- 1Sorbonne Universités, UPMC Univ Paris 06, CNRS, Laboratoire de Biologie du Développement de Villefranche-sur-mer (LBDV), Villefranche-sur-mer, France
- 2Université Côte d'Azur, CNRS, INSERM, Institute for Research on Cancer and Aging (IRCAN), Nice, France
The ability to perform muscle contractions is one of the most important and distinctive features of eumetazoans. As the sister group to bilaterians, cnidarians (sea anemones, corals, jellyfish, and hydroids) hold an informative phylogenetic position for understanding muscle evolution. Here, we review current knowledge on muscle function, diversity, development, regeneration and evolution in cnidarians. Cnidarian muscles are involved in various activities, such as feeding, escape, locomotion and defense, in close association with the nervous system. This variety is reflected in the large diversity of muscle organizations found in Cnidaria. Smooth epithelial muscle is thought to be the most common type, and is inferred to be the ancestral muscle type for Cnidaria, while striated muscle fibers and non-epithelial myocytes would have been convergently acquired within Cnidaria. Current knowledge of cnidarian muscle development and its regeneration is limited. While orthologs of myogenic regulatory factors such as MyoD have yet to be found in cnidarian genomes, striated muscle formation potentially involves well-conserved myogenic genes, such as twist and mef2. Although satellite cells have yet to be identified in cnidarians, muscle plasticity (e.g., de- and re-differentiation, fiber repolarization) in a regenerative context and its potential role during regeneration has started to be addressed in a few cnidarian systems. The development of novel tools to study those organisms has created new opportunities to investigate in depth the development and regeneration of cnidarian muscle cells and how they contribute to the regenerative process.
Introduction
Muscles, tissues specialized for contraction, are an essential component of the eumetazoan (all animals except sponges and placozoans) body. They are involved in various functions of the body and are well characterized in various vertebrate and main non-vertebrate models (reviewed in Schmidt-Rhaesa, 2007; Bryson-Richardson and Currie, 2008; Bentzinger et al., 2012; Andrikou and Arnone, 2015; Almada and Wagers, 2016). In bilaterians, muscles are rich in myofilaments (organized arrays composed principally of actin and myosin II) and present two basic types of cells: true muscle cells (myocytes) and myoepithelial cells. Myocytes are individual muscle cells, usually not anchored to the extracellular matrix (ECM), which during embryogenesis derive mainly (but not exclusively) from the mesoderm layer. In contrast, myoepithelial cells, which have a variety of embryological origins, are anchored to the ECM and are fully integrated into an epithelial tissue layer. Both of these muscle cell-types can be further defined as either striated or smooth, depending on the internal organization of the myofilaments. Visible striations represent repeating functional units of the muscle (the sarcomeres), which result from aligned rows of alternating antiparallel actin and myosin myofilaments, spaced by their supporting Z-discs. Conversely, in smooth muscles, the myofilaments are organized irregularly.
The diversity of muscle organizations is best characterized in mammals. There are four muscular organizations: two are striated, named skeletal and cardiac muscles; the other two are the smooth and myoepithelial muscles (Alberts et al., 2015). In skeletal muscles, myocytes fuse to form multinucleated syncytia called muscle fibers or myotubes. In contrast, cardiac and smooth muscles are composed of mononucleated muscle cells for which mechanical, chemical, and electrical coupling is possible via complex junctions (adherens and gap), forming the typical “intercalated disc” structures of cardiac muscles. Myoepithelial cells in mammals are generally found in glandular epithelia such as the mammary or salivary glands and display a double identity, smooth muscle and epithelial cell (Petersen and van Deurs, 1989). In non-vertebrate bilaterians, striated and smooth myocytes as well as myoepithelial muscles are also present (reviewed in Schmidt-Rhaesa, 2007). Smooth and striated muscle cells can either be mono- or multinucleated, as described for example in Drosophila (Susic-Jung et al., 2012). Although myogenesis, muscle physiology and muscle regeneration have been extensively described and studied in bilaterians (reviewed in Bryson-Richardson and Currie, 2008; Bentzinger et al., 2012; Andrikou and Arnone, 2015; Almada and Wagers, 2016), less is known about their evolutionary origin(s) (Dayraud et al., 2012; Steinmetz et al., 2012; Brunet et al., 2016) as well as function, development and plasticity of muscles in non-bilaterian animals.
A group of organisms that has attracted a long standing interest in this research area is the Cnidaria (Chapman et al., 1962; Quaglia, 1981; Seipel and Schmid, 2005, 2006). This group of animals, as the sister group to the bilaterian clade (Figure 1A, Chang et al., 2015; Zapata et al., 2015), holds a key phylogenetic position for understanding muscle evolution. The two main groups of the phylum Cnidaria are Anthozoa and Medusozoa (Figure 1B). Anthozoa (sea anemones, corals) are mostly sessile and are represented by individual or colony-forming polyps arising from the metamorphosis of a planula larva. Medusozoa (jellyfish, hydroids) form in some species a free-swimming medusa (jellyfish), in addition to the polyp and planula stages. Beside anthozoans and medusozoans, a group of parasites, myxozoans, have recently been formally identified as cnidarians on the basis of molecular phylogenies (Figure 1B) (Chang et al., 2015) and presence of cnidarian specific genes (Holland et al., 2011; Shpirer et al., 2014). They have been proposed to be the sister group to another cnidarian parasitic species, Polypodium hydriforme (Chang et al., 2015), forming the clade Endocnidozoa (Zrzavý and Hypša, 2003).
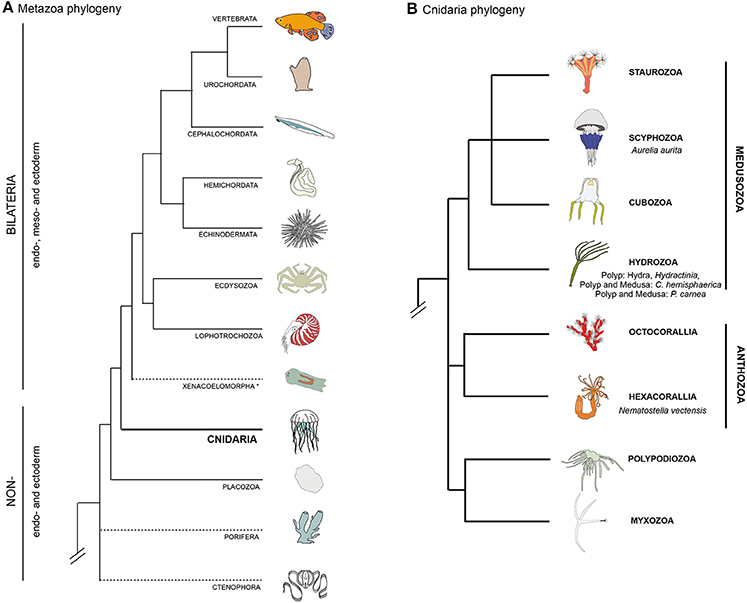
Figure 1. Bilaterian and cnidarian phyolgenies. (A) Metazoan phylogeny, highlighting the pivotal position of cnidarians as the sister group to extant bilaterian animals. The position of Ctenophora and Porifera (sponges) outside the Bilateria remains controversial (as indicated by dashed lines). (B) Cnidarian phylogeny showing the relationships between the main lineages based on recently published data (Chang et al., 2015; Zapata et al., 2015).
A handful of cnidarians has emerged in the past decades as experimental models in molecular, cell and developmental biology, providing insights into the evolution of developmental programs, including regeneration, stem cell biology and the evolution of key bilaterian traits (Kraus et al., 2007, 2016; Momose and Houliston, 2007; Amiel et al., 2009; Chera et al., 2009; Boehm et al., 2012; Layden et al., 2012; Röttinger et al., 2012; Sinigaglia et al., 2013; Leclère and Rentzsch, 2014; Abrams et al., 2015; Bradshaw et al., 2015; Helm et al., 2015; reviewed in Technau and Steele, 2011; Layden et al., 2016; Leclère et al., 2016; Rentzsch and Technau, 2016). The main, but not exclusive, cnidarian models are the medusozoan hydrozoans Hydra, Hydractinia, Podocoryna and Clytia (reviewed in Houliston et al., 2010; Galliot, 2012; Plickert et al., 2012; Gahan et al., 2016; Leclère et al., 2016) as well as the anthozoans Nematostella vectensis (reviewed in Layden et al., 2016; Rentzsch and Technau, 2016) and the coral Acropora (Shinzato et al., 2011; Hayward et al., 2015; Okubo et al., 2016).
Cnidarians display a broad variety of muscle organizations performing various functions. Unlike bilaterians, the main muscle cell type of cnidarians is the epitheliomuscular cell, a specialized epithelial cell containing smooth myofilaments, and which constitutes the principal building block of the two body layers (ectodermal and endodermal epithelia, also referred as epidermis and gastrodermis for both polyps and medusae, e.g., Brusca and Brusca, 2003; Schmidt-Rhaesa, 2007). The terms “epitheliomuscular cell” and “myoepithelial cell” are often used interchangeably (e.g., Brusca and Brusca, 2003). Some authors, however, apply morphology-based definitions: “epitheliomuscular cells” are exposed to both sides of the epithelium, while “myoepithelial cells” have reduced apical ends and are not exposed to the apical surface (e.g., Ruppert et al., 2004). Following most of the literature, here we simply define those terms taxonomically, using “epitheliomuscular cells” and “myoepithelial cells” when referring to the myofilaments-containing epithelial cells of, respectively, cnidarians and bilaterians. Other muscle types are also found in Cnidaria, such as the striated muscle of the medusa required for swimming. The complex life cycles and high regenerative capabilities found in Cnidaria involve a remarkable plasticity of muscle systems, which can take on different configurations during the life cycle of a given species (Figure 2). Cnidaria display both similarities and differences to its sister group, the bilaterians, with respect to muscle organization and cellular constituents. Additional data from cnidarian muscles can therefore provide important insights into their ontogeny, function and plasticity, in particular within an evolutionary framework. In this review, we discuss muscle diversity, function, development and regeneration in cnidarians. We conclude by proposing that cnidarians, in addition to increasing our understanding of metazoan muscle evolution, may also provide new insights into the development/regeneration and (re-) patterning of epitheliomuscular/myoepithelial cells, as well as into the role that muscle fibers play in the regeneration process.
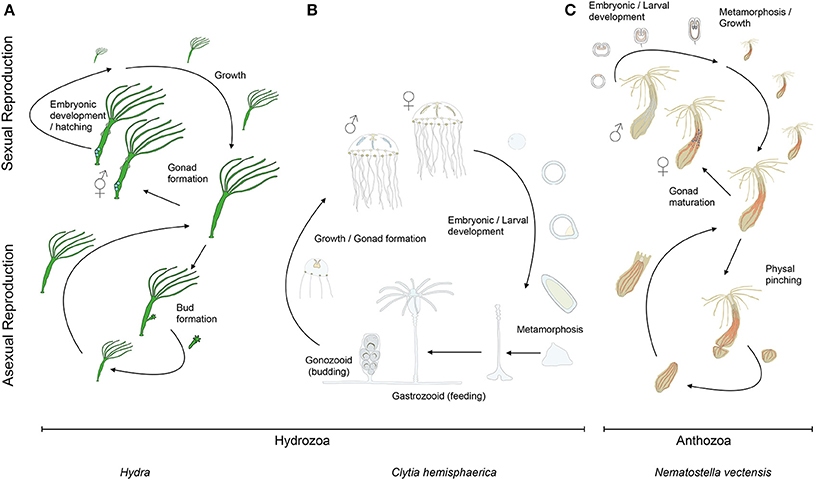
Figure 2. Cnidarian life cycles. The life cycles of (A) the solitary fresh water polyp Hydra, (B) the marine jellyfish Clytia (both hydrozoans) and (C) the anthozoan polyp Nematostella. At the lower part of the panels are indicated their asexual reproductive potentials (budding, physal pinching) that give rise to new (A) Hydra or (C) Nematostella polyps, or (B) juvenile Clytia medusae, respectively. Under harsh environmental conditions, gonads develop and sexual reproduction in (A) Hydra can occur. Depending on the species, Hydra can be gonochoric or hermaphroditic (represented here). After fertilization, embryonic development occurs within a solid capsule that, after hatching, frees a juvenile Hydra. (B) Clytia and (C) Nematostella are gonochoric and oocytes and sperm are released into the water column. After fertilization, embryonic development leads to the formation of swimming planula larvae that after metamorphosis develop into (B) a polyp colony for Clytia or (C) a solitary juvenile polyp for Nematostella.
Cnidarian Muscle Functions
Cnidarian muscles play crucial roles in locomotion, defense from predators (e.g., contracting and burying in crevices/sand), feeding and digestion through continuous peristaltic movements (Shimizu et al., 2004, Figure 3). In the following section we briefly review the described functions of muscles at each stage of the cnidarian life cycle and the known connections to the nervous system.
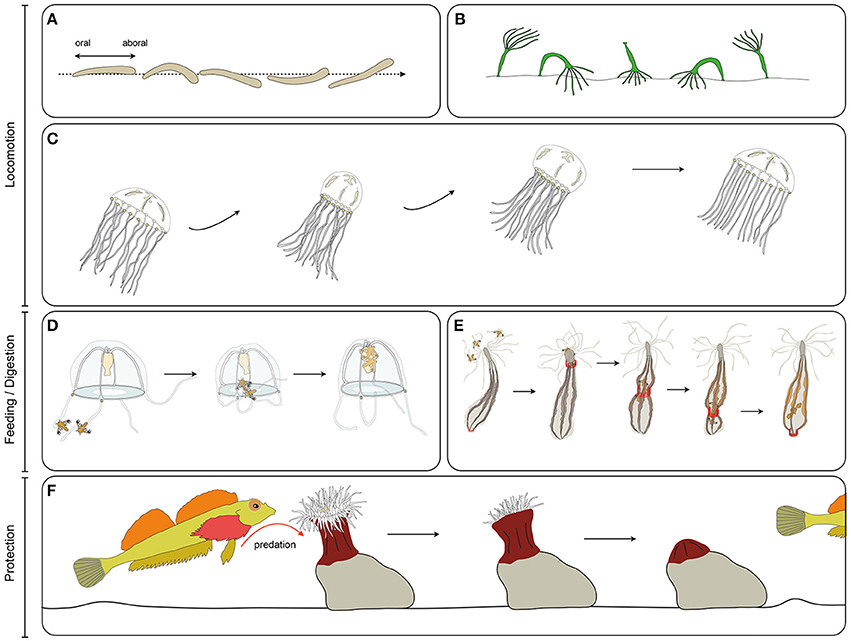
Figure 3. Cnidarian muscle functions. (A) Planula larva crawling, (B), Hydra polyp somersaulting, (C) jellyfish pulsation, (D) guided tentacle retraction of the jellyfish to bring the food toward the mouth, (E) digestive peristaltic movements of the polyp (red rings along the body column indicate circumferential muscle contractions), (F) protective retraction of the polyp in response to predation pressure.
Most of cnidarian muscle cells are epitheliomuscular and one distinctive feature of those cells compared to muscles cells of other animal groups is their multifunctionality. In Hydra for instance, endodermal epitheliomuscular cells participate in nutrient absorption during the digestion process (Buzgariu et al., 2015). Epitheliomuscular cells in the ectoderm of the foot produce vesicles containing an adhesive substance responsible for attachment to the substrate, while specialized epitheliomuscular ectodermal cells, the “battery cells,” function as supporting cells for the nematocytes (Hufnagel et al., 1985; Campbell, 1987). In many anthozoans, epitheliomuscular cells of the endodermal body wall host a large population of dinoflagellate symbionts, and take part in the digestive process, mixing the content of the gastrovascular cavity via beating of apical cilia and performing intracellular digestion (Hyman, 1940). Multifunctionality is thought to be an ancestral characteristic of epitheliomuscular/myoepithelial cells (Arendt, 2008). The inherent multifunctional potential of epitheliomuscular cells has been recently demonstrated in Hydra, whose epitheliomuscular cells displayed a remarkable functional plasticity (Wenger et al., 2016). The authors showed that in strains lacking nerve cells, the expression of several neurogenesis- and neurotransmission-specific genes was upregulated in epitheliomuscular cells, thus suggesting they could compensate for the loss of the nervous system by extending their multifunctionality.
Muscle Functions at the Planula Stage
At the planula stage, movement is mostly mediated by cilia beating of the ectodermal cells. However, in a number of species, bending along the oral-aboral axis to modulate the swimming direction is muscle dependent, such as in the hydrozoan Clava multicornis, in which muscles allow bending of the larvae for efficient phototaxis (Figure 3A; Piraino et al., 2011). How this coordinated behavior is regulated by the nervous system of the planula larva is not yet known.
Muscle Functions at the Polyp Stage
At the polyp stage, muscle contraction drives a wide variety of behavior: rhythmic contraction, mouth opening (Passano and McCullough, 1963; Carter et al., 2016), prey capture and handling (Miglietta and Tommasa, 2000), contracting or extending tentacle in order to regulate oxygen, waste and symbiont exposure (Bell et al., 2006), defense, escape (Figure 3F) and protection by retraction (Miglietta and Tommasa, 2000; Swain et al., 2015), peristaltic movements allowing fluid circulation within the body cavity and facilitating digestion (Figure 3E; Anctil et al., 2005), and locomotion. Hydra is notably able to move via a complex array of movements, including rare instances of somersaulting (alternative attachment and release of the foot and tentacles combined with contraction and extension of the body column, Figure 3B) (Trembley, 1744; Ewer and Fox, 1947). Many sea anemones (Actiniaria) are able to perform creeping using the muscles of their pedal disk (McClendon, 1906), their tentacles (Ross and Sutton, 1961) or to burrow using peristaltic movements (Williams, 2003). Some sea anemones are able to swim through sharp flexions of the column (Yentsch and Pierce, 1955; Ross and Sutton, 1967) or synchronous lashing of the tentacles (Josephson and March, 1966; Robson, 1966).
Polyps contract and extend efficiently even though their muscles are not organized in pairs of antagonists as in many bilaterian animals. In many cases, extension and retraction movements are performed by perpendicularly oriented muscles, as for example in the Hydra polyps: longitudinal ectodermal muscles are involved in contraction while endodermal circular muscles are involved in polyp extension.
All the above mentioned behaviors are regulated by the nervous system (reviewed in Galliot et al., 2009). Some involve rhythmic contraction of the body column. In Hydra, a pacemaker system regulates this process (Passano and McCullough, 1963, 1964; Kass-Simon et al., 2003; Ruggieri et al., 2004). It is constituted by a small subset of nerve cells connected by gap junctions located near the foot, and capable of synchronous firing (Takaku et al., 2014). Comparable pacemaker systems have been described in other cnidarian polyps, such as the hydrozoan Tubularia (Josephson and Uhrich, 1969; de Kruijf, 1977) and the swimming anthozoan sea anemone Stomphia (Robson, 1961, 1963). Chemical synapses between nerve cells and epitheliomuscular cells have been shown to be widespread in Cnidaria (Westfall et al., 1971; Westfall, 1973). They presumably contain neuropeptides and at least several components of the bilaterian neuromuscular junctions (Chapman et al., 2010).
Muscle Functions at the Medusa Stage
Medusae inhabit the water column, and move by means of passive drifting in the water currents, combined with active swimming. Muscles are not only used for propulsion, but also for many other functions such as catching prey, bringing food to the mouth (often showing a great amount of coordination with the movements of the bell and of the manubrium—stalk-like structure bearing the mouth – Figure 3D), digestion and dispersion of gametes (see for e.g., Passano, 1973; Bourmaud and Gravier-Bonnet, 2004). Rhythmic contraction and extension of the medusae bell are mediated differently; contraction of the medusa bell is the result of contraction of the striated muscle of the subumbrella, while viscoelastic (Alexander, 1964) and elastic properties (Demont and Gosline, 1988) of the medusa mesoglea (the thick layer of extracellular matrix located between the two epithelia) counteract muscle contraction and allow the bell to regain its original shape at each contraction cycle (Figure 3C).
Swimming efficiency has been studied in several species, and has been shown to depend on several parameters affecting hydrodynamics, such as the overall shape of the medusa, the disposition of the muscles in the subumbrella, the flexibility of the bell margin and the shape of the velum (bi-layered epithelium running around the rim of the bell, present in hydrozoan medusae, and reducing the size of the bell cavity opening) (Dabiri et al., 2005, 2010; Colin et al., 2012). Rhythmic contractions of the bell has been shown to be a very efficient process for underwater propulsion (Gemmell et al., 2013). A recent study recreated this configuration artificially using chemically dissociated rat heart muscle laid on ephyra (juvenile scyphozoan medusa) shaped silicone polymers (Nawroth et al., 2012). This demonstrated that the rhythmic contraction of the rat muscle cells activated by a periodic electrical stimulation, coupled to the elasticity of the polymer, is sufficient to generate efficient propulsion of the artificial jellyfish.
Even if medusae are generally considered to be “simple” organisms, coordination of muscle-based locomotion and integration of spatial information can be quite complex, at least in some species. For instance, several cubomedusae display courtship behaviors (Lewis and Long, 2005), perform obstacle avoidance (Garm et al., 2007) or even use terrestrial visual cues for navigation through mangroves forests (Garm et al., 2011). Similarly, the scyphomedusa Rhizostoma pulmo has recently been shown to actively swim countercurrent in response to current drift (Fossette et al., 2015). Directionality and propulsion can also result from the coordination of multiple individuals, as seen for instance in colonial siphonophores. In Nanomia bijuga, clonal medusoid individuals, termed nectophores, propel the colony, and developmental differences between them generate a division of labor that ultimately modulates locomotion (Costello et al., 2015).
Jellyfish contractions are regulated by a complex nervous system, including neural nets and concentrations of nerve cells at the bell margin called the nerve rings (reviewed in Satterlie, 2011). Pacemaker neurons regulating bell margin contractions have been described in cubozoan, scyphozoan and hydrozoan jellyfish (reviewed in Satterlie and Nolen, 2001; Mackie, 2004; Katsuki and Greenspan, 2013). How the photoreceptor systems control the swim pacemaker has started to be addressed in cubomedusae (Garm and Mori, 2009; Stöckl et al., 2011; Bielecki et al., 2013). In hydrozoan medusae, the contraction of striated muscle in the subumbrella is notably regulated by gap junctions, which electrically couple the muscle cells (Satterlie, 2008). This process has yet not been reported in other cnidarian groups. Finally, anatomical specialization of the nerve nets can allow for a fine tuning of movements: for example, in Aglantha, a complex and well-characterized neuromuscular system allows the jellyfish to swim either slowly or fast, thanks to different neural circuitries, modulating the escape response (reviewed in Mackie, 2004).
Cnidarian Muscle Types
The main, and in many species exclusive, muscle cell type in cnidarians is the epitheliomuscular cell. These cells have a typical polarized epithelial morphology, including apical cilia, with the specificity that myofibrils project from the basal side, aligning within the extracellular matrix of the tissue to provide its contractile property. In a few cnidarians, smooth muscles are found totally embedded in the mesoglea, having lost contact with the epithelia (see below for more details). Interestingly though, in most of the free-swimming medusae, muscles are composed of striated epitheliomusclar cells. Despite the few muscle cell types found in cnidarians, there is a wide diversity of muscle systems in this phylum. In this section we describe briefly the diversity of muscle organization and muscle cell types described in the major groups of cnidarians.
Muscle Systems in Hydrozoans
Most ectodermal and endodermal epithelial cells in hydrozoan planulae, polyps, and medusae are epitheliomuscular (West, 1978). Much of the available information about hydrozoan epitheliomuscular cells comes from anatomical and physiological studies on Hydra polyps. Hydra ectodermal and endodermal epitheliomuscular cells display, respectively, longitudinally and circularly oriented processes, called myonemes (Figures 4Aa,a'; Mueller, 1950). Ectodermal epitheliomuscular cells of Hydra are large columnar or cuboidal cells bearing two long myonemes oriented along the oral-aboral axis (David, 1973). These two myonemes of roughly cylindrical shape, composed of irregularly arranged myofilaments, are found in each ectodermal cell, as visualized by electron microscopy (West, 1978) or recently by LifeAct-GFP transgenic polyps (Seybold et al., 2016). Instead, endodermal epitheliomuscular cells are tall and columnar, have short muscle processes at the basal end and several flagella at the apical end. Their myonemes are oriented perpendicularly and have a structure similar to those found in the ectoderm, though more numerous (David, 1973; Seybold et al., 2016). Epitheliomuscular cells of the body column (both in the endoderm and ectoderm) divide continuously, thus displacing cells toward the oral (mouth) and aboral (foot) extremities where they are ultimately eliminated (Campbell, 1967).
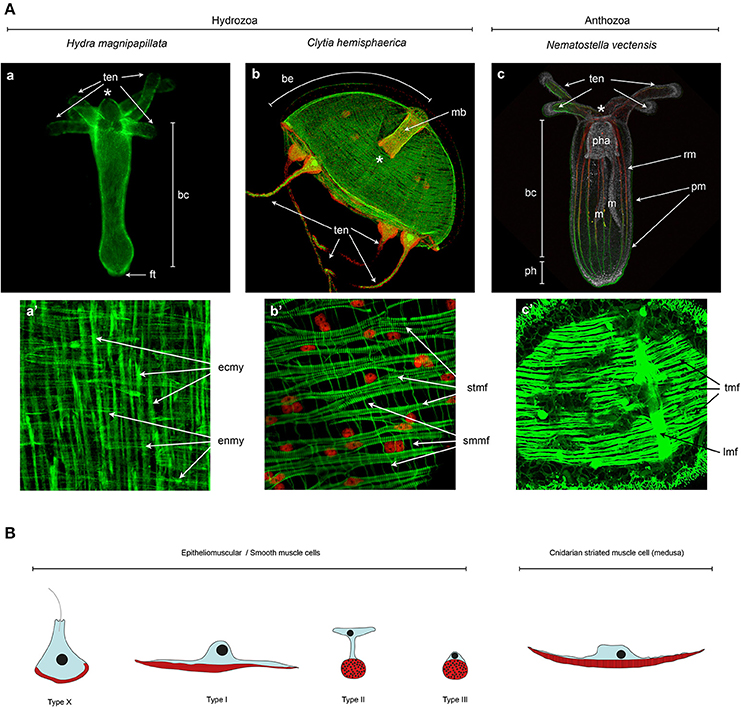
Figure 4. Cnidarian muscle diversity. (A) Muscle networks of (a,a') Hydra magnipapillata, (b,b') Clytia hemisphaerica jellyfish and (c,c') Nematostella vectensis. The upper panels show the muscle network of entire organisms and the lower panel magnification of certain body regions to highlight the orientation and fine structure of the muscles. (A) Living Lifeact:GFP transgene (Seybold et al., 2016) labeling actin filaments, (c) fixed MyHC1::mCherry (Renfer et al., 2010) transgene labeling the actin fibers of the retractor muscles, co-labeled with phalloidin. All other panels (a',b,b',c') are phalloidin stainings. Image labels are as follows: (*) mouth, (ten) tentacles, (bc) body column, (ft) foot, (be) bell, (mb) manubrium, (pha) pharynx, (m) mesentery, (ph) physa, (rm) retractor muscle, (pm) parietal muscle, (ecmy) ectodermal myonemes, (enmy) endodermal myonemes, (stmf) striated muscle fibers, (smmf) smooth-like muscle fibers, (tmf) transversal muscle fibers, (lmf) loongitudinal muscle fibers. (a,a') Image courtesy of Aufschnaiter and Hobmayer, (b,b') images from Kraus et al. (2015) and (c,c') Image from Amiel et al. (2015) as well as courtesy of Amiel. (B) Epitheliomuscular cell type diversity in Cnidaria. After Krasińska (1914) and Doumenc (1979) in Seipel and Schmid (2006), and Jahnel et al. (2014).
Each epitheliomuscular cell process of a Hydra polyp is in contact with the basal processes of several adjacent cells, thus forming a continuous muscle fiber network spanning the entire body (Mueller, 1950). It should be noted here that the term “muscle fiber” is generally associated to the multinucleated syncytia of skeletal muscles; following most of the literature on cnidarian muscle, we will use henceforth this term to indicate condensed actin filaments constituting the contractile elements of cnidarian muscles. Adjacent epitheliomuscular cells in Hydra are connected by septate and gap junctions; additionally, where the myoneme-containing regions of two adjacent cells come into contact, they form a characteristic and unique type of junction, which structurally resembles the intercalated discs found in vertebrate cardiac muscles: on the inner surface of each cell membrane is an irregular band of dense material through which the filaments of the myoneme itself pass (Haynes et al., 1968).
As a general rule, the muscle fibers of hydrozoan planula larvae and polyps are circularly arranged in the endoderm and longitudinally in the ectoderm (Hyman, 1940; Bouillon, 1993). Common parts of the polyp colony also harbor epitheliomuscular cells, such as the endodermal epitheliomuscular cells of the stolon in Podocoryna carnea (Buss et al., 2013). However, not all hydrozoan epithelial cells are epitheliomuscular. For instance, endodermal epithelial cells of the tentacles of many hydrozoan species are arranged in only one row of turgescent cells and do not contain myofilaments (Bouillon, 1993).
The main muscle of the hydrozoan medusae is the circular striated muscle found in the subumbrella (the inner layer of the bell—Figures 4Ab,b'), responsible for the rhythmic contraction of the bell, and composed of epitheliomuscular cells. As for smooth epithelial muscles, basally located striated myofilaments are connected between neighboring cells, forming a continuous circular muscle. Each epitheliomuscular cell contains about 30–50 sarcomeres, as for instance in the hydrozoan medusae Aglantha digitale (Singla, 1978). Sarcomeres in a relaxed state are approximately 1 μm long. As described in various hydrozoan species (e.g., Keough and Summers, 1976; Boelsterli, 1977; Singla, 1978), they are of very similar structure compared to those of vertebrate striated muscles, being separated by Z-discs and composed of ordered arrays of thick and thin filament areas forming denser A-bands and rarer I-bands. As in vertebrates, A-bands contain a central H-band and a M-line. An interesting deviation can be observed in Obelia medusae (Chapman, 1968), probably linked to their unusually flat shape. In these species, the striated myofilaments of the subumbrella are not oriented circularly but distributed in two perpendicularly oriented sets, generating a grid-like pattern. In addition to the subumbrella, in most hydrozoan medusae striated epitheliomuscular cells also constitute a contractile ring on the inner layer of the velum.
In hydrozoan medusae, while swimming is generally performed by the circular striated muscles, other behaviors are mostly mediated by the smooth muscles. Hydrozoan medusae are therefore rich in smooth epitheliomuscular cells (Bouillon, 1993) such as (i) the longitudinal muscle fibers of the tentacle ectoderm, (ii) the outer layer of the velum, and (iii) the radially oriented smooth muscle fibers of the subumbrella (the underside of the bell) that run from the manubrium to the bell margin and cover the striated muscle layer. In a few species, additional epitheliomuscular cells have been described that form (iv) the ring muscle fibers of the endodermal (gastrovascular) canal system and (v) the radiating muscle fibers of the exumbrella (the outer layer of the bell).
The anatomical details of medusa muscle systems differ greatly among species, an interesting case for evo-devo studies, still to be explored. Most strikingly, the organization of the smooth muscles covering the striated muscle of the subumbrella, responsible for the bending of the medusa, shows great variation: in many species, such as Clytia hemisphaerica, this layer covers the entire subumbrella, while in others, such as Podocoryna carnea, these radial smooth muscles are organized in four bundles, each covering one of the four radial canals (Seipel and Schmid, 2006).
Muscle Systems in Other Medusozoans
One of the most striking feature of the muscle systems of Scyphozoa, Cubozoa, and Staurozoa compared to Hydrozoa, is the quasi-absence of muscle fibers in the endoderm at the planula, polyp and medusa stages. Past (Hyman, 1940; Chapman, 1965; Chapman and Werner, 1972; Werner et al., 1976; Anderson and Schwab, 1981; Martin and Chia, 1982; Chia et al., 1984) and more recent studies (Chapman, 1999; Eggers and Jarms, 2007; Nakanishi et al., 2008) do not report any endodermal muscle fibers. However, Gold et al. (2015) convincingly describe poorly developed circular muscle fibers in the endoderm of the polyp tentacles of Aurelia. Smooth and striated myofilaments found in the ectoderm, are nevertheless structurally similar to those found in Hydrozoa (Anderson and Schwab, 1981; Chia et al., 1984). As in Hydrozoa, medusa stages in Scyphozoa and Cubozoa contains strong circular epitheliomuscular striated muscles lining the subumbrella (Hyman, 1940; Satterlie et al., 2005; Helm et al., 2015), often called the coronal muscles. Most medusae of these groups also contain radial smooth muscles lining parts of the subumbrella and longitudinal ectodermal epitheliomuscular smooth muscles in the tentacles (Hyman, 1940; Satterlie et al., 2005).
Polyp stages in Scyphozoa, Cubozoa, and Staurozoa have strong longitudinal muscles, of ectodermal origin. In many species, these muscles are constituted by myocytes completely embedded in the mesoglea, and thus not connected either to the ectoderm or the endoderm epithelia (Widersten, 1966; Werner et al., 1976; Chapman, 1978; Chia et al., 1984; Westlake and Page, 2016). In addition, smooth epitheliomuscular cells are present in the ectoderm of the polyp tentacles (Franc, 1993). In several cubozoan and scyphozoan species (Chapman, 1978; Chia et al., 1984; Golz, 1993) some ectodermal cells at the polyp stage display striated muscle fibers of unknown origin and function, which certainly deserve more attention.
Muscle Systems in Anthozoans
In Anthozoa, epitheliomuscular cells are present both in the ectoderm and the endoderm of planulae and polyps, the medusa stage being absent in this clade. Importantly, unlike in medusozoans where the major muscular components are localized in the ectoderm, muscles in anthozoans are more developed in the endoderm; most of the species possess in fact transversal (circumferential) and strong longitudinal endodermal muscles (Figures 4Ac,c').
Most anthozoan muscle cells are epitheliomuscular, containing smooth myofilaments. Loosely defined sarcomeres have been so far reported in the tentacles of only two sea anemones: Aiptasia diaphana and Stomphia coccinea (Amerongen and Peteya, 1980). True muscle cells totally embedded in the mesoglea have also been described in several anthozoans, such as the mesogleal sphincter musculature found in some Actiniaria and Zoantharia (Doumenc and Van Praët, 1987; Herberts, 1987; Swain et al., 2015).
Endodermal epitheliomuscular muscles in anthozoans can be generally classified into three types: (i) the circular musculature found throughout the body wall, (ii) the longitudinal parietal muscles positioned at the junction between the mesenteries (reproductive and digestive structures subdividing the gastric cavity into chambers) and the body wall, and (iii) the longitudinal retractor muscles located on one side of the mesenteries. In many anthozoan groups, the retractor muscles are arranged in a bilateral manner along the secondary body axis (called the directive axis), and constitute one of the landmarks of bilateral symmetry in these organisms (e.g., in Nematostella: Jahnel et al., 2014; Leclère and Rentzsch, 2014). Ectodermal muscles are for most anthozoans confined to the tentacles and the oral disc, except in some Ceriantharia, Antipatharia and Scleractinia that have longitudinal muscles in the body column ectoderm (Chevalier and Beauvais, 1987; Doumenc and Van Praët, 1987; Herberts, 1987; Tiffon, 1987; Van Praët et al., 1987).
Most descriptions of anthozoan muscles resulted from research on sea anemones (order Actiniaria) (reviewed in Doumenc and Van Praët, 1987). A recent description of the muscular system of the sea anemone Nematostella vectensis (Figures 4Ac,c') highlighted the existence of at least three different epitheliomuscular cell types (Figure 4B; Jahnel et al., 2014). Type I classical epitheliomuscular cells and type II epitheliomuscular cells, with elongated cytoplasmic bridges, constitute mainly the longitudinal component of the muscular system such as the parietal and retractor muscles (Figures 4A,B; Jahnel et al., 2014). Conversely, type III epitheliomuscular cells are basiepithelial muscle cells and are primarily encountered in the ectoderm of the tentacles. Many sea anemone species have extra sets of radial muscles: (i) ectodermal radial muscles in the oral disk, involved probably in mouth opening (Doumenc and Van Praët, 1987), (ii) radial muscles in the endodermal part of the mesenteries on the side opposite the retractor muscle (Doumenc, 1979), and (iii) radial muscles in the endoderm involved in pedal disk contraction (Doumenc and Van Praët, 1987). While ST myhc-positive cells are present in the oral disc of Nematostella (Renfer et al., 2010), a pedal disk is lacking and radial muscles in the endodermal part of the mesenteries have yet to be described in Nematostella.
Muscle Systems in Endocnidozoans
The musculature of the myxozoan Buddenbrockia plumatellae and of Polypodium hydriforme has recently been investigated (Raikova et al., 2007; Gruhl and Okamura, 2012). They represent a unique case within cnidarians, possessing only smooth muscle cells localized in the mesoglea and lacking epithelial muscle cells altogether. In particular, the worm-like parasite Buddenbrockia possesses four longitudinal non epithelial smooth muscles (Gruhl and Okamura, 2012) while Polypodium has a complex array of smooth myocytes located in different parts of the body (Raikova et al., 2007). Many other myxozoans species are even more extremely specialized to their parasitic life style, not possessing any muscle cells (Hartikainen et al., 2014).
Ontogeny of Cnidarian Muscles
Decades of developmental biology have taught us a great deal about striated muscle development in vertebrates and other bilaterian model systems, but little is known about smooth and myoepithelial muscles development. Similarly, studies on cnidarian muscle development have so far mainly focused on the epitheliomuscular striated muscles of the medusa, while the development of the predominant epithelial smooth muscle cell type and the myocyte type have so far been rather neglected.
Striated muscle development has been primarily studied in hydrozoan medusae. In most species, striated muscles of the subumbrella and the velum derive from the entocodon, a hydrozoan specific cell layer located in the early medusae buds, between the ectoderm and endoderm, considered by some authors to be homologous to the mesoderm of bilaterians (Boero et al., 1998; Seipel and Schmid, 2006), but see (Martindale et al., 2004; Burton, 2008) for an alternative opinion. In most hydrozoan species, this territory derives from the ectoderm (Boelsterli, 1977; Bouillon, 1993; Seipel and Schmid, 2006; Kraus et al., 2015). The work of Schmid and colleagues on the hydrozoan medusae Podocoryna carnea provided valuable data about cnidarian striated muscle differentiation and transdifferentiation (see below). The other medusozoan groups lack an entocodon and their striated muscles instead, differentiate from the ectoderm of the subumbrella. In fact, a recent study showed that the striated muscles of the scyphozoan Chrysaora are produced anew during ephyra formation (Helm et al., 2015).
Cnidarian epitheliomuscular cells reside in the ectodermal and/or endodermal epithelia. Their fate is probably specified during germ layer formation, but data are scarce, and it is still unclear what drives epithelial cells toward a epitheliomuscular fate in a given cnidarian, germ layer or body region. In a few species, epitheliomuscular cells derive from non-epithelial stem-cells, such as the interstitial stem cells (i-cells) of Hydractinia echinata (Müller et al., 2004; Künzel et al., 2010). I-cells are hydrozoan-specific stem-cells, capable of giving rise to multiple cell types, such as neurons, gametes, gland cells and nematocytes. It is worth noting that in Hydra, epitheliomuscular cells do not differentiate from i-cells, but solely from fate-restricted ectodermal and endodermal epithelial stem cells (Hobmayer et al., 2012).
Molecular Characterization of Muscles in Cnidarians
Myogenic Genes
The development of vertebrate skeletal muscles is fairly well characterized at the molecular level, while a few factors involved in vertebrate smooth muscle formation have been identified, such as Myocardin, SRF and Capsulin (a paralog of MyoR; Kumar and Owens, 2003; Wang et al., 2003). In contrast, little is known about the cellular and molecular characteristics of myoepithelial cell precursors as well as the mechanisms controlling the double “myo” and “epithelial” phenotype (Tamgadge et al., 2013).
A set of bHLH (basic helix-loop-helix) domain containing transcription factors, the Myogenic Regulatory Factors (MRFs), play key roles in vertebrate skeletal myoblast specification and differentiation. MRFs are notably able, when overexpressed, to transform fibroblasts into myoblasts (Davis et al., 1987). They are also present in non-vertebrate bilaterians where they similarly regulate specification and differentiation of striated muscles (reviewed in Andrikou and Arnone, 2015). The four vertebrate MRF paralogs—Myf5, MyoD, Mrf4, and Myogenin—resulted from vertebrate specific duplications; therefore, only one MRF ortholog, usually called MyoD, is found in most non-vertebrate bilaterian groups.
MRFs are part of a conserved myogenesis gene regulatory network, which includes the transcription factors Dach (Dachshund), Pax3, Pax7, Six1, Six4, as well as their co-factors Eya1 and Eya2 (Grifone et al., 2005; Christensen et al., 2008). MRFs are also able to induce differential transcription of specific mef2 splice variants, a MADS family transcription factor (Potthoff and Olson, 2007; Potthoff et al., 2007). While Mef2 governs expression of a set of downstream factors including Myocardin, a protein required for muscle development (Wang et al., 2001), Mef2 per se does not have myogenic activity, but cooperates transcriptionally to potentiate the effects of MRFs (Molkentin et al., 1995). Two other bHLH factors, MyoR (Myogenic Repressor) and Twist negatively regulate skeletal muscle differentiation by repressing MyoD activity (Spicer et al., 1996; Hebrok et al., 1997; Lu et al., 1999). A non-exhaustive list of the major bilaterian myogenic factors is shown in Figure 5 (reviewed in Bentzinger et al., 2012; Andrikou and Arnone, 2015). The set of Pax, bHLH, Six, Eya, Dach, and MADS transcription factors involved in myogenesis is conserved throughout Bilateria. However, the hierarchy of gene interactions has been reshuffled in some bilaterian groups, and some key myogenic factors were lost in some lineages during evolution, such as Pax3/7 in sea urchins (Andrikou et al., 2015). The general consensus is that MRFs play a crucial role in bilaterian muscle specification and differentiation (reviewed in Bentzinger et al., 2012; Andrikou and Arnone, 2015).
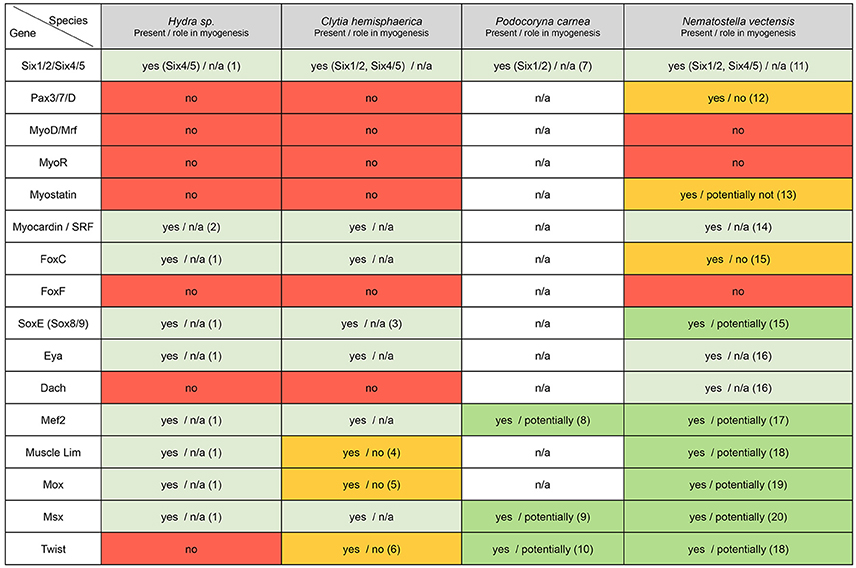
Figure 5. Cnidarian “muscle” gene repertoire. Overview of the cnidarian “muscle” gene repertoire in regard to known bilaterian myogenic factors. Cnidarians are represented by Hydra, Clytia, Podocoryna, and Nematostella. The potential role in myogenesis of a given gene in the indicated species has been assessed by functional studies if available or by published gene expression patterns, (n/a) no information available. References cited in this figure: (1) Chapman et al., 2010; (2) Hoffmann and Kroiher, 2001; (3) Jager et al., 2011; (4) Steinmetz et al., 2012; (5) Chiori et al., 2009; (6) Kraus et al., 2015; (7) Stierwald et al., 2004; (8) Spring et al., 2002; (9) Galle et al., 2005; (10) Spring et al., 2000; (11) Ryan et al., 2006; (12) Matus et al., 2007; (13) Saina and Technau, 2009; (14) Putnam et al., 2007; (15) Magie et al., 2005; (16) Nakanishi et al., 2015; (17) Genikhovich and Technau, 2011; (18) Martindale et al., 2004; (19) Ryan et al., 2007; (20) Ryan et al., 2006.
No MRFs have been identified in the published cnidarian genomes (Putnam et al., 2007; Chapman et al., 2010; Shinzato et al., 2011), while several orthologs to other bilaterian transcription factors and signaling components related to myogenesis were found (Figure 5). Thorough phylogenetic analyses showed that a previously reported MyoD putative ortholog from Podocoryna named JellyD1 (Müller et al., 2003), is indeed not related to the MyoD family of bHLH factors (Simionato et al., 2007). The absence of MRF orthologs in cnidarians raises the pivotal question of the developmental mechanisms underlying muscle formation in these organisms. An unbiased systematic analysis of genes regulating muscle formation would be particularly helpful.
The first extensive search for myogenic genes in cnidarians was carried out in the hydrozoan medusae Podocoryna. Volker Schmid and collaborators identified and characterized the bHLH transcription factor Twist, the MADS factor mef2, as well as the homeobox transcription factor msx, and showed that all three genes are expressed in the entocodon of the medusa bud and its derivatives, from which the smooth-like and striated muscles of the bell originate (Spring et al., 2000, 2002; Galle et al., 2005). While msx expression is downregulated in bilaterian striated muscles, striated muscles of the medusa maintain elevated levels of msx expression (Galle et al., 2005). The transcription factors twist and mef2 are also expressed in non-muscle tissues, thus suggesting they could play additional roles during jellyfish development (Spring et al., 2000, 2002).
In the sea anemone Nematostella, orthologs for nearly all of the main “myogenic genes” [Figure 5, with the exception of foxF (Santagata et al., 2012), myoR and myoD] have been identified. Among those genes potentially involved in muscle formation, only mef2 has been studied functionally (Genikhovich and Technau, 2011). Genikhovich and colleagues described several differentially expressed splice variants, and in particular one responsible for proper endoderm formation. Through a combination of TEM analysis and transgenic approaches, using a Myosin Heavy Chain promoter-driven mCherry [MyHC1::mCherry (Renfer et al., 2010) also called ST myhc::mCherry (Steinmetz et al., 2012)], the authors showed that longitudinal muscle formation is impaired in some NvMef2 splice-specific morphants (Genikhovich and Technau, 2011). However, given that the defects in endoderm formation appeared prior to the condensation of actin filaments that will form the retractor muscles, and also that direct binding of NvMef2 to the ST myhc promoter is not required for the expression of the myosin reporter, the direct role of NvMef2 is still unclear (Genikhovich and Technau, 2011). Therefore, the function of all potential myogenic factors during muscle specification and formation in cnidarians remains to be determined.
Structural Muscle Genes
The essential contractile machinery—alternation of actin thin filaments and Myosin II thick filaments—is conserved between cnidarians and bilaterians. However, contrary to bilaterians, actin paralogs specific for muscle and cytoplasm have not been reported from cnidarians (Fisher and Bode, 1989). ST myhc (“striated muscle” type II Myosin Heavy Chain) is present in the thick filaments of both smooth (Renfer et al., 2010; Steinmetz et al., 2012) and striated muscles (Schuchert et al., 1993; Aerne et al., 1996; Steinmetz et al., 2012) in several cnidarians, while NM myhc (“non-muscle” type II Myosin Heavy Chain) is expressed in either smooth-muscle and non-muscle cells in Clytia and Nematostella (Steinmetz et al., 2012). This situation resembles the arrangement found in most bilaterians for which ST myhc is used in fast contracting muscles, while NM myhc functions in slow contracting muscles (Brunet et al., 2016) and constitutes an important component of the cytoskeleton (Vicente-Manzanares et al., 2009).
Several actin or myosin regulators and binding partners characterizing bilaterian muscles (reviewed in Hooper and Thuma, 2005) were also found in cnidarians. Myosin Essential and Regulatory Light Chains, Myosin Light Chain-Kinase and Phosphatase, as well as the smooth muscle ATPase regulator Calponin are present in cnidarians genomes (Steinmetz et al., 2012) but have not been functionally characterized yet. Several Tropomyosin paralogs have also been described in cnidarians (Baader et al., 1993; López de Haro et al., 1994; Gröger et al., 1999, 2000; Fujinoki et al., 2002; Steinmetz et al., 2012), including one specific to the striated muscle cells of Podocoryna (Gröger et al., 1999, 2000). However, Troponins, important components of the striated muscle thin filaments, have to date not been found in any cnidarian genomes (Steinmetz et al., 2012). Finally, all major components of the Dystroglycan complex, a protein complex involved in anchoring muscle fibers to the extracellular matrix in many bilaterians, have been identified in cnidarian genomes (Adams and Brancaccio, 2015) and await functional characterization.
Sarcomeres consist of a succession of thin and thick filaments organized in arrays by proteins complexes located at the Z-disks and M-lines. Recent work investigated the evolution of the most conserved Z-disk proteins (Steinmetz et al., 2012). The authors could show that most conserved proteins present in both vertebrate and Drosophila Z-disks, such as α-Actinin, Muscle-LIM and ZASP/LDB3, were present in cnidarians. However, in Clytia medusae, in situ hybridization signal was not detected in striated muscles for orthologs of the Z-disk proteins (Muscle-LIM and ZASP/LDB3), or showed ubiquitous expression (α-Actinin). Conversely, clear orthologs of Titin, the large protein which links Z-disk to thick filaments in bilaterians, could not be found. Most of the proteins regulating the organization of the M-line have yet to be investigated in cnidarians. Orthologs of Obscurin/UNC-89, giant proteins involved in M-line alignment in diverse bilaterians (Benian et al., 1996; Katzemich et al., 2012), have been identified in Hydra, Clytia and Nematostella (Steinmetz et al., 2012) and appear to be broadly expressed in striated, smooth, and non-muscle-cells.
Origin and Evolution of Cnidarian Muscles
It is generally accepted that smooth epitheliomuscular cells of cnidarians are homologous to bilaterian smooth muscles and myoepithelial cells (Steinmetz et al., 2012). Epitheliomuscular cells are found in all cnidarian species, except for some highly derived parasitic groups (see Section Cnidarian Muscle Types), and most of the molecular components of smooth muscle myofilaments are conserved between Cnidaria and Bilateria (Steinmetz et al., 2012). The current lack of functional data, however, does not allow discriminating whether the same regulatory cascade in Cnidaria and Bilateria controls smooth muscle development.
A recent study concluded that the striated muscles found in hydrozoan medusae originated independently from those found in bilaterians (Steinmetz et al., 2012). As described in the previous section, available cnidarian genomes lack key striated muscle proteins, such as the Troponins and the Z-disks component Titin while others, such as muscle-LIM and LDB3, were found to be excluded from striated muscle tissue in Clytia medusae. The structural convergence between hydrozoan and bilaterian sarcomeres represents an interesting and well-supported hypothesis, nevertheless awaiting confirmation from other cnidarian species. A stimulating possibility would be that striated muscles appeared during cnidarian evolution in concomitance with the acquisition of the medusa stage, and thus with the functional requirement for a fast-contracting swimming muscle. More work is therefore needed to understand the evolutionary tinkering that produced so similar phenotypes with different sets of proteins.
Smooth myocytes, muscles cells that lost connection to the epithelia, and are therefore totally embedded in the mesoglea, likely originated several times within Cnidaria. They have only been described in a few disparate instances, such as the sphincter muscle of some Anthozoa (in Actiniaria and Zoantharia), the longitudinal ectodermal muscles of scyphozoan and cubozoan polyps and staurozoans, and they represent the sole muscle type described in the parasitic groups Myxozoa and Polypodium (see Section Cnidarian Muscle Types). The most parsimonious interpretation for this pattern is that they represent clade-specific adaptations. Indeed, phylogenetic reconstructions of Zoantharia (Swain et al., 2015) and Actiniaria (Rodriguez et al., 2014) support several convergent acquisitions of myocytes within these groups. Furthermore, acquisition of true myocytes and loss of epitheliomuscular cells in the myxozoan Buddenbrockia and in Polypodium are likely a direct consequence of the adoption of a parasitic life style.
Several losses of either striated or smooth muscle cell types were inferred in Cnidaria, often in relation to the evolution of their complex life cycles. For instance, the multiple evolutionary losses of the medusa stage in Hydrozoa led to likewise losses of striated muscles (Leclère et al., 2009). As a consequence, Hydra does not develop striated muscle at any stage of its simplified life cycle (Nawrocki et al., 2012). Similarly, many myxozoan species completely lost muscle cells following extreme adaptation to the parasitic life style (Hartikainen et al., 2014). Genomic data analyses are still scarce (Chapman et al., 2010; Chang et al., 2015), though, and it remains to be determined how these losses impacted the structural and regulatory muscle genes.
Muscle Plasticity and Regeneration in Cnidarians
While regeneration phenomena are widespread among metazoans, the regenerative capacity varies considerably within a given phylum and at the organ/tissue levels within an organism (Bely and Nyberg, 2010; Tiozzo and Copley, 2015). Although still quite variable within the phylum, cnidarians in general exhibit tremendous tissue plasticity and regeneration abilities (Figure 6). Our understanding about (i) muscle plasticity/muscle regeneration itself (at the tissue, cellular, and/or molecular levels), and (ii) the role that muscles play in the regenerative process of lost tissues or body parts is still sparse. In bilaterians, muscle regeneration is fueled by specific stem cells called satellite cells, however no such cells have yet been identified in cnidarians. The PaxD transcription factor Pax3/7, crucial for satellite cell activation and muscle regeneration/renewal in bilaterians (Konstantinides and Averof, 2014; reviewed in Dumont et al., 2015) has been retrieved from anthozoan genomes and further characterized in Nematostella (Figure 5). However, given its gene expression pattern in restricted regions of the ectoderm, it does not seem to be associated with a potential muscle renewal process (Matus et al., 2007). This rather limited set of evidence suggests that the cnidarian muscle regeneration process may differ from the one described in bilaterians. In this section we review current knowledge about muscle regeneration/plasticity in cnidarians and the potential role played by muscle cells during injury-response and remodeling processes.
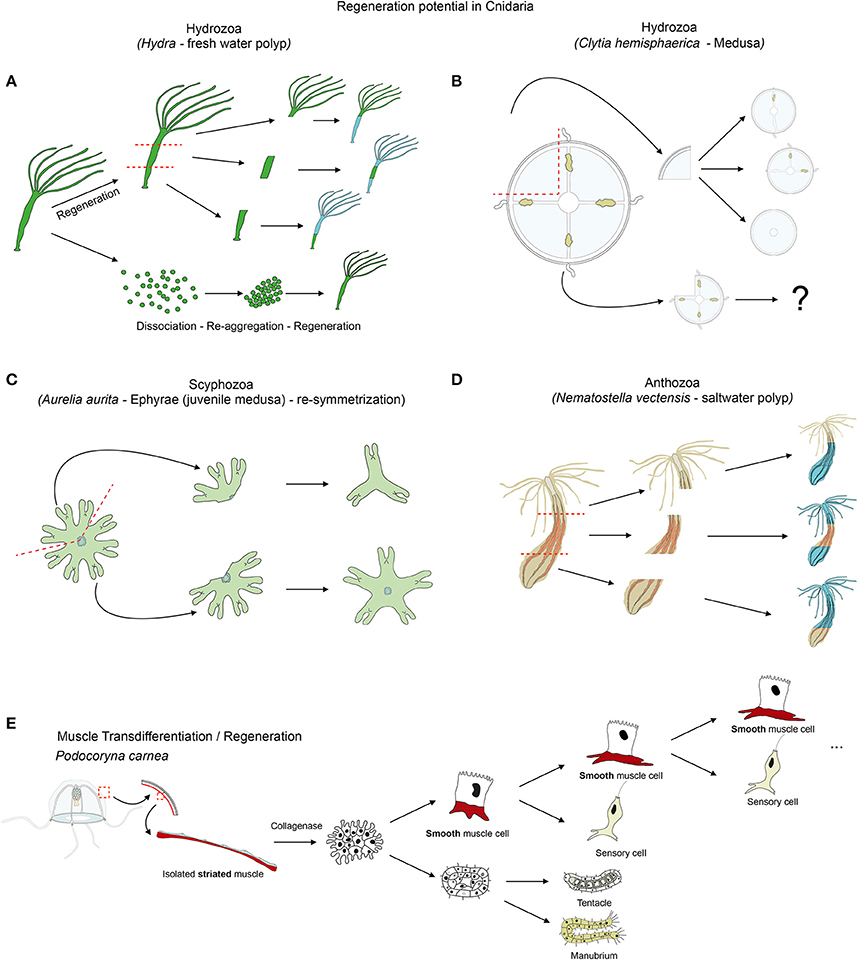
Figure 6. Cnidarian regeneration potential. Regenerative capacities of (A) Hydra, (B) Clytia medusa and (D) Nematostella as cnidarian representatives. (C) Illustrates the re-symmetrization process of juvenile medusa that is not regeneration per se, but allows a quick regain of the medusa functionality. (E) Illustrates the transdifferentiation and regeneration potential of striated muscle cells isolated from jellyfish and cultured in vitro. See text for further details.
Epithelial Muscle Plasticity in Hydra Polyps
A recent study on Hydra analyzed the repolarization of epithelial cells during the regenerative process (Seybold et al., 2016), taking advantage of the fact that dissociated Hydra cells are able of aggregating and regenerating a polyp. Hydra is indeed a classical model organism to study whole body regeneration. It can reform fully functional polyps when bisected (Trembley, 1744; Wittlieb et al., 2006), from small tissue pieces (Shimizu et al., 1993), isolated germ layers (Normandin, 1960; Kishimoto et al., 1996) and even from dissociated cell aggregates (Gierer et al., 1972; Technau et al., 2000; Seybold et al., 2016) (Figure 6A). The regenerative capacity of Hydra and the role of stem cells in this process have been extensively studied and reviewed elsewhere (Galliot and Schmid, 2002; Holstein et al., 2003; Bosch, 2007; Bosch et al., 2010; Galliot and Chera, 2010; Galliot and Ghila, 2010; Hobmayer et al., 2012).
Thanks to the development of a transgenic lifeact::GFP line (staining actin filaments in vivo, Figures 4Aa), Seybold et al. (2016) observed that polarized actin structures appeared progressively, in a cell autonomous manner, between 6 and 24 h post dissociation (hpd). Interestingly, the orientation of the reforming myonemes (in number of 2–3 per cell) appears to be polarized within a single cell, but randomly aligned to the myonemes in surrounding cells (Seybold et al., 2016). Only at about 48 hpd the myonemes of each cell align to form a coordinated muscle system. The polarization process takes place in a very comparable manner within the ectodermal and the endodermal epithelia, though the two muscle networks will ultimately be orthogonally oriented (Seybold et al., 2016). Further work is required to characterize the molecular mechanisms underlying not only the cellular autonomous repolarization of the myonemes in Hydra, but also how the individual cells communicate in order to form the polarized and coordinated muscle networks.
Striated Muscle Transdifferentiation in Podocoryna
Cellular plasticity plays a crucial role in most regenerative processes. The most extensive work aimed at understanding muscle plasticity in cnidarians, was carried out by Schmid and colleagues in the hydrozoan jellyfish Podocoryna carnea. In a seminal series of papers they detailed the remarkable transdifferentiation process able to convert isolated striated muscle cells to neuronal or smooth muscle fates (Figure 6E) and to ultimately regenerate a fully functional manubrium (reviewed in Schmid, 1988; Schmid et al., 1988; Brockes, 1994; Reber-Müller et al., 1995).
From the subumbrella of the medusa Podocoryna carnea, endodermal and striated muscle layers can be isolated and cultivated for weeks. Following collagenase treatment to disrupt muscle and ECM interaction, striated muscle cells transdifferentiate into smooth-like muscle cells (Schmid, 1978, 1992). They lose their striated myofibrils, develop a cilium and adopt a morphology that is similar to smooth muscle cells. This process is transcription and translation but not proliferation dependent (Schmid, 1975; Weber et al., 1987). Once transdifferentiated, those cells behave in a stem cell fashion, as they self-renew and give rise to a differentiated cell, following a strict pattern (Figure 6E). In fact, each subsequent division results in a nerve cell expressing the neurotransmitter FMRF-amide and a cycling smooth muscle cell (Alder and Schmid, 1987).
Combined with isolated endodermal cells of the umbrella, isolated striated muscle cells can regenerate a functional manubrium containing at least seven new cell types, including gametes (Schmid, 1974, 1976; Schmid et al., 1982). Further refinement of the cell separation protocol allowed Schmid and colleagues to obtain a fully regenerated manubrium from a pure population of destabilized (collagenase treated) striated muscle cells (Schmid and Alder, 1984). These transdifferentiation experiments were also successfully performed using striated muscles of other hydrozoan medusae (Schmid, 1978) albeit with lower efficiency than in Podocoryna carnea.
A number of genes expressed in Podocoryna striated muscle cells and whose expression is altered during transdifferentiation, have been characterized. While twist is likely not involved in this process (Spring et al., 2000), msx expression is downregulated in response to cellular dissociation and strongly reactivated during smooth-like muscle differentiation (Galle et al., 2005). bmp2/4 expression is initiated immediately after excision and bmp5/8 in the initial phase of the transdifferentiation process (Reber-Müller et al., 2006). Interestingly, expression of the Podocoryna Piwi homolog, cniwi, is upregulated during transdifferentiation (Seipel et al., 2004) and potentially involved in the potency (Van Wolfswinkel, 2014) of the muscle cells to become neurons. Although these data suggest an implication of the transcription factor Msx, the RNA-binding protein Cniwi and BMP signaling in the transformation potential of striated muscles in Podocoryna, no functional data is available.
Muscle Plasticity during Jellyfish Self-Repair
Following up on this in vitro work on Podocoryna, Lin and colleagues analyzed wound healing and remodeling of the striated muscle cells in toto, in the umbrella of the jellyfish Polyorchis penicillatus (Lin et al., 2000). After wounding, the striated muscles cells can lose their condensed actin fibers and dedifferentiate, enabling them to migrate toward the wound. During the migration process, which is dependent on intracellular calcium resources, the cells lose also their contractile ability while surrounding intact epitheliomuscular cells remain able to contract in response to a chemical stimulus (Lin et al., 2000). The dedifferentiation-migration response to wounding takes about 8–10 h and does not seem to involve cell proliferation. Once the dedifferentiated cells have filled up the wound site, they stop migrating and begin to re-differentiate and re-polarize, for finally becoming fully functional muscle cells within 24–48 h post injury (Lin et al., 2000).
In culture, the migrating striated muscle cells in Podocoryna induce a change in gene expression that is rapidly communicated to the non-migrating cells, thus allowing a coordinated tissue reorganization (Yanze et al., 1999). In addition to the transdifferentiation potential of striated muscle cells in vitro, these observations show the de- and re-differentiation capacity of the same muscle cell type in vivo.
Another hydrozoan jellyfish that has been used to understand medusa self-repair mechanism is Clytia hemisphaerica (previously named Phialidium hemisphaericum or Campanularia johnstoni). Differently sized fragments of the jellyfish umbrella are able to rapidly restore the bell shape by a “morphodynamic process” and subsequently reform, at various degrees, missing structures such as the canals, tentacles and gonads (Figure 6B; Schmid and Tardent, 1971; Schmid et al., 1976). The mechanisms by which Clytia medusae reform the missing structures and restore the bell shape are still unknown.
More recently, the scyphozoan Aurelia aurita was used to gain insights into a particular mechanism that enables injured ephrya (juvenile jellyfish) to rapidly regain a functional shape and pursue its development into adulthood (Abrams et al., 2015). In this specific case, the “healing” process does not involve cellular proliferation or apoptosis but a so-called symmetrization (term introduced by Abrams et al., 2015). This process reshapes the animal by reorganizing the existing parts, without reformation of the missing parts (Figure 6C). This self-repairing event is crucial to allow subsequent development of the damaged juvenile jellyfish into a radially symmetrical adult. Unlike during regeneration in other cnidarians (Chera et al., 2009; Passamaneck and Martindale, 2012; Amiel et al., 2015), inhibition of cellular proliferation or apoptosis does not affect the symmetrization process in Aurelia aurita (Abrams et al., 2015). Interestingly, inhibiting the muscle reconnection following injury using low doses of cytochalasin D (to avoid nonspecific actin dependent effects and to still allow contraction of the existing muscles) does not affect symmetrization (Abrams et al., 2015). However, further analyses using muscle relaxants, such as magnesium chloride or menthol causing the decrease of the pulsation frequency and the inhibition of symmetrization, revealed that contraction forces that are generated by the musculature network of the juvenile jellyfish are likely important for this process (Abrams et al., 2015). If this contraction dependent symmetrization process is specific to Aurelia aurita ephrya, or represents a general strategy for maintaining the swimming capacity in injured adult jellyfish, is currently unknown.
Muscle and Regeneration in Nematostella
Nematostella vectensis is emerging as a new regeneration model (Reitzel et al., 2007; Trevino et al., 2011; Passamaneck and Martindale, 2012; Bossert et al., 2013; DuBuc et al., 2014; Amiel et al., 2015), particularly well-suited to compare development and regeneration within the same organism (Burton and Finnerty, 2009; Layden et al., 2016). Recent studies have analyzed its basic regeneration capacity (Figure 6D; Reitzel et al., 2007; Amiel et al., 2015), establishing precise staging systems to analyze the regeneration process under physiological and perturbation conditions (Bossert et al., 2013; Amiel et al., 2015), and developing new in vivo tools to asses wound healing, pharynx formation and tissue tracing (Amiel et al., 2015). These studies have shown that cellular proliferation is induced at the amputation site and required for the regeneration process (Passamaneck and Martindale, 2012; Amiel et al., 2015). Additional work in Nematostella is required to identify stem and progenitor cells.
The muscle regeneration process in Nematostella was initially studied with a MyHC1::mCherry transgenic line labeling the retractor muscles (Renfer et al., 2010). Immediately after amputation, these muscles retracted from the wound site. Later in the process, numerous cells accumulated at the regenerating site expressing the MyHC1::mCherry transgene (in a non-polarized manner) suggesting active cellular differentiation and reorganization events in this region (Renfer et al., 2010). However, nothing is known about the cellular origin of the newly formed retractor muscle fibers, nor the cellular and molecular mechanisms underlying this process.
A recent study suggested that muscle contraction could play a role in regeneration of missing body parts (Bossert et al., 2013). In fact, contraction of the circumferential muscle fibers may be involved in reducing the size of the wound in isolated adult physa (the most aboral part of the polyp in burrowing actiniaria) and thus, potentially promoting the wound healing process and the reformation of oral structures. A detailed characterization of the oral regeneration process in juveniles shows a very stereotyped and dynamic behavior of the tissues during the regeneration program (Amiel et al., 2015), suggesting that muscle contractions may play a role also during later steps of regeneration. Additional analyses are required to understand the process of muscle fiber regeneration and repolarization as well as the role that muscles and muscle contractions play during wound healing and regeneration in Nematostella.
Conclusion and Perspectives
In this overview, we have introduced the cnidarians (Figure 1), a group of animals with diverse life cycles (Figure 2) and holding a key phylogenetic position as the sister group to bilaterians. Cnidarian muscles are composed of a set of epitheliomuscular cell types and, in some species, include additional, independently–evolved, striated muscles (Figure 4) and myocytes. The epitheliomuscular cells play a role in prey capture, locomotion or defense from predators (Figure 3). Intriguingly, cnidarians possess genes that are generally associated with muscle formation in bilaterians (e.g., Mef2), but lack classical myogenic regulatory factors such as MyoD (Figure 5) as well as terminal differentiation proteins typical of bilaterian striated muscles such as the Troponins and Titin. Cnidarians possess quite extraordinary regenerative capacities as they can regrow missing body parts from isolated fragments or in some species even from dissociated cells aggregations (Figure 6). Although the regenerative capacity has intrigued scientists for over 300 years, currently little is known about their capacity to reform/regenerate injured muscles. In order to better understand the similarities and differences of muscle plasticity in cnidarians, an emphasis has to be put on carrying out functional studies in existing as well as new models. Recent technological advances will be greatly beneficial for both aspects.
Open Questions in Cnidarian Muscle Development and Regeneration
As described above, muscle plasticity has only been studied in a handful of cnidarians and is to date rather descriptive. Work carried out in jellyfish suggest that de- and re-differentiation as well as cell migration might be involved in wound healing and reformation of striated muscle fiber network. The fact that cellular proliferation is not detected during this process raises the question about how cellular homeostasis is maintained. Are there undifferentiated precursors involved in the wound healing process as well? Which are the molecular signals inducing dedifferentiation process, cellular migration and its guidance, as well as the re-differentiation into striated muscles? While latter questions are rather medusa specific, there are also questions that are relevant to all cnidarians. How are the condensation and the polarization of the actin fibers controlled to reform a perfectly organized and integrated muscle network? What are the molecular cues responsible for myoneme polarization during re-aggregation/regeneration experiments in Hydra? What controls the suggested de- and re-differentiation of retractor muscle cells during oral regeneration in Nematostella? Does this anthozoan possess epithelial stem cells similar to the ones described in hydrozoans or do they possess multi-potent stem cells? What causes the condensation and orientation of the thick longitudinal retractor muscle fibers, compared to the thin circumferential muscle fibers, both residing in the endodermal epithelium? Are those dependent on signaling molecules released by the mesenteries, mechanical forces induced by mesenterial infoldings, or a combination of the two? Do “smooth-muscle-like” epitheliomuscular cells possess transdifferentiation potential similar to the ones described from striated jellyfish muscle cells? Answering this non-exhaustive catalog of open questions is not only important to provide new insights into cnidarian muscle plasticity, but will also help providing a better understanding of the mechanisms underlying initial cnidarian muscle development.
Understanding Muscle Polarization in Cnidarians
Epitheliomuscular cells associate to form condensed muscle fibers (e.g., muscle net in Hydra Figures 4Aa,a' or longitudinal muscle fibers in Nematostella, Figures 4Cc,c') in diverse orientations (Figures 4A–C). While recent work has nicely described the repolarization process in Hydra (Seybold et al., 2016), the molecular and/or mechanical signals that control the condensation and orientation/polarization of cnidarian muscle fibers during development are unknown. A study using transgenic Nematostella MyHC1::mCherry (Renfer et al., 2010) has shown that condensed muscle fibers of the retractor muscles appear progressively during the late planula-primary polyp transition (Jahnel et al., 2014). One intriguing aspect of muscle development in Nematostella is that the polarity of epitheliomuscular cells within the same endodermal epithelium varies according to their spatial coordinates. The myonemes localized in the portions of the body column in-between the mesenteries form the circumferential ring musculature, while those included at and in the mesenteries (parietal and retractor muscles) are oriented longitudinally (Figures 4Cc,c'; Jahnel et al., 2014). As the infolding of the endodermal epithelium is likely contributing to the formation of the mesenteries (Jahnel et al., 2014; Leclère and Rentzsch, 2014), it would be interesting to investigate the mechanical aspects of this process, by looking at the role that mechanical forces play on the orientation of the myonemes, or conversely, the role that longitudinal muscle fibers have on the guidance/formation of mesenteries.
Cnidarians as New Models to Study Myoepithelial Development/Plasticity
Studies on cnidarians (Figure 1) could help gaining insights into the evolution of the mesodermal germ layer (absent in cnidarians, but present in bilaterians) and thus about those tissues that in bilaterians are mesodermal derivatives, such as muscles (reviewed by Seipel and Schmid, 2006; Burton, 2008; Technau and Steele, 2011; Layden et al., 2016). The developmental program of cnidarian muscles is currently largely unknown and requires intense functional work. Thus, addressing this question has been initiated by studying the expression (Spring et al., 2000, 2002; Fritzenwanker et al., 2004; Martindale et al., 2004) and function (Genikhovich and Technau, 2011) of “mesodermal” genes (e.g., brachyury, mef2) or the gene regulatory networks controlling endomesoderm development (Röttinger et al., 2012). However, these studies have an undeniable bias, the implicit assumption being that cnidarian muscle cells are essentially similar to bilaterian muscles. If on one hand the hypothesis of an independent origin for cnidarian and bilaterian striated muscles has been taken into account (Steinmetz et al., 2012), on the other hand all other cnidarian muscle cell types have generically been considered as “smooth muscle cells” (Seipel and Schmid, 2005; Burton, 2008; Steinmetz et al., 2012). The latter statement is supported by the fact that they are mononucleated and express “smooth muscle” proteins such as Myosin Heavy Chain (Renfer et al., 2010).
It is however important to keep in mind that the embryological origin of cnidarian muscle cells is not the mesodermal germ layer, but either the endodermal or the ectodermal layer and, importantly, that cnidarian muscle cells are mostly epithelial. As for cnidarian epitheliomuscular cells, bilaterians myoepithelial cells originate from tissues of various developmental origins (Petersen and van Deurs, 1989; Schmidt-Rhaesa, 2007; Tamgadge et al., 2013). Furthermore, myoepithelial cells in mammalians are receiving increasing interest, because of their importance in processes such as gland development, growth and differentiation, in pathologies such as breast cancer (Silva et al., 2015) as well as their capacity to control tumorigenesis (Gudjonsson et al., 2005; reviewed in Deugnier et al., 2002; Sopel, 2010). Thus, it could be particularly fruitful to compare bilaterian myoepithelial and cnidarian epitheliomuscular cells, their developmental origin, the molecular or mechanical signals that control their polarization, condensation and organization into muscle nets, rings or fibers and how they regenerate after injury. The easy access to biological material offered by cnidarian models, combined with various modern approaches that are now routinely performed on these organisms make them very interesting models to investigate myoepithelium formation and regeneration.
Potential Roles of Muscles in Cnidarian Regeneration
Recent work suggests that muscle contraction could play a primary role during the regenerative process, by promoting wound healing in Nematostella (Bossert et al., 2013) and allowing juvenile scyphozoan jellyfish to reshape rapidly into a functional body, in a process recently called “symmetrization” (Abrams et al., 2015). Interestingly, mammary myoepithelial cells, in addition to their contractile function, preserve also the regenerative potential of the tissue and are able to modulate in an integrin mediated process the proliferation and differentiation of surrounding cells (Deugnier et al., 2006; Shackleton et al., 2006; Stingl et al., 2006; Sleeman et al., 2007; reviewed in Moumen et al., 2011). In planarians, which similarly to cnidarians display impressive regenerative capacities, several lines of evidence show that the vast majority of “position control genes,” (Reddien, 2011) such as wnt1 or notum (Adell et al., 2009; Petersen and Reddien, 2011), are not only responsible for the polarity and patterning events during regeneration, but are also expressed in the muscles cells (Witchley et al., 2013). These observations led to the hypothesis that planarian muscle cells provide positional information to the surrounding stem cells, thus promoting regional differentiation and body polarization (Witchley et al., 2013; reviewed in Cebrià, 2016). Along these lines, it would be particularly interesting to address whether cnidarian epitheliomuscular cells and/or muscle networks play a role during wound healing and subsequent regenerative processes by providing biochemical or biomechanical cues.
The development of new molecular tools in a handful of hydrozoan and anthozoan species has already provided new insights into several long-standing evolutionary and developmental questions (Houliston et al., 2010; Technau and Steele, 2011; Galliot, 2012; Nebel and Bosch, 2012; Plickert et al., 2012; Sinigaglia et al., 2013; Layden et al., 2016; Rentzsch and Technau, 2016). Those tools, in combination with “omics” and functional genomics approaches (Momose and Houliston, 2007; Rentzsch et al., 2008; Amiel et al., 2009; Chera et al., 2009; Genikhovich and Technau, 2009; Boehm et al., 2012; Röttinger et al., 2012; Layden et al., 2013; Lapébie et al., 2014; Bradshaw et al., 2015) as well as with the recently developed techniques for genome editing (Ikmi et al., 2014) are now opening new opportunities to functionally and thoroughly address the developmental and regenerative program of cnidarian muscles systems, but also the role(s) that epitheliomuscular cells, muscle fibers and muscle contraction can play on the regeneration process.
Author Contributions
All authors listed, have made substantial, direct and intellectual contribution to the work, and approved it for publication.
Conflict of Interest Statement
The authors declare that the research was conducted in the absence of any commercial or financial relationships that could be construed as a potential conflict of interest.
Acknowledgments
The authors thank Bert Hobmayer, Johanna Kraus, and Aldine Amiel for sharing unpublished and published images that were used in Figure 4. We are also grateful to Evelyn Houliston, Aldine Amiel, and Chiara Sinigaglia for comments on the manuscript and to the reviewers for their comments that helped improving the final version of the manuscript. LL is supported by the ANR (MEDUSEVO-ANR-13-PDOC-0016). ER is supported by an ATIP-Avenir award (Plan Cancer), a Marie-Curie Career Integration Grant (CIG-FP7 - 631665) as well as by the “Fondation ARC pour la Recherche sur le Cancer”.
References
Abrams, M. J., Basinger, T., Yuan, W., Guo, C.-L., and Goentoro, L. (2015). Self-repairing symmetry in jellyfish through mechanically driven reorganization. Proc. Natl. Acad. Sci. U.S.A. 112, E3365–E3373. doi: 10.1073/pnas.1502497112
Adams, J. C., and Brancaccio, A. (2015). The evolution of the dystroglycan complex, a major mediator of muscle integrity. Biol. Open 4, 1163–1179. doi: 10.1242/bio.012468
Adell, T., Saló, E., Boutros, M., and Bartscherer, K. (2009). Smed-Evi/Wntless is required for beta-catenin-dependent and -independent processes during planarian regeneration. Development 136, 905–910. doi: 10.1242/dev.033761
Aerne, B., Gröger, H., Schuchert, P., Spring, J., and Schmid, V. (1996). The polyp and its medusa: a molecular approach. Sci. Mar. 60, 7–16.
Alberts, B., Johnson, A., Lewis, J., Morgan, D., Raff, M., Roberts, K., et al. (2015). Molecular Biology of the Cell, 6th Edn. New York, NY: Garland Science.
Alder, H., and Schmid, V. (1987). Cell cycles and in vitro transdifferentiation and regeneration of isolated, striated muscle of jellyfish. Dev. Biol. 124, 358–369. doi: 10.1016/0012-1606(87)90488-X
Alexander, R. M. (1964). Visco-elastic properties of the mesogloea of jellyfish. J. Exp. Biol. 41, 363–369.
Almada, A. E., and Wagers, A. J. (2016). Molecular circuitry of stem cell fate in skeletal muscle regeneration, ageing and disease. Nat. Rev. Mol. Cell. Biol. 17, 267–279. doi: 10.1038/nrm.2016.7
Amerongen, H. M., and Peteya, D. J. (1980). Ultrastructural study of two kinds of muscle in sea anemones: the existence of fast and slow muscles. J. Morphol. 166, 145–154. doi: 10.1002/jmor.1051660203
Amiel, A., Leclère, L., Robert, L., Chevalier, S., and Houliston, E. (2009). Conserved functions for Mos in eumetazoan oocyte maturation revealed by studies in a cnidarian. Curr. Biol. 19, 305–311. doi: 10.1016/j.cub.2008.12.054
Amiel, A. R., Johnston, H. T., Nedoncelle, K., Warner, J. F., Ferreira, S., and Röttinger, E. (2015). Characterization of morphological and cellular events underlying oral regeneration in the sea anemone, Nematostella vectensis. Int. J. Mol. Sci. 16, 28449–28471. doi: 10.3390/ijms161226100
Anctil, M., Poulain, I., and Pelletier, C. (2005). Nitric oxide modulates peristaltic muscle activity associated with fluid circulation in the sea pansy Renilla koellikeri. J. Exp. Biol. 208, 2005–2017. doi: 10.1242/jeb.01607
Anderson, P. A. V., and Schwab, W. E. (1981). The organization and structure of nerve and muscle in the jellyfish Cyanea capillata (coelenterata; scyphozoa). J. Morphol. 170, 383–399. doi: 10.1002/jmor.1051700309
Andrikou, C., and Arnone, M. I. (2015). Too many ways to make a muscle: evolution of GRNs governing myogenesis. Zool. Anz. 256, 2–13. doi: 10.1016/j.jcz.2015.03.005
Andrikou, C., Pai, C.-Y., Su, Y.-H., and Arnone, M. I. (2015). Logics and properties of a genetic regulatory program that drives embryonic muscle development in an echinoderm. eLife 4:e07343. doi: 10.7554/eLife.07343
Arendt, D. (2008). The evolution of cell types in animals: emerging principles from molecular studies. Nat. Rev. Genet. 9, 868–882. doi: 10.1038/nrg2416
Baader, C. D., Schmid, V., and Schuchert, P. (1993). Characterization of a tropomyosin cDNA from the hydrozoan Podocoryne carnea. FEBS Lett. 328, 63–66. doi: 10.1016/0014-5793(93)80966-X
Bell, J. J., Shaw, C., and Turner, J. R. (2006). Factors controlling the tentacle and polyp expansion behaviour of selected temperate Anthozoa. J. Mar. Biol. Assoc. 86, 977–992. doi: 10.1017/S0025315406013956
Bely, A. E., and Nyberg, K. G. (2010). Evolution of animal regeneration: re-emergence of a field. Trends Ecol. Evol. 25, 161–170. doi: 10.1016/j.tree.2009.08.005
Benian, G. M., Tinley, T. L., Tang, X., and Borodovsky, M. (1996). The Caenorhabditis elegans gene unc-89, required for muscle M-line assembly, encodes a giant modular protein composed of Ig and signal transduction domains. J. Cell Biol. 132, 835–848. doi: 10.1083/jcb.132.5.835
Bentzinger, C. F., Wang, Y. X., and Rudnicki, M. A. (2012). Building muscle: molecular regulation of myogenesis. Cold Spring Harb. Perspect. Biol. 4:a008342. doi: 10.1101/cshperspect.a008342
Bielecki, J., Nachman, G., and Garm, A. (2013). Swim pacemaker response to bath applied neurotransmitters in the cubozoan Tripedalia cystophora. J. Comp. Physiol. A Neuroethol. Sens. Neural. Behav. Physiol. 199, 785–797. doi: 10.1007/s00359-013-0839-1
Boehm, A.-M., Khalturin, K., Anton-Erxleben, F., Hemmrich, G., Klostermeier, U. C., Lopez-Quintero, J. A., et al. (2012). FoxO is a critical regulator of stem cell maintenance in immortal Hydra. Proc. Natl. Acad. Sci. U.S.A. 109, 19697–19702. doi: 10.1073/pnas.1209714109
Boelsterli, U. (1977). An electron microscopic study of early developmental stages, myogenesis, oogenesis and cnidogenesis in the anthomedusa, Podocoryne carnea M. Sars. J. Morphol. 154, 259–289. doi: 10.1002/jmor.1051540206
Boero, F., Gravili, C., Pagliara, P., Piraino, S., Bouillon, J., and Schmid, V. (1998). The cnidarian premises of metazoan evolution: from triploblasty, to coelom formation, to metamery. Ital. J. Zool. 65, 5–9. doi: 10.1080/11250009809386722
Bosch, T. C. G. (2007). Why polyps regenerate and we don't: towards a cellular and molecular framework for Hydra regeneration. Dev. Biol. 303, 421–433. doi: 10.1016/j.ydbio.2006.12.012
Bosch, T. C. G., Anton-Erxleben, F., Hemmrich, G., and Khalturin, K. (2010). The Hydra polyp: nothing but an active stem cell community. Dev. Growth. Differ. 52, 15–25. doi: 10.1111/j.1440-169X.2009.01143.x
Bossert, P. E., Dunn, M. P., and Thomsen, G. H. (2013). A staging system for the regeneration of a polyp from the aboral physa of the anthozoan cnidarian Nematostella vectensis. Dev. Dyn. 242, 1320–1331. doi: 10.1002/dvdy.24021
Bouillon, J. (1993). “Classe des hydrozoaires,” in Traité de Zoologie: Cnidaires, Ctenaires, Vol. III Fascicule 2. ed P. P. Grassé (Paris: Masson), 29–416.
Bourmaud, C., and Gravier-Bonnet, N. (2004). Medusoid release and spawning in the life cycle of Macrorynchia philippina Kirchenpauer 1872 (Cnidaria, Hydrozoa, Aglaopheniidae). Hydrobiologia 530, 365–372. doi: 10.1007/s10750-004-2665-5
Bradshaw, B., Thompson, K., and Frank, U. (2015). Distinct mechanisms underlie oral versus aboral regeneration in the cnidarian Hydractinia echinata. eLife 4:e05506. doi: 10.7554/eLife.05506
Brockes, J. (1994). Cell differentiation: muscle escapes from a jelly mould. Curr. Biol. 4, 1030–1032. doi: 10.1016/S0960-9822(00)00235-9
Brunet, T., Fischer, A. H., Steinmetz, P. R., Lauri, A., Bertucci, P., and Arendt, D. (2016). The evolutionary origin of bilaterian smooth and striated myocytes. eLife 5:e19607. doi: 10.1101/064881
Bryson-Richardson, R. J., and Currie, P. D. (2008). The genetics of vertebrate myogenesis. Nat. Rev. Genet. 9, 632–646. doi: 10.1038/nrg2369
Burton, P. M. (2008). Insights from diploblasts; the evolution of mesoderm and muscle. J. Exp. Zool. 310, 5–14. doi: 10.1002/jez.b.21150
Burton, P. M., and Finnerty, J. R. (2009). Conserved and novel gene expression between regeneration and asexual fission in Nematostella vectensis. Dev. Genes Evol. 219, 79–87. doi: 10.1007/s00427-009-0271-2
Buss, L. W., Anderson, C., and Bolton, E. W. (2013). Muscular anatomy of the Podocoryna carnea hydrorhiza. PLoS ONE 8:e72221. doi: 10.1371/journal.pone.0072221
Buzgariu, W., Haddad, Al. S., Tomczyk, S., Wenger, Y., and Galliot, B. (2015). Multi-functionality and plasticity characterize epithelial cells in Hydra. Tissue Barriers 3:e1068908. doi: 10.1080/21688370.2015.1068908
Campbell, R. D. (1967). Tissue dynamics of steady state growth in Hydra littoralis. II. Patterns of tissue movement. J. Morphol. 121, 19–28. doi: 10.1002/jmor.1051210103
Campbell, R. D. (1987). Organization of the nematocyst battery in the tentacle of hydra: arrangement of the complex anchoring junctions between nematocytes, epithelial cells, and basement membrane. Cell. Tissue Res. 249, 647–655. doi: 10.1007/BF00217337
Carter, J. A., Hyland, C., Steele, R. E., and Collins, E.-M. S. (2016). Dynamics of mouth opening in Hydra. Biophys. J. 110, 1191–1201. doi: 10.1016/j.bpj.2016.01.008
Cebrià, F. (2016). Planarian body-wall muscle: regeneration and function beyond a simple skeletal support. Front. Cell Dev. Biol. 4:8. doi: 10.3389/fcell.2016.00008
Chang, E. S., Neuhof, M., Rubinstein, N. D., Diamant, A., Philippe, H., Huchon, D., et al. (2015). Genomic insights into the evolutionary origin of Myxozoa within Cnidaria. Proc. Natl. Acad. Sci. U.S.A. 112, 14912–14917. doi: 10.1073/pnas.1511468112
Chapman, D. M. (1965). Co-ordination in a scyphistoma. Integr. Comp. Biol. 5, 455–464. doi: 10.1093/icb/5.3.455
Chapman, D. M. (1968). A new type of muscle cell from the subumbrella of Obelia. J. Mar. Biol. Assoc. UK 48, 667–688. doi: 10.1017/S0025315400019226
Chapman, D. M. (1978). Microanatomy of the cubopolyp,Tripedalia cystophora. Helgol. Wiss. Meeresunters 31, 128–168. doi: 10.1007/BF02296994
Chapman, D. M. (1999). Microanatomy of the bell rim of Aurelia aurita (Cnidaria: Scyphozoa). Can. J. Zool. 77, 34–46. doi: 10.1139/z98-193
Chapman, D. M., Pantin, C. F., and Robson, E. A. (1962). Muscle in coelenterates. Rev. Can. Biol. 21, 267–278.
Chapman, D. M., and Werner, B. (1972). Structure of a solitary and a colonial species of Stephanoscyphus (Scyphozoa, Coronatae) with observations on periderm repair. Helgol. Wiss. Meeresunters 23, 393–421. doi: 10.1007/BF01625293
Chapman, J. A., Kirkness, E. F., Simakov, O., Hampson, S. E., Mitros, T., Weinmaier, T., et al. (2010). The dynamic genome of Hydra. Nature 464, 592–596. doi: 10.1038/nature08830
Chera, S., Ghila, L., Dobretz, K., Wenger, Y., Bauer, C., Buzgariu, W., et al. (2009). Apoptotic cells provide an unexpected source of Wnt3 signaling to drive Hydra head regeneration. Dev. Cell. 17, 279–289. doi: 10.1016/j.devcel.2009.07.014
Chevalier, J. P., and Beauvais, L. (1987). “Ordre des scléractiniaires” in Traité de Zoologie: Cnidaires, Ctenaires, Vol. III Fascicule 3, ed P. P. Grassé (Paris: Masson), 403–764.
Chia, F.-S., Amerongen, H. M., and Peteya, D. J. (1984). Ultrastructure of the neuromuscular system of the polyp of Aurelia aurita L., 1758 (Cnidaria, scyphozoa). J. Morphol. 180, 69–79. doi: 10.1002/jmor.1051800108
Chiori, R., Jager, M., Denker, E., Wincker, P., Da Silva, C., Le Guyader, H., et al. (2009). Are Hox genes ancestrally involved in axial patterning? Evidence from the hydrozoan Clytia hemisphaerica (Cnidaria). PLoS ONE 4:e4231. doi: 10.1371/journal.pone.0004231
Christensen, K. L., Patrick, A. N., and McCoy, E. L. (2008). The six family of homeobox genes in development and cancer. Adv. Cancer Res. 101, 93–126. doi: 10.1016/S0065-230X(08)00405-3
Colin, S. P., Costello, J. H., Dabiri, J. O., Villanueva, A., Blottman, J. B., Gemmell, B. J., et al. (2012). Biomimetic and live medusae reveal the mechanistic advantages of a flexible bell margin. PLoS ONE 7:e48909. doi: 10.1371/journal.pone.0048909
Costello, J. H., Colin, S. P., Gemmell, B. J., Dabiri, J. O., and Sutherland, K. R. (2015). Multi-jet propulsion organized by clonal development in a colonial siphonophore. Nat. Comm. 6:8158. doi: 10.1038/ncomms9158
Dabiri, J. O., Colin, S. P., Costello, J. H., and Gharib, M. (2005). Flow patterns generated by oblate medusan jellyfish: field measurements and laboratory analyses. J. Exp. Biol. 208, 1257–1265. doi: 10.1242/jeb.01519
Dabiri, J. O., Colin, S. P., Katija, K., and Costello, J. H. (2010). A wake-based correlate of swimming performance and foraging behavior in seven co-occurring jellyfish species. J. Exp. Biol. 213, 1217–1225. doi: 10.1242/jeb.034660
David, C. N. (1973). A quantitative method for maceration of Hydra tissue. Wilhelm Roux Arch. Entwickl. Mech. 171, 259–268. doi: 10.1007/BF00577724
Davis, R. L., Weintraub, H., and Lassar, A. B. (1987). Expression of a single transfected cDNA converts fibroblasts to myoblasts. Cell 51, 987–1000. doi: 10.1016/0092-8674(87)90585-X
Dayraud, C., Alié, A., Jager, M., Chang, P., Le Guyader, H., Manuel, M., et al. (2012). Independent specialisation of myosin II paralogues in muscle vs. non-muscle functions during early animal evolution: a ctenophore perspective. BMC Evol. Biol. 12:107. doi: 10.1186/1471-2148-12-107
de Kruijf, H. A. (1977). Bursting pacemaker activity in the solitary hydroid Tubularia solitaria. J. Exp. Biol. 68, 19–34.
Demont, M. E., and Gosline, J. M. (1988). Mechanics of jet propulsion in the hydromedusan jellyfish, Polyorchis penicillatus: II. Energetics of the jet cycle. J. Exp. Biol. 134, 333–345.
Deugnier, M.-A., Faraldo, M. M., Teulière, J., Thiery, J. P., Medina, D., and Glukhova, M. A. (2006). Isolation of mouse mammary epithelial progenitor cells with basal characteristics from the Comma-Dβ cell line. Dev. Biol. 293, 414–425. doi: 10.1016/j.ydbio.2006.02.007
Deugnier, M.-A., Teulière, J., Faraldo, M. M., Thiery, J. P., and Glukhova, M. A. (2002). The importance of being a myoepithelial cell. Breast Cancer Res. 4, 224–230. doi: 10.1186/bcr459
Doumenc, D. A. (1979). Structure et origine des systèmes squelettiques et neuromusculaires au cours de l'organogenèse des stades postlarvaires de l'actinie Cereus pedunculatus. Arch. Zool. Exp. Gen. 120, 431–476.
Doumenc, D. A., and Van Praët, M. (1987). “Ordre des actiniaires, ordre des ptychodactiniaires, ordre des corallimorphaires” in Traité de Zoologie: Cnidaires, Ctenaires, Vol. III Fascicule 3, ed P. P. Grassé (Paris: Masson), 257–401.
DuBuc, T. Q., Traylor-Knowles, N., and Martindale, M. Q. (2014). Initiating a regenerative response; cellular and molecular features of wound healing in the cnidarian Nematostella vectensis. BMC Biol. 12:24. doi: 10.1186/1741-7007-12-24
Dumont, N. A., Bentzinger, C. F., Sincennes, M.-C., and Rudnicki, M. A. (2015). Satellite cells and skeletal muscle regeneration. Compr. Physiol. 5, 1027–1059. doi: 10.1002/cphy.c140068
Eggers, N., and Jarms, G. (2007). The morphogenesis of ephyra in Coronatae (Cnidaria, Scyphozoa). Mar. Biol. 152, 495–502. doi: 10.1007/s00227-007-0719-8
Ewer, R. F., and Fox, H. M. (1947). On the functions and mode of action of the nematocysts of Hydra. J. Zool. 117, 365–376. doi: 10.1111/j.1096-3642.1947.tb00524.x
Fisher, D. A., and Bode, H. R. (1989). Nucleotide sequence of an actin-encoding gene from Hydra attenuata: structural characteristics and evolutionary implications. Gene 84, 55–64. doi: 10.1016/0378-1119(89)90139-X
Fossette, S., Gleiss, A. C., Chalumeau, J., Bastian, T., Armstrong, C. D., Vandenabeele, S., et al. (2015). Current-oriented swimming by jellyfish and its role in bloom maintenance. Curr. Biol. 25, 342–347. doi: 10.1016/j.cub.2014.11.050
Franc, A. (1993). “Classe des scyphozoaires” in Traité de Zoologie: Cnidaires, Ctenaires, Vol. III Fascicule 2, ed. P. P. Grassé (Paris: Masson), 597–884.
Fritzenwanker, J. H. J., Saina, M. M., and Technau, U. U. (2004). Analysis of forkhead and snail expression reveals epithelial-mesenchymal transitions during embryonic and larval development of Nematostella vectensis. Dev. Biol. 275, 389–402. doi: 10.1016/j.ydbio.2004.08.014
Fujinoki, M., Tomiyama, T., and Ishimoda-Takagi, T. (2002). Tropomyosin isoforms present in the sea anemone, Anthopleura japonica (Anthozoa, Cnidaria). J. Exp. Zool. 293, 649–663. doi: 10.1002/jez.10180
Gahan, J. M., Bradshaw, B., Flici, H., and Frank, U. (2016). The interstitial stem cells in Hydractinia and their role in regeneration. Curr. Opin. Genet. Dev. 40, 65–73. doi: 10.1016/j.gde.2016.06.006
Galle, S., Yanze, N., and Seipel, K. (2005). The homeobox gene Msx in development and transdifferentiation of jellyfish striated muscle. Int. J. Dev. Biol. 49, 961–967. doi: 10.1387/ijdb.052009sg
Galliot, B. (2012). Hydra, a fruitful model system for 270 years. Int. J. Dev. Biol. 56, 411–423. doi: 10.1387/ijdb.120086bg
Galliot, B., and Chera, S. (2010). The Hydra model: disclosing an apoptosis-driven generator of Wnt-based regeneration. Trends Cell Biol. 20, 514–523. doi: 10.1016/j.tcb.2010.05.006
Galliot, B., and Ghila, L. (2010). Cell plasticity in homeostasis and regeneration. Mol. Reprod. Dev. 77, 837–855. doi: 10.1002/mrd.21206
Galliot, B., Quiquand, M., Ghila, L., de Rosa, R., Miljkovic-Licina, M., and Chera, S. (2009). Origins of neurogenesis, a cnidarian view. Dev. Biol. 332, 2–24. doi: 10.1016/j.ydbio.2009.05.563
Galliot, B., and Schmid, V. (2002). Cnidarians as a model system for understanding evolution and regeneration. Int. J. Dev. Biol. 46, 39–48.
Garm, A., and Mori, S. (2009). Multiple photoreceptor systems control the swim pacemaker activity in box jellyfish. J. Exp. Biol. 212, 3951–3960. doi: 10.1242/jeb.031559
Garm, A., O'Connor, M., Parkefelt, L., and Nilsson, D.-E. (2007). Visually guided obstacle avoidance in the box jellyfish Tripedalia cystophora and Chiropsella bronzie. J. Exp. Biol. 210, 3616–3623. doi: 10.1242/jeb.004044
Garm, A., Oskarsson, M., and Nilsson, D.-E. (2011). Box jellyfish use terrestrial visual cues for navigation. Curr. Biol. 21, 798–803. doi: 10.1016/j.cub.2011.03.054
Gemmell, B. J., Costello, J. H., Colin, S. P., Stewart, C. J., Dabiri, J. O., Tafti, D., et al. (2013). Passive energy recapture in jellyfish contributes to propulsive advantage over other metazoans. Proc. Natl. Acad. Sci. U.S.A. 110, 17904–17909. doi: 10.1073/pnas.1306983110
Genikhovich, G., and Technau, U. (2009). The starlet sea anemone Nematostella vectensis: an anthozoan model organism for studies in comparative genomics and functional evolutionary developmental biology. Cold Spring Harb. Protoc. 2009, pdb.emo129–pdb.emo129. doi: 10.1101/pdb.emo129
Genikhovich, G., and Technau, U. (2011). Complex functions of Mef2 splice variants in the differentiation of endoderm and of a neuronal cell type in a sea anemone. Development 138, 4911–4919. doi: 10.1242/dev.068122
Gierer, A., Berking, S., Bode, H., David, C. N., Flick, K., Hansmann, G., et al. (1972). Regeneration of hydra from reaggregated cells. Nat. New Biol. 239, 98–101. doi: 10.1038/newbio239098a0
Gold, D. A., Nakanishi, N., Hensley, N. M., Cozzolino, K., Tabatabaee, M., Martin, M., et al. (2015). Structural and developmental disparity in the tentacles of the moon jellyfish Aurelia sp.1. PLoS ONE 10:e0134741. doi: 10.1371/journal.pone.0134741
Golz, R. (1993). Anchorage and retraction of nematocytes in the tentacles of the cubopolyp Carybdea marsupialis are mediated by a species-specific mesogleal support. Cell Tissue Res. 274, 173–180. doi: 10.1007/BF00327998
Grifone, R., Demignon, J., Houbron, C., Souil, E., Niro, C., Seller, M. J., et al. (2005). Six1 and Six4 homeoproteins are required for Pax3 and Mrf expression during myogenesis in the mouse embryo. Development 132, 2235–2249. doi: 10.1242/dev.01773
Gröger, H., Callaerts, P., Gehring, W. J., and Schmid, V. (1999). Gene duplication and recruitment of a specific tropomyosin into striated muscle cells in the jellyfish Podocoryne carnea. J. Exp. Zool. 285, 378–386.
Gröger, H., Callaerts, P., Gehring, W. J., and Schmid, V. (2000). Characterization and expression analysis of an ancestor-type Pax gene in the hydrozoan jellyfish Podocoryne carnea. Mech. Dev. 94, 157–169. doi: 10.1016/S0925-4773(00)00286-0
Gruhl, A., and Okamura, B. (2012). Development and myogenesis of the vermiform Buddenbrockia (Myxozoa) and implications for cnidarian body-plan evolution. EvoDevo 3:10. doi: 10.1186/2041-9139-3-10
Gudjonsson, T., Adriance, M. C., Sternlicht, M. D., Petersen, O. W., and Bissell, M. J. (2005). Myoepithelial cells: their origin and function in breast morphogenesis and neoplasia. J. Mammary Gland Biol. Neoplasia 10, 261–272. doi: 10.1007/s10911-005-9586-4
Hartikainen, H., Gruhl, A., and Okamura, B. (2014). Diversification and repeated morphological transitions in endoparasitic cnidarians (Myxozoa: Malacosporea). Mol. Phylogenet. Evol. 76, 261–269. doi: 10.1016/j.ympev.2014.03.010
Haynes, J. F., Burnett, A. L., and Davis, L. E. (1968). Histological and ultrastructural study of the muscular and nervous systems in Hydra. I. The muscular system and the mesoglea. J. Exp. Zool. 167, 283–293. doi: 10.1002/jez.1401670304
Hayward, D. C., Grasso, L. C., Saint, R., Miller, D. J., and Ball, E. E. (2015). The organizer in evolution-gastrulation and organizer gene expression highlight the importance of brachyury during development of the coral, Acropora millepora. Dev. Biol. 399, 337–347. doi: 10.1016/j.ydbio.2015.01.006
Hebrok, M., Füchtbauer, A., and Füchtbauer, E. M. (1997). Repression of muscle-specific gene activation by the murine Twist protein. Exp. Cell Res. 232, 295–303. doi: 10.1006/excr.1997.3541
Helm, R. R., Tiozzo, S., Lilley, M. K. S., Lombard, F., and Dunn, C. W. (2015). Comparative muscle development of scyphozoan jellyfish with simple and complex life cycles. EvoDevo 6:11. doi: 10.1186/s13227-015-0005-7
Herberts, C. (1987). “Ordre des Zoanthaires” in Traité de Zoologie: Cnidaires, Ctenaires, Vol. III Fascicule 3. ed P. P. Grassé (Paris: Masson), 783–810.
Hobmayer, B., Jenewein, M., Eder, D., Eder, M.-K., Glasauer, S., Gufler, S., et al. (2012). Stemness in Hydra - a current perspective. Int. J. Dev. Biol. 56, 509–517. doi: 10.1387/ijdb.113426bh
Hoffmann, U., and Kroiher, M. (2001). A possible role for the cnidarian homologue of serum response factor in decision making by undifferentiated cells. Dev. Biol. 236, 304–315. doi: 10.1006/dbio.2001.0335
Holland, J. W., Okamura, B., Hartikainen, H., and Secombes, C. J. (2011). A novel minicollagen gene links cnidarians and myxozoans. Proc. R. Soc. Lond. Biol. Sci. 278, 546–553. doi: 10.1098/rspb.2010.1301
Holstein, T. W., Hobmayer, E., and Technau, U. (2003). Cnidarians: an evolutionarily conserved model system for regeneration? Dev. Dyn. 226, 257–267. doi: 10.1002/dvdy.10227
Hooper, S. L., and Thuma, J. B. (2005). Invertebrate muscles: muscle specific genes and proteins. Physiol. Rev. 85, 1001–1060. doi: 10.1152/physrev.00019.2004
Houliston, E., Momose, T., and Manuel, M. (2010). Clytia hemisphaerica: a jellyfish cousin joins the laboratory. Trends Genet. 26, 159–167. doi: 10.1016/j.tig.2010.01.008
Hufnagel, L. A., Kass-Simon, G., and Lyon, M. K. (1985). Functional organization of battery cell complexes in tentacles of Hydra attenuata. J. Morphol. 184, 323–341. doi: 10.1002/jmor.1051840307
Ikmi, A., McKinney, S. A., Delventhal, K. M., and Gibson, M. C. (2014). TALEN and CRISPR/Cas9-mediated genome editing in the early-branching metazoan Nematostella vectensis. Nat. Comm. 5:5486. doi: 10.1038/ncomms6486
Jager, M., Quéinnec, E., Le Guyader, H., and Manuel, M. (2011). Multiple Sox genes are expressed in stem cells or in differentiating neuro-sensory cells in the hydrozoan Clytia hemisphaerica. EvoDevo 2:12. doi: 10.1186/2041-9139-2-12
Jahnel, S. M., Walzl, M., and Technau, U. (2014). Development and epithelial organisation of muscle cells in the sea anemone Nematostella vectensis. Front. Zool. 11:44. doi: 10.1186/1742-9994-11-44
Josephson, R. K., and March, S. C. (1966). The swimming performance of the sea-anemone Boloceroides. J. Exp. Biol. 44, 493–506.
Josephson, R. K., and Uhrich, J. (1969). Inhibition of pacemaker systems in the hydroid Tubularia. J. Exp. Biol. 50, 1–14.
Kass-Simon, G., Pannaccione, A., and Pierobon, P. (2003). GABA and glutamate receptors are involved in modulating pacemaker activity in Hydra. Comp. Biochem. Physiol. A Mol. Integr. Physiol. 136, 329–342. doi: 10.1016/S1095-6433(03)00168-5
Katsuki, T., and Greenspan, R. J. (2013). Jellyfish nervous systems. Curr. Biol. 23, R592–R594. doi: 10.1016/j.cub.2013.03.057
Katzemich, A., Kreisköther, N., Alexandrovich, A., Elliott, C., Schöck, F., Leonard, K., et al. (2012). The function of the M-line protein obscurin in controlling the symmetry of the sarcomere in the flight muscle of Drosophila. J. Cell Sci. 125, 3367–3379. doi: 10.1242/jcs.097345
Keough, E. M., and Summers, R. G. (1976). An ultrastructural investigation of the striated subumbrellar musculature of the anthomedusan, Pennaria tiarella. J. Morphol. 149, 507–525. doi: 10.1002/jmor.1051490405
Kishimoto, Y. Y., Murate, M. M., and Sugiyama, T. T. (1996). Hydra regeneration from recombined ectodermal and endodermal tissue. I. Epibolic ectodermal spreading is driven by cell intercalation. J. Cell Sci. 109, 763–772.
Konstantinides, N., and Averof, M. (2014). A common cellular basis for muscle regeneration in arthropods and vertebrates. Science 343, 788–791. doi: 10.1126/science.1243529
Krasińska, V. S. (1914). Beiträge zur Histologie der Medusen. Inaugural-Dissertation, Universität Zürich, Wilhelm Engelmann (Leipzig; Berlin).
Kraus, J. E. M., Fredman, D., Wang, W., Khalturin, K., and Technau, U. (2015). Adoption of conserved developmental genes in development and origin of the medusa body plan. EvoDevo 6, 67–15. doi: 10.1186/s13227-015-0017-3
Kraus, Y., Aman, A., Technau, U., and Genikhovich, G. (2016). Pre-bilaterian origin of the blastoporal axial organizer. Nat. Comm. 7:11694. doi: 10.1038/ncomms11694
Kraus, Y., Fritzenwanker, J. H., Genikhovich, G., and Technau, U. (2007). The blastoporal organiser of a sea anemone. Curr. Biol. 17, R874–R876. doi: 10.1016/j.cub.2007.08.017
Kumar, M. S., and Owens, G. K. (2003). combinatorial control of smooth muscle–specific gene expression. Arterioscler. Thromb. Vasc. Biol. 23, 737–747. doi: 10.1161/01.ATV.0000065197.07635.BA
Künzel, T., Heiermann, R., Frank, U., Müller, W., Tilmann, W., Bause, M., et al. (2010). Migration and differentiation potential of stem cells in the cnidarian Hydractinia analysed in eGFP-transgenic animals and chimeras. Dev. Biol. 348, 120–129. doi: 10.1016/j.ydbio.2010.08.017
Lapébie, P., Ruggiero, A., Barreau, C., Chevalier, S., Chang, P., Dru, P., et al. (2014). Differential responses to Wnt and PCP disruption predict expression and developmental function of conserved and novel genes in a cnidarian. PLoS Genet. 10:e1004590. doi: 10.1371/journal.pgen.1004590
Layden, M. J., Boekhout, M., and Martindale, M. Q. (2012). Nematostella vectensis achaete-scute homolog NvashA regulates embryonic ectodermal neurogenesis and represents an ancient component of the metazoan neural specification pathway. Development 139, 1013–1022. doi: 10.1242/dev.073221
Layden, M. J., Rentzsch, F., and Röttinger, E. (2016). The rise of the starlet sea anemone Nematostella vectensis as a model system to investigate development and regeneration. Wiley Interdiscip. Rev. Dev. Biol. 5, 408–428. doi: 10.1002/wdev.222
Layden, M. J., Röttinger, E., Wolenski, F. S., Gilmore, T. D., and Martindale, M. Q. (2013). Microinjection of mRNA or morpholinos for reverse genetic analysis in the starlet sea anemone, Nematostella vectensis. Nat. Protoc. 8, 924–934. doi: 10.1038/nprot.2013.009
Leclère, L., Copley, R. R., Momose, T., and Houliston, E. (2016). Hydrozoan insights in animal development and evolution. Curr. Opin. Genet. Dev. 39, 157–167. doi: 10.1016/j.gde.2016.07.006
Leclère, L., and Rentzsch, F. (2014). RGM regulates BMP-mediated secondary axis formation in the sea anemone Nematostella vectensis. Cell Rep. 9, 1921–1930. doi: 10.1016/j.celrep.2014.11.009
Leclère, L., Schuchert, P., Cruaud, C., Couloux, A., and Manuel, M. (2009). Molecular phylogenetics of Thecata (Hydrozoa, Cnidaria) reveals long-term maintenance of life history traits despite high frequency of recent character changes. Syst. Biol. 58, 509–526. doi: 10.1093/sysbio/syp044
Lewis, C., and Long, T. A. F. (2005). Courtship and reproduction in Carybdea sivickisi (Cnidaria: Cubozoa). Mar. Biol. 147, 477–483. doi: 10.1007/s00227-005-1602-0
Lin, Y. C., Grigoriev, N. G., and Spencer, A. N. (2000). Wound healing in jellyfish striated muscle involves rapid switching between two modes of cell motility and a change in the source of regulatory calcium. Dev. Biol. 225, 87–100. doi: 10.1006/dbio.2000.9807
López de Haro, M. S., Salgado, L. M., David, C. N., and Bosch, T. C. (1994). Hydra tropomyosin TROP1 is expressed in head-specific epithelial cells and is a major component of the cytoskeletal structure that anchors nematocytes. J. Cell. Sci. 107, 1403–1411.
Lu, J., Webb, R., Richardson, J. A., and Olson, E. N. (1999). MyoR: a muscle-restricted basic helix-loop-helix transcription factor that antagonizes the actions of MyoD. Proc. Natl. Acad. Sci. U.S.A. 96, 552–557. doi: 10.1073/pnas.96.2.552
Mackie, G. O. (2004). Central neural circuitry in the jellyfish Aglantha: a model “simple nervous system”. Neurosignals 13, 5–19. doi: 10.1159/000076155
Magie, C. R., Pang, K., and Martindale, M. Q. (2005). Genomic inventory and expression of Sox and Fox genes in the cnidarian Nematostella vectensis. Dev. Genes Evol. 215, 618–630. doi: 10.1007/s00427-005-0022-y
Martin, V. J., and Chia, F.-S. (1982). Fine structure of a scyphozoan planula, Cassiopeia xamnchana. Biol. Bull. 163, 320–328. doi: 10.2307/1541269
Martindale, M. Q., Pang, K., and Finnerty, J. R. (2004). Investigating the origins of triploblasty: “mesodermal” gene expression in a diploblastic animal, the sea anemone Nematostella vectensis (phylum, Cnidaria; class, Anthozoa). Development 131, 2463–2474. doi: 10.1242/dev.01119
Matus, D. Q., Pang, K., Daly, M., and Martindale, M. Q. (2007). Expression of Pax gene family members in the anthozoan cnidarian, Nematostella vectensis. Evol. Dev. 9, 25–38. doi: 10.1111/j.1525-142X.2006.00135.x
McClendon, J. F. (1906). On the locomotion of a sea anemone (Metridium marginatum). Biol. Bull. 10, 66–67. doi: 10.2307/1535667
Miglietta, M. P., and Tommasa, D. L. (2000). Approaches to the ethology of hydroids and medusae (Cnidaria, Hydrozoa). Sci. Mar. 64, 63–71. doi: 10.3989/scimar.2000.64s163
Molkentin, J. D., Black, B. L., Martin, J. F., and Olson, E. N. (1995). Cooperative activation of muscle gene expression by MEF2 and myogenic bHLH proteins. Cell 83, 1125–1136. doi: 10.1016/0092-8674(95)90139-6
Momose, T., and Houliston, E. (2007). Two oppositely localised frizzled RNAs as axis determinants in a cnidarian embryo. PLoS Biol. 5:e70. doi: 10.1371/journal.pbio.0050070
Moumen, M., Chiche, A., Cagnet, S., Petit, V., Raymond, K., Faraldo, M. M., et al. (2011). The mammary myoepithelial cell. Int. J. Dev. Biol. 55, 763–771. doi: 10.1387/ijdb.113385mm
Mueller, J. F. (1950). Some observations on the structure of Hydra, with particular reference to the muscular system. Trans. Am. Microsc. Soc. 69, 133. doi: 10.2307/3223402
Müller, P., Seipel, K., Yanze, N., Reber-Muller, S., Streitwolf-Engel, R., Stierwald, M., et al. (2003). Evolutionary aspects of developmentally regulated helix-loop-helix transcription factors in striated muscle of jellyfish. Dev. Biol. 255, 216–229. doi: 10.1016/S0012-1606(02)00091-X
Müller, W. A., Teo, R., and Frank, U. (2004). Totipotent migratory stem cells in a hydroid. Dev. Biol. 275, 215–224. doi: 10.1016/j.ydbio.2004.08.006
Nakanishi, N., Camara, A. C., Yuan, D. C., Gold, D. A., and Jacobs, D. K. (2015). Gene expression data from the moon jelly, Aurelia, provide insights into the evolution of the combinatorial code controlling animal sense organ development. PLoS ONE 10:e0132544. doi: 10.1371/journal.pone.0132544
Nakanishi, N., Yuan, D., and Jacobs, D. K. (2008). Early development, pattern, and reorganization of the planula nervous system in Aurelia (Cnidaria, Scyphozoa). Dev. Genes Evol. 218, 511–524. doi: 10.1007/s00427-008-0239-7
Nawrocki, A. M., Schuchert, P., and Cartwright, P. (2012). A novel mode of colony formation in a hydrozoan through fusion of sexually generated individuals. Curr. Biol. 22, 825–829. doi: 10.1016/j.cub.2012.03.026
Nawroth, J. C., Lee, H., Feinberg, A. W., Ripplinger, C. M., McCain, M. L., Grosberg, A., et al. (2012). A tissue-engineered jellyfish with biomimetic propulsion. Nat. Biotechnol. 30, 792–797. doi: 10.1038/nbt.2269
Nebel, A., and Bosch, T. C. G. (2012). Evolution of human longevity: lessons from Hydra. Aging 4, 730–731. doi: 10.18632/aging.100510
Normandin, D. (1960). Regeneration of Hydra from the endoderm. Science 132, 678. doi: 10.1126/science.132.3428.678
Okubo, N., Hayward, D. C., Forêt, S., and Ball, E. E. (2016). A comparative view of early development in the corals Favia lizardensis, Ctenactis echinata, and Acropora millepora - morphology, transcriptome, and developmental gene expression. BMC Evol. Biol. 16:48. doi: 10.1186/s12862-016-0615-2
Passamaneck, Y. J., and Martindale, M. Q. (2012). Cell proliferation is necessary for the regeneration of oral structures in the anthozoan cnidarian Nematostella vectensis. BMC Dev. Biol. 12:34. doi: 10.1186/1471-213X-12-34
Passano, L. M. (1973). Behavioral control systems in medusae: a comparison between hydro- and scyphomedusae. Pub. Seto Mar. Biol. Lab. 20, 615–645.
Passano, L. M., and McCullough, C. B. (1963). Pacemaker hierarchies controlling the behaviour of hydras. Nature 1999, 1174–1175. doi: 10.1038/1991174a0
Passano, L. M., and McCullough, C. B. (1964). Co-ordinating systems and behaviour in Hydra: I. Pacemaker system of the periodic contractions. J. Exp. Biol. 41, 643–664.
Petersen, C. P., and Reddien, P. W. (2011). Polarized notum activation at wounds inhibits Wnt function to promote planarian head regeneration. Science 332, 852–855. doi: 10.1126/science.1202143
Petersen, O. W., and van Deurs, B. (1989). Distinction between vascular smooth muscle cells and myoepithelial cells in primary monolayer cultures of human breast tissue. In Vitro Cell. Dev. Biol. 25, 259–266. doi: 10.1007/BF02628464
Piraino, S., Zega, G., Di Benedetto, C., Leone, A., Dell'Anna, A., Pennati, R., et al. (2011). Complex neural architecture in the diploblastic larva of Clava multicornis (Hydrozoa, Cnidaria). J. Comp. Neurol. 519, 1931–1951. doi: 10.1002/cne.22614
Plickert, G., Frank, U., and Müller, W. A. (2012). Hydractinia, a pioneering model for stem cell biology and reprogramming somatic cells to pluripotency. Int. J. Dev. Biol. 56, 519–534. doi: 10.1387/ijdb.123502gp
Potthoff, M. J., Arnold, M. A., McAnally, J., Richardson, J. A., Bassel-Duby, R., and Olson, E. N. (2007). Regulation of skeletal muscle sarcomere integrity and postnatal muscle function by Mef2c. Mol. Cell Biol. 27, 8143–8151. doi: 10.1128/MCB.01187-07
Potthoff, M. J., and Olson, E. N. (2007). MEF2: a central regulator of diverse developmental programs. Development 134, 4131–4140. doi: 10.1242/dev.008367
Putnam, N. H., Srivastava, M., Hellsten, U., Dirks, B., Chapman, J., Salamov, A., et al. (2007). Sea anemone genome reveals ancestral eumetazoan gene repertoire and genomic organization. Science 317, 86–94. doi: 10.1126/science.1139158
Quaglia, A. (1981). The muscular system of coelenterates. Ital. J. Zool. 48, 51–56. doi: 10.1080/11250008109438715
Raikova, E. V., Ibragimov, A. Y., and Raikova, O. I. (2007). Muscular system of a peculiar parasitic cnidarian Polypodium hydriforme: a phalloidin fluorescence study. Tissue Cell 39, 79–87. doi: 10.1016/j.tice.2007.01.003
Reber-Müller, S., Spissinger, T., Schuchert, P., Spring, J., and Schmid, V. (1995). An extracellular matrix protein of jellyfish homologous to mammalian fibrillins forms different fibrils depending on the life stage of the animal. Dev. Biol. 169, 662–672. doi: 10.1006/dbio.1995.1177
Reber-Müller, S., Streitwolf-Engel, R., Yanze, N., Schmid, V., Stierwald, M., Erb, M., et al. (2006). BMP2/4 and BMP5-8 in jellyfish development and transdifferentiation. Int. J. Dev. Biol. 50, 377–384. doi: 10.1387/ijdb.052085sr
Reddien, P. W. (2011). Constitutive gene expression and the specification of tissue identity in adult planarian biology. Trends Genet. 27, 277–285. doi: 10.1016/j.tig.2011.04.004
Reitzel, A., Burton, P., Krone, C., and Finnerty, J. (2007). Comparison of developmental trajectories in the starlet sea anemone Nematostella vectensis: embryogenesis, regeneration, and two forms of asexual fission. Invert. Biol. 126, 99–112. doi: 10.1111/j.1744-7410.2007.00081.x
Renfer, E., Amon-Hassenzahl, A., Steinmetz, P. R. H., and Technau, U. (2010). A muscle-specific transgenic reporter line of the sea anemone, Nematostella vectensis. Proc. Natl. Acad. Sci. U.S.A. 107, 104–108. doi: 10.1073/pnas.0909148107
Rentzsch, F., Fritzenwanker, J. H., Scholz, C. B., and Technau, U. (2008). FGF signalling controls formation of the apical sensory organ in the cnidarian Nematostella vectensis. Development 135, 1761–1769. doi: 10.1242/dev.020784
Rentzsch, F., and Technau, U. (2016). Genomics and development of Nematostella vectensis and other anthozoans. Curr. Opin. Genet. Dev. 39, 63–70. doi: 10.1016/j.gde.2016.05.024
Robson, E. A. (1961). The swimming response and its pacemaker system in the anemone Stomphia coccinea. J. Exp. Biol. 38, 685–694.
Robson, E. A. (1963). The nerve-net of a swimming anemone, Stomphia coccinea. J. Cell Sci. s3-104, 535–549.
Robson, E. A. (1966). “Swimming in Actiniaria,” in The Cnidaria and their evolution, ed W. J. Reese (New York, NY: Academic Press), 333–360.
Rodriguez, E., Barbeitos, M. S., Brugler, M. R., Crowley, L. M., Grajales, A., Gusmão, L., et al. (2014). Hidden among sea anemones: the first comprehensive phylogenetic reconstruction of the order Actiniaria (Cnidaria, Anthozoa, Hexacorallia) reveals a novel group of hexacorals. PLoS ONE 9:e96998. doi: 10.1371/journal.pone.0096998
Ross, D. M., and Sutton, L. (1961). The response of the sea anemone Calliactis parasitica to shells of the hermit crab Pagurus bernhardus. Proc. R. Soc. Lond. B. Biol. Sci. 155, 266–281. doi: 10.1098/rspb.1961.0070
Ross, D. M., and Sutton, L. (1967). Swimming sea anemones of Puget Sound: swimming of Actinostola new species in response to Stomphia coccinea. Science 155, 1419–1421. doi: 10.1126/science.155.3768.1419
Röttinger, E., Dahlin, P., and Martindale, M. Q. (2012). A Framework for the Establishment of a cnidarian gene regulatory network for “endomesoderm” specification: the inputs of ß-Catenin/TCF signaling. PLoS Genet. 8:e1003164. doi: 10.1371/journal.pgen.1003164
Ruggieri, R. D., Pierobon, P., and Kass-Simon, G. (2004). Pacemaker activity in hydra is modulated by glycine receptor ligands. Comp. Biochem. Physiol. A Mol. Integr. Physiol. 138, 193–202. doi: 10.1016/j.cbpb.2004.03.015
Ruppert, E. E., Fox, R. S. and Barnes, R. D. (2004). Invertebrate Zoology: A Functional Evolutionary Approach, 7th Edn. Belmont, CA: Thomson Brooks/Cole.
Ryan, J. F., Burton, P. M., Mazza, M. E., Kwong, G. K., Mullikin, J. C., and Finnerty, J. R. (2006). The cnidarian-bilaterian ancestor possessed at least 56 homeoboxes: evidence from the starlet sea anemone, Nematostella vectensis. Genome Biol. 7:R64. doi: 10.1186/gb-2006-7-7-r64
Ryan, J. F., Mazza, M. E., Pang, K., Matus, D. Q., Baxevanis, A. D., Martindale, M. Q., et al. (2007). Pre-bilaterian origins of the Hox cluster and the Hox code: evidence from the sea anemone, Nematostella vectensis. PLoS ONE 2:e153. doi: 10.1371/journal.pone.0000153
Saina, M., and Technau, U. (2009). Characterization of myostatin/gdf8/11 in the starlet sea anemone Nematostella vectensis. J. Exp. Zool. 312, 780–788. doi: 10.1002/jez.b.21304
Santagata, S., Resh, C., Hejnol, A., Martindale, M. Q., and Passamaneck, Y. J. (2012). Development of the larval anterior neurogenic domains of Terebratalia transversa (Brachiopoda) provides insights into the diversification of larval apical organs and the spiralian nervous system. EvoDevo 3:3. doi: 10.1186/2041-9139-3-3
Satterlie, R. A. (2008). Control of swimming in the hydrozoan jellyfish Aequorea victoria: subumbrellar organization and local inhibition. J. Exp. Biol. 211, 3467–3477. doi: 10.1242/jeb.018952
Satterlie, R. A. (2011). Do jellyfish have central nervous systems? J. Exp. Biol. 214, 1215–1223. doi: 10.1242/jeb.043687
Satterlie, R. A., and Nolen, T. G. (2001). Why do cubomedusae have only four swim pacemakers? J. Exp. Biol. 204, 1413–1419.
Satterlie, R. A., Thomas, K. S., and Gray, G. C. (2005). Muscle organization of the cubozoan jellyfish Tripedalia cystophora Conant 1897. Biol. Bull. 209, 154–163. doi: 10.2307/3593133
Schmid, V. (1974). Structural alterations in cultivated striated muscle cells from anthomedusae (Hydrozoa). A metaplasiaic event. Exp. Cell Res. 86, 193–198. doi: 10.1016/0014-4827(74)90672-7
Schmid, V. (1975). Cell transformation in isolated striated muscle of hydromedusae independent of DNA synthesis. Exp. Cell Res. 94, 401–408. doi: 10.1016/0014-4827(75)90506-6
Schmid, V. (1976). The transformational potential of striated muscle in hydromedusae. Dev. Biol. 49, 508–517. doi: 10.1016/0012-1606(76)90192-5
Schmid, V. (1978). Striated muscle: influence of an acellular layer on the maintenance of muscle differentiation in anthomedusa. Dev. Biol. 64, 48–59. doi: 10.1016/0012-1606(78)90059-3
Schmid, V. (1988). The potential for transdifferentiation and regeneration of isolated striated muscle of medusae in vitro. Cell Differ. 22, 173–182. doi: 10.1016/0045-6039(88)90009-7
Schmid, V. (1992). Transdifferentiation in Medusae. Int. Rev. Cytol. 142, 213–261. doi: 10.1016/S0074-7696(08)62077-X
Schmid, V., and Alder, H. (1984). Isolated, mononucleated, striated muscle can undergo pluripotent transdifferentiation and form a complex regenerate. Cell 38, 801–809. doi: 10.1016/0092-8674(84)90275-7
Schmid, V., Alder, H., Plickert, G., and Weber, C. (1988). Transdifferentiation from striated muscle of medusae in vitro. Cell Differ. 25, 137–146. doi: 10.1016/0922-3371(88)90110-4
Schmid, V., Schmid, B., Schneider, B., Stidwill, R., and Baker, G. (1976). Factors effecting manubrium-regeneration in hydromedusae (Coelenterata). Wilhelm Roux Arch. Entwickl. Mech. Org. 179, 41–56. doi: 10.1007/BF00857639
Schmid, V., and Tardent, P. (1971). The reconstitutional performances of the Leptomedusa Campanularia jonstoni. Mar. Biol. 8, 99–104. doi: 10.1007/BF00350924
Schmid, V., Wydler, M., and Alder, H. (1982). Transdifferentiation and regeneration in vitro. Dev. Biol. 92, 476–488. doi: 10.1016/0012-1606(82)90193-2
Schuchert, P., Müller, S. R., and Schmid, V. (1993). Life stage specific expression of a myosin heavy chain in the hydrozoan Podocoryne carnea. Differentiation 54, 11–18. doi: 10.1111/j.1432-0436.1993.tb00654.x
Seipel, K., and Schmid, V. (2005). Evolution of striated muscle: jellyfish and the origin of triploblasty. Dev. Biol. 282, 14–26. doi: 10.1016/j.ydbio.2005.03.032
Seipel, K., and Schmid, V. (2006). Mesodermal anatomies in cnidarian polyps and medusae. Int. J. Dev. Biol. 50, 589–599. doi: 10.1387/ijdb.062150ks
Seipel, K., Yanze, N., and Schmid, V. (2004). The germ line and somatic stem cell gene Cniwi in the jellyfish Podocoryne carnea. Int. J. Dev. Biol. 48, 1–7. doi: 10.1387/ijdb.15005568
Seybold, A., Salvenmoser, W., and Hobmayer, B. (2016). Sequential development of apical-basal and planar polarities in aggregating epitheliomuscular cells of Hydra. Dev. Biol. 412, 148–159. doi: 10.1016/j.ydbio.2016.02.022
Shackleton, M., Vaillant, F., Simpson, K. J., Stingl, J., Smyth, G. K., Asselin-Labat, M.-L., et al. (2006). Generation of a functional mammary gland from a single stem cell. Nature 439, 84–88. doi: 10.1038/nature04372
Shimizu, H., Koizumi, O., and Fujisawa, T. (2004). Three digestive movements in Hydra regulated by the diffuse nerve net in the body column. J. Comp. Physiol. A Neuroethol. Sens. Neural. Behav. Physiol. 190, 623–630. doi: 10.1007/s00359-004-0518-3
Shimizu, H., Sawada, Y., and Sugiyama, T. (1993). Minimum tissue size required for Hydra regeneration. Dev. Biol. 155, 287–296. doi: 10.1006/dbio.1993.1028
Shinzato, C., Shoguchi, E., Kawashima, T., Hamada, M., Hisata, K., Tanaka, M., et al. (2011). Using the Acropora digitifera genome to understand coral responses to environmental change. Nature 476, 320–323. doi: 10.1038/nature10249
Shpirer, E., Chang, E. S., Diamant, A., Rubinstein, N., Cartwright, P., and Huchon, D. (2014). Diversity and evolution of myxozoan minicollagens and nematogalectins. BMC Evol. Biol. 14:205. doi: 10.1186/s12862-014-0205-0
Silva, C. A. B., Martinez, E. F., Demasi, A. P. D., Altemani, A., da Silveira Bossonaro, J. P., Araújo, N. S., et al. (2015). Cellular senescence and autophagy of myoepithelial cells are involved in the progression of in situ areas of carcinoma ex-pleomorphic adenoma to invasive carcinoma. An in vitro model. J. Cell Commun. Signal. 9, 255–265. doi: 10.1007/s12079-015-0291-9
Simionato, E., Ledent, V., Richards, G., Thomas-Chollier, M., Kerner, P., Coornaert, D., et al. (2007). Origin and diversification of the basic helix-loop-helix gene family in metazoans: insights from comparative genomics. BMC Evol. Biol. 7:33. doi: 10.1186/1471-2148-7-33
Singla, C. L. (1978). Locomotion and neuromuscular system of Aglantha digitale. Cell Tissue Res. 188, 317–327. doi: 10.1007/BF00222640
Sinigaglia, C., Busengdal, H., Leclère, L., Technau, U., and Rentzsch, F. (2013). The bilaterian head patterning gene six3/6 controls aboral domain development in a cnidarian. PLoS Biol. 11:e1001488. doi: 10.1371/journal.pbio.1001488
Sleeman, K. E., Kendrick, H., Robertson, D., Isacke, C. M., Ashworth, A., and Smalley, M. J. (2007). Dissociation of estrogen receptor expression and in vivo stem cell activity in the mammary gland. J. Cell Biol. 176, 19–26. doi: 10.1083/jcb.200604065
Sopel, M. (2010). The myoepithelial cell: its role in normal mammary glands and breast cancer. Folia Morphol. 69, 1–14.
Spicer, D. B., Rhee, J., Cheung, W. L., and Lassar, A. B. (1996). Inhibition of myogenic bHLH and MEF2 transcription factors by the bHLH protein Twist. Science 272, 1476–1480. doi: 10.1126/science.272.5267.1476
Spring, J., Yanze, N., Jösch, C., Middel, A. M., Winninger, B., and Schmid, V. (2002). Conservation of Brachyury, Mef2, and Snail in the myogenic lineage of jellyfish: a connection to the mesoderm of Bilateria. Dev. Biol. 244, 372–384. doi: 10.1006/dbio.2002.0616
Spring, J., Yanze, N., Middel, A. M., Stierwald, M., Gröger, H., and Schmid, V. (2000). The mesoderm specification factor twist in the life cycle of jellyfish. Dev. Biol. 228, 363–375. doi: 10.1006/dbio.2000.9956
Steinmetz, P. R. H., Kraus, J. E. M., Larroux, C., Hammel, J. U., Amon-Hassenzahl, A., Houliston, E., et al. (2012). Independent evolution of striated muscles in cnidarians and bilaterians. Nature 487, 231–234. doi: 10.1038/nature11180
Stierwald, M., Yanze, N., Bamert, R. P., Kammermeier, L., and Schmid, V. (2004). The Sine oculis/Six class family of homeobox genes in jellyfish with and without eyes: development and eye regeneration. Dev. Biol. 274, 70–81. doi: 10.1016/j.ydbio.2004.06.018
Stingl, J., Eirew, P., Ricketson, I., Shackleton, M., Vaillant, F., Choi, D., et al. (2006). Purification and unique properties of mammary epithelial stem cells. Nature 439, 993–997. doi: 10.1038/nature04496
Stöckl, A. L., Petie, R., and Nilsson, D.-E. (2011). Setting the pace: new insights into central pattern generator interactions in box jellyfish swimming. PLoS ONE 6:e27201. doi: 10.1371/journal.pone.0027201
Susic-Jung, L., Hornbruch-Freitag, C., Kuckwa, J., Rexer, K.-H., Lammel, U., and Renkawitz-Pohl, R. (2012). Multinucleated smooth muscles and mononucleated as well as multinucleated striated muscles develop during establishment of the male reproductive organs of Drosophila melanogaster. Dev. Biol. 370, 86–97. doi: 10.1016/j.ydbio.2012.07.022
Swain, T. D., Schellinger, J. L., Strimaitis, A. M., and Reuter, K. E. (2015). Evolution of anthozoan polyp retraction mechanisms: convergent functional morphology and evolutionary allometry of the marginal musculature in order Zoanthidea (Cnidaria: Anthozoa: Hexacorallia). BMC Evol. Biol. 15:123. doi: 10.1186/s12862-015-0406-1
Takaku, Y., Hwang, J. S., Wolf, A., Böttger, A., Shimizu, H., David, C. N., et al. (2014). Innexin gap junctions in nerve cells coordinate spontaneous contractile behavior in Hydra polyps. Sci. Rep. 4:3573. doi: 10.1038/srep03573
Tamgadge, S., Tamgadge, A., and Satheesan, E. (2013). Myoepithelial cell–A morphologic diversity–A review. Res. Rev. J. Dent. 4, 5–13.
Technau, U., Laue, von, C. C., Rentzsch, F., Luft, S., Hobmayer, B., Bode, H. R., et al. (2000). Parameters of self-organization in Hydra aggregates. Proc. Natl. Acad. Sci. U.S.A. 97, 12127–12131. doi: 10.1073/pnas.97.22.12127
Technau, U., and Steele, R. E. (2011). Evolutionary crossroads in developmental biology: cnidaria. Development 138, 1447–1458. doi: 10.1242/dev.048959
Tiffon, J. (1987). “Ordre des Cérianthaires” in Traité de Zoologie: Cnidaires, Ctenaires, Vol. III Fascicule 3, ed P. P. Grassé (Paris: Masson), 211–256.
Tiozzo, S., and Copley, R. R. (2015). Reconsidering regeneration in metazoans: an evo-devo approach. Front. Ecol. Evol. 3:67. doi: 10.3389/fevo.2015.00067
Trembley, A. (1744). Mémoires pour servir à l'histoire d‘un genre de polypes d'eau douce, à bras en forme de cornes. Leiden: Chen Jean and Herman Verbeek.
Trevino, M., Stefanik, D. J., Rodriguez, R., Harmon, S., and Burton, P. M. (2011). Induction of canonical Wnt signaling by alsterpaullone is sufficient for oral tissue fate during regeneration and embryogenesis in Nematostella vectensis. Dev. Dyn. 240, 2673–2679. doi: 10.1002/dvdy.22774
Van Praët, M., Doumenc, D., and Pax, F. (1987). “Ordre des Antipathaires” in Traité de Zoologie: Cnidaires, Ctenaires, vol. III Fascicule 3, ed P. P. Grassé (Paris: Masson), 189–210.
Van Wolfswinkel, J. C. (2014). Piwi and potency: PIWI proteins in animal stem cells and regeneration. Integr. Comp. Biol. 54, 700–713. doi: 10.1093/icb/icu084
Vicente-Manzanares, M., Ma, X., Adelstein, R. S., and Horwitz, A. R. (2009). Non-muscle myosin II takes centre stage in cell adhesion and migration. Nat. Rev. Mol. Cell. Biol. 10, 778–790. doi: 10.1038/nrm2786
Wang, D., Chang, P. S., Wang, Z., Sutherland, L., Richardson, J. A., Small, E., et al. (2001). Activation of cardiac gene expression by myocardin, a transcriptional cofactor for serum response factor. Cell 105, 851–862. doi: 10.1016/S0092-8674(01)00404-4
Wang, Z., Wang, D.-Z., Pipes, G. C. T., and Olson, E. N. (2003). Myocardin is a master regulator of smooth muscle gene expression. Proc. Natl. Acad. Sci. U.S.A. 100, 7129–7134. doi: 10.1073/pnas.1232341100
Weber, C., Alder, H., and Schmid, V. (1987). In vitro transdifferentiation of striated muscle to smooth muscle cells of a medusa. Cell Differ. 20, 103–115. doi: 10.1016/0045-6039(87)90424-6
Wenger, Y., Buzgariu, W., and Galliot, B. (2016). Loss of neurogenesis in Hydra leads to compensatory regulation of neurogenic and neurotransmission genes in epithelial cells. Philos. Trans. R. Soc. Lond. B Biol. Sci. 371:20150040. doi: 10.1098/rstb.2015.0040
Werner, B., Chapman, D. M., and Cutress, C. E. (1976). Muscular and nervous systems of the cubopolyp (Cnidaria). Experientia 32, 1047–1049. doi: 10.1007/BF01933964
West, D. L. (1978). The epitheliomuscular cell of Hydra: Its fine structure, three-dimensional architecture and relation to morphogenesis. Tissue Cell 10, 629–646. doi: 10.1016/0040-8166(78)90051-4
Westfall, J. A. (1973). Ultrastructural evidence for neuromuscular systems in coelenterates. Integr. Comp. Biol. 13, 237–246. doi: 10.1093/icb/13.2.237
Westfall, J. A., Yamataka, S., and Enos, P. D. (1971). Ultrastructural evidence of polarized synapses in the nerve net of Hydra. J. Cell Biol. 51, 318–323.
Westlake, H. E., and Page, L. R. (2016). Muscle and nerve net organization in stalked jellyfish (Medusozoa: Staurozoa). J. Morphol. 278, 29–49. doi: 10.1002/jmor.20617
Widersten, B. (1966). On the development of septal muscles, supporting fibrils and periderm in the scyphistoma of semaeostome Scyphozoa. Arkiv. Zool. 18, 567–575.
Williams, R. B. (2003). Locomotory behaviour and functional morphology of Nemalostella vectensis (Anthozoa: Actiniaria: Edwardsiidae): a contribution to a comparative study of burrowing behaviour in athenarian sea anemones. Zool. Verhandel. 345, 437–484.
Witchley, J. N., Mayer, M., Wagner, D. E., Owen, J. H., and Reddien, P. W. (2013). Muscle cells provide instructions for planarian regeneration. Cell Rep. 4, 633–641. doi: 10.1016/j.celrep.2013.07.022
Wittlieb, J., Khalturin, K., Lohmann, J. U., Anton-Erxleben, F., and Bosch, T. C. G. (2006). Transgenic Hydra allow in vivo tracking of individual stem cells during morphogenesis. Proc. Natl. Acad. Sci. U.S.A. 103, 6208–6211. doi: 10.1073/pnas.0510163103
Yanze, N., Gröger, H., Müller, P., and Schmid, V. (1999). Reversible inactivation of cell-type-specific regulatory and structural genes in migrating isolated striated muscle cells of jellyfish. Dev. Biol. 213, 194–201. doi: 10.1006/dbio.1999.9347
Yentsch, C. S., and Pierce, D. C. (1955). “Swimming” anemone from Puget Sound. Science 122, 1231–1233. doi: 10.1126/science.122.3182.1231
Zapata, F., Goetz, F. E., Smith, S. A., Howison, M., Siebert, S., Church, S. H., et al. (2015). Phylogenomic analyses support traditional relationships within cnidaria. PLoS ONE 10:e0139068. doi: 10.1371/journal.pone.0139068
Keywords: cnidaria, muscle, myoepithelial cells, development, regeneration, evolution, epitheliomuscular cells
Citation: Leclère L and Röttinger E (2017) Diversity of Cnidarian Muscles: Function, Anatomy, Development and Regeneration. Front. Cell Dev. Biol. 4:157. doi: 10.3389/fcell.2016.00157
Received: 21 November 2016; Accepted: 30 December 2016;
Published: 23 January 2017.
Edited by:
Stefano Biressi, University of Trento, ItalyReviewed by:
Maja Adamska, Australian National University, AustraliaPatrick Steinmetz, University of Bergen, Norway
Copyright © 2017 Leclère and Röttinger. This is an open-access article distributed under the terms of the Creative Commons Attribution License (CC BY). The use, distribution or reproduction in other forums is permitted, provided the original author(s) or licensor are credited and that the original publication in this journal is cited, in accordance with accepted academic practice. No use, distribution or reproduction is permitted which does not comply with these terms.
*Correspondence: Lucas Leclère, bHVjYXMubGVjbGVyZUBvYnMtdmxmci5mcg==
Eric Röttinger, ZXJpYy5yb3R0aW5nZXJAdW5pY2UuZnI=