- 1Stem Cell Research Laboratory, Department of Biotechnology, Delhi Technological University, Delhi, India
- 2Department of Chemistry, University of Delhi, Delhi, India
Stem cells are defined by their capabilities to self-renew and give rise to various types of differentiated cells depending on their potency. They are classified as pluripotent, multipotent, and unipotent as demonstrated through their potential to generate the variety of cell lineages. While pluripotent stem cells may give rise to all types of cells in an organism, Multipotent and Unipotent stem cells remain restricted to the particular tissue or lineages. The potency of these stem cells can be defined by using a number of functional assays along with the evaluation of various molecular markers. These molecular markers include diagnosis of transcriptional, epigenetic, and metabolic states of stem cells. Many reports are defining the particular set of different functional assays, and molecular marker used to demonstrate the developmental states and functional capacities of stem cells. The careful evaluation of all these methods could help in generating standard identifying procedures/markers for them.
Introduction
Stem cells are unique in their capabilities to either self-renew or differentiate into various cell lineages. The most primitive stem cells have the potential to generate all the cell types in any organism and termed as pluripotent stem cells (PSCs) (Hanna J. H. et al., 2010). Whereas, multipotent and unipotent stem cells are defined to bear limited self-renewing capacity and these cells, differentiate into a particular tissue type or cell lineage. It is important to notice that cells derived from fertilized egg (zygote/blastomeres) have the potential to generate all the embryonic and extra-embryonic cells; potential referred to as totipotency and thus can give rise to the whole organism, however their developmental potential remains undefined in vitro (Kelly, 1977). Both human and mouse derived Embryonic Stem Cells (ESCs) are demonstrated to generate all types of cells, but lack potential to contribute to the extra-embryonic cells such as placenta. These cells are termed as PSCs and have various functional properties depending on their culture conditions. Another important class of stem cells is lineage specific multipotent stem cells [e.g., Hematopoietic Stem Cells (HSCs)] which have limited differentiation potential and develop only in their tissue/cell types. The multipotent stem cells do not differentiate into cell types of different tissue origin under normal physiological circumstances. The developmental potential of unipotent stem cells is further restricted and they remain able to give rise to only a single cell type (for example, blast forming unit-erythroid (BFU-E) may be differentiated into erythrocytes).
Thus, the traditional developmental dogma follows the differentiation of totipotent stem cells to PSCs, PSCs to multipotent stem cells, multipotent stem cells to unipotent stem cells and finally mature cells. Both the self-renewal capacity and differential potential are reduced during their journey from totipotent to mature cell state. However, the discovery of nuclear reprogramming methods such as somatic cell nuclear transfer method and use of transcriptional factors to induce pluripotency in any cell type are demonstrated as powerful tools to reverse this hierarchy (Gurdon, 1962; Kato and Tsunoda, 1993; Campbell et al., 1995, 1996; Wilmut et al., 1997; Kato et al., 1998; Wakayama et al., 1998; Wakayama and Yanagimachi, 1999; Takahashi and Yamanaka, 2006; Takahashi et al., 2007). These findings show that the particular state of a somatic cell can be reprogrammed to achieve a totipotent or pluripotent state. iPSC generated from patients have great potential in disease modeling and regenerative medicine (reviewed by Singh et al., 2015). It is clear that defining various fundamental levels of pluripotency states (e.g., naïve vs. prime etc.) remain central in developing various strategies for their clinical/research uses and therefore it is important to rigorously evaluate the different methods/molecular markers etc. reported so far for the various PSCs types.
A comprehensive review of all the functional assays defining the pluripotent states of stem cells would be of great importance to assess the functional applications and reprogramming efficiency of different methods and cell sources that are being explored both in clinical and research settings. Recently, many researchers have developed few alternative approaches such as in silico analysis to detect pluripotency or differentiation potential of any existing or new cell for clinical and research purposes (Sato et al., 2003; Sperger et al., 2003; Bhattacharya et al., 2004; Suárez-Farinas et al., 2005; Müller et al., 2008). It would be of great importance to have more concrete definitions and defining markers to demonstrate the significance of these in silico approaches and decide the clinical utility of the particular cell population that is to be used. Present article focuses on the various molecular markers and diagnostic strategies being used to define the exact state of any given cellular population that is assumed to be pluripotent or multipotent and may be used further in any relevant clinical/research regime. As discussed in the later sections, there are many molecular markers (including TFs e.g., OCT4, SOX4, NANOG etc.; micro RNAs, Transcriptional regulators and epigenetic chromosomal modifiers, etc.; discussed in detail in later sections) that are promptly used for a quick evaluation of cellular potency by most researchers/clinicians. Although, the complexity associated with the definition of the actual state of pluripotency (e.g., ground state, naïve, and prime states of pluripotency etc.) and the incapability of individual “pluripotency-defining molecular markers” which often remain doubtful, has raised the demand for identification of more conspicuous definitions and diagnostic tools. Since then, functional assays have remained the gold test for defining pluripotency of all types of stem cells in vertebrates. There are a variety of functional assays which differ both in the time and effort taken to perform them and the accuracy of the results obtained from these assays. The different studies done in mice, human, and other mammals have been useful to define the functional assay in different categories.
Pluripotent Stem Cells: The Definition
The standard definition of PSCs relies on the fact that they can produce all three germ cell layer cells and thus can give rise to almost all types of cells in the body (Table 1; Hanna J. H. et al., 2010). However, PSCs seem to lack capabilities of producing extra embryonic cells and thus may not contribute to the development of placental cells. These PSCs is therefore different from Totipotent stem cells (capable of giving rise to extra-embryonic tissue also) since they may not be developed into a complete organism. Another important feature for defining pluripotency has been their capabilities to self-renew themselves. However, recent studies revealed that PSCs can be maintained in self-renewing states in vitro indefinitely through a number of growth factors/exogenous signals indicating that the self renewing capacity may not be the defining hallmark of pluripotency (Nichols and Smith, 2012). Studies done by using various types of pluripotent cells indicated that pluripotency is more accurately defined as a transient and dynamic state in vitro and different types of PSCs can be derived from different stages of developing embryos in vertebrates. The pluripotency of these cells is reflected through the expression of TFs, assessment of genomic integrity, and their capability to differentiate robustly into all 3 cell lineages.
There are multiple types of PSCs (including rodents, humans and primates) that are typically classified on the basis of their origin from a specific tissue (Figure 1) such as various germline tumors, blastomeres, and inner cell mass of developing embryos (Box-1). Somatic cell reprogramming techniques gave alternative tools for generating PSCs both in mouse and humans. Somatic cells have been reported to become ESCs-like by Nuclear Transfer resulting into NT-ESCs (Wakayama et al., 2001; Tachibana et al., 2013; Yamada et al., 2014). Similarly, PSCs can be derived by inducing reprogramming through ectopic expression of a number of different TFs (Takahashi and Yamanaka, 2006). The ectopic expression of basal reprogramming factors (OCT4, KLF4, SOX2, and c-MYC) in the mouse fibroblasts led to their reprogramming into pluripotent cells, which were called as induced pluripotent stem cells (iPSCs; Takahashi and Yamanaka, 2006). The generation of these iPSCs came out as breakthrough as it gave a way of generating PSCs from somatic cells without having any requirement of ESCs which have associated ethical concerns. Since then, researchers all over the world have used this technique to reprogramme different somatic cell types into iPSCs (Yu et al., 2007). Many groups have also reported different approaches to reprogramming, which include the exclusion or replacement of one or more of the basal 4 reprogramming factors, along with the inclusion of different small molecules that have been reported to increase the reprogramming efficiency (Feng et al., 2009). IPSCs pose to have many applications in the stem cell therapies, drug toxicity assays, disease modeling, gene therapy, and hence they hold great importance (Singh et al., 2015).
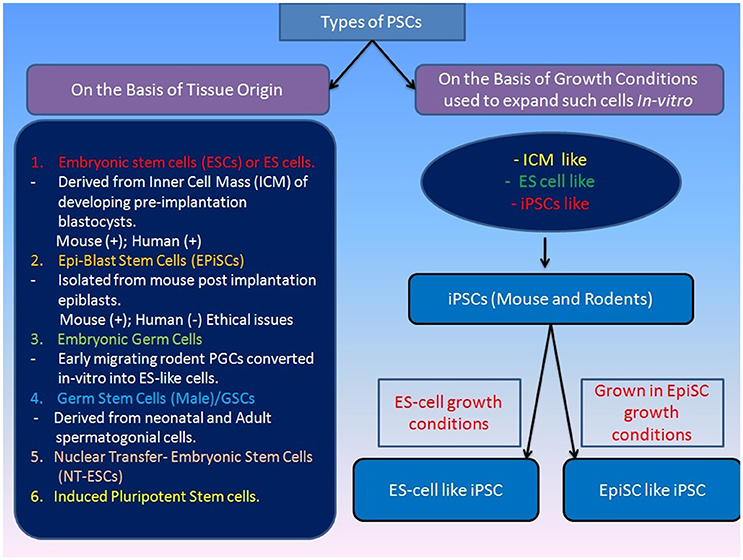
Figure 1. Classification of various types of pluripotenct stem cells on the basis of their origin. Various types of pluripotent stem cells can be classified on the basis of their origin or source from where they are taken and this would directly define their self-renewing and differentiation potential. Pluripotency of stem cells is also regulated through their culture microenvironment and they are often named on the basis of their similarity to the various types of previously established PSCs e.g., ICM-like or ES-like PSCs. [Mouse (+), Human (+) indicates derivation of ESCs inthese mammals; Human(−) indicates non-derivation of post-implantation EPIsc in humans].
Box 1. Multiple Types of PSCs in Mouse and Humans
Mouse pluripotent stem cells
Initially, Stevens and his colleagues demonstrated the formation of teratocarcinoma cells by using strain 129 mice model and thus in vitro methods for the propagation of ESCs derived from similar mice model studies was established (Stevens, 1958). Subsequently, methods for the propagation of immortalized non-transformed embryonic stem cell lines were demonstrated by growing cells derived from murine blastocysts preconditioned media with preformed teratocarcinoma stem cell line (Evans and Kaufman, 1981; Martin, 1981). This method of obtaining ESCs was followed by some researchers for the isolation of pluripotent cells lines from various types of sources (Thomson et al., 1995). Initial studies suggested that mouse ESCs could only be obtained from the embryo before implantation in the uterus. However, later reports established that rodent ESCs can be derived from the post-implantation embryo (epiblast tissue) that generates the embryo proper (Brons et al., 2007; Tesar et al., 2007). These cells are referred as EpiSCs (post-implantation epiblast-derived stem cells), and can be derived by culturing cells in chemically defined media along with other supplements (Brons et al., 2007; Tesar et al., 2007). For ethical reason similar EpiSCs are not attempted in humans so far.
Early migrating mouse PGCs are shown to have pluripotent ESC-like properties when grown in vitro under specific conditions and termed as embryonic germ cells (Matsui et al., 1992; Leitch et al., 2010). The mouse PGCs were grown up to 20 passages in the presence of “Steel Factor” (membrane-associated SF) and “Leukemia Inhibitory Factor” (LIF) and bFGF supporting their continued proliferation. The cells exhibit pluripotency through the generation of embryoid bodies and multiple differentiated cell phenotypes in monolayer culture and tumors in nude mice, and can also contribute to chimeras when injected into host blastocysts (Matsui et al., 1992; Leitch et al., 2010).
The successful establishment of ES-like cells has been reported from neonatal mouse testis exhibiting phenotypic similarities to ESCs/EGCs except in their genomic imprinting pattern (Kanatsu-Shinohara et al., 2004). In similar studies, adult unipotent germline stem cells (GSCs) was reported to be capable of differentiating into multiple cell types both by in vivo and in vitro assays, including germ cell contribution and transmission (Ko et al., 2009). There have been efforts to define marker profiling of ESCs and it is shown that ESCs are distinct from early inner cell mass (ICM) and closely resemble preimplantation epiblast (Boroviak et al., 2014).
Many studies have revealed manifestation of distinct features of various types of PSCs attributed to their origin and maintenance conditions (Figure 1). Depending on their origin, they are coined with different terms such as ESCs (established from pre-implanted embryos) and Epiblast stem cells or EpiSCs (generated from later embryonic epiblast stages; Figure 1; Brons et al., 2007; Tesar et al., 2007). They are also often termed as “naïve” (for ESCs) and “primed” (for EpiSCs) pluripotent stem cells to highlight their early and late phases of development (Nichols and Smith, 2009). The mouse “naïve” and “primed” ESCs differ in their capacity to form high-grade chimeras with naïve ESCs being able to form chimeras but primed ESC remain incapable. However, the difference in the chimera forming capabilities of naïve and primed PSCs could be attributed to the use of suboptimal regimes for chimera generation experiments and development of more defined protocols by addition of some regulatory factors (e.g., FGF-4) may improve the chimera forming efficiency of even primed PSCs in mouse at least (Joo et al., 2014). The conventional human PSCs (hESCs) look similar to EpiSC by their molecular markers thus called as “primed” ESCs. However, “naïve” pluripotency may not be evaluated in hESCs due to ethical restriction on the formation of human chimeras. Nevertheless, existence of a naïve state of pluripotency in hESCs has been reported recently, and it is believed that the pluripotency of hESCs also can be enhanced by genetic modification or optimized culture systems (Buecker et al., 2010; Hanna J. et al., 2010; Lengner et al., 2010; Xu et al., 2010; Gafni et al., 2013). The nonhuman primates ESCs have been assessed for naïve pluripotency showing their inability to form a chimera with pre-implantation embryos (Tachibana et al., 2012).
Evaluation of Pluripotency Through Molecular Marker Profiling and Functional Assays
Development of Various Molecular Diagnostics for PSCs
The pluripotency of cells is mainly governed by a number of molecular mechanisms which regulate the expression of genes responsible for the maintenance of primitive stages and repression of differentiation. There are a number of key molecules which sustain the self-renewal, but inhibit the genes for differentiation or keep them silent till external stimuli inducing differentiation activates them. Few important TFs are playing the crucial role in the maintenance of pluripotency, and it is their expression level which defines the pluripotency in various types of ESCs/EpiSCs (Figure 2).
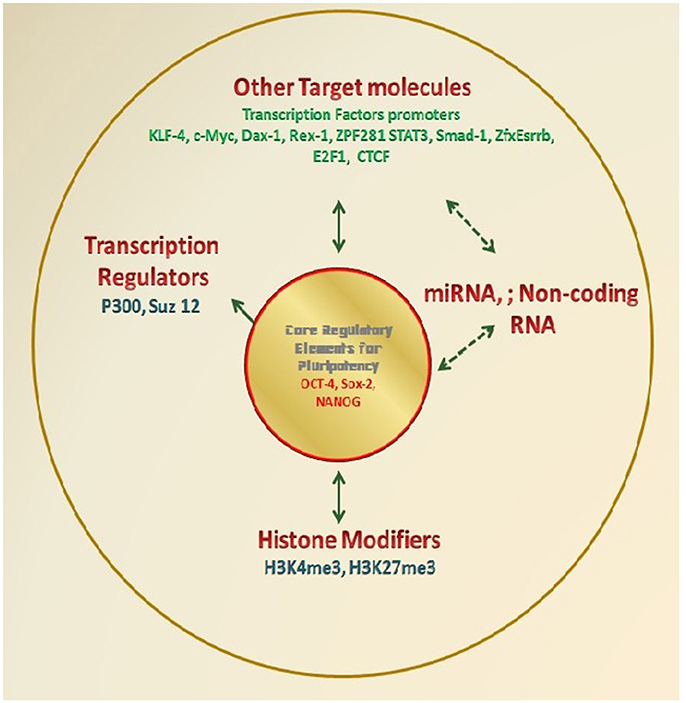
Figure 2. Molecular diagnostics for determination of Pluripotency. Various research groups have demonstrated the molecular markers especially TFs which essentially regulate the self-renewal and differentiation potential of PSCs. These factors either directly regulate renewal/ differentiation through their binding to the target gene and therefore regulating target gene expression; or they themselves get regulated and are the target for a number of other transcription regulating elements such as miTNA, Noncoding RNA, Histone modifiers. Various researchers have wisely used these factors as the marker to define the potential of any test cells as an alternate approach to the standard functional assays.
For example, the basal four reprogramming factors OCT4, SOX2, KLF4, and c-MYC (OSKM) were found to be able to induce somatic cells reprogramming and are often correlated with the pluripotency state of any cell population. All of these factors have their own important role in the generation and regulation of pluripotency in the PSCs. For example, OCT4 is the most important reprogramming factor of these four. Loss of OCT4 in embryos lacking Smad2 results into premature differentiation of epiblast which supports the role of OCT4 as an exclusive pluripotency marker and further suggests its crucial role in the development of pluripotency in ICM and its maintenance in ESCs (Waldrip et al., 1998). Similarly, SOX2 is important for various development processes such as maintenance of “stemness” in neural progenitor cells and its removal lead to their differentiation (Graham et al., 2003). The main role of SOX2 is the regulation of pluripotency and the determination of cell fate. KLF4 is a mediator of LIF-Stat3 signals and it helps OCT4 and SOX2 in the regulation of NANOG by binding to the promoter of NANOG which also plays a crucial role in the maintenance of pluripotency (Zhang et al., 2010). c-MYC has been responsible for the promotion of active chromatin environment, and enhancement of cell proliferation. It also has an important role in the enhancement of the transition that occurs from the initiation to elongation of the transcription. It has been found to be having an important role in the early stages of the induction as evidenced from the enhanced generation of partially reprogrammed cells, which have not yet turned on the genes for OCT4, SOX2, and KLF4 (Schmidt and Plath, 2012).
Further, it has been reported that some molecular markers such as stage specific embryonic antigen-1 (SSEA1) and alkaline phosphatase get induced in very early stages of reprogramming. Few of the SSEA1+ cell give rise to reprogrammed cells through the activation of OCT4, SOX2, and NANOG (Ho et al., 2011). Many reports demonstrate their particular expression profiles and genetic screening assays to define their essential roles in maintaining pluripotency in both mice and humans (Schöler et al., 1990; Nichols et al., 1998; Avilion et al., 2003; Chambers et al., 2003, 2007; Mitsui et al., 2003; Boyer et al., 2005; Loh et al., 2006; Takahashi and Yamanaka, 2006; Masui et al., 2007; Wang et al., 2012).
OCT4 interacts with other TFs to activate and repress gene expression in mouse ESCs (Pesce and Schöler, 2001). For example, OCT4 can heterodimerize with the SOX2 (HMG-box transcription factor) to regulate gene expression level of many genes in mouse ESCs (Yuan et al., 1995; Botquin et al., 1998; Nishimoto et al., 1999).
However, the role of NANOG in the induction and maintenance of pluripotency is not very clear. It may not be essential in mouse PSCs (Chambers et al., 2007). The low-level expression of NANOG (or even absent) in mouse EpiSCs indicates that it may not be the essential part of the core regulatory circuitry (Figure 2). However, it is reported to stabilize PSCs and required for the development of pluripotency in ICM in vivo (Silva et al., 2009). Its co-localization with OCT4/SOX2 indicates its potential role in the maintenance of pluripotency in both mice and humans. Reports show that the major role is owned by OCT4 in the maintenance and induction of pluripotency while under exceptional circumstances SOX2 and NANOG may either be replaced by other substitutes or removed without losing the pluripotency in PSCs.
Genome-scale location analysis for the identification of OCT4, SOX2, and NANOG (OSN) target genes revealed that they co-occupy a substantial portion of their target genes. Most common target genes encode for the transcriptional factors, and many of them are developmentally important homeodomain proteins (Boyer et al., 2005; Loh et al., 2006). These studies suggested a regulatory model for the maintenance of self-renewal potential and repressing differentiation by cooperative binding of OSN to their promoters and thus auto-regulates their function (Boyer et al., 2005; Loh et al., 2006).
Studies in mouse ESCs to understand regulatory network maintaining pluripotency defined target promoter for a number of TFs including OCT4, SOX2, NANOG (and others, e.g., KLF4, c-MYC, Dax1, Rex1, Zpf281, and Nac1). These results indicated two categories of promoters for the target genes on the basis of the number of TFs bound to them (TFs no <4 indicates inactive or repressed promoter; TFs no. >4 indicates promoter active in the pluripotent state but gets repressed during differentiation). Similar studies have been carried out by using chromatin immunoprecipitation coupled with ultra-high-throughput DNA sequencing (ChIP-seq) for identifying and mapping the locations of some important TFs (including STAT3, NANOG, OCT4, Smad1, SOX2, KLF4, Esrrb, Tcfcp2l1, Zfx, c-MYC, n-MYC, E2f1, and CTCF). Also, identification of 2 transcription regulators p300 and Suz12 also revealed activation of a substantial fraction of protein-coding, miRNA and non-coding RNA genes in ESCs. At the same time, OSN interacts with the promoters of the genes encoding lineage-specific regulators (Chen et al., 2008; Kim et al., 2008).
Parallel studies established histone modification signatures as crucial regulators of the gene expression in mammals. This is further supported by specific roles defined for the histone three lysine 4 and histone three lysine 27 trimethylations (H3K4me3 and H3K27me3, respectively) role in controlling gene regulation in ESCs (Lee et al., 2004; Boyer et al., 2005; Bernstein et al., 2006; Pan et al., 2007). The OSN are defined to bind promoters of many lineages regulatory genes, and thus, both active (H3K4me3) and repressive (H3K27me3) histone methyltransferase activities (a bivalent state) possessed by ESCs is believed to facilitate activation of developmental genes upon exit from pluripotency (Bernstein et al., 2006). This capability of OSN to activate self-renewal maintenance genes, while repressing lineage-specifying regulators (i.e., Differentiation) is a major indicator for the double hallmark features of ESCs.
PSCs are further classified as naïve or primed PSCs, and different molecular markers can be defined to identify these states among various types of PSCs. A set of diagnostic molecular signatures for mouse PSCs that delineate their proximity to the preimplantation ICM or post-implantation epiblast respectively has been proposed amongst the various pluripotent states. These distinct identification markers include X chromosome status in female cells, global levels of DNA methylation, OCT4 enhancer utilization, and expression levels of specific regulators' group defined as “naïve” TFs (for example a select group of TFs; KLF4, KLF2, Esrrb, Tfcp2l1, Tbx3, and Gbx2; Tesar et al., 2007; Bao et al., 2009; Guo et al., 2009; Silva et al., 2009; Dunn et al., 2014).
The set of above mentioned naïve TFs and Nanog are not expressed (or very low expression level) in primed PSCs. They are also capable of resetting primed PSCs in conjunction with naïve pluripotency culture conditions. This reflects a regulatory intersection between the naïve transcriptional network and epigenetic resetting of both the DNA methylome and X chromosome. Mouse ESCs derived from pre-implantation blastocysts exhibit a ground state which is defined as the configuration where the cells grow without depending on any exogenous signaling stimuli, at the molecular level when cultured along with the LIF and small molecule inhibitors of MEK and Gsk3 kinases (2i/LIF conditions). These conditions are proposed to stabilize the diagnostic signatures of pluripotency in ESCs (Ying et al., 2008; Marks et al., 2012; Leitch et al., 2013).
There are distinct molecular diagnostic markers exhibited by ESCs demonstrating their ground state, including the presence of two active X chromosomes in female cells, low levels of DNA methylation, high activity of OCT4 distal enhancer, and naïve transcription factor expression. On the other hand, use of FGF and ACTIVIN supports primed ESC state. While comparing with the naïve ESCs, primed EpiSC is defined to have inactivated forms of X-chromosome in female cells, a higher degree of DNA methylation, high activity of proximal enhancer elements for OCT4 gene and repression of naïve TFs.
There have been studies showing genome-wide DNA methylation maps for different stages of PSCs, and it is proposed that different molecular changes including DNA methylation be highly dynamic during mammalian embryogenesis. These developmental changes of ground state ESCs to EpiSCs (primed) in vitro also resembles the changes during in vivo maturation of preimplantation epiblast to post-implantation epiblast (Smith et al., 2012; Boroviak et al., 2014).
Furthermore, the fine line existing between naïve and primed states defining markers reveal a heterogeneous distribution of various diagnostic markers among these states. It is evident from the observation that both naïve PS markers (e.g., Chimera formation capability) and post-implantation Epiblast marker (e.g., High DNA methylation pattern) are exhibited by mouse PSCs (Leitch et al., 2013). Similarly, this molecular heterogeneity is observed in EpiSCs which are demonstrated to engraft into posterior epiblast and possess diagnostic markers of post-implantation stage indicating their primed PSC state (Wu et al., 2015). While, region-selective EpiSCs are also reported to harbor high cloning efficiency, indicating their naïve PS like properties. These variable shows the need to redefine the various states and their corresponding diagnostic markers more precisely and that seems to incorporate a greater spectrum of functional and molecular state markers. Similarly, the concept of ground state PSCs appears to be incompletely defined. It is indicated by the existing heterogeneity as demonstrated in gene expression profiles, flow cytometry and replating experiments in single cell studies (Kumar et al., 2014). This heterogeneity demonstrates the coexistence of distinct molecular/functional states in mouse ESCs (Kumar et al., 2014). This inherent metastability of PSCs is further reflected by existing heterogeneity among individual cells. Further, even much homogenous ground state cultures have been reported to bear variable TFs expression profile showing dynamic states of PSCs that is both time and space related (Morgani et al., 2013).
However, detailed information on the origin and consequence of this heterogeneity is yet to be developed and by that time PSC classification shall remain ambiguous due to the dynamic nature of pluripotency defining markers. It is important to be noticed that molecular markers may not strictly define the expected functional development of PSCs. For example, mouse PSCs were demonstrated not to get differentiated (an indication of functional pluripotency) but exhibit all the key molecular features of pluripotency such as expression of core TFs in the absence of DNA methylation and H3K27 methylation activities (Li et al., 1992; Okano et al., 1999; Tsumura et al., 2006; Chamberlain et al., 2008). This indicates that the pluripotency of any cell may only be decided by carefully comparing molecular markers and their capability to perform functionally.
Similar results are not available for the human ESCs which do not grow in the absence of DNA methylation gene DNMT1 and thus shown functional differences from the mouse ESCs (Liao et al., 2015). Further, naïve PSCs are not affected by the epigenetic regulatory factors which play important role in the primed PSC state.
Assessment of Pluripotency by Various Functional Assays
Since the discovery of stem cells various advancements in the study of pluripotency has been evolved from initial embryological experiments to the in vitro generation of an array of pluripotent states. Initial studies were done by using murine ESCs (Evans and Kaufman, 1981) that remain an amenable and convenient platform to study various developmental pathways and identification of the major regulating factors of sustenance of pluripotency. Subsequently, development of methods and identification of different other types of pluripotent cells, such as EG cells, EpiSCs, and IPSCs led to the comprehension of the existing methodologies (Figure 3). In 1998, human ESCs were defined for the first time (Thomson et al., 1998) and a wealth of existing information about mouse pluripotent states served as a reference point in defining human pluripotency. Recently, the advent of iPSCs and more advanced technical resources have provided some diagnostic tools for defining pluripotency as discussed above.
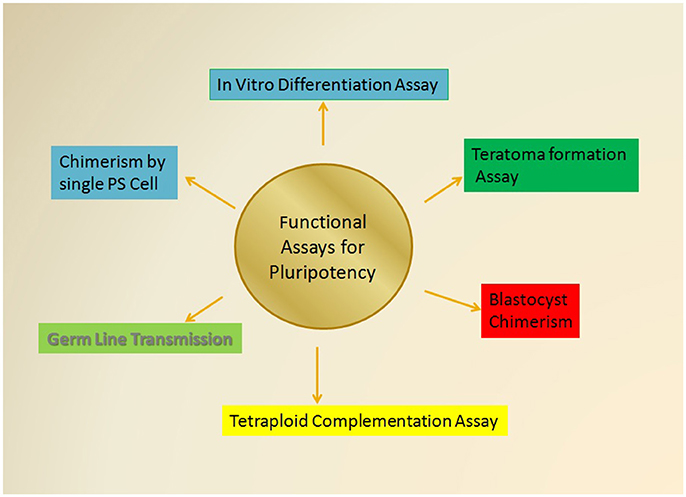
Figure 3. The Various functional assessment approaches for Pluripotency. The most stringent tests for the determination of pluripotent cells that includes the various functional assay that essentially rely on the capabilities of test pluripotent cell to self-renew and differentiate into all the three germ cell lineages including ectoderm, mesoderm, and endoderm as mentioned in the text. Some of these assays would also explore the ability of test cells to give rise the whole organism and give rise to a chimeric animal of desired characteristics.
The study of various types of PSCs and their capability to give different developmental states has led to the accumulation of many functional assays. These functional assays provide stringent quality assessment tools for the evaluation of pluripotency in both human and mice PSCs (Figure 3). These experimental assays are majorly categorized as follows: (i) in vitro differentiation into all three germ layers; (ii) capability to form teratomas; (iii) chimerism of blastocysts; (iv) tetraploid complementation; (v) transmission of germ line; and (vi) chimera formation by using single pluripotent cell.
In vitro Differentiation Assays
Pluripotency is defined as the ability to give rise to cells from all three germ layers of the body, which may be evaluated in vivo (teratoma formation assay), and/or in vitro, by inducing the differentiation of the ESCs into all three germ layers. Initially, it was defined via the demonstration of aggregate formation (embryoid bodies) in a hanging drop suspension culture method (Wobus et al., 1984). Since then, various protocols have been published showing the use of small molecules and growth factors to induce differentiation into all three germ layers (Table 1). However, in vitro differentiation is considered as the least stringent test to establish the pluripotency.
Generally, in vitro differentiation assays are performed by including stem cell culture (e.g., Embryoid bodies) in the media containing a cocktail of differentiation inducing cytokines, morphogens, or chemicals, and after that marker of a specific target, tissues are identified to establish their differentiation (Keller, 1995; Smith, 2001). For example, differentiation of neural tissues (neurospheres) from neural stem cells (Lee et al., 2000; Studer, 2001; Ferrari et al., 2010), cardiac bodies generation from cardiac stem cells and their further differentiation into cardiomyocytes, endothelial cells and smooth muscle cells (Taubenschmid and Weitzer, 2012; Hoebaus et al., 2013) and definitive endodermal differentiation from mouse ESCs (Borowiak et al., 2009; Zhu et al., 2009). Mouse ESCs aggregates are reported to resemble early stages of mouse embryonic differentiation (for 7–8 days) which spontaneously develop into cells of ecto-, meso-, and endodermal origins (Bader et al., 2001). These embryonic bodies are highly useful in studying cellular functions (by electrophysiology) and cell-cell interactions (by using immunofluorescence assays). Whereas, pluripotency assessment in human cells is inherently an intractable problem. As it is described above, pluripotency evaluation in animal (e.g., mouse, rat, primates, etc.) involves their verification through direct means i.e., PSCs are introduced into a developing embryo and their differentiation potential can be directly determined by evaluating the degree of chimerism and/or by determining the organism's viability that is derived from in vitro stem cells (Nagy et al., 1993). This may not be applicable in humans because of ethical reasons which would not allow these types of stringent experimentation in the human system; thus indirect evidence through biomarkers and correlative measures of differentiation potential are the next best level of evidence for human stem cell pluripotency. There are many pluripotency determining markers being used for human cells which are chosen by the existing evidence from other species. Defining pluripotency in human system may be erroneous due to: (1) availability of small number of markers and their use would erroneously validate potentially abnormal stem cells which may also express the similar limited set of markers as in normal stem cells, (2) despite some striking orthologous similarities, the parallel comparison of mechanistic insight from animals to the humans for the determination of pluripotent features is problematic due to species-specific differences in the cultured pluripotent phenotypes. In addition, there are evolutionary structural variations in the transcriptional communicating networks of human and animal cells. There are few techniques such as, in silico genome-wide methods (e.g., whole genome transcriptome profiles in combination with complex biomarker models) which may be useful in defining the ideal phenotype on a global scale (Skreb et al., 1971; Müller et al., 2008, 2011; discussed in a later section).
Teratoma Formation Assays
This test is commonly used for defining the pluripotency in mammals and being used for many decades (Stevens and Little, 1954; Stevens, 1958, 1970; Solter et al., 1970; Skreb et al., 1971; Evans and Kaufman, 1981). Moreover, it is a widely accepted method in stem cell research and banking (ISCBI, 2009; Gertow et al., 2011; Wesselschmidt, 2011). The process can be summarized as the injection/infusion of test cells (expected to be pluripotent) in animal models (mostly mice) into various anatomical sites such as subcutaneous, intramuscular, under the capsule of the kidney, or intra-testicular, of immunocompromised mice and assessment of their potential to develop a tumor. The pluripotency of the test cells is judged on the basis of their ability to develop a tumor with cells exhibiting characteristics of all three germ layers, namely ectoderm (nerve/skin), mesoderm (bone, cartilage/muscle), and endoderm (liver/gut; Brivanlou et al., 2003).
Although, used regularly and frequently, there have been various issues related to the significance of this assay to define pluripotency. The teratoma assay needs to be standardized regarding graft site, the age of animal/mice, a number of cells or graft size and the preparation cell details for various pluripotent cell lines. The formation of teratomas is invariably influenced by all these factors (Brivanlou et al., 2003; Hentze et al., 2009; Wesselschmidt, 2011). Recently, a systematic evaluation of some of these factors for two ESC lines has been proposed (Gropp et al., 2012). Further, the assay is also regarded as time, cost and labor intensive and raises ethical concerns (induce pain and suffering of the animals used) for the utilization of the assay.
Apart from these issues, there are other technical drawbacks such as inefficient histological analysis of teratomas, which may lead to counting even incompletely reprogrammed cells that generate masses with a superficial resemblance to teratomas yet lack terminal three-germ-layer differentiation (Chan et al., 2009). Also, there is a need for lineage tracing or analysis of donor cell-specific marker to distinguish between host and donor cells. This is important since most of the cell preparation uses matrices or scaffold materials which can elicit inflammation or foreign body reaction leading to the misinterpretation of tissue differentiation (RIKEN, 2014).
Chimeras of Blastocysts
The term chimera (originated from Greek Xíμαιρα “she-goat and sometimes named monster,” a fire-breathing creature bearing body parts of three different animals, including a lion, a serpent, and a goat) referred to a single organism generated from the fusion of two (or more) genetically distinct cells from a different origin. For most of the researcher generation of mouse embryonic chimeras works as a well-established tool for determining cell lineage commitment and pluripotency. Various successful reports demonstrating experimental procedures for chimeras' generation by combining two or more preimplantation embryos or, by introducing test cells (ESCs) into a host embryo. This Chimera production technique gained popularity for designing and generating knockout or knock in mice by using genetically modified ESCs.
Initially (the 1960s), experimental mouse chimera were generated by aggregating two or more whole 8-cell embryos that give rise to normal-sized mice consisting tissues with cells from both the parental embryos (Tarkowski, 1961; Mintz, 1962). Mouse chimera production was also reported by injecting isolated ICM cells into a host blastocyst cavity (Tarkowski, 1961; Gardner and Munro, 1974; Bradley et al., 1984). Later on, ESCs PGCs (Matsui et al., 1992) generated by somatic cell nuclear transfer ESCs (ntESCs) (Wakayama et al., 2001) and iPSCs (Okita et al., 2007) were also demonstrated to produce mice chimeras. Further, mouse chimeras are also reported by using tetraploid host embryos.
Apart from these conventional methods (e.g., aggregation of embryos or embryonic cells derived from the same developmental stage), mixing of embryonic cells from different developmental stages can also result in the chimeric offspring (Gearhart and Oster-Granite, 1981; Nagashima et al., 2004). For example, isolated ICMs are shown to produce chimeras when injected into the blastocoelic cavity of host blastocysts, and at the same time when introduced in 8-cell or morula stage embryos (Butler et al., 1987; Polzin et al., 1987; Roth et al., 1989; Nagy et al., 1990; Picard et al., 1990).
In order to identify the contribution of the parental cells in chimeric organism various genetic, biochemical and phenotypic markers are developed. For example, the distinct coat color pattern (a different strain of mice), electrophoretic variants of the housekeeping enzyme glucose-6-phosphate isomerase has frequently been used in earlier studies. In addition, DNA satellite markers (strain-specific and first generation genetic marker; Rossant et al., 1983) are well-reported for cell lineage tracking based on in situ hybridization of histological samples that would strike out the different origin of the chimera cells.
Histochemical staining methods to detect β-galactosidase enzyme (E. coli lacZ gene and the epifluorescent microscopic methods for the detection of green fluorescent protein are widely used markers. There are new genetic markers also such as microsatellites (short tandem repeats) being employed by a few researchers (Tachibana et al., 2012). Experimental embryonic chimeras have been reported in a number of species, including sheep (Tucker et al., 1974), rats (Mayer and Fritz, 1974), rabbits (Gardner and Munro, 1974), and cattle (Brem et al., 1984) and more recently in nonhuman primates (Tachibana et al., 2012). Despite the demonstration of ESCs or ESC-like cells expressing pluripotency markers from several species, the germline chimeras are reported only for the mouse and rat cells. Firstly, rat ESCs expressing typical pluripotency markers (e.g., differentiate into derivatives of all three germ layers) were reported to contribute germline chimeras (Li et al., 2008) and their reintroduction into early cleaving embryos was capable of inducing the chimera formation (Li et al., 2008). Recent advances in iPSC technology have been useful to generate PSCs from many species, including rats (Liao et al., 2009), cattle (Han et al., 2011), sheep (Liu et al., 2008, 2012), rhesus monkey (Liu et al., 2008), and pig (Ezashi et al., 2009; Wu et al., 2009). Recently, porcine iPSCs has been shown to exhibit chimera generating potential with multiple tissue contribution for the three germ layers (West et al., 2010). Generation of naïve-like porcine iPSCs has been reported with the capabilities of contributing many organs in the fetal chimera (such as head, atrium, ventricle, liver, brachial arch, limb bud; Fujishiro et al., 2013). In humans, embryonic chimerism is shown to occur by spontaneous aggregation of two different zygotes/embryos and due to lack of detectable features of chimerism, most of these conditions may not be diagnosed. Therefore, chimerism in human is generally reported by studies from either developmental anomalies or genotype/sex discordance (Boklage, 2006; Mascetti and Pedersen, 2016).
Experimental human chimerism has been successfully demonstrated by aggregation of fertilized embryos with unfertilized embryos (parthenogenesis or androgenetic) or by aggregation with the fertilized second polar body (Strain et al., 1995; Malan et al., 2006). Thus, these studies have firmly established the method for chimera production as a powerful tool, and it is conceivable from a number of experimental reports that high-quality PSCs would support the generation of high-grade chimera. This is assessed by extensive colonization of all embryonic cell lineages/tissues including germ line. On the other hand, comparatively reduced embryonic viability and small frequency of chimerism would indicate the relatively little potency of the test cells.
Tetraploid Complementation Assays
The tetraploid complementation assay is a technique used to construct genetically modified organisms, the study of mutation related consequence of embryonic development, and in the study of PSCs (Nagy et al., 1993; Kang et al., 2009).
This assay is based on the fact that the different lineage potency is displayed by the two cellular constituents of the (ESC ↔ Tetraploid embryo) chimera. On one hand, the ESCs are developed into all structures in the fetus, while the tetraploid cells produce the extraembryonic primitive endoderm tissue and the trophectoderm.
One can carefully select genotypically different ESCs and the tetraploid embryos (mutant versus wild type) to achieve completely segregated cells (mutant cells vs. wild-type cells) into two distinctive tissue compartments of the conceptus. The method involves the production of a tetraploid cell (4n chromosome) by fusing both the cells of a two-cell stage embryo by applying an electrical current. This results in a tetraploid cell which can divide and give rise to similar 4n daughter cells. Although, this tetraploid embryo may develop up to the blastocyst stage and can implant in the uterus wall generating extraembryonic tissue (placenta) but it usually cannot be developed into proper fetus due to its abnormal genetic composition. The tetraploid complementation assay would use a tetraploid embryo (either at the morula or blastocyst stage) that is mixed with normal 2n ESCs (i.e., Test cells) from a different organism to develop into the fetus. Fetus cells are exclusively derived from the ESC (test cells) while the tetraploid cells contribute to the generation of extra-embryonic tissues. Therefore, this ESC-tetraploid embryo chimera becomes a useful experimental tool to test the essentially required genes to function in the embryonic versus extra-embryonic tissues for the development of the concepts. This is one of the most stringent assays to check the pluripotency and developmental ability of the ES or iPSCs. The study of the manner in which development of an embryo occurs helps to determine the effects of a specific allele (wild type/mutant) on the development of the organism. Abnormal development of the embryo from mutant tetraploid cells/wild type ESC composition would indicate defects in the extra-embryonic tissues that are likely to be imposed by the mutation of the extra-embryonic endoderm and the trophectoderm only. Whereas, the development of an abnormal embryo from mutant ESCs (in the epiblast-derived tissues) and wild-type tetraploid cells (in the extra-embryonic tissues), would be visible in the embryonic tissues or the extra-embryonic mesoderm.
So, if there is a lethal mutation in the tetraploid cells, the abnormality in the development of the organism would be due to the defects in the extra-embryonic tissues. This allows us to target the specific gene of interest so that the mutation is removed (wild type) and the potential of a liveborn organism can be achieved.
Transmission of Germ Line
Germ line transmission is a process in which the ES derived cells of a chimera result in the formation of reproductive cells of the organism. As the germ cells are passed on to the inherited offspring, it is known as germ line transmission. The assessment of iPSCs for their capability for the formation of chimeras contributing to all of the three germ layers, trophectoderm and the gonads is done by studying the transmission of the germ line. iPSCs possess in vitro differentiation potential and morphological immunoreactivity but their plasticity still needs to be tested for the formation of chimera which is an important assay for pluripotency as the iPSCs derived from the transduction of Oct4, Sox2, Klf4, and c-Myc possessed many pluripotent characteristics but were unable to form chimeras which indicates partial reprogramming (Esteban et al., 2009). The capability of the chimera to breed and give rise to viable all-donor pluripotent stem cell-derived offspring is a direct indicator of their success and complete chimerism. The generation of live chimeric offspring validates the potential of the iPSCs for the application in stem cell therapies.
Technically, donor derived test PSCs are integrated into all tissues of the later stages of viable embryos and those results in the adult mice with chimeric tissue throughout their body including germ line tissues. Further breeding of these chimeric animals would lead to all donor PSCs-derived offspring (germ line transmission) which is a robust indicator of chromosomal integration and functional pluripotency.
Chimera Formation by Using Single Pluripotent Cell
Chimeric or entirely embryonic stem (ES) cell-derived mice may be produced by using Test cells into diploid (2n)/tetraploid (4n) host blastocysts respectively to demonstrate their pluripotency. As described by a number of successful experiments with ~10–15 ESCs can be injected into the host blastocyst. Since pluripotency stringently refers to the property of a single cell to produce chimeras due to its widespread contribution. Analysis of single PSCs-derived embryo would be of great significance in determining clonal capacities of the test PSCs (Wang and Jaenisch, 2004). However, single-cell chimerism and tetraploid complementation assays are referred almost definitive tools for determining pluripotency of any cell population, but as they suffer from higher failure rates a researcher may not be able to choose them as obvious tools.
Defining Human Pluripotent Cells on the Basis of Molecular and Functional Diagnostic Tools
Human pluripotent cells (conventional) may be defined on the basis of various molecular markers and functional assays developed as “Primed” embryonic stem cell state. Since it is shown to utilize the OCT4 proximal enhancer more preferentially, and significantly high levels of DNA methylation. It is reported to have inclined tendency to have an inactivated X chromosome in female cell lines (Hanna J. et al., 2010). There are reports regarding the assessment of human “naïve” PSCs by blastocyst chimerism (Takashima et al., 2014; Theunissen et al., 2014; Gafni et al., 2015). Despite ethical constraints on using human ESCs in these studies limited experiments done with both primed and altered human PSCs, which showed a little contribution in chimera production on their infusion into mouse pre-implantation embryos (Takashima et al., 2014; Gafni et al., 2015).
However, studies done by using primate naïve iPSCs have resulted to significant cross-species blastocyst chimerism. These primate naïve iPSCs were injected into mouse blastocysts resulting to the clonal contribution to the solid tissues (Fang et al., 2014).
Further studies to demonstrate interspecies chimerism by using primate ICM cells have not been successful in generating blastocyst chimerism showing their different properties from mouse ICM cells which readily produce chimeras (Tachibana et al., 2012). Chen et al. described low-grade contribution to all three germ layers by using altered primate PSCs (Chen et al., 2015). But the more stringent test like high-grade contribution and germline transmission are yet to be demonstrated to establish naïve pluripotency in primate ESCs.
The most of these studies indicate the distinct behavior of primate PSCs in chimeras studies. Therefore, interspecies chimerism of human cells into mouse embryos would require additional validation before its routine uses to assess human stem cell potency. Recently, hiPSCs and hESCs have been demonstrated to contribute to the normal mouse development by using a stage-matching approach which involved transplantation of hiPSCs/hESCs into gastrula-stage embryos. Both hiPSCs and hESCs were reported to form interspecies chimeras with high efficiency (Mascetti and Pedersen, 2016). The hiPSCs/hESCs well colonized the embryo and differentiate into all the three primary tissue layers. This tissue-specific fate post-transplantation defined the potential of hPSCs to possess pluripotent characteristics and provided mush awaited functional evidence that human-mouse interspecies developmental competency may be useful in defining their pluripotency by carefully matching their stage of development.
The human stem-cell potency is also assessed by comparing transcriptional and epigenetic molecular markers with the pluripotent cells in human preimplantation embryos. As discussed in earlier sections, the degree of DNA methylation and demethylation/remethylation in various PSCs is widely accepted as a diagnostic signature for pre-implantation and germline developmental stage derived cell in mammals (Deng et al., 2009). There are reports of studies revealing the degree of genomic methylation (hypomethylated vs. hypermethylated DNA) which shown hypomethylated genome human pre-implantation embryonic cells. Further, reports depicted a remethylated genome in ICM derived cells and maintenance of hypermethylated genome that is similar to mouse primed PSCs in establishing human ESCs (Guo et al., 2014; Smith et al., 2014). The epigenetic resetting is controlled by distinct regulatory molecules and their interactions with each other in various states of PSCs. Studies in mice naïve PSCs have highlighted a prominent role of TFs such as KLF4, VTFCP2L1, ESRRB, TBX3, and GBX2. Studies demonstrating single-cell RNA sequencing (RNA-Seq) analysis of human preimplantation embryos (epiblast) and hESCs to define gene expression signatures shown significant differences in their transcriptomes (1498 differentially expressing genes). Human preimplantation epiblast cells also express these molecules. On the other hand, hESCs did not express them showing similarities with mouse EpiSC states (Yan et al., 2013). A simple comparison of the expression levels of specific TFs in mice originated naïve cell with the human preimplantation epiblast cells may not be sufficient to define their similar state of development since some of these factors (e.g., KLF4) are absent in human epiblasts.
Further, the interspecies comparison of other molecular markers such as X chromosome inactivation timing and epigenetic erosion in prime human ESCs has raised the complexity (Silva et al., 2008; Okamoto et al., 2011; Anguera et al., 2012; O'Leary et al., 2012), and identification of hES (ground state or naïve PSCs) by comparing these molecular criteria with the mouse ground state PSCs is not completely acceptable and more specific marker may yet to be demonstrated for human PSCs. While these discrepancies are increasingly being observed by various research groups, a new concept of a metastable naïve state of pluripotency is being suggested (Chan et al., 2013; Valamehr et al., 2014; Ware et al., 2014). The uncertainty about the role of X chromosome inactivation is further reflected by studies from PSCs generated by Jaenisch and Smith Laboratories showing expression of mouse ground state specific TFs (Silva et al., 2008; Okamoto et al., 2011; Anguera et al., 2012; O'Leary et al., 2012; Takashima et al., 2014; Theunissen et al., 2014; Gafni et al., 2015). Declined DNA methylation pattern exhibited by PSCs derived by Smith laboratories indicated their embryonic preimplantation state, but other molecular markers such as activities of OCT4 distal enhancer, and limited information regarding the transgene-independent cell lines' characterization raise ambiguities about the stability of the reset state in these human pluripotent cells (Takashima et al., 2014). Therefore, due to these uncertainties among various molecular markers to define the exact developmental state and hence their pluripotency would be possible only after the direct derivation of ground state ESCs from human embryos. These should be accompanied by comprehensive experimental information regarding the transition of these cells from totipotent to pluripotent states in human and primates.
Bioinformatics and Computer-Based Methods for Pluripotency Determination
As described in earlier sections PSCs may be useful in various ways to understand human developmental stages, and personalized regenerative medicine for most of the incurable diseases. However, the direct functional assessment of their propensity is cumbersome and even may not be feasible every time. There are efforts reported from various research groups regarding the development of high-throughput production of human stem cells for use in regenerative medicine and standardization of pluripotency assays by deploying a number of approaches that promise to improve unbiased prediction of the utility of both human-induced PSCs and ESCs by using bioinformatics and gene expression profiling.
Genomic Analysis of PSCs
This is first method defined to evaluate the pluripotency of PSCs by gene expression profiling through DNA microarrays. It applied global molecular analysis approach for mapping the transcriptome of PSCs (Sato et al., 2003; Sperger et al., 2003; Bhattacharya et al., 2004; Suárez-Farinas et al., 2005) and has become a standard assay of pluripotency in many studies.
There are a number of algorithms that have been used to classify cell lines into similar transcriptional states. Muller et al. demonstrated a computational way (“Stem Cell Matrix”) that allows the user to the classify cultured human stem cells in various contexts of pluripotency, multipotency, and differentiated cell types and thus, PSCs may be distinguished from other stem cell types/differentiated cell types (Müller et al., 2008). There has been significant progress in the way these methods are being applied to define more subtle differences in PSCs. The earlier comparisons of iPSCs and ESCs indicated statistical differences between them (Maherali et al., 2008; Chin et al., 2009; Soldner et al., 2009), and which was visible even in their later stages with a significantly reduced level. Recent advancements established global similarities with small differences between iPSCs and hESCs (Marchetto et al., 2009; Bock et al., 2011; Lister et al., 2011) such as changes in gene expression signature in miRNA and long intergenic noncoding RNA along with mRNA (Lakshmipathy et al., 2010; Loewer et al., 2010; Stadtfeld et al., 2010). However, a more comprehensive study would be required to define the reasons behind these variations which may be due to differences in growth parameters/conditions, laboratory-to-laboratory variation (Newman and Cooper, 2010), heterogeneity in iPSC quality (Maherali et al., 2008), or small sample sizes (Chin et al., 2009).
One must be interested in developing such a method that may be useful in identifying the pluripotency of any individual cell or cell population. Since the gene expression profile is highly variable among different pluripotent cells, it may be difficult to establish such a signature expression profile, especially when the sample size used in these gene expression studies is relatively small and may not be sufficient to find consistent small observable differences among different PSCs (Bock et al., 2011). Advancement in the availabilities of curated databases with sufficiently large no. of samples should ensure the reliability of these approaches.
Pluritest
Pluritest presents one of the excellent examples that can quantify the extent to which experimental methodologies may perform in order to convert a parental cell toward the target cell (Müller et al., 2008). Since the assessment of the continuously expanding larger data sets by the researchers through the newer techniques such as machine learning may be helpful in classifying the various types of PSC lines available to the researchers. The Pluritest makes the exact same comparisons by using an algorithm that relies upon the use of training sets containing sufficiently large dataset including huge numbers of (undifferentiated, differentiated, normal, and abnormal) human stem cell lines and tissues. These databases with larger sample sizes are used to develop bioinformatics models for identifying stem cells' pluripotency on the basis of gene expression measurements done by DNA microarray (Müller et al., 2011). The bioinformatics model for these assessments is generated by using two important vectors that are calculated to categorize (i) pluripotent cells vs. differentiated cells, and (ii) abnormal vs. normal gene expression signature profiles. This calculation utilizes a large training set of almost 500 samples curated for microarray data quality and then consists results from microarray data originated from the studies done on various types of pluripotent cells e.g., hESCs, germ cell tumors, primary cell lines, and somatic tissues microarray analysis. Pluritest algorithm has been reported to distinguish the different test samples of germ cell tumors from hESCs independently as well as distinguish reprogrammed from partially reprogrammed iPSCs. Further, it is also useful in identifying parthenogenetic stem cell lines and separating them from hESCs which are assumed to be a manifestation of differences at imprinted loci. This ability could be helpful in distinguishing between abnormal and normal samples and also in their categorization as undifferentiated/differentiated cell stage.
The algorithm has also been reported to be useful for iPSCs characterization (MacArthur et al., 2012; Mariani et al., 2012; Nazor et al., 2012). Similar models for mouse ESCs are also developed showing their ability to calculate the reprogramming efficiency in response to NANOG overexpression (MacArthur et al., 2012; Mariani et al., 2012; Nazor et al., 2012). The algorithm may find the further application, and various other types of databases (e.g., epigenetic status or stem cell lines) may be examined to define valuable information (Williams et al., 2011). Although, the efficiency and sensitivity of these algorithms to determine abnormalities e.g., copy number variations or translocations is yet to be established. One approach to improving this quality may be the inclusion of genomic integrity tests and improving the variability of the training dataset used to construct the model.
Comparison of Epigenetic Profiles of PSCs
Despite inconsistencies monitoring epigenetics may be sensitive enough to define small changes and a more elaborated definition for their functional consequences may be developed. For example, together methylation mapping and gene expression signatures methodologies by these sophisticated algorithms may help in generating robust tools to infer the cell state. Bock and colleagues shown minor yet significant differences in DNA methylation and gene expression pattern in some IPS cell lines but not in ESCs lines through a number of statistical tests against preexisting datasets (Chin et al., 2009; Doi et al., 2009; Stadtfeld et al., 2010; Bock et al., 2011; Theunissen et al., 2014). As described in their studies by using a support vector machine learning algorithm to classify the data, including both from DNA methylation and gene expression data obtained from ESCs lines (20 lines) and iPSCs lines (12 lines) this method efficiently classifies ESCs cell lines. However, results with the iPSCs were of moderate importance only when trying to classify iPSCs lines. In brief, iPSCs specific gene signature was identified with great accuracy (81%) and specificity (91%) but with moderate sensitivity (61%). Considering the size of training datasets used (for combined methylation and gene expression) in these studies a significantly bigger dataset was used in similar methodologies employed by Pluritest and its larger data set for training set development in this method may also improve the predictions.
Newer Methods to Analyze the Differentiation Potential of PSCs: The Scorecard Approach
Since the right selection of most suitable undifferentiated cell (PSCs) is a key to determine the success of developing a desired cellular lineage for both the clinical and research purposes. Presently used labor intensive methods e.g., teratoma formation assay etc. are both time consuming and expensive. The different in silico approached discussed so far mostly focus on the identification and characterization of the status of differentiation in PSCs and may not be able to predict their ability to differentiate into various required lineage cells. Bock et al. (2011) have tried to fill this gap by demonstrating a method which predicts this ability of PSCs by using combined gene expression data and epigenetic measures with in vitro differentiation assays. In their report, firstly a deviation scorecard is generated to evaluate existing data for DNA methylation and gene expression profiles. These profiles are relative to a set of reference standard has lines which identify deviated lines as reported by outlier detection methods.
This analysis would result in a number of genes (outliers) that may be screened for their probable role in respective functional assay's performances (Boulting et al., 2011). For example, their group assessed the data to identify genes associated with an aberrant function for motor neuron when iPSCs is directed to get differentiated into neuronal lineages. These studies could highlight one putative gene GRM (glutamate receptor in a motor neuron), and thus, the particular cell line may be ruled out on the basis of this test result.
Further, a quantitative embryoid body assay through high-throughput counting methods was used to develop quick methods of highlighting the differentiation potential for PSCs and develop a respective scorecard for the same. In a non-directed embryoid differentiation assay (20 ESCs and 12 iPSC) RNA analysis was done to probe the expression level of 500 marker genes by Bock and colleagues. The results obtained from these studies were used to generate a quantitative gene expression profile from reference hESCs line derived embryoid bodies. As described in their reports, the lineage scorecard successfully detected and classified iPSCs lines based on their ability to differentiate into ISL1-positive motor neurons in direct differentiation assays.
In brief, prediction of differentiation fate in iPSCs could be possible by the integration of the multiple high content functional assay data. The linear scorecard approach is also beneficial in predicting the possible ability of a particular cell line into specific lineages on induction with appropriate factors/culture environments through the selection of specific gene sets and recalibration to reference standards. With the enhancement of data (number of lines screened increases) identified potential of this approach for the most frequent gene expression and epigenetic aberrations may be achieved. In addition, frequent use of these methods may benefit by reducing the cost and time taken by conventional assay to decide the most appropriate lines for a particular set of experiments or regime.
Conclusion
It is clear from the available information that pluripotency is a dynamic state, and a large number of molecular factors essentially regulate the fate of any developing cell. There are many different types of molecular markers such as TFs, their target gene promoters, miRNAs, noncoding RNAs, histone modifiers proteins, and their corresponding gene expression, which are being scrutinized by the global research community to define the level of pluripotency. However, there have been some discrepancies among these methods, and the reliability and authenticity of these diagnostics markers may be liable to vary for different cell types being evaluated by the researchers. Since these molecular diagnostic molecules are yet to be optimized for their ability to become more sensitive and accuracy of the prediction made on the basis of the various bioinformatics approaches would also be improved. Since then, only the functional assays of various types, e.g., teratoma formation assays, blastocyst chimerism, tetroid complementation assays, etc. would remain the gold standard for the determination of pluripotency. Recent advancements in the technology to get pluripotent cells by reprogramming (iPSCs) and more robust molecular techniques such as CHIP-seq, microarrays and enhanced computing methods, together all these would further enhance our understanding of the core regulatory elements essential for the maintenance and induction of pluripotency in various types of cells. Further, the capability to predict the most obvious outcome by in silico tools when a PSC line is allowed to differentiate would improve the clinical outcome in near future.
Author Contributions
All authors listed, have made substantial, direct and intellectual contribution to the work, and approved it for publication.
Funding
INSPIRE Faculty award is a prestigious award given to the candidates working in all the scientific fields. The award supports young and upcoming scientist with a fellowship and research grant for a max period of 5 years. VS is thankful to the DST and INSA for providing him an opportunity to work independently and develop as a scientific personnel.
Conflict of Interest Statement
The authors declare that the research was conducted in the absence of any commercial or financial relationships that could be construed as a potential conflict of interest.
Acknowledgments
We are thankful to the Honorable Chairman and the Honorable Vice- Chancellor of the Delhi Technological University, Shahbad Daulatpur, Bawana Road, Delhi-42, for support. VS particularly thanks the Department of Science and Technology and Indian National Science Academy (INSA), INDIA, for the research grant.
References
Ali, N. N., Edgar, A. J., Samadikuchaksaraei, A., Timson, C. M., Romanska, H. M., Polak, J. M., et al. (2002). Derivation of type II alveolar epithelial cells from murine embryonic stem cells. Tissue Eng. 8, 541–550. doi: 10.1089/107632702760240463
Anguera, M. C., Sadreyev, R., Zhang, Z., Szanto, A., Payer, B., Sheridan, S. D., et al. (2012). Molecular signatures of human induced pluripotent stem cells highlight sex differences and cancer genes. Cell Stem Cell 11, 75–90. doi: 10.1016/j.stem.2012.03.008
Avilion, A. A., Nicolis, S. K., Pevny, L. H., Perez, L., Vivian, N., and Lovell-Badge, R. (2003). Multipotent cell lineages in early mouse development depend on SOX2 function. Genes Dev. 17, 126–140. doi: 10.1101/gad.224503
Bader, A., Gruss, A., Höllrigl, A., Al-Dubai, H., Capetanaki, Y., and Weitzer, G. (2001). Paracrine promotion of cardiomyogenesis in embryoid bodies by LIF modulated endoderm. Differentiation 68, 31–43. doi: 10.1046/j.1432-0436.2001.068001031.x
Bao, S., Tang, F., Li, X., Hayashi, K., Gillich, A., Lao, K., et al. (2009). Epigenetic reversion of post-implantation epiblast to pluripotent embryonic stem cells. Nature 461, 1292–1295. doi: 10.1038/nature08534
Bernstein, B. E., Mikkelsen, T. S., Xie, X., Kamal, M., Huebert, D. J., Cuff, J., et al. (2006). A bivalent chromatin structure marks key developmental genes in embryonic stem cells. Cell 125, 315–326. doi: 10.1016/j.cell.2006.02.041
Bhattacharya, B., Miura, T., Brandenberger, R., Mejido, J., Luo, Y., Yang, A. X., Joshi, et al. (2004). Gene expression in human embryonic stem cell lines: unique molecular signature. Blood 103, 2956–2964. doi: 10.1182/blood-2003-09-3314
Bock, C., Kiskinis, E., Verstappen, G., Gu, H., Boulting, G., Smith, Z. D., et al. (2011). Reference maps of human ES and iPS cell variation enable high-throughput characterization of pluripotent cell lines. Cell 144, 439–452. doi: 10.1016/j.cell.2010.12.032
Boklage, C. E. (2006). Embryogenesis of chimeras, twins and anterior midline asymmetries. Hum. Reprod. 21, 579–591. doi: 10.1093/humrep/dei370
Boroviak, T., Loos, R., Bertone, P., Smith, A., and Nichols, J. (2014). The ability of inner-cell-mass cells to self-renew as embryonic stem cells following epiblast specification. Nat. Cell Biol. 16, 516–528. doi: 10.1038/ncb2965
Borowiak, M., Maehr, R., Chen, S., Chen, A. E., Tang, W., Fox, J. L., et al. (2009). Small molecules efficiently direct endodermal differentiation of mouse and human embryonic stem cells. Cell Stem Cell 4, 348–358. doi: 10.1016/j.stem.2009.01.014
Botquin, V., Hess, H., Fuhrmann, G., Anastassiadis, C., Gross, M. K., Vriend, G., et al. (1998). New POU dimer configuration mediates antagonistic control of an osteopontin preimplantation enhancer by Oct-4 and Sox-2. Genes Dev. 12, 2073–2090. doi: 10.1101/gad.12.13.2073
Boulting, G. L., Kiskinis, E., Croft, G. F., Amoroso, M. W., Oakley, D. H., Wainger, B. J., et al. (2011). A functionally characterized test set of human induced pluripotent stem cells. Nat. Biotechnol. 29, 279–286. doi: 10.1038/nbt.1783
Boyer, L. A., Lee, T. I., Cole, M. F., Johnstone, S. E., Levine, S. S., Zucker, J. P., et al. (2005). Core regulatory circuitry in human embryonic stem cells. Cell 122, 947–956. doi: 10.1016/j.cell.2005.08.020
Bradley, A., Evans, M., Kaufman, M. H., and Robertson, E. (1984). Formation of germ-line chimeras from embryo-derived teratocarcinoma cell lines. Nature 309, 255–256. doi: 10.1038/309255a0
Brem, G., Tenhumberg, H., and Krausslich, H. (1984). Chimerism in cattle through microsurgical aggregation of morulae. Theriogenology 122, 609–613. doi: 10.1016/0093-691X(84)90061-X
Brivanlou, A. H., Gage, F. H., Jaenisch, R., Jessell, T., Melton, D., and Rossant, J. (2003). Stem cells. Setting standards for human embryonic stem cells. Science 300, 913–916. doi: 10.1126/science.1082940
Brons, I. G., Smithers, L. E., Trotter, M. W., Rugg-Gunn, P., Sun, B., Chuva de Sousa Lopes, S. M., et al. (2007). Derivation of pluripotent epiblast stem cells from mammalian embryos. Nature 448, 191–195. doi: 10.1038/nature05950
Buecker, C., Chen, H. H., Polo, J. M., Daheron, L., Bu, L., Barakat, T. S., et al. (2010). A murine ESC-like state facilitates transgenesis and homologous recombination in human pluripotent stem cells. Cell Stem Cell 6, 535–546. doi: 10.1016/j.stem.2010.05.003
Butler, J. E., Anderson, G. B., BonDurant, R. H., Pashen, R. L., and Penedo, M. C. (1987). Production of ovine chimeras by inner cell mass transplantation. J. Anim. Sci. 65, 317–324. doi: 10.2527/jas1987.651317x
Buttery, L. D., Bourne, S., Xynos, J. D., Wood, H., Hughes, F. J., Hughes, S. P., et al. (2001). Differentiation of osteoblasts and in vitro bone formation from murine embryonic stem cells. Tissue Eng. 7, 89–99. doi: 10.1089/107632700300003323
Campbell, K. H. S., McWhir, J., Ritchie, W., and Wilmut, I. (1995). Production of live lambs following nuclear transfer of cultured embryonic disc cells. Theriogenology 43, 181–191. doi: 10.1016/0093-691X(95)92335-7
Campbell, K. H. S., Ritchie, W. A., McWhir, J., and Wilmut, I. (1996). Cloning farm animals by nuclear transfer: from cell cycles to cells. Embryo Transfer Newslett. 14, 12–17.
Chamberlain, S. J., Yee, D., and Magnuson, T. (2008). Polycomb repressive complex 2 is dispensable for maintenance of embryonic stem cell pluripotency. Stem Cells 26, 1496–1505. doi: 10.1634/stemcells.2008-0102
Chambers, I., Colby, D., Robertson, M., Nichols, J., Lee, S., and Tweedie, S. (2003). Functional expression cloning of Nanog, a pluripotency sustaining factor in embryonic stem cells. Cell 113, 643–655. doi: 10.1016/S0092-8674(03)00392-1
Chambers, I., Silva, J., Colby, D., Nichols, J., Nijmeijer, B., Robertson, M., et al. (2007). Nanog safeguards pluripotency and mediates germline development. Nature 450, 1230–1234. doi: 10.1038/nature06403
Chan, E. M., Ratanasirintrawoot, S., Park, I. H., Manos, P. D., Loh, Y. H., Huo, H., et al. (2009). Live cell imaging distinguishes bonafide human iPS cells from partially reprogrammed cells. Nat. Biotechnol. 27, 1033–1037. doi: 10.1038/nbt.1580
Chan, Y. S., Göke, J., Ng, J. H., Lu, X., Gonzales, K. A. U., Tan, C. P., et al. (2013). Induction of a human pluripotent state with distinct regulatory circuitry that resembles preimplantation epiblast. Cell Stem Cell 13, 663–675. doi: 10.1016/j.stem.2013.11.015
Chen, X., Xu, H., Yuan, P., Fang, F., Huss, M., Vega, V. B., et al. (2008). Integration of external signaling pathway with the core transcriptional network in embryonic stem cells. Cell 133, 1106–1117. doi: 10.1016/j.cell.2008.04.043
Chen, Y., Niu, Y., Li, Y., Ai, Z., Kang, Y., Shi, H., et al. (2015). Generation of cynomolgus monkey chimeric fetuses using embryonic stem cells. Cell Stem Cell 17, 116–124. doi: 10.1016/j.stem.2015.06.004
Chin, M. H., Mason, M. J., Xie, W., Volinia, S., Singer, M., Peterson, C., et al. (2009). Induced pluripotent stem cells and embryonic stem cells are distinguished by gene expression signatures. Cell Stem Cell 5, 111–123. doi: 10.1016/j.stem.2009.06.008
Colman, A. (2004). Making new beta cells from stem cells. Semin. Cell Dev. Biol. 15, 337–345. doi: 10.1016/j.semcdb.2004.02.003
Dani, C., Smith, A. G., Dessolin, S., Leroy, P., Staccini, L., Villageois, P., et al. (1997). Differentiation of embryonic stem cells into adipocytes in vitro. J. Cell Sci. 110, 1279–1285.
Deng, J., Shoemaker, R., Xie, B., Gore, A., LeProust, E. M., Antosiewicz-Bourget, J., et al. (2009). Targeted bisulfite sequencing reveals changes in DNA methylation associated with nuclear reprogramming. Nat. Biotechnol. 27, 353–360. doi: 10.1038/nbt.1530
Doi, A., Park, I. H., Wen, B., Murakami, P., Aryee, M. J., Irizarry, R., et al. (2009). Differential methylation of tissue- and cancer-specific CpG island shores distinguishes human induced pluripotent stem cells, embryonic stem cells and fibroblasts. Nat. Genet. 41, 1350–1353. doi: 10.1038/ng.471
Dunn, S. J., Martello, G., Yordanov, B., Emmott, S., and Smith, A. G. (2014). Defining an essential transcription factor program for naïve pluripotency. Science 344, 1156–1160. doi: 10.1126/science.1248882
Esteban, M. A., Xu, J., Yang, J., Peng, M., Qin, D., Li, W., et al. (2009). Generation of induced pluripotent stem cell lines from Tibetan miniature pig. J. Biol. Chem. 284, 17634–17640. doi: 10.1074/jbc.M109.008938
Evans, M. J., and Kaufman, M. H. (1981). Establishment in culture of pluripotential cells from mouse embryos. Nature 292, 154–156. doi: 10.1038/292154a0
Ezashi, T., Telugu, B. P., Alexenko, A. P., Sachdev, S., Sinha, S., and Roberts, R. M. (2009). Derivation of induced pluripotent stem cells from pig somatic cells. Proc. Natl. Acad. Sci. U.S.A. 106, 10993–10998. doi: 10.1073/pnas.0905284106
Fang, R., Liu, K., Zhao, Y., Li, H., Zhu, D., Du, Y., et al. (2014). Generation of naive induced pluripotent stem cells from rhesus monkey fibroblasts. Cell Stem Cell 15, 488–496. doi: 10.1016/j.stem.2014.09.004
Feng, B., Ng, J. H., Heng, J. C. D., and Ng, H. H. (2009). Molecules that promote or enhance reprogramming of somatic cells to induced pluripotent stem cells. Cell Stem Cell 4, 301–312. doi: 10.1016/j.stem.2009.03.005
Ferrari, D., Binda, E., De Filippis, L., and Vescovi, A. L. (2010). Isolation of neural stem cells from neural tissues using the neurosphere technique. Curr. Protoc. Stem Cell Biol. Chapter 2, Unit 2D. 6. doi: 10.1002/9780470151808.sc02d06s15
Fujishiro, S. H., Nakano, K., Mizukami, Y., Azami, T., Arai, Y., Matsunari, H., et al. (2013). Generation of naive-like porcine-induced pluripotent stem cells capable of contributing to embryonic and fetal development. Stem Cells Dev. 22, 473–482. doi: 10.1089/scd.2012.0173
Gafni, O., Weinberger, L., Mansour, A. A., Manor, Y. S., Chomsky, E., Ben-Yosef, D., et al. (2013). Derivation of novel human ground state naive pluripotent stem cells. Nature 504, 282–286. doi: 10.1038/nature12745
Gafni, O., Weinberger, L., Mansour, A. A., Manor, Y. S., Chomsky, E., Ben-Yosef, D., et al. (2015). Corrigendum: derivation of novel human ground state naive pluripotent stem cells. Nature 520:710. doi: 10.1038/nature14370
Gardner, R. L., and Munro, A. J. (1974). Successful construction of chimeric rabbit. Nature 250, 146–147. doi: 10.1038/250146a0
Gearhart, J., and Oster-Granite, M. L. (1981). Reproduction in a population of chimeric mice: relationship of chromosomal sex to functional germ cells and proportions of chimeric components in several tissues. Biol. Reprod. 24, 713–722. doi: 10.1095/biolreprod24.4.713
Gertow, K., Cedervall, J., Jamil, S., Ali, R., Imreh, M. P., Gulyas, M. B., et al. (2011). Early events in xenograft development from the human embryonic stem cell line HS181—resemblance with an initial multiple epiblast formation. PLoS ONE 6:27741. doi: 10.1371/journal.pone.0027741
Graham, V., Khudyakov, J., Ellis, P., and Pevny, L. (2003). SOX2 functions to maintain neural progenitor identity. Neuron 39, 749–765. doi: 10.1016/S0896-6273(03)00497-5
Gropp, M., Shilo, V., Vainer, G., Gov, M., Gil, Y., Khaner, H., et al. (2012). Standardization of the teratoma assay for analysis of pluripotency of human ESCs and biosafety of their differentiated progeny. PLoS ONE 7:45532. doi: 10.1371/journal.pone.0045532
Guo, G., Yang, J., Nichols, J., Hall, J. S., Eyres, I., Mansfield, W., et al. (2009). Klf4 reverts developmentally programmed restriction of ground state pluripotency. Development 136, 1063–1069. doi: 10.1242/dev.030957
Guo, H., Zhu, P., Yan, L., Li, R., Hu, B., Lian, Y., et al. (2014). The DNA methylation landscape of human early embryos. Nature 511, 606–610. doi: 10.1038/nature13544
Gurdon, J. B. (1962). The developmental capacity of nuclei taken from intestinal epithelium cells of feeding tadpoles. J. Embryol. Exp. Morphol. 10, 622–640.
Haar, J. L., and Ackerman, G. A. (1971). A phase and electron microscopic study of vasculogenesis and erythropoiesis in the yolk sac of the mouse. Anat. Rec. 170, 199–224. doi: 10.1002/ar.1091700206
Hamazaki, T., Iiboshi, Y., Oka, M., Papst, P. J., Meacham, A. M., Zon, L. I., et al. (2001). Hepatic maturation in differentiating embryonic stem cells in vitro. FEBS Lett. 497, 15–19. doi: 10.1016/S0014-5793(01)02423-1
Han, X., Han, J., Ding, F., Cao, S., Lim, S. S., Dai, Y., et al. (2011). Generation of induced pluripotent stem cells from bovine embryonic fibroblast cells. Cell Res. 21, 1509–1512. doi: 10.1038/cr.2011.125
Hanna, J. H., Saha, K., and Jaenisch, R. (2010). Pluripotency and cellular reprogramming: facts, hypotheses, unresolved issues. Cell 143, 508–525. doi: 10.1016/j.cell.2010.10.008
Hanna, J., Cheng, A. W., Saha, K., Kim, J., Lengner, C. J., Soldner, F., et al. (2010). Human embryonic stem cells with biological and epigenetic characteristics similar to those of mouse ESCs. Proc. Natl. Acad. Sci. U.S.A. 107, 9222–9227. doi: 10.1073/pnas.1004584107
Hentze, H., Soong, P. L., Wang, S. T., Phillips, B. W., Putti, T. C., and Dunn, N. R. (2009). Teratoma formation by human embryonic stem cells: evaluation of essential parameters for future safety studies. Stem Cell Res. 2, 198–210. doi: 10.1016/j.scr.2009.02.002
Hescheler, J., Fleischmann, B. K., Lentini, S., Maltsev, V. A., Rohwedel, J., Wobus, A. M., et al. (1997). Embryonic stem cells: a model to study structural and functional properties in cardiomyogenesis. Cardiovasc. Res. 36, 149–162. doi: 10.1016/S0008-6363(97)00193-4
Ho, R., Chronis, C., and Plath, K. (2011). Mechanistic insights into reprogramming to induced pluripotency. Cell Physiol. 226, 868–878. doi: 10.1002/jcp.22450
Hoebaus, J., Heher, P., Gottschamel, T., Scheinast, M., Auner, H., Walder, D., et al. (2013). Embryonic stem cells facilitate the isolation of persistent clonal cardiovascular progenitor cell lines, and leukemia inhibitor factor maintains their self-renewal and myocardial differentiation potential in vitro. Cells Tissues Organs 197, 249–268. doi: 10.1159/000345804
ISCBI (2009). Consensus guidance for banking and supply of human embryonic stem cell lines for research purposes. Stem Cell Rev. 5, 301–314. doi: 10.1007/s12015-009-9085-x
Jones, E. A., Tosh, D., Wilson, D. I., Lindsay, S., and Forrester, L. M. (2002). Hepatic differentiation of murine embryonic stem cells. Exp. Cell Res. 272, 15–22. doi: 10.1006/excr.2001.5396
Joo, J. Y., Choi, H. W., Kim, M. J., Zaehres, H., Tapia, N., Stehling, M., et al. (2014). Establishment of a primed pluripotent epiblast stem cell in FGF4-based conditions. Sci Rep. 4, 1–7. doi: 10.1038/srep07477
Kanatsu-Shinohara, M., Inoue, K., Lee, J., Yoshimoto, M., Ogonuki, N., Miki, H., et al. (2004). Generation of pluripotent stem cells from neonatal mouse testis. Cell 119, 1001–1012. doi: 10.1016/j.cell.2004.11.011
Kang, L., Wang, J., Zhang, Y., Kou, Z., and Gao, S. (2009). iPS cells can support full-term development of tetraploid blastocyst-complemented embryos. Cell Stem Cell 5, 135–138. doi: 10.1016/j.stem.2009.07.001
Kato, Y., Tani, T., Sotomaru, Y., Kurokawa, K., Kato, J.-Y., Doguchi, H., et al. (1998). Eight calves cloned from somatic cells of a single adult. Science 282, 2095–2098. doi: 10.1126/science.282.5396.2095
Kato, Y., and Tsunoda, Y. (1993). Totipotency and pluripotency of embryonic nuclei in the mouse. Mol. Reprod. Dev. 36, 276–278. doi: 10.1002/mrd.1080360230
Kaufman, D. S., Hanson, E. T., Lewis, R. L., Auerbach, R., and Thomson, J. A. (2001). Hematopoietic colony-forming cells derived from human embryonic stem cells. Proc. Natl. Acad. Sci. U.S.A. 98, 10716–10721. doi: 10.1073/pnas.191362598
Keller, G. (1995). In vitro differentiation of embryonic stem cells. Curr. Opin. Cell Biol. 7, 862–869. doi: 10.1016/0955-0674(95)80071-9
Kelly, S. J. (1977). Studies of the developmental potential of 4-and 8-cell stage blastomeres. J. Exp. Zool. 200, 365–376. doi: 10.1002/jez.1402000307
Kim, J., Chu, J., Shen, X., Wang, J., and Orkin, S. H. (2008). An extended transcriptional network for pluripotency of embryonic stem cells. Cell 132, 1049–1061. doi: 10.1016/j.cell.2008.02.039
Ko, K., Tapia, N., Wu, G., Kim, J. B., Bravo, M. J., Sasse, P., et al. (2009). Induction of pluripotency in adult unipotent germline stem cells. Cell Stem Cell 5, 87–96. doi: 10.1016/j.stem.2009.05.025
Kramer, J., Hegert, C., Guan, K., Wobus, A. M., Muller, P. K., and Rohwedel, J. (2000). Embryonic stem cell-derived chondrogenic differentiation in vitro: activation by BMP-2 and BMP-4. Mech. Dev. 92, 193–205. doi: 10.1016/S0925-4773(99)00339-1
Ku, H. T., Zhang, N., Kubo, A., O'Connor, R., Mao, M., Keller, G., et al. (2004). Committing embryonic stem cells to early endocrine pancreas in vitro. Stem Cells 22, 1205–1217. doi: 10.1634/stemcells.2004-0027
Kumar, R. M., Cahan, P., Shalek, A. K., Satija, R., DaleyKeyser, A. J., Li, H., et al. (2014). Deconstructing transcriptional heterogeneity in pluripotent stem cells. Nature 516, 56–61. doi: 10.1038/nature13920
Lakshmipathy, U., Davila, J., and Hart, R. P. (2010). miRNA in pluripotent stem cells. Regen. Med. 5, 545–555. doi: 10.2217/rme.10.34
Lee, J. H., Hart, S. R., and Skalnik, D. G. (2004). Histone deacetylase activity is required for embryonic stem cell differentiation. Genesis 38, 32–38. doi: 10.1002/gene.10250
Lee, S. H., Lumelsky, N., Studer, L., Auerbach, J. M., and McKay, R. D. (2000). Efficient generation of midbrain and hindbrain neurons from mouse embryonic stem cells. Nat. Biotechnol. 18, 675–679. doi: 10.1038/76536
Leitch, H. G., Blair, K., Mansfield, W., Ayetey, H., Humphreys, P., Nichols, J., et al. (2010). Embryonic germ cells from mice and rats exhibit properties consistent with a generic pluripotent ground state. Development 137, 2279–2287. doi: 10.1242/dev.050427
Leitch, H. G., McEwen, K. R., Turp, A., Encheva, V., Carroll, T., Grabole, N., et al. (2013). Naïve pluripotency is associated with global DNA hypomethylation. Nat. Struct. Mol. Biol. 20, 311–316. doi: 10.1038/nsmb.2510
Lengner, C. J., Gimelbrant, A. A., Erwin, J. A., Cheng, A. W., Guenther, M. G., Welstead, G. G., et al. (2010). Derivation of pre-X inactivation human embryonic stem cells under physiological oxygen concentrations. Cell 141, 872–883. doi: 10.1016/j.cell.2010.04.010
Li, E., Bestor, T. H., and Jaenisch, R. (1992). Target mutation of the DNA methyltransferase gene results in embryonic lethality. Cell 69, 915–926. doi: 10.1016/0092-8674(92)90611-F
Li, M., Pevny, L., Lovell-Badge, R., and Smith, A. (1998). Generation of purified neural precursors from embryonic stem cells by lineage selection. Curr. Biol. 8, 971–974. doi: 10.1016/S0960-9822(98)70399-9
Li, P., Tong, C., Mehrian-Shai, R., Jia, L., Wu, N., Yan, Y., et al. (2008). Germline competent embryonic stem cells derived from rat blastocysts. Cell 135, 1299–1310. doi: 10.1016/j.cell.2008.12.006
Liao, J., Cui, C., Chen, S., Ren, J., Chen, J., Gao, Y., et al. (2009). Generation of induced pluripotent stem cell lines from adult rat cells. Cell Stem Cell 4, 11–15. doi: 10.1016/j.stem.2008.11.013
Liao, J., Karnik, R., Gu, H., Ziller, M. J., Clement, K., Tsankov, A. M., et al. (2015). Targeted disruption of DNMT1, DNMT3A, and DNMT3 Binhuman embryonic stem cells. Nat. Genet. 47, 469–478. doi: 10.1038/ng.3258
Lin, R. Y., Kubo, A., Keller, G. M., and Davies, T. F. (2003). Committing embryonic stem cells to differentiate into thyrocytelike cells in vitro. Endocrinology 144, 2644–2649. doi: 10.1210/en.2002-0122
Lister, R., Pelizzola, M., Kida, Y. S., Hawkins, R. D., Nery, J. R., Hon, G., et al. (2011). Hotspots of aberrant epigenomic reprogramming in human induced pluripotent stem cells. Nature 471, 68–73. doi: 10.1038/nature09798
Liu, H., Zhu, F., Yong, J., Zhang, P., Hou, P., Li, H., et al. (2008). Generation of induced pluripotent stem cells from adult rhesus monkey fibroblasts. Cell Stem Cell 3, 587–590. doi: 10.1016/j.stem.2008.10.014
Liu, J., Balehosur, D., Murray, B., Kelly, J. M., Sumer, H., and Verma, P. J. (2012). Generation and characterization of reprogrammed sheep induced pluripotent stem cells. Theriogenology 77, 338–346. doi: 10.1016/j.theriogenology.2011.08.006
Loewer, S., Cabili, M. N., Guttman, M., Loh, Y. H., Thomas, K., and Park, I. H. (2010). Large intergenic non–coding RNA–RoR modulates reprogramming of human induced pluripotent stem cells. Nat. Genet. 42, 1113–1117. doi: 10.1038/ng.710
Loh, Y. H., Wu, Q., Chew, J. L., Vega, V. B., Zhang, W., Chen, X., et al. (2006). The Oct4 and Nanog transcription network regulates pluripotency in mouse embryonic stem cells. Nat. Genet. 38, 431–440. doi: 10.1038/ng1760
MacArthur, B. D., Sevilla, A., Lenz, M., Muller, F. J., Schuldt, B. M., Schuppert, A. A., et al. (2012). Nanog-dependent feedback loops regulate murine embryonic stem cell heterogeneity. Nat. Cell Biol. 14, 1139–1147. doi: 10.1038/ncb2603
Maherali, N., Ahfeldt, T., Rigamonti, A., Utikal, J., Cowan, C., and Hochedlinger, K. (2008). A high-efficiency system for the generation and study of human induced pluripotent stem cells. Cell Stem Cell 3, 340–345. doi: 10.1016/j.stem.2008.08.003
Malan, V., Vekemans, M., and Turleau, C. (2006). Chimera and other fertilization errors. Clin. Genet. 70, 363–373. doi: 10.1111/j.1399-0004.2006.00689.x
Marchetto, M. C., Yeo, G. W., Kainohana, O., Marsala, M., Gage, F. H., and Muotri, A. R. (2009). Transcriptional signature and memory retention of human-induced pluripotent stem cells. PLoS ONE 4:7076. doi: 10.1371/journal.pone.0007076
Mariani, J., Simonini, M. V., Palejev, D., Tomasini, L., Coppola, G., Szekely, A. M., et al. (2012). Modeling human cortical development in vitro using induced pluripotent stem cells. Proc. Natl. Acad. Sci. U.S.A. 109, 12770–12775. doi: 10.1073/pnas.1202944109
Marks, H., Kalkan, T., Menafra, R., Denissov, S., Jones, K., Hofemeister, H., et al. (2012). The transcriptional and epigenomic foundations of ground state pluripotency. Cell 149, 590–604. doi: 10.1016/j.cell.2012.03.026
Martin, G. R. (1981). Isolation of a pluripotent cell line from early mouse embryos cultured in medium conditioned by teratocarcinoma stem cells. Proc. Natl. Acad. Sci. U.S.A. 78, 7634–7638. doi: 10.1073/pnas.78.12.7634
Mascetti, V. L., and Pedersen, R. A. (2016). Human-mouse chimerism validates human stem cell pluripotency. Cell Stem Cell 18, 67–72. doi: 10.1016/j.stem.2015.11.017
Masui, S., Nakatake, Y., Toyooka, Y., Shimosato, D., Yagi, R., Takahashi, K., et al. (2007). Pluripotency governed by Sox2 via regulation of Oct3/4 expression in mouse embryonic stem cells. Nat. Cell Biol. 9, 625–635. doi: 10.1038/ncb1589
Matsui, Y., Zsebo, K., and Hogan, B. L. M. (1992). Derivation of pluripotential embryonic stem cells from murine primordial germ cells in culture. Cell 70, 841–847. doi: 10.1016/0092-8674(92)90317-6
Mayer, J. F. Jr., and Fritz, H. I. (1974). The culture of preimplantation rat embryos and the production of allophenic rats. J. Reprod. Fertil. 39, 1–9. doi: 10.1530/jrf.0.0390001
Min, J. Y., Yang, Y., Converso, K. L., Liu, L., Huang, Q., Morgan, J. P., et al. (2002). Transplantation of embryonic stem cells improves cardiac function in postinfarcted rats. J. Appl. Physiol. 92, 288–296.
Min, J. Y., Yang, Y., Sullivan, M. F., Ke, Q., Converso, K. L., Chen, Y., et al. (2003). Long-term improvement of cardiac function in rats after infarction by transplantation of embryonic stem cells. J. Thorac. Cardiovasc. Surg. 125, 361–369. doi: 10.1067/mtc.2003.101
Mintz, B. (1962). Experimental study of the developing mammalian egg: removal of the zona pellucida. Science 138, 594–595. doi: 10.1126/science.138.3540.594
Mitsui, K., Tokuzawa, Y., Itoh, H., Segawa, K., Murakami, M., Takahashi, K., et al. (2003). The homeoprotein Nanog is required for maintenance of pluripotency in mouse epiblast and ESCs. Cell 113, 631–642. doi: 10.1016/S0092-8674(03)00393-3
Morgani, S. M., Canham, M. A., Nichols, J., Sharov, A. A., Migueles, R. P., Ko, M. S., et al. (2013). Totipotent embryonic stem cells arise in ground-state culture conditions. Cell Rep. 3, 1945–1957. doi: 10.1016/j.celrep.2013.04.034
Müller, F. J., Laurent, L. C., Kostka, D., Ulitsky, I., Williams, R., Lu, C., et al. (2008). Regulatory networks define phenotypic classes of human stem cell lines. Nature 455, 401–405. doi: 10.1038/nature07213
Müller, F. J., Schuldt, B. M., Williams, R., Mason, D., Altun, G., Papapetrou, E. P., et al. (2011). A bioinformatic assay for pluripotency in human cells. Nat. Methods 8, 315–317. doi: 10.1038/nmeth.1580
Nagashima, H., Giannakis, C., Ashman, R. J., and Nottle, M. B. (2004). Sex differentiation and germ cell production in chimeric pigs produced by inner cell mass injection into blastocysts. Biol. Reprod. 70, 702–707. doi: 10.1095/biolreprod.103.022681
Nagy, A., Gócza, E., Diaz, E. M., Prideaux, V. R., Ivanyi, E., Markkula, M., et al. (1990). Embryonic stem cells alone are able to support fetal development in the mouse. Development 110, 815–821.
Nagy, A., Rossant, J., Nagy, R., Abramow-Newerly, W., and Roder, J. C. (1993). Derivation of completely cell culture-derived mice from early-passage embryonic stem cells. Proc. Natl. Acad. Sci. U.S.A. 90, 8424–8428. doi: 10.1073/pnas.90.18.8424
Nazor, K. L., Altun, G., Lynch, C., Tran, H., Harness, J. V., Slavin, I., et al. (2012). Recurrent variations in DNA methylation in human pluripotent stem cells and their differentiated derivatives. Cell Stem Cell 10, 620–634. doi: 10.1016/j.stem.2012.02.013
Newman, A. M., and Cooper, J. B. (2010). Lab-specific gene expression signatures in pluripotent stem cells. Cell Stem Cell 7, 258–262. doi: 10.1016/j.stem.2010.06.016
Nichols, J., and Smith, A. (2009). Naïve and primed pluripotent states. Cell Stem Cell 4, 487–492. doi: 10.1016/j.stem.2009.05.015
Nichols, J., and Smith, A. (2012). Pluripotency in the embryo and in culture. Cold Spring Harb. Perspect. Biol. 4:008128. doi: 10.1101/cshperspect.a008128
Nichols, J., Zevnik, B., Anastassiadis, K., Niwa, H., Klewe-Nebenius, D., and Chambers, I. (1998). Formation of pluripotent stem cells in the mammalian embryo depends on the POU transcription factor Oct4. Cell 95, 379–391. doi: 10.1016/S0092-8674(00)81769-9
Nir, S. G., David, R., Zaruba, M., Franz, W. M., and Itskovitz-Eldor, J. (2003). Human embryonic stem cells for cardiovascular repair. Cardiovasc. Res. 58, 313–323. doi: 10.1016/S0008-6363(03)00264-5
Nishimoto, M., Fukushima, A., Okuda, A., and Muramatsu, M. (1999). The gene for the embryonic stem cell coactivator UTF1 carries a regulatory element which selectively interacts with a complex composed of Oct-3/4 and Sox-2. Mol. Cell. Biol. 19, 5453–5465. doi: 10.1128/MCB.19.8.5453
Okamoto, I., Patrat, C., Thépot, D., Peynot, N., Fauque, P., Daniel, N., et al. (2011). Eutherian mammals use diverse strategies to initiate X-chromosome inactivation during development. Nature 472, 370–374. doi: 10.1038/nature09872
Okano, M., Bell, D. W., Habeer, D. A., and Li, E. (1999). DNA methyltransferases Dnmt3a and Dnmt3b are essential for de-novo methylation and mammalian development. Cell 99, 247–257. doi: 10.1016/S0092-8674(00)81656-6
Okita, K., Ichisaka, T., and Yamanaka, S. (2007). Generation of germline-competent induced pluripotent stem cells. Nature 448, 313–317. doi: 10.1038/nature05934
O'Leary, T., Heindryckx, B., Lierman, S., van Bruggen, D., Goeman, J. J., Vandewoestyne, M., et al. (2012). Tracking the progression of the human inner cell mass during embryonic stem cell derivation. Nat. Biotechnol. 30, 278–282. doi: 10.1038/nbt.2135
Pan, G., Tian, S., Nie, J., Yang, C., Ruotti, V., Wei, H., et al. (2007). Whole-genome analysis of histone H3 lysine 4 and lysine 27 methylation in human embryonic stem cells. Cell Stem Cell 1, 299–312. doi: 10.1016/j.stem.2007.08.003
Pesce, M., and Schöler, H. R. (2001). Oct-4: gatekeeper in the beginnings of mammalian development. Stem Cells 19, 271–278. doi: 10.1634/stemcells.19-4-271
Picard, L., Chartrain, I., King, W. A., and Betteridge, K. J. (1990). Production of chimeric bovine embryos and calves by aggregation of inner cell masses with morulae. Mol. Reprod. Dev. 27, 295–304. doi: 10.1002/mrd.1080270404
Polzin, V. J., Anderson, D. L., Anderson, G. B., BonDurant, R. H., Butler, J. E., Pashen, R. L., et al. (1987). Production of sheep-goat chimeras by inner cell mass transplantation. J. Anim. Sci. 65, 325–330. doi: 10.2527/jas1987.651325x
Reubinoff, B. E., Itsykson, P., Turetsky, T., Pera, M. F., Reinhartz, E., Itzik, A., et al. (2001). Neural progenitors from human embryonic stem cells. Nat. Biotechnol. 19, 1134–1140. doi: 10.1038/nbt1201-1134
RIKEN (2014). Report on STAP Cell Research Paper Investigation. Available online at: http://www3.riken.jp/stap/e/c13document52.pdf
Rohwedel, J., Maltsev, V., Bober, E., Arnold, H. H., Hescheler, J., and Wobus, A. M. (1994). Muscle cell differentiation of embryonic stem cells reflects myogenesis in vivo: developmentally regulated expression of myogenic determination genes and functional expression of ionic currents. Dev. Biol. 164, 87–101. doi: 10.1006/dbio.1994.1182
Rossant, J., Vijh, M., Siracusa, L. D., and Chapman, V. M. (1983). Identification of embryonic cell lineages in histological sections of M. musculus ↔ M. caroli chimaeras J. Embryol. Exp. Morphol. 73, 179–191.
Roth, T. L., Anderson, G. B., Bon Durant, R. H., and Pashen, R. L. (1989). Survival of sheep × goat hybrid inner cell masses after injection into ovine embryos. Biol. Reprod. 41, 675–682. doi: 10.1095/biolreprod41.4.675
Sato, N., Sanjuan, I. M., Heke, M., Uchida, M., Naef, F., and Brivanlou, A. H. (2003). Molecular signature of human embryonic stem cells and its comparison with the mouse. Dev. Biol. 260, 404–413. doi: 10.1016/S0012-1606(03)00256-2
Schmidt, R., and Plath, K. (2012). The roles of the reprogramming factors Oct4, Sox2 and Klf4 in resetting the somatic cell epigenome during induced pluripotent stem cell generation. Genome Biol. 13:251. doi: 10.1186/gb-2012-13-10-251
Schöler, H. R., Dressler, G. R., Balling, R., Rohdewohld, H., and Gruss, P. (1990). Oct-4: a germline-specific transcription factor mapping to the mouse t-complex. EMBO J. 9, 2185–2195.
Silva, J., Nichols, J., Theunissen, T. W., Guo, G., van Oosten, A. L., Barrandon, O., et al. (2009). Nanog is the gateway to the pluripotent ground state. Cell 138, 722–737. doi: 10.1016/j.cell.2009.07.039
Silva, S. S., Rowntree, R. K., Mekhoubad, S., and Lee, J. T. (2008). X-chromosome inactivation and epigenetic fluidity in human embryonic stem cells. Proc. Natl. Acad. Sci. U.S.A. 105, 4820–4825. doi: 10.1073/pnas.0712136105
Singh, V. K., Kalsan, M., Saini, A., Kumar, N., and Chandra, R. (2015). Induced pluripotent stem cells: applications in regenerative medicine, disease modelling and drug discovery. Front. Cell Dev. Biol. 2, 1–18. doi: 10.3389/fcell.2015.00002
Skreb, N., Svajger, A., and Levak-Svajger, B. (1971). Growth and differentiation of rat egg-cylinders under the kidney capsule. J. Embryol. Exp. Morphol. 25, 47–56.
Smith, A. G. (2001). Embryo-derived stem cells: of mice and men. Annu. Rev. Cell Dev. Biol. 17, 435–462. doi: 10.1146/annurev.cellbio.17.1.435
Smith, Z. D., Chan, M. M., Humm, K. C., Karnik, R., Mekhoubad, S., Regev, A., et al. (2014). DNA methylation dynamics of the human preimplantation embryo. Nature 511, 611–615. doi: 10.1038/nature13581
Smith, Z. D., Chan, M. M., Mikkelsen, T. S., Gu, H., Gnirke, A., Regev, A., et al. (2012). A unique regulatory phase of DNA methylation in the early mammalian embryo. Nature 484, 339–344. doi: 10.1038/nature10960
Soldner, F., Hockemeyer, D., Beard, C., Gao, Q., Bell, G. W., Cook, E. G., et al. (2009). Parkinson's disease patient-derived induced pluripotent stem cells free of viral reprogramming factors. Cell 136, 964–977. doi: 10.1016/j.cell.2009.02.013
Solter, D., Skreb, N., and Damjanov, I. (1970). Extrauterine growth of mouse egg-cylinders results in malignant teratoma. Nature 227, 503–504. doi: 10.1038/227503a0
Sperger, J. M., Chen, X., Draper, J. S., Antosiewicz, J. E., Chon, C. H., Jones, S. B., et al. (2003). Gene expression patterns in human embryonic stem cells and human pluripotent germ cell tumors. Proc. Natl. Acad. Sci. U.S.A. 100, 13350–13355. doi: 10.1073/pnas.2235735100
Stadtfeld, M., Apostolou, E., Akutsu, H., Fukuda, A., Follett, P., Natesan, S., et al. (2010). Aberrant silencing of imprinted genes on chromosome 12qF1 in mouse induced pluripotent stem cells. Nature 465, 175–181. doi: 10.1038/nature09017
Stevens, L. C. (1958). Studies on transplantable testicular teratomas of strain 129 mice. J. Natl. Cancer Inst. 20, 1257–1275.
Stevens, L. C. (1970). The development of transplantable teratocarcinomas from intratesticular grafts of pre- and postimplantation mouse embryos. Dev. Biol. 21, 364–382. doi: 10.1016/0012-1606(70)90130-2
Stevens, L. C., and Little, C. C. (1954). Spontaneous testicular teratomas in an inbred strain of mice. Proc. Natl. Acad. Sci. U.S.A. 40, 1080–1087. doi: 10.1073/pnas.40.11.1080
Strain, L., Warner, J. P., Johnston, T., and Bonthron, D. T. (1995). A human parthenogenetic chimaera. Nat. Genet. 11, 164–169. doi: 10.1038/ng1095-164
Studer, L. (2001). Stem cells with brainpower. Nat. Biotechnol. 19, 1117–1118. doi: 10.1038/nbt1201-1117
Suárez-Farinas, M., Noggle, S., Heke, M., Hemmati-Brivanlou, A., and Magnasco, M. O. (2005). Comparing independent microarray studies: the case of human embryonic stem cells. BMC Genomics 6:99. doi: 10.1186/1471-2164-6-99
Tachibana, M., Amato, P., Sparman, M., Gutierrez, N. M., Tippner-Hedges, R., Ma, H., et al. (2013). Human embryonic stem cells derived by somatic cell nuclear transfer. Cell 153, 1228–1238. doi: 10.1016/j.cell.2013.05.006
Tachibana, M., Sparman, M., Ramsey, C., Ma, H., Lee, H. S., and Penedo, M. C. (2012). Generation of chimeric rhesus monkeys. Cell 148, 285–295. doi: 10.1016/j.cell.2011.12.007
Takahashi, K., Tanabe, K., Ohnuki, M., Narita, M., Ichisaka, T., Tomoda, K., et al. (2007). Induction of pluripotent stem cells from adult human fibroblasts by defined factors. Cell 131, 861–872. doi: 10.1016/j.cell.2007.11.019
Takahashi, K., and Yamanaka, S. (2006). Induction of pluripotent stem cells from mouse embryonic and adult fibroblast cultures by defined factors. Cell 126, 663–676. doi: 10.1016/j.cell.2006.07.024
Takashima, Y., Guo, G., Loos, R., Nichols, J., Ficz, G., Krueger, F., et al. (2014). Resetting transcription factor control circuitry toward ground-state pluripotency in human. Cell 158, 1254–1269. doi: 10.1016/j.cell.2014.08.029
Tarkowski, A. K. (1961). Mouse chimeras developed from fused eggs. Nature 190, 857–860. doi: 10.1038/190857a0
Taubenschmid, J., and Weitzer, G. (2012). Mechanisms of cardiogenesis in cardiovascular progenitor cells. Int. Rev. Cell Mol. Biol. 293, 195–267. doi: 10.1016/b978-0-12-394304-0.00012-9
Tesar, P. J., Chenoweth, J. G., Brook, F. A., Davies, T. J., Evans, E. P., Mack, D. L., et al. (2007). New cell lines from mouse epiblast share defining features with human embryonic stem cells. Nature 448, 196–199. doi: 10.1038/nature05972
Theunissen, T. W., Powell, B. E., Wang, H., Mitalipova, M., Faddah, D. A., Reddy, J., et al. (2014). Systematic identification of culture conditions for induction and maintenance of naive human pluripotency. Cell Stem Cell 15, 471–487. doi: 10.1016/j.stem.2014.07.002
Thomson, J. A., Itskovitz-Eldor, J., Shapiro, S. S., Waknitz, M. A., Swiergiel, J. J., Marshall, V. S., et al. (1998). Embryonic stem cell lines derived from human blastocysts. Science 282, 1145–1147. doi: 10.1126/science.282.5391.1145
Thomson, J. A., Kalishman, J., Golos, T. G., Durning, M., Harris, C. P., Becker, R. A., et al. (1995). Isolation of a primate embryonic stem cell line. Proc. Natl. Acad. Sci. U.S.A. 92, 7844–7848. doi: 10.1073/pnas.92.17.7844
Tsumura, A., Hayakawa, T., Kumaki, Y., Takebayashi, S., Sakaue, M., Matsuoka, C., et al. (2006). Maintenance of self-renewal ability of mouse embryonic stem cells in the absence of DNA methyltransferases Dnmt1, Dnmt3a, and Dnmt3b. Genes Cells 11, 805–814. doi: 10.1111/j.1365-2443.2006.00984.x
Tucker, E. M., Moor, R. M., and Rowson, L. E. (1974). Tetraparental sheep chimaeras induced by blastomere transplantation. Changes in blood type with age. Immunology 26, 613–621.
Valamehr, B., Robinson, M., Abujarour, R., Rezner, B., Vranceanu, F., Le, T., et al. (2014). Platform for induction and maintenance of transgene-free hiPSCs resembling ground state pluripotent stem cells. Stem Cell Rep. 2, 366–381. doi: 10.1016/j.stemcr.2014.01.014
Wakayama, T., Perry, A. C. F., Zuccotti, M., Johnson, K. R., and Yanagimachi, R. (1998). Full-term development of mice from enucleated oocytes injected with cumulus cell nuclei. Nature 394, 369–374. doi: 10.1038/28615
Wakayama, T., Tabar, V., Rodriguez, I., Perry, A. C., Studer, L., and Mombaerts, P. (2001). Differentiation of embryonic stem cell lines generated from adult somatic cells by nuclear transfer. Science 292, 740–743. doi: 10.1126/science.1059399
Wakayama, T., and Yanagimachi, R. (1999). Cloning of male mice from adult tail-tip cells. Nat. Genet. 22, 127–128. doi: 10.1038/9632
Waldrip, W. R., Bikoff, E. K., Hoodless, P. A., Wrana, J. L., and Robert son, E. J. (1998). Smad2 signaling in extraembryonic tissues determines anterior-posterior polarity of the early mouse embryo. Cell 92, 797–808. doi: 10.1016/S0092-8674(00)81407-5
Wang, Z., and Jaenisch, R. (2004). At most three ES cells contribute to the somatic lineages of chimeric mice and of mice produced by ES-tetraploid complementation. Dev. Biol. 275, 192–201. doi: 10.1016/j.ydbio.2004.06.026
Wang, Z., Oron, E., Nelson, B., Razis, S., and Ivanova, N. (2012). Distinct lineage specification roles for NANOG, OCT4, and SOX2 in human embryonic stem cells. Cell Stem Cell 10, 440–454. doi: 10.1016/j.stem.2012.02.016
Ware, C. B., Nelson, A. M., Mecham, B., Hesson, J., Zhou, W., Jonlin, E. C., et al. (2014). Derivation of naive human embryonic stem cells. Proc. Natl. Acad. Sci. U.S.A. 111, 4484–4489. doi: 10.1073/pnas.1319738111
Wesselschmidt, R. L. (2011). The teratoma assay: an in vivo assessment of pluripotency. Methods Mol. Biol. 767, 231–241. doi: 10.1007/978-1-61779-201-4_17
West, F. D., Terlouw, S. L., Kwon, D. J., Mumaw, J. L., Dhara, S. K., Hasneen, K., et al. (2010). Porcine induced pluripotent stem cells produce chimeric offspring. Stem Cells Dev. 19, 1211–1220. doi: 10.1089/scd.2009.0458
Williams, R., Schuldt, B., and Müller, F. J. (2011). A guide to stem cell identification: progress and challenges in system-wide predictive testing with complex biomarkers. Bioessays 33, 880–890. doi: 10.1002/bies.201100073
Wilmut, I., Schnieke, A. E., McWhir, J., Kind, A. J., and Campbell, K. H. (1997). Viable offspring derived from fetal and adult mammalian cells. Nature 385, 810–813. doi: 10.1038/385810a0
Wobus, A. M., Holzhausen, H., Jäkel, P., and Schoneich, J. (1984). Characterization of a pluripotent stem cell line derived from a mouse embryo. Exp. Cell Res. 152, 212–219. doi: 10.1016/0014-4827(84)90246-5
Wu, J., Okamura, D., Li, M., Suzuki, K., Luo, C., Ma, L., et al. (2015). An alternative pluripotent state confers interspecies chimeric competency. Nature 521, 316–321. doi: 10.1038/nature14413
Wu, Z., Chen, J., Ren, J., Bao, L., Liao, J., Cui, C., et al. (2009). Generation of pig induced pluripotent stem cells with a drug-inducible system. J. Mol. Cell Biol. 1, 46–54. doi: 10.1093/jmcb/mjp003
Xu, Y., Zhu, X., Hahm, H. S., Wei, W., Hao, E., Hayek, A., et al. (2010). Revealing a core signaling regulatory mechanism for pluripotent stem cell survival and self-renewal by small molecules. Proc. Natl. Acad. Sci. U.S.A. 107, 8129–8134. doi: 10.1073/pnas.1002024107
Yamada, M., Johannesson, B., Sagi, I., Burnett, L. C., Kort, D. H., Prosser, R. W., et al. (2014). Human oocytes reprogram adult somatic nuclei of a type 1 diabetic to diploid pluripotent stem cells. Nature 510, 533–536. doi: 10.1038/nature13287
Yamada, T., Yoshikawa, M., Kanda, S., Kato, Y., Nakajima, Y., Ishizaka, S., et al. (2002a). In vitro differentiation of embryonic stem cells into hepatocyte-like cells identified by cellular uptake of indocyanine green. Stem Cells 20, 146–154. doi: 10.1634/stemcells.20-2-146
Yamada, T., Yoshikawa, M., Takaki, M., Torihashi, S., Kato, Y., Nakajima, Y., et al. (2002b). In vitro functional gut-like organ formation from mouse embryonic stem cells. Stem Cells 20, 41–49. doi: 10.1634/stemcells.20-1-41
Yan, L., Yang, M., Guo, H., Yang, L., Wu, J., Li, R., et al. (2013). Single-cell RNA-Seq profiling of human preimplantation embryos and embryonic stem cells. Nat. Struct. Mol. Biol. 20, 1131–1139. doi: 10.1038/nsmb.2660
Ying, Q. L., Wray, J., Nichols, J., Batlle-Morera, L., Doble, B., Woodgett, J., et al. (2008). The ground state of embryonic stem cell self-renewal. Nature 453, 519–523. doi: 10.1038/nature06968
Yu, J., Vodyanik, M. A., Smuga-Otto, K., Antosiewicz-Bourget, J., Frane, J. L., Tian, S., et al. (2007). Induced pluripotent stem cell lines derived from human somatic cells. Science 318, 1917–1920. doi: 10.1126/science.1151526
Yuan, H., Corbi, N., Basilico, C., and Dailey, L. (1995). Developmental-specific activity of the FGF-4 enhancer requires the synergistic action of Sox2 and Oct-3. Genes Dev. 9, 2635–2645. doi: 10.1101/gad.9.21.2635
Zhang, P., Andrianakos, R., Yang, Y., Liu, C., and Lu, W. (2010). Kruppel-like factor 4 (Klf4) prevents embryonic stem (ES) cell differentiation by regulating Nanog gene expression. J. Biol. Chem. 285, 9180–9189. doi: 10.1074/jbc.M109.077958
Zhang, S. C., Wernig, M., Duncan, I. D., Brustle, O., and Thomson, J. A. (2001). In vitro differentiation of transplantable neural precursors from human embryonic stem cells. Nat. Biotechnol. 19, 1129–1133. doi: 10.1038/nbt1201-1129
Zhu, S., Wurdak, H., Wang, J., Lyssiotis, C. A., Peters, E. C., Cho, C. Y., et al. (2009). A small molecule primes embryonic stem cells for differentiation. Cell Stem Cell 4, 416–426. doi: 10.1016/j.stem.2009.04.001
Keywords: pluripotency, iPSCs, teratoma, chimera, EpiSCs, EpiBlast, naïve, primed
Citation: Singh VK, Saini A, Kalsan M, Kumar N and Chandra R (2016) Describing the Stem Cell Potency: The Various Methods of Functional Assessment and In silico Diagnostics. Front. Cell Dev. Biol. 4:134. doi: 10.3389/fcell.2016.00134
Received: 18 May 2016; Accepted: 02 November 2016;
Published: 22 November 2016.
Edited by:
Prasanna R. Kolatkar, Qatar Biomedical Research Institute, QatarReviewed by:
Takahiko Hara, Tokyo Metropolitan Institute of Medical Science, JapanAkshay Bhinge, Genome Institute of Singapore, Singapore
Copyright © 2016 Singh, Saini, Kalsan, Kumar and Chandra. This is an open-access article distributed under the terms of the Creative Commons Attribution License (CC BY). The use, distribution or reproduction in other forums is permitted, provided the original author(s) or licensor are credited and that the original publication in this journal is cited, in accordance with accepted academic practice. No use, distribution or reproduction is permitted which does not comply with these terms.
*Correspondence: Vimal Kishor Singh, dmltX2tpc3NvckB5YWhvby5jby5pbg==; dmltYWxraXNob3JzaW5naEBnbWFpbC5jb20=; dmltYWxrc2luZ2hAZGNlLmFjLmlu