilton, L. K., Joppe, S. E., Cochard, L. M., and Fernandes, K. J. (2013). Aging and neurogenesis in the adult forebrain: what we have learned and where we should go from here. Eur. J. Neurosci. 37, 1978–1986. doi: 10.1111/ejn.12207
Hargus, G., Ehrlich, M., Arauzo-Bravo, M. J., Hemmer, K., Hallmann, A. L., Reinhardt, P., et al. (2014). Origin-dependent neural cell identities in differentiated human iPSCs in vitro and after transplantation into the mouse brain. Cell Rep. 8, 1697–1703. doi: 10.1016/j.celrep.2014.08.014
Harry, G. J. (2013). Microglia during development and aging. Pharmacol. Ther. 139, 313–326. doi: 10.1016/j.pharmthera.2013.04.013
Hassani, Z., O'Reilly, J., Pearse, Y., Stroemer, P., Tang, E., Sinden, J., et al. (2012). Human neural progenitor cell engraftment increases neurogenesis and microglial recruitment in the brain of rats with stroke. PLoS ONE 7:e50444. doi: 10.1371/journal.pone.0050444
Hawkins, K. E., Sharp, T. V., and McKay, T. R. (2013). The role of hypoxia in stem cell potency and differentiation. Regen. Med. 8, 771–782. doi: 10.2217/rme.13.71
Hicks, C., Stevanato, L., Stroemer, R. P., Tang, E., Richardson, S., and Sinden, J. D. (2013). In vivo and in vitro characterization of the angiogenic effect of CTX0E03 human neural stem cells. Cell Transplant. 22, 1541–1552. doi: 10.3727/096368912X657936
Huang, L., Wong, S., Snyder, E. Y., Hamblin, M. H., and Lee, J. P. (2014). Human neural stem cells rapidly ameliorate symptomatic inflammation in early-stage ischemic-reperfusion cerebral injury. Stem Cell Res. Ther. 5:129. doi: 10.1186/scrt519
Huang, X., Yuan, T., Tschannen, M., Sun, Z., Jacob, H., Du, M., et al. (2013). Characterization of human plasma-derived exosomal RNAs by deep sequencing. BMC Genomics 14:319. doi: 10.1186/1471-2164-14-319
Hussein, S. M., Puri, M. C., Tonge, P. D., Benevento, M., Corso, A. J., Clancy, J. L., et al. (2014). Genome-wide characterization of the routes to pluripotency. Nature 516, 198–206. doi: 10.1038/nature14046
Huttner, H. B., Bergmann, O., Salehpour, M., Racz, A., Tatarishvili, J., Lindgren, E., et al. (2014). The age and genomic integrity of neurons after cortical stroke in humans. Nat. Neurosci. 17, 801–803. doi: 10.1038/nn.3706
Ihrie, R. A., Shah, J. K., Harwell, C. C., Levine, J. H., Guinto, C. D., Lezameta, M., et al. (2011). Persistent sonic hedgehog signaling in adult brain determines neural stem cell positional identity. Neuron 71, 250–262. doi: 10.1016/j.neuron.2011.05.018
Imitola, J., Raddassi, K., Park, K. I., Mueller, F. J., Nieto, M., Teng, Y. D., et al. (2004). Directed migration of neural stem cells to sites of CNS injury by the stromal cell-derived factor 1α/CXC chemokine receptor 4 pathway. Proc. Natl. Acad. Sci. U.S.A. 101, 18117–18122. doi: 10.1073/pnas.0408258102
Iosif, R. E., Ahlenius, H., Ekdahl, C. T., Darsalia, V., Thored, P., Jovinge, S., et al. (2008). Suppression of stroke-induced progenitor proliferation in adult subventricular zone by tumor necrosis factor receptor 1. J. Cereb. Blood Flow Metab. 28, 1574–1587. doi: 10.1038/jcbfm.2008.47
Irvin, D. K., Nakano, I., Paucar, A., and Kornblum, H. I. (2004). Patterns of jagged1, jagged2, delta-like 1 and delta-like 3 expression during late embryonic and postnatal brain development suggest multiple functional roles in progenitors and differentiated cells. J. Neurosci. Res. 75, 330–343. doi: 10.1002/jnr.10843
Ito, K., and Suda, T. (2014). Metabolic requirements for the maintenance of self-renewing stem cells. Nat. Rev. Mol. Cell Biol. 15, 243–256. doi: 10.1038/nrm3772
Itoh, K., Maki, T., Lok, J., and Arai, K. (2015). Mechanisms of cell-cell interaction in oligodendrogenesis and remyelination after stroke. Brain Res. 1623, 135–149. doi: 10.1016/j.brainres.2015.04.039
Itoh, T., Satou, T., Nishida, S., Tsubaki, M., Hashimoto, S., and Ito, H. (2009). Expression of amyloid precursor protein after rat traumatic brain injury. Neurol. Res. 31, 103–109. doi: 10.1179/016164108X323771
Ivanovic, Z., and Vlaski-Lafarge, M. (eds.). (2016). Anaerobiosis and Stemness. (Boston, MA: Academic Press).
Jackson, E. L., Garcia-Verdugo, J. M., Gil-Perotin, S., Roy, M., Quinones-Hinojosa, A., VandenBerg, S., et al. (2006). PDGFR alpha-positive B cells are neural stem cells in the adult SVZ that form glioma-like growths in response to increased PDGF signaling. Neuron 51, 187–199. doi: 10.1016/j.neuron.2006.06.012
Jagielska, A., Lowe, A. L., Makhija, E., Wroblewsa, L., Guck, J., Franklin, R. M., et al. (2017). Mechanical strain promots oligodendrocytes differentiation by global changesof gene expression. Front. Cell. Neurosci. 11:93. doi: 10.3389/fncel.2017.00093
James, R. E., Hillis, J., Adorjan, I., Gration, B., Mundim, M. V., Iqbal, A. J., et al. (2016). Loss of galectin-3 decreases the number of immune cells in the subventricular zone and restores proliferation in a viral model of multiple sclerosis. Glia 64, 105–121. doi: 10.1002/glia.22906
Jensen, M. B., Yan, H., Krishnaney-Davison, R., Al Sawaf, A., and Zhang, S. C. (2013). Survival and differentiation of transplanted neural stem cells derived from human induced pluripotent stem cells in a rat stroke model. J. Stroke Cerebrovasc. Dis. 22, 304–308. doi: 10.1016/j.jstrokecerebrovasdis.2011.09.008
Jiang, Q., Zhang, Z. G., Ding, G. L., Zhang, L., Ewing, J. R., Wang, L., et al. (2005). Investigation of neural progenitor cell induced angiogenesis after embolic stroke in rat using MRI. Neuroimage 28, 698–707. doi: 10.1016/j.neuroimage.2005.06.063
Jiao, J., and Chen, D. F. (2008). Induction of neurogenesis in nonconventional neurogenic regions of the adult central nervous system by niche astrocyte-produced signals. Stem Cells 26, 1221–1230. doi: 10.1634/stemcells.2007-0513
Jin, K., Wang, X., Xie, L., Mao, X. O., Zhu, W., Wang, Y., et al. (2006). Evidence for stroke-induced neurogenesis in the human brain. Proc. Natl. Acad. Sci. U.S.A. 103, 13198–13202. doi: 10.1073/pnas.0603512103
Jin, K., Xie, L., Mao, X., Greenberg, M. B., Moore, A., Peng, B., et al. (2011). Effect of human neural precursor cell transplantation on endogenous neurogenesis after focal cerebral ischemia in the rat. Brain Res. 1374, 56–62. doi: 10.1016/j.brainres.2010.12.037
Jin, K., Zhu, Y., Sun, Y., Mao, X. O., Xie, L., and Greenberg, D. A. (2002). Vascular endothelial growth factor (VEGF) stimulates neurogenesis in vitro and in vivo. Proc. Natl. Acad. Sci. U.S.A. 99, 11946–11950. doi: 10.1073/pnas.182296499
Joseph D'Ercole, A., and Ye, P. (2008). Expanding the mind: insulin-like growth factor I and brain development. Endocrinology 149, 5958–5962. doi: 10.1210/en.2008-0920
Kalladka, D., Sinden, J., Pollock, K., Haig, C., McLean, J., Smith, W., et al. (2016). Human neural stem cells in patients with chronic ischaemic stroke (PISCES): a phase 1, first-in-man study. Lancet 388, 787–796. doi: 10.1016/S0140-6736(16)30513-X
Kam, M., Curtis, M. A., McGlashan, S. R., Connor, B., Nannmark, U., and Faull, R. L. (2009). The cellular composition and morphological organization of the rostral migratory stream in the adult human brain. J. Chem. Neuroanat. 37, 196–205. doi: 10.1016/j.jchemneu.2008.12.009
Karimi, M., Bahrami, S., Mirshekari, H., Basri, S. M., Nik, A. B., Aref, A. R., et al. (2016). Microfluidic systems for stem cell-based neural tissue engineering. Lab Chip 16, 2551–2571. doi: 10.1039/c6lc00489j
Katajisto, P., Dohla, J., Chaffer, C. L., Pentinmikko, N., Marjanovic, N., Iqbal, S., et al. (2015). Stem cells. Asymmetric apportioning of aged mitochondria between daughter cells is required for stemness. Science 348, 340–343. doi: 10.1126/science.1260384
Katsimpardi, L., Litterman, N. K., Schein, P. A., Miller, C. M., Loffredo, F. S., Wojtkiewicz, G. R., et al. (2014). Vascular and neurogenic rejuvenation of the aging mouse brain by young systemic factors. Science 344, 630–634. doi: 10.1126/science.1251141
Kazanis, I., Gorenkova, N., Zhao, J. W., Franklin, R. J., Modo, M., and Ffrench-Constant, C. (2013). The late response of rat subependymal zone stem and progenitor cells to stroke is restricted to directly affected areas of their niche. Exp. Neurol. 248, 387–397. doi: 10.1016/j.expneurol.2013.06.025
Kerever, A., Schnack, J., Vellinga, D., Ichikawa, N., Moon, C., Arikawa-Hirasawa, E., et al. (2007). Novel extracellular matrix structures in the neural stem cell niche capture the neurogenic factor fibroblast growth factor 2 from the extracellular milieu. Stem Cells 25, 2146–2157. doi: 10.1634/stemcells.2007-0082
Kerever, A., Yamada, T., Suzuki, Y., Mercier, F., and Arikawa-Hirasawa, E. (2015). Fractone aging in the subventricular zone of the lateral ventricle. J. Chem. Neuroanat. 66–67, 52–60. doi: 10.1016/j.jchemneu.2015.06.001
Kim, J. B., Ju, J. Y., Kim, J. H., Kim, T. Y., Yang, B. H., Lee, Y. S., et al. (2004). Dexamethasone inhibits proliferation of adult hippocampal neurogenesis in vivo and in vitro. Brain Res. 1027, 1–10. doi: 10.1016/j.brainres.2004.07.093
Kim, S. U., and de Vellis, J. (2005). Microglia in health and disease. J. Neurosci. Res. 81, 302–313. doi: 10.1002/jnr.20562
Kim, S., Lehtinen, M. K., Sessa, A., Zappaterra, M. W., Cho, S. H., Gonzalez, D., et al. (2010). The apical complex couples cell fate and cell survival to cerebral cortical development. Neuron 66, 69–84. doi: 10.1016/j.neuron.2010.03.019
Kirby, E. D., Kuwahara, A. A., Messer, R. L., and Wyss-Coray, T. (2015). Adult hippocampal neural stem and progenitor cells regulate the neurogenic niche by secreting VEGF. Proc. Natl. Acad. Sci. U.S.A. 112, 4128–4133. doi: 10.1073/pnas.1422448112
Kleine, T. O., and Benes, L. (2006). Immune surveillance of the human central nervous system (CNS): different migration pathways of immune cells through the blood-brain barrier and blood-cerebrospinal fluid barrier in healthy persons. Cytometry A 69, 147–151. doi: 10.1002/cyto.a.20225
Knobloch, M., Braun, S. M., Zurkirchen, L., von Schoultz, C., Zamboni, N., Arauzo-Bravo, M. J., et al. (2013). Metabolic control of adult neural stem cell activity by Fasn-dependent lipogenesis. Nature 493, 226–230. doi: 10.1038/nature11689
Knoth, R., Singec, I., Ditter, M., Pantazis, G., Capetian, P., Meyer, R. P., et al. (2010). Murine features of neurogenesis in the human hippocampus across the lifespan from 0 to 100 years. PLoS ONE 5:e8809. doi: 10.1371/journal.pone.0008809
Kojima, T., Hirota, Y., Ema, M., Takahashi, S., Miyoshi, I., Okano, H., et al. (2010). Subventricular zone-derived neural progenitor cells migrate along a blood vessel scaffold toward the post-stroke striatum. Stem Cells 28, 545–554. doi: 10.1002/stem.306
Kokaia, Z., Martino, G., Schwartz, M., and Lindvall, O. (2012). Cross-talk between neural stem cells and immune cells: the key to better brain repair? Nat. Neurosci. 15, 1078–1087. doi: 10.1038/nn.3163
Kokoeva, M. V., Yin, H., and Flier, J. S. (2005). Neurogenesis in the hypothalamus of adult mice: potential role in energy balance. Science 310, 679–683. doi: 10.1126/science.1115360
Kokovay, E., Goderie, S., Wang, Y., Lotz, S., Lin, G., Sun, Y., et al. (2010). Adult SVZ lineage cells home to and leave the vascular niche via differential responses to SDF1/CXCR4 signaling. Cell Stem Cell 7, 163–173. doi: 10.1016/j.stem.2010.05.019
Komura, T., Kato, K., Konagaya, S., Nakaji-Hirabayashi, T., and Iwata, H. (2015). Optimization of surface-immobilized extracellular matrices for the proliferation of neural progenitor cells derived from induced pluripotent stem cells. Biotechnol. Bioeng. 112, 2388–2396. doi: 10.1002/bit.25636
Kuhlmann, T., Miron, V., Cui, Q., Wegner, C., Antel, J., and Bruck, W. (2008). Differentiation block of oligodendroglial progenitor cells as a cause for remyelination failure in chronic multiple sclerosis. Brain 131(Pt 7), 1749–1758. doi: 10.1093/brain/awn096
Kulkarni, A., Ganesan, P., and O'Donnell, L. A. (2016). Interferon gamma: influence on neural stem cell function in neurodegenerative and neuroinflammatory disease. Clin. Med. Insights Pathol. 9(Suppl. 1), 9–19. doi: 10.4137/CPath.S40497
Kusuma, S., Shen, Y. I., Hanjaya-Putra, D., Mali, P., Cheng, L., and Gerecht, S. (2013). Self-organized vascular networks from human pluripotent stem cells in a synthetic matrix. Proc. Natl. Acad. Sci. U.S.A. 110, 12601–12606. doi: 10.1073/pnas.1306562110
Kyritsis, N., Kizil, C., Zocher, S., Kroehne, V., Kaslin, J., Freudenreich, D., et al. (2012). Acute inflammation initiates the regenerative response in the adult zebrafish brain. Science 338, 1353–1356. doi: 10.1126/science.1228773
Lassmann, H. (2003). Hypoxia-like tissue injury as a component of multiple sclerosis lesions. J. Neurol. Sci. 206, 187–191. doi: 10.1016/S0022-510X(02)00421-5
Laterza, C., Merlini, A., De Feo, D., Ruffini, F., Menon, R., Onorati, M., et al. (2013). iPSC-derived neural precursors exert a neuroprotective role in immune-mediated demyelination via the secretion of LIF. Nat. Commun. 4:2597. doi: 10.1038/ncomms3597
Le Belle, J. E., Orozco, N. M., Paucar, A. A., Saxe, J. P., Mottahedeh, J., Pyle, A. D., et al. (2011). Proliferative neural stem cells have high endogenous ROS levels that regulate self-renewal and neurogenesis in a PI3K/Akt-dependant manner. Cell Stem Cell 8, 59–71. doi: 10.1016/j.stem.2010.11.028
Lee, N., Batt, M. K., Cronier, B. A., Jackson, M. C., Bruno Garza, J. L., Trinh, D. S., et al. (2013). Ciliary neurotrophic factor receptor regulation of adult forebrain neurogenesis. J. Neurosci. 33, 1241–1258. doi: 10.1523/JNEUROSCI.3386-12.2013
Lehtinen, M. K., and Walsh, C. A. (2011). Neurogenesis at the brain-cerebrospinal fluid interface. Annu. Rev. Cell Dev. Biol. 27, 653–679. doi: 10.1146/annurev-cellbio-092910-154026
Li, D., Zhou, J., Chowdhury, F., Cheng, J., Wang, N., and Wang, F. (2011). Role of mechanical factors in fate decisions of stem cells. Regen. Med. 6, 229–240. doi: 10.2217/rme.11.2
Li, L., Candelario, K. M., Thomas, K., Wang, R., Wright, K., Messier, A., et al. (2014). Hypoxia inducible factor-1a(HIF-1a) is required for neural stem cell maintenance and vascular stability in the adult mouse SVZ. J. Neurosci. 34, 16713–16719. doi: 10.1523/JNEUROSCI.4590-13.2014
Li, L., Harms, K. M., Ventura, P. B., Lagace, D. C., Eisch, A. J., and Cunningham, L. A. (2010a). Focal cerebral ischemia induces a multilineage cytogenic response from adult subventricular zone that is predominantly gliogenic. Glia 58, 1610–1619. doi: 10.1002/glia.21033
Li, L., Walker, T. L., Zhang, Y., Mackay, E. W., and Bartlett, P. F. (2010b). Endogenous interferon gamma directly regulates neural precursors in the non-inflammatory brain. J. Neurosci. 30, 9038–9050. doi: 10.1523/JNEUROSCI.5691-09.2010
Li, M., Hale, J. S., Rich, J. N., Ransohoff, R. M., and Lathia, J. D. (2012). Chemokine CXCL12 in neurodegenerative diseases: an SOS signal for stem cell-based repair. Trends Neurosci. 35, 619–628. doi: 10.1016/j.tins.2012.06.003
Liddelow, S. A., Guttenplan, K. A., Clarke, L. E., Bennett, F. C., Bohlen, C. J., Schirmer, L., et al. (2017). Neurotoxic reactive astrocytes are induced by activated microglia. Nature 541, 481–487. doi: 10.1038/nature21029
Lie, D. C., Colamarino, S. A., Song, H. J., Desire, L., Mira, H., Consiglio, A., et al. (2005). Wnt signalling regulates adult hippocampal neurogenesis. Nature 437, 1370–1375. doi: 10.1038/nature04108
Lie, D. C., Dziewczapolski, G., Willhoite, A. R., Kaspar, B. K., Shults, C. W., and Gage, F. H. (2002). The adult substantia nigra contains progenitor cells with neurogenic potential. J. Neurosci. 22, 6639–6649.
Lim, D. A., Tramontin, A. D., Trevejo, J. M., Herrera, D. G., Garcia-Verdugo, J. M., and Alvarez-Buylla, A. (2000). Noggin antagonizes BMP signaling to create a niche for adult neurogenesis. Neuron 28, 713–726. doi: 10.1016/S0896-6273(00)00148-3
Liu, C., Teng, Z. Q., Santistevan, N. J., Szulwach, K. E., Guo, W., Jin, P., et al. (2010). Epigenetic regulation of miR-184 by MBD1 governs neural stem cell proliferation and differentiation. Cell Stem Cell 6, 433–444. doi: 10.1016/j.stem.2010.02.017
Liu, F., Benashski, S. E., Persky, R., Xu, Y., Li, J., and McCullough, L. D. (2012). Age-related changes in AMP-activated protein kinase after stroke. Age 34, 157–168. doi: 10.1007/s11357-011-9214-8
Liu, Q., Sanai, N., Jin, W. N., La Cava, A., Van Kaer, L., and Shi, F. D. (2016). Neural stem cells sustain natural killer cells that dictate recovery from brain inflammation. Nat. Neurosci. 19, 243–252. doi: 10.1038/nn.4211
Liu, X. S., Chopp, M., Zhang, R. L., Hozeska-Solgot, A., Gregg, S. C., Buller, B., et al. (2009). Angiopoietin 2 mediates the differentiation and migration of neural progenitor cells in the subventricular zone after stroke. J. Biol. Chem. 284, 22680–22689. doi: 10.1074/jbc.M109.006551
Liu, X., Wang, Q., Haydar, T. F., and Bordey, A. (2005). Nonsynaptic GABA signaling in postnatal subventricular zone controls proliferation of GFAP-expressing progenitors. Nat. Neurosci. 8, 1179–1187. doi: 10.1038/nn1522
Llorens-Martin, M., Torres-Aleman, I., and Trejo, J. L. (2009). Mechanisms mediating brain plasticity: IGF1 and adult hippocampal neurogenesis. Neuroscientist 15, 134–148. doi: 10.1177/1073858408331371
Lu, Z., and Kipnis, J. (2010). Thrombospondin 1—a key astrocyte-derived neurogenic factor. FASEB J. 24, 1925–1934. doi: 10.1096/fj.09-150573
Lu, Z., Elliott, M. R., Chen, Y., Walsh, J. T., Klibanov, A. L., Ravichandran, K. S., et al. (2011). Phagocytic activity of neuronal progenitors regulates adult neurogenesis. Nat. Cell Biol. 13, 1076–1083. doi: 10.1038/ncb2299
Luo, C. X., Jin, X., Cao, C. C., Zhu, M. M., Wang, B., Chang, L., et al. (2010). Bidirectional regulation of neurogenesis by neuronal nitric oxide synthase derived from neurons and neural stem cells. Stem Cells 28, 2041–2052. doi: 10.1002/stem.522
Luo, J., Shook, B. A., Daniels, S. B., and Conover, J. C. (2008). Subventricular zone-mediated ependyma repair in the adult mammalian brain. J. Neurosci. 28, 3804–3813. doi: 10.1523/JNEUROSCI.0224-08.2008
Macas, J., Nern, C., Plate, K. H., and Momma, S. (2006). Increased generation of neuronal progenitors after ischemic injury in the aged adult human forebrain. J. Neurosci. 26, 13114–13119. doi: 10.1523/JNEUROSCI.4667-06.2006
Madhavan, L., Ourednik, V., and Ourednik, J. (2006). Increased “vigilance” of antioxidant mechanisms in neural stem cells potentiates their capability to resist oxidative stress. Stem Cells 24, 2110–2119. doi: 10.1634/stemcells.2006-0018
Magnusson, J. P., Goritz, C., Tatarishvili, J., Dias, D. O., Smith, E. M., Lindvall, O., et al. (2014). A latent neurogenic program in astrocytes regulated by Notch signaling in the mouse. Science 346, 237–241. doi: 10.1126/science.346.6206.237
Mahad, D. H., Trapp, B. D., and Lassmann, H. (2015). Pathological mechanisms in progressive multiple sclerosis. Lancet Neurol. 14, 183–193. doi: 10.1016/S1474-4422(14)70256-X
Marcialis, M. A., Coni, E., Pintus, M. C., Ravarino, A., Fanos, V., Coni, C., et al. (2016). Introduction to embryonic and adult neural stem cells: from the metabolic circuits of the niches to the metabolome. J. Pediatric Neonatal. Individualized Med. 5. doi: 10.7363/050215
Marinigh, R., Lip, G. Y., Fiotti, N., Giansante, C., and Lane, D. A. (2010). Age as a risk factor for stroke in atrial fibrillation patients: implications for thromboprophylaxis. J. Am. Coll. Cardiol. 56, 827–837. doi: 10.1016/j.jacc.2010.05.028
Marques, F., Sousa, J. C., Coppola, G., Gao, F., Puga, R., Brentani, H., et al. (2011). Transcriptome signature of the adult mouse choroid plexus. Fluids Barriers CNS 8:10. doi: 10.1186/2045-8118-8-10
Marschallinger, J., Schaffner, I., Klein, B., Gelfert, R., Rivera, F. J., Illes, S., et al. (2015). Structural and functional rejuvenation of the aged brain by an approved anti-asthmatic drug. Nat. Commun. 6:8466. doi: 10.1038/ncomms9466
Marti-Fabregas, J., Romaguera-Ros, M., Gomez-Pinedo, U., Martinez-Ramirez, S., Jimenez-Xarrie, E., Marin, R., et al. (2010). Proliferation in the human ipsilateral subventricular zone after ischemic stroke. Neurology 74, 357–365. doi: 10.1212/WNL.0b013e3181cbccec
Martino, G. (2004). How the brain repairs itself: new therapeutic strategies in inflammatory and degenerative CNS disorders. Lancet Neurol. 3, 372–378. doi: 10.1016/S1474-4422(04)00771-9
Martino, G., and Pluchino, S. (2006). The therapeutic potential of neural stem cells. Nat. Rev. Neurosci. 7, 395–406. doi: 10.1038/nrn1908
Martino, G., Butti, E., and Bacigaluppi, M. (2014). Neurogenesis or non-neurogenesis: that is the question. J. Clin. Invest. 124, 970–973. doi: 10.1172/JCI74419
Martino, G., Pluchino, S., Bonfanti, L., and Schwartz, M. (2011). Brain regeneration in physiology and pathology: the immune signature driving therapeutic plasticity of neural stem cells. Physiol. Rev. 91, 1281–1304. doi: 10.1152/physrev.00032.2010
Meng, C., Zhang, J. C., Shi, R. L., Zhang, S. H., and Yuan, S. Y. (2015). Inhibition of interleukin-6 abolishes the promoting effects of pair housing on post-stroke neurogenesis. Neuroscience 307, 160–170. doi: 10.1016/j.neuroscience.2015.08.055
Menn, B., Garcia-Verdugo, J. M., Yaschine, C., Gonzalez-Perez, O., Rowitch, D., and Alvarez-Buylla, A. (2006). Origin of oligodendrocytes in the subventricular zone of the adult brain. J. Neurosci. 26, 7907–7918. doi: 10.1523/JNEUROSCI.1299-06.2006
Mercier, F., Kitasako, J. T., and Hatton, G. I. (2002). Anatomy of the brain neurogenic zones revisited: fractones and the fibroblast/macrophage network. J. Comp. Neurol. 451, 170–188. doi: 10.1002/cne.10342
Michailidou, I., de Vries, H. E., Hol, E. M., and van Strien, M. E. (2014). Activation of endogenous neural stem cells for multiple sclerosis therapy. Front. Neurosci. 8:454. doi: 10.3389/fnins.2014.00454
Minden, S. L., Frankel, D., Hadden, L. S., Srinath, K. P., and Perloff, J. N. (2004). Disability in elderly people with multiple sclerosis: An analysis of baseline data from the Sonya Slifka longitudinal multiple sclerosis study. NeuroRehabilitation 19, 55–67.
Mine, Y., Tatarishvili, J., Oki, K., Monni, E., Kokaia, Z., and Lindvall, O. (2013). Grafted human neural stem cells enhance several steps of endogenous neurogenesis and improve behavioral recovery after middle cerebral artery occlusion in rats. Neurobiol. Dis. 52, 191–203. doi: 10.1016/j.nbd.2012.12.006
Ming, G. L., and Song, H. (2005). Adult neurogenesis in the mammalian central nervous system. Annu. Rev. Neurosci. 28, 223–250. doi: 10.1146/annurev.neuro.28.051804.101459
Ming, G. L., and Song, H. (2011). Adult neurogenesis in the mammalian brain: significant answers and significant questions. Neuron 70, 687–702. doi: 10.1016/j.neuron.2011.05.001
Minger, S. L., Ekonomou, A., Carta, E. M., Chinoy, A., Perry, R. H., and Ballard, C. G. (2007). Endogenous neurogenesis in the human brain following cerebral infarction. Regen. Med. 2, 69–74. doi: 10.2217/17460751.2.1.69
Miranda, C. J., Braun, L., Jiang, Y., Hester, M. E., Zhang, L., Riolo, M., et al. (2012). Aging brain microenvironment decreases hippocampal neurogenesis through Wnt-mediated survivin signaling. Aging Cell 11, 542–552. doi: 10.1111/j.1474-9726.2012.00816.x
Molofsky, A. V., Slutsky, S. G., Joseph, N. M., He, S., Pardal, R., Krishnamurthy, J., et al. (2006). Increasing p16INK4a expression decreases forebrain progenitors and neurogenesis during ageing. Nature 443, 448–452. doi: 10.1038/nature05091
Monje, M. L., Toda, H., and Palmer, T. D. (2003). Inflammatory blockade restores adult hippocampal neurogenesis. Science 302, 1760–1765. doi: 10.1126/science.1088417
Moriyama, M., Fukuhara, T., Britschgi, M., He, Y., Narasimhan, R., Villeda, S., et al. (2011). Complement receptor 2 is expressed in neural progenitor cells and regulates adult hippocampal neurogenesis. J. Neurosci. 31, 3981–3989. doi: 10.1523/JNEUROSCI.3617-10.2011
Mosher, K. I., Andres, R. H., Fukuhara, T., Bieri, G., Hasegawa-Moriyama, M., He, Y., et al. (2012). Neural progenitor cells regulate microglia functions and activity. Nat. Neurosci. 15, 1485–1487. doi: 10.1038/nn.3233
Muller, F. J., Snyder, E. Y., and Loring, J. F. (2006). Gene therapy: can neural stem cells deliver? Nat. Rev. Neurosci. 7, 75–84. doi: 10.1038/nrn1829
Muratori, L., Ronchi, G., Raimondo, S., Geuna, S., Giacobini-Robecchi, M. G., and Fornaro, M. (2015). Generation of new neurons in dorsal root Ganglia in adult rats after peripheral nerve crush injury. Neural Plast. 2015:860546. doi: 10.1155/2015/860546
Niquet, J., Baldwin, R. A., Allen, S. G., Fujikawa, D. G., and Wasterlain, C. G. (2003). Hypoxic neuronal necrosis: protein synthesis-independent activation of a cell death program. Proc. Natl. Acad. Sci. U.S.A. 100, 2825–2830. doi: 10.1073/pnas.0530113100
Oh, J., Lee, Y. D., and Wagers, A. J. (2014). Stem cell aging: mechanisms, regulators and therapeutic opportunities. Nat. Med. 20, 870–880. doi: 10.1038/nm.3651
Ohab, J. J., and Carmichael, S. T. (2008). Poststroke neurogenesis: emerging principles of migration and localization of immature neurons. Neuroscientist 14, 369–380. doi: 10.1177/1073858407309545
Ohab, J. J., Fleming, S., Blesch, A., and Carmichael, S. T. (2006). A neurovascular niche for neurogenesis after stroke. J. Neurosci. 26, 13007–13016. doi: 10.1523/JNEUROSCI.4323-06.2006
Okamoto, M., Inoue, K., Iwamura, H., Terashima, K., Soya, H., Asashima, M., et al. (2011). Reduction in paracrine Wnt3 factors during aging causes impaired adult neurogenesis. FASEB J. 25, 3570–3582. doi: 10.1096/fj.11-184697
O'Keeffe, G. C., Tyers, P., Aarsland, D., Dalley, J. W., Barker, R. A., and Caldwell, M. A. (2009). Dopamine-induced proliferation of adult neural precursor cells in the mammalian subventricular zone is mediated through EGF. Proc. Natl. Acad. Sci. U.S.A. 106, 8754–8759. doi: 10.1073/pnas.0803955106
Oki, K., Tatarishvili, J., Wood, J., Koch, P., Wattananit, S., Mine, Y., et al. (2012). Human-induced pluripotent stem cells form functional neurons and improve recovery after grafting in stroke-damaged brain. Stem Cells 30, 1120–1133. doi: 10.1002/stem.1104
Osman, A. M., Neumann, S., Kuhn, H. G., and Blomgren, K. (2016). Caspase inhibition impaired the neural stem/progenitor cell response after cortical ischemia in mice. Oncotarget 7, 2239–2248. doi: 10.18632/oncotarget.6803
Paez-Gonzalez, P., Abdi, K., Luciano, D., Liu, Y., Soriano-Navarro, M., Rawlins, E., et al. (2011). Ank3-dependent SVZ niche assembly is required for the continued production of new neurons. Neuron 71, 61–75. doi: 10.1016/j.neuron.2011.05.029
Paez-Gonzalez, P., Asrican, B., Rodriguez, E., and Kuo, C. T. (2014). Identification of distinct ChAT+ neurons and activity-dependent control of postnatal SVZ neurogenesis. Nat. Neurosci. 17, 934–942. doi: 10.1038/nn.3734
Palmer, T. D., Willhoite, A. R., and Gage, F. H. (2000). Vascular niche for adult hippocampal neurogenesis. J. Comp. Neurol. 425, 479–494. doi: 10.1002/1096-9861(20001002)425:4<479::AID-CNE2>3.0.CO;2-3
Parent, J. M., Vexler, Z. S., Gong, C., Derugin, N., and Ferriero, D. M. (2002). Rat forebrain neurogenesis and striatal neuron replacement after focal stroke. Ann. Neurol. 52, 802–813. doi: 10.1002/ana.10393
Park, C. B., and Larsson, N. G. (2011). Mitochondrial DNA mutations in disease and aging. J. Cell Biol. 193, 809–818. doi: 10.1083/jcb.201010024
Pavlica, S., Milosevic, J., Keller, M., Schulze, M., Peinemann, F., Piscioneri, A., et al. (2012). Erythropoietin enhances cell proliferation and survival of human fetal neuronal progenitors in normoxia. Brain Res. 1452, 18–28. doi: 10.1016/j.brainres.2012.02.043
Pereira, L., Medina, R., Baena, M., Planas, A. M., and Pozas, E. (2015). IFN gamma regulates proliferation and neuronal differentiation by STAT1 in adult SVZ niche. Front. Cell. Neurosci. 9:270. doi: 10.3389/fncel.2015.00270
Perez-Asensio, F. J., Perpina, U., Planas, A. M., and Pozas, E. (2013). Interleukin-10 regulates progenitor differentiation and modulates neurogenesis in adult brain. J. Cell Sci. 126(Pt 18), 4208–4219. doi: 10.1242/jcs.127803
Plane, J. M., Whitney, J. T., Schallert, T., and Parent, J. M. (2008). Retinoic acid and environmental enrichment alter subventricular zone and striatal neurogenesis after stroke. Exp. Neurol. 214, 125–134. doi: 10.1016/j.expneurol.2008.08.006
Platel, J. C., Dave, K. A., Gordon, V., Lacar, B., Rubio, M. E., and Bordey, A. (2010). NMDA receptors activated by subventricular zone astrocytic glutamate are critical for neuroblast survival prior to entering a synaptic network. Neuron 65, 859–872. doi: 10.1016/j.neuron.2010.03.009
Pluchino, S., Cusimano, M., Bacigaluppi, M., and Martino, G. (2010). Remodelling the injured CNS through the establishment of atypical ectopic perivascular neural stem cell niches. Arch. Ital. Biol. 148, 173–183.
Pluchino, S., Gritti, A., Blezer, E., Amadio, S., Brambilla, E., Borsellino, G., et al. (2009). Human neural stem cells ameliorate autoimmune encephalomyelitis in non-human primates. Ann. Neurol. 66, 343–354. doi: 10.1002/ana.21745
Pluchino, S., Muzio, L., Imitola, J., Deleidi, M., Alfaro-Cervello, C., Salani, G., et al. (2008). Persistent inflammation alters the function of the endogenous brain stem cell compartment. Brain 131(Pt 10), 2564–2578. doi: 10.1093/brain/awn198
Pluchino, S., Quattrini, A., Brambilla, E., Gritti, A., Salani, G., Dina, G., et al. (2003). Injection of adult neurospheres induces recovery in a chronic model of multiple sclerosis. Nature 422, 688–694. doi: 10.1038/nature01552
Pluchino, S., Zanotti, L., Rossi, B., Brambilla, E., Ottoboni, L., Salani, G., et al. (2005). Neurosphere-derived multipotent precursors promote neuroprotection by an immunomodulatory mechanism. Nature 436, 266–271. doi: 10.1038/nature03889
Polentes, J., Jendelova, P., Cailleret, M., Braun, H., Romanyuk, N., Tropel, P., et al. (2012). Human induced pluripotent stem cells improve stroke outcome and reduce secondary degeneration in the recipient brain. Cell Transplant. 21, 2587–2602. doi: 10.3727/096368912X653228
Pollock, K., Stroemer, P., Patel, S., Stevanato, L., Hope, A., Miljan, E., et al. (2006). A conditionally immortal clonal stem cell line from human cortical neuroepithelium for the treatment of ischemic stroke. Exp. Neurol. 199, 143–155. doi: 10.1016/j.expneurol.2005.12.011
Popa-Wagner, A., Carmichael, S. T., Kokaia, Z., Kessler, C., and Walker, L. C. (2007). The response of the aged brain to stroke: too much, too soon? Curr. Neurovasc. Res. 4, 216–227. doi: 10.2174/156720207781387213
Pourabdolhossein, F., Gil-Perotin, S., Garcia-Belda, P., Dauphin, A., Mozafari, S., Tepavcevic, V., et al. (2017). Inflammatory demyelination induces ependymal modifications concomitant to activation of adult (SVZ) stem cell proliferation. Glia 65, 756–772. doi: 10.1002/glia.23124
Preston, M., and Sherman, L. S. (2011). Neural stem cell niches: roles for the hyaluronan-based extracellular matrix. Front. Biosci. 3, 1165–1179. doi: 10.2741/218
Qi, Y., Zhang, X. J., Renier, N., Wu, Z., Atkin, T., Sun, Z., et al. (2017). Combined small-molecule inhibition accelerates the derivation of functional cortical neurons from human pluripotent stem cells. Nat. Biotechnol. 35, 154–163. doi: 10.1038/nbt.3777
Quinones-Hinojosa, A., Sanai, N., Soriano-Navarro, M., Gonzalez-Perez, O., Mirzadeh, Z., Gil-Perotin, S., et al. (2006). Cellular composition and cytoarchitecture of the adult human subventricular zone: a niche of neural stem cells. J. Comp. Neurol. 494, 415–434. doi: 10.1002/cne.20798
Rabie, T., Kunze, R., and Marti, H. H. (2011). Impaired hypoxic response in senescent mouse brain. Int. J. Dev. Neurosci. 29, 655–661. doi: 10.1016/j.ijdevneu.2011.06.003
Rafalski, V. A., Mancini, E., and Brunet, A. (2012). Energy metabolism and energy-sensing pathways in mammalian embryonic and adult stem cell fate. J. Cell Sci. 125(Pt 23), 5597–5608. doi: 10.1242/jcs.114827
Reinhardt, P., Glatza, M., Hemmer, K., Tsytsyura, Y., Thiel, C. S., Hoing, S., et al. (2013). Derivation and expansion using only small molecules of human neural progenitors for neurodegenerative disease modeling. PLoS ONE 8:e59252. doi: 10.1371/journal.pone.0059252
Reiss, K., Mentlein, R., Sievers, J., and Hartmann, D. (2002). Stromal cell-derived factor 1 is secreted by meningeal cells and acts as chemotactic factor on neuronal stem cells of the cerebellar external granular layer. Neuroscience 115, 295–305. doi: 10.1016/S0306-4522(02)00307-X
Rist, J. M., and Franklin, R. J. (2008). Taking ageing into account in remyelination-based therapies for multiple sclerosis. J. Neurol. Sci. 274, 64–67. doi: 10.1016/j.jns.2008.04.027
Roger, V. L., Go, A. S., Lloyd-Jones, D. M., Benjamin, E. J., Berry, J. D., Borden, W. B., et al. (2012). Heart disease and stroke statistics–2012 update: a report from the American Heart Association. Circulation 125, e2–e220. doi: 10.1161/CIR.0b013e31823ac046
Rohatgi, R., Milenkovic, L., and Scott, M. P. (2007). Patched1 regulates hedgehog signaling at the primary cilium. Science 317, 372–376. doi: 10.1126/science.1139740
Ruckh, J. M., Zhao, J. W., Shadrach, J. L., van Wijngaarden, P., Rao, T. N., Wagers, A. J., et al. (2012). Rejuvenation of regeneration in the aging central nervous system. Cell Stem Cell 10, 96–103. doi: 10.1016/j.stem.2011.11.019
Ruiz de Almodovar, C., Lambrechts, D., Mazzone, M., and Carmeliet, P. (2009). Role and therapeutic potential of VEGF in the nervous system. Physiol. Rev. 89, 607–648. doi: 10.1152/physrev.00031.2008
Samanta, J., Grund, E. M., Silva, H. M., Lafaille, J. J., Fishell, G., and Salzer, J. L. (2015). Inhibition of Gli1 mobilizes endogenous neural stem cells for remyelination. Nature 526, 448–452. doi: 10.1038/nature14957
Sanai, N., Tramontin, A. D., Quinones-Hinojosa, A., Barbaro, N. M., Gupta, N., Kunwar, S., et al. (2004). Unique astrocyte ribbon in adult human brain contains neural stem cells but lacks chain migration. Nature 427, 740–744. doi: 10.1038/nature02301
Sandvig, I., Gadjanski, I., Vlaski-Lafarge, M., Buzanska, L., Loncaric, D., Sarnowska, A., et al. (2017). Strategies to enhance implantation and survival of stem cells after their injection in ischemic neural tissue. Stem Cells Dev. 26, 554–565. doi: 10.1089/scd.2016.0268
Santilli, G., Lamorte, G., Carlessi, L., Ferrari, D., Rota Nodari, L., Binda, E., et al. (2010). Mild hypoxia enhances proliferation and multipotency of human neural stem cells. PLoS ONE 5:e8575. doi: 10.1371/journal.pone.0008575
Scalfari, A., Neuhaus, A., Daumer, M., Ebers, G. C., and Muraro, P. A. (2011). Age and disability accumulation in multiple sclerosis. Neurology 77, 1246–1252. doi: 10.1212/WNL.0b013e318230a17d
Seib, D. R., Corsini, N. S., Ellwanger, K., Plaas, C., Mateos, A., Pitzer, C., et al. (2013). Loss of Dickkopf-1 restores neurogenesis in old age and counteracts cognitive decline. Cell Stem Cell 12, 204–214. doi: 10.1016/j.stem.2012.11.010
Seri, B., Garcia-Verdugo, J. M., Collado-Morente, L., McEwen, B. S., and Alvarez-Buylla, A. (2004). Cell types, lineage, and architecture of the germinal zone in the adult dentate gyrus. J. Comp. Neurol. 478, 359–378. doi: 10.1002/cne.20288
Shen, Q., Goderie, S. K., Jin, L., Karanth, N., Sun, Y., Abramova, N., et al. (2004). Endothelial cells stimulate self-renewal and expand neurogenesis of neural stem cells. Science 304, 1338–1340. doi: 10.1126/science.1095505
Shen, Q., Wang, Y., Kokovay, E., Lin, G., Chuang, S. M., Goderie, S. K., et al. (2008). Adult SVZ stem cells lie in a vascular niche: a quantitative analysis of niche cell-cell interactions. Cell Stem Cell 3, 289–300. doi: 10.1016/j.stem.2008.07.026
Shetty, A. K., and Hattiangady, B. (2016). Grafted subventricular zone neural stem cells display robust engraftment and similar differentiation properties and form new neurogenic niches in the young and aged hippocampus. Stem Cells Transl. Med. 5, 1204–1215. doi: 10.5966/sctm.2015-0270
Shetty, A. K., Hattiangady, B., and Shetty, G. A. (2005). Stem/progenitor cell proliferation factors FGF-2, IGF-1, and VEGF exhibit early decline during the course of aging in the hippocampus: role of astrocytes. Glia 51, 173–186. doi: 10.1002/glia.20187
Shimazu, K., Zhao, M., Sakata, K., Akbarian, S., Bates, B., Jaenisch, R., et al. (2006). NT-3 facilitates hippocampal plasticity and learning and memory by regulating neurogenesis. Learn. Mem. 13, 307–315. doi: 10.1101/lm.76006
Shyh-Chang, N., Daley, G. Q., and Cantley, L. C. (2013). Stem cell metabolism in tissue development and aging. Development 140, 2535–2547. doi: 10.1242/dev.091777
Siegenthaler, J. A., Ashique, A. M., Zarbalis, K., Patterson, K. P., Hecht, J. H., Kane, M. A., et al. (2009). Retinoic acid from the meninges regulates cortical neuron generation. Cell 139, 597–609. doi: 10.1016/j.cell.2009.10.004
Sierra, A., Tremblay, M. E., and Wake, H. (2014). Never-resting microglia: physiological roles in the healthy brain and pathological implications. Front. Cell. Neurosci. 8:240. doi: 10.3389/fncel.2014.00240
Sim, F. J., Zhao, C., Penderis, J., and Franklin, R. J. (2002). The age-related decrease in CNS remyelination efficiency is attributable to an impairment of both oligodendrocyte progenitor recruitment and differentiation. J. Neurosci. 22, 2451–2459.
Siqueira, I. R., Fochesatto, C., de Andrade, A., Santos, M., Hagen, M., Bello-Klein, A., et al. (2005). Total antioxidant capacity is impaired in different structures from aged rat brain. Int. J. Dev. Neurosci. 23, 663–671. doi: 10.1016/j.ijdevneu.2005.03.001
Smith, L. K., He, Y., Park, J. S., Bieri, G., Snethlage, C. E., Lin, K., et al. (2015). β2-microglobulin is a systemic pro-aging factor that impairs cognitive function and neurogenesis. Nat. Med. 21, 932–937. doi: 10.1038/nm.3898
Smith, S. C., Zhang, X., Zhang, X., Gross, P., Starosta, T., Mohsin, S., et al. (2015). GDF11 does not rescue aging-related pathological hypertrophy. Circ. Res. 117, 926–932. doi: 10.1161/CIRCRESAHA.115.307527
Sola, S., Morgado, A. L., and Rodrigues, C. M. (2013). Death receptors and mitochondria: two prime triggers of neural apoptosis and differentiation. Biochim. Biophys. Acta 1830, 2160–2166. doi: 10.1016/j.bbagen.2012.09.021
Song, J., Zhong, C., Bonaguidi, M. A., Sun, G. J., Hsu, D., Gu, Y., et al. (2012). Neuronal circuitry mechanism regulating adult quiescent neural stem-cell fate decision. Nature 489, 150–154. doi: 10.1038/nature11306
Sonntag, W. E., Ramsey, M., and Carter, C. S. (2005). Growth hormone and insulin-like growth factor-1 (IGF-1) and their influence on cognitive aging. Ageing Res. Rev. 4, 195–212. doi: 10.1016/j.arr.2005.02.001
Spalding, K. L., Bergmann, O., Alkass, K., Bernard, S., Salehpour, M., Huttner, H. B., et al. (2013). Dynamics of hippocampal neurogenesis in adult humans. Cell 153, 1219–1227. doi: 10.1016/j.cell.2013.05.002
Stein, L. R., and Imai, S. (2014). Specific ablation of Nampt in adult neural stem cells recapitulates their functional defects during aging. EMBO J. 33, 1321–1340. doi: 10.1002/embj.201386917
Stidworthy, M. F., Genoud, S., Li, W. W., Leone, D. P., Mantei, N., Suter, U., et al. (2004). Notch1 and Jagged1 are expressed after CNS demyelination, but are not a major rate-determining factor during remyelination. Brain 127(Pt 9), 1928–1941. doi: 10.1093/brain/awh217
Stolp, H. B., and Molnar, Z. (2015). Neurogenic niches in the brain: help and hindrance of the barrier systems. Front. Neurosci. 9:20. doi: 10.3389/fnins.2015.00020
Su, P., Zhang, J., Zhao, F., Aschner, M., Chen, J., and Luo, W. (2014). The interaction between microglia and neural stem/precursor cells. Brain Res. Bull. 109, 32–38. doi: 10.1016/j.brainresbull.2014.09.005
Suh, H., Deng, W., and Gage, F. H. (2009). Signaling in adult neurogenesis. Annu. Rev. Cell Dev. Biol. 25, 253–275. doi: 10.1146/annurev.cellbio.042308.113256
Sun, X., Tanaka, M., Kondo, S., Okamoto, K., and Hirai, S. (1998). Clinical significance of reduced cerebral metabolism in multiple sclerosis: a combined PET and MRI study. Ann. Nucl. Med. 12, 89–94.
Takahashi, K., Tanabe, K., Ohnuki, M., Narita, M., Ichisaka, T., Tomoda, K., et al. (2007). Induction of pluripotent stem cells from adult human fibroblasts by defined factors. Cell 131, 861–872. doi: 10.1016/j.cell.2007.11.019
Tatarishvili, J., Oki, K., Monni, E., Koch, P., Memanishvili, T., Buga, A. M., et al. (2014). Human induced pluripotent stem cells improve recovery in stroke-injured aged rats. Restor. Neurol. Neurosci. 32, 547–558. doi: 10.3233/RNN-140404
Tavazoie, M., Van der Veken, L., Silva-Vargas, V., Louissaint, M., Colonna, L., Zaidi, B., et al. (2008). A specialized vascular niche for adult neural stem cells. Cell Stem Cell 3, 279–288. doi: 10.1016/j.stem.2008.07.025
Taverna, E., Gotz, M., and Huttner, W. B. (2014). The cell biology of neurogenesis: toward an understanding of the development and evolution of the neocortex. Annu. Rev. Cell Dev. Biol. 30, 465–502. doi: 10.1146/annurev-cellbio-101011-155801
Tepavcevic, V., Lazarini, F., Alfaro-Cervello, C., Kerninon, C., Yoshikawa, K., Garcia-Verdugo, J. M., et al. (2011). Inflammation-induced subventricular zone dysfunction leads to olfactory deficits in a targeted mouse model of multiple sclerosis. J. Clin. Invest. 121, 4722–4734. doi: 10.1172/JCI59145
Thery, C., Zitvogel, L., and Amigorena, S. (2002). Exosomes: composition, biogenesis and function. Nat. Rev. Immunol. 2, 569–579. doi: 10.1038/nri855
Thored, P., Arvidsson, A., Cacci, E., Ahlenius, H., Kallur, T., Darsalia, V., et al. (2006). Persistent production of neurons from adult brain stem cells during recovery after stroke. Stem Cells 24, 739–747. doi: 10.1634/stemcells.2005-0281
Thored, P., Wood, J., Arvidsson, A., Cammenga, J., Kokaia, Z., and Lindvall, O. (2007). Long-term neuroblast migration along blood vessels in an area with transient angiogenesis and increased vascularization after stroke. Stroke 38, 3032–3039. doi: 10.1161/STROKEAHA.107.488445
Tirone, F., Farioli-Vecchioli, S., Micheli, L., Ceccarelli, M., and Leonardi, L. (2013). Genetic control of adult neurogenesis: interplay of differentiation, proliferation and survival modulates new neurons function, and memory circuits. Front. Cell. Neurosci. 7:59. doi: 10.3389/fncel.2013.00059
Tonchev, A. B., Yamashima, T., Zhao, L., Okano, H. J., and Okano, H. (2003). Proliferation of neural and neuronal progenitors after global brain ischemia in young adult macaque monkeys. Mol. Cell. Neurosci. 23, 292–301. doi: 10.1016/S1044-7431(03)00058-7
Tornero, D., Tsupykov, O., Granmo, M., Rodriguez, C., Gronning-Hansen, M., Thelin, J., et al. (2017). Synaptic inputs from stroke-injured brain to grafted human stem cell-derived neurons activated by sensory stimuli. Brain 40, 692–706. doi: 10.1093/brain/aww347
Tornero, D., Wattananit, S., Gronning Madsen, M., Koch, P., Wood, J., Tatarishvili, J., et al. (2013). Human induced pluripotent stem cell-derived cortical neurons integrate in stroke-injured cortex and improve functional recovery. Brain 136(Pt 12), 3561–3577. doi: 10.1093/brain/awt278
Trapp, B. D., and Stys, P. K. (2009). Virtual hypoxia and chronic necrosis of demyelinated axons in multiple sclerosis. Lancet Neurol. 8, 280–291. doi: 10.1016/S1474-4422(09)70043-2
Triaca, V., Tirassa, P., and Aloe, L. (2005). Presence of nerve growth factor and TrkA expression in the SVZ of EAE rats: evidence for a possible functional significance. Exp. Neurol. 191, 53–64. doi: 10.1016/j.expneurol.2004.08.034
Tropepe, V., Craig, C. G., Morshead, C. M., and van der Kooy, D. (1997). Transforming growth factor-alpha null and senescent mice show decreased neural progenitor cell proliferation in the forebrain subependyma. J. Neurosci. 17, 7850–7859.
Udo, H., Yoshida, Y., Kino, T., Ohnuki, K., Mizunoya, W., Mukuda, T., et al. (2008). Enhanced adult neurogenesis and angiogenesis and altered affective behaviors in mice overexpressing vascular endothelial growth factor 120. J. Neurosci. 28, 14522–14536. doi: 10.1523/JNEUROSCI.3673-08.2008
Ueki, T., Tanaka, M., Yamashita, K., Mikawa, S., Qiu, Z., Maragakis, N. J., et al. (2003). A novel secretory factor, Neurogenesin-1, provides neurogenic environmental cues for neural stem cells in the adult hippocampus. J. Neurosci. 23, 11732–11740.
Valerio, A., Ferrario, M., Dreano, M., Garotta, G., Spano, P., and Pizzi, M. (2002). Soluble interleukin-6 (IL-6) receptor/IL-6 fusion protein enhances in vitro differentiation of purified rat oligodendroglial lineage cells. Mol. Cell. Neurosci. 21, 602–615. doi: 10.1006/mcne.2002.1208
Vallieres, L., Campbell, I. L., Gage, F. H., and Sawchenko, P. E. (2002). Reduced hippocampal neurogenesis in adult transgenic mice with chronic astrocytic production of interleukin-6. J. Neurosci. 22, 486–492.
Villeda, S. A., Luo, J., Mosher, K. I., Zou, B., Britschgi, M., Bieri, G., et al. (2011). The ageing systemic milieu negatively regulates neurogenesis and cognitive function. Nature 477, 90–94. doi: 10.1038/nature10357
Villeda, S. A., Plambeck, K. E., Middeldorp, J., Castellano, J. M., Mosher, K. I., Luo, J., et al. (2014). Young blood reverses age-related impairments in cognitive function and synaptic plasticity in mice. Nat. Med. 20, 659–663. doi: 10.1038/nm.3569
Vrotsos, E. G., Kolattukudy, P. E., and Sugaya, K. (2009). MCP-1 involvement in glial differentiation of neuroprogenitor cells through APP signaling. Brain Res. Bull. 79, 97–103. doi: 10.1016/j.brainresbull.2009.01.004
Walton, N. M., Sutter, B. M., Laywell, E. D., Levkoff, L. H., Kearns, S. M., Marshall, G. P. II., et al. (2006). Microglia instruct subventricular zone neurogenesis. Glia 54, 815–825. doi: 10.1002/glia.20419
Wang, C., Zhang, M., Sun, C., Cai, Y., You, Y., Huang, L., et al. (2011). Sustained increase in adult neurogenesis in the rat hippocampal dentate gyrus after transient brain ischemia. Neurosci. Lett. 488, 70–75. doi: 10.1016/j.neulet.2010.10.079
Wang, J., Xie, L., Yang, C., Ren, C., Zhou, K., Wang, B., et al. (2015). Activated regulatory T cell regulates neural stem cell proliferation in the subventricular zone of normal and ischemic mouse brain through interleukin 10. Front. Cell. Neurosci. 9:361. doi: 10.3389/fncel.2015.00361
Wang, X., Fu, S., Wang, Y., Yu, P., Hu, J., Gu, W., et al. (2007). Interleukin-1βmediates proliferation and differentiation of multipotent neural precursor cells through the activation of SAPK/JNK pathway. Mol. Cell. Neurosci. 36, 343–354. doi: 10.1016/j.mcn.2007.07.005
Wang, Y., Imitola, J., Rasmussen, S., O'Connor, K. C., and Khoury, S. J. (2008). Paradoxical dysregulation of the neural stem cell pathway sonic hedgehog-Gli1 in autoimmune encephalomyelitis and multiple sclerosis. Ann. Neurol. 64, 417–427. doi: 10.1002/ana.21457
Wells, C. A., Mosbergen, R., Korn, O., Choi, J., Seidenman, N., Matigian, N. A., et al. (2013). Stemformatics: visualisation and sharing of stem cell gene expression. Stem Cell Res. 10, 387–395. doi: 10.1016/j.scr.2012.12.003
Widera, D., Mikenberg, I., Elvers, M., Kaltschmidt, C., and Kaltschmidt, B. (2006). Tumor necrosis factor alpha triggers proliferation of adult neural stem cells via IKK/NF-κB signaling. BMC Neurosci. 7:64. doi: 10.1186/1471-2202-7-64
Wilhelmsson, U., Faiz, M., de Pablo, Y., Sjoqvist, M., Andersson, D., Widestrand, A., et al. (2012). Astrocytes negatively regulate neurogenesis through the Jagged1-mediated Notch pathway. Stem Cells 30, 2320–2329. doi: 10.1002/stem.1196
Wu, M. D., Montgomery, S. L., Rivera-Escalera, F., Olschowka, J. A., and O'Banion, M. K. (2013). Sustained IL-1β expression impairs adult hippocampal neurogenesis independent of IL-1 signaling in nestin+ neural precursor cells. Brain Behav. Immun. 32, 9–18. doi: 10.1016/j.bbi.2013.03.003
Wyss-Coray, T. (2016). Ageing, neurodegeneration and brain rejuvenation. Nature 539, 180–186. doi: 10.1038/nature20411
Yang, R. F., Sun, L. H., Zhang, R., Zhang, Y., Luo, Y. X., Zheng, W., et al. (2015). Suppression of Mic60 compromises mitochondrial transcription and oxidative phosphorylation. Sci. Rep. 5:7990. doi: 10.1038/srep07990
Yao, B., Christian, K. M., He, C., Jin, P., Ming, G. L., and Song, H. (2016). Epigenetic mechanisms in neurogenesis. Nat. Rev. Neurosci. 17, 537–549. doi: 10.1038/nrn.2016.70
Yong, V. W., and Rivest, S. (2009). Taking advantage of the systemic immune system to cure brain diseases. Neuron 64, 55–60. doi: 10.1016/j.neuron.2009.09.035
Young, S. Z., Lafourcade, C. A., Platel, J. C., Lin, T. V., and Bordey, A. (2014). GABAergic striatal neurons project dendrites and axons into the postnatal subventricular zone leading to calcium activity. Front. Cell. Neurosci. 8:10. doi: 10.3389/fncel.2014.00010
Yuan, T., Liao, W., Feng, N. H., Lou, Y. L., Niu, X., Zhang, A. J., et al. (2013). Human induced pluripotent stem cell-derived neural stem cells survive, migrate, differentiate, and improve neurologic function in a rat model of middle cerebral artery occlusion. Stem Cell Res. Ther. 4, 73. doi: 10.1186/scrt224
Zhang, C., Cao, J., Li, X., Xu, H., Wang, W., Wang, L., et al. (2016). Treatment of multiple sclerosis by transplantation of neural stem cells derived from induced pluripotent stem cells. Sci. China Life Sci. 59, 950–957. doi: 10.1007/s11427-016-0114-9
Zhang, G., Li, J., Purkayastha, S., Tang, Y., Zhang, H., Yin, Y., et al. (2013). Hypothalamic programming of systemic ageing involving IKK-β, NF-κB and GnRH. Nature 497, 211–216. doi: 10.1038/nature12143
Zhang, L., Marsboom, G., Glick, D., Zhang, Y., Toth, P. T., Jones, N., et al. (2014). Bioenergetic shifts during transitions between stem cell states (2013 Grover Conference series). Pulm. Circ. 4, 387–394. doi: 10.1086/677353
Zhang, P., Li, J., Liu, Y., Chen, X., Lu, H., Kang, Q., et al. (2011). Human embryonic neural stem cell transplantation increases subventricular zone cell proliferation and promotes peri-infarct angiogenesis after focal cerebral ischemia. Neuropathology 31, 384–391. doi: 10.1111/j.1440-1789.2010.01182.x
Zhang, R. L., Chopp, M., Gregg, S. R., Toh, Y., Roberts, C., Letourneau, Y., et al. (2009). Patterns and dynamics of subventricular zone neuroblast migration in the ischemic striatum of the adult mouse. J. Cereb. Blood Flow Metab. 29, 1240–1250. doi: 10.1038/jcbfm.2009.55
Zhang, R. L., Chopp, M., Roberts, C., Wei, M., Wang, X., Liu, X., et al. (2012). Sildenafil enhances neurogenesis and oligodendrogenesis in ischemic brain of middle-aged mouse. PLoS ONE 7:e48141. doi: 10.1371/journal.pone.0048141
Zhang, R. L., Zhang, Z. G., Zhang, L., and Chopp, M. (2001). Proliferation and differentiation of progenitor cells in the cortex and the subventricular zone in the adult rat after focal cerebral ischemia. Neuroscience 105, 33–41. doi: 10.1016/S0306-4522(01)00117-8
Zhang, Y., Chopp, M., Liu, X. S., Katakowski, M., Wang, X., Tian, X., et al. (2016). Exosomes derived from mesenchymal stromal cells promote axonal growth of cortical neurons. Mol. Neurobiol. 54, 2659–2673. doi: 10.1007/s12035-016-9851-0
Zhang, Z., and Chopp, M. (2016). Neural stem cells and ischemic brain. J Stroke 18, 267–272. doi: 10.5853/jos.2016.00206
Zhao, C., Deng, W., and Gage, F. H. (2008). Mechanisms and functional implications of adult neurogenesis. Cell 132, 645–660. doi: 10.1016/j.cell.2008.01.033
Zheng, X., Boyer, L., Jin, M., Mertens, J., Kim, Y., Ma, L., et al. (2016). Metabolic reprogramming during neuronal differentiation from aerobic glycolysis to neuronal oxidative phosphorylation. Elife 5:e13374. doi: 10.7554/eLife.13374
Zhu, P., Hata, R., Cao, F., Gu, F., Hanakawa, Y., Hashimoto, K., et al. (2008). Ramified microglial cells promote astrogliogenesis and maintenance of neural stem cells through activation of Stat3 function. FASEB J. 22, 3866–3877. doi: 10.1096/fj.08-105908
Keywords: neural stem cells, microenvironment, plasticity, metabolism, inflammation, stroke, multiple sclerosis, aging
Citation: Ottoboni L, Merlini A and Martino G (2017) Neural Stem Cell Plasticity: Advantages in Therapy for the Injured Central Nervous System. Front. Cell Dev. Biol. 5:52. doi: 10.3389/fcell.2017.00052
Received: 01 February 2017; Accepted: 25 April 2017;
Published: 12 May 2017.
Edited by:
Ivana Gadjanski, University of Belgrade, SerbiaReviewed by:
Loic P. Deleyrolle, University of Florida, USAMarco Tatullo, Tecnologica Research Institute, Italy
Copyright © 2017 Ottoboni, Merlini and Martino. This is an open-access article distributed under the terms of the Creative Commons Attribution License (CC BY). The use, distribution or reproduction in other forums is permitted, provided the original author(s) or licensor are credited and that the original publication in this journal is cited, in accordance with accepted academic practice. No use, distribution or reproduction is permitted which does not comply with these terms.
*Correspondence: Gianvito Martino, bWFydGluby5naWFudml0b0Boc3IuaXQ=
†These authors have contributed equally to this work.